- 1Department of Psychiatry, Division of Neurosciences, Faculty of Medical Sciences, School of Medicine, Aristotle University of Thessaloniki, Thessaloniki, Greece
- 2VA Center of Excellence for Stress and Mental Health (CESAMH), VA San Diego Healthcare System, San Diego, CA, United States
- 3First Department of Pediatrics, Division of Endocrinology, Metabolism and Diabetes, School of Medicine, National and Kapodistrian University of Athens, “Aghia Sophia” Children’s Hospital, Athens, Greece
- 4Unit of Developmental & Behavioral Pediatrics, First Department of Pediatrics, School of Medicine, National and Kapodistrian University of Athens, “Aghia Sophia” Children’s Hospital, Athens, Greece
The dramatic fluctuations in energy demands by the rhythmic succession of night and day on our planet has prompted a geophysical evolutionary need for biological temporal organization across phylogeny. The intrinsic circadian timing system (CS) represents a highly conserved and sophisticated internal “clock,” adjusted to the 24-h rotation period of the earth, enabling a nyctohemeral coordination of numerous physiologic processes, from gene expression to behavior. The human CS is tightly and bidirectionally interconnected to the stress system (SS). Both systems are fundamental for survival and regulate each other’s activity in order to prepare the organism for the anticipated cyclic challenges. Thereby, the understanding of the temporal relationship between stressors and stress responses is critical for the comprehension of the molecular basis of physiology and pathogenesis of disease. A critical loss of the harmonious timed order at different organizational levels may affect the fundamental properties of neuroendocrine, immune, and autonomic systems, leading to a breakdown of biobehavioral adaptative mechanisms with increased stress sensitivity and vulnerability. In this review, following an overview of the functional components of the SS and CS, we present their multilevel interactions and discuss how traumatic stress can alter the interplay between the two systems. Circadian dysregulation after traumatic stress exposure may represent a core feature of trauma-related disorders mediating enduring neurobiological correlates of trauma through maladaptive stress regulation. Understanding the mechanisms susceptible to circadian dysregulation and their role in stress-related disorders could provide new insights into disease mechanisms, advancing psychochronobiological treatment possibilities and preventive strategies in stress-exposed populations.
Introduction
Living organisms consist of highly complex biological systems with the ability to preserve a complex dynamic balance state with a constant oscillation around an ideal homeostatic condition (nonequilibrium homeodynamic state) (1, 2). To achieve this, organisms have developed a highly sophisticated and multifaceted biological system, the so-called stress system (SS), which serves self-regulation and adaptability of the organism to ongoing intrinsic or extrinsic, real or perceived (i.e., subject-dependent value attribution), altering challenges or stimuli, defined as stressors (3). When stressors surpass a manageable severity or temporal verge, the initiated stress response redirects energy depending on the present needs to restore homeostasis (4–8). Thus, stress is defined as the state of threatened homeodynamic balance of the organism (6, 9). Repeated, ephemeral, and motivating stress states lead to adaptive responses and are fairly beneficial, while inadequate, aversive, excessive, or prolonged stress may surpass the natural regulatory capacity and adjustive resources of the organism and majorly affect adaptive responses leading to cacostasis (i.e., negatively altered homedynamic state, dyshomeostasis), and accumulated cacostatic load (6).
The understanding of the temporal relationship between stressors and physiological stress responses is crucial for the comprehension of the molecular basis of physiology and pathophysiology of disease. Biological processes always take place in an appropriate order, in order to synchronize required homeostatic mechanisms. As life on earth has evolved in the context of the earth’s rotation around its own axis, there was a geophysical evolutionary need for temporal organization and adjustment of internal activity and physiological processes to the dramatic fluctuations in energy demands by the constant rhythmic succession of night and day. This need has generated a highly conserved and sophisticated internal molecular “clock,” creating endogenous rhythmicity with a period adjusted to the 24-h rotation of our planet throughout phylogeny (10–12).
This intrinsic circadian (lat. circa diem – about a day) timing system (CS) creates an internal representation of the external Zeitraum (germ. time-space) and helps living organisms keep track of time from a centrally created circadian rhythm (13, 14). By orchestrating a dynamic milieu that oscillates with a 24-h rhythm, the CS coordinates physiological processes and rhythmic changes, from gene expression to behavior and prepares living organisms for the anticipated cyclic challenges, promoting homeostasis and environmental adaptation and creating an evolutionary advantage to optimize survival (15–18). In order to achieve this, the CS upregulates the SS before the organism’s active phase and turns it down again for the resting and restorative phases.
The CS and the SS are both fundamental for survival and regulate each other’s activity, through intimate reciprocal interactions with each other at multiple levels (19, 20). An intact communication between the CS and the SS is important for maintaining homeostasis and environmental adaptation (21–23). The SS is undoubtedly at the heart of circadian biology, mediating temporal signals and vice versa (24). Investigating the interactions between the two systems is essential to understand pathophysiological pathways mediating risk for disease, as dysregulation in either of these systems may lead to similar pathologic conditions (25).
In this review, following a general overview of the functional elements of the two systems, we present their multilevel interconnections, and discuss how excessive (i.e., traumatic) stress can affect the harmonic central and peripheral interplay between SS and CS.
The Human Stress System
The human SS consists of central and peripheral components. The central, critically interconnected components of the SS are mainly located in the hypothalamus and the brainstem, and include: (a) the parvocellular neurons of corticotropine-releasing-hormone (CRH), (b) the arginine-vasopressin (AVP) neurons of the hypothalamic paraventricular nuclei (PVN), (c) the CRH neurons of the paragigantocellular and parabranchial nuclei of the medulla and the locus caeruleus (LC), (d) the arcuate nucleus proopiomelanocortin-derived peptides alpha-melanocyte–stimulating hormone (MSH) and beta-endorphin, (e) other mostly noradrenergic (NE) cell groups in the medulla and pons (LC/NE system), and (f) the central nuclei of the autonomic nervous system (ANS) [cf. Figure 1]. These neuroanatomical loci communicate with each other, influencing their own activity, and interact with several other brain subsystems, such as the mesocortical/mesolimbic dopaminergic system, involved in reward and motivation and the amygdala central nuclei, generating fear and anger (6, 9).
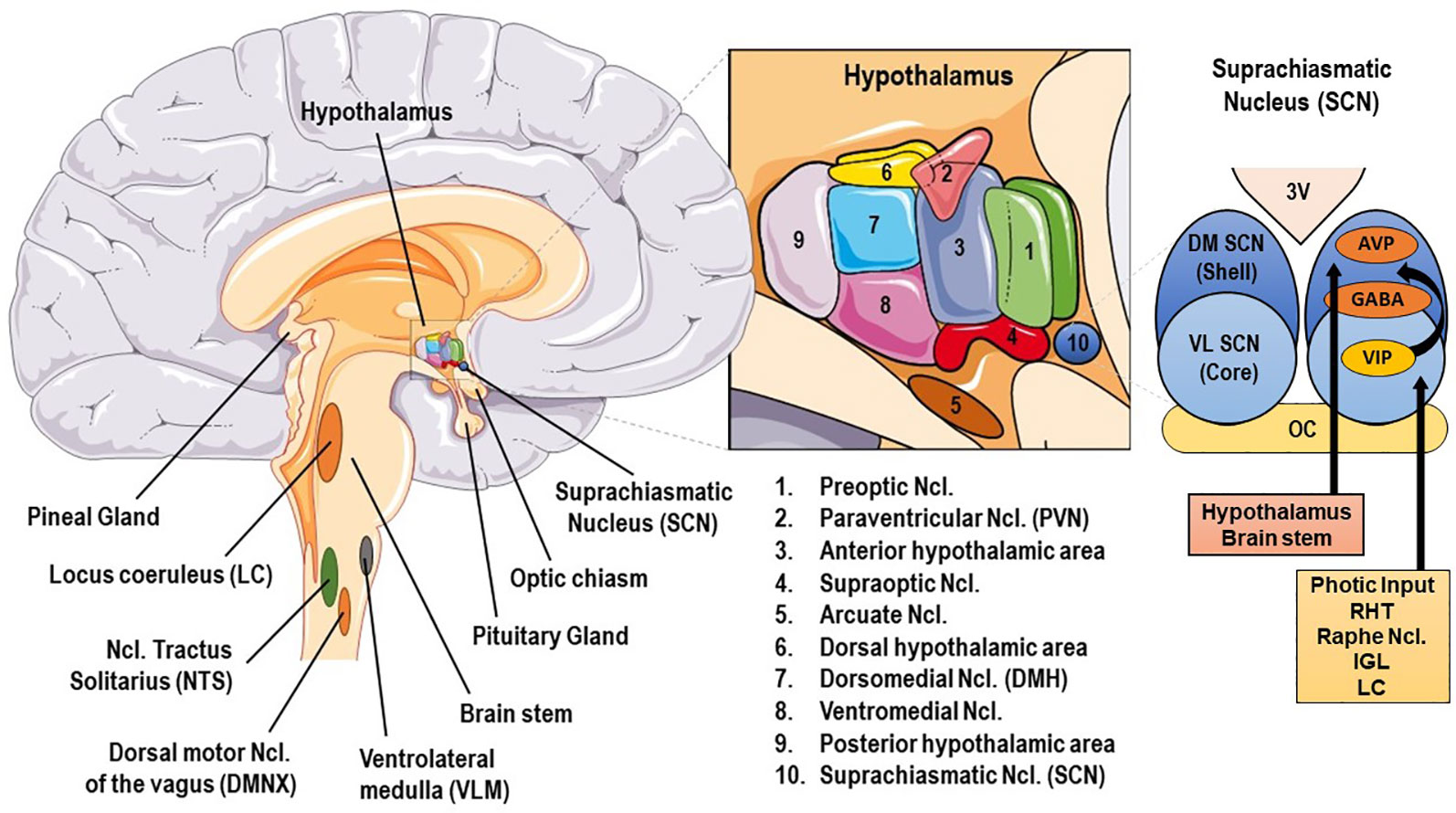
Figure 1 Basic anatomy of stress and circadian system related brain structures. AVP, arginine vasopressin; GABA, γ-aminobutyric acid; DM SCN, dorsomedial suprachiasmatic nucleus; IGL, thalamic intergeniculate leaflet; LC, locus caeruleus; RHT, retinohypothalamic tract; VIP, vasoactive intestinal peptide; VL SCN, ventrolateral SCN.
The peripheral components of the SS include: (a) the hypothalamic-pituitary-adrenal (HPA) axis and (b) the ANS comprised of (i) the sympathetic nervous system (SNS) and sympatho-adrenomedullary (SAM) system and (ii) the parasympathetic nervous system (PNS). The main terminal peripheral effector molecules of the SS are the HPA axis-regulated glucocorticoids (GCs; i.e., cortisol in humans), and the SAM-regulated catecholamines (Cas; i.e., NE and epinephrine). HPA axis and ANS have largely complementary actions throughout the body and are increasingly studied together (26), as integrated and interrelated components of an internal neural regulation system. Findings suggest that the appropriate regulation of the HPA-axis depends in part on ANS, especially on vagal influences (27).
When stressors exceed a certain severity or temporal threshold, stressor-related information initiates a complex stress response to induce remarkably consistent acute, normally adaptive, and time-limited microphysiologic, mesophysiologic, and macrophysiologic compensatory responses throughout several effector tissues (4–8, 28). Together, these responses represent a well-orchestrated and fine-tuned answer to challenge in both the central nervous system (CNS) and the somatic periphery (29).
The Autonomic Nervous System
The ANS, although not under overt voluntary direction (autonomous), plays a crucial role in the preservation of a homeodynamic balance by providing a rapidly responding control system for a plethora of physiological reactions to physical, emotional, and cognitive challenges (30, 31). It is especially the precise regulation of organ and tissue functions through fine-tuning of the ANS limbs that is crucial for optimal stress reactivity, adaptive responses, and health.
The exact ANS activity is fine-tuned through central and peripheral autonomic reflexes and feedback mechanisms (32). The central autonomic modulation does not simply rely on a monolithic network of brain regions, but is instead regulated by the central autonomic network (CAN), an internal central autonomic regulation system featuring certain task and division specificity (33). The CAN is additionally characterized by bilateral interconnections, parallel organization, state-dependent activity, and neurochemical complexity (30, 31, 34, 35). It includes the insular cortex, central nucleus of the amygdala, hypothalamus, periaqueductal gray matter, parabrachial complex, nucleus of the solitary tract (NTS), and ventrolateral medulla (VLM) (36, 37) [cf. Figure 2]. The insular cortex and amygdala mediate high-order autonomic control associated with cognitive perception and emotional responses through hypothalamic-brainstem pathways (30). NTS, PVN, and VLM contain a network of respiratory, cardiovagal, and vasomotor neurons, receiving afferent vagal sensory input from thoracic and abdominal viscera and other cranial nerves. These structures accordingly modulate the activity of preganglionic autonomic neurons. CAN dysregulation can be critically involved in stress-related disorders, as it may affect downstream autonomic centers, thereby altering peripheral ANS activity and cardiac function. CAN dysregulation (35, 38, 39) may affect downstream autonomic core centers, thereby altering peripheral ANS activity (39–41).
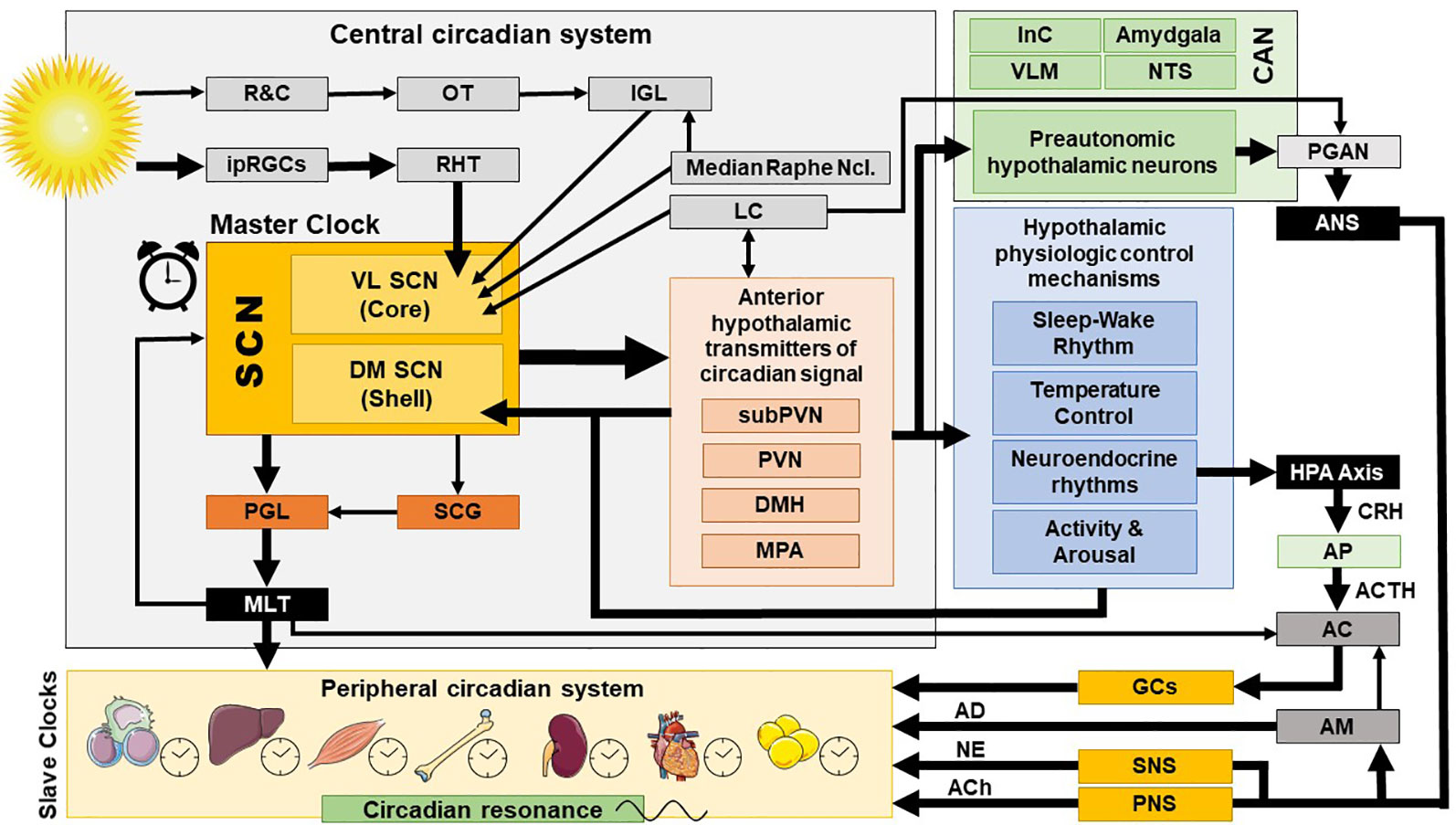
Figure 2 Central and peripheral circadian system and their interconnections. AC, adrenal cortex; ACh, acetylcholine; ACTH, adrenocorticotropic hormone; AD, adrenalin; AM, adrenal medulla; ANS, autonomic nervous system; AP, anterior pituitary; CAN, central autonomic network; CRH, corticotropin releasing hormone; DM SCN, dorsomedial SCN; DMH, dorsomedial hypothalamus; GCs, glucocorticoids; HPA axis, hypothalamic-pituitary-adrenal axis; InC, insular cortex; IGL, thalamic intergeniculate leaflet; ipRGC, intrinsically photosensitive retinal ganglion cells; LC, locus caeruleus; MLT, melatonin; MPA, medial preoptic area; NE, norepinephrine; NTS, nucleus of the solitary tract; OT, optic tract; PNS, parasympathetic nervous system; PGAN, preganglionic autonomic neurons; PGL, pineal gland; PVN, paraventricular nucleus; R&C, rodes and cones; RHT, retinohypothalamic tract; SCN, suprachiasmatic nucleus; SCG, superior cervical ganlia; SNS, sympathetic nervous system; subPVN, subparaventricular area; VL SCN, ventrolateral SCN; VLM, ventrolateral medulla.
Since the early 20th century, pragmatic and anatomic reasons has led to a common division of the ANS into two, or sometimes three peripheral tracts: the sympathetic, parasympathetic and, the largest one, the enteric autonomic division, although they practically mirror one larger control system (42, 43). Especially the separation into SNS and PNS has led to enormous misconceptions, the most serious being the view that the two divisions are somehow in opposition to each other. On the contrary, SNS and the PNS are rather in a dynamic interdependent state and act on different time scales but in concert and through numerous and multilevel, bidirectional interactions to control the abovementioned autonomic functions (44, 45), while autonomic dysregulation translates into decreased dynamic adaptability, increased morbidity and mortality (27, 30, 46, 47). In general, since both systems are tonically active, the PNS can both assist and antagonize SNS functions by withdrawing or increasing its activity (frequency of neuronal discharge), respectively. This ANS characteristic is of major importance and improves its ability to more precisely regulate an effector’s function.
The Sympathetic Nervous System
The SNS originates in brainstem nuclei and gives rise to preganglionic cholinergic (ACh) efferent fibers mostly projecting to postganglionic sympathetic ganglia. The long postganglionic neurons terminate outwards on effector tissues, mostly releasing NE. Alternatively, preganglionic neurons may also directly synapse with the modified postganglionic chromaffin cells of the adrenal medulla. A sympathetic activation, thus, principally releases NE (locally and to a lesser extent systematically from the adrenal medulla) or adrenaline (systematically from the adrenal medulla) together with other neuropeptides in the body (48). Sympathetic activation generally predominates during emergency (fight-or-flight) situations and during exercise, preparing the body for strenuous physical activity.
The Parasympathetic Nervous System
Whereas SNS activity depends on two peripheral branches (neural and adrenal), parasympathetic activity is displayed only by nerves. The preganglionic neurons of the PNS arise from numerous brainstem nuclei and from the spinal sacral region (S2–S4). The preganglionic ACh-axons are quite long and synapse with short postganglionic neurons within terminal ganglia close to or embedded to effector tissues. Accordingly, PNS actions are mostly more discrete and localized compared to the SNS, where a more diffuse and global discharge is probable. The preganglionic neurons that arise from the brainstem exit the CNS through the cranial nerves [N. occulomotorius (III); N. facialis (VII); N. glossopharyngeus (IX); N. vagus (X)]. The vagus nerve innervates the thoracic and abdominal viscera and has a major physiological significance, as approximately ¾ of all parasympathetic fibers originate from the vagus nerve (49). The PNS stress response is mainly activated by the nucleus ambiguus and the dorsal motor nucleus of the vagus nerve, possibly after NTS stimulation. The PNS generally predominates during resting conditions towards conserving and storing energy or regulating basic body functions (e.g., digestion, defecation, urination). Through its tonic properties, the PNS is vital especially under resting conditions, and is, therefore, particularly implicated in the development of cardiovascular diseases and other comorbidities (27, 50).
The Hypothalamus-Pituitary-Adrenal Axis
The HPA axis consists of the PVN, the pituitary corticotrophs and the zona fasciculata of the adrenal cortex, which, respectively, employ corticotropin-releasing hormone (CRH)/arginine vasopressin (AVP), adrenocorticotropic hormone (ACTH), and glucocorticoids (GCs, i.e., cortisol in humans) as their signalling effector molecules [cf. Figure 2]. CRH and AVP are released from the PVN into the hypophyseal system in response to stimulatory signals from higher regulatory centers (e.g., PFC) and reach the pituitary gland to stimulate the secretion of ACTH. ACTH reaches the cortex of the adrenal glands through release in the systemic circulation and stimulates both production and secretion of GCs. Systemically released GCs, in turn, besides their major actions, close a negative feedback loop by suppressing the activation of the PVN and the pituitary gland (6, 51).
Glucocorticoid Receptors and Signaling
GCs influence a myriad of physiologic functions and are essential for the activation, maintenance, and downregulation of the stress response. GCs mainly exert their pleiotropic effects through genomic, nongenomic, and mitochondrial actions of the intracellular cognate GC and mineralocorticoid receptors (GR, MR), which function as a ligand-activated transcription factors (4–9, 52–56). GR and MR are evolutionarily close, showing large homologies at their DNA-binding domain and sharing many responsive genes. Upon ligand-binding, the receptors dissociate from the interacting proteins (i.e., shock proteins and immunophillins), translocate to the nucleus, form homo- or hetero-dimmers and bind to specific DNA response elements located in the regulatory regions of thousands responsive genes, leading to their transactivation or transrepression (8, 52, 54–57). GR and MR have complementary actions with respect to HPA axis activity and reactivity (58). Altered GC-signaling, through dysregulations at different levels of the HPA axis, may greatly negatively affect the organisms’ physiology and could influence life expectancy, as seen in many complex behavioral and somatic disorders (e.g., depression, posttraumatic stress disorder, sleep disorders, chronic pain and fatigue syndromes, obesity, diabetes Type II and the metabolic syndrome, essential hypertension, atherosclerosis, osteoporosis, autoimmune inflammatory, and allergic disorders) (55, 59).
In humans, the glucocorticoid receptor (hGR) is encoded by the NR3C1 gene, which is located in the long arm of chromo-some 5 and consists of 10 exons. The alternative usage of exon 9α or 9β gives rise to the two main receptor isoforms, the classic hGRα and the hGRβ (8, 52, 54–57). Ubiquitarilly expressed in every tissue except the suprachiasmatic nucleus (SCN) of the hypothalamus, the hGRα is primarily localized in the cytoplasm of glucocorticoid target cells (57, 60). hGRβ, exclusively localized in the nucleus of certain cells (e.g., endothelial cells), acts mainly as negative regulator of hGRα transcriptional activity (61, 62). A growing body of evidence suggests that hGRβ has its own, hGRα-independent transcriptional activity and plays an important role in insulin signalling, inflammation, and carcinogenesis (63). The MR is encoded by the NR3C2 gene, is located on chromosome 4 and also consisting of 10 exons (64). MR is peripherally expressed in several tissues (e.g., adipose tissue, kidney, endothelium, macrophages) and exerts vital regulatory functions through its main endogenous MR ligand as part of the renin-angiotensin-aldosterone system, among others, in cell growth, renal and cardiovascular function, metabolism and immunity.
Of particular importance are the GR and MR effects in the CNS. While GR are expressed throughout the brain, MR are abundantly expressed in limbic brain structures involved in emotional processing, arousal and memory (i.e., hippocampus, amygdala, prefrontal cortex) thus exerting a basal inhibitory tone on GC secretion (65, 66). Interestingly, the MRs show a tenfold higher affinity to cortisol than GRs and are largely already occupied under basal cortisol levels, while GRs become gradually occupied through cortisol peak levels (e.g., circadian peak, acute stress) (58, 67), resulting in a regulative, MR-associated threshold for HPA axis activation and stress sensitivity (68). Thus, depending on receptor type, cell topology, tissue-specific expression, their specific ligands (e.g., aldosterone) or relevant enzymes (e.g., cortisol-inactivating enzyme 11β-hydroxysteroid dehydrogenase type 2, 11βHSD2), HPA axis activation differentially regulates the expression of various target genes with different transcriptional potencies in response to cortisol.
In addition, GC may also signal through protein-protein interactions between receptors and other important transcription factors, including the nuclear factor-κB (NF-κB), the activator protein-1 (AP-1), and the signal transducers and activators of transcription (STATs). However, perhaps even more importantly, GC exert also rapid, nongenomic actions, mediated by membrane-bound MRs and GRs that trigger the activation of kinase signal transduction pathways (8, 52, 54–57, 69). Membrane-bound MRs and GRs show lower GC affinity than intracellular receptors and are increasingly occupied only through higher cortisol concentrations, thus mainly playing a crucial role in translation of rapid GC pulses in the initial phase of HPA axis activation (70–72).
The Human Circadian System
Circadian molecular oscillations are independently generated in virtually every cell of living organisms, thus influencing molecular biological processes over the course of the day. However, it is the orchestration of these innumerable, diverging and tissue-specific peripheral oscillations into a main rhythmic symphony that is of vital importance for the promotion of homeostasis in higher organisms. The CS represents an extensive network of time-keeping mechanisms that creates and maintains this cellular and systemic rhythmicity, through temporal organization and coordination of many physiological and transcriptional oscillating processes throughout several structural levels in the organism (17, 18). In order to stay adjusted to the geophysical time, the CS receives continuously input by behavioral, hormonal, and environmental signals, a process called entrainment.
The mammalian CS is organized in a hierarchical manner with a central, pacemaking, and light-sensitive “master clock” in the CNS and a peripheral, subordinated multioscillator component (“slave clocks”), showing both top-down and bottom-up organization based on positive and negative endocrine, autonomic, and transcriptional regulatory feedback loops (15, 73–75). The CS has three main functions as (a) pacemaker through intrinsic and self-sustainable rhythm generation, (b) internal Zeitgeber (germ. time-giver) with a distinct rhythm output for peripheral synchronization, and (c) Zeitnehmer (germ. time-taker) continuously receiving time-shifting signals from external/secondary Zeitgebers (e.g, nutrition, light, sleep, social activity) for proper time entrainment of the intrinsic period to the environmental cycle (76).
The Central and Peripheral Circadian System
The central mammalian CS includes specialized signal transduction mechanisms in the retina, the retinohypothalamic tract (RHT), the suprachiasmatic nucleus (SCN), the superior cervical ganglia, the pineal gland (PGL), the thalamic intergeniculate leaflet (IGL), and the raphe nuclei (18, 77, 78) (cf. Figures 1 and 2). The SCN is a bilateral paired structure with high cell density, consisting of 50,000 neurons (in humans) displaying a synchronised rhythmic metabolic and electrical activity, and is located in the anterior hypothalamus directly over of the optic chiasm, next to the third ventricle. The SCN is the integrative “master clock” of the organism, by integrating its distinct primary pacemaker activity through intrinsic neural firing and all received environmental Zeitgeber cues to a main circadian rhythm (17, 18, 79–81). The most important Zeitgeber is light. The SCN receives photic input (photoentrainment) from the rod/cone photoreceptors and particularly from other nonimage-forming photosensitive cells in the retina, the intrinsically photosensitive retinal ganglion cells (ipRGCs) (77). These melanopsin-containing cells have been shown to be sensitive to light wavelengths (460–480 nm, i.e. blue light) different from the classical visual system (i.e., rod and cone cells) and they react slowly and tonically to luminance changes (77, 82–87). The photic input transmitted from the ipRGC through the retinohypothalamic tract to the SCN (88) and from there to the upper part of the thoracic spinal cord, the superior cervical ganglia and the PGL gland (89). The NPY-containing pathway from the IGL and the serotonergic pathway from the median raphe represent the two other main afferent projections to the SCN (78). Taken together, anatomical routes directly involved with the SCN are numerous, with up to 15 efferent and 35 afferent projections (78).
The peripheral, subordinated multioscillator component of the CS (“slave clocks”) show a similar, tissue-specific, self-sustained, and cell-autonomous rhythm generation machinery, regulating several functions of their residing tissues, with one essential difference to the central CS: These peripheral “slave clocks” do not exchange phase information and must therefore kept synchronized by the main integrative SCN rhythm via different pathways (16), which leads to a 4-h optimal phase synchronization delay of peripheral with respect to the central CS rhythm (90). This synchrony gets mostly lost without an input from the SCN (91), although other Zeitgebers, such as nutrient, temperature, and social cues, can also entrain peripheral clocks (92).
The Molecular Clockwork
In the past decades, mounting evidence has evolved our understanding from the first discovered clock gene (Period or PER) conserved from fruit flies to humans (93) to a complex molecular clockwork generated at the cellular level by molecular oscillators in all nucleus-containing cells of an organism (15, 74, 94). The intrinsic circadian rhythmicity of the biological clock is based on a core set of clock genes intertwined with an autoregulatory, delayed, interlocking transcriptional/translational feedback (TTFL) loop machinery, coupled to several auxiliary mechanisms and leading to mutual transcriptional activation and repression, ultimately maintaining an approximately 24-h oscillation, thus, reinforcing robustness and stability of the clock (14, 15, 74, 94–97).
Central among the core TTFL are the transcriptional activator “circadian locomotor output cycle kaput” (CLOCK), its heterodimer partner “brain-muscle-ARNT-like protein 1” (BMAL1), and the essential negative regulating circadian genes “Period 1, 2, and 3” (PER1-3) and “Cryptochrome 1 and 2” (CRY1/2) (98). The activated CLOCK/BMAL1 heterodimer binds to the enhancer box (E-box) response elements located in the promoter region and stimulates the transcription of PER1-3 and CRY1/2 at circadian dawn (circadian time 0, CT0). PER1-3 and CRY1/2 mRNA gets translated into proteins, which accumulate by the end of the circadian day (CT12). Over the course of the circadian night (CT12–CT0), inhibitory complexes of PER1-3 and CRY1/2 with the caseine kinase 1ϵ and δ, are phosphorylated and translocate from the cytoplasm into the nucleus and repress the transcriptional activity of the CLOCK/BMAL1 in the SCN, shutting down PER1-3/CRY1/2 transcription (99). After degradation of nuclear PER1-3/CRY1/2 complexes the next morning (CT0), the inhibition on CLOCK/BMAL1 transcriptional activity is released and thereby a new cycle starts over after approximately 24 h (79) [cf. Figure 3]. During the circadian day, PER1-3 and CRY1/2 transcription is high in the SCN, leading also to high SCN electrical activity. Besides this core negative feedback loop, there are also auxiliary feedback loops that stabilize the transcriptional activity of the core regulatory loop (94, 100–102). CLOCK/BMAL1 upregulates, for example, the expression of other clock-related proteins, such as the reverse viral erythroblastosis oncogene product α and β (REV-ERBα/β) and the retinoic acid receptor-related orphan receptor α (RORα), which, in turn, regulate BMAL1 expression. Genetic polymorphisms in these clock genes are responsible for a great distribution of entrained phases (chronotypes) between individuals, ranging from “larks” to “owls,” with most individuals falling between these extremes (103).
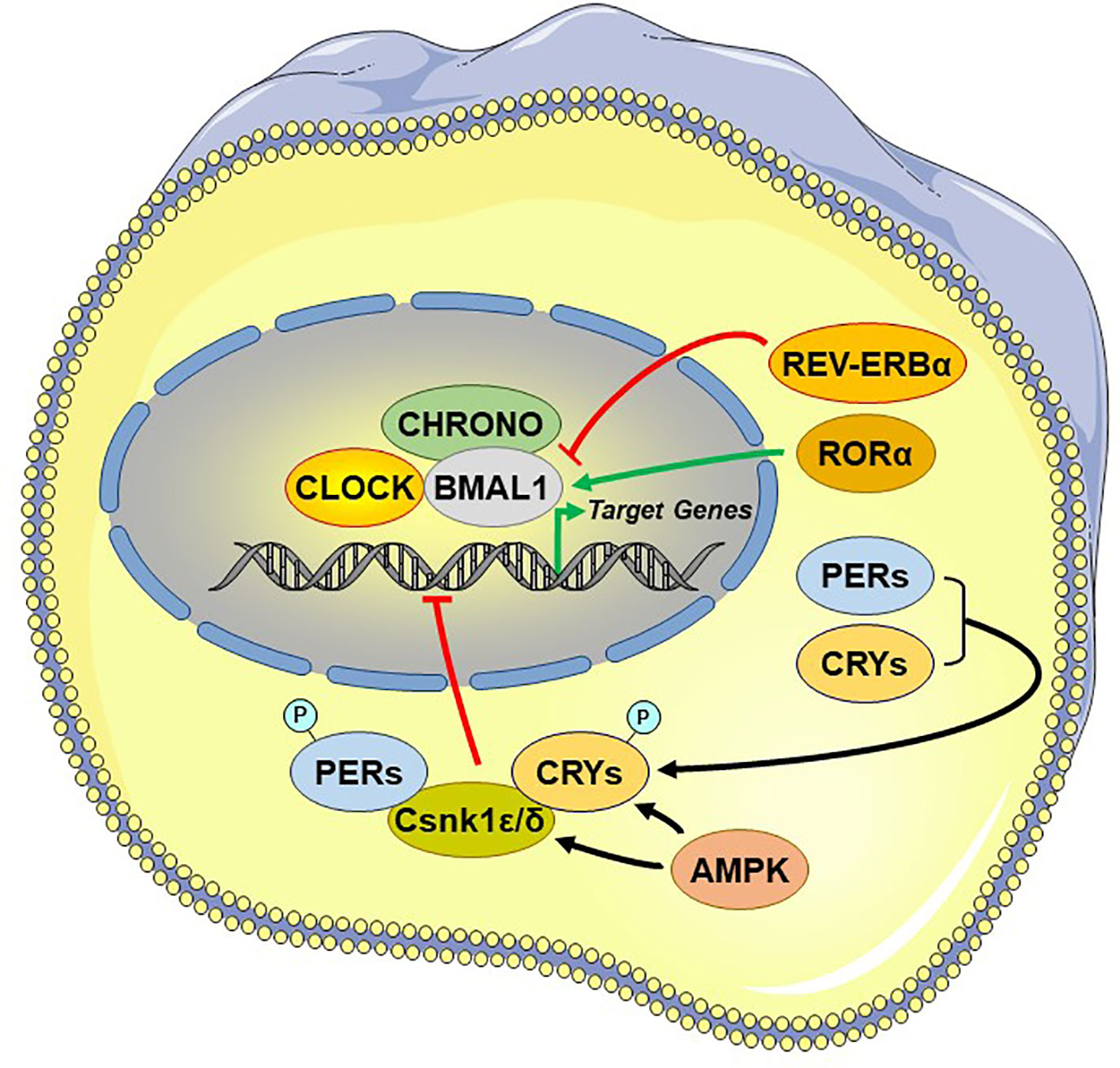
Figure 3 Principal and auxiliary transcriptional/translational feedback loops of the circadian system. AMPK, adenosine monophosphate (AMP)-activated protein kinase; BMAL1, brain-muscle-arnt-like protein 1; CHRONO, ChIP-derived repressor of network oscillator; CLOCK, circadian locomotor output cycle kaput; CRYs: cryptochromes; Csnk1ϵ/δ, casein kinase 1ϵ/δ; P, phosphate residue on the phosphorylated molecules; PERs, periods; RORα, retinoic acid receptor-related orphan nuclear receptor α. REV-ERBα, reverse viral erythroblastosis oncogene product alpha.
The transcription factors of both principal and auxiliary TTFLs can modulate the expression levels of many clock-responsive genes in various tissues, influencing a broad spectrum of physiologic functions, such as hormonal fluctuations, sleep/wakefulness, feeding, immune activity, thermoregulation, energy household, and glucose metabolism (14). These regulatory loops, receive adjustive input from related influencing systems. Besides the strongest circadian entrainment by light, other biological cues, such as nutrition and temperature, can also influence the activity of the clock system. For example, peripheral clocks can be influenced by food-related signals through adenosine monophosphate-activated protein kinase (AMPK), a tissue sensor and master regulator of energy balance, which phosphorylates Per1-3 and Cry1/2 leading to their degradation (104, 105). Similarly, temperature decrease can represent a strong circadian cue, as the cold-inducible RNA-binding protein CRBP accumulates under lower body temperature in peripheral clocks (but not in the SCN) and influences circadian gene expression (106).
Circadian System Interconnections and Effector Pathways
The superior robustness and resilience of the distinct intrinsic activity rhythm of the SCN is mainly preserved by the synchronization of SCN neurons through intercellular coupling to its neighbour cells in an action-potential-dependent manner (107). There are different kinds of SCN neurons containing different neuropeptides, such as arginin-vasopressin (AVP), vasoactive intestinal peptide (VIP), γ-amino-butyric-acid (GABA), glutamate, gastrin-releasing peptide, and somatostatin. This large variety of neuropeptides within the SCN ensures a rich diversity in signalling properties to effector targets (108). According to its neurocircuit topology, the SCN can be functionally divided into two subregions. The dorsomedial shell region primarily produces AVP and gets mainly innervated by the hypothalamus, while the ventrolateral core region primarily produces VIP and receives photic input [cf. Figure 1]. SCN output projections target many different brain regions and modulate the activity of downstream neurohumoral pathways in a rhythmic manner, herewith influencing a plethora of physiological processes (14, 16, 109). The most important effector targets of the SCN include: (i) hypothalamic centers associated with activity, temperature, and sleep regulation, such as the subparaventricular area (subPVN) and the dorsomedial nucleus of the hypothalamus (DMH) (110), (ii) preautonomic hypothalamic neurons, affecting vagal and sympathetic autonomic centers in brain stem and spinal cord and, thus, exerting circadian control throughout the body via ANS activity (80), and (iii) neuroendocrine hypothalamic centers responsible for hormone secretion (e.g., CRH synthesizing PVN parvocellular neurons) [cf. Figures 2 and 5]. The PVN is a significant integrating center for energy homeostasis and distribution center of circadian rhythmicity to the body, as its parvocellular neurons project to the median eminence to control the release of ACTH and thyroid-stimulating hormone (TSH) in the anterior pituitary (i.e., hypothalamic-pituitary-adrenal axis, HPA axis; hypothalamic-pituitary-thyroid axis, HPT axis), and also innervates the sympathetic limb of the ANS (22).
In addition, the central CS, exerts its synchronizing effects also through humoral (i.e., endocrine/paracrine) signals. The main effector of the central CS and essential synchronizing hormone is pineal melatonin (MLT) (111–114), whose secretion is strictly modulated by the SCN and sympathetic fibers originating from the superior cervical ganglia (112, 113, 115–117). Reversely, MLT is a direct modulator of the SCN neuron electrical activity (118, 119), as SCN expresses a high number of MLT receptors (MT) (120), while it also interacts with “clock” gene TTFLs in the SCN, and so modulates circadian rhythms and adjustment to environmental photoperiod changes (121). MLT modulates central and peripheral oscillators and related secondary molecular pathways mainly by cell-specific control through G-protein-coupled MLT membrane receptors MT1 and MT2 (118) and GABAergic mechanisms (119, 122, 123) [cf. Figure 4]. MT is broadly distributed in the body and are vital for immunomodulation, endocrine, reproductive and cardiovascular regulation, cancerogenesis, and aging. Additionally, MLT interacts with cytoplasmic factors (i.e. quinone-reductase-II/MT3 receptors, calmodulin) and nuclear receptors (i.e. retinoid acid receptor related orphan and Z receptors, ROR, RZR), while numerous other actions of MLT are receptor independent (e.g., radical scavenging) (114, 124–128). MLT concentration reaches high levels at night (plasma peak between 0200 h and 0400 h), overlapping with decreases in core body temperature, alertness, and performance (111, 113). The sharp elevation of nocturnal cerebrospinal fluid (CSF) MLT exerts substantial protective effects and is responsible for nocturnal tissue recovery after the daily free radical brain damage due to high oxygen utilization (129). These multifaceted chronobiotic regulatory actions have led to the recognition of MLT as one of the most pleiotropic biological signals in photoperiodic species (114, 130). On the other hand, it is important to note that the majority of laboratory mouse strains do not produce melatonin and thus challenge the importance of MLT in related animal findings (131).
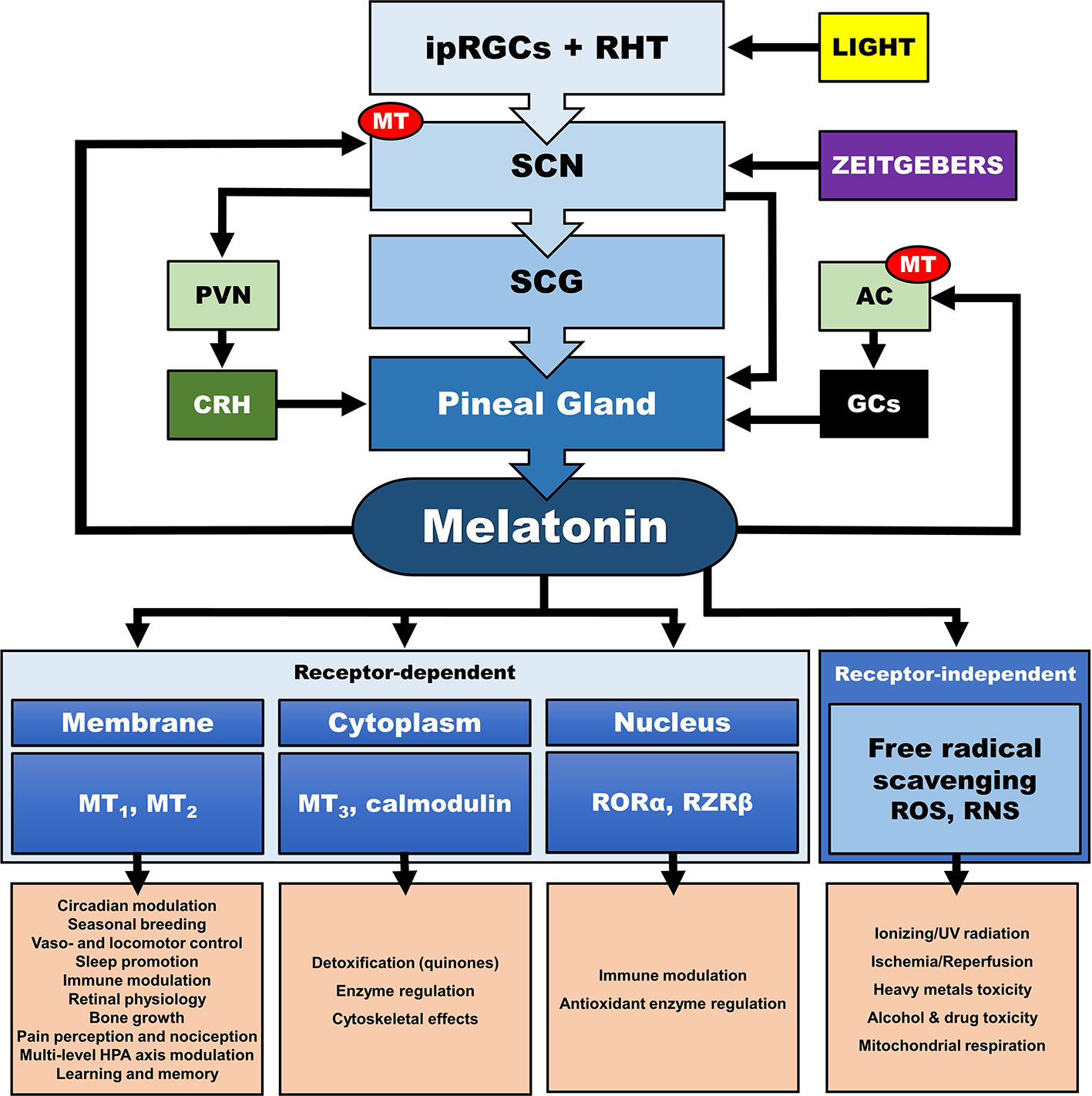
Figure 4 Multilevel interactions between the circadian system and the hypothalamic-pituitary-adrenal (HPA) axis. AC, adrenal cortex; CRH, corticotropin releasing hormone; GCs, glucocorticoids; ipRGC, intrinsically photosensitive retinal ganglion cells; MT, melatonin receptor; PVN, paraventricular nucleus; RHT, retinohypothalamic tract; RNS, reactive nitrogen species; RORα, retinoic acid receptor-related orphan receptor α; ROS, radical oxygen species; RZRβ, retinoid acid receptor related Z receptor β; SCN, suprachiasmatic nucleus; SCG, superior cervical ganlia.
Finally, sleep acts restorative in concert with the CS, but also independently, towards optimizing the internal temporal order (132). Sleep propensity and sleep stage timing, regulated through the subPVN and DMH, are bidirectionally associated with circadian gene expression in the SCN (133), but also strongly modulated by MLT levels (119, 134–138).
Interactions Between the Human Circadian and Stress System
The human CS and SS are closely and bidirectionally interconnected at multiple central and peripheral functional levels (19, 22, 23, 139–148). The circadian properties of the HPA axis are so distinct, that, along with MLT, GCs have been established as a robust measure of CS output activity. Additionally, MLT and GCs can also feedback at various levels and influence the main circadian rhythm themselves. Interestingly, the phase angle between CORT and MLT onset, the two major hormonal output signals of the CS and the HPA axis, has been identified as a potential useful biomarker in human stress-related research (149).
Influence of the CS on SS Activity and Reactivity
The HPA axis shows distinct circadian activity at rest with a robust diurnal oscillation of circulating GCs (i.e., cortisol, CORT) concentrations, rapidly rising in the middle of the biological night and peaking in the early morning, reaching their nadir before the habitual inactive phase onset (19, 141, 142, 150). SCN ablation completely abolishes the GC circadian rhythm, suggesting that HPA axis activity is driven by the central CS (151). In addition, the CS has a major influence on the ANS. Major human cardiovascular markers, such as heart rate, blood pressure, baroreflex, heart rate variability (vagal measure), plasma epinephrine, and norepinephrine levels (sympathetic measure) and their response to stressors exhibit robust circadian variations with a distinct peak of sympathetic activity and nadir of parasympathetic activity in the morning hours (152–157). By doing so, the HPA axis and SNS activity are believed to prepare the organism for the higher energetic demand associated with typical external and internal stressors of the waking phase (24).
Neurohumoral Interactions
The CS orchestrates the circadian activity and reactivity of the HPA axis through both hormonal and neuronal pathways. There are three main pathways of CS influence on the HPA axis: (i) direct SCN influence on HPA axis at the hypothalamic level, (ii) SCN innervation of the adrenal glands through indirect, multisynaptic autonomic innervation, and (iii) peripheral rhythms of local adrenal clocks, all three involved in the steroidogenic pathway and the ACTH-dependent transduction cascade in the zona glomerulosa and zona fasciculata of the adrenal gland (158) [cf. Figures 2 and 5]. The first pathway includes direct and indirect (through subPVN and DMH) neuronal projections of the SCN to CRH/AVP containing neurons of the medial parvocellular PVN modulating the circadian secretion of CRH and AVP (108, 146, 159, 160). Through the second pathway, the SCN transmits photic information via multisynaptic autonomic innervation (i.e., preganglionic intermediolateral projections to the spinal cord and splanchnic nerve innervation) to the adrenal medulla and from there through catecholamines to the cortex (161), thus both modulating the diurnal ACTH sensitivity of the adrenal cortex and stimulating the GC circadian release in light exposure conditions through an HPA axis-independent manner of direct interaction with the own peripheral rhythm of the adrenal gland (i.e., PER1 and StAR gene expression) (7, 140, 162–169). Interestingly, SCN neurons display connections to SNS and PNS, indicating that the SCN is not only essential for the physiologic autonomic diurnal fluctuations seen in humans (153, 155, 157), but also involved in both activation and deactivation of neuronal innervation of the adrenal in a circadian circle (80). The intrinsic circadian rhythm of adrenal glands in metabolic activity and GC release even in culture has been shown very early in literature (170), while clock genes expression was repeatedly reported in the following years (140, 164, 165, 171, 172). However, additional adrenal-intrinsic mechanisms depending on systemic cues, such as food-entrainable oscillators of the gland, could influence the diurnal rhythms of GC secretion (173, 174). Another very important mechanism for shaping the GC circadian rhythm is their own systemic levels, exerting a negative feedback regulation of ACTH release (175). The sensitivity of this feedback mechanism is highest during the trough point of the circadian glucocorticoid rhythm depending only MR at this time, while both MR and GR are involved at the GC peak-point lowest sensitivity (175). Finally, MLT, apart from its direct modulating effect on the SCN (176), has been also shown to directly influence GC production and release by the adrenal gland, as well as acetylation rhythms of GR, GR translocation to the nuclei and transcriptional activity (125, 172, 177, 178). MLT has been found to prevent adrenal response to ACTH (177, 179) and directly inhibit CORT production through MT1 adrenal receptor activation, possibly through their action on the Type II 3β-HSD (3β-Hydroxysteroid-dehydrogenase/Δ5-4 isomerase) enzyme activity, which catalyzes the biosynthesis of hormonal steroids through the oxidation and isomerization of Δ5-3β-hydroxysteroid precursors to Δ4-ketosteroids (180). Taken together, this illustrates the multilevel circadian “gating” control on the physiological GC secretion rhythm through SCN, HPA axis and ANS activity, GC and MLT levels, feeding and the robust intrinsic rhythm of the adrenal gland itself, involving clock gene expression in the metabolism and secretion of GCs (80, 140, 141).
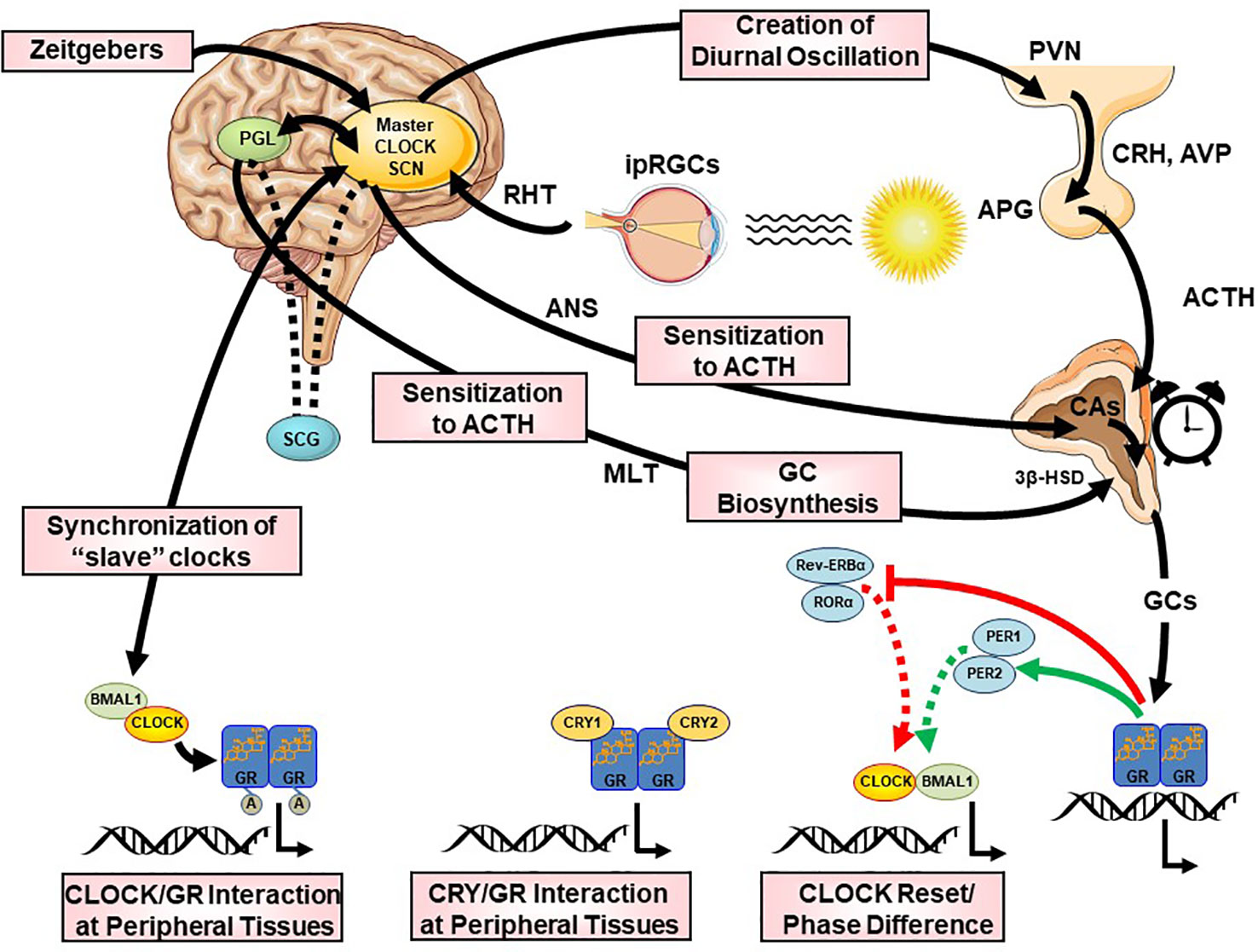
Figure 5 Multilevel interactions between the circadian system and the hypothalamic-pituitary-adrenal (HPA) axis. ACTH, adrenocorticotropic hormone; APG, anterior pituitary gland; AVP, arginine vasopressin; BMAL1, brain-muscle-arnt-like protein 1; CA, catecholamines; CLOCK, circadian locomotor output cycle kaput; CRH, corticotropin releasing hormone; CRYs, cryptochromes; HSD, hydroxysteroid dehydrogenase; ipRGC, intrinsically photosensitive retinal ganglion cells; GCs, glucocorticoids; GR, glucocorticoid receptor; PERs, periods; PVN, paraventricular nucleus; REV-ERBα, reverse viral erythroblastosis oncogene product alpha; RHT, retinohypothalamic tract; RORα, retinoic acid receptor-related orphan nuclear receptor alpha SCN, suprachiasmatic nucleus.
In addition, MLT acts directly through MT1/MT2 on the electrical activity in SCN neurons (118, 119) and interacts with the “clock” gene (PER1/2, CRY1/2, CLOCK, BMAL1, etc.) proteasome TTFL in the SCN, thus being crucial for circadian entrainment in photoperiodic species (121).
Molecular Interactions
The neurohumoral interactions between CS and SS described above, have further molecular underpinnings at the cellular level, where the GR plays a fundamental role. For example, findings suggest that the CLOCK/BMAL1 heterodimer behaves as a reverse-phase negative regulator of hGRα in the periphery, antagonizing the physiologic actions of diurnally fluctuating GCs. Through a region enclosed in the C-terminal part of the CLOCK protein, CLOCK/BMAL1 physically interacts with the ligand-binding domain of hGRα and acetylates the hGRα at multiple lysine residues, thereby reducing GR’s affinity to its cognate glucocorticoid response elements (GREs) and, thus, leading to decreased hGRα-induced transcriptional activity of glucocorticoid-responsive genes (144, 181–184). GR transactivational activity fluctuates in a circadian fashion and in reverse phase with CLOCK/BMAL1 mRNA expression (182) and leads to a higher hGRα acetylation and decreased tissue glucocorticoid sensitivity in the morning, mirroring the circadian pattern of serum CORT concentrations (183). In addition, a CLOCK-mediated posttranslational modification of hGRα is involved with the nuclear localization signal 1 (NL1), altering the cytoplasm-to-nucleus translocation of the receptor following ligand-induced activation, and indicates that the hGRα acetylation by CLOCK is linked to several molecular mechanisms (182). Moreover, Lamia and collaborators demonstrated that CRY1/2 interacted with the carboxyterminal domain of hGRα, thereby reducing the DNA-binding of the receptor and its transcriptional activity (185). Interestingly, the effect of a specific clock gene deletion on circulating GCs seems to depend on the specific TTFL missing member, suggesting that alteration of the positive or negative limb of the core clock feedback loop may have opposing effects on stress regulation. Accordingly, BMAL1 (TTFL positive limb gene) deletion leads to low adrenal ACTH sensitivity throughout the circadian circle, supporting constant low GC levels and insensitivity to acute stress (186). Genetic deletion of CRY1/2 (TTFL negative limb genes) leads to nonoscillating and elevated GC levels due to impaired feedback inhibition (185, 187). In contradistinction, the PER1/CRY1 complex reduces the maximal GR transactivation but not the efficacy of the receptor (184). Furthermore, CHRONO (ChIP-derived repressor of network oscillator), which is encoded by a BMAL-target gene, interacted with BMAL1, CRY2 and DEC2 and recruited the histone deacetylase 1 (HDAC1) to the transcriptional machinery, ultimately repressing the principal transcriptional loop (188). CHRONO is also able to acetylate the hinge region lysine cluster of GR, reducing its DNA-binding and thus indicating that this protein might play a fundamental role in the interaction of the CS with the SS (182, 188, 189). More recent in vitro and in vivo studies also showed that REVERBa, in interaction with heat-shock-protein (HSP) 90, influences the stability and nuclear localization of GR in the liver and provides another link between the CS, metabolism and glucocorticoid actions (190, 191). In addition, transcriptional cofactors of nuclear receptors (e.g., PGC1a) has recently been also implicated in circadian clock function (192), while interacting with the GR (193). Similarly, HSP, forming a dynamic complex with the GR in the cytoplasm (i.e., before GC binding and nuclear translocation), also display a circadian regulation through systemic circadian temperature changes, thus contributing to clock entrainment in peripheral tissues (194, 195). Finally, FKBP5, a chaperone protein of particular interest involved in directing activated GRs to the nucleus and implicated in a number of stress related psychiatric disorders, is also rhythmically expressed in most tissues (196), suggesting its involvement in circadian gating of GC signals.
Influence of the SS on the Central and Peripheral CS
Apart from the influence on many important biological processes, the rhythmic oscillations of the SS activity and especially the HPA axis and GC rhythmicity exert a vital synchronizing effect on the central and peripheral CS activity (19, 23, 92). GCs, through binding to the hGRα, can efficiently reset the activity of peripheral clocks (197–199), while they spare the SCN, which maintains its master intrinsic circadian rhythm, as it does not express GRs (158, 197). The attenuation of the peripheral clocks by the phase-shifting effects of the GCs is then normally restored by the influence of the SCN. However, the SS has to directly influence the SCN through an alternative pathway, as both stress exposure and exogenous GC application enhances AVP and VIP mRNA expression and release in the SCN (200, 201), while acute stress exposure also leads to an upregulation of Per1 and Per2 protein expression in the SCN (202). For example, CORT and CRH are suggested to directly modulate PGL activity and stimulate MLT synthesis, interfering in the daily adjustment of the light/dark cycle (179, 203–206). In addition, GCs play an important role in the adjustment of nutrition-related uncoupling between the central and peripheral CS, as their high secretion after feeding slows down the circadian uncoupling and restores proper phasing (173, 207). GCs are, thus, not just a downstreal hormonal output of central and peripheral clocks, but can also influence the CS itself and interact with other clock outputs toward a harmonious circadian regulation (141, 197), adding another interaction level between the stress and the circadian clock system. Alteration of the GC rhythm (e.g., through exogenous GC administration) can, thus, attenuate the central and peripheral circadian activity and vice versa (167, 208). Taken together, the SS through its effectors efficiently adjusts the circadian rhythm-linked output pathways of the body to properly respond to stressors, providing resistance to stress challenges in order to evade uncordinated circadian shifts (23).
Molecular Interactions
Diurnally circulating GCs vitally contribute to the development of the CS activity by adjusting the phase of peripheral oscillators (19, 148). GCs synchronizing effects mainly involve GR-related phase shifting of peripheral circadian expression of several clock-related genes (197, 209–216). All peripheral clocks express GR, which translocate into the nucleus after activation and modulate transcriptional activity of several clock genes (e.g., PER1/2) and transrepressing genes expressing transcription factors of the auxiliary TTFL (e.g., Rev-ERBα, RORα) through binding to functional GREs in their promoter region (217–222). PER1 contains GRs in its regulatory sequences, while GRs influence the expression of PER2 through binding to an intronic domain (218). GCs lead herewith to upregulation of these genes, causing a phase delay of peripheral clocks with respect to the SCN master clock (218). A genetically, functionally (e.g., adrenalectomy) or pharmacologically (i.e. externally administered corticosteroids) attenuated GC diurnal rhythm has been shown to be associated with abolished or shifted circadian clock gene (e.g., PER1/2) expression in several peripheral tissues (e.g., liver, preadipocytes, kidney, bronchial epithelial cells, pancreas, bone tissue, cornea, fibroblasts cardiac muscle tissue), despite the presence of an intact molecular oscillator (167, 197, 208, 209, 215, 216, 223–226). Even externally applied corticosteroids can entrain molecular oscillation in peripheral clocks (215, 227) and have been shown, for example, to speed up or slow down adaptation to a new light-dark schedule after jetlag-induced circadian desynchrony (198).
However, rhythmic GC signaling is also required for periodic clock gene expression in certain brain regions outside the SCN, suggesting an important role of the adrenal rhythm also for higher brain functions in key stress-system-related regions (228). Indeed, GR-mediated GC signaling is, for example, fundamental for the rhythmic expression of PER2 in the amygdala (213, 229), while adrenalectomy is shown to supress and extended GC exposure to increase PER gene expression in the PVN, bed nucleus of stria terminalis (BNST) and other limbic areas (219, 228, 230–232). GC-dependent circadian gene expression could even be indirectly involved in a GC feedback pathway to the SCN (233). For example, serotonergic projections of the raphe nucleus to the SCN involved in light entrainment (234) show a GC-dependent circadian transcription of tryptophan hydroxylase-2 (TH-2), an enzyme involved in serotonin synthesis (235).
Finally, the SAM/ANS constitutes another pathway in stress-induced peripheral circadian entrainment. Administration of adrenaline or noradrenaline has been shown to induce PER1/2 expression through the cAMP response element-binding protein (CREB) signalling pathway (236–238). Furthermore, GR-related GC effects and clock machinery also interact through a modulation of catecholamine biosynthesis and degradation, thus influencing time-of-day-dependent stress responses and further reinforcing the interaction between the CS and the SS (94, 239, 240). Catecholamine biosynthesis is both GC- and clock-regulated, as TH (i.e., the main synthesis pacemaker enzyme) is repressed by Rev-ERBα (241) and induced by the GR-activated the nuclear orphan receptor NURR1 (NR4A2) (242). Similarly, catecholamine degradation depends on the CLOCK/BMAL1-activated monoamine oxidase I (MAO-A) and the GR-regulated catechol-O-methyltransferase (COMT) (243).
Taken together, GC rhythms exert an accompanying circadian signal which consitutes an additional level of security to ensure proper circadian signalling input to the cell cycle oscillating machinery, while, on the other hand, peripheral clocks might gate this GR-specific input.
Stress and Circadian Misalignment
Chronodisruption and Sleep Dysregulation
The human CS enables the nyctohemeral organization and coordination of many temporal physiologic processes promoting homeostasis and environmental adaptation (18). A misalignment of the human circadian rhythm is associated with a critical loss of this harmonious biological timed order at different organizational levels, which is defined as chronodisruption (244–246). Chronodisruption-related cacostatic load with short- and long-term pathophysiologic and epigenetic consequences (245–247) can lead to a wide range of biological consequences in the organism (246, 248–255). Chronodisruption may gradually change the fundamental properties of brain systems regulating neuroendocrine, immune, and autonomic function and denotes a breakdown of appropriate biobehavioral adaptations to challenges with increased stress sensitivity and vulnerability to stress-related disorders (20, 256, 257).
In human research, chronodisruption has been tightly associated with sleep deprivation/dysregulation (SD) or phase shifting (i.e., jet-lag, swift-workers) (81, 132, 258). Sleep acts in concert with the central CS, but also independently towards an optimal internal temporal order (132). Specific sleep stages are closely related with specific clock gene expression in the SCN and are tightly ruled by the CS (81, 132, 258). SD has been associated with circadian-related gene expression alterations in humans (259–262). In addition, SD also relates to various HPA axis dysregulations (e.g., flattened CORT rhythm amplitude, blunted CORT awakening response (CAR), increased but also decreased diurnal CORT levels, higher CRH levels) and altered endocrine stress reactivity (e.g., attenuated pituitary ACTH reactivity, increased adrenocortical ACTH sensitivity) (257, 263–269), as well as to altered autonomic regulation with increased sympatho-adrenal and reduced vagal activity and blunted cardiovascular autonomic rhythmicity and autonomic reactivity (257, 270–273). Accordingly, chronodisruption in humans has been associated with increased risk for cardiovascular morbidity, metabolic consequences, inflammation, immune dysregulation, psychiatric disorders and even elevated cancer risk (226, 240, 274–280). Interestingly, even circadian gene polymorphisms have been associated with some similar consequences (281, 282).
Stress and Chronodisruption
In addition to other crucial circadian cues that can dysregulate circadian rhythms (e.g., SD, nutrition, light), stress can also lead to acute/reversible or sustained chronodisruption. Normally, after exposure to stressors, the SS can transiently override the CS creating a transient uncoupling of the central and peripheral circadian rhythm, through a hGR-related phase shift of peripheral clock-related genes (182, 197, 207, 212, 217, 221, 283). Thereby, the SCN is only indirectly influenced (198) and is, thus, able to maintain its master rhythm and restore its initial main phase to the periphery after stress termination (283, 284). Indeed, subacute stressors have been experimentally shown to have only transient impact on SCN-regulated rhythms in animal research (285, 286). However, the stability of the SCN clock appears to fade away after extensive acute or chronic physical, psychological, inflammatory, or metabolic stress (25). For example, in a study comparing single versus chronic social defeat across two weeks, single stress exposure advanced only the adrenal peripheral clock, while chronic stress also clocks in the CNS (287). Animal research provides additional evidence that chronic mild stress disrupts the regulated gene expression of several clock genes in several peripheral (287, 288), but also CNS tissues, including the hippocampus, amygdala, PFC (202, 286, 289, 290) and the SCN (283, 284, 291, 292). Chronic stress exposure in mice has been shown to alter the circadian properties of the HPA axis (293, 294), while extensive physical stress after surgery in humans leads to disturbances in MLT, CORT and core body temperature rhythms (295). In addition, numerous human and animal studies suggest that acute extensive and chronic stress can affect major sleep centers of the brain (202, 205, 288, 289, 293–296) and, thus, influence sleep physiology leading to both immediate and long-lasting sleep disruption (297–299).
Circadian-Phase-Dependent Stressor Effects
Apart from the physiological circadian activity of the SS, the stress responsiveness also displays diurnal sensitivity changes, probably through differential interference of the SCN to different brain areas (146, 159, 300). For example, acute psychological stress, involving higher brain areas and the limbic system, as well as acute physical external stress (i.e., restraint/immobilization, foot shock, shaking stress) exert the largest stress response during the rest phase (301, 302), when the HPA axis is less responsive, while acute physiological internal stress (i.e., oxidative stress, hypoglycaemia, hemorrhage), relayed to the PVN and brainstem, at the beginning of the activity phase (303, 304), when the HPA axis is most sensitive to stimulation (175). This appears reasonable, as acute physiological internal stress represents a greater threat during the active phase of animals, while acute external physical stressors (e.g., predator attack) during the inactive phase, while animals are asleep.
Interestingly, further experimental findings in animals suggest that repeated external stress exposure (i.e., chronic stress) has a more detrimental effect when applied during the inactive phase, (284, 305–308), while chronic psychosocial stress (i.e. social-defeat paradigm) shows inverse effects and exerts more detrimental effects during the active phase (307, 309) in animal research. These results jointly suggest that the effect of a stressor depends not only on the circadian phase of exposure, but also on the interaction of the circadian phase with the stressor type, as well as with the chronicity of the stressor (25, 310). For example, both physical and psychological stress at the beginning of the light phase leads to a phase advance, while at the beginning of the dark phase to a phase delay of PER2 expression in mice (286).
Chronodisruption and Traumatic Stress
The stress-related effects on internal rhythms described above have supported a recent research focus on the potential causal role of SD and chronodisruption in the acute pathophysiology and the development of long-term effects of traumatic stress exposure, suggesting that chronodisruption may represent a potential underlying neurobiologic link (311–315). The association between sleep and circadian disruption and psychopathology was first officially noted by Emil Kreapelin in 1883 (316) and has evolved through the years by numerous biological findings (317).
Traumatic stress exposure may cause both immediate and long-lasting SD (297–299), which may represent a central pathway mediating the enduring neurobiological correlates of trauma (297, 311, 312, 318–320) [cf. Figure 6]. For example, several human cohort studies have repeatedly suggested that early-life traumatic stress exposure is related to adult SD years later, including global (i.e., insomnia), but also other specific sleep pathologies, such as prolonged sleep onset latency, shortened total sleep time, decreased sleep efficiency, nightmare related distress, increased number of awakenings, sleep apnoea, and higher nocturnal activity (321–332). Such sleep dysregulation could further enhance maladaptive stress regulation and precipitate the neurobiological correlates of traumatic stress through impaired homeodynamic balance, resulting in the extensive symptomatology and comorbidity of trauma-related disorders (314, 333–350).
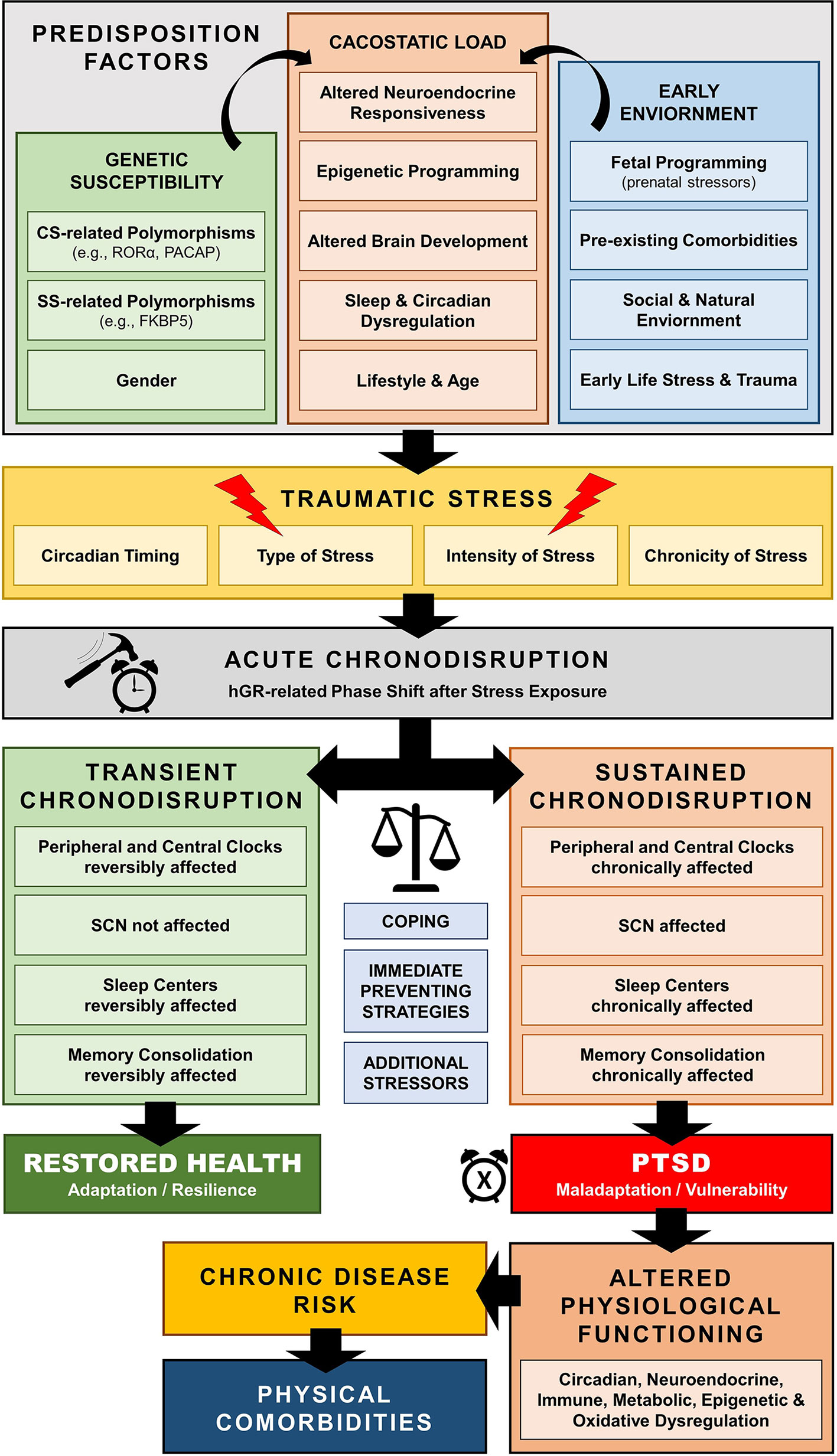
Figure 6 Schematic model of trauma-related chronodisruption as underlying biological pathway leading to posttraumatic stress disorder (PTSD) and PTSD-related comorbidities.
Posttraumatic Stress Disorder: When Time Stands Still
Posttraumatic stress disorder (PTSD) is classified in DSM-5 as a trauma- and stress-related disorder following a psychologically distressing event outside the range of usual human experience (351). Evidence of circadian dysregulation in PTSD mostly originates from sleep research findings. According to DSM-5, SD represents prominent clinical feature of the disorder with very high prevalence (312, 320, 351), and is often closely related to severity of PTSD psychopathology (352, 353) and resistant to first-line treatments (354–356).
SD in PTSD is associated with sleep-related arousal dysregulation (357) and include sleep avoidance, insomnia, nightmares, hyperarousal states, sleep terrors and nocturnal anxiety attacks, body-movement and breathing-related sleep disorders (311, 320, 358–362), with increased sympathovagal tone during rapid-eye-movement (REM) sleep, fragmented REM sleep patterns, and reduced REM theta activity (311–313, 318, 363–365). Similar findings have been in animal and human SD studies (366, 367). Interestingly, REM sleep disruption in the immediate aftermath of a trauma (311, 318, 319), as well as sleep impairment prior to traumatic stress exposure could represent risk factors for PTSD development (368, 369). SD prior to trauma have been specifically shown to be associated with a 2.5-fold increased risk of fulfilling PTSD criteria 3 months after a trauma in general population admitted to a hospital or after deployment in active military troops respectively (368, 369). SD after trauma thus represents a rather core than secondary feature of PTSD (297, 311, 312, 318–320, 370) and may be both a precipitating and perpetuating factor of the disorder (371–373).
Besides SD, traumatic stress also affects neural correlates of memory formation (374–376). Memory processing, formation and consolidation are directly influenced by sleep (377–387). Sleep promotes memory consolidation, particularly for emotionally salient information (383), while SD reduces the connectivity between amygdala and PFC (388) thus disrupting memory consolidation (389–393), as repeatedly shown in PTSD.
In addition to SD studies in PTSD, additional CS-related evidence on chronodisruption in PTSD originates from genetic, neuroendocrine, autonomic, and immune findings. For example, genome-wide association studies have also implicated to core circadian genes as PTSD candidate risk genes: pituitary adenylate cyclase-activating polypeptide (PACAP) and retinoid-related orphan receptor alpha (RORA-α) gene. PACAP is involved in phase resetting in response to light (394–396) and RORA-α is rhythmically expressed and regulates BMAL activity (397, 398). Furthermore, as immune system activity tightly follows circadian rhythms imposed by the SCN synchronisation (205, 399–405), our recent first report on the loss of the typical peripheral biphasic rhythm of IL-6 in combat stress exposed individuals (406), is of particular importance.
Further neuroendocrine findings in PTSD repeatedly show increased central CRH levels, altered HPA axis reactivity with enhanced negative feedback inhibition and blunted circadian CORT rhythm and CAR, while some studies—but not all—have shown decreased circulating concentrations of CORT (407–419). Similarly, patients with PTSD exhibit increased autonomic reactivity, elevated central and peripheral norepinephrine concentrations, higher basal heart rate, increased sympathovagal balance, blunted salivary alpha-amylase awakening response and, most importantly, blunted diurnal autonomic differences (341, 417, 420–427), suggesting central neuroautonomic dysregulation leading to higher cardiovascular risk in PTSD (415, 428, 429). In addition, disrupted MLT levels in the first 48 h after traumatic stress exposure were shown to be associated with a higher PTSD development risk (430).
Finally, PTSD has been frequently related to several other comorbidities, such as chronic fatigue syndrome (CFS) (431–434), fibromyalgia (435–439), rheumatoid arthritis (348), which all share a very similar underlying neuroendocrinological profile to PTSD (e.g., hypocortisolism, blunted diurnal CORT rhythm and HPA axis reactivity) (440–445) and have all been repeatedly associated with sustained chronodisruption (446–456).
Chronotherapeutic Implications for PTSD
Current evidence suggests that SD and CD may have a vital predispositional role in PTSD development (314), while their effective treatment could be associated with substantial improvement of overall PTSD symptomology (312, 457–459). Nevertheless, SD is still often clinically addressed as a secondary symptom in PTSD. Careful assessment and treatment of SD and CD should therefore be an integral part in PTSD management (356, 364, 371–373). Cognitive-behavioral sleep management in PTSD constitutes a widely acceptable and effective treatment option with durable gains and beneficial effects (356, 460–462). In addition, the antihypertensives α-1 adrenoreceptor antagonist prazosin and α-2 adrenoreceptor agonist clonidine, the synthetic cannabinoid receptor 1 and 2 agonist nabilone and the multilemodal antidepressant trazodone (i.e., serotonin-reuptake inhibitor, 5-HT2A receptor agonist, histamine H1 receptor antagonist, α-1 and α-2 adrenoreceptor antagonist) have been all shown to be effective pharmacological approaches for PTSD-related sleep disturbances and trauma-specific nightmares (463–467). Standard pharmacological sleep management in PTSD, however, may treat sleep quantity sufficiently, but often fail to improve daytime functioning and restore CD in PTSD (132, 468). Therefore, development of chronopharmacological interventions that would restore CS-related alterations and herethrough counteract changes in PTSD-related neurocircuitry could represent interesting novel therapeutic strategies (469–472).
Melatonergic Treatment
Recent experimental findings emphasize on a pleiotropic, but crucial role of MLT in mechanisms of sleep, cognition and memory, metabolism, pain, neuroimmunomodulation, stress endocrinology and physiology, circadian gene expression, oxidative stress, and epigenetics, thus suggesting a potentially beneficiary effect of an add-on melatonergic treatment in PTSD (374, 473). Numerous studies have repeatedly confirmed the efficacy of melatonergic treatment on almost every aspect of sleep disturbance, while preserving a benign side-effect profile and safety in both short- and long-term administration, with no efficacy wear-off, withdrawal effects or dependence risk (119, 474–480). MLT and melatonergic agonists, were found roboustly associated with (i) reduced sleep onset latency and increased sleep propensity, efficiency, quality, and total sleep duration in patients with insomnia, (ii) increased REM sleep percentage and continuity, normalization of sleep patterns, body-movement and breathing-related pathologies and improvements in subjective measures of daytime dysfunction in neuropsychiatric patients and (iii) advanced sleep/wake rhythm phase adjustment and sleep and wake-up propensity in healthy adults (119, 134, 135, 138, 474, 477, 478, 480–486). In addition, MLT is known to adjust and reset amplitude and phase of CNS (e.g., SCN, hippocampus, pituitary pars tuberalis) and peripheral (e.g., adrenal gland) circadian-related gene expression (172, 177, 178, 180, 487–489) and to moderate the circadian regulation of GR function (140, 141, 144, 183, 490). MLT also decreases hypothalamic CRH levels and inhibit the ACTH-stimulated CORT production in the primate and human adrenal gland (172, 177–180, 487–489), thus attenuating the adrenocortical secretory response in acute and chronic stress models (491–494). With respect to the ANS, MLT entrain disrupted autonomic rhythmicity by inhibiting central sympatho-adreno-medullary (SAM) outflow and shifting autonomic balance in favour of vagal activity (154, 495–498). Interestingly, research findings suggest a direct enhancing effect of melatonergic transmission in stimulus processing, memory consolidation, and conditional cued fear extinction, especially under stress (499–502). Finally, immediate melatonergic treatment directly after exposure to stress, normalizes the altered expression of Per 1 and Per 2 genes in hippocampal regions of rats, thus suggesting a possible immediate preventing properties (202). MLT has been shown to protect these hippocampal neurons from oxidative stress, by preventing GC-related toxicity through decrease of receptor translocation to nuclei in models of sleep deprivation and chronic stress (503–506). Taken together, MLT and melatonergic agents could therefore represent a promising adjuvant contribution to the clinical treatment and perhaps prevention of stress-related syndromes and comorbidities in mental disorders in general and PTSD in particular (124, 314, 471, 507–509).
Other Potential Treatment Possibilities
Further options for a pharmacological or nonpharmacological manipulation of the interplay between CS and SS in order to interfere in the pathophysiology of trauma-related disorders are of theoretical interest and deserve thorough further investigation through preclinical research and clinical confirmation. For example, exogenous application of GCs and GC-analogs in a time-of-day dependent fashion (i.e., as in immune therapy), could contribute to a reset of peripheral clocks (55, 144, 510) or even contribute to PTSD prevention if applied immediately after trauma exposure (511). On the other hand, pharmacological GR-antagonism has been found associated with insomnia symptoms improvement (512) and could also represent a potential approach.
As sleep promotes memory consolidation, particularly for emotionally salient information, sleep deprivation in the beginning of the resting phase directly after traumatic stress exposure may also decrease the risk of PTSD development (513), possibly through reduction of mPFC-amygdala connectivity (388, 390, 392). Furthermore, first findings suggest that casein kinase 1ϵ, a closely related clock components implicated in period determination, could represent a novel target of pharmacological inhibition, thus stabilizing the circadian clock against phase shift (472). Finally, it is important to mention, that selective serotonine reuptake inhibitors (SSRI), as first-line treatment option for PTSD, have been shown to exert additional, CS-related effects. In particular, fluoxetine treatment was shown to modulate the CS via phase advances of SCN neuronal firing (514) and also normalize disrupted circadian locomotor activity and hippocampal clock gene expression in a genetic mouse model of high trait anxiety and depression (515).
Conclusions
In Plato’s cosmology, as presented in the Timaeus, time is suggested to depend on the periodic regularity of movement, which is secured and defined by the planets (516). This periodic movement of our planet has contributed to the evolution of the internal time-keeping system, that creates and maintains cellular and systemic rhythmicity, through temporal organization of physiologic processes throughout several structural levels in the organism, the CS. The intrinsic rhythmicity of this system is based on a core set of clock genes involved with an autoregulatory transcriptional/translational feedback loop machinery. By rephrasing Plato’s words, we could, thus, state that human time depends on the periodic regularity of transcription, which is secured and defined by the clock genes. The award of the 2017 Nobel Prize in Physiology or Medicine to J.C. Hall, M. Rosbash and M. W. Young “for their discoveries of molecular mechanisms controlling the circadian rhythm” (517) is a testament to the fundamental importance of circadian clocks and the molecular complexity of behavior regulation.
However, over the past seven decades, modern society has cultivated a new, round-the-clock lifestyle, which enhances temporal misalignment between internal (i.e., central and peripheral) and geophysical circadian cycles. Given the close interconnection between the CS and the SS at various levels, internal desynchrony could synergistically contribute to the development of a higher stress sensitivity and vulnerability for stress-related disorders. Understanding the mechanisms susceptible to chronodisruption following toxic stress exposure and their role in a chronically dysregulated circadian network in stress-related disorders could provide new insights into disease mechanisms, advancing psychochronobiological treatment possibilities and enabling preventive strategies in stress-exposed populations (74, 312, 518).
Author Contributions
AA managed all literature searches. AA and NN wrote the first draft of the paper. VB, GC, and PP contributed with significant text passages and revised the draft for important intellectual content. All authors have contributed to, read and approved the final version of the manuscript.
Conflict of Interest
The authors declare that the research was conducted in the absence of any commercial or financial relationships that could be construed as a potential conflict of interest.
References
2. Ikegami T, Suzuki K. From a homeostatic to a homeodynamic self. Biosystems (2008) 91(2):388–400. doi: 10.1016/j.biosystems.2007.05.014
3. McEwen BS. Protective and damaging effects of stress mediators. N Engl J Med (1998) 338(3):171–9. doi: 10.1056/NEJM199801153380307
4. Tsigos C, Chrousos GP. Hypothalamic-pituitary-adrenal axis, neuroendocrine factors and stress. J Psychosom Res (2002) 53(4):865–71. doi: 10.1016/S0022-3999(02)00429-4
5. Elenkov IJ, Chrousos GP. Stress system–organization, physiology and immunoregulation. Neuroimmunomodulation (2006) 13(5-6):257–67. doi: 10.1159/000104853
6. Chrousos GP. Stress and disorders of the stress system. Nat Rev Endocrinol (2009) 5(7):374–81. doi: 10.1038/nrendo.2009.106
7. Ulrich-Lai YM, Herman JP. Neural regulation of endocrine and autonomic stress responses. Nat Rev Neurosci (2009) 10(6):397–409. doi: 10.1038/nrn2647
8. Nicolaides NC, Kyratzi E, Lamprokostopoulou A, Chrousos GP, Charmandari E. Stress, the stress system and the role of glucocorticoids. Neuroimmunomodulation (2015) 22(1-2):6–19. doi: 10.1159/000362736
9. Chrousos GP, Gold PW. The concepts of stress and stress system disorders. Overview of physical and behavioral homeostasis. JAMA (1992) 267(9):1244–52. doi: 10.1001/jama.267.9.1244
10. Panda S, Hogenesch JB, Kay SA. Circadian rhythms from flies to human. Nature (2002) 417(6886):329–35. doi: 10.1038/417329a
11. Paranjpe DA, Sharma VK. Evolution of temporal order in living organisms. J Circadian Rhythms (2005) 3(1):7. doi: 10.1186/1740-3391-3-7
12. Ko CH, Takahashi JS. Molecular components of the mammalian circadian clock. Hum Mol Genet (2006) 15(Spec No 2):R271–277. doi: 10.1093/hmg/ddl207
13. Hastings M, O’Neill JS, Maywood ES. Circadian clocks: regulators of endocrine and metabolic rhythms. J Endocrinol (2007) 195(2):187–98. doi: 10.1677/JOE-07-0378
14. Hastings MH, Maywood ES, Brancaccio M. Generation of circadian rhythms in the suprachiasmatic nucleus. Nat Rev Neurosci (2018) 19(8):453–69. doi: 10.1038/s41583-018-0026-z
15. Takahashi JS, Hong HK, Ko CH, McDearmon EL. The genetics of mammalian circadian order and disorder: implications for physiology and disease. Nat Rev Genet (2008) 9(10):764–75. doi: 10.1038/nrg2430
16. Dibner C, Schibler U, Albrecht U. The mammalian circadian timing system: organization and coordination of central and peripheral clocks. Annu Rev Physiol (2010) 72:517–49. doi: 10.1146/annurev-physiol-021909-135821
17. Moore RY. The suprachiasmatic nucleus and the circadian timing system. Prog Mol Biol Transl Sci (2013) 119:1–28. doi: 10.1016/B978-0-12-396971-2.00001-4
18. Saper CB. The central circadian timing system. Curr Opin Neurobiol (2013) 23(5):747–51. doi: 10.1016/j.conb.2013.04.004
19. Nader N, Chrousos GP, Kino T. Interactions of the circadian CLOCK system and the HPA axis. Trends Endocrinol Metab (2010) 21(5):277–86. doi: 10.1016/j.tem.2009.12.011
20. Morris CJ, Aeschbach D, Scheer FA. Circadian system, sleep and endocrinology. Mol Cell Endocrinol (2012) 349(1):91–104. doi: 10.1016/j.mce.2011.09.003
21. Buijs RM, Escobar C, Swaab DF. The circadian system and the balance of the autonomic nervous system. Handb Clin Neurol (2013) 117:173–91. doi: 10.1016/B978-0-444-53491-0.00015-8
22. Tsang AH, Barclay JL, Oster H. Interactions between endocrine and circadian systems. J Mol Endocrinol (2014) 52(1):R1–16. doi: 10.1530/JME-13-0118
23. Koch CE, Leinweber B, Drengberg BC, Blaum C, Oster H. Interaction between circadian rhythms and stress. Neurobiol Stress (2017) 6:57–67. doi: 10.1016/j.ynstr.2016.09.001
24. Gamble KL, Berry R, Frank SJ, Young ME. Circadian clock control of endocrine factors. Nat Rev Endocrinol (2014) 10(8):466–75. doi: 10.1038/nrendo.2014.78
25. Helfrich-Forster C. Interactions between psychosocial stress and the circadian endogenous clock. Psych J (2017) 6(4):277–89. doi: 10.1002/pchj.202
26. Licht CM, Vreeburg SA, van Reedt Dortland AK, Giltay EJ, Hoogendijk WJ, DeRijk RH, et al. Increased sympathetic and decreased parasympathetic activity rather than changes in hypothalamic-pituitary-adrenal axis activity is associated with metabolic abnormalities. J Clin Endocrinol Metab (2010) 95(5):2458–66. doi: 10.1210/jc.2009-2801
27. Thayer JF, Sternberg E. Beyond heart rate variability: vagal regulation of allostatic systems. Ann N Y Acad Sci (2006) 1088:361–72. doi: 10.1196/annals.1366.014
28. Kagias K, Nehammer C, Pocock R. Neuronal responses to physiological stress. Front Genet (2012) 3:222. doi: 10.3389/fgene.2012.00222
29. Joels M, Baram TZ. The neuro-symphony of stress. Nat Rev Neurosci (2009) 10(6):459–66. doi: 10.1038/nrn2632
30. Thayer JF, Lane RD. A model of neurovisceral integration in emotion regulation and dysregulation. J Affect Disord (2000) 61(3):201–16. doi: 10.1016/S0165-0327(00)00338-4
31. Critchley HD. Neural mechanisms of autonomic, affective, and cognitive integration. J Comp Neurol (2005) 493(1):154–66. doi: 10.1002/cne.20749
32. Ondicova K, Mravec B. Multilevel interactions between the sympathetic and parasympathetic nervous systems: a minireview. Endocr Regul (2010) 44(2):69–75. doi: 10.4149/endo_2010_02_69
33. Beissner F, Meissner K, Bar KJ, Napadow V. The autonomic brain: an activation likelihood estimation meta-analysis for central processing of autonomic function. J Neurosci (2013) 33(25):10503–11. doi: 10.1523/JNEUROSCI.1103-13.2013
34. Saper CB. The central autonomic nervous system: conscious visceral perception and autonomic pattern generation. Annu Rev Neurosci (2002) 25:433–69. doi: 10.1146/annurev.neuro.25.032502.111311
35. Saper CB. Central autonomic system. In: Paxinos G, editor. The Rat Nervous System. Elsevier: Amsterdam (2004). p. 761–96. doi: 10.1016/B978-012547638-6/50025-0
36. Agelink MW, Klimke A, Cordes J, Sanner D, Kavuk I, Malessa R, et al. A functional-structural model to understand cardiac autonomic nervous system (ANS) dysregulation in affective illness and to elucidate the ANS effects of antidepressive treatment. Eur J Med Res (2004) 9(1):37–50.
37. Thayer JF, Ahs F, Fredrikson M, Sollers JJ 3rd, Wager TD. A meta-analysis of heart rate variability and neuroimaging studies: Implications for heart rate variability as a marker of stress and health. Neurosci Biobehav Rev (2012) 36(2):747–56. doi: 10.1016/j.neubiorev.2011.11.009
38. Loewy AD, Spyer KM. Central Regulation of Autonomic Functions. Oxford: Oxford University Press (1990).
39. Davis AM, Natelson BH. Brain-heart interactions. The neurocardiology of arrhythmia and sudden cardiac death. Tex Heart Inst J (1993) 20(3):158–69.
40. Thayer JF, Lane RD. Claude Bernard and the heart-brain connection: further elaboration of a model of neurovisceral integration. Neurosci Biobehav Rev (2009) 33(2):81–8. doi: 10.1016/j.neubiorev.2008.08.004
41. Stiedl O, Youn J, Jansen RF. Cardiovascular conditioning: neural substrates. In: Koob GF, LeMoal M, Thompson RF, editors. Encyclopedia of Behavioral Neuroscience, vol. 1. Elsevier: Amsterdam (2010). p. 226–35. doi: 10.1016/B978-0-08-045396-5.00130-5
42. Furness JB. The organisation of the autonomic nervous system: peripheral connections. Auton Neurosci (2006) 130(1-2):1–5. doi: 10.1016/j.autneu.2006.05.003
43. Nilsson S. Comparative anatomy of the autonomic nervous system. Auton Neurosci (2011) 165(1):3–9. doi: 10.1016/j.autneu.2010.03.018
44. Shields RW Jr. Functional anatomy of the autonomic nervous system. J Clin Neurophysiol (1993) 10(1):2–13. doi: 10.1097/00004691-199301000-00002
45. Meyer M, Stiedl O. Self-affine fractal variability of human heartbeat interval dynamics in health and disease. Eur J Appl Physiol (2003) 90(3-4):305–16. doi: 10.1007/s00421-003-0915-2
46. Lipsitz LA, Goldberger AL. Loss of ‘complexity’ and aging. Potential applications of fractals and chaos theory to senescence. JAMA (1992) 267(13):1806–9. doi: 10.1001/jama.267.13.1806
47. Peng CK, Buldyrev SV, Hausdorff JM, Havlin S, Mietus JE, Simons M, et al. Non-equilibrium dynamics as an indispensable characteristic of a healthy biological system. Integr Physiol Behav Sci (1994) 29(3):283–93. doi: 10.1007/BF02691332
48. Schmidt RF, Thews G, Lang F. Physiologie des Menschen. Berlin: Springer (2000). doi: 10.1007/978-3-662-09346-7
50. Thayer JF, Lane RD. The role of vagal function in the risk for cardiovascular disease and mortality. Biol Psychol (2007) 74(2):224–42. doi: 10.1016/j.biopsycho.2005.11.013
51. Chrousos GP. Regulation and dysregulation of the hypothalamic-pituitary-adrenal axis. The corticotropin-releasing hormone perspective. Endocrinol Metab Clin North Am (1992) 21(4):833–58. doi: 10.1016/S0889-8529(18)30191-9
52. Chrousos GP, Charmandari E, Kino T. Glucocorticoid action networks–an introduction to systems biology. J Clin Endocrinol Metab (2004) 89(2):563–4. doi: 10.1210/jc.2003-032026
53. Charmandari E, Tsigos C, Chrousos G. Endocrinology of the stress response. Annu Rev Physiol (2005) 67:259–84. doi: 10.1146/annurev.physiol.67.040403.120816
54. Chrousos GP, Kino T. Intracellular glucocorticoid signaling: a formerly simple system turns stochastic. Sci STKE (2005) 2005(304):pe48. doi: 10.1126/stke.3042005pe48
55. Chrousos GP, Kino T. Glucocorticoid signaling in the cell. Expanding clinical implications to complex human behavioral and somatic disorders. Ann N Y Acad Sci (2009) 1179:153–66. doi: 10.1111/j.1749-6632.2009.04988.x
56. Nicolaides NC, Galata Z, Kino T, Chrousos GP, Charmandari E. The human glucocorticoid receptor: molecular basis of biologic function. Steroids (2010) 75(1):1–12. doi: 10.1016/j.steroids.2009.09.002
57. Oakley RH, Cidlowski JA. The biology of the glucocorticoid receptor: new signaling mechanisms in health and disease. J Allergy Clin Immunol (2013) 132(5):1033–44. doi: 10.1016/j.jaci.2013.09.007
58. de Kloet ER. Functional profile of the binary brain corticosteroid receptor system: mediating, multitasking, coordinating, integrating. Eur J Pharmacol (2013) 719(1-3):53–62. doi: 10.1016/j.ejphar.2013.04.053
59. Rohleder N, Wolf JM, Wolf OT. Glucocorticoid sensitivity of cognitive and inflammatory processes in depression and posttraumatic stress disorder. Neurosci Biobehav Rev (2010) 35(1):104–14. doi: 10.1016/j.neubiorev.2009.12.003
60. Lu NZ, Cidlowski JA. Translational regulatory mechanisms generate N-terminal glucocorticoid receptor isoforms with unique transcriptional target genes. Mol Cell (2005) 18(3):331–42. doi: 10.1016/j.molcel.2005.03.025
61. Bamberger CM, Bamberger AM, de Castro M, Chrousos GP. Glucocorticoid receptor beta, a potential endogenous inhibitor of glucocorticoid action in humans. J Clin Invest (1995) 95(6):2435–41. doi: 10.1172/JCI117943
62. Charmandari E, Chrousos GP, Ichijo T, Bhattacharyya N, Vottero A, Souvatzoglou E, et al. The human glucocorticoid receptor (hGR) beta isoform suppresses the transcriptional activity of hGRalpha by interfering with formation of active coactivator complexes. Mol Endocrinol (2005) 19(1):52–64. doi: 10.1210/me.2004-0112
63. Kino T, Su YA, Chrousos GP. Human glucocorticoid receptor isoform beta: recent understanding of its potential implications in physiology and pathophysiology. Cell Mol Life Sci (2009) 66(21):3435–48. doi: 10.1007/s00018-009-0098-z
64. Arriza JL, Simerly RB, Swanson LW, Evans RM. The neuronal mineralocorticoid receptor as a mediator of glucocorticoid response. Neuron (1988) 1(9):887–900. doi: 10.1016/0896-6273(88)90136-5
65. de Kloet ER, Otte C, Kumsta R, Kok L, Hillegers MH, Hasselmann H, et al. Stress and Depression: a Crucial Role of the Mineralocorticoid Receptor. J Neuroendocrinol (2016) 28(8). doi: 10.1111/jne.12379
66. Wingenfeld K, Otte C. Mineralocorticoid receptor function and cognition in health and disease. Psychoneuroendocrinology (2019) 105:25–35. doi: 10.1016/j.psyneuen.2018.09.010
67. de Kloet ER, Meijer OC, de Nicola AF, de Rijk RH, Joels M. Importance of the brain corticosteroid receptor balance in metaplasticity, cognitive performance and neuro-inflammation. Front Neuroendocrinol (2018) 49:124–45. doi: 10.1016/j.yfrne.2018.02.003
68. ter Heegde F, De Rijk RH, Vinkers CH. The brain mineralocorticoid receptor and stress resilience. Psychoneuroendocrinology (2015) 52:92–110. doi: 10.1016/j.psyneuen.2014.10.022
69. Ayroldi E, Cannarile L, Migliorati G, Nocentini G, Delfino DV, Riccardi C. Mechanisms of the anti-inflammatory effects of glucocorticoids: genomic and nongenomic interference with MAPK signaling pathways. FASEB J (2012) 26(12):4805–20. doi: 10.1096/fj.12-216382
70. Joels M, Karst H, DeRijk R, de Kloet ER. The coming out of the brain mineralocorticoid receptor. Trends Neurosci (2008) 31(1):1–7. doi: 10.1016/j.tins.2007.10.005
71. Henckens MJ, Pu Z, Hermans EJ, van Wingen GA, Joels M, Fernandez G. Dynamically changing effects of corticosteroids on human hippocampal and prefrontal processing. Hum Brain Mapp (2012) 33(12):2885–97. doi: 10.1002/hbm.21409
72. Joels M, Pasricha N, Karst H. The interplay between rapid and slow corticosteroid actions in brain. Eur J Pharmacol (2013) 719(1-3):44–52. doi: 10.1016/j.ejphar.2013.07.015
73. Edery I. Circadian rhythms in a nutshell. Physiol Genomics (2000) 3(2):59–74. doi: 10.1152/physiolgenomics.2000.3.2.59
74. Roenneberg T, Merrow M. The network of time: understanding the molecular circadian system. Curr Biol (2003) 13(5):R198–207. doi: 10.1016/S0960-9822(03)00124-6
75. Weinert D. Ontogenetic development of the mammalian circadian system. Chronobiol Int (2005) 22(2):179–205. doi: 10.1081/CBI-200053473
76. Bass J, Takahashi JS. Circadian integration of metabolism and energetics. Science (2010) 330(6009):1349–54. doi: 10.1126/science.1195027
77. Berson DM, Dunn FA, Takao M. Phototransduction by retinal ganglion cells that set the circadian clock. Science (2002) 295(5557):1070–3. doi: 10.1126/science.1067262
78. Morin LP. Neuroanatomy of the extended circadian rhythm system. Exp Neurol (2013) 243:4–20. doi: 10.1016/j.expneurol.2012.06.026
79. Czeisler CA, Duffy JF, Shanahan TL, Brown EN, Mitchell JF, Rimmer DW, et al. Stability, precision, and near-24-hour period of the human circadian pacemaker. Science (1999) 284(5423):2177–81. doi: 10.1126/science.284.5423.2177
80. Buijs RM, van Eden CG, Goncharuk VD, Kalsbeek A. The biological clock tunes the organs of the body: timing by hormones and the autonomic nervous system. J Endocrinol (2003) 177(1):17–26. doi: 10.1677/joe.0.1770017
81. Morris CJ, Aeschbach D, Scheer FAJL. Circadian system, sleep and endocrinology. Mol Cell Endocrinol (2012) 349(1):91–104. doi: 10.1016/j.mce.2011.09.003
82. Provencio I, Rodriguez IR, Jiang G, Hayes WP, Moreira EF, Rollag MD. A novel human opsin in the inner retina. J Neurosci (2000) 20(2):600–5. doi: 10.1523/JNEUROSCI.20-02-00600.2000
83. Cermakian N, Sassone-Corsi P. Environmental stimulus perception and control of circadian clocks. Curr Opin Neurobiol (2002) 12(4):359–65. doi: 10.1016/S0959-4388(02)00347-1
84. Hattar S, Liao HW, Takao M, Berson DM, Yau KW. Melanopsin-containing retinal ganglion cells: architecture, projections, and intrinsic photosensitivity. Science (2002) 295(5557):1065–70. doi: 10.1126/science.1069609
85. Van Gelder RN. Making (a) sense of non-visual ocular photoreception. Trends Neurosci (2003) 26(9):458–61. doi: 10.1016/S0166-2236(03)00211-X
86. Warman VL, Dijk DJ, Warman GR, Arendt J, Skene DJ. Phase advancing human circadian rhythms with short wavelength light. Neurosci Lett (2003) 342(1-2):37–40. doi: 10.1016/S0304-3940(03)00223-4
87. Panda S, Nayak SK, Campo B, Walker JR, Hogenesch JB, Jegla T. Illumination of the melanopsin signaling pathway. Science (2005) 307(5709):600–4. doi: 10.1126/science.1105121
88. Ohi K, Takashima M, Nishikawa T, Takahashi K. N-methyl-D-aspartate receptor participates in neuronal transmission of photic information through the retinohypothalamic tract. Neuroendocrinology (1991) 53(4):344–8. doi: 10.1159/000125740
89. Moller M, Baeres FM. The anatomy and innervation of the mammalian pineal gland. Cell Tissue Res (2002) 309(1):139–50. doi: 10.1007/s00441-002-0580-5
90. Schibler U, Gotic I, Saini C, Gos P, Curie T, Emmenegger Y, et al. Clock-Talk: Interactions between Central and Peripheral Circadian Oscillators in Mammals. Cold Spring Harb Symp Quant Biol (2015) 80:223–32. doi: 10.1101/sqb.2015.80.027490
91. Guo H, Brewer JM, Lehman MN, Bittman EL. Suprachiasmatic regulation of circadian rhythms of gene expression in hamster peripheral organs: effects of transplanting the pacemaker. J Neurosci (2006) 26(24):6406–12. doi: 10.1523/JNEUROSCI.4676-05.2006
92. Stratmann M, Schibler U. Properties, entrainment, and physiological functions of mammalian peripheral oscillators. J Biol Rhythms (2006) 21(6):494–506. doi: 10.1177/0748730406293889
93. Konopka RJ, Benzer S. Clock mutants of Drosophila melanogaster. Proc Natl Acad Sci U S A (1971) 68(9):2112–6. doi: 10.1073/pnas.68.9.2112
94. Zhang EE, Kay SA. Clocks not winding down: unravelling circadian networks. Nat Rev Mol Cell Biol (2010) 11(11):764–76. doi: 10.1038/nrm2995
95. Yagita K, Tamanini F, van Der Horst GT, Okamura H. Molecular mechanisms of the biological clock in cultured fibroblasts. Science (2001) 292(5515):278–81. doi: 10.1126/science.1059542
96. von Gall C, Weaver DR, Moek J, Jilg A, Stehle JH, Korf HW. Melatonin plays a crucial role in the regulation of rhythmic clock gene expression in the mouse pars tuberalis. Ann N Y Acad Sci (2005) 1040:508–11. doi: 10.1196/annals.1327.105
97. Gallego M, Virshup DM. Post-translational modifications regulate the ticking of the circadian clock. Nat Rev Mol Cell Biol (2007) 8(2):139–48. doi: 10.1038/nrm2106
98. Reppert SM, Weaver DR. Coordination of circadian timing in mammals. Nature (2002) 418(6901):935–41. doi: 10.1038/nature00965
99. Lee C, Etchegaray JP, Cagampang FR, Loudon AS, Reppert SM. Posttranslational mechanisms regulate the mammalian circadian clock. Cell (2001) 107(7):855–67. doi: 10.1016/S0092-8674(01)00610-9
100. Kiyohara YB, Tagao S, Tamanini F, Morita A, Sugisawa Y, Yasuda M, et al. The BMAL1 C terminus regulates the circadian transcription feedback loop. Proc Natl Acad Sci U S A (2006) 103(26):10074–9. doi: 10.1073/pnas.0601416103
101. Kondratov RV, Kondratova AA, Lee C, Gorbacheva VY, Chernov MV, Antoch MP. Post-translational regulation of circadian transcriptional CLOCK(NPAS2)/BMAL1 complex by CRYPTOCHROMES. Cell Cycle (2006) 5(8):890–5. doi: 10.4161/cc.5.8.2684
102. Padmanabhan K, Robles MS, Westerling T, Weitz CJ. Feedback regulation of transcriptional termination by the mammalian circadian clock PERIOD complex. Science (2012) 337(6094):599–602. doi: 10.1126/science.1221592
103. Roenneberg T, Wirz-Justice A, Merrow M. Life between clocks: daily temporal patterns of human chronotypes. J Biol Rhythms (2003) 18(1):80–90. doi: 10.1177/0748730402239679
104. Lamia KA, Sachdeva UM, DiTacchio L, Williams EC, Alvarez JG, Egan DF, et al. AMPK regulates the circadian clock by cryptochrome phosphorylation and degradation. Science (2009) 326(5951):437–40. doi: 10.1126/science.1172156
105. Jordan SD, Lamia KA. AMPK at the crossroads of circadian clocks and metabolism. Mol Cell Endocrinol (2013) 366(2):163–9. doi: 10.1016/j.mce.2012.06.017
106. Gotic I, Omidi S, Fleury-Olela F, Molina N, Naef F, Schibler U. Temperature regulates splicing efficiency of the cold-inducible RNA-binding protein gene Cirbp. Genes Dev (2016) 30(17):2005–17. doi: 10.1101/gad.287094.116
107. Welsh DK, Logothetis DE, Meister M, Reppert SM. Individual neurons dissociated from rat suprachiasmatic nucleus express independently phased circadian firing rhythms. Neuron (1995) 14(4):697–706. doi: 10.1016/0896-6273(95)90214-7
108. Buijs RM, Kalsbeek A. Hypothalamic integration of central and peripheral clocks. Nat Rev Neurosci (2001) 2(7):521–6. doi: 10.1038/35081582
109. Kalsbeek A, Perreau-Lenz S, Buijs RM. A network of (autonomic) clock outputs. Chronobiol Int (2006) 23(3):521–35. doi: 10.1080/07420520600651073
110. Aston-Jones G, Chen S, Zhu Y, Oshinsky ML. A neural circuit for circadian regulation of arousal. Nat Neurosci (2001) 4(7):732–8. doi: 10.1038/89522
111. Arendt J. Importance and relevance of melatonin to human biological rhythms. J Neuroendocrinol (2003) 15(4):427–31. doi: 10.1046/j.1365-2826.2003.00987.x
112. Macchi MM, Bruce JN. Human pineal physiology and functional significance of melatonin. Front Neuroendocrinol (2004) 25(3-4):177–95. doi: 10.1016/j.yfrne.2004.08.001
113. Arendt J. Melatonin and human rhythms. Chronobiol Int (2006) 23(1-2):21–37. doi: 10.1080/07420520500464361
114. Hardeland R, Cardinali DP, Srinivasan V, Spence DW, Brown GM, Pandi-Perumal SR. Melatonin–a pleiotropic, orchestrating regulator molecule. Prog Neurobiol (2011) 93(3):350–84. doi: 10.1016/j.pneurobio.2010.12.004
115. Nowak JZ, Zawilska JB. Melatonin and its physiological and therapeutic properties. Pharm World Sci (1998) 20(1):18–27. doi: 10.1023/A:1008688724058
116. Figueiro MG, Rea MS. The effects of red and blue lights on circadian variations in cortisol, alpha amylase, and melatonin. Int J Endocrinol (2010) 2010:829351. doi: 10.1155/2010/829351
117. Brainard GC, Hanifin JP, Warfield B, Stone MK, James ME, Ayers M, et al. Short-wavelength enrichment of polychromatic light enhances human melatonin suppression potency. J Pineal Res (2015) 58(3):352–61. doi: 10.1111/jpi.12221
118. Mason R, Brooks A. The electrophysiological effects of melatonin and a putative melatonin antagonist (N-acetyltryptamine) on rat suprachiasmatic neurones in vitro. Neurosci Lett (1988) 95(1-3):296–301. doi: 10.1016/0304-3940(88)90674-X
119. Srinivasan V, Pandi-Perumal SR, Trahkt I, Spence DW, Poeggeler B, Hardeland R, et al. Melatonin and melatonergic drugs on sleep: possible mechanisms of action. Int J Neurosci (2009) 119(6):821–46. doi: 10.1080/00207450802328607
120. Reppert SM, Weaver DR, Rivkees SA, Stopa EG. Putative melatonin receptors in a human biological clock. Science (1988) 242(4875):78–81. doi: 10.1126/science.2845576
121. Vriend J, Reiter RJ. Melatonin feedback on clock genes: a theory involving the proteasome. J Pineal Res (2015) 58(1):1–11. doi: 10.1111/jpi.12189
122. Niles L. Melatonin interaction with the benzodiazepine-GABA receptor complex in the CNS. Adv Exp Med Biol (1991) 294:267–77. doi: 10.1007/978-1-4684-5952-4_24
123. Golombek DA, Pevet P, Cardinali DP. Melatonin effects on behavior: possible mediation by the central GABAergic system. Neurosci Biobehav Rev (1996) 20(3):403–12. doi: 10.1016/0149-7634(95)00052-6
124. Hardeland R, Madrid JA, Tan DX, Reiter RJ. Melatonin, the circadian multioscillator system and health: the need for detailed analyses of peripheral melatonin signaling. J Pineal Res (2012) 52(2):139–66. doi: 10.1111/j.1600-079X.2011.00934.x
125. Slominski RM, Reiter RJ, Schlabritz-Loutsevitch N, Ostrom RS, Slominski AT. Melatonin membrane receptors in peripheral tissues: distribution and functions. Mol Cell Endocrinol (2012) 351(2):152–66. doi: 10.1016/j.mce.2012.01.004
126. Garcia JJ, Lopez-Pingarron L, Almeida-Souza P, Tres A, Escudero P, Garcia-Gil FA, et al. Protective effects of melatonin in reducing oxidative stress and in preserving the fluidity of biological membranes: a review. J Pineal Res (2014) 56(3):225–37. doi: 10.1111/jpi.12128
127. Reiter RJ, Tan DX, Galano A. Melatonin: exceeding expectations. Physiol (Bethesda) (2014) 29(5):325–33. doi: 10.1152/physiol.00011.2014
128. Manchester LC, Coto-Montes A, Boga JA, Andersen LP, Zhou Z, Galano A, et al. Melatonin: an ancient molecule that makes oxygen metabolically tolerable. J Pineal Res (2015) 59(4):403–19. doi: 10.1111/jpi.12267
129. Reiter RJ, Tan DX, Kim SJ, Cruz MH. Delivery of pineal melatonin to the brain and SCN: role of canaliculi, cerebrospinal fluid, tanycytes and Virchow-Robin perivascular spaces. Brain Struct Funct (2014) 219(6):1873–87. doi: 10.1007/s00429-014-0719-7
130. Pandi-Perumal SR, Srinivasan V, Maestroni GJ, Cardinali DP, Poeggeler B, Hardeland R. Melatonin: Nature’s most versatile biological signal? FEBS J (2006) 273(13):2813–38. doi: 10.1111/j.1742-4658.2006.05322.x
131. Kennaway DJ. Melatonin research in mice: a review. Chronobiol Int (2019) 36(9):1167–83. doi: 10.1080/07420528.2019.1624373
132. Zisapel N. Sleep and sleep disturbances: biological basis and clinical implications. Cell Mol Life Sci (2007) 64(10):1174–86. doi: 10.1007/s00018-007-6529-9
133. Lee ML, Swanson BE, de la Iglesia HO. Circadian timing of REM sleep is coupled to an oscillator within the dorsomedial suprachiasmatic nucleus. Curr Biol (2009) 19(10):848–52. doi: 10.1016/j.cub.2009.03.051
134. Dijk DJ, Cajochen C. Melatonin and the circadian regulation of sleep initiation, consolidation, structure, and the sleep EEG. J Biol Rhythms (1997) 12(6):627–35. doi: 10.1177/074873049701200618
135. Kunz D, Mahlberg R, Muller C, Tilmann A, Bes F. Melatonin in patients with reduced REM sleep duration: two randomized controlled trials. J Clin Endocrinol Metab (2004) 89(1):128–34. doi: 10.1210/jc.2002-021057
136. Rajaratnam SM, Middleton B, Stone BM, Arendt J, Dijk DJ. Melatonin advances the circadian timing of EEG sleep and directly facilitates sleep without altering its duration in extended sleep opportunities in humans. J Physiol (2004) 561(Pt 1):339–51. doi: 10.1113/jphysiol.2004.073742
137. Dubocovich ML. Melatonin receptors: role on sleep and circadian rhythm regulation. Sleep Med (2007) 8(Suppl 3):34–42. doi: 10.1016/j.sleep.2007.10.007
138. Pandi-Perumal SR, Srinivasan V, Spence DW, Cardinali DP. Role of the melatonin system in the control of sleep: therapeutic implications. CNS Drugs (2007) 21(12):995–1018. doi: 10.2165/00023210-200721120-00004
139. Chrousos GP. Ultradian, circadian, and stress-related hypothalamic-pituitary-adrenal axis activity–a dynamic digital-to-analog modulation. Endocrinology (1998) 139(2):437–40. doi: 10.1210/endo.139.2.5857
140. Oster H, Damerow S, Hut RA, Eichele G. Transcriptional profiling in the adrenal gland reveals circadian regulation of hormone biosynthesis genes and nucleosome assembly genes. J Biol Rhythms (2006) 21(5):350–61. doi: 10.1177/0748730406293053
141. Dickmeis T. Glucocorticoids and the circadian clock. J Endocrinol (2009) 200(1):3–22. doi: 10.1677/JOE-08-0415
142. Gan EH, Quinton R. Physiological significance of the rhythmic secretion of hypothalamic and pituitary hormones. Prog Brain Res (2010) 181:111–26. doi: 10.1016/S0079-6123(08)81007-2
143. Dickmeis T, Foulkes NS. Glucocorticoids and circadian clock control of cell proliferation: at the interface between three dynamic systems. Mol Cell Endocrinol (2011) 331(1):11–22. doi: 10.1016/j.mce.2010.09.001
144. Kino T, Chrousos GP. Circadian CLOCK-mediated regulation of target-tissue sensitivity to glucocorticoids: implications for cardiometabolic diseases. Endocr Dev (2011) 20:116–26. doi: 10.1159/000321232
145. Son GH, Chung S, Kim K. The adrenal peripheral clock: glucocorticoid and the circadian timing system. Front Neuroendocrinol (2011) 32(4):451–65. doi: 10.1016/j.yfrne.2011.07.003
146. Kalsbeek A, van der Spek R, Lei J, Endert E, Buijs RM, Fliers E. Circadian rhythms in the hypothalamo-pituitary-adrenal (HPA) axis. Mol Cell Endocrinol (2012) 349(1):20–9. doi: 10.1016/j.mce.2011.06.042
147. Leliavski A, Dumbell R, Ott V, Oster H. Adrenal Clocks and the Role of Adrenal Hormones in the Regulation of Circadian Physiology. J Biol Rhythms (2014) 30(1):20–34. doi: 10.1177/0748730414553971
148. Nicolaides NC, Charmandari E, Chrousos GP, Kino T. Circadian endocrine rhythms: the hypothalamic-pituitary-adrenal axis and its actions. Ann N Y Acad Sci (2014) 1318:71–80. doi: 10.1111/nyas.12464
149. Buckley TM, Schatzberg AF. A pilot study of the phase angle between cortisol and melatonin in major depression - a potential biomarker? J Psychiatr Res (2010) 44(2):69–74. doi: 10.1016/j.jpsychires.2009.06.012
150. Qian X, Droste SK, Lightman SL, Reul JMHM, Linthorst ACE. Circadian and ultradian rhythms of free glucocorticoid hormone are highly synchronized between the blood, the subcutaneous tissue, and the brain. Endocrinology (2012) 153(9):4346–53. doi: 10.1210/en.2012-1484
151. Moore RY, Eichler VB. Loss of a circadian adrenal corticosterone rhythm following suprachiasmatic lesions in the rat. Brain Res (1972) 42(1):201–6. doi: 10.1016/0006-8993(72)90054-6
152. Scheer FA, Van Doornen LJ, Buijs RM. Light and diurnal cycle affect autonomic cardiac balance in human; possible role for the biological clock. Auton Neurosci (2004) 110(1):44–8. doi: 10.1016/j.autneu.2003.03.001
153. Vandewalle G, Middleton B, Rajaratnam SM, Stone BM, Thorleifsdottir B, Arendt J, et al. Robust circadian rhythm in heart rate and its variability: influence of exogenous melatonin and photoperiod. J Sleep Res (2007) 16(2):148–55. doi: 10.1111/j.1365-2869.2007.00581.x
154. Dominguez-Rodriguez A, Abreu-Gonzalez P, Sanchez-Sanchez JJ, Kaski JC, Reiter RJ. Melatonin and circadian biology in human cardiovascular disease. J Pineal Res (2010) 49(1):14–22. doi: 10.1111/j.1600-079X.2010.00773.x
155. Kalsbeek A, Yi CX, la Fleur SE, Buijs RM, Fliers E. Suprachiasmatic nucleus and autonomic nervous system influences on awakening from sleep. Int Rev Neurobiol (2010) 93:91–107. doi: 10.1016/S0074-7742(10)93004-3
156. Shea SA, Hilton MF, Hu K, Scheer FA. Existence of an endogenous circadian blood pressure rhythm in humans that peaks in the evening. Circ Res (2011) 108(8):980–4. doi: 10.1161/CIRCRESAHA.110.233668
157. Portaluppi F, Tiseo R, Smolensky MH, Hermida RC, Ayala DE, Fabbian F. Circadian rhythms and cardiovascular health. Sleep Med Rev (2012) 16(2):151–66. doi: 10.1016/j.smrv.2011.04.003
158. Bartlang MS, Lundkvist GB. Stress and the central circadian clock. In: Fink G, editor, Stress: Neuroendocrinology and Neurobiology (2017). vol. 2. Academic Press p. 385–93. doi: 10.1016/B978-0-12-802175-0.00038-3
159. Kalsbeek A, Palm IF, La Fleur SE, Scheer FA, Perreau-Lenz S, Ruiter M, et al. SCN outputs and the hypothalamic balance of life. J Biol Rhythms (2006) 21(6):458–69. doi: 10.1177/0748730406293854
160. Kalsbeek A, Yi CX, Cailotto C, la Fleur SE, Fliers E, Buijs RM. Mammalian clock output mechanisms. Essays Biochem (2011) 49(1):137–51. doi: 10.1042/bse0490137
161. Buijs RM, Wortel J, Van Heerikhuize JJ, Feenstra MG, Ter Horst GJ, Romijn HJ, et al. Anatomical and functional demonstration of a multisynaptic suprachiasmatic nucleus adrenal (cortex) pathway. Eur J Neurosci (1999) 11(5):1535–44. doi: 10.1046/j.1460-9568.1999.00575.x
162. Clark BJ, Wells J, King SR, Stocco DM. The purification, cloning, and expression of a novel luteinizing hormone-induced mitochondrial protein in MA-10 mouse Leydig tumor cells. Characterization of the steroidogenic acute regulatory protein (StAR). J Biol Chem (1994) 269(45):28314–22.
163. Engeland WC, Arnhold MM. Neural circuitry in the regulation of adrenal corticosterone rhythmicity. Endocrine (2005) 28(3):325–32. doi: 10.1385/ENDO:28:3:325
164. Ishida A, Mutoh T, Ueyama T, Bando H, Masubuchi S, Nakahara D, et al. Light activates the adrenal gland: timing of gene expression and glucocorticoid release. Cell Metab (2005) 2(5):297–307. doi: 10.1016/j.cmet.2005.09.009
165. Oster H, Damerow S, Kiessling S, Jakubcakova V, Abraham D, Tian J, et al. The circadian rhythm of glucocorticoids is regulated by a gating mechanism residing in the adrenal cortical clock. Cell Metab (2006) 4(2):163–73. doi: 10.1016/j.cmet.2006.07.002
166. Ulrich-Lai YM, Arnhold MM, Engeland WC. Adrenal splanchnic innervation contributes to the diurnal rhythm of plasma corticosterone in rats by modulating adrenal sensitivity to ACTH. Am J Physiol Regul Integr Comp Physiol (2006) 290(4):R1128–1135. doi: 10.1152/ajpregu.00042.2003
167. Son GH, Chung S, Choe HK, Kim HD, Baik SM, Lee H, et al. Adrenal peripheral clock controls the autonomous circadian rhythm of glucocorticoid by causing rhythmic steroid production. Proc Natl Acad Sci U S A (2008) 105(52):20970–5. doi: 10.1073/pnas.0806962106
168. Clow A, Hucklebridge F, Stalder T, Evans P, Thorn L. The cortisol awakening response: more than a measure of HPA axis function. Neurosci Biobehav Rev (2010) 35(1):97–103. doi: 10.1016/j.neubiorev.2009.12.011
169. Chung S, Son GH, Kim K. Circadian rhythm of adrenal glucocorticoid: its regulation and clinical implications. Biochim Biophys Acta (2011) 1812(5):581–91. doi: 10.1016/j.bbadis.2011.02.003
170. Andrews RV, Folk GE Jr. Circadian Metabolic Patterns in Cultured Hamster Adrenal Glands. Comp Biochem Physiol (1964) 11:393–409. doi: 10.1016/0010-406X(64)90006-4
171. Bittman EL, Doherty L, Huang L, Paroskie A. Period gene expression in mouse endocrine tissues. Am J Physiol Regul Integr Comp Physiol (2003) 285(3):R561–569. doi: 10.1152/ajpregu.00783.2002
172. Torres-Farfan C, Mendez N, Abarzua-Catalan L, Vilches N, Valenzuela GJ, Seron-Ferre M. A circadian clock entrained by melatonin is ticking in the rat fetal adrenal. Endocrinology (2011) 152(5):1891–900. doi: 10.1210/en.2010-1260
173. Damiola F, Le Minh N, Preitner N, Kornmann B, Fleury-Olela F, Schibler U. Restricted feeding uncouples circadian oscillators in peripheral tissues from the central pacemaker in the suprachiasmatic nucleus. Genes Dev (2000) 14(23):2950–61. doi: 10.1101/gad.183500
174. Mendoza J. Circadian clocks: setting time by food. J Neuroendocrinol (2007) 19(2):127–37. doi: 10.1111/j.1365-2826.2006.01510.x
175. Jacobson L. Hypothalamic-pituitary-adrenocortical axis regulation. Endocrinol Metab Clin North Am (2005) 34(2):271–92. vii. doi: 10.1016/j.ecl.2005.01.003
176. Gillette MU, McArthur AJ. Circadian actions of melatonin at the suprachiasmatic nucleus. Behav Brain Res (1996) 73(1-2):135–9. doi: 10.1016/0166-4328(96)00085-X
177. Richter HG, Torres-Farfan C, Garcia-Sesnich J, Abarzua-Catalan L, Henriquez MG, Alvarez-Felmer M, et al. Rhythmic expression of functional MT1 melatonin receptors in the rat adrenal gland. Endocrinology (2008) 149(3):995–1003. doi: 10.1210/en.2007-1009
178. Valenzuela FJ, Torres-Farfan C, Richter HG, Mendez N, Campino C, Torrealba F, et al. Clock gene expression in adult primate suprachiasmatic nuclei and adrenal: is the adrenal a peripheral clock responsive to melatonin? Endocrinology (2008) 149(4):1454–61. doi: 10.1210/en.2007-1518
179. Campino C, Valenzuela FJ, Torres-Farfan C, Reynolds HE, Abarzua-Catalan L, Arteaga E, et al. Melatonin exerts direct inhibitory actions on ACTH responses in the human adrenal gland. Horm Metab Res (2011) 43(5):337–42. doi: 10.1055/s-0031-1271693
180. Torres-Farfan C, Richter HG, Rojas-Garcia P, Vergara M, Forcelledo ML, Valladares LE, et al. mt1 Melatonin receptor in the primate adrenal gland: inhibition of adrenocorticotropin-stimulated cortisol production by melatonin. J Clin Endocrinol Metab (2003) 88(1):450–8. doi: 10.1210/jc.2002-021048
181. Doi M, Hirayama J, Sassone-Corsi P. Circadian regulator CLOCK is a histone acetyltransferase. Cell (2006) 125(3):497–508. doi: 10.1016/j.cell.2006.03.033
182. Nader N, Chrousos GP, Kino T. Circadian rhythm transcription factor CLOCK regulates the transcriptional activity of the glucocorticoid receptor by acetylating its hinge region lysine cluster: potential physiological implications. FASEB J (2009) 23(5):1572–83. doi: 10.1096/fj.08-117697
183. Charmandari E, Chrousos GP, Lambrou GI, Pavlaki A, Koide H, Ng SS, et al. Peripheral CLOCK regulates target-tissue glucocorticoid receptor transcriptional activity in a circadian fashion in man. PloS One (2011) 6(9):e25612. doi: 10.1371/journal.pone.0025612
184. Han DH, Lee YJ, Kim K, Kim CJ, Cho S. Modulation of glucocorticoid receptor induction properties by core circadian clock proteins. Mol Cell Endocrinol (2014) 383(1-2):170–80. doi: 10.1016/j.mce.2013.12.013
185. Lamia KA, Papp SJ, Yu RT, Barish GD, Uhlenhaut NH, Jonker JW, et al. Cryptochromes mediate rhythmic repression of the glucocorticoid receptor. Nature (2011) 480(7378):552–6. doi: 10.1038/nature10700
186. Leliavski A, Shostak A, Husse J, Oster H. Impaired glucocorticoid production and response to stress in Arntl-deficient male mice. Endocrinology (2014) 155(1):133–42. doi: 10.1210/en.2013-1531
187. Yang S, Liu A, Weidenhammer A, Cooksey RC, McClain D, Kim MK, et al. The role of mPer2 clock gene in glucocorticoid and feeding rhythms. Endocrinology (2009) 150(5):2153–60. doi: 10.1210/en.2008-0705
188. Goriki A, Hatanaka F, Myung J, Kim JK, Yoritaka T, Tanoue S, et al. A novel protein, CHRONO, functions as a core component of the mammalian circadian clock. PloS Biol (2014) 12(4):e1001839. doi: 10.1371/journal.pbio.1001839
189. Robinson R. Chronos: stress makes the clock tick. PloS Biol (2014) 12(4):e1001838. doi: 10.1371/journal.pbio.1001838
190. Okabe T, Chavan R, Fonseca Costa SS, Brenna A, Ripperger JA, Albrecht U. REV-ERBalpha influences the stability and nuclear localization of the glucocorticoid receptor. J Cell Sci (2016) 129(21):4143–54. doi: 10.1242/jcs.190959
191. Caratti G, Iqbal M, Hunter L, Kim D, Wang P, Vonslow RM, et al. REVERBa couples the circadian clock to hepatic glucocorticoid action. J Clin Invest (2018) 128(10):4454–71. doi: 10.1172/JCI96138
192. Liu C, Li S, Liu T, Borjigin J, Lin JD. Transcriptional coactivator PGC-1alpha integrates the mammalian clock and energy metabolism. Nature (2007) 447(7143):477–81. doi: 10.1038/nature05767
193. Knutti D, Kaul A, Kralli A. A tissue-specific coactivator of steroid receptors, identified in a functional genetic screen. Mol Cell Biol (2000) 20(7):2411–22. doi: 10.1128/MCB.20.7.2411-2422.2000
194. Grad I, Picard D. The glucocorticoid responses are shaped by molecular chaperones. Mol Cell Endocrinol (2007) 275(1-2):2–12. doi: 10.1016/j.mce.2007.05.018
195. Reinke H, Saini C, Fleury-Olela F, Dibner C, Benjamin IJ, Schibler U. Differential display of DNA-binding proteins reveals heat-shock factor 1 as a circadian transcription factor. Genes Dev (2008) 22(3):331–45. doi: 10.1101/gad.453808
196. Yan J, Wang H, Liu Y, Shao C. Analysis of gene regulatory networks in the mammalian circadian rhythm. PloS Comput Biol (2008) 4(10):e1000193. doi: 10.1371/journal.pcbi.1000193
197. Balsalobre A, Brown SA, Marcacci L, Tronche F, Kellendonk C, Reichardt HM, et al. Resetting of circadian time in peripheral tissues by glucocorticoid signaling. Science (2000) 289(5488):2344–7. doi: 10.1126/science.289.5488.2344
198. Kiessling S, Eichele G, Oster H. Adrenal glucocorticoids have a key role in circadian resynchronization in a mouse model of jet lag. J Clin Invest (2010) 120(7):2600–9. doi: 10.1172/JCI41192
199. Nicolaides NC, Charmandari E, Kino T, Chrousos GP. Stress-Related and Circadian Secretion and Target Tissue Actions of Glucocorticoids: Impact on Health. Front Endocrinol (Lausanne) (2017) 8:70. doi: 10.3389/fendo.2017.00070
200. Larsen PJ, Vrang N, Moller M, Jessop DS, Lightman SL, Chowdrey HS, et al. The diurnal expression of genes encoding vasopressin and vasoactive intestinal peptide within the rat suprachiasmatic nucleus is influenced by circulating glucocorticoids. Brain Res Mol Brain Res (1994) 27(2):342–6. doi: 10.1016/0169-328X(94)90021-3
201. Engelmann M, Ebner K, Landgraf R, Wotjak CT. Swim stress triggers the release of vasopressin within the suprachiasmatic nucleus of male rats. Brain Res (1998) 792(2):343–7. doi: 10.1016/S0006-8993(98)00243-1
202. Koresh O, Kozlovsky N, Kaplan Z, Zohar J, Matar MA, Cohen H. The long-term abnormalities in circadian expression of Period 1 and Period 2 genes in response to stress is normalized by agomelatine administered immediately after exposure. Eur Neuropsychopharmacol (2012) 22(3):205–21. doi: 10.1016/j.euroneuro.2011.07.012
203. Kellner M, Yassouridis A, Manz B, Steiger A, Holsboer F, Wiedemann K. Corticotropin-releasing hormone inhibits melatonin secretion in healthy volunteers–a potential link to low-melatonin syndrome in depression? Neuroendocrinology (1997) 65(4):284–90. doi: 10.1159/000127186
204. Steiger A. Neurochemical regulation of sleep. J Psychiatr Res (2007) 41(7):537–52. doi: 10.1016/j.jpsychires.2006.04.007
205. Couto-Moraes R, Palermo-Neto J, Markus RP. The immune-pineal axis: stress as a modulator of pineal gland function. Ann N Y Acad Sci (2009) 1153:193–202. doi: 10.1111/j.1749-6632.2008.03978.x
206. Mazzoccoli G, Carughi S, Sperandeo M, Pazienza V, Giuliani F, Tarquini R. Neuro-endocrine correlations of hypothalamic-pituitary-thyroid axis in healthy humans. J Biol Regul Homeost Agents (2011) 25(2):249–57.
207. Le Minh N, Damiola F, Tronche F, Schutz G, Schibler U. Glucocorticoid hormones inhibit food-induced phase-shifting of peripheral circadian oscillators. EMBO J (2001) 20(24):7128–36. doi: 10.1093/emboj/20.24.7128
208. Koyanagi S, Okazawa S, Kuramoto Y, Ushijima K, Shimeno H, Soeda S, et al. Chronic treatment with prednisolone represses the circadian oscillation of clock gene expression in mouse peripheral tissues. Mol Endocrinol (2006) 20(3):573–83. doi: 10.1210/me.2005-0165
209. Burioka N, Takata M, Okano Y, Ohdo S, Fukuoka Y, Miyata M, et al. Dexamethasone influences human clock gene expression in bronchial epithelium and peripheral blood mononuclear cells in vitro. Chronobiol Int (2005) 22(3):585–90. doi: 10.1081/CBI-200062416
210. Fukuoka Y, Burioka N, Takata M, Ohdo S, Miyata M, Endo M, et al. Glucocorticoid administration increases hPer1 mRNA levels in human peripheral blood mononuclear cells in vitro or in vivo. J Biol Rhythms (2005) 20(6):550–3. doi: 10.1177/0748730405279866
211. Oishi K, Amagai N, Shirai H, Kadota K, Ohkura N, Ishida N. Genome-wide expression analysis reveals 100 adrenal gland-dependent circadian genes in the mouse liver. DNA Res (2005) 12(3):191–202. doi: 10.1093/dnares/dsi003
212. Yamamoto T, Nakahata Y, Tanaka M, Yoshida M, Soma H, Shinohara K, et al. Acute physical stress elevates mouse period1 mRNA expression in mouse peripheral tissues via a glucocorticoid-responsive element. J Biol Chem (2005) 280(51):42036–43. doi: 10.1074/jbc.M509600200
213. Segall LA, Perrin JS, Walker CD, Stewart J, Amir S. Glucocorticoid rhythms control the rhythm of expression of the clock protein, Period2, in oval nucleus of the bed nucleus of the stria terminalis and central nucleus of the amygdala in rats. Neuroscience (2006) 140(3):753–7. doi: 10.1016/j.neuroscience.2006.03.037
214. Gomez-Abellan P, Diez-Noguera A, Madrid JA, Lujan JA, Ordovas JM, Garaulet M. Glucocorticoids affect 24 h clock genes expression in human adipose tissue explant cultures. PloS One (2012) 7(12):e50435. doi: 10.1371/journal.pone.0050435
215. Pezuk P, Mohawk JA, Wang LA, Menaker M. Glucocorticoids as entraining signals for peripheral circadian oscillators. Endocrinology (2012) 153(10):4775–83. doi: 10.1210/en.2012-1486
216. Barnea M, Madar Z, Froy O. Dexamethasone induces high-amplitude rhythms in preadipocytes, but hinders circadian expression in differentiated adipocytes. Chronobiol Int (2013) 30(6):837–42. doi: 10.3109/07420528.2013.767824
217. Torra IP, Tsibulsky V, Delaunay F, Saladin R, Laudet V, Fruchart JC, et al. Circadian and glucocorticoid regulation of Rev-erbalpha expression in liver. Endocrinology (2000) 141(10):3799–806. doi: 10.1210/endo.141.10.7708
218. So AY, Bernal TU, Pillsbury ML, Yamamoto KR, Feldman BJ. Glucocorticoid regulation of the circadian clock modulates glucose homeostasis. Proc Natl Acad Sci U S A (2009) 106(41):17582–7. doi: 10.1073/pnas.0909733106
219. Conway-Campbell BL, Sarabdjitsingh RA, McKenna MA, Pooley JR, Kershaw YM, Meijer OC, et al. Glucocorticoid ultradian rhythmicity directs cyclical gene pulsing of the clock gene period 1 in rat hippocampus. J Neuroendocrinol (2010) 22(10):1093–100. doi: 10.1111/j.1365-2826.2010.02051.x
220. Surjit M, Ganti KP, Mukherji A, Ye T, Hua G, Metzger D, et al. Widespread negative response elements mediate direct repression by agonist-liganded glucocorticoid receptor. Cell (2011) 145(2):224–41. doi: 10.1016/j.cell.2011.03.027
221. Cheon S, Park N, Cho S, Kim K. Glucocorticoid-mediated Period2 induction delays the phase of circadian rhythm. Nucleic Acids Res (2013) 41(12):6161–74. doi: 10.1093/nar/gkt307
222. Landgraf D, McCarthy MJ, Welsh DK. Circadian clock and stress interactions in the molecular biology of psychiatric disorders. Curr Psychiatry Rep (2014) 16(10):483. doi: 10.1007/s11920-014-0483-7
223. van der Veen DR, Shao J, Xi Y, Li L, Duffield GE. Cardiac atrial circadian rhythms in PERIOD2::LUCIFERASE and per1:luc mice: amplitude and phase responses to glucocorticoid signaling and medium treatment. PloS One (2012) 7(10):e47692. doi: 10.1371/journal.pone.0047692
224. Fujihara Y, Kondo H, Noguchi T, Togari A. Glucocorticoids mediate circadian timing in peripheral osteoclasts resulting in the circadian expression rhythm of osteoclast-related genes. Bone (2014) 61:1–9. doi: 10.1016/j.bone.2013.12.026
225. Kolbe I, Dumbell R, Oster H. Circadian Clocks and the Interaction between Stress Axis and Adipose Function. Int J Endocrinol (2015) 2015:693204. doi: 10.1155/2015/693204
226. Oster H, Challet E, Ott V, Arvat E, de Kloet ER, Dijk DJ, et al. The Functional and Clinical Significance of the 24-Hour Rhythm of Circulating Glucocorticoids. Endocr Rev (2017) 38(1):3–45.
227. Kamagata M, Ikeda Y, Sasaki H, Hattori Y, Yasuda S, Iwami S, et al. Potent synchronization of peripheral circadian clocks by glucocorticoid injections in PER2::LUC-Clock/Clock mice. Chronobiol Int (2017) 34(8):1067–82. doi: 10.1080/07420528.2017.1338716
228. Lamont EW, Robinson B, Stewart J, Amir S. The central and basolateral nuclei of the amygdala exhibit opposite diurnal rhythms of expression of the clock protein Period2. Proc Natl Acad Sci U S A (2005) 102(11):4180–4. doi: 10.1073/pnas.0500901102
229. Segall LA, Milet A, Tronche F, Amir S. Brain glucocorticoid receptors are necessary for the rhythmic expression of the clock protein, PERIOD2, in the central extended amygdala in mice. Neurosci Lett (2009) 457(1):58–60. doi: 10.1016/j.neulet.2009.03.083
230. Takahashi S, Yokota S, Hara R, Kobayashi T, Akiyama M, Moriya T, et al. Physical and inflammatory stressors elevate circadian clock gene mPer1 mRNA levels in the paraventricular nucleus of the mouse. Endocrinology (2001) 142(11):4910–7. doi: 10.1210/endo.142.11.8487
231. Amir S, Lamont EW, Robinson B, Stewart J. A circadian rhythm in the expression of PERIOD2 protein reveals a novel SCN-controlled oscillator in the oval nucleus of the bed nucleus of the stria terminalis. J Neurosci (2004) 24(4):781–90. doi: 10.1523/JNEUROSCI.4488-03.2004
232. Su Y, van der Spek R, Foppen E, Kwakkel J, Fliers E, Kalsbeek A. Effects of adrenalectomy on daily gene expression rhythms in the rat suprachiasmatic and paraventricular hypothalamic nuclei and in white adipose tissue. Chronobiol Int (2015) 32(2):211–24. doi: 10.3109/07420528.2014.963198
233. Buijs RM, Escobar C. Corticosterone and activity: the long arms of the clock talk back. Endocrinology (2007) 148(11):5162–4. doi: 10.1210/en.2007-0926
234. Sage D, Ganem J, Guillaumond F, Laforge-Anglade G, Francois-Bellan AM, Bosler O, et al. Influence of the corticosterone rhythm on photic entrainment of locomotor activity in rats. J Biol Rhythms (2004) 19(2):144–56. doi: 10.1177/0748730403261894
235. Malek ZS, Sage D, Pevet P, Raison S. Daily rhythm of tryptophan hydroxylase-2 messenger ribonucleic acid within raphe neurons is induced by corticoid daily surge and modulated by enhanced locomotor activity. Endocrinology (2007) 148(11):5165–72. doi: 10.1210/en.2007-0526
236. Akiyama M, Minami Y, Nakajima T, Moriya T, Shibata S. Calcium and pituitary adenylate cyclase-activating polypeptide induced expression of circadian clock gene mPer1 in the mouse cerebellar granule cell culture. J Neurochem (2001) 78(3):499–508. doi: 10.1046/j.1471-4159.2001.00452.x
237. Travnickova-Bendova Z, Cermakian N, Reppert SM, Sassone-Corsi P. Bimodal regulation of mPeriod promoters by CREB-dependent signaling and CLOCK/BMAL1 activity. Proc Natl Acad Sci U S A (2002) 99(11):7728–33. doi: 10.1073/pnas.102075599
238. Terazono H, Mutoh T, Yamaguchi S, Kobayashi M, Akiyama M, Udo R, et al. Adrenergic regulation of clock gene expression in mouse liver. Proc Natl Acad Sci U S A (2003) 100(11):6795–800. doi: 10.1073/pnas.0936797100
239. Zhang R, Lahens NF, Ballance HI, Hughes ME, Hogenesch JB. A circadian gene expression atlas in mammals: implications for biology and medicine. Proc Natl Acad Sci U S A (2014) 111(45):16219–24. doi: 10.1073/pnas.1408886111
240. Albrecht U. Molecular Mechanisms in Mood Regulation Involving the Circadian Clock. Front Neurol (2017) 8:30. doi: 10.3389/fneur.2017.00030
241. Chung S, Lee EJ, Yun S, Choe HK, Park SB, Son HJ, et al. Impact of circadian nuclear receptor REV-ERBalpha on midbrain dopamine production and mood regulation. Cell (2014) 157(4):858–68. doi: 10.1016/j.cell.2014.03.039
242. Carpentier R, Sacchetti P, Segard P, Staels B, Lefebvre P. The glucocorticoid receptor is a co-regulator of the orphan nuclear receptor Nurr1. J Neurochem (2008) 104(3):777–89. doi: 10.1111/j.1471-4159.2007.05055.x
243. Hampp G, Ripperger JA, Houben T, Schmutz I, Blex C, Perreau-Lenz S, et al. Regulation of monoamine oxidase A by circadian-clock components implies clock influence on mood. Curr Biol (2008) 18(9):678–83. doi: 10.1016/j.cub.2008.04.012
244. Erren TC, Reiter RJ. Defining chronodisruption. J Pineal Res (2009) 46(3):245–7. doi: 10.1111/j.1600-079X.2009.00665.x
245. Erren TC, Reiter RJ. Revisiting chronodisruption: when the physiological nexus between internal and external times splits in humans. Naturwissenschaften (2013) 100(4):291–8. doi: 10.1007/s00114-013-1026-5
246. Zelinski EL, Deibel SH, McDonald RJ. The trouble with circadian clock dysfunction: Multiple deleterious effects on the brain and body. Neurosci Biobehav Rev (2014) 24(40C):80–101. doi: 10.1016/j.neubiorev.2014.01.007
247. Orozco-Solis R, Sassone-Corsi P. Epigenetic control and the circadian clock: Linking metabolism to neuronal responses. Neuroscience (2014) 264:76–87. doi: 10.1016/j.neuroscience.2014.01.043
248. Chrusciel P, Goch A, Banach M, Mikhailidis DP, Rysz J, Goch JH. Circadian changes in the hemostatic system in healthy men and patients with cardiovascular diseases. Med Sci Monit (2009) 15(10):RA203–208. doi: 10.12659/MSM.878203
249. Hill SM, Frasch T, Xiang S, Yuan L, Duplessis T, Mao L. Molecular mechanisms of melatonin anticancer effects. Integr Cancer Ther (2009) 8(4):337–46. doi: 10.1177/1534735409353332
250. Hoogerwerf WA. Role of biological rhythms in gastrointestinal health and disease. Rev Endocr Metab Disord (2009) 10(4):293–300. doi: 10.1007/s11154-009-9119-3
251. Kovac J, Husse J, Oster H. A time to fast, a time to feast: the crosstalk between metabolism and the circadian clock. Mol Cells (2009) 28(2):75–80. doi: 10.1007/s10059-009-0113-0
252. Reiter RJ, Tan DX, Erren TC, Fuentes-Broto L, Paredes SD. Light-mediated perturbations of circadian timing and cancer risk: a mechanistic analysis. Integr Cancer Ther (2009) 8(4):354–60. doi: 10.1177/1534735409352026
253. Scheer FA, Hilton MF, Mantzoros CS, Shea SA. Adverse metabolic and cardiovascular consequences of circadian misalignment. Proc Natl Acad Sci U S A (2009) 106(11):4453–8. doi: 10.1073/pnas.0808180106
254. Karatsoreos IN, Bhagat S, Bloss EB, Morrison JH, McEwen BS. Disruption of circadian clocks has ramifications for metabolism, brain, and behavior. Proc Natl Acad Sci U S A (2011) 108(4):1657–62. doi: 10.1073/pnas.1018375108
255. McEwen BS, Karatsoreos IN. Sleep Deprivation and Circadian Disruption: Stress, Allostasis, and Allostatic Load. Sleep Med Clin (2015) 10(1):1–10. doi: 10.1016/j.jsmc.2014.11.007
256. Weibel L, Maccari S, Van Reeth O. Circadian clock functioning is linked to acute stress reactivity in rats. J Biol Rhythms (2002) 17(5):438–46. doi: 10.1177/074873002237138
257. Meerlo P, Sgoifo A, Suchecki D. Restricted and disrupted sleep: effects on autonomic function, neuroendocrine stress systems and stress responsivity. Sleep Med Rev (2008) 12(3):197–210. doi: 10.1016/j.smrv.2007.07.007
258. Richardson GS. The human circadian system in normal and disordered sleep. J Clin Psychiatry (2005) 66 Suppl 9:3–9; quiz 42–3
259. Ackermann K, Plomp R, Lao O, Middleton B, Revell VL, Skene DJ, et al. Effect of sleep deprivation on rhythms of clock gene expression and melatonin in humans. Chronobiol Int (2013) 30(7):901–9. doi: 10.3109/07420528.2013.784773
260. Fonken LK, Aubrecht TG, Melendez-Fernandez OH, Weil ZM, Nelson RJ. Dim light at night disrupts molecular circadian rhythms and increases body weight. J Biol Rhythms (2013) 28(4):262–71. doi: 10.1177/0748730413493862
261. Moller-Levet CS, Archer SN, Bucca G, Laing EE, Slak A, Kabiljo R, et al. Effects of insufficient sleep on circadian rhythmicity and expression amplitude of the human blood transcriptome. Proc Natl Acad Sci U S A (2013) 110(12):E1132–1141. doi: 10.1073/pnas.1217154110
262. Bracci M, Manzella N, Copertaro A, Staffolani S, Strafella E, Barbaresi M, et al. Rotating-shift nurses after a day off: peripheral clock gene expression, urinary melatonin, and serum 17-beta-estradiol levels. Scand J Work Environ Health (2014) 40(3):295–304. doi: 10.5271/sjweh.3414
263. Rodenbeck A, Hajak G. Neuroendocrine dysregulation in primary insomnia. Rev Neurol (Paris) (2001) 157(11 Pt 2):S57–61. doi: 10.1016/S0167-8140(01)80800-8
264. Vgontzas AN, Bixler EO, Lin HM, Prolo P, Mastorakos G, Vela-Bueno A, et al. Chronic insomnia is associated with nyctohemeral activation of the hypothalamic-pituitary-adrenal axis: clinical implications. J Clin Endocrinol Metab (2001) 86(8):3787–94. doi: 10.1210/jcem.86.8.7778
265. Backhaus J, Junghanns K, Hohagen F. Sleep disturbances are correlated with decreased morning awakening salivary cortisol. Psychoneuroendocrinology (2004) 29(9):1184–91. doi: 10.1016/j.psyneuen.2004.01.010
266. Buckley TM, Schatzberg AF. On the interactions of the hypothalamic-pituitary-adrenal (HPA) axis and sleep: normal HPA axis activity and circadian rhythm, exemplary sleep disorders. J Clin Endocrinol Metab (2005) 90(5):3106–14. doi: 10.1210/jc.2004-1056
267. Sgoifo A, Buwalda B, Roos M, Costoli T, Merati G, Meerlo P. Effects of sleep deprivation on cardiac autonomic and pituitary-adrenocortical stress reactivity in rats. Psychoneuroendocrinology (2006) 31(2):197–208. doi: 10.1016/j.psyneuen.2005.06.009
268. Wright CE, Valdimarsdottir HB, Erblich J, Bovbjerg DH. Poor sleep the night before an experimental stress task is associated with reduced cortisol reactivity in healthy women. Biol Psychol (2007) 74(3):319–27. doi: 10.1016/j.biopsycho.2006.08.003
269. Novati A, Roman V, Cetin T, Hagewoud R, den Boer JA, Luiten PG, et al. Chronically restricted sleep leads to depression-like changes in neurotransmitter receptor sensitivity and neuroendocrine stress reactivity in rats. Sleep (2008) 31(11):1579–85. doi: 10.1093/sleep/31.11.1579
270. Smolensky MH, Hermida RC, Castriotta RJ, Portaluppi F. Role of sleep-wake cycle on blood pressure circadian rhythms and hypertension. Sleep Med (2007) 8(6):668–80. doi: 10.1016/j.sleep.2006.11.011
271. Palesh O, Zeitzer JM, Conrad A, Giese-Davis J, Mustian KM, Popek V, et al. Vagal regulation, cortisol, and sleep disruption in women with metastatic breast cancer. J Clin Sleep Med (2008) 4(5):441–9.
272. Mullington JM, Haack M, Toth M, Serrador JM, Meier-Ewert HK. Cardiovascular, inflammatory, and metabolic consequences of sleep deprivation. Prog Cardiovasc Dis (2009) 51(4):294–302. doi: 10.1016/j.pcad.2008.10.003
273. Ruger M, Scheer FA. Effects of circadian disruption on the cardiometabolic system. Rev Endocr Metab Disord (2009) 10(4):245–60. doi: 10.1007/s11154-009-9122-8
274. Ekstrand K, Bostrom PA, Arborelius M, Nilsson JA, Lindell SE. Cardiovascular risk factors in commercial flight aircrew officers compared with those in the general population. Angiology (1996) 47(11):1089–94. doi: 10.1177/000331979604701109
275. Filipski E, Li XM, Levi F. Disruption of circadian coordination and malignant growth. Cancer Causes Control (2006) 17(4):509–14. doi: 10.1007/s10552-005-9007-4
276. Fujino Y, Iso H, Tamakoshi A, Inaba Y, Koizumi A, Kubo T, et al. A prospective cohort study of shift work and risk of ischemic heart disease in Japanese male workers. Am J Epidemiol (2006) 164(2):128–35. doi: 10.1093/aje/kwj185
277. Sookoian S, Gemma C, Fernandez Gianotti T, Burgueno A, Alvarez A, Gonzalez CD, et al. Effects of rotating shift work on biomarkers of metabolic syndrome and inflammation. J Intern Med (2007) 261(3):285–92. doi: 10.1111/j.1365-2796.2007.01766.x
278. Stevens RG, Hansen J, Costa G, Haus E, Kauppinen T, Aronson KJ, et al. Considerations of circadian impact for defining ‘shift work’ in cancer studies: IARC Working Group Report. Occup Environ Med (2011) 68(2):154–62. doi: 10.1136/oem.2009.053512
279. Savvidis C, Koutsilieris M. Circadian rhythm disruption in cancer biology. Mol Med (2012) 18:1249–60. doi: 10.2119/molmed.2012.00077
280. Albrecht U. Circadian clocks and mood-related behaviors. Handb Exp Pharmacol (2013) 217:227–39. doi: 10.1007/978-3-642-25950-0_9
281. Scott EM, Carter AM, Grant PJ. Association between polymorphisms in the Clock gene, obesity and the metabolic syndrome in man. Int J Obes (Lond) (2008) 32(4):658–62. doi: 10.1038/sj.ijo.0803778
282. Sookoian S, Gemma C, Gianotti TF, Burgueno A, Castano G, Pirola CJ. Genetic variants of Clock transcription factor are associated with individual susceptibility to obesity. Am J Clin Nutr (2008) 87(6):1606–15. doi: 10.1093/ajcn/87.6.1606
283. Tahara Y, Aoyama S, Shibata S. The mammalian circadian clock and its entrainment by stress and exercise. J Physiol Sci (2017) 67(1):1–10. doi: 10.1007/s12576-016-0450-7
284. Bartlang MS, Savelyev SA, Johansson AS, Reber SO, Helfrich-Forster C, Lundkvist GB. Repeated psychosocial stress at night, but not day, affects the central molecular clock. Chronobiol Int (2014) 31(9):996–1007. doi: 10.3109/07420528.2014.940085
285. Meerlo P, van den Hoofdakker RH, Koolhaas JM, Daan S. Stress-induced changes in circadian rhythms of body temperature and activity in rats are not caused by pacemaker changes. J Biol Rhythms (1997) 12(1):80–92. doi: 10.1177/074873049701200109
286. Tahara Y, Shiraishi T, Kikuchi Y, Haraguchi A, Kuriki D, Sasaki H, et al. Entrainment of the mouse circadian clock by sub-acute physical and psychological stress. Sci Rep (2015) 5:11417. doi: 10.1038/srep11417
287. Razzoli M, Karsten C, Yoder JM, Bartolomucci A, Engeland WC. Chronic subordination stress phase advances adrenal and anterior pituitary clock gene rhythms. Am J Physiol Regul Integr Comp Physiol (2014) 307(2):R198–205. doi: 10.1152/ajpregu.00101.2014
288. Takahashi K, Yamada T, Tsukita S, Kaneko K, Shirai Y, Munakata Y, et al. Chronic mild stress alters circadian expressions of molecular clock genes in the liver. Am J Physiol Endocrinol Metab (2013) 304(3):E301–309. doi: 10.1152/ajpendo.00388.2012
289. Weber GF, Johnson BN, Yamamoto BK, Gudelsky GA. Effects of Stress and MDMA on Hippocampal Gene Expression. BioMed Res Int (2014) 2014:141396. doi: 10.1155/2014/141396
290. Logan RW, Edgar N, Gillman AG, Hoffman D, Zhu X, McClung CA. Chronic Stress Induces Brain Region-Specific Alterations of Molecular Rhythms that Correlate with Depression-like Behavior in Mice. Biol Psychiatry (2015) 78(4):249–58. doi: 10.1016/j.biopsych.2015.01.011
291. Jiang WG, Li SX, Zhou SJ, Sun Y, Shi J, Lu L. Chronic unpredictable stress induces a reversible change of PER2 rhythm in the suprachiasmatic nucleus. Brain Res (2011) 1399:25–32. doi: 10.1016/j.brainres.2011.05.001
292. Kinoshita C, Miyazaki K, Ishida N. Chronic stress affects PERIOD2 expression through glycogen synthase kinase-3beta phosphorylation in the central clock. Neuroreport (2012) 23(2):98–102. doi: 10.1097/WNR.0b013e32834e7ec2
293. Paredes SD, Sanchez S, Parvez H, Rodriguez AB, Barriga C. Altered circadian rhythms of corticosterone, melatonin, and phagocytic activity in response to stress in rats. Neuro Endocrinol Lett (2007) 28(4):489–95.
294. Christiansen S, Bouzinova EV, Palme R, Wiborg O. Circadian activity of the hypothalamic-pituitary-adrenal axis is differentially affected in the rat chronic mild stress model of depression. Stress (2012) 15(6):647–57. doi: 10.3109/10253890.2011.654370
295. Gogenur I, Ocak U, Altunpinar O, Middleton B, Skene DJ, Rosenberg J. Disturbances in melatonin, cortisol and core body temperature rhythms after major surgery. World J Surg (2007) 31(2):290–8. doi: 10.1007/s00268-006-0256-5
296. Pina G, Brun J, Tissot S, Claustrat B. Long-term alteration of daily melatonin, 6-sulfatoxymelatonin, cortisol, and temperature profiles in burn patients: a preliminary report. Chronobiol Int (2010) 27(2):378–92. doi: 10.3109/07420520903502234
297. Lavie P. Sleep disturbances in the wake of traumatic events. N Engl J Med (2001) 345(25):1825–32. doi: 10.1056/NEJMra012893
298. Touma C, Fenzl T, Ruschel J, Palme R, Holsboer F, Kimura M, et al. Rhythmicity in mice selected for extremes in stress reactivity: behavioural, endocrine and sleep changes resembling endophenotypes of major depression. PloS One (2009) 4(1):e4325. doi: 10.1371/journal.pone.0004325
299. Philbert J, Pichat P, Beeske S, Decobert M, Belzung C, Griebel G. Acute inescapable stress exposure induces long-term sleep disturbances and avoidance behavior: a mouse model of post-traumatic stress disorder (PTSD). Behav Brain Res (2011) 221(1):149–54. doi: 10.1016/j.bbr.2011.02.039
300. Atkinson HC, Wood SA, Kershaw YM, Bate E, Lightman SL. Diurnal variation in the responsiveness of the hypothalamic-pituitary-adrenal axis of the male rat to noise stress. J Neuroendocrinol (2006) 18(7):526–33. doi: 10.1111/j.1365-2826.2006.01444.x
301. Gattermann R, Weinandy R. Time of day and stress response to different stressors in experimental animals. Part I: Golden hamster (Mesocricetus auratus Waterhouse, 1839). J Exp Anim Sci (1996) 38(2):66–76.
302. Bernatova I, Key MP, Lucot JB, Morris M. Circadian differences in stress-induced pressor reactivity in mice. Hypertension (2002) 40(5):768–73. doi: 10.1161/01.HYP.0000036405.27562.02
303. Antoch MP, Kondratov RV, Takahashi JS. Circadian clock genes as modulators of sensitivity to genotoxic stress. Cell Cycle (2005) 4(7):901–7. doi: 10.4161/cc.4.7.1792
304. Fanjul-Moles ML, Lopez-Riquelme GO. Relationship between Oxidative Stress, Circadian Rhythms, and AMD. Oxid Med Cell Longev (2016) 2016:7420637. doi: 10.1155/2016/7420637
305. Rybkin II, Zhou Y, Volaufova J, Smagin GN, Ryan DH, Harris RB. Effect of restraint stress on food intake and body weight is determined by time of day. Am J Physiol (1997) 273(5 Pt 2):R1612–1622. doi: 10.1152/ajpregu.1997.273.5.R1612
306. Aslani S, Harb MR, Costa PS, Almeida OF, Sousa N, Palha JA. Day and night: diurnal phase influences the response to chronic mild stress. Front Behav Neurosci (2014) 8:82. doi: 10.3389/fnbeh.2014.00082
307. Bartlang MS, Oster H, Helfrich-Forster C. Repeated psychosocial stress at night affects the circadian activity rhythm of male mice. J Biol Rhythms (2015) 30(3):228–41. doi: 10.1177/0748730415576192
308. Fonken LK, Weber MD, Daut RA, Kitt MM, Frank MG, Watkins LR, et al. Stress-induced neuroinflammatory priming is time of day dependent. Psychoneuroendocrinology (2016) 66:82–90. doi: 10.1016/j.psyneuen.2016.01.006
309. Koch CE, Bartlang MS, Kiehn JT, Lucke L, Naujokat N, Helfrich-Forster C, et al. Time-of-day-dependent adaptation of the HPA axis to predictable social defeat stress. J Endocrinol (2016) 231(3):209–21. doi: 10.1530/JOE-16-0163
310. Kalsbeek A, Ruiter M, La Fleur SE, Van Heijningen C, Buijs RM. The diurnal modulation of hormonal responses in the rat varies with different stimuli. J Neuroendocrinol (2003) 15(12):1144–55. doi: 10.1111/j.1365-2826.2003.01112.x
311. Mellman TA, Hipolito MM. Sleep disturbances in the aftermath of trauma and posttraumatic stress disorder. CNS Spectr (2006) 11(8):611–5. doi: 10.1017/S1092852900013663
312. Germain A, Buysse DJ, Nofzinger E. Sleep-specific mechanisms underlying posttraumatic stress disorder: integrative review and neurobiological hypotheses. Sleep Med Rev (2008) 12(3):185–95. doi: 10.1016/j.smrv.2007.09.003
313. Germain A. Sleep disturbances as the hallmark of PTSD: where are we now? Am J Psychiatry (2013) 170(4):372–82. doi: 10.1176/appi.ajp.2012.12040432
314. Agorastos A, Kellner M, Baker DG, Otte C. When time stands still. An integrative review on the role of chronodisruption in PTSD. Curr Opin Psychiatry (2014) 27:385–92. doi: 10.1097/YCO.0000000000000079
315. Agorastos A, Pervanidou P, Chrousos GP, Kolaitis G. Early life stress and trauma: developmental neuroendocrine aspects of prolonged stress system dysregulation. Hormones (Athens) (2018) 17(4):507–20. doi: 10.1007/s42000-018-0065-x
316. Kraeplin E. Compendium der Psychiatrie zum Gebrauch fuer Studierende und Aerzte. Leipzig: Abel Verlag (1883).
317. Wulff K, Gatti S, Wettstein JG, Foster RG. Sleep and circadian rhythm disruption in psychiatric and neurodegenerative disease. Nat Rev Neurosci (2010) 11(8):589–99. doi: 10.1038/nrn2868
318. Mellman TA, Bustamante V, Fins AI, Pigeon WR, Nolan B. REM sleep and the early development of posttraumatic stress disorder. Am J Psychiatry (2002) 159(10):1696–701. doi: 10.1176/appi.ajp.159.10.1696
319. Mellman TA, Knorr BR, Pigeon WR, Leiter JC, Akay M. Heart rate variability during sleep and the early development of posttraumatic stress disorder. Biol Psychiatry (2004) 55(9):953–6. doi: 10.1016/j.biopsych.2003.12.018
320. Spoormaker VI, Montgomery P. Disturbed sleep in post-traumatic stress disorder: secondary symptom or core feature? Sleep Med Rev (2008) 12(3):169–84. doi: 10.1016/j.smrv.2007.08.008
321. Bader K, Schafer V, Schenkel M, Nissen L, Schwander J. Adverse childhood experiences associated with sleep in primary insomnia. J Sleep Res (2007) 16(3):285–96. doi: 10.1111/j.1365-2869.2007.00608.x
322. Koskenvuo K, Hublin C, Partinen M, Paunio T, Koskenvuo M. Childhood adversities and quality of sleep in adulthood: A population-based study of 26,000 Finns. Sleep Med (2010) 11(1):17–22. doi: 10.1016/j.sleep.2009.03.010
323. Greenfield EA, Lee C, Friedman EL, Springer KW. Childhood abuse as a risk factor for sleep problems in adulthood: evidence from a U.S. national study. Ann Behav Med (2011) 42(2):245–56. doi: 10.1007/s12160-011-9285-x
324. Schafer V, Bader K. Relationship between early-life stress load and sleep in psychiatric outpatients: a sleep diary and actigraphy study. Stress Health (2013) 29(3):177–89. doi: 10.1002/smi.2438
325. Baiden P, Fallon B, den Dunnen W, Boateng GO. The enduring effects of early-childhood adversities and troubled sleep among Canadian adults: a population-based study. Sleep Med (2015) 16(6):760–7. doi: 10.1016/j.sleep.2015.02.527
326. Kajeepeta S, Gelaye B, Jackson CL, Williams MA. Adverse childhood experiences are associated with adult sleep disorders: a systematic review. Sleep Med (2015) 16(3):320–30. doi: 10.1016/j.sleep.2014.12.013
327. Lind MJ, Aggen SH, Kendler KS, York TP, Amstadter AB. An epidemiologic study of childhood sexual abuse and adult sleep disturbances. Psychol Trauma (2016) 8(2):198–205. doi: 10.1037/tra0000080
328. Petrov ME, Davis MC, Belyea MJ, Zautra AJ. Linking childhood abuse and hypertension: sleep disturbance and inflammation as mediators. J Behav Med (2016) 39(4):716–26. doi: 10.1007/s10865-016-9742-x
329. Taylor DJ, Pruiksma KE, Hale WJ, Kelly K, Maurer D, Peterson AL, et al. Prevalence, Correlates, and Predictors of Insomnia in the US Army prior to Deployment. Sleep (2016) 39(10):1795–806. doi: 10.5665/sleep.6156
330. Thordardottir EB, Hansdottir I, Valdimarsdottir UA, Shipherd JC, Resnick H, Gudmundsdottir B. The Manifestations of Sleep Disturbances 16 Years Post-Trauma. Sleep (2016) 39(8):1551–4. doi: 10.5665/sleep.6018
331. Wang Y, Raffeld MR, Slopen N, Hale L, Dunn EC. Childhood adversity and insomnia in adolescence. Sleep Med (2016) 21:12–8. doi: 10.1016/j.sleep.2016.01.011
332. Steine IM, Winje D, Krystal JH, Bjorvatn B, Milde AM, Gronli J, et al. Cumulative childhood maltreatment and its dose-response relation with adult symptomatology: Findings in a sample of adult survivors of sexual abuse. Child Abuse Negl (2017) 65:99–111. doi: 10.1016/j.chiabu.2017.01.008
333. Boscarino JA. Diseases among men 20 years after exposure to severe stress: Implications for clinical research and medical care. Psychosom Med (1997) 59(6):605–14. doi: 10.1097/00006842-199711000-00008
334. Gorman JM, Sloan RP. Heart rate variability in depressive and anxiety disorders. Am Heart J (2000) 140(4 Suppl):77–83. doi: 10.1067/mhj.2000.109981
335. Wagner AW, Wolfe J, Rotnitsky A, Proctor SP, Erickson DJ. An investigation of the impact of posttraumatic stress disorder on physical health. J Trauma Stress (2000) 13(1):41–55. doi: 10.1023/A:1007716813407
336. Breslau N. Epidemiologic studies of trauma, posttraumatic stress disorder, and other psychiatric disorders. Can J Psychiatry (2002) 47(10):923–9. doi: 10.1177/070674370204701003
337. Mayou RA, Bryant B. Outcome 3 years after a road traffic accident. Psychol Med (2002) 32(4):671–5. doi: 10.1017/S0033291702005470
338. Vanitallie TB. Stress: a risk factor for serious illness. Metabolism (2002) 51(6 Suppl 1):40–5. doi: 10.1053/meta.2002.33191
339. Boscarino JA. Posttraumatic stress disorder and physical illness: results from clinical and epidemiologic studies. Ann N Y Acad Sci (2004) 1032:141–53. doi: 10.1196/annals.1314.011
340. Rohleder N, Karl A. Role of endocrine and inflammatory alterations in comorbid somatic diseases of post-traumatic stress disorder. Minerva Endocrinol (2006) 31(4):273–88.
341. Bedi US, Arora R. Cardiovascular manifestations of posttraumatic stress disorder. J Natl Med Assoc (2007) 99(6):642–9.
342. Kubzansky LD, Koenen KC, Spiro A 3rd, Vokonas PS, Sparrow D. Prospective study of posttraumatic stress disorder symptoms and coronary heart disease in the Normative Aging Study. Arch Gen Psychiatry (2007) 64(1):109–16. doi: 10.1001/archpsyc.64.1.109
343. Boscarino JA. A prospective study of PTSD and early-age heart disease mortality among Vietnam veterans: implications for surveillance and prevention. Psychosom Med (2008) 70(6):668–76. doi: 10.1097/PSY.0b013e31817bccaf
344. Jakupcak M, Luterek J, Hunt S, Conybeare D, McFall M. Posttraumatic stress and its relationship to physical health functioning in a sample of Iraq and Afghanistan War veterans seeking postdeployment VA health care. J Nerv Ment Dis (2008) 196(5):425–8. doi: 10.1097/NMD.0b013e31817108ed
345. O’Toole BI, Catts SV. Trauma, PTSD, and physical health: an epidemiological study of Australian Vietnam veterans. J Psychosom Res (2008) 64(1):33–40. doi: 10.1016/j.jpsychores.2007.07.006
346. Cohen BE, Marmar CR, Neylan TC, Schiller NB, Ali S, Whooley MA. Posttraumatic stress disorder and health-related quality of life in patients with coronary heart disease: findings from the Heart and Soul Study. Arch Gen Psychiatry (2009) 66(11):1214–20. doi: 10.1001/archgenpsychiatry.2009.149
347. Qureshi SU, Pyne JM, Magruder KM, Schulz PE, Kunik ME. The link between post-traumatic stress disorder and physical comorbidities: a systematic review. Psychiatr Q (2009) 80(2):87–97. doi: 10.1007/s11126-009-9096-4
348. Boscarino JA, Forsberg CW, Goldberg J. A twin study of the association between PTSD symptoms and rheumatoid arthritis. Psychosom Med (2010) 72(5):481–6. doi: 10.1097/PSY.0b013e3181d9a80c
349. Yaffe K, Vittinghoff E, Lindquist K, Barnes D, Covinsky KE, Neylan T, et al. Posttraumatic stress disorder and risk of dementia among US veterans. Arch Gen Psychiatry (2010) 67(6):608–13. doi: 10.1001/archgenpsychiatry.2010.61
350. Gupta MA. Review of somatic symptoms in post-traumatic stress disorder. Int Rev Psychiatry (2013) 25(1):86–99. doi: 10.3109/09540261.2012.736367
351. American Psychiatric Association. Diagnostic and Statistical Manual of Mental Disorders. 5th Edition. Washington DC (2013). doi: 10.1176/appi.books.9780890425596
352. Clum GA, Nishith P, Resick PA. Trauma-related sleep disturbance and self-reported physical health symptoms in treatment-seeking female rape victims. J Nerv Ment Dis (2001) 189(9):618–22. doi: 10.1097/00005053-200109000-00008
353. Nishith P, Resick PA, Mueser KT. Sleep difficulties and alcohol use motives in female rape victims with posttraumatic stress disorder. J Trauma Stress (2001) 14(3):469–79. doi: 10.1023/A:1011152405048
354. Zayfert C, DeViva JC. Residual insomnia following cognitive behavioral therapy for PTSD. J Trauma Stress (2004) 17(1):69–73. doi: 10.1023/B:JOTS.0000014679.31799.e7
355. Belleville G, Guay S, Marchand A. Persistence of sleep disturbances following cognitive-behavior therapy for posttraumatic stress disorder. J Psychosom Res (2011) 70(4):318–27. doi: 10.1016/j.jpsychores.2010.09.022
356. Schoenfeld FB, Deviva JC, Manber R. Treatment of sleep disturbances in posttraumatic stress disorder: a review. J Rehabil Res Dev (2012) 49(5):729–52. doi: 10.1682/JRRD.2011.09.0164
357. Mellman TA. Psychobiology of sleep disturbances in posttraumatic stress disorder. Ann N Y Acad Sci (1997) 821:142–9. doi: 10.1111/j.1749-6632.1997.tb48275.x
358. Pillar G, Malhotra A, Lavie P. Post-traumatic stress disorder and sleep-what a nightmare! Sleep Med Rev (2000) 4(2):183–200. doi: 10.1053/smrv.1999.0095
359. Krakow B, Melendrez D, Pedersen B, Johnston L, Hollifield M, Germain A, et al. Complex insomnia: insomnia and sleep-disordered breathing in a consecutive series of crime victims with nightmares and PTSD. Biol Psychiatry (2001) 49(11):948–53. doi: 10.1016/S0006-3223(00)01087-8
360. Harvey AG, Jones C, Schmidt DA. Sleep and posttraumatic stress disorder: a review. Clin Psychol Rev (2003) 23(3):377–407. doi: 10.1016/S0272-7358(03)00032-1
361. Maher MJ, Rego SA, Asnis GM. Sleep disturbances in patients with post-traumatic stress disorder: epidemiology, impact and approaches to management. CNS Drugs (2006) 20(7):567–90. doi: 10.2165/00023210-200620070-00003
362. Westermeyer J, Khawaja IS, Freerks M, Sutherland RJ, Engle K, Johnson D, et al. Quality of sleep in patients with posttraumatic stress disorder. Psychiatry (Edgmont) (2010) 7(9):21–7.
363. Kobayashi I, Boarts JM, Delahanty DL. Polysomnographically measured sleep abnormalities in PTSD: a meta-analytic review. Psychophysiology (2007) 44(4):660–9. doi: 10.1111/j.1469-8986.2007.537.x
364. Lamarche LJ, De Koninck J. Sleep disturbance in adults with posttraumatic stress disorder: a review. J Clin Psychiatry (2007) 68(8):1257–70. doi: 10.4088/JCP.v68n0813
365. Cowdin N, Kobayashi I, Mellman TA. Theta frequency activity during rapid eye movement (REM) sleep is greater in people with resilience versus PTSD. Exp Brain Res (2014) 232(5):1479–85. doi: 10.1007/s00221-014-3857-5
366. Murck H, Uhr M, Ziegenbein M, Kunzel H, Held K, Antonijevic IA, et al. Renin-angiotensin-aldosterone system, HPA-axis and sleep-EEG changes in unmedicated patients with depression after total sleep deprivation. Pharmacopsychiatry (2006) 39(1):23–9. doi: 10.1055/s-2006-931476
367. Shea JL, Mochizuki T, Sagvaag V, Aspevik T, Bjorkum AA, Datta S. Rapid eye movement (REM) sleep homeostatic regulatory processes in the rat: changes in the sleep-wake stages and electroencephalographic power spectra. Brain Res (2008) 1213:48–56. doi: 10.1016/j.brainres.2008.03.062
368. Bryant RA, Creamer M, O’Donnell M, Silove D, McFarlane AC. Sleep disturbance immediately prior to trauma predicts subsequent psychiatric disorder. Sleep (2010) 33(1):69–74. doi: 10.1093/sleep/33.1.69
369. Koffel E, Polusny MA, Arbisi PA, Erbes CR. Pre-deployment daytime and nighttime sleep complaints as predictors of post-deployment PTSD and depression in National Guard troops. J Anxiety Disord (2013) 27(5):512–9. doi: 10.1016/j.janxdis.2013.07.003
370. Koren D, Arnon I, Lavie P, Klein E. Sleep complaints as early predictors of posttraumatic stress disorder: a 1-year prospective study of injured survivors of motor vehicle accidents. Am J Psychiatry (2002) 159(5):855–7. doi: 10.1176/appi.ajp.159.5.855
371. Bajor LA, Ticlea AN, Osser DN. The Psychopharmacology Algorithm Project at the Harvard South Shore Program: an update on posttraumatic stress disorder. Harv Rev Psychiatry (2011) 19(5):240–58. doi: 10.3109/10673229.2011.614483
372. van Liempt S. Sleep disturbances and PTSD: a perpetual circle? Eur J Psychotraumatol (2012) 3. doi: 10.3402/ejpt.v3i0.19142
373. Ticlea AN, Bajor LA, Osser DN. Addressing sleep impairment in treatment guidelines for PTSD. Am J Psychiatry (2013) 170(9):1059. doi: 10.1176/appi.ajp.2013.13050641
374. Bob P, Fedor-Freybergh P. Melatonin, consciousness, and traumatic stress. J Pineal Res (2008) 44(4):341–7. doi: 10.1111/j.1600-079X.2007.00540.x
375. Henckens MJ, Hermans EJ, Pu Z, Joels M, Fernandez G. Stressed memories: how acute stress affects memory formation in humans. J Neurosci (2009) 29(32):10111–9. doi: 10.1523/JNEUROSCI.1184-09.2009
376. Woon FL, Sood S, Hedges DW. Hippocampal volume deficits associated with exposure to psychological trauma and posttraumatic stress disorder in adults: a meta-analysis. Prog Neuropsychopharmacol Biol Psychiatry (2010) 34(7):1181–8. doi: 10.1016/j.pnpbp.2010.06.016
377. Gorfine T, Zisapel N. Melatonin and the human hippocampus, a time dependent interplay. J Pineal Res (2007) 43(1):80–6. doi: 10.1111/j.1600-079X.2007.00446.x
378. Lyons LC, Green CL, Eskin A. Intermediate-term memory is modulated by the circadian clock. J Biol Rhythms (2008) 23(6):538–42. doi: 10.1177/0748730408325359
379. Ruby NF, Hwang CE, Wessells C, Fernandez F, Zhang P, Sapolsky R, et al. Hippocampal-dependent learning requires a functional circadian system. Proc Natl Acad Sci U S A (2008) 105(40):15593–8. doi: 10.1073/pnas.0808259105
380. Wagner U, Born J. Memory consolidation during sleep: interactive effects of sleep stages and HPA regulation. Stress (2008) 11(1):28–41. doi: 10.1080/10253890701408822
381. Diekelmann S, Wilhelm I, Born J. The whats and whens of sleep-dependent memory consolidation. Sleep Med Rev (2009) 13(5):309–21. doi: 10.1016/j.smrv.2008.08.002
382. Gerstner JR, Lyons LC, Wright KP Jr., Loh DH, Rawashdeh O, Eckel-Mahan KL, et al. Cycling behavior and memory formation. J Neurosci (2009) 29(41):12824–30. doi: 10.1523/JNEUROSCI.3353-09.2009
383. Gerstner JR, Yin JC. Circadian rhythms and memory formation. Nat Rev Neurosci (2010) 11(8):577–88. doi: 10.1038/nrn2881
384. Albrecht U. The circadian clock, reward, and memory. Front Mol Neurosci (2011) 4:41. doi: 10.3389/fnmol.2011.00041
385. Rawashdeh O, Maronde E. The hormonal Zeitgeber melatonin: role as a circadian modulator in memory processing. Front Mol Neurosci (2012) 5:27. doi: 10.3389/fnmol.2012.00027
386. Hinkelmann K, Muhtz C, Dettenborn L, Agorastos A, Moritz S, Wingenfeld K, et al. Association between cortisol awakening response and memory function in major depression. Psychol Med (2013) 43(11):2255–63. doi: 10.1017/S0033291713000287
387. Ruby NF, Fernandez F, Garrett A, Klima J, Zhang P, Sapolsky R, et al. Spatial memory and long-term object recognition are impaired by circadian arrhythmia and restored by the GABAAAntagonist pentylenetetrazole. PloS One (2013) 8(8):e72433. doi: 10.1371/journal.pone.0072433
388. Yoo SS, Gujar N, Hu P, Jolesz FA, Walker MP. The human emotional brain without sleep–a prefrontal amygdala disconnect. Curr Biol (2007) 17(20):R877–878. doi: 10.1016/j.cub.2007.08.007
389. Graves LA, Heller EA, Pack AI, Abel T. Sleep deprivation selectively impairs memory consolidation for contextual fear conditioning. Learn Mem (2003) 10(3):168–76. doi: 10.1101/lm.48803
390. Hagewoud R, Whitcomb SN, Heeringa AN, Havekes R, Koolhaas JM, Meerlo P. A time for learning and a time for sleep: the effect of sleep deprivation on contextual fear conditioning at different times of the day. Sleep (2010) 33(10):1315–22. doi: 10.1093/sleep/33.10.1315
391. Alkadhi K, Zagaar M, Alhaider I, Salim S, Aleisa A. Neurobiological consequences of sleep deprivation. Curr Neuropharmacol (2013) 11(3):231–49. doi: 10.2174/1570159X11311030001
392. Menz MM, Rihm JS, Salari N, Born J, Kalisch R, Pape HC, et al. The role of sleep and sleep deprivation in consolidating fear memories. Neuroimage (2013) 75:87–96. doi: 10.1016/j.neuroimage.2013.03.001
393. Zelinski EL, Tyndall AV, Hong NS, McDonald RJ. Persistent impairments in hippocampal, dorsal striatal, and prefrontal cortical function following repeated photoperiod shifts in rats. Exp Brain Res (2013) 224(1):125–39. doi: 10.1007/s00221-012-3293-3
394. Colwell CS, Michel S, Itri J, Rodriguez W, Tam J, Lelievre V, et al. Selective deficits in the circadian light response in mice lacking PACAP. Am J Physiol Regul Integr Comp Physiol (2004) 287(5):R1194–1201. doi: 10.1152/ajpregu.00268.2004
395. Ressler KJ, Mercer KB, Bradley B, Jovanovic T, Mahan A, Kerley K, et al. Post-traumatic stress disorder is associated with PACAP and the PAC1 receptor. Nature (2011) 470(7335):492–7. doi: 10.1038/nature09856
396. Dias BG, Ressler KJ. PACAP and the PAC1 receptor in post-traumatic stress disorder. Neuropsychopharmacology (2013) 38(1):245–6. doi: 10.1038/npp.2012.147
397. Amstadter AB, Sumner JA, Acierno R, Ruggiero KJ, Koenen KC, Kilpatrick DG, et al. Support for association of RORA variant and post traumatic stress symptoms in a population-based study of hurricane exposed adults. Mol Psychiatry (2013) 18(11):1148–9. doi: 10.1038/mp.2012.189
398. Logue MW, Baldwin C, Guffanti G, Melista E, Wolf EJ, Reardon AF, et al. A genome-wide association study of post-traumatic stress disorder identifies the retinoid-related orphan receptor alpha (RORA) gene as a significant risk locus. Mol Psychiatry (2013) 18(8):937–42. doi: 10.1038/mp.2012.113
399. Irwin M. Effects of sleep and sleep loss on immunity and cytokines. Brain Behav Immun (2002) 16(5):503–12. doi: 10.1016/S0889-1591(02)00003-X
400. Bryant PA, Trinder J, Curtis N. Sick and tired: Does sleep have a vital role in the immune system? Nat Rev Immunol (2004) 4(6):457–67. doi: 10.1038/nri1369
401. Lorton D, Lubahn CL, Estus C, Millar BA, Carter JL, Wood CA, et al. Bidirectional communication between the brain and the immune system: implications for physiological sleep and disorders with disrupted sleep. Neuroimmunomodulation (2006) 13(5-6):357–74. doi: 10.1159/000104864
402. Coogan AN, Wyse CA. Neuroimmunology of the circadian clock. Brain Res (2008) 1232:104–12. doi: 10.1016/j.brainres.2008.07.087
403. Imeri L, Opp MR. How (and why) the immune system makes us sleep. Nat Rev Neurosci (2009) 10(3):199–210. doi: 10.1038/nrn2576
404. Cermakian N, Lange T, Golombek D, Sarkar D, Nakao A, Shibata S, et al. Crosstalk between the circadian clock circuitry and the immune system. Chronobiol Int (2013) 30(7):870–88. doi: 10.3109/07420528.2013.782315
405. Curtis AM, Bellet MM, Sassone-Corsi P, O’Neill LA. Circadian Clock Proteins and Immunity. Immunity (2014) 40(2):178–86. doi: 10.1016/j.immuni.2014.02.002
406. Agorastos A, Hauger RL, Barkauskas DA, Lerman IR, Moeller-Bertram T, Snijders C, et al. Relations of combat stress and posttraumatic stress disorder to 24-h plasma and cerebrospinal fluid interleukin-6 levels and circadian rhythmicity. Psychoneuroendocrinology (2018) 100:237–45. doi: 10.1016/j.psyneuen.2018.09.009
407. Baker DG, West SA, Nicholson WE, Ekhator NN, Kasckow JW, Hill KK, et al. Serial CSF corticotropin-releasing hormone levels and adrenocortical activity in combat veterans with posttraumatic stress disorder. Am J Psychiatry (1999) 156(4):585–8.
408. Yehuda R. Current status of cortisol findings in post-traumatic stress disorder. Psychiatr Clin North Am (2002) 25341-368(2):vii. doi: 10.1016/S0193-953X(02)00002-3
409. Raison CL, Miller AH. When not enough is too much: the role of insufficient glucocorticoid signaling in the pathophysiology of stress-related disorders. Am J Psychiatry (2003) 160(9):1554–65. doi: 10.1176/appi.ajp.160.9.1554
410. Yehuda R, Golier JA, Kaufman S. Circadian rhythm of salivary cortisol in holocaust survivors with and without PTSD. Am J Psychiatry (2005) 162(5):998–U995. doi: 10.1176/appi.ajp.162.5.998
411. de Kloet CS, Vermetten E, Geuze E, Kavelaars A, Heijnen CJ, Westenberg HG. Assessment of HPA-axis function in posttraumatic stress disorder: pharmacological and non-pharmacological challenge tests, a review. J Psychiatr Res (2006) 40(6):550–67. doi: 10.1016/j.jpsychires.2005.08.002
412. Heim C, Nemeroff CB. Neurobiology of posttraumatic stress disorder. CNS Spectr (2009) 14(1 Suppl 1):13–24.
413. Bauer ME, Wieck A, Lopes RP, Teixeira AL, Grassi-Oliveira R. Interplay between neuroimmunoendocrine systems during post-traumatic stress disorder: a minireview. Neuroimmunomodulation (2010) 17(3):192–5. doi: 10.1159/000258721
414. Pace TW, Heim CM. A short review on the psychoneuroimmunology of posttraumatic stress disorder: from risk factors to medical comorbidities. Brain Behav Immun (2011) 25(1):6–13. doi: 10.1016/j.bbi.2010.10.003
415. Pitman RK, Rasmusson AM, Koenen KC, Shin LM, Orr SP, Gilbertson MW, et al. Biological studies of post-traumatic stress disorder. Nat Rev Neurosci (2012) 13(11):769–87. doi: 10.1038/nrn3339
416. Thomas KS, Bower JE, Williamson TJ, Hoyt MA, Wellisch D, Stanton AL, et al. Post-traumatic disorder symptoms and blunted diurnal cortisol production in partners of prostate cancer patients. Psychoneuroendocrinology (2012) 37(8):1181–90. doi: 10.1016/j.psyneuen.2011.12.008
417. van Liempt S, Arends J, Cluitmans PJ, Westenberg HG, Kahn RS, Vermetten E. Sympathetic activity and hypothalamo-pituitary-adrenal axis activity during sleep in post-traumatic stress disorder: a study assessing polysomnography with simultaneous blood sampling. Psychoneuroendocrinology (2013) 38(1):155–65. doi: 10.1016/j.psyneuen.2012.05.015
418. Wahbeh H, Oken BS. Salivary cortisol lower in posttraumatic stress disorder. J Trauma Stress (2013) 26(2):241–8. doi: 10.1002/jts.21798
419. Keeshin BR, Strawn JR, Out D, Granger DA, Putnam FW. Cortisol awakening response in adolescents with acute sexual abuse related posttraumatic stress disorder. Depress Anxiety (2014) 31(2):107–14. doi: 10.1002/da.22154
420. Yehuda R, Siever LJ, Teicher MH, Levengood RA, Gerber DK, Schmeidler J, et al. Plasma norepinephrine and 3-methoxy-4-hydroxyphenylglycol concentrations and severity of depression in combat posttraumatic stress disorder and major depressive disorder. Biol Psychiatry (1998) 44(1):56–63. doi: 10.1016/S0006-3223(98)80007-3
421. Geracioti TD Jr., Baker DG, Ekhator NN, West SA, Hill KK, Bruce AB, et al. CSF norepinephrine concentrations in posttraumatic stress disorder. Am J Psychiatry (2001) 158(8):1227–30. doi: 10.1176/appi.ajp.158.8.1227
422. Haley RW, Vongpatanasin W, Wolfe GI, Bryan WW, Armitage R, Hoffmann RF, et al. Blunted circadian variation in autonomic regulation of sinus node function in veterans with Gulf War syndrome. Am J Med (2004) 117(7):469–78. doi: 10.1016/j.amjmed.2004.03.041
423. Pole N. The psychophysiology of posttraumatic stress disorder: a meta-analysis. Psychol Bull (2007) 133(5):725–46. doi: 10.1037/0033-2909.133.5.725
424. Woodward SH, Arsenault NJ, Voelker K, Nguyen T, Lynch J, Skultety K, et al. Autonomic activation during sleep in posttraumatic stress disorder and panic: a mattress actigraphic study. Biol Psychiatry (2009) 66(1):41–6. doi: 10.1016/j.biopsych.2009.01.005
425. Thoma MV, Joksimovic L, Kirschbaum C, Wolf JM, Rohleder N. Altered salivary alpha-amylase awakening response in Bosnian War refugees with posttraumatic stress disorder. Psychoneuroendocrinology (2012) 37(6):810–7. doi: 10.1016/j.psyneuen.2011.09.013
426. Agorastos A, Boel JA, Heppner PS, Hager T, Moeller-Bertram T, Haji U, et al. Diminished vagal activity and blunted diurnal variation of heart rate dynamics in posttraumatic stress disorder. Stress (2013) 16(3):300–10. doi: 10.3109/10253890.2012.751369
427. Ulmer CS, Calhoun PS, Bosworth HB, Dennis MF, Beckham JC. Nocturnal blood pressure non-dipping, posttraumatic stress disorder, and sleep quality in women. Behav Med (2013) 39(4):111–21. doi: 10.1080/08964289.2013.813434
428. Boscarino JA. PTSD is a risk factor for cardiovascular disease: time for increased screening and clinical intervention. Prev Med (2012) 54(5):363–4. author reply 365. doi: 10.1016/j.ypmed.2012.01.001
429. Turner JH, Neylan TC, Schiller NB, Li Y, Cohen BE. Objective evidence of myocardial ischemia in patients with posttraumatic stress disorder. Biol Psychiatry (2013) 74(11):861–6. doi: 10.1016/j.biopsych.2013.07.012
430. McFarlane AC, Barton CA, Briggs N, Kennaway DJ. The relationship between urinary melatonin metabolite excretion and posttraumatic symptoms following traumatic injury. J Affect Disord (2010) 127(1-3):365–9. doi: 10.1016/j.jad.2010.05.002
431. Heim C, Nater UM, Maloney E, Boneva R, Jones JF, Reeves WC. Childhood Trauma and Risk for Chronic Fatigue Syndrome Association With Neuroendocrine Dysfunction. Arch Gen Psychiatry (2009) 66(1):72–80. doi: 10.1001/archgenpsychiatry.2008.508
432. Eglinton R, Chung MC. The relationship between posttraumatic stress disorder, illness cognitions, defence styles, fatigue severity and psychological well-being in chronic fatigue syndrome. Psychiatry Res (2011) 188(2):245–52. doi: 10.1016/j.psychres.2011.04.012
433. Nater UM, Maloney E, Heim C, Reeves WC. Cumulative life stress in chronic fatigue syndrome. Psychiatry Res (2011) 189(2):318–20. doi: 10.1016/j.psychres.2011.07.015
434. Dansie EJ, Heppner P, Furberg H, Goldberg J, Buchwald D, Afari N. The Comorbidity of Self-Reported Chronic Fatigue Syndrome, Post-Traumatic Stress Disorder, and Traumatic Symptoms. Psychosomatics (2012) 53(3):250–7. doi: 10.1016/j.psym.2011.08.007
435. Haviland MG, Morton KR, Oda K, Fraser GE. Traumatic experiences, major life stressors, and self-reporting a physician-given fibromyalgia diagnosis. Psychiatry Res (2010) 177(3):335–41. doi: 10.1016/j.psychres.2009.08.017
436. Dell’Osso L, Carmassi C, Consoli G, Conversano C, Ramacciotti CE, Musetti L, et al. Lifetime post-traumatic stress symptoms are related to the health-related quality of life and severity of pain/fatigue in patients with fibromyalgia. Clin Exp Rheumatol (2011) 29(6):S73–8.
437. Galek A, Erbsloh-Moller B, Kollner V, Kuhn-Becker H, Langhorst J, Petermann F, et al. Mental disorders in patients with fibromyalgia syndrome. Screening in centres of different medical specialties. Schmerz (2013) 27(3):296–304. doi: 10.1007/s00482-013-1323-0
438. Hauser W, Galek A, Erbsloh-Moller B, Kollner V, Kuhn-Becker H, Langhorst J, et al. Posttraumatic stress disorder in fibromyalgia syndrome: Prevalence, temporal relationship between posttraumatic stress and fibromyalgia symptoms, and impact on clinical outcome. Pain (2013) 154(8):1216–23. doi: 10.1016/j.pain.2013.03.034
439. Afari N, Ahumada SM, Wright LJ, Mostoufi S, Golnari G, Reis V, et al. Psychological trauma and functional somatic syndromes: a systematic review and meta-analysis. Psychosom Med (2014) 76(1):2–11. doi: 10.1097/PSY.0000000000000010
440. Parker AJ, Wessely S, Cleare AJ. The neuroendocrinology of chronic fatigue syndrome and fibromyalgia. Psychol Med (2001) 31(8):1331–45. doi: 10.1017/S0033291701004664
441. Adler GK, Manfredsdottir VF, Creskoff KW. Neuroendocrine abnormalities in fibromyalgia. Curr Pain Headache Rep (2002) 6(4):289–98. doi: 10.1007/s11916-002-0050-5
442. Tanriverdi F, Karaca Z, Unluhizarci K, Kelestimur F. The hypothalamo-pituitary-adrenal axis in chronic fatigue syndrome and fibromyalgia syndrome. Stress (2007) 10(1):13–25. doi: 10.1080/10253890601130823
443. Lanfranco F, Motta G, Minetto MA, Baldi M, Balbo M, Ghigo E, et al. Neuroendocrine alterations in obese patients with sleep apnea syndrome. Int J Endocrinol (2010) 2010:474518. doi: 10.1155/2010/474518
444. Karaca Z, Ismailogullari S, Korkmaz S, Cakir I, Aksu M, Baydemir R, et al. Obstructive sleep apnoea syndrome is associated with relative hypocortisolemia and decreased hypothalamo-pituitary-adrenal axis response to 1 and 250 mu g ACTH and glucagon stimulation tests. Sleep Med (2013) 14(2):160–4. doi: 10.1016/j.sleep.2012.10.013
445. Nijhof SL, Rutten JM, Uiterwaal CS, Bleijenberg G, Kimpen JL, Putte EM. The role of hypocortisolism in chronic fatigue syndrome. Psychoneuroendocrinology (2014) 42:199–206. doi: 10.1016/j.psyneuen.2014.01.017
446. Korszun A. Sleep and circadian rhythm disorders in fibromyalgia. Curr Rheumatol Rep (2000) 2(2):124–30. doi: 10.1007/s11926-000-0052-4
447. Zoli A, Lizzio MM, Ferlisi EM, Massafra V, Mirone L, Barini A, et al. ACTH, cortisol and prolactin in active rheumatoid arthritis. Clin Rheumatol (2002) 21(4):289–93. doi: 10.1007/s100670200076
448. Di Giorgio A, Hudson M, Jerjes W, Cleare AJ. 24-hour pituitary and adrenal hormone profiles in chronic fatigue syndrome. Psychosom Med (2005) 67(3):433–40. doi: 10.1097/01.psy.0000161206.55324.8a
449. Nater UM, Youngblood LS, Jones JF, Unger ER, Miller AH, Reeves WC, et al. Alterations in diurnal salivary cortisol rhythm in a population-based sample of cases with chronic fatigue syndrome. Psychosom Med (2008) 70(3):298–305. doi: 10.1097/PSY.0b013e3181651025
450. Mahdi AA, Fatima G, Das SK, Verma NS. Abnormality of circadian rhythm of serum melatonin and other biochemical parameters in fibromyalgia syndrome. Indian J Biochem Biophys (2011) 48(2):82–7.
451. Fatima G, Mahdi AA, Das SK, Anjum B, Verma NS, Kumar P, et al. Lack of Circadian Pattern of Serum TNF-alpha and IL-6 in Patients with Fibromyalgia Syndrome. Indian J Clin Biochem (2012) 27(4):340–3. doi: 10.1007/s12291-012-0205-z
452. Riva R, Mork PJ, Westgaard RH, Lundberg U. Comparison of the cortisol awakening response in women with shoulder and neck pain and women with fibromyalgia. Psychoneuroendocrinology (2012) 37(2):299–306. doi: 10.1016/j.psyneuen.2011.06.014
453. Fatima G, Das SK, Mahdi AA, Verma NS, Khan FH, Tiwari AM, et al. Circadian rhythm of serum cortisol in female patients with fibromyalgia syndrome. Indian J Clin Biochem (2013) 28(2):181–4. doi: 10.1007/s12291-012-0258-z
454. Gibbs JE, Ray DW. The role of the circadian clock in rheumatoid arthritis. Arthritis Res Ther (2013) 15(1):205. doi: 10.1186/ar4146
455. Kouri VP, Olkkonen J, Kaivosoja E, Ainola M, Juhila J, Hovatta I, et al. Circadian timekeeping is disturbed in rheumatoid arthritis at molecular level. PloS One (2013) 8(1):e54049. doi: 10.1371/journal.pone.0054049
456. Yoshida K, Hashimoto T, Sakai Y, Hashiramoto A. Involvement of the circadian rhythm and inflammatory cytokines in the pathogenesis of rheumatoid arthritis. J Immunol Res (2014) 2014:282495. doi: 10.1155/2014/282495
457. Krakow B, Hollifield M, Johnston L, Koss M, Schrader R, Warner TD, et al. Imagery rehearsal therapy for chronic nightmares in sexual assault survivors with posttraumatic stress disorder: a randomized controlled trial. JAMA (2001) 286(5):537–45. doi: 10.1001/jama.286.5.537
458. Raskind MA, Peskind ER, Kanter ED, Petrie EC, Radant A, Thompson CE, et al. Reduction of nightmares and other PTSD symptoms in combat veterans by prazosin: a placebo-controlled study. Am J Psychiatry (2003) 160(2):371–3. doi: 10.1176/appi.ajp.160.2.371
459. Germain A, Shear MK, Hall M, Buysse DJ. Effects of a brief behavioral treatment for PTSD-related sleep disturbances: a pilot study. Behav Res Ther (2007) 45(3):627–32. doi: 10.1016/j.brat.2006.04.009
460. Epstein DR, Babcock-Parziale JL, Haynes PL, Herb CA. Insomnia treatment acceptability and preferences of male Iraq and Afghanistan combat veterans and their healthcare providers. J Rehabil Res Dev (2012) 49(6):867–78. doi: 10.1682/JRRD.2011.06.0114
461. Margolies SO, Rybarczyk B, Vrana SR, Leszczyszyn DJ, Lynch J. Efficacy of a cognitive-behavioral treatment for insomnia and nightmares in Afghanistan and Iraq veterans with PTSD. J Clin Psychol (2013) 69(10):1026–42. doi: 10.1002/jclp.21970
462. Talbot LS, Maguen S, Metzler TJ, Schmitz M, McCaslin SE, Richards A, et al. Cognitive behavioral therapy for insomnia in posttraumatic stress disorder: a randomized controlled trial. Sleep (2014) 37(2):327–41. doi: 10.5665/sleep.3408
463. Warner MD, Dorn MR, Peabody CA. Survey on the usefulness of trazodone in patients with PTSD with insomnia or nightmares. Pharmacopsychiatry (2001) 34(4):128–31. doi: 10.1055/s-2001-15871
464. Fraser GA. The use of a synthetic cannabinoid in the management of treatment-resistant nightmares in posttraumatic stress disorder (PTSD). CNS Neurosci Ther (2009) 15(1):84–8. doi: 10.1111/j.1755-5949.2008.00071.x
465. Aurora RN, Zak RS, Auerbach SH, Casey KR, Chowdhuri S, Karippot A, et al. Best practice guide for the treatment of nightmare disorder in adults. J Clin Sleep Med (2010) 6(4):389–401.
466. Alao A, Selvarajah J, Razi S. The use of clonidine in the treatment of nightmares among patients with co-morbid PTSD and traumatic brain injury. Int J Psychiatry Med (2012) 44(2):165–9. doi: 10.2190/PM.44.2.g
467. Raskind MA, Peterson K, Williams T, Hoff DJ, Hart K, Holmes H, et al. A trial of prazosin for combat trauma PTSD with nightmares in active-duty soldiers returned from Iraq and Afghanistan. Am J Psychiatry (2013) 170(9):1003–10. doi: 10.1176/appi.ajp.2013.12081133
468. Pollack MH, Hoge EA, Worthington JJ, Moshier SJ, Wechsler RS, Brandes M, et al. Eszopiclone for the treatment of posttraumatic stress disorder and associated insomnia: a randomized, double-blind, placebo-controlled trial. J Clin Psychiatry (2011) 72(7):892–7. doi: 10.4088/JCP.09m05607gry
469. Marshall RD, Garakani A. Psychobiology of the acute stress response and its relationship to the psychobiology of post-traumatic stress disorder. Psychiatr Clin North Am (2002) 25(2):385–95. doi: 10.1016/S0193-953X(01)00005-3
470. Mendlewicz J. Disruption of the circadian timing systems: molecular mechanisms in mood disorders. CNS Drugs (2009) 23 (Suppl 2):15–26. doi: 10.2165/11318630-000000000-00000
471. Comai S, Gobbi G. Unveiling the role of melatonin MT2 receptors in sleep, anxiety and other neuropsychiatric diseases: a novel target in psychopharmacology. J Psychiatry Neurosci (2014) 39(1):6–21. doi: 10.1503/jpn.130009
472. Pilorz V, Cunningham PS, Jackson A, West AC, Wager TT, Loudon AS, et al. A Novel Mechanism Controlling Resetting Speed of the Circadian Clock to Environmental Stimuli. Curr Biol (2014) 24(7):766–73. doi: 10.1016/j.cub.2014.02.027
473. Agorastos A, Linthorst AC. Potential pleiotropic beneficial effects of adjuvant melatonergic treatment in posttraumatic stress disorder. J Pineal Res (2016) 61(1):3–26. doi: 10.1111/jpi.12330
474. Brzezinski A, Vangel MG, Wurtman RJ, Norrie G, Zhdanova I, Ben-Shushan A, et al. Effects of exogenous melatonin on sleep: a meta-analysis. Sleep Med Rev (2005) 9(1):41–50. doi: 10.1016/j.smrv.2004.06.004
475. Buscemi N, Vandermeer B, Hooton N, Pandya R, Tjosvold L, Hartling L, et al. The efficacy and safety of exogenous melatonin for primary sleep disorders. A meta-analysis. J Gen Intern Med (2005) 20(12):1151–8. doi: 10.1111/j.1525-1497.2005.0243.x
476. Sanchez-Barcelo EJ, Mediavilla MD, Tan DX, Reiter RJ. Clinical uses of melatonin: evaluation of human trials. Curr Med Chem (2010) 17(19):2070–95. doi: 10.2174/092986710791233689
477. Srinivasan V, Brzezinski A, Pandi-Perumal SR, Spence DW, Cardinali DP, Brown GM. Melatonin agonists in primary insomnia and depression-associated insomnia: are they superior to sedative-hypnotics? Prog Neuropsychopharmacol Biol Psychiatry (2011) 35(4):913–23. doi: 10.1016/j.pnpbp.2011.03.013
478. Ferracioli-Oda E, Qawasmi A, Bloch MH. Meta-analysis: melatonin for the treatment of primary sleep disorders. PloS One (2013) 8(5):e63773. doi: 10.1371/journal.pone.0063773
479. Hansen MV, Danielsen AK, Hageman I, Rosenberg J, Gogenur I. The therapeutic or prophylactic effect of exogenous melatonin against depression and depressive symptoms: a systematic review and meta-analysis. Eur Neuropsychopharmacol (2014) 24(11):1719–28. doi: 10.1016/j.euroneuro.2014.08.008
480. Laudon M, Frydman-Marom A. Therapeutic effects of melatonin receptor agonists on sleep and comorbid disorders. Int J Mol Sci (2014) 15(9):15924–50. doi: 10.3390/ijms150915924
481. Shamir E, Laudon M, Barak Y, Anis Y, Rotenberg V, Elizur A, et al. Melatonin improves sleep quality of patients with chronic schizophrenia. J Clin Psychiatry (2000) 61(5):373–7. doi: 10.4088/JCP.v61n0509
482. Arendt J. Melatonin: characteristics, concerns, and prospects. J Biol Rhythms (2005) 20(4):291–303. doi: 10.1177/0748730405277492
483. Nunes DM, Mota RM, Machado MO, Pereira ED, Bruin VM, Bruin PF. Effect of melatonin administration on subjective sleep quality in chronic obstructive pulmonary disease. Braz J Med Biol Res (2008) 41(10):926–31. doi: 10.1590/S0100-879X2008001000016
484. Cardinali DP, Srinivasan V, Brzezinski A, Brown GM. Melatonin and its analogs in insomnia and depression. J Pineal Res (2011) 52(4):365–75. doi: 10.1111/j.1600-079X.2011.00962.x
485. Kuriyama A, Honda M, Hayashino Y. Ramelteon for the treatment of insomnia in adults: a systematic review and meta-analysis. Sleep Med (2014) 15(4):385–92. doi: 10.1016/j.sleep.2013.11.788
486. McGrane IR, Leung JG, St Louis EK, Boeve BF. Melatonin therapy for REM sleep behavior disorder: a critical review of evidence. Sleep Med (2015) 16(1):19–26. doi: 10.1016/j.sleep.2014.09.011
487. Johnston JD, Tournier BB, Andersson H, Masson-Pevet M, Lincoln GA, Hazlerigg DG. Multiple effects of melatonin on rhythmic clock gene expression in the mammalian pars tuberalis. Endocrinology (2006) 147(2):959–65. doi: 10.1210/en.2005-1100
488. Nagy AD, Iwamoto A, Kawai M, Goda R, Matsuo H, Otsuka T, et al. Melatonin adjusts the expression pattern of clock genes in the suprachiasmatic nucleus and induces antidepressant-like effect in a mouse model of seasonal affective disorder. Chronobiol Int (2014) 32(4):447–57. doi: 10.3109/07420528.2014.992525
489. Ikeno T, Nelson RJ. Acute melatonin treatment alters dendritic morphology and circadian clock gene expression in the hippocampus of Siberian Hamsters. Hippocampus (2015) 25(2):142–8. doi: 10.1002/hipo.22358
490. Persengiev SP. Multiple domains of melatonin receptor are involved in the regulation of glucocorticoid receptor-induced gene expression. J Steroid Biochem Mol Biol (1999) 68(5-6):181–7. doi: 10.1016/S0960-0760(99)00029-1
491. Konakchieva R, Mitev Y, Almeida OF, Patchev VK. Chronic melatonin treatment and the hypothalamo-pituitary-adrenal axis in the rat: attenuation of the secretory response to stress and effects on hypothalamic neuropeptide content and release. Biol Cell (1997) 89(9):587–96. doi: 10.1111/j.1768-322X.1997.tb01036.x
492. Konakchieva R, Mitev Y, Almeida OF, Patchev VK. Chronic melatonin treatment counteracts glucocorticoid-induced dysregulation of the hypothalamic-pituitary-adrenal axis in the rat. Neuroendocrinology (1998) 67(3):171–80. doi: 10.1159/000054312
493. Pawlikowski M, Kolomecka M, Wojtczak A, Karasek M. Effects of six months melatonin treatment on sleep quality and serum concentrations of estradiol, cortisol, dehydroepiandrosterone sulfate, and somatomedin C in elderly women. Neuro Endocrinol Lett (2002) 23(Suppl 1):17–9.
494. Sejian V, Srivastava RS. Effects of Melatonin on Adrenal Cortical Functions of Indian Goats under Thermal Stress. Vet Med Int (2010) 2010:348919. doi: 10.4061/2010/348919
495. Wang M, Yokotani K, Nakamura K, Murakami Y, Okada S, Osumi Y. Melatonin inhibits the central sympatho-adrenomedullary outflow in rats. Jpn J Pharmacol (1999) 81(1):29–33. doi: 10.1254/jjp.81.29
496. Kitajima T, Kanbayashi T, Saitoh Y, Ogawa Y, Sugiyama T, Kaneko Y, et al. The effects of oral melatonin on the autonomic function in healthy subjects. Psychiatry Clin Neurosci (2001) 55(3):299–300. doi: 10.1046/j.1440-1819.2001.00866.x
497. Mutoh T, Shibata S, Korf HW, Okamura H. Melatonin modulates the light-induced sympathoexcitation and vagal suppression with participation of the suprachiasmatic nucleus in mice. J Physiol (2003) 547(Pt 1):317–32. doi: 10.1113/jphysiol.2002.028001
498. Juszczak K, Ziomber A, Machowska A, Furgala A, Dobrek L, Wyczolkowski M, et al. The ameliorating effect of exogenous melatonin on urinary bladder function in hyperosmolar bladder overactivity and its influence on the autonomic nervous system activity. Acta Med (Hradec Kralove) (2011) 54(2):63–8. doi: 10.14712/18059694.2016.20
499. Gorfine T, Yeshurun Y, Zisapel N. Nap and melatonin-induced changes in hippocampal activation and their role in verbal memory consolidation. J Pineal Res (2007) 43(4):336–42. doi: 10.1111/j.1600-079X.2007.00482.x
500. Conboy L, Tanrikut C, Zoladz PR, Campbell AM, Park CR, Gabriel C, et al. The antidepressant agomelatine blocks the adverse effects of stress on memory and enables spatial learning to rapidly increase neural cell adhesion molecule (NCAM) expression in the hippocampus of rats. Int J Neuropsychopharmacol (2009) 12(3):329–41. doi: 10.1017/S1461145708009255
501. Rimmele U, Spillmann M, Bartschi C, Wolf OT, Weber CS, Ehlert U, et al. Melatonin improves memory acquisition under stress independent of stress hormone release. Psychopharmacol (Berl) (2009) 202(4):663–72. doi: 10.1007/s00213-008-1344-z
502. Huang F, Yang Z, Liu X, Li CQ. Melatonin facilitates extinction, but not acquisition or expression, of conditional cued fear in rats. BMC Neurosci (2014) 15:86. doi: 10.1186/1471-2202-15-86
503. Quiros I, Mayo JC, Garcia-Suarez O, Hevia D, Martin V, Rodriguez C, et al. Melatonin prevents glucocorticoid inhibition of cell proliferation and toxicity in hippocampal cells by reducing glucocorticoid receptor nuclear translocation. J Steroid Biochem Mol Biol (2008) 110(1-2):116–24. doi: 10.1016/j.jsbmb.2008.02.009
504. Tongjaroenbuangam W, Ruksee N, Mahanam T, Govitrapong P. Melatonin attenuates dexamethasone-induced spatial memory impairment and dexamethasone-induced reduction of synaptic protein expressions in the mouse brain. Neurochem Int (2013) 63(5):482–91. doi: 10.1016/j.neuint.2013.08.011
505. Zhang L, Zhang HQ, Liang XY, Zhang HF, Zhang T, Liu FE. Melatonin ameliorates cognitive impairment induced by sleep deprivation in rats: role of oxidative stress, BDNF and CaMKII. Behav Brain Res (2013) 256:72–81. doi: 10.1016/j.bbr.2013.07.051
506. Ekthuwapranee K, Sotthibundhu A, Tocharus C, Govitrapong P. Melatonin ameliorates dexamethasone-induced inhibitory effects on the proliferation of cultured progenitor cells obtained from adult rat hippocampus. J Steroid Biochem Mol Biol (2015) 145:38–48. doi: 10.1016/j.jsbmb.2014.10.003
507. Pacchierotti C, Iapichino S, Bossini L, Pieraccini F, Castrogiovanni P. Melatonin in psychiatric disorders: a review on the melatonin involvement in psychiatry. Front Neuroendocrinol (2001) 22(1):18–32. doi: 10.1006/frne.2000.0202
508. Maldonado MD, Perez-San-Gregorio MA, Reiter RJ. The role of melatonin in the immuno-neuro-psychology of mental disorders. Recent Pat CNS Drug Discovery (2009) 4(1):61–9. doi: 10.2174/157488909787002564
509. Maldonado MD, Reiter RJ, Perez-San-Gregorio MA. Melatonin as a potential therapeutic agent in psychiatric illness. Hum Psychopharmacol (2009) 24(5):391–400. doi: 10.1002/hup.1032
510. Chrousos GP, Kino T. Glucocorticoid action networks and complex psychiatric and/or somatic disorders. Stress (2007) 10(2):213–9. doi: 10.1080/10253890701292119
511. Cohen H, Kaplan Z, Zohar J. [Can Post-Traumatic Stress Disorder Be Prevented with Glucocorticoids]? Harefuah (2016) 155(12):757–61.
512. Buckley T, Duggal V, Schatzberg AF. The acute and post-discontinuation effects of a glucocorticoid receptor (GR) antagonist probe on sleep and the HPA axis in chronic insomnia: a pilot study. J Clin Sleep Med (2008) 4(3):235–41.
513. Cohen S, Kaplan Z, Zohar J, Cohen H. Preventing sleep on the first resting phase following a traumatic event attenuates anxiety-related responses. Behav Brain Res (2017) 320:450–6. doi: 10.1016/j.bbr.2016.10.039
514. Sprouse J, Braselton J, Reynolds L. Fluoxetine modulates the circadian biological clock via phase advances of suprachiasmatic nucleus neuronal firing. Biol Psychiatry (2006) 60(8):896–9. doi: 10.1016/j.biopsych.2006.03.003
515. Schaufler J, Ronovsky M, Savalli G, Cabatic M, Sartori SB, Singewald N, et al. Fluoxetine normalizes disrupted light-induced entrainment, fragmented ultradian rhythms and altered hippocampal clock gene expression in an animal model of high trait anxiety- and depression-related behavior. Ann Med (2016) 48(1-2):17–27. doi: 10.3109/07853890.2015.1122216
517. Huang RC. The discoveries of molecular mechanisms for the circadian rhythm: The 2017 Nobel Prize in Physiology or Medicine. BioMed J (2018) 41(1):5–8. doi: 10.1016/j.bj.2018.02.003
Keywords: circadian system, circadian clocks, stress, trauma, HPA axis, autonomic nervous system, glucocorticoids, sleep
Citation: Agorastos A, Nicolaides NC, Bozikas VP, Chrousos GP and Pervanidou P (2020) Multilevel Interactions of Stress and Circadian System: Implications for Traumatic Stress. Front. Psychiatry 10:1003. doi: 10.3389/fpsyt.2019.01003
Received: 11 December 2018; Accepted: 19 December 2019;
Published: 28 January 2020.
Edited by:
Chad A. Bousman, University of Calgary, CanadaReviewed by:
Anna Blasiak, Jagiellonian University, PolandDominic Landgraf, Ludwig Maximilian University of Munich, Germany
Urs Albrecht, Université de Fribourg, Switzerland
Copyright © 2020 Agorastos, Nicolaides, Bozikas, Chrousos and Pervanidou. This is an open-access article distributed under the terms of the Creative Commons Attribution License (CC BY). The use, distribution or reproduction in other forums is permitted, provided the original author(s) and the copyright owner(s) are credited and that the original publication in this journal is cited, in accordance with accepted academic practice. No use, distribution or reproduction is permitted which does not comply with these terms.
*Correspondence: Agorastos Agorastos, YWFnb3Jhc3RAYXV0aC5ncg==