- 1Department of Neonatology, University Medical Center Utrecht, Utrecht University, Utrecht, Netherlands
- 2Department of Psychiatry, Amsterdam Neuroscience, Amsterdam UMC, Vrije Universiteit Amsterdam, Amsterdam, Netherlands
- 3Department of Anatomy & Neurosciences, Amsterdam Neuroscience, Amsterdam UMC, Vrije Universiteit Amsterdam, Amsterdam, Netherlands
The third trimester of pregnancy represents a sensitive phase for infant brain plasticity when a series of fast-developing cellular events (synaptogenesis, neuronal migration, and myelination) regulates the development of neural circuits. Throughout this dynamic period of growth and development, the human brain is susceptible to stress. Preterm infants are born with an immature brain and are, while admitted to the neonatal intensive care unit, precociously exposed to stressful procedures. Postnatal stress may contribute to altered programming of the brain, including key systems such as the hypothalamic–pituitary–adrenal axis and the autonomic nervous system. These neurobiological systems are promising markers for the etiology of several affective and social psychopathologies. As preterm birth interferes with early development of stress-regulatory systems, early interventions might strengthen resilience factors and might help reduce the detrimental effects of chronic stress exposure. Here we will review the impact of stress following premature birth on the programming of neurobiological systems and discuss possible stress-related neural circuits and pathways involved in resilience and vulnerability. Finally, we discuss opportunities for early intervention and future studies.
Introduction
The third trimester of pregnancy represents a sensitive phase for infant brain plasticity, as a series of fast-developing cellular events, such as synaptogenesis, neuronal migration, and myelination regulate the development of neural circuits (1). Throughout this period of growth and development, the human brain is highly susceptible to stress exposure. Very preterm infants are born with a neurobiological immature system and are precociously exposed to stressful procedures during weeks to months in the Neonatal Intensive Care Unit (NICU). The excessive and prolonged exposure to stress during NICU admission can exceed the infant's natural regulatory capacity, threatening the allostatic balance of the infant, and might permanently alter neuroendocrine, autonomic, cardiovascular, and neural responses (2), leading to persisting mental morbidity throughout the lifespan (3, 4).
Along with increased survival in extremely preterm born infants (EP; gestational age <28 weeks) due to continued progress in perinatal care (5, 6), the rates of dysfunction in the area of mental health and behavior have remained unchanged or even worsened during the last decades (7–10). With an increased risk for a wide spectrum of psychiatric disorders, the preterm phenotype is primarily represented by deficits in attention, executive functioning, and emotional symptoms. Importantly, whilst preterm birth is associated with a higher prevalence of psychiatric disorders, a large proportion of children remain relatively unaffected [e.g., (11)].
Here we will review the impact of stress following prematurity on the programming of neurobiological systems. We begin by giving a short overview of the different types of stressors observed in postnatal stress research, followed by the typical development of autonomic, endocrine, and top-down regulatory systems in the fetal period. We then turn to evidence that postnatal stress following prematurity has short- and long-term effects on brain development. Lastly, we discuss possible mechanisms by which postnatal adversity increases the risk for social and affective problems following prematurity. Throughout this review, we call attention to critical gaps and unanswered questions and make suggestions for future research elucidating the mechanisms linking postnatal stress, neurobiology, and future social and affective development. Where appropriate, we focus on providing evidence from human postnatal studies; however, we rely on prenatal and/or animal studies where investigations of critical questions in preterm individuals are lacking.
Sources of Stress After Premature Birth
In the current review, we define stressors as any “real or interpreted threat to the physiological or psychological integrity of an individual that results in physiological and/or behavioral responses” [(4), p. 508]. In full recognition of the fact that there are numerous categorizations and types of stressors, including the administration of synthetic corticosteroids (e.g., dexamethasone) and its detrimental impact on postnatal development [e.g., (12)], for the purpose of this review we divided postnatal stressors into physical, environmental, and maternal stimuli or events. It is important to note that the effects of neonatal stress might be confounded by prenatal factors causative of preterm birth, such as vascular disease and infections (13), and hence both mechanisms may coexist. Also, the abrupt loss of intrauterine neurotrophic support following preterm birth could have deleterious effects on developmental programming, with reported damage to oligodendrocytes in the developing nervous system [for a review see (14)]. These prenatal factors are both highly relevant but are beyond the scope of the current review.
Physical Stress
Physical stress of repetitive procedural pain occurs routinely in extremely preterm neonates who are admitted to the intensive care unit. Preterm infants and neonates show an increased physiological and behavioral sensitivity toward painful procedures, as their pain transmission and modulation are still underdeveloped [for a review see (15)]. Due to a disbalance between afferent excitatory neurotransmitters and the descending inhibitory neurotransmitters, this hypersensitivity to pain is exacerbated in preterm infants (16, 17). There are currently two main categorizations of pain-related stressors: (1) acute procedural, (2) and acute prolonged (18). Acute procedural stress is triggered by a specific noxious stimulus (19), such as a heel stick, whereas acute prolonged stress represents a longer time duration with a distinguishable beginning and expected endpoint, such as mechanical ventilation or surgery (20).
Although we currently only defined physical neonatal stress as painful procedures and interventions; medical complications, such as hypoxia, infections, and inflammation, could also be considered as extremely stressful for preterm-born individuals. Hence, the current description of physical stress is not exhaustive.
Environmental Postnatal Stress
The effects of the nursery environment on the preterm infant, such as sound levels and nursing interventions, have become an area of concern for research. Studies showed that noise levels often exceed the American Academy of Pediatrics-recommendation of 40–45-dB (21), with NICU sounds ranging from 50 to 90 dB (22). Continuous loud noise has deleterious physiological effects on preterm infants and induces stress behaviors. More specifically, excess auditory stimulations have been associated with decreased oxygen saturation, increased heart rate and blood pressure, and alterations in sleep-wake state [for a review see (23)]. To reduce these unfavorable environmental factors, recent effort has been put into investigating the effects of single-family rooms vs. open bay units [for a review see (24) and section Opportunities for Early Intervention].
Physicians and nurses have rated routine caregiving events in the NICU as stressful to preterm infants (25). Indeed, common nursing interventions, such as diaper changes, noise, and light, can elicit similar stress-like responses as with invasive procedures [e.g., salivary cortisol levels, crying, heart rate (26, 27)]. However, to date, the impact of caregiving-related stress on the developing brain is not thoroughly researched.
Maternal Care
Preterm infants generally experience atypical maternal care while admitted to the NICU, whilst maternal behavior toward their preterm infant plays a crucial role in the early regulation of the infant's stress responses (28). Both physical and emotional closeness might become obstructed, subsequently increasing feelings of separation and negatively impacting mother-infant attachment (29, 30). Interestingly, previous studies found that both mother's mental well-being and the physical conditions of the infant contribute independently to the degree of maternal attachment (31). More specifically, preterm infants may be regarded as less “rewarding social partners,” as their neurological immaturity negatively impacts their social responsiveness [e.g., less time in alert state (32–34)]. In other words, preterm infants might be less responsive to parental cues and show more negative expressions, which might negatively impact the quality of mother-infant interaction [for a review see (35)]. In turn, due to the circumstances, mothers of preterm infants tend to spend less time holding, talking to, and looking at their premature infant compared to mothers of full terms (36).
Pre- and Post-Natal Brain Development of Stress Systems and Brain Networks
Fetal brain development is characterized by large maturational changes in volume, as well as changes in microstructural and functional connectivity, which has broad implications for future development (37). These maturational changes are regulated by complex molecular and cellular processes, such as neurogenesis and neuronal migration, synaptogenesis, and axonal growth [for an overview of the spatial progression, the reader is referred to (38)]. The endocrine and autonomic stress-systems are fundamentally shaped during the fetal period. Here we will give a short overview of the spatiotemporal changes involved in the development of the autonomic nervous system (ANS), hypothalamic-pituitary-adrenal axis (HPA-axis), and stress-related brain networks, and their role in the fetal stress response (see Figure 1).
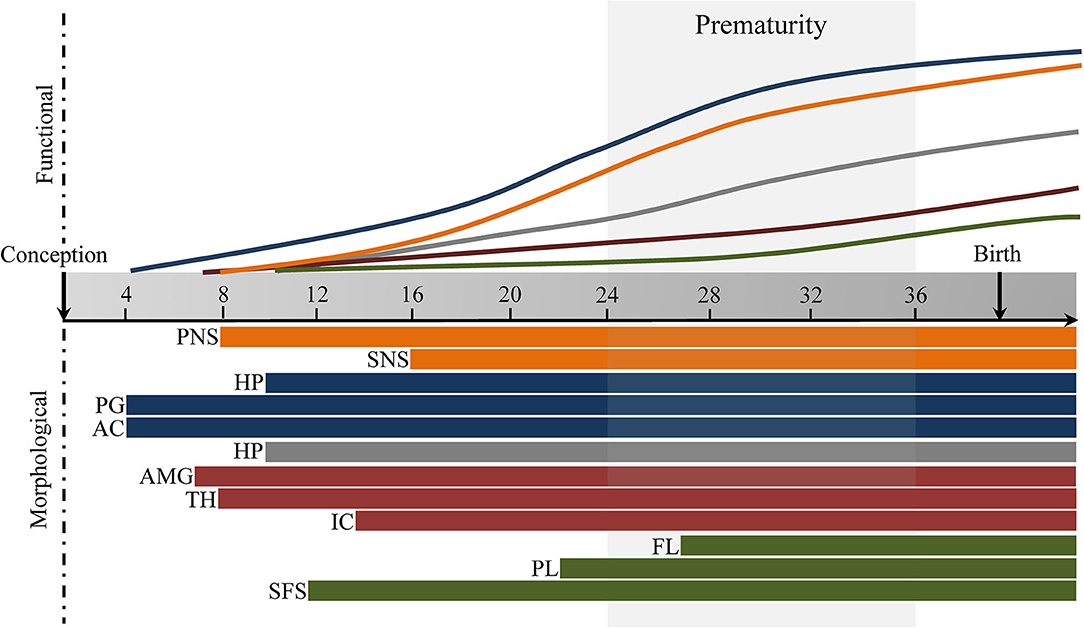
Figure 1. Fetal development of stress-response and stress-regulatory brain networks for illustration purposes. Autonomic Nervous System (orange), Hypothalamic-Pituitary-Adrenal axis (blue), Salience Network (red), Executive Control Network (green). PNS, parasympathetic nervous system, SNS, sympathetic nervous system, HPT, hypothalamus, PG, pituitary gland; AC, adrenal gland; HP, hippocampus; AMG, amygdala; TH, thalamus; IC, insular cortex; FL, frontal lobe; PL, parietal lobe; SFS, superior frontal sulcus. Timing based on literature reviewed under section Pre- and Post-natal Brain Development of Stress Systems and Brain Networks. Default Mode Network (gray).
Fetal Development of the Autonomic Nervous System (ANS)
The most immediate response to a stressor is modulated by the autonomic nervous system (ANS)—through its parasympathetic and sympathetic functions—which plays an important role in maintaining physiological homeostasis. The sympathetic discharge, during a “fight-or-flight” response, is accompanied by stimulation of the sympatho-adrenomedullary system, which is pivotal for rapid changes in physiological state, such as increased heart rate and blood pressure by catecholamine–induced excitation of the cardiovascular system (39, 40), whereas parasympathetic activation modulates the sympathetic system and restores the body to a restful state.
During the third trimester, the fetal ANS is changing rapidly (41). The maturational changes in the ANS are pivotal for the successful adaptation of the newborn to extrauterine life. The vagal nerve plays an important role in the parasympathetic regulation of autonomic functioning and subsequent socio-emotional function (42). There are two major components of the parasympathetic nervous system (PNS), namely, the unmyelinated vagal fibers [dorsal nucleus of the vagus (DMNX)] and the myelinated vagal system (nucleus ambiguus). At 9 weeks of gestation, the DMNX is distinguishable from the caudal brainstem, with the first differentiation into two subnuclei (i.e., dorsomedial and ventral) at 13 weeks. At 15–21 weeks, the subnuclei of the DMNX become more clearly visible, and the cytoarchitectonic differentiation of the DMNX is largely completed by 25 weeks (43, 44). Importantly, the phylogenetically primitive unmyelinated vagal nerve does not have many functions prior to birth, as it facilitates immobilization to deal with environmental challenges.
The nucleus ambiguus system develops later in fetal development, as it heavily depends on myelination. The nucleus ambiguus system is the primary vagal inhibitory pathway, facilitating an active vagal brake, modulating cardiac output by regulating inhibitory vagal control (45). Neurons in the nucleus ambiguus first appear at 8–9 weeks of gestation, with differentiation of neuronal subgroups appearing at 10 weeks of gestation (46). The number of myelinated vagus fibers increases rapidly from 24 weeks of gestation until the first year postpartum, indicating maturation of the parasympathetic branch of the ANS (47, 48). Advanced gestational age is accompanied by increased fetal heart rate variability (HRV) (49); these findings are in line with a rapid maturation of cardiac neuroregulatory activity and especially increasing parasympathetic tone in the last prenatal period (>32 weeks of gestation) (50).
ANS functioning is not solely mediated by the (un)myelinated vagus nerve, autonomic state is also regulated by sympathetic functioning. However, the maturation of the sympathoneural branch is less well-described. It is theorized that the sympathetic system develops before the NA, but after the DMNX (47). In line with this, research does indicate a steady maturational increase with growing fetal age from the late second into the early third trimester [~16–28 weeks of gestation (51, 52)].
Throughout most of gestation, the medulla (i.e., inner layer of adrenal gland) is not recognized as a distinct structure. At a later stage of development, after the first postnatal week, the adrenal medulla starts to form. Importantly, it takes 12–18 months for the medulla to become adult-like (53).
The fetal development of autonomic control is complex and difficult to disentangle, and important maturational milestones are reached during the transitional period from the second into the third trimester (see Figure 1). It is likely that exposure or experiences of stress during those important milestones have a potent effect on ANS development and function, which emphasizes the possible detrimental effects of preterm birth on the programming of ANS and subsequent stress-regulation [see paragraph on autonomic nervous system (ANS)].
Fetal Development of the Hypothalamic-Pituitary-Adrenal Axis (HPA-Axis)
Activation of the Hypothalamic-Pituitary-Adrenal axis (HPA-axis) results in the secretion of glucocorticoids, i.e., cortisol in humans, from the adrenal cortex (39), which acts on several organ systems to mobilize energy reserves. The emerging fetal HPA-axis undergoes large shifts in maturation and organization during the prenatal period. The fetal hypothalamus could be longitudinally subdivided into three zones, namely the midline, core, and lateral zones (54, 55). Early gestation (9–14 weeks of gestation) is distinguished by differentiation of the lateral hypothalamic zone, leading to the formation of the lateral hypothalamic area (LHA) and the perifornical hypothalamus. The hypothalamic core is differentiated around the second trimester, 18–33 weeks of gestation. The late second until third trimester (24–33 weeks of gestation) is characterized by advances in structural maturation of the periventricular (or midline) zone, followed by differentiation in (1) the suprachiasmatic [i.e., circadian clock under the strong influence of light/dark input (56)], (2) arcuate [i.e., sensor to modulate cortisol release (57)], and (3) paraventricular nuclei [i.e., promotes corticotrophin releasing hormone (CRH) and vasopressin (AVP) (58)]. These maturational changes extend into the third trimester. Around the postnatal period (immediate after birth), the major hypothalamic structures are clearly differentiated and resemble an adult-like form (54, 55).
The fetal pituitary gland seems to mature before the adrenal cortex. More specifically, the kidney-shaped anterior lobe of the pituitary gland, which is connected to the hypothalamus, starts to form from Rathke's pouch by 4–5 weeks of gestation. The first 12 weeks of gestation are characterized by major cellular differentiation (59), and by 21 weeks a further distinction can be made between the long and thin stalk region of the pituitary and the posterior lobe (60). Although the pituitary gland matures until the third postnatal month, after which it appears to be adult-like (61), fetal adrenocorticotrophin hormone (ACTH) is detectable by 8–10 weeks of gestation, peaking between the first and second trimester, after which it declines late in gestation (62).
Starting at the 4th weeks of gestation, the adrenal cortex (i.e., outer layer of adrenal gland) begins to form, and the morphology remains relatively stable after 10–12 weeks of gestation. Research suggests that the fetal adrenal cortex is unable to synthesize cortisol between 16 and 22 weeks of gestation, as the 3ß -hydroxysteroid dehydrogenase (3ß -HSD; converts pregnenolone to progesterone) enzyme is not expressed before the start of the third trimester (63). Hence, these findings indicate that the fetus is able to adapt to environmental changes and to maintain homeostasis, through glucocorticoid secretion, after 23 weeks of gestation. However, there is not much consensus on when exactly the fetal adrenal cortex is able to synthesize cortisol. More specifically, at 30 weeks of gestation, the fetal adrenal cortex resembles the elementary form of the adult adrenal cortex [(64), see (65), for review], and some studies suggest that the fetal adrenal cortex is unable to produce cortisol de novo until then (66), but instead uses the abundant placental progesterone. This would indicate that in absence of placental progesterone, the fetus might be unable to produce cortisol before 30 weeks of gestation.
The developmental trajectories of the HPA-axis that are established during the prenatal period could have lifelong consequences for future development (see Figure 1). Preterm-born infants are neuroendocrinologically immature, and their NICU stay associated with (multiple) stressful events might disturb the central regulation of HPA-axis. Therefore, prematurity might be characterized by the inability to maintain homeostasis in the face of acute stress [see Hypothalamic-Pituitary-Adrenal axis (HPA-axis)].
Fetal Development of the Stress-Related Neural Networks
There are three core neural networks that are implicated in the central response of stress, namely the (1) default mode network [DMN; which includes the posterior cingulate cortex (PCC), hippocampus, and parahippocampal cortex, amongst others (67)], the (2) salience network [SN; which includes the dorsal anterior cingulate cortex (ACC), frontoinsular cortex, amygdala, and several other (sub)cortical structures (68)], and (3) executive control network [ECN; which includes dorsolateral prefrontal and parietal regions (69)]. The ability to dynamically shift neural resources within these large-scale networks is theorized to facilitate adaptive responses to stress, and alterations in these networks possibly underlie phenotypic abnormalities (70). Interestingly, nodes within these networks start to develop in the fetal period, showing large morphological and functional changes throughout gestation. Below we will give a short overview of the typical maturational changes in the embryonic and fetal period (see Figure 1).
Default Mode Network
The hippocampus, a core node of the DMN, plays an important role in the regulation of the stress response due to its high expression of glucocorticoids and mineralocorticoids, thereby exerting negative feedback on the HPA-axis (71). As early as 9 weeks post-conception, four distinct hippocampal layers can be distinguished: intermediate zone, ventricular zone, hippocampal plate, and marginal zone (72). An unfolded hippocampus, along the medial surface of the temporal lobe, is present at 13 weeks of gestation. Throughout the following weeks, infolding of the hippocampus into the temporal lobe start as the dentate gyrus and cornu ammonis develop into an interlocking C shape. By 18–21 weeks of gestation, the hippocampus shows morphological maturity similar to that of the adult brain (73). The absolute volumes increase linearly from 14 to 22 weeks of gestation, with, relative to other brain regions, a faster growth from 14 to 17 weeks, ]but a slower growth from 18 until 22 weeks (73, 74). The dentate gyrus develops latest, showing a mature cytoarchitercute after 34 weeks of gestation (72). Compared to other brain structures, the hippocampus seems to be one of the earliest developing brain regions in humans. Importantly, the morphological development of the PCC and parahippcampal gyrus, both important components of the DMN, are not well-documented.
Important connections of the adult DMN are already present in the fetal period. For instance, some short-range pathways between the hippocampus and cortico-cortical regions, i.e., entorhinal cortex, are established as early as 19 weeks of gestation (75). Functional studies reported that from 19 weeks onwards, connectivity of the PCC became increasingly negative, which according to the authors, might serve a foundational role in the establishment of large-scale neural networks (76). Similarly, older (>35 weeks of gestation) but not younger fetuses showed a more synchronized positive functional connectivity between the PCC and medial PFC, and negative connectivity to the lateral prefrontal and parietal regions (77). Although the DMN becomes more synchronized across the first 2 years of life and achieve adult-like structures at the end of the first year, including increased connectivity between the PCC and hippocampus, the network is still rather immature in neonates (78–80). In sum, much of the foundation of the DMN is laid down in the early fetal and neonatal period. With the unfolding of several neuromaturational processes, disturbances in normative brain development, including an adverse extra-uterine environment, has likely far-reaching consequences. More studies are needed to elucidate the structural and functional milestones of the DMN and the impact of neonatal stress.
Salience Network
The amygdala, a core node of the SN, is a key component of the limbic system and is commonly implicated in emotional and behavioral regulation. This structure shows large morphological changes during fetal development. Differentiation of the amygdala nuclei continuous from the embryonic through the fetal period and neurogenesis is completed by birth (7.5–34 weeks gestation). More specifically, at 12 weeks of gestation migration of the neurons to the lateral amygdaloid nucleus are visible (81), and all major nuclei are formed by 15 weeks. The amygdala appears to be fully mature and functional at birth (81–83), and its connections are laid down early in gestation. Despite the absence of myelin, at a very early stage (i.e., 13–22 weeks of gestation) the amygdala establishes the first connections to several areas of the cortex (84, 85) with the appearance of association white matter fibers, such as the uncinate fasciculus [i.e., a major white matter fiber tract connecting the anterior temporal lobe and the amygdala to the lateral orbitofrontal cortex to the inferior frontal cortex (86)], appearing at around 15 weeks of gestation.
Although structural amygdala connectivity appears early in fetal development, these connections are predominantly short-range, with long-range tracts becoming more evident by term (87). Similarly, the functional connections of the amygdala stabilize early in fetal development. Late second and early third trimester (21st−26th weeks of gestation) are dominated by occipital and temporal connections, with a substantial increase in functional connectivity between the frontal and temporal lobes later in gestation [29–37 weeks of gestation (88)].
The thalamus is another core node of the salience network, which is a region that is strongly connected to the amygdala and involved in the regulation of stress, amongst others (89). From 8th week on, thalamic neurons show intensive morphological changes, with projection from the spinal cord to the thalamus. From 10 to 14 weeks of gestation, neuronal differentiation into several thalamic nuclei begins (90). Neurogenesis in the posterior medial thalamus extends into the late first and early second trimesters of pregnancy. By 26 weeks' gestation, the characteristic layers of the thalamus are visible, with obvious similarities to the adult brain. Thalamocortical pathways to the subplate neurons are evident at 17 weeks of gestation, but the thalamic projections to the cortical plate continue to develop later during the fetal period (24–32 weeks) (84, 85, 87, 91), reaching adult-like connections at 34 weeks of gestation.
It is well-known that subcortical structures demonstrate earlier maturation than the cerebral cortex. However, the insular cortex, also described as the “center of salience processing” (92), is among the first macroscopical structures that can be identified in the human fetal cortical development. Afif et al. (93) described the morphological stages of insular sulci and gyri maturation, with the first sulcus appearing at 13–17 weeks of gestation. Around 27–28 weeks of gestation, all insular sulci and gyri are in place and its structure is similar to its adult-like form. More specifically, by the end of the third trimester, the insula can be divided into two parts, the anterior insula (i.e., comprises three short gyri), and the posterior insula (i.e., comprises two long gyri). Radial migration pathways, between the ventricular zone and superior temporal region, were observed at 15 weeks of gestation, continuing to grow in number and thickness until 20 weeks of gestation (94). Further, by 26 weeks of gestation, migration pathways are regressing, and by 31 weeks of gestation the insular neuronal migration is fully completed. Importantly, similar connectivity is observed between 31 and 40 weeks of gestation, and it seems that several pathways observed in the fetal period (e.g., insular-parietal and insular-temporal pathways) are similar to those in adults.
The fact that several core nodes of the salience network mature before birth emphasizes the importance of early fetal development for functioning of the salience network. To date, there are only a few studies directly investigating early development of structural and functional connectivity within the salience network. One study did report synchronous activity of the anterior insula with anterior cingulate cortex in neonates, although quite primitive (95). Interestingly, however, while the neonatal brain consisted of large locally connected clusters, 1- and 2-year olds demonstrated more sophisticated distributed topology. Further, enhanced connectivity between the anterior insular and long-range prefrontal cortices and anterior cingulate cortex seemed adult-like in 1-year olds (with only a few changes in 2-year olds) (95). In line with this, other studies do suggest that connection strength increase with age, but only moderately, leading to still premature network topologies at the end of the first year (80) and second-year (96). Interestingly, recent studies were able to identify so-called “hubs,” which are highly connected regions, in the fetal period. Both the temporal lobe (97) and the insular cortex (76, 98) were found to be already highly connected before birth. In sum, key nodes of the salience network seem, on a morphological level, adult-like at birth, but the topological features of fetal brain network remain underdeveloped (see Figure 1). Changes in typical fetal development, such as preterm birth, might precociously impact the architectural characteristics of the immature SN.
Executive Control Network
Brain regions that serve a high-order function, such as the dorsomedial prefrontal cortex (DMPFC) and dorsal posterior parietal cortex (DPPC), which are all core nodes of the ECN, mature latest. The corticogenic events occur at different rates in different regions, and some cortical areas start to differentiate earlier in gestation than others. For instance, studies consistency showed a maturational lag in the frontal cortical regions, as the neural migration [i.e., as indicated by peak fractional anisotropy [26–30 weeks of gestation]] of the frontal lobe is preceded by the parietal lobe (21–25 weeks gestation) [e.g., (99, 100)]. Some studies suggest that the superior longitudinal fasciculus (SLF), a white matter tract connecting the superior parietal and superior frontal lobes and extending to the dorsal premotor and dorsolateral prefrontal regions, is completely absent in the fetal brain (84, 101). However, a recent study was able to reliably visualize the SLF at 26 weeks, suggesting that the SLF may start to develop in the second trimester, and continue to develop throughout gestation (102). In line with this, short- and long-range corticocortical association pathways could be observed at 22 weeks of gestation, and become more prominent throughout gestation (103).
Short-range cortico-cortical tracts emerge prior to gyrification in regions where sulci will later develop. The cortical plate starts to form in the human telencephalon around 7–10 weeks of gestation (104). The timing of the different types of sulci occurs hierarchically. Primary sulci start to appear as early as 10 weeks of gestation and continue to develop until the 28th week of gestation (105, 106). This is followed by the development of secondary and tertiary sulci. All the primary and most of the secondary sulci are believed to be present by 34 weeks of gestation (106, 107), whilst tertiary sulci appear around term [36–41 weeks of gestation (108)]. A core node of the ECN, the superior frontal sulcus, emerges between 22 and 24 weeks of gestation (109–111), and become clearly visible from ~27 weeks onwards (105, 106, 112).
Although the ECN is observable in fetuses and neonates (see Figure 1), it is still in a premature form at the end of the first postnatal year (79). However, the fetal period constitutes a time of vast development, and a study on functional connectivity found large differences in the ECN between younger and older fetuses. More specifically, they reported that older fetuses (i.e., 34 weeks of gestation) showed increased connectivity between the prefrontal areas and the parietal cortex, compared to younger fetuses (i.e., ~27 weeks of gestation) (76). In sum, using structural and functional connectivity and graph-theoretical analyses, studies were able to provide preliminary evidence for the emerging ECN in fetuses, highlighting the importance of the establishment of ECN nodes to the effect of functional and structural injuries typically sustained during premature birth. Importantly, the large variability in spatiotemporal development of nodes seem to indicate a developmental sequence starting from the DMN, to the SN, and finally the ECN, as the (sub)cortical nodes implicated in the DMN and SN appear to be maturing during the beginning of the first trimester, as opposed to the relatively delayed maturation of cortical nodes implicated in the ECN.
Neonatal Stress and Brain Development
More than 50 years ago, researchers expressed their concerns regarding the possible detrimental effects of neonatal stress on physiology and behavior, stating that early experience in animals, such as handling or electric shock, lead to changes in corticosterone response and emotionality [e.g., (113–115)]. Rodent studies gave rise to a large body of experimental studies reflecting the importance of early neonatal stress in regulating brain development (116, 117). The studies that have been conducted thus far found quite consistently that, similar to animal studies [e.g., (118)], exposure to neonatal stress is associated with alterations in several structures. For instance, using the Neonatal Infant Stressor Scale [NISS; cumulative stress score including physical and environmental stressors (25)], researchers found an association between high stress and decreased frontal and parietal brain volumes, as well as alterations in white matter microstructure in the temporal lobes in preterm born infants at term equivalent age (119). Similarly, the number of invasive procedures was associated with reduced white matter and subcortical gray matter maturation in preterm neonates. Moreover, greater invasive procedures were independently associated with reduced total brain volume, white matter diffusivity, and maturation of subcortical gray matter (e.g., thalamus, basal ganglia) (49, 120). Interestingly, Brummelte and colleagues could distinguish a period of increased vulnerability, namely early procedural pain [i.e., before scan 1 (median 32.1 weeks of gestation)] had a stronger association with white matter maturation compared to later stress (around term equivalent age), whereas subcortical gray matter showed sustained sensitivity toward neonatal stress (120). These results are in line with findings from another study, where they reported that functional and structural connectivity patterns were affected following exposure to early (i.e., from birth to scan 1), but not late neonatal stress [i.e., scan 1 to scan 2 [term-equivalent age]]. More specifically, early neonatal stress (e.g., heel lances, central line insertion) was associated with weaker structural (121) and functional (122) connectivity between the right insula and limbic system, and in the thalamocortical pathways. However, some inconsistencies remain, as researchers also reported no association between neonatal stress and hippocampal growth (123). With some ambiguity, these studies converge to reveal a developmental period of increased sensitivity to stress, subsequently affecting stress-regulatory networks. However, there have been no further studies to confirm the possible detrimental effects of postnatal stress on large-scale brain networks, and the possible increased risk of future social and affective functional impairment.
The adverse effects associated with exposure to postnatal physical stress appear to extend beyond the relationships observed in the neonatal period. For instance, a higher number of invasive procedures during NICU admission was related to abnormalities in white matter microstructure (e.g., superior white matter), as reflected by an increase in radial diffusivity at age 7 (124). Procedural pain was also observed to be related to abnormal maturation of brain volumes at school age, including the hippocampus, amygdala, thalamus, striatum, globus pallidus, and cerebellum (125, 126), and lower cortical thickness (127). Studies using magnetoencephalagraphy (MEG) found that differences in spontaneous gamma- to alpha-band oscillations in preterm-born school-age children were predicted by the number of invasive neonatal procedures, which, as suggested by the authors, might be attributed to alterations in thalamorcortical connectivity (128, 129). Hence, the abnormal maturation observed in the neonatal period seems to persist into childhood, affecting brain regions implicated in the regulatory capacity to future stressors (see Table 1 for an overview).
These findings are supported by evidence from fetal stress models demonstrating that full-term and preterm neonates exposed to prenatal stress showed alterations in brain development. More specifically, prenatal stress (e.g., maternal anxiety/depression) was associated with reductions in region-specific gray matter volume [i.e., PFC, temporal lobe (140)], alterations in white matter microstructure (e.g., amygdala, limbic system) (141–143), and reduced functional and structural connectivity between the amygdala, limbic, and frontal regions in infants (144–146). The effects of prenatal stress on brain development have been extensively reviewed elsewhere [see (147–149)].
Although changes in previously described brain regions have been consistently implicated in a wide range of behavioral problems in preterm born individuals [e.g., (150–155)], it remains elusive whether alterations in brain development might modulate the relationship between neonatal stress and future affective and social functioning.
Mechanisms Underlying Later Life Resilience and Vulnerability Following Prematurity
Autonomic Nervous System (ANS)
Ex utero third trimester development often leads to alterations in normal autonomic development in extremely preterm infants, which is essential for respiratory and cardiovascular homeostasis (156). General maturation of the ANS is often assessed using indices of heart rate, blood pressure, and respiratory rate. Studies showed an impaired autonomic maturation in preterm born neonates, as reflected by dampened sympathetic (e.g., low-frequency HRV) and parasympathetic (e.g., high-frequency HRV) tone (41, 50, 157, 158). Thus, far only one study investigated the sympatho-adrenomedullary system (SAM), with results indicating elevated sympathoadrenal tone (as indicated by increased levels of catecholamines) in preterm-born children (159). This increased release of catecholamines could be attributed to the reduced parasympathetic inhibition, as mentioned previously.
The autonomic development is altered during the neonatal period. Accordingly, an increasing body of evidence suggests that ANS dysfunction following prematurity persists into infancy (160, 161), childhood [(162, 163); but not all (164)], adolescence (165), and adulthood (166). This prolonged abnormal maturation of ANS functioning could be attributed to the amount of neonatal stress. More specifically, research showed that greater exposure to neonatal stress was related to dampening of ANS reactivity (130, 131).
Changes in central autonomic regulation in typically developing individuals limit the capacity to adequately respond to environmental changes, and have previously been implicated in psychiatric disorders (167–169). Consistent with this, ineffective vagal modulation has been implicated in dysfunctional emotion regulation in toddlers [e.g., (170)], children [e.g., (171)], and adults [e.g., (172)]. Interestingly, similar findings were observed in preterm infants, namely preterm born infants' degree of respiratory sinus arrhythmia (RSA) was positively associated with their social competence, whereas lower (mean) heart rate was associated with less behavioral problems and greater social competence (173). These findings indicate that functional deficiencies of the vagus, and more specifically the phylogenetically newer ventral vagal complex (VVC; i.e., part of the nucleus ambiguus and suppresses robust emotional reactions), might underlie difficulties in emotion regulation in preterm-born individuals (see Table 1 for an overview).
Although no further studies investigate the relationship between ANS functioning and outcome, these findings conform to the framework of the polyvagal theory, proposed by Porges (45, 174–176), which states that alterations in vagal tone and reactivity, and thus parasympathetic regulation, possibly lead to the development of psychiatric disorders in preterm-born individuals. The framework articulates three phylogenetic stages that underlie different behavioral responses, all associated with a distinct autonomic subsystem: (1) social communication [e.g., emotion (via ventral vagal complex)], (2) mobilization [e.g., fight-flight responses [via sympathetic-adrenal system]], and (3) immobilization [e.g., tonic immobility (via unmyelinated vagus)]. Alterations in these distinct subsystems possibly underlie the behavioral problems observed in preterm individuals. However, further research is needed to delineate the effects of preterm birth, and postnatal stress, on autonomic control and subsequent longitudinal brain and behavioral development.
Hypothalamic-Pituitary-Adrenal Axis (HPA-Axis)
A considerable array of research has found that neonatal adversity impacts neuroendocrine development. Studies reported, for instance, that more invasive procedures were associated with lower cortisol responses to a stressor [below 33 weeks post-conception (133); for a review see (177)]. Also, hyporeactivity to socio-emotional stress, as measured with the Face-to-Face Still-Face (FFSF) procedure (i.e., assesses socio-emotional regulation by rating negative emotionality, social engagement, and avoidance behavior), has been linked to the number of invasive procedures during NICU admission in 3-month old preterm infants (135). In children born very preterm, greater exposure to neonatal pain-related stress was associated with higher basal cortisol levels at 8 months (133) and 18 months (178), but lower basal, diurnal (132) and cumulative (hair) cortisol (134) at age 7–8 years. It is well-recognized that chronic stress can lead to downregulation of cortisol production [e.g., (179)], thereby reducing the detrimental effects of glucocorticoids. These alterations in HPA-axis functioning seem to persist into adulthood. More specifically, studies showed both decreased and increased HPA-axis responses (i.e., cortisol and ACTH) following acute stress, when compared to full-term controls (180, 181). These mixed results might be due to the developmental timing at which neonatal stress occurs, including age of onset, duration, and severity, affecting the effects of concurrent stress on the dynamically changing HPA-axis (see paragraph on fetal development of the hypothalamic-pituitary-adrenal axis).
The different developmental stages seem to be mirrored by a shift between hypo- and hyper-reactivity of the HPA-axis, with postnatal stress possibly altering the set-point of HPA-axis functioning in preterm-born individuals. This observation highlights the fact that both the type and magnitude of the stress-responses largely depends on the timing of the stressor. Moreover, exposure to postnatal stress at different points in the development of the HPA-axis might exhibit a different impact. Indeed, rodent studies showed that “early” maternal separation (i.e., 3–4 postnatal days) was associated with a hyper-responsivity to stress later in life, while “late” maternal separation (i.e., 7–8 or 11–12 postnatal days) showed an effect in the opposite direction (182, 183). These findings are in line with studies indicating that synthesis of CRH receptors is regionally distinct and age-specific. More specifically, CRH receptor density in rodents is highest during early postnatal days (i.e., 2–9), with CRHR1 mRNA levels increase to a maximal of 300–600% of adult levels (184). Perturbation of the profound changes in CRF system, due to extreme and chronic stress, might have long-lasting consequences for development. Hence, these critical developmental processes are extremely complex, and dependent on the timing of exposure, postnatal stress might have differential consequences. However, to date, this explanation for the mixed results of HPA-functioning in preterm born individuals remains speculative, and research is needed to delineate the possible time-specific effects of postnatal stress following preterm birth.
Surprisingly, altered HPA-axis functioning and its impact on (brain) development in ways that increase susceptibility to later stress-related disorders have not been extensively studied in preterm born individuals (see Table 1 for an overview). Thus far, higher basal cortisol in preterm born infants has been associated with poorer mother interactive behavior, as well as more problems in terms of emotional reactivity, anxiety, depression, and attention, amongst others (185). Similar findings were observed in preterm-born children, that is, increased HPA-reactivity was linked to more problems with attention, emotional reactivity, anxiety, depression, and negative mother-child interactions (186). Hence, converging evidence does suggest that HPA-axis functioning is a key mediator of developing psychopathology. Although the structural and functional development of neural networks is tightly linked to HPA-axis functioning, such that early life adversity sensitizes hippocampal-amygdala responses to acute stress (187, 188), to date, there are no studies that explored neural circuitry associated with HPA-dysfunction in preterm born individuals.
In sum, postnatal stress following prematurity lead to long-lasting changes in HPA-functioning, which, in turn, is associated with problem behavior (e.g., emotional reactivity, anxiety). Differences in HPA-functioning might be attributed to the timing of postnatal exposure, although extensive research is needed to disentangle the neurobiological mechanisms involved. Importantly, there is currently little research as to whether the altered patterns of HPA-axis functioning in the context of prematurity and postnatal stress are permanent, what epigenetic pathways might underlie the contradicting findings (see paragraph on epigenetic pathways), and the potential of prevention following postnatal interventions such as skin-to-skin contact (see paragraph on possibilities for early intervention).
Epigenetic Pathways
There is increasing evidence for the role of genetic and epigenetic variation in long-term effects of early life stress (189). It is suggested that epigenetic markers are developmentally sensitive to the quality of the pre- and post-natal environment and that early adversity produces lasting epigenetic modifications (190–192). Studies on the so-called “early-life programming” of the epigenetic regulation of gene transcription, have mainly focused on the serotonin transporter, due to its polymorphisms and role in mediating early stress and later life mental health, and glucocorticoids (GR), due to its negative feedback control on stress responsivity [for a review see (193)]. Importantly, most studies have focused on candidate genes related to serotonin and glucocorticoid functioning. Below we will review evidence from postnatal studies, but we will rely on prenatal studies where investigation in preterm individuals is lacking.
There are a few studies that investigated the influence of postnatal stress on SLC6A4 [i.e., a gene encoding the serotonin transporter (5-HTT)] promoter methylation. Studies reported an association between greater postnatal stress and lower SLC6A4 methylation in preterm-born infants (138, 139) and school-aged children with the COMT 158 Met/Met genotype (136). Importantly, authors suggested that SLC6A4 promoter methylation could not be attributed to preterm birth per se, rather, high levels of postnatal stress exposure altered the transcriptional functionality of 5-HTT (139, 194). In turn, greater SLC6A4 methylation predicted poorer stress-regulation in response to the still-face procedure at NICU discharge (194). Additionally, methylation at discharge was associated with greater negative emotionality and suboptimal socio-emotional regulation (137, 194). Thus far, only one study investigated the possible moderating role of SLC6A4 methylation on the relationship between NICU-stress and later brain development. More specifically, authors reported that preterm infants exposed to greater stress showed higher SLC6A4 methylation, which in turn was associated with reduced anterior temporal lobe (ATL) volumes (137).
Extensive scientific literature has repeatedly reported that changes in glucocorticoid receptor methylation (NR3C1) play a pivotal role in the regulation of the HPA-axis and the endocrine response to stress (195). Indeed, infants exposed to third-trimester prenatal stress, as measured by maternal emotional state, showed increased methylation of the NR3C1 gene (196, 197). Additionally, Oberlander and colleagues found that increased methylation of the NR3C1 was in turn associated with increased HPA-axis reactivity. Even though NR3C1 is tightly linked to stress vulnerability and resilience, studies investigating the epigenetic changes in NR3C1 following prematurity is limited. Few studies did investigate the role of prematurity in NR3C1 methylation, and findings were in line with studies on prenatal stress [for a review see (193, 198)], that is, preterm infants exposed to an adverse postnatal environment influenced NR3C1 methylation (199). Specifically, increased methylation of glucocorticoid receptor gene was observed in the first 4 days following preterm birth. However, findings remain inconsistent as studies demonstrated both decreased and increased DNA methylation of NR3C1 in high-risk preterm infants [i.e., scoring high on Neonatal Intensive Care Unit Network Neurobehavioral Scale (NNNS) or more medical problems] compared to low-risk preterm infants (200, 201).
Several other genes have been found imperative for the regulation of HPA-axis function, including the FKBP5 gene, which exerts an inhibitory role on GR signaling by modulating hormone-binding affinity (i.e., the strength of binding interaction) (202, 203), and the 11beta-hydroxysteroid dehydrogenase type 1 and 2 (11β-HSD), functions as a dehydrogenase which degrades cortisol to cortisone (11β-HSD2), and catalyzing the conversion of inactive cortisone to active cortisol (11β-HSD1) [for a review see (204)]. So far, only one study investigated FKBP5 gene transcription in relation to prematurity. Piyasena et al. (205) showed that preterm born infants had markedly lower methylation at FKBP5 compared to term-born infants. Importantly, these differences in FKBP5 methylation were resolved at 1 year of age. In line with this, a longitudinal epigenome-wide association study (EWAS) identified a total of 1,555 sites with significant differences in methylation in preterm born infants, but the majority of these differences did not persist into adulthood (206). Hence, these studies question whether the effects of prematurity and postnatal stress on DNA methylation persist across the life course.
11β-HSD1 is seen in the adult central nervous system and has previously been shown to influence HPA-axis regulation (207, 208), whereas 11β-HSD2 has a major central role in developmental programming due to the high expression in placenta and fetal tissue (209). As the function of 11β-HSD seems to reflect protection from the deleterious consequences of glucocorticoid overexposure, it has been suggested that 11β-HSD might function as a potential pathway in early life stress exposure and later outcome (210, 211). Studies indeed showed that prenatal stress was associated with downregulation of placental 11β-HSD2 gene encoding (212, 213), as well as lower and greater methylation of 11β-HSD2, respectively (214, 215). In turn, in rodents, 11β-HSD2−/− selectively determined programming of anxiety and depressive-like adult behavior (204, 211, 216). Similarly, 11β-HSD1−/− mice showed elevated basal corticosterone levels and exaggerated responses to stress (207, 208, 217). Increased levels of placental β-HSD1 mRNA were observed in mothers exposed to prenatal stress (218, 219), leading to increased glucocorticoid transport to the fetus. Although these findings could theoretically be extrapolated to a preterm population, as the assumed changes in 11β-HSD fail to protect the immature neurons from premature stress exposure, further investigation will be required to determine the degree to which changes in 11β-HSD are prevalent in preterm born individuals.
Only recently studies began to enhance our understanding of epigenetic changes following prematurity. Converging evidence suggests that preterm born individual show profound changes in glucocorticoid and serotonin transporter gene transcription, with some studies suggesting that these alterations can be specifically attributed to postnatal stress. Other genes involved in the regulation of HPA-axis, such as FKBP5 and 11β-HSD, have not been studied extensively. Importantly, epigenetic changes following prematurity might be non-persistent. Nonetheless, more studies are needed to further delineate the epigenetic changes following prematurity and the specific role of postnatal stress. There are several theoretical and methodological challenges in the field of behavioral epigenetics in preterm born individuals, including heterogeneity of the population (e.g., gestational age), lack of prospective longitudinal and epigenome-wide studies, small sample sizes, and inadequate control of confounders (e.g., race), amongst others.
Disruptions in the Neural Equilibrium
It is well-known that stress induces large-scale neural modulations and that extreme and prolonged stress trigger long-lasting changes in network balance. Both the salience network (SN) and the executive control network (ECN) are implicated in the adaptive regulation of stress, and these complex networks already originate in the fetal period (see paragraph on Fetal development of the stress-related neural networks). In the face of environmental challenges, via increased catecholamines, the SN is supposedly upregulated, facilitating increased vigilance and attentional reorienting, and autonomic-neuroendocrine control (220). On the contrary, the ECN, which is implicated in cognitive control processes and decision-making, is downregulated following stress (221). It is theorized that in the aftermath of stress, resources are allocated to the ECN, downregulating the SN (70). Disruptions in this neural equilibrium, possibly due to morphological alterations in prefrontal (222) and hippocampal (223) neurons following chronic stress, have been implicated in the pathogenesis of Post-Traumatic Stress Disorder (PTSD) (224, 225), depression (226, 227), anxiety (228, 229), bipolar disorder and schizophrenia (230, 231), amongst others.
Emotion Processing and Executive Functioning
A large number of studies have described a behavioral phenotype in preterm-born individuals constituting problems in the area of socio-emotional and executive functioning [for further details please see (232–234)], two higher-order cognitive phenotypes implicated in stress regulation (235, 236). The neural mechanisms underlying these behavioral phenotypes appear to be altered in preterm-born individuals. Although there are currently no studies investigating the role of postnatal stress on these stress-regulatory neural mechanisms, a growing number of studies does recognize the pivotal role of large-scale neural networks, rather than region-of-interest (ROI) based approaches, in preterm-born individuals.
Resting-state network studies showed an altered coupling between the SN and default mode network (DMN). The DMN comprises the medial prefrontal, posterior cingulate, precuneus, and bilateral angular gyrus (237), and exhibits low-frequency activity at rest, and has been proposed to be related to self-referential mental activity, including task-unrelated imagery and thoughts and self-reflection in preterm born individuals (238). Studies consistently reported a hypo-connectivity between the amygdala, mPFC, posterior cingulate cortex (PCC), anterior insula (AI), and the precuneus (pC) in preterm-born infants at term equivalent age (239), adolescents (240), and adults (241, 242). As suggested by the authors, the negative connectivity between the SN (i.e., AI/amygdala) and DMN (i.e., PCC/pC) could indicate an overactive inhibitory function of the PCC in modulating the left amygdala, greatly affecting their emotion processing (241). In line with this, a study reported that variability in the connectivity between the amygdala and other regions was predictive of greater internalizing symptoms at 2 years (239). Also, alterations in white matter microstructure involved in the SN and other networks, including the thalamus, inferior fronto-occipital fasciculus, and inferior/superior longitudinal fasciculus have been associated with internalizing symptoms in preterm born children aged 9–16 (243).
Preterm children also showed structural alteration in the anatomical organization of the cortico-basal ganglia-thalamo-cortical pathway (CBGTC). Especially connections between the thalamus, putamen, globus pallidus and caudate nucleus were weaker (151). These SN-specific nodes are disrupted in psychiatric diseases [e.g., PTSD, obsessive-compulsive disorder (OCD), schizophrenia (244)], dissociating multiple interconnected mental operations, such as processing affective content, decision making, and attention (245). Studies also reported significant smaller amygdala volumes in preterm-born infants at term equivalent age, compared to term born infants, and this altered amygdala development has been linked to maladaptive fear-processing (as measured by Unpredictable Mechanical Toy episodes) (246). A key limbic tract, the uncinate fascicle [i.e., temporo-amygdala-orbitofrontal network (247)], critical for social-emotional functions (86, 248), showed significant white matter reductions in preterm-born individuals [children; (249); adolescents (250)]. At present, it would be helpful to have a clearer understanding of how these neural patterns vary as a function of postnatal stress.
These limbic-cortical pathways are not only involved in socio-emotional behavior but also play a pivotal role in higher-order behavioral control. Studies repeatedly reported significant alterations in white matter microstructure, including the cingulum (i.e., connection between anterior cingulate cortex, dorsolateral prefrontal cortex, and inferior parietal lobe), fronto-occipital fascicles (i.e., bridging frontal-temporal-parietal-occipital lobe), fornix, corpus callosum, and superior longitudinal fasciculus, amongst others (153, 251–255). These white matter indices have been directly linked to alterations in executive functioning, that is, lower executive functioning was related to reductions in several white matter microstructure (e.g., inferior-fronto-occipital fascicles, cingulum, and superior longitudinal fasciculus) [Wisconsin Card Sorting Test (256); Test of Everyday Attention for Children (TEA-Ch) (257); Child Behavior Check List (CBCL) (153); Delis-Kaplan Executive Function Systems (D-KEFS) (258)]. Importantly, one study was unable to find an independent effect of preterm birth on white matter microstructure, and authors emphasized that postnatal factors, such as the degree of stress (i.e., days of mechanical ventilation), grossly impact postnatal brain development (259).
The alterations in connectivity are also observed on a functional level. Specifically, studies found that preterm born adults, compared to controls, showed increased activity in the middle temporal/occipital gyrus, posterior cingulate gyrus, and precuneus [go-/no-go task; (260); verbal fluency task (261)], which replicates previous findings (262). Additionally, during oddball trials (i.e., “odd” stimuli to control for low-frequency no-go stimuli), preterm-born young adults displayed attenuated brain activation in a fronto-parietal-cerebellar network. More recent studies disentangled the neural underpinnings of proactive vs. reactive cognitive control in preterm-born adults. Using the Not-X continuous performance test (CPT), authors reported (1) hypo-activation between the frontal pole and anterior cingulate gyrus, as well as the posterior cingulate gyrus and precuneus, and (2) hyper-activation between the posterior cingulate gyrus and precuneus, and the right lateral occipital cortex and angular gyrus (255). In other words, authors showed that preterm born adults exhibited more reactive behavioral control, rather than proactive. Recent research started to reveal the importance of these functional patterns, as well as the decoupling between the ECN and DMN, for the development of cognitive control (263) and emotion-regulation (264).
In sum, preterm born individuals show profound alteration in large-scale brain networks involved in emotion regulation and executive functioning, the SN and ECN, respectively. These changes possibly underlie individual differences in stress-sensitivity, as both large-scale networks, and its behavioral phenotype, have been implicated in adaptive stress responses (70). As mentioned previously, the DMN, SN, and ECN start to develop during gestation. Although these networks are still immature at birth, the formation of important pathways during gestation gives rise to potential points of vulnerability. The degree to which postnatal stress might underlie these extensive network changes in preterm born individuals remains elusive.
Opportunities for Early Intervention
Inadequate treatment of stressors in preterm infants have previously been associated with short- and long-term alterations in brain and behavior, greatly impacting their ability to maintain homeostasis (see paragraph on neonatal stress and brain development). This highlights the importance of adequately and promptly assessing stress in the newborn, and gave rise to the Newborn Individualized Developmental Care and Assessment Program (NIDCAP) (265, 266), which is a technique that uses detailed observations of infant behavior to provide caregivers and parents with recommendations on how to minimize stress. Developmental care theories postulate that one should actively observe the infant, during several caregiving procedures (e.g., collection of a blood sample), to assess the infant's efforts of self-regulation in response to stress. Based on such observations, both clinicians and families can make adjustments to optimize and adapt the traditionally delivered newborn intensive care to the infants' current needs. These adjustments can include interventions developed to increase self-regulation in the preterm infant. In full recognition of the fact that there are numerous interventions aimed at preventing or reducing postnatal stress, including the possible protective effects of fetal neurosteroids such as allopregnanolone, and its inhibiting properties in relation to the HPA-axis [(267, 268); for a review see (269)], for the purpose of this review, we decided to focus on non-pharmacological interventions as several analgesics have the potential to adversely impact the developing postnatal brain by altering neuronal processing [e.g., (270, 271)].
Skin-to-skin contact, also called kangaroo care, is a promising intervention possibly reducing infants' stress during NICU admission. For instance, research showed that preterm born infants exhibited lower basal stress levels (i.e., autonomic responses) during kangaroo care when compared to regular care (272, 273), as well as lower stress reactivity when exposed to physical stress (274–277), and improved white matter microstructural development (278). Kangaroo care also seems to affect HPA-axis functioning, as indicated by reduced saliva cortisol levels when exposed to a period of kangaroo care (279), as well as lower salivary cortisol reactivity at 1 month (280). However, kangaroo care seems to not bring about sustained reductions in salivary cortisol (280–282). Interestingly, research did show sustained effects on autonomic control. More specifically, one study found that kangaroo care accelerated the maturation of vagal tone between 32 and 37 weeks of gestation, as indicated by increased amplitude of RSA, positively affecting autonomic control (283). As suggested by the authors, kangaroo care might exert developmentally sensitive effects and should be administered during an appropriate developmental window to alter the maturational trajectories of systems that are currently developing. In other words, the timing of the intervention is pivotal for achieving optimal levels of physiological maturation. Although kangaroo care showed some positive effect on preterm infants' physiological stability and maturation, the degree to which these effects are long-term remains elusive.
Although inconclusive, there is some evidence for the positive effects of kangaroo care on attachment behavior and parental stress levels. More specifically, kangaroo care positively affected mother's mood, reduced parental stress levels, increased parental affiliative (e.g., touch) and attachment behavior when compared to standard care [e.g., (177, 272, 284, 285)]. These changes in parental care and behavior might, in turn, have positive effects on the preterm infant's stress-regulatory capacities [e.g., (286, 287)], which provide preliminary support for changing the biological organization of the stress system in preterm infants through parental-driven interventions.
There is growing evidence to promote not only the use of kangaroo care, but also the use of music, massage, co-bedding, and Family Nurture Interventions in extremely preterm infants (288–297). Despite the use of different measures to assess stress and pronounced differences in intervention, results from these studies largely indicated improvement in HPA-axis functioning and autonomic control, as indicated by lower cortisol levels and increase autonomic stability following the intervention. These auditory and tactile interventions can be viewed as environmental enrichment, possibly stimulating cortical plasticity and attenuating the stress response in preterm infants (298). Indeed, recent studies found encouraging, but preliminary, evidence for increased microstructural maturation at term-equivalent age for preterm infants exposed to music during their NICU stay (299), as well as a greater maturation of cardiac function (296). In the long-term, both Family Integrated Care, i.e., infant care provided by families by enhancing parental support and education (300), and Family Nurture Interventions, i.e., promotes calming interaction between mother and infants, seem to have a sustained positive effect of behavioral outcome, with more robust self-regulation skills and less negative emotionality at 18–21 months and 4–5 years of age, amongst others (297, 301, 302). Further research is warranted into the exact neurobiological pathways underlying the relationship between tactile and auditory interventions and preterm infant stress-regulation.
There are several reasons to hypothesize that single-family rooms (SFR) will reduce infant and parental stress, increase parental involvement, and subsequently improve infants' outcome. Nonetheless, scientific evidence on the benefits of SFR, vs. open bay, is at this point mixed and questions whether the change from open bay to SFR is justified [for a review see (24)]. Some studies reported positive benefits of private rooms, including less physiological stress, improved neurobehavioral development, and better long-term outcomes in preterm infants (303–305). On the contrary, studies also reported potential adverse effects in relation to SFR, that is, increased maternal stress (306, 307), altered infant cerebral development, and worse neurodevelopmental outcome (308), and no effects on infant salivary cortisol reactivity (309). Together, these findings suggest that consideration of the design and environment is important for the health and well-being of preterm infants admitted to the NICU, including noise control, parent-infant closeness, and parental involvement. However, much uncertainty remains regarding the design of the NICU environment, how much or what type of sensory stimulation would optimize brain development in preterm infants. It is important to realize that both neurosensory deprivation, a consequence of SFR, and neurosensory overexposure, a consequence of open ward, might be maladaptive (310).
In sum, a range of aspects of the physical environment is pivotal for stimulating development of the preterm infant. Research showed beneficial effects of parent-infant bonding, sensory stimulation, and the use of private family-rooms on mitigating postnatal stress in preterm infants. Moreover, these interventions seem to promote infant self-regulation, by adequately utilizing their neurophysiological modulatory system to safeguard oneself from excessive over-stimulation and arousal. Hence, appropriate tactile and auditory stimulation seems sufficient to induce improvement in self-regulation. Nonetheless, results are not consistent, and the degree to which these improvements are sustained remains inconclusive. Also, several methodological challenges, such as lack of standardization, possibly introduce confounding effects.
Concluding Remarks
In summary, the reviewed literature suggests that stress has both proximate and long-lasting detrimental effects on brain and behavior in preterm-born individuals. A key question is whether there are, so-called, “sensitive” periods of pre- and post-natal development during which stress-regulatory mechanisms are established. Indeed, research indicates that not only the HPA-axis and ANS are formed during the embryonic, fetal, and postnatal period, also large-scale brain networks implicated in the central response of stress including the DMN, SN, and ECN start to develop early on. This window of increased vulnerability offers an important clue for the cellular impairment that might underlie stress-sensitivity and long-term outcome in preterm born individuals.
A few studies have emerged to investigate the effects of “early” vs. “late” stress. Postnatal work indeed demonstrated that early stress has differential and long-lasting effects on brain development, compared to stress experienced around term-equivalent age. Importantly, some studies also suggested an independent effect of postnatal stress on brain development, rather than prematurity per se. The current review underscores increasing evidence that postnatal stress might persistently impact later functioning in part by affecting neurobiological systems, including the HPA-axis, ANS, large-scale brain networks, and gene expression. Also, an increasing number of intervention studies found preliminary evidence for the possible beneficial effects of parent-infant bonding, sensory stimulation, and family rooms on mitigating the detrimental effects of postnatal stress. However, the reviewed evidence is far from conclusive and there is a paucity of clinical studies on the subject of prematurity and stress resilience and vulnerability. We have only started to investigate the role of postnatal stress in resilience and vulnerability to developing psychiatric disorders following prematurity. Therefore, our schematic presentations of some proposed relations (see Box 1 and Figure 2) should not be considered comprehensive, rather, it should guide future research toward possible modulating factors between postnatal stress and future resilience or vulnerability in preterm born individuals.
Box 1. Unanswered questions.
1. Following premature birth, how do postnatal stressor type, timing, and duration translate to changes in neurobiological systems and subsequent outcome across the life course?
2. Which (combination of) neurobiological mechanisms underlie vulnerability or resilience following postnatal stress?
3. Are neurobiological changes following premature birth (i.e., physiological, neural network, and epigenetic) indicative of maladaptive functioning or do they also occur as a result of adaptive processes in stress-regulatory systems?
4. Can targeted and individualized neonatal interventions reverse morphological and functional remodeling of neurobiological systems and lead to improved outcomes? Also, when are these interventions able to buffer the effects of postnatal stress on neurobiological development to mitigate long-term risk for affective and social functioning?
5. What is the potential of identifying individual developmental trajectories, and the subsequent early identification of who is and who is not at risk following preterm birth and chronic stress exposure?
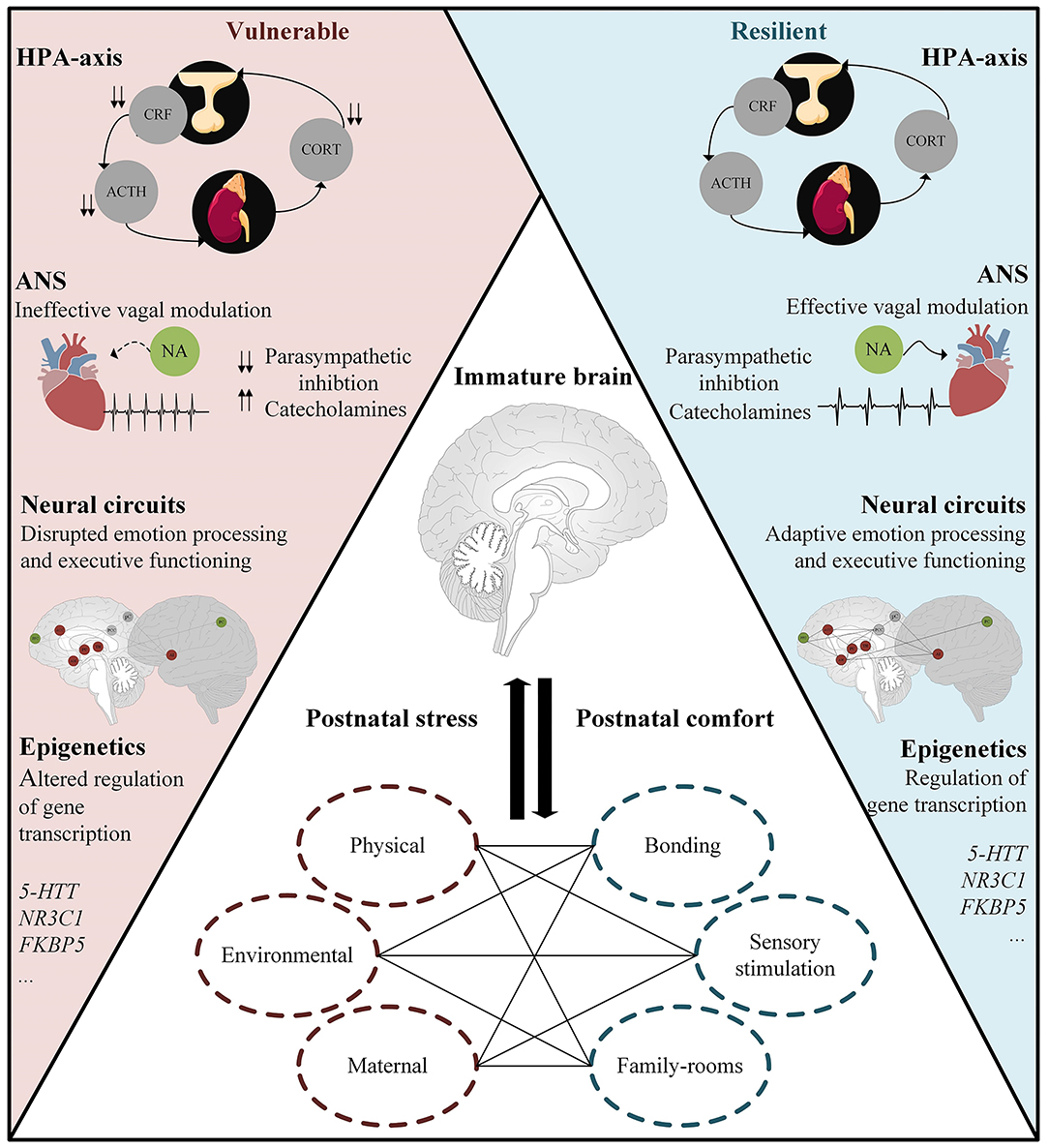
Figure 2. Resilient functioning in preterm born individuals exposed to chronic postnatal stress might be facilitated by; the ability to regulate and dampen stress responsivity; effective vagal modulation; homeostasis in large-scale neural networks underlying emotion processing and executive functioning; and adaptive regulation of gene transcription. Middle panel: Postnatal factors influencing brain maturation and, in turn, the onset/development of stress-related disorders such as anxiety and depression. ACTH, adrenocorticotropic hormone; Cort, cortisol; CRF, corticotropin-releasing factor; HPA-axis, hypothalamus–pituitary–adrenal axis; ANS, autonomic nervous system; NA, Nucleus Ambiguus; 5-HTT, serotonin transporter; NR3C1, glucocorticoid receptor; FKBP5, FK506 binding protein 5 and acts as a co-chaperone that modulates glucocorticoid receptor activity.
To better understand and reduce the impact of postnatal stress and prematurity on brain development, psychopathology, and possible mechanisms, one should consider integrating several additional issues into future studies (see Box 1 for unanswered questions). First, existing research has predominantly focused on the effects of physical stressors, such as skin-breaking procedures, rather than taking into account different stressor types, including maternal care and environmental stress. As pointed out previously, there is a great dependence of stressor type in characterizing risk factors. In other words, each stressor has distinct and possibly cumulative effects on later development of brain and behavior, and failing to account for stressor type might greatly impact results. Hence, there is a great need for systematic research on the possible detrimental effects of postnatal stress. These investigations should particularly focus on the possible long-term effects of postnatal stress on later development of brain and behavior, including adolescence and adulthood.
Second, it is important to note that only a minority of preterm born individuals will develop a psychiatric disorder. It has become clear that several neurobiological pathways might react differently to neonatal stress in resilient and vulnerable individuals. However, the specific factors that interact and account for these differences are still undetermined. Behavioral studies reported that positive parental behavior is favorable and predisposes preterm individuals to stress resilience (297, 301, 302, 311, 312). On a neurobiological level, detailed analyses of factors that account for and interact with variation in several moderating and mediating factors (e.g., HPA-axis, epigenetics), in both vulnerable and resilient individuals, is lacking. Such research would benefit from prospective longitudinal studies, with a systematic assessment of postnatal stress, and the association with changes in neurobiological systems and later affective and social functioning. For instance, as stress has a global effect on brain functioning, investigating large-scale brain networks gives a greater insight into the reorganization of connectivity patterns, rather than limiting analyses to predefined regions of the brain (313). To date, it remains unclear whether and how acute stressors relate to shifts in resource allocation between large-scale brain networks, and whether specific neural patterns might underlie resilience in preterm born individuals. Most importantly, how these networks dynamically unfold as a function of stress, and whether postnatal stress might alter these dynamics, remains unanswered.
Third, the ability to “bounce-back” is not viewed as a stable trait, rather, it is viewed as malleable and easily modified by interventions (314). Certain postnatal interventions appear to be beneficial in reducing the detrimental effects of postnatal stress, particularly interventions increasing parent-infant bonding (e.g., kangaroo care). However, considerable uncertainty remains as to whether these beneficial effects persist over time, and what, if any, neurobiological systems are remodeled.
Preterm born individuals have an increased risk for developing psychiatric sequelae, and this heightened vulnerability might have its origin in the postnatal exposures. Fortunately, research investigating the role of postnatal stress on later development in preterm born individuals has gathered momentum over the past two decades, and an increasing number of studies have focused on ways to diminish the detrimental effect of postnatal stress. Although the mechanisms that lead to resilient phenotypes is far from being fully determined, the current review identified several potential factors that might facilitate an adaptive stress response in the face of adversity. An increased understanding of the neurological, physiological, and epigenetic circuitry underlying resilience in preterm born individuals might be a starting point for the development of targeted and individualized intervention and prevention programs.
Author Contributions
FL drafted the paper. CV, MT, and MB provided critical revisions. All authors approved the final version of the paper prior to submission.
Funding
FL was supported by a grant from the Wilhelmina Children's Hospital (D-17-010007). The content is the sole responsibility of the authors and does not necessarily represent the official views of the funding agencies.
Conflict of Interest
The authors declare that the research was conducted in the absence of any commercial or financial relationships that could be construed as a potential conflict of interest.
References
1. Tau GZ, Peterson BS. Normal development of brain circuits. Neuropsychopharmacology. (2010) 35:147–68. doi: 10.1038/npp.2009.115
2. McEwen BS. Physiology and neurobiology of stress and adaptation: central role of the brain. Physiol Rev. (2007) 87:873–904. doi: 10.1152/physrev.00041.2006
3. Doom JR, Gunnar MR. Stress physiology and developmental psychopathology: past, present, and future. Dev Psychopathol. (2013) 25(4 Part 2):1359–73. doi: 10.1017/S0954579413000667
4. McEwen BS. Allostasis and allostatic load: implications for neuropsychopharmacology. Neuropsychopharmacology. (2000) 22:108–24. doi: 10.1016/S0893-133X(99)00129-3
5. de Waal CG, Weisglas-Kuperus N, van Goudoever JB, Walther FJ. Mortality, neonatal morbidity and two year follow-up of extremely preterm infants born in the netherlands in 2007. PLoS ONE. (2012) 7:e41302. doi: 10.1371/journal.pone.0041302
6. Wilson-Costello D, Friedman H, Minich N, Siner B, Taylor G, Schluchter M, et al. Improved neurodevelopmental outcomes for extremely low birth weight infants in 2000-2002. Pediatrics. (2007) 119:37–45. doi: 10.1542/peds.2006-1416
7. Aarnoudse-Moens CSH, Weisglas-Kuperus N, Van Goudoever JB, Oosterlaan J. Meta-analysis of neurobehavioral outcomes in very preterm and/or very low birth weight children. Pediatrics. (2009) 124:717–28. doi: 10.1542/peds.2008-2816
8. Anderson PJ. Neuropsychological outcomes of children born very preterm. Semin Fetal Neonatal Med. (2014) 19:90–6. doi: 10.1016/j.siny.2013.11.012
9. Burnett AC, Anderson PJ, Cheong J, Doyle LW, Davey CG, Wood SJ. Prevalence of psychiatric diagnoses in preterm and full-term children, adolescents and young adults: a meta-analysis. Psychol Med. (2011) 41:2463–74. doi: 10.1017/S003329171100081X
10. Burnett AC, Anderson PJ, Lee KJ, Roberts G, Doyle LW, Cheong JLY, et al. Trends in executive functioning in extremely preterm children across 3 birth eras. Pediatrics. (2018) 141:1–10. doi: 10.1542/peds.2017-1958
11. Johnson S, Marlow N. Preterm birth and childhood psychiatric disorders. Pediatric Res. (2011) 69(5 Part 2):22–8. doi: 10.1203/PDR.0b013e318212faa0
12. Maleab SN, Stonestreet BS. Steroids and injury to the developing brain: net harm or net benefit? Shadi Clin Perinatol. (2014) 31:191–208. doi: 10.1016/j.clp.2013.09.006
13. Goldenberg RL, Culhane JF, Iams JD, Romero R. Epidemiology and causes of preterm birth. Lancet. (2008) 371:75–84. doi: 10.1016/S0140-6736(08)60074-4
14. Shaw JC, Berry MJ, Dyson RM, Crombie GK, Hirst JJ, Palliser HK. Reduced neurosteroid exposure following preterm birth and its' contribution to neurological impairment: a novel avenue for preventative therapies. Front Physiol. (2019) 10:599. doi: 10.3389/fphys.2019.00599
15. Hatfield L. Neonatal pain: what′s age got to do with it? Surg Neurol Int. (2014) 5:479. doi: 10.4103/2152-7806.144630
16. Anand KJS. Clinical importance of pain and stress in preterm neonates. Biol Neonate. (1998) 73:1–9. doi: 10.1159/000013953
17. Fitzgerald M, Walker SM. Infant pain management: a developmental neurobiological approach. Nat Clin Pract Neurol. (2009) 5:35–50. doi: 10.1038/ncpneuro0984
18. Anand KJS. Pain assessment in preterm neonates. Pediatrics. (2007) 119:605–7. doi: 10.1542/peds.2006-2723
19. American Academy of Pediatrics/American Pain Society [APP/APS. The assessment and management of acute pain in infants, children, and adolescents. Pediatrics. (2001) 108:793–7. doi: 10.1542/peds.108.3.793
20. Puchalski M, Hummel P. The reality of neonatal pain. Adv Neonatal Care. (2002) 2:233–44. doi: 10.1016/S1536-0903(02)80059-5
21. Darcy AE, Hancock LE, Ware EJ. A descriptive study of noise in the neonatal intensive care unit: ambient levels and perceptions of contributing factors. Adv Neonatal Care. (2008) 8:165–75. doi: 10.1097/01.ANC.0000324341.24841.6e
22. Williams AL, van Drongelen W, Lasky RE. Noise in contemporary neonatal intensive care. J Acoust Soc Am. (2007) 121:2681–90. doi: 10.1121/1.2717500
23. Wachman EM, Lahav A. The effects of noise on preterm infants in the NICU. Arch Dis Childhood. (2011) 96:305–9. doi: 10.1136/adc.2009.182014
24. van Veenendaal NR, van Kempen AAMW, Franck LS, O'Brien K, Limpens J, van der Lee JH, et al. Hospitalising preterm infants in single family rooms versus open bay units: a systematic review and meta-analysis of impact on parents. EClinicalMedicine. (2020) 23:100388. doi: 10.1016/j.eclinm.2020.100388
25. Newnham CA, Inder TE, Milgrom J. Measuring preterm cumulative stressors within the NICU: the neonatal infant stressor scale. Early Hum Dev. (2009) 85:549–55. doi: 10.1016/j.earlhumdev.2009.05.002
26. Mörelius E, Hellström-Westas L, Carlén C, Norman E, Nelson N. Is a nappy change stressful to neonates? Early Hum Dev. (2006) 82:669–76. doi: 10.1016/j.earlhumdev.2005.12.013
27. Peng NH, Bachman J, Jenkins R, Chen C-H, Chang Y-C, Chang Y-S, et al. Relationships between environmental stressors and stress biobehavioral responses of preterm infants in NICU. J Perinat Neonatal Nurs. (2009) 23:363–71. doi: 10.1097/JPN.0b013e3181bdd3fd
28. Meaney MJ, Szyf M. Maternal care as a model for experience-dependent chromatin plasticity? Trends Neurosci. (2005) 28:456–63. doi: 10.1016/j.tins.2005.07.006
29. Nyström K, Axelsson K. Mothers' experience of being separated from their newborns. J Obstetric Gynecol Neonatal Nurs. (2002) 31:275–82. doi: 10.1111/j.1552-6909.2002.tb00049.x
30. Pennestri MH, Gaudreau H, Bouvette-Turcot AA, Moss E, Lecompte V, Atkinson L, et al. Attachment disorganization among children in neonatal intensive care unit: preliminary results. Early Hum Dev. (2015) 91:601–6. doi: 10.1016/j.earlhumdev.2015.07.005
31. Feldman R, Weller A, Leckman JF, Kuint J, Eidelman AI. The nature of the mother's tie to her infant: maternal bonding under conditions of proximity, separation, and potential loss. J Child Psychol Psychiatry Allied Disciplines. (1999) 40:929–39. doi: 10.1017/S0021963099004308
32. Eckerman CO, Oehler JM, Hannan TE, Molitor A. The development prior to term age of very prematurely born newborns' responsiveness in en face exchanges. Infant Behav Dev. (1995) 18:283–97. doi: 10.1016/0163-6383(95)90017-9
33. Hughes MB, Shults J, McGrath J, Medoff-Cooper B. Temperament characteristics of premature infants in the first year of life. J Dev Behav Pediatr. (2002) 23:430–5. doi: 10.1097/00004703-200212000-00006
34. Landry SH, Garner PW, Swank PR, Baldwin CD. Effects of maternal scaffolding during joint toy play with preterm and full?term infants. Merrill-Palmer Q. (1996) 42:177–99.
35. Korja R, Latva R, Lehtonen L. The effects of preterm birth on mother-infant interaction and attachment during the infant's first two years. Acta Obstetr Gynecol Scand. (2012) 91:164–73. doi: 10.1111/j.1600-0412.2011.01304.x
36. Davis DH, Thoman EB. The early social environment of premature and fullterm infants. Early Hum Dev. (1988) 17:221–32. doi: 10.1016/0378-3782(88)90010-2
37. Buss C, Entringer S, Wadhwa PD. Fetal programming of brain development: intrauterine stress and susceptibility to psychopathology. Sci Signal. (2012) 5:1–8. doi: 10.1126/scisignal.2003406
38. Ouyang M, Dubois J, Yu Q, Mukherjee P, Huang H. Delineation of early brain development from fetuses to infants with diffusion MRI and beyond. NeuroImage. (2019) 185:836–50. doi: 10.1016/j.neuroimage.2018.04.017
39. Joëls M, Baram TZ. The neuro-symphony of stress. Nat Rev Neurosci. (2009) 10:459–66. doi: 10.1038/nrn2632
40. McCorry LK. Physiology of the autonomic nervous system. Am J Pharm Educ. (2007) 71:1–11. doi: 10.5688/aj710478
41. Fyfe KL, Yiallourou SR, Wong FY, Odoi A, Walker AM, Horne RSC. The effect of gestational age at birth on post-term maturation of heart rate variability. Sleep. (2015) 38:1635–44. doi: 10.5665/sleep.5064
42. Field T, Diego M. Vagal activity, early growth and emotional development. Infant Behav Dev. (2008) 31:361–73. doi: 10.1016/j.infbeh.2007.12.008
43. Cheng G, Zhu H, Zhou X, Qu J, Ashwell KWS, Paxinos G. Development of the human dorsal nucleus of the vagus. Early Hum Dev. (2008) 84:15–27. doi: 10.1016/j.earlhumdev.2007.01.012
44. Cheng Z, Zhang H, Guo SZ, Wurster R, Gozal D. Differential control over postganglionic neurons in rat cardiac ganglia by NA and DmnX neurons: anatomical evidence. Am J Physiol Regul Integr Comp Physiol. (2004) 286:625–33. doi: 10.1152/ajpregu.00143.2003
45. Porges SW. Cardiac vagal tone: a physiological index of stress. Neurosci Biobehav Rev. (1995) 19:225–33. doi: 10.1016/0149-7634(94)00066-A
46. Brown JW. Prenatal development of the human nucleus ambiguous during theembryonic and early fetal periods. Am J Anatomy. (1990) 189:267–83. doi: 10.1002/aja.1001890310
47. Porges SW, Furman SA. The early development of the autonomic nervous system provides a neural platform for social behavior: a polyvagal perspective. Infant Child Dev. (2011) 20:106–18. doi: 10.1002/icd.688
48. Sachis PN, Armstrong DL, Becker LE, Bryan AC. Myelination of the human vagus nerve from 24 weeks postconceptional age to adolescence. J Neuropathol Exp Neurol. (1982) 41:466–72. doi: 10.1097/00005072-198207000-00009
49. Schneider J, Duerden EG, Guo T, Ng K, Hagmann P, Bickle Graz M, et al. Procedural pain and oral glucose in preterm neonates: brain development and sex-specific effects. Pain. (2018) 159:515–25. doi: 10.1097/j.pain.0000000000001123
50. Longin E, Gerstner T, Schaible T, Lenz T, König S. Maturation of the autonomic nervous system: differences in heart rate variability in premature vs. term infants. J Perinatal Med. (2006) 34:303–8. doi: 10.1515/JPM.2006.058
51. Schneider U, Bode F, Schmidt A, Nowack S, Rudolph A, Dölker EM, et al. Correction: developmental milestones of the autonomic nervous system revealed via longitudinal monitoring of fetal heart rate variability. PLoS ONE. (2018) 13:e0200799. doi: 10.1371/journal.pone.0200799
52. Kintraia PI, Zarnadze MG, Kintraia NP, Kashakashvili IG. Development of daily rhythmicity in heart rate and locomotor activity in the human fetus. J Circadian Rhythms. (2005) 3:1–12. doi: 10.1186/1740-3391-3-5
53. Crowder RE. The development of the adrenal gland in man, with special reference to origin and ultimate location of cell types and evidence in favor of the “cell migration” theory. Contrib Embryol. (1957) 36:193–210.
54. Koutcherov Y, Mai JK, Ashwell KWS, Paxinos G. Organization of human hypothalamus in fetal development. J Compar Neurol. (2002) 446:301–24. doi: 10.1002/cne.10175
55. Koutcherov Y, Mai JK, Paxinos G. Hypothalamus of the human fetus. J Chem Neuroanatomy. (2003) 26:253–70. doi: 10.1016/j.jchemneu.2003.07.002
56. Nader N, Chrousos GP, Kino T. Interactions of the circadian CLOCK system and the HPA axis nancy. Trends Endocrinol Metab. (2010) 21:277–86. doi: 10.1016/j.tem.2009.12.011
57. Leon-Mercado L, Chao DHM, Basualdo MdC, Kawata M, Escobar C, Buijs RM. The arcuate nucleus: a site of fast negative feedback for corticosterone secretion in male rats. ENeuro. (2017) 4:1–14. doi: 10.1523/ENEURO.0350-16.2017
58. Evanson NK, Herman JP. Role of paraventricular nucleus glutamate signaling in regulation of HPA axis stress responses. Interdiscipl Information Sci. (2015) 21:253–60. doi: 10.4036/iis.2015.B.10
59. Foyouzi N, Frisbæk Y, Norwitz ER. Pituitary gland and pregnancy. Obstetr Gynecol Clin N Am. (2004) 31:873–92. doi: 10.1016/j.ogc.2004.08.003
60. Ikeda H, Suzuki J, Sassano N, Nilzuma H. The development and morphogenesis of the human pituitary gland. Anat Embryol. (1988) 178:327–36. doi: 10.1007/BF00698663
61. Castillo M. Pituitary gland: development, normal appearances, and magnetic resonance imaging protocols. Top Magnetic Resonance Imaging. (2005) 16:259–68. doi: 10.1097/01.rmr.0000224682.91253.15
62. Mastorakos G, Ilias I. Maternal and fetal hypothalamic-pituitary-adrenal axes during pregnancy and postpartum. Ann NY Acad Sci. (2003) 997:136–49. doi: 10.1196/annals.1290.016
63. Narasaka T, Suzuki T, Moriya T, Sasano H. Temporal and spatial distribution of Corticosteroidogenic Enzymes Immunoreactivity in developing human adrenal. Mol Cell Endocrinol. (2001) 174:111–20. doi: 10.1016/S0303-7207(00)00445-7
64. Sucheston ME, Cannon MS. Development of zonular patterns in the human adrenal gland. J Morphol. (1968) 126:477–91. doi: 10.1002/jmor.1051260408
65. Ishimoto H, Jaffe RB. Development and function of the human fetal adrenal cortex: A key component in the feto-placental unit. Endocr Rev. (2011) 32:317–55. doi: 10.1210/er.2010-0001
66. Mesiano S, Jaffe RB. Developmental and functional biology of the primate fetal adrenal cortex. Endocrine Rev. (1997) 18:378–403. doi: 10.1210/edrv.18.3.0304
67. Alves PN, Foulon C, Karolis V, Bzdok D, Margulies DS, Volle E, et al. An improved neuroanatomical model of the default-mode network reconciles previous neuroimaging and neuropathological findings. Commun Biol. (2019) 2:370. doi: 10.1038/s42003-019-0611-3
68. Seeley WW, Menon V, Schatzberg AF, Keller J, Glover GH, Kenna H, et al. Dissociable intrinsic connectivity networks for salience processing and executive control. J Neurosci. (2007) 27:2349–56. doi: 10.1523/JNEUROSCI.5587-06.2007
69. Menon V. Large-scale brain networks and psychopathology: a unifying triple network model. Trends Cogn Sci. (2011) 15:483–506. doi: 10.1016/j.tics.2011.08.003
70. Hermans EJ, Henckens MJAG, Joels M, Fernandez G. Dynamic adaptation of large-scale brain networks in response to acute stressors. Trends Neurosci. (2014) 37:304–14. doi: 10.1016/j.tins.2014.03.006
71. Herman JP, McKlveen JM, Ghosal S, Kopp B, Wulsin A, Makinson R, et al. Regulation of the hypothalamic-pituitary- adrenocortical stress response. Comprehens Physiol. (2016) 6:603–21. doi: 10.1002/cphy.c150015
72. Arnold SE, Trojanowski JQ. Human fetal hippocampal development: I. Cytoarchitecture, myeloarchitecture, and neuronal morphologic features. J Compar Neurol. (1996) 367:274–92. doi: 10.1002/(SICI)1096-9861(19960401)367:2<274::AID-CNE9>3.0.CO;2-2
73. Kier EL, Kim JH, Fulbright RK, Bronen RA. Embryology of the human fetal hippocampus: Mr imaging, anatomy, and histology. Am J Neuroradiol. (1997) 18:525–32.
74. Ge X, Shi Y, Li J, Zhang Z, Lin X, Zhan J, et al. Development of the human fetal hippocampal formation during early second trimester. NeuroImage. (2015) 119:33–43. doi: 10.1016/j.neuroimage.2015.06.055
75. Hevner RF, Kinney HC. Reciprocal entorhinal-hippocampal connections established by human fetal midgestation. J Compar Neurol. (1996) 372:384–94. doi: 10.1002/(SICI)1096-9861(19960826)372:3<384::AID-CNE4>3.0.CO;2-Z
76. Thomason ME, Brown JA, Dassanayake MT, Shastri R, Marusak HA, Hernandez-Andrade E, et al. Intrinsic functional brain architecture derived from graph theoretical analysis in the human fetus. PLoS ONE. (2014) 9:e94423. doi: 10.1371/journal.pone.0094423
77. Thomason ME, Grove LE, Lozon TA, Vila AM, Ye Y, Nye MJ, et al. Age-related increases in long-range connectivity in fetal functional neural connectivity networks in utero. Dev Cogn Neurosci. (2015) 11:96–104. doi: 10.1016/j.dcn.2014.09.001
78. Gao W, Zhu H, Giovanello KS, Smith JK, Shen D, Gilmore JH, et al. Evidence on the emergence of the brain's default network from 2-week-old to 2-year-old healthy pediatric subjects. Proc Natl Acad Sci USA. (2009) 106:6790–5. doi: 10.1073/pnas.0811221106
79. Gao W, Lin W, Grewen K, Gilmore JH. Functional connectivity of the infant human brain: plastic and modifiable. Neuroscientist. (2017) 23:169–84. doi: 10.1177/1073858416635986
80. Gao W, Alcauter S, Elton A, Hernandez-Castillo CR, Smith JK, Ramirez J, et al. Functional network development during the first year: relative sequence and socioeconomic correlations. Cerebral Cortex. (2015) 25:2919–28. doi: 10.1093/cercor/bhu088
81. Nikolić I, Kostović I. Development of the lateral amygdaloid nucleus in the human fetus: transient presence of discrete cytoarchitectonic units. Anatomy Embryol. (1986) 174:355–60. doi: 10.1007/BF00698785
82. Humphrey T. The development of the human amygdala during early embryonic life. J Compar Neurol. (1868) 132:135–65. doi: 10.1002/cne.901320108
83. Ulfig N, Setzer M, Bohl J. Ontogeny of the human Amygdala. Ann NY Acad Sci. (2003) 985:22–33. doi: 10.1111/j.1749-6632.2003.tb07068.x
84. Huang H, Xue R, Zhang J, Ren T, Richards LJ, Yarowsky P, et al. Anatomical characterization of human fetal brain development with diffusion tensor magnetic resonance imaging. J Neurosci. (2009) 29:4263–73. doi: 10.1523/JNEUROSCI.2769-08.2009
85. Vasung L, Huang H, Jovanov-Milošević N, Pletikos M, Mori S, Kostović I. Development of axonal pathways in the human fetal fronto-limbic brain: histochemical characterization and diffusion tensor imaging. J Anatomy. (2010) 217:400–17. doi: 10.1111/j.1469-7580.2010.01260.x
86. Von Der Heide RJ, Skipper LM, Klobusicky E, Olson IR. Dissecting the uncinate fasciculus: disorders, controversies and a hypothesis. Brain. (2013) 136:1692–707. doi: 10.1093/brain/awt094
87. Takahashi E, Folkerth RD, Galaburda AM, Grant PE. Emerging cerebral connectivity in the human fetal brain: an MR tractography study. Cerebral Cortex. (2012) 22:455–64. doi: 10.1093/cercor/bhr126
88. Jakab A, Schwartz E, Kasprian G, Gruber GM, Prayer D, Schöpf V, et al. Fetal functional imaging portrays heterogeneous development of emerging human brain networks. Front Hum Neurosci. (2014) 8:852. doi: 10.3389/fnhum.2014.00852
89. Hsu DT, Kirouac GJ, Zubieta JK, Bhatnagar S. Contributions of the paraventricular thalamic nucleus in the regulation of stress, motivation, and mood. Front Behav Neurosci. (2014) 8:73. doi: 10.3389/fnbeh.2014.00073
90. Mojsilović J, Zečević N. Early development of the human thalamus: Golgi and Nissl study. Early Hum Dev. (1991) 27:119–44. doi: 10.1016/0378-3782(91)90033-Y
91. Wilkinson M, Kane T, Wang R, Takahashi E. Migration pathways of thalamic neurons and development of thalamocortical connections in humans revealed by diffusion MR tractography. Cerebral Cortex. (2017) 27:5683–95. doi: 10.1093/cercor/bhw339
92. Uddin LQ. Salience processing and insular cortical function and dysfunction. Nat Rev Neurosci. (2015) 16:55–61. doi: 10.1038/nrn3857
93. Afif A, Bouvier R, Buenerd A, Trouillas J, Mertens P. Development of the human fetal insular cortex: study of the gyration from 13 to 28 gestational weeks. Brain Struct Funct. (2007) 212:335–46. doi: 10.1007/s00429-007-0161-1
94. Das A, Takahashi E. Neuronal migration and axonal pathways linked to human fetal insular development revealed by diffusion MR tractography. Cerebral Cortex. (2018) 28:3555–63. doi: 10.1093/cercor/bhx224
95. Alcauter S, Lin W, Keith Smith J, Gilmore JH, Gao W. Consistent anterior-posterior segregation of the insula during the first 2 years of life. Cerebral Cortex. (2015) 25:1176–87. doi: 10.1093/cercor/bht312
96. Gao W, Alcauter S, Smith JK, Gilmore JH, Lin W. Development of human brain cortical network architecture during infancy. Brain Struct Funct. (2015) 220:1173–86. doi: 10.1007/s00429-014-0710-3
97. van den Heuvel MI, Turk E, Manning JH, Hect J, Hernandez-Andrade E, Hassan SS, et al. Hubs in the human fetal brain network. Dev Cogn Neurosci. (2018) 30:108–15. doi: 10.1016/j.dcn.2018.02.001
98. Turk E, van den Heuvel MI, Benders MJ, de Heus R, Franx A, Manning JH, et al. Functional connectome of the fetal brain. J Neurosci. (2019) 289:1–18. doi: 10.1523/JNEUROSCI.2891-18.2019
99. Rajagopalan V, Scott J, Habas PA, Kim K, Corbett-Detig J, Rousseau F, et al. Local tissue growth patterns underlying normal fetal human brain gyrification quantified in utero. J Neurosci. (2011) 31:2878–87. doi: 10.1523/JNEUROSCI.5458-10.2011
100. Trivedi R, Gupta RK, Husain N, Rathore RKS, Saksena S, Srivastava S, et al. Region-specific maturation of cerebral cortex in human fetal brain: diffusion tensor imaging and histology. Neuroradiology. (2009) 51:567–76. doi: 10.1007/s00234-009-0533-8
101. Huang H, Zhang J, Wakana S, Zhang W, Ren T, Richards LJ, et al. White and gray matter development in human fetal, newborn and pediatric brains. NeuroImage. (2006) 33:27–38. doi: 10.1016/j.neuroimage.2006.06.009
102. Khan S, Vasung L, Marami B, Rollins CK, Afacan O, Ortinau CM, et al. Fetal brain growth portrayed by a spatiotemporal diffusion tensor MRI atlas computed from in utero images. NeuroImage. (2019) 185:593–608. doi: 10.1016/j.neuroimage.2018.08.030
103. Huang H, Vasung L. Gaining insight of fetal brain development with diffusion MRI and histology. Int J Dev Neurosci. (2014) 32:11–22. doi: 10.1016/j.ijdevneu.2013.06.005
104. Meyer G, Schaaps JP, Moreau L, Goffinet AM. Embryonic and early fetal development of the human neocortex. J Neurosci. (2000) 20:1858–68. doi: 10.1523/JNEUROSCI.20-05-01858.2000
105. Chi JG, Dooling EC, Gilles FH. Gyral development of the human brain. Ann Neurol. (1977) 1:86–93. doi: 10.1002/ana.410010109
106. Garel C, Chantrel E, Elmaleh M, Brisse H, Sebag G. Fetal MRI: normal gestational landmarks for cerebral biometry, gyration and myelination. Child Nervous System. (2003) 19:422–5. doi: 10.1007/s00381-003-0767-4
107. Garel C, Chantrel E, Brisse H, Elmaleh M, Luton D, Oury JF, et al. Fetal cerebral cortex: normal gestational landmarks identified using prenatal MR imaging. Am J Neuroradiol. (2001) 22:184–9.
108. Kostovic I, Vasung L. Insights from in vitro fetal magnetic resonance imaging of cerebral development. Semin Perinatol. (2009) 33:220–33. doi: 10.1053/j.semperi.2009.04.003
109. Habas PA, Scott JA, Roosta A, Rajagopalan V, Kim K, Rousseau F, et al. Early folding patterns and asymmetries of the normal human brain detected from in utero MRI. Cerebral Cortex. (2012) 22:13–25. doi: 10.1093/cercor/bhr053
110. Nishikuni K, Ribas GC. Study of fetal and postnatal morphological development of the brain sulci: laboratory investigation. J Neurosur Pediatrics. (2013) 11:1–11. doi: 10.3171/2012.9.PEDS12122
111. Zhang Z, Hou Z, Lin X, Teng G, Meng H, Zang F, et al. Development of the fetal cerebral cortex in the second trimester: assessment with 7T postmortem MR imaging. Am J Neuroradiol. (2013) 34:1462–7. doi: 10.3174/ajnr.A3406
112. Clouchoux C, Kudelski D, Gholipour A, Warfield SK, Viseur S, Bouyssi-Kobar M, et al. Quantitative in vivo MRI measurement of cortical development in the fetus. Brain Struct Funct. (2012) 217:127–39. doi: 10.1007/s00429-011-0325-x
113. Ader R, Grota LJ. Effects of early experience on adrenocortical reactivity. Physiol Behav. (1969) 4:303–5. doi: 10.1016/0031-9384(69)90179-6
114. Levine S. Infantile experience and resistance to physiological stress. Science. (1957) 126:405–6. doi: 10.1126/science.126.3270.405
115. Levine S. Plasma-free corticosteroid response to electric shock in rats stimulated in infancy. Science. (1962) 135:795–6. doi: 10.1126/science.135.3506.795-a
116. Peters KL. Neonatal stress reactivity and cortisol. J Perinatal Neonatal Nurs. (1998) 11:45–59. doi: 10.1097/00005237-199803000-00007
117. Plotsky PM, Meaney MJ. Early, postnatal experience alters hypothalamic corticotropin-releasing factor (CRF) mRNA, median eminence CRF content and stress-induced release in adult rats. Mol Brain Res. (1993) 18:195–200. doi: 10.1016/0169-328X(93)90189-V
118. Nishi M, Horii-Hayashi N, Sasagawa T. Effects of early life adverse experiences on the brain: implications from maternal separation models in rodents. Front Neurosci. (2014) 8:166. doi: 10.3389/fnins.2014.00166
119. Smith GC, Gutovich J, Smyser C, Pineda R, Newnham C, Tjoeng TH, et al. Neonatal intensive care unit stress is associated with brain development in preterm infants. Ann Neurol. (2011) 70:541–9. doi: 10.1002/ana.22545
120. Brummelte S, Grunau RE, Chau V, Poskitt KJ, Brant R, Vinall J, et al. Procedural pain and brain development in premature newborns. Ann Neurol. (2012) 71:385–96. doi: 10.1002/ana.22267
121. Duerden EG, Grunau RE, Guo T, Foong J, Pearson A, Au-Young S, et al. Early procedural pain is associated with regionally-specific alterations in thalamic development in preterm neonates. J Neurosci. (2018) 38:878–86. doi: 10.1523/JNEUROSCI.0867-17.2017
122. Tortora D, Severino M, Di Biase C, Malova M, Parodi A, Minghetti D, et al. Early pain exposure influences functional brain connectivity in very preterm neonates. Front Neurosci. (2019) 13:899. doi: 10.3389/fnins.2019.00899
123. Duerden EG, Guo T, Dodbiba L, Chakravarty MM, Chau V, Poskitt KJ, et al. Midazolam dose correlates with abnormal hippocampal growth and neurodevelopmental outcome in preterm infants. Ann Neurol. (2016) 79:548–59. doi: 10.1002/ana.24601
124. Vinall J, Miller SP, Bjornson BH, Fitzpatrick KPV, Poskitt KJ, Brant R, et al. Invasive procedures in preterm children: brain and cognitive development at school age. Pediatrics. (2014) 133:412–21. doi: 10.1542/peds.2013-1863
125. Chau CMY, Ranger M, Bichin M, Park MTM, Amaral RSC, Chakravarty M, et al. Hippocampus, amygdala, and thalamus volumes in very preterm children at 8 years: neonatal pain and genetic variation. Front Behav Neurosci. (2019) 13:51. doi: 10.3389/fnbeh.2019.00051
126. Ranger M, Zwicker JG, Chau CMY, Park MTM, Chakravarthy MM, Poskitt K, et al. Neonatal pain and infection relate to smaller cerebellum in very preterm children at school age. J Pediatrics. (2015) 167:292–8.e1. doi: 10.1016/j.jpeds.2015.04.055
127. Ranger M, Chau CMY, Garg A, Woodward TS, Beg MF, Bjornson B, et al. Neonatal pain-related stress predicts cortical thickness at age 7 years in children born very preterm. PLoS ONE. (2013) 8:e76702. doi: 10.1371/journal.pone.0076702
128. Doesburg SM, Chau CM, Cheung TPL, Moiseev A, Ribary U, Herdman AT, et al. Neonatal pain-related stress, functional cortical activity and visual-perceptual abilities in school-age children born at extremely low gestational age. Pain. (2013) 154:1946–52. doi: 10.1016/j.pain.2013.04.009
129. Kozhemiako N, Nunes A, Vakorin VA, Chau CMY, Moiseev A, Ribary U, et al. Atypical resting state neuromagnetic connectivity and spectral power in very preterm children. J Child Psychol Psychiatry. (2019) 60:975–87. doi: 10.1111/jcpp.13026
130. Goffaux P, Lafrenaye S, Morin M, Patural H, Demers G, Marchand S. Preterm births: can neonatal pain alter the development of endogenous gating systems? Eur J Pain. (2008) 12:945–51. doi: 10.1016/j.ejpain.2008.01.003
131. Grunau RE, Oberlander TF, Whitfield MF, Fitzgerald C, Lee SK, Background A. Low birth weight neonates at 32 weeks' postconceptional age. Pediatrics. (2001) 107:105–12. doi: 10.1542/peds.107.1.105
132. Brummeltje S, Chau CMY, Cepeda IL, Degenhardt A, Weinberg J, Synnes AR, et al. Cortisol levels in former preterm children at school age are predicted by neonatal procedural pain-related stress. Psychoneuroendocrinology. (2015) 51:151–63. doi: 10.1016/j.psyneuen.2014.09.018
133. Grunau RE, Weinberg J, Whitfield MF. Neonatal procedural pain and preterm infant cortisol response to novelty at 8 months. Pediatrics. (2004) 114:1–16. doi: 10.1542/peds.114.1.e77
134. Grunau RE, Cepeda IL, Chau CMY, Brummelte S, Weinberg J, Lavoie PM, et al. Neonatal pain-related stress and NFKBIA genotype are associated with altered cortisol levels in Preterm boys at school age. PLoS ONE. (2013) 8:e73926. doi: 10.1371/journal.pone.0073926
135. Provenzi L, Giusti L, Fumagalli M, Tasca H, Ciceri F, Menozzi G, et al. Pain-related stress in the Neonatal Intensive Care Unit and salivary cortisol reactivity to socio-emotional stress in 3-month-old very preterm infants. Psychoneuroendocrinology. (2016) 72:161–5. doi: 10.1016/j.psyneuen.2016.07.010
136. Chau CMY, Ranger M, Sulistyoningrum D, Devlin AM, Obertander TF, Grunau RE. Neonatal pain and comt Val158Met genotype in relation to serotonin transporter (SLC6A4) promoter methylation in very preterm children at school age. Front Behav Neurosci. (2014) 8:409. doi: 10.3389/fnbeh.2014.00409
137. Fumagalli M, Provenzi L, De Carli P, Dessimone F, Sirgiovanni I, Giorda R, et al. From early stress to 12-month development in very preterm infants: preliminary findings on epigenetic mechanisms and brain growth. PLoS ONE. (2018) 13:e0190602. doi: 10.1371/journal.pone.0190602
138. Montirosso R, Provenzi L. Implications of epigenetics and stress regulation on research and developmental care of preterm infants. J Obstetric Gynecol Neonatal Nurs. (2015) 44:174–82. doi: 10.1111/1552-6909.12559
139. Provenzi L, Fumagalli M, Sirgiovanni I, Giorda R, Pozzoli U, Morandi F, et al. Pain-related stress during the neonatal intensive care unit stay and SLC6A4 methylation in very preterm infants. Front Behav Neurosci. (2015) 9:99. doi: 10.3389/fnbeh.2015.00099
140. Buss C, Davis EP, Muftuler TL, Head K, Sanman CA. High pregnancy anxiety during mid-gestation is associated with decreased gray matter density in 6-9 year-old children. Psychoneuroendocrinology. (2010) 35:141–53. doi: 10.1016/j.psyneuen.2009.07.010
141. Dean DC, Planalp EM, Wooten W, Kecskemeti SR, Adluru N, Schmidt CK, et al. Association of prenatal maternal depression and anxiety symptoms with infant white matter microstructure. JAMA Pediatrics. (2018) 172:973–81. doi: 10.1001/jamapediatrics.2018.2132
142. El Marroun H, Zou R, Muetzel RL, Jaddoe VW, Verhulst FC, White T, et al. Prenatal exposure to maternal and paternal depressive symptoms and white matter microstructure in children. Depression Anxiety. (2018) 35:321–9. doi: 10.1002/da.22722
143. Rifkin-Graboi A, Bai J, Chen H, Hameed WBR, Sim LW, Tint MT, et al. Prenatal maternal depression associates with microstructure of right amygdala in neonates at birth. Biol Psychiatry. (2013) 74:837–44. doi: 10.1016/j.biopsych.2013.06.019
144. Lautarescu A, Pecheva D, Nosarti C, Nihouarn J, Zhang H, Victor S, et al. Maternal prenatal stress is associated with altered uncinate fasciculus microstructure in premature neonates. Biol Psychiatry. (2020) 87:559–69. doi: 10.1016/j.biopsych.2019.08.010
145. Posner J, Cha J, Roy AK, Peterson BS, Bansal R, Gustafsson HC, et al. Alterations in amygdala-prefrontal circuits in infants exposed to prenatal maternal depression. Trans Psychiatry. (2016) 6:4–11. doi: 10.1038/tp.2016.146
146. Scheinost D, Kwon SH, Lacadie C, Sze G, Sinha R, Constable RT, et al. Prenatal stress alters amygdala functional connectivity in preterm neonates. NeuroImage Clin. (2016) 12:381–8. doi: 10.1016/j.nicl.2016.08.010
147. Sandman CA, Davis EP, Buss C, Glynn LM. Prenatal programming of human neurological function. Int J Peptides. (2011) 2011. doi: 10.1155/2011/837596
148. Scheinost D, Sinha R, Cross SN, Kwon SH, Sze G, Constable RT, et al. Does prenatal stress alter the developing connectome? Pediatric Res. (2017) 81:214–26. doi: 10.1038/pr.2016.197
149. Suri R, Lin AS, Cohen LS, Altshuler LL. Acute and long-term behavioral outcome of infants and children exposed in utero to either maternal depression or antidepressants: a review of the literature. J Clin Psychiatry. (2014) 75:e1142–52. doi: 10.4088/JCP.13r08926
150. Clark CAC, Woodward LJ, Horwood LJ, Moor S. Development of emotional and behavioral regulation in children born extremely preterm and very preterm: biological and social influences. Child Dev. (2008) 79:1444–62. doi: 10.1111/j.1467-8624.2008.01198.x
151. Fischi-Gómez E, Vasung L, Meskaldji DE, Lazeyras F, Borradori-Tolsa C, Hagmann P, et al. Structural brain connectivity in school-age preterm infants provides evidence for impaired networks relevant for higher order cognitive skills and social cognition. Cerebral Cortex. (2015) 25:2793–805. doi: 10.1093/cercor/bhu073
152. Healy E, Reichenberg A, Nam KW, Allin MPG, Walshe M, Rifkin L, et al. Preterm birth and adolescent social functioning-alterations in emotion-processing brain areas. J Pediatrics. (2013) 163:1596–604. doi: 10.1016/j.jpeds.2013.08.011
153. Loe IM, Lee ES, Feldman HM. Attention and internalizing behaviors in relation to white matter in children born preterm. J Dev Behav Pediatrics. (2013) 34:156–64. doi: 10.1097/DBP.0b013e3182842122
154. Rogers CE, Anderson PJ, Thompson DK, Kidokoro H, Wallendorf M, Treyvaud K, et al. Regional cerebral development at term relates to school-age social-emotional development in very preterm children. J Am Acad Child Adolesc Psychiatry. (2012) 51:181–91. doi: 10.1016/j.jaac.2011.11.009
155. Zubiaurre-Elorza L, Soria-Pastor S, Junque C, Sala-Llonch R, Segarra D, Bargallo N, et al. Cortical thickness and behavior abnormalities in children born preterm. PLoS ONE. (2012) 7:e42148. doi: 10.1371/journal.pone.0042148
156. Gordan R, Gwathmey JK, Xie L-H. Autonomic and endocrine control of cardiovascular function. World J Cardiol. (2015) 7:204. doi: 10.4330/wjc.v7.i4.204
157. Mulkey SB, Kota S, Swisher CB, Hitchings L, Metzler M, Wang Y, et al. Autonomic nervous system depression at term in neurologically normal premature infants. Early Hum Dev. (2018) 123:11–6. doi: 10.1016/j.earlhumdev.2018.07.003
158. Patural H, Pichot V, Jaziri F, Teyssier G, Gaspoz JM, Roche F, et al. Autonomic cardiac control of very preterm newborns: a prolonged dysfunction. Early Hum Dev. (2008) 84:681–7. doi: 10.1016/j.earlhumdev.2008.04.010
159. Johansson S, Norman M, Legnevall L, Dalmaz Y, Lagercrantz H, Vanpée M. Increased catecholamines and heart rate in children with low birth weight: perinatal contributions to sympathoadrenal overactivity. J Internal Med. (2007) 261:480–7. doi: 10.1111/j.1365-2796.2007.01776.x
160. Burtchen N, Myers MM, Lucchini M, Ordonez Retamar M, Rodriguez D, Fifer WP. Autonomic signatures of late preterm, early term, and full term neonates during early postnatal life. Early Hum Dev. (2019) 137. doi: 10.1016/j.earlhumdev.2019.06.012
161. Yiallourou SR, Witcombe NB, Sands SA, Walker AM, Horne RSC. The development of autonomic cardiovascular control is altered by preterm birth. Early Hum Dev. (2013) 89:145–52. doi: 10.1016/j.earlhumdev.2012.09.009
162. Rakow A, Katz-Salamon M, Ericson M, Edner A, Vanpée M. Decreased heart rate variability in children born with low birth weight. Pediatric Res. (2013) 74:339–43. doi: 10.1038/pr.2013.97
163. Urfer-Maurer N, Ludyga S, Stalder T, Brand S, Holsboer-Trachsler E, Gerber M, et al. Heart rate variability and salivary cortisol in very preterm children during school age. Psychoneuroendocrinology. (2018) 87:27–34. doi: 10.1016/j.psyneuen.2017.10.004
164. De Rogalski Landrot I, Roche F, Pichot V, Teyssier G, Gaspoz JM, Barthelemy JC, et al. Autonomic nervous system activity in premature and full-term infants from theoretical term to 7 years. Autonomic Neurosc Basic Clin. (2007) 136:105–9. doi: 10.1016/j.autneu.2007.04.008
165. Haraldsdottir K, Watson AM, Goss KN, Beshish AG, Pegelow DF, Palta M, et al. Impaired autonomic function in adolescents born preterm. Physiol Rep. (2018) 6:1–9. doi: 10.14814/phy2.13620
166. Mathewson KJ, Van Lieshout RJ, Saigal S, Morrison KM, Boyle MH, Schmidt LA. Autonomic functioning in young adults born at extremely low birth weight. Glob Pediatr Heal. (2015) 2:1–15. doi: 10.1177/2333794x15589560
167. Alvares GA, Quintana DS, Hickie IB, Guastella AJ. Autonomic nervous system dysfunction in psychiatric disorders and the impact of psychotropic medications: a systematic review and meta-analysis. J Psychiatry Neurosci. (2016) 41:89–104. doi: 10.1503/jpn.140217
168. Boyce WT, Quas J, Alkon A, Smider NA, Essex MJ, Kupfer DJ, et al. Autonomic reactivity and psychopathology in middle childhood. Br J Psychiatry. (2001) 179:144–50. doi: 10.1192/bjp.179.2.144
169. Svensson TH. Peripheral, autonomic regulation of locus coeruleus noradrenergic neurons in brain: putative implications for psychiatry and psychopharmacology. Psychopharmacology. (1987) 92:1–7. doi: 10.1007/BF00215471
170. Brooker RJ, Buss KA. Dynamic measures of RSA predict distress and regulation in toddlers. Dev Psychobiol. (2010) 52:372–82. doi: 10.1002/dev.20432
171. Hastings PD, Nuselovici JN, Utendale WT, Coutya J, McShane KE, Sullivan C. Applying the polyvagal theory to children's emotion regulation: social context, socialization, and adjustment. Biol Psychol. (2008) 79:299–306. doi: 10.1016/j.biopsycho.2008.07.005
172. Geisler FCM, Kubiak T, Siewert K, Weber H. Cardiac vagal tone is associated with social engagement and self-regulation. Biol Psychol. (2013) 93:279–86. doi: 10.1016/j.biopsycho.2013.02.013
173. Doussard-Roosevelt JA, Porges SW, Scanlon JW, Alemi B, Scanlon KB. Vagal regulation of heart rate in the prediction of developmental outcome for very low birth weight preterm infants. Child Dev. (1997) 68:173–86. doi: 10.2307/1131844
174. Porges SW. The polyvagal theory: phylogenetic substrates of a social nervous system. Int J Psychophysiol. (2001) 42:123–46. doi: 10.1016/S0167-8760(01)00162-3
175. Porges SW. Stress and parasympathetic control. Encycl Neurosci. (2009) 2:463–9. doi: 10.1016/B978-008045046-9.00099-1
176. Porges SW. The polyvagal theory: phylogenetic contributions to social behavior. In: Physiology and Behavior. Vol. 79. Elsevier Inc. (2003). p. 503–13. doi: 10.1016/S0031-9384(03)00156-2
177. Mörelius E, He HG, Shorey S. Salivary cortisol reactivity in preterm infants in neonatal intensive care: an integrative review. Int J Environ Res Public Health. (2016) 13:1–14. doi: 10.3390/ijerph13030337
178. Grunau RE, Haley DW, Whitfield MF, Weinberg J, Yu W, Thiessen P. Altered basal cortisol levels at 3 6, 8, and 18 months in infants born at extremely low gestational age. Methods. (2007) 150:151–6. doi: 10.1016/j.jpeds.2006.10.053
179. Fries E, Hesse J, Hellhammer J, Hellhammer DH. A new view on hypocortisolism. Psychoneuroendocrinology. (2005) 30:1010–6. doi: 10.1016/j.psyneuen.2005.04.006
180. Kaseva N, Wehkalampi K, Pyhälä R, Moltchanova E, Feldt K, Pesonen AK, et al. Blunted hypothalamic-pituitary-adrenal axis and insulin response to psychosocial stress in young adults born preterm at very low birth weight. Clin Endocrinol. (2014) 80:101–6. doi: 10.1111/cen.12251
181. Sullivan MC, Winchester SB, Bryce CI, Granger DA. Prematurity and perinatal adversity effects hypothalamic-pituitary-adrenal axis reactivity to social evaluative threat in adulthood. Dev Psychobiol. (2017) 59:976–83. doi: 10.1002/dev.21570
182. Van Oers HJJ, De Kloet ER, Levine S. Early vs. late maternal deprivation differentially alters the endocrine and hypothalamic responses to stress. Dev Brain Res. (1998) 111:245–52. doi: 10.1016/S0165-3806(98)00143-6
183. Van Oers HJJ, De Kloet ER, Whelan T, Levine S. Maternal deprivation effect on the infant's neural stress markers is reversed by tactile stimulation and feeding but not by suppressing corticosterone. J Neurosci. (1998) 18:10171–9. doi: 10.1523/JNEUROSCI.18-23-10171.1998
184. Avishai-Eliner S, Yi S-J, Baram TZ. Developmental profile of messenger RNA for the corticotropin-releasing hormone receptor in the rat limbic system. Dev Brain Res. (1996) 91:159–63. doi: 10.1016/0165-3806(95)00158-1
185. Brummelte S, Grunau RE, Zaidman-Zait A, Weinberg J, Nordstokke D, Cepeda IL. Cortisol levels in relation to maternal interaction and child internalizing behavior in preterm and full-term children at 18 months corrected age. Dev Psychobiol. (2011) 53:184–95. doi: 10.1002/dev.20511
186. Bagner DM, Sheinkopf SJ, Vohr BR, Lester BM. A preliminary study of cortisol reactivity and behavior problems in young children born premature. Dev Psychobiol. (2010) 52:574–82. doi: 10.1002/dev.20464
187. Fan Y, Pestke K, Feeser M, Aust S, Pruessner JC, Böker H, et al. Amygdala-hippocampal connectivity changes during acute psychosocial stress: joint effect of early life stress and oxytocin. Neuropsychopharmacology. (2015) 40:2736–44. doi: 10.1038/npp.2015.123
188. Seo D, Tsou KA, Ansell EB, Potenza MN, Sinha R. Cumulative adversity sensitizes neural response to acute stress: association with health symptoms. Neuropsychopharmacology. (2014) 39:670–80. doi: 10.1038/npp.2013.250
189. Murgatroyd C, Patchev AV, Wu Y, Micale V, Bockmühl Y, Fischer D, et al. Dynamic DNA methylation programs persistent adverse effects of early-life stress. Nat Neurosci. (2009) 12:1559–66. doi: 10.1038/nn.2436
190. Jawahar MC, Murgatroyd C, Harrison EL, Baune BT. Epigenetic alterations following early postnatal stress: a review on novel aetiological mechanisms of common psychiatric disorders. Clin Epigenetics. (2015) 7:1–13. doi: 10.1186/s13148-015-0156-3
191. Nagy C, Turecki G. Sensitive periods in epigenetics: bringing us closer to complex behavioral phenotypes. Epigenomics. (2012) 4:445–57. doi: 10.2217/epi.12.37
192. Roth TL, Sweatt JD. During sensitive periods of development. J Child Psychol Psychiatry. (2011) 52:398–408. doi: 10.1111/j.1469-7610.2010.02282.x
193. Palma-Gudiel H, Córdova-Palomera A, Eixarch E, Deuschle M, Fañanás L. Maternal psychosocial stress during pregnancy alters the epigenetic signature of the glucocorticoid receptor gene promoter in their offspring: a meta-analysis. Epigenetics. (2015) 10:893–902. doi: 10.1080/15592294.2015.1088630
194. Montirosso R, Provenzi L, Fumagalli M, Sirgiovanni I, Giorda R, Pozzoli U, et al. Serotonin transporter gene (SLC6A4) methylation associates with neonatal intensive care unit stay and 3-month-old temperament in preterm infants. Child Dev. (2016) 87:38–48. doi: 10.1111/cdev.12492
195. Liu PZ, Nusslock R. How stress gets under the skin: early life adversity and glucocorticoid receptor epigenetic regulation. Curr Genom. (2017) 19:653–64. doi: 10.2174/1389202919666171228164350
196. Hompes T, Izzi B, Gellens E, Morreels M, Fieuws S, Pexsters A, et al. Investigating the influence of maternal cortisol and emotional state during pregnancy on the DNA methylation status of the glucocorticoid receptor gene (NR3C1) promoter region in cord blood. J Psychiatric Res. (2013) 47:880–91. doi: 10.1016/j.jpsychires.2013.03.009
197. Oberlander TF, Weinberg J, Papsdorf M, Grunau R, Misri S, Devlin AM. Prenatal exposure to maternal depression, neonatal methylation of human glucocorticoid receptor gene (NR3C1) and infant cortisol stress responses. Epigenetics. (2008) 3:97–106. doi: 10.4161/epi.3.2.6034
198. Sosnowski DW, Booth C, York TP, Amstadter AB, Kliewer W. Maternal prenatal stress and infant DNA methylation: a systematic review. Dev Psychobiol. (2018) 60:127–39. doi: 10.1002/dev.21604
199. Kantake M, Yoshitake H, Ishikawa H, Araki Y, Shimizu T. Postnatal epigenetic modification of glucocorticoid receptor gene in preterm infants: a prospective cohort study. BMJ Open. (2014) 4:1–8. doi: 10.1136/bmjopen-2014-005318
200. Giarraputo J, DeLoach J, Padbury J, Uzun A, Marsit C, Hawes K, et al. Medical morbidities and DNA methylation of NR3C1 in preterm infants. Pediatric Res. (2017) 81:68–74. doi: 10.1038/pr.2016.185
201. Lester BM, Marsit CJ, Giarraputo J, Hawes K, Lagasse LL, Padbury JF. Neurobehavior related to epigenetic differences in preterm infants. Epigenomics. (2015) 7:1123–36. doi: 10.2217/epi.15.63
202. Binder EB. The role of FKBP5, a co-chaperone of the glucocorticoid receptor in the pathogenesis and therapy of affective and anxiety disorders. Psychoneuroendocrinology. (2009) 34:186–95. doi: 10.1016/j.psyneuen.2009.05.021
203. Zannas AS, Wiechmann T, Gassen NC, Binder EB. Gene-stress-epigenetic regulation of FKBP5: clinical and translational implications. Neuropsychopharmacology. (2016) 41:261–74. doi: 10.1038/npp.2015.235
204. Holmes MC, Sangra M, French KL, Whittle IR, Paterson J, Mullins JJ, et al. 11?-hydroxysteroid dehydrogenase type 2 protects the neonatal cerebellum from deleterious effects of glucocorticoids. Neuroscience. (2006) 137:865–73. doi: 10.1016/j.neuroscience.2005.09.037
205. Piyasena C, Cartier J, Provença N, Wiechmann T, Khulan B, Sunderasan R, et al. Dynamic changes in DNA methylation occur during the first Year of life in preterm infants. Front Endocrinol. (2016) 7:158. doi: 10.3389/fendo.2016.00158
206. Cruickshank MN, Oshlack A, Theda C, Davis PG, Martino D, Sheehan P, et al. Analysis of epigenetic changes in survivors of preterm birth reveals the effect of gestational age and evidence for a long term legacy. Genome Med. (2013) 5:96. doi: 10.1186/gm500
207. Carter RN, Paterson JM, Tworowska U, Stenvers DJ, Mullins JJ, Seckl JR, et al. Hypothalamic-pituitary-adrenal axis abnormalities in response to deletion of 11β-HSD1 is strain-dependent. J Neuroendocrinol. (2009) 21:879–87. doi: 10.1111/j.1365-2826.2009.01899.x
208. Harris HJ, Kotelevtsev Y, Mullins JJ, Seckl JR, Holmes MC, Endocrinology M, et al. Intracellular regeneration of glucocorticoids by 11β-hydroxysteroid dehydrogenase (11β-HSD)-1 plays a key role in regulation of the hypothalamic-pituitary-adrenal axis: analysis of 11β-HSD-1-deficient mice. Endocrinology. (2001) 142:114–20. doi: 10.1210/endo.142.1.7887
209. Cottrell EC, Seckl JR. Prenatal stress, glucocorticoids and the programming of adult disease. Front Behav Neurosci. (2009) 3:19. doi: 10.3389/neuro.08.019.2009
210. Maniam J, Antoniadis C, Morris MJ. Early-life stress, HPA axis adaptation, and mechanisms contributing to later health outcomes. Front Endocrinol. (2014) 5:73. doi: 10.3389/fendo.2014.00073
211. Wyrwoll CS, Holmes MC. Prenatal excess glucocorticoid exposure and adult affective disorders: a role for serotonergic and catecholamine pathways. Neuroendocrinology. (2012) 95:47–55. doi: 10.1159/000331345
212. O'Donnell D, Larocque S, Seckl JR, Meaney MJ. Postnatal handling alters glucocorticoid, but not mineralocorticoid messenger RNA expression in the hippocampus of adult rats. Mol Brain Res. (1994) 26:242–8. doi: 10.1016/0169-328X(94)90096-5
213. Seth S, Lewis AJ, Saffery R, Lappas M, Galbally M. Maternal prenatal mental health and placental 11β-HSD2 gene expression: initial findings from the mercy pregnancy and emotionalwellbeing study. Int J Mol Sci. (2015) 16:27482–96. doi: 10.3390/ijms161126034
214. Appleton AA, Armstrong DA, Lesseur C, Lee J, Padbury JF, Lester BM, et al. Patterning in placental 11-B hydroxysteroid dehydrogenase methylation according to prenatal socioeconomic adversity. PLoS ONE. (2013) 8:e74691. doi: 10.1371/journal.pone.0074691
215. Conradt E, Lester BM, Appleton AA, Armstrong DA, Marsit CJ. The roles of DNA methylation of NR3C1 and 11β-HSD2 and exposure to maternal mood disorder in utero on newborn neurobehavior. Epigenetics. (2013) 8:1321–9. doi: 10.4161/epi.26634
216. Wyrwoll C, Keith M, Noble J, Stevenson PL, Bombail V, Cormbie S, et al. Fetal brain 11β-hydroxysteroid dehydrogenase type 2 selectively determines programming of adult depressive-like behaviors and cognitive function, but not anxiety behaviors in male mice. Psychoneuroendocrinology. (2015) 59:59–70. doi: 10.1016/j.psyneuen.2015.05.003
217. Kotelevtsev Y, Holmes MC, Burchell A, Houston PM, Schmoll D, Jamieson P, et al. 11β-Hydroxysteroid dehydrogenase type 1 knockout mice show attenuated glucocorticoid-inducible responses and resist hyperglycemia on obesity or stress. Proc Natl Acad Sci USA. (1997) 94:14924–9. doi: 10.1073/pnas.94.26.14924
218. Mina TH, Räikkönen K, Riley SC, Norman JE, Reynolds RM. Maternal distress associates with placental genes regulating fetal glucocorticoid exposure and IGF2: role of obesity and sex. Psychoneuroendocrinology. (2015) 59:112–22. doi: 10.1016/j.psyneuen.2015.05.004
219. Räikkönen K, O'Reilly JR, Pesonen AK, Kajantie E, Villa P, Laivuori H, et al. Associations between maternal level of education and occupational status with placental glucocorticoid regeneration and sensitivity. Clin Endocrinol. (2014) 81:175–82. doi: 10.1111/cen.12412
220. Hermans EJ, Van Marle HJF, Ossewaarde L, Henckens MJAG, Qin S, Van Kesteren MTR, et al. Stress-related noradrenergic activity prompts large-scale neural network reconfiguration. Science. (2011) 334:1151–3. doi: 10.1126/science.1209603
221. Qin S, Hermans EJ, van Marle HJF, Luo J, Fernández G. Acute psychological stress reduces working memory-related activity in the dorsolateral prefrontal cortex. Biol Psychiatry. (2009) 66:25–32. doi: 10.1016/j.biopsych.2009.03.006
222. Arnsten AFT. Stress signalling pathways that impair prefrontal cortex structure and function. Nat Rev Neurosci. (2009) 10:410–22. doi: 10.1038/nrn2648
223. Magariños AM, García Verdugo JM, Mcewen BS. Chronic stress alters synaptic terminal structure in hippocampus. Proc Natl Acad Sci USA. (1997) 94:14002–8. doi: 10.1073/pnas.94.25.14002
224. Daniels JK, Mcfarlane AC, Bluhm RL, Moores KA, Richard Clark C, Shaw ME, et al. Switching between executive and default mode networks in posttraumatic stress disorder: alterations in functional connectivity. J Psychiatry Neurosci. (2010) 35:258–66. doi: 10.1503/jpn.090010
225. Akiki TJ, Averill CL, Wrocklage KM, Scott JC, Averill LA, Schweinsburg B, et al. Default mode network abnormalities in posttraumatic stress disorder: a novel network-restricted topology approach. Neuroimage. (2018) 176:489–98. doi: 10.1016/j.neuroimage.2018.05.005
226. Sheline YI, Barch DM, Price JL, Rundle MM, Vaishnavi SN, Snyder AZ, et al. The default mode network and self-referential processes in depression. Proc Natl Acad Sci USA. (2009) 106:1942–7. doi: 10.1073/pnas.0812686106
227. Zheng C, Zhang T. Synaptic plasticity-related neural oscillations on hippocampus-prefrontal cortex pathway in depression. Neuroscience. (2015) 292:170–80. doi: 10.1016/j.neuroscience.2015.01.071
228. Sylvester CM, Barch DM, Corbetta M, Power JD, Schlaggar BL, Luby JL. Resting state functional connectivity of the ventral attention network in children with a history of depression or anxiety. J Am Acad Child Adolesc Psychiatry. (2013) 52:1326. doi: 10.1016/j.jaac.2013.10.001
229. Zhao XH, Wang PJ, Li CB, Hu ZH, Xi Q, Wu WY, et al. Altered default mode network activity in patient with anxiety disorders: an fMRI study. Eur J Radiol. (2007) 63:373–8. doi: 10.1016/j.ejrad.2007.02.006
230. Manoliu A, Riedl V, Doll A, Bäuml JG, Mühlau M, Schwerthöffer D, et al. Insular dysfunction reflects altered between-network connectivity and severity of negative symptoms in schizophrenia during psychotic remission. Front Hum Neurosci. (2013) 7:216. doi: 10.3389/fnhum.2013.00216
231. Öngür D, Lundy M, Greenhouse I, Shinn AK, Menon V, Cohen BM, et al. Default mode network abnormalities in bipolar disorder and schizophrenia. Psychiatry Res Neuroimaging. (2010) 183:59–68. doi: 10.1016/j.pscychresns.2010.04.008
232. Aarnoudse-Moens CSH, Smidts DP, Oosterlaan J, Duivenvoorden HJ, Weisglas-Kuperus N. Executive function in very preterm children at early school age. J Abnorm Child Psychol. (2009) 37:981–93. doi: 10.1007/s10802-009-9327-z
233. Montagna A, Nosarti C. Socio-emotional development following very preterm birth: pathways to psychopathology. Front Psychol. (2016) 7:80. doi: 10.3389/fpsyg.2016.00080
234. Taylor HG, Clark CAC. Executive function in children born preterm: risk factors and implications for outcome. Semin Perinatol. (2016) 40:520–9. doi: 10.1053/j.semperi.2016.09.004
235. Lam S, Dickerson SS, Zoccola PM, Zaldivar F. Emotion regulation and cortisol reactivity to a social-evaluative speech task. Psychoneuroendocrinology. (2009) 34:1355–62. doi: 10.1016/j.psyneuen.2009.04.006
236. Williams PG, Suchy Y, Rau HK. Individual differences in executive functioning: implications for stress regulation. Ann Behav Med. (2009) 37:126–40. doi: 10.1007/s12160-009-9100-0
237. Raichle ME, MacLeod AM, Snyder AZ, Powers WJ, Gusnard DA, Shulman GL. A default mode of brain function. Proc Natl Acad Sci USA. (2001) 98:676–82. doi: 10.1073/pnas.98.2.676
238. Spreng RN, Mar RA, Kim ASN. The common neural basis of autobiographical memory, prospection, navigation, theory of mind, and the default mode: a quantitative meta-analysis. J Cogn Neurosci. (2009) 21:489–510. doi: 10.1162/jocn.2008.21029
239. Rogers CE, Sylvester CM, Mintz C, Kenley JK, Shimony JS, Barch DM, et al. Neonatal amygdala functional connectivity at rest in healthy and preterm infants and early internalizing symptoms. J Am Acad Child Adolesc Psychiatry. (2017) 56:157–66. doi: 10.1016/j.jaac.2016.11.005
240. Johns CB, Lacadie C, Vohr B, Ment LR, Scheinost D. Amygdala functional connectivity is associated with social impairments in preterm born young adults. NeuroImage Clin. (2019) 21:101626. doi: 10.1016/j.nicl.2018.101626
241. Papini C, White TP, Montagna A, Brittain PJ, Froudist-Walsh S, Kroll J, et al. Altered resting-state functional connectivity in emotion-processing brain regions in adults who were born very preterm. Psychol Med. (2016) 46:3025–39. doi: 10.1017/S0033291716001604
242. White TP, Symington I, Castellanos NP, Brittain PJ, Froudist Walsh S, Nam KW, et al. Dysconnectivity of neurocognitive networks at rest in very-preterm born adults. NeuroImage Clin. (2014) 4:352–65. doi: 10.1016/j.nicl.2014.01.005
243. Liao XM, Yang XD, Jia J, Li JT, Xie XM, Su YA, et al. Blockade of corticotropin-releasing hormone receptor 1 attenuates early-life stress-induced synaptic abnormalities in the neonatal hippocampus. Hippocampus. (2014) 24:528–40. doi: 10.1002/hipo.22254
244. Peters SK, Dunlop K, Downar J. Cortico-striatal-thalamic loop circuits of the salience network: a central pathway in psychiatric disease and treatment. Front Syst Neurosci. (2016) 10:104. doi: 10.3389/fnsys.2016.00104
245. Pessoa L. A network model of the emotional brain. Trends Cogn Sci. (2017) 21:357–71. doi: 10.1016/j.tics.2017.03.002
246. Cismaru AL, Gui L, Vasung L, Lejeune F, Barisnikov K, Truttmann A, et al. Altered amygdala development and fear processing in prematurely born infants. Front Neuroanatomy. (2016) 10:55. doi: 10.3389/fnana.2016.00055
247. Catani M, Dell'Acqua F, Thiebaut de Schotten M. A revised limbic system model for memory, emotion and behaviour. Neurosci Biobehav Rev. (2013) 37:1724–37. doi: 10.1016/j.neubiorev.2013.07.001
248. Coad BM, Postans M, Hodgetts CJ, Muhlert N, Graham KS, Lawrence AD. Structural connections support emotional connections: Uncinate Fasciculus microstructure is related to the ability to decode facial emotion expressions. Neuropsychologia. (2020) 145:1–12. doi: 10.1016/j.neuropsychologia.2017.11.006
249. Constable RT, Ment LR, Vohr BR, Kesler SR, Fulbright RK, Lacadie C, et al. Prematurely born children demonstrate white matter microstructural differences at 12 years of age, relative to term control subjects: an investigation of group and gender effects. Pediatrics. (2008) 121:306–16. doi: 10.1542/peds.2007-0414
250. Giménez M, Junqué C, Vendrell P, Narberhaus A, Bargalló N, Botet F, et al. Abnormal orbitofrontal development due to prematurity. Neurology. (2006) 67:1818–22. doi: 10.1212/01.wnl.0000244485.51898.93
251. Caldinelli C, Froudist-Walsh S, Karolis V, Tseng CE, Allin MP, Walshe M, et al. White matter alterations to cingulum and fornix following very preterm birth and their relationship with cognitive functions. NeuroImage. (2017) 150:373–82. doi: 10.1016/j.neuroimage.2017.02.026
252. Counsell SJ, Edwards AD, Chew ATM, Anjari M, Dyet LE, Srinivasan L, et al. Specific relations between neurodevelopmental abilities and white matter microstructure in children born preterm. Brain. (2008) 131:3201–8. doi: 10.1093/brain/awn268
253. Dodson CK, Travis KE, Ben-Shachar M, Feldman HM. White matter microstructure of 6-year old children born preterm and full term. NeuroImage Clin. (2017) 16:268–75. doi: 10.1016/j.nicl.2017.08.005
254. Eikenes L, Løhaugen GC, Brubakk AM, Skranes J, Håberg AK. Young adults born preterm with very low birth weight demonstrate widespread white matter alterations on brain DTI. NeuroImage. (2011) 54:1774–85. doi: 10.1016/j.neuroimage.2010.10.037
255. Olsen A, Dennis EL, Evensen KAI, Husby Hollund IM, Løhaugen GCC, Thompson PM, et al. Preterm birth leads to hyper-reactive cognitive control processing and poor white matter organization in adulthood. NeuroImage. (2018) 167:419–28. doi: 10.1016/j.neuroimage.2017.11.055
256. Skranes J, Lohaugen GC, Martinussen M, Indredavik MS, Dale AM, Haraldseth O, et al. White matter abnormalities and executive function in children with very low birth weight. NeuroReport. (2009) 20:263–6. doi: 10.1097/WNR.0b013e32832027fe
257. Murray AL, Thompson DK, Pascoe L, Leemans A, Inder TE, Doyle LW, et al. White matter abnormalities and impaired attention abilities in children born very preterm. NeuroImage. (2016) 124:75–84. doi: 10.1016/j.neuroimage.2015.08.044
258. Vollmer B, Lundequist A, Mårtensson G, Nagy Z, Lagercrantz H, Smedler AC, et al. Correlation between white matter microstructure and executive functions suggests early developmental influence on long fibre tracts in preterm born adolescents. PLoS ONE. (2017) 12:e0178893. doi: 10.1371/journal.pone.0178893
259. Bonifacio SL, Glass HC, Chau V, Berman JI, Xu D, Brant R, et al. Extreme premature birth is not associated with impaired development of brain microstructure. J Pediatrics. (2010) 157:726–32.e1. doi: 10.1016/j.jpeds.2010.05.026
260. Lawrence EJ, Rubia K, Murray RM, McGuire PK, Walshe M, Allin M, et al. The neural basis of response inhibition and attention allocation as mediated by gestational age. Hum Brain Mapp. (2009) 30:1038–50. doi: 10.1002/hbm.20564
261. Kalpakidou AK, Allin MPG, Walshe M, Giampietro V, McGuire PK, Rifkin L, et al. Functional neuroanatomy of executive function after neonatal brain injury in adults who were born very preterm. PLoS ONE. (2014) 9:e113975. doi: 10.1371/journal.pone.0113975
262. Nosarti C, Rubia K, Smith AB, Frearson S, Williams SC, Rifkin L, et al. Altered functional neuroanatomy of response inhibition in adolescent males who were born very preterm. Dev Med Child Neurol. (2006) 48:265–71. doi: 10.1017/S0012162206000582
263. Cocchi L, Zalesky A, Fornito A, Mattingley JB. Dynamic cooperation and competition between brain systems during cognitive control. Trends Cogn Sci. (2013) 17:493–501. doi: 10.1016/j.tics.2013.08.006
264. Ochsner KN, Silvers JA, Buhle JT. Review and evolving model of the cognitive control of emotion. Ann NY Acad Sci. (2012) 1251:E1–24. doi: 10.1111/j.1749-6632.2012.06751.x
265. Als H, Lawhon G, Brown E, Gibes R, Duffy FH, McAnulty G, et al. Individualized behavioral and environmental care for the very low birth weight preterm infant at high risk for bronchopulmonary dysplasia: neonatal intensive care unit and developmental outcome. Pediatrics. (1986) 78:1123–32.
266. Westrup B. Newborn individualized developmental care and assessment program (NIDCAP) - family-centered developmentally supportive care. Early Hum Dev. (2007) 83:443–9. doi: 10.1016/j.earlhumdev.2007.03.006
267. Brunton PJ, McKay AJ, Ochçdalski T, Piastowska A, Rçbas E, Lachowicz A, et al. Central opioid inhibition of neuroendocrine stress responses in pregnancy in the rat is induced by the neurosteroid allopregnanolone. J Neurosci. (2009) 29:6449–60. doi: 10.1523/JNEUROSCI.0708-09.2009
268. Misztal T, Młotkowska P, Marciniak E, Misztal A. Allopregnanolone reduces neuroendocrine response to acute stressful stimuli in sheep. J Endocrinol. (2020) 244:201–11. doi: 10.1530/JOE-19-0376
269. Brunton PJ, Russell JA, Hirst JJ. Allopregnanolone in the brain: protecting pregnancy and birth outcomes. Prog Neurobiol. (2014) 113:106–36. doi: 10.1016/j.pneurobio.2013.08.005
270. Durrmeyer X, Vutskits L, Anand KJS, Rimensberger PC. Use of analgesic and sedative drugs in the NICU: integrating clinical trials and laboratory data. Pediatric Res. (2010) 67:117–27. doi: 10.1203/PDR.0b013e3181c8eef3
271. Ng E, Taddio A, Ohlsson A. Intravenous midazolam infusion for sedation of infants in the neonatal intensive care unit. Cochrane Database Syst Rev. (2012) 2012:CD002052. doi: 10.1002/14651858.CD002052.pub2
272. Cho ES, Kim SJ, Kwon MS, Cho H, Kim EH, Jun EM, et al. The effects of Kangaroo care in the Neonatal Intensive Care Unit on the physiological functions of preterm infants, maternal-infant attachment, and maternal stress. J Pediatric Nurs. (2016) 31:430–8. doi: 10.1016/j.pedn.2016.02.007
273. Lee J, Bang K-S. The effects of Kangaroo care on maternal self-esteem and premature infants' physiological stability. Korean J Women Health Nurs. (2011) 17:454. doi: 10.4069/kjwhn.2011.17.5.454
274. Castral TC, Warnock F, Leite AM, Haas VJ, Scochi CGS. The effects of skin-to-skin contact during acute pain in preterm newborns. Eur J Pain. (2008) 12:464–71. doi: 10.1016/j.ejpain.2007.07.012
275. Cong X, Cusson RM, Walsh S, Hussain N, Ludington-Hoe SM, Zhang D. Effects of skin-to-skin contact on autonomic pain responses in preterm infants. J Pain. (2012) 13:636–45. doi: 10.1016/j.jpain.2012.02.008
276. Ludington-Hoe SM, Hosseini RB. Skin-to-skin contact analgesia for preterm infant heel stick. AACN Clin Issues. (2005) 16:373–87. doi: 10.1097/00044067-200507000-00010
277. Lyngstad LT, Tandberg BS, Storm H, Ekeberg BL, Moen A. Does skin-to-skin contact reduce stress during diaper change in preterm infants? Early Hum Dev. (2014) 90:169–72. doi: 10.1016/j.earlhumdev.2014.01.011
278. Milgrom J, Newnham C, Anderson PJ, Doyle LW, Gemmill AW, Lee K, et al. Early sensitivity training for parents of preterm infants: Impact on the developing brain. Pediatr Res. (2010) 67:330–5. doi: 10.1203/PDR.0b013e3181cb8e2f
279. Gitau R, Modi N, Gianakoulopoulos X, Bond C, Glover V, Stevenson J. Acute effects of maternal skin-to-skin contact and massage on saliva cortisol in preterm babies. J Reprod Infant Psychol. (2002) 20:83–8. doi: 10.1080/02646830220134595
280. Mörelius E, Örtenstrand A, Theodorsson E, Frostell A. A randomised trial of continuous skin-to-skin contact after preterm birth and the effects on salivary cortisol, parental stress, depression, and breastfeeding. Early Hum Dev. (2015) 91:63–70. doi: 10.1016/j.earlhumdev.2014.12.005
281. Miles R, Cowan F, Glover V, Stevenson J, Modi N. A controlled trial of skin-to-skin contact in extremely preterm infants. Early Hum Dev. (2006) 82:447–55. doi: 10.1016/j.earlhumdev.2005.11.008
282. Mitchell AJ, Hall RW. Does daily Kangaroo care provide sustained pain and stress. J Neonatal Perinatal Med. (2014) 6:45–52. doi: 10.3233/NPM-1364212
283. Feldman R, Eidelman AI. Skin-to-skin contact (Kangaroo care) accelerates autonomic and neurobehavioural maturation in preterm infants. Dev Med Child Neurol. (2003) 45:274–81. doi: 10.1111/j.1469-8749.2003.tb00343.x
284. Athanasopoulou E, Fox JRE. Effects of Kangaroo mother care on maternal mood and interaction patterns between parents and their preterm, low birth weight infants: a systematic review. Infant Mental Health J. (2014) 35:245–62. doi: 10.1002/imhj.21444
285. Feldman R, Eidelman AI, Sirota L, Weller A. Comparison of skin-to-skin (Kangaroo) and traditional care: parenting outcomes and preterm infant development. Pediatrics. (2002) 110:16–26. doi: 10.1542/peds.110.1.16
286. Habersaat S, Pierrehumbert B, Forcada-Guex M, Nessi J, Ansermet F, Müller-Nix C, et al. Early stress exposure and later cortisol regulation: impact of early intervention on mother–infant relationship in preterm infants. Psychol Trauma Theory Res Pract Policy. (2014) 6:457–64. doi: 10.1037/a0033878
287. Mooney-Leber SM, Brummelte S. Neonatal pain and reduced maternal care: early-life stressors interacting to impact brain and behavioral development. Neuroscience. (2017) 342:21–36. doi: 10.1016/j.neuroscience.2016.05.001
288. Acolet D, Modi N, Giannakoulopoulos X, Bond C, Weg W, Clow A, et al. Changes in plasma cortisol and catecholamine concentrations in response to massage in preterm infants. Arch Dis Child. (1993) 68:29–31. doi: 10.1136/adc.68.1_Spec_No.29
289. Arnon S, Diamant C, Bauer S, Regev R, Sirota G, Litmanovitz I. Maternal singing during kangaroo care led to autonomic stability in preterm infants and reduced maternal anxiety. Acta Paediatrica Int J Paediatrics. (2014) 103:1039–44. doi: 10.1111/apa.12744
290. Campbell-Yeo ML, Johnston CC, Joseph KS, Feeley N, Chambers CT, Barrington KJ, et al. Co-bedding between preterm twins attenuates stress response after heel lance: results of a randomized trial. Clin J Pain. (2014) 30:598–604. doi: 10.1097/AJP.0000000000000015
291. Qiu J, Jiang Y-F, Li F, Tong Q-H, Rong H, Cheng R. Effect of combined music and touch intervention on pain response and β-endorphin and cortisol concentrations in late preterm infants. BMC Pediatrics. (2017) 17:38. doi: 10.1186/s12887-016-0755-y
292. Schwilling D, Vogeser M, Kirchhoff F, Schwaiblmair F, Boulesteix AL, Schulze A, et al. Live music reduces stress levels in very low-birthweight infants. Acta Paediatr Int J Paediatr. (2015) 104:360–7. doi: 10.1111/apa.12913
293. Smith SL, Lux R, Haley S, Slater H, Beechy J, Moyer-Mileur LJ. The effect of massage on heart rate variability in preterm infants. J Perinatol. (2013) 33:59–64. doi: 10.1038/jp.2012.47
294. Standley JM. A meta-analysis of the efficacy of music therapy for premature infants. J Pediatric Nurs. (2002) 17:107–13. doi: 10.1053/jpdn.2002.124128
295. Welch MG, Hofer MA, Brunelli SA, Stark RI, Andrews HF, Austin J, et al. Family nurture intervention (FNI): methods and treatment protocol of a randomized controlled trial in the NICU. BMC Pediatr. (2012) 12:14. doi: 10.1186/1471-2431-12-14
296. Porges SW, Davila MI, Lewis GF, Kolacz J, Okonmah-Obazee S, Hane AA, et al. Autonomic regulation of preterm infants is enhanced by family nurture intervention. Dev Psychobiol. (2019) 61:942–52. doi: 10.1002/dev.21841
297. Welch MG, Firestein MR, Austin J, Hane AA, Stark RI, Hofer MA, et al. Family nurture intervention in the Neonatal Intensive Care Unit improves social-relatedness, attention, and neurodevelopment of preterm infants at 18 months in a randomized controlled trial. J Child Psychol Psychiatry Allied Discipl. (2015) 56:1202–11. doi: 10.1111/jcpp.12405
298. Shoemark H, Hanson-Abromeit D, Stewart L. Constructing optimal experience for the hospitalized newborn through neuro-based music therapy. Front Hum Neurosci. (2015) 9:487. doi: 10.3389/fnhum.2015.00487
299. Sa de Almeida J, Lordier L, Zollinger B, Kunz N, Bastiani M, Gui L, et al. Music enhances structural maturation of emotional processing neural pathways in very preterm infants. NeuroImage. (2019) 207:116391. doi: 10.1016/j.neuroimage.2019.116391
300. O'Brien K, Bracht M, Robson K, Ye XY, Mirea L, Cruz M, et al. Evaluation of the Family Integrated Care model of neonatal intensive care: a cluster randomized controlled trial in Canada and Australia. BMC Pediatrics. (2015) 15:210. doi: 10.1186/s12887-015-0527-0
301. Church PT, Grunau RE, Mirea L, Petrie J, Soraisham AA, Synnes A, et al. Family Integrate Care (FICare): positive impact on behavioural outcomes at 18 months. Early Hum Dev. (2020) 151:105196. doi: 10.1016/j.earlhumdev.2020.105196
302. Welch MG, Barone JL, Porges SW, Hane AA, Kwon KY, Ludwig RJ, et al. Family nurture intervention in the NICU increases autonomic regulation in mothers and children at 4-5 years of age: follow-up results from a randomized controlled trial. PLoS ONE. (2020) 15:e0236930. doi: 10.1371/journal.pone.0236930
303. Lester BM, Hawes K, Abar B, Sullivan M, Miller R, Bigsby R, et al. Single-Family room care and neurobehavioral and medical outcomes in preterm infants. Pediatrics. (2014) 134:754–60. doi: 10.1542/peds.2013-4252
304. Örtenstrand A, Westrup B, Broström EB, Sarman I, Åkerström S, Brune T, et al. The Stockholm neonatal family centered care study: effects on length of stay and infant morbidity. Pediatrics. (2010) 125, e278–e285. doi: 10.1542/peds.2009-1511
305. Vohr B, McGowan E, McKinley L, Tucker R, Keszler L, Alksninis B. Differential effects of the single-family room Neonatal Intensive Care Unit on 18- to 24-month bayley scores of preterm infants betty. J Pediatrics. (2017) 108:42–8. doi: 10.1016/j.jpeds.2017.01.056
306. Erdeve O, Arsan S, Canpolat FE, Ertem IO, Karagol BS, Atasay B, et al. Does individual room implemented family- centered care contribute to mother-infant interaction in preterm deliveries necessitating neonatal intensive care unit hospitalization? Am J Perinatol. (2009) 26:159–64. doi: 10.1055/s-0028-1095186
307. Pineda RG, Stransky KE, Rogers C, Duncan MH, Smith GC, Neil J, et al. The single-patient room in the NICU: maternal and family effects. J Perinatol. (2012) 32:545–51. doi: 10.1038/jp.2011.144
308. Pineda RG, Neil J, Dierker D, Smyser CD, Wallendorf M, Kidokoro H, et al. Alterations in brain structure and neurodevelopmental outcome in preterm infants hospitalized in different neonatal intensive care unit environments. J Pediatrics. (2014) 164, 52–60.e2. doi: 10.1016/j.jpeds.2013.08.047
309. Mörelius E, Broström EB, Westrup B, Sarman I, Örtenstrand A. The Stockholm Neonatal Family-Centered Care Study: effects on salivary cortisol in infants and their mothers. Early Hum Dev. (2012) 88:575–81. doi: 10.1016/j.earlhumdev.2011.12.033
310. Jobe AH. A risk of sensory deprivation in the neonatal intensive care unit. J Pediatrics. (2014) 164:1265–7. doi: 10.1016/j.jpeds.2014.01.072
311. Faure N, Habersaat S, Harari MM, Müller-Nix C, Borghini A, Ansermet F, et al. Maternal sensitivity: a resilience factor against internalizing symptoms in early adolescents born very preterm? J Abnormal Child Psychol. (2017) 45:671–80. doi: 10.1007/s10802-016-0194-0
312. Poehlmann-Tynan J, Gerstein ED, Burnson C, Weymouth L, Bolt DM, Maleck S, et al. Risk and resilience in preterm children at age 6. Dev Psychopathol. (2014) 760:843–58. doi: 10.1017/S095457941400087X
313. Sporns O. Contributions and challenges for network models in cognitive neuroscience. Nat Neurosci. (2014) 17:652–60. doi: 10.1038/nn.3690
Keywords: prematurity, stress, hypothalamus-pituitary-adrenal axis, autonomic nervous system, large-scale brain networks, epigenetics, resilience
Citation: Lammertink F, Vinkers CH, Tataranno ML and Benders MJNL (2021) Premature Birth and Developmental Programming: Mechanisms of Resilience and Vulnerability. Front. Psychiatry 11:531571. doi: 10.3389/fpsyt.2020.531571
Received: 31 January 2020; Accepted: 01 December 2020;
Published: 08 January 2021.
Edited by:
Livio Provenzi, Neurological Institute Foundation Casimiro Mondino (IRCCS), ItalyReviewed by:
Ruth Eckstein Grunau, University of British Columbia, CanadaMorgan Firestein, Columbia University Irving Medical Center, United States
Copyright © 2021 Lammertink, Vinkers, Tataranno and Benders. This is an open-access article distributed under the terms of the Creative Commons Attribution License (CC BY). The use, distribution or reproduction in other forums is permitted, provided the original author(s) and the copyright owner(s) are credited and that the original publication in this journal is cited, in accordance with accepted academic practice. No use, distribution or reproduction is permitted which does not comply with these terms.
*Correspondence: Manon J. N. L. Benders, bS5iZW5kZXJzQHVtY3V0cmVjaHQubmw=