- 1Department of Biology, Khorramabad Branch, Islamic Azad University, Khorramabad, Iran
- 2Pediatric Department, Mashhad University of Medical Sciences, Mashhad, Iran
- 3Nervous System Stem Cells Research Center, Semnan University of Medical Sciences, Semnan, Iran
- 4Cellular and Molecular Research Center, Qom University of Medical Sciences, Qom, Iran
- 5Department of Medical Microbiology, Faculty of Medicine, Shahed University, Tehran, Iran
- 6Laboratory Department, Buraimi Hospital, Buraimi, Oman
Microplastics (MPs) are particles with a diameter of <5 mm. The disposal of plastic waste into the environment poses a significant and pressing issue concern globally. Growing worry has been expressed in recent years over the impact of MPs on both human health and the entire natural ecosystem. MPs impact the feeding and digestive capabilities of marine organisms, as well as hinder the development of plant roots and leaves. Numerous studies have shown that the majority of individuals consume substantial quantities of MPs either through their dietary intake or by inhaling them. MPs have been identified in various human biological samples, such as lungs, stool, placenta, sputum, breast milk, liver, and blood. MPs can cause various illnesses in humans, depending on how they enter the body. Healthy and sustainable ecosystems depend on the proper functioning of microbiota, however, MPs disrupt the balance of microbiota. Also, due to their high surface area compared to their volume and chemical characteristics, MPs act as pollutant absorbers in different environments. Multiple policies and initiatives exist at both the domestic and global levels to mitigate pollution caused by MPs. Various techniques are currently employed to remove MPs, such as biodegradation, filtration systems, incineration, landfill disposal, and recycling, among others. In this review, we will discuss the sources and types of MPs, the presence of MPs in different environments and food, the impact of MPs on human health and microbiota, mechanisms of pollutant adsorption on MPs, and the methods of removing MPs with algae and microbes.
Introduction
There is growing concern among researchers and environmentalists regarding the impact of microplastics (MPs) on human health and aquatic ecosystems. They can be found in both freshwater and marine ecosystems, although the significance of freshwater environments is often overlooked and underreported in comparison to marine ecosystems (1, 2). MPs are particles with a diameter of <5 millimeters (3). The term “MPs” was introduced nearly two decades ago by Thompson et al. (4) in their research on marine plastic contamination in the United Kingdom. Since then, MPs have garnered the interest of the academic community, governmental bodies, non-governmental organizations, and various other stakeholders. In the last century, global plastic production has reached 320 million tons a year, and more than 40 percent of it is used as single-use packaging. One hundred ninety-two coastal nations produced 275 million metric tons (MT) of plastic garbage in 2010, and this figure reached 350 million tons in 2017 (5–8). In 2013, China manufactured around 63.0 million metric tons of plastic, representing the largest share of global plastic production. When this figure is aggregated with the plastic output of other nations in Asia, the collective plastic production amounts to around 114.0 million tons (9). The European Union emerged as the second most significant region in terms of plastic production, generating approximately 50.0 million tons (10). Furthermore, it is anticipated that the amount of plastic trash produced will reach around 250 million metric tons by 2025, placing further strain on systems for managing plastic garbage (11). The disposal of plastic waste into the environment poses a significant and pressing issue. Atmospheric elements, including abrasion, wave action, mild oxidation, and ultraviolet radiation, along with microbial activity, contribute to the degradation of plastic fragments into micro and nanoparticles (12, 13). There has been a notable rise in public apprehension regarding the issue of MP pollution in recent years. MPs can spread various unsettling deterrents through the environment in addition to acting as a poisonous deterrent. MPs impact the feeding and digestive capabilities of marine organisms, as well as hinder the development of plant roots and leaves (14–18). When plastics are introduced into the environment, they undergo degradation, resulting in the formation of smaller fragments that have the potential to enter the food chain directly or introduce potentially hazardous substances into it (19, 20). Most individuals ingest substantial quantities of MPs and even nanoplastic particles from their diet, particularly from consuming fish and other types of seafood (21). So far, MPs have been discovered in various food items, including drinking water, sugar, seafood, canned tuna, honey, and table salt (22, 23). Numerous nations have established goals to eradicate or decrease specific items like plastic bags and waste to combat plastic pollution (24, 25). In May 2018, the European Commission implemented a new plan that prohibited various single-use plastic items and imposed more stringent rules on others. Sixty nations have implemented prohibitions or levies on disposable plastics (26, 27). The generation and dispersion of plastic waste in marine environments are progressively escalating, leading to a corresponding increase in its accumulation at both surface and seabed levels (28). Plastic in aquatic environments disrupts vital conditions, leading to adverse impacts on the socio-economic aspects of industries such as tourism, trawling, shipping, and fish farming (29, 30). The resilient and enduring nature of MPs renders them prevalent in aquatic environments as a form of marine pollution, serving as a conduit for transmitting pollutants to aquatic organisms (31, 32). The diminutive dimensions of MPs result in their ingestion by various marine organisms, causing disturbances in their physiological processes. This subsequently permeates through the food chain, giving rise to adverse health effects in humans (33, 34). Exposure to MPs induces oxidative stress, microbial dysbiosis, and persistent inflammation within the human organism. These particles have also been associated with cancer and may also impact the development of the fetus (35–37). The number of articles published in PubMed with the Keyword microplastics [Title/Abstract] from 2012 to 22 March 2024 is shown in Figure 1.
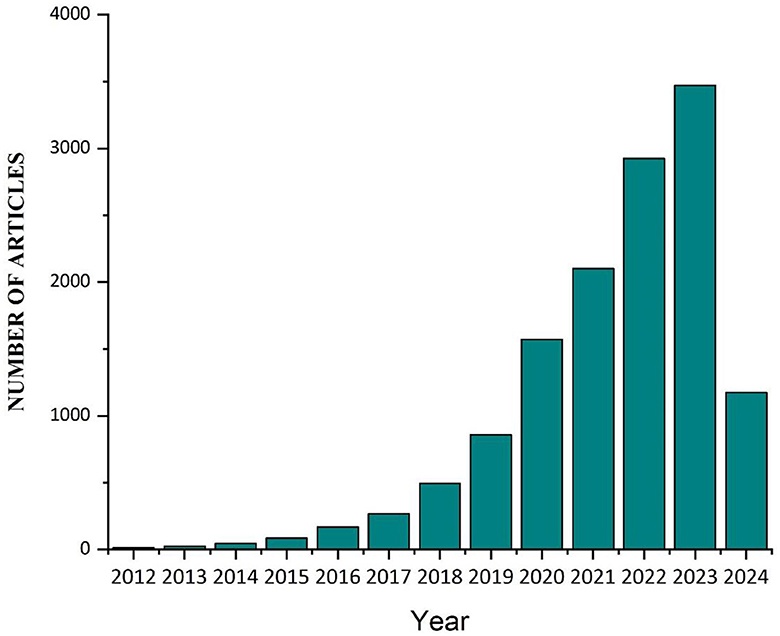
Figure 1. The number of articles published on the MPs updated to 22 March 2024. The expanding number of papers is indicative of the scientific community's and researchers' increased interest in and awareness of the problem of MP contamination. The number of papers has been steadily increasing, which highlights the relevance of tackling MP pollution in different ecosystems and the developing character of research on this topic.
The objective of this investigation is to acquire an extensive comprehension of MPs, encompassing their origin and assortment, along with exploring the significance of MPs in different environments and examining their influence on human health, as well as their impact on the microbiome and the degradation of MPs by algae, fungi, and bacteria.
Types and sources of microplastics
MP particles are categorized into many categories and vary in composition, size, color, and thickness. Plastic particles were classified as macro, meso, micro, and nano, respectively, based on their size ranges: more than 25 mm, between 5 and 25 mm, 1 to 5 mm, and 1 nm to 1 micrometer (38–40) (Figure 2). Research demonstrates two main sources of MPs: primary and secondary. Primary MPs are discharged into our ecosystems directly by tires, personal care items, marine coatings, and synthetic textile goods. Conversely, secondary MPs come from bigger plastics broken down into smaller pieces by mechanical abrasion or UV exposure. These larger plastics include fishing nets, garbage cans, and tire wear (41, 42). MP particles, with an average size of 0.25 mm, are commonly utilized in industrial shot-blasting abrasives and cosmetic treatments. Granules and powders, which are particles of MP diameters, are undoubtedly used in a variety of applications (43, 44). Primary MPs being released into the environment straight from home factories and sewerage. Skin care product MP beads are deposited in the environment due to being transported through the sewage system with sewage and not being adequately eliminated. An average of 700,000 fibers are released from 6 kg of synthetic garments containing MPs in a single wash (45–47). MPs enter the environment through pellets used as raw material for plastic products in industrial applications. Additionally,sewage releases MPs used in dentistry and pharmaceutical containers into the environment. Primary MPs are challenging to remove from aquatic systems because of their smaller size and lack of knowledge (28, 48). There has also been evidence of raw food contamination from contact with plastic chopping boards (49). The amount of MPs released from plastic materials may be influenced by several variables, including physical stress and heat. A polypropylene (PP) baby milk bottle heated from 25 to 90 degrees Celsius emitted between 0.6 and 55 million MP particles per liter (50). It is believed that MPs, which have also been recovered from surgical environments, are a result of the widespread usage of plastics in healthcare settings (51). There have also been reports of MPs falling from home objects. MPs have been found to have leaked from plastic tea filter bags, throwaway cups, and food containers (52–54). The prevalent MPs found in the environment include polyethylene (PE), PP, polyvinyl chloride (PVC), and polystyrene (PS) (55). Table 1 compiles the physical characteristics and origins of prevalent MPs (56).
Microplastics in different environments
MPs in marine environments
MP contamination has been documented in various ecosystems, including aquatic, terrestrial, and atmospheric environments (64–66). The passage describes the inevitable exposure of MPs in the human body due to the transfer of these particles through the aquatic food chain (67, 68). Most plastics have a density similar to that of water, causing plastic particles to either float or remain suspended in water and be carried by the current. According to a study, it was approximated that plastic constitutes 60 to 80 percent of marine debris (69). Elevated levels of plastic particles have been detected in coastal regions, fishing equipment, and semi-enclosed seas (70). Carson et al.' (71) research revealed that the levels of MP in the North Pacific gyre varied between 85 and 184 items per square kilometer. Lusher and colleagues (72) conducted a study in which they gathered and characterized 2,315 plastic particles from surface waters in the northeastern Atlantic Ocean. Their findings revealed that 89% of the collected particles were smaller than 5 mm, suggesting that MPs are the predominant form of plastic pollution in quantity (72). In the Yellow Sea of China, the mean concentration of MPs in the surface water was recorded at 545 ± 282 particles per cubic meter. Additionally, sea cucumbers, a commonly consumed food along China's coast, were found to ingest a higher quantity of MPs compared to other marine organisms in the same region (73). Meteorological events such as precipitation and severe weather can also influence the distribution and fate of MPs in the ocean to a certain degree (74). Hitchcock examined fluctuations in the prevalence of MPs in surface water in the context of a storm event and determined that the highest concentration of MPs reached 17,833 cubic meters at the height of the storm (75). The primary contributors to plastic fragments in marine ecosystems are activities that occur on land. The presence of MPs exhibited a notable positive association with population density and urban/suburban expansion. Additionally, MPs transported by coastal currents tend to amass near urban industrial hubs along the coastlines (76, 77). Furthermore, plastics are introduced into the marine ecosystem through direct means such as sewage overflows, sewage discharges, and shipping operations (78). MP contamination resulting from fishing activities is frequently underestimated. Large quantities of plastic materials are utilized in fishing equipment, including ropes, nets, and pots. Furthermore, the presence of fiber plastic waste can be attributed to the mechanical wear and tear of fishing nets and ropes during operational use (79). One research study found that the amount of plastic debris present on the surface of the ocean is significantly less than the total amount of plastic debris that enters the sea, as the majority of plastic waste ultimately settles on the ocean floor (80). After MPs are introduced into aquatic ecosystems, they do not solely remain as individual particles. Plastic particles that are suspended or floating in water engage with other substances or organisms through absorption, accumulation, and phagocytosis, leading to the vertical displacement of plastic particles within the water column (81). Furthermore, MP sedimentation is reversible, as even MPs located in deep water bodies or sediment can rise to the surface due to biological processes or water movement (82). The possibility that MP pollution might harm ecosystem health is one of the main worries (83). MPs' minuscule size makes them easy for marine animals to consume. When MP contaminants are eaten by smaller marine species (primary consumers, such as zooplanktons), then transferred to secondary consumers, such as big fish, and finally tertiary consumers, such as people, the food chain, or food web, is seriously disrupted (84). Meanwhile, when MPs reach the food chain, marine animals may end up eating additional pollutants and dangerous substances (such as poisonous heavy metal species) that stick to their surfaces or become lodged in their mass (85). The health of both people and marine life is at risk from these very toxic MPs. That is, the hydrophobic pollutants in seawater may stick to plastic debris in a typical environmental setting (86). Furthermore, MP pollution may have a significant impact on the biodiversity of the impacted ecosystems. MPs have the power to modify habitat structures, interfere with ecosystem functions, and affect an organism's ability to reproduce and behave (87, 88). For instance, MP buildup in aquatic environments can suffocate and smother benthic creatures, such as corals and shellfish, preventing them from growing and developing normally (89, 90).
MPs in soil
Previous research has primarily concentrated on the dispersion of MPs in marine environments, but recent studies have underscored the significance of MPs in terrestrial ecosystems (91). MPs have been detected in soils across the globe, and the yearly influx of MPs into soil ecosystems may exceed that of the world's oceans (92). Agriculture represents a significant contributor to the presence of soil MPs, with an annual release of hundreds of thousands of tons of these particles into agricultural land (93). MPs have the potential to infiltrate agricultural soil via various pathways, such as the application of plastic mulch, compost, and irrigation. This can result in the buildup of numerous MPs, ranging from tens to hundreds per kilogram of soil (94, 95). Each year in Germany, an estimated 0.035 to 2.2 trillion MPs are introduced into agricultural soil through composting (96). The utilization of sludge resulted in the presence of 1,000–4,000 MP particles per kilogram of soil in farmland soil in Europe (97). Roughly 20 million hectares of agricultural land globally utilize plastic mulch, with China representing the majority share at approximately 90%. Removing the mulching film from agricultural fields requires significant labor and time, leading to instances where the films or their remnants are deliberately or inadvertently left behind in the farmland (94). MPs can potentially impact the biophysical characteristics of soil, including soil structure, pH levels, fertility, nutrient content, microbial activity, and the formation of water-stable aggregates (98). Currently, there is limited information regarding the potential response of plants to the presence of MPs. Recent research by de Souza Machado et al. (98) has confirmed that the addition of MPs changes the physical properties of soil, thereby affecting its hydrodynamics and microbial activity. This study also shows that the impact of MPs on the soil depends on the shape and size of the MP particles (94, 98). MPs build up in soils and affect terrestrial ecosystems, the soil biota, nutrient cycling, and soil biodiversity. When micro- and mesofauna in the soil are exposed to MPs, it can negatively impact their growth, reproduction, and overall ecosystem function. These pollutants can also be absorbed by plants, generating worries about human health consequences through the food chain (99, 100).
MPs in freshwater
As previously stated, a significant portion of marine plastic originates from terrestrial sources, with rivers serving as the primary conduit for transporting plastics of diverse dimensions (101). The attention toward MPs in freshwater ecosystems is a relatively new development, with initial research published in the past 15 years. MPs have rapidly emerged as a prevalent form of pollution. It is not unusual for studies in freshwater environments to detect and document the presence of MPs at all sampling locations and frequently in all samples collected (102–104). The broadening of research attention to encompass freshwater ecosystems is paramount, given the recognized significance of rivers in the conveyance of MPs, especially toward marine habitats (105). MPs found in freshwater environments originate from diverse sources. Studies have indicated spatial associations between the types of MPs in a particular location and human activities. Significant contributors include industrial discharges, urban waterways, and effluents from wastewater treatment plants in the vicinity (55). Both direct and diffuse sources of pollution contribute substantial quantities of MPs to river ecosystems (106, 107). Scholars have approximated that the yearly release of MPs into adjacent rivers from an industrial manufacturing facility may reach a maximum of 95.5 tons (108). Since high tidal forces, among other mechanical consequences, worsen plastic disintegration in the marine environment, the freshwater environment is not susceptible to these forces, leading most experts to conclude that secondary MPs are primarily found in the latter. These are not all primary MPs, however, as examination of the morphology and particle size of MPs in freshwater environments has revealed that many are secondary MPs created by the breakdown of bigger plastics (109). Since secondary MPs can enter the freshwater ecosystem through various routes and can be formed either before or after entering freshwater, pinpointing a specific source can be challenging (110). An excellent illustration of secondary MPs introduced into the environment consists of synthetic fibers that become dislodged from clothing during the washing process. An average 6 kg wash load of acrylic fabric is estimated to release between 140,000 and 730,000 microfibers (47). The microfibers turn into secondary MPs before being released into the surroundings. However, most secondary MPs are created following their introduction into the environment via biological, photodegradation, or mechanical abrasion. They are then carried to the freshwater environment by wind, surface runoff, and other activities (105, 111).
MPs in the atmosphere
Although polymers with higher densities have been recorded in atmospheric deposition/air mass sampling, the variety of polymer types detected in atmospheric samples described thus far does not clearly distinguish between lesser or greater density (112). Most MPs found in the atmosphere are microfibers with minor amounts of foam, film, and fragments. These MPs are mainly at the micron scale (113, 114). MPs found in urban air are frequently linked to high levels of human activity, with primary sources stemming from the incineration of waste, degradation of synthetic textiles, tire abrasion, industrial processes, and urban particulate matter (115, 116). The atmosphere is crucial in facilitating the movement of MPs. Additionally, the movement of air in the atmosphere and the processes of wet and dry deposition are significant mechanisms through which MPs originating from land-based sources may enter and impact other environmental compartments. These processes can influence the dynamics of plastic pollution as it moves between different ecosystems (112, 117). Furthermore, the distribution of atmospheric MPs is influenced by patterns of rainfall and heat cycles (118). This indicates that the movement and preservation of plastics in the air vary depending on the current weather patterns over various periods or geographical areas. The research above, has established the widespread presence of MPs in the atmosphere and the potential for terrestrial MPs to be carried into marine environments. However, the extent to which atmospheric transportation contributes to pollution in aquatic and terrestrial ecosystems remains uncertain. Additional investigation is necessary to explore the transportation mechanisms and their correlation with meteorological factors (119–121).
MPs in human food items
The widespread presence of MPs in oceans and seas suggests that products derived from these marine environments may also contain significant amounts of MPs. Numerous studies have reported the occurrence of MPs in aquatic organisms, particularly in seafood such as crabs, fish, and clams (122, 123). Likewise, sea salt, a non-living product derived from the sea, has also been documented to contain MPs (124, 125). As per the research conducted by Danapoulos et al. (126), most studies have detected MP contamination in seafood, with reported MPs content typically being <1 MP particle per gram (126). In a study, Jin et al. (127) found that aquatic food items such as fish and bivalves exhibit varying levels of MPs, with concentrations ranging from 0 to 10.5 items per gram for bivalves and 0 to 20 items per individual for fish. These same authors reported that drinking water and salt are also a pathway of MPs exposure to humans, with concentrations ranging from 0–61 particles/L in tap water, from 0–3,074 MPs/L in bottled water, and from 0–13,629 particles/kg for salt (127, 128). MPs have also been detected in various food sources, including vegetables (6.4 particles/100 g), honey (1,992–9,752 particles/kg), sugar (249 ± 130 particles/kg), cereals (5.7 particles/100 g), fruits (5.2 particles/100 g), beers (152 ± 50.97 particles/L), dairy products (8.1 particles/100 g), meats (9.6 particles/100 g), energy drinks (14 ± 5.79 particles/L), tea (11 ± 5.26 particles/L), and soft drinks (40 ± 24.53 particles/L) (129–134). Sure researchers argue that the consumption of MPs through bottled water is typically higher than tap water (128, 135). A compilation of research results from multiple studies regarding the presence of MPs in seafood is presented in Table 2 (122, 136, 137, 139–143).
The impact of MPs on human health
As a burgeoning area of research, MPs necessitate further investigation to comprehensively understand their effects on both organisms and human health (145). Plastic production has significantly increased in recent years, with projections indicating an additional 33 billion tons of plastic will be generated by 2050, adding to the current level of approximately 370 million tons produced in 2019 (146). This remarkable surge in plastic production also serves as a cautionary reminder of the substantial volume of plastic waste being deposited into the environment (147). MPs have been discovered to possess the capacity to impact human health (148–150). Research has indicated that MPs have the potential to infiltrate the human body via ingestion of water and food, as well as inhalation of airborne particles (151). Also, MPs have been identified in various human biological samples, such as lungs, placenta, stool, sputum, liver, breast milk, and blood (148, 152). MPs can cause various illnesses in humans, depending on how they enter the body (Figure 3) (153). They may release harmful chemicals into the body, which may result in a variety of health concerns, including cancer, developmental disorders, and problems with reproduction (154, 155). Additionally, it has been discovered that MPs aid in developing antibiotic resistance. This is due to the possibility that MPs may operate as a breeding ground for bacteria that will eventually develop antibiotic resistance. Given that antibiotic resistance is already a major worldwide health problem, this might have substantial ramifications for human health (156, 157). Ingestion is the primary way that MPs are consumed. One of the main ways that people consume MPs is via eating seafood that has been polluted; eating sea salt can also cause one to consume MPs. The human body also obtains MPs from drinks, tap water, and bottled water. According to a recent study, MPs can enter the body by eating fruits and vegetables (158, 159). Inhaling dust from both indoor and outdoor sources also facilitates the entrance of MPs into the human body; the majority of MPs identified in dust come from synthetic fabrics, aerosols, and tires (160, 161). The direct penetration of MPs through the dermal layer is uncommon due to the delicate nature of the skin membrane. However, there have been documented instances of MPs entering through hair follicles, sweat glands, and skin lesions such as cuts or wounds (162). Recent research has indicated the presence of MPs in human fecal matter, providing evidence for the ingestion of MPs by humans (134, 163). The results of research on the effects of MPs on the human body are displayed in Table 3. The extent of penetration of MPs into the organs or lungs is influenced by the size of the MPs (135). The lungs or cells will directly absorb MPs that are a few microns in size through cellular uptake; more prominent MPs (up to 130 microns) can still reach tissues through paracellular uptake; MPs larger than 150 microns are not absorbed (135, 203). Hence, it can be inferred that MPs have the as well as to impact human health directly. In the following, we will examine the implications of MPs on human health, including the specific organs and tissues that may be affected.
Lung damage
Previous research has identified the existence of synthetic fibers in human lung tissue samples. However, there is a scarcity of studies that have utilized chemical analysis techniques, such as micro-Raman spectroscopy and Fourier transform infrared spectroscopy, to definitively confirm the presence of MPs in the lungs (36, 204). The potential for inhaling MPs has been emphasized, with studies documenting the presence of MPs smaller than 5 μm in air samples (205). It is still uncertain whether MP particles can infiltrate and persist in the respiratory system of the general public due to environmental exposure, as opposed to the sustained levels observed in industrial environments. MPs are engineered to possess durability, making them resistant to lung degradation, which may result in their potential accumulation over time, contingent upon their aerodynamic diameter and the body's respiratory defenses (164, 206). Smaller-sized MPs can induce respiratory discomfort and cytotoxic and inflammatory effects when they penetrate the human respiratory system (164, 165, 207–209). Particles of 50 nm in size of PS have been shown to have cytotoxic and genotoxic effects on pulmonary epithelial cells (166). Interstitial fibrosis, inflammatory and fibrotic changes in the bronchial and peribronchial tissue (chronic bronchitis), and asthma-like bronchial reactions are a few symptoms of a synthetic particle entering the body. These symptoms have been seen in textile industry workers who are in close contact with acrylic, polyester, and nylon fibers (210). In comparison to bigger particles (202–535 nm), the smaller particles (64 nm) produced a considerably higher neutrophil influx in the lungs, according to research examining proinflammatory responses in rats to different sizes of PS particles (167). Evidence suggests that MPs may also spread to other tissues after being swallowed or breathed. For example, research reported that fluorescent PS microspheres given intraperitoneally to mice were discovered in the spleen 10 days later (211).
Colorectal cancer
Colorectal cancer (CRC), which ranks as the third most prevalent cancer globally with 1.9 million cases reported in 2020, is on the rise among individuals under the age of 50 (212, 213). This rise is believed to be influenced by an environmental factor, and MPs have been investigated as a potential catalyst for this shift (170). CRC is associated with intestinal microbiota and their interaction with the mucosa (170). The current equilibrium between the gut bacteria and the mucus layer may be altered by MPs that enter the diet and make their way to the colon. Consequently, they may also modify the colonocytes' exposure to different potentially detrimental elements of the gut microbiota, thus impacting the occurrence of CRC. Moreover, colonocyte cells may be directly exposed to MP-associated carcinogens, raising the risk because MPs tend to adsorb hydrophobic compounds from the environment (170, 214). The discovery of significant MP concentrations in stool samples indicates that most MPs pass straight through the small intestine and into the colon. Newly discovered evidence of MPs in human blood and tissue samples indicates that not all MPs ingested are excreted directly through the gastrointestinal (GI) tract. Instead, considering that the dispersion of particles in the respiratory tract is largely dependent on particle size, it is probable that size may also impact the deposition, distribution, and, or aggregation of MPs at this location (171). Furthermore, surface-associated chemicals and, or porosity may affect how MPs are absorbed, distributed, and, or trafficked throughout the GI system. Because of the makeup of their polymers (PS, for example), most plastics have hydrophobic surfaces. MPs' chemical surface allows them to bind charged molecules and ions (including toxic metals) through electrostatic interactions, adsorb hydrophobic compounds (some of which are carcinogens), adhere microorganisms (some of which may be pathogens), and simply cause a local inflammatory response (which may lead to non-genotoxic carcinogenesis) (170). MPs eaten are likely to fragment due to a combination of factors, including GI fluids and mechanical pressures inside the GI lumen (215–220). Furthermore, the colon's chemical milieu, such as pH, may alter the properties of harmful chemical adsorption. This is probably especially important when it comes to ingesting MPs that have weathered. A few smaller MPs known as bile-associated MPs pass through the colon after being absorbed in the small intestine and ending in bile (221, 222). MPs will touch the loosely adhering outer mucous layer, which serves as the colon's initial line of defense as they transit through the body. This layer's hydrophobic domains can bind MPs, some of which may then be shed due to the GI tract's physiological peristaltic processes, which cause this layer to constantly turnover (223). The intestinal epithelium is probably similarly protected from MPs by the inner mucus layer, which typically serves as a barrier to shield the underlying epithelial cells from harmful substances and germs. When MPs penetrate the inner layer of the colonic mucus, they may serve as rafts for the growth of biofilms, which are intricate bacterial communities known to be significant regulators of gut health (170, 224). The hypothesis that bacterial adhesion to MPs in situ may offer a platform for early biofilm formation is supported by evidence of bacteria-rich biofilms on the surface of MPs recovered from seawater (225). Two recent investigations have examined MPs' potential for carcinogenicity, and both have concluded that there is probably a connection (172, 226). However, as Domenech et al. (226) note, most research has used short-term rodent trials or in vitro models, making it impossible to draw firm conclusions. Toxins produced by carcinogenic bacteria may be delivered to the colonic epithelium by MPs. This is demonstrated by developing a genotoxin by Escherichia coli (E. coli) in the colon, which is linked to an increased risk of CRC (173, 227). Although E. Coli typically lives in the intestinal lumen, research showing that E. coli can attach to MPs in an aquaculture model implies that they can also bind to MPs in the colon (228, 229). If this is the case, MPs containing pks+ E. coli have the potential to serve as a means of transporting these genotoxic bacteria to the colonic epithelium surface. However, evidence indicates that this process may rely on the absence of a fully intact inner mucus layer (230). Put simply, the relative abundance of pks+ E. coli next to the colonic epithelium may rise in response to a shift in the relative abundance of bacterial species (dysbiosis) that break down colonic mucus (231). Mice subjected to continuous exposure to MPs in their drinking water for 6 weeks also exhibited imbalanced microbial communities in the colon (174, 175). Given the growing body of evidence linking colonic microbiota to the development of CRC, this becomes increasingly significant (232, 233). Although the pro-carcinogenic mechanisms of these different bacterial species vary, it is plausible that their association with MPs could increase the delivery of the corresponding bacterial toxins to the colonic epithelium. This would support the theory that carcinogenesis in otherwise healthy colons may be caused by long-term storage of toxic bacteria (233, 234). Furthermore, research has demonstrated that MPs, specifically PE, can induce adverse effects in human intestinal cells, such as heightened oxidative stress and reduced cell viability (176).
Inflammatory bowel disease
Following ingesting food and liquids, the gut, the most fundamental part of the human digestive system, carries out the crucial processes of digestion and nutrient absorption. Intestinal cells are exposed to various substances, poisons, and possible pollutants throughout this process. The intestinal barrier, which is made up of several components, has a variety of roles in immunological homeostasis and in preventing the entry of pathogens and toxins (235). Collectively, these elements work toward maintaining the proper operation of the digestive system and play a role in maintaining overall immune balance. As a result, the intestinal damage caused by contaminants poses a substantial threat to human wellbeing and is a matter of public apprehension. There is mounting evidence indicating that the disturbance or impairment of the intestinal barrier may play a role in the development of inflammatory bowel disease (IBD) and other systemic conditions (236–238). IBD is a non-specific, chronic GI illness that is typified by an immune response that is not normal. The two main types of IBD are Crohn's disease and ulcerative colitis (239, 240). The involvement of environmental variables in the development of IBD has been progressively validated by epidemiological research (241). A recent investigation revealed that the presence of MPs in the fecal matter of individuals with IBD was notably elevated at 41.8 items per gram of dry matter, in comparison to the lower concentration of 28.0 items per gram of dry matter in healthy subjects. Furthermore, a direct relationship was observed between the concentration of MPs and the severity of the disease (178). These findings imply that MPs have a significant role in the onset and course of IBD as an environmental factor. The fundamental processes behind the association between MP exposure and IBD are still unknown despite a lack of studies in this area (235). To ascertain the impact of MPs on the development of IBD, it is imperative to gain a comprehensive understanding of cytokines and their interplay with the pathogenesis of IBD (242). After cells are exposed to plastic particles, higher production levels of the cytokine interleukin 6 (IL-6) are seen. Furthermore, individuals with IBD exhibit elevated levels of IL-6 production by CD4+ T-cells and macrophages (167, 179, 243–246). The presence of MPs in the GI tract can disrupt the equilibrium of the intestinal immune system, leading to non-specific immune stress and impacting the integrity of the intestinal barrier (247). Liu et al. (248) conducted a study wherein they observed that 20 μg/mL polystyrene microplastics (PS-MPs) decreased the expression of TJ-related genes ZO-1 and Occludin and had a proinflammatory effect on colonic cell model Caco-2 cells. These findings suggest that PS-MPs can directly impact the structure and function of intestinal cells (248). Li et al. (243) discovered that feeding mice 600 μg/day PE-MPs for 5 weeks may raise the level of IL-1α in the serum and activate the signal pathways for TLR4/AP-1 (Toll-like receptor 4/Activated protein 1) and TLR4/IRF5 (Interferon Regulatory Factor 5) (243). Proinflammatory transcription factors that can stimulate inflammatory macrophage polarization, cell death, and cytokine production are activated by these pathways (249, 250). In the research conducted by Sun et al. (251), mice were given PE-MPs orally at doses of 0, 0.002, and 0.2 μg/g/d for 30 days. The investigators noted elevated levels of IL-1β and IL-6 in the high-dose group, suggesting that PE-MPs may induce a mild inflammatory reaction in the colon (251). Notwithstanding the absence of PS-MP accumulation in the gut, Rawle et al. (252) subjected mice to 80 μg/kg/d PS-MPs for 4 weeks and discovered substantial transcriptional alterations linked to inflammation in the colon (252).
MPs and ocular surface
Research conducted in vitro demonstrated that human corneal and conjunctival epithelial cell lines could absorb MP particles made of PS, which would then gather around the nuclei of the cells. These particles were cytotoxic, as evidenced by the reduced cell viability and proliferation indicators (180). To investigate the effects of MP exposure on the ocular surface in mouse models, test animals were given 2.5 μL of a topical slurry containing 1 mg/mL of either 50 nm or two μm PS-MPs three times a day, without anesthesia, over 2–4 weeks. The control group was administered a normal saline treatment, while a separate group, referred to as the standard group, did not undergo any interventions (180). Weekly assessment of ocular surface fluorescein staining revealed a notable increase in staining within the test group, while no such increase was observed in the control or regular group. Interestingly, the mice receiving normal saline delivery showed intermittent punctate staining. The storage conditions of the normal saline, likely in a plastic container, were not specified, and there was no indication of pre-testing the normal saline solution for MPs. The production of tear film was examined weekly using a phenol red thread test. A decrease in the production of tears was observed, and this reduction in tear secretion persisted throughout the study in both treatment groups. Further evidence of a progressive build-up of MP particles in the lower conjunctival sac was provided by stereo-fluorescence microscopy. After the research, an analysis of ex vivo tissues revealed that, compared to the control group, the goblet cells in the lower lid had shrunk in size and density. In addition, there was a downregulation of proliferation-related markers (p63, Ki-67, and K14) in the treatment groups compared to the controls. In both treatment groups, the arrangement of lacrimal gland acini was different from that of the standard and control groups. There have also been reports of inflammatory cells between acini and time-dependent elevation of inflammatory factors and cytokines (IL-1α, IL1-β, and IL-6). Mice given the fluid containing 50 nm MP particles showed more excellent apoptosis rates than those given the suspension containing two μm MP particles (180). When particulate matter 2.5 (PM2.5) environmental pollutants, which may contain MPs, are exposed to the murine ocular surface, the result is decreased tear volume, a slower break-up of the tear film, and the loss of corneal epithelial microvilli and corneal desmosomes (181). Elevated concentrations of Tumor necrosis factor alpha (TNF-α) and Nuclear factor kappa B (NF-κB) p65 (Ser-536 phosphorylation) on the ocular surface indicated the presence of ocular surface abnormalities resembling those observed in individuals with dry eye disease (182). A recent multicenter cohort study conducted in China involving 387 individuals diagnosed with dry eye disease observed that areas with elevated levels of PM2.5 were associated with more severe Ocular Surface Disease Index (OSDI) scores, greater incidence of meibomian gland dysfunction, and elevated concentrations of IL-8 and IL-6 (183).
MPs and male fertility
Numerous environmental pollutants have the potential to function as endocrine-disrupting chemicals (EDCs), imitating the actions of natural steroid hormones and disrupting endocrine processes through various mechanisms (184). Recently, there has been a notable focus on plastic additives, plasticizers, and emerging contaminants of concern (CECs), such as personal care products, pharmaceuticals, food additives, natural and synthetic hormones, and micro- and nano-sized. These substances are being released into the environment either directly or indirectly (184). In this regard, substances such as bisphenols, phthalates, poly- and perfluorinated alkyl substances, and others are widely utilized in the manufacturing of everyday consumer products, leading to their frequent release into the environment as waste (185). The adverse effects of EDCs include oxidative stress-induced tissue damage leading to apoptosis, developmental abnormalities, impaired gamete quality, metabolic disorders, neurotoxicity, and epigenetic changes due to in-utero exposure (185–189, 253). Research has indicated that exposure to MPs can lead to abnormalities in the structure of testicular and sperm cells, reduced sperm viability, and disruption of the endocrine system in male individuals (190). The harm inflicted by MPs on the male reproductive system may result in reproductive dysfunction and reduced fertility (191). The impact of the plasticizer bisphenol A (BPA) on spermatogenesis is multifaceted, involving central and local effects. It influences steroid biosynthesis, triggers apoptosis in germ and Sertoli cells, disrupts the initial stage of spermatogenesis, hinders the development of the blood-testis barrier, and alters the expression patterns of non-coding RNA, consequently impacting sperm quality (184). Various results regarding male reproductive effects have been documented about the method of exposure, levels of exposure, duration of exposure, and developmental stage. Research conducted on humans has compared levels of BPA in urine with semen parameters, suggesting a potential correlation between BPA exposure and decreased semen quality (192–194). For instance, Pollards et al. (193) demonstrated an increased exposure to BPA correlated with atypical sperm tail structure in a group of 161 men between the ages of 18 and 40 who did not have recognized subfertility. Omran et al. (194) documented an inverse relationship between urinary BPA concentrations and antioxidant levels, as well as semen quality parameters such as morphology, motility, and concentration. Additionally, they observed a positive correlation between BPA levels and DNA damage, as well as lipid peroxidation in seminal plasma. Finally, a potential association between the presence of BPA/phthalates metabolites in urine and sperm characteristics was examined, indicating a higher level of exposure to EDCs in individuals with reduced fertility compared to the broader population (195).
Effects of MPs on microbiota
Healthy and sustainable ecosystems depend on the proper functioning of microbiota, with the diversity and quantity of microorganisms within a healthy microbiota believed to remain relatively constant (254). The microbiota comprises microorganisms that exhibit symbiotic, pathogenic, or commensal relationships. In multicellular organisms, the intestinal microbiota plays a crucial role in preventing diseases by creating a protective barrier against potential pathogens and enhancing GI physiology and mucosal immunity (255, 256). The utilization of mice as a model to investigate the effects of MPs on microbiota in organisms revealed that the absorption of PE led to an elevation in inflammatory markers such as IL-1β, IL-6, IL-8, and IL-10. Additionally, it resulted in a reduction in colon mucin expression, disturbance in lipopolysaccharide (LPS) metabolism, and an increase in the amino acid metabolism pathway of the microflora by modifying the composition of intestinal microflora (251). The microbiome plays a crucial role in preventing the introduction of novel bacterial strains from the surrounding environment through colonization. Disruption of this protective barrier could potentially facilitate the colonization of pathogens and contribute to the onset of disease (257). An instance of this is when Chinese mitten crabs (Eriocheir sinensis) were subjected to MPs, leading to the upregulation of immune-related genes and a reduction in the population of Firmicutes and Bacteroidetes, which are recognized as the predominant bacterial species in the GI tract (258). Members of Parliament MPs have been found to disrupt the balance of gut microbiota and induce inflammation in the intestines by promoting the growth of Proteobacteria and increasing LPS production in Danio rerio, a widely used aquatic model organism (259). Moreover, MPs have the potential to elevate the levels of reactive oxygen species (ROS) within various microorganisms, such as Danio rerio and Sparus aurata Linnaeus, by influencing the composition of bacterial populations in their microbiota, including Proteobacteria, Fusobacteria, Bacteroidetes, and Firmicutes (260, 261). Scholars have also documented the adverse effects of MPs on the growth and regeneration of epithelial cells in the intestinal tract of Danio rerio, a vertebrate species, through the reduction of Pseudomonas and Aeromonas populations (262). Within marine ecosystems, sediments serve as a primary reservoir of organic carbon, with the microbiota inhabiting these sediments playing a significant role in the biogeochemical processes and nutrient cycling within the ecosystem. Disturbing data indicates that MPs are disrupting the equilibrium of microbial communities in marine sediments. Seeley et al. (263) found that MPs have antibacterial properties that support certain types of bacteria, such as sulfate reducers, while hindering others like nitrifiers. Additionally, their research suggests that MPs could serve as a carbon source for specific microbial communities in sediment, such as Acidobacteria, Bacteroidetes, and Chloroflexi (264). Microorganisms functioning as decomposers play a crucial role in circulating organic compounds and energy within the soil ecosystem. However, introducing MPs into this ecosystem disrupts the equilibrium of bacterial populations. Research indicates that certain bacteria, such as Rhodococcus ruber and Actinomadura sp., can utilize MPs as a source of energy. However, the degradation process of MPs by these bacteria can lead to the release of harmful compounds like phthalates, which can adversely affect soil biota (260, 265). Moreover, due to the high hydrophobic nature of MPs, certain environmental pollutants like antibiotics and heavy metals tend to adhere to their surface through adsorption. The hazardous compound mixtures found in MPs have the potential to exert a more substantial influence on microbiota compared to the MP particles alone. A recent research study indicates that heavy metal concentrations found in MP particles are significantly higher, ranging from 10 to 100 times greater than those typically observed in the surrounding local environment (266). Furthermore, the microbiota balance is affected by the form, composition, and concentration of MPs. In this study, Sun et al. (91) examined the impacts of different concentrations and compositions of spherical MPs (150 μm) on the bacterial community within soil. The results indicated that the polymer structure's composition plays a significant role in influencing bacterial reactions within the soil environment (91).
Mechanisms of pollutant adsorption on MPs
MPs serve as absorbers of pollutants in various environments because of their elevated surface area relative to their volume and their unique chemical characteristics (267, 268). When MPs break down into smaller plastic particles, more of their surface area is exposed, increasing their chemical reactivity, which might improve the adsorption of pollutants on MPs. Environmental factors that can have a substantial impact on the kinetics of contaminant adsorption onto MPs include weathering, UV, pH, and the hydrophobicity of persistent organic pollutants (POPs) (269). The efficiency of MP treatment and other emerging contaminants coexisting in the aquatic environment may be affected by MPs with adsorbed contaminants (c-MPs). However, studies on the mechanisms of contaminant adsorption on MPs, the fate and transport of MPs with adsorbed contaminants, and the effectiveness of MP treatment are often lacking. Per- and poly-fluoroalkyl substances (PFAS), one of the growing pollutants, have become a greater threat to human health due to their extensive use, manufacture, and resilience to environmental degradation (267, 270). Of all the perfluorinated compounds (PFCs), perfluorooctanoic acid (PFOA), and perfluorooctane sulfonate (PFOS) are particularly concerning due to their high stability, unclear destination, and frequent discovery in the environment, animals, and even human bodies (271). It's possible that newly identified pollutants of concern, such as per- and poly-fluoroalkyl substances (PFAS) polycyclic aromatic hydrocarbons (PAHs) and polychlorinated biphenyls (PCBs), will be adsorbed on MPs. Due to the widespread discovery of PFAS in drinking water, surface water, and wastewater treatment facilities, as well as their resistance to degradation and chemical stability, PFAS has recently been a significant source of worry (2, 272–281). PFAS consists of two primary categories, namely PFOA and PFOS, which have garnered escalating concern due to their adverse impacts on both public health and the environment (282). MPs readily absorb other POPs and pollutants (such as EDCs, PBDEs, and PPCPs) in aqueous conditions due to their hydrophobic nature. Because of their vast surface area and hydrophobicity, MPs may adsorb contaminants, which might lead to pollutants associated with MPs being released into the environment. The process of adsorption and desorption of pollutants onto and from MPs is intricate within diverse environmental settings due to a combination of dynamic variables including the characteristics of MPs (such as composition, structure, binding energy, and surface properties), the medium in which they are released (including pH, temperature, salinity, and ionic strength), and factors related to contamination (such as solubility, redox state, charges, and stability) (283–286). For instance, when benzo(a)pyrene is adsorbed onto PVC MPs, it exhibits a time- and dose-dependent adsorption kinetics that results in heightened toxicity levels compared to both unaltered MPs and benzo(a)pyrene in isolation. This underscores the substantial function of MPs as carriers for organic pollutants within sediment environments and highlights the potential synergistic impact of pollutant-absorbing MPs (287, 288). Under different environmental conditions, the pollutants could influence MPs' transition into byproducts including plastic particles, however, this information isn't documented in the literature. Regarding the processes of pollutants adsorbed on hydrophobic adsorbents, hydrophobic interaction, electrostatic repulsion and attraction, pore obstruction, and site competition may be the main mechanisms involved in c-MPs (289–292). MPs' hydrophobicity (KOW) and weathering/aging processes are the mechanisms by which contaminants adsorb onto them (293). Depending on the kind of MP, such as PE, PS, PP, and PVC, the adsorption/desorption kinetics may vary; PE (rubbery polymer PE) has greater adsorption than that of other forms of MPs (64). Even at high temperatures, a PFAS molecule with a negatively charged head and a hydrophobic C–F chain remains chemically stable (294, 295). The processes by which PFAS adhere to MPs may entail electrostatic and hydrophobic interactions, which play a significant role in the adsorption of PFAS onto various adsorbent substrates (296). Hydrogen bonding and covalent bonding can also be observed in the interactions between PFAS and adsorbents (296). The occurrence of either electrostatic repulsion or electrostatic interaction is dependent on the surface charge of adsorbents, with repulsion taking place when the adsorbent surface carries a negative charge, and interaction occurring when the surface charge is positive. For short-chain PFAS, electrostatic interactions seem to play a primary role, while longer PFAS tend to adsorb through hydrophobic interactions, promoting the formation of molecular aggregates of PFAS on the active surface of the adsorbent (297–299). The presence of organic matter (OM) in the environment can impact the adsorption of both long- and short-chain PFAS on MPs due to the complexation of PFAS with OM or co-sorption (296, 300). As a result, PFAS adsorption on OM in the presence of MPs may happen as a result of hydrophobic or electrostatic interactions between PFAS and OM-adsorbed MP surfaces (294, 296). The research found that OM inhibited the sorption of PFOA on active carbon fiber, but no discernible sorption happened when the quantity of OM was increased to 500 mg L−1 (301). The findings back up the competitive sorption between OM and PFOA as well as OM's pore-blocking of active carbon fiber. Through the clarification of the processes involved in the adsorption of pollutants onto MPs, a deeper comprehension can be gained regarding the destiny, movement, and environmental consequences of pollutants associated with MPs (267, 268, 292, 302).
New methods of removing MPs
Numerous policies and initiatives exist at both the domestic and global levels to mitigate pollution caused by MPs. On a worldwide scale, the United Nations has initiated efforts to combat MP pollution by launching the Clean Seas campaign, which aims to eliminate primary sources of plastic and MP pollution in the oceans (303). The campaign is centered on advocating for the decrease and eradication of disposable plastics, enhancing waste disposal practices, and raising public consciousness (303, 304). In Europe, the European Union has enforced a prohibition on using MPs in personal care items, including facial cleansers and toothpaste (305, 306). The EU has also suggested implementing a prohibition on disposable plastics, encompassing items like utensils and drinking straws (307). Various strategies are used in MP removal and elimination procedures to address the problem of MP contamination (308). Studies on the degradation of MPs have advanced with a specific emphasis on biological and non-biological methodologies. The utilization of microorganisms such as algae, bacteria, and fungi for the degradation of MPs is viewed as a promising method for cost-efficient and environmentally friendly treatment strategies (309). The process of wastewater treatment is essential for the effective capture and removal of MPs from wastewater before its release into aquatic environments. Sophisticated treatment methods such as membrane filtration and activated sludge systems are utilized for this objective (308). Various filtration systems such as sand filters, mesh screens, and activated carbon filters are employed to capture larger MP particles from water sources. In regions characterized by elevated levels of MP accumulation, floating boom systems may be utilized to confine and retrieve floating plastic waste, which encompasses MPs. One commonly employed approach involves manually extracting visible plastic waste from rivers, shorelines, and beaches as part of clean-up efforts. Moreover, novel technologies such as electrocoagulation, magnetic nano adsorbents, and ultrasonic treatment are currently under investigation for their potential to improve the efficiency of removing MPs (310). The process of biological decomposition of MPs involves the presence of numerous enzymes (311, 312). Various extracellular enzymes such as lipases, esterases, laccases, lignin peroxidases, and manganese peroxidases are crucial in the degradation of MPs. These enzymes enhance the hydrophilicity of MPs and transform them into carbonyl or alcohol residues (313). Hydrolase enzymes, including esterases, lipases, and cutinases, facilitate the degradation of MPs on plastic surfaces by promoting chain cleavage reactions. These enzymes are unable to penetrate the polymer matrix. However, they exert their catalytic activity on the surface, leading to the development of fissures. The resulting monomers are absorbed into the cytoplasm of microorganisms and subsequently participate in various metabolic pathways (309). In the following, we will discuss novel methods for MP removal by algae, fungi, and bacteria.
Algae in the degradation of MPs
Microalgae, along with their enzymes and toxins, have demonstrated efficacy in the enzymatic degradation of polymeric substances (314–316). One primary benefit is that they do not necessitate a high carbon source for their growth in contrast to bacterial systems, and they are well-suited to a diverse range of environments where most MPs are found (317). Microalgae have been observed to attach to plastic surfaces within wastewater streams, which leads to the initiation of plastic degradation through the secretion of ligninolytic and exopolysaccharide enzymes. Primarily, these polymers function as a carbon reservoir, augmenting cellular proteins, and carbohydrates, thereby enhancing the growth rate. Most recently, the surface deterioration or disintegration of low-density PE sheets due to algal colonization has been detected through scanning electron microscopy (SEM) (318). Algal biodegradation primarily occurs through, including hydrolysis, corrosion, fouling, penetration, and other processes (315). Phormidium lucidum and Oscillatoria subbrevis were identified as capable of inhabiting the surface of low-density polyethylene and breaking it down without the need for prooxidative additives or prior treatment (319). The compound BPA, which exhibits estrogenic properties and is frequently present in polymers, was decomposed through a collaborative effort involving various bacteria and algae species such as Chlorella fusca var. vacuolate, Stephanodiscus hantzschii, Chlorella vulgaris, and Chlamydomonas mexicana (320–322). Recent advancements in various biotechnological methods have enabled the development of genetically modified microalgal cell factories that can produce and release enzymes necessary for the degradation of plastics (323). The green microalgae Chlamydomonas reinhardtii underwent genetic modification to express Polyethylene terephthalate (PET) hydrolase, an enzyme capable of breaking down PET films and terephthalic acid (324). A comparable alteration was effectively implemented in P. tricornutum, resulting in the production of PET hydrolase that exhibited catalytic efficacy toward PET and the copolymer polyethylene terephthalate glycol (PETG) (314). In conclusion, microalgae may use plastic monomers as a carbon source by producing degrading enzymes, and because they are simple to grow, they have the potential to be effective MP degraders (325).
Fungal degradation of MPs
The fungi encompass various organisms that primarily function as saprotrophs, opportunistic parasites, or obligate parasites. They exhibit remarkable adaptability and are capable of thriving in a variety of habitats, including aquatic and terrestrial ecosystems, across a range of environmental conditions. In addition to their ability to withstand harmful chemicals and metals, these organisms exhibit a wide array of external enzymes and natural surfactants, such as hydrophobins, which can break down intricate polymers into basic monomers. This process enables them to serve as a supplier of electrons and carbon for microorganisms, thereby aiding in the breakdown and conversion of complex pollutants into simpler forms (326, 327). The primary genera linked to the decomposition of various polymer varieties like PE, PET, and PP consist of Cladosporium, Aspergillus niger, Zalerion maritimum, and Penicillium simplicissimum (328–330). These microorganisms utilize MPs as their exclusive carbon source after the breakdown enable by extracellular enzymes. They facilitate the creation of various chemical bonds characterized by carboxyl, carbonyl, and ester functional groups while reducing their hydrophobic nature. The deterioration of PU material was observed in multiple fungal species, including Cladosporium pseudocladosporioides, Aspergillus tubingensis, Aspergillus fumigatus, Penicillium chrysogenum, and Fusarium solani, and in strains of Pestalotiopsis microspora (331–334). In most instances, serine hydrolase serves as a crucial factor in the process of PU degradation. The breakdown of high-density PE in marine coastal environments by two fungal strains, Aspergillus tubingensis VRKPT1 and Aspergillus flavus VRKPT2, was found to be approximately 6.02 ± 0.2% and 8.51 ± 0.1%, respectively (330). In a recent study, Kunlere et al. (335) documented the effective breakdown of low-density PE by Aspergillus flavus and Mucor circinelloides strains obtained from a municipal landfill. Before biodegradation, the MPs, specifically PE, can be subjected to pretreatment using substances like sodium hydroxide and nitric acid. This process has been observed to enhance the biodegradation rate of PE by the fungus Aspergillus niger (336). Thermal oxidation at 80°C for 15 days was necessary to induce degradation in low-density PE facilitated by Penicillium pinophilum and Aspergillus niger, resulting in degradation levels of 0.57% and 0.37% respectively following a 30-month incubation period (337). Likewise, Lysinibacillus spp. and Aspergillus spp. exhibited a biodegradation rate of 29.5% for UV-irradiated polymer films and 15.8% for non-UV-irradiated polymer films (338).
Bacterial degradation of MPs
Various research investigations have been carried out utilizing bacteria to break down MPs. Bacteria with the ability to break down MPs have been identified in multiple environments, such as sludge, wastewater, polluted sediments, compost, municipal landfills, and even in extreme climates like Antarctic soils, mangrove areas, and marine sediments (339, 340). Additionally, microorganisms capable of degrading MPs have been identified within the GI microbiota of earthworms. It's commonly known that microorganisms that reside in contaminated areas frequently learn how to activate the enzyme system that breaks down MPs (341). Both individual bacterial strains and mixed bacterial communities can be employed to degrade MPs. Nevertheless, using pure cultures provides numerous benefits in the degradation process, serving as a practical method for investigating the metabolic pathways associated with this process. Furthermore, the influence of environmental elements such as pH, temperature, substrate properties, and surfactants on the degradation process can be more readily observed (342). The initial investigation into MP biodegradation by microorganisms was carried out by Cacciari et al. (343), who utilized a combination of Pseudomonas stutzeri, Pseudomonas chlororaphis, and Vibrio sp. to facilitate the degradation of PP. Similarly, the study also found that the inclusion of starch was observed to enhance the biodegradability capacity. Subsequent studies by Arkatkar et al. (344), as well as Fontanella et al. (345), documented the biodegradation of PP through the utilization of a mixed culture comprising Pseudomonas stutzeri, Rhodococcus rhodochrous, Bacillus subtilis, and B. flexus. In a research investigation by Auta et al. (346) B. gottheilii caused weight reductions of 6.2%, 3.0%, 3.6%, and 5.8% for PE, PET, PP, and PS MPs, respectively (347). Several other bacteria linked to the degradation of PP were identified, such as Pseudomonas, Bacillus, Chelatococcus, and Lysinibacillus fusiformis. These bacteria were isolated from diverse environments, including mangrove habitats, cow dung, compost, and land polluted with plastic waste. The intestinal microbiota of various arthropods such as Plodia interpunctella (Indian meal moth), Tenebrio molitor (mealworms), and Galleria mellonella (wax moths) have been documented to contain microorganisms with the ability to biodegrade MPs (348–350). In a research investigation by Yang et al. (348), a bacterial strain known as Exiguobacterium sp. was extracted from the intestinal tracts of mealworms, demonstrating the capacity to create biofilm structures and break down PS material. Effective degradation of low-density PE was achieved through the utilization of bacterial strains such as Pseudomonas aeruginosa and Microbacterium paraoxydans, resulting in degradation rates of approximately 61.0% and 50.5%, respectively, over 2 months under incubation conditions (351). Likewise, it has been documented that the biofilm produced by Pseudomonas sp. AKS2 can break down low-density PE by approximately 5 ± 1% over a 45-day incubation period without the need for any prior treatment (352). Similarly, the breakdown of PE was documented through the isolation of Rhodococcus ruber C208, with a degradation rate of 0.86% per week (353). The microorganisms obtained from the GI tract consisted of Firmicutes and Actinobacteria genera. These microorganisms were individually investigated and found to possess the capability to break down low-density PE MPs, leading to the release of volatile compounds such as docosane, eicosane, and tricosane. A collaboration between Pseudomonas and Enterobacter bacteria found in cow dung resulted in a weight reduction of up to 15% over 120 days (354). Numerous marine hydrocarbon-degrading bacteria, including Alcanivorax borkumensis, have demonstrated proficiency in breaking down alkanes, alkyl cycloalkanes, isoprenoid hydrocarbons, and branched aliphatic compounds (355). The study was conducted using the identical strain that had previously demonstrated the ability to form biofilms on low-density PE when exposed to hexadecane, pyruvate, and yeast extract, as well as on low-density PE films (356). Various actinomycetes, such as Streptomyces and Rhodococcus ruber, were also found to play a role in the biodegradation of PE (357). In the context of MP degradation, it was observed that Pseudomonas accounted for 21% of the bacterial genera involved, while Bacillus constituted approximately 15%. Additionally, combining these two genera contributed to 17% of the total bacterial population associated with this process (358).
Conclusion
MPs are any type of plastic piece with a length of <5 millimeters, which has become one of the main challenges for the environment and public health. Studies have shown that MPs are found in various ecosystem environments, including marine, air, soil, and freshwater environments, and may enter the food chain. The influences of MPs on oceanic life and other ecosystems are significant, including ingestion by marine animals, interference with their reproductive systems, and even death. The harmful effects of MPs on human health are also severe. Studies have shown that MPs can be influential in various diseases and health complications, including damage to the lungs, eyes, brain, GI system, skin, male fertility, etc. They could discharge dangerous substances into the body, which might lead to several health issues, such as cancer, developmental difficulties, and reproduction issues. In addition, healthy and sustainable ecosystems depend on the proper functioning of microbiota; MPs cause damage to microbiota and upset their balance, which is a severe issue. However, new methods have been proposed to remove MPs, which include the use of algae, fungi, and bacteria. These approaches offer the potential to remove MPs from various environments and can be regarded as viable strategies for addressing this issue. A comprehensive comprehension of the source, classifications, impacts, and remedies associated with MPs is imperative to develop enhanced approaches for mitigating their adverse consequences on the environment and human health, thereby facilitating positive outcomes in this domain. The creation and implementation of strict environmental regulations aimed at managing and reducing MP contamination need to be a top priority for legislators. A few examples of these policies would be to outlaw single-use plastics in specific industries, provide incentives for recycling and reusing recyclable materials, enact laws to reduce the number of MPs released into the environment and promote the widespread use of biodegradable plastics. Scientists and researchers contribute significantly to our growing knowledge of the origins, distribution, and effects of MPs. Research projects examining non-plastic alternatives, creating cutting-edge technologies for MP identification and removal, carrying out long-term studies evaluating the health and environmental impacts of MP pollution, and lessening the harm that MPs cause to the ecosystem should all receive funding and support.
Author contributions
AY: Investigation, Software, Writing – original draft. SH: Writing – original draft. PS: Writing – original draft. HA: Conceptualization, Project administration, Supervision, Writing – review & editing. HK: Conceptualization, Project administration, Supervision, Writing – review & editing.
Funding
The author(s) declare that no financial support was received for the research, authorship, and/or publication of this article.
Conflict of interest
The authors declare that the research was conducted in the absence of any commercial or financial relationships that could be construed as a potential conflict of interest.
Publisher's note
All claims expressed in this article are solely those of the authors and do not necessarily represent those of their affiliated organizations, or those of the publisher, the editors and the reviewers. Any product that may be evaluated in this article, or claim that may be made by its manufacturer, is not guaranteed or endorsed by the publisher.
References
1. Tirkey A, Upadhyay LSB. Microplastics: an overview on separation, identification and characterization of microplastics. Mar Pollut Bull. (2021) 170:112604. doi: 10.1016/j.marpolbul.2021.112604
2. Sun J, Dai X, Wang Q, Van Loosdrecht MC Ni BJ. Microplastics in wastewater treatment plants: Detection, occurrence and removal. Water Res. (2019) 152:21–37. doi: 10.1016/j.watres.2018.12.050
3. Hartmann NB, Huffer T, Thompson RC, Hassellöv M, Verschoor A, Daugaard AE, et al. Are we speaking the same language? Recommendations for a definition and categorization framework for plastic debris. Environ Sci Technol. (2019) 53:1039–47. doi: 10.1021/acs.est.8b05297
4. Thompson RC, Olsen Y, Mitchell RP, Davis A, Rowland SJ, John AWG, et al. Lost at sea: where is all the plastic? Science. (2004) 304:838–838. doi: 10.1126/science.1094559
5. Rafey A, Siddiqui FZ. A review of plastic waste management in India–challenges and opportunities. Int J Environ Analyt Chem. (2023) 103:3971–87. doi: 10.1080/03067319.2021.1917560
6. Ryu HW, Kim DH, Jae J, Lam SS, Park ED, Park Y-K. Recent advances in catalytic co-pyrolysis of biomass and plastic waste for the production of petroleum-like hydrocarbons. Bioresour Technol. (2020) 310:123473. doi: 10.1016/j.biortech.2020.123473
7. Brooks AL, Wang S, Jambeck JR. The Chinese import ban and its impact on global plastic waste trade. Sci Adv. (2018) 4:eaat0131. doi: 10.1126/sciadv.aat0131
8. Ul Hassan HM, Ali Q, Rahman MA, Kamal M, Tanjin S, Farooq U, et al. Growth pattern, condition and prey-predator status of 9 fish species from the Arabian Sea (Baluchistan and Sindh), Pakistan. Egyptian J Aquatic Biol Fisher. (2020) 24:281–92. doi: 10.21608/ejabf.2020.97439
9. Ryan PG, A. brief history of marine litter research. Mar Anthropog Litter. (2015) 2015:1–25. doi: 10.1007/978-3-319-16510-3_1
10. Osman AI, Hosny M, Eltaweil AS, Omar S, Elgarahy AM, Farghali M, et al. Microplastic sources, formation, toxicity and remediation: a review. Environ Chem Lett. (2023) 21:2129–69. doi: 10.1007/s10311-023-01593-3
11. Focardi A, Moore LR, Raina JB, Seymour JR, Paulsen IT, Tetu SG. Plastic leachates impair picophytoplankton and dramatically reshape the marine microbiome. Microbiome. (2022) 10:179. doi: 10.1186/s40168-022-01369-x
12. Lin Z, Jin T, Zou T, Xu L, Xi B, Xu D, et al. Current progress on plastic/microplastic degradation: fact influences and mechanism. Environ Pollut. (2022) 304:119159. doi: 10.1016/j.envpol.2022.119159
13. Liu C, Bunditsakulchai P, Zhuo Q. Impact of COVID-19 on food and plastic waste generated by consumers in Bangkok. Sustainability. (2021) 13:8988. doi: 10.3390/su13168988
14. Walters LJ, Craig CA, Dark E, Wayles J, Encomio V, Coldren G, et al. Quantifying spatial and temporal trends of microplastic pollution in surface water and in the eastern oyster crassostrea virginica for a dynamic florida estuary. Environments. (2022) 9:131. doi: 10.3390/environments9100131
15. Cole M, Lindeque P, Fileman E, Halsband C, Galloway TS. The impact of polystyrene microplastics on feeding, function and fecundity in the marine copepod Calanus helgolandicus. Environ Sci Technol. (2015) 49:1130–7. doi: 10.1021/es504525u
16. Wright SL, et al. Microplastic ingestion decreases energy reserves in marine worms. Current Biol. (2013) 23:R1031–R1033. doi: 10.1016/j.cub.2013.10.068
17. Lian J, Liu W, Meng L, Wu J, Chao L, Zeb A, et al. Foliar-applied polystyrene nanoplastics (PSNPs) reduce the growth and nutritional quality of lettuce (Lactuca sativa L). Environ Pollut. (2021) 280:116978. doi: 10.1016/j.envpol.2021.116978
18. Pflugmacher S, Sulek A, Mader H, Heo J, Noh JH, Penttinen OP, et al. The influence of new and artificial aged microplastic and leachates on the germination of Lepidium sativum L. Plants. (2020) 9:339. doi: 10.3390/plants9030339
19. Edwards S, León-Zayas R, Ditter R, Laster H, Sheehan G, Anderson O, et al. Microbial consortia and mixed plastic waste: pangenomic analysis reveals potential for degradation of multiple plastic types via previously identified PET degrading bacteria. Int J Mol Sci. (2022) 23:5612. doi: 10.3390/ijms23105612
20. Chen JY-S, Lee Y-C, Walther BA. Microplastic contamination of three commonly consumed seafood species from Taiwan: a pilot study. Sustainability. (2020) 12:9543. doi: 10.3390/su12229543
21. Wagner M, Lambert S. Freshwater Microplastics: Emerging Environmental Contaminants? New York: Springer Nature. (2018). doi: 10.1007/978-3-319-61615-5
22. Galloway T, et al. Plastics additives and human health: a case study of Bisphenol A (Bpa). Plast Environ. (2018) 47:131. doi: 10.1039/9781788013314-00131
23. Shin M-Y, Lee S, Choi H, Jeong D-I, Moon H-B, Kim S. Placental and lactational transfer of decabromodiphenyl ether and 2, 2′, 4, 4′-tetrabromodiphenyl ether in dam-offspring pairs of Sprague-Dawley rats. Food Chem. Toxicol. (2017) 102:198–203. doi: 10.1016/j.fct.2017.01.027
24. Sonnemann G, Valdivia S. Medellin declaration on marine litter in life cycle assessment and management: facilitated by the forum for sustainability through life cycle innovation (FSLCI) in close cooperation with La Red Iberoamericana de Ciclo de Vida (RICV) on wednesday 14 of June (2017) Int J Life Cycle Assess (2017) 22:1637–9. doi: 10.1007/s11367-017-1382-z
25. Borrelle SB, Ringma J, Law KL, Monnahan CC, Lebreton L, McGivern A, et al. Predicted growth in plastic waste exceeds efforts to mitigate plastic pollution. Science. (2020) 369:1515–8. doi: 10.1126/science.aba3656
26. Kochanska E, Wozniak K, Nowaczyk A, Piedade PJ, Lavorato MLd, Almeida AM, et al. Global ban on plastic and what next? Are consumers ready to replace plastic with the second-generation bioplastic? Results of the snowball sample consumer research in China, Western and Eastern Europe, North America and Brazil. Int J Environ Res Public Health. (2022) 19:13970. doi: 10.3390/ijerph192113970
27. Alberghini L, Truant A, Santonicola S, Colavita G, Giaccone V. Microplastics in fish and fishery products and risks for human health: a review. Int J Environ Res Public Health. (2022) 20:789. doi: 10.3390/ijerph20010789
28. Issac M, Kandasubramanian NB. Effect of microplastics in water and aquatic systems. Environ Sci Pollut Res. (2021) 28:19544–62. doi: 10.1007/s11356-021-13184-2
29. Henderson L, Green C. Making sense of microplastics? Public understandings of plastic pollution. Mar Pollut Bull. (2020) 152:110908. doi: 10.1016/j.marpolbul.2020.110908
30. Thushari GGN, Senevirathna JDM. Plastic pollution in the marine environment. Heliyon. (2020) 6:e047009. doi: 10.1016/j.heliyon.2020.e04709
31. Choy CA, Robison BH, Gagne TO, Erwin B, Firl E, Halden RU, et al. The vertical distribution and biological transport of marine microplastics across the epipelagic and mesopelagic water column. Sci Rep. (2019) 9:7843. doi: 10.1038/s41598-019-44117-2
32. Rodrigues JP, et al. Significance of interactions between microplastics and POPs in the marine environment: a critical overview. TrAC. (2019) 111:252–60. doi: 10.1016/j.trac.2018.11.038
33. Li J, et al. Effects of microplastics on higher plants: a review. Bull Environ Contam Toxicol. (2022) 109:241–265. doi: 10.1007/s00128-022-03566-8
34. Cózar A, Echevarría F, González-Gordillo JI, Irigoien X, Úbeda B, Hernández-León S, et al. Plastic debris in the open ocean. Proc Natl Acad Sci. (2014) 111:10239–10244. doi: 10.1073/pnas.1314705111
35. Ragusa A, Svelato A, Santacroce C, Catalano P, Notarstefano V, Carnevali O, et al. Plasticenta: First evidence of microplastics in human placenta. Environ Int. (2021) 146:106274. doi: 10.1016/j.envint.2020.106274
36. Pauly JL, Stegmeier SJ, Allaart HA, Cheney RT, Zhang PJ, Mayer AG, et al. Inhaled cellulosic and plastic fibers found in human lung tissue. Cancer Epidemiol Biomark Prev. (1998) 7:419–28.
37. Paul MB, Fahrenson C, Givelet L, Herrmann T, Loeschner K, Böhmert L, et al. Beyond microplastics-investigation on health impacts of submicron and nanoplastic particles after oral uptake in vitro. Microplastics Nanoplast. (2022) 2:16. doi: 10.1186/s43591-022-00036-0
38. Gontard N, et al. Recognizing the long-term impacts of plastic particles for preventing distortion in decision-making. Nat. Sustainab. (2022) 5:472–478. doi: 10.1038/s41893-022-00863-2
39. Lee J, Lee JS, Jang YC, Hong SY, Shim WJ, Song YK, et al. Distribution and size relationships of plastic marine debris on beaches in South Korea. Arch Environ Contam Toxicol. (2015) 69:288–98. doi: 10.1007/s00244-015-0208-x
40. Gigault J, Halle AT, Baudrimont M, Pascal P-Y, Gauffre F, Phi T-L, et al. Current opinion: what is a nanoplastic? Environ Pollut. (2018) 235:1030–4. doi: 10.1016/j.envpol.2018.01.024
41. De-la-Torre GE, Dioses-Salinas DC, Pizarro-Ortega CI, Saldaña-Serrano M. Global distribution of two polystyrene-derived contaminants in the marine environment: a review. Marine Pollut Bull. (2020) 161:111729. doi: 10.1016/j.marpolbul.2020.111729
42. Wang T, Li B, Zou X, Wang Y, Li Y, Xu Y, et al. Emission of primary microplastics in mainland China: invisible but not negligible. Water Res. (2019) 162:214–24. doi: 10.1016/j.watres.2019.06.042
43. Sharma S, Chatterjee S. Microplastic pollution, a threat to marine ecosystem and human health: a short review. Environ Sci Pollut Res. (2017) 24:21530–47. doi: 10.1007/s11356-017-9910-8
44. Ng EL, Lwanga EH, Eldridge SM, Johnston P, Hu HW, Geissen V, et al. An overview of microplastic and nanoplastic pollution in agroecosystems. Sci Total Environ. (2018) 627:1377–88. doi: 10.1016/j.scitotenv.2018.01.341
45. Sun Q, Li J, Wang C, Chen A, You Y, Yang S, et al. Research progress on distribution, sources, identification, toxicity, and biodegradation of microplastics in the ocean, freshwater, and soil environment. Front Environ Sci Eng. (2022) 16:1. doi: 10.1007/s11783-021-1429-z
46. Kalčíková G, Alič B, Skalar T, Bundschuh M, Gotvajn AŽ. Wastewater treatment plant effluents as source of cosmetic polyethylene microbeads to freshwater. Chemosphere. (2017) 188:25–31. doi: 10.1016/j.chemosphere.2017.08.131
47. Napper IE, Thompson RC. Release of synthetic microplastic plastic fibres from domestic washing machines: effects of fabric type and washing conditions. Marine Pollut Bull. (2016) 112:39–45. doi: 10.1016/j.marpolbul.2016.09.025
48. JohnJ, et al. Microplastics in mangroves and coral reef ecosystems: a review. Environ Chem Lett. (2022) 2022:1–20. doi: 10.1007/s10311-021-01326-4
49. Habib RZ, Kindi RA, Salem FA, Kittaneh WF, Poulose V, Iftikhar SH, et al. Microplastic contamination of chicken meat and fish through plastic cutting boards. Int J Environ Res Public Health. (2022) 19:13442. doi: 10.3390/ijerph192013442
50. Li D, Shi Y, Yang L, Xiao L, Kehoe DK, Gun'ko YK, et al. Microplastic release from the degradation of polypropylene feeding bottles during infant formula preparation. Nat Food. (2020) 1:746–54. doi: 10.1038/s43016-020-00171-y
51. Field DT, Green JL, Bennett R, Jenner LC, Sadofsky LR, Chapman E, et al. Microplastics in the surgical environment. Environ Int. (2022) 170:107630. doi: 10.1016/j.envint.2022.107630
52. Fadare OO, Wan B, Guo L-H, Zhao L. Microplastics from consumer plastic food containers: are we consuming it? Chemosphere. (2020) 253:126787. doi: 10.1016/j.chemosphere.2020.126787
53. Chen H, Xu L, Yu K, Wei F, Zhang M. Release of microplastics from disposable cups in daily use. Sci Total Enviro. (2023) 854:158606. doi: 10.1016/j.scitotenv.2022.158606
54. Mei T, Wang J, Xiao X, Lv J, Li Q, Dai H, et al. Identification and evaluation of microplastics from tea filter bags based on Raman imaging. Foods. (2022) 11:2871. doi: 10.3390/foods11182871
55. Li W, Zu B, Yang Q, Guo J, Li J. Sources, distribution, and environmental effects of microplastics: a systematic review. RSC Adv. (2023) 13:15566–74. doi: 10.1039/D3RA02169F
56. Andrady AL. Microplastics in the marine environment. Marine Pollut Bull. (2011) 62:1596–1605. doi: 10.1016/j.marpolbul.2011.05.030
57. Carlini G, Kleine K. Advancing the international regulation of plastic pollution beyond the United Nations Environment Assembly resolution on marine litter and microplastics. Rev Eur Compar Int Environ Law. (2018) 27:234–244. doi: 10.1111/reel.12258
58. Ghosh S, Sinha JK, Ghosh S, Vashisth K, Han S, Bhaskar R. Microplastics as an emerging threat to the global environment and human health. Sustainability. (2023) 15:10821. doi: 10.3390/su151410821
59. von Knoch M, Sprecher C, Barden B, Saxler G, Löer F, Wimmer M. Size and shape of commercially available polyethylene particles for in-vitro and in-vivo-experiments. Z Orthop Grenzgebiete. (2004) 142:366–70. doi: 10.1055/s-2004-822589
60. Savrik S, et al. Characterization of poly (vinyl chloride) powder produced by emulsion polymerization. J Thermal Anal Calorimetry. (2010) 101:801–806. doi: 10.1007/s10973-010-0942-2
61. Busico V, Cipullo R. Microstructure of polypropylene. Progr Polymer Sci. (2001) 26:443–533. doi: 10.1016/S0079-6700(00)00046-0
62. Yoon DY, Sundararajan PR, Flory PJ. Conformational characteristics of polystyrene. Macromolecules. (1975) 8:776–783. doi: 10.1021/ma60048a019
63. Palmer RJ. Polyamides, plastics. In: Kirk-Othmer Encyclopedia of Chemical Technology. Wiley Online Library (2000). doi: 10.1002/0471238961.1612011916011213.a01
64. Alimi OS, Budarz JF, Hernandez LM, Tufenkji N. Microplastics and nanoplastics in aquatic environments: aggregation, deposition, and enhanced contaminant transport. Environ Sci Technol. (2018) 52:1704–24. doi: 10.1021/acs.est.7b05559
65. Truong T-N-S, Strady E, Kieu-Le T-C, Tran Q-V, Le T-M-T, Thuong Q-T. Microplastic in atmospheric fallouts of a developing Southeast Asian megacity under tropical climate. Chemosphere. (2021) 272:129874. doi: 10.1016/j.chemosphere.2021.129874
66. Zhang S, Wang J, Yan P, Hao X, Xu B, Wang W, et al. Non-biodegradable microplastics in soils: a brief review and challenge. J Hazard Mater. (2021) 409:124525. doi: 10.1016/j.jhazmat.2020.124525
67. Tang Y, Liu Y, Chen Y, Zhang W, Zhao J, He S, Yang C, Zhang T, Tang C, Zhang C, Yang Z. A review: Research progress on microplastic pollutants in aquatic environments. Sci Total Environ. (2021) 766:142572. doi: 10.1016/j.scitotenv.2020.142572
68. Zarus GM, et al. A review of data for quantifying human exposures to micro and nanoplastics and potential health risks. Sci Total Environ. (2021) 756:144010. doi: 10.1016/j.scitotenv.2020.144010
69. Bejgarn S, MacLeod M, Bogdal C, Breitholtz M. Toxicity of leachate from weathering plastics: an exploratory screening study with Nitocra spinipes. Chemosphere. (2015) 132:114–9. doi: 10.1016/j.chemosphere.2015.03.010
70. Cai M, He H, Liu M, Li S, Tang G, Wang W, et al. Lost but can't be neglected: huge quantities of small microplastics hide in the South China Sea. Sci Total Environ. (2018) 633:1206–16. doi: 10.1016/j.scitotenv.2018.03.197
71. Carson HS, Lamson MR, Nakashima D, Toloumu D, Hafner J, Maximenko N, et al. Tracking the sources and sinks of local marine debris in Hawai ‘i. Mar Environ Res. (2013) 84:76–83. doi: 10.1016/j.marenvres.2012.12.002
72. Lusher AL, Tirelli V, O'Connor I, Officer R. Microplastics in Arctic polar waters: the first reported values of particles in surface and sub-surface samples. Sci Rep. (2015) 5:14947. doi: 10.1038/srep14947
73. Li, Q, X. Sun, Progress on microplastics research in the Yellow Sea, China. Anthropocene Coasts, (2020) 3:43–52. doi: 10.1139/anc-2018-0033
74. Cheung CK, Not HC. Impacts of extreme weather events on microplastic distribution in coastal environments. Sci Total Environ. (2023) 904:166723. doi: 10.1016/j.scitotenv.2023.166723
75. Hitchcock JN. Storm events as key moments of microplastic contamination in aquatic ecosystems. Sci Total Environ. (2020) 734:139436. doi: 10.1016/j.scitotenv.2020.139436
76. Yonkos LT, Friedel EA, Perez-Reyes AC, Ghosal S, Arthur CD. Microplastics in four estuarine rivers in the Chesapeake Bay, USA. Environ Sci Technol. (2014) 48:14195–202. doi: 10.1021/es5036317
77. Collins C, Hermes J. Modelling the accumulation and transport of floating marine micro-plastics around South Africa. Marine Pollut Bull. (2019) 139:46–58. doi: 10.1016/j.marpolbul.2018.12.028
78. Schernewski G, Radtke H, Robbe E, Haseler M, Hauk R, Meyer L, et al. Emission, transport, and deposition of visible plastics in an estuary and the Baltic Sea—A monitoring and modeling approach. Environ Manage. (2021) 68:860–81. doi: 10.1007/s00267-021-01534-2
79. Xue B, Zhang L, Li R, Wang Y, Guo J, Yu K, et al. Underestimated microplastic pollution derived from fishery activities and “hidden” in deep sediment. Environ Sci Technol. (2020) 54:2210–7. doi: 10.1021/acs.est.9b04850
80. Kooi M, Primpke S, Mintenig SM, Lorenz C, Gerdts G, Koelmans AA. Characterizing the multidimensionality of microplastics across environmental compartments. Water Res. (2021) 202:117429. doi: 10.1016/j.watres.2021.117429
81. Li Y, Zhang H, Tang C. A review of possible pathways of marine microplastics transport in the ocean. Anthropocene Coasts. (2020) 3:6–13. doi: 10.1139/anc-2018-0030
82. Wang X, Bolan N, Tsang DCW, Sarkar B, Bradney L, Li Y, et al. review of microplastics aggregation in aquatic environment: Influence factors, analytical methods, and environmental implications. J Hazard Mater. (2021) 402:123496. doi: 10.1016/j.jhazmat.2020.123496
83. Prata JC, et al. A One Health perspective of the impacts of microplastics on animal, human and environmental health. Sci Total Environ. (2021) 777:146094. doi: 10.1016/j.scitotenv.2021.146094
84. Mamun AA, Prasetya TAE, Dewi IR. Ahmad M. Microplastics in human food chains: food becoming a threat to health safety. Sci Total Environ. (2023) 858:159834. doi: 10.1016/j.scitotenv.2022.159834
85. He S, Jia M, Xiang Y, Song B, Xiong W, Cao J, et al. Biofilm on microplastics in aqueous environment: Physicochemical properties and environmental implications. J Hazard Mater. (2022) 424:127286. doi: 10.1016/j.jhazmat.2021.127286
86. Ren Z, Gui X, Xu X, Zhao L, Qiu H. Cao X. Microplastics in the soil-groundwater environment: aging, migration, and co-transport of contaminants–a critical review. J Hazard Mater. (2021) 419:126455. doi: 10.1016/j.jhazmat.2021.126455
87. Hu D, Shen M, Zhang Y, Li H. Zeng G. Microplastics and nanoplastics: would they affect global biodiversity change?. Environ Sci Pollut Res. (2019) 26:19997–20002. doi: 10.1007/s11356-019-05414-5
88. Agathokleous E, et al. Ecological risks in a ‘plastic'world: a threat to biological diversity?. J Hazard Mater. (2021) 417:126035. doi: 10.1016/j.jhazmat.2021.126035
89. Ding J, Li J, Sun C, Jiang F, He C, Zhang M, et al. An examination of the occurrence and potential risks of microplastics across various shellfish. Sci Total Environ. (2020) 739:139887. doi: 10.1016/j.scitotenv.2020.139887
90. Raju P, Santhanam P, Perumal P. Impacts of microplastics on marine organisms: present perspectives and the way forward. Egyptian J Aquatic Res. (2022) 48:205–209. doi: 10.1016/j.ejar.2022.03.001
91. Sun Y, Duan C, Cao N, Li X, Li X, Chen Y, et al. Effects of microplastics on soil microbiome: The impacts of polymer type, shape, and concentration. Sci Total Environ. (2022) 806:150516. doi: 10.1016/j.scitotenv.2021.150516
92. Wang F, et al. Effects of microplastics on soil properties: current knowledge and future perspectives. J Hazardous Mater. (2022) 424:127531. doi: 10.1016/j.jhazmat.2021.127531
93. Zhou B, Wang J, Zhang H, Shi H, Fei Y, Huang S, et al. Microplastics in agricultural soils on the coastal plain of Hangzhou Bay, east China: Multiple sources other than plastic mulching film. J Hazard Mater. (2020) 388:121814. doi: 10.1016/j.jhazmat.2019.121814
94. Yang L, et al. Microplastics in soil: a review on methods, occurrence, sources, and potential risk. Sci Total Environ. (2021) 780:146546. doi: 10.1016/j.scitotenv.2021.146546
95. Katsumi N, et al. Accumulation of microcapsules derived from coated fertilizer in paddy fields. Chemosphere. (2021) 267:129185. doi: 10.1016/j.chemosphere.2020.129185
96. Weithmann N, et al. Organic fertilizer as a vehicle for the entry of microplastic into the environment. Sci Adv. (2018) 4:eaap8060. doi: 10.1126/sciadv.aap8060
97. Zubris KAV, Richards BK. Synthetic fibers as an indicator of land application of sludge. Environ. Pollut. (2005) 138:201–211. doi: 10.1016/j.envpol.2005.04.013
98. de Souza Machado AA, Lau CW, Kloas W, Bergmann J, Bachelier JB, Faltin E, et al. Microplastics can change soil properties and affect plant performance. Environ. Sci. Technol. (2019) 53:6044–6052. doi: 10.1021/acs.est.9b01339
99. Tibbett M, Fraser TD, Duddigan S. Identifying potential threats to soil biodiversity. PeerJ. (2020) 8:e9271. doi: 10.7717/peerj.9271
100. de Souza Machado AA, Lau CW, Till J, Kloas W, Lehmann A, Becker R, et al. Impacts of microplastics on the soil biophysical environment. Environ Sci Technol. (2018) 52:9656–9665. doi: 10.1021/acs.est.8b02212
101. Chauhan JS, Semwal D, Nainwal M, Badola N, Thapliyal P. Investigation of microplastic pollution in river Alaknanda stretch of Uttarakhand. Environ Dev Sustain. (2021) 63:16819–16833. doi: 10.1007/s10668-021-01388-y
102. Liu Y, Zhang J, Cai C, He Y, Chen L, Xiong X, et al. Occurrence and characteristics of microplastics in the Haihe River: an investigation of a seagoing river flowing through a megacity in northern China. Environ Pollut. (2020) 262:114261. doi: 10.1016/j.envpol.2020.114261
103. Shruti VC, Jonathan MP, Rodriguez-Espinosa PF, Rodríguez-González F. Microplastics in freshwater sediments of atoyac river basin, puebla city, Mexico. Sci Total Environ. (2019) 654:154–63. doi: 10.1016/j.scitotenv.2018.11.054
104. Yin L, Wen X, Du C, Jiang J, Wu L, Zhang Y, et al. Comparison of the abundance of microplastics between rural and urban areas: a case study from East Dongting Lake. Chemosphere. (2020) 244:125486. doi: 10.1016/j.chemosphere.2019.125486
105. Talbot R, Chang H. Microplastics in freshwater: a global review of factors affecting spatial and temporal variations. Environ Pollut. (2022) 292:118393. doi: 10.1016/j.envpol.2021.118393
106. Kabir AHME, Sekine M, Imai T, Yamamoto K, Kanno A, Higuchi T. Assessing small-scale freshwater microplastics pollution, land-use, source-to-sink conduits, and pollution risks: Perspectives from Japanese rivers polluted with microplastics. Sci Total Environ. (2021) 768:144655. doi: 10.1016/j.scitotenv.2020.144655
107. Wang Z, Zhang Y, Kang S, Yang L, Shi H, Tripathee L, et al. Research progresses of microplastic pollution in freshwater systems. Sci Total Environ. (2021) 795:148888. doi: 10.1016/j.scitotenv.2021.148888
108. Lechner A, Ramler D. The discharge of certain amounts of industrial microplastic from a production plant into the River Danube is permitted by the Austrian legislation. Environ Pollut. (2015) 200:159–60. doi: 10.1016/j.envpol.2015.02.019
109. Fan Y, Zheng K, Zhu Z, Chen G, Peng X. Distribution, sedimentary record, and persistence of microplastics in the Pearl River catchment, China. Environ Pollut. (2019) 251:862–70. doi: 10.1016/j.envpol.2019.05.056
110. Lasee S, Mauricio J, Thompson WA, Karnjanapiboonwong A, Kasumba J, Subbiah S, et al. Microplastics in a freshwater environment receiving treated wastewater effluent. Integr Environ Assess Manag. (2017) 13:528–32. doi: 10.1002/ieam.1915
111. Li C, Busquets R, Campos LC. Assessment of microplastics in freshwater systems: a review. Sci Total Environ. (2020) 707:135578. doi: 10.1016/j.scitotenv.2019.135578
112. Zhang Y, et al. Atmospheric microplastics: A review on current status and perspectives. Earth-Sci Rev. (2020) 203:103118. doi: 10.1016/j.earscirev.2020.103118
113. Chen G, et al. An overview of analytical methods for detecting microplastics in the atmosphere. TrAC. (2020) 130:115981. doi: 10.1016/j.trac.2020.115981
114. Liu K, Wang X, Fang T, Xu P, Zhu L, Li D. Source and potential risk assessment of suspended atmospheric microplastics in Shanghai. Sci Total Environ. (2019) 675:462–71. doi: 10.1016/j.scitotenv.2019.04.110
115. Evangeliou N, Grythe H, Klimont Z, Heyes C, Eckhardt S, Lopez-Aparicio S, et al. Atmospheric transport is a major pathway of microplastics to remote regions. Nat Commun. (2020) 11:3381. doi: 10.1038/s41467-020-17201-9
116. Liu F, Vianello A, Vollertsen J. Microplastics in urban and highway stormwater retention ponds. Sci Total Environ. (2019) 671:992–1000. doi: 10.1016/j.scitotenv.2019.03.416
117. Liu K, Wu T, Wang X, Song Z, Zong C, Wei N, et al. Consistent transport of terrestrial microplastics to the ocean through atmosphere. Environ Sci Technol. (2019) 53:10612–9. doi: 10.1021/acs.est.9b03427
118. Purwiyanto AIS, Prartono T, Riani E, Naulita Y, Cordova MR, Koropitan AF. The deposition of atmospheric microplastics in Jakarta-Indonesia: the coastal urban area. Mar Pollut Bull. (2022) 174:113195. doi: 10.1016/j.marpolbul.2021.113195
119. Chen G, Feng Q, Wang J. Mini-review of microplastics in the atmosphere and their risks to humans. Sci Total Environ. (2020) 703:135504. doi: 10.1016/j.scitotenv.2019.135504
120. Wright SL, Ulke J, Font A, Chan KLA, Kelly FJ. Atmospheric microplastic deposition in an urban environment and an evaluation of transport. Environ Int. (2020) 136:105411. doi: 10.1016/j.envint.2019.105411
121. Trainic M, Flores JM, Pinkas I, Pedrotti ML, Lombard F, Bourdin G, et al. Airborne microplastic particles detected in the remote marine atmosphere. Commun Earth Environ. (2020) 1:64. doi: 10.1038/s43247-020-00061-y
122. Van Cauwenberghe L, Janssen CR. Microplastics in bivalves cultured for human consumption. Environ Pollut. (2014) 193:65–70. doi: 10.1016/j.envpol.2014.06.010
123. Li J, Yang D, Li L, Jabeen K, Shi H. Microplastics in commercial bivalves from China. Environmental pollution. (2015) 207:190–5. doi: 10.1016/j.envpol.2015.09.018
124. Yang D, Shi H, Li L, Li J, Jabeen K, Kolandhasamy P. Microplastic pollution in table salts from China. Environ Sci Technol. (2015) 49:13622–7. doi: 10.1021/acs.est.5b03163
125. Zhang Q, Xu EG Li J, Chen Q, Ma L, Zeng EY, Shi H, et al. review of microplastics in table salt, drinking water, and air: direct human exposure. Environ Sci Technol. (2020) 54:3740–51. doi: 10.1021/acs.est.9b04535
126. Danopoulos E, Jenner LC, Twiddy M, Rotchell JM. Microplastic contamination of seafood intended for human consumption: a systematic review and meta-analysis. Environ Health Perspect. (2020) 128:126002. doi: 10.1289/EHP7171
127. Jin M, Wang X, Ren T, Wang J, Shan J. Microplastics contamination in food and beverages: Direct exposure to humans. J Food Sci. (2021) 86:2816–37. doi: 10.1111/1750-3841.15802
128. Oßmann BE, Sarau G, Holtmannspötter H, Pischetsrieder M, Christiansen SH, Dicke W. Small-sized microplastics and pigmented particles in bottled mineral water. Water Res. (2018) 141:307–16. doi: 10.1016/j.watres.2018.05.027
129. Kwon JH, Kim JW, Pham TD, Tarafdar A, Hong S, Chun SH, et al. Microplastics in food: a review on analytical methods and challenges. Int J Environ Res Public Health. (2020) 17:6710. doi: 10.3390/ijerph17186710
130. Bai CL, Liu LY, Hu YB, Zeng EY, Guo Y. Microplastics: A review of analytical methods, occurrence and characteristics in food, and potential toxicities to biota. Science of The Total Environment. (2022) 806:150263. doi: 10.1016/j.scitotenv.2021.150263
131. Rubio-Armendáriz C, Alejandro-Vega S, Paz-Montelongo S, Gutiérrez-Fernández ÁJ, Carrascosa-Iruzubieta CJ. Hardisson-de la Torre A. Microplastics as emerging food contaminants: a challenge for food safety International Journal of Environmental Research and Public Health. (2022) 19:1174. doi: 10.3390/ijerph19031174
132. Huang Y, Chapman J, Deng Y, Cozzolino D. Rapid measurement of microplastic contamination in chicken meat by mid infrared spectroscopy and chemometrics: A feasibility study. Food Control. (2020) 113:107187. doi: 10.1016/j.foodcont.2020.107187
133. Conti GO, Ferrante M, Banni M, Favara C, Nicolosi I, Cristaldi A, et al. Micro-and nano-plastics in edible fruit and vegetables. The first diet risks assessment for the general population. (2020) 187:109677. doi: 10.1016/j.envres.2020.109677
134. Shruti VC, Pérez-Guevara F, Elizalde-Martínez I, Kutralam-Muniasamy G. First study of its kind on the microplastic contamination of soft drinks, cold tea and energy drinks-Future research and environmental considerations. Science of the total environment. (2020) 726:138580. doi: 10.1016/j.scitotenv.2020.138580
135. Cox K, D. et al. Human consumption of microplastics Environ Sci Technol. (2019) 53:7068–74. doi: 10.1021/acs.est.9b01517
136. Ghosh GC, Akter SM, Islam RM, Habib A, Chakraborty TK, Zaman S, et al. Microplastics contamination in commercial marine fish from the Bay of Bengal. Regional Studies in Marine Science. (2021) 44:101728. doi: 10.1016/j.rsma.2021.101728
137. Cabansag JB, Olimberio RB, Villanobos ZM. Microplastics in some fish species and their environs in Eastern Visayas, Philippines. Mar Pollut Bull. (2021) 167:112312. doi: 10.1016/j.marpolbul.2021.112312
138. Zhang L, Xie Y, Zhong S, Liu J, Qin Y, Gao P. Microplastics in freshwater and wild fishes from Lijiang River in Guangxi, Southwest China. Science of the Total Environment. (2021) 755:142428. doi: 10.1016/j.scitotenv.2020.142428
139. Sun D, Wang J, Xie S, Tang H, Zhang C, Xu G, et al. Characterization and spatial distribution of microplastics in two wild captured economic freshwater fish from north and west rivers of Guangdong province. Ecotoxicol Environ Saf. (2021) 207:111555. doi: 10.1016/j.ecoenv.2020.111555
140. Jonathan MP, Sujitha SB, Rodriguez-Gonzalez F, Villegas LE, Hernández-Camacho CJ, Sarkar SK. Evidences of microplastics in diverse fish species off the Western Coast of Pacific Ocean, Mexico. Ocean Coast Manag. (2021) 204:105544. doi: 10.1016/j.ocecoaman.2021.105544
141. Sathish MN, Jeyasanta I, Patterson J. Microplastics in salt of Tuticorin, southeast coast of India. Arch Environ Contam Toxicol. (2020) 79:111–21. doi: 10.1007/s00244-020-00731-0
142. Karuppasamy PK, Ravi A, Vasudevan L, Elangovan MP, Mary PD, Vincent SG, et al. Baseline survey of micro and mesoplastics in the gastro-intestinal tract of commercial fish from Southeast coast of the Bay of Bengal. Mar Pollut Bull. (2020) 153:110974. doi: 10.1016/j.marpolbul.2020.110974
143. Cho Y, Shim WJ, Jang M, Han GM, Hong SH. Nationwide monitoring of microplastics in bivalves from the coastal environment of Korea. Environmental Pollution. (2021) 270:116175. doi: 10.1016/j.envpol.2020.116175
144. Keshavarzifard M, Vazirzadeh A, Sharifinia M. Occurrence and characterization of microplastics in white shrimp, Metapenaeus affinis, living in a habitat highly affected by anthropogenic pressures, northwest Persian Gulf. Mar Pollut Bull. (2021) 169:112581. doi: 10.1016/j.marpolbul.2021.112581
145. Sanjay K, Mridula R, Rajesh K, Suyani N, Ahamed RA, Pratiksha KS. Impact of microplastics on aquatic organisms and human health: a review. Int J Environ Sci Nat Resour. (2020) 26:59–64. doi: 10.19080/IJESNR.2020.26.556184
146. Galloway TS. Micro-and nano-plastics and human health. Marine Anthropogenic Litter 2015:343–366. doi: 10.1007/978-3-319-16510-3_13
147. Evode N, Qamar SA, Bilal M, Barceló D, Iqbal HMN. Plastic waste and its management strategies for environmental sustainability. Case Stud Chem Environ Eng. (2021) 4:100142. doi: 10.1016/j.cscee.2021.100142
148. Yee MS Hii LW Looi CK Lim WM Wong SF Kok YY Tan BK Wong CY Leong Leong CO Impact of microplastics and nanoplastics on human health. Nanomaterials. (2021) 11:496. doi: 10.3390/nano11020496
149. Ebrahimi P, Abbasi S, Pashaei R, Bogusz A, Oleszczuk P. Investigating impact of physicochemical properties of microplastics on human health: a short bibliometric analysis and review. Chemosphere. (2022) 289:133146. doi: 10.1016/j.chemosphere.2021.133146
150. Bošković N, Joksimović D, Bajt O. Microplastics in mussels from the Boka Kotorska Bay (Adriatic Sea) and impact on human health. Food Chem Toxicol. (2023) 173:113641. doi: 10.1016/j.fct.2023.113641
151. Huang W, Song B, Liang J, Niu Q, Zeng G, Shen M, et al. Microplastics and associated contaminants in the aquatic environment: a review on their ecotoxicological effects, trophic transfer, and potential impacts to human health. J Hazardous Mater. (2021) 405:124187. doi: 10.1016/j.jhazmat.2020.124187
152. Yu H, Zhang Y, Tan W, Zhang Z. Microplastics as an emerging environmental pollutant in agricultural soils: effects on ecosystems and human health. Front Environ Sci. (2022) 10:217. doi: 10.3389/fenvs.2022.855292
153. Das A. The emerging role of microplastics in systemic toxicity: involvement of reactive oxygen species (ROS). Sci Total Environ. (2023) 2023:165076. doi: 10.1016/j.scitotenv.2023.165076
154. Jaishankar M, Tseten T, Anbalagan N, Mathew BB, Beeregowda KN. Toxicity, mechanism and health effects of some heavy metals. Interdisc Toxicol. (2014) 7:60. doi: 10.2478/intox-2014-0009
155. Segovia-Mendoza M, Nava-Castro KE, Palacios-Arreola MI, Garay-Canales C, Morales-Montor J. How microplastic components influence the immune system and impact on children health: Focus on cancer. Birth Defects Res. (2020) 112:1341–61. doi: 10.1002/bdr2.1779
156. Shi J, Wu D, Su Y, Xie B. Selective enrichment of antibiotic resistance genes and pathogens on polystyrene microplastics in landfill leachate. Sci Total Environ. (2021) 765:142775. doi: 10.1016/j.scitotenv.2020.142775
157. Dong H, Chen Y, Wang J, Zhang Y, Zhang P, Li X, et al. Interactions of microplastics and antibiotic resistance genes and their effects on the aquaculture environments. J Hazard Mater. (2021) 403:123961. doi: 10.1016/j.jhazmat.2020.123961
158. Guo JJ, Huang XP, Xiang L, Wang YZ, Li YW, Li H, et al. Source, migration and toxicology of microplastics in soil. Environ Int. (2020) 137:105263. doi: 10.1016/j.envint.2019.105263
159. Aydin RB, Yozukmaz A, Sener I, Temiz F, Giannetto D. Occurrence of microplastics in most consumed fruits and vegetables from Turkey and public risk assessment for consumers. Life. (2023) 13:1686. doi: 10.3390/life13081686
160. Lehner R, Weder C, Petri-Fink A, Rothen-Rutishauser B. Emergence of nanoplastic in the environment and possible impact on human health. Environ Sci Technol. (2019) 53:1748–1765. doi: 10.1021/acs.est.8b05512
161. Stapleton P. Toxicological considerations of nano-sized plastics. AIMS Environ Sci. (2019) 6:367. doi: 10.3934/environsci.2019.5.367
162. EOM SH, et al. Potential toxicity of polystyrene nano-and microplastics to human skin barrier function. 한국생물공학회 학술대회 (2022) 2022:187–187.
163. Schwabl P, Köppel S, Königshofer P, Bucsics T, Trauner M, Reiberger T, et al. Detection of various microplastics in human stool: a prospective case series. Ann Intern Med. (2019) 171:453–7. doi: 10.7326/M19-0618
164. Prata JC. Airborne microplastics: consequences to human health? Environ Pollut. (2018) 234:115–26. doi: 10.1016/j.envpol.2017.11.043
165. Hurley K, Reeves EP, Carroll TP, McElvaney NG. Tumor necrosis factor-α driven inflammation in alpha-1 antitrypsin deficiency: a new model of pathogenesis and treatment. Expert Rev Respir Med. (2016) 10:207–22. doi: 10.1586/17476348.2016.1127759
166. Paget V, Dekali S, Kortulewski T, Grall R, Gamez C, Blazy K, et al. Specific uptake and genotoxicity induced by polystyrene nanobeads with distinct surface chemistry on human lung epithelial cells and macrophages. PLoS ONE. (2015) 10:e0123297. doi: 10.1371/journal.pone.0123297
167. Brown DM, Wilson MR, MacNee W, Stone V, Donaldson K. Size-dependent proinflammatory effects of ultrafine polystyrene particles: a role for surface area and oxidative stress in the enhanced activity of ultrafines. Toxicol Appl Pharmacol. (2001) 175:191–9. doi: 10.1006/taap.2001.9240
168. Kai H, Kato Y, Toyosato R. Nishizawa M. Microplastics in air: are we breathing it in?. Curr Opin Environ Sci Health. (2018) 1:1–5.
169. Wright SL, Kelly FJ. Plastic and human health: a micro issue? Environ Sci Technol. (2017) 51:6634–6647. doi: 10.1021/acs.est.7b00423
170. Li S, Keenan JI, Shaw IC, Frizelle FA. Could microplastics be a driver for early onset colorectal cancer? Cancers. (2023) 15:3323. doi: 10.3390/cancers15133323
171. Lei L, Wu S, Lu S, Liu M, Song Y, Fu Z, et al. Microplastic particles cause intestinal damage and other adverse effects in zebrafish Danio rerio and nematode Caenorhabditis elegans. Sci Total Environ. (2018) 619:1–8. doi: 10.1016/j.scitotenv.2017.11.103
172. Baj J, Dring JC, Czeczelewski M, Kozyra P, Forma A, Flieger J, et al. Derivatives of plastics as potential carcinogenic factors: the current state of knowledge. Cancers. (2022) 14:4637. doi: 10.3390/cancers14194637
173. Iyadorai T, Mariappan V, Vellasamy KM, Wanyiri JW, Roslani AC, Lee GK, et al. Prevalence and association of pks+ Escherichia coli with colorectal cancer in patients at the University Malaya Medical Centre, Malaysia. PLoS ONE. (2020) 15:e0228217. doi: 10.1371/journal.pone.0228217
174. Lu L, Wan Z, Luo T, Fu Z, Jin Y. Polystyrene microplastics induce gut microbiota dysbiosis and hepatic lipid metabolism disorder in mice. Sci Total Environ. (2018) 631:449–58. doi: 10.1016/j.scitotenv.2018.03.051
175. Jin Y, et al. Impacts of polystyrene microplastic on the gut barrier, microbiota and metabolism of mice. Sci Total Environ. (2019) 649:308–17. doi: 10.1016/j.scitotenv.2018.08.353
176. Herrala M, Huovinen M, Järvelä E, Hellman J, Tolonen P, Lahtela-Kakkonen M, et al. Micro-sized polyethylene particles affect cell viability and oxidative stress responses in human colorectal adenocarcinoma Caco-2 and HT-29 cells. Sci Total Environ. (2023) 867:161512. doi: 10.1016/j.scitotenv.2023.161512
177. Schmidt C, Lautenschlaeger C, Collnot E-M, Schumann M, Bojarski C, Schulzke J-D, et al. Nano-and microscaled particles for drug targeting to inflamed intestinal mucosa—A first in vivo study in human patients. J Controlled Release (2013) 165:139–45. doi: 10.1016/j.jconrel.2012.10.019
178. Yan Z, Liu Y, Zhang T, Zhang F, Ren H, Zhang Y. Response to Comment on “Analysis of Microplastics in Human Feces Reveals a Correlation between Fecal Microplastics and Inflammatory Bowel Disease Status”. Environ Sci Technol. (2022) 56:12779–80. doi: 10.1021/acs.est.2c05327
179. Huang J-N, Wen B, Zhu J-G, Zhang Y-S, Gao J-Z, Chen Z-Z. Exposure to microplastics impairs digestive performance, stimulates immune response and induces microbiota dysbiosis in the gut of juvenile guppy (Poecilia reticulata). Sci Total Environ. (2020) 733:138929. doi: 10.1016/j.scitotenv.2020.138929
180. Zhou X, Wang G, An X, Wu J, Fan K, Xu L, et al. Polystyrene microplastic particles: In vivo and in vitro ocular surface toxicity assessment. Environ Pollut. (2022) 303:119126. doi: 10.1016/j.envpol.2022.119126
181. Tan G, Li J, Yang Q, Wu A, Qu DY, Wang Y, et al. Air pollutant particulate matter 2.5 induces dry eye syndrome in mice. Sci Rep. (2018) 8:17828. doi: 10.1038/s41598-018-36181-x
182. Wu D, Lim BXH, Seah I, Xie S, Jaeger JE, Symons RK, et al. Impact of microplastics on the ocular surface. Int J Mol Sci. (2023) 24:3928. doi: 10.3390/ijms24043928
183. Hao R, Zhang M, Zhao L, Liu Y, Sun M, Dong J, et al. Impact of air pollution on the ocular surface and tear cytokine levels: A multicenter prospective cohort study. Front Med. (2022) 9:909330. doi: 10.3389/fmed.2022.909330
184. D'Angelo SR. Meccariello, Microplastics: a threat for male fertility. Int J Environ Res Public Health. (2021) 18:2392. doi: 10.3390/ijerph18052392
185. Chianese R, Troisi J, Richards S, Scafuro M, Fasano S, Guida M, et al. Bisphenol A in reproduction: epigenetic effects. Curr Med Chem. (2018) 25:748–770. doi: 10.2174/0929867324666171009121001
186. Ho S-M, Cheong A, Adgent MA, Veevers J, Suen AC, Tam NVC. Environmental factors, epigenetics, and developmental origin of reproductive disorders. Reprod Toxicol. (2017) 68:85–104. doi: 10.1016/j.reprotox.2016.07.011
187. D'Angelo S, Scafuro M, Meccariello R. BPA and nutraceuticals, simultaneous effects on endocrine functions. Endocr Metab Immune Disorders-Drug Targets. (2019) 19:594–604. doi: 10.2174/1871530319666190101120119
188. Santoro A, Mele E, Marino M, Viggiano A, Nori SL, Meccariello R. The complex interplay between endocannabinoid system and the estrogen system in central nervous system and periphery. Int J Mol Sci. (2021) 22:972. doi: 10.3390/ijms22020972
189. Santoro A, Chianese R, Troisi J, Richards S, Nori SL, Fasano S, et al. Neuro-toxic and reproductive effects of BPA. Curr Neuropharmacol. (2019) 17:1109–32. doi: 10.2174/1570159X17666190726112101
190. He Y, Yin R. The reproductive and transgenerational toxicity of microplastics and nanoplastics: a threat to mammalian fertility in both sexes. J Appl Toxicol. (2023) 44:66–85. doi: 10.1002/jat.4510
191. Grechi N, Franko R, Rajaraman R, St⊠ckl JB, Trapphoff T, Dieterle S, et al. Microplastics are present in women's and cows' follicular fluid and polystyrene microplastics compromise bovine oocyte function in vitro. bioRxiv, 2022:2022.11. 04.514813. (2022). doi: 10.1101/2022.11.04.514813
192. Radwan M, Wielgomas B, Dziewirska E, Radwan P, Kałużny P, Klimowska A. Urinary bisphenol A levels and male fertility. Am J Men Health. (2018) 12:2144–2151. doi: 10.1177/1557988318799163
193. Pollard SH, Cox KJ, Blackburn BE, Wilkins DG, Carrell DT, Stanford JB, et al. Male exposure to bisphenol A (BPA) and semen quality in the Home Observation of Periconceptional Exposures (HOPE) cohort. Reprod Toxicol. (2019) 90:82–7. doi: 10.1016/j.reprotox.2019.08.014
194. Omran GA, Gaber HD, Mostafa NAM, Abdel-Gaber RM, Salah EA. Potential hazards of bisphenol A exposure to semen quality and sperm DNA integrity among infertile men. (2018) 81:188–95. doi: 10.1016/j.reprotox.2018.08.010
195. Caporossi L, Alteri A, Campo G, Paci E, Tranfo G, Capanna S, et al. Cross sectional study on exposure to BPA and phthalates and semen parameters in men attending a fertility center. (2020) 17:489. doi: 10.3390/ijerph17020489
196. Jeong B, Baek JY, Koo J, Park S, Ryu Y-K, Kim K-S, et al. Maternal exposure to polystyrene nanoplastics causes brain abnormalities in progeny. (2022) 426:127815. doi: 10.1016/j.jhazmat.2021.127815
197. Liu X, Zhao Y, Dou J, Hou Q, Cheng J, Jiang X. Bioeffects of inhaled nanoplastics on neurons and alteration of animal behaviors through deposition in the brain. (2022) 22:1091–9. doi: 10.1021/acs.nanolett.1c04184
198. Li Y, Wang C, Zong S, Qi J, Dong X, Zhao W, et al. The trigeminal pathway dominates the nose-to-brain transportation of intact polymeric nanoparticles: evidence from aggregation-caused quenching probes. (2019) 15:686–702. doi: 10.1166/jbn.2019.2724
199. Schirinzi GF, Pérez-Pomeda I, Sanchís J, Rossini C, Farré M, Barceló D. Cytotoxic effects of commonly used nanomaterials and microplastics on cerebral and epithelial human cells. (2017) 159:579–87. doi: 10.1016/j.envres.2017.08.043
200. Grafmueller S, Manser P, Diener L, Diener P-A, Maeder-Althaus X, Maurizi L, et al. Bidirectional transfer study of polystyrene nanoparticles across the placental barrier in an ex vivo human placental perfusion model. (2015) 123:1280–6. doi: 10.1289/ehp.1409271
201. Xu X, Wang S, Gao F, Li J, Zheng L, Sun C, et al. Marine microplastic-associated bacterial community succession in response to geography, exposure time, and plastic type in China's coastal seawaters. Mar Pollut Bull. (2019) 145:278–86. doi: 10.1016/j.marpolbul.2019.05.036
202. Goodman KE, Hare JT, Khamis ZI, Hua T, Sang QX. Exposure of human lung cells to polystyrene microplastics significantly retards cell proliferation and triggers morphological changes. Chem Res Toxicol. (2021) 34:1069–81. doi: 10.1021/acs.chemrestox.0c00486
203. Bouwmeester H, Hollman PC, Peters RJ. Potential health impact of environmentally released micro-and nanoplastics in the human food production chain: experiences from nanotoxicology. Environ. Sci. Technol. (2015) 49:8932–8947. doi: 10.1021/acs.est.5b01090
204. Amato-Lourenço LF, Carvalho-Oliveira R, Ribeiro G Jr, Galvão LdS, Ando RA, Mauad T. Presence of airborne microplastics in human lung tissue. J Hazardous Mater. (2021) 416:126124. doi: 10.1016/j.jhazmat.2021.126124
205. Jenner LC, Rotchell JM, Bennett RT, Cowen M, Tentzeris V, Sadofsky LR. Detection of microplastics in human lung tissue using μFTIR spectroscopy. Sci Total Environ. (2022) 831:154907. doi: 10.1016/j.scitotenv.2022.154907
206. Law B, Bunn W, Hesterberg T. Solubility of polymeric organic fibers and manmade vitreous fibers in gambles solution. Inhal Toxicol. (1990) 2:321–39. doi: 10.3109/08958379009145261
207. Dehghani S, Moore F, Akhbarizadeh R. Microplastic pollution in deposited urban dust, Tehran metropolis, Iran. Environ Sci Pollut Res. (2017) 24:20360–71. doi: 10.1007/s11356-017-9674-1
208. Rezaei M, Riksen MJPM, Sirjani E, Sameni A, Geissen V. Wind erosion as a driver for transport of light density microplastics. Sci Total Environ. (2019) 669:273–81. doi: 10.1016/j.scitotenv.2019.02.382
209. Dong T, Zhang Y, Ma P, Zhang Y, Bernardini P, Ding M, et al. Charge measurement of cosmic ray nuclei with the plastic scintillator detector of DAMPE Astroparticle Physics. (2019) 105:31–6. doi: 10.1016/j.astropartphys.2018.10.001
210. Campanale C, Massarelli C, Savino I, Locaputo V, Uricchio VF. A detailed review study on potential effects of microplastics and additives of concern on human health. Int J Environ Res Public Health. (2020) 17:1212. doi: 10.3390/ijerph17041212
211. Eyles J, Bramwell VW, Williamson ED, Alpar HO. Microsphere translocation and immunopotentiation in systemic tissues following intranasal administration. Vaccine. (2001) 19:4732–4742. doi: 10.1016/S0264-410X(01)00220-1
212. Eng C, Jacome AA, Agarwal R, Hayat MH, Byndloss MX, Holowatyj AN, et al. A comprehensive framework for early-onset colorectal cancer research. Lancet Oncol. (2022) 23:e116–28. doi: 10.1016/S1470-2045(21)00588-X
213. Collaborative RE, Zaborowski AM, Abdile A, Adamina M, Aigner F, d'Allens L. Characteristics of early-onset vs late-onset colorectal cancer: a review. JAMA Surg. (2021) 156:865–874.
214. Wiśniowska E. Włodarczyk-Makuła M, The effect of selected acidic or alkaline chemical agents amendment on leachability of selected heavy metals from sewage sludge. Sci Total Environ. (2018) 633:463–9. doi: 10.1016/j.scitotenv.2018.03.163
215. Dawson AL, Kawaguchi S, King CK, Townsend KA, King R, Huston WM, et al. Turning microplastics into nanoplastics through digestive fragmentation by Antarctic krill. Nat Commun. (2018) 9:1001. doi: 10.1038/s41467-018-03465-9
216. Andrady AL. The plastic in microplastics: a review. Marine Pollut. Bull. (2017) 119:12–22. doi: 10.1016/j.marpolbul.2017.01.082
217. Luo H, Zhao Y, Li Y, Xiang Y, He D, Pan X. Aging of microplastics affects their surface properties, thermal decomposition, additives leaching and interactions in simulated fluids. Sci Total Environ. (2020) 714:136862. doi: 10.1016/j.scitotenv.2020.136862
218. Enders K, Lenz R, Beer S, Stedmon CA. Extraction of microplastic from biota: recommended acidic digestion destroys common plastic polymers. ICES J Marine Sci. (2017) 74:326–331. doi: 10.1093/icesjms/fsw173
219. Kaczmarek H, Kowalonek J, Szalla A, Sionkowska A. Surface modification of thin polymeric films by air-plasma or UV-irradiation. Surface Sci. (2002) 507:883–8. doi: 10.1016/S0039-6028(02)01367-5
220. Pospíšil J, Pilař, Billingham NC, Marek A, Horák Z, S. Nešpurek S. Factors affecting accelerated testing of polymer photostability. Polymer Degrad Stab. (2006) 91:417–422. doi: 10.1016/j.polymdegradstab.2005.01.049
221. Jani PU, Nomura T, Yamashita F, Takakura Y, Florence AT, Hashida M. Biliary excretion of polystyrene microspheres with covalently linked FITC fluorescence after oral and parenteral administration to male Wistar rats. J Drug Target. (1996) 4:87–93. doi: 10.3109/10611869609046266
222. Ridlon JM, Kang D, Hylemon PB, Bajaj JS. Bile acids and the gut microbiome. Current Opin Gastroenterol. (2014) 30:332–338. doi: 10.1097/MOG.0000000000000057
223. Lai SK, Wang Y-Y, Hanes J. Mucus-penetrating nanoparticles for drug and gene delivery to mucosal tissues. Adv Drug Deliv Rev. (2009) 61:158–71. doi: 10.1016/j.addr.2008.11.002
224. De Weirdt R, Van de Wiele T. Micromanagement in the gut: microenvironmental factors govern colon mucosal biofilm structure and functionality. NPJ Biofilms Microb. (2015) 1:1–6. doi: 10.1038/npjbiofilms.2015.26
225. Tziourrou P, Bourikas K, Karapanagioti HK. Measuring the size and the charge of microplastics in aqueous suspensions with and without microorganisms using a zeta-sizer meter. in Proceedings of the 2nd International Conference on Microplastic Pollution in the Mediterranean Sea, Springer (2020). doi: 10.1007/978-3-030-45909-3_39
226. Domenech J, Annangi B, Marcos R, Hernández A, Catalán J. Insights into the potential carcinogenicity of micro-and nano-plastics. Mutat Res. (2023) 791:108453. doi: 10.1016/j.mrrev.2023.108453
227. Nougayrède J-P, Homburg S, Taieb F, Boury M, Brzuszkiewicz E, Gottschalk G. Escherichia coli induces DNA double-strand breaks in eukaryotic cells. Science. (2006) 313:848–851. doi: 10.1126/science.1127059
228. Huang JY, Lee SM, Mazmanian SK. The human commensal Bacteroides fragilis binds intestinal mucin. Anaerobe. (2011) 17:137–41. doi: 10.1016/j.anaerobe.2011.05.017
229. Hou J, Lei Z, Cui L, Hou Y, Yang L, An R, et al. Polystyrene microplastics lead to pyroptosis and apoptosis of ovarian granulosa cells via NLRP3/Caspase-1 signaling pathway in rats. Ecotoxicol Environ Saf. (2021) 212:112012. doi: 10.1016/j.ecoenv.2021.112012
230. Reuter C, Alzheimer M, Walles H, Oelschlaeger TA. An adherent mucus layer attenuates the genotoxic effect of colibactin. Cell Microbiol. (2018) 20:e12812. doi: 10.1111/cmi.12812
231. Glover JS, Ticer TD, Engevik MA. Characterizing the mucin-degrading capacity of the human gut microbiota. Sci Rep. (2022) 12:8456. doi: 10.1038/s41598-022-11819-z
232. Dejea CM, Wick EC, Hechenbleikner EM, White JR, Welch JLM, Rossetti BJ, et al. Microbiota organization is a distinct feature of proximal colorectal cancers. Proc Nat Acad Sci. (2014) 111:18321–6. doi: 10.1073/pnas.1406199111
233. Gagnaire A, Nadel B, Raoult D, Neefjes J, Gorvel J-P. Collateral damage: insights into bacterial mechanisms that predispose host cells to cancer. Nat Rev Microbiol. (2017) 15:109–28. doi: 10.1038/nrmicro.2016.171
234. Tjalsma H, Boleij A, Marchesi JR, Dutilh BE, A. bacterial driver–passenger model for colorectal cancer: beyond the usual suspects. Nat Rev Microbiol. (2012) 10:575–82. doi: 10.1038/nrmicro2819
235. Ji J Wu X Li X Zhu Y Effects Effects of microplastics in aquatic environments on inflammatory bowel disease. Environ Res. (2023) 82:115974. doi: 10.1016/j.envres.2023.115974
236. Yang S, Liu T, Cheng Y, Bai Y, Liang G. Immune cell infiltration as a biomarker for the diagnosis and prognosis of digestive system cancer. Cancer Sci. (2019) 110:3639–3649. doi: 10.1111/cas.14216
237. Saez A, Gomez-Bris R, Herrero-Fernandez B, Mingorance C, Rius C, Gonzalez-Granado JM. Innate lymphoid cells in intestinal homeostasis and inflammatory bowel disease. International J Molec Sci. (2021) 22:7618. doi: 10.3390/ijms22147618
238. Axelrad JE Cadwell KH Colombel J-F Shah Shah SC The role of gastrointestinal pathogens in inflammatory bowel disease: a systematic review. Therap Adv Gastroenterol. (2021) 14:17562848211004493. doi: 10.1177/17562848211004493
239. Berre CL, Ananthakrishnan AN, Danese S, Singh S, Peyrin-Biroulet L. Ulcerative colitis and Crohn's disease have similar burden and goals for treatment. Clin Gastroenterol Hepatol. (2020) 18:14–23. doi: 10.1016/j.cgh.2019.07.005
240. Siegel CA, Yang F, Eslava S, Cai Z. Treatment pathways leading to biologic therapies for ulcerative colitis and Crohn's disease in the United States. Clin Transl Gastroenterol. (2020) 11:523. doi: 10.14309/ctg.0000000000000128
241. Carreras-Torres R, Ibáñez-Sanz G, Obón-Santacana M, Duell EJ, Moreno V. Identifying environmental risk factors for inflammatory bowel diseases: a Mendelian randomization study. Sci Rep. (2020) 10:19273. doi: 10.1038/s41598-020-76361-2
242. Remmelts M Effect Effect of nano-and microplastics on the human immune system and their influence on inflammatory bowel disease. 2021.
243. Li B, Ding Y, Cheng X, Sheng D, Xu Z, Rong Q, et al. Polyethylene microplastics affect the distribution of gut microbiota and inflammation development in mice. Chemosphere. (2020) 244:125492. doi: 10.1016/j.chemosphere.2019.125492
244. Neurath, M.F, Cytokines in inflammatory bowel disease. Nature Reviews Immunology, (2014) 14:329–342. doi: 10.1038/nri3661
245. Han S, Bang J, Choi D, Hwang J, Kim T, Oh Y, et al. Surface pattern analysis of microplastics and their impact on human-derived cells. ACS Applied Polymer Materials, (2020) 2:4541–4550. doi: 10.1021/acsapm.0c00645
246. Forte M, Iachetta G, Tussellino M, Carotenuto R, Prisco M, De Falco M, et al. Polystyrene nanoparticles internalization in human gastric adenocarcinoma cells. Toxicology in Vitro. (2016) 31:126–36. doi: 10.1016/j.tiv.2015.11.006
247. Almeida AS, de Souza CB. The Impact of Microplastic on Human Health. Current Biotechnology, (2021) 10:158–167. doi: 10.2174/2211550111666211221120852
248. Liu S, Wu X, Gu W, Yu J, Wu B. Influence of the digestive process on intestinal toxicity of polystyrene microplastics as determined by in vitro Caco-2 models. Chemosphere. (2020) 256:127204. doi: 10.1016/j.chemosphere.2020.127204
249. Fukata M Abreu M TLR4 signalling in the intestine in health and disease. Biochemical Society Transactions, (2007) 35:1473–1478. doi: 10.1042/BST0351473
250. Ding Y, Liu P, Chen Z-L, Zhang S-J, Wang Y-Q, Cai X, et al. Emodin attenuates lipopolysaccharide-induced acute liver injury via inhibiting the TLR4 signaling pathway in vitro and in vivo. Front Pharmacol. (2018) 9:962. doi: 10.3389/fphar.2018.00962
251. Sun H, Chen N, Yang X, Xia Y, Wu D. Effects induced by polyethylene microplastics oral exposure on colon mucin release, inflammation, gut microflora composition and metabolism in mice. Ecotoxicol Environ Saf. (2021) 220:112340. doi: 10.1016/j.ecoenv.2021.112340
252. Rawle DJ, Dumenil T, Tang B, Bishop CR, Yan K, Le TT, et al. Microplastic consumption induces inflammatory signatures in the colon and prolongs a viral arthritis. Sci Total Environ. (2022) 809:152212. doi: 10.1016/j.scitotenv.2021.152212
253. Singh S, Li SS. Epigenetic effects of environmental chemicals bisphenol A and phthalates. Int J Mol Sci. (2012) 13:10143–53. doi: 10.3390/ijms130810143
254. Lyte M. Microbial endocrinology: an ongoing personal journey. Microbial Endocrinology: Interkingdom Signaling in Infectious Disease and Health. (2016) 2016:1–24. doi: 10.1007/978-3-319-20215-0_1
255. Hou K, Wu ZX, Chen XY, Wang JQ, Zhang D, Xiao C, et al. Microbiota in health and diseases. Sig Transduct Target Ther. (2022) 7:135. doi: 10.1038/s41392-022-00974-4
256. Yarahmadi A, Afkhami H. The role of microbiomes in gastrointestinal cancers: new insights. Front Oncol. (2024) 13:1344328. doi: 10.3389/fonc.2023.1344328
257. Parsaeimehr A, Miller CM, Ozbay G. Microplastics and their interactions with microbiota. Heliyon. (2023) 9:e15104. doi: 10.1016/j.heliyon.2023.e15104
258. Liu Z, Yu P, Cai M, Wu D, Zhang M, Chen M, et al. Effects of microplastics on the innate immunity and intestinal microflora of juvenile Eriocheir sinensis. Sci Total Environ. (2019) 685:836–46. doi: 10.1016/j.scitotenv.2019.06.265
259. Qiao R, Deng Y, Zhang S, Wolosker MB, Zhu Q, Ren H, et al. Accumulation of different shapes of microplastics initiates intestinal injury and gut microbiota dysbiosis in the gut of zebrafish. Chemosphere. (2019) 236:124334. doi: 10.1016/j.chemosphere.2019.07.065
260. Chen, M.-M, Nie F-H., Qamar A, Zhu D.-H., Hu Y, Zhang M, et al. Effects of microplastics on microbial community in zhanjiang mangrove sediments. Bull Environ Contamin Toxicol. (2022) 108:867–877. doi: 10.1007/s00128-021-03429-8
261. Han Y, Zhang X, Liu P, Xu S, Chen D, Liu JN, et al. Microplastics exposure causes oxidative stress and microbiota dysbiosis in planarian Dugesia japonica. Environ Sci Pollut Res. (2022) 29:28973–83. doi: 10.1007/s11356-022-18547-x
262. Cheesman SE, Neal JT, Mittge E, Seredick BM, Guillemin K. Epithelial cell proliferation in the developing zebrafish intestine is regulated by the Wnt pathway and microbial signaling via Myd88. Proc Natl Acad Sci. USA (2011) 108:4570–4577. doi: 10.1073/pnas.1000072107
263. Seeley ME, Song B, Passie P, Hale RC. Microplastics affect sedimentary microbial communities and nitrogen cycling. Nat Commun. (2020) 11:2372. doi: 10.1038/s41467-020-16235-3
264. Zhu M, Yin H, Yuan Y, Liu H, Qi X, Ren Y, et al. Discrepancy strategies of sediment abundant and rare microbial communities in response to floating microplastic disturbances: study using a microcosmic experiment. Sci Total Environ. (2022) 835:155346. doi: 10.1016/j.scitotenv.2022.155346
265. de Souza Machado AA, Kloas W, Zarfl C, Hempel S, Rillig MC. Microplastics as an emerging threat to terrestrial ecosystems. Global Change Biol. (2018) 24:1405−1416. doi: 10.1111/gcb.14020
266. Yan W, Hamid N, Deng S, Jia P-P, Pei S. D-Individual and combined toxicogenetic effects of microplastics and heavy metals (Cd, Pb, and Zn) perturb gut microbiota homeostasis and gonadal development in marine medaka (Oryzias melastigma). J Hazard Mater. (2020) 397:122795. doi: 10.1016/j.jhazmat.2020.122795
267. Joo SH, Liang Y, Kim M, Byun J, Choi H. Microplastics with adsorbed contaminants: Mechanisms and Treatment. Environ Challenges. (2021) 3:100042. doi: 10.1016/j.envc.2021.100042
268. Costigan E, Collins A, Hatinoglu MD, Bhagat K, MacRae J, Perreault F. Adsorption of organic pollutants by microplastics: Overview of a dissonant literature. J Hazard Mater Adv. (2022) 6:100091. doi: 10.1016/j.hazadv.2022.100091
269. Antunes J, Frias JGL, Micaelo AC, Sobral P. Resin pellets from beaches of the Portuguese coast and adsorbed persistent organic pollutants. Estuarine, Coastal and Shelf Science (2013) 130:62–9. doi: 10.1016/j.ecss.2013.06.016
270. Podder A, Sadmani AHMA, Reinhart D, Chang N-B, Goel Per R. and poly-fluoroalkyl substances (PFAS) as a contaminant of emerging concern in surface water: a transboundary review of their occurrences and toxicity effects. J Hazard Mater. (2021) 419:126361. doi: 10.1016/j.jhazmat.2021.126361
271. Wang Y, Zhang P, Pan G, Chen H. Ferric ion mediated photochemical decomposition of perfluorooctanoic acid (PFOA) by 254 nm UV light. J Hazard Mater. (2008) 160:181–6. doi: 10.1016/j.jhazmat.2008.02.105
272. Boiteux V, Dauchy X, Bach C, Colin A, Hemard J, Sagres V, et al. Concentrations and patterns of perfluoroalkyl and polyfluoroalkyl substances in a river and three drinking water treatment plants near and far from a major production source. Sci Total Environ. (2017) 583:393–400. doi: 10.1016/j.scitotenv.2017.01.079
273. Xiao F. Perfluorooctanoic acid (PFOA) and perfluorooctanesulfonic acid (PFOS) in surface waters, sediments, soils and wastewater–A review on concentrations and distribution coefficients. Chemosphere. (2013) 91:725–732. doi: 10.1016/j.chemosphere.2013.02.024
274. Post GB, Louis JB, Lippincott RL. Procopio NA. Occurrence of perfluorinated compounds in raw water from New Jersey public drinking water systems. Environ Sci Technol.(2013) 47:13266–75. doi: 10.1021/es402884x
275. Zhang X, Lohmann R, Dassuncao C, Hu XC, Weber AK, Vecitis CD, et al. Source attribution of poly-and perfluoroalkyl substances (PFASs) in surface waters from Rhode Island and the New York Metropolitan Area. Environ Sci Technol Lett. (2016) 3:316–21. doi: 10.1021/acs.estlett.6b00255
276. McCormick AR, et al. Microplastic in surface waters of urban rivers: concentration, sources, and associated bacterial assemblages. Ecosphere. (2016) 7:e01556. doi: 10.1002/ecs2.1556
277. Lv X, Dong Q, Zuo Z, Liu Y, Huang X, Wu W-M. Microplastics in a municipal wastewater treatment plant: Fate, dynamic distribution, removal efficiencies, control strategies. J Clean Prod. (2019) 225:579–86. doi: 10.1016/j.jclepro.2019.03.321
278. Giannico OV, Baldacci S, Basile FC, Pellegrino A, Desiante F, Franco E, et al. PCDD/Fs and PCBs in hen eggs from a contaminated area in Italy: a 9 years spatio-temporal monitoring study. Food Addit Contamin Part A. (2023) 40:294–304. doi: 10.1080/19440049.2022.2157051
279. Giannico OV, Baldacci S, Desiante F, Basile FC, Franco E, Fragnelli GR, et al. PCDD/Fs and PCBs in Mytilus galloprovincialis from a contaminated area in Italy: the role of mussel size, temperature and meteorological factors. Food Addit Contamin Part A. (2022) 39:1123–35. doi: 10.1080/19440049.2022.2059108
280. Giannico OV, Fragnelli GR, Baldacci S, Desiante F, Pellegrino A, Basile FC, et al. Dioxins and PCBs contamination in milk and dairy products from Province of Taranto (Puglia Region, Southern Italy): a six years spatio-temporal monitoring study. Annali dell'Istituto Superiore di Sanità. (2021) 57:236. doi: 10.4415/ANN_21_03_06
281. Giannico OV, Desiante F, Basile FC, Franco E, Baldacci S, Fragnelli GR, et al. Dioxins and PCBs contamination in mussels from Taranto (Ionian Sea, Southern Italy): a seven years spatio-temporal monitoring study. Annali dell'Istituto Superiore di Sanità. (2020) 56:452–61. doi: 10.4415/ANN_20_04_07
282. Ng A, Weerakoon D, Lim E, Padhye LP. Fate of environmental pollutants. Water Environ Res. (2019) 91:1294–1325. doi: 10.1002/wer.1225
283. Verla AW, Enyoh CE, Verla EN, Nwarnorh KO. Microplastic–toxic chemical interaction: a review study on quantified levels, mechanism and implication. SN Appl Sci. (2019) 1:1–30. doi: 10.1007/s42452-019-1352-0
284. Yu F, Yang C, Zhu Z, Bai X. Ma J. Adsorption behavior of organic pollutants and metals on micro/nanoplastics in the aquatic environment. Sci Total Environ. (2019) 694:133643. doi: 10.1016/j.scitotenv.2019.133643
285. Wang F, Zhang M, Sha W, Wang Y, Hao H, Dou Y, et al. Sorption behavior and mechanisms of organic contaminants to nano and microplastics. Molecules. (2020) 25:1827. doi: 10.3390/molecules25081827
286. Li J, Liu H, Chen JP. Microplastics in freshwater systems: A review on occurrence, environmental effects, and methods for microplastics detection. Water Res. (2018) 137:362–74. doi: 10.1016/j.watres.2017.12.056
287. Gomiero A, Strafella P, Pellini G, Salvalaggio V, Fabi G. Comparative effects of ingested PVC micro particles with and without adsorbed benzo (a) pyrene vs spiked sediments on the cellular and sub cellular processes of the benthic organism. Hediste Diversicolor. (2018) 5:99. doi: 10.3389/fmars.2018.00099
288. Caruso G. Microplastics as vectors of contaminants. Mar Pollut Bull. (2019) 146:921–4. doi: 10.1016/j.marpolbul.2019.07.052
289. Ma B, Xue W, Hu C, Liu H, Qu J, Li L. Characteristics of microplastic removal via coagulation and ultrafiltration during drinking water treatment. Chem Eng J. (2019) 359:159–67. doi: 10.1016/j.cej.2018.11.155
290. Ma B, Xue W, Ding Y, Hu C, Liu H, Qu J. Removal characteristics of microplastics by Fe-based coagulants during drinking water treatment. J Environ Sci. (2019) 78:267–75. doi: 10.1016/j.jes.2018.10.006
291. Enfrin M, Lee J, Le-Clech P, Dumée LF. Kinetic and mechanistic aspects of ultrafiltration membrane fouling by nano-and microplastics. J Membr Sci. (2020) 601:117890. doi: 10.1016/j.memsci.2020.117890
292. Lim C, Kim N, Lee J, Yoon Y. Potential of adsorption of diverse environmental contaminants onto microplastics. Water. (2022) 14:4086. doi: 10.3390/w14244086
293. Wang F, Wong CS, Chen D, Lu X, Wang F, Zeng EY. Interaction of toxic chemicals with microplastics: a critical review. Water Res. (2018) 139:208–19. doi: 10.1016/j.watres.2018.04.003
294. Du Z, Deng S, Bei Y, Huang Q, Wang B, Huang J, et al. Adsorption behavior and mechanism of perfluorinated compounds on various adsorbents—A review. J Hazard Mater. (2014) 274:443–54. doi: 10.1016/j.jhazmat.2014.04.038
295. Vecitis CD, Park H, Cheng J, Mader BT, Michael R. Hoffmann Treatment technologies for aqueous perfluorooctanesulfonate (PFOS) and perfluorooctanoate (PFOA). Front Environ Sci Eng China. (2009) 3:129–51. doi: 10.1007/s11783-009-0022-7
296. Gagliano E, Sgroi M, Falciglia PP, Vagliasindi FGA, Roccaro P. Removal of poly-and perfluoroalkyl substances (PFAS) from water by adsorption: Role of PFAS chain length, effect of organic matter and challenges in adsorbent regeneration. Water Res. (2020) 171:115381. doi: 10.1016/j.watres.2019.115381
297. Deng S, Zhang Q, Nie Y, Wei H, Wang B, Huang J, et al. Sorption mechanisms of perfluorinated compounds on carbon nanotubes. Environ Pollut. (2012) 168:138–44. doi: 10.1016/j.envpol.2012.03.048
298. Deng S, Zheng YQ, Xu FJ, Wang B, Huang J, Yu G. Highly efficient sorption of perfluorooctane sulfonate and perfluorooctanoate on a quaternized cotton prepared by atom transfer radical polymerization. Chem Eng J. (2012) 193:154–60. doi: 10.1016/j.cej.2012.04.005
299. Zaggia A, Conte L, Falletti L, Fant M. Chiorboli A. Use of strong anion exchange resins for the removal of perfluoroalkylated substances from contaminated drinking water in batch and continuous pilot plants. Water Res. (2016) 91:137–46. doi: 10.1016/j.watres.2015.12.039
300. Ateia M, Zheng T, Calace S, Tharayil N, Pilla S. Karanfil T. Sorption behavior of real microplastics (MPs): insights for organic micropollutants adsorption on a large set of well-characterized MPs. Sci Total Environ. (2020) 720:137634. doi: 10.1016/j.scitotenv.2020.137634
301. Wang Y, Niu J, Li Y, Zheng T, Xu Y, Liu Y. Performance and mechanisms for removal of perfluorooctanoate (PFOA) from aqueous solution by activated carbon fiber. RSC Adv. (2015) 5:86927–86933. doi: 10.1039/C5RA15853B
302. Liu Q, Wu H, Chen J, Guo B, Zhao X, Lin H, et al. Adsorption mechanism of trace heavy metals on microplastics and simulating their effect on microalgae in river. Environ Res. (2022) 214:113777. doi: 10.1016/j.envres.2022.113777
303. Usman S, Razis AFA, Shaari K, Azmai MNA, Saad MZ, Isa NM, et al. The burden of microplastics pollution and contending policies and regulations. J Environ Res Public Health. (2022) 19:6773. doi: 10.3390/ijerph19116773
304. Nikiema J, Asiedu Z. A review of the cost and effectiveness of solutions to address plastic pollution. Environ Sci Pollut Res. (2022) 29:24547–73. doi: 10.1007/s11356-021-18038-5
305. Lee, M, Kim HJN. COVID-19 pandemic and microplastic pollution. Nanomaterials. (2022) 12:851. doi: 10.3390/nano12050851
306. Guzik, M, Czerwińska-Ledwig O, Piotrowska AJC. Compositions of abrasive cosmetics from Polish manufacturers. Cosmetics. (2023) 10:67. doi: 10.3390/cosmetics10020067
307. Grosso MJWM. Research, It's all about plastics. London, England: SAGE Publications Sage UK. (2022). p. 607–608. doi: 10.1177/0734242X221093821
308. Tang KH, Hadibarata T. Microplastics removal through water treatment plants: its feasibility, efficiency, future prospects and enhancement by proper waste management. Environ Challenges. (2021) 5:100264. doi: 10.1016/j.envc.2021.100264
309. Anand U, Dey S, Bontempi E, Ducoli S, Vethaak AD, Dey A, et al. Biotechnological methods to remove microplastics: a review. Environ Chem Lett. (2023) 21:1787–1810. doi: 10.1007/s10311-022-01552-4
310. Kim KT, Park S. Enhancing microplastics removal from wastewater using electro-coagulation and granule-activated carbon with thermal regeneration. Processes. (2021) 9:617. doi: 10.3390/pr9040617
311. Padervand M, Lichtfouse E, Robert D, Wang C. Removal of microplastics from the environment: a review. Environ Chem Lett. (2020) 18:807–28. doi: 10.1007/s10311-020-00983-1
312. Bacha A-U-R, Nabi I, Zhang L. Mechanisms and the engineering approaches for the degradation of microplastics. ACS Es T Eng. (2021) 1:1481–1501. doi: 10.1021/acsestengg.1c00216
313. Taniguchi I, Yoshida S, Hiraga K, Miyamoto K, Kimura Y, Oda K. Biodegradation of PET: current status and application aspects. ACS Catal. (2019) 9:4089–4105. doi: 10.1021/acscatal.8b05171
314. Moog D, Schmitt J, Senger J, Zarzycki J, Rexer K-H, Linne U, et al. Using a marine microalga as a chassis for polyethylene terephthalate (PET) degradation. Microb Cell Factor. (2019) 18:1–15. doi: 10.1186/s12934-019-1220-z
315. Chia WY, Tang DYY, Khoo KS, Lup ANK. Chew KW. Nature's fight against plastic pollution: Algae for plastic biodegradation and bioplastics production. Environ Sci Ecotechnol. (2020) 4:100065. doi: 10.1016/j.ese.2020.100065
316. Manzi HP, Abou-Shanab RAI, Jeon B-H, Wang J. Salama E-S. Algae: A frontline photosynthetic organism in the microplastic catastrophe. Trends Plant Sci. (2022) 27:1159–72. doi: 10.1016/j.tplants.2022.06.005
317. Yan N, Fan C, Chen Y, Hu Z. The potential for microalgae as bioreactors to produce pharmaceuticals. Int J Mol Sci. (2016) 17:962. doi: 10.3390/ijms17060962
318. Sanniyasi E, Gopal RK, Gunasekar DK. Raj PP. Biodegradation of low-density polyethylene (LDPE) sheet by microalga, Uronema africanum Borge. Sci Rep. (2021) 11:17233. doi: 10.1038/s41598-021-96315-6
319. Sarmah P, Rout J. Efficient biodegradation of low-density polyethylene by cyanobacteria isolated from submerged polyethylene surface in domestic sewage water. Environ Sci Pollut Res. (2018) 25:33508–20. doi: 10.1007/s11356-018-3079-7
320. Hirooka T, Nagase H, Uchida K, Hiroshige Y, Ehara Y, Nishikawa J-i, et al. Biodegradation of bisphenol A and disappearance of its estrogenic activity by the green alga Chlorella fusca var. vacuolata. (2005) 24:1896–901. doi: 10.1897/04-259R.1
321. Li R, Chen G-Z, Tam NFY, Luan T-G, Shin PKS, Cheung SG, et al. Toxicity of bisphenol A and its bioaccumulation and removal by a marine microalga Stephanodiscus hantzschii. (2009) 72:321–8. doi: 10.1016/j.ecoenv.2008.05.012
322. Ji MK, Kabra AN, Choi J, Hwang JH, Kim JR, Abou-Shanab RA, et al. Biodegradation of bisphenol A by the freshwater microalgae Chlamydomonas mexicana and Chlorella vulgaris. Ecol Eng. (2014) 73:260–9. doi: 10.1016/j.ecoleng.2014.09.070
323. Shen M, Zeng G, Zhang Y, Wen X, Song B. Tang W. Can biotechnology strategies effectively manage environmental (micro) plastics? Sci Total Environ. (2019) 697:134200. doi: 10.1016/j.scitotenv.2019.134200
324. Kim JW, Park SB, Tran QG, Cho DH, Choi DY, Lee YJ, et al. Functional expression of polyethylene terephthalate-degrading enzyme (PETase) in green microalgae. Microb Cell Fact. (2020) 19:1–9. doi: 10.1186/s12934-020-01355-8
325. Priya AK, Jalil AA, Dutta K, Rajendran S, Vasseghian Y, Karimi-Maleh H, et al. Algal degradation of microplastic from the environment: mechanism, challenges, and future prospects. Algal Res. (2022) 67:102848. doi: 10.1016/j.algal.2022.102848
326. Olicón-Hernández DR, González-López J, Aranda E. Overview on the biochemical potential of filamentous fungi to degrade pharmaceutical compounds. Front Microbiol. (2017) 8:256025. doi: 10.3389/fmicb.2017.01792
327. Sánchez C. Fungal potential for the degradation of petroleum-based polymers: an overview of macro-and microplastics biodegradation. Biotechnol Adv. (2020) 40:107501. doi: 10.1016/j.biotechadv.2019.107501
328. Paço A, Duarte K, da Costa JP, Santos PS, Pereira R, Pereira ME, et al. Biodegradation of polyethylene microplastics by the marine fungus Zalerion maritimum. Sci Total Environ. (2017) 586:10–5. doi: 10.1016/j.scitotenv.2017.02.017
329. de Oliveira TA, Barbosa R, Mesquita AB, Ferreira JH, de Carvalho LH, Alves TS. Fungal degradation of reprocessed PP/PBAT/thermoplastic starch blends. J Mater Res Technol. (2020) 9:2338–49. doi: 10.1016/j.jmrt.2019.12.065
330. Devi RS, Kannan VR, Nivas D, Kannan K, Chandru S, Antony AR. Biodegradation of HDPE by Aspergillus spp. from marine ecosystem of Gulf of Mannar, India. Mar Pollut Bull. (2015) 96:32–40. doi: 10.1016/j.marpolbul.2015.05.050
331. Khan S, Nadir S, Shah ZU, Shah AA, Karunarathna SC, Xu J, et al. Biodegradation of polyester polyurethane by Aspergillus tubingensis. Environm Pollu. (2017) 225:469–80. doi: 10.1016/j.envpol.2017.03.012
332. Álvarez-Barragán J, Domínguez-Malfavón L, Vargas-Suárez M, González-Hernández R, Aguilar-Osorio G, Loza-Tavera H. Biodegradative activities of selected environmental fungi on a polyester polyurethane varnish and polyether polyurethane foams. Appl Environ Microbiol. (2016) 82:5225–35. doi: 10.1128/AEM.01344-16
333. Magnin A, Pollet E, Phalip V, Avérous L. Evaluation of biological degradation of polyurethanes. Biotechnol Adv. (2020) 39:107457. doi: 10.1016/j.biotechadv.2019.107457
334. Russell JR, Huang J, Anand P, Kucera K, Sandoval AG, Dantzler KW, et al. Biodegradation of polyester polyurethane by endophytic fungi. Appl Environ Microbiol. (2011) 77:6076–84. doi: 10.1128/AEM.00521-11
335. Kunlere IO, Fagade OE, Nwadike BI. Biodegradation of low density polyethylene (LDPE) by certain indigenous bacteria and fungi. Int J Environ Stud. (2019) 76:428–40. doi: 10.1080/00207233.2019.1579586
336. Nwachukwu S, Obidi O, Odocha C. Occurrence and recalcitrance of polyethylene bag waste in Nigerian soils. Afr J Biotechnol. (2010) 9:6096–104.
337. Volke-Sepúlveda T, Saucedo-Castañeda G, Gutiérrez-Rojas M, Manzur A, Favela-Torres E. Thermally treated low density polyethylene biodegradation by Penicillium pinophilum and Aspergillus niger. J Appl Polym Sci. (2002) 83:305–14. doi: 10.1002/app.2245
338. Esmaeili A, Pourbabaee AA, Alikhani HA, Shabani F, Esmaeili E. Biodegradation of low-density polyethylene (LDPE) by mixed culture of Lysinibacillus xylanilyticus and Aspergillus niger in soil. PLoS ONE. (2013) 8:e71720. doi: 10.1371/journal.pone.0071720
339. Mehmood CT, Qazi IA, Hashmi I, Bhargava S, Deepa S. Biodegradation of low density polyethylene (LDPE) modified with dye sensitized titania and starch blend using Stenotrophomonas pavanii. Int Biodeterior Biodegr. (2016) 113:276–86. doi: 10.1016/j.ibiod.2016.01.025
340. Awasthi AK, Tan Q, Li J. Biotechnological potential for microplastic waste. Trends Biotechnol. (2020) 38:1196–9. doi: 10.1016/j.tibtech.2020.03.002
341. Yuan J, Ma J, Sun Y, Zhou T, Zhao Y, Yu F. Microbial degradation and other environmental aspects of microplastics/plastics. Sci Total Environ. (2020) 715:136968. doi: 10.1016/j.scitotenv.2020.136968
342. Janssen PH, Yates PS, Grinton BE, Taylor PM, Sait M. Improved culturability of soil bacteria and isolation in pure culture of novel members of the divisions Acidobacteria, Actinobacteria, Proteobacteria, and Verrucomicrobia. Appl Environ Microbiol. (2002) 68:2391–6. doi: 10.1128/AEM.68.5.2391-2396.2002
343. Cacciari I, Quatrini P, Zirletta G, Mincione E, Vinciguerra V, Lupattelli P, et al. Isotactic polypropylene biodegradation by a microbial community: physicochemical characterization of metabolites produced. Appl Environ Microbiol. (1993) 59:3695–700. doi: 10.1128/aem.59.11.3695-3700.1993
344. Arkatkar A, Juwarkar AA, Bhaduri S, Uppara PV, Doble M. Growth of Pseudomonas and Bacillus biofilms on pretreated polypropylene surface. Int Biodeterior Biodegr. (2010) 64:530–6. doi: 10.1016/j.ibiod.2010.06.002
345. Fontanella S, Bonhomme S, Brusson JM, Pitteri S, Samuel G, Pichon G, et al. Comparison of biodegradability of various polypropylene films containing pro-oxidant additives based on Mn, Mn/Fe or Co. Polym Degrad Stab. (2013) 98:875–84. doi: 10.1016/j.polymdegradstab.2013.01.002
346. Auta HS, Emenike CU, Fauziah SH. Screening of Bacillus strains isolated from mangrove ecosystems in Peninsular Malaysia for microplastic degradation. Environ Pollut. (2017) 231:1552–9. doi: 10.1016/j.envpol.2017.09.043
347. Auta HS, Emenike CU, Jayanthi B, Fauziah SH. Growth kinetics and biodeterioration of polypropylene microplastics by Bacillus sp, Rhodococcus sp. isolated from mangrove sediment. (2018) 127:15–21. doi: 10.1016/j.marpolbul.2017.11.036
348. Yang Y, Yang J, Wu WM, Zhao J, Song Y, Gao L, et al. Biodegradation and mineralization of polystyrene by plastic-eating mealworms: part 2. Role of gut microorganisms. (2015) 49:12087–12093. doi: 10.1021/acs.est.5b02663
349. Yang J, Yang Y, Wu WM, Zhao J, Jiang L. Evidence of polyethylene biodegradation by bacterial strains from the guts of plastic-eating waxworms. Environ Sci Technol. (2014) 48:13776–84. doi: 10.1021/es504038a
350. Kong HG, Kim HH, Chung JH, Jun J, Lee S, Kim HM, et al. The Galleria mellonella hologenome supports microbiota-independent metabolism of long-chain hydrocarbon beeswax. Cell Rep. (2019) 26:2451–64. doi: 10.1016/j.celrep.2019.02.018
351. Rajandas H, Parimannan S, Sathasivam K, Ravichandran M, Yin LS, A. novel FTIR-ATR spectroscopy based technique for the estimation of low-density polyethylene biodegradation. Polym Test. (2012) 31:1094–9. doi: 10.1016/j.polymertesting.2012.07.015
352. Tribedi P, Sil AK. Low-density polyethylene degradation by Pseudomonas sp. AKS2 biofilm. Environ Sci Pollut Res. (2013) 20:4146–53. doi: 10.1007/s11356-012-1378-y
353. Sivan A, Szanto M, Pavlov V. Biofilm development of the polyethylene-degrading bacterium Rhodococcus ruber. Appl Microbiol Biotechnol. (2006) 72:346–52. doi: 10.1007/s00253-005-0259-4
354. Skariyachan S, Taskeen N, Kishore AP, Krishna BV, Naidu G. Novel consortia of Enterobacter and Pseudomonas formulated from cow dung exhibited enhanced biodegradation of polyethylene and polypropylene. J Environ Manage. (2021) 284:112030. doi: 10.1016/j.jenvman.2021.112030
355. Davoodi SM, Miri S, Taheran M, Brar SK, Galvez-Cloutier R, Martel R. Bioremediation of unconventional oil contaminated ecosystems under natural and assisted conditions: a review. Environ Sci Technol. (2020) 54:2054–67. doi: 10.1021/acs.est.9b00906
356. Delacuvellerie A, Cyriaque V, Gobert S, Benali S, Wattiez R. The plastisphere in marine ecosystem hosts potential specific microbial degraders including Alcanivorax borkumensis as a key player for the low-density polyethylene degradation. J Hazard Mater. (2019) 380:120899. doi: 10.1016/j.jhazmat.2019.120899
357. Sivan A. New perspectives in plastic biodegradation. Curr Opin Biotechnol. (2011) 22:422–6. doi: 10.1016/j.copbio.2011.01.013
Keywords: microplastics, human health, sources, biodegradation, environment
Citation: Yarahmadi A, Heidari S, Sepahvand P, Afkhami H and Kheradjoo H (2024) Microplastics and environmental effects: investigating the effects of microplastics on aquatic habitats and their impact on human health. Front. Public Health 12:1411389. doi: 10.3389/fpubh.2024.1411389
Received: 02 April 2024; Accepted: 13 May 2024;
Published: 06 June 2024.
Edited by:
Renata Sisto, National Institute for Insurance against Accidents at Work (INAIL), ItalyReviewed by:
Orazio Valerio Giannico, Local Health Authority of Taranto, ItalyMirmajid Farhadi, Consultant, Khorramabad, Iran
Copyright © 2024 Yarahmadi, Heidari, Sepahvand, Afkhami and Kheradjoo. This is an open-access article distributed under the terms of the Creative Commons Attribution License (CC BY). The use, distribution or reproduction in other forums is permitted, provided the original author(s) and the copyright owner(s) are credited and that the original publication in this journal is cited, in accordance with accepted academic practice. No use, distribution or reproduction is permitted which does not comply with these terms.
*Correspondence: Hamed Afkhami, hamedafkhami70@gmail.com; Hadis Kheradjoo, hadiskheradjoo@gmail.com
†These authors share first authorship