Microbiological Aspects of Root Canal Infections and Disinfection Strategies: An Update Review on the Current Knowledge and Challenges
- 1Discipline of Endodontology, Division of Restorative Dental Sciences, Faculty of Dentistry, The University of Hong Kong, Hong Kong, China
- 2Division of Oral Diseases, Department of Dental Medicine, Karolinska Institute, Huddinge, Sweden
The oral cavity is the habitat of several hundreds of microbial taxa that have evolved to coexist in multispecies communities in this unique ecosystem. By contrast, the internal tissue of the tooth, i.e., the dental pulp, is a physiologically sterile connective tissue in which any microbial invasion is a pathological sign. It results in inflammation of the pulp tissue and eventually to pulp death and spread of inflammation/infection to the periradicular tissues. Over the past few decades, substantial emphasis has been placed on understanding the pathobiology of root canal infections, including the microbial composition, biofilm biology and host responses to infections. To develop clinically effective treatment regimens as well as preventive therapies, such extensive understanding is necessary. Rather surprisingly, despite the definitive realization that root canal infections are biofilm mediated, clinical strategies have been focused more on preparing canals to radiographically impeccable levels, while much is left desired on the debridement of these complex root canal systems. Hence, solely focusing on “canal shaping” largely misses the point of endodontic treatment as the current understanding of the microbial aetiopathogenesis of apical periodontitis calls for the emphasis to be placed on “canal cleaning” and chemo-mechanical disinfection. In this review, we dissect in great detail, the current knowledge on the root canal microbiome, both in terms of its composition and functional characteristics. We also describe the challenges in root canal disinfection and the novel strategies that attempt to address this challenge. Finally, we provide some critical pointers for areas of future research, which will serve as an important area for consideration in Frontiers in Oral Health.
Introduction
Root canal infections are caused by microorganisms that have penetrated the dental pulp and colonized the root canal system. As these microbial communities and their metabolic by-products rapidly gain access to periradicular tissues via apical or lateral foramina, they trigger a series of inflammatory responses. These responses cause the lysis of soft and hard periradicular tissues mostly as a result of the proteolytic activity of immune cells (neutrophils, macrophages, and mast cells) and the recruitment of osteoclasts [1]. This ultimately creates a bony cavity filled with cellular debris, cholesterol crystals, osteoclasts, fibroblasts, and various proportions of immune cells according to the level of inflammation [2]. Dead bacteria, and in some instances also live bacteria, are also found [3]. Such periradicular lesion triggered by the microbial communities within the root canal system is termed apical periodontitis. Apical periodontitis represents the main cause of dental emergency interventions [4, 5], and its exacerbated forms may spread to nearby facial spaces further leading to severe, life-threatening complications [6]. Reportedly, endodontic infections are responsible for nearly 7,000 hospitalizations per year in the US only [7]. On top of these adverse infectious evolutions, there is evidence supporting associations between chronic endodontic infections and systemic conditions such as diabetes mellitus or cardiovascular diseases [8–10].
Despite substantial progresses of modern endodontics with regards to mechanical instrumentation of radicular spaces, root canal infections, and their associated apical periodontitis lesions remain remarkably prevalent [11]. In fact, a recent systematic review has even reported an increase in the prevalence of apical periodontitis lesions during the last 8–9 years, reportedly due to inadequate endodontic and restorative treatments [12]. In Europe, the prevalence of apical periodontitis reaches 34–61% of individuals and affects between 2.8 and 4.2% of teeth, figures that increase in aging populations [13, 14]. Whereas, such infectious apical lesions can heal following root canal treatment, procedures that have not satisfactorily eliminated the microbial communities colonizing the root canal system are deemed to perpetuate persistent apical lesions [15–17]. Among Western populations, between 41 and 59% of individuals are estimated to have undergone at least one endodontic treatment, yet 24–65% of these treated root canals remain associated with radiographic evidence of persistent apical periodontitis [13, 14].
The ultimate goal of root canal treatment is to prevent the development of apical periodontitis, by removing infected and/or inflamed pulpal tissues and by creating the aseptic intraradicular conditions compatible with periradicular healing, if a lesion already exists. In essence, root canal treatment aims at eradicating the infection and further preventing microorganisms from re-penetrating the root canal system. In this perspective, a thorough understanding of the microbial-driven etiology of apical periodontitis, along with the antimicrobial challenges faced within root canals, is the foundation of the endodontic practice and provides the outline for effective therapy.
In this review, we focus on the latest microbial identification methods, based on high-throughput DNA sequencing to characterize the intra-radicular microbiota, and current antimicrobial approaches to tackle intra-radicular biofilms. We finally outline areas where further research may be warranted to improve our current understanding of the microbial ecology within root canals and its impact on oral and systemic health.
Microbial Etiopathogenesis of Apical Periodontitis
Decades of research have established that the presence of bacteria in the root canal system is elemental for the development of apical periodontitis [18–20]. Although bacteria play an indispensable role in the etiopathogenesis, the pulp is generally guarded by layers of mineralized tissues, which help protect and maintain pulpal health and integrity. Bacterial invasion from the oral environment is only possible when there is a breach of the surrounding dentine, enamel and/or cementum, permitting entry of bacteria and its by-products, contaminating the originally sterile pulp. In fact, there are many potential pathways that may allow bacteria to invade the root canal system, the most common of which is dental caries. Other common routes of infection include cracks, trauma, exposed dentinal tubules, and iatrogenic causes [21].
As the root canal infection progresses, the pulpal status evolves from a healthy to a diseased state, eventually marked by necrosis. Although a vital pulp presents with a plethora of defense mechanisms to prevent its demise, an injured or necrotic pulp is much more vulnerable to bacterial invasion as the damaged vascular innervation and reduced number of viable cells strip it from its ability to carry out reparative and protective reactions [22, 23]. Given the low compliance nature of the pulpal environment and limited collateral circulation, the host's local defense systems are readily overwhelmed by the pathogenic challenge and this ultimately leads to necrosis [24]. The bacteria present in saliva, carious biofilms, and dental plaque morph into opportunistic microbes as they rapidly occupy the evolving ecological niche of the root canal system, forming multi-species communities. Their pathogenicity is driven by virulence factors such as lipopolysaccharides, proteases, exotoxin, and other secreted or structural components, with the former playing dominant role in the development of apical periodontitis [25]. Bacteria and their by-products then infiltrate the periradicular tissues through apical and lateral foramina, instigating inflammation and mineralized tissue resorption [26].
Root canal diseases are instigated by polymicrobial infections. The microbial composition of these bacterial communities evolves over time, fueled by the evolving root canal ecology and inter-species interactions. During the initial phases of the infection, the pulp presents with higher oxygen tension and an abundance of nutrients from oral cavity, allowing facultative bacteria to thrive. This also leads to greater bacterial counts and overall diversity [27]. On the other hand, the more carbohydrate depleted yet protein rich, anaerobic environment that present in more established infections, particularly in apical depths of the root canal, favors asaccharolytic, obligate anaerobes [28, 29]. Microbial succession is a dynamic process that can also be affected by iatrogenic alterations to the pulpal ecology, such as introduction of root canal disinfectants and changes to oxygen tension during the commencement of therapy. This may add to the selective pressure of specific species that thrive in harsh environments and have the potential to form persistent infections [30]. Several possible mechanisms that enable bacteria to persist include their ability to form biofilm, penetrate and remain in dentinal tubules, survive in nutrient depleted environments, possess virulence factors that can alter the host response and exhibit resistance to antimicrobial agents [29].
Apical Periodontitis as a Biofilm-Mediated Disease
Our current understanding points toward the cumulative pathogenicity of a multi-species microbial community acting as a unit and the main driver in the pathogenesis of apical periodontitis [31]. These mixed infections primarily present in the root canal in the form of biofilms, which are not only found adhering to the intraradicular dentinal walls, ramifications and isthmuses, but also occasionally present on extraradicular root surfaces [32]. Ricucci and Siqueira [32] reported that biofilms were found in the overwhelming majority of canals associated with apical periodontitis, particularly those with large apical lesions. They can tolerate chemo-mechanical disinfection strategies and hide in anatomical complexities, contributing to recalcitrant root canal infections [33]. The microbial diversity contributes to their resilience against antimicrobial agents and is associated with persistent apical periodontitis [34, 35]. Furthermore, not only do the bacteria themselves contribute to the destructive processes of root canal diseases, but the components of the biofilm matrix can also encourage inflammation [36]. The root canal environment may similarly impact both the biofilm extracellular matrix and cellular composition. This was highlighted in a recent study which reported that biofilm age and exposure to collagen, saliva, and serum, differentially influenced the exopolysaccharide and protein content, as well as the number of colony forming units in monospecies E. faecalis biofilm models [37].
Biofilms are sessile communities of bacterial aggregates suspended in an extra-cellular fluid phase. These intraradicular biofilms are characterized by microbial microcolonies embedded in a self-produced matrix of extracellular polysaccharides, phosphoproteins, and eDNA infiltrated by fluid channels that allow circulation of nutrients and metabolites [38]. The matrix of extracellular polymeric substance (EPS) plays an important role in the form and function of biofilm, such as providing structural stability, enabling intercellular interactions, acting as a nutrient reservoir and offering protection against external stressors [39]. Furthermore, a key difference between biofilm bacteria and their planktonic counterparts is that they possess an altered phenotype which allows them to exhibit different characteristics such as enhanced pathogenicity and survival capabilities [40, 41].
In general, biofilm interactions can result in relationships of various dynamics that exert an impact on species selection. Positive relationships such as mutualism and commensalism promote the growth of certain species by way of synergistic nutritional webs, environment modification, genetic exchange, and so on. In contrast, negative interactions including competition for resources and amensalism deter the survival of others [42]. Furthermore, the close proximity and cell density of multiple microbial species enables for complex interactions that can alter the gene expression of the biofilm bacteria, which affects the biofilm characteristics and how it functions as a unit. Horizontal gene transfer may improve the overall biofilm survival by the inter-species exchange of genetic information responsible for virulence properties, adaptation to environment and resistance to environmental stresses and host defenses [43]. Quorum-sensing is another method of inter-microbial communication and involves the production and excretion of autoinducers, followed by activation of receptors which trigger or suppress specific genes that regulate virulence factors and biofilm formation amongst other things [41]. Overall, this results in biofilm-specific behaviors, which may not only enhance their pathogenicity but also heighten their antimicrobial resistance.
Biofilms are known to exhibit a superior tolerance to antimicrobial agents whilst compared to the more vulnerable planktonic equivalents, with some studies reporting a 1,000-fold difference [44, 45]. Apart from transfer of genetic material and cell-cell signaling, there are other advantages of the biofilm lifestyle that enhance the resistance against antimicrobial agents. Bacteria in biofilms benefit from the added protection from the EPS matrix, which shields them from the infiltration of antimicrobial agents [38]. Also, components of the biofilm matrix also can chemically react with antimicrobial agents to neutralize them [44]. Furthermore, biofilm bacteria exhibit reduced growth rates which prevent rapid uptake of antimicrobial agents [46]. Environmental stressors, such as nutrient scarcity and the exposure to antimicrobials, may induce gene expression for more resistant phenotypes and elicit a state of dormancy to give way to repopulation of antimicrobial-tolerant persister cells that can survive root canal treatment [47, 48].
Microbial Profiles of Root Canal Infections
The term microbiota defines a collection of microorganisms that thrive within a defined ecosystem. The oral ecosystem harbors one of the richest microbiota of the human body, by far dominated by the domain Bacteria; although Archaea, fungi, viruses and Protozoa may also be found [49, 50]. To date, no <775 species-level bacterial taxa have been cataloged on the human oral microbiome database (HOMD) [51]. Because root canal infections most often occur as a sequel to caries or trauma, the bacterial communities that initially colonize the pulpal space result from an ecological selection of the oral microbiota [52, 53]. The composition of the endodontic microbiota therefore derives from a compositional shift of oral communities, driven by the specific ecological conditions of infected root canals.
Endodontic infections can be didactically subdivided into three categories evocative of the time when microorganisms entered the pulpal space [54]. Primary endodontic infections are caused by microorganisms involved in initial pulp invasion and subsequent colonization of necrotic tissues. Secondary endodontic infections are caused by microorganisms introduced into the root canal secondarily to professional intervention, either iatrogenically during operative procedures, or later by coronal percolation via micro-leaking restorations. Persistent endodontic infections are caused by microorganisms that are part of either a primary or secondary infection, that resisted chemo-mechanical debridement procedures and survived within the scarce environment of treated root canals. Of note, because persistent and secondary infections remain clinically challenging to distinguish, they tend to be regrouped under the same pathological entity [55].
Targeted Microbial Identification in Root Canal Infections
For many years, knowledge of the root canal microbiota originated mostly from culture techniques and closed-ended molecular approaches such as fluorescent in situ hybridization (FISH), DNA-DNA hybridization checkerboards or PCR and its variations [56–59]. These approaches permitted to identify sets of bacterial taxa that appeared distinct between root canal infection types. Primary infections appeared dominated by 40–50 Gram-negative strictly anaerobic species of the genera Fusobacterium, Prevotella, Porphyromonas, Tannerella, Treponema [55, 60, 61]. Persistent/secondary infections seemed to harbor less diverse microbial communities composed of 10–20 taxa, mostly Gram-positive facultative anaerobes including species of Streptococcus, Lactobacillus, Actinomyces, and Enterococcus [17, 62]. Enterococcus faecalis particularly was frequently retrieved from persistent/secondary infections and yet remained rarely detected in primary infections [63–65]. These observations demonstrated the ability of E. faecalis to cope with the bleak conditions of treated root canals, and advocated for an important pathogenic role in secondary infections [66].
Together, conventional approaches provided a substantial body of information on the microbial composition and diversity of the different endodontic infections, albeit undermined by inherent limitations. Whereas, culture techniques fail to identify the as-yet-uncultivated microbiota, the pre-selection of primers and probes typical of closed-ended molecular methods skews microbial identification toward pre-targeted species and precludes the detection of less studied or “unexpected” taxa.
Next-Generation Profiling of the Intraradicular Microbiome
During the last decade, next-generation sequencing (NGS) technologies, that allow the high-throughput sequencing of DNA, have found unquestionable relevance to study complex microbial communities [67, 68]. In endodontics, microbial identification has mostly relied on the partial sequencing of the 16S ribosomal RNA (16S rRNA) gene [69]. This gene bares the advantages of being ubiquitous among bacteria and to combine slowly-evolving (conserved) regions, that can be employed to detect virtually all taxa in a sample, along with fast-evolving (variable) regions, that differ between taxa and become valuable targets for bacterial identification [70].
Typically, 16S amplicons that span one or several variable regions are sequenced thousands of times in parallel (5,000–50,000 coverage depth), and then employed to infer bacterial taxonomy by comparison with reference databases. NGS profiling of root canal communities unveiled a previously-hidden microbial diversity, substantially expanding the catalog of bacteria identified above 400 taxa on average [69]. Furthermore, such important diversity was similarly observed in both primary and persistent/secondary infections, which is contrasting with classical reports [71–75]. As a result of this important diversity, bacterial taxa were no longer unequivocally specific to an infection type, rather, the same taxa tended to be identified in all root canal infections, albeit in different abundances. The root canal microbiota was therefore deemed to be compositionally unspecific, yet differentially abundant in each infection type. Table 1 provides an overview of the main characteristics and findings of the NGS-based studies included herein.
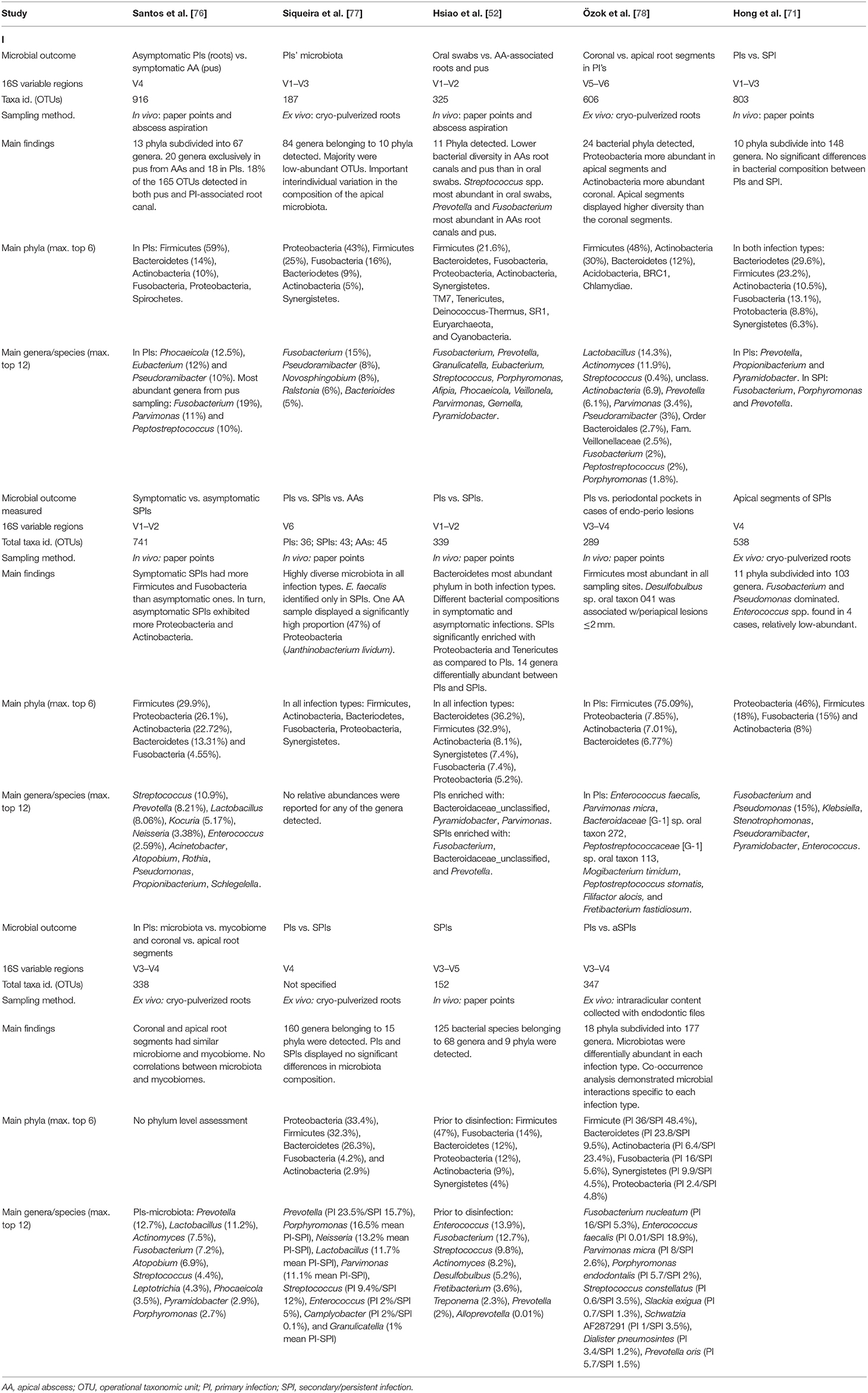
Table 1. Overview of the studies included that investigated the root canal microbiota by means of 16S rRNA gene next-generation sequencing.
Overall, Firmicutes, Bacteroidetes, Proteobacteria, Actinobacteria, and Fusobacteria were the most abundant phyla detected in root canal infections, regardless of the type [69]. At lower taxonomic levels, primary infections were frequently confirmed to harbor higher abundances (>5%) of previously identified genera such as Fusobacterium, Pseudoramibacter, Porphyromonas, Prevotella, Lactobacillus, Bacteroides, or Ralstonia [77, 78, 80, 82]. These taxa were frequently detected along with strictly anaerobic, proteolytic Firmicutes such as Pseudoramibacter alactolyticus, Parvimonas micra and asaccharolytic species of Dialister, such as D. pneumosintes or D. invisus. NGS studies also disclosed the involvement of previously little-known taxa, such as the Clostridales species Mogibacterium timidum, seemingly enriched in primary infections [75, 80].
In turn, persistent/secondary infections appeared enriched with Synergistetes representatives including Pyramidobacter piscolens and Fretibacterium fastidiosum [75, 81, 83]. Other genera detected in increased abundances in persistent/secondary infections included Streptococcus, Prevotella, Lactobacillus, Desulfobulbus, Kocuria, Neisseria, and Enterococcus [75, 79, 83]. The role of E. faecalis in persistent/secondary infections has been challenged by NGS reports. Unlike classical studies that reported prevalence values of E. faecalis in cases of treated root canals reaching up to 90%, the species was detected in significantly lower frequencies (~30%) by 16S-sequencing in persistent/secondary infection [72, 74, 75, 81, 83]. Nonetheless, when present, the species could reach intriguingly high abundance ranging from 14 up to 90% [75, 81, 83]. Because evidence shows that E. faecalis is more frequently retrieved from root canals after multiple visits or temporarily left open, it has been purported that the species is a secondary opportunistic colonizer in treated root canals rather than a persister from unsuccessfully treated primary infections [84, 85]. In such case, one could speculate that treated root canals displaying remarkably high abundance of E. faecalis may reflect secondary infections as opposed to persistent ones, that would in turn be associated with a higher microbial diversity. In this line, it has been shown using in vitro biofilm models that when opportunistically present among other common oral species, it can grow in a predominant manner within the biofilm [86].
Few studies have also attempted to discriminate the microbiota of symptomatic and asymptomatic presentations, both in cases of primary or persistent/secondary infections. Overall, the microbiota of symptomatic primary root canal infections tended to be more diverse than asymptomatic ones [73, 76]. This trend appears reversed in persistent/secondary infections, in which decreased diversity was associated with asymptomatic clinical presentations [73, 79]. These results are nonetheless to be considered with caution, as the comparison of samples collected from root canals or obtained by pus aspiration may raise some methodological concerns [76].
Taken together, NGS-based community profiling has confirmed the involvement of previously identified species, and further expanded the list to a myriad of low abundant taxa with yet-poorly understood clinical relevance. From an ecological standpoint, however, every taxon in a mixed consortium is important to detect regardless of its abundance, as any taxa may act as keystone species and potentiate the pathogenicity of the entire community [53, 87]. In this perspective, the relatively short length of the 16S amplicons used by current 16S rRNA-sequencing approaches (roughly 400 bp) is an important limitation, as it limits taxonomic profiling to the genus level [88]. Although advances in long read technologies are expected to soon allow the full length-sequencing of the 16S rRNA gene and improve taxonomic resolution [89, 90], significant taxonomic and functional characteristics would remain concealed at the strain-level [91]. One element of answer may stem from shotgun metagenomics, that can re-assemble complete metagenomes to identify strain-level changes and predict the functional genetic potential of microbial communities [92]. Besides, to better grasp the metabolic processes and functional networks that underlie pathogenicity, assessment of the microbial genes that are actively transcribed (metatranscriptomics) and/or translated (metaproteomics) is also necessary [93, 94].
Insights Into the Metaproteome of Root Canal Communities
Because proteins are function effectors, proteomic approaches that examine the collection and abundance of proteins within microbial communities, i.e., the metaproteome, inform on the ongoing biological processes. Thus far, only few studies have relied on metaproteomics to functionally explore the processes engaged in bacterial cells during endodontic infections [95–98]. Although these studies remain preliminary due to the limited number of samples (between 7 and 20 per study), and the considerable variations in sampling methods (pus aspiration from abscesses, intra-radicular sampling with paper points, apical lesions, and root fragments resected during surgery), their findings provided invaluable and novel insights into the mechanisms of bacterial survival and virulence within root canals. Intriguingly, housekeeping processes were among the most abundant functional categories regardless of the sample type [96–98]. This indicates the presence of metabolically active bacteria in both necrotized and treated root canals, and further supports the concept of active bacteria able to invade periradicular tissues, as evidenced by analyses of surgically retrieved apical lesions [97].
Aside from housekeeping functions, several other proteins were related to pathogenic processes. Abundantly detected enzymes and adhesins, such as fibrinogen-binding protein, fibronectin-binding A domain, polysaccharide lyases or glycosyltransferase I (exopolysaccharide producing enzyme), are testimony of bacterial adhesion and biofilm formation [99–101]. Equally crucial to pathogenicity, are the numerous proteolytic enzymes detected including collagenases, metalloproteases, serine proteases, and extracellular peptidases [95–98].
During the course of an infection, proteolytic activities serve to break-down peptides and acquire amino acids, to degrade connective tissues for host invasion or to cleave antibodies and complement molecules for immune evasion [102–104]. Also, a series of antibiotic-resistance factors have also been recurrently detected in endodontic communities. These typically included TetRA, conferring resistance to tetracycline, β-lactam-degrading enzymes (β-lactamase), multidrug efflux pumps (MdtB), and multiple transcriptional regulators of antibiotic-resistance genes (AsnC-type, LacL-type, and QseB-type) [95–98]. Because systemic antibiotics become indispensable when endodontic infections spread into topical tissues or systemically, these findings are clinically troublesome [105, 106]. Finally, numerous stress response proteins including the chaperonins GroEL, DnaK, or HslU along with the nucleotide excision repair complex UvrABC were common findings [95–98]. This is unsurprising as expression of stress response proteins is often co-regulated with virulence factors and antibiotic resistance genes.
Importantly though, in addition to increasing tolerance limits, stress response proteins may also exhibit cytotoxicity and potentiate pathogenicity by promoting the secretion of pro-inflammatory cytokines [107, 108]. The proinflammatory response in the pulpal and periradicular region is an important histopathological hallmark of endodontic infections, that may take far beyond this review to discuss. Worth highlighting here, is the significance of the receptor activator of NF-κB ligand-osteoprotegerin system in the periradicular osteolysis that may accompany endodontic infections [109], which may in turn be differentially regulated depending on the endodontic diagnosis [110].
Clinical Implications of Root Canal Infections
Challenges in Eliminating Root Canal Biofilms
While the understanding of the microbial complexity of root canal infections has substantially improved over the past decade. On the other hand, although the success rates of root canal treatments have been very favorable, they have not experienced the same extent of improvement, specifically in teeth which had a necrotic pulp with or without a periradicular lesion pre-operatively [111, 112]. From a microbiological standpoint, this is unsurprising—infected teeth have densely colonizing biofilms that are strongly adherent to the dentin, posing challenges in removal. However, an additional, yet exemplary challenge appears in the form of anatomical complexities in the root canal system—inter and intra-canal communications/isthmi, lateral and accessory canals, apical delta—are a norm rather than an exception. Therefore, there are two key challenges in removing root canal biofilms: microbiological and anatomical.
As discussed earlier, biofilms are inherently tolerant to antimicrobial agents. Biofilms are surface-attached communities where the bacterial and/or fungal cells are held together in a highly cohesive matrix, which presents a diffusion limitation to antimicrobials. Therefore, for an antimicrobial agent to effectively kill the microbial cells, it should be able to dissolve and disrupt the matrix. Alternatively, this matrix should be disrupted by mechanical means to allow antimicrobial chemicals to reach the cells housed inside the biofilm matrix. While this appears simple in theory, the clinical practicalities are complicated by the rather “blind” area of the root canal system that we work in and the complex myriad of inter-communicating pathways which are never accessible to mechanical instrumentation.
Despite such knowledge of the so-called biofilm entity, it is notable that most of the reported studies on anti-biofilm agents, including traditional disinfectants such as sodium hypochlorite or antimicrobials such as chlorhexidine and calcium hydroxide, do not characterize the effects on biofilm composition. Such as the constituents of the self-produced EPS, which includes various extra-polysaccharides, eDNA, and phosphoproteins. The widely reported experiments in this regard, include counting the number of microbes after treatments using the plate count method or qPCR and characterizing the “architecture” of biofilms using three-dimensional imaging approaches such as confocal laser scanning microscopy. However, such studies on confocal microscopic examination routinely use fluorophores that stain the live and dead microbial cells but not the matrix. While one may assume that the disinfectants should have likely disrupted the matrix to reach the microbial cells, this should be demonstrated with experimental evidence. Furthermore, such an assumption may not be true. Tawakoli et al. [113] characterized the effects of 5% sodium hypochlorite, 17% EDTA, 3% hydrogen peroxide, and 2% chlorhexidine on a three-species biofilm and reported that sodium hypochlorite eradicated the stainable matrix and bacteria in cultured biofilms, while chlorhexidine and hydrogen peroxide merely reduced bacterial cell volumes without dissolving the matrix [113]. Ali et al. [114] demonstrated that a natural molecule trans-cinnamaldehyde could kill E. faecalis biofilm cells as effectively as sodium hypochlorite. Confocal microscopic characterization demonstrated largely “red” areas in the treated biofilm, implying complete bacterial killing [114]. However, when the same authors characterized the polysaccharide matrix component, they discovered that trans-cinnamaldehyde could not eliminate the matrix.
The above summary results in a call for action. The authors of this review strongly recommend that when traditional or novel disinfectants are tested on biofilms, both the cellular and extracellular content of the biofilm must be studied to provide comprehensive information on anti-biofilm effects. Such characterization of the extracellular content maybe performed using biochemical methods [114, 115] or by using matrix-component specific fluorophores for confocal microscopic imaging [37]. Furthermore, the use of multi-species as well as aged-biofilm models may better represent the biofilms found in infected root canals, which could enhance the applicability of in vitro research to the clinical situation.
From the context of anatomical challenges, while instrumentation of the root canal with stainless steel or nickel titanium hand or engine-driven instruments may help in removing biofilms from root canal walls, much is required to eliminate biofilms and reduce microbial loads from the anatomical eccentricities [33, 116]. The goal of activated irrigation is to mechanically flush the irrigating solutions such as sodium hypochlorite into such eccentricities in addition to chemically enhancing their ability to dissolve organic matter [117, 118]. In this regard, there has been wide confusion over the use of agitation and activation strategies. While this is certainly important, such clarification of terminology is not within the scope of this review. That said, several means of “dynamic” irrigation such as ultrasonic, sonic, multisonic, apical negative pressure, and laser have been interrogated to demonstrate their ability on eliminating biofilms and/or killing bacterial cells. Indeed, such adjunctive strategies are considered to improve irrigant infiltration into anatomical complexities [119].
One systematic review concluded that ultrasonic activation of irrigants with ultrasonic results in superior bacterial reduction from the root canal systems compared to other methods of irrigant activation and conventional syringe irrigation [120]. By contrast, another systematic review reported that there was insufficient evidence to conclude that ultrasonics resulted in superior bacterial reduction from root canals [121]. New multisonic approaches such as Gentlewave (Sonendo, Laguna Hills, CA, USA) have been shown to significantly reduce bacterial counts compared to ultrasonic [122]. That said, none of the studies that investigated the activation strategies reported the effects of activation on biofilm matrices or truly complex multi-species biofilms. Furthermore, the substantial heterogeneity in terms of irrigant concentration, duration, activation cycles preclude meaningful extrapolation to provide clinically relevant conclusions.
Contemporary Root Canal Disinfection Strategies
Root Canal Irrigants
Irrigants are used to flush out debris, lubricate the canal and aid the elimination of root canal infection. Sodium hypochlorite is the most commonly used irrigant in endodontics due to its antimicrobial and tissue dissolving properties [123–125]. It has been suggested that 0.5 and 5% sodium hypochlorite were equally effective in reducing bacterial load when evaluated using culture studies [123]. However, other studies suggest the removal of E. faecalis in both biofilm and planktonic forms with sodium hypochlorite may be concentration dependent, with higher concentrations resulting in better outcomes [126, 127]. On the other hand, high concentrations of sodium hypochlorite, such as 5 or 9%, may result in the disintegration of the organic dentine matrix [128]. Such concentrations may be severely caustic to the apical tissues, particularly if extruded out the apical foramen [129]. Furthermore, needle irrigation with sodium hypochlorite may only be able to eliminate the bacterial residing in the more superficial regions of dentinal tubules [130]. Other than concentration, other factors can also affect its antimicrobial efficacy such as irrigant refreshment, volume, exposure time, flow, wall shear stress created and the method of delivery [131].
Bisbiguanide solutions are also commonplace in irrigation strategies, such as chlorhexidine digluconate, which is a frequently used disinfectant in endodontics. Chlorhexidine has been advocated as a final rinse irrigant due to its broad-spectrum antimicrobial activity, substantivity and its ability to inhibit collagen degradation [125, 132–134]. However, several drawbacks of its use include the incapacity to degrade organic tissue and a potential deleterious impact on periapical health outcomes, hence its use throughout endodontic treatment, particularly alongside sodium hypochlorite, is not recommended [135]. Furthermore, although chlorhexidine's ability to eradicate E. faecalis has been demonstrated in several studies [136], such effects appears to be limited to planktonic cells and its efficacy decreases in the presence of mature biofilm structures, permitting biofilm recovery [137, 138]. Although a recent meta-analysis of randomized controlled trials comparing sodium hypochlorite and chlorhexidine concluded that their antimicrobial efficacy was comparable, the authors highlighted the heterogeneity in bacterial detection methods within the included studies and also did not address the antibiofilm efficacy of the two irrigants [139]. It has been suggested the addition of 0.2% cetrimide, a cationic surfactant agent, may be able to act synergistically with 2% chlorhexidine to enhance antibacterial efficacy and improve substantivity [140]. Other emerging antiseptic solutions include alexidine and octenidine hydrochloride, which have been shown to exhibit antimicrobial effects similar to and greater than sodium hypochlorite, respectively [141].
Chelating agents are used in irrigation protocols to dissolve smear layer, remove mineralized debris and calcifications, and disrupt biofilm. Various acidic chelators exist, including such as ethylenediaminetetraacetic acid (EDTA), maleic acid, citric acid, and phosphoric acid [142, 143]. EDTA in particular has been recommended as an chelating irrigant due to its capacity to predictably remove the smear layer [125, 142, 144] and detach biofilm cells [145]. Pairing it with sodium hypochlorite has been shown to enhance the antibiofilm effects against E. faecalis biofilm [146]. A disadvantage is that excessive use may heavily demineralize dentine and weaken the root structure [147]. When coupled with sodium hypochlorite, it resulted in the loss of the mineral encapsulation and subsequent dissolution of the exposed collagen fibers [148]. The antibiofilm effects of EDTA and other chelators requires more investigation, using complex biofilm models.
Irrigants that combine chelating agents with antimicrobials have been developed in order to provide “one-stop” solutions for root canal disinfection. Several commercially available combination irrigants include BioPure® MTAD® (Dentsply Tulsa Dental Specialties, Tulsa, OK, USA), QMix® 2in1 (Dentsply Tulsa Dental Specialties), SmearOFF™ (Vista™ Dental Products, Racine, WI, USA). MTAD®, a mix of doxycycline, citric acid and a detergent, was developed as a final rinse irrigant [149]. It has been shown to possess antibacterial activity against E. faecalis [149, 150]. However, evidence suggests that it may not be superior than a final rinse of sodium hypochlorite and EDTA [151]. Qmix®, a formulation of chlorhexidine, EDTA and a detergent, was reported to be more effective than 2% chlorhexidine and MTAD® against planktonic and biofilm forms of E. faecalis [152]. Its antibacterial effectiveness against E. faecalis was similarly reported on by recent systematic review of in vitro studies [153]. The recently developed SmearOFF™ likewise contains chlorhexidine, EDTA and a surfactant, however an in vitro study suggested it had poor antibiofilm properties against E. faecalis [141].
Unlike the current final rinse protocols and solutions that utilize strong chelating agents such as EDTA, formulations combining antimicrobials with weak chelators offer the option of continuous chelation, which would be used throughout the cleaning and shaping process. EDTA is not only a strong cation chelator, but it also unfavorably reacts with sodium hypochlorite and reduces the chlorine content [154]. Therefore, a weak chelating agent, etidronic acid, also known as 1-hydroxyethylidene-1,1-bisphosphonate or HEBP, has been combined with sodium hypochlorite to form a solution for continuous chelation [155]. The suggested concentration ranges between 7 and 18% [154, 156]. When the combination of HEBP and sodium hypochlorite was applied to root dentine, it was able to remove the smear layer without excessive decalcification [156], although a recent study reported that it negatively affected the dentine nanohardness [157]. This solution also appears to possess commendable antibiofilm activity against E. faecalis biofilm [158] but this may be influenced by its short-term storage ability [159]. A clinical study evaluated a recently developed commercially available continuous chelator i.e., Dual Rinse® HEDP (Medcem GmbH, Weinfelden, Switzerland) and reported comparable antimicrobial activity to sodium hypochlorite used in isolation [160].
Recent studies have proposed clodronate, also a first-generation bisphosphonate, as another candidate for continuous chelation. Clodronate combined with sodium hypochlorite was found to effective in removing the smear layer [161]. It appeared to be better than solutions with etidronate at dissolving organic tissue [162]. However, evidence of its antimicrobial and antibiofilm efficacy has yet to be reported in the literature.
Intracanal Medicaments
The placement of an interappointment dressing has been advocated to provide a continuous supply of antimicrobial agents, restrict bacterial growth, and create a barrier for bacteria recolonization. Historically, a variety of intracanal medicaments have been suggested including calcium hydroxide, iodine potassium iodide, eugenol, formocresol, phenolic compounds, and various antibiotics [163]. Whilst several of these medicaments have fallen out of favor due to concerns of potential mutagenic and allergenic effects, calcium hydroxide remains as a first line intracanal medicament for necrotic cases with established infections [135, 164]. Multiple classic studies have shown its ability to reduce bacterial load due to its highly alkaline, tissue dissolving, and antimicrobial properties [124, 165, 166].
However, the efficacy of calcium hydroxide has also been questioned, particularly against bacteria implicated in recalcitrant root canal infections, such as E. faecalis and Candida albicans [167–170]. It also exhibits limited ability to adequately disinfect dentinal tubules [171] and has the potential to weaken the root structure if used over long periods of time [172]. There is conflicting and insufficient evidence on modifying calcium hydroxide with chlorohexidine to improve its antibacterial effects [173–175]. On the other hand, Zehnder et al. [176] reported that a combination of calcium hydroxide and sodium hypochlorite exhibited significantly better tissue dissolving properties and antimicrobial efficacy compared to mixtures using saline. The same study also found favorable properties with calcium hydroxide-chlorhexidine paste, although the effects were relatively short-lived [176].
A range of antibiotics and their combinations have been proposed as alternative intracanal medicaments since the mid-twentieth century [177]. More recently, triple antibiotic paste (TAP), consisting of metronidazole, ciprofloxacin, and minocycline, has been enthusiastically advocated for root canal disinfection prior to regenerative endodontic strategies [178, 179] due to its reportedly superior antibiofilm properties and ability to disinfect dentinal tubules compared to calcium hydroxide [180–182]. However, an in vitro study found TAP was only effective against E. faecalis biofilm and not against Candida albicans biofilm [170]. Furthermore, some guidelines challenge the evidence supporting its routine use, especially given the risks dentine discolouration, antibiotic resistance [164] and potential detriment to stem cells [183].
Antibiotics have also been combined with anti-inflammatory drugs in order to provide a dual therapeutic effect. Ledermix® paste (Lederle Laboratories, Seefeld, Germany), which consists of triamcinolone and a demeclocycline, has been proposed as an intracanal medicament for symptomatic teeth and concurrent endodontic and periodontal lesions due to the corticosteroid component [184]. However, Ledermix® on its own has limited antibacterial and antibiofilm effects against E. faecalis [169]. Some have suggested combining Ledermix® with calcium hydroxide to augment the antibacterial efficacy [169, 185], although this remains controversial with other studies refuting such benefits [186, 187].
Novel and Advanced Disinfection Therapies
Nanoparticles
In the last decade, nanoparticle-based disinfection therapies have generated significant enthusiasm within the endodontic research community. Nanoparticles measure between 1 and 100 nm [188]. Their miniscule dimensions and large surface area to volume ratio mean they exhibit greater antimicrobial activity than their macroscale counterparts and are able to better infiltrate biofilm. They can also be functionalized with other compounds, such as photosensitizers, bioactive molecules, and drugs, to produce synergistic effects [189].
Silver nanoparticles are the most widely investigated metallic nanoparticle in the field of root canal disinfection strategies owing to their antibiofilm, antimicrobial, and antifungal properties [190]. A study found that a silver nanoparticle-based irrigant had antibiofilm activity comparable to 2% chlorhexidine and 5% sodium hypochlorite [191]. However, the antimicrobial efficacy of silver nanoparticles may be contact and time-dependent, hence their application as intracanal medicaments or root canal sealers may be more appropriate [189, 192]. There are concerns regarding its potential to cause dentine discolouration [193] and produce cytotoxic and inflammatory reactions from the host tissues [194]. Other antimicrobial metallic nanoparticles that have been investigated as root canal irrigants include zinc oxide, magnesium oxide, titanium dioxide, and iron oxide [195, 196].
Chitosan is an organic polysaccharide that is derived from chitin, a natural component found in shrimp and crab shells [197]. Chitosan nanoparticles exhibit excellent biocompatibility as well as promising antibiofilm and antimicrobial properties [198, 199]. Chitosan nanoparticles were found to potently kill planktonic and biofilm E. faecalis cells [200]. Studies have demonstrated its capacity to remove the smear layer [199, 201] and stabilize dentine by providing inhibiting collagenase degradation [202]. Chitosan nanoparticles have also been functionalized with photosensitizers such as Rose Bengal and methylene blue to enhance antibiofilm efficacy via photodynamic activation [203, 204].
Intracanal medicaments with calcium hydroxide nanoparticles have been developed to help improve its diffusivity and antibacterial activity [205]. Studies have reported that nano-calcium hydroxide was able to penetrate deeper into dentinal tubules and was more effective against E. faecalis compared to conventional calcium hydroxide [205–207]. These novel intracanal medicaments were also found to have less detrimental on the fracture resistance of the root dentine [205]. Nanosized calcium silicate compounds have also been investigated as novel intracanal disinfectants by acting as drug delivery vehicles allowing the sustained release of antimicrobial compounds [208, 209]. Mesoporous calcium silicate nanoparticles combined with silver nanoparticles were able to inhibit the growth of both planktonic and biofilm forms of E. faecalis [209, 210]. Studies have also suggested their applicability as novel root canal sealers due to their bioactive and osteogenic properties [211–214]. Other nanoparticles with sustained antibiofilm activity that have been explored as root canal sealers include quaternary ammonium polyethyleneimine [215] and quaternary ammonium methacrylate nanoparticles [216].
Light Activated Disinfection
In general, disinfection strategies that utilize laser or light can be divided into three groups: direct laser irradiation, photodynamic therapy, and laser activated irrigation [217]. Research into traditional forms of laser endodontics, such as direct laser irradiation, have fallen out of favor because of detrimental thermal effects on the root dentine [218] and a lack of in vivo microbiological evidence to support its use as a root canal disinfectant strategy [219]. Furthermore, irrigation with 1% sodium hypochlorite had a more remarkable antibacterial effect in root canals infected with E. faecalis when compared to direct laser irradiation [220]. Instead, the focus has shifted toward laser activated irrigation and photodynamic therapies, both of which appear to be of promising value as disinfection adjuncts.
Laser activated irrigation harnesses the ability of certain wavelengths to react with water molecules to instigate the cavitation effect. This produces bubble expansion followed by implosions and shock waves, resulting in turbulent flow that helps distribute the irrigants into anatomical complexities [221]. An in vitro study reported that Erbium:YAG (Er:YAG) laser activated irrigation was better at eliminating E. faecalis biofilms than ultrasonic activated or syringe irrigation [222]. A variation of laser activated strategies is photon-induced photoacoustic streaming (PIPS). Whilst it also operates using the principles of hydrodynamic cavitation, the main difference is that the laser tip is specially designed to allow it to be positioned the pulp chamber without needing to advance into the root canal system [223]. Several studies have shown PIPS was effective at removing biofilm and the smear layer [224, 225]. Dentinal tubule disinfection was more favorable using PIPS compared to ultrasonic irrigation and Er:YAG laser activation, however none were unable completely eliminate bacterial biofilm and tissue remnants [226].
Photodynamic therapy involves the use of light at a specific wavelength that interacts with a photo-absorbing photosensitizer to excite its electronic layers to a so-called triplet state. In this state, the photosensitizer may react with molecular oxygen and generate reactive oxygen species that elicit an antibacterial effect by degrading microbial lipids, proteins, and DNA [227, 228]. Several options exist for photosensitizers such as methylene blue [204], Rose bengal-conjugated chitosan [229], toluidine blue [230], and indocyanine green [231]. Studies have highlighted the antibiofilm potential of photodynamic therapy for multiple species biofilms [232, 233]. Photoactivated rose bengal-conjugated chitosan was also able to inactivate endotoxins in vivo [229]. Notably, Curcumin, the compound responsible for the yellow hue of turmeric or Curcuma longa, was found to effectively eliminate biofilm bacteria when applied as a photosensitizer [234]. A systematic review concluded that although there was a paucity of clinical studies, the available evidence suggests photodynamic therapy is able to effectively reduce the microbial load and can be a worthy adjunct to current disinfection protocols [235].
Natural Compounds (Phytotherapy)
Natural therapeutic options have sparked the interest of researchers in the search of novel root canal disinfectants. A variety of herbal and plant derived compounds have been identified as potential root canal disinfectants owing to a range of favorable attributes including antibacterial, antifungal, and biocompatible properties. Some of these compounds are found in commonly encountered herbs and spices. Cinnamomum zeylanicum, Syzygium aromaticum, and Ocimum sanctum, also known as cinnamon, clove and holy basil, each showed antimicrobial activity against E. faecalis in both planktonic and biofilm forms [236]. The essential oil constituents, particularly eugenol, may be responsible for some of the antimicrobial properties of these herbal compounds [236]. Specifically, trans-cinnamaldehyde, a phenylpropanoid compound, commonly found in cinnamon, was recently reported to eradicate biofilm cells as potently as sodium hypochlorite and more importantly prevented repopulation of bacteria [114], in contrast to chlorhexidine which could not prevent biofilm recovery [138]. This is a significant finding as this is the first evidence that a natural molecule can kill biofilm cells and prevent reinfection with the same potency as sodium hypochlorite in clinically relevant time frames.
Other plant extracts whose applications traditionally have roots in from Ayurvedic, Chinese or other historical forms medicines include berberine, Morinda citrifolia and Triphala. Berberine, a quaternary ammonium salt found in a variety of plants, was reported to be more efficacious against a multi-species biofilm model when compared to 5.25% sodium hypochlorite and 2% chlorhexidine [237]. Triphala is a mixture of the fruits from three plant species Emblica officinalis, Terminalia bellirica, and Terminalia chebula. It was proposed as an herbal alternative to conventional irrigants owing to its ability to completely inhibit E. faecalis biofilm formation [150]. The juice from the Morinda citrifolia, colloquially known as noni juice, exhibited greater antimicrobial efficacy against E. faecalis in dentinal tubules than calcium hydroxide. However, 2% chlorhexidine reigned superior in regard to the antimicrobial effect [238].
Propolis is the resinous product of bees derived from sap and other botanical material. Kandaswamy et al. [238] also reported that propolis had comparable inhibitory effects against E. faecalis when compared to Morinda citrifolia. When applied as an intracanal medicament, it was found to have better antimicrobial activity against E. faecalis compared to calcium hydroxide [239]. Propolis-loaded nanoparticles exhibited low cytotoxicity and antimicrobial efficacy against E. faecalis, Streptococcus mutans and Candida albicans [240].
Antimicrobial Peptides
Antimicrobial peptides have recently gained attention for their potential therapeutic applications in disinfection. This diverse group of biomolecules are involved in various host-defense mechanisms and are also responsible for immunomodulation [241]. In general, these peptides disrupt and destabilize the bacterial membrane integrity and cellular functions, eventually leading to cell death. This enables their broad-spectrum antimicrobial activity including drug-resistant strains [241, 242]. A myriad of antimicrobial peptides have been reported to possess promising antibiofilm and antimicrobial efficacy against various microorganisms implicated in root canal infections. One of the first antimicrobial peptides to be discovered and subsequently gain attention for its potential biomedical applications is nisin [243]. Nisin used as an irrigant was found to reduce E. faecalis growth and disrupt its biofilm structure [244]. Peptide GH12 exhibited multimode mechanisms against E. faecalis by influencing its genetic expression and virulence whilst also proving to be bactericidal in vitro and ex vivo [245]. Peptide DJK-5, with or without chlorhexidine was shown to have efficacy against multispecies biofilms [246]. When mixed with EDTA, peptide DJK-5 likewise exhibited superior antibacterial properties against E. faecalis biofilms compared to another peptide 1,018 [247], which when combined with chlorhexidine, exhibited a less pronounced antibacterial effect and required longer periods of exposure [246]. The latter peptide nonetheless has the potential to modulate the host immune response and inflammation, which may provide another dimension of benefit [248].
Conclusions and Call for Future Research
This review focused on the microbial aspect of root canal infections and disinfection strategies, with focus on intra-radicular microbiological challenges. Omics-approaches have considerably enhanced our appreciation of the taxonomic diversity within root canal communities, and to a lesser extent, of the functional and metabolic pathways of bacterial survival within root canals. These aspects can be further addressed by metatranscriptomic, metaproteomic, and metabolomic approaches [93] that may well reveal altered functional profiles in disease-associated communities that underpin pathogenicity.
Such high-throughput approaches would additionally benefit from standardization efforts, in sampling and controls' design for instance. This would facilitate inter-studies comparisons and help dodging methodological pitfalls [249]. Also, further investigations may gain relevance by attaching clinical issues to the community-based investigations, rather than remaining solely descriptive of microbial communities. As examples, could we exploit microbiome data from deep caries or exposed pulps in order to guide treatment strategy toward vital pulp approaches or pulpectomy? Could the root canal microbiota have a prognostic value on periradicular healing? Why is it that pulpal inflammation and periradicular disease may sometimes develop unnoticed by the patient, and in other instances assume excruciating clinical presentations that may lead to life-threatening infectious complications? Whereas the state of the local immune system was shown to contribute to curbing the process [250, 251], little is known of the microbiological factors responsible for acute infections.
As mounting evidence supports associations between endodontic infections and systemic conditions such as diabetes mellitus or cardiovascular diseases [8–10], could we identify microbial signatures able to predict such systemic outcomes? Lastly, in order to combat the biofilm-mediated etiopathogenesis of root canal infections, future research may be directed toward novel anti-biofilm strategies alongside targeting the microbial impetus, such as quorum sensing inhibition, EPS dispersion, biofilm disassembly, and inhibition of signaling systems and macromolecule synthesis, which may pave the way to a variety of novel endodontic disinfection therapies [252]. This may pave the way for the development of more holistic, and potentially more powerful, endodontic disinfection therapies. Addressing these still-open questions may contribute to provide a framework for improving diagnostic procedures, therapeutic regimens and reduce the incidence of adverse clinical evolutions.
Author Contributions
PNe and GB: conception, design, manuscript—editing, and final draft. JW, DM, and PNä: literature review and manuscript preparation—original draft. All authors contributed to the article and approved the submitted version.
Conflict of Interest
The authors declare that the research was conducted in the absence of any commercial or financial relationships that could be construed as a potential conflict of interest.
References
1. Nair PN. Apical periodontitis: a dynamic encounter between root canal infection and host response. Periodontol 2000. (1997) 13:121–48. doi: 10.1111/j.1600-0757.1997.tb00098.x
2. Lin LM, Huang GT-J. Pathobiology of apical periodontitis. In: Berman LH, Hargreaves KM, RotsteinI, editor. Cohen's Pathways of the Pulp. 11 ed. St. Louis, MO: Elsevier (2013). p. 630-59.
3. Nair PN. Pathogenesis of apical periodontitis and the causes of endodontic failures. Crit Rev Oral Biol Med. (2004) 15:348–81. doi: 10.1177/154411130401500604
4. Sindet-Pedersen S, Petersen JK, Gotzsche PC. Incidence of pain conditions in dental practice in a Danish county. Commun Dent Oral Epidemiol. (1985) 13:244–6. doi: 10.1111/j.1600-0528.1985.tb01914.x
5. Widstrom E, Pietila I, Nilsson B. Diagnosis and treatment of dental emergencies in two Finnish cities. Commun Dent Health. (1990) 7:173–8.
6. Dorn SO, Cheung GS-P. Managment of endodontic emergencies. In: Hargreaves KM, Berman LH, Rotstein I, editors. Cohen's Pathways of the Pulp. 11 ed. St. Louis, MI: Elsevier (2016). p. 706–21.
7. Shah AC, Leong KK, Lee MK, Allareddy V. Outcomes of hospitalizations attributed to periapical abscess from 2000 to 2008: a longitudinal trend analysis. J Endod. (2013) 39:1104–10. doi: 10.1016/j.joen.2013.04.042
8. Pasqualini D, Bergandi L, Palumbo L, Borraccino A, Dambra V, Alovisi M, et al. Association among oral health, apical periodontitis, CD14 polymorphisms, and coronary heart disease in middle-aged adults. J Endod. (2012) 38:1570–7. doi: 10.1016/j.joen.2012.08.013
9. Segura-Egea JJ, Martin-Gonzalez J, Castellanos-Cosano L. Endodontic medicine: connections between apical periodontitis and systemic diseases. Int Endod J. (2015) 48:933–51. doi: 10.1111/iej.12507
10. Aminoshariae A, Kulild JC, Mickel A, Fouad AF. Association between systemic diseases and endodontic outcome: a systematic review. J Endod. (2017) 43:514–9. doi: 10.1016/j.joen.2016.11.008
11. Persoon IF, Ozok AR. Definitions and Epidemiology of Endodontic Infections. Curr Oral Health Rep. (2017) 4:278–85. doi: 10.1007/s40496-017-0161-z
12. Jakovljevic A, Nikolic N, Jacimovic J, Pavlovic O, Milicic B, Beljic-Ivanovic K, et al. Prevalence of apical periodontitis and conventional nonsurgical root canal treatment in general adult population: an updated systematic review and meta-analysis of cross-sectional studies published between 2012 and 2020. J Endod. (2020) 46:1371–86. e1378. doi: 10.1016/j.joen.2020.07.007
13. Jimenez-Pinzon A, Segura-Egea JJ, Poyato-Ferrera M, Velasco-Ortega E, Rios-Santos JV. Prevalence of apical periodontitis and frequency of root-filled teeth in an adult Spanish population. Int Endod J. (2004) 37:167–73. doi: 10.1111/j.0143-2885.2004.00759.x
14. Lopez-Lopez J, Jane-Salas E, Estrugo-Devesa A, Castellanos-Cosano L, Martin-Gonzalez J, Velasco-Ortega E, et al. Frequency and distribution of root-filled teeth and apical periodontitis in an adult population of Barcelona, Spain. Int Dent J. (2012) 62:40–6. doi: 10.1111/j.1875-595X.2011.00087.x
15. Lin LM, Skribner JE, Gaengler P. Factors associated with endodontic treatment failures. J Endod. (1992) 18:625–7. doi: 10.1016/S0099-2399(06)81335-X
16. Sundqvist G, Figdor D, Persson S, Sjogren U. Microbiologic analysis of teeth with failed endodontic treatment and the outcome of conservative re-treatment. Oral Surg Oral Med Oral Pathol Oral Radiol Endod. (1998) 85:86–93. doi: 10.1016/S1079-2104(98)90404-8
17. Pinheiro ET, Gomes BP, Ferraz CC, Sousa EL, Teixeira FB, Souza-Filho FJ. Microorganisms from canals of root-filled teeth with periapical lesions. Int Endod J. (2003) 36:1–11. doi: 10.1046/j.1365-2591.2003.00603.x
18. Kakehashi S, Stanley HR, Fitzgerald RJ. The effects of surgical exposures of dental pulps in germ-free and conventional laboratory rats. Oral Surg Oral Med Oral Pathol. (1965) 20:340–9. doi: 10.1016/0030-4220(65)90166-0
19. Moller AJ, Fabricius L, Dahlen G, Ohman AE, Heyden G. Influence on periapical tissues of indigenous oral bacteria and necrotic pulp tissue in monkeys. Scand J Dent Res. (1981) 89:475–84. doi: 10.1111/j.1600-0722.1981.tb01711.x
20. Nair PN. Light and electron microscopic studies of root canal flora and periapical lesions. J Endod. (1987) 13:29–39. doi: 10.1016/S0099-2399(87)80089-4
21. Siqueira JF Jr, and Rôças IN. Microbiology of endodontic infections. In: Hargreaves KM, Berman LB, editors. Cohen's Pathways of the Pulp. 11 ed. St. Louis, MO: Elsevier (2016). p. 599–629.
23. Fouad AF, Levin LG. Pulpal reactions to caries dental procedures. In: Hargreaves KM, Berman LB, editors. Cohen's Pathways of the Pulp. 11 ed. St. Louis, MO: Elsevier (2016). p. 573–99.
24. Heyeraas KJ. Pulpal hemodynamics and interstitial fluid pressure: balance of transmicrovascular fluid transport. J Endodontics. (1989) 15:468–72. doi: 10.1016/S0099-2399(89)80026-3
25. Siqueira JF Jr, and Rôças IN. Bacterial pathogenesis and mediators in apical periodontitis. Brazil Dental J. (2007) 18:267–80. doi: 10.1590/S0103-64402007000400001
26. Tani-Ishii N, Wang CY, Tanner A, Stashenko P. Changes in root canal microbiota during the development of rat periapical lesions. Oral Microbiol Immunol. (1994) 9:129–35. doi: 10.1111/j.1399-302X.1994.tb00048.x
27. Fabricius L, Dahlén G, Holm SE, Möller AJR. Influence of combinations of oral bacteria on periapical tissues of monkeys. Eur J Oral Sci. (1982) 90:200–6. doi: 10.1111/j.1600-0722.1982.tb00728.x
28. Fabricius L, Dahlen G, Ohman AE, Moller AJ. Predominant indigenous oral bacteria isolated from infected root canals after varied times of closure. Scand J Dent Res. (1982) 90:134–44. doi: 10.1111/j.1600-0722.1982.tb01536.x
29. Siqueira JF Jr, and Rôças IN. Clinical implications and microbiology of bacterial persistence after treatment procedures. J Endod. (2008) 34:1291–301 e1293. doi: 10.1016/j.joen.2008.07.028
30. Haapasalo M, Shen YA, Ricucci D. Reasons for persistent and emerging post-treatment endodontic disease. Endodontic Topics. (2008) 18:31–50. doi: 10.1111/j.1601-1546.2011.00256.x
31. Siqueira JF Jr, and Rôças IN. Community as the unit of pathogenicity: an emerging concept as to the microbial pathogenesis of apical periodontitis. Oral Surg Oral Med Oral Pathol Oral Radiol Endod. (2009) 107:870–8. doi: 10.1016/j.tripleo.2009.01.044
32. Ricucci D, Siqueira JF Jr. Biofilms and apical periodontitis: study of prevalence and association with clinical and histopathologic findings. J Endod. (2010) 36:1277–88. doi: 10.1016/j.joen.2010.04.007
33. Vera J, Siqueira JF Jr, Ricucci D, Loghin S, Fernández N, Flores B, et al. One- versus two-visit endodontic treatment of teeth with apical periodontitis: a histobacteriologic study. J Endod. (2012) 38:1040–52. doi: 10.1016/j.joen.2012.04.010
34. Lee KW, Periasamy S, Mukherjee M, Xie C, Kjelleberg S, Rice SA. Biofilm development and enhanced stress resistance of a model, mixed-species community biofilm. ISME J. (2014) 8:894–907. doi: 10.1038/ismej.2013.194
35. Sanchez-Sanhueza G, Bello-Toledo H, Gonzalez-Rocha G, Goncalves AT, Valenzuela V, Gallardo-Escarate C. Metagenomic study of bacterial microbiota in persistent endodontic infections using Next-generation sequencing. Int Endod J. (2018) 51:1336–48. doi: 10.1111/iej.12953
36. Ramirez T, Shrestha A, Kishen A. Inflammatory potential of monospecies biofilm matrix components. Int Endod J. (2019) 52:1020–7. doi: 10.1111/iej.13093
37. Ali IAA, Cheung BPK, Yau JYY, Matinlinna JP, Levesque CM, Belibasakis GN, et al. The influence of substrate surface conditioning and biofilm age on the composition of Enterococcus faecalis biofilms. Int Endod J. (2020) 53:53–61. doi: 10.1111/iej.13202
38. Donlan RM, Costerton JW. Biofilms: survival mechanisms of clinically relevant microorganisms. Clin Microbiol Rev. (2002) 15:167–93. doi: 10.1128/CMR.15.2.167-193.2002
39. Flemming HC, Wingender J. The biofilm matrix. Nat Rev Microbiol. (2010) 8:623–33. doi: 10.1038/nrmicro2415
40. Costerton JW, Lewandowski Z, Caldwell DE, Korber DR, Lappin-Scott HM. Microbial biofilms. Annu Rev Microbiol. (1995) 49:711–45. doi: 10.1146/annurev.mi.49.100195.003431
41. Parsek MR, Greenberg EP. Sociomicrobiology: the connections between quorum sensing and biofilms. Trends Microbiol. (2005) 13:27–33. doi: 10.1016/j.tim.2004.11.007
42. Siqueira JF Jr, and Rôças IN. Microbiology of apical periodontitis. In: Orstavik D, editor. Essential Endodontology: Prevention Treatment of Apical Periodontitis. 3rd ed. Oxford: Wiley-Blackwell (2019). p. 91–142.
43. Burmolle M, Ren D, Bjarnsholt T, Sorensen SJ. Interactions in multispecies biofilms: do they actually matter? Trends Microbiol. (2014) 22:84–91. doi: 10.1016/j.tim.2013.12.004
44. Nichols WW, Evans MJ, Slack MP, Walmsley HL. The penetration of antibiotics into aggregates of mucoid and non-mucoid Pseudomonas aeruginosa. J Gen Microbiol. (1989) 135:1291–303. doi: 10.1099/00221287-135-5-1291
45. Wilson M. Susceptibility of oral bacterial biofilms to antimicrobial agents. J Med Microbiol. (1996) 44:79–87. doi: 10.1099/00222615-44-2-79
46. Del Pozo JL, Patel R. The challenge of treating biofilm-associated bacterial infections. Clin Pharmacol Ther. (2007) 82:204–9. doi: 10.1038/sj.clpt.6100247
47. Evans M, Davies JK, Sundqvist G, Figdor D. Mechanisms involved in the resistance of Enterococcus faecalis to calcium hydroxide. Aust Endod J. (2001) 27:115. doi: 10.1111/j.1747-4477.2001.tb00474.x
48. Wood TK, Knabel SJ, Kwan BW. Bacterial persister cell formation and dormancy. Appl Environ Microbiol. (2013) 79:7116–21. doi: 10.1128/AEM.02636-13
49. Avila M, Ojcius DM, Yilmaz O. The oral microbiota: living with a permanent guest. DNA Cell Biol. (2009) 28:405–11. doi: 10.1089/dna.2009.0874
50. Costalonga M, Herzberg MC. The oral microbiome and the immunobiology of periodontal disease and caries. Immunol Lett. (2014) 162:22–38. doi: 10.1016/j.imlet.2014.08.017
51. Dewhirst FE, Chen T, Izard J, Paster BJ, Tanner AC, Yu WH, et al. The human oral microbiome. J Bacteriol. (2010) 192:5002–17. doi: 10.1128/JB.00542-10
52. Hsiao WW, Li KL, Liu Z, Jones C, Fraser-Liggett CM, Fouad AF. Microbial transformation from normal oral microbiota to acute endodontic infections. BMC Genomics. (2012) 13:345. doi: 10.1186/1471-2164-13-345
53. Zehnder M, Belibasakis GN. On the dynamics of root canal infections-what we understand and what we don't. Virulence. (2015) 6:216–22. doi: 10.4161/21505594.2014.984567
54. Siqueira JF Jr. Endodontic infections: concepts, paradigms, and perspectives. Oral Surg Oral Med Oral Pathol Oral Radiol Endod. (2002) 94:281–93. doi: 10.1067/moe.2002.126163
55. Siqueira JF Jr, and Rocas IN. Exploiting molecular methods to explore endodontic infections: part 2–redefining the endodontic microbiota. J Endod. (2005) 31:488–98. doi: 10.1097/01.don.0000157990.86638.49
56. Gatti JJ, Dobeck JM, Smith C, White RR, Socransky SS, Skobe Z. Bacteria of asymptomatic periradicular endodontic lesions identified by DNA-DNA hybridization. Endod Dent Traumatol. (2000) 16:197–204. doi: 10.1034/j.1600-9657.2000.016005197.x
57. Siqueira JF Jr, and Rocas IN. Polymerase chain reaction-based analysis of microorganisms associated with failed endodontic treatment. Oral Surg Oral Med Oral Pathol Oral Radiol Endod. (2004) 97:85–94. doi: 10.1016/S1079-2104(03)00353-6
58. Fernandes Cdo C, Rechenberg DK, Zehnder M, Belibasakis GN. Identification of synergistetes in endodontic infections. Microb Pathog. (2014) 73:1–6. doi: 10.1016/j.micpath.2014.05.001
59. Zehnder M, Rechenberg DK, Thurnheer T, Luthi-Schaller H, Belibasakis GN. FISHing for gutta-percha-adhered biofilms in purulent post-treatment apical periodontitis. Mol Oral Microbiol. (2017) 32:226–35. doi: 10.1111/omi.12166
60. Munson MA, Pitt-Ford T, Chong B, Weightman A, Wade WG. Molecular and cultural analysis of the microflora associated with endodontic infections. J Dent Res. (2002) 81:761–6. doi: 10.1177/0810761
61. Rocas IN, Siqueira JF Jr. Root canal microbiota of teeth with chronic apical periodontitis. J Clin Microbiol. (2008) 46:3599–606. doi: 10.1128/JCM.00431-08
62. Sakamoto M, Siqueira JF Jr, Rocas IN, Benno Y. Molecular analysis of the root canal microbiota associated with endodontic treatment failures. Oral Microbiol Immunol. (2008) 23:275–81. doi: 10.1111/j.1399-302X.2007.00423.x
63. Molander A, Reit C, Dahlen G, Kvist T. Microbiological status of root-filled teeth with apical periodontitis. Int Endod J. (1998) 31:1–7. doi: 10.1046/j.1365-2591.1998.t01-1-00111.x
64. Sedgley C, Nagel A, Dahlen G, Reit C, Molander A. Real-time quantitative polymerase chain reaction and culture analyses of Enterococcus faecalis in root canals. J Endod. (2006) 32:173–7. doi: 10.1016/j.joen.2005.10.037
65. Zoletti GO, Siqueira JF Jr, and Santos KR. Identification of Enterococcus faecalis in root-filled teeth with or without periradicular lesions by culture-dependent and-independent approaches. J Endod. (2006) 32:722–6. doi: 10.1016/j.joen.2006.02.001
66. Stuart CH, Schwartz SA, Beeson TJ, Owatz CB. Enterococcus faecalis: its role in root canal treatment failure and current concepts in retreatment. J Endod. (2006) 32:93–8. doi: 10.1016/j.joen.2005.10.049
67. Yarza P, Yilmaz P, Pruesse E, Glockner FO, Ludwig W, Schleifer KH, et al. Uniting the classification of cultured and uncultured bacteria and archaea using 16S rRNA gene sequences. Nat Rev Microbiol. (2014) 12:635–45. doi: 10.1038/nrmicro3330
68. Goodwin S, Mcpherson JD, Mccombie WR. Coming of age: ten years of next-generation sequencing technologies. Nat Rev Genet. (2016) 17:333–51. doi: 10.1038/nrg.2016.49
69. Manoil D, Al-Manei K, Belibasakis GN. A systematic review of the root canal microbiota associated with apical periodontitis: lessons from next-generation sequencing. Proteomics Clin Appl. (2020) 14:e1900060. doi: 10.1002/prca.201900060
70. Kuczynski J, Lauber CL, Walters WA, Parfrey LW, Clemente JC, Gevers D, et al. Experimental and analytical tools for studying the human microbiome. Nat Rev Genet. (2011) 13:47–58. doi: 10.1038/nrg3129
71. Hong BY, Lee TK, Lim SM, Chang SW, Park J, Han SH, et al. Microbial analysis in primary and persistent endodontic infections by using pyrosequencing. J Endod. (2013) 39:1136–40. doi: 10.1016/j.joen.2013.05.001
72. Vengerfeldt V, Spilka K, Saag M, Preem JK, Oopkaup K, Truu J, et al. Highly diverse microbiota in dental root canals in cases of apical periodontitis (data of illumina sequencing). J Endod. (2014) 40:1778–83. doi: 10.1016/j.joen.2014.06.017
73. Tzanetakis GN, Azcarate-Peril MA, Zachaki S, Panopoulos P, Kontakiotis EG, Madianos PN, et al. Comparison of bacterial community composition of primary and persistent endodontic infections using pyrosequencing. J Endod. (2015) 41:1226–33. doi: 10.1016/j.joen.2015.03.010
74. Keskin C, Demiryurek EO, Onuk EE. Pyrosequencing analysis of cryogenically ground samples from primary and secondary/persistent endodontic infections. J Endod. (2017) 43:1309–16. doi: 10.1016/j.joen.2017.03.019
75. Bouillaguet S, Manoil D, Girard M, Louis J, Gaia N, Leo S, et al. Root microbiota in primary and secondary apical periodontitis. Front Microbiol. (2018) 9:2374. doi: 10.3389/fmicb.2018.02374
76. Santos AL, Siqueira JF Jr, Rocas IN, Jesus EC, Rosado AS, Tiedje JM. Comparing the bacterial diversity of acute and chronic dental root canal infections. PLoS ONE. (2011) 6:e28088. doi: 10.1371/journal.pone.0028088
77. Siqueira JF Jr, Alves FR, Rocas IN. Pyrosequencing analysis of the apical root canal microbiota. J Endod. (2011) 37:1499–503. doi: 10.1016/j.joen.2011.08.012
78. Ozok AR, Persoon IF, Huse SM, Keijser BJ, Wesselink PR, Crielaard W, et al. Ecology of the microbiome of the infected root canal system: a comparison between apical and coronal root segments. Int Endod J. (2012) 45:530–41. doi: 10.1111/j.1365-2591.2011.02006.x
79. Anderson AC, Al-Ahmad A, Elamin F, Jonas D, Mirghani Y, Schilhabel M, et al. Comparison of the bacterial composition and structure in symptomatic and asymptomatic endodontic infections associated with root-filled teeth using pyrosequencing. PLoS ONE. (2013) 8:e84960. doi: 10.1371/journal.pone.0084960
80. Gomes BP, Berber VB, Kokaras AS, Chen T, Paster BJ. Microbiomes of endodontic-periodontal lesions before and after chemomechanical preparation. J Endod. (2015) 41:1975–84. doi: 10.1016/j.joen.2015.08.022
81. Siqueira JF Jr, Antunes HS, Rocas IN, Rachid CT, Alves FR. Microbiome in the apical root canal system of teeth with post-treatment apical periodontitis. PLoS ONE. (2016) 11:e0162887. doi: 10.1371/journal.pone.0162887
82. Persoon IF, Buijs MJ, Ozok AR, Crielaard W, Krom BP, Zaura E, et al. The mycobiome of root canal infections is correlated to the bacteriome. Clin Oral Investig. (2017) 21:1871–81. doi: 10.1007/s00784-016-1980-3
83. Zandi H, Kristoffersen AK, Orstavik D, Rocas IN, Siqueira JF Jr, and Enersen M. Microbial analysis of endodontic infections in root-filled teeth with apical periodontitis before and after irrigation using pyrosequencing. J Endod. (2018) 44:372–8. doi: 10.1016/j.joen.2017.11.019
84. Siren EK, Haapasalo MP, Ranta K, Salmi P, Kerosuo EN. Microbiological findings and clinical treatment procedures in endodontic cases selected for microbiological investigation. Int Endod J. (1997) 30:91–5. doi: 10.1111/j.1365-2591.1997.tb00680.x
85. Zehnder M, Guggenheim B. The mysterious appearance of enterococci in filled root canals. Int Endod J. (2009) 42:277–87. doi: 10.1111/j.1365-2591.2008.01537.x
86. Thurnheer T, Belibasakis GN. Incorporation of staphylococci into titanium-grown biofilms: an in vitro “submucosal” biofilm model for peri-implantitis. Clin Oral Implants Res. (2016) 27:890–95. doi: 10.1111/clr.12715
87. Hajishengallis G, Lamont RJ. Dancing with the stars: how choreographed bacterial interactions dictate nososymbiocity and give rise to keystone pathogens, accessory pathogens, and pathobionts. Trends Microbiol. (2016) 24:477–89. doi: 10.1016/j.tim.2016.02.010
88. Wade WG, Prosdocimi EM. Profiling of oral bacterial communities. J Dent Res. (2020) 99:621–29. doi: 10.1177/0022034520914594
89. Schloss PD, Jenior ML, Koumpouras CC, Westcott SL, Highlander SK. Sequencing 16S rRNA gene fragments using the PacBio SMRT DNA sequencing system. PeerJ. (2016) 4:e1869. doi: 10.7717/peerj.1869
90. Callahan BJ, Wong J, Heiner C, Oh S, Theriot CM, Gulati AS, et al. High-throughput amplicon sequencing of the full-length 16S rRNA gene with single-nucleotide resolution. Nucleic Acids Res. (2019) 47:e103. doi: 10.1093/nar/gkz569
91. Quince C, Walker AW, Simpson JT, Loman NJ, Segata N. Shotgun metagenomics, from sampling to analysis. Nat Biotechnol. (2017) 35:833–44. doi: 10.1038/nbt.3935
92. Bowers RM, Kyrpides NC, Stepanauskas R, Harmon-Smith M, Doud D, Reddy TBK, et al. Minimum information about a single amplified genome (MISAG) and a metagenome-assembled genome (MIMAG) of bacteria and archaea. Nat Biotechnol. (2017) 35:725–31. doi: 10.1038/nbt.3893
93. Bostanci N, Grant M, Bao K, Silbereisen A, Hetrodt F, Manoil D, et al. Metaproteome and metabolome of oral microbial communities. Periodontol 2000. (2020) 85:46–81. doi: 10.1111/prd.12351
94. Duran-Pinedo AE. Metatranscriptomic analyses of the oral microbiome. Periodontol 2000. (2020) 85:28–45. doi: 10.1111/prd.12350
95. Nandakumar R, Madayiputhiya N, Fouad AF. Proteomic analysis of endodontic infections by liquid chromatography-tandem mass spectrometry. Oral Microbiol Immunol. (2009) 24:347–52. doi: 10.1111/j.1399-302X.2009.00520.x
96. Provenzano JC, Siqueira JF Jr, Rocas IN, Domingues RR, Paes Leme AF, Silva MR. Metaproteome analysis of endodontic infections in association with different clinical conditions. PLoS ONE. (2013) 8:e76108. doi: 10.1371/journal.pone.0076108
97. Provenzano JC, Antunes HS, Alves FR, Rocas IN, Alves WS, Silva MR, et al. Host-bacterial interactions in post-treatment apical periodontitis: a metaproteome analysis. J Endod. (2016) 42:880–5. doi: 10.1016/j.joen.2016.02.013
98. Francisco PA, Delboni MG, Lima AR, Xiao Y, Siqueira WL, Gomes B. Proteomic profile of root canal contents in teeth with post-treatment endodontic disease. Int Endod J. (2019) 52:451–60. doi: 10.1111/iej.13021
99. Lewis K. Riddle of biofilm resistance. Antimicrob Agents Chemother. (2001) 45:999–1007. doi: 10.1128/AAC.45.4.999-1007.2001
100. Bowen WH, Koo H. Biology of Streptococcus mutans-derived glucosyltransferases: role in extracellular matrix formation of cariogenic biofilms. Caries Res. (2011) 45:69–86. doi: 10.1159/000324598
101. Theilacker C, Sava I, Sanchez-Carballo P, Bao Y, Kropec A, Grohmann E, et al. Deletion of the glycosyltransferase bgsB of Enterococcus faecalis leads to a complete loss of glycolipids from the cell membrane and to impaired biofilm formation. BMC Microbiol. (2011) 11:67. doi: 10.1186/1471-2180-11-67
102. Sundqvist G. Pathogenicity and virulence of black-pigmented gram-negative anaerobes. FEMS Immunol Med Microbiol. (1993) 6:125–37. doi: 10.1111/j.1574-695X.1993.tb00315.x
103. Jansen HJ, Van Der Hoeven JS, Walji S, Goertz JH, Bakkeren JA. The importance of immunoglobulin-breakdown supporting the growth of bacteria in oral abscesses. J Clin Periodontol. (1996) 23:717–23. doi: 10.1111/j.1600-051X.1996.tb00600.x
104. Potempa J, Banbula A, Travis J. Role of bacterial proteinases in matrix destruction and modulation of host responses. Periodontol 2000. (2000) 24:153–92. doi: 10.1034/j.1600-0757.2000.2240108.x
105. Han YW, Wang X. Mobile microbiome: oral bacteria in extra-oral infections and inflammation. J Dent Res. (2013) 92:485–91. doi: 10.1177/0022034513487559
106. Siqueira JF Jr, and Rocas IN. Microbiology and treatment of acute apical abscesses. Clin Microbiol Rev. (2013) 26:255–73. doi: 10.1128/CMR.00082-12
107. Goulhen F, Grenier D, Mayrand D. Oral microbial heat-shock proteins and their potential contributions to infections. Crit Rev Oral Biol Med. (2003) 14:399–412. doi: 10.1177/154411130301400603
108. Henderson B, Allan E, Coates AR. Stress wars: the direct role of host and bacterial molecular chaperones in bacterial infection. Infect Immun. (2006) 74:3693–706. doi: 10.1128/IAI.01882-05
109. Belibasakis GN, Rechenberg DK, Zehnder M. The receptor activator of NF-kappaB ligand-osteoprotegerin system in pulpal and periapical disease. Int Endod J. (2013) 46:99–111. doi: 10.1111/j.1365-2591.2012.02105.x
110. Rechenberg DK, Bostanci N, Zehnder M, Belibasakis GN. Periapical fluid RANKL and IL-8 are differentially regulated in pulpitis and apical periodontitis. Cytokine. (2014) 69:116–9. doi: 10.1016/j.cyto.2014.05.014
111. Ng YL, Mann V, Rahbaran S, Lewsey J, Gulabivala K. Outcome of primary root canal treatment: systematic review of the literature - part 1. Effects of study characteristics on probability of success. Int Endod J. (2007) 40:921–39. doi: 10.1111/j.1365-2591.2007.01322.x
112. Restrepo-Restrepo FA, Canas-Jimenez SJ, Romero-Albarracin RD, Villa-Machado PA, Perez-Cano MI, Tobon-Arroyave SI. Prognosis of root canal treatment in teeth with preoperative apical periodontitis: a study with cone-beam computed tomography and digital periapical radiography. Int Endod J. (2019) 52:1533–46. doi: 10.1111/iej.13168
113. Tawakoli PN, Ragnarsson KT, Rechenberg DK, Mohn D, Zehnder M. Effect of endodontic irrigants on biofilm matrix polysaccharides. Int Endod J. (2017) 50:153–60. doi: 10.1111/iej.12604
114. Ali IAA, Cheung BPK, Matinlinna J, Lévesque CM, Neelakantan P. Trans-cinnamaldehyde potently kills Enterococcus faecalis biofilm cells and prevents biofilm recovery. Microb Pathog. (2020) 149:104482. doi: 10.1016/j.micpath.2020.104482
115. Liu Y, Naha PC, Hwang G, Kim D, Huang Y, Simon-Soro A, et al. Topical ferumoxytol nanoparticles disrupt biofilms and prevent tooth decay in vivo via intrinsic catalytic activity. Nat Commun. (2018) 9:2920. doi: 10.1038/s41467-018-05342-x
116. Neelakantan P, Romero M, Vera J, Daood U, Khan AU, Yan A, et al. Biofilms in endodontics-current status and future directions. Int J Mol Sci. (2017) 18:1748. doi: 10.3390/ijms18081748
117. Lee OYS, Khan K, Li KY, Shetty H, Abiad RS, Cheung GSP, et al. Influence of apical preparation size and irrigation technique on root canal debridement: a histological analysis of round and oval root canals. Int Endod J. (2019) 52:1366–76. doi: 10.1111/iej.13127
118. Cheung AWT, Lee AHC, Cheung GSP. Clinical efficacy of activated irrigation in endodontics: a focused review. Restor Dent Endod. (2021) 46:1–16. doi: 10.5395/rde.2021.46.e10
119. European Society of Endodontology. Quality guidelines for endodontic treatment: consensus report of the European Society of Endodontology. Int Endod J. (2006) 39:921–30. doi: 10.1111/j.1365-2591.2006.01180.x
120. Nagendrababu V, Jayaraman J, Suresh A, Kalyanasundaram S, Neelakantan P. Effectiveness of ultrasonically activated irrigation on root canal disinfection: a systematic review of in vitro studies. Clin Oral Investig. (2018) 22:655–70. doi: 10.1007/s00784-018-2345-x
121. Căpută PE, Retsas A, Kuijk L, Chávez De Paz LE, Boutsioukis C. Ultrasonic irrigant activation during root canal treatment: a systematic review. J Endod. (2019) 45:31–44 e13. doi: 10.1016/j.joen.2018.09.010
122. Zhang D, Shen Y, De La Fuente-Núñez C, Haapasalo M. In vitro evaluation by quantitative real-time PCR and culturing of the effectiveness of disinfection of multispecies biofilms in root canals by two irrigation systems. Clin Oral Investig. (2019) 23:913–20. doi: 10.1007/s00784-018-2515-x
123. Byström A, Sundqvist G. Bacteriologic evaluation of the effect of 0.5 percent sodium hypochlorite in endodontic therapy. Oral Surg Oral Med Oral Pathol. (1983) 55:307–12. doi: 10.1016/0030-4220(83)90333-X
124. Hasselgren G, Olsson B, Cvek M. Effects of calcium hydroxide and sodium hypochlorite on the dissolution of necrotic porcine muscle tissue. J Endod. (1988) 14:125–7. doi: 10.1016/S0099-2399(88)80212-7
126. Clegg MS, Vertucci FJ, Walker C, Belanger M, Britto LR. The effect of exposure to irrigant solutions on apical dentin biofilms in vitro. J Endod. (2006) 32:434–7. doi: 10.1016/j.joen.2005.07.002
127. Retamozo B, Shabahang S, Johnson N, Aprecio RM, Torabinejad M. Minimum contact time and concentration of sodium hypochlorite required to eliminate Enterococcus faecalis. J Endod. (2010) 36:520–3. doi: 10.1016/j.joen.2009.12.005
128. Marending M, Luder HU, Brunner TJ, Knecht S, Stark WJ, Zehnder M. Effect of sodium hypochlorite on human root dentine–mechanical, chemical and structural evaluation. Int Endod J. (2007) 40:786–93. doi: 10.1111/j.1365-2591.2007.01287.x
129. Hülsmann M, Hahn W. Complications during root canal irrigation – literature review and case reports. Int Endod J. (2000) 33:186–93. doi: 10.1046/j.1365-2591.2000.00303.x
130. Wong DT, Cheung GS. Extension of bactericidal effect of sodium hypochlorite into dentinal tubules. J Endod. (2014) 40:825–9. doi: 10.1016/j.joen.2013.09.045
131. Boutsioukis C, Van Der Sluis LWM. Syringe irrigation: blending endodontics and fluid dynamics. In: Basrani B, editor. Endodontic Irrigation. Cham: Springer (2015). p. 45–64.
132. Rolla G, Loe H, Schiott CR. Retention of chlorhexidine in the human oral cavity. Arch Oral Biol. (1971) 16:1109–16. doi: 10.1016/0003-9969(71)90215-9
133. Zamany A, Safavi K, Spångberg LSW. The effect of chlorhexidine as an endodontic disinfectant. Oral Surg Oral Med Oral Pathol Oral Radiol Endod. (2003) 96:578–81. doi: 10.1016/S1079-2104(03)00168-9
134. Osorio R, Yamauti M, Osorio E, Ruiz-Requena ME, Pashley D, Tay F, et al. Effect of dentin etching and chlorhexidine application on metalloproteinase-mediated collagen degradation. Eur J Oral Sci. (2011) 119:79–85. doi: 10.1111/j.1600-0722.2010.00789.x
135. Ng YL, Mann V, Gulabivala K. A prospective study of the factors affecting outcomes of nonsurgical root canal treatment: part 1: periapical health. Int Endod J. (2011) 44:583–609. doi: 10.1111/j.1365-2591.2011.01872.x
136. Portenier I, Waltimo TMT, Haapasalo M. Enterococcus faecalis – the root canal survivor and ‘star' in post-treatment disease. Endodontic Topics. (2003) 6:135–59. doi: 10.1111/j.1601-1546.2003.00040.x
137. Shen Y, Stojicic S, Haapasalo M. Antimicrobial efficacy of chlorhexidine against bacteria in biofilms at different stages of development. J Endod. (2011) 37:657–61. doi: 10.1016/j.joen.2011.02.007
138. Shen Y, Zhao J, De La Fuente-Núñez C, Wang Z, Hancock REW, Roberts CR, et al. Experimental and theoretical investigation of multispecies oral biofilm resistance to chlorhexidine treatment. Sci Rep. (2016) 6:27537. doi: 10.1038/srep27537
139. Ruksakiet K, Hanak L, Farkas N, Hegyi P, Sadaeng W, Czumbel LM, et al. Antimicrobial efficacy of chlorhexidine and sodium hypochlorite in root canal disinfection: a systematic review and meta-analysis of randomized controlled trials. J Endod. (2020) 46:1032–41 e1037. doi: 10.1016/j.joen.2020.05.002
140. Baca P, Mendoza-Llamas ML, Arias-Moliz MT, Gonzalez-Rodriguez MP, Ferrer-Luque CM. Residual effectiveness of final irrigation regimens on Enteroccus faecalis-infected root canals. J Endod. (2011) 37:1121–3. doi: 10.1016/j.joen.2011.05.003
141. Srivastava S. Antibiofilm efficacy of 0.1% Octenidine, SmearOFF, 1% Alexidine and 5.25% Sodium Hypochlorite against E. faecalis biofilm formed on tooth substrate. IAIM. (2019) 6:1–8.
142. Garberoglio R, Becce C. Smear layer removal by root canal irrigants. A comparative scanning electron microscopic study. Oral Surg Oral Med Oral Pathol. (1994) 78:359–67. doi: 10.1016/0030-4220(94)90069-8
143. Ferrer-Luque CM, Arias-Moliz MT, González-Rodríguez MP, Baca P. Antimicrobial activity of maleic acid and combinations of cetrimide with chelating agents against Enterococcus faecalis biofilm. J Endod. (2010) 36:1673–5. doi: 10.1016/j.joen.2010.06.009
144. Torabinejad M. Winter 2011 Endodontics: Colleagues for Excellence Newsletter - Root Canal Irrigants and Disinfectants. American Association of Endodontists (2011). Available online at: https://f3f142zs0k2w1kg84k5p9i1o-wpengine.netdnassl.com/specialty/wp-content/uploads/sites/2/2017/07/rootcanalirrigantsdisinfectants.pdf (accessed October 21, 2021).
145. De Almeida J, Hoogenkamp M, Felippe WT, Crielaard W, Van Der Waal SV. Effectiveness of EDTA and modified salt solution to detach and kill cells from Enterococcus faecalis biofilm. J Endod. (2016) 42:320–3. doi: 10.1016/j.joen.2015.11.017
146. Ozdemir HO, Buzoglu HD, Calt S, Stabholz A, Steinberg D. Effect of ethylenediaminetetraacetic acid and sodium hypochlorite irrigation on Enterococcus faecalis biofilm colonization in young and old human root canal dentin: in vitro study. J Endod. (2010) 36:842–6. doi: 10.1016/j.joen.2010.01.008
147. Calt S, Serper A. Time-dependent effects of EDTA on dentin structures. J Endod. (2002) 28:17–9. doi: 10.1097/00004770-200201000-00004
148. Rath PP, Yiu CKY, Matinlinna JP, Kishen A, Neelakantan P. The effects of sequential and continuous chelation on dentin. Dent Mater. (2020) 36:1655–65. doi: 10.1016/j.dental.2020.10.010
149. Torabinejad M, Shabahang S, Aprecio RM, Kettering JD. The antimicrobial effect of MTAD: an in vitro investigation. J Endod. (2003) 29:400–3. doi: 10.1097/00004770-200306000-00005
150. Prabhakar J, Senthilkumar M, Priya MS, Mahalakshmi K, Sehgal PK, Sukumaran VG. Evaluation of antimicrobial efficacy of herbal alternatives (Triphala and green tea polyphenols), MTAD and 5% sodium hypochlorite against Enterococcus faecalis biofilm formed on tooth substrate: an in vitro study. J Endod. (2010) 36:83–6. doi: 10.1016/j.joen.2009.09.040
151. Kho P, Baumgartner JC. A comparison of the antimicrobial efficacy of NaOCl/Biopure MTAD versus NaOCl/EDTA against Enterococcus faecalis. J Endod. (2006) 32:652–5. doi: 10.1016/j.joen.2005.11.004
152. Zhang R, Chen M, Lu Y, Guo X, Qiao F, Wu L. Antibacterial and residual antimicrobial activities against Enterococcus faecalis biofilm: a comparison between EDTA chlorhexidine, cetrimide, MTAD and QMix. Sci Rep. (2015) 5:12944. doi: 10.1038/srep12944
153. Lim BSH, Parolia A, Chia MSY, Jayaraman J, Nagendrababu V. Antimicrobial efficacy of QMix on Enterococcus faecalis infected root canals: a systematic review of in vitro studies. Restor Dent Endod. (2020) 45:e23. doi: 10.5395/rde.2020.45.e23
154. Zehnder M, Schmidlin P, Sener B, Waltimo T. Chelation in root canal therapy reconsidered. J Endod. (2005) 31:817–20. doi: 10.1097/01.don.0000158233.59316.fe
155. Neelakantan P, Varughese AA, Sharma S, Subbarao CV, Zehnder M, De-Deus G. Continuous chelation irrigation improves the adhesion of epoxy resin-based root canal sealer to root dentine. Int Endod J. (2012) 45:1097–102. doi: 10.1111/j.1365-2591.2012.02073.x
156. Lottanti S, Gautschi H, Sener B, Zehnder M. Effects of ethylenediaminetetraacetic, etidronic and peracetic acid irrigation on human root dentine and the smear layer. Int Endod J. (2009) 42:335–43. doi: 10.1111/j.1365-2591.2008.01514.x
157. Ulusoy ÖI, Manti AS, Çelik B. Nanohardness reduction and root dentine erosion after final irrigation with ethylenediaminetetraacetic, etidronic and peracetic acids. Int Endod J. (2020) 53:1549–58. doi: 10.1111/iej.13372
158. Arias-Moliz MT, Ordinola-Zapata R, Baca P, Ruiz-Linares M, Garcia Garcia E, Hungaro Duarte MA, et al. Antimicrobial activity of Chlorhexidine, Peracetic acid and Sodium hypochlorite/etidronate irrigant solutions against Enterococcus faecalis biofilms. Int Endod J. (2015) 48:1188–93. doi: 10.1111/iej.12424
159. Zollinger A, Mohn D, Zeltner M, Zehnder M. Short-term storage stability of NaOCl solutions when combined with Dual Rinse HEDP. Int Endod J. (2018) 51:691–6. doi: 10.1111/iej.12875
160. Ballal NV, Gandhi P, Shenoy PA, Shenoy Belle V, Bhat V, Rechenberg DK, et al. Safety assessment of an etidronate in a sodium hypochlorite solution: randomized double-blind trial. Int Endod J. (2019) 52:1274–82. doi: 10.1111/iej.13129
161. Wright PP, Cooper C, Kahler B, Walsh LJ. From an assessment of multiple chelators, clodronate has potential for use in continuous chelation. Int Endod J. (2020) 53:122–34. doi: 10.1111/iej.13213
162. Wright PP, Scott S, Kahler B, Walsh LJ. Organic tissue dissolution in clodronate and etidronate mixtures with sodium hypochlorite. J Endod. (2020) 46:289–94. doi: 10.1016/j.joen.2019.10.020
163. Gergely JM, Difiore PM. Intracanal medication in endodontic treatment: a survey of endodontic programs. Gen Dent. (1993) 41:328–31.
164. Galler KM, Krastl G, Simon S, Van Gorp G, Meschi N, Vahedi B, et al. European Society of Endodontology position statement: revitalization procedures. Int Endod J. (2016) 49:717–23. doi: 10.1111/iej.12629
165. Byström A, Sunvqvist G. The antibacterial action of sodium hypochlorite and EDTA in 60 cases of endodontic therapy. Int Endod J. (1985) 18:35–40. doi: 10.1111/j.1365-2591.1985.tb00416.x
166. Sjögren U, Figdor D, Spångberg L, Sundqvist G. The antimicrobial effect of calcium hydroxide as a short-term intracanal dressing. Int Endod J. (1991) 24:119–25. doi: 10.1111/j.1365-2591.1991.tb00117.x
167. Love RM. Enterococcus faecalis –a mechanism for its role in endodontic failure. Int Endod J. (2001) 34:399–405. doi: 10.1046/j.1365-2591.2001.00437.x
168. Upadya M, Shrestha A, Kishen A. Role of efflux pump inhibitors on the antibiofilm efficacy of calcium hydroxide, chitosan nanoparticles, light-activated disinfection. J Endod. (2011) 37:1422–6. doi: 10.1016/j.joen.2011.06.017
169. Plutzer B, Zilm P, Ratnayake J, Cathro P. Comparative efficacy of endodontic medicaments and sodium hypochlorite against Enterococcus faecalis biofilms. Aust Dent J. (2018) 63:208–16. doi: 10.1111/adj.12580
170. Zancan RF, Calefi PHS, Borges MMB, Lopes MRM, De Andrade FB, Vivan RR, et al. Antimicrobial activity of intracanal medications against both Enterococcus faecalis and Candida albicans biofilm. Microsc Res Tech. (2019) 82:494–500. doi: 10.1002/jemt.23192
171. Haapasalo M, Orstavik D. In vitro infection and disinfection of dentinal tubules. J Dent Res. (1987) 66:1375–9. doi: 10.1177/00220345870660081801
172. Andreasen JO, Farik B, Munksgaard EC. Long-term calcium hydroxide as a root canal dressing may increase risk of root fracture. Dental Traumatol. (2002) 18:134–7. doi: 10.1034/j.1600-9657.2002.00097.x
173. Basrani B, Tjäderhane L, Santos JM, Pascon E, Grad H, Lawrence HP, et al. Efficacy of chlorhexidine- and calcium hydroxide containing medicaments against Enterococcus faecalis in vitro. Oral Surg Oral Med Oral Pathol Oral Radiol Endod. (2003) 96:618–24. doi: 10.1016/S1079-2104(03)00166-5
174. Manzur A, González AM, Pozos A, Silva-Herzog D, Friedman S. Bacterial quantification in teeth with apical periodontitis related to instrumentation and different intracanal medications: a randomized clinical trial. J Endod. (2007) 33:114–8. doi: 10.1016/j.joen.2006.11.003
175. Ghabraei S, Bolhari B, Sabbagh MM, Afshar MS. Comparison of antimicrobial effects of triple antibiotic paste and calcium hydroxide mixed with 2% chlorhexidine as intracanal medicaments against Enterococcus faecalis biofilm. J Dent. (2018) 15:151–60.
176. Zehnder M, Grawehr M, Hasselgren G, Waltimo T. Tissue-dissolution capacity and dentin-disinfecting potential of calcium hydroxide mixed with irrigating solutions. Oral Surg Oral Med Oral Pathol Oral Radiol Endod. (2003) 96:608–13. doi: 10.1016/S1079-2104(03)00157-4
177. Grossman LI. Polyantibiotic treatment of pulpless teeth. J Am Dent Assoc. (1951) 43:265–78. doi: 10.14219/jada.archive.1951.0213
178. Hoshino E, Kurihara-Ando N, Sato I, Uematsu H, Sato M, Kota K, et al. In-vitro antibacterial susceptibility of bacteria taken from infected root dentine to a mixture of ciprofloxacin, metronidazole and minocycline. Int Endod J. (1996) 29:125–30. doi: 10.1111/j.1365-2591.1996.tb01173.x
179. American Association of Endodontists. AAE Clinical Considerations for a Regenerative Procedure. Chicago, IL (2018). Available online at: https://www.aae.org/specialty/wp-content/uploads/sites/2/2018/06/ConsiderationsForRegEndo_AsOfApril2018.pdf (accessed October 20, 2020).
180. Sabrah AH, Yassen GH, Gregory RL. Effectiveness of antibiotic medicaments against biofilm formation of Enterococcus faecalis and Porphyromonas gingivalis. J Endod. (2013) 39:1385–9. doi: 10.1016/j.joen.2013.05.003
181. Moradi Eslami L, Vatanpour M, Aminzadeh N, Mehrvarzfar P, Taheri S. The comparison of intracanal medicaments, diode laser and photodynamic therapy on removing the biofilm of Enterococcus faecalis and Candida albicans in the root canal system (ex-vivo study). Photodiagnosis Photodyn Ther. (2019) 26:157–61. doi: 10.1016/j.pdpdt.2019.01.033
182. Zargar N, Rayat Hosein Abadi M, Sabeti M, Yadegari Z, Akbarzadeh Baghban A, Dianat O. Antimicrobial efficacy of clindamycin and triple antibiotic paste as root canal medicaments on tubular infection: an in vitro study. Aust Endod J. (2019) 45:86–91. doi: 10.1111/aej.12288
183. Sabrah AHA, Yassen GH, Spolnik KJ, Hara AT, Platt JA, Gregory RL. Evaluation of residual antibacterial effect of human radicular dentin treated with triple and double antibiotic pastes. J Endod. (2015) 41:1081–4. doi: 10.1016/j.joen.2015.03.001
184. Abbott PV, Salgado JC. Strategies for the endodontic management of concurrent endodontic and periodontal diseases. Aust Dent J. (2009) 54 (Suppl. 1):S70–85. doi: 10.1111/j.1834-7819.2009.01145.x
185. Abbott PV, Hume WR, Heithersay GS. Effects of combining Ledermix® and calcium hydroxide pastes on the diffusion of corticosteroid and tetracycline through human tooth roots in vitro. Dent Traumatol. (1989) 5:188–92. doi: 10.1111/j.1600-9657.1989.tb00358.x
186. Athanassiadis M, Jacobsen N, Parashos P. The effect of calcium hydroxide on the steroid component of Ledermix and Odontopaste. Int Endod J. (2011) 44:1162–9. doi: 10.1111/j.1365-2591.2011.01940.x
187. Athanassiadis M, Jacobsen N, Nassery K, Parashos P. The effect of calcium hydroxide on the antibiotic component of Odontopaste and Ledermix paste. Int Endod J. (2013) 46:530–7. doi: 10.1111/iej.12021
188. European Commission. Commission Recommendation of 18 October 2011 on the Definition of Nanomaterial. Brussels (2011). Available online at: http://data.europa.eu/eli/reco/2011/696/oj (accessed October 10, 2020).
189. Shrestha A, Kishen A. Antibacterial nanoparticles in endodontics: a review. J Endod. (2016) 42:1417–26. doi: 10.1016/j.joen.2016.05.021
190. Yin IX, Zhang J, Zhao IS, Mei ML, Li Q, Chu CH. The antibacterial mechanism of silver nanoparticles and its application in dentistry. Int J Nanomed. (2020) 15:2555–62. doi: 10.2147/IJN.S246764
191. De Almeida J, Cechella BC, Bernardi AV, De Lima Pimenta A, Felippe WT. Effectiveness of nanoparticles solutions and conventional endodontic irrigants against Enterococcus faecalis biofilm. Indian J Dent Res. (2018) 29:347–51. doi: 10.4103/ijdr.IJDR_634_15
192. Teixeira A, Vidal C, Tornavoi D, Oliveira-Santos C, Schiavon M, Reis A. Incorporating antimicrobial nanomaterial and its effect on the antimicrobial activity, flow and radiopacity of endodontic sealers. Eur Endod J. (2017) 2:1–6. doi: 10.5152/eej.2017.16029
193. Moazami F, Sahebi S, Ahzan S. Tooth discoloration induced by imidazolium based silver nanoparticles as an intracanal irrigant. J Dent. (2018) 19:280–6.
194. Gomes-Filho JE, Silva FO, Watanabe S, Cintra LT, Tendoro KV, Dalto LG, et al. Tissue reaction to silver nanoparticles dispersion as an alternative irrigating solution. J Endod. (2010) 36:1698–702. doi: 10.1016/j.joen.2010.07.007
195. Beyth N, Houri-Haddad Y, Domb A, Khan W, Hazan R. Alternative antimicrobial approach: nano-antimicrobial materials. Evid Based Complement Alternat Med. (2015) 2015:246012. doi: 10.1155/2015/246012
196. Monzavi A, Eshraghi S, Hashemian R, Momen-Heravi F. In vitro and ex vivo antimicrobial efficacy of nano-MgO in the elimination of endodontic pathogens. Clin Oral Investig. (2015) 19:349–56. doi: 10.1007/s00784-014-1253-y
197. Rabea EI, Badawy ME, Stevens CV, Smagghe G, Steurbaut W. Chitosan as antimicrobial agent: applications and mode of action. Biomacromolecules. (2003) 4:1457–65. doi: 10.1021/bm034130m
198. Kishen A, Shi Z, Shrestha A, Neoh KG. An investigation on the antibacterial and antibiofilm efficacy of cationic nanoparticulates for root canal disinfection. J Endod. (2008) 34:1515–20. doi: 10.1016/j.joen.2008.08.035
199. Silva PV, Guedes DF, Nakadi FV, Pecora JD, Cruz-Filho AM. Chitosan: a new solution for removal of smear layer after root canal instrumentation. Int Endod J. (2013) 46:332–8. doi: 10.1111/j.1365-2591.2012.02119.x
200. Shrestha A, Shi Z, Neoh KG, Kishen A. Nanoparticulates for antibiofilm treatment and effect of aging on its antibacterial activity. J Endod. (2010) 36:1030–5. doi: 10.1016/j.joen.2010.02.008
201. Del Carpio-Perochena A, Bramante CM, Duarte MA, De Moura MR, Aouada FA, Kishen A. Chelating and antibacterial properties of chitosan nanoparticles on dentin. Restor Dent Endod. (2015) 40:195–201. doi: 10.5395/rde.2015.40.3.195
202. Kishen A, Shrestha S, Shrestha A, Cheng C, Goh C. Characterizing the collagen stabilizing effect of crosslinked chitosan nanoparticles against collagenase degradation. Dent Mater. (2016) 32:968–77. doi: 10.1016/j.dental.2016.05.005
203. Shrestha A, Hamblin MR, Kishen A. Characterization of a conjugate between Rose Bengal and chitosan for targeted antibiofilm and tissue stabilization effects as a potential treatment of infected dentin. Antimicrob Agents Chemother. (2012) 56:4876–84. doi: 10.1128/AAC.00810-12
204. Camacho-Alonso F, Julián-Belmonte E, Chiva-García F, Martínez-Beneyto Y. Bactericidal efficacy of photodynamic therapy and chitosan in root canals experimentally infected with Enterococcus faecalis: an in vitro study. Photomed Laser Surg. (2017) 35:184–9. doi: 10.1089/pho.2016.4148
205. Sireesha A, Jayasree R, Vidhya S, Mahalaxmi S, Sujatha V, Kumar TSS. Comparative evaluation of micron- and nano-sized intracanal medicaments on penetration and fracture resistance of root dentin - an in vitro study. Int J Biol Macromol. (2017) 104:1866–73. doi: 10.1016/j.ijbiomac.2017.05.126
206. Dianat O, Saedi S, Kazem M, Alam M. Antimicrobial activity of nanoparticle calcium hydroxide against Enterococcus faecalis: an in vitro study. Iran Endod J. (2015) 10:39–43.
207. Zand V, Mokhtari H, Hasani A, Jabbari G. Comparison of the penetration depth of conventional and nano-particle calcium hydroxide into dentinal tubules. Iran Endod J. (2017) 12:366–70. doi: 10.22037/iej.v12i3.16421
208. Fan W, Wu D, Ma T, Fan B. Ag-loaded mesoporous bioactive glasses against Enterococcus faecalis biofilm in root canal of human teeth. Dent Mater J. (2015) 34:54–60. doi: 10.4012/dmj.2014-104
209. Fan W, Wu Y, Ma T, Li Y, Fan B. Substantivity of Ag-Ca-Si mesoporous nanoparticles on dentin and its ability to inhibit Enterococcus faecalis. J Mater Sci Mater Med. (2016) 27:16. doi: 10.1007/s10856-015-5633-x
210. Fan W, Wu D, Tay FR, Ma T, Wu Y, Fan B. Effects of adsorbed and templated nanosilver in mesoporous calcium-silicate nanoparticles on inhibition of bacteria colonization of dentin. Int J Nanomed. (2014) 9:5217–30. doi: 10.2147/IJN.S73144
211. Mohn D, Zehnder M, Imfeld T, Stark WJ. Radio-opaque nanosized bioactive glass for potential root canal application: evaluation of radiopacity, bioactivity and alkaline capacity. Int Endod J. (2010) 43:210–7. doi: 10.1111/j.1365-2591.2009.01660.x
212. Fan W, Wu C, Han P, Zhou Y, Xiao Y. Porous Ca–Si-based nanospheres: a potential intra-canal disinfectant-carrier for infected canal treatment. Mater Lett. (2012) 81:16–9. doi: 10.1016/j.matlet.2012.04.142
213. Zhu J, Liang R, Sun C, Xie L, Wang J, Leng D, et al. Effects of nanosilver and nanozinc incorporated mesoporous calcium-silicate nanoparticles on the mechanical properties of dentin. PLoS ONE. (2017) 12:e0182583. doi: 10.1371/journal.pone.0182583
214. Leng D, Li Y, Zhu J, Liang R, Zhang C, Zhou Y, et al. The antibiofilm activity and mechanism of nanosilver- and nanozinc-incorporated mesoporous calcium-silicate nanoparticles. Int J Nanomedicine. (2020) 15:3921–36. doi: 10.2147/IJN.S244686
215. Abramovitz I, Wisblech D, Zaltsman N, Weiss EI, Beyth N. Intratubular antibacterial effect of polyethyleneimine nanoparticles: an ex vivo study in human teeth. J Nanomater. (2015) 2015:1–5. doi: 10.1155/2015/980529
216. Seung J, Weir MD, Melo MAS, Romberg E, Nosrat A, Xu HH, et al. A modified resin sealer: physical and antibacterial properties. J Endod. (2018) 44:1553–7. doi: 10.1016/j.joen.2018.06.016
217. Olivi G. Laser use in endodontics: evolution from direct laser irradiation to laser-activated irrigation. J Laser Dent. (2013) 21:58–71.
218. Anić I, Tachibana H, Masumoto K, Qi P. Permeability, morphologic and temperature changes of canal dentine walls induced by Nd: YAG. CO2 and argon lasers. Int Endod J. (1996) 29:13–22. doi: 10.1111/j.1365-2591.1996.tb01354.x
219. Leonardo MR, Guillén-Carías MG, Pécora JD, Ito IY, Silva LAB. Er:YAG laser: antimicrobial effects in the root canals of dogs' teeth with pulp necrosis and chronic periapical lesions. Photomed Laser Surg. (2005) 23:295–9. doi: 10.1089/pho.2005.23.295
220. Yavari HR, Rahimi S, Shahi S, Lotfi M, Barhaghi MH, Fatemi A, et al. Effect of Er, Cr: YSGG laser irradiation on Enterococcus faecalis in infected root canals. Photomed Laser Surg. (2010) 1 (28 Suppl.):S91–6. doi: 10.1089/pho.2009.2539
221. Matsumoto H, Yoshimine Y, Akamine A. Visualization of irrigant flow and cavitation induced by Er:YAG laser within a root canal model. J Endod. (2011) 37:839–43. doi: 10.1016/j.joen.2011.02.035
222. Neelakantan P, Cheng CQ, Mohanraj R, Sriraman P, Subbarao C, Sharma S. Antibiofilm activity of three irrigation protocols activated by ultrasonic, diode laser or Er:YAG laser in vitro. Int Endod J. (2015) 48:602–10. doi: 10.1111/iej.12354
223. Olivi G, Divito E. Review photoacoustic: endodontics using PIPS™: experimental background and clinical protocol. J Laser Health Acad. (2011) 1:22–5.
224. Ordinola-Zapata R, Bramante CM, Aprecio RM, Handysides R, Jaramillo DE. Biofilm removal by 6% sodium hypochlorite activated by different irrigation techniques. Int Endod J. (2014) 47:659–66. doi: 10.1111/iej.12202
225. Golob BS, Olivi G, Vrabec M, El Feghali R, Parker S, Benedicenti S. Efficacy of photon-induced photoacoustic streaming in the reduction of Enterococcus faecalis within the root canal: different settings and different sodium hypochlorite concentrations. J Endod. (2017) 43:1730–5. doi: 10.1016/j.joen.2017.05.019
226. Peters OA, Bardsley S, Fong J, Pandher G, Divito E. Disinfection of root canals with photon-initiated photoacoustic streaming. J Endod. (2011) 37:1008–12. doi: 10.1016/j.joen.2011.03.016
227. Dai T, Huang YY, Hamblin MR. Photodynamic therapy for localized infections–state of the art. Photodiagnosis Photodyn Ther. (2009) 6:170–88. doi: 10.1016/j.pdpdt.2009.10.008
228. Ali IAA, Neelakantan P. Light activated disinfection in root canal treatment-a focused review. Dent J. (2018) 6:31. doi: 10.3390/dj6030031
229. Shrestha A, Friedman S, Torneck CD, Kishen A. Bioactivity of photoactivated functionalized nanoparticles assessed in lipopolysaccharide-contaminated root canals in vivo. J Endod. (2018) 44:104–10. doi: 10.1016/j.joen.2017.08.021
230. Nielsen HK, Garcia J, Væth M, Schlafer S. Comparison of riboflavin and toluidine blue o as photosensitizers for photoactivated disinfection on endodontic and periodontal pathogens in vitro. PLoS ONE. (2015) 10:e0140720. doi: 10.1371/journal.pone.0140720
231. Afkhami F, Akbari S, Chiniforush N. Entrococcus faecalis elimination in root canals using silver nanoparticles, photodynamic therapy, diode laser, or laser-activated nanoparticles: an in vitro study. J Endod. (2017) 43:279–82. doi: 10.1016/j.joen.2016.08.029
232. Fimple JL, Fontana CR, Foschi F, Ruggiero K, Song X, Pagonis TC, et al. Photodynamic treatment of endodontic polymicrobial infection in vitro. J Endod. (2008) 34:728–34. doi: 10.1016/j.joen.2008.03.011
233. De Oliveira BP, Aguiar CM, Camara AC, De Albuquerque MM, Correia AC, Soares MF. The efficacy of photodynamic therapy and sodium hypochlorite in root canal disinfection by a single-file instrumentation technique. Photodiagnosis Photodyn Ther. (2015) 12:436–43. doi: 10.1016/j.pdpdt.2015.05.004
234. Neelakantan P, Cheng CQ, Ravichandran V, Mao T, Sriraman P, Sridharan S, et al. Photoactivation of curcumin and sodium hypochlorite to enhance antibiofilm efficacy in root canal dentin. Photodiagnosis Photodyn Ther. (2015) 12:108–14. doi: 10.1016/j.pdpdt.2014.10.011
235. Chrepa V, Kotsakis GA, Pagonis TC, Hargreaves KM. The effect of photodynamic therapy in root canal disinfection: a systematic review. J Endod. (2014) 40:891–8. doi: 10.1016/j.joen.2014.03.005
236. Gupta A, Duhan J, Tewari S, Sangwan P, Yadav A, Singh G, et al. Comparative evaluation of antimicrobial efficacy of Syzygium aromaticum, Ocimum sanctum and Cinnamomum zeylanicum plant extracts against Enterococcus faecalis: a preliminary study. Int Endod J. (2013) 46:775–83. doi: 10.1111/iej.12058
237. Donyavi Z, Arabestani MR, Dastan D, Esmaeilzadeh M, Shahsavand N. Comparison of antimicrobial effect of berberine as an endodontic irrigant with that of other common root canal irrigants on three microorganisms involved in persistent endodontic infections. J Mol Biol Res. (2018) 8:1008–12. doi: 10.5539/jmbr.v8n1p153
238. Kandaswamy D, Venkateshbabu N, Gogulnath D, Kindo AJ. Dentinal tubule disinfection with 2% chlorhexidine gel, propolis, morinda citrifolia juice, 2% povidone iodine, calcium hydroxide. Int Endod J. (2010) 43:419–23. doi: 10.1111/j.1365-2591.2010.01696.x
239. Zare Jahromi M, Toubayani H, Rezaei M. Propolis: a new alternative for root canal disinfection. Iran Endod J. (2012) 7:127–33.
240. Abdel Raheem IA, Abdul Razek A, Elgendy AA, Saleh NM, Shaaban MI, Abd El-Hady FK. Design, evaluation and antimicrobial activity of egyptian propolis-loaded nanoparticles: intrinsic role as a novel and naturally based root canal nanosealer. Int J Nanomed. (2019) 14:8379–98. doi: 10.2147/IJN.S219577
241. Wang Z, Shen Y, Haapasalo M. Antibiofilm peptides against oral biofilms. J Oral Microbiol. (2017) 9:1327308. doi: 10.1080/20002297.2017.1327308
242. Wimley WC, Hristova K. Antimicrobial peptides: successes, challenges and unanswered questions. J Membr Biol. (2011) 239:27–34. doi: 10.1007/s00232-011-9343-0
243. Pepperney A, Chikindas ML. Antibacterial peptides: opportunities for the prevention and treatment of dental caries. Probiotics Antimicrob Proteins. (2011) 3:68–96. doi: 10.1007/s12602-011-9076-5
244. Kajwadkar R, Shin JM, Lin GH, Fenno JC, Rickard AH, Kapila YL. High-purity nisin alone or in combination with sodium hypochlorite is effective against planktonic and biofilm populations of Enterococcus faecalis. J Endod. (2017) 43:989–94. doi: 10.1016/j.joen.2017.01.034
245. Li Y, Wang Y, Chen X, Jiang W, Jiang X, Zeng Y, et al. Antimicrobial peptide GH12 as root canal irrigant inhibits biofilm and virulence of Enterococcus faecalis. Int Endod J. (2020) 53:948–61. doi: 10.1111/iej.13296
246. Huang X, Haapasalo M, Wang Z, Hancock REW, Wei X, Shen Y. Effect of long-term exposure to peptides on mono- and multispecies biofilms in dentinal tubules. J Endod. (2019) 45:1522–8. doi: 10.1016/j.joen.2019.09.003
247. Ye WH, Yeghiasarian L, Cutler CW, Bergeron BE, Sidow S, Xu HHK, et al. Comparison of the use of d-enantiomeric and l-enantiomeric antimicrobial peptides incorporated in a calcium-chelating irrigant against Enterococcus faecalis root canal wall biofilms. J Dent. (2019) 91:103231. doi: 10.1016/j.jdent.2019.103231
248. Pletzer D, Coleman SR, Hancock RE. Anti-biofilm peptides as a new weapon in antimicrobial warfare. Curr Opin Microbiol. (2016) 33:35–40. doi: 10.1016/j.mib.2016.05.016
249. Kim D, Hofstaedter CE, Zhao C, Mattei L, Tanes C, Clarke E, et al. Optimizing methods and dodging pitfalls in microbiome research. Microbiome. (2017) 5:52. doi: 10.1186/s40168-017-0267-5
250. Fouad AF, Burleson J. The effect of diabetes mellitus on endodontic treatment outcome: data from an electronic patient record. J Am Dent Assoc. (2003) 134:43–51; quiz 117–8. doi: 10.14219/jada.archive.2003.0016
251. Marending M, Peters OA, Zehnder M. Factors affecting the outcome of orthograde root canal therapy in a general dentistry hospital practice. Oral Surg Oral Med Oral Pathol Oral Radiol Endod. (2005) 99:119–24. doi: 10.1016/j.tripleo.2004.06.065
Keywords: biofilm, disinfection, irrigation, microbiome, metaproteome, metatranscriptome
Citation: Wong J, Manoil D, Näsman P, Belibasakis GN and Neelakantan P (2021) Microbiological Aspects of Root Canal Infections and Disinfection Strategies: An Update Review on the Current Knowledge and Challenges. Front. Oral. Health 2:672887. doi: 10.3389/froh.2021.672887
Received: 26 February 2021; Accepted: 17 May 2021;
Published: 25 June 2021.
Edited by:
Ulvi Kahraman Gürsoy, University of Turku, FinlandReviewed by:
Christopher John Nile, Newcastle University, United KingdomGeorgios Charalampakis, National and Kapodistrian University of Athens, Greece
Copyright © 2021 Wong, Manoil, Näsman, Belibasakis and Neelakantan. This is an open-access article distributed under the terms of the Creative Commons Attribution License (CC BY). The use, distribution or reproduction in other forums is permitted, provided the original author(s) and the copyright owner(s) are credited and that the original publication in this journal is cited, in accordance with accepted academic practice. No use, distribution or reproduction is permitted which does not comply with these terms.
*Correspondence: Prasanna Neelakantan, prasanna@hku.hk
†These authors have contributed equally to this work and share first authorship