Direct air capture of CO2: from insights into the current and emerging approaches to future opportunities
- 1Department of Chemical and Biomolecular Engineering, Case Western Reserve University, Cleveland, OH, United States
- 2Energy Science and Technology Directorate, Oak Ridge National Laboratory, Oak Ridge, TN, United States
- 3Department of Chemistry, Texas A&M University, College Station, TX, United States
- 4Department of Materials Science and Engineering, Texas A&M University, College Station, TX, United States
- 5Department of Chemical and Biomolecular Engineering, Clemson University, Clemson, SC, United States
The rapid development of direct air capture (DAC) technologies has become critical in order to remove CO2 from the atmosphere and limit global warming to a maximum of 1.5°C. In this perspective, we provide a mini review of the current research on the emerging liquid- and solid-based sorbent materials to capture CO2, summarize the existing challenges of DAC technologies, and suggest future research directions to accelerate the development of DAC systems. In particular, the desired properties for a breakthrough sorbent that efficiently captures CO2 from the air and releases it for sequestration are described.
1. Introduction
The growing global reliance on fossil fuels for energy and material production has resulted in record-high carbon dioxide (CO2) emissions in the atmosphere (Kumar et al., 2015; Sanz-Pérez et al., 2016). To mitigate the potential impact on climate change, ~10 gigatons of CO2/year needs to be removed to limit global warming to a maximum of 1.5°C by 2050 according to multiple reports (World Resources Institute, 2002; Philander, 2012; Ozkan, 2021; IEA, 2022). Direct air capture (DAC), a process that captures CO2 directly from air is critical to negative emissions technologies (NETs), and plays a significant role in achieving net zero emissions by the 2050 Scenario, among other CO2 removal (CDR) approaches (National Academies of Sciences, 2019; IEA, 2021). According to the International Energy Agency (IEA) projection for achieving net-zero emissions by 2050 Scenario, the CDR removal target by DAC is ~980 Mt CO2 per year (House et al., 2011). However, capturing CO2, particularly from the air is a challenging and energy-intensive process, because CO2 in the atmosphere is highly diluted, ca. 400 ppm (0.04%), when compared to the CO2 concentration in a flue gas stream emitted from a power station, cement or natural gas plant (≈4–15%). Further, a significant amount of CO2 must be removed from the atmosphere and sequestered to have a quantifiable positive impact on the environment, and thus the technological challenge is formidable.
In this perspective, we provide an overview of the current approaches of DAC systems and emerging sorbent materials associated with CO2 capture from dilute streams. Sorbents typically capture CO2 at ambient temperatures and are regenerated by releasing the captured CO2 at elevated temperatures (e.g., by applying convective heat) or using alternative heat sources (e.g., microwave) (Gomez-Rueda et al., 2022), as well as non-thermal techniques such as electrochemical (Stern and Hatton, 2014; Voskian and Hatton, 2018; Rahimi et al., 2020), ultrasound (Ying et al., 2014) and pressure-swing (Wiheeb et al., 2016). Lackner and co-workers introduced a moisture-swing process for DAC where the sorbent absorbs CO2 when dry and releases it when wet (Lackner, 2009; Wang T. et al., 2011; Shi et al., 2020). Sorbents can be liquid, solid, or a hybrid material that has both liquid-like and solid-like components. The development of sorbents requires the consideration of numerous performance parameters, such as high CO2 capacity, selectivity, fast sorption/desorption kinetics, and chemical stability under variable temperature and humidity. Furthermore, industry-related challenges such as the operational cost and energy demand can only be addressed when an efficient sorbent material is integrated into a DAC system. Thus, a molecular-level understanding of sorption kinetics, thermodynamics, and structure-property relations are imperative for the discovery of a breakthrough sorbent. Further, research focusing on alternative regeneration techniques is essential to reduce the overall energy consumption and cost of CO2 removed, i.e., joule per ton of extracted CO2 in a DAC system.
2. Current status and challenges
In general, CO2 capture or removal from a mixture stream process includes adsorption, absorption, and/or membrane-based separation. Adsorption and absorption-based processes are typically considered more promising for directly capturing of CO2 at ultradilute conditions (McQueen et al., 2021). In an absorption-based process, CO2 dissolves in a liquid solvent such as an aqueous amine, aqueous sodium hydroxide, ionic liquid (IL), or a mixture of solvents via physisorption or chemisorption (Mahmoudkhani and Keith, 2009; Kumar et al., 2020). Alternatively, in an adsorption-based process CO2 is chemisorbed or physisorbed onto a solid surface that has chemical functionality for CO2-philicity, such as in the case of zeolites, metal organic frameworks, or covalent organic frameworks (Choi et al., 2011). CO2 binding processes are exothermic in nature and requires active cooling to maintain CO2 capacity. This challenge is mitigated to some extent due to distributed heat of the solid surface material and the density of sites in adsorption. Thermodynamically, the affinity between the sorbent and the target gas molecule is quantified through the heat of absorption or the isosteric heat of adsorption (Builes et al., 2013). Recent studies comparing the energetics of temperature swing vs. pressure swing CO2 separation processes for a generic adsorbent (with a heat of adsorption of −65 kJ/mol) found the temperature swing adsorption to be more efficient under dilute CO2 concentrations (i.e., 50% removal from feed and 95% product purity) (Lackner, 2013; Lively and Realff, 2016). Conversely, pressure swing adsorption is more efficient for bulk gas separations, as pressurizing the inlet feed with low CO2 concentration makes the process energetically costly.
Even with temperature swing processes, both adsorption and absorption processes consume large amounts of thermal energy during the sorbent regeneration, in addition to the energy required to move air through the sorbents. In particular, the trade-off between the strength of CO2 molecular interactions with the sorbent and the required regeneration energy remains a challenge. An aqueous solution of calcium hydroxide is an effective sorbent for capturing CO2 from the air due to its high affinity to CO2. For example, pilot plant of Carbon Engineering has the capacity to remove 1 Mt of CO2/year (requires 8.81 GJ per ton of CO2 removed) by using an aqueous-sorbent (Keith et al., 2018). First, air is passed through a series of filters to remove any particles and pollutants. Next, the purified air flows into a contactor, a container filled with a solution of potassium hydroxide (KOH). As the air passes through the contactor, the CO2 in the air reacts with KOH to form potassium carbonate (K2CO3) and water. The aqueous solution is then regenerated for reuse by heating at 900°C to release the captured CO2. Table 1 summarizes the key comparisons for DAC plants around the world, including Carbon Engineering (Ozkan et al., 2022).
In contrast to absorption and adsorption processes, membrane-based CO2 separation works on the principle of preferential permeation, where the target gas molecules diffuse across the membrane film under isothermal conditions. The gas molecule can permeate through the membrane via different mechanisms including size sieving, solution diffusion, surface diffusion, ion and facilitated transport. For CO2 separations from air using a membrane, combination of these diffusion mechanisms is needed since there is a lack of high-pressure driving force to transport CO2 across the membrane by solution diffusion alone. Few recent studies explored facilitated transport membranes for DAC (Lee and Gurkan, 2021; Matsuoka et al., 2021; Nabity et al., 2021; Lee et al., 2022). Lee et al. (2022) prepared a thin film of poly(ionic liquid) (PIL)–IL impregnated graphene oxide membrane supported on a poly(ethersulfone)/poly(ethylene terephthalate) substrate, and reported excellent CO2 permanence of 3,923 GPU and CO2/N2 selectivity of 1,200 under 410 ppm CO2 with a 1 bar feed gas (CO2/N2/H2O mixture) at 40% RH and 22°C. In this example, helium was used as the sweep gas on the effluent side which limits the purity of the separated CO2 and the post-process application. For post-sequestering purposes, higher purity CO2 is needed and the study showed the separation performance of the membrane decreases under vacuum conditions on the effluent side due to the need to reinforce the membrane further. However, the purity requirement does not have to meet prior targets set (i.e., > 95%) for CO2 removal from post-combustion flue gas. While the recent reports are encouraging to further develop facilitated transport membranes for DAC, they also suggest the need of an integrated approach based on multiple membrane modules or integration with other separation units. Another emerging approach alternative to temperature swing processes is the Faradaic electro-swing process developed by Hatton and co-workers where the CO2 binding is regulated by electroactive species [e.g., amine sorbents (Wang M. et al., 2020) and quinone (Gurkan et al., 2015) carriers] (Voskian and Hatton, 2019). Recently, they demonstrated a bench scale, solid-state Faradaic electro-swing reactive adsorption unit that achieved > 90% Faradaic efficiency with a CO2 feed < 0.1%, demonstrating a work of 40–90 kJ per mole of CO2 captured, representing favorable thermodynamics comparison to most other thermally regenerated adsorbents (Voskian and Hatton, 2018).
In the following sections, we focus on the conventional and emerging sorbents based on absorption and adsorption-based processes that have demonstrated promising CO2 capture capabilities at ultradilute (400 ppm) conditions.
2.1. Conventional sorbents for CO2 capture
To date, most of the research on DAC has been focused on hydroxide- or amine-based aqueous sorbents due to their efficacy in capturing CO2 under ultradilute conditions (Sanz-Pérez et al., 2016; Sodiq et al., 2023). The hydroxide based solvents need 900°C for regeneration with energy requirement of 6–9 GJ/tCO2–equivalent to the energy utilized by an average household in the US for 2–3 months (Baciocchi et al., 2006; National Academies of Sciences, 2019; McQueen et al., 2021; Lebling et al., 2022). Besides this substantial energy requirement, sorbent loss incurred during the sorption/desorption process via evaporation and degradation is of significant concern. Further, water loss in the air contactor of solvent-based carbon capture systems can also significantly limit their deployment and techonomic effectiveness, particularly in dry climates (Rosa et al., 2021; An et al., 2022). On the other hand, porous solid-supported amines present higher stability under moisture in ambient air, lower heat capacities (≈1–1.5 J/g K compared to the CO2-loaded monoethanolamine ≈4 J/g K) (Weiland et al., 1997; McQueen et al., 2021), and mild regeneration temperatures (≈50–120°C) (Wang et al., 2015). However, when compared to liquid sorbents, amine supported solid sorbents are limited by their slow sorption kinetics, limited CO2 capacities at low partial pressures, and poor cyclability (absorption-desorption cycles with maintained capacity) (Wang Q. et al., 2011; Lai et al., 2021). The following sections discuss the improvements made over conventional sorbents in consideration of these challenges.
2.2. Emerging liquid sorbents
Aqueous amino acids have been investigated for carbon capture, which are relatively non-volatile, environmentally friendly, and regenerable using mild heating (≈100°C). For instance, Custelcean and co-workers reported amino acid-based sorbent with a capacity of 0.7 mol of CO2 per mol of aqueous solution at ambient air conditions (Custelcean et al., 2019). The CO2-saturated bicarbonate species in the solution were then crystallized using a 2,6-pyridine-bis(iminonoguanidine) (PyBIG) to form a solid hydrated carbonate in an aqueous solution. Heating the solid carbonate produced at a comparatively mild temperature (120°C) removes most of the bound CO2 and water via the reaction mechanisms shown in Figure 1A. In general, amino acids with a highly basic functional group are desirable for CO2 capture (Ramezani et al., 2022), but the reported amino acids present lower cyclic capacities typically in the range of 0.12–0.4 mol CO2/mol sorbent (Recker et al., 2022).
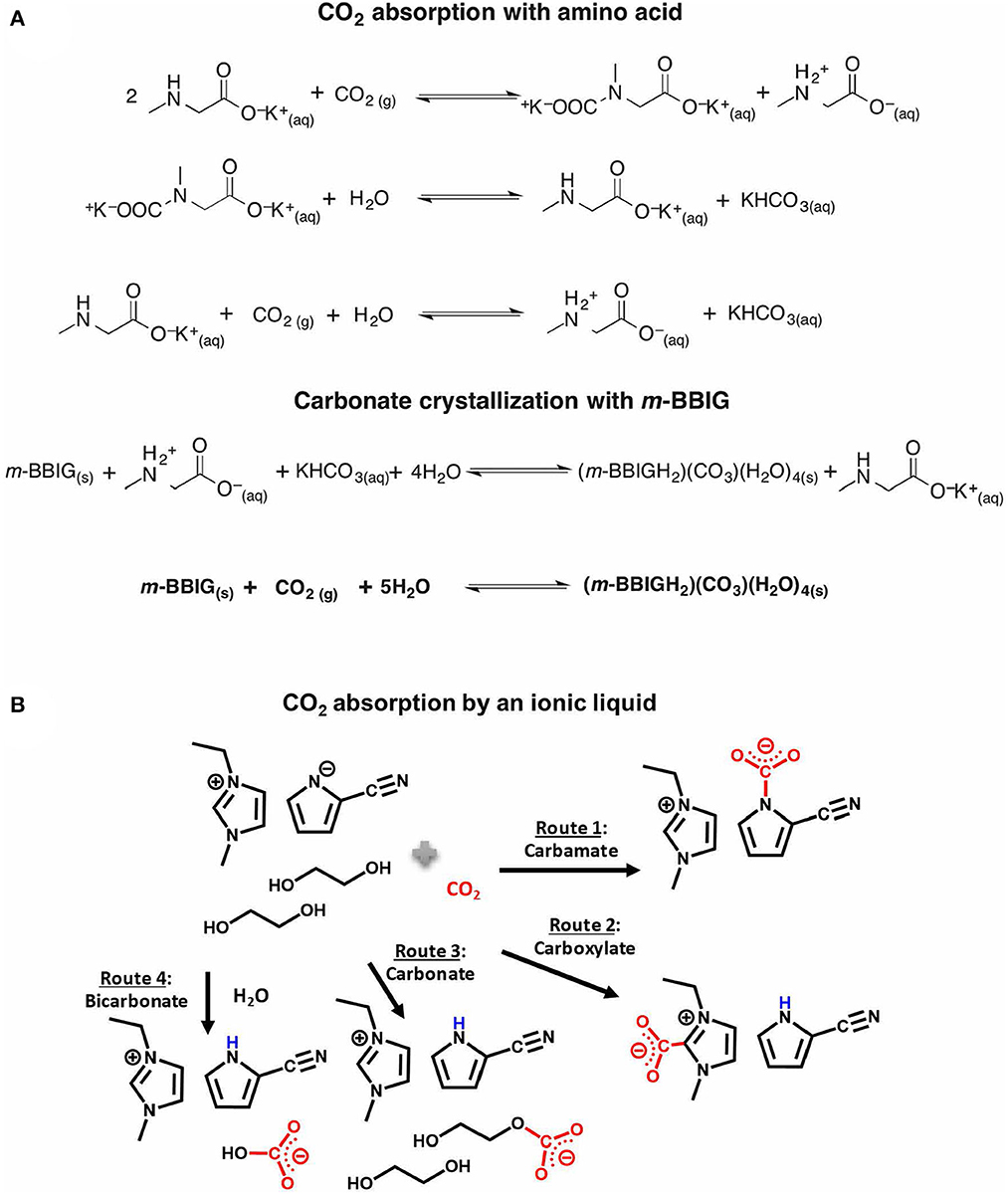
Figure 1. (A) CO2 capture reaction mechanism with aqueous amino acids. Reproduced with permission (Custelcean et al., 2019). Copyright 2019, American Chemical Society. (B) Proposed reaction mechanism routes between CO2 molecules and DES. Reproduced with permission (Lee et al., 2021) under CC-BY-NC-ND license.
Researchers have also explored the use of amino acid derived ILs as an alternative liquid sorbent for CO2 capture. ILs are unequivocally versatile materials for CO2 capture with negligible volatility and high chemical stability (Zhang et al., 2012). For instance, Gurkan et al. synthesized an amino acid-based IL, trihexyl(tetradecyl)phosphonium prolinate, ([P66614][Pro]), which exhibited a CO2 capacity of ~0.9 mol CO2/mol IL in low partial pressure region at 22°C (Gurkan et al., 2010a). A significant disadvantage of amine-functionalized ILs for CO2 capture application is their high viscosity leading to slow sorption kinetics. In this regard, Schneider, Brennecke, and co-workers developed aprotic heterocyclic anion (AHA) ILs for CO2 capture and showed that the viscosity of ILs remained unchanged before and after the CO2 absorption while maintaining a 1:1 CO2 sorption stoichiometry (Gurkan et al., 2010b; Seo et al., 2014). In addition, the reaction enthalpy (~ 50 kJ/mol) was lower than conventional amines such that regeneration was possible at temperatures < 100°C, giving a reasonable working capacity (capacity difference between absorption and desorption). However, the demonstrated AHA ILs had low gravimetric CO2 capacities (~9%) due to their large molar mass. Although there has been extensive research on the use of ILs for capture and utilization of pure CO2 (Aghaie et al., 2018; Shukla et al., 2019), studies on their application in DAC have been limited (Yang and Dai, 2021).
Similar to ILs, deep eutectic solvents (DESs) are known to have significant physisorption capacity for CO2 (García et al., 2015; Trivedi et al., 2016). DESs are mixtures of two or more components that form stable, low-melting-eutectics and are typically composed of a hydrogen bond acceptor, such as a halide salt, and a hydrogen bond donor (Smith et al., 2014; Hansen et al., 2021). Recent studies demonstrated functionalized DESs and eutectics that demonstrate CO2 chemisorption capacity, similar to amine functionalized ILs (Zhang et al., 2018; Yan et al., 2020; Lee et al., 2021; Klemm et al., 2023). Lee et al. reported a functionalized DES for CO2 capture that overcomes the limitations of traditional ILs, such as high viscosity and poor gravimetric CO2 capacity at low partial pressures (Lee et al., 2021). In that study, a reactive IL, 1-ethyl-3-methylimidazolium 2-cyanopyrrolide, was used as a hydrogen bond acceptor component and ethylene glycol (EG) was the hydrogen bond donor. The 1:2 molar mixture of IL:EG showed a capacity of 0.85 mol CO2/mol solvent at 1 bar of CO2 and 0.31 mol CO2/mol solvent at 410 ppm of CO2 at 25°C. Here, it is worth noting that the gravimetric CO2 capacity of the DES (2.7 mol CO2/kg sorbent) was higher than that of the neat IL (2.1 mol CO2/kg sorbent) at 410 ppm CO2. This was attributed to carbonate formation with the deprotonated EG, in the presence of the pyrrolide anion, which was stabilized by the hydrogen bonding network (Figure 1B). This study demonstrates that while the H-bonding network in DESs may increase viscosity, it can also modulate the CO2 interactions with the sorbent, leading to an alternative reaction route as compared to neat IL. To tune functionalized DESs for DAC beyond the capture capacity, thermal and oxidative stability upon cycling must be established, since some of the parent compounds are volatile (unlike ILs).
2.3. Emerging solid sorbents
Traditional amine functionalized sorbents such as zeolites (Fu et al., 2022), mesoporous silicas (Shi et al., 2020) (Figure 2A), and moisture-swing resins/polymers (Wang et al., 2017; Wang T. et al., 2020) have been investigated for CO2 capture, yet they have considerable shortcomings for DAC. This includes low CO2 capacities, slow kinetics, diffusion-limited sorption, small surface area, and poor multi-cycle capacity, all of which significantly impact the sorbent material's overall performance. In this context, MOFs are considered superior to conventional solid sorbents because of their highly accessible surface area and adjustable chemical functionality (Sadiq et al., 2020). Choi et al. (2012) developed ethylene diamine (ED) modified ED-Mg/DOBDC (Figure 2B), which showed a capacity of 1.5 mmol/g under ambient air conditions together with excellent thermal stability and regenerability. The amine groups grafted on the open metal sites introduced additional sites for chemisorption, which improved the CO2 capacity compared to the parent MOF (1.35 mmol/g). Similarly, McDonald et al. (2012) demonstrated that alkylamine-loaded Mg2(dobpdc) have exceptional CO2 uptake capacity (2 mmol/g) under 390 ppm at 25°C (Figure 2C). This improvement in the CO2 uptake at very low partial pressure was ascribed to the interaction of electrophilic carbon of CO2 with the nitrogen electron pair in diamine.
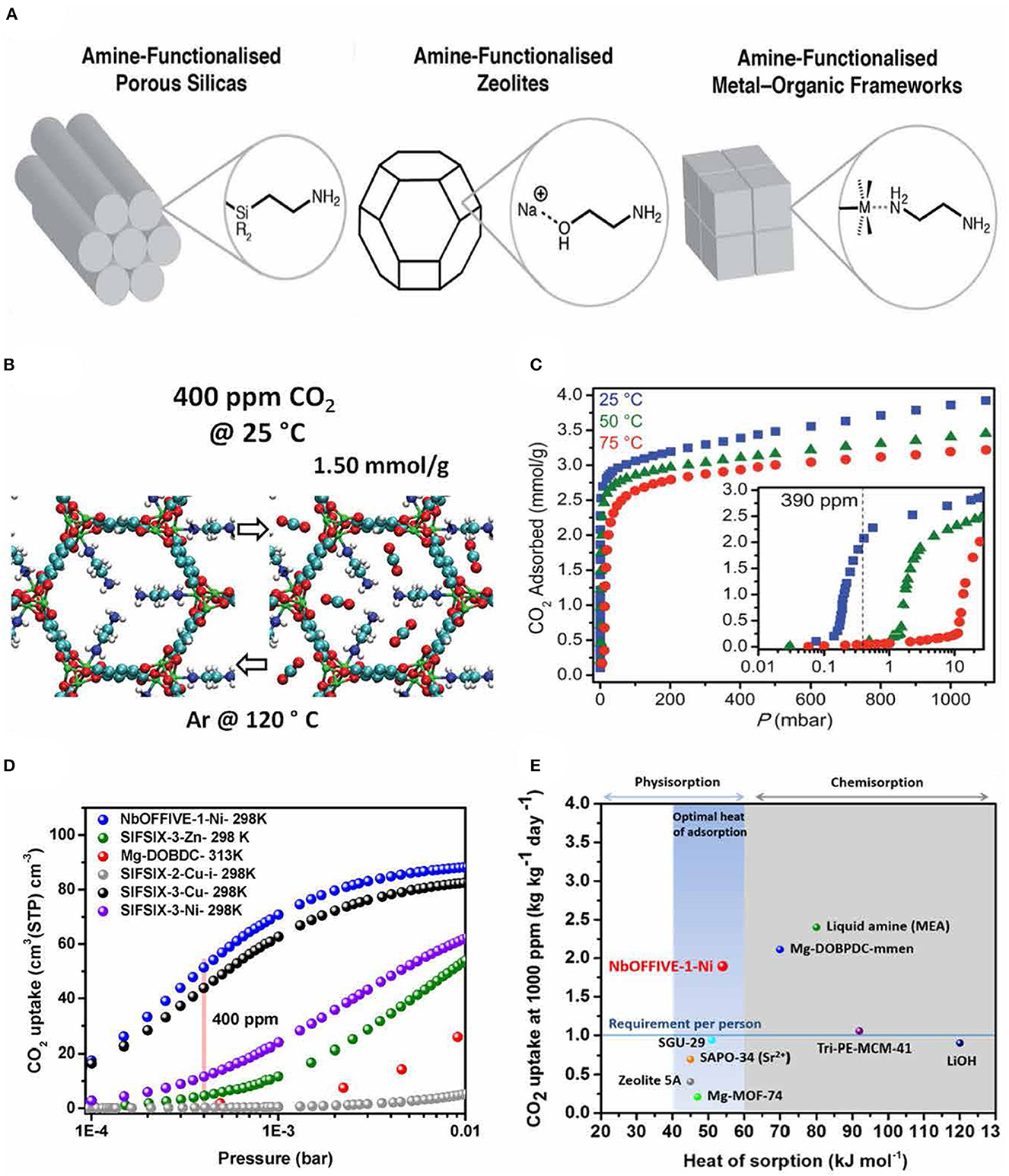
Figure 2. (A) Schematics of amine-functionalized solid adsorbents for CO2 capture. Reproduced with permission (Forse and Milner, 2021). Copyright 2021, Royal Society of Chemistry. (B) CO2 uptake of MOF (Mg/DOBDC) under ambient air conditions. Reproduced with permission (Choi et al., 2012). Copyright 2012, American Chemical Society. (C) Adsorption of CO2 in MOF [Mg2(dobpdc)] in the pressure range of 1–1,000 mbar. Reproduced with permission (McDonald et al., 2012). Copyright 2012, American Chemical Society. (D) CO2 uptake of NbOFFIVE-1-Ni MOF at low pressure and 25°C and (E) CO2 heat of adsorption in comparison with other benchmark materials. Reproduced with permission (Bhatt et al., 2016). Copyright 2016, American Chemical Society.
Eddaoudi and coworkers synthesized a MOF, NbOFFIVE-1-Ni, which exhibited excellent CO2 sorption capacity (1.3 mmol/g) under 400 ppm CO2 at 25°C due to the favorable interactions of CO2 with the fluorine centers of the MOF, with a regeneration energy of 54 kJ/mol (Figures 2D, E) (Bhatt et al., 2016). Alternatively, Darunte et al. illustrated that the CO2 capacity of a simple MOF [MIL-101(Cr)] could be improved by incorporating amine molecules into the MOF pores (Darunte et al., 2016). Accordingly, tris(2-amino ethyl) (TREN)-loaded MIL-101(Cr) exhibited eight-times higher CO2 capacity (2.8 mmol/g) than the corresponding pristine MOF (0.35 mmol/g) at 0.4 mbar (400 ppm CO2 in He) and 25°C. The composite sample, however, showed a significant loss in cyclic capacity due to the excessive amount of TREN loading (more than the available unsaturated coordination sites). Therefore, future research on MOFs for DAC should focus on stability under various temperature and humidity conditions, cycling capacity, high CO2 selectivity, and cost-efficient scalability.
3. The way forward: key matrix for a breakthrough sorbent
Fundamental studies aiming to develop structure-property-performance relations are still needed to further develop sorbents for widespread application in DAC. In addition, computational studies, for example utilizing machine learning, are needed to accelerate sorbent discovery (Mohan et al., 2022). For example, simulations can provide insight into material properties that maximize CO2 sorption (Guan et al., 2022) as well as CO2 binding sites and their corresponding thermodynamics and kinetics (Qazvini et al., 2021; Ahmad et al., 2022). In the following sections, we discuss the significance of individual properties of the sorbents that make up the key matrix for a breakthrough sorbent which would significantly expedite the development of DAC technologies to help meet the global goal of decreasing atmospheric CO2 levels.
3.1. Sorption, kinetics, and diffusion
Sorbents with high basicity can increase affinity to CO2 which is an acidic gas. However, strong binding of CO2, such as with primary and secondary amines relative to tertiary amines for example, also increases the overall energy required to regenerate the sorbent. Therefore, both the enthalpy of the reaction (recommended ≈ −49 to 65 kJ mol−1) (Lively and Realff, 2016; Yang and Dai, 2021) and the CO2 capacity must be considered when designing a sorbent for DAC. Similarly, there is a trade-off between capacity and adsorption rate for solid sorbents. Grafting of CO2-philic moieties such as amine, hydroxy, sulphonate, imidazole, triazine, and imine groups onto solid sorbent pore surfaces to improve CO2 capacity (Petrovic et al., 2021) can result in blocking of the pore openings and prevents CO2 diffusion. In a solid adsorbent, gas diffusion can be tuned by modification of pore openings and creating a high surface-to-volume ratio to facilitate the accessibility to the active surface area.
For liquid sorbents, gas diffusion is impacted by the viscosity. Mota-Martinez et al. examined the impact of physiochemical and transport properties of CO2 capture solvents in terms of the cost of the overall separation process for the emission mitigation purposes. They report that viscosity is a critical parameter determining the absorber size and the associated operational and capital costs (Mota-Martinez et al., 2017). For example, the aqueous amine benchmark solvent with a viscosity of 2.5 cP requires a 50 m tall absorber column whereas a solvent with a viscosity of 16 cP requires 133 m in height for the absorber. For post-combustion CO2 capture, to maximize the efficiency of CO2 capture process, it is generally recommended to keep the viscosity of a CO2-capturing solvent under 5 cP when operating in an absorber column (Song et al., 2017). However, similar analysis are not yet available for emerging DAC solvents. In complement to designing novel liquid sorbents with low viscosity, packaging sorbents, e.g., via encapsulation, is an attractive strategy for enhancing performance. Provided a capsule shell that is permeable to CO2 is used, encapsulation can increase the effective surface area of the liquid sorbent and lead to more rapid gas uptake. Capsules of IL can be prepared by impregnation of a hollow carbon shell using a co-surfactant (Moya et al., 2018), extrusion (Zhang and Cai, 2012), or interfacial polymerization (Weiss and Abu-Reziq, 2017). For example, Pentzer, Gurkan, and coworkers used interfacial polymerization in IL-in-water or IL-in-oil emulsions to prepare capsules a core of IL and polyurea-based shell (Huang et al., 2019; Luo et al., 2019; Gaur et al., 2021). The same group encapsulated a task specific IL for the chemisorption of CO2 and performed breakthrough and regeneration experiments; the capsules were stable under humid conditions, outperformed zeolites at low pressure, and were stable through the multiple absorption-desorption cycles (Lee et al., 2020). Thus, in addition to enhancing the physical properties of liquid sorbents, e.g., by decreasing viscosity, such composites can give access to new structures with various knobs to tune performance related to capacity, selectivity, and regeneration.
3.2. CO2 selectivity
Selective capture of CO2 from atmospheric air is crucial since molecules such as N2, H2O, and O2 can also be physisorbed. Further, volatile organic components can permanently bind to active sites that would otherwise be available to bind CO2, leading to a decrease in the overall CO2 capacity of a sorbent. It is important to note that CO2 capacity of liquid sorbents generally improves under moist conditions, since the interaction between CO2 and water can also lead to the formation of carbonic acid and increase the CO2 capacity (Avelar Bonilla et al., 2019). However, the presence of co-adsorbed moisture consumes additional thermal energy during the solvent regeneration. This is because water has a high heat capacity (4.2 J/g K), and the presence of moisture in the sorbent will consume more energy for regeneration (Quang et al., 2015). Additionally, in the context of adsorptive processes, water has higher selectivity compared to CO2 toward sorption sites, thus hindering CO2 sorption at the amine sites (Shaik et al., 2022). Recently, Young et al. (2021) proposed a mechanistic isotherm model for amine-containing sorbents that successfully predicted CO2 co-adsorption performance in humid conditions on Lewatit® VP OC 1065 (a commercially available benchmark sorbent comparable to first-generation sorbent used by Climeworks for DAC process). However, the applicability of such accurate co-adsorption models to other types of amine-functionalized sorbents, especially under high relative humidity representing real-world condition remains unclear and requires further development to uncover potential opportunities for enhancing the overall efficiency of a DAC process. Finally, high-purity CO2 must be obtained so that it can be further sequestered or utilized as a feedstock to produce renewable fuels or value added chemicals. Therefore, to produce highly pure CO2 from a mixture stream, pore openings of solid sorbent materials should be tuned according to the kinetic diameter of CO2 (3.3 Å) together with high chemical affinity toward CO2.
3.3. Regeneration energy
The regeneration of sorbents in DAC currently requires energy in the range of 6–10 GJ/tCO2 and 4–6 GJ/tCO2 for liquid and solid sorbents, respectively (Ozkan et al., 2022). As mentioned earlier, the regeneration energy is related to the heat capacity and the reaction enthalpy of CO2 binding. To make DAC cost-effective, developing sorbents that can be regenerated using energy similar to the flue gas CO2 capture process (≈ 2 GJ/tCO2) is crucial (Zhang et al., 2016). Furthermore, theoretical calculations estimate that the energy requirement to produce a highly concentrated stream of CO2 (>90% purity) is 20 kJ/mol CO2 under ≈400 ppm of CO2 concentration (House et al., 2011). However, DAC technologies are currently not as efficient, with typical energy requirements of ~400 kJ/mol CO2, assuming an efficiency of 5% for DAC system (House et al., 2011). Thus, increasing the CO2 capture efficiency, ideally above 20%, is a critical target for making DAC more economically sustainable. Additionally, the high regeneration temperature requirement (e.g., 900°C for hydroxide-based solvents) (Keith et al., 2018; National Academies of Sciences, 2019) poses a challenge in terms of thermal energy and heating rate efficacy; necessitating the need for alternative energy sources and the targeted delivery of energy (i.e., overcome reliance on bulk convective heating). In this context, electrical energy can be utilized to regenerate sorbents through techniques such as microwave (MW) and induction-based heating, which offer rapid dielectric heating rates compared to conventional thermal heating (Wilcox, 2020; Mohd Pauzi et al., 2022). Ozkan et al. (2022) demonstrated that the use of electricity for both liquid and solid sorbent regeneration yields a lower thermal energy equivalent for DAC. In addition, MW based-heating has several potential benefits compared to conductive heating methods, including energy efficiency, rapid heating rates, and the ability to provide instantaneous dielectric heating without heat transfer limitations (Gomez-Rueda et al., 2022). Lee et al. (2023) recently demonstrated the susceptibility of IL sorbents to dielectric heating and rapid CO2 desorption. These benefits may help to reduce the overall energy consumption, regeneration rate, and cost of DAC operations. However, further research is needed to fully understand the potential of MW assisted regeneration method and its impacts on sorbent stability and absorption-desorption cyclability for DAC.
3.4. Thermal stability and oxidative degradation
Cyclic capacity is dependent on the thermal and oxidative stability of a sorbent. Oxidative degradation can be particularly problematic in amine-based CO2 capture systems where the material is exposed to oxygen; amines can undergo chemical reactions in the presence of O2 resulting in the formation of undesired byproducts, such as ammonia and amine-derived carbonates (Spietz et al., 2018; Vevelstad et al., 2022). Indeed, oxidative and thermal degradation in amines leads to significant CO2 capacity loss (Vevelstad et al., 2022). In addition, degradation of solvent can increase corrosion, foaming, and fouling in a CO2 capture unit, thus decreasing the lifetime of a system and increasing the cost of the CO2 capture process (Saeed et al., 2018). Using a thermally stable solvent can help to minimize the need for frequent solvent replacement and reduce the overall cost of the process, as well as minimize environmental impacts. Recently, we reported on the oxidative and thermal degradation mechanism of a functionalized IL where its superior stability, compared to conventional CO2 capture solvents, was shown to maintain its high capacity (Lee et al., 2023). Hence, developing sorbent materials with minimal thermal degradation and excellent oxidative stability is essential for the development of practical DAC technologies. For most of the emerging sorbents, detailed stability and cyclability studies are scarce and thus their practicality cannot be fully assessed.
3.5. Design and cost
DAC technology has an estimated cost of $264–1,000 per tCO2 assuming 75% air capture efficiency and 95% CO2 purity (National Academies of Sciences, 2019), which is still significantly higher than the cost of CO2 capture from flue gas ($50–100/tCO2) (Lebling et al., 2022). It is important to note that the current capture efficiency of DAC technology is <10% (Zeman, 2007; Long-Innes and Struchtrup, 2022), which significantly impacts the cost, making the debate to achieve the highly ambitious target of $100–300/tCO2 viability skeptical (Küng et al., 2023). Since, DAC is an emerging technology, it is imperative to comprehensively explore the general energy requirements and economic feasibility, as well as the environmental viability of a breakthrough sorbent. An optimized design of an air-sorbent contactor column is critical to efficiently remove CO2 from the air, especially in the case of powder sorbents. However, driving large volumes of atmospheric air and the associated pressure drop in a sorbent unit incur a substantial operational cost (Zolfaghari et al., 2022). Thus, developing an effective contactor to process the large volume of air will also help lower the overall cost of capturing CO2. Recent studies have indicated that fossil fuels remain a cost-effective energy source, but their use may also render the CO2 capture process ineffective as it releases significant amounts of CO2 (Terlouw et al., 2021). Therefore, for net zero emissions, in a DAC unit that utilizes fossil fuel-derived thermal energy, the downstream emissions should be captured while controlling the upstream emissions (Ozkan et al., 2022). On the other hand, integrating renewable energy resources such as wind and solar to a DAC unit would enable a cost-effective and environmentally friendly solution for reducing the current atmospheric CO2 concentration (Zolfaghari et al., 2022).
3.6. Environmental impacts of sorbents
Environmental assessment of sorbents in DAC technologies is often overlooked. To fully evaluate DAC feasibility, it is crucial to consider the environmental impacts of sorbent production, disposal, and lifecycle costs (Leonzio et al., 2022). For example, sodium hydroxide used in DAC process is corrosive and generates toxic chlorine gas during production (Realmonte et al., 2019). ILs, on the hand, are relatively benign, in particular due to their negligible volatility, but require proper disposal (de Jesus and Maciel Filho, 2022). A recent study linked the presence of high levels of imidazolium-based cations in soil (i.e., landfill site sampled in United Kingdom) to human health issues such as primary biliary cholangitis which is a chronic autoimmune liver disease (Abdelghany et al., 2020). Likewise, MOF synthesis often involves solvothermal strategies that use toxic solvents (Kumar et al., 2019; He et al., 2022). To address these issues, further research should focus on developing environmental friendly synthesis routes for emerging CDR sorbents that avoid the use of hazardous solvents and explore innovative techniques like microwave-assisted synthesis (Thomas-Hillman et al., 2018), electrosynthesis (Al-Kutubi et al., 2015), or 3D printing (Lieu et al., 2022). For MOF synthesis, these approaches are also shown to reduce synthesis time, promote rapid growth, and enable homogeneous packing assemblies as additional benefits.
4. Conclusions
In this perspective, we have highlighted the emerging sorbents to capture CO2 directly from the air. Most current research focuses on improving CO2 working capacity sorption kinetics and does not typically address cyclability or the design of scalable DAC infrastructure. In the development of new sorbents, computational studies identifying descriptor for molecular design and approaches that accelerate sorbent discovery and prediction of properties are required. These will likely require multiscale modeling aimed at interrogating both bulk sorbent properties as well as detailed CO2 binding energetics (Alizadeh et al., 2021; Heydari Dokoohaki and Zolghadr, 2021; Malik et al., 2021). The field is still in search of a breakthrough sorbent that possess high selectivity, high working capacity under varied humidity and temperature conditions, improved thermal and oxidative stability and cyclability. Even with a breakthrough sorbent, the critical factor in ensuring the successful net and sustainable removal of CO2 is integrating the DAC system with low-carbon energy sources and establishing the lifetime of the sorbent, including appropriate operating conditions and stability to CO2 uptake-release cycles. Therefore, there is also the need to develop technology platforms that transfer energy efficiently. Taken together, intensive research focus, government policy and support, and private industry funding are needed to overcome the technological challenges of developing viable DAC systems to operate at a net-zero cost.
Data availability statement
The original contributions presented in the study are included in the article, further inquiries can be directed to the corresponding author.
Author contributions
MZ and BG created the first draft of the manuscript. All authors contributed to the revisions.
Funding
This study was supported by the U.S. Department of Energy, Office of Science, Basic Energy Sciences under award number DE-SC0022214. BG acknowledges funding from Research Corporation for Science Advancement (award number: 27704) through the Scialog: Negative Emissions Science.
Conflict of interest
The authors declare that the research was conducted in the absence of any commercial or financial relationships that could be construed as a potential conflict of interest.
Publisher's note
All claims expressed in this article are solely those of the authors and do not necessarily represent those of their affiliated organizations, or those of the publisher, the editors and the reviewers. Any product that may be evaluated in this article, or claim that may be made by its manufacturer, is not guaranteed or endorsed by the publisher.
References
Abdelghany, T. M., Leitch, A. C., Nevjestić, I., Ibrahim, I., Miwa, S., Wilson, C., et al. (2020). Emerging risk from “environmentally-friendly” solvents: interaction of methylimidazolium ionic liquids with the mitochondrial electron transport chain is a key initiation event in their mammalian toxicity. Food Chem. Toxicol. 145, 111593. doi: 10.1016/j.fct.2020.111593
Aghaie, M., Rezaei, N., and Zendehboudi, S. (2018). A systematic review on CO2 capture with ionic liquids: current status and future prospects. Renew. Sustain. Energy Rev. 96, 502–525. doi: 10.1016/j.rser.2018.07.004
Ahmad, N., Qian, W., Sun, P., Wang, X., Zhang, K., Xu, X., et al. (2022). Understanding the side chain effect on regulation of CO2 Capture by amino acid-based deep eutectic solvents. J. Mol. Liq. 368, 120660. doi: 10.1016/j.molliq.2022.120660
Alizadeh, V., Esser, L., and Kirchner, B. (2021). How is CO2 absorbed into a deep eutectic solvent? J. Chem. Phys. 154, 94503. doi: 10.1063/5.0038093
Al-Kutubi, H., Gascon, J., Sudhölter, E. J. R., and Rassaei, L. (2015). Electrosynthesis of metal-organic frameworks: challenges and opportunities. ChemElectroChem 2, 462–474. doi: 10.1002/celc.201402429
An, K., Farooqui, A., and McCoy, S. T. (2022). The impact of climate on solvent-based direct air capture systems. Appl. Energy 325, 119895. doi: 10.1016/j.apenergy.2022.119895
Avelar Bonilla, G. M., Morales-Collazo, O., and Brennecke, J. F. (2019). Effect of water on CO2 capture by aprotic heterocyclic anion (AHA) ionic liquids. ACS Sustain. Chem. Eng. 7, 16858–16869. doi: 10.1021/acssuschemeng.9b04424
Baciocchi, R., Storti, G., and Mazzotti, M. (2006). Process design and energy requirements for the capture of carbon dioxide from air. Chem. Eng. Process. Process Intensif. 45, 1047–1058. doi: 10.1016/j.cep.2006.03.015
Bhatt, P. M., Belmabkhout, Y., Cadiau, A., Adil, K., Shekhah, O., Shkurenko, A., et al. (2016). Fine-tuned fluorinated MOF addresses the needs for trace CO2 removal and air capture using physisorption. J. Am. Chem. Soc. 138, 9301–9307. doi: 10.1021/jacs.6b05345
Builes, S., Sandler, S. I., and Xiong, R. (2013). Isosteric heats of gas and liquid adsorption. Langmuir 29, 10416–10422. doi: 10.1021/la401035p
Carbon Engineering (2023). Available online at: https://carbonengineering.com/our-technology/ (accessed May 5, 2023).
Choi, S., Drese, J. H., Eisenberger, P. M., and Jones, C. W. (2011). Application of amine-tethered solid sorbents for direct CO2 capture from the ambient air. Environ. Sci. Technol. 45, 2420–2427. doi: 10.1021/es102797w
Choi, S., Watanabe, T., Bae, T. H., Sholl, D. S., and Jones, C. W. (2012). Modification of the Mg/DOBDC MOF with amines to enhance CO 2 adsorption from ultradilute gases. J. Phys. Chem. Lett. 3, 1136–1141. doi: 10.1021/jz300328j
Climeworks (2023). Available online at: https://climeworks.com/ (accessed May 5, 2023).
Custelcean, R., Williams, N. J., Garrabrant, K. A., Agullo, P., Brethomé, F. M., Martin, H. J., et al. (2019). Direct air capture of CO2 with aqueous amino acids and solid bis-iminoguanidines (BIGs). Ind. Eng. Chem. Res. 58, 23338–23346. doi: 10.1021/acs.iecr.9b04800
Darunte, L. A., Oetomo, A. D., Walton, K. S., Sholl, D. S., and Jones, C. W. (2016). Direct air capture of CO2 using amine functionalized MIL-101(Cr). ACS Sustain. Chem. Eng. 4, 5761–5768. doi: 10.1021/acssuschemeng.6b01692
de Jesus, S. S., and Maciel Filho, R. (2022). Are ionic liquids eco-friendly? Renew. Sustain. Energy Rev. 157, 112039. doi: 10.1016/j.rser.2021.112039
Forse, A. C., and Milner, P. J. (2021). New chemistry for enhanced carbon capture: beyond ammonium carbamates. Chem. Sci. 12, 508–516. doi: 10.1039/D0SC06059C
Fu, D., Park, Y., and Davis, M. E. (2022). Confinement effects facilitate low-concentration carbon dioxide capture with zeolites. Proc. Natl. Acad. Sci. U. S. A. 119, e2211544119. doi: 10.1073/pnas.2211544119
García, G., Aparicio, S., Ullah, R., and Atilhan, M. (2015). Deep eutectic solvents: physicochemical properties and gas separation applications. Energy Fuels 29, 2616–2644. doi: 10.1021/ef5028873
Gaur, S. S., Edgehouse, K. J., Klemm, A., Wei, P., Gurkan, B., Pentzer, E. B., et al. (2021). Capsules with polyurea shells and ionic liquid cores for CO2 capture. J. Polym. Sci. 59, 2980–2989. doi: 10.1002/pol.20210342
Giving Green Earth (2023). Available online at: https://www.givinggreen.earth/carbon-offsets-research/climeworks (accessed May 5, 2023).
Global Thermostat (2023). Available online at: https://www.globalthermostat.com/ (accessed May 5, 2023).
Gomez-Rueda, Y., Verougstraete, B., Ranga, C., Perez-Botella, E., Reniers, F., Denayer, J. F. M., et al. (2022). Rapid temperature swing adsorption using microwave regeneration for carbon capture. Chem. Eng. J. 446, 137345. doi: 10.1016/j.cej.2022.137345
Guan, J., Huang, T., Liu, W., Feng, F., Japip, S., Li, J., et al. (2022). Design and prediction of metal organic framework-based mixed matrix membranes for CO2 capture via machine learning. Cell Reports Phys. Sci. 3, 100864. doi: 10.1016/j.xcrp.2022.100864
Gurkan, B., Goodrich, B. F., Mindrup, E. M., Ficke, L. E., Massel, M., Seo, S., et al. (2010b). Molecular design of high capacity, low viscosity, chemically tunable ionic liquids for CO2 capture. J. Phys. Chem. Lett. 1, 3494–3499. doi: 10.1021/jz101533k
Gurkan, B., Simeon, F., and Hatton, T. A. (2015). Quinone reduction in ionic liquids for electrochemical CO2 separation. ACS Sustain. Chem. Eng. 3, 1394–1405. doi: 10.1021/acssuschemeng.5b00116
Gurkan, B. E., de la Fuente, J. C., Mindrup, E. M., Ficke, L. E., Goodrich, B. F., Price, E. A., et al. (2010a). Equimolar CO2 absorption by anion-functionalized ionic liquids. J. Am. Chem. Soc. 132, 2116–2117. doi: 10.1021/ja909305t
Hansen, B. B., Spittle, S., Chen, B., Poe, D., Zhang, Y., Klein, J. M., et al. (2021). Deep eutectic solvents: a review of fundamentals and applications. Chem. Rev. 121, 1232–1285. doi: 10.1021/acs.chemrev.0c00385
He, Q., Zhan, F., Wang, H., Xu, W., Wang, H., Chen, L., et al. (2022). Recent progress of industrial preparation of metal–organic frameworks: synthesis strategies and outlook. Mater. Today Sustain. 17, 100104. doi: 10.1016/j.mtsust.2021.100104
Heydari Dokoohaki, M., and Zolghadr, A. R. (2021). Significant improvement in CO2 absorption by deep eutectic solvents as immobilized sorbents: computational analysis. J. Phys. Chem. B 125, 10035–10046. doi: 10.1021/acs.jpcb.1c03367
House, K. Z., Baclig, A. C., Ranjan, M., Van Nierop, E. A., Wilcox, J., Herzog, H. J., et al. (2011). Economic and energetic analysis of capturing CO2 from ambient air. Proc. Natl. Acad. Sci. U. S. A. 108, 20428–20433. doi: 10.1073/pnas.1012253108
Huang, Q., Luo, Q., Wang, Y., Pentzer, E., and Gurkan, B. (2019). Hybrid ionic liquid capsules for rapid CO2 capture. Ind. Eng. Chem. Res. 58, S1–S7. doi: 10.1021/acs.iecr.9b00314
IEA (2021). Net Zero by 2050. Paris: IEA. Available online at: https://www.iea.org/reports/net-zero-by-2050, License: CC BY 4.0.
IEA (2022). Direct Air Capture. Paris: IEA. Available online at: https://www.iea.org/reports/direct-air-capture, License: CC BY 4.0.
Infinitree LLC (2023). http://www.infinitreellc.com/ (accessed May 5, 2023).
Keith, D. W., Holmes, G., St. Angelo, D., and Heidel, K. (2018). A process for capturing CO2 from the atmosphere. Joule 2, 1573–1594. doi: 10.1016/j.joule.2018.05.006
Klemm, A., Vicchio, S. P., Bhattacharjee, S., Cagli, E., Park, Y., Zeeshan, M., et al. (2023). Impact of hydrogen bonds on CO2 binding in eutectic solvents: an experimental and computational study toward sorbent design for CO2 capture. ACS Sustain. Chem. Eng. 11, 3740–3749. doi: 10.1021/acssuschemeng.2c06767
Kumar, A., Madden, D. G., Lusi, M., Chen, K. J., Daniels, E. A., Curtin, T., et al. (2015). Direct air capture of CO2 by physisorbent materials. Angew. Chemie Int. Ed. 54, 14372–14377. doi: 10.1002/anie.201506952
Kumar, D. R., Rosu, C., Sujan, A. R., Sakwa-Novak, M. A., Ping, E. W., Jones, C. W., et al. (2020). Alkyl-aryl amine-rich molecules for CO2 removal via direct air capture. ACS Sustain. Chem. Eng. 8, 10971–10982. doi: 10.1021/acssuschemeng.0c03706
Kumar, P., Anand, B., Tsang, Y. F., Kim, K. H., Khullar, S., Wang, B., et al. (2019). Regeneration, degradation, and toxicity effect of MOFs: opportunities and challenges. Environ. Res. 176, 108488. doi: 10.1016/j.envres.2019.05.019
Küng, L., Aeschlimann, S., Charalambous, C., McIlwaine, F., Young, J., Shannon, N., et al. (2023). Roadmap for Achieving Scalable, Safe, and Low-Cost Direct Air Carbon Capture and Storage. Preprint.
Lackner, K. S. (2009). Capture of carbon dioxide from ambient Air. Eur. Phys. J. Spec. Top. 176, 93–106. doi: 10.1140/epjst/e2009-01150-3
Lackner, K. S. (2013). The thermodynamics of direct air capture of carbon dioxide. Energy 50, 38–46. doi: 10.1016/j.energy.2012.09.012
Lai, J. Y., Ngu, L. H., and Hashim, S. S. A. (2021). review of CO2 adsorbents performance for different carbon capture technology processes conditions. Greenh. Gases Sci. Technol. 11, 1076–1117. doi: 10.1002/ghg.2112
Lebling, K., Leslie-Bole, H., Byrum, Z., and Bridgwater, L. (2022). Direct Air Capture: 6 Things To Know. Washington, DC: World Resources Institute.
Lee, Y. Y., Cagli, E., Klemm, A., Park, Y., Dikki, R., Kidder, M. K., et al. (2023). Microwave regeneration and thermal and oxidative stability of imidazolium cyanopyrrolide ionic liquid for direct air capture of carbon dioxide. ChemSusChem. 2013, e202300118. doi: 10.1002/cssc.202300118
Lee, Y. Y., Edgehouse, K., Klemm, A., Mao, H., Pentzer, E., Gurkan, B., et al. (2020). Capsules of reactive ionic liquids for selective capture of carbon dioxide at low concentrations. ACS Appl. Mater. Interfaces 12, 19184–19193. doi: 10.1021/acsami.0c01622
Lee, Y. Y., and Gurkan, B. (2021). Graphene oxide reinforced facilitated transport membrane with poly(ionic liquid) and ionic liquid carriers for CO2/N2 separation. J. Memb. Sci. 638, 119652. doi: 10.1016/j.memsci.2021.119652
Lee, Y. Y., Penley, D., Klemm, A., Dean, W., and Gurkan, B. (2021). Deep eutectic solvent formed by imidazolium cyanopyrrolide and ethylene glycol for reactive CO2 separations. ACS Sustain. Chem. Eng. 9, 1090–1098. doi: 10.1021/acssuschemeng.0c07217
Lee, Y. Y., Wickramasinghe, N. P., Dikki, R., Jan, D. L., and Gurkan, B. (2022). Facilitated transport membrane with functionalized ionic liquid carriers for CO2/N2, CO2/O2, and CO2/Air separations. Nanoscale 14, 12638–12650. doi: 10.1039/D2NR03214G
Leonzio, G., Mwabonje, O., Fennell, P. S., and Shah, N. (2022). Environmental performance of different sorbents used for direct air capture. Sustain. Prod. Consum. 32, 101–111. doi: 10.1016/j.spc.2022.04.004
Lieu, W. Y., Fang, D., Tay, K. J., Li, X. L., Chu, W. C., Ang, Y. S., et al. (2022). Progress on 3D-printed metal-organic frameworks with hierarchical structures. Adv. Mater. Technol. 7, 2200023. doi: 10.1002/admt.202200023
Lively, R. P., and Realff, M. J. (2016). On thermodynamic separation efficiency: adsorption processes. AIChE J. 62, 3699–3705. doi: 10.1002/aic.15269
Long-Innes, R., and Struchtrup, H. (2022). Thermodynamic loss analysis of a liquid-sorbent direct air carbon capture plant. Cell Reports Phys. Sci. 3, 100791. doi: 10.1016/j.xcrp.2022.100791
Luo, Q., Wang, Y., Chen, Z., Wei, P., Yoo, E., Pentzer, E., et al. (2019). Pickering emulsion-templated encapsulation of ionic liquids for contaminant removal. ACS Appl. Mater. Interfaces 11, 9612–9620. doi: 10.1021/acsami.8b21881
Mahmoudkhani, M., and Keith, D. W. (2009). Low-energy sodium hydroxide recovery for CO2 capture from atmospheric air-thermodynamic analysis. Int. J. Greenh. Gas Control 3, 376–384. doi: 10.1016/j.ijggc.2009.02.003
Malik, A., Dhattarwal, H. S., and Kashyap, H. K. (2021). Distinct solvation structures of CO2 and SO2 in reline and ethaline deep eutectic solvents revealed by aimd simulations. J. Phys. Chem. B 125, 1852–1860. doi: 10.1021/acs.jpcb.0c09824
Matsuoka, A., Taniguchi, S., Kamio, E., and Matsuyama, H. (2021). Fundamental investigation of the rate-determining step of CO2 permeation through ion gel membranes containing amino-acid ionic liquid as the CO2 carrier. Ind. Eng. Chem. Res. 60, 7397–7405. doi: 10.1021/acs.iecr.1c00615
McDonald, T. M., Lee, W. R., Mason, J. A., Wiers, B. M., Hong, C. S., Long, J. R., et al. (2012). Capture of carbon dioxide from air and flue gas in the alkylamine-appended metal-organic framework Mmen-Mg 2(Dobpdc). J. Am. Chem. Soc. 134, 7056–7065. doi: 10.1021/ja300034j
McQueen, N., Gomes, K. V., McCormick, C., Blumanthal, K., Pisciotta, M., Wilcox, J. A., et al. (2021). Review of direct air capture (DAC): scaling up commercial technologies and innovating for the future. Prog. Energy 3, 32001. doi: 10.1088/2516-1083/abf1ce
Mohan, M., Demerdash, O., Simmons, B. A., Smith, J. C., Kidder, M. K., Singh, S., et al. (2022). Accurate prediction of carbon dioxide capture by deep eutectic solvents using quantum chemistry and a neural network. Green Chem. 25, 3309–3724. doi: 10.1039/D2GC04425K
Mohd Pauzi, M. M., Azmi, N., and Lau, K. K. (2022). Emerging solvent regeneration technologies for CO2 capture through offshore natural gas purification processes. Sustainability 14, 4350. doi: 10.3390/su14074350
Mota-Martinez, M. T., Hallett, J. P., and Mac Dowell, N. (2017). Solvent selection and design for CO2 capture-how we might have been missing the point. Sustain. Energy Fuels 1, 2078–2090. doi: 10.1039/C7SE00404D
Moya, C., Alonso-Morales, N., de Riva, J., Morales-Collazo, O., Brennecke, J. F., and Palomar, J. (2018). Encapsulation of ionic liquids with an aprotic heterocyclic anion (AHA-IL) for CO2 capture: preserving the favorable thermodynamics and enhancing the kinetics of absorption. J. Phys. Chem. B 122, 2616–2626. doi: 10.1021/acs.jpcb.7b12137
Nabity, J., Aaron, R., and Wickham, D. (2021). “Integrated system modelling for spacecraft atmospheric revitalization using a supported ionic liquid membrane,” in 50th International Conference on Environmental Systems.
National Academies of Sciences, Medicine and Engineering (2019). Negative Emissions Technologies and Reliable Sequestration: A Research Agenda.
Ozkan, M. (2021). Direct air capture of CO2: a response to meet the global climate targets. MRS Energy Sustain. 8, 51–56. doi: 10.1557/s43581-021-00005-9
Ozkan, M., Nayak, S. P., Ruiz, A. D., and Jiang, W. (2022). Current status and pillars of direct air capture technologies. iScience 25, 103990. doi: 10.1016/j.isci.2022.103990
Petrovic, B., Gorbounov, M., and Masoudi Soltani, S. (2021). Influence of surface modification on selective CO2 adsorption: a technical review on mechanisms and methods. Microporous Mesoporous Mater. 312, 110751. doi: 10.1016/j.micromeso.2020.110751
Philander, S. G. (2012). Center for Climate and Energy Solutions. SAGE Publications, Inc. doi: 10.4135/9781452218564
Qazvini, O. T., Babarao, R., and Telfer, S. G. (2021). Selective capture of carbon dioxide from hydrocarbons using a metal-organic framework. Nat. Commun. 12, 197. doi: 10.1038/s41467-020-20489-2
Quang, D. V., Dindi, A., Rayer, A. V., Hadri, N. E., Abdulkadir, A., and Abu-Zahra, M. R. (2015). Effect of moisture on the heat capacity and the regeneration heat required for CO2 capture process using pei impregnated mesoporous precipitated silica. Greenh. Gases Sci. Technol. 5, 91–101. doi: 10.1002/ghg.1427
Rahimi, M., Catalini, G., Hariharan, S., Wang, M., Puccini, M., Hatton, T. A., et al. (2020). Carbon dioxide capture using an electrochemically driven proton concentration process. Cell Reports Phys. Sci. 1, 100033. doi: 10.1016/j.xcrp.2020.100033
Ramezani, R., Mazinani, S., and Di Felice, R. (2022). State-of-the-art of CO2capture with amino acid salt solutions. Rev. Chem. Eng. 38, 273–299. doi: 10.1515/revce-2020-0012
Realmonte, G., Drouet, L., Gambhir, A., Glynn, J., Hawkes, A., Köberle, A. C., et al. (2019). An inter-model assessment of the role of direct air capture in deep mitigation pathways. Nat. Commun. 10, 1–12. doi: 10.1038/s41467-019-10842-5
Recker, E. A., Green, M., Soltani, M., Paull, D. H., Mcmanus, G. J., Davis, J. H., et al. (2022). Direct air capture of CO2via ionic liquids derived from “waste” amino acids. ACS Sustain. Chem. Eng. 10, 11885–11890. doi: 10.1021/acssuschemeng.2c02883
Rosa, L., Sanchez, D. L., Realmonte, G., Baldocchi, D., and D'Odorico, P. (2021). The water footprint of carbon capture and storage technologies. Renew. Sustain. Energy Rev. 138, 110511. doi: 10.1016/j.rser.2020.110511
Sadiq, M. M., Batten, M. P., Mulet, X., Freeman, C., Konstas, K., Mardel, J. I., et al. (2020). A pilot-scale demonstration of mobile direct air capture using metal-organic frameworks. Adv. Sustain. Syst. 4, 2000101. doi: 10.1002/adsu.202000101
Saeed, I. M., Alaba, P., Mazari, S. A., Basirun, W. J., Lee, V. S., Sabzoi, N., et al. (2018). Opportunities and challenges in the development of monoethanolamine and its blends for post-combustion CO2 capture. Int. J. Greenh. Gas Control 79, 212–233. doi: 10.1016/j.ijggc.2018.11.002
Sanz-Pérez, E. S., Murdock, C. R., Didas, S. A., and Jones, C. W. (2016). Direct capture of CO2 from ambient air. Chem. Rev. 116, 11840–11876. doi: 10.1021/acs.chemrev.6b00173
Seo, S., Quiroz-Guzman, M., Desilva, M. A., Lee, T. B., Huang, Y., Goodrich, B. F., et al. (2014). Chemically tunable ionic liquids with aprotic heterocyclic anion (AHA) for CO2 capture. J. Phys. Chem. B 118, 5740–5751. doi: 10.1021/jp502279w
Shaik, A., Benneker, A., and McCoy, S. (2022). Achieving net negative CO2 emissions with sorbent direct air capture under different energy inputs and climate variations. 16th International Conference on Greenhouse Gas Control Technologies, GHGT-16. Lyon, France. doi: 10.2139/ssrn.4285081
Shi, X., Xiao, H., Azarabadi, H., Song, J., Wu, X., Chen, X., et al. (2020). Sorbents for the direct capture of CO2 from ambient air. Angew. Chemie Int. Ed. 59, 6984–7006. doi: 10.1002/anie.201906756
Shukla, S. K., Khokarale, S. G., Bui, T. Q., and Mikkola, J. P. T. (2019). Ionic liquids: potential materials for carbon dioxide capture and utilization. Front. Mater. 6, 42. doi: 10.3389/fmats.2019.00042
Smith, E. L., Abbott, A. P., and Ryder, K. S. (2014). Deep eutectic solvents (DESs) and their applications. Chem. Rev. 114, 11060–11082. doi: 10.1021/cr300162p
Sodiq, A., Abdullatif, Y., Aissa, B., Ostovar, A., Nassar, N., El-Naas, M., et al. (2023). review on progress made in direct air capture of CO2. Environ. Technol. Innov. 29, 102991. doi: 10.1016/j.eti.2022.102991
Song, D., Seibert, A. F., and Rochelle, G. T. (2017). “Effect of liquid viscosity on mass transfer for packings,” in Kister Distill. Symp. 2017 - Top. Conf. 2017 AIChE Spring Meet. 13th Glob. Congr. Process Saf., Vol. 63, 268–279.
Spietz, T., Chwoła, T., Krótki, A., Tatarczuk, A., Wiecław-Solny, L., Wilk, A., et al. (2018). Ammonia emission from CO2 capture pilot plant using aminoethylethanolamine. Int. J. Environ. Sci. Technol. 15, 1085–1092. doi: 10.1007/s13762-017-1475-z
Stern, M. C., and Hatton, T. A. (2014). Bench-scale demonstration of CO2 capture with electrochemically-mediated amine regeneration. RSC Adv. 4, 5906–5914. doi: 10.1039/c3ra46774k
Terlouw, T., Treyer, K., Bauer, C., and Mazzotti, M. (2021). Life cycle assessment of direct air carbon capture and storage with low-carbon energy sources. Environ. Sci. Technol. 55, 11397–11411. doi: 10.1021/acs.est.1c03263
Thomas-Hillman, I., Laybourn, A., Dodds, C., and Kingman, S. W. (2018). Realising the environmental benefits of metal-organic frameworks: recent advances in microwave synthesis. J. Mater. Chem. A 6, 11564–11581. doi: 10.1039/C8TA02919A
Trivedi, T. J., Lee, J. H., Lee, H. J., Jeong, Y. K., and Choi, J. W. (2016). Deep eutectic solvents as attractive media for CO2 capture. Green Chem. 18, 2834–2842. doi: 10.1039/C5GC02319J
Vevelstad, S. J., Buvik, V., Knuutila, H. K., Grimstvedt, A., and da Silva, E. F. (2022). Important aspects regarding the chemical stability of aqueous amine solvents for CO2 capture. Ind. Eng. Chem. Res. 61, 15737–15753. doi: 10.1021/acs.iecr.2c02344
Voskian, S., and Hatton, T. A. (2018). “Faradaic electro-swing reactive adsorption for carbon capture at low concentrations,” in 14th Greenhouse Gas Control Technologies Conference Melbourne, 21–26.
Voskian, S., and Hatton, T. A. (2019). Faradaic electro-swing reactive adsorption for CO2 capture. Energy Environ. Sci. 12, 3530–3547. doi: 10.1039/C9EE02412C
Wang, J., Huang, H., Wang, M., Yao, L., Qiao, W., Long, D., et al. (2015). Direct capture of low-concentration CO2 on mesoporous carbon-supported solid amine adsorbents at ambient temperature. Ind. Eng. Chem. Res. 54, 5319–5327. doi: 10.1021/acs.iecr.5b01060
Wang, M., Herzog, H. J., and Hatton, T. A. (2020). CO2 capture using electrochemically mediated amine regeneration. Ind. Eng. Chem. Res. 59, 7087–7096. doi: 10.1021/acs.iecr.9b05307
Wang, Q., Luo, J., Zhong, Z., and Borgna, A. (2011). CO2 capture by solid adsorbents and their applications: current status and new trends. Energy Environ. Sci. 4, 42–55. doi: 10.1039/C0EE00064G
Wang, T., Hou, C., Ge, K., Lackner, K. S., Shi, X., Liu, J., et al. (2017). Spontaneous cooling absorption of CO2 by a polymeric ionic liquid for direct air capture. J. Phys. Chem. Lett. 8, 3986–3990. doi: 10.1021/acs.jpclett.7b01726
Wang, T., Lackner, K. S., and Wright, A. (2011). Moisture swing sorbent for carbon dioxide capture from ambient air. Environ. Sci. Technol. 45, 6670–6675. doi: 10.1021/es201180v
Wang, T., Wang, X., Hou, C., and Liu, J. (2020). Quaternary functionalized mesoporous adsorbents for ultra-high kinetics of CO2 capture from air. Sci. Rep. 10, 1–8. doi: 10.1038/s41598-020-77477-1
Weiland, R. H., Dingman, J. C., and Cronin, D. B. (1997). Heat capacity of aqueous monoethanolamine, diethanolamine, N-methyldiethanolamine, and N-methyldiethanolamine-based blends with carbon dioxide. J. Chem. Eng. Data 42, 1004–1006. doi: 10.1021/je960314v
Weiss, E., and Abu-Reziq, R. (2017). Ionic liquid-based polymeric microreactors and their applicability. J. Mater. Sci. 52, 10637–10647. doi: 10.1007/s10853-017-1236-x
Wiheeb, A. D., Helwani, Z., Kim, J., and Othman, M. R. (2016). Pressure swing adsorption technologies for carbon dioxide capture. Sep. Purif. Rev. 45, 108–121. doi: 10.1080/15422119.2015.1047958
Wilcox, J. (2020). An electro-swing approach. Nat. Energy 5, 121–122. doi: 10.1038/s41560-020-0554-4
World Resources Institute (2002). Available online at: https://www.wri.org/initiatives/carbon-removal
Yan, H., Zhao, L., Bai, Y., Li, F., Dong, H., Wang, H., et al. (2020). Superbase ionic liquid-based deep eutectic solvents for improving CO2 absorption. ACS Sustain. Chem. Eng. 8, 2523–2530. doi: 10.1021/acssuschemeng.9b07128
Yang, Z., and Dai, S. (2021). Challenges in engineering the structure of ionic liquids towards direct air capture of CO2. Green Chem. Eng. 2, 342–345. doi: 10.1016/j.gce.2021.08.003
Ying, J., Eimer, D. A., Mathisen, A., Sørensen, H., and Haugen, H. A. (2014). Intensification of CO2 stripping from amine solutions by ultrasonic. Energy Proc. 63, 781–786. doi: 10.1016/j.egypro.2014.11.087
Young, J., García-Díez, E., Garcia, S., and Van Der Spek, M. (2021). The impact of binary water- CO2 isotherm models on the optimal performance of sorbent-based direct air capture processes. Energy Environ. Sci. 14, 5377–5394. doi: 10.1039/D1EE01272J
Zeman, F. (2007). Energy and material balance of CO2 capture from ambient air. Environ. Sci. Technol. 41, 7558–7563. doi: 10.1021/es070874m
Zhang, K., Hou, Y., Wang, Y., Wang, K., Ren, S., Wu, W., et al. (2018). Efficient and reversible absorption of CO2 by functional deep eutectic solvents. Energy Fuels 32, 7727–7733. doi: 10.1021/acs.energyfuels.8b01129
Zhang, W., Liu, H., Sun, Y., Cakstins, J., Sun, C., Snape, C. E., et al. (2016). Parametric study on the regeneration heat requirement of an amine-based solid adsorbent process for post-combustion carbon capture. Appl. Energy 168, 394–405. doi: 10.1016/j.apenergy.2016.01.049
Zhang, X., and Cai, Y. (2012). Octadecyltrichlorosilane (OTS)-coated ionic liquid drops: micro-reactors for homogenous catalytic reactions at designated interfaces. Beilstein J. Nanotechnol. 3, 33–39. doi: 10.3762/bjnano.3.4
Zhang, X., Zhang, X., Dong, H., Zhao, Z., Zhang, S., Huang, Y., et al. (2012). Carbon capture with ionic liquids: overview and progress. Energy Environ. Sci. 5, 6668–6681. doi: 10.1039/c2ee21152a
Keywords: eutectic solvents, ionic liquids, carbon capture, zeolites, metal organic frameworks, capsules, climate change, negative emissions
Citation: Zeeshan M, Kidder MK, Pentzer E, Getman RB and Gurkan B (2023) Direct air capture of CO2: from insights into the current and emerging approaches to future opportunities. Front. Sustain. 4:1167713. doi: 10.3389/frsus.2023.1167713
Received: 16 February 2023; Accepted: 29 May 2023;
Published: 15 June 2023.
Edited by:
Eric C. D. Tan, National Renewable Energy Laboratory (DOE), United StatesReviewed by:
Sean McCoy, University of Calgary, CanadaYuan Jiang, Pacific Northwest National Laboratory (DOE), United States
Copyright © 2023 Zeeshan, Kidder, Pentzer, Getman and Gurkan. This is an open-access article distributed under the terms of the Creative Commons Attribution License (CC BY). The use, distribution or reproduction in other forums is permitted, provided the original author(s) and the copyright owner(s) are credited and that the original publication in this journal is cited, in accordance with accepted academic practice. No use, distribution or reproduction is permitted which does not comply with these terms.
*Correspondence: Burcu Gurkan, beg23@case.edu