- 1Vista Magnet Middle School, Vista, CA, United States
- 2Division of Biological Sciences, University of California, San Diego, La Jolla, CA, United States
- 3Department of Physics, University of California, San Diego, La Jolla, CA, United States
- 4Biology Department, Swarthmore College, Swarthmore, PA, United States
The growing possibilities of space travel are quickly moving from science fiction to reality. However, to realize the dream of long-term space travel, we must understand how these conditions affect biological and physiological processes. Planarians are master regenerators, famous for their ability to regenerate from very small parts of the original animal. Understanding how this self-repair works may inspire regenerative therapies in humans. Two studies conducted aboard the International Space Station (ISS) showed that planarian regeneration is possible in microgravity. One study reported no regenerative defects, whereas the other study reported behavioral and microbiome alterations post-space travel and found that 1 of 15 planarians regenerated a Janus head, suggesting that microgravity exposure may not be without consequences. Given the limited number of studies and specimens, further microgravity experiments are necessary to evaluate the effects of microgravity on planarian regeneration. Such studies, however, are generally difficult and expensive to conduct. We were fortunate to be sponsored by the Student Spaceflight Experiment Program (SSEP) to investigate how microgravity affects regeneration of the planarian species Dugesia japonica on the ISS. While we were unable to successfully study planarian regeneration within the experimental constraints of our SSEP Mission, we systematically analyzed the cause for the failed experiment, leading us to propose a modified protocol. This work thus opens the door for future experiments on the effects of microgravity on planarian regeneration on SSEP Missions as well as for more advanced experiments by professional researchers.
Introduction
Space travel and inhabitation capture the human imagination. They also pose biological and engineering challenges that are not encountered on Earth. The National Center for Earth and Space Science Education (NCESSE) runs the Student Spaceflight Experiment Program (SSEP) for students from different communities, including Grades 5–12, community colleges, and universities “to inspire the next generation of scientists and engineers” (http://ssep.ncesse.org). A key aspect of the program is to have students work like “real scientists,” and be involved in all parts of the scientific process, including conceptualization and design of a scientific experiment, participating in a two-step proposal competition, and performing the actual experiment while learning to work within financial and experimental constraints (see Appendix for more information on the details of this specific mission and team). Students design an experiment that can be from a number of fields, including geosciences, biology, physics, and physiology, which can be completely contained within a “MixStix mini-laboratory” consisting of a proprietary Teflon fluids mixing enclosure (FME) tube (Nanoracks). This enclosure can hold up to three separate sample materials, separated by clamps that can be opened to allow mixing of the components (http://nanoracks.com/products/mixstix/). The SSEP experiments had to be designed such that they could be conducted with a single interaction (unclamping) between the astronauts and the tube on a specific date (limited to 5 possible options) and allow for an initial dormancy period (approximately 2 weeks) while the experiments were transported to the ISS.
Since its inception in 2010, the SSEP program has conducted ten Space Missions. Biological specimens used in these missions have included various species of insects, worms, echinoderms and fish. The life cycles of many of these species allow for experiments to be conducted by placing eggs or larvae, often in a dormant state, in the FME tubes. Development can then be initiated in space, such as by the switch from cold to ambient storage, to determine the effects of microgravity on these processes. The outcomes of these studies, however, remain largely unknown, because student teams primarily report at the Annual SSEP Conference prior to the flight experiment and thus post-flight results are often not publically available. According to the SSEP website, 76 biological experiments have been presented at the Annual SSEP conference between 2012 and 2017. However, of these, only about a quarter reported results and about half of those were inconclusive due to limited time, tube space or sample size.
To the best of our knowledge, the results of only one biological SSEP experiment have been published (Warren et al., 2013). In this study, the authors used an earlier version of the FME mini-lab system with Caenorhabditis elegans to study the effect of the transcription factor DAF-16 on previously reported genomic expression changes induced by spaceflight in C. elegans. While the study was unsuccessful, partially due to incorrect activation of the experiment in space, it established that nematode viability could be maintained in the FME system for a maximum of 3 weeks (Warren et al., 2013).
Our experiment, as part of the 2017 SSEP Mission 11, aimed to test the effect of microgravity on the regeneration of freshwater planarians of the species Dugesia japonica. To fulfill the SSEP requirements, the experiment was designed such that planarians were amputated on Earth and the resulting tail pieces were kept dormant at 4°C during transportation to the ISS, where they were moved to ambient temperature to initiate regeneration in microgravity. Regeneration was terminated by the astronauts after 2–4 weeks by releasing a fixing agent, formaldehyde, from the second compartment. Upon sample retrieval, the microgravity sample was compared with Earth laboratory controls.
Studying invertebrate regeneration is a popular experiment in schools because students can readily engage with this fascinating phenomenon. Planarians are particularly interesting, because they can regenerate a complete animal, including eyes and a fairly complicated brain, from small fragments of the original body within 7–12 days (Morgan, 1989; Cebrià, 2007). While this process is quite robust, regeneration can be influenced by molecular (Reddien et al., 2005), chemical (Best and Morita, 1982; Hagstrom et al., 2015, 2016) and physical factors (Marsh and Beams, 1952; Brondsted and Brondsted, 1961; Novikov et al., 2008).
To the best of our knowledge, only three studies have investigated the role of gravity in planarian regeneration. While one study found that extended exposure to simulated microgravity is generally detrimental to planarian health (Adell et al., 2014), others have shown that planarians are able to regenerate fully under these conditions (Gorgiladze, 2008; Morokuma et al., 2017). Notably, while Adell et al. conducted their experiments on Earth using a random positioning machine to mimic space conditions, Gorgiladze and Morokuma et al. performed their experiments on board the ISS. It is also worth noting that lethality in the simulated microgravity experiments only occurred under specific rotation conditions (i.e., 60°/s but not 10°/s) and after long-term exposure (13 days) (Adell et al., 2014), which may not be representative of actual space conditions. Furthermore, the planarian species differed in each of the three studies, complicating direct comparisons of the results, especially since species-specific sensitivities to spatial confinement have been reported (Carter et al., 2015). Adell et al. used Schmidtea mediterranea, Gorgiladze used Dugesia tigrina, and Morokuma et al. used D. japonica, the same planarian species used in this study. While neither study on board the ISS found planarians incapable of regeneration, differences in the findings nevertheless exist. Gorgiladze reported no regeneration defects monitoring 60 planarians. In contrast, Morokuma et al. found 1 of 15 planarians regenerated into a two-headed animal, which is a rare event that the authors never observed in the approximate 15,000 planarians they cultured in the laboratory over the past 5 years. Similarly, we have never observed such a phenotype in our toxicology screens (Hagstrom et al., 2015), which also comprise thousands of D. japonica planarians. Furthermore, Morokuma et al showed long-term behavioral and microbiotic changes that were attributed to space travel (Morokuma et al., 2017). Taken together, the discrepancies in the existing studies call for further experiments to determine the effects of microgravity on planarian regeneration and physiology. However, these experiments are expensive and difficult to realize. The SSEP, thus, provides a unique opportunity to address these kinds of intriguing questions by facilitating and engaging aspiring young scientists in the scientific process.
In this paper, we report our experiences conducting a planarian regeneration experiment within SSEP. Because of the lack of published SSEP experiments, we could not build upon prior work to optimize our experimental protocol. Correspondence with the SSEP Director at the 2017 Annual SSEP Conference revealed that all previous planarian regeneration experiments had failed. Because our experiment was similarly unsuccessful, our goal is to explain why it failed and how it could be improved to allow for future successful studies within the experimental constraints imposed by the SSEP program.
Materials and Methods
Animals
Asexual planarians of the species D. japonica were used for the experiments. Planarians were maintained in bulk in Instant Ocean (IO)-water, fed organic chicken or beef liver once a week and cleaned twice a week, as previously described (Hagstrom et al., 2018). Animals were starved for at least 5 days before being used for an experiment. Animals used for experiments were 6.9 ±1.1 mm (mean ± standard deviation) in length. To induce regeneration, intact animals were amputated between the auricles and pharynx with an ethanol-sterilized razor blade as in Hagstrom et al. (2015). The heads were returned to the animal stocks and the tails were allowed to heal for 1 h before loading into the tubes.
Fluids Mixing Enclosure (FME) Type 2
The Type 2 Fluids Mixture Enclosure (FME) Mark II Mini Laboratory was used for SSEP Mission 11 to the ISS. The silicone tube is 170 mm long with an outer diameter of 13 mm and an inner diameter of 9.5 mm. The Type 2 FME tube can be subdivided into two or three separate compartments through the use of clamps. The total volume of sample the Type 2 FME can hold is 9.2 ml. For the planarian experiments, each FME tube was split into two compartments: an 8 ml compartment containing the animals and a 1 ml compartment containing 37% formaldehyde (Fisher Scientific, Waltham, MA). NCESSE shipped 5 FME Mini-lab Kits to each participating community. Three were reserved for the selected flight (1 tube) and ground (2 tubes) truth experiments.
Pre-experiment: Regeneration Test at 4°C
To support the experimental proposal, we conducted an initial test to determine the degree of regeneration at 4°C of D. japonica planarians. This test was performed using 5 ml plastic culture tubes (Falcon, Corning, NY), since FME tubes were not available prior to proposal approval. Ten D. japonica tails were placed in a parafilm-sealed tube in 4°C refrigeration in the dark. A second set of 10 D. japonica tails were prepared the same way and placed at room temperature (RT) in the dark. The tubes were filled approximately to the top, but without an exact measurement of the volume added, thus leaving varying amounts (up to 20%) of air. After 2 weeks, both samples were compared using a Leica S6 trinocular stereo microscope (Wetzlar, Germany) equipped with a Basler A601f camera (Basler, Germany). This comparison revealed that all planarians were able to survive 2-week enclosure at either temperature and that minimal regeneration occurred during incubation at 4°C (Figures 1A,B).
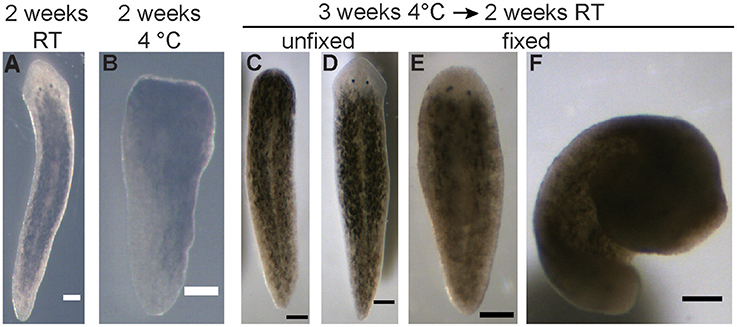
Figure 1. Pre-flight experiments on planarian viability and regeneration. (A) Representative image of a regenerated planarian, from an original tail piece, stored in a sealed 5 ml culture tube for 2 weeks at RT. (B) Representative image of a planarian tail piece which had not regenerated after being stored in a sealed 5 ml culture tube for 2 weeks at 4°C. (C,D) Either 5 or 10 planarian tail pieces were stored in the FME tubes for 3 weeks at 4°C, followed by an additional 2 weeks at RT. (C) Representative image of a planarian which failed to regenerate during this time as no blastema has formed. (D) Representative image of a successfully regenerated planarian. (E,F) Representative images of planarians that were fixed following storage in the FME tubes. While some planarians were fixed extended (E), others curled up (F). Scale bars: 0.25 mm.
Pre-experiment: Viability Test in FME Tubes
During the experimental design phase, one FME tube was prepared with 5 tails and one FME tube was prepared with 10 tails to determine how many worm pieces could survive in a single FME tube. A critical difference from the actual flight experiment was that formaldehyde was not placed in the second volume of the FME tube, since only the effect of lack of oxygen on worm viability was tested. Both FME tubes were kept at 4°C for 3 weeks and then moved to ambient temperature. After 2 more weeks at ambient temperature, the tube containing 5 worms was emptied and the worms evaluated (Figures 1C–E). In this tube, 4 worms were found alive with 3 out of the 4 regenerating normal. The 4th worm had a closed wound but no blastema (Figure 1C). The worms in the second FME tube were fixed by adding 1 ml formaldehyde. The planarians were analyzed the following day after incubation overnight at 4°C. All 10 worms were still present. Six of them had regenerated 2 eyes and 4 had no eyes. Thus, while lack of oxygen appeared to affect their ability to regenerate properly, planarians were able to survive 5 weeks in the FME tube without formaldehyde in the second chamber.
Pre-launch Preparation
The FME tubes were rinsed thoroughly with IO-water in the laboratory. On July 24, 2017, 30 D. japonica were decapitated between the auricles and the pharynx with an ethanol-sterilized razor blade in IO-water. The animals were allowed to close the wound for 1 h. Pre-chilled (4°C) Crystal Geyser (CG) spring water was aerated by pouring back and forth between two glass beakers at least 5 times. Ten D. japonica tails were added to each of the three FME tubes with 8 ml of pre-chilled CG water. CG-water was used instead of IO-water because it was commercially available.
One milliliter of 37% formaldehyde was added to the second chamber of each FME tube so the planarians could be fixed, and thus end the experiment, after sufficient time for regeneration in microgravity. The two ground truth FME tubes were sealed and stored horizontally at 4°C for 25 days. The flight experiment FME tube was placed in a Cold Shipping Package at 2–8°C for transport to Nanoracks in Houston. The FME was refrigerated at approximately 2–4°C until handover to NASA. This allowed the experiment to be kept in a dormant state until arrival on the ISS.
Flight Experiment on the ISS
SpaceX CRS-12 launched from Space Launch Complex 39 at Cape Canaveral Air Force Station, Florida on August 14, 2017. The mini-labs were captured by the ISS on August 16, 2017 with subsequent unloading to ambient temperature (21–24°C) on August 17, 2017. The two ground truth experiments in the laboratory were transferred to ambient temperature in the dark on the same day.
After 3 weeks in microgravity, an ISS crew member performed the U-14d interaction on September 4, 2017 by opening the clamp that separated the planarians and formaldehyde, and shaking vigorously for 5 s to release the formaldehyde, thus terminating and preserving the experiment in microgravity. The two ground truth experiments in the laboratory were treated using similar actions on the same day.
Post-Flight Analysis
On the day of harvesting, the liquid was poured into Petri dishes for analysis. All planarians in both ground truth and flight experiments had disintegrated. Images of the disintegrated pieces were taken with a Leica KL300 LED dissecting microscope and Point Gray Flea3 color camera (FLIR Systems Inc., Wilsonville, OR).
Post-flight: FME Viability Test
To assay which factors may have contributed to worm death during the experiment, additional post-flight experiments were performed. New FME tubes were set up as described in Table 1. Specifically, we assayed the effects of the presence of formaldehyde, presence of air, and tube type. Of note, due to the high cost of FME tubes, when possible and appropriate, certain conditions were tested repeatedly in the same FME tube, with extensive washing before loading new worms. When repeating conditions which contained formaldehyde, the original formaldehyde compartment was left intact. For the tube type experiments, we used 5 ml culture tubes (VWR International, Radnor, PA) containing 6 tails and 5 ml of CG spring water and 9 ml glass borosilicate tubes (Corning, Corning, NY) containing 10 tails and 9 ml spring water to keep the worm:volume ratio relatively constant. Experiments were stored at 4°C for 2–3 weeks and then, when possible, moved to RT for an additional 2–3 weeks (5 weeks total). Planarian viability was checked by eye every 3–4 days.
Results
The goal of this study was to analyze the effect of microgravity on planarian head regeneration. Ideally, planarians would be amputated on board the ISS and then their regeneration documented. This, however, was not possible within the constraints of the SSEP, which only allowed a single interaction of the astronauts with the experiment. Therefore, we designed an experimental protocol where the worms would be amputated on Earth before loading into 8 ml pre-chilled spring water on one side of the FME tube and adding 1 ml of 37% formaldehyde on the other side, separated by a clamp (Figures 3A,B and section Materials and Methods). Because the Mission 11 SSEP timeline consisted of an initial 2.5 week period at 4°C for transport of the flight FME tubes to Nanoracks and the ISS, the amputated planarians needed to be dormant or in stasis until arrival on the ISS. While it was expected that regeneration would be delayed at colder temperatures (Brondsted and Brondsted, 1961), it was uncertain whether we could sufficiently delay it for such a long time. Furthermore, the regenerating planarians needed to be able to survive without oxygen for 2.5 weeks at 4°C, followed by an additional 2–4 weeks at RT on board the ISS before fixation with formaldehyde would occur to stop the experiment. Given these experimental constraints, we conducted several pre-flight experiments to assay (a) the planarians' ability to survive and regenerate in an enclosed tube for such a long time, and (b) the extent of regeneration occurring during storage at 4°C.
Pre-flight Experiments
To test whether planarian regeneration could be put in a dormant state, we conducted a comparative study of regeneration at 4°C and RT, as described in Materials and Methods. First, we evaluated how long the regenerating worms were able to survive without oxygen. Under these conditions, all planarians survived in enclosed tubes for 2 weeks at either 4°C or RT. While some studies have shown limited survival in enclosed conditions, with worms disintegrating within 5 days at a density of 0.2 worms/ml at 10 or 20°C without any oxygen (Morokuma et al., 2017), our preliminary experiments using 2 worms/ml at 4°C and RT have shown that 2 week enclosure was possible. Moreover, a comparison of 10 D. japonica tails stored at 4°C vs. at RT for 2 weeks, under otherwise identical conditions, showed that regeneration was sufficiently delayed, so the 2-weeks transport time to the ISS would not lead to significant regeneration. Planarians stored at 4°C showed only a small blastemal tissue and no eyes whereas worms stored at RT had fully regenerated (Figures 1A,B). Further, we confirmed that at least 10 regenerating tail pieces could survive enclosed in the FME tubes when stored for 3 weeks at 4°C, followed by 2 weeks at RT (Figures 1C–E and section Materials and Methods). However, some of the enclosed animals did show regeneration defects (Figure 1C).
Finally, we confirmed that the one-step fixation of the regenerated tails using formaldehyde would work for our purposes, since standard planarian fixation protocols contain a mucus-removal step before the administration of formaldehyde or other fixatives (Umesono et al., 1997; Pearson et al., 2009). While some of the planarians remained straight (Figure 1E), others curled up during fixation (Figure 1F), making imaging difficult. However, in those cases we were still able to manually determine whether the tails had fully regenerated and quantify the number of eyes. We therefore deemed the protocol adequate given the experimental constraints imposed by the mission, which prohibited a multiple step fixation procedure.
Flight and Ground Control Experiments
On July 24, 2017, two ground control and one flight experiment were prepared in the FME tubes as described in Materials and Methods (Figure 2). The flight experiment was sent to Nanoracks, LLC and the ISS according to the timeline in Figure 2E. The ground truth controls were kept on the ground at 4°C in the dark and the necessary actions (movement to RT and unclamping of the tube) were performed on the same days as in the flight experiment. Of note, the original launch was scheduled for August 10, but was delayed until August 14. This caused the planarians to be stored 5 additional days at 4°C, which was not planned for in the original experiment.
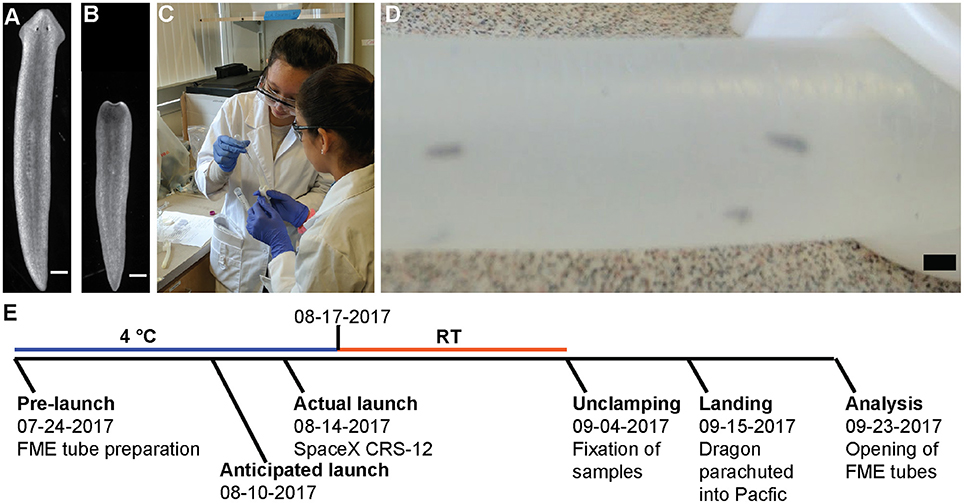
Figure 2. Setup and timeline of experiment. (A,B) Representative pictures of planarians (A) before loading or (B) after being cut. Scale bar: 0.5 mm. (C) Two of the girls load planarians into the FME tubes. Written informed consent from the student's parents was obtained for use of this image. (D) Worms inside the FME tube. Scale bar: 2 cm (E) Schematic of experimental time line. A detailed description of the flight schedule can be found on the SSEP website.
On September 23, 2017, the FME tubes were opened and analyzed. The liquid from each FME mini-lab was poured into small Petri dishes for harvesting and analysis. In all experiments, the planarians were dead and had completely disintegrated into small pieces (Figure 3). Interestingly, the planarian fragments in the ground truth experiments were larger compared to the flight experiment (compare Figures 3C,D with Figure 3E). Of note, we observed some disintegration in the ground truth experiments during the initial movement to RT suggesting death may have occurred before the flight experiments were exposed to microgravity. It is therefore possible that the difference in worm fragment size of Earth and flight experiments is a consequence of the multi-g forces experienced during space launch and return.
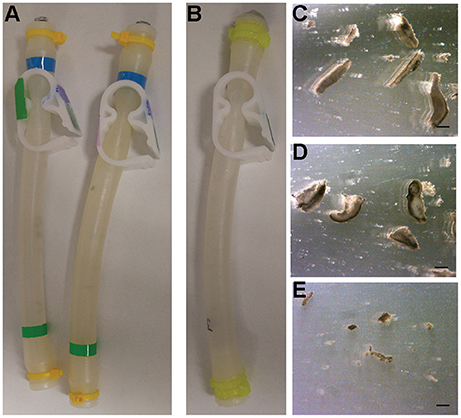
Figure 3. Results from Ground and Flight experiments. (A) Ground control FME tubes before opening. (B) Flight FME tube returned from space before opening. (C–E) Representative images of disintegrated planarians from (C,D) ground controls or (E) the flight experiment. Scale bars: 0.1 mm.
The failure of the experiment, including the ground-truth control, was surprising given that our pre-flight ground control experiments indicated that the worms were able to survive 5 weeks in the FME mini-lab. However, these preliminary experiments had been conducted without formaldehyde in the second chamber, raising the possibility that the fumes from the formaldehyde affected the planarian's ability to survive in the FME tubes in the real experiment. Although the clamp separated the liquid and no leakage was observed, as confirmed by post-flight tests using food coloring (Figure 4G), it may not have separated the fumes.
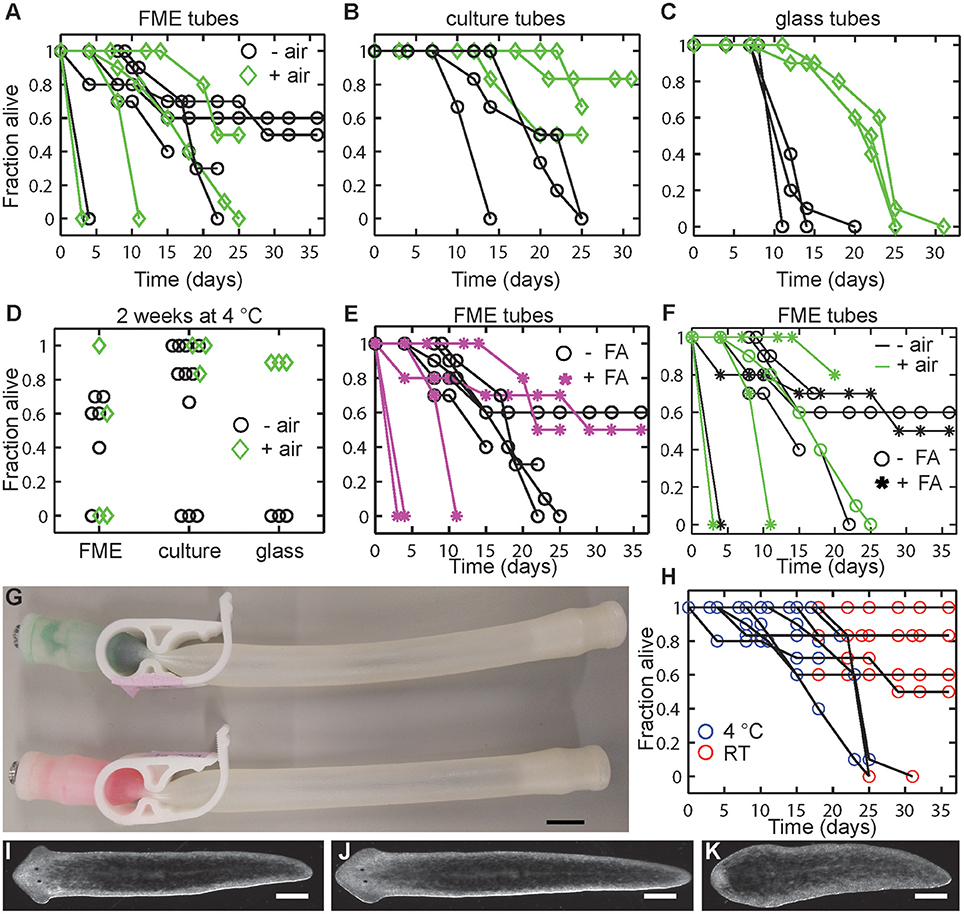
Figure 4. Post-flights tests on planarian viability. (A–C) Planarian viability is increased in tubes which contain air (green diamonds) compared to tubes without air (black circles) in (A) FME tubes, (B) culture tubes, or (C) glass tubes. (D) Considerable variability exists within the different set ups. Graph shows a comparison of all data collected in each tube type after 2 weeks (14–17 days) at 4°C and compares tubes with and without air (green diamonds and black circles, respectively). (E) Addition of formaldehyde (FA) generally negatively affects planarian viability. (F) Comparison of all FME tube experiments (data from A,E) comparing with and without air (green or black color, respectively) and with and without FA (stars and circles, respectively). (G) No leakage of liquid was found between FME compartments. Food coloring and formaldehyde was added to the left compartment of the FME tube and IO water to the right compartment. Image was taken after the tubes were stored at 4°C for 2 weeks (17 days). No leakage of the formaldehyde/food coloring mixture was observed. Scale bar: 1 cm. (H) Moving planarians from 4°C (blue) to RT (red) generally does not affect viability. (I–K) Representative images of regenerated planarians which survived 5 week enclosure (2 weeks at 4°C and 3 weeks at RT) in either a (I) FME or (J) culture tube. (K) Representative image of a planarian which failed to regenerate properly after 3 weeks at 4°C and 2 weeks at RT. Image is from FME tube, condition 2. Scale bar: 0.5 mm.
Post-flight Experiments
As the planarians in both the ground control and flight experiments had disintegrated over the course of the experiment, post-flight experiments were performed to determine how long planarians could survive under these conditions and whether different factors (presence of formaldehyde, presence of air, or tube type) could affect viability Thus, using new FME tubes, different conditions were set up in the same manner as the real experiment with the alterations listed in Table 1. Conditions 2 and 5 contained 1 ml 37% formaldehyde in the second chamber while in the remaining tubes the second chamber was left empty. To determine whether lack of oxygen caused the worms to die, condition 5 was set up with only 6 ml of CG spring water (and 2 ml of air) in the 8 ml chamber. Moreover, to test whether both the lack of oxygen and presence of formaldehyde caused the observed worm lethality, Condition 6 was set up in an FME tube without the clamp to give only one chamber containing 10 amputated tails, 8 ml of CG spring water and approximately 1.2 ml of air. Lastly, to determine if components of the FME tube were detrimental to planarian health, control experiments were performed with 5 ml culture tubes or with 9 ml glass borosilicate tubes using the same worm:volume ratio as in our FME experiments. For air tests, controls were prepared in culture tubes with 3.75 ml CG water and 1.25 ml air or in glass tubes with 8 ml spring water and 1 ml air. All tubes were stored horizontally. The viability of the worms was checked by eye every 3–4 days.
Our post-flight experiments (Figure 4) show that the presence of air (“+ air” in Figure 4) in the various enclosures generally increased planarian long-term viability. While little differences were observed between the - air and + air conditions in the first week at 4°C, at longer times worms without air in all tube types died more rapidly (Figures 4A–C). Notably, tube type/material appears to affect the results as we observed the largest variability in the data in the FME tubes (Figure 4A), followed by the culture tubes (Figure 4B), and then the glass tubes (Figure 4C, see Figure 4D for direct comparison). The positive effect of air in the tube on worm viability was least obvious in the FME tubes, as in some cases the animals in the - air conditions lived significantly longer than the animals in the + air conditions. However, in the culture (Figure 4B) and glass tubes (Figure 4C), planarians stored in tubes with air survived significantly longer than those without air. For example, in the glass tubes, all worms in tubes without air died within 20 days, while worms in glass tubes with air only showed about 40% death in the same time frame. Thus, while the general trend in the data suggests that presence of some air in the FME tubes promotes planarian viability, it is not a guarantee for planarian viability over the course of the experiment.
Additionally, we found that the presence of formaldehyde generally negatively impacts planarian viability in the FME tubes (Figures 4E,F). In 3 of the 5 tests, addition of formaldehyde caused rapid death (within 11 days). This is not due to leakage of the formaldehyde liquid into the planarian chamber as potential leakage through the clamp was checked by tapping on the tube before the formaldehyde was added. Moreover, we found no leakage between the two chambers after 2 weeks of storage (Figure 4G). However, it is still possible that formaldehyde vapors may have been able to leak into the second chamber. Again, as observed in the +/– air tests, the variability in the FME tube data makes it difficult to draw strong conclusions.
Finally, and importantly for future experimental design as elaborated on in the Discussion, we observed that planarian viability stayed relatively constant when the tubes were moved to RT (Figure 4H). This suggests that the initial storage conditions at 4°C to induce dormancy are the main determinant of whether the planarians are able to survive. Generally, if the animals were able to survive this 2–3 week period at 4°C, they were able to persist and regenerate in the subsequent storage at RT (Figures 4I–K). Similarly to our pre-flight tests, we also found that some worms had regeneration defects (no blastema, small blastema or abnormal eyes) following regeneration at RT. These defects were more prevalent in tubes which had been stored at 4°C for 3 weeks rather than only 2 weeks and in the FME tubes when compared to the culture tubes (Figure 4K and Table 2).
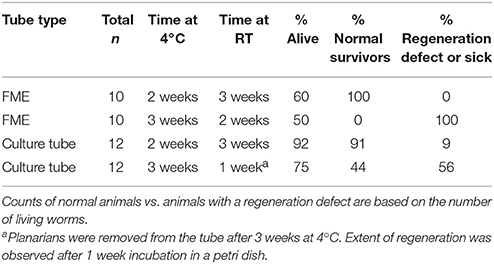
Table 2. Lethality and regeneration defects in planarians stored for 2 or 3 weeks at 4°C in either FME or culture tubes.
Discussion
The parameters set within the SSEP made it challenging to create a successful microgravity experiment using live biological samples. Despite the pre-launch tests that indicated that the experimental conditions were viable, all planarians (flight and ground truth) disintegrated over the 43 days of the experiment. Our post-flight ground-control experiments suggest that a combination of the extended storage at 4°C due to the launch slip and the lack of oxygen were likely the largest factors contributing to this outcome.
Extended Storage at 4°C
Our pre- and post-flight experiments have shown that planarian regeneration can be put into a dormant state at 4°C. However, we found increased death and a significantly higher percentage of planarians with regeneration defects upon longer cold storage, suggesting extended cold storage negatively affected worm health (Table 2). The launch slip, necessitating the experiment to be kept at 4°C for 5 more days than planned, therefore may be the main reason for the negative experimental outcomes. From the time our flight experiment was prepared to the time it arrived on the ISS was 25 days. Generally, such a long transportation time is not ideal for biological experiments. It has been previously emphasized that late loading and early retrieval are optimal to maximize organismal health for life science experiments conducted in space (Hughes-Fulford, 2004; National Research Council of the National Academies, 2011; Warren et al., 2013). Travel time was considerably shorter in the non-SSEP planarian ISS experiments, with launch occurring within 12–14 or 31 h for the Gorgiladze (2008) and Morokuma et al. (2017) studies, respectively. Thus, these significantly shorter transportation times may be responsible for why their planarians were viable and regenerated while ours disintegrated. In fact, in the case of studying the effect of microgravity on regeneration, the ideal experiment would be to amputate the planarians on board the ISS and not on Earth (Morokuma et al., 2017). The study by Gorgiladze (2008) has thus come closest to these ideal conditions.
Lack of Oxygen and Air to Water Ratio
Interestingly, we observed a highly variable effect of lack of oxygen on our test planarians; while a few tubes showed hardly any effects, others showed significant or complete death under the exact same experimental conditions (Figures 4A–C). It was more difficult to eliminate all air in the FME and culture tubes compared to the glass tubes, thus small air bubbles may have been present in some of the samples, contributing to the variability in the data. However, compared to the air volume in the + air conditions, these bubbles are negligible and thus cannot explain why some – air conditions outperformed the + air conditions in the FME-tubes. The previously reported experiments with C. elegans similarly found that chance largely dictated whether the nematodes survived long-term storage in the FME tubes (Warren et al., 2013).
Morokuma et al. (2017) concluded that the optimal condition for 30-day survival of intact planarians at 20°C was a 50–50 air-to-water ratio and that using 100% water and no air resulted in complete lethality of intact planarians within 5 days. On the other hand, experiments with G. tigrina were viable for the duration of the 10 day flight in tubes without additional oxygen (Gorgiladze, 2008). Of note, in our no-air setups using regenerating tail pieces stored at 4°C, initial deaths begin to appear between 5 and 8 days though complete lethality was not always observed, even up to 36 days. Our original experimental setup contained no air due to concerns that planarians may crawl out of the water and dry out, which has been observed in other instances (Hagstrom et al., 2015). However, this did not appear to be an issue in our post-flight tests when volume was left for air.
Planarian Density
Related to the considerations above is the question of how many planarians should be used pertube. There is a trade-off between using more worms and thus getting more reliable data and compromising the individual's health by increasing the population density. Morokuma et al. (2017) used a density of 1 worm/2.5 ml Poland Spring water and 2.5 ml air for their initial water-air ratio tests and experiments with intact planarians, but in their regeneration experiments, 15 amputated pieces (heads, trunks, and tails) were placed in a single tube, resulting in a density of 1 worm piece/1.7 ml Poland Spring water/1.7 ml air. Gorgiladze (2008) reported using 1 trunk piece/2 ml fresh water. In contrast, we used nearly double that density by placing 1 tail in 0.8 ml CG water. Previous experiments (Carter et al., 2015) have shown that the carrying capacity of D. japonica, the species used in this study and by Morokuma et al. (2017), is twice that of D. tigrina, used by Gorgiladze (2008), suggesting that we should be able to use higher densities without compromising worm viability. The reason for choosing this higher worm density was based on two considerations: (1) Our preliminary experiments revealed no difference in worm health when comparing 5 vs. 10 worms in the same volume of water. (2) To account for the possibility that some planarians may die over the course of the experiment, 10 worms increased the likelihood of obtaining survivors upon space travel completion. Of note, while we were restricted to a single FME tube with 9.2 ml maximum volume in this SSEP study, the other two studies were able to use one 50 ml (Morokuma et al., 2017) and six 20 ml tubes (Gorgiladze, 2008), respectively.
Other Considerations That May Improve The Success Rate of Live Biological SSEP Experiments
The most important change which we expect to significantly improve the success rate of biological experiments, besides shorter transportation times, is a requirement to report the SSEP project results and make this information publicly available. This will allow future research to build upon the experience of prior experiments. We therefore suggest that the SSEP requires a short summary write-up of the results of all participating teams that can then be posted on its website.
There are a few other issues that would be worth exploring for future experiments. Biological samples for SSEP are currently transported in a specialized cold package, FedEx Temp-Assure, which keeps the shipment at a constant temperature of 2–8°C. Such variance in temperature during transport for the flight experiment is suboptimal. Similarly, the temperature of the ground truth experiment likely also fluctuated, since the samples were stored in a common laboratory refrigerator or cold room (post-flight experiments), respectively. In both cases, opening of the door will lead to temporarily higher temperatures. Morokuma et al. (2017) used a temperature-controlled portable incubator for their experiment. While such an incubator is likely outside the budget for SSEP Missions, it would be worthwhile to conduct pre-flight experiments to evaluate the effect of temperature fluctuations on the samples.
Thirdly, the currently limited options for timing of crew interactions is not ideal to track planarian regeneration. Crew interactions were limited to only 5 options provided by the SSEP: day of arrival on the ISS, 2 days post-arrival, 2 weeks prior to undock, 5 days prior to undock, and 2 days prior to undock. For the purposes of studying planarian regeneration, which takes about 7 days, only the 2 week prior to undock interaction was appropriate, although this can vary from 2 to 4 weeks of microgravity exposure. Adding this broad time window to potential delays in launch or transportation thus can significantly alter the timeline of the experiment and cause experimental milestones to deviate from initial plans. A crew interaction of 7 days post-arrival would be ideal to increase the viability of the experiment and minimize the time the live worms are in the enclosed FME mini lab. Furthermore, longer regeneration times may also obscure any possible regeneration delays that may occur as a consequence of microgravity exposure.
Proposed New Working Protocol
Based on our post-flight experiments, we propose that the transport time at 4°C should be minimized as much as possible. Since this is not always under the control of the individual research teams, we also suggest a few modifications to optimize the current protocol. We propose that addition of air in the FME set-up would provide a greater chance of success. This would mean using 6 ml CG in the 8 ml compartment. Due to the smaller volume of water, one could also adjust the amount of formaldehyde in the second compartment, thus saving resources. Due to the constraints of the SSEP experimental timelines, the addition of formaldehyde to the mini-lab is necessary to be able to stop the experiment mid-flight. This would, thus, decrease the time the worms are enclosed and halt regeneration after a sufficient period. While having a generally negative effect on planarian health, our post-flight tests indicate that some planarians can survive in the FME tube with formaldehyde in the second chamber (Figures 4E,F). Although we observed some regeneration defects in the ground controls under these conditions, the prevalence of defects can still be compared between Space and Earth samples to decipher the effects of microgravity on planarian regeneration. Since the viability of the worms seems to be largely affected by chance, we suggest still using 10 D. japonica tails for each experiment to maximize the chance that enough will survive to provide conclusive data. Because of this randomness, it would also be highly beneficial if teams could send two FME tubes to the ISS instead of the current one. Executing a single experiment is not customary or sufficient in most sciences. Therefore, allowing for two tubes would also incorporate the concept of experimental replicates, thus more closely mimicking professional science conditions.
In summary, our data suggests that using these proposed changes (addition of air, 2 tubes instead of 1) and a shorter experimental timeline (ideally ≤ 2 weeks at 4°C and 1 week at RT under microgravity conditions) would greatly improve the chance of obtaining meaningful data. Importantly, the proposed changes increase the chances of successful regeneration in the controls, which is a necessity for accurate interpretations as a high defect rate in ground control experiments would obscure the interpretation of defects observed in the space samples.
Value of Project For Middle School Student Scientists
The value of the SSEP is clearly (1) the potential gathering of new knowledge about the effects of microgravity on various processes, and (2) the impact this program has on student development and future trajectories. Although our study on the effect of microgravity on planarian regeneration was inconclusive, the SSEP did offer us an invaluable journey to experience the work of “real scientists.” We learned to critically design an experiment within financial, logistical, and technical constraints to explore an outstanding scientific question, “How does microgravity affect planarian regeneration?”, and were provided with the unique opportunity to have our experiment performed on board the ISS.
Our hands-on experience through this STEM education initiative opened our eyes to what science has to offer and has changed our views by allowing us to dig deeper, giving hands on experience and insight into what “real” science looks like, and discover not only how, but why many things work together and in harmony. Furthermore, SSEP greatly impacted our career plans, teaching us the importance and value of the team experience, which allowed us to work collaboratively with not only students but professionals, and improved our writing and presentation skills. We also learned to appreciate when students have the same mindset, and strive for different perspectives and views. Further remarks from the professional researchers' perspectives can be found in the Appendix.
Author Contributions
Vista SSEP Mission 11 Team: designed the original space experiment; Vista SSEP Mission 11 Team, DH, and E-MC: conducted experiments. All authors co-wrote the paper.
Funding
This work was supported by the Burroughs Wellcome Fund Career Awards at the Scientific Interface and NSF CAREER Grant 1555109 (to E-MC).
Conflict of Interest Statement
The authors declare that the research was conducted in the absence of any commercial or financial relationships that could be construed as a potential conflict of interest.
Acknowledgments
The students thank their parents: Rachel and Mike Currington, Jennifer and Ralph Wagner, Brenda and Brent Ansell, Angelica Becaria and Carlos Camacho, and the Principal of Vista Magnet Middle School, Anne Green, Dan Hendricks from the Open Source Maker Lab, NanoRacks LLC, NCESSE, and SSEP, for making this experience possible. The authors thank Rob Steele and Catherine Crouch for comments on the manuscript, and Stacy Hamel, Flight Operations Manager of SSEP and Education Program Manager of NCESSE, for providing extra FME tubes for the post-flight experiments.
References
Adell, T., Saló, E., Van Loon, J. J. W. A., and Auletta, G. (2014). Planarians sense simulated microgravity and hypergravity. Biomed Res. Int. 2014:679672. doi: 10.1155/2014/679672
Best, J. B., and Morita, M. (1982). Planarians as a model system for in vitro teratogenesis studies. Teratog. Carcinog. Mutagen. 2, 277–291. doi: 10.1002/1520-6866(1990)2:3/4 < 277::AID-TCM1770020309>3.0.CO;2-8
Brondsted, A., and Brondsted, H. V. (1961). Influence of temperature on rate of regeneration in the time-graded regeneration field in planarians. Development 9, 159–166.
Carter, J. A., Lind, C. H., Truong, M. P., and Collins, E.-M. S. (2015). To each his own. J. Stat. Phys. 161, 250–272. doi: 10.1007/s10955-015-1310-1
Cebrià, F. (2007). Regenerating the central nervous system: how easy for planarians! Dev. Genes Evol. 217, 733–748. doi: 10.1007/s00427-007-0188-6
Gorgiladze, G. I. (2008). Regenerative capacity of the planarian Girardia tigrina and the snail Helix lucorum exposed to microgravity during an orbital flight on board the International Space Station. Dokl. Biol. Sci. 421, 244–247. doi: 10.1134/S0012496608040078
Hagstrom, D., Cochet-Escartin, O., and Collins, E.-M. S. (2016). Planarian brain regeneration as a model system for developmental neurotoxicology. Regeneration 3, 65–77. doi: 10.1002/reg2.52
Hagstrom, D., Cochet-Escartin, O., Zhang, S., Khuu, C., and Collins, E.-M. S. (2015). Freshwater planarians as an alternative animal model for neurotoxicology. Toxicol. Sci. 147, 270–285. doi: 10.1093/toxsci/kfv129
Hagstrom, D., Zhang, S., Ho, A., Tsai, E. S., Radić, Z., Jahromi, A., et al. (2018). Planarian cholinesterase: molecular and functional characterization of an evolutionarily ancient enzyme to study organophosphorus pesticide toxicity. Arch. Toxicol. 92, 1161–1176. doi: 10.1007/s00204-017-2130-7
Hughes-Fulford, M. (2004). Lessons learned about spaceflight and cell biology experiments. J. Gravit. Physiol. 11, 105–109.
Marsh, G., and Beams, H. W. (1952). Electrical control of morphogenesis in regenerating Dugesia tigrina. I. Relation of axial polarity to field strength. J. Cell. Comp. Physiol. 39, 191–213. doi: 10.1002/jcp.1030390203
Morgan, T. H. (1989). Experimental studies of the regeneration of Planaria maculata. Arch. Entwickelungs. Org. 7, 364–397. doi: 10.1007/BF02161491
Morokuma, J., Durant, F., Williams, K. B., Finkelstein, J. M., Blackiston, D. J., Clements, T., et al. (2017). Planarian regeneration in space: persistent anatomical, behavioral, and bacteriological changes induced by space travel. Regeneration 4, 85–102. doi: 10.1002/reg2.79
National Research Council of the National Academies (2011). Recapturing a Future for Space Exploration: Life and Physical Sciences Research for a New Era. Washington, DC: National Academies Press.
Novikov, V. V., Sheiman, I. M., and Fesenko, E. E. (2008). Effect of weak static and low-frequency alternating magnetic fields on the fission and regeneration of the planarian dugesia (Girardia) tigrina. Bioelectromagnetics 29, 387–393. doi: 10.1002/bem.20407
Pearson, B. J., Eisenhoffer, G. T., Gurley, K. A., Rink, J. C., Miller, D. E., and Sánchez Alvarado, A. (2009). Formaldehyde-based whole-mount in situ hybridization method for planarians. Dev. Dyn. 238, 443–450. doi: 10.1002/dvdy.21849
Reddien, P. W., Bermange, A. L., Murfitt, K. J., Jennings, J. R., and Sánchez Alvarado, A. (2005). Identification of genes needed for regeneration, stem cell function, and tissue homeostasis by systematic gene perturbation in planaria. Dev. Cell 8, 635–649. doi: 10.1016/j.devcel.2005.02.014
Umesono, Y., Watanabe, K., and Agata, K. (1997). A planarian orthopedia homolog is specifically expressed in the branch region of both the mature and regenerating brain. Dev. Growth Differ. 39, 723–727. doi: 10.1046/j.1440-169X.1997.t01-5-00008.x
Warren, P., Golden, A., Hanover, J., Love, D., Shephard, F., and Szewczyk, N. J. (2013). Evaluation of the fluids mixing enclosure system for life science experiments during a commercial Caenorhabditis elegans spaceflight experiment. Adv. Sp. Res. 51, 2241–2250. doi: 10.1016/j.asr.2013.02.002
Appendix
Remarks on the Journey of the Mission 11 Team from the professional researchers' perspective.
Following the spirit of the SSEP program to give students a “real scientists' experience” and as part of this special issue aimed at highlighting the contribution of “Women in Science”, this article was jointly written by the 5 female students (aged 11–13), their female teacher, and us, their professional female mentors, with the aim to accomplish two things: (1) to report why planarian regeneration experiments conducted within the constraints of these educational programs are prone to fail. (2) To document the young students' journey of persistence and discovery, and the importance of mentorship on the path to becoming a scientist. Notably, by co-writing this article, the students were also introduced to the process of scientific writing. Below we provide some context on the background of this collaboration and our experiences as mentors to these students.
For the 2017 SSEP Mission 11, student teams from participating communities were given 9 weeks to design and propose experiments which, if selected, would fly in low Earth orbit aboard the ISS to test the effects of microgravity. Each community's flight experiment was selected through a formal two-step proposal review process (see SSEP website for details). Our team of 5 female students was selected for SSEP Mission 11 in the Vista, CA community. One of these students had previously led a team of two that applied for SSEP Mission 8 but their proposal, although being the top proposal from the school, was denied at the school administration level. Importantly, the original team leader persevered, recruited four more girls, and tried again, leading to the work reported here.
By engaging in a community-wide competition via submission of a 2000+ word research proposal, the students learned the value and necessity of strong writing and communication skills in science. The program further encouraged student teams to reach out to local and international researchers for advice and mentorship, thus connecting K-12 science education to the professional scientific community. Finally, the students were provided the opportunity to travel to the Kennedy Space Center to watch the SpaceX CRS-12 launch with their experiment on board, and to present to a scientific community at the annual SSEP National Conference at the Smithsonian's National Air and Space Museum in Washington, D.C.
The connection between the authors of this study was first established in 2015, when the two “founder students” sought advice for their original proposal for SSEP Mission 8. The students' perseverance and enthusiasm, as well as the passionate dedication of their teacher, has fueled this collaboration since and ultimately culminated in this article.
Through this joint article, we wanted to teach them that a lot can be learned from scientific failures and that publishing such knowledge can be beneficial to future research and the scientific community – in addition to introducing them with a “hands-on” experience to writing a scientific paper.
To us, as professional female scientists, the opportunity to mentor these young female students was particularly important. So far, these students report that they “have never felt any restrictions on going into science because of [their] gender”. Research experiences and mentorship opportunities, such as those provided by the SSEP, aim to foster this sense of inclusion in the sciences so that these students hopefully will never experience exclusion. The drive, curiosity, and perseverance of these young student scientists is a testimony to what our next generation of scientists, both male and female, can accomplish, especially with the help of passionate mentors, and an inspiration to every professional scientist.
Keywords: Dugesia japonica, International Space Station, FME tubes, NASA, microgravity, regeneration, school student scientists, SSEP
Citation: Vista SSEP Mission 11 Team, Hagstrom D, Bartee C and Collins E-MS (2018) Studying Planarian Regeneration Aboard the International Space Station Within the Student Space Flight Experimental Program. Front. Astron. Space Sci. 5:12. doi: 10.3389/fspas.2018.00012
Received: 17 January 2018; Accepted: 16 April 2018;
Published: 07 May 2018.
Edited by:
Jennifer Lynn Ross, University of Massachusetts Amherst, United StatesReviewed by:
C. S. Unnikrishnan, Tata Institute of Fundamental Research, IndiaJoshua Shaevitz, Princeton University, United States
Copyright © 2018 Vista SSEP Mission 11 Team, Hagstrom, Bartee and Collins. This is an open-access article distributed under the terms of the Creative Commons Attribution License (CC BY). The use, distribution or reproduction in other forums is permitted, provided the original author(s) and the copyright owner are credited and that the original publication in this journal is cited, in accordance with accepted academic practice. No use, distribution or reproduction is permitted which does not comply with these terms.
*Correspondence: Eva-Maria S. Collins, ZWNvbGxpbjNAc3dhcnRobW9yZS5lZHU=
†Vista SSEP Mission 11 Team, Evie Currington, Isabella Ansell, Sydney Wagner, Isabel Camacho, and Charlotte Currington. They designed the original space experiment, executed and analyzed experiments, and co-wrote the manuscript.