- Helio Research, La Crescenta, CA, United States
Observational evidence for the origin of active region magnetic fields has been sought from published information on extended solar cycles, statistical distributions of active regions and ephemeral regions, helioseismology results, positional relationships to supergranules, and fine-scale magnetic structure of active regions and their sunspots during their growth. Statistical distributions of areas of ephemeral and active regions blend together to reveal a single power law. The shape of the size distribution in latitude of all active regions is independent of time during the solar cycle, yielding further evidence that active regions of all sizes belong to the same population. Elementary bipoles, identified also by other names, appear to be the building blocks of active regions; sunspots form from elementary bipoles and are therefore deduced to develop from the photosphere downward, consistent with helioseismic detection of downflows to 3–4 Mm below sunspots as well as long-observed downflows from chromospheric/coronal arch filaments into sunspots from their earliest appearance. Time-distance helioseismology has been effective in revealing flows related to sunspots to depths of 20 Mm. Ring diagram analysis shows a statistically significant preference for upflows to precede major active region emergence and downflows after flux emergence but both are often observed together or not detected. From deep-focus helioseismic techniques for seeking magnetic flux below the photosphere prior major active regions, there is evidence of acoustic travel-time perturbation signatures rising in the limited range of depths of 42–75 Mm but these have not been verified or found at more shallow depths by helioseismic holographic techniques. The development of active regions from clusters of elementary bipoles appears to be the same irrespective of how much flux an active region eventually develops. This property would be consistent with the magnetic fields of large active regions being generated in the same way and close the same depth as small active regions in a shallow zone below the photosphere. All evidence considered together, understanding the origins of the magnetic fields of solar cycles boils down to learning how and where elementary bipoles are generated beneath the photosphere.
Introduction
Ample and excellent reviews have been made about the many theories and models of solar cycles that place the initial development of active regions within the convection zone (Cattaneo and Hughes, 2001) or at the base of the convection zone (reviews in Fisher et al., 2002; Ossendrijver, 2003; Charbonneau, 2010, 2014). A comparison of the advantages and disadvantages of both categories of models has been made by Brandenburg (2005a,b). The chains of reasoning behind various suggested origins are also well described in Kosovichev et al. (2013) and Jarboe et al. (2017). Relatively few theories have proposed origins for the magnetic flux of solar cycles in the subsurface shear layer discovered by helioseismology (Brandenburg, 2006; Kosovichev et al., 2013) or closer to the photosphere (Jarboe et al., 2017).
Until recently, little attention was given to the implications and relevancy of the extended solar cycle to the origin of the magnetic fields of active regions. This has been justified by an assumption for the Sun has different mechanisms for producing a large-scale dynamo and a small-scale dynamo close to the photosphere such as the local dynamo modeled by Kitiashvili et al. (2015). However, recently Cameron et al. (2017) and Cameron et al. (2018) have proposed the extended cycle, with its shallow component, is consistent with the Babcock-Leighton model (Babcock, 1961; Leighton, 1964, 1969 and ensuing papers).
Without delving into implications for solar cycle theory, Cliver (2015) has thoroughly reviewed the observational studies on which the extended solar cycle is based. The extended solar cycle treats both large and small scale bipolar regions as parts of a single population and both as parts of the global solar cycles.
While there are hundreds of papers on newly emerging magnetic flux of active regions in the visual domain, most authors of these papers assume that there is an absence of evidence of the origin of the magnetic fields of active regions below the photosphere. However, there are some direct observations which provide clues or evidence of the general depth of origin of the new magnetic fields of solar cycles. In this review, these observations, seldom-considered in the context of the origin of active regions, are discussed as well as the observational results coming from helio seismology. The objective is to bring more observational information together that bears on the most-likely depths at which the magnetic flux of active regions originates.
The Extended Solar Cycle
Like other major insights into the solar cycle, the gradual discovery of “the extended solar cycle,” was made possible by instrumental achievements. The recognition of a component of the solar cycle in the form of small active regions gradually emerged in the 1970s from studies, reviewed in Cliver (2015), of high resolution magnetograms produced by the original magnetograph built for the McMath-Pierce Telescope at Kitt Peak National Solar Observatory. Until then, the small, ephemeral active regions were already known but could be largely ignored as insignificant contributors to the magnetic flux of solar cycles. In the new Kitt Peak magnetograms, the ephemeral active regions outnumbered the reported active regions by several factors; the ephemeral regions were much broader in their latitude distribution than the reported active regions (Harvey and Martin, 1973). A next study of ephemeral active regions (Harvey et al. (1975) extended the overlap of successive cycles 6–8 years, more at the beginning of the 11-year cycles than at the end of the cycles. The name, “extended solar cycle” was introduced in a summary paper by Wilson et al. (1988) which also mentioned a possible relationship to torsional oscillations (Howard and Labonte, 1980).
Recent analyses of the extended solar cycle have demonstrated how the approximate 11-year cycles stretch to nearly a full 22 years (McIntosh et al., 2014; McIntosh and Leamon, 2017, this volume). Using x-ray bright points as proxies for ephemeral regions, McIntosh et al. (2014) confirmed the existence of ephemeral regions in the polar regions initially shown in Harvey et al. (1975). McIntosh et al. (2014) favored the idea that the torsional oscillation, already thought to begin in the polar regions, was another manifestation of the extended solar cycle. This appeared to confirm extension of each solar cycle to nearly a full 22 years although Cliver (2015) raises questions about the true beginning of the solar cycle and more conservatively suggests the duration of recent solar cycles is in the range of 18–22 years. Although the indirect method of detecting ephemeral regions by the proxy of X-ray bright points is a statistically valid approach, the true start of the ephemeral regions of the new cycle in the polar region remains uncertain because there is no compelling reason for every ephemeral region to lead to one or more X-ray bright points. Also, as Cliver (2015) pointed out, the increase in coronal emission during the early rise of the solar cycle recorded by Altrock (1997) might be more closely related to the development of the polar crown filament(s) than small-scale bipoles at high latitudes.
Less discussion and analyses have been about the true ends of solar cycles near the equator although this has been addressed by McIntosh et al. (2014). If we extrapolate from butterfly diagrams, each solar cycle ends by the time of solar maximum or before; new cycles are thought to begin concurrent with the reversal of the polar magnetic fields, which is often 1–2 year after solar maximum and 1–2 years different in time in the northern and southern hemispheres. With about a 2-year uncertainty, it is not a stretch to estimate the duration of solar cycles in the last century to be 20–22 years and possibly longer in some solar cycles.
Ephemeral regions without sunspots and small active regions with sunspots have never been considered to have a deep origin. This raises the question: Does the small percentage of large active regions with major sunspots, present during about half of each ~22 year extended solar cycle, have an origin much deeper than the shallow ephemeral active regions that are present throughout the whole ~22-year extended cycles?
The Distribution of Active Regions by Size or Magnetic Flux
An important question has been: Is there more than one population of active regions? In her PhD thesis, Harvey (1993a) thoroughly re-addressed this question. Her analyses of thousands of ephemeral regions and active regions from Kitt Peak magnetograms provided a definitive confirmation of the earlier findings by Harvey et al. (1975) of no significant physical differences between ephemeral active regions and active regions; both are parts of a single population of bipolar magnetic features.
To illustrate this important conclusion, copies of two of the original illustrations of the statistics on which K. L. Harvey's conclusion is based, are shown in Figure 1. Figure 1A is the distribution of the sizes of ephemeral active regions (Harvey, 1993b). The measurement of areas of active regions were chosen because areas are linearly proportional to active region magnetic flux excluding sunspots (Schrijver and Harvey, 1994) and are much more quickly and reliably measured over long intervals than magnetic flux.
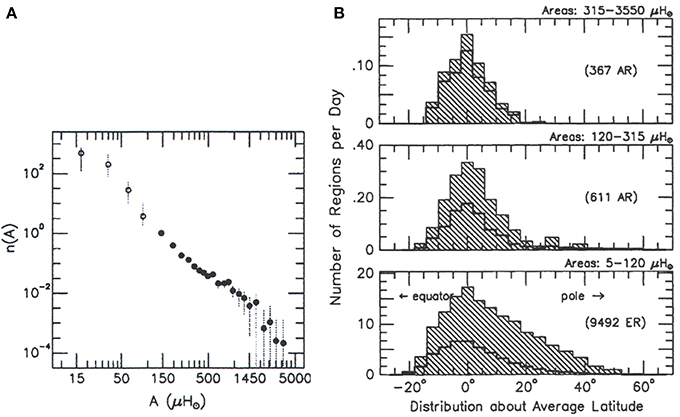
Figure 1. (A) Ephemeral active regions (o) and active regions (•) blend smoothly into a single curve providing evidence that both are parts of a single population. Vertical lines indicate the estimated error. (B) Ephemeral regions (ER) and active regions (AR) of all sizes, measured in millionths of a hemisphere (μH⊙), are centered at the same latitude for data from the same time interval, again providing evidence that they are parts of a single population of features on the Sun. The hatched histogram is the corrected region counts and the heavy histogram, the actual counts of regions. © ASP. Reproduced with permission.
Figure 1A shows how the distribution of the areas of ephemeral active regions blend smoothly into the distribution of larger active regions as further illustrated by Harvey and Zwaan (1993) and summarized by Howard (1994). Harvey and Zwaan (1993) were first to characterize the size distribution of bipolar active regions as following a power law for both ephemeral active regions and active regions with areas greater than 520 Mm2. A log normal distribution of umbral areas of sunspots had already been established by Bogdan et al. (1988). Parnell et al. (2009) analyzed small-scale flux emergence using data from Hinode/SOT. Combining these new observations with other existing data sets, they found the power law distribution applies to over five decades in the size distribution of emerging active regions.
Figure 1B by Harvey (1993b) reveals the shape of the size distribution of small to large-scale bipolar active regions. Harvey (1993a,b); and Harvey and Zwaan (1993) found that the shape of the size distribution of active regions was independent of the phase of the solar cycle. A similar conclusion about the shape of the size distribution of umbral areas of sunspots over several solar cycles had been drawn by Bogdan et al. (1988).
As indicated in Figures 1A,B, the vast majority of magnetic flux in active regions is from small-scale bipoles. Thornton and Parnell (2011) suggested that the rate of flux emergence is independent of the solar cycle and consistent with a turbulent dynamo throughout the convection zone. In contrast, the findings of Harvey (1993a) and Bogdan et al. (1988) together reveal that only the shape of the size distribution is independent of solar cycle; the quantity and rate of emergence of new magnetic flux vary significantly with the changing latitude and size distribution within each extended ~22-year solar cycle.
Overall, the above cited analyses, provide evidence that:
1. Whatever mechanism(s) cause the active regions of the solar cycle, it is ever-present and changes its amplitude as a function of latitude and time over approximate 22-year cycles that overlap in time by about 11 years.
2. At all times during the solar cycle, new small-scale bipolar regions are dominant over large-scale active regions in both number and magnetic flux.
3. As parts of the same population, large active regions are expected to be greater amplitude versions of whatever creates the huge population of small active regions.
Because large active regions are parts of the same population as ephemeral active regions, it is logical for the large active regions to have relatively shallow origins similar to that of ephemeral regions and small active regions.
Solar Seimological Evidence of Active Region Features Below the Photosphere
Early helioseismic observations of large sunspots detected them at depths down 20 Mm below the photosphere (Braun and Lindsay, 2000, 2001; Kosovichev et al., 2002). Higher resolution helioseismic imaging of subsurface structure such as by Kosovichev (2009), Kosovichev et al. (2009) and Zhao et al. (2010) have shown much more detailed subsurface sunspot structure. Down drafts are seen from the surface down to depths of 3–4 Mm and additional components of upward flows to depths from about 5–10 Mm below the photosphere. They appear to be components of a larger, more complex, circulatory system of flows. In the moat around sunspots, flows carrying moving magnetic features, are in a shallow layer extending to a depth of 1 Mm (Kosovichev, 2011, 2012 reviews).
At visual wavelengths, the helioseismic observations are consistent with downflows within the umbra of sunspots observed at the surface, with inward flows into the umbra from superpenumbral fibrils in the high Hα chromosphere, and with outward horizontal flows in the penumbra fibrils, and outward flows in the moat at the photosphere below the superpenumbral fibrils.
If the magnetic fields, that subsequently form sunspots, emerge from deep below the photosphere, it is expected they should have some relationship to subphotospheric supergranulation or other subsurface features or flows prior to the detection of active region magnetic flux at the photosphere. Kosovichev et al. (2009) analyzed surface plasma flows in a large emerging active region. They found strong localized upflows and downflows at the initial phase of emergence, but they found no evidence in the supergranulation of large-scale flows that could indicate the future appearance of a large scale magnetic structure. In observations relatively far from disk center, Toriumi et al. (2012) report horizontal divergent flow before flux emergence of active region NOAA AR11081 but its large distance, from disk center, makes the relative timing of the new flux and origin of these flows questionable; divergent flows of supergranules exist all of the time. In analyses beneath three active regions, including sound-speed inversion results and the distribution of deep-focus travel-time anomalies, Kosovichev and Duvall (2011) found diverging roots of magnetic structures but no clear connection between the three active regions in the depth of 0–48 Mm.
Komm et al. (2008) used ring-diagram analysis to study 13 large active regions before and after flux emergence. Overall, they found a weak but statistically significant trend for upflows before flux emergence and downflows after flux emergence. However, the results are mixed with both upflows and downflows being observed in some cases and in two cases, neither upflows nor downflows were observed. Although they studied only large emerging active regions, the signals are weak in part due to the low temporal resolution and a ring size of 15°. One can say that the results are promising with future improvements in resolution. The well-known clustering of new flux at the sites of previously emerged flux is also significant. With a lack of temporal resolution better than many hours to 1 day, it will be difficult to sort out the individual effects of clusters of magnetic flux emergence of varying sizes and close in time, latitude, and longitude.
From helioseismic acoustic travel-time perturbations signatures, Ilonidis et al. (2011, 2013), and Kosovichev et al., 2016) found evidence of rising perturbations presumed to be discrete magnetic flux concentrations in the depth range of 42–75 Mm preceding 5 major active regions. Rising speeds of the perturbations on the order of 1 km s−1 was achieved in all 5 cases by separately analyzing more narrow subsurface layers and calculating the speed from the difference in time that features were seen in lower to higher layers. Unfortunately, this technique is not applicable to more shallow depths. Therefore, the estimates of how much earlier flux might be detected at depth before it emerges at the photosphere are approximate. The time differences between first detection below the photosphere and at visual wavelengths at the photosphere have been of the order of hours and times, between peak signals below the photosphere and maximum flux emerged at the photosphere, have been of the order of 1–2 days. They calculated that speeds of emergence in the range of 0.3–0.6 km s−1 should be found for anticipated, correlated features rising close to photosphere. The authors have not commented on the prevalence of such perturbations below the active region belt although they found no evidence of similar features below areas of the quiet Sun.
Other helioseismic techniques applicable to depths of 20 Mm below the photosphere have not confirmed the existence of precursor magnetic fields or flows described in the preceding paragraph. Braun (2012) commented that the results of Ilonidis et al. (2011) were not reproduced when he applied helioseismic holography below the same regions. This prompted a rebuttal by Ilonidis et al. (2012b).
The collaborating teams in the papers by Leka et al. (2013); Barnes et al. (2014), and Birch et al. (2018) also rigorously employed the technique of helioseismic holography used by Braun (2012) and developed by Lindsay and Braun (2000) to statistically look for evidence of precursors before the emergence of 100 active regions. Birch et al. (2018) applied this technique with the statistical rigor spelled out in Leka et al. (2013). They investigated subsurface, pre-emergent zones for a little more than 1 day (27.7 h) prior to the sample of 100 active regions and also selected an equal number of control areas where new magnetic flux was minimal or below the threshold of detection. Birch et al. (2018) found weak but statistically significant signatures in the average subsurface flows and the apparent wave speed within the 1-day interval before the first appearance of the new magnetic flux of the active regions. They specifically ruled-out precursors with spatially-extended flows greater than ~15 m s−1, within 20 Mm below the photosphere. Hence, they did not confirm the previously promising calculations of the elongated and long-enduring features rising with average velocities of 0.3–0.6 km in the zone from 0 to 20 Mm below active regions. This leaves open questions about the nature of the features observed from the several applications of the deep focus technique (Ilonidis et al., 2011, 2012a, 2013; Kosovichev et al., 2016).
Barnes et al. (2014) performed more detailed analyses of the same statistical data sets in which Birch et al. (2018) above applied discriminant analysis. The Barnes et al. results are consistent with Birch et al. finding a weak but statistically significant subsurface precursor to active region emergences, during at least a day before the start of emergence and not found in the control sample. They clarify that their results are consistent with flows converging on the forthcoming site of emergence which could be interpreted simply as emergence occurring preferentially at the boundaries between supergranules. (The diverging flows from the centers of supergranules would be converging flows relative to the boundaries between supergranules). The possible preference for active regions to emerge or preferentially grow at the boundaries of supergranules is already backed-up by observations as discussed in the next section.
Barnes et al. also found the average unsigned magnetic flux at the surface was the best discriminator between their primary data sample and their control sample. To this reader, this discriminator is consistent with the well-observed recurrence of newly emerging magnetic flux within or very close to previous sites of newly emerged magnetic flux of active regions. The apparent affinity of new magnetic flux for sites of previously emerged magnetic flux is so common, that it would be very difficult to find any examples of major emerging active regions that are not extremely close to recently emerged magnetic flux. Due to this clustering, the pre-emergent sample of Barnes et al. could not avoid having more residual magnetic flux than their control sample.
The study of Barnes et al. (2014) is a subtle substantiation of the tight clustering of new magnetic flux at sites of previous magnetic flux emergence. Active region clustering is important to understanding the solar cycle. The statistical tendency of new flux to occur close to previously emerged flux could be part of the explanation of why the shape of the size distribution of active regions of all sizes is the same (Figure 1B) and does not change over the solar cycle (Harvey and Zwaan, 1993). If or when a new narrow band of emergence is established at high latitudes at the outset of a next solar cycle, it would tend to remain the preferred site of emerging flux - thereby resulting in its increased amplitude at approximately the same latitude in addition to the slow, equatorward migration of new active regions; concurrently the dispersal of decaying flux from the initial band of small active regions by random supergranulation flows would also result in a slow broadening in latitude of the band of emergence. These two patterns are consistently observed as solar cycles develop.
While the statistical results of Barnes et al. are not useful in predicting specific sites where magnetic flux will emerge for a given active region, they are a forward step in pointing to the direction of where helioseismology techniques might be refined to further search for unique subsurface flows preceding the appearance of active regions at the photosphere. Where surface flows increase in relation to small amounts of emerging magnetic flux, subsurface flows might also strengthen and lead to more magnetic flux generation than at locations more devoid of magnetic flux.
From helioseismic studies, we learn the depth of origin of the magnetic fields of active regions is still extremely difficult to ascertain in spite of the very substantial progress that helioseismology has made to date. If the current rate of progress continues with higher spatial and temporal resolution, we can anticipate definitive forthcoming answers to the question about the depths of origin of the magnetic fields of active regions.
To date, helioseismic studies are consistent with shallow sunspots and lack convincing evidence that they form at any other depth than in the shallow zone where they are observed within 20 Mm below the photosphere.
The Appearance of New Active Regions Relative to Supergranules
Another way to seek information on the origin of new active regions is to see where they first appear relative to supergranules. Depending on their depth of origin, one might expect some consistency in where they appear relative to supergranules at the solar surface or network magnetic fields at the boundaries and vertices between supergranules. Martin (1990) followed the evolution of a limited sample of ephemeral regions and small active regions in movies of limited-field video-magnetograms from the Big Bear Solar Observatory and noticed that they can occur in network magnetic fields or adjacent to network magnetic fields or in areas free of network. The more comprehensive statistical study of Harvey (1993a) showed ephemeral region sites to be random with respect to supergranules.
Born (1974) studied 63 examples of sunspots and/or arch filament formation in newly emerging active regions in relation to chromospheric cells (supergranules). While arch filaments first studied by Bruzek (1967, 1969) are now known to appear slightly later than new flux at the photosphere, this is still relevant information. Born observed that arch filaments evolve along chromospheric cell borders (supergranule cells) and are rooted in their borders, specifically not in the interiors of supergranules. He observed that pores also form along cell borders. This is consistent with the above findings of Martin (1990) and Harvey (1993a) if those new ephemeral regions, that originate within supergranules, are transported rapidly to the supergranule boundaries. Downflows are well known along the borders of supergranules as well as the upflows that arise in the middles of these cells. Observations like those of Born (1974), which show early signs of new active regions at these sites of downflows, are inconsistent with concepts of the new flux of active regions steadily rising from below at the same sites. However, such observations would not be contradictory to new active region flux if it originated from downward flows from the chromosphere or corona.
The pattern of downflows in the centers of sunspots are somewhat similar to the down flows at the vertices of supergranules. It therefore seems possible for early, small concentrations of flux, forming a new sunspot, to drift into coincidence with the boundaries and vertices between multiple supergranules due to observed supergranular flows, consistent with the above-cited observations. The downdrafts at the vertices between a few supergranules then might serve to sustain the strong downflows flows of a young sunspot and possibly also aid in amplifying those down flows such as observed by Ravindra (2006), Kubo and Shimizu (2007), and Li et al. (2010).
Sunspot Dynamics as Clues to Their Depth and Origin
A toroidal-like pattern of flows is most clear in and around nearly symmetric, round sunspots. The direction is downward in the umbra and inner border of the penumbra consistent with coronal rain into sunspots known since the early days of sunspot observations. At the chromospheric level, flows in penumbral fibrils and some super-penumbral fibrils are inward toward the umbra while at the photospheric level, flows are outward in the penumbra. It should be noted that these toroidal-like flows, associated with sunspots at the photosphere and above, have the opposite sense from the toroidal flows within supergranules which is upward in the centers of supergranules and downward at their borders. Sunspot dynamics, therefore, seem to be incompatible with the dynamics of supergranules unless they develop at the sites of downflows at the borders or vertices between supergranules as first shown by Born (1974). This raises the question: if the magnetic fields that form sunspots emerge from deep below the photosphere, shouldn't they develop toroidal flow patterns similar to supergranules rather than the opposite?
The above question is resolved, if we understand that sunspots are secondary magnetic flux patterns that develop after the initial appearance of the new magnetic flux of active regions as discussed in the next section.
The downflows in sunspots are observed from their beginning to be coincident with downflows of arch filaments which can be as high as 50 km s−1 (Bruzek, 1967, 1969). This is consistent with helioseismic observations cited above revealing down drafts to 5 Mm beneath large sunspots. Together these observations from above and below the photosphere are compelling evidence that sunspots are not the initial sites of new flux appearing at the photosphere but rather are sites of the coalescence of flux that has already emerged such as shown by Strous and Zwaan (1999). Apparently, while growing, they are in a state of convective collapse (Zwaan, 1978; Spruit, 1979; Spruit and Zweibel, 1979); this process of concentrating magnetic flux accounts for their fields strengths growing to a few thousand Gauss in contrast to hundreds of Gauss for areas of plage without sunspots.
The observations cited in this section imply that sunspots must grow from the photosphere downward rather than rising from below; downflows in their centers, and their association with falling mass in arch filaments upon their initial appearance and throughout their early growth, confirm this.
Elementary Bipoles and Associated Features
Numerous authors have found that the magnetic flux appearing in new active regions consists of tiny dot-like features of both polarities streaming away from apparent, small source areas. If the spatial resolution of magnetograms is sufficiently high, around 1–2 arc seconds, the tiny magnetic dots are seen to appear as a succession bipolar pairs whose poles move directly apart from each other at speeds of a few kilometers per second. To reflect their fundamental nature, Martin (1990) chose the name “elementary bipoles” and the acronym “eBiPs.” This is the term used in this paper.
The earliest paper on elementary bipoles was by Vrabec (1974) who called them MMI for “moving magnetic features—inward. (toward a sunspot).” He used this name to distinguish MMI from MMO “moving magnetic features—outward” which he had recorded in the same set of images revealing MMI. In a movie of his magnetograms, he showed that MMI were very similar to MMO and explained that MMO were the same as the moving magnetic features (MMFs) away from sunspots as previously described by Harvey and Harvey (1973).
Strous (1994) and Strous and Zwaan (1999) described elementary bipoles as seen in magnetograms and continuum images, and Hα images taken with the Lockheed SOUP filter at the Swedish Solar Observatory. They called them flux emergence events and showed small magnetic features (Figure 2) with the similar sizes and the flow patterns as discussed independently by Vrabec (1974) and Martin (1990). Another independent observation was by Bernasconi et al. (2002) who described features they named Moving Dipolar Features (MDFs) as seen in magnetograms from a balloon born observatory, “The Flare Genesis Experiment.” This balloon-borne experiment carried into outer-space a vector magnetograph equipped with a tunable Fabry-Perot narrow-band filter. These authors describe “peculiar moving dipolar features in the emerging flux that flowed into sunspots and supergranule boundaries.” This is a good description of eBiPs.
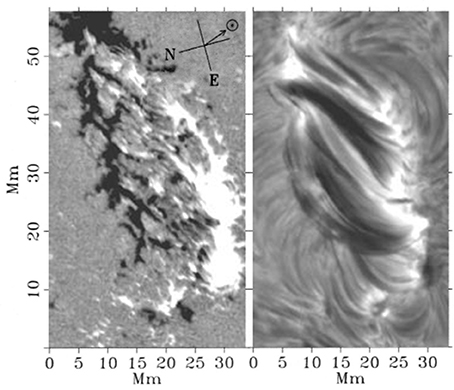
Figure 2. The newest appearing elementary bipoles (eBiPs) are pairs of tiny black and white dots that are slightly elongated in the direction of motion. Most of the white, positive-polarity eBiPs move SE while most of the black ones move NW directly away from their corresponding white pole. Apparent reversals to this pattern are those in which an eBiP is cancelling (disappearing by reconnection) with a neighboring eBiP of opposite polarity. On the right is a corresponding Hα image showing dark arch filaments that span pairs of eBiPs of opposite polarity. Illustration from Strous and Zwaan (1999) using images they recorded at the Swedish Solar Observatory on 1989 July 29. © AAS. Reproduced with permission.
Bernasconi et al. (2002) saw eBiPs at three distinct locations in a growing active region; their example was like the largest example in Figure 9 by Martin (1990) which also shows 3 separate groups of eBiPs in a newly developing active region. Strous and Zwaan (1999) performed power spectral analyses and found a preferred cluster spacing of about 8 Mm within the large group of eBiPs in the new active region they studied in detail. The larger-scale clustering of emerging flux, without the fine detail discussed in this section, is a well-known phenomenon called “nests” of emerging active regions by Gaizauskas et al. (1983).
Most relevant to the topic of this paper is the fact that eBiPs appear to be the same and to evolve similarly irrespective of how many contribute to a group or how many groups of them appear during the growth of an active region Martin (1990). Together, the papers on eBiPs cited herein show that the larger the number of eBiPs per cluster and the larger the number of clusters of eBiPs that develop within an active region, the larger the region can become in both area and in magnetic flux. Large regions are generally known to be complex and it is primarily nesting that makes them complex (Gaizauskas et al., 1983).
From vector magnetograms, Martinez-Pillet et al. (1998) made historically early measurements of the magnetic field strength of the horizontal magnetic field that connected small, newly emerging bipoles seen in line-of-sight magnetograms of relatively high spatial resolution. They found the field strengths to be in the range of 200–600 Gauss for bipoles assumed here to be the same as eBiPs.
Figure 2 by Strous and Zwaan (1999) illustrates the eBiPs in a new active region when only 8 h old. In an interval of 1.5 h between 10:42 and 12:14 UT, this active region, NOAA 5617, Strous and Zwaan (1999) measured 111 eBiPs that occurred in loose clusters. The pairing and clustering of the elementary bipoles is not nearly as clear in the single magnetogram in Figure 2 as in movies of eBiPs.
The arch filaments seen in the Hα image on the right in Figure 2 are the highest visible arches and span the area of the eBiPs; they apparently obscure shorter ones joining the newer eBiPs below these. Each of the initial arches form in concert with a pair of EBiPs linking the opposite polarity poles to the two ends of an arch filament. The arch filaments are another means of identifying clusters of eBiPs.
The pairs of eBiPs and the arch filaments, at the time shown in Figure 2, have a similar orientation and are consistent with the Hale polarity pattern for the solar cycle (23) but have an unusually high inclination of 37° counterclockwise with respect to the solar equator at the time of early appearance; this is 11° larger than the tilt of the active region sunspots. Both measures of tilt were in the opposite sense of the average tilt of about 5–10° clockwise for the axes of active regions (Howard, 1994).
Those eBiPs, that have high angles with respect to the majority in a growing group, tend to have shorter lifetimes as bipolar entities because cancellation (interpreted as magnetic reconnection) reconfigures them such that their fields disappear from line-of-sight magnetograms. Cancellation is interpreted as magnetic reconnection at or very close to the photosphere (Litvinenko, 1999; Litvinenko et al., 2007). The line-of-sight flux is transformed into horizontal flux that rises into the corona where it is likely to become invisible from losing mass when rising. Martin (1990) showed examples in which some eBiPs appear nearly orthogonal to the previous ones or even reversed in orientation. Because cancellation results in the apparent demise of the more oddly oriented eBiPs, it can play a large role in determining the overall east-west orientation of groups of eBiPs and conformance with the Hale pattern of active region and sunspot polarities.
The occurrence of many eBiPs in small clusters is a reason to question the depth of their origin in all cases. The groups with just a few eBiPs are expected to be shallow in origin. However, the groups with just a few eBiPs appear no different than the groups with tens to hundreds of eBiPs that appear in similar small clusters. If they came from different depths in the convection zone, why would they all appear the same after interacting with the magnetic fields of convection cells? This leads to reasoning that, whatever causes the large groups of eBiPs, is likely to be the same shallow process that creates the small ones.
Similarities Between Moving Magnetic Features and Elementary Bipoles
There is just one class of features on the Sun in several ways like elementary bipoles. They are “moving magnetic features” (MMFs) around the periphery of sunspot penumbrae Ma et al. (2015) mentioned in the section on Sunspot Dynamics as Clues to their Depth and Origin. MMFs are tiny knots of magnetic flux of both polarities. They look like elementary bipoles and are roughly the same size as elementary bipoles, about an arc second in diameter. They occur in opposite polarity pairs usually with the outer pole having the polarity of the associated sunspot (Zhang et al., 2003). However, instead of the poles moving in opposite directions from each other like elementary bipoles, the MMF poles move together as a unit of opposite polarity pairs approximately radially away from the perimeter of a sunspot penumbra (Harvey and Harvey, 1973).
Magnetograms reveal MMFs to be knots of magnetized plasma. They cancel and merge like other magnetic features on the Sun. Like elementary bipoles, a sufficient collection of moving magnetic features of the same polarity can merge to form small sunspots (Li et al., 2015). Such mergers confirm that these sunspots are not different than small sunspots formed by clusters of elementary bipoles.
From the whole range of properties of MMFs a question is: Are MMFs clues to the origin of elementary bipoles? The sites of MMFs indicate they are possible secondary products of the toroidal-like flows associated with the upper parts of sunspots. Following downflows in the centers of sunspots, MMFs might originate from a subsurface part of the return horizontal and then upward flow of some of the plasma circulating in magnetic toroidal-like flow pattern of sunspots confirmed by helioseismology; they would be related to a fraction of the flow that is diverted outward rather than inward toward the umbra. MMFs have been associated with penumbral fibrils since the first observations of them by Sheeley (1969). Some observations show MMFs can originate within the penumbra rather than at the penumbral boundary Li et al. (2010). At the penumbral perimeter they seem to be enhanced; possibly their flux becomes more vertical in the line-of-sight outside of the influence of the nearly horizontal fields in the penumbra or possibly there is a flux amplification process at the perimeter. The relevance here is that MMFs represent substantial quantities of magnetic flux not previously seen.
If elementary bipoles are analogous to MMFs, this is a basis to conjecture that emerging elementary bipoles could be related to unidentified or invisible downflows -not necessarily at their specific sites but in their close vicinity. Numerous and varied kinds of downflows are known. As discussed in Section The Appearance of New Active Regions Relative to Supergranules, there are downflows at the vertices of supergranules. There are downflows with many types of dynamic events such as in flare loops, in the legs of erupting filaments, in surges, and in all kinds of phenomena under the name, “jets”; all could penetrate the photosphere. Additionally, we especially cannot rule out invisible plasma downflows with higher temperatures and lower densities than visible downflows associated with various solar features. Several examples of the frequent occurrence of high-speed local mass downflows on the solar surface are described by Shimizu et al. (2008) from observations with the Solar Optical Telescope on board the Hinode satellite and low-density, supersonic downflows into sunspots are reported by Samanta et al. (2017) to be statistically common in transition-region spectra from IRIS. From the small areas observed, it is likely that future studies of other areas and larger areas will lead to more new information about these high-speed downflows and possible associated phenomena.
At present, I offer no specific hypothesis for a mechanism to initiate elementary bipoles. However, based on their similarity in size and varying number to moving magnetic features, and the reported high frequency of high-speed local downflows from SOT on Hinode, I will suggest the origins of elementary bipoles could be bi-products of a not-yet-identified but common pattern of plasma flows downward into the photosphere.
Discussion and Conclusions
The observations reviewed and discussed here together provide consistent evidence that the magnetic fields of active regions, small and large, have shallow origins beneath the photosphere.
What is required for the formation of the magnetic fields of elementary bipoles are small-scale currents. Those currents could be associated with plasma flows originating close to the photosphere, either above or below it. The second requirement is a repeating or multiplying factor to account for the varying number of elementary bipoles, from one to a few for ephemeral regions and up to hundreds of elementary bipoles in major emerging flux regions.
In the absence of any definitive depth from helioseismology techniques, it is timely to seek new observations and theories to account for the manifestations of new magnetic fields of the active region population of the solar cycle due to processes close to the photosphere as well as down to the subsurface shear layer.
Author Contributions
The author confirms being the sole contributor of this work and approved it for publication.
Funding
This writing of this paper was not funded by any agency or contributor other than author's institution, Helio Research.
Conflict of Interest Statement
The author declares that the research was conducted in the absence of any commercial or financial relationships that could be construed as a potential conflict of interest.
Acknowledgments
Thank you to L. H. Strous for permission to re-publish the images in Figure 2 from the Swedish Solar Telescope on La Palma in the Canary Islands.
References
Altrock, R. C. (1997). An ‘extended solar cycle' as observed in Fe XIV. Solar Phys. 170, 411–423. doi: 10.1023/A:1004958900477
Babcock, H. W. (1961). The topology of the sun's magnetic field and the 22 year cycle. Astrophys. J. 133, 572–587. doi: 10.1086/147060
Barnes, G., Birch, A. C., Leka, K. D., and Braun, D. C. (2014). Helioseismology of pre-emerging active regions, III. Statistical analysis. Astrophys. J. 786:19. doi: 10.1088/0004-637X/786/1/19
Bernasconi, P. N., Rust, D. M., and Geogoulis, M. K. (2002). Moving dipolar features in an emerging flux region. Solar Phys. 209, 119–139. doi: 10.1023/A:1020943816174
Birch, A. C., Braun, D. C., Leka, K. D., Barnes, G., and Javornik, B. (2018). Helioseismology of pre-emerging active regions, II: average emergence properties. arXiv:1303.1391v1 [astro-ph.SR]
Bogdan, T. J., Gilman, P. A., Lerche, I., and Howard, R. (1988). Distribution of sunspot umbral areas 1917–1982. Astrophys. J. 327, 451–456. doi: 10.1086/166206
Born, R. (1974). First phase of active regions and their relationship to the chromospheric network. Solar Phys. 38, 127–131. doi: 10.1007/BF00161830
Brandenburg, A. (2005a). The case for a distributed solar dynamo shaped by near-surface shear. Astrophys. J. 625, 539–547. doi: 10.1086/429584
Brandenburg, A. (2005b). Distributed versus tachocline dynamos. arXiv:astro-ph/0512638v1 (December 29, 2005).
Brandenburg, A. (2006). “Location of the solar dynamo and near-surface shear,” in Solar MHD Theory and Observations: A High Spatial Resolution Perspective, ASP Conference Series, eds H. Uitenbroek, J. Leibacher, and R. F. Stein (San Francisco, CA), 121–126.
Braun, D. C. (2012). Comment on “detection of emerging sunspot regions in the solar interior.” Science 336:296. doi: 10.1126/science.1215425
Braun, D. C., and Lindsay, C. (2000). Helioseismic holography of active-region subphotospheres - (invited review). Solar Phys. 192, 285–305. doi: 10.1023/A:1005287419566
Braun, D. C., and Lindsay, C. (2001). “Seismic holography of the solar interior and far side,” in Recent Insights into the Physics of the Sun and Heliosphere: Highlights from SOHO and Other Space Missions, Proceedings of IAU Symposium, eds P. Brekke, B. Fleck, and J. B. Gurman (San Francisco, CA: ASP), 167–169.
Bruzek, A. (1967). On arch filament systems in spotgroups. Solar Phys. 2, 451–461. doi: 10.1007/BF00146493
Cameron, R. H., Dikpati, M., and Brandenburg, A. (2017). The global solar dynamo. Space Sci. Rev. 210, 367–395.
Cameron, R. H., Duvall, T. L., Schssler, M., and Schunker, H. (2018) Observing modeling the poloidal toroidal fields of the solar dynamo. A&A 609 doi: 10.1051/0004-6361/201731481
Cattaneo, F. and Hughes, D. W. (2001). Solar dynamo theory: a new look at the origin of small-scale magnetic fields. Astron. Geophys. 42, 3.18–3.22. doi: 10.1046/j.1468-4004.2001.42318.x
Charbonneau, P. (2010). Dynamo models of the solar cycle. Living Rev. Solar Phys. 7:3. doi: 10.12942/lrsp-2010-3
Charbonneau, P. (2014). Solar dynamo theory. Ann. Rev. Astron. Astrophys. 52, 251–290. doi: 10.1146/annurev-astro-081913-040012
Cliver, E. W. (2015). “The extended cycle of solar activity and the sun's 22-yr magnetic cycle,” in The Solar Activity Cycle, Space Science Series of ISSI, eds A. Balogh, H. Hudson, K. Petrovay, and R. Steiger (New York, NY: Springer), 169–189.
Fisher, G. H., Fan, Y., Longcope, D. W., Linton, M. G., and Pevtsov, A. A. (2002). The solar dynamo and emerging flux - (Invited Review). Solar Phys. 192, 119–139. doi: 10.1023/A:1005286516009
Gaizauskas, V., Harvey, K. L., Harvey, J. W., and Zwaan, C. (1983). Large-scale patterns formed by solar active regions during the ascending phase of cycle 21. Astrophys. J. 265, 1056–1065. doi: 10.1086/160747
Harvey, K., and Harvey, J. (1973). Observations of moving magnetic features near sunspots. Solar Phys. 28, 61–71. doi: 10.1007/BF00152912
Harvey, K. L. (1993b). “Properties of emerging bipolar active regions,” in The Magnetic and Velocity Fields of Solar Active Regions, ASP Conference Series, eds H. Zirin, G. Ai, and H. Wang (San Francisco, CA), 488–491.
Harvey, K. L., and Martin, S. F. (1973). Ephemeral active regions. Solar Phys. 32, 389–402. doi: 10.1007/BF00154951
Harvey, K. L., and Zwaan, C. (1993). Properties and emergence patterns of bipolar active regions. Solar Phys. 148, 85–118. doi: 10.1007/BF00675537
Harvey, K. L., Harvey, J.W., and Martin, S. F. (1975). Ephemeral active regions in 1970 and 1973. Solar Phys. 40, 87–102.
Howard, R. (1994). “Active regions on the sun, in solar active region evolution: comparing models with observations,” in Proceedings of the Fourteenth (14th) International Summer Workshop, National Solar Observatory 30 August−3 September 1993, eds K. S. Balasubramaniam and G. W. Simon (Fourteenth Sunspot, NM; San Francisco, CA), 1–16.
Howard, R., and Labonte, B. J. (1980). The sun is observed to be a torsional oscillator with a period of 11 years. Astrophys. J. 239, L33–L36. doi: 10.1086/183286
Ilonidis, S., Zhao, J., and Hartlep, T. (2013). Helioseismic investigation of emerging magnetic flux in the solar convection zone. Astrophys. J. 777:138. doi: 10.1088/0004-637X/777/2/138
Ilonidis, S., Zhao, J., and Kosovichev, A. G. (2011). Helioseismic detection of emerging magnetic flux. Science 333:399.
Ilonidis, S., Zhao, J., and Kosovichev, A. G. (2012a). “Helioseismic detection of emerging magnetic flux,” in Progress in Solar/Stellar Physics with Helio- and Asteroseismology, ASP Conference Series, Vol. 462, eds H. Shibahashi, M. Takata, and A. E. Lynas-Gray (San Francisco, CA), 283–291.
Ilonidis, S., Zhao, J., and Kosovichev, A. G. (2012b). Response to “Comment on Helioseismic Detection of Emerging Magnetic Flux, Sci. 336:296. doi: 10.1126/science.1215539
Jarboe, T. R., Benedict, T. E., Everson, C. J., Hansen, C. J., Hossack, A. C., Morgan, K. D., et al. (2017). Evidence for a Shallow Thin Magnetic Structure and Solar Dynamo: The Driver of Torsional Oscillations, arXiv:1712.01247v1, astro-ph.SR.
Kitiashvili, I. N., Kosovichev, A. G., Mansour, N. N., and Wray, A. A. (2015). Realistic modeling of local dynamo processes on the sun. Astrophys. J. 809:84. doi: 10.1088/0004-637X/809/1/84
Komm, R., Morita, S., Howe, R., and Hill, F. (2008). Astrophys. J. 672, 1254–1265. doi: 10.1086/523998
Kosovichev, A. G. (2009). Photospheric and subphotospheric dynamics of emerging magnetic flux. Space Sci. Rev. 144, 175–195. doi: 10.1007/s11214-009-9487-8
Kosovichev, A. G. (2011). “Advances in global and local helioseismology: an introductory review,” in The Pulsations of the Sun and the Stars, Lecture Notes in Physics,Vol. 832 (Berlin; Heidelberg: Springer-Verlag), 3.
Kosovichev, A. G. (2012). Local helioseismology of sunspots: current status and perspectives. Solar Phys. 279, 323–348. doi: 10.1007/s11207-012-9996-6
Kosovichev, A. G., and Duvall, T. L. Jr. (2011). “Investigation of a sunspot complex by time-distance helioseismology,” in The Physics of the Sun and Star Spots, Proceedings of IAU Symposium, eds D. P. Choudhary and K. G. Strassmeier (Cambridge, UK: Cambridge University Press), 320–324.
Kosovichev, A. G., Duvall, T. L., and Zhao, J. (2002). “Helioseismic observations of subphotospheric dynamics of sunspots and developing active regions,” in SOLMAG 2002. Proceedings of the Magnetic Coupling of the Solar Atmosphere Euroconference and IAU Colloquium 188, 11–15 June 2002, Santorini, Greece, ed H. Sawaya-Lacoste. ESA SP-505 (Noordwijk: ESA Publications Division), 79–82.
Kosovichev, A. G., Pipin, V. V., and Zhao, J. (2013). “Helioseismic constraints and a paradigm shift in the solar dynamo,” in ASP Conference Series, Vol. 479, 395–407.
Kosovichev, A., Zhao, J., and Ilonidis, S. (2016). Local helioseismology of emerging active regions: a case study. arXiv:160704987v1 [astro-ph.SR]
Kosovichev, A., Zhao, J., Sekii, T., Nagashima, K., and Mitra-Kraev, U. (2009). “Recent progress and future directions for helioseismology,” in The Second Hinode Science Meeting: beyond Discovery-Toward Understanding, Proceedings of a Meeting Held 29 September–3 October 2008, San Francisco, CA: PASP Conf. Series, Vol. 415, eds B. Lites, M. Cheung, T. Magara, J. Mariska, and K. Reeves (Boulder, CO: National Center for Atmospheric Research), 399–404.
Kubo, M., and Shimizu, T. (2007). “Magnetic correspondence between moving magnetic features and penumbral magnetic fields,” in New Solar Physics with Solar-B Mission, ASP Conference Series, Vol. 369, eds K. Shibata, S. Nagata, and T. Sakurai (San Francisco,CA), 145–152.
Leighton, R. B. (1964). Transport of magnetic fields on the sun. Astrophys. J. 140, 1547–1562. doi: 10.1086/148058
Leighton, R. B. (1969). A magneto-kinematic model of the solar cycle. Astrophys. J. 156, 1–26. doi: 10.1086/149943
Leka, K. D., Barnes, G., Birch, A. C., Gonzalez-Hernandez, I., Dunn, T., Javornik, G., et al. (2013). Helioseismology of pre-emerging active regions, I. overview, data, and target selection criteria. Astrophys. J. 762:130. doi: 10.1088/0004-637X/762/2/130
Li, K.-J., Shen, Y.-D., Yang, L.-H., and Jiang, Y.-C. (2010). A study of the hinode observations of the bipolar moving magnetic features in sunspot penumbrae. Chin. Astron. Astrophys. 34, 142–153. doi: 10.1016/j.chinastron.2010.04.005
Lindsay, C., and Braun, D. C. (2000). Basic principles of solar acoustic holography - (Invited Review). Solar Phys. 192, 261–284. doi: 10.1023/A:1005227200911
Litvinenko, Y. (1999). Photospheric magnetic reconnection and cancelling magnetic features on the Sun. Astrophys. J. 515, 435–440. doi: 10.1086/307001
Litvinenko, Y., Chae, J., and Park, S.-Y. (2007). Flux pile-up magnetic reconnection in the solar photosphere. Astrophys. J. 662, 1302–1308. doi: 10.1086/518115
Li, X., Yang, Z., and Zhang, H. (2015). Formation of pores associated with the inflow of moving magnetic features. Astrophys. J. 807, 160–173. doi: 10.1088/0004-637X/807/2/160
Ma, L., Zhou, W., Zhou, G., and Zhang, J. (2015). The evolution of arch filament systems and moving magnetic features around a sunspot. A & A 583:A110. doi: 10.1051/0004-6361/201424872
Martinez-Pillet, V., Lites, B. W., and Skumanich, A. (1998). Vector magnetic fields of emerging solar flux. ESASP 417, 259–261
Martin, S. F. (1990). Elementary bipoles of active regions and ephemeral active regions, Societa Astronomica Italiana. Memorie 61, 293–315.
McIntosh, S. W., and Leamon, R. J. (2017). Deciphering solar magnetic activity: spotting solar cycle 25. Front. Astron. Space Sci. 4:4. doi: 10.3389/fspas.2017.00004
McIntosh, S. W., Wang, X., Leamon, R. J., Davey, A. R., Howe, R., Krista, L. D., et al. (2014). Deciphering solar magnetic activity. I. On the relationship between the sunspot cycle and the evolution of small magnetic features. Astrophys. J. 792:12. doi: 10.1088/0004-637X/792/1/12
Ossendrijver, M. (2003). “The solar dynamo: a challenge for theory and observations,” in Current Theoretical Models and Hi-Res Obs: Preparing for ATST, ASP Conference Series, Vol. 286, eds A. A. Pevtsov and H. Uitenbroek (San Francisco, CA), 97–112.
Parnell, C. E., DeForest, C. R., Hagenaar, H. J., Johnston, B. A., Lamb, D. A., and Welsch, B. T. (2009). A power-law distribution of solar magnetic fields over more than five decades in flux. Astrophys. J. 698, 75–82. doi: 10.1088/0004-637X/698/1/75
Ravindra, B. (2006). Moving magnetic features in and out of penumbral filaments. Solar Phys. 237, 297–319. doi: 10.1007/s11207-006-0144-z
Samanta, T., Tian, H., and Choudhary, D. P. (2017). Statistical investigation of supersonic downflows in the transition region above sunspot. arXiv:1804.05054.
Schrijver, C. J., and Harvey, K. L. (1994). The photospheric magnetic flux budget. Solar Phys. 150, 1–18. doi: 10.1007/BF00712873
Sheeley, N. R. (1969). The evolution of the photospheric network. Solar Phys. 9, 347–357. doi: 10.1007/BF02391657
Shimizu, T., Lites, B. W., Katsukawa, Y., Ichimoto, K., Suematsu, Y., Tsuneta, S., et al. (2008). Frequent occurrence of high-speed local mass downflows on the solar surface. Astrophys. J. 680, 1467–1476. doi: 10.1086/588775
Spruit, H. (1979). Convective collapse of flux tubes. Solar Phys. 61, 363–378. doi: 10.1007/BF00150420
Spruit, H. C., and Zweibel, E. G. (1979). Convective instability of thin flux tubes. Solar Phys. 62, 15–22. doi: 10.1007/BF00150128
Strous, L. H. (1994). “Dynamics of small magnetic elements in a growing active region,” in Solar Surface Magnetism, NATO Advanced Science Institutes, Series C, eds R. J. Rutten and C. J. Schrijver (Dordrecht: Kluwer Academic Publishers), 73–90.
Strous, L. H., and Zwaan, C. (1999). Phenomena in an emerging active region. II. properties of the dynamic small-scale structure. Astrophys. J. 527, 435–444. doi: 10.1086/308071
Thornton, L. M., and Parnell, C. E. (2011). Small-scale flux emergence using hinode/SOT. Solar Phys. 269,13–40. doi: 10.1007/s11207-010-9656-7
Toriumi, S., Hayashi, K., and Yokoyama, T. (2012). Detection of the Horizontal Divergent Flow (HDF) as a precursor of sunspot emergence. Astrophys. J. 751, 154–163. doi: 10.1088/0004-637X/751/2/154
Vrabec, D. (1974). “Streaming magnetic features near sunspots, chromospheric fine structure,” IAU Symposium 56, Surfer's Paradise, QLD Australia, 3–7 September 1973, ed. R. Grant Athay (Dordrecht; Boston, MA: Reidel), 201–231.
Wilson, P. R., Altrock, R. C., Harvey, K. L., Martin, S. F., and Snodgrass, H. B. (1988). The extended solar activity cycle. Nature 333, 748–750. doi: 10.1038/333748a0
Zhang, J., Solanki, S. K., and Wang, J. (2003). On the nature of moving magnetic feature pairs around sunspots. A&A 399, 755–761. doi: 10.1051/0004-6361:20021807
Zhao, J., Kosovichev, A. G., and Sekii, T. (2010). High-resolution helioseismic imaging of subsurface structures and flows of a solar active region observed by Hinode. Astrophys. J. 708:304313. doi: 10.1088/0004-637X/708/1/304
Keywords: solar cycle, emerging magnetic flux, sunspots, pores, ephemeral regions
Citation: Martin SF (2018) Observational Evidence of Shallow Origins for the Magnetic Fields of Solar Cycles. Front. Astron. Space Sci. 5:17. doi: 10.3389/fspas.2018.00017
Received: 05 January 2018; Accepted: 30 April 2018;
Published: 28 May 2018.
Edited by:
Scott William McIntosh, National Center for Atmospheric Research (UCAR), United StatesReviewed by:
Luca Bertello, National Solar Observatory, United StatesRobert James Leamon, University of Maryland, College Park, United States
Copyright © 2018 Martin. This is an open-access article distributed under the terms of the Creative Commons Attribution License (CC BY). The use, distribution or reproduction in other forums is permitted, provided the original author(s) and the copyright owner are credited and that the original publication in this journal is cited, in accordance with accepted academic practice. No use, distribution or reproduction is permitted which does not comply with these terms.
*Correspondence: Sara F. Martin, c2FyYUBoZWxpb3Jlc2VhcmNoLm9yZw==