- Department of Chemistry, School of Life Sciences, University of Sussex, Brighton, United Kingdom
Monocyclic aromatic hydrocarbons such as benzene, toluene and xylene are thought to play an important role as precursors to the formation of polycyclic aromatic hydrocarbons (PAHs) and their methylated counterparts in a range of astrophysical environments. Benzene has been detected in two carbon rich objects and models have predicted that it could also be present in the interstellar medium (ISM). It has hence been speculated that small aromatic molecules are present in molecular clouds in the ISM, although they have not been detected to date. If they are present in the ISM, they are likely to exist in water-ice dominated icy mantles on the surface of dust grains.
We present a laboratory study of benzene, toluene and two xylene isomers (ortho- and para-xylene) in the presence of water ice on a carbonaceous model dust grain surface (highly oriented pyrolytic graphite, HOPG). Temperature programmed desorption (TPD) shows how the desorption of the molecules is affected by the presence of water ice. The importance of these data for astrophysical situations is demonstrated by the use of TPD-derived kinetic parameters to generate a simple model of desorption in dense molecular clouds on an astrophysical timescale. Since benzene, toluene and xylene have not been detected in water-dominated icy mantles to date, desorption has been simulated in a range of different water-containing environments to show the different behaviour expected depending on ice composition. The simulations demonstrate how future observations of aromatic molecules in dense molecular clouds at known temperatures could reveal which environments the molecules are in. Data from these experiments are also used to predict the behaviour of other, larger, aromatic molecules such as PAHs. Reflection absorption infrared spectroscopy (RAIRS) is also used to record the infrared spectra of the small molecules in different water ice configurations. These spectra can be used to aid identification of these icy aromatics in future observations, such as those that will be possible with the James Webb Space Telescope (JWST). In all cases, spectra of mixed ices consisting of the aromatic molecule and amorphous water ice show evidence of interactions between the water ice and the aromatic species.
Introduction
In circumstellar and interstellar environments, monocyclic aromatic molecules such as benzene, toluene and xylene are thought to play an important role as precursors to the formation of polycyclic aromatic hydrocarbons (PAHs) and their methylated counterparts (Frenklach and Feigelson, 1989; Contreras and Salama, 2013). PAHs are ubiquitous in the interstellar medium (ISM) where they account for up to 20% of the galactic carbon and are believed to be carriers of the diffuse interstellar bands (DIBs) and aromatic infrared bands (Tielens, 2008). Indeed, there is a large body of literature that focuses on the gas phase infrared spectra of PAHs in a bid to aid the assignments of the UIRs and DIBs to PAH spectra, see for example (Mackie et al., 2015; Peeters et al., 2017; Mattioda et al., 2020). PAH emission has also been detected in protostellar disks (Geers et al., 2006) and in protoplanetary nebulae (Cox et al., 2016). Fullerenes have also been postulated in various regions of space (Cami et al., 2010; Sellgren et al., 2010) and C60+ has been confirmed as a carrier of two DIBs (Campbell et al., 2015). In addition, methyl derivatives of aromatic molecules are thought to be responsible for the 3.4–3.6 µm unidentified infrared (UIR) emission band, assigned to the vibrational excitation of the CH3 stretch (Shan et al., 1991).
Gas phase benzene has been detected in two carbon-rich objects (CRL 618 (Cernicharo et al., 2001) and SMP LMC 11 (Bernard-Salas et al., 2006)) at the transition between the asymptotic giant branch (AGB) and planetary nebula stages. In the circumstellar envelope of these protoplanetary nebulae, complex organic chemistry (Blommaert et al., 2005), driven by ultra-violet (UV) radiation and shocks, forms larger molecules such as PAHs (Frenklach and Feigelson, 1989). Protoplanetary nebulae are thought to have short lifetimes of around 1,000 years, with benzene being produced within the first 500 years (Woods et al., 2003).
Benzene formation is often thought to be the rate limiting step in the formation of PAHs (Contreras and Salama, 2013). Benzene synthesis routes have been proposed in dense interstellar clouds (McEwan et al., 1999), protoplanetary nebulae (Woods et al., 2002; Woods et al., 2003) and on dust grains in protostellar disks (Woods and Willacy, 2007). Photodissociation of carbonaceous grains in the ISM can also lead to the production of smaller PAHs and benzene (Duley et al., 2014). Laboratory investigations of benzene formation on icy mantles in the ISM from propargyl alcohol (Sivaraman et al., 2014) and the ethynyl radical and 1,3-butadiene (Jones et al., 2011) have also been undertaken. More recent experiments have also shown that benzene can be synthesised in the solid phase via galactic cosmic ray exposure of low temperature acetylene ices on Titan’s surface (Abplanalp et al., 2019). Gas-phase formation of toluene from the deuterated ethynyl radical and isoprene has also been successful (Dangi et al., 2013). Calculations on the formation of toluene from ethylene and propylene (Kaiser et al., 2001) suggest that toluene, as well as ortho- and para-xylene, should be present in the circumstellar shell of CRL 618, although these molecules have not currently been detected in space.
Although they have not yet been detected, it is thought that PAHs and smaller aromatics such as benzene, toluene and xylene, could also be present in water-dominated icy mantles on dust grains in dense molecular clouds (Geers et al., 2009). Icy mantles are comprised of up to 70% water, depending on the astrophysical environment. Laboratory studies (Collings et al., 2004; Burke et al., 2015) have shown that the dominant water component of the ices has a large effect on the thermal processing behaviour of these ices. In particular, smaller molecules such as CO and CO2 are trapped within the water ice beyond their natural sublimation temperature and are released at the water amorphous to crystalline phase transition (so called volcano desorption) or when the bulk of the water desorbs from the surface (codesorption) (Noble et al., 2012; Edridge et al., 2013; Collings et al., 2014). Larger molecules such as benzene, toluene and xylene are expected to give rise to complex behaviour in the presence of water ice. In particular, the ability of these molecules to both mix and phase separate in the presence of water means that understanding their interactions and thermal processing in the presence of water ice in astrophysical conditions is important.
Previous experimental studies of the thermal processing of benzene and water in model interstellar ices have used temperature programmed desorption (TPD) (Souda, 2004; Bahr and Kempter, 2007; Thrower et al., 2009) to quantify benzene desorption. The interaction between benzene and water ice at 24 K has also been probed using vacuum ultraviolet and infrared spectroscopy (Dawes et al., 2018). These results show that in mixed ices with water, benzene is trapped above its natural desorption temperature until water desorption begins. Recent theoretical studies using classical molecular dynamics and quantum calculations have also studied the interaction between a range of PAHs, including benzene, and amorphous and crystalline water ice surfaces (Michoulier et al., 2018a; Michoulier et al., 2018b; Michoulier et al., 2020) and have found that the crystalline water ice surface gave higher adsorption energies for the PAHs than the amorphous water ice surface. The calculations also showed that there are fewer adsorption sites on the amorphous water ice surface compared to on the crystalline water ice, leading to the more rapid saturation of these sites by PAHs. Following saturation, the PAHs then form clusters on the amorphous water ice surface.
Here, we present a laboratory study of the thermal processing of a series of monocyclic aromatic hydrocarbons, benzene, toluene and two xylene isomers (ortho and para, o- and p-) shown in Figure 1, on a carbonaceous model dust grain surface (highly oriented pyrolytic graphite–HOPG). TPD is used to show how the thermal behaviour of these molecules is affected by the presence of water ice. The importance of these experimental results for astrophysical situations is demonstrated by the inclusion of kinetic parameters, derived from TPD data (Salter et al., 2018), in a simple model of thermal processing and desorption in dense molecular clouds on astrophysical timescales. Since benzene, toluene and xylene have not been detected in water-dominated icy mantles to date, desorption has been simulated in a range of different water-containing environments in order to show the different behaviours depending on the ice composition. Infrared spectra have also been recorded for a range of different ice configurations consisting of the aromatic molecule and amorphous or crystalline water ice. These spectra can potentially be used to aid identification of these icy aromatics in future observations, such as those that will be possible with the James Webb Space Telescope (JWST). Finally, the data from these experiments using small aromatic molecules is also used to predict the desorption behaviour of other, larger, aromatic molecules such as PAHs. To the best of our knowledge, this is the first experimental investigation of the temperature programmed desorption of toluene and o- and p-xylene adsorbed in the presence of amorphous solid water (ASW) and crystalline ice (CI) at cryogenic temperatures.
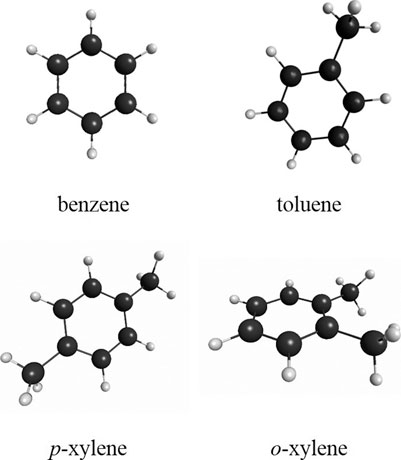
FIGURE 1. Schematic showing the structure of the four small aromatic molecules, benzene, toluene, o-xylene and p-xylene.
Materials and Methods
Experiments were performed in two stainless steel ultrahigh vacuum (UHV) chambers. Benzene and toluene experiments were undertaken in chamber 1 and xylene data were recorded in chamber 2. A HOPG surface was used as a carbonaceous dust grain analogue in both cases. The surface was cleaned before each experiment by annealing to 250 K. Surface cleanness was confirmed by the absence of any desorption products in TPD experiments with no dose. A closed-cycle helium refrigerator was used in both chambers to cool the sample to a base temperature of between 25 and 30 K.
In order to study the effect of water ice on the adsorption and desorption behaviour of benzene, toluene and xylene, model ices were grown in situ on the HOPG surface. The ice configurations investigated are shown in Figure 2 and comprise the molecule of interest adsorbed in either a layered ice on top of ASW or CI or in a mixed ice comprised of ASW and the aromatic molecule. The different ice configurations allow the interactions of the aromatic molecules with different types of water ice to be studied in detail.
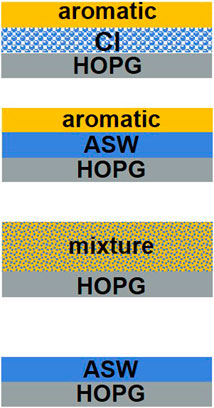
FIGURE 2. Schematic showing the different ice configurations investigated. The ice configurations were (from top to bottom) layered ices consisting of the aromatic molecule adsorbed on top of CI and on top of ASW and binary mixtures of the aromatic molecule with ASW. The bottom schematic shows the pure water system which was also studied to provide a reference for the TPD data.
Benzene (Sigma Aldrich, purity 99.9%), toluene (Sigma Aldrich, purity 99.9%), p-xylene (Sigma Aldrich, purity 99.5%), o-xylene (Sigma Aldrich, purity 99.5%) and deionised water were purified by repeated freeze-pump-thaw cycles. Ices were grown on the HOPG surface via background deposition using high-precision leak valves. Doses were recorded in Langmuir (Lm) where 1 Lm = 10–6 mbar s. For the layered and mixed ice systems, 50 Lm of water was deposited onto the HOPG surface at base temperature to form ASW. This exposure gives a water layer around 11 nm thick and was chosen to ensure complete coverage of the HOPG surface, with no exposed substrate. To form CI, the HOPG surface was either held at 135 K during deposition of water (chamber 1), or water was deposited at base temperature and then annealed to 137 K for 3 min (chamber 2). Reflection absorption infrared spectroscopy (RAIRS) was used to check the crystallinity of the water ice. For the layered ices, exposures of 5–150 Lm were dosed, giving rise to ice thicknesses of approximately 0.3–10 nm of the aromatic molecule on top of the water ice. Mixed ices were co-deposited from two high precision leak valves simultaneously. The exact composition of the mixed ices was determined using mass spectrometry recorded during dosing.
TPD experiments were carried out using a linear heating rate of 0.5 K s−1. The desorbing gas phase species were recorded using a quadrupole mass spectrometer (Hiden Analytical). Infrared data were collected in the reflection mode using a Thermo-Nicolet infrared spectrometer coupled to a liquid nitrogen cooled mercury cadmium telluride detector. A spectrum typically consists of the co-addition of 256 scans using a resolution of 2 cm−1 for the xylene experiments and 4 cm−1 for the benzene and toluene experiments.
Results
Thermal Desorption Data
Figure 3 shows the results of TPD experiments for benzene, toluene, o-xylene and p-xylene adsorbed on HOPG at 25–30 K in different layered and mixed ices with water. Since water is the main constituent of ices in a wide range of astrophysical environments, it is important to look at the effect of water on the small aromatic molecules investigated here. The spectra shown in Figure 3 are for the aromatic molecules adsorbed in layered ices on top of either CI or ASW and for mixed ices consisting of 91% ASW and 9% of the aromatic molecule. In all cases, similar amounts of the aromatic molecule are present in each experiment: 5 Lm (∼0.2–0.3 nm) of the aromatic molecule is adsorbed on top of 50 Lm (∼11 nm) of ASW or CI. The bottom panel of Figure 3 shows a TPD spectrum for desorption of 11 nm (50 Lm) of pure ASW from the HOPG surface for comparison. In this spectrum, it is clear that there is a low temperature shoulder on the leading edge, before the main water desorption peak. This corresponds to the ASW to CI phase transition (Smith et al., 1997). When the phase transition occurs, molecules that are trapped within the ASW pore structure are released in what is termed volcano desorption. This phenomenon has been observed for many molecules (Collings et al., 2004; Burke and Brown, 2010). In all experiments, the amount of water on the surface was kept constant to avoid any variations in the TPD spectra due to differing amounts of water. For example, changing the amount of water present would affect the desorption temperature and amount trapped within the water ice pores and hence desorbing during volcano and codesorption events.
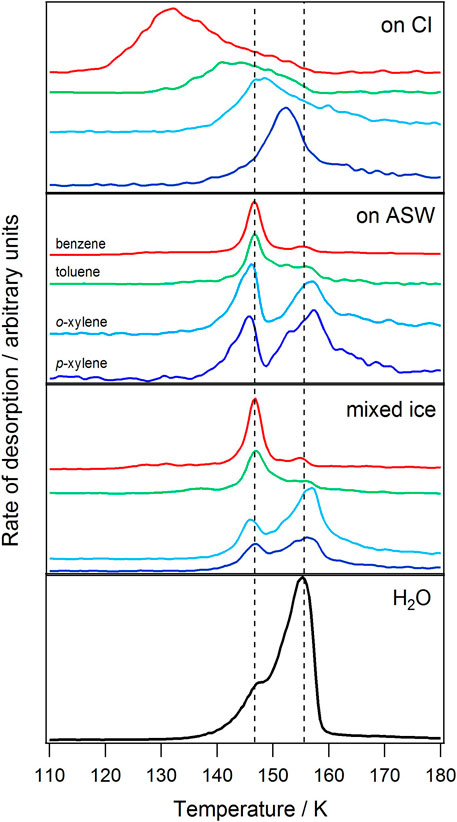
FIGURE 3. TPD spectra of 5 Lm of benzene, toluene, o-xylene and p-xylene in different ice configurations, shown in Figure 2, deposited on HOPG between 25 and 30 K. From top to bottom: layered ices consisting of the aromatic molecule adsorbed on top of 50 Lm CI, on top of 50 Lm ASW, and binary mixtures of the aromatic molecule (9%) with ASW (total 55 Lm). The bottom panel shows the desorption of pure water from HOPG.
It is clear from Figure 3 that the desorption of the aromatic molecules is strongly affected by the presence of water ice and that the exact ice configuration is very important. For adsorption on CI, the behaviour of all four molecules is similar to that observed for the molecules adsorbed directly on the HOPG surface, reported previously (Salter et al., 2018). For o- and p-xylene in particular, adsorption on CI does not affect the desorption of the molecules in any noticeable way. For benzene and toluene, the profile of the desorption of thin layers of the aromatic molecule from the CI surface is similar to that from HOPG, although the desorption temperature is slightly lower from CI than from HOPG (∼6 K lower for benzene and ∼11 K lower for toluene), suggesting a slightly weaker interaction with the CI surface than with the bare HOPG surface.
The desorption of all four molecules from ASW layered and mixed ices is very different to that from CI and shows the large effect the presence of ASW can have on the desorption of these molecules. For the layered ices, the TPD spectra for all of the molecules (Figure 3) are dominated by volcano desorption that occurs as the ASW to CI phase transition occurs. Codesorption is also observed for all molecules, concurrent with the main desorption peak of the water, with the amount of codesorption increasing with increasing molecular size (Table 1). The exception to this is p-xylene where the high temperature codesorption peak is not assigned to the codesorption of the molecule with water, but instead is assigned to the desorption of the p-xylene from the surface of the HOPG following the desorption of the bulk of the water (so called surface desorption from HOPG–Table 1). This assignment was confirmed by additional experiments where p-xylene was layered on top of amorphous D2O. The peak temperature for the desorption of D2O is ∼10 K higher than that of H2O (Smith et al., 2011) and hence if the high temperature peak for p-xylene was due to codesorption then it would shift up in temperature in the presence of D2O. The peak position remains unchanged in the presence of D2O, hence the assignment of the high temperature p-xylene peak observed in the ASW layers to desorption directly from the bare HOPG surface. The trapping of the aromatic molecules benzene, toluene and o- and p-xylene by ASW, as shown in Figure 3, will clearly affect their desorption characteristics in astrophysical ices. The trapping of benzene in layered ASW ices has previously been observed by Thrower et al. (2009). Experiments have not previously been reported in the literature concerning the trapping of toluene and xylene in ASW.
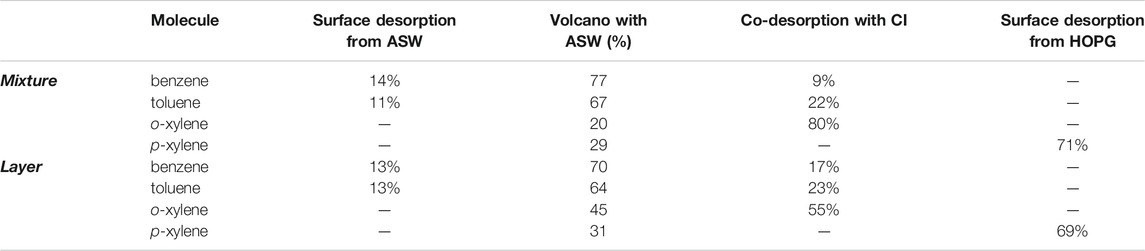
TABLE 1. The percentage of each desorption component from ASW mixed and layered ices containing mostly water and a small amount of the aromatic molecule of interest. The percentages are calculated from the integrated areas under the TPD peaks (9–17% data for the mixed ices, and up to 10 Lm of the molecule on a 50 Lm layer of ASW).
Although not clearly visible in Figure 3, benzene and toluene also show the desorption of a small amount of the molecule directly from the ASW surface. Figure 4 shows this effect more clearly for benzene, where the assignments of each of the TPD features for benzene from a layered ASW ice are also clearly labelled. The exact amount of desorption of each type is shown in Table 1, with benzene and toluene showing desorption from the ASW surface as well as volcano and codesorption.
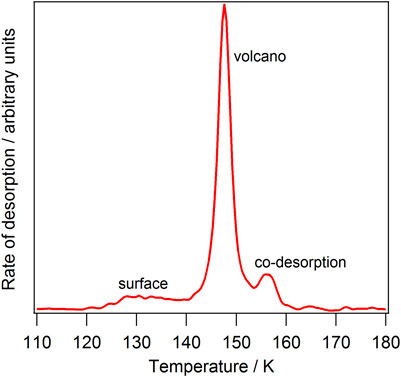
FIGURE 4. TPD spectrum of 5 Lm of benzene adsorbed in a layered configuration on top of 50 Lm ASW, showing the assignment of each TPD feature.
Figure 3 shows that the mixed ices show similar behaviour to the layered ices, with volcano and codesorption being observed for benzene, toluene and o-xylene, as for the layered ASW ices. As for the layered ices, the high temperature peak for p-xylene is also assigned to surface desorption from the HOPG surface following the desorption of the bulk of the water ice. For benzene and toluene, the desorption behaviour of layered and mixed ices is very similar, with similar proportions of the molecules desorbing as volcano and codesorption events as shown in Table 1. However, Figure 3 and Table 1 clearly show that for the o-xylene isomer the proportion of the molecule desorbing as so-called volcano desorption is considerably reduced in the mixed ice compared to in the layered ice. It is likely that this difference between benzene/toluene and o-xylene is due to the smaller size of the benzene and toluene compared to the xylene molecule, which means that they are more mobile when the ice is heated. For p-xylene, the behaviour in the layered and mixed ices is also very similar, with the p-xylene not being influenced very much by the presence of water ice. Regardless of the subtleties of the behaviour of the individual ice configurations, it is clear that the desorption of all of the ices is different in the presence of amorphous and crystalline water ice.
Infrared Data
Infrared data were also recorded for the layered and mixed ices. Infrared data can be of use for comparing with measured observational data (such as that which will be recorded by JWST in the future) and hence for helping to identify the nature and environment of astrophysical ices. Figure 5 shows surface infrared spectra for the four aromatic molecules in the pure ice and in a binary mixed ice with ASW. All data are recorded at base temperature (25–30 K) and show the changes in the infrared spectra that arise due to the presence of water ice. For the pure ices, band assignments have been previously made (Salter et al., 2018) and are not given here. For toluene, p- and o-xylene the spectra shown are those for a 1:1 mixture of the aromatic molecule with ASW which corresponds to 50 Lm of each species (11 nm ASW thickness plus ∼3 nm of the aromatic). Whilst these ice concentrations are considerably larger than those likely to be found in astrophysical environments, this mixture is shown as it exhibits a good signal to noise ratio in the spectra. Lower percentage ices were also investigated and all show the same infrared spectra. For benzene, ices with lower percentages of the aromatic molecule show different spectra compared to those at higher percentages. Hence the benzene mixed ice spectrum seen in Figure 5 corresponds to a 15% mixture of benzene with ASW, with a total ASW and benzene thickness of around 60 nm (300 Lm total dose). For all of the aromatic molecules studied, the pure ice spectrum shown in Figure 5 for comparison is for the same amount of the molecule as is present in the mixed ice to prevent any coverage effects affecting the comparisons.
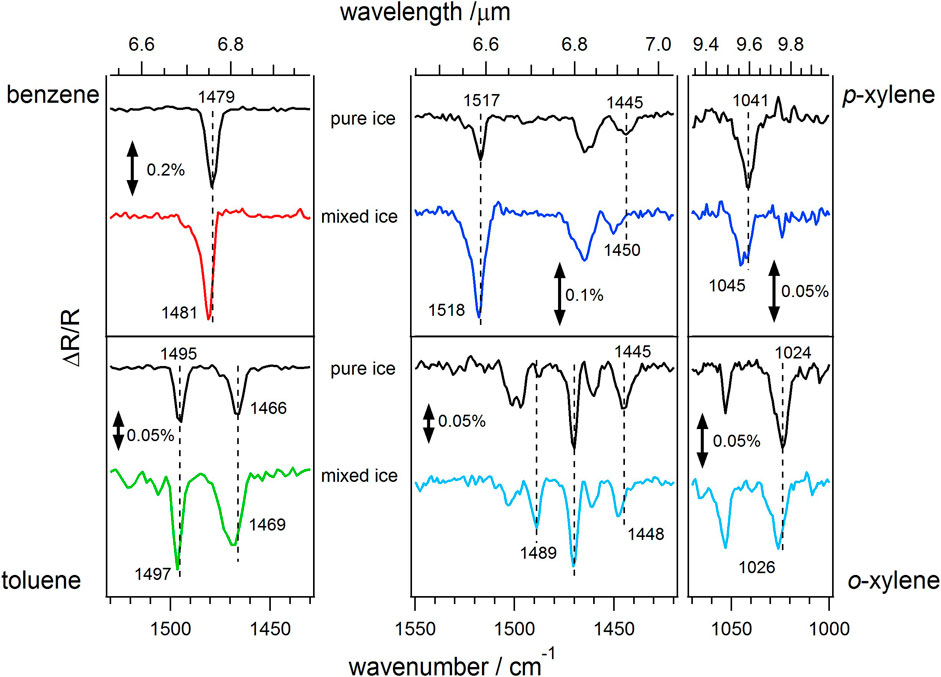
FIGURE 5. RAIR spectra of 50 Lm pure aromatic ices, and binary mixed aromatic and ASW ices, deposited between 25 and 30 K. For toluene, o-xylene and p-xylene, the spectra are for a 1:1 mixture of the aromatic molecule with ASW, 100 Lm total. The benzene spectrum is a 15% mixture with ASW, 300 Lm total. The left-hand panel shows benzene and toluene, top and bottom respectively, in the region between 1,530 and 1,430 cm−1, which contains the aromatic C=C stretching modes. The right-hand panels show p-xylene and o-xylene, top and bottom respectively. These show the regions between 1,550 and 1,420 cm−1, which contains the aromatic C=C stretching and methyl deformation modes, and the region between 1,070 and 1,000 cm−1 which contain the methyl rocking modes.
For benzene, the spectra in Figure 5 show a small blue shift of the C=C aromatic mode in the presence of ASW, from 1,479 cm−1 to 1,481 cm−1, due to the interaction of the benzene ring with ASW. This observation is in agreement with that of Thrower et al. (2009), who saw evidence of hydrogen bonding between benzene and ASW. A similar blue shift is also seen in Figure 5 for the C=C mode of toluene, from 1,495 cm−1 for pure toluene to 1,497 cm−1 for toluene in the mixed ice and this blue shift can again be assigned to an interaction between the toluene and the ASW. As seen in Figure 5 for p-xylene, a similar small blue shift is seen in the C=C aromatic mode (1,517 cm−1 for pure p-xylene and 1,518 cm−1 for p-xylene in the ASW mixture). However, a larger shift is seen for the CH3 modes at 1,445 cm−1 and 1,041 cm−1 for pure p-xylene. These modes blue shift to 1,450 cm−1 and 1,045 cm−1, respectively, in the presence of ASW, suggesting a larger interaction between the CH3 groups of the xylene and the ASW than between the benzene ring on the xylene and the ASW. The same effect is also observed for o-xylene (Figure 5), with the C=C modes at 1,489 cm−1 and 1,470 cm−1 shifting very little, if at all, in the presence of ASW. However, as seen for p-xylene, the CH3 modes at 1,445 cm−1 and 1,024 cm−1 for the pure o-xylene are blue shifted by 2–3 cm−1 in the presence of ASW, again suggesting that the interaction between the o-xylene and the ASW occurs primarily via the CH3 groups.
Discussion and Astrophysical Implications
Dense Molecular Clouds and Simple Model of Desorption From Astrophysical Ices
PAHs are ubiquitous in the ISM in the gas phase and it is also very likely they will be present in icy dust grain mantles (Allamandola et al., 1999). In dense molecular clouds, ice mantles on dust grains will be subject to many types of radiation, including UV and proton radiation. Ruiterkamp et al., 2005 have shown that the half-life of benzene within ice mantles of H2O, CO and CO2, subject to UV and proton radiation, is larger than the average lifetime for dense clouds. It is therefore possible that aromatic molecules on dust grains could survive into the hot core phase. Assuming that this is the case, it is hence important to understand the temperature at which they are released into the gas phase.
We can model the astrophysical desorption processes occurring in these regions, based on our experimental data. Although it is not a comprehensive model, it is able to give estimates of desorption temperatures and timescales under interstellar conditions. However, several assumptions need to be made to ensure that the results are astrophysically relevant. These concern the heating rate and sample thickness.
The simple model we employ is based on that of (Viti et al., 2004) and uses information gained from TPD spectra using leading edge analysis (Burke and Brown, 2010). We are able to extract the desorption order, desorption energy and the pre-exponential factor with the aid of the Polanyi-Wigner equation:
where ν is the pre-exponential factor, θ is the surface coverage, n is the desorption order, Edes is the desorption energy, t is time, R is the gas constant and T is the temperature. This equation is also used to find the rate of desorption in the model. TPD spectra are used to derive values for the kinetic parameters n, Edes and ν. Kinetic parameters for benzene, toluene, o-xylene and p-xylene relevant to the models in this publication, as well as for ASW and CI (Fraser et al., 2001), are given in Table 2. More details of the data analysis of the systems described in the results section are given elsewhere (Salter et al., 2018).
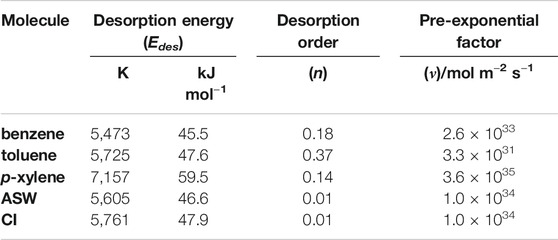
TABLE 2. Kinetic parameters derived from experimental data and used in the model for astrophysical ices. Parameters for benzene, toluene and p-xylene are those reported by Salter et al. (2018) and ASW and CI are those reported by Fraser et al. (2001).
Laboratory experiments occur on a much shorter timescale than processes in the ISM. Also, in the laboratory a linear timescale is used, 0.5 Ks−1. However, a non-linear heating rate is much more appropriate for astrophysical environments. To simulate the thermal processing of ices in the interstellar medium, in the vicinity of hot cores, we use the heating rate described in the equation below (Viti et al., 2004):
where T is the temperature of a star, t the time in years, and A and B are constants that depend on the temperature and mass of the star. For a 5 M◉ star, A = 4.856 × 10–2 and B = 0.6255 (Viti et al., 2004). In this paper we use 5 M◉ in our model since the two environments where benzene has been detected, CRL 618 (Cernicharo et al., 2001) and SMP LMC 11 (Bernard-Salas et al., 2006), are both in the transition stage between AGB and protoplanetary nebulae surrounding stars between 1 and 10 M◉. PAH emission has also been detected towards T Tauri and Herbig Ae/Be stars which are up to 8 M◉ (Geers et al., 2006). The thickness of the ice mantle on the dust grain is also an important consideration in the model. Based on estimates of ices accreted on dust grains, a suitable thickness to use for interstellar ices is 0.3 µm which corresponds to 9.5 × 1021 molecules m−2 (Brown and Bolina, 2007). This is much thicker than the ices in our laboratory experiments.
It is very unlikely that icy mantles would consist of only one molecule, especially if the molecule in question is one of the aromatics studied here. Instead, aromatic molecules would exist in small fractions (approximately 1% of the total) amongst a rich molecular composition. Water is one of the most abundant molecules on dust grains (van Dishoeck et al., 2013) and has been observed in CRL 618 (Herpin and Cernicharo, 2000), therefore it is important to consider how these molecules would behave in a water-rich ice mantle. As discussed in the results section, the particular ice environment affects the desorption temperature and profile of these aromatic molecules and hence the same effects would be expected to be present for ices in an astrophysical environment.
The simplest of the aromatic-water ice systems is the aromatic molecule on top of a layer of CI. To model the desorption of the aromatic molecule in this environment, the kinetic parameters for multilayer desorption of the relevant molecule from HOPG are used (Salter et al., 2018), given in Table 2. Although the aromatic molecule only accounts for 1% of the total ice, this still equates to 9.5 × 1019 molecules m−2 which is in the multilayer regime, therefore it is appropriate to use kinetic parameters for multilayer desorption. Multilayer desorption is also independent of the substrate and therefore values for desorption from HOPG (Salter et al., 2018) are appropriate. The top panel of Figure 6 shows the results of the simulation for a layer of the aromatic molecule on top of a thick (approximately 0.3 µm) layer of CI. It is clear that only one desorption event is observed in this system. The temperatures at which the molecules desorb on astrophysical timescales is much lower than the desorption temperatures observed in the laboratory. In the top panel of Figure 6, benzene and toluene have both completely desorbed by 86 K, and the xylene isomers desorb at 103 and 106 K, for o- and p-xylene respectively. This is in contrast to laboratory data where desorption temperatures for the four aromatic molecules on CI range from 131 K for benzene to 152 K for p-xylene, Figure 3.
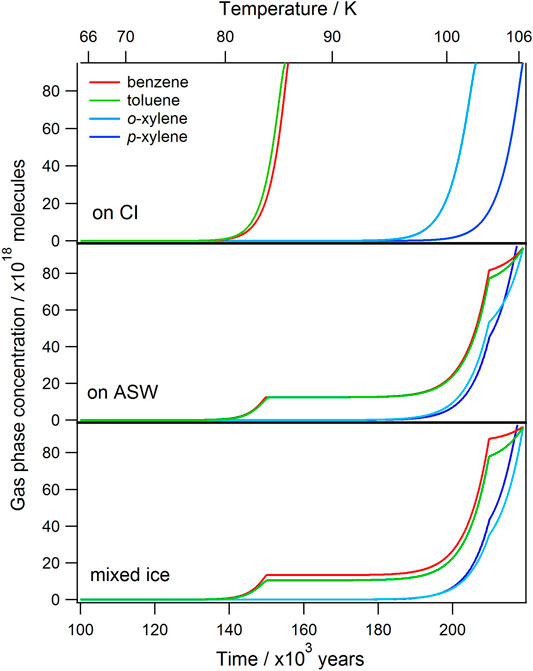
FIGURE 6. Gas phase concentration profiles for 1% of benzene, toluene, o-xylene and p-xylene in different water ice configurations, using astrophysical timescales for a 5 M◉ star. The top panel shows the data from a layered CI ice, the middle panel from a layered ASW ice, and the bottom from a mixed ASW ice.
To simulate how an aromatic ice in layered and mixed ASW systems would behave we have to account for how much of the desorption is due to surface, volcano and co-desorption events. The percentage of each desorption component, taken from the integrated areas under the TPD peaks, is given in Table 1. For the mixed ice systems, these were calculated using data for 9–17% mixtures of the molecule in ASW. For the molecule on top of an ASW layer, these values were calculated using data for the lowest exposures (up to 10 Lm) of the molecule adsorbed on top of a 50 Lm layer of ASW. From Table 1, and from the data in Figure 3, we can see that volcano desorption is dominant in layered and mixed ices for both benzene and toluene, accounting for between 64 and 77%, whereas it accounts for a smaller proportion of the xylene isomer desorption. To model the components of the ice which are due to volcano and co-desorption we use the kinetic parameters for ASW and CI (Fraser et al., 2001), given in Table 2. To model the surface component of desorption from ASW in the interstellar ice, the kinetic parameters for multilayer desorption of the molecule from HOPG are used, as for layered CI ices.
The middle and bottom panels of Figure 6 show the results of the simulation for 1% of the aromatic molecules in an ASW layered and mixed ice, respectively. The mixed and layered ices show similar profiles with only subtle differences, which are due to the contributions from each desorption event, given in Table 1. The desorption temperatures for the molecules in layered ASW and mixed ices, like the CI layered ices, are much lower for astrophysical timescales compared to those observed in the laboratory. However, unlike CI layered ices, several desorption events are observed and these correspond to the equivalent events observed in the experimental data, as described earlier. Benzene and toluene desorb in three phases corresponding to pure ice desorption, volcano desorption with ASW and co-desorption with CI. A large proportion of benzene and toluene is not released until volcano desorption occurs between 97 and 104 K, due to the molecules being trapped in the water ice pores. This is in contrast to the behaviour of benzene and toluene in layered CI systems, which desorb at lower temperatures (approximately 20 K earlier) and on shorter timescales. The xylene isomers exhibit two desorption events, as detailed in Table 1. They also begin to desorb at later times, and higher temperatures, approximately 40,000 years later than benzene and toluene. The gas phase profiles of the xylene isomers are quite similar, with the two desorption events occurring at similar temperatures. In all three environments, the temperature and timescale difference between benzene and toluene and the two xylene isomers is quite pronounced, showing how the small change in molecular weight can have a large impact on thermal desorption. In the ASW layered and mixed ices, the xylene isomers remain trapped in the icy mantles on dust grains for longer timescales and until higher temperatures, compared to benzene and toluene.
These simulations demonstrate how future observations of aromatic molecules in dense molecular clouds at known temperatures, or times, could reveal information about the environment that the aromatic molecules are found in. Although real astrophysical ices will be much more complicated than binary ices, the model still provides a useful comparison. If gas-phase benzene and toluene are detected at earlier times, this would imply that they come from layered CI systems. The behaviour of the aromatic molecules in ASW layered and mixed ice systems are only subtly different and so it may be difficult to identify which of these two environments they are in. The desorption profile of p-xylene is similar for all three studied systems, therefore it would also be difficult to distinguish which environment it is in.
Categorisation of Molecules
In Thermal desorption data we showed how small changes in molecular weight and structure can affect the thermal desorption behaviour of small aromatic molecules, especially in the presence of water ice. Previously introduced categories based on the thermal desorption behaviour of small volatile molecules (Collings et al., 2004) and complex organic molecules (COMs) (Burke et al., 2015) in water-rich ices can also be used to classify the aromatic molecules studied here. These categorisations help us to understand different molecular types within a wider context and also help towards developing models for molecules in astrophysical environments.
The original classifications of (Collings et al., 2004) were CO-like, water-like, or intermediate species. These show volcano and co-desorption as well as monolayer and multilayer desorption, a single co-desorption event, and volcano and co-desorption with a small monolayer, respectively. More recently introduced classifications (Burke et al., 2015) are described as complex intermediate or complex water-like species. Complex intermediate species are defined by their weak interaction with water, resulting in mainly volcano desorption with small amounts of co-desorption and other additional events. These species also have a lower desorption temperature in pure ice than water. On the other hand, complex water-like species have higher desorption temperatures in pure ices due to their ability to form hydrogen bonds. In mixed ices, co-desorption is the principal event due to limited trapping within the water ice. For higher percentages of the molecule within a mixed ice, the crystallisation of water ice itself may be affected.
In mixed water-ice systems, benzene and toluene behave as complex intermediate species; they are dominated by volcano desorption due to the trapping of the molecule within the pore network of ASW, and exhibit only a weak interaction with water. They also have small monolayer and co-desorption events. The behaviour of the xylene isomers is more difficult to assign, as they behave partly as complex intermediate and partly as complex water-like molecules. Due to their size, they are not able to penetrate the ASW pore network to the same extent as benzene and toluene. However volcano desorption is still observed.
The polarity and size of aromatic molecules clearly has an effect on their desorption in the presence of H2O and hence on their categorisation. However, for binary mixtures containing only a few percent of the aromatic molecule, size rather than polarity is the dominant factor. We predict that as the number of aromatic rings increases, it will be more difficult for the molecule to be trapped within the water ice. The size of the volcano desorption peak will decrease and the thermal desorption behaviour will be dominated by a single desorption event, either coincident with water or occurring at a higher temperature. Currently, we are not able to confirm our predictions as there have been no TPD studies on larger aromatic molecules in water ices. However, TPD data of pure naphthalene, coronene, ovalene and fullerene adsorbed onto an HOPG surface show that as the size of the PAH increases so does the desorption temperature and binding energy (Zacharia et al., 2004; Ulbricht et al., 2006). This is a trend that we have also observed in our data, shown in Table 2.
Using Infrared Spectra to Identify the Ice Environment
The data in Figure 5 show that the infrared spectra of the aromatic molecules studied here are all affected by the presence of water ice, showing evidence of interactions between the molecules and the water ice. This is in agreement with recent theoretical studies which have shown evidence for the changing vibrational signature of PAHs on water ice (Clark and Benoit, 2019). This interaction between the aromatic molecule and the water ice most likely occurs via the dangling OH species which are found on the ASW surface, as shown by Michoulier et al. (2020). Whilst infrared spectra for the aromatic molecules investigated here are unchanged in the presence of CI, bands are shifted in the presence of ASW as seen in Figure 5 and described in Infrared data. Hence with the high infrared spectral resolution expected with JWST it is anticipated that it will be possible to determine whether aromatic molecules such as those investigated here are found in environments containing CI or ASW.
Conclusion
We have studied the thermal desorption behaviour of four small aromatic molecules, benzene, toluene, o-xylene and p-xylene, in the presence of water on layered CI and ASW ices, and in a mixed ASW ice. TPD results show that the desorption behaviour of these four molecules is strongly affected by the particular water ice environment. In binary layered ASW and mixed ASW ices, all four molecules are trapped to some extent in the ASW ice, and therefore their desorption is dictated by the water ice. The desorption of the aromatic molecules from a CI layer shows only one desorption event, with benzene and toluene desorbing before CI, and the xylene isomers desorbing at a similar temperature to CI. Based on the experimental data, a simple thermal desorption model of benzene, toluene and xylene in interstellar ices has been simulated utilising astrophysical timescales. The temperatures and timescales of the gas phase concentration of the aromatic molecules derived from the simulation could help aid the identification of the environment the molecules are found in. Gas phase benzene and toluene from layered CI ices will be detected at lower temperatures and on shorter timescales than from ASW containing ice configurations. In comparison, the xylene isomers may be detected at higher temperatures and on longer timescales. Information about the ice environment can also be obtained from infrared data, and may offer a useful comparison for measured observational data. RAIRS shows that the presence of ASW affects the infrared spectra of the aromatic molecules, with differences observed for the mixed ices compared to those in layered configurations.
Data Availability Statement
The raw data supporting the conclusions of this article will be made available by the authors, without undue reservation.
Author Contributions
The initial conception of the research idea, the day to day supervision of the research and the conception and writing of the programme used to model the data under astrophysical conditions was done by WB. Benzene and toluene experimental data were recorded by JS. The ortho- and para-xylene experimental data were recorded by TS and LB. The manuscript was written by TS with editing undertaken by WB. All authors contributed to the article and approved the submitted version.
Funding
This work was funded by the STFC under Grant No. ST/M000869/1. The STFC also funded a post-doctoral fellowship for TS. under this grant code. JS. thanks the University of Sussex for a PhD studentship.
Conflict of Interest
The authors declare that the research was conducted in the absence of any commercial or financial relationships that could be construed as a potential conflict of interest.
References
Abplanalp, M. J., Frigge, R., and Kaiser, R. I. (2019). Low-temperature synthesis of polycyclic aromatic hydrocarbons in titan’s surface ices and on airless bodies. Sci. Adv. 5, eaaw5841. doi:10.1126/sciadv.aaw5841
Allamandola, L. J., Hudgins, D. M., and Sandford, S. A. (1999). Modeling the unidentified infrared emission with combinations of polycyclic aromatic hydrocarbons. Astrophys. J. 511, L115–L119. doi:10.1086/311843
Bahr, S., and Kempter, V. (2007). Interaction of benzene with amorphous solid water adsorbed on polycrystalline Ag. J. Chem. Phys. 127, 074707. doi:10.1063/1.2759914
Bernard-Salas, J., Peeters, E., Sloan, G. C., Cami, J., Guiles, S., and Houck, J. R. (2006). The spitzer IRS spectrum of SMP LMC 11. Astrophys. J. 652, L29–L32. doi:10.1086/509777
Blommaert, J. A. D. L., Cami, J., Szczerba, R., and Barlow, M. J. (2005). Late stages of stellar evolution. Space Sci. Rev. 119, 215–243. doi:10.1007/s11214-005-8057-y
Brown, W. A., and Bolina, A. S. (2007). Fundamental data on the desorption of pure interstellar ices. Mon. Not. R. Astron. Soc. 374, 1006–1014. doi:10.1111/j.1365-2966.2006.11216.x
Burke, D. J., and Brown, W. A. (2010). Ice in space: surface science investigations of the thermal desorption of model interstellar ices on dust grain analogue surfaces. Phys. Chem. Chem. Phys. 12, 5947. doi:10.1039/b917005g
Burke, D. J., Puletti, F., Woods, P. M., Viti, S., Slater, B., and Brown, W. A. (2015). Trapping and desorption of complex organic molecules in water at 20 K. J. Chem. Phys. 143, 164704. doi:10.1063/1.4934264
Cami, J., Bernard-Salas, J., Peeters, E., and Malek, S. E. (2010). Detection of C60 and C70 in a young planetary nebula. Science 329, 1180–1182. doi:10.1126/science.1192035
Campbell, E. K., Holz, M., Gerlich, D., and Maier, J. P. (2015). Laboratory confirmation of C60+ as the carrier of two diffuse interstellar bands. Nature 523, 322–323. doi:10.1038/nature14566
Cernicharo, J., Heras, A. M., Tielens, A. G. G. M., Pardo, J. R., Herpin, F., Guélin, M., et al. (2001). Infrared space observatory’s discovery of C4H2, C6H2, and benzene in CRL 618. Astrophys. J. 546, L123–L126. doi:10.1086/318871
Clark, V. H. J., and Benoit, D. M. (2019). The vibrational signatures of polyaromatic hydrocarbons on an ice surface. Proc. Int. Astron. Union 15, 468–470. doi:10.1017/S174392131900944X
Collings, M. P., Anderson, M. A., Chen, R., Dever, J. W., Viti, S., Williams, D. A., et al. (2004). A laboratory survey of the thermal desorption of astrophysically relevant molecules. Mon. Not. R. Astron. Soc. 354, 1133–1140. doi:10.1111/j.1365-2966.2004.08272.x
Collings, M. P., Dever, J. W., and McCoustra, M. R. S. (2014). The interaction of carbon monoxide with model astrophysical surfaces. Phys. Chem. Chem. Phys. 16, 3479. doi:10.1039/c3cp54024c
Contreras, C. S., and Salama, F. (2013). Laboratory investigations of polycyclic aromatic hydrocarbon formation and destruction in the circumstellar outflows of carbon stars. Astrophys. J. Suppl. Ser. 208, 6. doi:10.1088/0067-0049/208/1/6
Cox, N. L. J., Pilleri, P., Berné, O., Cernicharo, J., and Joblin, C. (2016). Polycyclic aromatic hydrocarbons and molecular hydrogen in oxygen-rich planetary nebulae: the case of NGC 6720. Mon. Not. R. Astron. Soc. Lett. 456, L89–L93. doi:10.1093/mnrasl/slv184
Dangi, B. B., Parker, D. S. N., Kaiser, R. I., Jamal, A., and Mebel, A. M. (2013). A combined experimental and theoretical study on the gas-phase synthesis of toluene under single collision conditions. Angew. Chem. Int. Ed. 52, 7186–7189. doi:10.1002/anie.201302344
Dawes, A., Pascual, N., Mason, N. J., Gärtner, S., Hoffmann, S. V., and Jones, N. C. (2018). Probing the interaction between solid benzene and water using vacuum ultraviolet and infrared spectroscopy. Phys. Chem. Chem. Phys. 20, 15273–15287. doi:10.1039/C8CP01228H
Duley, W. W., Zaidi, A., Wesolowski, M. J., and Kuzmin, S. (2014). Small molecules from the decomposition of interstellar carbons. Mon. Not. R. Astron. Soc. 447, 1242–1246. doi:10.1093/mnras/stu2508
Edridge, J. L., Freimann, K., Burke, D. J., and Brown, W. A. (2013). Surface science investigations of the role of CO2 in astrophysical ices. Philos. Trans. R. Soc. A Math. Phys. Eng. Sci. 371, 20110578. doi:10.1098/rsta.2011.0578
Fraser, H. J., Collings, M. P., McCoustra, M. R. S., and Williams, D. A. (2001). Thermal desorption of water ice in the interstellar medium. Mon. Not. R. Astron. Soc. 327, 1165–1172. doi:10.1046/j.1365-8711.2001.04835.x
Frenklach, M., and Feigelson, E. D. (1989). Formation of polycyclic aromatic hydrocarbons in circumstellar envelopes. Astrophys. J. 341, 372. doi:10.1086/167501
Geers, V. C., Augereau, J.-C., Pontoppidan, K. M., Dullemond, C. P., Visser, R., Kessler-Silacci, J. E., et al. (2006). C2D Spitzer-IRS spectra of disks around T Tauri stars. Astron. Astrophys. 459, 545–556. doi:10.1051/0004-6361:20064830
Geers, V. C., van Dishoeck, E. F., Pontoppidan, K. M., Lahuis, F., Crapsi, A., Dullemond, C. P., et al. (2009). Lack of PAH emission toward low-mass embedded young stellar objects. Astron. Astrophys. 495, 837–846. doi:10.1051/0004-6361:200811001
Herpin, F., and Cernicharo, J. (2000). O-bearing molecules in carbon-rich proto–planetary objects. Astrophys. J. 530, L129–L132. doi:10.1086/312507
Jones, B. M., Zhang, F., Kaiser, R. I., Jamal, A., Mebel, A. M., Cordiner, M. A., et al. (2011). Formation of benzene in the interstellar medium. Proc. Natl. Acad. Sci. 108, 452–457. doi:10.1073/pnas.1012468108
Kaiser, R. I., Nguyen, T. L., Le, T. N., and Mebel, A. M. (2001). An ab initio investigation of reactions of carbon atoms, C(3Pj), with C2H4 and C3H6 in the interstellar medium. Astrophys. J. 561, 858–863. doi:10.1086/323261
Mackie, C. J., Peeters, E., Bauschlicher, C. W., and Cami, J. (2015). Characterizing the infrared spectra of small, neutral, fully dehydrogenated polycyclic aromatic hydrocarbons. Astrophys. J. 799, 131. doi:10.1088/0004-637X/799/2/131
Mattioda, A. L., Hudgins, D. M., Boersma, C., Bauschlicher, C. W., Ricca, A., Cami, J., et al. (2020). The NASA ames PAH IR spectroscopic database: the laboratory spectra. Astrophys. J. Suppl. Ser. 251, 22. doi:10.3847/1538-4365/abc2c8
McEwan, M. J., Scott, G. B. I., Adams, N. G., Babcock, L. M., Terzieva, R., and Herbst, E. (1999). New H and H2 reactions with small hydrocarbon ions and their roles in benzene synthesis in dense interstellar clouds. Astrophys. J. 513, 287–293. doi:10.1086/306861
Michoulier, E., Ben Amor, N., Rapacioli, M., Noble, J. A., Mascetti, J., Toubin, C., et al. (2018a). Theoretical determination of adsorption and ionisation energies of polycyclic aromatic hydrocarbons on water ice. Phys. Chem. Chem. Phys. 20, 11941–11953. doi:10.1039/C8CP01175C
Michoulier, E., Noble, J. A., Simon, A., Mascetti, J., and Toubin, C. (2018b). Adsorption of PAHs on interstellar ice viewed by classical molecular dynamics. Phys. Chem. Chem. Phys. 20, 8753–8764. doi:10.1039/C8CP00593A
Michoulier, E., Toubin, C., Simon, A., Mascetti, J., Aupetit, C., and Noble, J. A. (2020). Perturbation of the surface of amorphous solid water by the adsorption of polycyclic aromatic hydrocarbons. J. Phys. Chem. C 124, 2994–3001. doi:10.1021/acs.jpcc.9b09499
Noble, J. A., Congiu, E., Dulieu, F., and Fraser, H. J. (2012). Thermal desorption characteristics of CO, O2 and CO2 on non-porous water, crystalline water and silicate surfaces at submonolayer and multilayer coverages. Mon. Not. R. Astron. Soc. 421, 768–779. doi:10.1111/j.1365-2966.2011.20351.x
Peeters, E., Bauschlicher, C. W., Allamandola, L. J., Tielens, A. G. G. M., Ricca, A., and Wolfire, M. G. (2017). The PAH emission characteristics of the reflection nebula NGC 2023. Astrophys. J. 836, 198. doi:10.3847/1538-4357/836/2/198
Ruiterkamp, R., Peeters, Z., Moore, M. H., Hudson, R. L., and Ehrenfreund, P. (2005). A quantitative study of proton irradiation and UV photolysis of benzene in interstellar environments. Astron. Astrophys. 440, 391–402. doi:10.1051/0004-6361:20042090
Salter, T. L., Stubbing, J. W., Brigham, L., and Brown, W. A. (2018). A TPD and RAIRS comparison of the low temperature surface behavior of benzene, toluene, and xylene on graphite. J. Chem. Phys. 149, 164705. doi:10.1063/1.5051134
Sellgren, K., Werner, M. W., Ingalls, J. G., Smith, J. D. T., Carleton, T. M., and Joblin, C. (2010). C60 in reflection nebulae. Astrophys. J. 722, L54–L57. doi:10.1088/2041-8205/722/1/L54
Shan, J., Suton, M., and Lee, L. C. (1991). 3.3 micron emission from ultraviolet excitation of some aromatic molecules. Astrophys. J. 383, 459. doi:10.1086/170803
Sivaraman, B., Mukherjee, R., Subramanian, K. P., and Banerjee, S. B. (2014). Benzene Formation on interstellar icy mantles containing propargyl alcohol. Astrophys. J. 798, 72. doi:10.1088/0004-637X/798/2/72
Smith, R. S., Huang, C., Wong, E. K. L., and Kay, B. D. (1997). The molecular volcano: abrupt CCl4 desorption driven by the crystallization of amorphous solid water. Phys. Rev. Lett. 79, 909–912. doi:10.1103/PhysRevLett.79.909
Smith, R. S., Matthiesen, J., Knox, J., and Kay, B. D. (2011). Crystallization kinetics and excess free energy of H2O and D2O nanoscale films of amorphous solid water. J. Phys. Chem. A. 115, 5908–5917. doi:10.1021/jp110297q
Souda, R. (2004). Interactions of water with pyridine and benzene studied by TOF-SIMS. J. Phys. Chem. B 108, 283–288. doi:10.1021/jp035996p
Thrower, J. D., Collings, M. P., Rutten, F. J. M., and McCoustra, M. R. S. (2009). Thermal desorption of C6H6 from surfaces of astrophysical relevance. J. Chem. Phys. 131, 244711. doi:10.1063/1.3267634
Tielens, A. G. G. M. (2008). Interstellar polycyclic aromatic hydrocarbon molecules. Annu. Rev. Astron. Astrophys. 46, 289–337. doi:10.1146/annurev.astro.46.060407.145211
Ulbricht, H., Zacharia, R., Cindir, N., and Hertel, T. (2006). Thermal desorption of gases and solvents from graphite and carbon nanotube surfaces. Carbon N. Y. 44, 2931–2942. doi:10.1016/j.carbon.2006.05.040
van Dishoeck, E. F., Herbst, E., and Neufeld, D. A. (2013). Interstellar water chemistry: from laboratory to observations. Chem. Rev. 113, 9043–9085. doi:10.1021/cr4003177
Viti, S., Collings, M. P., Dever, J. W., McCoustra, M. R. S., and Williams, D. A. (2004). Evaporation of ices near massive stars: models based on laboratory temperature programmed desorption data. Mon. Not. R. Astron. Soc. 354, 1141–1145. doi:10.1111/j.1365-2966.2004.08273.x
Woods, P. M., Millar, T. J., Herbst, E., and Zijlstra, A. A. (2003). The chemistry of protoplanetary nebulae. Astron. Astrophys. 402, 189–199. doi:10.1051/0004-6361:20030215
Woods, P. M., Millar, T. J., Zijlstra, A. A., and Herbst, E. (2002). The synthesis of benzene in the proto–planetary nebula CRL 618. Astrophys. J. 574, L167–L170. doi:10.1086/342503
Woods, P. M., and Willacy, K. (2007). Benzene Formation in the inner regions of protostellar disks. Astrophys. J. 655, L49–L52. doi:10.1086/511680
Keywords: polycyclic aromatic hydrocarbons, water ice, temperature programmed desorption, small aromatic molecules, infrared spectroscopy, graphitic grains, desorption
Citation: Salter TL, Stubbing JW, Brigham L and Brown WA (2021) Using Laboratory Investigations to Aid the Identification of Small Aromatic Molecules in Water-Containing Astrophysical Ices. Front. Astron. Space Sci. 8:644277. doi: 10.3389/fspas.2021.644277
Received: 20 December 2020; Accepted: 12 February 2021;
Published: 24 March 2021.
Edited by:
Piero Ugliengo, University of Turin, ItalyReviewed by:
Albert Rimola, Autonomous University of Barcelona, SpainMarzio Rosi, University of Perugia, Italy
Copyright © 2021 Salter, Stubbing, Brigham and Brown. This is an open-access article distributed under the terms of the Creative Commons Attribution License (CC BY). The use, distribution or reproduction in other forums is permitted, provided the original author(s) and the copyright owner(s) are credited and that the original publication in this journal is cited, in accordance with accepted academic practice. No use, distribution or reproduction is permitted which does not comply with these terms.
*Correspondence: Wendy A. Brown, dy5hLmJyb3duQHN1c3NleC5hYy51aw==
†Present address: Tara L. Salter, Department of Earth Science and Engineering, Imperial College London, London, United KingdomJames W. Stubbingv, Surrey Space Centre, University of Surrey, Guilford, Surrey, United Kingdom