- Istituto Nazionale di Astrofisica (INAF)–Osservatorio Astronomico di Capodimonte, Naples, Italy
Refractory dust grains have an important role to play in the chemistry of star and planet-forming regions. Their surfaces interact with interstellar gas and act as a catalyst for the formation of simple and complex molecules in space. Several mechanisms have been invoked to explain how molecular hydrogen is formed in reactions on dust grain surfaces in different regions of space. In this article, we give an overview of our understanding of the laboratory experiments, conducted over the last 20 years, that deal with H2 formation on interstellar grain analogs in space simulated conditions.
1. Introduction
The interstellar medium (ISM) is the tenuous gas and dust that pervades space between the stars in a galaxy. Interstellar gas is the major component that accounts for 99% of the interstellar mass, of which 70% is hydrogen either in atomic or molecular form (Ferriere, 2001). Solid refractory dust, which is made up of either silicates or carbonaceous materials, forms the remaining 1% of the interstellar mass. Molecular hydrogen (H2) is by far the most abundant molecule in the Universe and it plays a crucial role in star formation. It also directly or indirectly contributes to all the reactions involved in the formation of simple and complex molecules in the ISM.
Naturally, a lot of interest lies in the understanding of how H atoms convert to H2 in different environments of space. In regions of low gas density and temperatures, H2 cannot form efficiently in the gas phase by the association of two H atoms. Therefore, the need to explore an alternative route of molecule formation becomes crucial to explain the observed abundances. Van de Hulst (1948) was the first to propose a significant role of dust grains in molecule formation. Dust grain surfaces can impart catalytic effect by (i) acting as a site for atoms to rapidly diffuse and react; (ii) acting as the third body to dissipate the recombination energy; (iii) reducing the reaction activation barriers. Based on the rate constant calculations of formation and destruction processes of H2 in interstellar space, it was suggested that recombination of H atoms on dust grain surface could proceed efficiently between 5 and 20 K (Gould and Salpeter, 1963). To explore the possibility of extending an efficient recombination of H atoms on the grain surface beyond 20 K, calculations were made with a more realistic model of interstellar grain having lattice defects or impurity sites rather than regular grain surface (Hollenbach and Salpeter, 1971). The H2 formation rate, calculated for the dust grain catalyzed reaction, was found to be compatible with the observed H2 abundances in diffuse ISM. A large number of experimental studies was also devoted to the understanding of H2 formation. However, their relevance to astrophysical conditions is not easy to derive since these studies were not performed on realistic interstellar grain analog surfaces and conditions encountered in space. For an account of those studies, see Pirronello et al. (2000). It was only in the late 1990s that laboratory experiments were performed on well-characterized interstellar grain analogs in simulated space conditions to examine the nuances of gas-grain chemistry relating to H2 formation. For a systematic review on H2 formation on interstellar dust grains, see Vidali (2013) and Wakelam et al. (2017). The aim of the present paper is to outline relevant laboratory experiments that discuss the catalytic role of the refractory dust grains in H2 formation on different grain temperatures found in varied regions of space.
2. Refractory Interstellar Dust Grains
Refractory dust grains are primarily formed in the circumstellar envelope of evolved stars during the asymptotic giant branch phase. The chemical composition of the dust is determined by the composition of the evolved stars producing it. There are two main classes of grains whose composition depends on the C/O abundance ratio in the stellar atmosphere. The carbon-rich outflows (C/O>1) lead to the production of carbonaceous grains (e.g., amorphous carbon, silicon carbide, titanium carbide, etc.). In oxygen-rich envelopes (C/O <1), silicates (olivine and pyroxene composition) and other metal oxides (e.g., MgO, FeO, Fe3O4, Al2O3) are formed. Other sources of refractory grains are the nova and supernova ejecta and R Coronae Borealis variable stars.
During the journey of dust grains through the ISM, they interact with cosmic rays, UV photons, atoms which induce many effects such as amorphization, sputtering, and implantation. As the dust traverses through dense and UV-shielded molecular clouds, various simple molecules (mainly H2O) accrete on them forming icy mantles. Observational evidence suggest that silicates, carbonaceous grains and water ice mantles are predominantly amorphous in the ISM. According to interstellar dust models, silicate grains are mainly sub-micron in size, while carbon grains range in size from nano to sub-micron (Draine, 2003; Jones et al., 2013). Krugel (2007) and Draine (2010) study provide a detailed review of the structure and composition of dust.
3. Preparation of Laboratory Dust Analogs
Laboratory dust analogs are prepared with the aim to understand how dust grains are formed, destroyed and altered in different astrophysical environments, and how these processes affect their spectral properties. Realistic analogs, which simulate the chemical composition and properties of the cosmic dust, have also been considered to investigate the role of dust grain surfaces for molecule formation. Different methods, such as arc discharge, laser ablation, chemical vapor deposition, flame synthesis, ion sputtering, sol-gel, have been applied in the laboratory for the production of dust analogs. The prepared analogs are thoroughly characterized by different techniques among which wet chemical analysis, scanning electron microscope, energy dispersive x-ray and x-ray diffraction are commonly used. A detailed report on the preparation and characterization of silicate and carbonaceous grain analogs can be found in Colangeli et al. (2003), Henning (2010), and Jäger et al. (2015).
4. Reaction Mechanisms Between Adsorbate and Surface
Physisorption and chemisorption are the two types of interactions that are important to consider between an atom and a surface. The former refers to weak and long range van der Waals forces that involve binding energy of the order of tens of meV. In the second case, there are strong and localized chemical bonds with a binding energy of the order of a few eV. Formation of H2 on surfaces can occur through three main mechanisms namely Langmuir–Hinshelwood (LH), Eley–Rideal (ER), and Harris–Kasemo (HK, commonly known as hot atom). In LH mechanism, at first atoms are thermally accommodated on the surface, then they diffuse (via thermal hopping or quantum tunneling) and react to form molecules at the surface temperature. In the other two processes, atoms from the gas phase directly (for ER) or indirectly (for HK) hit other pre-adsorbed atoms on the surface. The HK is considered as an intermediate mechanism between LH and ER.
In the case of H2 formation in space, H atoms can remain physisorbed on the grain surface until its temperature is low enough to prevent desorption. This happens in the cold interstellar clouds where grain temperatures are typically <20 K. However, in these conditions H atoms are mobile enough to initiate reactions mainly through LH mechanism. Besides, in warmer environments such as photodissociation regions (PDRs), while physisorption fails to hold H atoms on the grain surface, chemisorbed H atoms that remain on the surface take part in formation reaction. In this regard, atoms are immobile and LH pathway can be fairly ruled out. Atoms from the gas phase with enough energy are needed to overcome the activation barrier to react on the surface, and molecule formation takes place via ER and HK mechanism. In the sub-sections that follow, we describe the H2 formation in laboratory experiments performed at low and high grain surface temperatures.
4.1. H2 Formation at Low Grain Surface Temperature
The first set of experiments to investigate H2 formation on a dust grain analog was performed on a natural, polycrystalline olivine sample (Pirronello et al., 1997a,b). Together with the prompt formation of molecular hydrogen during irradiation of the surface by H atoms, the temperature programmed desorption (TPD) technique was applied to measure the recombination efficiency of H and D atoms (possessing low kinetic energies) that were still on the surface for a range of dust grain temperatures (<20 K) encountered in the ISM (see Figure 1). The HD formation efficiency was found to be lower than the other theoretical model based estimates. The efficiency decreases with increasing sample temperature due to a short residence time of physisorbed H/D atoms at high temperatures. This aspect is also demonstrated by the trend with temperature of the sticking probability of H atoms on a dust analog surface (see Figure 2). HD formation on olivine followed a second order kinetics at low coverages of H and D atoms. This means that atoms continued to remain localized on the surface without recombining till the surface temperature was raised. HD formation experiments were also carried out on amorphous carbon grains (prepared using the arc-discharge method) in the experimental conditions identical to the previous set (Pirronello et al., 1999). HD recombination efficiency was found to be higher in amorphous carbon grains than the silicates (Figure 1). The authors attributed this difference to the variation in surface morphology. A rate equation model, reproducing the above experimental data, confirmed that H2 molecules can form efficiently only at low temperatures i.e., in the range 6–10 K for olivine and 10–20 K for amorphous carbon grains (Katz et al., 1999). Identical experiments of HD formation, as discussed above, were performed on amorphous olivine with different compositions prepared by laser ablation of mixed oxides used in varying proportions (Perets et al., 2007; Vidali et al., 2007, 2009). Amorphous silicate grains were found to be a more efficient catalyst for H2 formation than polycrystalline olivine because H2 formation efficiency is extended and shifted to higher grain temperature in the case of amorphous silicates. It was also suggested that H2 molecules thermalize to the grain temperature before desorption. In another study, HD formation on single crystal olivine was found to be less effective than both amorphous and polycrystalline silicates (He et al., 2011). An interesting class of laboratory experiments that concerns H2 formation at low dust grain temperature are reactions on amorphous water ice mantles covering the refractory dust grains. It has been shown that such surfaces at 8 K possess shallow and deep adsorption sites that allows trapped H atoms to wait for the arrival of other H atoms from the gas phase in the low flux conditions of interstellar clouds and facilitate H2 formation (Watanabe et al., 2010). Although H2 formation on interstellar ices has been a topic of much interest, it is beyond the scope of the present article (see, for example, Watanabe and Kouchi (2008) and Hama and Watanabe (2013) for a review on this subject.
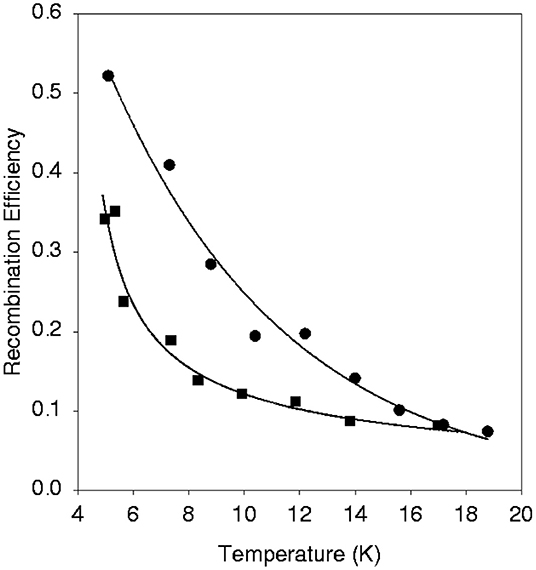
Figure 1. HD recombination efficiency as a function of temperature on a surface of amorphous carbon grains (top, filled circles) and crystalline olivine (bottom, filled squares). Lines are guides to the eye. From Pirronello et al. (1999), reproduced with permission from Astronomy & Astrophysics, ©ESO.
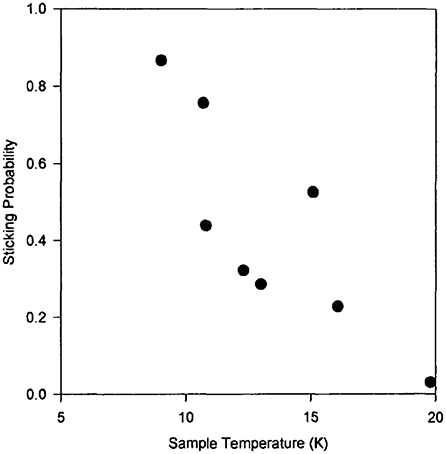
Figure 2. Sticking probability of H atoms on amorphous carbon sample as a function of the sample temperature. From Pirronello et al. (2000), reproduced with permission of the Cambridge University Press through PLSclear.
The process of H2 formation raises an important concern about the fate of the 4.5 eV energy released during H atom recombination. In other words, we need to understand how this energy is distributed between a grain surface and the nascent molecule ejecting from the surface. TPD and resonance enhanced multiphoton ionization (REMPI) spectroscopy were simultaneously applied to study energy partitioning as a function of sample temperature during D2 formation on amorphous silicate films (Lemaire et al., 2010). The films were prepared by electron beam evaporation of a San Carlos olivine on a gold coated substrate. The D2 molecules, desorbed promptly just after formation, were detected in high excited states (vibrational level = 4, rotational level = 2). It was inferred that the morphology of the surface influences the internal energy of the formed molecules. In fact, D2 molecules ejected from the crystalline sample were found to have a lower rotational energy distribution than those desorbed from amorphous samples of varying stoichiometry prepared by laser ablation technique (Gavilan et al., 2014). Similar sort of experiments were also performed on highly oriented pyrolitic graphite (HOPG), an idealized representation of an interstellar grain surface, between 15 and 50 K (Creighan et al., 2006; Islam et al., 2007; Latimer et al., 2008). In these experiments, HD molecules that were leaving the surface upon formation were detected in the vibrationally excited state up to the 7th level. Such investigations are important not only to look for observational signatures during H2 formation in space, but also to develop accurate theoretical models.
4.2. H2 Formation at High Grain Surface Temperature
For H2 formation at high dust grain temperatures, reaction mechanisms that involve tightly bound chemisorbed H atoms on graphite and highly defected carbon/ silicate surface were theoretically proposed (Cazaux and Tielens, 2004; Cuppen and Herbst, 2005). Experimentally, adsorption behavior of H (D) atoms on HOPG was investigated and recombinative desorption of H2 (D2) showed a double peak in the TPD spectra (Zecho et al., 2002a). The desorption activation energy of H (D) on the surface was estimated to be ~ 0.6 (0.9) eV. Scanning tunneling microscopy (STM) images revealed that H2 recombination is possible from two hydrogen dimer states on HOPG (Hornekær et al., 2006b). These dimer states were identified as the origin of the unusual double peak in TPD traces for hydrogen on graphite. Moreover, activation barriers of ~ 1.2 and ~ 1.6 eV were calculated for H2 formation. STM images further revealed that H atoms have a preferential sticking on HOPG on those sites where an H atom is already pre-adsorbed, resulting in the formation of large clusters that favor H2 formation (Hornekær et al., 2006a). In another study, HD formation via ER or HK abstraction was investigated after exposure of H atoms with kinetic energies of 2,000 K on deuterated HOPG at 150 K (Zecho et al., 2002b). Depending on the coverage of the chemisorbed D atoms on the surface, the abstraction cross-section varied between 4 and 17 2. Concerning the partitioning of 4.5 eV, the kinetic energy of H2/D2 molecules formed by laser assisted associative desorption of H/D atoms on HOPG at room temperature showed a broad distribution with a peak at ~ 1.3 eV (Baouche et al., 2006).
H2 formation on amorphous carbonaceous surface with aliphatic C–H bonds was initially ignored since the binding energies of H atoms were considered very high (~ 4 eV). Mennella (2006) has experimentally estimated an activation barrier of 6 meV (70 K) for H atoms to form the aliphatic CH2, 3 groups on hydrogen free nano-sized amorphous carbon grains. The barrier is much lower than the typical values of few tenths of an eV derived for H atoms on graphite (Hornekær et al., 2006b). On such hydrogenated amorphous carbon surface, HD formation has been observed for a range of surface temperatures 13–300 K, and for impinging D atoms between 80 and 300 K through ER mechanism (Mennella, 2008). At the aliphatic CH2, 3 groups, H/D exchange occurs through a two-step reaction sequence: (1) dehydrogenation via H atom abstraction with the formation of an HD molecule and (2) deuteration with the addition of a D atom. Figure 3 (red curve a) depicts the intensity decrease of the C–H stretching feature of aliphatic CH2, 3 groups and the simultaneous increase of the corresponding aliphatic C–D bonds during the process. The net result of the exchange reaction sequence is formation of an HD molecule. This process was found to be barrierless at low surface temperatures, while an activation barrier of 130 K was found for surface above 100 K. An abstraction cross-section of 0.03 2 was evaluated for H atoms (300 K) impinging on the analog sample at room temperature. Furthermore, the nascent HD molecules formed in the aliphatic sites should not be in a highly excited state as exoenergeticity of formation reaction is reduced by ~4 eV which is necessary to break the C–H bond. H2 formation is not specific to H atom chemisorbed on aliphatic carbon sites. In fact, H2 formation was observed in experiments carried out on hydrogenated aromatic carbon material with varying complexity starting from a simple polyaromatic hydrocarbon molecule (coronene film) (Mennella et al., 2012; Thrower et al., 2012) to complex carbon soots with a marked aromatic character (Mennella, 2011). In these cases, an initial carbon aromatic sp2 to aliphatic sp3 rehybridization reaction was followed by the same exchange reactions as those observed in the aliphatic carbon sample. VUV photolysis of hydrogenated amorphous carbon was suggested to be a feasible route to H2 formation (Alata et al., 2014). However, this mechanism alone can not explain the catalytic role of carbon grains and can only be a marginal source of H2 in the absence of efficient H atom addition process.
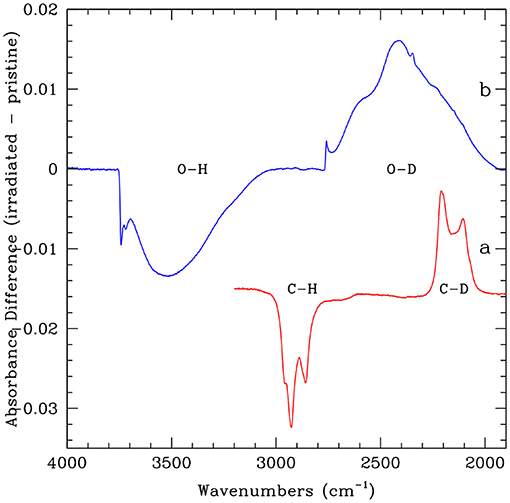
Figure 3. The evolution of IR spectrum of hydrogenated nano sized carbon grains after irradiation of 1 × 1019 D atoms cm−2 (red curve a). The spectral evolution of Mg-rich hydroxylated amorphous silicate grains after irradiation of 3.8 × 1018 D atoms cm−2 is also reported (blue curve b). The spectra are shown after subtraction of the initial spectrum and are shifted in ordinate for the sake of clarity.
H2 formation involving chemisorbed H atoms has recently been investigated using Mg-rich hydroxylated amorphous silicates (Mennella and Suhasaria, 2019). The dust grain analogs prepared by laser ablation of an oxide mixture showed a broad infrared band resulting from chemisorbed hydroxyl groups. These hydroxylated silicate surfaces were exposed to D atoms with an energy of a few tens of meV. The catalytic H2 formation was a result of the exchange reaction similar to those on hydrogenated carbon surfaces. Figure 3 (blue curve b) shows a reduction in the number of O–H stretching modes due to the abstraction of already bonded H atoms in O–H groups of silicates. At the same time, new O–D bonds are formed on silicate grains. In the process, HD molecules are formed via exchange reactions.
5. Final Remarks
Laboratory investigations are crucial to the understanding of the physico-chemical processes involved in H2 formation. They have demonstrated how weakly adsorbed H atoms on refractory grain analogs at temperatures <20 K migrate to another H atom and recombine to H2 via Langmuir–Hinshelwood mechanism. It is also evident that the ejected H2 molecules after formation on such grain analogs are likely to be vibrationally hot but rotationally cold. In the limited number of studies that concerns H2 formation involving chemisorbed H atoms, LH mechanism was found to be inefficient at higher grain temperature and H2 formation relies on ER/HK mechanism. Laboratory investigations have also indicated that varying carbon based structures altering from a simple aliphatic to an aromatic to a mixed aliphatic–aromatic system catalyze H2 molecules. This occurs through an exchange reaction when H atoms are already pre-adsorbed on them. The role of hydroxylated amorphous silicates as catalysts for H2 formation has also been highlighted in a recent study. It is apparent that so far, most laboratory investigations have focused either on physisorption or chemisorption formation mechanism. However, the presence of H2 in a wide variety of space environments hints to the fact that the two formation mechanism are not discrete but they should be operating at the same time. Thus, there is a need for a systematic approach that underlines the intertwining of the two formation mechanisms.
Author Contributions
All authors contributed to the article and approved the submitted version.
Conflict of Interest
The authors declare that the research was conducted in the absence of any commercial or financial relationships that could be construed as a potential conflict of interest.
Acknowledgments
We thank V. Pirronello for a careful reading of the manuscript and helpful comments and suggestions. We also thank N. Ferrigno for helping us in the editing of the manuscript. This work has been supported by Istituto Nazionale di Astrofisica (INAF).
References
Alata, I., Cruz-Diaz, G. A., Caro, G. M. M., and Dartois, E. (2014). Vacuum ultraviolet photolysis of hydrogenated amorphous carbons-I. interstellar H2 and CH4 formation rates. Astron. Astrophys. 569:A119. doi: 10.1051/0004-6361/201323118
Baouche, S., Gamborg, G., Petrunin, V. V., Luntz, A. C., Baurichter, A., and Hornekær, L. (2006). High translational energy release in H2 (D2) associative desorption from H (D) chemisorbed on C (0001). J. Chem. Phys. 125:084712. doi: 10.1063/1.2220565
Cazaux, S., and Tielens, A. G. G. M. (2004). H2 formation on grain surfaces. Astrophys. J. 604:222. doi: 10.1086/381775
Colangeli, L., Henning, T., Brucato, J. R., Clément, D., Fabian, D., Guillois, O., et al. (2003). The role of laboratory experiments in the characterisation of silicon-based cosmic material. Astron. Astrophys. Rev. 11, 97–152. doi: 10.1007/s00159-002-0017-x
Creighan, S. C., Perry, J. S. A., and Price, S. D. (2006). The rovibrational distribution of H2 and HD formed on a graphite surface at 15-50 K. J. Chem. Phys. 124:114701. doi: 10.1063/1.2174878
Cuppen, H. M., and Herbst, E. (2005). Monte carlo simulations of H2 formation on grains of varying surface roughness. Monthly Notices R. Astron. Soc. 361, 565–576. doi: 10.1111/j.1365-2966.2005.09189.x
Draine, B. T. (2003). Interstellar dust grains. Annu. Rev. Astron. Astrophys. 41, 241–289. doi: 10.1146/annurev.astro.41.011802.094840
Draine, B. T. (2010). Physics of the Interstellar and Intergalactic Medium. Princeton and Oxford: Princeton University Press. doi: 10.2307/j.ctvcm4hzr
Ferriere, K. M. (2001). The interstellar environment of our galaxy. Rev. Mod. Phys. 73:1031. doi: 10.1103/RevModPhys.73.1031
Gavilan, L., Lemaire, J. L., Vidali, G., Sabri, T., and Jæger, C. (2014). The formation of molecular hydrogen on silicate dust analogs: the rotational distribution. Astrophys. J. 781:79. doi: 10.1088/0004-637X/781/2/79
Gould, R. J., and Salpeter, E. E. (1963). The interstellar abundance of the hydrogen molecule. I. Basic processes.: 1963. Astrophys. J. 138:393. doi: 10.1086/147654
Hama, T., and Watanabe, N. (2013). Surface processes on interstellar amorphous solid water: adsorption, diffusion, tunneling reactions, and nuclear-spin conversion. Chem. Rev. 113, 8783–8839. doi: 10.1021/cr4000978
He, J., Frank, P., and Vidali, G. (2011). Interaction of hydrogen with surfaces of silicates: single crystal vs. amorphous. Phys. Chem. Chem. Phys. 13, 15803–15809. doi: 10.1039/c1cp21601e
Henning, T. (2010). “Laboratory astrophysics of cosmic dust analogues,” in Astromineralogy Vol. 815, ed T. Henning (Heidelberg; Berlin: Springer), 313–329. doi: 10.1007/978-3-642-13259-9_8
Hollenbach, D., and Salpeter, E. E. (1971). Surface recombination of hydrogen molecules. Astrophys. J. 163:155. doi: 10.1086/150754
Hornekær, L., Rauls, E., Xu, W., Šljivančanin, Ž., Otero, R., Stensgaard, I., et al. (2006a). Clustering of chemisorbed H (D) atoms on the graphite (0001) surface due to preferential sticking. Phys. Rev. Lett. 97:186102. doi: 10.1103/PhysRevLett.97.186102
Hornekær, L., Šljivančanin, Ž., Xu, W., Otero, R., Rauls, E., Stensgaard, I., et al. (2006b). Metastable structures and recombination pathways for atomic hydrogen on the graphite (0001) surface. Phys. Rev. Lett. 96:156104. doi: 10.1103/PhysRevLett.96.156104
Islam, F., Latimer, E. R., and Price, S. D. (2007). The formation of vibrationally excited HD from atomic recombination on cold graphite surfaces. J. Chem. Phys. 127:064701. doi: 10.1063/1.2754684
Jäger, C., Gail, H.-P., Rietmeijer, F. J., Nuth, J. A., Mutschke, H., and Mennella, V. (2015). “Formation of nanoparticles and solids”, in Laboratory Astrochemistry: From Molecules through Nanoparticles to Grains, eds S. Schlemmer, H. Mutschke, T. Giesen, and C. Jäger (Wiley), 419–500. doi: 10.1002/9783527653133.ch7
Jones, A. P., Fanciullo, L., Köhler, M., Verstraete, L., Guillet, V., Bocchio, M., et al. (2013). The evolution of amorphous hydrocarbons in the ISM: dust modelling from a new vantage point. Astron. Astrophys. 558:A62. doi: 10.1051/0004-6361/201321686
Katz, N., Furman, I., Biham, O., Pirronello, V., and Vidali, G. (1999). Molecular hydrogen formation on astrophysically relevant surfaces. Astrophys. J. 522:305. doi: 10.1086/307642
Krugel, E. (2007). An Introduction to the Physics of Interstellar Dust. New york and London: Taylor & Francis. doi: 10.1201/b15897
Latimer, E. R., Islam, F., and Price, S. D. (2008). Studies of HD formed in excited vibrational states from atomic recombination on cold graphite surfaces. Chem. Phys. Lett. 455, 174–177. doi: 10.1016/j.cplett.2008.02.105
Lemaire, J.-L., Vidali, G., Baouche, S., Chehrouri, M., Chaabouni, H., and Mokrane, H. (2010). Competing mechanisms of molecular hydrogen formation in conditions relevant to the interstellar medium. Astrophys. J. Lett. 725:L156. doi: 10.1088/2041-8205/725/2/L156
Mennella, V. (2006). Activation energy of C-H bond formation in carbon grains irradiated with hydrogen atoms. Astrophys. J. Lett. 647:L49. doi: 10.1086/507296
Mennella, V. (2008). HD formation by abstraction of H/D chemisorbed in carbon grains with D/H atoms under simulated interstellar conditions. Astrophys. J. Lett. 684:L25. doi: 10.1086/592001
Mennella, V. (2011). “Interaction of atomic hydrogen with carbon grains,” in PAHs and the Universe, Vol. 46, eds C. Joblin and A. G. G. M.Tielens. (Cambridge: EDP Sciences), 393–398. doi: 10.1051/eas/1146040
Mennella, V., Hornekær, L., Thrower, J., and Accolla, M. (2012). The catalytic role of coronene for molecular hydrogen formation. Astrophys. J. Lett. 745:L2. doi: 10.1088/2041-8205/745/1/L2
Mennella, V., and Suhasaria, T. (2019). H2 formation on Mg-rich amorphous silicates. Int. Astron. Union. 15, 109–113. doi: 10.1017/S1743921319008834
Perets, H. B., Lederhendler, A., Biham, O., Vidali, G., Li, L., Swords, S., et al. (2007). Molecular hydrogen formation on amorphous silicates under interstellar conditions. Astrophys. J. Lett. 661:L163. doi: 10.1086/518862
Pirronello, V., Biham, O., Liu, C., Shen, L., and Vidali, G. (1997a). Efficiency of molecular hydrogen formation on silicates. Astrophys. J. Lett. 483:L131. doi: 10.1086/310746
Pirronello, V., Biham, O., Manico, G., Roser, J., and Vidali, G. (2000). “Laboratory studies of molecular hydrogen formation on surfaces of astrophysical interest,” in Molecular Hydrogen in Space, eds F. C. Combes and G. Pineau des Forets (Cambridge: Cambridge University Press), 71. doi: 10.1017/CBO9780511564635.012
Pirronello, V., Liu, C., Roser, J. E., and Vidali, G. (1999). Measurements of molecular hydrogen formation on carbonaceous grains. Astron. Astrophys. 344, 681–686.
Pirronello, V., Liu, C., Shen, L., and Vidali, G. (1997b). Laboratory synthesis of molecular hydrogen on surfaces of astrophysical interest. Astrophys. J. Lett. 475:L69. doi: 10.1086/310464
Thrower, J. D., Jørgensen, B., Friis, E. E., Baouche, S., Mennella, V., Luntz, A. C., et al. (2012). Experimental evidence for the formation of highly superhydrogenated polycyclic aromatic hydrocarbons through H atom addition and their catalytic role in H2 formation. Astrophys. J. 752:3. doi: 10.1088/0004-637X/752/1/3
Vidali, G. (2013). H2 formation on interstellar grains. Chem. Rev. 113, 8762–8782. doi: 10.1021/cr400156b
Vidali, G., Li, L., Roser, J. E., and Badman, R. (2009). Catalytic activity of interstellar grains: formation of molecular hydrogen on amorphous silicates. Adv. Space Res. 43, 1291–1298. doi: 10.1016/j.asr.2008.12.019
Vidali, G., Pirronello, V., Li, L., Roser, J., Manico, G., Congiu, E., et al. (2007). Analysis of molecular hydrogen formation on low-temperature surfaces in temperature programmed desorption experiments. J. Phys. Chem. A 111, 12611–12619. doi: 10.1021/jp0760657
Wakelam, V., Bron, E., Cazaux, S., Dulieu, F., Gry, C., Guillard, P., et al. (2017). H2 formation on interstellar dust grains: the viewpoints of theory, experiments, models and observations. Mol. Astrophys. 9, 1–36. doi: 10.1016/j.molap.2017.11.001
Watanabe, N., Kimura, Y., Kouchi, A., Chigai, T., Hama, T., and Pirronello, V. (2010). Direct measurements of hydrogen atom diffusion and the spin temperature of nascent H2 molecule on amorphous solid water. Astrophys. J. Lett. 714:L233. doi: 10.1088/2041-8205/714/2/L233
Watanabe, N., and Kouchi, A. (2008). Ice surface reactions: a key to chemical evolution in space. Prog. Surf. Sci. 83, 439–489. doi: 10.1016/j.progsurf.2008.10.001
Zecho, T., Güttler, A., Sha, X., Jackson, B., and Küppers, J. (2002a). Adsorption of hydrogen and deuterium atoms on the (0001) graphite surface. J. Chem. Phys. 117, 8486–8492. doi: 10.1063/1.1511729
Keywords: laboratory astrochemistry, dust analogs, molecular hydrogen, surface chemistry, interstellar medium
Citation: Suhasaria T and Mennella V (2021) Catalytic Role of Refractory Interstellar Grain Analogs on H2 Formation. Front. Astron. Space Sci. 8:655883. doi: 10.3389/fspas.2021.655883
Received: 19 January 2021; Accepted: 06 April 2021;
Published: 07 May 2021.
Edited by:
Ryan C. Fortenberry, University of Mississippi, United StatesReviewed by:
Martin Robert Stewart McCoustra, Heriot-Watt University, United KingdomGianfranco Vidali, Syracuse University, United States
Copyright © 2021 Suhasaria and Mennella. This is an open-access article distributed under the terms of the Creative Commons Attribution License (CC BY). The use, distribution or reproduction in other forums is permitted, provided the original author(s) and the copyright owner(s) are credited and that the original publication in this journal is cited, in accordance with accepted academic practice. No use, distribution or reproduction is permitted which does not comply with these terms.
*Correspondence: Vito Mennella, dml0by5tZW5uZWxsYUBpbmFmLml0