The Role of Terahertz and Far-IR Spectroscopy in Understanding the Formation and Evolution of Interstellar Prebiotic Molecules
- 1Centre for Astrophysics and Planetary Science, School of Physical Sciences, University of Kent, Canterbury, United Kingdom
- 2Institute for Nuclear Research (Atomki), Debrecen, Hungary
- 3School of Electronic Engineering and Computer Science, Queen Mary University of London, London, United Kingdom
- 4Microwave Electronics Laboratory, Department of Microtechnology and Nanoscience, Chalmers University of Technology, Göteborg, Sweden
Stellar systems are often formed through the collapse of dense molecular clouds which, in turn, return copious amounts of atomic and molecular material to the interstellar medium. An in-depth understanding of chemical evolution during this cyclic interaction between the stars and the interstellar medium is at the heart of astrochemistry. Systematic chemical composition changes as interstellar clouds evolve from the diffuse stage to dense, quiescent molecular clouds to star-forming regions and proto-planetary disks further enrich the molecular diversity leading to the evolution of ever more complex molecules. In particular, the icy mantles formed on interstellar dust grains and their irradiation are thought to be the origin of many of the observed molecules, including those that are deemed to be “prebiotic”; that is those molecules necessary for the origin of life. This review will discuss both observational (e.g., ALMA, SOFIA, Herschel) and laboratory investigations using terahertz and far-IR (THz/F-IR) spectroscopy, as well as centimeter and millimeter spectroscopies, and the role that they play in contributing to our understanding of the formation of prebiotic molecules. Mid-IR spectroscopy has typically been the primary tool used in laboratory studies, particularly those concerned with interstellar ice analogues. However, THz/F-IR spectroscopy offers an additional and complementary approach in that it provides the ability to investigate intermolecular interactions compared to the intramolecular modes available in the mid-IR. THz/F-IR spectroscopy is still somewhat under-utilized, but with the additional capability it brings, its popularity is likely to significantly increase in the near future. This review will discuss the strengths and limitations of such methods, and will also provide some suggestions on future research areas that should be pursued in the coming decade exploiting both space-borne and laboratory facilities.
1 Interstellar Chemistry: From Principles to Practice
The idea of active chemistry occurring within interstellar space is a relatively new one. Historically, interstellar temperatures and pressures were considered to be far too low to permit atoms or ions to come into contact with each other and overcome a reaction activation energy barrier. However, since the first evidence of interstellar molecular species arose in the late 1930s, the scientific community has come to recognize that the interstellar medium is home to a plethora of structurally diverse molecules, with different environments displaying their own characteristic chemistry. A more complete description of the history of astrochemistry may be found in the work of Feldman (2001).
Matter in the interstellar medium is composed of around 99% atomic or molecular hydrogen and 1% carbonaceous or silicate dust grains. This matter is cycled through a number of phases each displaying a characteristic chemistry (Figure 1). The smallest over-densities result in the formation of diffuse clouds, which possess particle densities of 102–103 cm−3 and kinetic temperatures of a few hundred K (Smith 1992). Diffuse clouds are relatively transparent to visible light and are continuously exposed to the interstellar radiation field which results in the dissociation and ionization of molecular material. As such, these clouds are dominated by the presence of atoms and ions, particularly H and H+, although a few molecular species do survive (Snow and Bierbaum 2008). Indeed, the first molecules to be detected in interstellar space were detected in diffuse interstellar media via optical spectroscopy (Smith 1992; Feldman 2001; Larsson et al., 2012). With the notable exception of the formation of H2 (Wakelam et al., 2017), most of the chemistry within diffuse interstellar clouds is mediated by gas-phase reactions (Geppert and Larsson 2013).
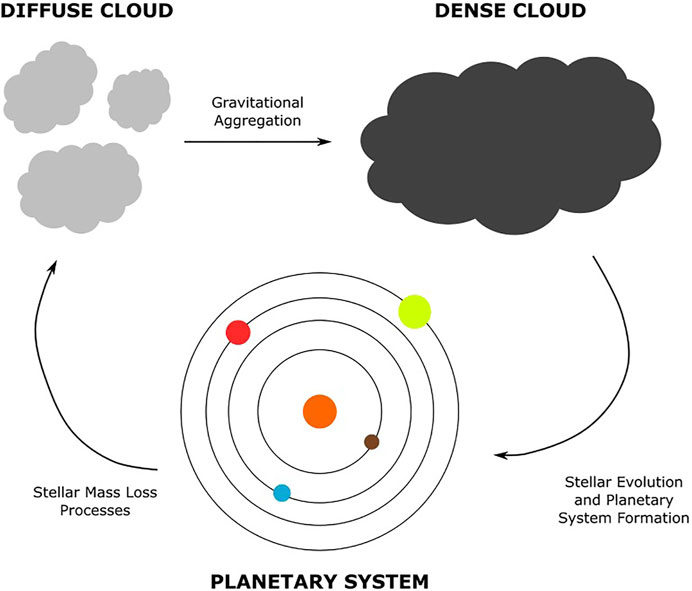
FIGURE 1. Matter in the interstellar medium is cycled through various phases: the aggregation of matter results in optically transparent diffuse clouds evolving into dense, dark molecular clouds. These clouds are considered to be “stellar nurseries” in which localized gravitational collapse results in the formation of a proto-star, with further accretion of material occurring via magneto-hydrodynamic flows onto the growing star. Within a few million years, the stellar object evolves into a pre-main sequence star surrounded by a proto-planetary disk. Collisions within the disk result in the accretion of dust grains which slowly grow to form planetesimals, thus taking the first steps towards the formation of a planetary system. Once the star’s nuclear fuel has been exhausted, it may follow one of two routes depending on the stellar mass: low-mass stars undergo several mass-loss processes in which molecular material is dispersed back into the interstellar medium leaving behind a remnant white dwarf, whereas high-mass stars instead explode as supernovae with their cores eventually becoming either neutron stars or black holes.
Accumulation of gaseous material within diffuse clouds may occur as a result of random perturbations, stellar winds, or more violent phenomena such as supernovae-induced shock waves. As diffuse clouds begin to accumulate matter, the proportion of molecular material (mainly H2) increases. This is accompanied by a diminishing of the proportion of ionized species present: for example, the dominant form of carbon changes to the atomic form from C+ and the number of free electrons also decreases (Snow and Bierbaum 2008). Thus, the accumulation of matter results in an evolution of so-called “atomic” diffuse clouds to “molecular” diffuse clouds.
Further accumulation of material as a result of gravitational attractions results in the formation of a dense interstellar cloud (also known as a dark interstellar cloud). These clouds are, as their name suggests, characterized by higher particle number densities of up to 106 cm−3 and are the primary location of stellar formation. The increase in density results in a concomitant increase in cloud opacity. As such, the far- and vacuum-UV components of the interstellar radiation field are attenuated at the edges of the cloud, resulting in an interior with a temperature of 7–20 K (Galli et al., 2002). The higher particle densities of these dark clouds enhance the efficiency of reactions in the gas phase, particularly those between ions and molecules, and contribute to the formation of several new species including complex organic molecules (Bergin and Tafalla 2007; Öberg 2016; Arumainayagam et al., 2019).
At the low temperatures encountered within the interiors of dense interstellar clouds, many species are able to freeze-out onto the surfaces of the carbonaceous and silicate dust grains, thus forming an icy mantle. This icy mantle is typically composed of two zones (Figure 2): a lower polar layer which results from the deposition and synthesis (via hydrogenation reactions) of polar molecules such as H2O, NH3, and also CH4; and an upper apolar layer characterized by the presence of CO and related molecules such as CO2 and CH3OH (Larsson et al., 2012; Öberg 2016).
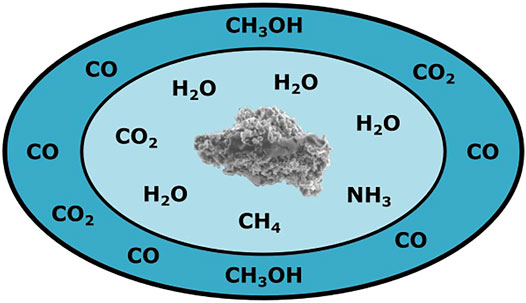
FIGURE 2. Median structure of icy grain mantles within dense interstellar clouds. Shown in the image, a diagram (not to scale) of a typical icy mantle divided into two zones: a lower polar layer rich in H2O molecules and hydrogenated heteroatoms, and an upper apolar layer largely consisting of CO and related molecules. More detailed information can be found in the work of Öberg (2016).
These molecular icy grain mantles play host to a rich chemistry mediated by several phenomena and which may result in the formation of complex molecules. For instance, galactic cosmic rays are not attenuated by the boundaries of the dense cloud, and so are able to penetrate through to the cloud core and induce chemistry within the icy layers. Interactions between these cosmic rays and gas-phase H2 results in the emission of Lyman-α photons which may induce photochemistry. Photochemistry in icy grain mantles may also be induced by lower wavelength photons which enter the cloud via less dense regions. Thermal chemistry may also occur in ices proximal to a heat source, such as a nascent star.
In this section, we review the chemistry which occurs within diffuse and dense interstellar clouds with a particular focus on reactions involving ionic species, and those which result in the formation of complex organic molecules. We should note that, in the literature, the term “complex organic molecule” typically refers to a saturated molecule which may be conceivably formed by geological or biochemical processes. However, for the purposes of this review, we extend this definition to include the unsaturated polyatomic carbon chains which have been detected in astrophysical settings. An understanding of interstellar chemistry is an important prerequisite for a discussion of the experimental and observational techniques used in astrochemistry, and although mentions of detections via terahertz and far-IR (THz/F-IR) spectroscopy and related techniques will be made, further details on such techniques will be given in the subsequent section. Reviews on the chemistry occurring during the various other phases of the astrochemical cycle (Figure 1) are also available elsewhere (van Dishoeck and Blake 1998; Williams and Hartquist 1999; Larsson et al., 2012; Geppert and Larsson 2013; van Dishoeck 2014).
1.1 Diffuse Interstellar Clouds: Overview of Ion Chemistry
As mentioned previously, matter within most diffuse interstellar clouds is primarily composed of atomic and ionic material, with a significantly smaller number of molecules also being present due to the high rates of photon and cosmic ray induced dissociation and ionization processes. Consequentially, the chemistry within these clouds is dominated by gas-phase reactions including those involving or producing ions. At the edges of the diffuse cloud, where stellar UV radiation is more intense, photo-ionization of carbon atoms produces C+, while within the diffuse cloud interior cosmic ray driven chemistry is more dominant and allows for the ionization of H and H2 (Smith 1992). The formation of H2+ in particular is important, as this species is the direct precursor to H3+:
For a long time, the formation and presence of H3+ within diffuse clouds were highly controversial due to its suspected high rate of dissociative recombination mediated by electrons emitted from the photo-ionization of carbon atoms (Larson et al., 2000; Larsson et al., 2008). However, its direct observation in diffuse clouds (McCall et al., 1998) combined with the discovery of a new method of recombination (Kokoouline and Greene 2003) confirmed its presence and role within interstellar chemistry: H3+ efficiently donates a proton to other atoms and molecules due to the low proton affinity of H2. The resultant protonated species is reactive, and is thus capable of triggering various chains of ion-molecule reactions. For instance, the reaction with CO produces HCO+, while the reaction with atomic oxygen produces OH+:
OH+ may also be formed as a result of a two-step process starting with the charge-transfer reaction between atomic oxygen and H+ to yield O+, which subsequently reacts with H2 to furnish OH+ (Yamamoto 2017). The formation of OH+ is the first step towards the formation of interstellar gas-phase H2O. The reaction of OH+ with one moiety of H2 yields H2O+, and the reaction with a second moiety produces H3O+ (Larsson et al., 2012). The H3O+ molecular ion is isoelectronic with NH3 and so is a stable species which resists further reactions with H2 (Geppert and Larsson 2013). Instead, it undergoes dissociative recombination with electrons to yield either OH (∼60% of outcomes) or H2O (∼25% of outcomes).
The detections of OH+, H2O+, and H3O+ within diffuse interstellar environments have been made using ground- and space-based telescopes working in the THz/F-IR range, such as the Atacama Pathfinder Experiment (APEX) and the HIFI instrument aboard the Herschel Space Observatory (for more detail, refer to Section 2), thus confirming their contribution to chemistry in such settings (Gerin et al., 2010a; Gupta et al., 2010; Wyrowski et al., 2010; Wiesemeyer et al., 2016). A reaction scheme analogous to the formation of H2O from OH+ has also been invoked to explain the formation of HCl within diffuse clouds starting from Cl+. Such a reaction scheme has been validated by the detection of the relevant intermediate species HCl+ and H2Cl+ by the HIFI instrument (Lis et al., 2010; De Luca et al., 2012).
Reactions leading to the formation of carbon-bearing molecules within diffuse interstellar media are more challenging to explain compared to the oxygen and chlorine chemistry outlined above. Indeed, the formation of CH+, one of the earliest known interstellar molecules (Douglas and Herzberg 1941), is still not completely understood and several simulations have underestimated its observed abundance within diffuse clouds (e.g., van Dishoeck and Black 1986; Pan et al., 2004; Godard et al., 2009). It is apparent that the formation of CH+ cannot rely on the reaction between H2 and C+ due to the considerable endothermicity of this reaction (Myers et al., 2015; Valdivia et al., 2017; Mosely et al., 2021; Plašil et al., 2021). In light of this, alternative mechanisms have been suggested to account for its presence within the diffuse interstellar medium. For instance, it has been suggested that the radiative association of C+ and H2 to yield CH2+ with the concomitant emission of a photon is the first step towards interstellar CH+, as the photo-dissociation of the former may lead to the latter (Yamamoto 2017). However, the rate at which radiative association occurs is fairly low (Graff et al., 1983; Barinovs and van Hemert 2006) and indeed the primary reaction pathway of CH2+ within diffuse interstellar media is the reaction with H2 which furnishes CH3+. As such, the potential contribution of this reaction network in accounting for interstellar CH+ abundances is probably low.
Another proposed formation route for CH+ is the direct reaction between C+ and H2 if the endothermicity of the reaction is overcome by some mechanism which causes a localized warming effect which raises the temperature to a few thousand K. Initially, it was thought that this localized warming could be achieved in regions of the diffuse cloud processed by magneto-hydrodynamic shock waves (Elitzur and Watson 1980; Draine and Katz 1986). However, such processes are now thought to be an unlikely source of CH+ as the radial velocity for this molecule and for CH have been observed to be similar. Among other ideas, gas heating caused by turbulences within the cloud has also been found to be unsatisfactory in accounting for the observed abundances of CH+ (Godard and Cernicharo 2013), as have reactions between C2+ and H2 in X-ray dominated regions of the diffuse cloud (Plašil et al., 2021). Alfvén waves have also been suggested as a potential source of CH+ (Federman et al., 1996), however, to the best of the authors’ knowledge, this has been neither corroborated nor firmly rejected. As such, the elusive source of CH+ within diffuse interstellar media remains an unanswered question. There thus exists a strong motivation to address this problem, as the formation of CH+ may be an important step in the synthesis of significantly more complex carbon-based molecules such as fullerenes and polycyclic aromatic hydrocarbons (PAHs), which are suspected of being the primary carriers of observed diffuse interstellar bands and which may bear relevance to the synthesis of prebiotic molecules (Taylor and Duley 1997).
With regards to molecular detections in diffuse interstellar clouds, a significant number have been made using methods and techniques which exploit the submillimeter, millimeter, and centimeter regions of the electromagnetic spectrum, including THz/F-IR spectroscopy (Snow and McCall 2006; Yamamoto 2017). Indeed, such techniques are the preferred method for detecting polyatomic molecules, as these molecules are difficult to identify using optical spectroscopy (as was done historically) due to the pre-dissociative nature of their excited states. The HIFI instrument aboard the Herschel Space Observatory in particular has proven to be invaluable to the detection and mapping of molecules in diffuse interstellar media, having provided much information on the chemistry of hydrogen-, carbon-, oxygen-, nitrogen-, sulfur-, fluorine-, and chlorine-bearing molecules within diffuse clouds (Gerin et al., 2010a, 2010b, 2012; Neufeld et al., 2010a, 2010b, 2012a; Langer et al., 2010; Persson et al., 2010; Sonnentrucker et al., 2010; Monje et al., 2011, 2013; De Luca et al., 2012; Esplugues et al., 2014; Schilke et al., 2014; Indriolo et al., 2015; Jacob et al., 2020).
Other instruments and telescopes have also aided greatly in the elucidation of the chemical complexity of the diffuse interstellar medium. For example, APEX, the Atacama Large Millimeter/Submillimeter Array (ALMA), and the GREAT instrument aboard the Stratospheric Observatory for Infrared Astronomy (SOFIA) have all been used in the detection of sulfur-bearing molecules, including isotopologues (Menten et al., 2011; Neufeld et al., 2012b, 2015; Muller et al., 2017). Perhaps the most exciting of all has been the series of recent ALMA observations of complex molecules. The work of Thiel et al. (2017), for instance, investigated the presence of prebiotic complex organic molecules within diffuse media and detected several species, including CH3OH, CH3CN, CH3CHO, HC3N, and CHONH2. Such results are of immense consequence, as they imply that certain molecular precursors to life either form early on in the astrochemical cycle (Figure 1), or else survive a process of expansion from denser interstellar media.
1.2 Dense (Dark) Interstellar Clouds: Overview of Ion Chemistry
Condensation of diffuse clouds results in the formation of dense (dark) clouds. During this process, the dominant material is converted from atomic and ionic species to molecular ones. For instance, H2 becomes the dominant form of hydrogen, while the dominant form of carbon changes sequentially from C+, to C, and finally to CO (Snow and Bierbaum 2008; Larsson et al., 2012). As such, these structures have also been appropriately dubbed molecular clouds. In their review, Arumainayagam et al. (2019) highlighted the chemical productivity of dense clouds and identified three milieux for astrochemical reactions within them. The first of these is gas-phase chemistry, which includes radical-radical, radical-neutral, and ion-neutral reactions. The second is chemistry occurring on bare carbonaceous or silicate dust grain surfaces, while the third includes all chemical reactions occurring via energetic and non-energetic processing of icy grain mantles. In this sub-section, we will largely limit ourselves to a brief overview of ion-molecule reactions which occur in the gas-phase within dense interstellar clouds.
Gas-phase ion-neutral reactions within dense interstellar clouds are favorable, barrierless chemical processes. The source of the ionic species within the cloud is somewhat dependent upon the location of the reaction within the cloud structure. Within the densest regions (surrounding the cloud core), ionization of molecules is caused almost exclusively by penetrating galactic cosmic rays. However, within the less dense regions of the clumpy cloud structure, far- and vacuum-UV components of the interstellar radiation field are the primary cause of molecular ionization via photon-induced electron loss processes. Furthermore, in the presence of OB stars, irradiation of molecular material by X-rays may also contribute to the formation of ionic species.
Cosmic ray induced ionization of H2 leads indirectly to the formation of H3+ as was described for diffuse interstellar clouds. The low proton affinity of H2 allows H3+ to be an efficient proton donor to other species (Larsson et al., 2012). For example, the reaction with N2 yields N2H+, which is frequently used as a tracer for N2 within interstellar space when observing with radio telescopes, since N2 does not possess a permanent dipole moment. Ionized hydrogen, primarily in the form of H+, readily undergoes reactions with other atoms such as nitrogen atoms. The resultant product NH+ may undergo subsequent hydrogenation reactions with H2 to sequentially form NH2+, NH3+, and NH4+ (Geppert and Larsson 2013). Finally, dissociative recombination with an incident electron efficiently affords gas-phase NH3 (Öjekull et al., 2004).
Interestingly, the analogous reaction pathway to the formation of gas-phase CH4 is not thought to be significant within dense clouds. This is because once CH5+ is formed, the dominant dissociative recombination mechanism involves the simultaneous loss of two hydrogen atoms, creating CH3 rather than CH4 (Semaniak et al., 1998; Viti et al., 2000). The formation of CH4 and several other carbon-based molecules within the interstellar medium is instead likely to rely on other mechanisms, such as hydrogenation reactions at the surfaces of dust grains (Qasim et al., 2020).
A large number of carbon-based complex organic molecules are known to exist within dense clouds. Until recently, it was thought that these molecules formed within the solid phase in icy grain mantles and were released into the gas phase upon thermal or photo-desorption. However, a growing body of literature has documented the presence of such gas-phase complex organic molecules within starless and pre-stellar cores, where such desorption processes do not take place. Scibelli and Shirley (2020), for instance, have detected CH3OH and CH3CHO in several such environments and have suggested that the radicals and molecules responsible for the formation of these species may be formed in the ice phase. The release of energy upon their formation allows them to sublimate into the gas phase (a process termed reactive desorption) whereupon they react to form these product molecules.
Another region of dense clouds which may host a rich gas-phase chemistry leading to the formation of complex organic molecules is the edge of the cloud: the photo-dissociation region (PDR). In this region, the cloud interacts with the intense UV components of the interstellar radiation field resulting in the desorption of solid-phase molecules and an increase in the occurrence of gas-phase processes. Using the IRAM 30 m telescope, Cuadrado et al. (2017) were able to perform a millimeter line survey towards the edge of the Orion Bar PDR and successfully detected a plethora of complex molecules including carboxylic acids, aldehydes, alkenes, and sulfur-containing molecules. Soma et al. (2018) reported similar results. The production and survival of complex molecules in such an environment is significant from the perspective of astrobiology and, although their exact formation mechanisms are still not completely understood, it is likely that a non-negligible contribution comes from gas-phase reactions.
Of course, other processes leading to the formation of complex organic molecules within dense interstellar clouds are also known. Garrod et al. (2008), for instance, demonstrated that the warm up of icy grain mantles previously processed by galactic cosmic rays under cold conditions yielded various complex organic molecules in abundances which matched those documented observationally, while Balucani et al. (2015) invoked radiative association as a gas-phase process leading to their formation. An interesting mechanism which has been suggested to account for the formation of complex organic molecules is that of non-diffusive surface reactions, which have been experimentally shown to yield such species in reasonably high abundances (Fedoseev et al., 2015, 2017; Chuang et al., 2016; Ioppolo et al., 2021).
The presence and distribution of complex, potentially prebiotic molecules within the interstellar medium have been extensively probed using various ground- and space-based observatories. Perhaps the most productive of these studies, however, have been those which have made use of the ALMA for molecular detections (Belloche et al., 2016, 2019; Garrod et al., 2017; Jørgensen et al., 2018; Calcutt et al., 2019; Willis et al., 2020). The impact of ALMA observations on the detection of interstellar molecules has been massive: first detections of prebiotic molecules which were previously controversial or unconfirmed, such as urea (NH2CONH2), have been achieved (Belloche et al., 2019), and more information has been gleaned on interstellar chemical processes such as deuteration (Belloche et al., 2016).
To conclude this sub-section, we provide an overview of two especially fruitful ongoing observational projects which include THz/F-IR, millimeter, and centimeter frequencies in their operational ranges: the GOTHAM and ARKHAM projects. These projects are run using the Robert C. Byrd 100 m Green Bank Telescope in the United States and have provided significant data demonstrating the presence of complex organic molecules within interstellar space, with a particular focus on aromatic species. Although operating in a similar fashion, the projects themselves have somewhat different objectives.
The GOTHAM project is primarily concerned with establishing a chemical inventory of the Taurus Molecular Cloud, one of the nearest dense clouds, by performing high-sensitivity wide-band spectral line surveys. Such work is motivated by the fact that benzonitrile (c-C6H5CN) was recently discovered in this pre-stellar source (McGuire et al., 2018a), contrasting with a working hypothesis which postulates that larger interstellar aromatic molecules are produced in the circumstellar regions of post-AGB stars and subsequently break down into smaller sub-units such as benzene (c-C6H6) under energetic processing (Tielens 2008): so-called “top-down” chemistry. However, these results suggest that reactions between smaller precursor molecules may also contribute to the formation of larger aromatics within pre-stellar regions through a “bottom-up” chemistry approach.
GOTHAM observations of the Taurus Molecular Cloud, combined with laboratory-generated spectra and computational models, have successfully identified many molecules, some of which had not yet been described in the interstellar medium. The unsaturated molecules detected within this dense cloud may play a pivotal role in the formation of larger aromatic molecules, as well as other species relevant to the origins of life. Early work from GOTHAM identified propargyl cyanide (HCCCH2CN) and the cyanopolyyne HC11N in the Taurus Molecular Cloud (McGuire et al., 2020; Loomis et al., 2021). Cyclic molecules have also been discovered, including 1-cyanocyclopentadiene and 2-cyanocyclopentadiene (Burkhardt et al., 2021a; McCarthy et al., 2021; Lee et al., 2021a). Other cyano and isocyano species have also been detected (Xue et al., 2020; Lee et al., 2021b).
The ARKHAM project is focused on investigating whether the chemistry observed in the Taurus Molecular Cloud is also found in other pre-stellar and proto-stellar sources so as to assess whether or not the resultant molecules survive the birth and evolution of a proto-star. Results from the project have shown that benzonitrile has been detected in four additional sources: Serpens 1A, Serpens 1B, Serpens 2, and MC27/L1521F (Burkhardt et al., 2021b). Such detections illustrate that aromatic chemistry is favorable and extensive throughout the very earliest stages of star formation. Interestingly, the observed abundances of benzonitrile exceeded those predicted by models, despite these models being well-suited to other large carbon-based molecules such as cyanopolyynes. It is therefore probable that the formation mechanisms of other aromatic species in pre-stellar sources differ from those of linear molecules and are not yet well understood.
1.3 The Link to Prebiotic Chemistry
Joint observational and laboratory investigations are key to deciphering the exact mechanisms of formation of the various complex organic molecules observed within interstellar and circumstellar media. To date, these studies have largely relied on a “bottom-up” chemical perspective in which large complex molecules are produced from simpler starting molecules undergoing some form of processing (such as photolysis or radiolysis). However, as has been demonstrated by the GOTHAM and ARKHAM projects, the formation of certain molecules (such as aromatic species) is likely to be dependent on some combination of “bottom-up” and “top-down” chemistry (McGuire et al., 2020): that is to say that they are formed both from the joining together of smaller molecules, ions, and radicals, as well as the break-up of larger structures (Figure 3).
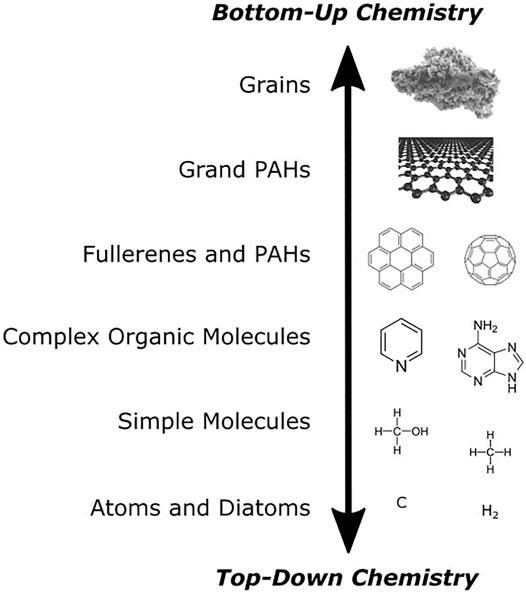
FIGURE 3. Schematic diagram exhibiting models of “top-down” and “bottom-up” chemistry using carbon molecules as an example. In the “bottom-up” perspective, the association of atoms and simple molecules allows for the formation of larger molecules such as CH4 and CH3OH. Further additions to the molecular structure may result in the formation of a complex organic molecule, such as an aromatic molecule or a PAH. Two-dimensional extension of the PAH structure produces graphite, which itself may be considered the basis of (sub-)micron sized interstellar carbonaceous dust grains. The “top-down” chemical perspective is essentially the reverse of this, in which larger molecular structures are broken up as a result of energetic processing, thus yielding simpler molecules.
PAHs are perhaps the best class of interstellar molecules to exemplify the contributions of “top-down” and “bottom-up” astrochemistry due to the fact that they are thought to be relatively ubiquitous in interstellar media, and also because they are able to undergo many chemical transformations yielding a plethora of molecular structures with various sizes, complexities, and arrangements of the aromatic rings, as well as different chemical characteristics such as the inclusion of heteroatoms and the addition of functional groups (such as amino or carboxylic moieties). Indeed, such chemical transformations are thought to be key to the formation of very small grains and fullerenes which result from the respective agglomeration and destruction of PAHs, as well as serving as a carbon feedstock for the production of molecules relevant to biology (Tielens 2008; Groen et al., 2012).
For instance, when mixed with H2O and subjected to energetic processing (a scenario which is plausible within icy grain mantles in the dense interstellar medium), PAHs have been shown to incorporate oxygen into their molecular structure and display new functional groups such as alcohols, ethers, and ketones (Cook et al., 2015). The addition of a ketone moiety to the PAH is biologically significant, as this provides the base structure for the vitamin K chemical family. Incorporation of heteroatoms (such as oxygen and nitrogen) into the ring structure of the PAH as a result of the energetic processing of mixed icy grain mantles is also possible (Materese et al., 2015), and the resultant heterocyclic molecules are also of great biological significance with nitrogen-containing heterocycles such as pyrimidine and purine being the building blocks for nucleobases.
Pyrimidine has yet to be formally detected within the interstellar medium, however it possesses many similar physico-chemical characteristics to PAHs and so is expected to exist to some extent within icy grain mantles. The UV irradiation of frozen pyrimidine in the presence of H2O, CH3OH, NH3, and CH4 has been shown to yield the nucleobases uracil, cytosine, and thymine (Nuevo et al., 2009, 2012, 2014; Bera et al., 2016). Similarly, the irradiation of purine in ices containing NH3 and H2O leads to the formation of the nucleobases guanine and adenine (Bera et al., 2017; Materese et al., 2017, 2018). Although the idea of an extended lifetime for these nucleobases within interstellar and near-Earth environments has been called into question (Peeters et al., 2003), if there exists some plausible mechanism by which they survive the stellar evolution phases of the astrochemical cycle depicted in Figure 1, then it is possible that they may have been delivered to the early Earth via meteoritic or cometary impacts, thus providing the starting material for the so-called “RNA World Hypothesis”.
The RNA World Hypothesis (Orgel 2004; Bernhardt 2012; Neveu et al., 2013; Higgs and Lehman 2015) hypothesizes that RNA or RNA-like molecules mediated the necessary information processing and metabolic transformations required for life to emerge from the early Earth’s prebiotic environment. Systems based on RNA could act as a precursor to the significantly more complex system of RNA, DNA, and proteins on which current life is based, and it has been recognized that the ribonucleotide co-enzymes now used by many proteins may in fact be molecular fossils from an RNA-based metabolism (White 1976; Raffaelli 2011). The existence of naturally occurring ribozyme catalysts, such as self-splicing introns and the ribonuclease P catalyst (Kruger et al., 1982; Doudna and Cech 2002), along with the fact that ribosomal RNA actually catalyzes the formation of peptide bonds in the ribosome (Lang et al., 2008), has lent some weight to this hypothesis. However, much is still unknown as to the exact mechanisms by which life could arise in the RNA World, and so the hypothesis remains one among many seeking to explain the origins of biology from chemistry.
The “top-down” aspect of PAH astrochemistry has also been considered by previous studies (Merino et al., 2014; Dartois et al., 2020). For instance, Merino et al. (2014) demonstrated experimentally that hydrogen atom interaction with graphene on top of SiC at elevated temperatures triggers an erosion process which results in the ejection of PAH-like flakes. Such results offer an alternative and efficient synthetic pathway for the formation of gas-phase PAHs in the interstellar medium. In the envelopes of evolved stars, SiC condenses to form grains whereupon thermal annealing by the proximal star results in an elemental segregation and the synthesis of a carbon-rich surface (Frenklach et al., 1989; Merino et al., 2014). Exposure of this surface to hydrogen atoms is likely in this environment, and the elevated temperatures (>1000 K) permit a thermally assisted etching effect to take place, thus resulting in the desorption of PAHs.
The dissociation of PAHs themselves into smaller molecules is also a possible process, and has been invoked to explain the interstellar presence of fullerenes, particularly C60. Experimental and modelling studies have shown that PAHs containing 60-66 carbon atoms may be completely dehydrogenated by UV photons to a graphene-like structure, after which the structure folds into a cage. Photon-induced shrinking of the structure then proceeds via the rate-limiting loss of C2 units until the C60 fullerene structure is attained (Berné and Tielens 2012; Zhen et al., 2014; Berné et al., 2015). The need to further characterize this “top-down” chemistry, particularly as it relates to other smaller carbon-based molecular species which may be relevant to prebiotic chemistry, was highlighted by van Dishoeck (2017).
2 The Use of THz/F-IR Spectroscopy in Astrochemistry
THz/F-IR frequencies cover the region of the electromagnetic spectrum ranging between 0.3–10 THz (10-333 cm−1), although the upper boundary is somewhat arbitrary and some sources consider it to be as high as 30 THz (Dhillon et al., 2017). Since, by some popular definitions, the THz/F-IR range begins at a wavelength of 1 mm and proceeds to shorter wavelengths, it is sometimes also referred to as the submillimeter band. The main advantages of performing spectroscopy at such frequencies are the possibility of resolving rotational and ro-vibrational spectral transitions of gaseous molecular species, and the potential to probe long-range interactions between molecules in the solid phase in terms of low-energy intra- and intermolecular modes (Brown and Carrington 2003; Profeta and Scandolo 2011; Townes and Schawlow 2013). Thus, THz/F-IR spectroscopy makes for a unique tool in the detection of gases and ices in interstellar clouds and circumstellar disks against the widespread dust continuum via a number of distinct absorption and emission spectral signatures.
For example, the H2O molecule (whose energy transitions are well-defined) is an asymmetric rotor with an irregular set of energy levels characterized by the rotational quantum number J and the pseudo-quantum numbers Ka and Kc (which replace the quantum number K used when considering symmetric rotors). These pseudo-quantum numbers represent hypothetical quantum states for the asymmetric rotor were it to assume the geometry of a prolate and oblate rotor, respectively (Cooke and Ohring 2012). Depending on the orientation of the nuclear spins of the constituent hydrogen atoms, the H2O molecule is either ortho- (if the spins of the hydrogen atoms are parallel) or para- (if the spins are anti-parallel). Each excitation or de-excitation of electrons from one energy level to another results in the propagation of photons of a certain energy (i.e., frequency). Figure 4 shows the energy levels of ortho- and para-H2O in terms of their pseudo-quantum numbers as detected by the Herschel Space Observatory. In this section, we provide a detailed description of the technical fundamentals of THz/F-IR spectroscopy, as well as an overview of its current applications to astrochemistry.
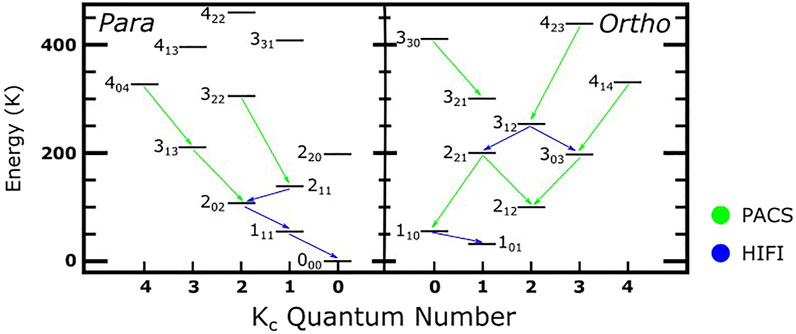
FIGURE 4. Energy level diagram of ortho- and para-H2O showing the transitions observed by the PACS and HIFI instruments aboard the Herschel Space Observatory. Further information may be found in the work of van Dishoeck et al. (2013).
2.1 Spectrometer and Detector Technology
THz/F-IR laboratory spectroscopy measurements may be acquired using several different methods: Fourier-transform IR spectroscopy (Griffiths and de Haseth 1986), spectroscopy based on photo-mixing (Preu et al., 2011) or parametric conversion (Kawase et al., 1996; Kiessling et al., 2013), backward-wave oscillator spectroscopy (Medvedev et al., 2010), chirped-pulse Fourier-transform spectroscopy (Park and Field 2016), time-domain spectroscopy (Auston, 1975; van Exter et al., 1989; Mittleman et al., 1998), and cascaded frequency multiplication (Drouin et al., 2005; Li and Yao 2010). These methods are all based on the detection of a continuous wave, broadband source, or pulsed THz/F-IR radiation illuminating gaseous or solid (i.e., ice) molecular samples of a species, and having varying frequency spectral ranges, sensitivities, and performances. For example, chirped-pulse spectrometers have a frequency bandwidth of up to 100 GHz (Park and Field 2016), while backward oscillation and time-domain spectrometers have frequency ranges of up to 2.1 THz and 7.5 THz, respectively (Komandin et al., 2013; Allodi et al., 2014).
THz/F-IR time-domain spectroscopy can combine the generation of a THz/F-IR pulse in a zinc telluride crystal via opto-electronic rectification or from plasma filamentation in air using (in both cases) a femtosecond laser with a delay line and opto-electronic detection method in order to record the time domain trace (Allodi et al., 2013). This kind of spectroscopy has been widely used for gas-phase spectral line characterization in vacuum chambers (Kilcullen et al., 2015), in the study of refractive indices (Giuliano et al., 2019), and in the study of intermolecular transitions in astrophysical ice analogues, where the THz/F-IR signal is reflected by or transmitted through the gas or ice. Although it is able to provide critical information regarding the frequency of the spectral transition and the absorption coefficient, it does have a few disadvantages including slow acquisition times and a fine-tuning requirement (Trofimov and Varentsova 2016). As such, these instruments are appropriate only for laboratory settings, and other detectors are usually found in observatories.
One form of THz/F-IR spectroscopy which has found widespread use within laboratory astrochemistry settings is that based on cascaded frequency multiplications (Drouin et al., 2005; Li and Yao 2010). This technology is based on using a sweep synthesizer as the source of radiation, which is used in a phase-locked continuous wave mode during measurements and which has a low phase-noise output. A filter (typically an yttrium iron garnet) is swept simultaneously to this synthesizer so as to suppress any frequency spurs. Frequency sextuplers allow for the production of millimeter radiation having a few milliwatts of power, which is then amplified via the use of microwave integrated circuit amplifiers to generate millimeter radiation with a few hundred milliwatts of power. Cascaded doublers or triplers then follow these amplifiers and generate the submillimeter (i.e., THz/F-IR) wavelength radiation.
Incoherent or direct detector arrays are commonly used in THz/F-IR observatories combined with multiplexer readout systems. They require a higher sensitivity than the photon background noise (such as the microwave background) and high pixel counts. The main technologies used are bolometers (exploiting the neutron transmutation doping process or implanted silicon), superconducting transition-edge-sensed bolometers (TESBs), and kinetic inductance detectors (KIDs) (Farrah et al., 2019). Prior to the development of TESBs and KIDs, bolometers operating at temperatures of 300 K or lower were used in observatories. Incoming radiation results in a change in the detector temperature which is read out as a change in its resistivity. Standard bolometers, however, are limited in their total pixel number which has led to the development and preferential use of other technologies, including TESBs and KIDs.
A TESB consists of a superconducting film operating near its superconducting transition temperature (Henderson et al., 2016; Thornton et al., 2016). The signal is seen as a current through a resistive film at a low temperature of around 100 mK (Suzuki et al., 2016). TESBs have high sensitivities which have allowed them to find use in many space-based instruments, as shown in Table 1. KIDs have a similar sensitivity to TESBs but operate below their superconducting transition temperature; below 300 mK (Griffin et al., 2016). The inductance of the detector material is increased due to broken Cooper pairs from incident photons on the superconducting film. In a resonant circuit, the shift in inductance causes a change in the resonant frequency which may be detected (Day et al., 2003).
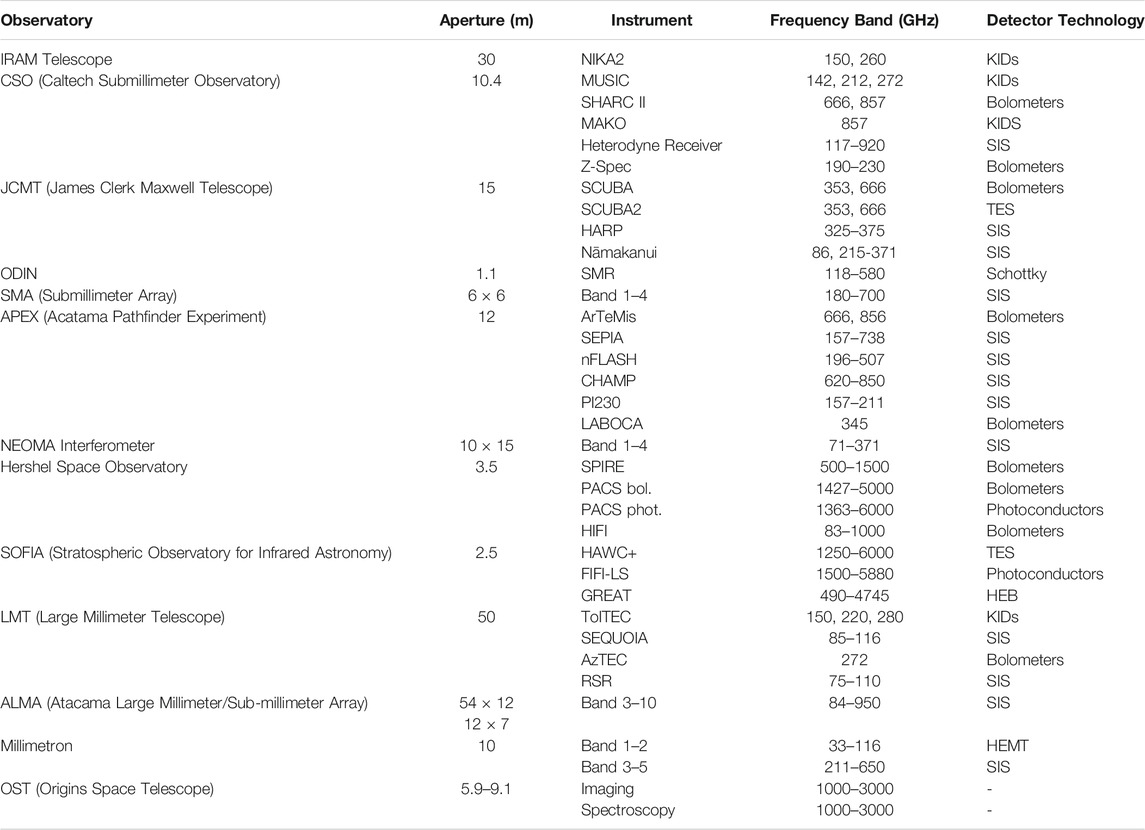
TABLE 1. Examples of the sensitivities achieved by selected observatories operating in the THz/F-IR range.
Coherent systems used in astronomy are based on the heterodyne detection principle: the THz/F-IR high-frequency (RF) signals from molecules in space are down-converted to a lower frequency band typically of a few GHz known as the intermediate frequency (IF). The IF is easily measurable by sensitive back-ends operating at low frequency (such as power meters, spectrum analyzers, digital spectrometers, etc.). The device used for detection and down-conversion is a mixer which requires the input of a local oscillator (LO) signal at the same frequency as the studied RF signal. LO generators need to have narrow linewidths, low noise, high stability, and a broad tunability with sufficient output power to couple to the mixer. In the THz/F-IR domain, multiple chains based on Schottky diodes and quantum cascade lasers (>3 THz) are most commonly used (Valavanis et al., 2019).
There are three main types of mixers used in radio telescopes: superconductor-insulator-superconductor (SIS) diodes (Kojima et al., 2017), hot electron bolometers (HEBs) (Burke et al., 1999), and Schottky diodes (Maestrini et al., 2010). State of the art SIS diodes and HEBs offer the best system noise temperature performances at around 5–10 times the quantum noise limit. These mixers require only a few microwatts of LO power to operate and are very common for instruments with frequency ranges higher than 1 THz and between 1–6 THz for SIS diodes and HEBs, respectively. SIS diode mixers offer wider IF bandwidths and better sensitivities than do HEBs. However, SIS diodes require cooling to 4 K thus necessitating the use of a complex cryostat. On the other hand, Schottky mixers operate at temperatures above 70 K, a frequency range of up to 3 THz, and an IF bandwidth wider than 8 GHz. Their main disadvantages are their lower sensitivity which is between 30–50 times the quantum noise limit, and their milliwatt LO power requirement (Wilson et al., 2008).
The high-resolution spectroscopy possible when using a coherent detector in telescope facilities is achieved by using a digital technique with real-time fast Fourier-transform (FFT) so as to produce high-resolution spectral data. This idea was first proposed by Weinreb (1961) and has been improved upon over the past 60 years in terms of acquisition speed, frequency bandwidth, power efficiency, cost, and size. Nowadays, three main digital systems are used in astronomy to sample the signal waveform at set time intervals: opto-acoustic spectrometers, digital autocorrelation spectrometers (Emrich 1997), and FFT spectrometers (Klein et al., 2012; Price 2016).
Opto-acoustic spectrometers are based on the diffraction of the signal on a Bragg crystal illuminated by a laser beam and detected by charge-coupled devices. This technique was used in the HIFI instrument aboard the Herschel Space Observatory (Siebertz et al., 2007). The main drawback of this spectrometer is its size, which defines its precision and spectral resolution. Digital autocorrelation spectrometers are based on the multiplication of a signal by a delayed version of the same signal using a series of delays. Spectrum measurement is achieved after the application of a Fourier transformation. Such spectrometers can have a bandwidth of a few GHz with a spectral resolution on the scale of one-hundredth of a kHz. Lastly, FFT spectrometers are based on field-programmable gate array (FPGA) chips. The FPGAs are combined with analogue-to-digital converters having a high data sampling rate of a few GHz samples per second. Their numerous spectral channels decompose the incoming RF signal into small sections giving an instantaneous FFT of a few kHz, as seen on the APEX telescope (Klein et al., 2006).
2.2 Telescope Facilities
Since the construction of the first radio telescope in 1937 (Kraus 1988), several telescopes operating in the THz/F-IR range have been successfully built and used by the astronomical community. A principal advantage of performing observations in the THz/F-IR range is the high sensitivity to low-abundance molecules within star-forming regions. Furthermore, observed emission and absorption features can be mapped for all areas within the stellar environment, such as snowlines where ice desorption processes begin to occur.
Progress in the sensitivity of detector technology over the past 50 years has permitted the investigation of interstellar molecular clouds at various wavelengths which has allowed us to improve our understanding of space chemistry. Powerful telescopes have been used with imaging and high spectral resolution instruments combined with large dish antennae. These telescopes have been instrumental in major scientific breakthroughs, such as the discovery of new molecules in the interstellar medium and elucidating the mechanisms for planetary system formation. A summary of the most important telescopes operating in the THz/F-IR range is given in Table 1.
In general, two varieties of observatory exist: ground-based (sometimes also referred to as atmosphere-based) and space-borne telescopes. Ground-based observatories are installed either on the ground at high altitudes or in airborne facilities. Atmospheric transmission has a direct impact on the sensitivity of these observatories at THz/F-IR frequencies since atmospheric molecules (especially H2O) present strong absorption lines over these frequencies. As such, high atmospheric H2O content limits atmospheric transmission, thus making observation heavily dependent upon weather conditions and altitude: drier sites to install ground-based telescopes are found at higher altitudes (Figure 5). Brief descriptions of the ground-based ALMA and space-borne Herschel Space Observatory now follow.
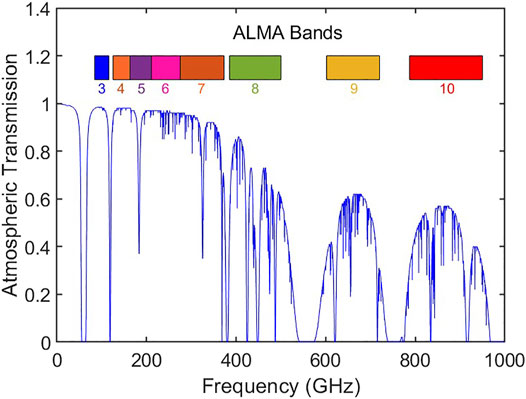
FIGURE 5. Zenith atmospheric transmission of H2O vapor of the ALMA telescope with precipitable H2O vapors of 0.5 mm simulated from the Atmospheric Transmission at Microwaves model described by Pardo et al. (2001). Further information may be found in the work of Maiolino (2008).
ALMA is a ground-based international astronomy facility built over an area of 6596 m2 at an altitude of 5000 m located at Llano de Chajnanto in the Atacama Desert, Chile. The 66 high-precision antennae making up the facility are either 12 m (54 of them) or 7 m (12 of them) in diameter. These sizes, combined with the area of the array, give a spatial resolution of 0.2–0.004 arcseconds (1 arcsecond = 1/3,600 degrees). ALMA is composed of ten receivers using Schottky local oscillator sources and SIS diode mixers cooled to 4 K which give up to 16 GHz bandwidth with a spectral resolution of a few kHz. The covered frequency bands range from 31.3–950 GHz in ten windows (Figure 5) with a maximum 8 GHz IF. The lower frequency Bands 1 and 2 are not yet operational. The number of spectral channels available is 4096 per IF. For Bands 3–8, receivers operate in single sideband detection mode acquiring both H and V polarization while Bands 9 and 10 operate in double sideband mode.
Space-based telescopes are not limited by atmospheric attenuations in the way that ground-based observatories are, but do have limitations connected with the size of their antenna aperture. The Herschel Space Observatory was commissioned by the European Space Agency and launched in 2009. The telescope was composed of a 3.5 m diameter dish which was passively cooled by liquid helium at 4 K. Overt time, the liquid helium reserves necessary for detector cooling were gradually depleted, and the end of the telescope’s operations came about in April 2013.
Three scientific instruments were on board the Herschel Space Observatory: a high-spectral resolution heterodyne spectrometer called HIFI (Heterodyne Instrument for the Far-Infrared), an imaging photometer called PACS (Photodetector Camera Array), and a medium resolution grating spectrometer called SPIRE (Spectral and Photometric Imaging Receiver). THz/F-IR frequencies were covered by the HIFI instrument from 408-1908 GHz separated in 12 windows using HEB and SIS diode mixer technology. PACS was composed of a camera integral field spectrometer combined with an imaging photometer operating between 1.427-6 THz and bolometers at frequencies centered around 1.873 THz, 2.997 THz, and 4.282 THz (Rosenthal et al., 2002). Finally, the SPIRE instrument had three main photometers at 600 GHz, 856 GHz, and 119 GHz, as well as a two-band imaging Fourier-transform spectrometer with bolometers operating between 447–1550 GHz (Griffin et al., 2010).
Amongst the key scientific objectives of the Herschel Space Observatory was an investigation of the formation of stellar and planetary systems, and increasing our understanding of the physics and chemistry of the interstellar medium, our Solar System, and extra-galactic galaxies. The HIFI instrument detected more than 100,000 spectral features in a single spectrum (Bergin et al., 2010). Through the Water in Star-Forming Regions with Herschel (WISH) program, many molecules such as H2O, CO, and O2 were detected in various astrophysical environments (Goldsmith et al., 2011). Molecules and radicals including H2O and OH– were detected further our and deeper in proto-planetary disks than before from surveys of THz/F-IR spectral lines using PACS (Fedele et al., 2013). Cold water reservoirs were discovered in two extra-solar planet-forming disks (TW Hydrae) through the detection of H2O gas produced by UV photo-desorption of the ice made by HIFI (Hogerheidje et al., 2011). H2O vapor was also detected in a pre-stellar core at the early stage of stellar formation using HIFI observations combined with models of the UV photo-desorption processes (Casselli et al., 2012).
Ground-based and space-borne observatories operating in the THz/F-IR range, including those listed in Table 1, have aided astronomers in elucidating the chemistry of interstellar space by allowing them to obtain spectroscopic information of molecular species (colloquially termed “molecular fingerprints”). Indeed, the improvement of technology throughout these past decades has allowed for the definitive identification of many new molecular signatures, largely through observation of their rotational emission spectra. Figure 6 shows the comparison of spectra acquired by ALMA Band 10 and the HIFI instrument aboard the Herschel Space Observatory while observing the NGC 6334I region (McGuire et al., 2018b). As can be seen, the number of spectral lines attributed to CO and CH3OH which are resolved by ALMA Band 10 is significantly higher than that detected by HIFI (Zernickel et al., 2012).
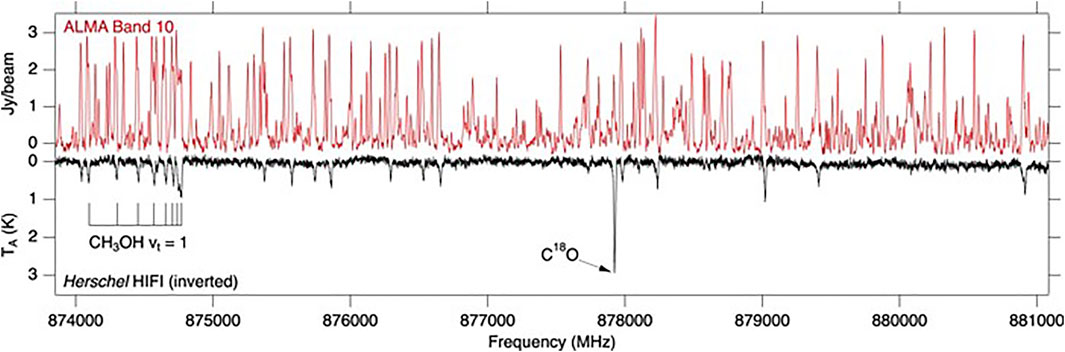
FIGURE 6. Comparison of the spectra acquired by ALMA Band 10 and HIFI while observing the NGC 6334I region. Figure taken from McGuire et al. (2018b) and reproduced with permission from the AAS.
Computer modelling and laboratory measurements of molecular spectra are often needed to assign observed spectral lines to particular molecular species. This is especially true in the case of complex organic molecules such as isopropyl cyanide ((CH3)2CHCN), which was the first branched carbon-chain molecule to be detected and which was found in Sgr B2 using the ALMA Band 3 (Belloche et al., 2014). Later, CH3OCH2OH and CH3Cl were observed for the first time by ALMA Bands 6 and 7 in NGC 6334I, and by ALMA Band 7 in IRAS 16293-2422, respectively (Fayolle et al., 2017; McGuire et al., 2017). However, many molecules and radical species may not be observed in the gaseous phase but may play a central role in the formation of observed gaseous species as a result of their presence and reactions in solid icy grain mantles. The use of laboratory THz/F-IR measurements in astrochemical research, particularly as it pertains to solid-phase chemistry, will be elaborated on in the next sub-section.
2.3 Laboratory Astrochemistry Using THz/F-IR Spectroscopy
Over 200 molecules have been detected and spectroscopically resolved in interstellar regions within our galaxy, and more than 60 have been observed in extra-galactic molecular clouds (Müller et al., 2005), ranging in complexity from simple diatomics to the more structurally complex fullerenes and PAHs. An overarching aim of laboratory astrochemistry is to be able to understand the physico-chemical processes which occur in these astrophysical environments so as to better comprehend the chemical reactions which lead to the formation of such molecules. Such work is motivated by the knowledge that such reactions can result in the production of various molecules and minerals. For instance, interstellar biomolecules which may form in dense, quiescent molecular clouds may be subsequently incorporated into the stellar and planetary systems which evolve from these clouds and thus play a defining role in the emergence of life within these systems (Watanabe and Kouchi, 2002; Jones et al., 2011; Fedoseev et al., 2017; Chuang et al., 2020; Sandford et al., 2020; Ioppolo et al., 2021). The synthesis of smaller, volatile molecules in astrophysical environments is equally important, as such molecules may contribute to the development of planetary and lunar atmospheres or transient exospheres (Milillo et al., 2016; Teolis and Waite 2016). Additionally, condensation processes and grain alterations contribute to the formation of minerals which are significant to the geological and geochemical evolution of planets and moons, including graphite, corundum, moissanite, and forsterite (Frenklach et al., 1989; Hazen et al., 2008; Hazen and Ferry 2010).
The unambiguous identification of such molecules by infrared and radio telescopes (including those which operate in the THz/F-IR range) relies on the availability and completeness of gas- and solid-phase spectroscopic databases at the corresponding frequencies for a great variety of molecules. Such information is obtained by performing classical laboratory measurements (Wlodarczak 1995; Smith 2011; Cataldo et al., 2013a; Allodi et al., 2013; Widicus-Weaver 2019; Mifsud et al., 2021). Presently, the two most commonly referenced catalogues are the Cologne Database for Molecular Spectroscopy (Müller et al., 2001; Endres et al., 2016) and the Jet Propulsion Laboratory (Pickett et al., 1998) catalogues, which give the frequency and amplitude of several molecular transitions. Two accessible databases listing vibrational transitions at IR wavelengths are also available: the HITRAN database (Rothman et al., 2009) and the EXOMOL line list.
Broadly speaking, laboratory astrochemistry studies are largely concerned with investigating reactions which occur in either the gas phase or the condensed (ice) phase. Experiments are typically performed using high or ultra-high vacuum chambers. For instance, a novel method of performing gas-phase spectroscopy is to make use of a heterodyne radiometer so as to observe the absorption features of various gaseous species within the chamber, thus functioning in a similar fashion to several telescope facilities. Two laboratory broadband emission THz/F-IR radiometers based on heterodyne detection methods have been developed: the first is based on Schottky-barrier technology combined with a FFT digital spectrometer operating between 80 and 110 GHz (Wehres et al., 2017), while the second is based on cryo-cooled SIS diode mixer technology operating between 270 and 290 GHz (Wehres et al., 2018). By using such instruments, the spectroscopic features of pyridine and CH3CN have been successfully measured and matched with analytical simulations. Similarly, a Schottky-based receiver has been used in combination with a FFT digital spectrometer and vacuum chamber to observe the emission line of N2O at 355.6 GHz (Parkes et al., 2018).
Laboratory experiments concerned with the solid phase also require the use of high or ultra-high vacuum chambers, wherein interstellar ice analogues may be synthesized on cold substrates via the direct or background deposition of dosed gases or vapors, or the effusive evaporation of refractories. The deposited ices are then processed in such a way as to simulate astrophysical conditions: atom (or free radical) additions represent non-energetic pathways towards molecule formation (Linnartz et al., 2015; Chuang et al., 2016; Potapov et al., 2017; Ioppolo et al., 2021); bombardment with energetic charged particles (i.e., electrons and ions) simulates ice interactions with galactic cosmic rays, the solar wind, or planetary magnetospheric plasmas (Baragiola et al., 1999; Dalton et al., 2013; Ding et al., 2013; Boamah et al., 2014; Boduch et al., 2015; Fulvio et al., 2018; Ioppolo et al., 2020; Garozzo et al., 2010); irradiation with photons (both ionizing and non-ionizing) simulates extra-terrestrial photochemistry (Bernstein et al., 2002; Lo et al., 2014; Öberg 2016; Mullikin et al., 2018); energetic shocks simulate collisions between interstellar or Solar System bodies (Goldman et al., 2010; Martins et al., 2013), and thermal processing simulates the chemical and structural changes induced within interstellar icy grain mantles proximal to nascent stars, or in Solar System bodies as they approach perihelion (Ehrenfreund et al., 1999; Kaňuchová et al., 2017).
The changes in the physico-chemical properties of the ice analogues induced by such processing have been traditionally monitored using mid-IR or UV-VIS spectroscopy (Mason et al., 2006; Boersma et al., 2014). This has been advantageous in that such laboratory work is complementary to observational work using space-borne telescopes working within these ranges of the electromagnetic spectrum, such as the Spitzer Space Telescope and the Hubble Space Telescope. Although not yet widely used within laboratory settings, the utility and applicability of THz/F-IR spectroscopy is being increasingly recognized due to its ability to detect lower frequency molecular vibrations, as well as intermolecular interactions and translational vibrations within solid lattices (Bertie 1968; Bertie and Jacobs 1977; Kulesa 2011; McIntosh et al., 2012). The remainder of this sub-section will be devoted to a discussion on the application of THz/F-IR spectroscopy to laboratory studies of astrophysical ice analogues.
THz/F-IR spectra differ depending upon the structural morphology of the ice (e.g., amorphous or crystalline solid phases), as demonstrated by Moore and Hudson (1992, 1994, 1995). For instance, recent studies investigating CO2 in H2O and CH3OH ice matrices at various temperatures have revealed that the measured THz/F-IR spectra are especially sensitive to the degree of segregation within the ice structure. Results have shown that CO2 roaming during warming of the ice results in a localized accumulation, or segregation. When mixed with crystalline H2O ice, this CO2 segregation results in a disruption of the hydrogen bonding network between adjacent H2O molecule layers, causing the H2O spectral resonance features to be shifted, distorted, or attenuated (Allodi et al., 2014). On the other hand, CO2 absorption bands were observed to become narrower and more defined when segregation from CH3OH ice occurred as a result of warming (McGuire et al., 2016).
Such work clearly demonstrates the sensitivity of THz/F-IR spectroscopy not only to the nature of the chemical species present within the ice, but also to the physical structure of the ice. Given that the nature of the observed spectral absorption features is dependent upon the temperature, degree of crystallinity, segregation, and thermal history of the ice, THz/F-IR spectroscopy appears to be highly suitable for assessing not only the composition and temperature characteristics of astrophysical ices, but also their morphology (Ioppolo et al., 2014). To date, relatively few of the observed molecules have been investigated using THz/F-IR spectroscopy. Amorphous solid H2O, for instance, presents a spectral line at 1 THz. CH3OH, CH3CHO, and (CH3)2CO have been found to have a broad feature at around 4 THz, while in HCOOH and CH3COOH this broad absorption feature is centered at about 7 THz (Ioppolo et al., 2014).
Laboratory THz/F-IR spectroscopy has also been used in the analysis of more complex molecular structures such as PAHs. Although these molecules are believed to be ubiquitous in interstellar and circumstellar media (Tielens 2008; Kwok and Zhang 2011), few single structures have been positively identified (McGuire et al. 2021). Systematic analysis of a series of PAHs, as well as their hydrogenated and alkylated derivatives, has revealed that the THz/F-IR spectra of these molecules display so-called “Jumping Jack” modes which correspond to the in-plane vibrations around the central molecular core (Cataldo et al., 2013b). As such, these spectra provide crucial information related to the number of fused aromatic rings present within the molecule, and are thus valuable in identifying the individual molecular carriers of PAH diffuse interstellar absorption bands. Other complex molecules, including those which may be of relevance to prebiotic chemistry, have also been studied using THz/F-IR spectroscopy (Widicus-Weaver et al., 2005; Carroll et al., 2010).
Recently, a new laboratory-based method for observing desorption mechanisms occurring within the interstellar medium and star-forming regions has been developed. Studying such desorption has been traditionally performed using mid-IR spectrophotometers and quadrupole mass spectrometers, however this has the drawback of not being able to detect ions and radicals and is difficult to relate to observations made using radio telescopes (Fraser et al., 2002; Collings et al., 2003; Fuchs et al., 2009; Muñoz-Caro et al., 2010). In principle, it is possible to desorb (either via thermal or non-thermal mechanisms) ices formed of pure or layered molecules and observe the resultant gas phase in the THz/F-IR frequency range. One way to do this is to passively observe the emission of those molecules during desorption in an astrochemical chamber equipped with a heterodyne radiometer (Auriacombe et al., 2015, 2016); another is by performing microwave absorption spectroscopy. The region in which desorbing molecules are observed may be directly above the ice, and the relevant spectral transitions may be detected with a HEB (see Figure 2 in Yocum et al., 2019). Alternatively, a chirped-pulse Fourier-transform spectrometer can be used to observe the desorption occurring inside a waveguide (Theulé et al., 2020). These emerging techniques are capable of bringing new insights and possibilities to our understanding of the desorption processes in star-forming regions.
Although the use of THz/F-IR spectroscopy is growing within laboratory-based astrochemical research (driven by developments in the relevant technologies and an increase in the commercial availability of the necessary spectrometers), much of the current work takes place at large-scale facilities. A complete and thorough description of the research conducted using THz/F-IR radiation at such facilities would go beyond the scope of this review, and we instead direct the interested reader to other works (e.g., Neil 2014). However, it is worthwhile to take some time to briefly describe the Free Electron Lasers for Infrared Experiments (FELIX) facility located in Nijmegen, the Netherlands, where an ultrahigh-vacuum chamber has been installed as the Laboratory Ice Surface Astrophysics (LISA) end-station thus permitting the characterization of astrophysical ice analogues which have been processed as a result of THz/F-IR irradiation.
The FELIX facility is home to four free electron lasers which provide short-pulsed coherent light covering the microwave to mid-IR regions of the electromagnetic spectrum. The FELIX-1 and FELIX-2 free electron laser beamlines respectively operate over the ∼65–330 cm−1 and ∼225–3300 cm−1 ranges and are capable of providing bursts of micro-pulses with selective and tunable wavelengths whose duration may be readily controlled. These beamlines are therefore highly suited to providing THz/F-IR (as well as mid-IR) radiation for use in laboratory studies of astrophysical ice analogues, which are prepared in the LISA end-station.
The LISA is an ultrahigh-vacuum chamber with nominal base pressure in the region of a few 10−10 mbar containing an elongated rectangular gold-coated deposition substrate which may be cooled down to 15 K using a closed-cycle helium cryostat head with a cooled compressor. The gold-coated substrate is manipulated by means of a xyz-linear translator allowing for the irradiation of different spots of the ice during a single experiment, as well as a rotary stage mostly employed for alignment purposes. Pure gases and gas mixtures are pre-prepared in a dosing line prior to being injected into the main chamber via an all-metal leak valve, wherein background deposition of the gases occurs to form the astrophysical ice analogues. Such background deposition of gases onto the deposition substrate allows for a more uniform ice deposition, and the resultant ice analogue may be used in systematic studies. Ices processed by mid-IR or THz/F-IR irradiation delivered by the FELIX beamlines may be monitored in two ways: mid-IR reflection-absorption spectroscopy may be used to monitor any physico-chemical effects induced as a result of the ice stimulation, and quadrupole mass spectrometry may be used to monitor species which desorb thermally from the bulk parent ice.
The FELIX-LISA facility has found regular use in effectively characterizing the physico-chemical changes induced in astrophysical ice analogues as a result of stimulation by incident irradiation. For instance, Noble et al. (2020) investigated the effect of resonant irradiation of amorphous solid H2O using mid-IR radiation supplied by the FELIX-2 beamline. Their results indicated that irradiation with wavelengths corresponding to the mid-IR stretching, bending, and libration modes of amorphous H2O resulted in a wide-ranging structural rearrangement of the ice into a crystalline-like form due to vibrational relaxation of the intermolecular hydrogen bonding network. Results such as these, combined with the experimental capabilities of large-scale facilities such as FELIX, make future dedicated studies looking into the irradiation of lower frequency THz/F-IR modes of astrophysical ice analogues especially attractive.
As has been demonstrated, the application of THz/F-IR spectroscopy to observational and laboratory astrochemistry has the potential to unravel much information related to the structure and reactivity of molecules in space. To date, however, THz/F-IR experiments at large-scale facilities such as FELIX tasked with investigating the chemistry of interstellar and Solar System ice analogues have not been explored to their fullest potential due to the fact that the dedicated pump-probe experiments required to study such chemistry are not available at the time of writing. The present LISA end-station configuration comprises an FTIR spectrophotometer with an extended spectral range to the far-IR. Such a system may be used to time-resolve transient events within the ice structure (such as diffusion of molecules through different ice layers and segregation events in mixed ices) at millisecond timescales.
To fully benefit from the unique capabilities of a free electron laser (i.e., a wide-range tunability, high peak power, and a controllable repetition rate), THz/F-IR time-domain spectroscopy with single-shot detection techniques should be employed to extend time resolutions to a few tens of picoseconds (Redlich et al., 2003; Perakis et al., 2013; Shalit et al., 2013; Mead et al., 2019). With such a configuration, free electron laser THz/F-IR radiation could be used to “pump” (i.e., inject energy into) inter- and intramolecular vibrations of solid species that, having been excited by the sudden excess of energy, will subsequently rearrange selectively or diffuse within the ice and possibly react with other species. Diffusion and reaction of molecules could be triggered and controlled by operating a free electron laser across its spectral range and could then be monitored by means of a THz/F-IR single-shot spectrometer in the solid phase, while desorbed species could be monitored by implementing a broadband emission THz/F-IR radiometer as described previously. The combination of a free electron laser and new advanced techniques in laboratory astrochemistry thus has the potential to reveal unprecedented details on fundamental phenomena which could play important roles in the formation of complex prebiotic molecules in space, and would thus expand our understanding of the relevant extra-terrestrial physics and chemistry.
Experimental work such as that proposed to be performed at the FELIX-LISA facility on broadband emission THz/F-IR radiometry would also contribute much to our interpretation of observational data. The importance of commissioning new space-borne observational facilities operating in the THz/F-IR was highlighted in the recently published Pathways to Discovery in Astronomy and Astrophysics for the 2020s roadmap (National Academies of Sciences, Engineering, and Medicine, 2021), particularly in light of the fact that the Space Infrared Telescope for Cosmology and Astrophysics (SPICA) is no longer being considered for launch. Interestingly, the far-IR is one of the spectral ranges where astronomical data with sub-arcsecond resolution is not currently available: a fact which is somewhat at odds with the potential usefulness of spectroscopic measurements in this range (Linz et al., 2021). A number of space-based observatories, such as the Far-Infrared Spectroscopic Surveyor (FIRSS) and the Terahertz Exploration and Zooming-In for Astrophysics (THEZA) telescopes have also been proposed to fill in this gap (Gurvits et al., 2021; Rigopoulou et al., 2021), and the acquisition of laboratory reference data will doubtlessly aid in further characterizing the molecular composition of the cosmos.
3 Conclusion and Recommendations for Future Work
In summary, we have provided a brief overview of the chemistry occurring in both diffuse and dense (dark) interstellar clouds with a focus on ion-molecule reactions in the gas phase which are relevant to the formation of complex prebiotic molecules, as well as how THz/F-IR techniques have aided in the elucidation of these reactions and how such reactions may relate to the RNA World Hypothesis on the abiogenic origins of life. We have also provided a review on current THz/F-IR spectrometer and detector technology in both laboratory and observatory settings, as well as their present and potential future applications to laboratory solid-phase astrochemistry. We conclude by emphasizing that further progress in THz/F-IR astrochemistry has the potential to provide great insight to several fundamental astrochemical processes and should therefore be pursued.
Author Contributions
DM, PH, AM, OA, and SI wrote the manuscript and all authors were responsible for corrections and improvements.
Funding
Our research has benefitted from support provided by the Europlanet 2024 RI, which has received funding from the European Union’s Horizon 2020 Research Innovation Program under grant agreement No. 871149.
Conflict of Interest
The authors declare that the research was conducted in the absence of any commercial or financial relationships that could be construed as a potential conflict of interest.
Publisher’s Note
All claims expressed in this article are solely those of the authors and do not necessarily represent those of their affiliated organizations, or those of the publisher, the editors and the reviewers. Any product that may be evaluated in this article, or claim that may be made by its manufacturer, is not guaranteed or endorsed by the publisher.
Acknowledgments
DM is the grateful recipient of a University of Kent Vice-Chancellor’s Research Scholarship. AM thanks Queen Mary University of London for doctoral funding. SI acknowledges the Royal Society for financial support.
References
Allodi, M. A., Baragiola, R. A., Baratta, G. A., Barucci, M. A., Blake, G. A., Boduch, P., et al. (2013). Complementary and Emerging Techniques for Astrophysical Ices Processed in the Laboratory. Space Sci. Rev. 180, 101–175. doi:10.1007/s11214-013-0020-8
Allodi, M. A., Ioppolo, S., Kelley, M. J., McGuire, B. A., and Blake, G. A. (2014). The Structure and Dynamics of Carbon Dioxide and Water Containing Ices Investigated via THz and Mid-IR Spectroscopy. Phys. Chem. Chem. Phys. 16, 3442. doi:10.1039/c3cp53767f
Arumainayagam, C. R., Garrod, R. T., Boyer, M. C., Hay, A. K., Bao, S. T., Campbell, J. S., et al. (2019). Extraterrestrial Prebiotic Molecules: photochemistry vs. Radiation Chemistry of Interstellar Ices. Chem. Soc. Rev. 48, 2293–2314. doi:10.1039/c7cs00443e
Auriacombe, O., Fraser, H., Ellison, B., Ioppolo, S., and Rea, S. (2016). Terahertz Desorption Emission Spectroscopy (THz DES) – ALMA in the Lab. Paper presented Am. Astronomical Soc. Meet. 228, 104–203.
Auriacombe, O., Fraser, H., Ellison, B., and Rea, S. (2015). Desorption Emission Spectroscopy Using THz Radiometry (THz DES). IET Conf. Proc. 3, 1. doi:10.1049/ic.2015.0093
Auston, D. H. (1975). Picosecond Optoelectronic Switching and Gating in Silicon. Appl. Phys. Lett. 26, 101–103. doi:10.1063/1.88079
Balucani, N., Ceccarelli, C., and Taquet, V. (2015). Formation of Complex Organic Molecules in Cold Objects: The Role of Gas-phase Reactions. Mon. Not. R. Astron. Soc. 449, L16–L20. doi:10.1093/mnrasl/slv009
Baragiola, R. A., Atteberry, C. L., Bahr, D. A., and Jakas, M. M. (1999). Solid-state Ozone Synthesis by Energetic Ions. Nucl. Instr. Methods Phys. Res. Section B: Beam Interactions Mater. Atoms 157, 233–238. doi:10.1016/s0168-583x(99)00431-0
Barinovs, Ğ., and van Hemert, M. C. (2006). CH+ Radiative Association. ApJ 636, 923–926. doi:10.1086/498080
Belloche, A., Garrod, R. T., Müller, H. S. P., Menten, K. M., Medvedev, I., Thomas, J., et al. (2019). Re-exploring Molecular Complexity with ALMA (ReMoCA): Interstellar Detection of Urea. Astron. Astrophys. 628, 10. doi:10.1051/0004-6361/201935428
Belloche, A., Garrod, R. T., Müller, H. S. P., and Menten, K. M. (2014). Detection of a Branched Alkyl Molecule in the Interstellar Medium: Iso -propyl Cyanide. Science 345, 1584–1587. doi:10.1126/science.1256678
Belloche, A., Müller, H. S. P., Garrod, R. T., and Menten, K. M. (2016). Exploring Molecular Complexity with ALMA (EMoCA): Deuterated Complex Organic Molecules in Sagittarius B2(N2). Astron. Astrophys. 587, 91. doi:10.1051/0004-6361/201527268
Bera, P. P., Nuevo, M., Materese, C. K., Sandford, S. A., and Lee, T. J. (2016). Mechanisms for the Formation of Thymine under Astrophysical Conditions and Implications for the Origin of Life. J. Chem. Phys. 144, 144308. doi:10.1063/1.4945745
Bera, P. P., Stein, T., Head-Gordon, M., and Lee, T. J. (2017). Mechanisms of the Formation of Adenine, Guanine, and Their Analogues in UV-Irradiated Mixed NH3:H2O Molecular Ices Containing Purine. Astrobiology 17, 771–785. doi:10.1089/ast.2016.1614
Bergin, E. A., Phillips, T. G., Comito, C., Crockett, N. R., Lis, D. C., Schilke, P., et al. (2010). Herschel observations of EXtra-ordinary Sources (HEXOS): The Present and Future of Spectral Surveys with Herschel/HIFI. A&A 521, L20. doi:10.1051/0004-6361/201015071
Bergin, E. A., and Tafalla, M. (2007). Cold Dark Clouds: The Initial Conditions for star Formation. Annu. Rev. Astron. Astrophys. 45, 339–396. doi:10.1146/annurev.astro.45.071206.100404
Berné, O., Montillaud, J., and Joblin, C. (2015). Top-down Formation of Fullerenes in the Interstellar Medium. Astron. Astrophys. 577, A133. doi:10.1051/0004-6361/201425338
Berné, O., and Tielens, A. G. G. M. (2012). Formation of Buckminsterfullerene (C60) in Interstellar Space. Proc. Nat. Acad. Sci. USA 109, 401. doi:10.1073/pnas.1114207108
Bernhardt, H. S. (2012). The RNA World Hypothesis: the Worst Theory of the Early Evolution of Life (Except for All the Others). Biol. Direct 7, 23. doi:10.1186/1745-6150-7-23
Bernstein, M. P., Dworkin, J. P., Sandford, S. A., Cooper, G. W., and Allamandola, L. J. (2002). Racemic Amino Acids from the Ultraviolet Photolysis of Interstellar Ice Analogues. Nature 416, 401–403. doi:10.1038/416401a
Bertie, J. E. (1968). Far-infrared Spectra of the Ices. Appl. Spectrosc. 22, 638. doi:10.1366/000370268774384164
Bertie, J. E., and Jacobs, S. M. (1977). Far-infrared Absorption by Ices Ih and Ic at 4.3 °K and the Powder Diffraction Pattern of Ice Ic. J. Chem. Phys. 67, 2445. doi:10.1063/1.435218
Boamah, M. D., Sullivan, K. K., Shulenberger, K. E., Soe, C. M., Jacob, L. M., Yhee, F. C., et al. (2014). Low-energy Electron-Induced Chemistry of Condensed Methanol: Implications for the Interstellar Synthesis of Prebiotic Molecules. Faraday Discuss. 168, 249–266. doi:10.1039/c3fd00158j
Boduch, P., Dartois, E., de Barros, A. L. F., da Silveira, E. F., Domaracka, A., Lv, X.-Y., et al. (2015). Radiation Effects in Astrophysical Ices. J. Phys. Conf. Ser. 629, 012008. doi:10.1088/1742-6596/629/1/012008
Boersma, C., Bauschlicher, C. W., Ricca, A., Mattioda, A. L., Cami, J., Peeters, E., et al. (2014). The NASA Ames PAH IR Spectroscopic Database Version 2.00: Updated Content, Web Site, and On(off)line Tools. ApJS 211, 8. doi:10.1088/0067-0049/211/1/8
Brown, P. J., and Carrington, A. (2003). Rotational Spectroscopy of Diatomic Molecules. Cambridge: Cambridge University Press.
Burke, P. J., Schoelkopf, R. J., Prober, D. E., Skalare, A., Karasik, B. S., Gaidis, M. C., et al. (1999). Mixing and Noise in Diffusion and Phonon Cooled Superconducting Hot-Electron Bolometers. J. Appl. Phys. 85, 1644–1653. doi:10.1063/1.369299
Burkhardt, A. M., Lee, K. L. K., Changala, P. B., Shingledecker, C. N., Cooke, I. R., Loomis, R. A., et al. (2021a). Discovery of the Pure Polycyclic Aromatic Hydrocarbon Indene (C-C9h8) with GOTHAM Observations of TMC-1. ArXiv [Preprint]. Available at: https://arxiv.org/abs/2104.15117 (Accessed May 16, 2021).
Burkhardt, A. M., Loomis, R. A., Shingledecker, C. N., Lee, K. L. K., Remijan, A. J., McCarthy, M. C., et al. (2021b). Ubiquitous Aromatic Carbon Chemistry at the Earliest Stages of star Formation. Nat. Astron. 5, 181–187. doi:10.1038/s41550-020-01253-4
Calcutt, H., Willis, E. R., Jørgensen, J. K., Bjerkeli, P., Ligterink, N. F. W., Coutens, A., et al. (2019). The ALMA-PILS Survey: Propyne (CH3CCH) in IRAS 16293-2422. Astron. Astrophys. 631, 137. doi:10.1051/0004-6361/201936323
Carroll, P. B., Drouin, B. J., and Widicus Weaver, S. L. (2010). The Submillimeter Spectrum of Glycolaldehyde. ApJ 723, 845–849. doi:10.1088/0004-637x/723/1/845
Casselli, P., Keto, E., Bergin, E. A., Tafalla, M., Aikawa, Y., Douglas, T., et al. (2012). First Detection of Water Vapor in a Pre-stellar Core. Astrophys. J. Lett. 759, L37.
Cataldo, F., Angelini, G., Aníbal García-Hernández, D., and Manchado, A. (2013b). Far Infrared (Terahertz) Spectroscopy of a Series of Polycyclic Aromatic Hydrocarbons and Application to Structure Interpretation of Asphaltenes and Related Compounds. Spectrochimica Acta A: Mol. Biomol. Spectrosc. 111, 68–79. doi:10.1016/j.saa.2013.03.077
Cataldo, F., García-Hernández, D. A., and Manchado, A. (2013a). Far- and Mid-infrared Spectroscopy of Complex Organic Matter of Astrochemical Interest: Coal, Heavy Petroleum Fractions and Asphaltenes. Mon. Not. R. Astron. Soc. 429, 3025–3039. doi:10.1093/mnras/sts558
Chuang, K.-J., Fedoseev, G., Ioppolo, S., van Dishoeck, E. F., and Linnartz, H. (2016). H-atom Addition and Abstraction Reactions in Mixed CO, H2CO and CH3OH Ices - an Extended View on Complex Organic Molecule Formation. Mon. Not. R. Astron. Soc. 455, 1702–1712. doi:10.1093/mnras/stv2288
Chuang, K. J., Fedoseev, G., Qasim, D., Ioppolo, S., Jäger, C., Henning, T., et al. (2020). Formation of Complex Molecules in Translucent Clouds: Acetaldehyde, Vinyl Alcohol, Ketene, and Ethanol via ‘non-Energetic’ Processing of C2H2 Ice. Astron. Astrophys. 635, 199. doi:10.1051/0004-6361/201937302
Collings, M. P., Dever, J., Fraser, H., and McCoustra, M. R. S. (2003). Laboratory Studies of the Interaction of Carbon Monoxide with Water Ice. Astrophys. Space Sci. 285, 663. doi:10.1023/a:1026144806831
Cook, A. M., Ricca, A., Mattioda, A. L., Bouwman, J., Roser, J., Linnartz, H., et al. (2015). Photochemistry of Polycyclic Aromatic Hydrocarbons in Cosmic Water Ice: The Role of PAH Ionization and Concentration. ApJ 799, 14. doi:10.1088/0004-637x/799/1/14
Cooke, S. A., and Ohring, P. (2012). Decoding Pure Rotational Molecular Spectra for Asymmetric Molecules. J. Spectrosc. 2013, 698392.
Cuadrado, S., Goicoechea, J. R., Cernicharo, J., Fuente, A., Pety, J., and Tercero, B. (2017). Complex Organic Molecules in Strongly UV-Irradiated Gas. Astron. Astrophys. 603, 124. doi:10.1051/0004-6361/201730459
Dalton, J. B., Cassidy, T., Paranicas, C., Shirley, J. H., Prockter, L. M., and Kamp, L. W. (2013). Exogenic Controls on Sulfuric Acid Hydrate Production at the Surface of Europa. Planet. Space Sci. 77, 45–63. doi:10.1016/j.pss.2012.05.013
Dartois, E., Charon, E., Engrand, C., Pino, T., and Sandt, C. (2020). Mechanochemical Synthesis of Aromatic Infrared Band Carriers. A&A 637, A82. doi:10.1051/0004-6361/202037725
Day, P. K., LeDuc, H. G., Mazin, B. A., Vayonakis, A., and Zmuidzinas, J. (2003). A Broadband Superconducting Detector Suitable for Use in Large Arrays. Nature 425, 817–821. doi:10.1038/nature02037
De Luca, M., Gupta, H., Neufeld, D., Gerin, M., Teyssier, D., Drouin, B. J., et al. (2012). Herschel/Hifi Discovery of Hcl + in the Interstellar Medium. ApJ 751, L37. doi:10.1088/2041-8205/751/2/l37
Dhillon, S. S., Vitiello, M. S., Linfield, E. H., Davies, A. G., Hoffmann, M. C., Booske, J., et al. (2017). The 2017 Terahertz Science and Technology Roadmap. J. Phys. D: Appl. Phys. 50, 043001. doi:10.1088/1361-6463/50/4/043001
Ding, J. J., Boduch, P., Domaracka, A., Guillous, S., Langlinay, T., Lv, X. Y., et al. (2013). Implantation of Multiply Charged Sulfur Ions in Water Ice. Icarus 226, 860–864. doi:10.1016/j.icarus.2013.07.002
Doudna, J. A., and Cech, T. R. (2002). The Chemical Repertoire of Natural Ribozymes. Nature 418, 222–228. doi:10.1038/418222a
Douglas, A. E., and Herzberg, G. (1941). Note on CH^{+} in Interstellar Space and in the Laboratory. ApJ 94, 381. doi:10.1086/144342
Draine, B. T., and Katz, N. (1986). Magnetohydrodynamic Shocks in Diffuse Clouds. II - Production of CH(+), OH, CH, and Other Species. ApJ 310, 392. doi:10.1086/164693
Drouin, B. J., Maiwald, F. W., and Pearson, J. C. (2005). Application of Cascaded Frequency Multiplication to Molecular Spectroscopy. Rev. Scientific Instr. 76, 093113. doi:10.1063/1.2042687
Ehrenfreund, P., Kerkhof, O., Schutte, W. A., Boogert, A. C. A., Gerakines, P. A., Dartois, E., et al. (1999). Laboratory Studies of Thermally Processed H2O-CH3OH-CO2 Ice Mixtures and Their Astrophysical Implications. Astron. Astrophys. 350, 240.
Elitzur, M., and Watson, W. D. (1980). Interstellar Shocks and Molecular CH/+/in Diffuse Clouds. ApJ 236, 172. doi:10.1086/157730
Emrich, A. (1997). “Autocorrelation Spectrometers for Spaceborne (Sub) Millimeter Astronomy,” in The Far Infrared and Submillimeter Universe. Editor A. Wilson (Noordwijk: NTRS), 361.
Endres, C. P., Schlemmer, S., Schilke, P., Stutzki, J., and Müller, H. S. P. (2016). The Cologne Database for Molecular Spectroscopy, CDMS, in the Virtual Atomic and Molecular Data Centre, VAMDC. J. Mol. Spectrosc. 327, 95–104. doi:10.1016/j.jms.2016.03.005
Esplugues, G. B., Viti, S., Goicoechea, J. R., and Cernicharo, J. (2014). Modelling the sulphur Chemistry Evolution in Orion KL. Astron. Astrophys. 567, 95. doi:10.1051/0004-6361/201323010
Farrah, D., Sminth, K. E., Ardila, D., Bradford, C. M., DiPirro, M. J., Ferkinhoff, C., et al. (2019). Review: Far-Infrared Instrumentation and Technological Development for the Next Decade. J. Astron. Telesc. Instrum. Syst. 5, 020901. doi:10.1117/1.jatis.5.2.020901
Fayolle, E. C., Öberg, K. I., Öberg, K. I., Jørgensen, J. K., Altwegg, K., Calcutt, H., et al. (2017). Protostellar and Cometary Detections of Organohalogens. Nat. Astron. 1, 703–708. doi:10.1038/s41550-017-0237-7
Fedele, D., Bruderer, S., van Dishoeck, E. F., Carr, J., Herczeg, G. J., Salyk, C., et al. (2013). Digit Survey of Far-Infrared Lines from Protoplanetary Disks. Astron. Astrophys. 559, 77. doi:10.1051/0004-6361/201321118
Federman, S. R., Rawlings, J. M. C., Taylor, S. D., and Williams, D. A. (1996). Synthesis of Interstellar CH+ without OH. Mon. Not. R. Astron. Soc. 279, L41–L46. doi:10.1093/mnras/279.3.l41
Fedoseev, G., Chuang, K.-J., Ioppolo, S., Qasim, D., Dishoeck, E. F. v., and Linnartz, H. (2017). Formation of Glycerol through Hydrogenation of CO Ice under Prestellar Core Conditions. ApJ 842, 52. doi:10.3847/1538-4357/aa74dc
Fedoseev, G., Cuppen, H. M., Ioppolo, S., Lamberts, T., and Linnartz, H. (2015). Experimental Evidence for Glycolaldehyde and Ethylene Glycol Formation by Surface Hydrogenation of CO Molecules under Dense Molecular Cloud Conditions. Mon. Not. R. Astron. Soc. 448, 1288–1297. doi:10.1093/mnras/stu2603
Feldman, P. A. (2001). Molecular Astronomy from a Canadian Perspective: The Early Years. Can. J. Phys. 79, 89–100. doi:10.1139/p01-002
Fraser, H. J., Collings, M. P., and McCoustra, M. R. S. (2002). Laboratory Surface Astrophysics experiment. Rev. Scientific Instr. 73, 2161–2170. doi:10.1063/1.1470232
Frenklach, M., Carmer, C. S., and Feigelson, E. D. (1989). Silicon Carbide and the Origin of Interstellar Carbon Grains. Nature 339, 196–198. doi:10.1038/339196a0
Fuchs, G. W., Cuppen, H. M., Ioppolo, S., Romanzin, C., Bisschop, S. E., Andersson, S., et al. (2009). Hydrogenation Reactions in Interstellar CO Ice Analogues. A&A 505, 629–639. doi:10.1051/0004-6361/200810784
Fulvio, D., Baratta, G. A., Sivaraman, B., Mason, N. J., da Silveira, E. F., de Barros, A. L. F., et al. (2018). Ion Irradiation of N2O Ices and NO2:N2O4 Ice Mixtures: First Steps to Understand the Evolution of Molecules with the N–O Bond in Space. Mon. Not. R. Astron. Soc. 483, 381. doi:10.1093/mnras/sty3081
Galli, D., Walmsley, M., and Gonçalves, J. (2002). The Structure and Stability of Molecular Cloud Cores in External Radiation fields. A&A 394, 275–284. doi:10.1051/0004-6361:20021125
Garozzo, M., Fulvio, D., Kaňuchová, Z., Palumbo, M. E., and Strazzulla, G. (2010). The Fate of S-Bearing Species after Ion Irradiation of Interstellar Icy Grain Mantles. Astron. Astrophys. 509, 67. doi:10.1051/0004-6361/200913040
Garrod, R. T., Belloche, A., Müller, H. S. P., and Menten, K. M. (2017). Exploring Molecular Complexity with ALMA (EMoCA): Simulations of Branched Carbon-Chain Chemistry in Sgr B2(N). Astron. Astrophys. 601, 48. doi:10.1051/0004-6361/201630254
Garrod, R. T., Weaver, S. L. W., and Herbst, E. (2008). Complex Chemistry in Star‐forming Regions: An Expanded Gas‐Grain Warm‐up Chemical Model. ApJ 682, 283–302. doi:10.1086/588035
Geppert, W. D., and Larsson, M. (2013). Experimental Investigations into Astrophysically Relevant Ionic Reactions. Chem. Rev. 113, 8872–8905. doi:10.1021/cr400258m
Gerin, M., De Luca, M., Black, J., Goicoechea, J. R., Herbst, E., Neufeld, D. A., et al. (2010a). Interstellar OH+, H2O+ and H2O+ along the Sight-Line to G10.6-0.4. A&A 518, L110. doi:10.1051/0004-6361/201014576
Gerin, M., Kaźmierczak, M., Jastrzebska, M., Falgarone, E., Hily-Blant, P., Godard, B., et al. (2010b). The Tight Correlation of CCH and C-C3h2 in Diffuse and Translucent Clouds. Astron. Astrophys. 525, 116. doi:10.1051/0004-6361/201015050
Gerin, M., Levrier, F., Falgarone, E., Godard, B., Hennebelle, P., Le Petit, F., et al. (2012). Hydride Spectroscopy of the Diffuse Interstellar Medium: New Clues on the Gas Fraction in Molecular Form and Cosmic ray Ionization Rate in Relation to H 3 +. Phil. Trans. R. Soc. A. 370, 5174–5185. doi:10.1098/rsta.2012.0023
Giuliano, B. M., Gavdush, A. A., Müller, B., Zaytsev, K. I., Grassi, T., Ivlev, A. V., et al. (2019). Broadband Spectroscopy of Astrophysical Ice Analogues I: Direct Measurement of the Complete Refractive index of CO Ice Using Terahertz Time-Domain Spectroscopy. Astron. Astrophys. 629, 112. doi:10.1051/0004-6361/201935619
Godard, B., and Cernicharo, J. (2013). A Complete Model of CH+ Rotational Excitation Including Radiative and Chemical Pumping Processes. Astron. Astrophys. 550, 8. doi:10.1051/0004-6361/201220151
Godard, B., Falgarone, E., and Pineau des Forêts, G. (2009). Models of Turbulent Dissipation Regions in the Diffuse Interstellar Medium. A&A 495, 847–867. doi:10.1051/0004-6361:200810803
Goldman, N., Reed, E. J., Fried, L. E., William Kuo, I.-F., and Maiti, A. (2010). Synthesis of Glycine-Containing Complexes in Impacts of Comets on Early Earth. Nat. Chem 2, 949–954. doi:10.1038/nchem.827
Goldsmith, P. F., Liseau, R., Bell, T. A., Black, J. H., Chen, J.-H., Hollenbach, D., et al. (2011). Herschel measurements of Molecular Oxygen in Orion. ApJ 737, 96. doi:10.1088/0004-637x/737/2/96
Graff, M. M., Moseley, J. T., and Roueff, E. (1983). Resonant and Nonresonant Processes in the Formation of CH(+) by Radiative Association. ApJ 269, 796. doi:10.1086/161088
Griffin, M. J., Abergel, A., Abreu, A., Ade, P. A. R., André, P., Augueres, J.-L., et al. (2010). TheHerschel-SPIRE Instrument and its In-Flight Performance. A&A 518, L3. doi:10.1051/0004-6361/201014519
Griffin, M. J., Baselmans, J., Baryshev, A., Doyle, S., Grim, M., Hargrave, P., et al. (2016). “SPACEKIDS: Kinetic Inductance Detectors for Space Applications,” in Proc. SPIE 9914, Millimeter, Submillimeter, and Far-Infrared Detectors and Instrumentation for Astronomy VIII (Springer), 991407. doi:10.1117/12.2231100
Griffiths, P., and de Haseth, J. (1986). Fourier Transform Infrared Spectroscopy. New York City (NY): John Wiley & Sons.
Groen, J., Deamer, D. W., Kros, A., and Ehrenfreund, P. (2012). Polycyclic Aromatic Hydrocarbons as Plausible Prebiotic Membrane Components. Orig. Life Evol. Biosph. 42, 295–306. doi:10.1007/s11084-012-9292-3
Gupta, H., Rimmer, P., Pearson, J. C., Yu, S., Herbst, E., Harada, N., et al. (2010). Detection of OH+ and H2O+ towards Orion KL. A&A 521, L47. doi:10.1051/0004-6361/201015117
Gurvits, L. I., Paragi, Z., Casasola, V., Conway, J., Davelaar, J., Falcke, H., et al. (2021). THEZA: TeraHertz Exploration and Zooming-In for Astrophysics. Exp. Astron. 51, 559–594. doi:10.1007/s10686-021-09714-y
Hazen, R. M., and Ferry, J. M. (2010). Mineral Evolution: Mineralogy in the Fourth Dimension. Elements 6, 9–12. doi:10.2113/gselements.6.1.9
Hazen, R. M., Papineau, D., Bleeker, W., Downs, R. T., Ferry, J. M., McCoy, T. J., et al. (2008). Mineral Evolution. Am. Mineral. 93, 1693–1720. doi:10.2138/am.2008.2955
Henderson, S. W., Stevens, J. R., Amiri, M., Austermann, J., Beall, J. A., Chaudhuri, S., et al. (2016). “Readout of Two-Kilopixel Transition-Edge Sensor Arrays for Advanced ACTPol,” in Proc. SPIE 9914, Millimeter, Submillimeter, and Far-Infrared Detectors and Instrumentation for Astronomy VIII (Springer), 9914. doi:10.1117/12.2233895
Higgs, P. G., and Lehman, N. (2015). The RNA World: Molecular Cooperation at the Origins of Life. Nat. Rev. Genet. 16, 7–17. doi:10.1038/nrg3841
Hogerheijde, M. R., Bergin, E. A., Brinch, C., Cleeves, L. I., Fogel, J. K., Blake, G. A., et al. (2011). Detection of the Water Reservoir in a Forming Planetary System. Science 334, 338–340. doi:10.1126/science.1208931
Indriolo, N., Neufeld, D. A., Gerin, M., Schilke, P., Benz, A. O., Winkel, B., et al. (2015). Herschel survey of Galactic Oh+, H2O+, and H3O+: Probing the Molecular Hydrogen Fraction and Cosmic-ray Ionization Rate. ApJ 800, 40. doi:10.1088/0004-637x/800/1/40
Ioppolo, S., Fedoseev, G., Chuang, K.-J., Cuppen, H. M., Clements, A. R., Jin, M., et al. (2021). A Non-energetic Mechanism for glycine Formation in the Interstellar Medium. Nat. Astron. 5, 197–205. doi:10.1038/s41550-020-01249-0
Ioppolo, S., Kaňuchová, Z., James, R. L., Dawes, A., Jones, N. C., Hoffmann, S. V., et al. (2020). Vacuum Ultraviolet Photoabsorption Spectroscopy of Space-Related Ices: 1 keV Electron Irradiation of Nitrogen- and Oxygen-Rich Ices. Astron. Astrophys. 641, 154. doi:10.1051/0004-6361/201935477
Ioppolo, S., McGuire, B. A., Allodi, M. A., and Blake, G. A. (2014). THz and Mid-IR Spectroscopy of Interstellar Ice Analogs: Methyl and Carboxylic Acid Groups. Faraday Discuss. 168, 461–484. doi:10.1039/c3fd00154g
Jacob, A. M., Menten, K. M., Wyrowski, F., Winkel, B., and Neufeld, B. A. (2020). Extending the View of ArH+ Chemistry in Diffuse Clouds. Astron. Astrophys. 643, 91. doi:10.1051/0004-6361/202039197
Jones, B. M., Bennett, C. J., and Kaiser, R. I. (2011). Mechanistical Studies on the Production of Formamide (H2Ncho) within Interstellar Ice Analogs. ApJ 734, 78. doi:10.1088/0004-637x/734/2/78
Jørgensen, J. K., Müller, H. S. P., Calcutt, H., Coutens, A., Drozdovskaya, M. N., Öberg, K. I., et al. (2018). The ALMA-PILS Survey: Isotopic Composition of Oxygen-Containing Complex Organic Molecules toward IRAS 16293-2422B. Astron. Astrophys. 620, 170. doi:10.1051/0004-6361/201731667
Kaňuchová, Z., Boduch, P., Domaracka, A., Palumbo, M. E., Rothard, H., and Strazzulla, G. (2017). Thermal and Energetic Processing of Astrophysical Ice Analogues Rich in SO2. Astron. Astrophys. 604, 68. doi:10.1051/0004-6361/201730711
Kawase, K., Sato, M., Taniuchi, T., and Ito, H. (1996). Coherent Tunable THz‐wave Generation from LiNbO3 with Monolithic Grating Coupler. Appl. Phys. Lett. 68, 2483–2485. doi:10.1063/1.115828
Kelvin Lee, K. L., Changala, P. B., Loomis, R. A., Burkhardt, A. M., Xue, C., Cordiner, M. A., et al. (2021a). Interstellar Detection of 2-cyanocyclopentadiene, C5H5CN, a Second Five-Membered Ring toward TMC-1. ApJL 910, L2. doi:10.3847/2041-8213/abe764
Kelvin Lee, K. L., Loomis, R. A., Burkhardt, A. M., Cooke, I. R., Xue, C., Siebert, M. A., et al. (2021b). Discovery of Interstellar Trans-cyanovinylacetylene (HC ≡ CCH = CHC ≡ N) and Vinylcyanoacetylene (H2C = CHC3N) in GOTHAM Observations of TMC-1. ApJ 908, L11. doi:10.3847/2041-8213/abdbb9
Kiessling, J., Breunig, I., Schunemann, P. G., Buse, K., and Vodopyanov, K. L. (2013). High Power and Spectral Purity Continuous-Wave Photonic THz Source Tunable from 1 to 4.5 THz for Nonlinear Molecular Spectroscopy. New J. Phys. 15, 105014. doi:10.1088/1367-2630/15/10/105014
Kilcullen, P., Hartley, I. D., Jensen, E. T., and Reid, M. (2015). Terahertz Time Domain Gas-phase Spectroscopy of Carbon Monoxide. J. Infrared Milli. Terahz. Waves 36, 380–389. doi:10.1007/s10762-014-0139-z
Klein, B., Hochgürtel, S., Krämer, I., Bell, A., Meyer, K., and Güsten, R. (2012). High-resolution Wide-Band Fast Fourier Transform Spectrometers. A&A 542, L3. doi:10.1051/0004-6361/201218864
Klein, B., Philipp, S. D., Güsten, R., Krämer, I., and Samtleben, D. (2006). A New Generation of Spectrometers for Radio Astronomy: Fast Fourier-Transform Spectrometer. Proc. SPIE 6275, 62751. doi:10.1117/12.670831
Kojima, T., Kroug, M., Uemizu, K., Niizeki, Y., Takahashi, H., and Uzawa, Y. (2017). Performance and Characterization of a Wide if SIS-Mixer-Preamplifier Module Employing High-J C SIS Junctions. IEEE Trans. Thz Sci. Technol. 7, 694–703. doi:10.1109/tthz.2017.2758260
Kokoouline, V., and Greene, C. H. (2003). Unified Theoretical Treatment of Dissociative Recombination of D3h Triatomic Ions: Applications to H3+ and D3+. Phys. Rev. A. 68, 012703. doi:10.1103/physreva.68.012703
Komandin, G. A., Chuchupal, S. V., Lebedev, S. P., Goncharov, Y. G., Korolev, A. F., Porodinkov, O. E., et al. (2013). BWO Generators for Terahertz Dielectric Measurements. IEEE Trans. Thz Sci. Technol. 3, 440–444. doi:10.1109/tthz.2013.2255914
Kruger, K., Grabowski, P. J., Zaug, A. J., Sands, J., Gottschling, D. E., and Cech, T. R. (1982). Self-splicing RNA: Autoexcision and Autocyclization of the Ribosomal RNA Intervening Sequence of tetrahymena. Cell 31, 147–157. doi:10.1016/0092-8674(82)90414-7
Kulesa, C. (2011). Terahertz Spectroscopy for Astronomy: From Comets to Cosmology. IEEE Trans. Terahertz Sci. Technol. 1, 232–240. doi:10.1109/tthz.2011.2159648
Kwok, S., and Zhang, Y. (2011). Mixed Aromatic-Aliphatic Organic Nanoparticles as Carriers of Unidentified Infrared Emission Features. Nature 479, 80–83. doi:10.1038/nature10542
Lang, K., Erlacher, M., Wilson, D. N., Micura, R., and Polacek, N. (2008). The Role of 23S Ribosomal RNA Residue A2451 in Peptide Bond Synthesis Revealed by Atomic Mutagenesis. Chem. Biol. 15, 485–492. doi:10.1016/j.chembiol.2008.03.014
Langer, W. D., Velusamy, T., Pineda, J. L., Goldsmith, P. F., Li, D., and Yorke, H. W. (2010). C+ detection of Warm Dark Gas in Diffuse Clouds. A&A 521, L17. doi:10.1051/0004-6361/201015088
Larson, Å., Djurić, N., Zong, W., Greene, C. H., Orel, A. E., Al-Khalili, A., et al. (2000). Resonant Ion-Pair Formation in Electron Collisions with HD+ and OH+. Phys. Rev. A. 62, 042707. doi:10.1103/physreva.62.042707
Larsson, M., Geppert, W. D., and Nyman, G. (2012). Ion Chemistry in Space. Rep. Prog. Phys. 75, 066901. doi:10.1088/0034-4885/75/6/066901
Larsson, M., McCall, B. J., and Orel, A. E. (2008). The Dissociative Recombination of H3+ - a Saga Coming to an End? Chem. Phys. Lett. 462, 145–151. doi:10.1016/j.cplett.2008.06.069
Li, W., and Yao, J. (2010). Investigation of Photonically Assisted Microwave Frequency Multiplication Based on External Modulation. IEEE Trans. Microwave Theor. Techn. 58, 3259–3268. doi:10.1109/tmtt.2010.2075671
Linnartz, H., Ioppolo, S., and Fedoseev, G. (2015). Atom Addition Reactions in Interstellar Ice Analogues. Int. Rev. Phys. Chem. 34, 205–237. doi:10.1080/0144235x.2015.1046679
Linz, H., Beuther, H., Gerin, M., Goicoechea, J. R., Helmich, F., Krause, O., et al. (2021). Bringing High Spatial Resolution to the Far-Infrared. Exp. Astron. 51, 661–697. doi:10.1007/s10686-021-09719-7
Lis, D. C., Pearson, J. C., Neufeld, D. A., Schilke, P., Müller, H. S. P., Gupta, H., et al. (2010). Herschel/HIFI Discovery of Interstellar Chloronium (H2Cl+). A&A 521, L9. doi:10.1051/0004-6361/201014959
Lo, J.-I., Chou, S.-L., Peng, Y.-C., Lin, M.-Y., Lu, H.-C., and Cheng, B.-M. (2014). Photochemistry of Solid Interstellar Molecular Samples Exposed to Vacuum-Ultraviolet Synchrotron Radiation. J. Electron Spectrosc. Relat. Phenomena 196, 173–176. doi:10.1016/j.elspec.2013.12.014
Loomis, R. A., Burkhardt, A. M., Shingledecker, C. N., Charnley, S. B., Cordiner, M. A., Herbst, E., et al. (2021). An Investigation of Spectral Line Stacking Techniques and Application to the Detection of HC11N. Nat. Astron. 5, 188–196. doi:10.1038/s41550-020-01261-4
Maestrini, A., Thomas, B., Wang, H., Jung, C., Treuttel, J., Jin, Y., et al. (2010). Schottky Diode-Based Terahertz Frequency Multipliers and Mixers. Comptes Rendus Physique 11, 480–495. doi:10.1016/j.crhy.2010.05.002
Maiolino, R. (2008). Prospects for AGN Studies with ALMA. New Astron. Rev. 52, 339–357. doi:10.1016/j.newar.2008.06.012
Martins, Z., Price, M. C., Goldman, N., Sephton, M. A., and Burchell, M. J. (2013). Shock Synthesis of Amino Acids from Impacting Cometary and Icy Planet Surface Analogues. Nat. Geosci 6, 1045–1049. doi:10.1038/ngeo1930
Mason, N. J., Dawes, A., Holtom, P. D., Mukerji, R. J., Davis, M. P., Sivaraman, B., et al. (2006). VUV Spectroscopy and Photo-Processing of Astrochemical Ices: An Experimental Study. Faraday Discuss. 133, 311. doi:10.1039/b518088k
Materese, C. K., Nuevo, M., McDowell, B. L., Buffo, C. E., and Sandford, S. A. (2018). The Photochemistry of Purine in Ice Analogs Relevant to Dense Interstellar Clouds. ApJ 864, 44. doi:10.3847/1538-4357/aad328
Materese, C. K., Nuevo, M., and Sandford, S. A. (2015). N- Ando-Heterocycles Produced from the Irradiation of Benzene and Naphthalene in H2O/NH3-Containing Ices. ApJ 800, 116. doi:10.1088/0004-637x/800/2/116
Materese, C. K., Nuevo, M., and Sandford, S. A. (2017). The Formation of Nucleobases from the Ultraviolet Photoirradiation of Purine in Simple Astrophysical Ice Analogues. Astrobiology 17, 761–770. doi:10.1089/ast.2016.1613
McCall, B. J., Geballe, T. R., Hinkle, K. H., and Oka, T. (1998). Detection of H 3 + in the Diffuse Interstellar Medium toward Cygnus OB2 No. 12. Science 279, 1910–1913. doi:10.1126/science.279.5358.1910
McCarthy, M. C., Lee, K. L. K., Loomis, R. A., Burkhardt, A. M., Shingledecker, C. N., Charnley, S. B., et al. (2021). Interstellar Detection of the Highly Polar Five-Membered Ring Cyanocyclopentadiene. Nat. Astron. 5, 176–180. doi:10.1038/s41550-020-01213-y
McGuire, B. A., Brogan, C. L., Hunter, T. R., Remijan, A. J., Blake, G. A., Burkhardt, A. M., et al. (2018b). First Results of an ALMA Band 10 Spectral Line Survey of NGC 6334I: Detections of Glycolaldehyde (HC(O)CH2OH) and a New Compact Bipolar Outflow in HDO and CS. ApJ 863, L35. doi:10.3847/2041-8213/aad7bb
McGuire, B. A., Burkhardt, A. M., Kalenskii, S., Shingledecker, C. N., Remijan, A. J., Herbst, E., et al. (2018a). Detection of the Aromatic Molecule Benzonitrile ( C -C 6 H 5 CN) in the Interstellar Medium. Science 359, 202–205. doi:10.1126/science.aao4890
McGuire, B. A., Burkhardt, A. M., Loomis, R. A., Shingledecker, C. N., Kelvin Lee, K. L., Charnley, S. B., et al. (2020). Early Science from GOTHAM: Project Overview, Methods, and the Detection of Interstellar Propargyl Cyanide (HCCCH2CN) in TMC-1. ApJ 900, L10. doi:10.3847/2041-8213/aba632
McGuire, B. A., Ioppolo, S., Allodi, M. A., and Blake, G. A. (2016). THz Time-Domain Spectroscopy of Mixed CO2-CH3OH Interstellar Ice Analogs. Phys. Chem. Chem. Phys. 18, 20199–20207. doi:10.1039/c6cp00632a
McGuire, B. A., Loomis, R. A., Burkhardt, A. M., Lee, K. L. K., Shingledecker, C. N., Charnley, S. B., et al. (2021). Detection of Two Interstellar Polycyclic Aromatic Hydrocarbons via Spectral Matched Filtering. Science 371, 1265–1269. doi:10.1126/science.abb7535
McGuire, B. A., Shingledecker, C. N., Willis, E. R., Burkhardt, A. M., El-Abd, S., Motiyenko, R. A., et al. (2017). ALMA Detection of Interstellar Methoxymethanol (CH3OCH2OH). Astrophys. J. Lett. 851, 2. doi:10.3847/2041-8213/aaa0c3
McIntosh, A. I., Yang, B., Goldup, S. M., Watkinson, M., and Donnan, R. S. (2012). Terahertz Spectroscopy: A Powerful New Tool for the Chemical Sciences? Chem. Soc. Rev. 41, 2072–2082. doi:10.1039/c1cs15277g
Mead, G., Katayama, I., Takeda, J., and Blake, G. A. (2019). An Echelon-Based Single Shot Optical and Terahertz Kerr Effect Spectrometer. Rev. Scientific Instr. 90, 053107. doi:10.1063/1.5088377
Medvedev, I. R., Neese, C. F., Plummer, G. M., and De Lucia, F. C. (2010). Submillimeter Spectroscopy for Chemical Analysis with Absolute Specificity. Opt. Lett. 35, 1533. doi:10.1364/ol.35.001533
Menten, K. M., Wyrowski, F., Belloche, A., Güsten, R., Dedes, L., and Müller, H. S. P. (2011). Submillimeter Absorption from SH+, a New Widespread Interstellar Radical, 13CH+ and HCl. Astron. Astrophys. 525, 77. doi:10.1051/0004-6361/201014363
Merino, P., Švec, M., Martinez, J. I., Jelinek, P., Lacovig, P., Dalmiglio, M., et al. (2014). Graphene Etching on SiC Grains as a Path to Interstellar Polycyclic Aromatic Hydrocarbons Formation. Nat. Commun. 5, 3054. doi:10.1038/ncomms4054
Mifsud, D. V., Kaňuchová, Z., Herczku, P., Ioppolo, S., Juhász, Z., Kovács, S. T. S., et al. (2021). Sulfur Ice Astrochemistry: A Review of Laboratory Studies. Space Sci. Rev. 217, 14. doi:10.1007/s11214-021-00792-0
Milillo, A., Plainaki, C., De Angelis, E., Mangano, V., Massetti, S., Mura, A., et al. (2016). Analytical Model of Europa's O2 Exosphere. Planet. Space Sci. 130, 3–13. doi:10.1016/j.pss.2015.10.011
Mittleman, D. M., Jacobsen, R. H., Neelamani, R., Baraniuk, R. G., and Nuss, M. C. (1998). Gas Sensing Using Terahertz Time-Domain Spectroscopy. Appl. Phys. B: Lasers Opt. 67, 379–390. doi:10.1007/s003400050520
Monje, R. R., Emprechtinger, M., Phillips, T. G., Lis, D. C., Goldsmith, P. F., Bergin, E. A., et al. (2011). Herschel/Hifi Observations of Hydrogen Fluoride toward Sagittarius B2(M). ApJ 734, L23. doi:10.1088/2041-8205/734/1/l23
Monje, R. R., Lis, D. C., Roueff, E., Gerin, M., De Luca, M., Neufeld, D. A., et al. (2013). Hydrogen Chloride in Diffuse Interstellar Clouds along the Line of Sight to W31C (G10.6-0.4). ApJ 767, 81. doi:10.1088/0004-637x/767/1/81
Moore, M. H., and Hudson, R. L. (1994). Far-infrared Spectra of Cosmic-type Pure and Mixed Ices. Astron. Astrophys. Suppl. Ser. 103, 45.
Moore, M. H., and Hudson, R. L. (1995). Far-infrared Spectral Changes Accompanying Proton Irradiation of Solids of Astrochemical Interest. Radiat. Phys. Chem. 45, 779. doi:10.1016/0969-806X(94)00099-6
Moore, M. H., and Hudson, R. L. (1992). Far-infrared Spectral Studies of Phase Changes in Water Ice Induced by Proton Irradiation. ApJ 401, 353. doi:10.1086/172065
Mosely, E. R., Draine, B. T., Tomida, K., and Stone, J. M. (2021). Turbulent Dissipation, CH+ Abundance, H2 Line Luminosities, and Polarization in the Cold Neutral Medium. Mon. Not. R. Astron. Soc. 500, 3290. doi:10.1093/mnras/staa3384
Müller, H. S. P., Schlöder, F., Stutzki, J., and Winnewisser, G. (2005). The Cologne Database for Molecular Spectroscopy: A Useful Tool for Astronomers and Spectroscopists. J. Mol. Struct. 742, 215. doi:10.1016/j.molstruc.2005.01.027
Müller, H. S. P., Thorwith, S., Roth, D. A., and Winnewisser, G. (2001). The Cologne Database for Molecular Spectroscopy. CDMS. Astron. Astrophys. 370, L49. doi:10.1051/0004-6361:20010367
Muller, S., Müller, H. S. P., Black, J. H., Gerin, M., Combes, F., Curran, S., et al. (2017). Detection of CH+, SH+, and Their 13C- and 34S-Isotopologues toward PKS 1830-211. Astron. Astrophys. 606, 109. doi:10.1051/0004-6361/201731405
Mullikin, E., van Mulbregt, P., Perea, J., Kasule, M., Huang, J., Buffo, C., et al. (2018). Condensed-Phase Photochemistry in the Absence of Radiation Chemistry. ACS Earth Space Chem. 2, 863–868. doi:10.1021/acsearthspacechem.8b00027
Muñoz-Caro, G. M., Jiménez-Escobar, A., Martín-Gago, J. Á., Rogero, C., Atienza, C., Puertas, S., et al. (2010). New Results on thermal and Photodesorption of CO Ice Using the Novel InsterStellar Astrochemistry Chamber (ISAC). Astron. Astrophys. 522, 108. doi:10.1051/0004-6361/200912462
Myers, A. T., McKee, C. F., and Li, P. S. (2015). The CH+ Abundance in Turbulent, Diffuse Molecular Clouds. Mon. Not. R. Astron. Soc. 453, 2747. doi:10.1093/mnras/stv1782
National Academies of Sciences, Engineering, and Medicine (2021). Pathways to Discovery in Astronomy and Astrophysics for the 2020s. Washington DC: The National Academies Press. doi:10.17226/26141
Neil, G. R. (2014). Accelerator Sources for THz Science: A Review. J. Infrared Milli Terahz Waves 35, 5–16. doi:10.1007/s10762-013-9999-x
Neufeld, D. A., Falgarone, E., Gerin, M., Godard, B., Herbst, E., Pineau des Forêts, G., et al. (2012b). Discovery of Interstellar Mercapto Radicals (SH) with the GREAT Instrument on SOFIA. A&A 542, L6. doi:10.1051/0004-6361/201218870
Neufeld, D. A., Godard, B., Gerin, M., Pineau des Forêts, G., Bernier, C., Falgarone, E., et al. (2015). Sulfur-bearing Molecules in Diffuse Molecular Clouds: New Results from SOFIA/GREAT and the IRAM 30 M Telescope. Astron. Astrophys. 577, 49. doi:10.1051/0004-6361/201425391
Neufeld, D. A., Goicoechea, J. R., Sonnentrucker, P., Black, J. H., Pearson, J., Yu, S., et al. (2010a). Herschel/HIFI Observations of Interstellar OH+and H2O+towards W49N: a Probe of Diffuse Clouds with a Small Molecular Fraction. A&A 521, L10. doi:10.1051/0004-6361/201015077
Neufeld, D. A., Roueff, E., Snell, R. L., Lis, D., Benz, A. O., Bruderer, S., et al. (2012a). Herschel observations of Interstellar Chloronium. ApJ 748, 37. doi:10.1088/0004-637x/748/1/37
Neufeld, D. A., Sonnentrucker, P., Phillips, T. G., Lis, D. C., De Luca, M., Goicoechea, J. R., et al. (2010b). Strong Absorption by Interstellar Hydrogen fluoride:Herschel/HIFI Observations of the Sight-Line to G10.6-0.4 (W31C). A&A 518, L108. doi:10.1051/0004-6361/201014523
Neveu, M., Kim, H.-J., and Benner, S. A. (2013). The "Strong" RNA World Hypothesis: Fifty Years Old. Astrobiology 13, 391–403. doi:10.1089/ast.2012.0868
Noble, J. A., Cuppen, H. M., Coussan, S., Redlich, B., and Ioppolo, S. (2020). Infrared Resonant Vibrationally Induced Restructuring of Amorphous Solid Water. J. Phys. Chem. C 124, 20864–20873. doi:10.1021/acs.jpcc.0c04463
Nuevo, M., Materese, C. K., and Sandford, S. A. (2014). The Photochemistry of Pyrimidine in Realistic Astrophysical Ices and the Production of Nucleobases. ApJ 793, 125. doi:10.1088/0004-637x/793/2/125
Nuevo, M., Milam, S. N., Sandford, S. A., Elsila, J. E., and Dworkin, J. P. (2009). Formation of Uracil from the Ultraviolet Photo-Irradiation of Pyrimidine in Pure H2O Ices. Astrobiology 9, 683–695. doi:10.1089/ast.2008.0324
Nuevo, M., Milam, S. N., and Sandford, S. A. (2012). Nucleobases and Prebiotic Molecules in Organic Residues Produced from the Ultraviolet Photo-Irradiation of Pyrimidine in NH3and H2O+ NH3Ices. Astrobiology 12, 295–314. doi:10.1089/ast.2011.0726
Öberg, K. I. (2016). Photochemistry and Astrochemistry: Photochemical Pathways to Interstellar Complex Organic Molecules. Chem. Rev. 116, 9631. doi:10.1021/acs.chemrev.5b00694
Öjekull, J., Andersson, P. U., Någård, M. B., Pettersson, J. B. C., Derkatch, A. M., Neau, A., et al. (2004). Dissociative Recombination of NH4+ and ND4+ Ions: Storage Ring Experiments and Ab Initio Molecular Dynamics. J. Chem. Phys. 120, 7391. doi:10.1063/1.1669388
Orgel, L. E. (2004). Prebiotic Chemistry and the Origin of the RNA World. Crit. Rev. Biochem. Mol. Biol. 39, 99–123. doi:10.1080/10409230490460765
Pan, K., Federman, S. R., Cunha, K., Smith, V. V., and Welty, D. E. (2004). Cloud Structure and Physical Conditions in Star‐forming Regions from Optical Observations. I. Data and Component Structure. Astrophys J. Suppl. S 151, 313–343. doi:10.1086/381805
Pardo, J. R., Cernicharo, J., and Serabyn, E. (2001). Atmospheric Transmission at Microwaves (ATM): an Improved Model for Millimeter/submillimeter Applications. IEEE Trans. Antennas Propagat. 49, 1683–1694. doi:10.1109/8.982447
Park, G. B., and Field, R. W. (2016). Perspective: The First Ten Years of Broadband Chirped Pulse Fourier Transform Microwave Spectroscopy. J. Chem. Phys. 144, 200901. doi:10.1063/1.4952762
Parkes, S., Dunstan, M., Scott, P., Dillon, D., Spark, A., Ellison, B., et al. (2018). “A Wideband Spectrometer in the Microsemi RTG4 FPGA,” in Paper Presented at Data Systems in Aerospace Conference, DASIA 2018 (Oxford, UK: IEEE).
Peeters, Z., Botta, O., Charnley, S. B., Ruiterkamp, R., and Ehrenfreund, P. (2003). The Astrobiology of Nucleobases. ApJ 593, L129–L132. doi:10.1086/378346
Perakis, F., Borek, J. A., and Hamm, P. (2013). Three-dimensional Infrared Spectroscopy of Isotope-Diluted Ice Ih. J. Chem. Phys. 139, 014501. doi:10.1063/1.4812216
Persson, C. M., Black, J. H., Cernicharo, J., Goicoechea, J. R., Hassel, G. E., Herbst, E., et al. (2010). Nitrogen Hydrides in Interstellar Gas. A&A 521, L45. doi:10.1051/0004-6361/201015105
Pickett, H. M., Poynter, R. L., Cohen, E. A., Delitsky, M. L., Pearson, J. C., and Müller, H. S. P. (1998). Submillimeter, Millimeter, and Microwave Spectral Line Catalog. J. Quantitative Spectrosc. Radiative Transfer 60, 883–890. doi:10.1016/s0022-4073(98)00091-0
Plašil, R., Rednyk, S., Kovalenko, A., Tran, T. D., Roučka, Š., Dohnal, P., et al. (2021). Experimental Study on the CH+ Formation from Doubly Charged Carbon and Molecular Hydrogen. Astrophys. J. 910, 155. doi:10.3847/1538-4357/abe86c
Potapov, A., Jäger, C., Henning, T., Jonusas, M., and Krim, L. (2017). The Formation of Formaldehyde on Interstellar Carbonaceous Grain Analogs by O/H Atom Addition. ApJ 846, 131. doi:10.3847/1538-4357/aa85e8
Preu, S., Döhler, G. H., Malzer, S., Wang, L. J., and Gossard, A. C. (2011). Tunable, Continuous-Wave Terahertz Photomixer Sources and Applications. J. Appl. Phys. 109, 061301. doi:10.1063/1.3552291
Price, D. C. (2016). Spectrometers and Polyphase Filterbanks in Radio Astronomy. ArXiv [Preprint]. Available at: https://arxiv.org/abs/1607.03579 (Accessed April 24, 2021).
Profeta, G., and Scandolo, S. (2011). Far-infrared Spectrum of Ice Ih: A First-Principles Study. Phys. Rev. B 84, 024103. doi:10.1103/physrevb.84.024103
Qasim, D., Fedoseev, G., Chuang, K.-J., He, J., Ioppolo, S., van Dishoeck, E. F., et al. (2020). An Experimental Study of the Surface Formation of Methane in Interstellar Molecular Clouds. Nat. Astron. 4, 781–785. doi:10.1038/s41550-020-1054-y
Raffaelli, N. (2011). “Nicotinamide Coenzyme Synthesis: A Case of Ribonucleotide Emergence or a Byproduct of the RNA World?,” in Origins of Life: The Primal Self-Organization. Editors R. Egel, D. H. Lankenau, and A. Y. Mulkidjanian (Heidelberg: Springer), 185–208. doi:10.1007/978-3-642-21625-1_9
Redlich, B., van der Meer, L., Zacharias, H., Meijer, G., and von Helden, G. (2003). FEL Induced Dynamics of Small Molecules on Surfaces: N2O on NaCl(100). Nucl. Instrum. Methods Phys. Res. A. 507, 556–560. doi:10.1016/b978-0-444-51417-2.50126-7
Rigopoulou, D., Pearson, C., Ellison, B., Wiedner, M., Okada, V. O., Tan, B. K., et al. (2021). The Far-Infrared Spectroscopic Surveyor (FIRSS). Exp. Astron. 51, 699–728. doi:10.1007/s10686-021-09716-w
Rosenthal, D., Beeman, J. W., Geis, N., Grözinger, U., Hönle, R., Katterloher, R. O., et al. (2002). Stressed Ge:Ga Detector Arrays for PACS and FIFI LS. Far-IR, Sub-mm and Mm Detector Technology Workshop. Monterey, CA: Springer.
Rothman, L. S., Gordon, I. E., Barbe, A., Benner, D. C., Bernath, P. F., Birk, M., et al. (2009). The HITRAN 2008 Molecular Spectroscopic Database. J. Quantitative Spectrosc. Radiative Transfer 110, 533–572. doi:10.1016/j.jqsrt.2009.02.013
Sandford, S. A., Nuevo, M., Bera, P. P., and Lee, T. J. (2020). Prebiotic Astrochemistry and the Formation of Molecules of Astrobiological Interest in Interstellar Clouds and Protostellar Disks. Chem. Rev. 120, 4616–4659. doi:10.1021/acs.chemrev.9b00560
Schilke, P., Neufeld, D. A., Müller, H. S. P., Comito, C., Bergin, E. A., Lis, D. C., et al. (2014). Ubiquitous Argonium (ArH+) in the Diffuse Interstellar Medium: A Molecular Tracer of Almost Purely Atomic Gas. Astron. Astrophys. 566, 29. doi:10.1051/0004-6361/201423727
Scibelli, S., and Shirley, Y. (2020). Prevalence of Complex Organic Molecules in Starless and Prestellar Cores within the Taurus Molecular Cloud. ApJ 891, 73. doi:10.3847/1538-4357/ab7375
Semaniak, J., Larson, A., Le Padellec, A., Stromholm, C., Larsson, M., Rosen, S., et al. (1998). Dissociative recombination and excitation of CH5+: Absolute cross sections and branching fractions. ApJ 498, 886–895. doi:10.1086/305581
Shalit, A., Perakis, F., and Hamm, P. (2013). Two-dimensional Infrared Spectroscopy of Isotope-Diluted Low Density Amorphous Ice. J. Phys. Chem. B 117, 15512–15518. doi:10.1021/jp4053743
Siebertz, O., Schmülling, F., Gal, C., Schloeder, F., Hartogh, P., Natale, V., et al. (2007). The Wide-Band Spectrometer (WBS) for the HIFI Instrument of Herschel. Proc. ISSTT 135, 1.
Smith, D. (1992). The Ion Chemistry of Interstellar Clouds. Chem. Rev. 92, 1473–1485. doi:10.1021/cr00015a001
Smith, I. W. M. (2011). Laboratory Astrochemistry: Gas-phase Processes. Annu. Rev. Astron. Astrophys. 49, 29–66. doi:10.1146/annurev-astro-081710-102533
Snow, T. P., and Bierbaum, V. M. (2008). Ion Chemistry in the Interstellar Medium. Annu. Rev. Anal. Chem. 1, 229–259. doi:10.1146/annurev.anchem.1.031207.112907
Snow, T. P., and McCall, B. J. (2006). Diffuse Atomic and Molecular Clouds. Annu. Rev. Astron. Astrophys. 44, 367–414. doi:10.1146/annurev.astro.43.072103.150624
Soma, T., Sakai, N., Watanabe, Y., and Yamamoto, S. (2018). Complex Organic Molecules in Taurus Molecular Cloud-1. ApJ 854, 116. doi:10.3847/1538-4357/aaa70c
Sonnentrucker, P., Neufeld, D. A., Phillips, T. G., Gerin, M., Lis, D. C., De Luca, M., et al. (2010). Detection of Hydrogen Fluoride Absorption in Diffuse Molecular Clouds with Herschel/HIFI: an Ubiquitous Tracer of Molecular Gas. A&A 521, L12. doi:10.1051/0004-6361/201015082
Suzuki, T., Khosropanah, P., Ridder, M. L., Hijmering, R. A., Gao, J. R., Akamatsu, H., et al. (2016). Development of Ultra-low-noise TES Bolometer Arrays. J. Low Temp. Phys. 184, 52–59. doi:10.1007/s10909-015-1401-z
Taylor, S. D., and Duley, W. W. (1997). The Formation of Long Carbon Chains in Diffuse Clouds. Mon. Not. R. Astron. Soc. 286, 344–348. doi:10.1093/mnras/286.2.344
Teolis, B. D., and Waite, J. H. (2016). Dione and Rhea Seasonal Exospheres Revealed by Cassini CAPS and INMS. Icarus 272, 277–289. doi:10.1016/j.icarus.2016.02.031
Theulé, P., Endres, C., Hermanns, M., Bossa, J. B., and Potapov, A. (2020). High-resolution Gas-phase Spectroscopy of Molecules Desorbed from an Ice Surface: A Proof-Of-Principle Study. ACS Earth Space Chem. 4, 86. doi:10.1021/acsearthspacechem.9b00246
Thiel, V., Belloche, A., Menten, K. M., Garrod, R. T., and Müller, H. S. P. (2017). Complex Organic Molecules in Diffuse Clouds along the Line of Sight to Sagittarius B2. A&A 605, L6. doi:10.1051/0004-6361/201731495
Thornton, R. J., Ade, P. A. R., Aiola, S., Angilè, F. E., Amiri, M., Beall, J. A., et al. (2016). The Atacama Cosmology Telescope: The Polarization-Sensitive Actpol Instrument. ApJS 227, 21. doi:10.3847/1538-4365/227/2/21
Tielens, A. G. G. M. (2008). Interstellar Polycyclic Aromatic Hydrocarbon Molecules. Annu. Rev. Astron. Astrophys. 46, 289–337. doi:10.1146/annurev.astro.46.060407.145211
Trofimov, V., and Varentsova, S. (2016). Essential Limitations of the Standard THz TDS Method for Substance Detection and Identification and a Way of Overcoming Them. Sensors 16, 502. doi:10.3390/s16040502
Valavanis, A., Auriacombe, O., Rawlings, T., Han, Y., Rea, S., Crook, M., et al. (2019). Waveguide-integrated THz Quantum-cascade Lasers for Atmospheric Research Satellite Payloads. Proc. IEEE Mtt-s IWS 1, 1. doi:10.1109/ieee-iws.2019.8803875
Valdivia, V., Godard, B., Hennebelle, P., Gerin, M., Lesaffre, P., and Le Bourlot, J. (2017). Origin of CH+ in Diffuse Molecular Clouds. Astron. Astrophys. 600, 114. doi:10.1051/0004-6361/201629905
van Dishoeck, E. F. (2014). Astrochemistry of Dust, Ice and Gas: Introduction and Overview. Faraday Discuss. 168, 9–47. doi:10.1039/c4fd00140k
van Dishoeck, E. F. (2017). Astrochemistry: Overview and Challenges. Proc. IAU 13, 3–22. doi:10.1017/s1743921317011528
van Dishoeck, E. F., and Black, J. H. (1986). Comprehensive Models of Diffuse Interstellar Clouds - Physical Conditions and Molecular Abundances. ApJS 62, 109. doi:10.1086/191135
van Dishoeck, E. F., and Blake, G. A. (1998). Chemical Evolution of star-forming Regions. Annu. Rev. Astron. Astrophys. 36, 317–368. doi:10.1146/annurev.astro.36.1.317
van Dishoeck, E. F., Herbst, E., and Neufeld, D. A. (2013). Interstellar Water Chemistry: From Laboratory to Observations. Chem. Rev. 113, 9043–9085. doi:10.1021/cr4003177
van Exter, M., Fattinger, C., and Grischkowsky, D. (1989). High‐brightness Terahertz Beams Characterized with an Ultrafast Detector. Appl. Phys. Lett. 55, 337–339. doi:10.1063/1.101901
Viti, S., Williams, D. A., and O’Neill, P. T. (2000). Hydrocarbons in Diffuse and Translucent Clouds. Astron. Astrophys. 354, 1062.
Wakelam, V., Bron, E., Cazaux, S., Dulieu, F., Gry, C., Guillard, P., et al. (2017). H 2 Formation on Interstellar Dust Grains: The Viewpoints of Theory, Experiments, Models and Observations. Mol. Astrophysics 9, 1–36. doi:10.1016/j.molap.2017.11.001
Watanabe, N., and Kouchi, A. (2002). Efficient Formation of Formaldehyde and Methanol by the Addition of Hydrogen Atoms to CO in H2O-CO Ice at 10 K. Astrophys. J. 571, 173. doi:10.1086/341412
Wehres, N., Heyne, B., Lewen, F., Hermanns, M., Schmidt, B., Endres, C., et al. (2017). 100 GHz Room-Temperature Laboratory Emission Spectrometer. Proc. IAU 13, 332–345. doi:10.1017/s1743921317007803
Wehres, N., Maßen, J., Borisov, K., Schmidt, B., Lewen, F., Graf, U. U., et al. (2018). A Laboratory Heterodyne Emission Spectrometer at Submillimeter Wavelengths. Phys. Chem. Chem. Phys. 20, 5530–5544. doi:10.1039/c7cp06394f
White, H. B. (1976). Coenzymes as Fossils of an Earlier Metabolic State. J. Mol. Evol. 7, 101–104. doi:10.1007/bf01732468
Widicus Weaver, S. L., Butler, R. A. H., Drouin, B. J., Petkie, D. T., Dyl, K. A., De Lucia, F. C., et al. (2005). Millimeter‐Wave and Vibrational State Assignments for the Rotational Spectrum of Glycolaldehyde. Astrophys J. Suppl. S 158, 188–192. doi:10.1086/429292
Widicus Weaver, S. L. (2019). Millimeterwave and Submillimeterwave Laboratory Spectroscopy in Support of Observational Astronomy. Annu. Rev. Astron. Astrophys. 57, 79–112. doi:10.1146/annurev-astro-091918-104438
Wiesemeyer, H., Güsten, R., Heyminck, S., Hübers, H. W., Menten, K. M., Neufeld, D. A., et al. (2016). Far-infrared Study of Tracers of Oxygen Chemistry in Diffuse Clouds. Astron. Astrophys. 585, 76. doi:10.1051/0004-6361/201526473
Williams, D. A., and Hartquist, T. W. (1999). The Chemistry of star-forming Regions. Acc. Chem. Res. 32, 334–341. doi:10.1021/ar970114o
Willis, E. R., Garrod, R. T., Belloche, A., Müller, H. S. P., Barger, C. J., Bonfard, M., et al. (2020). Exploring Molecular Complexity with ALMA (EMoCA): Complex Isocyanides in Sgr B2(N). Astron. Astrophys. 636, 29. doi:10.1051/0004-6361/201936489
Wilson, T. L., Rohlfs, K., and Hüttemeister, S. (2008). Tools of Radio Astronomy. Heidelberg: Springer.
Wlodarczak, G. (1995). Rotational Spectroscopy and Astrochemistry. J. Mol. Struct. 347, 131–142. doi:10.1016/0022-2860(95)08541-3
Wyrowski, F., Menten, K. M., Gusten, R., and Belloche, A. (2010). First Interstellar Detection of OH+. Astron. Astrophys. 518, 26. doi:10.1051/0004-6361/201014364
Xue, C., Willis, E. R., Loomis, R. A., Kelvin Lee, K. L., Burkhardt, A. M., Shingledecker, C. N., et al. (2020). Detection of Interstellar HC4NC and an Investigation of Isocyanopolyyne Chemistry under TMC-1 Conditions. ApJ 900, L9. doi:10.3847/2041-8213/aba631
Yamamoto, S. (2017). Introduction to Astrochemistry: Chemical Evolution from Interstellar Clouds to Star and Planet Formation. Tokyo: Springer.
Yocum, K. M., Smith, H. H., Todd, E. W., Mora, L., Gerakines, P. A., Milam, S. N., et al. (2019). Millimeter/Submillimeter Spectroscopic Detection of Desorbed Ices: A New Technique in Laboratory Astrochemistry. J. Phys. Chem. A. 123, 8702–8708. doi:10.1021/acs.jpca.9b04587
Zernickel, A., Schilke, P., Schmiedeke, A., Lis, D. C., Brogan, C. L., Ceccarelli, C., et al. (2012). Molecular Line Survey of the High-Mass star-forming Region NGC 6334I with Herschel/HIFI and the Submillimeter Array. Astron. Astrophys. 546, 87. doi:10.1051/0004-6361/201219803
Keywords: terahertz spectroscopy, far-IR spectroscopy, astrochemistry, interstellar chemistry, prebiotic chemistry, review
Citation: Mifsud DV, Hailey PA, Traspas Muiña A, Auriacombe O, Mason NJ and Ioppolo S (2021) The Role of Terahertz and Far-IR Spectroscopy in Understanding the Formation and Evolution of Interstellar Prebiotic Molecules. Front. Astron. Space Sci. 8:757619. doi: 10.3389/fspas.2021.757619
Received: 12 August 2021; Accepted: 08 November 2021;
Published: 29 November 2021.
Edited by:
Ashraf-Ali, University of Maryland, College Park, United StatesReviewed by:
Cristina Puzzarini, University of Bologna, ItalyEric Herbst, University of Virginia, United States
Copyright © 2021 Mifsud, Hailey, Traspas Muiña, Auriacombe, Mason and Ioppolo. This is an open-access article distributed under the terms of the Creative Commons Attribution License (CC BY). The use, distribution or reproduction in other forums is permitted, provided the original author(s) and the copyright owner(s) are credited and that the original publication in this journal is cited, in accordance with accepted academic practice. No use, distribution or reproduction is permitted which does not comply with these terms.
*Correspondence: Duncan V. Mifsud, duncanvmifsud@gmail.com
†ORCID: Duncan V. Mifsud, 0000-0002-0379-354X; Perry A. Hailey, 0000-0002-8121-9674; Alejandra Traspas Muiña, 0000-0002-4304-2628; Olivier Auriacombe, 0000-0002-5810-8650; Nigel J. Mason, 0000-0002-4468-8324; Sergio Ioppolo, 0000-0002-2271-1781