- Department of Physics and Astronomy, University College London, London, United Kingdom
The desire to characterize and model the atmospheres of the many extrasolar planets that have been discovered over the last three decades is a major driver of current astronomy. However, this goal is impacted by the lack of spectroscopic data on the molecules in question. As most atmospheres that can be studied are hot, some surprisingly so, this activity requires spectroscopic information not readily available from laboratory studies. This article will review the current status of available molecular spectroscopic data, usually presented as line lists, for studies of exoplanet atmospheres and, indeed, the atmospheres of other astronomical objects hotter than the Earth such as brown dwarfs, cool stars and even sunspots. Analysis of exoplanet transit spectra and the calculation of the relevant opacities often require huge datasets comprising billions of individual spectroscopic transitions. Conversely, the newly-developed high-resolution Doppler-shift spectroscopy technique has proved to be a powerful tool for detecting molecular species in exoplanet atmospheres, but relies on the use of smaller, highly accurate line lists. Methods of resolving issues arising from the competing demands of completeness versus accuracy for line lists are discussed.
1 Introduction
Almost 5,000 exoplanets have now been positively identified with many more candidate planets awaiting confirmation, and with both ground-based and space-borne missions regularly detecting new planets. These discoveries have sparked significant activity on characterizing these planets through their spectra. Broadly speaking there are three methods of observing the spectrum of an exoplanet: directly imaging the planet, transit spectroscopy which involves observing light from the host star as a planet passes in front (and behind) it, and high resolution Doppler shift spectroscopy.
These techniques all have different characteriztics in terms of which planets are amenable to being observed, how the observational data are obtained, what information can be obtained and, of importance for this article, the requirements in terms of laboratory data needed to interpret observations. We run the ExoMol project (Tennyson and Yurchenko, 2012) which aims to provide molecular line lists, and increasingly other data, for studies of exoplanets and other astronomical atmospheres. In this paper we review the provision of available spectroscopic data for characterization of exoplanet atmospheres. There are already a number of reviews which discuss this situation for studies involving transit spectroscopy (Tinetti et al., 2013; Tennyson and Yurchenko, 2017; Tennyson and Yurchenko, 2018; Madhusudhan, 2019) so here while we summarize the current situation regarding transit spectroscopy and direct imaging, we concentrate particularly on the laboratory data required for the newer method of high-resolution Doppler-shift spectroscopy (HRDSS), also known as high-resolution cross-correlation spectroscopy.
2 Detecting Molecules on Exoplanets
The original detection of molecules on exoplanets were based on transit spectroscopy at infrared wavelengths for hot Jupiter exoplanets (Tinetti et al., 2007; Swain et al., 2008). These detections of respectively water and methane in hot objects (temperatures were generally assumed to be over 1000 K) using relatively low-resolution space telescopes relied on the detection of broad spectral features. Modeling these features requires significant quantities of laboratory data (Yurchenko et al., 2014) since the spectra of hot molecules in the infrared consists of many (106–109) individual absorption lines. Stimulated by these observations a number of groups started providing comprehensive lists of molecular transitions (line lists) appropriate for modeling molecular spectra at elevated temperatures. Besides ExoMol, the TheoRets project (Rey et al., 2016) and NASA Ames (eg., Huang et al., 2017) provide comprehensive, computed line lists for a variety of molecules while the MoLLIST project of Bernath (2020) attempts to address this problem from a more experimental standpoint. These line lists, which can be very large, are often pre-processed into cross sections representing opacities for ease of use in forward models and spectroscopic retrievals (Freedman et al., 2014; Min, 2017; Allard et al., 2000; Goyal et al., 2018; Yurchenko et al., 2018a; Tennyson and Yurchenko, 2018; Chubb et al., 2021; Gharib-Nezhad et al., 2021; Grimm et al., 2021).
The importance of these activities can be gauged from attempts to determine C/O ratios in exoplanetary atmospheres. At the time of the initial detections of methane in short-period hot Jupiter exoplanets with temperatures typically in the 1,000–2000 K range, spectral modeling of methane relied on the existing laboratory measurements of methane spectra which were almost entirely performed at room temperature. A consequence of this was that atmospheric retrievals tended to indicate high C/O ratios due to overestimated amounts of methane detected as at room temperature higher-lying rotation-vibrations levels are not populated, leading to the opacity being underestimated. The need for a reliable methane line list or opacity has sparked a range of studies (Yurchenko and Tennyson, 2014; Rey et al., 2014; Yurchenko et al., 2014; Rey et al., 2017; Yurchenko et al., 2017; Wong et al., 2019; Hargreaves et al., 2020). The status of comprehensive line lists for methane and other molecules important in exoplanets has been discussed elsewhere (Tennyson et al., 2020). The careful use of observational data and modern, comprehensive line lists is leading to reliable abundance determinations and C/O ratios, see Line et al., 2021.
The transit spectroscopy technique is only applicable to planets whose orbit leads to them passing in front of (and behind) their host star as viewed from the Solar System. Obviously this criterion is not satisfied by most exoplanets but in fact missions have concentrated on finding transiting exoplanets which as a result make up well over half of those exoplanets detected up to now. Figure 1 (Top) shows an example of the detection of water in the gas giant exoplanet Wasp-127b by Spake et al. (2020). Water is relatively easy to detect by this methodology (Tinetti et al., 2012; Tsiaras et al., 2018; Fraine et al., 2014; Tsiaras et al., 2019). It is also much easier to see a large exoplanet in a nearby orbit transiting so the sample of transiting exoplanets has a strong observational bias towards short period and hot planets.
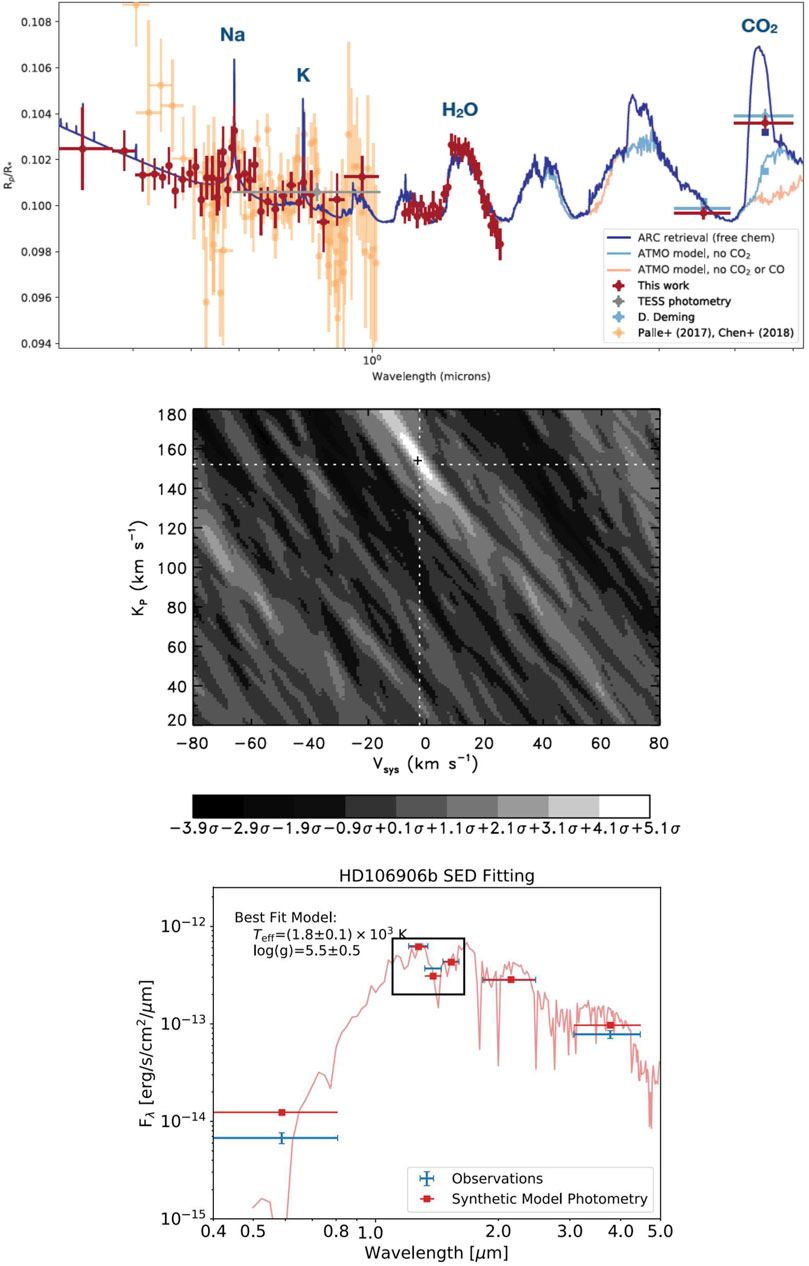
FIGURE 1. Detection of water in exoplanets: Top, Transmission (Hubble Space Telescope and Spitzer) spectrum of transiting exoplanet Wasp-127b (reproduced from Figure 18 of Spake et al., 2020); Middle, high-resolution detection of water in with significance of 4.8σ (reproduced from Figure 3 of Birkby et al., 2013); Bottom, first 1.4 μm water band photometric measurement for HD 106906 by Zhou et al., 2020. (©AAS. Reproduced with permission.)
Conversely, direct imaging relies on being able to separate the spectral signature of a planet from that of its host star. This is most easily done for nearby stars with planets in large orbits. Planets that orbit far from their star should be cool as they receive only weak starlight. However, in this case such planets would also be faint and hard to detect. Young planets, however, are formed hot due to the heating generated by gravitational collapse. As a result observation and characterization of directly imaged planets has largely concentrated on very young (a few tens of millions years old) planets. Progress is being made on the characterization of young, self-luminous giant planets at wide orbital separations from their host stars by direct imaging; an example of a recent detection of water in directly imaged exoplanet HD 106906b by Zhou et al. (2020) is given in Figure 1 (Bottom).
In fact, the most secure detections of molecules thus far have not been made by transit spectroscopy or direct imaging. Observations using these methods have relied on use of relatively low resolution methods resulting in spectra showing broad features rather than individual transitions. While these broad features can be associated with certain molecules, the results are open to alternative explanations, see Blain et al. (2021) for an example. The HRDSS method pioneered by Snellen and co-workers (Snellen, 2014) involves recording spectra of the combined star and exoplanetary system over the entire orbit of the exoplanet, which does not have to be transiting. Molecular signatures can then be identified using the combination of line positions and appropriate Doppler shifts. This technique has been used to make a number of molecular detections at 5σ level or better (Birkby, 2018; Brogi and Line, 2019). Figure 1 (Middle) shows the detection of water in hot Jupiter exoplanet HD 189733b by Birkby et al. (2013). In this case the figure confirming the detection does not look like a standard spectrum but instead takes the form of a cross-correlation diagram obtained using a particular set of molecular transitions and appropriate Doppler shifts.
The HRDSS method is powerful but requires high resolution laboratory data to be successful, see Hoeijmakers et al. (2015) for example. Typically to be useful, wavelengths, λ, need to be known with a resolving power
3 High Resolution Molecular Line Lists
Given that theory alone cannot provide line positions accurate enough for HRDSS studies, it is necessary to find an alternative procedure. For temperate planets the HITRAN database (Gordon et al., 2022), which largely comprises data from laboratory measurements, provides a good starting point. However, for hotter exoplanets or ones with exotic atmospheres one has to move beyond this towards computed line lists. Using experimental data to improve the underlying potential energy surface used in a nuclear motion calculations is a standard procedure, see Tennyson (2012), but not one which yields the required accuracy for HRDSS. We have therefore adopted an alternative approach based on use of the MARVEL (measured accurate rotation vibration energy levels) procedure.
MARVEL was originally developed by Furtenbacher et al. (2007) to address the challenge of obtaining a consistent and accurate set of empirical energy levels for the main isotopologues of water (Tennyson et al., 2014a). The MARVEL algorithm has subsequently been improved (Furtenbacher and Császár, 2012; Tóbiás et al., 2019), largely to improve the treatment of uncertainties. Put simply, the procedure involves assembling assigned transitions with uncertainties from all high resolution spectroscopy experiments which are then inverted to give empirical energy levels using the MARVEL procedure, which is available as an online tool. In practice a considerable amount of data cleaning is usually required before final answers are obtained. The empirical energy levels obtained using MARVEL are then validated against the appropriate line list allowing the reliability of those energy levels characterized by only one or two transitions to be assessed. The resulting empirical energy levels can then be used to substitute those calculated in a computed line list, which are then assigned the uncertainty obtained in the MARVEL process. This approach not only guarantees that a line list reproduces the observed transitions accurately, but results in many other, as yet unmeasured, transitions being predicted with the accuracy of high resolution spectroscopy. The replacement of energy levels in the full line list also ensures the retention of the original line list completeness.
A recent example of this appropach is provided by a MARVEL study on formaldehyde (CH2O) by Al-Derzi et al., 2021 who compiled a list of 16,403 non-redundant observed transitions taken from 43 experimental sources. Using MARVEL gave 5,029 empirical energy levels which were validated by comparing with the predictions of the AYTY ExoMol line list (Al-Refaie et al., 2015). Substituting these empirical energy levels back into this line list yields 367,779 transition frequencies with accuracy good enough to be used for HRDSS astronomical studies. This represents a more than twenty-fold increase compared to the original experimental measurements.
Thus the basic strategy for computing a high accuracy line list is clear. Step 1 is to compute a line list using the best available potential curves or surfaces, which should be tuned to the experimental data if possible. Step 2 is to use available empirical energy levels obtained from a MARVEL study to replace computed ones where possible. This already gives a significant improvement for many predicted transitions. The NASA Ames group have also used MARVEL energy levels in this fashion (Huang et al., 2019b). However, this strategy can be further improved on (Bowesman et al., 2021; McKemmish et al., 2022). Table 1 summarizes MARVEL studies on molecules of interest for exoplanets studies.
We note that MARVEL is an active procedure which means that when new data become available they can simply be added to an existing compilation and the process re-run. As can be seen from Table 1 a number of updates to existing MARVEL compilations have been published. Indeed experiments are now being designed and performed to explicitly update available MARVEL compilations by, for example, performing measurements for transitions critical for improving the network (Tobias et al., 2020; Diouf et al., 2021).
The traditional method of representing the energy levels obtained from high resolution molecular spectroscopy is via so-called effective Hamiltonians which use rotational and other constants to represent the observed levels. Effective Hamiltonians have a proven record of providing compact and highly accurate representations of molecular energy levels. However, there are two issues with their use. Firstly, while they accurately interpolate between observed data, their extrapolation properties are known to be problematic in many cases (Polyansky et al., 1994; Furtenbacher et al., 2020a). Secondly, they often struggle to accurately represent so-called resonance states: ones which are perturbed by interactions with states of the same symmetry but belonging to another vibrational or vibronic state. Representing states in resonance using effective Hamiltonians usually requires many parameters and a significant amount of extra experimental data. While MARVEL uses (approximate) quantum number assignments for states, these are actually just a labelling scheme which makes no assumptions about the underlying physics of the state involved. MARVEL simply obtains a value for the observed energy level and its behavior is not affected by any unexpected or resonant interactions.
However, one issue with using MARVEL is that it may not give complete coverage: levels may be missing because transitions to them have yet to be observed. Effective Hamiltonians can be used to fill in these missing energy levels, see the recent study of AlO by Bowesman et al. (2021) for example. Bowesman et al. (2021) also found that the observed minus calculated energy levels can also give information allowing further improvement to be achieved for yet to be observed transitions involving to be observed states.
Figure 2 shows the coverage of the MARVEL data in providing accurate transition frequencies for water by using the ExoMol POKAZATEL line list for
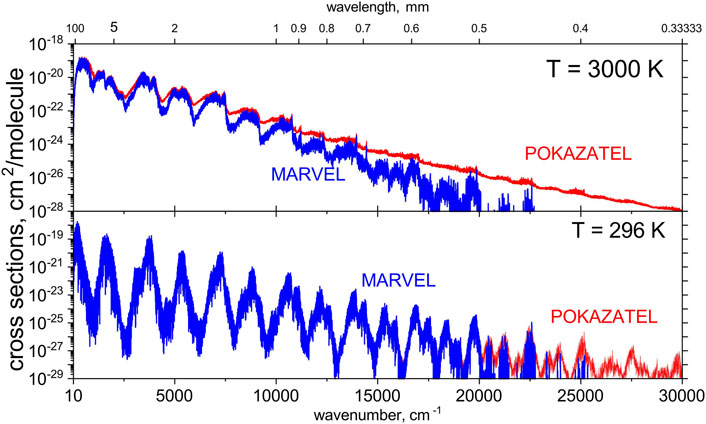
FIGURE 2. Contribution of MARVEL states to the spectra of
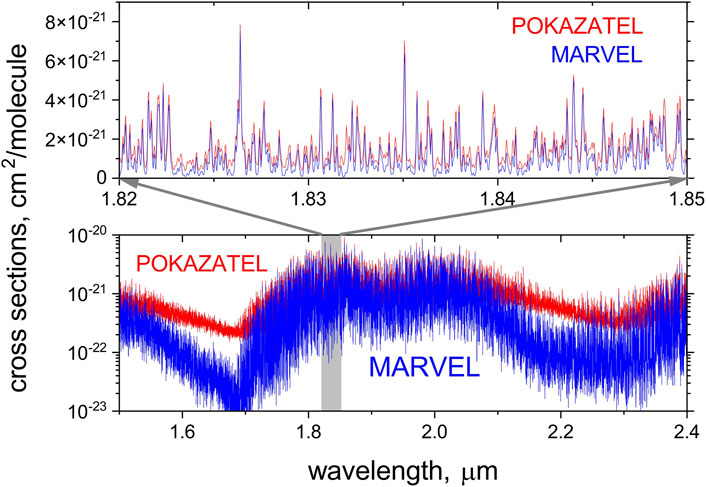
FIGURE 3. Same as Figure 2 but at higher resolution (R = 45,000): contribution of MARVEL states to the spectra of
Very recently Zhang et al., 2021 reported the first detection in an exoplanet of an isotopically substituted molecule, an isotopologue, in this case 13CO. As isotopic abundances vary across the Universe, the abundance of isotopologues will also vary and their observation has the capability of yielding significant extra information about exoplanets; an increasing number of such studies can be anticipated. Although a number of MARVEL studies have provided energy levels for isotopologues, see Table 1, it is true to say that there is always significantly less information available for these minor species than for the most abundant or parent isotopologue.
Polyansky et al. (2017) showed that it was possible to use the observed minus calculated residues in the energy levels of the main (parent) isotopologue to improve the predicted energy levels of the minor isotopologues. This procedure, which was further improved by Furtenbacher et al. (2020c), can greatly increase the number of levels and hence transitions which are known to high accuracy for the minor isotopologues. The effectiveness of this procedure for obtaining what are termed pseudo-experimental energy levels has been shown by a recent independent high resolution spectroscopy study of
4 Data Formats and Uncertainties
The ExoMol project has a well-developed data structure (Tennyson et al., 2013b; Tennyson et al., 2020) which is designed to give a compact representation of what are very large datasets. This data structure, which can be read by post-processing programs such as ExoCross (Yurchenko et al., 2018a) and the effective Hamiltonian program PGOPHER (Western, 2017), is based about the use of two files. A states file which contains all the energy levels of the molecule (isotopologue) in question plus associated meta data such as quantum numbers, and a trans file which is simply a list of two state numbers plus an Einstein A coefficient. This structure means that it is easy to incorporate improved energy levels from a MARVEL project as it is only the states file that needs to be updated; doing this automatically updates the transition wavenumbers which are computed as energy differences (upper minus lower) from the states file. In the current way ExoMol handles its datasets, updating the states file with, for example, improved energy levels represents an update of the underlying line list rather than a new line list. The update becomes a new version with version number given by the date in YYYYMMDD format.
One important thing missing from the original ExoMol format was any consideration of uncertainties. This was done to conserve space but in an era where the data are being used to both give comprehensive opacities and to model high resolution spectra it has become important to indicate the uncertainty with which the wavenumber (or wavelength) of a given transition is predicted. Therefore in the latest release of the ExoMol database, Tennyson et al. (2020) revised the data format to allow an uncertainty to be included for each energy level. This update is still in the process of implementation but our plan is that this will become a compulsory part of the ExoMol format. At present there is no corresponding uncertainty in the intensities (or Einstein A coefficients). Our judgement is that at present the benefit of including this extra information does not justify the further increase in size of already very large datasets which would be required to implement it.
5 Conclusion
The provision of spectroscopic data for studies of exoplanets and other hot astronomical atmospheres is being driven by the demands of astronomers both modeling and observing various heavenly bodies. In particular, important developments in techniques have driven the demand that molecular line lists that should not only be complete, an essential requirement for producing accurate opacity functions, but at least for strong lines, they should also be accurate. In this paper we describe measures being taken to ensure that line lists meet these dual requirements. This has meant the upgrading of many ExoMol line lists, see Bowesman et al., 2021 for example, to include available high accuracy empirical energy levels obtained using the MARVEL procedure; a process we refer to as refactoring. This process is still ongoing.
With modern technological advances in astrophysical exploration allowing studies of increasingly complex phenomena in atmospheres of exoplanets, it is becoming possible to study non-local thermodynamic equilibrium (non-LTE) effects (Fisher and Heng, 2019) which are already well-known elsewhere, such as in the spectra of stars, planets, comets and interstellar medium (ISM) (López-Puertas and Taylor, 2001). Non-LTE spectroscopy is based on modeling of rotational, vibrational and electronic populations and therefore introduces special requirements both on the molecular data and spectral simulation software, such as, for example, provision of ro-vibronic assignment or non-Boltzmann populations, with non-LTE methods of different levels of complexity ranging from simplified bi-temperature models (Pannier and Laux, 2019; Clark and Yurchenko, 2021) to sophisticated non-LTE radiative transfer calculations (Funke et al., 2012). This line of research is been actively explored by ExoMol (Wright et al., 2021).
The ExoMol database contains approximately 700 billion lines. Even once refactoring is complete it can be anticipated that only a small minority of these lines, maybe several tens of millions, will be determined with spectroscopic accuracy. By and large these lines constitute the most important and strongest lines for each molecule. Separately identifying these lines can be important for purposes such as line assignment in well-resolved spectra. For this reason we have updated our spectral modeling program ExoCross (Yurchenko et al., 2018a) to allow accuracy of predicted line positions to be a selection criterion when generating model spectra. We are also in the process of creating an interactive database, ExoMolHR, which will allow astronomers and others to search for high resolution data contained in the ExoMol database.
Author Contributions
All authors listed have made a substantial, direct, and intellectual contribution to the work and approved it for publication.
Funding
The project has been supported by ERC Advanced Investigator Awards 267219 (2011-16) and 883830 (2020-25), as well as STFC as part of the UCL consolidated grants (ST/M001334/1 and ST/R000476/1) in the intervening years.
Conflict of Interest
The authors declare that the research was conducted in the absence of any commercial or financial relationships that could be construed as a potential conflict of interest.
Publisher’s Note
All claims expressed in this article are solely those of the authors and do not necessarily represent those of their affiliated organizations or those of the publisher, the editors, and the reviewers. Any product that may be evaluated in this article, or claim that may be made by its manufacturer, is not guaranteed or endorsed by the publisher.
Acknowledgments
We thank Tony Lynas-Gray for many helpful comments on the manuscript and the many talented scientists who have worked with us during the ExoMol project.
References
AlDerzi, A. R., Furtenbacher, T., Yurchenko, S. N., Tennyson, J., and Császár, A. G. (2015). MARVEL Analysis of the Measured High-Resolution Spectra of 14NH3. J. Quant. Spectrosc. Radiat. Transf. 161, 117–130. doi:10.1016/j.jqsrt.2015.03.034
Al-Derzi, A. R., Yurchenko, S. N., Tennyson, J., Melosso, M., Jiang, N., Puzzarini, C., et al. (2021). MARVEL Analysis of the Measured High-Resolution Spectra of Formaldehyde. J. Quant. Spectrosc. Radiat. Transf. 266, 107563. doi:10.1016/j.jqsrt.2021.107563
Al-Refaie, A. F., Yurchenko, S. N., Yachmenev, A., and Tennyson, J. (2015). ExoMol Line Lists - VIII: A Variationally Computed Line List for Hot Formaldehyde. Mon. Not. R. Astron. Soc. 448, 1704–1714. doi:10.1093/mnras/stv091
Allard, F., Hauschildt, P. H., and Schwenke, D. (2000). TiO and H2O Absorption Lines in Cool Stellar Atmospheres. Astrophys. J. 540, 1005–1015. doi:10.1086/309366
Barton, E. J., Hill, C., Yurchenko, S. N., Tennyson, J., Dudaryonok, A., and Lavrentieva, N. N. (2017). Pressure Dependent Water Absorption Cross Sections for Exoplanets and Hot Other Atmospheres. J. Quant. Spectrosc. Radiat. Transf. 187, 453–460. doi:10.1016/j.jqsrt.2016.10.024
Bernath, P. F. (2020). MoLLIST: Molecular Line Lists, Intensities and Spectra. J. Quant. Spectrosc. Radiat. Transf. 240, 106687. doi:10.1016/j.jqsrt.2019.106687
Birkby, J. L. (2018). Spectroscopic Direct Detection of Exoplanets. Handbook of Exoplanets, 1485–1508. doi:10.1007/978-3-319-55333-7_16
Birkby, J. L., de Kok, R. J., Brogi, M., de Mooij, E. J. W., Schwarz, H., et al. (2013). Detection of Water Absorption in the Day Side Atmosphere of HD 189733 B Using Ground-Based High-Resolution Spectroscopy at 3.2 μm. Mon. Not. R. Astron. Soc. 436, L35–L39. doi:10.1093/mnrasl/slt107
Blain, D., Charnay, B., and Bezard, B. (2021). 1D Atmospheric Study of the Temperate Sub-Neptune K2-18b. Astron. Astrophys. 646, A15. doi:10.1051/0004-6361/202039072
Bowesman, C. A., Shuai, M., Yurchenko, S. N., and Tennyson, J. (2021). A High Resolution Line List for AlO. Mon. Not. R. Astron. Soc. 508, 3181–3193. doi:10.1093/mnras/stab2525
Brogi, M., and Line, M. R. (2019). Retrieving Temperatures and Abundances of Exoplanet Atmospheres with High-Resolution Cross-Correlation Spectroscopy. Astrophys. J. 157, 114. doi:10.3847/1538-3881/aaffd3
Chubb, K. L., Joseph, M., Franklin, J., Choudhury, N., Furtenbacher, T., Császár, A. G., et al. (2018a). MARVEL Analysis of the Measured High-Resolution Spectra of C2H2. J. Quant. Spectrosc. Radiat. Transf. 204, 42–55. doi:10.1016/j.jqsrt.2017.08.018
Chubb, K. L., Naumenko, O. V., Keely, S., Bartolotto, S., MacDonald, S., Mukhtar, M., et al. (2018b). MARVEL Analysis of the Measured High-Resolution Rovibrational Spectra of H2S. J. Quant. Spectrosc. Radiat. Transf. 218, 178–186. doi:10.1016/j.jqsrt.2018.07.012
Chubb, K. L., Rocchetto, M., Yurchenko, S. N., Min, M., Waldmann, I., Barstow, J. K., et al. (2021). The ExoMolOP Database: Cross-Sections and K-Tables for Molecules of Interest in High-Temperature Exoplanet Atmospheres. Astron. Astrophys. 646, A21. doi:10.1051/0004-6361/202038350
Clark, V. H. J., and Yurchenko, S. N. (2021). Modelling the Non-local Thermodynamic Equilibrium Spectra of Silylene (SiH2). Phys. Chem. Chem. Phys. 23, 11990–12004. doi:10.1039/D1CP00839K
Darby-Lewis, D., Tennyson, J., Lawson, K. D., Yurchenko, S. N., Stamp, M. F., Shaw, A., et al. (2018). Synthetic Spectra of BeH, BeD and BeT for Emission Modelling in JET Plasmas. J. Phys. B: Mol. Opt. Phys. 51, 185701. doi:10.1088/1361-6455/aad6d0
Diouf, M. L., Tobias, R., Simko, I., Cozijn, F. M. J., Salumbides, E. J., Ubachs, W., et al. (2021). Network-Based Design of Near-Infrared Lamb-Dip Experiments and the Determination of Pure Rotational Energies of H218O at kHz Accuracy. J. Phys. Chem. Ref 50 023106. doi:10.1063/5.0052744
Fisher, C., and Heng, K. (2019). How Much Information Does the Sodium Doublet Encode? Retrieval Analysis of Non-LTE Sodium Lines at Low and High Spectral Resolutions. Astrophysical J. 881, 25. doi:10.3847/1538-4357/ab29e8
Fraine, J., Deming, D., Benneke, B., Knutson, H., Jordan, A., Espinoza, N., et al. (2014). Water Vapour Absorption in the clear Atmosphere of a Neptune-sized Exoplanet. Nature 513, 526. doi:10.1038/nature13785
Freedman, R. S., Lustig-Yaeger, J., Fortney, J. J., Lupu, R. E., Marley, M. S., and Lodders, K. (2014). Gaseous Mean Opacities for Giant Planet and Ultracool dwarf Atmospheres over a Range of Metallicities and Temperatures. Astrophys. J. Suppl. 214, 25. doi:10.1088/0067-0049/214/2/25
Funke, B., López-Puertas, M., García-Comas, M., Kaufmann, M., Höpfner, M., and Stiller, G. (2012). GRANADA: A Generic RAdiative traNsfer AnD Non-LTE Population Algorithm. J. Quant. Spectrosc. Radiat. Transf. 113, 1771–1817. doi:10.1016/j.jqsrt.2012.05.001
Furtenbacher, T., Coles, P. A., Tennyson, J., Yurchenko, S. N., Yu, S., Drouin, B., et al. (2020a). Empirical Rovibational Energy of Ammonia up to 7500 Cm−1. J. Quant. Spectrosc. Radiat. Transf. 251, 107027. doi:10.1016/j.jqsrt.2020.107027
Furtenbacher, T., and Császár, A. G. (2012). MARVEL: Measured Active Rotational-Vibrational Energy Levels. II. Algorithmic Improvements. J. Quant. Spectrosc. Radiat. Transf. 113, 929–935. doi:10.1016/j.jqsrt.2012.01.005
Furtenbacher, T., Császár, A. G., and Tennyson, J. (2007). MARVEL: Measured Active Rotational-Vibrational Energy Levels. J. Mol. Spectrosc. 245, 115–125. doi:10.1016/j.jms.2007.07.005
Furtenbacher, T., Horváth, M., Koller, D., Sólyom, P., Balogh, A., Balogh, I., et al. (2019). MARVEL Analysis of the Measured High-Resolution Rovibronic Spectra and Definitive Ideal-Gas Thermochemistry of the 16O2 Molecule. J. Phys. Chem. Refdata 48 023101. doi:10.1063/1.5083135
Furtenbacher, T., Szabó, I., Császár, A. G., Bernath, P. F., Yurchenko, S. N., and Tennyson, J. (2016). Experimental Energy Levels and Partition Function of the 12C2 Molecule. Astrophys. J. Suppl. 224, 44. doi:10.3847/0067-0049/224/2/44
Furtenbacher, T., Szidarovszky, T., Fábri, C., and Császár, A. G. (2013a). MARVEL Analysis of the Rotational–Vibrational States of the Molecular Ions H2D+ and D2H+. Phys. Chem. Chem. Phys. 15, 10181–10193. doi:10.1039/c3cp44610g
Furtenbacher, T., Szidarovszky, T., Mátyus, E., Fábri, C., and Császár, A. G. (2013b). Analysis of the Rotational–Vibrational States of the Molecular Ion. J. Chem. Theor. Comput. 9, 5471–5478. doi:10.1021/ct4004355
Furtenbacher, T., Tóbiás, R., Tennyson, J., Polyansky, O. L., and Császár, A. G. (2020b). A Database of Validated Rovibrational Experimental Transitions and Empirical Energy Levels of O. J. Phys. Chem. Ref. 49, 033101. doi:10.1063/5.0008253
Furtenbacher, T., Tóbiás, R., Tennyson, J., Polyansky, O. L., Kyuberis, A. A., Ovsyannikov, R. I., et al. (2020c). A Database of Validated Rovibrational Experimental Transitions and Empirical Energy Levels Part II. O and O with an Update to O. J. Phys. Chem. Ref 49, 043103. doi:10.1063/5.0030680
Gharib-Nezhad, E., Iyer, A. R., Line, M. R., Freedman, R. S., Marley, M. S., and Batalha, N. E. (2021). EXOPLINES: Molecular Absorption Cross-Section Database for Brown Dwarf and Giant Exoplanet Atmospheres. Astrophys. J. Suppl. 254, 34. doi:10.3847/1538-4365/abf504
Gordon, I. E., Rothman, L. S., Hargreaves, R. J., Hashemi, R., Karlovets, E. V., Skinner, F. M., et al. (2022). The Hitran2020 Molecular Spectroscopic Database. J. Quant. Spectrosc. Radiat. Transf. 277, 107949. doi:10.1016/j.jqsrt.2021.107949
Goyal, J. M., Mayne, N., Sing, D. K., Drummond, B., Tremblin, P., Amundsen, D. S., et al. (2018). A Library of ATMO Forward Model Transmission Spectra for Hot Jupiter Exoplanets. Mon. Not. R. Astron. Soc. 473, 5158–5185. doi:10.1093/mnras/stx3015
Grimm, S. L., Malik, M., Kitzmann, D., Guzmán-Mesa, A., Hoeijmakers, H. J., Fisher, C., et al. (2021). HELIOS-K 2.0 and an Open-Source Opacity Database for Exoplanetary Atmospheres. Astrophys. J. Suppl. 253, 30. doi:10.3847/1538-4365/abd773
Hargreaves, R. J., Gordon, I. E., Rey, M., Nikitin, A. V., Tyuterev, V. G., Kochanov, R. V., et al. (2020). An Accurate, Extensive, and Practical Line List of Methane for the HITEMP Database. Astrophys. J. Suppl. 247, 55. doi:10.3847/1538-4365/ab7a1a
Hoeijmakers, H. J., de Kok, R. J., Snellen, I. A. G., Brogi, M., Birkby, J. L., et al. (2015). A Search for TiO in the Optical High-Resolution Transmission Spectrum of HD 209458b: Hindrance Due to Inaccuracies in the Line Database. Astron. Astrophys. 575, A20. doi:10.1051/0004-6361/201424794
Huang, X., Schwenke, D. W., Freedman, R. S., and Lee, T. J. (2017). Ames-2016 Line Lists for 13 Isotopologues of CO2: Updates, Consistency, and Remaining Issues. J. Quant. Spectrosc. Radiat. Transf. 203, 224–241. doi:10.1016/j.jqsrt.2017.04.026
Huang, X., Schwenke, D. W., and Lee, T. J. (2019a). Isotopologue Consistency of Semi-empirically Computed Infrared Line Lists and Further Improvement for Rare Isotopologues: CO2 and SO2 Case Studies. J. Quant. Spectrosc. Radiat. Transf. 230, 222–246. doi:10.1016/j.jqsrt.2019.03.002
Huang, X., Schwenke, D. W., and Lee, T. J. (2019b). Quantitative Validation of Ames IR Intensity and New Line Lists for 32/33/34S16O2, 32S18O2 and 16O32S18O. J. Quant. Spectrosc. Radiat. Transf. 225, 327–336. doi:10.1016/j.jqsrt.2018.11.039
Line, M., Brogi, M., Bean, J., Gandhi, S., Zalesky, J., Parmentier, V., et al. (2021). A Precise Carbon and Oxygen Abundance Determination in a Hot jupiter Atmosphere. Nature 598, 580–584.
López-Puertas, M., and Taylor, F. W. (2001). Non-LTE Radiative Transfer in the Atmosphere,, Vol. 3. World Scientific.
Madhusudhan, N. (2019). Exoplanetary Atmospheres: Key Insights, Challenges, and Prospects. Ann. Rev. Astron. Astrophys 57, 617–663. doi:10.1146/annurev-astro-081817-051846
McKemmish, L. K., Borsovszky, J., Goodhew, K. L., Sheppard, S., Bennett, A. F. V., Martin, A. D. J., et al. (2018). MARVEL Analysis of the Measured High-Resolution Spectra of 90Zr16O. Astrophys. J. 867, 33. doi:10.3847/1538-4357/aadd19
McKemmish, L. K., Masseron, T., Hoeijmakers, J., Pérez-Mesa, V. V., Grimm, S. L., Yurchenko, S. N., et al. (2019). ExoMol Molecular Line Lists – XXXIII. The Spectrum of Titanium Oxide. Mon. Not. R. Astron. Soc. 488, 2836–2854. doi:10.1093/mnras/stz1818
McKemmish, L. K., Masseron, T., Sheppard, S., Sandeman, E., Schofield, Z., Furtenbacher, T., et al. (2017). MARVEL Analysis of the Measured High-Resolution Spectra of 48Ti16O. Astrophys. J. Suppl. 228, 15. doi:10.3847/1538-4365/228/2/15
McKemmish, L. K., Syme, A. M., Borsovszky, J., Yurchenko, S. N., Tennyson, J., Furtenbacher, T., et al. (2020). Incorportating New Experiments in Diatomic Spectral Databases: An Update to the 12C2 MARVEL Database and ExoMok Line List of 12C2. Mon. Not. R. Astron. Soc. 497, 1081–1097. doi:10.1093/mnras/staa1954
McKemmish, L. K., Syme, A. M., Bowesman, C. A., Kefela, K., Yurchenko, S. N., and Tennyson, J. (2022). A Combined Approach to Generating Line Lists for High Accuracy Studies of Exoplanets and Other Hot Astronomical Objects. Mon. Not. R. Astron. Soc.
Min, M. (2017). Random Sampling Technique for Ultra-fast Computations of Molecular Opacities for Exoplanet Atmospheres. Astron. Astrophys. 607, A9. doi:10.1051/0004-6361/201731612
Pannier, E., and Laux, C. O. (2019). RADIS: A Nonequilibrium Line-By-Line Radiative Code for CO2 and HITRAN-like Database Species. J. Quant. Spectrosc. Radiat. Transf. 222-223, 12–25. doi:10.1016/j.jqsrt.2018.09.027
Pavlenko, Y. V., Yurchenko, S. N., McKemmish, L. K., and Tennyson, J. (2020). Analysis of the TiO Isotopologues in Stellar Optical Spectra. Astron. Astrophys. 42, A77. doi:10.1051/0004-6361/202037863
Polyansky, O. L., Jensen, P., and Tennyson, J. (1994). A Spectroscopically Determined Potential Energy Surface for the Ground State of O: a New Level of Accuracy. J. Chem. Phys. 101, 7651–7657.
Polyansky, O. L., Kyuberis, A. A., Lodi, L., Tennyson, J., Ovsyannikov, R. I., and Zobov, N. (2017). ExoMol Molecular Line Lists XIX: High Accuracy Computed Line Lists for O and O. Mon. Not. R. Astron. Soc. 466, 1363–1371. doi:10.1093/mnras/stw3125
Polyansky, O. L., Kyuberis, A. A., Zobov, N. F., Tennyson, J., Yurchenko, S. N., and Lodi, L. (2018). ExoMol Molecular Line Lists XXX: a Complete High-Accuracy Line List for Water. Mon. Not. R. Astron. Soc. 480, 2597–2608. doi:10.1093/mnras/sty1877
Qu, Q., Cooper, B., Yurchenko, S. N., and Tennyson, J. (2021). A Spectroscopic Model for the Low-Lying Electronic States of NO. J. Chem. Phys. 154, 074112. doi:10.1063/5.0038527
Rey, M., Nikitin, A. V., Babikov, Y. L., and Tyuterev, V. G. (2016). TheoReTS – an Information System for Theoretical Spectra Based on Variational Predictions from Molecular Potential Energy and Dipole Moment Surfaces. J. Mol. Spectrosc. 327, 138–158. doi:10.1016/j.jms.2016.04.006
Rey, M., Nikitin, A. V., and Tyuterev, V. G. (2014). Theoretical Hot Methane Line List up T=2000 K for Astrophysical Applications. Astrophys. J. 789, 2. doi:10.1088/0004-637X/789/1/2
Rey, M., Nikitin, A. V., and Tyuterev, V. G. (2017). Accurate Theoretical Methane Line Lists in the Infrared up to 3000 K and Quasi-Continuum Absorption/Emission Modeling for Astrophysical Applications. Astrophys. J. 847, 105. doi:10.3847/1538-4357/aa8909
Serindag, D. B., Snellen, I. A. G., and Mollière, P. (2021). Measuring Titanium Isotope Ratios in Exoplanet Atmospheres. Astron. Astrophys. 645, A90. doi:10.1051/0004-6361/202039135
Sinitsa, L. N., Serdyukov, V. I., Polovtseva, E. R., Bykov, A. D., and Scherbakov, A. P. (2021). LED-based Fourier Transform Spectroscopy of H217O in the Range of 17000-20000 cm−1. 5ν, 5ν + δ and 6ν Resonance Polyads. J. Quant. Spectrosc. Radiat. Transf. 271, 107749. doi:10.1016/j.jqsrt.2021.107749
Snellen, I. (2014). High-dispersion Spectroscopy of Extrasolar Planets: from CO in Hot Jupiters to in Exo-Earths. Phil. Trans. R. Soc. Lond. A 372, 20130075. doi:10.1098/rsta.2013.0075
Spake, J. J., Sing, D. K., Wakeford, H. R., Nikolov, N., Mikal-Evans, T., Deming, D., et al. (2020). Abundance Measurements of H2O and Carbon-Bearing Species in the Atmosphere of WASP-127b Confirm its Supersolar Metallicity. Mon. Not. R. Astron. Soc. 500, 4042–4064. doi:10.1093/mnras/staa3116
Swain, M. R., Vasisht, G., and Tinetti, G. (2008). The Presence of Methane in the Atmosphere of an Extrasolar Planet. Nature 452, 329–331. doi:10.1038/nature06823
Syme, A.-M., and McKemmish, L. K. (2020). Experimental Energy Levels of 12C14N through MARVEL Analysis. Mon. Not. R. Astron. Soc. 499, 25–39. doi:10.1093/mnras/staa2791
Tennyson, J. (2012). Accurate Variational Calculations for Line Lists to Model the Vibration Rotation Spectra of Hot Astrophysical Atmospheres. Wires Comput. Mol. Sci. 2, 698–715. doi:10.1002/wcms.94
Tennyson, J., Bernath, P. F., Brown, L. R., Campargue, A., Carleer, M. R., Császár, A. G., et al. (2009). IUPAC Critical Evaluation of the Rotational-Vibrational Spectra of Water Vapor. Part I. Energy Levels and Transition Wavenumbers for O and O. J. Quant. Spectrosc. Radiat. Transf. 110, 573–596. doi:10.1016/j.jqsrt.2009.02.014
Tennyson, J., Bernath, P. F., Brown, L. R., Campargue, A., Carleer, M. R., Császár, A. G., et al. (2010). IUPAC Critical Evaluation of the Rotational-Vibrational Spectra of Water Vapor. Part II. Energy Levels and Transition Wavenumbers for HD16O, HD17O, and O. J. Quant. Spectrosc. Radiat. Transf. 111, 2160–2184. doi:10.1016/j.jqsrt.2010.06.012
Tennyson, J., Bernath, P. F., Brown, L. R., Campargue, A., Carleer, M. R., Császár, A. G., et al. (2013a). IUPAC Critical Evaluation of the Rotational-Vibrational Spectra of Water Vapor. Part III. Energy Levels and Transition Wavenumbers for O. J. Quant. Spectrosc. Radiat. Transf. 117, 29–80. doi:10.1016/j.jqsrt.2012.10.002
Tennyson, J., Bernath, P. F., Brown, L. R., Campargue, A., Császár, A. G., Daumont, L., et al. (2014a). A Database of Water Transitions from Experiment and Theory (IUPAC Technical Report). Pure Appl. Chem. 86, 71–83. doi:10.1515/pac-2014-5012
Tennyson, J., Bernath, P. F., Brown, L. R., Campargue, A., Császár, A. G., Daumont, L., et al. (2014b). IUPAC Critical Evaluation of the Rotational-Vibrational Spectra of Water Vapor. Part IV. Energy Levels and Transition Wavenumbers for O, O and O. J. Quant. Spectrosc. Radiat. Transf. 142, 93–108. doi:10.1016/j.jqsrt.2014.03.019
Tennyson, J., Hill, C., and Yurchenko, S. N. (2013b). Data Structures for ExoMol: Molecular Line Lists for Exoplanet and Other Atmospheres. in” international conference on atomic and molecular data and their applications ICAMDATA-2012 (AIP, New York) 1545, 186–195. doi:10.1063/1.4815853
Tennyson, J., and Yurchenko, S. N. (2012). ExoMol: Molecular Line Lists for Exoplanet and Other Atmospheres. Mon. Not. R. Astron. Soc. 425, 21–33. doi:10.1111/j.1365-2966.2012.21440.x
Tennyson, J., and Yurchenko, S. N. (2017). Laboratory Spectra of Hot Molecules: Data Needs for Hot Super-earth Exoplanets. Mol. Astrophys. 8, 1–18. doi:10.1016/j.molap.2017.05.002
Tennyson, J., and Yurchenko, S. N. (2018). The ExoMol Atlas of Molecular Opacities. Atoms 6, 26. doi:10.3390/atoms6020026
Tennyson, J., Yurchenko, S. N., Al-Refaie, A. F., Clark, V. H. J., Chubb, K. L., Conway, E. K., et al. (2020). The 2020 Release of the ExoMol Database: Molecular Line Lists for Exoplanet and Other Hot Atmospheres. J. Quant. Spectrosc. Radiat. Transf. 255, 107228. doi:10.1016/j.jqsrt.2020.107228
Tinetti, G., Encrenaz, T., and Coustenis, A. (2013). Spectroscopy of Planetary Atmospheres in Our Galaxy. Astron. Astrophys. Rev. 21, 1–65. doi:10.1007/s00159-013-0063-6
Tinetti, G., Tennyson, J., Griffiths, C. A., and Waldmann, I. (2012). Water in Exoplanets. Phil. Trans. R. Soc. Lond. A 370, 2749–2764. doi:10.1098/rsta.2011.0338
Tinetti, G., Vidal-Madjar, A., Liang, M.-C., Beaulieu, J.-P., Yung, Y., Carey, S., et al. (2007). Water Vapour in the Atmosphere of a Transiting Extrasolar Planet. Nature 448, 169–171. doi:10.1038/nature06002
Tóbiás, R., Furtenbacher, T., Császár, A. G., Naumenko, O. V., Tennyson, J., Flaud, J.-M., et al. (2018). Critical Evaluation of Measured Rotational-Vibrational Transitions ofFour Sulphur Isotopologues of S16O2. J. Quant. Spectrosc. Radiat. Transf. 208, 152–163. doi:10.1016/j.jqsrt.2018.01.006
Tobias, R., Furtenbacher, T., Simko, I., Csaszar, A. G., Diouf, M. L., Cozijn, F. M. J., et al. (2020). Spectroscopic-network-assisted Precision Spectroscopy and its Application to Water. Nat. Comms. 11, 1708. doi:10.1038/s41467-020-15430-6
Tóbiás, R., Furtenbacher, T., Tennyson, J., and Császár, A. G. (2019). Accurate Empirical Rovibrational Energies and Transitions of O. Phys. Chem. Chem. Phys. 21, 3473–3495. doi:10.1039/c8cp05169k
Tsiaras, A., Waldmann, I. P., Tinetti, G., Tennyson, J., and Yurchenko, S. N. (2019). Water Vapour in the Atmosphere of the Habitable-Zone Eight-Earth-Mass Planet K2 18b. Nat. Astron. 3, 1086–1091. doi:10.1038/s41550-019-0878-9
Tsiaras, A., Waldmann, I. P., Zingales, T., Rocchetto, M., Morello, G., Damiano, M., et al. (2018). A Population Study of Gaseous Exoplanets. Astron. J. 155, 156. doi:10.3847/1538-3881/aaaf75
Wang, Y., Owens, A., Tennyson, J., and Yurchenko, S. N. (2020). MARVEL Analysis of the Measured High-Resolution Rovibronic Spectra of the Calcium Monohydroxide Radical (CaOH). Astrophys. J. Suppl. 248, 9. doi:10.3847/1538-4365/ab85cb
Western, C. M. (2017). PGOPHER: A Program for Simulating Rotational, Vibrational and Electronic Spectra. J. Quant. Spectrosc. Radiat. Transf. 186, 221–242. doi:10.1016/j.jqsrt.2016.04.010
Wong, A., Bernath, P. F., Rey, M., Nikitin, A. V., and Tyuterev, V. G. (2019). Atlas of Experimental and Theoretical High-Temperature Methane Cross Sections from T = 295 to 1000 K in the Near-Infrared. Astrophys. J. Suppl. 240, 4. doi:10.3847/1538-4365/aaed39
Wong, A., Yurchenko, S. N., Bernath, P., Mueller, H. S. P., McConkey, S., and Tennyson, J. (2017). ExoMol Line List XXI: Nitric Oxide (NO). Mon. Not. R. Astron. Soc. 470, 882–897. doi:10.1093/mnras/stx1211
Wright, S. O. M., Waldmann, I., and Yurchenko, S. N. (2021). Non-Local thermal Equilibrium Spectra of Atmospheric Molecules of Exoplanets. Astrophys. J. Submitted.
Yurchenko, S. N., Al-Refaie, A. F., and Tennyson, J. (2018a). ExoCross: A General Program for Generating Spectra from Molecular Line Lists. Astron. Astrophys. 614, A131. doi:10.1051/0004-6361/201732531
Yurchenko, S. N., Amundsen, D. S., Tennyson, J., and Waldmann, I. P. (2017). A Hybrid Line List for CH4 and Hot Methane Continuum. Astron. Astrophys. 605, A95. doi:10.1051/0004-6361/201731026
Yurchenko, S. N., and Tennyson, J. (2014). ExoMol Line Lists IV: The Rotation-Vibration Spectrum of Methane up to 1500 K. Mon. Not. R. Astron. Soc. 440, 1649–1661. doi:10.1093/mnras/stu326
Yurchenko, S. N., Tennyson, J., Bailey, J., Hollis, M. D. J., and Tinetti, G. (2014). Spectrum of Hot Methane in Astronomical Objects Using a Comprehensive Computed Line List. Proc. Nat. Acad. Sci. 111, 9379–9383. doi:10.1073/pnas.1324219111
Yurchenko, S. N., Williams, H., Leyland, P. C., Lodi, L., and Tennyson, J. (2018b). ExoMol Line Lists XXVIII: The Rovibronic Spectrum of AlH. Mon. Not. R. Astron. Soc. 479, 1401–1411. doi:10.1093/mnras/sty1524
Zhang, Y., Snellen, I. A. G., Bohn, A. J., Molliere, P., Ginski, C., Hoeijmakers, H. J., et al. (2021). The 13CO-rich Atmosphere of a Young Accreting Super-Jupiter. Nature 595, 370. doi:10.1038/s41586-021-03616-x
Keywords: opacity, databases, exoplanets, brown dwarfs, cool stars, Doppler shift spectroscopy, transit spectroscopy, ExoMol
Citation: Tennyson J and Yurchenko SN (2022) High Accuracy Molecular Line Lists for Studies of Exoplanets and Other Hot Atmospheres. Front. Astron. Space Sci. 8:795040. doi: 10.3389/fspas.2021.795040
Received: 14 October 2021; Accepted: 16 November 2021;
Published: 03 January 2022.
Edited by:
Rudolf von Steiger, University of Bern, SwitzerlandReviewed by:
Xinchuan Huang, NASA Ames Research Center, United StatesCopyright © 2022 Tennyson and Yurchenko. This is an open-access article distributed under the terms of the Creative Commons Attribution License (CC BY). The use, distribution or reproduction in other forums is permitted, provided the original author(s) and the copyright owner(s) are credited and that the original publication in this journal is cited, in accordance with accepted academic practice. No use, distribution or reproduction is permitted which does not comply with these terms.
*Correspondence: Jonathan Tennyson, ai50ZW5ueXNvbkB1Y2wuYWMudWs=