- 1Loker Hydrocarbon Research Institute and Department of Chemistry, University of Southern California, Los Angeles, CA, United States
- 2Department of Organic Chemistry, Instituto de Química, Universidade Federal do Rio de Janeiro, Rio de Janeiro, Brazil
The role and relevance of methanol in the origin and structure of the RNA world is discussed. Methanol is a pivotal, renewable, and regenerable source from which almost all chemical materials, simple or complex, can be accessed. Olefins and carbonyl compounds, amines and amino acids, peptides and polypeptides, and the molecular building blocks in the initial stages of the biological evolution to life’s origin are obtained through methanol as a source material by its chemical transformation. The formation of methanol, whether in stellar and interstellar media, in deep sea-bottom hot hydrothermal vents or from geothermal sources, results from CO2 hydrogenation. It is the basic reaction, setting the stage for the formation of fundamental “organic” building blocks for the formation of simple prebiotic cells to subsequent biological evolution to cells. The important observation of many organics– hydrocarbons and ions including the large expanse of methane and methanol in the interstellar medium and stellar peripheries is a clear indication of “stellar reductive processes” and ensuing reactions shedding light on the probable significant role of extraterrestrial methanol as the basic source material toward a multi-step transformation into complex life molecules such as RNA.
1 Introduction
In this perspective, the relevance and significance of C1 molecules, especially the key role of methanol in the formation of simple to complex organic molecules, in the interstellar medium (ISM) is discussed. Since the presence of many of these molecules in ISM and their role as leading sources to signature chemical units for life’s architecture such as amino acids, proteins, nucleic bases, and nucleic acids in the terrestrial world are well-known, the probability of a similar scenario in the astrochemical world is discussed. Following the introduction (Section 1), a brief discussion of extraterrestrial molecules and molecular ions, especially relevant C1 units (Section 2) is presented. In Section 3, the formation of methanol in the stellar and interstellar media as well as the proposed routes/constraints for its formation (Section 3.1), transformation of methanol to hydrocarbons (MTHC) and its relevance in terrestrial and astrochemical world (Section 3.2), and the significance of methanol as a key synthon to access complex organic molecules and eventually to RNA and DNA (Section 3.3) is presented. The concluding Section 4 emphasizes the intermediacy of methanol as a reactive C1 building block and its key role in protocols that would lead to the formation of fundamental building blocks for the entry to the RNA world and its subsequent biological evolution to primitive cells.
Chemistry plays a central role in every feature of human life, its environment, and its multi-faceted society (Shaik, 2003; Bertozzi, 2015; Roth, 2015; Mahaffy et al., 2019). Behind life and its processes, along with hydrogen and oxygen, the most important element which plays the biggest role is carbon. Miller & Urey (1959) pioneering studies in search of primordial chemistry in the mid-20th century rejuvenated serious discussions about the scientific understanding of nature and the source of origin of life and life molecules. Their classic experiment (Miller, 1953) was conducted using a mixture of simple precursor molecules—hydrogen, ammonia, methane, and water—under electric discharge or conditions thought to be similar to those of the primordial soup (Oparin, 1924; Haldane, 1929; Oparin, 1952) which showed the formation of varied amino acids, the building blocks to polypeptides and eventually proteins, essential to the formation of living systems. Recent observations indicate that the Earth’s original atmosphere and its chemical composition might have been different from Miller’s conditions. However, the results from his studies provide a significant insight into the primordial molecular interaction and chemical aspects of the evolutionary process toward further terrestrial life.
In Miller’s spark experiment, the gas mixture containing the reactive product molecules HCN, aldehydes, or ketones was subsequently allowed to disperse into the aqueous phase where they underwent Strecker synthesis to yield amino acids such as glycine and other compounds. Later, further simulation studies with the permutation combination of similar experimental conditions showed even the possibility of extraterrestrial formation of many other building blocks such as sugars and nucleotides, besides amino acids (Horst et al., 2012). In the following decades, with the discovery of the structure of nucleic acids and the development of modern biology sparked by the revolutionary achievement of Watson & Crick (1953), there has been a giant leap in our understanding of biological systems and our quest to unravel the essential and historical question of ages—origin and evolution of life from inanimate precursor molecules.
In our search for the carbon source of these precursor molecules, the possible role of the simplest C1 molecules such as carbon dioxide (CO2), methane (CH4), or its oxygenate methanol (CH3OH) and their derivatives has been a theme of serious discussion in the last few decades. It is now quite clear that under suitable conditions as in the liquid water lakes or in the depth of the oceans of our Earth, seeding the simplest inanimate precursor molecules such as methane, methanol, and ammonia can lead to fundamental building blocks of life such as amino acids, proteins, and sugars, as revealed by Miller’s pioneering studies (Miller, 1953; Miller & Urey, 1959). The relevance and significance of C1 molecules, especially methanol as the source material for many higher organic and biological molecules including the unique multifunctional biopolymer, the RNA, will be discussed in the ensuing sections.
2 Extraterrestrial molecules and molecular ions
Nucleosynthesis of carbon and its transformations in stellar and interstellar media (ISM) is well known. The formation and transformation of molecules and molecular ions play an important role in the constitution and vitality of the interstellar medium. Interstellar medium is rich in C1 compounds and ions such as CH4, its protonated ion CH5+ and its oxygenated CH3OH, HCHO, CO, CO2, etc. Astronomers have already identified over 250 molecular species as interstellar molecules (Yamamoto, 2017; McCarthy & McGuire, 2021; Woon, 2021; Guélin and Cernicharo, 2022). In the ISM, the carbon atom remains in its ionized form C+, its dominant form in the gas phase (Williams, 2005; Liszt, 2011). As expected, this species and its hydrogenated and oxygenated forms contribute to a subsequent chemical evolution to rich C1 chemistry. White et al. (1999), Oka (2015) studied the presence and spectral characteristics of CH5+, the parent C1 carbonium ion, with reference to related studies by Olah et al. (2009) in the development of non-classical carbonium ion chemistry through his pioneering efforts in identifying this fundamental species and its related ions along with a wide array of classical carbocations (carbenium ions) under terrestrial conditions in the condensed-state in super acid media.
Contrary to earlier thoughts that an interstellar medium poses an unsafe atmosphere for the formation of organic species and their survival, many molecules and ions (over 200), cyclic as well as open chain hydrocarbons and their derivatives have been discovered since the last five decades (Herbst & van Dishoeck 2009; Tielens, 2013; McGuire, 2022). Well established by now, both methane and its mono oxygenate methanol are among the most abundant molecular species in interstellar clouds and stellar peripheries in the observable galaxies spanning many billions of miles (Harvey-Smith & Cohen, 2006; Heward, 2006; Whittet et al., 2011). In fact, it was found that in high-mass protostellar object W33A, the abundance of methanol (CH3OH) is significant, exceeding that of both CO and CO2 (Gibb et al., 2000). Detection of various carbocations and their terrestrial analogs starting from CH5+ (methanium ion) in Titan’s upper atmosphere through Cassini–Huygens mission using the ion neutral mass spectrometer (INMS) and the Cassini plasma spectrometer (CAPS) instruments on board (Ali et al., 2013; Puzzarini et al., 2014; Ali et al., 2015) not only sheds light on the ongoing abiotic organic synthesis in an extraterrestrial world—in the ISM and on the surface of other planets and stars, but also its remarkable relationship to non-classical carbonium ion chemistry similar to that observed in the terrestrial world. Studies of the aerosol contents in Titan’s thermosphere–ionosphere by negative ion measurements by the CAPS′ Electron Spectrometer (ELS) showed the formation of unsaturated anion carbon chains and positive ion measurements using the ion neutral mass spectrometer (INMS) indicate cation formations of many aromatic hydrocarbons (Sittler et al., 2020).
The discovery of many polyaromatic entities such as C7H11+, C11H9+, C15H11+, etc., on the surface of Titan discussed by Ali et al. (2013, 2015) and their formation as vital intermediates toward more complex systems starting with simple molecular entities such as CH5+ formed outside the terrestrial perimeter emphasizes the role and the scope of astrosynthesis, which leads to a molecular evolution from simple systems to complex systems (Puzzarini, 2017). The relevance of an RNA–world hypothesis to the origin of life is further supported by the current understanding of the basic elements for life architecture such as proteins (of which amino acids are the simple precursors) and nucleic acids (both DNA and RNA, of which, the phosphate backbone with ribose and nucleic bases are properly integrated) (Figure 1) as well as the presence of their source chemical entities in terrestrial, stellar, and interstellar media.
Apart from the Earth, in the other three so-called terrestrial planets- Mercury, Venus, and Mars, presence of organics has been reported. For example, a surface reflectance study with the mercury laser altimeter (MLA) on board the MESSENGER spacecraft on permanently shadowed areas near Mercury’s north pole indicated the presence of surface water-ice in the optically bright regions (bright deposits) and a surface layer of complex organic material in the dark regions (dark deposits), which might have originated from impacts of comets or volatile-rich asteroids (Newmann et al., 2013). A recent re-analysis of the data from a large probe neutral mass spectrometer (LNMS) on board the Pioneer Venus Multiprobe (Pioneer 13) during its mission in 1978 showed the presence of traces of several chemical species related to anaerobic phosphorus metabolism (phosphine), anoxygenic photosynthesis (nitrite), and the nitrogen cycle in Venus’ clouds (Mogul et al., 2021). Reports from the SAM (Sample Analysis at Mars) data showed conclusive evidence for the presence of organic compounds, both aliphatic and aromatic including thiophene in samples drilled out from Mars’ Gale Crater (Lyons et al., 2005; Kite et al., 2017; Eigenbrode et al., 2018; ten Kate, 2018). Despite the fact that no conclusive evidence for extraterrestrial biology has been obtained so far, all these findings underscore our statement in one of our previous reports (Olah et al., 2016a) as noted by Puzzarini (Puzzarini et al., 2017), “Of particular interest to us is the remarkable detection of varied carbocations and their similarity with their terrestrial analogs. The proven similarity with our terrestrial studied chemistry provides the first scientific evidence that our Earth is not a unique celestial body for producing the chemical building blocks.” All these observations suggest that these ions and molecules define the future of chemical and biological complexity and activity in the interstellar medium.
3 Relevance and significance of methanol in stellar and interstellar media
Methanol is one of the most significant, highly versatile chemicals with innumerable applications—as a synthetic feedstock for various commodity chemicals, as fuel, and as a source material in fuel cells and pharmaceuticals. Its terrestrial and extraterrestrial presence suggests its adept role in the tellural and the astrochemical world. Parent C1 hydrocarbon methane, the major ingredient of Earth’s natural gas and the terrestrial hydrogenation product of CO2 (Sabatier’s methanation reaction) (Sabatier et al., 1902; Fujita et al., 1993; Wang et al., 2011Fechete, 2016) is also present in Mars’ atmosphere in conspicuous amounts with seasonal variation (Webster et al., 2018). The partially hydrogenated intermediates formic acid, formaldehyde, and methanol are key synthons in many organic synthetic reactions. The indisputable role of these signature substances, especially methanol in the molecular evolution to cells’ building blocks such as sugars, nucleic bases, and proteins following known synthetic pathways is manifested by many studies in recent years. This motivates us for further exploration of astrochemical evolution in the stellar and interstellar territory.
3.1 Formation of methanol in stellar and interstellar media
Though homogeneous and heterogeneous reductions of CO2 to methanol are already established under terrestrial conditions, the exact extraterrestrial conditions (and the suggested pathways) for the formation of vast methanol clouds in stellar and interstellar regions are yet to be revealed. The overall reduction process (methanation) is exothermic (ΔH298K = −39.4 kcal/mol, Scheme 1, Eq. 1) but the initiation of the process needs optimal conditions such as high pressure, high temperature, and a suitable catalyst to overcome the kinetic barrier. At the initial stage, the reduction process can proceed through deoxygenation to CO (reverse water gas shift reaction, Scheme 1, Eq. 2) (Porosoff et al., 2016) or even direct hydrogenation to formic acid (HCOOH) if it meets the appropriate conditions. Both of these initiation steps (Scheme 1, Eqs 2, 3) are endothermic processes. However, after the initiation at the region of high temperature and pressure in the interstellar medium and the dispersal to the interstellar medium with drastic reduction in concentration and temperature, the probability for the controlled reduction process in a thin layer of interstellar CO2 dust grains to methanol by a diffused thin layer of hydrogen (similar to terrestrial RWGS reaction) is worth considering. Various possible steps during CO2 hydrogenation are shown in Scheme 1. The first organic acid detected in the interstellar medium was formic acid. A dense cloud of formic acid (HCOOH) was located not only in the dark cloud (e.g., L 134 N) (Irvine et al., 1988; Irvine et al., 1990) but also inside the hot cores (hot star-forming cores) in molecular clouds, namely, Sagittarius B2, Orion KL, and W51 ((Liu et al., 2001; Remijan et al., 2004; Tercero et al., 2018; Li et al., 2020). Detection of compounds such as methylenimine (H2C=NH), intermediate hydrogenation products from HCN in the interstellar atmosphere in cogent amounts also reveals the hydrogenation process prevalent there (Dickens et al., 1997). Individual hydrogenation experiment with both solid HCN and CH2NH leads to the formation of methylamine suggesting the possibility of CH2NH being the active intermediate during HCN hydrogenation to CH3NH2. This shows the significance of the incessant hydrogenation process occurring in ISM (Theule et al., 2011).
The major route for the formation of methanol would be the successive hydrogenation of CO on the surfaces of interstellar dust grains. (Tielens & Whittet, 1997; Watanabe et al., 2004; Hidaka et al., 2009). Using a cold (30 K) atomic hydrogen beam, Watanabe et al. (2004) investigated the hydrogenation of CO on pure solid CO and CO–H2O mixed ice at temperatures below 20 K and noticed that it proceeds efficiently producing methanol in both cases. Upon exposure to cold H atoms, CO is consumed with successive hydrogenation first to HCHO and subsequently CH3OH, leading to a mixture of both HCHO and CH3OH below 12 K. H∙ has good mobility which favor the formation of reduction products HCHO and CH3OH (Bredehöft, 2020). Simulation studies also show that molecules such as CH3OH, H3O, and CO2 can be formed in the solid state in the ISM without the need for energetic processing such as thermal, UV/cosmic ray processing (i.e., through H-atom addition) (Ioppolo et al., 2011). The transition of CO2 from a solid form (dry ice, below 194.5 K) to a gaseous form at and below the ambient pressure suggests a much longer lifetime as solid and reactive interaction period for CO2 with associated molecules in the interstellar ice grains during various events at varying temperatures compared to those for CO, which has a much lower freezing point (81.5 K, ambient pressure) but a greater reactivity once it is formed.
For a successive hydrogen addition on CO to occur at very low temperatures, quantum mechanical tunneling through the activation barrier is suggested and the formation of methanol occurs at the warm-up period and is then released when the dust is warmed by radiation from the newly formed star. Garrod et al. (2006) studied a gas-phase model that included the simulation of the surface formation of methanol on ice grains similar to the formation of molecular hydrogen. They also utilized a gas–grain code suggesting a mechanism for desorption of methanol following the warming-up due to exothermic chemical reactions. This can be a viable route for the emulation of the observed abundance of the gas-phase methanol as well as many gas-phase species in the dark and cold molecular clouds such as the well-known Taurus TMC1-CP (Soma et al., 2015). Since the calculated activation barriers for step 1 and step 3 (Scheme 2) are high, they are proposed to occur by quantum mechanical tunneling reactions (Woon, 2002). Though the formation of ∙CH2OH in the third step (3b) is energetically viable and more exothermic than that of CH3O∙ (3a), its probability is lower as it has a higher activation barrier (Tielens & Whittet, 1997; Woon, 2002; Osamura et al., 2005). However, it is possible that favorable reaction conditions, which can reduce the activation barrier, may also arise as a result of many astrophysical events in the interstellar medium.
Methane absorbed on the surface of interstellar space ice/dust grains, can facilitate methanol formation in an otherwise extreme dilution of space as it can also act as a reductant at appropriate temperature–pressure conditions developing over time during stellar events as observed in our extensively studied single step bi-reforming/oxidative bi-reforming reactions, under terrestrial conditions though such a comparison needs to be justified by further experimental studies (Olah et al., 2013; Olah et al., 2015; Olah and Mathew, 2015). Carbon oxides synthesized by the interaction of carbon and oxygen already formed in the interiors of stars are ejected to ISM and undergo subsequent hydrogenation on the surface of space ice/dust grains to give methanol as observed in telluric chemistry. Recently, we achieved direct glycol-assisted CO2 hydrogenation to methanol using heterogeneous Cu/ZnO/Al2O3 with high efficacy, further underscoring the relevance of our direct CO2-methanol concept (Sen et al., 2021) in telluric chemistry. It emphasizes the significance of the hypothesis of similar possibilities in the interstellar medium.
A spectral survey of the star forming region W51 e1/e2, mainly in the hot cores showed the signs of about 105 molecules and their isotopic species (Kalenskii and Johansson, 2010). These include CO, CS, HCN, CH3OCH3, CH3COCH3, CH3OCHO, C2H5OH, CH3CN, CH3OH, H2O, and SO2 along with various molecular ions such as N2H+, HCO+, and HCS+. However, in the condensed phase of interstellar dust grains, CO, CO2, and H2O are the most abundant molecules. CO2 has been identified in many interstellar sites such as dense ISM clouds, YSOs (young stellar objects) (Ehrenfreund & Charnley, 2000), and comets (Irvine et al., 2000; Bibring et al., 2015). Interestingly, the widely accepted route for CO2 formation is the energetic processing of carbon monoxide with water acting as the oxygen donor on dust grain surfaces (Bredehöft, 2020). It reveals the interstellar water gas shift reaction (WGSR) prevalent in the stellar/interstellar medium. Therefore, it is quite reasonable to suggest that the stellar equivalent of WGSR occurs at stellar peripheries and reverse water gas shift (RWGS) reactions occur on dust grain surfaces in the interstellar medium. Other products observed during energetic processing studies {UV light (Milligan & Jacox, 1971; Allamandola et al., 1988; Watanabe & Kouchi, 2002; Watanabe et al., 2007), slow and fast electron beams (Yamamoto et al., 2004; Bennett et al., 2011; Petrik et al., 2014a; Petrik et al., 2014b; Schmidt et al., 2019); X-ray (Laffon et al., 2010)}, mimicking processes under radiative conditions (such as cosmic radiations) in the interstellar medium include formaldehyde, formic acid, and methanol. As early as over half a century ago, many researchers were able to detect ammonia (Cheung et al., 1968), methanol (Ball et al., 1970), formic acid (Zuckerman et al., 1971), and formaldehyde (Snyder et al., 1969) in the interstellar space where high energy radiation is imminent, hence the formation of amino acids in interstellar space is well explicable. Wollin and Ericson (Wollin & Ericson, 1971) were able to isolate a series of amino acids by mixing ammonia, methanol, and formic acid/formaldehyde (all in gaseous form, detected in interstellar medium) at 115°C and subjecting the resulting product mixture to UV irradiation (275 W) at 35°C in a trial to identify the products in an astrochemical context, though the conditions and atmospheres do not hold an exact similarity.
Oxygen insertion into methane has been manifested to be an alternate pathway for the formation of methanol as well as complex organic molecules (COMs) on stellar/interstellar ice dust particles (Bergner et al., 2017). In condensed systems, oxygen insertion into CH4 has been demonstrated by the photolysis of oxygenating compounds at very low temperatures (9–25 K) under appropriate conditions such as HOF in a CH4 matrix under high vacuum of ∼10−7 Torr (Appleman et al., 1989), N2O or O3 in to CH4 in an Ar matrix (Parnis et al., 1993; Lugez et al., 1994), etc. UV–visible irradiation (broad-band) of argon matrices containing 2% N2O and 10% of methane resulted in the formation of CH3OH as the dominant photoproduct from the reaction of methane with the first excited atomic oxygen, O(1D) (Parnis et al., 1993). Similarly, oxygen insertion in the C-H bond with O(1D) generated by ozone during photolysis of CH4/O3 in a low-temperature argon matrix gave CH3OH (Lugez et al., 1994) as the major product as noted in the case of silane (SiH4) and ozone (Withnall & Andrews, 1988). It is quite intriguing to note that the vibrational spectrum of the product mixture obtained by the irradiation of a mixture of CH3OH (0.1% in Ar) and ozone (0.5% in Ar) at 11 K, showed the formation of CO, CH2O, H2O, and H2O2 revealing further probable oxidation and oxidative dissociation pathways under ISM-like conditions (Scheme 3). Methanol oxygenation can occur either by oxygen insertion into the C-H bond or O-H bond, C-H insertion leads to dihydroxy methane or methyl hydroperoxide, which decomposes to formaldehyde and water.
Knowing the significance of methane, mono-oxygenate methanol and its role as a key synthon toward many pivotal substrates for their molecular evolution to complex biological molecules; it is worthwhile to discuss a few prominent reactions, which are suggested as fundamental routes and a practical approach to their access.
3.2 Methanol-to-hydrocarbon process
Methanol-to-hydrocarbon (MTHC) process can be considered as fundamental in the transformation of methanol to complex organic molecules (COMs). Methanol is spotted in many regions of the interstellar medium (vide supra). Vast areas of the methanol cloud spread into billions of square miles in the interstellar medium similar to the one as a cloudy bridge of methanol, which surrounds Sagittarius B2, is 288 billion miles wide in the milky way Galaxy itself (Harvey-Smith & Cohen, 2006; Heward, 2006; Whittet et al., 2011). Therefore, it is quite rational in viewing its astrochemical role as a prominent molecular synthon in the formation of COMs in the interstellar medium. In our terrestrial (telluric) hydrocarbon chemistry, methanol-to-hydrocarbon (MTHC) transformations over acidic zeolites (HZSM-5) by the Mobil research team (Miesel et al., 1976; Chang & Lang, 1977; Rollmann, 1982) as well as via a bifunctional acid−base catalysis by Olah (1983); Olah et al. (1984); Olah & Prakash (2009) have been established in the 1970s, 1980s, and later (Scheme 4). Gasoline shortage in the 1970s led to pioneering efforts by many research groups to develop a methodology for synthetic fuels (synfuels). The simple and convenient commercially developed catalytic process for the conversion of methanol to hydrocarbons (gasoline with high octane numbers, 90–95) using shape-selective zeolite catalysts was first announced by Mobil Chemicals in 1976 (Miesel et al., 1976). Gasoline of superior quality has been produced in much higher yield by this method compared to the nature of the product obtained by Fischer–Tropsch (FT) chemistry. These studies showed that not only methanol, but many hetero-organic compounds could also lead to hydrocarbons with high efficacy (Chang & Silvestri, 1977; Chang & Silvestri, 1987). Further studies (Liang et al., 1990) showed that SAPO catalysts drive this reaction with high conversion and yield. In general, methanol-to-hydrocarbon (MTHC) processes (Scheme 3) include methanol-to-olefin (MTO), methanol-to-gasoline (MTG), and methanol-to-aromatics (MTA) processes.
As more and more secrets of interstellar space and prevailing molecular composition thereof unfold, it is no wonder that processes such as AMTO (astro methanol to olefin process similar to the terrestrial methanol to olefin process) can also occur. Computational studies showed that the conversion of methanol to ethylene can occur through the protolytic cracking of CH3OH2+ in the presence of H3+ to give CH5+, a dominant astrochemical species in ISM (Olah et al., 1969; White and Tang, 1999; Oka, 2015), followed by a radiation-induced cleavage to CH2 singlet and exergonic dimerization to ethylene. The reaction of H3+, also a dominant species in ISM (Oka, 2013; Oka, 2019), with CH3OH2+ to form CH5+ is shown to be exothermic by 40.3 kcal/mol (Figure 2, Eq 4; ΔH = −41.7 kcal/mol by our earlier calculation- Olah et al., 2016b), suggesting that the AMTO route for the formation of ethylene from methanol is overall thermodynamically favorable. Similar to the observed CH5+, protonation of CH3OH to CH3OH2+ also helps with stabilization against radiative dissociation to some extent. In our computational studies of the various steps shown with good probability for their involvement in the AMTO process, thermodynamic analysis at temperatures 298.15 and 10 K considering both the terrestrial and ISM thermal environments showed that for the conversion of methanol to ethylene, the pathway involving astrochemical protolytic cleavage of CH3OH2+ promoted by interaction of H3+ to give CH5+- methanium ion, the simplest carbonium ion (Sefcik et al., 1974; Schreiner et al., 1993; Müller et al., 1997) can also be feasible. This pathway (Figure 2; Eq. 4) seems less probable due to charge−charge repulsion than the direct conversion of methanol to singlet methylene.
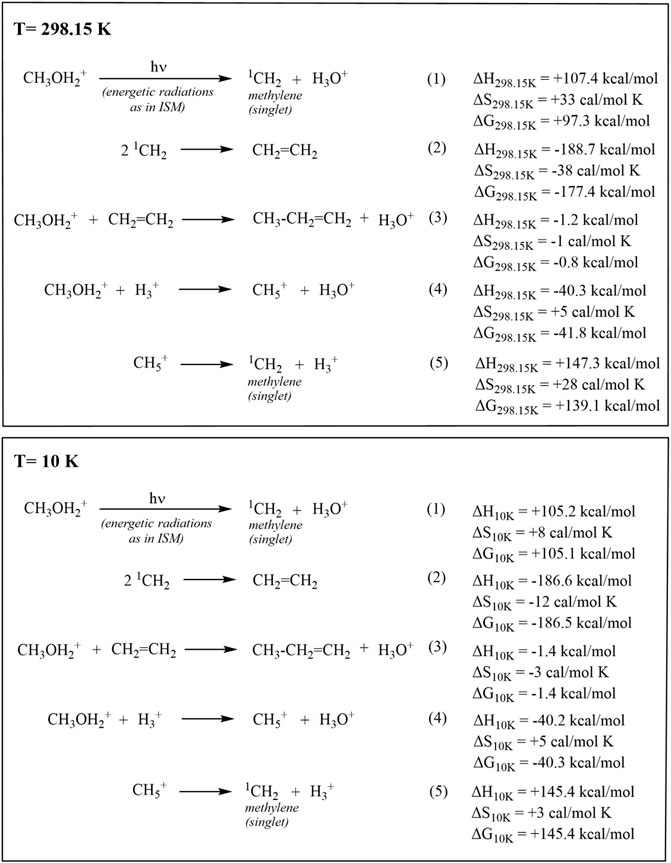
FIGURE 2. Probable pathways involved in the formation of ethylene and propylene by the “Astrochemical methanol to olefins (AMTO)” process in the ISM, the comparison of thermodynamic parameters at 298.15 and 10 K.
It should be pointed out that the laboratory microwave spectra for protonated methanol, CH3OH2+, and protonated methane, CH5+ could not be obtained due to their irregularity from the effect of “fluxional” long-amplitude quantum motions over multiple minima on the potential energy surface. Therefore, search of these ions by rotational spectroscopy in the interstellar medium is still challenging (Puzzarini, 2017). However, it lays out a feasible connection of the chemistry of fluxonial methanium ion with methanol conversions and future studies can shed more light on the probability of these steps.
One can argue that, under extraterrestrial conditions such as varying temperatures (extremely low to high) and high-energy radiation, the feasibility of these reactions fluctuates considerably, however, optimal circumstances leading to favorable conditions may also arise intermittently. Although the radiative decomposition of the neutral form as well as the formation of protonated and clustered forms is an unavoidable process, the recurring formation of methanol can help to maintain its concentration without much depreciation. In fact, laboratory experiments approximating the composition of such systems show that the imperfection in dust grains are active sites promoting the association of CH3OH2+ with neutral CH3OH or even H2 and CH4 (association of CH3OH with H3+ or CH5+ is also another probable course) via weak bonding interactions.
Results from the gas-phase ion cyclotron and vibrational spectroscopic studies of such clusters underscore the possibility of cluster formation (Kebarle et al., 1967; Kebarle et al., 1972; Hiraoka & Kebarle, 1975; Lau et al., 1982; Masamura, 2001; Witt et al., 2008). This shows that under suitable extraterrestrial conditions, a single carbon-containing building block can produce a large variety of hydrocarbons and their heteroatom derivatives. Therefore, as mentioned earlier, radiation induced monomolecular dehydration of methanol or “astrochemical methanol to olefins (AMTO)” process under the influence of highly energetic cosmic rays on suitable associated methanol dust or ice particles prevalent in the interstellar medium (ISM) through methyloxonium ion intermediate (CH3OH2+) can be envisaged as a viable route to ethylene and propylene (Figure 2).
At both the terrestrial and ISM thermal conditions, the cleavage of CH3OH2+ to give singlet methylene is an endothermic process (Figure 2; Eq. 1, equivalent to Eqs 4,5; ΔH298.15 K = +107.4 kcal/mol and ΔH10 K = +105.2 kcal/mol), while its dimerization to form ethylene is spontaneous, a highly exothermic process (Figure 2; Eq. 2; ΔH298.15 K = −188.7 kcal/mol and ΔH10 K = −186.6 kcal/mol). The structures, energies, and zero-point vibrational energies (ZPEs) of the structures were computed at the CCSD(T)/aug-cc-pVTZ level.
3.3 Methanol, a key synthon toward complex organic molecules
Among the discoveries of an astrochemical recipe for the formation of various molecular forms identified in space, the formation of formaldehyde, methanol, glycolaldehyde, and glycerol, are crucial precursors and molecular backbones for the chemical evolution to form complex biological molecules, under prestellar core conditions has been achieved in recent years. Complex organic molecules (COMs) are formed in the interstellar medium by the interaction of interstellar ice grains with the ionizing radiations from galactic cosmic rays and the internal UV photon field in the cold molecular clouds containing the stellar nurseries/star-forming regions and planetary systems (Abplanalp & Kaiser, 2019). The interstellar ice mantles are comprised of molecules mainly H2O, CO2, CO, and CH3OH with smaller amounts of HCHO, CH4, NH3, and HCOOH, of which, CH3OH is present comparatively in significant amounts as methanol clouds (Gibb et al., 2004). Also, catalytic metal oxides/minerals (Duley, 1976; Goumans et al., 2007) and silicate grains (Jones, 2007) present in the ice dust can act as suitable catalysts for many astrochemical transformations. Therefore, our proposal of the possibility of an astrochemical MTHC reaction in the interstellar medium is based on these facts, though it needs to be further substantiated with results from more experimental studies.
Though smaller molecules such as carbon monoxide is very volatile and concentration in space is significantly low, at very low temperatures in ISM (10–25 K) they remain frozen on dust grains and accumulate creating a dense molecular cloud and seeding the formation of bigger molecules by cryogenic hydrogenation occurring in ISM. The veracity of this process has been demonstrated by mimicking hydrogenation of carbon monoxide by cryogenic hydrogen bombardment in the laboratory that resulted in the formation of formaldehyde, methanol, glycolaldehyde, and glycerol among others (Fuchs et al., 2009; Fedoseev et al., 2015; Fedoseev et al., 2017). This creates the roadmap to extraterrestrial, biologically relevant sugar molecules such as ribose and others as detected recently in carbonaceous chondrites, which include some of the most primitive meteorites (Furukawa et al., 2019). Recent studies by Kurokawa et al. (2022) have shown that surface materials of asteroids having 3.1 μm absorption features (indicative of ammoniated phyllosilicates) and carbonaceous chondrites (CCs) can originate from different regions of the same source, a single water–rock-differentiated parent body. Now, it is a generally accepted concept based on the results from numerous laboratory studies by astrochemical simulations, which imply that similar surface reactions by accumulation or coalescence of smaller species under the impact of cosmic rays, vacuum ultraviolet (VUV) photons, and thermal events prevailing in stellar and prestellar core conditions lead to the evolution of complex molecules (Charnley & Rodgers, 2008; Garrod et al., 2008; Herbst & van Dishoeck, 2009; Vasyunin, & Herbst, 2013; Walsh et al., 2014a; Walsh et al., 2014b; Linnartz et al., 2015; Öberg, 2016; Sewilo et al., 2019).
As mentioned earlier, experiments have shown that quantum mechanical tunneling can process many surface reactions, seemingly non-viable under ordinary conditions due to a high-activation barrier (Hama & Watanabe, 2013). Interaction of the H atom with CH3OH leading to the formation of ∙CH2OH and H2 assisted by quantum mechanical tunneling can also be considered (Goumans & Kästner, 2010; Cuppen et al., 2017; Simonciˇ et al., 2020). Radiolysis of methanol leads to ∙CH2OH, which on dimerization yields ethylene glycol (Harris et al., 1995; Bergantini et al., 2018). Formation of glycolaldehyde can be considered as the result of recombination of ∙CH2OH and ∙HCO (Butscher et al., 2015).
The aforementioned results from the studies of Fedoseev et al. (2017) suggest that under an atmosphere which allow the reaction to continue, there is a great probability for the formation of higher sugar alcohols, sugar molecules including stream of ribose, and more complex molecules, which helps to provide answers to the questions on chemical evolution of complex biogenic molecules from cosmic origins (Scheme 5). Chemical ingredients for RNA and DNA, the basic structural motifs in the phenomenon of life—the amino acids, nucleobases, phosphates, and nucleosides are found in meteorites (Saladino et al., 2015; Saladino et al., 2016). Initial dimerization of formaldehyde to glycoladehyde through a free radical process though slow can occur under high energy radiations in the ISM. The reaction then turns autocatalytic and continues as glycoladehyde in trace quantities can catalyze the next aldol reaction to form glyceraldehyde from formaldehyde and glycoladehyde (Socha et al., 1980). With isomerization of glyceraldehyde to dihydroxy acetone and further aldol reaction, the chain grows to aldotetroses and then to ribose, the key building block of ribonucleic acid. Researchers proposed the formation of molecules of the (OH−) type on interstellar oxide grains (Duley et al., 1978). Interstellar oxide grains which might include basic oxides such as CaO or MgO (Duley et al., 1978); corresponding hydroxides with ice particles in dust grains) can promote the base catalyzed formose reaction (Breslow, 1959) as generally studied (Scheme 6). While the formation of COMs through the recombination of reactive carbonaceous radicals during the CO to CH3OH hydrogenation route is highly likely, we also suggested earlier that methanol itself, being very reactive C1 oxygenate, can undergo the AMTO process forming olefins, which can provide aldehydes, amides, amino acids, etc., by known chemical transformations under varying conditions. The interstellar medium also provides varying conditions which may match up with the required parameters to drive these reactions. Formaldehyde can undergo the Cannizzaro reaction, the redox disproportionation to form methanol (and formic acid) and stepwise formose reaction (Orgel, 2000) to form sugars and Strecker reaction to form amino acids under appropriate conditions. The interstellar medium with a molecular mixture of heterosubstituted molecules such as HCN, NH3, HCHO, HCONH2, and CH3OH (highly reactive intermediate) among interstellar ice dust particles with mineral granules can act as a continuous functional laboratory consisting of high temperature “ovens” and cryogenic “dewars” based on various galactic phenomena occurring in different regions. Therefore, astro MTO (methanol to olefin), MTHC (methanol to hydrocarbons), FTT (Fischer–Tropsch type), Cannizzaro and formose reactions, as well as the transformation of formamide to nucleobases are all probable under appropriate varying conditions of ISM developing intermittently and contribute to the cosmic origin of complex biogenic molecules (Olah et al., 2017). Identification of organic molecules such as acetaldehyde (CH3CHO), formamide (NH2CHO), and methyl formate (HCOOCH3) achieved by remote observations at radio wavelengths also underscore the prevalent chemical processes in the ISM (Biver & Bockelée-Morvan, 2019).
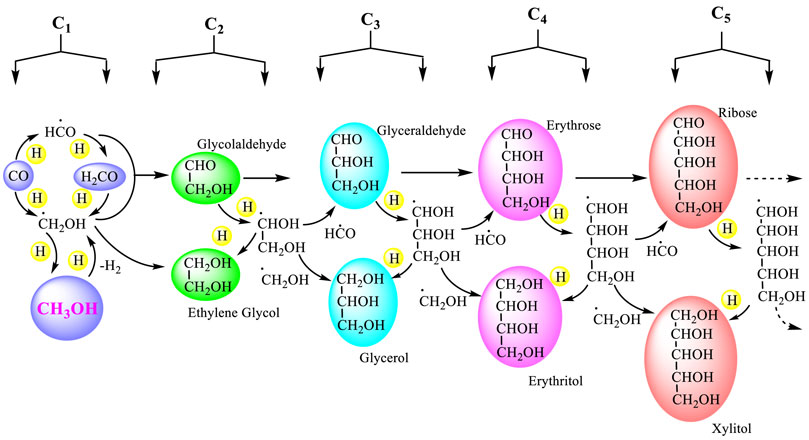
SCHEME 5. Astrohydrogenation of CO on interstellar dust grain surface, formation of methanol (wood alcohol), and the buildup of higher sugar alcohols and sugars in the interstellar medium (Fedoseev et al., 2017; Fedoseev et al., 2017).
Radiolysis studies of frozen methanol using heavy cosmic rays and energetic solar particle analogs show dissociation of CH3OH to molecular species H2CO, CH2OH, CH4, CO, CO2, HCO, and HCOOCH3 (de Barros et al., 2011). From HCHO (H2CO) by radiation-induced reductive or base-catalyzed transformation, the formation of aldoses and polyalcohols from methanol can be envisaged. By the pioneering efforts of many astronomers, over 240 organic molecular species (McGuire, 2022) in neutral, ionic, and radical forms, simple (as water and ammonia) and complex were so far observed in the extraterrestrial world with the help of various onboard analytical instruments of spacecrafts such as the Cassini−Huygens spacecraft from the surface of Titan (the Moon of Saturn) and the Philae Lander that landed on comet 67P/Churyumov–Gerasimenko (Ali et al., 2015; Bibring et al., 2015) in the course of their visits. Also, albeit their presence in low concentration, at low-temperature regions, we suggest the possibility that many species in the molecular clouds (the densest interstellar clouds by ISM standards) remain in associated forms which would undergo the desired reactions as right conditions emerge. Indeed, the formation of COMs in ISM must be a slow and long process, not stipulated to a definite time period.
The interstellar medium witnesses many astrophysical events including meteorite impacts with celestial bodies. Studies showed that a significant portion of soluble organic matter observed in carbonaceous meteorites can indeed originate from organic ices, derived from dense molecular clouds (Danger et al., 2021). For example, laboratory impact experiments showed that glycolaldehyde found in the ISM, as well as glycolaldehyde mixed with montmorillonite clay, when subjected to reverberated shocks (∼5 to >25 GPa), resulted in the formation of a mixture of important biologically significant molecules such as ethylene glycol, threose, and erythrose (McCaffrey et al., 2014). Meteorite impact with planetary bodies is proposed as a possible route to their delivery as the course of their origin on Earth and other planets. Recently, it has been shown that the catalytic synthesis of polyribonucleic acids from ribonucleoside triphosphates is possible on prebiotic rock glasses, which resemble the rock on Hadean Earth surface formed by volcanic activities and impacts (Jerome et al., 2022). It is probable that such rock materials, silicates, and minerals in dust grains in the interstellar medium can catalyze similar chemical transformations resulting in molecular evolution to complex systems such as RNA from simple systems.
The vast expanse of methanol observed on interstellar clouds or protoplanetary space dust shows the efficacy of the continuous formation of methanol in space by both methane oxygen insertion as well as CO2/CO hydrogenation (Schemes 1, 2, 3, 5). Similar to water transport by comets and meteorites to the Earth while it was still young, the transportation of methanol and these astrochemicals and their transformation to more complex prebiotic molecules and protocells would have occurred under Earth’s favorable (Goldilocks) conditions over a long period (Chyba & Sagan, 1997; Pizzarello & Shock, 2010; Ehrenfreund et al., 2011). The close resemblance of these molecular species and their chemistry with terrestrial chemistry supports the transport–transformation process also and help to unveil the pathways involved in various astrochemical transformations. It is important to consider the possibility that the simplest inanimate precursor molecules such as methane, the reactive monooxygenate methanol, CO2, ammonia etc., under favorable catalytic conditions, can react and seed into fundamental life building blocks such as amino acids, sugars, proteins, nucleic acids. and bases over a long geological time span. The chemical transformation of simple substrates with active functional groups to complex systems without catalysts and at elevated temperatures is well studied. Studies have confirmed the gas-phase formation of formamide, a key source material in the formation of nucleobases, in the star-forming region in interstellar clouds (Codella et al., 2017). Results from emission studies in the Protostellar shock regions L1157-B1 and L1157-B2 suggest them as significant sources of HNCO and NH2CHO (Mendoza et al., 2014). For example, thermal decomposition of formamide in vacuum leads to a mixture of nucleic bases purine, cytosine, adenine, as well as hypoxanthine, pterin, urea, urocanic acid, glycine, alanine, and norvaline (Enchev et al., 2021). The formation of the nucleic bases, uracil and thymine, was observed on heating formamide at 160°C for a day (Petera et al., 2021); similar events though varying kinetically, are envisaged in galactic chimneys.
Though many prebiotic precursors are observed in the ISM, it does not prove any sign of extraterrestrial life. However, their presence manifests the prevailing abiotic astrochemical protocols leading to their formation. For example, the CO2−H2 hydrogenation, the most significant among the events occurring in deep sea alkaline hydrothermal vents as observed in the “Lost City” exposed through the pioneering studies by Martin et al. (2008); Martin et al. (2014); Lane (2015); Jordan et al. (2019) shed light on probable initial stages of the molecular evolution that can instigate stepwise biological evolution to primitive cells. Experiments mimicking hydrothermal vents showed that the minerals such as olivine, serpentinite, Ni or Co containing pyrite and zeolite sediments present in the vents possess catalytic properties similar to those of enzymes and therefore can serve as potential catalysts for CO2−H2 to methanol (CH3OH), the reactive intermediate and a key synthon toward molecular evolution (Barge et al., 2015; Roldan et al., 2015)., Presence of similar catalytic sites though in minute quantities is a possible scenario in dust grains in the ISM too which triggers similar chemical transformations as mentioned previously. Therefore, the relevance and significance of methanol as a key synthetic motif (methanol economy concept- Olah et al., 2014; Olah & Mathew, 2015; Olah et al., 2018) in astrochemical organic synthesis cannot be ignored, especially in light of detection of the large expanse of extraterrestrial methanol.
4 Conclusion
In this brief perspective, based on the detection of methanol, the reactive C1 oxygenate in various regions of the interstellar medium, we summarized the possible routes for its continuous formation and key role as a source material for molecular evolution to multi-carbon complex organic and biogenic molecules, including proteins, nucleic bases, and nucleic acids, thus its significance in the RNA world. The close analogy of extra-terrestrially observed molecules and ions with terrestrial hydrocarbon ions and molecules suggests not only resemblance in plausible pathways for their formations, but also can help us to answer many unanswered questions on the molecular evolution in extraterrestrial world- interstellar medium, stars, planets, moons, and their atmospheres. Though the constituents of these atmospheres vary, the existence of these molecular and ionic species must have originated by the interaction of high energy cosmic rays and radiations on elements and subsequent chemical transformations taking place on the surface of space dust (ice) and in celestial bodies as well as the transport–transformation process via comets and meteorites. The CO2/CO hydrogenation for the formation of methanol can be viewed as the probable basic step, which lays out subsequent protocols for the formation of fundamental building blocks toward the entry to the RNA world and subsequent biological evolution to primitive cells. Therefore, it is quite rational to propose that whether under suitable extraterrestrial conditions or deep sea thermal vent conditions, formation of all signature intermediates such as amino acids, sugars, nucleic bases etc., involves the intermediacy of methanol as the reactive C1 building block. We anticipate that the key role of methanol in the evolution of prebiotic molecules and in the RNA world will be more manifested from the results of ongoing and future studies in the area.
Data availability statement
The original contributions presented in the study are included in the article/Supplementary Material; further inquiries can be directed to the corresponding authors.
Author contributions
All authors listed have made a substantial, direct, and intellectual contribution to the work and approved it for publication.
Acknowledgments
Support of our work by the Loker Hydrocarbon Research Institute is gratefully acknowledged.
Conflict of interest
The authors declare that the research was conducted in the absence of any commercial or financial relationships that could be construed as a potential conflict of interest.
Publisher’s note
All claims expressed in this article are solely those of the authors and do not necessarily represent those of their affiliated organizations, or those of the publisher, the editors, and the reviewers. Any product that may be evaluated in this article, or claim that may be made by its manufacturer, is not guaranteed or endorsed by the publisher.
References
Abplanalp, M. J., and Kaiser, R. I. (2019). On the formation of complex organic molecules in the interstellar medium: Untangling the chemical complexity of carbon monoxide–hydrocarbon containing ice analogues exposed to ionizing radiation via a combined infrared and reflectron time-of-flight analysis. Phys. Chem. Chem. Phys. 21, 16949–16980. doi:10.1039/c9cp01793c
Ali, A., Sittler, E. C., Chornay, D., Rowe, B. R., and Puzzarini, C. (2013). Cyclopropenyl cation – The simplest huckel's aromatic molecule – And its cyclic methyl derivatives in Titan's upper atmosphere. Planet. Space Sci. 87, 96–105. doi:10.1016/j.pss.2013.07.007
Ali, A., Sittler, E. C., Chornay, D., Rowe, B. R., and Puzzarini, C. (2015). Organic chemistry in Titan׳s upper atmosphere and its astrobiological consequences: I. Views towards Cassini plasma spectrometer (CAPS) and ion neutral mass spectrometer (INMS) experiments in space. Plan. Space Sci. 109−110, 46–63. doi:10.1016/j.pss.2015.01.015
Allamandola, L. J., Sandford, S. A., and Valero, G. J. (1988). Photochemical and thermal evolution of interstellar/precometary ice analogs. Icarus 76, 225–252. doi:10.1016/0019-1035(88)90070-x
Appleman, E. H., Downs, A. J., and Gardner, C. J. (1989). Matrix photochemistry of hypofluorous acid, HOF: Oxygen atom transfer and other reactions. J. Phys. Chem. 93, 598–608. doi:10.1021/j100339a021
Ball, J. H., Gottlieb, C. A., Lilley, A. E., and Radford, H. E. (1970). Detection of methyl alcohol in Sagittarius. Astrophys. J. 162, L203. doi:10.1086/180654
Barge, L. M., Abedian, Y., Russell, M. J., Doloboff, I. J., Cartwright, J. H. E., Kidd, R. D., et al. (2015). From chemical gardens to fuel cells: Generation of electrical potential and current across self-assembling iron mineral membranes. Angew. Chem. Int. Ed. 54, 8184–8187. doi:10.1002/anie.201501663
Bennett, C. J., Hama, T., Kim, Y. S., Kawasaki, M., and Kaiser, R. I. (2011). Laboratory studies on the formation of formic acid (HCOOH) in interstellar and cometary ices. Astrophys. J. 727, 27. doi:10.1088/0004-637x/727/1/27
Bergantini, A., Góbi, S., Abplanalp, M. J., and Kaiser, R. I. (2018). A mechanistical study on the formation of dimethyl ether (CH3OCH3) and ethanol (CH3CH2OH) in methanol-containing ices and implications for the chemistry of star-forming regions. Astrophys. J. 852, 70. doi:10.3847/1538-4357/aa9ce2
Bergner, J. B., Oberg, K. L., and Rajappan, M. (2017). methanol formation via oxygen insertion chemistry in ices. Astrophys. J. 845, 29. doi:10.3847/1538-4357/aa7d09
Bertozzi, C. R. (2015). The centrality of chemistry. ACS Cent. Sci. 1, 1–2. doi:10.1021/acscentsci.5b00090
Bibring, J.-P., Taylor, M. G. G. T., Alexander, C., Auster, U., Biele, J., Finzi, A. E., et al. (2015). Philae's first days on the comet. Science 349, 493. doi:10.1126/science.aac5116
Biver, N., and Bockelée-Morvan, D. (2019). Complex organic molecules in comets from remote-sensing observations at millimeter wavelengths. ACS Earth Space Chem. 3, 1550–1555. doi:10.1021/acsearthspacechem.9b00130
Bredehöft, J. H. (2020). CO2: A small ubiquitous molecule with a lot of astrochemical debate attached. Front. Astron. Space Sci. 7, 33. doi:10.3389/fspas.2020.00033
Breslow, R. (1959). On the mechanism of the formose reaction. Tetrahedron Lett. 1, 22–26. doi:10.1016/s0040-4039(01)99487-0
Butscher, T., Duvernay, F., Theule, P., Danger, G., Carissan, Y., Hagebaum-Reignier, D., et al. (2015). Formation mechanism of glycolaldehyde and ethylene glycol in astrophysical ices from HCO· and ·CH2OH recombination: An experimental study. Mon. Not. R. Astron. Soc. 453, 1587–1596. doi:10.1093/mnras/stv1706
Chang, C. D., and Silvestri, A. J. (1977). The conversion of methanol and other O-compounds to hydrocarbons over zeolite catalysts. J. Catal. 47, 249–259. doi:10.1016/0021-9517(77)90172-5
Charnley, S. B., and Rodgers, S. D. (2008). Interstellar reservoirs of cometary matter. Space Sci. Rev. 138, 59–73. doi:10.1007/s11214-008-9331-6
Cheung, A. C., Rank, D. M., Townes, C. H., Thornton, D. D., and Welch, W. (1968). Detection of NH3Molecules in the interstellar medium by their microwave emission. Phys. Rev. Lett. 21, 1701–1705. doi:10.1103/physrevlett.21.1701
Chyba, C. F., and Sagan, C. (1997). in Comets and the origin and evolution of life; thomas (New York: Springer), 147–173.
Codella, C., Ceccarelli, C., Caselli, P., Balucani, N., Barone, V., Fontani, F., et al. (2017). Seeds of Life in Space (SOLIS) II. Formamide in protostellar shocks: Evidence for gas-phase formation. Astron. Astrophys. 605, L3. doi:10.1051/0004-6361/201731249
Cuppen, H. M., Walsh, C., Lamberts, T., Semenov, D., Garrod, R. T., Penteado, S., et al. (2017). Grain surface models and data for Astrochemistry. Space Sci. Rev. 212, 1–58. doi:10.1007/s11214-016-0319-3
Danger, G., Vinogradoff, V., Matzka, M., Viennet, J-C., Remusat, L., Bernard, S., et al. (2021). Exploring the link between molecular cloud ices and chondritic organic matter in laboratory. Nat. Commun. 12, 3538. doi:10.1038/s41467-021-23895-2
de Barros, A. L. F., Domaracka, A., Andrade, D. P. P., Boduch, P., Rothard, H., da Silveira, E. F., et al. (2011). Radiolysis of frozen methanol by heavy cosmic ray and energetic solar particle analogues. Mon. Not. R. Astron. Soc. 418, 1363–1374. doi:10.1111/j.1365-2966.2011.19587.x
Dickens, J. E., Irvine, W. M., DeVries, C. H., and Ohishi, M. (1997). Hydrogenation of interstellar molecules: A survey for methylenimine (CH2NH). Astrophys. J. 479, 307–312. doi:10.1086/303884
Duley, W. W., Millar, T. J., and Williams, D. A. (1978). Molecule production on interstellar oxide grains. Mon. Not. R. Astron. Soc. 185, 915–926. doi:10.1093/mnras/185.4.915
Duley, W. W. (1976). Origin of the diffuse interstellar absorption bands. II: Evidence for MgO and CaO solids in the interstellar medium. Astrophys. Space Sci. 45, 253–259. doi:10.1007/bf00642662
Ehrenfreund, P., and Charnley, S. B. (2000). Organic molecules in the interstellar medium, comets, and meteorites: A voyage from dark clouds to the early earth. Annu. Rev. Astron. Astrophys. 38, 427–483. doi:10.1146/annurev.astro.38.1.427
Ehrenfreund, P., Spaans, M., and Holm, N. G. (2011). The evolution of organic matter in space. Phil. Trans. R. Soc. A 369, 538–554. doi:10.1098/rsta.2010.0231
Eigenbrode, J. L., Summons, R. E., Steele, A., Freissinet, C., Millan, M., Navarro-González, R., et al. (2018). Organic matter preserved in 3-billion-year-old mudstones at Gale crater, Mars. Science 360, 1096–1101. doi:10.1126/science.aas9185
Enchev, V., Angelov, I., Dincheva, I., Stoyanova, N., Slavova, S., Rangelov, M., et al. (2021). Chemical evolution: From formamide to nucleobases and amino acids without the presence of catalyst. J. Biomol. Struct. Dyn. 39, 5563–5578. doi:10.1080/07391102.2020.1792986
Fechete, I. (2016). Paul Sabatier – the father of the chemical theory of catalysis. C. R. Chim. 19, 1374–1381. doi:10.1016/j.crci.2016.08.006
Fedoseev, G., Chuang, K.-J., Ioppolo, S., Qasim, D., van Dishoeck, E. F., Linnartz, H., et al. (2017). formation of glycerol through hydrogenation of CO ice under prestellar core conditions. Astrophys. J. 842, 52. doi:10.3847/1538-4357/aa74dc
Fedoseev, G., Cuppen, H. M., Ioppolo, S., Lamberts, T., and Linnartz, H. (2015). Experimental evidence for glycolaldehyde and ethylene glycol formation by surface hydrogenation of CO molecules under dense molecular cloud conditions. Mon. Not. R. Astron. Soc. 448, 1288–1297.
Fuchs, G. W., Cuppen, H. M., Ioppolo, S., Romanzin, C., Bisschop, S. E., Andersson, S., et al. (2009). Hydrogenation reactions in interstellar CO ice analogues- A combined experimental/theoretical approach. Astron. Astrophys. 505, 629–639. doi:10.1051/0004-6361/200810784
Fujita, S., Nakamura, M., Doi, T., and Takezawa, N. (1993). Mechanisms of methanation of carbon dioxide and carbon monoxide over nickel/alumina catalysts. Appl. Catal. A General 104, 87–100. doi:10.1016/0926-860x(93)80212-9
Furukawa, Y., Chikaraishi, Y., Ohkouchi, N., Ogawa, N. O., Glavin, D. P., Dworkin, J. P., et al. (2019). Extraterrestrial ribose and other sugars in primitive meteorites. Proc. Natl. Acad. Sci. U. S. A. 116, 24440–24445. doi:10.1073/pnas.1907169116
Garrod, R., Park, I. H., Casellic, P., and Herbst, E. (2006). Are gas-phase models of interstellar chemistry tenable? The case of methanol. Faraday Discuss. 133, 51. doi:10.1039/b516202e
Garrod, R. T., Weaver, S. L. W., and Herbst, E. (2008). Complex chemistry in star-forming regions-an expanded gas-grain warm-up chemical model. Astrophys. J. 682, 283–302. doi:10.1086/588035
Gibb, E. L., Whittet, D. C. B., Boogert, A. C. A., and Tielens, A. G. G. M. (2004). Interstellar ice: The infrared space observatory legacy. Astrophys. J. Suppl. Ser. 151, 35–73. doi:10.1086/381182
Gibb, E. L., Whittet, D. C. B., Schutte, W. A., Boogert, A. C. A., Chiar, J. E., Ehrenfreund, P., et al. (2000). An inventory of interstellar ices toward the embedded protostar W33A. Astrophys. J. 536, 347–356. doi:10.1086/308940
Goumans, T. P. M., and Kästner, J. (2010). Hydrogen-atom tunneling could contribute to H2 formation in space. Angew. Chem. Int. Ed. 49, 7350–7352. doi:10.1002/anie.201001311
Goumans, T. P. M., Wander, A., Catlow, C. R. A., and Brown, W. A. (2007). Silica grain catalysis of methanol formation. Mon. Not. R. Astron. Soc. 382, 1829–1832. doi:10.1111/j.1365-2966.2007.12491.x
Guélin, M., and Cernicharo, J. (2022). Organic molecules in interstellar space: Latest advances. Front. Astron. Space Sci. 9, 787567. doi:10.3389/fspas.2022.787567
Hama, T., and Watanabe, N. (2013). Surface processes on interstellar amorphous solid water: Adsorption, diffusion, tunneling reactions, and nuclear-spin conversion. Chem. Rev. 113, 8783–8839. doi:10.1021/cr4000978
Harris, T. D., Lee, D. H., Blumberg, M. Q., and Arumainayagam, C. R. (1995). Electron-induced reactions in methanol ultrathin films studied by temperature-programmed desorption: A useful method to study radiation chemistry. J. Phys. Chem. 99, 9530–9535. doi:10.1021/j100023a035
Harvey-Smith, L., and Cohen, R. J. (2006). Discovery of large-scale methanol and hydroxyl maser filaments in W3(OH). Mon. Not. R. Astron. Soc. 371, 1550–1558. doi:10.1111/j.1365-2966.2006.10806.x
Herbst, E., and van Dishoeck, E. F. (2009). Complex organic interstellar molecules. Ann. Rev. Astron. Astrophys. 47, 427–480. doi:10.1146/annurev-astro-082708-101654
Heward, A. (2006). Upgraded MERLIN spies cloud of alcohol spanning 288 billion miles. PN 06/14 (NAM7). London, United Kingdom: Royal Astronomical Society.
Hidaka, H., Watanabe, M., Kouchi, A., and Watanabe, N. (2009). Reaction routines in the CO–H2CO-dn–CH3OH-dm system clarified from H(D) exposure of solid formaldehyde at low temperatures. Astrophys. J. 702, 291–300. doi:10.1088/0004-637x/702/1/291
Hiraoka, K., and Kebarle, P. (1975). Energetics, stabilities, and possible structures of CH5+(CH4)n clusters from gas phase study of equilibriums CH5+(CH4)n-1 + CH4 = CH5+(CH4)n for n = 1-5. J. Am. Chem. Soc. 97, 4179–4183. doi:10.1021/ja00848a005
Hörst, S. M., Yelle, R. V., Buch, A., Carrasco, N., Cernogora, G., Dutuit, O., et al. (2012). formation of amino acids and nucleotide bases in a titan atmosphere simulation experiment. Astrobiology 12, 809–817. doi:10.1089/ast.2011.0623
Ioppolo, S., Cuppen, H. M., and Linnartz, H. (2011). surface formation routes of interstellar molecules: Hydrogenation reactions in simple ices. Rend. Fis. Acc. Lincei 22, 211. doi:10.1007/s12210-011-0135-3
Irvine, W. M., Avery, L. W., Friberg, P., Matthews, H. E., and Ziurys, L. M. (1988). Newly detected molecules in dense interstellar clouds. Astrophys. Lett. Commun. 26, 167–180.
Irvine, W. M., Friberg, P., Kaifu, N., Matthews, H. E., Minh, Y. C., Ohishi, M., et al. (1990). Detection of formic acid in the cold, dark cloud L 134N. Astron. Astrophys. 229, L9–L12.
Irvine, W. M., Schloerb, F. P., Crovisier, J., Fegley, B., and Mumma, M. J. (2000). “Comets: A link between interstellar and nebular chemistry,” in Protostars and planets IV. Editors V. Mannings, A. P. Boss, and S. S. Russell (Tucson, AZ: University of Arizona Press), 1159–1200.
Jerome, C. A., Kim, H-J., Mojzsis, S. J., Benner, S. A., and Blondi, E. (2022). Catalytic synthesis of polyribonucleic acid on prebiotic rock glasses. Astrobiology 22, 629–636. doi:10.1089/ast.2022.0027
Jones, A. P. (2007). The mineralogy of cosmic dust: Astromineralogy. Eur. J. Mineral. 19, 771–782. doi:10.1127/0935-1221/2007/0019-1766
Jordan, S. F., Rammu, H., Zheludev, I. N., Hartley, A. M., Maréchal, A., Lane, N., et al. (2019). Promotion of protocell self-assembly from mixed amphiphiles at the origin of life. Nat. Ecol. Evol. 3, 1705–1714. doi:10.1038/s41559-019-1015-y
Kalenskii, S. V., and Johansson, L. E. B. (2010). Spectral Survey of the Star-Forming Region W51 e1/e2 at 3 mm. Astron. Rep. 54, 1084–1104. doi:10.1134/s1063772910120036
Kebarle, P., and Franklin, J. L. (1972). “Higher order reactions – ion clusters and ion solvation,” in Ion−Molecule reactions (New York: Springer), Vol. 1.
Kebarle, P., Haynes, R. N., and Collins, J. G. (1967). Competitive Solvation of the Hydrogen Ion by Water and Methanol Molecules Studied in the Gas Phase, 89, 5753–5757.J. Am. Chem. Soc.
Kite, E. S., Gao, P., Goldblatt, C., Mischna, M. A., Mayer, D. P., Yung, Y. L., et al. (2017). Methane bursts as a trigger for intermittent lake-forming climates on post-Noachian Mars. Nat. Geosci. 10, 737–740. doi:10.1038/ngeo3033
Kurokawa, H., Shibuya, T., Sekine, Y., Ehlmann, B. L., Usui, F., Kikuchi, S., et al. (2022). Distant formation and differentiation of outer main belt asteroids and carbonaceous chondrite parent bodies. AGU Adv. 3, e2021AV000568. doi:10.1029/2021AV000568
Laffon, C., Lasne, J., Bournel, F., Schulte, K., Lacombe, S., Parent, P., et al. (2010). Photochemistry of carbon monoxide and methanol in water and nitric acid hydrate ices: A nexafs study. Phys. Chem. Chem. Phys. 12, 10865. doi:10.1039/c0cp00229a
Lau, Y. K., Ikuta, S., and Kebarle, P. (1982). Thermodynamics and kinetics of the gas-phase reactions H3O+(H2O)n-1 + water = H3O+(H2O)n. J. Am. Chem. Soc. 104, 1462–1469. doi:10.1021/ja00370a002
Li, J., Wang, J., Qiao, H., Quan, D., Fang, M., Du, F., et al. (2020). Mapping observations of complex organic molecules around Sagittarius B2 with the ARO 12 m telescope. Mon. Not. R. Astron. Soc. 492, 556–565. doi:10.1093/mnras/stz3337
Liang, J., Li, H., Zhao, S., Guo, W., Wang, R., Yang, M., et al. (1990). Characteristics and performance of SAPO-34 catalyst for methanol-to-olefin conversion. Appl. Catal. 64, 31–40. doi:10.1016/s0166-9834(00)81551-1
Linnartz, H., Ioppolo, S., and Fedoseev, G. (2015). Atom addition reactions in interstellar ice analogues. Int. Rev. Phys. Chem. 34, 205–237. doi:10.1080/0144235x.2015.1046679
Liszt, H. S. (2011). How does C+ recombine in diffuse molecular gas? Astron. Astrophys. 527, A45. doi:10.1051/0004-6361/201015824
Liu, S-Y., Mehringer, D. M., and Snyder, L. E. (2001). Observations of formic acid in hot molecular cores. Astrophys. J. 552, 654–663. doi:10.1086/320563
Lugez, C., Schriver, A., Levant, R., and Schriver-Mazzouli, L. A. (1994). A matrix-isolation infrared spectroscopic study of the reactions of methane and methanol with ozone. Chem. Phys. 181, 129–146. doi:10.1016/0301-0104(94)85021-6
Lyons, J. R., Manning, C., and Nimmo, F. (2005). Formation of methane on Mars by fluid–rock interaction in the crust. Geophys. Res. Lett. 32, L13201. doi:10.1029/2004gl022161
Mahaffy, P. G., Ho, F. M., Haack, J. A., and Brush, E. J. (2019). Can chemistry Be a central science without systems thinking? J. Chem. Educ. 96, 2679–2681. doi:10.1021/acs.jchemed.9b00991
Martin, M., Baross, J., Kelley, D., and Russell, M. J. (2008). Hydrothermal vents and the origin of life. Nat. Rev. Microbiol. 6, 805–814. doi:10.1038/nrmicro1991
Martin, W. F., Sousa, F. L., and Lane, N. (2014). Energy at life's origin. Science 344, 1092–1093. doi:10.1126/science.1251653
Masamura, M. (2001). Ab initio molecular orbital study on the structures and energetics of CH3OH(H2O)n and CH3SH (H2O)n in the gas phase. J. Comput. Chem. 22, 125–131. doi:10.1002/1096-987x(20010115)22:1<125::aid-jcc12>3.0.co;2-0
McCaffrey, V. P., Zellner, N. E. B., Waun, C. M., Bennett, E. R., and Earl, E. K. (2014). Reactivity and survivability of glycolaldehyde in simulated meteorite impact experiments. Orig. Life Evol. Biosph. 44, 29–42. doi:10.1007/s11084-014-9358-5
McCarthy, M. C., and McGuire, B. A. (2021). Aromatics and cyclic molecules in molecular clouds: A new dimension of interstellar organic chemistry. J. Phys. Chem. A 125, 3231–3243. doi:10.1021/acs.jpca.1c00129
McGuire, B. A. (2022). Census of interstellar, circumstellar, extragalactic, protoplanetary disk, and exoplanetary molecules. Astrophys. J. Suppl. Ser. 259 (30), 1–51. doi:10.3847/1538-4365/ac2a48
Meisel, S. L., McCullough, J. P., Lechthaler, C. H., and Weisz, P. B. (1976). Gasoline from methanol in one step. Chemtech 6, 86–89.
Mendoza, E., Lefloch, B., Lopez-Sepulcre, A., Ceccarelli, C., Codella, C., Boechat-Roberty, H. M., et al. (2014). Molecules with a peptide link in protostellar shocks: A comprehensive study of L1157. Mon. Not. R. Astron. Soc. 445, 151–161. doi:10.1093/mnras/stu1718
Miller, S. L. (1953). A production of amino acids under possible primitive earth conditions. Science 117, 528–529. doi:10.1126/science.117.3046.528
Miller, S. L., and Urey, H. C. (1959). Organic Compound Synthesis on the Primitive Earth: Several questions about the origin of life have been answered, but much remains to be studied. Science 130, 245–251. doi:10.1126/science.130.3370.245
Milligan, D. E., and Jacox, M. E. (1971). Infrared spectrum and structure of intermediates in the reaction of OH with CO. J. Chem. Phys. 54, 927–942. doi:10.1063/1.1675022
Mogul, R., Limaye, S. S., Way, M. J., and Cordova, J. A. (2021). Venus' mass spectra show signs of disequilibria in the middle clouds. Geophys. Res. Lett. 48, e2020GL091327. doi:10.1029/2020gl091327
Müller, H., Kutzelnigg, W., Noga, J., and Klopper, W. (1997). CH5+: The story goes on. An explicitly correlated coupled-cluster study. J. Chem. Phys. 106, 1863–1869. doi:10.1063/1.473340
Newmann, G. A., Cavanaugh, J. F., Sun, X., Mozarico, E. M., Smith, D. E., Zuber, M. T., et al. (2013). Bright and dark polar deposits on Mercury: Evidence for surface volatiles. Science 339, 296–300. doi:10.1126/science.1229764
Öberg, K. I. (2016). Photochemistry and Astrochemistry: Photochemical pathways to interstellar complex organic molecules. Chem. Rev. 116, 9631–9663. doi:10.1021/acs.chemrev.5b00694
Oka, T. (2019). H3+, the ideal probe for in situ measurement of the Galactic cosmic rays. Phil. Trans. R. Soc. A 377, 20180402. doi:10.1098/rsta.2018.0402
Oka, T. (2013). Interstellar H3+. Interstellar H3+. Chem. Rev. 113, 8738–8761. doi:10.1021/cr400266w
Oka, T. (2015). Taming CH5+, the “enfant terrible” of chemical structures. Science 347, 1313–1314. doi:10.1126/science.aaa6935
Olah, G. A. (1983). Bifunctional acid-base catalyzed conversion of hetero-substituted methanes into olefins. WO 1983000483 A1. U.S. Pat. 4, 373–109.
Olah, G. A., Doggweiler, H., Felberg, J. D., Frohlich, S., Grdina, M. J., Karpeles, R., et al. (1984). Onium Ylide chemistry. 1. Bifunctional acid-base-catalyzed conversion of heterosubstituted methanes into ethylene and derived hydrocarbons. The onium ylide mechanism of the C1 .fwdarw. C2 conversion. J. Am. Chem. Soc. 106, 2143–2149. doi:10.1021/ja00319a039
Olah, G. A., Goeppert, A., Czaun, M., Mathew, T., May, R. B., Prakash, G. K. S., et al. (2015). Single step Bi-reforming and oxidative Bi-reforming of methane (natural gas) with steam and carbon dioxide to metgas (CO-2H2) for methanol synthesis: Self-sufficient effective and exclusive oxygenation of methane to methanol with oxygen. J. Am. Chem. Soc. 137, 8720–8729. doi:10.1021/jacs.5b02029
Olah, G. A., Goeppert, A., and Prakash, G. K. S. (2018). Beyond oil and gas: The methanol economy, 3rd updated and enlarged ed.; Wiley VCH: Weinheim, Germany.
Olah, G. A., Klopman, G., and Schlosberg, R. H. (1969). Chemistry in super acids. III." protonation of alkanes and the intermediacy of alkanonium ions, pentacoordinated carbon cations of the CH5+ type. Hydrogen exchange, protolytic cleavage, hydrogen abstraction, and polycondensation of methane, ethane, 2, 2-dimethylpropane (neopentane), and 2, 2, 3, 3-tetramethylbutane in FSO3H-SbF5 (“Magic acid”) solution. J. Am. Chem. Soc. 91, 3261–3268. doi:10.1021/ja01040a029
Olah, G. A., and Mathew, T. (2015). A life of magic chemistry: Autobiographical reflections including post-nobel prize years and the methanol economy, 2nd updated ed.; John Wiley & Sons, New York, Chapters 13−15.
Olah, G. A., Mathew, T., and Prakash, G. K. S. (2017). Chemical formation of methanol and hydrocarbon (“Organic”) derivatives from CO2 and H2 – carbon sources for subsequent biological cell evolution and life’s origin. J. Am. Chem. Soc. 139, 566–570. doi:10.1021/jacs.6b10230
Olah, G. A., Mathew, T., and Prakash, G. K. S. (2016b). Relevance and significance of extraterrestrial abiological hydrocarbon chemistry. J. Am. Chem. Soc. 138, 6905–6911. doi:10.1021/jacs.6b03136
Olah, G. A., Mathew, T., Rasul, G., and Prakash, G. K. S. (2016a). Chemical aspects of astrophysically observed extraterrestrial methanol, hydrocarbon derivatives, and ions. J. Am. Chem. Soc. 138, 1717–1722. doi:10.1021/jacs.6b00343
Olah, G. A., and Prakash, G. K. S. (2009). Efficient and selective conversion of CO2 to methanol, dimethyl ether and derived products. U.S. Pat. 7, 293–B2.
Olah, G. A., Prakash, G. K. S., Goeppert, A., Czaun, M., and Mathew, T. (2013). Self-sufficient and exclusive oxygenation of methane and its source materials with oxygen to methanol via metgas using oxidative Bi-reforming. J. Am. Chem. Soc. 135, 10030–10031. doi:10.1021/ja405439c
Olah, G. A., Prakash, G. K. S., and Mathew, T. (2014). “Anthropogenic carbon cycle and the methanol economy (Part XXX),” in Across conventional lines–the sixth decade and the methanol economy (Singapore: World Scientific), 3.
Olah, G. A., Prakash, G. K. S., Molnar, Á., and Sommer, J. (2009). Superacid chemistry, 2nd ed.; John Wiley & Sons: Hoboken, New Jersey.
Orgel, L. E. (2000). Self-organizing biochemical cycles. Proc. Natl. Acad. Sci. U. S. A. 97, 12503–12507. doi:10.1073/pnas.220406697
Osamura, Y., Roberts, H., and Herbst, E. (2005). The gas-phase deuterium fractionation of formaldehyde. Astrophys. J. 621, 348–358. doi:10.1086/427474
Parnis, J. M., Hoover, L. E., Fridgen, T. D., and Lafleur, R. D. (1993). Stabilization of the primary products of O (1D) reactions with CO, CO2, CH4, and other hydrocarbons in cryogenic matrices. J. Phys. Chem. 97, 10708–10711. doi:10.1021/j100143a030
Petera, L., Mrazikova, K., Nejdl, L., Zemankova, K., Vaculovicova, M., Pastorek, A., et al. (2021). Prebiotic route to thymine from formamide—a combined experimental–theoretical study. Molecules 26, 2248. doi:10.3390/molecules26082248
Petrik, N. G., Monckton, R. J., Koehler, S. P. K., and Kimmel, G. A. (2014b). Distance-dependent radiation chemistry: Oxidation versus hydrogenation of CO in electron-irradiated H2O/CO/H2O ices. J. Phys. Chem. C 118, 27483–27492. doi:10.1021/jp509785d
Petrik, N. G., Monckton, R. J., Koehler, S. P. K., and Kimmel, G. A. (2014a). Electron-stimulated reactions in layered CO/H2O films: Hydrogen atom diffusion and the sequential hydrogenation of CO to methanol. J. Chem. Phys. 140, 204710. doi:10.1063/1.4878658
Pizzarello, S., and Shock, E. (2010). The organic composition of carbonaceous meteorites: The evolutionary story ahead of biochemistry. Cold Spring Harb. Perspect. Biol. 2, a002105. doi:10.1101/cshperspect.a002105
Porosoff, M. D., Yan, B., and Chen, J. G. (2016). Catalytic reduction of CO2 by H2 for synthesis of CO, methanol and hydrocarbons: Challenges and opportunities. Energy Environ. Sci. 9, 62–73. doi:10.1039/c5ee02657a
Puzzarini, C., Ali, A., Biczysko, M., and Barone, V. (2014). Accurate spectroscopic characterization of protonated oxirane: A potential prebiotic species in Titan’s atmosphere. Astrophys. J. 792, 118. doi:10.1088/0004-637x/792/2/118
Puzzarini, C. (2017). Astronomical complex organic molecules: Quantum chemistry meets rotational spectroscopy. Int. J. Quantum Chem. 117, 129–138. doi:10.1002/qua.25284
Puzzarini, C., Baiardi, A., Bloino, J., Barone, V., Murphy, T. E., Drew, H. D., et al. (2017). Spectroscopic characterization of key aromatic and heterocyclic molecules: A route toward the origin of life. Astron. J. 154, 82. doi:10.3847/1538-3881/aa7d54
Remijan, A., Shiao, Y.-S., Friedel, D. N., Meier, D. S., and Snyder, L. E. (2004). A survey of large molecules of biological interest toward selected high-mass star-forming regions. Astrophys. J. 617, 384–398. doi:10.1086/425266
Roldan, A., Hollingsworth, N., Roffey, A., Islam, H.-U., Goodall, J. B. M., Catlow, C. R. A., et al. (2015). Bio-inspired CO2conversion by iron sulfide catalysts under sustainable conditions. Chem. Commun. 51, 7501–7504. doi:10.1039/c5cc02078f
Roth, D. L. (2015). Several centuries of centrality. ACS Cent. Sci. 1, 103–105. doi:10.1021/acscentsci.5b00198
Sabatier, P., Senderens, J. B., and Hebd, C. R. (1902). Direct hydrogenation of oxides of carbon in presence of various finely divided metals. Séances Acad. Sci. Paris 134, 689–691.
Saladino, R., Carota, E., Botta, G., Kapralov, M., Timoshenko, G. N., Rozanov, A., et al. (2016). First evidence on the role of heavy ion irradiation of meteorites and formamide in the origin of biomolecules. Orig. Life Evol. Biosph. 46, 515–521. doi:10.1007/s11084-016-9495-0
Saladino, R., Carota, E., Botta, G., Kapralov, M., Timoshenko, G. N., Rozanov, A. Y., et al. (2015). Meteorite-catalyzed syntheses of nucleosides and of other prebiotic compounds from formamide under proton irradiation. Proc. Natl. Acad. Sci. U. S. A. 112, E2746–E2755. doi:10.1073/pnas.1422225112
Schmidt, F., Swiderek, P., and Bredehöft, J. H. (20191974–1986). Formation of formic acid, formaldehyde, and carbon dioxide by electron-induced chemistry in ices of water and carbon monoxide. ACS Earth Space Chem. 3, 1974–1986. doi:10.1021/acsearthspacechem.9b00168
Schreiner, P. R., Kim, S.-J., Schaefer, H. F., and Schleyer, P. v. R. (1993). CH5+: The never-ending story or the final word? J. Chem. Phys. 99, 3716–3720. doi:10.1063/1.466147
Sefcik, M. D., Henis, J. M. S., and Gaspar, P. P. (1974). The methanium ion, CH5+. Evidence for the structure of a nonclassical ion from reaction studies by ion cyclotron resonance spectroscopy. J. Chem. Phys. 61, 4321–4328. doi:10.1063/1.1681738
Sen, R., Koch, C. J., Galvan, V., Entesari, N., Goeppert, A., Prakash, G. K. S., et al. (2021). Glycol assisted efficient conversion of CO2 captured from air to methanol with a heterogeneous Cu/ZnO/Al2O3 catalyst. J. CO2 Util. 54, 101762. doi:10.1016/j.jcou.2021.101762
Sewiło, M., Charnley, S. B., Schilke, P., Taquet, V., Oliveira, J. M., Shimonishi, T., et al. (2019). Complex organic molecules in star-forming regions of the magellanic clouds. ACS Earth Space Chem. 3, 2088–2109. doi:10.1021/acsearthspacechem.9b00065
Shaik, S. (2003). Chemistry—a central pillar of human culture. Angew. Chem. Int. Ed. 42, 3208–3215. doi:10.1002/anie.200330038
Simonciˇ cˇ, M. D., Krasnokutski, S. S., Henning, Th., and Jäger, C. (2020). Sensitivity of gas-grain chemical models to surface reaction barriers, Effect from a key carbon-insertion reaction, C + H2 → CH2. Astron. Astrophys. 637, A72.
Sittler, E. C., Cooper, J. F., Sturner, S. J., and Ali, A. (2020). Titan's ionospheric chemistry, fullerenes, oxygen, galactic cosmic rays and the formation of exobiological molecules on and within its surfaces and lakes. Icarus 344, 113246. doi:10.1016/j.icarus.2019.03.023
Snyder, L. E., Buhl, D., Zuckerman, B., and Palmer, P. (1969). Microwave detection of interstellar formaldehyde. Phys. Rev. Lett. 22, 679–681. doi:10.1103/physrevlett.22.679
Socha, R. F., Weiss, A. H., and Sakharov, M. M. (1980). Autocatalysis in the formose reaction. React. Kinet. Catal. Lett. 14 (2), 119–128. doi:10.1007/bf02061275
Soma, T., Sakai, N., Watanabe, Y., and Yamamoto, S. (2015). Methanol in the starless core, Taurus molecular cloud-1. Astrophys. J. 802, 74. doi:10.1088/0004-637X/802/2/74
ten Kate, I. L. (2018). Organic molecules on Mars. Science 360, 1068–1069. doi:10.1126/science.aat2662
Tercero, B., Cuadrado, S., López, A., Brouillet, N., Despois, D., Cernicharo, J., et al. (2018). Chemical segregation of complex organic O-bearing species in Orion KL. Astron. Astrophys. 620, L6. doi:10.1051/0004-6361/201834417
Theule, P., Borget, F., Mispelaer, F., Danger, G., Duvernay, F., Guillemin, J. C., et al. (2011). Hydrogenation of solid hydrogen cyanide HCN and methanimine CH2NH at low temperature. Astron. Astrophys. 534, A64. doi:10.1051/0004-6361/201117494
Tielens, A. G. G. M. (2013). The molecular Universe. Rev. Mod. Phys. 85, 1021–1081. doi:10.1103/RevModPhys.85.1021
Tielens, A. G. G. M., and Whittet, D. C. B. (1997). in Molecules in astrophysics: Probes & processes. Editor E. F. van Dishoeck (Dordrecht: Kluwer), 45.
Vasyunin, A. I., and Herbst, E. (2013). Reactive desorption and radiative association as possible drivers of complex molecule formation in the cold interstellar medium. Astrophys. J. 769, 34. doi:10.1088/0004-637x/769/1/34
Walsh, C., Herbst, E., Nomura, H., Millar, T. J., and Weaver, S. W. (2014a). Complex organic molecules along the accretion flow in isolated and externally irradiated protoplanetary disks. Faraday Discuss. 168, 389–421. doi:10.1039/c3fd00135k
Walsh, C., Millar, T. J., Nomura, H., Herbst, E., Weaver, S. W., Aikawa, Y., et al. (2014b). Complex organic molecules in protoplanetary disks. Astron. Astrophys. 563, A33. doi:10.1051/0004-6361/201322446
Wang, W., Wang, S., Ma, X., and Gong, J. (2011). Recent advances in catalytic hydrogenation of carbon dioxide. Chem. Soc. Rev. 40, 3703. doi:10.1039/c1cs15008a
Watanabe, N., and Kouchi, A. (2002). Measurements of conversion rates of CO to CO2 in ultraviolet-induced reaction of D2O(H2O)/CO amorphous ice. Astrophys. J. 567, 651–655. doi:10.1086/338491
Watanabe, N., Mouri, O., Nagaoka, A., Chigai, T., Kouchi, A., Pirronello, V., et al. (2007). Laboratory simulation of competition between hydrogenation and photolysis in the chemical evolution of H2O-CO ice mixtures. ApJ. 668, 1001–1011. doi:10.1086/521421
Watanabe, N., Nagaoka, A., Shiraki, T., and Kouchi, A. (2004). Hydrogenation of CO on pure solid CO on and CO-H2O mixed ice. Astrophys. J. 616, 638–642. doi:10.1086/424815
Watson, J. D., and Crick, F. H. C. (1953). Molecular structure of nucleic acids: A structure for deoxyribose nucleic acid. Nature 171, 737–738. doi:10.1038/171737a0
Webster, C. R., Mahaffy, P. R., Atreya, S. K., Moores, J. E., Flesch, G. J., Malespin, C., et al. (2018). Background levels of methane in Mars’ atmosphere show strong seasonal variations. Science 360, 1093–1096. doi:10.1126/science.aaq0131
White, E. T., Tang, J., and Oka, T. C. H5+ (1999). CH 5+ : The infrared spectrum observed. Science 284, 135–137. doi:10.1126/science.284.5411.135
Whittet, D. C. B., Cook, A. M., Herbst, E., Chiar, J. E., and Shenoy, S. S. (2011). Observational constraints on methanol production in interstellar and preplanetary ices. Astrophys. J. 742, 28. doi:10.1088/0004-637x/742/1/28
Williams, D. A. (2005). Gas and dust in the interstellar medium. J. Phys. Conf. Ser. 6, 1–17. doi:10.1088/1742-6596/6/1/001
Withnall, R., and Andrews, L. (1988). Matrix reactions of methylsilanes and oxygen atoms. J. Phys. Chem. 92, 594–602. doi:10.1021/j100314a006
Witt, A., Ivanov, S. D., Forbert, H., and Marx, D. (2008). Microsolvation of protonated methane: Structures and energetics of CH5+(H2)n. J. Phys. Chem. A 112, 12510–12517. doi:10.1021/jp8054069
Wollin, G., and Ericson, D. B. (1971). Amino-acid synthesis from gases detected in interstellar space. Nature 233, 615–616. doi:10.1038/233615a0
Woon, D. E. (2002). Modeling gas-grain chemistry with quantum chemical cluster calculations. 1. Heterogeneous hydrogenation of CO and H2CO on icy grain mantles. Astrophys. J. 569, 541–548. doi:10.1086/339279
Woon, D. E. (2021). The astrochymist. Available at: http://www.astrochymist.org/
Yamamoto, S., Beniya, A., Mukai, K., Yamashita, Y., and Yoshinobu, J. (2004). Low-energy electron-stimulated chemical reactions of CO in water ice. Chem. Phys. Lett. 388, 384–388. doi:10.1016/j.cplett.2004.03.030
Yamamoto, S. (2017). Introduction to Astrochemistry, Astronomy and astrophysics. Tokyo, Japan: Springer.
Keywords: interstellar medium (ISM), complex organic molecules (COMs), astro methanol-to-olefin (AMTO) synthesis, carbonaceous meteorites, quantum mechanical tunneling, the Methanol Economy
Citation: Mathew T, Esteves PM and Prakash GKS (2022) Methanol in the RNA world: An astrochemical perspective. Front. Astron. Space Sci. 9:809928. doi: 10.3389/fspas.2022.809928
Received: 05 November 2021; Accepted: 05 July 2022;
Published: 10 August 2022.
Edited by:
Ashraf-Ali, University of Maryland, College Park, United StatesReviewed by:
David Van Vranken, University of California, Irvine, United StatesRanajit Talukdar, University of Colorado Boulder, United States
Copyright © 2022 Mathew, Esteves and Prakash. This is an open-access article distributed under the terms of the Creative Commons Attribution License (CC BY). The use, distribution or reproduction in other forums is permitted, provided the original author(s) and the copyright owner(s) are credited and that the original publication in this journal is cited, in accordance with accepted academic practice. No use, distribution or reproduction is permitted which does not comply with these terms.
*Correspondence: Thomas Mathew, dG1hdGhld0B1c2MuZWR1; G. K. Surya Prakash, Z3ByYWthc2hAdXNjLmVkdQ==