Estimating the Coronal Supra-Arcade Downflow Radio Emission: From Centimeter Through Submillimeter Wavelengths
- 1Centro de Rádio Astronomia e Astrofísica Mackenzie (CRAAM), Universidade Presbiteriana Mackenzie, São Paulo, Brazil
- 2Instituto de Astronomía y Física del Espacio (IAFE-UBA), Consejo Nacional de Investigaciones Científicas y Técnicas (CONICET), Buenos Aires, Argentina
- 3Instituto de Astronomía Teórica y Experimental (IATE), Consejo Nacional de Investigaciones Científicas y Técnicas, Córdoba, Argentina
- 4Observatorio Astronómico de Córdoba (OAC), Universidad Nacional de Córdoba (UNC), Córdoba, Argentina
- 5NAT—Núcleo de Astrofísica, Universidade Cidade de São Paulo, São Paulo, Brazil
Supra-arcade downflows (SADs) are infrequent, wiggly, and low-emission structures observed to descend through the solar corona, mostly in EUV and soft X-ray frequencies. Based on their physical characteristics, SADs have been interpreted as low-density bubbles and are related to magnetic reconnection processes during long-term erupting flares. In this work, we use numerical MHD simulations to compute flux density maps, which are convolved with telescope beams to synthesize images with the aim to assess the expected SAD emission in radio wavelengths. We assume that the emission is thermal bremsstrahlung from a fully ionized plasma and without any appreciable gyroresonance contribution since magnetic fields are of the order of 10 G. We find that SAD emission should be optically thin in the frequency range of [10–1,000] GHz, and the spatially integrated flux should be larger than 1 Jy. We conclude, therefore, that SADs consistently are less bright than the surrounding fan and that observing SADs in radio frequencies between [0.5–1,000] GHz is feasible with present instrumentation. The observing strategies are proposed, including the instruments that can be used. Moreover, since the emission is, for the most part, optically thin, the flux density is proportional to temperature, density, and line-of-sight depth and when combined with EUV and soft X-ray images may allow a better density and temperature determination of SADs.
1 Introduction
In EUV and soft X-ray observations, coronal supra-arcade downflows (SADs) are seen as wiggly, low-emission structures leaving extended dark wakes during their descending motions toward the solar surface till they finally stop and gradually disappear. Often SAD descending motions resemble tadpoles swimming upstream through the fluid interstices that oppose less resistance. SADs have usually been detected during the early decay phases of long-term eruptive flares producing coronal mass ejections occurring many times during the first hour after the peak flare intensity. The SAD typical lifetime is of a few minutes. Today there exists a general consensusthat SADs are subdense cavities of plasma, with densities lower than their surroundings and, thus, having an intrinsically lower brightness (Innes et al., 2003b). SADs have been reported in several events (McKenzie and Hudson, 1999; McKenzie and Savage, 2009; Savage and McKenzie, 2011; McKenzie and Savage, 2011; Warren et al., 2011; Hanneman and Reeves, 2014; Chen et al., 2017) and using different instruments and observational techniques: direct images, with TRACE in EUV and XRT/Hinode and AIA/SDO in soft X-rays; and spectra with SUMER/SOHO in EUV. SADs are observed to descend from the upper part of the fan, a region that lies above the post-flare arcade, which is formed after the eruption, and it is also known in the literature as post-eruption supra-arcade; plasma sheet; thermal halo; supra-arcade fan; or just fan (hereafter used, see the sketch of Figure 1). The fan is a dynamical region exhibiting turbulent motions with a relatively high plasma β parameter (≳ 1; McKenzie, 2013; Freed and McKenzie, 2018), is bright in EUV and soft X-ray wavelengths, providing the necessary contrast for SAD detection; and is also hotter and denser than the external coronal medium by roughly an order of magnitude. SADs are observed in limb flares and generally when the fan is viewed face-on (i.e., perpendicular to the arcade axis, see Figure 1), whereas almost not in edge-on views. On the other hand, soft X-ray observations have provided evidence that the bright plasma of the fan surrounds a current sheet (Liu et al., 2013; Warren et al., 2018) that, in line with the classical model of eruptive flares, is expected to form behind the coronal mass ejection. In addition, there is observational evidence that the current sheet extends high in the corona with the fan just covering the lower part of it.
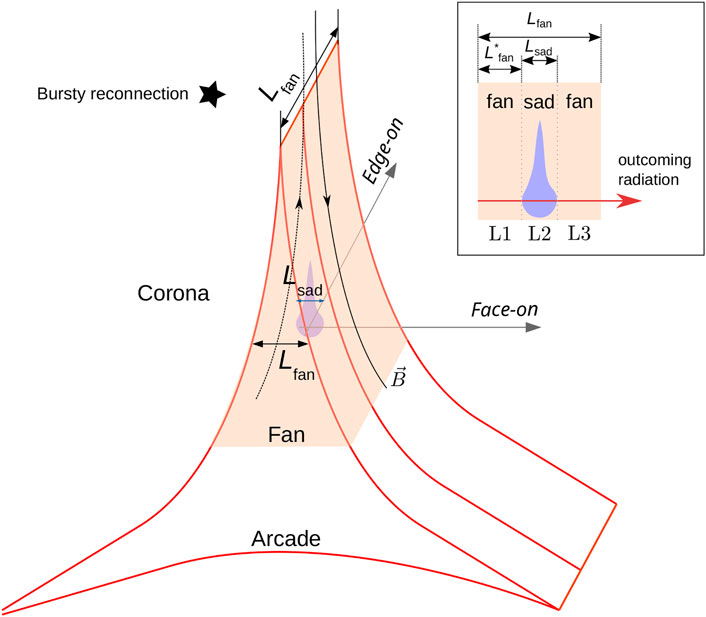
FIGURE 1. Schematic scenario of a SAD (blue feature) embedded in the bright, hot, and dense fan plasma (shaded region). Below the fan lies the post-flare arcade, and the external coronal medium surrounds it. The fan in addition embeds the expected supra-arcade current-sheet (dark lines with arrows). The fan is represented as viewed by an observer to the limb with two particular line-of-sights of interest represented, the edge-on and face-on views. Given that the fan is a thin, linear, and long structure along the edge-on direction, when the fan is viewed edge-on, the line-of-sight depth Lfan is greater than when viewed face-on. (Inset) Sketches a three-layer scheme used to compute the outcoming radiation considering a SAD embedded in the fan for an observer in the arrow direction.
Despite the fact that several models of SADs have been proposed, the SAD origin and dynamics are not yet completely understood; therefore, the mechanism of their driving physical process is still open to further research. Based on observations and theoretical considerations, a common point among all proposed models is the association of possible SAD origins with post-eruption magnetic reconnection processes occurring inside the fan or in its upper coronal medium (Figure 1). Some models (Linton et al., 2009; Longcope et al., 2009; Scott et al., 2013; Guo et al., 2014; Longcope et al., 2018), have pointed out to reconnection downflows descending through the fan as the driven mechanism: considering the standard model of erupting flares, behind the coronal mass ejection, magnetic field lines of opposite polarities are swept together forming the supra-arcade current sheet where the lines reconnect. As a result, part of the reconnection outflows is propelled downward, via magnetic tension, to the already-formed underlying arcade, likely in the form of descending magnetic flux tubes or loops. With this picture in mind, Linton et al. (2009), see also Linton and Longcope (2006), carried out 3D MHD simulations considering an intermittent burst of patchy reconnection in a Y-type current sheet, which supported the idea that SADs are descending reconnected flux tubes. Then, Savage et al. (2012) interpreted SADs as subdense wakes left by shrinking reconnected flux tubes by the observational analysis of one event. In turn, Guo et al. (2014), see also Innes et al. (2014), proposed that reconnected flux tubes in their retracting motions through the inhomogenous fan generate interfaces of plasma where Rayleigh–Taylor instabilities are developed and subsequently generate the SAD phenomenon. In these models, SADs are triggered in the current sheet neighborhood, that is, inside the fan or in its upper coronal region. A common issue with the reconnection downflow interpretation of SADs is the several-time reported discrepancy between the measured SAD speeds (often smaller than 200 km s−1) and the theoretical reconnection downflow speeds, expected to be the Alfvén speed (∼1,000 km s−1) of the fan. Among the possible explanations to overcome this speed mismatch, it was proposed that drag forces could act on the descending motion of reconnection downflows (Longcope et al., 2018), slowing them down.
In contrast with the abovementioned reconnection downflow interpretation of SADs, Costa et al. (2009), Schulz et al. (2010), Maglione et al. (2011), Cécere et al. (2012), Cécere et al. (2015), and Zurbriggen et al. (2016) have assumed SADs to be generated by spontaneous, intermittent, and bursty magnetic reconnection processes taking place somewhere in the turbulent-like fan or in its upper coronal region. One of these bursty reconnection events injects energy in a localized fast way, resembling a spark or explosive event, generating shock and rarefaction nonlinear waves, leading to the formation of a subdense cavity, whose structure supports the external pressure because it is hotter than its surroundings, and which is then observed in EUV and soft X-rays as a low-emission structure moving down along the bright fan. These spontaneous reconnection drivers are considered local processes, which could be independent of the overall supra-arcade current sheet. A common characteristic of simulated SADs by Costa et al. (2009) and their subsequent works is that SADs are hotter than the fan. However, some observational studies (Hanneman and Reeves, 2014; Reeves et al., 2017; Xue et al., 2020) have pointed out that SADs are hotter than the upper coronal medium, but they do not seem to be hotter than the fan. This temperature discrepancy between SADs and the fan is due to simplifying assumptions on our modeling, which may be overcome by considering a more complex fan scenario. In addition, a questionable point related to the scenario proposed by Zurbriggen et al. (2016) is that the simulated SADs exhibited lack of elongated tadpole-like shapes during their descent; instead, they showed a more compact shape which opposes several observational reports. This issue is a consequence of considering explicitly anisotropic thermal conduction in the MHD simulations.
Finally, given that SADs are not usually detected during eruptive flares, an important question still remaining to be answered by observations and models is whether SADs are an infrequent phenomenon, and thus, rarely observed, or are they a common phenomenon usually not detected for some reason. SADs have been mainly detected and studied in EUV and soft X-ray frequencies, whereas in radio wavelengths, only indirect evidence have been reported, and, as far as we know, no attempt has been made to detect them directly. In this work, we compute the expected thermal bremsstrahlung emission produced by SADs in wavelengths from centimeter to submillimeter using the numerical MHD models presented by Guo et al. (2014), Cécere et al. (2015), and Zurbriggen et al. (2016). Moreover, we synthesize maps by convolving the telescope beams of the Atacama Large Millimeter/Submillimeter Array (ALMA) in Chajnantor (Chile) and the Karl G. Jansky Very Large Array (VLA) in Socorro (United States) with the MHD simulations of SADs carried out by Zurbriggen et al. (2016). The goal of this work is to motivate and identify the best strategy to observe in centimeter – submillimeter wavelengths these illusive structures triggered during long-term solar erupting flares.
2 Review of Observations
EUV and soft X-ray observations have reported that the fan is hotter and denser than the background coronal plasma in approximately an order of magnitude, with fan temperatures in the ∼[10–20] MK range and ion number densities of ∼109 cm−3. In an observational study, Hanneman and Reeves (2014) measured plasma temperatures for the fan, SADs, and coronal background in four flare events using differential emission measures, briefly reporting that the peak temperatures of SADs are in the ∼[2–12] MK range, with just one SAD being hotter than 10 MK; the fan temperatures are ∼10 MK, except a temperature measurement of 20 MK, but having high uncertainty and background temperatures of ∼[2–3] MK. In addition, in the literature, we find that SADs have been detected at heights ∼[40–150] Mm above the solar surface, with an average height of ∼80 Mm; descend distances of ∼[10–20] Mm; have downward speeds in the ∼[50–500] km s−1 range, but with a relatively low average speed of ∼130 km s−1; with SAD sizes between ∼[1–10] Mm; and have lifetimes of around ∼[5–10] min, although there have been few extreme exceptions. On the other hand, the emission contrast between the dark structure and surrounding bright fan is an essential parameter during SAD detection, where, for example, values of ∼[2–4] were reported by Xue et al. (2020), see their Figure 4D. Less observational data about SAD properties are available in wavelengths other than EUV and soft X-rays. Among all limb flare events reported in the literature with SAD detection or any signature of their presence, four events extensively studied occurred on 21 April 2002 on the NOAA active region (AR) 9906 (e.g., see Innes et al., 2003a,b; Verwichte et al., 2005); 22 October 2011 on NOAA AR 11314 (McKenzie, 2013; Hanneman and Reeves, 2014; Reeves et al., 2017; Xue et al., 2020; Li et al., 2021); 19 July 2012 on NOAA AR 11520 (Liu, 2013; Liu et al., 2013; Innes et al., 2014); and 10 September 2017 on NOAA AR 12673 (Longcope et al., 2018; Warren et al., 2018; Cai et al., 2019; Hayes et al., 2019; Yu et al., 2020). While the fan view for the first two events was face-on, the view for the third and fourth ones was edge-on. On the other hand, the fan is a linear-like, long column of plasma along the current sheet direction (edge-on view, see the sketch of Figure 1) with ∼[3–30] Mm depths, and it is noted that it is considerably thinner in the perpendicular direction (face-on view).
So far, there has not been any direct SAD detection in radio or microwaves at any frequency. On the other hand, some observational studies of limb flaring events (Asai et al., 2004; Chen et al., 2015; Yu et al., 2020), preferably in edge-on views, revealed temporal and spatial correlations between EUV recurring downflow motions in the fan and subsequent detection of impulsive radio emission bursts on the top and/or leg of the lower arcade. In any case, the downflows had a counterpart in radio wavelengths. Assuming the reconnection downflow interpretation of SADs, these authors interpreted the radio impulsive emissions as a consequence of the presence of SADs.

TABLE 1. Summary of relevant mean characteristics of different numerical MHD models. Lsad is the SAD size; nsad, ρsad, and Tsad are the SAD (inner) average ion number densities, equivalent mass density, and temperature, respectively; nfan, ρfan, and Tfan are the corresponding fan (outer) values; and Bfan [G] represents the mean magnetic field intensity of the fan. Data were taken from literature and here referred as model 1 from Zurbriggen et al. (2016); models 2 – 3 from Cécere et al. (2015); and models 4 – 5 from Guo et al. (2014).
3 Modeling the Expected Emission
3.1 Supra-Arcade Downflow Models Description
In order to calculate the thermal bremsstrahlung emissions of fans and SADs, we need to know their plasma temperatures and densities. Given that these data are not always provided by the models, we circumscribe to the numerical MHD scenarios presented by Guo et al. (2014), Cécere et al. (2015), and Zurbriggen et al. (2016). Table 1 summarizes the relevant mean characteristics of each model: SAD size Lsad; SAD (inner) ion number density nsad (also equivalent mass density ρsad) and temperature Tsad; and fan (outer) ion number density nfan, temperature Tfan, and mean magnetic field intensity Bfan. Comparing the values of the models listed in Table 1 and those reported by observations in Section 2, it is to be noted that all models predict SAD temperatures higher than the observationally reported ones, whereas the modeled fan temperatures and densities are in agreement with those in observations, except the fan densities of models 2 and 3 that seem to be a bit high.
Following the work of Costa et al. (2009) and motivated by the turbulent-like description of the fan provided by McKenzie (2013), Cécere et al. (2015) carried out 3D ideal MHD simulations to model a turbulent and dynamical fan as the medium where SADs descend. In order to generate this, a combination of tearing-mode and Kelvin–Helmholtz instabilities was used. It was argued that to obtain SADs compatible with observations, the activation of spontaneous bursty reconnection processes is required and that SADs support the external pressure because they are hotter than their surroundings. Moreover, for contrast requirements, Cécere et al. (2015) suggested that there must be a closed relation between SAD sizes and fan widths that should be satisfied for SADs to be observable. For this reason, SADs of two different characteristic sizes were generated with Lsad = 4 Mm and Lsad = 12 Mm (models 2 and 3 in Table 1, respectively).
Considering the scenario presented by Cécere et al. (2015), the 2D MHD simulations of Zurbriggen et al. (2016) considered the particular case of small SADs (L ≈ 2 Mm), where thermal conduction is expected to be more efficient and SADs fade away much faster than their typical lifetime. In this case, a properly turbulent fan was generated using a stirring force source. Figure 2 shows images of density (Figure 2A) and temperature (Figure 2B) maps. The images display three SADs, two strongly faded away due to the thermal diffusion, and the third one at the bottom left corner with coordinates (x, y) = (−3, −6) × 108 cm and size ≈2 Mm ≡ 2.8″ is the most intense. This SAD has a mean density ρsad ≈ 5.1 × 10−15 g cm−3, while the fan is almost three times denser, ρfan ≈ 1.2 × 10−14 g cm−3. On the other hand, the SAD inner temperature Tsad ≈ 19.2 MK is three times greater than the outer Tfan ≈ 7 MK. This model (model 1 in Table 1) represents the case of a fan viewed face-on.
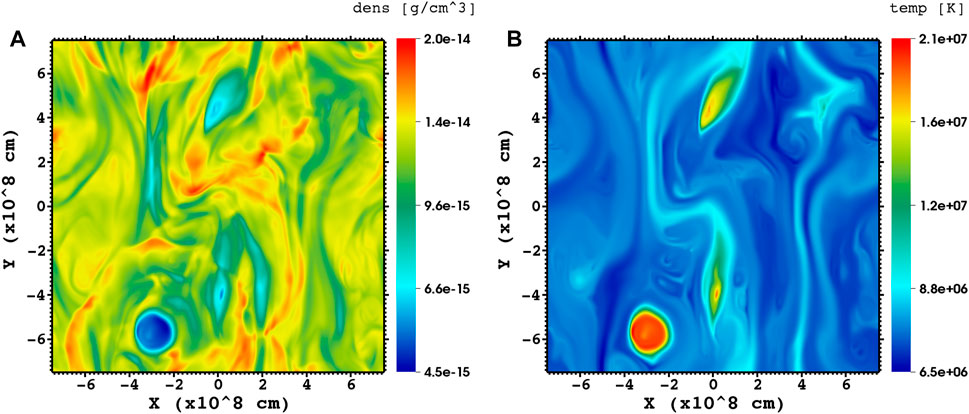
FIGURE 2. 2D MHD numerical simulation of SADs descending through a turbulent fan explicitly considering thermal conduction (presented in Zurbriggen et al., 2016). (A) Density values are shown. (B) Corresponding temperature. This model represents a fan viewed face-on. It is to be noted that 108 cm ≡ 1 Mm.
Guo et al. (2014), performing 3D-resistive MHD simulations, generated SADs by means of Rayleigh–Taylor instabilities associated with shrinking motions of magnetic flux tubes. In their scenario, using constant magnetic resistivity, SADs have characteristic sizes within [6′′ –
3.2 Expected Total Flux
Hereafter, we will refer to models summarized in Table 1 as model 1 to the smallest SAD (here considered) taken from the study by Zurbriggen et al. (2016); models 2 and 3 to the small and big SADs simulated by Cécere et al. (2015); and models 4 and 5 to the extreme sizes (6″ and 15″) reported by Guo et al. (2014).
In order to compute the (free–free) thermal bremsstrahlung emission, we use the expression
where kB is the Boltzmann constant and c is the speed of light. Τν is the optical depth at frequency ν, which is approximated by Dulk (1985)
where κν is the thermal bremsstrahlung opacity, L is the plasma line-of-sight depth considered, and G(T, ν) is the Gaunt factor that we obtain numerically from van Hoof et al. (2014). In addition, ne and ni are the number densities of free electrons and ions of specie i, respectively, and Zi is the atomic number of specie i. As we consider a fully ionized, ideal plasma with a solar abundance of 70.7% H + 27.4% He + 1.9% heavier elements (Prialnik, 2000), we infer that
From Eq. 2, we compute the thermal bremsstrahlung opacities κν as a function of the observing frequency ν for the SADs and fans of models 1–5. Figure 3 shows the obtained results. Color-continuous lines represent the SAD opacities, while color-dashed lines represent the corresponding fan opacities, which in general are larger in values. For fans and SADs, the regime should be optically thin greater than 10 GHz. However, in some cases, that will be analyzed later, the transition from optically thick to thin regimes may happen at frequencies below 1 GHz.
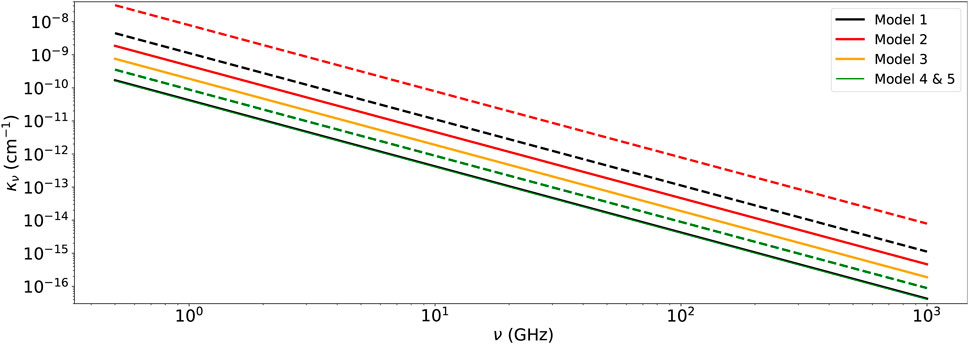
FIGURE 3. Mean thermal bremsstrahlung opacities κν for the different models (identified by colors) in the frequency range [0.5–1,000] GHz. Continuous lines correspond to inner (SAD) opacities, while dashed lines correspond to outer (fan) opacities. Fan opacities for models 2 and 3 are equal; the same happens for models 4 and 5. It is to be noted that SAD opacities for models 1, 4, and 5 are similar (lowest superimposed continuous lines).
We can now estimate the integrated flux density emitted by the whole SAD using Eq. 1 and the mean values from Table 1. The intrinsically inhomogenous dynamics of the fan implies that random fluctuations cancel out when integrated over the line-of-sight, and therefore the use of mean values is appropriate for computing the outgoing radiation observed on Earth. In the calculation, we assume that all sources are cylindrical with a diameter equal to the SAD size, and the solid angle
The emerging flux density from L1, after traversing the SAD (L2) and outer fan (L3) layers, is
where
Finally, for the outer layer, we have
On the other hand, the emerging radiation when observing the SAD direction has only the fan contribution (a one-layer calculation)
where τfan(ν) is the opacity of the whole fan line-of-sight depth Lfan.
Figure 4 displays the resulting integrated flux densities and contrasts Q = Ffan(ν)/Fsad(ν), dividing Eq. 7 by Eq. 3. The results are displayed for the edge-on (Figures 4A,B) and face-on (Figures 4C,D) views of the fan, with line-of-sight depths Lfan = 30 Mm and Lfan = 5 Mm, respectively. It is to be noted that the flux density is almost constant for frequencies ν ≳ 5 GHz, where the spectrum is optically thin. The SAD integrated flux densities Fsad(ν) are in the range
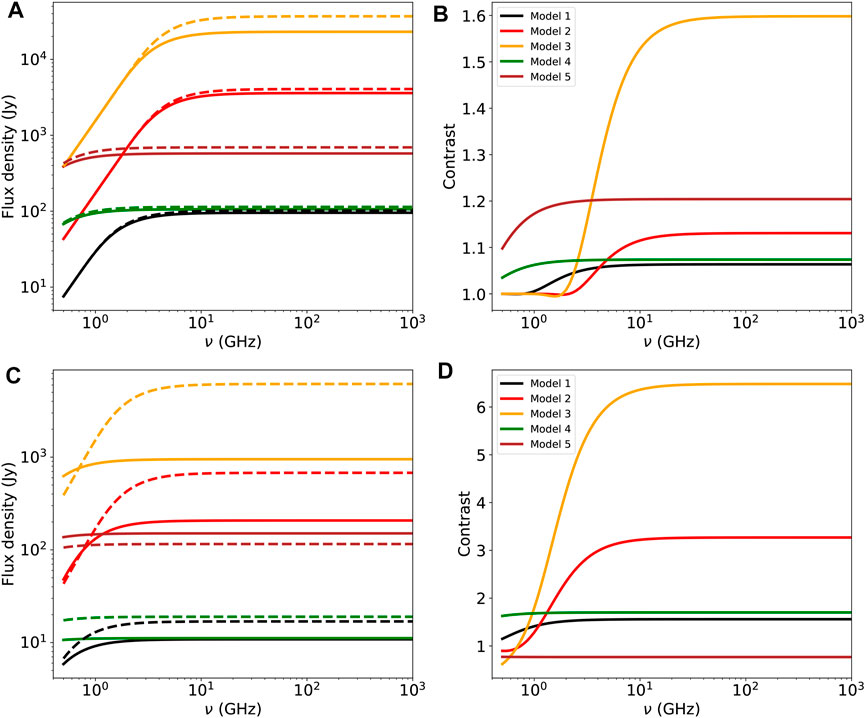
FIGURE 4. Left panels show the expected flux density spectra incoming from the SADs (continuous lines) and for the fan (dashed lines). Right panels show the fan-SAD contrasts (flux density quotient between the fan and SADs). (A,B) Results for the fan viewed edge-on. (C,D) Results for the fan viewed face-on.
3.3 Gyroresonance
In the previous calculations of Section 3.2, we did consider gyroresonance radiation, an emission mechanism that depends on the magnetic field intensity, in addition to the temperature and density. The gyroresonance opacity at frequency ν depends on the harmonic number s = ν/νB, where νB = 2.8 × 106B, with B the magnetic field intensity in G. The opacity at a given harmonic number τs (e.g., Casini et al., 2017) is proportional to
3.4 2D Synthesized Radio Maps
Here, we use model 1 to synthesize images that we expect to observe with the present radio telescopes. Since model 1 is 2D, we do not include the fan contribution in front of or behind the SAD as was carried out previously in Section 3.2; instead, every grid cell is thought of as a homogenous source with a line-of-sight depth L = 5 Mm. This value for the line-of-sight depth is deduced from the fact that model 1 accounts for a fan viewed face-on. The solid angle Ω = δx2/AU2 is considered, which is defined by the grid resolution (δx = δy = 5 × 106 cm) of the simulation. Using Eq. 1, we obtain the flux density emitted by every grid cell, and then to simulate observations, the resulting flux map is convolved with representations of instrument beams. In this case, due to the small size of the SAD, an instrument with high spatial resolution is needed to separate the SAD emission from the background fan. Nowadays, there are only two instruments with arcsec spatial resolution in radio wavelengths and capable of observing the Sun: the VLA and ALMA. Both are interferometers with synthesized beams of the order of 1 arcsec and sensitivities below 1 mJy.
We compute the emissions for the VLA microwave band S (3 GHz) and also for the ALMA bands: 3 (100 GHz), 6 (230 GHz), and 9 (720 GHz). Since the spectrum is mostly optically thin, and aiming to display results for a low and high frequency in the thermal bremsstrahlung–opacity domain [0.5–1,000] GHz considered in Figure 3, we show in Figure 5 the synthesized maps for the VLA band S at 3 GHz (Figures 5A,B) and the ALMA band 3 at 100 GHz (Figures 5C,D). Left panels represent the flux densities with the simulation grid spatial resolution, while the right panels show the resulting flux densities convolved with the two instrument beams, at the top using the VLA band S with a half-power beamwidth HPBW = 0.65″ and at the bottom using the ALMA band 3 with HPBW = 0.3″. It is to be noted that the fan emission is always brighter than the SAD, with a contrast Q ≈ 6. On the other hand, the convolved maps depend more on the spatial resolution (instrument beam) than on the flux density. For small SADs, such as that of model 1, using instruments with HPBW > 1″ will result in SADs spatially unresolved from the background fan emission. In terms of sensitivity, the expected flux detected by the instruments is in the order of or above 1 mJy/pixel.
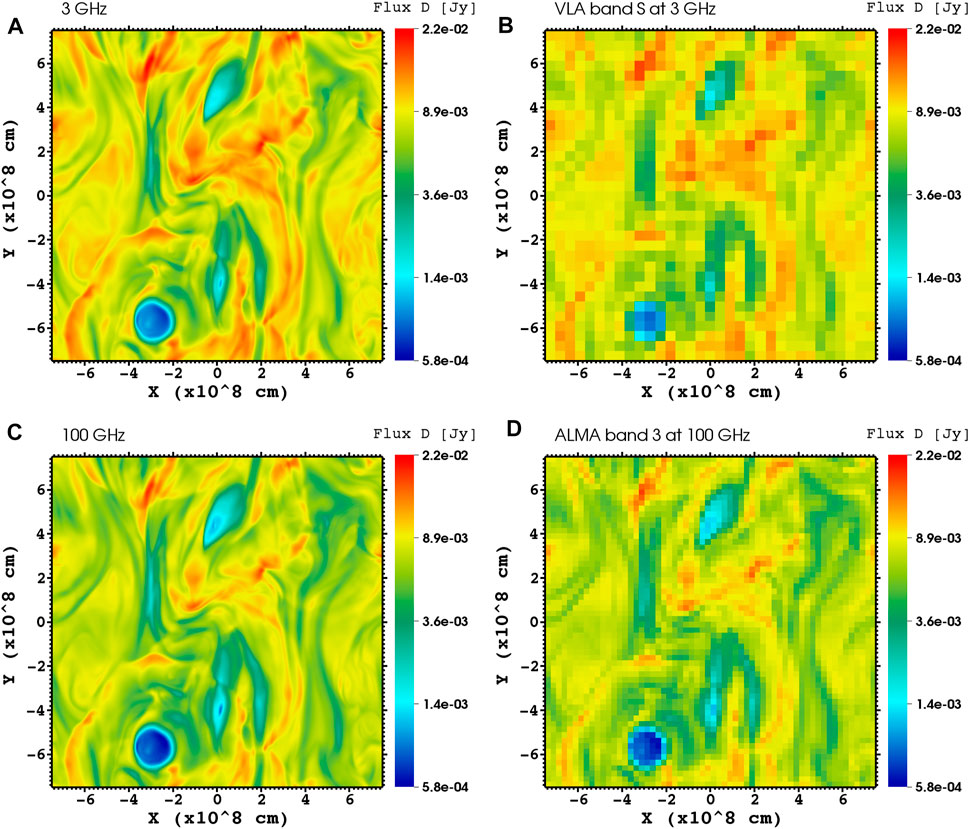
FIGURE 5. (A) Expected flux density at 3 GHz obtained from an MHD simulation, and the flux density convolved with the VLA-band S beam of HPBW = 0.65″ (B). (C) Simulated flux density at 100 GHz and the flux density convolved with the ALMA-band 3 beam of HPBW = 0.3″ (D). It is to be noted that 108 cm ≡ 1 Mm.
In the 2D scenario, the order of magnitude for the emission contrast Q between the fan and SADs can be estimated straightforwardly in the optically thin regime. As was pointed out previously, the contrast depends on the model considered, for example, for models 1–3, the fan temperatures are roughly half of the SAD ones, whereas the fan densities are roughly twice of the SAD ones. Due to the fact that we are considering the optically thin regime, the opacity κν ∝ n2ν−2T−3/2 (Eq. 2); furthermore, the emission in the Rayleigh–Jeans approximation can be written as Fν ∝ Tν2κνLΩ ≡ n2T−1/2LΩ; and thus, for these models, we obtain an order-of-magnitude contrast
This number is consistent with the contrast displayed by the flux densities in Figure 5. In addition, and as a support to the 2D-scenario results, the approximate contrast of Eq. 8 is of the same order as that reported by Guo et al. (2014) in their Figures 2D,E, where 2D slices of the expected emissions were shown to be corresponding to face-on views of the SDO/AIA Fe XXI and Fe XXIV channels, respectively. Finally, if we repeat the simple contrast calculation of Eq. 8, but instead using model 2 (see the 2D slices in density and temperature displayed in Figure 5 by Cécere et al. (2015), we again obtain a similar value. However, the contrast decrease is considerable when the plasma line-of-sight depth is taken into account, as illustrated in Figure 4.
3.5 Observational Strategies
It is evident from the flux density spectra displayed in Figure 4 that observations with edge-on views produce lower contrasts than with face-on views, making SAD detection more difficult, which worsens in the optically thick regime. Since the emission from microwaves to submillimeter wavelengths is mostly optically thin, the best strategy to observe SADs is when the fan line-of-sight is over the dark limb and when the fan is viewed face-on. On the other hand, according to the models analyzed in this work, the spatially integrated flux emitted by SADs (Figures 4A–C) can be as low as ≈ 1 Jy and as high as
4 Final Remarks
So far, SADs have been elusive structures likely produced by magnetic reconnection processes during solar flares. They have been detected only in EUV and soft X-ray emission, implying that currently known SAD characteristics, such as their temperatures, densities, and sizes, have been inferred using this wavelength range. The same thermal bremsstrahlung phenomenon that produces emission in EUV and soft X-ray should also produce emission in radio wavelengths. However, no direct observation of SADs in radio has been reported yet. Instead, in radio only were reported signatures of magnetic reconnection processes assumed to be responsible for SAD triggering, and also spatial/temporal correlations of SAD signatures with a burst of impulsive microwave emissions. Thus, the use of radio observations for SAD detection may help improve the estimations of SAD characteristics.
In order to contribute to the understanding of SAD nature, in this study, we produced spatially integrated spectra and synthesized images at selected radio frequencies. The spectra were obtained utilizing numerical MHD models of SADs based on the bursty localized reconnection interpretation by Costa et al. (2009) and subsequent works and the reconnection downflow interpretation by Guo et al. (2014). Here, we demonstrated that the fan emission should be detectable with current radio observatories and that the SADs should consistently be less bright than the surrounding fan. In the frequency range analyzed, [0.5–1,000] GHz, the spectra of SADs and fans show that the thermal bremsstrahlung emission greater than 10 GHz is mostly optically thin, and therefore proportional to the temperature, density, and line-of-sight depth. Furthermore, the gyroresonance contribution to the flux density is negligible. The fan emission and SAD sizes have a strong influence in their measured contrast Q. The contrast is a parameter that describes the detectability of a SAD, meaning that it is indistinguishable from the ambient-fan emission when it is close to unity. The fact that the contrast is not sufficiently high may be a reason why SADs are barely detected during eruptive events, particularly when the fan is viewed edge-on (as shown in Figure 4).
Despite our results being model-dependent, a strategic plan to increase chances of SAD detection in radio wavelengths is to first search for post-flare magnetic arcades over the limb, where a face-on view of the fan will be mostly desired; and second, to simultaneously observe at centimeter and millimeter wavelengths. Imaging SADs with arcsec resolution simultaneously at different radio wavelengths would be an excellent diagnostic to constraint models, with the VLA and ALMA interferometers being the best instruments to achieve this goal. Moreover, a combination of radio, EUV, and soft X-ray observations will enrich the description and deepen our understanding of the physical processes.
It is known that magnetic reconnection is in the origin of a great variety of phenomena: flares, CMEs, etc. Some of these phenomena are weak and, therefore, difficult to detect, as is the case with SADs. The SAD models used in this work consider that magnetic reconnection occurs at coronal heights, which is in line with the above-the-looptop hard X-ray source scenario (also known as Masuda flares, Masuda et al., 1994; Krucker et al., 2008) In order to fully understand the reconnection process and its consequences, we need to complete the SAD picture. Radio observations can be a key to having a more detailed image of them.
Data Availability Statement
The original contributions presented in the study are included in the article/Supplementary Material, further inquiries can be directed to the corresponding author.
Author Contributions
EZ, CC, and MC contributed to the conception and design of the study. CC carried out the analytical calculations, EZ performed the synthetic images, and CS assessed the radio emission mechanisms. AC contributed to the model and result discussions. EZ and CC led the manuscript writing. All the authors contributed to manuscript revision and read and approved the submitted version.
Funding
The research leading to these results has received funding from CAPES grant 88881.310386/2018-01, FAPESP grant 2013/24155-3. EZ, AC, and MC acknowledge support by CONICET grant number PIP No. 11220200103150CO.
Conflict of Interest
The authors declare that the research was conducted in the absence of any commercial or financial relationships that could be construed as a potential conflict of interest.
Publisher’s Note
All claims expressed in this article are solely those of the authors and do not necessarily represent those of their affiliated organizations, or those of the publisher, the editors and the reviewers. Any product that may be evaluated in this article, or claim that may be made by its manufacturer, is not guaranteed or endorsed by the publisher.
Acknowledgments
EZ is grateful to the FAPESP to have financed this research by the grant 2018/25177-4. GC thanks CNPq for support with a Productivity Research Fellowship. The authors are also grateful to Mackenzie Research Funding Mackpesquisa for the received support. GC is correspondent researcher of the Consejo Nacional de Investigaciones Científicas y Técnicas (CONICET) for the Instituto de Astronomía y Física del Espacio (IAFE), Argentina. MC and AC are members of the Carrera del Investigador Científico (CONICET). MC acknowledges support from ANPCyT under the grant PICT No. 2016-2480. MC also acknowledges support from the SECYT-UNC grant no. 33620180101147CB. We also thank the VisIt team–graphical tool (Harrison and Krishnan, 2012). The authors thank the editor and the referees for valuable and constructive comments and suggestions that helped improving this work significantly.
References
Asai, A., Yokoyama, T., Shimojo, M., and Shibata, K. (2004). Downflow Motions Associated with Impulsive Nonthermal Emissions Observed in the 2002 July 23 Solar Flare. ApJ 605, L77–L80. doi:10.1086/420768
Cai, Q., Shen, C., Raymond, J. C., Mei, Z., Warmuth, A., Roussev, I. I., et al. (2019). Investigations of a Supra-arcade Fan and Termination Shock above the Top of the Flare-Loop System of the 2017 September 10 Event. Mon. Not. Roy. Astron. Soc. 489, 3183–3199. doi:10.1093/mnras/stz2167
Casini, R., White, S. M., and Judge, P. G. (2017). Magnetic Diagnostics of the Solar Corona: Synthesizing Optical and Radio Techniques. Space Sci. Rev. 210, 145–181. doi:10.1007/s11214-017-0400-6
Cécere, M., Schneiter, M., Costa, A., Elaskar, S., and Maglione, S. (2012). Simulation of Descending Multiple Supra-arcade Reconnection Outflows in Solar Flares. ApJ 759, 79. doi:10.1088/0004-637X/759/2/79
Cécere, M., Zurbriggen, E., Costa, A., and Schneiter, M. (2015). 3D MHD Simulation of Flare Supra-Arcade Downflows in a Turbulent Current Sheet Medium. ApJ 807, 6. doi:10.1088/0004-637X/807/1/6
Chen, B., Bastian, T. S., Shen, C., Gary, D. E., Krucker, S., and Glesener, L. (2015). Particle Acceleration by a Solar Flare Termination Shock. Science 350, 1238–1242. doi:10.1126/science.aac8467
Chen, X., Liu, R., Deng, N., and Wang, H. (2017). Thermodynamics of Supra-arcade Downflows in Solar Flares. A&A 606, A84. doi:10.1051/0004-6361/201629893
Costa, A., Elaskar, S., Fernandez, C. A., and Martinez, G. (2009). Simulation of Dark Lanes in post-flare Supra-arcade. Mon. Not. Roy. Astron. Soc. 400, L85–L89. doi:10.1111/j.1745-3933.2009.00769.x
Dulk, G. A. (1985). Radio Emission from the Sun and Stars. Annu. Rev. Astron. Astrophys. 23, 169–224. doi:10.1146/annurev.aa.23.090185.001125
Freed, M. S., and McKenzie, D. E. (2018). Quantifying Turbulent Dynamics Found within the Plasma Sheets of Multiple Solar Flares. ApJ 866, 29. doi:10.3847/1538-4357/aadee4
Gary, D. E., Chen, B., Dennis, B. R., Fleishman, G. D., Hurford, G. J., and Krucker, S. (2018). Microwave and Hard X-Ray Observations of the 2017 September 10 Solar Limb Flare. Astrophys. J. 863, 83. doi:10.3847/1538-4357/aad0ef
Guo, L. J., Huang, Y., Bhattacharjee, A., and Innes, D. E. (2014). Rayleigh-Taylor Type Instabilities in the Reconnection Exhaust Jet as a Mechanism for Supra-arcade Downflows in the Sun. Astrophys. J. Lett. 796, L29. doi:10.1088/2041-8205/796/2/l29
Hanneman, W. J., and Reeves, K. K. (2014). Thermal Structure of Current Sheets and Supra-arcade Downflows in the Solar Corona. Astrophys. J. 786, 95. doi:10.1088/0004-637X/786/2/95
Harrison, C., and Krishnan, H. (2012). “Python’s Role in VisIt,” in 11th Python in Science Conf. (Scipy 2012). Editors A. Ahmadia, J. Millman, and S. van der, 23–29. doi:10.25080/Majora-54c7f2c8-00d
Hayes, L. A., Gallagher, P. T., Dennis, B. R., Ireland, J., Inglis, A., and Morosan, D. E. (2019). Persistent Quasi-Periodic Pulsations during a Large X-Class Solar Flare. Astrophys. J. 875, 33. doi:10.3847/1538-4357/ab0ca3
Innes, D. E., Guo, L. J., Bhattacharjee, A., Huang, Y. M., and Schmit, D. (2014). Observations of Supra-arcade Fans: Instabilities at the Head of Reconnection Jets. Astrophys. J. 796, 27. doi:10.1088/0004-637X/796/1/27
Innes, D. E., McKenzie, D. E., and Wang, T. (2003a). Observations of 1000 Km S−1 Doppler Shifts in 107 K Solar Flare Supra-arcade. Solar Phys. 217, 267–279. doi:10.1023/b:sola.0000006874.31799.bc
Innes, D. E., McKenzie, D. E., and Wang, T. (2003b). SUMER Spectral Observations of post-flare Supra-arcade Inflows. Solar Phys. 217, 247–265. doi:10.1023/b:sola.0000006899.12788.22
Kerdraon, A., and Delouis, J.-M. (1997). “The Nançay Radioheliograph,” in Coronal Physics from Radio and Space Observations. Editor G. Trottet (Springer Berlin Heidelberg), 192–201. doi:10.1007/BFb0106458
Krucker, S., Battaglia, M., Cargill, P. J., Fletcher, L., Hudson, H. S., MacKinnon, A. L., et al. (2008). Hard X-ray Emission from the Solar corona. Astron. Astrophys. Rev. 16, 155–208. doi:10.1007/s00159-008-0014-9
Li, Z. F., Cheng, X., Ding, M. D., Reeves, K. K., Kittrell, D., Weber, M., et al. (2021). Thermodynamic Evolution of Solar Flare Supra-arcade Downflows. Astrophys. J. 915, 124. doi:10.3847/1538-4357/ac043e
Linton, M. G., Devore, C. R., and Longcope, D. W. (2009). Patchy Reconnection in a Y-type Current Sheet. Earth, Planets, and Space 61, 573–576. doi:10.1186/bf03352925
Linton, M. G., and Longcope, D. W. (2006). A Model for Patchy Reconnection in Three Dimensions. Astrophys. J. 642, 1177–1192. doi:10.1086/500965
Liu, R. (2013). Dynamical Processes at the Vertical Current Sheet behind an Erupting Flux Rope. Mon. Not. Roy. Astron. Soc. 434, 1309–1320. doi:10.1093/mnras/stt1090
Liu, W., Chen, Q., and Petrosian, V. (2013). Plasmoid Ejections and Loop Contractions in an Eruptive M7.7 Solar Flare: Evidence of Particle Acceleration and Heating in Magnetic Reconnection Outflows. Astrophys. J. 767, 168. doi:10.1088/0004-637X/767/2/168
Longcope, D., Unverferth, J., Klein, C., McCarthy, M., and Priest, E. (2018). Evidence for Downflows in the Narrow Plasma Sheet of 2017 September 10 and Their Significance for Flare Reconnection. Astrophys. J. 868, 148. doi:10.3847/1538-4357/aaeac4
Longcope, D. W., Guidoni, S. E., and Linton, M. G. (2009). Gas-dynamic Shock Heating of Post-flare Loops Due to Retraction Following Localized, Impulsive Reconnection. Astrophys. J. Lett. 690, L18–L22. doi:10.1088/0004-637x/690/1/l18
Maglione, L. S., Schneiter, E. M., Costa, A., and Elaskar, S. (2011). Simulation of Dark Lanes in post-flare Supra-arcades. III. A 2D Simulation. Astron. Astrophys. 527, L5. doi:10.1051/0004-6361/201015934
Masuda, S., Kosugi, T., Hara, H., Tsuneta, S., and Ogawara, Y. (1994). A Loop-Top Hard X-ray Source in a Compact Solar Flare as Evidence for Magnetic Reconnection. Nature 371, 495–497. doi:10.1038/371495a0
McKenzie, D. E., and Hudson, H. S. (1999). X-Ray Observations of Motions and Structure above a Solar Flare Arcade. Astrophys. J. Lett. 519, L93–L96. doi:10.1086/312110
McKenzie, D. E., and Savage, S. L. (2011). Distribution Functions of Sizes and Fluxes Determined from Supra-arcade Downflows. Astrophys. J. Lett. 735, L6. doi:10.1088/2041-8205/735/1/L6
McKenzie, D. E., and Savage, S. L. (2009). Quantitative Examination of Supra-arcade Downflows in Eruptive Solar Flares. Astrophys. J. 697, 1569–1577. doi:10.1088/0004-637X/697/2/1569
McKenzie, D. E. (2013). Turbulent Dynamics in Solar Flare Sheet Structures Measured with Local Correlation Tracking. Astrophys. J. 766, 39. doi:10.1088/0004-637X/766/1/39
Prialnik, D. (20002000). An Introduction to the Theory of Stellar Structure and Evolution”. Cambridge: University Press. doi:10.1080/00107514.2011.580371
Reeves, K. K., Freed, M. S., McKenzie, D. E., and Savage, S. L. (2017). An Exploration of Heating Mechanisms in a Supra-arcade Plasma Sheet Formed after a Coronal Mass Ejection. Astrophys. J. 836, 55. doi:10.3847/1538-4357/836/1/55
Savage, S. L., and McKenzie, D. E. (2011). Quantitative Examination of a Large Sample of Supra-arcade Downflows in Eruptive Solar Flares. Astrophys. J. 730, 98. doi:10.1088/0004-637X/730/2/98
Savage, S. L., McKenzie, D. E., and Reeves, K. K. (2012). Re-interpretation of Supra-arcade Downflows in Solar Flares. Astrophys. J. Lett. 747, L40. doi:10.1088/2041-8205/747/2/L40
Schulz, W., Costa, A., Elaskar, S., and Cid, G. (2010). Simulation of Dark Lanes in post-flare Supra-arcades - II. A Contribution to the Remote Sensing of the Coronal Magnetic Field. Mon. Not. Roy. Astron. Soc. 407, L89–L93. doi:10.1111/j.1745-3933.2010.00911.x
Scott, R. B., Longcope, D. W., and McKenzie, D. E. (2013). Peristaltic Pumping Near Post-coronal Mass Ejection Supra-arcade Current Sheets. Astrophys. J. 776, 54. doi:10.1088/0004-637X/776/1/54
Selhorst, C. L., Silva-Válio, A., and Costa, J. E. R. (2008). Solar Atmospheric Model over a Highly Polarized 17 GHz Active Region. Astron. Astrophys. 488, 1079–1084. doi:10.1051/0004-6361:20079217
van Haarlem, M. P., Wise, M. W., Gunst, A. W., Heald, G., McKean, J. P., Hessels, J. W. T., et al. (2013). LOFAR: The LOw-Frequency ARray. Astron. Astrophys. 556. doi:10.1051/0004-6361/201220873
van Hoof, P. A. M., Williams, R. J. R., Volk, K., Chatzikos, M., Ferland, G. J., Lykins, M., et al. (2014). Accurate Determination of the Free-free Gaunt Factor - I. Non-relativistic Gaunt Factors. Mon. Not. Roy. Astron. Soc. 444, 420–428. doi:10.1093/mnras/stu1438
Verwichte, E., Nakariakov, V. M., and Cooper, F. C. (2005). Transverse Waves in a post-flare Supra-arcade. Astron. Astrophys. 430, L65–L68. doi:10.1051/0004-6361:200400133
Warren, H. P., Brooks, D. H., Ugarte-Urra, I., Reep, J. W., Crump, N. A., and Doschek, G. A. (2018). Spectroscopic Observations of Current Sheet Formation and Evolution. Astrophys. J. 854, 122. doi:10.3847/1538-4357/aaa9b8
Warren, H. P., O’Brien, C. M., Sheeley, J., and Neil, R. (2011). Observations of Reconnecting Flare Loops with the Atmospheric Imaging Assembly. Astrophys. J. 742, 92. doi:10.1088/0004-637X/742/2/92
White, S. M. (2004). “Coronal Magnetic Field Measurements through Gyroresonance Emission,”. Astrophysics and Space Science Library. Editors D. E. Gary, and C. U. Keller, 314, 89. doi:10.1007/1-4020-2814-8_5
Xue, J., Su, Y., Li, H., and Zhao, X. (2020). Thermodynamical Evolution of Supra-arcade Downflows. Astrophys. J. 898, 88. doi:10.3847/1538-4357/ab9a3d
Yu, S., Chen, B., Reeves, K. K., Gary, D. E., Musset, S., Fleishman, G. D., et al. (2020). Magnetic Reconnection during the Post-impulsive Phase of a Long-Duration Solar Flare: Bidirectional Outflows as a Cause of Microwave and X-Ray Bursts. Astrophys. J. 900, 17. doi:10.3847/1538-4357/aba8a6
Keywords: sun: corona, sun: flares, sun: magnetic fields, sun: radio radiation, MHD—instabilities
Citation: Zurbriggen E, Giménez De Castro CG, Costa A, Cécere M and Selhorst CL (2022) Estimating the Coronal Supra-Arcade Downflow Radio Emission: From Centimeter Through Submillimeter Wavelengths. Front. Astron. Space Sci. 9:832607. doi: 10.3389/fspas.2022.832607
Received: 10 December 2021; Accepted: 02 March 2022;
Published: 26 April 2022.
Edited by:
Masumi Shimojo, National Astronomical Observatory of Japan (NINS), JapanReviewed by:
Debi Prasad Choudhary, California State University, Northridge, United StatesDavid McKenzie, National Aeronautics and Space Administration (NASA), United States
Copyright © 2022 Zurbriggen, Giménez De Castro, Costa, Cécere and Selhorst. This is an open-access article distributed under the terms of the Creative Commons Attribution License (CC BY). The use, distribution or reproduction in other forums is permitted, provided the original author(s) and the copyright owner(s) are credited and that the original publication in this journal is cited, in accordance with accepted academic practice. No use, distribution or reproduction is permitted which does not comply with these terms.
*Correspondence: Ernesto Zurbriggen, ernesto.zurbriggen@craam.mackenzie.br
†ORCID ID: Ernesto Zurbriggen, orcid.org/0000-0002-3480-7107; C. Guillermo Giménez De Castro, orcid.org/0000-0002-8979-3582; Andrea Costa, orcid.org/0000-0002-4369-7392; Mariana Cécere, orcid.org/0000-0002-9844-0033