Planned Science and Scientific Discovery in Equatorial Aeronomy
- Department of Earth and Atmospheric Sciences, Cornell University, Ithaca, NY, United States
This paper discusses the relationship between planning and discovery in science using examples drawn from equatorial aeronomy in general and research at the Jicamarca Radio Observatory in particular. The examples reveal a pattern of discoveries taking place despite rather than because of careful planning.
1 Introduction
Edward Lorenz is famous because of a shortcut he took in 1961. Integrating a small system of differential equations numerically, he initialized the calculations using values from the middle of a prior run to save time. Surprisingly, the solution he found departed rapidly from the prior tabulated result. He traced the discrepancy to miniscule roundoff errors in the values he used for initial conditions. (His computer stored floating point numbers to six decimal places, but he had only printed and then rekeyed them to three). That small changes in the initial conditions could lead so quickly to drastic changes in the behavior of the system defied common sense. The finding led to chaos theory, raised deep questions about determinism, and doomed prospects for long-range weather forecasting, Lorenz’s original problem (Lorenz, 1963).
Undoubtedly, other investigators had encountered similar phenomena in the early days of numerical computing but dismissed them, concentrating instead on the immediate problem at hand. Lorenz is remarkable for having set aside the comparatively routine task before him in favor of getting to the bottom of the “error.” His keen judgment, and his freedom to move “off task,” led to one of the most important scientific results of the 20th century with impacts beyond Lorenz’s discipline.
History is full of scientific endeavors which were important because they did not go according to plan. A short list of examples includes Rutherford’s discovery of the nucleus, Penzias’ and Wilson’s discovery of cosmic background radiation, and the Michelson Morley experiment. Space physics and aeronomy is no exception, and it is worth recounting a few more modest but more contemporary examples as reminders of how plans gone wrong remain hallmarks of scientific discovery.
2 Examples From Equatorial Aeronomy and Space Physics
Built at about the same time as the Arecibo Observatory, the Jicamarca Radio Observatory was constructed outside Lima, Peru, to demonstrate the possibility of studying the upper atmosphere through the scattering of electromagnetic waves from free electrons (Gordon, 1958). This was to be a cost-effective way of learning about the environment that new spacecraft were being designed to inhabit. For a detailed history of early developments at Jicamarca, see (Woodman et al., 2019).
The motivation for Jicamarca’s location was its proximity to the magnetic equator where it would be relatively inexpensive to build a flat antenna to illuminate the ionosphere at an angle normal to the earth’s magnetic field. Early intuition about the scattering mechanism held that different ion species,gyrating about the magnetic field lines, would give distinct peaks in the autocorrelation function of the backscattered signal. The ionosphere would in effect become a giant ion mass spectrometer. The required sensitivity of the radar was expected to be substantial, and so a design employing 5 MW transmitter power and an antenna field with a 300 m-squared area was selected. The tests were not expected to take very long.
During the build-out of Jicamarca, extensive experiments were performed, and both the theory of scattering from thermal electron fluctuations in a plasma (now known as incoherent scatter) and the experimental techniques required to observe it underwent substantial development. The ion gyroresonances were not observed however. As (Farley, 1964; Dougherty, 1964) would show independently, the gyroresonances are largely destroyed by ion Coulomb collisions. Even when the Coulomb collision frequency is much less than the ion gyrofrequency, the gyroresonance cannot be observed if the deviation of the electron position over a gyroperiod is comparable to the radar wavelength divided by 4π. Later, Farley (Farley, 1967) would observe the gyroresonance for H+ ions [see also (Rodrigues et al., 2007)], but the O+ gyroresonance cannot be detected given nominal ionospheric conditions.
So early experiments at Jicamarca did not go according to plan, but were they a failure? Hardly. To begin with, the experiments provided a basis for much deeper quantitative understanding of incoherent scatter and the practical methods required to make it useful. These include an understanding of the interpretation of the scattering cross section, practical means of measuring absolute electron densities, and theory and methods for inferring ion composition and electron and ion temperatures from the autocorrelation function (Bowles et al., 1962; Bowles, 1963; Farley, 1966). Within a few years, the incoherent scatter technique had fulfilled its promises and could be used to measure the most important state parameters of ionospheric plasmas. The techniques were equally applicable at non-equatorial sites. ISR remains the most incisive tool for ground-based remote sensing of the ionosphere.
Had construction waited on a more complete knowledge of ISR theory, it is unlikely that an incoherent scatter radar would have been built at the magnetic equator. It is fortunate that it was because this led directly to an enormous range of discoveries in aeronomy that would otherwise have been greatly delayed. Some of these discoveries are at the center of contemporary research in equatorial aeronomy, space physics, and space weather.
2.1 The Temperature Ratio Problem
By the 1980s, plasma state parameter estimates including electron and ion temperature, ion composition, plasma drift, and electron number density were being measured at ISR facilities around the world and deposited in databases. The procedure involved measuring and fitting the scattered signal autocorrelation function to ISR theory. An inconsistency appeared in the results for Jicamarca, however, were the electron-to-ion temperature ratio at night was found to be consistently less than unity. This is not physically reasonable. There seemed to be a problem, but was it with the experiment or with ISR theory?
Other facilities were not reporting difficulties with the temperature ratio, and archival data from early measurements at Jicamarca did not exhibit the problem either, so it was assumed that a bias had crept into the modern experiment. A painstaking analysis of errors and biases in the methodology did not reveal a mistake, however (Pingree, 1990). Furthermore, new experiments showed that the problem nearly vanished when the angle between the radar beam and the perpendicular-to-B direction was increased. Eventually, records were unearthed indicating that the temperature ratio problem had always existed at Jicamarca but had been artificially “corrected” in the database (Clark et al., 1976)! Indeed, the temptation to simply “correct” the problem in the modern era was strong too.
The temperature radio problem ultimately pointed to a subtle problem with ISR theory at small magnetic aspect angles arising from the neglect of electron Coulomb collisions (Sulzer and Gonzalez, 1999; Woodman, 2004; Kudeki and Milla, 2011; Milla and Kudeki, 2011). The effect of Coulomb collisions on the ISR spectrum at small magnetic aspect angles is difficult to capture and has not been formulated in closed form, and numerical estimates of the experimental effects are expensive to calculate and store. There remains no completely satisfactory resolution to the problem, although interim methods allow us to infer electron and ion temperatures from ISR autocorrelation function measurements sufficiently well for most intents and purposes so long as the magnetic aspect angle is not too small.
More importantly, the problem is now being investigated using a variety of avante garde methods including large particle-in-cell (PIC) simulations, producing fundamental insights into transport properties in kinetic plasmas and effective means of exploring them [see (Longley et al., 2018; Longley et al., 2019)]. The research will very likely be more impactful in the end than the ionospheric energy balance problem that motivated electron and ion temperature ratio measurements in the first place.
2.2 Ionospheric Irregularities
Due to its frequency and its equatorial geometry, Jicamarca immediately encountered intense, ubiquitous, and unexpected radar “clutter.” Figure 1 shows a typical range-time-intensity plot illustrating coherent backscatter observed at Jicamarca over a 24-h period. The enhanced backscatter comes from plasma density irregularities excited by different mechanisms including neutral turbulence and plasma instabilities. Coherent scatter signifies free energy in the upper atmosphere and is a distinctive telltale of space weather.
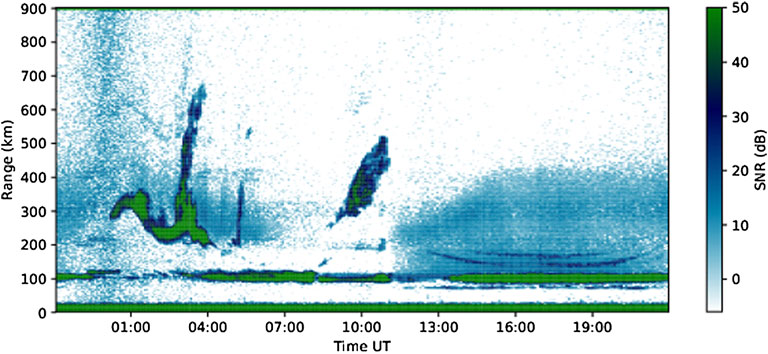
FIGURE 1. Range time intensity plot for September 14, 2010, showing backscatter signal-to-noise ratio versus altitude and time. Note that UT = LT + 5 h. Cyan hues mainly represent incoherent scatter whereas blue and green hues represent coherent scatter (see text).
Incoherent scatter cannot be measured in regions of strong clutter which comes through the main lobe and/or the sidelobes of all of Jicamarca’s antenna pointing positions. This prohibits a number of desirable experiments from being performed. The scientific tradeoff of the clutter is favorable, however, since coherent scatter offers keen insights into important processes in equatorial aeronomy and space weather that might have gone unnoticed otherwise.
Near 100 km altitude, the equatorial electrojet, a strong current system confined to low magnetic latitudes, flows. The current gives rise to both configuration-space and phase-space instabilities, gradient drift and Farley Buneman instabilities respectively (Farley, 1963; Simon, 1963). The resulting plasma density irregularities are strongly magnetic field aligned, as are irregularities in the F region and above. The two instabilities are also closely coupled and described by a unified dispersion relation (Fejer and Kelley, 1980).
The irregularities can be studied in detail near the magnetic equator where it is straightforward to distinguish echoes from different altitudes and where interferometry and imaging methods can be applied. Primary gradient drift waves can be observed at Jicamarca using interferometry and aperture-synthesis imaging, for example, (Kudeki et al., 1982; Hysell and Chau, 2002). Following their discovery at Jicamarca, similar instabilities were found to operate the auroral zone and, later, at middle latitudes. Farley Buneman instability has important implications outside equatorial aeronomy, altering the conductance of the auroral E region important for MI coupling (Liu et al., 2016) and causing heating in the solar chromosphere [e.g., (Madsen et al., 2013)].
Below the electrojet irregularities are intermittent irregularities in the mesosphere. These represent fluctuations in the index of refraction driven by mesospheric turbulence and exaggerated by the presence of free electrons in the D region. While they are not unique to the equatorial zone, they can be readily observed at Jicamarca due to the 50 MHz frequency and the high sensitivity of the facility (Woodman and Guillén, 1974). Neutral turbulence can be observed at Jicamarca in the mesosphere, stratosphere, and troposphere, and the discovery gave rise to the field of MST-radar techniques. Using these techniques, it is possible to measure neutral wind speeds, turbulence parameters, and turbulent fine structure (Fukao et al., 1974; Sheth et al., 2006; Guo et al., 2007; Lee et al., 2019).
Above the electrojet and throughout the F region, ionospheric interchange instability driven by free energy in the postsunset bottomside F region density gradient produces deep deformations in the bottomside that can penetrate into the topside, producing towering plumes of coherent backscatter (see Figure 1). The phenomenon underlies equatorial spread F (ESF) which is among the earliest space weather effects detected (Booker and Wells, 1938). The association with interchange instability enjoyed considerable speculation but was not established until Woodman and La Hoz (Woodman and La Hoz, 1976) produced definitive range-time-intensity (RTI) radar imagery of the process. (The authors reportedly needed to justify the recent purchase of an expensive new Versatec printer and invented RTI-style figures for this paper! The figures were very persuasive and remain the standard means of presentation).
ESF remains the cause célèbre of equatorial aeronomy as it disrupts modern radio communication, navigation, and imaging systems. Despite the fact that the underlying physics appears to be well understood, accurate forecasts remain elusive (Woodman, 2009). Jicamarca contributes to the research by measuring simultaneously both the causes (background ionospheric structure, vertical, and east-west plasma drifts via ISR) and the effects (irregularity morphology via coherent scatter and aperture synthesis imaging).
The interchange instabilities responsible for ESF are very similar to the E × B and current convective instabilities that create irregularities in the auroral F region, i.e., the irregularities monitored by the SuperDARN radar network. (The predecessor to SuperDARN, STARE, was an effort to infer high-latitude convection patterns from auroral electrojet echoes on the basis of earlier experiences from Jicamarca). Some of the other irregularities in Figure 1, meanwhile, are unique to the equatorial zone. Their discoveries were contrary to orthodoxy and deserve special attention.
2.2.1 150-km Echoes
Balsley (1964) identified another persistent source of radar clutter in the daytime valley region between about 140 and 170 km altitude in the early days of Jicamarca. (Royrvik and Miller, 1981; Royrvik, 1982) would associate the clutter with field-aligned plasma density irregularities, but it was not until a decade later that mysterious “150-km echoes” would receive serious scientific attention. (Mysterious because they exist in a homogeneous stratum of the ionosphere where gradient drift-type instability should not occur). (Kudeki and Fawcett, 1993) investigated the layers with a new, high-resolution mode and found them to be highly structured, exhibiting a stunning necklace shape that plunged with decreasing solar zenith angle. Most remarkably, the Doppler shifts of the echoes seemed to match the vertical plasma drifts. This suggested a means of measuring ionospheric dynamics using relatively low power radar systems going forward. The correspondence between the 150-km echo Doppler shifts at zenith with the vertical plasma drifts was established by (Woodman and Villanueva, 1995). Later, (Chau and Woodman, 2004) would show that both the vertical and zonal plasma drifts could be estimated accurately on the basis of line-of-sight 150-km echo Doppler shift measurements.
The source of the echoes remained a mystery for many more years. Two important clues helped expedite the research. One was the discovery of two distinct types of echoes—one spectrally narrow and field-aligned, and the other broad like a naturally enhanced ion line (Chau, 2004; Chau and Kudeki, 2013). The other was the observation that the layer height and intensity reacted to solar flares (Reyes, 2012) [see also (Pedatella et al., 2019)]. The echoes were clearly not due to some variant of gradient drift instability.
A pivotal finding came from (Oppenheim and Dimant, 2016) who identified energetic photoelectrons as the likely source of free energy behind the echoes. Their simulations reproduced narrow and broad spectral features and predicted both enhanced ion and electron lines. The authors tentatively associated their findings with upper hybrid instability (Basu et al., 1982; Jasperse et al., 1995). That association was made more explicitly by (Longley et al., 2020) who pointed out that the gaps in the echo necklace structure could be explained by cyclotron damping where the upper hybrid frequency matches an electron gyroharmonic frequency. As shown by (Lehmacher et al., 2020), this implies that not only plasma drifts but also plasma density profiles can be inferred from the 150-km echoes using low-power radar.
2.2.2 Bottom-Type Layers
Woodman and La Hoz (1976) identified several types of coherent scatter echoes associated with postsunset F-region instability. Among these were “bottom-type” or thin scattering layers that serve as precursors for intense plume events. The layers do not have signatures in ionograms, total electron content (TEC) measurements, radio scintillations, or airglow imagery and were neglected by the aeronomy and space-weather communities. Since “ESF” is a term taken from ionospheric sounding, if a phenomenon does not affect ionograms, it is not regarded as ESF. The bottom-type layers were just another unfortunate source of clutter.
For decades after their discovery, it was taken for granted that bottom-type layers signified marginal collisional interchange instability. There are several problems with this explanation, however. First, the layers exhibit little or no vertical development over time whereas interchange instabilities are convective instabilities and require vertical development. Second, the intensity of the layers does not vary directly with the strength of the background zonal electric field as is expected for the ionospheric interchange instability (Zargham and Seyler, 1987). Thirdly, the layers form at the base of the F region, near the valley, rather than in the steepest part of the bottomside where the growth rate for interchange instability is greatest.
Three clues shed light on the significance of the layers. The first was that fine structure in backscatter from the layers often exhibits horizontal striations (Hysell, 1992). This might be expected for gradient drift-type instability driven by zonal winds near a horizontal density gradient. The second was that the layers are not continuous but are patchy with horizontal scales of tens to hundreds of km (Hysell et al., 2005) (see Figure 2). The third was that they exist at altitudes and times where the plasma flow is westward–opposite the direction of the zonal neutral winds. Vertical shear flow predominates in the postsunset bottomside ionosphere, and associated with the shear flow is strong vertical current that is, usually neglected in stability analysis (Haerendel et al., 1992).
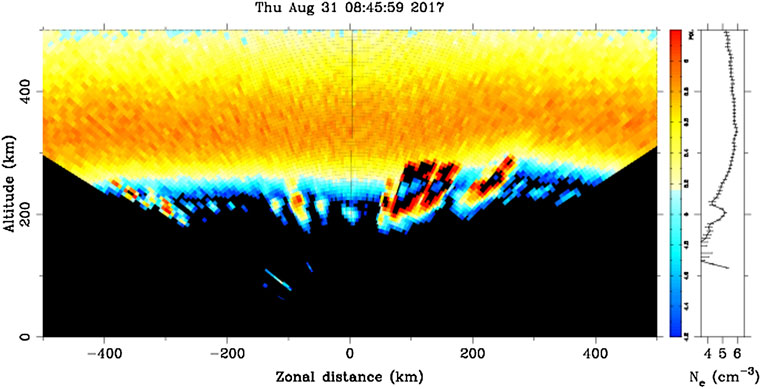
FIGURE 2. ALTAIR radar images showing bottom-type layers at the base of the F region just prior to the onset of ESF. The data were acquired during NASA project WINDY (Hysell et al., 2020).
Hysell and Kudeki (2004) showed that the aforementioned vertical current destabilizes the ionosphere to irregularities propagating at angles intermediate between the vertical and the horizon. The instability has a larger growth rate than the ionospheric interchange instability but is confined to a narrow stratum. The instability causes the bottomside to be corrugated and unstable to wind-driven gradient drift instability in the ascending phases, explaining the bottom-type layers. Most importantly, the irregularities precondition the ionosphere to interchange instability which can grow more rapidly than it would otherwise. Numerical simulations show that this auxiliary instability is required for ESF depletions and radar plumes to develop and penetrate the topside as quickly after sunset as they do (Hysell et al., 2022).
2.2.3 High-Altitude Echoes
In 2008, a problem was discovered with some of Jicamarca’s routine ISR experiments. Noise estimates were being calculated using samples from distant range gates above about 1,500 km. At night, the noise estimates would sometimes become anomalously large. It appeared that the distant range gates were being contaminated by signals of some kind. Further investigation showed that intense scattering layers were present at very high altitudes, most often between midnight and sunrise. At the time, a different method for estimating noise was introduced. The layers did not receive special attention and eventually disappeared.
However, the high-altitude echoes returned during the next solar minimum, and this time they were investigated further in a series of experiments beginning in 2018 and continuing to the present (Derghazarian et al., 2021). It was found that, during low solar flux conditions, the echoes are common in the pre-dawn sector between about 1,500 and 2,200 km altitude. The echoes exhibit Doppler shifts between about ±150 m/s and zonal drift rates of a few tens of m/s determined by multi-beam experiments. They are not obviously related to ESF.
Most importantly, the echoes exhibit sidebands upshifted and downshifted from the carrier by the lower hybrid frequency for protons. This is a remarkable result that is, not well understood. One candidate mechanism is lower hybrid drift instability (Krall and Liewer, 1971; Gladd, 1975), a streaming instability similar to modified two-stream instability, excited in this case by ion diamagnetic drift in the vicinity of existing plasma density irregularities. Another candidate is linear mode conversion and/or resonance instability in the vicinity of existing irregularities driven by lightning-induced whistlers (Lee and Kuo, 1984). The former mechanism is related to one invoked to explain small-scale irregularities in ESF (Huba and Ossakow, 1981). The latter mechanism is similar to one invoked to explain explosive spread F (Liao et al., 1989). In either case, pre-existing irregularities are required to bootstrap the instability. The source of these could be ESF although this is merely speculation at this point.
The high-altitude echoes represent frontier science in equatorial aeronomy with overtones for adjacent research domains like lightning research and even magnetic reconnection. For many years, however, they were just an overlooked source of radar clutter. In fact, the high-altitude echoes were recognized in the early days of Jicamarca experiments but were neglected because of more pressing problems making ISR experiments work.
3 Conclusion
This paper reviewed a number of discoveries in equatorial aeronomy and space physics going back to the 1960s. In each case, the discoveries came from projects and experiments that did not go according to plan. These were not merely serendipitous discoveries. Nor should they be considered negative outcomes of hypothesis tests. Rather, in most cases, they were the byproducts of the failures of assumptions that were not in doubt. Gyroresonances were supposed to be part of the incoherent backscatter spectrum. Coulomb collisions were supposed to be negligible. ESF was supposed to be driven entirely by ion interchange instability. The equatorial valley region and the inner plasmasphere were supposed to be stable. Pursuing the problems furthermore required deliberate departures from planned research. In some cases, this occurred only after long delays.
How can research be structured to make allowances for plans gone wrong and subsequent off-plan excursions? The premise seems to be at odds with contemporary trends which favor decadal planning for funding agencies, meticulous planning in research proposals, and long-range research plans for applicants for even junior positions. Perhaps, as Eisenhower famously said, plans are useless, but planning is indispensable. The important point is that it is essential to have the freedom to pivot when plans fail and to pursue the discoveries which may lie beneath.
Data Availability Statement
The datasets presented in this study can be found in online repositories. The names of the repository/repositories and accession number(s) can be found below: http://millstonehill.haystack.mit.edu/.
Author Contributions
The author confirms being the sole contributor of this work and has approved it for publication.
Funding
This work was supported by the award NNX15AL02G from the National Aeronautics and Space Administration. The Jicamarca Radio Observatory is a facility of the Instituto Geofisíco del Perú operated with support from the NSF award AGS-1732209 through Cornell.
Conflict of Interest
The author declares that the research was conducted in the absence of any commercial or financial relationships that could be construed as a potential conflict of interest.
The reviewer MM declared a past co-authorship with the author DH to the handling editor.
Publisher’s Note
All claims expressed in this article are solely those of the authors and do not necessarily represent those of their affiliated organizations, or those of the publisher, the editors and the reviewers. Any product that may be evaluated in this article, or claim that may be made by its manufacturer, is not guaranteed or endorsed by the publisher.
Acknowledgments
The author is indebted to Prof. Juha Vierinen who acquired and provided the data shown in Figure 1 and to Prof. Jorge Chau for his helpful suggestions.
References
Balsley, B. B. (1964). Evidence of a Stratified Echoing Region at 150 Kilometers in the Vicinity of the Magnetic Equator during Daylight Hours. J. Geophys. Res. 69, 1925–1930. doi:10.1029/jz069i009p01925
Basu, B., Chang, T., and Jasperse, J. R. (1982). Electrostatic Plasma Instabilities in the Daytime Lower Ionosphere. Geophys. Res. Lett. 9, 68–71. doi:10.1029/gl009i001p00068
Booker, H. G., and Wells, H. W. (1938). Scattering of Radio Waves by theF-Region of the Ionosphere. J. Geophys. Res. 43, 249. doi:10.1029/te043i003p00249
Bowles, K. L. (1963). Measuring Plasma Density of the Magnetosphere. Science 139, 389–391. doi:10.1126/science.139.3553.389
Bowles, K. L., Ochs, G. R., and Green, J. L. (1962). On the Absolute Intensity of Incoherent Scatter Echoes from the Ionosphere. J. Res. Natl. Bur. Stan. Sect. D. Rad. Prop. 66D, 395. doi:10.6028/jres.066d.041
Chau, J. L., and Kudeki, E. (2013). Discovery of Two Distinct Types of Equatorial 150 Km Radar Echoes. Geophys. Res. Lett. 40, 4509–4514. doi:10.1002/grl.50893
Chau, J. L. (2004). Unexpected Spectral Characteristics of VHF Radar Signals from 150-km Region over Jicamarca. Geophys. Res. Lett. 31, L23803. doi:10.1029/2004GL021620
Chau, J. L., and Woodman, R. F. (2004). Daytime Vertical and Zonal Velocities from 150-km Echoes: Their Relevance toF-Region Dynamics. Geophys. Res. Lett. 31, a–n. doi:10.1029/2004GL020800
Clark, W. L., McClure, J. P., and Van Zandt, T. E. (1976). “Description and Catalog of Ionospheric F Region Data, Jicamarca Radio Observatory (November 1966 – April, 1969),” in Tech. Rep. UAG-53, World Data Center A for Solar-Terrestrial Physics.
Derghazarian, S., Hysell, D. L., Kuyeng, K., and Milla, M. A. (2021). High Altitude Echoes from the Equatorial Topside Ionosphere during Solar Minimum. J. Geophys. Res. Space Phys. 126. doi:10.1029/2020JA028424
Dougherty, J. P. (1964). Model Fokker-Planck Equation for a Plasma and its Solution. Phys. Fluids 7, 1788. doi:10.1063/1.2746779
Farley, D. T. (1963). A Plasma Instability Resulting in Field-Aligned Irregularities in the Ionosphere. J. Geophys. Res. 68, 6083–6097. doi:10.1029/jz068i022p06083
Farley, D. T. (1966). A Theory of Incoherent Scattering of Radio Waves by a Plasma: 4. The Effect of Unequal Ion and Electron Temperatures. J. Geophys. Res. 71, 4091–4098. doi:10.1029/jz071i017p04091
Farley, D. T. (1967). Proton Gyroresonance Observed in Incoherent Scattering from the Ionosphere. Phys. Fluids 10, 1584–1586. doi:10.1063/1.1762326
Farley, D. T. (1964). The Effect of Coulomb Collisions on Incoherent Scattering of Radio Waves by a Plasma. J. Geophys. Res. 69, 1972402–1973200. doi:10.1029/jz069i001p00197
Fejer, B. G., and Kelley, M. C. (1980). Ionospheric Irregularities. Rev. Geophys. 18, 401. doi:10.1029/rg018i002p00401
Fukao, S., Sato, T., Kato, S., Harper, R. M., Woodman, R. F., and Gordon, W. E. (1974). Mesospheric Winds and Waves over Jicamarca on May 23–24, 1974. J. Geophys. Res. 84, 4379–4386.
Gladd, N. T. (1975). The Lower Hybrid Drift Instability and the Modified Two Stream Instability in High Density Theta Pinch Environments. Plasma Phys. 18, 27–40.
Gordon, W. (1958). Incoherent Scattering of Radio Waves by Free Electrons with Applications to Space Exploration by Radar. Proc. IRE 46, 1824–1829. doi:10.1109/jrproc.1958.286852
Guo, L., Lehmacher, G., Kudeki, E., Akgiray, A., Sheth, R., and Chau, J. L. (2007). Turbulent Kinetic Energy Dissipation Rate and Eddy Diffusivity Study in the Tropical Mesosphere Using Jicamarca Radar Data. Adv. Space Res. 40. doi:10.1016/j.asr.2007.05.068
Haerendel, G., Eccles, J. V., and Çakir, S. (1992). Theory for Modeling the Equatorial Evening Ionosphere and the Origin of the Shear in the Horizontal Plasma Flow. J. Geophys. Res. 97, 1209. doi:10.1029/91ja02226
Huba, J. D., and Ossakow, S. L. (1981). On 11-cm Irregularities during Equatorial spreadF. J. Geophys. Res. 86, 829. doi:10.1029/ja086ia02p00829
Hysell, D. L., and Chau, J. L. (2002). Imaging Radar Observations and Nonlocal Theory of Large-Scale Plasma Waves in the Equatorial Electrojet. Ann. Geophys. 20, 1167–1179. doi:10.5194/angeo-20-1167-2002
Hysell, D. L., and Kudeki, E. (2004). Collisional Shear Instability in the equatorialFregion Ionosphere. J. Geophys. Res. 109. doi:10.1029/2004JA010636
Hysell, D. L., Larsen, M. F., Swenson, C. M., Barjatya, A., Wheeler, T. F., Sarango, M. F., et al. (2005). Onset Conditions for Equatorial spreadFdetermined during EQUIS II. Geophys. Res. Lett. 32, L24104. doi:10.1029/2005GL024743
Hysell, D. L., Fang, T. W., and Fuller-Rowell, T. J. (2022). Modeling Equatorial F-Region Ionospheric Instability Using a Regional Irregularity Model and WAM-IPE. J. Geophys. Res.
Hysell, D. L. (1992). On the Hierarchy of Processes Contributing to Equatorial Spread F. Ph.D. Thesis. Ithaca, N. Y: Cornell Univ.
Hysell, D. L., Rao, S., Groves, K. M., and Larsen, M. F. (2020). Radar Investigation of Postsunset Equatorial Ionospheric Instability over Kwajalein during Project WINDY. J. Geophys. Res. Space Phys. 125. doi:10.1029/2020ja027997
Jasperse, J. R., Basu, B., Retterer, J. M., Decker, D. T., and Chang, T. (1995). “High Frequency Electrostatic Plasma Instabilities and Turbulence Layers in the Lower Ionosphere,” in Space Plasmas: Coupling between Small and Medium Scale Processes (Geophysical Monograph 86 (American Geophysical Union), 77–94. doi:10.1029/gm086p0077
Krall, N. A., and Liewer, P. C. (1971). Low-frequency Instabilities in Magnetic Pulses. Phys. Rev. A. 4, 2094–2103. doi:10.1103/PhysRevA.4.2094
Kudeki, E., Farley, D. T., and Fejer, B. G. (1982). Long Wavelength Irregularities in the Equatorial Electrojet. Geophys. Res. Lett. 9, 684–687. doi:10.1029/gl009i006p00684
Kudeki, E., and Fawcett, C. D. (1993). High Resolution Observations of 150 Km Echoes at Jicamarca. Geophys. Res. Lett. 20, 1987–1990. doi:10.1029/93gl01256
Kudeki, E., and Milla, M. A. (2011). Incoherent Scatter Spectral Theories-Part I: A General Framework and Results for Small Magnetic Aspect Angles. IEEE Trans. Geosci. Remote Sensing 49 (1), 315–328. doi:10.1109/tgrs.2010.2057252
Lee, K., Kudeki, E., Reyes, P. M., Lehmacher, G. A., and Milla, M. (2019). Mesospheric Wind Estimation with the Jicamarca MST Radar Using Spectral Mainlobe Identification. Radio Sci. 54, 1222–1239. doi:10.1029/2019RS006892
Lee, M. C., and Kuo, S. P. (1984). Production of Lower Hybrid Waves and Field-Aligned Plasma Density Striations by Whistlers. J. Geophys. Res. 89 (A12), 10873–10880. 10. doi:10.1029/JA089iA12p10873
Lehmacher, G. A., Wu, H., Kudeki, E., Reyes, P. M., Hysell, D. L., and Milla, M. (2020). Height Variation of Gaps in 150‐km Echoes and Whole Atmosphere Community Climate Model Electron Densities Suggest Link to Upper Hybrid Resonance. J. Geophys. Res. Space Phys. 125. doi:10.1029/2019JA027204
Liao, C. P., Freidberg, J. P., and Lee, M. C. (1989). Explosive Spread F Caused by Lightning-Induced Electromagnetic Effects. J. Atmos. Terrestrial Phys. 51, 751–758. doi:10.1016/0021-9169(89)90032-9
Liu, J., Wang, W., Oppenheim, M., Dimant, Y., Wiltberger, M., and Merkin, S. (2016). Anomalous Electron Heating Effects on the E Region Ionosphere in TIEGCM. Geophys. Res. Lett. 43, 2351–2358. doi:10.1002/2016GL068010
Longley, W. J., Oppenheim, M. M., and Dimant, Y. S. (2019). Nonlinear Effects of Electron‐Electron Collisions on ISR Temperature Measurements. J. Geophys. Res. Space Phys. 124, 6313–6329. doi:10.1029/2019JA026753
Longley, W. J., Oppenheim, M. M., Fletcher, A. C., and Dimant, Y. S. (2018). ISR Spectra Simulations with Electron-Ion Coulomb Collisions. J. Geophys. Res. Space Phys. 123, 2990–3004. doi:10.1002/2017JA025015
Longley, W. J., Oppenheim, M. M., Pedatella, N. M., and Dimant, Y. S. (2020). The Photoelectron‐Driven Upper Hybrid Instability as the Cause of 150‐km Echoes. Geophys. Res. Lett. 47. doi:10.1029/2020GL087391
Lorenz, E. N. (1963). Deterministic Nonperiodic Flow. J. Atmos. Sci. 20, 130–141. doi:10.1175/1520-0469(1963)020<0130:dnf>2.0.co;2
Madsen, C. A., Dimant, Y. S., Oppenheim, M. M., and Fontenla, J. M. (2013). The Multi-Species Farley-Buneman Instability in the Solar Chromosphere. ApJ 783. doi:10.1088/0004–637X/783/2/128
Milla, M. A., and Kudeki, E. (2011). Incoherent Scatter Spectral Theories-Part II: Modeling the Spectrum for Modes Propagating Perpendicular to B. IEEE Trans. Geosci. Remote Sensing 49 (1), 329–345. doi:10.1109/tgrs.2010.2057253
Oppenheim, M. M., and Dimant, Y. S. (2016). Photoelectron‐induced Waves: A Likely Source of 150 Km Radar Echoes and Enhanced Electron Modes. Geophys. Res. Lett. 43, 3637–3644. doi:10.1002/2016GL068179
Pedatella, N. M., Chau, J. L., Vierinen, J., Qian, L., Reyes, P., Kudeki, E., et al. (2019). Solar Flare Effects on 150‐km Echoes Observed over Jicamarca: WACCM‐X Simulations. Geophys. Res. Lett. 46, 10951–10958. 10. doi:10.1029/2019GL084790
Pingree, J. E. (1990). Incoherent Scatter Measurements and Inferred Energy Fluxes in the Equarotial F-Region Ionosphere. Ithaca, N. Y: Ph.D. thesis, Cornell Univ.
Reyes, P. M. (2012). Solar Flare Effects Observed over Jicamarca during MST-ISR Experiments. Master’s thesis, University of Illinois at Urbana-Champaign.
Rodrigues, F. S., Nicolls, M. J., Woodman, R., Hysell, D. L., Chau, J. L., and González, S. A. (2007). Ion Gyroresonance Observations at Jicamarca Revisited. Geophys. Res. Lett. 34, a–n. doi:10.1029/2007GL029680
Royrvik, O. (1982). Drift and Aspect Sensitivity of Scattering Irregularities in the Upper Equatorial E-Region. J. Geophys. Res. 87, 8338.
Royrvik, O., and Miller, K. L. (1981). Nonthermal Scattering of Radio Waves Near 150 Km above Jicamarca, Peru. J. Geophys. Res. 86, 180.
Sheth, R., Kudeki, E., Lehmacher, G., Sarango, M., Woodman, R., Chau, J., et al. (2006). A High-Resolution Study of Mesospheric fine Structure with the Jicamarca MST Radar. Ann. Geophys. 24, 1281–1293. doi:10.5194/angeo-24-1281-2006
Simon, A. (1963). Instability of a Partially Ionized Plasma in Crossed Electric and Magnetic fields. Phys. Fluids 6, 382. doi:10.1063/1.1706743
Sulzer, M. P., and Gonzalez, S. (1999). The Effect of Electron Coulomb Collisions on the Incoherent Scatter Spectrum in the F Region at Jicamarca. J. Geophys. Res. 104, 552. 22,535–22. doi:10.1029/1999ja900288
Woodman, R. F., Farley, D. T., Balsley, B. B., and Milla, M. A. (2019). The Early History of the Jicamarca Radio Observatory and the Incoherent Scatter Technique. Hist. Geo Space Sci. doi:10.5194/hgss-10-245-2019
Woodman, R. F., and Guillén, A. (1974). Radar Observations of Winds and Turbulence in the Stratosphere and Mesosphere. J. Atmos. Sci. 31, 493–505. doi:10.1175/1520-0469(1974)031<0493:roowat>2.0.co;2
Woodman, R. F., and La Hoz, C. (1976). Radar Observations ofFregion Equatorial Irregularities. J. Geophys. Res. 81, 5447–5466. doi:10.1029/ja081i031p05447
Woodman, R. F. (2004). On a Proper Electron Collision Frequency for a Fokker-Planck Collision Model with Jicamarca Applications. J. Atmos. Solar-Terrestrial Phys. 6617, 1521–1541. doi:10.1016/j.jastp.2004.07.001
Woodman, R. F. (2009). Spread F - an Old Equatorial Aeronomy Problem Finally Resolved? Ann. Geophys. 27, 1915–1934. doi:10.5194/angeo-27-1915-2009
Woodman, R. F., and Villanueva, F. (1995). “Comparison of Electric fields Measured at F Region Heights with 150 Km Irregularity Drift Measurements,” in Proceedings of the Ninth International Symposium on Equatorial Aeronomy, Indonesia, March 20-24 (Bali). [Dataset].
Keywords: equatorial aeronomy, incoherent scatter, radar, instability, irregularity, discovery science, serendipity
Citation: Hysell DL (2022) Planned Science and Scientific Discovery in Equatorial Aeronomy. Front. Astron. Space Sci. 9:883050. doi: 10.3389/fspas.2022.883050
Received: 24 February 2022; Accepted: 11 March 2022;
Published: 14 April 2022.
Edited by:
Jorge Luis Chau, Leibniz Institute of Atmospheric Physics (LG), GermanyReviewed by:
Marco Milla, Pontifical Catholic University of Peru, PeruCopyright © 2022 Hysell. This is an open-access article distributed under the terms of the Creative Commons Attribution License (CC BY). The use, distribution or reproduction in other forums is permitted, provided the original author(s) and the copyright owner(s) are credited and that the original publication in this journal is cited, in accordance with accepted academic practice. No use, distribution or reproduction is permitted which does not comply with these terms.
*Correspondence: D. L. Hysell, david.hysell@cornell.edu