- 1Laboratory Astrophysics Group of the Max Planck Institute for Astronomy at the Friedrich Schiller University Jena, Institute of Solid State Physics, Jena, Germany
- 2Max Planck Institute for Astronomy, Heidelberg, Germany
The study of exoplanetary atmospheres extends the frontiers of astronomy, astrophysics, and astrochemistry. Moreover, studies of exoplanets as being linked to the search for extraterrestrial life and other habitable planets are of interest not only for scientists, but for a much wider public audience. There is much evidence that clouds exist and are common in the exoplanetary atmospheres at high temperatures. Their origin can be gas-phase condensation of silicate materials and other refractory materials. Clouds have a major impact on the planets’ observable properties. Models describing atmospheres of exoplanets and brown dwarfs point to the necessity of including nanometer-to micrometer-sized grains of silicates. Observational mid-IR spectra have also provided tentative evidence of silicate grain absorption. Thus, silicates seem to be the first target for future astronomical observations of cloudy atmospheres and for laboratory studies supporting these observations. However, high-temperature laboratory studies of optical and structural properties of refractory materials, including silicates, and of gas-grain and grain surface chemistry needed for the decoding of astronomical spectra and for the development of reliable atmospheric models present practically uncharted territory. The aim of our paper is to review previous studies of optical and chemical properties of silicate materials and to emphasize the importance and perspective of high-temperature measurements of laboratory analogues of atmospheric silicate grains for exoplanet atmosphere characterization. This is particularly important in the light of new advanced astronomical instruments, which, as we expect, will bring comprehensive information on exoplanetary atmospheres.
Exoplanets and their atmospheres
The Nobel Prize in Physics 2019 was given for the first discovery of an extrasolar planet crowning the last two decades during which we progressed from the first exoplanet discovered to a collection of more than 4000 exoplanets with a great diversity in their parameters, such as the planetary masses, radii, temperatures, and orbits. The study of the structure, composition, and formation history of exoplanets and physical-chemical processes taking place in their atmospheres and interiors is one of the most intriguing and quickly developing research directions.
Exoplanetary atmospheres, whose spectral signatures can be measured by photometric and spectroscopic methods using Earth and space observatories, are among the main targets for recent and future astronomical observations. Such observations provide comprehensive information on the physical properties of the atmospheres, such as temperature, pressure, and density; and chemical ones, such as elemental and molecular composition. They allow for modelling chemical networks and connect them to the exoplanetary formation conditions and evolution processes. The exoplanets, whose atmospheres are available for characterization, are divided into several groups according to their mass and effective temperature, such as hot Jupiters (T ∼ 1300–3000 K), warm gas giants (T ∼ 500–1500 K), hot Neptunes (T ∼ 700–1200 K), and temperate super-Earths (T ∼ 500 K) (Madhusudhan et al., 2014). One more set of targets for extrasolar atmospheric observations is brown dwarfs as their atmospheres present the best analogs to the atmospheres of exoplanets due to the similarities in the temperature and mass leading to likely similar radiative, convective, hydrostatic, and chemical properties (Madhusudhan et al., 2014).
The observations and properties of exoplanetary atmospheres are very nicely described in a number of review papers (Madhusudhan et al., 2014; Crossfield, 2015; Kreidberg and DeegBelmonte, 2018; Helling, 2019). We refer to these papers for a detailed overview of the different methodologies currently available to characterize exoplanetary atmospheres. These atmospheres can be chemically extremely rich consisting of various elements, such as H, He, Si, Fe, Mg, O, C etc., and molecules, such as H2, H2O, CO, CH4, N2, NH3, SiO, H2S, Fe(OH)2, and Mg(OH)2 (Tsiaras et al., 2018; Helling, 2019; Roudier et al., 2021). These atoms, molecules and potentially ions are the starting point for the particle (grain) formation through gas-phase condensation and the particle formation is a prerequisite for the formation of atmospheric clouds. Condensation occurs when gas-phase molecules react, combine and form solid particles (condensation nucleus) that may subsequently grow due to further gas-nuclei reactions (condensation of gas-phase species on nucleus) and collisions between particles (Helling, 2019; Samra et al., 2022). There is now much evidence that clouds exist and are common in exoplanet and brown dwarf atmospheres (Burgasser et al., 2010; Marley et al., 2012; Mackwell et al., 2013; Manjayacas et al., 2014; Wakeford and Sing, 2015; Heng, 2016; Pinhas and Madhusudhan, 2017). They strongly influence the temperature, atmospheric properties and dynamics of planets and can present suitable environments for the formation of complex organic and prebiotic molecules. Thus, understanding of the formation, composition, optical, and structural properties, and gas-phase and gas-grain chemistry of clouds is crucial for developing reliable atmospheric models and searching for extraterrestrial life.
Grains forming in high-temperature atmospheres of exoplanets and brown dwarfs may include silicates, such as SiO2, MgSiO3, Mg2SiO4, MgFeSiO4, Fe2SiO4; metal oxides, such as TiO2, Al2O3, MgO, FeO; and others, for example, Fe, CaTiO3, FeS (Helling, 2019). Silicates present one of the most important groups of materials relevant to exoplanetary atmospheres. Models describing atmospheres of exoplanets and brown dwarfs at different temperatures from 500 to 2000 K include grains of silica SiO2, pyroxenes MgxFe1-xSiO3 (with x from 1 to 0), and olivines MgxFe2-xSiO4 (with x from 2 to 0) (Seager and Sasselov, 2000; Helling et al., 2006; Helling et al., 2008; Burgasser et al., 2010; Visscher et al., 2010; Morley et al., 2012; Wakeford and Sing, 2015; Molliere et al., 2017; Pinhas and Madhusudhan, 2017; Kitzmann and Heng, 2018). There is also tentative evidence of absorption and scattering of silicate grains in the mid-IR spectra (Cushing et al., 2006; Helling et al., 2006; Looper et al., 2008) and near-IR spectra (Lecavelier des Etangs et al., 2008; Looper et al., 2008). Examples of the silicate grains detection and modelling are shown in Figure 1.
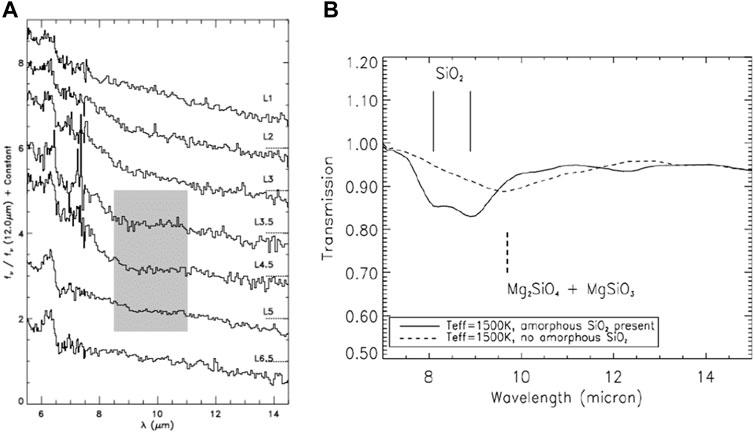
FIGURE 1. (A) IR Spitzer spectra of various exoplanetary atmospheres. The gray box indicates the wavelength range of the silicate absorption (Si-O stretching, 9–11 μm) and objects that exhibit the plateau. Reproduced with permission from (Cushing et al., 2006) © AAS. (B) Absorption transmission spectrum for the model with (solid line) and without (dashed line) formation of SiO2. Reproduced with permission from (Helling et al., 2006) © ESO.
There is an open question—if dust grains present in exoplanetary atmospheres may originate in and be brought from the parent (to planetary systems) astrochemical environments, such as planet-forming disks or prestellar cores. It would be in principle possible for the first generation of dust grains. However, as discussed in (Helling, 2019) gravitational settling should bring grains to the regions of higher temperatures (closer to the planetary surface) where the grains may change their material composition and finally evaporate enriching the upper atmosphere via convection of grain elements and leading to the in situ formation of the next generation of atmospheric dust grains.
Optical properties of dust grains
Room and low-temperature optical properties have been studied for a variety of refractory materials including various types of silicates (see, e.g., the Heidelberg—Jena–St. Petersburg–Database of Optical Constants). However, the temperatures of the majority of known exoplanets are much higher than room temperature. Citing a recent paper devoted to optical properties of potential solid-state materials in exoplanetary atmospheres: “In most cases, however, the refractive index has been measured at room temperature or in environments resembling the cold interstellar medium. Data for temperatures more related to the atmospheres of brown dwarfs or extrasolar giant planets are rarely available and measurements for these cases are strongly required” (Kitzmann and Heng, 2018). The White Paper 2020 describing the needs for laboratory studies aimed at understanding exoplanetary atmospheres stresses the importance of measurements of optical properties of various classes of materials (particles) in broad temperature, pressure and spectral ranges (Fortney et al., 2019).
Indeed, there are not many studies of optical properties of refractory materials at high-temperatures relevant to exoplanet atmospheres. We can mention the measurements of 1) natural single crystals of olivine Mg1.8Fe0.2SiO4 in the UV-mid-IR spectral range at temperatures from 300 to 1700 K (Shankland et al., 1979) and olivine Mg1.8Fe0.2SiO4 and enstatite MgSiO3 in the mid-IR range at temperatures from 10 to 973 K (Zeidler et al., 2015), 2) oxides, such as Al2O3, MgAl2O4, and SiO2, in the mid-IR range from 300 to 900 K (Zeidler et al., 2013), 3) silicon in the UV-VIS range from room temperature up to 800 K (Weakliem and Redfield, 1979; Jellison and Modine, 1994), 4) amorphous magnesium silicate smokes (Hallenbeck et al., 1998) and amorphous Mg-rich pyroxene particles (Brucato et al., 1999) embedded after annealing at temperatures between 1000 and 1300 in KBr pellets and measured ex situ in the mid-IR spectral range, and crystalline silicates produced by high-temperature (∼1000 K) annealing of amorphous silicates, such as MgSiO3, Mg2SiO4, and Fe2SiO4 (Fabian et al., 2000; Roskosz et al., 2011; Sabri et al., 2014). Having in mind possible carbon-rich exoplanetary atmospheres (Helling et al., 2017), it is worth to mention also high-temperature measurements of carbonaceous species, such as hydrogenated amorphous carbon films (Chou, 1992), diamond-like carbon films (Akkerman et al., 1996; Cheng et al., 1997), and nano-crystalline carbon in silicon oxycarbide (Rosenburg et al., 2018).
Thus, as the reader can see, the ranges of the measured species and temperatures are very limited. Silicate samples studied include a few types of natural single crystals, silicate grains mixed with KBr and measured ex situ and crystalline silicates. There is only a handful of in situ high-temperature (up to about 1000 K) measurements of spectra for crystalline silicate grains, the most reliable analogs of atmospheric siliceous particles. Regarding ex situ analysis, it may lead to unexpected and “undesirable” chemical reactions in the sample with the ambient environment after exposing the sample to air. These reactions may change the composition and structure of the sample and harm the analysis.
As shown by experimental and theoretical studies, optical properties are clearly a function of temperature due to structural and chemical changes in refractory materials (see, e.g., (Hallenbeck et al., 1998; Jäger et al., 1998; Zeidler et al., 2013; Sabri et al., 2014; Zeidler et al., 2015; Zamirri et al., 2019; Guiu et al., 2021)). As an example, in Figure 2 we present the temperature-dependent reflection spectra of an enstatite sample from (Zeidler et al., 2015) and the IR spectra of amorphous (room temperature) and crystalline (annealed at 1150 K) magnesium silicate grains from (Sabri et al., 2014), where spectral changes are clearly visible. Temperature-dependent effects include, in general, appearance/disappearance and shift and broadening of spectral lines and changes in the general slopes of spectra. Thus, the usage of room temperature optical data can lead to crucial uncertainties and incorrect results for models describing exoplanetary atmospheres.
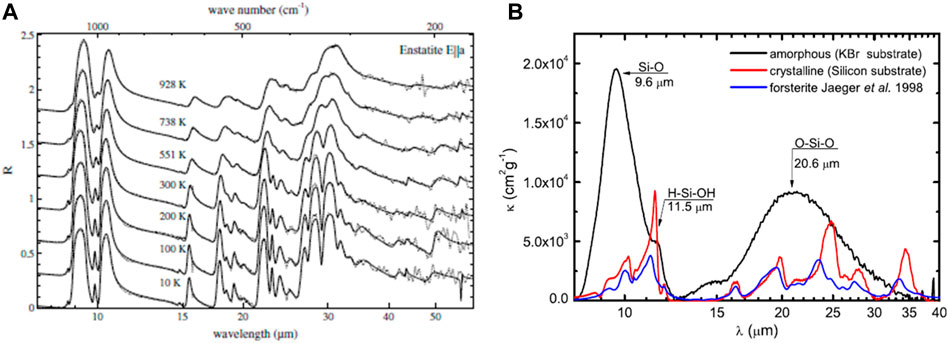
FIGURE 2. (A) Temperature-dependent reflection spectra of the enstatite sample. Reproduced with permission from (Zeidler et al., 2015) © AAS. (B) IR spectra of amorphous and crystalline (annealed at 1150 K) magnesium silicate grains. Reproduced with permission from (Sabri et al., 2014) © AAS.
As silicates seem to be the first target for future astronomical observations of cloudy atmospheres, we suppose that future laboratory measurements should focus, first of all, on the main types of silicate grains included into models, such as MgSiO3, Mg2SiO4, MgFeSiO4, and Fe2SiO4. The temperature range (limit) is defined by the possibility of creating high-temperature experimental conditions and by the vaporization temperatures of silicate materials of about 2000 K (Ben Younes et al., 1999; Costa et al., 2017; Davies et al., 2020) (depending on the composition of the material). Thus, the desired temperature range for potential studies would be 300–2000 K. As the next steps, we would consider a broader look at silicate types with varying Mg/Fe ratio, such as pyroxenes MgxFe1−xSiO3 and olivines Mg2xFe2−2xSiO4, studies of metal oxides, such as TiO2 and Al2O3, and studies of carbon grains. Having the optical properties of these “simple” species known, we may think about studying their mixtures, going step by step from simple to more complex samples.
Importance of the porosity of dust grains
As we know from the analysis of cometary dust particles (Harmon et al., 1997; Fulle et al., 2000; Hörz et al., 2006; Fulle and Blum, 2017), models (Ossenkopf, 1993; Suyama et al., 2008; Wada et al., 2009; Kataoka et al., 2013; Tazaki et al., 2016), and laboratory experiments (Wurm and Blum, 1998; Krause and Blum, 2004; Jäger et al., 2008; Sabri et al., 2014; Jäger et al., 2015; Blum, 2018) dust grains in interstellar and circumstellar media form grain aggregates having a very high porosity (up to 90%). As for exoplanetary atmospheres, it was shown that the micro-porosity of cloud particles arising from the organization of grain monomers affects the structure and material composition of clouds and has to be taken into account by modelling clouds (Samra et al., 2020).
High porosity means the existence of a very large surface of grain aggregates (Potapov et al., 2020a). To demonstrate high porosity and large surface of grain aggregates, we show in Figure 3 the structure of a numerically simulated dust aggregate and a high-resolution electron microscopy image of amorphous MgSiO3 grain aggregates produced in the laboratory. According to the laboratory experiments, high porosity is a characteristic of both major studied types of dust particles, siliceous and carbonaceous grains. Regarding atmospheric particles, it was discussed that a large surface area of porous particles leads them to efficiently grow higher in the atmosphere as compared to compact particles and influences the spectral characteristics of clouds in exoplanetary atmospheres (Samra et al., 2020).
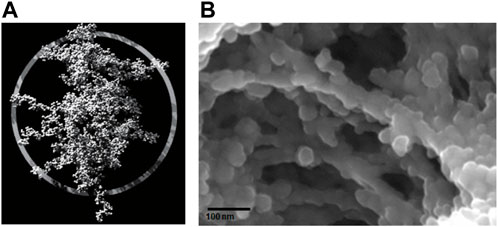
FIGURE 3. (A) Numerically simulated dust aggregate. Reproduced with permission from (Wada et al., 2009) © AAS. (B) High-resolution electron microscopy image of MgSiO3 grain aggregates produced by gas-phase condensation of nm-sized grains and their subsequent deposition onto a substrate. Reproduced with permission from (Potapov et al., 2019) © AAS. The aggregates are characterized by high porosity and large surface area.
Surface properties influence (and in some cases define) desorption, adsorption, mobility, and reactivity of volatile atomic and molecular species on grains [see (Potapov and McCoustra, 2021) for a review]. The catalytic role of dust grain surfaces is discussed in the next section. The main point of the porosity is that high porosity means fluffiness of the dust and existence of a large surface area of dust grains. Fluffiness leads to a more efficient growth of grain aggregates as compared to compact particles. Large surface area provides more sites (as compared to the smaller surface area in the case of non-porous compact dust grains) for adsorption of gas-phase species, their subsequent reactions between each other and with surface atoms and functional groups, and catalysis of these reactions.
Thus, the other uncertainties for atmospheric models include the effect of the porosity and a corresponding large surface area of grains on the optical properties, gas-grain interactions, and surface chemistry. To understand the real physical-chemical picture of planetary atmospheres, laboratory experiments and models have to deal with reliable dust grain analogues, such as porous grain aggregates presented in Figure 3.
The fractal growth of particles, such as silica particles, various types of amorphous silicate grains (pyroxenes and olivines), amorphous hydrogenated and non-hydrogenated carbon grains, into highly porous aggregates in low-temperature environments have been demonstrated by the laboratory experiments (see the references at the beginning of this section). However, regarding exoplanet atmospheres an important point is the growth of grains at high temperatures. Open questions are—what is the influence of the temperature on the porosity of grain aggregates? Would grain monomers tend to aggregate producing more compact or less compact particles as compared to low-temperature conditions? Only high-temperature in situ studies may answer these questions. This is exactly what could be done in the laboratory—deposition of nm-sized grain monomers on a substrate at high temperatures and comparison of the structure of high- and-low temperature deposits (see Section 5 for laboratory experimental techniques).
Gas-grain and grain surface chemistry: Catalytic formation of molecules on silicate surfaces
Gas-phase chemical processes at physical conditions relevant to the exoplanetary atmospheres have been studied by a number of groups (Gavilan et al., 2018; Hörst et al., 2018; Venot et al., 2018; Fleury et al., 2019; He et al., 2019). Regarding gas-grain and grain surface chemical studies relevant to high-temperature atmospheres, this direction of research presents practically uncharted territory.
The catalytic role of dust grains except being a meeting place for reactants includes dissipation of energy excess released in molecular bond formation, lowering of diffusion and reaction activation barriers, and direct participation of grain atoms or functional groups in surface reactions. There are a number of low-temperature studies on the formation of molecules on silicate and carbon surfaces: H2 (Pirronello et al., 1997a; Pirronello et al., 1997b; Katz et al., 1999; Pirronello et al., 1999; Perets et al., 2007; Vidali et al., 2007; Lemaire et al., 2010; Gavilan et al., 2012; Gavilan et al., 2014; Wakelam et al., 2017), H2O (Jing et al., 2011; Dulieu et al., 2013; He and Vidali, 2014), CO and CO2 (Mennella et al., 2004; Mennella et al., 2006; Raut et al., 2012; Fulvio et al., 2014; Sabri et al., 2015; Shi et al., 2015), H2CO (Potapov et al., 2017), and NH4+NH2COO− (Potapov et al., 2019; Potapov et al., 2020b). In all these studies, the catalytic effect of the grain surface has been clearly shown.
There is also a handful of high-temperature chemical studies involving silicate grain surfaces. The catalytic role of olivines MgxFe2-xSiO4 with different Mg/Fe ratios in the high-temperature (160°C) formation of purines and pyrimidines from formamide was studied (Saladino et al., 2005). It was demonstrated that olivines favour formamide conversion into various pyrimidine derivatives including two nucleobases, uracil and cytosine. The synthesis of methane, water, and ammonia, and also complex organic molecules of prebiotic interest, such as methylamine and acetonitrile, from CO, N2, and H2 was studied on the surface of amorphous silicates at 500–900 K (Hill and Nuth, 2003) demonstrating a great catalytic efficiency of silicates. These results provide examples of possible synthesis of prebiotic molecules in high-temperature environments. It is worth mentioning the study of the role of porous silica environments in prebiotic organic transformations, especially from amino acids to peptides to proteins, on the early Earth (Navrotsky et al., 2021) and studies of the formation of organic compounds in meteorites (acting as catalysts) at high temperatures (Anders et al., 1973; Rotelli et al., 2016). There are also chemical applications of the catalytic properties of silicate materials at high temperatures (e.g., (Fazlinia and Sheikh, 2018; Ramanathan and Subramaniam, 2018; Bawaked and Narasimharao, 2020)). However, their relation to astrochemical processes is not always direct and needs careful investigation.
Thus, high-temperature gas-grain and grain surface chemistry involving silicate grains may provide routes to complex molecular species including prebiotic molecules. In addition, energetic processing (e.g., UV photons and high-energy electrons) may considerably change the composition and structure of the atmosphere [e.g., formation of atmospheric hazes through photochemistry (Hörst et al., 2018)] and may trigger gas-grain and grain surface reactions, resulting in the formation of more complex species [as it is well-know from low-temperature studies, see, e.g., (Arumainayagam et al., 2019) for a review].
Such processes are of great relevance to the processes taking place in the atmospheres of exoplanets and have to be studied in detail using reliable laboratory analogues of atmospheric dust particles, such as porous silicate grains described above. We need to investigate gas-grain and grain surface chemistry involving silicate grains and various gas-phase molecules, at the first step, molecules detected in exoplanet atmospheres (McGuire, 2022), such as OH, CO, TiO, H2O, HCN, CO2, NH3, C2H2, and CH4, as well as their mixtures. These studies will also lead to a better understanding of the grain growth/dissipation and depletion of elements.
Laboratory experimental techniques
Cosmic dust analogues are typically produced using gas-phase condensation techniques, which mimic astrophysical condensation processes of dust grains (Colangeli et al., 1995; Jäger et al., 2008; Mate et al., 2014; Sabri et al., 2014; Martinez et al., 2018; Martinez et al., 2020). To perform the experimental studies discussed above, an experimental setup allowing simultaneous deposition and in situ measurements of dust grains and gas-phase molecules has to be developed. It can be similar to that used in the experiments simulating the formation of porous cosmic dust grains in the Laboratory Astrophysics Group of the Max Planck Institute for Astronomy in Jena (Jäger et al., 2003; Jäger et al., 2008; Jäger et al., 2009; Sabri et al., 2014; Jäger et al., 2016; Potapov et al., 2018a; Potapov et al., 2018b). In the laboratory in Jena, laser ablation of targets, such as graphite, MgSi, FeSi or MgFeSi, in quenching atmospheres of He/H2 or He/O2 is used to produce nanometre-sized carbon and silicate grains. These grains are extracted from the ablation chamber, deposited in a separate chamber onto a substrate fixed on a cryocooler (for low-temperature studies) and probed in situ by Fourier transform infrared (FTIR) spectroscopy. The grains aggregate on the substrate forming a layer of porous fractal particles. An additional gas dosing line connected on one side to the deposition chamber and on the other side to a gas inlet system allows for deposition of mixtures of gases or (if required) for filling the chamber with gas mixtures at different pressures.
Following the schematic of the setup in Jena, a setup for exoplanetary dust studies should include a system of vacuum chambers to produce and deposit nanoparticles, a laser for laser ablation, an FTIR spectrometer for detection of species in the solid-state, a mass spectrometer for detection of species in the gas-phase, a UV source and an electron gun for triggering the solid-state chemistry, and a gas inlet system. A deposition chamber should allow for high-temperature measurements of solid-state samples. Closed-cycle cryocoolers allowing for measurements in the temperature range from 3 to 1000 K exist. Using such a cryocooler would be the easiest way for many laboratories to switch from low-temperature to high-temperature measurements.
However, to go beyond 1000 K, development of a deposition chamber with a heater instead of a cryocooler is needed. The following requirements for the chamber could be imagined: water cooling of the chamber walls due to the temperature limits of chamber optical windows, and an access to the substrate from two or three sides to be able to perform spectroscopic measurements in the reflection mode (as thermally stable metal substrates should be used) and to control the deposit thickness. Additional question is the grain formation, deposition and measurements at high pressures of gases (a few to a few tens of bar) relevant to exoplanet atmospheres. It makes sense to make both the deposition and ablation chambers suitable for such experiments.
Such a setup will allow for spectral measurements of solid deposits and for gas-grain and grain surface studies discussed in the sections above. It would be also possible to study the gas-phase condensation of grain monomers in atmospheres of different gases consisting of aforementioned detected molecules and their mixtures and influence of the quenching atmosphere composition on the grain growth process at high temperatures.
To study the porosity of dust aggregates deposited at high temperature (see Section 3), ex situ techniques providing structural characterization of samples, such as transmission electron microscopy (TEM) and scanning electron microscopy (SEM), should be also used. An example of a high-resolution TEM image of grain aggregates produced by gas-phase condensation of nm-sized grains and their subsequent deposition onto a substrate is presented in the right part of Figure 3.
Observations
The astronomical and astrophysical societies expect that newly developed and future advanced instruments, such as the James Webb Space Telescope (Gardner et al., 2006) (JWST) launched on 25th of December 2021, the European Extremely Large Telescope (Brandl et al., 2008; Davies et al., 2010) (EELT) planned to be in exploitation from 2027, the Atmospheric Remote-sensing Infrared Exoplanet Large-survey (Pascale et al., 2018) (Ariel) to be delivered for launch in 2029, and the Origins Space Telescope (Wiedner et al., 2021) proposed for 2035, will bring comprehensive information about the physics and chemistry of exoplanet atmospheres. In the following, we will focus on JWST as its first observational data are expected from the second half of 2022 onward. In the first year of operation JWST will be used to study the atmospheres of more than 50 exoplanets (Birkmann et al., 2022).
JWST will provide the first high signal-to-noise near- and mid-IR spectra of exoplanetary atmospheres. In the recent years, a number of studies of JWST’s capabilities for the characterization of exoplanets have been presented [e.g., (Beichman et al., 2014; Greene et al., 2016; Molliere et al., 2017; Schlawin et al., 2018) and a review (Madhusudhan, 2019)]. Importantly for the topic of this review is that JWST will be able to probe the 10- and 20-micron features of silicate clouds. Figure 4 shows results of simulations demonstrating that small silicate grains in the atmospheres of hot Jupiters are detectable with the JWST MIRI [mid-IR instrument (Rieke et al., 2015)] in planetary transits (Molliere et al., 2017). The spectral signatures of dust grains at these wavelengths are strongly dependent on the physical properties of the dust grains such as porosity, grain shape and size (Samra et al., 2020). The wide wavelength coverage of the JWST instrument, covering multiple spectral bands of the same dust species, will be crucial to correctly identify the different species and to break any degeneracies, in very much the same way as has been demonstrated by mid-infrared studies of propoplanetary disks [e.g., (Juhasz et al., 2010)]. The expected gain in signal-to-noise and overall wavelength coverage with JWST in comparison to current ground and space based observatories, will make it possible to distinguish between different silicate species and grain shapes and sizes, placing far greater constraints on the cloud formation and atmospheric dynamics in hot gas giants. The JWST instruments will also allow for the identification of molecular species that form in the exoplanetary atmospheres including those presenting on the surface of dust grains as their spectral signatures corresponding to vibrational modes lay in the IR spectral region (Greene et al., 2016).
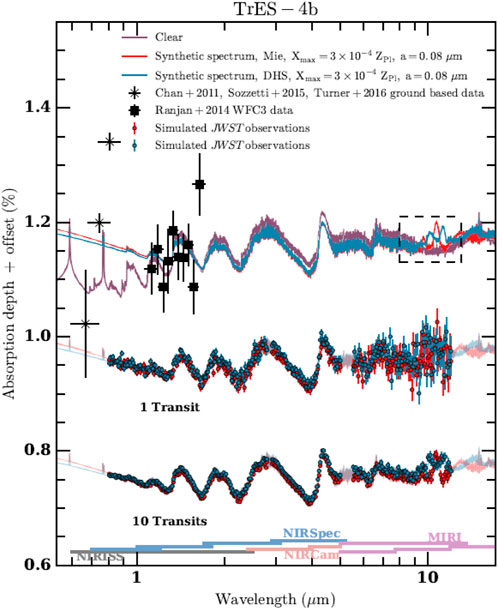
FIGURE 4. Simulated JWST transit observations for the hot Jupiter TrES-4b. Shown in this figure are 2 models with different silicate cloud particles and one model without clouds. The dashed box at 10 μm highlights the silicates cloud features due to Mg2SiO4 resonances for different dust grain models. Also shown in the figure are current observations with HST and ground based instruments (see (Molliere et al., 2017) for details and references). Reproduced with permission from (Molliere et al., 2017) © ESO.
Using the experimental data discussed in the previous sections as the basis for the atmospheric chemistry models, the expected high data quality should allow for a realistic comparison between laboratory measurements and observations, which should allow us to place tight constraints in the physics of cloud formations and chemistry in exoplanet atmospheres.
Conclusions
With this short review, we would like to stress that astronomical observations, atmospheric modelling, and laboratory experimental studies should go hand in hand to provide us with reliable analysis of observations, predictions of observables, and understanding of the chemical pathways to detected species. New laboratory facilities allowing for high-temperature in situ studies of dust grains relevant to exoplanetary atmospheres should be developed. The primary goal of future laboratory studies is to answer two main questions:
1) What are the optical properties of different types of silicate grains in the near- and mid-IR spectral range (the range of new advanced telescopes) at high temperatures? To answer this question, we need high-temperature (300–2000 K) measurements of optical properties of different types of silicate grains, such as MgSiO3, Mg2SiO4, MgFeSiO4, and Fe2SiO4. The main objective in this case will be providing the exoplanet community with unique sets of experimental optical data for silicate grains in a broad range of temperatures and wavelengths.
2) How do dust grains interact with the molecular gas at high temperatures? To answer this question, we need to investigate gas-grain and grain surface chemistry involving silicate grains and gas-phase molecules detected in exoplanet atmospheres, such as OH, CO, TiO, H2O, HCN, CO2, NH3, C2H2, and CH4, and their mixtures. The main objective in this case will be providing the exoplanet and astrochemistry community with a list of possible reactions, reaction products, and reaction networks caused by the gas-grain interaction at the physical conditions relevant to exoplanetary atmospheres.
Author contributions
All authors listed have made a substantial, direct, and intellectual contribution to the work and approved it for publication.
Conflict of interest
The authors declare that the research was conducted in the absence of any commercial or financial relationships that could be construed as a potential conflict of interest.
Publisher’s note
All claims expressed in this article are solely those of the authors and do not necessarily represent those of their affiliated organizations, or those of the publisher, the editors and the reviewers. Any product that may be evaluated in this article, or claim that may be made by its manufacturer, is not guaranteed or endorsed by the publisher.
References
Akkerman, Z. L., Efstathiadis, H., and Smith, F. W. (1996). Thermal stability of diamondlike carbon films. J. Appl. Phys. 80 (5), 3068–3075. doi:10.1063/1.363167
Anders, E., Hayatsu, R., and Studier, M. H. (1973). Organic compounds in meteorites. Science 182 (4114), 781–790. doi:10.1126/science.182.4114.781
Arumainayagam, C. R., Garrod, R. T., Boyer, M. C., Hay, A. K., Bao, S. T., Campbell, J. S., et al. (2019). Extraterrestrial prebiotic molecules: Photochemistry vs. radiation chemistry of interstellar ices. Chem. Soc. Rev. 48 (8), 2293–2314. doi:10.1039/c7cs00443e
Bawaked, S., and Narasimharao, K. (2020). Structural and catalytic properties of copper silicate nanomaterials. Sci. Rep. 10 (1), 518. doi:10.1038/s41598-020-57502-z
Beichman, C., Benneke, B., Knutson, H., Smith, R., Lagage, P. O., Dressing, C., et al. (2014). Observations of transiting exoplanets with the James Webb space telescope (JWST). Publ. Astron. Soc. Pac. 126 (946), 1134–1173. doi:10.1086/679566
Ben Younes, M. E., Gregoire, D. C., and Chakrabarti, C. L. (1999). Vaporization and removal of silica for the direct analysis of geological materials by slurry sampling electrothermal vaporization-inductively coupled plasma-mass spectrometry. J. Anal. At. Spectrom. 14 (11), 1703–1708. doi:10.1039/a903269j
Birkmann, S. M., Ferruit, P., Giardino, G., Nielsen, L. D., García Muñoz, A., al, E., et al. (2022). The near-infrared spectrograph (NIRSpec) on the James Webb space telescope IV. Capabilities and predicted performance for exoplanet characterization. Astron. Astrophys. 661, A83. doi:10.1051/0004-6361/202142592
Blum, J. (2018). Dust evolution in protoplanetary discs and the formation of planetesimals what have we learned from laboratory experiments? Space Sci. Rev. 214 (2), 52. doi:10.1007/s11214-018-0486-5
Brandl, B., Lenzen, R., Pantin, E., Glasse, A., Blommaert, J., and al, e. (2008). Metis: The mid-infrared E-ELT imager and spectrograph. Proc. SPIE 7014, 70141N
Brucato, J. R., Colangeli, L., Mennella, V., Palumbo, P., and Bussoletti, E. (1999). Mid-infrared spectral evolution of thermally annealed amorphous pyroxene. Astron Astrophys. 348 (3), 1012
Burgasser, A. J., Simcoe, R. A., Bochanski, J. J., Saumon, D., Mamajek, E. E., Cushing, M. C., et al. (2010). Clouds in the coldest Brown dwarfs: Fire spectroscopy of ross 458c. Astrophys. J. 725 (2), 1405–1420. doi:10.1088/0004-637x/725/2/1405
Cheng, C. L., Lin, J. C., and Chang, H. C. (1997). The absolute absorption strength and vibrational coupling of CH stretching on diamond C(111). J. Chem. Phys. 106 (17), 7411–7421. doi:10.1063/1.473701
Chou, L. H. (1992). Hydrogenated amorphous-carbon films prepared by plasma-enhanced chemical-vapor deposition. J. Appl. Phys. 72 (5), 2027–2035. doi:10.1063/1.351631
Colangeli, L., Mennella, V., Palumbo, P., Rotundi, A., and Bussoletti, E. (1995). Mass extinction coefficients of various submicron amorphous-carbon grains - tabulated values from 40 Nm to 2 mm. Astron Astrophys. Sup 113 (3), 561
Costa, G. C. C., Jacobson, N. S., and Fegley, B. (2017). Vaporization and thermodynamics of forsterite-rich olivine and some implications for silicate atmospheres of hot rocky exoplanets. Icarus 289, 42–55. doi:10.1016/j.icarus.2017.02.006
Crossfield, I. J. M. (2015). Observations of exoplanet atmospheres. Publ. Astron. Soc. Pac. 127 (956), 941–960. doi:10.1086/683115
Cushing, M. C., Roellig, T. L., Marley, M. S., Saumon, D., Leggett, S. K., Kirkpatrick, J. D., et al. (2006). A Spitzer infrared spectrograph spectral sequence of M, L, and T dwarfs. Astrophys. J. 648 (1), 614–628. doi:10.1086/505637
Davies, E. J., Carter, P. J., Root, S., Kraus, R. G., Spaulding, D. K., Stewart, S. T., et al. (2020). Silicate melting and vaporization during rocky planet formation. JGR. Planets 125 (2). doi:10.1029/2019je006227
Davies, R., Ageorges, N., Barl, L., Bedin, L., Bender, R., and al, e. (2010). Micado: The E-ELT adaptive optics imaging camera. Proc. SPIE 7735, 77352A. doi:10.1117/12.856379
Dulieu, F., Congiu, E., Noble, J., Baouche, S., Chaabouni, H., Moudens, A., et al. (2013). How micron-sized dust particles determine the chemistry of our Universe. Sci. Rep. 3, 1338. doi:10.1038/srep01338
Fabian, D., Jager, C., Henning, T., Dorschner, J., and Mutschke, H. (2000). Steps toward interstellar silicate mineralogy V. Thermal evolution of amorphous magnesium silicates and silica. Astron Astrophys. 364 (1), 282
Fazlinia, A., and Sheikh, S. (2018). Metal silicates: Efficient catalysts for the three-component preparation of 2-amino-5-oxo-4-aryl-4, 5-dihydroindeno[1, 2-b]pyran-3-carbonitrile derivatives. Main. Group Metal. Chem. 41 (3-4), 47–52. doi:10.1515/mgmc-2017-0042
Fleury, B., Gudipati, M. S., Henderson, B. L., and Swain, M. (2019). Photochemistry in hot H-2-dominated exoplanet atmospheres. Astrophys. J. 871 (2), 158. doi:10.3847/1538-4357/aaf79f
Fortney, J., Robinson, T. D., Domagal-Goldman, S., Del Genio, A. D., Gordon, I. E., Gharib-Nezhad, E., et al. (2019). astro-ph.EP, 07064. doi:10.48550/arXiv.1905.07064
Fulle, M., and Blum, J. (2017). Fractal dust constrains the collisional history of comets. Mon. Not. R. Astron. Soc. 469, S39–S44. doi:10.1093/mnras/stx971
Fulle, M., Levasseur-Regourd, A. C., McBride, N., and Hadamcik, E. (2000). In situ dust measurements from within the coma of 1P/Halley: First-order approximation with a dust dynamical model. Astron. J. 119 (4), 1968–1977. doi:10.1086/301285
Fulvio, D., Brieva, A. C., Cuylle, S. H., Linnartz, H., Jäger, C., and Henning, T. (2014). A straightforward method for Vacuum-Ultraviolet flux measurements: The case of the hydrogen discharge lamp and implications for solid-phase actinometry. Appl. Phys. Lett. 105 (1), 014105. doi:10.1063/1.4887067
Gardner, J. P., Mather, J. C., Clampin, M., Doyon, R., Greenhouse, M. A., Hammel, H. B., et al. (2006). The James Webb space telescope. Space Sci. Rev. 123 (4), 485–606. doi:10.1007/s11214-006-8315-7
Gavilan, L., Carrasco, N., Hoffmann, S. V., Jones, N. C., and Mason, N. J. (2018). Organic aerosols in anoxic and oxic atmospheres of earth-like exoplanets: VUV-MIR spectroscopy of CHON tholins. Astrophys. J. 861 (2), 110. doi:10.3847/1538-4357/aac8df
Gavilan, L., Lemaire, J. L., and Vidali, G. (2012). Are molecule-covered dust grains efficient catalysts of H2 formation in the cold ISM? Mon. Not. R. Astron. Soc. 424 (4), 2961–2970. doi:10.1111/j.1365-2966.2012.21463.x
Gavilan, L., Lemaire, J. L., Vidali, G., Sabri, T., and Jaeger, C. (2014). the formation of molecular hydrogen on silicate dust analogs: The rotational distribution. Astrophys. J. 781 (2), 79. doi:10.1088/0004-637x/781/2/79
Greene, T. P., Line, M. R., Montero, C., Fortney, J. J., Lustig-Yaeger, J., and Luther, K. (2016). Characterizing transiting exoplanet atmospheres with jwst. Astrophys. J. 817 (1), 17. doi:10.3847/0004-637x/817/1/17
Guiu, J. M., Escatllar, A. M., and Bromley, S. T. (2021). How does temperature affect the infrared vibrational spectra of nanosized silicate dust? ACS Earth Space Chem. 5 (4), 812–823. doi:10.1021/acsearthspacechem.0c00341
Hallenbeck, S. L., Nuth, J. A., and Daukantas, P. L. (1998). Mid-infrared spectral evolution of amorphous magnesium silicate smokes annealed in vacuum: Comparison to cometary spectra. Icarus 131 (1), 198–209. doi:10.1006/icar.1997.5854
Harmon, J. K., Ostro, S. J., Benner, L. A. M., Rosema, K. D., Jurgens, R. F., Winkler, R., et al. (1997). Radar detection of the nucleus and coma of comet hyakutake (C/1996 B2). Science 278 (5345), 1921–1924. doi:10.1126/science.278.5345.1921
He, C., Horst, S. M., Lewis, N. K., Moses, J. I., Kempton, E. M. R., Marley, M. S., et al. (2019). Gas phase chemistry of cool exoplanet atmospheres: Insight from laboratory simulations. ACS Earth Space Chem. 3 (1), 39–50. doi:10.1021/acsearthspacechem.8b00133
He, J., and Vidali, G. (2014). Experiments of water formation on warm silicates. Astrophys. J. 788 (1), 50. doi:10.1088/0004-637x/788/1/50
Helling, C. (2019). Exoplanet clouds. Annu. Rev. Earth Planet. Sci. 47, 583–606. doi:10.1146/annurev-earth-053018-060401
Helling, C., Thi, W. F., Woitke, P., and Fridlund, M. (2006). Detectability of dirty dust grains in Brown dwarf atmospheres. Astron. Astrophys. 451 (2), L9–L12. doi:10.1051/0004-6361:20064944
Helling, C., Tootill, D., Woitke, P., and Lee, G. (2017). Dust in Brown dwarfs and extrasolar planets V. Cloud formation in carbon- and oxygen-rich environments. Astron. Astrophys. 603, A123. doi:10.1051/0004-6361/201629696
Helling, C., Woitke, P., and Thi, W. F. (2008). Dust in Brown dwarfs and extra-solar planets - I. Chemical composition and spectral appearance of quasi-static cloud layers. Astron. Astrophys. 485 (2), 547–560. doi:10.1051/0004-6361:20078220
Heng, K. (2016). A cloudiness index for transiting exoplanets based on the sodium and potassium lines: Tentative evidence for hotter atmospheres being less cloudy at visible wavelengths. Astrophys. J. 826 (1), L16. doi:10.3847/2041-8205/826/1/l16
Hill, H. G. M., and Nuth, J. A. (2003). The catalytic potential of cosmic dust: Implications for prebiotic chemistry in the solar nebula and other protoplanetary systems. Astrobiology 3 (2), 291–304. doi:10.1089/153110703769016389
Hörst, S. M., He, C., Lewis, N. K., Kempton, E. M. R., Marley, M. S., Morley, C. V., et al. (2018). Haze production rates in super-Earth and mini-Neptune atmosphere experiments. Nat. Astron. 2 (4), 303–306. doi:10.1038/s41550-018-0397-0
Hörz, F., Bastien, R., Borg, J., Bradley, J. P., Bridges, J. C., Brownlee, D. E., et al. (2006). Impact features on Stardust: Implications for comet 81P/Wild 2 dust. Science 314 (5806), 1716–1719. doi:10.1126/science.1135705
Jäger, C., Dorschner, J., Mutschke, H., Posch, T., and Henning, T. (2003). Steps toward interstellar silicate mineralogy - VII. Spectral properties and crystallization behaviour of magnesium silicates produced by the sol-gel method. Astron. Astrophys. 408 (1), 193–204. doi:10.1051/0004-6361:20030916
Jäger, C., Huisken, F., Mutschke, H., Jansa, I. L., and Henning, T. H. (2009). formation of polycyclic aromatic hydrocarbons and carbonaceous solids in gas-phase condensation experiments. Astrophys. J. 696 (1), 706–712. doi:10.1088/0004-637x/696/1/706
Jäger, C. (2015). “Laboratory approach to gas-phase condensation of particles,” in Laboratory astrochemistry. Editors S. Schlemmer, and T. Giesen (WeinheimJäger C: Wiley-VCHMutschke H.), 447.
Jäger, C., Molster, F. J., Dorschner, J., Henning, T., Mutschke, H., and Waters, L. B. F. M. (1998). Steps toward interstellar silicate mineralogy. IV. The crystalline revolution. Astron Astrophys. 339, 904.
Jäger, C., Mutschke, H., Henning, T., and Huisken, F. (2008). Spectral properties of gas-phase condensed fullerene-like carbon nanoparticles from far-ultraviolet to infrared wavelengths. Astrophys. J. 689 (1), 249–259. doi:10.1086/592729
Jäger, C., Sabri, T., Wendler, E., and Henning, T. (2016). Ion-Induced processing of cosmic silicates: A possible formatION pathway to gems. Astrophys. J. 831, 66. doi:10.3847/0004-637x/831/1/66
Jellison, G. E., and Modine, F. A. (1994). Optical functions of silicon at elevated-temperatures. J. Appl. Phys. 76 (6), 3758–3761. doi:10.1063/1.357378
Jing, D. P., He, J., Brucato, J., De Sio, A., Tozzetti, L., and Vidali, G. (2011). ON water formation in the interstellar medium: Laboratory study of the O plus D reaction on surfaces. Astrophys. J. 741 (1), L9. doi:10.1088/2041-8205/741/1/l9
Juhasz, A., Bouwman, J., Henning, T., Acke, B., van den Ancker, M. E., Meeus, G., et al. (2010). Dust evolution inprotoplanetary disks around herbig Ae/Be stars-the Spitzer view. Astrophys. J. 721 (1), 431–455. doi:10.1088/0004-637x/721/1/431
Kataoka, A., Tanaka, H., Okuzumi, S., and Wada, K. (2013). Fluffy dust forms icy planetesimals by static compression. Astron. Astrophys. 557, L4. doi:10.1051/0004-6361/201322151
Katz, N., Furman, I., Biham, O., Pirronello, V., and Vidali, G. (1999). Molecular hydrogen formation on astrophysically relevant surfaces. Astrophys. J. 522 (1), 305–312. doi:10.1086/307642
Kitzmann, D., and Heng, K. (2018). Optical properties of potential condensates in exoplanetary atmospheres. Mon. Not. R. Astron. Soc. 475 (1), 94–107. doi:10.1093/mnras/stx3141
Krause, M., and Blum, J. (2004). Growth and form of planetary seedlings: Results from a sounding rocket microgravity aggregation experiment. Phys. Rev. Lett. 93 (2), 021103. doi:10.1103/physrevlett.93.021103
Kreidberg, L. (2018). Exoplanet atmosphere measurements from transmission spectroscopy and other planet star combined light observations. Handbook of exoplanets. Editors H. J. Deeg, and J. A. Belmonte (Cham, Schweiz: Springer).
Lecavelier des Etangs, A. L. D., Pont, F., Vidal-Madjar, A., and Sing, D. (2008). Rayleigh scattering in the transit spectrum of HD189733b. Astron. Astrophys. 481 (2), L83–L86. doi:10.1051/0004-6361:200809388
Lemaire, J. L., Vidali, G., Baouche, S., Chehrouri, M., Chaabouni, H., and Mokrane, H. (2010). Competing mechanisms of molecular hydrogen formation in conditions relevant to the interstellar medium. Astrophys. J. 725 (2), L156–L160. doi:10.1088/2041-8205/725/2/l156
Looper, D. L., Kirkpatrick, J. D., Cutri, R. M., Barman, T., Burgasser, A. J., Cushing, M. C., et al. (2008). Discovery of two nearby peculiar L dwarfs from the 2MASS proper-motion survey: Young or metal-rich? Astrophys. J. 686 (1), 528–541. doi:10.1086/591025
Mackwell, S. J., Simon-Miller, A. A., Harder, J. W., and Bullock, M. A. (2013). Clouds and hazes in exoplanet atmospheres. Tucson, Arizona: University of Arizona Press, 367.
Madhusudhan, N. (2019). Exoplanetary atmospheres: Key insights, challenges, and prospects. Annu. Rev. Astron. Astrophys. 5757, 617–663. doi:10.1146/annurev-astro-081817-051846
Madhusudhan, N., Knutson, H., Fortney, J. J., and Barman, T. (2014). Protostars and planets VI. Editors H. Beuther, R. S. Klessen, and C. P. Dullemond (Tucson, Arizona: The University of Arizona Press), 739
Manjayacas, E., Bonnefoy, M., Schlieder, J. E., Allard, F., Rojo, P., Goldman, B., et al. (2014). New constraints on the formation and settling of dust in the atmospheres of young M and L dwarfs. Astron. Astrophys. 564, A55. doi:10.1051/0004-6361/201323016
Marley, M. S., Saumon, D., Cushing, M., Ackerman, A. S., Fortney, J. J., and Freedman, R. (2012). Masses, radii, and cloud properties of the hr 8799 planets. Astrophys. J. 754 (2), 135. doi:10.1088/0004-637x/754/2/135
Martinez, L., Lauwaet, K., Santoro, G., Sobrado, J. M., Pelaez, R. J., Herrero, V. J., et al. (2018). Precisely controlled fabrication, manipulation and in-situ analysis of Cu based nanoparticles. Sci. Rep. 8, 7250. doi:10.1038/s41598-018-25472-y
Martinez, L., Santoro, G., Merino, P., Accolla, M., Lauwaet, K., Sobrado, J., et al. (2020). Prevalence of non-aromatic carbonaceous molecules in the inner regions of circumstellar envelopes. Nat. Astron. 4 (1), 97–105. doi:10.1038/s41550-019-0899-4
Mate, B., Tanarro, I., Moreno, M. A., Jimenez-Redondo, M., Escribano, R., and Herrero, V. J. (2014). Stability of carbonaceous dust analogues and glycine under UV irradiation and electron bombardment. Faraday Discuss. 168, 267–285. doi:10.1039/c3fd00132f
McGuire, B. A. (2022). 2021 census of interstellar, circumstellar, extragalactic, protoplanetary disk, and exoplanetary molecules. Astrophys. J. Suppl. Ser. 259 (2), 30. doi:10.3847/1538-4365/ac2a48
Mennella, V., Baratta, G. A., Palumbo, M. E., and Bergin, E. A. (2006). Synthesis of CO and CO2 molecules by UV irradiation of water ice-covered hydrogenated carbon grains. Astrophys. J. 643 (2), 923–931. doi:10.1086/502965
Mennella, V., Palumbo, M. E., and Baratta, G. A. (2004). Formation of CO and CO2 molecules by ion irradiation of water ice-covered hydrogenated carbon grains. Astrophys. J. 615 (2), 1073–1080. doi:10.1086/424685
Molliere, P., van Boekel, R., Bouwman, J., Henning, T., Lagage, P. O., and Min, M. (2017). Observing transiting planets with JWST Prime targets and their synthetic spectral observations. Astron. Astrophys. 600, A10. doi:10.1051/0004-6361/201629800
Morley, C. V., Fortney, J. J., Marley, M. S., Visscher, C., Saumon, D., and Leggett, S. K. (2012). Neglected clouds in T and Y dwarf atmospheres. Astrophys. J. 756 (2), 172. doi:10.1088/0004-637x/756/2/172
Navrotsky, A., Hervig, R., Lyons, J., Seo, D. K., Shock, E., and Voskanyan, A. (2021). Cooperative formation of porous silica and peptides on the prebiotic Earth. Proc. Natl. Acad. Sci. U. S. A. 118 (2), e2021117118. doi:10.1073/pnas.2021117118
Ossenkopf, V. (1993). Dust coagulation in dense molecular clouds - the formation of fluffy aggregates. Astron Astrophys. 280 (2), 617
Pascale, E., Bezawada, N., Barstow, J., Beaulieu, J.-P., Bowles, N., and al, e. (2018). The ARIEL space mission. Proc. SPIE 10698, 106980H.
Perets, H. B., Lederhendler, A., Biham, O., Vidali, G., Li, L., Swords, S., et al. (2007). Molecular hydrogen formation on amorphous silicates under interstellar conditions. Astrophys. J. 661 (2), L163–L166. doi:10.1086/518862
Pinhas, A., and Madhusudhan, N. (2017). On signatures of clouds in exoplanetary transit spectra. Mon. Not. R. Astron. Soc. 471 (4), 4355–4373. doi:10.1093/mnras/stx1849
Pirronello, V., Biham, O., Liu, C., Shen, L. O., and Vidali, G. (1997). Efficiency of molecular hydrogen formation on silicates. Astrophys. J. 483 (2), L131–L134. doi:10.1086/310746
Pirronello, V., Liu, C., Roser, J. E., and Vidali, G. (1999). Measurements of molecular hydrogen formation on carbonaceous grains. Astron Astrophys. 344 (2), 681–686.
Pirronello, V., Liu, C., Shen, L. Y., and Vidali, G. (1997). Laboratory synthesis of molecular hydrogen on surfaces of astrophysical interest. Astrophys. J. 475 (1), L69–L72. doi:10.1086/310464
Potapov, A., Jäger, C., and Henning, T. (2020). Ice coverage of dust grains in cold astrophysical environments. Phys. Rev. Lett. 124, 221103. doi:10.1103/physrevlett.124.221103
Potapov, A., Jäger, C., Henning, T., Jonusas, M., and Krim, L. (2017). the formation of formaldehyde on interstellar carbonaceous grain analogs by O/H atom addition. Astrophys. J. 846 (2), 131. doi:10.3847/1538-4357/aa85e8
Potapov, A., Jäger, C., and Henning, T. (2018). Temperature programmed desorption of water ice from the surface of amorphous carbon and silicate grains as related to planet-forming disks. Astrophys. J. 865 (1), 58. doi:10.3847/1538-4357/aad803
Potapov, A., Jäger, C., and Henning, T. (2020). Thermal formation of ammonium carbamate on the surface of laboratory analogs of carbonaceous grains in protostellar envelopes and planet-forming disks. Astrophys. J. 894, 110. doi:10.3847/1538-4357/ab86b5
Potapov, A., and McCoustra, M. R. S. (2021). Physics and chemistry on the surface of cosmic dust grains: A laboratory view. Int. Rev. Phys. Chem. 40, 299–364. doi:10.1080/0144235x.2021.1918498
Potapov, A., Mutschke, H., Seeber, P., Henning, T., and Jäger, C. (2018). Low-temperature optical properties of interstellar and circumstellar icy silicate grain analogs in the mid-infrared spectral region. Astrophys. J. 861, 84. doi:10.3847/1538-4357/aac6d3
Potapov, A., Theule, P., Jäger, C., and Henning, T. (2019). Evidence of surface catalytic effect on cosmic dust grain analogs: The ammonia and carbon dioxide surface reaction. Astrophys. J. 878, L20. doi:10.3847/2041-8213/ab2538
Ramanathan, A., and Subramaniam, B. (2018). Metal-incorporated mesoporous silicates: Tunable catalytic properties and applications. Molecules 23 (2), 263. doi:10.3390/molecules23020263
Raut, U., Fulvio, D., Loeffler, M. J., and Baragiola, R. A. (2012). Radiation synthesis of carbon dioxide in ice-coated carbon: Implications for interstellar grains and icy moons. Astrophys. J. 752 (2), 159. doi:10.1088/0004-637x/752/2/159
Rieke, G. H., Wright, G. S., Boker, T., Bouwman, J., Colina, L., Glasse, A., et al. (2015). The mid-infrared instrument for the James Webb space telescope, I: Introduction. Publ. Astron. Soc. Pac. 127 (953), 584–594. doi:10.1086/682252
Rosenburg, F., Ionescu, E., Nicoloso, N., and Riedel, R. (2018). High-temperature Raman spectroscopy of nano-crystalline carbon in silicon oxycarbide. Materials 11 (1), 93. doi:10.3390/ma11010093
Roskosz, M., Gillot, J., Capet, F., Roussel, P., and Leroux, H. (2011). A sharp change in the mineralogy of annealed protoplanetary dust at the glass transition temperature. Astron. Astrophys. 529, A111. doi:10.1051/0004-6361/201016244
Rotelli, L., Trigo-Rodriguez, J. M., Moyano-Cambero, C. E., Carota, E., Botta, L., Di Mauro, E., et al. (2016). The key role of meteorites in the formation of relevant prebiotic molecules in a formamide/water environment. Sci. Rep. 6, 38888. doi:10.1038/srep38888
Roudier, G. M., Swain, M. R., Gudipati, M. S., West, R. A., Estrela, R., and Zellem, R. T. (2021). Disequilibrium chemistry in exoplanet atmospheres observed with the hubble space telescope. Astron. J. 162 (2), 37. doi:10.3847/1538-3881/abfdad
Sabri, T., Baratta, G. A., Jäger, C., Palumbo, M. E., Henning, T., Strazzulla, G., et al. (2015). A laboratory study of ion-induced erosion of ice-covered carbon grains. Astron. Astrophys. 575, A76. doi:10.1051/0004-6361/201425154
Sabri, T., Gavilan, L., Jager, C., Lemaire, J. L., Vidali, G., Mutschke, H., et al. (2014). Interstellar silicate analogs for grain-surface reaction experiments: Gas-phase condensation and characterization of the silicate dust grains. Astrophys. J. 780 (2), 180. doi:10.1088/0004-637x/780/2/180
Saladino, R., Crestini, C., Neri, V., Brucato, J. R., Colangeli, L., Ciciriello, F., et al. (2005). Synthesis and degradation of nucleic acid components by formamide and cosmic dust analogues. Chembiochem 6 (8), 1368–1374. doi:10.1002/cbic.200500035
Samra, D., Helling, C., and Birnstiel, T. (2022). Mineral snowflakes on exoplanets and Brown dwarfs Coagulation and fragmentation of cloud particles with HyLandS. Astron. Astrophys. 663, A47. doi:10.1051/0004-6361/202142651
Samra, D., Helling, C., and Min, M. (2020). Mineral snowflakes on exoplanets and Brown dwarfs: Effects of micro-porosity, size distributions, and particle shape. Astron Astrophys. 639, A107. doi:10.1051/0004-6361/202037553
Schlawin, E., Greene, T. P., Line, M., Fortney, J. J., and Rieke, M. (2018). Clear and cloudy exoplanet forecasts for JWST: Maps, retrieved composition, and constraints on formation with MIRI and NIRCam. Astron. J. 156 (1), 40. doi:10.3847/1538-3881/aac774
Seager, S., and Sasselov, D. D. (2000). Theoretical transmission spectra during extrasolar giant planet transits. Astrophys. J. 537 (2), 916–921. doi:10.1086/309088
Shankland, T. J., Nitsan, U., and Duba, A. G. (1979). Optical-absorption and radiative heat-transport in olivine at high-temperature. J. Geophys. Res. 84 (4), 1603–1610. doi:10.1029/jb084ib04p01603
Shi, J., Grieves, G. A., and Orlando, T. M. (2015). Vacuum ultraviolet photon-stimulated oxidation of buried ice: Graphite grain interfaces. Astrophys. J. 804 (1), 24. doi:10.1088/0004-637x/804/1/24
Suyama, T., Wada, K., and Tanaka, H. (2008). Numerical simulation of density evolution of dust aggregates in protoplanetary disks. I. Head-on collisions. Astrophys. J. 684 (2), 1310–1322. doi:10.1086/590143
Tazaki, R., Tanaka, H., Okuzumi, S., Kataoka, A., and Nomura, H. (2016). Light scattering by fractal dust aggregates. I. Angular dependence of scattering. Astrophys. J. 823 (2), 70. doi:10.3847/0004-637x/823/2/70
Tsiaras, A., Waldmann, I. P., Zingales, T., Rocchetto, M., Morello, G., Damiano, M., et al. (2018). A population study of gaseous exoplanets. Astron. J. 155 (4), 156. doi:10.3847/1538-3881/aaaf75
Venot, O., Benilan, Y., Fray, N., Gazeau, M. C., Lefevre, F., Es-Sebbar, E., et al. (2018). VUV-absorption cross section of carbon dioxide from 150 to 800 K and applications to warm exoplanetary atmospheres. Astron. Astrophys. 609, A34. doi:10.1051/0004-6361/201731295
Vidali, G., Pirronello, V., Li, L., Roser, J., Manico, G., Congiu, E., et al. (2007). Analysis of molecular hydrogen formation on low-temperature surfaces in temperature programmed desorption experiments. J. Phys. Chem. A 111 (49), 12611–12619. doi:10.1021/jp0760657
Visscher, C., Lodders, K., and Fegley, B. (2010). Atmospheric chemistry in giant planets, Brown dwarfs, and low-mass dwarf stars. Iii. Iron, magnesium, and silicon. Astrophys. J. 716 (2), 1060–1075. doi:10.1088/0004-637x/716/2/1060
Wada, K., Tanaka, H., Suyama, T., Kimura, H., and Yamamoto, T. (2009). Collisional growth conditions for dust aggregates. Astrophys. J. 702 (2), 1490–1501. doi:10.1088/0004-637x/702/2/1490
Wakeford, H. R., and Sing, D. K. (2015). Transmission spectral properties of clouds for hot Jupiter exoplanets. Astron. Astrophys. 573, A122. doi:10.1051/0004-6361/201424207
Wakelam, V., Bron, E., Cazaux, S., Dulieu, F., Gry, C., Guillard, P., et al. (2017). H-2 formation on interstellar dust grains: The viewpoints of theory, experiments, models and observations. Mol. Astrophys. 9, 1–36. doi:10.1016/j.molap.2017.11.001
Weakliem, H. A., and Redfield, D. (1979). Temperature-dependence of the optical-properties of silicon. J. Appl. Phys. 50 (3), 1491–1493. doi:10.1063/1.326135
Wiedner, M. C., Aalto, S., Armus, L., Bergin, E., Birkby, J., Bradford, C. M., et al. (2021). Origins space telescope: From first light to life. Exp. Astron. (Dordr). 51 (3), 595–624. doi:10.1007/s10686-021-09782-0
Wurm, G., and Blum, J. (1998). Experiments on preplanetary dust aggregation. Icarus 132 (1), 125–136. doi:10.1006/icar.1998.5891
Zamirri, L., Escatllar, A. M., Guiu, J. M., Ugliengo, P., and Bromley, S. T. (2019). What can infrared spectra tell us about the crystallinity of nanosized interstellar silicate dust grains? ACS Earth Space Chem. 3 (10), 2323–2338. doi:10.1021/acsearthspacechem.9b00157
Zeidler, S., Mutschke, H., and Posch, T. (2015). Temperature-dependent infrared optical constants of olivine and enstatite. Astrophys. J. 798 (2), 125. doi:10.1088/0004-637x/798/2/125
Keywords: exoplanetary atmospheres, atmospheric clouds, dust grains, silicates, laboratory experiments
Citation: Potapov A and Bouwman J (2022) Importance of laboratory experimental studies of silicate grains for exoplanet atmosphere characterization. Front. Astron. Space Sci. 9:912302. doi: 10.3389/fspas.2022.912302
Received: 05 April 2022; Accepted: 30 September 2022;
Published: 12 October 2022.
Edited by:
Jerome Lasne, IMT Lille Douai, FranceReviewed by:
Dipen Sahu, Physical Research Laboratory, IndiaPanayotis Lavvas, Groupe de Spectrométrie Moléculaire et Atmosphérique (GSMA), France
Copyright © 2022 Potapov and Bouwman. This is an open-access article distributed under the terms of the Creative Commons Attribution License (CC BY). The use, distribution or reproduction in other forums is permitted, provided the original author(s) and the copyright owner(s) are credited and that the original publication in this journal is cited, in accordance with accepted academic practice. No use, distribution or reproduction is permitted which does not comply with these terms.
*Correspondence: Alexey Potapov, YWxleGV5LnBvdGFwb3ZAdW5pLWplbmEuZGU=; Jeroen Bouwman, Ym91d21hbkBtcGlhLmRl