- 1Office of Space Research and Technology, Academy of Athens, Athens, Greece
- 2Institute for Particle Physics and Astrophysics, Zurich, Switzerland
- 3Applied Physics Laboratory, The Johns Hopkins University, Laurel, MD, United States
- 4NASA Goddard Space Flight Center, Greenbelt, MD, United States
- 5IFIR/UNR-CONICET, Santa Fe, Argentina
- 6TRIDENT—BERDICHEVSKY, Greenbelt, MD, United States
- 7INFN sez Milano-Bicocca, Milano, Italy
- 8Physics Institute, University of Bern, Bern, Switzerland
- 9Astronomy Department, Boston University, Boston, MA, United States
- 10Department of Physics and Astronomy, University of Iowa, Iowa City, IA, United States
- 11Laboratoire d’ Astrophysique de Bordeaux, University Bordeaux, Pessac, France
- 12Kavli Institute for Astrophysics and Space Research and Department of Physics, Massachusetts Institute of Technology, Cambridge, MA, United States
- 13Max-Planck-Institut für Sonnensystemforschung, Göttingen, Germany
- 14Southwest Research Institute, San Antonio, TX, United States
- 15Columbia University, New York, NY, United States
The recently published Interstellar Probe (ISP) study report describes a pragmatic mission concept with a launch window that starts in 2036 and is expected to reach several hundreds of astronomical units past the heliopause within a time frame of ≥50 years (https://interstellarprobe.jhuapl.edu/Interstellar-Probe-MCR.pdf). Following the ISP report, this paper, that will also be accessible from the Bulletin of the AAS (BAAS) in the framework of the Decadal Survey for Solar and Space Physics (Heliophysics) 2024–2033 (Dialynas et al., A future Interstellar Probe on the dynamic heliosphere and its interaction with the very local interstellar medium: In-situ particle and fields measurements and remotely sensed ENAs, 2022a), aims to highlight the importance of studying the physics of the interactions pertaining to the expanding solar wind that meets the plasma, gas and dust flows of the very local interstellar medium, forming the complex and vast region of our astrosphere. We focus on three fundamental open science questions that reveal the dynamical nature of the heliosphere A) Where are the heliosphere boundaries and how thick is the heliosheath B) Is there a “missing” pressure component towards exploring the dynamics of the global heliosheath and its interaction with the very local interstellar medium C) Why does the shape and size of the global heliosphere appear different in different Energetic Neutral Atom energies? We argue that these questions can only be addressed by exploiting a combination of in-situ charged particle, plasma waves and fields measurements with remotely sensed Energetic Neutral Atoms that can be measured simultaneously from the instruments of a future Interstellar Probe mission, along its trajectory from interplanetary space through the heliosheath and out to the very local interstellar medium.
1 Introduction
Launched in 1977, the Voyager 1 and Voyager 2 (V1 and V2) missions surveyed the interplanetary space for 27–30 years (Figure 1A). Their measurements (Figure 1B; see also review article from Dialynas et al. (2022b)) showed a general decrease in ∼0.14–0.22 MeV ion intensities as the inverse square of the radial distance from the Sun with a minimum at about the year 2000, followed by a gradual increase thereafter. These measurements revealed also for the first time the evolution of the outward propagating solar wind through the interplanetary space, together with the effects of Solar Particle Events (SEPs) and Corotating Interaction Regions (CIRs), from one AU (1 Astronomical Unit = 150 × 106 km) up to the termination shock. Higher energy ions (Figure 1C) showed a general increase in interplanetary space, presenting intensity dropouts in anti-correlation with the solar activity over the solar cycle (Figure 1D).
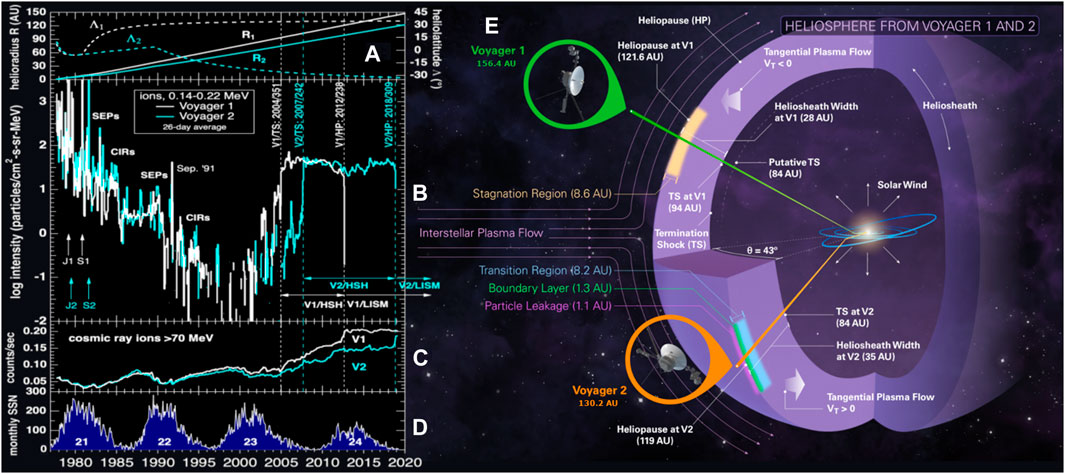
FIGURE 1. (A) The trajectories of V1 and V2 since their launch in 1977, through interplanetary space, the termination shock and heliopause until 2020, together with the LECP measurements of (B) 0.14–0.22 MeV ions and (C)
The heliosheath, the domain of Pickup Ions (PUIs) and Anomalous Cosmic Rays (ACRs) between the termination shock and the heliopause, is characterized as a “reservoir” of charged particles; ions and electrons mainly from the solar wind that are quasi-trapped in a large-scale magnetic field. Similar reservoirs are also found throughout the inner Solar System (
Several key open science questions drive the need for more detailed in-situ particle and fields measurements and remotely sensed Energetic Neutral Atom (ENA) observations from a future Interstellar Probe (ISP; McNutt et al., 2022; Brandt et al., 2022b) mission to:
• Understand the physics of the large scale reservoir that constitutes the heliosheath.
• Unveil the processes that govern the transport and acceleration of particles inside the heliosheath.
• Understand the locations and/or the physical processes that occur in the “walls” of our solar bubble, such as the termination shock and heliopause.
• Characterize the interaction of the heliosheath with the Very Local Interstellar Medium (VLISM) and the structure and physics of the VLISM itself.
• Finally, understand the force balance that controls our Sun’s astrosphere, together with its shape and size.
2 In-situ ions and remotely sensed ENAs
The crossings of V1 and V2 from the termination shock in 2004 and 2007, respectively, at distances of ∼94 (Decker et al., 2005; Stone et al., 2005) and ∼84 AU (Decker et al., 2008; Stone et al., 2008) led to the discovery of the previously unknown reservoir of ions and electrons that constitute the heliosheath. The V1 and V2 crossings of the heliopause (although other interpretations exist, e.g., Fisk and Gloeckler (2014); Fisk and Gloeckler (2022)), in 2012 (Burlaga et al., 2013; Gurnett et al., 2013; Krimigis et al., 2013; Stone et al., 2013) and 2018 (Burlaga et al., 2019; Gurnett and Kurth, 2019; Krimigis et al., 2019; Richardson et al., 2019; Stone et al., 2019) at ∼122 AU and ∼119 AU, respectively, pinpointed the extent of the upwind heliosphere’s expansion into the VLISM and its rough symmetry. The Voyagers also showed for the first time that the heliosheath alone filters ∼65% of the very high energy Galactic Cosmic Rays (GCR), providing an immense shield that protects our Solar System from the harsh galactic radiation (overall ∼25% of GCRs reach 1 AU; Figure 1C). Both crossings of the heliopause were associated with a virtual depletion of particles of solar origin at all levels, an abrupt increase of GCR, magnetic field and plasma density upstream at the heliopause, whereas the temperature was found higher than expected. All parameters were previously significantly underestimated in most models of the heliosphere.
Apart from the similarities between the V1 and V2 crossings, some substantial and puzzling differences were also identified (Krimigis et al., 2019) (Figure 1E). For example, the V1 crossing of the heliopause was associated with the discovery of a flow stagnation region that was observed before the boundary, possibly due to flux tube interchange instability at the boundary (Krimigis et al., 2013). The use of Low Energy Charged Particle (LECP; Krimigis et al., 1977) measurements in V1, revealed the existence of a radial inflow of ∼40–139 keV ions within the heliosheath, for ∼9–10 AU before the heliopause crossing and a radial outflow over a spatial scale of ∼28 AU past the heliopause (Dialynas et al., 2021), that corresponds to an ion population leaking from the heliosheath into interstellar space; although a different interpretation for these measurements may indicate that V1 is still surveying the heliosheath (Fisk and Gloeckler, 2022). Unlike V1, which found two interstellar flux tubes that had invaded the heliosheath with strong anticorrelations in GCRs, V2 found no similar precursors to the heliopause (Stone et al., 2019). Clearly, the interstellar space upstream at the heliopause is not a “calm sea” of rarefied plasma and fields (e.g., Gurnett et al., 2021, and references therein).
The Voyager in-situ observations/findings, which guide the requirements (baseline) for the particle and fields measurements from a future ISP mission, were complemented by global images using remotely sensed Energetic Neutral Atoms (ENAs), revealing a number of previously unanticipated heliospheric structures (Figure 4). The Interstellar Boundary Explorer (IBEX; McComas et al., 2009) measurements at ∼1 AU (
3 Where are the heliosphere boundaries and how thick is the heliosheath?
In-situ measurements of
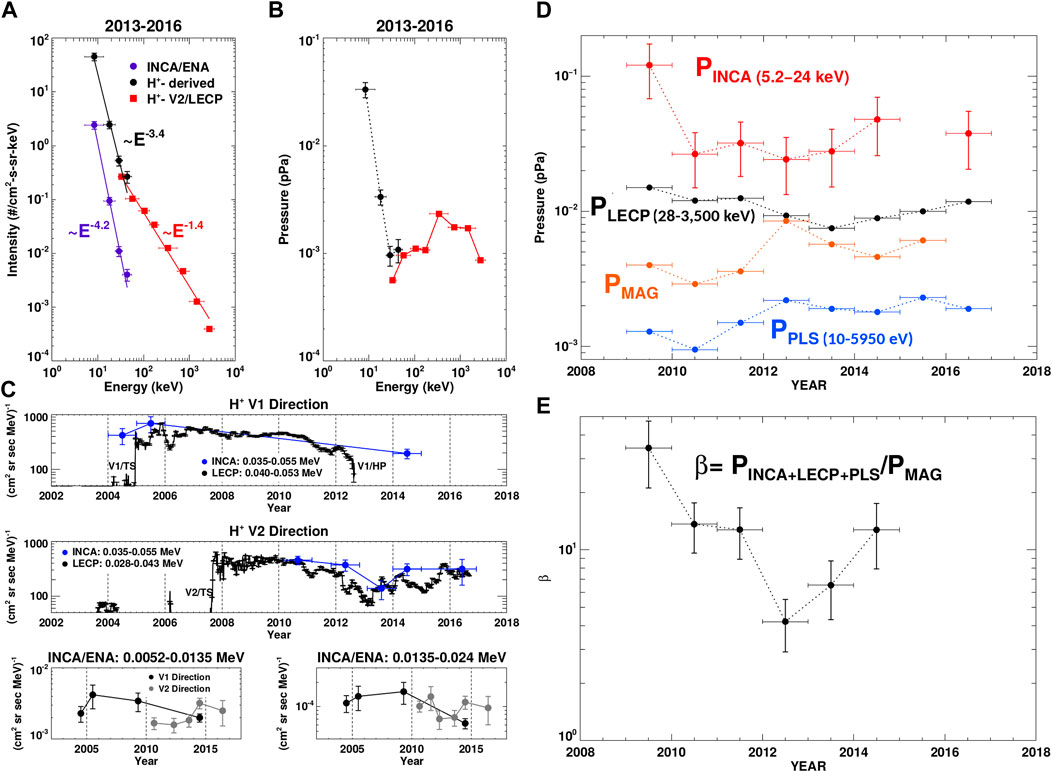
FIGURE 2. (A) Average 5.2–55 keV ENA energy spectra around the V2 pixel, as measured by Cassini/INCA and the deduced H+ spectra (LV2 = 35.2 AU and nH = 0.12 cm−3) compared with in-situ ∼28–3,500 keV ion energy spectra as measured by V2/LECP. (B) The corresponding 5.2–3,500 keV H+ partial pressure in pPa per each energy bin (in keV). (C) INCA/ENAs compared directly with in-situ LECP ion histories (Dialynas et al., 2017b; Dialynas et al., 2019). (D) Partial pressure of ∼5.2–24 keV ENA-derived H+ enclosing the V2 pixel (INCA), 28–3,500 keV H+ (V2/LECP),
Although both steady-state (Izmodenov and Alexashov, 2015) and time-dependent (Izmodenov and Alexashov, 2020) kinetic magnetohydrodynamic (MHD) simulations show that it is not possible to obtain the heliosheath thickness as measured by Voyager with the same set of data-driven boundary conditions, adding electron thermal conductivity and under the assumption that the plasma flow is isothermal (Izmodenov et al., 2014), the observed distances to the termination shock and heliopause are obtained, when thermal pressure is decreased in the heliosheath. The use of a κ-distribution (Heerikhuisen et al., 2008) also results in reduced pressure, but as shown by the combination of LECP and INCA measurements (Dialynas et al., 2019), a single κ-distribution underestimates the partial pressure that lies in the ∼5.2–24 keV energy range. A thin heliosheath may also be obtained due to charge-exchange losses of PUIs in the heliosheath (Opher et al., 2020) or loss of a fraction of ACRs whose energy comes from the solar wind (Guo et al., 2018) or due to time-dependent instabilities (Borovikov and Pogorelov, 2014).
4 A “missing” pressure component toward exploring the dynamics of the global heliosheath and its interaction with the VLISM?
The V1 and V2 respective crossings of the termination shock, showed that the non-thermal plasma dominates the dynamics of the heliosheath (Roelof et al., 2010) (Figures 2D,E), a fact that was verified by the V1and2/LECP and plasma measurements (Decker et al., 2015), the combination of Voyager and INCA/ENAs (Krimigis et al., 2010; Dialynas et al., 2019) together with MHD models (Opher et al., 2020). Recent calculations (Rankin et al., 2019; Dialynas et al., 2020) on the total effective pressure in the heliosphere and comparison with IBEX observations (Schwadron et al., 2014) provided additional evidence. The shocked thermal plasma downstream of the termination shock remained supersonic, as only 20% of the solar wind energy density went into heating the thermal ions, whereas ∼65% was transferred into heating PUIs and ∼15% transferred to the
The in-situ measurements from the Voyagers and remotely sensed Cassini/INCA ENAs showed that the heliosheath consists of a reservoir of superthermal particles and weak magnetic fields, characterized by plasma with β values that are always ≫1 and mostly
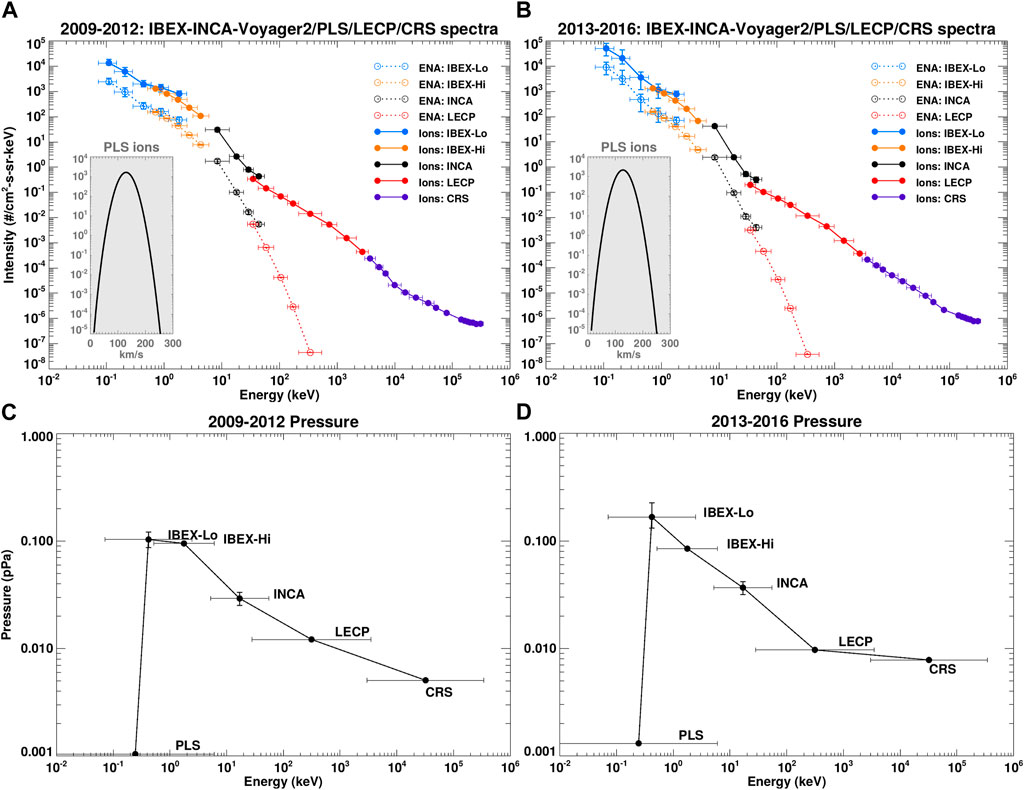
FIGURE 3. Average 0.11–55 keV ENA energy spectra (IBEX, INCA) in the pixels enclosing the position of V2, together with the deduced H+ spectra (LHS = 35 AU and nH = 0.12 cm−3), and the 0.028–344 MeV ion energy spectra measured in-situ (LECP, CRS) for (A) 2009–2012 and (B) 2013–2016. The inlays indicate the 10 to 5,950 eV ion intensities derived from fits to the PLS data with an isotropic Maxwellian. The ∼28–540 keV LECP measurements are converted to ENAs, providing a prediction for the upcoming IMAP. If IMAP observed similar energy, this would indicate that these ENAs are the result of charge-exchange interactions inside the heliosheath. Possible differences would enable the search for different source and/or acceleration mechanisms (C, D) The average 10 eV to 344 MeV H+ partial pressures inside the heliosheath, within the energy ranges covered by each different instrument (PLS, IBEX-Hi, IBEX-Lo, INCA, LECP and CRS) using the measurements in panels (A) and (B) (details in (Dialynas et al., 2020).
A recent analysis (Gkioulidou et al., 2022) showed the existence of an energy-dependent discrepancy between models and the 0.52–55 keV measured spectra, indicating that the termination shock may not accelerate the PUIs to sufficient energies. Thus, further acceleration inside the heliosheath via additional physical processes is required to account for the observed ENA fluxes by IBEX and INCA. The dynamic properties of
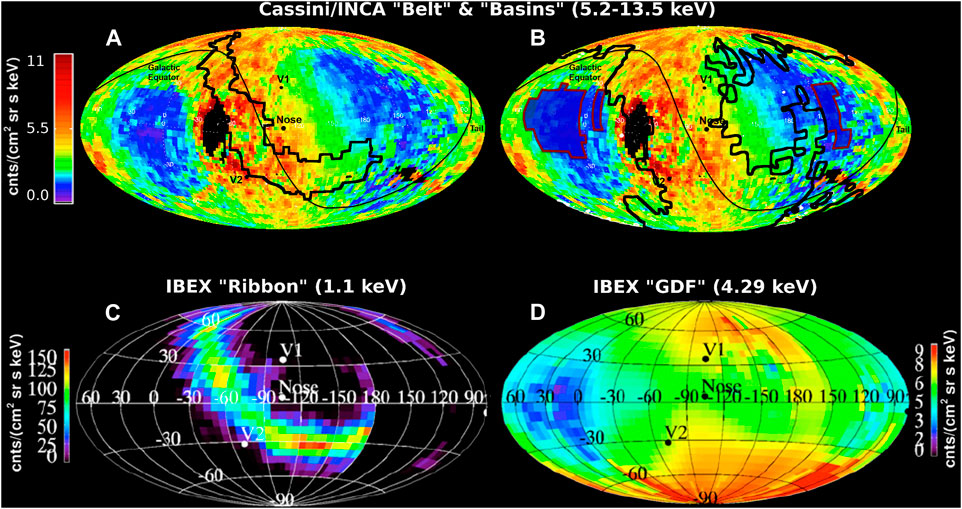
FIGURE 4. (Top) Image of heliospheric ENAs in the range of ∼5.2 to 13.5 keV, in ecliptic coordinates showing the “Belt” and the “Basins”, as detailed in the text. The black outlines at each image enclose the regions of maximum ENA intensities of (A) IBEX-Hi at ∼1.1 keV and (B) IBEX-Hi at ∼4.29 keV (Dialynas et al., 2013) (Bottom) Image of heliospheric ENAs from IBEX at (C) ∼1.1 keV showing the “Ribbon” and (D) ∼4.29 keV showing the ENA fluxes from the GDF (Schwadron et al., 2011).
5 Why does the shape and size of the global heliosphere appear different in different ENA energies?
All measurements described above show clear evidence of a “deflating” and “inflating” heliosphere following the pressure changes of the solar cycle (Dialynas et al., 2017b). However, the detailed physics that explains the interaction of the heliosphere with the VLISM is still an open question and debated in the literature (e.g., Dialynas et al., 2022b; Kleimann et al., 2022).
Since the V1and2 became interstellar missions, it was identified that the interstellar flow is not the primary driver of the interaction of the heliosphere with the VLISM, but rather it is the pressure of the interstellar magnetic field that mainly configures the heliosheath, as was initially suggested by Cassini/INCA-Voyager studies (Krimigis et al., 2009; Dialynas et al., 2013). The use of 5.2–55 keV ENA data from Cassini/INCA combined with V1 (
Other studies (McComas et al., 2013) using IBEX-Hi ENAs show a tail that forms due to the presence of fast/slow wind, where both the external dynamic and magnetic pressures strongly affect the heliosphere, producing an “intermediate configuration”, that remains consistent with a long tail configuration that may extend out to several 1000s of AU. Recent interpretations (McComas et al., 2020), based on modeling of the 0.52–6 keV IBEX-Hi and 58–88 keV SOHO/HSTOF measurements (Czechowski et al., 2020) support the notion of the traditional (long tail) comet-type configuration (heliotail extending to ∼20,000 AU).
Advanced MHD models for the global heliosphere (Opher et al., 2015; Kornbleuth et al., 2018) argue that the magnetic tension of the solar magnetic field plays a crucial role in organizing the solar wind into two jet-like structures, producing a “croissant-like” shape for the heliosphere, where the distance to the heliopause towards the tail and nose is on the same order. Including the thermal ions and PUIs as separate plasmas (Opher et al., 2020) results in a more deflated heliosphere, as a result of energy loss of PUIs in the heliosheath due to charge-exchange. Other models also demonstrate confinement by the solar magnetic field but remain consistent with a tail that is elongated (Izmodenov and Alexashov, 2015; Pogorelov et al., 2015). The comparison (Kornbleuth et al., 2021) of the Boston University-croissant (Michael et al., 2021) and Moscow long-tail (Izmodenov and Alexashov, 2020) models showed collimation of the heliosheath plasma by the solar magnetic field and identical solutions up to at least ∼300 AU downwind. The HelMod model (Boschini et al., 2019, 2020) toward explaining the evolution of GCRs in the heliosphere acknowledged the dominant role of the interstellar magnetic field and obtained the V1/V2 crossings of the termination shock and heliopause using a dimensionless stagnation pressure that corresponds to a diamagnetic bubble-like heliosphere.
6 Key points and recommendations
Following the ISP study report (https://interstellarprobe.jhuapl.edu/Interstellar-Probe-MCR.pdf; McNutt et al., 2022; Brandt et al., 2022b), a pragmatic mission concept (launch window starts in 2036), traveling well beyond the heliopause, and in anticipation of the IMAP mission (McComas et al., 2018) at ∼1 AU (launch in 2025) acting as a high-res “guide” to ISP, this paper highlighted the importance of studying the physics of the interactions pertaining to the expanding solar wind that meets the plasma, gas and dust flows of the VLISM, forming the complex and vast region of our astrosphere (see also Brandt et al., 2022a; Dialynas et al., 2022a; DeMajistre et al., 2022; Hill et al., 2022; Lavraud et al., 2022; Mostafavi et al., 2022; Opher et al., 2022; Provornikova et al., 2022; Sokół et al., 2022; Sterken et al., 2022). We focused on three fundamental open science questions that can only be addressed by exploiting a combination of in-situ charged particle, plasma waves and fields measurements with remotely sensed ENAs, that are measured simultaneously on the future ISP mission.
In Section 3 we showed that there is currently a disconnect between the observations and heliosphere models towards explaining the fundamental force balance, responsible for the formation of the entire bubble and -especially- the locations of the termination shock and heliopause through the solar cycle, and lack of simultaneous in-situ particle, fields and ENA measurements at the same energies, the combination of which can provide invaluable input toward exploring the structure of the heliosheath and serve as long-term precursors to the heliopause and termination shock locations (e.g., review articles Dialynas et al., 2022b; Kleimann et al., 2022). We argue that the combination of in-situ particle and fields measurements with remotely sensed ENAs over the evolving solar cycle is required, in order to identify a) the way(s) that multi-scale solar wind structures propagate and evolve in the outer heliosphere, b) the resulting plasma flows inside the heliosheath, c) the role of charge exchange, d) the changes in the termination shock and heliopause locations in response to pressure pulses, shocks, and waves in the solar wind.
In Section 4 we showed that the shape of the ion energy spectra plays a critical role toward determining the acceleration mechanisms inside the heliosheath, while the combined use of in-situ ion distributions, magnetic fields and remotely sensed ENAs can constrain/provide accurate estimates of the heliosheath thickness, the interstellar neutral H density and magnetic field upstream of the heliopause, and delineate the components of the ion pressure in the heliosheath. Imaging the heliosphere in ENAs, essentially translates to imaging the pressure of the global heliosheath, whereas the combination of ENAs with in-situ particle spectra, providing differential pressure profiles, can reveal the force balance that forms our solar bubble (e.g., review article Dialynas et al., 2022b). We argue that ENA imaging over a broad energy range and from a changing vantage point (inside and ultimately outside the Heliosphere) over at least a solar cycle is required. Synergistic use of in-situ ion measurements from inside the heliosheath over a significant fraction of the solar cycle are also required to investigate the dynamical force balance. At the same time, the total charge density (via the electron density, e.g., from a plasma wave -PWS- instrument; Gurnett et al., 2013; Gurnett and Kurth, 2019; Kurth and Gurnett, 2020) is important in order to know that all the ions are accounted for.
In Section 5 we showed that interpretations based on different ENA energies reveal non-conclusive views for the global configuration of the heliosphere: it is consistent with a stretched bubble when looking at ∼10 eV to ∼2 keV ENAs (IBEX-Lo), but it becomes consistent with the traditional long tail (comet-type) configuration when looking at ∼0.5 to 6 keV ENAs (IBEX-Hi). The heliosphere is consistent with a stretched bubble of a few 100s of AU at 5.2–55 keV ENAs (INCA; combined with
7 Short discussion on missing measurements
The science goals that became the focus of the present study are only a small part of the fundamental questions posed by numerous works (referenced throughout the manuscript), detailing on the measurements of V1, V2, Cassini, IBEX and New Horizons missions concerning the physics of the heliosphere, that drive the requirements of a future ISP mission.
Undoubtedly, V1 and V2 made substantial discoveries and posed new and exciting questions about the interactions of the heliosphere with the VLISM, being the only missions to date that have transcended through the boundaries of our solar bubble (now surveying through interstellar space). However, because the Voyagers were initially designed to perform planetary flybys, due to instrument limitations (e.g., partial charged particle observations and/or insufficient magnetic field resolution, etc.), they have also left several science questions unresolved. On the other hand, relying on measurements from the New Horizons mission (currently located at ∼55 AU from the Sun) would not suffice, as it includes a rather limited scientific payload (e.g., it does not carry a magnetometer or a plasma wave instrument), whereas depending on its power budget the spacecraft may not provide measurements well beyond the termination shock and/or (especially) close (or beyond) the heliopause. An ISP would become the only spacecraft to carry the necessary instrumentation to provide in-situ charged particle and fields measurements, alongside remotely sensed ENAs throughout the heliosphere, with no energy gaps and with sufficient resolution.
Here we will only briefly discuss the required measurements from a future ISP mission, but we highlight that the details of the baseline ISP mission architecture, trajectory options, required measurements, example payload instruments and comparison with active and past missions can be thoroughly reviewed in the published ISP report (https://interstellarprobe.jhuapl.edu/Interstellar-Probe-MCR.pdf) and the recently published works of McNutt et al. (2022) and Brandt et al. (2022b), Brandt et al. (2023), and references therein.
The determination of plasma flow velocities, densities, temperatures and magnetic fields is critically important for understanding the acceleration of particles in the solar wind and the helioseath, the physics at the termination shock and the heliopause, plasma wave generation and propagation, wave-particle interactions, plasma turbulence, magnetic reconnection, etc., but also to determine the important charge fraction of the VLISM, the shocks of solar origin (e.g., Gurnett et al., 2021) and/or the very weak emissions that are barely detectable from Voyager/PWS (e.g., Ocker et al., 2021), together with its overall thermal properties (e.g., see review articles by Dialynas et al. (2022b); Kleimann et al. (2022); Richardson et al. (2022)). Such studies would require a combination of high time and energy resolution plasma measurements from ∼3 eV/q (with high signal-to-noise ratio; SNR) up to PUI energies, with a plasma wave detector that would measure the emissions around the electron plasma frequency, and high sensitivity that would allow for independent measurements of the plasma density and temperature in the VLISM (
Distinguishing between different acceleration processes of ACRs and determining their source, together with their relation to singly charged PUIs (e.g., see review article by Giacalone et al. (2022)), requires measuring the intensities and anisotropies of protons, He, Li-Be-B, C, N, O, Ne as well as other heavy ions within the energy range of 100s of keV to ∼100 MeV/nuc. At the same time, the Voyager missions demonstrated that further measurements of the intensities and anisotropies of GCRs are needed, to study their sources, modulation by the heliospheric shielding, solar dynamics over the solar cycle, together with the properties of the unshielded GCR spectra in the ISM, including rare species and isotopes that were not measured in V1 and V2.
In direct response to the science questions posed in this paper, it should be highlighted that, to date, there have been no in-situ ion measurements at a very crucial part of the ion distribution that contributes substantially to the ion processes in the heliosheath and the heliopause, i. e., PUI energies up to about 28 keV, corresponding to energies between the V2/PLS energy range (V1/PLS failed during the 1980s) and the V2/LECP (V1/LECP takes measurements at the energy range beyond ∼40 keV). As explained in his paper, because PUIs carry a substantial part of the particle pressure inside the heliosheath, their role in the dynamics of the outer heliosphere and the VLISM is dominant. Noteably, even if New Horizons reaches well beyond the termination shock, its instrumentation is not designed to measure multiple and heavier species of PUIs. Thus, determining the role of the thermal plasma, PUIs and energetic particles in the interaction between the heliosheath plasma and the VLISM (the relation of ACRs to singly charged PUIs, etc.), highly relies on the existence of a future ISP mission. Furthermore, measuring the intensities and distributions of energetic charged particles (of keV energies) beyond the heliopause (that drop to a level of ∼1% of that in the heliosheath) requires significantly improved SNR measurements (compared to the Voyagers), a factor that depends on the design of the corresponding detector on the future ISP. Such higher sensitivity instrumentation is imperative to explore the state of the VLISM.
From the perspective of remotely sensed ENA measurements, it should be noted that after the end of the Cassini mission (15-Sep.-2017), a
Data availability statement
The present work does not involve a new analysis of any Cassini/MIMI observations, Voyager 1 and 2 measurements or other spacecraft data. The measurements included in Figures 1–4 can be acquired from the repositories that are indicated by the corresponding manuscripts, as referenced in each Figure caption, i.e., doi:10.1088/0004-637X/778/1/40, doi:10.1038/s41550-017-0115, doi:10.1029/2019GL083924, doi:10.3847/2041-8213/abcaaa, doi:10.1007/s11214-022-00889-0, doi:10.1038/s41550-019-0927-4, doi:10.1088/0004-637X/731/1/56.
Author contributions
All authors have equally contributed to all aspects of the manuscript. The manuscript represents their contribution to the Decadal Survey for Solar and Space Physics (Heliophysics) 2024–2033, that will also be accessible from the Bulletin of the AAS (BAAS): Dialynas et al., A future Interstellar Probe on the dynamic heliosphere and its interaction with the very local interstellar medium: In-situ particle and fields measurements and remotely sensed ENAs, 2022a. All authors listed have made a substantial, direct, and intellectual contribution to the work and approved it for publication.
Funding
This work was supported at JHU/APL by NASA under contracts NAS5 97271, NNX07AJ69G, and NNN06AA01C and by subcontract at the Office for Space Research and Technology. Veerle J. Sterken received funding from the European Union’s Horizon 2020 research and innovation programme under grant agreement N°851544-ASTRODUST. Open access funding provided by ETH Zurich.
Acknowledgments
The authors are grateful to all Cassini/MIMI (http://cassini-mimi.jhuapl.edu/), Voyager 1 & Voyager 2 (LECP, CRS, PLS, PWS and MAG; https://voyager.jpl.nasa.gov/mission/), SHIELD DRIVE Science Center (https://shielddrivecenter.com/) and Interstellar Probe (https://interstellarprobe.jhuapl.edu/) teams for useful discussions that made this work possible.
Conflict of interest
The authors declare that the research was conducted in the absence of any commercial or financial relationships that could be construed as a potential conflict of interest.
Publisher’s note
All claims expressed in this article are solely those of the authors and do not necessarily represent those of their affiliated organizations, or those of the publisher, the editors and the reviewers. Any product that may be evaluated in this article, or claim that may be made by its manufacturer, is not guaranteed or endorsed by the publisher.
References
Borovikov, S. N., and Pogorelov, N. V. (2014). Voyager 1 near the heliopause. Astrophysical J. Lett. 783, L16. doi:10.1088/2041-8205/783/1/L16
Boschini, M. J., Della Torre, S., Gervasi, M., Grandi, D., Jóhannesson, G., La Vacca, G., et al. (2020). Inference of the local interstellar spectra of cosmic-ray nuclei Z ≤ 28 with the GALPROP-HELMOD framework. ApJS 250, 27. doi:10.3847/1538-4365/aba901
Boschini, M. J., Della Torre, S., Gervasi, M., La Vacca, G., and Rancoita, P. G. (2019). The HELMOD model in the works for inner and outer heliosphere: From AMS to Voyager probes observations. Adv. Space Res. 64, 2459–2476. doi:10.1016/j.asr.2019.04.007
Brandt, P. C., Bale, S., DeMajistre, R., Dialynas, K., Eriksson, S., Fields, B., et al. (2022a). Exploration of the heliosphere and the very local interstellar medium by an Interstellar Probe. Decadal Survey for Solar and Space Physics (Heliophysics) 2024-2033.
Brandt, P. C., Provornikova, E. A., Cocoros, A., Turner, D., DeMajistre, R., Runyon, K., et al. (2022b). Interstellar probe: Humanity’s exploration of the galaxy begins. Acta Astronaut. 199, 364–373. doi:10.1016/j.actaastro.2022.07.011
Brandt, P. C., Provornikova, E., Bale, S. D., Cocoros, A., DeMajistre, R., Dialynas, K., et al. (2023). Future exploration of the outer heliosphere and very local interstellar medium by interstellar probe. Space Sci. Rev., (In press).
Burlaga, L. F., Ness, N. F., Berdichevsky, D. B., Park, J., Jian, L. K., Szabo, A., et al. (2019). Magnetic field and particle measurements made by Voyager 2 at and near the heliopause. Nat. Astron. 3, 1007–1012. doi:10.1038/s41550-019-0920-y
Burlaga, L. F., Ness, N. F., and Stone, E. C. (2013). Magnetic field observations as voyager 1 entered the heliosheath depletion region. Science 341, 147–150. doi:10.1126/science.1235451
Czechowski, A., Bzowski, M., Sokół, J. M., Kubiak, M. A., Heerikhuisen, J., Zirnstein, E. J., et al. (2020). Heliospheric structure as revealed by the 3-88 keV H ENA spectra. ApJ 888, 1. doi:10.3847/1538-4357/ab5b14
Decker, R. B., Krimigis, S. M., Roelof, E. C., Hill, M. E., Armstrong, T. P., Gloeckler, G., et al. (2008). Mediation of the solar wind termination shock by non-thermal ions. Nature 454, 67–70. doi:10.1038/nature07030
Decker, R. B., Krimigis, S. M., Roelof, E. C., Hill, M. E., Armstrong, T. P., Gloeckler, G., et al. (2005). Voyager 1 in the foreshock, termination shock, and heliosheath. Science 309, 2020–2024. doi:10.1126/science.1117569
Decker, R. B., Krimigis, S. M., Roelof, E. C., and Hill, M. E. (2012). No meridional plasma flow in the heliosheath transition region. Nature 489, 124–127. doi:10.1038/nature11441
Decker, R. B., Krimigis, S. M., Roelof, E. C., and Hill, M. E. (2015). Recent particle measurements from Voyagers 1 and 2. J. Phys. Conf. Ser. 577, 012006. doi:10.1088/1742-6596/577/1/012006
DeMajistre, R., Brandt, P. C., Mitchell, D. G., McNutt, R., Roelof, E. C., Provornikova, E., et al. (2022). Sensing the shape, dynamics and global structure of the heliosphere. Decadal Survey for Solar and Space Physics (Heliophysics) 2024-2033.
Dialynas, K., Brandt, P. C., Burlaga, L., Berdichevsky, D. B., Decker, R. B., Della Torre, S., et al. (2022a). A future Interstellar Probe on the dynamic heliosphere and its interaction with the very local interstellar medium: In-situ particle and fields measurements and remotely sensed ENAs. Decadal Survey for Solar and Space Physics (Heliophysics) 2024-2033.
Dialynas, K., Galli, A., Dayeh, M. A., Cummings, A. C., Decker, R. B., Fuselier, S. A., et al. (2020). Combined ∼10 eV to ∼344 MeV particle spectra and pressures in the heliosheath along the voyager 2 trajectory. ApJL 905, L24. doi:10.3847/2041-8213/abcaaa
Dialynas, K., Krimigis, S. M., Decker, R. B., and Hill, M. E. (2021). Ions measured by Voyager 1 outside the Heliopause to ∼ 28 AU and implications thereof. Astrophysical J. 917, 42. doi:10.3847/1538-4357/ac071e
Dialynas, K., Krimigis, S. M., Decker, R. B., Hill, M., Mitchell, D. G., Hsieh, K. C., et al. (2022b). The structure of the global heliosphere as seen by in-situ ions from the Voyagers and remotely sensed ENAs from Cassini. Space Sci. Rev. 218, 21. doi:10.1007/s11214-022-00889-0
Dialynas, K., Krimigis, S. M., Decker, R. B., and Mitchell, D. G. (2019). Plasma pressures in the heliosheath from Cassini ENA and voyager 2 measurements: Validation by the voyager 2 heliopause crossing. GRL 46, 7911–7919. doi:10.1029/2019GL083924
Dialynas, K., Krimigis, S. M., Mitchell, D. G., Decker, R. B., and Roelof, E. C. (2017a). Response times of Cassini/INCA 5.2 keV ENAs and Voyager ions in the heliosheath over the solar cycle. J. Phys. Conf. Ser. 900, 012005. doi:10.1088/1742-6596/900/1/012005
Dialynas, K., Krimigis, S. M., Mitchell, D. G., Decker, R. B., and Roelof, E. C. (2017b). The bubble-like shape of the heliosphere observed by Voyager and Cassini. Nat. Astron. 1, 0115. doi:10.1038/s41550-017-0115
Dialynas, K., Krimigis, S. M., Mitchell, D. G., Roelof, E. C., and Decker, R. B. (2013). A three-coordinate system (ecliptic, galactic, ISMF) spectral analysis of heliospheric ENA emissions using Cassini/INCA measurements. Astrophysical J. 778, 40. doi:10.1088/0004-637X/778/1/40
Fisk, L. A., and Gloeckler, G. (2022). Global structure and dominant particle acceleration mechanism of the heliosheath: Definitive conclusions. Astrophysical J. 927, 73. doi:10.3847/1538-4357/ac4d2f
Fisk, L. A., and Gloeckler, G. (2014). On whether or not voyager 1 has crossed the heliopause. Astrophysical J. 789, 41. doi:10.1088/0004-637X/789/1/41
Galli, A., Wurz, P., Fichtner, H., Futaana, Y., and Barabash, S. (2019). An empirical model of energetic neutral Atom imaging of the heliosphere and its implications for future heliospheric missions at great heliocentric distances. Astrophysical J. 886, 70. doi:10.3847/1538-4357/ab4e94
Galli, A., Wurz, P., Schwadron, N. A., Kucharek, H., Möbius, E., Bzowski, M., et al. (2017). The downwind hemisphere of the heliosphere: Eight years of IBEX-lo observations. ApJ 851, 2. doi:10.3847/1538-4357/aa988f
Galli, A., Wurz, P., Schwadron, N. A., Kucharek, H., Möbius, E., Bzowski, M., et al. (2016). The roll-over of heliospheric neutral hydrogen below 100 eV: Observations and implications. ApJ 821, 107. doi:10.3847/0004-637X/821/2/107
Giacalone, J., Fahr, H., Fichtner, H., Florinski, V., Heber, B., Hill, M. E., et al. (2022). Anomalous cosmic Rays and heliospheric energetic particles. Space Sci. Rev. 218, 22. doi:10.1007/s11214-022-00890-7
Gkioulidou, M., Opher, M., Kornbleuth, M., Dialynas, K., Giacalone, J., Richardson, J. D., et al. (2022). On the energization of Pickup ions downstream of the heliospheric termination shock by comparing 0.52-55 keV observed energetic neutral Atom spectra to ones inferred from proton hybrid simulations. Astrophysical J. Lett. 931, L21. doi:10.3847/2041-8213/ac6beb
Guo, X., Florinski, V., and Wang, C. (2018). Effects of anomalous cosmic Rays on the structure of the outer heliosphere. Astrophysical J. 859, 157. doi:10.3847/1538-4357/aabf42
Gurnett, D. A., Kurth, W. S., Burlaga, L. F., and Ness, N. F. (2013). In situ observations of interstellar plasma with voyager 1. Science 341, 1489–1492. doi:10.1126/science.1241681
Gurnett, D. A., and Kurth, W. S. (2019). Plasma densities near and beyond the heliopause from the Voyager 1 and 2 plasma wave instruments. Nat. Astron. 3, 1024–1028. doi:10.1038/s41550-019-0918-5
Gurnett, D. A., Kurth, W. S., Stone, E. C., Cummings, A. C., Heikkila, B., Lal, N., et al. (2021). A foreshock model for interstellar shocks of solar origin: Voyager 1 and 2 observations. AJ 161, 11. doi:10.3847/1538-3881/abc337
Heerikhuisen, J., Pogorelov, N. V., Florinski, V., Zank, G. P., and le Roux, J. A. (2008). The effects of a κ-distribution in the heliosheath on the global heliosphere and ENA flux at 1 AU. Astrophysical J. 682, 679–689. doi:10.1086/588248
Hill, M., Allen, R. C., Brandt, P. C., Decker, R. B., Gkioulidou, M., Krimigis, S. M., et al. (2022). Cosmic Rays measurements on an interstellar Probe: A bold approach to concluding the second century of space aged science. Decadal Survey for Solar and Space Physics (Heliophysics) 2024-2033.
Izmodenov, V. V., and Alexashov, D. B. (2020). Magnitude and direction of the local interstellar magnetic field inferred from Voyager 1 and 2 interstellar data and global heliospheric model. Astronomy Astrophysics 633, L12. doi:10.1051/0004-6361/201937058
Izmodenov, V. V., Alexashov, D. B., and Ruderman, M. S. (2014). Electron thermal conduction as a possible physical mechanism to make the inner heliosheath thinner. Astrophysical J. Lett. 795, L7. doi:10.1088/2041-8205/795/1/L7
Izmodenov, V. V., and Alexashov, D. B. (2015). Three-dimensional kinetic-MHD model of the global heliosphere with the heliopause-surface fitting. ApJ-S 220, 32. doi:10.1088/0067-0049/220/2/32
Izmodenov, V. V., Malama, Y. G., and Ruderman, M. S. (2008). Modeling of the outer heliosphere with the realistic solar cycle. Adv. Space Res. 41, 318–324. doi:10.1016/j.asr.2007.06.033
Kleimann, J., Dialynas, K., Fraternale, F., Galli, A., Heerikhuisen, J., Izmodenov, V., et al. (2022). The structure of the large-scale heliosphere as seen by current models. Space Sci. Rev. 218, 36. doi:10.1007/s11214-022-00902-6
Kornbleuth, M., Opher, M., Baliukin, I., Gkioulidou, M., Richardson, J. D., Zank, G. P., et al. (2021). The development of a split-tail heliosphere and the role of non-ideal processes: A comparison of the BU and Moscow models. Astrophysical J. 923, 179. doi:10.3847/1538-4357/ac2fa6
Kornbleuth, M., Opher, M., Michael, A. T., and Drake, J. F. (2018). Globally distributed energetic neutral Atom maps for the “croissant” heliosphere. Astrophysical J. 865, 84. doi:10.3847/1538-4357/aadbac
Krimigis, S. M., Armstrong, T. P., Axford, W. I., Bostrom, C. O., Fan, C. Y., Gloeckler, G., et al. (1977). The Low energy charged particle (LECP) experiment on the voyager spacecraft. SSRv 21, 329–354. doi:10.1007/BF00211545
Krimigis, S. M., Decker, R. B., Hill, M. E., Armstrong, T. P., Gloeckler, G., Hamilton, D. C., et al. (2003). Voyager 1 exited the solar wind at a distance of 85AU from the Sun. Nature 426, 45–48. doi:10.1038/nature02068
Krimigis, S. M., Decker, R. B., Roelof, E. C., Hill, M. E., Armstrong, T. P., Gloeckler, G., et al. (2013). Search for the exit: Voyager 1 at heliosphere’s border with the galaxy. Science 341, 144–147. doi:10.1126/science.1235721
Krimigis, S. M., Decker, R. B., Roelof, E. C., Hill, M. E., Bostrom, C. O., Dialynas, K., et al. (2019). Energetic charged particle measurements from Voyager 2 at the heliopause and beyond. Nat. Astron. 3, 997–1006. doi:10.1038/s41550-019-0927-4
Krimigis, S. M., Mitchell, D. G., Roelof, E. C., and Decker, R. B. (2010). “ENA (E>5 keV) images from Cassini and voyager “ground truth”: Suprathermal pressure in the heliosheath,” in Pickup ions throughout the heliosphere and beyondof American Institute of physics conference series. Editors J. Le Roux, G. P. Zank, A. J. Coates, and V. Florinski, 1302, 79–85. doi:10.1063/1.3529994
Krimigis, S. M., Mitchell, D. G., Roelof, E. C., Hsieh, K. C., and McComas, D. J. (2009). Imaging the interaction of the heliosphere with the interstellar medium from Saturn with Cassini. Science 326, 971–973. doi:10.1126/science.1181079
Krimigis, S. M., Roelof, E. C., Decker, R. B., and Hill, M. E. (2011). Zero outward flow velocity for plasma in a heliosheath transition layer. Nature 474, 359–361. doi:10.1038/nature10115
Kurth, W. S., and Gurnett, D. A. (2020). Observations of a radial density gradient in the very local interstellar medium by voyager 2. Astrophysical J. Lett. 900, L1. doi:10.3847/2041-8213/abae58
Lavraud, B., Opher, M., Dialynas, K., Turner, D. L., Eriksson, S., Provornikova, E., et al. (2022). What is the heliopause? Importance of magnetic reconnection and measurement requirements. Decadal Survey for Solar and Space Physics (Heliophysics) 2024-2033.
McComas, D. J., Allegrini, F., Bochsler, P., Bzowski, M., Christian, E. R., Crew, G. B., et al. (2009). Global observations of the interstellar interaction from the interstellar boundary explorer (IBEX). Science 326, 959–962. doi:10.1126/science.1180906
McComas, D. J., Bzowski, M., Dayeh, M. A., DeMajistre, R., Funsten, H. O., Janzen, P. H., et al. (2020). Solar cycle of imaging the global heliosphere: Interstellar boundary explorer (IBEX) observations from 2009-2019. Astrophysical J. Suppl. Ser. 248, 26. doi:10.3847/1538-4365/ab8dc2
McComas, D. J., Dayeh, M. A., Funsten, H. O., Heerikhuisen, J., Janzen, P. H., Reisenfeld, D. B., et al. (2018). Heliosphere responds to a large solar wind intensification: Decisive observations from IBEX. ApJL 856, L10. doi:10.3847/2041-8213/aab611
McComas, D. J., Dayeh, M. A., Funsten, H. O., Livadiotis, G., and Schwadron, N. A. (2013). The heliotail revealed by the interstellar boundary explorer. Astrophysical J. 771, 77. doi:10.1088/0004-637X/771/2/77
McComas, D. J., Zirnstein, E. J., Bzowski, M., Dayeh, M. A., Funsten, H. O., Fuselier, S. A., et al. (2017). Seven years of imaging the global heliosphere with IBEX. ApJS 229, 41. doi:10.3847/1538-4365/aa66d8
McNutt, R. L., Wimmer-Schweingruber, R. F., Gruntman, M., Krimigis, S. M., Roelof, E. C., Brandt, P. C., et al. (2022). Interstellar probe - destination: Universe. Acta Astronaut. 196, 13–28. doi:10.1016/j.actaastro.2022.04.001
Michael, A. T., Opher, M., Tóth, G., Tenishev, V., and Drake, J. F. (2021). The impact of kinetic neutrals on the heliotail. Astrophysical J. 906, 37. doi:10.3847/1538-4357/abc953
Mostafavi, P., Allen, R. C., Brandt, P. C., Burlaga, L., Chen, T. Y., Decker, R., et al. (2022). Shock waves propagation beyond the heliosphere: How far does the Sun’s influence extend into the interstellar medium?. Decadal Survey for Solar and Space Physics (Heliophysics) 2024-2033.
Ocker, S. K., Cordes, J. M., Chatterjee, S., Gurnett, D. A., Kurth, W. S., and Spangler, S. R. (2021). Persistent plasma waves in interstellar space detected by Voyager 1. Nat. Astron. 5, 761–765. doi:10.1038/s41550-021-01363-7
Opher, M., Drake, J. F., Zieger, B., and Gombosi, T. I. (2015). Magnetized jets driven by the sun: The structure of the heliosphere revisited. ApJL 800, L28. doi:10.1088/2041-8205/800/2/L28
Opher, M., Richardson, J., Giacalone, J., Kornbleuth, M., Brandt, P. C., Provornikova, E., et al. (2022). Our heliospheric shield, a case of a habitable astrosphere: An imperative need to revisit this region with in-situ measurements with modern instrumentation. Decadal Survey for Solar and Space Physics (Heliophysics) 2024-2033.
Opher, M., Loeb, A., Drake, J., and Toth, G. (2020). A small and round heliosphere suggested by magnetohydrodynamic modelling of pick-up ions. Nat. Astron. 4, 675–683. doi:10.1038/s41550-020-1036-0
Pogorelov, N. V., Borovikov, S. N., Heerikhuisen, J., and Zhang, M. (2015). The heliotail. ApJL 812, L6. doi:10.1088/2041-8205/812/1/L6
Provornikova, E., Brandt, P., Opher, M., Lavraud, B., Roelof, E.C., Izmodenov, V.V., et al. (2022). Structured solar wind from the sun to the boundary of the heliosphere: Required measurements and simulation tools. Decadal Survey for Solar and Space Physics (Heliophysics) 2024-2033.
Rankin, J. S., McComas, D. J., Richardson, J. D., and Schwadron, N. A. (2019). Heliosheath properties measured from a voyager 2 to voyager 1 transient. ApJ 883, 101. doi:10.3847/1538-4357/ab3d9d
Reisenfeld, D. B., Bzowski, M., Funsten, H. O., Fuselier, S. A., Galli, A., Janzen, P. H., et al. (2016). Tracking the solar cycle through IBEX observations of energetic neutral Atom flux variations at the heliospheric Poles. ApJ 833, 277. doi:10.3847/1538-4357/833/2/277
Reisenfeld, D. B., Bzowski, M., Funsten, H. O., Heerikhuisen, J., Janzen, P. H., Kubiak, M. A., et al. (2021). A three-dimensional map of the heliosphere from IBEX. ApJS 254, 40. doi:10.3847/1538-4365/abf658
Richardson, J. D., Belcher, J. W., Garcia-Galindo, P., and Burlaga, L. F. (2019). Voyager 2 plasma observations of the heliopause and interstellar medium. Nat. Astron. 3, 1019–1023. doi:10.1038/s41550-019-0929-2
Richardson, J. D., Burlaga, L. F., Elliott, H., Kurth, W. S., Liu, Y. D., and von Steiger, R. (2022). Observations of the outer heliosphere, heliosheath, and interstellar medium. Space Sci. Rev. 218, 35. doi:10.1007/s11214-022-00899-y
Richardson, J. D., Kasper, J. C., Wang, C., Belcher, J. W., and Lazarus, A. J. (2008). Cool heliosheath plasma and deceleration of the upstream solar wind at the termination shock. Nature 454, 63–66. doi:10.1038/nature07024
Roelof, E. C. (2015). Charged particle energization and transport in reservoirs throughout the heliosphere: 1. Solar energetic particles. J. Phys. Conf. Ser. 642, 012023. doi:10.1088/1742-6596/642/1/012023
Roelof, E. C., Krimigis, S. M., Mitchell, D. G., Decker, R. B., Richardson, J. D., Gruntman, M., et al. (2010). “Implications of generalized rankine-hugoniot conditions for the PUI population at the voyager 2 termination shock,” in Pickup ions throughout the heliosphere and beyondof American Institute of physics conference series. Editors J. Le Roux, G. P. Zank, A. J. Coates, and V. Florinski, 1302, 133–141. doi:10.1063/1.3529960
Scherer, K., Dialynas, K., Fichtner, H., Galli, A., and Roussos, E. (2022). The properties of 0.11 keV-344 MeV ion spectra in the inner heliosheath using regularized κ-distributions. Astronomy Astrophysics 664, A132. doi:10.1051/0004-6361/202243449
Schwadron, N. A., Allegrini, F., Bzowski, M., Christian, E. R., Crew, G. B., Dayeh, M., et al. (2011). Separation of the interstellar boundary explorer Ribbon from globally distributed energetic neutral Atom flux. ApJ 731, 56. doi:10.1088/0004-637X/731/1/56
Schwadron, N. A., Moebius, E., Fuselier, S. A., McComas, D. J., Funsten, H. O., Janzen, P., et al. (2014). Separation of the Ribbon from globally distributed energetic neutral Atom flux using the first five years of IBEX observations. Astrophysical J. Suppl. Ser. 215, 13. doi:10.1088/0067-0049/215/1/13
Sokół, J. M., Dayeh, M. A., Dialynas, K., DeMajistre, R., Elliott, H., Fuselier, S. A., et al. (2022). Measurements Beyond 1 au are Necessary for Exploration of the Outer Heliosphere and Local Interstellar Medium. Decadal Survey for Solar and Space Physics (Heliophysics) 2024-2033.
Sterken, V., Hunziker, S., Dialynas, K., Herbst, K., Li, A., Baalmann, L.R., et al. (2022). Synergies between interstellar dust and heliospheric science with an Interstellar Probe. Decadal Survey for Solar and Space Physics (Heliophysics) 2024-2033.
Stone, E. C., Cummings, A. C., Heikkila, B. C., and Lal, N. (2019). Cosmic ray measurements from Voyager 2 as it crossed into interstellar space. Nat. Astron. 3, 1013–1018. doi:10.1038/s41550-019-0928-3
Stone, E. C., Cummings, A. C., McDonald, F. B., Heikkila, B. C., Lal, N., and Webber, W. R. (2008). An asymmetric solar wind termination shock. Nature 454, 71–74. doi:10.1038/nature07022
Stone, E. C., Cummings, A. C., McDonald, F. B., Heikkila, B. C., Lal, N., and Webber, W. R. (2005). Voyager 1 explores the termination shock region and the heliosheath beyond. Science 309, 2017–2020. doi:10.1126/science.1117684
Stone, E. C., Cummings, A. C., McDonald, F. B., Heikkila, B. C., Lal, N., and Webber, W. R. (2013). Voyager 1 observes low-energy galactic cosmic Rays in a region depleted of heliospheric ions. Science 341, 150–153. doi:10.1126/science.1236408
Zirnstein, E. J., Dayeh, M. A., McComas, D. J., and Sokół, J. M. (2020). Distance to the energetic neutral hydrogen source from the heliotail. ApJ 897, 138. doi:10.3847/1538-4357/ab9605
Keywords: interstellar probe, heliosphere, heliosheath, Voyager, Cassini, energetic neutral atoms, very local interstellar medium, solar wind
Citation: Dialynas K, Sterken VJ, Brandt PC, Burlaga L, Berdichevsky DB, Decker RB, Della Torre S, DeMajistre R, Galli A, Gkioulidou M, Hill ME, Krimigis SM, Kornbleuth M, Kurth W, Lavraud B, McNutt R, Mitchell DG, Mostafavi PS, Nikoukar R, Opher M, Provornikova E, Roelof EC, Rancoita PG, Richardson JD, Roussos E, Sokół JM, La Vacca G, Westlake J and Chen TY (2023) A future interstellar probe on the dynamic heliosphere and its interaction with the very local interstellar medium: In-situ particle and fields measurements and remotely sensed ENAs. Front. Astron. Space Sci. 10:1061969. doi: 10.3389/fspas.2023.1061969
Received: 05 October 2022; Accepted: 16 February 2023;
Published: 06 March 2023.
Edited by:
Gian Luca Delzanno, Los Alamos National Laboratory (DOE), United StatesReviewed by:
Kaijun Liu, Southern University of Science and Technology, ChinaStas Barabash, Swedish Institute of Space Physics, Sweden
Copyright © 2023 Dialynas, Sterken, Brandt, Burlaga, Berdichevsky, Decker, Della Torre, DeMajistre, Galli, Gkioulidou, Hill, Krimigis, Kornbleuth, Kurth, Lavraud, McNutt, Mitchell, Mostafavi, Nikoukar, Opher, Provornikova, Roelof, Rancoita, Richardson, Roussos, Sokół, La Vacca, Westlake and Chen. This is an open-access article distributed under the terms of the Creative Commons Attribution License (CC BY). The use, distribution or reproduction in other forums is permitted, provided the original author(s) and the copyright owner(s) are credited and that the original publication in this journal is cited, in accordance with accepted academic practice. No use, distribution or reproduction is permitted which does not comply with these terms.
*Correspondence: V. J. Sterken, dnN0ZXJrZW5AZXRoei5jaA==; K. Dialynas, a2RpYWx5bmFzQHBoeXMudW9hLmdy