- 1George Mason University, Fairfax, VA, United States
- 2Goddard Space Flight Center, National Aeronautics and Space Administration, Greenbelt, MD, United States
- 3University of Minnesota Twin Cities, StPaul, MN, United States
- 4Laboratory for Atmospheric and Space Physics, University of Colorado Boulder, Boulder, CO, United States
- 5University of Colorado Boulder, Boulder, CO, United States
- 6The University of IA, Iowa City, IA, United States
The drivers and atmospheric impacts of energetic electron precipitation are not yet well understood. Further, electron precipitation is often poorly represented in atmospheric modeling. Additional investigations of the drivers and impacts of electron precipitation are needed to improve models and space weather forecasting requirements. To accurately represent the troposphere through the ionosphere in model simulations, it is vital to account for the chemistry accurately. Electron precipitation is a frequent, yet often ignored middle to high latitude forcing that can have dramatic effects on the middle and upper atmosphere. Over the past decade, several electron precipitation data sets have been developed, however, validation has been difficult due to the lack of independent observations of electron fluxes. Additionally, the limited number of satellites making measurements of global magnetospheric wave activity in concert with the resulting electron precipitation restricts our ability to accurately capture the drivers simultaneously with the precipitation. Accurate characterization of the drivers is needed for physics-based magnetosphere modeling. Likewise, accurate precipitating electron fluxes and relative energies are needed to improve our atmospheric modeling studies. Finally, in order to properly validate and improve our current modeling efforts, observations of atmospheric composition are necessary.
Introduction
Energetic electron precipitation (EEP) is a process by which energetic electrons deposit their energy into the atmosphere. The deposition altitude depends on the energy of the electron along with the density of the atmosphere (see Figure 1). Low energy electrons (<30 keV) deposit their energy into the thermosphere. These electrons can have direct impacts on the thermosphere and ionosphere by altering chemical composition (Rees et al., 1983) as well as increasing neutral density through ion heating (Zhu et al., 2022). Impacts within this region are a concern to space weather users who are impacted by things such as satellite drag. Low energy electrons precipitate mainly through acceleration from Alfvén waves, quasi-static potential structures, and wave scattering of the plasma sheet electrons.
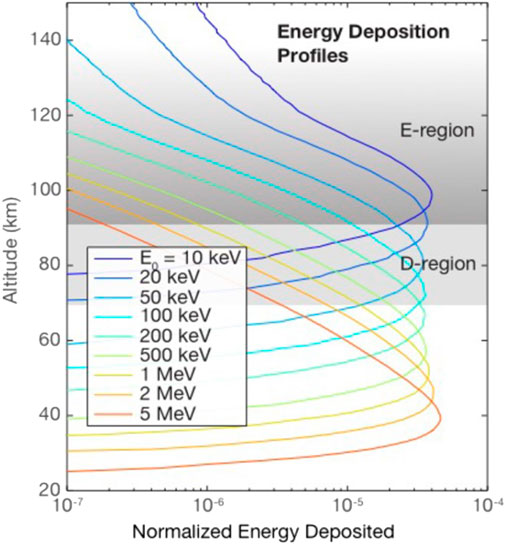
FIGURE 1. Energy deposition profiles of electrons colored by energy. Courtesy of Robert Marshall, CU Boulder/ASEN.
Medium energy electrons (30–300 keV) are lost to the mesosphere and cause significant production of hydroxyl and odd nitrogen. During the polar winter, the odd nitrogen can be controlled dynamically resulting in air rich in odd nitrogen descending into the stratosphere where ozone destruction can occur (Randall et al., 2007). High energy electrons (>300 keV) can penetrate directly to the upper stratosphere, causing the production of odd nitrogen through the ionization of nitrogen and oxygen molecules. Odd nitrogen participates in catalytic chemical reactions resulting in the destruction of ozone (Thorne, 1980; Solomon et al., 1981; 1982). Both the medium and high energy EEP create issues for space weather users including increased radiation. While many case studies have been published investigating short-term impacts on the mesosphere and stratosphere, long-term changes due to the continual bombardment of electrons have not been fully explored. Furthermore, the indirect impacts of ozone loss from EEP are unclear. Some research suggests that EEP-induced ozone loss can contribute to changes in tropospheric weather systems (Rozanov et al., 2005; Seppälä et al., 2009). Other research argues the possibility of impacts on the ionosphere through the modification of gravity waves (Salminen et al., 2019; Asikainen et al., 2020).
To further our understanding of the impacts of EEP on the atmosphere and ionosphere, our research community needs more complete and better-validated data sets. Most current low Earth Orbit (LEO) data sets of EEP make use of Polar-orbiting Operational Environmental Satellites (POES) Medium Energy Proton Electron Detector (MEPED) observations. However, even though these data sets all make use of the POES observations, there is substantial variability among them, especially during active geomagnetic storm periods (Nesse Tyssøy et al., 2021). Moreover, all the data sets utilized the Polar-orbiting Operational Environmental Satellites (POES) Medium Energy Proton and Electron Detector (MEPED) observations. Figure 2 (supplementary section) shows ionization rates from eight different POES-based electron data sets during April of 2010. The figure highlights that there are significant differences between the data set with the lowest ionization (ApEEP) and the highest (MP15). In order to validate these processed data sets, we need in situ data within the atmosphere. In the short-term sensitivity studies of how the different inputs affect the model results can be informative.
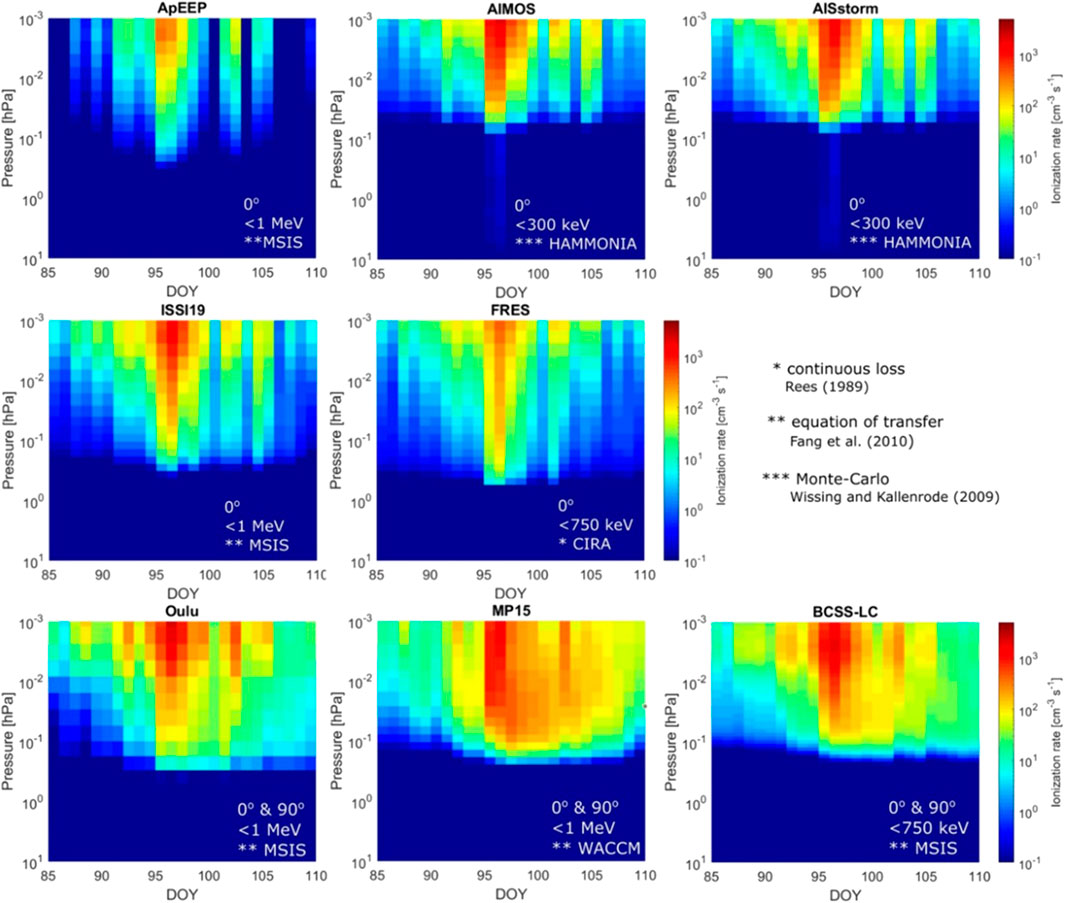
FIGURE 2. Latitude corrected hemispheric mean poleward of 45oS for the eight ionization rate estimate. The legend in each panel lists the detector(s), Upper energy limit, background atmosphere and ionization rate method applied (Nesse Tyssøy et al., 2021).
Another area that needs further examination is the drivers of electron precipitation. Understanding the interdependence and relative importance of the drivers of electron precipitation is essential to accurately predict space weather and in turn further our understanding of the impact of space weather on atmospheric chemistry (Andersson et al., 2014; Mironova et al., 2015; Meredith et al., 2017). For electrons ranging from tens of keV up to relativistic (>1 MeV) energies, it is thought that the dominant cause of radiation belt electron loss to the atmosphere is through wave-particle interactions in the magnetosphere. The primary waves thought to be responsible for the loss of radiation belt electrons are chorus waves, plasmaspheric hiss, electromagnetic ion cyclotron (EMIC) waves, and ULF waves. Each of these wave modes precipitates electrons at different energies, magnetic local times and L-shells (see reviews by Ripoll et al., 2020; Thorne, 2010; Brito et al., 2020). Our current approach to directly observing waves and the subsequent precipitation relies on combining satellite missions and/or ground-based wave detectors with electron flux data from Low Earth Orbiting (LEO) satellites, balloons and/or CubeSats through magnetic conjunctions (e.g., Halford et al., 2015; Clilverd et al., 2017; Shumko et al., 2021). For instance, the POES satellites (in LEO) can measure precipitating electrons in the bounce loss cone, however simultaneous in situ wave observations are needed from equatorial satellites where the waves are generated. These equatorial satellites are often unable to resolve electrons in the loss cone, hence collaborative observations, such as those mentioned above, are required. Additionally, more modeling and validation are required to improve physics-based models that can successfully forecast both the wave generation and subsequent particle precipitation.
Recent work has uncovered significant information regarding the creation, observation, modeling and validating of EEP. Despite modern advancements, much work remains to fully understand the drivers, impacts, and how to accurately model and forecast these events in the future. Case studies investigating active geomagnetic time periods using atmospheric models such as the Whole Atmosphere Community Climate Model (WACCM) have struggled to accurately represent middle atmospheric chemistry before, during and after these events (Pettit et al., 2021). The accuracy is further compounded during periods of exceptional dynamics in the stratosphere and mesosphere such as sudden stratospheric warming events. Figure 3 illustrates this by displaying model simulation results using WACCM forced with the MEPED Precipitating Electron (MPE) data set (Pettit et al., 2021) during the 2004 Northern Hemisphere winter. Observations showed exceptional amounts of odd nitrogen reaching the stratosphere due to atmospheric wave activity that enhanced descent from the mesosphere to the stratosphere. As shown in the figure, however, the model underestimates the stratospheric odd nitrogen changes in the upper stratosphere at 40 km altitude. This likely results from some combination of incorrect atmospheric dynamics and errors in odd nitrogen production, despite the fact that medium energy electron ionization was included in this case.
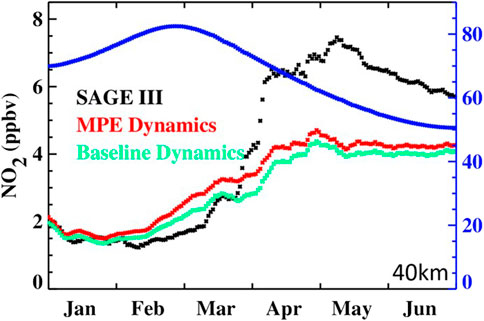
FIGURE 3. Comparisons between SD-WACCM simulation with improvement dynamics and the solar occulation instrument SAGE III of NO2. WACCM output corresponds to satelite measurement times and locations. The green line indicates a simulation without the inclusion of higher energy electron precipitation (>30 KeV), while the red line indicates the simluation using the MPE forcing. The blue line shows the measurement latitudes and are referenced on the right vertical axis.
Currently, most investigations of the atmospheric impacts of EEP use a version of the POES MEPED-derived data. Despite having several satellites providing coverage, a very important region is missing due to the failure of the NOAA16 satellite in 2013. Figure 4 (supplementary section) demonstrates this by showing an arbitrary day that is representative of all days of observations using the POES MEPED data. It should be clear from the figure that we have very limited observations around the noon sector for l-shells two through 6. This particular sector is a very active precipitation region for chorus wave activity. Thus, any simulations using the POES MEPED data will subsequently miss precipitation from chorus wave activity, resulting in possible underestimation of atmospheric ionization.
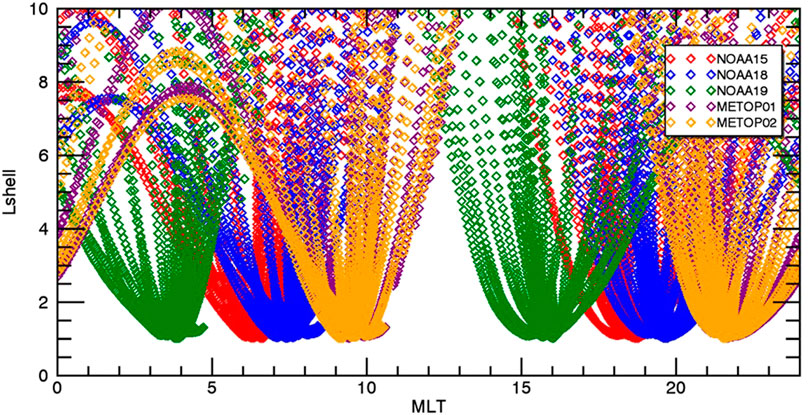
FIGURE 4. Measurement locations of all satelites currently measuring data for a single day in 2017 as a function of magnetic local time and I-shell. Due to limited changes in orbit, the day is represantative of all daily measurement of the MEPED data.
Science priorities and path to successful understanding of the drivers and impacts of EEP
To summarize, while improvements have been made with regard to investigating the drivers of EEP, further work is needed in the creation of EEP data sets, the validation of the data sets, and the modeling efforts that include electron precipitation. In particular, validation of the available electron precipitation data sets is needed to verify accurate electron flux precipitation in the bounce loss cone at a wide range of energies. The validation of the EEP data sets is necessary to help constrain the model and data error bars with our analysis to ensure we are correctly capturing the relevant physics. Additionally, validation of the magnetospheric wave activity driving the precipitation would be vital to identify the important causes of the precipitation. Furthermore, once we have confidence in our estimations of electron precipitation, atmospheric modeling efforts need to be supported to estimate the impacts of EEP on the atmospheric system. Lastly, observational data is needed to compare our modeling results with satellite observations of a variety of important chemicals such as NO, OH and ozone. This would include the further funding of the limited observations we currently have in the mesosphere and lower thermosphere region, which include the Microwave Limb Sounder (MLS) and the Sounding of the Atmosphere using Broadband Emission Radiometry (SABER). These instruments are necessary to confirm the chemistry and temperature changes from EEP from modeling studies and help verify they are accurate. The upcoming Geospace Dynamics Constellation (GDC) mission would help further our understanding of low-energy electron precipitation by direct measurements of the electrons with energies from tens of eV through 30 keV along with measurements of the neutral density to observe effects on satellite drag.
The following are proposed science goals along with decadal length solutions using space-based instrumentation, high-performance computational computing, and current available database resources to achieve our goals.
1) Improve our understanding of the drivers that affect EEP and implement these drivers in empirical and physics-based models.
- In order to fully resolve both the drivers and the subsequent electron precipitation, we would need a space-based constellation of satellites whose orbit would include measurements along magnetic field lines from the wave source regions near the magnetic equator to the bounce loss cone, measuring both magnetospheric waves and precipitating particles. In the absence of such a mission, we would have to rely on investigations using conjunctions of satellites that measure magnetospheric waves such as the Van Allen Probes [RBSP], Arase and ground-based VLF stations along with LEO satellites, such as POES, that measure particle fluxes. While past conjunctions exist, RBSP is no longer functioning leaving only a few satellite-based and ground-based VLF stations as our only magnetospheric wave detectors moving forward. Furthering our understanding of the wave drivers would improve empirical and physics-based modeling efforts that could generate internally derived electron precipitation without the need for data. Validation could be performed using current electron precipitation and wave data sets. Accurate physics-based models could then be used in operational forecasting of space weather events to improve mitigation strategies.
2) Creation of event-specific and long-term trapped and precipitating electron fluxes over all relevant energy ranges, validated at all L-shells and MLTs using current and future space-based missions. Additionally, the creation of data-driven fluxes using machine learning techniques for use in both operational forecasting and long-term future climate simulation studies.
- For low-energy electrons (<30 keV), the support of the GDC mission would be extremely beneficial for global observations of low-energy precipitation in the ionosphere and thermosphere. For higher energy electrons, several electron data sets have been produced primarily using the MEPED instrument aboard POES, however, validation is difficult due to the lack of independent observations of electron fluxes in the atmosphere. Further support is needed to help validate precipitation data sets using the variety of data we currently have. Moreover, using more than 2 decades worth of POES MEPED data, models based on machine learning could be trained to generate precipitation maps for time periods when data is not available. These could be used for long-term future climate simulations as well as for operational forecasting of space weather events. They would also be useful to estimate precipitating fluxes in gap regions where the NOAA16 satellite previously measured.
3) Successfully model both event-specific and long-term impacts of EEP on the various layers of the atmosphere from the troposphere through the top of the ionosphere.
- Once we understand the drivers of EEP fully, along with having accurate, global precipitation data, we can use the data for input into a variety of numerical models. Global climate models such as WACCM and ionospheric/thermospheric models such as Global Ionosphere and Thermosphere Model (GITM) rely on accurate electron inputs to drive the chemistry and dynamics of the simulations. We propose further efforts in computational resources and model development to understand all the effects EEP has on the various layers of the atmosphere. Furthermore, to validate model simulations, observations are needed for comparison. Thus, we propose the continued funding of the TIMED SABER instrument as well as the UARS MLS instrument in order to continue our measurements of the mesosphere and lower thermosphere. Finally, the funding of the upcoming GDC mission would be paramount in understanding the detection of low-energy electrons along with the impact on thermospheric composition and the potential effects on satellite drag. Given the scale and coverage of the GDC mission, we would have unprecedented data on both thermospheric chemistry and low-energy electrons.
To conclude, the field of electron precipitation has come a long way over the past decade, but with further funding, our understanding could substantially improve over the next decade. With the support of the new space-based mission GDC along with continued support of SABER and MLS, and our continued efforts in model and data set development, we could finally have a fully resolved understanding of the drivers and impacts of EEP (Miyoshi et al., 2018).
Data availability statement
The original contributions presented in the study are included in the article/supplementary material, further inquiries can be directed to the corresponding author.
Author contributions
SE: Helped draft the original manuscript and added much of the magnetosphere wave sections. CR: Helped with editing and contributed to atmospheric chemistry/observation sections. AH: Helped significantly with editing and improving the manuscript. AJ: Helped with editing. KG-S: Helped with editing.
Conflict of interest
The authors declare that the research was conducted in the absence of any commercial or financial relationships that could be construed as a potential conflict of interest.
Publisher’s note
All claims expressed in this article are solely those of the authors and do not necessarily represent those of their affiliated organizations, or those of the publisher, the editors and the reviewers. Any product that may be evaluated in this article, or claim that may be made by its manufacturer, is not guaranteed or endorsed by the publisher.
References
Andersson, M. E., Verronen, P. T., Rodger, C. J., Cliverd, M. A., and Seppälä, A. (2014). Missing driver in the Sun-Earth connection from energetic electron precipitation impacts mesospheric ozone. Nat. Comm. 5, 5197. doi:10.1038/ncomms6197
Asikainen, T., Salminen, A., Maliniemi, V., and Mursula, K. (2020). Influence of enhanced planetary wave activity on the polar vortex enhancement related to energetic electron precipitation. J. Geophys. Res. Atmos. 125, e2019JD032137. doi:10.1029/2019JD032137
Brito, Thiago V., Halford, Alexa J., and Elkington, Scot R. (2020). “Chapter 2 - ultralow frequency-wave induced losses,” in The dynamic loss of earth's radiation belts, Allison N. Jaynes, and Maria E. Usanova (Elsevier, Amsterdam, Netherlands), 29–48. doi:10.1016/B978-0-12-813371-2.00002-0
Clilverd, M. A., Rodger, C. J., McCarthy, M., Millan, R., Blum, L. W., Cobbett, N., et al. (2017). Investigating energetic electron precipitation through combining ground-based and balloon observations. J. Geophys. Res. Space Phys. 122, 534–546. doi:10.1002/2016JA022812
Halford, A. J., McGregor, S. L., Murphy, K. R., Millan, R. M., Hudson, M. K., Woodger, L. A., et al. (2015). BARREL observations of an ICME-shock impact with the magnetosphere and the resultant radiation belt electron loss. J. Geophys. Res. Space Phys. 120, 2557–2570. doi:10.1002/2014JA020873
Meredith, N. P., Horne, R. B., Sandberg, I., Papadimitriou, C., and Evans, H. D. R. (2017). Extreme relativistic electron fluxes in the Earth’s outer radiation belt: Analysis of INTEGRAL IREM data. Space weather 15, 917–933. doi:10.1002/2017SW001651
Mironova, I. A., Aplin, K. L., Arnold, F., Bazilevskaya, G. A., Harrison, R. G., Krivolutsky, A. A., et al. (2015). Energetic particle influence on the earth’s atmosphere. Space Sci. Rev. 194 (1-4), 1–96. doi:10.1007/s11214-015-0185-4
Miyoshi, Y., Hori, T., Shoji, M., Teramoto, M., Chang, T.-F., Segawa, T., et al. (2018). The ERG science center. Earth Planets Space 70 (1), 96. doi:10.1186/s40623-018-0867-8
Nesse Tyssøy, H., Sinnhuber, M., Asikainen, T., Bender, S., Clilverd, M. A., Funke, B., et al. (2021). HEPPA III intercomparison experiment on electron precipitation impacts: 1. Estimated ionization rates during a geomagnetic active period in April 2010. J. Geophys. Res. Space Phys. 126, e2021JA029128. doi:10.1029/2021JA029128
Pettit, J. M., Randall, C. E., Peck, E. D., and Harvey, V. L. (2021). A new MEPED-based precipitating electron data set. J. Geophys. Res. Space Phys. 126, e2021JA029667. doi:10.1029/2021JA029667
Randall, C. E., Harvey, V. L., Singleton, C. S., Bailey, S. M., Bernath, P. F., Codrescu, M., et al. (2007). Energetic particle precipitation effects on the Southern Hemisphere stratosphere in 1992–2005. J. Geophys. Res. 112, D08308. doi:10.1029/2006JD007696
Rees, M. H., Emery, B. A., Roble, R. G., and Stamnes, K. (1983). Neutral and ion gas heating by auroral electron precipitation. J. Geophys. Res. 88, 6289–6300. doi:10.1029/ja088ia08p06289
Ripoll, J.-F., Claudepierre, S. G., Ukhorskiy, A. Y., Colpitts, C., Li, X., Fennell, J., et al. (2020). Particle dynamics in the Earth's radiation belts: Review of current research and open questions. J. Geophys. Res. Space Phys. 125 (5), e2019JA026735. doi:10.1029/2019JA026735
Rozanov, E., Callis, L., Schlesinger, M., Yang, F., Andronova, N., and Zubov, V. (2005). Atmospheric response to NOy source due to energetic electron precipitation. Geophys. Res. Lett. 32, L14811. doi:10.1029/2005GL023041
Salminen, A., Asikainen, T., Maliniemi, V., and Mursula, K. (2019). Effect of energetic electron precipitation on the northern polar vortex: Explaining the QBO modulation via control of meridional circulation. J. Geophys. Res. Atmos. 124, 2018JD029296–5821. doi:10.1029/2018JD029296
Seppälä, A., Randall, C. E., Clilverd, M. A., Rozanov, E., and Rodger, C. J. (2009). Geomagnetic activity and polar surface air temperature variability. J. Geophys. Res. 114, A10312. doi:10.1029/2008JA014029
Shumko, M., Gallardo-Lacourt, B., Halford, A. J., Liang, J., Blum, L. W., Donovan, E., et al. (2021). A strong correlation between relativistic electron microbursts and patchy aurora. Geophys. Res. Lett. 48, e2021GL094696. doi:10.1029/2021GL094696
Solomon, S., Crutzen, P. J., and Roble, R. G. (1982). Photochemical coupling between the thermosphere and the lower atmosphere: 1. Odd nitrogen from 50 to 120 km. J. Geophys. Res. Oceans 87 (C9), 7206–7220. doi:10.1029/JC087iC09p07206
Solomon, S., Rusch, D. W., Gérard, J. C., Reid, G. C., and Crutzen, P. J. (1981). The effect of particle precipitation events on the neutral and ion chemistry of the middle atmosphere: II. Odd hydrogen. Planet. Space Sci. 8, 885–893. doi:10.1016/0032-0633(81)90078-7
Thorne, R. M. (2010). Radiation belt dynamics: The importance of wave particle interactions. Geophys. Res. Lett. 37 (22), L22107. doi:10.1029/2010GL044990
Thorne, R. M. (1980). The importance of energetic particle precipitation on the chemical composition of the middle atmosphere. Pure Appl. Geophys. 118, 128–151. doi:10.1007/BF01586448
Keywords: upper atmosphere, middle atmosphere, composition and chemistry, magnetosphere, electron precipitation, satellite observations
Citation: Pettit J, Elliott S, Randall C, Halford A, Jaynes A and Garcia-Sage K (2023) Investigation of the drivers and atmospheric impacts of energetic electron precipitation. Front. Astron. Space Sci. 10:1162564. doi: 10.3389/fspas.2023.1162564
Received: 09 February 2023; Accepted: 13 March 2023;
Published: 30 March 2023.
Edited by:
Noora Partamies, The University Centre in Svalbard, NorwayReviewed by:
Monika Szelag, Finnish Meteorological Institute, FinlandCopyright © 2023 Pettit, Elliott, Randall, Halford, Jaynes and Garcia-Sage. This is an open-access article distributed under the terms of the Creative Commons Attribution License (CC BY). The use, distribution or reproduction in other forums is permitted, provided the original author(s) and the copyright owner(s) are credited and that the original publication in this journal is cited, in accordance with accepted academic practice. No use, distribution or reproduction is permitted which does not comply with these terms.
*Correspondence: Joshua Pettit, am9zaHVhLnBldHRpdEBjb2xvcmFkby5lZHU=