- Center for Theoretical Astrophysics and Cosmology, Institute for Computational Science, University of Zurich, Zurich, Switzerland
The characterisation of giant exoplanets is crucial to constrain giant planet formation and evolution theory and for putting the solar-system’s giant planets in perspective. Typically, mass-radius (M-R) measurements of moderately irradiated warm Jupiters are used to estimate the planetary bulk composition, which is an essential quantity for constraining giant planet formation, evolution and structure models. The successful launch of the James Webb Space Telescope (JWST) and the upcoming ARIEL mission open a new era in giant exoplanet characterisation as atmospheric measurements provide key information on the composition and internal structure of giant exoplanets. In this review, we discuss how giant planet evolution models are used to infer the planetary bulk composition, and the connection between the compositions of the interior and atmosphere. We identify the important theoretical uncertainties in evolution models including the equations of state, atmospheric models, chemical composition, interior structure and main energy transport processes. Nevertheless, we show that atmospheric measurements by JWST and ARIEL and the accurate determination of stellar ages by PLATO can significantly reduce the degeneracy in the inferred bulk composition. Furthermore, we discuss the importance of evolution models for the characterisation of direct-imaged planets. We conclude that giant planet theory has a critical role in the interpretation of observation and emphasise the importance of advancing giant planet theory.
1 Introduction
The study of giant exoplanets gives a unique peek into the formation of planets, because their composition is linked to their form history (e.g., Mousis et al., 2009; Helled et al., 2014; Johansen and Lambrechts, 2017; Ginzburg and Chiang, 2020). Since the first discovery of the hot Jupiter 51 Peg b (Mayor and Queloz, 1995), there have been over a thousand detections of giant exoplanets with diverse masses, sizes, and equilibrium temperatures (see Figure 1). Most observed giant exoplanets are hot Jupiters, but a fraction are so-called warm giants with equilibrium temperatures below ∼1000 K. Warm giants are particularly interesting objects, since, compared to hot Jupiters, they are superior for characterisation. This is because hot Jupiters are inflated by a poorly understood mechanism (e.g., Fortney and Nettelmann, 2010; Weiss et al., 2013; Baraffe et al., 2014; Fortney et al., 2021; Sarkis et al., 2021), and therefore their interiors are more difficult to characterise. The large observed radii of giant exoplanets imply the presence of massive hydrogen-helium envelopes, which can progressively contract and cool as the planets evolve in time (e.g., Hubbard, 1977; Burrows et al., 2001). This implies that any effort to characterise these planets must rely on theoretical (numerical) models that simulate the planetary evolution.
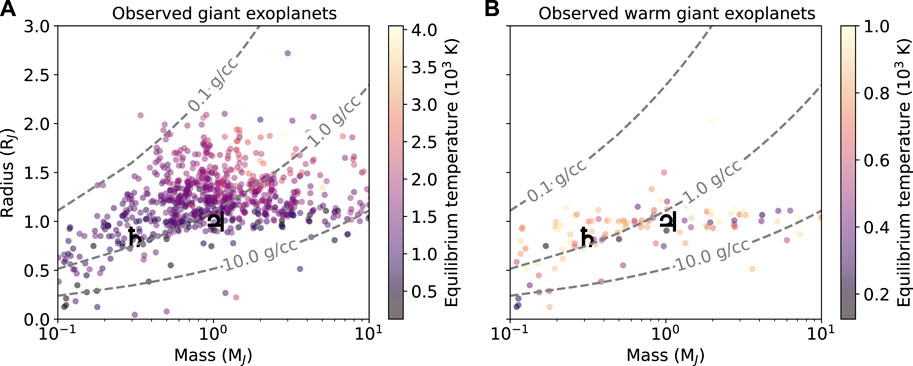
FIGURE 1. Masses and radii of giant exoplanets with masses between 0.1 ≤ MJ ≤ 10 (A) and equilibrium temperatures below 1000 K (B). The dashed lines show lines of constant density. Jupiter () and Saturn (
) are shown with their respective symbols. Error bars are omitted for readability. The data was taken from the NASA Exoplanet Archive.
The combination of evolution models with measurements of planetary radius, mass, and stellar age enables the estimation of the planetary bulk composition, which is, in the case of giant planets, represented by the heavy-element mass (e.g., Guillot et al., 2006; Fortney et al., 2007; Guillot, 2008; Miller and Fortney, 2011; Mordasini, 2014; Thorngren et al., 2016). This is done by comparing the observed radius to the one predicted by evolution models. In order to include the measurement uncertainties, often the bulk metallicity is inferred as a posterior distribution. Since giant planets cool and contract, their evolution path directly affects their characterisation: At a given observed planetary age, a different planetary radius would be predicted, yielding a different estimate of the composition. Furthermore, a determination of the bulk composition of giant planets relies on accurate measurements of the planetary radius, mass and stellar ages. Otherwise, the inferred heavy-element mass is highly uncertain. While planetary masses and radii are often somewhat well constrained, stellar ages are not well-determined and have uncertainties of a few Gyr. The upcoming PLATO mission (Rauer et al., 2014) is expected to accurately determine stellar ages, which is crucial for constraining the planetary composition, in particular for young planets that cool and shrink rapidly.
Furthermore, JWST (Gardner et al., 2006) and the upcoming ARIEL mission (Tinetti et al., 2018) will push exoplanet characterisation to the next level by determining the atmospheric composition of many giant exoplanets. To-date only several hot Jupiter atmospheres have been studied (e.g., Madhusudhan et al., 2014; Welbanks et al., 2019; Ben-Yami et al., 2020; Line et al., 2021), with recent detection of both water (Rustamkulov et al., 2022) and carbon dioxide (The JWST Transiting Exoplanet Community Early Release Science Team et al., 2022). Future JWST and ARIEL observations will provide a more complete picture of the atmospheric compositions of hot Jupiters. They will also observe warm Jupiters, whose interior models have much less theoretical uncertainties compared to hot Jupiters and are therefore superior targets for interior characterisation. Therefore, the characterisation of warm giant exoplanets is essential to better understand the origins of giant planets (Teske et al., 2019; Turrini et al., 2021; Knierim et al., 2022).
Additionally, masses and radii of exoplanets are now more accurately determined by observations. This is in part due to more precise radial velocity and transit measurements, but also thanks to improved information of the host stars from the GAIA mission (Gaia Collaboration et al., 2016). Therefore, exoplanetary science is now in an era at which theoretical uncertainties are no longer negligible and can affect the data interpretation. In order to interpret this current and upcoming wealth of data, a robust theoretical foundation and knowledge of how giant planets evolve are required.
In this review, we summarise the current state-of-the art in warm giant planet characterisation, and discuss the challenges due to theoretical uncertainties of evolution models. We discuss the role of evolution models in the interpretation of upcoming PLATO, JWST and ARIEL measurements, and show that these missions will significantly improve our understanding of giant exoplanets.
2 Giant planet evolution models
Giant planet evolution models are constructed assuming that the planets are spherically symmetric and in hydrostatic equilibrium. The equations of planetary evolution are then written as a coupled set of partial differential equations describing the mass, momentum and energy conservation, and the transport of chemical elements and energy (e.g., Kippenhahn et al., 2012). In most models, energy transport is assumed to be by radiation (and conduction) or convection, however there is also the possibility of double-diffusive energy transport (Wood et al., 2013; Radko et al., 2014). The Ledoux criterion (Ledoux, 1947) is used locally to determine the dominant energy mechanism. Convection is usually modelled with the mixing-length theory (Böhm-Vitense, 1958). A good approximation is that rigorous convection leads to (nearly) adiabatic planetary interiors. The missing pieces are an appropriate equation of state, a description of the opacity, and atmospheric boundary conditions.
Since there is no general analytical solution to the evolution equations they must be solved numerically (e.g., Henyey et al., 1965; Guillot and Morel, 1995; Burrows et al., 1997; Vazan et al., 2015; Thorngren et al., 2016). A popular open-source code is Modules for Experiments in Stellar Astrophysics (MESA; Paxton et al. (2011); Paxton et al., 2013; Paxton et al., 2015; Paxton et al., 2018; Paxton et al., 2019); Jermyn et al. (2022)), which is also suitable to calculate the evolution of planets (e.g., Mankovich et al., 2016; Berardo and Cumming, 2017; Cumming et al., 2018; Müller et al., 2020b; Malsky and Rogers, 2020). Recently, Müller and Helled (2021) used MESA to calculate an extensive grid of giant planet evolution models, and developed a python module to generate cooling tracks by interpolation.
2.1 Theoretical uncertainties in giant planet evolution models
Giant planet evolution models have to make a range of assumptions, such as the equations of state (hereafter EoS) for the different elements and their distribution in the interior, the opacity and the atmospheric model, as well as what metal is used to represent the heavy elements. These choices influence the cooling, and therefore the predictions from the evolution models (such as radius or luminosity) to various degrees (Guillot, 1999). In the following sections, we discuss the key assumptions and parameters that affect giant planet evolution models, and show how they can influence the interpretation of exoplanetary data.
2.1.1 Equations of state and atmospheric models
Typically, giant planet evolution models involve three different equations of state that are combined with the ideal-mixing law: hydrogen (H), helium (He) and a heavy element (Z). There are several hydrogen-helium equations of state that are often used in the modelling of giant planets (Saumon et al., 1995; Militzer and Hubbard, 2013; Becker et al., 2014; Chabrier et al., 2019). Since giant planets are hydrogen-dominated in composition, the uncertainties in the H EoS have important implications for the interior modelling of giant planets (Helled et al., 2020; Howard et al., 2023). For example, switching between the one from Saumon et al. (1995) to Chabrier et al. (2019) leads to predicted radii that are smaller by up to ∼10% (Müller et al., 2020a). In addition, non-ideal H-He interactions that are often neglected are non-negligible and can change the predicted radius by up to 8%, especially at younger ages (Howard and Guillot, 2023).
A common simplification of evolution models is that they are limited to representing all the heavy elements with one component. This is often water, a type of rock such as olivine or SiO2, or a water-rock mixture (see, e.g., More et al., 1988; Thompson, 1990; Mazevet et al., 2019). However, in reality, giant planets are not expected to contain a single heavy element or rock-type, but rather a combination. The uncertainty from the heavy-element composition also affects the predicted radius, likely by a few percent (e.g., Baraffe et al., 2008; Vazan et al., 2015). This is usually most significant for lower-mass giant planets, with masses below
Since giant planets cool down by radiating energy from their atmospheres, the opacity and the atmospheric model are crucial for the evolution. Unfortunately, these parameters are not well constrained. For the opacity, different contributions must be considered: molecular, grains and clouds. A commonly used molecular opacity is from Freedman et al. (2014) which also includes the effect of heavy elements. This is particularly important for irradiated planets with enriched atmospheres. However, this also means that if the atmospheric composition is unknown, the molecular opacity is not well determined. It is also not known whether clouds or grains are present and how efficient they are at blocking infrared radiation as clouds could trap heat in the interior and slow down cooling (Vazan et al., 2013; Mordasini, 2014; Poser et al., 2019). An additional complication is that most giant exoplanets observed today are highly irradiated and have an unknown albedo. A variety of models exist to account for the instellation, including semi-gray and full atmospheric models (Fortney et al., 2007; Guillot, 2010; Parmentier and Guillot, 2014). The uncertainties linked to the opacity are particularly large for young planets due to their fast contraction. In that case, differences in opacity can significantly affect the estimates of the planetary parameters. Overall, the uncertainties in opacities and atmospheric models probably lead to ∼10% uncertainty in the predicted planetary radius (Valsecchi et al., 2015; Müller et al., 2020a), but are potentially even larger for young planets.
2.1.2 Primordial state, energy transport, and distribution of elements
For young giant planets (∼1–10 Myr), an additional complication is that the primordial internal structure and its thermal state are unknown and likely depends on the formation history (e.g., Baraffe et al., 2003; Marley et al., 2007; Spiegel and Burrows, 2012). If giant planets are fully convective shortly after their formation, this issue is resolved after a few 10 Myr, since different initial configurations converge to the same cooling track (e.g., Marley et al., 2007; Berardo and Cumming, 2017; Berardo et al., 2017; Cumming et al., 2018). However, if young giant planets are not fully convective, the primordial state will significantly affect the planetary contraction. Recent formation models predict that young giant planets are expected to have composition gradients and therefore may not be fully convective, which complicates the situation (e.g., Helled and Stevenson, 2017; Lozovsky et al., 2017; Valletta and Helled, 2020; Stevenson et al., 2022). Indeed, studies of Jupiter and Saturn suggest that parts of their interiors are not fully convective today (e.g., Debras and Chabrier, 2019; Mankovich and Fuller, 2021).
If young giant planets are not mostly convective, the initial conditions are not lost as quickly and the cooling is slower. Therefore, the luminosity at young ages is lower than predicted from fully convective models. After a few Gyr, the predicted luminosity would be higher, since the energy transport is less efficient (Leconte and Chabrier, 2012; Wood et al., 2013; Radko et al., 2014) and there is more primordial heat trapped inside the planet. This introduces an additional uncertainty to evolution models. For example, Kurokawa and Inutsuka (2015) showed that old giant planets cooling by double-diffusive convection are inflated by ∼10%, which would mean an increase in their luminosity by ∼20%. In addition to the heat transport mechanism, the radius of a giant planet is also influenced by the distribution of chemical elements in the interior. The two extremes are that all heavy elements are in the core (core-envelope structure) or homogeneously distributed. The latter generally results in a smaller planet by a few percent (e.g., Baraffe et al., 2008; Vazan et al., 2013; Müller et al., 2020a).
Lastly, it is also possible that extraneous events, such as giant impacts, contribute to the inflation of giant planets. These are usually not included in evolution models due to their stochastic nature. However, unless collisions are frequent and violent, which is unlikely after a few Gyr, the energy deposited during the impacts is quickly re-radiated, and the planets are only inflated for a short time, up to a few 104 years (Müller and Helled, 2023). Nevertheless, collisions could be important for the interpretation of individual giant planets.
3 Warm giant exoplanet characterisation
3.1 Mass-metallicity trends of warm giant planets
Thorngren et al. (2016) used evolution models to infer the metallicity of 47 warm giant planets (20M⊕ < M < 20MJ) with moderate instellation fluxes (F* < 2 × 108 erg s−1 cm−2). The main results from the study were that there is 1) a correlation between the heavy-element mass of a planet and its total mass, and 2) a strong relation between the metal-enrichment of a planet (Z/Z*, where Z* is the metallicity of the host star) and its mass. They found that the mass-scaling was approximately
Teske et al. (2019) performed a slightly different study by focusing on whether different host-star heavy elements are correlated with the bulk heavy-element mass of warm giants. Their findings suggest that the stellar metallicity is not correlated with the planetary residual metallicity, i.e., the residual metallicity that is not explained by the trend with planetary mass. Using different evolution models, Müller et al. (2020a) later independently inferred a qualitative mass-metallicity correlation in agreement with the results from Thorngren et al. (2016). However, they also showed that different model parameters, such as EoS and opacity, can have a large influence on the inferred bulk metallicity of giant planets. A similar mass-metallicity trend was also found by Müller and Helled (2023) who analysed warm giants in the Ariel mission reference sample (Edwards and Tinetti, 2022). Their results suggest a lower heavy-element mass for a given planetary mass compared to Thorngren et al. (2016), and also a less statistically significant correlation. It is fair to say that currently it is unclear to what extent, if any, trend exists between the planetary heavy-element mass and planetary mass. This is in particular due to the many theoretical uncertainties associated with the models used for the data interpretation. Therefore, there is a risk of over-interpreting the comparisons between predictions from formation models with the inferred mass-metallicity trends from observations.
The inferred mass-metallicity correlation serves as an important constraint for planet formation models, and can be used to test planet formation theory. The observation that the metal enrichment decreases with planetary mass is in qualitative agreement with the core-accretion model (e.g., Pollack et al., 1996), in which a heavy-element core accretes large amounts of metal-poor gas as the planet grows. Planetary population synthesis models (Mordasini et al., 2014) also yield a similar power-law, however with different exponents. It has also been suggested that the observed metal-enrichment can be explained if it traces the final assembly of giant planets, where the heavy elements are predominantly accreted from a planetesimal disks with large gaps (Hasegawa et al., 2018). One of the major unresolved problems is that the large heavy-element masses of some giant exoplanets cannot be explained by standard formation models. Formation models suggest that the core masses of giant planets are limited to a few 10M⊕ (e.g., Pollack et al., 1996; Helled et al., 2014; Bitsch et al., 2018). Since the accreted gas likely has a stellar metallicity, the additional metal enrichment has to come from different sources, such as planetesimal accretion (Mousis et al., 2009; Shibata and Ikoma, 2019; Shibata et al., 2020), pebble accretion (Johansen and Lambrechts, 2017), and collisions between planetary embryos (Ginzburg and Chiang, 2020).
4 Connection to future observations
In this section, we use the evolution models from Müller and Helled (2021) to demonstrate how upcoming observations can improve the characterisation of giant exoplanets (Section 4.1 and Section 4.2) and the importance of evolution models in determining the mass of direct-imaged planets (Section 4.3).
4.1 PLATO: the importance of accurate stellar age measurements
Stellar ages are currently often only determined within a few Gyr, which makes the inferred composition of giant exoplanets degenerate. This is demonstrated in Figure 2A for three exoplanets that cover the typical mass-range of warm giants (Kepler 16b, Kepler 167e and K2 144b). The coloured lines show the calculated evolution of the three planets for different bulk metallicities. By comparing the observed radii to the one from the evolution models, it is clear that the large uncertainty of the stellar age causes a degeneracy in the inferred composition. Kepler 16b, for example, has a very precisely determined radius, but its composition still cannot be clearly determined due to its large age uncertainty. This is where the PLATO mission will clearly improve giant exoplanet characterisation: It will determine stellar ages to within an accuracy of ∼ 10%, breaking the degeneracy in the inferred heavy-element mass and reduce its uncertainty by about a factor of two (Müller and Helled, 2023).
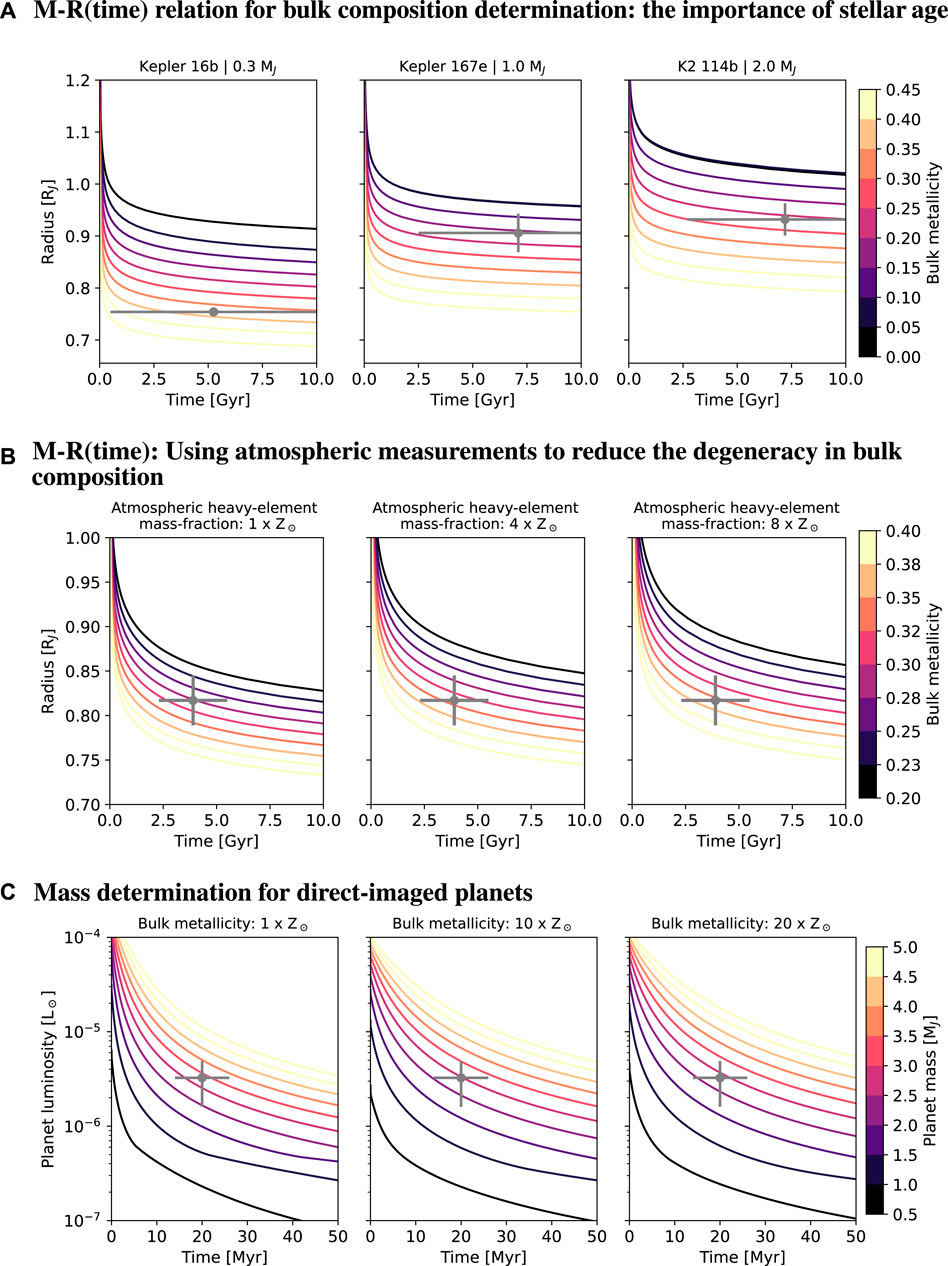
FIGURE 2. (A) Radius evolution for different bulk metallicites shown together with three exoplanets (Kepler 16b, Kepler 167e, K2 114b). The large age uncertainties cause a degeneracy in the inferred composition. (B) Radius evolution for different bulk (coloured lines) and atmospheric (columns) heavy-element mass-fraction shown together with the exoplanet NGTS 11b. Measuring the atmospheric metallicity breaks the degeneracy in the inferred composition. (C) Luminosity evolution for different masses (coloured lines) and bulk metallicites (columns) shown together with the directly imaged exoplanet 51 Eri b. The inferred mass of the planet depends on the assumed composition.
4.2 JWST and ariel: atmospheric measurements and the connection to the bulk composition
Measuring the chemical composition of giant planets’ atmospheres promises a new dawn in giant exoplanets characterisation: Knowledge of the atmospheric composition can reveal information on the planetary interior and origin (Burrows, 2014; Teske et al., 2019; Turrini et al., 2021; Edwards et al., 2022; Helled et al., 2022). Although the connection between the atmospheric composition and that of the planetary bulk is challenging and is yet to be determined (e.g., Helled et al., 2022), in the case of warm Jupiters the planets can be better characterised. In particular, it was clearly shown that atmospheric measurements can break the degeneracy in determining the planetary bulk composition and it is expected that measurements of warm Jupiter atmospheres can reduce the uncertainty of the bulk composition by at least a factor of four (Müller and Helled, 2023). We demonstrate this in Figure 2B, where we simulate the evolution of NGTS-11 b for various bulk and atmospheric heavy-element mass-fractions (atmospheric metallicity). Depending on the assumed atmospheric metallicity, the observed age and radius of NGTS-11 b matches cooling curves with a different heavy-element fraction. Therefore, the determination of a planet’s atmospheric composition clearly improves the planet’s characterisation. JWST will further improve the planetary characterisation since white-light curves can constrain the planetary transit depths to very high precision. This will significantly reduce the uncertainties on the planetary radius and therefore on the bulk composition.
However, a key question remains: What is the link between the atmospheric and the bulk composition? We know that the solar system gas giants likely have different interior and atmospheric compositions (e.g., Helled, 2018). Characterising the atmospheres of many giant exoplanets will further constrain the internal structure and will reveal how well-mixed they are (Thorngren and Fortney, 2019). It should be noted, however, that the measurements only trace the very upper atmospheres and it is unclear whether and how this composition changes with depth. Finally, since the mass of the outermost atmosphere is very small and the outermost part of the atmosphere is radiative, the measured atmospheric composition could be a result of a recent “pollution”, for example, by accreting a small object which vaporised in the atmosphere. It is therefore crucial to better understand under what conditions the atmospheric composition can be linked to the bulk composition.
4.3 Characterisation of direct-imaged planets
Another important application for evolution models is the mass-determination of exoplanets that are detected by direct imaging (e.g., Mordasini et al., 2017). This is achieved by using the planet’s thermal emissions and comparing it to evolution models. Direct-imaged exoplanets are commonly younger than 1 Gyr and orbit at large radial distances. This allows probing a completely different regime than the usual mass-radius measurements, and therefore provides additional constraints on planet formation theory. Earlier mass-characterisations used tables of planetary isochrones (e.g., Baraffe et al., 2003). However, in addition to the mass there are many other parameters that influence the planet’s luminosity, for example, its composition. It is therefore important to use evolution models that go beyond only accounting for the planetary mass to estimate the mass of direct-imaged exoplanets. In Figure 2C we show the luminosity as a function of time for evolution models between 0.5 − 5MJ and different bulk metallicites. The observed luminosity and the planet’s age are shown in the same figure. It is evident that the inferred mass depends on the assumed composition.
Most evolution models assume a hot-start formation scenario (Baraffe et al., 2003; Marley et al., 2007). While currently hot-starts are the expected formation pathway in the core accretion framework (Berardo and Cumming, 2017; Berardo et al., 2017; Cumming et al., 2018), it is important to note that cold- (Marley et al., 2007; Fortney et al., 2008) and warm-start scenarios would (Spiegel and Burrows, 2012; Marleau and Cumming, 2014) yield significantly different mass estimates.
5 Conclusion
Advanced evolution models are crucial for the characterisation of giant exoplanets. Evolution models provide information of the planetary bulk composition using measurements of mass and radius (with planetary age), connect the atmospheric composition with that of the bulk, and determine the planetary mass of direct-imaged planets. There are theoretical uncertainties associated with the models that can affect the data interpretation, and taking full advantage of future observations requires the determination of the most realistic parameters for evolution models, which is work in progress. Advances in giant exoplanet characterisation are expected with the accurate determination of stellar ages from PLATO and atmospheric measurements from JWST and ARIEL as well as ground-based observations. In particular, future measurements will constrain the bulk heavy-element mass of giant exoplanets and reveal information on the link between the atmospheres and the interiors of giant exoplanets.
Author contributions
SM was the main author of the article and prepared the figures. RH made significant contributions and edits to the text. Both authors approved the article for publication.
Funding
We acknowledge support from Swiss National Science Foundation (SNSF) grant 200020_188460.
Acknowledgments
We thank Daniel Thorngren and an anonymous referee for valuable comments. We acknowledge additional support from the National Centre for Competence in Research “PlanetS” supported by SNSF. This research used data from the NASA Exoplanet Archive, which is operated by the California Institute of Technology, under contract with the National Aeronautics and Space Administration under the Exoplanet Exploration Program. Extensive use was made of the python packages numpy (Harris et al., 2020), matplotlib (Hunter, 2007) and jupyter (Kluyver et al., 2016).
Conflict of interest
The authors declare that the research was conducted in the absence of any commercial or financial relationships that could be construed as a potential conflict of interest.
Publisher’s note
All claims expressed in this article are solely those of the authors and do not necessarily represent those of their affiliated organizations, or those of the publisher, the editors and the reviewers. Any product that may be evaluated in this article, or claim that may be made by its manufacturer, is not guaranteed or endorsed by the publisher.
References
Baraffe, I., Chabrier, G., Barman, T. S., Allard, F., and Hauschildt, P. H. (2003). Evolutionary models for cool Brown dwarfs and extrasolar giant planets. The case of HD 209458. A&A 402, 701–712. doi:10.1051/0004-6361:20030252
Baraffe, I., Chabrier, G., and Barman, T. (2008). Structure and evolution of super-Earth to super-Jupiter exoplanets. I. Heavy element enrichment in the interior. A&A 482, 315–332. doi:10.1051/0004-6361:20079321
Baraffe, I., Chabrier, G., Fortney, J., and Sotin, C. (2014). “Planetary internal structures,” in Protostars and planets VI. Editors H. Beuther, R. S. Klessen, C. P. Dullemond, and T. Henning (University of Arizona Press), 763. doi:10.2458/azu_uapress_9780816531240-ch033
Becker, A., Lorenzen, W., Fortney, J. J., Nettelmann, N., Schöttler, M., and Redmer, R. (2014). Ab initio equations of state for hydrogen (H-REOS.3) and helium (He-REOS.3) and their implications for the interior of Brown dwarfs. Astrophysical J. Suppl. Ser. 215, 21. doi:10.1088/0067-0049/215/2/21
Ben-Yami, M., Madhusudhan, N., Cabot, S. H. C., Constantinou, S., Piette, A., Gandhi, S., et al. (2020). Neutral Cr and V in the atmosphere of ultra-hot jupiter WASP-121 b. Astrophysical J. 897, L5. doi:10.3847/2041-8213/ab94aa
Berardo, D., and Cumming, A. (2017). Hot-start giant planets form with radiative interiors. Astrophysical J. 846, L17. doi:10.3847/2041-8213/aa81c0
Berardo, D., Cumming, A., and Marleau, G.-D. (2017). The evolution of gas giant entropy during formation by runaway accretion. Astrophysical J. 834, 149. doi:10.3847/1538-4357/834/2/149
Bitsch, B., Morbidelli, A., Johansen, A., Lega, E., Lambrechts, M., and Crida, A. (2018). Pebble-isolation mass: Scaling law and implications for the formation of super-Earths and gas giants. A&A 612, A30. doi:10.1051/0004-6361/201731931
Böhm-Vitense, E. (1958). Über die Wasserstoffkonvektionszone in Sternen verschiedener Effektivtemperaturen und Leuchtkräfte. Mit 5 Textabbildungen. Z. für Astrophysi 46, 108.
Burrows, A. S. (2014). Spectra as windows into exoplanet atmospheres. Proc. Natl. Acad. Sci. 111 (35), 12601–12609.
Burrows, A., Hubbard, W. B., Lunine, J. I., and Liebert, J. (2001). The theory of brown dwarfs and extrasolar giant planets. Rev. Mod. Phys. 73 (3), 719–765.
Burrows, A., Marley, M., Hubbard, W. B., Lunine, J. I., Guillot, T., and Saumon, D. (1997). A nongray theory of extrasolar giant planets and brown dwarfs. ApJ 491 (2), 856–875.
Chabrier, G., Mazevet, S., and Soubiran, F. (2019). A new equation of state for dense hydrogen-helium mixtures. Astrophysical J. 872, 51. doi:10.3847/1538-4357/aaf99f
Cumming, A., Helled, R., and Venturini, J. (2018). The primordial entropy of Jupiter. Mon. Notices R. Astronomical Soc. 477, 4817–4823. doi:10.1093/mnras/sty1000
Debras, F., and Chabrier, G. (2019). New models of jupiter in the context of juno and galileo. Astrophysical J. 872, 100. doi:10.3847/1538-4357/aaff65
Edwards, B., Changeat, Q., Tsiaras, A., Hou Yip, K., Al-Refaie, A. F., Anisman, L., et al. (2022). Exploring the ability of HST WFC3 G141 to uncover trends in populations of exoplanet atmospheres through a homogeneous transmission survey of 70 gaseous planets. arXiv e-prints , arXiv:2211.00649:10.48550/arXiv.2211.00649.
Edwards, B., and Tinetti, G. (2022). The Ariel target list: The impact of TESS and the potential for characterising multiple planets within a system. arXiv e-prints , arXiv:2205.05073.
Fortney, J. J., Dawson, R. I., and Komacek, T. D. (2021). Hot Jupiters: Origins, structure, atmospheres. J. Geophys. Res. (Planets) 126, e06629. doi:10.1029/2020JE006629
Fortney, J. J., Marley, M. S., and Barnes, J. W. (2007). Planetary radii across five orders of magnitude in mass and stellar insolation: Application to transits. Astrophysical J. 659, 1661–1672. doi:10.1086/512120
Fortney, J. J., Marley, M. S., Saumon, D., and Lodders, K. (2008). Synthetic spectra and colors of young giant planet atmospheres: Effects of initial conditions and atmospheric metallicity. Astrophysical J. 683, 1104–1116. doi:10.1086/589942
Fortney, J. J., and Nettelmann, N. (2010). The interior structure, composition, and evolution of giant planets. Space Sci. Rev. 152, 423–447. doi:10.1007/s11214-009-9582-x
Freedman, R. S., Lustig-Yaeger, J., Fortney, J. J., Lupu, R. E., Marley, M. S., and Lodders, K. (2014). Gaseous mean opacities for giant planet and ultracool dwarf atmospheres over a range of metallicities and temperatures. Astrophysical J. Suppl. Ser. 214, 25. doi:10.1088/0067-0049/214/2/25
Gardner, J. P., Mather, J. C., Clampin, M., Doyon, R., Greenhouse, M. A., Hammel, H. B., et al. (2006). The James Webb Space telescope. Space Sci. Rev. 123, 485–606. doi:10.1007/s11214-006-8315-7
Gaia Collaboration Prusti, T., de Bruijne, J. H. J., Brown, A. G. A., Vallenari, A., Babusiaux, C., Bailer-Jones, C. A. L., et al. (2016). The Gaia mission. A&A 595, A1. doi:10.1051/0004-6361/201629272
Ginzburg, S., and Chiang, E. (2020). Heavy-metal Jupiters by major mergers: Metallicity versus mass for giant planets. MNRAS 498, 680–688. doi:10.1093/mnras/staa2500
Guillot, T. (1999). Interiors of giant planets inside and outside the solar system. Science 286, 72–77. doi:10.1126/science.286.5437.72
Guillot, T., and Morel, P. (1995). Cepam: A code for modeling the interiors of giant planets. A&AS 109, 109–123.
Guillot, T. (2010). On the radiative equilibrium of irradiated planetary atmospheres. A&A 520, A27. doi:10.1051/0004-6361/200913396
Guillot, T., Santos, N. C., Pont, F., Iro, N., Melo, C., and Ribas, I. (2006). A correlation between the heavy element content of transiting extrasolar planets and the metallicity of their parent stars. A&A 453, L21–L24. doi:10.1051/0004-6361:20065476
Guillot, T. (2008). The composition of transiting giant extrasolar planets. Phys. Scr. 130, 014023. doi:10.1088/0031-8949/2008/T130/014023
Harris, C. R., Millman, K. J., van der Walt, S. J., Gommers, R., Virtanen, P., Cournapeau, D., et al. (2020). Array programming with NumPy. Nature 585, 357–362. doi:10.1038/s41586-020-2649-2
Hasegawa, Y., Bryden, G., Ikoma, M., Vasisht, G., and Swain, M. (2018). The origin of the heavy-element content trend in giant planets via core accretion. Astrophysical J. 865, 32. doi:10.3847/1538-4357/aad912
Helled, R., Bodenheimer, P., Podolak, M., Boley, A., Meru, F., Nayakshin, S., et al. (2014). “Giant planet formation, evolution, and internal structure,” in Protostars and planets VI. Editors H. Beuther, R. S. Klessen, C. P. Dullemond, and T. Henning (University of Arizona Press), 643. doi:10.2458/azu_uapress_9780816531240-ch028
Helled, R., Mazzola, G., and Redmer, R. (2020). Understanding dense hydrogen at planetary conditions. Nat. Rev. Phys. 2, 562–574. doi:10.1038/s42254-020-0223-3
Helled, R., and Stevenson, D. (2017). The fuzziness of giant planets’ cores. Astrophysical J. 840, L4. doi:10.3847/2041-8213/aa6d08
Helled, R. (2018). The interiors of jupiter and Saturn. 175. doi:10.1093/acrefore/9780190647926.013.175
Helled, R., Werner, S., Dorn, C., Guillot, T., Ikoma, M., Ito, Y., et al. (2022). Ariel planetary interiors white paper. Exp. Astron. 53, 323–356. doi:10.1007/s10686-021-09739-3
Henyey, L., Vardya, M. S., and Bodenheimer, P. (1965). Studies in stellar evolution. III. The calculation of model envelopes. Astrophysical J. 142, 841. doi:10.1086/148357
Howard, S., and Guillot, T. (2023). Accounting for non-ideal mixing effects in the hydrogen-helium equation of state. arXiv e-prints , arXiv:2302.07902. doi:10.48550/arXiv.2302.07902
Howard, S., Guillot, T., Bazot, M., Miguel, Y., Stevenson, D. J., Galanti, E., et al. (2023). Jupiter’s interior from Juno: Equation-of-state uncertainties and dilute core extent. arXiv e-prints , arXiv:2302.09082. doi:10.48550/arXiv.2302.09082
Hubbard, W. B. (1977). The jovian surface condition and cooling rate. Icarus 30, 305–310. doi:10.1016/0019-1035(77)90164-6
Hunter, J. D. (2007). Matplotlib: A 2d graphics environment. Comput. Sci. Eng. 9, 90–95. doi:10.1109/MCSE.2007.55
Jermyn, A. S., Bauer, E. B., Schwab, J., Farmer, R., Ball, W. H., Bellinger, E. P., et al. (2022). Modules for Experiments in stellar Astrophysics (MESA): Time-dependent convection, energy conservation, automatic differentiation, and infrastructure. arXiv e-prints , arXiv:2208.03651.
Johansen, A., and Lambrechts, M. (2017). Forming planets via pebble accretion. Annu. Rev. Earth Planet. Sci. 45, 359–387. doi:10.1146/annurev-earth-063016-020226
Kippenhahn, R., Weigert, A., and Weiss, A. (2012). Stellar structure and evolution. Springer-Verlag Berlin Heidelberg. doi:10.1007/978-3-642-30304-3
Kluyver, T., Ragan-Kelley, B., Pérez, F., Granger, B., Bussonnier, M., Frederic, J., et al. (2016). “Jupyter notebooks - a publishing format for reproducible computational workflows,” in Positioning and power in academic publishing: Players, agents and agendas. Editors F. Loizides, and B. Scmidt (Netherlands: IOS Press), 87–90.
Knierim, H., Shibata, S., and Helled, R. (2022). Constraining the origin of giant exoplanets via elemental abundance measurements. A&A 665, L5. doi:10.1051/0004-6361/202244516
Kurokawa, H., and Inutsuka, S.-i. (2015). On the radius anomaly of hot Jupiters: Reexamination of the possibility and impact of layered convection. Astrophysical J. 815, 78. doi:10.1088/0004-637X/815/1/78
Leconte, J., and Chabrier, G. (2012). A new vision of giant planet interiors: Impact of double diffusive convection. A&A 540, A20. doi:10.1051/0004-6361/201117595
Ledoux, P. (1947). Stellar models with convection and with discontinuity of the mean molecular. Astrophysical J. 105, 305. doi:10.1086/144905
Line, M. R., Brogi, M., Bean, J. L., Gandhi, S., Zalesky, J., Parmentier, V., et al. (2021). A solar C/O and sub-solar metallicity in a hot Jupiter atmosphere. Nature 598, 580–584. doi:10.1038/s41586-021-03912-6
Lozovsky, M., Helled, R., Rosenberg, E. D., and Bodenheimer, P. (2017). Jupiter’s formation and its primordial internal structure. Astrophysical J. 836, 227. doi:10.3847/1538-4357/836/2/227
Madhusudhan, N., Crouzet, N., McCullough, P. R., Deming, D., and Hedges, C. (2014). H2O abundances in the atmospheres of three hot Jupiters. Astrophysical J. 791, L9. doi:10.1088/2041-8205/791/1/L9
Malsky, I., and Rogers, L. A. (2020). Coupled thermal and compositional evolution of photo evaporating planet envelopes. arXiv e-prints , arXiv:2002.06466.
Mankovich, C., Fortney, J. J., and Moore, K. L. (2016). Bayesian evolution models for jupiter with helium rain and double-diffusive convection. Astrophysical J. 832, 113. doi:10.3847/0004-637X/832/2/113
Mankovich, C., and Fuller, J. (2021). A diffuse core in Saturn revealed by ring seismology. arXiv e-prints , arXiv:2104.13385.
Marleau, G. D., and Cumming, A. (2014). Constraining the initial entropy of directly detected exoplanets. MNRAS 437, 1378–1399. doi:10.1093/mnras/stt1967
Marley, M. S., Fortney, J. J., Hubickyj, O., Bodenheimer, P., and Lissauer, J. J. (2007). On the luminosity of young Jupiters. Astrophysical J. 655, 541–549. doi:10.1086/509759
Mayor, M., and Queloz, D. (1995). A Jupiter-mass companion to a solar-type star. Nature 378, 355–359. doi:10.1038/378355a0
Mazevet, S., Licari, A., Chabrier, G., and Potekhin, A. Y. (2019). Ab initio based equation of state of dense water for planetary and exoplanetary modeling. A&A 621, A128. doi:10.1051/0004-6361/201833963
Militzer, B., and Hubbard, W. B. (2013). Ab initio equation of state for hydrogen-helium mixtures with recalibration of the giant-planet mass-radius relation. Astrophysical J. 774, 148. doi:10.1088/0004-637X/774/2/148
Miller, N., and Fortney, J. J. (2011). The heavy-element masses of extrasolar giant planets, revealed. Astrophysical J. 736, L29. doi:10.1088/2041-8205/736/2/L29
Mordasini, C. (2014). Grain opacity and the bulk composition of extrasolar planets: II. An analytical model for grain opacity in protoplanetary atmospheres⋆. A&A 572, A118. doi:10.1051/0004-6361/201423702
Mordasini, C., Klahr, H., Alibert, Y., Miller, N., and Henning, T. (2014). Grain opacity and the bulk composition of extrasolar planets: I. Results from scaling the ISM opacity⋆. A&A 566, A141. doi:10.1051/0004-6361/201321479
Mordasini, C., Marleau, G. D., and Mollière, P. (2017). Characterization of exoplanets from their formation. III. The statistics of planetary luminosities. A&A 608, A72. doi:10.1051/0004-6361/201630077
More, R. M., Warren, K. H., Young, D. A., and Zimmerman, G. B. (1988). A new quotidian equation of state (QEOS) for hot dense matter. Phys. Fluids 31, 3059–3078. doi:10.1063/1.866963
Mousis, O., Marboeuf, U., Lunine, J. I., Alibert, Y., Fletcher, L. N., Orton, G. S., et al. (2009). Determination of the minimum masses of heavy elements in the envelopes of jupiter and Saturn. Astrophysical J. 696, 1348–1354. doi:10.1088/0004-637X/696/2/1348
Müller, S., Ben-Yami, M., and Helled, R. (2020a). Theoretical versus observational uncertainties: Composition of giant exoplanets. Astrophysical J. 903, 147. doi:10.3847/1538-4357/abba19
Müller, S., Helled, R., and Cumming, A. (2020b). The challenge of forming a fuzzy core in Jupiter. A&A 638, A121. doi:10.1051/0004-6361/201937376
Müller, S., and Helled, R. (2021). Synthetic evolution tracks of giant planets. Mon. Notices R. Astronomical Soc. 507, 2094–2102. doi:10.1093/mnras/stab2250
Müller, S., and Helled, R. (2023). Towards a new era in giant exoplanet characterisation. A&A 669, A24. doi:10.1051/0004-6361/202244827
Parmentier, V., and Guillot, T. (2014). A non-grey analytical model for irradiated atmospheres. I. Derivation. A&A 562, A133. doi:10.1051/0004-6361/201322342
Paxton, B., Bildsten, L., Dotter, A., Herwig, F., Lesaffre, P., and Timmes, F. (2011). Modules for Experiments in stellar Astrophysics (MESA). Astrophysical J. Suppl. Ser. 192, 3. doi:10.1088/0067-0049/192/1/3
Paxton, B., Cantiello, M., Arras, P., Bildsten, L., Brown, E. F., Dotter, A., et al. (2013). Modules for Experiments in stellar Astrophysics (MESA): Planets, oscillations, rotation, and massive stars. Astrophysical J. Suppl. Ser. 208, 4. doi:10.1088/0067-0049/208/1/4
Paxton, B., Marchant, P., Schwab, J., Bauer, E. B., Bildsten, L., Cantiello, M., et al. (2015). Modules for Experiments in stellar Astrophysics (MESA): Binaries, pulsations, and explosions. Astrophysical J. Suppl. Ser. 220, 15. doi:10.1088/0067-0049/220/1/15
Paxton, B., Schwab, J., Bauer, E. B., Bildsten, L., Blinnikov, S., Duffell, P., et al. (2018). Modules for Experiments in stellar Astrophysics (MESA): Convective boundaries, element diffusion, and massive star explosions. Astrophysical J. Suppl. Ser. 234, 34. doi:10.3847/1538-4365/aaa5a8
Paxton, B., Smolec, R., Schwab, J., Gautschy, A., Bildsten, L., Cantiello, M., et al. (2019). Modules for Experiments in stellar Astrophysics (MESA): Pulsating variable stars, rotation, convective boundaries, and energy conservation. Astrophysical J. Suppl. Ser. 243, 10. doi:10.3847/1538-4365/ab2241
Pollack, J. B., Hubickyj, O., Bodenheimer, P., Lissauer, J. J., Podolak, M., and Greenzweig, Y. (1996). formation of the giant planets by concurrent accretion of solids and gas. Icarus 124, 62–85. doi:10.1006/icar.1996.0190
Poser, A. J., Nettelmann, N., and Redmer, R. (2019). The effect of clouds as an additional opacity source on the inferred metallicity of giant exoplanets. Atmosphere 10, 664. doi:10.3390/atmos10110664
Radko, T., Bulters, A., Flanagan, J. D., and Campin, J. M. (2014). Double-diffusive recipes. Part I: Large-scale dynamics of thermohaline staircases. J. Phys. Oceanogr. 44, 1269–1284. doi:10.1175/JPO-D-13-0155.1
Rauer, H., Catala, C., Aerts, C., Appourchaux, T., Benz, W., Brandeker, A., et al. (2014). The PLATO 2.0 mission. Exp. Astron. 38, 249–330. doi:10.1007/s10686-014-9383-4
Rustamkulov, Z., Sing, D. K., Mukherjee, S., May, E. M., Kirk, J., Schlawin, E., et al. (2022). Early Release science of the exoplanet WASP-39b with JWST NIRSpec PRISM. arXiv e-prints , arXiv:2211.10487:10.48550/arXiv.2211.10487.
Sarkis, P., Mordasini, C., Henning, T., Marleau, G. D., and Mollière, P. (2021). Evidence of three mechanisms explaining the radius anomaly of hot Jupiters. A&A 645, A79. doi:10.1051/0004-6361/202038361
Saumon, D., Chabrier, G., and van Horn, H. M. (1995). An equation of state for low-mass stars and giant planets. Astrophysical J. 99, 713. doi:10.1086/192204
Shibata, S., Helled, R., and Ikoma, M. (2020). The origin of the high metallicity of close-in giant exoplanets. Combined effects of resonant and aerodynamic shepherding. A&A 633, A33. doi:10.1051/0004-6361/201936700
Shibata, S., and Ikoma, M. (2019). Capture of solids by growing proto-gas giants: Effects of gap formation and supply limited growth. Mon. Notices R. Astronomical Soc. 487, 4510–4524. doi:10.1093/mnras/stz1629
Spiegel, D. S., and Burrows, A. (2012). Spectral and photometric diagnostics of giant planet formation scenarios. Astrophysical J. 745, 174. doi:10.1088/0004-637X/745/2/174
Stevenson, D. J., Bodenheimer, P., Lissauer, J. J., and D’Angelo, G. (2022). Mixing of condensable constituents with H-He during the formation and evolution of jupiter. PSJ 3, 74. doi:10.3847/PSJ/ac5c44
The JWST Transiting Exoplanet Community Early Release Science Team Ahrer, E.-M., Alderson, L., Batalha, N. M., Batalha, N. E., Bean, J. L., et al. (2022). Identification of carbon dioxide in an exoplanet atmosphere. arXiv e-prints, arXiv:2208.11692. doi:10.48550/arXiv.2208.11692
Teske, J. K., Thorngren, D., Fortney, J. J., Hinkel, N., and Brewer, J. M. (2019). Do metal-rich stars make metal-rich planets? New insights on giant planet formation from host star abundances. AJ 158, 239. doi:10.3847/1538-3881/ab4f79
Thompson, S. L. (1990). ANEOS analytic equations of state for shock physics codes input manual. doi:10.2172/6939284
Thorngren, D., and Fortney, J. J. (2019). Connecting giant planet atmosphere and interior modeling: Constraints on atmospheric metal enrichment. Astrophysical J. 874, L31. doi:10.3847/2041-8213/ab1137
Thorngren, D. P., Fortney, J. J., Murray-Clay, R. A., and Lopez, E. D. (2016). The mass-metallicity relation for giant planets. Astrophysical J. 831, 64. doi:10.3847/0004-637X/831/1/64
Tinetti, G., Drossart, P., Eccleston, P., Hartogh, P., Heske, A., Leconte, J., et al. (2018). A chemical survey of exoplanets with ARIEL. Exp. Astron. 46, 135–209. doi:10.1007/s10686-018-9598-x
Turrini, D., Schisano, E., Fonte, S., Molinari, S., Politi, R., Fedele, D., et al. (2021). Tracing the formation history of giant planets in protoplanetary disks with carbon, oxygen, nitrogen, and sulfur. Astrophysical J. 909, 40. doi:10.3847/1538-4357/abd6e5
Valletta, C., and Helled, R. (2020). Giant planet formation models with a self-consistent treatment of the heavy elements. Astrophysical J. 900, 133. doi:10.3847/1538-4357/aba904
Valsecchi, F., Rappaport, S., Rasio, F. A., Marchant, P., and Rogers, L. A. (2015). Tidally-driven roche-lobe overflow of hot Jupiters with MESA. Astrophysical J. 813, 101. doi:10.1088/0004-637X/813/2/101
Vazan, A., Helled, R., Kovetz, A., and Podolak, M. (2015). Convection and mixing in giant planet evolution. Astrophysical J. 803, 32. doi:10.1088/0004-637X/803/1/32
Vazan, A., Kovetz, A., Podolak, M., and Helled, R. (2013). The effect of composition on the evolution of giant and intermediate-mass planets. Mon. Notices R. Astronomical Soc. 434, 3283–3292. doi:10.1093/mnras/stt1248
Weiss, L. M., Marcy, G. W., Rowe, J. F., Howard, A. W., Isaacson, H., Fortney, J. J., et al. (2013). The mass of KOI-94d and a relation for planet radius, mass, and incident flux. Astrophysical J. 768, 14. doi:10.1088/0004-637X/768/1/14
Welbanks, L., Madhusudhan, N., Allard, N. F., Hubeny, I., Spiegelman, F., and Leininger, T. (2019). Mass-metallicity trends in transiting exoplanets from atmospheric abundances of H2O, Na, and K. Astrophysical J. 887, L20. doi:10.3847/2041-8213/ab5a89
Keywords: planets and satellites: gaseous planets, formation, evolution, interiors, composition, characterisation
Citation: Müller S and Helled R (2023) Warm giant exoplanet characterisation: current state, challenges and outlook. Front. Astron. Space Sci. 10:1179000. doi: 10.3389/fspas.2023.1179000
Received: 03 March 2023; Accepted: 21 April 2023;
Published: 10 May 2023.
Edited by:
Steve B. Howell, National Aeronautics and Space Administration, United StatesReviewed by:
Daniel Thorngren, Johns Hopkins University, United StatesJun Yang, Peking University, China
Copyright © 2023 Müller and Helled. This is an open-access article distributed under the terms of the Creative Commons Attribution License (CC BY). The use, distribution or reproduction in other forums is permitted, provided the original author(s) and the copyright owner(s) are credited and that the original publication in this journal is cited, in accordance with accepted academic practice. No use, distribution or reproduction is permitted which does not comply with these terms.
*Correspondence: Simon Müller, c2ltb25hbmRyZXMubXVlbGxlckB1emguY2g=