- 1Institute of Animal Rearing Technologies, Lithuanian University of Health Sciences, Kaunas, Lithuania
- 2Microbiology and Virology Institute, Lithuanian University of Health Sciences, Kaunas, Lithuania
- 3Department of Physiology and Anatomy, Lithuanian University of Health Sciences, Kaunas, Lithuania
- 4Department of Food Safety and Quality, Lithuanian University of Health Sciences, Kaunas, Lithuania
- 5Institute of Food Safety, Animal Health and Environment BIOR, Riga, Latvia
- 6Department of Food Science and Technology, Kaunas University of Technology, Kaunas, Lithuania
The aim of this study was to apply a combination of the microbial starters Lactobacillus uvarum LUHS245, Lactobacillus casei LUHS210, Pediococcus acidilactici LUHS29, and Pediococcus pentosaceus LUHS183 for feed fermentation and to evaluate the influence of fermentation on feed acidity and microbiological characteristics, as well as on the piglet feces microbiota, health, and growth performance. Additionally, mycotoxin biotransformation was analyzed, including masked mycotoxins, in feed and piglet feces samples. The 36-day experiment was conducted using 25-day-old Large White/Norwegian Landrace (LW/NL) piglets with an initial body weight of 6.9–7.0 kg, which were randomly distributed into two groups (in each 100 piglets): control group, fed with basal diet (based on barley, wheat, potato protein, soybean protein concentrate, and whey powder), and treated group, fed with fermented feed at 500 g kg−1 of total feed. Compared to a commercially available lactic acid bacteria (LAB) combination, the novel LAB mixture effectively reduced feed pH (on average pH 3.65), produced a 2-fold higher content of L(+) lactic acid, increased viable LAB count [on average 8.8 log10 colony-forming units (CFU) g−1], and led to stable feed fermentation during the entire test period (36 days). Fecal microbiota analysis showed an increased number of probiotic bacteria in the treated group, particularly Lactobacillus, when compared with the control group at the end of experiment. This finding indicates that fermented feed can modify microbial profile change in the gut of pigs. In treated piglets' blood (at day 61), the serum high-density lipoprotein (HDL) cholesterol and triglycerides (TG) were significantly higher, but the levels of T4, glucose, K, alkaline phosphatase (AP), and urea were significantly decreased (p ≤ 0.05) compared with the control group. Mycotoxin analysis showed that alternariol monomethyl ether (AME) and altenuene were found in 61-day-old control piglets' feces and in fermented feed samples. However, AME was not found in treated piglets' feces. Feed fermentation with the novel LAB combination is a promising means to modulate piglets' microbiota, which is essential to improve nutrient absorption, growth performance, and health parameters. The new LAB composition suggests a novel dietary strategy to positively manipulate fermented feed chemicals and bio-safety and the piglet gut microbial ecology to reduce antimicrobials use in pig production and increase local feed stock uses and economical effectiveness of the process.
Introduction
Veterinary drugs are widely used in animal production. This use has become a problem because pathogens develop resistance to antimicrobials, and the drugs can reach the soil and water through the animal excreta and act as serious environment pollutants (1–3). Research has suggested a myriad of nutritional strategies to improve animal health, productivity, and production quality. Most of these methods use feed supplements (plant and/or microbial) that stimulate a suitable intestinal ecosystem. Modification of intestinal microbiota is important for the health status of the pigs (4). Many lactic acid bacteria (LAB) are desirable intestinal microorganisms that can survive at surfaces of the gastrointestinal tract. Most of the LAB can ferment carbohydrates and reduce pH, an action that leads to a more acidic environment and suppression of pathogenic bacteria growth (5). Some LAB strains possess antimicrobial properties because they have the ability to produce substances [bacteriocins or bacteriocin-like inhibitory substances (BLIS)] that have the capacity to inhibit pathogens and that make them more specific anti-pathogenic agents (6). Our previous studies showed that Lactobacillus uvarum LUHS245, Lactobacillus casei LUHS210, Pediococcus acidilactici LUHS29, and Pediococcus pentosaceus LUHS183 strains inhibit a variety of pathogenic and opportunistic microorganisms in vitro (7). For instance, LUHS245 strain showed antimicrobial activity against methicillin-resistant Staphylococcus aureus, LUHS 210 and LUHS29 showed antimicrobial activity against Salmonella enterica, while hemp seed fermented with LUHS183 and LUHS245 showed inhibition of Pasteurella multocida (8).
There are published results about the effects of viable LAB on pigs' zootechnical parameters, intestinal microbiota, and gut health (9). Additionally, LAB strains have anti-infectious properties, e.g., reduction of Salmonella and enterotoxigenic Escherichia coli colonization (10). LAB-mediated fermentation can reduce toxins in feed and, during the fermentation, some of the microbial starters excrete enzymes that may transform mycotoxins into non-toxic compounds. However, fermentation can lead to the formation of masked mycotoxins, and special attention must be paid to control these processes. Additionally, LAB might lead to metabolic disorders in the host (11).
During the LAB metabolism, excreted lactic acid, in most of the cases, is a combination of the L-(+)- and D-(−) isomers. D(−) lactic acid cannot be metabolized by mammals; for this reason, it can cause acidosis, i.e., a disturbance in the acid–alkali balance (12). Therefore, in this study, we used LAB starters (previously tested in vitro) with antimicrobial properties against pathogenic and opportunistic strains. We examined whether this activity altered the piglet microbiota, an action that might improve the animals' health and productivity. In this study, we hypothesized that administration of a LAB combination with antimicrobial characteristics may reduce pathogenic and opportunistic strain concentration in intestine of piglets. Furthermore, modifying intestinal microbiota might improve piglets' health and growth performance.
The aim of this study was to apply a combination of the microbial starters L. uvarum LUHS245, L. casei LUHS210, P. acidilactici LUHS29, and P. pentosaceus LUHS183 for feed fermentation and to evaluate the influence of fermentation on feed acidity and microbiological characteristics, as well as on the piglet feces microbiota, health, and growth performance. Additionally, mycotoxin biotransformation was analyzed, including masked mycotoxins, in feed and piglet feces samples.
Materials and Methods
The whole experiment principal scheme is shown in Figures 1A,B.
Fermented Feed Preparation and Analysis
LAB Strains Used for Feed Fermentation
The L. uvarum LUHS245, L. casei LUHS210, P. acidilactici LUHS29, and P. pentosaceus LUHS183 strains were obtained from the Lithuanian University of Health Sciences collection (Kaunas, Lithuania). Our previous studies showed that the abovementioned strains inhibit various pathogenic and opportunistic microorganisms and are suitable for fermentation of various cereal substrates (5, 7, 13, 14). The abovementioned LAB strains were stored at −80°C in a Microbank system (Pro-Lab Diagnostics, UK) and separately propagated in de Man-Rogosa-Sharpe (MRS) broth (CM 0359, Oxoid Ltd., Hampshire, UK) at 30 ± 3°C for 48 h before their use for feed fermentation.
Feed Fermentation
The feed (composition: crude protein–19.00%, crude fiber–3.15%, crude oil and fats–6.51%, lysine–1.45%, methionine–0.55%, tryptophan–0.26%, threonine–0.93%, Ca–0.90%, total P–0.59%, and Na–0.20%), water, and LAB strain (equal parts of each strain by volume) suspension (3% from dry matter of feed mass, v/m), containing 8.9 log10 CFU ml−1, was fermented at 30 ± 2°C for 18 h. The final moisture content of the feed was 60 g 100 g−1. The moisture content was determined by drying the samples at 103 ± 2°C to a constant weight (15). Whole fermented feed mass (100%) was divided into two parts (30 and 70%, by mass): 70% of the fermented feed was used for piglet feeding, and 30% of fermented feed was used as a starter for additional feed fermentation cycles (Figure 1A). Non-fermented feed samples were analyzed as the control. In addition, a commercial LAB combination for feed fermentation was tested: Lactobacillus plantarum 1k2079, P. pentosaceus 1k2103, and Lactococcus lactis 1k2082 (H. Wilhelm Schaumann GmbH, Pinneberg, Germany). With the commercially available and newly developed LAB composition, fermented feed samples were analyzed every 18 h during the first 6 days to compare its pH and viable LAB counts (the main parameters of fermentation). Further, from the fifth day of fermentation, analyses were performed every 5 days.
Evaluation of Fermented Feed Acidity and Microbiological Parameters
The pH of samples was measured and recorded using a pH electrode (PP-15; Sartorius, Goettingen, Germany). Concentrations of L(+) and D(−) lactic acid isomers were determined with a specific Megazyme assay Kit (Megazyme Int., Bray, Ireland).
Evaluation of the LAB count was performed according the ISO 15214:1998 method (16), described in detail by Bartkiene et al. (13). The number of microorganisms was counted and expressed as log10 of colony-forming units per gram (CFU g−1). All results are expressed as the mean of three determinations.
In vivo Experiment With Piglets
Animals and Housing
All animal procedures were conducted according to the EU Directive (17) of the European Parliament and of Council from 22 September 2010 on the protection of animals used for scientific purposes and Requirements for the Keeping, Maintenance, and Use of Animals Intended for Science and Education Purposes, approved by the order of the Lithuanian Director of the State Food and Veterinary Service (31/10/2012, No. B1-866) [(18); Figure 1B]. The study was conducted at a pig farm in the Klaipeda district (Kontvainiai, Lithuania) and at the Institute of Animal Rearing Technologies, Lithuanian University of Health Sciences (Kaunas, Lithuania). A 36-day experiment was conducted using 25-day-old Large White/Norwegian Landrace (LW/NL) piglets (100 piglets in each group) with an initial body weight of 6.9–7.0 kg. The weaner piglets were kept in a section with two climate zones. The first had a heated concrete floor (36°C) and roof on it, and the second had plastic piglet floors and optimum ventilated air and temperature for the active period. Drinking water and compound liquid feed were available ad libitum throughout the trial. Antibiotic treatment was not applied.
Experimental Design and Diets
The piglets were distributed into two groups (each of 100 animals), and samples (feces and blood) from 10 animals per group were collected. Two dietary treatments were compared: (i) non-fermented basal diet and (ii) fermented basal diet. Fermented feed comprised 500 g kg−1 of total feed; it was included in the diet of treated group beginning at day 25 of life until day 61. Evaluation of piglets' growth performance was performed by testing 100 piglets from each group. The basal feed was formulated according to the nutritional requirements prescribed in the Nutrient Requirements of Swine (19). The feed composition and nutritional value are shown in Table 1. Dietary contents were analyzed according to the AOAC recommendations (20).
Metagenomics and Microbial Profiling Analysis
Before the experiment, feces from 25-day-old control and treated piglets were collected from 10 piglets per group. The same procedure, using 10 piglets per group, was used at the end of the experiment (day 61 of the piglets' life) to generate representative samples of feces content from both animal groups. The DNA from each sample was kept in DNA/RNA Shield 1:10 (R1100-250, Zymo Research, USA) at −70°C before DNA extraction. DNA was extracted with a fecal DNA MiniPrep kit (D6010, Zymo Research, USA). Library preparation, metagenomic sequencing, and taxonomic characterization of reads was performed as described previously (21). ZymoBIOMICS Microbial Community Standard (D6300, Zymo Research, USA) was used as a microbiome profiling quality control. The results of taxonomic classification were visualized using the interactive online platform (https://genome-explorer.com).
Microbiological Analysis of Fecal Samples
The piglets' fecal samples were collected before and after the experiment, stored in vials (+4°C) with a transport medium (Fecal Enteric Plus, Oxoid, Basingstoke, UK), and analyzed on the same day. MRS agar was used to determine the LAB count in the feces. Violet Red Bile Glucose (VRBG) agar (Oxoid Ltd., Basingstoke, United Kingdom) was used to determine the total count of enterobacteria (TCE). Plate Count Agar (Biolife Italiana Srl, Milan, Italy) was used to determine the total aerobic and facultative anaerobic bacteria count (TCM) in the feces. The results are expressed as a log10 of CFU g−1 of a sample.
Blood Analysis
Piglets were bled (10 animals from each group) from the jugular vein into vacuum blood tubes (BD Vacutiner, United Kingdom) before the morning feeding. Tubes with clot activator were used for biochemical examination. Blood biochemical variables were evaluated before and after the experiment (on days 25 and 61 of the piglets' life). The following parameters were included: aspartate aminotransferase (AST), cholesterol (mmol), high-density lipoprotein cholesterol (HDL-C), low-density lipoprotein (LDL) cholesterol, triglycerides (TG), phosphorus (IP), magnesium (Mg), potassium (K), sodium (Na), triiodothyronine (T3), thyroxine (T4), immunoglobulin IgG, vitamin B12, alanine aminotransferase (ALT), albumin (ALB), total protein (TP), iron (Fe), glucose (GLU), calcium (Ca), creatinine analyzed by the Jaffe method (CREA), alkaline phosphatase (AP), and urea. They were analyzed with an automatic biochemistry analyzer “SIEMENS ADVIA 1800” (Siemens Healthcare GmbH, Germany) and immunochemical analyses [triiodothyronine (T3) and thyroxine (T4)] by analyzer “SIEMENS ADVIA Centaur XP” (Siemens Healthcare GmbH, Germany) in the accredited laboratory “Anteja” (Klaipeda, Lithuania).
Evaluation of Piglets' Growth Performance
Group body weight (BW) was recorded on days 25, 32, 39, 46, 53, and 61 of age using an electronic weighing system (model type: IT1000, SysTec GmbH Bergheim, Germany). The feed efficiency (FE) was determined from feed intake and BW, which was recorded on the same days as BW using a WEDA (Dammann & Westerkamp GmbH, Germany) automated feeding system that has an electronic flowmeter and weighing system.
High-Performance Liquid Chromatography Coupled to Time of Flight High-Resolution Mass Spectrometry (HPLC-TOF-HRMS) for Mycotoxin Analysis
The standards of beauvericin (BEA, ≥95%), enniatin A (ENN A, ≥99%), enniatin A1 (ENN A1, ≥99%), enniatin B (ENN B, ≥99%), enniatin B1 (ENN B1, ≥99%), meleagrin (MEL, ≥98%), cytochalasin A (CCA, ≥98%), cytochalasin B (CCB, ≥98%), cytochalasin C (CCC, ≥99%), cytochalasin D (CCD, ≥95%), cytochalasin E (CCE, ≥98%), cytochalasin J (CCJ, ≥95%), cytochalasin H (CCH, ≥95%), 15-acetyldeoxynivalenol (15-AcDON, ≥99%), 3-acetyldeoxynivalenol (3-AcDON, ≥99%), tentoxin (TNX, ≥99%), citreoviridin (CVD, ≥95%), stachybotrylactam (SBL, ≥95%), alternariol monomethyl ether (AME, ≥98%), dihydrochalasin B (DTC B, ≥98%), and fusaric acid (FA, ≥98%) were purchased from Cayman Chemical (Ann Arbor, MI, USA). Deoxynivalenol (DON, 98.3%), aflatoxins (AFB1, AFB2, AFG1, AFG2, ≥99%), aflatoxin M1, aflatoxin Ro (aflatoxicol), HT-2 toxin (HT-2, 99%), T-2 toxin (T-2, 99%), sterigmatocystin (STC, 99.7%), zearalenone (ZEN, 99,66%), ochratoxin A (OTA, 99%), fumonisins B1 and B2 (FB1, 98%; FB2, 97.5%), fusarenon-X (FUS-X, 99.4%), and deoxynivalenol-3-glucoside (D3G, 96%) were acquired from Romer Labs (Tulln, Austria). Neosolaniol (NEO, 99%), anisomycin (ANC, 98.9%), T-2 toxin tetraol (T-2TET, >99%), apicidin (API, >99%), ansamitocin P3 (AN P3, 99%), altenuene (ALT, 99.3%), alternariol (AOH, >98%), cerulenin (CER, 98%), chaetocin (CTC, 99%), 15-acetoxyscirpenol (15-AcS, >98%), T-2 toxin triol (T-2TRI, 99%), fumonisin B3 (FB3, 99%), myriocin (MYR, 99%), brefeldin A (BRF A, 99.9%), 17-dimethylaminoethylamino-17-demethoxygeldanamycin (17-DMAG, 99.4%), altertoxin I (ATX I, 99%), 17-(allylamino)-17-demetoxygeldanamycin (17-AAG, 99%), aflatoxicol (AFL, 99%), chaetoglobosin A (CHG A, 99%), verruculogen (VCL, >99%), wortmannin (WTM, 99%), helvolic acid (HA, 99%), ochratoxin B (OTB, 99%), destruxin A (DTX A, 99%), destruxin B (DTX B, 99%), paxilline (PXL, 99%), penitrem A (PN A, >99%), gliotoxin (GTX, >99%), curvularin (CVL, 99%), bafilomycin A1 (BFA1, >99%), and bafilomycin B1 (BFB1, >99%) were purchased from Fermentek (Jerusalem, Israel), while mycophenolic acid (MPA, >99%), penicillic acid (PA, >99%), and roquefortine-C (ROQ-C, >99%) were purchased from Santa Cruz Biotechnology (Dallas, TX, USA).
Standard stock solutions of all mycotoxins were prepared in acetonitrile, methanol, or their mixtures with DMSO, with the exception of BEA and enniatins that were kept in DMF. The spiking solutions and calibration standards were prepared by serial dilution of stock solutions and were stored in UV-protected glassware at 4°C (22).
The samples were prepared using a modified QuEChERS method. HPLC-TOF-HRMS analysis was performed on an UltiMate 3000 (Thermo Fisher Scientific, USA) HPLC system coupled to a Compact Q-ToF time-of-flight mass spectrometer (Bruker, Germany). Chromatographic separation was performed on a reversed-phase analytical column (Kinetex C18, 1.7 μm, 100 Å, 50 × 3.00 mm; Phenomenex, USA) at a 0.35 ml min−1 flow rate. The analysis was performed in positive full scan mode for all mycotoxins over the m/z scanning range from 50 to 1,000. The mass extraction window applied for quantification purposes was set to ±5 ppm at 10,000 full with at half maximum (FWHM) resolution. Data acquisition was controlled by HyStar 3.2. software (Bruker Daltonik GmbH, Bremen, Germany), and data analysis was performed with QuantAnalysis 4.3. software (Bruker Daltonik GmbH, Bremen, Germany).
Statistical Analysis
In order to evaluate the influence of fermentation on feed characteristics, data were subjected to analysis of variance (ANOVA) and paired t-test column statistics. Comparisons were considered significant when p ≤ 0.05. All feed sample analytical experiments were performed in triplicate (n = 3). ANOVA was also performed to assess the effects of treatment with fermented feed on piglet' parameters. When the ANOVA indicated a significant treatment effect, the means were separated using Duncan's multiple range tests. In the tables, piglets' sample results are presented as mean values with pooled standard errors (n = 10).
Results and Discussion
Characteristics of Fermented Feed
pH and LAB Count for Feed Fermented With Commercial and Novel LAB Combinations
The changes in fermented feed pH and LAB count during the fermentation with the commercial or novel LAB combinations are presented in Figure 2. The feed fermentation process was performed according to the scheme in Figure 1A, and pure LAB cultures were not added during the experimental period. From 18 to 144 h of fermentation, in most of the cases (except samples after 90-h fermentation), the newly developed LAB combination significantly reduced the feed pH compared to the commercial combination (average pH was 3.65 and 3.84, respectively; Figure 2C). This same tendency was noted during the entire 35-day experimental period (35 days). On average, the pH for samples fermented with the commercial LAB combination was 3.86, while it was 3.66 with the newly developed LAB combination (Figure 2D).
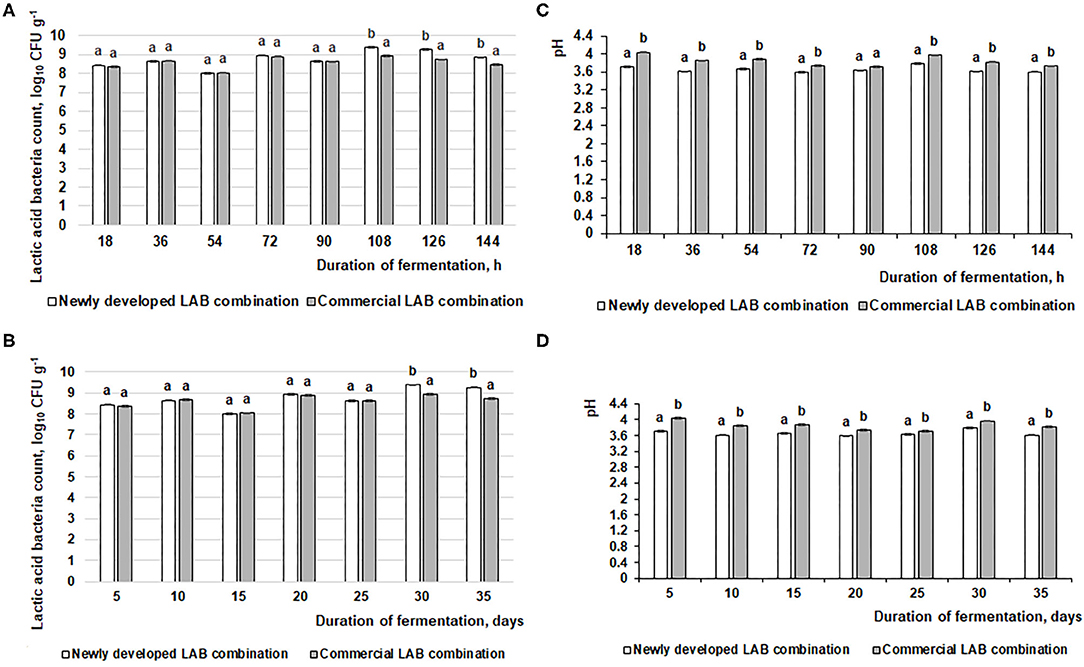
Figure 2. Changes in pH and lactic acid bacteria (LAB) count (log10 CFU g−1) during the feed fermentation. (A,C) LAB count and pH of the feed after 18, 36, 54, 72, 90, 108, 126, and 144 h. (B,D) LAB count and pH of the feed after 5, 10, 15, 20, 25, 30, and 35 days. Data are expressed at the mean ± standard deviation (n = 10). Data were statistically compared with a paired t-test and column statistics; p ≤ 0.05 was considered significant. Each parameter, means followed by different letters are significantly different (p ≤ 0.05).
When comparing the viable LAB count in fermented feed samples, after 18, 36, 54, 72, and 90 h of fermentation, there were significant differences between the LAB counts in feed samples fermented with the commercial or newly developed LAB combination. On average, the LAB count in fermented feed was 8.5 log10 CFU g−1 (Figure 2A). After 108, 126, and 144 h, the LAB count was significantly higher in feed samples fermented with the newly developed compared to the commercial LAB combination (on average, 9.2 log10 CFU g−1 and 8.7 log10 CFU g−1, respectively). There was a similar tendency for this measure during the entire 35-day evaluated period: 8.6 log10 CFU g−1 in feed fermented with the commercial LAB combination and 8.8 log10 CFU g−1 for feed fermented with the novel combination (Figure 2B).
LAB are popular microorganisms used for fermented liquid and solid-state feed preparations to reduce the pH of fermentable substrates by converting carbohydrates to organic acids (23). In fermented feed, the inhibition of pathogenic and opportunistic strains is explained by evaluating the number of Enterobacteriaceae and molds. High content of LAB with anti-pathogenic characteristics may have a positive influence on the microbial population in the intestine. It was published that Lactobacillus brevis, Lactobacillus acidophilus, Lactobacillus reuteri, and L. plantarum, can reduce E. coli in the intestine of weaned piglets (24). LAB are natural inhabitants of the intestine that can survive in gastrointestinal tract and perform nutritional compounds' degradation activity. Their possibility to adherence to gastrointestinal tract surfaces may reduce colonization of pathogens (25). In feed fermentations, the main metabolite of LAB is lactic acid, the concentration of which should be above 150 mmol L−1 to inhibit endogenous pathogens in the fermentable substrate (26). However, lactic and acetic acid—and the ethanol concentrations—should be controlled in fermented feed to avoid causing undesirable palatability of end products and/or acidosis (27). Furthermore, a decline in pH may partially unbalance the secretion of hydrochloric acid in the stomach of young piglets. It can reduce the stomach's ability to digest and absorb feed and kill off pathogens (28). Fermented feed pH, one from the most important fermented feed quality indicators, allows experimenters to evaluate the nutritional value and biosafety of the end product to suppress pathogenic bacteria (29). pH is a very important fermentation indicator and should be monitored to control the fermented feed preparation process. Optimal fermentation conditions occur when the fermentable substrate pH is 4.0–5.0; such a pH does not indicate overfermentation or uncontrolled fermentation (23, 30). Finally, desirable fermented feed characteristics are predominantly live probiotics, desirable technological microorganism metabolites and prebiotics, low counts of endogenous pathogens, and good sensory properties (23) According to this study, the abovementioned fermented feed characteristics were obtained by using the scheme shown in Figure 1, in which pure starter cultures are used in only the initial stage of the process.
L(+) and D(−) Lactic Acid Isomers Concentration in Fermented Feed
L(+) and D(−) lactic acid isomer concentration and the L/D ratio in fermented feed samples are shown in Figure 3. Feed samples fermented with the novel LAB combination exhibited a 2-fold higher L(+) lactic acid isomer concentration and a 1.36-fold higher L/D ratio (compared with the feed samples fermented with the commercial LAB combination). Lactate is a major end product of LAB; however, increased lactic acid concentrations are often established in the feces of mammals that show some diseases. In farm animals, lactic acidosis is caused by an imbalance in lactic acid concentration (31, 32). This phenomenon increases mortality and reduces survival in neonatal pigs and humans. Therefore, lactate metabolism plays an important role in maintaining the host animal's health. Lactic acid can be metabolized in vitro into acetate and propionate in pig cecal digesta (33). L-lactic acidosis is the most common cause of metabolic acidosis in the critical care factor. It has been associated with a significant increase in mortality. L-lactic acidosis is defined by a blood L-lactate level of >5 mmol L−1 (34). D-lactic acid is the geometric isomer of L-lactate (a body metabolite). It is a metabolic end product of the intestinal flora (35). D-lactic acid is widely distributed within a body, and the concentration of D isomer is correlated with various diseases. Increases of D-lactic acid concentration can be influenced by short bowel syndrome, ischemia, and bacterial infection. D-lactic acid is potentially a feed quality indicator, which may indicate contamination with bacteria that causes undesirable changes in quality and taste (36).
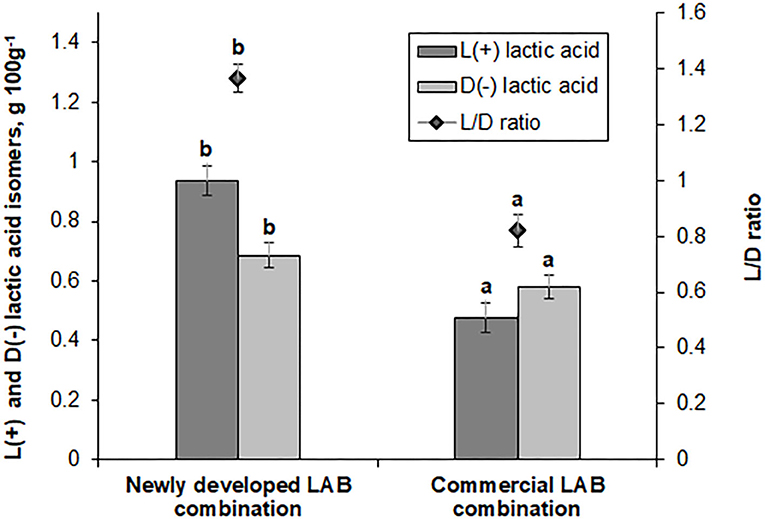
Figure 3. L(+) and D(−) lactic acid isomer concentrations (g 100 g−1) and ratio (L/D) in feed samples fermented with commercial or newly developed lactic acid bacteria (LAB) combinations. Data are expressed as the mean ± standard deviation (n = 10). Data were statistically compared with the paired t-test and column statistics. For each parameter, means followed by different letters are significantly different (p ≤ 0.05).
Influence of Fermented Feed on Pigs' Parameters
Microbial Profiles of Pig Feces
From 41,000 to 61,000 metagenomic sequence reads were obtained and analyzed (depending on the sample and period of investigation). Before the experiment, the most prevalent genus in both piglet groups was Prevotella: 33% and 25% from all of the genera in the control and experimental groups, respectively. The other most prevalent genera included Barnesiella, Alloprevotella, Bacteroides, Clostridium, Escherichia, and Lactobacillus. Such data are consistent with the findings from other authors who investigated microbiota in young piglets (2, 37–40). Overall, 179 and 151 genera with a prevalence ≥0.01% were detected in the fecal DNA of control and experimental animals, respectively (Supplementary Files 1, 2).
After the experiment (day 61), there were significant differences among microbial profiles between the groups, although the number and variety of genera remained very similar (153 and 152 genera with a prevalence ≥0.01% in the control and experimental groups, respectively). The most prevalent genus in both groups was still Prevotella, but the prevalence of Lactobacillus was 6-fold higher in the experimental compared to control animals (23.7 vs. 3.9%). The differences of microbial profiles between the groups are presented in Figure 4 and Supplementary Files 3, 4.
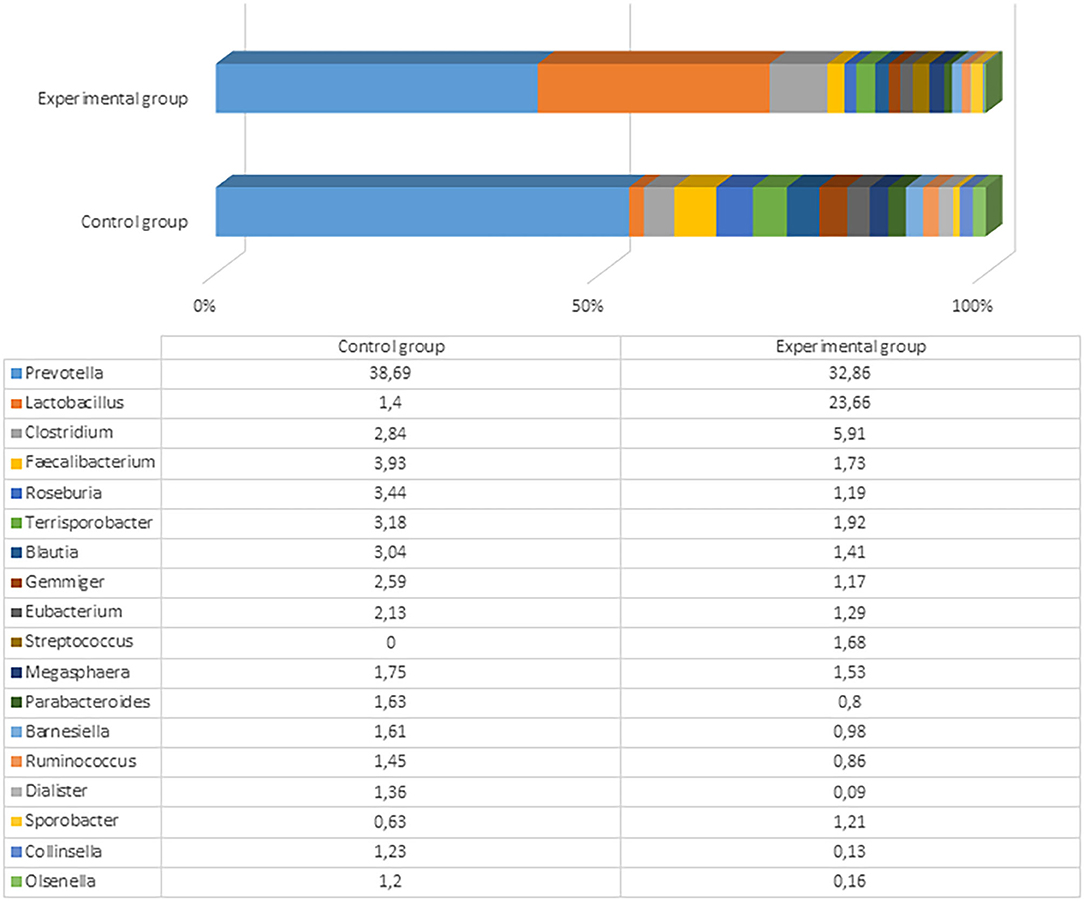
Figure 4. The most prevalent microbiota at a genus level (the prevalence at least 1% from all bacteria in control or either experimental group) in the fecal content of pig feces after the experiment (61st day).
The variety of the most prevalent bacteria (>1%) at the genus level was not very high: Only Prevotella, Lactobacillus, Clostridium, Faecalibacterium, Roseburia, Terrisporobacter, Blautia, and some other genera reached this level. Besides the higher Lactobacillus prevalence in the experimental pigs, there were higher numbers of Clostridium and Streptococcus in experimental pigs' fecal content compared with the control group. Among Clostridium, more than 60 species were detected in the experimental group, with the highest prevalence being of Clostridium cellulovorans (4.08%), Clostridium celatum (0.38%), and Clostridium quinii (0.31%). C. cellulovorans is a mesophilic and anaerobic cellulolytic bacterium that utilizes cellulose and hemicelluloses composed of xylose, fructose, galactose, and mannose (41). However, there is no clear information regarding functions of this species in the pig gut. Seven Streptococcus species were detected in the experimental group of pigs, including Streptococcus lutetiensis, Streptococcus gallolyticus, Streptococcus danielleae, Streptococcus equinus, Streptococcus macedonicus, Streptococcus porcorum, and Streptococcus equi. No streptococci were detected in the control animals at the end of the experiment. The fecal content in the control group contained higher amounts of Faecalibacterium, Roseburia, Ruminococcus, Terrisporobacter, Blautia, and some other microorganisms, although those differences were not very large between the groups. These distinctions were probably associated with high content of Lactobacillus in the experimental group that rendered a lower relative (percent) content of other bacterial genera. Clostridia from the genera Roseburia, Blautia, and Ruminococcus can help prevent pathogen colonization of the pig gastrointestinal tract by pathogens (42). Therefore, the microbial composition in the control group of pigs was also appropriate.
The most prevalent bacterial species in the control pigs was Prevotella copri (21.81%), followed by Faecalibacterium prausnitzii (3.91%). Comparatively, the experimental group was predominated by Lactobacillus amylovorus (19.39%), followed by different Prevotella spp., including P. copri (18.59%), Prevotella stercorea (4.64%), and Prevotella oralis (3.43%). The bacterial species variety and difference between the groups are presented in Figure 5 and Supplementary Files 5, 6.
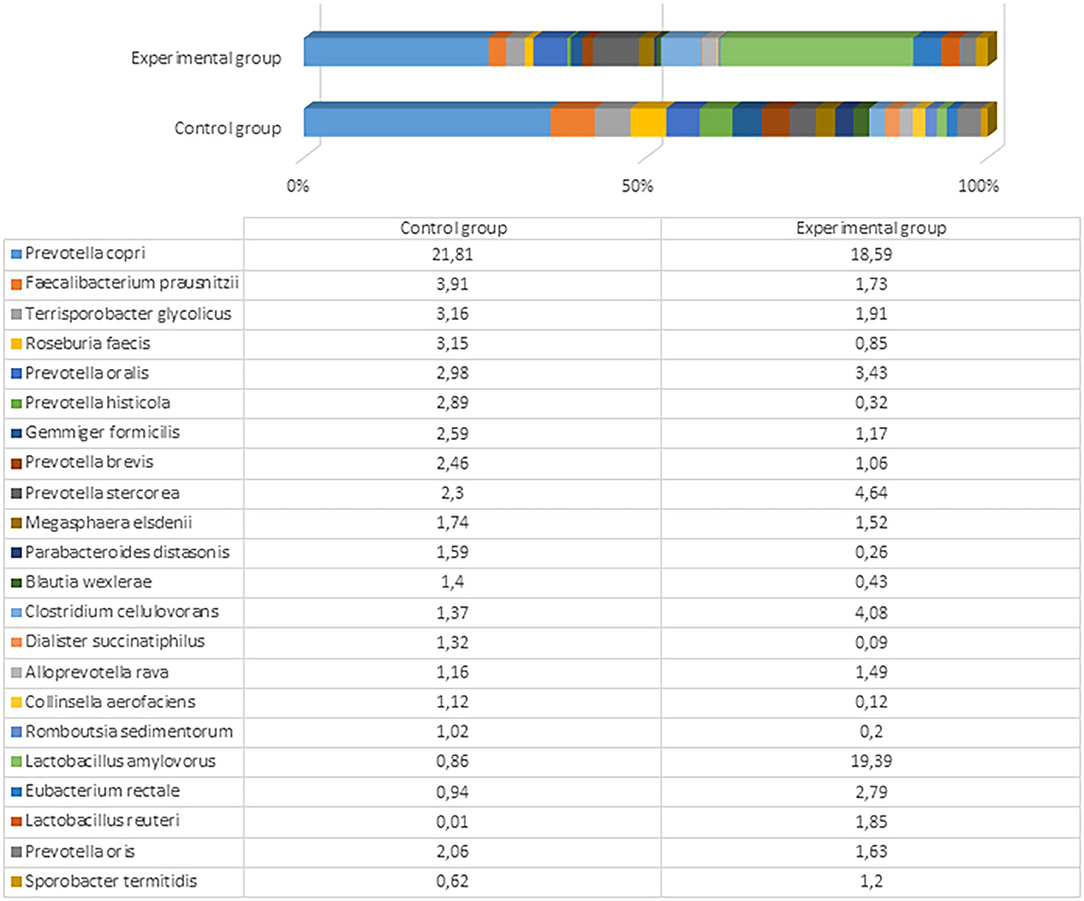
Figure 5. The most prevalent bacterial species (the prevalence at least 1% from all bacteria in control or either experimental group) in the fecal content of pig feces after the experiment (61st day).
L. amylovorus is present in the intestines of piglets and exhibits several potential probiotic properties, including antimicrobial activity against enteric pathogens, in both in vitro and in vivo assays (43). We detected a marked difference in the amount of this bacterium in pigs fed with fermented and conventional feed. Although other microbiota detected in both experimental and control groups can be treated as normal or even probiotic, there were large differences in Lactobacillus that are known and recognized as beneficial and crucial microorganisms (i.e., to ensure good health) in the gut of pigs fed with treated food. Dietary changes can greatly shape the structure and function of gut microbiota. Various fermented feeds have been reported to exert beneficial effects on the pig microbiota during different growth states. The most common change is an increase in the LAB concentration, particularly in the stomach and small intestine (44). Moran et al. (45) reported that the ratio of LAB to coliform bacteria in the lower gut of pigs weaned using fermented liquid feed is shifted in favor of LAB. In contrast, this ratio is shifted in favor of the coliforms in piglets fed with dried feed. Another significant change in the microbial population of the gastrointestinal tract after feeding with fermented feed is an increase in the number of yeast cells (23). Yeast can bind enterobacteria surfaces and thereby block the binding of these bacteria to the gut epithelium (46). There are also opposite findings, where the fermented feed tends to decrease the population of LAB and anaerobic bacteria in general, especially in the large intestine, and increase the pH of the lower gut (26). Urlings et al. (47) hypothesized that fewer nutrients, including vitamins and amino acids, reach the large intestine, and this deficit promotes less microbial development and an increase in pH in the lower part of the gastrointestinal tract. A decrease in feed intake and body weight gain reportedly occurs due to impaired palatability after fermentation (44). The differences in those findings compared to this study might be associated with different microorganisms used for feed fermentation, the technological process, and distinct microbiome studies, as only recent achievements in molecular biology have allowed researchers to explore the microbiome more deeply, particularly regarding probiotic anaerobic bacteria. Previous studies were based on culturable methods; therefore, multiple species, including unculturable bacteria, were probably underestimated.
Influence of Fermented Feed on LAB, Total Enterobacteria Count (TEC), and Mold/Yeast M/Y Count in Piglets' Feces
The influence of fermented feed on TEC, LAB, and M/Y count in piglets' feces is shown in Table 2. TEC in the control and treated group feces decreased from day 25 to 61 (by 35.5 and 43.2%, respectively). However, TEC was significantly lower in the treated compared to the control group at the end of the experiment. There were no significant differences in the LAB count between groups; the average content was 7.2 log10 CFU g−1. The M/Y count was significantly higher (18.4%) at the beginning of the experiment in the treated compared with the control group. The same tendency occurred at the end of the experiment (M/Y was 9.6% higher compared with the control group). However, the difference between the M/Y count at the end of experiment was 1.9 times lower compared with the counts at the beginning of experiment. Demecková et al. (48) reported a lower number of coliforms and higher LAB count in the feces of piglets from sows fed with fermented liquid feed compared to piglets from sows fed with non-fermented or dry feed. In another study, there were fewer coliform bacteria in pigs that received a fermented feed diet, while differences in the number of LAB in the small intestine of differently fed pigs were not significant (44). A low pH, high lactic acid concentration, and high numbers of LAB in fermented feed are believed to be responsible for the decrease of enteropathogens (49). The fermented feed decreases pH and stimulates proteolytic activity in stomach, which is an important barrier against pathogens. These phenomena reduce the growth of undesirable pathogenic bacteria in the lower small intestine, cecum, and colon (50). Low pH plays a vital role in the inhibition of Enterobacteriaceae (51). In addition, it is important to use starter cultures that possess antimicrobial activity against gram-negative microorganisms (52). Our results are consistent with Väkeväinen et al. (53), who determined that both L. lactis A1MS3 and P. pentosaceus S0l10 possess antimicrobial properties. Starter cultures noticeably decrease Enterobacteriaceae, leading to a microbiologically safer end product. The pH decrease and increase of LAB and yeasts counts during the fermentation were in accordance with previous findings (54). At the end of the experiment, our results were similar with Nowak et al. (55), who reported that levels of yeast and molds in the cecal digesta are reduced. LAB are considered to be beneficial intestinal bacteria, whereas coliforms and Salmonella are considered to be major bacteria that often cause gut health problems such as diarrhea, especially in younger animals. Our results are consistent with Upadhaya et al. (56), who demonstrated that the LAB population is weakly influenced by fermented feed but the coliform population is significantly reduced. These finding indicate that gut microbiota is positively influenced by feed fermentation.
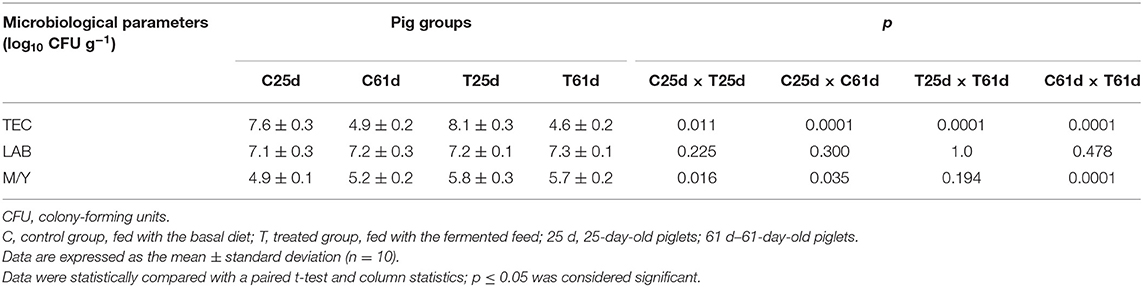
Table 2. Microbiological parameters [total enterobacteria count (TEC), lactic acid bacteria (LAB), and mold/yeast (M/Y) ratio] for feces from 25- and 61-day-old pigs.
Piglet Blood Parameters
Piglet blood parameters are shown in Table 3. There were significantly higher serum ALB, T4, and Fe concentrations, as well as lower serum hepatic enzyme AST activity and LDL cholesterol, K, Ca, vitamin B12, and urea concentrations in the treated piglet blood samples before the feeding experiment. At day 61, serum HDL cholesterol and TG were significantly higher, and T4, glucose, K, AP, and urea were decreased (p ≤ 0.05) in blood from piglets fed with fermented compared with the control feed. Our study is consistent with Dong et al. (57), who reported that fermented feed improves the hematological profile and serum concentrations of total protein, albumin, and globulin and reduces serum triglyceride and cholesterol in weaned piglets. Increased serum glucose level in pigs supplemented with fermented feed was reported (58). The plasma protein concentration shows factors affecting the state of health: the hormone balance, nutritional status, water balance, etc. (59). The positive effect of P. acidilactici FT28 was consistent with a decreased serum TG concentration. Joysowal et al. (60) also observed a lower serum TG level by supplementing species-specific P. acidilactici and L. acidophilus in grower-finisher pigs. Blood analyses showed that the fermented feed increased glucose and decreased urea concentrations, data that are indicative of alterations in metabolism associated with the diet (61). Our findings are in agreement with Tretola et al. (61), namely, that these changes are due to the higher digestibility of the starchy feed and their higher glycemic index. Additionally, glucose—irrespective of insulin levels—decreases hepatic amino nitrogen conversion, an action that reduces the plasma nitrogen urea concentration (62).
Piglet Growth Performance
Average daily gain (ADG) and FE for the piglets are shown in Figure 6. The ADG was significantly higher at day 61 in the treated compared with the control piglets (0.546 vs. 0.455 kg, respectively). However, there were distinct tendencies during the different time points. At day 46, there was no difference in ADG between the groups, while at day 53, the control group ADG was higher than the treated group. In most of the cases, FE was higher in the control group piglets (except at day 53) compared with the treated group (1.63 and 1.53 kg, respectively). In modern swine production, fermented feed has been included to reduce the use of antibiotic growth promoters (63) and decrease feed price by using food processing by-products (64). During the fermentation process, most of the antinutritional factors are degraded, macronutrients are converted to lower-molecular-weight and more digestible compounds, and probiotics and their desirable metabolites occur in fermentable substrate (47, 65). The use of feed with a high content of viable desirable microorganisms increases the bioavailability of feed and improves pigs' digestibility and overall gastrointestinal functions (44), reduces the risk of diarrhea (66), and benefits pigs' health and growth performance (67). The use of fermented feed can reduce the feed cost in animal production (68). Supplementation with fermented feed increases organic acids and short-chain fatty acid concentrations in the hindgut (49) and improves intestinal functions, all of which further improve performance (69). Finally, feed fermentation with selected starters increases the nutritional quality and utilization of feed and provides health-related microorganisms that exert growth-promoting effects in the animals.
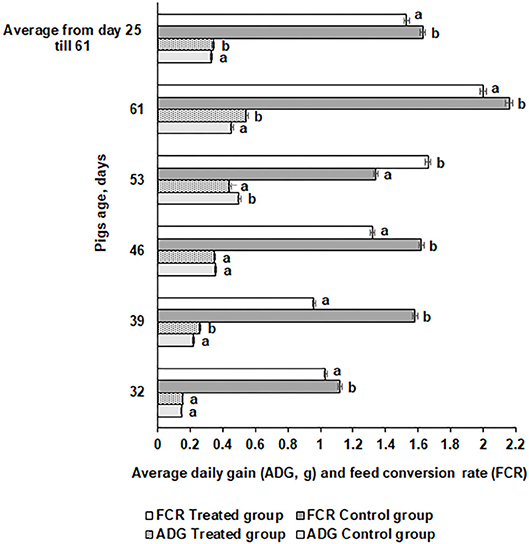
Figure 6. Average daily gain (ADG) and feed conversion rate (FCR) for the pigs. Data are expressed as the mean ± standard deviation (n = 10). Data were statistically compared with a paired t-test and column statistics. Means followed by different letters are significantly different (p ≤ 0.05).
In vivo Mycotoxins Bioconversion
The mycotoxin concentration (μg kg−1) in feed (basal and fermented) and piglets' fecal samples (control and treated groups on days 25 and 61) is shown in Table 4. AME was found in day 61 control feces and in fermented feed samples. However, AME was not found in feces from treated pigs. Alternaria fungi produce many secondary metabolites (more than 70); however, the most known are AOH, AME, tenuazonic acid (TeA), and TNX (70). While AME is mutagenic in vitro, there is limited evidence for AME carcinogenicity (71). AME has strong antifungal activity (72, 73) and exhibits genotoxic potential (74). Notably, AME is readily hydroxylated by pig hepatic microsomes (74). While AME is genotoxic at high micromolar concentrations in vitro (75), the cumulative concentration at the highest applied extract dose was calculated to be 54 nM, ~1,000-fold lower than what would be needed to impair DNA integrity (76).
Another secondary metabolite, ALT, showed a similar tendency to AME in the tested samples. Specifically, ALT was found in day 61 control group piglets' feces and in fermented feed samples. Alternaria mycotoxins contaminate cereal and may impact animal health, but data on its mammalian metabolism are scarce (77). The Alternaria mycotoxins often contaminate feed, a phenomenon that leads to a challenge for risk assessment. Some Alternaria mycotoxins possess estrogenic properties, which, together with other compounds such as ALT, iso-altenuene (iso-ALT), or altenuisol (ATL), can form the dibenzo-α-pyrone group of Alternaria toxins. Synergistic and cumulative effects might increase the toxicological effect of separate compounds. Thus, it is unclear which mechanism of action exerts a significant impact for adverse outcomes. This effect particularly applies to the estrogenic activity of dibenzo-α-pyrones, as growth-stimulating effects triggered by endocrine disruption are obviously only of potential relevance in sub-cytotoxic and sub-genotoxic concentrations. Finally, numerous Alternaria produce large quantities and varieties of toxins. Given this diverse mixture, it might lead to an overlay of distinct bioactivities depending on the qualitative and quantitative compositions of the exposure. The naturally occurring composition of Alternaria toxins might contain contrary effects and possess weak estrogenic activity (e.g., AOH, AME, and their respective metabolites) or anti-estrogenic activity (76).
FB1 is the most abundant and documented fumonisin toxin; it is produced by more than 30 species (78). FB1 is nephrotoxic and hepatotoxic (79, 80) and exhibits deleterious effects on animal health (81). Other clinical diseases induced by FB1 are leukoencephalomalacia, pulmonary edema, cardiac dysfunction (82), carcinogenesis (83), neural tube defects (84), and disruption of the intestines and immune system (85). Contamination levels of FB1 in feed are strictly regulated (86, 87). However, the mechanisms associated with FB1 toxicity remain unclear (81). FB1 reduces the concentration of ceramide and sphingomyelin and increases the levels of Sa and sphingolipid terminal products. For this reason, the Sa/So ratio is called a biomarker of FB1 exposure in animals (88, 89). There is a correlation between sphingolipids and the changes of other lipids (sterols and fatty acids). In the adipose tissue of rats, Cers4 is a potent target of endogenous lipid metabolism modulators (90), insulin, and/or changes in phospholipid transfer protein activity (91). Moreover, FA elongase 1 activity, which is included in both saturated and monounsaturated FA synthesis, is regulated by sphingolipid metabolism products (92). Sphingomyelins are also included in the post-translational processes of master regulators of FA and cholesterol metabolism (93). Kinome and transcriptome profiles of piglets exposed to FB1 showed that most of the effects of the mycotoxin are mediated by the influence on ceramide concentration (94). This mechanism of action induces the reduction of integrin-mediated cell-matrix adhesion, an inflammatory response, and alters the expression of genes included in cholesterol and FA homeostasis (95).
ROQ-C is a typical mycotoxin for Northern and Western European countries. It is frequently found in grass silages (96). A concentration of 25,000 μg kg−1 has no toxicological effect on sheep (97). However, there is a relationship between dairy cow diseases (paralysis, ketosis, and inappetence) and ROQ-C levels in feed at 25,000 μg kg−1 (98). Pigs are very sensitive to mycotoxins. Due to their high consumption of cereals, pigs are exposed to these toxins, as well as chronic contamination. Mycotoxins modulate the immune response of pigs, an action that leads to non-resistance to infectious diseases and lower vaccine efficacy. Furthermore, mycotoxins indirectly affect animal productivity (99). In EU, six mycotoxins, as feed contaminants, are reglemented: aflatoxins (AF), OTA, fumonisins (FB), ZEN, and trichothecenes (principally DON, T-2 and HT-2 toxins) (100). Notably, ROQ-C is not on the regulated mycotoxins list.
TNX was found in both basal and fermented feed (25.4 and 109.7 μg kg−1, respectively), as well as in 61-day-old treated piglet feces (22.4 μg kg−1). Till now, there are no regulations on Alternaria toxins in feed. AOH, AME, TeA, iso-TaA, ATXs, tentoxin (TEN), and ALT have been identified and chemically characterized. By increasing the sensitivity of the analytic techniques, several other Alternaria toxins have been identified. Information about Alternaria toxins in feed, their changes during the technological processes, and other factors is scarce. There is no information published about absorption, distribution, and excretion of Alternaria mycotoxins in animals. It was published that TEN is not mutagenic in bacteria. However, no data are available for Alternaria toxins, including in vivo genotoxicity or carcinogenicity. There is not enough knowledge about the possible effects of Alternaria toxins on farm, as well as about the occurrence of these mycotoxins in feed and, for this reason, to assess the risk regarding Alternaria toxins for animal health is not possible (101). However, the presence of emerging, masked, modified, etc. mycotoxins revealed by new analytical methods can also increase the health risk for pigs. Currently, very few studies document the occurrence and toxicity of these toxins. Finally, there is a need to determine the risk they represent in pig production (102).
Another compound, 15-AcDON, was found only in fermented feed samples (66.7 μg kg−1). Various factors can alter a mycotoxin's chemical structure. One of the factors is their hydrolysis to free DON or deepoxy-deoxynivalenol (DOM-1) by the intestinal microflora (103). In animals, mycotoxin metabolic detoxification has been described as the deep oxidation of DON, with the participation of intestinal microflora (102, 104) to DOM-1. This mechanism occurs in pigs and other animals. Furthermore, during DON biotransformation, it is conjugated with glucuronides, sulfonates, or glutathione (105). Glucuronidation involves UDP-glucuronosyltransferase activity and can occur in intestinal microsomes (104). Epoxidation is not very significant in pigs; however, glucuronidation is a very important factor of DON metabolism (106, 107). Conjugation of mycotoxins in animals contribute to the formation of DON-3,8,15-glucuronides (107). Enzyme-catalyzed glucuronidation is a slow process that is strongly influenced on the animal species (108). Modified DON forms are scarcely reported. European regulations limit the maximum permissible levels of major mycotoxins in feed also on animal age (for example, 900–12,000 μg kg−1 for DON) (109, 110). Finally, the risk for animals to be influenced by modified mycotoxins may be very high, and it is very important to start DON analog regulation in feed. Fusarium fungi that produce DON are separated into two sub-groups, according to chemotype: 3Ac-DON and 15-AcDON, these chemotypes may generate acetylated derivatives (111). In 2010, the Expert Committee of FAO/WHO for food additives published that acetyl derivatives of DON, also, should be controlled. In 2017, the European Food Safety Authority published report on hazards for animals by DON and its acetylated/modified derivatives in feed, where cereals are mentioned as the main risk source. Recent studies published that safety/toxicity of masked mycotoxins highly depends on the toxin type and the exposure (112). It was published that toxicity of the DON and its acetylated derivatives (3Ac-DON and 15Ac-DON) are potentially different. The first barrier for contaminants is an intestinal epithelium, which is highly sensitive to mycotoxins, particularly DON. It was reported that 3Ac-DON is less toxic than DON and that DON is less toxic than 15Ac-DON. The latter compound lowered the protective functions of the intestinal epithelium; however, such an influence of 3Ac-DON and DON on epithelium was not established. These findings were confirmed in ex vivo and in vivo studies (113).
Finally, mycotoxin biotransformation mechanism can be influenced by many factors (absolute concentration of mycotoxin, mycotoxin profile in intestine, dietary composition, and conditions, etc.), of which the microorganisms profile of the digestive tract is very important (103). Regulation applies only to the parent compounds and, unfortunately, does not include modified forms that are commonly present in feed. Abovementioned forms are a big challenge for the scientific community, namely, because no data are currently available on the toxicity and relations with other mycotoxins in vivo.
Conclusions
Compared to a commercially available LAB composition, the novel LAB composition effectively reduced feed pH, produced a 2-fold higher L(+) lactic acid content, increased the viable LAB count (on average 8.8 log10 CFU g−1), and led to stable feed fermentation during the 36-day experimental period. Fecal microbiota analysis showed an increased number of probiotic bacteria, particularly Lactobacillus, in the treated compared with the control group at the end of experiment. These data indicate that fermented feed can modify microbial profile change in the gut of pigs. Furthermore, fermented feed improved the hematological profile of the treated piglets. Mycotoxin analysis revealed that AME and ALT were found in 61-day-old control group piglets' feces and in fermented feed samples. However, AME was absent from treated piglet feces. Finally, feed fermentation with new LAB strain combination is very promising as a piglet microbiota modulation factor to improve nutrient absorption, growth performance, and health parameters. We also described a promising technology to increase local feed stock uses and make the process more economically feasible.
Data Availability Statement
All datasets generated for this study are included in the article/Supplementary Material.
Ethics Statement
All animal procedures were conducted according to the EU Directive (12) of the European Parliament and of Council from 22 September 2010 on the protection of animals used for scientific purposes and Requirements for the Keeping, Maintenance and Use of Animals Intended for Science and Education Purposes, approved by the order of the Lithuanian Director of the State Food and Veterinary Service (31/10/2012, No. B1-866) (13). This study was conducted at a pig farm in the Klaipeda district (Kontvainiai, Lithuania) and at the Institute of Animal Rearing Technologies, Lithuanian University of Health Sciences (Kaunas, Lithuania).
Author Contributions
The LUHS group conceived the study, participated in its design and coordination, participated in interpretation of the findings, and drafted the manuscript. The KTU group participated in the animal studies, statistical analysis, and interpretation of the findings in the manuscript. The BIOR group developed the method for mycotoxin (including masking mycotoxins) determination in feed and fecal samples and performed the analysis. All authors (LV, MR, VL, VS, PZ, EZ, VB, IP, IR, SB, DK, EM, AD, RG, and EB) read and approved the final manuscript.
Conflict of Interest
The authors declare that the research was conducted in the absence of any commercial or financial relationships that could be construed as a potential conflict of interest.
Acknowledgments
The authors gratefully acknowledge the EUREKA Network Project E!13309 SUSFEETECH and COST Action CA18101 SOURDOugh biotechnology network toward novel, healthier and sustainable food and bIoproCesseS.
Supplementary Material
The Supplementary Material for this article can be found online at: https://www.frontiersin.org/articles/10.3389/fvets.2020.528990/full#supplementary-material
Supplementary File 1. Control group before experiment genera.
Supplementary File 2. Experimental group before experiment genera.
Supplementary File 3. Control group after experiment genera.
Supplementary File 4. Experimental group after experiment genera.
Supplementary File 5. Control group after experiment species.
Supplementary File 6. Experimental group after experiment species.
References
1. Tasho RP, Cho JY. Veterinary antibiotics in animal waste, its distribution in soil and uptake by plants: a review. Sci Total Environ. (2016) 563–564:366–76. doi: 10.1016/j.scitotenv.2016.04.140
2. Wang X, Tsai T, Deng F, Wei X, Chai J, Knapp J, et al. Longitudinal investigation of the swine gut microbiome from birth to market reveals stage and growth performance associated bacteria. Microbiome. (2019) 7:109. doi: 10.1186/s40168-019-0721-7
3. Quaik S, Embrandiri A, Ravindran B, Hossain K, Al-Dhabi NA, Arasu MV, et al. Veterinary antibiotics in animal manure and manure laden soil: scenario and challenges in Asian countries. J King Saud Univ Sci. (2020) 32:1300–5. doi: 10.1016/j.jksus.2019.11.015
4. Jeong YD, Ko HS, Hosseindoust A, Choi YH, Chae BJ, Yu DJ, et al. Lactobacillus-based fermentation product and lactose level in the feed for weanling pigs: effects on intestinal morphology, microbiota, gas emission, and targeted intestinal coliforms. Livestock Sci. (2019) 227:90–6. doi: 10.1016/j.livsci.2019.06.018
5. Bartkiene E, Lele V, Sakiene V, Zavistanaviciute P, Ruzauskas M, Bernatoniene J, et al. Improvement of the antimicrobial activity of lactic acid bacteria in combination with berries/fruits and dairy industry by-products. J Sci Food Agric. (2019) 99:3992–4002. doi: 10.1002/jsfa.9625
6. Alcántara C, Bäuerl C, Revilla-Guarinos A, Pérez-Martínez G, Monedero V, Zúñiga M. Peptide and amino acid metabolism is controlled by an OmpR-family response regulator in Lactobacillus casei. Mol Microbiol. (2016) 100:25–41. doi: 10.1111/mmi.13299
7. Bartkiene E, Lele V, Ruzauskas M, Domig KJ, Starkute V, Zavistanaviciute P, et al. Lactic acid bacteria isolation from spontaneous sourdough and their characterization including antimicrobial and antifungal properties evaluation. Microorganisms. (2020) 8:64. doi: 10.3390/microorganisms8010064
8. Bartkiene E, Zokaityte E, Lele V, Sakiene V, Zavistanaviciute P, Klupsaite D, et al. Technology and characterisation of whole hemp seed beverages prepared from ultrasonicated and fermented whole seed paste. Int J Food Sci Technol. (2020) 55:406–19. doi: 10.1111/ijfs.14285
9. Cho JH, Zhao PY, Kim IH. Probiotics as a dietary additive for pigs: a review. J Anim and Vete Adv. (2011) 10:2127–34. doi: 10.3923/javaa.2011.2127.2134
10. Callaway TR, Edrington TS, Anderson RC, Harvey RB, Genovese KJ, Kennedy CN, et al. Probiotics, prebiotics and competitive exclusion for prophylaxis against bacterial disease. Anim Health Res Rev. (2008) 9:217–25. doi: 10.1017/S1466252308001540
11. Pajarillo EAB, Chae JP, Balolong MP, Kim HB, Park C-S, Kang D-K. Effects of probiotic Enterococcus faecium NCIMB 11181 administration on swine fecal microbiota diversity and composition using barcoded pyrosequencing. Anim Feed Sci Technol. (2015) 201:80–8. doi: 10.1016/j.anifeedsci.2015.01.011
12. Jastrzebski Z, Zychowska M, Konieczna A, Ratkowski W, Radzimiński Ł. Changes in the acid-base balance and lactate concentration in the blood in amateur ultramarathon runners during a 100-km run. Biol Sport. (2015) 32:261–5. doi: 10.5604/20831862.1163372
13. Bartkiene E, Bartkevics V, Krungleviciute V, Pugajeva I, Zadeike D, Juodeikiene G. Lactic acid bacteria combinations for wheat sourdough preparation and their influence on wheat bread quality and acrylamide formation. J Food Sci. (2017) 82:2371–8. doi: 10.1111/1750-3841.13858
14. Bartkiene E, Bartkevics V, Lele V, Pugajeva I, Zavistanaviciute P, Mickiene R, et al. A concept of mould spoilage prevention and acrylamide reduction in wheat bread: application of lactobacilli in combination with a cranberry coating. Food Control. (2018) 91:284–93. doi: 10.1016/j.foodcont.2018.04.019
15. ICC Standard Methods. 105/2 Determination of Crude Protein in Cereals and Cereal Products for Food and for Feed | ICC - International Association for Cereal Science and Technology (1994).
16. ISO 15214:1998. Microbiology of Food and Animal Feeding Stuffs — Horizontal Method for the Enumeration of Mesophilic Lactic Acid Bacteria — Colony-Count Technique at 30 Degrees C. Geneva: International Organization for Standardization ISO Central Secretariat (1998).
17. Directive 2010/63/EU of the European Parliament and of the Council of 22 September 2010 on the Protection of Animals Used for Scientific Purposes Text with EEA relevance. 276, 32010L0063 (2010) p. 33–76.
18. Valstybes Žinios. VMVT direktoriaus isakymas “Del Mokslo ir mokymo tikslais naudojamu gyvunu laikymo, prieŽiuros ir naudojimo reikalavimu patvirtinimo” Vilnius, Lithuania: Lithuanian Director of the State Food and Veterinary Service. B1-866 (2012) p. 1–58.
19. Nutrient Requirements of Swine: Eleventh Revised Edition. National Reaserch Counsil. Washington, DC: National Academic Press (2012).
21. Merkeviciene L, Ruzauskaite N, Klimiene I, Siugzdiniene R, Dailidaviciene J, Virgailis M, et al. Microbiome and antimicrobial resistance genes in microbiota of cloacal samples from European herring gulls (Larus argentatus). J Vet Res. (2017) 61:27–35. doi: 10.1515/jvetres-2017-0004
22. Reinholds I, Bogdanova E, Pugajeva I, Bartkevics V. Mycotoxins in herbal teas marketed in latvia and dietary exposure assessment. Food Additiv Contamin Part B Surveill. (2019) 12:199–208. doi: 10.1080/19393210.2019.1597927
23. Missotten JA, Michiels J, Degroote J, De Smet S. Fermented liquid feed for pigs: an ancient technique for the future. J Anim Sci Biotechnol. (2015) 6:4. doi: 10.1186/2049-1891-6-4
24. Liu H, Ji HF, Zhang DY, Wang SX, Wang J, Shan DC, et al. Effects of Lactobacillus brevis preparation on growth performance, fecal microflora and serum profile in weaned pigs. Lives Sci. (2015) 178:251–4. doi: 10.1016/j.livsci.2015.06.002
25. Messaoudi S, Manai M, Kergourlay G, Prévost H, Connil N, Chobert J-M, et al. Lactobacillus salivarius: bacteriocin and probiotic activity. Food Microbiol. (2013) 36:296–304. doi: 10.1016/j.fm.2013.05.010
26. Winsen RL, van Urlings BAP, Lipman LJA, Snijders JMA, Keuzenkamp D, Verheijden JHM, et al. Effect of fermented feed on the microbial population of the gastrointestinal tracts of pigs. Appl Environ Microbiol. (2001) 67:3071–6. doi: 10.1128/AEM.67.7.3071-3076.2001
27. Silva TC, da Silva LD, da Santos EM, Oliveira JS, Perazzo AF. Importance of the fermentation to produce high-quality silage. Ferment Proc. (2017) doi: 10.5772/64887. Available online at: file:///C:/Users/Elena/Downloads/52326%20(1).pdf
28. Kenny M, Smidt H, Mengheri E, Miller B. Probiotics – do they have a role in the pig industry? J Animal Biosci. (2011) 5:462–70. doi: 10.1017/S175173111000193X
29. Pringsulaka O, Rueangyotchanthana K, Suwannasai N, Watanapokasin R, Amnueysit P, Sunthornthummas S, et al. In vitro screening of lactic acid bacteria for multi-strain probiotics. Livest Sci. (2015) 174:66–73. doi: 10.1016/j.livsci.2015.01.016
30. Canibe N, Jensen BB. Fermented liquid feed—microbial and nutritional aspects and impact on enteric diseases in pigs. Anim Feed Sci Tech. (2012) 173:17–40. doi: 10.1016/j.anifeedsci.2011.12.021
31. Nabuurs MJ, Van De Weijgert EJ, Grootendorst AF, Niewold TA. Oedema disease is associated with metabolic acidosis and small intestinal acidosis. Res Vet Sci. (2001) 70:247–53. doi: 10.1053/rvsc.2001.0468
32. Wagerle LC, Kumar SP, Belik J, Delivoria-Papadopoulos M. Blood-brain barrier to hydrogen ion during acute metabolic acidosis in piglets. J Appl Physiol. (1988) 65:776–81. doi: 10.1152/jappl.1988.65.2.776
33. Ito T, Miyamoto H, Kumagai Y, Udagawa M, Shinmyo T, Mori K, et al. Thermophile-fermented compost extract as a possible feed additive to enhance fecundity in the laying hen and pig: modulation of gut metabolism. J Biosci Bioeng. (2016) 121:659–64. doi: 10.1016/j.jbiosc.2015.10.014
34. Kamel KS, Oh MS, Halperin ML. L-lactic acidosis: pathophysiology, classification, and causes; emphasis on biochemical and metabolic basis. Kidney Int. (2020) 97:75–88. doi: 10.1016/j.kint.2019.08.023
35. Su Y, Li B, Zhu W-Y. Fecal microbiota of piglets prefer utilizing dl-lactate mixture as compared to d-lactate and l-lactate in vitro. Anaerobe. (2013) 19:27–33. doi: 10.1016/j.anaerobe.2012.11.006
36. Satomura T, Hayashi J, Sakamoto H, Nunoura T, Takaki Y, Takai K, et al. d-Lactate electrochemical biosensor prepared by immobilization of thermostable dye-linked d-lactate dehydrogenase from Candidatus Caldiarchaeum subterraneum. J Biosci Bioeng. (2018) 126:425–30. doi: 10.1016/j.jbiosc.2018.04.002
37. Gresse R, Chaucheyras Durand F, Dunière L, Blanquet-Diot S, Forano E. Microbiota composition and functional profiling throughout the gastrointestinal tract of commercial weaning piglets. Microorganisms. (2019) 7:343. doi: 10.3390/microorganisms7090343
38. Correa-Fiz F, Blanco-Fuertes M, Navas MJ, Lacasta A, Bishop RP, Githaka N, et al. Comparative analysis of the fecal microbiota from different species of domesticated and wild suids. Sci Rep. (2019) 9:13616. doi: 10.1038/s41598-019-49897-1
39. Yang Q, Huang X, Zhao S, Sun W, Yan Z, Wang P, et al. Structure and function of the fecal microbiota in diarrheic neonatal piglets. Front Microbiol. (2017) 8:502. doi: 10.3389/fmicb.2017.00502
40. Xu J, Xu C, Chen X, Cai X, Yang S, Sheng Y, et al. Regulation of an antioxidant blend on intestinal redox status and major microbiota in early weaned piglets. Nutrition. (2014) 30:584–9. doi: 10.1016/j.nut.2013.10.018
41. Dredge R, Radloff SE, van Dyk JS, Pletschke BI. Lime pretreatment of sugar beet pulp and evaluation of synergy between ArfA, ManA and XynA from clostridium cellulovorans on the pretreated substrate. 3 Biotech. (2011) 1:151–9. doi: 10.1007/s13205-011-0019-3
42. Argüello H, Estellé J, Leonard FC, Crispie F, Cotter PD, O'Sullivan O, et al. Influence of the intestinal microbiota on colonization resistance to Salmonella and the shedding pattern of naturally exposed pigs. mSystems. (2019) 4:e00021–19. doi: 10.1128/mSystems.00021-19
43. Konstantinov SR, Smidt H, Akkermans ADL, Casini L, Trevisi P, Mazzoni M, et al. Feeding of Lactobacillus sobrius reduces Escherichia coli F4 levels in the gut and promotes growth of infected piglets. FEMS Microbiol Ecol. (2008) 66:599–607. doi: 10.1111/j.1574-6941.2008.00517.x
44. Canibe N, Jensen BB. Fermented and nonfermented liquid feed to growing pigs: effect on aspects of gastrointestinal ecology and growth performance. J Anim Sci. (2003) 81:2019–31. doi: 10.2527/2003.8182019x
45. Moran CAM, Scholten RHJ, Tricarico JM, Brooks PH, Verstegen MWA. Fermentation of wheat: effects of backslopping different proportions of pre-fermented wheat on the microbialand chemical composition. Arch Animal Nutr. (2006) 60:158–69. doi: 10.1080/17450390600562700
46. Mul AJ, Perry FG. The role of fructooligosaccharides in animal nutrition. In: Garnsworthy PC, Cole DJA, editors. Recent Advances in Animal Nutrition. Nottingham: Nottingham University Press (1994). p. 57–79.
47. Urlings HAP, Mug AJ, Klooster AT van ‘t, Bijker PGH, Logtestijn JG, van Gils LG. Microbial and nutritional aspects of feeding fermented feed (poultry by-products) to pigs. Vet Q. (1993) 15:146–51. doi: 10.1080/01652176.1993.9694394
48. Demecková V, Kelly D, Coutts AGP, Brooks PH, Campbell A. The effect of fermented liquid feeding on the faecal microbiology and colostrum quality of farrowing sows. Int J Food Microbiol. (2002) 79:85–97. doi: 10.1016/S0168-1605(02)00182-4
49. Missotten JAM, Michiels J, Ovyn A, Smet SD, Dierick NA. Fermented liquid feed for pigs. Arch Anim Nutr. (2010) 64:437–66. doi: 10.1080/1745039X.2010.512725
50. Brooks PH. Fermented liquid feed for pigs. CAB reviews: perspectives in agriculture. Vet Sci. (2008) 3:1–18. doi: 10.1079/PAVSNNR20083073
51. Dinardo FR, Minervini F, De Angelis M, Gobbetti M, Gänzle MG. Dynamics of enterobacteriaceae and lactobacilli in model sourdoughs are driven by pH and concentrations of sucrose and ferulic acid. Food Sci Tehnol. (2019) 114:108394. doi: 10.1016/j.lwt.2019.108394
52. García-Cano I, Velasco-Pérez L, Rodríguez-Sanoja R, Sánchez S, Mendoza-Hernández G, Llorente-Bousquets A, et al. Detection, cellular localization and antibacterial activity of two lytic enzymes of Pediococcus acidilactici ATCC 8042. J Appl Microbiol. (2011) 111:607–15. doi: 10.1111/j.1365-2672.2011.05088.x
53. Väkeväinen K, Hernández J, Simontaival A-I, Severiano-Pérez P, Díaz-Ruiz G, von Wright A, et al. Effect of different starter cultures on the sensory properties and microbiological quality of Atole agrio, a fermented maize product. Food Control. (2020) 109:106907. doi: 10.1016/j.foodcont.2019.106907
54. Greppi A, Rantsiou K, Padonou W, Hounhouigan J, Jespersen L, Jakobsen M, et al. Determination of yeast diversity in ogi, mawè, gowé and tchoukoutou by using culture-dependent and -independent methods. Int J Food Microbiol. (2013) 165:84–8. doi: 10.1016/j.ijfoodmicro.2013.05.005
55. Nowak P, Kasprowicz-Potocka M, Zaworska A, Nowak W, Stefańska B, Sip A, et al. The effect of eubiotic feed additives on the performance of growing pigs and the activity of intestinal microflora. Arch Anim Nutr. (2017) 71:455–69. doi: 10.1080/1745039X.2017.1390181
56. Upadhaya SD, Park JW, Lee JH, Kim IH. Efficacy of β-mannanase supplementation to corn–soya bean meal-based diets on growth performance, nutrient digestibility, blood urea nitrogen, faecal coliform and lactic acid bacteria and faecal noxious gas emission in growing pigs. Arch Anim Nutr. (2016) 70:33–43. doi: 10.1080/1745039X.2015.1117697
57. Dong X, Zhang N, Zhou M, Tu Y, Deng K, Diao Q. Effects of dietary probiotics on growth performance, faecal microbiota and serum profiles in weaned piglets. Anim Prod Sci. (2013) 54:616–21. doi: 10.1071/AN12372
58. Kumar S, Verma AK, Mondal SK, Gupta M, Patil AK, Jangir BL. Effect of live saccharomyces cerevisiae feeding on serum biochemistry in early weaned cross bred piglets. Vet World. (2012) 5:663–6. doi: 10.5455/vetworld.2012.663-666
59. Dowarah R, Verma AK, Agarwal N, Singh P, Singh BR. Selection and characterization of probiotic lactic acid bacteria and its impact on growth, nutrient digestibility, health and antioxidant status in weaned piglets. PLoS ONE. (2018) 13:e0192978. doi: 10.1371/journal.pone.0192978
60. Joysowal M, Saikia B, Dowarah R, Tamuly S, Kalita D, Choudhury K. Effect of probiotic pediococcus acidilactici FT28 on growth performance, nutrient digestibility, health status, meat quality, and intestinal morphology in growing pigs. Vet World. (2018) 11:1669–76. doi: 10.14202/vetworld.2018.1669-1676
61. Tretola M, Ottoboni M, Luciano A, Rossi L, Baldi A, Pinotti L. Former food products have no detrimental effects on diet digestibility, growth performance and selected plasma variables in post-weaning piglets. Ital J Anim Sci. (2019) 18:987–96. doi: 10.1080/1828051X.2019.1607784
62. Newman MA, Zebeli Q, Eberspächer E, Grüll D, Molnar T, Metzler-Zebeli BU. Transglycosylated starch improves insulin response and alters lipid and amino acid metabolome in a growing pig model. Nutrients. (2017) 9:291. doi: 10.3390/nu9030291
63. Plumed-Ferrer C, Wright AV. Fermented pig liquid feed: nutritional, safety and regulatory aspects. J Appl Microbiol. (2009) 106:351–68. doi: 10.1111/j.1365-2672.2008.03938.x
64. Wang J, Han Y, Zhao J, Zhou Z, Fan H. Consuming fermented distillers' dried grains with solubles (DDGS) feed reveals a shift in the faecal microbiota of growing and fattening pigs using 454 pyrosequencing. J Integr Agric. (2017) 16:900–10. doi: 10.1016/S2095-3119(16)61523-X
65. Kiarie E, Bhandari S, Scott M, Krause DO, Nyachoti CM. Growth performance and gastrointestinal microbial ecology responses of piglets receiving saccharomyces cerevisiae fermentation products after an oral challenge with Escherichia coli (K88). J Anim Sci. (2011) 89:1062–78. doi: 10.2527/jas.2010-3424
66. Kiers JL, Meijer JC, Nout MJR, Rombouts FM, Nabuurs MJA, Meulen JVD. Effect of fermented soya beans on diarrhoea and feed efficiency in weaned piglets. J Appl Microbiol. (2003) 95:545–52. doi: 10.1046/j.1365-2672.2003.02011.x
67. Mukherjee R, Chakraborty R, Dutta A. Role of fermentation in improving nutritional quality of soybean meal — a review. Asian-Australas J Anim Sci. (2015) 29:1523–9. doi: 10.5713/ajas.15.0627
68. Shi C, Zhang Y, Yin Y, Wang C, Lu Z, Wang F, et al. Amino acid and phosphorus digestibility of fermented corn-soybean meal mixed feed with Bacillus subtilis and Enterococcus faecium fed to pigs. J Anim Sci. (2017) 95:3996–4004. doi: 10.2527/jas.2017.1516
69. Niba AT, Beal JD, Kudi AC, Brooks PH. Bacterial fermentation in the gastrointestinal tract of non-ruminants: influence of fermented feeds and fermentable carbohydrates. Trop Anim Health Prod. (2009) 41:1393–407. doi: 10.1007/s11250-009-9327-6
70. Arcella D, Eskola M, Ruiz JAG. Dietary exposure assessment to Alternaria toxins in the European population. EFSA J. (2016) 14:e04654. doi: 10.2903/j.efsa.2016.4654
71. Scott PM, Zhao W, Feng S, Lau BP-Y. Alternaria toxins alternariol and alternariol monomethyl ether in grain foods in Canada. Mycotoxin Res. (2012) 28:261–6. doi: 10.1007/s12550-012-0141-z
72. Hussain H, Krohn K, Ullah Z, Draeger S, Schulz B. Bioactive chemical constituents of two endophytic fungi. Biochem Syst Ecol. (2007) 35:898–900. doi: 10.1016/j.bse.2007.04.011
73. Bensassi F, Gallerne C, el Dein OS, Hajlaoui MR, Bacha H, Lemaire C. Mechanism of alternariol monomethyl ether-induced mitochondrial apoptosis in human colon carcinoma cells. Toxicology. (2011) 290:230–40. doi: 10.1016/j.tox.2011.09.087
74. Pfeiffer E, Schebb NH, Podlech J, Metzler M. Novel oxidative in vitro metabolites of the mycotoxins alternariol and alternariol methyl ether. Mol Nutr Food Res. (2007) 51:307–16. doi: 10.1002/mnfr.200600237
75. Schwarz C, Kreutzer M, Marko D. Minor contribution of alternariol, alternariol monomethyl ether and tenuazonic acid to the genotoxic properties of extracts from alternaria alternata infested rice. Toxicol Lett. (2012) 214:46–52. doi: 10.1016/j.toxlet.2012.08.002
76. Aichinger G, Krüger F, Puntscher H, Preindl K, Warth B, Marko D. Naturally occurring mixtures of alternaria toxins: anti-estrogenic and genotoxic effects in vitro. Arch Toxicol. (2019) 93:3021–31. doi: 10.1007/s00204-019-02545-z
77. Puntscher H, Hankele S, Tillmann K, Attakpah E, Braun D, Kütt M-L, et al. First insights into alternaria multi-toxin in vivo metabolism. Toxicol Lett. (2019) 301:168–78. doi: 10.1016/j.toxlet.2018.10.006
78. Norhasima WMW, Abdulamir AS, Bakar FA, Son R, Norhafniza A. The health and toxic adverse effects of fusarium fungal mycotoxin, fumonisins, on human population. Am J Infect Dis. (2009) 5:273–81. doi: 10.3844/ajidsp.2009.273.281
79. Voss KA, Chamberlain WJ, Bacon CW, Herbert RA, Walters DB, Norred WP. Subchronic feeding study of the mycotoxin fumonisin B1 in B6C3F1 mice and fischer 344 rats. Fundam Appl Toxicol. (1995) 24:102–10. doi: 10.1006/faat.1995.1012
80. Humphreys SH, Carrington C, Bolger M. A quantitative risk assessment for fumonisins B1 and B2 in US corn. Food Addit Contam. (2001) 18:211–20. doi: 10.1080/02652030010021486
81. Régnier M, Polizzi A, Lukowicz C, Smati S, Lasserre F, Lippi Y, et al. The protective role of liver X receptor (LXR) during fumonisin B1-induced hepatotoxicity. Arch Toxicol. (2019) 93:505–17. doi: 10.1007/s00204-018-2345-2
82. Haschek WM, Gumprecht LA, Smith G, Tumbleson ME, Constable PD. Fumonisin toxicosis in swine: an overview of porcine pulmonary edema and current perspectives. Environm Health Perspect. (2001) 109(Suppl. 2):251–7. doi: 10.1289/ehp.01109s2251
84. Marasas WFO, Riley RT, Hendricks KA, Stevens VL, Sadler TW, Gelineau-van Waes J, et al. Fumonisins disrupt sphingolipid metabolism, folate transport, and neural tube development in embryo culture and in vivo: a potential risk factor for human neural tube defects among populations consuming fumonisin-contaminated maize. J Nutr. (2004) 134:711–6. doi: 10.1093/jn/134.4.711
85. Grenier B, Bracarense A-PFL, Schwartz HE, Trumel C, Cossalter A-M, Schatzmayr G, et al. The low intestinal and hepatic toxicity of hydrolyzed fumonisin B1 correlates with its inability to alter the metabolism of sphingolipids. Biochem Pharmacol. (2012) 83:1465–73. doi: 10.1016/j.bcp.2012.02.007
86. Commission Regulation (EC) No 1126/2007 of 28 September 2007 amending Regulation (EC) No 1881/2006 Setting Maximum Levels for Certain Contaminants in Foodstuffs as Regards Fusarium Toxins in Maize and Maize Products. Official Journal of the European Union. (2007) 255. p. 14–7.
87. Commission Recommendation of 17 August 2006 on the Presence of Deoxynivalenol Zearalenone Ochratoxin A T-2 and HT-2 and Fumonisins in Products Intended for Animal Feeding. Text with EEA Relevance) (2006/576/EC). Official Journal of the European Union (2006) 49. p. 7–9.
88. Wang E, Norred WP, Bacon CW, Riley RT, Merrill AH. Inhibition of sphingolipid biosynthesis by fumonisins. Implications for diseases associated with fusarium moniliforme. J Biol Chem. (1991) 266:14486–90.
89. Loiseau N, Polizzi A, Dupuy A, Therville N, Rakotonirainy M, Loy J, et al. New insights into the organ-specific adverse effects of fumonisin B1: comparison between lung and liver. Arch Toxicol. (2015) 89:1619–29. doi: 10.1007/s00204-014-1323-6
90. Bonzón-Kulichenko E, Schwudke D, Gallardo N, Moltó E, Fernández-Agulló T, Shevchenko A, et al. Central leptin regulates total ceramide content and sterol regulatory element binding protein-1C proteolytic maturation in rat white adipose tissue. Endocrinology. (2009) 150:169–78. doi: 10.1210/en.2008-0505
91. Rosenthal EA, Ronald J, Rothstein J, Rajagopalan R, Ranchalis J, Wolfbauer G, et al. Linkage and association of phospholipid transfer protein activity to LASS4. J Lipid Res. (2011) 52:1837–46. doi: 10.1194/jlr.P016576
92. Ohno Y, Suto S, Yamanaka M, Mizutani Y, Mitsutake S, Igarashi Y, et al. ELOVL1 production of C24 acyl-CoAs is linked to C24 sphingolipid synthesis. Proc Natl Acad Sci USA. (2010) 107:18439–44. doi: 10.1073/pnas.1005572107
93. Scheek S, Brown MS, Goldstein JL. Sphingomyelin depletion in cultured cells blocks proteolysis of sterol regulatory element binding proteins at site 1. Proc Natl Acad Sci USA. (1997) 94:11179–83. doi: 10.1073/pnas.94.21.11179
94. Régnier M, Gourbeyre P, Pinton P, Napper S, Laffite J, Cossalter A-M, et al. Identification of signaling pathways targeted by the food contaminant FB1: transcriptome and kinome analysis of samples from pig liver and intestine. Mol Nutr Food Res. (2017) 61. doi: 10.1002/mnfr.201700433. Available online at: https://onlinelibrary.wiley.com/doi/epdf/10.1002/mnfr.201700433
95. Gronemeyer H, Gustafsson J-A, Laudet V. Principles for modulation of the nuclear receptor superfamily. Nat Rev Drug Discov. (2004) 3:950–64. doi: 10.1038/nrd1551
96. McElhinney C, Danaher M, Elliott C, O'Kiely P. Mycotoxin occurrence on baled and pit silages collected in Co. Meath. Irish Agr Food Res. (2015) 54:87–97. doi: 10.1515/ijafr-2015-0010
97. Tüller G, Armbruster G, Wiedenmann S, Hänichen T, Schams D, Bauer J. Occurrence of roquefortine in silage — toxicological relevance to sheep. J Anim Physiol Anim Nutr. (1998) 80:246–9. doi: 10.1111/j.1439-0396.1998.tb00536.x
98. Häggblom P. Isolation of roquefortine C from feed grain. Appl Environ Microbiol. (1990) 56:2924–6. doi: 10.1128/AEM.56.9.2924-2926.1990
99. Klasing KC. Nutrition and the immune system. Br Poult Sci. (2007) 48:525–37. doi: 10.1080/00071660701671336
100. Bennett JW, Klich M. Mycotoxins. Clin Microbiol Rev. (2003) 16:497–516. doi: 10.1128/CMR.16.3.497-516.2003
101. Scientific Opinion on the risks for public health related to the presence of zearalenone in food. EFSA J. (2011) 9:2197. doi: 10.2903/j.efsa.2011.2197
102. Pierron A, Mimoun S, Murate LS, Loiseau N, Lippi Y, Bracarense A-PFL, et al. Intestinal toxicity of the masked mycotoxin deoxynivalenol-3-β-d-glucoside. Arch Toxicol. (2016) 90:2037–46. doi: 10.1007/s00204-015-1592-8
103. Bryła M, Waśkiewicz A, Ksieniewicz-Wozniak E, Szymczyk K, Jedrzejczak R. Modified fusarium mycotoxins in cereals and their products—metabolism, occurrence, and toxicity: an updated review. Molecules. (2018) 23:963. doi: 10.3390/molecules23040963
104. Wu Q, Dohnal V, Huang L, Kuca K, Yuan Z. Metabolic pathways of trichothecenes. Drug Metab Rev. (2010) 42:250–67. doi: 10.3109/03602530903125807
105. Wen J, Mu P, Deng Y. Mycotoxins: cytotoxicity and biotransformation in animal cells. Toxicol Res. (2016) 5:377–87. doi: 10.1039/C5TX00293A
106. Nagl V, Woechtl B, Schwartz-Zimmermann HE, Hennig-Pauka I, Moll W-D, Adam G, et al. Metabolism of the masked mycotoxin deoxynivalenol-3-glucoside in pigs. Toxicol Lett. (2014) 229:190–7. doi: 10.1016/j.toxlet.2014.06.032
107. Uhlig S, Ivanova L, Fæste CK. Enzyme-assisted synthesis and structural characterization of the 3-, 8-, and 15-glucuronides of deoxynivalenol. J Agric Food Chem. (2013) 61:2006–12. doi: 10.1021/jf304655d
108. Maul R, Warth B, Schebb NH, Krska R, Koch M, Sulyok M. In vitro glucuronidation kinetics of deoxynivalenol by human and animal microsomes and recombinant human UGT enzymes. Arch Toxicol. (2015) 89:949–60. doi: 10.1007/s00204-014-1286-7
109. Commission Regulation (EC) No. 1881/2006 of 19 December 2006 Setting Maximum Levels for Certain Contaminants in Foodstuffs. Official Journal of the European Union (2006) p. 5–24.
110. Commission Recommendation (EU) 2016/1319 of 29 July 2016 Amending Recommendation 2006/576/EC as regards Deoxynivalenol Zearalenone and Ochratoxin A in Pet Food. Official Journal of the European Union (2016). p. 4863.
111. Zhang H, Zhang Z, van der Lee T, Chen WQ, Xu J, Xu JS, et al. Population genetic analyses of fusarium asiaticum populations from barley suggest a recent shift favoring 3ADON producers in Southern China. Phytopathology. (2010) 100:328–36. doi: 10.1094/PHYTO-100-4-0328
112. Dellafiora L, Galaverna G, Righi F, Cozzini P, Dall'Asta C. Assessing the hydrolytic fate of the masked mycotoxin zearalenone-14-glucoside – A warning light for the need to look at the “maskedome.” Food Chem Toxicol. (2017) 99:9–16. doi: 10.1016/j.fct.2016.11.013
Keywords: fermentation, feed, piglets, microbiota, blood parameters, growth performance, mycotoxins
Citation: Vadopalas L, Ruzauskas M, Lele V, Starkute V, Zavistanaviciute P, Zokaityte E, Bartkevics V, Pugajeva I, Reinolds I, Badaras S, Klupsaite D, Mozuriene E, Dauksiene A, Gruzauskas R and Bartkiene E (2020) Combination of Antimicrobial Starters for Feed Fermentation: Influence on Piglet Feces Microbiota and Health and Growth Performance, Including Mycotoxin Biotransformation in vivo. Front. Vet. Sci. 7:528990. doi: 10.3389/fvets.2020.528990
Received: 22 January 2020; Accepted: 10 September 2020;
Published: 16 October 2020.
Edited by:
Andrea Serra, University of Pisa, ItalyReviewed by:
Inkyung Park, Animal Biosciences and Biotechnology Laboratory (USDA-ARS), United StatesYosra Ahmed Soltan, Alexandria University, Egypt
Copyright © 2020 Vadopalas, Ruzauskas, Lele, Starkute, Zavistanaviciute, Zokaityte, Bartkevics, Pugajeva, Reinolds, Badaras, Klupsaite, Mozuriene, Dauksiene, Gruzauskas and Bartkiene. This is an open-access article distributed under the terms of the Creative Commons Attribution License (CC BY). The use, distribution or reproduction in other forums is permitted, provided the original author(s) and the copyright owner(s) are credited and that the original publication in this journal is cited, in accordance with accepted academic practice. No use, distribution or reproduction is permitted which does not comply with these terms.
*Correspondence: Elena Bartkiene, ZWxlbmEuYmFydGtpZW5lQGxzbXVuaS5sdA==