- 1Division of Molecular Biology and Human Genetics, Faculty of Medicine and Health Sciences, Department of Science and Innovation - National Research Foundation Centre of Excellence for Biomedical Tuberculosis Research, South African Medical Research Council Centre for Tuberculosis Research, Stellenbosch University, Cape Town, South Africa
- 2Disease Investigations, San Diego Zoo Global, San Diego, CA, United States
- 3Veterinary Wildlife Services, Kruger National Park, Skukuza, South Africa
Cases of tuberculosis (TB) resulting from infection with Mycobacterium tuberculosis complex (MTBC) have been recorded in captive white (Ceratotherium simum) and black (Diceros bicornis) rhinoceros. More recently, cases have been documented in free-ranging populations of both species in bovine tuberculosis (bTB) endemic areas of South Africa. There is limited information on risk factors and transmission patterns for MTBC infections in African rhinoceros, however, extrapolation from literature on MTBC infections in other species and multi-host systems provides a foundation for understanding TB epidemiology in rhinoceros species. Current diagnostic tests include blood-based immunoassays but distinguishing between subclinical and active infections remains challenging due to the lack of diagnostic techniques. In other species, demographic risk factors for MTBC infection include sex and age, where males and adults are generally at higher risk than females and younger individuals. Limited available historical information reflects similar age- and sex-associated patterns for TB in captive black and white rhinoceros, with more reports of MTBC-associated disease in black rhinoceros than in white rhinoceros. The degree of MTBC exposure in susceptible wildlife depends on their level of interaction, either directly with other infected individuals or indirectly through MTBC contaminated environments, which is dependent on the presence and abundance of infected reservoir hosts and the amount of MTBC shed in their excreta. Captive African rhinoceros have shown evidence of MTBC shedding, and although infection levels are low in free-ranging rhinoceros, there is a risk for intraspecies transmission. Free-ranging rhinoceros in bTB endemic areas may be exposed to MTBC from other infected host species, such as the African buffalo (Syncerus caffer) and greater kudu (Tragelaphus strepsiceros), through shared environmental niches, and resource co-utilization. This review describes current knowledge and information gaps regarding the epidemiology of TB in African rhinoceros.
Introduction
Tuberculosis (TB) is a chronic infectious disease that affects a broad range of host species (1). It is caused by members of a group of closely related pathogenic mycobacteria known as the Mycobacterium tuberculosis complex (MTBC) (1). Bovine tuberculosis (bTB) is caused by Mycobacterium bovis (M. bovis), which is known to infect livestock as well as captive and free-ranging wildlife species (2, 3). For most wildlife, however, little is known about susceptibility to MTBC infection, pathogenesis, and its impact on affected populations.
Rhinoceros are iconic species which are under threat due to habitat destruction and heavy poaching pressure. Cases of TB resulting from infection with M. tuberculosis and M. bovis have been recorded in captive, semi-captive (maintained in private reserves and more intensively managed), and free-ranging rhinoceros worldwide (4–19); TB was implicated as the cause of death in some of these cases.
Kruger National Park (KNP) and Hluhluwe–iMfolozi Park (HiP) are home to large populations of free-ranging black (Diceros bicornis) and white (Ceratotherium simum) rhinoceros in South Africa. As of 2016, HiP housed ~1,500 white rhinoceros and 360 black rhinoceros (20). In 2017, the Kruger National Park contained ~5,150 white rhinoceros and 500 black rhinoceros (21), at which time the global populations totaled 20,300 white rhinoceros and 5,200 black rhinoceros (22). The HiP and KNP populations have been and continue to be central to the “Integrated Strategic Management of Rhinoceros” plan, introduced by the South African Department of Environmental Affairs (23, 24). Part of this strategy relies on the translocation of rhinoceros from the feeder populations in these parks to newly developing rhinoceros safeguarding strongholds around the country. However, KNP and HiP are endemic for bTB, and these rhinoceros populations share habitat ranges and various resources with M. bovis–infected wildlife (over 20 species in KNP), including important bTB maintenance hosts such as African buffaloes (Syncerus caffer) (2), and greater kudu (Tragelaphus strepsiceros) (25–27). The identification of disease in free-ranging wildlife is often challenging due to limited resources and access to these populations for diagnostic testing, and it was only with the increase in poaching and associated veterinary interventions that evidence of MTBC infection in white and black rhinoceros in KNP was discovered (9, 19).
Although M. bovis and M. tuberculosis have not been considered an immediate threat to the world's African rhinoceros populations, the potential impact of these pathogens on their health and conservation is largely unknown. Because bTB is a World Organization for Animal Health (OIE) and nationally notifiable disease (1), animals with M. bovis infection are subject to regulatory requirements which limit their movements between populations; this hampers conservation efforts that are reliant on translocation of rhinoceros from bTB-endemic to bTB-free areas.
Since the discovery of M. bovis infection in the free-ranging rhinoceros populations in KNP (13, 23), knowledge gaps regarding the risk of MTBC infection, intra- and inter-species transmission, and disease progression in these species have become apparent. This review describes the current knowledge regarding TB in African rhinoceros and provides information on epidemiological aspects of this disease in other relevant species, especially free-ranging populations, to improve understanding of the disease and inform management strategies.
Assessment of Infection Risk in African Rhinoceros
With any infectious disease, the risk of becoming infected is based on an individual's susceptibility when exposed to an infectious dose of the etiological agent, as well as the likelihood of exposure to the pathogen (28). These factors provide a foundation for investigating infection risks and will be discussed as they pertain to M. tuberculosis and M. bovis infection in black and white rhinoceros.
Outcomes and Detection of MTBC Infections: Epidemiological Implications
MTBC infections are typically chronic and once disease occurs, usually progressive. In various human and animal hosts, infections have been observed to move between various stages in a dynamic host-pathogen interaction network, where clinical manifestations of the infection vary between stages (29–32). Following infection, the host's innate immune system may eliminate the mycobacteria. If the mycobacteria are not eliminated, T helper type-1 cell-mediated immune (CMI) responses develop and are followed by the T helper type-2 humoral immune response through B-lymphocyte activation and an increase in circulating antibodies (33–35). Immunological responses of the host play a key role in determining the outcome of infection, and in humans, these include latent, incipient and subclinical stages (30). These stages have not been clearly defined in animal hosts, but subclinical MTBC infections have been reported in a variety of species (36–39). Although controversial, there is speculation that latent infection of animals with M. bovis may also occur (35, 40). These complex and dynamic interactions between host and pathogen can lead to elimination of infection, or an asymptomatic stage in which the mycobacteria are either dormant or result in localized disease, or progression to active disease, which has been observed in a multitude of species (29, 30, 41, 42).
Although there is a paucity of information on outcomes of MTBC infection in rhinoceros, a hypothesized scenario is shown in Figure 1; however, further investigation is required to verify these stages. Most cases of TB in zoos have only been detected once disease is sufficiently advanced to detect clinical signs, resulting in the death of the rhinoceros either due to euthanasia or disease complications (Supplementary Table 1) (4–6, 17). However, a study involving three experimentally M. bovis-infected white rhinoceros, monitored serially over 2 years, suggests that although immunological responses could be detected, the animals appeared to contain, and potentially eliminate, the infection (43, 44). Similarly, in the limited cases of natural M. bovis infection in white rhinoceros, pathological lesions were localized in lymph nodes or other tissues (19). The outcome of infection appears to be more complex than simply a progression to disease, based on these observations as well as reports of immunological responses (without evidence of disease) in rhinoceros that have been exposed to other known TB cases (7, 13, 15). In addition, decreases in immunocompetence as a result of comorbidities, drought, capture/transport–induced stress, increased age, or other factors may be associated with greater susceptibility to disease as sequelae of acute infection or activation of subclinical infection in rhinoceros, although tools to identify these stages need to be developed.
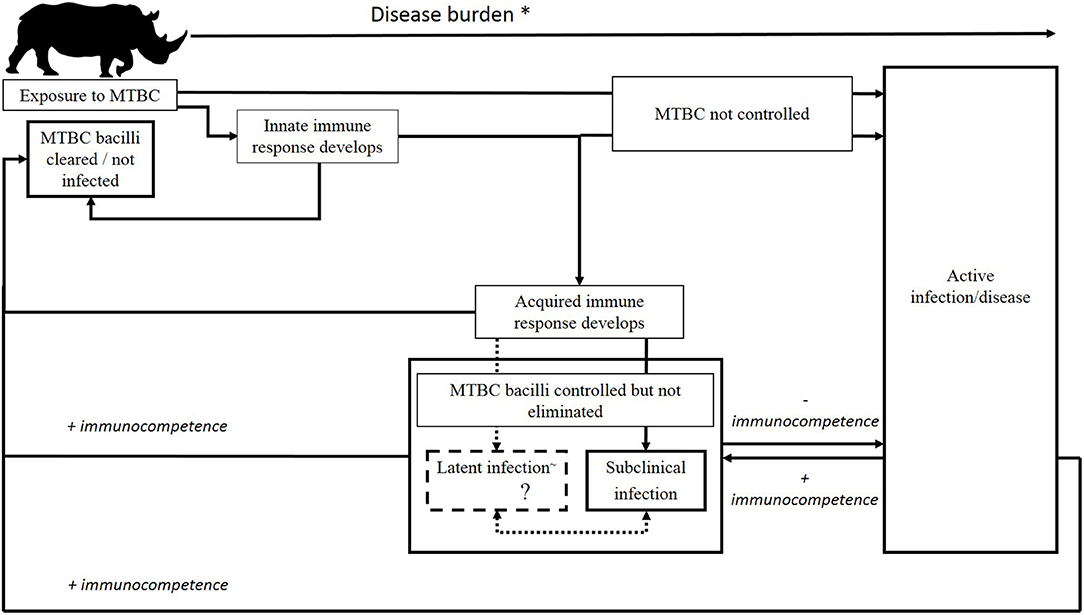
Figure 1. Possible outcomes of M. bovis infection in African rhinoceros. After initial exposure, MTBC may be eliminated by the host's immune response, persist as a subclinical or latent~ infection, or progress to active infection/disease. Following the establishment of subclinical or latent infection, the host's immune response may clear the MTBC, or infection may persist in this form, either naturally progressing in a slow or rapid fashion to active tuberculosis, or cycling through subclinical and latent states, before development into symptomatic disease or eventual eradication of the infection by the host's adaptive immune response. *Rising disease burden implies an increase in abundance of TB and/or MTBC biomarkers, immunological changes characteristic of stage of infection, and increasing pathology, with a declining recovery prognosis. ~Although controversial, there is speculation that latent infection of animals with M. bovis may occur. More research is required.
In order to characterize the epidemiology of TB in a population, it is important to have accurate diagnostic methods that can distinguish between various stages of infection and disease, since animals in different stages of infection may present with altered levels of transmission risk (45). Risk factors associated with acquiring an infection may be different from those that increase the likelihood of disease progression, or the maintenance of a subclinical or latent infection (28, 30). This important distinction may have implications for understanding the epidemiology of TB and could impact subsequent management decisions.
In vivo and in vitro indirect detection methods for early MTBC infection primarily rely on the detection of TB-specific adaptive immune responses of the host, including the tuberculin skin test (TST) and MTBC antigen stimulated cytokine assays (46, 47). In addition, serological assays for the detection of host-specific antibodies to MTBC antigens have been useful for diagnosis of TB in certain animal species (48–50), although they are considered unreliable for TB diagnosis in humans (51). In rhinoceros, the TST is unreliable due to cross-reactivity with environmental mycobacteria (7, 13, 52); therefore, a white rhinoceros whole blood MTBC antigen-specific interferon-gamma release assay (IGRA) for M. bovis infection has recently been developed (44, 53). Serological assays for the detection of antigen-specific antibodies have also been shown to be useful for the diagnosis of MTBC infection in rhinoceros (15, 18). However, the use of these indirect immunological diagnostic assays alone may not distinguish between recent infection, latent/incipient/subclinical infection, and active disease.
One way to overcome the challenges posed by these indirect tests is to directly detect the pathogen by mycobacterial culture and nucleic acid amplification tests (NAATs) (54, 55). Mycobacterial culture and speciation are useful as both pre- and post-mortem diagnostic tests for MTBC infection. Ante-mortem samples obtained for culture include bronchoalveolar, tracheal and gastric lavages, as well as nasal and fecal swabs, although culture of tissue obtained during necropsy may be more sensitive for detection of bacilli. Although this method is highly specific, culture of ante-mortem samples has low sensitivity, which may be related to the site of infection and whether the individual is shedding at the time of sampling (56). For example, in a study evaluating shedding in three experimentally M. bovis-infected rhinoceros, only one of 36 tracheal lavage samples collected monthly over a 2-year period was M. bovis culture positive (43). In humans and recently in wildlife, mycobacterial culture has been supplemented with NAATs including the automated GeneXpert MTB/RIF Ultra qPCR assay (Ultra) (57, 58). This rapid ancillary test may enable the direct detection of MTBC DNA in some tissues (59), as well as animal respiratory samples (57). Regardless, the direct detection of MTBC organisms alone also does not provide information on the host's stage of infection or disease. The presence and classification of lesions detected by macroscopic and microscopic examination provides important information for staging disease, although this is primarily used post-mortem (43, 60).
Molecular methods such as spoligotyping, mycobacterial interspersed repetitive unit-variable number of tandem repeat (MIRU-VNTR) genotyping, or whole genome sequencing of M. bovis isolates may be useful not just for diagnosis, but also for tracing the origin of MTBC infection in multi-host systems (26, 61–64). The application of these techniques in rhinoceros and other free-ranging wildlife, though useful, is challenging due to limited samples. Nonetheless, these techniques have been employed to investigate the distribution and transmission of MTBC strains in some wildlife multi-host systems (63), including between brushtail-possums (Trichosurus volpecula) (65), badgers (65, 66), deer (67) or African buffaloes (68) and cattle at the livestock/wildlife interface (65–68). Therefore, use of both direct and indirect detection methods should be included in investigations of transmission in rhinoceros.
Investigating Susceptibility of Black and White Rhinoceros to Infection With M. tuberculosis and M. bovis
As a result of the popularity of rhinoceros for zoological exhibition, they have historically been globally distributed through importation. Reports of TB in captive rhinoceros in zoological gardens worldwide date back to the late 1800's. Historical cases of bTB and TB in black and white rhinoceros are summarized in Figure 2. While TB is still considered a rare occurrence in domestic perissodactyls (69), these cases provide evidence for susceptibility of black and white rhinoceros.
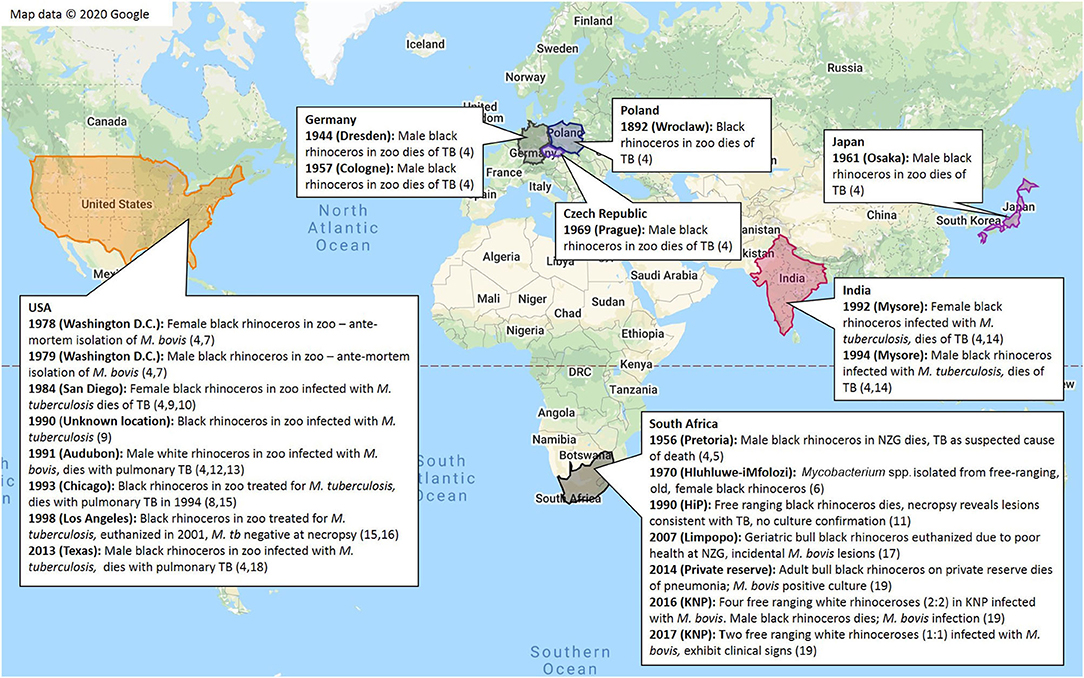
Figure 2. Summary of globally recorded historical cases of tuberculosis in black rhinoceros (D. bicornis) and white rhinoceros (C. simum) (4–19)*. *This data was located in the Rhinoceros Resource Center (RRC) literature database, or through extensive web searches with (9) as a guide.
According to limited information, most TB cases have been recorded in black rhinoceros, with an apparent paucity of cases identified in white rhinoceros (4–19). This observation may be related to differences in numbers, demographics, housing or management of these species in zoological collections, exposure to other infected animals, employees or visitors, differences in species-specific susceptibility to the different pathogens, or the impact of individual or species-specific co-morbidities on immunocompetence and TB susceptibility. Black rhinoceros in captivity are known to suffer from a variety of syndromes (70); afflicted individuals may have compromised immunity that increases their susceptibility to TB, which could explain the apparent higher prevalence in this species compared to white rhinoceros.
Most reports of TB in captive rhinoceros have been caused by infection with M. tuberculosis, with only a few caused by M. bovis. This is hypothesized to be a result of a high level of exposure of captive animals to M. tuberculosis through direct or indirect interactions with humans (especially in high TB burden countries), while in captivity or during exportation (4) (Supplementary Table 1). Another explanation might be differences in virulence of M. tuberculosis and M. bovis in rhinoceros, although limited studies suggest that M. bovis has greater virulence in other species, such as mice and goats, compared to M. tuberculosis (71, 72).
The susceptibility of white rhinoceros to M. bovis was studied in experimentally infected animals (43, 44). The results confirmed susceptibility to infection and potential to shed bacilli, albeit based on the detection of viable M. bovis in only one of 36 tracheal lavage samples collected over the course of the study. None of the individuals developed clinical signs or evidence of disease based on gross and histological examination, although M. bovis DNA was detected by PCR in lung tissues of two animals at necropsy (43). The immune response kinetics and pathological findings suggested that the rhinoceros were able to contain and possibly clear M. bovis infection (44). This observation is consistent with the historical lack of TB cases in white rhinoceros, which may be due to the ability to contain and clear the infection before the onset of disease. However, it should be noted that the response of rhinoceros to experimental inoculation in this study may not reflect naturally occurring infection, which could occur through one or more exposure events over time, each with variable numbers of MTBC bacilli. Additionally, the individuals in this study were not subject to stressful conditions and were young (4–7 years old); all individuals had adapted to living in managed care by the time of initial infection, and were not exposed to the seasonal variations in food availability that they might have been if free-ranging. It is unknown whether the lack of disease development in these white rhinoceros was a consequence of low susceptibility to disease resulting from M. bovis infection, or the conditions associated with the experimental infection. In contrast, a naturally M. bovis infected 29-year-old white rhinoceros in a zoo developed weight loss, cough and nasal discharge and succumbed to the infection (13), demonstrating that this species can develop disease.
In contrast to white rhinoceros, evidence of TB disease in black rhinoceros has been reported more frequently. An elderly (estimated 35–40 years old) black rhinoceros, euthanized due to loss of condition, had small non-encapsulated pulmonary granulomas associated with M. bovis infection (17). Similarly, the free-ranging black rhinoceros in KNP, infected with M. bovis, had evidence of significant pulmonary pathological changes (9). Interestingly, the first case was in an elderly animal and the second rhinoceros case was discovered after a prolonged period of drought. However, cases of M. bovis-associated disease in zoo black rhinoceros have been reported in animals that were ~20–25 years old (7). In addition, post-mortem pulmonary changes consistent with M. tuberculosis disease were observed in multiple zoo black rhinoceros aged 13–33 years old (14, 15, 18). The reports of clinical signs and presence of changes post-mortem in black rhinoceros infected with M. bovis or M. tuberculosis suggests this species may be more prone to TB disease.
Assessing Risk Factors for Infection and Transmission Patterns of MTBC in African Rhinoceros Populations
Demographic Risk Factors
There is limited literature that characterizes risk factors of MTBC exposure and transmission patterns in free-ranging black and white rhinoceros populations, likely a result of the logistical and technical difficulties associated with disease surveillance and sporadic cases in these animals. Therefore, extrapolation from literature on TB in other species and multi-host systems may aid in understanding the epidemiology of MTBC in African rhinoceros populations. Demographic risk factors for MTBC infection, such as sex and age, have been described in humans, mice, cattle, and limited species of wildlife (48, 49, 73–82). Results from these studies may inform hypotheses regarding demographic patterns of MTBC infection in rhinoceros.
In many TB-susceptible species, sex is considered a risk factor for infection (48, 49, 73–78). In humans, the global male to female ratio for individuals that develop TB is 2:1 (73). While this has partially been due to socioeconomic and cultural barriers in access to healthcare (74), inherent biological characteristics are also implicated. Sex-based differences in susceptibility are usually observed in adults, but not in children or adolescents (83). This suggests that the relative male: female difference in susceptibility is related to the effect of steroid sex hormones and their regulatory activities on immune cells (84).
Both testosterone and progesterone are immunosuppressive. These hormones impair macrophage activation and may increase TB susceptibility (85, 86). In contrast, estrogen is a pro-inflammatory mediator that stimulates the production of tumor necrosis factor alpha (TNF-α) (87), and interacts with the IFN-γ promoter (88). In mice, increased susceptibility to TB has been observed in post-adolescent males relative to post-adolescent females, with this difference partially mitigated by castration (84).
In various wildlife species, studies have shown a higher frequency of MTBC infection in males, which could be linked to hormonal differences, but behavioral differences may also play a role. In a cohort of free-ranging African elephants (Loxodonta africana) tested in KNP, overall TB seroprevalence was higher in males than in females (48). Another study reported a higher risk for both bTB infection and disease in male badgers (Meles meles) than in females (75). An epidemiological study of white-tailed deer (Odocoileus virginianus) in Michigan also reported a higher odds of being bTB test-positive in males compared to females (76). This study also reported a dramatic effect of sex on the association of increasing age with positive TB test status. In fawns and yearlings, no significant difference in TB incidence between males and females was found; however, in age groups of 2 years and above, males were increasingly more likely to test positive for TB than females of the same age class. This is a similar trend to what is observed in humans, and may be due either to sex-based hormonal differences, or to the contrasting social/reproductive behavior between mature males and females (76, 89). Therefore, it may be difficult to separate risk factors associated with hormonal and behavioral differences in adults.
Most wildlife epidemiological studies report a higher risk of bTB in males than in females; however, this association does not strictly hold true for all species. Studies of different populations of wild boar, for example, have yielded conflicting results with respect to the association between sex and bTB risk. One study on wild boars in Portugal (82) reported a significantly higher bTB incidence in female than males in all age groups. In a different wild boar population in Spain (81), studies showed a significantly higher bTB prevalence in males. Studies of wild boar populations in France (77) and Italy (78) reported no significant association between sex and TB risk. Variable findings from different populations of the same species illustrate the complexity of determining sex-associated bTB risk. Hormone-derived TB susceptibility of a species may be largely conserved between different multi-host systems; however, sex may also be a mediator of pathogen exposure due to sex-related differences in social, reproductive, and territorial behavior as well as movement patterns. The degree to which such sex-related factors alter rhinoceros' exposure to MTBC may depend on the unique characteristics of dispersal and transmission in that particular host system (89).
Most historical reports of TB in captive rhinoceros have occurred in black rhinoceros males (Figure 2 and Supplementary Table 1). It is unknown whether this observation represents a true species and sex predilection for TB, or whether reports are biased for other reasons, such as the skewed natal sex ratios in captive black rhinoceros (90), or a disproportionate number of black rhinoceros and/ or males kept in zoos. However, available records show a preference for import and exhibition of female white rhinoceros over males, and no substantial preferential import of male vs. female black rhinoceros for exhibition during the twentieth century (4). Records for USA, Mexico, and Australia show a near-equivalent sex ratio of black rhinoceros currently kept in captivity (91, 92), and the sex ratio of white rhinoceros in captivity in Canada, the USA, Mexico, Chile, and Singapore is substantially skewed toward females (93). These records also indicate that a higher number of white rhinoceros are kept in captivity compared to black rhinoceros; there are currently 278 white rhinoceros in captivity in Canada, the USA, Mexico, Chile, and Singapore (93), compared to 96 black rhinoceros in the USA, Mexico, and Australia (91, 92). These data do not support an apparent bias toward males or black rhinoceros in captivity, which suggests that reports (Figure 2 and Supplementary Table 1) may reflect a true increased risk for TB in these groups. While the absolute historical numbers of rhinoceros housed in captivity globally are unknown, and therefore cannot be used to draw conclusions on TB risk in rhinoceros, these observations provide avenues for further investigation into species and sex-specific susceptibility.
In humans, TB occurs in individuals of all ages, although the highest burden is in men past adolescence (≥15 years old) (73). Human susceptibility to TB shows an increase with age, which may be due to age-related effects on the immune system or possibly the outcome of multiple exposures over time (79). A similar age-related bTB trend has been observed in cattle, with a peak in incidence after 12 months of age (80). Adult warthogs (Phacochoerus africanus) and African elephants (>25 years old) in bTB endemic regions also showed a higher seroprevalence than their younger counterparts (48, 49). Increasing age had the greatest effect on TB disease risk in a meerkat population in South Africa (94).
While most studies in wildlife show increasing bTB prevalence with age, studies conducted in the Iberian Peninsula report higher prevalence in juvenile wild boar than adults in high-prevalence multi-host systems (81, 95). This could be due to higher susceptibility in juveniles compared to fully-grown adults in this species, possibly related to immunological maturity, or age-related changes in behavior that result in increased exposure to the pathogen. Interestingly, historically reported cases of TB in captive African rhinoceros appear to have occurred exclusively in adults (Figure 2 and Supplementary Table 1). These observations suggest that infection can take years to manifest in these species (18), or that there is an increase in susceptibility and/or repeat exposures with age. Based on other species, it is likely that both sex and age are risk factors for MTBC exposure and infection in black and white rhinoceros.
Transmission of M. bovis in Multi-Host Systems
Investigation of TB transmission has been limited in free-ranging rhinoceros until recently because of a lack of diagnostic assays and paucity of samples. Therefore, characterization of transmission depends largely on extrapolation using patterns observed in other multi-host systems. Some of the predictors for persistence and transmission of a pathogen within a multi-host system appear to be related to patterns of movement, migration, and different modes of interactions between host species (28, 96, 97). Importantly, the presence of an infected reservoir species in the system has been shown to increase the risk of spill-over to other susceptible hosts. Wildlife reservoir hosts for bTB are present globally, including African buffaloes in South Africa (27) [and possibly other areas in Africa where the species occurs (98, 99)], greater kudu in South Africa (27), brush-tailed possums (T. volpecula) in New Zealand (100), European wild boar (Sus scrofa), red deer and fallow deer (Dama dama) in Spain (95), white-tailed deer (O. virginianus) in the USA (101), elk (Cervus canadensis) (102) and American bison (Bison bison) (103) in Canada, and European badgers (M. meles) in the United Kingdom (97). In wildlife populations with M. bovis, there are numerous examples of intra- and inter-species transmission (27, 104–107). Direct intra-species M. bovis transmission can occur through respiratory droplets in social species like African buffaloes (107) or through antagonistic or territorial behaviors like those that occur between white-tailed deer (76).
The mechanism of inter-species M. bovis transmission to herbivores is largely unknown but has been attributed to indirect interactions through contamination of pastures, feed, or browse with MTBC shed by infected hosts. Various studies have demonstrated that infected hosts shed M. bovis into the environment (106, 108). In one study, intranasal administration of M. bovis to calves resulted in intermittent shedding for up to 38 weeks (108). A European study of infected wild boar and red deer demonstrated shedding by oronasal, bronchial-alveolar, fecal and urinary routes (106). In that study, 83% of wild ungulates with bTB had mycobacteria isolated in at least one type of excretion, which suggests a high level of shedding into the environment. In a study in Spain, interactions between four different species (cattle, domestic pigs, red deer, and wild boar) in a bTB endemic system found that although there was a low percentage of direct interactions between these species, there was a high percentage of indirect interactions over the 3-day time frame investigated, suggesting a high risk of indirect transmission (109). A similar study in France detected a high frequency of indirect interactions between badgers, wild boar, and red deer at waterholes and baited locations (110). Therefore, environmental contamination may present risks for transmission to susceptible hosts sharing the same resources as infected individuals. However, in addition to the presence of an infected host that is shedding, the pathogen must remain viable in the environment for enough time to encounter the susceptible host.
Routes of Transmission of M. bovis to Rhinoceros in Sub-Saharan Africa
Predicting routes of transmission of MTBC requires an understanding of patterns of shedding, movement patterns, social behavior, and resource utilization of susceptible hosts in relation to infected hosts, and the persistence of the pathogen in a contaminated environment.
Wildlife Maintenance Hosts as a Source of M. bovis Infection in African Rhinoceros in Sub-Saharan Africa
Domestic livestock (such as cattle) are implicated as bTB maintenance hosts where spillover into wildlife occurs (25, 111). However, since KNP and HiP have perimeter fencing in place to prevent disease interactions between wildlife and cattle, transmission from livestock is unlikely to be a major mode of M. bovis infection acquisition in rhinoceros in these areas. In South Africa, the African buffalo is a recognized bTB reservoir host that is implicated in the spill-over of M. bovis to other susceptible hosts, both directly, and indirectly through shedding into the environment (27, 68, 105, 112). There is evidence that greater kudu can also be maintenance hosts in the Kruger National Park and possibly in other bTB endemic areas where the species occurs (27). Since these large herbivorous hosts are often found in similar ranges and utilize the same resources as white and black rhinoceros, interactions between these species are likely to occur. These interactions may be a potential route for transmission of M. bovis to African rhinoceros.
According to recent biodiversity statistics, KNP African rhinoceros share the park with an estimated 37,130 African buffaloes (21). Similarly, HiP has a buffalo population of ~3,500 (113). African buffaloes are socially organized into herds, which can be as large as 1,000 individuals (112, 114). A study that investigated seasonal movements and habitat use by these animals revealed home ranges varying between 73 and 601 km2 (115). Due to the size of their home ranges, interactions with other species (including rhinoceros), particularly at aggregation points such as water sources or shared feeding areas, are likely to occur at a relatively regular frequency. Dispersal events, though less frequent in adult females, occur in adults of both sexes of buffalo (116). Natal dispersal events occur at least once in most adult male buffaloes, and can be driven in both sexes by seasonal (water and nutrient) or social resource limitations (117). Additionally, bTB disease may influence individual health and body condition in buffaloes, which could indirectly impact dispersal events (118). The resulting frequency of dispersal events may influence the probability of pathogen exposure opportunities resulting in spillover from buffaloes to other susceptible species, including white and black rhinoceros in bTB endemic areas.
Investigation of preferred vegetation and habitat of buffaloes showed the strongest association with open to closed herbaceous vegetation on temporarily flooded land, closed shrubs, open shrubs or with 40–65% crown cover (115). The white rhinoceros, like the African buffalo, is a grazing species (119, 120). Their vegetation preference closely mirrors that of buffaloes. In wet months, white rhinoceros may concentrate their grazing in the short grass-dominated grasslands, while in the dry seasons, they move to tall grass grasslands, with a general preference for shaded grasses. Thickets are generally rejected in favor of open grassland vegetation. The black rhinoceros is a browsing species, and their vegetation preference has less in common with that of African buffaloes. They tend to associate closely with thickets (closed shrubland or low forest areas) for access to food (120). For this reason, pathogen exposure interactions with buffaloes due to aggregation at shared feeding areas may be more likely to occur in white rhinoceros than in black rhinoceros.
Available statistics indicate that there are between 11,200 and 17,300 greater kudu in KNP (21). For HiP, a recent estimate of the greater kudu population was not found. The greater kudu is a browsing species of antelope that is socially organized into small bachelor, cow, or mixed herds, typically of fewer than ten individuals (121–123). The home ranges of these herds are typically small and stable, and male home ranges often overlap; the greater kudu social system appears to be based on absolute social dominance (according to age) and territoriality is not evident in this species. Due to their small and stable home ranges, interactions with other species (even indirect) are likely to be less frequent than those observed in buffaloes, who range more widely. However, black rhinoceros share their vegetation preference of thickets or more woody, covered vegetation with this species (124); as a result, indirect interactions with infected kudu (e.g., via shedding of M. bovis through fistulated lymph nodes in kudu leading to contamination of vegetation during browsing) may be an important mode of bTB transmission to black rhinoceros. Buffaloes, greater kudus, black and white rhinoceros share water pans, which may also increase the frequency of interactions within and between these species (125–128).
Overall, the wide ranges traversed by buffaloes on a seasonal basis and the potential for contamination of browse by infected kudu, coupled with evidence supporting their integral roles as maintenance hosts for bTB, support the potential for M. bovis transmission to white and black rhinoceros (115, 129). While overlapping vegetation preference, mud wallow usage, and ranges may be important infection predictors, indirect transmission of M. bovis from maintenance hosts may not be the only risk factor for infection of rhinoceros. Risk factors for infection should be considered as part of a multi-dimensional network, with the potential for transmission from other infected species, or possibly intra-species transmission.
Intra-Species Transmission of MTBC in Rhinoceros
Initial M. bovis infection in free-ranging rhinoceros in KNP was likely a result of spread from African buffaloes or other infected wildlife species, since the strains of M. bovis isolated from rhinoceros cases were the same as those identified in other KNP wildlife, based on comparison of spoligotypes in different studies (19, 64). However, it is unclear whether these infections were the immediate result of inter- or intra-species transmission in rhinoceros. Although rhinoceros have been translocated extensively, it is interesting that the only reported cases of bTB in rhinoceros in South Africa are in animals that originated from or spent time in parks with M. bovis-infected reservoir hosts (9, 17, 19). Therefore, further investigation is needed to determine if there is a risk of intra-species spread in rhinoceros.
As with inter-species transmission, the risk of intra-species transmission is dependent on whether the infected host is shedding MTBC, and the frequency of interactions between shedding and susceptible individuals, either directly or indirectly through utilization of shared resources. Evidence suggests that rhinoceros can shed MTBC into their environment in respiratory secretions, or at least that mycobacteria are present in the respiratory system of infected individuals (43, 130). Necropsies of two black rhinoceros in an Indian zoo revealed the presence of acid-fast organisms and large volumes of purulent material in the lungs (14). One of these rhinoceroses was sneezing and had a yellow muco-purulent nasal discharge in the days before its death. M. tuberculosis has also been isolated from nasal secretions from an infected black rhinoceros in a zoo which had diagnosed TB in several different species of animals (16). In addition, M. tuberculosis was isolated from a gastric lavage sample of a captive black rhinoceros with pulmonary disease, which suggests that like in humans, infected material may be coughed up and swallowed, leading to potential shedding of mycobacteria in feces (8, 15). These observations suggest the possibility that infected rhinoceros may transmit MTBC in secretions, presenting a risk for spread to other animals, and possibly humans, that are in close prolonged contact, such as in a zoo setting.
In free-ranging African rhinoceros, characteristics of social organization may inform the frequency of interactions between shedding and susceptible individuals. Adult female rhinoceros tend to occupy home ranges of up to 70 km2, whereas adult bulls are often territorial, and occupy ranges up to 40 km2 with little to no overlap, although young bulls may share their territory (131, 132). Young adult females generally range more widely than males, then settle into a similar, smaller home range to have their first calf (131). Home ranges are typically based on permanent water sources and food availability. African rhinoceros may move beyond their usual home ranges during dry periods and peak mating months (124, 133). Although there are some cases in which social groups have been observed in black rhinoceros (134), they tend to be more solitary. In general, cohesive social groups of white rhinoceros are mostly pairs—these can be adolescent-adolescent, cow-adolescent, cow-cow, and cow-calf pairs (132). However, social groups of up to ten individuals have been observed in white rhinoceros in the KNP (134). As density of white rhinoceros in a home range increases, the range occupied by individual cows or territorial bulls decreases. Groups of up to four adult cows with their offspring generally have smaller home ranges than solitary cows (132).
Behavioral characteristics of rhinoceros may also influence the risk of intra-species transmission of pathogens. Territorial behavior is prominent in adult bulls, with frequent olfactory territorial marking, or urine “spraying.” Additionally, both defecation and urination are ritualized in territorial bulls, using specific locations, called “middens,” scattered around the territory (131, 133, 135). Therefore, if M. bovis is excreted in feces, like in wild boar and red deer (106), middens might serve as a contaminated site where MTBC bacteria persist. In both black and white rhinoceros, mud wallowing is a behavior practiced more frequently during summer, and in the heat of the day, but can occur at any time (133). Therefore, shared use of wallows may be a potential source of intra-species transmission of M. bovis excreted in respiratory secretions or feces.
In summary, both direct and indirect interactions occur between individual rhinoceros, with direct contacts likely occurring at a higher frequency in white rhinoceros. Social exchanges, as well as overlap of ranges, and shared utilization of water sources, middens, and mud wallows are likely to result in indirect interfaces that carry potential for pathogen transmission. However, the apparent low prevalence and lack of disseminated disease in affected free-ranging populations of rhinoceros suggest that the risk of intra-species transmission of MTBC is lower than in captive rhinoceros (19), due to differences in duration and frequency of contact.
Environmental Contamination as a Route for Indirect Transmission of M. bovis
For indirect transmission of bTB to occur, an area, shared by the recipient host, must be contaminated with MTBC by an infected individual (109). Several studies have successfully isolated pathogenic mycobacterial DNA from various environmental substrates, including water, soil, sediments, and grass; this finding supports the possibility of a M. bovis-contaminated environment as a source of exposure for rhinoceros (136). Recipient host exposure risk likely increases with an increase in the mycobacterial load shed by infected hosts into the environment.
Factors affecting persistence of M. bovis in the environment have been investigated but are still poorly understood. Because of the low sensitivity associated with culture of MTBC from the environment, qPCR specific for MTBC DNA has been adapted as a supplementary quantification method (136, 137), and 16S rRNA has also been used as a proxy for viable MTBC (138). In addition, the type of samples collected within a system also appears to influence detection of M. bovis. For example, using PCR, MTBC DNA has been more frequently recovered in sediments around waterholes than in water in environments with M. bovis-infected hosts (139).
Seasonal changes in environmental conditions appear to influence persistence of M. bovis in the environment. Both air and soil temperatures affect detection of MTBC DNA in environmental samples (136, 137, 139). Soil concentrations of M. bovis DNA were higher in spring compared to all other seasons in the Iberian Peninsula (139). Regardless of soil type, M. bovis DNA concentrations were higher when air and soil temperatures were moderate (averaging ~15°C and 17°C, respectively), and with greater soil moisture content (~50%) in spring, compared to higher air and soil temperatures (maximum averages 32.6 and 26.4°C, respectively) and lower soil moisture content (~2%) in summer in this region (139). A study in Michigan (USA) showed that the persistence of M. bovis (measured by PCR and culture) in contaminated environmental substrates, which varied between 4 weeks and 6 months, was shortened by exposure to high ambient temperatures, increased intensity of solar radiation, and higher loss of substrate moisture through evapotranspiration (136). In addition, when soil was spiked with M. bovis, persistence was longer at 4°C than at 22°C (137). This was in agreement with field studies that showed that M. bovis persisted longer in soil in autumn/winter than in summer in Michigan and New Zealand (140, 141), though in these cases it is not clear whether this was only correlated to temperature, or a combination of variables associated with certain seasons. One previous study done under controlled conditions presented contradictory findings related to the correlation of M. bovis persistence with temperature; M. bovis persisted longer in spiked soils at 37°C than at 4°C (138), highlighting some of the continued knowledge gaps related to persistence of M. bovis in the environment. Physico-chemical properties in soil, such as the proportional contribution of clay, silt, sand and organic matter to overall composition, as well as pH, and mineral content (137), may also affect M. bovis persistence in the environment. Presence of shade has also been associated with the persistence of environmental M. bovis, likely due to maintenance of higher water content and moderate temperatures of the soil and vegetation (142). Additionally, reduced ultraviolet radiation in shade results in less cell stress and fewer genetic mutations, improving bacterial survival (142). These areas may play a role in exposure to M. bovis because resting rhinoceros and other species often occupy shady areas during the hottest times of the day, which may promote concentration of bacteria shed in secretions.
In addition to abiotic factors, there are other biological reservoirs that are ubiquitous in the environment and may play a vital role in environmental persistence and subsequent transmission of MTBC. MTBC bacilli have been isolated from free-living amoeba, found frequently in soil (143). It has also been discovered that earthworms (Lumbricus terrestris) can disseminate M. bovis from contaminated animal feces to the surrounding soil through casting egestion (144). These worms can shed bacteria for up to 4 days after initial ingestion of contaminated feces. The presence of these organisms in areas where grazing by M. bovis-infected and susceptible hosts occurs may promote exposure through indirect interactions.
It is hypothesized that, due to the influence of environmental variables on the ability of M. bovis to persist in the environment, there may be a seasonal variation in bTB transmission risk. Environmental persistence of M. bovis under cold and wet conditions, as well as seasonal changes in the presence of shade and vegetation, may influence M. bovis exposure risk in rhinoceros; however further studies are needed to determine pathogen persistence in the natural habitats of these species.
Discussion
The Importance of MTBC Infections in African Rhinoceros
Today, the largest free-ranging populations of African rhinoceros in South Africa are located in bTB endemic areas. While poaching of African rhinoceros for their horns is currently the most substantial threat to their conservation (23, 24), the presence of bTB in rhinoceros presents a considerable barrier to conservation due to the inability to translocate animals from bTB endemic areas to safeguarding areas that are bTB free. Without the tools to screen rhinoceros in endemic areas for M. bovis infection, regulations prevent translocation to bTB-free areas. Individuals that cannot be moved for safeguarding purposes are then exposed to risk of mortality resulting from poaching incidents. Although bTB is not currently recognized as a major cause of morbidity or mortality or a threat to rhinoceros population health, understanding the epidemiology and pathogenesis of this disease in rhinoceros will provide a foundation for studying the impact of bTB on these species. African rhinoceros populations in zoos around the world may also be at risk of MTBC infection, as individuals in these populations have exhibited morbidity and mortality (4–19). Based on limited case reports, both M. tuberculosis and M. bovis infect black and white rhinoceros, although the epidemiology and sources of these infections may differ. In addition to the impact on individual rhinoceros health, these infections may also result in spread to other animals, as well as humans, in the zoo environment (12, 13, 130). Therefore, it is essential to investigate the epidemiology of MTBC infections in rhinoceros in various settings to inform the most appropriate disease management and control strategies.
Hypothesized TB Risk Factors
As in other species, it is expected that the risk of MTBC infection in rhinoceros will be based on factors influencing susceptibility of the individual and exposure to the MTBC (28).
M. tuberculosis vs. M. bovis
Most reported cases of TB in captive rhinoceros resulted from infection with M. tuberculosis, with only a few cases caused by M. bovis. This is likely due to differing levels of exposure to each pathogen according to its prevalence, as well as the likelihood of interaction with an infected host, including both other animals and humans. TB affects human populations worldwide, and most human cases are caused by infection with M. tuberculosis (145). Captive rhinoceros may therefore have a higher likelihood of exposure to M. tuberculosis than M. bovis through their prolonged close contact with infected human caregivers. In these cases, transmission may occur through aerosols or through a contaminated environment, and possibly both.
While M. bovis can cause TB in humans, the pathogen is less efficient than M. tuberculosis at propagating through human hosts (146). This animal-adapted MTBC species predominantly occurs in livestock and wildlife populations, and is maintained in certain endemic areas by susceptible host populations (3). Free-ranging rhinoceros in bTB endemic areas may be exposed to M. bovis through infected hosts or a contaminated environment; the latter is likely to occur more frequently, especially for inter-species transmission, as free-ranging animals are less likely to have close prolonged contact with other infected host species than they are to share aggregation points in their environments (e.g., water sources) with these species. Exposure to M. tuberculosis is less likely in free-ranging populations than in captive rhinoceros, as they have little to no interaction with humans, which are the most affected by this pathogen.
Species-Specific Susceptibility
The more frequently reported occurrence of TB disease in captive black rhinoceros than in white rhinoceros, from the case series outlined in Figure 2 and Supplementary Table 1, lends credence to the hypothesis that black rhinoceros are more prone to TB disease than white rhinoceros. However, this observation may be due to other confounding factors, including inapparent differences in numbers of black and white rhinoceros in captivity, and differences in M. tuberculosis or M. bovis exposure. Disease surveillance and reporting biases also exist because not all institutions that house rhinoceros conduct post-mortem TB surveillance or have equal diagnostic capabilities, not all cases of TB in rhinoceros are recorded in the literature, and non-cases are often not reported (147). Nevertheless, the data presented provides foundational hypotheses for further evaluation of TB risk in black and white rhinoceros.
Sex as a Risk Factor for MTBC Infection and TB
Limited available data from scientific reports on rhinoceros show more cases of TB in males compared to females (Figure 2 and Supplementary Table 1). It is unknown whether this observation represents a true sex predilection for TB, or whether reports are biased toward males for other reasons. That said, a proposed hypothesis is that African rhinoceros males have a higher susceptibility to TB than females, and this is further supported by males in other species tending to have higher rates of TB compared to females (refer to section Demographic Risk Factors).
In free-ranging African rhinoceros populations in South Africa, it is hypothesized that females have a higher exposure to M. bovis due to their wider home range, and more consistent association in cohesive groups than males, and it is unknown how this may contribute to the overall TB risk of males and females in these populations. More controlled investigation in free-ranging populations is required to test these hypotheses.
Age as a Risk Factor for MTBC Infection and TB
All recorded TB and bTB cases (Figure 2 and Supplementary Table 1) have occurred in adults. One explanation for this observation is the chronic and recurring nature of this disease. It is possible that young individuals are just as susceptible to MTBC infection as older individuals, but that the disease takes extended time to clinically manifest. An alternative explanation is that diminishing immunocompetence with age (as occurs with age-based hormonal changes in humans as well as the immune effects of old age) could render adults more susceptible to infection and onset of disease than young rhinoceros. It is possible that this observation is attributed not only to age-based changes in susceptibility, but is also due to age-related changes in exposure to either M. tuberculosis and M. bovis due to translocation of captive rhinoceros for management and breeding (Figure 2 and Supplementary Table 1) (4). Based on this limited information, and age-related TB risk trends observed in most other species, it is hypothesized that susceptibility to MTBC infection and disease progression with exposure increases with age in black and white rhinoceros species.
In free-ranging populations of African rhinoceros in SA, incidence of M. bovis is expected to increase with age, due to more intense, and consistent exposure of adults in their smaller, more “settled” home ranges compared to that of the more nomadic young, and the accumulation of repeat exposures over their lifetime. The prevalence of M. bovis may also be higher in older animals, reflecting the chronic nature of disease in these long-lived species. The impact of increasing age on the overall TB risk of these populations is still unknown, and requires more investigation.
Environmental and Seasonal Factors Affecting Exposure to and Transmission of M. bovis in Free-Ranging Rhinoceros in South Africa
The characteristic closer association of black rhinoceros with closed, shady environments than white rhinoceros may increase exposure to M. bovis in this species; this is expected to occur as a result of their sharing of this habitat with the suspected bTB maintenance host, the greater kudu, as well as the longer persistence of M. bovis in shady (vs. irradiated) conditions. Conversely, the more frequent association of white rhinoceros with soil-associated bTB reservoirs and African buffaloes (a prominent bTB maintenance host) with whom they share their environment and food source, is likely to increase their M. bovis exposure. Additionally, because the white rhinoceros is considered a more social species than the black rhinoceros, it is hypothesized that M. bovis risk resulting from intra-species interactions will be comparatively higher in white rhinoceros. Overall, because of the higher population of buffaloes (the maintenance host with which white rhinoceros is expected to more frequently associate) than greater kudus (with which the black rhinoceros is expected to more frequently associate), as well as the expected occurrence of more intra-species interactions in white rhinoceros, it is hypothesized that there will be a higher risk M. bovis exposure in white rhinoceros populations than in black rhinoceros populations in KNP and HiP.
In free-ranging African rhinoceros populations in South Africa, it is hypothesized that there is an association between seasonal fluctuations in environmental conditions, rhinoceros spatial patterns, and M. bovis infection risk. During hot or dry periods, the M. bovis exposure of rhinoceros (and other species) may increase due to increased aggregation of M. bovis-infected and susceptible hosts at water sources. During hotter periods, specifically, the aggregation of infected and susceptible hosts in shady areas and/or mud wallows may be associated with increased M. bovis exposure. Seasonal fluctuations in soil-associated bTB reservoirs such as free -living M. bovis in the soil, as well as earthworms and amoeba, may also be associated with seasonal changes in incidence M. bovis infection in African rhinoceros and other animals.
Future Research
The most pressing concern related to TB in African rhinoceros is the acquisition of knowledge and the development of tools to inform surveillance and control strategies for the disease, as well as conservation plans.
The development of sophisticated diagnostic tools may allow for early detection of infection; this would enable earlier interventions that could improve the prognosis of infected individuals and mitigate the spread of the infection in captive and free-ranging systems. Of particular consideration is logistical feasibility and fitness-for-purpose of a test. Capture and immobilization of rhinoceros, especially in free-ranging populations, is extremely costly and is a source of stress for the animal. Therefore, the use of a test like the TST, in addition to being unreliable in this species, would be ill-advised, as it involves immobilization for both administration and interpretation of the test on separate occasions, increasing the cost and the stress for the rhinoceros undergoing testing. Development of blood-based cytokine release assays for bTB in African rhinoceros is currently ongoing; these tests require a single capture and immobilization, and once validated, may be reliable, cost effective diagnostic methods for bTB in rhinoceros.
Coordinated studies in captive populations may help to clarify demographic factors (e.g., age, sex, species) as risks for M. bovis infection and disease progression in African rhinoceros. This would involve ante-mortem monitoring for MTBC infection, as well as thorough post-mortem exams that include histopathology and ancillary diagnostic tests. Because zoological facilities tend to keep curated medical records, retrospective, longitudinal data may already be available to address these knowledge gaps. Careful, standardized data curation across institutions could inform and enumerate a study population and be used to identify cases and non-cases. In any such study, attention to confounding factors such as differences in exposure to MTBC based on animal origin, movement history, and TB prevalence in human populations should be considered.
In South Africa, population-based epidemiological studies of bTB in free-ranging African rhinoceros populations are currently ongoing. Findings from such studies could help identify major drivers of bTB infection in free-ranging populations as well as identify low risk individuals, which would have immediate benefit to current conservation and translocation efforts. This knowledge could be applied to inform management decisions for these populations, e.g., to minimize the probability of moving a false-negative infected animal out of bTB endemic areas and inadvertently spreading bTB to other areas. Such studies may also aid in identifying important bTB risk mitigation opportunities aimed to decrease continued spread of bTB in black and white rhinoceros living in these fragile ecosystems.
Conclusion
This review has focused on available literature that could help to characterize the risk posed by MTBC (including M. bovis and M. tuberculosis) to African rhinoceros species. It has also drawn attention to major knowledge gaps pertaining to TB in rhinoceros. By identifying and systematically addressing each of these knowledge gaps, advances will inform management decisions for conservation of African rhinoceros, and South African biodiversity.
Author Contributions
RD, MM, WG, and CW reviewed available literature and wrote and edited the manuscript. PB edited the manuscript. RD and MM generated Figures 1, 2. All authors read and approved the final manuscript.
Funding
RD, WG, and MM were partially funded by the South African government through the South African Medical Research Council, Stellenbosch University Faculty of Medicine and Health Sciences, and National Research Foundation South African Research Chair Initiative (SARChI grant 86949). WG was supported by the National Geographic Society (NGS-61089C-19) and Wildlife Disease Association crowdfunding campaign through Experiment.com and American Association of Zoo Veterinarians Wild Animal Health Fund (S005651). The content and conclusions of this review are the sole responsibility of the authors and do not represent the official views of the funders. The funder's only role was for financial assistance.
Conflict of Interest
The authors declare that the research was conducted in the absence of any commercial or financial relationships that could be construed as a potential conflict of interest.
The reviewer BB declared a past co-authorship with one of the authors PB to the handling editor.
Acknowledgments
We thank Stellenbosch University for their efforts in providing access to literary and internet resources for staff and students that are working remotely during the COVID-19 crisis. We also thank the Veterinary Wildlife Services staff for assistance with collecting samples from Kruger National Park rhinoceros.
Supplementary Material
The Supplementary Material for this article can be found online at: https://www.frontiersin.org/articles/10.3389/fvets.2020.580476/full#supplementary-material
References
1. World Organization for Animal Health. Terrestrial Animal Health Code. (2019). Available online at: https://www.oie.int/fileadmin/Home/eng/Health_standards/tahc/current/chapitre_bovine_tuberculosis.pdf (accessed August 25, 2020).
2. Michel AL, Bengis RG, Keet DF, Hofmeyr M, Klerk LM de, Cross PC, et al. Wildlife tuberculosis in South African conservation areas: implications and challenges. Vet Microbiol. (2006) 112:91–100. doi: 10.1016/j.vetmic.2005.11.035
3. Corner LAL. The role of wild animal populations in the epidemiology of tuberculosis in domestic animals: how to assess the risk. Vet Microbiol. (2006) 112:303–12. doi: 10.1016/j.vetmic.2005.11.015
4. Rookmaaker LC. The Rhinoceros in Captivity: A List of 2439 Rhinoceroses Kept From Roman Times to 1994. Amsterdam: Kugler Publications, SPB Academic Publishing (1998).
5. Hofmeyr CFB. Two hundred and eighty four autopsies at the national zoological gardens, Pretoria. J Afr Vet Assoc. (1956) 27:263–82.
6. Keep M, Basson P. Mycobacteriosis in a black rhinoceros (Diceros bicornis Linnaeus 1758). J Afr Vet Assoc. (1973) 44:285–6.
7. Mann P, Bush M, Janssen D, Frank E, Montali R. Clinicopathologic correlations of tuberculosis in large zoo mammals. J Am Vet Med Assoc. (1981) 179:1123–9.
8. Barbiers R. Mycobacterium tuberculosis in a black rhinoceros (Diceros bicornis). Proc Am Assoc Zoo Vet Annu Meet. (1994) 1994:171–2.
9. Miller MA, Michel A, van Helden P, Buss P. Tuberculosis in rhinoceros: an underrecognized threat? Transbound Emerg Dis. (2017) 64:1071–8. doi: 10.1111/tbed.12489
10. Witte C. Personal Communication Reporting Internal Database Search for 1984 TB Case in a Black Rhinoceros in San Diego (2020).
11. Morar D, Schreuder J, Mény M, van Kooten PJS, Tijhaar E, Michel AL, et al. Towards establishing a rhinoceros-specific interferon-gamma (IFN-γ) assay for diagnosis of tuberculosis. Transbound Emerg Dis. (2013) 60:60–6. doi: 10.1111/tbed.12132
12. Dalovisio JR, Stetter M, Mikota-Wells S. Rhinoceros' rhinorrhea: cause of an outbreak of infection due to airborne Mycobacterium bovis in zookeepers. Clin Infect Dis. (1992) 15:598–600. doi: 10.1093/clind/15.4.598
13. Stetter M, Mikota S, Gutter A, Monterroso E, Dalovisio J, Degraw C, et al. Epizootic of Mycobacterium bovis in a zoologic park. J Am Vet Med Assoc. (1995) 207:1618–21.
15. Duncan A, Lyashchenko K, Greenwald R, Miller M, Ball R. Application of elephant TB stat-pak assay and mapia (multi-antigen print immunoassay) for detection of tuberculosis and monitoring of treatment in black rhinoceros (Diceros bicornis). J Zoo Wildl Med. (2009) 40:781–5. doi: 10.1638/2009-0044.1
16. Oh P, Granich R, Scott J, Sun B, Joseph M, Stringfield C, et al. Human exposure following Mycobacterium tuberculosis infection of multiple animal species in a Metropolitan Zoo. J Emerg Infect Dis. (2002) 8:1290–3. doi: 10.3201/eid0811.020302
17. Espie IW, Hlokwe TM, van Pittius NCG, Lane E, Tordiffe ASW, Michel AL, et al. Pulmonary infection due to Mycobacterium bovis in a black rhinoceros (Diceros bicornis minor) in South Africa. J Wildl Dis. (2009) 45:1187–93. doi: 10.7589/0090-3558-45.4.1187
18. Miller MA, Greenwald R, Lyashchenko KP. Potential for serodiagnosis of tuberculosis in black rhinoceros (Diceros bicornis). J Zoo Wildl Med. (2015) 46:100–4. doi: 10.1638/2014-0172R1.1
19. Miller MA, Buss P, Parsons SDC, Roos E, Chileshe J, Goosen WJ, et al. Conservation of white rhinoceroses threatened by bovine tuberculosis, South Africa, 2016–2017. J Emerg Infect Dis. (2018) 24:3. doi: 10.3201/eid2412.180293
20. KZN Nature Conservation Board. Ezemvelo KZN Wildlife Annual Report: 2016/2017. Ezemvelo KZN Wildlife (2017). Available online at: https://provincialgovernment.co.za/entity_annual/371/2017-kwazulu-natal-ezemvelo-kzn-wildlife-annual-report.pdf (accessed August 25, 2020).
21. SANParks Scientific Services. Kruger National Park: Biodiversity Statistics. (2011). Available online at: https://www.sanparks.org/parks/kruger/conservation/scientific/ff/biodiversity_statistics.php (accessed August 26, 2020).
22. International Rhino Foundation: Annual report 2017. International Rhino Foundation (2017). Available online at: https://rhinos.org/wp-content/uploads/2018/09/Annual-2017.pdf (accessed May 20, 2020).
23. Department of Environment Forestry and Fisheries. Minister Edna Molewa leads implementation of integrated strategic management of rhinoceros in South Africa. (2014). Available online at: https://www.environment.gov.za/mediarelease/molewa_integratedstrategicmanagement_rhinoceros (accessed August 18, 2020).
24. Department of Environmental Affairs (DEA). Department of Environmental Affairs Highlights Progress on the Implementation of the Integrated Strategic Management of Rhinoceros. (2018). Available online at: https://www.environment.gov.za/progressonimplementationofintegratedstrategicmanagementofrhinoceros (accessed August 25, 2020).
25. Bengis R, Keet D, Michel A, Kriek N. Tuberculosis, caused by Mycobacterium bovis, in a kudu (Tragelaphus strepsiceros) from a commercial game farm in the Malelane area of the Mpumalanga province, South Africa. Onderstepoort J Vet Res. (2001) 68:239–41.
26. Michel AL, Coetzee ML, Keet DF, Maré L, Warren R, Cooper D, et al. Molecular epidemiology of Mycobacterium bovis isolates from free-ranging wildlife in South African game reserves. Vet Microbiol. (2009) 133:335–43. doi: 10.1016/j.vetmic.2008.07.023
27. Renwick AR, White PCL, Bengis RG. Bovine tuberculosis in southern African wildlife: a multi-species host–pathogen system. Epidemiol Infect. (2007) 135:529–40. doi: 10.1017/S0950268806007205
28. Jakob-Hoff RM, OIE-World Organization for Animal Health, International Union for Conservation of Nature and Natural Resources, , editors. Manual of Procedures for Wildlife Disease Risk Analysis. Paris: OIE (2014).
29. Domingo M, Vidal E, Marco A. Pathology of bovine tuberculosis. Res Vet Sci. (2014) 97:S20–9. doi: 10.1016/j.rvsc.2014.03.017
30. Drain PK, Bajema KL, Dowdy D, Dheda K, Naidoo K, Schumacher SG, et al. Incipient and subclinical tuberculosis: a clinical review of early stages and progression of infection. Clin Microbiol Rev. (2018) 31:e00021–18. doi: 10.1128/CMR.00021-18
31. Flynn JL, Gideon HP, Mattila JT, Lin PL. Immunology studies in non-human primate models of tuberculosis. Immunol Rev. (2015) 264:60–73. doi: 10.1111/imr.12258
32. Palmer MV, Thacker TC, Rabideau MM, Jones GJ, Kanipe C, Vordermeier HM, et al. Biomarkers of cell-mediated immunity to bovine tuberculosis. Vet Immunol Immunopathol. (2020) 220:109988. doi: 10.1016/j.vetimm.2019.109988
33. Mogues T, Goodrich ME, Ryan L, LaCourse R, North RJ. The relative importance of T cell subsets in immunity and immunopathology of airborne Mycobacterium tuberculosis infection in mice. J Exp Med. (2001) 193:271–280. doi: 10.1084/jem.193.3.271
34. Myllymäki H, Bäuerlein CA, Rämet M. The zebrafish breathes new life into the study of tuberculosis. Front Immunol. (2016) 7:196. doi: 10.3389/fimmu.2016.00196
35. Pollock JM, Neill SD. Mycobacterium bovis infection and tuberculosis in cattle. Vet J. (2002) 163:115–27. doi: 10.1053/tvjl.2001.0655
36. Gill WP, Harik NS, Whiddon MR, Liao RP, Mittler JE, Sherman DR. A replication clock for Mycobacterium tuberculosis. Nat Med. (2009) 15:211–4. doi: 10.1038/nm.1915
37. Diedrich CR, Mattila JT, Klein E, Janssen C, Phuah J, Sturgeon TJ, et al. Reactivation of latent tuberculosis in cynomolgus macaques infected with SIV is associated with early peripheral T cell depletion and not virus load. PLoS ONE. (2010) 5:e9611. doi: 10.1371/journal.pone.0009611
38. Gallagher J, Monies R, Gavier-Widen M, Rule B. Role of infected, non-diseased badgers in the pathogenesis of tuberculosis in the badger. Vet Rec. (1998) 142:710–4. doi: 10.1136/vr.142.26.710
39. Centre for Food Security and Public Health. Zoonotic Tuberculosis in Mammals, Including Bovine and Caprine Tuberculosis. The Centre for Food Security and Public Health (2019). Available online at: http://www.cfsph.iastate.edu/Factsheets/pdfs/bovine_tuberculosis.pdf (accessed May 20, 2020).
40. Sabio y García J, Bigi MM, Klepp LI, García EA, Blanco FC, Bigi F. Does Mycobacterium bovis persist in cattle in a non-replicative latent state as Mycobacterium tuberculosis in human beings? Vet Microbiol. (2020) 247:108758. doi: 10.1016/j.vetmic.2020.108758
41. Cadena AM, Fortune SM, Flynn JL. Heterogeneity in tuberculosis. Nat Rev Immunol. (2017) 17:691–702. doi: 10.1038/nri.2017.69
42. Vallejo R, García Marín JF, Juste RA, Muñoz-Mendoza M, Salguero FJ, Balseiro A. Immunohistochemical characterization of tuberculous lesions in sheep naturally infected with Mycobacterium bovis. BMC Vet Res. (2018) 14:154. doi: 10.1186/s12917-018-1476-2
43. Michel AL, Lane EP, de Klerk-Lorist L-M, Hofmeyr M, van der Heijden EMDL, Botha L, et al. Experimental Mycobacterium bovis infection in three white rhinoceroses (Ceratotherium simum): susceptibility, clinical and anatomical pathology. PLoS ONE. (2017) 12:e0179943. doi: 10.1371/journal.pone.0179943
44. Parsons SDC, Morar-Leather D, Buss P, Hofmeyr J, McFadyen R, Rutten VPMG, et al. The kinetics of the humoral and interferon-gamma immune responses to experimental Mycobacterium bovis infection in the white rhinoceros (Ceratotherium simum). Front Immunol. (2017) 8:1831. doi: 10.3389/fimmu.2017.01831
45. Walzl G, McNerney R, du Plessis N, Bates M, McHugh TD, Chegou NN, et al. Tuberculosis: advances and challenges in development of new diagnostics and biomarkers. Lancet Infect Dis. (2018) 18:e199–210. doi: 10.1016/S1473-3099(18)30111-7
46. Chambers M. Review of the diagnosis and study of tuberculosis in non-bovine wildlife species using immunological methods. Transbound Emerg Dis. (2009) 56:215–27. doi: 10.1111/j.1865-1682.2009.01076.x
47. Pollock JM, Welsh MD, McNair J. Immune responses in bovine tuberculosis: towards new strategies for the diagnosis and control of disease. Vet Immunol Immunopathol. (2005) 108:37–43. doi: 10.1016/j.vetimm.2005.08.012
48. Kerr T, Waal C, Buss P, Hofmeyr J, Lyashchenko K, Miller M. Seroprevalence of Mycobacterium tuberculosis complex in free-ranging African elephants (Loxodonta africana) in Kruger National Park, South Africa. J Wildl Dis. (2019) 55:923–7. doi: 10.7589/2018-12-292
49. Roos E, Olea-Popelka F, Buss P, de Klerk L-M, Cooper D, Helden P, et al. Seroprevalence of Mycobacterium bovis infection in warthogs (Phacochoerus africanus) in bovine tuberculosis-endemic regions of South Africa. Transbound Emerg Dis. (2018) 65:1182–9. doi: 10.1111/tbed.12856
50. Miller MA. Chapter 2—Current diagnostic methods for tuberculosis in zoo animals. In: Fowler ME, Miller RE, editors. Zoo and Wild Animal Medicine. 6th ed. Saint Louis, MO: W.B. Saunders (2008), 10–19. doi: 10.1016/B978-141604047-7.50005-1
51. World Health Organization. Commercial Serodiagnostic Tests for Diagnosis of Tuberculosis. (2011). Available online at: https://www.who.int/tb/publications/tb-serodiagnostic-policy/en/ (accessed August 25, 2020).
52. Godfrey RW, Dresser BL, Campbell BJ. Tuberculosis testing of captive rhinoceros. Proc Am Assoc Zoo Vet Annu Meet. (1990) 312.
53. Chileshe J, Roos EO, Goosen WJ, Buss P, Hausler G, Rossouw L, et al. An interferon-gamma release assay for the diagnosis of the Mycobacterium bovis infection in white rhinoceros (Ceratotherium simum). Vet Immunol Immunopathol. (2019) 217:109931. doi: 10.1016/j.vetimm.2019.109931
54. Lu P-L, Yang Y-C, Huang SC, Jenh Y-S, Lin Y-C, Huang H-H, et al. Evaluation of the bactec MGIT 960 system in combination with the MGIT TBc identification test for detection of Mycobacterium tuberculosis Complex in respiratory specimens. J Clin Microbiol. (2011) 49:2290–2. doi: 10.1128/JCM.00571-11
55. Maas M, Michel A, Rutten VPMG. Facts and dilemmas in diagnosis of tuberculosis in wildlife. Comp Immunol Microbiol Infect Dis. (2013) 36:269–85. doi: 10.1016/j.cimid.2012.10.010
56. Drewe JA, Tomlinson AJ, Walker NJ, Delahay RJ. Diagnostic accuracy and optimal use of three tests for tuberculosis in live badgers. PLoS ONE. (2010) 5:e11196. doi: 10.1371/journal.pone.0011196
57. Goosen WJ, Kerr TJ, Kleynhans L, Warren RM, van Helden PD, Persing DH. The Xpert MTB/RIF ultra assay detects Mycobacterium tuberculosis F complex DNA in white rhinoceros (Ceratotherium simum) and African elephants (Loxodonta africana). Sci Rep. (2020) 10:14482. doi: 10.1038/s41598-020-71568-9
58. Hlokwe TM, Mogano RM. Utility of xpert® MTB/RIF ultra assay in the rapid diagnosis of bovine tuberculosis in wildlife and livestock animals from South Africa. Prev Vet Med. (2020) 177:104980. doi: 10.1016/j.prevetmed.2020.104980
59. Kerr T, Gumbo R, Goosen W, Rogers P, Last R, Miller M. Novel techniques for detection of Mycobacterium bovis infection in a cheetah. Emerg Infect Dis J. (2020) 26:630. doi: 10.3201/eid2603.191542
60. Gupta M, Lobo FD, Adiga DSA, Gupta A. A histomorphological pattern analysis of pulmonary tuberculosis in lung autopsy and surgically resected specimens. Pathol Res Int. (2016) 2016:8132741. doi: 10.1155/2016/8132741
61. Cunha MV, Matos F, Canto A, Albuquerque T, Alberto JR, Aranha JM, et al. Implications and challenges of tuberculosis in wildlife ungulates in Portugal: a molecular epidemiology perspective. Res Vet Sci. (2012) 92:225–35. doi: 10.1016/j.rvsc.2011.03.009
62. Guimaraes AMS, Zimpel CK. Mycobacterium bovis: from genotyping to genome sequencing. Microorganisms. (2020) 8:667. doi: 10.3390/microorganisms8050667
63. Price-Carter M, Brauning R, De Lisle GW, Livingstone P, Neill M, Sinclair J, et al. Whole genome sequencing for determining the source of Mycobacterium bovis infections in livestock herds and wildlife in New Zealand. Front Vet Sci. (2018) 5:272. doi: 10.3389/fvets.2018.00272
64. Dippenaar A, Parsons SDC, Miller MA, Hlokwe T, Gey van Pittius NC, Adroub SA, et al. Progenitor strain introduction of Mycobacterium bovis at the wildlife-livestock interface can lead to clonal expansion of the disease in a single ecosystem. Infect Genet Evol. (2017) 51:235–8. doi: 10.1016/j.meegid.2017.04.012
65. Crispell J, Zadoks RN, Harris SR, Paterson B, Collins DM, De-Lisle GW, et al. Using whole genome sequencing to investigate transmission in a multi-host system: bovine tuberculosis in New Zealand. BMC Genomics. (2017) 18:180. doi: 10.1186/s12864-017-3569-x
66. Biek R, O'Hare A, Wright D, Mallon T, Mccormick C, Orton RJ, et al. Whole genome sequencing reveals local transmission patterns of Mycobacterium bovis in sympatric cattle and badger populations. PLoS Pathog. (2012) 8:e1003008. doi: 10.1371/journal.ppat.1003008
67. Crispell J, Cassidy S, Kenny K, Mcgrath G, Warde S, Cameron H, et al. Mycobacterium bovis genomics reveals transmission of infection between cattle and deer in Ireland. Microb Genomics. (2020) 6:1–8. doi: 10.1099/mgen.0.000388
68. Sichewo PR, Hlokwe TM, Etter EMC, Michel AL. Tracing cross species transmission of Mycobacterium bovis at the wildlife/livestock interface in South Africa. BMC Microbiol. (2020) 20:49. doi: 10.1186/s12866-020-01736-4
69. Pesciaroli M, Alvarez J, Boniotti MB, Cagiola M, Di Marco V, Marianelli C, et al. Tuberculosis in domestic animal species. Res Vet Sci. (2014) 97:S78–85. doi: 10.1016/j.rvsc.2014.05.015
70. Dennis PM, Funk JA, Rajala-Schultz PJ, Blumer ES, Miller RE, Wittum TE, et al. A review of some of the health issues of captive black rhinoceroses (Diceros bicornis). J Zoo Wildl Med. (2007) 38:509–17. doi: 10.1638/MS05-012.1
71. Bezos J, Casal C, Díez-Delgado I, Romero B, Liandris E, Álvarez J, et al. Goats challenged with different members of the Mycobacterium tuberculosis complex display different clinical pictures. Vet Immunol Immunopathol. (2015) 167:185–9. doi: 10.1016/j.vetimm.2015.07.009
72. Dong H, Lv Y, Sreevatsan S, Zhao D, Zhou X. Differences in pathogenicity of three animal isolates of Mycobacterium species in a mouse model. PLoS ONE. (2017) 12:e0183666. doi: 10.1371/journal.pone.0183666
74. Uplekar M, Rangan S, Ogden J. Gender and Tuberculosis Control: Towards a Strategy for Research and Action. World Health Organization (1999).
75. Graham J, Smith GC, Delahay RJ, Bailey T, McDonald RA, Hodgson D. Multi-state modelling reveals sex-dependent transmission, progression and severity of tuberculosis in wild badgers. Epidemiol Infect. (2013) 141:1429–36. doi: 10.1017/S0950268812003019
76. O'Brien DJ, Schmitt SM, Fierke JS, Hogle SA, Winterstein SR, Cooley TM, et al. Epidemiology of Mycobacterium bovis in free-ranging white-tailed deer, Michigan, USA, 1995–2000. Prev Vet Med. (2002) 54:47–63. doi: 10.1016/S0167-5877(02)00010-7
77. Zanella G, Durand B, Hars J, Moutou F, Garin-Bastuji B, Duvauchelle A, et al. Mycobacterium bovis in wildlife in France. J Wildl Dis. (2008) 44:99–108. doi: 10.7589/0090-3558-44.1.99
78. Di Marco V, Mazzone P, Capucchio MT, Boniotti MB, Aronica V, Russo M, et al. Epidemiological significance of the domestic black pig (Sus scrofa) in maintenance of bovine tuberculosis in Sicily. J Clin Microbiol. (2012) 50:1209. doi: 10.1128/JCM.06544-11
79. Byng-Maddick R, Noursadeghi M. Does tuberculosis threaten our ageing populations? BMC Infect Dis. (2016) 16:119. doi: 10.1186/s12879-016-1451-0
80. Brooks-Pollock E, Conlan AJK, Mitchell AP, Blackwell R, McKinley TJ, Wood JL. Age-dependent patterns of bovine tuberculosis in cattle. J Vet Res. (2013) 44:97. doi: 10.1186/1297-9716-44-97
81. Santos N, Correia-Neves M, Ghebremichael S, Källenius G, Svenson SB, Almeida V. Epidemiology of Mycobacterium bovis infection in wild boar (Sus scrofa) from Portugal. J Wildl Dis. (2009) 45:1048–61. doi: 10.7589/0090-3558-45.4.1048
82. Madeira S, Manteigas A, Ribeiro R, Otte J, Fonseca AP, Caetano P, et al. Factors that influence Mycobacterium bovis infection in red deer and wild boar in an epidemiological risk area for tuberculosis of game species in Portugal. Transbound Emerg Dis. (2017) 64:793–804. doi: 10.1111/tbed.12439
83. Neyrolles O, Quintana-Murci L. Sexual inequality in tuberculosis. PLoS Med. (2009) 6:e1000199. doi: 10.1371/journal.pmed.1000199
84. Bini EI, Mata Espinosa D, Marquina Castillo B, Barrios Payán J, Colucci D, Cruz AF, et al. The influence of sex steroid hormones in the immunopathology of experimental pulmonary tuberculosis. PLoS ONE. (2014) 9:e93831. doi: 10.1371/journal.pone.0093831
85. D'Agostino P, Milano S, Barbera C, Di Bella G, La Rosa M, Ferlazzo V, et al. Sex hormones modulate inflammatory mediators produced by macrophages. Ann N Acad Sci. (1999) 876:426–9. doi: 10.1111/j.1749-6632.1999.tb07667.x
86. Rook GAW, Hernandez-Pando R, Dheda K, Teng Seah G. IL-4 in tuberculosis: implications for vaccine design. Trends Immunol. (2004) 25:483–8. doi: 10.1016/j.it.2004.06.005
87. Zuckerman SH, Bryan-Poole N, Evans GF, Short L, Glasebrook AL. In vivo modulation of murine serum tumour necrosis factor and interleukin-6 levels during endotoxemia by oestrogen agonists and antagonists. Immunol. (1995) 86:18–24.
88. Fox HS, Bond BL, Parslow TG. Estrogen regulates the IFN-gamma promoter. J Immunol. (1991) 146:4362.
89. Vanderwaal K, Enns EA, Picasso C, Packer C, Craft ME. Evaluating empirical contact networks as potential transmission pathways for infectious diseases. J R Soc Interface. (2016) 13:20160166. doi: 10.1098/rsif.2016.0166
90. Dennis PM, Rajala-Schultz PJ, Funk JA, Blumer ES, Miller RE, Wittum TE, et al. Risk factors associated with a skewed natal sex ratio in captive black rhinoceroses (Diceros bicornis) in the United States. J Zoo Wildl Med. (2007) 38:533–9. doi: 10.1638/MS05-011.1
91. Ferrie GM. AZA Regional studbook: eastern black rhinoceros (Diceros bicornis michaeli). Silver Springs, MD: Association of Zoos and Aquariums (2020).
93. Capiro J. AZA Regional Studbook: Southern White Rhinoceros (Ceratotherium simum simum). Silver Springs, MD: Association of Zoos and Aquariums (2020).
94. Patterson S, Drewe JA, Pfeiffer DU, Clutton-Brock TH. Social and environmental factors affect tuberculosis related mortality in wild meerkats. J Anim Ecol. (2017) 86:442–50. doi: 10.1111/1365-2656.12649
95. Gortázar C, Torres MJ, Vicente J, Acevedo P, Reglero M, de la Fuente J, et al. Bovine tuberculosis in doñana biosphere reserve: the role of wild ungulates as disease reservoirs in the last Iberian lynx strongholds. PLoS ONE. (2008) 3:e2776. doi: 10.1371/journal.pone.0002776
96. Rogers LM, Delahay R, Cheeseman CL, Langton S, Smith GC, Clifton-Hadley RS. Movement of badgers (Meles meles) in a high-density population: individual, population and disease effects. Proc Biol Sci. (1998) 265:1269–76. doi: 10.1098/rspb.1998.0429
97. Vicente J, Delahay RJ, Walker NJ, Cheeseman CL. Social organization and movement influence the incidence of bovine tuberculosis in an undisturbed high-density badger (Meles meles) population. J Anim Ecol. (2007) 76:348–60. doi: 10.1111/j.1365-2656.2006.01199.x
98. Kalema-Zikusoka G, Bengis RG, Michel AL, Woodford MH. A preliminary investigation of tuberculosis and other diseases in African buffalo (Syncerus caffer) in Queen Elizabeth National Park, Uganda. Onderstepoort J Vet Res. (2005) 72:145–51. doi: 10.4102/ojvr.v72i2.210
99. Clifford DL, Kazwala RR, Sadiki H, Roug A, Muse EA, Coppolillo PC, et al. Tuberculosis infection in wildlife from the Ruaha ecosystem Tanzania: implications for wildlife, domestic animals, and human health. Epidemiol Infect. (2013) 141:1371–81. doi: 10.1017/S0950268813000836
100. Caley P, Hickling GJ, Cowan PE, Pfeiffer DU. Effects of sustained control of brushtail possums on levels of Mycobacterium bovis infection in cattle and brushtail possum populations from Hohotaka, New Zealand. N Z Vet J. (1999) 47:133–42. doi: 10.1080/00480169.1999.36130
101. Schmitt SM, Fitzgerald SD, Cooley TM, Bruning-Fann CS, Sullivan L, Berry D, et al. Bovine tuberculosis in free-ranging white-tailed deer from Michigan. J Wildl Dis. (1997) 33:749–58. doi: 10.7589/0090-3558-33.4.749
102. Lees VW, Copeland S, Rousseau P. Bovine tuberculosis in elk (Cervus elaphus manitobensis) near Riding Mountain National Park, Manitoba, from 1992 to 2002. Can Vet J Rev Veterinaire Can. (2003) 44:830–1.
103. Nishi JS, Shury T, Elkin BT. Wildlife reservoirs for bovine tuberculosis (Mycobacterium bovis) in Canada: strategies for management and research. Vet Microbiol. (2006) 112:325–38. doi: 10.1016/j.vetmic.2005.11.013
104. Barasona JA, Vicente J, Díez-Delgado I, Aznar J, Gortázar C, Torres MJ. Environmental presence of Mycobacterium tuberculosis complex in aggregation points at the wildlife/livestock Interface. Transbound Emerg Dis. (2017) 64:1148–58. doi: 10.1111/tbed.12480
105. Musoke J, Hlokwe T, Marcotty T, du Plessis BJA, Michel AL. Spillover of Mycobacterium bovis from wildlife to livestock, South Africa. J Emerg Infect Dis. (2015) 21:448–51. doi: 10.3201/eid2103.131690
106. Santos N, Almeida V, Gortázar C, Correia-Neves M. Patterns of Mycobacterium tuberculosis-complex excretion and characterization of super-shedders in naturally-infected wild boar and red deer. Vet Res. (2015) 46:129. doi: 10.1186/s13567-015-0270-4
107. Michel AL, de Klerk L-M, van Pittius NCG, Warren RM, van Helden PD. Bovine tuberculosis in African buffaloes: observations regarding Mycobacterium bovis shedding into water and exposure to environmental mycobacteria. BMC Vet Res. (2007) 3:23. doi: 10.1186/1746-6148-3-23
108. Neill S, Hanna J, O'Brien J, McCracken R. Excretion of Mycobacterium bovis by experimentally infected cattle. Vet Rec. (1988) 123:340. doi: 10.1136/vr.123.13.340
109. Cowie CE, Hutchings MR, Barasona JA, Gortázar C, Vicente J, White PCL. Interactions between four species in a complex wildlife: livestock disease community: implications for Mycobacterium bovis maintenance and transmission. Eur J Wildl Res. (2016) 62:51–64. doi: 10.1007/s10344-015-0973-x
110. Payne A, Philipon S, Hars J, Dufour B, Gilot-Fromont E. Wildlife interactions on baited places and waterholes in a French area infected by bovine tuberculosis. Front Vet Sci. (2017) 3:122. doi: 10.3389/fvets.2016.00122
111. de Garine-Wichatitsky M, Caron A, Kock R, Tschopp R, Munyeme M, Hofmeyr M, et al. A review of bovine tuberculosis at the wildlife–livestock–human interface in sub-Saharan Africa. Epidemiol Infect. (2013) 141:1342–56. doi: 10.1017/S0950268813000708
112. de Vos V, Bengis RG, Kriek NPJ, Michel A, Keet DF, Raath JP. The epidemiology of tuberculosis in free-ranging African buffalo (Syncerus caffer) in the Kruger National Park, South Africa. Onderstepoort J Vet Res. (2001) 68:119–30.
113. Hlokwe TM, Jenkins AO, Streicher EM, Venter EH, Cooper D, Godfroid J, et al. Molecular characterization of Mycobacterium bovis isolated from African buffaloes (Syncerus caffer) in Hluhluwe-iMfolozi park in KwaZulu-Natal, South Africa. Onderstepoort J Vet Res. (2011) 78:6. doi: 10.4102/ojvr.v78i1.232
114. Hughes K, Fosgate GT, Budke CM, Ward MP, Kerry R, Ingram B. Modeling the spatial distribution of African buffalo (Syncerus caffer) in the Kruger National Park, South Africa. PLoS ONE. (2017) 12:e0182903. doi: 10.1371/journal.pone.0182903
115. Roug A, Muse EA, Clifford DL, Larsen R, Paul G, Mathayo D, et al. Seasonal movements and habitat use of African buffalo in Ruaha National Park, Tanzania. BMC Ecol. (2020) 20:6. doi: 10.1186/s12898-020-0274-4
116. Olff H, Ritchie ME, Prins HHT. Global environmental controls of diversity in large herbivores. Nature. (2002) 415:901–4. doi: 10.1038/415901a
117. Spaan RS, Epps CW, Ezenwa VO, Jolles AE. Why did the buffalo cross the park? resource shortages, but not infections, drive dispersal in female African buffalo (Syncerus caffer). Ecol Evol. (2019) 9:5651–63. doi: 10.1002/ece3.5145
118. Caron A, Cross PC, du Toit JT. Ecological implications of bovine tuberculosis in African buffalo herds. Ecol Appl. (2003) 13:1338–45. doi: 10.1890/02-5266
119. Pienaar DJ. Habitat preference of the white rhino in the Kruger National Park. In: Symposium on “Rhinos as Game Ranch animals.” Onderstepoort (1994).
120. Roche C. Preliminary findings of a home range, diet and midden significance in a black rhino bull. Ecol J. (2001) 3:29–33.
121. Owen-Smith N. Assessing the foraging efficiency of a large herbivore, the kudu. S-Afr Tydskr Natuurnav. (1979) 9.
122. du Toit JT. Home range—body mass relations: a field study on African browsing ruminants. Oecologia. (1990) 85:301–3. doi: 10.1007/BF00319416
123. Perrin MR. The social organization of the greater kudu Tragelaphus strepsiceros (Pallas 1766). Trop Zoo. (1999) 12:169–208. doi: 10.1080/03946975.1999.10539388
124. Mukinya JG. Density, distribution, population structure and social organization of the black rhinoceros in Masai Mara Game Reserve. Afr J Ecol. (1973) 11:385–400. doi: 10.1111/j.1365-2028.1973.tb00100.x
125. Owen-Smith RN. Megaherbivores: The Influence of Very Large Body Size on Ecology. New York, NY: Cambridge University Press (1988). doi: 10.1017/CBO9780511565441
126. Valeix M, Fritz H, Matsika R, Matsvimbo F, Madzikanda H. The role of water abundance, thermoregulation, perceived predation risk and interference competition in water access by African herbivores. Afr J Ecol. (2008) 46:402–10. doi: 10.1111/j.1365-2028.2007.00874.x
127. Bennitt E, Bonyongo MC, Harris S. Behaviour-related scalar habitat use by cape buffalo (Syncerus caffer caffer). PLoS ONE. (2015) 10:e0145145. doi: 10.1371/journal.pone.0145145
128. Hutchins M, Kreger MD. Rhinoceros behaviour: implications for captive management and conservation. Int Zoo Yearb. (2006) 40:150–73. doi: 10.1111/j.1748-1090.2006.00150.x
129. Caron A, Cornelis D, Foggin C, Hofmeyr M, de Garine-Wichatitsky M. African buffalo movement and zoonotic disease risk across transfrontier conservation areas, southern Africa. J Emerg Infect Dis. (2016) 22:277–80. doi: 10.3201/eid2202.140864
130. Sternberg Lewerin S, Eld K, Bölske G, Olsson S-L, Röken B, Ghebremichael S, et al. Outbreak of Mycobacterium tuberculosis infection among captive Asian elephants in a Swedish zoo. Vet Rec. (2005) 156:171. doi: 10.1136/vr.156.6.171
131. Adcock K, Hansen HB, Lindemann H. Lessons from the introduced black rhino population in Pilanesberg National Park. Pachyderm. (1998) 26:40–51.
132. Roche C. Notes on territory and home range size of white rhinoceros in the southern Timbavati. CCA Ecol J. (2000) 2:130–3.
133. Owen-Smith NR. The social system of the white rhincoceros. In: Conference on the Behaviour of Ungulates and its Relation to Management. (1974). Available online at: http://www.rhinoresourcecenter.com/pdf_files/146/1463354044.pdf?view (accessed May 20, 2020).
134. Buss PE. Personal Communication-Observed Social Habits of the African Rhinoceros in the Kruger National Park (2020).
135. Seidel DP, Linklater WL, Kilian W, du Preez P, Getz WM. Mesoscale movement and recursion behaviors of Namibian black rhinos. Mov Ecol. (2019) 7:34. doi: 10.1186/s40462-019-0176-2
136. Adams A, Bolin SR, Fine AE, Bolin CA, Kaneene JB. Comparison of PCR versus culture for detection of Mycobacterium bovis after experimental inoculation of various matrices held under environmental conditions for extended periods. J Appl Environ Microbiol. (2013) 79:6501–6. doi: 10.1128/AEM.02032-13
137. Barbier E, Rochelet M, Gal L, Boschiroli ML, Hartmann A. Impact of temperature and soil type on Mycobacterium bovis survival in the environment. PLoS ONE. (2017) 12:e0176315. doi: 10.1371/journal.pone.0176315
138. Young JS, Gormley E, Wellington EMH. Molecular detection of Mycobacterium bovis and Mycobacterium bovis BCG (Pasteur) in soil. J Appl Environ Microbiol. (2005) 71:1946–52. doi: 10.1128/AEM.71.4.1946-1952.2005
139. Santos N, Santos C, Valente T, Gortázar C, Almeida V, Correia-Neves M. Widespread environmental contamination with Mycobacterium tuberculosis complex revealed by a molecular detection protocol. PLoS ONE. (2015) 10:e0142079. doi: 10.1371/journal.pone.0142079
140. Jackson R, de Lisle GW, Morris RS. A study of the environmental survival of Mycobacterium bovis on a farm in New Zealand. N Z Vet J. (1995) 43:346–52. doi: 10.1080/00480169./1995.35918
141. Fine AE, O'Brien DJ, Winterstein SR, Kaneene JB. An effort to isolate Mycobacterium bovis from environmental substrates during investigations of bovine tuberculosis transmission sites (cattle farms and wildlife areas) in Michigan, USA. ISRN Vet Sci. (2011) 2011:1–11. doi: 10.5402/2011/787181
142. Rodríguez-Hernández E, Pizano-Martínez OE, Canto-Alarcón G, Flores-Villalva S, Quintas-Granados LI, Milián-Suazo F. Persistence of Mycobacterium bovis under environmental conditions: is it a real biological risk for cattle? Rev Med Microbiol. (2016) 27:20–4. doi: 10.1097/MRM.0000000000000059
143. Sanchez-Hidalgo A, Obregón-Henao A, Wheat W, Jackson M, Gonzalez-Juarrero M. Mycobacterium bovis hosted by free-living-amoebae permits their long-term persistence survival outside of host mammalian cells and remain capable of transmitting disease to mice. Environ Microbiol. (2017) 19:4010–21. doi: 10.1111/1462-2920.13810
144. Barbier E, Chantemesse B, Dequaire-Rochelet M, Fayolle L, Bollache L, Boschiroli M-L, et al. Rapid dissemination of M. bovis from cattle dung to soil by the earthworm Lumbricus terrestris. Vet Microbiol. (2016) 186:1–7. doi: 10.1016/j.vetmic.2016.01.025
145. Heemskerk D, Caws M, Marais B, Farrar J. Tuberculosis in Adults and Children. Cham: Springer International Publishing (2015). doi: 10.1007/978-3-319-19132-4
146. Behr MA, Gagneux S. The rise and fall of the Mycobacterium tuberculosis complex. In: Tibayrenc M, editor. Genetics and Evolution of Infectious Disease. London: Elsevier (2011). p. 651–67. doi: 10.1016/B978-0-12-384890-1.00024-8
Keywords: epidemiology, Mycobacterium bovis, rhinoceros, TB risk, TB transmission, tuberculosis
Citation: Dwyer RA, Witte C, Buss P, Goosen WJ and Miller M (2020) Epidemiology of Tuberculosis in Multi-Host Wildlife Systems: Implications for Black (Diceros bicornis) and White (Ceratotherium simum) Rhinoceros. Front. Vet. Sci. 7:580476. doi: 10.3389/fvets.2020.580476
Received: 06 July 2020; Accepted: 05 October 2020;
Published: 04 November 2020.
Edited by:
Mario Santoro, University of Naples Federico II, ItalyReviewed by:
Alexandre Caron, Institut National de la Recherche Agronomique (INRA), FranceAna M. S. Guimaraes, University of São Paulo, Brazil
Nuno Santos, University of Porto, Portugal
Brianna R. Beechler, Oregon State University, United States
Copyright © 2020 Dwyer, Witte, Buss, Goosen and Miller. This is an open-access article distributed under the terms of the Creative Commons Attribution License (CC BY). The use, distribution or reproduction in other forums is permitted, provided the original author(s) and the copyright owner(s) are credited and that the original publication in this journal is cited, in accordance with accepted academic practice. No use, distribution or reproduction is permitted which does not comply with these terms.
*Correspondence: Michele Miller, bWlsbGVyQHN1bi5hYy56YQ==