- 1Department of Animal Public Health, Shanghai Veterinary Research Institute, Chinese Academy of Agricultural Sciences, Shanghai, China
- 2College of Veterinary Medicine, Yangzhou University, Yangzhou, China
- 3Jiangsu Co-innovation Center for Prevention and Control of Important Animal Infectious Diseases and Zoonosis, Yangzhou, China
Inappropriate use of antibiotics has accelerated to the emergence of multidrug-resistant bacteria, becoming a major health threat. Moreover, bacterial biofilms contribute to antibiotic resistance and prolonged infections. Bacteriophage (phage) therapy may provide an alternative strategy for controlling multidrug-resistant bacterial infections. In this study, a broad-host-range phage, SHWT1, with lytic activity against multidrug-resistant Salmonella was isolated, characterized and evaluated for the therapeutic efficacy in vitro and in vivo. Phage SHWT1 exhibited specific lytic activity against the prevalent Salmonella serovars, such as Salmonella Pullorum, Salmonella Gallinarum, Salmonella Enteritidis, and Salmonella Typhimurium. Morphological analysis showed that phage SHWT1 was a member of the family Siphoviridae and the order Caudovirales. Phage SHWT1 had a latent period of 5 min and burst size of ~150 plaque-forming units (PFUs)/cell. The phage was stable from pH 3-12 and 4–65°C. Phage SHWT1 also showed capacity to lyse Salmonella planktonic cells and inhibit the biofilm formation at optimal multiplicity of infection (MOI) of 0.001, 0.01, 0.1, and 100, respectively. In addition, phage SHWT1 was able to lyse intracellular Salmonella within macrophages. Genome sequencing and phylogenetic analyses revealed that SHWT1 was a lytic phage without toxin genes, virulence genes, antibiotic resistance genes, or significant genomic rearrangements. We found that phage SHWT1 could successfully protect mice against S. enteritidis and S. typhimurium infection. Elucidation of the characteristics and genome sequence of phage SHWT1 demonstrates that this phage is a potential therapeutic agent against the salmonellosis caused by multidrug-resistant Salmonella.
Introduction
Salmonella is a Gram-negative bacterium that is an important pathogen of humans and animals. Salmonella can cause numerous diseases, ranging from a self-limiting gastroenteritis to typhoid-like disease to systemic infections, known as salmonellosis. In addition, Salmonella is a leading etiological agent associated with outbreaks of food-borne illness worldwide, commonly resulting in global economic losses (1–3). Animals, including poultry are a main reservoir for Salmonella, such as the prevalent Salmonella serovars Salmonella Pullorum (S. Pullorum), Salmonella Gallinarum (S. Gallinarum), Salmonella Enteritidis (S. Enteritidis) and Salmonella Typhimurium (S. Typhimurium) (4–10). Some of these serovars can lead to serious zoonotic diseases via contaminated foods such as poultry and eggs.
Traditionally, antibiotics are used to prevent and treat Salmonella infections. However, the preventive antibiotics administration can accelerate the emergence of multidrug-resistant bacteria, including Salmonella (4, 11, 12). In addition, Salmonella can form biofilms on biotic or abiotic surfaces, which contribute to antibiotic resistance and prolonged infections. The increasing prevalence of multidrug-resistant bacteria means antibiotic treatments are becoming ineffective (13, 14). Consequently, there is an urgent need to develop alternative approaches to prevent and control salmonellosis caused by multidrug-resistant Salmonella.
Bacteriophages (phages) are specific viruses found in diverse environments that target and kill bacteria, and since their discovery, phages have been used to treat bacterial infections (15). Due to their wide distribution, it is easier to obtain and isolate phages than to discover new antibiotics. Moreover, phages are highly specific and only eliminate target bacteria without destroying the normal microbial flora. Thus, phages have received renewed attention as an alternative therapeutic option against infectious bacterial diseases due to the rise in antibiotic resistance (16–18). Phage therapy has been effectively applied to control bacterial infections of humans, animals, and plants as well as being used as a biological control for food-borne pathogens (19–23). Various phages with specific activity against Salmonella have been isolated and characterized (24–27). Moreover, commercial phage products, such as Salmonelex and SalmoFresh, have been approved as food-processing aids to control Salmonella in food products (28, 29).
However, there are some challenges with phage therapy, including the low efficacy, narrow host range, and emergence of phage-resistant bacteria (30, 31). Therefore, it is necessary to continuously search and characterize new phages with broad host range and high lytic capacity for further applications. In this study, a broad-host-spectrum phage, SHWT1, with lytic activity against multidrug-resistant Salmonella was isolated. Genome sequence analysis and characterization of phage SHWT1 was conducted. Furthermore, the efficiency of phage SHWT1 against Salmonella planktonic cells, biofilm formation, intracellular Salmonella within macrophages in vitro and Salmonella infection in vivo were investigated to explore potential use of the phage for biocontrol of salmonellosis.
Materials and Methods
Bacterial Strains and Growth Conditions
The bacterial strains, containing Salmonella, Escherichia coli, and Staphylococcus aureus were isolated from chicken farms in eastern China through selective medium culture and PCR confirmation in this study and our previous studies (32, 33). These bacteria were used for phage isolation and host range determination. Salmonella and Escherichia coli were routinely grown at 37°C in Luria-Bertani (LB) broth (pH 7.2-7.4) with shaking or on LB agar. Staphylococcus aureus was culture in Tryptic Soy broth (TSB) or on plates containing Tryptic Soy agar (TSA).
Antibiotic Susceptibility
Susceptibility of the bacterial isolates to 18 different antibiotics was determined by the standard Kirby-Bauer test, with data analyzed according to guidelines of the Clinical and Laboratory Standards Institute (CLSI) (34).
Isolation and Purification of Bacteriophages
Wastewater samples were collected from chicken farms in eastern China. Isolation and purification of phages were performed as described previously (35). Briefly, particulate matter in the wastewater samples was removed by centrifugation at 1,000 g. The supernatant was mixed with an S. Pullorum SP01 culture and incubated for 12 h at 37°C to enrich for phages. The enriched culture was centrifuged at 7,000 g for 10 min at 4°C, and supernatant was filtered using a 0.22-μm filter membrane (Millipore, USA) to remove bacteria. The filtrate was inoculated with the indicator S. Pullorum SP01 and seeded on soft agar plates according to the double-agar overlay method. Purified phages were obtained by performing a plaque assay six times. Phages were subsequently concentrated in SM buffer (100 mM NaCl, 8 mM MgSO4, 50 mM Tris-HCl) and stored at 4°C until further analyses.
Determination of Phage Host Range
The bacterial isolates were tested for their susceptibility to phages using spotting methods as previously described (36). Then, the host range of phage were confirmed by double-agar overlay methods to avoid the so-called lysis from without (37).
Phage Morphology
Phage morphology was determined by transmission electron microscopy (TEM). Briefly, purified phage was dropped on acopper grid and negatively stained with 2% phosphotungstic acid. The grids were then observed under a FEI T12 transmission electron microscope (FEI, Ltd, Hillsboro, OR, USA).
Optimal Multiplicity of Infection (MOI) of Phage
The optimal MOI of phage was determined as previously described (38) with some modifications. The bacteria were grown to exponential phase and harvested. The pellets were suspended and adjust to OD600 nm as 0.2 (~1 ×108 clone-forming units (CFUs)/mL) with an Eppendorf BioSpectrometerBasic Spectrophotometer (Eppendorf AG, Germany). The fresh culture of bacteria were mixed with equal volumes of diluted phage (1 ×103-1 ×1011 plaque-forming units (PFUs)/mL) at the MOI as 0.00001, 0.0001, 0.001, 0.01, 0.1, 1, 10, and 100. Mixtures were cultured at 37°C for 3 h, then phage titer determination was performed using the double-layer plate method. Experiments were performed independently three times.
Adsorption Rate and One-Step Growth Curve Assay
The adsorption ability of phage was determined as previously described (39) with some modifications. Briefly, the phage was mixed with host strain S. Pullorum SP01 (1 ×108 CFUs/mL) at MOI of 0.1, and incubated at 37°C. The samples were taken at 1, 3, 5, and 10 min, and centrifuged at 10,000 g (4°C) to remove the absorbed phages. The titers of unabsorbed phages in the supernatant were determined after serial dilution. Finally, the percentages of phage adsorption at different time points were calculated. The adsorption rate constant (k value) of phage were calculated as previously described (40). Experiments were performed independently three times.
A one-step growth curve assay was performed as previously described with some modifications (38). The phage was mixed with exponential phase S. Pullorum SP01 (1 ×108 CFUs/mL) at the MOI of 0.1, and was incubated at 37°C for 5 min to allow maximum adsorption of phage. Subsequently, the mixture was centrifuged at 10,000 g (4°C) for 10 min to remove unabsorbed phage. Then, the supernatant was discarded, and the pellets were washed and suspended with LB broth. These samples were incubated at 37°C and taken at indicated time points. The phage titers were measured using the double-layer agar method. Experiments were performed independently three times.
Thermal and pH Stability
For thermal stability testing, the phage suspension was incubated at 4, 37, 40, 45, 50, 55, 60, 65, 70, 75, 80, and 85°C, respectively. Samples were taken after 20, 40, and 60 min and the phage titers were determined. In addition, the phage suspension was stored at room temperature (25°C) and phage titers were monitored for 4 weeks.
To investigate pH stability, phages were incubated at 37°C for 1 h in LB medium at pH ranging from 3 to 12 (adjusted with HCl or NaOH for acidic or alkaline, respectively). Phage titer from each pH were determined by the double-layered agar plate method as previously described (41). Experiments were repeated three times.
Bacterial Challenge Test With Planktonic Cells
The bacterial challenge test was performed according to the protocol previously reported with minor changes (42). Representative Salmonella strains were grown to an OD600 nm of 0.2, and were infected with phage SHWT1 at the optimal MOI. Bacterial growth was determined by monitoring the optical density at 2 h intervals. Meanwhile, the number of bacterial cells were also determined by serial dilutions and plating.
Determination of Bacterial Biofilm Susceptibility to Phage SHWT1
The effects of phage SHWT1 on biofilm formation by Salmonella strains were tested referring to previous studies (42, 43). Bacterial cultures with or without phage SHWT1 were dispensed in 96-well plates and cultured without shaking at 37°C for 24 or 48 h. Planktonic bacterial cells were removed and biofilms were stained with 200 μL crystal violet (0.1%, W/V) for 20 min. After rinsing and air-drying, the biofilms were solubilized, and the absorbance was measured at 595 nm. Given that most bacteria in clinical infections reside in biofilms, we tested the roles of phage SHWT1 on the number of bacteria within the biofilm as described previously (44). After 24 h incubation, the wells were washed twice with PBS to remove the planktonic bacterial cells. Then, the biofilms at the wells were disrupted, following by suspended and mixed in PBS. These biofilm suspensions were serial diluted and the bacteria were enumerated by plating. Experiments were performed three times.
In addition, the capacity to disrupt the bacterial biofilm was determined as previously study (42, 43). The Salmonella biofilms were developed for 24 h at 37°C as mentioned above. The wells were then washed twice and treated with phage SHWT1 or SM buffer control. After incubation, the supernatants were removed and wells were washed twice. Determination of biofilms and enumeration of bacteria within the biofilm were performed as mentioned above.
Evaluation of the Intracellular Lytic Activity of Phage SHWT1
The lytic activity of phage SHWT1 against intracellular Salmonella were evaluated as described previously (45) with some modifications. In brief, the avian macrophage HD11 monolayers were washed with Dulbecco's modified Eagle's medium (DMEM) without fetal bovine serum (FBS), followed by infection with Salmonella strains at a multiplicity of infection (MOI) of 10 for 1 h at 37°C to allow maximum uptake. The infected cells were treated with DMEM containing gentamicin (100 μg/mL) for 1 h to kill extracellular bacteria. Then, the phage SHWT1 (108 PFUs/well) was added into the cells for another 12 h treatment. The cells were washed and lysed with 0.5% Triton X-100, and the intracellular bacteria were enumerated by plating on LB plates.
Genome Sequencing and Analysis
Phage DNA was extracted using the Phage Genome DNA Quick Extraction Kit (Zhuangmeng International Biology Gene Technology co., Ltd), and whole-genome sequencing was performed at the Shanghai Personal Biotechnology Co., Ltd on the Illumina NovaSeq PE150 platform with ~22,978-fold coverage. High-quality paired-end reads were assembled using A5-MiSeq v20160825 (https://arxiv.org/abs/1401.5130) and SPAdes v3.12.0 (http://cab.spbu.ru/files/release3.12.0/manual.html), and the genome sequence was proofread using software MUMmer v3.1 (http://mummer.sourceforge.net/) (46) and Pilon v1.18 (https://github.com/broadinstitute/pilon) (47). Potential open reading frames (ORFs) were predicted using GeneMarkS (48). Genome annotation was analyzed using RAST (https://rast.nmpdr.org/), HHpre (https://toolkit.tuebingen.mpg.de/#/tools/hhpred), BLAST and Conserved Domain Identify of NCBI. The Virulence Factor Database (http://www.mgc.ac.cn/VFs/main.htm) and Comprehensive Antibiotic Resistance Database (https://card.mcmaster.ca/) were queried to retrieve the toxic genes, virulence genes and antibiotic resistance genes in the phage genome. tRNAscan-SE search program (https://lowelab.ucsc.edu/tRNAscan-SE/) was used to identify putative tRNAs (49). A circular representation of the genome of phage SHWT1 was generated using BRIG software. Comparisons and phylogenetic analysis of the genome of phage SHWT1 with other phages were conducted with NCBI BLASTN algorithm (http://blast.ncbi.nlm.nih.gov). The complete genome sequence of phage SHWT1 has been deposited in the GenBank database under accession number MT740291.1.
Ethics Statement
The animal experiments were conducted in strict accordance with the guidelines of the Humane Treatment of Laboratory Animals and were approved by the Animal Care and Use Committee at the Shanghai Veterinary Research Institute (permit No: SHVRI- SV-20201014-04).
Efficacy of Phage Therapy for Salmonella Infection Using Mouse Model
To evaluate the therapeutic efficacy of phage SHWT1 in vivo, 6 week-old specific-pathogen-free BALB/c mice were infected with S. Enteritidis SE12 or S. Typhimurium SAT52 by oral administration. At the 6 or 12 h post-infection, mice were treated with a single dose (1.6 ×1010 PFUs) of phage SHWT1 by oral administration. As control groups, mice were challenged with PBS and received only the phage. The mortality of mice was monitored daily for 21 days.
Statistical Analyses
Statistical analyses were conducted using the GraphPad Software package. Multivariate comparisons were analyzed by using one-way and two-way analysis of variance (ANOVA). Values were considered significant when P < 0.05.
Results
Determination of the Antibiotic Sensitivity Profile of Bacterial Isolates
Over the study period, various bacteria were isolated and identified by PCR, including Salmonella, Escherichia coli, and Staphylococcus aureus. Determination of antibiotic sensitivity revealed the isolates had different antibiotic resistance profiles. In general, the isolates were resistant to 6–18 antibiotics (Supplementary Table 1), which indicated that the isolates were multidrug-resistant.
Isolation, Purification, and Host Range Determination of Phages
Sixty-eight lytic phages infecting S. Pullorum were isolated and purified from the wastewater samples collected from chicken farms in eastern China. These phage isolates produced clear plaques with diameters ranging from 2.0 ± 0.3 to 8.0 ± 0.8 mm against S. Pullorum strain SP01. Lytic activity of the phages against various bacterial strains was analyzed to screen phages with broad host range. The phages showed lytic activity against three to nine different serovars of Salmonella. Among them, phage SHWT1 had the broadest host range, with the capacity to lyse nine serovars of Salmonella at different lytic rates. However, no lytic activity was observed against the other species tested in this study, including Escherichia coli and Staphylococcus aureus (Table 1). When infecting the prevalent Salmonella serovars S. Pullorum, S. Gallinarum, S. Enteritidis, S. Typhimurium, phage SHWT1 formed clear plaques with diameters of 3.0 ± 0.4 to 8.0 ± 0.8 mm (Figure 1A). Phage SHWT1 was therefore selected for further analysis.
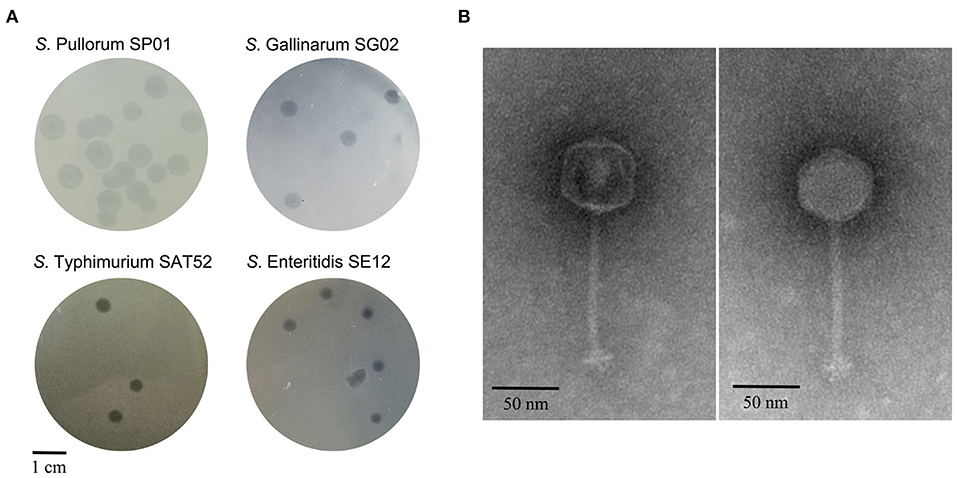
Figure 1. Morphology of phage SHWT1. (A) Plaques morphologies of phage SHWT1. Phage SHWT1 formed clear plaques with well-defined boundaries on bacterial lawns of S. Pullorum, S. Gallinarum, S. Enteritidis, and S. Typhimurium. Scale bar, 1 cm. (B) Transmission electron micrographs of phage SHWT1. Scale bar, 50 nm.
Morphology of Phage SHWT1
TEM analysis of purified SHWT1 particles revealed a structure comprising a polyhedral head with diameter 52.8 ± 2.3 nm and a non-contractile tail of 122.0 ± 2.8 nm (Figure 1B). Based on morphological features, phage SHWT1 belongs to the family Siphoviridae in the order Caudovirales according to the guidelines of the International Committee on Taxonomy of Viruses (50).
Determination of Optimal MOI of Phage SHWT1
Host Salmonella strains were infected with phage SHWT1 at various ratios, and the phage titer was tested to determine the optimal MOI. Based on the maximum phage titer obtained, the optimal MOIs of phage SHWT1 to S. Pullorum, S. Gallinarum, S. Enteritidis and S. Typhimurium, and were 0.001, 0.01, 100, and 0.1, respectively (Table 2).
One-Step Growth Curve
We examined the adsorption ability of phage SHWT1 with host S. Pullorum SP01. The results showed that phage SHWT1 had an adsorption rate of 87.2% within 1 min, 93.6% within 3 min, 99% within 5 and 10 min, indicating that saturation of adsorption was reached to 99% after 5 min (Figure 2A). This result indicated the high and rapidly adsorption rate of phage SHWT1. The adsorption rate constant (k value) of SHWT1 to host S. Pullorum SP01 was 8.8 ± 0.5 ×10−9 mL/min for the 5 min time point.
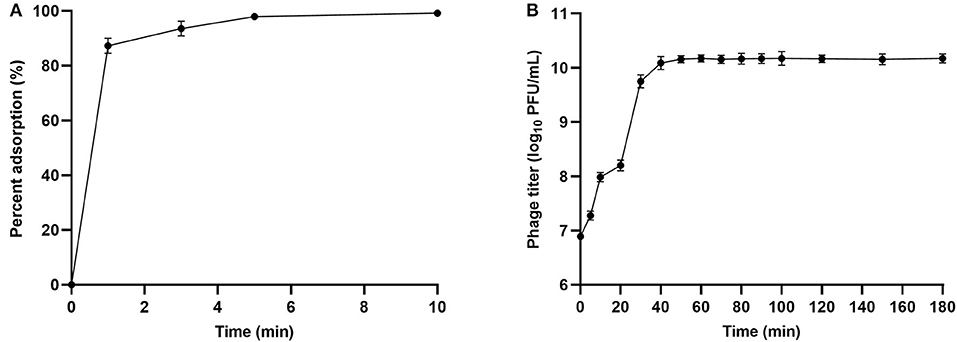
Figure 2. Adsorption rate and one-step growth curve of phage SHWT1. (A) Adsorption rate. Adsorption of phage SHWT1 to S. Pullorum SP01 was expressed as a percentage of the total phages added. (B) One-step growth curve of phage SHWT1 on S. Pullorum SP01.
The one-step growth curve produced for phage SHWT1 on S. Pullorum SP01 showed a latent period of ~5 min and a burst period of 35 min, with an average burst size of 146.6 ± 10.8 PFUs/cell. The titer of phage SHWT1 reached stationary phase after 50 min, with a titer of 1010 PFU/mL (Figure 2B).
Thermal Stability and pH Sensitivity of Phage SHWT1
Thermal stability tests indicated that phage SHWT1 survived incubation for 1 h at 4 to 65°C. The phage titer continuously decreased when the incubation temperature was above 70°C. Moreover, the phage was inactivated within 20 min in 80°C thermal condition (Figure 3A). Phage SHWT1 maintained activity over 4 weeks when stored at room temperature (Figure 3B), demonstrating its ability to tolerate normal temperature environments and its suitability for potential future applications.
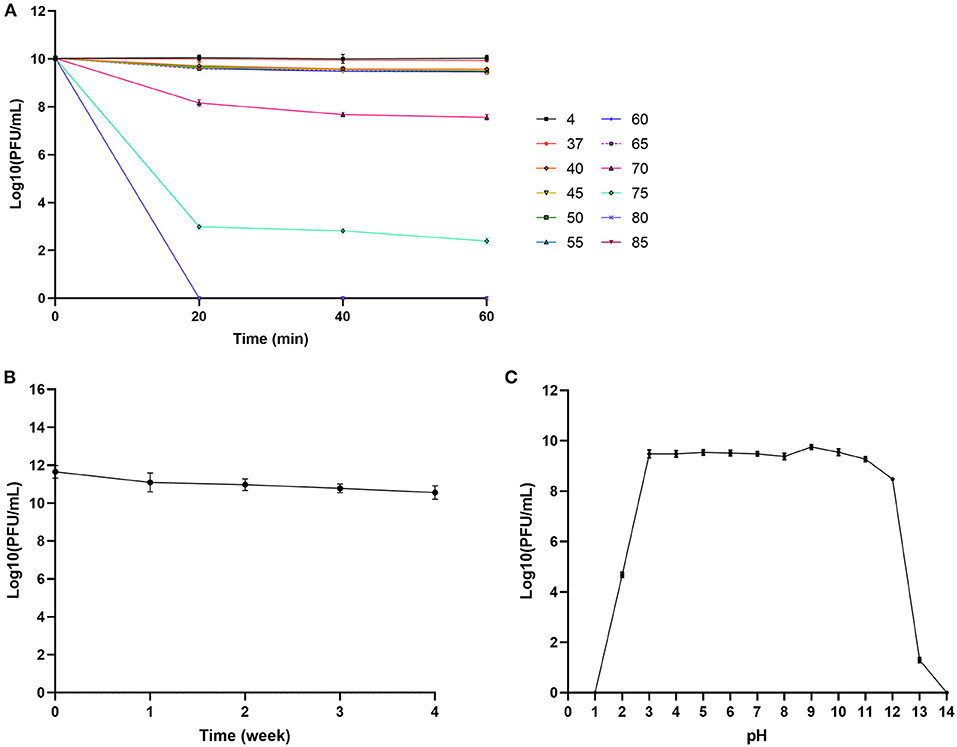
Figure 3. Stability of phage SHWT1 under different temperature and pH. (A) Thermal stability. Phage SHWT1 was stable from 4 to 65°C, but its stability decreased above 70°C. (B) Room temperature storage test. Phage SHWT1 maintained its activity for 4 weeks when stored at room temperature. (C) pH stability. Phage SHWT1 exhibited pH stability range from 3 to 12. Values are means of three repeats with standard deviations.
pH sensitivity tests showed that SHWT1 maintained activity at pH 3 to pH 12, but the titers dramatically decreased at pH 2 and pH 13. No surviving phages were observed at pH 1 or pH 14 (Figure 3C).
Inhibition of Salmonella Planktonic Cells by Phage SHWT1 in vitro
To evaluate the antibacterial effect of phage SHWT1 in vitro, the different serovars of Salmonella were treated with SHWT1 at optimal MOIs. The bacterial planktonic cells growth was efficiently inhibited by SHWT1 (P < 0.01 and P < 0.001) (Figure 4A). Moreover, phage SHWT1 treatment lead to significant reductions in the number of living bacterial cells of each Salmonella serovars (P < 0.05 and P < 0.01) (Figure 4B). These experiments generally corroborated the conclusions that phage SHWT1 was able to lyse the Salmonella planktonic cells.
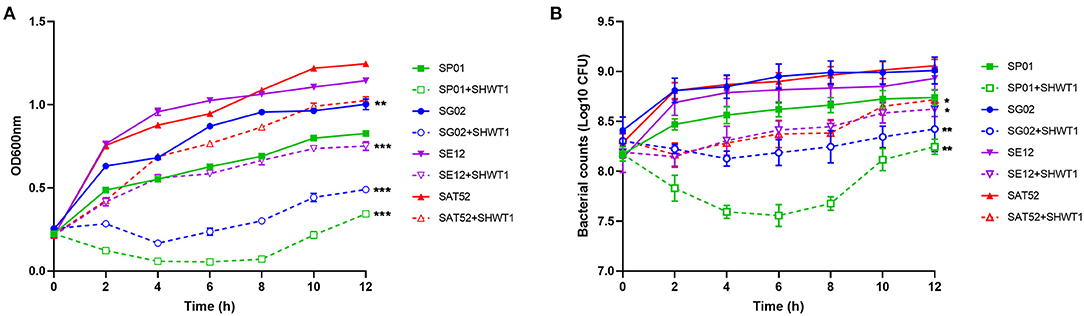
Figure 4. Inhibition of Salmonella planktonic cells by phage SHWT1 in vitro. Each Salmonella were incubated with or without phage SHWT1. The growth (A) and bacterial counts (B) of the Salmonella were significantly inhibited by phage SHWT1. Statistical significance was assessed using two-way ANOVA (*P < 0.05; **P < 0.01; ***P < 0.001).
Control of Biofilm Formation by Phage SHWT1
Given that most bacteria in clinical infections reside in biofilms, the activity of phage SHWT1 in inhibiting biofilm formation was determined by crystal violet staining in 96-well plates. Biofilm formation by S. Pullorum, S. Gallinarum, S. Enteritidis, and S. Typhimurium was significantly inhibited when treating with phage SHWT1 compared with the negative control (P < 0.05 and P < 0.01) (Figure 5A). The bacterial numbers within the biofilms were estimated in a similar experiment. Consistently, treatment of phage SHWT1 led to the significant reductions in viable counts of Salmonella within biofilm (P < 0.05 and P < 0.01) (Figure 5B). In addition, the capacity to disrupt biofilms of phage SHWT1 was tested. We found that phage SHWT1 was able to eliminate the formed biofilm and lyse the bacterial cells (P < 0.05 and P < 0.01) (Figures 5C,D).
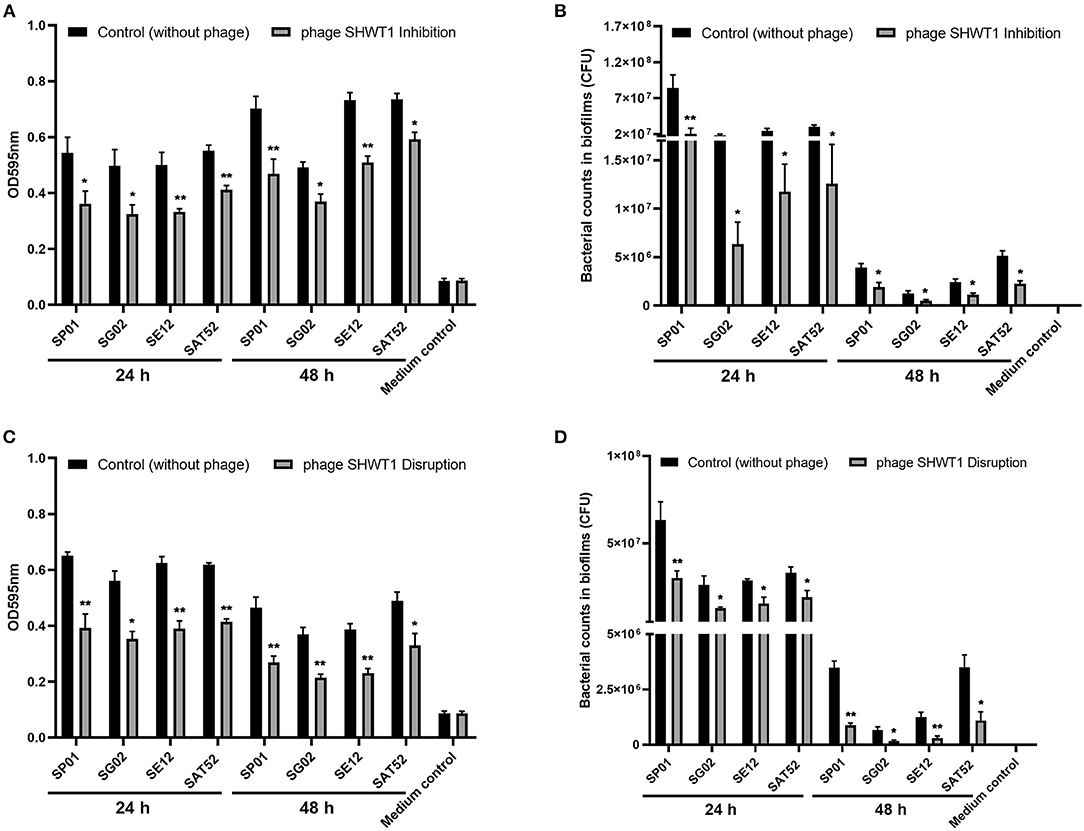
Figure 5. The effect of phage SHWT1 on biofilm formation by Salmonella was tested as described in “Materials and Methods.” The biofilm formation (A) and bacterial counts within biofilm (B) were significant inhibited when treating with phage SHWT1. Moreover, the phage SHWT1 could significant eliminate the formed biofilm (C) and lyse the bacterial cells (D). Statistical significance was assessed using one-way ANOVA (*P < 0.05; **P < 0.01).
Intracellular Lytic Activity of Phage SHWT1
The lytic activity of phage SHWT1 against the intracellular Salmonella was evaluated, and result in terms of percent survival was shown in Figure 6. After 12 h of phage treatment, S. Pullorum, S. Gallinarum, S. Enteritidis, and S. Typhimurium showed 87.6, 61.7, 57.9, and 59.9% reductions in intracellular survival, respectively (P < 0.01 and P < 0.001). This observation indicated that phage SHWT1 was able to lyse intracellular Salmonella of macrophages.
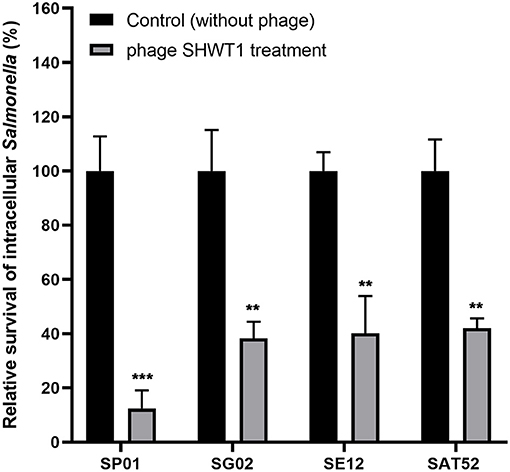
Figure 6. Phage SHWT1 eliminates intracellular Salmonella within macrophages. Chicken macrophage HD11 cells were infected with each Salmonella, and the cells were incubated with phage SHWT1 for 12 h to kill the intracellular Salmonella. The relative survival of intracellular Salmonella in HD11 cells was expressed compared with the untreatment group. Statistical significance was assessed using one-way ANOVA (**P < 0.01; ***P < 0.001).
Genomic and Phylogenetic Analysis
The genome of phage SHWT1 comprises 40,629 bp of double-stranded DNA, with a GC content of 49.83%. Putative ORFs were predicted using GeneMarkS, and 56 ORFs were identified (Supplementary Table 2), with 18 ORFs present on the positive strand and 38 ORFs on the negative strand. Among the 56 ORFs, only 21 (37.5%) had annotated functions, while 35 ORFs (62.5%) were assigned as hypothetical proteins. The identified ORFs were categorized into four functional groups, including DNA replication/modification (primase/helicase, DNA binding protein, DNA polymerase, DNA cytosine methyltransferase), structural components (tail spike protein, tape measure protein, tail protein, neck protein, head-tail joining protein, head protein, coat protein, head morphogenesis protein), packaging module (portal protein, terminase large subunit), and host lysis (lysozyme) (Figure 7A). There was no tRNA gene in phage SHWT1, suggesting that this phage is completely dependent on the host tRNA for protein synthesis. Toxin genes, virulence genes, and antibiotic resistance genes were not detected in the genome of phage SHWT1 through the genomic sequence analysis using online database. In addition, no putative integrase genes were identified, indicating that phage SHWT1 could not integrate into the host bacterial genome and might be lytic in nature (39). Thus, phage SHWT1 is potentially a safe agent for controlling Salmonella infection.
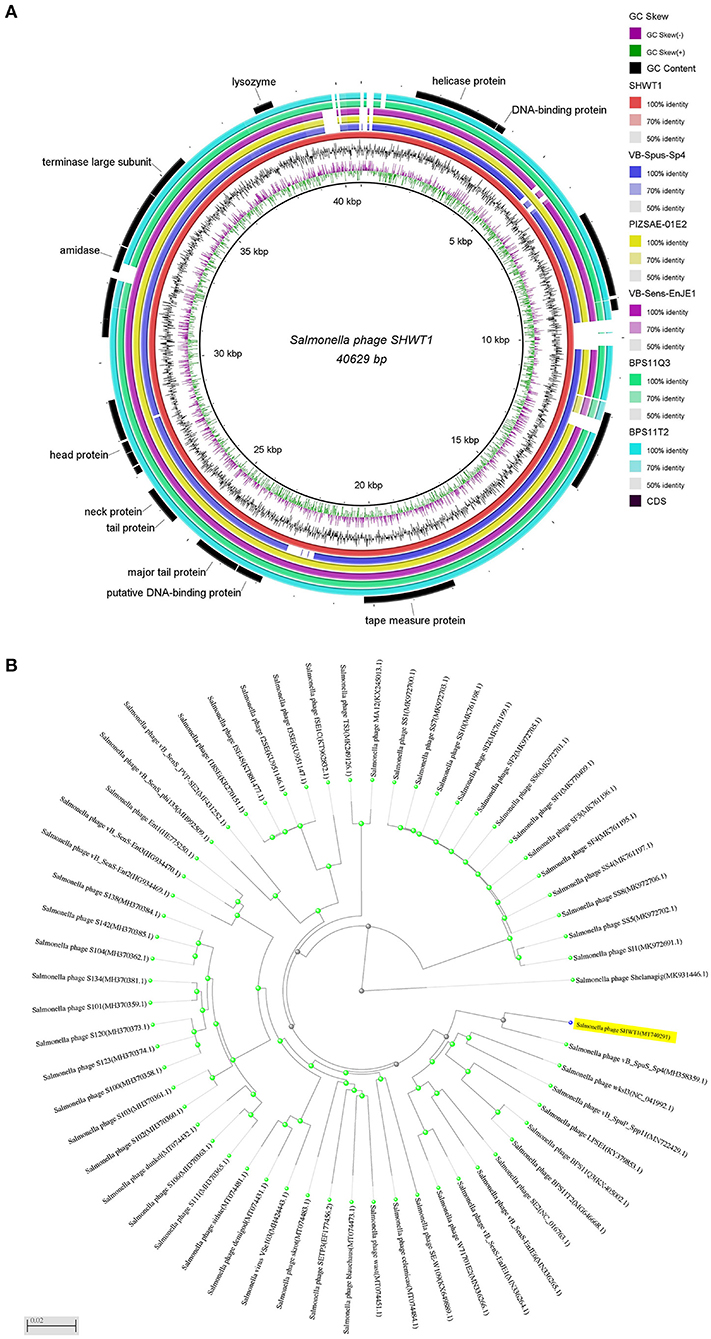
Figure 7. Genomic and phylogenetic analysis of phage SHWT1. (A) Genome map of phage SHWT1. The genomic organization of phage SHWT1 was compared to Salmonella phages vB-SpuS-Sp4, PIZSAE-01E2, vB-SenS-EnJE1, BPS11Q3, and BPS11T2. Genome arrangements were divided into several circles. The two inner circles represent the GC skew of G-C/G+C as purple and green and GC content, respectively. The full length genome of phage SHWT1 were showed as red circle. The coincident regions of other Salmonella phages were displayed, and the blank was the non-coincident region. The outermost circle represents the important functional proteins of phage SHWT1. (B) Phylogenetic analysis of Salmonella phages. Phylogenetic trees were constructed based on whole genome sequences of Salmonella phages by NCBI BLASTN algorithm. GenBank accession numbers are also provided. Phage SHWT1 is closely related to phage vB-SpuS-Sp4.
Whole genome sequence analysis revealed that phage SHWT1 is a member of the subfamily Guernseyvirinae, genus Jerseyvirus. The genome of phage SHWT1 was homologous to those of other Salmonella phages. Phage SHWT1 was most closely related to phage vB-SpuS-Sp4 (GenBank accession no. MH358359.1), with a 97% sequence similarity based on 90% query coverage, which formed a distinct branch within the clade (Figure 7B). The genome of phage vB-SpuS-Sp4 has been sequenced and is a 43,614 bp circular DNA, with 67 predicted ORFs. However, the characteristics of phage vB-SpuS-Sp4 are unknown. Other phages showed an average sequence similarity below 93% with SHWT1.
The Therapeutic Effect of Phage SHWT1
The therapeutic efficacy of phage SHWT1 against the S. Enteritidis and S. Typhimurium infection in a mouse model was tested. The results showed that reduced mortality was observed when phage treatment was introduced. The survival rate of S. Enteritidis infection group is 40%, however, all the mice survival by the phage SHWT1 therapy. Moreover, phage SHWT1 could rescue 40 and 80% mice from S. Typhimurium infection when treatment at 6 or 12 h post-infection. The health and survival of phage control group was same to the PBS control group (Figure 8), indicating the safety of phage SHWT1.
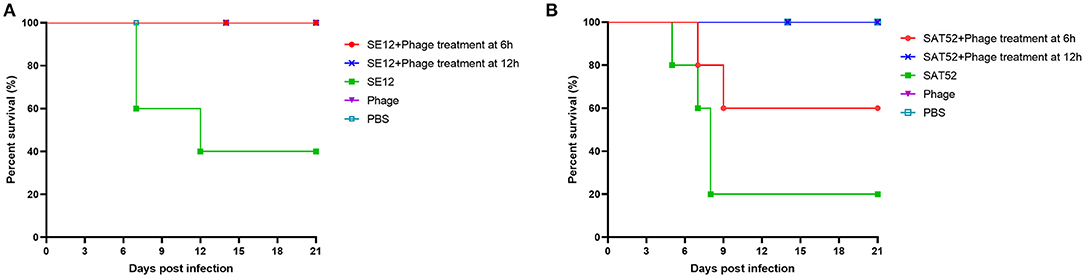
Figure 8. The therapeutic effect of phage SHWT1 against Salmonella infection. Mice were orally infected with S. Enteritidis SE12 (A) or S. Typhimurium SAT52 (B). Then, mice were treatment with phage SHWT1 at 6 or 12 h post-infection. Mice injected with Salmonella, phage or PBS only was used as control groups. The survival rates of mice were monitored. The phage SHWT1 successfully cures S. Enteritidis and S. Typhimurium infections in mice.
Discussion
With the development and spread of antibiotic-resistant bacteria, it is imperative to explore novel or alternative therapeutics against bacterial infections. The worldwide abundance, safety, high specificity, and environmentally friendly characteristics of phages render them ideal candidates to combat drug-resistant bacterial infections. The concept of phage therapy for controlling bacterial infections has been widely accepted (16–18). However, although numerous studies have described the applications of phages to inhibit bacterial infections, the relatively narrow lytic spectrum of phages is an important challenge to their further application (30, 31). Consequently, it is necessary to isolate novel and sensitive phages and determine their physiological and genomic characteristics to enrich the phage arsenal.
In this study, 68 lytic phages infecting Salmonella were isolated from wastewater of poultry farms in eastern China. Bacteriophages belonging to different families, including Myoviridae, Siphoviridae, Microviridae, and Podoviridae, are widely distributed in wastewater due to the presence of a vast array of host microorganisms (42, 51). Among the 68 lytic phages, a broad-host-spectrum phage, SHWT1, against various serovars of Salmonella was identified and characterized. Morphological and genome analysis showed that phage SHWT1 was a member of the family Siphoviridae, subfamily Guernseyvirinae, genus Jerseyvirus. Biological characteristics such as the latent period, burst size of phage, and stability in different environments are key factors for therapeutic applications of phages. A short latent period and high burst size were reported to facilitate effective killing of bacteria (52). Phage SHWT1 had a short latent period (5 min) and an average burst size of 146.6 ± 10.8 PFUs/cell, suggesting its potential as a bacterial treatment. Environmental pH and temperature affect phage activity and stability, thus it is essential that these parameters are tested to determine environment setups for phage application. Phage SHWT1 retained lytic activity for at least 60 min at temperatures ranging between 4 and 65°C. The stability of phage SHWT1 at room temperature for a month, suggested its suitability for practical applications. Salmonella infections commonly occur in the intestine where the pH is acidic. The efficacy of phage therapy via oral administration might be poor if the phage is unable to survive in the low gastric pH environment. Thus, it is necessary to determine the pH stability of the phage. Phage SHWT1 was stable at pH 3 to 12. These temperature and pH assays demonstrated that phage SHWT1 was tolerant to heat and extreme pH conditions, and these are appealing and unique characteristics for potential applications of the phage.
As a natural biological antagonist of bacteria, having a broad host range is one of the most important criteria in phage selection and application (53). Phage SHWT1 could lyse nine serovars of Salmonella including the prevalent serovars S. Pullorum, S. Gallinarum, S. Enteritidis, and S. Typhimurium. No lytic activity was observed against the tested bacterial strains of normal intestinal flora. Different host ranges have been reported for Salmonella phages of the family Siphoviridae (35, 36). A previous study indicated that Siphoviridae phages were considered as restricted-host-range bacteriophages (54). In contrast, it has been reported that some Siphoviridae phages simultaneously infect and lyse various strains of one species or many related bacteria (55, 56). The bacterial reduction test in this study indicated that growth of multidrug-resistant Salmonella was constantly inhibited for at least 8 h in the presence of phage SHWT1 at optimal MOIs. However, most antibiotics, including Erythromycin, Roxithromycin, Clindamycin, Cephradine, Spectinomycin, Doxycycline at 2 × minimum inhibitory concentration could not significantly reduce the viable cell density of multidrug-resistant S. Pullorum, S. Gallinarum, S. Enteritidis, and S. Typhimurium (data not shown). In addition, the ability to kill Salmonella within macrophages suggests the potential of phage SHWT1 therapies. Ideally, the therapeutic evaluation in vivo model is also needed for candidates for phage therapy. Previous studies have shown the bacterial infection can be cured by treatment with phage in experimentally or naturally infected animals (35, 57). Thus, we carried out the evaluation of the therapeutic efficacy of SHWT1 in vivo infection test. Although the efficacy of phage therapy by oral administration was poor due to the inactivation of phage in the stomach extreme pH conditions, our phage SHWT1 successfully protected mice against S. Enteritidis and S. Typhimurium infection. This is attribute to the acid tolerance characteristics of phage SHWT1. These results indicated that the effective therapeutic effect of phage SHWT1 for the multidrug-resistant Salmonella infection.
Biofilms provide a favorable environment for pathogens and contribute to multidrug resistance and persistent bacterial infections. Thus, biofilm formation is a significant problem as it renders bacterial infections more difficult to control (13, 14). Phages damage biofilms through various mechanisms, and these have been applied as biotechnologies in the treatment of infections and on the surfaces of medical devices (13, 58–60). Phage SHWT1 could inhibit biofilm formation and bacterial counts with biofilm of Salmonella. Furthermore, we found that phage SHWT1 was able to disrupt the Salmonella biofilms. However, the mechanism of action remains to be elucidated. This phage might directly degrade biofilms by killing bacteria during colonization onto the surface or it may act via an as yet unknown degradation mechanism. Previous studies similarly indicated that phages of the family Podoviridae not only inhibited bacterial growth but also decreased biofilm formation (36, 61, 62).
Phages acquire and contribute genes to other phage genomes as well as to bacterial genomes, and such genes are powerful factors in the evolution, physiology, and virulence of the host bacteria (31). Complete genome sequencing, annotation, and alignment demonstrated that phage SHWT1 possessed crusted functional regions, including domains for DNA replication/modification, structural components, packaging module, and host lysis; this was similar to other Salmonella phages (63). Since the geographical locations of these phages are different, the high homology might arise from the complex evolutionary relationships with their common host Salmonella. The genome of phage SHWT1 showed highest similarity to that of phage vB-SpuS-Sp4, which characteristics was unknown. The only characterized related phage wksl3 exhibited similar host range compared with phage SHWT1 (64), which might due to the homology of tail spike protein. However, there were some genomic rearrangements and distinct gene regions between these two phages. This indicated that SHWT1 was a novel Salmonella phage. Annotation of the genome of phage SHWT1 identified some important proteins for antibacterial activity, including lysozyme, which are essential proteins involved in host cell lysis (65, 66). Spread of antibiotic-resistant genes virulence genes via mobile genetic elements, such as plasmid, transposon, phage, contributes to the increase in multidrug-resistance and virulence of bacterial pathogen. Evidences had suggested that phage probably be a potential reservoir for the antibiotic-resistant genes, virulence genes acquisition and dissemination (31). Thus, characterization of phage genome sequences could be helpful in excluding the phages carrying harmful genes from phage therapy. In this study, toxin genes, virulence genes, antibiotic resistance genes, and integrase genes were not observed in the genome of phage SHWT1, demonstrating the potential safety of this phage if used as a therapeutic agent against Salmonella infection.
Conclusion
In summary, a lytic phage, SHWT1, against multidrug-resistant Salmonella was isolated and characterized. Phage SHWT1 tolerates a range of pH and temperatures, and is able to effectively inhibit the growth, biofilm formation, bacterial counts within biofilm of the prevalent Salmonella serovars. Moreover, phage SHWT1 exhibits lytic activity against the intracellular Salmonella within macrophages. Genome analysis of phage SHWT1, herein, provides fundamental research for functional studies and supports the application of this phage in biocontrol. Especially, phage SHWT1 could successfully protect mice against S. Enteritidis and S. Typhimurium infection. With the prohibition of the use of antibiotics in poultry farm, phage SHWT1 has potential as an alternative therapeutic agent against salmonellosis caused by multidrug-resistant Salmonella.
Data Availability Statement
The complete genome sequence of phage SHWT1 has been deposited in the GenBank database under accession number MT740291.1.
Ethics Statement
The animal experiments were conducted in strict accordance with the guidelines of the Humane Treatment of Laboratory Animals and were approved by the Animal Care and Use Committee at the Shanghai Veterinary Research Institute (permit No: SHVRI- SV-20201014-04).
Author Contributions
SW conceived the project. ZY isolated phages. CT, YZ, YW, HZ, and DA performed experiments to characterize phage SHWT1. CT wrote the original manuscript draft. TL, MT, JQ, CD, and SG analyzed and discussed the experimental results. SW and SY directed the experiments, funded the research, and edited the manuscript. All authors contributed to the article and approved the submitted version.
Funding
This research was funded by the National Key Research and Development Program of China (Grant Nos.: 2016YFD0500800 and 2018YFD0500500), the Scientific and Technical Innovation Project of the Chinese Academy of Agricultural Sciences, China (Grant No.: SHVRI-ASTIP-2014-8), and the Guangxi key research and development plan (Grant No.: AB18221074).
Conflict of Interest
The authors declare that the research was conducted in the absence of any commercial or financial relationships that could be construed as a potential conflict of interest.
Acknowledgments
The authors would like to acknowledge veterinarians for the sample collection, and Shanghai Personal Biotechnology Company for genome sequencing.
Supplementary Material
The Supplementary Material for this article can be found online at: https://www.frontiersin.org/articles/10.3389/fvets.2021.683853/full#supplementary-material
References
1. Coburn B, Grassl GA, Finlay BB. Salmonella, the host and disease: a brief review. Immunol Cell Biol. (2007) 85:112–8. doi: 10.1038/sj.icb.7100007
2. Majowicz SE, Musto J, Scallan E, Angulo FJ, Kirk M, O'brien SJ, et al. The global burden of nontyphoidal Salmonella gastroenteritis. Clin Infect Dis. (2010) 50:882–9. doi: 10.1086/650733
3. Alyousef MK, Al-Sayed AA, Al Afif A, Alamoudi U, Leblanc JM, Leblanc R. A pain in the neck: Salmonella spp. as an unusual cause of a thyroid abscess. A case report and review of the literature. Bmc Infect Dis. (2020) 20:436. doi: 10.1186/s12879-020-05161-w
4. Medalla F, Gu W, Mahon BE, Judd M, Folster J, Griffin PM, et al. Estimated incidence of antimicrobial drug-resistant nontyphoidal Salmonella infections, United States, 2004-2012. Emerg Infect Dis. (2016) 23:29–37. doi: 10.3201/eid2301.160771
5. Gu D, Wang Z, Tian Y, Kang X, Meng C, Chen X, et al. Prevalence of salmonella isolates and their distribution based on whole-genome sequence in a chicken slaughterhouse in Jiangsu, China. Front Vet Sci. (2020) 7:29. doi: 10.3389/fvets.2020.00029
6. Wang J, Li J, Liu F, Cheng Y, Su J. Characterization of Salmonella enterica Isolates from Diseased Poultry in Northern China between 2014 and 2018. Pathogens. (2020) 9:95. doi: 10.3390/pathogens9020095
7. Xu Y, Zhou X, Jiang Z, Qi Y, Ed-Dra A, Yue M. Epidemiological investigation and antimicrobial resistance profiles of salmonella isolated from breeder chicken hatcheries in Henan, China. Front Cell Infect Microbiol. (2020) 10:497. doi: 10.3389/fcimb.2020.00497
8. Liu Y, Jiang J, Ed-Dra A, Li X, Peng X, Xia L, et al. Prevalence and genomic investigation of Salmonella isolates recovered from animal food-chain in Xinjiang, China. Food Res Int. (2021) 142:110198. doi: 10.1016/j.foodres.2021.110198
9. Yu X, Zhu H, Bo Y, Li Y, Zhang Y, Liu Y, et al. Prevalence and antimicrobial resistance of Salmonella enterica subspecies enterica serovar Enteritidis isolated from broiler chickens in Shandong Province, China, 2013-2018. Poult Sci. (2021) 100:1016-23. doi: 10.1016/j.psj.2020.09.079
10. Zhao X, Ju Z, Wang G, Yang J, Wang F, Tang H, et al. Prevalence and antimicrobial resistance of salmonella isolated from dead-in-shell chicken embryos in Shandong, China. Front Vet Sci. (2021) 8:581946. doi: 10.3389/fvets.2021.581946
11. Parveen S, Taabodi M, Schwarz JG, Oscar TP, Harter-Dennis J, White DG. Prevalence and antimicrobial resistance of Salmonella recovered from processed poultry. J Food Prot. (2007) 70:2466–2472. doi: 10.4315/0362-028x-70.11.2466
12. Jajere SM. A review of Salmonella enterica with particular focus on the pathogenicity and virulence factors, host specificity and antimicrobial resistance including multidrug resistance. Vet World. (2019) 12:504–21. doi: 10.14202/vetworld.2019.504-521
13. Hall-Stoodley L, Stoodley P. Biofilm formation and dispersal and the transmission of human pathogens. Trends Microbiol. (2005) 13:7–10. doi: 10.1016/j.tim.2004.11.004
14. Winkelstroter LK, Teixeira FBD, Silva EP, Alves VF, De Martinis ECP. Unraveling Microbial Biofilms of Importance for Food Microbiology. Microb Ecol. (2014) 68:35–46. doi: 10.1007/s00248-013-0347-4
15. Carlton RM. Phage therapy: past history and future prospects. Arch Immunol Ther Exp. (1999) 47:267–74
16. Burrowes B, Harper DR, Anderson J, Mcconville M, Enright MC. Bacteriophage therapy: potential uses in the control of antibiotic-resistant pathogens. Expert Rev Anti Infect Ther. (2011) 9:775–85. doi: 10.1586/eri.11.90
17. Ghannad MS, Mohammadi A. Bacteriophage: time to re-evaluate the potential of phage therapy as a promising agent to control multidrug-resistant bacteria. Iran J Basic Med Sci. (2012) 15:693–701.
18. Huss P, Raman S. Engineered bacteriophages as programmable biocontrol agents. Curr Opin Biotechnol. (2020) 61:116–21. doi: 10.1016/j.copbio.2019.11.013
19. Garcia P, Martinez B, Obeso JM, Rodriguez A. Bacteriophages and their application in food safety. Lett Appl Microbiol. (2008) 47:479–85. doi: 10.1111/j.1472-765X.2008.02458.x
20. Lopez-Cuevas O, Castro-Del Campo N, Leon-Felix J, Gonzalez-Robles A, Chaidez C. Characterization of bacteriophages with a lytic effect on various Salmonella serotypes and Escherichia coli O157:H7. Can J Microbiol. (2011) 57:1042–51. doi: 10.1139/w11-099
21. Gigante A, Atterbury RJ. Veterinary use of bacteriophage therapy in intensively-reared livestock. Virol J. (2019) 16:155. doi: 10.1186/s12985-019-1260-3
22. Kakasis A, Panitsa G. Bacteriophage therapy as an alternative treatment for human infections. A comprehensive review. Int J Antimicrob Agents. (2019) 53:16–21.doi: 10.1016/j.ijantimicag.2018.09.004
23. Zbikowska K, Michalczuk M, Dolka B. The use of bacteriophages in the poultry industry. Animals. (2020) 10:872. doi: 10.3390/ani10050872
24. Carey-Smith GV, Billington C, Cornelius AJ, Hudson JA, Heinemann JA. Isolation and characterization of bacteriophages infecting Salmonella spp. FEMS Microbiol Lett. (2006) 258:182–6.doi: 10.1111/j.1574-6968.2006.00217.x
25. O'flynn G, Coffey A, Fitzgerald GF, Ross RP. The newly isolated lytic bacteriophages st104a and st104b are highly virulent against Salmonella enterica. J Appl Microbiol. (2006) 101:251–9. doi: 10.1111/j.1365-2672.2005.02792.x
26. Ahiwale SS, Bankar AV, Tagunde SN, Zinjarde S, Ackermann HW, Kapadnis BP. Isolation and characterization of a rare waterborne lytic phage of Salmonella enterica serovar Paratyphi B. Can J Microbiol. (2013) 59:318–23. doi: 10.1139/cjm-2012-0589
27. Huang C, Virk SM, Shi J, Zhou Y, Willias SP, Morsy MK, et al. Isolation, characterization, and application of bacteriophage LPSE1 against Salmonella enterica in ready to eat (RTE) Foods. Front Microbiol. (2018) 9:1046. doi: 10.3389/fmicb.2018.01046
28. Goodridge LD, Bisha B. Phage-based biocontrol strategies to reduce foodborne pathogens in foods. Bacteriophage. (2011) 1:130–7.doi: 10.4161/bact.1.3.17629
29. Sukumaran AT, Nannapaneni R, Kiess A, Sharma CS. Reduction of Salmonella on chicken meat and chicken skin by combined or sequential application of lytic bacteriophage with chemical antimicrobials. Int J Food Microbiol. (2015) 207:8–15. doi: 10.1016/j.ijfoodmicro.2015.04.025
30. Hyman P, Abedon ST. Bacteriophage host range and bacterial resistance. Adv Appl Microbiol. (2010) 70:217–48. doi: 10.1016/S0065-2164(10)70007-1
31. Chaturongakul S, Ounjai P. Phage-host interplay: examples from tailed phages and Gram-negative bacterial pathogens. Front Microbiol. (2014) 5:442. doi: 10.3389/fmicb.2014.00442
32. Wang S, Liu X, Xu X, Zhao Y, Yang D, Han X, et al. Escherichia coli type III secretion system 2 (ETT2) is widely distributed in avian pathogenic Escherichia coli isolates from Eastern China. Epidemiol Infect. (2016) 144:2824–30. doi: 10.1017/S0950268816000820
33. Wang S, Yang D, Wu X, Yi Z, Wang Y, Xin S, et al. The ferric uptake regulator represses Type VI secretion system function by binding directly to the clpV promoter in Salmonella enterica serovar typhimurium. Infect Immun. (2019) 87:e00562–00519. doi: 10.1128/IAI.00562-19
34. Institute CLS. Performance standards for antimicrobial susceptibility testing. Clin Lab Stand Instit Wayne. (2018) 31:76–9.
35. Tang F, Zhang P, Zhang Q, Xue F, Ren J, Sun J, et al. Isolation and characterization of a broad-spectrum phage of multiple drug resistant Salmonella and its therapeutic utility in mice. Microb Pathog. (2019) 126:193–8. doi: 10.1016/j.micpath.2018.10.042
36. Islam MS, Hu Y, Mizan MFR, Yan T, Nime I, Zhou Y, et al. Characterization of Salmonella phage LPST153 that effectively targets most prevalent Salmonella serovars. Microorganisms. (2020) 8:1089. doi: 10.3390/microorganisms8071089
38. Danis-Wlodarczyk K, Olszak T, Arabski M, Wasik S, Majkowska-Skrobek G, Augustyniak D, et al. Characterization of the newly isolated lytic bacteriophages KTN6 and KT28 and their efficacy against pseudomonas aeruginosa biofilm. PLoS ONE. (2015) 10:e0127603. doi: 10.1371/journal.pone.0127603
39. Al-Zubidi M, Widziolek M, Court EK, Gains AF, Smith RE, Ansbro K, et al. Identification of novel bacteriophages with therapeutic potential that target Enterococcus faecalis. Infect Immun. (2019) 87:e00512–9. doi: 10.1128/IAI.00512-19
40. Hyman P, Abedon ST. Practical methods for determining phage growth parameters. Methods Mol Biol. (2009) 501:175–202. doi: 10.1007/978-1-60327-164-6_18
41. Peng Q, Yuan Y. Characterization of a newly isolated phage infecting pathogenic Escherichia coli and analysis of its mosaic structural genes. Scient Rep. (2018) 8:8086. doi: 10.1038/s41598-018-26004-4
42. Adnan M, Ali Shah MR, Jamal M, Jalil F, Andleeb S, Nawaz MA, et al. Isolation and characterization of bacteriophage to control multidrug-resistant Pseudomonas aeruginosa planktonic cells and biofilm. Biologicals. (2020) 63:89–96. doi: 10.1016/j.biologicals.2019.10.003
43. Wang S, Yang D, Wu X, Wang Y, Wang D, Tian M, et al. Autotransporter MisL of Salmonella enterica serotype Typhimurium facilitates bacterial aggregation and biofilm formation. FEMS Microbiol Lett. (2018) 365:142. doi: 10.1093/femsle/fny142
44. Dickey J, Perrot V. Adjunct phage treatment enhances the effectiveness of low antibiotic concentration against Staphylococcu saureus biofilms in vitro. PLoS ONE. (2019) 14:e0209390. doi: 10.1371/journal.pone.0209390
45. Zhang LL, Sun LC, Wei RC, Gao Q, He T, Xu CF, et al. Intracellular Staphylococcus aureus control by virulent bacteriophages within MAC-T bovine mammary epithelial cells. Antimicrob Agents Chemother. (2017) 61:e01990–6. doi: 10.1128/AAC.01990-16
46. Kurtz S, Phillippy A, Delcher AL, Smoot M, Shumway M, Antonescu C, et al. Versatile and open software for comparing large genomes. Genome Biol. (2004) 5:R12. doi: 10.1186/gb-2004-5-2-r12
47. Walker BJ, Abeel T, Shea T, Priest M, Abouelliel A, Sakthikumar S, et al. Pilon: an integrated tool for comprehensive microbial variant detection and genome assembly improvement. PLoS ONE. (2014) 9:e112963. doi: 10.1371/journal.pone.0112963
48. Besemer J, Lomsadze A, Borodovsky M. GeneMarkS: a self-training method for prediction of gene starts in microbial genomes. Implications for finding sequence motifs in regulatory regions. Nucleic Acids Res. (2001) 29:2607–18. doi: 10.1093/nar/29.12.2607
49. Lowe TM, Chan PP. tRNAscan-SE On-line: integrating search and context for analysis of transfer RNA genes. Nucleic Acids Res. (2016) 44:W54–7. doi: 10.1093/nar/gkw413
50. Ackermann HW. 5500 Phages examined in the electron microscope. Arch Virol. (2007) 152:227–43. doi: 10.1007/s00705-006-0849-1
51. Grabow WOK. Bacteriophages: update on application as models for viruses in water. Water Sa. (2001) 27:251–68. doi: 10.4314/wsa.v27i2.4999
52. Abedon ST, Herschler TD, Stopar D. Bacteriophage latent-period evolution as a response to resource availability. Appl Environ Microbiol. (2001) 67:4233–41. doi: 10.1128/aem.67.9.4233-4241.2001
53. Sharma S, Chatterjee S, Datta S, Prasad R, Dubey D, Prasad RK, et al. Bacteriophages and its applications: an overview. Folia Microbiol. (2017) 62:17–55. doi: 10.1007/s12223-016-0471-x
54. Wichels A, Biel SS, Gelderblom HR, Brinkhoff T, Muyzer G, Schutt C. Bacteriophage diversity in the North Sea. Appl Environ Microbiol. (1998) 64:4128–33. doi: 10.1128/AEM.64.11.4128-4133.1998
55. Hamdi S, Rousseau GM, Labrie SJ, Tremblay DM, Kourda RS, Ben Slama K, et al. Characterization of two polyvalent phages infecting Enterobacteriaceae. Sci Rep. (2017) 7:40349. doi: 10.1038/srep40349
56. Shahin K, Bouzari M, Wang R. Isolation, characterization and genomic analysis of a novel lytic bacteriophage vB_SsoS-ISF002 infecting shigella sonnei and shigella flexneri. J Med Microbiol. (2018) 67:376–86. doi: 10.1099/jmm.0.000683
57. Dallal MMS, Nikkhahi F, Alimohammadi M, Douraghi M, Rajabi Z, Foroushani AR, et al. Phage therapy as an approach to control Salmonella enterica serotype enteritidis infection in mice. Rev Soc Bras Med Trop. (2019) 52:e20190290. doi: 10.1590/0037-8682-0290-2019
58. Hughes KA, Sutherland IW, Jones MV. Biofilm susceptibility to bacteriophage attack: the role of phage-borne polysaccharide depolymerase. Microbiology-Uk. (1998) 144:3039–47. doi: 10.1099/00221287-144-11-3039
59. Azeredo J, Sutherland IW. The use of phages for the removal of infectious biofilms. Curr Pharm Biotechnol. (2008) 9:261–6. doi: 10.2174/138920108785161604
60. Parasion S, Kwiatek M, Gryko R, Mizak L, Malm A. Bacteriophages as an alternative strategy for fighting biofilm development. Polish J Microbiol. (2014) 63:137–45.
61. Dakheel KH, Rahim RA, Neela VK, Al-Obaidi JR, Hun TG, Isa MNM, et al. Genomic analyses of two novel biofilm-degrading methicillin-resistant Staphylococcu saureus phages. BMC Microbiol. (2019) 19:114. doi: 10.1186/s12866-019-1484-9
62. Islam MS, Zhou Y, Liang L, Nime I, Liu K, Yan T, et al. Application of a phage cocktail for control of Salmonella in foods and reducing biofilms. Viruses. (2019) 11:841. doi: 10.3390/v11090841
63. Switt AIM, Orsi RH, Den Bakker HC, Vongkamjan K, Altier C, Wiedmann M. Genomic characterization provides new insight into Salmonella phage diversity. Bmc Genomics. (2013) 14:481. doi: 10.1186/1471-2164-14-481
64. Kang HW, Kim JW, Jung TS, Woo GJ. wksl3, a new biocontrol agent for salmonella enterica serovars enteritidis and typhimurium in foods: characterization, application, sequence analysis, and oral acute toxicity study. Appl Environ Microbiol. (2013) 79:1956-68. doi: 10.1128/AEM.02793-12
65. Krupovic M, Bamford DH. Holin of bacteriophage lambda: structural insights into a membrane lesion. Mol Microbiol. (2008) 69:781–3. doi: 10.1111/j.1365-2958.2008.06335.x
Keywords: Salmonella, bacteriophage, characteristics, biofilm, genome, multidrug-resistant
Citation: Tao C, Yi Z, Zhang Y, Wang Y, Zhu H, Afayibo DJA, Li T, Tian M, Qi J, Ding C, Gao S, Wang S and Yu S (2021) Characterization of a Broad-Host-Range Lytic Phage SHWT1 Against Multidrug-Resistant Salmonella and Evaluation of Its Therapeutic Efficacy in vitro and in vivo. Front. Vet. Sci. 8:683853. doi: 10.3389/fvets.2021.683853
Received: 22 March 2021; Accepted: 14 May 2021;
Published: 10 June 2021.
Edited by:
Min Yue, Zhejiang University, ChinaCopyright © 2021 Tao, Yi, Zhang, Wang, Zhu, Afayibo, Li, Tian, Qi, Ding, Gao, Wang and Yu. This is an open-access article distributed under the terms of the Creative Commons Attribution License (CC BY). The use, distribution or reproduction in other forums is permitted, provided the original author(s) and the copyright owner(s) are credited and that the original publication in this journal is cited, in accordance with accepted academic practice. No use, distribution or reproduction is permitted which does not comply with these terms.
*Correspondence: Shengqing Yu, eXVzQHNodnJpLmFjLmNu; Shaohui Wang, c2h3YW5nMDgyN0AxMjYuY29t