- 1College of Veterinary Medicine, University of Missouri, Columbia, MO, United States
- 2Department of Veterinary Medicine and Surgery, College of Veterinary Medicine, University of Missouri, Columbia, MO, United States
- 3Comparative Internal Medicine Laboratory, College of Veterinary Medicine, University of Missouri, Columbia, MO, United States
- 4University of Missouri Metagenomics Center, University of Missouri, Columbia, MO, United States
- 5Department of Veterinary Pathobiology, College of Veterinary Medicine, University of Missouri, Columbia, MO, United States
Deviations from a core airway microbiota have been associated with the development and progression of asthma as well as disease severity. Pet cats represent a large animal model for allergic asthma, as they spontaneously develop a disease similar to atopic childhood asthma. This study aimed to describe the lower airway microbiota of asthmatic pet cats and compare it to healthy cats to document respiratory dysbiosis occurring with airway inflammation. We hypothesized that asthmatic cats would have lower airway dysbiosis characterized by a decrease in richness, diversity, and alterations in microbial community composition including identification of possible pathobionts. In the current study, a significant difference in airway microbiota composition was documented between spontaneously asthmatic pet cats and healthy research cats mirroring the finding of dysbiosis in asthmatic humans. Filobacterium and Acinetobacter spp. were identified as predominant taxa in asthmatic cats without documented infection based on standard culture and could represent pathobionts in the lower airways of cats. Mycoplasma felis, a known lower airway pathogen of cats, was identified in 35% of asthmatic but not healthy cats.
This article has been published alongside “Temporal changes of the respiratory microbiota as cats transition from health to experimental acute and chronic allergic asthma” (1).
Introduction
In humans, asthma is used to describe a group of inflammatory airway diseases with distinct phenotypes based on the clinical manifestation of the disease and underpinned by specific endotypes or mechanistic pathways (2). Interactions between the host immune system and microbiome have recently been recognized as important contributors to asthmatic endotypes, driving studies of the composition and structure of the airway microbiota of asthmatic patients to develop a deeper understanding of such a complex disease (3–6). While the relationship among phenotypes, endotypes, and the respiratory microbiota is not completely understood (7–9), the respiratory microbiota is known to play critical roles in the development, regulation, and maintenance of a healthy immune system (10–12).
Further, dysbiosis or alterations in the composition of the microbial community can contribute to immune dysregulation, potentially influencing the development of disease as well as response to treatment (7, 13–15). In a healthy environment, equilibrium or homeostasis exists, and triggers such as environmental irritants may lead to pathogen colonization or alterations in microbial community composition that cause low-grade inflammation, allowing for bacterial replication, epithelial damage, and recruitment of immune cells, contributing to a vicious cycle of inflammation. It is unclear if the dysbiosis documented in asthma occurs as a result of inflammation or secondary bacterial infection, or if dysbiosis occurs first, triggering inflammation, and providing a niche for opportunistic pathogens or pathobionts to thrive, or both (16).
Differences in microbial community composition between spontaneously asthmatic and healthy airways of humans (5, 17) and horses (18, 19) as well as experimentally induced asthma in mice (20) and cats (1) have been documented. Since cats can experimentally or spontaneously develop airway eosinophilia, airway hyperresponsiveness, and airway remodeling analogous to human allergic asthma, they serve as an important large animal model for the type 2 high endotype (21, 22). In this study, we aimed to characterize the lower airway microbiota of pet cats with spontaneous allergic asthma and compare it to that of healthy cats. We hypothesized that, as in humans, spontaneously asthmatic pet cats would have decreased richness and diversity and significant alterations in the microbial community, and the potential emergence of possible pathobionts compared to healthy cats.
Materials and methods
Ethics statement
All studies were performed in accordance with the Guide for the Use and Care of Laboratory Animals and were approved by the University of Missouri Institutional Animal Care and Use Committee (MU IACUC protocol #7891).
Cats
Client-owned pet cats presenting to the University of Missouri Veterinary Health Center between 2015 and 2019 that were diagnosed with asthma and had bronchoalveolar lavage fluid (BALF) samples banked were retrospectively enrolled. Diagnostic criteria for feline asthma included a history of clinical signs such as cough and respiratory distress, thoracic radiographs with evidence of a bronchial pattern and lung hyperinflation, and BALF cytology containing >7% eosinophils (23). Signalment, home environment (indoor vs. outdoor), clinical signs, peripheral eosinophil count, heartworm test results, serum allergy testing, thoracic radiographic pattern, and BALF cytology report were extracted from the medical record when available. A clinical severity score was assigned based upon a previously published (24) three-point scoring system, namely, 1 = mild (cough alone); 2 = moderate (wheezing or exercise intolerance); or 3 = severe (respiratory distress episodes).
Banked BALF samples from eleven healthy research cats were used as controls. Control cats were from a research colony (Comparative Internal Medicine Laboratory, University of Missouri, Columbia, MO). Cats were housed in large runs with elevated platforms for climbing and enrichment toys. Access to food and clean drinking water was provided ad libitum. There were 6 female and 5 male cats from 2 different litters and they were housed according to sex. Cats were determined to be healthy by the absence of respiratory clinical signs, a normal physical examination by a board-certified veterinary internal medicine specialist, and a lack of cytologic evidence of infection or inflammation from BALF samples. Euthanasia was not an endpoint of the study; all cats were subsequently adopted into private homes.
Sample collection
All cats, control and client-owned, were anesthetized and carefully intubated using a sterile 3.5–4 French endotracheal tube. To collect BALF, a 20 ml aliquot of sterile saline was instilled and aspirated via a sterile 8 French red rubber catheter that was threaded through the endotracheal tube until it was gently wedged in the distal airway. Immediately after collection, all samples were placed on ice and transported to the laboratory. Promptly after collection, samples were centrifuged to pellet bacterial cells. The supernatant was discarded and pellets were resuspended in 800 μl of lysis buffer adapted from Yu et al. (4% sodium dodecyl sulfate, 50 mM EDTA, 500 mM NaCl, and 50 mM Tris–HCl pH 8.0) (25). All the samples were banked at −80 °C until the end of the study, and DNA was extracted as a single batch.
DNA extraction, 16S rRNA library preparation, sequencing, and informatics
DNA from BALF was extracted using the column method as previously described (26, 27). Library construction and sequencing were completed at the University of Missouri DNA Core facility as previously described (26). Assembly, filtering, binning, and annotation of DNA sequences were performed at MU Informatics with Quantitative Insights Into Microbial Ecology 2 (QIIME 2) v2021.2 (28). Sequences were trimmed from the Illumina adapters with cutadapt (29). Using DADA2 (30), trimmed forward and reverse reads were truncated to 150 base pairs, paired, and then denoised into unique sequences called Amplicon Sequence Variants (ASVs). A feature table containing the frequency of each ASV per sample was rarefied to 11,562 total features per sample, maximizing the number of subsampled features per sample and a total number of samples retained for further analysis. Samples with a total feature number of <1,488 were omitted from downstream analyses. A taxonomy was assigned to each unique ASV with a sklearn algorithm (31) using the QIIME2-provided 99% non-redundant SILVA v132 reference database of the 515F/806R region of the 16S rRNA gene (28, 32). Code used for sequence processing can be accessed at https://github.com/mubioinformatics/nf-qimme2 and https://github.com/ericsson-lab/spontaneous_asthma. Alpha diversity analysis, principal coordinate analysis (PCoA), and one-way permutational analysis of variance (PERMANOVA) of Jaccard distances (1/4 root-transformed data) were performed using the vegan v2.5-7 (33) package within R v4.1.2 (34).
Statistical analysis
Statistical analysis was performed using Sigma Plot 14.0 (Systat Software Inc., Carlsbad, CA) and R v4.1.2. Normality was first tested using the Shapiro–Wilk method, and equal variance was tested using the Brown–Forsyth method. Fischer's exact tests were utilized to determine associations. Mann–Whitney Rank sum tests were used to test for differences between sample sites in coverage, richness, and relative abundance of all taxa at the level of family detected at > 0.5% of samples. Results are presented as mean ± SEM. PCoA was used to visualize the relatedness of samples. Enhanced Volcano (35) was utilized to generate a volcano plot to visualize which taxa had undergone the most significant changes. Results were considered statistically significant for p-values ≤ 0.05.
Results
Cats
Twenty-six cats, 12 females and 14 males, all altered, weighing 4.9 ± 0.19 kg (mean ± SEM) from a variety of breeds including domestic short hair (n = 18), domestic long hair (3), Siamese (2), Persian, (1), Maine Coon (1), and Himalayan (1) were enrolled. Clinical signs on presentation were reported to be acute (<2 weeks; 6) or chronic (>2 weeks; 20) and included cough (15), wheeze (6), respiratory distress (5), increased respiratory effort in combination with the increased respiratory rate (5), increased respiratory effort alone (3), and exercise intolerance (3). Cats were categorized as having mild (12), moderate (7), or severe (7) signs based on the clinical severity score. Ten cats also had upper airway signs, including nasal discharge (5), sneezing (3), and stridor (2). In addition to asthma, the cats with upper airway signs were also diagnosed with chronic rhinitis (3), nasopharyngeal stenosis (2), and nasal carcinoma (1). None of the cats tested for heartworm antibodies were positive (n = 16). BALF culture results were negative in 21/24 and were not performed in 2/26 cats. In 3 cats, culture was positive for Stenotrophomonas maltophilia (Pseudomonas maltophilia), Pseudomonas putida, and an unidentified Pasteurella spp. and Streptococcus spp. Pasteurella multocida, and Pseudomonas aeruginosa. Recent drug administration, defined as within 14 days prior to sampling, included antibiotics (4), corticosteroids (4), or both (2). The 11 control cats were significantly younger than the asthmatic cats (2.7 ± 0.1 vs. 5.2 ± 0.6 years, respectively; p = 0.016). BALF eosinophil counts in healthy cats ranged from 2.5% to 6.5% (mean ± SEM; 4.6 ± 0.5%) and were significantly lower compared to asthmatic cats, which ranged from 11.1% to 71.5% (37.8 ± 3.8; p < 0.001). Individual cat data are summarized in Supplementary Table 1.
Coverage and richness in BALF are significantly lower and diversity higher in asthmatic vs. healthy cats
Samples of BALF from asthmatic cats had significantly lower depth of coverage than healthy cats (mean ± SEM of 2,172 ± 92 and 7,441 ± 216 sequences/sample, respectively, p < 0.001), as well as richness (33 ± 1 and 50 ± 1, respectively; p = 0.019; Figures 1A,B). Asthmatic cats exhibited higher diversity than healthy cats using Shannon (2.05 ± 9.19 and 1.24 ± 0.05, respectively; p = 0.0061) and Simpson (0.70 ± 0.05 and 0.44 ± 0.02, respectively; p < 0.001; Figures 1C,D).
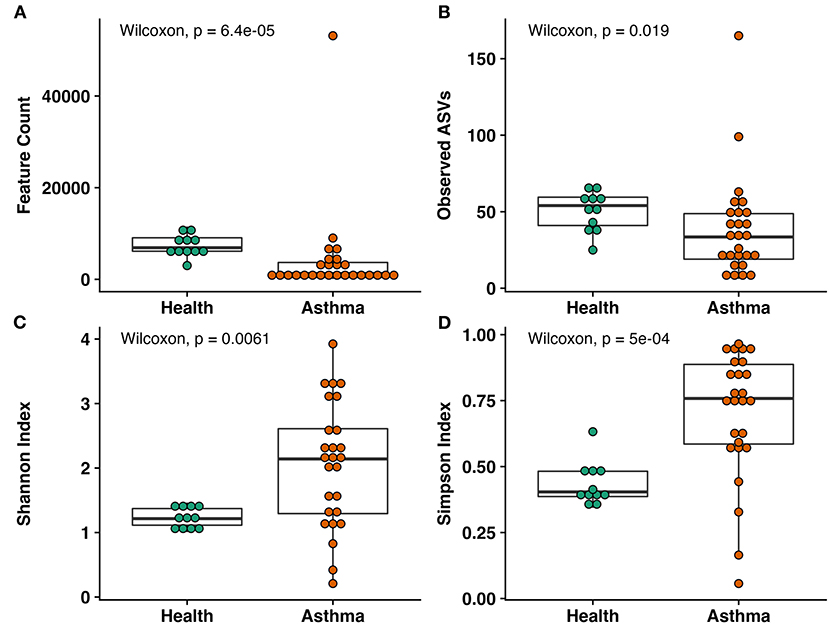
Figure 1. Alpha diversity metrics including (A) total observed features, (B) within sample richness, (C) Shannon Index, and (D) Simpson Index. Significant decreases in coverage and richness were observed in asthma compared to healthy cats. Within sample diversity was significantly increased in asthmatic cats compared to healthy cats. Wilcoxon rank sum test, p-values indicated.
Microbial community composition is significantly altered in asthmatic cats
Principal coordinate analysis (PCoA), used to assess the β-diversity of microbial communities, showed no overlap between healthy and asthmatic cats (Figure 2). One-way PERMANOVA confirmed a significant difference in the microbial community composition between the two groups (p = 0.0001; F = 10.03). Although the airways were predominated by the phyla Proteobacteria, Bacteroidetes, and Firmicutes in healthy and asthmatic cats, there were significant differences in the relative abundance of these taxa between groups. In healthy compared to asthmatic cats, changes were primarily attributed to a decreased relative abundance of Pseudomonadaceae (phylum Proteobacteria) from 78.3% ± 7.6% to 4.4% ± 0.3% (mean ± SEM); p < 0.001), and an increased relative abundance of Moraxellaceae (phylum Proteobacteria) from 3.0% ± 0.4% to 24.2% ± 0.1% (p < 0.001), Weeksellaceae (phylum Bacteroidetes) from 0.1% ± 0.01% to 52.4% ± 2.2 % (p < 0.001) and Chitinophagaceae (phylum Bacteroidetes) from 3.9% ± 1.0% to 22.5% ± 1.2 % (p < 0.001) (Figure 3). A volcano plot was used to identify the phyla and families that had a significant (p < 0.05) and at least a 2-fold change in relative abundance between health and asthma (Figure 4); 13 families met these criteria (Figure 5). The relative abundance of these taxa, as well as any taxa that were present in any cat at > 40% at any time point are summarized in Table 1.
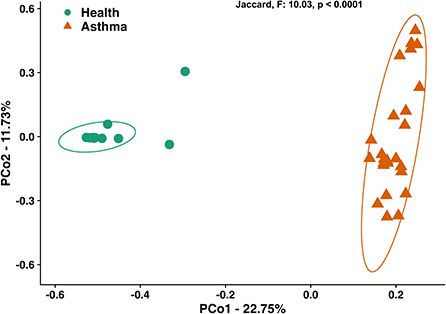
Figure 2. Principal coordinate analysis of Jaccard similarity index showing significant differences (p < 0.0001; F = 10.03) in microbial community composition between healthy and spontaneously asthmatic cats. Circles represent healthy cats; triangles represent asthmatic cats and ellipses represent 95% CIs.
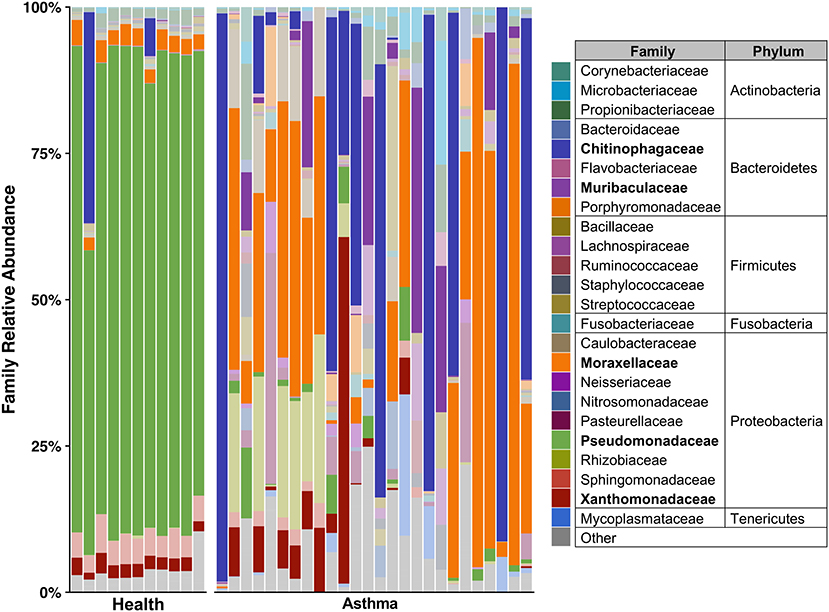
Figure 3. Taxa present in bronchoalveolar lavage fluid—mean relative abundance of taxa present at > 5% in bronchoalveolar lavage fluid collected from healthy and cats with spontaneous asthma. Families with a relative abundance of <5% are grouped into “Other.” Families with >40% relative abundance in at least one sample are bolded in the legend and represented by a darker color in the plot.
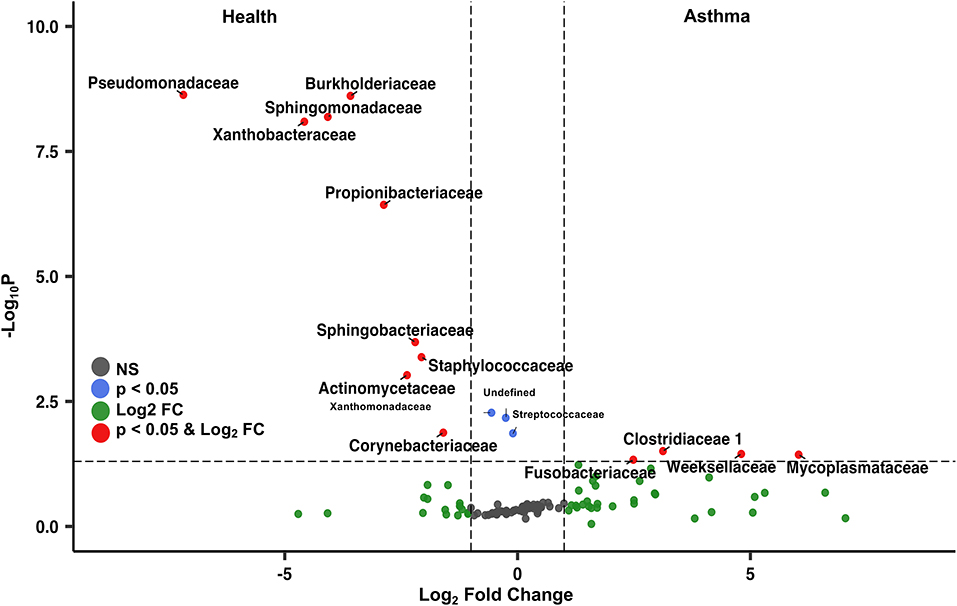
Figure 4. Volcano plot highlighting the most abundant families in the lower airways that were significantly different (p < 0.05) and underwent at least a 2-fold change in abundance in asthmatic pet cats compared to healthy cats. The taxa on the top left were more abundant in health, whereas the taxa on the right were more abundant in asthmatic pet cats.
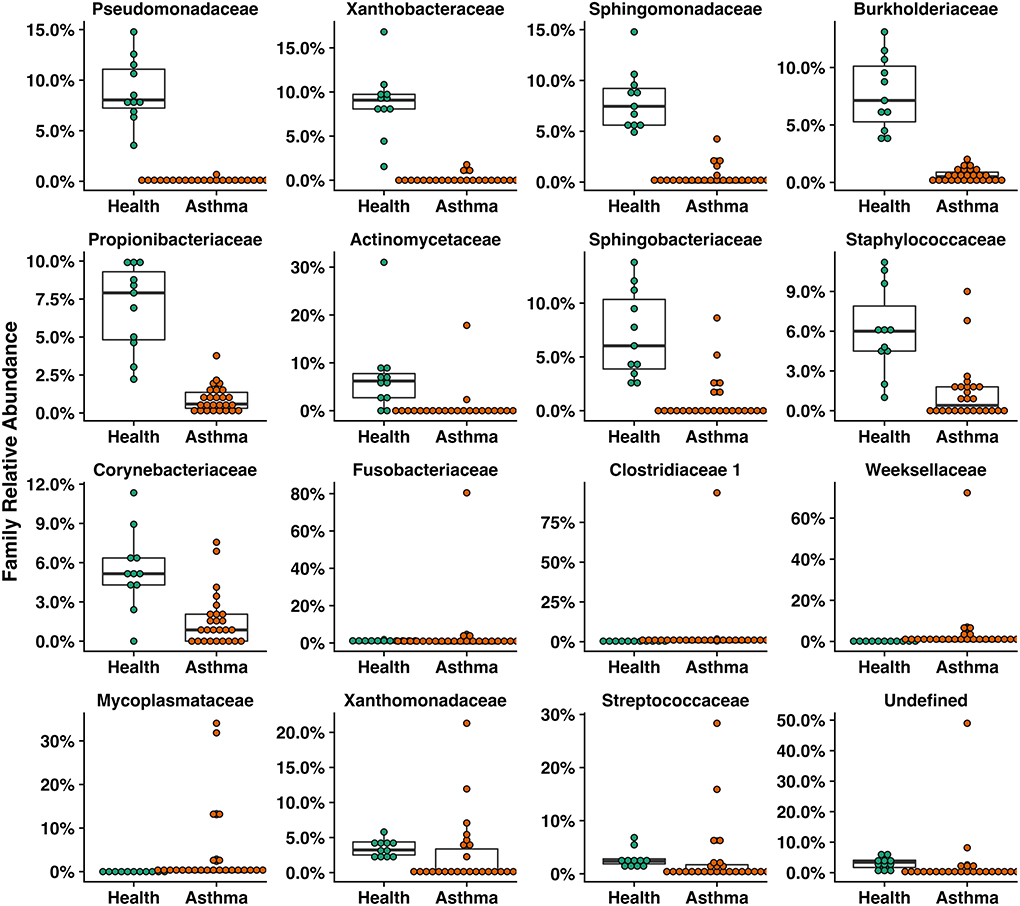
Figure 5. Box plots of families that were significantly different including those with a greater than two-fold change or were significantly different in healthy compared to asthmatic pet cats.
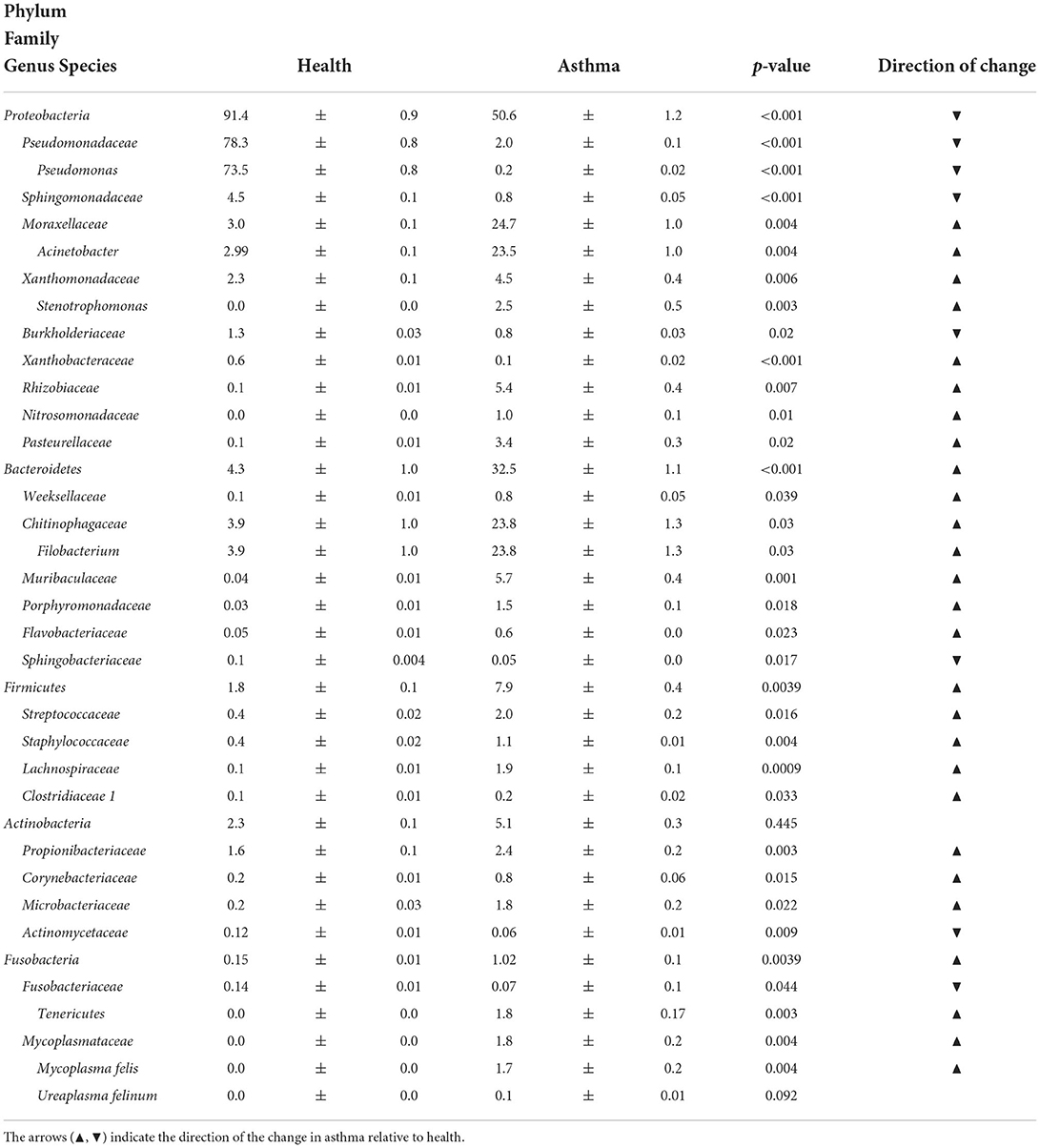
Table 1. Relative abundance (mean ± SEM%) of taxa present in any BALF sample at a minimum of 0.5% in either health or asthma that underwent at least a 2-fold and significant change (p < 0.05) when comparing healthy and asthmatic cats or comprised more than 40% relative abundance in any sample.
Intra-Group variation in community composition within asthmatic cats was observed
In comparison to healthy cats, in which Pseudomonadaceae (phylum Proteobacteria) was the dominating taxon, asthmatic cats had a more variable composition of their microbial communities. Sixty-one percent of asthmatic cats (16/26) had one predominant taxon, comprising more than 40% of the population. Relative abundance of Filobacterium spp. (family Chitinophagaceae) ranged from 48.1% to 97.1% in eight cats, Acinetobacter spp. (family Moraxellaceae) ranged from 42.4% to 90.4% in six cats, and there was one cat each with relative abundances of 59.2% Stenotrophomonas spp. (family Xanthomonadaceae) and Pasteurellaceae at 39.5%, respectively. The latter two cats had positive BALF cultures in agreement with the predominant taxa at the taxonomic level of ASV and family respectively (Supplementary Table 2). Additionally, four other cats had a predominance of Acinetobacter spp. ranging from 25.2% and 37.3% relative abundance.
Potential pathogens were detected in asthmatic cats
Filobacterium spp. were sequenced in 2/11 (18%) healthy cats with relative abundances of 6.4 and 36.0% compared to 12/26 (46%) asthmatic cats, with a relative abundances of 2.2 to 97.1%. Family Mycoplasmataceae, was not sequenced in any healthy cat but was sequenced in 11/26 (42%) of asthmatic cats with relative abundance ranging from 0.1 to 24.2%, including the three cats with positive BALF cultures. Annotated to the ASV level, 5/11 cats had Mycoplasma felis, 2/11 had Ureaplasma felinum, and 4/11 had both taxa identified.
Discussion
Spontaneous feline asthma, estimated to affect 1–5% of the pet cat population (36), is driven by T helper 2 cells, resulting in airway eosinophilia, airway hyperresponsiveness, and airway remodeling. It most closely resembles childhood-onset (type 2 high) asthma in humans (2) and has been proposed as a large animal model with relevance to One Health (22). The microbial composition and community structure of the lower airways of humans significantly differ between asthmatic and healthy states (17, 37) supporting the concept that respiratory dysbiosis occurs in human asthma. Similarly, in this study, respiratory dysbiosis was noted in spontaneously asthmatic cats and was characterized by decreased richness, increased α-diversity, and changes in the microbial community composition, including intra-group heterogeneity of predominating taxa and presence of taxa likely to be pathobionts or opportunistic pathogens. The respiratory microbiota may be influenced by environmental contributors (38), medications [antibiotics (39) or corticosteroids (40)], or even perturbations at distant mucosal sites such as the gut (41, 42). It is still unclear if altered microbial community composition is caused by or contributes to airway inflammation, but knowledge of altered bacterial populations in asthmatic airways could open the door for novel targeted therapies. Comprehensive characterization of microbial communities in the respiratory tract is only possible with sequencing, as evidenced by the high proportion of negative cultures in asthmatic cats corresponding to rich sequenced taxa. Compared to healthy cats and in parallel with human asthmatics (9, 17, 43), richness in asthmatic cats decreased. Many studies of human asthmatics cite inconsistent changes [increases (44, 45) or decreases (46)] in airway bacterial diversity as a key feature of dysbiosis compared with health. Diversity metrics vary across studies and include numbers of unique taxa present in each sample reflecting richness and evenness (α-diversity; Shannon and Simpson indices) or how many different taxa are shared between samples (β-diversity; Jaccard and Bray Curtis dis(similarity) indices). In spontaneously asthmatic cats compared to healthy cats, the Shannon and Simpson indices showed significantly increased alpha diversity. Taking into consideration the influence that different bacteria taxa may have on the microenvironment (e.g., microbial factors leading to disruption of immune maturation), β-diversity might provide a better insight into understanding the lower airway microbiota when comparing healthy and diseased states, especially when considering what taxa are significantly altered in disease or present in health and absent in disease. These changes in diversity may provide insight into which organisms are supportive of a “healthy” environment, potentially providing an avenue for intervention, in the way of replenishing or supporting the taxa associated with health. Using the Jaccard dissimilarity index, healthy and asthmatic cats had no overlap in microbial communities, and asthmatic cats had near obliteration of the Pseudomonadaceae family (phylum Proteobacteria), the predominant taxa found in healthy feline airways (27, 47). It is unclear if alterations in microbial community composition set the stage to initiate allergic airway inflammation culminating in spontaneous disease or if allergic asthma results in respiratory dysbiosis, further exacerbating and perpetuating the aberrant immune response. Certain identified pathobionts gaining a foothold in asthmatic airways, including Filobacterium and Acinetobacter spp., were noted in the current study. Additionally, Mycoplasma felis, a known lower airway pathogen of cats (48, 49) was identified in 35% of asthmatic but not in healthy cats, supporting that in addition to pathobionts, some opportunistic bacteria may also contribute to the respiratory dysbiosis observed in this study.
Asthma is a multifactorial heterogenous inflammatory airway disease classified both according to phenotype (clinical presentation) and endotype (distinct mechanistic pathways) (2, 50). While not universally recognized, two phenotypes are noted in cats, namely, cough variant only or cough in combination with clinical signs of airflow obstruction, including wheeze or episodic respiratory distress (51). Spontaneous feline asthma is orchestrated by T-helper 2 cells with allergen-specific IgE, leading to airway hyperresponsiveness and structural changes of the airways (22), modeling the atopic phenotype and T2 high endotype described in humans (2). Endotype characterization is important for therapy and prognosis and necessitates an understanding of a number of determinants, including the microbiome (50, 52, 53). Initial studies of the respiratory microbiota in humans showed the microbial composition and community structure of the lower airways were significantly different between asthmatic and healthy humans; however, these studies considered asthma as a single entity (17, 37, 54). More recently, the microbiota composition has been associated with disease severity and specific inflammatory pathways in humans with asthma underscoring the importance of recognizing phenotypic and endotypic characteristics (7, 55–57). Whether cats with different phenotypes have yet unrecognized differences in endotypes deserves further study with a more thorough immunologic evaluation but could be supported by the heterogeneity between bacterial taxa within the asthmatic cats of this study. The respiratory microbiota has been shown to help maintain mucosal homeostasis (10, 11) with microbiota being dynamic and competitive as they interact with the mucosal immune system (16). In health, the respiratory microbiota may directly inhibit pathogens from causing disease by a number of mechanisms, including competition for nutrients, biofilm formation, disruption of signaling molecules, direct bactericidal activity, and spatial occlusion (58). When homeostasis is disrupted by an infection, steroids, antibiotics, or other environmental triggers, a dysbiotic state can be established, which can foster a permissive environment for certain organisms to thrive and contribute to that dysbiotic state (10, 16). Certain commensal organisms exerting specific effects on the host mucosal immune system associated with the development of clinical disease are termed pathobionts. It has been postulated that treating dysbiosis by shifting the microbial community composition toward “health” or a homeostatic state may provide a more holistic approach than using antimicrobials to target an organism identified via standard culture techniques (16). While dysbiosis was demonstrated in all the asthmatic cats in this cohort, only 3 had positive bacterial cultures, highlighting the poor utility of this test alone to understand the impact of microbes in this lower airway disease of cats.
The majority of cats (69%) enrolled in this study had a predominance by sequencing of either Filobacterium spp. or Acinetobacter spp. Filobacterium spp., sometimes referred to as cilia-associated respiratory bacillus (CARB), has been recognized as a lower airway pathogen in several animal species (59–64). The presence of organisms consistent with CARB identified by light and electron microscopy was noted in a cat with an anesthetic death having bronchitis and bronchiolitis as well as in healthy cats (65), and in a larger group of cats with chronic bronchitis35. Identifying CARB as part of the healthy cat microbiota in a low relative abundance (3.9% ± 1.0%) and documenting the significant increase in relative abundance in asthmatic cats (23.8% ± 1.3%, p = 0.03), supports the concept of Filobacterium spp. as a pathobiont. Similarly, Acinetobacter spp., which has been detected in healthy research cats (66) and cats with experimentally induced asthma (1), were noted to have a significant increase in relative abundance when comparing healthy to asthmatic cats in the current study (2.99% ± 0.1% and 23.5% ± 1.0%, respectively; p = 0.004). Like Filobacterium spp., Acinetobacter spp. may be a pathobiont of the feline lower airways and perhaps changes in the lower airway microenvironment secondary to inflammation set the stage for them to thrive and potentially contribute to the pathogenesis of asthma. Mycoplasmas are considered commensals of the upper airways of cats and play a role in secondary infections of the upper respiratory tract and conjunctiva (67, 68). However, Mycoplasma spp. has not been detected in the lower airways of healthy cats using standard culture (69), PCR (23, 66), or microbiota analysis (1, 27, 47). In humans, infections with Mycoplasma can precede the onset of asthma, exacerbate symptoms and complicate the management of the disease, (70, 71) and successful treatment has led to improvement of lung function in asthmatic patients (72). While several studies have estimated the prevalence of Mycoplasma to be between 15%(48) and 35% (49) in cats with respiratory disease, Mycoplasma spp. has not been detected using sequencing in a cohort of research cats before or after experimental asthma induction (1). In contrast, it was detected in 9/26 (35%) of spontaneously asthmatic cats in the current study; 6 of which had moderate to severe clinical signs. While not technically fitting the definition of a pathobiont, as Mycoplasma is not a commensal symbiont of the lower airways, it stands to reason that either Mycoplasma is a true opportunistic infection, or it may populate the lower airways via extension from the upper airways to take advantage of an altered commensal environment (73). Considering the associations between Mycoplasma spp. detection and asthma in humans and the high prevalence of this study, testing for Mycoplasma in asthmatic cats is recommended as targeted therapy may improve clinical outcomes.
This study demonstrates dysbiosis of the lower respiratory microbiota in pet cats with spontaneous asthma and represents the first step in exploring the role that some taxa may play in the pathophysiology of feline asthma. Understanding of the precise effects of the lower airway microbiota on pulmonary health and the mechanisms by which it can influence or regulate the immune system is limited. Future studies including larger cohorts of pet cats with spontaneous asthma, by characterizing the microbiota with complimentary metabolomics and immune assays could further our knowledge in understanding the mechanisms by which the microbiota interacts with the host immune system. A larger cohort could allow for further characterization of the potential influence of steroids, antibiotics, or other comorbid conditions may have on the respiratory microbiota of asthmatic pet cats. This in turn may allow the development of novel therapeutic or management strategies in asthmatic cats which may serve as a large animal model for type 2 high asthma in humans.
Data availability statement
The datasets presented in this study can be found in online repositories. The names of the repository/repositories and accession number(s) can be found in the article/Supplementary material.
Ethics statement
The animal study was reviewed and approved by University of Missouri Animal Care and Use Committee.
Author contributions
AV-P participated in the conception and design of the study, sample collection, DNA extraction, data analysis, interpretation, and drafted the manuscript. AE participated in the conception and design of the study, interpreted sequence data, and helped to draft the manuscript. ZM participated in the data analysis and contributed to the drafting of the manuscript. CR participated in the development of the animal model, the conception and design of the study, sample collection, data analysis, and helped to draft the manuscript. HR assisted with DNA extraction, sample collection, and study coordination. All authors contributed to the article and approved the submitted version.
Acknowledgments
The authors wish to acknowledge Andrew Reid for his assistance in clinical data gathering.
Conflict of interest
The authors declare that the research was conducted in the absence of any commercial or financial relationships that could be construed as a potential conflict of interest.
Publisher's note
All claims expressed in this article are solely those of the authors and do not necessarily represent those of their affiliated organizations, or those of the publisher, the editors and the reviewers. Any product that may be evaluated in this article, or claim that may be made by its manufacturer, is not guaranteed or endorsed by the publisher.
Supplementary material
The Supplementary Material for this article can be found online at: https://www.frontiersin.org/articles/10.3389/fvets.2022.930385/full#supplementary-material
References
1. Vientós-Plotts AI, Ericsson AC, McAdams ZL, Rindt H, Reinero CR. Temporal changes of the respiratory microbiota as cats transition from health to experimental acute and chronic allergic asthma. Front Vet Sci. (2022) 9:983375. doi: 10.3389/fvets.2022.983375
2. Kuruvilla ME, Lee FE-H, Lee GB. Understanding asthma phenotypes, endotypes, and mechanisms of disease. Clin Rev Allergy Immunol. (2019) 56:219–33. doi: 10.1007/s12016-018-8712-1
3. Barcik W, Boutin RCT, Sokolowska M, Finlay BB. The role of lung and gut microbiota in the pathology of asthma. Immunity. (2020) 52:241–55. doi: 10.1016/j.immuni.2020.01.007
4. Gollwitzer ES, Marsland BJ. Microbiota abnormalities in inflammatory airway diseases - potential for therapy. Pharmacol Ther. (2014) 141:32–9. doi: 10.1016/j.pharmthera.2013.08.002
5. Huang YJ, Boushey HA. The microbiome in asthma. J Allergy Clin Immunol. (2015) 135:25–30. doi: 10.1016/j.jaci.2014.11.011
6. Marsland BJ, Yadava K, Nicod LP. The airway microbiome and disease. Chest. (2013) 144:632–7. doi: 10.1378/chest.12-2854
7. Chung KF. Potential role of the lung microbiome in shaping asthma phenotypes. Ann Am Thorac Soc. (2017) 14(Suppl_5):S326–31. doi: 10.1513/AnnalsATS.201702-138AW
8. Huang YJ, Nariya S, Harris JM, Lynch SV, Choy DF, Arron JR, et al. The airway microbiome in patients with severe asthma: Associations with disease features and severity. J Allergy Clin Immunol. (2015) 136:874–84. doi: 10.1016/j.jaci.2015.05.044
9. Kozik AJ, Huang YJ. The microbiome in asthma: role in pathogenesis, phenotype, and response to treatment. Ann Allergy Asthma Immunol. (2019) 122:270–5. doi: 10.1016/j.anai.2018.12.005
10. de Steenhuijsen Piters WA, Sanders EA, Bogaert D. The role of the local microbial ecosystem in respiratory health and disease. Philos Trans R Soc Lond B Biol Sci. (2015) 370:20140294. doi: 10.1098/rstb.2014.0294
11. Koppen IJN, Bosch A, Sanders EAM, van Houten MA, Bogaert D. The respiratory microbiota during health and disease: a paediatric perspective. Pneumonia (Nathan). (2015) 6:90–100. doi: 10.15172/pneu.2015.6/656
12. Murdoch DR. How recent advances in molecular tests could impact the diagnosis of pneumonia. Expert Rev Mol Diagn. (2016) 16:533–40. doi: 10.1586/14737159.2016.1156536
13. Lira-Lucio JA, Falfán-Valencia R, Ramírez-Venegas A, Buendía-Roldán I, Rojas-Serrano J, Mejía M, et al. Lung microbiome participation in local immune response regulation in respiratory diseases. Microorganisms. (2020) 8:8071059. doi: 10.3390/microorganisms8071059
14. Shukla SD, Budden KF, Neal R, Hansbro PM. Microbiome effects on immunity, health and disease in the lung. Clin Transl Immunology. (2017) 6:e133. doi: 10.1038/cti.2017.6
15. Carr TF, Alkatib R, Kraft M. Microbiome in mechanisms of asthma. Clin Chest Med. (2019) 40:87–96. doi: 10.1016/j.ccm.2018.10.006
16. Hakansson AP, Orihuela CJ, Bogaert D. Bacterial-Host interactions: physiology and pathophysiology of respiratory infection. Physiol Rev. (2018) 98:781–811. doi: 10.1152/physrev.00040.2016
17. Hilty M, Burke C, Pedro H, Cardenas P, Bush A, Bossley C, et al. Disordered microbial communities in asthmatic airways. PLoS ONE. (2010) 5:e8578. doi: 10.1371/journal.pone.0008578
18. Bullone M, Lavoie JP. The equine asthma model of airway remodeling: from a veterinary to a human perspective. Cell Tissue Res. (2020) 380:223–36. doi: 10.1007/s00441-019-03117-4
19. Bond SL, Timsit E, Workentine M, Alexander T, Léguillette R. Upper and lower respiratory tract microbiota in horses: bacterial communities associated with health and mild asthma (inflammatory airway disease) and effects of dexamethasone. BMC Microbiol. (2017) 17:184. doi: 10.1186/s12866-017-1092-5
20. Alessandrini F, Musiol S, Schneider E, Blanco-Perez F, Albrecht M. Mimicking Antigen-Driven asthma in rodent models-how close can we get? Front Immunol. (2020) 11:575936. doi: 10.3389/fimmu.2020.575936
21. Norris Reinero CR, Decile KC, Berghaus RD, Williams KJ, Leutenegger CM, Walby WF, et al. An experimental model of allergic asthma in cats sensitized to house dust mite or bermuda grass allergen. Int Arch Allergy Immunol. (2004) 135:117–31. doi: 10.1159/000080654
22. Reinero CR, DeClue AE, Rabinowitz P. Asthma in humans and cats: is there a common sensitivity to aeroallegens in shared environments? Environ Res. (2009) 109:634–40. doi: 10.1016/j.envres.2009.02.001
23. Padrid PA, Feldman BF, Funk K, Samitz EM, Reil D, Cross CE. Cytologic, microbiologic, and biochemical analysis of bronchoalveolar lavage fluid obtained from 24 healthy cats. Am J Vet Res. (1991) 52:1300–7.
24. van Eeden ME, Vientós-Plotts AI, Cohn LA, Reinero CR. Serum allergen-specific IgE reactivity: is there an association with clinical severity and airway eosinophilia in asthmatic cats? J Feline Med Surg. (2020) 22:1129–36. doi: 10.1177/1098612X20907178
25. Yu Z, Morrison M. Improved extraction of PCR-quality community DNA from digesta and fecal samples. Biotechniques. (2004) 36:808–12. doi: 10.2144/04365ST04
26. Ericsson AC, Personett AR, Grobman ME, Rindt H, Reinero CR. Composition and predicted metabolic capacity of upper and lower airway microbiota of healthy dogs in relation to the fecal microbiota. PLoS ONE. (2016) 11:e0154646. doi: 10.1371/journal.pone.0154646
27. Vientos-Plotts AI, Ericsson AC, Rindt H, Grobman ME, Graham A, Bishop K, et al. Dynamic changes of the respiratory microbiota and its relationship to fecal and blood microbiota in healthy young cats. PLoS ONE. (2017) 12:e0173818. doi: 10.1371/journal.pone.0173818
28. Bolyen E, Rideout JR, Dillon MR, Bokulich NA, Abnet CC, Al-Ghalith GA, et al. Reproducible, interactive, scalable and extensible microbiome data science using QIIME 2. Nat Biotechnol. (2019) 37:852–7. doi: 10.1038/s41587-019-0209-9
29. Martin M. Cutadapt removes adapter sequences from high-throughput sequencing reads. EMBnetjournal. (2011) 17:10. doi: 10.14806/ej.17.1.200
30. Callahan BJ, Mcmurdie PJ, Rosen MJ, Han AW, Johnson AJA, Holmes SP. DADA2: High-resolution sample inference from Illumina amplicon data. Nat Methods. (2016) 13:581–3. doi: 10.1038/nmeth.3869
31. Pedregosa F, Varoquaux G, Gramfort A, Michel V, Thirion B, Grisel O, et al. Scikit-learn: Machine Learning in Python. J Mach Learn Res. (2011) 12:2825–30.
32. Quast C, Pruesse E, Yilmaz P, Gerken J, Schweer T, Yarza P, et al. The SILVA ribosomal RNA gene database project: improved data processing and web-based tools. Nucleic Acids Res. (2013) 41:D590–6. doi: 10.1093/nar/gks1219
33. Jari Oksanen FGB, Michael Friendly, Roeland Kindt, Pierre Legendre, Dan McGlinn PRM R. B., O'Hara Gavin L., Simpson Peter Solymos M., Henry H. Stevens ESHW. vegan: Community ecology package. 2.5-7 ed2006. p. R package.
34. R Core Team. R: A Language and Environment for Statistical Computing. Vienna, Austria: R Foundation for Statistical Computing (2020).
35. Blighe K RS, Lewis M. EnhancedVolcano: Publication-ready volcano plots with enhanced colouring and labeling. 1.12.0 ed2018.
37. Huang YJ, Charlson ES, Collman RG, Colombini-Hatch S, Martinez FD, Senior RM. The role of the lung microbiome in health and disease. a national heart, lung, and blood institute workshop report. Am J Respir Crit Care Med. (2013) 187:1382–7. doi: 10.1164/rccm.201303-0488WS
38. Dickson RP, Martinez FJ, Huffnagle GB. The role of the microbiome in exacerbations of chronic lung diseases. Lancet (London, England). (2014) 384:691–702. doi: 10.1016/S0140-6736(14)61136-3
39. Fastrès A, Taminiau B, Vangrinsven E, Tutunaru AC, Moyse E, Farnir F, et al. Effect of an antimicrobial drug on lung microbiota in healthy dogs. Heliyon. (2019) 5:e02802. doi: 10.1016/j.heliyon.2019.e02802
40. Denner DR, Sangwan N, Becker JB, Hogarth DK, Oldham J, Castillo J, et al. Corticosteroid therapy and airflow obstruction influence the bronchial microbiome, which is distinct from that of bronchoalveolar lavage in asthmatic airways. J Allergy Clin Immunol. (2016) 137:1398–405 e3. doi: 10.1016/j.jaci.2015.10.017
41. Enaud R, Prevel R, Ciarlo E, Beaufils F, Wieërs G, Guery B, et al. The gut-lung axis in health and respiratory diseases: a place for inter-organ and inter-kingdom crosstalks. Front Cell Infect Microbiol. (2020) 10:9. doi: 10.3389/fcimb.2020.00009
42. Raftery AL, Tsantikos E, Harris NL, Hibbs ML. Links between inflammatory bowel disease and chronic obstructive pulmonary disease. Front Immunol. (2020) 11:2144. doi: 10.3389/fimmu.2020.02144
43. Huang YJ. The respiratory microbiome and innate immunity in asthma. Curr Opin Pulm Med. (2015) 21:27–32. doi: 10.1097/MCP.0000000000000124
44. Turek EM, Cox MJ, Hunter M, Hui J, James P, Willis-Owen SAG, et al. Airway microbial communities, smoking and asthma in a general population sample. EBioMedicine. (2021) 71:103538. doi: 10.1016/j.ebiom.2021.103538
45. Huang YJ, Nelson CE, Brodie EL, Desantis TZ, Baek MS, Liu J, et al. Airway microbiota and bronchial hyperresponsiveness in patients with suboptimally controlled asthma. J Allergy Clin Immunol. (2011) 127:372–81 e1-3. doi: 10.1016/j.jaci.2010.10.048
46. Russell SL, Gold MJ, Hartmann M, Willing BP, Thorson L, Wlodarska M, et al. Early life antibiotic-driven changes in microbiota enhance susceptibility to allergic asthma. EMBO Rep. (2012) 13:440–7. doi: 10.1038/embor.2012.32
47. Vientos-Plotts AI, Ericsson AC, Rindt H, Reinero CR. Oral probiotics alter healthy feline respiratory microbiota. Front Microbiol. (2017) 8:1287. doi: 10.3389/fmicb.2017.01287
48. Reed N, Simpson K, Ayling R, Nicholas R, Gunn-Moore D. Mycoplasma species in cats with lower airway disease: improved detection and species identification using a polymerase chain reaction assay. J Feline Med Surg. (2012) 14:833–40. doi: 10.1177/1098612X12451796
49. Schulz BS, Richter P, Weber K, Mueller RS, Wess G, Zenker I, et al. Detection of feline Mycoplasma species in cats with feline asthma and chronic bronchitis. J Feline Med Surg. (2014) 16:943–9. doi: 10.1177/1098612X14524969
50. Agache I, Akdis CA. Endotypes of allergic diseases and asthma: an important step in building blocks for the future of precision medicine. Allergol Int. (2016) 65:243–52. doi: 10.1016/j.alit.2016.04.011
51. Trzil JE, Masseau I, Webb TL, Chang CH, Dodam JR, Liu H, et al. Intravenous adipose-derived mesenchymal stem cell therapy for the treatment of feline asthma: a pilot study. J Feline Med Surg. (2016) 18:981–90. doi: 10.1177/1098612X15604351
52. Zou XL, Wu JJ, Ye HX, Feng DY, Meng P, Yang HL, et al. Associations between gut microbiota and asthma endotypes: a cross-sectional study in south china based on patients with newly diagnosed asthma. J Asthma Allergy. (2021) 14:981–92. doi: 10.2147/JAA.S320088
53. Shukla SD, Shastri MD, Chong WC, Dua K, Budden KF, Mahmood MQ, et al. Microbiome-focused asthma management strategies. Curr Opin Pharmacol. (2019) 46:143–9. doi: 10.1016/j.coph.2019.06.003
54. Raedler D, Schaub B. Immune mechanisms and development of childhood asthma. Lancet Respiratory Med. (2014) 2:647–56. doi: 10.1016/S2213-2600(14)70129-8
55. Abdel-Aziz MI, Brinkman P, Vijverberg SJH, Neerincx AH, Riley JH, Bates S, et al. Sputum microbiome profiles identify severe asthma phenotypes of relative stability at 12 to 18 months. J Allergy Clin Immunol. (2021) 147:123–34. doi: 10.1016/j.jaci.2020.04.018
56. Zhang Q, Cox M, Liang Z, Brinkmann F, Cardenas PA, Duff R, et al. Airway microbiota in severe asthma and relationship to asthma severity and phenotypes. PLoS ONE. (2016) 11:e0152724. doi: 10.1371/journal.pone.0152724
57. Wang J, Chai J, Zhang L, Zhang L, Yan W, Sun L, et al. Microbiota associations with inflammatory pathways in asthma. Clin Exp Allergy. (2021) 52:697–705. doi: 10.1111/cea.14089
58. Man WH, de Steenhuijsen Piters WA, Bogaert D. The microbiota of the respiratory tract: gatekeeper to respiratory health. Nat Rev Microbiol. (2017) 15:259–70. doi: 10.1038/nrmicro.2017.14
59. Ganaway JR, Spencer TH, Moore TD, Allen AM. Isolation, propagation, and characterization of a newly recognized pathogen, cilia-associated respiratory bacillus of rats, an etiological agent of chronic respiratory disease. Infect Immun. (1985) 47:472–9. doi: 10.1128/iai.47.2.472-479.1985
60. Goto K, Nozu R, Takakura A, Matsushita S, Itoh T. Detection of cilia-associated respiratory bacillus in experimentally and naturally infected mice and rats by the polymerase chain reaction. Exp Anim. (1995) 44:333–6. doi: 10.1538/expanim.44.333
61. Ike F, Sakamoto M, Ohkuma M, Kajita A, Matsushita S, Kokubo T. Filobacterium rodentium gen. nov, sp nov, a member of Filobacteriaceae fam nov within the phylum Bacteroidetes; includes a microaerobic filamentous bacterium isolated from specimens from diseased rodent respiratory tracts. Int J Syst Evol Microbiol. (2016) 66:150–7. doi: 10.1099/ijsem.0.000685
62. Caniatti M, Crippa L, Giusti M, Mattiello S, Grilli G, Orsenigo R, et al. Cilia-associated respiratory (CAR) bacillus infection in conventionally reared rabbits. Zentralbl Veterinarmed B. (1998) 45:363–71. doi: 10.1111/j.1439-0450.1998.tb00805.x
63. Oros J, Fernandez A, Rodriguez JL, Franklin CL, Matsushita S, Poveda JB. Association of cilia-associated respiratory (CAR) bacillus with natural chronic tracheitis in goats. J Comp Pathol. (1997) 117:289–94. doi: 10.1016/S0021-9975(97)80025-4
64. Nietfeld JC, Franklin CL, Riley LK, Zeman DH, Groff BT. Colonization of the tracheal epithelium of pigs by filamentous bacteria resembling cilia-associated respiratory bacillus. J Vet Diagn Invest. (1995) 7:338–42. doi: 10.1177/104063879500700307
65. Ramos-Vara JA, Franklin C, Miller MA. Bronchitis and bronchiolitis in a cat with cilia-associated respiratory bacillus-like organisms. Vet Pathol. (2002) 39:501–4. doi: 10.1354/vp.39-4-501
66. Randolph JF, Moise NS, Scarlett JM, Shin SJ, Blue JT, Corbett JR. Prevalence of mycoplasmal and ureaplasmal recovery from tracheobronchial lavages and of mycoplasmal recovery from pharyngeal swab specimens in cats with or without pulmonary disease. Am J Vet Res. (1993) 54:897–900.
67. Low HC, Powell CC, Veir JK, Hawley JR, Lappin MR. Prevalence of feline herpesvirus 1, Chlamydophila felis, and Mycoplasma spp DNA in conjunctival cells collected from cats with and without conjunctivitis. Am J Vet Res. (2007) 68:643–8. doi: 10.2460/ajvr.68.6.643
68. Chandler JC, Lappin MR. Mycoplasmal respiratory infections in small animals: 17 cases (1988-1999). J Am Anim Hosp Assoc. (2002) 38:111–9. doi: 10.5326/0380111
69. Lee-Fowler T. Feline respiratory disease: what is the role of Mycoplasma species? J Feline Med Surg. (2014) 16:563–71. doi: 10.1177/1098612X14539087
70. MacDowell AL, Bacharier LB. Infectious triggers of asthma. Immunol Allergy Clin North Am. (2005) 25:45–66. doi: 10.1016/j.iac.2004.09.011
71. Lieberman D, Lieberman D, Printz S, Ben-Yaakov M, Lazarovich Z, Ohana B, et al. Atypical pathogen infection in adults with acute exacerbation of bronchial asthma. Am J Respir Crit Care Med. (2003) 167:406–10. doi: 10.1164/rccm.200209-996OC
72. Kraft M, Cassell GH, Pak J, Martin RJ. Mycoplasma pneumoniae and Chlamydia pneumoniae in asthma: effect of clarithromycin. Chest. (2002) 121:1782–8. doi: 10.1378/chest.121.6.1782
Keywords: respiratory microbiota, inflammatory airway disease, large animal model, 16S rRNA gene, translational research
Citation: Vientós-Plotts AI, Ericsson AC, McAdams ZL, Rindt H and Reinero CR (2022) Respiratory dysbiosis in cats with spontaneous allergic asthma. Front. Vet. Sci. 9:930385. doi: 10.3389/fvets.2022.930385
Received: 07 May 2022; Accepted: 04 July 2022;
Published: 08 September 2022.
Edited by:
Valentín Pérez, Universidad de León, SpainReviewed by:
Vanessa L. Hale, The Ohio State University, United StatesMuhammad Ishfaq, Huanggang Normal University, China
Copyright © 2022 Vientós-Plotts, Ericsson, McAdams, Rindt and Reinero. This is an open-access article distributed under the terms of the Creative Commons Attribution License (CC BY). The use, distribution or reproduction in other forums is permitted, provided the original author(s) and the copyright owner(s) are credited and that the original publication in this journal is cited, in accordance with accepted academic practice. No use, distribution or reproduction is permitted which does not comply with these terms.
*Correspondence: Carol R. Reinero, cmVpbmVyb2NAbWlzc291cmkuZWR1