- 1College of Animal Science and Technology, Henan University of Science and Technology, Luoyang, Henan, China
- 2Anqiu County Animal Husbandry Development Center, Weifang, Shandong, China
- 3The Kay Laboratory of High Quality Livestock and Poultry Germplasm Resources and Genetic Breeding of Luoyang, Luoyang, Henan, China
This study aimed to provide evidence for the effects of isobutyric acid on the intestinal mucosal barrier and gut microbiota in weaned piglets. In this study, 30 piglets were divided into two groups: one group was fed a standard diet (CON group), and the other group was fed a diet supplemented with 0.5% isobutyric acid (IB group) for 21 days. The results showed that isobutyric acid significantly increased (p < 0.05) serum immunity and antioxidant capacity in weaned piglets. In small intestine of piglets, the ratio of villus height to crypt depth was significantly increased (p < 0.05). Administration of isobutyric acid also increased (p < 0.05) the expression of genes related to intestinal mucosal barrier function. Cecal microbiota analysis revealed that isobutyric acid significantly increased (p < 0.05) the abundance of the Eubacterium coprostanoligenes group. Untargeted serum metabolomics analysis indicated that the top three categories of metabolites were lipids and lipid-like molecules, organic acids and derivatives, and organic heterocyclic compounds. Additionally, in longissimus thoracis muscle, isobutyric acid significantly increased (p < 0 0.05) intramuscular fat and triglyceride content compared with the CON group. Overall, isobutyric acid can improve small intestinal mucosal barrier function, and may influence the fat deposition through the regulation of serum metabolites in weaned piglets.
1 Introduction
Weaning is a challenge for piglets, which are prone to intestinal stress due to their underdeveloped digestive system and changes in diet and environment (1). Damage to the intestinal barrier can affect paracellular permeability, disrupt tight junctions, and increase the risk of bacterial translocation and intestinal infection, subsequently leading to intestinal stress (2). During the weaning phase, the proteins in solid feeds neutralize the piglets’ gastric acids, leading to an increase in the pH of the piglets’ digestive tract and a decrease in the activity of digestive enzymes (3). During this period, piglets take less food, which subsequently leads to less nutrients being digested and absorbed and an increased risk of diarrhea (4, 5). Over the past few decades, the farming industry has addressed this problem by adding antibiotics to feed rations (6). With the banning of antibiotics in the livestock industry, a number of feed additives with beneficial effects were gradually used in the pig industry.
Meats are rich in proteins, amino acids, vitamins and trace elements needed by the human body to provide energy. Pork is the most widely consumed meat globally, and holds a significant place in human dietary culture (7). In recent years, there has been a growing demand for pork quality (8). In contrast, high-quality meats are more favored by consumers. The muscle with higher intramuscular fat content often exhibits lower shear force and higher scoring marble pattern, representing higher meat quality. Intramuscular fat is defined as the fat located between muscle fiber bundles. Physiologically, intramuscular fat is considered to have similar functions as other fat depots in the body, such as energy storage (9). As a food, intramuscular fat greatly enhances the texture and flavor of pork, satisfying consumer demands while also attracting more purchases. The intramuscular fat content is a key indicator for evaluating the quality of pork. The urgent problem that needs to be solved in the pig farming industry is how to increase the intramuscular fat content of pork and improve its quality.
Gut microbiota is an important environmental factor that affects energy harvest from the diet and energy storage in the host. Studies of GF and conventionalized mice revealed that the microbiota promotes absorption of monosaccharides from the gut lumen, with resulting induction of de novo hepatic lipogenesis (10). Metagenomic sequencing revealed that high-fat pigs had a higher abundance of Archaeal species with methanogenesis functions, leading to more-efficient fat deposition, while low-fat pigs had higher abundances of butyrate-producing bacteria species that improved the formation of SCFAs, especially butyrate, thus alleviating fat deposition in pigs (11). Increased oxidative stress associated with fat consumption may alter the bacterial composition and the expression of lipogenic genes. GSH-Px levels were significantly lower in high-fat pigs, which are more susceptible to oxidative stress than low-fat pigs, and hence more susceptible to obesity (12).
Short-chain fatty acids (SCFA) are important metabolic regulators as energy-providing substrates. The composition of dietary fatty acids affects the digestion, absorption and metabolism of nutrients (13). Branched chain fatty acids (BCFA) are primarily saturated fatty acids with one or more methyl branches on the carbon chain, which are components of common food fats (14). BCFA reduced the incidence of necrotizing enterocolitis and increased the intestinal anti-inflammatory cytokine IL-10, and altered gastrointestinal microbial ecology in a neonatal rat model (15). Isobutyric acid is one of the microbial breakdown products of indigestible carbohydrates (16). Exogenous supplementation with butyric acid or butyrate improves intestinal barrier function and promotes intestinal health in weaned piglets (17). Addition of fatty acid blend products to maternal rations improves offspring performance (18). Several studies have shown that short-chain fatty acid levels increase in obese individuals and decrease after weight loss (19). This suggests that increased levels of short-chain fatty acids increase the amount of energy obtained from the diet.
Straight-chain fatty acid modulate the composition of gut microbiota, and are thought to affect the intestinal health; however, very little is known about BCFA influence on intestinal mucosal barrier function and intramuscular fat deposition in weaned piglets. Although isobutyric acid has been reported as a dietary additive for ruminants (20, 21), its application in weaned piglets remains limited, and its effects on the intestinal mucosal barrier and gut microbiota of weaned piglets are not yet well understood. Therefore, this study aims to investigate the impact of dietary supplementation with isobutyric acid on the intestinal mucosal barrier function of weaned piglets.
2 Materials and methods
2.1 Ethics approval
The animal study was approved by the Institutional Animal Ethics Committee of Henan University of Science and Technology (approval 2022-02-023).
2.2 Animals and experimental design
All procedures involving the use of animals were performed in accordance with the animal protection and welfare guidelines established by the Chinese Ministry of Agriculture. The rearing trial was conducted at Henan University of Science and Technology experimental animal farm in Luoyang city, Henan province. In this study, 30 weaned pigs (Large White pigs) with an average body weight of 10 ± 2 kg were reared at the unified farm of Henan University of Science and Technology in Luoyang City, Henan Province, and fed with suckling pig compound feed twice a day, with free drinking water. After 10 days of acclimatization to the environment, the pigs were divided into two treatment groups: a control group (CON group, fed with basal diet) and an isobutyric acid group (IB group, fed a diet with 0.5% isobutyric acid added to the basal diet). Supplementary Table S2 shows the composition and nutrients of the basal diet performed by National Research Council nutrient requirements (61). Isobutyric acid (Macklin I914990, Shanghai, China) was purchased from the Shanghai Macklin Biochemical Technology Company. The trial period was 21 days. At the end of the experiment, three pigs were randomly selected from each group. The pigs were fasted for 24 h and euthanized with an anesthetic injection given into the ear vein with an overdose of Alfaxan (0.7 mg/kg), and blood samples were collected. After slaughter, approximately 200 g of longissimus thoracis (LT) and backfat tissue at the fifth to sixth rib was promptly collected, immediately preserved in liquid nitrogen, and then stored in a refrigerator set at −80°C. Each intestinal segments (duodenum, jejunum, ileum, and cecum) were also isolated and collected in a refrigerator at −80°C.
2.3 Analysis of serum biochemistry, immunoglobulin, and antioxidative index
The activity of total antioxidant capacity (T-AOC) and glutathione S-transferase (GST), and superoxide dismutase (SOD) and malondialdehyde (MDA) concentration in serum was measured using biochemical methods following manufacturer’s instructions provided for each reagent kit (Beijing Solarbio Science & Technology Co., Ltd., Beijing, China). Briefly, the content of MDA was determined by the thiobarbituric acid reaction. The activity of T-AOC, GST, and SOD were assayed using the Fe3+-Tripyridyltriazine, the dithiodinitrobenzoic acid colorimetric, and xanthine oxidase, respectively. The concentrations of leptin (LEP), cholecystokinin (CCK), growth hormone (GH), glucocorticoid receptor (GR), Ghrelin, adiponectin (ADP), and insulin (INS) in serum were determined by the commercial ELISA kit (Wuhan ColorfulGene Biological Technology Co., LTD., Wuhan, China). And the concentrations of immunoglobulin A (Ig A), immunoglobulin G (Ig G), and immunoglobulin M (Ig M) in serum were determined by the commercial ELISA kit (Wuhan ColorfulGene Biological Technology Co., LTD.). All procedures were performed according to the manufacturer’s instructions.
2.4 Histological analysis
For morphology, the abdomen was opened after slaughter, and the duodenum, jejunum, and ileum were removed and rinsed with phosphate buffer solution (PBS), and the intestinal segments were preserved in 4% paraformaldehyde solution before embedding, and consecutive segments of 5 μm thickness were counterstained with hematoxylin and eosin (H&E) (22).
Morphological changes were observed under a DM300 light microscope from Leica (Germany). For analysis, 10 crypts were randomly selected from different parts of the section, and their crypt depths and corresponding villus heights, the number of goblet cells were measured and their ratios were calculated using ImageJ v1.8.0 software.
2.5 Determination of meat quality
After slaughter, backfat thickness, marbling score, pH, meat color, IMF content, and the triglyceride (TG) content of muscle and backfat were measured. The average backfat thickness of the first rib, last lumbar spine, and last rib were used to define the backfat thickness. The marbling score was determined from a cross-sectional slice of the muscle at the first lumbar spine and ranged from 0 to 5, with higher scores indicating more abundant IMF content. The color of LT was determined using OPTO-STAR (Germany, Denmark), and the meat color parameters included L* (lightness), a* (intensity), and b* (yellowness).
The pH value of LT was determined 40–50 min after slaughter (HI99163, HANNA, Italy). The IMF content was measured using a Soxhlete extractor (SX-360, OPSIS, Sweden). Triglyceride content was determined using a triglyceride kit (Beijing Solarbio Science & Technology Co., Ltd., Beijing, China), following manufacturer’s instructions.
2.6 Analysis of the microbial composition and diversity of the cecal contents
Bacterial DNA from cecal contents was extracted using Fecal DNA Extraction Kit Solarbio D2700-50 (Solarbio Bio-Tek, Beijing, China) according to manufacturer instructions. Extracted DNA was assessed using 1% agarose gel electrophoresis and Nanodrop-2000 (Thermo Scientific). The barcode primers were 341 F(5’-CCTACGGGNGGCWGCAG-3′), and 805 5′-R(5GACTACHVGGGTATCTAATCC-3′). These primers were used to amplify the V3–V4 hypervariable region of the 16S rRNA. The PCR products were quantified using the QuantiFluor™ -ST Blue fluorescent Quantitative System (Promega), followed by Illumina PE250 library construction and Illumina PE250 sequencing. The PE reads obtained by Illumina PE250 sequencing were first assembled according to the overlap relationship, and sequence quality was controlled and filtered simultaneously. After distinguishing the samples, OTU cluster and taxonomic analyses were performed. The sequencing depth was determined based on taxonomic information, and statistical analyses of the community structure were performed at each taxonomic level (23). The sequence data can be found in National Center for Biotechnology Information (accession number PRJNA1246593). The correlation analysis was conducted between gut microbiota and intestinal morphology, IMF, TG.
2.7 Untargeted metabolomics assays
Relevant experimental methods and procedures for metabolomics performed upon serum can be found in previous studies (24, 25). Briefly, 200 mg serum samples were mixed with 2-chlorophenylalanine (4 ppm) methanol (−20°C) and ground by a high-throughput tissue grinder for 90 s at 60 Hz. The samples were centrifuged at 4°C for 10 min at 12,000 rpm, and the supernatant was filtered through 0.22 μm membrane to obtain the prepared samples for LC–MS. Twenty microlitre from each sample were taken to the quality control samples, and the rest of the samples were used for LC–MS detection.
The pre-processed data were converted to the Statistics Analysis files and then processed using SIMCA software (V16.0.2, Sartorius Stedim Data Analytics AB, Umea, Sweden). Orthogonal projections to latent structures- discriminant analysis (OPLS-DA) was conducted as a supervised method to identify the significant variables with discriminatory capability, and validity of the OPLS-DA model was corroborated by a permutation test with a 200-time repetition. The variable importance in the projection (VIP) value of each variable from the OPLS-DA model was computed to demonstrate its contribution to the classification. Metabolites with the VIP value>1 were further analyzed in Student’s t-test at univariate level to evaluate the significance of each metabolite. Metabolites with p value<0.05 and fold change >1.5 were regarded to have statistical significance. To assess the differentially expressed metabolites, the volcano plot was made for the two groups. What’s more, Pearson correlation coefficient was calculated to analyze the correlation between all metabolites and the results were shown in the correlation heatmap. Furthermore, the Kyoto Encyclopedia of Genes and Genomes (KEGG) annotation analysis as well as the pathway analysis (including enrichment analysis and network analysis) was conducted for identification of the differentially expressed metabolites. The sequence data can be found in China National Center for Bioinformation (accession number PRJCA038200). The correlation analysis was conducted serum metabolism and IMF, TG.
2.8 Total RNA extraction and qPCR
TRIzol reagent (Servicebio, China) was used to extract total RNA from intestine and LT, and a spectrophotometer (NanoDrop 2000, Thermo Fisher) was used to determine the absorbance of RNA at 260 nm and 280 nm to ensure the purity and concentration of RNA. The RNA was then reverse-transcribed using the SweScript RT I First Strand cDNA Synthesis Kit (Servicebio, China) and random primers. QPCR was performed using a Bio-Rad CFX Connect PCR fluorescence quantitative PCR instrument with SYBR Green qPCR Master Mix and gene-specific primers (Supplementary Table S1), and relative expression was calculated using the 2−ΔΔCt method (26).
2.9 Western blot analysis
The protein expression levels of longissimus thoracis FASN, ACC, LPL, HSL, FABP4, β-actin were determined. The total proteins were isolated using the RIPA buffer with PMSF and a phosphatase inhibitor. The protein concentration was quantified using the bicinchoninic acid (BCA) protein assay kit (Servicebio, China). Protein separation was conducted by 8% (12%) sodium dodecyl sulfate-polyacrylamidegel electrophoresis (SDS-PAGE). The separated proteins were transferred to the polyvinylidene fluoride (PVDF) membrane by a wet tank transfer blotting system (Bio-Rad Laboratories). The PVDF membrane containing proteins was cut with a blade according to the location of the target protein and blocked using 5% skimmed milk for 30 min at room temperature. Then, the membrane was incubated with specific primary antibodies overnight at 4°C, followed by secondary antibodies for 2 h at room temperature. The blots were quantified using the Gel imaging system software (Image Lab 5.2, Bio-Rad).
2.10 Statistical analysis
Statistical analyses were performed using SPSS 22.0. GraphPad software (9.0), and Origin 2022 were used for correlation analysis and data visualization, and p values less than 0.05 were considered significant. Growth performance was analyzed by one-way ANOVA, and other experimental results were compared by t-test. Data are represented in tables and graphs as mean and standard deviation (SD) (n = 3).
3 Results
3.1 Effects of isobutyric acid supplementation on serum physiochemical parameters, immunoglobulin level, and antioxidative index in weaned piglets
The effects of dietary isobutyric acid on serum physiochemical parameters in weaned piglets are present in Table 1. Isobutyric acid administration caused a decrease in serum ADP (p < 0.01). Compared to the CON group, a diet with 0.5% isobutyric acid supplementation increased serum immunoglobulin A (Ig A) (p < 0.05) and immunoglobulin G (Ig G) (p < 0.05) levels (Figure 1).
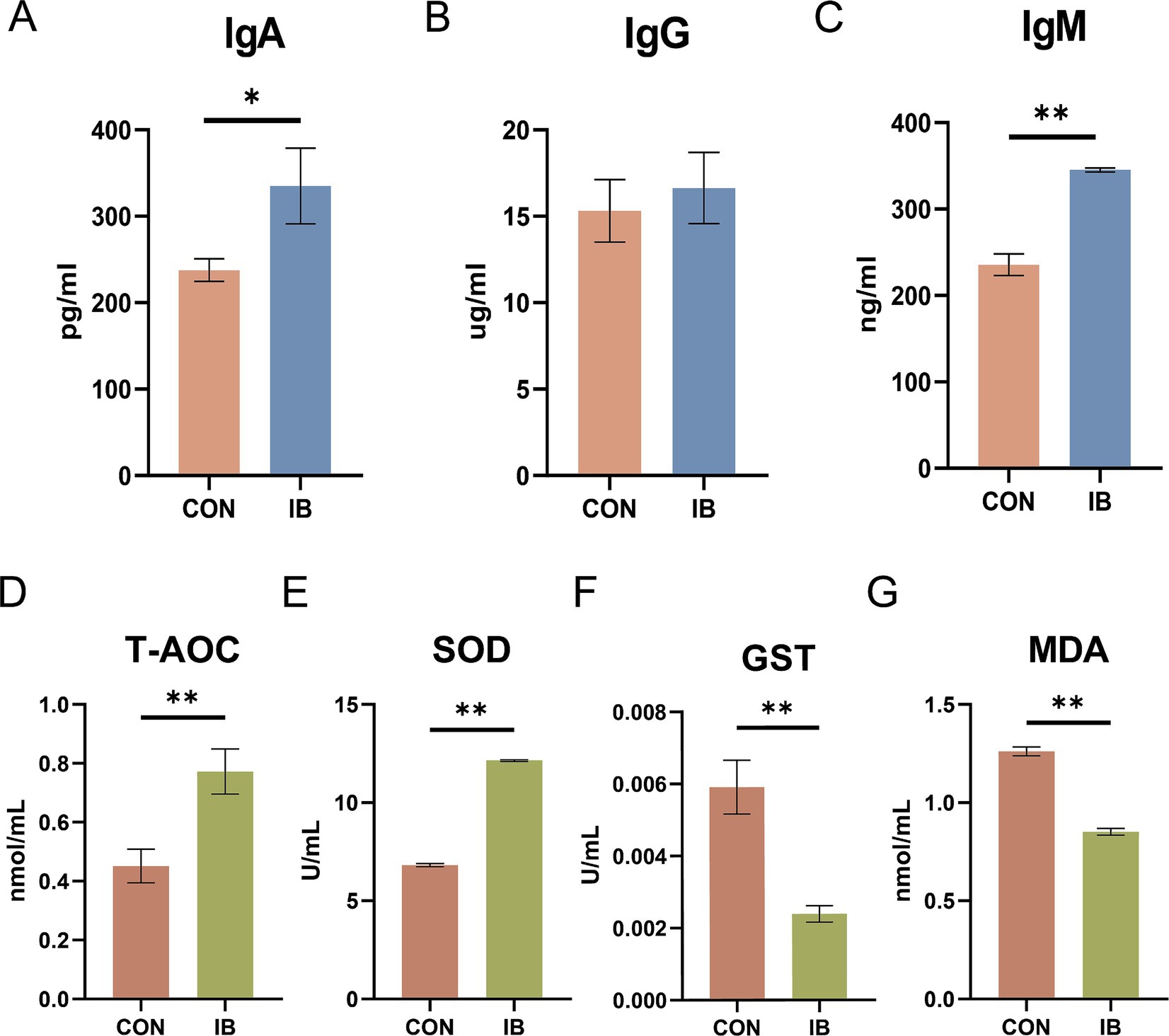
Figure 1. Effect of isobutyric acid on immunoglobulin level and antioxidant capacity of weaned piglets. (A) Immunoglobulin A (Ig A); (B) Immunoglobulin G (Ig G); (C) Immunoglobulin M (Ig M); (D) Total antioxidant capacity (T-AOC); (E) Superoxide dismutase (SOD); (F) Glutathione S-transferase (GST); (G) Malondialdehyde (MDA).* p < 0.05 and ** p < 0.01.
As presented in Figure 1, piglets fed diets containing 0.5% isobutyric acid had higher total antioxidant capacity (T-AOC) (p < 0.05) level. Moreover, isobutyric acid treatments exhibited a decrease in serum glutathione S-transferase (GST) activity (p < 0.05) but an increase in serum superoxide dismutase (SOD) activity (p < 0.01). We also investigated the serum malondialdehyde (MDA) level. As expected, a diet supplemented with 0.5% isobutyric acid significantly reduced the serum MDA concentration (p < 0.01). Our findings indicated that isobutyric acid could enhance immunity and antioxidant capacity in weaned piglets.
3.2 Effect of isobutyric acid on small intestinal mucosal barrier function in weaned piglets
Representative images of HE staining are shown as the picture (Figure 2A). We found that isobutyric acid increased villus height (VH) and decreased crypt depth (CD) in duodenum (Figures 2B,C). Compared with CON group, we observed isobutyric acid significantly reduced CD (p < 0.05) in jejunum and ileum (Figure 2C). Interestingly, the HE staining only showed a significantly increasing of VH (p < 0.05) in ileum (Figure 2B). In addition, we calculated the ratio of villus height to crypt depth (VCR) in each part of the small intestine. The results showed that the VCR was significantly increased in all parts of the small intestine (p < 0.05, p < 0.01) compared to the CON group (Figure 2D). We also calculated the number of goblet cells (GCs). And we found the GCs in each intestinal segment was significantly higher than CON group (p < 0.05) (Figure 2E).
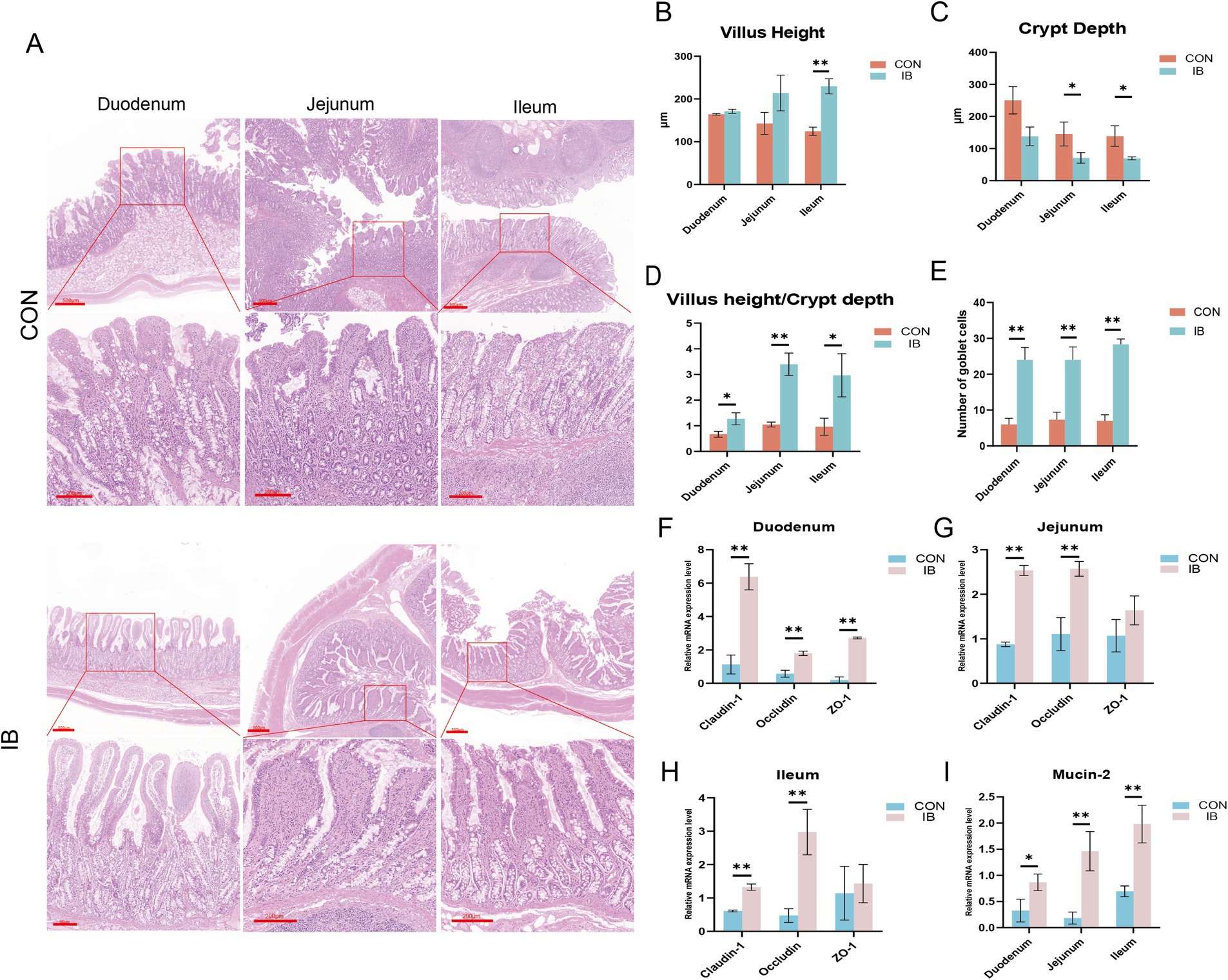
Figure 2. Effect of isobutyric acid on small intestinal mucosal barrier function. (A) HE staining shows the morphology of various segments of the intestine; (B) Villus height of the duodenum, jejunum and ileum; (C) Crypt depth of the duodenum, jejunum and ileum; (D) Ratio of villus height to crypt depth of the duodenum, jejunum and ileum; (E) Number of goblet cells of the duodenum, jejunum and ileum; (F) The relative mRNA expression levels of Claudin-1, Occludin and ZO-1 in duodenum between the CON and IB groups; (G) Jejunum; (H) Ileum; (I) The relative mRNA expression levels of MUC2 in duodenum, jejunum and ileum. * p < 0.05 and ** p < 0.01.
The significant differences in small intestinal morphology between the two groups made us wanna explore the effects of isobutyric acid on the mucosal barrier of the small intestine. To demonstrate whether dietary supplementation with isobutyric acid positively affects the small intestinal barrier, we measured the genes related to tight junction protein, including Claudin-1, Occludin and Zonula occludens-1 (ZO-1). Compared with the CON group, the expression of Claudin-1, Occludin and ZO-1 were significantly upregulated (p < 0.01) in duodenum in the IB group (Figure 2F). The results also showed that the expression of Claudin-1 and Occludin are significantly upregulated (p < 0.01) in jejunum (Figure 2G), as well as in ileum (Figure 2H). Meanwhile, we further measured the expression of mucin-2 (MUC2). MUC2 is the major glycoprotein in intestinal mucus. The expression of MUC2 showed significantly upregulated (p < 0.05) in all small intestinal segments (Figure 2I). These findings indicated that dietary isobutyric acid improves small intestinal mucosal barrier function, and promotes intestinal health in piglets.
3.3 Effect of isobutyric acid on meat quality and lipid traits in LT of weaned piglets
Compared with the CON group, we confirmed the better meat quality in IB group, as evidenced by the higher marbling score indice (p < 0.05). In addition, isobutyric acid significantly increased intramuscular fat (IMF) (p < 0.05) and triglyceride (TG) content (p < 0.05) in longissimus thoracis (LT) muscle (Table 2). And growth performance are shown in Supplementary Table S3.
Having demonstrated the elevation of lipid traits in LT on weaned piglets, we next sought to examine the expression of genes related to lipid metabolism. As shown in Figure 3, the expression of fatty acid synthase (FASN) and acetyl-CoA carboxylase (ACC) were significantly upregulated (p < 0.05), whereas the expression of peroxisome proliferator-activated receptor α (PPARα) and hormone-sensitive lipase (HSL) were significantly downregulated (p < 0.05). And the expression of sterol regulatory element binding protein 1c (SREBP-1c) (p < 0.01) and diacylglycerol-O-acyltransferase 1 (DGAT1) (p < 0.05) were significantly upregulated, whereas the expression level of fatty acid transport protein 1 (FATP1), fatty acid-binding protein 4 (FABP4) and lipoprotein lipase (LPL) were significantly downregulated (p < 0.05).
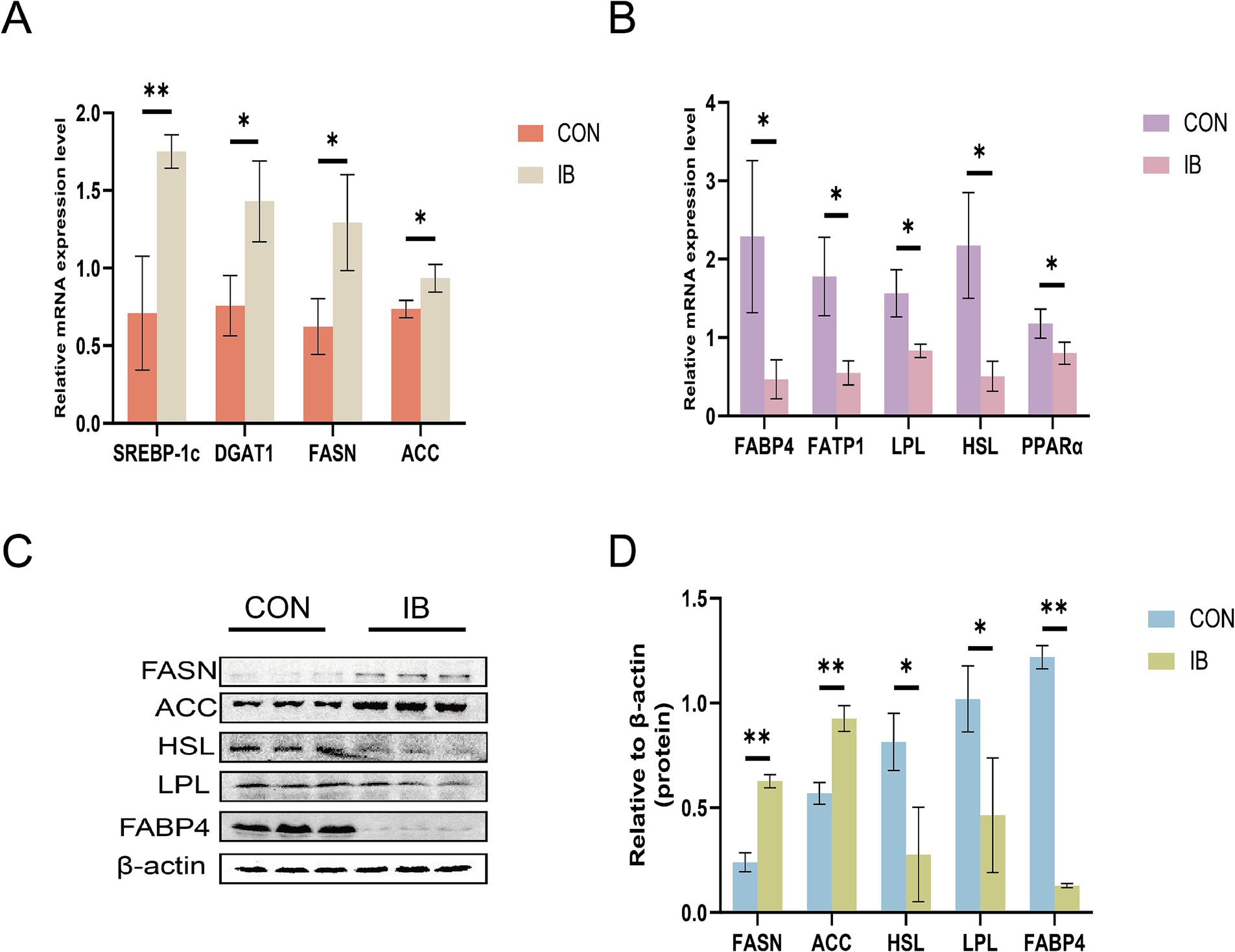
Figure 3. Effect of isobutyric acid on the expression of genes and proteins involved in lipid metabolism. (A) Effect of isobutyric acid on the relative mRNA expression of lipid synthesis; (B) Effect of isobutyric acid on the relative mRNA expression of lipid breakdown and transport; (C) Western blot showing the protein level; (D) Bar plots showing the densitometric values. * p < 0.05 and ** p < 0.01.
3.4 Effect of isobutyric acid on gut microbiota in weaned pigs
We detected and screened 659,837 sequences. OTU analysis of non-repetitive sequences with 97% similarity obtained 817 OTUs, and the rarefaction curve tended to be flat (Supplementary Figures S1A,B). The result indicated that the amount of sequencing for each sample in this study is sufficient. The results of Alpha diversity analysis showed no significant difference in the IB group compared with the CON group, including the Ace index, Chao1 index, Shannon index and Simpson index (Supplementary Figure S1C). Principal component analysis (PCA) revealed clear separation between the microbial communities between the CON and IB groups (Figure 4A). Venn diagrams displayed that 176 genera and 284 species overlapped between the CON and IB groups (Figures 4B,C).
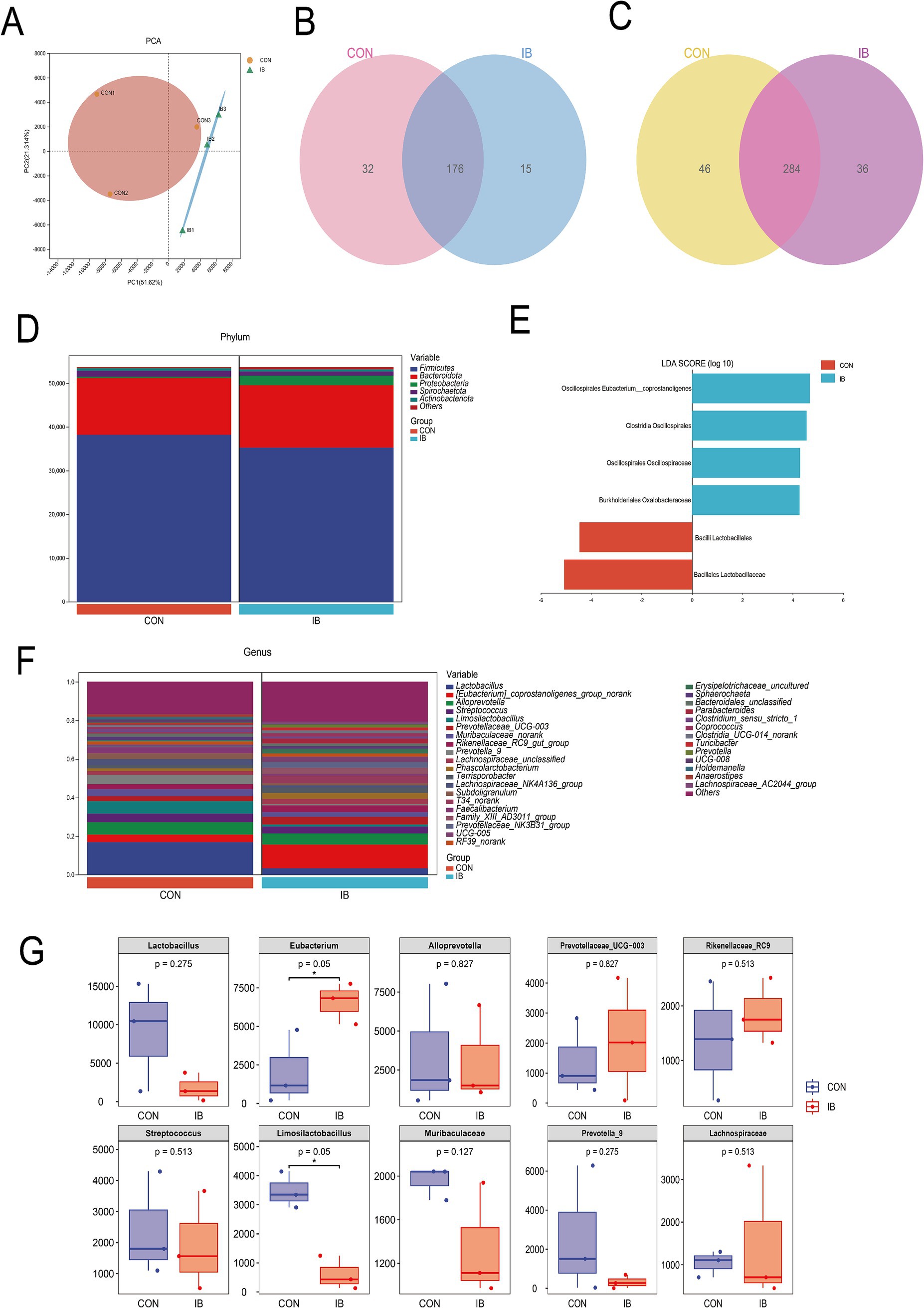
Figure 4. Diversity analysis between CON and IB group. (A) Principal component analysis (PCA); (B) Venn at the genus level; (C) Venn at the species level; (D) The proportion of bacteria at the phylum level; (E) Linear discriminant analysis Effect Size (LEfSe); (F) The proportion of the top 20 bacteria at the genus level; (G) The top 10 bacterial abundance at the genus level in boxplot.
Gut microbiota at the phylum level consisted mostly of Firmicutes, Bacteroidota, Proteobacteria, Spirochaetota and Actinobacteriota. Firmicutes and Bacteroidota represented more than 90% relative abundance (Figure 4D). To further understand the detailed variation in gut microbiota, the relative abundance changes at the genus level were analyzed (Figure 4F). Feeding a diet with isobutyric acid supplementation significantly increased the relative abundance of [Eubacterium]_coprostanoligenes (p < 0.05) (Figure 4G). [Eubacterium]_coprostanoligenes belongs to Eubacteriaceae, Firmicutes. Increased levels of Eubacterium may in favor of maintenance of intestinal homeostasis. Limosilactobacillus was significantly decreased (p < 0.05) in the IB group. These findings showed that isobutyric acid has a regulatory role in balancing probiotics and pathogenic bacteria in gut microbiota. Furthermore, Linear discriminant analysis Effect Size (LEfSe) was used to determine bacteria with significant differences between the CON and IB groups (Figure 4E). Firmicutes phylum occupied a dominant position in both groups, including three species from the order Oscillospirales and two species from the order Lactobacillales. In general, these changes induced by isobutyric acid help promote intestinal health.
3.5 Correlation analysis between gut microbiota and intestinal morphology, IMF, TG
To further investigate the effect of cecal microbial composition on gut morphology, we correlated all microorganisms detected with gut morphology indicators. The results showed that Solobacterium, UCG-002, UCG-005 were significantly and positively correlated with the VCR (p < 0.05) of small intestinal segments, as well as Lactobacillus_mucosae_LM1, Lactobacillus_salivarius, Lactobacillus_amylovorus, Lactobacillus_reuteri were significantly positively correlated with CD (p < 0.05) (Figures 5A–C). We further explored the relationship between the cecal microbial composition and lipid traits. We found that Solobacterium showed a significant positive correlation with IMF, whereas [Eubacterium]_coprostanoligenes_group, T34, Family_XIII_AD3011_group, Prevotellaceae_NK3B31_group. Prevotella, UCG-005, Parabacteroides showed significant positive correlation with muscle TG content (Figure 5D).
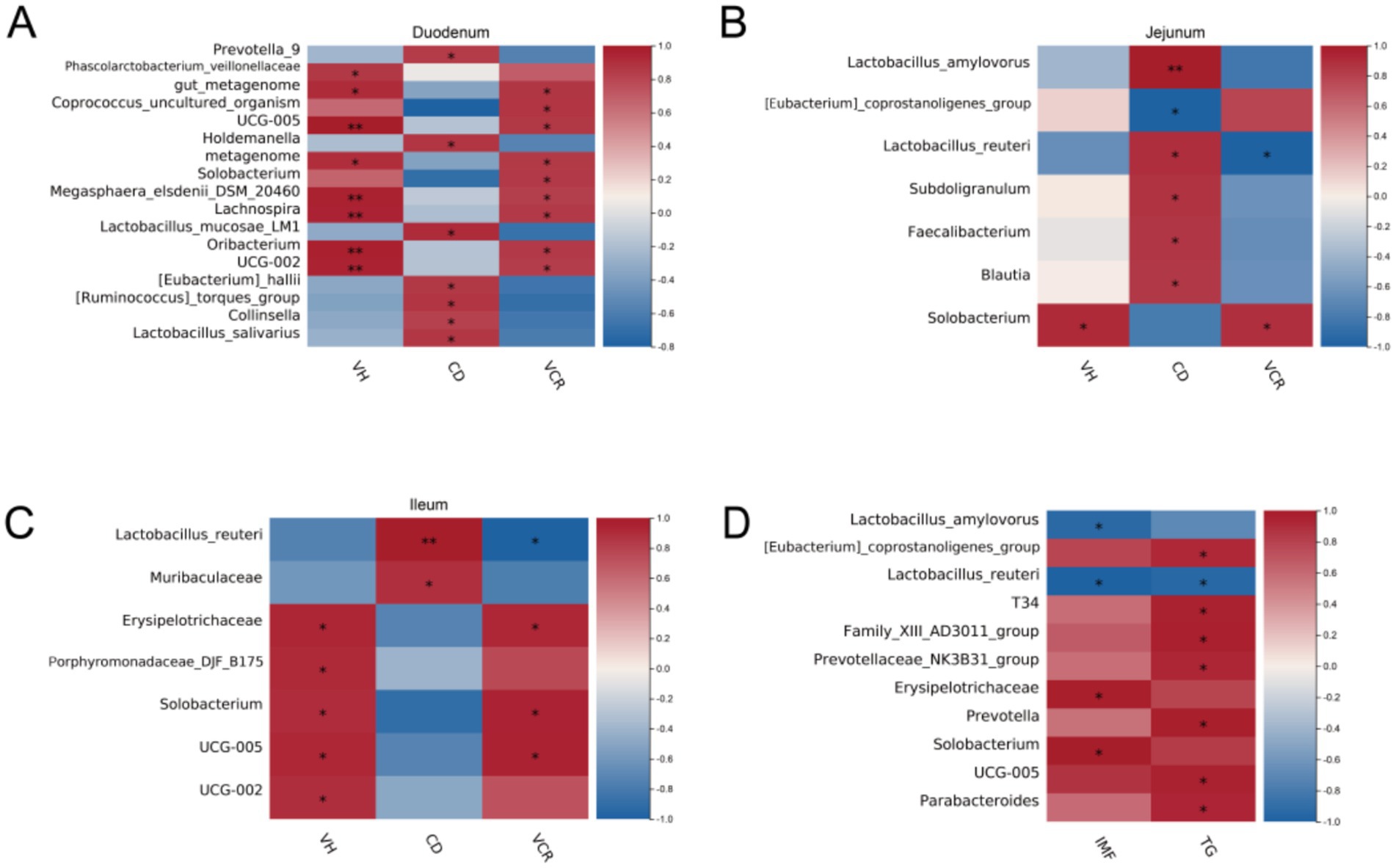
Figure 5. Correlation analysis on cecal microbiota. (A) Heat map of the correlation between the differential bacteria and intestinal morphology in duodenum; (B) Heat map of the correlation between the differential bacteria and intestinal morphology in jejunum; (C) Heat map of the correlation between the differential bacteria and intestinal morphology in ileum; (D) Heat map of the correlation between the differential bacteria and lipid traits in LT.
3.6 Untargeted metabolomics analysis of serum
Subsequently, serum untargeted metabolomics analysis showed that there were significant differences in serum metabolites between the two groups (Figure 6A), of which 706 metabolites were significantly up-regulated (p < 0.05) and 415 metabolites were significantly down-regulated (p < 0.05) (Figure 6B). The top three classes with the highest proportion were Lipids and lipid−like molecules, Organic acids and derivatives, and Organoheterocyclic compounds (Figure 6C). In this study, the top 20 serum metabolites were selected for differential analysis, of which 15 were significantly up-regulated (p < 0.05) and 5 were significantly down-regulated (p < 0.05) (Figure 6D). The six most abundant differential serum metabolites were Alpha-ketoisovaleric acid, 1-Hydroxy-2-naphthoic acid, D-Phenylalanine, and 2-Hydroxybutyric acid, 2-Hydroxy-3-methylbutyric acid, and 4-Acetylbutyrate, among which 1-Hydroxy-2-naphthoic acid and 4-Acetylbutyrate were significantly down-regulated (p < 0.05), the remaining four were significantly up-regulated (p < 0.05) (Figure 6E). KEGG pathway enrichment analysis showed that Serum differential metabolites were significantly enriched (p < 0.05) in Biosynthesis of unsaturated fatty acids, Choline metabolism in cancer, and Glycerolipid metabolism and other pathways (Figures 6F,G). Through metabolic network analysis, Glycerophospholipid metabolism, Biosynthesis of unsaturated fatty acids, Sphingolipid signaling pathway, and Phospholipase D were found signaling pathways are the core pathways of differential metabolites (Figure 6H).
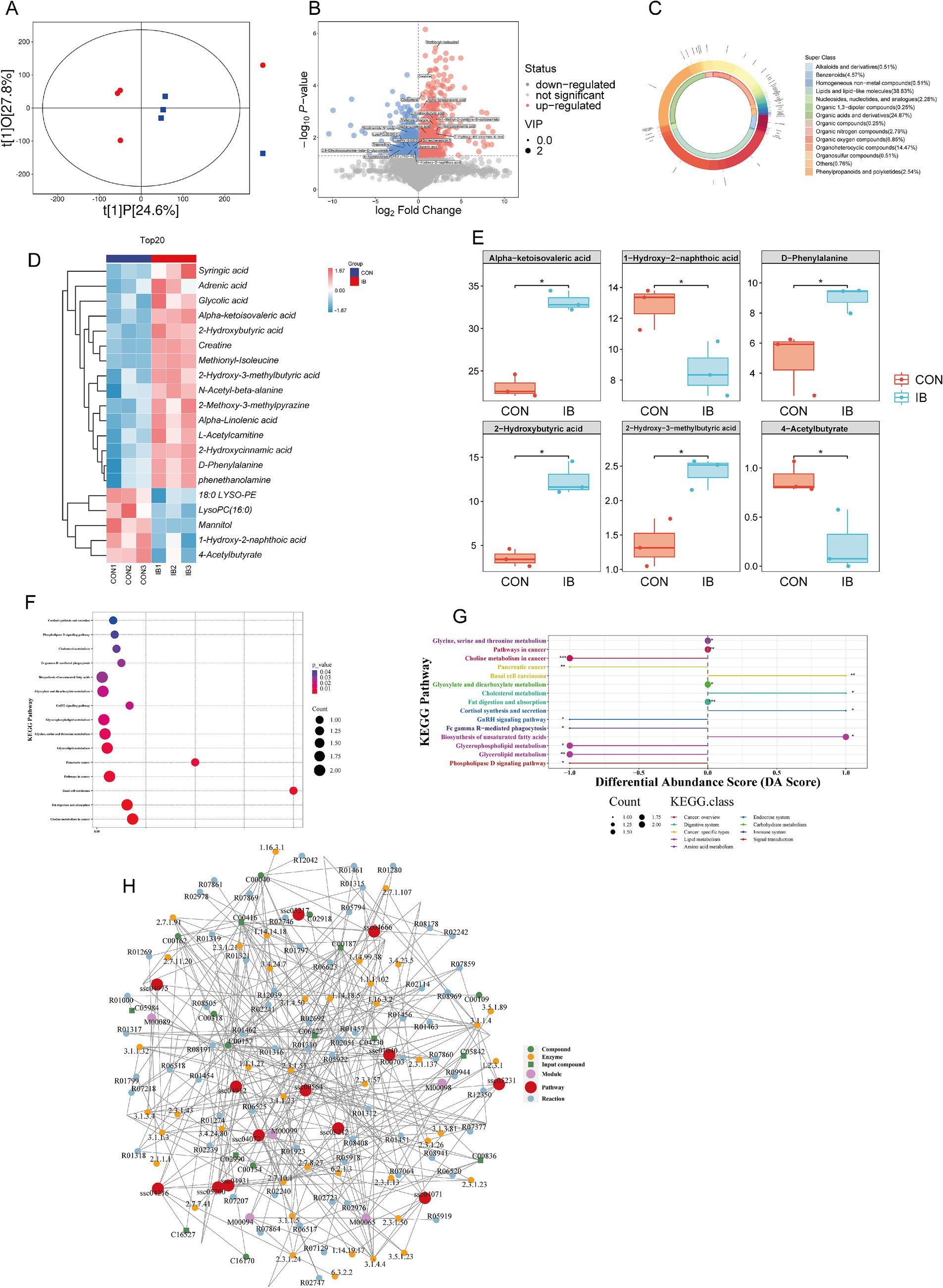
Figure 6. Effect of isobutyric acid on serum metabolites. (A) OPLS-DA; (B) Volcano plot of serum metabolites; (C) Donut plot of metabolite classification and proportion; (D) Heatmap of the top 20 serum metabolites; (E) The top six serum metabolites in boxplot. (F) KEGG enrichment bubble for differential metabolites; (G) Differential abundance score for differential metabolites; (H) Network analysis for differential metabolites.
3.7 Correlation analysis between serum metabolism and IMF, TG
In order to further ensure the accuracy of analysis and screen out the core metabolites that may affect lipid traits, we simulated the weighted gene correlation network analysis (WGCNA) algorithm commonly used in transcriptome analysis and tried to apply it to metabolomics analysis. The core metabolites were expected to be screened by modular classification of metabolism and association analysis by extracting module eigenvalues and lipid traits. In this study, the soft threshold was set as 12 (Figure 7A), and serum metabolites were divided into 89 modules according to their abundance characteristics (Figure 7B). The correlation between the eigenvalues of each module and lipid traits was analyzed. A total of 27 modules with strong correlation (R > 0.7) were screened out (Figure 7C). Four modules with the highest correlation were screened out and 105 serum metabolites were extracted from them (Figure 7D). Subsequently, correlation analysis between all serum metabolites and lipid traits was performed, and 40 serum metabolites with significant associations with lipid traits were obtained (Figure 7E). The results of WGCNA analysis and the results of correlation analysis were intersected, six potential core serum metabolites SM (d18:0/16:0), Hydrogen phosphate, Zedoarondiol, Syringic acid, 7-methyladenine, and Normetanephrine that might affect lipid traits were obtained (Figure 7F).
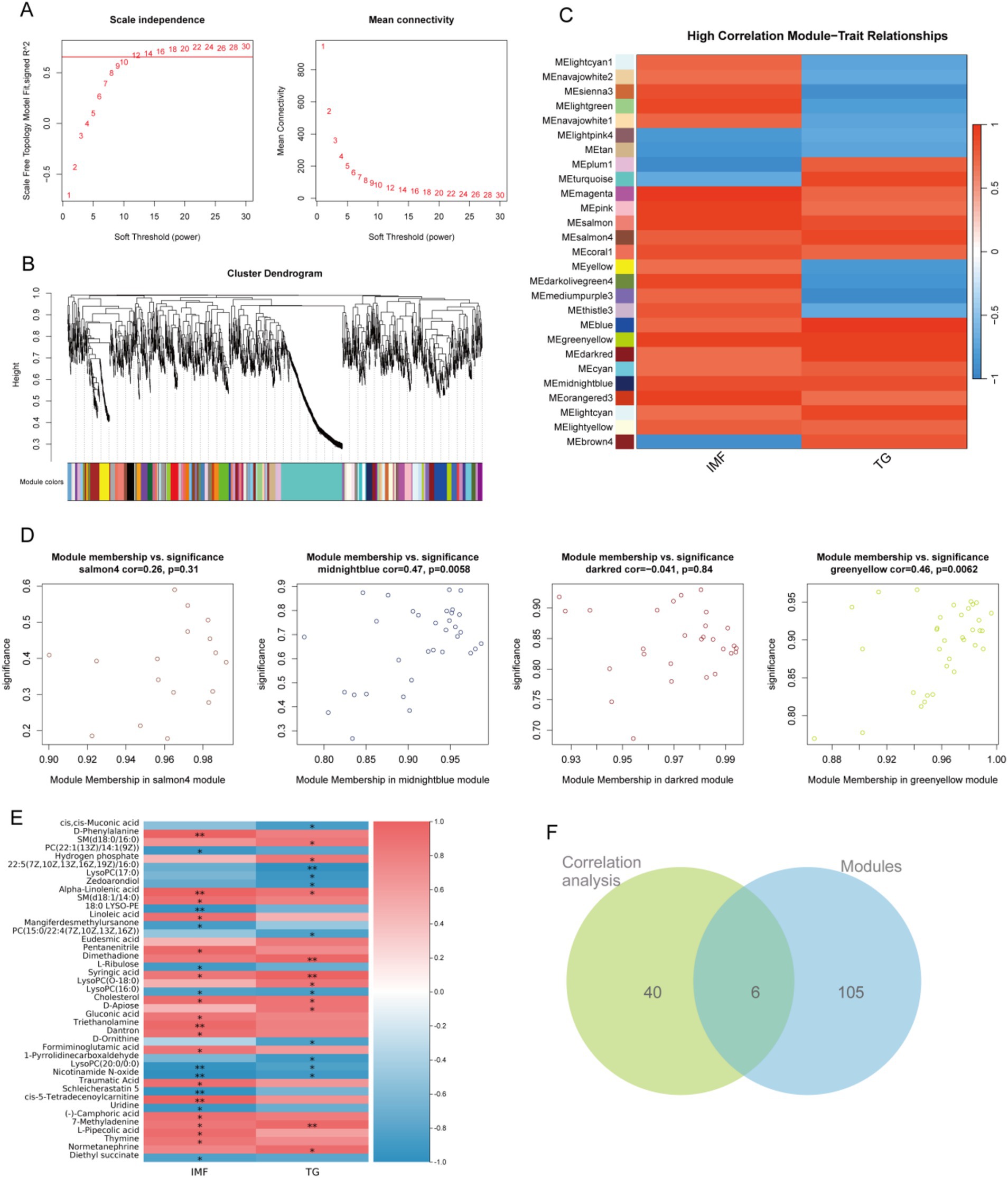
Figure 7. Weighted gene co-expression network analysis (WGCNA). (A) Scale independence (left) and mean connectivity (right) analysis for choosing the soft threshold in establishing the WGCNA network; (B) Gene clustering and identification of gene modules using WGCNA; (C) Heatmap of the relationship between gene modules and lipid traits. The relationships were assessed by calculating the Pearson correlation coefficients between the module eigengenes and lipid traits (IMF and TG); (D) Four core modules that are highly correlated with lipid traits; (E) Heatmap of the relationship between serum metabolites and lipid traits; (F) Venn of modules and association analysis.
4 Discussion
Isobutyric acid is used as an organic acidifier in piglets’ diet in the present study. Isobutyric acid, also known as 2-methylpropionic acid, is one of the main energy providers for intestinal epithelial cells (27). Adding isobutyric acid to chicken diets can promote intestinal integrity and alter the composition of cecal microbiota (28). We found isobutyric acid could improve serum antioxidant ability and immunity, and promote intestinal mucosal barrier function, which may be related to the change of the composition of the cecal microbiota. Feeding gluconic acid influences the composition and activity of the intestinal microflora and may improve growth performance of piglets after weaning (29). Interestingly, we also found isobutyric acid increased IMF and muscular TG in LT of weaned piglets. Therefore, the supplementation with isobutyric acid may enhance intestinal mucosal barrier function and further improve meat quality.
Weaning stress is a major contributor to oxidative stress in blood of piglets. Malondialdehyde is the second reaction product of lipid peroxidation. MDA content can be used as the main product to measure the degree of oxidative stress (30). The activities of SOD and GST reflect the antioxidant status of the body (31). Isobutyric acid strengthened the stress resistance of weaned piglets, obviously increased T-AOC level and SOD activity (p < 0.01). For further verification of the effect of the isobutyric acid on oxidative stress, we analyzed the level of GST and MDA. In the current study, a diet with 0.5% isobutyric acid showed a significant decrease (p < 0.01) in the activity of GST and content of serum MDA. Previous studies showed that treatment with acetate and butyrate could reverse the production of MDA but increase levels of SOD in glomerular mesangial cells (32). Oral Epigallocatechin-3-gallate increase the production of propionate and butyrate, with an increases of T-AOC and SOD in mice (33). Therefore, it is necessary to investigate the changes of serum metabolites between IB group and CON group.
Immunoglobulins have antibody activity and play an important role in defending disease. We found Ig A significantly increased (p < 0.05) in IB group. Ig A can prevent pathogens from adhering to and invading mucosal epithelial cells, reducing the risk of infection (34). The result also showed an upregulated (p < 0.01) in Ig M. As the primary antibody of the initial immune response, Ig M is found mostly in the blood, which can activate the complement system and facilitate pathogen clearance (35). Therefore, feeding a diet supplemented with isobutyric acid can improve immunity in weaned piglets.
From birth to post-weaning, the piglets’ intestine undergoes radial changes from crypt stem cells to the villous surface and changes in transport function from the duodenum to the distal colon (36, 37). The mucus, villi, and tight junctions in the intestinal lumen together form the first barrier controlling the movement of materials in and out of the intestine (33). MUC2 released by goblet cells is an important component of the mucus layer in the intestinal lumen (38). Tight junctions are located below the bottom of the microvilli. ZO-1, Claudin-1 and Occludin form a histone scaffold together, which is important component in maintaining the integrity of the intestinal mucosal barrier (39). We further confirmed that isobutyric acid increased the expression of Claudin-1 and Occludin in jejunum and ileum. Overall, these data demonstrated that isobutyric acid has a positive effect on intestinal morphology and mucosal barrier function in weaned piglets.
Microorganisms play an essential role in maintaining intestinal homeostasis (40). Probiotics and pathogenic bacteria in the intestinal tract constrain and regulate each other with the intestinal environment to uphold mucosal integrity and barrier function (41). Changes in diet can alter the composition of the intestinal flora, leading to changes in bacterial metabolites and the phenotype of the animal (42). In this study, we examined the composition of the gut microbiota. Our research results confirmed that the addition of isobutyric acid in the diet affects the composition and abundance of gut microbiota, leading to partial changes in the intestinal microbiota structure of weaned piglets. Firmicutes and Bacteroidetes, which together account for more than 90% of the total bacterial abundance in the cecum microbiota, are two major phyla. After the addition of isobutyric acid to the diet, the relative abundance of Firmicutes decreased, while Bacteroidetes and Verrucomicrobia increased. At the genus level, most bacteria belong to the phylum Firmicutes and produce short-chain fatty acids, especially acetate and butyrate (43). Acetate can induce immune responses to resist harmful bacteria, and when potentially harmful bacteria are present, acetate can trigger the production of Ig A in the colon, which binds to bacteria and inhibits their colonization and invasion in the mucosal layer (44). Butyrate is the preferred energy source for intestinal epithelial cells, and 75% of the energy of the intestinal mucosa comes from its metabolism through β-oxidation of hydroxybutyryl-CoA (45).
In order to further substantiate the potential link between intestinal morphology and cecal microbiota, we conducted a correlation analysis. The results showed that [Eubacterium]_coprostanoligenes, T34, Family_XIII_AD3011, Prevotellaceae_NK3B31, Prevotella, Solobacterium, UCG-005 and Parabacteroides were significantly positively correlated (p < 0.05) with VCR in small intestinal segments. Bai et al. observed that AUF1 stabilizes MUC2 mRNA in goblet cells, and the increase of [Eubacterium]_coprostanoligenes_group induced MUC2 mRNA depends on AUF1 expression (46).
Meanwhile, the correlation analysis between lipid traits and cecal microbiota showed Solobacterium and Erysipelotrichaceae were significantly positively correlated (p < 0.05) with IMF, and [Eubacterium]_coprostanoligenes, T34, Family_XIII_AD3011, Prevotellaceae_NK3B31, Prevotella, UCG-005 and Parabacteroides were significantly positively correlated with muscle TG. We found that these strains shared commonalities in their products. Most of their metabolites are short-chain fatty acids and short-chain fatty acid salts, especially butyric acid and butyrate, which are consistent with the changes in the intestinal flora structure (47). [Eubacterium]_coprostanoligenes_group, a cholesterol-reducing anaerobe in feces, has been found decreased in the HFD-induced hyperlipidemic rats and could generate beneficial SCFAs and have beneficial effects on dyslipidemia (48, 49). Previous study had shown that [Eubacterium]_coprostanoligenes_group can decompose cholesterol into sterols that cannot be absorbed in the intestine and is finally excreted with feces (50). In addition, [Eubacterium]_coprostanoligenes_group is considered to be the pivotal genus in the fecal microecosystem mediating the effect of HFD on dyslipidemia through sphingosine (51). This consistency may be one of the reasons why isobutyric acid enhances intestinal mucosal barrier function and promotes intramuscular fat deposition.
The dietary supplementation with 0.5% isobutyric acid increased the marbling score of muscle tissue of piglets. IMF is positively correlation with the meat quality, including the color, texture and flavor. As food, higher IMF substantially enhances the overall satisfaction of consumers (52). Our results showed that isobutyric acid elevated the IMF and TG in LT. Like other studies using short-chain fatty acid as a dietary additive, Huang et al. found that maternal butyrate supplementation lead to ectopic lipid accumulation in skeletal of offspring (18). Studies have found that the downregulation of adiponectin is interrelated with the tendency of pigs to accumulate fat, which is associated with our results (53). Adiponectin, an adipocyte-derived hormone, plays an important role in lipid metabolism and glucose homeostasis (54). In our study, isobutyric acid may regulate fat deposition through the de novo fatty acid synthesis (DNL) pathway. ACC and FASN are the key metabolic enzyme of the DNL pathway (55). We found that isobutyric acid significantly increased the expression of ACC and FASN in LT, which may be the cause of the IMF deposition we observed in the IB group. Moreover, isobutyric acid had a significantly inhibitory effect on LPL, FABP4 and HSL., which are key genes involved in cellular fatty acid uptake and the rate-limiting gene for lipolysis. In addition, butyrate could enhance the formation of adipocytes by 10–20% and mRNA expression of adipocyte markers by 20–200% in procine SVF undergoing adipocyte differentiation (56).
Serum metabolomics could comprehensively analyze and identify the serum metabolites by using mass spectrometry. Untargeted serum metabolomics technologies have a wide range of applications in medicine, including the identification of disease markers, the analysis of pathological mechanisms and the screening of clinical animal models. However, fewer studies have used the serum metabolome to analyze serum markers associated with intramuscular fat in weaned piglets. In our study, isobutyric acid significantly altered (p < 0.05) the composition of serum metabolites in weaned piglets especially Lipids and lipid−like molecules, Organic acids and derivatives, and Organoheterocyclic compounds. KEGG showed a significantly enriched (p < 0.05) pathway related to lipid metabolism, for instance Biosynthesis of unsaturated fatty acids, Choline metabolism in cancer, and Glycerolipid metabolism and other pathways. And Glycerophospholipid metabolism is one of the core pathway of differential metabolites through metabolic network analysis. Glycerophospholipid metabolism is the process of biosynthesis, catabolism and transformation of intracellular glycerophospholipids. Glycerophospholipids are one of the main components of cell membranes and are involved in intracellular signaling, energy storage, and cellular recognition. Glycerophospholipid metabolism may be a potential regulator of the promotion of intramuscular fat deposition (30). Another core pathway is Phospholipase D signaling pathway. Phospholipase D hydrolyses phosphatidylcholine to produce phosphatidic acid and free choline. Phosphatidic acid can not only be converted to diacylglycerol by phosphatidic acid phosphohydrolase, but also deacylated by phospholipase A to produce lysophosphatidic acid (57). To summarize, these serum metabolites may affect the IMF content and meat quality by Glycerophospholipid metabolism and Phospholipase D signaling pathway.
The results of the correlation analysis showed that Alpha-Linolenic acid, Syringic acid, 7-methylidene and cholesterol are positively correlated with IMF and TG. Alpha-Linolenic acid is a polyunsaturated fatty acid, and animals cannot synthesize by themselves, must be taken in through dietary intake. Alpha-Linolenic acid inhibits inflammatory responses, boosts immunity and antioxidant ability (58). In the current study, isobutyric acid improve antioxidant capacities may be related to the upregulation of Alpha-Linolenic acid. Syringic acid is a phenolic compound that plays an important role in regulating fat metabolism and lowering the lipid content of the liver (49). Furthermore, we intersected the results derived from the WGCNA with the results of the correlation analysis, and we obtained six potential core serum metabolites. In addition to Syringic acid and Normetanephrine, we discovered four other related metabolites, such as SM (d18:0/16:0), Hydrogen phosphate, Zedoarondiol and 7-methylidene. Previous studies showed different doses of phosphate can regulate fat metabolism by affecting the expression of lipogenic and lipolysis genes (59). Sphingolipid metabolism may be associated with excess body fat (60). These results may explain the diet supplementation with isobutyric acid promote IMF deposition in weaned piglets.
In summary, the findings of our study demonstrate that diet supplementation with 0.5% isobutyric acid could promote intestinal mucosal barrier function and antioxidant ability, probably by changing the composition of cecal microbiota. Moreover, we speculated isobutyric acid may increase the deposition of IMF by regulating the composition of cecal microbiota, and subsequently regulating the serum metabolism to influence fat deposition in LT. Therefore, isobutyric acid may be a resultful addition in piglets diets to improve small intestinal mucosal barrier function. Meanwhile, isobutyric acid also promoted meat quality to improved nutritional value of pork in the window period.
5 Conclusion
In summary, the findings of our study demonstrate that diet supplementation with 0.5% isobutyric acid could promote intestinal mucosal barrier function and antioxidant ability, probably by changing the composition of cecal microbiota. Moreover, we speculated isobutyric acid may increase the deposition of IMF by regulating the composition of cecal microbiota, and subsequently regulating the serum metabolis to influence fat deposition in LT (Figure 8). Therefore, isobutyric acid may be a resultful addition in piglets diets to improve small intestinal mucosal barrier function. Meanwhile, isobutyric acid also promoted meat quality to improved nutritional value of pork in the window period. However, it is essential to acknowledge that our studies may not reflect long-term effects, necessitating further chronic studies.
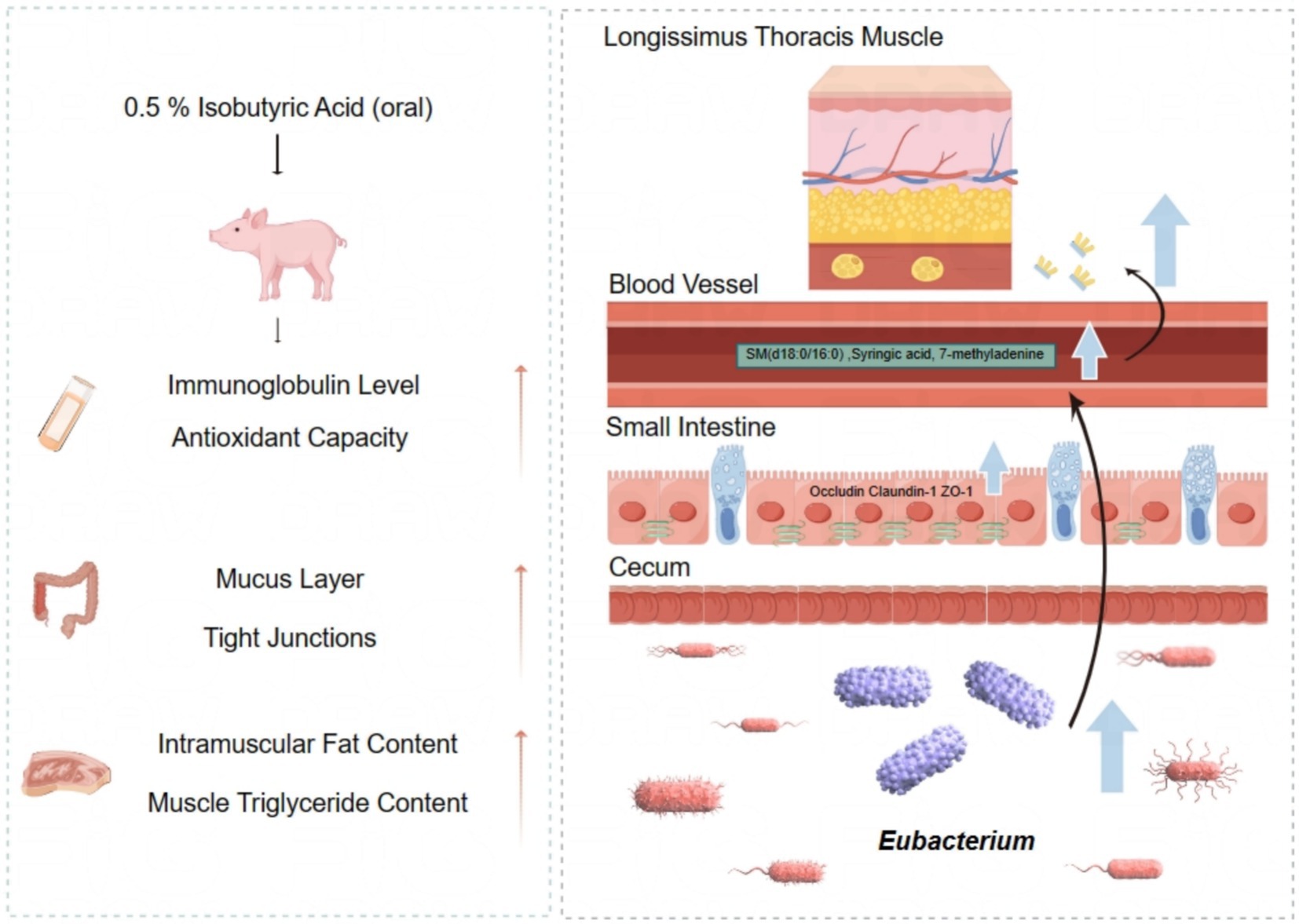
Figure 8. Effect of isobutyric acid on intestinal mucosal barrier function and meat quality of weaned piglets.
Data availability statement
The original contributions presented in the study are included in the article/Supplementary material, further inquiries can be directed to the corresponding author.
Ethics statement
The animal studies were approved by the Institutional Animal Ethics Committee of Henan University of Science and Technology. The studies were conducted in accordance with the local legislation and institutional requirements. Written informed consent was obtained from the owners for the participation of their animals in this study.
Author contributions
BW: Conceptualization, Data curation, Formal analysis, Investigation, Project administration, Software, Writing – original draft, Writing – review & editing. JH: Conceptualization, Investigation, Software, Writing – original draft. YC: Funding acquisition, Investigation, Writing – original draft. HW: Conceptualization, Investigation, Methodology, Writing – original draft. KS: Formal analysis, Investigation, Writing – original draft. XJ: Conceptualization, Investigation, Software, Writing – review & editing. XC: Methodology, Software, Supervision, Writing – original draft. YZ: Methodology, Writing – original draft. SJ: Writing – review & editing. LS: Investigation, Writing – original draft. KL: Methodology, Writing – original draft. ZS: Supervision, Writing – review & editing. FW: Conceptualization, Investigation, Software, Writing – original draft, Writing – review & editing.
Funding
The author(s) declare that financial support was received for the research and/or publication of this article. This research was funded by the grants from Program of the National Natural Science Foundation of China (U1804118), Henan Province natural science Foundation project (252300420177), a Program of the Henan Province Higher Education Key Teacher Training (2018GGJS052), and Science and technology project of the Education Department of Henan Province (222102110088).
Acknowledgments
We sincerely thank Shanghai Macklin Biochemical Technology Company for providing isobutyric acid and Luoyang Yiluan Agricultural and Livestock Development Co. for providing Large White pigs.
Conflict of interest
The authors declare that the research was conducted in the absence of any commercial or financial relationships that could be construed as a potential conflict of interest.
Generative AI statement
The author(s) declare that no Gen AI was used in the creation of this manuscript.
Publisher’s note
All claims expressed in this article are solely those of the authors and do not necessarily represent those of their affiliated organizations, or those of the publisher, the editors and the reviewers. Any product that may be evaluated in this article, or claim that may be made by its manufacturer, is not guaranteed or endorsed by the publisher.
Supplementary material
The Supplementary material for this article can be found online at: https://www.frontiersin.org/articles/10.3389/fvets.2025.1565216/full#supplementary-material
SUPPLEMENTARY FIGURE S1 | Diversity analysis in two groups. (A) Rankabundance. (B) Rarefaction curves. (C) Alpha diversity analysis.
SUPPLEMENTARY TABLE S1 | Primers used for gene expression analysis via RT-qPCR.
SUPPLEMENTARY TABLE S2 | Composition and nutrient levels of the basal diet.
SUPPLEMENTARY TABLE S3 | Effect of isobutyric acid on growth performance of weaned piglets.
References
1. Wang, Y, Li, J, Wang, H, Mi, Y, Xue, Y, Li, J, et al. Effects of essential oil coated with glycerol monolaurate on growth performance, intestinal morphology, and serum profiles in weaned piglets. Anim Biosci. (2023) 36:753–60. doi: 10.5713/ab.22.0261
2. Kuo, WT, Odenwald, MA, Turner, JR, and Zuo, L. Tight junction proteins occludin and ZO-1 as regulators of epithelial proliferation and survival. Ann N Y Acad Sci. (2022) 1514:21–33. doi: 10.1111/nyas.14798
3. Ren, Z, Zhang, X, Fan, H, Yu, Y, Yao, S, Wang, Y, et al. Effects of different dietary protein levels on intestinal aquaporins in weaned piglets. J Anim Physiol Anim Nutr (Berl). (2023) 107:541–55. doi: 10.1111/jpn.13732
4. Lallès, JP, Bosi, P, Janczyk, P, Koopmans, SJ, and Torrallardona, D. Impact of bioactive substances on the gastrointestinal tract and performance of weaned piglets: a review. Animal. (2009) 3:1625–43. doi: 10.1017/S175173110900398X
5. Maito, CD, Melo, ADB, de Oliveira, ACDF, Genova, JL, Engracia Filho, JR, de Macedo, REF, et al. Simultaneous feeding of calcium butyrate and tannin extract decreased the incidence of diarrhea and proinflammatory markers in weaned piglets. Anim Biosci. (2022) 35:87–95. doi: 10.5713/ab.21.0011
6. Baran, A, Kwiatkowska, A, and Potocki, L. Antibiotics and bacterial resistance-a short story of an endless arms race. Int J Mol Sci. (2023) 24:5777. doi: 10.3390/ijms24065777
7. Cheng, Y, Song, M, Zhu, Q, Azad, MAK, Gao, Q, and Kong, X. Dietary betaine addition alters carcass traits, meat quality, and nitrogen metabolism of Bama Mini-pigs. Front Nutr. (2021) 8:728477. doi: 10.3389/fnut.2021.728477
8. Zhu, Q, Azad, MAK, Dong, H, Li, C, Li, R, Cheng, Y, et al. Sow-offspring diets supplemented with probiotics and Synbiotics are associated with Offspring’s growth performance and meat quality. Int J Mol Sci. (2023) 24:7668. doi: 10.3390/ijms24087668
9. Tan, Z, and Jiang, H. Molecular and cellular mechanisms of intramuscular fat development and growth in cattle. Int J Mol Sci. (2024) 25:2520. doi: 10.3390/ijms25052520
10. Bäckhed, F, Ding, H, Wang, T, Hooper, LV, Koh, GY, Nagy, A, et al. The gut microbiota as an environmental factor that regulates fat storage. Proc Natl Acad Sci USA. (2004) 101:15718–23. doi: 10.1073/pnas.0407076101
11. Zhao, G, Xiang, Y, Wang, X, Dai, B, Zhang, X, Ma, L, et al. Exploring the possible link between the gut microbiome and fat deposition in pigs. Oxidative Med Cell Longev. (2022) 2022:1–13. doi: 10.1155/2022/1098892
12. Boyer-Diaz, Z, Morata, P, Aristu-Zabalza, P, Gibert-Ramos, A, Bosch, J, and Gracia-Sancho, J. Oxidative stress in chronic liver disease and portal hypertension: potential of DHA as nutraceutical. Nutrients. (2020) 12:2627. doi: 10.3390/nu12092627
13. Tang, Q, Li, W, Ren, Z, Ding, Q, Peng, X, Tang, Z, et al. Different fatty acid supplementation in low-protein diets regulate nutrient utilization and lipid and amino acid metabolism in weaned pigs model. Int J Mol Sci. (2023) 24:8501. doi: 10.3390/ijms24108501
14. Ran-Ressler, RR, Sim, D, O’Donnell-Megaro, AM, Bauman, DE, Barbano, DM, and Brenna, JT. Branched chain fatty acid content of United States retail Cow’s Milk and implications for dietary intake. Lipids. (2011) 46:569–76. doi: 10.1007/s11745-011-3530-8
15. Ran-Ressler, RR, Khailova, L, Arganbright, KM, Adkins-Rieck, CK, Jouni, ZE, Koren, O, et al. Branched chain fatty acids reduce the incidence of necrotizing enterocolitis and alter gastrointestinal microbial ecology in a neonatal rat model. PLoS One. (2011) 6:e29032. doi: 10.1371/journal.pone.0029032
16. Macfarlane, S, and Macfarlane, GT. Regulation of short-chain fatty acid production. Proc Nutr Soc. (2003) 62:67–72. doi: 10.1079/PNS2002207
17. Grilli, E, Tugnoli, B, Foerster, CJ, and Piva, A. Butyrate modulates inflammatory cytokines and tight junctions components along the gut of weaned pigs. J Anim Sci. (2016) 94:433–6. doi: 10.2527/jas.2015-9787
18. Huang, Y, Gao, S, Chen, J, Albrecht, E, Zhao, R, and Yang, X. Maternal butyrate supplementation induces insulin resistance associated with enhanced intramuscular fat deposition in the offspring. Oncotarget. (2017) 8:13073–84. doi: 10.18632/oncotarget.14375
19. Meyer, RK, Lane, AI, Weninger, SN, Martinez, TM, Kangath, A, Laubitz, D, et al. Oligofructose restores postprandial short-chain fatty acid levels during high-fat feeding. Obesity (Silver Spring). (2022) 30:1442–52. doi: 10.1002/oby.23456
20. Wang, C, Liu, Q, Zhang, YL, Pei, CX, Zhang, SL, Wang, YX, et al. Effects of isobutyrate supplementation on ruminal microflora, rumen enzyme activities and methane emissions in Simmental steers. J Anim Physiol Anim Nutr (Berl). (2015) 99:123–31. doi: 10.1111/jpn.12191
21. Wang, C, Liu, Q, Zhang, YL, Pei, CX, Zhang, SL, Guo, G, et al. Effects of isobutyrate supplementation in pre- and post-weaned dairy calves diet on growth performance, rumen development, blood metabolites and hormone secretion. Animal. (2017) 11:794–801. doi: 10.1017/S1751731116002093
22. Wang, K, Cao, G, Zhang, H, Li, Q, and Yang, C. Effects of clostridium butyricum and Enterococcus faecalis on growth performance, immune function, intestinal morphology, volatile fatty acids, and intestinal flora in a piglet model. Food Funct. (2019) 10:7844–54. doi: 10.1039/C9FO01650C
23. Li, D, Wang, P, Wang, P, Hu, X, and Chen, F. Gut microbiota promotes production of aromatic metabolites through degradation of barley leaf fiber. J Nutr Biochem. (2018) 58:49–58. doi: 10.1016/j.jnutbio.2018.05.001
24. Liu, W, Chen, L, Miao, K, You, Y, Li, J, Lu, J, et al. Identification and validation of diagnostic biomarkers for intrahepatic cholestasis of pregnancy based on untargeted and targeted metabolomics analyses of urine metabolite profiles. BMC Pregnancy Childbirth. (2023) 23:828. doi: 10.1186/s12884-023-06102-6
25. Wang, H, Meng, L, and Mi, L. Effects of Leymus chinensis hay and alfalfa hay on growth performance, rumen microbiota, and untargeted metabolomics of meat in lambs. Front Vet Sci. (2023) 10:1256903. doi: 10.3389/fvets.2023.1256903
26. Zheng, H, Zhao, Q, Chen, J, Lu, J, Li, Y, and Gao, H. Gastrointestinal microbiome of ARDS patients induces neuroinflammation and cognitive impairment in mice. J Neuroinflammation. (2023) 20:166. doi: 10.1186/s12974-023-02825-7
27. Sun, M, Wu, W, Liu, Z, and Cong, Y. Microbiota metabolite short chain fatty acids, GPCR, and inflammatory bowel diseases. J Gastroenterol. (2017) 52:1–8. doi: 10.1007/s00535-016-1242-9
28. Khan, RU, Naz, S, Raziq, F, Qudratullah, Q, Khan, NA, Laudadio, V, et al. Prospects of organic acids as safe alternative to antibiotics in broiler chickens diet. Environ Sci Pollut Res Int. (2022) 29:32594–604. doi: 10.1007/s11356-022-19241-8
29. Biagi, G, Piva, A, Moschini, M, Vezzali, E, and Roth, FX. Effect of gluconic acid on piglet growth performance, intestinal microflora, and intestinal wall morphology. J Anim Sci. (2006) 84:370–8. doi: 10.2527/2006.842370x
30. Li, Z, Liu, S, Zhao, Y, Wang, J, and Ma, X. Compound organic acid could improve the growth performance, immunity and antioxidant properties, and intestinal health by altering the microbiota profile of weaned piglets. J Anim Sci. (2023) 101:skad196. doi: 10.1093/jas/skad196
31. Chen, MY, Duan, YL, Zhu, Y, Wang, JH, Hu, QB, Guo, SS, et al. Responses of intestinal morphology, immunity, antioxidant status and cecal microbiota to the mixture of glycerol monolaurate and cinnamaldehyde in laying hens. Poult Sci. (2024) 103:103645. doi: 10.1016/j.psj.2024.103645
32. Huang, W, Guo, HL, Deng, X, Zhu, TT, Xiong, JF, Xu, YH, et al. Short-chain fatty acids inhibit oxidative stress and inflammation in mesangial cells induced by high glucose and lipopolysaccharide. Exp Clin Endocrinol Diabetes. (2017) 125:98–105. doi: 10.1055/s-0042-121493
33. Wu, Z, Huang, S, Li, T, Li, N, Han, D, Zhang, B, et al. Gut microbiota from green tea polyphenol-dosed mice improves intestinal epithelial homeostasis and ameliorates experimental colitis. Microbiome. (2021) 9:184. doi: 10.1186/s40168-021-01115-9
34. He, JW, Zhou, XJ, Lv, JC, and Zhang, H. Perspectives on how mucosal immune responses, infections and gut microbiome shape IgA nephropathy and future therapies. Theranostics. (2020) 10:11462–78. doi: 10.7150/thno.49778
35. Keyt, BA, Baliga, R, Sinclair, AM, Carroll, SF, and Peterson, MS. Structure, function, and therapeutic use of IgM antibodies. Antibodies (Basel). (2020) 9:53. doi: 10.3390/antib9040053
36. Tang, X, Xiong, K, Fang, R, and Li, M. Weaning stress and intestinal health of piglets: a review. Front Immunol. (2022) 13:1042778. doi: 10.3389/fimmu.2022.1042778
37. Sun, W, Chen, Z, Huang, Z, Wan, A, Zhou, M, and Gao, J. Effects of dietary traditional Chinese medicine residues on growth performance, intestinal health and gut microbiota compositions in weaned piglets. Front Cell Infect Microbiol. (2023) 13:1283789. doi: 10.3389/fcimb.2023.1283789
38. Liu, Y, Yu, Z, Zhu, L, Ma, S, Luo, Y, Liang, H, et al. Orchestration of MUC2 – the key regulatory target of gut barrier and homeostasis: a review. Int J Biol Macromol. (2023) 236:123862. doi: 10.1016/j.ijbiomac.2023.123862
39. Horowitz, A, Chanez-Paredes, SD, Haest, X, and Turner, JR. Paracellular permeability and tight junction regulation in gut health and disease. Nat Rev Gastroenterol Hepatol. (2023) 20:417–32. doi: 10.1038/s41575-023-00766-3
40. Xun, W, Ji, M, Ma, Z, Deng, T, Yang, W, Hou, G, et al. Dietary emodin alleviates lipopolysaccharide-induced intestinal mucosal barrier injury by regulating gut microbiota in piglets. Anim Nutr. (2023) 14:152–62. doi: 10.1016/j.aninu.2023.05.004
41. Vicentini, FA, Keenan, CM, Wallace, LE, Woods, C, Cavin, JB, Flockton, AR, et al. Intestinal microbiota shapes gut physiology and regulates enteric neurons and glia. Microbiome. (2021) 9:210. doi: 10.1186/s40168-021-01165-z
42. Wu, T, Shi, Y, Zhang, Y, Zhang, M, Zhang, L, Ma, Z, et al. Lactobacillus rhamnosus LB1 alleviates Enterotoxigenic Escherichia coli-induced adverse effects in piglets by improving host immune response and anti-oxidation stress and restoring intestinal integrity. Front Cell Infect Microbiol. (2021) 11:724401. doi: 10.3389/fcimb.2021.724401
43. Fu, X, Liu, Z, Zhu, C, Mou, H, and Kong, Q. Nondigestible carbohydrates, butyrate, and butyrate-producing bacteria. Crit Rev Food Sci Nutr. (2019) 59:S130–52. doi: 10.1080/10408398.2018.1542587
44. Imoto, S, and Namioka, S. Nutritive value of acetate in growing pigs. J Anim Sci. (1983) 56:858–66. doi: 10.2527/jas1983.564858x
45. Hamer, HM, Jonkers, D, Venema, K, Vanhoutvin, S, Troost, FJ, and Brummer, RJ. Review article: the role of butyrate on colonic function. Aliment Pharmacol Ther. (2008) 27:104–19. doi: 10.1111/j.1365-2036.2007.03562.x
46. Bai, D, Zhao, J, Wang, R, Du, J, Zhou, C, Gu, C, et al. Eubacterium coprostanoligenes alleviates chemotherapy-induced intestinal mucositis by enhancing intestinal mucus barrier. Acta Pharm Sin B. (2024) 14:1677–92. doi: 10.1016/j.apsb.2023.12.015
47. Wan Saudi, WS, and Sjöblom, M. Short-chain fatty acids augment rat duodenal mucosal barrier function. Exp Physiol. (2017) 102:791–803. doi: 10.1113/EP086110
48. Wan, Y, Tong, W, Zhou, R, Li, J, Yuan, J, Wang, F, et al. Habitual animal fat consumption in shaping gut microbiota and microbial metabolites. Food Funct. (2019) 10:7973–82. doi: 10.1039/C9FO01490J
49. Si, X, Shang, W, Zhou, Z, Shui, G, Lam, SM, Blanchard, C, et al. Gamma-aminobutyric acid enriched Rice bran diet attenuates insulin resistance and balances energy expenditure via modification of gut microbiota and short-chain fatty acids. J Agric Food Chem. (2018) 66:881–90. doi: 10.1021/acs.jafc.7b04994
50. Yang, G, Hong, E, Oh, S, and Kim, E. Non-viable Lactobacillus johnsonii JNU3402 protects against diet-induced obesity. Food Secur. (2020) 9:1494. doi: 10.3390/foods9101494
51. Wei, W, Jiang, W, Tian, Z, Wu, H, Ning, H, Yan, G, et al. Fecal g. Streptococcus and g. Eubacterium_coprostanoligenes_group combined with sphingosine to modulate the serum dyslipidemia in high-fat diet mice. Clin Nutr. (2021) 40:4234–45. doi: 10.1016/j.clnu.2021.01.031
52. Hou, J, Ji, X, Chu, X, Shi, Z, Wang, B, Sun, K, et al. Comprehensive lipidomic analysis revealed the effects of fermented Morus alba L. intake on lipid profile in backfat and muscle tissue of Yuxi black pigs. J Anim Physiol Anim Nutr (Berl). (2024) 108:764–77. doi: 10.1111/jpn.13932
53. De Rosa, A, Monaco, ML, Nigro, E, Scudiero, O, D’Andrea, M, Pilla, F, et al. Tissue-specific downregulation of the adiponectin “system”: possible implications for fat accumulation tendency in the pig. Domest Anim Endocrinol. (2013) 44:131–8. doi: 10.1016/j.domaniend.2012.11.001
54. da Silva Rosa, SC, Liu, M, and Sweeney, G. Adiponectin synthesis, secretion and extravasation from circulation to interstitial space. Physiology (Bethesda). (2021) 36:134–49. doi: 10.1152/physiol.00031.2020
55. Yao, Z, Gong, Y, Chen, W, Shao, S, Song, Y, Guo, H, et al. Upregulation of WDR6 drives hepatic de novo lipogenesis in insulin resistance in mice. Nat Metab. (2023) 5:1706–25. doi: 10.1038/s42255-023-00896-7
56. Li, G, Yao, W, and Jiang, H. Short-chain fatty acids enhance adipocyte differentiation in the stromal vascular fraction of porcine adipose tissue. J Nutr. (2014) 144:1887–95. doi: 10.3945/jn.114.198531
57. Jenkins, GM, and Frohman, MA. Phospholipase D: a lipid centric review. Cell Mol Life Sci. (2005) 62:2305–16. doi: 10.1007/s00018-005-5195-z
58. Kim, OY, and Song, J. Important roles of linoleic acid and α-linolenic acid in regulating cognitive impairment and neuropsychiatric issues in metabolic-related dementia. Life Sci. (2024) 337:122356. doi: 10.1016/j.lfs.2023.122356
59. Imi, Y, Yabiki, N, Abuduli, M, Masuda, M, Yamanaka-Okumura, H, and Taketani, Y. High phosphate diet suppresses lipogenesis in white adipose tissue. J Clin Biochem Nutr. (2018) 63:181–91. doi: 10.3164/jcbn.17-141
60. Green, CD, Maceyka, M, Cowart, LA, and Spiegel, S. Sphingolipids in metabolic disease: the good, the bad, and the unknown. Cell Metab. (2021) 33:1293–306. doi: 10.1016/j.cmet.2021.06.006
61. National Research Council. (2012). Nutrient Requirements of Swine. 11th ed. Washington, DC: National Academies Press; Available at: https://doi.org/10.17226/13298
Keywords: isobutyric acid, intestinal mucosal barrier function, gut microbiota, intramuscular fat, serum metabolome, weaned piglets
Citation: Wang B, Hou J, Cao Y, Wei H, Sun K, Ji X, Chu X, Zhang Y, Jiang S, Shi L, Liu K, Song Z and Wen F (2025) Dietary isobutyric acid supplementation improves intestinal mucosal barrier function and meat quality by regulating cecal microbiota and serum metabolites in weaned piglets. Front. Vet. Sci. 12:1565216. doi: 10.3389/fvets.2025.1565216
Edited by:
Shengyu Xu, Sichuan Agricultural University, ChinaReviewed by:
Gang Shu, Sichuan Agricultural University, ChinaYingying Liu, Hunan Institute of Animal Husbandry and Veterinary Medicine, China
Copyright © 2025 Wang, Hou, Cao, Wei, Sun, Ji, Chu, Zhang, Jiang, Shi, Liu, Song and Wen. This is an open-access article distributed under the terms of the Creative Commons Attribution License (CC BY). The use, distribution or reproduction in other forums is permitted, provided the original author(s) and the copyright owner(s) are credited and that the original publication in this journal is cited, in accordance with accepted academic practice. No use, distribution or reproduction is permitted which does not comply with these terms.
*Correspondence: Fengyun Wen, bmV3d2Z5dW5AaGF1c3QuZWR1LmNu