- 1Space Sciences Laboratory, University of California Berkeley, Berkeley, CA, United States
- 2School of Physics and Astronomy, University of Leicester, Leicester, United Kingdom
- 3NASA Goddard Space Flight Center, Greenbelt, MD, United States
- 4Center for Space Physics, Boston University, Boston, MA, United States
- 5University of Colorado, Boulder, CO, United States
- 6Space Science Center, University of New Hampshire, Durham, NH, United States
- 7University of Iowa, Iowa, IA, United States
- 8Montana State University, Bozeman, MT, United States
- 9Predictive Science Inc, San Diego, CA, United States
- 10Southwest Research Institute, Boulder, CO, United States
- 11Deep Space Exploration Laboratory, University of Science and Technology of China, Hefei, China
- 12University of Kiel, Kiel, Germany
- 13Johns Hopkins University Applied Physics Laboratory, Laurel, MD, United States
- 14Southwest Research Institute, San Antonio, TX, United States
- 15University of Texas at San Antonio, San Antonio, TX, United States
This perspective article discusses the knowledge gaps and open questions regarding the solar and interplanetary drivers of space weather conditions experienced at Mars during active and quiescent solar periods, and the need for continuous, routine observations to address them. For both advancing science and as part of the strategic planning for human exploration at Mars by the late 2030s, now is the time to consider a network of upstream space weather monitors at Mars. Our main recommendations for the heliophysics community are the following: 1. Support the advancement for understanding heliophysics and space weather science at ∼1.5 AU and continue the support of planetary science payloads and missions that provide such measurements. 2. Prioritize an upstream Mars L1 monitor and/or areostationary orbiters for providing dedicated, continuous observations of solar activity and interplanetary conditions at ∼1.5 AU. 3. Establish new or support existing 1) joint efforts between federal agencies and their divisions and 2) international collaborations to carry out #1 and #2.
1 Introduction: Current status and motivation
Both critical infrastructure and human explorers, either in orbit or on the surface, are vulnerable to ill effects from space weather hazards. Based on decades’ worth of routine and detailed observations on Earth, we have an increasingly comprehensive understanding of how solar activity and the local interplanetary conditions impact the geospace environment. In contrast to Earth, Mars is weakly shielded by its “hybrid magnetosphere,” with both induced and intrinsic magnetospheric features resulting from the planet’s tenuous atmosphere and inhomogeneous crustal magnetic fields (DiBraccio et al., 2018). Thus, the space weather impacts and effects on human explorers and supporting infrastructure (communications, power systems, habitat, etc.), both in the orbit around and on the surface of Mars, will be different from the terrestrial experience in many aspects.
During the last two solar cycles, observations from the orbit and ground by Mars Global Surveyor (MGS), Mars Odyssey (ODY), Mars Express (MEX), Mars Science Laboratory (MSL), Mars Atmosphere and Volatile Evolution (MAVEN), InSight, etc. have indicated that Mars can be significantly affected by solar activity (e.g., Crider et al., 2005; Zeitlin et al., 2010; Jakosky et al., 2015; Lee et al., 2018; Sánchez-Cano et al., 2019). However, the characteristics of space weather phenomena at Mars’ location, as well as the extent of their effects and impacts on the entire Martian system, warrant further investigations that require the availability of dedicated, routine space weather observations. Many important questions remain, such as “What are the effects of space weather drivers such as solar flares, coronal mass ejections (CMEs), high-speed streams (HSS), stream interaction regions (SIRs), solar energetic particles (SEPs), the heliospheric current sheet (HCS), and the nominal solar wind on the Martian system (magnetosphere, ionosphere, and surface)?”, and “What are the planetary responses to space weather, and how may this impact infrastructure that supports human explorers in orbit and at the surface?”
Near-Earth space weather monitors (e.g., ACE, SOHO, SDO, and GOES) have been providing a rich data set of observations of the Sun and the influences of its activity on the interplanetary conditions around the geospace environment while providing real-time “beacon” information for space weather forecasting. Such observations have been tremendously valuable for the development of “Sun-to-mud” models and modeling frameworks for use as scientific research tools (e.g., via NASA/CCMC) and for space weather forecasting (e.g., by NOAA/SWPC).
Our understanding of the interplanetary conditions at Mars’ heliocentric distances (1.38–1.66 AU; hereafter ∼1.5 AU) has relied on Earth-based observations for periods when Mars is in opposition or in the Parker spiral alignment with Earth that occurs about every 2 years (e.g., Crider et al., 2005; Palmerio et al., 2021; 2022; Thampi et al., 2021) and on measurements by planetary science missions at Mars (MGS, ODY, MEX, MSL, and MAVEN) (Crider et al., 2005; Delory et al., 2012; Hassler et al., 2014; Jakosky et al., 2015; Lee et al., 2018) or at ∼1.5 AU en route to the outer planets (e.g., Davies et al., 2021). Although these measurements constrain observation gaps, the temporal resolution of the data can be low and/or irregular. For example, MAVEN and MEX together have provided some of the most comprehensive sets of observations of the upstream interplanetary conditions at Mars, but due to their precessing, elliptical orbits, the interplanetary conditions are only sampled when their apoapsis segments are located outside the Martian bow shock. Thus, there can be periods from tens of minutes up to many months when upstream conditions are not observed (see, e.g., Figure 2 in Lee et al., 2017). The solar wind coverage for MAVEN was ∼38% in 2014–2019 and ∼20% in 2019–2021, and for MEX (without magnetic field measurements), about ∼53% in 2014–2021. Meanwhile, the Earth-based observations are not reliable when Mars is in superior conjunction (i.e., on the far side of the Sun, as seen from Earth).
A dedicated network of upstream space weather monitors must be a priority for heliophysics space research over the next decade in order to gain a deeper, more detailed understanding of the space environment at ∼1.5 AU, the Martian response to the varying interplanetary conditions, and the evolution of space weather conditions between 1 and ∼1.5 AU. The knowledge gained will not only expand and deepen our scientific understanding of solar and interplanetary drivers that influence the space environment around Mars but will also prepare us for sending humans to Mars by the 2030s.
2 Knowledge and observation gaps
2.1 On interplanetary structures and energetic particles at ∼1.5 AU
Detailed studies of interplanetary structures (CMEs, SIRs, HCS, and HSSs), their associated shocks, and energetic particles at Mars orbit have been very limited thus far due to the lack of continuous upstream observations at Mars. Fundamental research on interplanetary structures and particles at ∼1.5 AU is important for deepening our understanding of the solar-heliospheric origins of the interplanetary conditions experienced at Mars while helping to expand our heliophysics science knowledge that has mostly been based on research that utilizes observations obtained within 1 AU. As the solar wind structures propagate, compressions (rarefactions) form as faster streams run into (away from) slower streams emitted earlier (after) along the same radial line. Such dynamic interactions between the fast and slow parcels continue to develop beyond 1 AU, and shocks in the SIR compressions become fully formed between 2 and 3 AU (Gosling, 1995).
Researchers have been utilizing planetary science mission data sets to infer information about space weather activity at Mars, such as evidence of an interplanetary CME (ICME) impact via ionospheric radar sounder measurements that reveals a compressed magnetosphere (Figure 12a in Palmerio et al., 2021), an ICME shock arrival via the detection of energetic storm particles (ESPs) using a SEP detector (Figure 3b in Lee et al., 2018), or the occurrence of a SEP proton event based on increased background counts of a non-SEP instrument (Delory et al., 2012). When direct measurements are available—e.g., solar wind moments from MEX and MAVEN; solar irradiance, interplanetary magnetic field (IMF), and SEP measurements from MAVEN; and surface radiation by MSL (Barabash et al., 2006; Hassler et al., 2012; Connerney et al., 2015; Eparvier et al., 2015; Halekas et al., 2015; Larson et al., 2015)—observation gaps in the data and/or lower temporal data resolution have prohibited in-depth studies, such as those who seek to understand the physical differences of a given interplanetary structure observed at 1 AU and at Mars (e.g. Geyer et al., 2021; Palmerio et al., 2022).
Figure 1 shows an example of a missed opportunity to directly study in detail an ICME structure (shock, sheath, and/or ejecta) at Mars that is associated with the 10 September 2017 solar activity. Figures 1D–G reveal the sparse solar wind and IMF observations by MAVEN and MEX during the ICME impact. Evidence for the ICME shock (dashed line labeled ‘S’) was inferred from a signature of ESPs (Figure 1B, spike marked by ‘S’ dashed line), while evidence for the ICME was inferred from the Forbush-like decrease in the MSL/RAD surface radiation dose rates (Figure 1H). Because of significant gaps in the IMF data (Figure 1G; components not shown), it was not possible to determine which part of the ICME structure impacted Mars. Furthermore, Figure 1C illustrates the limitations to the MAVEN/SEP data for studying the acceleration and transport of E
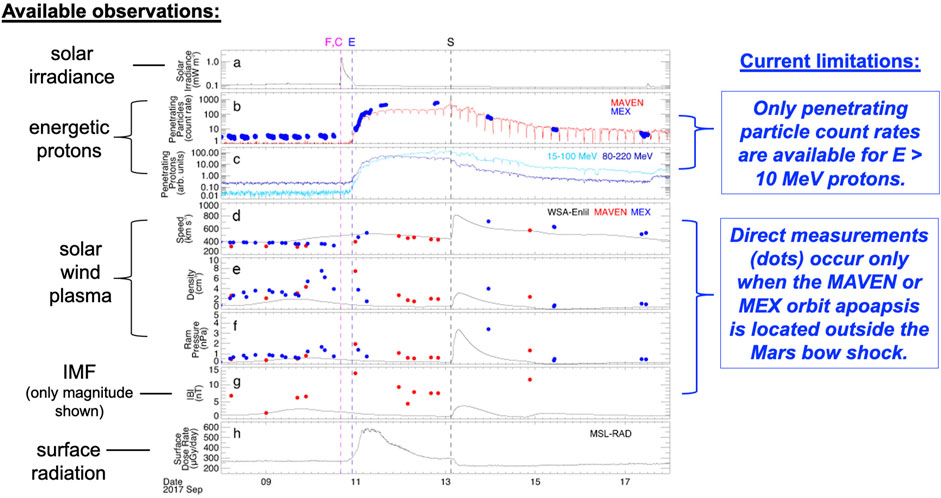
FIGURE 1. Example of a missed opportunity to study in detail an ICME at Mars due to observation gaps during the September 2017 event period. The MAVEN apoapsis location entered the Mars bow shock, while the observation coverage was limited for Mars Express (MEX) due to power restrictions. The figure is adapted from Lee et al. (2018).
2.2 On solar irradiance variability at ∼1.5 AU
At Mars, solar irradiance is modulated by both the solar variations themselves (time scales from seconds to decades) and the variable Sun–Mars distance and is the primary energy source driving the Martian atmosphere. In addition to heating and regulating the thermosphere, solar irradiance also ionizes the constituents to create the ionosphere. Episodic increases in irradiance caused by flares are one of the main space weather drivers. When Mars is far in longitude from Earth, these flares and new active regions cannot be observed from Earth and consequently cannot produce warnings for Mars. During an X-class flare event, for example, the electron densities in the ionosphere can increase up to an order of magnitude at low altitudes (Mendillo et al., 2006) and affect the ability of an orbiting radar to penetrate through the ionosphere to see the surface, while increased thermospheric temperatures (Jain et al., 2018; Thiemann et al., 2018) can impact orbital maneuvers and control.
The MAVEN Extreme Ultraviolet Monitor (EUVM; Eparvier et al., 2015) has been continuously measuring irradiance at Mars (e.g., Figure 1A) and demonstrating the benefit of direct observations of the solar irradiance input to the Martian system. However, when MAVEN is located behind Mars or when the spacecraft is reoriented for data downlinks to Earth, EUVM is unable to observe any flare activity that occurs on the Mars-facing side of the solar disk. Near-continuous monitoring of solar UV irradiance at Mars is critical for understanding the Martian atmospheric dynamics on all time scales, especially when Mars and Earth are on opposite sides of the Sun, rendering Earth-based observations useless.
2.3 Open questions
The following questions are compelling from a fundamental science perspective but are also highly relevant for human exploration and infrastructure at Mars.
• What are the average interplanetary conditions (solar irradiance, solar wind plasma and fields, SEPs, and GCR background radiation) for stronger and weaker solar cycles at ∼1.5 AU?
• How efficiently do SIR-related compression regions undergo transition to shocks from 1 AU to ∼1.5 AU? Under what conditions can SIRs be more impactful than ICMEs in driving magnetospheric (e.g., Gruesbeck et al., 2021) and ionospheric disturbances?
• What is the nature of the evolution of ICMEs, ICME-driven shocks, and the associated SEPs as they propagate from the Sun out to ∼1.5 AU?
• How often are the interplanetary structures at ∼1.5 AU a confluence of structure types, e.g., a CME merged behind a SIR (near/not near a HCS), interacting CMEs, etc.? How do the merged structures affect SEP acceleration, transport, and space weather impacts on Mars?
• What ICME and/or SIR characteristics are responsible for the observed (or unobserved) variability in the surface radiation? Can these also be the cause of observed surface magnetic field fluctuations (e.g., Mittelholz et al., 2021)?
• How much does the background GCR radiation at ∼1.5 AU get modulated over time scales of a solar modulation? How far do the different interplanetary structure types (ICMEs, SIRs, or merged CME–SIR structures) play a role in these changes (e.g., Schwadron et al., 2018; Freiherr von Forstner et al., 2020; Geyer et al., 2021; Guo et al., 2021)?
• What SEP energy range(s) and with what intensity are most hazardous for the instruments and crews in Mars’ orbit or at the surface? What precursor observations may serve as a warning (e.g., Posner and Strauss, 2020)?
• Which types of SEPs can penetrate the deepest into Mars’ ionosphere to cause the most extended radar blackouts (e.g., Sánchez-Cano et al., 2019)? For example, during an SEP event, diffuse auroral emissions originating from ∼60 km on the nightside have been attributed both to SEP electrons (Schneider et al., 2018) and SEP protons (Nakamura et al., 2022), but observations are insufficient to constrain this. Do the enhanced crustal magnetic field regions at Mars provide some shielding from SEPs at the surface?
• What are the physical changes induced by solar flares in the Martian atmosphere? How will these changes impact the technology and assets that are planned for human exploration to Mars?
• What are the space weather implications of near-radial IMF on the hybrid magnetosphere? How often do radial IMF conditions occur? During such conditions, the IMF no longer drapes around Mars to form a magnetic barrier and the solar wind flow can penetrate much deeper into the ionosphere (e.g., Chang et al., 2020).
3 Need for dedicated space weather observations at Mars
The planetary and heliophysics divisions of several world-wide space agencies are recognizing the importance and need for having upstream monitoring and observations of the Sun and their influence on the interplanetary conditions at Mars. For example, the NASA Heliophysics Division expanded the Heliophysics System Observatory (HSO) fleet of missions by funding the ESCAPADE mission, which has two spacecraft in elliptical orbits (apoapsis ∼8,000 km) to investigate solar wind energy flow into the Mars plasma system to provide simultaneous upstream and downstream measurements, and transitioned MSL/RAD to provide routine, near-real-time information (SEPs and dose rate progression) at Mars’ surface. Meanwhile, there is an ongoing collaboration between the NASA Moon to Mars (M2M) Space Weather Analysis Office and the MAVEN mission team to develop data products (event catalogs and data sets) that are of relevance to the analysis and validation of predictions of space weather at Mars. Submitted as an invite-only Phase 2 proposal to the ESA Medium-size mission of opportunity, the “Mars Magnetosphere Atmosphere Ionosphere and Space-weather Science” concept has two spacecraft orbiting Mars (one with orbit apoapsis ∼10,000 km) to investigate the dynamic response of the magnetosphere, ionosphere, and thermosphere system to space weather activity (Sanchez-Cano et al., 2022). For the recent Planetary Science Decadal Survey, NASA HQ explored the Planetary Mission Concept Study “Mars Orbiters for Surface, Atmosphere, and Ionosphere Connections” (Lillis et al., 2021), which have observing platforms that will measure upstream conditions at ∼17,000 km altitude.
In the 2023–2032 Planetary Decadal Survey Final Report, there is no clear mention about advancing fundamental research on Mars space weather science for future human exploration at Mars despite the need for these observations. To address the open science questions of relevance for heliophysics and as part of the strategic planning for human exploration at Mars by the late 2030s, now is the time for upstream monitors at Mars to be prioritized by NASA and other space agencies.
3.1 Prioritization of a Mars L1 monitor
Measurements from high-heritage instruments located ∼1.1 million km upstream at Mars L1 (see Figure 2) would provide continuous, unobstructed coverage of solar UV irradiance, solar activity, and interplanetary conditions, and enable new detailed studies for addressing the open science questions listed in §2.3. The instrument suite would include EUV/soft X-ray sensors, proton/electron electrostatic analyzers, magnetometers, and energetic particle and radiation detectors.
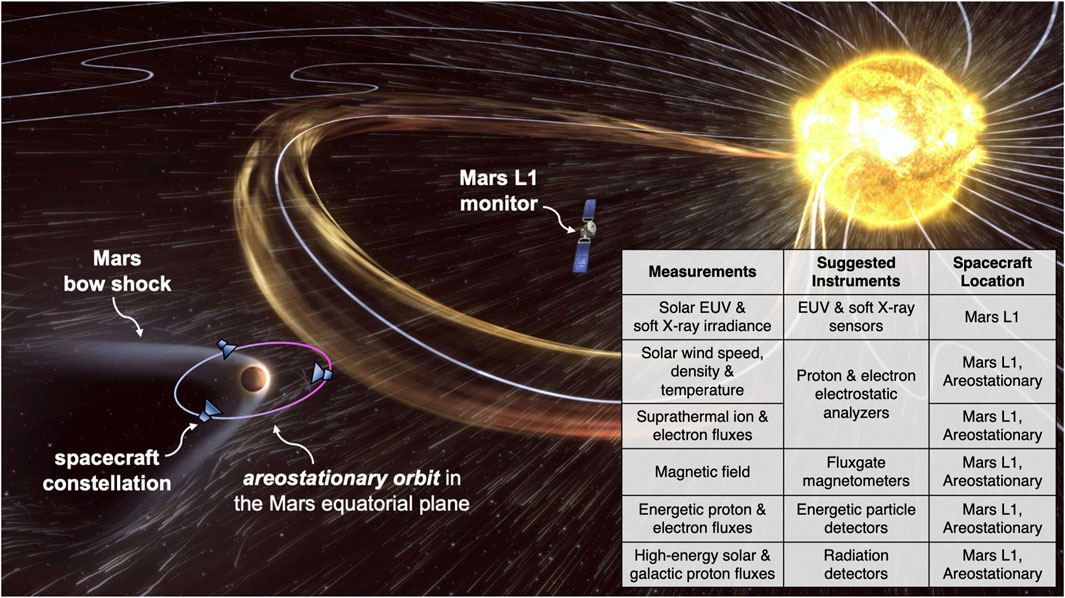
FIGURE 2. Concept (not shown to scale) of monitors at Mars L1 (upstream at ∼1.1 million km) and in areostationary orbit (the Mars-equivalent of geostationary orbit; ∼17,000 km altitude) situated in the Mars equatorial plane during a Mars-directed ICME event. A suggestion for instruments and their measurements along with the observation location is listed in the table. The image is adapted from NASA/GSFC.
Beacon data from this L1 instrument suite can be used to provide advance warning of extreme Mars-impacting solar events that are potentially hazardous to space- and ground-based assets, especially when considering the lag times for Earth-based warnings to reach Mars or when direct communication with Earth cannot occur for several weeks due to solar conjunction. For example, a fast CME (v ∼1,500 km/s) would be detected within ∼12 min at L1 before impacting the planet, thereby providing the necessary time for safety shutdown of electronic systems. Meanwhile, the mitigation of human-related radiation hazards based solely on this beacon data set would be a challenge. However, the detection of energetic particles between 10 s MeV and a few hundred MeV at Mars L1 could give essential data inputs for validating and testing nowcasting systems of Mars orbital and surface radiation levels (Guo et al., 2021) and providing radiation warnings for spacecraft in transit to Mars based on a two-tier warning system at Earth and Mars (Posner and Strauss, 2020).
3.2 Prioritization of areostationary orbiters
The Mars equivalent of the geostationary orbit is the areostationary orbit. Located at ∼17,000 km above the surface, an areostationary orbiter would spend
3.3 Mars L1 monitor plus areostationary orbiters
This constellation would provide a coordinated set of observations needed to address the observation and knowledge gaps discussed in this perspective article. As part of the HSO spacecraft fleet, the constellation would enable investigations of large-scale variabilities in the interplanetary conditions observed near Mars and would set in place a solar storm warning system that will be critical for protecting human explorers, their technology, and infrastructures at Mars.
4 Discussion
Real-time space weather forecasting at Mars is currently very challenging because, among other factors, it needs a continuous monitoring platform of the interplanetary conditions to provide timely and accurate space weather information. At the moment, the most available measurements of the Mars-facing Sun and upstream solar wind at Mars occur when Mars and Earth are in apparent opposition or perfectly aligned along the Parker spiral such that Earth-based assets can be used. The challenge arises when both planets are not closely aligned, which happens for ∼1.5 Earth years. In those situations, Mars does not have a sufficiently accurate monitor of the Sun and local space environment. Therefore, the analysis of space weather drivers and effects on the Martian environment can be extremely difficult. Currently, these depend on the upstream observations taken, in the best of the cases, during the reduced periods in which the spacecraft is located outside the Martian bowshock (e.g. Lee et al., 2018).
The future of heliophysics science at Mars requires new upstream space weather observations at Mars L1 and in areostationary orbits in combination with assets at lower altitudes and those at the surface to understand the effects and impacts of space weather activity. The constellation of coordinated multi-point measurements would not only enable us to better understand the nature of the interplanetary conditions at the variable Mars heliocentric distances—and their impacts on the dynamic Martian system—but these observations would also help us build upon the current space weather modeling and forecasting capabilities for deep-space conditions. In addition, the mission architecture and development activities for the upstream monitors would foster cross-divisional and cross-agency capabilities and establish new joint international efforts for planetary and human exploration to Mars. With the current efforts to send human explorers to the Moon and within a decade out to Mars, the need is now for continuous upstream space weather monitors at ∼1.5 AU.
Data availability statement
The original contributions presented in the study are included in the article/Supplementary Material; further inquiries can be directed to the corresponding author.
Author contributions
BS-C, GD, MM, SZ, PC, ED, CS, RF, RR, EP, BL, JL, BE, JG, RA, SV, RW, and HE all contributed significant ideas and/or text to the article at various stages of drafting. They also contributed significantly to the revising and editing of the article.
Acknowledgments
COL acknowledges support from NASA LWS Grant 80NSSC21K1325. B.S-C. acknowledges support through the STFC Ernest Rutherford Fellowship ST/V004115/1. CL, GD, PC, SX, JL, MM, and RR are partially supported by the MAVEN project funded through the NASA Mars Exploration Program. SX, BL, and CL acknowledge support from NASA HGI Grant 80NSSC21K073. SX also acknowledges support from NASA MDAP Grant 80NSSC17K0455. BE acknowledges support from NASA SMD/Heliophysics and HEOMD/AES under the JPL subcontract 1273039 to SwRI. CL and BE also acknowledge support from NASA MDAP Grant 80NSSC19K1224. EP acknowledges the NSF PREEVENTS program, Grant ICER-1854790. ED is supported by NASA Grants 80NSSC19K0914 and 80NSSC20K0431. RW acknowledges support from the NASA grant 80NSSC19K0914 and partial support from the NASA STEREO grant 80NSSC20K0431. RF acknowledges the NSF AGS PRF Grant. MAVEN and MSL data can be accessed at the Planetary Plasma Interactions (PPI) Node of NASA’s Planetary Data System (PDS) database. MX data are openly available at ESA’s Planetary Science Archive (PSA) database. COL would like to thank the reviewer, Arik Posner, for his comments and suggestions on how to improve the content of this perspective article.
Conflict of interest
EP was employed by Predictive Science Inc.
The remaining authors declare that the research was conducted in the absence of any commercial or financial relationships that could be construed as a potential conflict of interest.
The handling editor declared a shared affiliation with the author GD at the time of the review.
Publisher’s note
All claims expressed in this article are solely those of the authors and do not necessarily represent those of their affiliated organizations, or those of the publisher, the editors, and the reviewers. Any product that may be evaluated in this article, or claim that may be made by its manufacturer, is not guaranteed or endorsed by the publisher.
References
Barabash, S., Lundin, R., Andersson, H., Brinkfeldt, K., Grigoriev, A., Gunell, H., et al. (2006). The analyzer of space plasmas and energetic atoms (ASPERA-3) for the Mars express mission. Space Sci. Rev. 126, 113–164. doi:10.1007/s11214-006-9124-8
Chang, Q., Xu, X., Xu, Q., Wang, J., Xu, J., Ye, Y., et al. (2020). The demagnetization of the venusian ionosphere under nearly flow-aligned interplanetary magnetic fields. Astrophys. J. 900, 63. doi:10.3847/1538-4357/aba62a
Connerney, J. E. P., Espley, J., Lawton, P., Murphy, S., Odom, J., Oliversen, R., et al. (2015). The MAVEN magnetic field investigation. Space Sci. Rev. 195, 257–291. doi:10.1007/s11214-015-0169-4
Crider, D. H., Espley, J., Brain, D. A., Mitchell, D. L., Connerney, J. E. P., and Acuña, M. H. (2005). Mars Global Surveyor observations of the Halloween 2003 solar superstorm’s encounter with Mars. J. Geophys. Res. (Space Phys. 110, A09S21. doi:10.1029/2004JA010881
Davies, E. E., Forsyth, R. J., Winslow, R. M., Möstl, C., and Lugaz, N. (2021). A Catalog of Interplanetary Coronal Mass Ejections Observed by Juno between 1 and 5.4 au. Astrophys. J. 923, 136. doi:10.3847/1538-4357/ac2ccb
Delory, G. T., Luhmann, J. G., Brain, D., Lillis, R. J., Mitchell, D. L., Mewaldt, R. A., et al. (2012). Energetic particles detected by the electron reflectometer instrument on the Mars global surveyor, 1999-2006. Space weather. 10, S06003. doi:10.1029/2012SW000781
DiBraccio, G. A., Luhmann, J. G., Curry, S. M., Espley, J. R., Xu, S., Mitchell, D. L., et al. (2018). The twisted configuration of the martian magnetotail: MAVEN observations. Geophys. Res. Lett. 45, 4559–4568. doi:10.1029/2018GL077251
Eparvier, F. G., Chamberlin, P. C., Woods, T. N., and Thiemann, E. M. B. (2015). The solar extreme ultraviolet monitor for MAVEN. Space Sci. Rev. 195, 293–301. doi:10.1007/s11214-015-0195-2
Freiherr von Forstner, J. L., Guo, J., Wimmer-Schweingruber, R. F., Dumbović, M., Janvier, M., Démoulin, P., et al. (2020). Comparing the properties of ICME-induced Forbush decreases at Earth and Mars. J. Geophys. Res. (Space Phys. 125, e27662. doi:10.1029/2019JA027662
Geyer, P., Temmer, M., Guo, J., and Heinemann, S. G. (2021). Properties of stream interaction regions at Earth and Mars during the declining phase of SC 24. Astron. Astrophys. 649, A80. doi:10.1051/0004-6361/202040162
Gosling, J. T. (1995). Solar wind corotating interaction regions: The third dimension. Rev. Geophys. 33, 597–601. doi:10.1029/95RG00125
Gruesbeck, J. R., Espley, J. R., Lee, C. O., and Curry, S. M. (2021). A generalized magnetospheric disturbance index: Initial application to Mars using MAVEN observations. J. Geophys. Res. (Space Phys. 126, e29479. doi:10.1029/2021JA029479
Guo, J., Zeitlin, C., Wimmer-Schweingruber, R. F., Hassler, D. M., Ehresmann, B., Rafkin, S., et al. (2021). Radiation environment for future human exploration on the surface of Mars: The current understanding based on MSL/RAD dose measurements. Astron. Astrophys. Rev. 29, 8. doi:10.1007/s00159-021-00136-5
Halekas, J. S., Taylor, E. R., Dalton, G., Johnson, G., Curtis, D. W., McFadden, J. P., et al. (2015). The solar wind ion analyzer for MAVEN. Space Sci. Rev. 195, 125–151. doi:10.1007/s11214-013-0029-z
Hassler, D. M., Zeitlin, C., Wimmer-Schweingruber, R. F., Böttcher, S., Martin, C., Andrews, J., et al. (2012). The radiation assessment detector (RAD) investigation. Space Sci. Rev. 170, 503–558. doi:10.1007/s11214-012-9913-1
Hassler, D. M., Zeitlin, C., Wimmer-Schweingruber, R. F., Ehresmann, B., Rafkin, S., Eigenbrode, J. L., et al. (2014). Mars’ surface radiation environment measured with the Mars science laboratory’s curiosity rover. Science 343, 1244797. doi:10.1126/science.1244797
Jain, S. K., Deighan, J., Schneider, N. M., Stewart, A. I. F., Evans, J. S., Thiemann, E. M. B., et al. (2018). Martian thermospheric response to an X8.2 solar flare on 10 september 2017 as seen by MAVEN/IUVS. Geophys. Res. Lett. 45, 7312–7319. doi:10.1029/2018GL077731
Jakosky, B. M., Grebowsky, J. M., Luhmann, J. G., Connerney, J., Eparvier, F., Ergun, R., et al. (2015). MAVEN observations of the response of Mars to an interplanetary coronal mass ejection. Science 350, 0210. doi:10.1126/science.aad0210
Larson, D. E., Lillis, R. J., Lee, C. O., Dunn, P. A., Hatch, K., Robinson, M., et al. (2015). The MAVEN solar energetic particle investigation. Space Sci. Rev. 195, 153–172. doi:10.1007/s11214-015-0218-z
Lee, C. O., Hara, T., Halekas, J. S., Thiemann, E., Chamberlin, P., Eparvier, F., et al. (2017). MAVEN observations of the solar cycle 24 space weather conditions at Mars. J. Geophys. Res. Space Phys. 122, 2768–2794. doi:10.1002/2016JA023495
Lee, C. O., Jakosky, B. M., Luhmann, J. G., Brain, D. A., Mays, M. L., Hassler, D. M., et al. (2018). Observations and impacts of the 10 september 2017 solar events at Mars: An overview and synthesis of the initial results. Geophys. Res. Lett. 45, 8871–8885. doi:10.1029/2018GL079162
Lillis, R. J., Mitchell, D., Montabone, L., Heavens, N., Harrison, T., Stuurman, C., et al. (2021). Mosaic: A satellite constellation to enable groundbreaking Mars climate system science and prepare for human exploration. Planet. Sci. J. 2, 211. doi:10.3847/PSJ/ac0538
Mendillo, M., Withers, P., Hinson, D., Rishbeth, H., and Reinisch, B. (2006). Effects of solar flares on the ionosphere of Mars. Science 311, 1135–1138. doi:10.1126/science.1122099
Mittelholz, A., Johnson, C. L., Fillingim, M., Joy, S. P., Espley, J., Halekas, J., et al. (2021). Space weather observations with InSight. Geophys. Res. Lett. 48, e95432. doi:10.1029/2021GL095432
Montabone, L., Heavens, N., Alvarellos, J. L., Aye, M., Babuscia, A., Barba, N., et al. (2021). Observing Mars from areostationary orbit: Benefits and applications. Bull. AAS 53. doi:10.3847/25c2cfeb.0cdca220
Nakamura, Y., Terada, N., Leblanc, F., Rahmati, A., Nakagawa, H., Sakai, S., et al. (2022). Modeling of diffuse auroral emission at Mars: Contribution of MeV protons. J. Geophys. Res. (Space Phys. 127, e29914. doi:10.1029/2021JA029914
Palmerio, E., Kilpua, E. K. J., Witasse, O., Barnes, D., Sánchez-Cano, B., Weiss, A. J., et al. (2021). CME magnetic structure and IMF preconditioning affecting SEP transport. Space weather. 19, e2020SW002654. doi:10.1029/2020SW002654
Palmerio, E., Lee, C. O., Richardson, I. G., Nieves-Chinchilla, T., Dos Santos, L. F. G., Gruesbeck, J. R., et al. (2022). CME evolution in the structured heliosphere and effects at Earth and Mars during solar minimum. Space weather. 20, e2022SW003215. doi:10.1029/2022SW003215
Posner, A., and Strauss, R. D. (2020). Warning time analysis from SEP simulations of a two-tier REleASE system applied to Mars exploration. Space weather. 18, e02354. doi:10.1029/2019SW002354
Sánchez-Cano, B., Blelly, P.-L., Lester, M., Witasse, O., Cartacci, M., Orosei, R., et al. (2019). Origin of the extended Mars radar blackout of september 2017. J. Geophys. Res. (Space Phys. 124, 4556–4568. doi:10.1029/2018JA026403
Sanchez-Cano, B., Lester, M., Leblanc, F., Andrews, D., and Opgenoorth, H. (2022). “The M-MATISSE mission: Mars magnetosphere ATmosphere ionosphere and surface SciencE,” in 44th COSPAR scientific assembly, 44, 421. Held 16-24 July.
Schneider, N. M., Jain, S. K., Deighan, J., Nasr, C. R., Brain, D. A., Larson, D., et al. (2018). Global aurora on Mars during the september 2017 space weather event. Geophys. Res. Lett. 45, 7391–7398. doi:10.1029/2018GL077772
Schwadron, N. A., Rahmanifard, F., Wilson, J., Jordan, A. P., Spence, H. E., Joyce, C. J., et al. (2018). Update on the worsening particle radiation environment observed by CRaTER and implications for future human deep-space exploration. Space weather. 16, 289–303. doi:10.1002/2017SW001803
Thampi, S. V., Krishnaprasad, C., Nampoothiri, G. G., and Pant, T. K. (2021). The impact of a stealth CME on the Martian topside ionosphere. Mon. Not. Roy. Astron. Soc. 503, 625–632. doi:10.1093/mnras/stab494
Thiemann, E. M. B., Andersson, L., Lillis, R., Withers, P., Xu, S., Elrod, M., et al. (2018). The Mars topside ionosphere response to the X8.2 solar flare of 10 september 2017. Geophys. Res. Lett. 45, 8005–8013. doi:10.1029/2018GL077730
Keywords: CMEs, SEPs, GCRs, charged particle radiation, solar wind, space weather, Mars, human exploration
Citation: Lee CO, Sánchez-Cano B, DiBraccio GA, Mayyasi M, Xu S, Chamberlin P, Davies E, Scolini C, Filwett RJ, Ramstad R, Palmerio E, Lynch BJ, Luhmann JG, Ehresmann B, Guo J, Allen RC, Vines S, Winslow R and Elliott H (2023) Heliophysics and space weather science at ∼1.5 AU: Knowledge gaps and need for space weather monitors at Mars. Front. Astron. Space Sci. 10:1064208. doi: 10.3389/fspas.2023.1064208
Received: 07 October 2022; Accepted: 09 January 2023;
Published: 10 February 2023.
Edited by:
Alexa (She/Her/Hers) Jean Halford, National Aeronautics and Space Administration, United StatesReviewed by:
Arik Posner, National Aeronautics and Space Administration (NASA), United StatesCopyright © 2023 Lee, Sánchez-Cano, DiBraccio, Mayyasi, Xu, Chamberlin, Davies, Scolini, Filwett, Ramstad, Palmerio, Lynch, Luhmann, Ehresmann, Guo, Allen, Vines, Winslow and Elliott. This is an open-access article distributed under the terms of the Creative Commons Attribution License (CC BY). The use, distribution or reproduction in other forums is permitted, provided the original author(s) and the copyright owner(s) are credited and that the original publication in this journal is cited, in accordance with accepted academic practice. No use, distribution or reproduction is permitted which does not comply with these terms.
*Correspondence: Christina O. Lee, Y2xlZUBzc2wuYmVya2VsZXkuZWR1