- 1Air Force Research Laboratory, Space Vehicles Directorate, Kirtland AFB, Albuquerque, NM, United States
- 2Department of Physics and Astronomy, University of New Mexico, Albuquerque, NM, United States
- 3Space Dynamics Laboratory, Utah State University Research Foundation, North Logan, UT, United States
- 4Department of Engineering Physics, Air Force Institute of Technology, Dayton, OH, United States
- 5Fourth State Communications, Albuquerque, NM, United States
Multi-instrument studies have recently shed new light on the morphology of sporadic E, especially intense sporadic E. Here we present simultaneous observations of dense sporadic E (
1 Introduction
In recent years, numerous studies have shed new light on the morphology of sporadic E (
The GNSS-observed structures may be related to the quasi-periodic (QP) echoes observed by very high frequency (VHF) coherent scatter radars (Yamamoto et al., 1994; Chu and Wang, 1997; Hysell and Burcham, 2000; Saito et al., 2006). QP echoes, however, are primarily observed at nighttime, when elongated fronts of field-aligned irregularities (FAIs) can exist, and they are typically observed to propagate to the southwest (in the Northern Hemisphere). The nighttime coupling between the E and F layers is also believed to produce the observed mid-latitude spread F, which is often coincident with VHF observations of QP echoes (Tsunoda and Cosgrove, 2001; Haldoupis et al., 2003). QP echoes are observed to have spatial scale sizes similar to the fronts measured by GNSS tomography and the LWA. However, the fronts observed by Maeda and Heki (2014) and others are a common feature of both daytime and nighttime Es. Moreover, they are observed to move in many directions—not just southwestward. Although it is possible that these two types of front-like
Sun et al. (2020) combined GNSS tomography with two ionosondes and two VHF radars to observe a case of strong daytime Es, during which two front-like events passed over southern China. As shown by the GNSS tomography, the front-like events move over the region, and both the ionosondes and the radars observed an approaching and then departing structure in range. This movement manifested in the range–time–intensity (RTI) plots from the VHF radars as a “V” shape. For the ionosondes, the approaching structure had a peak frequency of at least 17 MHz, indicating an exceptional event. The results clearly showed that the front-like structure was associated with irregular three dimensional (3D) structure, capable of creating backscatter at both HF and VHF frequencies over a range of angles. Patra et al. (2012) reported a similarly shaped range-time structure, as observed from the Gadanki radar at 53 MHz. The described “U”-shaped structure was coincident with a strong
Larsen (2000) proposed that the same wind shear that forms
Much of the aforementioned modeling work on
The technique described by Obenberger et al. (2021) allows us to track intense
In this paper, we present a Digisonde Portable Sounder 4D (DPS4D) and passive LWA radio telescope observations captured over a six-month period centered on the summer of 2022. We examine strong
2 Description of the experiment
The observations for this experiment were carried out during the summer of 2022. The experiment itself was largely a follow-on study to Obenberger et al. (2021), which showed that
2.1 DPS4D observations/analysis
For this study, we deployed a DPS4D (see Reinisch et al. (2008) and Reinisch et al. (2009) for more details on the DPS4D) to a Pecos County property in Ft. Stockton, TX (30.888 N; 102.832 W). We operated the DPS4D to produce one ionogram every 5 minutes (288 ionograms per day) at frequencies between 2 and 20 MHz, using both ordinary mode (O-mode) and extraordinary mode (X-mode) polarizations. We operated the DPS4D receive array in the standard equilateral triangle configuration, but, due to space constraints, we reduced the size of the triangle from 60 m on a side to 30 m, as suggested by the DPS4D manual (https://digisonde.com/pdf/Digisonde4DManual_LDI-web.pdf). This configuration preserved our ability to distinguish off-zenith reflections and acquire estimates of the azimuthal directions of returns.
Aside from a few short periods of power outages, the DPS4D in Ft. Stockton operated continuously from 13 April 2022 until 17 October 2022. Both the processed Portable Network Graphics (PNG) and Routine Scientific Format (RSF) files were archived for the entire data collection window, totaling approximately 80 GB of data. The RSF files, which contain the raw ionograms (power as a function of range and frequency) and the angle of arrival information, were used for the analysis presented in this paper. The PNG files, which show the plotted ionograms and the angle of arrival information, were useful primarily for quick looks at the data.
The RSF ionograms were processed to remove radio frequency interference (RFI). This was carried out using a simple spectral subtraction technique. Since RFI is generally a narrow band feature and occurs at all ranges equally, we simply subtracted the median spectra from each ionogram. The median was used because it largely ignores the radar returns, which are generally limited to a few range bins per frequency. The left panel of Figure 1 shows a raw O-mode ionogram before the background is removed, and the center panel shows the same ionogram after removal.
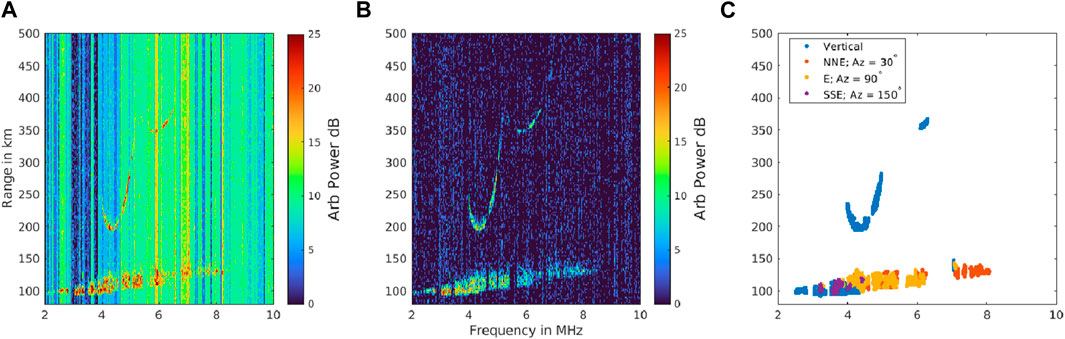
Figure 1. Ionogram produced by the Ft. Stockton DPS4D on 18 June 2022. (A) Raw ionogram, (B) ionogram with median spectrum removed, and (C) identified returns from the ionogram, with colors indicating their azimuthal direction.
Once the noise is reduced, we can search for
Additionally, the right panel in Figure 1 shows the estimated angle of arrivals from the returns. The DPS4D records the angle of arrival by beamforming in seven directions, and the direction of a given return is determined by the beam in which it appears the brightest. The first beam is vertical (at zenith), and the other six are at zenith angles of 30
2.2 LWA observations/analysis
At the time of the experiment, two LWA stations were operational in central New Mexico. The first station, LWA1, is co-located with the Very Large Array at 34.0689 N, 107.6283 W (Ellingson et al., 2013). The second station, LWA-SV is located at Sevilleta National Wildlife Refuge with a latitude and longitude of 34.3484 N and 106.8858 W, respectively (Cranmer et al., 2017). LWA-SV is approximately 75 km northeast of LWA1 and 540 km northwest of Ft. Stockton. Each station consists of 256 dual-polarization bent dipole antennas pseudo-randomly spaced over a 100 × 110 m ellipse with one or more additional outrigger antennas for calibration.
Both LWA stations are capable of beamforming and all-sky imaging. For this paper, we utilized the all-sky imaging capabilities, where the raw voltages from each antenna are cross-correlated and imaged to produce all-sky maps. For more details on LWA all-sky imaging, see Obenberger et al. (2015). The LWA all-sky imager (LASI) at LWA1 uses 100 kHz of bandwidth and can be tuned anywhere in the 10–88 MHz usable frequency range. Typically, LASI is tuned to 38.1 MHz, and all of the LWA observations used in this paper were at 38.1 MHz. LWA-SV has an upgraded version of LASI, called Orville, which uses a new graphical processing unit (GPU)-based correlator (Varghese et al., 2021). This new imager is capable of capturing 19.8 MHz of bandwidth, which is initially channelized into 198 bins (100 kHz each). The full-resolution images are kept for 2 weeks, but frequency-averaged images with six bins are stored indefinitely. During this experiment, the imager was centered at 30 MHz and imaged from approximately 20 to 40 MHz. Both stations produce 5-second averaged snapshots, resulting in 720 images per hour.
As demonstrated by Obenberger et al. (2021), both LWA stations can image and geolocate discrete clouds of Es. Obenberger et al. (2021) showed that at least some of the observed emissions come from unintentional anthropogenic noise, likely originating from power lines and other sources of broadband radio emissions. However, we note that it is currently unclear whether this accounts for all of the observed emissions. Assuming that the altitude of the
The intention of this experiment was to operate both LWA stations throughout the entire period of time during which the DPS4D was deployed to Ft. Stockton. However, due to maintenance issues at both stations, continuous co-observations did not occur. LWA-SV began experiencing cooling issues in early July and missed the majority of July. Similarly, LWA1 experienced computer hardware failures in mid-July and missed the second half of the month through the end of August. Therefore, most of the LWA observations presented in this paper occurred in May and June, with a few exceptions. We also note that the LWA was not observing the emissions from the DPS4D. The DPS4D is only transmitting up to 20 MHz, just below the lowest frequencies observed by the LWA. The LWA observations are 100% passive, utilizing broadband anthropogenic noise that scatters off intense
Before an all-sky image from either station can be used to detect sporadic E, the background sky needs to be removed. To achieve this, we produce a library of all-sky images at every local sidereal time (LST). This library is created by stacking nearly 100 images for a given 5 s LST window, each image from a different day. We then take the median across all the images at a given LST to produce a “model” of the background sky. We use the median because it largely ignores outliers caused by intermittent solar bursts, lightning, and other sources of RFI. After building the LST library, we then used it to remove the background sky from any given image.
Figure 2 shows an example of LWA-SV all-sky image from 18 May 2022 at 1800 UT, captured at 34.9 MHz. It also shows the median background image at the same LST and the result of subtracting the median image from the image obtained on 18 May. As can be seen, the constant galactic emission is removed, leaving only the transient phenomenon. The excess emission to the south and southeast is from Es, but the emission to the north is from local RFI, namely, a noisy inverter on a nearby recreational vehicle parked at the Sevilleta National Wildlife Refuge. Other features, such as the sun (just southeast of the zenith) and scintillating point sources, were removed by zeroing the pixels within 3
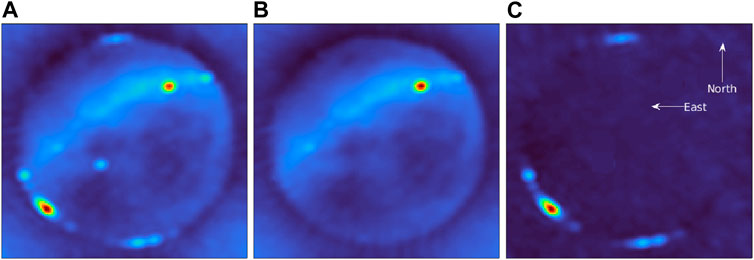
Figure 2. (A) LWA-SV all-sky image captured on 18 May 2022 at 1800 UT, centered at 34.9 MHz. (B) Median background all-sky image for the same LST (02:38 LST) and frequency, similar to the image on the left. (C) LWA-SV image after subtraction of the median background model.
After the background sky is removed, the search for
The final step in the
Figure 3 shows an example of
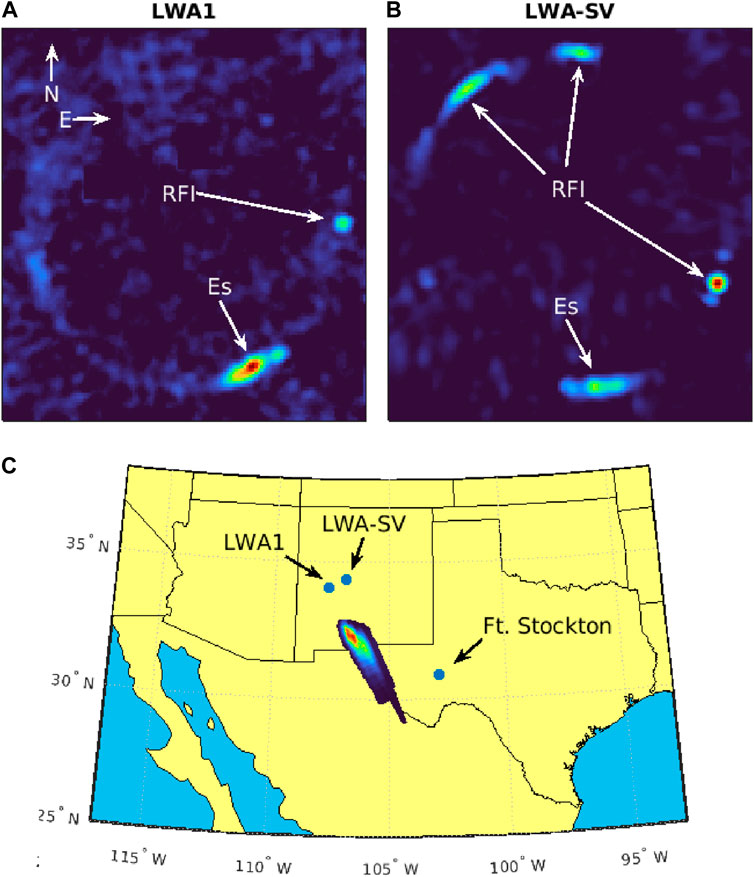
Figure 3. (A) Background-subtracted all-sky image from LWA1 on 6 June 2022 at 1300 UT, showing
Parallax observations from two or more stations greatly reduce the positioning error, especially when
2.3 HF receiver
In addition to the DPS4D at Fort Stockton, we also operated a wide-band HF receiver at LWA-SV. This receiver utilizes a software-defined radio, a magnetic loop antenna, and a large storage RAID system to record signals in the 2–22 MHz range. As described by Obenberger et al. (2023), the receiver is comprised of a magnetic loop antenna, an Ettus ×300 Universal Software Radio Peripheral (USRP), a GPS-synced clock, a PC, and 160 TB RAID. The receiver was configured to listen to the Fort Stockton DPS4D and produce oblique ionograms. Due to the overheating issues at LWA-SV, the receiver was only operated during the first part of the experiment, being shut down on June 06.
The goal of the receiver was to measure the impacts of
3 Results
In this section, we describe the results of the experiment. The primary objective of the experiment was to quantify the LWA’s ability to identify and characterize Es. However, we have also found that LWA observations have enabled a better understanding of the common features detected in the ionosonde observations of Es.
3.1 Accuracy of LWA Es tracking
In this section, we test the accuracy of the LWA radio telescopes to observe
where
To determine the accuracy of the LWA for identifying sporadic E, we compare the instances where LWA-SV did and did not observe emission toward Ft. Stockton to DPS4D measurements of Es. We can use the method described in Section 2.1 to find instances of
Since we are trying to determine the LWA’s ability to accurately measure
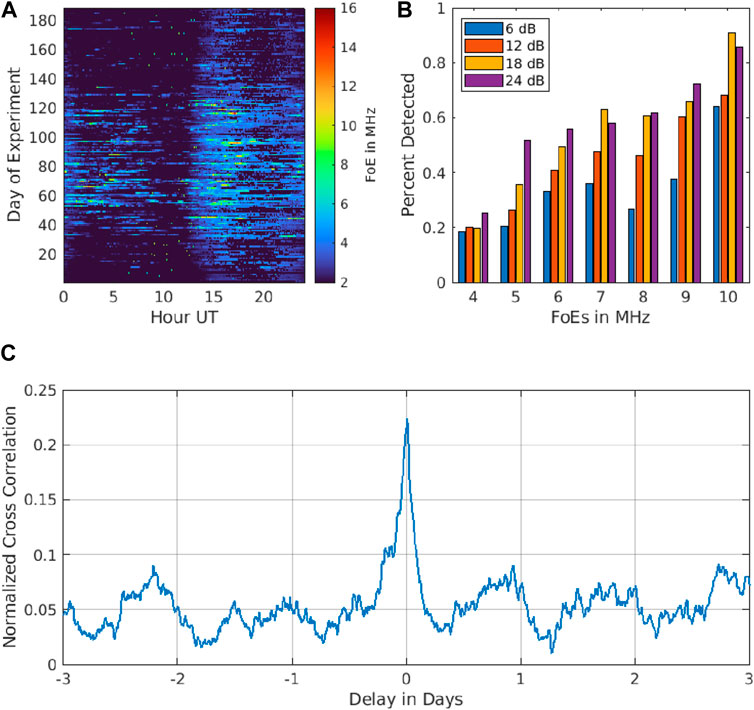
Figure 4. (A) foE measured for each hour of each day of the experiment window. (B) Percent of measured
Using a simple binary system, we assign a value of 1 when the DPS4D measurement detects
Although we assume that the DPS4D is “truth,” we must keep in mind that
The false positive rate, on the other hand, remains
To show the correlation in time, we can also run a cross-correlation between the occurrence of
The peak normalized correlation value of 0.22 is lower than what would be expected for all
It is also worth noting that the morphology of the
3.2 Off-zenith scatter from intense Es
As Figure 4 demonstrates, we observed numerous instances of high
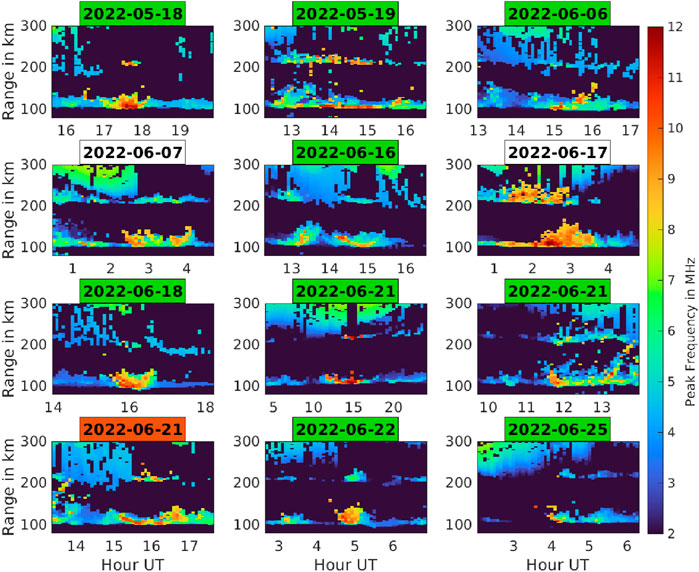
Figure 5. DPS4D range vs. time plots showing the evolution of
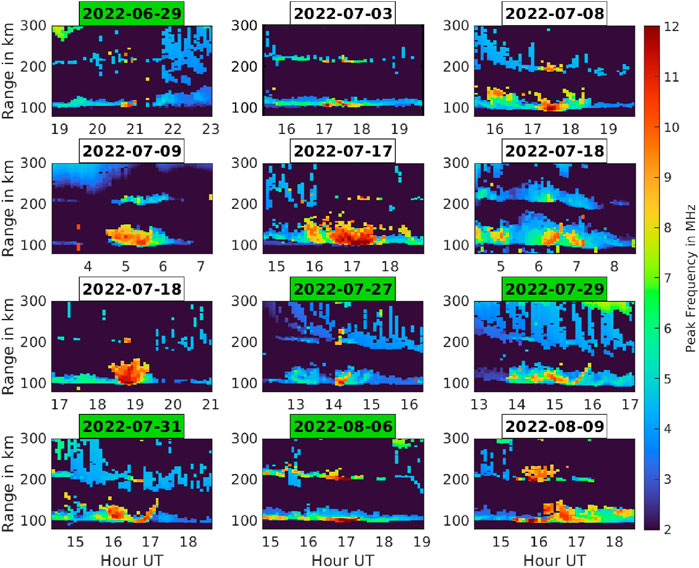
Figure 6. DPS4D range vs. time plots showing the evolution of
Upon examination of Figures 5, 6, common features can be observed in many of the intense
Naively, one might assume that the “V” shape is indicative of the vertical movement of
In most of these cases, the azimuthal direction of the intense
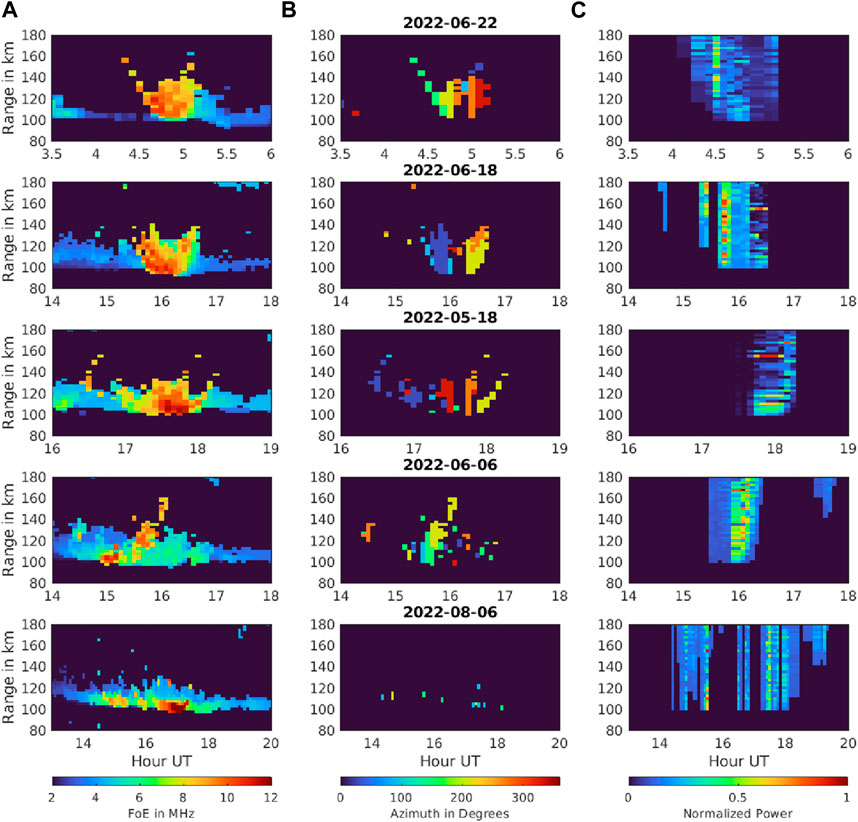
Figure 7.
In the following subsections, we will discuss the angle of arrival measurements from the DPS4D; however, we note that although the cables used for the DPS4D were phase-matched in the laboratory, no calibration was performed in the field. Moreover, the beam pattern of a four-element array includes many strong side-lobes. Although the small array may be suitable for strong point sources, a spread
3.2.1 22 June 2022
We will first analyze a nighttime
The right panel in Figure 7 shows the LWA-measured sky power as a function of time and range from the Ft. Stockton DPS4D. We note that the altitude of the LWA-measured
Comparing the LWA and DPS4D measurements, we can see that they are measuring the same structure, and both the timing of arrival and departure and the apparent velocity toward the DPS4D are strikingly similar, indicating that they are the same structure. This structure appears to be on top of an existing
3.2.2 18 June 2022
On 18 June 2022, a daytime event occurred near 1600 UT (1100 LT). Similar to the 22 June event, the disturbance appears to be a high density modulation superimposed on an existing
As in the case of 22 June, the DPS4D observes a “V” structure that approaches, reaches zenith, and then moves away at a speed of 65 m/s. Again, similar to the case of 22 June, the structure is observed off-zenith and moves from one side of the sky to the other. We note that at 1610 UT (1110 LT), the dominant beam is the one at the zenith, which is not represented in the azimuth plot in Figure 7. Furthermore, the only time an
3.2.3 18 May 2022
During this observation, a relatively strong 4–5 MHz
3.2.4 6 June 2022
A structure is observed between 1500 and 1630 UT (1000 and 1130 LT). In this case, neither the LWA nor the DPS4D observed the structure’s approach; however, both detected its departure (a partial “V”). The observations shown in the second-to-last row of Figure 7 indicate that the structure mostly formed as it was moving away from the Ft. Stockton area at an apparent speed of 37 m/s. As with the previous cases, the structure appears to be a modulation superimposed on an existing
3.2.5 6 August 2022
The strong event observed on 6 August 2022 serves as a counterpoint to the overall trend where a moving
This event is worth noting because it contained the highest
3.3 Es and daytime spread F
As mentioned in Section 2.3, an HF receiver was operated at LWA-SV to measure the impacts of
3.3.1 18 May 2022
We can first analyze how the oblique ionograms from 18 May 2024 are affected by the passing of the intense sporadic E structure discussed in Section 3.2.3. Since the
Figure 8 shows a progression of ionograms made on the oblique link at the time the intense
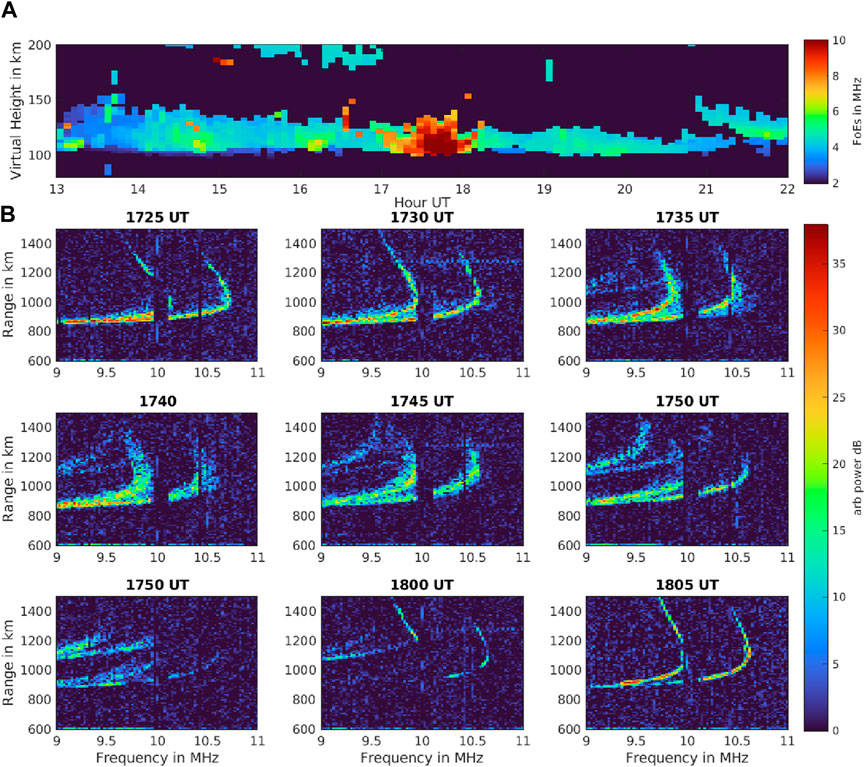
Figure 8. (A) RTF plot from the Ft. Stockton DPS4D on 18 May 2022. (B) [nine panels] Oblique ionograms measured between the Ft. Stockton DPS4D and an HF receiver at LWA-SV. Spread F and blanketing conditions can be observed, coincidingt with the passage of intense Es.
3.3.2 6 June 2022
Similar to the 18 May event, the 6 June event described in Section 3.2.4 had a distinct
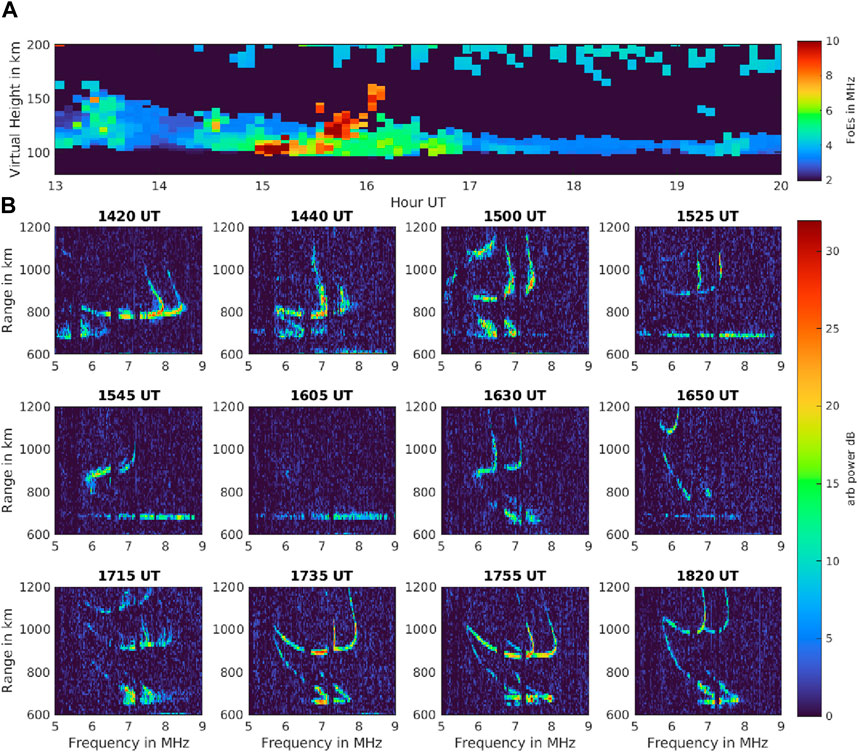
Figure 9. (A) RTF plot from the Ft. Stockton DPS4D on 6 June 2022. (B) [12 panels] Oblique ionograms measured between the Ft. Stockton DPS4D and an HF receiver at LWA-SV. Spread F and blanketing conditions can be observed, coinciding with the passage of intense Es.
3.3.3 19 May 2022
While the 18 May and 6 June events had a discrete
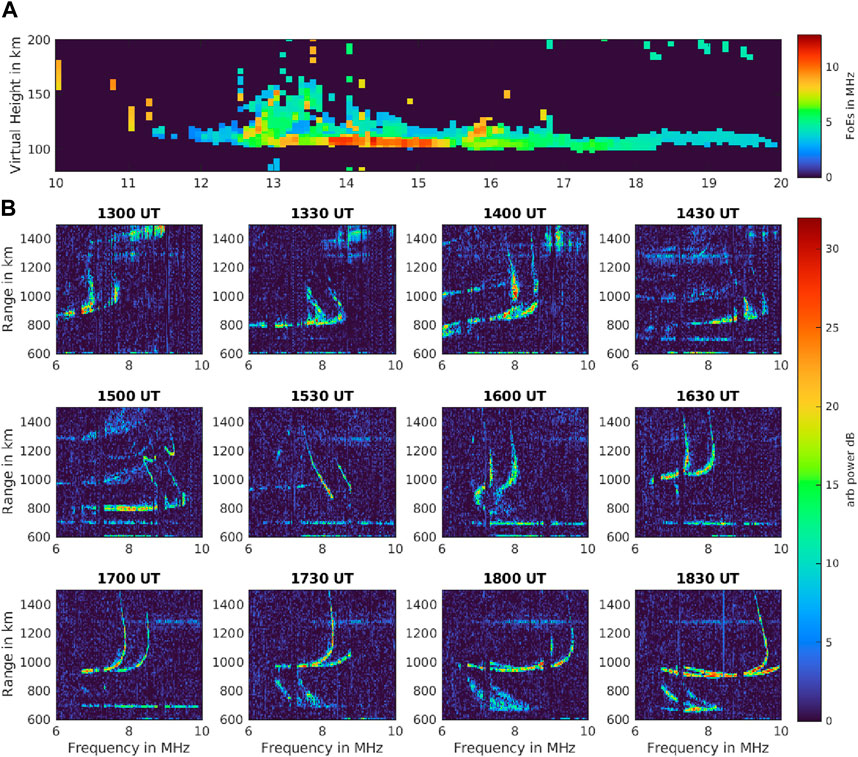
Figure 10. (A) RTF plot from the Ft. Stockton DPS4D on 19 May 2022. (B) [12 panels] Oblique ionograms measured between the Ft. Stockton DPS4D and an HF receiver at LWA-SV. Spread F and blanketing conditions can be observed, coinciding with the occurrence of intense Es.
3.3.4 7 May 2022
The previous observations utilize the vertical Es soundings from the Ft. Stockton DPS4D to identify
Figure 11 shows the geolocation data from both the LWA radio telescopes and the corresponding oblique ionograms from the same time. The red line represents the straight line path between the Ft. Stockton DPS4D and the Sevilleta receiver; the HF propagation can be assumed to follow this path. As shown in the figure, the passage of the intense
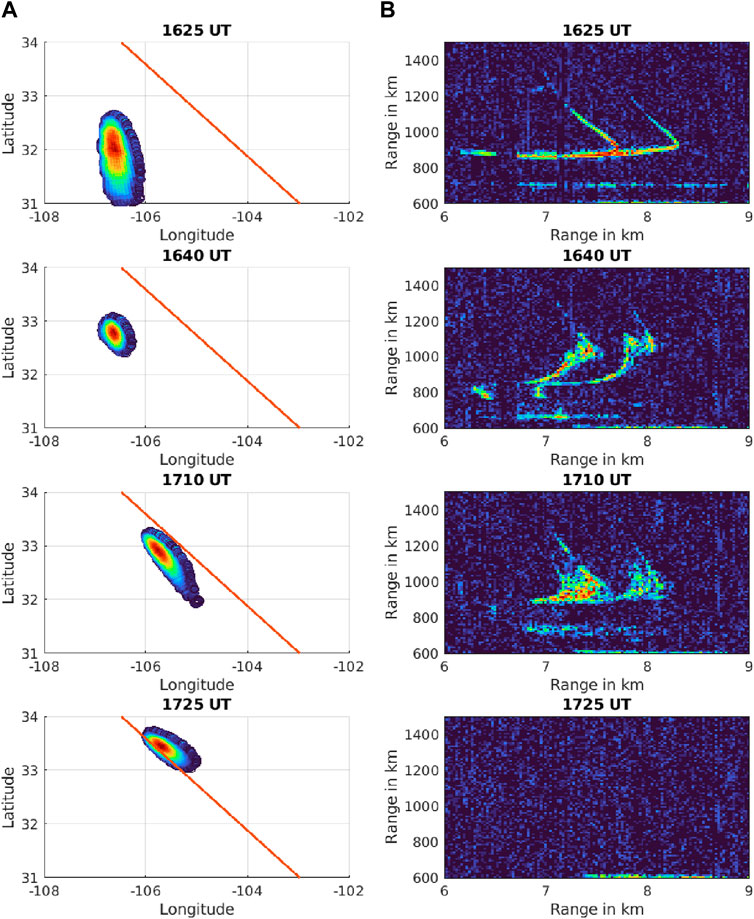
Figure 11. (A) Four snapshots of an intense
Since the LWAs imaged the
4 Discussion
The cases of intense
Comparing VHF coherent scatter measurements of
Alternatively, the corrugation responsible for the off-zenith backscatter (spread
The “V”-shaped structures reported in this study are strikingly similar to those observed by the VHF radars in Patra et al. (2012) and Sun et al. (2020). While these two examples are from VHF observations, it is possible that mid-latitude stations of the Super Dual Auroral Radar Network (SuperDARN) also detect backscatter from intense
The daytime events described by Patra et al. (2012) and Sun et al. (2020) were also coincident with the scintillation of trans-ionospheric RF links. Although no scintillation receivers were deployed during our experiment, spread F conditions were observed to be associated with the
The daytime mid-latitude spread F presented in this study, however, was not caused by these exclusively nighttime events. Assuming that daytime
As discussed in the introduction, KH instabilities almost certainly occur within
Although the majority of the observations presented in this study are too close to the horizon to resolve any front-like shapes, Obenberger et al. (2021) showed that the LWA observed structures that are observed at higher elevation angles are often front-like, similar to the GNSS tomography observations reported by Maeda and Heki (2014). Furthermore, the tens of m/s velocities measured by Obenberger et al. (2021) and this study are consistent with GNSS tomography observations. It is possible that the structures reported in this study and those reported by Maeda and Heki (2014) (and subsequent studies) are caused by KH billows or Ekman spirals. Fortunately, a sufficient number of GNSS receivers were co-located with the observations made by Sun et al. (2020), and not surprisingly, a strong
The structures reported in Maeda and Heki (2014), Patra et al. (2012), Sun et al. (2020), and in this study may be a more general version of the irregularities that trigger the FAIs associated with QP echoes. Comparing the observations of QP echoes with GNSS tomography or a passive VHF system, such as the LWA, would be beneficial for understanding this mechanism. There may be other sources of turbulence within
It is not particularly surprising that
Finally, it is worth discussing exactly what the LWA is observing. Obenberger et al. (2021) concluded that at least some of the emissions detected by the LWA had to originate from broadband RFI generated by devices connected to the electrical grid. This type of RFI is often detected coming from noisy power lines and their components, such as transformers. It is worth questioning, however, whether this is the only source. Platonov and Fleishman (2002) described how a turbulent plasma can radiate broadband RF when suprathermal electrons are present through a process called resonant transition radiation (RTR). In this study, suprathermal electrons with energies on the order of an eV radiate RF as they pass through the regions of changing electron density. Another possibility is that Langmuir waves might be generated and potentially converted to electromagnetic emission. Oppenheim and Dimant (2016) showed that photoelectrons can trigger a bump-on-tail instability in the F1 layer to produce 150 km echoes, often observed by powerful VHF radars perpendicular to the geomagnetic field.
An example of HF/VHF emissions in the E region may have already been found; Obenberger et al. (2014b) and later works have shown that large meteors can produce a broadband glow. Furthermore, Obenberger et al. (2020) examined how anion oxidation could produce a spectrum of suprathermal electrons capable of radiating RTR in a turbulent plasma or possibly even generating Langmuir waves. Given that turbulent
5 Conclusion
In this study, we presented coincident observations of
Data availability statement
The raw data supporting the conclusions of this article will be made available by the authors, without undue reservation.
Author contributions
KO: conceptualization, data curation, formal analysis, funding acquisition, investigation, methodology, project administration, resources, software, supervision, validation, visualization, and writing – original draft. CA: data curation, formal analysis, software, and writing – review and editing. JC: conceptualization, funding acquisition, supervision, and writing – review and editing. ED: conceptualization, data curation, formal analysis, resources, software, and writing – review and editing. JD: data curation, resources, software, and writing – review and editing. JE: conceptualization and writing – review and editing. DE: conceptualization and writing – review and editing. CF: formal analysis, funding acquisition, supervision, validation, and writing – review and editing. JH: funding acquisition, resources, supervision, validation, and writing – review and editing. GT: conceptualization, funding acquisition, resources, supervision, and writing – review and editing.
Funding
The author(s) declare that financial support was received for the research and/or publication of this article. This research was funded by the NASA Space Weather Science Applications Operations 2 Research program under Grant 20-SWO2R20-2-0007. This research was also sponsored in part by the Air Force Office of Scientific Research (AFOSR) Lab Task 23RVCOR002.
Acknowledgments
The authors thank referees for their insightful comments. The authors would like to thank Mickey Perry and Pecos County, Texas, for allowing AFRL to deploy a DPS4D on country property. The authors would also like to thank Dwayne Bonham and Benny Walker of the Pecos County Amateur Radio Club for assistance during the experiment.
Conflict of interest
Author CT was employed by the Fourth State Communications.
The remaining authors declare that the research was conducted in the absence of any commercial or financial relationships that could be construed as a potential conflict of interest.
Generative AI statement
The author(s) declare that no Generative AI was used in the creation of this manuscript.
Publisher’s note
All claims expressed in this article are solely those of the authors and do not necessarily represent those of their affiliated organizations, or those of the publisher, the editors and the reviewers. Any product that may be evaluated in this article, or claim that may be made by its manufacturer, is not guaranteed or endorsed by the publisher.
Author disclaimer
The views expressed are those of the authors and do not reflect the official guidance or position of the United States Government, the Department of Defense, or of the United States Air Force. The appearance of external hyperlinks does not constitute endorsement by the United States Department of Defense (DoD) of the linked websites, or the information, products, or services contained therein. The DoD does not exercise any editorial, security, or other control over the information you may find at these locations.
References
Bernhardt, P. A. (2002). The modulation of sporadic-e layers by kelvin–helmholtz billows in the neutral atmosphere. J. Atmos. Solar-Terrestrial Phys. 64, 1487–1504. doi:10.1016/S1364-6826(02)00086-X
Bui, M. X., Hysell, D. L., and Larsen, M. F. (2023). Midlatitude sporadic e-layer horizontal structuring modulated by neutral instability and mixing in the lower thermosphere. J. Geophys. Res. Space Phys. 128, e2022JA030929. doi:10.1029/2022JA030929
Chu, Y.-H., and Wang, C.-Y. (1997). Interferometry observations of three-dimensional spatial structures of sporadic e irregularities using the chung-li vhf radar. Radio Sci. 32, 817–832. doi:10.1029/96RS03578
Cranmer, M. D., Barsdell, B. R., Price, D. C., Dowell, J., Garsden, H., Dike, V., et al. (2017). Bifrost: a python/c++ framework for high-throughput stream processing in astronomy. J. Astronomical Instrum. 06, 1750007. doi:10.1142/S2251171717500076
Ejiri, M. K., Nakamura, T., Tsuda, T. T., Nishiyama, T., Abo, M., Takahashi, T., et al. (2019). Vertical fine structure and time evolution of plasma irregularities in the es layer observed by a high-resolution ca+ lidar. Earth Planets Space 3, 3. doi:10.1186/s40623-019-0984-z
Ellingson, S. W., Taylor, G. B., Craig, J., Hartman, J., Dowell, J., Wolfe, C. N., et al. (2013). The lwa1 radio telescope. IEEE Trans. Antennas Propag. 61, 2540–2549. doi:10.1109/TAP.2013.2242826
Haldoupis, C., Kelley, M. C., Hussey, G. C., and Shalimov, S. (2003). Role of unstable sporadic-e layers in the generation of midlatitude spread f. J. Geophys. Res. Space Phys. 108. doi:10.1029/2003JA009956
Haldoupis, C., Meek, C., Christakis, N., Pancheva, D., and Bourdillon, A. (2006). Ionogram height–time–intensity observations of descending sporadic e layers at mid-latitude. J. Atmos. solar-terrestrial Phys. 68, 539–557. doi:10.1016/j.jastp.2005.03.020
Hu, L., Yue, X., and Ning, B. (2017). Development of the beidou ionospheric observation network in China for space weather monitoring. Space weather. 15, 974–984. doi:10.1002/2017SW001636
Huba, J. D. (2023). Resolution of the equatorial spread f problem: revisited. Front. Astronomy Space Sci. 9. doi:10.3389/fspas.2022.1098083
Hussey, G. C., Schlegel, K., and Haldoupis, C. (1998). Simultaneous 50-mhz coherent backscatter and digital ionosonde observations in the midlatitude e region. J. Geophys. Res. Space Phys. 103, 6991–7001. doi:10.1029/97JA03089
Hysell, D. L., and Burcham, J. D. (2000). The 30-mhz radar interferometer studies of midlatitude e region irregularities. J. Geophys. Res. Space Phys. 105, 12797–12812. doi:10.1029/1999JA000411
Hysell, D. L., and Larsen, M. F. (2021). Vhf imaging radar observations and theory of banded midlatitude sporadic e ionization layers. J. Geophys. Res. Space Phys. 126, e2021JA029257. doi:10.1029/2021JA029257
Hysell, D. L., Nossa, E., Larsen, M. F., Munro, J., Smith, S., Sulzer, M. P., et al. (2012). Dynamic instability in the lower thermosphere inferred from irregular sporadic e layers. J. Geophys. Res. Space Phys. 117. doi:10.1029/2012JA017910
Hysell, D. L., Nossa, E., Larsen, M. F., Munro, J., Sulzer, M. P., and González, S. A. (2009). Sporadic e layer observations over arecibo using coherent and incoherent scatter radar: Assessing dynamic stability in the lower thermosphere. J. Geophys. Res. Space Phys. 114. doi:10.1029/2009JA014403
Kunduri, B. S. R., Erickson, P. J., Baker, J. B. H., Ruohoniemi, J. M., Galkin, I. A., and Sterne, K. T. (2023). Dynamics of mid-latitude sporadic-e and its impact on hf propagation in the north american sector. J. Geophys. Res. Space Phys. 128. doi:10.1029/2023JA031455
Larsen, M. F. (2000). A shear instability seeding mechanism for quasiperiodic radar echoes. J. Geophys. Res. Space Phys. 105, 24931–24940. doi:10.1029/1990
Maeda, J., and Heki, K. (2014). Two-dimensional observations of midlatitude sporadic e irregularities with a dense gps array in japan. Radio Sci. 49, 28–35. doi:10.1002/2013RS005295
Maeda, J., and Heki, K. (2015). Morphology and dynamics of daytime mid-latitude sporadic-e patches revealed by gps total electron content observations in Japan. Earth Planets Space 67, 89. doi:10.1186/s40623-015-0257-4
Martinis, C., Baumgardner, J., Mendillo, M., Wroten, J., Coster, A., and Paxton, L. (2015). The night when the auroral and equatorial ionospheres converged. J. Geophys. Res. Space Phys. 120, 8085–8095. doi:10.1002/2015JA021555
Obenberger, K., Taylor, G., Hartman, J., Clarke, T., Dowell, J., Dubois, A., et al. (2015). Monitoring the sky with the prototype all-sky imager on the lwa1. J. Astronomical Instrum. 04, 1550004. doi:10.1142/S225117171550004X
Obenberger, K. S., Dowell, J., Fallen, C. T., Holmes, J. M., Taylor, G. B., and Varghese, S. S. (2021). Using broadband radio noise from power-lines to map and track dense Es structures. Radio Sci. 56, e2020RS007169. doi:10.1029/2020RS007169
Obenberger, K. S., Dugick, F. K. D., and Bowman, D. C. (2023). Remote sensing small explosives with an ionospheric radar. Earth Space Sci. 10, e2022EA002737. doi:10.1029/2022EA002737
Obenberger, K. S., Holmes, J. M., Ard, S. G., Dowell, J., Shuman, N. S., Taylor, G. B., et al. (2020). Association between meteor radio afterglows and optical persistent trains. J. Geophys. Res. Space Phys. 125, e2020JA028053. doi:10.1029/2020JA028053
Obenberger, K. S., Taylor, G. B., Hartman, J. M., Dowell, J., Ellingson, S. W., Helmboldt, J. F., et al. (2014b). Detection of radio emission from fireballs. Astrophysical J. 788, L26. doi:10.1088/2041-8205/788/2/l26
Oppenheim, M. M., and Dimant, Y. S. (2016). Photoelectron-induced waves: a likely source of 150 km radar echoes and enhanced electron modes. Geophys. Res. Lett. 43, 3637–3644. doi:10.1002/2016GL068179
Patra, A. K., Chaitanya, P. P., and Bhattacharyya, A. (2012). On the nature of radar backscatter and 250 MHz scintillation linked with an intense daytime Es patch. J. Geophys. Res. Space Phys. 117. doi:10.1029/2011JA016981
Platonov, K. Y., and Fleishman, G. D. (2002). Transition radiation in media with random inhomogeneities. Physics-Uspekhi 45, 235–291. doi:10.1070/pu2002v045n03abeh000952
Reinisch, B. W., Galkin, I. A., Khmyrov, G. M., Kozlov, A. V., Bibl, K., Lisysyan, I. A., et al. (2009). New digisonde for research and monitoring applications. Radio Sci. 44. doi:10.1029/2008RS004115
Reinisch, B. W., Galkin, I. A., Khmyrov, G. M., Kozlov, A. V., Lisysyan, I. A., Bibl, K., et al. (2008). Advancing digisonde technology: the dps-4d. AIP Conf. Proc. 974, 127–143. doi:10.1063/1.2885022
Saito, S., Yamamoto, M., Hashiguchi, H., and Maegawa, A. (2006). Observation of three-dimensional structures of quasi-periodic echoes associated with mid-latitude sporadic-e layers by mu radar ultra-multi-channel system. Geophys. Res. Lett. 33. doi:10.1029/2005GL025526
Sun, W., Ning, B., Hu, L., Yue, X., Zhao, X., Lan, J., et al. (2020). The evolution of complex Es observed by multi instruments over low-latitude China. J. Geophys. Res. Space Phys. 125, e2019JA027656. doi:10.1029/2019JA027656
Sun, W., Ning, B., Yue, X., Li, G., Hu, L., Chang, S., et al. (2018). Strong sporadic e occurrence detected by ground-based gnss. J. Geophys. Res. Space Phys. 123, 3050–3062. doi:10.1002/2017JA025133
Taylor, C. A., Dowell, J., Taylor, G. B., Obenberger, K. S., Chastain, S. I., Verastegui, J., et al. (2025). Design and commissioning of an lwa swarm station: the long wavelength array – north arm. Journal of Astronomical Instrumentation under review.
Tsunoda, R. T., and Cosgrove, R. B. (2001). Coupled electrodynamics in the nighttime midlatitude ionosphere. Geophys. Res. Lett. 28, 4171–4174. doi:10.1029/2001GL013245
Varghese, S. S., Dowell, J., Obenberger, K. S., Taylor, G. B., and Malins, J. (2021). Broadband imaging to study the spectral distribution of meteor radio afterglows. J. Geophys. Res. Space Phys. 126, e2021JA029296. doi:10.1029/2021JA029296
Woodman, R. F. (2009). Spread f – an old equatorial aeronomy problem finally resolved? Ann. Geophys. 27, 1915–1934. doi:10.5194/angeo-27-1915-2009
Keywords: sporadic E, Kelvin–Helmholtz instability, mid-latitude spread F, HF propagation, ionosonde, ionosphere
Citation: Obenberger KS, Taylor CA, Colman JJ, Dao E, Dowell J, Eccles JV, Emmons DJ, Fallen CT, Holmes JM and Taylor GB (2025) Simultaneous observations of irregular sporadic E structures using the LWA and a DPS4D. Front. Astron. Space Sci. 12:1564037. doi: 10.3389/fspas.2025.1564037
Received: 20 January 2025; Accepted: 28 March 2025;
Published: 29 April 2025.
Edited by:
Evgeny V. Mishin, Boston College, United StatesReviewed by:
Eliana Nossa, The Aerospace Corporation, United StatesBingkun Yu, Deep Space Exploration Laboratory, China
Copyright © 2025 Obenberger, Taylor, Colman, Dao, Dowell, Eccles, Emmons, Fallen, Holmes and Taylor. This is an open-access article distributed under the terms of the Creative Commons Attribution License (CC BY). The use, distribution or reproduction in other forums is permitted, provided the original author(s) and the copyright owner(s) are credited and that the original publication in this journal is cited, in accordance with accepted academic practice. No use, distribution or reproduction is permitted which does not comply with these terms.
*Correspondence: K. S. Obenberger, YWZybC5ydmJvcmdtYWlsYm94QHVzLmFmLm1pbA==