- 1NASA Goddard Space Flight Center, Greenbelt, MD, United States
- 2Department of Astronomy, University of Maryland, College Park, MD, United States
- 3Heliophysics Science Division, NASA Goddard Space Flight Center, Greenbelt, MD, United States
- 4Department of Physics and Astornomy, University of Leicester, Leicester, United Kingdom
- 5LATMOS-IPSL, CNRS, UVSQ Paris-Saclay, Sorbonne Université, Guyancourt, France
Highly charged ions in the solar wind undergo charge exchange with neutral atoms in the Earth’s exosphere, particularly within the magnetosheath and cusps. This solar wind charge exchange process generates X-rays, which are expected to be crucial for imaging Earth’s dayside magnetosphere in the upcoming Lunar Environment Heliospheric X-ray Imager (LEXI) and Solar Wind Magnetosphere Ionosphere Link Explorer (SMILE) missions. A key parameter in this process is the density of neutral hydrogen in the Earth’s exosphere. This study estimates the exospheric density during solar maximum using soft X-ray data from the XMM-Newton astrophysics observatory. We used the Open Geospace Global Circulation Model (OpenGGCM), a global MHD model, to calculate plasma density, velocity, and temperature, and then extracted the exospheric density from the soft X-ray data by deconvolving plasma contributions. Based on five XMM-Newton observations during the solar maximum period from 2000 to 2003, we estimate the exospheric density at the 10
1 Introduction
The interaction between the solar wind and Earth’s magnetospheric system is an important topic in heliophysics. Many fundamental plasma physics processes occurring near Earth, including magnetic reconnection, have been studied primarily using in situ measurements. While these in situ measurements from heliophysics space observatories have revealed details of local-scale magnetopause reconnection properties (Escoubet et al., 2001; Angelopoulos, 2009; Burch et al., 2016), the global characteristics of dayside reconnection and its impact on the geospace system remain unclear. Recently, an innovative technique for visualizing Earth’s magnetospheric system using soft (0.1–2.0 keV) X-ray emission has been proposed (Collier et al., 2009). The Lunar Environment Heliospheric X-ray Imager (LEXI; Walsh et al., 2024) and the Solar wind–Magnetosphere–Ionosphere Link Explorer (SMILE; Branduardi-Raymont et al., 2018) are designed to utilize this technique.
When encountering Earth, the incoming solar wind slows and diverts around the magnetosphere after passing through the bow shock, eventually reaching the magnetopause, which is typically located around 10 Earth radii (
Highly charged solar wind ions, such as
In this study, we selected five observations during solar maximum from the XMM-Newton data archive to estimate neutral densities at the 10
In Section 2, we describe our methodology for extracting exospheric density. Section 3 presents the details of our analysis for one of the events and the results from the full sample. In Section 4, we compare our findings with previous studies and discuss limitations. Finally, Section 5 summarizes our study.
2 Methodology
We estimated the exospheric neutral density at the 10
XMM-Newton (hereafter XMM) follows a highly elliptical orbit with an apogee of 114,000 km, a perigee of 7,000 km, an orbital inclination of approximately −40
For event selection, we utilized a list of XMM observations affected by exospheric SWCX emissions (Carter et al., 2011). From this list, we searched for observations where XMM captured SWCX emissions from the dayside magnetosheath under stable solar wind and interplanetary magnetic field (IMF) conditions—i.e., conditions under which a global MHD model provides reliable predictions of the dayside magnetosheath. We excluded observations with bright or extended X-ray sources, particularly those larger than 500 arcseconds in the XMM FOV, as their signals may persist even after filtering and contaminate near-Earth SWCX signals. Additionally, we discarded soft proton flaring observations, in which energetic protons directly enter the telescope and contaminate the entire X-ray dataset, including SWCX signals (Walsh et al., 2014). After this rigorous selection process, five XMM observations were chosen. For more details on the selection process, refer to Jung et al. (2022). The selected events span from 2000 to 2003, and their corresponding XMM observation numbers are listed in Table 1, along with the estimated neutral density at 10
XMM’s primary objective is to study cosmic X-ray sources, and astrophysicists typically remove various background signals from the observed data. These background signals fall into three categories: photons, particles, and electronic noise (Read and Ponman, 2003; Carter and Read, 2007). The photon background includes astrophysical sources, such as thermal emission and emission from unresolved cosmological sources, as well as SWCX from both the heliosphere and exosphere. In our study, we utilized the SWCX background components from the exosphere to estimate
To obtain the SWCX count rates
We simulated magnetosheath conditions—specifically plasma number density, velocity, and temperature—for each event using the OpenGGCM MHD model. OpenGGCM simulates the interaction between the solar wind and Earth’s magnetosphere by solving resistive MHD equations on a stretched Cartesian grid. The model’s inputs are solar wind parameters and IMF data from a solar wind monitor, while its outputs include plasma density, velocity, temperature, and electromagnetic fields within the simulation domain. The simulation domain in this study extends from 25
We derived the exospheric neutral density (
where
Here,
The term
In deriving Equation 1, Jung et al. (2022) assumed that the exospheric neutral density is spherically symmetric and inversely proportional to the cube of the radial distance from Earth:
3 XMM case studies
Figure 1A shows the location of XMM on 23 July 2000 when the telescope FOV passes through the dayside magnetosheath. The locations of the magnetopause (red) and bow shock (yellow) were calculated using the models of Shue et al. (1998) and Jelínek et al. (2012), respectively, based on solar wind/IMF conditions from NASA OMNI data. XMM started at the orange dot and moved slightly along the short blue line (almost invisible due to the small motion of XMM During the period of interest), and the black line denotes telescope pointing. During the period of our interest, XMM moved from the coordinates (10.3, −3.5, −15.0) to (10.7, −2.4, −15.5)
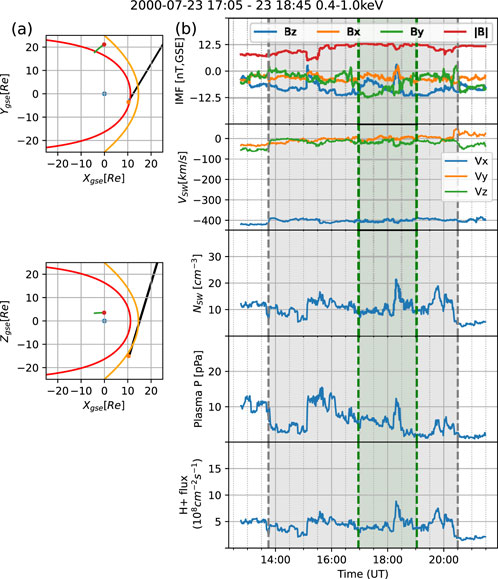
Figure 1. (a) XMM location (blue line starting from orange dot), its pointing direction (black), and the Geotail orbit (green) projected on the GSE XY (top) and XZ (bottom) planes, on 23 July 2000. The starting location of XMM and Geotail are shown as orange and red dots, respectively. The yellow and red curves show the bow shock (Jelínek et al., 2012) and the magnetopause (Shue et al., 1998), respectively. (b) Solar wind and IMF conditions during the XMM observation (green area) and during the Geotail magnetosheath crossing (gray area), obtained from OMNI data. The IMF, solar wind velocity, number density, plasma pressure (=nkT), and solar wind proton flux
We ran an OpenGGCM simulation to calculate the potential reaction rate Q. To validate the model result, we compared the OpenGGCM results with Geotail (Nishida, 1994) in situ measurements in Figure 2. In top three panels, plasma number density
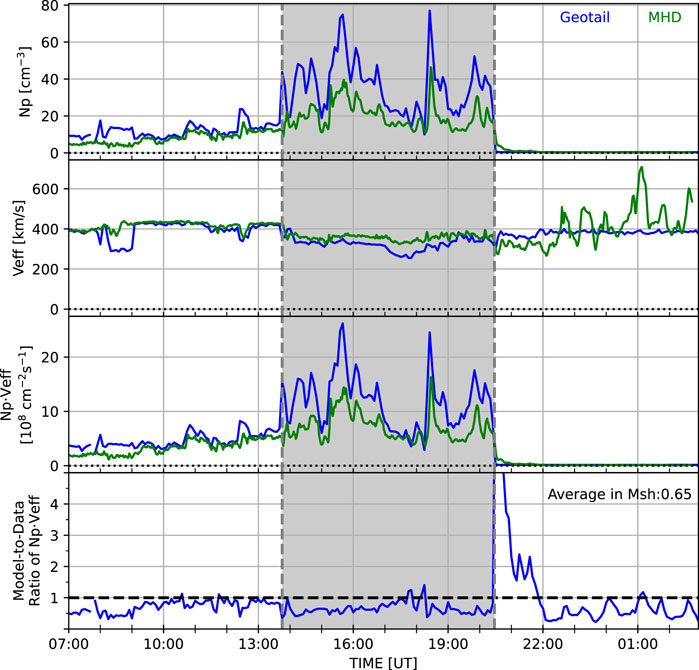
Figure 2. Comparison of the OpenGGCM results (green) with the geotail plasma observation (blue) on 23 July 2000. From top to bottom, the IMF, solar wind velocity, density, plasma pressure, and proton flux are shown. The gray shaded area indicates when Geotail passed through the magnetosheath. The dashed horizontal black line in the bottom panel shows where the model-to-data ratio equals 1.
We also estimated the error in Q due to differences between the modeled and observed magnetosheath boundaries. If the simulated magnetosheath is thicker (or thinner) than observed, the instrument’s line of sight (LOS) within the magnetosheath will be extended (or shortened), resulting in the accumulation of more (or fewer) SWCX photons along the LOS, which could lead to an overestimation or underestimation of Q. We applied the technique of Jung et al. (2022) to calculate this error in Q. This technique adjusts the locations of the magnetopause and bow shock based on boundary crossing observations, and estimates the largest error in Q by calculating the discrepancy between Q values obtained using modeled and adjusted magnetosheath. Figure 2 shows that the boundary crossings of Geotail, marked by the endpoints of the gray shaded area, matches well with the model predictions. As a result, the maximum error in Q for this event was 1.1%.
Figure 3 presents the solar wind flux, the modeled potential reaction rate
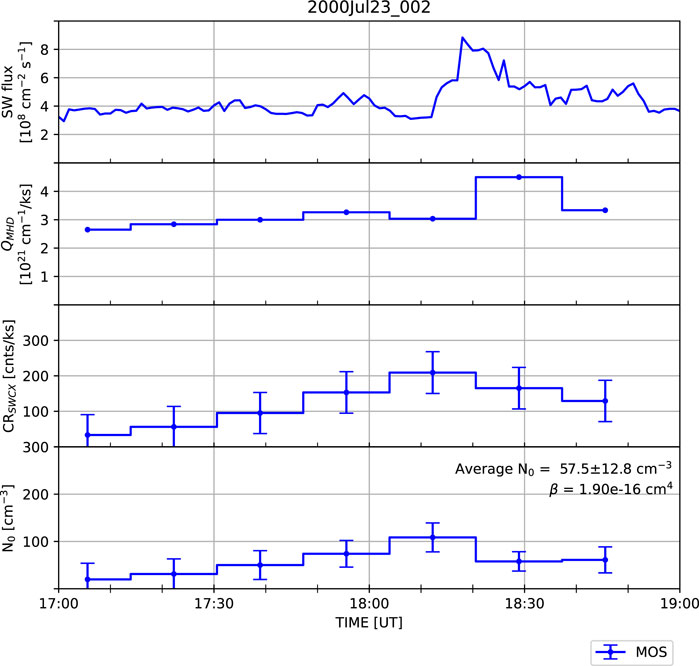
Figure 3. Neutral density estimates for 23 July 2000 event. From top to bottom, solar wind flux, modeled potential reaction rate
The error in
By applying this approach, we obtained the average neutral density of 60.8
In the first panel of Figure 3, the solar wind flux was mostly steady, with an increase around 18:20 UT. Potential reaction rate Q also follows the same pattern, as expected in Equation 2. SWCX count rates show a similar pattern but the increase happens a bit earlier, around 18:05 UT. The discrepancy between the peaks of
We applied similar techniques to four additional XMM observations. Table 1 shows these observation dates, XMM observation number, effective scale factor
4 Discussion
Our exospheric neutral density estimates are consistent with previous X-ray studies. Connor and Carter (2019) derived neutral densities at 10
Recent Lyman-
However, our results differ from those based on Energetic Neutral Atom (ENA) observations. ENAs form when solar wind protons exchange electrons with exospheric hydrogen atoms. Fuselier et al. (2010), Fuselier et al. (2020) derived neutral densities at 10
The influence of solar activity on exospheric density remains an active area of investigation. Geocoronal observations from the Two Wide-angle Imaging Neutral-atom Spectrometers (TWINS) spacecraft suggest higher densities at 3–8
Our study builds on Jung et al. (2022), who derived a density of 36.8
These results indicate a trend of higher exospheric densities during solar maximum compared to solar minimum, consistent with TWINS observations. However, given the overlapping error bars, the difference between solar maximum and minimum values appears modest, suggesting a minimal solar cycle impact on exospheric density. Further statistical studies are needed to confirm variations in neutral density across the solar cycle.
5 Conclusion
We estimated exospheric neutral density at the subsolar location at 10
The soft X-ray telescopes onboard the LEXI and SMILE missions will study the global interaction between the solar wind and Earth’s magnetospheric system by imaging the magnetosheath boundary and its motion in soft X-rays. The density of exospheric hydrogen is a critical parameter influencing the strength of magnetosheath SWCX signals. Numerical simulations by Sibeck et al. (2018) demonstrated that magnetosheath SWCX signals can be readily distinguished from other soft X-ray background emission if the exospheric density is at least 22
Previous mission studies (e.g., Connor et al., 2021; Sun et al., 2019; Samsonov et al., 2023) have commonly used 25
Data availability statement
The datasets presented in this study can be found in online repositories. The names of the repository/repositories and accession number(s) can be found below: https://github.com/jjung11/XMM2025.
Author contributions
JJ: Conceptualization, Data curation, Formal Analysis, Investigation, Methodology, Project administration, Resources, Software, Validation, Visualization, Writing – original draft, Writing – review and editing. HC: Conceptualization, Funding acquisition, Project administration, Supervision, Writing – review and editing. JC: Conceptualization, Data curation, Methodology, Supervision, Validation, Writing – review and editing. DK: Resources, Writing – review and editing. CP: Methodology, Resources, Writing – review and editing.
Funding
The author(s) declare that financial support was received for the research and/or publication of this article. This work was supported by the NSF grant AGS-1928883 and the NASA grants, 80NSSC18K1043 and 80NSSC20K1670, 80MSFC20C0019, WBS 516741.01.24.01.03, and WBS 791926.02.04.02.26. JAC is supported by Royal Society grant DHF∖R1∖211,068.
Conflict of interest
The authors declare that the research was conducted in the absence of any commercial or financial relationships that could be construed as a potential conflict of interest.
Generative AI statement
The author(s) declare that no Gen AI was used in the creation of this manuscript.
Publisher’s note
All claims expressed in this article are solely those of the authors and do not necessarily represent those of their affiliated organizations, or those of the publisher, the editors and the reviewers. Any product that may be evaluated in this article, or claim that may be made by its manufacturer, is not guaranteed or endorsed by the publisher.
References
Baliukin, I. I., Bertaux, J.-L., Quémerais, E., Izmodenov, V., and Schmidt, W. (2019). Swan/soho lyman-α mapping: the hydrogen geocorona extends well beyond the moon. J. Geophys. Res. Space Phys. 124, 861–885. doi:10.1029/2018ja026136
Branduardi-Raymont, G., Wang, C., Dai, L., Donovan, E., Li, L., and Sembay, S. (2018). Smile definition study report. ESA/SCI 2018, 1. doi:10.5270/esa.smile.definition_study_report-2018-12
Burch, J., Moore, T., Torbert, R., and Giles, B.-h. (2016). Magnetospheric multiscale overview and science objectives. Space Sci. Rev. 199, 5–21. doi:10.1007/978-94-024-0861-4_2
Carter, J., and Read, A. (2007). The xmm-Newton epic background and the production of background blank sky event files. Astronomy and Astrophysics 464, 1155–1166. doi:10.1051/0004-6361:20065882
Carter, J., Sembay, S., and Read, A. (2010). A high charge state coronal mass ejection seen through solar wind charge exchange emission as detected by xmm–Newton. Mon. Notices R. Astronomical Soc. 402, 867–878. doi:10.1111/j.1365-2966.2009.15985.x
Carter, J., Sembay, S., and Read, A. (2011). Identifying xmm-Newton observations affected by solar wind charge exchange–part ii. Astronomy and Astrophysics 527, A115. doi:10.1051/0004-6361/201015817
Collier, M., Abbey, T., Bannister, N., Carter, J., Choi, M., Cravens, T., et al. (2009). The lunar x-ray observatory (lxo)/magnetosheath explorer in x-rays (magex). AIP Conf. Proc. Am. Inst. Phys. 1156, 105–111. doi:10.1063/1.3211802
Connor, H., and Carter, J. A. (2019). Exospheric neutral hydrogen density at the nominal 10 RE subsolar point deduced from XMM-Newton X-ray observations. J. Geophys. Res. Space Phys. 124, 1612–1624. doi:10.1029/2018ja026187
Connor, H., Raeder, J., Sibeck, D., and Trattner, K. (2015). Relation between cusp ion structures and dayside reconnection for four imf clock angles: openggcm-ltpt results. J. Geophys. Res. Space Phys. 120, 4890–4906. doi:10.1002/2015ja021156
Connor, H., Raeder, J., and Trattner, K. (2012). Dynamic modeling of cusp ion structures. J. Geophys. Res. Space Phys. 117. doi:10.1029/2011ja017203
Connor, H., Sibeck, D., Collier, M., Baliukin, I., Branduardi-Raymont, G., Brandt, P., et al. (2021). Soft x-ray and ena imaging of the earth’s dayside magnetosphere. J. Geophys. Res. Space Phys. 126, e2020JA028816. doi:10.1029/2020ja028816
Connor, H., Zesta, E., Fedrizzi, M., Shi, Y., Raeder, J., Codrescu, M. V., et al. (2016). Modeling the ionosphere-thermosphere response to a geomagnetic storm using physics-based magnetospheric energy input: openggcm-ctim results. J. Space Weather Space Clim. 6, A25. doi:10.1051/swsc/2016019
Connor, H., Zesta, E., Ober, D., and Raeder, J. (2014). The relation between transpolar potential and reconnection rates during sudden enhancement of solar wind dynamic pressure: openggcm-ctim results. J. Geophys. Res. Space Phys. 119, 3411–3429. doi:10.1002/2013ja019728
Cramer, W. D., Raeder, J., Toffoletto, F., Gilson, M., and Hu, B. (2017). Plasma sheet injections into the inner magnetosphere: two-way coupled openggcm-rcm model results. J. Geophys. Res. Space Phys. 122, 5077–5091. doi:10.1002/2017ja024104
Cravens, T., Robertson, I., and Snowden, S. (2001). Temporal variations of geocoronal and heliospheric x-ray emission associated with the solar wind interaction with neutrals. J. Geophys. Res. Space Phys. 106, 24883–24892. doi:10.1029/2000ja000461
Cucho-Padin, G., Kameda, S., and Sibeck, D. G. (2022). The earth’s outer exospheric density distributions derived from procyon/laica uv observations. J. Geophys. Res. Space Phys. 127, e2021JA030211. doi:10.1029/2021ja030211
de la Calle, I. (2021). Users guide of the xmm-Newton science analysis system. 16.0, 2021 (ESA: XMM-Newton SOC)
Dimmock, A., and Nykyri, K. (2013). The statistical mapping of magnetosheath plasma properties based on themis measurements in the magnetosheath interplanetary medium reference frame. J. Geophys. Res. Space Phys. 118, 4963–4976. doi:10.1002/jgra.50465
Escoubet, C., Fehringer, M., and Goldstein, M. (2001). IntroductionThe cluster mission. Ann. Geophys. Copernic. GmbH 19, 1197–1200. doi:10.5194/angeo-19-1197-2001
Ferdousi, B., and Raeder, J. (2016). Signal propagation time from the magnetotail to the ionosphere: openggcm simulation. J. Geophys. Res. Space Phys. 121, 6549–6561. doi:10.1002/2016ja022445
Ferdousi, B., Raeder, J., Zesta, E., Cramer, W., and Murphy, K. (2021). Association of auroral streamers and bursty bulk flows during different states of the magnetotail: a case study. J. Geophys. Res. Space Phys. 126, e2021JA029329. doi:10.1029/2021ja029329
Fuselier, S., Dayeh, M., Galli, A., Funsten, H., Schwadron, N., Petrinec, S., et al. (2020). Neutral atom imaging of the solar wind-magnetosphere-exosphere interaction near the subsolar magnetopause. Geophys. Res. Lett. 47, e2020GL089362. doi:10.1029/2020gl089362
Fuselier, S., Funsten, H., Heirtzler, D., Janzen, P., Kucharek, H., McComas, D., et al. (2010). Energetic neutral atoms from the earth’s subsolar magnetopause. Geophys. Res. Lett. 37. doi:10.1029/2010gl044140
Jansen, F., Lumb, D., Altieri, B., Clavel, J., Ehle, M., Erd, C., et al. (2001). Xmm-Newton observatory-i. the spacecraft and operations. Astronomy and Astrophysics 365, L1–L6. doi:10.1051/0004-6361:20000036
Jelínek, K., Němeček, Z., and Šafránková, J. (2012). A new approach to magnetopause and bow shock modeling based on automated region identification. J. Geophys. Res. Space Phys. 117. doi:10.1029/2011ja017252
Jensen, J. B., Raeder, J., Maynard, K., and Cramer, W. D. (2017). Particle precipitation effects on convection and the magnetic reconnection rate in earth’s magnetosphere. J. Geophys. Res. Space Phys. 122, 11–413. doi:10.1002/2017ja024030
Jung, J., Connor, H., Dimmock, A., Sembay, S., Read, A., and Soucek, J. (2023). Mshpy23: a user-friendly, parameterized model of magnetosheath conditions. Phys. Earth Planet. Interiors 8, 89–104. doi:10.26464/epp2023065
Jung, J., Connor, H. K., Carter, J. A., Koutroumpa, D., Pagani, C., and Kuntz, K. (2022). Solar minimum exospheric neutral density near the subsolar magnetopause estimated from the xmm soft x-ray observations on 12 november 2008. J. Geophys. Res. Space Phys. 127, e2021JA029676. doi:10.1029/2021ja029676
Kameda, S., Ikezawa, S., Sato, M., Kuwabara, M., Osada, N., Murakami, G., et al. (2017). Ecliptic north-south symmetry of hydrogen geocorona. Geophys. Res. Lett. 44, 11–706. doi:10.1002/2017gl075915
Kavosi, S., Spence, H., Fennell, J., Turner, D., Connor, H., and Raeder, J. (2018). Mms/feeps observations of electron microinjections due to kelvin-helmholtz waves and flux transfer events: a case study. J. Geophys. Res. Space Phys. 123, 5364–5378. doi:10.1029/2018ja025244
King, J., and Papitashvili, N. (2005). Solar wind spatial scales in and comparisons of hourly wind and ace plasma and magnetic field data. J. Geophys. Res. Space Phys. 110. doi:10.1029/2004ja010649
Koutroumpa, D., Lallement, R., Kharchenko, V., Dalgarno, A., Pepino, R., Izmodenov, V., et al. (2006). Charge-transfer induced euv and soft x-ray emissions in the heliosphere. Astronomy and Astrophysics 460, 289–300. doi:10.1051/0004-6361:20065250
[Dataset] Nishida, A. (1994). The geotail mission. Geophys. Res. Lett. 21, 2871–2873. doi:10.1029/94gl01223
Oliveira, D. M., and Raeder, J. (2015). Impact angle control of interplanetary shock geoeffectiveness: a statistical study. J. Geophys. Res. Space Phys. 120, 4313–4323. doi:10.1002/2015ja021147
Raeder, J., Larson, D., Li, W., Kepko, E. L., and Fuller-Rowell, T. (2008). Openggcm simulations for the themis mission. Space Sci. Rev. 141, 535–555. doi:10.1007/s11214-008-9421-5
Raeder, J., McPherron, R., Frank, L., Kokubun, S., Lu, G., Mukai, T., et al. (2001). Global simulation of the geospace environment modeling substorm challenge event. J. Geophys. Res. Space Phys. 106, 381–395. doi:10.1029/2000ja000605
Read, A. M., and Ponman, T. J. (2003). The xmm-Newton epic background: production of background maps and event files. Astronomy and Astrophysics 409, 395–410. doi:10.1051/0004-6361:20031099
Samsonov, A., Branduardi-Raymont, G., Sembay, S., Read, A., Sibeck, D., and Rastaetter, L. (2023). Simulation of the smile soft x-ray imager response to a southward interplanetary magnetic field turning. Earth Planet. Phys. 8, 39–46. doi:10.26464/epp2023058
Shi, Y., Zesta, E., Connor, H., Su, Y.-J., Sutton, E., Huang, C., et al. (2017). High-latitude thermosphere neutral density response to solar wind dynamic pressure enhancement. J. Geophys. Res. Space Phys. 122, 11–559. doi:10.1002/2017ja023889
Shue, J.-H., Song, P., Russell, C., Steinberg, J., Chao, J., Zastenker, G., et al. (1998). Magnetopause location under extreme solar wind conditions. J. Geophys. Res. Space Phys. 103, 17691–17700. doi:10.1029/98ja01103
Sibeck, D., Collier, M., and Silveira, M. (2021). Neutral densities in the outer exosphere near the subsolar magnetopause. Geophys. Res. Lett. 48, e2021GL093383. doi:10.1029/2021gl093383
Sibeck, D. G., Allen, R., Aryan, H., Bodewits, D., Brandt, P., Branduardi-Raymont, G., et al. (2018). Imaging plasma density structures in the soft x-rays generated by solar wind charge exchange with neutrals. Space Sci. Rev. 214, 79–124. doi:10.1007/s11214-018-0504-7
Snowden, S., Collier, M., and Kuntz, K. (2004). Xmm-Newton observation of solar wind charge exchange emission. Astrophysical J. 610, 1182–1190. doi:10.1086/421841
Sun, T., Wang, C., Sembay, S., Lopez, R., Escoubet, C., Branduardi-Raymont, G., et al. (2019). Soft x-ray imaging of the magnetosheath and cusps under different solar wind conditions: mhd simulations. J. Geophys. Res. Space Phys. 124, 2435–2450. doi:10.1029/2018ja026093
Turner, M. J., Abbey, A., Arnaud, M., Balasini, M., Barbera, M., Belsole, E., et al. (2001). The european photon imaging camera on xmm-Newton: the mos cameras. Astronomy and Astrophysics 365, L27–L35. doi:10.1051/0004-6361:20000087
Walsh, B., Kuntz, K., Busk, S., Cameron, T., Chornay, D., Chuchra, A., et al. (2024). The lunar environment heliophysics x-ray imager (lexi) mission. Space Sci. Rev. 220, 37–27. doi:10.1007/s11214-024-01063-4
Walsh, B., Kuntz, K., Collier, M., Sibeck, D., Snowden, S., and Thomas, N. (2014). Energetic particle impact on x-ray imaging with xmm-Newton. Space weather. 12, 387–394. doi:10.1002/2014sw001046
Whittaker, I. C., and Sembay, S. (2016). A comparison of empirical and experimental o7+, o8+, and o/h values, with applications to terrestrial solar wind charge exchange. Geophys. Res. Lett. 43, 7328–7337. doi:10.1002/2016gl069914
Zhang, Y., Sun, T., Wang, C., Ji, L., Carter, J. A., Sembay, S., et al. (2022). Solar wind charge exchange soft x-ray emissions in the magnetosphere during an interplanetary coronal mass ejection compared to its driven sheath. Astrophysical J. Lett. 932, L1. doi:10.3847/2041-8213/ac7521
Zoennchen, J., Nass, U., and Fahr, H. (2015). Terrestrial exospheric hydrogen density distributions under solar minimum and solar maximum conditions observed by the twins stereo mission. Ann. Geophys. Copernic. GmbH 33, 413–426. doi:10.5194/angeo-33-413-2015
Keywords: exospheric neutral density, solar maximum, XMM-Newton observations, soft X-ray emission, solar wind charge exchange
Citation: Jung J, Connor HK, Carter JA, Koutroumpa D and Pagani C (2025) Exospheric neutral density at the 10
Received: 30 January 2025; Accepted: 26 March 2025;
Published: 17 April 2025.
Edited by:
Orenthal Tucker, Goddard Space Flight Center, National Aeronautics and Space Administration, United StatesReviewed by:
Thomas Cravens, University of Kansas, United StatesTianran Sun, Chinese Academy of Sciences (CAS), China
Copyright © 2025 Jung, Connor, Carter, Koutroumpa and Pagani. This is an open-access article distributed under the terms of the Creative Commons Attribution License (CC BY). The use, distribution or reproduction in other forums is permitted, provided the original author(s) and the copyright owner(s) are credited and that the original publication in this journal is cited, in accordance with accepted academic practice. No use, distribution or reproduction is permitted which does not comply with these terms.
*Correspondence: Jaewoong Jung, amFld29vbmcuanVuZ0B1cmkuZWR1
†Present address: Jaewoong Jung, Department of Physics, University or Rhode Island, Kingston, RI, United States