- Department of Biochemistry and Molecular Biology, Colorado State University, Fort Collins, CO, United States
Platelets are anucleate cellular fragments derived from megakaryocytes (MKs) and α-granules constitute their most numerous membrane-bound compartments. These granules play a role in platelet aggregation to form a hemostatic plug but also contain numerous cargo proteins with key functions in angiogenesis, inflammation, wound healing and cancer. Human genetic disorders that cause deficiencies in the biogenesis of platelet α-granules manifest with prolonged bleeding. The initial studies on platelets and MKs from these patients provided a first glimpse into the biosynthesis of α-granules as a membrane trafficking problem. Significant progress in the field has been made in recent years in part due to the creation of iPSC-derived megakaryocytic cells capable of releasing functional platelets, thus overcoming the limitations of working with primary MKs. The emerging model indicates that sorting and recycling endosomes are key intermediate stations traversed by α-granule cargo on their way to the α-granule. Here we describe the different trafficking pathways used by α-granule proteins and elaborate on their commonalities. Similar to other lysosome-related organelles, most of the proteins involved in the biogenesis of α-granules are ubiquitously expressed and we discuss NBEAL2 as a factor highly expressed in MKs that likely diverts this machinery to make α-granules. Importantly, understanding the trafficking pathways involved in the making of the α-granule has an impact not only on platelet biology but may also illuminate the broader lysosome-related organelle field.
Introduction
Platelets are anucleate cells fundamental for normal hemostasis that contain two types of lysosome-related organelles (LROs): α- and dense (δ-) granules (Blair and Flaumenhaft, 2009). Platelets exert their function in part by releasing the content of these granules in a regulated fashion. (Joshi and Whiteheart, 2017; Jonnalagadda et al., 2012). Each platelet has only 2-8 δ-granules that carry polyphosphate and small molecules such as serotonin, adenosine diphosphate (ADP), adenosine triphosphate (ATP), calcium and zinc (Frojmovic and Milton, 1982; Muller et al., 2009). Alpha-granules on the other hand are more numerous, averaging 40 per platelet, and they contain a variety of protein cargo including not only factors involved in blood clotting (e.g., von Willebrand Factor (vWF) and Factor V (FV)), but also in angiogenesis (e.g., Vascular Endothelial Growth Factor (VEGF), Endostatin, Thrombospondin-1 and Platelet Factor 4 (PF4), inflammation (chemokines such as CXCL1 and Interleukin-8), wound healing (e.g., VEGF and Fibroblast Growth Factor) and cancer (e.g., Platelet-Derived Growth Factor (PDGF), VEGF and RANTES) (Blair and Flaumenhaft, 2009; Frojmovic and Milton, 1982; Pluthero and Kahr, 2023; Sharda and Flaumenhaft, 2018).
Platelets derive from megakaryocytes (MKs), which primarily reside in the bone marrow, and it is in these cells that both α- and δ-granules are synthesized and packaged into proplatelets that are subsequently released into circulation (Patel et al., 2005; Italiano et al., 1999; Hartwig and Italiano, 2003). Primary MKs are not amenable for either cell biology or biochemical analyses, which has been a major obstacle in the study of platelet granule biogenesis. In 2014 however, the creation of an iPSC-derived megakaryocytic cell line (imMKCL) that recapitulates the biogenesis of platelet granules provided a new tool to the field (Nakamura et al., 2014; Ito et al., 2018). Since then, new players and pathways have been discovered and the sorting and recycling endosomes in MKs have been established as key hubs of the α-granule biosynthesis machine (Ambrosio et al., 2022; Ambrosio and Di Pietro, 2019; Lo et al., 2020).
Platelet α-granules are round or ellipsoidal, 200–500 nm in diameter, their lumen is acidic, with an average pH of 5.2, and they contain a heterogeneous population of protein cargo (Italiano et al., 2008; Sehgal and Storrie, 2007; Yao and Kahr, 2025; Pokrovskaya et al., 2020). Both luminal and transmembrane α-granule proteins are either synthesized by the MK, such as P-Selectin, PF4 and vWF, or endocytosed like Fibrinogen, FV, immunoglobulins and albumin (Blair and Flaumenhaft, 2009; Cramer et al., 1989). An endocytic pathway to α-granules is also present in platelets (Behnke, 1992), demonstrated by the increase in accumulation of some endocytosed α-granule proteins as platelets age (Heilmann et al., 1994).
Here, we elaborate on the established knowledge of the intracellular trafficking pathways and machinery used by α-granule proteins to reach the α-granule. We describe mutations in membrane trafficking proteins that underlie platelet α-granule biogenesis phenotypes associated with human genetic disorders manifesting with prolonged bleeding. We also discuss how the ubiquitous sorting endosomal retrieval and recycling machinery is repurposed in MKs to synthesize α-granules, an emergent feature for cells specialized in the biogenesis of LROs. And finally, we comment on how the field is utilizing the knowledge gained about α-granules and their biogenesis to start engineering designer platelets with potential clinical uses.
Transport to α-granules of plasma proteins taken up by megakaryocytes
The first evidence that indicated α-granules originate from the endolysosomal system came from electron microscopy images following the path and kinetics of gold-labeled bovine serum albumin (BSA) taken up by cultured primary MKs (Heijnen et al., 1998). The authors visualized BSA first populating endosomes, followed by multi-vesicular bodies (MVBs) and finally reaching α-granules. Interestingly, they noticed BSA first in MVBs with typical morphology (MVBI) and then a special type of MVB that contained not only internal vesicles but also electron-dense material (MVBII), which is considered an intermediate maturation compartment between MVBI and the α-granule. Like albumin, immunoglobulin G and other luminal α-granule proteins reflect the composition and concentration of plasma, indicating they are likely acquired by fluid-phase endocytosis (George, 1990; Handagama and Bainton, 1989; Handagama et al., 1989).
Fibrinogen is endocytosed, both in MKs and platelets, bound to its receptor integrin αIIbβ3 with evidence for this process to be both clathrin dependent or independent (Behnke, 1992; Handagama et al., 1993; Gao et al., 2018). The intracellular traffic of αIIbβ3 is regulated by Arf6 and, consistently, the uptake and storage of Fibrinogen is decreased ∼50% in platelets from Arf6 deficient mice (Huang et al., 2016). Additionally, αIIbβ3 recycles from endosomes back to the plasma membrane. In particular, integrin β3 contains a sorting signal in its cytosolic domain that is bound by the endosomal adaptor SNX17 and this suggests that, similar to other integrins, αIIbβ3 is a cargo of the Commander endosomal retrieval and recycling pathway (see “The α-granule biogenesis machinery” section for details) (Tseng et al., 2014; Ghai et al., 2013; Butkovic et al., 2024; McNally et al., 2017).
Another well studied endocytosed protein present in α-granules is FV. Unlike Fibrinogen, FV cannot be endocytosed by platelets but only by megakaryocytes during a specific stage of their differentiation (Bouchard et al., 2005). The endocytosis of FV from plasma is clathrin- and Ca2+-dependent and involves LRP-1 and an unidentified, specific FV receptor (Bouchard et al., 2008). It has been shown Galectin-8 also mediates the uptake of FV. Galectin-8 binds FV and it has been proposed it transfers FV to LRP-1 or cross-links FV to integrins (Zappelli et al., 2012). Consistently, Galectin-8 has been shown to bind both LRP-1 and αIIbβ3 and exert its function by protein-sugar and protein-protein interactions (Zick, 2022; Levy et al., 2006). Remarkably, LRP-1 also contains a SNX17 sorting signal in its cytosolic domain and it has been shown to be a cargo of the Commander pathway in other cell types (Butkovic et al., 2024).
Transport to α-granules of proteins synthesized by megakaryocytes
Alpha-granule proteins, either soluble or transmembrane, traverse the endoplasmic reticulum (ER) and Golgi apparatus where they are post-translationally modified, however their topology, size and individual characteristics are unlikely satisfied by a single route to the α-granule (Blair and Flaumenhaft, 2009; Yao and Kahr, 2025). Also, there is no known universal sorting signal associated with α-granule proteins that would mediate transport to α-granules. Immunoelectron microscopy images of Golgi-associated vesicles containing α-granule proteins led to the conclusion that α-granule cargo was delivered from the Golgi directly to MVBs (Blair and Flaumenhaft, 2009; Cramer et al., 1989). However, live cell fluorescence microscopy analysis of imMKCL cells showed that newly synthesized α-granule proteins traffic through endosomes before reaching MVBs (Ambrosio et al., 2022; Ambrosio and Di Pietro, 2019). In particular, after leaving the Golgi, the luminal α-granule protein PF4 traverses the Rab5 sorting endosome followed by the Rab11 recycling endosome before reaching a Rab7 MVB and finally arriving at α-granules (Ambrosio and Di Pietro, 2019).
Despite the recent advances described above, the traffic of luminal, MK-made proteins to the α-granule is not completely understood. Nevertheless, evidence obtained from several groups studying various cargoes suggests these proteins must be actively retained by MKs (Chanzu et al., 2021; Lykins et al., 2025; Lo et al., 2018). However, how these luminal cargoes are linked to the cytosolic α-granule biogenesis machinery remains unknown. The current consensus idea is that α-granule luminal proteins either concentrate on electrostatic “sponges” or self-aggregate to reach the α-granule (Chanzu et al., 2021; Lykins et al., 2025; Cramer et al., 1985; Blagoveshchenskaya et al., 2002; Wagner et al., 1991). The proteoglycan serglycin (SG) is an example of an electrostatic “sponge”. Binding to SG is required for the packaging of small positively charged chemokines into α-granules which is evident by the reduction of PF4, PDGF, and β-Thromboglobulin in SG deficient mouse platelets (Chanzu et al., 2021; Lykins et al., 2025; Woulfe et al., 2008). Also, Thrombospondin-1, that contains a positively charged heparin-binding domain, has been shown to highly colocalize with SG by structured illumination microscopy of human platelets (Pluthero and Kahr, 2023). SG is a small polypeptide (18 kDa) produced in both hematopoietic and non-hematopoietic cells and decorated with tissue-specific glycosaminoglycan chains with different sulfation patterns (Kolset and Gallagher, 1990; Schick et al., 2001; Kolset and Pejler, 2011). It can accommodate several PF4 molecules that interact electrostatically with its sulfated glycans chains (Lord et al., 2017).
Supporting the importance of the electrostatic “sponge” mechanism, it has been shown SG deficient MK are unable to retain PF4, which is then secreted to the interstitial fluid of the bone marrow altering bone marrow morphology and affecting the homeostasis of the hematopoietic stem cell niche (Chanzu et al., 2021; Lykins et al., 2025). Importantly, although at significantly lower levels than SG deficient MK, wild type MK also release PF4 in the bone marrow (Chanzu et al., 2021; Bruns et al., 2014; Lambert et al., 2007). Intramedullary PF4 has a negative paracrine effect on megakaryopoiesis and it is endocytosed and stored in α-granules in, at least in part, an LRP-1-dependent pathway (Bruns et al., 2014; Lambert et al., 2009; Lambert et al., 2015). Additionally, a hydrophilic loop present in PF4 and other chemokines has been proposed to target these molecules to the α-granule but no receptor or mechanism has been elucidated (El Golli et al., 2005).
An example of self-aggregation as a proposed mechanism aiding α-granule targeting comes from vWF. This large, multimeric glycoprotein is found in eccentric internal structures within α-granules that resemble tubules formed by vWF in another LRO: the Weibel-Palade body (WPB) in endothelial cells (Cramer et al., 1985; Huang et al., 2008). The heterologous expression of vWF can induce the formation of storage organelles in cells that contain a regulated secretory pathway, potentially through an aggregation event that involves its propolypeptide (Blagoveshchenskaya et al., 2002; Wagner et al., 1991). Although, the existence of a putative targeting sequence within its propolypeptide has not been discarded (Wagner et al., 1991). Multimerin, a FV binding protein, is another large glycoprotein proposed to be sorted by homoaggregation (Hayward et al., 1999). Similar to vWF, in resting platelets Multimerin accumulates in the peripheral, electron-lucent zone of α-granules complexed to FV (Hayward et al., 1995).
Several of the transmembrane proteins present on the α-granule limiting membrane are also expressed on the plasma membrane of both MK and platelets (Berger et al., 1996). This is the case of integrins such as αIIbβ3, α2β1 or α6β1, the Thrombospondin-1 receptor CD36, the tetraspanin CD9, the adhesion molecule PECAM1, and GPIb-IX-V, the receptor for vWF (Berger et al., 1996; Cramer et al., 1990; Maynard et al., 2010; Berger et al., 1993; Cramer et al., 1994). In some instances, these proteins are known to be the endocytic receptors for luminal α-granule components, e.g., αIIbβ3 is the receptor for extracellular Fibrinogen (Handagama et al., 1993), and it has been speculated they use the same endocytic pathway as plasma proteins to reach the α-granule (Berger et al., 1996). Interestingly, many of these plasma membrane proteins are known integrin β3 and β1 interactors (Miao et al., 2001; Berditchevski, 2001; Jackson, 2003), which suggests they coexist on the same plasma membrane domains and they probably use the same endocytic carriers. On the other hand, other transmembrane proteins like CD40 ligand (CD40L) and P-Selectin, which are also known to bind integrins (Hassan et al., 2022; Andre et al., 2002a; Takada et al., 2023), are stored in α-granules and relocate to the plasma membrane upon platelet activation and granule fusion with the plasma membrane (Andre et al., 2002b).
The transport of P-Selectin to both the α-granule and WPB in endothelial cells, has been studied by several groups (Ambrosio et al., 2022; Lo et al., 2020; Harrison-Lavoie et al., 2006; Hartwell et al., 1998; Modderman et al., 1998). P-Selectin is a single pass transmembrane protein that contains several sorting signals in its cytosolic domain, including signals that mediate endocytosis and binding to the endosomal adaptor SNX17 (Ghai et al., 2013; Modderman et al., 1998; Blagoveshchenskaya et al., 1999). In MKs, P-Selectin recycles from endosomes to the plasma membrane before being stored in α-granules in a process that depends on its cytosolic domain and the Commander retrieval and recycling pathway (Ambrosio et al., 2022). Interestingly, in endothelial cells, a pathway from the plasma membrane to WPB has also been described (Harrison-Lavoie et al., 2006). However, the complete removal of P-Selectin cytosolic domain does not impede its localization to α-granules, demonstrated by experiments using platelets of transgenic mice expressing a truncated version of P-Selecting missing its cytosolic domain. When activated, these platelets expose the same amount of plasma membrane P-Selectin and show a similar level of platelet-leukocyte interaction as wild-type platelets (Hartwell et al., 1998). Similarly, a P-Selectin molecule missing its cytosolic domain is able to reach WPB in human umbilical vein endothelial cells (HUVEC) (Harrison-Lavoie et al., 2006). These pieces of evidence indicate the P-Selectin extracellular domain (equivalent to the α-granule lumen) contains determinants for α-granule/WPB localization.
The α-granule biogenesis machinery
Similar to other LROs like melanosomes or δ-granules, most of the known proteins involved in making α-granules are ubiquitously expressed and involved in essential membrane trafficking pathways (Sharda and Flaumenhaft, 2018; Bultema et al., 2012; Ambrosio and Di Pietro, 2017; Ambrosio et al., 2012). The discovery that mutations in VPS33B or VPS16B (VIPAS39, VIPAR, SPE-39) are responsible for arthrogryposis, renal dysfunction, and cholestasis (ARC) syndrome provided the first evidence that the biogenesis of α-granules depends on membrane trafficking machinery (Urban et al., 2012; Lo et al., 2005; Gissen et al., 2004). Patients with this autosomal recessive multisystem disorder present with abnormal bleeding due to α-granule deficiency among other severe clinical symptoms (Yao and Kahr, 2025).
VPS33B belongs to Sec1/Munc18 (SM) protein family that regulate soluble N-ethylmaleimide–sensitive factor attachment protein receptor (SNARE) function (Lo et al., 2005). Members of the SM family contribute to the efficiency and accuracy of membrane fusion events mediated by SNARE proteins by acting as templates of these reactions (Baker and Hughson, 2016). VPS33B and VPS16B form a stable complex that does not contain other proteins, in contrast to paralogs VPS33A and VPS16A, which associate with each other and additional proteins into tethering complexes known as HOPS and CORVET (Ambrosio and Di Pietro, 2019; Liu et al., 2023; Hunter et al., 2018; Shvarev et al., 2022). However, VPS33B and VPS16B have been proposed to associate with a 2:3 stoichiometry that would allow simultaneous interaction with two SNARE bundles or SNAREpins (Liu et al., 2023).
VPS33B deficiency in imMKCL cells results in degradation of α-granule proteins in lysosomes, demonstrating the VPS16B-VPS33B complex role is to ensure the accuracy of α-granule protein transport (Ambrosio and Di Pietro, 2019). VPS33B localizes to sorting endosomes where it binds the SNARE protein Syntaxin 12 (Stx12) and the coiled-coil protein CCDC22, one of the components of the Commander complex (Ambrosio and Di Pietro, 2019). The 16 subunit Commander complex is composed of the Retriever sub-complex (VPS26C, VPS35L and VPS29), the dodecameric COMMD/CCDC22/CCDC93 (CCC) sub-complex, and DENND10 (Butkovic et al., 2024). In non-specialized cell types, Commander engages SNX17 and its bound transmembrane protein cargoes, rescuing them from a late endosome/lysosome degradative pathway and instead recycling these proteins to the plasma membrane (Butkovic et al., 2024; McNally et al., 2017). This is also true in MKs but there may be an additional MK-specific Commander function mediating transport to MVBII/α-granules as seen for another LRO: the melanosome (Bajpai et al., 2023). The FERM domain of SNX17 recognizes transmembrane proteins that contain a ØxNxx[Y/F] sorting signal in their cytosolic domain (where Ø is a hydrophobic residue and x is any residue) such as integrins β1 and β3, P-Selectin and LRP-1 (Ghai et al., 2013). Accordingly, a dominant negative version of SNX17 causes P-Selectin retention in endosomes (Ambrosio et al., 2022). VPS33B interactors Stx12 and COMMD3, a member of the CCC complex, are needed for normal α-granule biogenesis. Also important, Stx12 and the CCDC22 subunit of CCC compete for binding to VPS33B, suggesting a hand-off mechanism in which the fusion of vesicles containing α-granule cargo with endosomal compartments is coupled with the retrieval machinery thus ensuring cargo proteins escape lysosomal degradation (Ambrosio et al., 2022). The WASH complex is required for endosomal deposition of F-actin and cargo trafficking and is a crucial component of the Commander pathway (Simonetti and Cullen, 2019). In agreement, it was recently reported that MK- and platelet-specific WASH deficient mice have a selective reduction of αIIbβ3 expression with a concomitant αIIbβ3 mislocalization to internal membranes and a delay in Fibrinogen uptake (Schurr et al., 2023).
NBEAL2 is the only other known protein that when mutated causes α-granule biogenesis defects both in mice and humans. Mutations in NBEAL2 were identified in Gray Platelet Syndrome (GPS) patients, whose platelets appear gray on electron microscopy images due to the absence of α-granules (Albers et al., 2011; Gunay-Aygun et al., 2011; Kahr et al., 2011). In contrast with VPS33B and VPS16B, NBEAL2 is mainly expressed in hematopoietic cells and therefore GPS symptoms affect the hematopoietic system and include thrombocytopenia, excessive bleeding and myelofibrosis (Yao and Kahr, 2025).
NBEAL2 is a multidomain, large, cytosolic protein and belongs to a group of 9 Beige and Chediak-Higashi syndrome (BEACH) domain containing proteins (Pereira and Gershlick, 2024). Members of this group have been associated with processes that involve membrane trafficking such as regulation of vesicles fusion and fission, autophagy and antigen cross-presentation (Cullinane et al., 2013; Simonsen et al., 2004; Theisen et al., 2018). Alphafold modelling of these proteins predicts they share an alpha-solenoid/beta-propeller molecular structure which indicates they may have a protocoatomer origin and function as membrane coat proteins (Pankiv et al., 2024). Generally, coat proteins are master membrane trafficking regulators involved in cargo selection and concentration, membrane deformation, differentiation of subdomains within organelles and association of carriers with both the cytoskeleton and the acceptor organelle (Bonifacino and Lippincott-Schwartz, 2003). Consistently, pulldown experiments show NBEAL2 binds P-Selectin using both human platelets extracts and over-expressed recombinant proteins in non-specialized cells (Lo et al., 2020; Lo et al., 2018). Also, NBEAL2 has been reported to associate with the ER protein SEC22B and deletion of this ER protein from imMKCL cells results in failure of α-granule formation (Lo et al., 2020). Additionally, a proximity ligation screening identified Dock7, Sec16a, and Vac14 as NBEAL2 interactors (Mayer et al., 2018). Immunofluorescence microscopy of primary MKs show NBEAL2 colocalizes with P-Selectin and SEC22B, pointing towards a role of ER contact sites in α-granule biogenesis (Lo et al., 2020). HeLa cells stably expressing recombinant NBEAL2 show it associates predominantly with Rab11 and Rab38 compartments, suggesting endosomal localization (Pankiv et al., 2024).
NBEAL2 deficient mice MKs do not retain soluble α-granule cargo, either endocytosed (Fibrinogen) or MK-made (PF4) (Lykins et al., 2025; Lo et al., 2018). The release by MK of α-granule proteins, such as growth factors and cytokines, into the bone marrow explains the myelofibrosis presented in GPS patients (Yao and Kahr, 2025). P-Selectin on the other hand, is not completely depleted from NBEAL2 deficient mouse platelets, indicating NBEAL2 functions downstream VPS16B/VPS33B in the α-granule biogenesis pathway (Kahr et al., 2013).
Current model for the transport of α-granule proteins in megakaryocytes
Proteins stored in α-granules are made by the MK or by other cells, are soluble or transmembrane, range in size from very large to very small, and perform many different functions in several different pathways (Blair and Flaumenhaft, 2009). However, they all converge in α-granules, which suggests they contain common trafficking labels that target them to this organelle. Most of the known trafficking machinery involved in the α-granule biogenesis pathways are proteins ubiquitously expressed (Ambrosio et al., 2022; Ambrosio and Di Pietro, 2019). Not only that, in MKs these proteins both perform essential cargo sorting functions and make α-granules, pointing towards the existence of a MK-specific factor that coordinates these two processes. NBEAL2 is well positioned to perform this task. It is highly expressed in hematopoietic cells and RNAseq of differentiated imMKCL cells shows its upregulation compared to undifferentiated cells (Ambrosio et al., 2022; Yao and Kahr, 2025). Consistently, GPS patients present with mostly hematopoietic phenotypes (Yao and Kahr, 2025). It has been recently proposed that NBEAL2 functions as an endosomal membrane coat protein (Pereira and Gershlick, 2024; Pankiv et al., 2024). Several reports support this idea. First, in agreement with the cargo selection and concentration function of coat proteins, NBEAL2 binds P-Selectin (Lo et al., 2020; Lo et al., 2018). Second, NBEAL2 deficient MKs secrete luminal α-granule cargo (Lykins et al., 2025; Lo et al., 2018). We speculate NBEAL2 may specify endosomal subdomains where α-granule cargo is both retrieved to avoid secretion and directed towards α-granules (Figure 1).
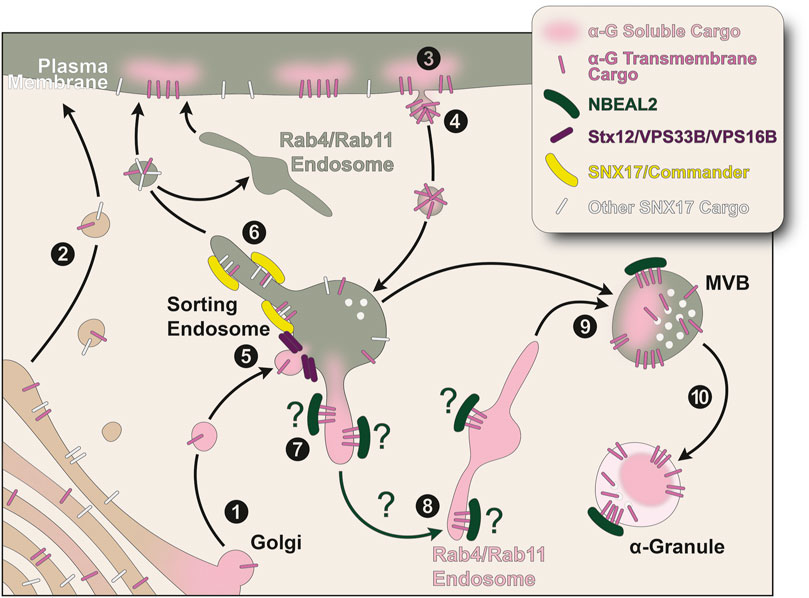
Figure 1. Model for transport of α-granule proteins in megakaryocytes. All MK-synthesized α-granule proteins traverse the ER and the Golgi. (1) Presumably, luminal α-granule proteins homoaggregate and/or concentrate in subdomains of the Golgi apparatus where they are packaged in carriers destined to sorting endosomes. Transmembrane α-granule proteins may also be included in these vesicles. Alternatively, (2) transmembrane proteins enter a default secretory pathway to the plasma membrane and, like luminal α-granule cargo, (3) reach the sorting endosome by endocytosis. (4) We speculate α-granule transmembrane proteins containing similar sorting signals are incorporated in plasma membrane patches and endocytosed in the same transport carriers. (5) Normal fusion of vesicles containing α-granule proteins with endosomes requires the SNARE protein Stx12 and the VPS33B-VPS16B complex. The sorting endosome is the main hub for the transport of α-granule proteins. (6) The endosomal retrieval and recycling Commander complex is responsible for both corralling α-granule cargo away from a degradative fate in lysosomes and the recycling of transmembrane proteins containing a sorting signal in their cytosolic domain that is recognized by SNX17. Sorting endosomes are also the precursor organelle of lysosomes and δ-granules (not depicted), therefore α-granule cargo must be segregated in a process potentially mediated by NBEAL2. (7, 8) We hypothesize NBEAL2 facilitates cargo transfer by binding the cytosolic domain of transmembrane proteins to generate cargo protein carriers destined for α-granules. These tubules and vesicles are Rab4/Rab11-labeled compartments, recycling endosomes that have been repurposed in these cells presumably to enrich and refine the proteins that reach the α-granule. (9) α-granule proteins reach MVBs providing the content for MVBI, containing vesicles but lacking electron dense luminal material, to mature into MVBII, containing both vesicles and electron dense luminal material. The MVBI-MVBII transition is not depicted for simplicity. (10) The maturation of MVBII is the final step in the biogenesis of α-granules. α-G: α-granule; MVB: multi-vesicular body.
The high volume of MK-synthesized α-granule cargo in itself is probably a driving force for the creation of α-granules. The luminal aggregation of very large multimeric proteins like vWF or Multimerin and the protein clusters generated by the electrostatic “sponge” SG could aid in the formation of specialized endosomal subdomain (Chanzu et al., 2021; Lykins et al., 2025; Blagoveshchenskaya et al., 2002; Wagner et al., 1991; Hayward et al., 1999; Hayward et al., 1995). But how do these luminal, high-protein concentration domains communicate with the trafficking machinery on the cytosolic side of the membrane? A common denominator across all different α-granule proteins’ types is their direct or indirect association with integrins (Miao et al., 2001; Berditchevski, 2001; Jackson, 2003; Hassan et al., 2022; Andre et al., 2002a; Takada et al., 2023). Several integrins are present on the α-granule limiting membrane and their luminal/extracellular domains interact with a host of other α-granule cargo, both soluble and transmembrane (Cramer et al., 1990; Maynard et al., 2010). Interestingly, integrins β1 and β3 share some of the same sorting signals in their cytosolic domains as P-Selectin and LRP-1: a receptor involved in the trafficking of FV and PF4 known to interact with integrins (Ghai et al., 2013; Hu et al., 2007; Rabiej et al., 2016). In agreement, a recent report indicates the luminal domain of P-Selectin binds integrins (Takada et al., 2023). An integrin-piggyback mechanism could explain the fact that the cytosolic domain of P-Selectin is not required to grant this protein α-granule localization (Hartwell et al., 1998).
We propose a model in which α-granule proteins must traverse the sorting endosome (Figure 1). MK-synthesized luminal proteins likely concentrate and leave the Golgi in sorting endosome-targeted carriers (Figure 1, step 1) while MK-made transmembrane proteins have the option of reaching the sorting endosome directly or by endocytosis from the plasma membrane (Figure 1, steps 1–4). Similarly, luminal α-granule cargo taken up by megakaryocytes is endocytosed in a receptor dependent or independent way. Several proteins present on the limiting membrane of the α-granule contain the same sorting signals in their cytosolic domains and their extracellular domains interact with integrins, which allows us to speculate they localize to the same plasma membrane patches and are endocytosed in the same transport carriers (Figure 1, steps 3 and 4). To avoid lysosomal degradation, α-granule proteins require a VPS16B/VPS33B-mediated membrane fusion step (Figure 1, step 5) and the retrieval from a degradative fate by the Commander complex (Figure 1, step 6) (Ambrosio et al., 2022; Ambrosio and Di Pietro, 2019). This complex is also responsible for the recycling to the plasma membrane of transmembrane proteins containing a sorting signal in their cytosolic domain that is recognized by SNX17, such as the α-granule proteins integrins β1 and β3, P-Selectin, and the FV and PF4 endocytic receptor LRP-1. We hypothesize NBEAL2 may be instrumental in concentrating both luminal and transmembrane α-granule cargo in Rab4/Rab11 endosomal subdomains redirecting the recycling machinery towards the α-granule biogenesis pathway (Figure 1, steps 7–9) instead of the conventional route to the cell surface. In this way α-granule proteins reach MVBs (Figure 1, step 9). We theorize MVBIIs do not necessarily originate from conventional MVBs. They contain dense proteinaceous material and intra-luminal vesicles (ILVs) decorated with CD63, P-Selectin and αIIbβ3 that resemble more ILVs found in WPBs than conventional MVBs destined for lysosomal degradation (Heijnen et al., 1998; Streetley et al., 2019; Heijnen, 2019). Finally, these MVBIIs mature into α-granules (Figure 1, step 10).
Learning about the biogenesis of α-granules not only has an impact on the understanding of hematopoietic and cardiovascular diseases but also on the ability to manipulate the content of platelet α-granules with potential clinical applications. Great progress has been made in the generation of MKs and platelets from induced pluripotent stem cells (iPSCs) (Nakamura et al., 2014; Ito et al., 2018; Borger et al., 2016; Feng et al., 2014; Liu et al., 2015). Recently, a patient suffering from alloimmune platelet transfusion refractoriness was successfully transfused with tailored platelets generated using her own iPSCs (Sugimoto et al., 2022). Excitingly, it has been shown that the content of in vitro grown MK α-granules can be modified by adding proteins to the culture medium that are then endocytosed by the MKs (Zhang and Newman, 2019; Poncz et al., 2024). Moreover, platelets derived from these MKs effectively release these ectopic proteins, opening the door to the use of designer platelets as a new method for the delivery of therapeutics in human disease (Poncz et al., 2024).
Author contributions
AA: Writing – original draft, Writing – review and editing, Conceptualization, Data curation, Formal Analysis, Investigation, Resources, Visualization. Santiago SD: Writing – original draft, Writing – review and editing, Conceptualization, Data curation, Formal Analysis, Funding acquisition, Investigation, Project administration, Supervision.
Funding
The author(s) declare that financial support was received for the research and/or publication of this article. Grant R01HL151988 supported both authors, research included in this article and this review.
Conflict of interest
The authors declare that the research was conducted in the absence of any commercial or financial relationships that could be construed as a potential conflict of interest.
Generative AI statement
The authors declare that no Generative AI was used in the creation of this manuscript.
Publisher’s note
All claims expressed in this article are solely those of the authors and do not necessarily represent those of their affiliated organizations, or those of the publisher, the editors and the reviewers. Any product that may be evaluated in this article, or claim that may be made by its manufacturer, is not guaranteed or endorsed by the publisher.
References
Albers, C. A., Cvejic, A., Favier, R., Bouwmans, E. E., Alessi, M. C., Bertone, P., et al. (2011). Exome sequencing identifies NBEAL2 as the causative gene for gray platelet syndrome. Nat. Genet. 43 (8), 735–737. doi:10.1038/ng.885
Ambrosio, A. L., Boyle, J. A., and Di Pietro, S. M. (2012). Mechanism of platelet dense granule biogenesis: study of cargo transport and function of Rab32 and Rab38 in a model system. Blood 120 (19), 4072–4081. doi:10.1182/blood-2012-04-420745
Ambrosio, A. L., and Di Pietro, S. M. (2017). Storage pool diseases illuminate platelet dense granule biogenesis. Platelets 28 (2), 138–146. doi:10.1080/09537104.2016.1243789
Ambrosio, A. L., and Di Pietro, S. M. (2019). Mechanism of platelet alpha-granule biogenesis: study of cargo transport and the VPS33B-VPS16B complex in a model system. Blood Adv. 3 (17), 2617–2626. doi:10.1182/bloodadvances.2018028969
Ambrosio, A. L., Febvre, H. P., and Di Pietro, S. M. (2022). Syntaxin 12 and COMMD3 are new factors that function with VPS33B in the biogenesis of platelet alpha-granules. Blood 139 (6), 922–935. doi:10.1182/blood.2021012056
Andre, P., Nannizzi-Alaimo, L., Prasad, S. K., and Phillips, D. R. (2002b). Platelet-derived CD40L: the switch-hitting player of cardiovascular disease. Circulation 106 (8), 896–899. doi:10.1161/01.cir.0000028962.04520.01
Andre, P., Prasad, K. S., Denis, C. V., He, M., Papalia, J. M., Hynes, R. O., et al. (2002a). CD40L stabilizes arterial thrombi by a beta3 integrin--dependent mechanism. Nat. Med. 8 (3), 247–252. doi:10.1038/nm0302-247
Bajpai, V. K., Swigut, T., Mohammed, J., Naqvi, S., Arreola, M., Tycko, J., et al. (2023). A genome-wide genetic screen uncovers determinants of human pigmentation. Science. 381 (6658), eade6289. doi:10.1126/science.ade6289
Baker, R. W., and Hughson, F. M. (2016). Chaperoning SNARE assembly and disassembly. Nat. Rev. Mol. Cell Biol. 17 (8), 465–479. doi:10.1038/nrm.2016.65
Behnke, O. (1992). Degrading and non-degrading pathways in fluid-phase (non-adsorptive) endocytosis in human blood platelets. J. Submicrosc. Cytol. Pathol. 24 (2), 169–178.
Berditchevski, F. (2001). Complexes of tetraspanins with integrins: more than meets the eye. J. Cell Sci. 114 (Pt 23), 4143–4151. doi:10.1242/jcs.114.23.4143
Berger, G., Caen, J. P., Berndt, M. C., and Cramer, E. M. (1993). Ultrastructural demonstration of CD36 in the alpha-granule membrane of human platelets and megakaryocytes. Blood 82 (10), 3034–3044. doi:10.1182/blood.v82.10.3034.bloodjournal82103034
Berger, G., Masse, J. M., and Cramer, E. M. (1996). Alpha-granule membrane mirrors the platelet plasma membrane and contains the glycoproteins Ib, IX, and V. Blood 87 (4), 1385–1395. doi:10.1182/blood.v87.4.1385.bloodjournal8741385
Blagoveshchenskaya, A. D., Hannah, M. J., Allen, S., and Cutler, D. F. (2002). Selective and signal-dependent recruitment of membrane proteins to secretory granules formed by heterologously expressed von Willebrand factor. Mol. Biol. Cell 13 (5), 1582–1593. doi:10.1091/mbc.01-09-0462
Blagoveshchenskaya, A. D., Hewitt, E. W., and Cutler, D. F. (1999). A complex web of signal-dependent trafficking underlies the triorganellar distribution of P-selectin in neuroendocrine PC12 cells. J. Cell Biol. 145 (7), 1419–1433. doi:10.1083/jcb.145.7.1419
Blair, P., and Flaumenhaft, R. (2009). Platelet alpha-granules: basic biology and clinical correlates. Blood Rev. 23 (4), 177–189. doi:10.1016/j.blre.2009.04.001
Bonifacino, J. S., and Lippincott-Schwartz, J. (2003). Coat proteins: shaping membrane transport. Nat. Rev. Mol. Cell Biol. 4 (5), 409–414. doi:10.1038/nrm1099
Borger, A. K., Eicke, D., Wolf, C., Gras, C., Aufderbeck, S., Schulze, K., et al. (2016). Generation of HLA-universal iPSC-derived megakaryocytes and platelets for Survival under refractoriness Conditions. Mol. Med. 22, 274–285. doi:10.2119/molmed.2015.00235
Bouchard, B. A., Meisler, N. T., Nesheim, M. E., Liu, C. X., Strickland, D. K., and Tracy, P. B. (2008). A unique function for LRP-1: a component of a two-receptor system mediating specific endocytosis of plasma-derived factor V by megakaryocytes. J. Thromb. Haemost. 6 (4), 638–644. doi:10.1111/j.1538-7836.2008.02894.x
Bouchard, B. A., Williams, J. L., Meisler, N. T., Long, M. W., and Tracy, P. B. (2005). Endocytosis of plasma-derived factor V by megakaryocytes occurs via a clathrin-dependent, specific membrane binding event. J. Thromb. Haemost. 3 (3), 541–551. doi:10.1111/j.1538-7836.2005.01190.x
Bruns, I., Lucas, D., Pinho, S., Ahmed, J., Lambert, M. P., Kunisaki, Y., et al. (2014). Megakaryocytes regulate hematopoietic stem cell quiescence through CXCL4 secretion. Nat. Med. 20 (11), 1315–1320. doi:10.1038/nm.3707
Bultema, J. J., Ambrosio, A. L., Burek, C. L., and Di Pietro, S. M. (2012). BLOC-2, AP-3, and AP-1 proteins function in concert with Rab38 and Rab32 proteins to mediate protein trafficking to lysosome-related organelles. J. Biol. Chem. 287 (23), 19550–19563. doi:10.1074/jbc.M112.351908
Butkovic, R., Walker, A. P., Healy, M. D., McNally, K. E., Liu, M., Veenendaal, T., et al. (2024). Mechanism and regulation of cargo entry into the Commander endosomal recycling pathway. Nat. Commun. 15 (1), 7180. doi:10.1038/s41467-024-50971-0
Chanzu, H., Lykins, J., Wigna-Kumar, S., Joshi, S., Pokrovskaya, I., Storrie, B., et al. (2021). Platelet alpha-granule cargo packaging and release are affected by the luminal proteoglycan, serglycin. J. Thromb. Haemost. 19 (4), 1082–1095. doi:10.1111/jth.15243
Cramer, E. M., Berger, G., and Berndt, M. C. (1994). Platelet alpha-granule and plasma membrane share two new components: CD9 and PECAM-1. Blood 84 (6), 1722–1730. doi:10.1182/blood.v84.6.1722.1722
Cramer, E. M., Debili, N., Martin, J. F., Gladwin, A. M., Breton-Gorius, J., Harrison, P., et al. (1989). Uncoordinated expression of fibrinogen compared with thrombospondin and von Willebrand factor in maturing human megakaryocytes. Blood 73 (5), 1123–1129. doi:10.1182/blood.v73.5.1123.1123
Cramer, E. M., Meyer, D., le Menn, R., and Breton-Gorius, J. (1985). Eccentric localization of von Willebrand factor in an internal structure of platelet alpha-granule resembling that of Weibel-Palade bodies. Blood 66 (3), 710–713. doi:10.1182/blood.v66.3.710.bloodjournal663710
Cramer, E. M., Savidge, G. F., Vainchenker, W., Berndt, M. C., Pidard, D., Caen, J. P., et al. (1990). Alpha-granule pool of glycoprotein IIb-IIIa in normal and pathologic platelets and megakaryocytes. Blood 75 (6), 1220–1227. doi:10.1182/blood.v75.6.1220.bloodjournal7561220
Cullinane, A. R., Schaffer, A. A., and Huizing, M. (2013). The BEACH is hot: a LYST of emerging roles for BEACH-domain containing proteins in human disease. Traffic 14 (7), 749–766. doi:10.1111/tra.12069
El Golli, N., Issertial, O., Rosa, J. P., and Briquet-Laugier, V. (2005). Evidence for a granule targeting sequence within platelet factor 4. J. Biol. Chem. 280 (34), 30329–30335. doi:10.1074/jbc.M503847200
Feng, Q., Shabrani, N., Thon, J. N., Huo, H., Thiel, A., Machlus, K. R., et al. (2014). Scalable generation of universal platelets from human induced pluripotent stem cells. Stem Cell Rep. 3 (5), 817–831. doi:10.1016/j.stemcr.2014.09.010
Frojmovic, M. M., and Milton, J. G. (1982). Human platelet size, shape, and related functions in health and disease. Physiol. Rev. 62 (1), 185–261. doi:10.1152/physrev.1982.62.1.185
Gao, W., Shi, P., Chen, X., Zhang, L., Liu, J., Fan, X., et al. (2018). Clathrin-mediated integrin αIIbβ3 trafficking controls platelet spreading. Platelets 29 (6), 610–621. doi:10.1080/09537104.2017.1353682
George, J. N. (1990). Platelet immunoglobulin G: its significance for the evaluation of thrombocytopenia and for understanding the origin of alpha-granule proteins. Blood 76 (5), 859–870. doi:10.1182/blood.v76.5.859.bloodjournal765859
Ghai, R., Bugarcic, A., Liu, H., Norwood, S. J., Skeldal, S., Coulson, E. J., et al. (2013). Structural basis for endosomal trafficking of diverse transmembrane cargos by PX-FERM proteins. Proc. Natl. Acad. Sci. U. S. A. 110 (8), E643–E652. doi:10.1073/pnas.1216229110
Gissen, P., Johnson, C. A., Morgan, N. V., Stapelbroek, J. M., Forshew, T., Cooper, W. N., et al. (2004). Mutations in VPS33B, encoding a regulator of SNARE-dependent membrane fusion, cause arthrogryposis-renal dysfunction-cholestasis (ARC) syndrome. Nat. Genet. 36 (4), 400–404. doi:10.1038/ng1325
Gunay-Aygun, M., Falik-Zaccai, T. C., Vilboux, T., Zivony-Elboum, Y., Gumruk, F., Cetin, M., et al. (2011). NBEAL2 is mutated in gray platelet syndrome and is required for biogenesis of platelet alpha-granules. Nat. Genet. 43 (8), 732–734. doi:10.1038/ng.883
Handagama, P., Scarborough, R. M., Shuman, M. A., and Bainton, D. F. (1993). Endocytosis of fibrinogen into megakaryocyte and platelet alpha-granules is mediated by alpha IIb beta 3 (glycoprotein IIb-IIIa) [published erratum appears in Blood 1993 Nov 1;82(9):2936]. Blood 82 (1), 135–138. doi:10.1182/blood.v82.1.135.bloodjournal821135
Handagama, P. J., and Bainton, D. F. (1989). Incorporation of a circulating protein into alpha granules of megakaryocytes. Blood Cells 15 (1), 59–72.
Handagama, P. J., Shuman, M. A., and Bainton, D. F. (1989). Incorporation of intravenously injected albumin, immunoglobulin G, and fibrinogen in guinea pig megakaryocyte granules. J. Clin. Invest 84 (1), 73–82. doi:10.1172/JCI114173
Harrison-Lavoie, K. J., Michaux, G., Hewlett, L., Kaur, J., Hannah, M. J., Lui-Roberts, W. W., et al. (2006). P-selectin and CD63 use different mechanisms for delivery to Weibel-Palade bodies. Traffic 7 (6), 647–662. doi:10.1111/j.1600-0854.2006.00415.x
Hartwell, D. W., Mayadas, T. N., Berger, G., Frenette, P. S., Rayburn, H., Hynes, R. O., et al. (1998). Role of P-selectin cytoplasmic domain in granular targeting in vivo and in early inflammatory responses. J. Cell Biol. 143 (4), 1129–1141. doi:10.1083/jcb.143.4.1129
Hartwig, J., and Italiano, J. (2003). The birth of the platelet. J. Thromb. Haemost. 1 (7), 1580–1586. doi:10.1046/j.1538-7836.2003.00331.x
Hassan, G. S., Salti, S., and Mourad, W. (2022). Novel functions of integrins as receptors of CD154: their role in inflammation and Apoptosis. Cells 11 (11), 1747. doi:10.3390/cells11111747
Hayward, C. P., Furmaniak-Kazmierczak, E., Cieutat, A. M., Moore, J. C., Bainton, D. F., Nesheim, M. E., et al. (1995). Factor V is complexed with multimerin in resting platelet lysates and colocalizes with multimerin in platelet alpha-granules. J. Biol. Chem. 270 (33), 19217–19224. doi:10.1074/jbc.270.33.19217
Hayward, C. P., Song, Z., Zheng, S., Fung, R., Pai, M., Masse, J. M., et al. (1999). Multimerin processing by cells with and without pathways for regulated protein secretion. Blood 94 (4), 1337–1347. doi:10.1182/blood.v94.4.1337
Heijnen, H. F., Debili, N., Vainchencker, W., Breton-Gorius, J., Geuze, H. J., and Sixma, J. J. (1998). Multivesicular bodies are an intermediate stage in the formation of platelet alpha-granules. Blood 91 (7), 2313–2325. doi:10.1182/blood.v91.7.2313.2313_2313_2325
Heijnen, H. F. G. (2019). WPBs and alpha-granules: more and more look-alike? Blood 133 (25), 2634–2636. doi:10.1182/blood-2019-02-900878
Heilmann, E., Hynes, L. A., Friese, P., George, I. N., Burstein, S. A., and Dale, G. L. (1994). Dog platelets accumulate intracellular fibrinogen as they age. J. Cell Physiol. 161 (1), 23–30. doi:10.1002/jcp.1041610104
Hu, K., Wu, C., Mars, W. M., and Liu, Y. (2007). Tissue-type plasminogen activator promotes murine myofibroblast activation through LDL receptor-related protein 1-mediated integrin signaling. J. Clin. Invest 117 (12), 3821–3832. doi:10.1172/JCI32301
Huang, R. H., Wang, Y., Roth, R., Yu, X., Purvis, A. R., Heuser, J. E., et al. (2008). Assembly of Weibel-Palade body-like tubules from N-terminal domains of von Willebrand factor. Proc. Natl. Acad. Sci. U. S. A. 105 (2), 482–487. doi:10.1073/pnas.0710079105
Huang, Y., Joshi, S., Xiang, B., Kanaho, Y., Li, Z., Bouchard, B. A., et al. (2016). Arf6 controls platelet spreading and clot retraction via integrin αIIbβ3 trafficking. Blood 127 (11), 1459–1467. doi:10.1182/blood-2015-05-648550
Hunter, M. R., Hesketh, G. G., Benedyk, T. H., Gingras, A. C., and Graham, S. C. (2018). Proteomic and biochemical Comparison of the cellular interaction Partners of human VPS33A and VPS33B. J. Mol. Biol. 430 (14), 2153–2163. doi:10.1016/j.jmb.2018.05.019
Italiano, J. E., Lecine, P., Shivdasani, R. A., and Hartwig, J. H. (1999). Blood platelets are assembled principally at the ends of proplatelet processes produced by differentiated megakaryocytes. J. Cell Biol. 147 (6), 1299–1312. doi:10.1083/jcb.147.6.1299
Italiano, J. E., Richardson, J. L., Patel-Hett, S., Battinelli, E., Zaslavsky, A., Short, S., et al. (2008). Angiogenesis is regulated by a novel mechanism: pro- and antiangiogenic proteins are organized into separate platelet alpha granules and differentially released. Blood 111 (3), 1227–1233. doi:10.1182/blood-2007-09-113837
Ito, Y., Nakamura, S., Sugimoto, N., Shigemori, T., Kato, Y., Ohno, M., et al. (2018). Turbulence Activates platelet biogenesis to enable clinical Scale ex vivo production. Cell 174 (3), 636–648. doi:10.1016/j.cell.2018.06.011
Jackson, D. E. (2003). The unfolding tale of PECAM-1. FEBS Lett. 540 (1-3), 7–14. doi:10.1016/s0014-5793(03)00224-2
Jonnalagadda, D., Izu, L. T., and Whiteheart, S. W. (2012). Platelet secretion is kinetically heterogeneous in an agonist-responsive manner. Blood 120 (26), 5209–5216. doi:10.1182/blood-2012-07-445080
Joshi, S., and Whiteheart, S. W. (2017). The nuts and bolts of the platelet release reaction. Platelets 28 (2), 129–137. doi:10.1080/09537104.2016.1240768
Kahr, W. H., Hinckley, J., Li, L., Schwertz, H., Christensen, H., Rowley, J. W., et al. (2011). Mutations in NBEAL2, encoding a BEACH protein, cause gray platelet syndrome. Nat. Genet. 43 (8), 738–740. doi:10.1038/ng.884
Kahr, W. H., Lo, R. W., Li, L., Pluthero, F. G., Christensen, H., Ni, R., et al. (2013). Abnormal megakaryocyte development and platelet function in Nbeal2(-/-) mice. Blood 122 (19), 3349–3358. doi:10.1182/blood-2013-04-499491
Kolset, S. O., and Gallagher, J. T. (1990). Proteoglycans in haemopoietic cells. Biochim. Biophys. Acta 1032 (2-3), 191–211. doi:10.1016/0304-419x(90)90004-k
Kolset, S. O., and Pejler, G. (2011). Serglycin: a structural and functional chameleon with wide impact on immune cells. J. Immunol. 187 (10), 4927–4933. doi:10.4049/jimmunol.1100806
Lambert, M. P., Meng, R., Xiao, L., Harper, D. C., Marks, M. S., Kowalska, M. A., et al. (2015). Intramedullary megakaryocytes internalize released platelet factor 4 and store it in alpha granules. J. Thromb. Haemost. 13 (10), 1888–1899. doi:10.1111/jth.13069
Lambert, M. P., Rauova, L., Bailey, M., Sola-Visner, M. C., Kowalska, M. A., and Poncz, M. (2007). Platelet factor 4 is a negative autocrine in vivo regulator of megakaryopoiesis: clinical and therapeutic implications. Blood 110 (4), 1153–1160. doi:10.1182/blood-2007-01-067116
Lambert, M. P., Wang, Y., Bdeir, K. H., Nguyen, Y., Kowalska, M. A., and Poncz, M. (2009). Platelet factor 4 regulates megakaryopoiesis through low-density lipoprotein receptor-related protein 1 (LRP1) on megakaryocytes. Blood 114 (11), 2290–2298. doi:10.1182/blood-2009-04-216473
Levy, Y., Auslender, S., Eisenstein, M., Vidavski, R. R., Ronen, D., Bershadsky, A. D., et al. (2006). It depends on the hinge: a structure-functional analysis of galectin-8, a tandem-repeat type lectin. Glycobiology 16 (6), 463–476. doi:10.1093/glycob/cwj097
Liu, R. J. Y., Al-Molieh, Y., Chen, S. Z., Drobac, M., Urban, D., Chen, C. H., et al. (2023). The Sec1-Munc18 protein VPS33B forms a uniquely bidirectional complex with VPS16B. J. Biol. Chem. 299 (6), 104718. doi:10.1016/j.jbc.2023.104718
Liu, Y., Wang, Y., Gao, Y., Forbes, J. A., Qayyum, R., Becker, L., et al. (2015). Efficient generation of megakaryocytes from human induced pluripotent stem cells using food and drug administration-approved pharmacological reagents. Stem Cells Transl. Med. 4 (4), 309–319. doi:10.5966/sctm.2014-0183
Lo, B., Li, L., Gissen, P., Christensen, H., McKiernan, P. J., Ye, C., et al. (2005). Requirement of VPS33B, a member of the Sec1/Munc18 protein family, in megakaryocyte and platelet alpha-granule biogenesis. Blood 106 (13), 4159–4166. doi:10.1182/blood-2005-04-1356
Lo, R. W., Li, L., Leung, R., Pluthero, F. G., and Kahr, W. H. A. (2018). NBEAL2 (Neurobeachin-Like 2) is required for retention of cargo proteins by alpha-granules during their production by megakaryocytes. Arterioscler. Thromb. Vasc. Biol. 38 (10), 2435–2447. doi:10.1161/ATVBAHA.118.311270
Lo, R. W., Li, L., Pluthero, F. G., Leung, R., Eto, K., and Kahr, W. H. A. (2020). The endoplasmic reticulum protein SEC22B interacts with NBEAL2 and is required for megakaryocyte alpha-granule biogenesis. Blood 136 (6), 715–725. doi:10.1182/blood.2019004276
Lord, M. S., Cheng, B., Farrugia, B. L., McCarthy, S., and Whitelock, J. M. (2017). Platelet factor 4 binds to Vascular proteoglycans and controls both growth factor Activities and platelet activation. J. Biol. Chem. 292 (10), 4054–4063. doi:10.1074/jbc.M116.760660
Lykins, J., Becker, I. C., Camacho, V., Alfar, H. R., Park, J., Italiano, J., et al. (2025). Serglycin controls megakaryocyte retention of platelet factor 4 and influences megakaryocyte fate in bone marrow. Blood Adv. 9 (1), 15–28. doi:10.1182/bloodadvances.2024012995
Mayer, L., Jasztal, M., Pardo, M., Aguera de Haro, S., Collins, J., Bariana, T. K., et al. (2018). Nbeal2 interacts with Dock7, Sec16a, and Vac14. Blood 131 (9), 1000–1011. doi:10.1182/blood-2017-08-800359
Maynard, D. M., Heijnen, H. F., Gahl, W. A., and Gunay-Aygun, M. (2010). The alpha-granule proteome: novel proteins in normal and ghost granules in gray platelet syndrome. J. Thromb. Haemost. 8 (8), 1786–1796. doi:10.1111/j.1538-7836.2010.03932.x
McNally, K. E., Faulkner, R., Steinberg, F., Gallon, M., Ghai, R., Pim, D., et al. (2017). Retriever is a multiprotein complex for retromer-independent endosomal cargo recycling. Nat. Cell Biol. 19 (10), 1214–1225. doi:10.1038/ncb3610
Miao, W. M., Vasile, E., Lane, W. S., and Lawler, J. (2001). CD36 associates with CD9 and integrins on human blood platelets. Blood 97 (6), 1689–1696. doi:10.1182/blood.v97.6.1689
Modderman, P. W., Beuling, E. A., Govers, L. A., Calafat, J., Janssen, H., Von dem Borne, A. E., et al. (1998). Determinants in the cytoplasmic domain of P-selectin required for sorting to secretory granules. Biochem. J. 336 (Pt 1), 153–161. doi:10.1042/bj3360153
Muller, F., Mutch, N. J., Schenk, W. A., Smith, S. A., Esterl, L., Spronk, H. M., et al. (2009). Platelet polyphosphates are proinflammatory and procoagulant mediators in vivo. Cell 139 (6), 1143–1156. doi:10.1016/j.cell.2009.11.001
Nakamura, S., Takayama, N., Hirata, S., Seo, H., Endo, H., Ochi, K., et al. (2014). Expandable megakaryocyte cell lines enable clinically applicable generation of platelets from human induced pluripotent stem cells. Cell Stem Cell 14 (4), 535–548. doi:10.1016/j.stem.2014.01.011
Pankiv, S., Dahl, A. K., Aas, A., Andersen, R. L., Brech, A., Holland, P., et al. (2024). BEACH domain proteins function as cargo-sorting adaptors in secretory and endocytic pathways. J. Cell Biol. 223 (12), e202408173. doi:10.1083/jcb.202408173
Patel, S. R., Hartwig, J. H., and Italiano, J. E. (2005). The biogenesis of platelets from megakaryocyte proplatelets. J. Clin. Invest 115 (12), 3348–3354. doi:10.1172/JCI26891
Pereira, C., and Gershlick, D. C. (2024). BEACH domain proteins in membrane trafficking and disease. J. Cell Biol. 223 (12), e202410147. doi:10.1083/jcb.202410147
Pluthero, F. G., and Kahr, W. H. A. (2023). Evaluation of human platelet granules by structured illumination laser fluorescence microscopy. Platelets 34 (1), 2157808. doi:10.1080/09537104.2022.2157808
Pokrovskaya, I. D., Yadav, S., Rao, A., McBride, E., Kamykowski, J. A., Zhang, G., et al. (2020). 3D ultrastructural analysis of alpha-granule, dense granule, mitochondria, and canalicular system arrangement in resting human platelets. Res. Pract. Thromb. Haemost. 4 (1), 72–85. doi:10.1002/rth2.12260
Poncz, M., Zaitsev, S. V., Ahn, H., Kowalska, M. A., Bdeir, K., Dergilev, K. V., et al. (2024). Packaging of supplemented urokinase into alpha granules of in vitro-grown megakaryocytes for targeted nascent clot lysis. Blood Adv. 8 (14), 3798–3809. doi:10.1182/bloodadvances.2024012835
Rabiej, V. K., Pflanzner, T., Wagner, T., Goetze, K., Storck, S. E., Eble, J. A., et al. (2016). Low density lipoprotein receptor-related protein 1 mediated endocytosis of β1-integrin influences cell adhesion and cell migration. Exp. Cell Res. 340 (1), 102–115. doi:10.1016/j.yexcr.2015.11.020
Schick, B. P., Gradowski, J. F., and San Antonio, J. D. (2001). Synthesis, secretion, and subcellular localization of serglycin proteoglycan in human endothelial cells. Blood 97 (2), 449–458. doi:10.1182/blood.v97.2.449
Schurr, Y., Reil, L., Spindler, M., Nieswandt, B., Machesky, L. M., and Bender, M. (2023). The WASH-complex subunit Strumpellin regulates integrin αIIbβ3 trafficking in murine platelets. Sci. Rep. 13 (1), 9526. doi:10.1038/s41598-023-36387-8
Sehgal, S., and Storrie, B. (2007). Evidence that differential packaging of the major platelet granule proteins von Willebrand factor and fibrinogen can support their differential release. J. Thromb. Haemost. 5 (10), 2009–2016. doi:10.1111/j.1538-7836.2007.02698.x
Sharda, A., and Flaumenhaft, R. (2018). The life cycle of platelet granules. F1000Res 7, 236. doi:10.12688/f1000research.13283.1
Shvarev, D., Schoppe, J., Konig, C., Perz, A., Fullbrunn, N., Kiontke, S., et al. (2022). Structure of the HOPS tethering complex, a lysosomal membrane fusion machinery. Elife 11, e80901. doi:10.7554/eLife.80901
Simonetti, B., and Cullen, P. J. (2019). Actin-dependent endosomal receptor recycling. Curr. Opin. Cell Biol. 56, 22–33. doi:10.1016/j.ceb.2018.08.006
Simonsen, A., Birkeland, H. C., Gillooly, D. J., Mizushima, N., Kuma, A., Yoshimori, T., et al. (2004). Alfy, a novel FYVE-domain-containing protein associated with protein granules and autophagic membranes. J. Cell Sci. 117 (Pt 18), 4239–4251. doi:10.1242/jcs.01287
Streetley, J., Fonseca, A. V., Turner, J., Kiskin, N. I., Knipe, L., Rosenthal, P. B., et al. (2019). Stimulated release of intraluminal vesicles from Weibel-Palade bodies. Blood 133 (25), 2707–2717. doi:10.1182/blood-2018-09-874552
Sugimoto, N., Kanda, J., Nakamura, S., Kitano, T., Hishizawa, M., Kondo, T., et al. (2022). iPLAT1: the first-in-human clinical trial of iPSC-derived platelets as a phase 1 autologous transfusion study. Blood 140 (22), 2398–2402. doi:10.1182/blood.2022017296
Takada, Y. K., Simon, S. I., and Takada, Y. (2023). The C-type lectin domain of CD62P (P-selectin) functions as an integrin ligand. Life Sci. Alliance 6 (7), e202201747. doi:10.26508/lsa.202201747
Theisen, D. J., Davidson, J. Tt, Briseno, C. G., Gargaro, M., Lauron, E. J., Wang, Q., et al. (2018). WDFY4 is required for cross-presentation in response to viral and tumor antigens. Science 362 (6415), 694–699. doi:10.1126/science.aat5030
Tseng, H. Y., Thorausch, N., Ziegler, T., Meves, A., Fassler, R., and Bottcher, R. T. (2014). Sorting nexin 31 binds multiple β integrin cytoplasmic domains and regulates β1 integrin surface levels and stability. J. Mol. Biol. 426 (18), 3180–3194. doi:10.1016/j.jmb.2014.07.003
Urban, D., Li, L., Christensen, H., Pluthero, F. G., Chen, S. Z., Puhacz, M., et al. (2012). The VPS33B-binding protein VPS16B is required in megakaryocyte and platelet alpha-granule biogenesis. Blood 120 (25), 5032–5040. doi:10.1182/blood-2012-05-431205
Wagner, D. D., Saffaripour, S., Bonfanti, R., Sadler, J. E., Cramer, E. M., Chapman, B., et al. (1991). Induction of specific storage organelles by von Willebrand factor propolypeptide. Cell 64 (2), 403–413. doi:10.1016/0092-8674(91)90648-i
Woulfe, D. S., Lilliendahl, J. K., August, S., Rauova, L., Kowalska, M. A., Abrink, M., et al. (2008). Serglycin proteoglycan deletion induces defects in platelet aggregation and thrombus formation in mice. Blood 111 (7), 3458–3467. doi:10.1182/blood-2007-07-104703
Yao, H. H. Y., and Kahr, W. H. A. (2025). Molecular basis of platelet granule defects. J. Thromb. Haemost. 23 (2), 381–393. doi:10.1016/j.jtha.2024.11.016
Zappelli, C., van der Zwaan, C., Thijssen-Timmer, D. C., Mertens, K., and Meijer, A. B. (2012). Novel role for galectin-8 protein as mediator of coagulation factor V endocytosis by megakaryocytes. J. Biol. Chem. 287 (11), 8327–8335. doi:10.1074/jbc.M111.305151
Zhang, N., and Newman, P. J. (2019). Packaging functionally important plasma proteins into the alpha-granules of human-induced pluripotent stem cell-derived megakaryocytes. J. Tissue Eng. Regen. Med. 13 (2), 244–252. doi:10.1002/term.2785
Keywords: platelet α-granule, megakaryocytes, organelle biogenesis, bleeding disorders, NBEAL2, VPS33B/VPS16B, intracellular protein transport
Citation: Ambrosio AL and Di Pietro SM (2025) The winding road to platelet α-granules. Front. Cell Dev. Biol. 13:1584059. doi: 10.3389/fcell.2025.1584059
Received: 26 February 2025; Accepted: 04 April 2025;
Published: 16 April 2025.
Edited by:
Cédric Delevoye, INSERM U1151 Institut Necker Enfants Malades, FranceReviewed by:
Francisco Rivero, University of Hull, United KingdomWalter Kahr, University of Toronto, Canada
Copyright © 2025 Ambrosio and Di Pietro. This is an open-access article distributed under the terms of the Creative Commons Attribution License (CC BY). The use, distribution or reproduction in other forums is permitted, provided the original author(s) and the copyright owner(s) are credited and that the original publication in this journal is cited, in accordance with accepted academic practice. No use, distribution or reproduction is permitted which does not comply with these terms.
*Correspondence: Santiago M. Di Pietro, c2FudGlhZ28uZGlwaWV0cm9AY29sb3N0YXRlLmVkdQ==