- 1Department of Endocrinology, Chengdu Shuangliu Hospital of Traditional Chinese Medicine, Chengdu, China
- 2Key Laboratory of Qinghai-Tibetan Plateau Animal Genetic Resource Reservation and Utilization, Sichuan Province and Ministry of Education, Southwest Minzu University, Chengdu, China
The skeletal muscle, one of the largest tissues in mammals, plays a crucial role in maintaining body movement and energy metabolism. Dysfunction or damage to the skeletal muscle can lead to various muscle diseases, such as muscular dystrophy, myasthenia, and others. The myofiber presents the fundamental structural unit of the skeletal muscle, and research on its structure and biological function is of great significance. Here, we review the latest progress in the structural and functional aspects of the myofiber, focusing on myofibril, the sarcoplasmic reticulum, mitochondria, and the cytoskeleton. The basic properties and dynamic interactions of a large number of muscle proteins have been described in detail, including the scaffold construction of core protein components and the fine-tuning of secondary protein components with functional redundancy. This overview provides new insights into skeletal muscle pathophysiology.
1 Introduction
The skeletal muscle, the most abundant tissue in vertebrates (accounting for ∼40% of total body weight), is primarily composed of myofibers with minor contributions from adipose, vascular, nervous, and connective tissues. Recent advances in single-cell sequencing, however, have unveiled its intricate cellular diversity, including multinucleated myofibers, muscle stem cells, endothelial cells, immune cells, adipocytes, neurocytes, and other mononuclear cell populations (Cai et al., 2023a). This cellular heterogeneity not only defines the tissue architecture but also enables efficient communication strategies between cell types to facilitate the exchange of biological information, thereby maintaining skeletal muscle homeostasis (Cai et al., 2023b; Krauss et al., 2017). Beyond its structural complexity, the skeletal muscle plays indispensable roles in basic mammalian body functions such as locomotion, respiration, and metabolism. These functions depend on a tightly orchestrated developmental program: in vertebrates, skeletal muscle ontogeny requires successive phases of fetal, postnatal, and adult myogenesis (Salvatore et al., 2014; Greggio et al., 2017; Egan and Zierath, 2013; Relaix et al., 2021). Central to this process are the main waves of myogenesis during in utero development, when myogenic progenitor cells located on the peripheral edge of the dermomyotome extend downward to form the myotome, which rapidly differentiates into spindle mononuclear myoblasts (Deries and Thorsteinsdottir, 2016). Following migration to muscle formation sites, these myoblasts undergo proliferation, differentiation, and fusion to assemble multinucleated, contractile myofibers (Buckingham et al., 2003). Postnatally, skeletal muscle growth shifts focus: myofiber numbers remain largely constant, with growth instead driven by hypertrophy and the regenerative activity of satellite cells in response to damage (Yin et al., 2013). Underpinning these developmental and regenerative processes is a well-established transcriptional hierarchy including master regulators such as Pax3, Pax7, myogenic regulatory factors (MRFs), and the myocyte enhancer factor 2 (MEF2) family (Bryson-Richardson and Currie, 2008; Asfour et al., 2018). While this transcriptional framework is well-characterized, the structural and functional complexity of myofibers—particularly their subcellular components—remains incompletely understood. To address this gap, we review key myofiber structures such as myofibrils, the sarcoplasmic reticulum (SR), mitochondria, and the cytoskeleton, focusing on their molecular architecture and functional mechanisms to advance our understanding of skeletal myogenesis.
2 Structure and composition of the skeletal myofiber
Skeletal muscle functionality hinges on the specialized organization of myofibers, which are multinucleated syncytia unique to terrestrial animals. These elongated cells, spanning centimeters in length and 10–100 µm in diameter, are surrounded by connective tissues (endomysium, perimysium, and epimysium) that stabilize the muscle architecture and transmit mechanical forces (Powell et al., 2002). However, the functional essence of myofibers resides in their internal components: myofibrils, dominating the cytoplasmic space; these tightly packed bundles of actin and myosin filaments form repeating sarcomeres—the contractile units responsible for muscle shortening; SR, a specialized endoplasmic reticulum enveloping myofibrils; the SR stores and releases calcium ions to regulate excitation–contraction coupling; mitochondria: positioned near the sarcolemma and between myofibrils; mitochondria generate ATP through oxidative phosphorylation, sustaining energy-demanding contractions; cytoskeleton: composed of costameres, intermediate filament (IF), and microtubules; this network maintains sarcomere alignment, distributes mechanical stress, and anchors organelles such as the nuclei and mitochondria. These components collaborate dynamically: myofibrils drive contraction, the SR synchronizes calcium signaling, mitochondria fuel activity, and the cytoskeleton integrates structural integrity.
Myofibers also harbor specialized structures critical to their function: the neuromuscular junctions (NMJs) for nerve signal transmission; myotendinous junctions (MTJs) for force transmission to tendons; and cilia, which have recently been implicated in mechanosensory signaling (Brooks et al., 2023; Ng et al., 2021). Notably, myofiber size adapts dynamically through radial hypertrophy (increase in diameter) or longitudinal growth (addition of sarcomeres in series), although the molecular drivers of these processes remain incompletely characterized. Surrounding the myofibers is a hierarchical network of connective tissues. From the innermost to the outermost, these include the following: endomysium, a thin layer of collagen and proteoglycans enveloping individual myofibers; perimysium, a thicker sheath encircling bundles of myofibers (fascicles), rich in blood vessels and nerves; and epimysium, a dense collagenous membrane encasing the entire muscle. These layers serve dual structural and functional roles: they transmit mechanical forces during contraction, anchor NMJs and MTJs, and provide a scaffold for satellite cell-mediated regeneration (Cretoiu et al., 2018). Intriguingly, recent studies suggest that connective tissues—not myofibers themselves—bear the majority of mechanical loads during muscle activity (Gillies and Lieber, 2011). Connective tissues externally reinforce this system, but the molecular interplay within myofibers ultimately dictates muscle performance and adaptability.
2.1 Myofibril
It is easily observable under the electron microscope that myofibers filled with myofibrils are aligned along the longitudinal axis of the cell body. The myofibril has a diameter of approximately 1 μm and presents a periodic striped structure alternating between light and dark, in which, each I-band (light area) is divided into two parts by a Z-line and each A-band (dark area) is divided into two portions by an M-line within the H zone. The segment of myofibrils between two adjacent Z lines is commonly described as a sarcomere, which is the smallest contractile unit of the striated muscle (Figure 1). On the meta-microscale, the sarcomere primarily consists of thin and thick myofilaments that are perpendicular to the Z-line: the actin, tropomyosin, and troponin complexes (troponin T, I, and C, respectively) are organized into thin myofilaments that are anchored in Z-lines, while the myosins are assembled into thick myofilaments in the A-band that overlap with thin myofilaments (Ertbjerg and Puolanne, 2017). These structures form the basis for the “sliding filament theory” of skeletal muscle contraction: muscle contraction entails the thin myofilaments sliding over the thick myofilaments into the A-band, causing the shortening of the I-band and H zone, while the widths of the Z-line, A-band, and M-line remain unchanged (Huxley and Niedergerke, 1954; Huxley and Hanson, 1954). Specifically, myosin heads bind actin at a ∼45° angle, forming the acto-myosin crossbridges, which pull actin filaments toward the M-line through ATP hydrolysis-driven conformational changes, resulting in sarcomere shortening. Additionally, approximately 30% of crossbridges maintain passive muscle tension even during relaxation, mediated by the spring-like domains of structural titin in coordination with residual crossbridge interactions to preserve myofiber elasticity (Al-Khayat, 2013). In addition, other myofilament-associated proteins such as myosin binding protein-C (MyBP-C), nebulin, and obscurin have also been proposed to play important structural and regulatory functions during the assembly of sarcomeres.
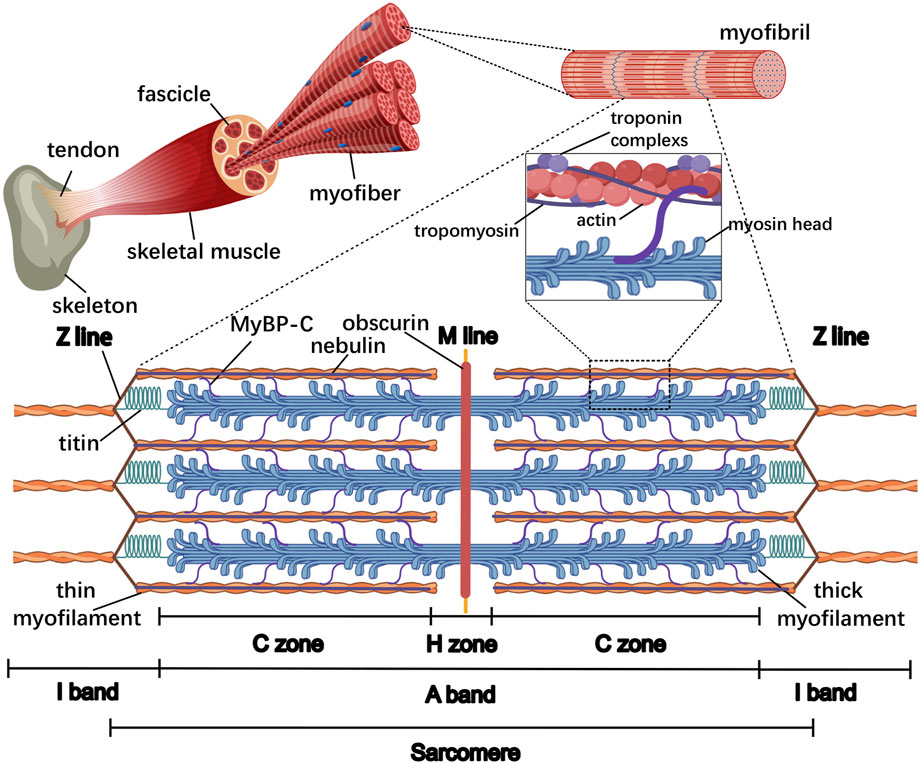
Figure 1. General organization of the sarcomere in the skeletal muscle. From the inside to the outside, the skeletal muscle comprises the myofiber, fascicle, and whole muscle, respectively. Within the myofiber, the myofibrils are aligned along the longitudinal axis of the cell body and almost occupy the entire intracellular volume, and the sarcomere is the smallest contractile unit of the myofiber. The major lines and bands are indicated, as well as myofilament-associated proteins such as thin and thick myofilaments, MyBP-C, titin, nebulin, and obscurin. The thin and thick myofilaments overlap at the C zone in the A-band where 7–9 MyBP-C stripes connect with both myofilaments; titin is directed toward its N-terminus at the Z-line and its C-terminus at the M-line; nebulin is aligned with its C terminus in the Z-line; obscurin is oriented with its N-terminus in the M-line and its C-terminus bound to the SR.
For instance, MyBP-C is a myofilament protein binding to thin and thick myofilaments maintaining the ordered arrangement of the sarcomere and cross-bridge cycling (van Dijk et al., 2014). The interaction of MyBP-C with both myofilaments is restricted to the C zone, where actin and myosin overlap in the A-band, and MyBP-C is arranged in 7–9 transverse stripes at 43-nm intervals (Luther and Craig, 2011). MyBP-C consists of a series of immunoglobin (Ig) and fibronectin III (Fn)-like domains, and the MyBP-C N-terminal binds to actin and myosin head, while the MyBP-C C-terminal binds with meromyosin and titin (Squire et al., 2003). In addition, accumulating evidence has implicated MyBP-C in the regulation of myofilament Ca2+ sensitivity and enzymatic activity of myosin (van Dijk et al., 2014).
As the largest known polypeptide, titin spans the length of half of the sarcomere, anchoring its N-terminus and C-terminal at the Z-line and M-line, respectively. The C-terminals of two titin molecules overlap on both sides of the M-line, forming a continuous titin filament harboring tight binding sites for a variety of sarcomeric proteins, which functions as a scaffold, mechanical sensor, and signaling mediator. For example, within the Z-line, titin N-terminus interacts with proteins such as α-actinin and telethonin to maintain the structural integrity and resist high mechanical stress (Young et al., 1998; Mues et al., 1998). Similarly, titin C-terminal binds with several proteins at the M-line, including Muscle RING finger-1 (MURF-1), myomesin, and M-protein, functioning as scaffolding structures for A-band assembly (Witt et al., 2005). Titin is normally regarded as a “molecular spring” that generates passive tension, and the elements responsible for this elasticity primarily reside in the I-band region of titin, including tandemly arranged Ig-domains, PEVK motifs, and N2A element (Linke et al., 1998; Linke et al., 2002; Granzier and Labeit, 2006; Granzier and Labeit, 2005). Unlike the elastic I band region of titin, the A-band region of titin is inextensible and provides a scaffold for myosin and MyBP-C, therefore determining the length and structure of thick myofilaments (Whiting et al., 1989). Moreover, as its I-band and M-line region contain Ser/Thr kinase domains, titin may be involved in signaling transduction from myofibrils to other compartments of myofiber, contributing to muscle adaptation in conditions of mechanical stress changes (Tskhovrebova and Trinick, 2008; Puchner et al., 2008).
Ultrastructural results with monoclonal antibodies have established that nebulin is oriented with its N-terminus at the tips of thin myofilaments in the I-band and its C-terminus at the Z-line, whose configuration both stabilizes the thin myofilaments and regulates the Z-line structure (Wright et al., 1993; Wang and Wright, 1988; Witt et al., 2006). Actually, nebulin is non-extensible because nebulin epitopes maintain a fixed distance from the Z-line, regardless of sarcomere stretching (Kruger et al., 1991). Numerous studies indicate that nebulin can bind to various proteins, including actin and myosin components, as well as proteins associated with its unique N- and C-terminal regions, especially for the central super repeats of nebulin, through which nebulin laterally interacts with the thin myofilament proteins actin, tropomyosin, and troponin complexes, functioning as the scaffold to control thin myofilament length (Kruger et al., 1991; Labeit et al., 1991). In addition, nebulin’s super-repeat region also binds to myosin, which may inhibit actomyosin ATPase activity and the sliding velocity of actin on myosin in a calcium-/calmodulin-dependent manner (Root and Wang, 1994). Differing in sequence from the central super repeats, the N-terminal sequence carries glutamate residues and can interact with tropomodulin to specify thin myofilament length, whereas the C-terminus is rich in serine residues, and the SH3 domain can directly bind to Z-line protein myopalladin and CapZ and titin to mediate the myofibrillar assembly and mechanochemical signaling (Mcelhinny et al., 2001; Wear et al., 2003; Bang et al., 2001; Kontrogianni-Konstantopoulos et al., 2009).
In contrast to MyBP-C, titin, and nebulin presenting within sarcomeres, obscurin wraps around myofilaments over the M-line and Z-line, contributing to their assembly and integration with other sarcoplasmic elements such as the SR (Kontrogianni-Konstantopoulos et al., 2003). Given its structure composing of tandem adhesion modules and signaling domains, obscurin can associate tightly and periodically with the myofilaments and the periphery of the myofibril. Specifically, the N-terminal Ig domain of obscurin binds directly to myofibrillar components such as titin, myomesin, and MyBP-C, while obscurin tightly connects with the SR component small ankyrin-1 (sAnk1) through the C-terminus to its last Ig-like domain (Kontrogianni-Konstantopoulos and Bloch, 2005; Porter et al., 2005). Other kinetic studies showed that in addition to structural support function, obscurin has a possible involvement in RhoA- and Ca2+-mediated signaling pathways via its Rho-GEF/PH and IQ motif, and the presence of obscurin at the NMJ is consistent with this, suggesting its direct or indirect involvement in signal transduction at the synaptic terminal (Fukuzawa et al., 2005; Young et al., 2001; Carlsson et al., 2008). Initial subcellular distribution of obscurin studies using antibodies indicated that obscurin briefly accumulates at the Z-line and primarily concentrates at the M-line during embryogenesis; however, it eventually loses Z-line distribution in later developmental stages (Young et al., 2001). Actually, multiple alternatively spliced forms of obscurin exist in distinct sarcomeric locations, such as obscurin A, obscurin B, and several additional variants that differ in the combination of C-terminal structural domain elements and localize to the M-line, A/I junction, and Z-line at the muscle resting state, respectively (Bowman et al., 2007). Notably though, when muscles are stretched, obscurin can redistribute to different locations along the sarcomere length (Bowman et al., 2007). Considering the preferential integration of these obscurin isoforms with certain regions of the sarcomere, it further implies specialized functions of these isoforms during myofibrillogenesis and at maturity. Collectively, myofibrillogenesis depends on the coordinated assembly and integration of a large number of myofilament-associated proteins into the sarcomeres. These molecules may interact with several protein ligands to function as scaffolds, regulate signaling cascades, and localize them to particular sites within or surrounding sarcomeres to coordinate sarcomeric arrangement.
2.2 Sarcoplasmic reticulum
The skeletal muscle contains a specialized endoplasmic reticulum network called SR, which is responsible for protein and calcium homeostasis regulation. Highly precise views under electron microscopes (EMs) showed that the SR consists of tubules and cisternae around each myofibril, in which the thin tubules are named longitudinal SR (l-SR) and are positioned around the A- and I-band, and its enriched Ca2+ ATPase (SERCA) is responsible for removing Ca2+ from the cytoplasm to the lumen; the tubule edges merge into the terminal cisternae at the boundaries of the A- and I-band and collectively form the triad structure, with the transverse tubules (TT) originating from the sarcolemma, maintaining excitation–contraction coupling (ECC) in the skeletal muscle (Rossi et al., 2022a) (Figure 2; Table 1). Within the muscle field, ECC is defined as the process linking the sarcolemma depolarization induced by motor–neuron stimulation and Ca2+ release from the SR. In particular, there are two Ca2+ channel proteins dihydropyridine receptor (DHPR) and ryanodine receptor 1 (RyR1) located on the TT and terminal cisternae region facing the TT terminal junctional SR (j-SR), respectively. Following sarcolemma depolarization, the DHPR undergoes conformational changes and directly transmits signals to open the RyR1 to trigger Ca2+ release into the myoplasm, thereby initiating muscle contraction (Barone et al., 2015). In fact, DHPR and RyR1 tightly wound together to form the core of the macromolecular complex known as the Ca2+ release unit, which includes numerous interacting proteins, and each of them influences the entire ECC process (Dulhunty, 2006). These proteins include TT biogenesis proteins amphiphysin 2/bridging integrator-1 (BIN1), caveolin 3 (CAV3), and dysferlin (DYSF); triad formation proteins mitsugumins (MG), junctophilin (JPH), myotubularin (MTM1), triadin, junctin, and the junctional sarcoplasmic reticulum protein 1 (JP-45); and Ca2+-binding proteins calsequestrin (CASQ), sarcalumenin (SAR), and HRC (Figure 2). For instance, BIN1 is highly expressed in the skeletal muscle and capable of driving membrane tubulation during TT maturation, in which BIN1 works separately or in cooperation with a GTPase dynamin 2 (DNM2) (Lee et al., 2002; Cowling et al., 2017). BIN1 knockout mouse myofibers present abnormal TTs and deficient ECC, and DNM2 downregulation improved its motor and histopathological phenotypes, suggesting that the balance between BIN1 and DNM2 is necessary for TT maturation, which is mediated by the interaction of the BIN1 SH3 domain with the proline-rich domain of DNM2 (Silva-Rojas et al., 2022; Fujise et al., 2021; Takei et al., 1999). As a member of the integral membrane protein family, CAV3 is not only implicated in caveolae membrane formation at the sarcolemma but also localizes at the developing TTs, and its deletion leads to TT membrane disorder with lateral loss. However, CAV3 is undetectable in the mature TTs, suggesting that CAV3 may be involved in the early development of the TT system in the skeletal muscle (Tang et al., 1996; Parton et al., 1997; Galbiati et al., 2001). DYSF mainly localizes to the sarcolemma for membrane fusion and repair, and DYSF-deficient muscle shows subsarcolemmal vacuoles contiguous with the TT system (Selcen et al., 2001). Similarly to mice deficient in CAV3, DYSF mouse mutants display dilated and longitudinally oriented TTs, and CAV3 and DYSF show partial colocalization and interaction in developing TT system, suggesting a specific role of DYSF in TT biogenesis (Hernandez-Deviez et al., 2006; Matsuda et al., 2001). Although the precise mechanism is not yet clear, there is a hypothesis that DYSF may facilitate the fusion of vesicles containing CAV3 with TTs (Klinge et al., 2010). These findings suggest that TT biogenesis requires advanced mechanisms of membrane fusion, and additional factors involved in TT formation are needed.
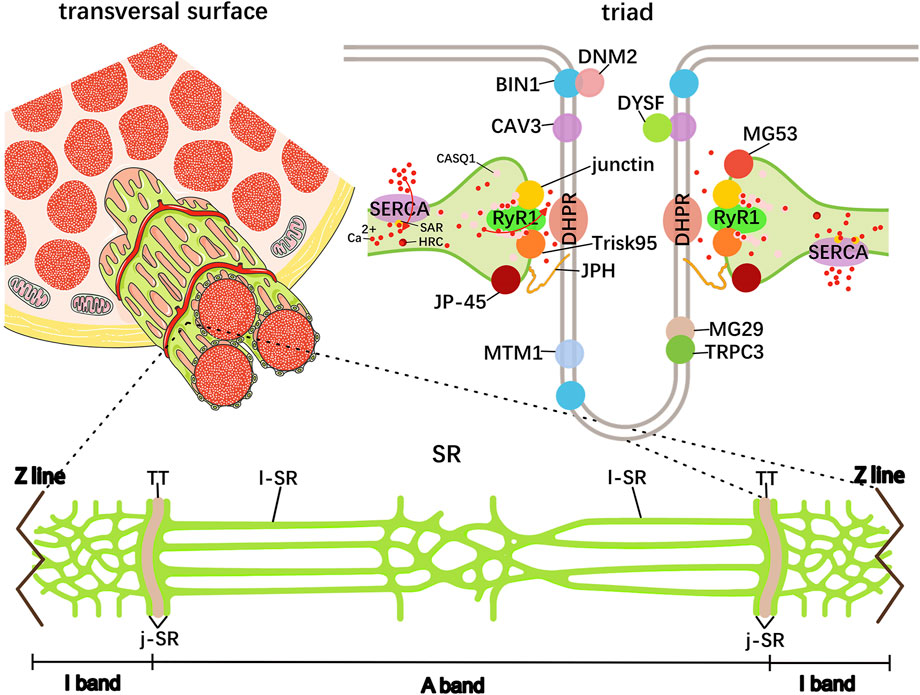
Figure 2. Schematic representation of skeletal muscle SR. The elaborated SR network consists of tubules and cisternae around each myofibril shown in green. The central TT and the terminal cisternae element on both sides collectively form the triad structure, in which many protein–protein interactions are responsible for Ca2+ cycling and storage. These proteins include dihydropyridine receptor (DHPR), ryanodine receptor 1 (RyR1), TT biogenesis proteins amphiphysin 2/bridging integrator-1 (BIN1), caveolin 3 (CAV3), and dysferlin (DYSF); triad formation proteins mitsugumins (MG), transient receptor potential cation channel type 3 (TRPC3), junctophilin (JPH), myotubularin (MTM1), triadin, junctin, and the junctional sarcoplasmic reticulum protein 1 (JP-45); and Ca2+-binding proteins calsequestrin (CASQ), sarcalumenin (SAR), and HRC.
An abundance of membrane proteins are involved in triad formation, including the maturation of SR terminal cisternae and the connection between TT and SR. A notable example is the mitsugumin 29 (MG29), a member of the synaptophysin family that appears preferentially at the SR membrane and is then transferred to the triad proteins during the early stages of skeletal myogenesis (Takeshima et al., 1998; Komazaki et al., 1999). Analysis of MG29-deficient mice exhibited an accumulation of transport vesicles and incomplete formation of triad and SR networks, suggesting that MG29 may facilitate the docking and fusion of transport vesicles, processes that lead to the creation of the SR network and TT to form the triad (Nishi et al., 1999; Komazaki et al., 2001). Moreover, MG29-deficient mice showed impaired store-operated Ca2+ entry (SOCE) in the skeletal muscle, in which MG29 directly interacts with canonical-type transient receptor potential cation channel type 3 (TRPC3) on TT membranes to regulate Ca2+ transients during skeletal muscle contraction (Woo et al., 2015). Another synaptophysin, MG53 (also termed TRIM72), contributes to intracellular vesicle translocation and accumulation, and it has been recognized as an essential component of the membrane repair machinery in the striated muscle (Cai et al., 2009a; Whitson et al., 2021). It has been suggested that MG53 can interact with CAV3 and DYSF to facilitate membrane repair at injury sites by forming a molecular complex (Cai et al., 2009b).
In the skeletal muscle, JPHs (JPH1 and JPH2) provide a structural basis for physiological coupling between the plasma membrane and SR: they bridge the TT membrane through their cytosolic N-terminal domain with the j-SR via their C-terminal transmembrane domain (Takeshima et al., 2000). Apart from their structural functions, JPHs can also interact with various proteins such as DHPR, RyR, CAV3, triadin, and CASQ, serving as a scaffold for assembling Ca2+-release complexes to ensure effective ECC (Perni and Beam, 2022; Golini et al., 2011).
MTM1 is a phosphoinositide phosphatase localized at triads, and in phosphoinositide metabolism, MTM1 can specifically dephosphorylate phosphatidyl-inositol-3-phosphate (PtdIns3P) and phosphatidyl-inositol-3,5-bisphosphate (PtdIns(3,5) P2), which mediate endocytosis and membrane trafficking (Hnia et al., 2012). Moreover, MTM1 knockout in animal models leads to disruption of triad morphology and Ca2+ homeostasis, while its overexpression causes membrane stack accumulation in the subsarcolemmal region, indicating the delicate balanced expression of MTM1 in triad formation (Al-Qusairi et al., 2009; Dowling et al., 2009; Buj-Bello et al., 2008).
Triadin is an integral span membrane protein family localized at the triad, and all isoforms share common N-terminal transmembrane domains but have different C-terminal segments (Marty et al., 2009; Oddoux et al., 2009). The classic isoform of approximately 95 kDa (Trisk 95) is located at the terminal cisternae of the skeletal muscle, and its knockout mice model presented abnormal triads and Ca2+ homeostasis, but no significant changes were observed in ECC (Shen et al., 2007). It has been demonstrated that Trisk 95 is able to interact with RyR1, JPH1, and Ca2+-binding protein CASQ1 to maintain the triad organization and intracellular Ca2+ homeostasis regulation (Shen et al., 2007; Rossi et al., 2022b; Lee et al., 2001). Similarly, a membrane-spanning protein structurally homologous to triadin, named junctin, is an alternative splicing product of the aspartate β-hydroxylase gene (Dulhunty et al., 2009). Junctin and Trisk 95 both interact with RyR1 and CASQ1, anchoring CASQ1 near RyR1; however, their binding sites on RyR1 and CASQ1 and the detailed functional effects are extremely different. For example, in contrast to one same binding site (KEKE motifs) on Trisk 95 for RyR1 and CASQ1, there are multiple binding sites on junctin for RyR1 and CASQ1. Due to the presence of multiple RyR1/junctin and junctin/CASQ1 binding sites, compared to Trisk 95, junctin could assure transmittance of signals from CASQ1 to RyR1 (Beard et al., 2008; Dulhunty et al., 2017). Moreover, another integral membrane protein JP-45 is highly enriched in j-SR, which interacts with DHPR via its N-terminal domain and with CASQ1 through its C-terminal segment (Anderson et al., 2003). A substantial body of research indicates that both overexpression and ablation of JP-45 leads to a reduction in voltage-dependent Ca2+-release, which may be closely correlated with changes in the collaboration between JP-45 and DHPR/CASQ1 (Anderson et al., 2006; Gouadon et al., 2006; Yasuda et al., 2013).
It is well-known that Ca2+ is preserved in the SR and mainly buffered by the low-affinity and high-capacity Ca2+ binding protein CASQ to polymerize into elongated linear polymers under physiological conditions (Wang et al., 1998; Perni et al., 2013). Actually, CASQ is localized exclusively in the j-SR by binding to RyR, triadin, and junctin, as well as creating a complex three-dimensional cross-linked network. In mammals, the CASQ1 isoform is exclusively expressed in the fast-twitch skeletal muscle, while the CASQ2 isoform is found in the slow-twitch skeletal muscle and heart; especially, CASQ1 is capable of storing 80% of the Ca2+ in the SR (Beard et al., 2004; Murphy et al., 2009). It has become increasingly clearer that CASQ1 interacts with RyR, triadin, and junctin in a Ca2+-dependent manner, which is attributed to changes in the Ca2+ concentration that induces a conformational change in CASQ1: CASQ1 maximally inhibits the RyR1 when the free Ca2+ concentration reaches 1 mM, and the inhibition gradually diminishes as the Ca2+ concentration is altered, while CASQ1 dissociates from the RyR1 complex at Ca2+≤ 100 μM or≥ 5 mM (Wang and Michalak, 2020; Royer and Rios, 2009). Recently, new functions of CASQ1 have been proposed, including regulation of SOCE by binding to STIM1/OraiI and ER stress responses interacting with IRE1α in the skeletal muscle (Michelucci et al., 2020; Wang et al., 2019). Similar to the j-SR, the l-SR also contains Ca2+ binding proteins, and the most plentiful one is SAR. It has been demonstrated that SAR colocalizes and directly interacts with SERCA, enhancing SERCA complex stability to pump Ca2+ into the lumen of the SR to initiate muscle relaxation (Yoshida et al., 2005; Conte et al., 2023). Another secondary SR Ca2+-binding protein is HRC, which is far less abundant than CASQs but structurally similar to CASQs, functioning as Ca2+ buffer. Actually, the local and rapid changes of Ca2+ levels within the SR may result in different multimer forms of HRC and affect its interactions with other SR components (Suk et al., 1999). It has been suggested that HRC could interact with either the RyR or SERCA complex to manage Ca2+ release and uptake, respectively. As the SR Ca2+ increases, the HRC/SERCA interaction gradually diminishes, causing the detachment of SERCA from HRC. The released HRC then regulates Ca2+ release through the RyR complex, while locally reduced SR Ca2+ concentration facilitates the reconnection of HRC/SERCA, resulting in the reuptake of cytoplasmic Ca2+ (Arvanitis et al., 2011). However, current research studies on HRC mainly focus on the cardiac muscle, and the HRC/triadin and HRC/SERCA interactions and their functional implications on Ca2+-uptake and release in skeletal muscle remain unclear.
2.3 Mitochondria
As a highly metabolic tissue, the skeletal muscle relies on mitochondria to convert substrates into ATP via oxidative phosphorylation. This ATP powers critical processes such as myofibrillar contraction (through myosin ATPase activity), ion transport (e.g., Ca2+ reuptake by the SR), and cellular homeostasis. Beyond energy production, the mitochondria are central to metabolite biogenesis, cell-cycle regulation, and apoptosis/autophagy signaling (Mcbride et al., 2006; Dominy and Puigserver, 2013; Tilokani et al., 2018; Palmer et al., 2011; Ploumi et al., 2017). Actually, mitochondrial function is dynamically regulated by cycles of fission (division), fusion (merging), and motility—collectively termed mitochondrial dynamics (Ni et al., 2015). These processes depend on coordinated nuclear gene expression, mtDNA transcription, and protein import (Ploumi et al., 2017). Dysregulation of fission/fusion disrupts mitochondrial membrane integrity, exacerbating apoptosis and autophagy through pathways such as Bax/Bcl-2-imbalance and mPTP opening (Jeong and Seol, 2008; Xian and Liou, 2021).
The mitochondria in myofibers exhibit distinct morphological and functional specialization based on their subcellular localization: the sub-sarcolemmal mitochondria (SSM) reside beneath the sarcolemma and are usually spherical in form with few branches, supplying ATP to sarcolemmal pumps (e.g., Na+/K+-ATPase); the perinuclear mitochondria (PNM) are in proximity to nuclei/capillaries and have structural similarities with SSM, supplying ATP to the nuclear pores, and both SSM and PNM are termed peripheral mitochondria (PM); the intra-myofibrillar mitochondria (IFM) are located between myofibrils and have a close contact with the SR displaying reticular network structure, which specialize in ATP production for contractile machinery (myofibrils) and SR Ca2+-ATPase (SERCA) (Vincent et al., 2019; Swalsingh et al., 2022). IFM exhibit higher oxidative enzyme activity, respiratory chain complex density, and nucleotide metabolism proteins compared to SSM, aligning with their role in sustaining contraction (Ferreira et al., 2010).
Emerging studies demonstrate that mitochondrial subpopulations diverge in stress responses and signaling. For instance, some studies demonstrated that IFM possess higher levels of cytochrome c/apoptosis-inducing factor (AIF) release and lower membrane potential, ROS production rate, and Bax-to-Bcl-2 ratio compared with SSM (Adhihetty et al., 2005). Considering its greater oxidative enzyme activities and respiration rates, IFM may represent the larger pro-apoptotic protein pool in the skeletal muscle. A recent spatial distribution study of mitochondrial enzymes showed that complexes responsible for membrane potential (complex IV) and ATP (complex V) production are preferentially located in PM and IFM, respectively, and it has been postulated that membrane potential generated in PM can be directly distributed to IFM through mitochondrial connections, promoting ATP production (Willingham et al., 2021). It has also been reported that IFM is structurally and functionally associated with SR, and their contact sites are referred to mitochondria-associated membranes (MAMs) for exchanging Ca2+. In the mitochondria, Ca2+ influx increases ATP production by enhancing the activity of mitochondrial oxidases, while the exceeding physiological needs of Ca2+ can promote the opening of the mitochondrial permeability transition pore (mPTP), thereby triggering apoptosis or necrotic cell death (Rossi et al., 2019; Matuz-Mares et al., 2022). Therefore, mitochondrial subcellular specialization is tightly linked with skeletal muscle quality and function.
2.4 Cytoskeleton
In the skeletal muscle, the cytoskeleton serves as a cellular scaffold determining the morphology of myofibers and transmitting mechanical force between myofibrils and extracellular skeleton. In addition to myofibrils themselves, the cytoskeleton component also includes costameres, IF, and microtubule. Costameres are positioned underneath the sarcolemma and vertical to the longitudinal axis of myofibers, connecting the sarcomere to sarcolemma via the Z-line and M-line. Along with assembling and stabilizing the sarcomere, costameres also regulate the interaction between the cytoskeleton and the extracellular matrix (ECM), all of which are based on its intricate protein composition. Current evidence supports the existence of major costameric proteins including the dystrophin–glycoprotein complex (DGC) and the vinculin–talin–integrin system (Figure 3). The DGC connects the cytoskeletal actin and the laminin via dystrophin and dystrophin-associated glycoprotein (DAG) interactions, respectively, in which the cytoskeletal protein dystrophin binds to cytoplasmic actin at its N-terminus, whereas its C-terminus is associated with DAGs that can be classified as cytoplasmic, supramembrane, and extracellular subcomplex (Blake et al., 2002; Sweeney and Barton, 2000). The cytoplasmic subcomplex comprises the α-dystrobrevin (α-DB) and syntrophin (syn), which are directly linked to dystrophin C-terminus. The supramembrane subcomplex includes glycosylated transmembrane protein sarcoglycan (SG) and sarcospan (SP), which need to be correctly assembled into a complex with dystrophin for maintaining sarcolemma integrity. For the extracellular subcomplex, the primary component α-dystroglycan (α-DG) strengthens the physical linkage between the ECM and the basement membrane components via binding to laminin-2 in the ECM and β-dystroglycan (β-DG) in the sarcolemma. The β-DG can also interact with the dystrophin, thus establishing the connection between the actin-based cytoskeleton and the ECM. Hence, it is clear that dystrophin serves a pivotal role in DGC system, whose absence or dysfunction leads to increased sarcolemma fragility, thereby weakening its functions to protect against mechanical stress (Jaka et al., 2015). Similarly, the vinculin–talin–integrin system is also fundamental for facilitating actin cytoskeleton interactions with the surrounding matrix, especially in skeletal muscle cell adhesion and signaling (Mukund and Subramaniam, 2020). Here, the dimeric protein talin links vinculin with integrin: on the one hand, talin binds to the cytoplasmic tails of integrins to activate ECM adhesion; on the other hand, the interaction between vinculin and talin provides the connection of actin filaments to the sarcolemma, reinforcing the adhesion site. Moreover, the complex can also participate in signal transduction pathways, which influence various cellular responses, such as changes in gene expression and cell behavior.
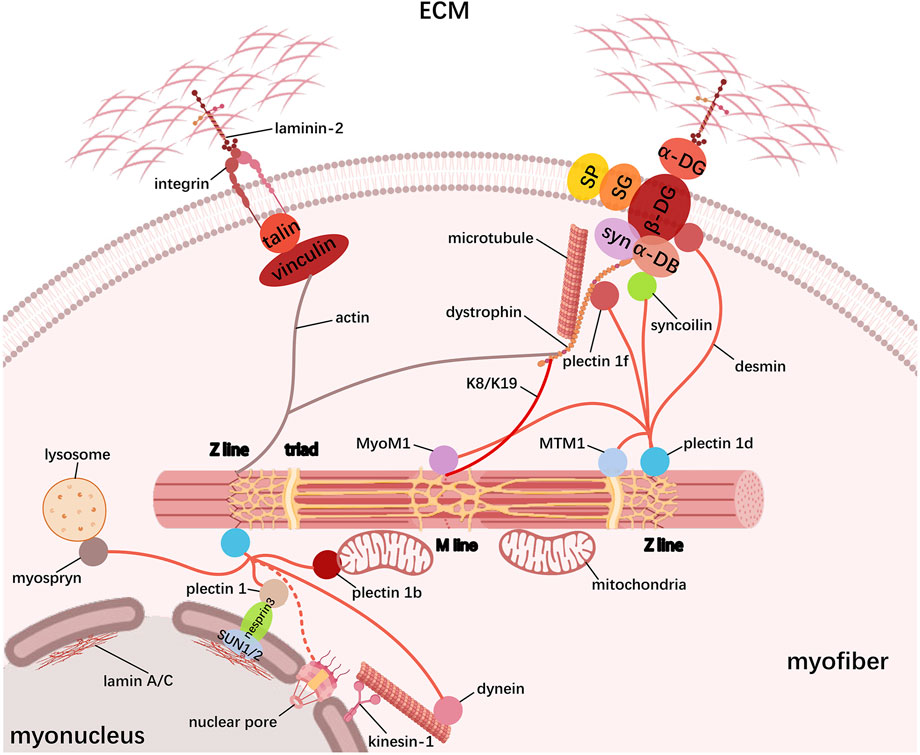
Figure 3. Schematic representation of the cytoskeleton in myofibers. Skeletal muscle cytoskeleton comprises myofibrils, costameres, IF, and microtubules. Costameres mainly contain the dystrophin–glycoprotein complex (DGC) and the vinculin–talin–integrin complex that bidirectionally link the ECM to myofibrils; IF proteins, especially desmin, tether the contractile myofibril to the sarcolemma and other organelles including myonucleus, mitochondria, SR, and lysosome, which forms the transverse fixation system of the muscle together with the costameres; microtubules form a grid containing longitudinal and transverse components across the myofiber to promote muscle maturation.
Another cytoskeletal element, IF, has been demonstrated to tether the contractile myofibrillar apparatus to the sarcolemma and other organelles such as the myonucleus, mitochondria, SR, and lysosome, which forms the transverse fixation system of the muscle together with the costameres (Figure 3). Based on similarities among their sequence and structure, IF proteins are grouped into six types, namely, types I and II (keratin), type III (desmin, vimentin, glial fibrillary acidic protein (GFAP), and peripherin), type IV (neurofilament-like protein), type V (lamin A/C), and type VI (large-molecular weight proteins such as nestin, synemin, paranemin, and syncoilin or a special group for CP49/phakinin and filensin/CP115), and the list is still growing (Hnia et al., 2015; Costa et al., 2004; Eldirany et al., 2021). During myogenesis, myoblasts initially express vimentin and nestin, while desmin is induced early in myogenic differentiation and then integrated into the pre-existing network of vimentin, and the mature skeletal muscle mainly contains desmin and keratin (Schweitzer et al., 2001). These filamentous structures form extended IF networks around the Z-line of the myofibrils, thereby linking adjacent myofibrils together, especially desmin and keratin (K8 and K19), which are linked to sarcolemma through costameric components. In addition, desmin knockout in mice causes disruption in the nuclear position, myofibril alignment, mitochondrial function, and even NMJ and MTJ integrity, indicating a more prominent role of desmin in maintaining cytoskeletal stability (Capetanaki et al., 1997; Castanon et al., 2013). Actually, molecular studies demonstrate that desmin can bind or copolymerize with IF proteins vimentin, nestin, synemin, paranemin, and syncoilin and widely interact with other functional proteins. In early myogenesis, nestin co-polymerizes with vimentin and later on with desmin to form filaments, whereas vimentin completely disappears and nestin is selectively expressed only in the NMJ and MTJ in mature skeletal myofibers (Romero et al., 2013; Hol and Capetanaki, 2017; Cizkova et al., 2009). Synemin is another desmin-associated molecule and is considered to link the heteropolymeric IFs to the sarcolemma by directly interacting with desmin/vimentin, α-actinin, α-dystrobrevin, and vinculin (a microfilament-associated protein) (Sun et al., 2008; Etienne-Manneville, 2018). For an IF component of developing skeletal muscle, paranemin has been shown to be required for desmin filaments organization, but not nestin or synemin (Schweitzer et al., 2001). Unlike the majority of IF proteins that form filaments by homopolymerization or heteropolymerization, syncoilin is a non-filament-forming member that binds directly to desmin and α-dystrobrevin, thus linking desmin to the sarcolemma and contributing to the lateral force transmission in the skeletal muscle. Except for being located near the sarcolemma, syncoilin is enriched at the NMJ and MTJ and around the nuclei in mature skeletal myofibers (Moorwood, 2008; Blake and Martin-Rendon, 2002). In the skeletal muscle, IFs are also located in the nuclei as lamins, mainly represented by lamin A/C, to form a nucleoskeleton beneath the inner nuclear membrane and hold mechanosignals. LINC complex (SUN1/2-nesprin3) on the nuclear membrane binds to lamin A/C at the inner nuclear membrane (through SUN1/2) and to desmin-binding protein plectin 1 at the outer nuclear membrane (through nesprin3), thus allowing the association of the nucleoskeleton with the cytoskeleton (Etienne-Manneville, 2018). Furthermore, via the nuclear pores, cytoplasmic IFs may directly connect lamins, but it remains to be determined in the skeletal muscle (Lockard and Bloom, 1993; Georgatos et al., 1987; Capetanaki et al., 2007). Desmin filaments have also been suggested to connect other cell components such as lysosome (via the myospryn), SR (via the MTM1), microtubule (via the dynein), and M-line (via the MyoM1) (Hnia et al., 2015), (Kielbasa et al., 2011). Especially, the desmin-binding protein plectins are responsible for linking desmin IF networks with nuclei (via the plectin 1), mitochondria (via the plectin 1b), Z-line (via the plectin 1 day), and costamere (via the plectin 1f) (Castanon et al., 2013), (Carlsson et al., 2000; Konieczny et al., 2008). These desmin interactions are likely to coordinate the activity of specific kinases and phosphatases, thus regulating signal transduction.
In addition to the costamere and IF, myofibers also contain microtubules to transmit mechanical force, which form a grid containing longitudinal and transverse components across the fiber. During myogenesis, myoblast fusion, nuclear localization to the cell periphery, and assembly of the sarcomere require microtubules to ensure proper myofiber structure and function (Lucas and Cooper, 2023). It is well known that microtubule assembly nucleates at the microtubule-organizing center (MTOC), and MTOC transferring from the centrosomal to non-centrosomal location is a marker of differentiation (Sanchez and Feldman, 2017). During skeletal muscle differentiation, mononuclear myoblasts cease proliferation and elongation to form myoblasts with fusogenic capability, attributed to the MTOC switching from centrosomes to nuclear membranes and bi-polar extension of microtubules toward the cell (Sanchez and Feldman, 2017; Clark et al., 2002). Myoblast fusion occurs at the tips of elongated myoblasts through close contacting and merging of the cell membranes; hence, the failure of myoblast elongation can lead to a lack of fusion (Kim et al., 2015). As fusion proceeds, the nucleus of a newly fused myoblast rapidly migrates to the central myotube nuclei, which is mediated by dynein motor–Par6 complex that connects the nuclear envelope with microtubules (Bone and Starr, 2016; Starr, 2017). Then, these nuclei spread apart and are evenly spaced throughout the myotube, and the process is currently thought to be regulated by a variety of mechanisms: the kinesin-1 motor-nesprin1/2 complex links the nuclei to the microtubules and transports the nuclei along the microtubules; the kinesin-1 motor-microtubule-associated protein 7 (MAP7) complex drives the sliding of anti-parallel microtubules that extend outward from adjacent nuclei, thereby pushing neighboring nuclei apart; Nesprin-α recruits centrosomal protein Akap450 to the nuclear envelope independently of kinesin to nucleate microtubules (Starr, 2017; Gimpel et al., 2017). Eventually, the nuclei move from the center of the myofiber to the sarcolemma in a desmin-dependent process; except for some nuclei that migrate to the NMJ and anchor there, the remaining nuclei are evenly spaced around the myofiber periphery (Bone and Starr, 2016). Moreover, microtubules bound by myosin-binding protein Muscle RING finger-2 (MURF-2) provide a scaffold for the association of sarcomeric myosin with the A-band portion of titin, thereby generating the mature A band during myofibril assembly (Pizon et al., 2002; Pizon et al., 2005). In a similar manner, another microtubule-binding protein dystrophin serves as the scaffold for microtubule growth, resulting in microtubules aligning with dystrophin in both longitudinal and transverse directions of the sarcomere (Prins et al., 2009). Currently, research on microtubule organization within myofibers is continually deepening, such as its involvement in fiber type-dependent, Golgi apparatus relevance, mitochondrial trafficking, and primary cilia signaling (Ng et al., 2021; Oddoux et al., 2013; Giovarelli et al., 2020).
3 Conclusion
Skeletal myogenesis is a lifelong process in mammals, governed by molecular mechanisms that remain both intricately orchestrated and incompletely resolved. While foundational regulators—including cyclin/CDK complexes, chromatin modifiers, and myogenic transcription factors—have been mapped, their spatiotemporal coordination during myofiber formation and adaptation is still emerging (Wu and Yue, 2024). Central to this process is the exquisite architectural organization of skeletal myofibers, where myofibrils, SR, mitochondria, and the cytoskeleton coalesce into a contractile machine. Myofibrils drive force generation, the SR and mitochondria synchronize excitation–energy coupling, and the cytoskeleton integrates mechanical and biochemical signals. This structural precision enables rapid, adaptable responses to physiological demands, but it also hinges on dynamic protein networks whose complexity we are only beginning to unravel.
In this review, we synthesized current knowledge on the structural and functional hierarchy of skeletal myofibers, emphasizing how core components (myofibrils, SR, mitochondria, and cytoskeleton) collaborate to enable contraction and homeostasis. Key findings include the following: myofibrils rely on scaffold proteins (e.g., titin and nebulin) for sarcomere assembly and minor regulators (e.g., α-actinin and telethonin) for stability under stress; SR–mitochondria crosstalk at mitochondria-associated membranes (MAMs) fine-tunes calcium signaling to balance ATP production and apoptosis; cytoskeletal networks (desmin and microtubules) distribute mechanical load and anchor organelles, linking contractile activity to nuclear reprogramming. Despite these advances, critical gaps persist. For example, the biological roles and molecular mechanisms of muscle protein components in skeletal myogenesis remain largely unknown, including what their signaling roles are within the myofiber architecture, what is the order of their interactions, how they are regulated and how they, in turn, regulate gene expression and homeostasis in myofibers; what mechanisms prioritize radial hypertrophy versus longitudinal growth during adaptation, and how do mechanical cues interface with transcriptional programs? Future progress will require leveraging emerging tools such as cryo-electron tomography to resolve subcellular structures in situ, single-cell multi-omics to deconvolve myonuclear specialization, and 3D biomimetic models to recapitulate niche-specific signaling. Building upon these methodological advances, further investigation of the nature of the dynamic and extraordinarily complicated interplay among muscle proteins using sophisticated molecular biology methods will have far-reaching implications on mammalian skeletal muscle pathophysiology.
Author contributions
JZ: writing – review and editing. XD: writing – review and editing. WF: writing – review and editing. HZ: writing – review and editing. BY: conceptualization, data curation, formal analysis, funding acquisition, investigation, methodology, project administration, resources, software, supervision, validation, visualization, writing – original draft, and writing – review and editing.
Funding
The author(s) declare that financial support was received for the research and/or publication of this article. The project was supported by The Fundamental Research Funds for the Central Universities, Southwest Minzu University (ZYN2025026); The Sichuan Provincial Administration of Traditional Chinese Medicine, Traditional Chinese Medicine Research Special Project (2024MS414); The Chengdu Medical Research Project (2024313); and The Sichuan Provincial Administration of Traditional Chinese Medicine, Traditional Chinese Medicine Research Special Project (2024MS313).
Conflict of interest
The authors declare that the research was conducted in the absence of any commercial or financial relationships that could be construed as a potential conflict of interest.
Generative AI statement
The author(s) declare that no Generative AI was used in the creation of this manuscript.
Publisher’s note
All claims expressed in this article are solely those of the authors and do not necessarily represent those of their affiliated organizations, or those of the publisher, the editors and the reviewers. Any product that may be evaluated in this article, or claim that may be made by its manufacturer, is not guaranteed or endorsed by the publisher.
References
Adhihetty, P. J., Ljubicic, V., Menzies, K. J., and Hood, D. A. (2005). Differential susceptibility of subsarcolemmal and intermyofibrillar mitochondria to apoptotic stimuli. Am. J. Physiol. Cell Physiol. 289 (4), C994–C1001. doi:10.1152/ajpcell.00031.2005
Al-Khayat, H. A. (2013). Three-dimensional structure of the human myosin thick filament: clinical implications. Glob. Cardiol. Sci. Pract. 2013 (3), 280–302. doi:10.5339/gcsp.2013.36
Al-Qusairi, L., Weiss, N., Toussaint, A., Berbey, C., Messaddeq, N., Kretz, C., et al. (2009). T-tubule disorganization and defective excitation-contraction coupling in muscle fibers lacking myotubularin lipid phosphatase. Proc. Natl. Acad. Sci. U. S. A. 106 (44), 18763–18768. doi:10.1073/pnas.0900705106
Anderson, A. A., Altafaj, X., Zheng, Z., Wang, Z. M., Delbono, O., Ronjat, M., et al. (2006). The junctional SR protein JP-45 affects the functional expression of the voltage-dependent Ca2+ channel Cav1.1. J. Cell Sci. 119 (Pt 10), 2145–2155. doi:10.1242/jcs.02935
Anderson, A. A., Treves, S., Biral, D., Betto, R., Sandona, D., Ronjat, M., et al. (2003). The novel skeletal muscle sarcoplasmic reticulum JP-45 protein. Molecular cloning, tissue distribution, developmental expression, and interaction with alpha 1.1 subunit of the voltage-gated calcium channel. J. Biol. Chem. 278 (41), 39987–39992. doi:10.1074/jbc.M305016200
Arvanitis, D. A., Vafiadaki, E., Sanoudou, D., and Kranias, E. G. (2011). Histidine-rich calcium binding protein: the new regulator of sarcoplasmic reticulum calcium cycling. J. Mol. Cell Cardiol. 50 (1), 43–49. doi:10.1016/j.yjmcc.2010.08.021
Asfour, H. A., Allouh, M. Z., and Said, R. S. (2018). Myogenic regulatory factors: the orchestrators of myogenesis after 30 years of discovery. Exp. Biol. Med. (Maywood) 243 (2), 118–128. doi:10.1177/1535370217749494
Bang, M. L., Mudry, R. E., Mcelhinny, A. S., Trombitas, K., Geach, A. J., Yamasaki, R., et al. (2001). Myopalladin, a novel 145-kilodalton sarcomeric protein with multiple roles in Z-disc and I-band protein assemblies. J. Cell Biol. 153 (2), 413–427. doi:10.1083/jcb.153.2.413
Barone, V., Randazzo, D., Del, R. V., Sorrentino, V., and Rossi, D. (2015). Organization of junctional sarcoplasmic reticulum proteins in skeletal muscle fibers. J. Muscle Res. Cell Motil. 36 (6), 501–515. doi:10.1007/s10974-015-9421-5
Beard, N. A., Laver, D. R., and Dulhunty, A. F. (2004). Calsequestrin and the calcium release channel of skeletal and cardiac muscle. Prog. Biophys. Mol. Biol. 85 (1), 33–69. doi:10.1016/j.pbiomolbio.2003.07.001
Beard, N. A., Wei, L., Cheung, S. N., Kimura, T., Varsanyi, M., and Dulhunty, A. F. (2008). Phosphorylation of skeletal muscle calsequestrin enhances its Ca2+ binding capacity and promotes its association with junctin. Cell Calcium 44 (4), 363–373. doi:10.1016/j.ceca.2008.01.005
Blake, D. J., and Martin-Rendon, E. (2002). Intermediate filaments and the function of the dystrophin-protein complex. Trends Cardiovasc Med. 12 (5), 224–228. doi:10.1016/s1050-1738(02)00166-4
Blake, D. J., Weir, A., Newey, S. E., and Davies, K. E. (2002). Function and genetics of dystrophin and dystrophin-related proteins in muscle. Physiol. Rev. 82 (2), 291–329. doi:10.1152/physrev.00028.2001
Bone, C. R., and Starr, D. A. (2016). Nuclear migration events throughout development. J. Cell Sci. 129 (10), 1951–1961. doi:10.1242/jcs.179788
Bowman, A. L., Kontrogianni-Konstantopoulos, A., Hirsch, S. S., Geisler, S. B., Gonzalez-Serratos, H., Russell, M. W., et al. (2007). Different obscurin isoforms localize to distinct sites at sarcomeres. FEBS Lett. 581 (8), 1549–1554. doi:10.1016/j.febslet.2007.03.011
Brooks, S. V., Guzman, S. D., and Ruiz, L. P. (2023). Skeletal muscle structure, physiology, and function. Handb. Clin. Neurol. 195, 3–16. doi:10.1016/B978-0-323-98818-6.00013-3
Bryson-Richardson, R. J., and Currie, P. D. (2008). The genetics of vertebrate myogenesis. Nat. Rev. Genet. 9 (8), 632–646. doi:10.1038/nrg2369
Buckingham, M., Bajard, L., Chang, T., Daubas, P., Hadchouel, J., Meilhac, S., et al. (2003). The formation of skeletal muscle: from somite to limb. J. Anat. 202 (1), 59–68. doi:10.1046/j.1469-7580.2003.00139.x
Buj-Bello, A., Fougerousse, F., Schwab, Y., Messaddeq, N., Spehner, D., Pierson, C. R., et al. (2008). AAV-mediated intramuscular delivery of myotubularin corrects the myotubular myopathy phenotype in targeted murine muscle and suggests a function in plasma membrane homeostasis. Hum. Mol. Genet. 17 (14), 2132–2143. doi:10.1093/hmg/ddn112
Cai, C., Masumiya, H., Weisleder, N., Matsuda, N., Nishi, M., Hwang, M., et al. (2009a). MG53 nucleates assembly of cell membrane repair machinery. Nat. Cell Biol. 11 (1), 56–64. doi:10.1038/ncb1812
Cai, C., Wan, P., Wang, H., Cai, X., Wang, J., Chai, Z., et al. (2023a). Transcriptional and open chromatin analysis of bovine skeletal muscle development by single-cell sequencing. Cell Prolif. 56 (9), e13430. doi:10.1111/cpr.13430
Cai, C., Weisleder, N., Ko, J. K., Komazaki, S., Sunada, Y., Nishi, M., et al. (2009b). Membrane repair defects in muscular dystrophy are linked to altered interaction between MG53, caveolin-3, and dysferlin. J. Biol. Chem. 284 (23), 15894–15902. doi:10.1074/jbc.M109.009589
Cai, C., Yue, Y., and Yue, B. (2023b). Single-cell RNA sequencing in skeletal muscle developmental biology. Biomed. Pharmacother. 162, 114631. doi:10.1016/j.biopha.2023.114631
Capetanaki, Y., Bloch, R. J., Kouloumenta, A., Mavroidis, M., and Psarras, S. (2007). Muscle intermediate filaments and their links to membranes and membranous organelles. Exp. Cell Res. 313 (10), 2063–2076. doi:10.1016/j.yexcr.2007.03.033
Capetanaki, Y., Milner, D. J., and Weitzer, G. (1997). Desmin in muscle formation and maintenance: knockouts and consequences. Cell Struct. Funct. 22 (1), 103–116. doi:10.1247/csf.22.103
Carlsson, L., Li, Z. L., Paulin, D., Price, M. G., Breckler, J., Robson, R. M., et al. (2000). Differences in the distribution of synemin, paranemin, and plectin in skeletal muscles of wild-type and desmin knock-out mice. Histochem Cell Biol. 114 (1), 39–47. doi:10.1007/s004180000158
Carlsson, L., Yu, J. G., and Thornell, L. E. (2008). New aspects of obscurin in human striated muscles. Histochem Cell Biol. 130 (1), 91–103. doi:10.1007/s00418-008-0413-z
Castanon, M. J., Walko, G., Winter, L., and Wiche, G. (2013). Plectin-intermediate filament partnership in skin, skeletal muscle, and peripheral nerve. Histochem Cell Biol. 140 (1), 33–53. doi:10.1007/s00418-013-1102-0
Cizkova, D., Soukup, T., and Mokry, J. (2009). Expression of nestin, desmin and vimentin in intact and regenerating muscle spindles of rat hind limb skeletal muscles. Histochem Cell Biol. 131 (2), 197–206. doi:10.1007/s00418-008-0523-7
Clark, P., Dunn, G. A., Knibbs, A., and Peckham, M. (2002). Alignment of myoblasts on ultrafine gratings inhibits fusion in vitro. Int. J. Biochem. Cell Biol. 34 (7), 816–825. doi:10.1016/s1357-2725(01)00180-7
Conte, E., Dinoi, G., Imbrici, P., De Luca, A., and Liantonio, A. (2023). Sarcoplasmic reticulum Ca(2+) buffer proteins: a focus on the yet-to-Be-explored role of sarcalumenin in skeletal muscle health and disease. Cells 12 (5), 715. doi:10.3390/cells12050715
Costa, M. L., Escaleira, R., Cataldo, A., Oliveira, F., and Mermelstein, C. S. (2004). Desmin: molecular interactions and putative functions of the muscle intermediate filament protein. Braz J. Med. Biol. Res. 37 (12), 1819–1830. doi:10.1590/s0100-879x2004001200007
Cowling, B. S., Prokic, I., Tasfaout, H., Rabai, A., Humbert, F., Rinaldi, B., et al. (2017). Amphiphysin (BIN1) negatively regulates dynamin 2 for normal muscle maturation. J. Clin. Invest 127 (12), 4477–4487. doi:10.1172/JCI90542
Cretoiu, D., Pavelescu, L., Duica, F., Radu, M., Suciu, N., and Cretoiu, S. M. (2018). Myofibers. Adv. Exp. Med. Biol. 1088, 23–46. doi:10.1007/978-981-13-1435-3_2
Deries, M., and Thorsteinsdottir, S. (2016). Axial and limb muscle development: dialogue with the neighbourhood. Cell Mol. Life Sci. 73 (23), 4415–4431. doi:10.1007/s00018-016-2298-7
Dominy, J. E., and Puigserver, P. (2013). Mitochondrial biogenesis through activation of nuclear signaling proteins. Cold Spring Harb. Perspect. Biol. 5 (7), a015008. doi:10.1101/cshperspect.a015008
Dowling, J. J., Vreede, A. P., Low, S. E., Gibbs, E. M., Kuwada, J. Y., Bonnemann, C. G., et al. (2009). Loss of myotubularin function results in T-tubule disorganization in zebrafish and human myotubular myopathy. PLoS Genet. 5 (2), e1000372. doi:10.1371/journal.pgen.1000372
Dulhunty, A., Wei, L., and Beard, N. (2009). Junctin - the quiet achiever. J. Physiol. 587 (Pt 13), 3135–3137. doi:10.1113/jphysiol.2009.171959
Dulhunty, A. F. (2006). Excitation-contraction coupling from the 1950s into the new millennium. Clin. Exp. Pharmacol. Physiol. 33 (9), 763–772. doi:10.1111/j.1440-1681.2006.04441.x
Dulhunty, A. F., Wei-Lapierre, L., Casarotto, M. G., and Beard, N. A. (2017). Core skeletal muscle ryanodine receptor calcium release complex. Clin. Exp. Pharmacol. Physiol. 44 (1), 3–12. doi:10.1111/1440-1681.12676
Egan, B., and Zierath, J. R. (2013). Exercise metabolism and the molecular regulation of skeletal muscle adaptation. Cell Metab. 17 (2), 162–184. doi:10.1016/j.cmet.2012.12.012
Eldirany, S. A., Lomakin, I. B., Ho, M., and Bunick, C. G. (2021). Recent insight into intermediate filament structure. Curr. Opin. Cell Biol. 68, 132–143. doi:10.1016/j.ceb.2020.10.001
Ertbjerg, P., and Puolanne, E. (2017). Muscle structure, sarcomere length and influences on meat quality: a review. Meat Sci. 132, 139–152. doi:10.1016/j.meatsci.2017.04.261
Etienne-Manneville, S. (2018). Cytoplasmic intermediate filaments in cell biology. Annu. Rev. Cell Dev. Biol. 34, 1–28. doi:10.1146/annurev-cellbio-100617-062534
Ferreira, R., Vitorino, R., Alves, R. M., Appell, H. J., Powers, S. K., Duarte, J. A., et al. (2010). Subsarcolemmal and intermyofibrillar mitochondria proteome differences disclose functional specializations in skeletal muscle. Proteomics 10 (17), 3142–3154. doi:10.1002/pmic.201000173
Fujise, K., Okubo, M., Abe, T., Yamada, H., Nishino, I., Noguchi, S., et al. (2021). Mutant BIN1-Dynamin 2 complexes dysregulate membrane remodeling in the pathogenesis of centronuclear myopathy. J. Biol. Chem. 296, 100077. doi:10.1074/jbc.RA120.015184
Fukuzawa, A., Idowu, S., and Gautel, M. (2005). Complete human gene structure of obscurin: implications for isoform generation by differential splicing. J. Muscle Res. Cell Motil. 26 (6-8), 427–434. doi:10.1007/s10974-005-9025-6
Galbiati, F., Engelman, J. A., Volonte, D., Zhang, X. L., Minetti, C., Li, M., et al. (2001). Caveolin-3 null mice show a loss of caveolae, changes in the microdomain distribution of the dystrophin-glycoprotein complex, and t-tubule abnormalities. J. Biol. Chem. 276 (24), 21425–21433. doi:10.1074/jbc.M100828200
Georgatos, S. D., Weber, K., Geisler, N., and Blobel, G. (1987). Binding of two desmin derivatives to the plasma membrane and the nuclear envelope of avian erythrocytes: evidence for a conserved site-specificity in intermediate filament-membrane interactions. Proc. Natl. Acad. Sci. U. S. A. 84 (19), 6780–6784. doi:10.1073/pnas.84.19.6780
Gillies, A. R., and Lieber, R. L. (2011). Structure and function of the skeletal muscle extracellular matrix. Muscle Nerve 44 (3), 318–331. doi:10.1002/mus.22094
Gimpel, P., Lee, Y. L., Sobota, R. M., Calvi, A., Koullourou, V., Patel, R., et al. (2017). Nesprin-1α-Dependent microtubule nucleation from the nuclear envelope via Akap450 is necessary for nuclear positioning in muscle cells. Curr. Biol. 27 (19), 2999–3009.e9. doi:10.1016/j.cub.2017.08.031
Giovarelli, M., Zecchini, S., Martini, E., Garre, M., Barozzi, S., Ripolone, M., et al. (2020). Drp1 overexpression induces desmin disassembling and drives kinesin-1 activation promoting mitochondrial trafficking in skeletal muscle. Cell Death Differ. 27 (8), 2383–2401. doi:10.1038/s41418-020-0510-7
Golini, L., Chouabe, C., Berthier, C., Cusimano, V., Fornaro, M., Bonvallet, R., et al. (2011). Junctophilin 1 and 2 proteins interact with the L-type Ca2+ channel dihydropyridine receptors (DHPRs) in skeletal muscle. J. Biol. Chem. 286 (51), 43717–43725. doi:10.1074/jbc.M111.292755
Gouadon, E., Schuhmeier, R. P., Ursu, D., Anderson, A. A., Treves, S., Zorzato, F., et al. (2006). A possible role of the junctional face protein JP-45 in modulating Ca2+ release in skeletal muscle. J. Physiol. 572 (Pt 1), 269–280. doi:10.1113/jphysiol.2005.104406
Granzier, H. L., and Labeit, S. (2005). Titin and its associated proteins: the third myofilament system of the sarcomere. Adv. Protein Chem. 71, 89–119. doi:10.1016/S0065-3233(04)71003-7
Granzier, H. L., and Labeit, S. (2006). The giant muscle protein titin is an adjustable molecular spring. Exerc Sport Sci. Rev. 34 (2), 50–53. doi:10.1249/00003677-200604000-00002
Greggio, C., Jha, P., Kulkarni, S. S., Lagarrigue, S., Broskey, N. T., Boutant, M., et al. (2017). Enhanced respiratory chain supercomplex formation in response to exercise in human skeletal muscle. Cell Metab. 25 (2), 301–311. doi:10.1016/j.cmet.2016.11.004
Hernandez-Deviez, D. J., Martin, S., Laval, S. H., Lo, H. P., Cooper, S. T., North, K. N., et al. (2006). Aberrant dysferlin trafficking in cells lacking caveolin or expressing dystrophy mutants of caveolin-3. Hum. Mol. Genet. 15 (1), 129–142. doi:10.1093/hmg/ddi434
Hnia, K., Ramspacher, C., Vermot, J., and Laporte, J. (2015). Desmin in muscle and associated diseases: beyond the structural function. Cell Tissue Res. 360 (3), 591–608. doi:10.1007/s00441-014-2016-4
Hnia, K., Vaccari, I., Bolino, A., and Laporte, J. (2012). Myotubularin phosphoinositide phosphatases: cellular functions and disease pathophysiology. Trends Mol. Med. 18 (6), 317–327. doi:10.1016/j.molmed.2012.04.004
Hol, E. M., and Capetanaki, Y. (2017). Type III intermediate filaments desmin, glial fibrillary acidic protein (GFAP), vimentin, and peripherin. Cold Spring Harb. Perspect. Biol. 9 (12), a021642. doi:10.1101/cshperspect.a021642
Huxley, A. F., and Niedergerke, R. (1954). Structural changes in muscle during contraction; interference microscopy of living muscle fibres. Nature 173 (4412), 971–973. doi:10.1038/173971a0
Huxley, H., and Hanson, J. (1954). Changes in the cross-striations of muscle during contraction and stretch and their structural interpretation. Nature 173 (4412), 973–976. doi:10.1038/173973a0
Jaka, O., Casas-Fraile, L., Lopez, D. M. A., and Saenz, A. (2015). Costamere proteins and their involvement in myopathic processes. Expert Rev. Mol. Med. 17, e12. doi:10.1017/erm.2015.9
Jeong, S. Y., and Seol, D. W. (2008). The role of mitochondria in apoptosis. BMB Rep. 41 (1), 11–22. doi:10.5483/bmbrep.2008.41.1.011
Kielbasa, O. M., Reynolds, J. G., Wu, C. L., Snyder, C. M., Cho, M. Y., Weiler, H., et al. (2011). Myospryn is a calcineurin-interacting protein that negatively modulates slow-fiber-type transformation and skeletal muscle regeneration. FASEB J. 25 (7), 2276–2286. doi:10.1096/fj.10-169219
Kim, J. H., Jin, P., Duan, R., and Chen, E. H. (2015). Mechanisms of myoblast fusion during muscle development. Curr. Opin. Genet. Dev. 32, 162–170. doi:10.1016/j.gde.2015.03.006
Klinge, L., Harris, J., Sewry, C., Charlton, R., Anderson, L., Laval, S., et al. (2010). Dysferlin associates with the developing T-tubule system in rodent and human skeletal muscle. Muscle Nerve 41 (2), 166–173. doi:10.1002/mus.21166
Komazaki, S., Nishi, M., Kangawa, K., and Takeshima, H. (1999). Immunolocalization of mitsugumin29 in developing skeletal muscle and effects of the protein expressed in amphibian embryonic cells. Dev. Dyn. 215 (2), 87–95. doi:10.1002/(SICI)1097-0177(199906)215:2<87::AID-DVDY1>3.0.CO;2-Y
Komazaki, S., Nishi, M., Takeshima, H., and Nakamura, H. (2001). Abnormal formation of sarcoplasmic reticulum networks and triads during early development of skeletal muscle cells in mitsugumin29-deficient mice. Dev. Growth Differ. 43 (6), 717–723. doi:10.1046/j.1440-169x.2001.00609.x
Konieczny, P., Fuchs, P., Reipert, S., Kunz, W. S., Zeold, A., Fischer, I., et al. (2008). Myofiber integrity depends on desmin network targeting to Z-disks and costameres via distinct plectin isoforms. J. Cell Biol. 181 (4), 667–681. doi:10.1083/jcb.200711058
Kontrogianni-Konstantopoulos, A., Ackermann, M. A., Bowman, A. L., Yap, S. V., and Bloch, R. J. (2009). Muscle giants: molecular scaffolds in sarcomerogenesis. Physiol. Rev. 89 (4), 1217–1267. doi:10.1152/physrev.00017.2009
Kontrogianni-Konstantopoulos, A., and Bloch, R. J. (2005). Obscurin: a multitasking muscle giant. J. Muscle Res. Cell Motil. 26 (6-8), 419–426. doi:10.1007/s10974-005-9024-7
Kontrogianni-Konstantopoulos, A., Jones, E. M., Van Rossum, D. B., and Bloch, R. J. (2003). Obscurin is a ligand for small ankyrin 1 in skeletal muscle. Mol. Biol. Cell 14 (3), 1138–1148. doi:10.1091/mbc.e02-07-0411
Krauss, R. S., Joseph, G. A., and Goel, A. J. (2017). Keep your friends close: cell-cell contact and skeletal myogenesis. Cold Spring Harb. Perspect. Biol. 9 (2), a029298. doi:10.1101/cshperspect.a029298
Kruger, M., Wright, J., and Wang, K. (1991). Nebulin as a length regulator of thin filaments of vertebrate skeletal muscles: correlation of thin filament length, nebulin size, and epitope profile. J. Cell Biol. 115 (1), 97–107. doi:10.1083/jcb.115.1.97
Labeit, S., Gibson, T., Lakey, A., Leonard, K., Zeviani, M., Knight, P., et al. (1991). Evidence that nebulin is a protein-ruler in muscle thin filaments. FEBS Lett. 282 (2), 313–316. doi:10.1016/0014-5793(91)80503-u
Lee, E., Marcucci, M., Daniell, L., Pypaert, M., Weisz, O. A., Ochoa, G. C., et al. (2002). Amphiphysin 2 (Bin1) and T-tubule biogenesis in muscle. Science 297 (5584), 1193–1196. doi:10.1126/science.1071362
Lee, H. G., Kang, H., Kim, D. H., and Park, W. J. (2001). Interaction of HRC (histidine-rich Ca(2+)-binding protein) and triadin in the lumen of sarcoplasmic reticulum. J. Biol. Chem. 276 (43), 39533–39538. doi:10.1074/jbc.M010664200
Linke, W. A., Kulke, M., Li, H., Fujita-Becker, S., Neagoe, C., Manstein, D. J., et al. (2002). PEVK domain of titin: an entropic spring with actin-binding properties. J. Struct. Biol. 137 (1-2), 194–205. doi:10.1006/jsbi.2002.4468
Linke, W. A., Stockmeier, M. R., Ivemeyer, M., Hosser, H., and Mundel, P. (1998). Characterizing titin's I-band Ig domain region as an entropic spring. J. Cell Sci. 111 (Pt 11), 1567–1574. doi:10.1242/jcs.111.11.1567
Lockard, V. G., and Bloom, S. (1993). Trans-cellular desmin-lamin B intermediate filament network in cardiac myocytes. J. Mol. Cell Cardiol. 25 (3), 303–309. doi:10.1006/jmcc.1993.1036
Lucas, L., and Cooper, T. A. (2023). Insights into cell-specific functions of microtubules in skeletal muscle development and homeostasis. Int. J. Mol. Sci. 24 (3), 2903. doi:10.3390/ijms24032903
Luther, P. K., and Craig, R. (2011). Modulation of striated muscle contraction by binding of myosin binding protein C to actin. Bioarchitecture 1 (6), 277–283. doi:10.4161/bioa.1.6.19341
Marty, I., Faure, J., Fourest-Lieuvin, A., Vassilopoulos, S., Oddoux, S., and Brocard, J. (2009). Triadin: what possible function 20 years later? J. Physiol. 587 (Pt 13), 3117–3121. doi:10.1113/jphysiol.2009.171892
Matsuda, C., Hayashi, Y. K., Ogawa, M., Aoki, M., Murayama, K., Nishino, I., et al. (2001). The sarcolemmal proteins dysferlin and caveolin-3 interact in skeletal muscle. Hum. Mol. Genet. 10 (17), 1761–1766. doi:10.1093/hmg/10.17.1761
Matuz-Mares, D., Gonzalez-Andrade, M., Araiza-Villanueva, M. G., Vilchis-Landeros, M. M., and Vazquez-Meza, H. (2022). Mitochondrial calcium: effects of its imbalance in disease. Antioxidants (Basel) 11 (5), 801. doi:10.3390/antiox11050801
Mcbride, H. M., Neuspiel, M., and Wasiak, S. (2006). Mitochondria: more than just a powerhouse. Curr. Biol. 16 (14), R551–R560. doi:10.1016/j.cub.2006.06.054
Mcelhinny, A. S., Kolmerer, B., Fowler, V. M., Labeit, S., and Gregorio, C. C. (2001). The N-terminal end of nebulin interacts with tropomodulin at the pointed ends of the thin filaments. J. Biol. Chem. 276 (1), 583–592. doi:10.1074/jbc.M005693200
Michelucci, A., Boncompagni, S., Pietrangelo, L., Takano, T., Protasi, F., and Dirksen, R. T. (2020). Pre-assembled Ca2+ entry units and constitutively active Ca2+ entry in skeletal muscle of calsequestrin-1 knockout mice. J. Gen. Physiol. 152 (10), e202012617. doi:10.1085/jgp.202012617
Moorwood, C. (2008). Syncoilin, an intermediate filament-like protein linked to the dystrophin associated protein complex in skeletal muscle. Cell Mol. Life Sci. 65 (19), 2957–2963. doi:10.1007/s00018-008-8306-9
Mues, A., van der Ven, P. F., Young, P., Furst, D. O., and Gautel, M. (1998). Two immunoglobulin-like domains of the Z-disc portion of titin interact in a conformation-dependent way with telethonin. FEBS Lett. 428 (1-2), 111–114. doi:10.1016/s0014-5793(98)00501-8
Mukund, K., and Subramaniam, S. (2020). Skeletal muscle: a review of molecular structure and function, in health and disease. Wiley Interdiscip. Rev. Syst. Biol. Med. 12 (1), e1462. doi:10.1002/wsbm.1462
Murphy, R. M., Larkins, N. T., Mollica, J. P., Beard, N. A., and Lamb, G. D. (2009). Calsequestrin content and SERCA determine normal and maximal Ca2+ storage levels in sarcoplasmic reticulum of fast- and slow-twitch fibres of rat. J. Physiol. 587 (2), 443–460. doi:10.1113/jphysiol.2008.163162
Ng, D., Ho, U. Y., and Grounds, M. D. (2021). Cilia, centrosomes and skeletal muscle. Int. J. Mol. Sci. 22 (17), 9605. doi:10.3390/ijms22179605
Ni, H. M., Williams, J. A., and Ding, W. X. (2015). Mitochondrial dynamics and mitochondrial quality control. Redox Biol. 4, 6–13. doi:10.1016/j.redox.2014.11.006
Nishi, M., Komazaki, S., Kurebayashi, N., Ogawa, Y., Noda, T., Iino, M., et al. (1999). Abnormal features in skeletal muscle from mice lacking mitsugumin29. J. Cell Biol. 147 (7), 1473–1480. doi:10.1083/jcb.147.7.1473
Oddoux, S., Brocard, J., Schweitzer, A., Szentesi, P., Giannesini, B., Brocard, J., et al. (2009). Triadin deletion induces impaired skeletal muscle function. J. Biol. Chem. 284 (50), 34918–34929. doi:10.1074/jbc.M109.022442
Oddoux, S., Zaal, K. J., Tate, V., Kenea, A., Nandkeolyar, S. A., Reid, E., et al. (2013). Microtubules that form the stationary lattice of muscle fibers are dynamic and nucleated at Golgi elements. J. Cell Biol. 203 (2), 205–213. doi:10.1083/jcb.201304063
Palmer, C. S., Osellame, L. D., Stojanovski, D., and Ryan, M. T. (2011). The regulation of mitochondrial morphology: intricate mechanisms and dynamic machinery. Cell Signal 23 (10), 1534–1545. doi:10.1016/j.cellsig.2011.05.021
Parton, R. G., Way, M., Zorzi, N., and Stang, E. (1997). Caveolin-3 associates with developing T-tubules during muscle differentiation. J. Cell Biol. 136 (1), 137–154. doi:10.1083/jcb.136.1.137
Perni, S., and Beam, K. (2022). Junctophilins 1, 2, and 3 all support voltage-induced Ca2+ release despite considerable divergence. J. Gen. Physiol. 154 (9), e202113024. doi:10.1085/jgp.202113024
Perni, S., Close, M., and Franzini-Armstrong, C. (2013). Novel details of calsequestrin gel conformation in situ. J. Biol. Chem. 288 (43), 31358–31362. doi:10.1074/jbc.M113.507749
Pizon, V., Gerbal, F., Diaz, C. C., and Karsenti, E. (2005). Microtubule-dependent transport and organization of sarcomeric myosin during skeletal muscle differentiation. EMBO J. 24 (21), 3781–3792. doi:10.1038/sj.emboj.7600842
Pizon, V., Iakovenko, A., Van Der Ven, P. F., Kelly, R., Fatu, C., Furst, D. O., et al. (2002). Transient association of titin and myosin with microtubules in nascent myofibrils directed by the MURF2 RING-finger protein. J. Cell Sci. 115 (Pt 23), 4469–4482. doi:10.1242/jcs.00131
Ploumi, C., Daskalaki, I., and Tavernarakis, N. (2017). Mitochondrial biogenesis and clearance: a balancing act. FEBS J. 284 (2), 183–195. doi:10.1111/febs.13820
Porter, N. C., Resneck, W. G., O'Neill, A., Van Rossum, D. B., Stone, M. R., and Bloch, R. J. (2005). Association of small ankyrin 1 with the sarcoplasmic reticulum. Mol. Membr. Biol. 22 (5), 421–432. doi:10.1080/09687860500244262
Powell, C. A., Smiley, B. L., Mills, J., and Vandenburgh, H. H. (2002). Mechanical stimulation improves tissue-engineered human skeletal muscle. Am. J. Physiol. Cell Physiol. 283 (5), C1557–C1565. doi:10.1152/ajpcell.00595.2001
Prins, K. W., Humston, J. L., Mehta, A., Tate, V., Ralston, E., and Ervasti, J. M. (2009). Dystrophin is a microtubule-associated protein. J. Cell Biol. 186 (3), 363–369. doi:10.1083/jcb.200905048
Puchner, E. M., Alexandrovich, A., Kho, A. L., Hensen, U., Schafer, L. V., Brandmeier, B., et al. (2008). Mechanoenzymatics of titin kinase. Proc. Natl. Acad. Sci. U. S. A. 105 (36), 13385–13390. doi:10.1073/pnas.0805034105
Relaix, F., Bencze, M., Borok, M. J., Der Vartanian, A., Gattazzo, F., Mademtzoglou, D., et al. (2021). Perspectives on skeletal muscle stem cells. Nat. Commun. 12 (1), 692. doi:10.1038/s41467-020-20760-6
Romero, N. B., Mezmezian, M., and Fidzianska, A. (2013). Main steps of skeletal muscle development in the human: morphological analysis and ultrastructural characteristics of developing human muscle. Handb. Clin. Neurol. 113, 1299–1310. doi:10.1016/B978-0-444-59565-2.00002-2
Root, D. D., and Wang, K. (1994). Calmodulin-sensitive interaction of human nebulin fragments with actin and myosin. Biochemistry 33 (42), 12581–12591. doi:10.1021/bi00208a008
Rossi, A., Pizzo, P., and Filadi, R. (2019). Calcium, mitochondria and cell metabolism: a functional triangle in bioenergetics. Biochim. Biophys. Acta Mol. Cell Res. 1866 (7), 1068–1078. doi:10.1016/j.bbamcr.2018.10.016
Rossi, D., Lorenzini, S., Pierantozzi, E., Van Petegem, F., Osamwonuyi, A. D., and Sorrentino, V. (2022b). Multiple regions within junctin drive its interaction with calsequestrin-1 and its localization to triads in skeletal muscle. J. Cell Sci. 135 (2), jcs259185. doi:10.1242/jcs.259185
Rossi, D., Pierantozzi, E., Amadsun, D. O., Buonocore, S., Rubino, E. M., and Sorrentino, V. (2022a). The sarcoplasmic reticulum of skeletal muscle cells: a labyrinth of membrane contact sites. Biomolecules 12 (4), 488. doi:10.3390/biom12040488
Royer, L., and Rios, E. (2009). Deconstructing calsequestrin. Complex buffering in the calcium store of skeletal muscle. J. Physiol. 587 (Pt 13), 3101–3111. doi:10.1113/jphysiol.2009.171934
Salvatore, D., Simonides, W. S., Dentice, M., Zavacki, A. M., and Larsen, P. R. (2014). Thyroid hormones and skeletal muscle--new insights and potential implications. Nat. Rev. Endocrinol. 10 (4), 206–214. doi:10.1038/nrendo.2013.238
Sanchez, A. D., and Feldman, J. L. (2017). Microtubule-organizing centers: from the centrosome to non-centrosomal sites. Curr. Opin. Cell Biol. 44, 93–101. doi:10.1016/j.ceb.2016.09.003
Schweitzer, S. C., Klymkowsky, M. W., Bellin, R. M., Robson, R. M., Capetanaki, Y., and Evans, R. M. (2001). Paranemin and the organization of desmin filament networks. J. Cell Sci. 114 (Pt 6), 1079–1089. doi:10.1242/jcs.114.6.1079
Selcen, D., Stilling, G., and Engel, A. G. (2001). The earliest pathologic alterations in dysferlinopathy. Neurology 56 (11), 1472–1481. doi:10.1212/wnl.56.11.1472
Shen, X., Franzini-Armstrong, C., Lopez, J. R., Jones, L. R., Kobayashi, Y. M., Wang, Y., et al. (2007). Triadins modulate intracellular Ca(2+) homeostasis but are not essential for excitation-contraction coupling in skeletal muscle. J. Biol. Chem. 282 (52), 37864–37874. doi:10.1074/jbc.M705702200
Silva-Rojas, R., Nattarayan, V., Jaque-Fernandez, F., Gomez-Oca, R., Menuet, A., Reiss, D., et al. (2022). Mice with muscle-specific deletion of Bin1 recapitulate centronuclear myopathy and acute downregulation of dynamin 2 improves their phenotypes. Mol. Ther. 30 (2), 868–880. doi:10.1016/j.ymthe.2021.08.006
Squire, J. M., Luther, P. K., and Knupp, C. (2003). Structural evidence for the interaction of C-protein (MyBP-C) with actin and sequence identification of a possible actin-binding domain. J. Mol. Biol. 331 (3), 713–724. doi:10.1016/s0022-2836(03)00781-2
Starr, D. A. (2017). Muscle development: nucleating microtubules at the nuclear envelope. Curr. Biol. 27 (19), R1071–R1073. doi:10.1016/j.cub.2017.08.030
Suk, J. Y., Kim, Y. S., and Park, W. J. (1999). HRC (histidine-rich Ca2+ binding protein) resides in the lumen of sarcoplasmic reticulum as a multimer. Biochem. Biophys. Res. Commun. 263 (3), 667–671. doi:10.1006/bbrc.1999.1432
Sun, N., Critchley, D. R., Paulin, D., Li, Z., and Robson, R. M. (2008). Human alpha-synemin interacts directly with vinculin and metavinculin. Biochem. J. 409 (3), 657–667. doi:10.1042/BJ20071188
Swalsingh, G., Pani, P., and Bal, N. C. (2022). Structural functionality of skeletal muscle mitochondria and its correlation with metabolic diseases. Clin. Sci. (Lond) 136 (24), 1851–1871. doi:10.1042/CS20220636
Sweeney, H. L., and Barton, E. R. (2000). The dystrophin-associated glycoprotein complex: what parts can you do without? Proc. Natl. Acad. Sci. U. S. A. 97 (25), 13464–13466. doi:10.1073/pnas.011510597
Takei, K., Slepnev, V. I., Haucke, V., and De Camilli, P. (1999). Functional partnership between amphiphysin and dynamin in clathrin-mediated endocytosis. Nat. Cell Biol. 1 (1), 33–39. doi:10.1038/9004
Takeshima, H., Komazaki, S., Nishi, M., Iino, M., and Kangawa, K. (2000). Junctophilins: a novel family of junctional membrane complex proteins. Mol. Cell 6 (1), 11–22. doi:10.1016/s1097-2765(00)00003-4
Takeshima, H., Shimuta, M., Komazaki, S., Ohmi, K., Nishi, M., Iino, M., et al. (1998). Mitsugumin29, a novel synaptophysin family member from the triad junction in skeletal muscle. Biochem. J. 331 (Pt 1), 317–322. doi:10.1042/bj3310317
Tang, Z., Scherer, P. E., Okamoto, T., Song, K., Chu, C., Kohtz, D. S., et al. (1996). Molecular cloning of caveolin-3, a novel member of the caveolin gene family expressed predominantly in muscle. J. Biol. Chem. 271 (4), 2255–2261. doi:10.1074/jbc.271.4.2255
Tilokani, L., Nagashima, S., Paupe, V., and Prudent, J. (2018). Mitochondrial dynamics: overview of molecular mechanisms. Essays Biochem. 62 (3), 341–360. doi:10.1042/EBC20170104
Tskhovrebova, L., and Trinick, J. (2008). Giant proteins: sensing tension with titin kinase. Curr. Biol. 18 (24), R1141–R1142. doi:10.1016/j.cub.2008.10.035
van Dijk, S. J., Bezold, K. L., and Harris, S. P. (2014). Earning stripes: myosin binding protein-C interactions with actin. Pflugers Arch. 466 (3), 445–450. doi:10.1007/s00424-013-1432-8
Vincent, A. E., White, K., Davey, T., Philips, J., Ogden, R. T., Lawless, C., et al. (2019). Quantitative 3D mapping of the human skeletal muscle mitochondrial network. Cell Rep. 26 (4), 996–1009.e4. doi:10.1016/j.celrep.2019.01.010
Wang, K., and Wright, J. (1988). Architecture of the sarcomere matrix of skeletal muscle: immunoelectron microscopic evidence that suggests a set of parallel inextensible nebulin filaments anchored at the Z line. J. Cell Biol. 107 (6 Pt 1), 2199–2212. doi:10.1083/jcb.107.6.2199
Wang, Q., Groenendyk, J., Paskevicius, T., Qin, W., Kor, K. C., Liu, Y., et al. (2019). Two pools of IRE1α in cardiac and skeletal muscle cells. FASEB J. 33 (8), 8892–8904. doi:10.1096/fj.201802626R
Wang, Q., and Michalak, M. (2020). Calsequestrin. Structure, function, and evolution. Cell Calcium 90, 102242. doi:10.1016/j.ceca.2020.102242
Wang, S., Trumble, W. R., Liao, H., Wesson, C. R., Dunker, A. K., and Kang, C. H. (1998). Crystal structure of calsequestrin from rabbit skeletal muscle sarcoplasmic reticulum. Nat. Struct. Biol. 5 (6), 476–483. doi:10.1038/nsb0698-476
Wear, M. A., Yamashita, A., Kim, K., Maeda, Y., and Cooper, J. A. (2003). How capping protein binds the barbed end of the actin filament. Curr. Biol. 13 (17), 1531–1537. doi:10.1016/s0960-9822(03)00559-1
Whiting, A., Wardale, J., and Trinick, J. (1989). Does titin regulate the length of muscle thick filaments? J. Mol. Biol. 205 (1), 263–268. doi:10.1016/0022-2836(89)90381-1
Whitson, B. A., Tan, T., Gong, N., Zhu, H., and Ma, J. (2021). Muscle multiorgan crosstalk with MG53 as a myokine for tissue repair and regeneration. Curr. Opin. Pharmacol. 59, 26–32. doi:10.1016/j.coph.2021.04.005
Willingham, T. B., Ajayi, P. T., and Glancy, B. (2021). Subcellular specialization of mitochondrial form and function in skeletal muscle cells. Front. Cell Dev. Biol. 9, 757305. doi:10.3389/fcell.2021.757305
Witt, C. C., Burkart, C., Labeit, D., Mcnabb, M., Wu, Y., Granzier, H., et al. (2006). Nebulin regulates thin filament length, contractility, and Z-disk structure in vivo. EMBO J. 25 (16), 3843–3855. doi:10.1038/sj.emboj.7601242
Witt, S. H., Granzier, H., Witt, C. C., and Labeit, S. (2005). MURF-1 and MURF-2 target a specific subset of myofibrillar proteins redundantly: towards understanding MURF-dependent muscle ubiquitination. J. Mol. Biol. 350 (4), 713–722. doi:10.1016/j.jmb.2005.05.021
Woo, J. S., Hwang, J. H., Huang, M., Ahn, M. K., Cho, C. H., Ma, J., et al. (2015). Interaction between mitsugumin 29 and TRPC3 participates in regulating Ca(2+) transients in skeletal muscle. Biochem. Biophys. Res. Commun. 464 (1), 133–139. doi:10.1016/j.bbrc.2015.06.096
Wright, J., Huang, Q. Q., and Wang, K. (1993). Nebulin is a full-length template of actin filaments in the skeletal muscle sarcomere: an immunoelectron microscopic study of its orientation and span with site-specific monoclonal antibodies. J. Muscle Res. Cell Motil. 14 (5), 476–483. doi:10.1007/BF00297210
Wu, J., and Yue, B. (2024). Regulation of myogenic cell proliferation and differentiation during mammalian skeletal myogenesis. Biomed. Pharmacother. 174, 116563. doi:10.1016/j.biopha.2024.116563
Xian, H., and Liou, Y. C. (2021). Functions of outer mitochondrial membrane proteins: mediating the crosstalk between mitochondrial dynamics and mitophagy. Cell Death Differ. 28 (3), 827–842. doi:10.1038/s41418-020-00657-z
Yasuda, T., Delbono, O., Wang, Z. M., Messi, M. L., Girard, T., Urwyler, A., et al. (2013). JP-45/JSRP1 variants affect skeletal muscle excitation-contraction coupling by decreasing the sensitivity of the dihydropyridine receptor. Hum. Mutat. 34 (1), 184–190. doi:10.1002/humu.22209
Yin, H., Price, F., and Rudnicki, M. A. (2013). Satellite cells and the muscle stem cell niche. Physiol. Rev. 93 (1), 23–67. doi:10.1152/physrev.00043.2011
Yoshida, M., Minamisawa, S., Shimura, M., Komazaki, S., Kume, H., Zhang, M., et al. (2005). Impaired Ca2+ store functions in skeletal and cardiac muscle cells from sarcalumenin-deficient mice. J. Biol. Chem. 280 (5), 3500–3506. doi:10.1074/jbc.M406618200
Young, P., Ehler, E., and Gautel, M. (2001). Obscurin, a giant sarcomeric Rho guanine nucleotide exchange factor protein involved in sarcomere assembly. J. Cell Biol. 154 (1), 123–136. doi:10.1083/jcb.200102110
Keywords: skeletal myofiber, myofibril, sarcoplasmic reticulum, mitochondria, cytoskeleton
Citation: Zhao J, Du X, Fang W, Zhu H and Yue B (2025) Architecture and molecular machinery of skeletal myofibers: a systematic review of the structure–function relationships. Front. Cell Dev. Biol. 13:1602607. doi: 10.3389/fcell.2025.1602607
Received: 30 March 2025; Accepted: 29 April 2025;
Published: 20 May 2025.
Edited by:
Joseph William Landry, Virginia Commonwealth University, United StatesReviewed by:
Ramy K. A. Sayed, Sohag University, EgyptMatthew J. Gage, University of Massachusetts Lowell, United States
Copyright © 2025 Zhao, Du, Fang, Zhu and Yue. This is an open-access article distributed under the terms of the Creative Commons Attribution License (CC BY). The use, distribution or reproduction in other forums is permitted, provided the original author(s) and the copyright owner(s) are credited and that the original publication in this journal is cited, in accordance with accepted academic practice. No use, distribution or reproduction is permitted which does not comply with these terms.
*Correspondence: Binglin Yue, eXVlYmluZ2xpbjEyM0AxNjMuY29t; Hongxia Zhu, d2w0MjA4NjY2OUAxNjMuY29t