- 1Department of Microbiology, Faculty of Science and Informatics, University of Szeged, Szeged, Hungary
- 2MTA-SZTE “Lendület” Fungal Pathogenicity Mechanisms Research Group, Department of Microbiology, University of Szeged, Szeged, Hungary
- 3Department of Biochemistry and Molecular Biology, Faculty of Science and Informatics, University of Szeged, Szeged, Hungary
Mucormycosis is a life-threatening opportunistic infection caused by certain members of the fungal order Mucorales. This infection is associated with high mortality rate, which can reach nearly 100% depending on the underlying condition of the patient. Treatment of mucormycosis is challenging because these fungi are intrinsically resistant to most of the routinely used antifungal agents, such as most of the azoles. One possible mechanism of azole resistance is the drug efflux catalyzed by members of the ATP binding cassette (ABC) transporter superfamily. The pleiotropic drug resistance (PDR) transporter subfamily of ABC transporters is the most closely associated to drug resistance. The genome of Mucor circinelloides encodes eight putative PDR-type transporters. In this study, transcription of the eight pdr genes has been analyzed after azole treatment. Only the pdr1 showed increased transcript level in response to all tested azoles. Deletion of this gene caused increased susceptibility to posaconazole, ravuconazole and isavuconazole and altered growth ability of the mutant. In the pdr1 deletion mutant, transcript level of pdr2 and pdr6 significantly increased. Deletion of pdr2 and pdr6 was also done to create single and double knock out mutants for the three genes. After deletion of pdr2 and pdr6, growth ability of the mutant strains decreased, while deletion of pdr2 resulted in increased sensitivity against posaconazole, ravuconazole and isavuconazole. Our result suggests that the regulation of the eight pdr genes is interconnected and pdr1 and pdr2 participates in the resistance of the fungus to posaconazole, ravuconazole and isavuconazole.
Introduction
Mucorales species are ubiquitous saprophytes (Hoffmann et al., 2013), but several species are also known as opportunistic human pathogens, which can cause a life-threatening infection commonly called as mucormycosis. The most common mucormycosis-causing agent is Rhizopus delemar (also referred to as R. arrhizus var. delemar and R. oryzae) (Walther et al., 2019), which can cause mucormycosis worldwide, followed by Lichtheimia, Apophysomyces, Rhizomucor, Mucor and Cunninghamella species (Prakash and Chakrabarti, 2019). Besides the pathogenesis of R. delemar, those of Lichtheimia corymbifera and Mucor circinelloides, have also been studied (Riley et al., 2016; Skiada et al., 2018; Jeong et al., 2019). Clinical manifestations of mucormycosis include rhino-cerebral, pulmonary, cutaneous, and disseminated forms in immunocompromised patients (Skiada et al., 2018; Jeong et al., 2019). These infections are generally associated with rapid progression and high mortality rates (i.e. from 20 to 100% depending on the underlying condition, the clinical presentation, and the treatment), furthermore, their diagnosis and treatment are often difficult (Riley et al., 2016; Skiada et al., 2018). The immunocompromised state due to malignancy (Ibrahim et al., 2012; Riley et al., 2016; Skiada et al., 2018) or immunosuppressive treatments (Bakr et al., 2008; Ibrahim et al., 2012), diabetic ketoacidosis (Roden et al., 2005; Rammaert et al., 2012; Roilides et al., 2012), corticosteroid treatment (Prabhu and Patel, 2004), malnutrition (Sun and Singh, 2011), burn and trauma (Riley et al., 2016) are the main risk factors for the infection. For the treatment of mucormycosis, international guidelines recommend systemic antifungal therapy in combination with surgical intervention (Caramalho et al., 2017; Skiada et al., 2018). The first-choice antifungal agent against Mucorales fungi is amphotericin B. Besides, application of two triazoles, posaconazole and isavuconazole are approved for second-line or salvage therapy (Riley et al., 2016). However, the spectrum of applicable drugs is extremely narrow as these fungi are intrinsically resistant to most of the routinely used antifungal agents including most azoles (Almyroudis et al., 2007; Drogari-Apiranthitou et al., 2012; Riley et al., 2016).
The specific target of azoles is a cytochrome P450 enzyme, the lanosterol 14α-demethylase enzyme (Cyp51 or Erg11), which catalyzes an intermediate step of the ergosterol biosynthesis, the conversion of lanosterol to 4,4-dimethyl-cholesta-8,14,24-trienol. Ergosterol is the main sterol component of the fungal cell membrane. Blocking of its synthesis causes accumulation of toxic 14α-methyl sterols, which alter the stability and permeability of the cell membrane (Odds et al., 2003). Development of antifungal resistance is a complex process and depends on either host- and microbial related factors (White et al., 1998). In the case of azoles, the resistance mechanisms most frequently include alterations in ergosterol biosynthetic enzymes and up-regulation of multidrug transporters (Cowen et al., 2015).
Expression of drug efflux pumps, which belong to the ATP-binding cassette (ABC) or the major facilitator superfamily (MFS) transporters, was found to a major factor contributing in the fungal resistance to azoles in several human pathogenic fungi (Prasad and Rawal, 2014; Holmes et al., 2016; Lopez-Ribot et al., 2017; Sharma and Chowdhary, 2017). In Candida albicans, two ABC transporters belonging to the pleiotropic drug resistance (PDR) protein class of ABC transporter superfamily, Candida drug resistance 1 (Cdr1) and Cdr2 were found to have the primary role in the azole resistance (Rocha et al., 2017). Cdr genes responsible for azole efflux have also been identified and characterized in other Candida species (Rocha et al., 2017). Overexpression of the Cryptococcus neoformans antifungal resistance 1 (AFR1) protein, which also belongs to the PDR family, led to resistance to fluconazole and an increased virulence in mouse models (Chang et al., 2018). In the case of Aspergillus fumigatus, the role of the ABC transporter Cdr1B in itraconazole, voriconazole and posaconazole resistance was proven (Fraczek et al., 2013; Hagiwara et al., 2017), but other ABC transporter genes were also found to be upregulated after azole treatment (Lopez-Ribot et al., 2017).
Little is known about the background of azole resistance in mucormycosis-causing fungi. It is assumed that specific amino acid substitutions in one of the Cyp51 enzymes can affect the sensitivity of these fungi to several azoles, such as fluconazole or voriconazole (Caramalho et al., 2017). However, the role of drug efflux pumps in azole resistance have not been examined in this fungal group. M. circinelloides belongs to the order Mucorales and it is a widely used model organism in genetic and molecular biological studies, among others on light sensing (Corrochano and Garre, 2010; Vellanki et al., 2018; Yu and Fischer, 2019; Navarro et al., 2020), molecular regulation and signal processes (Lee et al., 2015; Ruiz-Vázquez et al., 2015; Calo et al., 2017; Lax et al., 2020), morphogenesis (Lee et al., 2015; Moriwaki-Takano et al., 2020; Vellanki et al., 2020), carotenogenesis (Zhang et al., 2016; Alcalde et al., 2019) and pathogenicity (Li et al., 2011; López-Fernández et al., 2018; Patiño-Medina et al., 2019). In this study, transcription of the eight genes of M. circinelloides possibly encoding PDR-type ABC transporters was analyzed and three of them (named as pdr1, pdr2 and pdr6) were knocked out by CRISPR-Cas9 mutagenesis. Using the knock-out mutants, the effect of these transporters on the azole resistance was examined.
Materials and Methods
Strains, Media, and Growth Conditions
Mucor circinelloides strain MS12 (leuA- and pyrG-) (Benito et al., 1992) was used in the study. For nucleic acid extraction from Mucor, 106 sporangiospores were plated onto solid minimal medium (YNB; 10 g glucose, 0.5 g yeast nitrogen base without amino acids (Sigma-Aldrich), 1.5 g (NH4)2SO4, 1.5 g sodium glutamate and 20 g agar per liter) supplemented with leucine and/or uracil (0.5 mg/ml) if required. In some cases, RNA extraction was performed after cultivation in 30 ml RPMI-1640 without agar (Biosera); the inoculum size was 104 sporangiospores/ml. Fungal cultures were grown for 4 days under continuous light at 25°C.
To test the effect of azoles on the gene expression, after cultivating the fungus in 10 ml liquid RPMI-1640 for 24 h at 25°C, it was treated with the corresponding azoles and incubated for another 16 h before the RNA extraction. Ketoconazole (Alfa Aesar), itraconazole (Across Organics), fluconazole (Alfa Aesar), ravuconazole (Sigma Aldrich) and isavuconazole (Sigma Aldrich) were applied in a final concentration of 8 μg/ml, while the final concentration of posaconazole (Sigma Aldrich) was 2 μg/ml. Anaerobic growth was performed in a BBL GasPak Anaerobic System (Becton Dickinson) at 25°C. For the antifungal susceptibility test, Candida krusei ATCC 6258 was used as a reference strain. After cultivating Mucor in liquid RPMI-1640 for 24 h at 25°C, it was treated with the corresponding azole (i.e., ketoconazole, itraconazole, fluconazole, posaconazole, ravuconazole or isavuconazole), and incubated for another 16 h before RNA extraction.
To measure the colony diameters of the strains, 104 sporangiospores were inoculated onto the center of solid YNB plates. The diameter of the colonies was measured daily after incubating the plates at 25°C using the MS12 strain as the growth control. In the experiment, we measured the colony diameter of two colonies using three biological and two technical replicates.
Molecular Techniques
General procedures for plasmid DNA preparation, cloning and transformation of E. coli DH5α were performed by following standard methods (Sambrook et al., 1989). Plasmid DNA was isolated using the PureYield Plasmid Miniprep System (Promega).
Genomic DNA and RNA samples were purified from mycelia using the ZR Fungal/Bacterial DNA MiniPrepTM (Zymo Research) and the Quick-RNA MiniPrep kit (Zymo Research), respectively, according to the manufacturers’ instructions. Genes were amplified by PCR using the Phusion Flash High-Fidelity PCR Master Mix (Thermo Scientific) and the primers presented in Supplementary Table 1. Primer and oligonucleotide sequences were designed using the M. circinelloides CBS277.49v2.0 genome database (DoE Joint Genome Institute; http://genome.jgi-psf.org/Mucci2/Mucci2.home.html) (Corrochano et al., 2016).
Sequence Analysis
Sequencing was commercially performed by LGC Genomics (Berlin, Germany). Amino acid sequences obtained from M. circinelloides CBS277.49v2.0 genome database and Cryptococcus neoformans Afr1 (UniProtKB: Q8X0Z3) were aligned using the ClustalW program (Higgins et al., 1996). BLAST searches were performed at the site of the National Center for Biotechnology Information (NCBI) (https://blast.ncbi.nlm.nih.gov/Blast.cgi.
Programs used to analyze the amino acid sequences of the putative PDR proteins were accessed throught the Swiss Expasy Server (http://www.expasy.ch) (Gasteiger et al., 2005). Domain search and prediction were performed using the Motif Scan (MyHits) program (Pagni et al., 2007).
Homologous sequences of Cryptococcus neoformans var. neoformans JEC21 AFR1 were searched on the JGI MycoCosm portal (https://mycocosm.jgi.doe.gov/mycocosm/home) (Grigoriev et al., 2014). Sequences with at least 50% identity were retrieved and hits from non-public genomes were removed. The remaining sequences were clustered by using MMseqs2 v. bbd564172bd55d9e6acd1170e59790c37157a21b (Steinegger and Söding, 2017). The sensitivity of the cascaded clustering was set to 7.5 applying the cluster reassign mode. Maximum Likelihood analysis was conducted with the cluster comprising of 853 sequences including the AFR1 protein and the 8 examined PDR proteins from M. circinelloides CBS 277.49 (for the sequences included see Supplementary Table 2). Sequences were aligned using MAFFT v. 7.453 (Katoh and Standley, 2013) with the E-INS-i iterative refinement method. Poorly aligned regions were removed by using trimAl v. 1.2rev57 (Capella-Gutierrez et al., 2009) with the -automated1 option. Phylogenetic reconstruction was carried out by using IQ-TREE v. 1.6.12 (Nguyen et al., 2015) with the LG4M+R6 model determined by the inbuilt model selection tool (Kalyaanamoorthy et al., 2017). Branch supports were calculated with 5000 ultrafast bootstrap (Hoang et al., 2018).
Real-Time Quantitative Reverse Transcription PCR Analysis
Reverse transcription was carried out with the Maxima H Minus First Strand cDNA Synthesis Kit (Thermo Scientific) using random hexamer and oligo (dT)18 primers, following the instructions of the manufacturer. The qRT-PCR experiments were performed in a CFX96 real-time PCR detection system (Bio-Rad) using the Maxima SYBR Green qPCR Master Mix (Thermo Scientific) and the primers presented in Supplementary Table 1. The relative quantification of the copy number and the gene expression was achieved with the 2-ΔΔCt method (Livak and Schmittgen, 2001) using the actin gene of M. circinelloides as a reference (Nagy et al., 2014). Experiments were performed in biological and technical triplicates.
Design of the gRNAs and Construction of the Disruption Cassettes to Disrupt the pdr Genes by the CRISPR-Cas9 Method
The protospacer sequences designed to target the DNA cleavage in pdr1 (CBS277.49v2.0 genome database Protein Id: 48059), pdr2 (CBS277.49v2.0 genome database Protein Id: 83305) and pdr6 (CBS277.49v2.0 genome database Protein Id: 146716) genes were the followings, 5′- tttatgaacttgtgtatgaa - 3′, 5′- tgctgatttcgagcgtatcac -3′ and 5’- ctatattgctcaagtcatca -3’, respectively. Using these sequences, the Alt-R CRISPR crRNA and Alt-RCRISPR-Cas9 tracrRNA molecules were designed and purchased from Integrated DNA Technologies (IDT). To form the crRNA:tracrRNA duplexes (i.e. the gRNAs), the Nuclease-Free Duplex Buffer (IDT) was used according to the instructions of the manufacturer. Genome editing strategies followed the set-up described earlier (Nagy et al., 2017). Homology direct repair (HDR) was applied for all gene disruptions following the strategy described previously (Nagy et al., 2017). Disruption cassettes functioning also as the template DNA for the HDR were constructed by PCR using the Phusion Flash High-Fidelity PCRMaster Mix (Thermo Scientific). At first, two fragments, upstream from start codon and downstream from stop codons of the targeted gene and the M. circinelloides pyrG gene (CBS277.49v2.0 genome database Protein Id: 36136) or M. circinelloides leuA gene (CBS277.49v2.0 genome database Protein Id: 33992) along with its own promoter and terminator sequences were amplified using gene specific primer pairs (see Supplementary Table 1). The amplified fragments were fused in a subsequent PCR using nested primers (see Supplementary Table 1); the ratio of the fragments in the reaction was 1:1:1.
Construction of the pPdr1compI Plasmid Used to Complement the Deletion of the pdr1 Gene
The leuA gene (CBS277.49v2.0 genome database ID: Mucci.e_gw1.2.132.1) using gene specific primers was amplified from the genomic DNA of the MS12 strain (Supplementary Table 1) and ligated into pJET1.2/blunt plasmid (Thermo Scientific) to create the plasmid pJet+leuA. The pdr1 gene was amplified together with its own promoter and terminator regions using gene specific primers (Supplementary Table 1) and the resulting fragment was also ligated into pJet1.2/blunt vector to create the plasmid pJet+pdr1. Then, pdr1 together with its regulatory regions was cut from this plasmid by the enzyme NotI and ligated at the same restriction site of pJet+leuA to construct pPdr1compl plasmid (Supplementary Figure 1A). This plasmid was used to introduce to the MS12- Δpdr1 to create MS12-Δpdr1+pPdr1compl strains. The complemented pdr1 gene was amplified by PCR using Mc48059P7 and Mc48059P8 primers (Supplementary Figure 1B and Supplementary Table 1).
Transformation Experiments
For the CRISPR-Cas9-mediated gene knock out and the complementation of the Δpdr1 gene, the PEG-mediated protoplast transformation method was used according to van Heeswijck and Roncero (1984). Protoplasts were prepared as described earlier (Nagy et al., 1994). For the gene disruption by CRISPR-Cas9 method, 5 μg template DNA (disruption cassette), 10 μM gRNA and 10 μM Cas9 nuclease were added to the protoplasts in one transformation reaction as described by Nagy et al. (2019). To complement the deletion of the pdr1, 5 μg pPdr1compl plasmid DNA was added into one transformation reaction. In each case, transformants were selected on solid YNB medium by the complementation of the uracil and/or leucine auxotrophy of the MS12 strain. From each primary transformant, monosporangial colonies were formed under selective conditions. Deletion of genes was confirmed by sequencing in all cases while the absence of the transcripts of the deleted genes was proven by qRT-PCR.
Measurement of Energy-Dependent Rhodamine 6G Efflux
Measurement of R6G efflux was analyzed according to Gbelska et al. (2017). Sporangiospores of the strains (107) were inoculated in 10 ml YNB and incubated in a fridge for 16 h. The spores were then incubated for 4 h at 25°C under continuous shaking at 220 rpm. Germinating spores were collected by centrifuging the samples for 10 min at 4°C at 2000×g. The pellets were washed two times with ice-cold PBS buffer and 2-deoxy-D-glucose and R6G were added to the cells in final concentrations of 2 mM and 100 µM, respectively. Fungal cells were incubated for 2 h at 25°C in dark, washed two times with ice cold PBS and finally resuspended in PBS. Then, D-glucose were added to the samples, in a final concentration of 2 nM. After incubation for 10, 20 and 30 min, 400 µl of the samples were centrifuged for 2 min at 8000×g and 100 µl of the supernatants were transferred into a 96-wells black plate. The fluorescence signal was measured using a fluorimeter (FLUOstar OPTIMA, BMG Labtech) where the excitation filter was 520BP1 and the emission filter was 590-10. Experiments were performed in biological and technical triplicates. To determine the R6G concentration, a standard curve was generated using the following concentrations: 0, 100, 250, 500 and 1000 nM.
Susceptibility Tests
Sensitivity of the fungal strains to different antifungal agents, and H2O2 was examined in a 96-well microtiter plate assay. The susceptibility test was performed according to the CLSI recommendation (Rex and Clinical, 2008) in three biological replicates. Ketoconazole (Alfa Aesar), itraconazole (Across Organics), fluconazole (Alfa Aesar), ravuconazole (Sigma Aldrich), posaconazole (Sigma Aldrich), isavuconazole (Sigma Aldrich), amphotericin B (Sigma Aldrich), hygromycin B (Sigma Aldrich), terbinafine (Sigma Aldrich), micafungin (Sigma Aldrich) and cyclohexymide (PanReac AppliChem) were dissolved in DMSO to prepare the stock. These stocks were then diluted with liquid RPMI-1640 medium. Final concentrations of ketoconazole, itraconazole, fluconazole, terbinafine and micafungin in the wells ranged from 0.125 to 64 μg/ml while those of ravuconazole and isavuconazole ranged from 0.125 to 16 μg/ml. In the case of posaconazole and amphotericin B, the range was 0.125 to 8 μg/ml. Cyclohexymide were diluted in RPMI-1640 to obtain a range of 250-0,78 μg/ml. Hydrogen peroxide was diluted in liquid RPMI-1640 to prepare a stock solution of 100 mM. Final concentrations of the hydrogen peroxide in the wells ranged from 0 to10 mM. Inocula were prepared and diluted in liquid RPMI-1640. Plates were incubated for 48 h at 25°C.
Statistical Analysis
All measurements were performed in at least two technical and three biological replicates. Significance was calculated with paired t-test or One-way Anova using the Graph Pad Prism 7 program. P values less than 0.05 were considered as statistically significant.
Results
Characteristic Features of PDR Proteins of Mucor circinelloides
In the M. circinelloides genome database (DoE Joint Genome Institute; M. circinelloides CBS277.49v2.0; http://genome.jgi-psf.org/Mucci2/Mucci2.home.html), eight potential PDR proteins were found by amino acid similarity search using the amino acid sequence of Cryptococcus neoformans Afr1 (UniProtKB: Q8X0Z3) and Candida albicans Cdr1p (UniProtKB: P43071). The identity of amino acid sequences was between 36.8 and 39.6%. Despite the low identity values, the characteristic motifs of the 8 putative Pdr proteins could be identified. Protein ID, location and amino acid length are shown in Table 1. In all eight putative PDR proteins, the amino acid motifs characteristic to the PDR family, such as the first and second Walker-A and B sequences, the first and second ABC signature motifs (Posteraro et al., 2003) and the conserved PDR specific NBD1 (T/SL/FLK/RT/V/II/L) and NBD2 (TLLN/DC/VL/R) motifs (Rawal et al., 2016) were identified (Supplementary Figure 2). PDR/CDR ABC motifs (Gauthier et al., 2003) were also identified in all eight putative PDR proteins using the motif scan (MyHits) program (Table 1).
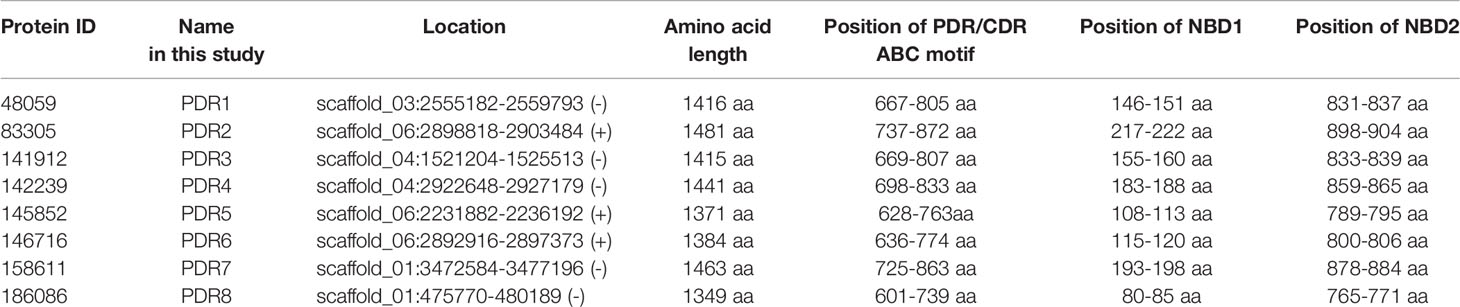
Table 1 The eight identified pleiotropic drug resistance protein genes and their location in the genome of M. circinelloides.
Comparing the amino acid sequences,PDR6 and PDR7 proved to be the most similar proteins followed by PDR2 and PDR5 showing an amino acid identity of 82.79 and 82.49%, respectively.
A phylogeny was inferred from 853 amino acid sequences of PDR proteins representing the five main fungal phyla. A simplified view of the resulting tree is shown in Figure 1 while the whole version can be found in Supplementary Figure 3. On this tree, PDR transporters of fungi belonging to the Chytridiomycota, Zoopagomycota and Mucoromycota (including the examined PDR1-8 of M. circinelloides) can be found in the clades, which clearly correspond to the phylogenetic position of these fungal groups. At the same time, Ascomycota and Basidiomycota sequences grouped together in various clades corresponding to different subfamilies of PDR proteins. Our results support the monophyly of Mucoromycota PDR proteins, which form a sister group of the Dicarya PDRs. Within the latter group the clade containing the C. neoformans Afr1 protein proved to be the closest to the Mucoromycota clade. Within the Mucoromycota clade, two main clades can be discriminated, among which the clade A contains the M. circinelloides PDR1, 6, 7 and 8 proteins while the clade B includes PDR2, 3, 4 and 5. Within the two main clades, proteins of a same species can be positioned in various subclades indicating several gene duplications and a diversification of the pdr genes in this fungal group.
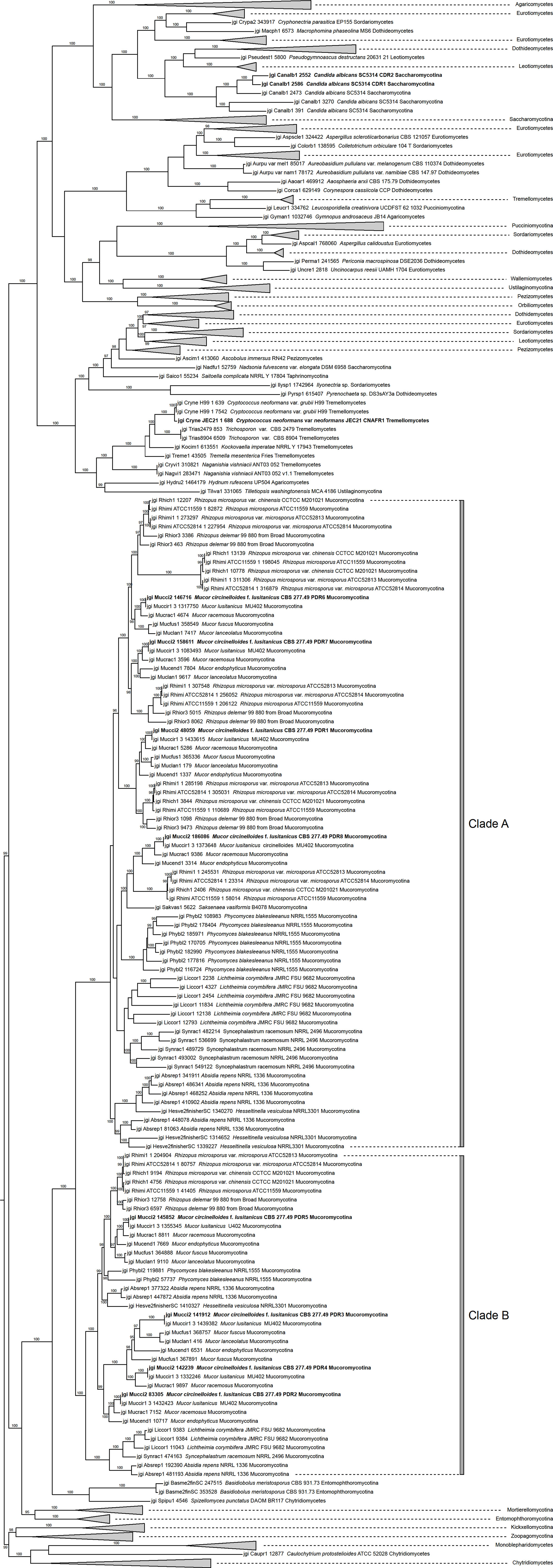
Figure 1 Phylogenetic tree of PDR proteins inferred from 853 amino acid sequences using Maximum Likelihood method. The eight M. circinelloides Pdr proteins, C. neoformand CnAfr1 and the C. albicans Cdr1 and Cdr2 are indicated with bold characters. Numbers above or below branches are ultrafast bootstrap supports. Only values greater than 95% are shown.
Relative Transcript Levels of the pdr Genes After Azole Treatment
In general, pdr genes responded differently to the tested azoles (Figure 2). Itraconazole had the widest effect on pdr expression upregulating five of the eight genes, i.e., pdr1, pdr2, pdr3, pdr5 and pdr6. Three genes (pdr1, pdr5 and pdr6) displayed increased transcript levels in response to ketoconazole, while fluconazole treatment resulted in increased transcript level for pdr1, pdr3, pdr4 and pdr7. Transcript level of pdr1, pdr2, pdr3 and pdr5 increased significantly after isavuconazole treatment. From the eight genes, only pdr1 had significantly increased transcript levels in response to all azoles (Figure 2). Interestingly only this gene responded with higher transcript level to posaconazole. Treatments with ketoconazole, itraconazole, fluconazole, posaconazole and ravuconazole resulted in decreased transcript levels for pdr7 and pdr8. Pdr4 only responded to treatment with fluconazole and ravuconazole, while the pdr6 transcript level significantly increased after ketoconazole and itraconazole treatments. A heat-map was generated to summarize the result of the qRT-PCR experiments (Figure 3). It can be seen that pdr1, pdr3, pdr4 and pdr6 are generally upregulated in response to azole treatments while pdr2, pdr5 and especially pdr7 and pdr8 are rather downregulated by the azoles.
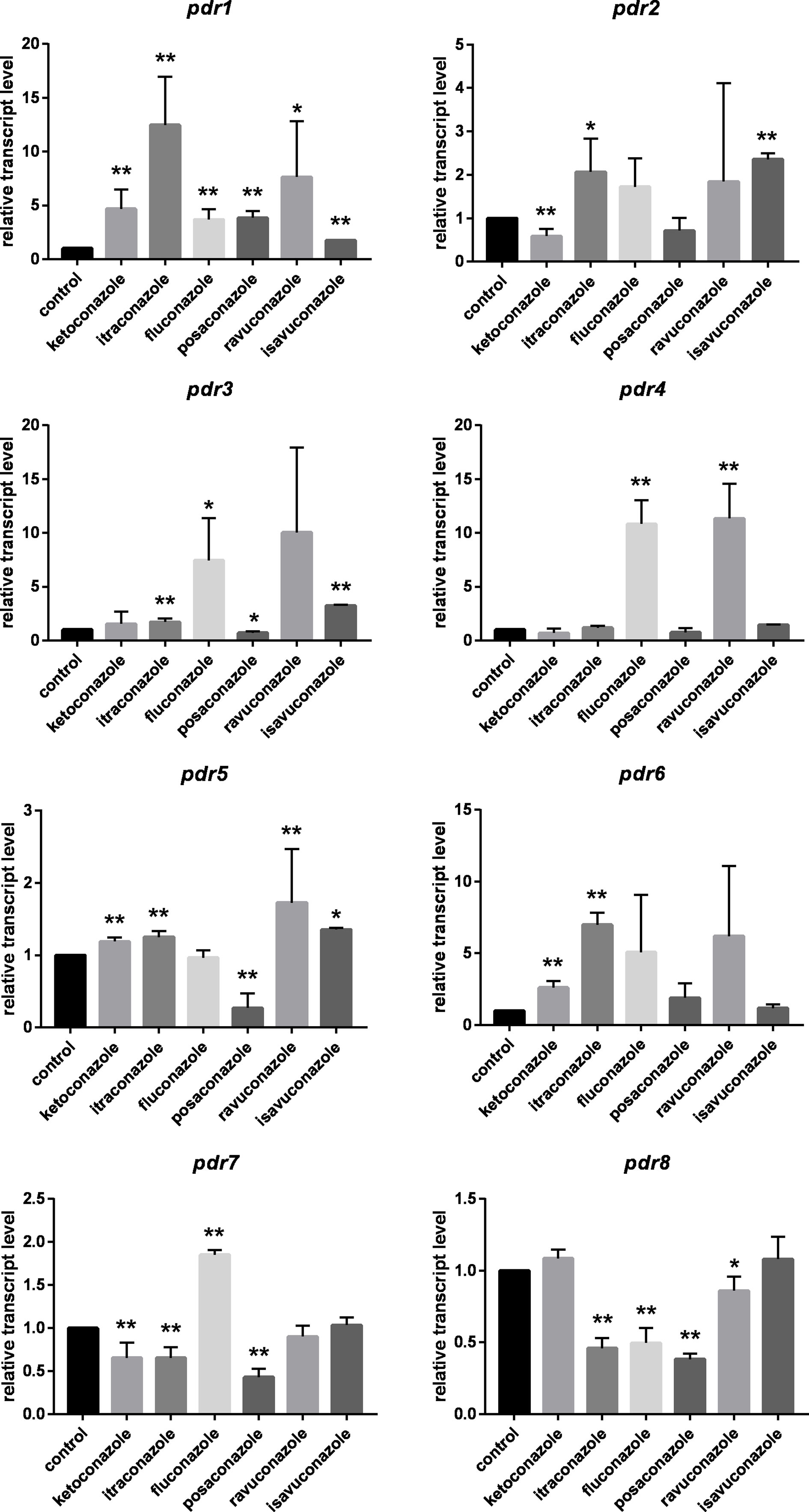
Figure 2 Relative transcript levels of the pdr genes of M. circinelloides after azole treatment. MS12 was grown in RPMI-1640 liquid media at 25°C; transcript level of each gene measured in the untreated control was taken as 1. The presented values are averages of three independent experiments; error bars indicate standard deviation. Relative transcript values followed by * and ** significantly differed from the untreated control according to the paired t-test (*p < 0.05 and **p < 0.01).
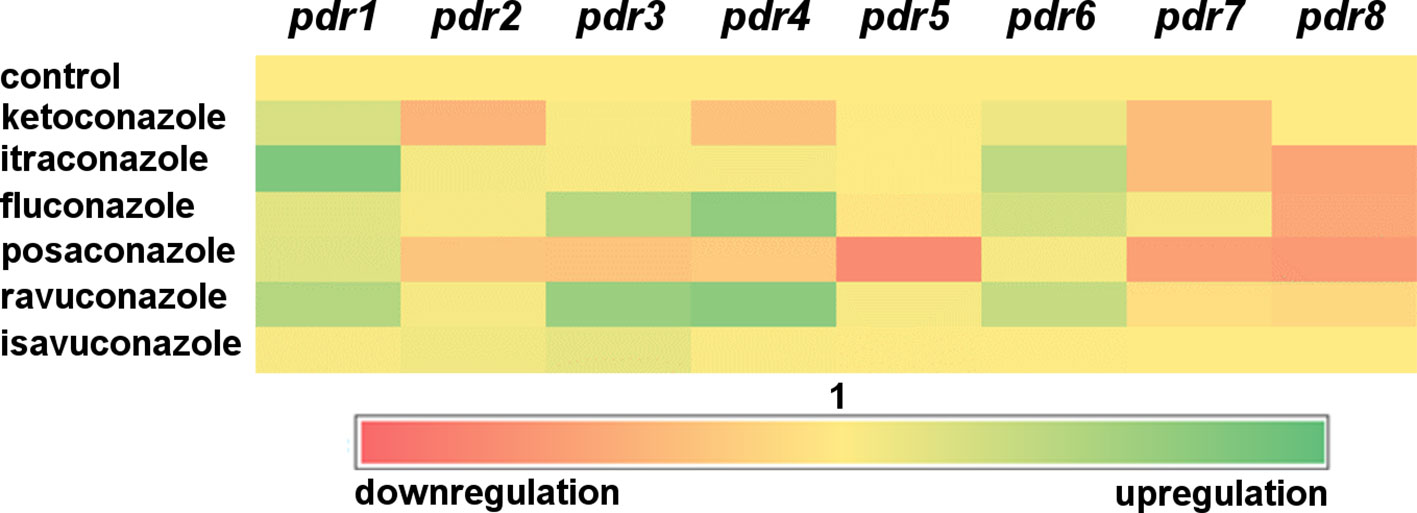
Figure 3 Heat-map of transcript level of pdr genes in response to exposure to azoles. Red and green colors indicate down- and upregulation, respectively, while yellow corresponds to the transcription activity of the control.
Relative Transcript Levels of pdr Genes Under Anaerobic Condition
Morphological dimorphism is a characteristic feature of M. circinelloides. In the absence of oxygen, filamentous growth of the fungus switches to a yeast-like form. MS12 was grown in liquid RPMI-1640 at 25°C for 24 h. Then, the culture was transferred into an oxygen-free environment containing 10% CO2. RNA was extracted after incubating the fungus anaerobically for 240 min. Pdr1 and pdr3 displayed significantly decreased transcript levels under anaerobiosis compared to their transcript levels measured in aerobic environment (Figure 4). Contrarily, the transcript level of pdr5 significantly increased under anaerobiosis (Figure 4).
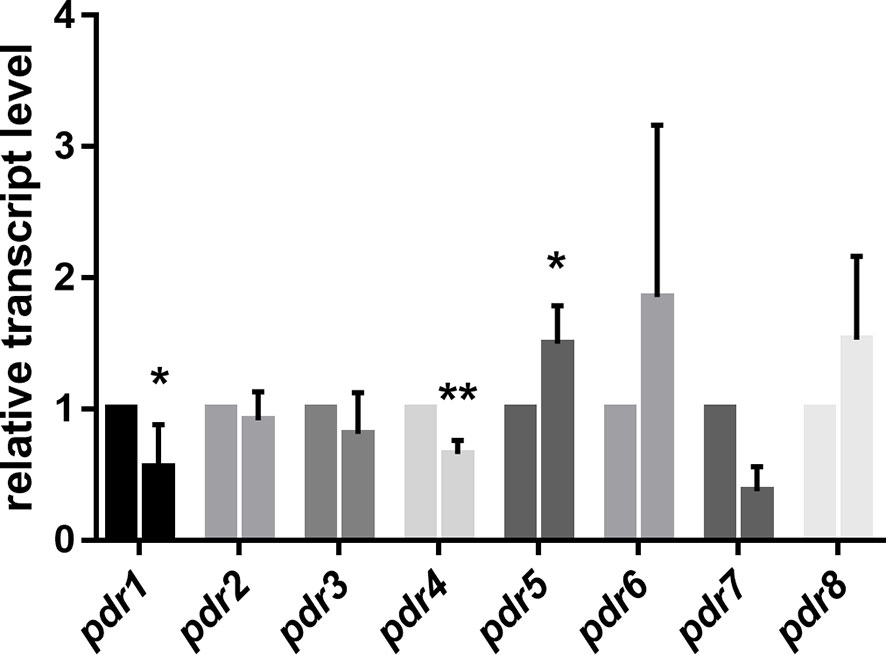
Figure 4 Relative transcript levels of pdr genes under anaerobic condition. Transcript level of each gene was measured under aerobic condition was taken as 1. The presented values are averages of three independent experiments; error bars indicate standard deviation. For RNA extraction, fungal strains were cultivated on YNB for two days at 25°C. Relative transcript values followed by * and ** significantly differed from the value taken as 1 according to the paired t-test (*p < 0.05 and **p < 0,01).
Knock Out of the pdr1 Gene Using the CRISPR-Cas9 Method
Based on the qPCR experiment, deletion of pdr1 gene was assigned for gene knock out by using the CRISPR-Cas9 technique. For deletion of pdr1, transformation and the genome editing frequencies were 4/105 protoplasts and 100%, respectively, as demonstrated by PCR analysis of the isolated colonies amplifying the expected fragments (Supplementary Table 3, Supplementary Figure 4). Mutants proved to be mitotically stable retaining the integrated fragment even after 10 cultivation cycles. From the transformation experiment two isolates (MS12-Δpdr1/1 and 2) were selected for the further experiments.
Relative Transcript Levels of the Intact pdr Genes in the Δpdr1 Knock Out Mutants
qRT-PCR analysis proved the absence of the transcript of the deleted pdr1 gene and revealed that the relative transcript levels of pdr2 and pdr6 changed significantly in the mutant (Figure 5A).
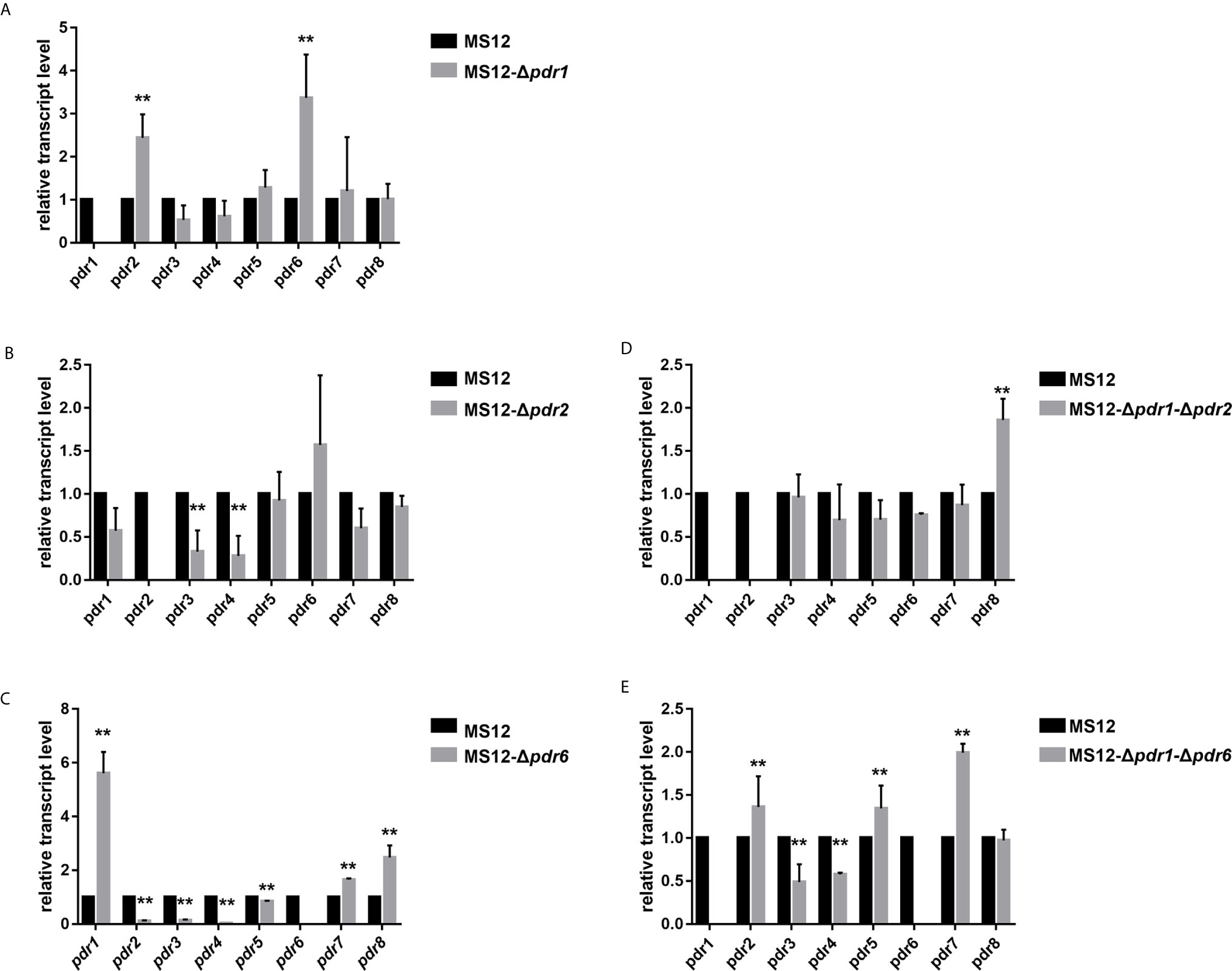
Figure 5 Relative transcript levels of pdr genes in the MS12-Δpdr1/1 (A), MS12-Δpdr2 (B), MS12-Δpdr6 (C) MS12-Δpdr1-Δpdr2 (D) and MS12-Δpdr1-Δpdr6 (E) mutant compared to that in the original strain. Transcript level of each gene measured in the strain MS12 (black column) was taken as 1. The presented values are averages of three independent experiments (error bars indicate standard deviation). For RNA extraction, fungal strains were cultivated on YNB for two days at 25°C. Relative transcript levels significantly different from the value taken as 1 according to the paired t-test are indicated with * or ** (*p < 0.05, **p < 0.01).
Deletion of pdr2 and pdr6 and Creation of Δpdr1-Δpdr2 and Δpdr1-Δpdr6 Double Knock out Mutants
Based on the qRT-PCR analysis of the MS12-Δpdr1 mutant strain, deletion of pdr2 and pdr6 gene was performed using the CRISPR-Cas9 system to create single and double mutants. For the MS12-Δpdr2 and MS12-Δpdr6 single mutants, transformation frequencies were 12 and 10 colonies per 105 protoplasts, respectively while the genome editing efficiency was 83% and 100%, respectively (Supplementary Table 3, Supplementary Figure 5). To create Δpdr1-Δpdr2 -and Δpdr1 Δpdr6 double mutants, MS12-Δpdr1 was used as the recipient strain. Transformation frequency was 8 and 3 colonies and the genome editing efficiency was 75% and 66.66% for MS12-Δpdr1-Δpdr2 and MS12-Δpdr1-Δpdr6, respectively (Supplementary Table 3). Two isolates from each the transformation experiments were analyzed by PCR and the expected fragments were amplified (Supplementary Figure 6).
Relative Transcript Levels of the Intact pdr Genes in the MS12-Δpdr2 and MS12-Δpdr6 Single-, and the MS12-Δpdr1-Δpdr2 and MS12-Δpdr1-Δpdr6 Double Knock Out Mutants
qRT-PCR analysis proved the absence of the transcripts of the deleted genes in all mutants. It also revealed that the transcript level of pdr3 and pdr4 significantly decreased in the MS12-Δpdr2 strain (Figure 5B). At the same time, deletion of the pdr6 gene led to significantly increased relative transcript levels for pdr1, pdr7 and pdr8, while the relative transcript level of the other pdr genes significantly decreased (Figure 5C).
After simultaneous deletion of pdr1 and pdr2, the transcript level of pdr8 significantly increased in the mutants (Figure 5D). In the MS12-Δpdr1-Δpdr6 mutant, the transcript levels of pdr2, pdr3, pdr5, pdr7 significantly increased, while those of pdr4 and pdr8 significantly decreased (Figure 5E).
Effect of the pdr1, pdr2, and pdr6 Gene Deletions on the Colony Growth of the Mutant Strains
Colony growth of the single (i.e., MS12-Δpdr1, MS12-Δpdr2 and MS12-Δpdr6) and double pdr mutants (i.e., MS12-Δpdr1-Δpdr2 and MS12-Δpdr1-Δpdr6) significantly decreased compared to the parental MS12 strain on the third and fourth days of cultivation (Figure 6). When deletion of the pdr1 gene was complemented by transforming pPdr1compl plasmid into the MS12-Δpdr1 strain, growth ability of the resulting MS12-Δpdr1+pPdr1compl strain proved to be similar to that of the original MS12 strain (Figure 6A).
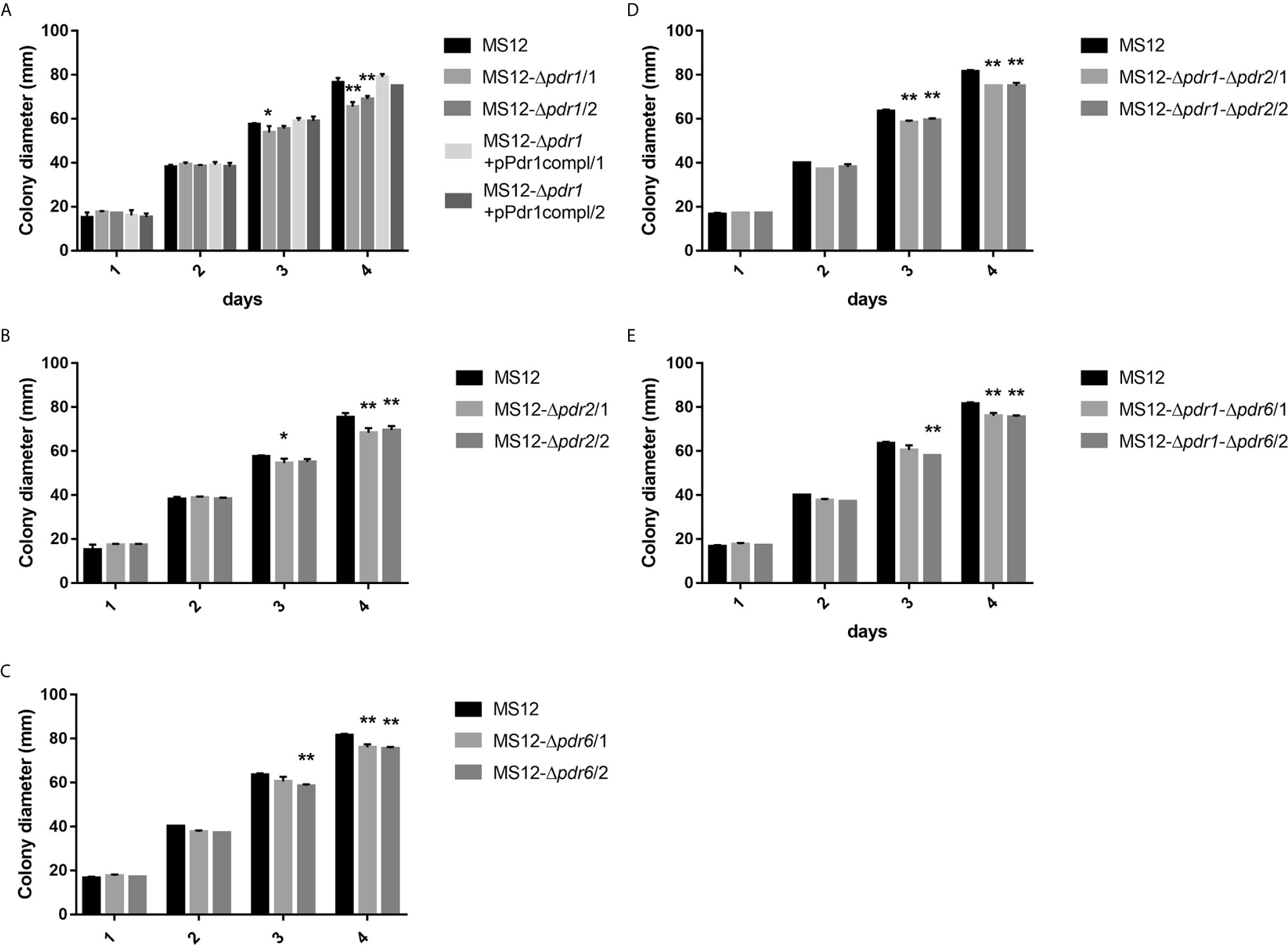
Figure 6 Colony diameters of the pdr deletion mutants and the original MS12 strain of Mucor circinelloides at 25°C on YNB medium. (A) shows the colony diameter of pdr1 deleted and complemented strains, (B, C) show the colony diameter of the single pdr2 and pdr6 knock out mutants, while (D, E) show the colony diameter of the double knock out strains. The presented values are averages; colony diameters were measured during three independent cultivation (error bars indicate standard deviation). Values followed by * and ** significantly differed from the corresponding value of the MS12 strain according to the two-way Anova (*p < 0.05; **p < 0.01).
Measurement of the R6G Efflux Activity of the Tested Strains
To examine the drug efflux activity of the strains, R6G efflux in the parental and the pdr mutant strains were compared. Twenty min after R6G uptake, MS12-Δpdr1 and MS12-Δpdr2 mutants showed significantly decreased efflux activity than the parental strain (Figure 7). After 30 min, efflux activity of all mutants, except MS12-Δpdr6, significantly decreased (Figure 7).
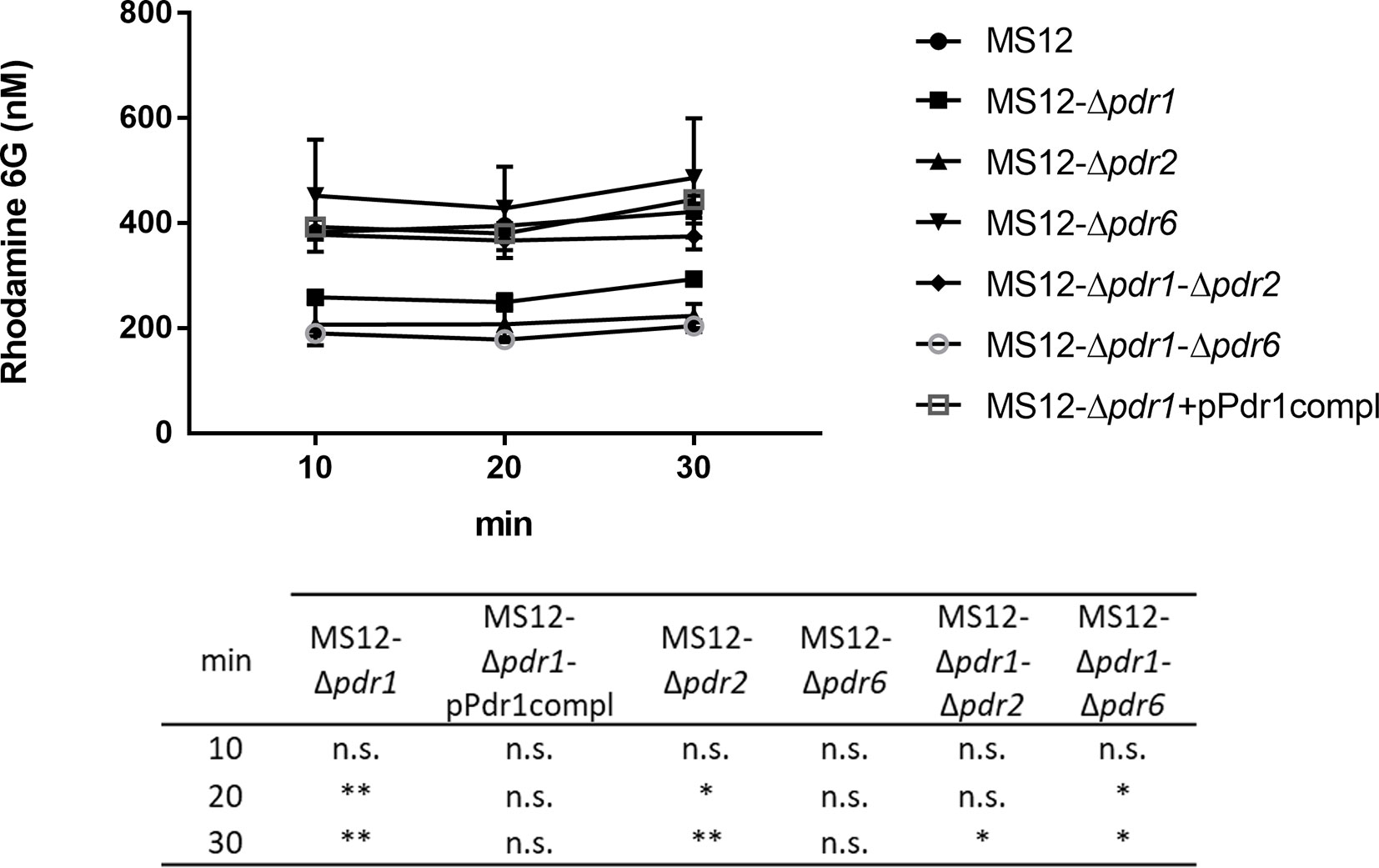
Figure 7 Measurement of the R6G efflux activity. After uptake of rhodamine 6G, fungal cells were resuspended in PBS supplemented with 2 nM of D-glucose. Values followed by * and ** significantly differed from the corresponding value of the MS12 strain according to the two-way Anova (*p < 0.05; **p < 0.01), n.s. is non-significant.
Effect of the Gene Knock Out on the Susceptibility to Different Antifungal Agents and Hydrogen Peroxide Under Aerobic and Anaerobic Conditions
Sensitivity of the MS12-Δpdr1, MS12-Δpdr2, MS12-Δpdr6, MS12-Δpdr1-Δpdr2 and MS12-Δpdr1-Δpdr6 mutants and the MS12 strain were determined to different antifungal agents and hydrogen peroxide (H2O2) by using the broth microdilution method under aerobic and anaerobic conditions and the minimal inhibitory concentration (MIC) of different azoles was determined. Sensitivity of the mutants did not differ from that of the original MS12 strain to amphotericin B, hygromycin B, terbinafine, micafungin, ketoconazole, fluconazole, cycloheximide and H2O2 under both aerobic and anaerobic conditions. All mutants showed, however, increased susceptibility to posaconazole and ravuconazole, while knock out of pdr1 resulted in increased sensitivity to isavuconazole under anaerobic condition as well (Table 2). After complementation of the pdr1 deletion, susceptibility of MS12-Δpdr1+pPdr1compl strains to all tested azoles was similar to that of the original MS12 strain.
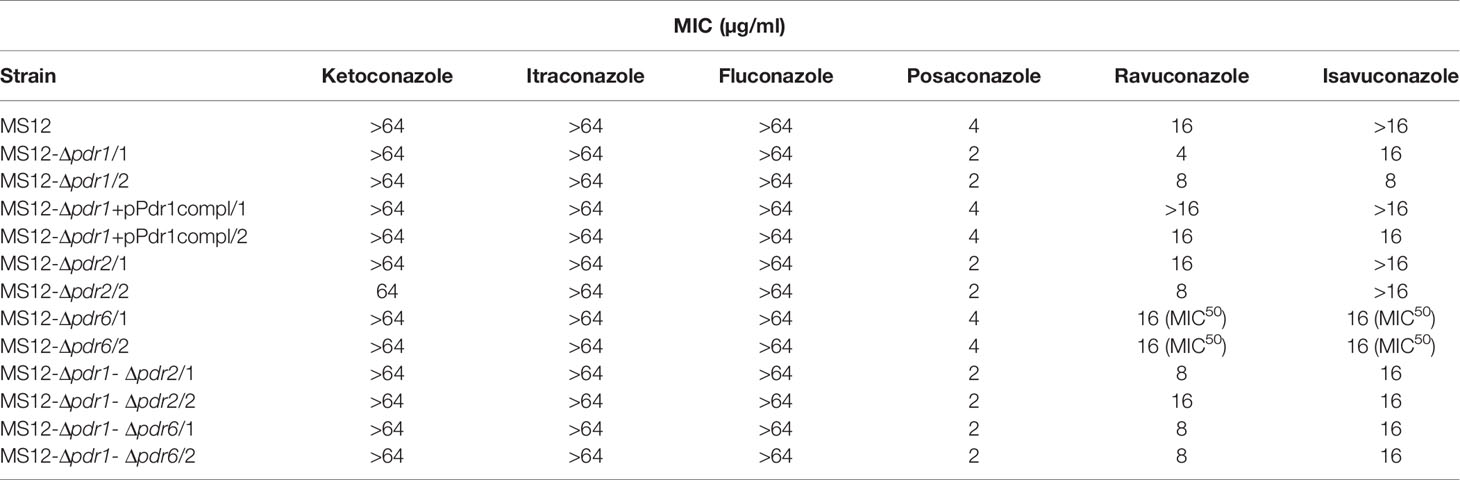
Table 2 Minimal inhibitory concentrations (MIC) of the azoles (µg/ml) against the mutants and the parental M. circinelloides MS12 strain under aerobic condition.
Under anaerobic condition, knock out of pdr2 resulted in decreased sensitivity to ketoconazole compared to the parental strain, while susceptibility of the MS12-Δpdr1 and MS12-Δpdr2 mutants increased to itraconazole, ravuconazole and isavuconazole. Deletion of pdr1 and pdr2 had no effect on the sensitivity to posaconazole under anaerobiosis. Knock out of pdr6 had no effect on the susceptibility to the different azoles at all under anaerobiosis (Table 3).
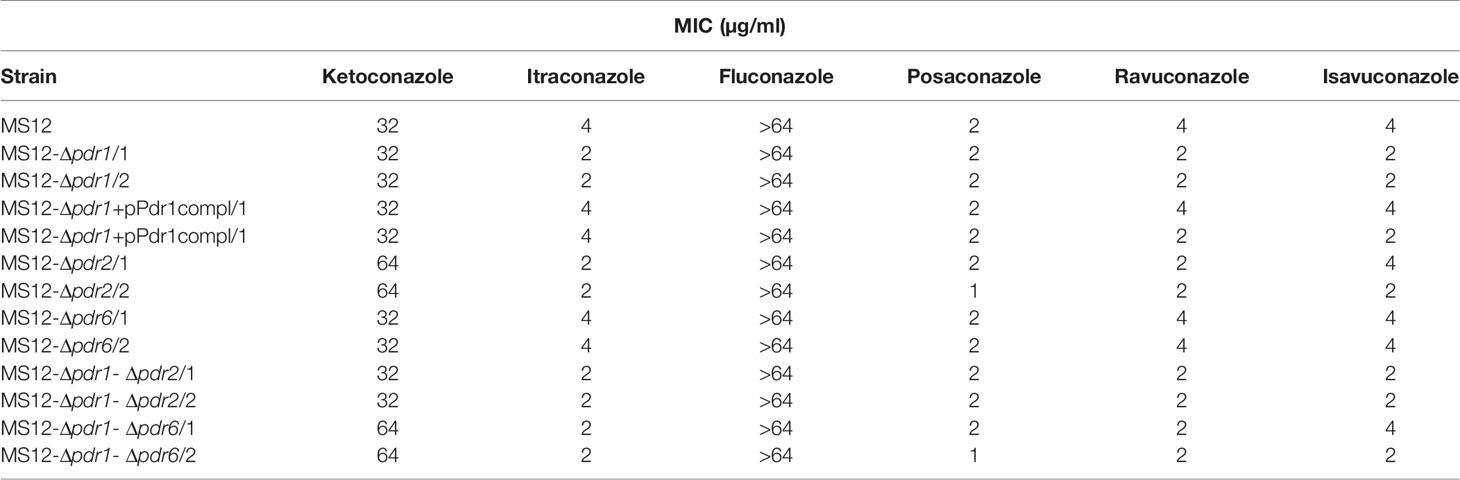
Table 3 Minimal inhibitory concentrations (MIC) of the azoles (µg/ml) against the transformants and the parental M. circinelloides MS12 strain under anaerobic condition.
Discussion
Treatment of mucormycosis is looked upon as challenging and aggravated by the fact that Mucorales species are resistant to most azole antifungals. The molecular mechanism of their innate resistance to short-tailed azoles was described, but yet not completely clarified (Caramalho et al., 2017). Resistance mechanisms of various Candida and Aspergillus species have been characterized with more details (Cuenca-Estrella, 2014). The main target of azoles is the lanosterol-demethylase enzyme (i.e. Cyp51 or Erg11), which plays a crucial role in the ergosterol biosynthesis of yeasts and molds (Monk et al., 2020). Overexpression of and point mutations in the cyp51 gene can cause increased resistance to different azoles used in the clinic and agriculture (Parker et al., 2014). Besides lanosterol-demethylase, ABC- and MFS transporters are involved in the mechanism of azole resistance (Fraczek et al., 2013; Cuenca-Estrella, 2014; Gonçalves et al., 2016; Rocha et al., 2017). In C. albicans, the MFS-transporter MDR1 contribute to the fluconazole, voriconazole and ketoconazole resistance (Morschhäuser, 2010), but does not confer resistance to posaconazole, isavuconazole or itraconazole (Sanglard and Coste, 2015).
ABC transporters constitute one of the largest transporter superfamily. These proteins often consist of multiple subunits (i.e., of the transmembrane domain [TMD] and the nucleotide binding domain [NBD]). The transport is driven by ATP hydrolysis (Wilkens, 2015). ATP is the substrate of NBD, which has some conserved motifs, such as Walker-A, Walker-B and the ABC-signature (Seeger and van Veen, 2009; Lamping et al., 2010; Holmes et al., 2016). The sequence of TMD is more diverse reflecting to the diversity of the transported substrate (Seeger and van Veen, 2009). Within the ABC transporters, the PDR subfamily is the most closely associated to drug resistance (Cannon et al., 2009). In the M. circinelloides genome database, eight putative PDR transporter coding genes were identified. The abovementioned motifs characteristic to ABC transporters were identified in each of them.
Analysis of the promoter regions of the eight Mucor genes did not reveal the presence of the motifs described as PDRE (TCCG/aC/tGG/cA) or CDRE (CGGA(A/T)ATCGGATATTTTTTTT) elements. These motifs were originally found and characterized in Saccharomyces (Mamnun et al., 2002) and Candida (de Micheli et al., 2002) as the binding sites of transcription factors regulating the expression of the pdr genes. If such binding sites are present, the consensus sequences established for Ascomycota cannot be applied to find them, maybe because of the large evolutionary distance.
Phylogenetic analysis of PDR transporters indicated the monophyly of Mucoromycota PDRs; although, within the Mucoromycota clade various gene duplication events can be assumed. Based on a phylogenetic analysis of 78 PDR proteins, Lamping et al. (2010) previously discerned 10 clades of PDR transporters within the Dicarya, among which four clades (i.e. clades B, G, H1a and H1b) contained PDRs of both Ascomycota and Basidiomycota fungi. None of the involved Mucoromycota sequences grouped together with any of the Dikarya PDRs.
Mucor pdr genes show similarity to the C. neoformans Afr1 gene, which was also strengthen by the phylogenetic analysis of the fungal PDR genes Afr1 is involved in the resistance of Cryptococcus to fluconazole (Sanguinetti et al., 2006). Overexpression of Afr1 increased the azole resistance indicating that upregulation of the gene may play a role in the fluconazole resistance (Posteraro et al., 2003; Sanguinetti et al., 2006; Morschhäuser, 2010). In our experiments, transcription level of all M. circinelloides pdr genes altered after treatment with either of the tested azoles, although only that of the pdr1 increased in response to all of them.
Mucorales species are generally resistant to fluconazole and voriconazole, while isavuconazole and itraconazole were found to have in vitro species-specific activity (Dannaoui, 2017). International guidelines for the treatment of mucormycosis suggest first-line antifungal therapy with lipid formulations of amphotericin B (AMB), whereas posaconazole (Cornely et al., 2014) is recommended and isavuconazole (Ashkenazi-Hoffnung et al., 2020) was successfully used as salvage therapy. In our study, posaconazole treatment affected the transcription of pdr1 only while isavuconazole significantly increased the transcript level of pdr1, pdr2, pdr3 and pdr5.
Deletion of pdr1 increased the sensitivity of the mutants to posaconazole and isavuconazole. Although it was found to be upregulated by isavuconazole, deletion of pdr2 did not affect the susceptibility to this compound. At the same time, MS12-Δpdr2 mutants showed increased susceptibility to posaconazole similarly to the MS12-Δpdr1 strains.
Fluconazole treatment affected the transcription of various genes including pdr1 but neither single nor double deletion mutants generated in this study displayed altered susceptibility to this drug. This result is in agreement with the observations that, in addition to active transport mechanisms, alterations in the lanosterol-demethylase gene (Edlind et al., 2001; Sionov et al., 2012; Leonardelli et al., 2016; Caramalho et al., 2017) and/or a novel Zn2-Cys6 transcription factor may also underlie the fluconazole resistance of these fungi (Hagiwara et al., 2017).
qRT-PCR analysis revealed highly similar transcription patterns for pdr1 and pdr6 after azole treatment. At the same time, deletion of pdr1 caused significantly increased transcript level of pdr2 and pdr6, while the relative transcript level of pdr1, pdr7 and pdr8 significantly increased in the MS12-Δpdr6 mutant. These results suggest that regulation of pdr genes is highly interconnected and coordinated. It can also be assumed that the increased activity of certain pdr genes may compensate the lack of the deleted genes. A similar phenomenon was previously described for the three HMG-CoA reductase isogenes in M. circinelloides (Nagy et al., 2019). Functional linkages of genes involved in antifungal resistance was observed in Saccharomyces cerevisiae where deletion of two multidrug transporter genes, yor1 and snq2 caused an increased expression of pdr5, while the lack of pdr5 and snq2 induced yor1 expression (Kolaczkowska et al., 2008).
Susceptibility testing of the deletion mutants suggests that pdr1 and pdr2 may have role in the protection from the effect of posaconazole, isavuconazole and ravuconazole and this function is affected by the oxygen level of the environment. At the same time, deletion of pdr6 had no significant effect on the azole resistance of the fungus. Deletion of pdr1 and pdr2 resulted decreased efflux activity in all tested mutant strains, while the efflux activity of MS12-Δpdr6 strain was similar to the parental MS12 strain.
Our results suggest that the tested pdr genes involved in different degrees in the resistance to the different azoles. In C. glabrata Cdr1 and Cdr2 also had a different effect on the resistance to azoles (Sanglard et al., 2001). In A. fumigatus deletion of abcA and abcB/cdr1B transporters, which show the highest similarity to S. cerevisiae pdr5, resulted in increased susceptibility to voriconazole, but this effect was more pronounced in case of the ΔabcB/cdr1B mutant (Paul et al., 2013). In Aspergillus oryzae, deletion of atrG belonging to the PDR subfamily, caused increased azole susceptibility, while its simultaneous deletion with atrA conferred azole hypersensitivity (Miura et al., 2018).
In the absence of oxygen, filamentous growth of M. circinelloides switches to a yeast-like form and the fungus produces energy by fermentation (Nagy et al., 2014). These morphological and metabolic changes are necessary associated to serious changes in the expression of numerous genes. Transporters and other membrane associated proteins are expected to be affected by these processes. Under anaerobic condition, the transcript levels of pdr1 and pdr4 decreased, while the transcript level of pdr5 increased significantly compared to the aerobic condition. The transcript levels of other pdr genes did not change significantly. The growth ability of the created mutants somewhat decreased compared to the parental strain. This phenomenon can be explained by the fact that the function of PDR transporters in not limited to the drug export. In S. cerevisiae Aus1p and Pdr11p are required for import of exogenous sterols for anaerobic growth (Wilcox et al., 2002; Reiner et al., 2006; Kohut et al., 2011; Zavrel et al., 2013) and simultaneous disruption of the encoding genes proved to be lethal under anaerobiosis (Wilcox et al., 2002). S. cerevisiae Pdr5 and Yor1 play role in the externalization of lipids (Decottignies et al., 1998; Pomorski et al., 2003). In C. albicans, Cdr1 plays role in the drug efflux and, along with Cdr2 and Cdr3, involved in the externalization of lipids (Smriti et al., 2002; Tsao et al., 2009).
Conclusion
M. circinelloides has eight genes encoding PDR transporters. Transcription analysis of these genes suggests that their regulation is interconnected and affected by the different azoles. The genes pdr1 and pdr2 seem to participate in the resistance or reduced sensitivity to posaconazole, ravuconazole and isavuconazole. It is also clear, that resistance to these azoles and azole resistance in general cannot be completely explained by the activity of the tested PDR proteins. Other mechanisms, such as special regulatory processes, multiple transporter genes and alterations in the lanosterol-demethylase gene might also be involved.
Data Availability Statement
The original contributions presented in the study are included in the article/Supplementary Material. Further inquiries can be directed to the corresponding author.
Author Contributions
GN (1st Author) and TP designed the approach and the experiments, participated in the experimental work, managed the study, and wrote the manuscript. SKi, RV, KB, CS, HM, ZN, and GN (10th Author) participated in the experimental work. SKo, LB, and CV participated in the data analysis, evaluation of the results and drafting the manuscript. All authors contributed to the article and approved the submitted version.
Funding
This study was supported by the “Lendület” Grant of the Hungarian Academy of Sciences (LP2016-8/2016), the project GINOP-2.3.2-15-2016-00035 and the NKFI project K131796. GN is grateful for support of the Premium Postdoctoral Fellowship Program of the Hungarian Academy of Sciences (460050). LB was supported by the János Bolyai Research Scholarship (BO/00522/19/8) of the Hungarian Academy of Sciences.
Conflict of Interest
The authors declare that the research was conducted in the absence of any commercial or financial relationships that could be construed as a potential conflict of interest.
Supplementary Material
The Supplementary Material for this article can be found online at: https://www.frontiersin.org/articles/10.3389/fcimb.2021.660347/full#supplementary-material
References
Alcalde, E., Cerdá-Olmedo, E., Al-Babili, S. (2019). Apocarotenoids produced from β-carotene by dioxygenases from Mucor circinelloides. Microbiology 165, 433–438. doi: 10.1128/AAC.00452-07
Almyroudis, N. G., Sutton, D. A., Fothergill, A. W., Rinaldi, M. G., Kusne, S. (2007). In vitro susceptibilities of 217 clinical isolates of zygomycetes to conventional and new antifungal agents. Antimicrob. Agents Chemother. 51, 2587–2590. doi: 10.1128/AAC.00452-07
Ashkenazi-Hoffnung, L., Bilavsky, E., Levy, I., Grisaru, G., Sadot, E., Ben-Ami, R., et al. (2020). Isavuconazole As Successful Salvage Therapy for Mucormycosis in Pediatric Patients. Pediatr. Infect. Dis. J. 39, 718–724. doi: 10.1097/INF.0000000000002671
Bakr, A., Wafa, E., Fouda, A., Elagroudy, A., Gheith, O., Sobh, M., et al. (2008). Successful treatment of mucormycosis in a renal allograft recipient. Clin. Exp. Nephrol. 12, 207–210. doi: 10.1007/s10157-008-0028-7
Benito, E. P., Díaz-Mínguez, J. M., Iturriaga, E. A., Campuzano, V., Eslava, A. P. (1992). Cloning and sequence analysis of the Mucor circinelloides pyrG gene encoding orotidine-5’-monophosphate decarboxylase: use of pyrG for homologous transformation. Gene 116, 59–67. doi: 10.1016/0378-1119(92)90629-4
Calo, S., Nicolás, F. E., Lee, S. C., Vila, A., Cervantes, M., Torres-Martinez, S., et al. (2017). A non-canonical RNA degradation pathway suppresses RNAi-dependent epimutations in the human fungal pathogen Mucor circinelloides. PloS Genet. 13, e1006686. doi: 10.1371/journal.pgen.1006686
Cannon, R. D., Lamping, E., Holmes, A. R., Niimi, K., Baret, P. V., Keniya, M. V., et al. (2009). Efflux-mediated antifungal drug resistance. Clin. Microbiol. Rev. 22, 291–321. doi: 10.1128/CMR.00051-08
Capella-Gutiérrez, S., Silla-Martínez, J. M., Gabaldón, T. (2009). trimAl: a tool for automated alignment trimming in large-scale phylogenetic analyses. Bioinformatics 25, 1972–1973. doi: 10.1093/bioinformatics/btp348
Caramalho, R., Tyndall, J. D. A., Monk, B. C., Larentis, T., Lass-Flörl, C., Lackner, M. (2017). Intrinsic short-tailed azole resistance in mucormycetes is due to an evolutionary conserved aminoacid substitution of the lanosterol 14α-demethylase. Sci. Rep. 7, 15898. doi: 10.1038/s41598-017-16123-9
Chang, M., Sionov, E., Khanal Lamichhane, A. K., Kwon-Chung, K. J., Chang, Y. C. (2018). Roles of three Cryptococcus neoformans and Cryptococcus gattii efflux pump-coding genes in response to drug treatment. Antimicrob. Agents Chemother. 62, e01751–17. doi: 10.1128/AAC.01751-17
Cornely, O. A., Arikan-Akdagli, S., Dannaoui, E., Groll, A. H., Lagrou, K., Chakrabarti, A., et al. (2014). ESCMID and ECMM joint clinical guidelines for the diagnosis and management of mucormycosis 2013. Clin. Microbiol. Infect. 20 Suppl 3, 5–26. doi: 10.1111/1469-0691.12371
Corrochano, L. M., Garre, V. (2010). Photobiology in the Zygomycota: multiple photoreceptor genes for complex responses to light. Fungal Genet. Biol. 47, 893–899. doi: 10.1016/j.fgb.2010.04.007
Corrochano, L. M., Kuo, A., Marcet-Houben, M., Polaino, S., Salamov, A., Villalobos-Escobedo, J. M., et al. (2016). Expansion of Signal Transduction Pathways in Fungi by Extensive Genome Duplication. Curr. Biol. 26, 1577–1584. doi: 10.1016/j.cub.2016.04.038
Cowen, L. E., Sanglard, D., Howard, S. J., Rogers, P. D., Perlin, D. S. (2015). Mechanisms of antifungal drug resistance. Cold Spring Harbor Perspect. Med. 5, 1–22. doi: 10.1101/cshperspect.a019752
Cuenca-Estrella, M. (2014). Antifungal drug resistance mechanisms in pathogenic fungi: from bench to bedside. Clin. Microbiol. Infect. 20, 54–59. doi: 10.1111/1469-0691.12495
Dannaoui, E. (2017). Antifungal resistance in mucorales. Int. J. Antimicrob. Agents 50, 617–621. doi: 10.1016/j.ijantimicag.2017.08.010
de Micheli, M., Bille, J., Schueller, C., Sanglard, D. (2002). A common drug-responsive element mediates the upregulation of the Candida albicans ABC transporters Cdr1 and Cdr2, two genes involved in antifungal drug resistance. Mol. Microbiol. 43, 1197–1214. doi: 10.1046/j.1365-2958.2002.02814.x
Decottignies, A., Grant, A. M., Nichols, J. W., de Wet, H., McIntosh, D. B., Goffeau, A. (1998). ATPase and multidrug transport activities of the overexpressed yeast ABC protein Yor1p. J. Biol. Chem. 273, 12612–12622. doi: 10.1074/jbc.273.20.12612
Drogari-Apiranthitou, M., Mantopoulou, F.-D., Skiada, A., Kanioura, L., Grammatikou, M., Vrioni, G., et al. (2012). In vitro antifungal susceptibility of filamentous fungi causing rare infections: synergy testing of amphotericin B, posaconazole and anidulafungin in pairs. J. Antimicrob. Chemother. 67, 1937–1940. doi: 10.1093/jac/dks137
Edlind, T. D., Henry, K. W., Metera, K. A., Katiyar, S. K. (2001). Aspergillus fumigatus CYP51 sequence: potential basis for fluconazole resistance. Med. Mycol. 39, 299–302. doi: 10.1080/mmy.39.3.299.302
Fraczek, M. G., Bromley, M., Buied, A., Moore, C. B., Rajendran, R., Rautemaa, R., et al. (2013). The cdr1B efflux transporter is associated with non-cyp51a-mediated itraconazole resistance in Aspergillus fumigatus. J. Antimicrob. Chemother. 68, 1486–1496. doi: 10.1093/jac/dkt075
Gasteiger, E., Hoogland, C., Gattiker, A., Duvaud, S., Wilkins, M. R., Appel, R. D., et al. (2005). “Protein Identification and Analysis Tools on the ExPASy Server,” in The Proteomics Protocols Handbook Springer Protocols Handbooks., ed. Walker, J. M. (Totowa, NJ: Humana Press), 571–607. doi: 10.1385/1-59259-890-0:571
Gauthier, C., Weber, S., Alarco, A.-M., Algawi, O., Daoud, R., Georges, E., et al. (2003). Functional Similarities and Differences between Candida albicans Cdr1p and Cdr2p Transporters. Antimicrob. Agents Chemother. 47, 1543–1554. doi: 10.1128/AAC.47.5.1543-1554.2003
Gbelska, Y., Toth Hervay, N., Dzugasova, V., Konecna, A. (2017). Measurement of Energy-dependent Rhodamine 6G Efflux in Yeast Species. Bio-protocol 7, e2428. doi: 10.21769/BioProtoc.2428
Gonçalves, S. S., Souza, A. C. R., Chowdhary, A., Meis, J. F., Colombo, A. L. (2016). Epidemiology and molecular mechanisms of antifungal resistance in Candida and Aspergillus. Mycoses 59, 198–219. doi: 10.1111/myc.12469
Grigoriev, I. V., Nikitin, R., Haridas, S., Kuo, A., Ohm, R., Otillar, R., et al. (2014). MycoCosm portal: gearing up for 1000 fungal genomes. Nucleic Acids Res. 42, 699–704. doi: 10.1093/nar/gkt1183
Hagiwara, D., Miura, D., Shimizu, K., Paul, S., Ohba, A., Gonoi, T., et al. (2017). A Novel Zn2-Cys6 Transcription Factor AtrR Plays a Key Role in an Azole Resistance Mechanism of Aspergillus fumigatus by Co-regulating cyp51A and cdr1B Expressions. PloS Pathog. 13, e1006096. doi: 10.1371/journal.ppat.1006096
Higgins, D. G., Thompson, J. D., Gibson, T. J. (1996). Using CLUSTAL for multiple sequence alignments. Methods Enzymol. 266, 383–402. doi: 10.1016/s0076-6879(96)66024-8
Hoang, D. T., Chernomor, O., von Haeseler, A., Minh, B. Q., Vinh, L. S. (2018). UFBoot2: Improving the ultrafast bootstrap approximation. Mol. Biol. Evol. 35, 518–522. doi: 10.1093/molbev/msx281
Hoffmann, K., Pawłowska, J., Walther, G., Wrzosek, M., de Hoog, G. S., Benny, G. L., et al. (2013). The family structure of the Mucorales: a synoptic revision based on comprehensive multigene-genealogies. Persoonia 30, 57–76. doi: 10.3767/003158513X666259
Holmes, A. R., Cardno, T. S., Strouse, J. J., Ivnitski-Steele, I., Keniya, M. V., Lackovic, K., et al. (2016). Targeting efflux pumps to overcome antifungal drug resistance. Future Med. Chem. 8, 1485–1501. doi: 10.4155/fmc-2016-0050
Ibrahim, A. S., Spellberg, B., Walsh, T. J., Kontoyiannis, D. P. (2012). Pathogenesis of Mucormycosis. Clin. Infect. Dis. 54, S16–S22. doi: 10.1093/cid/cir865
Jeong, W., Keighley, C., Wolfe, R., Lee, W. L., Slavin, M. A., Kong, D. C. M., et al. (2019). The epidemiology and clinical manifestations of mucormycosis: a systematic review and meta-analysis of case reports. Clin. Microbiol. Infect. 25, 26–34. doi: 10.1016/j.cmi.2018.07.011
Kalyaanamoorthy, S., Minh, B. Q., Wong, T. K. F., von Haeseler, A., Jermiin, L. S. (2017). ModelFinder: fast model selection for accurate phylogenetic estimates. Nat. Methods 14, 587–589. doi: 10.1038/nmeth.4285
Katoh, K., Standley, D. M. (2013). MAFFT multiple sequence alignment software version 7: improvements in performance and usability. Mol. Biol. Evol. 30, 772–780. doi: 10.1093/molbev/mst010
Kohut, P., Wüstner, D., Hronska, L., Kuchler, K., Hapala, I., Valachovic, M. (2011). The role of ABC proteins Aus1p and Pdr11p in the uptake of external sterols in yeast: Dehydroergosterol fluorescence study. Biochem. Biophys. Res. Commun. 404, 233–238. doi: 10.1016/j.bbrc.2010.11.099
Kolaczkowska, A., Kolaczkowski, M., Goffeau, A., Moye-Rowley, W. S. (2008). Compensatory activation of the multidrug transporters Pdr5p, Snq2p, and Yor1p by Pdr1p in Saccharomyces cerevisiae. FEBS Lett. 582, 977–983. doi: 10.1016/j.febslet.2008.02.045
Lamping, E., Baret, P. V., Holmes, A. R., Monk, B. C., Goffeau, A., Cannon, R. D. (2010). Fungal PDR transporters: Phylogeny, topology, motifs and function. Fungal Genet. Biol. 47, 127–142. doi: 10.1016/j.fgb.2009.10.007
Lax, C., Pérez-Arques, C., Navarro-Mendoza, M. I., Cánovas-Márquez, J. T., Tahiri, G., Pérez-Ruiz, J. A., et al. (2020). Genes, pathways, and mechanisms involved in the virulence of Mucorales. Genes 11, 317. doi: 10.3390/genes11030317
Lee, S. C., Li, A., Calo, S., Inoue, M., Tonthat, N. K., Bain, J. M., et al. (2015). Calcineurin orchestrates dimorphic transitions, antifungal drug responses and host-pathogen interactions of the pathogenic mucoralean fungus Mucor circinelloides. Mol. Microbiol. 97, 844–865. doi: 10.1111/mmi.13071
Leonardelli, F., Macedo, D., Dudiuk, C., Cabeza, M. S., Gamarra, S., Garcia-Effron, G. (2016). Aspergillus fumigatus Intrinsic Fluconazole Resistance Is Due to the Naturally Occurring T301I Substitution in Cyp51Ap. Antimicrob. Agents Chemother. 60, 5420–5426. doi: 10.1128/AAC.00905-16
Li, C. H., Cervantes, M., Springer, D. J., Boekhout, T., Ruiz-Vazquez, R. M., Torres-Martinez, S. R., et al. (2011). Sporangiospore size dimorphism is linked to virulence of Mucor circinelloides. PloS Pathog. 7, e1002086. doi: 10.1371/journal.ppat.1002086
Livak, K. J., Schmittgen, T. D. (2001). Analysis of relative gene expression data using real-time quantitative PCR and the 2(-Delta Delta C(T)) Method. Methods 25, 402–408. doi: 10.1006/meth.2001.1262
López-Fernández, L., Sanchis, M., Navarro-Rodríguez, P., Nicolás, F. E., Silva-Franco, F., Guarro, J., et al. (2018). Understanding Mucor circinelloides pathogenesis by comparative genomics and phenotypical studies. Virulence 9, 707–720. doi: 10.1080/21505594.2018.1435249
Lopez-Ribot, J. L., Wiederhold, N. P., Patterson, T. F. (2017). “Fungal Drug Resistance: Azoles,” in Antimicrobial Drug Resistance, eds. Mayers, D. L., Sobel, J. D., Ouellette, M., Kaye, K. S., Marchaim, D. (Cham: Springer International Publishing), 397–405. doi: 10.1007/978-3-319-46718-4_27
Mamnun, Y. M., Pandjaitan, R., Mahé, Y., Delahodde, A., Kuchler, K. (2002). Thw yeast zinc finger regulators Pdr1p and Pdr3p control pleiotropic drug resistance (PDR) as homo- and heterodimers in vivo. Mol. Microbiol. 45, 1429–1440. doi: 10.1046/j.1365-2958.2002.03262.x
Miura, D., Sugiyama, K., Ito, A., Ohba-Tanaka, A., Tanaka, M., Shintani, T., et al. (2018). The PDR-type ABC transporters AtrA and AtrG are involved in azole drug resistance in Aspergillus oryzae. Biosci. Biotechnol. Biochem. 82, 1840–1848. doi: 10.1080/09168451.2018.1497941
Monk, B. C., Sagatova, A. A., Hosseini, P., Ruma, Y. N., Wilson, R. K., Keniya, M. V. (2020). Fungal Lanosterol 14α-demethylase: A target for next-generation antifungal design. Biochim. Biophys. Acta (BBA) - Proteins Proteomics 1868, 140206. doi: 10.1016/j.bbapap.2019.02.008
Moriwaki-Takano, M., Iwakura, R., Hoshino, K. (2020). Dimorphic mechanism on cAMP mediated signal pathway in Mucor circinelloides. Appl. Biochem. Biotechnol. doi: 10.1007/s12010-020-03342-6
Morschhäuser, J. (2010). Regulation of multidrug resistance in pathogenic fungi. Fungal Genet. Biol. 47, 94–106. doi: 10.1016/j.fgb.2009.08.002
Nagy, Á., Vágvölgyi, CS., Balla, É., Ferenczy, L. (1994). Electrophoretic karyotype of Mucor circinelloides. Curr. Genet. 26, 45–48. doi: 10.1007/BF00326303
Nagy, G., Farkas, A., Csernetics, Á., Bencsik, O., Szekeres, A., Nyilasi, I., et al. (2014). Transcription of the three HMG-CoA reductase genes of Mucor circinelloides. BMC Microbiol. 14:93. doi: 10.1186/1471-2180-14-93
Nagy, G., Szebenyi, C., Csernetics, Á., Vaz, A. G., Tóth, E. J., Vágvölgyi, C., et al. (2017). Development of a plasmid free CRISPR-Cas9 system for the genetic modification of Mucor circinelloides. Sci. Rep. 7, 16800. doi: 10.1038/s41598-017-17118-2
Nagy, G., Vaz, A. G., Szebenyi, C., Takó, M., Tóth, E. J., Csernetics, Á., et al. (2019). CRISPR-Cas9-mediated disruption of the HMG-CoA reductase genes of Mucor circinelloides and subcellular localization of the encoded enzymes. Fungal Genet. Biol. 129, 30–39. doi: 10.1016/j.fgb.2019.04.008
Navarro, E., Niemann, N., Kock, D., Dadaeva, T., Gutiérrez, G., Engelsdorf, T., et al. (2020). The DASH-type cryptochrome from the fungus Mucor circinelloides is a canonical CPD-photolyase. Curr. Biol. 30, 4483–4490. doi: 10.1016/j.cub.2020.08.051
Nguyen, L-T., Schmidt, H. A., von Haeseler, A., Minh, B. Q. (2015). IQ-TREE: A fast and effective stochastic algorithm for estimating maximum likelihood phylogenies. Mol. Biol. Evol. 32, 268–274. doi: 10.1093/molbev/msu300
Odds, F. C., Brown, A. J. P., Gow, N. A. R. (2003). Antifungal agents: mechanisms of action. Trends Microbiol. 11, 272–279. doi: 10.1016/s0966-842x(03)00117-3
Pagni, M., Ioannidis, V., Cerutti, L., Zahn–Zabal, M., Jongeneel, C. V., Hau, J., et al. (2007). MyHits: improvements to an interactive resource for analyzing protein sequences. Nucleic Acids Res. 35, 433–437. doi: 10.1093/nar/gkm352
Parker, J. E., Warrilow, A. G. S., Price, C. L., Mullins, J. G. L., Kelly, D. E., Kelly, S. L. (2014). Resistance to antifungals that target CYP51. J. Chem. Biol. 7, 143–161. doi: 10.1007/s12154-014-0121-1
Patiño-Medina, J. A., Reyes-Mares, N. Y., Valle-Maldonado, M. I., Jácome-Galarza, I. E., Pérez-Arques, C., Nuñez-Anita, R. E., et al. (2019). Heterotrimeric G-alpha subunits Gpa11 and Gpa12 define a transduction pathway that control spore size and virulence in Mucor circinelloides. PloS One 14, e0226682. doi: 10.1371/journal.pone.0226682
Paul, S., Diekema, D., Moye-Rowley, W. S. (2013). Contributions of Aspergillus fumigatus ATP-Binding Cassette Transporter Proteins to Drug Resistance and Virulence. Eukaryot Cell 12, 1619–1628. doi: 10.1128/EC.00171-13
Pomorski, T., Lombardi, R., Riezman, H., Devaux, P. F., van Meer, G., Holthuis, J. C. M. (2003). Drs2p-related P-type ATPases Dnf1p and Dnf2p are required for phospholipid translocation across the yeast plasma membrane and serve a role in endocytosis. Mol. Biol. Cell 14, 1240–1254. doi: 10.1091/mbc.e02-08-0501
Posteraro, B., Sanguinetti, M., Sanglard, D., Sorda, M. L., Boccia, S., Romano, L., et al. (2003). Identification and characterization of a Cryptococcus neoformans ATP binding cassette (ABC) transporter-encoding gene, CnAFR1, involved in the resistance to fluconazole. Mol. Microbiol. 47, 357–371. doi: 10.1046/j.1365-2958.2003.03281.x
Prabhu, R. M., Patel, R. (2004). Mucormycosis and entomophthoramycosis: a review of the clinical manifestations, diagnosis and treatment. Clin. Microbiol. Infect. 10 Suppl 1, 31–47. doi: 10.1111/j.1470-9465.2004.00843.x
Prakash, H., Chakrabarti, A. (2019). Global Epidemiology of Mucormycosis. J. Fungi (Basel) 5, 26. doi: 10.3390/jof5010026
Prasad, R., Rawal, M. K. (2014). Efflux pump proteins in antifungal resistance. Front. Pharmacol. 5, 202. doi: 10.3389/fphar.2014.00202
Rammaert, B., Lanternier, F., Zahar, J.-R., Dannaoui, E., Bougnoux, M.-E., Lecuit, M., et al. (2012). Healthcare-associated mucormycosis. Clin. Infect. Dis. 54 Suppl 1, S44–S54. doi: 10.1093/cid/cir867
Rawal, M., Banerjee, A., Shah, A., Khan, M. F., Sen, S., Saxena, A. K., et al. (2016). Newly identified motifs in Candida albicans Cdr1 protein nucleotide binding domains are pleiotropic drug resistance subfamily-specific and functionally asymmetric. Sci. Rep. 6, 27132. doi: 10.1038/srep27132
Reiner, S., Micolod, D., Zellnig, G., Schneiter, R. (2006). A genomewide screen reveals a role of mitochondria in anaerobic uptake of sterols in yeast. Mol. Biol. Cell 17, 90–103. doi: 10.1091/mbc.e05-06-0515
Rex, J., Clinical (2008). Reference method for broth dilution antifungal susceptibility testing of filamentous fungi : Approved standard. undefined. Available at: http://www.paper/Reference-method-for-broth-dilution-antifungal-of-%3A-Rex-Clinical/cb65d2f9705fc1efdbd0ed2f9b3bf1cccd6836b5 (Accessed January 6, 2021).
Riley, T. T., Muzny, C. A., Swiatlo, E., Legendre, D. P. (2016). Breaking the Mold: A Review of Mucormycosis and Current Pharmacological Treatment Options. Ann. Pharmacother. 50, 747–757. doi: 10.1177/1060028016655425
Rocha, M. F. G., Bandeira, S. P., de Alencar, L. P., Melo, L. M., Sales, J. A., Paiva, M. de A.N., et al. (2017). Azole resistance in Candida albicans from animals: Highlights on efflux pump activity and gene overexpression. Mycoses 60, 462–468. doi: 10.1111/myc.12611
Roden, M. M., Zaoutis, T. E., Buchanan, W. L., Knudsen, T. A., Sarkisova, T. A., Schaufele, R. L., et al. (2005). Epidemiology and outcome of zygomycosis: a review of 929 reported cases. Clin. Infect. Dis. 41, 634–653. doi: 10.1086/432579
Roilides, E., Kontoyiannis, D. P., Walsh, T. J. (2012). Host defenses against zygomycetes. Clin. Infect. Dis. 54, S61–S66. doi: 10.1093/cid/cir869
Ruiz-Vázquez, R. M., Nicolás, F. E., Torres-Martínez, S., Garre, V. (2015). Distinct RNAi Pathways in the Regulation of Physiology and Development in the Fungus Mucor circinelloides. Adv. Genet. 91, 55–102. doi: 10.1016/bs.adgen.2015.07.002
Sambrook, J., Fritsch, E. F., Maniatis, T. (1989). Molecular cloning: a laboratory manual (Cold Spring Harbor, N.Y: Cold Spring Harbor Laboratory).
Sanglard, D., Coste, A. T. (2015). Activity of Isavuconazole and Other Azoles against Candida Clinical Isolates and Yeast Model Systems with Known Azole Resistance Mechanisms. Antimicrob. Agents Chemother. 60, 229–238. doi: 10.1128/AAC.02157-15
Sanglard, D., Ischer, F., Bille, J. (2001). Role of ATP-Binding-Cassette Transporter Genes in High-Frequency Acquisition of Resistance to Azole Antifungals in Candida glabrata. Antimicrob. Agents Chemother. 45, 1174–1183. doi: 10.1128/AAC.45.4.1174-1183.2001
Sanguinetti, M., Posteraro, B., La Sorda, M., Torelli, R., Fiori, B., Santangelo, R., et al. (2006). Role of AFR1, an ABC Transporter-Encoding Gene, in the In Vivo Response to Fluconazole and Virulence of Cryptococcus neoformans. Infect. Immun. 74, 1352–1359. doi: 10.1128/IAI.74.2.1352-1359.2006
Seeger, M. A., van Veen, H. W. (2009). Molecular basis of multidrug transport by ABC transporters. Biochim. Biophys. Acta 1794, 725–737. doi: 10.1016/j.bbapap.2008.12.004
Sharma, C., Chowdhary, A. (2017). Molecular bases of antifungal resistance in filamentous fungi. Int. J. Antimicrob. Agents 50, 607–616. doi: 10.1016/j.ijantimicag.2017.06.018
Sionov, E., Chang, Y. C., Garraffo, H. M., Dolan, M. A., Ghannoum, M. A., Kwon-Chung, K. J. (2012). Identification of a Cryptococcus neoformans cytochrome P450 lanosterol 14α-demethylase (Erg11) residue critical for differential susceptibility between fluconazole/voriconazole and itraconazole/posaconazole. Antimicrob. Agents Chemother. 56, 1162–1169. doi: 10.1128/AAC.05502-11
Skiada, A., Lass-Floerl, C., Klimko, N., Ibrahim, A., Roilides, E., Petrikkos, G. (2018). Challenges in the diagnosis and treatment of mucormycosis. Med. Mycol. 56, S93–S101. doi: 10.1093/mmy/myx101
Smriti, null., Krishnamurthy, S., Dixit, B. L., Gupta, C. M., Milewski, S., Prasad, R. (2002). ABC transporters Cdr1p, Cdr2p and Cdr3p of a human pathogen Candida albicans are general phospholipid translocators. Yeast 19, 303–318. doi: 10.1002/yea.818
Steinegger, M., Soding, J. (2017). MMseqs2 enables sensitive protein sequence searching for the analysis of massive data sets. Nat. Biotechnol. 35, 1026–1028. doi: 10.1038/nbt.3988
Sun, H.-Y., Singh, N. (2011). Mucormycosis: its contemporary face and management strategies. Lancet Infect. Dis. 11, 301–311. doi: 10.1016/S1473-3099(10)70316-9
Tsao, S., Rahkhoodaee, F., Raymond, M. (2009). Relative contributions of the Candida albicans ABC transporters Cdr1p and Cdr2p to clinical azole resistance. Antimicrob. Agents Chemother. 53, 1344–1352. doi: 10.1128/AAC.00926-08
van Heeswijck, R., Roncero, M. I. G. (1984). High frequency transformation of Mucor with recombinant plasmid DNA. Carlsberg Res. Commun. 49, 691. doi: 10.1007/BF02907500
Vellanki, S., Navarro-Mendoza, M. I., Garcia, A. E., Murcia, L., Perez-Arques, C., Garre, V., et al. (2018). Mucor circinelloides: Growth, Maintenance and Genetic Manipulation. Curr. Protoc. Microbiol. 49, e53. doi: 10.1002/cpmc.53
Vellanki, S., Billmyre, R. B., Lorenzen, A., Campbell, M., Turner, B., Huh, E. Y., et al. (2020). A novel resistance pathway for calcineurin inhibitors in the human-pathogenic Mucorales Mucor circinelloides. mBio 11, e02949–e02919. doi: 10.1128/mBio.02949-19
Walther, G., Wagner, L., Kurzai, O. (2019). Updates on the Taxonomy of Mucorales with an Emphasis on Clinically Important Taxa. J. Fungi (Basel) 5, 106. doi: 10.3390/jof5040106
White, T. C., Marr, K. A., Bowden, R. A. (1998). Clinical, cellular, and molecular factors that contribute to antifungal drug resistance. Clin. Microbiol. Rev. 11, 382–402. doi: 10.1128/CMR.11.2.382
Wilcox, L. J., Balderes, D. A., Wharton, B., Tinkelenberg, A. H., Rao, G., Sturley, S. L. (2002). Transcriptional profiling identifies two members of the ATP-binding cassette transporter superfamily required for sterol uptake in yeast. J. Biol. Chem. 277, 32466–32472. doi: 10.1074/jbc.M204707200
Wilkens, S. (2015). Structure and mechanism of ABC transporters. F1000Prime Rep. 7, 14. doi: 10.12703/P7-14
Yu, Z., Fischer, R. (2019). Light sensing and responses in fungi. Nat. Rev. Microbiol. 17, 25–36. doi: 10.1038/s41579-018-0109-x
Zavrel, M., Hoot, S. J., White, T. C. (2013). Comparison of sterol import under aerobic and anaerobic conditions in three fungal species, Candida albicans, Candida glabrata, and Saccharomyces cerevisiae. Eukaryot Cell 12, 725–738. doi: 10.1128/EC.00345-12
Zhang, Y., Navarro, E., Cánovas-Márquez, J. T., Almagro, L., Chen, H., Chen, Y. Q., et al. (2016). A new regulatory mechanism controlling carotenogenesis in the fungus Mucor circinelloides as a target to generate β-carotene over-producing strains by genetic engineering. Microb. Cell Fact 15, 99. doi: 10.1186/s12934-016-0493-8
Keywords: Mucor, pleiotropic drug resistance, CRISPR-Cas9, azole, ABC transporter
Citation: Nagy G, Kiss S, Varghese R, Bauer K, Szebenyi C, Kocsubé S, Homa M, Bodai L, Zsindely N, Nagy G, Vágvölgyi C and Papp T (2021) Characterization of Three Pleiotropic Drug Resistance Transporter Genes and Their Participation in the Azole Resistance of Mucor circinelloides. Front. Cell. Infect. Microbiol. 11:660347. doi: 10.3389/fcimb.2021.660347
Received: 29 January 2021; Accepted: 19 March 2021;
Published: 14 April 2021.
Edited by:
Dominique Sanglard, University of Lausanne, SwitzerlandReviewed by:
Miguel Cacho Teixeira, University of Lisbon, PortugalRichard Cannon, University of Otago, New Zealand
Copyright © 2021 Nagy, Kiss, Varghese, Bauer, Szebenyi, Kocsubé, Homa, Bodai, Zsindely, Nagy, Vágvölgyi and Papp. This is an open-access article distributed under the terms of the Creative Commons Attribution License (CC BY). The use, distribution or reproduction in other forums is permitted, provided the original author(s) and the copyright owner(s) are credited and that the original publication in this journal is cited, in accordance with accepted academic practice. No use, distribution or reproduction is permitted which does not comply with these terms.
*Correspondence: Gábor Nagy, bmFneWdhYjg2QGdtYWlsLmNvbQ==