- 1Arundeep Akshay Urja Pvt. Ltd. Gorakhpur, Uttar Pradesh, India
- 2School of Applied Sciences and Technology (SAST), Gujarat Technological University, Ahmedabad, Gujarat, India
- 3Department of Zoology, Gargi College, University of Delhi, Delhi, India
- 4Faculty of Life Sciences, Mandsaur University, Mandsaur, Madhya Pradesh, India
- 5Centre of Research for Development (CR4D), Parul University, Vadodara, Gujarat, India
The use of nanomaterials in several fields of science has undergone a revolution in the last few decades. It has been reported by the National Institutes of Health (NIH) that 65% and 80% of infections are accountable for at least 65% of human bacterial infections. One of their important applications in healthcare is the use of nanoparticles (NPs) to eradicate free-floating bacteria and those that form biofilms. A nanocomposite (NC) is a multiphase stable fabric with one or three dimensions that are much smaller than 100 nm, or systems with nanoscale repeat distances between the unique phases that make up the material. Using NC materials to get rid of germs is a more sophisticated and effective technique to destroy bacterial biofilms. These biofilms are refractory to standard antibiotics, mainly to chronic infections and non-healing wounds. Materials like graphene and chitosan can be utilized to make several forms of NCs, in addition to different metal oxides. The ability of NCs to address the issue of bacterial resistance is its main advantage over antibiotics. This review highlights the synthesis, characterization, and mechanism through which NCs disrupt Gram-positive and Gram-negative bacterial biofilms, and their relative benefits and drawbacks. There is an urgent need to develop materials like NCs with a larger spectrum of action due to the rising prevalence of human bacterial diseases that are multidrug-resistant and form biofilms.
1 Introduction
The extensive association of the encapsulated immobile microorganisms that cling to the solid surface with the aid of a self-generated hydrated extracellular matrix is called biofilm. These biofilms are often found on damp surfaces forming a slippery covering. The environment is not always favourable outside the bacterial cell, such as in the biological system. There is always a chance that the bacteria would encounter host immune cells (phagocytes) and their product in the form of antibodies. In the non-biological system, bacteria get exposed to hostile environments such as heat, cold, and UV light. This matrix is composed of extracellular polymeric substances such as carbohydrates and exopolysaccharides (Prosser et al., 1987; Lawrence et al., 1991), which accompanies the adhering of the giant bacterial framework together, thereby providing strength and protection from these extreme biological and non-biological factors. The microscopic examination of the biofilm is tedious because dehydration causes the matrix to collapse. This biofilm comprises 82%–85% of the extracellular matrix and 15%–20% of the microbial consortium (Cowan 2011). Initially, Antony van Leeuwenhoek investigated the biofilm from his dental plaque and observed that sessile bacteria contribute to biofilm formation. Later, it was found that the aggregation pattern of the bacteria depends on the different bacterial species. One bacterial species can form a network with other bacterial species (Costerton et al., 1999). Fundamentally, through Brownian motion, the planktonic bacteria encounter the exposed surface, where they are subjected to the abhorrent electrostatic force. This immotile mass of cells is nutritionally deficient; hence, some of these sessile cells skip the matrix and become motile to access the nutrients readily and reproduce expeditiously. The cells in the motile phase are also called plankton.
It is now understood that approximately 40%–80% of bacterial cells on earth can form biofilms (Flemming and Wuertz, 2019). Bacterial biofilms are embedded communities of bacteria apprehended together by a self-produced polymer matrix mainly consisting of polysaccharide proteins and extracellular nucleic acid. In the human body, biofilm is found over the skin, mucosa, and teeth. The best-known example of biofilm is plaque. It is shown that biofilms are found in implantable medical devices. Devices that partly penetrate the skin, such as a central venous catheter, can become coated with bacterial biofilms. It is found on fully implanted devices such as artificial hip or knee joints. The biofilm of Escherichia coli is frequently found on urinary catheters. The studies also revealed that biofilm forms inside the patients having chronic lung infection, cystic fibrosis, and lung damage (Kostakioti et al., 2013). There are various biofilm-creating pathogens that are related to chronic upper respiratory infections (Pseudomonas aeruginosa) (Koch and Hoiby, 1993; Govan and Deretic, 1996), urinary tract infections (UTIs) (uropathogenic E. coli [UPEC], Klebsiella pneumoniae), periodontitis (mixed biofilms of Streptococcus mutans and other bacteria), and catheter-induced and other device-associated infections (E. coli, Enterococcus faecalis, and others) (Venditti et al., 1993; Ferrieres et al., 2007; Jacobsen et al., 2008; Kuramitsu and Wang, 2011). The strains of S. epidermidis and Streptococcus aureus adhere to the ocular infections (Hou et al., 2012).
2 Biofilm-related infections
The skin is the largest organ in the human body and is populated by distinct microbes like viruses, bacteria, and fungi (Byrd et al., 2018). The distribution of these distinct microbes depends on not only the skin microhabitat and texture (scorched, damp, and oily) but also the microenvironment of the skin (acidic or nutritionally deficient environment).
As illustrated in Figure 1, biofilms are associated with various human diseases. The primary concern is the face, chest, and abdomen. Dental plaque, otitis media, and chronic rhinosinusitis and chronic tonsillitis are the predominant diseases in the face and neck region of the human body. Dental plaques are seen after the aging of the disease as biofilm was established well enough between teeth and gums. Dental plaque is a biofilm that is both physically and functionally structured. The plaque has a complex microbial makeup that, in good health, builds in an organized manner and is largely stable over time (microbial homeostasis) (Marsh, 2006). Next to this, chronic tonsillitis in the mouth lies near the junction of the mouth and neck. This disease is mainly found in growing children aged between 5 and 15 years. It is primarily due to Streptococcus bacteria; a repository of infection in wet and warm folds of the tonsils is a major source for its reoccurrence (Bakar et al., 2018). Otitis media is a middle ear infection that occurs as a result of cold, sore throat, or respiratory infection (Niedzielski et al., 2021), while chronic rhinosinusitis is associated with nasal congestion, mucus discharge from the nose, facial pain, and pressure along with a decreased sense of smell (Karunasagar et al., 2018) and is common in both adults and children. For this, the first line of treatment is nasal sprays. Moving towards the chest, cystic fibrosis and endocarditis are the dominant bacterial diseases. Endocarditis is an infection of the heart’s inner lining (endocardium), usually involving the heart valves. It occurs when germs from elsewhere in the body travel through the blood and attach to the damaged areas of the heart. People with damaged or artificial heart valves or other heart conditions are mostly at risk. Streptococci and Staphylococci are the main pathogens for such infections (Lerche et al., 2021; Martin et al., 2021). In cystic fibrosis, a lung infection caused by P. aeruginosa, great tolerance to antibiotics and resistance to phagocytosis were reported (Høiby et al., 2010). These infections often last for decades. Anti-inflammatory medications along with mucus-thinning drugs can enhance the power of antibiotics in such cases. Finally, in the abdomen area, we have a major concern with biliary tract infection, kidney stones, and vaginosis. In the case of biliary tract infection and kidney stones, insoluble solids of large dimensions are formed that obstruct the passage and disrupt normal functioning. The pathogenesis of brown pigment gallstones, the blockage of biliary stents, and biliary parasitic infestations are related to the formation of bacterial biofilms. E. coli and other enteric organisms attach themselves to the surface of an ulcerated biliary mucosa or implanted biomaterials to form a bacterial biofilm. Crystals of calcium bilirubin and other biliary sediments accumulate around the bacterial microcolonies and glycoproteins, resulting in thick and often calcified biofilms obstructing the normal bile flow (Sung et al., 1992). Urinary tract stones are induced by infection with urease-positive pathogens and affect dialysis systems, including peritoneal and central venous catheters (Soto, 2014). In the lower abdomen, vaginal biofilms have the maximum number of cases due to the presence of biofilm-protecting bacteria from antibiotic treatment and serve as a reservoir for the regrowth of pathogens. Vaginal biofilms play a key role not only in bacterial vaginosis pathogenesis but also in its treatment failure and recurrence (Machado et al., 2015). Lactobacillus species is one of the major causes of this disease. In osteomyelitis, non-organ-related biofilm formation is clearly evident in bones. In this condition, inflammation of bones in the legs, arm, or spine is observed. Infection in this case can reach bones by traveling through the bloodstream or spreading from nearby tissue or due to injury. Smokers and diabetic individuals are more prone to it. Staphylococcus is a major cause of such infection. Artificial joints like hip replacement, metal implants such as screws, and bedsores are other major factors contributing to osteomyelitis.
3 Chronic wounds and biofilms
Wounds that last for more than 45 days lead to the formation of chronic wounds, which is an impending clinical problem. These wounds are unable to heal because of disordered physiological processes and wound environments drastically affected by many microbes. These polymicrobial associations involve the arduous interaction between the organisms leading to the pathogenesis of bacterial biofilm-associated infections (Verbrugghe et al., 2021). Several types of communications occur between the microbes associated with biofilms. This association can be cooperative, competitive, or antagonistic (Nadell et al., 2016). There are certain chemicals released by both Gram-positive and Gram-negative bacteria, making it possible for the bacteria to communicate with each other, such as Gram-negative bacteria releasing homoserine lactones or quinolones. Similarly Gram-positive bacteria release short peptides (Sturme et al., 2002). Bacterial biofilms not only offer resistance against antibiotics but also offer resistance against ultraviolet light and heavy metals. Micronutrients and wound exudates, which contribute to the optimal condition of the wound, are breeding hubs for multiple microbes forming biofilms. Approximately 60% of chronic wounds contain biofilms (Omar et al., 2017). As per reports, infection persistence and polymicrobial association are the leading factors for frequent infection. Generally, chronic wounds are primarily classified into three classes, i.e., pressure ulcers, venous leg ulcers (VLUs), and diabetic foot ulcers (DFUs), whereas the secondary class of chronic wound consists of cancer ulcers, inflammatory ulcers, and ischemic ulcers. Microbial profiling of this wound reveals the prevalence of Serratia, Pseudomonas, Staphylococcus, and Enterobacter.
3.1 Pressure ulcer
Decubitus ulcers or bedsores notoriously known as pressure ulcers have not been studied immensely. Elderly people who shifted to long-term care facilities (LTCFs) are more prone to this type of ulcer, which is commonly seen in medical conditions. These ulcers are formed in bed-rest patients, by the pressure generated on the skin because of friction and the body weight for a longer period. Such initial pressure for approximately 1–2 h leads to pressure-induced cell deformation causing cell death. Pressure ulcers if untreated for a long time can also infect soft tissues, causing osteomyelitis and may even lead to death (in 39.7% of patients) (Rennert et al., 2009). P. aeruginosa, S. aureus, coagulase-negative Staphylococcus (CoNS), and E. faecalis are prominent microbes associated with pressure ulcers (Suleman and Percival, 2015). According to the National Pressure Ulcer Advisory Panel (NPUAP) and the European Pressure Ulcer Advisory Panel (EPUAP), pressure ulcers are categorized into four stages of wound infection, i.e., stage I (non-blanchable erythema), stage II (partial thickness), stage III (full thickness skin loss), and stage IV (full thickness tissue loss) (Panel, 2014). Medical sectors are under huge economic strain because of the increased cost of treatment of pressure ulcers, i.e., approximately £1,214 (category 1) to £14,108 (category IV) (Dealey et al., 2012). The peptide nucleic acid-based fluorescence in situ hybridization (PNA-FISH) studies by Kirketerp-Møller and colleagues on pressure ulcers revealed the existence of biofilms in it. Polymicrobial biofilm in association with S. aureus and C. albicans in this ulcer has been responsible for resistance to vancomycin (Harriott and Noverr, 2009).
3.2 Venous leg ulcer
Fazli and his colleagues used PNA-FISH to figure out that the VLU had biofilm (Fazli et al., 2011). P. aeruginosa is the persistent and prime bacterium responsible for chronic VLU. A leg ulcer is a long-lasting sore that usually develops on the inside of the leg, between the knee and the ankle. Its symptoms include pain, itching, swelling, and discoloration. The hardened skin around the ulcer and the sore may produce a discharge with a foul smell. There are two major causes for its development. It develops after a minor injury due to persistent high pressure in the veins of the legs. When there is high pressure in the veins of the lower leg, the veins become scarred and blocked, resulting in blood pooling in the legs. This is called venous insufficiency. The increased pressure and fluid buildup prevent nutrients and oxygen from reaching tissues. The lack of nutrients causes damage to the tissue and wound formation. Improvement of blood flow in the legs is a temporary method of treatment, and this internal environment facilitates the pathogens to develop a sustained colony as biofilm.
3.3 Diabetic foot ulcer
Approximately 15%–25% of the patients suffering from diabetes mellitus are prone to developing DFU (Armstrong et al., 2017). Diabetic foot complications are the most common cause of non-traumatic lower extremity amputations. A round, red crater in the skin bordered by thickened callused skin is the identifying feature of this disease. Poor control of blood sugar, foot deformities, bad foot care, shoes that do not fit right, peripheral neuropathy, poor circulation, and dry skin are the root causes. Slow recovery is one of the biggest problems with getting rid of this disease. This makes it easier for bacteria to take over the affected area, which causes biofilm to form. Routine practice for treatment includes optimizing diabetes control to reduce neuropathic and vascular complications. Implementing MADADORE (M = metabolic/medication; A = assessment; D = debridement; A = antibiotics; D = dressing; O = offloading; R = referral; E = education) is now being talked about in the medical community as a way to control it (Lazzarini et al., 2019).
4 Therapeutic strategies against bacterial biofilms
Several opportunistic pathogens evoke skin infection by the formation of biofilm, which is a crucial virulence factor. Traditional therapies are inefficient for treating the obstinate factor of biofilm and antibiotic tolerance. Biofilm forms the protective covering around the bacteria that prevents the intake of antibacterial therapies. Nanoparticle-based curative therapies proved to be pivotal in counteracting these robust biofilms, thereby making the entry of the drugs feasible to the site of infection. Different forms of nanoparticles (liposomes, lipid nanoparticles, and metal oxide nanoparticles) have direct applications in treating skin infections (Lin et al., 2021).
5 Nanocomposites and its varieties
The continuous demand of humans for more sophisticated technology for self-betterment brings forth a new class of materials where two different materials combine in such a way as to become a far different or an entirely new material. Such materials are called composite materials. In the present scenario, exploiting the exciting properties of nanomaterials at a very large scale is practically considered to design nanocomposites (Cho et al., 2014; Kandiah et al., 2015). The term “nanocomposites” was first coined by Komarneni, 1992; Harriott and Noverr, 2009). Nanocomposites are defined as a class of materials that contain at least one phase with constituents in the nanometer (1 nm = 10–9 m) domain. Additionally, as dimensions reach the nanometer level, interactions at phase interfaces become largely improved, and this is important to enhance the material’s properties. In this context, the surface area/volume ratio of reinforcement materials employed in the preparation of nanocomposites is crucial to the understanding of their structure–property relationships. The varieties of nanocomposites include polymer-based and non-polymer-based nanocomposites (Figure 2).
5.1 Polymer-based nanocomposites
Composites made up of polymer matrix and filler (clay, nanotubes, nanoparticles, etc.) with at least one dimension less than 100 nm are called polymer nanocomposites. Scratch-resistant transparent amorphous thermoplastic coating is the latest example of the modern application of polymer-based nanocomposite materials due to the small size of the fillers. Unlike traditional micron-filled composites, these novel fillers often alter the properties of the entire polymer matrix and, at the same time, impart new functionality because of their chemical composition and nanoscale size.
5.1.1 Polymer/ceramic nanocomposites
When the single ceramic layer of nano range is homogeneously dispersed in a polymer matrix, it is called polymer/ceramic nanocomposites. Due to dipole–dipole interaction, ceramic layers tend to align parallel. An extensively used technique for polymer/ceramic nanocomposite is the sol-gel process. However, intercalation of a single polymer chain is the most suitable method to obtain ordered layered structures that can be acquired by polymerizing a monomer with a ceramic. A drastic change has been obtained in the properties of such composites due to a large number of polymer–ceramic interfaces at the nano-level and ordered polymer chains in the surroundings.
5.1.2 Polymer/layered silicate nanocomposites
Polymer/layered silicate (PLS) nanocomposites are broadly classified as intercalated nanocomposites, flocculated nanocomposites, and exfoliated nanocomposites. Intercalated nanocomposites bear a regular fashion of polymer chain insertion into silicate layers with a distance of a few nanometers, regardless of the polymer-to-clay ratio. Here, the monomer is in a solution where silicate layers are swollen within. The polymerization is initiated between layers, expanding and dispersing the clays into the polymer (Kumar et al., 2009). An advantage of this method is the tethering effect, which allows nano-clays with a chemically active surface to link polymer chains during polymerization (Hussain et al., 2006). Flocculated nanocomposites consist of intercalated and stacked silicate layers flocculated (the process of clay aggregate into clot-like masses or precipitate into small lumps) to some extent due to the hydroxylated edge–edge interaction of the silicate layers. Exfoliated nanocomposites are composed of single silicate layers separated in the polymer matrix by average distances governed by the type of clay loading. To fabricate such nanocomposites, the process involves the use of solvent, in which polymer or prepolymer is soluble, to promote the exfoliation of layered silicate into single layers, and it is possible to break the weak forces that stack the layers together. Then, the polymer is easily absorbed onto the laminated sheets. After solvent evaporation, a sandwich of polymer is obtained by sheet reassembly (Alexandre and Dubois, 2000, Hussain et al., 2006). However, this process reveals commercial handling problems due to the solvent’s high costs and phase separation of the synthesized product from the solvent (Gao, 2004; 2013). Processing equipment and processing conditions are important parameters for clay dispersion. Along with this, polymer molecular weight also regulates clay dispersion. Polymers with a high molecular weight enhance clay dispersion.
5.1.3 Polymer/polymer nanocomposites
It is assumed that appropriate scaling (near the nano range) of polymer can practically be achieved by employing block copolymers. The basic requirements for these nanocomposites are control over dispersion, morphology, and adhesion between immiscible phases. Strong mechanical stirring provides a good dispersion of polymer A in an immiscible polymer B. Block copolymers can be synthesized by various chemical methods opted as per requirement (Parhi et al., 2022; Sharwani et al., 2022).
5.1.4 Inorganic/organic polymer nanocomposites
Metal clusters of nano range dispersed in a polymer matrix formed Metal/polymer nanocomposites. Owing different properties by nanoparticles are beneficial to incorporate them into a matrix material, e.g., a polymer solution or melt, or an elastomer. Zerovalent metal nanoparticles can readily be dispersed in an inert polymer thin film matrix. The ‘bottom-up’ approach to the synthesis of metal nanoparticles has largely relied on the colloidal route involving the reduction of metal ions by appropriate chemical reagents and stabilization by suitable surfactants. For thin film generation of composite metal/polymer, plasma-enhanced vapor deposition of polymer and simultaneous sputtering of any metal is well employed at low-temperature processing (Haghparasti and Shahri, 2018).
5.1.5 Inorganic/organic hybrid nanocomposites
This group of nanocomposites bears both organic and inorganic components as constituents. Both homogeneous and heterogeneous systems are observed containing at least one component in the nano range. Again, the nature of the interface (based on the type of bonds, i.e., weak or strong) has been used to divide these materials into two distinct classes. In class I, both constituents are embedded and only weak bonds (hydrogen, van der Waals, or ionic bonds) give cohesion to the whole structure, while in class II materials, the constituents are linked together through strong chemical bonds (covalent or iono-covalent bonds). Indeed, the mild synthetic conditions offered by the sol-gel process (metallo-organic precursors, organic solvents, low processing temperatures, and processing versatility of the colloidal state) allow the mixing of inorganic and organic components at the nanometric scale. Due to the anti-corrosive nature of certain metal nanocomposites, there has been a profound application as an antibacterial coating in biomedical devices (Owhal et al., 2021). The stable mechanical and biological properties make hybrid nanocomposites beneficial in orthopedic implants. GC-induced implant failure and osseointegration failure can be cured with the aid of the organic–inorganic hybrid Lbl coating (Liu et al., 2013). This hybrid nanocomposite has a potential bactericidal role on MRSA and E. coli.
5.2 Non-polymer-based nanocomposites
Ceramics have good wear resistance and high thermal and chemical stability. However, they are brittle. To overcome this limitation, ceramic–matrix nanocomposites (metal/ceramic and ceramic/ceramic) have been receiving attention along with metal/metal nanocomposites.
5.2.1 Metal/metal nanocomposites
Alloys were discovered and developed during the era of human civilization. New alloys continue to be sought after to meet future demands. Nanocrystalline alloys can be made using numerous techniques. However, numerous properties, including porosity, impurity, texture, and grain size distribution, are very essential and determined by the processing procedure. Kappa-carrageenan (CRG) synthesized by green synthesis has proved to be a potent antimicrobial agent for removing bacterial biofilms. CRG has direct application in the biomedical field and food packaging industry (Goel et al., 2019). In the case of alloy nanoparticles, the combination of gold and palladium is popular since these metals are miscible at any ratio. New qualities can be produced by simply combining two different metal nanoparticles together or using the sol-gel method. The main restriction, however, is an accumulation of components that reduces surface area. A few strategies, such as gas fluidization and the addition of an external force like vibration, sound waves, and magnetic excitation, are used.
5.2.2 Metal/ceramic nanocomposites
Titanium-based nanocomposites are resistant to corrosion and have good biocompatibility. Dental implants have been using titanium with great success. Dental crowning is the prevalent implant, thereby protecting tooth from later damages. Ceramics, on the other hand, have improved strength. Calcium phosphate released near an implant improves bone apposition (Toussi et al., 2018). Metal/ceramic nanocomposite materials are used in high-temperature applications involving strength, stiffness, crack resistance, and other properties associated with structural materials in the aerospace industry. Carefully, agglomerate-free particles of the nano range will be synthesized via the sol-gel method. Partial hydrogen reduction of ceramic and metallic oxide mixture will be carried out at a specific temperature, yielding pre-sintering powder. Furthermore, optimization of processing parameters (temperature and pressure), which are mostly physical, is applied to obtain the desired product.
5.2.3 Ceramic/ceramic nanocomposites
Biomaterials like artificial joint implants are one of the major thrust areas for ceramic nanocomposites. The ceramic-ceramic implants made of zirconia-toughened alumina nanocomposites have a potential lifespan of more than 30 years. Chemical methods include the sol-gel process, colloidal and precipitation approaches, and template synthesis. A large variety of parameters affecting the sol-gel process, such as type of solvent, timing, pH, precursor, and water/metal ratio, allow the versatile control of structural and chemical properties of the final oxide materials (Ennas et al., 1998).
5.3 Green nanocomposites
The use of chemicals have attracted attention from the public since they affect not just researchers and businesses but also the environment. To reduce this global concern, scientists have developed new ways to minimize it. In this context, natural products have played a vital role in fulfilling it. Mostly, plant parts and microbes are utilized for this purpose. Extracts of these have multiple roles, such as media for reactions, reducing agents, and capping agents. They are cheap as compared to chemicals, are easily available, and are easy to use—factors that contribute to the versatility of this technique, leading to the term “green.” Among various green nanoformulations, green nanocomposites have gained attention. To maintain a sustainable environment, green nanocomposites have played an important role through various applications in automotive, construction (Presting and Konig, 2003; Sharwani et al., 2022), packaging (Zare et al., 2019), and medical science (Bibi et al., 2021). They are based on eco-friendly materials. The biodegradable polymers generated provide solutions to the issues caused by synthetic and plastic waste. Green composites are composed of plant fibers and natural resins. We need to use synthetic polymers and/or additives as complements to green polymers. Green nanocomposites (either nanoscale natural macromolecules or biopolymers containing nanoparticles) are a class of scaffolds with acceptable biomedical properties (drug delivery and cardiac, nerve, bone, and cartilage as well as skin tissue engineering), enabling one to achieve the required level of skin regeneration and wound healing (Pathania et al., 2022).
6 Antimicrobial activity of nanocomposites against bacterial biofilms
Biofilm is a major problem in the field of medicine; it consists of a complex process that involves the attachment of bacteria to the surface, proliferation, extracellular polymeric substance (EPS) production, small colonies formation, and ultimately mature biofilm diffusion (Riau et al., 2019; Elshaarawy et al., 2020). Biofilm is a complex biological structure, consisting of a wide range of bacteria, which involves the association of an extracellular polymer matrix (EPM) with microorganism cultures. The bacteria respond to environmental cues that induce the synthesis of EPS. As a result, if the formation of EPS can be inhibited or prevented, then biofilm formation will also be restricted (Chávez de Paz et al., 2011; Riau et al., 2019). Bacterial biofilm formation is a major area of concern in the medical field as it can resist harsh conditions (Dokoshi et al., 2020) and is ineffective to traditional antibiotic therapies as compared to planktonic bacteria (Wu et al., 2015). Moreover, the majority of infections that are hard to treat are caused by biofilms, including urinary tract infections, catheter infections, and dental plaque formation (Lewis, 2001). Therefore, there is an urgent need to develop novel methods against antibacterial-resistant biofilm (Xu et al., 2021). However, nanoparticles are also not much effective against antibiotic resistance mechanisms (Wang et al., 2017). To overcome the resistance of biofilm against conventional antibiotic therapy, antimicrobial-loaded nanoparticles alone or combined with other substances could enhance the bactericidal activity of nanomaterials. This includes killing the pathogens effectively without harming other cells or causing any adverse effects on living cells (Thambirajoo et al., 2021). The use of nanotechnology in medicine is known as targeting. The goal of this strategy is to deliver medicines, diagnostics, including imaging agents, or both using nanoparticles (NPs) to target specific diseases and disorders. Targeting NP treatments are getting a lot of attention for identifying, treating, and managing neurodegenerative disorders. They have distinctive characteristics for targeting and for bridging the blood–brain barrier (BBB), which is well recognized. The characteristics of nanomaterials are different from conventional antimicrobial agents, which gives a new path for preventing and eradicating biofilm formation (Yuan et al., 2020). These nanocomposites are observed to have improved antimicrobial activity against resistant bacteria. Phytochemicals, which are antibiotics derived from plants, offer a viable alternative to conventional antibiotics. Their ineffectiveness in treating biofilm infections, however, is due to their limited solubility in aqueous media. Cross-linked polymer nanocomposite “sponges” were made with phytochemicals and incorporated for the treatment of bacterial biofilms. In addition, it was also demonstrated that nanocomposites with less hydrophobic phytochemicals have more potent antibiofilm efficacy (Li et al., 2019).
6.1 Nanocomposites targeting Gram-positive bacterial biofilm
There are various Gram-positive bacteria such as S. aureus and S. epidermidis that impede the healing of wounds (Roy et al., 2020). Studies reported that nanocomposites are more effective than pure NPs; for example, CuO embedded with Zn nanocomposite showed increased antibacterial activity compared with pure CuO or ZnO (Malka et al., 2013). Moreover, TiO2-doped CuO and Li-doped MgO also have a significant antibacterial effect than pure MgO and TiO2 (Rao et al., 2013). Similarly, CeO2-CdO nanocomposites also had antimicrobial activity against Gram-negative bacteria (Magdalane et al., 2017). Furthermore, nanocomposite Mn and Fe ion-doped ZnO NPs have been observed to have higher antibacterial activity against a wide range of bacteria such as S. aureus, Bacillus subtilis, Proteus mirabilis, and E. coli compared with ZnO (Unal et al., 2018; Bahari et al., 2019). It was also seen that nanocomposites with metal oxides significantly improve the antimicrobial action through some external factor induction (Hsueh, 2010). Apart from this, a combination of three metal oxides like TiO2-ZnO-MgO and CuZnFe oxide nanomaterials have higher antibacterial activity in inhibiting biofilm synthesis (Alzahrani et al., 2018). CuZnFe oxide NPs were found to be more effective against E. faecalis than ZnO NPs but less effective than CuO (Alzahrani et al., 2018). Thus, the nanocomposite with the combination of three NPs affected the biofilm formation to a higher degree than alone (Alzahrani et al., 2018). The mechanism behind the metal oxide NPs is that oxide causes the slow release of metal ions, which disrupt cellular processes by penetrating the cell membrane (Hayden et al., 2012). Metallic NPs cause severe oxidative stress through the generation of reactive oxygen species (ROS). The ROS level is in turn correlated with the antibacterial activity due to its damaging effect to the bacterial cell membrane, disrupting the nucleic acids, inhibiting the enzyme, and thus obstructing the biofilm formation (Ali et al., 2019; Song et al., 2019). Some studies report the effects of different nanocomposites such as biodegradable crosslinked polymer-stabilized oil-in-water, effective against bacterial biofilm (Landis et al., 2018). Nanocomposites are observed to be effective against the antibiotic-resistant bacterial biofilm of Gram-positive bacteria. A study has reported that a nanocomposite with immobilized ciprofloxacin results in the reduction of biofilm formation in S. aureus of up to 85%. This nanocomposite has higher antibacterial activity against Gram-positive bacteria compared with Gram-negative bacteria (Rumyantceva et al., 2021). This showed that nanocomposites have better antibacterial properties and are effective in treating Gram-positive bacteria such as S. aureus (Xu et al., 2021). Furthermore, it was observed that the dendritic collagen matrix embedded dexamethasone-silver nanoparticle biofilm was formed, which involved S. aureus (Ramyaa Shri et al., 2021). Another study reported that using Legistromia speciosa floral extract, the synthesis of graphene oxide-silver nanocomposite (GO-Ag) inhibited the Gram-negative (E. cloacae) biofilm formation (Kulshrestha et al., 2017). Moreover, combining graphene quantum dots (GQDs) and OH– radicals was observed to inhibit the biofilm formation of S. aureus by damaging the intercellular components (Sun et al., 2014). Polymethyl methacrylate (PMMA) incorporation into CNTs could also reduce the infections caused by microbes, such as S. mutans, S. aureus, and C. albicans, by affecting microbial adherence (Kim et al., 2019). Nanocomposite GO with Ag impedes the growth of S. aureus by preventing cell mitosis of bacterial cells (Singh et al., 2018). These studies indicated that nanocomposites have excellent antibacterial ability against Gram-positive bacteria. Thus, it can be used as a novel antimicrobial agent for treatment.
6.2 Nanocomposites targeting Gram-negative bacterial biofilm
Nanocomposites are efficient against the development of resistant biofilms by Gram-negative bacteria as well as Gram-positive bacteria in various combinations. Same as Gram-positive bacteria, dendritic collagen matrix embedded dexamethasone-silver nanoparticles inhibit biofilm formation in the Gram-negative bacteria K. pneumoniae (Ramyaa Shri et al., 2021). A recent study has also observed that the AMP@PDA@AgNP nanocomposite has an inhibitory effect against Gram-negative bacteria, and E. coli and P. aeruginosa biofilm was higher than Gram-positive S. aureus. Furthermore, biofilm destruction in Gram-negative bacteria was also observed to be significantly different when compared with control (Xu et al., 2021). Moreover, nanocomposite immobilized with an antibiotic, ciprofloxacin, leads to reduced E. coli biofilm formation, and approximately 71% of its activity was even more effective compared with antibiotic alone (Rumyantceva et al., 2021). There are various studies that reported that, just like Gram-negative bacteria, there are various crosslinked polymer-stabilized oil-in-water and graphene oxide-silver nanocomposites that have antibacterial activity against Gram-negative bacteria biofilm formation (Kulshrestha et al., 2017; Landis et al., 2018). It has been seen that nanocomposites eradicated Gram-negative bacteria, including P. aeruginosa, E. coli, and E. cloacae complex in the biofilms (Li et al., 2019). These results indicated that nanocomposites are a better and novel approach for targeting resistant biofilm formation due to the wide range of Gram-negative bacteria. Apart from this, there were nanocomposites of copper, silver, or zinc NPs, embedded with zeolite, that were not effective against V. cholera biofilm formation, which showed that these bacteria have a resistance mechanism for these nanocomposites (Meza-Villezcas et al., 2019). Furthermore, the nanocomposite of silver NPs modified with curcumin causes oxidative stress through a hole in the membrane leading to antibacterial activity against B. subtilis and E. coli (Song et al., 2019). Similar to Gram-positive bacteria, metal oxides have antibacterial properties. For instance, mixing zinc and magnesium oxide with hydroxypropyl methylcellulose prevented P. mirabilis from forming a biofilm (Iribarnegaray et al., 2019). Another nanocomposite of octenyl succinic anhydride modified HA (OSA-HA) encapsulated antibiotic peptides, and poly D,L-lactic-co-glycolic acid (PLGA) disseminated the P. aeruginosa biofilm formation in lung mucus lining (Kłodzińska et al., 2019). Carbon NPs with an iron oxide nanocomposite have an antibacterial effect through the cell membrane, protein, and DNA degradation of E. coli (Alimardani et al., 2019). As we have already discussed, metal oxides have antibacterial activity, and ZnO, CuO, MgO, and TiO2 doped with other metal nanoparticles have higher antibacterial activity against Gram-negative such as E. coli than any of them in pure form (Malka et al., 2013; Rao et al., 2013). Furthermore, the combination of three metal oxide nanoparticles such as CuZnFe oxides reduces E. coli biofilm formation ability more than individual oxides of metal alone (Alzahrani et al., 2018). These oxides release metals slowly, which can penetrate the cell membrane and affect the cellular processes (Hayden et al., 2012). The toxic effect of ZnO can be minimized significantly by doping iron or Mg, which is safe for mammalian cells (Vidic et al., 2013; Ravichandran et al., 2015). In addition, nickel oxide also showed antibacterial activity against the P. aeruginosa biofilm. The mechanism behind the antibacterial activity of NiO is that it affects DNA replication and protein synthesis and alters the cell membrane, which also leads to cell death (Maruthupandy et al., 2020). A study reported that the ZnO/CdS nanocomposite impedes P. aeruginosa biofilm formation and also reduces the biofilm microbial population (Gholap et al., 2020). Moreover, the addition of Ag and Au in nanocomposites enhances their antibacterial potential against resistant E. coli (Matai et al., 2014); for example, Ag-SiO2 and Ag/Fe3O4 nanocomposites have increased antibacterial ability against E. coli (Mosselhy et al., 2017; Chang et al., 2018). The nanocomposite of ZnO NPs doped with Mn and Fe has also increased antibacterial activity against a wide range of bacteria such as E. coli, K. pneumoniae, S. typhi, P. aeruginosa, and P. mirabilis (Sharma et al., 2016; Bahari et al., 2019). Thus, as discussed previously, these nanocomposites with metal oxides have improved the efficacy of bacterial biofilm treatment through ROS generation and targeting bacterial DNA and proteins. Therefore, these results showed that choosing nanocomposites for biofilm treatment is the better way to treat biofilm-related infections.
7 Detection and characterization of bacterial biofilm
The World Health Organization (WHO) has previously raised concerns regarding bacterial biofilms in water pollution (Wingender and Flemming, 2011) and antibiotic resistance (Talebi et al., 2019), emphasizing the significance of developing novel and sensitive biofilm characterization strategies that can also be used to screen drugs that are specifically suited for biofilms. In this condition, innovative sensing techniques help deal with the biofilm problem using different approaches (Parlak and Richter-Dahlfors, 2020). Researchers have focused on the sensors and methods that identify the development of bacterial biofilms.
7.1 Sensors to investigate biofilm processes
The dynamics of certain indicators (such as oxygen level, pH, ions, and metabolite concentrations) within bacterial biofilms have been tracked using a variety of sensing techniques. The most effective method for a given application depends on several variables, including the type of target and the biofilm’s level of resistance to intrusive operations. For instance, highly sensitive and dependable electrochemical sensors are based on microelectrodes. To take the measurement, they must contact and pierce the biofilm, potentially endangering the biofilm’s structural integrity. Conversely, optical methods utilizing planar optodes and tiny particles are less intrusive. The biofilm’s oxygen and pH levels have been monitored using nanoparticles. Planar optodes, which are polymeric films embedded with oxygen-sensitive luminescent probes, and labeled micro- and nanoparticles have been employed in optical imaging systems based on confocal and fluorescence microscopy to monitor changes in oxygen and pH in the biofilm. Planar optodes, which are 2D polymer films on which the cells cling to form the biofilm, are unable to reveal information inside the biofilm. In contrast, micro- and nanoparticles doped with fluorescent or luminescent dyes, which are sensitive to changes in pH or oxygen levels in the biofilm, can be dispersed inside the EPS matrix, providing 3D mapping of the oxygen concentration and pH inside the biofilm. In situ studies of the biofilm can be conducted with less stress due to their non-destructive nature. The detection of heavy metal (Fe3+, Cu2+, Zn2+, and Hg2+) adsorption by bacterial biofilms that is pertinent to bioremediation applications has also been done using fluorescent probes (Funari and Shen, 2022).
The challenges of detecting biofilm in complex food samples, such as the characterization of biofilm formation mechanisms, identification of microbial metabolic activities, diagnosis of potential food pathogens, and sanitation monitoring of food processing equipment, have been taken on by optical nanosensors (Pu et al., 2021). Recently, nanosensors have been developed for biofilm optical detection with high sensitivity and great spatial resolution at nanoscale scopes that can convert biological information into optical signals (Talebi et al., 2019). Nanosensors, which are usually referred to as nanoprobes and nanozymes in microbiological detection due to their varied optical properties, can be tuned and designed for bacterium sensing and chemical recognition. The primary focus of nanosensors is on their capacity to transform chemical data from biomolecules in a microscopic environment into signals appropriate for detection. As a result, fluorescent, SERS, and colorimetric nanosensors make up the majority of optical nanosensors (Talebi et al., 2019).
Funari et al. have demonstrated that the utilization of localized surface plasmon resonance (LSPR) is done for label-free, in situ, real-time monitoring of E. coli biofilm formation on a gold mushroom-shaped nanoplasmonic substrate. By tracking the wavelength change in the LSPR resonance peak with fine temporal precision, the LSPR sensor can detect the traces of biofilm growth in real time (Funari et al., 2018). This sensor functions to analyze how different medications, such as kanamycin and ampicillin, as well as rifapentine, affect biofilm formation, preventing cell adhesion (Funari et al., 2018). LSPR-based technology is flexible and easy to use, making it a great tool for biofilm characterization and drug screening. It can be applied to a wide range of therapeutically relevant bacteria and provides an invaluable tool for drug screening and biofilm characterization (Funari et al., 2018).
There are recent advancements in biofilm prevention and control by using active antibiofilm nanocoatings. Antibiofilm tactics based on antimicrobial substances that eliminate infections and prevent their growth or interfere with the molecular pathways underlying the biofilm-associated rise in resistance and tolerance have been developed (Balaure and Grumezescu, 2020). These substances, which come in a variety of chemical forms, work in a variety of ways to target important bacterial metabolic pathways, cellular components including cell walls and membranes, or processes that underlie various stages of the biofilm life cycle. Eventually, smart antibiofilm coatings have been introduced that release their antimicrobial payload when necessary and are activated by a variety of triggers, including changes in the environment’s pH or temperature or enzymatic triggers.
Oxygen-sensitive luminescent nanosensors that can be incorporated into biofilms to study oxygen penetration, distribution, and antibiotic efficacy were successfully tested (Jewell et al., 2019). Sensors were used to track how antibiotics affect metabolism in biofilms made from clinical isolates. It not only provides a non-disruptive method for imaging and detecting oxygen in biofilms but also shows how to adapt a nanoparticle-based sensing platform to measure a variety of ions and small-molecule analytes (Jewell et al., 2019).
7.2 Quorum sensing disruption/molecular pathway inhibition of quorum sensing molecules
Antibiotic resistance among bacteria has evolved because of profound antibiotic stress. Likewise, P. aeruginosa evolve an elevated level of resistance to antibacterial agents via quorum sensing (QS) (Sadoq et al., 2023). With the advancement of antiviral therapies, the bacterial infection can be constrained without offending its resistance. In the phenomenon called QS, the bacteria correspond to each other in a density-dependent manner. This communication between the bacteria can be interrupted by disarming quorum-sensing systems. Such a strategy would save an ample amount of investment in the pharmaceutical industry. The Rhll/R system of P. aeruginosa is involved in the formation of biofilm. Molecular docking studies have shown the capability of silver, zinc, and copper oxide-based interactions with the acyl-homoserine-lactone synthases (LasI and RhII), thereby impeding the synthesis of functional signaling molecules. There are some regulatory proteins (LasR and RhIR) as well, which can bind to the nanoparticles, reducing the expression of QS-controlled genes (Sadoq et al., 2023).
These nanoparticles thwart the process of biofilm formation. Biofilm-based infections can be cured by intruding on the QS signaling cascade. pH, signal flow rate, and nutrient availability regulate QS-mediated biofilm function (Mishra et al., 2023). QS works by binding signaling molecules to the microbial receptors, instigating the activation of the gene network. Histidine kinase receptors sense the QS signals regulating gene expression in Gram-positive bacteria, which is nothing but the two-component regulating system, whereas receptive cell regulatory proteins after attaching to AHLs regulate the gene network in Gram-negative bacteria (Kalia, 2013).
Microbiology, nanotechnology, and optical technology are all involved in the multidisciplinary field of study on the imaging, identification, and chemical characterization of biofilms using optical nanosensors. Future studies on biofilm control will have a theoretical foundation due to the data collected by optical nanosensors, which include information on changes in biochemical contents as well as their spatial distribution and morphology.
8 Potential combating strategies
Microbial evolution has led to bacterial biofilms gaining resistance to broad-spectrum antibiotics, which is the main cause of morbidity and mortality. To overcome the challenge of these drug-resistant superbugs, several antimicrobial nanocomposites act as weapons (Roy et al., 2018). Various anti-biofilm molecules tested so far may include herbal active compounds, chelating agents, peptide antibiotics, antibiotics, and synthetic chemical compounds as summarized in Figure 3.
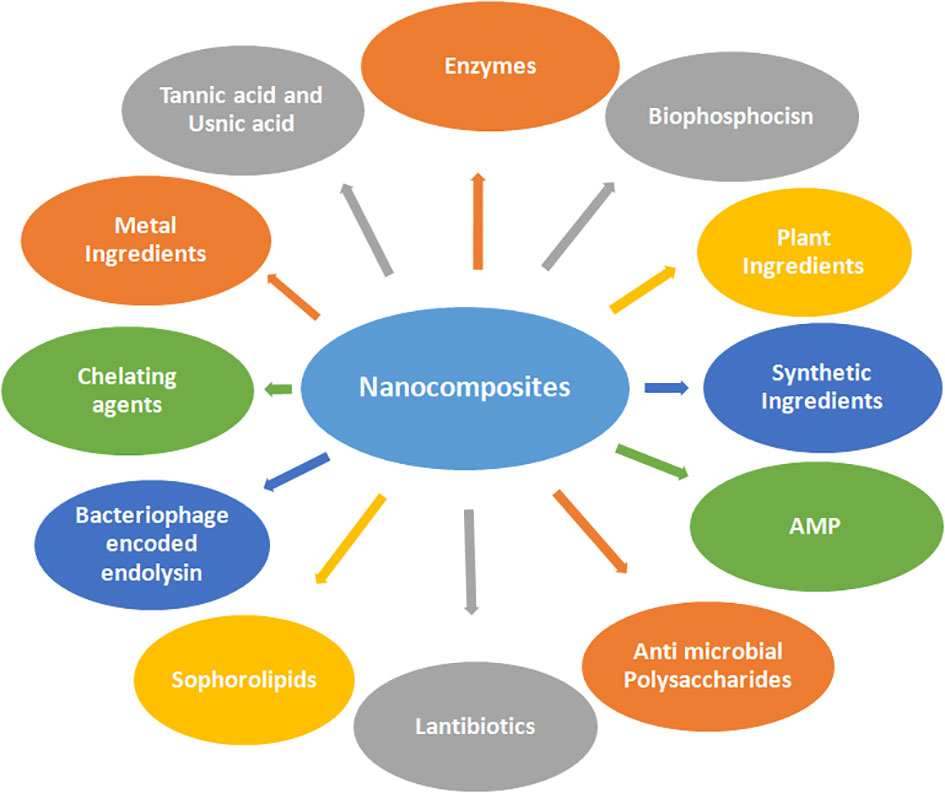
Figure 3 Various anti-biofilm molecules tested so far include herbal active compounds, chelating agents, peptide antibiotics, antibiotics, and synthetic chemical compounds involving nanocomposites.
8.1 Enzymes
They are a good choice to attack the unique target sites either in bacterial cell walls or non-selectively by generating bactericidal molecules. The antimicrobial properties of enzymes are attributed to their tendencies to aggregate or incorporate polymeric nanocomposites, which have potential in human therapeutics (Wu et al, 2017). Dispersin B (glycoside hydrolase), along with cefamandole nafate, is found to be a highly promising tool for preventing bacterial colonization of medical devices against S. epidermidis. Deoxyribonuclease I is also a potent anti-biofilm agent that acts by degrading extracellular DNA present in the matrix and rendering the matrix weaker and more susceptible to antimicrobials. DNase acts as a better anti-biofilm agent in individual biofilm formation than against the mixed species biofilms (Donelli et al., 2007; Sharma and Singh, 2018).
8.2 Biophosphocins
They are also known as nubiotics, which act as novel antimicrobials for enhanced killing of antibiotic-resistant and biofilm-forming bacteria. These are small protonated deoxynucleotide molecules that cause depolarization of bacterial cell membrane and also penetration of bacterial biofilms leading to death of encased bacteria (Wong et al., 2015).
8.3 Plant ingredients
The most abundant polyphenol found in green tea (Camella sinesis) is epigallocatechin gallate (EGCG) (Vidigal et al., 2014). Ellagic acid, esculetin, and fisetin are natural ingredients of dietary plants where fisetin was found to inhibit biofilm formation by S. aureus and S. dysgalactiae in a better manner than esculetin and ellagic acid. Esculetin, in turn, is found to be more efficient in preventing biofilms by S. aureus than ellagic acid. Ellagic acid prevents biofilm formation by S. dysgalactiae. Ellagic acid is also obtained from green tea while sources for esculetin are Santolina oblongifolia, Tagetes lucida, and Alchemilla speciosa, and those for fisetin are Vitis vinifera, Cucumis sativa, Fragaria ananassa, Allium cepa, Solanum lycopersicum, and Malus domestica (Durig et al., 2020).
Chitosan (a polysaccharide), curcumin (a beta diketone), eugenol (a phenol), and linoleic acid (omega-6-fatty acid) are antibacterial while berberine and resperine suppress biofilm formation in K. pneumoniae. The source of curcumin is Curcuma longa; that of eugenol is Syzigium aromaticum and Ocimum plants; that of linoleic acid is Coptis chinensis, Berberis vulgaris, B. aquifolium, B. aristata, and Hydrastis canadensis; that of berberine is Berberis aristata, B. vulgaris, and B. aquifolium; and that of Resperine is Rauwolfia serpentine and R. vomitoria (Magesh et al., 2013). Quercetin is obtained from Usnea longissima and it acts against biofilms of K. pneumoniae, Campylobacter violaccum, and P. aeruginosa (Gopu et al., 2015).
8.4 Synthetic ingredients
The synthetic halogenated Furanone (F-56) compound is derived from natural Furanone. It is produced by Australian macro alga Delisea pulchra and causes detachment of biomass of E. coli and P. aeruginosa (Hentzer et al., 2002).
8.5 Antimicrobial peptides
A previous study demonstrated the effect of an antimicrobial peptide derived from the stomach of the toad, Buforin-II, which enters the cell and binds to the nucleic acid to kill it without any membrane permeabilization (Cho et al., 2009). A small lytic peptide PTP-7 was found to be capable of diffusing into deeper layers of S. aureus biofilms to kill the bacteria effectively, thus providing a very good alternative to antibiotics for controlling infections related to bacterial biofilms (Kharidia and Liang, 2011). Two antibacterial peptides, cecropin P1 and PR-39, can be obtained from the intestine of a pig. Cecropin can kill E. coli in association with more peptides while PR-39 is capable of killing wild-type E. coli (Boman et al., 1993). Indolicidin was isolated from bovine neutrophils and found to inhibit E. coli DNA synthesis, making this antimicrobial agent of significant use in human therapeutics (Subbalakshmi and Sitaram, 1998). The antibiotic microcin B17 is a bactericidal peptide that acts as a poison for the DNA replication enzyme DNA gyrase, hence killing the bacteria (Vizan et al., 1991). Similarly, lytic peptide (PTP-7), a synthetic analog from Gaegurin 5; LL-37, a human cationic host defense peptide; PMAP-23, a cathelicidin-derived peptide identified from porcine leukocytes; and sushi peptides, derived from the sushi-3 domain of factor C, which is an LPS-sensitive serine protease of horseshow crab coagulation cascade, are potent anti-biofilm agents. Peptide 1018 is a small peptide that has the potential to eradicate Acinetobacter baumannii, E. coli, K. pneumoniae, P. aeruginosa, S. aureus, and Salmonella typhimurium biofilms at high doses and even checks the formation of biofilms (Fuente-Núñez et al., 2014). This peptide is derived from bovine host defense peptide bactenecins found in neutrophil granules.
8.6 Antimicrobial polysaccharides
CFT073 group II capsular polysaccharide is produced by extraintestinal E. coli of phylogenetic group B2 or D. These have the potential to inhibit biofilm formation by E. faecalis, E. coli, K. pneumoniae, P. aeruginosa, S. aureus, and S. epidermidis (Rendueles et al., 2013). Two additional anti-biofilm polysaccharides, Pel and Psl, were identified in a similar study and were found to be effective against E. coli and Staphylococcus epidermidis biofilms (Qin et al., 2009; Liang, 2015).
8.7 Lantibiotics
Gallidermin, which is obtained from Staphylococcus gallinarum, is found to prevent biofilm formation by S. aureus and S. epidermidis (Saising et al., 2012). Subtilin is another antibiotic that is considered similar to Nisin. Both are known to destroy bacterial biofilms by creating membrane pores in S. simulans and B. subtilis cells. Subtilin is sourced from B. subtilis Strain ATCC6633, Nisin is sourced from Lactococcus lactis, and Epidermin is sourced from S. epidermidis (Parisot et al., 2008).
8.8 Sophorolipids
These are the category of hydrophobic or hydrophilic biosurfactants that are produced on microbial cells or excreted extracellularly. Their antimicrobial property is attributed to the tendency to disrupt biofilms and the prevention of biofilm formation (Rienzo et al., 2015). Colistin is a polymixin E, obtained from Paenibacillus polymyxa, and is known to check biofilm formation against S. aureus for cystic fibrosis infections in lungs (Vidigal et al., 2014), while polymixin B is another biosurfactant and has the potential to combat multidrug-resistant bacteria and their biofilms (Park et al., 2011).
8.9 Chelating agents
An interesting study reported that chelating agents can disrupt the architecture of biofilms to destabilize bacterial membranes (Shadia and Aeron, 2014). Sodium citrate, tetrasodium EDTA, and disodium EDTA with tigecycline or gentamicin could reduce biofilms of Staphylococcus species and P. aeruginosa.
8.10 Bacteriophage-encoded endolysin
A bacteriophage-encoded enzyme endolysin, Ply C, can potentially act against the biofilms of Streptococcus (Shen et al., 2013). Ply C acts as a cell wall hydrolase enzyme to destroy the static and dynamic biofilms by diffusing through the matrix. It interacts with the cell surface carbohydrate that is peptidoglycan of the cell wall and has one enzyme active domain (EAD) to hydrolyze peptidoglycan bonds and another cell binding domain (CBD) in it (King et al., 2021).
8.11 Metal ingredients
Silver and iodine are employed as antimicrobials to help suppress the biofilms in chronic wounds (Percival et al., 2014). Octenidine hydrochloride, chlorhexidine, and cadexomer iodine polyhexamethylenebiguanide are also known antimicrobial agents. Gold nanoparticles integrated with graphitic carbon nitride (g-C3N4) have bactericidal effects against drug-resistant E. coli and S. aureus when combined with hydrogen peroxide (Wang et al., 2017). This has not only reduced bacterial infections but also accelerated wound healing rate.
8.12 Tannic acid and usnic acid
Tannic acid was found to inhibit surface colonization in S. aureus (Payne et al, 2013) while usnic acid is a natural secondary lichen metabolite that acts as an antimicrobial agent for inhibiting the formation of bacterial biofilms even on polymeric surfaces (Francolini et al, 2004) and also inhibits the virulent morphological traits of Candida albicans (Nithyanand et al, 2015).
9 Advantages of nanocomposites
One advantage of nanocomposites is their antimicrobial activity against Gram-negative planktonic bacteria and Gram-negative bacterial biofilms. These nanocomposites are capable of eradicating 90% of bacteria in biofilms and preventing infectious bacterial diseases. Nano-carriers show great anti-biofilm potential due to their small size and strong permeability. Nanocomposites can act on the formation and the diffusion stage of biofilms. They target biofilms, destroy their extracellular polymeric substances, and enhance biofilm permeability for antimicrobial substances. They improve the antibacterial ability of antibacterial drugs in the biofilm. This is acquired by protecting the loaded drug and controlling the release of antimicrobial substances. Therefore, polymer nanocomposites show superior mechanical properties, structural and thermal stability, promising electrical conductivity, and noise damping. They are corrosion-resistant and allow low permeability of fluids. They have lower density as compared to ceramic or metallic materials. They have low filter content and are easy to manufacture.
Epigallactocatechin gallate (EGCG) in patients with cystic fibrosis not only inhibited the formation of biofilms of Stenotrophomonas maltophilia but also reduced the number of viable cells in young and mature biofilms, thereby displaying promising therapeutic properties against S. maltophilia infection in cystic fibrosis (Vidigal et al., 2014). It shows a reduction in cell viability in mature biofilms, exhibiting its bactericidal properties. Ellagic acid, esculetin, and fisetin can be used to form antimicrobial drugs against a large number of infections. Chitosan, curcumin, eugenol, linoleic acid, berberine, and resperine-based nanocomposite drugs can fight against respiratory tract infections, urinary tract infections, and septicemia, especially in immunocompromised individuals. This is carried out by controlling and suppressing biofilm formation in K. pneumoniae (Magesh et al., 2013). Quercetin serves as a novel QS-based antimicrobial drug to manage food-borne pathogens (Gopu et al., 2015).
Synthetic halogenated furanone (F56) compounds can be used for the development of novel antibiotic, antimicrobial, or antipathogenic agents that hinder cell-to-cell communication in bacteria, making them less virulent and more sensitive to such therapies (Hentzer et al., 2002). These nano enzymes are more stable and cheaper than natural enzymes. Due to their small size, cells can easily take them up, and penetration into tissues and diffusion through the extracellular matrix allow them to reach atherosclerotic plaques, tumors, and biofilms (Benoit and Koo, 2016). The multifunctional nanocomposites can be exploited for their innovative biomedical applications to treat biofilm-related infections and also prevent oxidative stress. A nanocomposite formed from a V2O5 nanowire (glutathione peroxidase) and MnO2 nanoparticle [superoxide dismutase (SOD) and catalase] was found to be effective against removing ROS in a rodent inflammation model (Huang et al., 2016). These catalytic nanomaterials having enzyme-like properties lead to the disruption of biofilms, hence preventing neurodegeneration, tumor prevention, and wound healing. Ceria-based nanocomposites can mimic SOD and have anti-inflammatory and neuroprotective activities (Hirst et al., 2009) besides protecting cardiac progenitor cells and preventing retinal degeneration (Chen et al., 2006), in-stent coatings (Son et al., 2015), and reducing injury and ischemia in stroke (Kim et al., 2021).
10 Toxicity of nanocomposites
Nanotoxicology is a specialized branch that addresses the issues related to the toxicity of nanoparticles, nanocomposites, and other nanomaterials. It deals with the study of the adverse effects of engineered nanomaterials or nanoparticles on living organisms. The fragment of nanocomposites is found during their synthesis, application, and disposal. The workers associated with nanocomposite cutting, drilling, and sanding of nanocomposites have a high risk of developing occupational diseases. Nanocomposite fragments in the nano- and microscale are released due to reasons such as photodegradation, incineration, mechanical stress, thermal stress, and interaction with the liquids (Pacheco and Buzea, 2021). The airborne nature of the nanoparticles and nanofibers shows potential toxicity due to inhalation. It has been revealed that the main toxic effects induced by TiO2 are triggered by oxidative stress, which leads to cell necrosis and DNA damage (Han et al., 2020). The size and physical properties of NPs are partly responsible for NP toxicity, with particles with smaller sizes and higher stability being more interactive with lung cells (Bengalli et al., 2019). Although iron oxide nanoparticles are used in biomedical applications, numerous reports have shown that they also exhibit toxicity to normal healthy cells. Similarly, the cytotoxicity of superparamagnetic iron oxide nanoparticles (SPIONs) to actin cytoskeleton modulation, gene expression profile alteration, iron homeostasis disturbance, impaired alterations in signaling pathways, cell regulation, DNA damage, and oxidative stress has been demonstrated (Singh et al., 2010). A literature survey also reported that the combination of silver and iron oxide nanoparticles as nanocomposites holds exclusive and unique antimicrobial activities (Trang et al., 2017). Even though iron oxide nanoparticles are used in biomedical applications, numerous reports showed that they also exhibit toxicity towards normal healthy cells. To create safe nanocomposites, it is important to determine the types of nanomaterials that pose a health risk and to limit their use or mitigate their toxicity.
11 Challenges of antibiotic resistance biofilm
Antibacterial resistance presents difficulties for modern medicine because the spread and emergence of resistant bacteria have reduced the efficiency of medicines. Bacteria can resist antibiotic effects in several ways, such as altering the antibiotic’s target site, destroying or altering the antibiotic, efflux of antibiotics through efflux transporters, and reducing the influx of antibiotics by reducing membrane permeability (Munita and Arias, 2016). A substantial number of antibiotics on the market right now are useless because they lack the necessary minimum bactericidal concentration (MBC) and minimum inhibitory concentration (MIC), making them ineffective against infections. The disruption of desired bacterial biofilms’ EPS matrix is accomplished through newer, or matrix-centered, tactics, such as controlling microbial metabolism, matrix-degrading enzyme, photodynamic therapy, naturally occurring chemicals, QS, and nanotechnology.
Since they can be used as an alternative to treat infections caused by biofilm and multidrug resistance, nanoparticles are of utmost importance (Pelgrift and Friedman, 2013). Their nano-formulations can overcome many of the limits and limitations of conventional therapy. These formulations can pass through biological barriers. In the past, several nanoparticles, including metal nanoparticles, green nanoparticles, and many other combinations, have been utilized as antibacterial and anti-biofilm agents (Khan et al., 2021). Numerous studies on the eradication of bacterial biofilm communities based on nanoparticles have been published (Kulshrestha et al., 2014). Nanoparticles with strong antibacterial capabilities, such as copper, oxide, silver, zinc, and quantum dots, have been shown to have potential in the fight against biofilms (Zaidi et al., 2017). By raising oxidative stress, promoting cytoplasmic leakage, and denaturing the metabolic proteins, nanoparticles carrying ROS harm bacteria’s cell membranes and cell walls (Shoji and Chen, 2020). It causes changed cellular functioning and has an impact on bacterial cells’ physiological processes (Zaidi et al., 2017). Numerous in vivo experiments showed that nanoparticles can be utilized to combat a wide range of Gram-positive and Gram-negative bacterial strains with little cell toxicity and great compatibility (Li et al., 2016; Qayyum and Khan, 2016). It results in the potential use of nanoparticles as a treatment for bacterial infections (Khan et al., 2020). Kulshrestha and his coworkers reported CaF2-NP’s suppressive effect on genes associated with virulence factors, such as vicR, ftf, gtfC, come, and spam of S. mutans, and demonstrated enzymatic activity inhibition associated with cell adhesion, glucan synthesis, acid production, QS, and acid tolerance (Kulshrestha et al., 2016). The capacity of currently available antibiotics to treat infections that are currently resistant to accessible current therapies may be improved by inhibiting biofilm resistance. However, more research is required to completely understand the mechanism of antibiotic resistance in biofilms. As discussed above, keeping in mind the toxicity effects of nanocomposites, a strict balance has to be maintained while using nanocomposites to create new therapeutic approaches.
12 Conclusion
Biofilms are organized and complex communities in which bacteria communicate with each other and gain tremendous advantages. They spread over the outer and inner parts of the entire human body including organs and bones. Few to hundreds of bacterial species are associated with this phenomenon. Traditional antibiotics have covered a large period in medical history, but the ultimate solution to this issue remains unanswered. Moving forward, through the application of nubiotics, enzymes, natural products, antimicrobial peptide, etc., along with various formulations, novel pathways toward treatment have been created but still had limitations. In the present era of nanotechnology, many promising nanomaterials have been suggested as potential candidates. These nanocomposites are a new emerging class of compounds of biomaterials that have a hybrid property of participating ingredient molecules/groups. This feature is unique and can be harvested for this problem as it has an open arena to manipulate the toxicological and other relevant issues related to the candidate molecule. It is thus suggested that with this tool for the treatment of biofilm infection, more sensitive methods via a synergistic approach can be designed.
Author contributions
AV, AW, and RS designed, conceived, wrote, and edited the manuscript. AV, ND, and RS prepared the figures. ND, NP, VU, and AM helped in writing and editing. AV, AW, and RS critically reviewed the manuscript. All authors contributed to the article and approved the submitted version.
Conflict of interest
Author AV was employed by Arundeep Akshay Urja Pvt. Ltd. Gorakhpur, Uttar Pradesh, India.
The remaining authors declare that the research was conducted in the absence of any commercial or financial relationships that could be construed as a potential conflict of interest.
Publisher’s note
All claims expressed in this article are solely those of the authors and do not necessarily represent those of their affiliated organizations, or those of the publisher, the editors and the reviewers. Any product that may be evaluated in this article, or claim that may be made by its manufacturer, is not guaranteed or endorsed by the publisher.
References
Alexandre, M., Dubois, P. (2000). Polymer-layered silicate nanocomposites: Preparation, properties and uses of a new class of materials. Materials Sci. Engineering: R: Rep. 28 (1–2), 1–63. doi: 10.1016/S0927-796X(00)00012-7
Ali, K., Ahmed, B., Ansari, S. M., Saquib, Q., Al-Khedhairy, A. A., Dwivedi, S., et al. (2019). Comparative in situ ROS mediated killing of bacteria with bulk analogue, eucalyptus leaf extract (ELE)-capped and bare surface copper oxide nanoparticles. Mater SciEng C Mater Biol. Appl. 100, 747–758. doi: 10.1016/j.msec.2019.03.012
Alimardani, V., Abolmaali, S. S., Borandeh, S. (2019). Antifungal and antibacterial properties of graphene-based nanomaterials: A mini-review. J. Nanostruct. 9 (3), 402–413. doi: 10.22052/JNS.2019.03.002
Alzahrani, K. E., Niazy, A. A., Alswieleh, A. M., Wahab, R., El-Toni, A. M., Alghamdi, H. S. (2018). Antibacterial activity of trimetal (CuZnFe) oxide nanoparticles. Int. J. Nanomedicine 13, 77–87. doi: 10.2147/IJN.S154218
Armstrong, D. G., Boulton, A. J. M., Bus, S. A. (2017). Diabetic foot ulcers and their recurrence. N Engl. J. Med. 376 (24), 2367–2375. doi: 10.1056/NEJMra1615439
Bahari, A., Roeinfard, M., Ramzannezhad, A., Khodabakhshi, M., Mohseni, M. (2019). Nanostructured features and antimicrobial properties of Fe3O4/ZnO nanocomposites. Natl. Acad. Sci. letters. 42, 9–12. doi: 10.1007/s40009-018-0667-5
Bakar, M. A., McKimm, J., Haque, S. Z., Majumder, M. A. A., Haque, M. (2018). Chronic tonsillitis and biofilms: A brief overview of treatment modalities. J. Inflammation Res. 11, 329–337. doi: 10.2147/JIR.S162486
Balaure, P. C., Grumezescu, A. M. (2020). Recent advances in surface nanoengineering for biofilm prevention and control. part II: Active, combined active and passive, and smart bacteria-responsive antibiofilm nanocoatings. Nanomaterials 10, 1527. doi: 10.3390/nano10061230
Bengalli, R., Ortelli, S., Blosi, M., Costa, A., Mantecca, P., Fiandra, L. (2019). In vitro toxicity of TiO2:SiO2 nanocomposites with different photocatalytic properties. Nanomaterials (Basel). 9 (7), 1041. doi: 10.3390/nano9071041
Benoit, D., Koo, H. (2016). Targeted, triggered drug delivery to tumor and biofilm microenvironments. Nanomedicine. 11, 873–879. doi: 10.2217/nnm-2016-0014
Bibi, H., Iqbal, M., Wahab, H., Öztürk, M., Ke, F., Iqbal, Z., et al. (2021). Green synthesis of multifunctional carbon coated copper oxide nanosheets and their photocatalytic and antibacterial activities. Sci. Rep. 11 (1), 1–11. doi: 10.1038/s41598-021-90207-5
Boman, H. G., Agerberth, B., Boman, A. (1993). Mechanisms of action on escherichia coli of cecropin P1 and PR-39, two antibacterial peptides from pig intestine. Infect. Immun. 61, 2978–2984. doi: 10.1128/iai.61.7.2978-2984.1993
Byrd, A. L., Belkaid, Y., Segre, J. A. (2018). The human skin microbiome. Nat. Rev. Microbiol. 16 (3), 143–155. doi: 10.1038/nrmicro.2017.157
Chang, M., Lin, W.-S., Xiao, W., Chen, Y. (2018). Antibacterial effects of magnetically-controlled Ag/Fe3O4 nanoparticles. Materials (Basel) 11, 659. doi: 10.3390/ma11050659
Chávez de Paz, L. E., Resin, A., Howard, K. A., Sutherland, D. S., Wejse, P. L. (2011). Antimicrobial effect of chitosan nanoparticles on streptococcus mutans biofilms. Appl. Environ. Microbiol. 77, 3892–3895. doi: 10.1128/AEM.02941-10
Chen, J. P., Patil, S., Seal, S., McGinnis, J. F. (2006). Rare earth nanoparticles prevent retinal degeneration induced by intracellular peroxides. Nat. Nanotechol. 1, 142–150. doi: 10.1038/nnano.2006.91
Cho, S., Lee, J. S., Jun, J., Kim, S. G., Jang, J. (2014). Fabrication of water-dispersible and highly conductive PSS-doped PANI/graphene nanocomposites using a high-molecular weight PSS dopant and their application in H2S detection. Nanoscale 6 (24), 15181–15195. doi: 10.1039/c4nr04413d
Cho, J. H., Sung, B. H., Kim, S. C. (2009). Buforins: Histone H2A-derived antimicrobial peptides from toad stomach. Biochim. Et Biophys. Acta 1788, 1564–1569. doi: 10.1016/j.bbamem.2008.10.025
Costerton, J. W., Stewart, P. S., Greenberg, E. P. (1999). Bacterial biofilms: A common cause of persistent infections. Science 284, 5418, 1318–1322. doi: 10.1126/science.284.5418.1318
Cowan, T. (2011). Biofilms and their management: From concept to clinical reality. J. Wound Care 20 (5), 220–226. doi: 10.12968/jowc.2011.20.5.220
Dealey, C., Posnett, J., Walker, A. (2012). The cost of pressure ulcers in the united kingdom. J. Wound Care 21 (6), 261–266. doi: 10.12968/jowc.2012.21.6.261
Dokoshi, T., Zhang, L.-J., Li, F., Nakatsuji, T., Butcher, A., Yoshida, H., et al. (2020). Hyaluronan degradation by cemip regulates host defense against staphylococcus aureus skin infection. Cell Rep. 30, 61–68.e4. doi: 10.1016/j.celrep.2019.12.001
Donelli, G., Francolini, I., Romoli, D., Guaglianone, E., Piozzi, A., Ragunath, C., et al. (2007). Synergistic activity of dispersin b and cefamandolenafate in inhibition of staphylococcal biofilm growth on polyurethanes. Antimicrob. Agents Chemother. 51, 2733–2740. doi: 10.1128/AAC.01249-06
Durig, A., Kouskoumvekaki, I., Vejborg, R. M., Klemm, P. (2020). Chemoinformatics-assisted development of new anti-biofilm compounds. Appl. MicrobiolBiotechnol 87, 309–317. doi: 10.1007/s00253-010-2471-0
Elshaarawy, R. F. M., Ismail, L. A., Alfaifi, M. Y., Rizk, M. A., Eltamany, E. E., Janiak, C. (2020). Inhibitory activity of biofunctionalized silver-capped n-methylated water-soluble chitosan thiomer for microbial and biofilm infections. Int. J. BiolMacromol. 152, 709–717. doi: 10.1016/j.ijbiomac.2020.02.284
Ennas, G., Mei, A., Musinu, A., Piccaluga, G., Pinna, G., Solinas, S. (1998). Sol–gel preparation and characterization of Ni–SiO2 nanocomposites. J. Non-Crystalline Solids 232–234, 587–593. doi: 10.1016/S0022-3093(98)00430-X
Fazli, M., Bjarnsholt, T., Kirketerp-Møller, K., Jørgensen, A., Andersen, C. B., Givskov, M., et al. (2011). Quantitative analysis of the cellular inflammatory response against biofilm bacteria in chronic wounds. Wound Repair Regener. 19 (3), 387–391. doi: 10.1111/j.1524-475X.2011.00681.x
Ferrieres, L., Hancock, V., Klemm, P. (2007). Specific selection for virulent urinary tract infections Escherichia coli strains during catheter-associated biofilm formation. FEMS Immunol. Med. Microbiol. 51, 212–219. doi: 10.1111/j.1574-695X.2007.00296.x
Flemming, H. C., Wuertz, S. (2019). Bacteria and archaea on earth and their abundance in biofilms. Nat. Rev. Microbiol. 17, 247–260. doi: 10.1038/s41579-019-0158-9
Francolini, I., Norris, P., Piozzi, A., Donelli, G., Stoodley, P. (2004). Usnic acid, a natural antimicrobial agent able to inhibit bacterial biofilm formation on polymer surfaces. Antimicrob. Agents Chemother. 48, 4360–4365. doi: 10.1128/AAC.48.11.4360-4365.2004
Fuente-Núñez, C., Reffuveille, F., Haney, E. F., Straus, S. K., Hancock, R. E. W. (2014). Broad-spectrum anti-biofilm peptide that targets a cellular stress response. PloS Pathog. 10. doi: 10.3389/fmicb.2017.01867
Funari, R., Bhalla, N., Chu, K.-Y., Soderstrom, B., Shen, A. Q. (2018). Nanoplasmonics for real-time and label-free monitoring of microbial biofilm formation. ACS Sens. 3, 1499–1509. doi: 10.1021/acssensors.8b00287
Funari, R., Shen, A. Q. (2022). Detection and characterization of bacterial biofilms and BiofilmBased sensors. ACS Sens. 7, 347–357. doi: 10.1021/acssensors.1c02722
Gao, F. (2004). Clay/polymer composites: The story. Materials Today 7 (11), 50–55. doi: 10.1016/S1369-7021(04)00509-7
Gao, Q., Han, J., Ma, Z. (2013). Polyamidoamine dendrimers-capped carbon dots/Au nanocrystal nanocomposites and its application for electrochemical immunosensor. Biosensors bioelectronics 49, 323–328. doi: 10.1016/j.bios.2013.05.048
Gholap, H., Gholap, A., Patil, R. (2020). ZnO/CdS nanocomposite: An anti-microbial and anti-biofilm agent. Adv. Microbiol. 10, 166–179. doi: 10.4236/aim.2020.104013
Goel, A., Meher, M. K., Gupta, P., Gulati, K., Pruthi, V., Poluri, K. M. (2019). Microwave assisted κ-carrageenan capped silver nanocomposites for eradication of bacterial biofilms. Carbohydr. Ploymers 206, 854–862. doi: 10.1016/j.carbpol.2018.11.033
Gopu, V., Meena, C. K., Shetty, P. H. (2015). Quercetin influences quorum sensing in food borne bacteria: In-vitro and in-silico evidence. PloS One 10, e0134684. doi: 10.1371/journal.pone.0134684
Govan, J. R., Deretic, V. (1996). Microbial pathogenesis in cystic fibrosis: Mucoid And. Microbiol. Rev. 60, 539–574. doi: 10.1128/mr.60.3.539-574.1996
Høiby, N., Ciofu, O., Bjarnsholt, T. (2010). Pseudomonas aeruginosa biofilms in cystic fibrosis. Future Microbiol. 5 (11), 1663–1674. doi: 10.2217/fmb.10.125
Haghparasti, Z., Shahri, M. M. (2018). Green synthesis of water-soluble nontoxic inorganic polymer nanocomposites containing silver nanoparticles using white tea extract and assessment of their in vitro antioxidant and cytotoxicity activities. Mater Sci. Eng. C 87, 139–148. doi: 10.1016/j.msec.2018.02.026
Han, B., Pei, Z., Shi, L., Wang, Q., Li, C., Zhang, B., et al. (2020). TiO2 nanoparticles caused DNA damage in lung and extra-pulmonary organs through ROS-activated FOXO3a signaling pathway after intratracheal administration in rats. Int. J. Nanomedicine. 15, 6279–6294. doi: 10.2147/IJN.S254969
Harriott, M. M., Noverr, M. C. (2009). Candida albicans and staphylococcus aureus form polymicrobial biofilms: Effects on antimicrobial resistance. Antimicrob. Agents Chemother. 53 (9), 3914–3922. doi: 10.1128/AAC.00657-09
Hayden, S. C., Zhao, G., Saha, K., Phillips, R. L., Li, X., Miranda, O. R., et al. (2012). Aggregation and interaction of cationic nanoparticles on bacterial surfaces. J. Am. Chem. Soc. 134, 6920–6923. doi: 10.1021/ja301167y
Hentzer, M., Riedel, K., Rasmussen, T. B., Heydorn, A., Anderson, J. B., Parsck, M. R., et al. (2002). Inhibition of quorum sensing in pseudomonas aeruginosa biofilm bacteria by a halogenated furanone compound. Microbiology 148, 87–102. doi: 10.1099/00221287-148-1-87
Hirst, S.M., Karakoti, A.S., Tyler, R.D., Sriranganathan, N., Seal, S., Reilly, C.M., et al (2009). Anti-inflammatory Properties of Cerium Oxide Nanoparticles. Nanotherapeutics 5 (24), 2848–2856. doi: 10.1002/smll.200901048
Hou, W., Sun, X., Wang, Z., Zhang, Y. (2012). Biofilm-forming capacity of staphylococcus epidermidis, staphylococcus aureus, and pseudomonas aeruginosa from ocular infections. Invest. Ophthalmol. Vis. Sci. 53 (9), 5624–5631. doi: 10.1167/iovs.11-9114
Hsueh, P.-R. (2010). New Delhi metallo-ß-lactamase-1 (NDM-1): An emerging threat among enterobacteriaceae. J. Formos Med. Assoc. 109, 685–687. doi: 10.1016/s0929-6646(10)60111-8
Huang, Y., Liu, Z., Liu, C., Ju, E., Zhang, Y., Ren, J., et al. (2016). Self-assembly of multi-nanozymes to mimic an intracellular antioxidant defense system. Angew. Chem. 55, 6646–6650. doi: 10.1002/anie.201600868
Hussain, F., Hojjati, M., Okamoto, M., Gorga, R. E. (2006). Review article: Polymer-matrix nanocomposites, processing, manufacturing, and application: An overview. J. Composite Materials 40 (17), 1511–1575. doi: 10.1177/0021998306067321
Iribarnegaray, V., Navarro, N., Robino, L., Zunino, P., Morales, J., Scavone, P. (2019). Magnesium-doped zinc oxide nanoparticles alter biofilm formation of Proteus mirabilis. Nanomedicine (Lond). 14, 1551–1564. doi: 10.2217/nnm-2018-0420
Jacobsen, S. M., Stickler, D. J., Mobley, H. L., Shirtliff, M. E. (2008). Complicated catheter-associated urinary tract infections due to And. ClinMicrobiol Rev. 21, 26–59. doi: 10.1128/CMR.00019-07
Jewell, M. P., Galyean, A. A., Kirk Harris, J., Zemanick, E. T., Cash, K. J. (2019). Luminescent nanosensors for ratiometric monitoring of three-dimensional oxygen gradients in laboratory and clinical pseudomonas aeruginosa biofilms. Appl. Environ. Microbiol. 85, e01116–e01119. doi: 10.1128/AEM.01116-19
Kalia, V. C. (2013). Quorum sensing inhibitors: An overview. Biotechnol. Adv. 31 (2), 224–245. doi: 10.1016/j.biotechadv.2012.10.004
Kandiah, K., Venkatachalam, R., Wang, C., Valiyaveettil, S., Ganesan, K. (2015). In vitro and preliminary in vivo toxicity screening of high-surface-area TiO2-chondroitin-4-sulfate nanocomposites for bone regeneration application. Colloids surfaces B Biointerfaces 128, 347–356. doi: 10.1016/j.colsurfb.2015.02.027
Karunasagar, A., Garag, S. S., Appannavar, S. B., Kulkarni, R. D., Naik, A. S. (2018). Bacterial biofilms in chronic rhinosinusitis and their implications for clinical management. Indian J. Otolaryngol Head Neck Surg. 70 (1), 43–48. doi: 10.1007/s12070-017-1208-0
Khan, F., Pham, D. T. N., Kim, Y. (2020). Alternative strategies for the application of aminoglycoside antibiotics against the biofilmforming human pathogenic bacteria. ApplMicrobiolBiotechnol 104, 1955–1976. doi: 10.1007/s00253-020-10360-1
Khan, J., Tarar, S. M., Gul, I., Nawaz, U., Arshad, M. (2021). Challenges of antibiotic resistance biofilms and potential combating strategies: a review. 3 Biotech. 11, 169. doi: 10.1007/s13205-021-02707-w
Kharidia, R., Liang, J. F. (2011). The activity of a small lytic peptide PTP-7 on staphylococcus aureus biofilms. J. Microbiol. 49, 663–668. doi: 10.1007/s12275-011-1013-5
Kim, C. K., Kim, T., Choi, I., Soh, M., Kim, D., Kim, Y., et al. (2021). Ceria nanoparticles that can protect against ischemic stroke. Angew. Chem. 51, 11039–11043. doi: 10.1002/anie.201203780
Kim, K.-I., Kim, D.-A., Patel, K. D., Shin, U. S., Kim, H.-W., Lee, J.-H., et al. (2019). Carbon nanotube incorporation in PMMA to prevent microbial adhesion. Sci. Rep. 9, 4921. doi: 10.1038/s41598-019-41381-0
King, H., Castro, S. A., Pohane, A. A., Scholte, C. M., Fischetti, V. A., Korotkova, N., et al. (2021). Molecular basis for recognition of the group a carbohydrate backbone by the PlyC streptococcal bacteriophage endolysin. Biochem. J. 478 (12), 2385–2397. doi: 10.1042/BCJ20210158
Kłodzińska, S. N., Wan, F., Jumaa, H., Claus, S., Thomas, R., Hanne, N. (2019). Utilizing nanoparticles for improving anti-biofilm effects of azithromycin: A head-to-head comparison of modified hyaluronic acid nanogels and coated poly (lactic-co-glycolic acid) nanoparticles. J. Colloid Interface Sci. 555, 595–606. doi: 10.1016/j.jcis.2019.08.006
Koch, C., Hoiby, N. (1993). Pathogenesis of cystic fibrosis. Lancet 341, 1065–1069. doi: 10.1016/0140-6736(93)92422-p
Komarneni, S. (1992). Feature article. Nanocomposites. J. Materials Chem. 2 (12), 1219–1230. doi: 10.1039/JM9920201219
Kostakioti, M., Hadjifrangiskou, M., Hultgren, S. J. (2013). Bacterial biofilms: development, dispersal, and therapeutic strategies in the dawn of the postantibiotic era. Cold Spring HarbPerspect Med. 3 (4), a010306. doi: 10.1101/cshperspect.a010306
Kulshrestha, S., Khan, S., Hasan, S., Khan, M. E., Misba, L., Khan, A. U. (2016). Calcium fluoride nanoparticles induced suppression of streptococcus mutans biofilm: An in vitro and in vivo approach. Appl. Microbiol. Biotechnol. 100, 1901–1914. doi: 10.1007/s00253-015-7154-4
Kulshrestha, S., Khan, S., Meena, R., Khan, A. U. (2014). A graphene/ zinc oxide nanocomposite film protects dental implant surfaces against cariogenic Streptococcusmutans. Biofouling 30, 1281–1294. doi: 10.1080/08927014.2014.983093
Kulshrestha, S., Qayyum, S., Khan, A. U. (2017). Antibiofilm efficacy of green synthesized graphene oxide-silver nanocomposite using lagerstroemia speciosa floral extract: A comparative study on inhibition of gram-positive and gram-negative biofilms. Microbial Pathogenesis 103, 167–177. doi: 10.1016/j.micpath.2016.12.022
Kumar, A. P., Depan, D., Tomer, N. S., Singh, R. P. (2009). Nanoscale particles for polymer degradation and stabilization–trends and future perspectives. Prog. Polymer Sci. 34 (6), 479–515. doi: 10.1016/j.progpolymsci.2009.01.002
Kuramitsu, H. K., Wang, B. Y. (2011). The whole is greater than the sum of its parts: Dental plaque bacterial interactions can affect the virulence properties of cariogenic. Am. J. Dent. 24, 153–154.
Landis, R. F., Li, C.-H., Gupta, A., Lee, Y.-W., Yazdani, M., Ngernyuang, N., et al. (2018). Biodegradable nanocomposite antimicrobials for the eradication of multidrug-resistant bacterial biofilms without accumulated resistance. J. Am. Chem. Soc 140 (19), 6176–6182. doi: 10.1021/jacs.8b03575
Lawrence, J., Korber, D., Hoyle, B., Costerton., J. W., Caldwell, D. (1991). Optical sectioning of microbial biofilms. J Bacteriol 173, 20, 6558–6567. doi: 10.1128/jb.173.20.6558-6567.1991
Lazzarini, P., Malindu, F., Jaap, V. N. (2019). Diabetic foot ulcers: Is remission a realistic goal? Endocrinol. Today 8 (2), 22–26.
Lerche, C. J., Schwartz, F., Theut, M., Fosbøl, E. L., Iversen, K., Bundgaard, H., et al. (2021). Anti-biofilm approach in infective endocarditis exposes new treatment strategies for improved outcome. Front. Cell Dev. Biol. 9. doi: 10.3389/fcell.2021.643335
Lewis, K. (2001). Riddle of biofilm resistance. Antimicrob. Agents Chemother. 45 (4), 999–1007. doi: 10.1128/AAC.45.4.999-1007.2001
Liang, Z. X. (2015). The expanding roles of c-di-GMP in the biosynthesis of exopolysaccharides and secondary metabolites. Natural Product Rep. 32, 663–683. doi: 10.1039/C4NP00086B
Li, C.-H., Chen, X., Landis, R. F., Geng, Y., Makabenta, J. M., Lemnios, W., et al. (2019). Phytochemical-based nanocomposites for the treatment of bacterial biofilms. ACS Infect. Dis. 5, 1590–1596. doi: 10.1021/acsinfecdis.9b00134
Li, Y. J., Harroun, S. G., Su, Y. C. (2016). Synthesis of self-assembled spermidine-carbon quantum dots effective against multidrug resistant bacteria. AdvHealthc Mater 5 (19), 2545–2554. doi: 10.1002/adhm.201600297
Lin, Y.-K., Yang, S.-C., Hsu, C.-Y., Sung, J.-T., Fang, J.-Y. (2021). The antibiofilm nanosystems for improved infection inhibition of microbes in skin. Biosens Bioelectron 26, 21, 6392. doi: 10.3390/molecules26216392
Liu, N., Chen, X., Ma, Z. (2013). Ionic liquid functionalized graphene/Au nanocomposites and its application for electrochemical immunosensor. Biosensors bioelectronics 48, 33–38. doi: 10.1016/j.bios.2013.03.080
Machado, D., Castro, J., Palmeira-de-Oliveira, A., Martinez-de-Oliveira, J., Cerca, N. (2015). Bacterial vaginosis biofilms: Challenges to current therapies and emerging solutions. Front. Microbiol. 6, 1528. doi: 10.2217/fmb.10.125
Magdalane, C. M., Kaviyarasu, K., Vijaya, J. J., Jaykumar, C., Maaza, M., Jayaraj, B. (2017). Photocatalytic degradation effect of malachite green and catalytic hydrogenation by UV-illuminated CeO2/CdO multilayered nanoplatelet arrays: Investigation of antifungal and antimicrobial activities. J. Photochem. Photobiol. B. 169, 110–123. doi: 10.1016/j.jphotobiol.2017.03.008
Magesh, H., Kumar, A., Alam, A., Sekar, U., Sumantran, V. N., Vaidyanathan, R. (2013). Identification of natural compounds which inhibit biofilm formation in clinical isolates of klebsiella pneumoniae. Indian J. ExpBiol 51, 764–772.
Malka, E., Perelshtein, I., Lipovsky, A., Shalom, Y., Naparstek, L., Perkas, N., et al. (2013). Eradication of multi-drug resistant bacteria by a novel zn-doped CuO nanocomposite. Small. 9, 4069–4076. doi: 10.1002/smll.201301081
Marsh, P. D. (2006). Dental plaque as a biofilm and a microbial community – implications for health and disease. BMC Oral. Health 6 (Suppl 1), S14. doi: 10.1186/1472-6831-6-S1-S14
Martin, I., Waters, V., Grasemann, H. (2021). Approaches to targeting bacterial biofilms in cystic fibrosis airways. Int. J. Mol. Sci. 22 (4), 2155. doi: 10.3390/ijms22042155
Maruthupandy, M., Rajivgandhi, G. N., Quero, F., Li, W.-J. (2020). Anti-quorum sensing and anti-biofilm activity of nickel oxide nanoparticles against Pseudomonas aeruginosa. J. Environ. Chem. Eng. 8, 104533. doi: 10.1016/j.jece.2020.104533
Matai, I., Sachdev, A., Dubey, P., Kumar, S. U., Bhushan, B., Gopinath, P. (2014). Antibacterial activity and mechanism of Ag-ZnO nanocomposite on s. aureus and GFP-expressing antibiotic resistant E. coli. Colloids Surf B Biointerfaces. 115, 359–367. doi: 10.1016/j.colsurfb.2013.12.005
Meza-Villezcas, A., Gallego-Hernández, A. L., Yildiz, F. H., Jaime-Acuña, O. E., Raymond-Herrera, O., Huerta-Saquero, A. (2019). Effect of antimicrobial nanocomposites on vibrio cholerae lifestyles: Pellicle biofilm, planktonic and surface-attached biofilm. PloS One 14, e0217869. doi: 10.1371/journal.pone.0217869
Mishra, S., Gupta, A., Upadhye, V., Singh, S. C., Sinha, R. P., Häder, D.-P. (2023). Therapeutic strategies against biofilm infections. Life 13 (1), 172. doi: 10.3390/life13010172
Mosselhy, D. A., Granbohm, H., Hynönen, U., Ge, Y., Palva, A., Nordström, K., et al. (2017). Nanosilver-silica composite: Prolonged antibacterial effects and bacterial interaction mechanisms for wound dressings. Nanomaterials (Basel). 7, E261. doi: 10.3390/nano7090261
Munita, J. M., Arias, C. A. (2016). Mechanisms of antibiotic resistance. MicrobiolSpectr 4, VMBF–0016–2015. doi: 10.1128/microbiolspec.VMBF-0016-2015
Nadell, C. D., Drescher, K., Foster, K. R. (2016). Spatial structure, cooperation and competition in biofilms. Nat. Rev. Microbiol. 14 (9), 589–600. doi: 10.1038/nrmicro.2016.84
Niedzielski, A., Chmielik, L. P., Stankiewicz, T. (2021). The formation of biofilm and bacteriology in otitis media with effusion in children: A prospective cross-sectional study. Int. J. Environ. Res. Public Health 18 (7), 3555. doi: 10.3390/ijerph18073555
Nithyanand, P., Shafreen, R. M. B., Muthamil, S., Pandian, S. K. (2015). Usnic acid inhibits biofilm formation and virulent morphological traits of Candida albicans. Microbiological Res. 179, 20–28. doi: 10.1016/j.micres.2015.06.009
Omar, A., Wright, J. B., Schultz, G., Burrell, R., Nadworny, P. (2017). Microbial biofilms and chronic wounds. Microorganisms 5 (1), 9. doi: 10.3390/microorganisms5010009
Owhal, A., Pingale, A. D., Belgamwar, S. U., Rathore, J. S. (2021). A brief manifestation of anti-bacterial nanofiller reinforced coatings against the microbial growth based novel engineering problems. Materials Today: Proc. 47, 3320–3330. doi: 10.1016/j.matpr.2021.07.151
Pacheco, I., Buzea, C. (2021). “Nanomaterials and nanocomposites: Classification and toxicity,” in Handbook of nanomaterials and nanocomposites for energy and environmental applications. Eds. Kharissova, O. V., Torres-Martínez, L. M., Kharisov, B. I. (Cham: Springer). doi: 10.1007/978-3-030-36268-3_1
Panel, National Pressure Ulcer Advisory Panel, European Pressure Ulcer Advisory Panel and Pan Pacific Pressure Injury Alliance (2014). Prevention and Treatment of Pressure Ulcers: Clinical Practice Guideline Haesler, E. Eds. (Osborne Park, Western Australia: Cambridge Media).
Parhi, B., Bharatiya, D., Swain, S. K. (2022). Effect of polycaprolactone on physicochemical, biological, and mechanical properties of polyethylene oxide and polyamino acids nano block copolymers. J. Appl. Polym Sci. 139 (19), 52116. doi: 10.1002/app.52116
Parisot, J., Carey, S., Breukink, E., Chan, W. C., Narbad, A., Bonev, B. (2008). Molecular mechanism of target recognition by subtilin, a class I lanthionine antibiotic. Antimicrob. Agents Chemother. 52, 612–618. doi: 10.1128/AAC.00836-07
Park, J. H., Lee, J. H., Cho, M. H., Herzberg, M., Lee, J. (2021). Acceleration of protease effect on staphylococcus aureus biofilm dispersal. FEMS Microbiol. Lett. 335, 31–38. doi: 10.1111/j.1574-6968.2012.02635.x
Park, S. C., Park, Y., Hahm, K. S. (2011). The role of antimicrobial peptides in preventing multidrug-resistant bacterial infections and biofilm formation. Int. J. MolSci 12, 5971–5992. doi: 10.3390/ijms12095971
Parlak, O., Richter-Dahlfors, A. (2020). Bacterial sensing and biofilm monitoring for infection diagnostics. Macromol. Biosci. 20, 2000129. doi: 10.1002/mabi.202000129
Pathania, D., Sharma, M., Thakur, P., Chaudhary, V., Kaushik, A., Furukawa, H., et al. (2022). Exploring phytochemical composition, photocatalytic, antibacterial, and antifungal efficacies of au NPs supported by cymbopogon flexuosus essential oil. Nature 12, 1, 1–15. doi: 10.1038/s41598-022-15899-9
Payne, D. E., Martin, N. R., Parzych, K. R., Rickard, A. H., Underwood, A., Boles, B. R. (2013). Tannic acid inhibits staphylococcus aureus surface colonization in an IsaA-dependent manner. Infect. Immun. 81, 496–504. doi: 10.1128/IAI.00877-12
Pelgrift, R. Y., Friedman, A. J. (2013). Nanotechnology as a therapeutic tool to combat microbial resistance. Adv. Drug Delivery Rev. 65, 1803–1815. doi: 10.1016/j.addr.2013.07.011
Percival, S. L., Finnegan, S., Donelli, G., Vuotto, C., Rimmer, S., Lipsky, B. A. (2014). Antiseptics for treating infected wounds: Efficacy on biofilms and effect of pH. Crit. Rev. Microbiol. 42 (2), 293–309. doi: 10.3109/1040841X.2014.940495
Presting, H., König, U. (2003). Future nanotechnology developments for automotive applications. Materials Sci. Engineering: C 23 (6–8), 737–741. doi: 10.1016/j.msec.2003.09.120
Prosser, B., Taylor, D., Dix, B. A., Cleeland, R. (1987). Method of evaluating effects of antibiotics on bacterial biofilm. Antimicrob. Agents Chemother. 31 (10), 1502–1506. doi: 10.1128/AAC.31.10.1502
Pu, H., Xu, Y., Sun, D.-W., Wei, Q., Li, X. (2021). Optical nanosensors for biofilm detection in the food industry: Principles, applications and challenges. Crit. Rev. Food Sci. Nutr. 61, 2107–2124. doi: 10.1080/10408398.2020.1808877
Qayyum, S., Khan, A. U. (2016). Biofabrication of broad range antibacterial and antibiofilm silver nanoparticles. IET Nanobiotechnol 10 (05), 349–357. doi: 10.1049/iet-nbt.2015.0091
Qin, Z., Yang, L., Qu, D., Molin, S., Tolker-Nielsen, T. (2009). Pseudomonas aeruginosa extracellular products inhibit staphylococcal growth, and disrupt established biofilms produced by Staphylococcus epidermidis. Microbiology 155, 2148–2156. doi: 10.1099/mic.0.028001-0
Ramyaa Shri, K., Narasimhan, S. P. S., Murugesan, R., Narayan, S. (2021). Fabrication of dexamethasone-silver nanoparticles entrapped dendrimer collagen matrix nanoparticles for dental applications. Biointerface Res. Appl. Chem. 11, 14935–14955. doi: 10.33263/BRIAC116.1493514955
Rao, Y., Wang, W., Tan, F., Cai, Y., Lu, J., Qiao, X. (2013). Influence of different ions doping on the antibacterial properties of MgO nanopowders. Appl. Surface Sci. 284, 726–731. doi: 10.1016/j.apsusc.2013.08.001
Ravichandran, K., Rathi, R., Baneto, M., Karthika, K., Rajkumar, P. V., Sakthivel, B., et al. (2015). Effect of Fe+F doping on the antibacterial activity of ZnO powder. Ceramics Int. 41, 3390–3395. doi: 10.1016/j.ceramint.2014.10.121
Rendueles, O., Kaplan, J. B., Ghigo, J. M. (2013). Antibiofilm polysaccharides. Environ. Microbiol. 15, 334–346. doi: doi : 10.1111/j.1462-2920.2012.02810.x
Rennert, R., Golinko, M., Yan, A., Flattau, A., Tomic-Canic, M., Brem, H. (2009). Developing and evaluating outcomes of an evidence-based protocol for the treatment of osteomyelitis in stage IV pressure ulcers: a literature and wound electronic medical record database review. Wound Manage. Prev. 55 (3), 42.
Riau, A. K., Aung, T. T., Setiawan, M., Yang, L., Yam, G. H. F., Beuerman, R. W., et al. (2019). Surface immobilization of nano-silver on polymeric medical devices to prevent bacterial biofilm formation. Pathogens. 8, E93. doi: 10.3390/pathogens8030093
Rienzo, M. A. D., Banat, I. M., Dolman, B., Winterburn, J., Martin, P. J. (2015). Sophorolipidbiosurfactants: Possible uses as antibacterial and antibiofilm agent. N Biotechnol. 7, 720–726. doi: 10.1016/j.nbt.2015.02.009
Roy, S., Santra, S., Das, A., Dixith, S., Sinha, M., Ghatak, S., et al. (2020). Staphylococcus aureus biofilm infection compromises wound healing by causing deficiencies in granulation tissue collagen. Ann. Surg. 271, 1174–1185. doi: 10.1097/SLA.0000000000003053
Roy, R., Tiwari, M., Donelli, G., Tiwari, V. (2018). Strategies for combating bacterial biofilms: A focus on anti-biofilm agents and their mechanisms of action. Virulence. 9 (1), 522–554. doi: 10.1080/21505594.2017.1313372
Rumyantceva, V., Rumyantceva, V., Andreeva, Y., Tsvetikova, S., Radaev, A., Vishnevskaya, M., et al. (2021). Magnetically controlled carbonate nanocomposite with ciprofloxacin for biofilm eradication. Int. J. Mol. Sci. 22, 6187. doi: 10.3390/ijms22126187
Sadoq, B.-E., Britel, M. R., Bouajaj, A., Touhami, A., Touhami, F., Maurady, A. (2023). In silico study of anti-quorum sensing activity of silver, zinc oxide, and copper oxide nanoparticles against pseudomonas aeruginosa. Res. Square Priprint PPR. doi: 10.21203/rs.3.rs-2453123/v1. PPR595175.
Saising, J., Dube, L., Ziebandt, A. K., Voravuthikunchai, S. P., Nega, M., Gotz, F. (2012). Activity of gallidermin on staphylococcus aureus and staphylococcus epidermidis biofilms. Antimicrob. Agents Chemother. 56, 5804–5810. doi: 10.1128/AAC.01296-12
Shadia, M. A. A., Aeron, A. (2014). Bacterial biofilm: Dispersal and inhibition strategies. SAJ Biotechnol. 1, 105. doi: 10.18875/2375-6713.1.105
Sharma, N., Jandaik, S., Kumar, S., Chitkara, M., Inderjit Singh Sandhu, I. S. (2016). Synthesis, characterisation and antimicrobial activity of manganese- and iron-doped zinc oxide nanoparticles. J. ExpNanaosci 11 (1), 54–71. doi: 10.1080/17458080.2015.1025302
Sharma, K., Singh, A. P. (2018). Antibiofilm effect of DNase against single and mixed species biofilm. Foods. 7 (3), 42. doi: 10.3390/foods7030042
Sharwani, A. A., Narayanan, K. B., Khan, M. E., Han, S. S. (2022). Photocatalytic degradation activity of goji berry extract synthesized silver-loaded mesoporous zinc oxide (Ag@ ZnO) nanocomposites under simulated solar light irradiation. Sci. Rep. 12 (1), 1–18. doi: 10.1038/s41598-022-14117-w
Shen, Y., Koller, T., Kreikemeyer, B., Nelson, D. C. (2013). Rapid degradation of Streptococcus pyogenes biofilms by PlyC, a bacteriophage-encoded endolysin. Antimicrob. Agents Chemother. 68, 1818–1824. doi: 10.1093/jac/dkt104
Shoji, M. M., Chen, A. F. (2020). Biofilms in periprosthetic joint infections: A review of diagnostic modalities, current treatments, and future directions. J. Knee Surg. 33, 119–131. doi: 10.1055/s-0040-1701214
Singh, N., Jenkins, G. J. S., Asadi, R., Doak, S. H. (2010). Potential toxicity of superparamagnetic iron oxide nanoparticles (SPION). Nano Rev. 1, 5358. doi: 10.3402/nano.v1i0.5358
Singh, R., Smitha, M. S., Karuppiah, S., Singh, S. P. (2018). Enhanced bioactivity of GO-Fe3O4nanocomposite against pathogenic bacterial strains. Int. J. Nanomedicine 13, 63–66. doi: 10.2147/IJN.S125004
Song, Z., Wu, Y., Wang, H., Han, H. (2019). Synergistic antibacterial effects of curcumin modified silver nanoparticles through ROS-mediated pathways. Mater SciEng C Mater Biol. Appl. 99, 255–263. doi: 10.1016/j.msec.2018.12.053
Son, D., Lee, J., Lee, D. J., Ghaffari, R., Yun, S., Kim, S. J., et al. (2015). Bioresorbable electronic stent integrated with therapeutic nanoparticles for endovascular diseases. ACS Nano 9, 5937–5946. doi: 10.1021/acsnano.5b00651
Soto, S. M. (2014). Importance of biofilms in urinary tract infections: New therapeutic approaches. Adv. Biol. 2014, 13. doi: 10.1155/2014/543974. Article ID 543974.
Sturme, M. H., Kleerebezem, M., Nakayama, J., Akkermans, A. D., Vaughan, E. E., De Vos, W. M. (2002). Cell to cell communication by autoinducing peptides in gram-positive bacteria. Antonie Van Leeuwenhoek 81 (1), 233–243. doi: 10.1023/a:1020522919555
Subbalakshmi, C., Sitaram, N. (1998). Mechanism of antimicrobial action of indolicidin. FEMS Microbiol. Lett. 160, 91–96. doi: 10.1111/j.1574-6968.1998.tb12896.x
Suleman, L., Percival, S. L. (2015). Biofilm-infected pressure ulcers: Current knowledge and emerging treatment strategies. AdvExp Med. Biol. 831, 29–43. doi: 10.1007/978-3-319-09782-4_3
Sun, H., Gao, N., Dong, K., Jinsong Ren, J., Qu, X. (2014). Graphene quantum dots-Band-Aids used for wound disinfection. ACS Nano 8, 6202–6210. doi: 10.1021/nn501640q
Sung, J. Y., Leung, J. W. C., Costerton, J. W. (1992). A new biofilm story in biliary tract infection. Int. Biodeterior. Biodegrad. 30 (2–3), 155–165. doi: 10.1016/0964-8305(92)90060-2
Talebi, B. A., Rizvanov, A. A., Haertlé, T., Blatt, N. L. (2019). World health organization report: Current crisis of antibiotic resistance. BioNanoScience 9, 778–788. doi: 10.1007/s12668-019-00658-4
Thambirajoo, M., Maarof, M., Lokanathan, Y., Katas, H., Ghazalli, N. F., Tabata, Y., et al. (2021). Potential of nanoparticles integrated with antibacterial properties in preventing biofilm and antibiotic resistance. Antibiotics(Basel). 10, 1338. doi: 10.3390/antibiotics10111338
Toussi, C. A., Ezatpour, H. R., Haddadnia, J., Shiri, J. G. (2018). Effect of using different metal and ceramic materials as restorations on stress distribution around dental implants: A comparative finite element study. Mater Res Express 5, 11, 115403. doi: 10.1088/2053-1591/aadc55
Trang, V. T., Tam, L. T., Quy, N. V., Huy, T. Q., Thuy, N. T., Tri, D. Q., et al. (2017). Functional iron oxide–silver hetero-nanocomposites: Controlled synthesis and antibacterial activity. J. Electronic Materials 46, 3381–3389. doi: 10.1007/s11664-017-5314-2
Unal, S., Ekren, N., Sengil, A. Z., Oktar, F. N., Irmak, S., Oral, O., et al. (2018). Synthesis, characterization, and biological properties of composites of hydroxyapatite and hexagonal boron nitride. J. BioMed. Mater Res. B Appl. Biomater. 106, 2384–2392. doi: 10.1002/jbm.b.34046
Venditti, M., Biavasco, F., Varaldo, P. E., Macchiarelli, A., De Biase, L., Marino, B., et al. (1993). Catheter-related endocarditis due to glycopeptide-resistant In a transplanted heart. Clin. Infect. Dis. 17, 524–525. doi: 10.1093/CLINIDS/17.3.524
Verbrugghe, E., Boyen, F., Gaastra, W., Bekhuis, L., Leyman, B., Parys, A. V., et al. (2021). The complex interplay between stress and bacterial infections in animals. Vet. Microbiol1 55 (2-4), 115–127. doi: 10.1016/j.vetmic.2011.09.012
Vidic, J., Stankic, S., Haque, F., Ciric, D., Goffic, R. L., Vidy, A., et al. (2013). Selective antibacterial effects of mixed ZnMgO nanoparticles. J. Nanopart Res. 15, 1595. doi: 10.1007/s11051-013-1595-4
Vidigal, P. G., Musken, M., Becker, K. A., Haussler, S., Wingender, J., Steinmann, E., et al. (2014). Effects of green tea compound epigallocatechin-3 gallate against Stenotrophomonas maltophilia infection and biofilm. PloS One 9, e92876. doi: 10.1371/journal.pone.0092876
Vizan, J. L., Hernandez-Chico, C., Castillo, I., Moreno, F. (1991). The antibiotic microcin B17 is a DNA gyrase Poison: Characterisation of the mode of inhibition. EMBO J. 10, 467–476. doi: 10.1006/jmbi.2001.4562
Wang, Z., Dong, K., Liu, Z., Zhang, Y., Chen, Z., Sun, H., et al. (2017). Activation of biologically relevant levels of reactive oxygen species by au/g-C3N4 hybrid nanozyme for bacteria killing and wound disinfection. Biomaterials. 113, 145–157. doi: 10.1016/j.biomaterials.2016.10.041
Wang, L., Hu, C., Shao, L. (2017). The antimicrobial activity of nanoparticles: present situation and prospects for the future. Int. J. Nanomedicine 12, 1227–1249. doi: 10.2147/IJN.S121956
Wingender, J., Flemming, H.-C. (2011). Biofilms in drinking water and their role as reservoir for pathogens. Int. J. Hyg. Environ. Health 214, 417–423. doi: 10.1016/j.ijheh.2011.05.009
Wong, J. P., DiTullio, P., Parkinson, S. (2015). Bisphosphocins: novel antimicrobials for enhanced killing of drug-resistant and biofilm-forming bacteria. Future Microbiol. 10 (11), 1751–1758. doi: 10.2217/fmb.15.70
Wu, X., Kwon, S.-J., Kim, J., Kane, R. S., Dordick, J. S., Kwon, S.-J., et al. (2017). Biocatalytic nanocomposites for combating bacterial pathogens. Annu. Rev. Chem. biomolecular engineering. 8, 87–113. doi: 10.1146/annurev-chembioeng-060816-101612
Wu, H., Moser, C., Wang, H.-Z., Høiby, N., Song, Z.-J. (2015). Strategies for combating bacterial biofilm infections. Int. J. Oral. Sci. 7, 1–7. doi: 10.1038/ijos.2014.65
Xu, J., Li, Y., Wang, H., Zhu, M., Feng, W., Liang, G. (2021). Enhanced antibacterial and anti-biofilm activities of antimicrobial peptides modified silver nanoparticles. Int. J. Nanomedicine. 16, 4831–4846. doi: 10.2147/IJN.S315839
Yuan, Z., Lin, C., He, Y., Tao, B., Chen, M., Zhang, J., et al. (2020). Near-infrared light-triggered nitric-Oxide-Enhanced photodynamic therapy and low-temperature photothermal therapy for biofilm elimination. ACS Nano. 14, 3546–3562. doi: 10.1021/acsnano.9b09871
Zaidi, S., Misba, L., Khan, A. U. (2017). Nano-therapeutics: a revolution in infection control in post antibiotic era. Nanomedicine 13 (07), 2281–2301. doi: 10.1016/j.nano.2017.06.015
Zare, M., Namratha, K., Alghamdi, S., Mohammad, Y. H. E., Hezam, A., Zare, M., et al. (2019). Novel green biomimetic approach for synthesis of ZnO-Ag nanocomposite; antimicrobial activity against food-borne pathogen, biocompatibility and solar photocatalysis. Sci. Rep. 9 (1), 1–15. doi: 10.1038/s41598-019-44309-w
Keywords: nanocomposite, biofilm, human disease, bacteria, infections
Citation: Varma A, Warghane A, Dhiman NK, Paserkar N, Upadhye V, Modi A and Saini R (2023) The role of nanocomposites against biofilm infections in humans. Front. Cell. Infect. Microbiol. 13:1104615. doi: 10.3389/fcimb.2023.1104615
Received: 21 November 2022; Accepted: 30 January 2023;
Published: 28 February 2023.
Edited by:
Tanmay Sarkar, West Bengal State Council of Technical Education, IndiaReviewed by:
Payal Gupta, Graphic Era University, IndiaRinarani Ray, Maulana Abul Kalam Azad University of Technology, India
Copyright © 2023 Varma, Warghane, Dhiman, Paserkar, Upadhye, Modi and Saini. This is an open-access article distributed under the terms of the Creative Commons Attribution License (CC BY). The use, distribution or reproduction in other forums is permitted, provided the original author(s) and the copyright owner(s) are credited and that the original publication in this journal is cited, in accordance with accepted academic practice. No use, distribution or reproduction is permitted which does not comply with these terms.
*Correspondence: Rashmi Saini, ZHIucmFzaG1pc2FpbmlAZ21haWwuY29t