- 1Amity Institute of Biotechnology, Amity University Maharashtra, Mumbai, India
- 2Murdoch Children’s Research Institute, Parkville, VIC, Australia
- 3Department of Pediatrics, University of Melbourne, Parkville, VIC, Australia
- 4Amity Centre of Excellence in Astrobiology, Amity University Maharashtra, Mumbai, India
- 5Center for Drug Discovery and Development (CD3), Amity Institute of Biotechnology, Amity University Maharashtra, Mumbai, India
Helicobacter pylori is a stomach-dwelling bacterium with a crude global prevalence of nearly 45% in adults and 35% in children and adolescents. Chronic H. pylori infection and the resulting inflammation are major causes of gastritis, peptic ulcer disease and gastric cancer. Since its discovery in 1982, various animal models have been proposed to recreate the specific pathophysiological interactions between H. pylori and the human host. These infection models have been instrumental in dissecting the key drivers of H. pylori colonization, persistence and mediators of host immune responses. However, a comprehensive understanding of the molecular triggers for malignant transformation of the gastric mucosa is still lacking. Vaccine development in this area has stalled, as promising candidates identified through animal studies have failed in advanced human clinical trials. Currently, H. pylori eradication is heavily reliant on different antimicrobial agents. As with other bacterial pathogens, the growing antimicrobial resistance in H. pylori remains a major challenge, making eradication therapy increasingly complex and prolonged, over time. Recent drug approvals have mostly been for newer combinations of conventional antibiotics and proton pump inhibitors. Thus, the development of novel treatments and innovative models are crucial for advancing the drug development pipeline. This review encompasses the development and recent advances in animal and non-animal models of H. pylori gastric infection and its applications in investigating novel therapeutics and vaccine candidates.
Introduction
The microaerobic Helicobacter pylori is a Gram-negative, spiral rod-shaped bacterium that predominantly colonizes the human gastric mucosal surface and is associated with acute and chronic gastritis, peptic ulcers, and other upper gastrointestinal disorders (Aihara et al., 2014; Chen et al., 2024). Recent estimates of H. pylori colonization show a crude global prevalence of nearly 45% in adults and 35% in children and adolescents (Chen et al., 2024). Despite the high prevalence, in 80%-85% of cases, the bacteria’s existence is unrelated to any clinically symptomatic disease (Elbehiry et al., 2023). However, individuals with H. pylori-associated chronic gastritis have a 1%-3% chance of developing gastric cancer (Uemura et al., 2001) and a 10%–20% chance of developing peptic ulcers (Peek, 2008). The World Health Organization (WHO) has designated H. pylori infection as a class I carcinogen due to its ability to cause gastric adenocarcinoma (stomach cancer) and mucosa-associated lymphoid tissue (MALT) lymphoma (Vogiatzi et al., 2007). Presently, gastric cancer ranks as the fifth most common cancer worldwide and the fifth leading cause of cancer-related deaths (Bray et al., 2024).
The prevalence of H. pylori varies between and within countries, but it is generally estimated to range from 30% to 50% in developed countries and from 60% to 80% in underdeveloped regions (Chen et al., 2024; Poddar, 2019). These estimates are based on stratified variables like geography, age, socioeconomic status, and ethnicity, which result in significant regional and national variations. In India, the prevalence of H. pylori infection has been reported to be relatively high, with studies indicating that up to 80% of children under the age of 10 may be infected (Poddar and Yachha, 2007). The high prevalence of H. pylori infection in India can be attributed to factors such as poor sanitation, overcrowding, and lack of access to clean water (Thirumurthi and Graham, 2012). H. pylori is typically acquired very early on in childhood via oral-oral, fecal-oral or iatrogenic routes of transmission (Brown, 2000). Having evolved with the human host for thousands of years (Linz et al., 2007), these bacteria demonstrate a remarkable ability to survive in the harsh acidic environment of the stomach, localizing within the gastric mucosal layer during chronic infection (Figure 1) and potentially persisting for the host’s lifetime. H. pylori have an arsenal of different colonization and virulence factors that facilitate its persistence within the gastric niche. Bacterial factors such as urease production, chemotactic motility, and the capacity to adjust to the changing gastric environment, all contribute to its ability to survive in the stomach for decades (Yamaoka, 2010). However, the more severe disease states are associated with presence of the cytotoxin-associated gene A (cagA) and vacuolating cytotoxin A (vacA) genes, both which encode for corresponding polymorphic cytotoxins that are injected and secreted by H. pylori and contribute significantly to its pathogenesis (Jones et al, 2010). H. pylori can further produce diverse classes of lytic enzymes, including lipases, phospholipases, and proteases, which degrade gastric mucus by altering its viscosity and hydrophobicity (Asante et al., 1997; Celli et al., 2009). The compromised mucus barrier allows H. pylori to closely associate with the gastric epithelium while making the latter more susceptible to gastric acid. It has recently been proposed that biofilm development also contributes to chronic colonization (Carron et al., 2006; Cole et al., 2004).
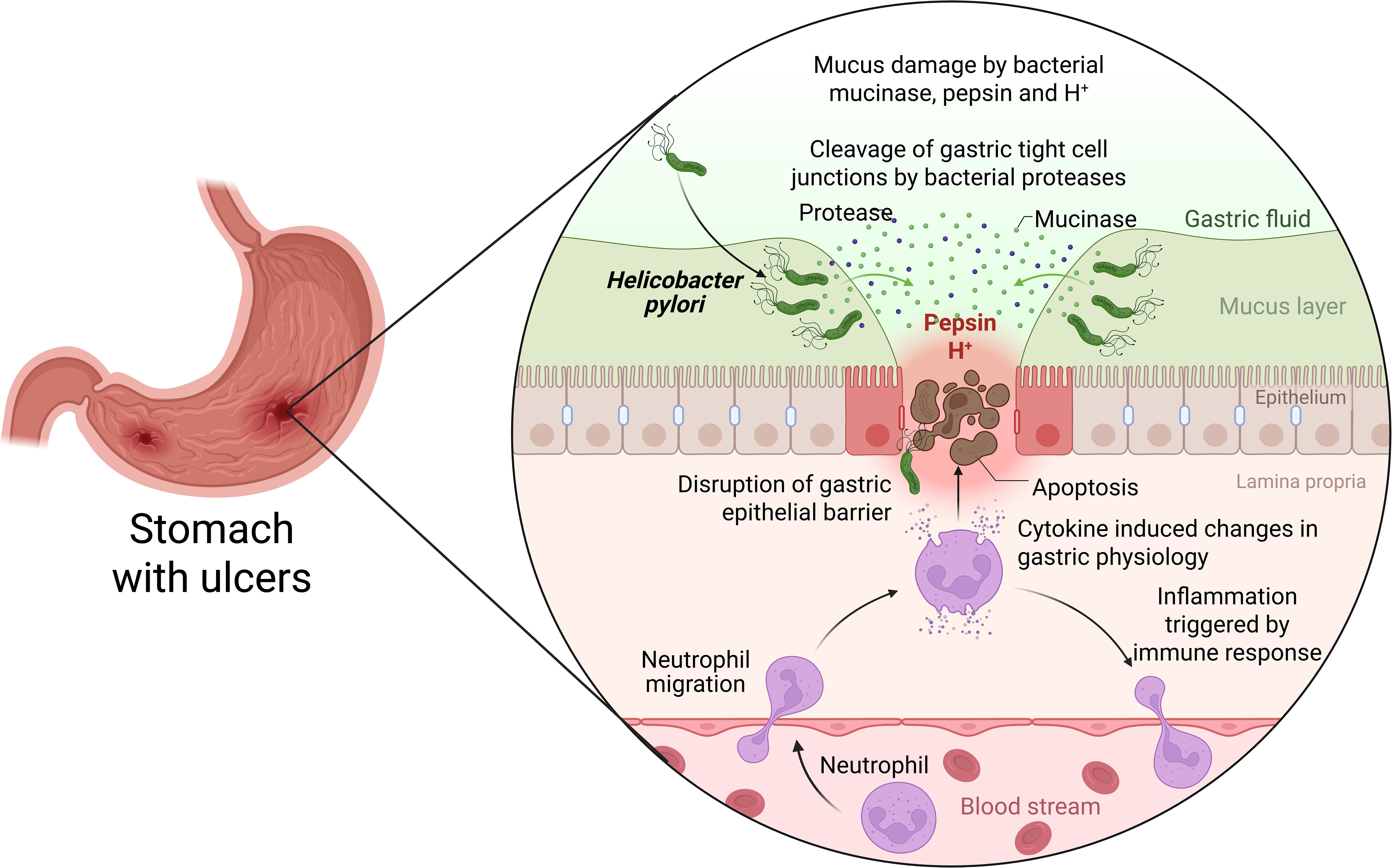
Figure 1. Pathogenesis of H. pylori infection within the gastric epithelium. Three main stages of H. pylori pathogenesis can be studied: i) bacterial attachment to and colonization of the stomach mucosa; ii) the host’s immune response and H. pylori’s immune-evasive mechanisms; and iii) the pathological consequences of chronic infection (Elbehiry et al., 2023) Created with BioRender.
H. pylori and the gastric precancerous cascade
Gastric cancer is the most serious pathological consequence of H. pylori infection, although exact steps leading up to it are poorly understood. While gastric cancer can be broadly divided into diffuse and intestinal types, it is the latter that is most commonly associated with H. pylori infection (Uemura et al., 2001; Hanada and Graham, 2014). The progression of chronic gastritis towards the development of the gastric ulcers and eventually, gastric cancer, is associated with mucosal pH variations (Brawner et al, 2014) and distinct, sequential changes within the tissue. This precancerous process takes place, presumably over several decades, before any clinical presentation of cancer. First delineated by Pelayo Correa in 1992 (Correa, 1992), specific stages of the precancerous cascade have been refined over the years. The ‘Correa Cascade’ describes the transition of the gastric mucosa from chronic active gastritis → chronic atrophic gastritis → intestinal metaplasia → dysplasia and ultimately, invasive carcinoma (Correa, 2013). This process is triggered by H. pylori infection and sustained by the resultant chronic inflammation. It was initially proposed that eradication of H. pylori till the stage of intestinal metaplasia can successfully halt the progression towards gastric cancer. However, more recent evidence suggests that while H. pylori eradication is still the most important method for preventing gastric cancer globally, its effect on established gastric intestinal metaplasia is limited (Cheng et al., 2023; Zhu et al., 2024). Thus, early and successful eradication of H. pylori is critical for the prevention of gastric cancer.
Current challenges in treatment of H. pylori: rise in antimicrobial resistance
The sole and current therapy for H. pylori infection in humans is the use of antibiotics (Suzuki et al., 2022). The triple and quadruple therapy regimens are used as the first line of defense against H. pylori infection depending on local resistance profiles and treatment guidelines. According to worldwide recommendations, the most commonly used first-line treatment for H. pylori infection involves a ‘triple therapy’ that includes a proton pump inhibitor (PPI) combined with two broad spectrum antibiotics (amoxicillin, clarithromycin, levofloxacin, and metronidazole) for 7 to 14 days (Chey et al., 2024). However, because of the prevalence of antibiotic resistance, the eradication rates of therapy have declined to <80% in many countries (Jiang et al., 2022; Aumpan et al., 2023). The addition of Bismuth to the PPI-antibiotic combination constitutes the ‘quadruple therapy’ regimen that is being increasingly used as a first line treatment in areas with high resistance (Chey et al., 2024).
Antimicrobial resistance is acknowledged as a significant public health issue with worldwide implications, particularly considering that the rate of advent of multidrug resistant bacteria has vastly outpaced and the slow discovery of novel antibiotics (Ortiz et al., 2019; Aumpan et al., 2023). Antimicrobial resistance may be more prevalent in developing nations than in high-income countries, even though statistics are scarce in low- and middle-income countries (Jaka et al., 2018; Ortiz et al., 2019). Despite concerted stewardship efforts, antimicrobials are increasingly being consumed worldwide, often without appropriate prescription or compliance (Zarauz et al., 2022; Ventola, 2015). Global antimicrobial usage grew by at least 35% in the last ten years, with only a handful of countries responsible for 75% of this growth (Collaborators, 2024). In many nations with significant antimicrobial usage, the high rates of self-medication and the availability of antimicrobials over the counter are of particular concern (Ortiz et al., 2019). In addition to variations in antibiotic resistance rates, there can be differences in the prevalence of the infection within and across different topographical regions within a country (Jiang et al., 2022).
Within the context of H. pylori eradication therapy, the results of a recent meta-analysis study released in 2018 show that the rates of primary and secondary resistance to levofloxacin, metronidazole, and clarithromycin have already reached alarming levels (>15%) in nearly all WHO areas (Shah et al, 2021). This has led to the failure rate of triple therapy rising to more than 20% in many regions of the world (Shah et al, 2021). Considering the poor prognosis of gastric cancer and the significant morbidity and costs associated with earlier stages of H. pylori pathologies (such as peptic ulcer disease), there is a critical need to create novel therapeutic approaches to combat antimicrobial resistance in H. pylori. Even though H. pylori has been excluded from the most recent iteration of WHO’s priority pathogen list for urgent drug development, the report acknowledges the increasing complexity of treatment and associated adverse effects and failure rates, which necessitates a renewed focus by researchers and drug developers, alike.
Here in this review, we have discussed various animal models, including knock-out and transgenic models, available for the study of various stages of H. pylori pathophysiology (Figure 1). We have further emphasized the ex vivo models such as gastric and intestinal organoids along with their advantages and limitations that can be a valuable addition to the resources that can be utilized for the development of novel therapeutics and vaccine candidates.
Animal models
While animal models have been instrumental in identifying some of the key features of H. pylori pathogenesis that underpins chronic gastritis and the development of ulcers, the exact triggers for gastric carcinogenesis remain under investigation (Ansari and Yamaoka, 2022). The natural history of infection in animals is unknown, and H. pylori does not readily infect the gastric mucosa of animals, despite the fact that it is well adapted to colonize the human stomach (Go, 2002). Investigations during early infection in humans are often hindered by the natural pathophysiology of the disease as gastric cancer takes decades to develop due to the complicated interactions between H. pylori and the stomach epithelium (Correa and Piazuelo, 2008). The pathogenesis of H. pylori infection and the immunological responses brought on by this bacterium have been difficult to ascertain due to the close co-evolution of this pathogen with its human host. However, animal models must be used in order to fully understand the role of host’s microenvironment in various H. pylori-induced disease states, including gastric cancer. These models have indeed been very helpful in understanding the pathophysiology of H. pylori colonization (Peek, 2008) with an emphasis on the comprehension of host immune responses and the natural history of infection (Figure 2). Animals like pigs, rodents, mice, Mongolian gerbils, and guinea pigs have all been mentioned as potential reservoirs for H. pylori (Peek, 2008). Thus, appropriate animal models must be used to enable a thorough understanding of the role of the host’s microenvironment that sustains and drives H. pylori-induced inflammation and gastric cancer. Although these animal models have been beneficial in understanding the host, bacterial, and environmental variables involved in gastritis and gastric carcinogenesis, no single model stands as the definitive standard for mimicking natural human infection (Table 1).
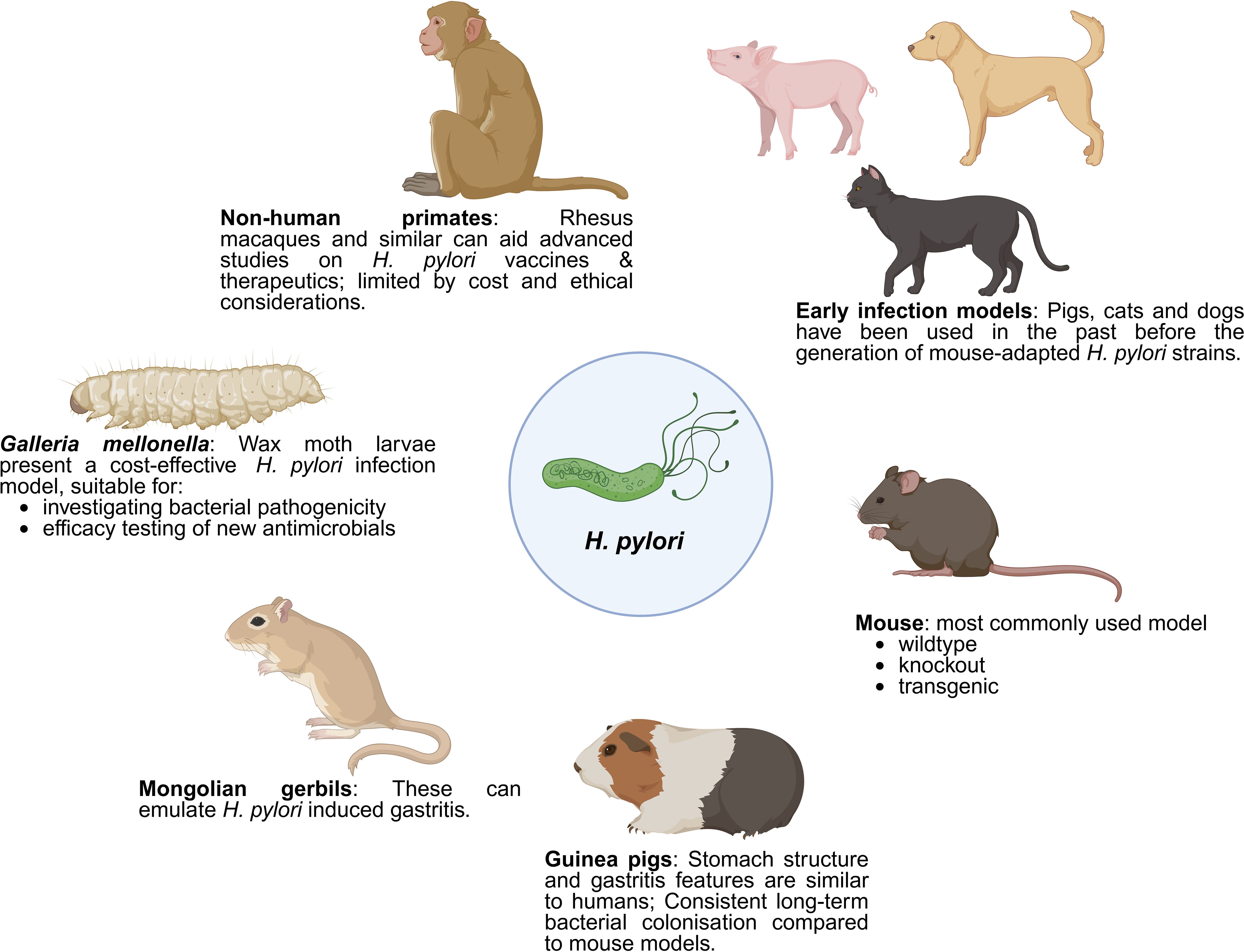
Figure 2. Various in vivo models for the study of H. pylori pathogenesis. This figure illustrates various animal models employed in the study of H. pylori infection and its pathogenesis. Early infection models, including pigs, cats, and dogs, were initially used before the development of mouse-adapted H. pylori strains. Mice remain the most commonly used model, available in wildtype, knockout, and transgenic varieties. Mongolian gerbils are effective in emulating H. pylori-induced gastritis, while guinea pigs, with stomach structures and gastritis features similar to humans, allow for consistent, long-term bacterial colonization studies. Non-human primates, such as rhesus macaques, are useful for advanced studies on H. pylori vaccines and therapeutics, despite limitations due to cost and ethical considerations. Wax moth larvae (Galleria mellonella) present a cost-effective infection model, suitable for investigating bacterial pathogenicity and testing new antimicrobials. Created with BioRender.
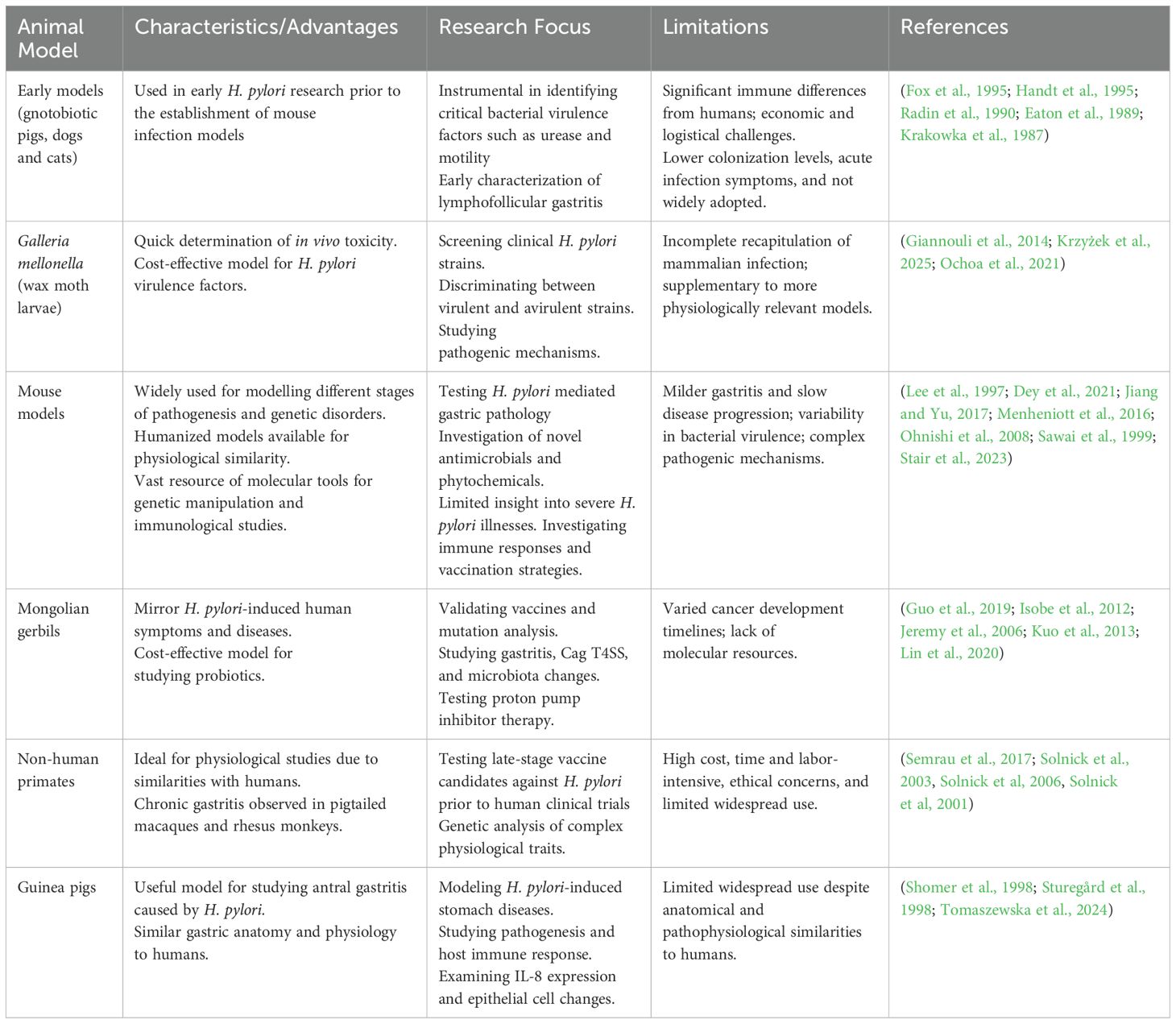
Table 1. Summary of different in vivo models used to study H. pylori pathogenesis with its advantages, limitations and applications in research.
Early animal models
Gnotobiotic piglets were probably the first animals to undergo successful infection with H. pylori (Lambert et al., 1987; Krakowka et al., 1987; Eaton et al, 1989). While these animals have been instrumental in studying the role of urease and motility in the mediation of H. pylori infection induced pathology, key immune features observed in this model can vary significantly from humans. Despite its early applications, researchers have moved away from using this model, likely due to economic limitations and the need for specialized breeding and experimental facilities.
Beagle dogs, both gnotobiotic pups and conventional animals, have been investigated for experimental H. pylori infection. Gnotobiotic pups, infected at 7 days of age, showed successful H. pylori colonization for at least a month post-infection, albeit at a lower level compared to humans (Radin et al., 1990). Gastric lesions were observed upon gross examination with microscopic evidence of immune cell infiltration into the gastric lamina propria. Antibodies specific to H. pylori were developed in conventional beagle dogs that were infected between four and six months of age and observed for up to 24 weeks (Rossi et al., 1999). Animals with an acute infection experienced vomiting and diarrhea. This was followed by polymorphonuclear cell infiltration and subsequent development of gastritis with epithelial changes linked to the development of MALT lymphoma in humans. Even though the pathophysiology of H. pylori infection in dogs closely resembles that of humans, this model has not been adopted more widely. Similarly, there are not many reported studies on feline infections. The initial descriptions of feline H. pylori infection demonstrated the development of lymphofollicular gastritis (Fox et al., 1995; Handt et al., 1995). According to a vaccination study, cats that received the urease antigen orally were protected against the H. pylori challenge (Handt et al., 1995). However, there have not been wider studies with this host species other than reports of isolation and characterization of non-H. pylori Helicobacter isolates.
Galleria mellonella larvae
Insects can be employed to quickly determine in vivo toxicity and efficacy of novel antimicrobial compounds, which makes it possible to conduct more targeted mammalian testing. Galleria mellonella is a wax moth present throughout the world and its larvae have been used as animal model in several research studies for over two decades (Tsai et al, 2016). The larvae of G. mellonella are being utilized more frequently as miniature hosts to study the pathogenesis and virulence components of many bacterial and fungal human pathogens from humans despite the fact that they do not recapitulate all elements of mammalian infection. This has the following benefits: i) adaptation to the human physiological temperature (37°C), ii) presence of a well-characterized phagocytic system, and iii) availability of a comprehensive transcriptome and immune gene repertoire. Several pathogens, including Pseudomonas aeruginosa, Staphylococcus aureus, Acinetobacter baumannii, Klebsiella pneumoniae, and Campylobacter jejuni, have been shown to be pathogenic in G. mellonella larvae (Tsai et al, 2016). Due to their susceptibility to H. pylori infection and relatively lower thresholds of legal or ethical considerations, G. mellonella larvae have been proposed as a simple in vivo model for the study of H. pylori virulence factors and pathogenic pathways (Giannouli et al., 2014). The experimental paradigm that has been developed can be helpful for screening a relatively large number of clinical H. pylori strains and for correlating the disease state of patients with the virulence of these strains (Giannouli et al., 2014). Recently this model was utilized to demonstrate biofilm formation by multidrug resistant H. pylori strain during its exposure to stress caused by clarithromycin (Krzyżek et al., 2025). The G. mellonella larval infection model recapitulates important aspects of H. pylori pathophysiology and is cost-effective in comparison to mammalian infection models. While it could be useful for the initial evaluation of the impact of H. pylori virulence parameters factors on particular cellular functions, it is unable to completely substitute the well-established and more physiologically relevant in vivo models in the analysis of the complex pathogenic mechanisms underlying H. pylori-related human disease (Giannouli et al., 2014; Ochoa et al., 2021) and the efficacy of therapeutics (Almeida Furquim de Camargo et al., 2020). In summary, the G. mellonella model may lessen reliance on mammalian infection models based on its ability to differentiate between virulent and non-virulent H. pylori isolates, determine putative genes associated with virulence through genome-wide association studies and identify novel molecular targets for antimicrobial therapy.
Mouse models
Mice have been a crucial model organism to study different human infectious diseases and genetic disorders. The predominantly used wildtype mouse strains in H. pylori infection are C57BL/6 and BALB/c, followed by less commonly used Swiss albino, ICR and Kunming mice (Ansari and Yamaoka, 2022; Wei et al., 2019; Kimura et al., 1998). These models are used to study the effects of H. pylori colonization and to test the efficacy of different antimicrobials, phytochemicals, and probiotics. The bacterial strain used to infect these mice presents another important facet to the infection as mice do not get colonized by H. pylori as readily as humans (Salama et al, 2013). It is interesting to note that before H. pylori infection models were developed, mouse infection models and vaccination trials extensively employed a feline Helicobacter isolate named H. felis. H. felis lacks the cag pathogenicity island and produces severe atrophic gastritis in C57BL/6 mice, but only mild disease in BALB/c mice (Zhang and Moss, 2012). In 1997, Lee et al., introduced the first standardized mouse infection model of H. pylori with the Sydney strain SS1 derived from an individual with duodenal ulcers (Lee et al., 1997). The original strain was cag+ vac+ (PMSS1) but underwent modification during experimental infection to yield the type 4 secretion system (T4SS) deficient SS1 strain widely used in infection studies nowadays. The parental PMSS1 strain is often used to study the effect of a functional cagPAI system (Arnold et al., 2011) although it has been reported that this genetic island is susceptible to genetic rearrangement and disruption leading to variability in bacterial virulence features (Dyer et al., 2018). Overall, H. pylori infection in wildtype mice frequently results in milder gastritis or slowly progressive illnesses, and these models offer less insight into the toxicity of clinical H. pylori strains. Furthermore, while these wildtype mice infected with H. pylori and H. felis develop lymphocytic gastritis, they generally do not advance to more severe conditions such as peptic ulcers or stomach cancer (Zhang and Moss, 2012).
To compensate for the deficiencies of the wildtype mouse models, several knockout and transgenic mice have been developed over the years. These have incrementally paved the way for the identification of critical host factors implicated in H. pylori pathogenesis and the development of gastric cancer. Some examples are reviewed below.
H. pylori infection-induced gastric inflammation is largely mediated by IFN-gamma and double-knockout mice have been demonstrated to allow longer colonization by strains that are unable to infect wildtype mice (Sawai et al., 1999). Chronic infection is typically associated with significantly lower inflammation in this model which has been used for many vaccine studies. Similar observations have been made in mice deficient in TNF-alpha signaling (Yamamoto et al., 2004).
H. pylori infection has a synergistic effect on the development of gastric cancer in individuals with IL-1β gene polymorphisms with the most severe gastric pathology being observed in patients with both host and bacterial high-risk genotypes (El-Omar et al., 2000). IL-1 receptor knockout mice have lower gastritis scores and associated pathology markers such as nitric oxide production (Huang et al., 2013). Gastrokine-2 is an anti-inflammatory protein produced in the gastric epithelium and its deletion drives premalignant gastric inflammation and tumor progression in mice that is accelerated by H. pylori infection (Menheniott et al., 2016). Furthermore, GKN-2 expression is progressively lost during the progression of gastric cancer, and it plays a causal role in its development (Chung Nien Chin et al., 2020). During early H. pylori infection, Fas-antigen mediated apoptosis depletes gastric parietal and chief cells which are then replaced by metaplastic glandular lineages resistant to Fas-apoptosis. This has been modelled in Fas antigen–deficient (lpr) mice that develop invasive stomach lesions post H. pylori infection (Cai et al., 2005).
Transgenic mice are genetically engineered mice that harbor gene insertions from different species. Humanized insulin/Gastrin (INS-GAS) transgenic mice are frequently used to model stomach cancer as they have high circulating levels of pancreatic gastrin (Jiang and Yu, 2017). These mice spontaneously develop atrophic gastritis and intestinal metaplasia which then progress to corpus-centric cancer. PMSS1 infection in IN-GAS mice results in invasive carcinoma whereas SS1 infection causes only dysplasia (Lofgren et al., 2011; Stair et al., 2023), thus demonstrating the importance of bacterial factors in determining disease progression. Transgenic expression of H. pylori CagA in mice has been shown to induce gastrointestinal and hematopoietic neoplasms (Ohnishi et al., 2008).
Mongolian Gerbils
Mongolian gerbils are small rodents that show similar symptoms to humans, such as appetite and weight loss, and recount many attributes of H. pylori-induced gastric colonization, inflammation, ulcers, and cancers (Noto et al., 2016). After it was discovered that Mongolian gerbils can mirror several characteristics of H. pylori-induced human stomach inflammation and cancer, these rodents captured considerable interest and attracted a lot of attention, particularly for vaccine studies (Jeremy et al., 2006; Guo et al., 2019; Lv et al., 2014; Noto et al., 2016; Wu et al., 2008). However, the timeline for cancer development varies greatly between studies and appears to be contingent on the infecting H. pylori strain. Gerbil infection by a modified H. pylori strain with an inducible T4SS has demonstrated that while an infectious trigger can be instrumental for cancer development, cancer progression does not necessarily depend on the persistent presence of the infectious agent (Lin et al., 2020). Comparative genomic studies in this model have shown that the genomes of three strains recovered from infected gerbil stomachs showed mutations in babA, tlpB, and gltS genes, all of which are linked to host adaptation (Suzuki et al., 2019).
This model has also been used in the study of probiotics in H. pylori eradication (Kuo et al., 2013; Zheng et al., 2024). Probiotics have been shown to inhibit H. pylori growth, adhesion, and the production of virulence factors in vitro. In the gerbil infection model, probiotics have been demonstrated to prevent the colonization of H. pylori (Zheng et al., 2024; Kuo et al., 2013; Isobe et al., 2012). This model has also demonstrated that the presence of H. pylori and related inflammation can alter microbiome composition in the non-inflamed regions of the gastrointestinal tract (Heimesaat et al., 2014). While the Mongolian gerbil infection model has been instrumental in dissecting the critical determinants mediating host-pathogen interactions during H. pylori infection, the lack of molecular resources has stymied its wider application within the research community.
Guinea pigs
The guinea pig stomach structure is akin to that of humans with a dietary requirement for vitamin C like humans and other primates. H. pylori infection in this model, first described in the late 1990’s, produces an inflammatory response driven by IL-8 secretion by gastric epithelial cells (Sturegård et al., 1998; Kleanthous et al, 1998). Infections with either the rodent adapted SS1 strain or clinical H. pylori isolates from gastric biopsies were able to establish lasting colonization and induce seroconversion in infected animals. Chronic infection was associated with the development of antral gastritis and lymphoid follicles like that of H. pylori-infected humans. Epithelial cell proliferation as evidenced by the presence of a large population of Ki67-positive gastric cells, is readily observed in this model (Gonciarz et al., 2019). This model has been used in a recent study delineating the role of H. pylori in driving metabolic syndrome (Tomaszewska et al., 2024). Despite the similarities with human gastric anatomy and H. pylori related pathophysiology, this model has not been widely used.
Non-human primates
Non-human primates, such as macaques, can be a suitable model for H. pylori infections due to their physiological and anatomical similarities with humans. Rhesus macaques (Macaca mulatta) acquire H. pylori in the stomach mucosa and experience chronic gastritis (Solnick et al., 2003, Solnick et al., 2001) and glandular atrophy, the precursor to stomach cancer (Dubois et al., 1999). However, it remains unclear if macaques naturally harbor H. pylori and act as a reservoir in wildlife or whether they acquire the bacteria upon contact with humans, after capture (Solnick et al., 2003, Solnick et al., 2006). Non-human primates are mostly used in testing vaccines against H. pylori (Lee, 2001). The genetic and physiological parallels between primates and humans make this model appealing and practical for research on H. pylori-related gastritis, but the high cost, time and labor requirements along with ethical considerations prevent its widespread use in Helicobacter research (Barnhill et al, 2016).
Non-animal (ex vivo) models
The preceding sections discuss various in vivo infection models that facilitate extended bacterial exposure in the host, proving crucial in identifying key factors driving H. pylori pathogenesis. However, these animal models do not precisely mirror the pathophysiological responses observed in humans (Table 1). Additionally, the large numbers of animals required have raised concerns about animal welfare and cost (Burkitt et al., 2017). In vitro studies using the widely available gastric cancer cell lines, on the other hand, do not have the complex cellular and architectural details of the intact gastric epithelium (Wagner et al., 1994; Birkness et al., 1996; Saberi et al., 2018). However, they do provide a more controlled environment for studying the intricate details of how hosts and pathogens interact.
Organoids are simplified, tissue-engineered in vitro model systems that replicate various features of the complex structures and function found in corresponding biological tissues (Chakrabarti and Zavros, 2020; Hofer and Lutolf, 2021; Idowu et al, 2022). Organoids can be generated from pluripotent or tissue-resident stem cells, whether embryonic or adult, or from differentiated cells isolated from healthy or diseased tissues (Hofer and Lutolf, 2021). More recently, organoids derived from primary cells have gained traction as suitable models for ex vivo studies, primarily due to their ability to closely mimic human gastrointestinal physiology. Recent advancements in gastric organoid models to study H. pylori infection could reduce an overdependence on ‘abnormal’ cancer cell lines and enhance the exploration of the initial stages of gastric carcinogenesis (Figure 3), thereby facilitating a deeper understanding of its pathogenesis (Idowu et al, 2022).
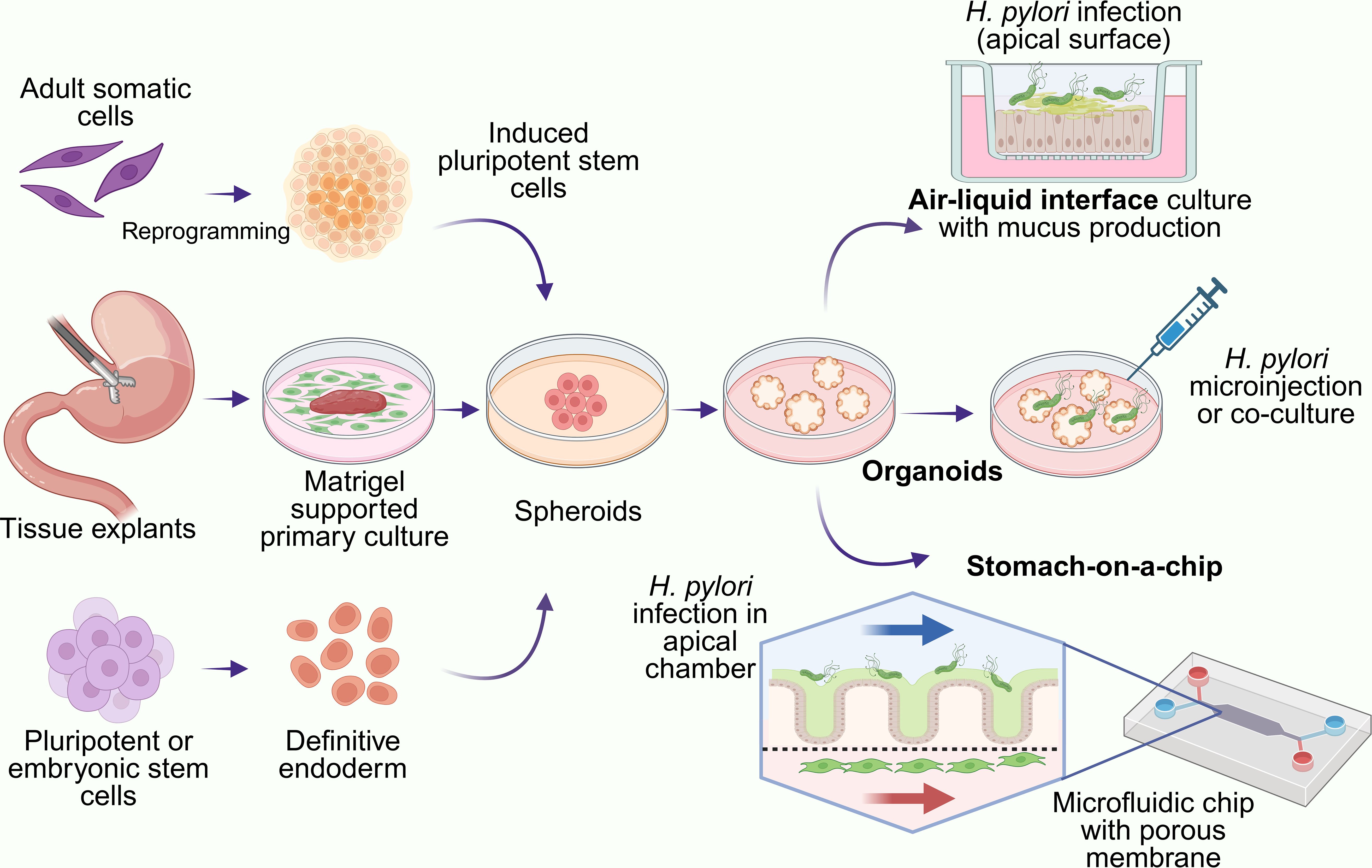
Figure 3. Organoid-based models of H. pylori infection. Gastric organoids can be derived from mouse or human tissue explants, adult somatic cells or stem cells. Created with BioRender.
Gastric organoids
Induced pluripotent stem cells (iPSC) are derived from adult somatic cells that undergo genetic reprogramming to attain a state akin to embryonic stem cells, achieved through the enforced expression of specific genes and factors crucial for maintaining pluripotency (Ye et al, 2013). Organoid models, a relatively recent advancement in three-dimensional (3D) cell cultivation systems, can be derived from iPSCs by controlled differentiation steps (Pellegrino and Gutierrez, 2021; Seidlitz et al, 2021). Gastric organoids can effectively mimic the cellular diversity and architectural complexity of the stomach, encompassing various epithelial cell types like mucous-secreting cells, chief cells, parietal cells, and enteroendocrine cells (Seidlitz et al, 2021). Given that the stomach serves as the primary site for H. pylori colonization and infection in humans, gastric organoids have emerged as invaluable tools for modeling infection and related gastric diseases such as cancer or ulcers (Ku et al., 2022). Compared to immortalized gastric cancer cell lines, organoid cultures offer a closer recapitulation of in vivo conditions, particularly in studying interactions between H. pylori and the apical-junctional complex (Uotani et al., 2019).
Modeling H. pylori infection in gastric organoids
Upon infection, gastric organoids recapitulate key aspects of H. pylori-induced diseases, including inflammation, epithelial damage, and dysregulation of tissue homeostasis (Idowu et al, 2022; Jeong et al., 2023; Seidlitz et al, 2021). A study by McCracken et al. demonstrated that microinjection of H. pylori into human iPSC-derived gastric organoids led to enhanced epithelial cell proliferation (McCracken et al., 2011). This finding further implied that H. pylori infection stimulates the proliferation of gastric epithelial cells, which might contribute to tissue repair mechanisms or pathological changes associated with chronic infection. During infection, CagA was injected into organoid cells via the T4SS and phosphorylated CagA bound to the Src homology 2 (SH2) domain-containing tyrosine phosphatase 2 (SHP-2) (Higashi et al, 2002). Such binding resulted in the activation of the Ras-extracellular signal-regulated kinase (Erk) signaling pathway, promoting cell proliferation, migration and survival. A similar study provided insights into the role of the Cag-SHP-2 interaction in H. pylori-induced gastric carcinogenesis using a similar gastric organoid model (Higashi et al, 2002).
Organoids infected with H. pylori exhibit alterations in glandular morphology, release of pro-inflammatory cytokines, and activation of signaling pathways associated with host defense and immune responses (Aguilar et al., 2022; Chakrabarti et al., 2021). Gastric organoid models have provided insights into the mechanisms underlying host-pathogen interactions during H. pylori infection, including bacterial adhesion, invasion, and modulation of host cell signaling pathways (Chakrabarti et al., 2021; Chakrabarti and Zavros, 2020). Due to the fact that organoids can be infected with H. pylori either by direct exposure to the bacterium or by incubation with specific bacterial components, gastric organoids have emerged as valuable tools for studying H. pylori infection and its pathogenesis.
Intestinal organoids
Intestinal organoids, also known as enteroids or colonoids, are derived from intestinal stem cells and mimic the cellular composition and architecture of the intestine (Lee et al., 2018; Mahe et al., 2013; Ohki et al., 2020). Although H. pylori primarily infects the stomach, studies suggest that it can transiently colonize the duodenum and colon (Fujimori, 2021). A model of mouse intestinal organoids generated from isolated intestinal crypts demonstrated the role of gastric hormones in inflammation and repair thus showing that endocrine cells within these organoids closely resemble those in the gut (Ohki et al., 2020). Given the roles played by gastric hormones in H. pylori-associated disease manifestation, intestinal organoids can be infected with H. pylori to study its interaction with the intestinal epithelium and investigate potential extra-gastric effects of the infection (Becher et al., 2010; Walduck and Becher, 2012) including alterations in gut microbiota composition and gastrointestinal symptoms such as diarrhea and irritable bowel syndrome (Tan and Goh, 2012; Tohumcu et al., 2024; Cui et al., 2025).
Co-culture of organoid and immune cells
To recapitulate the host immune response to H. pylori infection, researchers can co-culture organoids with immune cells such as macrophages, dendritic cells, and T cells. Co-culture systems grow stomach organoids with immune cells from the same host. This helps us learn more about the roles that innate and adaptive immune cells play in H. pylori infection (Idowu et al., 2022). Sebrell et al., 2019, investigated the recruitment of dendritic cells during H. pylori infection using human gastric organoids co-cultured with monocyte-derived dendritic cells and cataloged the various chemokines that were expressed in response to infection (Sebrell et al., 2019). The effect of H. pylori infection on cytokine production by innate immune cells using co-cultured gastric organoids and macrophages from infected mice has been studied as well (Suarez et al., 2019). These studies revealed a remarkable increase in cytokine production in Nod1-deficient cells, particularly when both macrophages and organoids lacked Nod1, suggesting that functional Nod1 suppresses cytokine production (Suarez et al., 2019).
Additionally, changes in mucin and antimicrobial peptide expression resulting from activation of innate and adaptive immunity have been investigated in these models. The bactericidal activity of mucus in a two-dimensional mucosoid culture model was demonstrated where it also acts as a physical barrier against H. pylori attachment (Boccellato et al., 2019). A co-culture of mucosoid organoids offers the potential to explore the effects of CD4+ T cell subsets and innate lymphoid cells on epithelial antimicrobial activity and offers opportunities to generate vaccinated organoids to test specific cytokines or hormone’s roles in protective responses as well as its associated mechanisms. Research employing co-culture organoids containing immune cells from the same host sheds light on the interaction between H. pylori and the host immune system during infection and advances our knowledge of the immunopathogenesis of diseases brought on by H. pylori.
Organoid-on-a-chip
Patient and stem cell derived gastrointestinal organoids have become an important tool for the study of H. pylori associated pathophysiology. Further advancements in these models have concentrated on integrating microfluidics to regulate the movement of cells, signaling molecules, and physical stimuli through channels and membranes that are bolstered by an extracellular matrix (ECM) constituent (Moses et al., 2021). These additional features introduce a significant layer of complexity, enhancing the resemblance of these models to the native structure and function of the stomach. The stomach-on-a-chip model is based on a sandwich’ structure that consists of two microchannels divided by a porous membrane to simulate the mucosal and basal surfaces of the stomach epithelium. Jeong et al., 2023 have described a human stomach micro-physiological system (hsMPS)-on-a-chip with epithelial cells derived from human antral organoids and primary mesenchymal stromal cells extracted from stomach tissue, co-cultivated under controlled flow (Jeong et al., 2023). This model recreated the maturation of gastric epithelial cells, leading to the formation of a mesh‐like mucus layer with mucus protective peptides and functional epithelial junctional complexes that exhibited gastroprotective effects against H. pylori. Although this model has been proposed as a platform for evaluating the antibacterial drug candidates, the porous membrane used for barrier separation can be prone to non-specific absorption of small molecules which can hinder the study. Another elegant model of the human stomach-on-a-chip demonstrated long-term growth of cannulated gastric organoids with biochemical agents delivered through the lumen using a peristaltic pump (Lee et al., 2018). This system recreated the rhythmic stretch and contraction of the organoid, reminiscent of gastric motility. Overall, with recent advances in stem cell technology and 3D matrices for supporting organotypic cultures, there is significant potential for these ex vivo models in identifying and validating novel therapeutic and vaccine targets (Li et al., 2022), while reducing an over-reliance on animal models.
Advantages and limitations
Animal models
The use of animal models has greatly advanced our understanding of the critical determinants of H. pylori pathology and factors involved in mediating host response to the infection, particularly the many drivers of the different phases leading to the development of gastric cancer (Ansari and Yamaoka, 2022). These models have also enabled the testing of many antimicrobials and vaccines over the years. Although early research on H. pylori infection was conducted mostly on non-rodent models, the isolation and generation of the mouse-adapted H. pylori strain has made the mouse model the cornerstone of H. pylori infection studies. However, there are several limitations to using mice as a surrogate for human pathophysiology and anatomy. Mouse metabolic processes are much faster compared to humans, and they can tolerate higher doses of most administered substances due to a quicker rate of kidney filtration and excretion (Sharma and McNeill, 2009). Furthermore, the mouse stomach is quite distinct from the human organ. Mice have a squamous glandular epithelium rather than the oxyntic type found in humans and typically display gastritis features within the stomach corpus as opposed to the antral gastritis observed more typically in humans, following H. pylori infection. Stomach cancer is also not easily modeled in this host without interfering with the expression of specific genes. Mongolian gerbils and guinea pigs have been suggested as alternatives, but they have not had the same success as the mouse models, perhaps due to the lack of molecular resources and relevant expertise.
Non-animal models
In recent years, the complexity of non-animal models, particularly 3D organoids, has increased tremendously, putting these at par with traditional animal models. In some instances, human organoids are superlative to animal models with the added benefit of being more efficient and cost-effective. The 3Rs’ (replace, reduce and refine) objectives that underpin the ethical frameworks governing animal research in most countries today can be complemented by an increased reliance on non-animal models in the coming years. These models can further encompass in silico simulations, which can inform subsequent in vitro or animal studies, as well as advanced 2D/3D cell cultures and ex vivo tissue explant cultures. This review focuses specifically on the emerging popularity and application of 3D organoid models within the H. pylori research community.
Organoids derived from genetically modified stem cells or patient-derived iPSCs allow for the investigation of host genetic factors influencing susceptibility to H. pylori infection and disease outcomes. Current iPSC derived gastric organoids closely resemble the cellular composition and architecture of the stomach. Technological advances have made it possible to generate adult stem cell-derived organoids from tissues obtained from a healthy or diseased donor (Pellegrino and Gutierrez, 2021). Donor-matched organoids have diverse applications in the development of precision medicine, particularly for gastric cancer, which continues to have a poor five-year survival rate. Furthermore, gastric organoids can be adapted for high-throughput screening assays to identify novel therapeutics targeting H. pylori infection and associated diseases (Du et al., 2020; Lukonin et al, 2021; Li et al., 2022). Organoid-based screening platforms enable the evaluation of drug efficacy, toxicity, and mode of action in a scalable and cost-effective manner. The added dimension of immune cell co-culture allows for the investigation of both innate and adaptive immune responses to H. pylori infection, providing a more detailed understanding of host-pathogen interactions. This approach permits the study of immune cell recruitment, activation, and function within the context of the gastric or intestinal epithelium during H. pylori infection and provides opportunities to study vaccine-induced immune responses against H. pylori.
Obvious limitations of these models are related to the availability and accessibility to the organoid model of interest besides the difficulties pertaining to the optimization of culture conditions and the reproducibility of experimental results (Eicher et al, 2018; Pang et al., 2022). Adult stem cells and iPSCs from genetically and phenotypically well-characterized donor pools may be difficult to source. Culture media for any organoid must be carefully optimized to support the growth and differentiation of stem cells while maintaining the physiological relevance of the model (Sato et al., 2009). Achieving the appropriate balance of growth factors, signaling molecules, and nutrients is crucial for the long-term maintenance of organoid cultures, while also avoiding the common pitfall of batch variation in media components. The composition and stiffness of the extracellular matrix (ECM) used for embedding gastric organoids can influence their growth, differentiation, and functionality. Optimization of ECM components, such as Matrigel® or synthetic hydrogels, is necessary to better mimic the native gastric microenvironment (Pang et al., 2022). Furthermore, most organoids lack vasculature which can lead to necrosis of underlying cells and hypoxia-induced stress responses unrelated to infection pathophysiology.
Summary
Ever since the association between H. pylori infection and the development of gastritis, ulcers, and ultimately gastric cancer was first identified, considerable progress has been made in understanding the complex interplay between bacterial, host, and environmental factors that affect the course of disease. Currently, clinical presentations of H. pylori related gastritis and ulcers can be effectively managed with triple or quadruple antimicrobial therapy in most cases. However, the rising emergence of antibiotic resistance among H. pylori clinical isolates is a grave concern and gastric cancer remains a leading cause of cancer-related deaths. Despite decades of efforts and the continually elevated prevalence of the bacteria in developing parts of the worldwide, there is still no vaccine available against H. pylori. The eradication of H. pylori remains a key issue because of several factors. The emergence of antibiotic-resistant strains, complex treatment regimens combined with poor compliance, high infection and reinfection rates, and limitations in diagnostic methods all contribute to the difficulty in successfully achieving bacterial eradication. Additionally, host factors such as immune response and genetic predisposition influence the treatment outcomes. Consequently, further research is imperative to develop novel antibiotics, identify biomarkers for early detection and treatment prediction, and explore non-antibiotic therapies.
Animal models play a crucial role in advancing our understanding of H. pylori pathogenesis, testing new drugs, and developing vaccines. They aid in the translation of preclinical research into clinical practice by offering insights into host-pathogen interactions, bacterial virulence, and the safety and effectiveness of possible treatments. This review has summarized advanced models available to researchers for investigating new therapeutics and vaccines against H. pylori, addressing a significant unmet need, particularly in regions where H. pylori is endemic. Studies in animal models have been instrumental in dissecting the correlates of protection and make the case for targeting H. pylori mediated inflammation to prevent or treat the pathological outcomes rather than aiming for complete eradication of the bacteria (Sarkar et al., 2023). Despite the lack of progress on H. pylori vaccines due to its complex relationship with the human host, mouse and non-human primate models remain invaluable resources for further studies. On the other hand, the precise environments for studying H. pylori infection and pathogenesis afforded by ex vivo models support high-throughput screening of therapeutics while reducing the reliance on animal testing and adhering to ethical principles. Due to their advantages over conventional in vitro procedures in terms of polarization, longevity, amenability, and accessibility, the use of gastric organoids has increased our understanding of H. pylori infection. Consequently, previously unattainable in other models, key features of chemotaxis, the intracellular effects of H. pylori virulence factors, interactions with the apical-junctional complex, innate immune activation, and the initiation of inflammation by H. pylori have been unraveled using gastric organoids.
Future perspectives
Future research on H. pylori infection and its associated diseases should include both animal and non-animal systems, to address current challenges and unserved needs. The development of novel therapeutics is vitally important, particularly in view of the increasing prevalence of antibiotic-resistant H. pylori strains. Researchers should focus on identifying new antimicrobial compounds and exploring alternative therapeutic strategies, such as bacteriophage therapy, antimicrobial peptides, and host-targeted approaches. The assimilation of precision medicine, using donor-matched organoids and advanced ex vivo models, can facilitate personalized treatment regimens and improve therapeutic outcomes. These models can also aid in high-throughput screening of potential therapeutics, accelerating the discovery and development process. Furthermore, considering the increasing numbers of aging populations around the world, the identification of biomarkers for early detection of gastric cancer and prediction of treatment response will be crucial in reducing the associated mortality. Therefore, continued collaboration between multidisciplinary research teams and the integration of cutting-edge technologies will be essential for overcoming the persistent challenges in H. pylori eradication and improving global health outcomes in the coming decades.
Author contributions
SP: Data curation, Writing – original draft, Writing – review & editing. SY: Data curation, Writing – original draft, Writing – review & editing. RJ: Conceptualization, Data curation, Supervision, Writing – review & editing. VR: Conceptualization, Data curation, Supervision, Writing – original draft, Writing – review & editing. SS: Conceptualization, Data curation, Resources, Supervision, Visualization, Writing – review & editing.
Funding
The author(s) declare that financial support was received for the research and/or publication of this article. Contributions by SP, RJ and VR are supported by the Science & Engineering Research Board (SERB), Department of Science and Technology (DST), Government of India, (GOI) New Delhi (SRG/2022/001512).
Acknowledgments
The authors acknowledge the infrastructural support extended by Amity University Maharashtra, Mumbai, Maharashtra, India. SS and SY acknowledge the infrastructure support received from the Murdoch Children’s Research Institute and The University of Melbourne.
Conflict of interest
The authors declare the research was conducted in the absence of any commercial or financial relationships that could be construed as a potential conflict of interest.
The author(s) declared that they were an editorial board member of Frontiers, at the time of submission. This had no impact on the peer review process and the final decision.
Generative AI statement
The author(s) declare that no Generative AI was used in the creation of this manuscript.
Publisher’s note
All claims expressed in this article are solely those of the authors and do not necessarily represent those of their affiliated organizations, or those of the publisher, the editors and the reviewers. Any product that may be evaluated in this article, or claim that may be made by its manufacturer, is not guaranteed or endorsed by the publisher.
References
Aguilar, C., Pauzuolis, M., Pompaiah, M., Vafadarnejad, E., Arampatzi, P., Fischer, M., et al. (2022). Helicobacter pylori shows tropism to gastric differentiated pit cells dependent on urea chemotaxis. Nat. Commun. 13, 5878 doi: 10.1038/s41467-022-33165-4
Aihara, E., Closson, C., Matthis, A. L., Schumacher, M. A., Engevik, A. C., Zavros, Y., et al. (2014). Motility and chemotaxis mediate the preferential colonization of gastric injury sites by Helicobacter pylori. PloS Pathog 10, e1004275. doi: 10.1371/journal.ppat.1004275
Almeida Furquim de Camargo, B., Soares Silva, D. E., Noronha da Silva, A., Campos, D. L., MaChado Ribeiro, T. R., Mieli, M. J., et al. (2020). New Silver(I) Coordination Compound Loaded into Polymeric Nanoparticles as a Strategy to Improve In Vitro Anti-Helicobacter pylori Activity. Mol. Pharm. 17, 2287–2298. doi: 10.1021/acs.molpharmaceut.9b01264
Ansari, S., Yamaoka, Y. (2022). Animal models and helicobacter pylori infection. J. Clin. Med. 11. doi: 10.3390/jcm11113141
Arnold, I. C., Lee, J. Y., Amieva, M. R., Roers, A., Flavell, R. A., Sparwasser, T., et al. (2011). Tolerance rather than immunity protects from Helicobacter pylori-induced gastric preneoplasia. Gastroenterology 140, 199–209. doi: 10.1053/j.gastro.2010.06.047
Asante, M., Ahmed, H., Patel, P., Davis, T., Finlayson, C., Mendall, M., et al. (1997). Gastric mucosal hydrophobicity in duodenal ulceration: role of Helicobacter pylori infection density and mucus lipids. Gastroenterology 113, 449–454. doi: 10.1053/gast.1997.v113.pm9247463
Aumpan, N., Issariyakulkarn, N., Mahachai, V., Graham, D., Yamaoka, Y., Vilaichone, R. K. (2023). Management of Helicobacter pylori treatment failures: A large population-based study (HP treatment failures trial). PloS One 18, e0294403. doi: 10.1371/journal.pone.0294403
Barnhill, A., Joffe, S., Miller, F. G. (2016). The ethics of infection challenges in primates. Hastings Cent Rep. 46, 20–26. doi: 10.1002/hast.2016.46.issue-4
Becher, D., Deutscher, M. E., Simpfendorfer, K. R., Wijburg, O. L., Pederson, J. S., Lew, A. M., et al. (2010). Local recall responses in the stomach involving reduced regulation and expanded help mediate vaccine-induced protection against Helicobacter pylori in mice. Eur. J. Immunol. 40, 2778–2790. doi: 10.1002/eji.200940219
Birkness, K. A., Gold, B. D., White, E. H., Bartlett, J. H., Quinn, F. D. (1996). In vitro models to study attachment and invasion of Helicobacter pylori. Ann. N Y Acad. Sci. 797, 293–295. doi: 10.1111/j.1749-6632.1996.tb52983.x
Boccellato, F., Woelffling, S., Imai-Matsushima, A., Sanchez, G., Goosmann, C., Schmid, M., et al. (2019). Polarised epithelial monolayers of the gastric mucosa reveal insights into mucosal homeostasis and defence against infection. Gut 68, 400–413. doi: 10.1136/gutjnl-2017-314540
Brawner, K. M., Morrow, C. D., Smith, P. D. (2014). Gastric microbiome and gastric cancer. Cancer J. 20, 211–216. doi: 10.1097/PPO.0000000000000043
Bray, F., Laversanne, M., Sung, H., Ferlay, J., Siegel, R. L., Soerjomataram, I., et al. (2024). Global cancer statistics 2022: GLOBOCAN estimates of incidence and mortality worldwide for 36 cancers in 185 countries. CA Cancer J. Clin. 74, 229–263. doi: 10.3322/caac.21834
Brown, L. M. (2000). Helicobacter pylori: epidemiology and routes of transmission. Epidemiol. Rev. 22, 283–297. doi: 10.1093/oxfordjournals.epirev.a018040
Burkitt, M. D., Duckworth, C. A., Williams, J. M., Pritchard, D.M. (2017). Helicobacter pylori-induced gastric pathology: insights from in vivo and ex vivo models. Dis. Models Mech. 10, 89–104. doi: 10.1242/dmm.027649
Cai, X., Stoicov, C., Li, H., Carlson, J., Whary, M., Fox, J. G., et al. (2005). Overcoming Fas-mediated apoptosis accelerates Helicobacter-induced gastric cancer in mice. Cancer Res. 65, 10912–10920. doi: 10.1158/0008-5472.CAN-05-1802
Carron, M. A., Tran, V. R., Sugawa, C., Coticchia, J. M. (2006). Identification of Helicobacter pylori biofilms in human gastric mucosa. J. Gastrointest Surg. 10, 712–717. doi: 10.1016/j.gassur.2005.10.019
Celli, J. P., Turner, B. S., Afdhal, N. H., Keates, S., Ghiran, I., Kelly, C. P., et al. (2009). Helicobacter pylori moves through mucus by reducing mucin viscoelasticity. Proc. Natl. Acad. Sci. U S A 106, 14321–14326. doi: 10.1073/pnas.0903438106
Chakrabarti, J., Koh, V., So, J. B. Y., Yong, W. P., Zavros, Y. (2021). A preclinical human-derived autologous gastric cancer Organoid/Immune cell Co-culture model to predict the efficacy of targeted therapies. JoVE (Journal Visualized Experiments) 173, e61443. doi: 10.3791/61443
Chakrabarti, J., Zavros, Y. (2020).Generation and use of gastric organoids for the study of Helicobacter pylori pathogenesis in Methods in cell biology (Elsevier). Available online at: https://www.sciencedirect.com/science/article/abs/pii/S0091679X20300753.
Chen, Y. C., Malfertheiner, P., Yu, H. T., Kuo, C. L., Chang, Y. Y., Meng, F. T., et al. (2024). Global prevalence of helicobacter pylori infection and incidence of gastric cancer between 1980 and 2022. Gastroenterology 166, 605–619. doi: 10.1053/j.gastro.2023.12.022
Cheng, H. C., Yang, Y. J., Yang, H. B., Tsai, Y. C., Chang, W. L., Wu, C. T., et al. (2023). Evolution of the Correa’s cascade steps: A long-term endoscopic surveillance among non-ulcer dyspepsia and gastric ulcer after H. pylori eradication. J. Formos Med. Assoc 122, 400–410. doi: 10.1016/j.jfma.2022.11.008
Chey, W. D., Howden, C. W., Moss, S. F., Morgan, D. R., Greer, K. B., Grover, S., et al. (2024). ACG clinical guideline: treatment of helicobacter pylori infection. Am. J. Gastroenterol. 119, 1730–1753. doi: 10.14309/ajg.0000000000002968
Chung Nien Chin, S., O’Connor, L., Scurr, M., Busada, J. T., Graham, A. N., Alipour Talesh, G., et al. (2020). Coordinate expression loss of GKN1 and GKN2 in gastric cancer via impairment of a glucocorticoid-responsive enhancer. Am. J. Physiol. Gastrointest Liver Physiol. 319, G175–Gg88. doi: 10.1152/ajpgi.00019.2020
Cole, S. P., Harwood, J., Lee, R., She, R., Guiney, D. G. (2004). Characterization of monospecies biofilm formation by Helicobacter pylori. J. Bacteriol 186, 3124–3132. doi: 10.1128/JB.186.10.3124-3132.2004
Collaborators, GBD (2021). Antimicrobial Resistance. 2024. Global burden of bacterial antimicrobial resistance 1990-2021: a systematic analysis with forecasts to 2050. Lancet 404, 1199–1226. doi: 10.1016/S0140-6736(24)01867-1
Correa, P. (1992). Human gastric carcinogenesis: a multistep and multifactorial process–First American Cancer Society Award Lecture on Cancer Epidemiology and Prevention. Cancer Res. 52, 6735–6740.
Correa, P., Piazuelo, M. B. (2008). Natural history of Helicobacter pylori infection. Dig Liver Dis. 40, 490–496. doi: 10.1016/j.dld.2008.02.035
Cui, S., Liu, X., Han, F., Zhang, L., Bu, J., Wu, S., et al. (2025). Helicobacter pylori CagA+ strains modulate colorectal pathology by regulating intestinal flora. BMC Gastroenterol. 25, 54. doi: 10.1186/s12876-025-03631-6
Dey, T. K., Karmakar, B. C., Sarkar, A., Paul, S., Mukhopadhyay, A. K. (2021). A mouse model of helicobacter pylori infection. . Methods Mol. Biol. 2283, 131–151. doi: 10.1007/978-1-0716-1302-3_14
Du, Y., Li, X., Niu, Q., Mo, X., Qui, M., Ma, T. (2020). Development of a miniaturized 3D organoid culture platform for ultra-high-throughput screening. J. Mol. Cell Biol. 12, 630–643. doi: 10.1093/jmcb/mjaa036
Dubois, A., Berg, D. E., Incecik, E. T., Fiala, N., Heman-Ackah, L. M., Del Valle, J., et al. (1999). Host specificity of Helicobacter pylori strains and host responses in experimentally challenged nonhuman primates. Gastroenterology 116, 90–96. doi: 10.1016/S0016-5085(99)70232-5
Dyer, V., Brüggemann, H., Sörensen, M., Kühl, A. A., Hoffman, K., Brinkmann, V., et al. (2018). Genomic features of the Helicobacter pylori strain PMSS1 and its virulence attributes as deduced from its in vivo colonisation patterns. Mol. Microbiol 110, 761–776. doi: 10.1111/mmi.2018.110.issue-5
Eaton, K. A., Morgan, D. R., Krakowka, S. (1989). Campylobacter pylori virulence factors in gnotobiotic piglets. Infect. Immun. 57, 1119–1125. doi: 10.1128/iai.57.4.1119-1125.1989
Eicher, A. K., Berns, H.M., wells, j. M. (2018). Translating developmental principles to generate human gastricáOrganoids. Cell. Mol. gastroenterology hepatology 5, 353–363. doi: 10.1016/j.jcmgh.2017.12.014
Elbehiry, A., Marzouk, E., Aldubaib, M., Abalkhail, A., Anagreyyah, S., Anajirih, N., et al. (2023). Helicobacter pylori infection: current status and future prospects on diagnostic, therapeutic and control challenges. Antibiotics (Basel) 12(191). doi: 10.3390/antibiotics12020191
El-Omar, E. M., Carrington, M., Chow, W. H., McColl, K. E., Bream, J. H., Young, H. A., et al. (2000). Interleukin-1 polymorphisms associated with increased risk of gastric cancer. Nature 404, 398–402. doi: 10.1038/35006081
Fox, J. G., Batchelder, M., Marini, R., Yan, L., Handt, L., Li, X., et al. (1995). Helicobacter pylori-induced gastritis in the domestic cat. Infect. Immun. 63, 2674–2681. doi: 10.1128/iai.63.7.2674-2681.1995
Fujimori, S. (2021). Progress in elucidating the relationship between Helicobacter pylori infection and intestinal diseases. World J. Gastroenterol. 27, 8040–8046. doi: 10.3748/wjg.v27.i47.8040
Giannouli, M., Palatucci, A. T., Rubino, V., Ruggiero, G., Romano, M., Triassi, M., et al. (2014). Use of larvae of the wax moth Galleria mellonella as an in vivo model to study the virulence of Helicobacter pylori. BMC Microbiol 14, 228. doi: 10.1186/s12866-014-0228-0
Go, M. F. (2002). Review article: natural history and epidemiology of Helicobacter pylori infection. Aliment Pharmacol. Ther. 16 Suppl 1, 3–15. doi: 10.1046/j.1365-2036.2002.0160s1003.x
Gonciarz, W., Krupa, A., Hinc, K., Obuchowski, M., Moran, A. P., Gajewski, A., et al. (2019). The effect of Helicobacter pylori infection and different H. pylori components on the proliferation and apoptosis of gastric epithelial cells and fibroblasts. PloS One 14, e0220636. doi: 10.1371/journal.pone.0220636
Guo, L., Hong, D., Wang, S., Zhang, F., Tang, F., Wu, T., et al. (2019). gTherapeutic protection against H. pylori infection in Mongolian gerbils by oral immunization with a tetravalent epitope-based vaccine with polysaccharide adjuvant. Front. Immunol. 10, 1185. doi: 10.3389/fimmu.2019.01185
Hanada, K., Graham, D. Y. (2014). Helicobacter pylori and the molecular pathogenesis of intestinal-type gastric carcinoma. Expert Rev. Anticancer Ther. 14, 947–954. doi: 10.1586/14737140.2014.911092
Handt, L. K., Fox, J. G., Stalis, I. H., Rufo, R., Lee, G., Linn, J., et al. (1995). Characterization of feline Helicobacter pylori strains and associated gastritis in a colony of domestic cats. J. Clin. Microbiol 33, 2280–2289. doi: 10.1128/jcm.33.9.2280-2289.1995
Heimesaat, M. M., Fischer, A., Plickert, R., Wiedemann, T., Loddenkemper, C., Göbel, U. B., et al. (2014). Helicobacter pylori induced gastric immunopathology is associated with distinct microbiota changes in the large intestines of long-term infected Mongolian gerbils. PloS One 9, e100362. doi: 10.1371/journal.pone.0100362
Higashi, H., Tsutsumi, R., Higashi, S., Sugiyama, T., Takeshi, A., Masahiro, H. (2002). SHP-2 tyrosine phosphatase as an intracellular target of Helicobacter pylori CagA protein. Science 295, 683–686. doi: 10.1126/science.1067147
Hofer, M., Lutolf, m. P. (2021). Engineering organoids. Nat. Rev. Materials 6, 402–420. doi: 10.1038/s41578-021-00279-y
Huang, F. Y., Chan, A. O., Lo, R. C., Rashid, A., Wong, D. K., Cho, C. H., et al. (2013). Characterization of interleukin-1β in Helicobacter pylori-induced gastric inflammation and DNA methylation in interleukin-1 receptor type 1 knockout (IL-1R1(-/-)) mice. Eur. J. Cancer 49, 2760–2770. doi: 10.1016/j.ejca.2013.03.031
Idowu, S., Bertrand, P. P., Walduck, A. K. (2022). Gastric organoids: Advancing the study of H. pylori pathogenesis and inflammation. Helicobacter 27, e12891. doi: 10.1111/hel.12891
Isobe, H., Nishiyama, A., Takano, T., Higuchi, W., Nakagawa, S., Taneike, I., et al. (2012). Reduction of overall Helicobacter pylori colonization levels in the stomach of Mongolian gerbil by Lactobacillus johnsonii La1 (LC1) and its in vitro activities against H. pylori motility and adherence. Biosci Biotechnol. Biochem. 76, 850–852. doi: 10.1271/bbb.110921
Jaka, H., Rhee, J. A., Östlundh, L., Smart, L., Peck, R., Mueller, A., et al. (2018). The magnitude of antibiotic resistance to Helicobacter pylori in Africa and identified mutations which confer resistance to antibiotics: systematic review and meta-analysis. BMC Infect. Dis. 18, 193. doi: 10.1186/s12879-018-3099-4
Jeong, H. J., Park, J. H., Kang, J. H., Sabaté Del Río, J., Kong, S. H., Park, T. E. (2023). Organoid-based human stomach micro-physiological system to recapitulate the dynamic mucosal defense mechanism. Adv. Sci. (Weinh) 10, e2300164. doi: 10.1002/advs.202300164
Jeremy, A. H., Du, Y., Dixon, M. F., Robinson, P. A., Crabtree, J. E. (2006). Protection against Helicobacter pylori infection in the Mongolian gerbil after prophylactic vaccination. Microbes Infect. 8, 340–346. doi: 10.1016/j.micinf.2005.06.025
Jiang, F., Guo, C. G., Cheung, K. S., Li, B., Law, S. Y. K., Leung, W. K. (2022). Age of eradication and failure rates of clarithromycin-containing triple therapy for Helicobacter pylori: A 15-year population-based study. Helicobacter 27, e12893. doi: 10.1111/hel.12893
Jiang, Y., Yu, Y. (2017). Transgenic and gene knockout mice in gastric cancer research. Oncotarget 8, 3696–3710. doi: 10.18632/oncotarget.12467
Jones, K. R., Whitmire, J. M., Merrell, D. S. (2010). A tale of two toxins: helicobacter pylori cagA and vacA modulate host pathways that impact disease. Front. Microbiol 1, 115. doi: 10.3389/fmicb.2010.00115
Kimura, N., Ariga, M., Icatlo, F. C., Jr., Kuroki, M., Ohsugi, M., Ikemori, Y., et al. (1998). A euthymic hairless mouse model of Helicobacter pylori colonization and adherence to gastric epithelial cells in vivo. Clin. Diagn. Lab. Immunol. 5, 578–582. doi: 10.1128/CDLI.5.4.578-582.1998
Kleanthous, H., Lee, C. K., Monath, T. P. (1998). Vaccine development against infection with Helicobacter pylori. Br. Med. Bull. 54, 229–241. doi: 10.1093/oxfordjournals.bmb.a011673
Krakowka, S., Morgan, D. R., Kraft, W. G., Leunk, R. D. (1987). Establishment of gastric Campylobacter pylori infection in the neonatal gnotobiotic piglet. Infect. Immun. 55, 2789–2796. doi: 10.1128/iai.55.11.2789-2796.1987
Krzyżek, P., Dudek, B., Brożyna, M., Krzyżanowska, B., Junka, A. (2025). Galleria mellonella larvae as a model for Helicobacter pylori biofilm formation under antibiotic stress. Microb Pathog 198, 107121. doi: 10.1016/j.micpath.2024.107121
Ku, C.-C., Wuputra, K., Pan, J.-B., Li, C.-P., Liu, C.-J., Liu, Y.-C., et al. (2022). Generation of human stomach cancer iPSC-derived organoids induced by helicobacter pylori infection and their application to gastric cancer research. Cells 11, 184. doi: 10.3390/cells11020184
Kuo, C. H., Wang, S. S., Lu, C. Y., Hu, H. M., Kuo, F. C., Weng, B. C., et al. (2013). Long-term use of probiotic-containing yogurts is a safe way to prevent helicobacter pylori: based on a Mongolian gerbil’s model. Biochem. Res. Int. 2013, 594561. doi: 10.1155/2013/594561
Lambert, J. R., Borromeo, M., Pinkard, K. J., Turner, H., Chapman, C. B., Smith, M. L. (1987). Colonization of gnotobiotic piglets with Campylobacter Pyloridis–an animal model? J. Infect. Dis. 155, 1344. doi: 10.1093/infdis/155.6.1344
Lee, C. K. (2001). Vaccination against Helicobacter pylori in non-human primate models and humans. Scand. J. Immunol. 53, 437–442. doi: 10.1046/j.1365-3083.2001.00911.x
Lee, K. K., McCauley, H. A., Broda, T. R., Kofron, M. J., Wells, J. M., Hong, C. I. (2018). Human stomach-on-a-chip with luminal flow and peristaltic-like motility. Lab. Chip 18, 3079–3085. doi: 10.1039/C8LC00910D
Lee, A., O’Rourke, J., De Ungria, M. C., Robertson, B., Daskalopoulos, G., Dixon, M. F. (1997). A standardized mouse model of Helicobacter pylori infection: introducing the Sydney strain. Gastroenterology 112, 1386–1397. doi: 10.1016/S0016-5085(97)70155-0
Li, X., Fu, G., Zhang, L., Guan, R., Tang, P., Zhang, J., et al. (2022). Assay establishment and validation of a high-throughput organoid-based drug screening platform. Stem Cell Res. Ther. 13, 219. doi: 10.1186/s13287-022-02902-3
Lin, A. S., McClain, M. S., Beckett, A. C., Caston, R. R., Harvey, M. L., Brea Dixon, A. M., et al. (2020). Temporal control of the helicobacter pylori cag type IV secretion system in a Mongolian gerbil model of gastric carcinogenesis. mBio 11, e01296-20. doi: 10.1128/mBio.01296-20
Linz, B., Balloux, F., Moodley, Y., Manica, A., Liu, H., Roumagnac, P., et al. (2007). An African origin for the intimate association between humans and Helicobacter pylori. Nature 445, 915–918. doi: 10.1038/nature05562
Lofgren, J. L., Whary, M. T., Ge, Z., Muthupalani, S., Taylor, N. S., Mobley, M., et al. (2011). Lack of commensal flora in Helicobacter pylori-infected INS-GAS mice reduces gastritis and delays intraepithelial neoplasia. Gastroenterology 140, 210–220. doi: 10.1053/j.gastro.2010.09.048
Lukonin, I., Zinner, M., Liberali, P. (2021). Organoids in image-based phenotypic chemical screens. Exp. Mol. Med. 53, 1495–1502. doi: 10.1038/s12276-021-00641-8
Lv, X., Yang, J., Song, H., Li, T., Guo, L., Xing, Y., et al. (2014). Therapeutic efficacy of the multi-epitope vaccine CTB-UE against Helicobacter pylori infection in a Mongolian gerbil model and its microRNA-155-associated immuno-protective mechanism. Vaccine 32, 5343–5352. doi: 10.1016/j.vaccine.2014.07.041
Mahe, M. M., Aihara, E., Schumacher, M. A., Zavros, Y., Montrose, M. H., Helmrath, M. A., et al. (2013). Establishment of gastrointestinal epithelial organoids. Curr. Protoc. Mouse Biol. 3, 217–240. doi: 10.1002/9780470942390.2013.3.issue-4
McCracken, K. W., Howell, J. C., Wells, J. M., Jason R, S. (2011). Generating human intestinal tissue from pluripotent stem cells in vitro. Nat. Protoc. 6, 1920–1928. doi: 10.1038/nprot.2011.410
Menheniott, T. R., O’Connor, L., Chionh, Y. T., Däbritz, J., Scurr, M., Rollo, B. N., et al. (2016). Loss of gastrokine-2 drives premalignant gastric inflammation and tumor progression. J. Clin. Invest. 126, 1383–1400. doi: 10.1172/JCI82655
Moses, S. R., Adorno, J. J., Palmer, A. F., Song, J. W. (2021). Vessel-on-a-chip models for studying microvascular physiology, transport, and function in vitro. Am. J. Physiol. Cell Physiol. 320, C92–c105. doi: 10.1152/ajpcell.00355.2020
Noto, J. M., Romero-Gallo, J., Piazuelo, M. B., Peek, R. M. (2016). The Mongolian gerbil: A robust model of helicobacter pylori-induced gastric inflammation and cancer. Methods Mol. Biol. 1422, 263–280. doi: 10.1007/978-1-4939-3603-8_24
Ochoa, S., Fernández, F., Devotto, L., France Iglesias, A., Collado, L. (2021). Virulence assessment of enterohepatic Helicobacter species carried by dogs using the wax moth larvae Galleria mellonella as infection model. Helicobacter 26, e12808. doi: 10.1111/hel.12808
Ohki, J., Sakashita, A., Aihara, E., Inaba, A., Uchiyama, H., Matsumoto, M., et al. (2020). Comparative analysis of enteroendocrine cells and their hormones between mouse intestinal organoids and native tissues. Biosci Biotechnol. Biochem. 84, 936–942. doi: 10.1080/09168451.2020.1713043
Ohnishi, N., Yuasa, H., Tanaka, S., Sawa, H., Miura, M., Matsui, A., et al. (2008). Transgenic expression of Helicobacter pylori CagA induces gastrointestinal and hematopoietic neoplasms in mouse. Proc. Natl. Acad. Sci. U S A 105, 1003–1008. doi: 10.1073/pnas.0711183105
Ortiz, V., Estevez-Ordonez, D., Montalvan-Sanchez, E., Urrutia-Argueta, S., Israel, D., Krishna, U. S., et al. (2019). Helicobacter pylori antimicrobial resistance and antibiotic consumption in the low-resource Central America setting. Helicobacter 24, e12595. doi: 10.1111/hel.2019.24.issue-4
Pang, M.-J., Burclaff, J. R., Jin, R., Adkins-Threats, M., Osaki, L. H., Han, Y., et al. (2022). Gastric organoids: progress and remaining challenges. Cell. Mol. gastroenterology hepatology 13, 19–33. doi: 10.1016/j.jcmgh.2021.09.005
Peek, R. M. (2008). Helicobacter pylori infection and disease: from humans to animal models. Dis. Model Mech. 1, 50–55. doi: 10.1242/dmm.000364
Pellegrino, E., Gutierrez, M. G. (2021). Human stem cell-based models for studying host-pathogen interactions. Cell. Microbiol. 23, e13335. doi: 10.1111/cmi.13335
Poddar, U. (2019). Helicobacter pylori: a perspective in low- and middle-income countries. Paediatr. Int. Child Health 39, 13–17. doi: 10.1080/20469047.2018.1490100
Poddar, U., Yachha, S. K. (2007). Helicobacter pylori in children: an Indian perspective. Indian Pediatr. 44, 761–770.
Radin, M. J., Eaton, K. A., Krakowka, S., Morgan, D. R., Lee, A., Otto, G., et al. (1990). Helicobacter pylori gastric infection in gnotobiotic beagle dogs. Infect. Immun. 58, 2606–2612. doi: 10.1128/iai.58.8.2606-2612.1990
Rossi, G., Rossi, M., Vitali, C. G., Fortuna, D., Burroni, D., Pancotto, L., et al. (1999). A conventional beagle dog model for acute and chronic infection with Helicobacter pylori. Infect. Immun. 67, 3112–3120. doi: 10.1128/IAI.67.6.3112-3120.1999
Saberi, S., Pournasr, B., Farzaneh, Z., Esmaeili, M., Hosseini, M. E., Baharvand, H., et al. (2018). A simple and cost-efficient adherent culture platform for human gastric primary cells, as an in vitro model for Helicobacter pylori infection. Helicobacter 23, e12489. doi: 10.1111/hel.2018.23.issue-4
Salama, N. R., Hartung, M. L., Müller, A. (2013). Life in the human stomach: persistence strategies of the bacterial pathogen Helicobacter pylori. Nat. Rev. Microbiol 11, 385–399. doi: 10.1038/nrmicro3016
Sarkar, S., Talesh, G. A., menheniott, T. R., sutton, p. (2023). Targeting host sulphonyl urea receptor 2 can reduce severity of helicobacter pylori associated gastritis. Gastro Hep Adv. 2, 721–732. doi: 10.1016/j.gastha.2023.03.007
Sato, T., Vries, R. G., Snippert, H. J., Wetering, M. V., Barker, N., Stange, D. E., et al. (2009). Single Lgr5 stem cells build crypt-villus structures in vitro without a mesenchymal niche. Nature 459, 262–265. doi: 10.1038/nature07935
Sawai, N., Kita, M., Kodama, T., Tanahashi, T., Yamaoka, Y., Tagawa, Y., et al. (1999). Role of gamma interferon in Helicobacter pylori-induced gastric inflammatory responses in a mouse model. Infect. Immun. 67, 279–285. doi: 10.1128/IAI.67.1.279-285.1999
Sebrell, T. A., Hashimi, M., Sidar, B., Wilkinson, R. A., Kirpotina, L., Quinn, M. T., et al. (2019). A novel gastric spheroid co-culture model reveals chemokine-dependent recruitment of human dendritic cells to the gastric epithelium. Cell. Mol. gastroenterology hepatology 8, 157–71. e3. doi: 10.1016/j.jcmgh.2019.02.010
Seidlitz, T., Koo, B.-K., Stange, D. E. (2021). Gastric organoids—an in vitro model system for the study of gastric development and road to personalized medicine. Cell Death Differentiation 28, 68–83. doi: 10.1038/s41418-020-00662-2
Semrau, A., Gerold, S., Frick, J. S., Iglauer, F. (2017). Non-invasive detection and successful treatment of a Helicobacter pylori infection in a captive rhesus macaque. Lab. Anim 51, 208–211. doi: 10.1177/0023677216669179
Shah, S. C., Iyer, P. G., Moss, S. F. (2021). AGA clinical practice update on the management of refractory helicobacter pylori infection: expert review. Gastroenterology 160, 1831–1841. doi: 10.1053/j.gastro.2020.11.059
Sharma, V., McNeill, J. H. (2009). To scale or not to scale: the principles of dose extrapolation. Br. J. Pharmacol. 157, 907–921. doi: 10.1111/j.1476-5381.2009.00267.x
Shomer, N. H., Dangler, C. A., Whary, M. T., Fox, J. G. (1998). Experimental Helicobacter pylori infection induces antral gastritis and gastric mucosa-associated lymphoid tissue in Guinea pigs. Infect. Immun. 66, 2614–2618. doi: 10.1128/IAI.66.6.2614-2618.1998
Solnick, J. V., Chang, K., Canfield, D. R., Parsonnet, J. (2003). Natural acquisition of Helicobacter pylori infection in newborn rhesus macaques. J. Clin. Microbiol 41, 5511–5516. doi: 10.1128/JCM.41.12.5511-5516.2003
Solnick, J. V., Fong, J., Hansen, L. M., Chang, K., Canfield, D. R., Parsonnet, J. (2006). Acquisition of Helicobacter pylori infection in rhesus macaques is most consistent with oral-oral transmission. J. Clin. Microbiol 44, 3799–3803. doi: 10.1128/JCM.01482-06
Solnick, J. V., Hansen, L. M., Canfield, D. R., Parsonnet, J. (2001). Determination of the infectious dose of Helicobacter pylori during primary and secondary infection in rhesus monkeys (Macaca mulatta). Infect. Immun. 69, 6887–6892. doi: 10.1128/IAI.69.11.6887-6892.2001
Stair, M. I., Winn, C. B., Burns, M. A., Holcombe, H., Artim, S. C., Ge, Z., et al. (2023). Effects of chronic Helicobacter pylori strain PMSS1 infection on whole brain and gastric iron homeostasis in male INS-GAS mice. Microbes Infect. 25, 105045. doi: 10.1016/j.micinf.2022.105045
Sturegård, E., Sjunnesson, H., Ho, B., Willén, R., Aleljung, P., Ng, H. C., et al. (1998). Severe gastritis in Guinea-pigs infected with Helicobacter pylori. J. Med. Microbiol 47, 1123–1129. doi: 10.1099/00222615-47-12-1123
Suarez, G., Romero-Gallo, J., Piazuelo, M. B., Sierra, J. C., Delgado, A. G., Washington, M. K., et al. (2019). Nod1 Imprints Inflammatory and Carcinogenic Responses toward the Gastric Pathogen Helicobacter pylori. Cancer Res. 79, 1600–1611. doi: 10.1158/0008-5472.CAN-18-2651
Suzuki, S., Kusano, C., Horii, T., Ichijima, R., Ikehara, H. (2022). The ideal helicobacter pylori treatment for the present and the future. Digestion 103, 62–68. doi: 10.1159/000519413
Suzuki, R., Satou, K., Shiroma, A., Shimoji, M., Teruya, K., Matsumoto, T., et al. (2019). Genome-wide mutation analysis of Helicobacter pylori after inoculation to Mongolian gerbils. Gut Pathog 11, 45. doi: 10.1186/s13099-019-0326-5
Tan, H.-J., Goh, K.-L. (2012). Extragastrointestinal manifestations of Helicobacter pylori infection: facts or myth? A critical review. J. Digestive Dis. 13, 342–349. doi: 10.1111/j.1751-2980.2012.00599.x
Thirumurthi, S., Graham, D. Y. (2012). Helicobacter pylori infection in India from a western perspective. Indian J. Med. Res. 136, 549–562.
Tohumcu, E., Kaitsas, F., Bricca, L., Ruggeri, A., Gasbarrini, A., Cammarota, G., et al. (2024). Helicobacter pylori and the human gastrointestinal microbiota: A multifaceted relationship. . Antibiotics (Basel) 13–584. doi: 10.3390/antibiotics13070584
Tomaszewska, A., Gonciarz, W., Rechcinski, T., Chmiela, M., Kurdowska, A. K., Krupa, A. (2024). Helicobacter pylori components increase the severity of metabolic syndrome and its hepatic manifestations induced by a high fat diet. Sci. Rep. 14, 5764. doi: 10.1038/s41598-024-56308-7
Tsai, C. J., Loh, J. M., Proft, T. (2016). Galleria mellonella infection models for the study of bacterial diseases and for antimicrobial drug testing. Virulence 7, 214–229. doi: 10.1080/21505594.2015.1135289
Uemura, N., Okamoto, S., Yamamoto, S., Matsumura, N., Yamaguchi, S., Yamakido, M., et al. (2001). Helicobacter pylori infection and the development of gastric cancer. N Engl. J. Med. 345, 784–789. doi: 10.1056/NEJMoa001999
Uotani, T., Murakami, K., Uchida, T., Tanaka, S., Nagashima, H., Zeng, X.-L., et al. (2019). Changes of tight junction and interleukin-8 expression using a human gastroid monolayer model of Helicobacter pylori infection. Helicobacter 24, e12583. doi: 10.1111/hel.2019.24.issue-3
Ventola, C. L. (2015). The antibiotic resistance crisis: part 1: causes and threats. P t 40, 277–283.
Vogiatzi, P., Cassone, M., Luzzi, I., Lucchetti, C., Otvos, L., Jr., Giordano, A. (2007). Helicobacter pylori as a class I carcinogen: physiopathology and management strategies. J. Cell Biochem. 102, 264–273. doi: 10.1002/jcb.v102:2
Wagner, S., Beil, W., Mai, U. E., Bokemeyer, C., Meyer, H. J., Manns, M. P. (1994). Interaction between Helicobacter pylori and human gastric epithelial cells in culture: effect of antiulcer drugs. Pharmacology 49, 226–237. doi: 10.1159/000139238
Walduck, A. K., Becher, o. (2012). Leptin, CD4+ Treg and the prospects for vaccination against H. pylori infection. Front. Immunol. 3, 29262. doi: 10.3389/fimmu.2012.00316
Wei, X., Feng, X. P., Wang, L. Y., Huang, Y. Q., Liang, L. L., Mo, X. Q., et al. (2019). Improved method for inducing chronic atrophic gastritis in mice. World J. Gastrointest Oncol. 11, 1115–1125. doi: 10.4251/wjgo.v11.i12.1115
Wu, C., Shi, Y., Guo, H., Zou, W. Y., Guo, G., Xie, Q. H., et al. (2008). Protection against Helicobacter pylori infection in Mongolian gerbil by intragastric or intramuscular administration of H. pylori multicomponent vaccine. Helicobacter 13, 191–199. doi: 10.1111/j.1523-5378.2008.00609.x
Yamamoto, T., Kita, M., Ohno, T., Iwakura, Y., Sekikawa, K., Imanishi, J. (2004). Role of tumor necrosis factor-alpha and interferon-gamma in Helicobacter pylori infection. Microbiol Immunol. 48, 647–654. doi: 10.1111/j.1348-0421.2004.tb03474.x
Yamaoka, Y. (2010). Mechanisms of disease: Helicobacter pylori virulence factors. Nat. Rev. Gastroenterol. Hepatol 7, 629–641. doi: 10.1038/nrgastro.2010.154
Ye, L., Swingen, C., Zhang, J. (2013). Induced pluripotent stem cells and their potential for basic and clinical sciences. Curr. Cardiol. Rev. 9, 63–72. doi: 10.2174/157340313805076278
Zarauz, J. M., Zafrilla, P., Ballester, P., Cerda, B. (2022). Study of the drivers of inappropriate use of antibiotics in community pharmacy: request for antibiotics without a prescription, degree of adherence to treatment and correct recycling of leftover treatment. Infect. Drug Resist. 15, 6773–6783. doi: 10.2147/IDR.S375125
Zhang, S., Moss, S. F. (2012). Rodent models of Helicobacter infection, inflammation, and disease. Methods Mol. Biol. 921, 89–98. doi: 10.1007/978-1-62703-005-2_12
Zheng, Y., Zhang, S., Zhang, T., Teng, X., Ling, X., Li, B., et al. (2024). A Bifidobacterium animalis subsp. lactis strain that can suppress Helicobacter pylori: isolation, in vitro and in vivo validation. Lett. Appl. Microbiol 77(1), ovae005. doi: 10.1093/lambio/ovae005
Keywords: Helicobacter pylori, animal models, antimicrobial resistance, gastritis, peptic ulcer disease, gastric cancer, gastric organoids
Citation: Patil S, Yu S, Jobby R, Ravichandran V and Sarkar S (2025) A critical review on In Vivo and Ex Vivo models for the investigation of Helicobacter pylori infection. Front. Cell. Infect. Microbiol. 15:1516237. doi: 10.3389/fcimb.2025.1516237
Received: 24 October 2024; Accepted: 21 April 2025;
Published: 14 May 2025.
Edited by:
Maurizio Sanguinetti, Catholic University of the Sacred Heart, ItalyReviewed by:
Paweł Krzyżek, Wroclaw Medical University, PolandKatie A. Lloyd, University of Chester, Chester, United Kingdom
Mahmoud Mohammed Bendary, Port Said University, Egypt
Copyright © 2025 Patil, Yu, Jobby, Ravichandran and Sarkar. This is an open-access article distributed under the terms of the Creative Commons Attribution License (CC BY). The use, distribution or reproduction in other forums is permitted, provided the original author(s) and the copyright owner(s) are credited and that the original publication in this journal is cited, in accordance with accepted academic practice. No use, distribution or reproduction is permitted which does not comply with these terms.
*Correspondence: Renitta Jobby, cmVuaXR0YTdAZ21haWwuY29t; Vinothkannan Ravichandran, dnJ2aW5vdGhhbkBnbWFpbC5jb20=; Sohinee Sarkar, c29oaW5lZS5zYXJrYXJAbWNyaS5lZHUuYXU=