- Biosynthesis and Bio Transformation Center, School of Life Sciences and Medicine, Shandong University of Technology (SDUT), Zibo, China
Antimicrobial peptides (AMPs) are critical effectors of innate immunity, presenting a compelling alternative to conventional antibiotics amidst escalating antimicrobial resistance. Their broad-spectrum efficacy and inherent low resistance development are countered by production challenges, including limited yields and proteolytic degradation, which restrict their clinical translation. While chemical synthesis offers precise structural control, it is often prohibitively expensive and complex for large-scale production. Heterologous expression systems provide a scalable, cost-effective platform, but necessitate optimization. This review comprehensively examines established and emerging AMP production strategies, encompassing fusion protein technologies, molecular engineering approaches, rational peptide design, and post-translational modifications, with an emphasis on maximizing yield, bioactivity, stability, and safety. Furthermore, we underscore the transformative role of artificial intelligence, particularly machine learning algorithms, in accelerating AMP discovery and optimization, thereby propelling their expanded therapeutic application and contributing to the global fight against drug-resistant infections.
1 Introduction
Antibiotic resistance, a rapidly escalating global health crisis, stands as one of the most significant challenges facing global health today, with an estimated 4.9 million deaths attributed to it in 2019 alone (Yang et al., 2018; Haney et al., 2019; Bellavita et al., 2023; Darby et al., 2023; Helmy et al., 2023; Salam et al., 2023; Almutairy, 2024; Viebrock et al., 2024). Bacterial resistance to antibiotics develops through intrinsic mechanisms (drug inactivation, target alteration, and efflux pumps) or is acquired via spontaneous mutations and horizontal gene transfer (Laws et al., 2019; Romo and Quirós, 2019). The emergence of antibiotic-resistant bacterial strains, first observed in 1940 with penicillin-resistant Staphylococcus aureus (Foster, 2017) has continuously escalated the global burden of antimicrobial resistance. The 2022 Global Burden of Disease study found that six bacteria— Escherichia coli (E. coli), Staphylococcus aureus (S. aureus), Klebsiella pneumoniae (K. pneumoniae), Streptococcus pneumoniae (S. pneumoniae), Acinetobacter baumannii (A. baumannii), and Pseudomonas aeruginosa (P. aeruginosa) were responsible for 73% of deaths from antimicrobial resistance in 2019, emphasizing the need for urgent medical solutions (Murray et al., 2022; Ho et al., 2024). With antibiotic resistance on the rise, the development of alternative antimicrobial therapies ideally with broad-spectrum efficacy, low toxicity, novel mechanisms, and new targets has become imperative (Murugaiyan et al., 2022; Aparicio-Blanco et al., 2024). Natural AMPs (AMPs) leveraging their multifunctional activity against diverse pathogens and minimal propensity for resistance evolution, exemplify pioneering solutions to transcend the limitations of conventional antibiotics (James et al., 2019; Margit Mahlapuu and Ekblom, 2020; Dwivedi et al., 2024; Purohit et al., 2024). AMPs are small, potent, broad-spectrum antimicrobials found across diverse life forms, from microbes to higher eukaryotes (Thomas and Antony, 2024). AMPs are generally short peptides, typically comprising around 100 amino acid residues with molecular weights under 5,000 Da, although some can be larger, reaching 130-150 residues (Hancock and Diamond, 2000; Zasloff, 2002; Mukesh Pasupuleti and Malmsten, 2012; Viruly et al., 2023). Gramicidin, derived from Bacillus brevis, was the first identified AMP (Yang and Yousef, 2018). Since its discovery, numerous other natural peptides with broad-spectrum activity against various pathogens (bacteria, viruses, fungi, and protozoa) have been identified (Datta and Roy, 2021). The Antimicrobial Peptide Database (APD; http://aps.unmc.edu/AP), which includes over 3,000 natural AMPs, serves as a crucial resource for identifying innovative therapeutic candidates (Wang et al., 2022a; Cresti et al., 2024). Similarly, the Data Repository of AMPss (DRAMP; http://dramp.cpu-bioinfor.org/), which provides a significantly larger database of over 30,000 AMP entries, including experimentally validated data on stability, hemolytic activity, and cytotoxicity. Based on their structural characteristics, AMPs, can be categorized into different groups, including linear peptides, cyclic peptides, and those containing disulfide bridge (Koehbach and Craik, 2019; Odunitan et al., 2023). These molecules often adopt distinct secondary and tertiary structures, frequently featuring post-translational modifications that are essential for their biological activity (Xuan et al., 2023; Vincenzi et al., 2024a). Many of these peptides require specific metal ions, such as calcium or magnesium, to maintain their active conformation and for optimal antimicrobial activity (Ngoc et al., 2023; Adriana et al., 2024). These structural and functional adaptations allow AMPs to disrupt microbial membranes through mechanisms such as the barrel-stave (transmembrane pores), toroidal pore (peptide-lipid cooperative pores), and carpet models (surface lysis) (Melo et al., 2009; Cruz et al., 2013) (Li et al., 2021b). As illustrated in Figure 1, cationic AMPs initially bind bacterial membranes via electrostatic attraction to anionic phospholipids. At high concentrations, this interaction causes direct membrane destabilization, whereas lower concentrations promote endocytic entry (Cudic and Otvos, 2002). Following internalization, AMPs target cytoplasmic enzymes, DNA, and RNA, disrupting replication and metabolic processes (Cudic and Otvos, 2002) (Steinstraesser et al., 2011; Haney et al., 2017; Divyashree et al., 2020). This multifaceted activity, including immunomodulatory effects, distinguishes them from traditional antibiotics (Torres et al., 2019). These properties have led to the Food and Drug Administration (FDA) approval of several peptide-based therapeutics, some of which are summarized in Table 1, while AMPs in various clinical phases are detailed in Table 2. Despite their potential, significant challenges remain in translating AMPs from research to clinical application. Despite their therapeutic potential, significant hurdles impede the transition of AMPs from research to clinical application (Marsian and Lomonossoff, 2016; De Oliveira et al., 2023). A primary challenge lies in producing therapeutically viable AMPs at scale (Jia et al., 2017). Large-scale production is essential not only to generate sufficient quantities for clinical use but also to enable thorough characterization of AMP composition, structure, function, and mechanisms of action. Such comprehensive characterization is critical for understanding interactions with target pathogens and optimizing efficacy and safety. Early AMP research relied on isolating these peptides from natural sources like cecropins from silk moth, Hyalophora cecropia to assess their activity (Qu et al., 1982; Bulet and Stocklin, 2005) and melatinin from bee venom (Apis milifera) (Smith et al., 1988). Extracting AMPs directly from natural sources, while yielding highly active molecules, presents several challenges. These include low, stress-dependent concentrations, slow and costly processing, potential environmental impact, and product impurities (Deng et al., 2017; Mazurkiewicz-Pisarek et al., 2023). In contrast, chemical synthesis offers high yields and purity, but it is expensive, especially for peptides requiring post-translational modifications. Furthermore, synthesizing longer peptides (over 50 residues) is complex and prone to sequence errors due to the stepwise addition of amino acids (Boutin et al., 2019; Wibowo and Zhao, 2019). These limitations highlight the need for cost-effective, efficient methods to produce large quantities of high-purity, therapeutically viable peptides. Heterologous production, involving the cloning of AMP-coding genes into expression vectors and their introduction into host organisms, offers a scalable and cost-effective strategy to meet this demand (Tripathi and Shrivastava, 2019; Lei et al., 2021). However, careful selection of the expression system is crucial, as the choice of host organism can significantly impact yield, cost-effectiveness, and the biological activity of the produced AMP (Ji et al., 2017; Muthunayake et al., 2020a). Wild-type E. coli, for example, is commonly used but unsuitable for cysteine-rich AMPs due to its inability to efficiently form disulfide bonds (Kim et al., 2020). Similarly, yeast species like Pichia pastoris are being explored for their ability to perform post-translational modifications essential for proteins and extracellular secretion (Garcia-Ortega et al., 2020). Another challenge in AMP development lies in their inherent instability and susceptibility to degradation (Luong et al., 2020). Optimizing these peptides is inherently complex, as improvements to one property (e.g., antimicrobial potency) often come at the expense of others (e.g., safety or stability). For example, structural attributes necessary for bacterial membrane disruption can inadvertently increase toxicity toward host cells, limiting therapeutic utility (Sarah et al., 2005; Kryczka and Boncela, 2018; Wu et al., 2024).
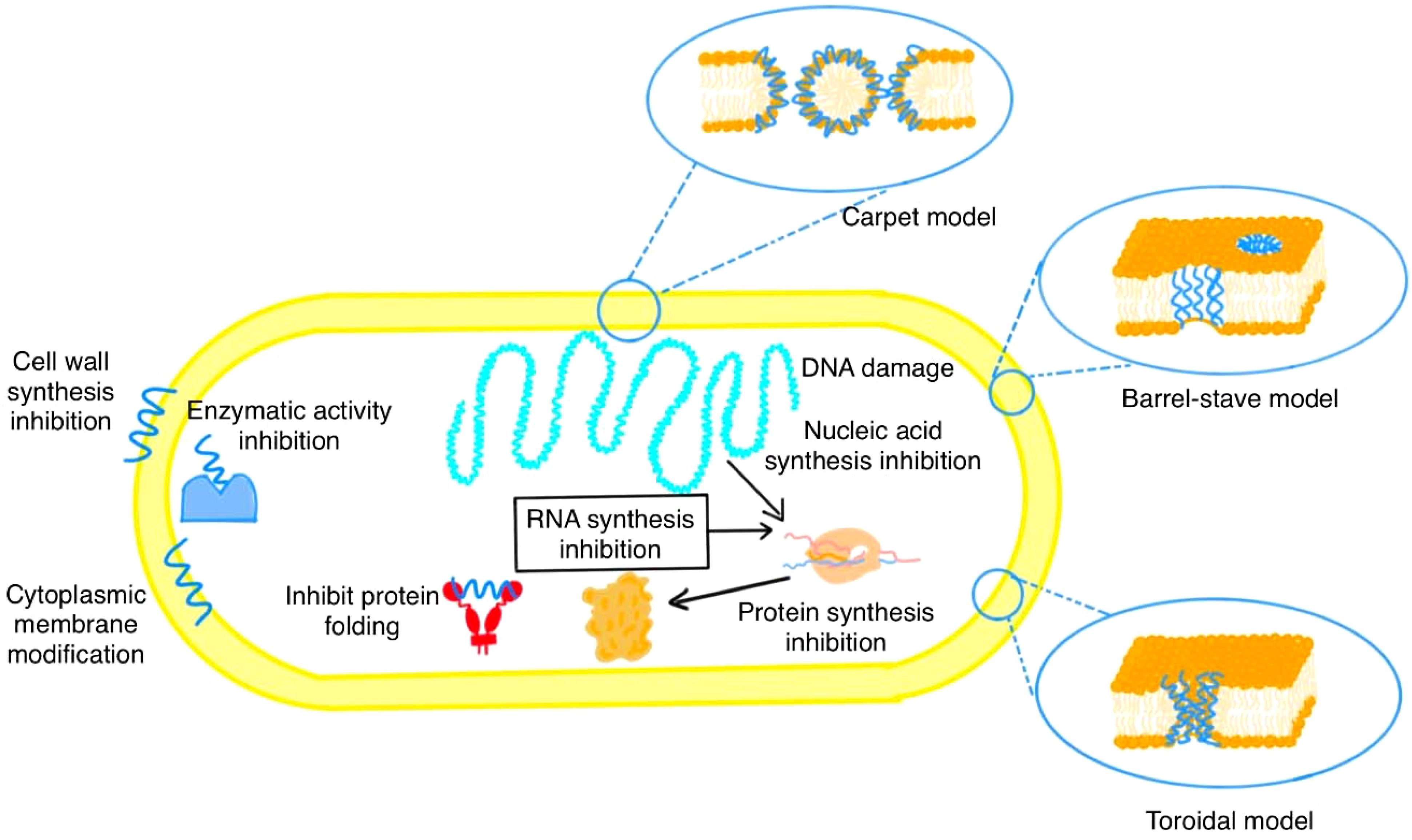
Figure 1. Proposed mechanisms of antimicrobial peptide (AMP) action. AMPs target bacterial cells through various mechanisms, including disruption of the cell membrane and wall. These include (i) membrane pore formation via the Barrel-stave, Toroidal, and Carpet models, (ii) inhibition of cell wall synthesis, and (iii) modification of the cytoplasmic membrane. Intracellularly, AMPs affect (iv) enzymatic activity, (v) protein folding, (vi) protein synthesis, and (vii) nucleic acid/RNA synthesis, as well as inducing microbial DNA damage.
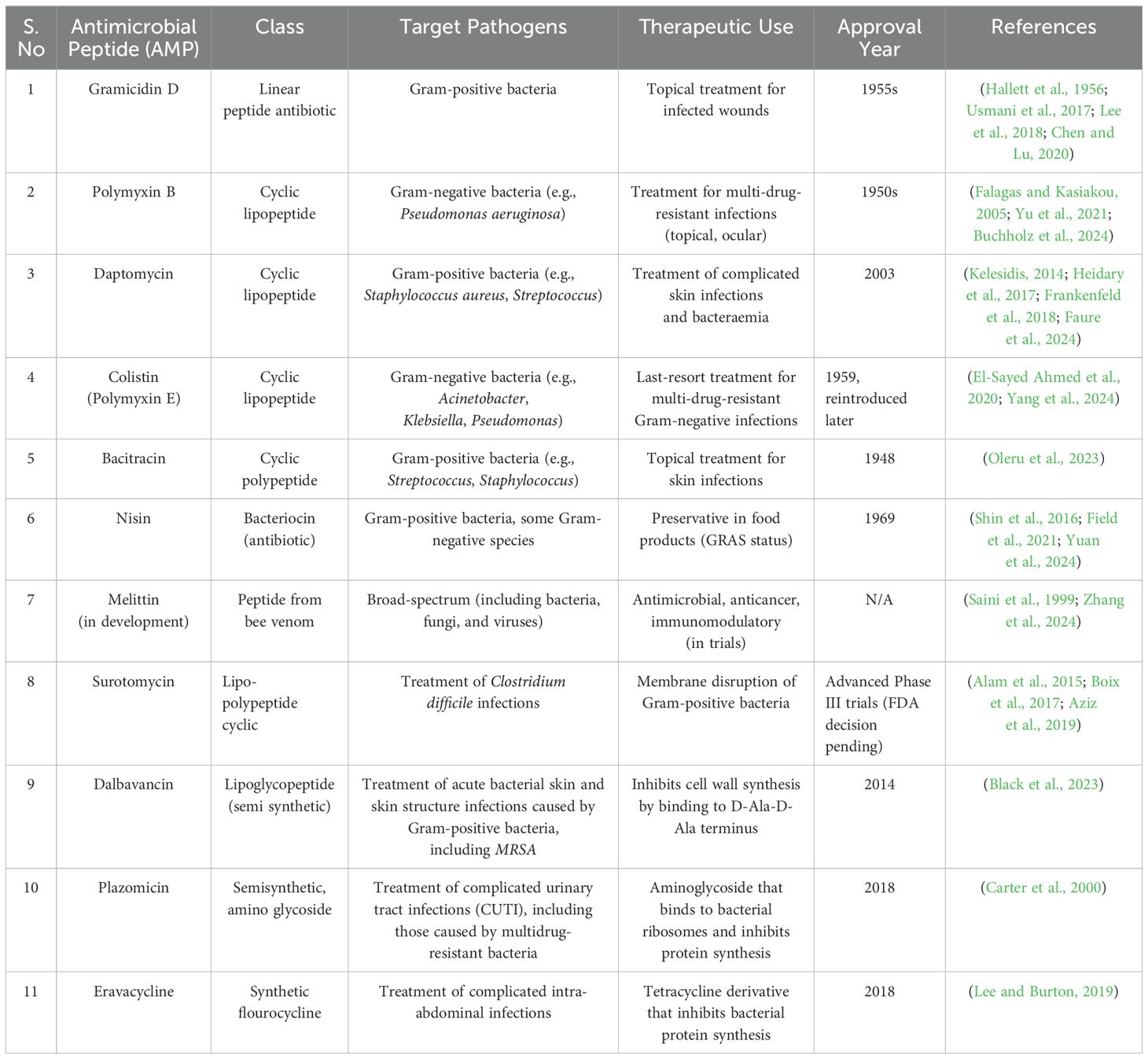
Table 1. FDA-Approved Antimicrobial Peptides (AMPs), Their classes, target pathogens, and therapeutic uses.
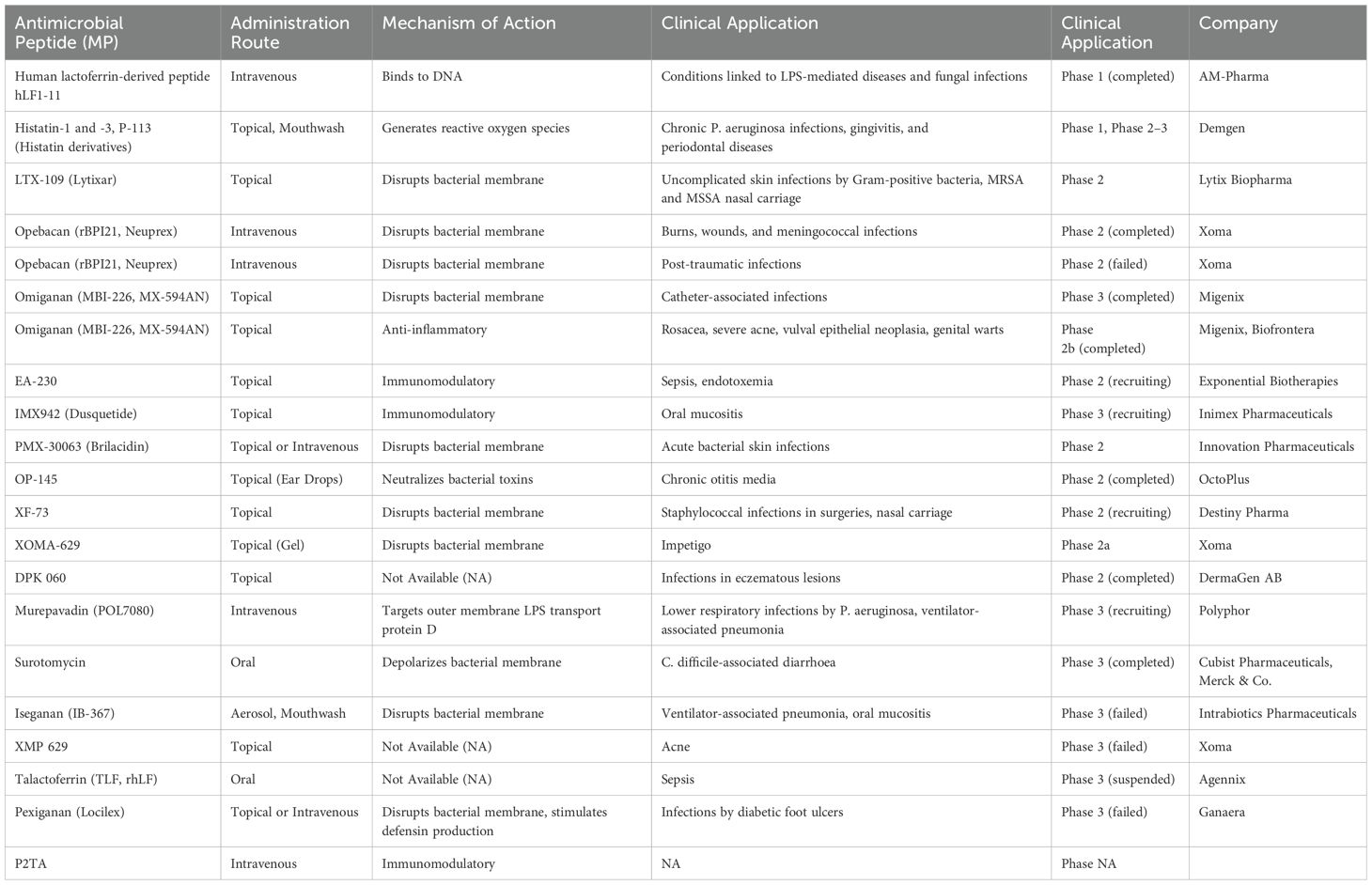
Table 2. Overview of antimicrobial peptides in clinical development: mechanisms, applications, and current trial status.
Another challenge in AMP development lies in their inherent instability and susceptibility to degradation Another challenge in AMP development is optimization for therapeutic use. Optimizing these peptides is inherently complex, as improvements to one property (e.g., antimicrobial potency) often come at the expense of others (e.g., safety or stability) (Luong et al., 2020; Wang et al., 2022b). For instance, hydrophobicity and amphipathicity, crucial for disrupting bacterial membranes, can also increase cytotoxicity (Boto et al., 2018; Wu et al., 2024). Similarly, while cost-effective linear l-peptides are susceptible to proteolysis, more stable, modified peptides are expensive to produce (Silva et al., 2011; Chow et al., 2019). Strategies such as cyclization, incorporation of unnatural amino acids, and terminal modifications have been employed to address these limitations. Numerous reviews have explored various facets of AMPs, including sequence, structure, activity, selectivity, toxicity, mechanisms of target and host cell interaction, and optimization strategies (Silva et al., 2011; Torres et al., 2019; Ting et al., 2020a; Mitchell et al., 2021). This review provides a comprehensive overview of established AMP production methods, including chemical synthesis and recombinant heterologous expression, aiming for high yield and purity. We also summarize key AMP design and optimization strategies crucial for enhancing the therapeutic effectiveness of these promising molecules.
2 Antimicrobial peptide synthesis
Initial AMP isolation involved low-yield extraction from substantial quantities of natural biological materials (Brand et al., 2013). Large-scale AMP production is now feasible through chemical synthesis or recombinant expression. While recombinant methods are generally preferred for longer peptides (>50 residues) due to cost-effectiveness (Guzmán et al., 2007), chemical synthesis remains essential for peptides requiring specific modifications or exhibiting toxicity in recombinant systems (often necessitating fusion proteins) (Ma et al., 2023). Although chemical synthesis allows precise sequence control, heterologous production is a more scalable approach for obtaining larger quantities of modified peptides. The advantages and challenges of each method are analyzed below.
2.1 Chemical synthesis of peptides
Chemical AMP synthesis offers advantages over natural extraction by enabling precise sequence modification, including incorporation of non-natural amino acids, for enhanced activity and stability studies (Haney et al., 2017). Peptides can be chemically synthesized via solution-phase or solid-phase methods (Kim and Mcalpine, 2013; Ollivier et al., 2017). Solid-phase peptide synthesis (SPPS) is often preferred for modified peptides due to simplified production and purification (Jaradat, 2018; Boutin et al., 2019). In SPPS, the growing peptide chain is anchored to a solid support (e.g., polystyrene resin) via a cleavable linker. Iterative deprotection and coupling reactions extend the chain, with wash steps removing excess reagents and by-products. Final cleavage from the resin yields the purified target peptide (Figure 2) (Coin et al., 2007; Lacroix and Li-Chan, 2014). Efficient peptide synthesis is crucial for high-throughput cell-based assays. SPOT synthesis, an automated and cost-effective method (López-Pérez et al., 2017), addresses this by directly generating peptides on a porous membrane, enabling both production and protein-protein interaction screening. This in situ synthesis involves sequential addition of protected amino acids, followed by deprotection and cleavage (Coin et al., 2007; Nandhini et al., 2022). Fmoc (9-fluorenylmethyloxycarbonyl) and Boc (tert-butoxycarbony) are the two most popular choices for protecting the N (α) group during this process (Noki et al., 2024). While both are effective, the Fmoc strategy is often preferred due to its milder deprotection conditions and the ability to simultaneously cleave both the linker and side chain protecting groups (Behrendt et al., 2016; Giesler et al., 2020). Synthesizing longer peptides, particularly AMPss exceeding 50 amino acids, remains challenging despite advancements in SPPS synthesis. Native chemical ligation provides an alternative by joining peptide fragments through a thioester-cysteine reaction, forming a native peptide bond (Yan and Dawson, 2001; Wan and Danishefsky, 2007; Bondalapati et al., 2016; Cistrone et al., 2019; Tian et al., 2022). This bond formation proceeds via trans thio esterification followed by an S-to-N acyl shift. Native chemical ligation (NCL), while useful, requires N-terminal cysteine, a residue with low natural abundance (∼1.7% in human proteins) and often unsuitable distribution for ligation. Figure 3 illustrates the principles of native chemical ligation (NCL) and desulfurization, along with examples of commonly used auxiliaries. Traditionally, NCL proceeds linearly from C- to N-terminus, developments such as desulfurization and auxiliary-mediated ligation overcome these limitations (Figure 3A) (Macmillan, 2006; Zuo et al., 2015; Agouridas et al., 2019; Sun and Brik, 2019; Kent, 2021). The Kent group pioneered the use of chemical auxiliaries, or non-native thiol modifications, for peptide ligation at Xaa-Gly and Gly-Xaa junctions. Their method employed a removable N-terminal oxyalkyl moiety, proceeding through a six-membered ring intermediate, and cleaved using zinc (Canne et al., 1996). Drawing upon Dawson’s auxiliary-mediated ligation strategy, Danishefsky’s group devised a cysteine-free native chemical ligation technique applicable to the synthesis of both N- and O-linked glycopeptides (Offer et al., 2002; Wu et al., 2006). Aimoto and colleagues introduced a photolabile auxiliary that is compatible with and cleavable under mild conditions for polypeptide synthesis (Kawakami and Aimoto, 2003). The Wong group introduced sugar-assisted ligation as a specific application of auxiliary-mediated ligation (Côté et al., 2007). A thio-sugar auxiliary, incorporated as a glycosyl amino acid during solid-phase peptide synthesis, facilitates amide bond formation near the ligation site via thiol exchange with a peptidyl thioester. Subsequent desulfurization removes the auxiliary (Yan and Dawson, 2001). Successfully synthesized via a two-step native chemical ligation/desulfurization approach, the AMPs murepavadin displayed potent activity against P. aeruginosa clinical isolates (Chaudhuri et al., 2021). An auxiliary developed by Seitz et al. facilitates ligation at sterically hindered junctions, enabling the synthesis of antimicrobial proteins such as DCD-1L and opistoporin-2. This SPPS-compatible auxiliary is cleavable under mild basic conditions (Loibl et al., 2015). The utility of these advancements has been demonstrated in the synthesis of a 120-amino acid peptide containing eight MUC5AC tandem repeats, achieved via the ligation of two 60-residue segments (Trunschke et al., 2022). These developments highlight the continuous evolution of auxiliary-mediated NCL, expanding its applicability for the synthesis of structurally complex peptides and proteins. These and other studies clearly demonstrate that the auxiliary-mediated approach effectively extends native chemical ligation, enabling the synthesis of complex, functionalized proteins with enhanced structural and functional diversity.
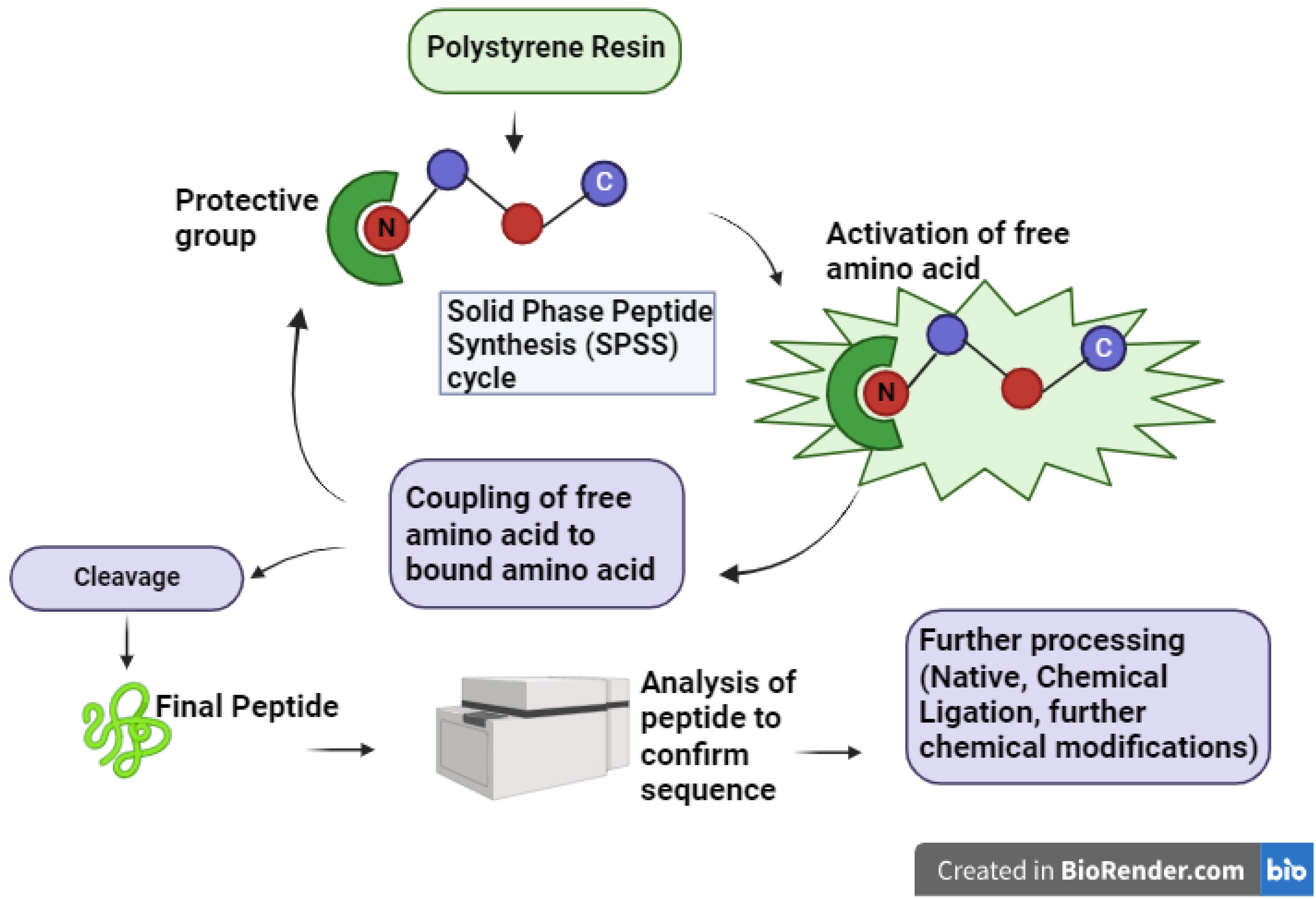
Figure 2. Overview of the Solid Phase Peptide Synthesis (SPPS) cycle. This schematic illustrates the essential steps in SPPS, starting with the activation of free amino acids and their sequential coupling to resin-bound amino acids. Following peptide elongation, the peptide is cleaved from the resin, analyzed to verify the sequence, and subjected to further processing, such as native chemical ligation or additional chemical modifications, to obtain the final, desired peptide product.
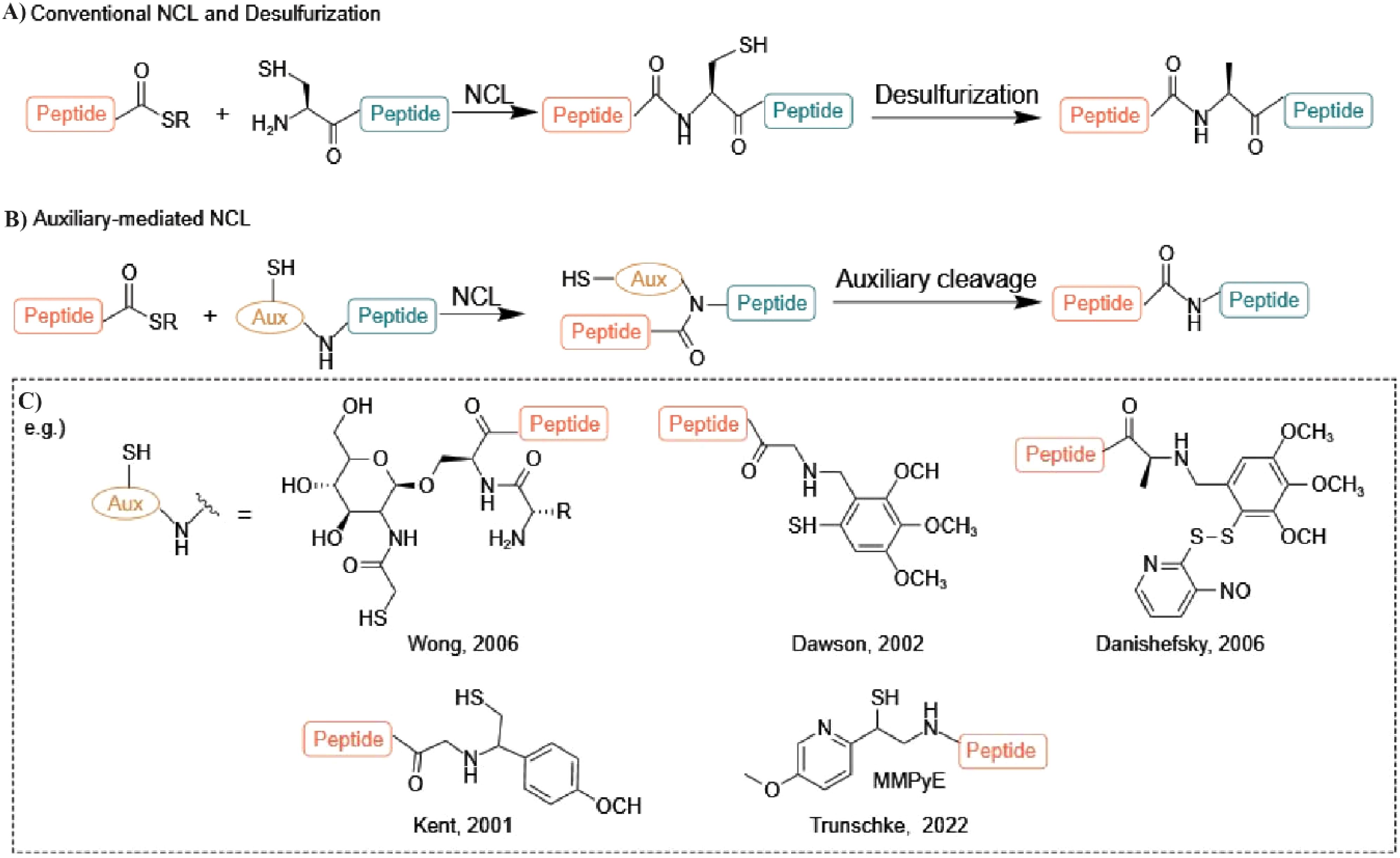
Figure 3. (A) Schematic representation of native chemical ligation (NCL) and desulfurization, illustrating native peptide bond formation through trans-thioesterification and S-to-N acyl shift mechanisms. (B) Auxiliary-mediated NCL, facilitating ligation followed by cleavage for peptide synthesis. (C) Examples of common auxiliaries introduced by Wong [1], Kent [2], Danishefsky [3], and MPyE auxiliary, developed by Trunschke et al. [4], enabling NCL at sterically hindered junctions and expanding the scope of peptide synthesis.
Expressed protein ligation is a strategy that combines recombinant protein production with native chemical ligation to overcome NCL’s size limitations. This involves ligating a recombinant protein thioester and a synthetic peptide with an N-terminal cysteine (Muir et al., 1998). Native chemical ligation has enabled successful synthesis of complex peptides and proteins, including siglec-7 (Izumi et al., 2014), human interleukin-6 glycoprotein (Reif et al., 2014), and anti-microbial peptide caenopore-5 (Medini et al., 2015). For a more comprehensive understanding of these synthetic approaches, readers are encouraged to refer to the relevant literature (Chalker, 2013; Fernández-Tejada et al., 2015; Raibaut et al., 2015; Bondalapati et al., 2016; Thompson and Muir, 2020; Wang and Cole, 2020). This approach is particularly useful in chemical and molecular biology for preparing proteins with defined post-translational modifications, such as glycosylation.
2.2 Heterologous expression system and strategies to upscale the AMP production
Heterologous expression involves introducing AMP genes into non-native hosts—such as bacteria, yeast, plant, or insect cells—to leverage their rapid growth and synthesis machinery (Figure 4). This approach has transformed these organisms into versatile biofactories, enabling scalable AMP synthesis (Hood, 1997). Below, we explore key heterologous systems (Table 3), highlighting their unique advantages and applications for AMP production.
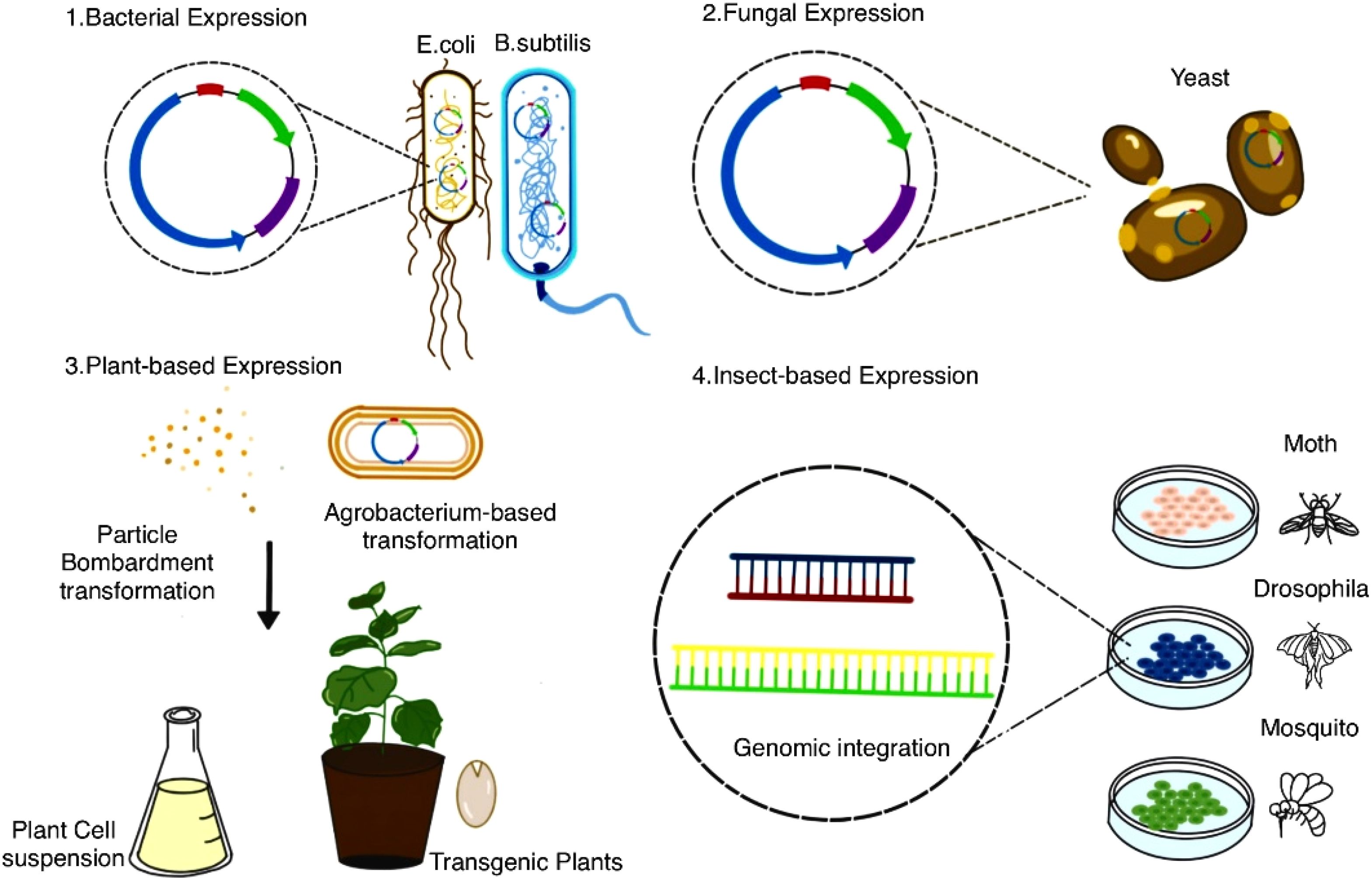
Figure 4. Expression systems for recombinant protein production. This diagram illustrates various expression systems used for recombinant protein production. (1) Bacterial expression utilizes bacteria, while Yeast expression relies on yeast cells for protein production. (2) Plant expression encompasses techniques like Agrobacterium gene transformation and particle bombardment transformation, enabling the creation of transgenic plants or plant cell suspensions. (3) Insect expression involves common insect hosts for genomic integration and protein expression. These systems represent diverse approaches for producing recombinant proteins in different biological hosts.
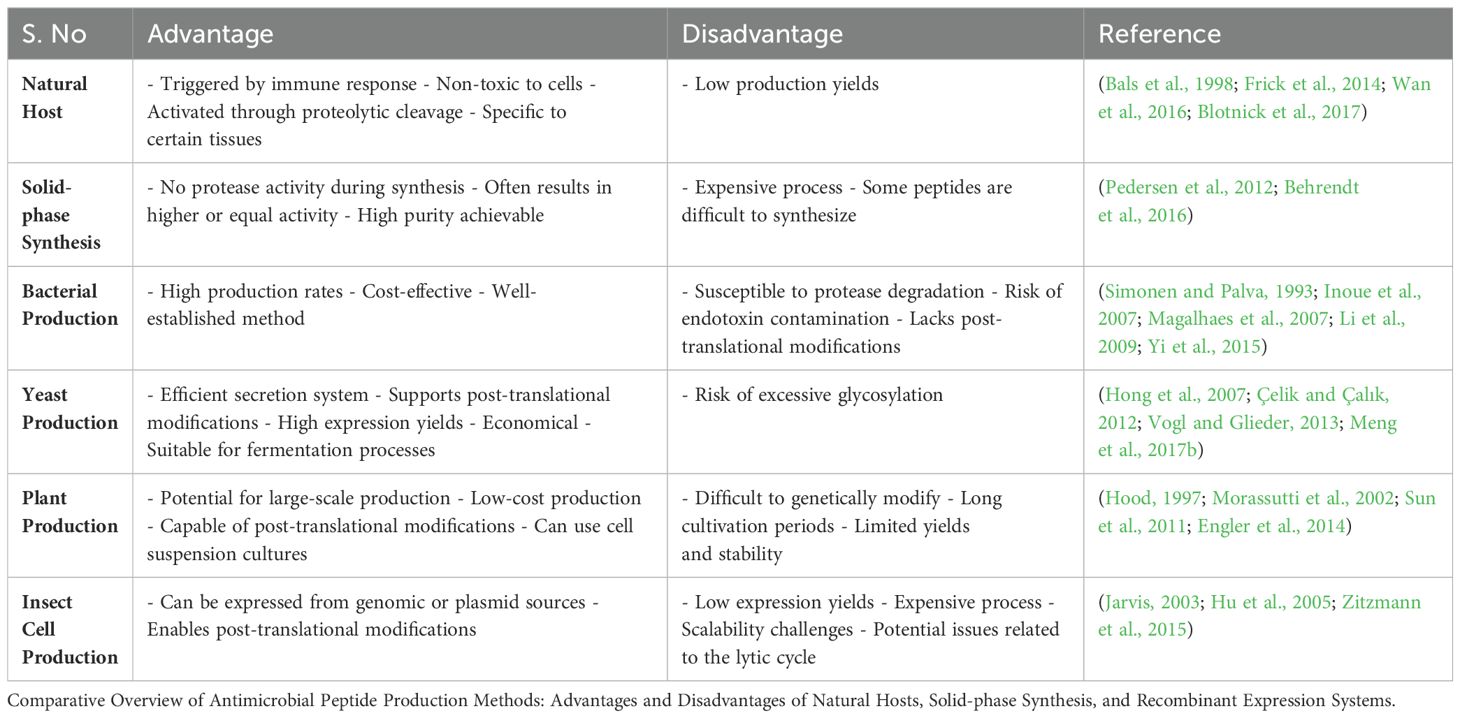
Table 3. Comparison of antimicrobial peptide production systems: natural hosts vs. recombinant methods.
2.2.1 Bacterial expression system
Bacteria are popular hosts for heterologous expression due to their rapid growth, cost-effectiveness, and efficient production (Zerbs et al., 2009; Deng et al., 2017; Burdette et al., 2018). However, some bacterial systems face limitations in secreting AMPs, forming disulfide bonds, and performing complex modifications like glycosylation (Zhang et al., 2019; Pratama et al., 2021). Various bacteria, including Corynebacterium (Salim et al., 1997), Streptomyces (Xu and Zhang, 2014; Myronovskyi and Luzhetskyy, 2019), and Pseudomonas (Loeschcke and Thies, 2015) can serve as hosts for heterologous expression. These hosts, in conjunction with different promoters and vectors, offer a range of options for peptide production. This section focuses on the most common bacterial hosts: E. coli, Bacillus subtilis.
Escherichia coli is a bacterial workhorse, widely employed for efficiently producing recombinant peptides, proteins, and membrane proteins (Parachin et al., 2012a; Wibowo and Zhao, 2019). However, producing complex molecules, especially small, positively charged peptides, pushes E. coli to its limits. These peptides are vulnerable to degradation, contamination, and misfolding due to the bacterium’s limited post-translational modification capabilities (Terpe, 2006; Chen, 2012; Kaur et al., 2018). To overcome these challenges, selecting an appropriate E. coli strain is crucial (Hayat et al., 2018). Genetically engineered strains, (Table 4) optimized for protein production, offer advantages like enhanced mRNA stability, cytoplasmic disulfide bond formation, reduced protease activity, and compatibility with challenging gene expression. These advantages can significantly improve peptide expression (Baneyx, 1999; Berkmen, 2012). For example, a study comparing the production of two recombinant defensins (HD5 and LAP) in E. coli strains BL21 and Origami B found that HD5 production was significantly higher in BL21, with superior quality and stability. This highlights how strain selection can profoundly impact recombinant protein production (López-Cano et al., 2022).
Producing AMPs in bacteria like E. coli often involves fusing the AMP to a carrier protein (Li et al., 2007; Pavelka et al., 2024). This strategy has proven particularly useful for AMPs that exhibit high toxicity to bacterial hosts, such as challenging ones like the potent cathelicidin LL-37 (Pavelka et al., 2024), protegrin-1 (Azari et al., 2020a) and the modified bovine lactoferricin known as LfcinB-W10 (Feng et al., 2010), by shielding them from premature breakdown within the bacterial cell.
To optimize recombinant expression in E. coli, careful selection of both the bacterial strain and expression vector is essential, guided by a thorough understanding of the target peptides characteristics. This includes considering the desired level of basal expression determined by the vector’s promoter (Lozano Terol et al., 2021). The choice of expression vector significantly impacts recombinant protein production in E. coli. Key considerations include the promoter system, which directly influences expression levels. Popular choices include the lac, tac, and lacUV5 promoters (utilized in vectors like pGEX, pMAL, and pUC), as well as the powerful T7 promoter found in the pET system (Rosano and Ceccarelli, 2014; Tucci et al., 2016; Du et al., 2021). Additionally, some vectors incorporate fusion tags to simplify purification or detection. Careful selection of these elements is crucial for optimizing yield and functionality (Zhang et al., 2022).
Bacillus subtilis (B. subtilis), a Gram-positive, endotoxin-free bacterium, offers a safer alternative to E. coli as a recombinant protein expression system (Schallmey et al., 2004). Its well-characterized protein secretion machinery makes it particularly suitable for the production and secretion of AMPs. Researchers explored the potential of B. subtilis and its efficient secretion pathways for producing recombinant AMPs and AMP fusion proteins extracellularly (Harwood and Cranenburgh, 2008; Taguchi et al., 2015; Rosales-Mendoza et al., 2016; Yang et al., 2016; Neef et al., 2021) (Fu et al., 2018; Zhang et al., 2018). A recent study reported the successful production of the cecropin A-melittin mutant in B. subtilis, with a yield of 159 mg/l of purified CAM-W (Ji et al., 2017). Two separate studies highlight the successful production of AMPs in Bacillus subtilis WB800N. One study (Zhang et al., 2015) produced the fungal defensin plectasin by fusing its gene with SUMO, 6xHis, and sacB signal peptide sequences within the pGJ148 shuttle plasmid. This method yielded 41 mg/L of fusion protein, which upon purification and SUMO protease digestion, resulted in 5.5 mg/L of 94% pure, biologically active plectasin. Another study successfully expressed and secreted PR-FO, a different AMPs, achieving yields of 16 mg/L and 23 mg/L of fusion protein using SPamyQ and SPsacB signal peptides, respectively (Zhang et al., 2020). Bacillus subtilis presents a promising system for industrial applications due to its beneficial characteristics. Recent publications have explored various approaches to enhance and optimize its use in these contexts (Westers et al., 2004; Kovács, 2019; Su et al., 2020; Lei et al., 2021; Put et al., 2024).
2.2.2 Yeast based expression system
Yeast offers a robust eukaryotic platform for AMPs (AMP) production, combining advantages absent in bacterial systems. Unlike prokaryotes, yeast integrates efficient secretion systems that minimize toxicity by exporting AMP extracellularly, while enabling essential post-translational modifications (PTMs) like glycosylation to enhance peptide stability and bioactivity (Vieira Gomes et al., 2018a; Kumar and Kumar, 2019a; Zhao et al., 2024).
Producing AMPs in non-native hosts presents several challenges. AMP production poses challenges due to their toxicity to expression systems (e.g., E. coli), instability, protease susceptibility, and post-translational modification requirements (Ingham and Moore, 2007; Da Cunha et al., 2017). While E. coli can be engineered for AMP production, the process can be costly and time-consuming, leading researchers to explore alternative expression systems (Schreiber et al., 2017). Yeast presents a compelling alternative, combining beneficial aspects of both eukaryotic and prokaryotic systems. These advantages include efficient protein secretion, post-translational modifications, and rapid growth (Mattanovich et al., 2012b; Parachin et al., 2012a; Schreiber et al., 2017; Vieira Gomes et al., 2018a; Lee et al., 2008; Kwak, 2024). Saccharomyces cerevisiae, first employed for recombinant protein production in the 1980s, remains a widely utilized host due to its well-characterized genetics, physiology, and fermentation processes, along with its GRAS status (Mattanovich et al., 2012b; Kastberg et al., 2022a). Pichia pastoris and Saccharomyces cerevisiae are the two most widely adopted yeasts for recombinant protein production, offering advantages such as adaptability to large-scale fermentation and the ability to produce biologically active eukaryotic proteins (Tran et al., 2017). The Saccharomyces cerevisiae expression system has been successfully employed for the production of various therapeutic proteins, including vaccines against hepatitis B and hantavirus, as well as insulin and other human hormones (Tomoko et al., 1985; Hahm and Chung, 2001; Antoniukas et al., 2006; Dimiceli et al., 2006; Baeshen et al., 2014). Traditional yeast expression systems, while widely used, present limitations such as fermentative metabolism, hyper glycosylation, and potentially low protein yields (Wagner and Alper, 2016; Wang et al., 2017; Baghban et al., 2019). The limitations of traditional yeast expression systems have led to the development of alternative systems utilizing unconventional yeasts. Among these, Pichia pastoris (P. pastoris) has emerged as a preferred host for heterologous protein expression (Zhu et al., 2019). This is reflected in the dramatic increase in studies utilizing P. pastoris in recent years (Bill, 2014; Wagner and Alper, 2016; Baghban et al., 2019). Unlike S. cerevisiae, which diverts significant carbon towards ethanol production, P. pastoris efficiently uses carbon for growth, leading to high biomass, and channels its resources towards recombinant protein production (Vieira Gomes et al., 2018a; Walker and Pretorius, 2018; Karbalaei et al., 2020a; Parapouli et al., 2020; Barone et al., 2023; Popova et al., 2023). Proteolytic degradation of recombinant proteins in K. phaffii (P. pastoris) typically occurs during vesicular transport or after secretion into the extracellular space, leading to reduced yields and compromised activity of the target protein (Werten et al., 2019; Raschmanová et al., 2021). To address protease-related challenges in K. phaffii, protease-deficient strains like SMD1163, SMD1165, and SMD1168 have been developed. These strains exhibit significantly reduced or eliminated protease activity due to targeted gene deletions. However, this advantage comes at the cost of reduced growth rates, lower transformation efficiencies, and decreased viability compared to wild-type strains (Daly and Hearn, 2005; Karbalaei et al., 2020a). Both constitutive and inducible promoters are employed for heterologous gene expression in yeast. However, inducible promoters are generally preferred due to their ability to fine-tune expression levels, leading to higher product yields (Kastberg et al., 2022a). Despite its popularity, offers a limited selection of expression vectors compared to other yeast or bacterial systems. Commonly used vectors for AMP production rely on the methanol-inducible AOX1 promoter, necessitating a two-step process: initial high cell density growth followed by methanol-induced protein expression. However, high yields are achievable with this system, as demonstrated by NZ2114 production reaching 2390 mg/L (Zhang et al., 2014). Table 5 summarizes various AMPs produced using Pichia pastoris as a heterologous host.
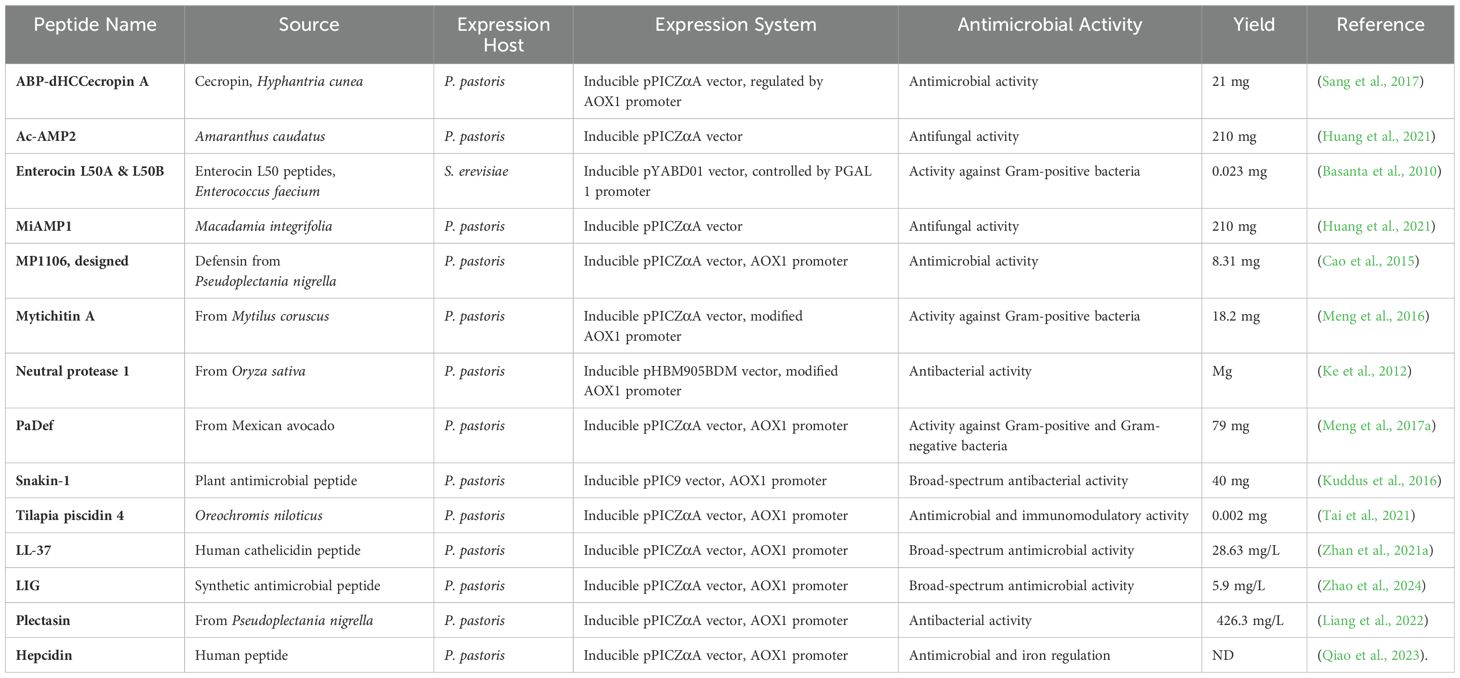
Table 5. Production of antimicrobial peptides in pichia pastoris and Saccharomyces cerevisiae: yield, activity, and expression systems.
Microbial systems, while advantageous for biopharmaceutical production, face challenges due to high-mannose N-glycosylation (Amann et al., 2019; Kulagina et al., 2021). This modification can impact protein half-life and potentially trigger immune responses (Chung et al., 2022). Glycoengineering is often needed to optimize the therapeutic potential of P. pastoris-produced proteins. Genetically engineered yeasts can now perform human-like N-glycosylation, including terminal sialic acid addition (Wildt and Gerngross, 2005; De Pourcq et al., 2010; De Wachter et al., 2021). The engineered yeast strain YSH44, devoid of endogenous glycosylation machinery, was employed to establish a humanized glycosylation pathway. This involved introducing genes encoding key enzymes for human N-glycosylation, including mannosidases I and II, N-acetylglucosaminyltransferases I and II, and a UDP-GlcNAc transporter. This engineered pathway enables the production of proteins bearing the human-like oligosaccharide GlcNAc2Man3GlcNAc2 (Logez et al., 2012; Felber et al., 2014).
2.2.3 Plants
Plants are emerging as a promising platform for producing various biomolecules, including AMPs. Producing recombinant AMPs in plants aims to achieve high AMP concentrations and enhance disease resistance in transgenic plants (Abranches et al., 2005; Nawrot et al., 2014; Zou et al., 2023; Chekan et al., 2024). Plants possess the cellular machinery necessary for essential post-translational modifications, including glycosylation, disulfide bond formation, and proteolytic cleavage (Webster and Thomas, 2012; Tschofen et al., 2016; Margolin et al., 2020). These modifications are crucial for the activity of various proteins, including AMPs (Pouresmaeil and Azizi-Dargahlou, 2023). Additionally, the presence of chaperones similar to those found in animals ensures proper protein folding, further supporting their suitability as a production platform (Shinmyo and Kato, 2010; Da Cunha et al., 2014; Pouresmaeil and Azizi-Dargahlou, 2023). Plant-based heterologous protein production, while advantageous, faces challenges due to differences in glycosylation patterns compared to mammalian cells (Gomord et al., 2010; Karki et al., 2021b). Plant-specific N-glycans, characterized by the presence of mannose, α-1,3-fucose, and β-1,2-xylose, can trigger immunogenic responses in humans (Strasser, 2016; Srisangsung et al., 2024). This poses a potential concern for the use of plant-derived therapeutics, as pre-existing antibodies against these glycans in human serum could lead to adverse immune reactions. Therefore, mitigating plant-specific glycosylation is crucial for the successful development of plant-based platforms for therapeutic protein production, particularly for heterologous AMP production (Shanmugaraj et al., 2021a; Dubey et al., 2023). To address the challenges of plant-specific glycosylation, researchers are developing glyco-engineered plant cell lines. These engineered lines, often generated using RNAi or CRISPR/Cas9 technology, lack the enzymes α-1,3-FucT and β-1,2-XylT, enabling the production of humanized proteins (Mercx et al., 2017; Karki et al., 2021a) as shown in Figure 5. AMP production in plants can be achieved through stable or transient transgene expression in whole plants or plant cell suspensions, utilizing either plastid or nuclear transformation (Hoelscher et al., 2022; Raimondo et al., 2023; Chaudhary et al., 2024). Stable transformation integrates foreign genes into the plant genome (nuclear or plastid), enabling heritable trait transfer (Bélanger et al., 2024). Jin et al. engineered two different plant species, Arabidopsis thaliana and Medicago sativa, to produce recombinant β-gallinacin-3, a cysteine-rich AMPs (Jin et al., 2018). In M. sativa, transgenic plants were generated after codon optimization and vector construction. Subsequent analysis revealed that the minimum inhibitory concentrations against three bacterial strains were 32, 16, and 128 μg/mL, respectively (Jin et al., 2022). This highlights the potential of plant systems for producing functional AMPs. Similar strategies, such as expressing a CBD-dermasptin B-1 fusion peptide in tobacco hairy roots, have also demonstrated enhanced antimicrobial activity against phytopathogens, particularly Alternaria alternata, compared to the unmodified peptide (Shams et al., 2019). These findings underscore the potential of plant-based expression systems for improving the efficacy of AMPs against specific targets. While advantageous for cost-effective peptide production due to seed-based accumulation, nuclear transformation in plants necessitates transgene containment strategies to mitigate the risk of outcrossing with native species (Horn et al., 2004). Chloroplast-based expression, or transplastomic expression, offers a promising alternative for producing peptides (Hoelscher et al., 2022). Molina et al. successfully produced high levels of a viral antigenic peptide in tobacco chloroplasts, demonstrating this approach’s potential for vaccine development (Molina et al., 2004). Transplastomic expression offers stable transgene inheritance, high protein yields, and multigenic expression capabilities. While lacking glycosylation pathways, chloroplasts facilitate proper protein folding and disulfide bond formation, enabling functional peptide production (Egelkrout et al., 2012; Abiri et al., 2016; Moustafa et al., 2016). Maternal inheritance further reduces transgene escape. Although stable transformation was once a standard method for biomolecule production, its lengthy timeline, particularly for establishing transgenic plants, prompted the search for faster alternatives. This led to the emergence of transient heterologous expression as a more efficient method for producing recombinant peptides (Marsian and Lomonossoff, 2016; Liu et al., 2018). Transient expression offers a faster and more flexible approach to producing heterologous peptides, partly because it avoids the complexities of long-term gene integration and expression (Egelkrout et al., 2012; Clark and Maselko, 2020). Established plant cell transformation protocols, particularly biolistic, contribute significantly to the widespread adoption of transient expression (Anami et al., 2013). This method employs DNA-coated microprojectiles to effectively deliver genetic material into plant cells, facilitating the generation of transgenic plants across a wide range of species, including challenging monocotyledons (Su et al., 2023). Agroinfiltration presents an effective alternative for delivering recombinant vectors into plant cells. This technique utilizes Agrobacterium tumefaciens, a bacterium with a unique ability to transfer genes into a host plant’s genome (Gelvin, 2017). For example, researchers were able to introduce a gene encoding the antifungal peptide Rs-AFP2 from radish (Raphanus sativus) into rice (Oryza sativa L. cv. Pusa basmati 1). This successful transfer holds promising implications for improving fungal disease resistance in rice (Jha and Chattoo, 2010). Plant-based AMP production has been achieved using various parts, including the successful expression of human lactoferrin (hLF) in tobacco hairy roots (Shanmugaraj et al., 2021a). Carrot suspension cell cultures served as the platform for producing taliglucerase-α, marking a milestone, as this first plant-derived pharmaceutical protein production method eliminates the need for further processing to modify glycosylation patterns after protein synthesis. The efficacy of taliglucerase alfa has been clinically proven (Manniello et al., 2021). A number of authoritative articles on strategies for heterologous expression of AMPs in plants have been reviewed elsewhere (Stączek et al., 2023).
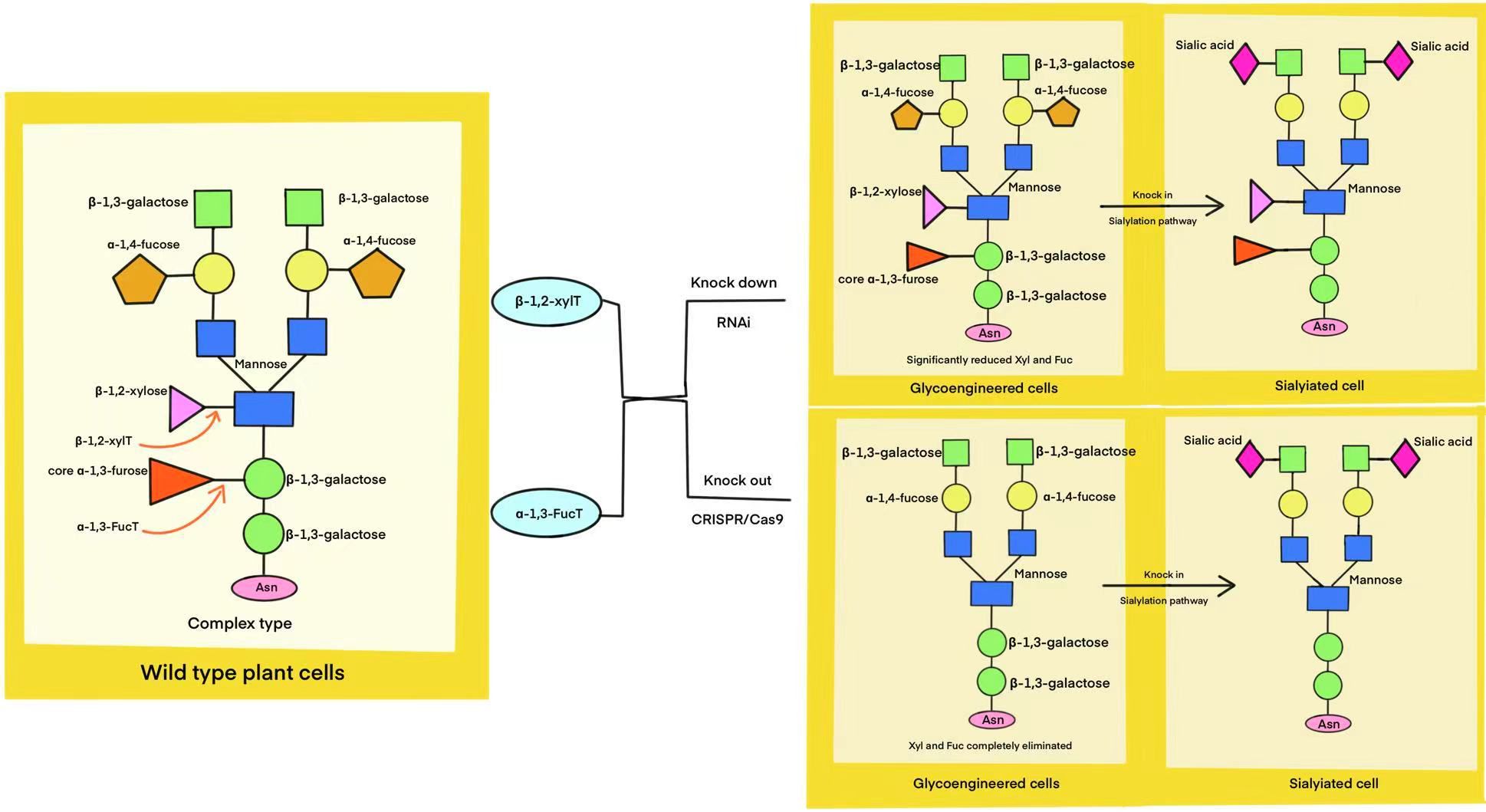
Figure 5. Glycoengineering strategies for humanizing plant N-glycans. This diagram illustrates the process of modifying wild-type plant cells to generate human-like N-glycan structures. By knocking down (RNAi) or knocking out (CRISPR/Cas9) specific genes, glycosylation pathways are altered to reduce undesired plant-specific modifications. Further genetic engineering, such as the introduction of the human sialylation pathway, allows for the incorporation of sialic acids, resulting in fully humanized N-glycans.
2.2.4 Insect cells
Insect-derived AMPs, offer a promising solution to bacterial resistance. Insects produce a diverse array of AMPs, including attacins, lebocins, and moricins, with the first identified AMP, cecropin, discovered in insects in 1980. The baculovirus expression system, utilizing insect cells, has a long and established history in producing a variety of heterologous proteins, including AMPs (Berger and Poterszman, 2015; Van Oers et al., 2015; Santry et al., 2017). Since Smith’s ground breaking 1983 study showcasing the use of Autographa californica multiple nucleopolyhedrovirus for heterologous protein production (Smith et al., 1983), the baculovirus expression system has evolved into a powerful protein production tool. This progress is driven by improvements in recombinant virus isolation, cell culture methods, and glycoprotein modification strategies (Kost et al., 2005; Joshi et al., 2021) (Kost et al., 2005; Joshi et al., 2021). Recombinant baculoviruses are proving useful beyond the production of important bioactive products, emerging as versatile tools for gene delivery and gene therapy applications (Wang et al., 2024). While insect cells offer several advantages for recombinant production, including efficient post-translational modifications and folding While insect cells offer several advantages for recombinant production, including efficient post-translational modifications and folding (Fabrick and Hull, 2017), their glycosylation capabilities are limited (Contreras-Gómez et al., 2014). Notably, they lack the enzymes required for terminal galactose and sialic acid addition (Palomares et al., 2021), which can impact the function of some recombinant proteins (Betenbaugh et al., 1996; Fernandes et al., 2021). Insect cell lines like Sf9 and Tni, while capable of N-glycosylation, produce simpler paucimannosidic structures due to the trimming activity of their FDL-encoded β-N-acetylglucosamines. To overcome this limitation, various attempts have been made. One approach involves introducing mammalian genes into insect cells, equipping them to build more complex glycans. For example, the engineered Sfβ4GalT cell line produces a human glycosyltransferase, enabling the addition of galactose to proteins (Jarvis, 2003; Chavez-Pena and Kamen, 2018). Another attempt focuses on disabling the gene responsible for the trimming enzyme. Silencing the fdl gene in BmN4-SID1 cells via RNA interference is one such approach, which has led to the production of glycoproteins with more complex N-glycan structures (Chavez-Pena and Kamen, 2018). Ongoing efforts aim to engineer insect cells to produce therapeutic peptides with improved glycosylation for enhanced effectiveness.
The CRISPR-Cas9 system was successfully employed to edit the fdl gene in Sf9 and BmN insect cells. This involved expressing Cas9 with the IE1 promoter and introducing specific guide RNAs (sgRNAs) with the DmU6:96Ab and BmU6-2 promoters (Mabashi-Asazuma and Jarvis, 2017b). An engineered insect cell line, based on Sf9 cells, expresses five mammalian glycosyltransferases. This allows the cells to produce recombinant proteins with complex, terminally sialylated N-glycans, resulting in mammalian-like glycosylation and higher overall glycosylation levels compared to proteins produced by the original Sf9 or High Five cells (Legardinier et al., 2005; Meng et al., 2016). Baculovirus-insect cell systems offer an effective alternative for producing AMPs, particularly those toxics to bacteria or fungi. This approach bypasses limitations of prokaryotic expression systems. For example, it has successfully produced human β-defensin- (Valore et al., 1998), the N-terminal portion of bovine lactoferrin (Valore et al., 1998) and snakin-1 (Zitzmann et al., 2017) and osmotin, a pathogenesis-related plant protein (Zitzmann et al., 2017). While producing the AMPs gloverin from the wax moth in microbial systems has proven challenging due to its inherent antibacterial activity, a study successfully achieved high yields using Drosophila cells. This suggests that insect cells may be a more suitable platform for producing insect-derived AMPs (Ikemura, 1985). Recently, scalable batch and perfusion processes were developed for producing BR033, a cecropin-like AMPs from Lucilia sericata, using Sf-9 insect cells (Käßer et al., 2022). While insect cell systems offer advantages like post-translational modifications, but their cost-effectiveness compared to established microbial platforms for large-scale production needs further consideration (Plotkin and Kudla, 2011). Table 6 summarizes various AMPs expressed in insects.
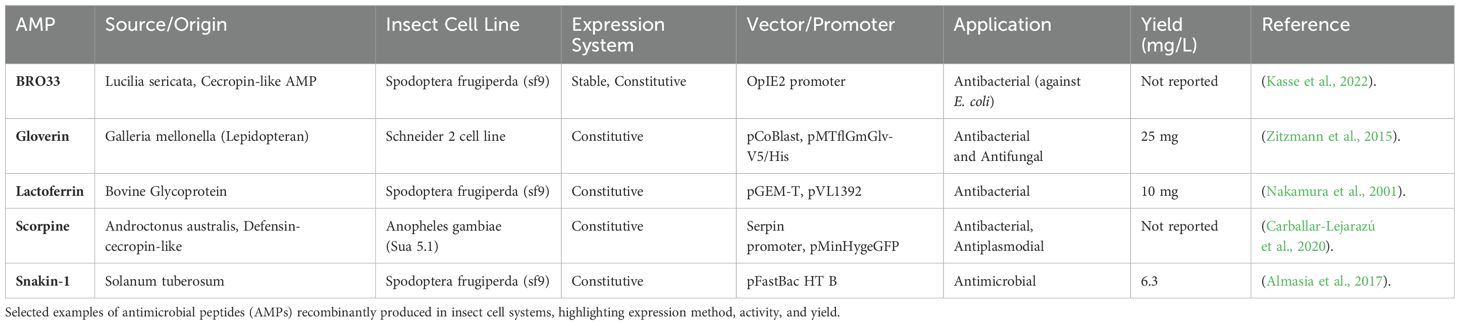
Table 6. Production of antimicrobial peptides in insect cell lines: expression systems, application, and yield.
3 Advancements in AMPs production, using heterologous systems
The ability to generate substantial quantities of pure AMPs through heterologous expression has revolutionized research in this field, offering a potent combination of cost-effectiveness, streamlined production, and straightforward purification. Figure 6 illustrates the key strategies for enhancing AMP expression (e.g., tandem multimeric expression, fusion tags, hybridization), while Table 7 summarizes representative AMPs produced via these methods, their yields, and purification efficiencies. Below, we explore these strategies and their implications for future advancements.
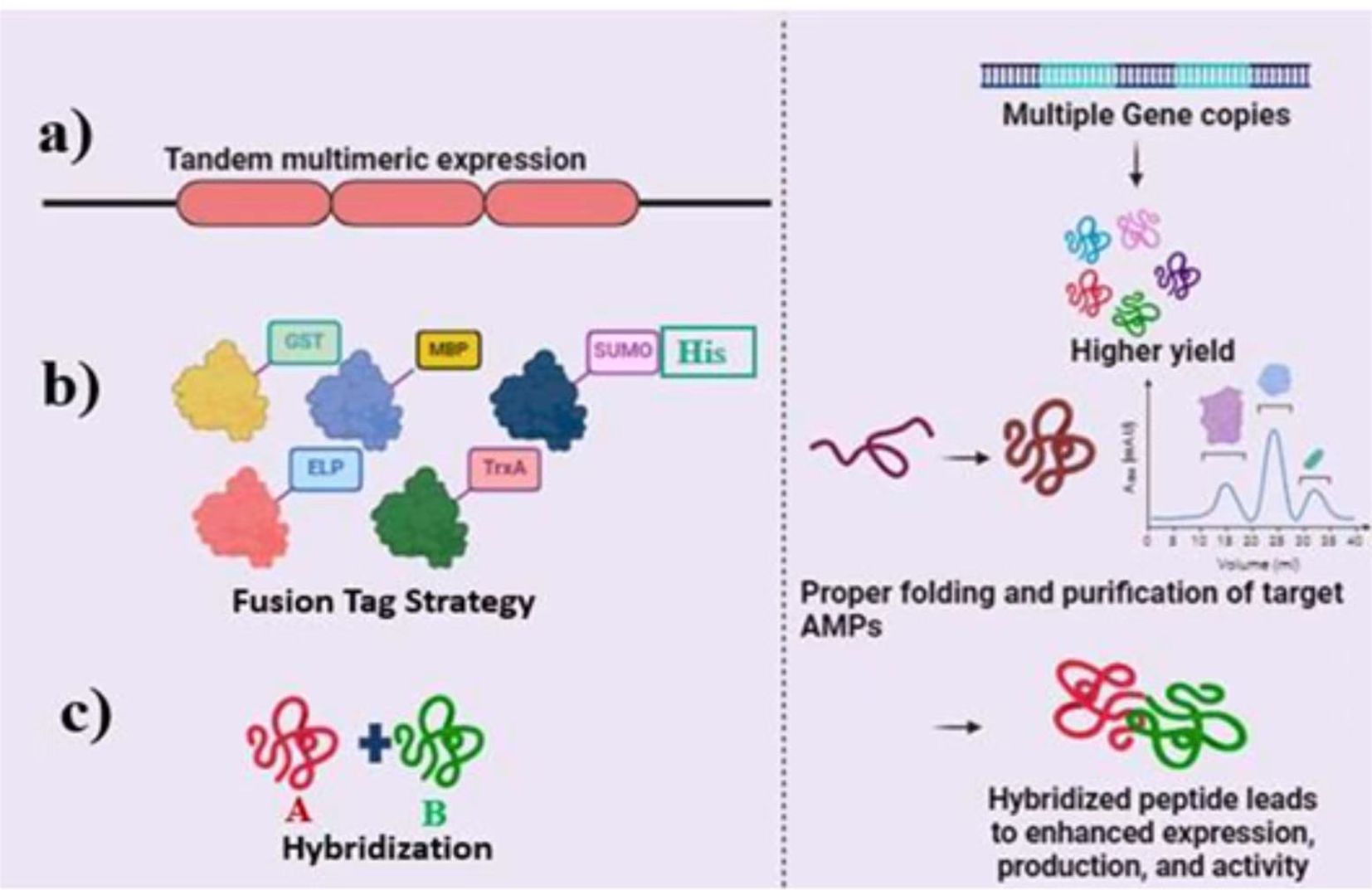
Figure 6. Advanced strategies for optimizing the expression and production of antimicrobial peptides (AMPs). (a) Tandem multimeric expression leverages multiple gene copies to significantly boost AMP yield. (b) Fusion tag strategy employs various tags (e.g., GST, MBP, SUMO, His) to enhance the solubility, purification, and correct folding of AMPs, ensuring higher production efficiency. (c) Peptide hybridization combines distinct peptide fragments to form hybrid AMPs, improving expression levels, biological activity, and overall peptide functionality. These strategies collectively enhance the yield, folding, and purification of active, high-quality AMPs.
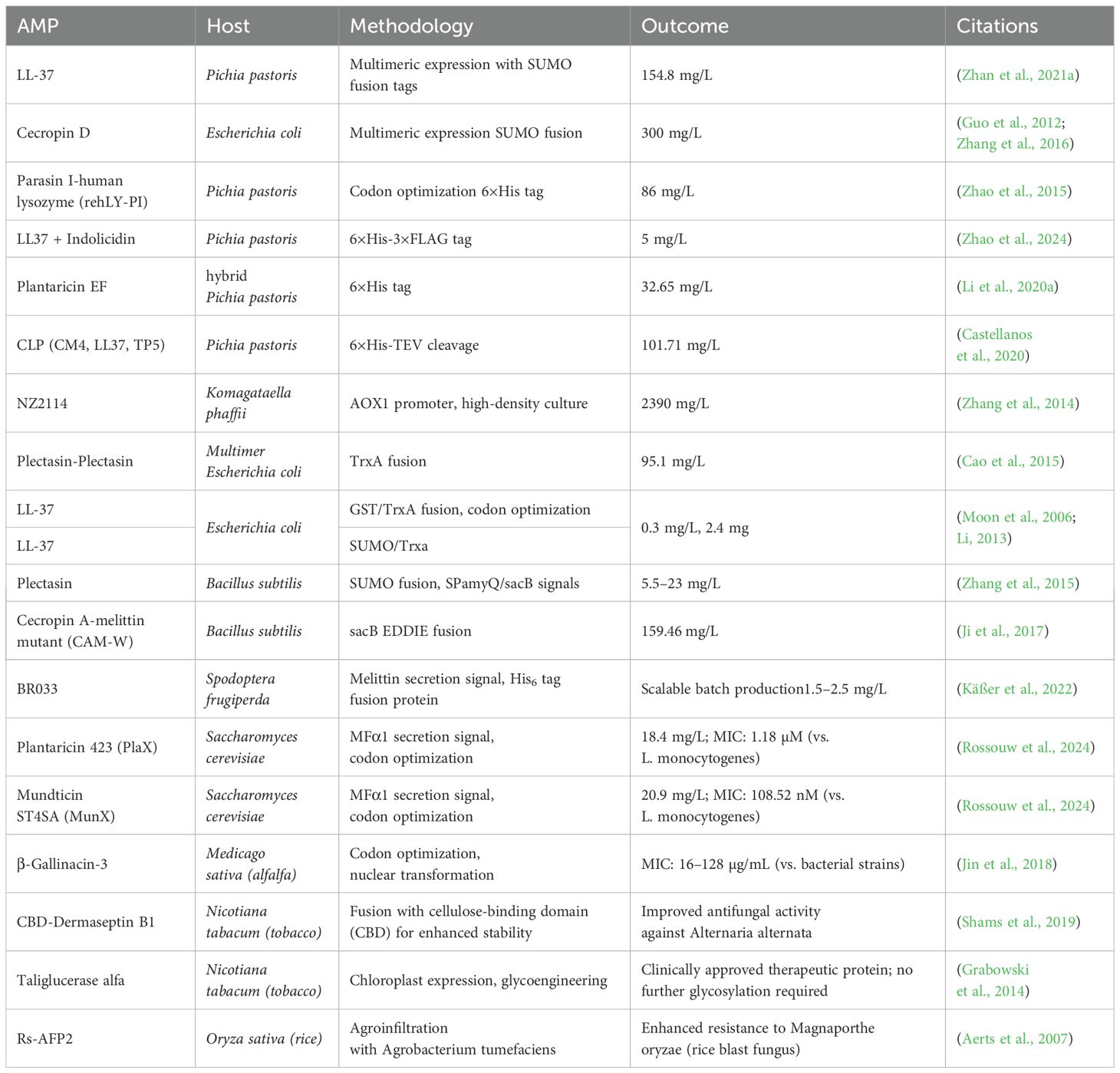
Table 7. Expression systems and methodologies for antimicrobial peptides: yields, outcomes, and host-specific strategies.
3.1 Optimization of codon usage
The genetic code, while nearly universal, exhibits a fascinating quirk called “codon usage bias”. This means that even though multiple codons can code for the same amino acid (due to flexibility at the third nucleotide, or wobble position), organisms tend to favor certain codons over others. This preference for specific codons has been observed across all genomes studied (Hershberg and Petrov, 2008; Chaney and Clark, 2015). Codons used more frequently are deemed preferred or optimal, while less favored ones are termed non-optimal or rare. For instance, mammals tend to favor C or G nucleotides at the wobble position, while budding yeast show a preference for A or U, likely reflecting differences in underlying mutational biases between these organisms (Duret, 2000; Quax et al., 2015). Once viewed as insignificant, codon usage bias is now recognized as a vital layer of genetic regulation. The preference for certain synonymous codons, even within the same gene, can significantly impact both protein levels and structure (Gustafsson et al., 2012; Zhou et al., 2016). Crucial genes, like those for ribosomal proteins, often prefer optimal codons across diverse organisms (Paremskaia et al., 2024). The relationship between codon usage and gene expression is increasingly evident, with genome-wide studies revealing a strong association between codon bias and protein levels. This connection is further supported by experimental manipulations, where optimizing codon usage has resulted in marked increases in protein production across diverse organisms (Peng et al., 2012).
Codon optimization makes genes easier for organisms to read by matching their DNA sequence to the organism’s preferred codons. This process is usually done using specialized computer software (Aguirre-López et al., 2017). Although most results are far more modest, it has been shown that codon optimization can boost protein expression by as much as 1000-fold (Kapust et al., 2002). Species like E. coli and yeast have distinct codon preferences due to the amount of tRNAs in their respective genome. Peng and his colleagues have demonstrated that employing codon optimization enhanced the expression of human beta-defensin-2 (hbd2) by a factor of nine, which resulted in a final concentration of 130 mg/L, surpassing the expression levels of human beta-defensin-5 & 6 (hbd5 and hbd6) in E. coli (Chadani et al., 2010). Moreover, codons encoding arginine have been found to greatly affect the production of heterologous proteins in E. coli. As a result, it is commonly suggested to replace the AGG or AGA codon for arginine with CGT in the target gene (Baghbeheshti et al., 2021). Additional stop codons are added in E. coli and yeast employing codon optimization for upscaling the production of AMPs. For example, in E. coli, the sequence TAATGA is commonly employed as a termination codon rather than merely TAA or TGA. This modification helps prevent the production of insufficient amounts of AMP products that can occur due to incorrect translational termination (Zhou et al., 2009). Researchers achieved the successful production of two beneficial bacteriocins, namely plantaricin 423 and mundticin ST4SA, using yeast (S. cerevisiae) (Rezaei et al., 2020). By optimizing the genes of mundticin ST4SA, the yeast demonstrated enhanced efficacy in combating pathogenic microorganisms. Nevertheless, the above-discussed examples help us to understand and explore the routes to efficiently produce and upscale the production of AMPs.
3.2 Tandem multimeric expression
One effective strategy to enhance the production of AMPs is through tandem multimeric expression (Thakker et al., 2011; Shen et al., 2012; Öztürk et al., 2017). This method involves introducing multiple copies of the AMP gene into a host cell, leading to the production of multiple AMP units linked together. This approach, unlike expressing single-unit AMPs, can significantly increase the overall yield of the desired AMP (Zhang et al., 2011). This higher gene dosage leads to increased transcription and, consequently, a higher yield of the desired peptide. The success of this strategy is evident in several studies (Table 8). For instance, increasing the copy number of the CM4 gene in E. coli BL21 significantly enhanced its production. By using pET32 with n 1-8 copies of AMP (CM4), the yield reached 68 mg/L, almost four times higher compared to using a single gene copy (Zhou et al., 2009).Similarly, increasing the gene copy number of ABP-dHC-cecropin A in E. coli from one to three resulted in a dramatic yield increase from 0 to 300 mg/L (Panteleev et al., 2017). These examples clearly demonstrate the potential of tandem multimeric expression for enhancing AMP production. This strategy also successfully produced a tetramer fusion of S3 and SΔ3 AMPs in E. coli BL21 for cost-effective large-scale production of AMPs. Using 8 gene fragment copies, P. pastoris released 146 mg/L of plectasin, an AMP with antibacterial action against S. suis and S. aureus (Seo et al., 2012). These studies demonstrate that both transcription and translational levels increased by employing this strategy. High expression levels due to tandem multimeric genes were seen not only in E. coliand P. pastoris, but also in B. subtilis. Additionally, using a tandem multimeric approach, B. subtilis significantly increased Tachyplesin expression levels (Holaskova et al., 2015). Although the tandem multimeric technique is an effective way to obtain high production of AMPs, the appropriate heterologous expression system must be selected for each AMP. It is also important to stress that there is no linear relationship between the number of copies of a gene and the amount of target protein expressed (Xu et al., 2005; Corrales-García et al., 2020). For instance, soluble expression of hBD2 in E. coli yielded conflicting results with 1–4 gene copies, cells containing only one or two joined hBD2 genes (instead of the more common four joined hBD2 genes) achieved a higher level of expression using the pGEX-4T-2 construct, whereas cells with fewer hBD2 gene copies showed a higher growth rate, suggesting that abundant AMP expression may strain the host cells (Almaaytah et al., 2018; Brady et al., 2019). For these reasons, optimizing AMP production in a heterologous expression system requires identifying the optimal gene copy number for a given AMP.
3.3 Hybridization expression approach
The hybridization expression strategy is a new method for increasing AMP expression and introducing unique characteristics and synergetic effect. The idea of hybridization expression was employed to combine two AMPs that possess complimentary yet unique characteristics. This fusion resulted in the development of a novel AMP that exhibits improved antimicrobial efficacy while minimizing harm to host cells (Feng et al., 2014). The use of cleavable tags, such as those removable by enzymatic or chemical treatment, or even self-cleaving tags like inteins, allows for the retrieval of the original peptide sequences after purification. These restored peptides can then be utilized together or further separated for individual applications. Numerous studies have highlighted the production fusion AMPS. Cecropins, one of the known AMPs composed of 31-39 amino acids, are frequently used in combination with other AMPs because of their ability to kill both Gram-negative and Gram-positive bacteria (Chen et al., 2009). A novel hybrid AMP, LF15-CA8, comprising amino acid sequences from cecropin A and LfcinB, demonstrated enhanced antibacterial activity against S. aureus without causing hemolytic activity (Wang, 2008). Cecropin A (1-8)-magainin2 (1-12) (CA-MA) was synthesized in P. pastoris, resulting in a higher yield and exhibited antibacterial activity against a diverse range of microorganisms, including yeasts as well as Gram-positive and Gram-negative bacteria. The peptide cecropin AD, composed of cecropin A and cecropin D, was synthesized in B. subtilis with a much higher yield of 30.6 mg/L. It exhibited antibacterial activity against S. aureus and E. coli (Zhan et al., 2021a).
LL37, the only member of the human cathelicidin family, is extensively found in several human organs and physiological fluids (Hoelscher et al., 2022). LL37 is of significant research interest due to its roles in immunological regulation, immune cell attraction, and wound healing (Engelberg and Landau, 2020; Yang et al., 2020; Ridyard and Overhage, 2021). Several studies demonstrated that the helical structural region consisting of 17-29 residues played a crucial role in LL37’s biological functionality (Engelberg and Landau, 2020; Kielkopf et al., 2020). For example, the hybrid peptide LL-37_Renalexin combines LL-37 and Renalexin, resulting in potent, broad-spectrum antimicrobial activity (Narh et al., 2024), while the hybrid peptide LL-37Tα1, derived from LL-37 and Tα1, acts as a potent anti-endotoxin without hemolytic or cytotoxic activity (Ahmad et al., 2019). Thus, the hybridization expression approach shows great promise for upscaling the AMP production and potent antimicrobial activity.
3.4 Fusion tag expression
The choice of host organism significantly influences the production yield of AMPs. While insects, plants, yeast, and bacteria are explored for recombinant AMP production, bacterial (Li, 2011a) and yeast systems (Pipiya et al., 2023) typically offer higher yields. However, AMP production in heterologous hosts faces challenges. Their small size, positive charge, and susceptibility to degradation contribute to cytotoxicity and complicate purification processes in heterologous hosts (Teixeira et al., 2020a; Zainal Baharin et al., 2021; Du et al., 2022; Cesaro et al., 2023; Tang et al., 2023). Intracellular expression of AMPs, often lacking signal peptides, necessitates cell lysis for extraction and purification (Muthunayake et al., 2020a). Fusion protein systems present a common strategy to overcome the challenges associated with AMP production (López-Otín and Matrisian, 2007; Parachin et al., 2012a; Deng et al., 2017). This approach has proven particularly advantageous in bacterial expression systems, enhancing both AMP expression levels and facilitating downstream purification (Costa et al., 2014). Carrier proteins, such as GST, TrxA, and SUMO, act as artificial pro-segments, enhancing solubility, aiding in proper folding, and simplifying purification (Wood, 2014). Once the fusion protein is expressed and purified, tags like GFP and 6 × His, incorporated into the AMP sequence, further facilitate detection and purification (Figure 6b). For instance, Thioredoxin, a fusion protein derived from E. coli, enhances the solubility of expressed proteins and has been successfully employed in the expression of various AMPs, including epinecidin (Jeyarajan et al., 2024), Snakin-2 (Herbel et al., 2015), stomoxyn ZH1 (Elhag et al., 2017), plectasin (Jing et al., 2010) and Human α-defensin 6 (Wang et al., 2010). GST fusion proteins offer a simple purification method using affinity chromatography with immobilized glutathione, allowing for quick isolation from crude lysates (Zhou et al., 2020). Several studies highlight the effectiveness of GST fusion in enhancing AMP expression. For instance, a GST-Cecropin B fusion expressed in Pichia pastoris showed improved bioactivity and yield (Skosyrev et al., 2003). Similarly, in E. coli, a GST-Melittin fusion achieved a high yield of 3.5 g/L with 90% purity (De Beer and Vivier, 2008). These findings demonstrate that GST fusion not only enhances the expression of recombinant peptides but also simplifies their purification. While GST fusion proteins often result in soluble expression, achieving optimal solubility may require fine-tuning various factors, including the choice of host strain and optimization of temperature and pH (Lavallie et al., 1993, 2000). Elastin-like polypeptides are versatile fusion carriers that facilitate efficient expression and purification of target proteins due to their unique properties and the strategic incorporation of specific cleavage sites (Varanko et al., 2020). Numerous AMPs, often cationic in nature, have been successfully produced using ELPs (Pereira et al., 2021). For example, CM4 and HβD4 were produced in a bacterial system using an ELP-intein strategy, achieving high antimicrobial activity and a final product yield of 1.84 mg/L (Ghidey et al., 2020). While intein-based approaches have shown promise for maximizing yields, as seen with Pa-MAP2 (Malakhov et al., 2004), chemical cleavage has also demonstrated high yields, such as the 90 mg/L achieved for Hep25 (Butt et al., 2005). Notably, fusing AMPs to ELPs in Nicotiana benthamiana resulted in high yields and significantly enhanced antimicrobial activity against Staphylococcus epidermidis compared to E. coli expression systems (Luengo et al., 2018). SUMO has emerged as a highly effective fusion carrier for recombinant protein production, enhancing both folding and solubility (Azari et al., 2020a; Park et al., 2021a). Its unique protease, SUMO protease 1, recognizes the tertiary structure of the SUMO tag, ensuring precise cleavage and yielding target proteins with native N-termini (Butt et al., 2005; Lee et al., 2008). This system has facilitated the successful expression of challenging proteins, including AMPs like PR-39 (Azari et al., 2020a), Cecropin B (Park et al., 2021a), LL37 (Zhan et al., 2021a), and others (Hoelscher et al., 2022). These findings underscore the importance of considering fusion tag properties and protein-tag interactions when selecting optimal tags for AMP synthesis and purification.
3.5 Signal peptide-based secretion
Secreting recombinant proteins directly into the growth medium offers significant advantages over intracellular expression. This strategy minimizes aggregation, reduces host cell toxicity, promotes proper protein folding, and simplifies purification (Freudl, 2018; Duan et al., 2019). Signal peptides, short sequences at the beginning of some proteins, act as “zip codes,” directing them to the cellular export machinery. This guidance ensures efficient transport and prevents premature folding (Yu et al., 2022). Highlighting the impact of signal peptides on protein secretion, a recent study successfully expressed a modified alpha toxin, H35L, as a fusion protein with an NSP4 signal peptide (NSP4_ATH35L) in E. coli. By modifying the secretion system through domain swapping and altering the dsbA and pelB signal peptides, the researchers achieved a 3.5-fold increase in the yield of secreted ATH35L (Han et al., 2021). Researchers have also harnessed the efficient SPYncM signal peptide in B. subtilis to boost protein secretion. By combining this signal peptide with a dual-promoter system (PHpaII–PgsiB) instead of the typical single promoter, they achieved a remarkable yield of 1.74g/L, demonstrating the potential of tandem promoters for substantial production increases (Gattu et al., 2023). Maeno et al. developed an innovative approach for producing apidaecins using Streptomyces lividans (Maeno et al., 1993).
By fusing the AMPs to a protease inhibitor with a cleavable linker, they achieved efficient secretion and simplified purification. This method effectively addressed the challenges of degradation and complex purification often associated with recombinant peptide production (Domhan et al., 2019). The α-factor preproleader has proven highly versatile for functional protein expression in yeast. This system has successfully produced a wide range of proteins, including fungal proteins, green fluorescent proteins, vaccines, (e.g., human insulin), and various AMPs (e.g., LL-37) (Kjeldsen et al., 2002; Fitzgerald and Glick, 2014; Zhan et al., 2021a). Moreover, it has been shown that the increase in the positive charge in the N-domain and high hydrophobicity in the H-domains are potent for efficient secretion system. Furthermore, selecting the desired signal peptide for protein production commonly relies on methods like high-throughput screening or single-specific verification (Zhao et al., 2021). Nevertheless, more work needs to be done in this domain to enhance their yield and maximize their antibacterial activity.
4 AMP modifications and in-vivo effectiveness
While promising antimicrobial agents, AMPs face challenges in therapeutic applications. Their effectiveness is often limited by protease degradation, binding to serum proteins, and susceptibility to physiological conditions. Additionally, high therapeutic doses can lead to cytotoxicity and hemolysis (López-Otín and Matrisian, 2007). To overcome these limitations, researchers are developing novel peptides with enhanced antibacterial properties and reduced side effects. These efforts focus on modifying naturally occurring AMP sequences using strategies like cyclization, nanoparticle formulations, and incorporation of uncommon amino acids. Chemical synthesis via SPPS is crucial for these modifications, enabling precise sequence control, improved stability, and exploration of structure-activity relationships (Al Musaimi et al., 2022).
4.1 Incorporation of unusual amino acids
Chemical synthesis of AMPs offers advantages over natural extraction, enabling precise sequence modification via solid-phase peptide synthesis (Andreu et al., 1985; Pazgier et al., 2006; Pandit and Klauda, 2012; Guan et al., 2019). This facilitates modulating antibacterial potency, exploring structure-activity relationships, and incorporating non-natural amino acids for enhanced activity and stability. For instance, ornithine, 2,4-diaminobutyric acid, and 2,3-diaminopropionic acid can replace lysine to adjust amino acid side chain length (Kumar et al., 2018; Ting et al., 2020a). Vogel et al. showed that substituting lysine with diaminopimelic acid in tryptophan-rich peptides enhanced their antimicrobial activity against E. coli. This four-fold increase in efficacy is likely attributed to greater membrane permeabilization (Arias et al., 2018). Cationic peptides incorporating unnatural amino acids demonstrate broad-spectrum antimicrobial activity alongside enhanced resistance to proteolytic degradation. These peptides effectively combat a wide range of pathogens, including multidrug-resistant bacteria, while maintaining stability in biological environments (Glibowicka et al., 2022). Petraccone and colleagues developed a series of cationic synthetic peptides incorporating unnatural amino acids, such as 2-naphthyl-L-alanine and S-tert-butylthio-L-cysteine. These modifications resulted in enhanced antimicrobial activity across a broad spectrum of pathogens while also significantly improving proteolytic stability (Oliva et al., 2018).
Building on this approach, LTX-109, a synthetic tripeptide, exemplifies how unnatural amino acid incorporation can optimize antimicrobial efficacy and structural stability (Nilsson Anna et al., 2014). Featuring a non-canonical tryptophan residue flanked by two arginine residues, LTX-109 (Figure 7 (1)) enhances membrane disruption and protease resistance, reinforcing the potential of synthetic modifications in AMP design. Similarly, dipeptide calpain inhibitors have been modified with unnatural amino acids containing fluoromethyl ketone and aldehyde moieties at the C-terminus of Cbz-protected dipeptides, exhibited notable bacteriostatic activity against Chlamydia trachomatis, but this effect was temporary, with bacterial growth resuming after treatment (Figure 7 (2) and (3)) (Itoh et al., 2019).Together, these studies emphasize the crucial role of unnatural amino acids in designing next-generation AMPs with enhanced potency, stability, and resistance to enzymatic degradation.
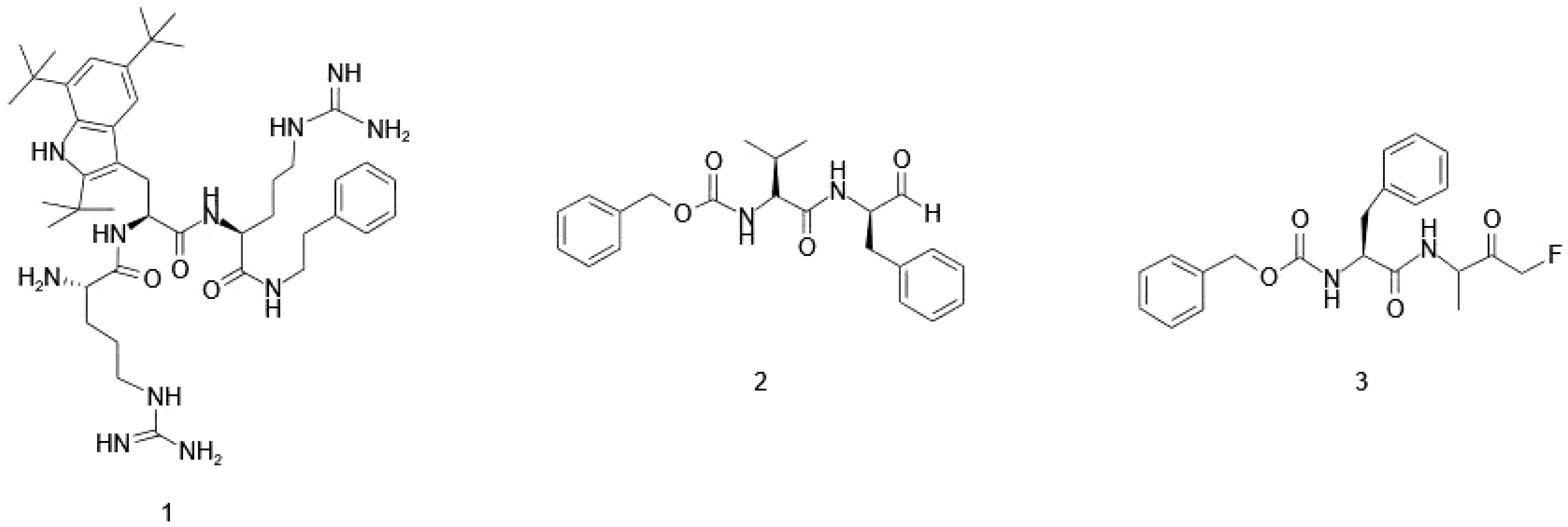
Figure 7. Incorporation of unnatural amino acids for the enhancement of therapeutic peptides. (1) LTX-109: A tri-peptide consisting of a lipophilic, unnatural tryptophan residue flanked by two arginines, illustrating the success of using unnatural amino acids to improve the activity of antimicrobial peptides (AMPs). (2) and (3) Cbz-protected dipeptide calpain inhibitors: Hiromatsu and co-workers repurposed these inhibitors by incorporating unnatural C-terminal amino acids, specifically with fluoromethyl ketone and aldehyde groups, enhancing their inhibitory potency and selectivity.
Amino acid substitution with proteinogenic L-residues is a common strategy to enhance AMP activity and selectivity. For example, modifying the natural AMP magainin II led to the development of pexiganan, a potent broad-spectrum analogue (Portelinha et al., 2021). This improved activity resulted from substituting specific neutral and anionic amino acids with cationic and hydrophobic residues (Gottler and Ramamoorthy, 2009). Several synthetic AMPs based on L-amino acid substitutions, such as iseganan (protegrin analogue), omiganan (indolicidin analogue), and P113 (histatin analogue), have progressed to late-stage clinical trials.
Substituting L-amino acids with D-amino acids is a promising strategy to enhance the stability and activity of AMPs. Studies have shown that this modification can maintain antimicrobial activity while enhancing protease resistance (Shao et al., 2019; Hasan et al., 2021). For example, a D-enantiomer of the wasp venom AMP, MPI, showed significantly increased protease resistance compared to its L-amino acid counterpart (Jia et al., 2017; Kumar et al., 2018). Furthermore, D-enantiomers of short synthetic AMPs, D-JK-5 and D-JK-6, exhibited increased proteolytic stability and retained anti-biofilm activity against a range of pathogens. However, it’s important to note that D-amino acid substitutions can also impact other AMP properties. Partial substitutions may disrupt α-helicity, while complete substitution creates an enantiomer with potentially different properties (Ting et al., 2020a). For instance, in a study by Hodges et al., D-amino acid substitutions in the AMP V681 led to decreased helical content and increased hemolytic activity, although the reasons for the latter remained unclear (Mant et al., 2019). This approach allows researchers to investigate the impact of these modifications on peptide stability, activity, and structure.
4.2 Cyclization
Macrocyclic peptides show promise as AMPs due to their enhanced stability, reduced flexibility, and improved antibacterial activity and selectivity (Vinogradov et al., 2019; Ting et al., 2020a). This is exemplified by naturally occurring cyclic AMPs like human β-defensins (hBDs), which exhibit these beneficial properties (Fernandez-Reyes et al., 2010; Nyembe et al., 2023). Furthermore, several FDA-approved systemically bioactive AMP medications, including tyrothricin, bacitracin, polymyxin B and E, daptomycin, and gramicidin S, are also cyclic peptides, highlighting the clinical relevance of this peptide architecture (Wang, 2012; Kurpet and Chwatko, 2022; Garcia Jimenez et al., 2023). Moreover, over the past five years, the FDA has granted approval to 17 macrocyclic drugs, further emphasizing their crucial contribution to modern therapeutics (Du et al., 2025). Due to their synthetic accessibility, macrocyclic peptides, are increasingly studied for their antimicrobial potential. Both the peptide sequence and the method of cyclization play crucial roles in optimizing antimicrobial activity while minimizing human toxicity. The potential of macrocyclic drugs for a variety of clinical applications has been emphasized in recent reviews (Garcia Jimenez et al., 2023). This section examines various chemical strategies employed to cyclize AMPs, aiming to enhance their antimicrobial activity and stability while minimizing hemolytic activity. Ghadiri and colleagues designed small, cyclic peptides made of alternating units of L-Trp and D-Leu. These peptides spontaneously form tube-like structures within cell membranes and show potent activity against both Gram-positive MRSA and Gram-negative E. coli bacteria. However, these cyclic peptides also exhibited hemolytic activity (Lai et al., 2022). Head-to-tail cyclization has been investigated to enhance the antimicrobial activity of peptides. Cyclized Arg/Trp-rich peptides exhibited significantly greater potency against B. subtilis and E. coli compared to their linear counterparts, albeit with increased hemolysis (Gan et al., 2021). Similarly, a head-to-tail cyclized peptide composed of alternating hydrophilic and hydrophobic residues exhibited greater activity against MRSA than its linear form (Mohammed et al., 2022). Researchers developed a cyclic AMPs by introducing two cysteine residues into cathelicidin-BF15-a3, a peptide derived from snake venom. This modification, enabled formation of a disulfide bond, yielding a highly potent peptide effective against P. aeruginosa and A. baumannii, with low toxicity to red blood cells, high stability in living organisms, and a reduced likelihood of inducing bacterial resistance (Mwangi et al., 2019). Hancock and colleagues examined the impact of different cyclization methods, including head-to-tail, side chain-to-tail, and disulfide bond formation, on the properties of the AMPs IDR-1018 (Figure 8). Notably, the side chain-to-tail cyclized variant demonstrated potent anti-inflammatory activity and effectively reduced bacterial burden in a mouse skin infection model (Eckert et al., 2006a, 2006b; De La Fuente-Núñez et al., 2014; Etayash et al., 2020).
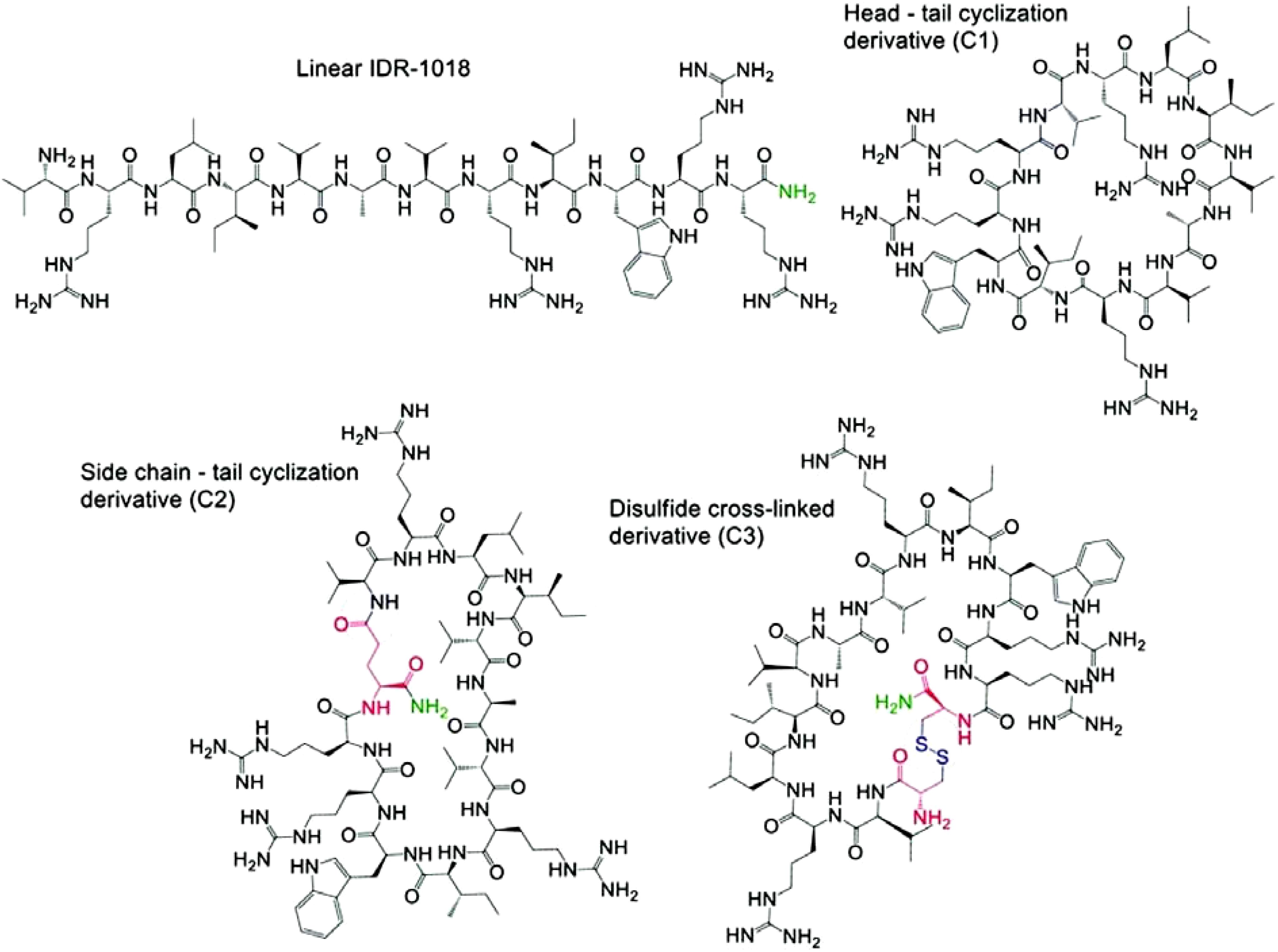
Figure 8. Structural derivatives of linear IDR-1018 peptide. The figure shows various cyclic and cross-linked derivatives of the linear IDR-1018 peptide. (Top) The Linear IDR-1018 structure is shown as the starting point. (Right) The Head-tail cyclization derivative (C1) forms a cyclic structure by linking the N- and C-terminal ends. (Bottom Left) The Side chain-tail cyclization derivative (C2) forms a cyclic structure by connecting the side chain with the peptide tail. (Bottom Right) The Disulfide cross-linked derivative (C3) features a disulfide bond that connects two side chains, stabilizing the structure. These modifications aim to enhance the peptide's stability and bioactivity.
4.3 Terminal/side chain modifications
N-terminal acetylation and C-terminal amidation (Figure 9) are frequently employed strategies to enhance peptide stability by mitigating proteolytic degradation (Ting et al., 2020a). N-terminal acetylation, a ubiquitous modification in both eukaryotic and prokaryotic cells, effectively inhibits aminopeptidase activity, thereby increasing peptide half-life (Parks and Escalante-Semerena, 2022). However, this modification in concomitantly reduces the net positive charge of the peptide, which may lead to diminished antimicrobial potency (Crusca et al., 2011; Nishita et al., 2017; Baeriswyl et al., 2019). A recent study found that AMPs derived from the MreB protein, a key component of the bacterial cytoskeleton, showed promising activity against a range of microbes. Specifically, the peptide fragment MreB1-9, with its +4 net charge, effectively targeted both Gram-positive and Gram-negative bacteria, as well as the fungal pathogen C. albicans. However, N-terminal acetylation while preserving antifungal activity, reduced its effectiveness against certain bacterial species like P. aeruginosa and S. aureus (Nishita et al., 2017). C-terminal amidation, a common modification in natural AMPs, influences their structure and function (Wang, 2012). While N-terminal acetylation can have mixed effects, C-terminal amidation generally enhances the antimicrobial activity of membrane-disrupting peptides. This is likely because it stabilizes their helical structure at the membrane interface, leading to more effective membrane disruption and pore formation (Dennison et al., 2009; Dennison and Phoenix, 2011; Irudayam and Berkowitz, 2012). For example, studies on aurein peptides demonstrate that C-terminal amidation enhances their antimicrobial activity, likely by promoting a stable alpha-helical structure that improves membrane disruption (Sarah et al., 2012; Mura et al., 2016). N-terminal acetylation and C-terminal amidation can have varying effects on antimicrobial activity. However, combining these modifications significantly enhances the stability of AMPs against enzymatic degradation. For example, this dual modification increased the stability of a human apolipoprotein B-derived AMP by more than fourfold in the presence of serum proteins (Oliva et al., 2018). Similarly, modified tachyplesin I showed greater stability than its unmodified form (Kuzmin et al., 2017; Oliva et al., 2018). Additionally, glycosylation and PEGylation of amino acid side chain are employed to increase the ability of peptides to be absorbed and tolerated by the body (Lalonde and Durocher, 2017; Li et al., 2021a). These modifications also reduce immune response likelihood, reduce the rate at which peptides are eliminated by the kidneys, and prolong the time that peptides remain in circulation when administered intravenously (Hagedorn et al., 2010). The utilization of glycoengineering technology facilitates the customization of glycan alterations, hence improving specificity, stability, and antibacterial activity (Correa et al., 2020; Lepeltier et al., 2020; Hadjicharalambous et al., 2022). Moreover, the bioavailability of peptides can be increased by conjugation with large stable proteins. For example, combining the peptide infestin-4 with human albumin extends its half-life from 20 minutes to 276 minutes, significantly prolonging its circulation in the blood (Chen and Jiang, 2023).
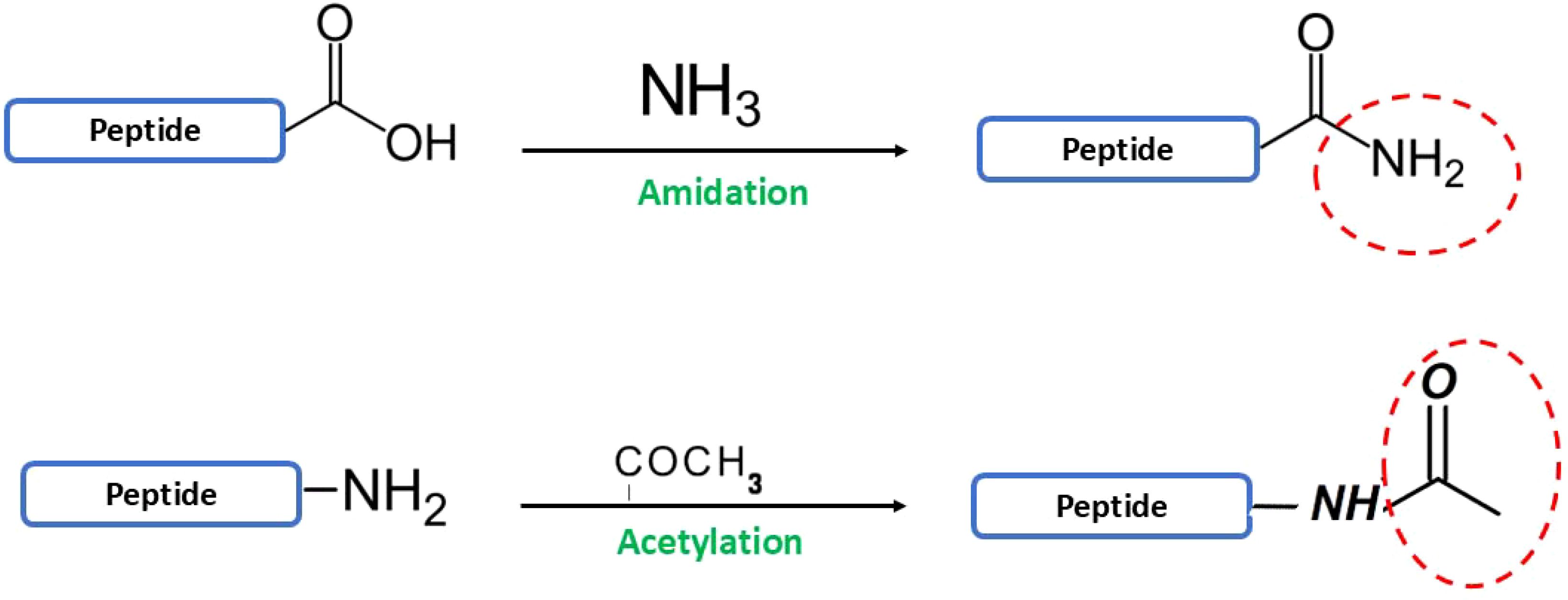
Figure 9. Peptide modification strategies: Amidation and Acetylation. The figure illustrates two common post-synthetic peptide modifications: (Top) Amidation, where the C-terminal hydroxyl group (-OH) of a peptide is converted into an amide group (-NH2) through reaction with ammonia (NH3). (Bottom) Acetylation, where the N-terminal amine group (-NH2) of a peptide is modified by the addition of an acetyl group (-COCH3), enhancing peptide stability and functionality. These modifications are crucial for optimizing the activity and stability of peptides.
4.4 Nanoparticle formulations
Recently, the use of nanoparticles (NPs) conjugated with AMPs has gained tremendous attention. Conjugating AMPs to NPs is a highly effective technique for improving the stability of these peptides in host cells, reducing their toxicity, and enhancing their antimicrobial activity. This approach also serves as a promising strategy for targeted drug administration, making AMP-NP conjugates good platforms for delivering drugs to specific targets (Moradi et al., 2016; Sánchez-López et al., 2020; Teixeira et al., 2020a). Furthermore, they do not require transfection as they are internalized within the cytoplasm via endocytosis and are independent of efflux pumps (Le et al., 2017). One of the distinctive physicochemical properties of nanomaterials is their large surface area which helps in the adsorption of more and more AMPs and also prevents their aggregation (Ji et al., 2021). In addition to their inherent antibacterial properties, nanoparticles (NPs) such as silver, zinc oxide, and titanium dioxide have been extensively studied for their effectiveness against a broad spectrum of microbes, including antibiotic-resistant strains. For instance, silver nanoparticles (AgNPs) have demonstrated significant antimicrobial activity by interacting with bacterial cell membranes, leading to cell damage and death. A notable example of an AMPs (AMP) conjugated with silver nanoparticles (AgNPs) causing bacterial cell death involves the AMP indolicidin. Researchers have conjugated indolicidin to AgNPs, resulting in a nanocomposite that exhibits enhanced antibacterial activity against pathogens such as Escherichia coli and Staphylococcus aureus. The conjugation enhances the peptide’s stability and facilitates its interaction with bacterial membranes. Upon contact, the indolicidin-AgNP complex disrupts the integrity of the bacterial cell membrane, leading to leakage of cellular contents and ultimately causing cell death. This synergistic effect leverages both the membrane-disrupting properties of indolicidin and the inherent antimicrobial characteristics of AgNPs, offering a potent strategy to combat bacterial infection (Pal et al., 2019). Due to their small size, large surface area, and strong targeting capabilities, AMPs encapsulated within these nanostructures are in high demand for developing advanced antimicrobial therapies. The combination of AMPs with NPs not only enhances antimicrobial efficacy but also offers a promising strategy to overcome the limitations of traditional antibiotics.
Additionally, NPs, such as silver, zinc oxide, and titanium dioxide, possess inherent antibacterial properties, which is advantageous to the conjugated AMP system. Moreover, a substantial amount of recently published work has shown the use of silicon-derived nano systems and silver and gold NPs for efficient AMP delivery (Besinis et al., 2014; Shameli et al., 2024). Because of their small size, large surface area, and strong targeting capability, AMPs encapsulated with nanostructures seem to have a great demand in the current context.
4.5 post-translational modifications
Several studies have been conducted in this field and it highlights the significance of post-translational modifications (PTMs) in enhancing the productivity and anti-microbial activities of the AMP. Moreover, AMPs require metal ions or PTMs to adopt proper amphiphilic structures, which are mandatory for their antimicrobial activity (Gan et al., 2021). Glycan structure plays a crucial role in promoting the dimerization of glycopeptides in solution. This dimerization effectively increases the valency of the glycopeptide, thereby amplifying its antimicrobial potency (Behren and Westerlind, 2019). One such example includes an antiretroviral drug, enfuvirtide, that is shown to be glycosylated with sialic acid residues, and it has demonstrated improved half-life without affecting the sensitivity and effectiveness towards its target.
Further findings indicated that glycosylation in P. pastoris, contributed to the enhanced antibacterial efficacy of the AMP products when compared to those produced in E. coli. In addition, P. pastoris has shown superiority over both E. coli cells and S. cerevisiae in various settings. The purified recombinant CHP demonstrated antimicrobial action against both Gram-positive and Gram-negative bacteria, as well as against certain fungi. Both E. coli and P. pastoris were seen to produce PaDef at elevated levels. However, only the products generated by P. pastoris demonstrated notable antibacterial action (Karbalaei et al., 2020a). Furthermore, it was observed that P. pastoris and S. cerevisiae both successfully expressed EnterocinL50A and EnterocinL50B. However, studies have shown that the bioactivities of the AMPs (AMP) products obtained from P. pastoris were 6 and 60 times higher, respectively, compared to those from S. cerevisiae. (Basanta et al., 2010). However, glycosylation does not always boost the antimicrobial activity of AMPs because the glycans attached may reduce the hydrophobicity and hence altogether positive charge of the peptides, which has an influence on their interactions and integration into the anionic membranes of the host (Salam et al., 2023). A deeper exploration of how glycosylation affects the function of AMPs is crucial for advancing this field of study.
4.6 AI and machine learning for AMP discovery and optimization
The convergence of increased computing power, abundant data, accessible neural networks, and advanced AI algorithms has propelled artificial intelligence to prominence in science and medicine (Ting et al., 2020a; Wang, 2012; Pasrija et al., 2022). Machine learning, in particular, offers a powerful approach for optimizing AMP sequences by leveraging extensive training data (Lin et al., 2020b; Pasrija et al., 2022; Pérez De Lastra et al., 2024). Various machine learning algorithms, including SVM, fuzzy KNN, RF, and NN, have been developed for this purpose (Lin et al., 2020a; Kamran et al., 2022; Xu et al., 2023). For a comprehensive overview of these methods in the context of AMPs, readers are directed to recent reviews (Wu et al., 2019; Jukič and Bren, 2022; Santos-Júnior et al., 2024).
In AMP research, the Quantitative Structure–Activity Relationship (QSAR) model is frequently employed to predict peptide activity by analyzing their chemical structures and molecular features (Wu et al., 2019; Keyvanpour and Shirzad, 2021; Jukič and Bren, 2022). QSAR modelling has been successfully employed to optimize AMP properties (Taboureau, 2010). For instance, Ding et al. demonstrated that substituting hydrophobic amino acids with less lipophilic residues can reduce hemolytic activity without compromising antimicrobial activity (Biasizzo and Kopitar-Jerala, 2020). Applied a QSAR model to uncover peptide motifs capable of forming silver nanoparticles, broadening its use beyond antimicrobial functions. Furthermore virtual screening using QSAR modelling has emerged as a powerful approach for discovering and optimizing AMPs (Vincenzi et al., 2024a). For example, a computational approach utilizing a QSAR model to screen a virtual library of peptide structures successfully identified promising candidates with enhanced antibiofilm activity. This highly accurate model (85% success rate) led to the discovery of peptide 3002, which demonstrated an eightfold increase in eradicating established MRSA biofilms in vitro compared to its parent peptide (Haney et al., 2017; Mansour, 2018). Using Mutator, a web-based computational tool for optimizing AMPs selectivity, Tossi and colleagues identified Dadapin-1 (Waghu et al., 2020). While computer-aided design shows promise for developing enhanced antibiofilm peptides, further refinement of predictive models is anticipated with the increasing availability of active peptide sequences in databases (Reymond et al., 2010).
Virtual screening of vast molecular libraries within chemical space accelerates the discovery of novel AMPs, identifying promising candidates for synthesis and testing (Reymond et al., 2010; Kale et al., 2023). One successful example is the work of the Reymond group, who have utilized this approach to discover and optimize bicyclic peptides—a class of molecules with high stability due to their constrained structure. Their research led to the identification of compounds 27b and 62b, which exhibited potent activity against multi-drug resistant P. aeruginosa and other clinically relevant Gram-negative bacteria (Di Bonaventura et al., 2017). In a separate study, they screened a virtual library of over 4.6 million bicyclic peptides, leading to the identification of bp56, a potent and stable compound active against MDR strains of A. baumannii and P. aeruginosa (Di Bonaventura et al., 2018). The Reymond group also employed a similar approach to enhance the activity of the AMPs dendrimer G3KL. This resulted in the discovery of T7, a dendrimer with significantly improved efficacy against a panel of Gram-negative bacteria, including MDR P. aeruginosa and K. pneumoniae strains (Siriwardena et al., 2018).
5 Conclusion
AMPs hold significant promise as a novel solution to the growing global threat of antibiotic resistance. Their broad-spectrum activity against bacteria, fungi, and viruses, coupled with their unique mechanisms of action, positions them as a valuable alternative to conventional antibiotics. While challenges remain in production and optimization, advancements in genetic engineering, chemical synthesis, and rational design are paving the way for AMPs to become viable therapeutic agents. Overcoming toxicity and stability hurdles through innovative research strategies, including structural modifications and targeted delivery systems, is crucial for realizing the full clinical potential of AMPs. Continued interdisciplinary efforts in microbiology, medicinal chemistry, and synthetic biology will be essential to refine AMP design and unlock their potential to combat drug-resistant infections effectively. The future of AMPs as a powerful tool in human healthcare hinges on continued research and development, ultimately offering hope for a new era of effective antimicrobial therapies.
Author contributions
MS: Visualization, Writing – original draft, Data curation, Formal analysis, Investigation. YL: Writing – review & editing. CW: Writing – review & editing, Software. FH: Formal analysis, Writing – review & editing. JZ: Formal analysis, Investigation, Validation, Funding acquisition, Supervision, Writing – review & editing. PX: Conceptualization, Funding acquisition, Methodology, Resources, Supervision, Formal analysis, Investigation, Validation, Writing – review & editing.
Funding
The author(s) declare that financial support was received for the research and/or publication of this article. This work was supported by the China Scholarship Council [202301040010].
Acknowledgments
MS gratefully acknowledges the financial support from China Scholarship Council bearing Ref. NO. 2018DFH007663 at Shandong University of Technology, Zibo China.
Conflict of interest
The authors declare that the research was conducted in the absence of any commercial or financial relationships that could be construed as a potential conflict of interest.
Generative AI statement
The author(s) declare that no Generative AI was used in the creation of this manuscript.
Publisher’s note
All claims expressed in this article are solely those of the authors and do not necessarily represent those of their affiliated organizations, or those of the publisher, the editors and the reviewers. Any product that may be evaluated in this article, or claim that may be made by its manufacturer, is not guaranteed or endorsed by the publisher.
References
Abiri, R., Valdiani, A., Maziah, M., Shaharuddin, N. A., Sahebi, M., Yusof, Z. N. B., et al. (2016). A critical review of the concept of transgenic plants: insights into pharmaceutical biotechnology and molecular farming. Curr. Issues Mol. Biol. 18, 21–42. doi: 10.21775/cimb.018.021
Abranches, R., Marcel, S., Arcalis, E., Altmann, F., Fevereiro, P., Stoger, E. (2005). Plants as bioreactors: A comparative study suggests that Medicago truncatula is a promising production system. J. Biotechnol. 120, 121–134. doi: 10.1016/j.jbiotec.2005.04.026
Adriana, M., Aleksandra, M., Denise, B., Kinga, G., Joanna, W., Aleksandra, H., et al. (2024). Zn(II) and cu(II) coordination enhances the antimicrobial activity of piscidin 3, but not that of piscidins 1 and 2. Inorganic Chem. 63, 12958–12968. doi: 10.1021/acs.inorgchem.4c01659
Aerts, A. M., François, I. E. J. A., Meert, E. M. K., Li, Q.-T., Cammue, B. P. A., Thevissen, K. (2007). The Antifungal Activity of RsAFP2, a Plant Defensin from Raphanus sativus, Involves the Induction of Reactive Oxygen Species in Candida albicans. J. Mol. Microbiol. Biotechnol. 13, 243–247. doi: 10.1159/000104753
Agouridas, V., El Mahdi, O., Diemer, V., Cargoët, M., Monbaliu, J.-C. M., Melnyk, O. (2019). Native chemical ligation and extended methods: mechanisms, catalysis, scope, and limitations. Chem. Rev. 119, 7328–7443. doi: 10.1021/acs.chemrev.8b00712
Aguirre-López, B., Cabrera, N., De Gómez-Puyou, M. T., Perez-Montfort, R., Gómez-Puyou, A. (2017). The importance of arginine codons AGA and AGG for the expression in E. coli of triosephosphate isomerase from seven different species. Biotechnol. Rep. 13, 42–48. doi: 10.1016/j.btre.2017.01.002
Ahmad, B., Hanif, Q., Xubiao, W., Lulu, Z., Shahid, M., Dayong, S., et al. (2019). Expression and purification of hybrid LL-37Tα1 peptide in pichia pastoris and evaluation of its immunomodulatory and anti-inflammatory activities by LPS neutralization. Front. Immunol. 10. doi: 10.3389/fimmu.2019.01365
Alam, M. Z., Wu, X., Mascio, C., Chesnel, L., Hurdle, J. G. (2015). Mode of Action and Bactericidal Properties of Surotomycin against Growing and Nongrowing Clostridium difficile. Antimicrobial Agents Chemotherapy 59, 5165–5170. doi: 10.1128/AAC.01087-15
Almaaytah, A., Qaoud, M. T., Abualhaijaa, A., Al-Balas, Q., Alzoubi, K. H. J. I., Resistance, D. (2018). Hybridization and antibiotic synergism as a tool for reducing the cytotoxicity of antimicrobial peptides. Infection Drug Resistance, 835–847. doi: 10.2147/IDR.S166236
Almasia, N. I., Molinari, M. P., Maroniche, G. A., Nahirñak, V., Barrios Barón, M. P., Taboga, O. A., et al. (2017). Successful production of the potato antimicrobial peptide Snakin-1 in baculovirus-infected insect cells and development of specific antibodies. BMC Biotechnol. 17, 1–11. doi: 10.1186/s12896-017-0401-2
Al Musaimi, O., Lombardi, L., Williams, D. R., Albericio, F. (2022). Strategies for improving peptide stability and delivery. Pharmaceuticals 15, 1283. doi: 10.3390/ph15101283
Almutairy, B. (2024). Extensively and multidrug-resistant bacterial strains: case studies of antibiotics resistance. Front. Microbiol. 15, 1381511. doi: 10.3389/fmicb.2024.1381511
Amann, T., Schmieder, V., Faustrup Kildegaard, H., Borth, N., Andersen, M. R. (2019). Genetic engineering approaches to improve posttranslational modification of biopharmaceuticals in different production platforms. Biotechnol. Bioengineering 116, 2778–2796. doi: 10.1002/bit.v116.10
Anami, S., Njuguna, E., Coussens, G., Aesaert, S., Van Lijsebettens, M. (2013). Higher plant transformation: principles and molecular tools. Int. J. Dev. Biol. 57, 483–494. doi: 10.1387/ijdb.130232mv
Andreu, D., Merrifield, R. B., Steiner, H., Boman, H. G. (1985). N-terminal analogues of cecropin A: synthesis, antibacterial activity, and conformational properties. Biochemistry 24, 1683–1688. doi: 10.1021/bi00328a017
Antoniukas, L., Grammel, H., Reichl, U. (2006). Production of hantavirus Puumala nucleocapsid protein in Saccharomyces cerevisiae for vaccine and diagnostics. J. Biotechnol. 124, 347–362. doi: 10.1016/j.jbiotec.2005.12.028
Aparicio-Blanco, J., Vishwakarma, N., Lehr, C.-M., Prestidge, C. A., Thomas, N., Roberts, R. J., et al. (2024). Antibiotic resistance and tolerance: What can drug delivery do against this global threat? Drug Delivery Trans. Res. 14, 1725–1734. doi: 10.1007/s13346-023-01513-6
Arias, M., Piga, K. B., Hyndman, M. E., Vogel, H. J. (2018). Improving the activity of trp-rich antimicrobial peptides by arg/lys substitutions and changing the length of cationic residues. Biomolecules 8, 19. doi: 10.3390/biom8020019
Azari, M., Asad, S., Mehrnia, M. R. (2020a). Heterologous production of porcine derived antimicrobial peptide PR-39 in Escherichia coli using SUMO and intein fusion systems. Protein Expression Purification 169, 105568. doi: 10.1016/j.pep.2020.105568
Aziz, M., Desai, M., Fatima, R., Thoguluva, C. V., Eid, A., Jackson, M., et al. (2019). Surotomycin (A Novel Cyclic Lipopeptide) vs. Vancomycin for the Treatment of Clostridioides difficile Infection: A Systematic Review and Meta-analysis. Curr. Clin. Pharmacol. 14, 166–174. doi: 10.2174/1574884714666190328162637
Baeriswyl, S., Gan, B.-H., Siriwardena, T. N., Visini, R., Robadey, M., Javor, S., et al. (2019). X-ray crystal structures of short antimicrobial peptides as pseudomonas aeruginosa lectin B complexes. ACS Chem. Biol. 14, 758–766. doi: 10.1021/acschembio.9b00047
Baeshen, N. A., Baeshen, M. N., Sheikh, A., Bora, R. S., Ahmed, M. M. M., Ramadan, H. A. I., et al. (2014). Cell factories for insulin production. Microbial Cell Factories 13, 141. doi: 10.1186/s12934-014-0141-0
Baghban, R., Farajnia, S., Rajabibazl, M., Ghasemi, Y., Mafi, A., Hoseinpoor, R., et al. (2019). Yeast expression systems: overview and recent advances. Mol. Biotechnol. 61, 365–384. doi: 10.1007/s12033-019-00164-8
Baghbeheshti, S., Hadadian, S., Eidi, A., Pishkar, L., Rahimi, H. (2021). Effect of flexible and rigid linkers on biological activity of recombinant tetramer variants of S3 antimicrobial peptide. Int. J. Pept. Res. Ther. 27, 457–462. doi: 10.1007/s10989-020-10095-7
Bals, R., Wang, X., Zasloff, M., Wilson, J. M. (1998). The peptide antibiotic LL-37/hCAP-18 is expressed in epithelia of the human lung where it has broad antimicrobial activity at the airway surface. Proc. Natl. Acad. Sci. 95, 9541–9546. doi: 10.1073/pnas.95.16.9541
Baneyx, F. (1999). Recombinant protein expression in Escherichia coli. Curr. Opin. Biotechnol. 10, 411–421. doi: 10.1016/S0958-1669(99)00003-8
Barone, G. D., Emmerstorfer-Augustin, A., Biundo, A., Pisano, I., Coccetti, P., Mapelli, V., et al. (2023). Industrial production of proteins with Pichia pastoris—Komagataella phaffii. Biomolecules 13, 441. doi: 10.3390/biom13030441
Basanta, A., Gómez-Sala, B., Sánchez, J., Diep, D. B., Herranz, C., Hernández, P. E., et al. (2010). Use of the yeast Pichia pastoris as an expression host for secretion of enterocin L50, a leaderless two-peptide (L50A and L50B) bacteriocin from Enterococcus faecium L50. Appl. Environ. Microbiol. 76, 3314–3324. doi: 10.1128/AEM.02206-09
Behren, S., Westerlind, U. (2019). Glycopeptides and-mimetics to detect, monitor and inhibit bacterial and viral infections: recent advances and perspectives. Molecules 24, 1004. doi: 10.3390/molecules24061004
Behrendt, R., White, P., Offer, J. (2016). Advances in Fmoc solid-phase peptide synthesis. J. Pept. Sci. 22 (1), 4–27. doi: 10.1002/psc.2836
Bélanger, J. G., Copley, T. R., Hoyos-Villegas, V., Charron, J.-B., O’donoughue, L. (2024). A comprehensive review of in planta stable transformation strategies. Plant Methods 20, 79. doi: 10.1186/s13007-024-01200-8
Bell, J. C., Kowalczykowski, S. C. (2016). RecA: regulation and mechanism of a molecular search engine. Trends Biochem. Sci. 41, 491–507. doi: 10.1016/j.tibs.2016.04.002
Bellavita, R., Braccia, S., Galdiero, S., Falanga, A. (2023). Glycosylation and lipidation strategies: Approaches for improving antimicrobial peptide efficacy. Pharmaceuticals 16, 439. doi: 10.3390/ph16030439
Berger, I., Poterszman, A. (2015). Baculovirus expression: old dog, new tricks. Bioengineered 6, 316–322. doi: 10.1080/21655979.2015.1104433
Berkmen, M. (2012). Production of disulfide-bonded proteins in Escherichia coli. Protein Expression Purification 82, 240–251. doi: 10.1016/j.pep.2011.10.009
Besinis, A., De Peralta, T., Handy, R. D. (2014). The antibacterial effects of silver, titanium dioxide and silica dioxide nanoparticles compared to the dental disinfectant chlorhexidine on Streptococcus mutans using a suite of bioassays. Nanotoxicology 8, 1–16. doi: 10.3109/17435390.2012.742935
Betenbaugh, M. J., Ailor, E., Whiteley, E., Hinderliter, P., Hsu, T.-A. (1996). Chaperone and foldase coexpression in the baculovirus-insect cell expression system. Cytotechnology 20, 149–159. doi: 10.1007/BF00350396
Biasizzo, M., Kopitar-Jerala, N. (2020). Interplay between NLRP3 inflammasome and autophagy. Front. Immunol. 11, 591803. doi: 10.3389/fimmu.2020.591803
Bill, R. M. (2014). Playing catch-up with Escherichia coli: using yeast to increase success rates in recombinant protein production experiments. Front. Microbiol. 5, 85. doi: 10.3389/fmicb.2014.00085
Binder, J. R., Conant, L. L., Humphries, C. J., Fernandino, L., Simons, S. B., Aguilar, M., et al. (2016). Toward a brain-based componential semantic representation. Cogn. Neuropsychol. 33, 130–174. doi: 10.1080/02643294.2016.1147426
Black, C. E., Gregory, E., Wilson, N., Shoemaker, D. M., Haas, G. A., Wark, K., et al. (2023). 1178. The dalba diary: A retrospective review of dalbavancin use, clinical outcomes, and treatment adherence challenges at an academic medical center. Open Forum Infect. Dis. 10. doi: 10.1093/ofid/ofad500.1018
Blotnick, E., Sol, A., Muhlrad, A. (2017). Histones bundle F-actin filaments and affect actin structure. PloS One 12, e0183760. doi: 10.1371/journal.pone.0183760
Boix, V., Fedorak, R. N., Mullane, K. M., Pesant, Y., Stoutenburgh, U., Jin, M., et al. (2017). Primary outcomes from a phase 3, randomized, double-blind, active-controlled trial of surotomycin in subjects with clostridium difficile infection. Open Forum Infect. Dis. 4. doi: 10.1093/ofid/ofw275
Bondalapati, S., Jbara, M., Brik, A. (2016). Expanding the chemical toolbox for the synthesis of large and uniquely modified proteins. Nat. Chem. 8, 407–418. doi: 10.1038/nchem.2476
Boto, A., Pérez de la Lastra, J. M., González, C. C. (2018). The road from host-defense peptides to a new generation of antimicrobial drugs. Molecules 23. doi: 10.3390/molecules23020311
Boutin, J. A., Tartar, A. L., Van Dorsselaer, A., Vaudry, H. (2019). General lack of structural characterization of chemically synthesized long peptides. Protein Sci. 28, 857–867. doi: 10.1002/pro.v28.5
Brady, D., Grapputo, A., Romoli, O., Sandrelli, F. (2019). Insect cecropins, antimicrobial peptides with potential therapeutic applications. Int. J. Mol. Sci. 20, 5862. doi: 10.3390/ijms20235862
Brand, G. D., Santos, R. C., Arake, L. M., Silva, V. G., Veras, L. M. C., Costa, V., et al. (2013). The skin secretion of the amphibian phyllomedusa nordestina: A source of antimicrobial and antiprotozoal peptides. Molecules 18, 7058–7070. doi: 10.3390/molecules18067058
Buchholz, K. R., Reichelt, M., Johnson, M. C., Robinson, S. J., Smith, P. A., Rutherford, S. T., et al. (2024). Potent activity of polymyxin B is associated with long-lived super-stoichiometric accumulation mediated by weak-affinity binding to lipid A. Nat. Commun. 15, 4733. doi: 10.1038/s41467-024-49200-5
Bulet, P., Stocklin, R. (2005). Insect antimicrobial peptides: structures, properties and gene regulation. Protein Pept. Lett. 12, 3–11. doi: 10.2174/0929866053406011
Burdette, L. A., Leach, S. A., Wong, H. T., Tullman-Ercek, D. (2018). Developing Gram-negative bacteria for the secretion of heterologous proteins. Microbial Cell Factories 17, 196. doi: 10.1186/s12934-018-1041-5
Butt, T. R., Edavettal, S. C., Hall, J. P., Mattern, M. R. (2005). SUMO fusion technology for difficult-to-express proteins. Protein Expression Purification 43, 1–9. doi: 10.1016/j.pep.2005.03.016
Canne, L. E., Bark, S. J., Kent, S. B. H. (1996). Extending the applicability of native chemical ligation. J. Am. Chem. Soc. 118, 5891–5896. doi: 10.1021/ja960398s
Cao, X., Zhang, Y., Mao, R., Teng, D., Wang, X., Wang, J. (2015). Design and recombination expression of a novel plectasin-derived peptide MP1106 and its properties against Staphylococcus aureus. Appl. Microbiol. Biotechnol. 99, 2649–2662. doi: 10.1007/s00253-014-6077-9
Carballar-Lejarazú, R., Ogaugwu, C., Tushar, T., Kelsey, A., Pham, T. B., Murphy, J., et al. (2020). Next-generation gene drive for population modification of the malaria vector mosquito, Anopheles Gambiae. Proc. Natl. Acad. Sci. 117, 22805–22814. doi: 10.1073/pnas.2010214117
Carter, A. P., Clemons, W. M., Brodersen, D. E., Morgan-Warren, R. J., Wimberly, B. T., Ramakrishnan, V. (2000). Functional insights from the structure of the 30S ribosomal subunit and its interactions with antibiotics. Nature 407, 340–348. doi: 10.1038/35030019
Castellanos, N., Diez, G. G., Antúnez-Almagro, C., Bressa, C., Bailén, M., González-Soltero, R., et al. (2020). Key bacteria in the gut microbiota network for the transition between sedentary and active lifestyle. Microorganisms 8, 785. doi: 10.3390/microorganisms8050785
Çelik, E., Çalık, P. (2012). Production of recombinant proteins by yeast cells. Biotechnol. Adv. 30, 1108–1118. doi: 10.1016/j.biotechadv.2011.09.011
Cesaro, A., Lin, S., Pardi, N., de la Fuente-Nunez, C. (2023). Advanced delivery systems for peptide antibiotics. Advanced Drug Delivery Rev. 196, 114733. doi: 10.1016/j.addr.2023.114733
Chadani, Y., Ono, K., Ozawa, S. I., Takahashi, Y., Takai, K., Nanamiya, H., et al. (2010). Ribosome rescue by Escherichia coli ArfA (YhdL) in the absence of trans-translation system. Mol. Microbiol. 78, 796–808. doi: 10.1111/j.1365-2958.2010.07375.x
Chalker, J. M. (2013). Prospects in the total synthesis of protein therapeutics. Chem. Biol. Drug Design 81, 122–135. doi: 10.1111/cbdd.2012.81.issue-1
Chaney, J. L., Clark, P. L. (2015). Roles for synonymous codon usage in protein biogenesis. Annu. Rev. Biophysics 44, 143–166. doi: 10.1146/annurev-biophys-060414-034333
Chaudhary, S., Ali, Z., Mahfouz, M. (2024). Molecular farming for sustainable production of clinical-grade antimicrobial peptides. Plant Biotechnol. J. 22, 2282–2300. doi: 10.1111/pbi.14344
Chaudhuri, D., Ganesan, R., Vogelaar, A., Dughbaj, M. A., Beringer, P. M., Camarero, J. A. (2021). Chemical synthesis of a potent antimicrobial peptide murepavadin using a tandem native chemical ligation/desulfurization reaction. J. Organic Chem. 86, 15242–15246. doi: 10.1021/acs.joc.1c01858
Chavez-Pena, C., Kamen, A. A. (2018). RNA interference technology to improve the baculovirus-insect cell expression system. Biotechnol. Adv. 36, 443–451. doi: 10.1016/j.biotechadv.2018.01.008
Chekan, J. R., Mydy, L. S., Pasquale, M. A., Kersten, R. D. (2024). Plant peptides – redefining an area of ribosomally synthesized and post-translationally modified peptides. Natural Product Rep. 41, 1020–1059. doi: 10.1039/D3NP00042G
Chen, R. (2012). Bacterial expression systems for recombinant protein production: E. coli and beyond. Biotechnol. Adv. 30, 1102–1107. doi: 10.1016/j.biotechadv.2011.09.013
Chen, N., Jiang, C. (2023). Antimicrobial peptides: Structure, mechanism, and modification. Eur. J. Medicinal Chem. 255, 115377. doi: 10.1016/j.ejmech.2023.115377
Chen, C. H., Lu, T. K. (2020). Development and challenges of antimicrobial peptides for therapeutic applications. Antibiotics 9, 24. doi: 10.3390/antibiotics9010024
Chen, X., Zhu, F., Cao, Y., Qiao, S. (2009). Novel expression vector for secretion of cecropin AD in Bacillus subtilis with enhanced antimicrobial activity. Antimicrobial Agents Chemotherapy 53, 3683–3689. doi: 10.1128/AAC.00251-09
Cheng, J., Ahmad, B., Raza, M. A., Guo, H., Ahmat, M., Wei, X., et al. (2023). Yeast expressed hybrid peptide CLP abridged pro-inflammatory cytokine levels by endotoxin neutralization. Microorganisms 11, 131. doi: 10.3390/microorganisms11010131
Chow, H. Y., Zhang, Y., Matheson, E., Li, X. (2019). Ligation technologies for the synthesis of cyclic peptides. Chem. Rev. 119, 9971–10001. doi: 10.1021/acs.chemrev.8b00657
Chung, Y. H., Church, D., Koellhoffer, E. C., Osota, E., Shukla, S., Rybicki, E. P., et al. (2022). Integrating plant molecular farming and materials research for next-generation vaccines. Nat. Rev. materials 7, 372–388. doi: 10.1038/s41578-021-00399-5
Cistrone, P. A., Bird, M. J., Flood, D. T., Silvestri, A. P., Hintzen, J. C., Thompson, D. A., et al. (2019). Native chemical ligation of peptides and proteins. Curr. Protoc. Chem. Biol. 11, e61. doi: 10.1002/cpch.v11.1
Clark, M., Maselko, M. (2020). Transgene biocontainment strategies for molecular farming. Front. Plant Sci. 11. doi: 10.3389/fpls.2020.00210
Coin, I., Beyermann, M., Bienert, M. (2007). Solid-phase peptide synthesis: from standard procedures to the synthesis of difficult sequences. Nat. Protoc. 2, 3247–3256. doi: 10.1038/nprot.2007.454
Contreras-Gómez, A., Sánchez-Mirón, A., García-Camacho, F., Molina-Grima, E., Chisti, Y. (2014). Protein production using the baculovirus-insect cell expression system. Biotechnol. Prog. 30, 1–18. doi: 10.1002/btpr.1842
Corrales-García, L. L., Serrano-Carreón, L., Corzo, G. (2020). Improving the heterologous expression of human β-defensin 2 (HBD2) using an experimental design. Protein Expression purification 167, 105539. doi: 10.1186/s13007-024-01200-8
Correa, M. G., Martínez, F. B., Vidal, C. P., Streitt, C., Escrig, J., De Dicastillo, C. L. (2020). Antimicrobial metal-based nanoparticles: A review on their synthesis, types and antimicrobial action. Beilstein J. Nanotechnology 11, 1450–1469. doi: 10.3762/bjnano.11.129
Costa, S., Almeida, A., Castro, A., Domingues, L. (2014). Fusion tags for protein solubility, purification and immunogenicity in Escherichia coli: the novel Fh8 system. Front. Microbiol. 5, 63. doi: 10.3389/fmicb.2014.00063
Côté, A. P., El-Kaderi, H. M., Furukawa, H., Hunt, J. R., Yaghi, O. M. (2007). Reticular synthesis of microporous and mesoporous 2D covalent organic frameworks. J. Am. Chem. Soc. 129, 12914–12915. doi: 10.1021/ja0751781
Cresti, L., Cappello, G., Pini, A. (2024). Antimicrobial peptides towards clinical application—A long history to be concluded. Int. J. Mol. Sci. 25. doi: 10.3390/ijms25094870
Crusca, E., Jr., Rezende, A. A., Marchetto, R., Mendes-Giannini, M. J. S., Fontes, W., Castro, M. S., et al. (2011). Influence of N-terminus modifications on the biological activity, membrane interaction, and secondary structure of the antimicrobial peptide hylin-a1. Pept. Sci. 96, 41–48. doi: 10.1002/bip.21454
Cruz, J., Mihailescu, M., Wiedman, G., Herman, K., Searson, P. C., Wimley, W. C., et al. (2013). A Membrane-Translocating Peptide Penetrates into Bilayers without Significant Bilayer Perturbations. Biophys. J. 104, 2419–2428. doi: 10.1016/j.bpj.2013.04.043
Cudic, M., Otvos, L., Jr (2002). Intracellular targets of antibacterial peptides. Curr. Drug Targets 3, 101–106. doi: 10.2174/1389450024605445
Da Cunha, N. B., Cobacho, N. B., Viana, J. F. C., Lima, L. A., Sampaio, K. B. O., Dohms, S. S. M., et al. (2017). The next generation of antimicrobial peptides (AMPs) as molecular therapeutic tools for the treatment of diseases with social and economic impacts. Drug Discovery Today 22, 234–248. doi: 10.1016/j.drudis.2016.10.017
Da Cunha, N. B., Vianna, G. R., Da Almeida Lima, T., Rech, E. (2014). Molecular farming of human cytokines and blood products from plants: Challenges in biosynthesis and detection of plant-produced recombinant proteins. Biotechnol. J. 9, 39–50. doi: 10.1002/biot.201300062
Daly, R., Hearn, M. T. W. (2005). Expression of heterologous proteins in Pichia pastoris: a useful experimental tool in protein engineering and production. J. Mol. Recognition 18, 119–138. doi: 10.1002/(ISSN)1099-1352
Darby, E. M., Trampari, E., Siasat, P., Gaya, M. S., Alav, I., Webber, M. A., et al. (2023). Molecular mechanisms of antibiotic resistance revisited. Nat. Rev. Microbiol. 21, 280–295. doi: 10.1038/s41579-022-00820-y
Datta, S., Roy, A. (2021). Antimicrobial peptides as potential therapeutic agents: A review. Int. J. Pept. Res. Ther. 27, 555–577. doi: 10.1007/s10989-020-10110-x
De Beer, A., Vivier, M. (2008). Vv-AMP1, a ripening induced peptide from Vitis vinifera shows strong antifungal activity. BMC Plant Biol. 8, 1–16. doi: 10.1186/1471-2229-8-75
De La Fuente-Núñez, C. S., Reffuveille, F., Haney, E. F., Straus, S. K., Hancock, R. E. (2014). Broad-spectrum anti-biofilm peptide that targets a cellular stress response. PloS Pathog. 10, e1004152. doi: 10.1371/journal.ppat.1004152
Deng, T., Ge, H., He, H., Liu, Y., Zhai, C., Feng, L., et al. (2017). The heterologous expression strategies of antimicrobial peptides in microbial systems. Protein Expression Purification 140, 52–59. doi: 10.1016/j.pep.2017.08.003
Dennison, S. R., Harris, F., Bhatt, T., Singh, J., Phoenix, D. A. (2009). The effect of C-terminal amidation on the efficacy and selectivity of antimicrobial and anticancer peptides. Mol. Cell. Biochem. 332, 43–50. doi: 10.1007/s11010-009-0172-8
Dennison, S. R., Phoenix, D. A. (2011). Influence of C-terminal amidation on the efficacy of modelin-5. Biochemistry 50, 1514–1523. doi: 10.1021/bi101687t
De Oliveira, K. B. S., Leite, M. L., Cunha, V. A., Da Cunha, N. B., Franco, O. L. (2023). Challenges and advances in antimicrobial peptide development. Drug Discovery Today 28, 103629. doi: 10.1016/j.drudis.2023.103629c
De Pourcq, K., De Schutter, K., Callewaert, N. (2010). Engineering of glycosylation in yeast and other fungi: current state and perspectives. Appl. Microbiol. Biotechnol. 87, 1617–1631. doi: 10.1007/s00253-010-2721-1
De Wachter, C., Van Landuyt, L., Callewaert, N. (2021). Engineering of yeast glycoprotein expression. Adv. Biochem. Engineering/Biotechnology 175, 93–135. doi: 10.1007/10_2018_69
Di Bonaventura, I., Baeriswyl, S., Capecchi, A., Gan, B.-H., Jin, X., Siriwardena, T. N., et al. (2018). An antimicrobial bicyclic peptide from chemical space against multidrug resistant Gram-negative bacteria. Chem. Commun. 54, 5130–5133. doi: 10.1039/C8CC02412J
Di Bonaventura, I., Jin, X., Visini, R., Probst, D., Javor, S., Gan, B.-H., et al. (2017). Chemical space guided discovery of antimicrobial bridged bicyclic peptides against Pseudomonas aeruginosa and its biofilms. Chem. Sci. 8, 6784–6798. doi: 10.1039/C7SC01314K
Dimiceli, L., Pool, V., Kelso, J. M., Shadomy, S. V., Iskander, J., Team, V.A.E.R.S (2006). Vaccination of yeast sensitive individuals: review of safety data in the US vaccine adverse event reporting system (VAERS). Vaccine 24, 703–707. doi: 10.1016/j.vaccine.2005.07.069
Divyashree, M., Mani, K. M., Reddy, D., Kumavath, R., Ghosh, P., Azevedo, V., et al. (2020). Clinical Applications of Antimicrobial Peptides (AMPs): Where do we Stand Now? Protein Pept. Lett. 27, 120–134. doi: 10.2174/0929866526666190925152957
Doherty, A. J., Ashford, S. R., Brannigan, J. A., Wigley, D. B. (1995). A superior host strain for the over-expression of cloned genes using the T7 promoter based vectors. Nucleic Acids Res. 23, 2074–2075. doi: 10.1093/nar/23.11.2074
Domhan, C., Uhl, P., Kleist, C., Zimmermann, S., Umstätter, F., Leotta, K., et al. (2019). Replacement of l-amino acids by d-amino acids in the antimicrobial peptide ranalexin and its consequences for antimicrobial activity and biodistribution. Molecules 24, 2987. doi: 10.3390/molecules24162987
Du, M., Hou, Z., Liu, L., Xuan, Y., Chen, X., Fan, L., et al. (2022). 1Progress, applications, challenges and prospects of protein purification technology. Front. Bioengineering Biotechnol. 10. doi: 10.3389/fbioe.2022.1028691
Du, F., Liu, Y.-Q., Xu, Y.-S., Li, Z.-J., Wang, Y.-Z., Zhang, Z.-X., et al. (2021). Regulating the T7 RNA polymerase expression in E. coli BL21 (DE3) to provide more host options for recombinant protein production. Microbial Cell Factories 20, 189. doi: 10.3389/fbioe.2022.1028691
Du, Y., Semghouli, A., Wang, Q., Mei, H., Kiss, L., Baecker, D., et al. (2025). FDA-approved drugs featuring macrocycles or medium-sized rings. Archiv der Pharmazie 358, e2400890. doi: 10.1002/ardp.202400890
Duan, G., Ding, L., Wei, D., Zhou, H., Chu, J., Zhang, S., et al. (2019). Screening endogenous signal peptides and protein folding factors to promote the secretory expression of heterologous proteins in Pichia pastoris. J. Biotechnol. 306, 193–202. doi: 10.1016/j.jbiotec.2019.06.297
Dubey, K. K., Kumar, A., Baldia, A., Rajput, D., Kateriya, S., Singh, R., et al. (2023). Biomanufacturing of glycosylated antibodies: Challenges, solutions, and future prospects. Biotechnol. Adv. 69, 108267. doi: 10.1016/j.biotechadv.2023.108267
Dumon-Seignovert, L., Cariot, G., Vuillard, L. (2004). The toxicity of recombinant proteins in Escherichia coli: a comparison of overexpression in BL21(DE3), C41(DE3), and C43(DE3). Protein Expression purification 37, 203–206. doi: 10.1016/j.pep.2004.04.025
Duret, L. (2000). tRNA gene number and codon usage in the C. elegans genome are co-adapted for optimal translation of highly expressed genes. Trends Genet. 16, 287–289. doi: 10.1016/S0168-9525(00)02041-2
Dwivedi, M., Parmar, M. D., Mukherjee, D., Yadava, A., Yadav, H., Saini, N. P. (2024). Biochemistry, mechanistic intricacies, and therapeutic potential of antimicrobial peptides: an alternative to traditional antibiotics. Curr. medicinal Chem. 31, 6110–6139. doi: 10.2174/0109298673268458230926105224
Eckert, R., He, J., Yarbrough, D. K., Qi, F., Anderson, M. H., Shi, W. (2006a). Targeted killing of streptococcus mutans by a pheromone-guided &x201c;Smart&x201d; antimicrobial peptide. Antimicrobial Agents Chemotherapy 50, 3651–3657. doi: 10.1128/AAC.00622-06
Eckert, R., Qi, F., Yarbrough, D. K., He, J., Anderson, M. H., Shi, W. (2006b). Adding selectivity to antimicrobial peptides: rational design of a multidomain peptide against Pseudomonas spp. Antimicrobial Agents Chemotherapy 50, 1480–1488. doi: 10.1128/AAC.50.4.1480-1488.2006
Egelkrout, E., Rajan, V., Howard, J. A. (2012). Overproduction of recombinant proteins in plants. Plant Sci. 184, 83–101. doi: 10.1016/j.plantsci.2011.12.005
Elhag, O., Zhou, D., Song, Q., Soomro, A. A., Cai, M., Zheng, L., et al. (2017). Screening, expression, purification and functional characterization of novel antimicrobial peptide genes from hermetia illucens (L.). PloS One 12, e0169582. doi: 10.1371/journal.pone.0169582
El-Sayed Ahmed, M.-G., Zhong, L.-L., Shen, C., Yang, Y., Doi, Y., Tian, G.-B. (2020). Colistin and its role in the Era of antibiotic resistance: an extended review (2000–2019). Emerging Microbes Infections 9, 868–885. doi: 10.1080/22221751.2020.1754133
Engelberg, Y., Landau, M. (2020). The Human LL-37(17-29) antimicrobial peptide reveals a functional supramolecular structure. Nat. Commun. 11, 3894. doi: 10.1038/s41467-020-17736-x
Engler, C., Youles, M., Gruetzner, R., Ehnert, T.-M., Werner, S., Jones, J. D., et al. (2014). A golden gate modular cloning toolbox for plants. ACS synthetic Biol. 3, 839–843. doi: 10.1021/sb4001504
Etayash, H., Pletzer, D., Kumar, P., Straus, S. K., Hancock, R. E. (2020). Cyclic derivative of host-defense peptide IDR-1018 improves proteolytic stability, suppresses inflammation, and enhances in vivo activity. J. medicinal Chem. 63, 9228–9236. doi: 10.1021/acs.jmedchem.0c00303
Fabrick, J. A., Hull, J. J. (2017). Transient expression and cellular localization of recombinant proteins in cultured insect cells. J. visualized experiments. doi: 10.3791/55756
Falagas, M. E., Kasiakou, S. K. (2005). Colistin: the revival of polymyxins for the management of multidrug-resistant gram-negative bacterial infections. Clin. Infect. Dis. 40, 1333–1341. doi: 10.1086/429323
Fathi-Roudsari, M., Akhavian-Tehrani, A., Maghsoudi, N. (2016). Comparison of three escherichia coli strains in recombinant production of reteplase. Avicenna J. Med. Biotechnol. 8, 16–22.
Faure, A., Manuse, S., Gonin, M., Grangeasse, C., Jault, J.-M., Orelle, C. (2024). Daptomycin avoids drug resistance mediated by the BceAB transporter in Streptococcus pneumoniae. Microbiol. Spectr. 12, e03638–e03623. doi: 10.1128/spectrum.03638-23
Felber, M., Pichler, H., Ruth, C. (2014). Strains and molecular tools for recombinant protein production in Pichia pastoris. Methods Mol. Biol. 1152, 87–111. doi: 10.1007/978-1-4939-0563-8_5
Feng, X., Liu, C., Guo, J., Bi, C., Cheng, B., Li, Z., et al. (2010). Expression and Purification of an Antimicrobial Peptide, Bovine Lactoferricin Derivative LfcinB-W10 in Escherichia á coli. Curr. Microbiol. 60, 179–184. doi: 10.1007/s00284-009-9522-8
Feng, X.-J., Xing, L.-W., Liu, D., Song, X.-Y., Liu, C.-L., Li, J., et al. (2014). Design and high-level expression of a hybrid antimicrobial peptide LF15-CA8 in Escherichia coli. J. Ind. Microbiol. Biotechnol. 41, 527–534. doi: 10.1007/s10295-013-1382-3
Fernandes, A., Piotrowski, Y., Williamson, A., Frade, K., Moe, E. (2021). Studies of multifunctional DNA polymerase I from the extremely radiation resistant Deinococcus radiodurans: Recombinant expression, purification and characterization of the full-length protein and its large fragment. Protein Expression Purification 187, 105925. doi: 10.1016/j.pep.2021.105925
Fernandez-Reyes, M., Diaz, D., de la Torre, B. G., Cabrales-Rico, A., Valles-Miret, M., Jiménez-Barbero, J., et al. (2010). Lysine N ϵ-trimethylation, a tool for improving the selectivity of antimicrobial peptides. J. medicinal Chem. 53, 5587–5596. doi: 10.1021/jm100261r
Fernández-Tejada, A., Brailsford, J., Zhang, Q., Shieh, J.-H., Moore, M. A. S., Danishefsky, S. J. (2015). “Total Synthesis of Glycosylated Proteins,” in Protein Ligation and Total Synthesis, I, ed. Ed. Liu, L. (Springer International Publishing, Cham), 1–26.
Field, D., Considine, K., O’connor, P. M., Ross, R. P., Hill, C., Cotter, P. D. (2021). Bio-engineered nisin with increased anti-staphylococcus and selectively reduced anti-lactococcus activity for treatment of bovine mastitis. Int. J. Mol. Sci. 22, 3480. doi: 10.3390/ijms22073480
Fitzgerald, I., Glick, B. S. (2014). Secretion of a foreign protein from budding yeasts is enhanced by cotranslational translocation and by suppression of vacuolar targeting. Microbial Cell Factories 13, 125. doi: 10.1186/s12934-014-0125-0
Foster, T. J. (2017). Antibiotic resistance in Staphylococcus aureus. Current status and future prospects. FEMS Microbiol. Rev. 41, 430–449. doi: 10.1093/femsre/fux007
Frankenfeld, C., Mittal, S., Melendez, Y., Mendez-Vigo, L., Lamp, K. C., Keller, K. N., et al. (2018). Daptomycin: a comparison of two intravenous formulations. Drug design Dev. Ther. 12, 1953–1958. doi: 10.2147/DDDT.S167010
Freudl, R. (2018). Signal peptides for recombinant protein secretion in bacterial expression systems. Microbial Cell Factories 17, 52. doi: 10.1186/s12934-018-0901-3
Frick, P. J., Ray, J. V., Thornton, L. C., Kahn, R. E. (2014). Can callous-unemotional traits enhance the understanding, diagnosis, and treatment of serious conduct problems in children and adolescents? A comprehensive review. psychol. Bull. 140, 1. doi: 10.1037/a0033076
Fu, G., Liu, J., Li, J., Zhu, B., Zhang, D. (2018). Systematic screening of optimal signal peptides for secretory production of heterologous proteins in Bacillus subtilis. J. Agric. Food Chem. 66, 13141–13151. doi: 10.1021/acs.jafc.8b04183
Gan, B. H., Gaynord, J., Rowe, S. M., Deingruber, T., Spring, D. R. (2021). The multifaceted nature of antimicrobial peptideAMPss: current synthetic chemistry approaches and future directions. Chem. Soc. Rev. 50, 7820–7880. doi: 10.1039/D0CS00729C
Garcia Jimenez, D., Poongavanam, V., Kihlberg, J. (2023). Macrocycles in drug discovery-Learning from the past for the future. J. Medicinal Chem. 66, 5377–5396. doi: 10.1021/acs.jmedchem.3c00134
Garcia-Ortega, X., I Sala, J. A., Seguí, J. L. M., Barranco, F. V., Nieto-Taype, M. A. (2020). Continuous cultivation as a tool toward the rational bioprocess development with pichia pastoris cell factory. Front. Bioengineering Biotechnol. 8. doi: 10.3389/fbioe.2020.00632
Gattu, R., Ramesh, S. S., Nadigar, S., Ramesh, S. J. A. (2023). Conjugation as a tool in therapeutics: Role of amino acids/peptides-bioactive (including Heterocycles) hybrid molecules in treating infectious diseases. Antibiotics (Basel Switzerland) 12, 532. doi: 10.3390/antibiotics12030532
Gelvin, S. B. (2017). Integration of agrobacterium T-DNA into the plant genome. Annu. Rev. Genet. 51, 195–217. doi: 10.1146/annurev-genet-120215-035320
Ghidey, M., Islam, S. A., Pruett, G., Kearney, C. M. (2020). Making plants into cost-effective bioreactors for highly active antimicrobial peptides. New Biotechnol. 56, 63–70. doi: 10.1016/j.nbt.2019.12.001
Giesler, R. J., Erickson, P. W., Kay, M. S. (2020). Enhancing native chemical ligation for challenging chemical protein syntheses. Curr. Opin. Chem. Biol. 58, 37–44. doi: 10.1016/j.cbpa.2020.04.003
Glibowicka, M., He, S., Deber, C. M. (2022). Enhanced proteolytic resistance of cationic antimicrobial peptideAMPss through lysine side chain analogs and cyclization. Biochem. Biophys. Res. Commun. 612, 105–109. doi: 10.1016/j.bbrc.2022.04.113
Goffin, P., Dehottay, P. (2017). Complete Genome Sequence of Escherichia coli BLR(DE3), a recA-Deficient Derivative of E. coli BL21(DE3). Genome announcements 5. doi: 10.1128/genomeA.00441-17
Gomord, V., Fitchette, A.-C., Menu-Bouaouiche, L., Saint-Jore-Dupas, C., Plasson, C., Michaud, D., et al. (2010). Plant-specific glycosylation patterns in the context of therapeutic protein production. Plant Biotechnol. J. 8, 564–587. doi: 10.1111/j.1467-7652.2009.00497.x
Gottler, L. M., Ramamoorthy, A. (2009). Structure, membrane orientation, mechanism, and function of pexiganan — A highly potent antimicrobial peptide designed from magainin. Biochim. Biophys. Acta (BBA) - Biomembranes 1788, 1680–1686. doi: 10.1016/j.bbamem.2008.10.009
Grabowski, G. A., Golembo, M., Shaaltiel, Y. (2014). Taliglucerase alfa: An enzyme replacement therapy using plant cell expression technology. Mol. Genet. Metab. 112, 1–8. doi: 10.1016/j.ymgme.2014.02.011
Guan, Q., Huang, S., Jin, Y., Campagne, R., Alezra, V., Wan, Y. (2019). Recent advances in the exploration of therapeutic analogues of gramicidin S, an old but still potent antimicrobial peptideAMPs. J. medicinal Chem. 62, 7603–7617. doi: 10.1021/acs.jmedchem.9b00156
Guo, C., Huang, Y., Zheng, H., Tang, L., He, J., Xiang, L., et al. (2012). Secretion and activity of antimicrobial peptide cecropin D expressed in Pichia pastoris. Exp. Ther. Med. 4, 1063–1068. doi: 10.3892/etm.2012.719
Gustafsson, C., Minshull, J., Govindarajan, S., Ness, J., Villalobos, A., Welch, M. (2012). Engineering genes for predictable protein expression. Protein Expression purification 83, 37–46. doi: 10.1016/j.pep.2012.02.013
Guzmán, F., Barberis, S., Illanes, A. C. (2007). Peptide synthesis: chemical or enzymatic. Electronic J. Biotechnol. 10, 279–314. doi: 10.2225/vol10-issue2-fulltext-13
Hadjicharalambous, A., Bournakas, N., Newman, H., Skynner, M. J., Beswick, P. (2022). Antimicrobial and cell-penetrating peptides: understanding penetration for the design of novel conjugate antibiotics. Antibiotics (Basel Switzerland) 11, 1636. doi: 10.3390/antibiotics11111636
Hagedorn, I., Schmidbauer, S., Pleines, I., Kleinschnitz, C., Kronthaler, U., Stoll, G., et al. (2010). Factor XIIa inhibitor recombinant human albumin Infestin-4 abolishes occlusive arterial thrombus formation without affecting bleeding. Circulation 121, 1510–1517. doi: 10.1161/CIRCULATIONAHA.109.924761
Hahm, M. S., Chung, B. H. (2001). Secretory expression of human growth hormone inSaccharomyces cerevisiae using three different leader sequences. Biotechnol. Bioprocess Eng. 6, 306–309. doi: 10.1007/BF02931995
Hallett, J. W., Wolkowicz, M. I., Leopold, I. H. (1956). Ophthalmic use of neosporin*. Am. J. Ophthalmol. 41, 850–853. doi: 10.1016/0002-9394(56)91781-0
Han, Y., Zhang, M., Lai, R., Zhang, Z. (2021). Chemical modifications to increase the therapeutic potential of antimicrobial peptides. Peptides 146, 170666. doi: 10.1016/j.peptides.2021.170666
Hancock, R. E. W., Diamond, G. (2000). The role of cationic antimicrobial peptides in innate host defences. Trends Microbiol. 8, 402–410. doi: 10.1016/S0966-842X(00)01823-0
Haney, E. F., Mansour, S. C., Hancock, R. E. W. (2017). “Antimicrobial Peptides: An Introduction,” in Antimicrobial Peptides: Methods and Protocols. Ed. Hansen, P. R. (Springer New York, New York, NY), 3–22.
Haney, E. F., Straus, S. K., Hancock, R. E. (2019). Reassessing the host defense peptide landscape. Front. Chem. 7, 435645. doi: 10.3389/fchem.2019.00043
Harwood, C. R., Cranenburgh, R. (2008). Bacillus protein secretion: an unfolding story. Trends Microbiol. 16, 73–79. doi: 10.1016/j.tim.2007.12.001
Hasan, C. M., Dutta, D., Nguyen, A. N. T. (2021). Revisiting antibiotic resistance: mechanistic foundations to evolutionary outlook. Antibiotics (Basel) 11. doi: 10.3390/antibiotics11010040
Hata, J., Arima, H., Rothwell, P. M., Woodward, M., Zoungas, S., Anderson, C., et al. (2013). Effects of visit-to-visit variability in systolic blood pressure on macrovascular and microvascular complications in patients with type 2 diabetes mellitus: the ADVANCE trial. Circulation 128, 1325–1334. doi: 10.1161/CIRCULATIONAHA.113.002717
Hayat, S. M. G., Farahani, N., Golichenari, B., Sahebkar, A. (2018). Recombinant protein expression in escherichia coli (E.coli): what we need to know. Curr. Pharm. design 24, 718–725. doi: 10.2174/1381612824666180131121940
Heidary, M., Khosravi, A. D., Khoshnood, S., Nasiri, M. J., Soleimani, S., Goudarzi, M. (2017). Daptomycin. J. Antimicrobial Chemotherapy 73, 1–11. doi: 10.1093/jac/dkx349
Helmy, Y. A., Taha-Abdelaziz, K., Hawwas, H.A.E.-H., Ghosh, S., Alkafaas, S. S., Moawad, M. M., et al. (2023). Antimicrobial resistance and recent alternatives to antibiotics for the control of bacterial pathogens with an emphasis on foodborne pathogens. Antibiotics 12, 274. doi: 10.3390/antibiotics12020274
Herbel, V., Schäfer, H., Wink, M. (2015). Recombinant production of snakin-2 (an antimicrobial peptide from tomato) in E. coli and analysis of its bioactivity. Molecules (Basel Switzerland) 20, 14889–14901. doi: 10.3390/molecules200814889
Hershberg, R., Petrov, D. (2008). Selection on codon bias. Annu. Rev. Genet. 42, 287–299. doi: 10.1146/annurev.genet.42.110807.091442
Ho, C. S., Wong, C. T., Aung, T. T., Lakshminarayanan, R., Mehta, J. S., Rauz, S., et al. (2024). Antimicrobial resistance: a concise update. Lancet Microbe. doi: 10.1016/j.lanmic.2024.07.010
Hoelscher, M. P., Forner, J., Calderone, S., Krämer, C., Taylor, Z., Loiacono, F. V., et al. (2022). Expression strategies for the efficient synthesis of antimicrobial peptides in plastids. Nat. Commun. 13, 5856. doi: 10.1038/s41467-022-33516-1
Holaskova, E., Galuszka, P., Frebort, I., Oz, M. T. (2015). Antimicrobial peptide production and plant-based expression systems for medical and agricultural biotechnology. Biotechnol. Adv. 33, 1005–1023. doi: 10.1016/j.biotechadv.2015.03.007
Hong, S., Lim, S., Li, A. G., Lee, C., Lee, Y. S., Lee, E.-K., et al. (2007). Smad7 binds to the adaptors TAB2 and TAB3 to block recruitment of the kinase TAK1 to the adaptor TRAF2. Nat. Immunol. 8, 504–513. doi: 10.1038/ni1451
Hood, L. (1997). The solar cycle variation of total ozone: Dynamical forcing in the lower stratosphere. J. Geophysical Research: Atmospheres 102, 1355–1370. doi: 10.1007/978-1-4615-4729-7_11
Horn, M. E., Woodard, S. L., Howard, J. A. (2004). Plant molecular farming: systems and products. Plant Cell Rep. 22, 711–720. doi: 10.1007/s00299-004-0767-1
Hu, M., Li, P., Song, L., Jeffrey, P. D., Chernova, T. A., Wilkinson, K. D., et al. (2005). Structure and mechanisms of the proteasome-associated deubiquitinating enzyme USP14. EMBO J. 24, 3747–3756. doi: 10.1038/sj.emboj.7600832
Huang, Y., Gao, L., Lin, M., Yu, T. (2021). Recombinant expression of antimicrobial peptides in Pichia pastoris: A strategy to inhibit the Penicillium expansum in pears. Postharvest Biol. Technol. 171, 111298. doi: 10.1016/j.postharvbio.2020.111298
Ikemura, T. (1985). Codon usage and tRNA content in unicellular and multicellular organisms. Mol. Biol. Evol. 2, 13–34. doi: 10.1093/oxfordjournals.molbev.a040335
Ingham, A. B., Moore, R. J. (2007). Recombinant production of antimicrobial peptides in heterologous microbial systems. Biotechnol. Appl. Biochem. 47, 1–9. doi: 10.1042/BA20060207
Inoue, H., Lin, L., Lee, X., Shao, Z., Mendes, S., Snodgrass-Belt, P., et al. (2007). Inhibition of the leucine-rich repeat protein LINGO-1 enhances survival, structure, and function of dopaminergic neurons in Parkinson's disease models. Proc. Natl. Acad. Sci. 104, 14430–14435. doi: 10.1073/pnas.0700901104
Irudayam, S. J., Berkowitz, M. L. (2012). Binding and reorientation of melittin in a POPC bilayer: Computer simulations. Biochim. Biophys. Acta (BBA) - Biomembranes 1818, 2975–2981. doi: 10.1016/j.bbamem.2012.07.026
Itoh, R., Soejima, T., Hiromatsu, K. (2019). Anti-chlamydial activities of cell-permeable hydrophobic dipeptide-containing derivatives. J. Infection Chemotherapy 25, 987–994. doi: 10.1016/j.jiac.2019.05.024
Izumi, M., Otsuki, A., Nishihara, M., Okamoto, R., Kajihara, Y. (2014). Chemical synthesis of a synthetic analogue of the sialic acid-binding lectin siglec-7. ChemBioChem 15, 2503–2507. doi: 10.1002/cbic.201402494
James, M., Xue, H., Ren, L., Zhi-Ye, Z. (2019). Antimicrobial peptides: new hope in the war against multidrug resistance. Zoological Res. 40, 488–505. doi: 10.24272/j.issn.2095-8137.2019.062
Jaradat, D. S. M. M. (2018). Thirteen decades of peptide synthesis: key developments in solid phase peptide synthesis and amide bond formation utilized in peptide ligation. Amino Acids 50, 39–68. doi: 10.1007/s00726-017-2516-0
Jarvis, D. L. (2003). Developing baculovirus-insect cell expression systems for humanized recombinant glycoprotein production. Virology 310, 1–7. doi: 10.1016/S0042-6822(03)00120-X
Jeyarajan, S., Peter, A. S., Sathyan, A., Ranjith, S., Kandasamy, I., Duraisamy, S., et al. (2024). Expression and purification of epinecidin-1 variant (Ac-Var-1) by acid cleavage. Appl. Microbiol. Biotechnol. 108, 176. doi: 10.1007/s00253-024-13017-5
Jha, S., Chattoo, B. B. (2010). Expression of a plant defensin in rice confers resistance to fungal phytopathogens. Transgenic Res. 19, 373–384. doi: 10.1007/s11248-009-9315-7
Ji, Z., Guo, W., Sakkiah, S., Liu, J., Patterson, T. A., Hong, H. (2021). Nanomaterial databases: data sources for promoting design and risk assessment of nanomaterials. Nanotoxicology 11, 1599. doi: 10.3390/nano11061599
Ji, S., Li, W., Baloch, A. R., Wang, M., Li, H., Cao, B., et al. (2017). Efficient biosynthesis of a Cecropin A-melittin mutant in Bacillus subtilis WB700. Sci. Rep. 7, 40587. doi: 10.1038/srep40587
Jia, F., Wang, J., Peng, J., Zhao, P., Kong, Z., Wang, K., et al. (2017). D-amino acid substitution enhances the stability of antimicrobial peptide polybia-CP. Acta Biochim. Biophys. Sin. (Shanghai) 49, 916–925. doi: 10.1093/abbs/gmx091
Jin, L., Wang, Y., Liu, X., Peng, R., Lin, S., Sun, D., et al. (2022). Codon optimization of chicken β Gallinacin-3 gene results in constitutive expression and enhanced antimicrobial activity in transgenic Medicago sativa L. Gene 835, 146656. doi: 10.1016/j.gene.2022.146656
Jin, L., Wang, Y., Xu, N., Wang, D., Liu, X., Peng, R., et al. (2018). Expression and activity analysis of β Gallinacin-3 in Arabidopsis. Protein Expression Purification 144, 1–4. doi: 10.1016/j.pep.2017.09.006
Jing, X.-L., Luo, X.-G., Tian, W.-J., Lv, L.-H., Jiang, Y., Wang, N., et al. (2010). High-level expression of the antimicrobial peptide plectasin in escherichia coli. Curr. Microbiol. 61, 197–202. doi: 10.1007/s00284-010-9596-3
Jones, M. P., Lawrie, A. C., Huynh, T. T., Morrison, P. D., Mautner, A., Bismarck, A., et al. (2019). Agricultural by-product suitability for the production of chitinous composites and nanofibers utilising Trametes versicolor and Polyporus brumalis mycelial growth. Process Biochem. 80, 95–102. doi: 10.1016/j.procbio.2019.01.018
Joshi, P. R. H., Venereo-Sanchez, A., Chahal, P. S., Kamen, A. A. (2021). Advancements in molecular design and bioprocessing of recombinant adeno-associated virus gene delivery vectors using the insect-cell baculovirus expression platform. Biotechnol. J. 16, e2000021. doi: 10.1002/biot.202000021
Jukič, M., Bren, U. (2022). Machine learning in antibacterial drug design. Front. Pharmacol. 13, 864412. doi: 10.3389/fphar.2022.864412
Käßer, L., Rotter, M., Coletta, L., Salzig, D., Czermak, P. (2022). Process intensification for the continuous production of an antimicrobial peptide in stably-transformed Sf-9 insect cells. Sci. Rep. 12, 1086. doi: 10.1038/s41598-022-04931-7
Kale, B., Clyde, A., Sun, M., Ramanathan, A., Stevens, R., Papka, M. E. (2023). “ChemoGraph: interactive visual exploration of the chemical space,” in Computer Graphics Forum (Wiley Online Library) 40 (3), 13–24. doi: 10.1111/cgf.14807
Kamran, M., Ullah, B., Ahmad, M., Sabri, M. M. S. (2022). Application of KNN-based isometric mapping and fuzzy c-means algorithm to predict short-term rockburst risk in deep underground projects. Front. Public Health 10, 1023890. doi: 10.3389/fpubh.2022.1023890
Kaplan, J. T., Gimbel, S. I., Harris, S. (2016). Neural correlates of maintaining one’s political beliefs in the face of counterevidence. Sci. Rep. 6, 39589. doi: 10.1038/srep39589
Kapust, R. B., Routzahn, K. M., Waugh, D. S. (2002). Processive degradation of nascent polypeptides, triggered by tandem AGA codons, limits the accumulation of recombinant tobacco etch virus protease in Escherichia coli BL21 (DE3). Protein Expression purification 24, 61–70. doi: 10.1006/prep.2001.1545
Karbalaei, M., Rezaee, S. A., Farsiani, H. (2020a). Pichia pastoris: A highly successful expression system for optimal synthesis of heterologous proteins. J. Cell. Physiol. 235, 5867–5881. doi: 10.1002/jcp.v235.9
Karki, U., Fang, H., Guo, W., Unnold-Cofre, C., Xu, J. (2021a). Cellular engineering of plant cells for improved therapeutic protein production. Plant Cell Rep. 40, 1087–1099. doi: 10.1007/s00299-021-02693-6
Karki, U., Fang, H., Guo, W., Unnold-Cofre, C., Xu, J. (2021b). Cellular engineering of plant cells for improved therapeutic protein production. Plant Cell Rep. 40, 1087–1099. doi: 10.1007/s00299-021-02693-6
Kasse, R. M., Geise, N. R., Sebti, E., Lim, K., Takacs, C. J., Cao, C., et al. (2022). Combined effects of uniform applied pressure and electrolyte additives in lithium-metal batteries. ACS Appl. Energy Materials 5, 8273–8281. doi: 10.1021/acsaem.2c00806
Kastberg, L. L. B., Ard, R., Jensen, M. K., Workman, C. T. (2022a). Burden imposed by heterologous protein production in two major industrial yeast cell factories: identifying sources and mitigation strategies. Front. Fungal Biol. 3. doi: 10.3389/ffunb.2022.827704
Kaur, J., Kumar, A., Kaur, J. (2018). Strategies for optimization of heterologous protein expression in E. coli: Roadblocks and reinforcements. Int. J. Biol. Macromolecules 106, 803–822. doi: 10.1016/j.ijbiomac.2017.08.080
Kawakami, T., Aimoto, S. (2003). A photoremovable ligation auxiliary for use in polypeptide synthesis. Tetrahedron Lett. 44, 6059–6061. doi: 10.1016/S0040-4039(03)01463-1
Ke, Y., Huang, W.-Q., Li, J.-Z., Xie, M.-Q., Luo, X.-C. (2012). Enzymatic characteristics of a recombinant neutral protease I (rNpI) from Aspergillus oryzae expressed in Pichia pastoris. J. Agric. Food Chem. 60, 12164–12169. doi: 10.1021/jf303167r
Kelesidis, T. (2014). The Interplay between Daptomycin and the Immune System. Front. Immunol. 5. doi: 10.3389/fimmu.2014.00052
Kent, S. B. H. (2021). “Characterization of protein molecules prepared by total chemical synthesis,” in Total chemical synthesis of proteins (WILEY-VCH GmbH: John Wiley & Sons, Ltd), 1–15.
Keyvanpour, M. R., Shirzad, M. B. (2021). An analysis of QSAR research based on machine learning concepts. Curr. Drug Discovery Technol. 18, 17–30. doi: 10.2174/1570163817666200316104404
Khankal, R., Luziatelli, F., Chin, J. W., Frei, C. S., Cirino, P. C. (2008). Comparison between Escherichia coli K-12 strains W3110 and MG1655 and wild-type E. coli B as platforms for xylitol production. Biotechnol. Lett. 30, 1645–1653. doi: 10.1007/s10529-008-9720-7
Kielkopf, C. L., Bauer, W., Urbatsch, I. L. (2020). Purification of fusion proteins by affinity chromatography on glutathione resin. Cold Spring Harbor Protoc. 2020, pdb.prot102202. doi: 10.1101/pdb.prot102202
Kim, K., Choe, D., Lee, D.-H., Cho, B.-K. (2020). Engineering biology to construct microbial chassis for the production of difficult-to-express proteins. Int. J. Mol. Sci. 21, 990. doi: 10.3390/ijms21030990
Kim, H.-K., Chun, D.-S., Kim, J.-S., Yun, C.-H., Lee, J.-H., Hong, S.-K., et al. (2006). Expression of the cationic antimicrobial peptide lactoferricin fused with the anionic peptide in Escherichia coli. Appl. Microbiol. Biotechnol. 72, 330–338. doi: 10.1007/s00253-005-0266-5
Kim, J. M., Jang, S. A., Yu, B. J., Sung, B. H., Cho, J. H., Kim, S. C. (2008). High-level expression of an antimicrobial peptide histonin as a natural form by multimerization and furin-mediated cleavage. Appl. Microbiol. Biotechnol. 78, 123–130. doi: 10.1007/s00253-007-1273-5
Kim, S. J., Mcalpine, S. R. (2013). Solid phase versus solution phase synthesis of heterocyclic macrocycles. Molecules (Basel Switzerland) 18, 1111–1121. doi: 10.3390/molecules18011111
Kjeldsen, T., Ludvigsen, S., Diers, I., Balschmidt, P., Sørensen, A. R., Kaarsholm, N. C. (2002). Engineering-enhanced protein secretory expression in yeast with application to insulin*. J. Biol. Chem. 277, 18245–18248. doi: 10.1074/jbc.C200137200
Koehbach, J., Craik, D. J. (2019). The vast structural diversity of antimicrobial peptides. Trends Pharmacol. Sci. 40, 517–528. doi: 10.1016/j.tips.2019.04.012
Kopanic, R. J., Schal, C. (1999). Coprophagy facilitates horizontal transmission of bait among cockroaches (Dictyoptera: blattellidae). Environ. Entomology 28, 431–438. doi: 10.1093/ee/28.3.431
Kost, T. A., Condreay, J. P., Jarvis, D. L. (2005). Baculovirus as versatile vectors for protein expression in insect and mammalian cells. Nat. Biotechnol. 23, 567–575. doi: 10.1038/nbt1095
Kovács, Á.T. (2019). Bacillus subtilis. Trends Microbiol. 27, 724–725. doi: 10.1016/j.tim.2019.03.008
Kryczka, J., Boncela, J. (2018). Cell migration related to MDR—Another impediment to effective chemotherapy? Molecules 23, 331. doi: 10.3390/molecules23020331
Kuddus, M. R., Rumi, F., Tsutsumi, M., Takahashi, R., Yamano, M., Kamiya, M., et al. (2016). Expression, purification and characterization of the recombinant cysteine-rich antimicrobial peptide snakin-1 in Pichia pastoris. Protein Expression purification 122, 15–22. doi: 10.1016/j.pep.2016.02.002
Kulagina, N., Besseau, S., Godon, C., Goldman, G. H., Papon, N., Courdavault, V. (2021). Yeasts as biopharmaceutical production platforms. Front. Fungal Biol. 2. doi: 10.3389/ffunb.2021.733492
Kumar, P., Kizhakkedathu, J. N., Straus, S. K. (2018). Antimicrobial peptides: diversity, mechanism of action and strategies to improve the activity and biocompatibility in vivo. Biomolecules 8. doi: 10.3390/biom8010004
Kumar, R., Kumar, P. (2019a). Yeast-based vaccines: New perspective in vaccine development and application. FEMS Yeast Res. 19. doi: 10.1093/femsyr/foz007
Kurpet, K., Chwatko, G. (2022). S100 proteins as novel therapeutic targets in psoriasis and other autoimmune diseases. Molecules (Basel Switzerland) 27, 6640. doi: 10.3390/molecules27196640
Kuzmin, D. V., Emelianova, A. A., Kalashnikova, M. B., Panteleev, P. V., Ovchinnikova, T. V. (2017). Effect of N- and C-terminal modifications on cytotoxic properties of antimicrobial peptide tachyplesin I. Bull. Exp. Biol. Med. 162, 754–757. doi: 10.1007/s10517-017-3705-2
Kwak, S. J. F. (2024). Therapeutic applications of native and engineered saccharomyces yeasts. Fermentation 10, 51. doi: 10.3390/fermentation10010051
Lacroix, I. M., Li-Chan, E. C. (2014). Peptide array on cellulose support—a screening tool to identify peptides with dipeptidyl-peptidase IV inhibitory activity within the sequence of α-lactalbumin. Int. J. Mol. Sci. 15, 20846–20858. doi: 10.3390/ijms151120846
Lai, Z., Yuan, X., Chen, H., Zhu, Y., Dong, N., Shan, A. (2022). Strategies employed in the design of antimicrobial peptideAMPss with enhanced proteolytic stability. Biotechnol. Adv. 59, 107962. doi: 10.1016/j.biotechadv.2022.107962
Lalonde, M.-E., Durocher, Y. (2017). Therapeutic glycoprotein production in mammalian cells. J. Biotechnol. 251, 128–140. doi: 10.1016/j.jbiotec.2017.04.028
Lavallie, E. R., Diblasio, E. A., Kovacic, S., Grant, K. L., Schendel, P. F., Mccoy, J. M. (1993). A thioredoxin gene fusion expression system that circumvents inclusion body formation in the E. coli cytoplasm. Bio/technology (Nature Publishing Company) 11, 187–193. doi: 10.1038/nbt0293-187
Lavallie, E. R., Lu, Z., Diblasio-Smith, E. A., Collins-Racie, L. A., Mccoy, J. M. (2000). “[21] Thioredoxin as a fusion partner for production of soluble recombinant proteins in Escherichia coli,” in Methods in enzymology (Elsevier), 322–340.
Laws, M., Shaaban, A., Rahman, K. M. (2019). Antibiotic resistance breakers: current approaches and future directions. FEMS Microbiol. Rev. 43, 490–516. doi: 10.1093/femsre/fuz014
Le, C.-F., Fang, C.-M., Sekaran, S. D. (2017). Intracellular targeting mechanisms by antimicrobial peptides. Antimicrobial Agents chemotherapy 61. doi: 10.1128/aac.02340-02316
Lee, Y. R., Burton, C. E. (2019). Eravacycline, a newly approved fluorocycline. Eur. J. Clin. Microbiol. Infect. Dis. 38, 1787–1794. doi: 10.1007/s10096-019-03590-3
Lee, J., Kim, M., Cho, J., Kim, S. (2002). Enhanced expression of tandem multimers of the antimicrobial peptide buforin II in Escherichia coli by the DEAD-box protein and trxB mutant. Appl. Microbiol. Biotechnol. 58, 790–796. doi: 10.1007/s00253-002-0962-3
Lee, C. D., Sun, H. C., Hu, S. M., Chiu, C. F., Homhuan, A., Liang, S. M., et al. (2008). An improved SUMO fusion protein system for effective production of native proteins. Protein Sci. 17, 1241–1248. doi: 10.1110/ps.035188.108
Lee, M.-T., Yang, P.-Y., Charron, N. E., Hsieh, M.-H., Chang, Y.-Y., Huang, H. W. (2018). Comparison of the effects of daptomycin on bacterial and model membranes. Biochemistry 57, 5629–5639. doi: 10.1021/acs.biochem.8b00818
Legardinier, S., Klett, D., Poirier, J.-C., Combarnous, Y., Cahoreau, C. (2005). Mammalian-like nonsialyl complex-type N-glycosylation of equine gonadotropins in Mimic™ insect cells. Glycobiology 15, 776–790. doi: 10.1093/glycob/cwi060
Lei, M., Jayaraman, A., Van Deventer, J. A., Lee, K. (2021). Engineering selectively targeting antimicrobial peptides. 23, 339–357. doi: 10.1146/annurev-bioeng-010220-095711
Lepeltier, E., Rijo, P., Rizzolio, F., Popovtzer, R., Petrikaite, V., Assaraf, Y. G., et al. (2020). Nanomedicine to target multidrug resistant tumors. Drug Resistance Updates 52, 100704. doi: 10.1016/j.drup.2020.100704
Li, Y. (2011a). Recombinant production of antimicrobial peptides in Escherichia coli: a review. Protein Expression purification 80, 260–267. doi: 10.1016/j.pep.2011.08.001
Li, Y. (2013). Production of human antimicrobial peptide LL-37 in Escherichia coli using a thioredoxin–SUMO dual fusion system. Protein Expression Purification 87, 72–78. doi: 10.1016/j.pep.2012.10.008
Li, Z., Cheng, Q., Guo, H., Zhang, R., Si, D. (2020b). Expression of hybrid peptide EF-1 in pichia pastoris, its purification, and antimicrobial characterization. Molecules 25, 5538. doi: 10.3390/molecules25235538
Li, S., Mccraw, A. J., Gardner, R. A., Spencer, D. I., Karagiannis, S. N., Wagner, G. K. (2021a). Glycoengineering of therapeutic antibodies with small molecule inhibitors. Antibodies (Basel Switzerland) 10, 44. doi: 10.3390/antib10040044
Li, J., Shang, L., Lan, J., Chou, S., Feng, X., Shi, B., et al. (2020a). Targeted and intracellular antibacterial activity against S. agalactiae chimeric peptides based pheromone cell-penetrating peptides. 12, 44459–44474. doi: 10.1021/acsami.0c12226
Li, S., Wang, Y., Xue, Z., Jia, Y., Li, R., He, C., et al. (2021b). The structure-mechanism relationship and mode of actions of antimicrobial peptides: A review. Trends Food Sci. Technol. 109, 103–115. doi: 10.1016/j.tifs.2021.01.005
Li, X., Xing, Y., Jiang, Y., Ding, Y., Li, W. (2009). Antimicrobial activities of ZnO powder-coated PVC film to inactivate food pathogens. Int. J. Food Sci. Technol. 44, 2161–2168. doi: 10.1111/j.1365-2621.2009.02055.x
Li, B.-C., Zhang, S.-Q., Dan, W.-B., Chen, Y.-Q., Cao, P. (2007). Expression in Escherichia coli and purification of bioactive antibacterial peptide ABP-CM4 from the Chinese silk worm, Bombyx mori. Biotechnol. Lett. 29, 1031–1036. doi: 10.1007/s10529-007-9351-4
Li, J. F., Zhang, J., Zhang, Z., Kang, C. T., Zhang, S. Q. (2011). SUMO Mediating Fusion Expression of Antimicrobial Peptide CM4 from two Joined Genes in Escherichia coli. Curr. Microbiol. 62, 296–300. doi: 10.1007/s00284-010-9705-3
Liang, X., Jiang, H., Si, X., Xin, Q., Meng, D., Chen, P., et al. (2022). Boosting expression level of plectasin in recombinant Pichia pastoris via 2A self-processing peptide assembly. Appl. Microbiol. Biotechnol. 106, 3669–3678. doi: 10.1007/s00253-022-11942-x
Lin, Y.-P., Frye, A. M., Nowak, T. A., Kraiczy, P. (2020b). New insights into CRASP-mediated complement evasion in the Lyme disease enzootic cycle. Front. Cell. infection Microbiol. 10, 1. doi: 10.3389/fcimb.2020.00001
Lin, S., Zhu, C., Li, H., Chen, Y., Liu, S. (2020a). Potent in vitro and in vivo antimicrobial activity of semisynthetic amphiphilic γ-mangostin derivative LS02 against Gram-positive bacteria with destructive effect on bacterial membrane. Biochim. Biophys. Acta (BBA)-Biomembranes 1862, 183353. doi: 10.1016/j.bbamem.2020.183353
Liu, P.-F., Wang, Y., Ulrich, R. G., Simmons, C. W., Vandergheynst, J. S., Gallo, R. L., et al. (2018). Leaf-encapsulated vaccines: agroinfiltration and transient expression of the antigen staphylococcal endotoxin B in radish leaves. J. Immunol. Res. 2018, 3710961. doi: 10.1155/2018/3710961
Lobstein, J., Emrich, C. A., Jeans, C., Faulkner, M., Riggs, P., Berkmen, M. (2012). SHuffle, a novel Escherichia coli protein expression strain capable of correctly folding disulfide bonded proteins in its cytoplasm. Microbial Cell factories 11, 56. doi: 10.1186/1475-2859-11-56
Loeschcke, A., Thies, S. (2015). Pseudomonas putida—a versatile host for the production of natural products. Appl. Microbiol. Biotechnol. 99, 6197–6214. doi: 10.1007/s00253-015-6745-4
Logez, C., Alkhalfioui, F., Byrne, B., Wagner, R. (2012). Preparation of Pichia pastoris expression plasmids. Methods Mol. Biol. 866, 25–40. doi: 10.1007/978-1-61779-770-5_3
Loibl, S. F., Harpaz, Z., Seitz, O. (2015). A type of auxiliary for native chemical peptide ligation beyond cysteine and glycine junctions. Angewandte Chemie Int. Edition 54, 15055–15059. doi: 10.1002/anie.201505274
López-Cano, A., Martínez-Miguel, M., Guasch, J., Bastardas, I. R., I Giralt, A. A., Garcia-Fruitos, E. (2022). Exploring the impact of the recombinant Escherichia coli strain on defensins antimicrobial activity: BL21 versus Origami strain. Microbial Cell factories (Print) 21. doi: 10.1186/s12934-022-01803-7
López-Otín, C., Matrisian, L. M. (2007). Emerging roles of proteases in tumour suppression. Nat. Rev. Cancer 7, 800–808. doi: 10.1038/nrc2228
López-Pérez, P. M., Grimsey, E., Bourne, L., Mikut, R., Hilpert, K. (2017). Screening and optimizing antimicrobial peptides by using SPOT-synthesis. Front. Chem. 5. doi: 10.3389/fchem.2017.00025
Lozano Terol, G., Gallego-Jara, J., Sola Martínez, R. A., Martínez Vivancos, A., Cánovas Díaz, M., De Diego Puente, T. (2021). Impact of the expression system on recombinant protein production in escherichia coli BL21. Front. Microbiol. 12. doi: 10.3389/fmicb.2021.682001
Luengo, T. M., Kityk, R., Mayer, M. P., Rüdiger, S. G. (2018). Hsp90 breaks the deadlock of the Hsp70 chaperone system. Mol. Cell 70, 545–552. e549. doi: 10.1016/j.molcel.2018.03.028
Luong, H. X., Thanh, T. T., Tran, T. H. (2020). Antimicrobial peptide–Advances in development of therapeutic applications. Life Sci. 260, 118407. doi: 10.1016/j.lfs.2020.118407
Ma, W., Liu, H., Li, X. (2023). Chemical synthesis of peptides and proteins bearing base-labile post-translational modifications: evolution of the methods in four decades. ChemBioChem 24, e202300348. doi: 10.1002/cbic.202300348
Mabashi-Asazuma, H., Jarvis, D. L. (2017b). CRISPR-Cas9 vectors for genome editing and host engineering in the baculovirus–insect cell system. Proc. Natl. Acad. Sci. 114, 9068–9073. doi: 10.1073/pnas.1705836114
Macmillan, D. (2006). Evolving strategies for protein synthesis converge on native chemical ligation. Angewandte Chemie Int. Edition 45, 7668–7672. doi: 10.1002/anie.200602945
Maeno, M., Taguchi, S., Momose, H. (1993). Production of antibacterial peptide ‘Apidaecin’ Using the secretory expression system of streptomyces. Bioscience Biotechnology Biochem. 57, 1206–1207. doi: 10.1271/bbb.57.1206
Magalhaes, J. V., Liu, J., Guimaraes, C. T., Lana, U. G., Alves, V. M., Wang, Y.-H., et al. (2007). A gene in the multidrug and toxic compound extrusion (MATE) family confers aluminum tolerance in sorghum. Nat. Genet. 39, 1156–1161. doi: 10.1038/ng2074
Makino, T., Skretas, G., Georgiou, G. (2011). Strain engineering for improved expression of recombinant proteins in bacteria. Microbial Cell factories 10, 32. doi: 10.1186/1475-2859-10-32
Malakhov, M. P., Mattern, M. R., Malakhova, O. A., Drinker, M., Weeks, S. D., Butt, T. R. (2004). SUMO fusions and SUMO-specific protease for efficient expression and purification of proteins. J. Struct. Funct. Genomics 5, 75–86. doi: 10.1023/B:JSFG.0000029237.70316.52
Manniello, M., Moretta, A., Salvia, R., Scieuzo, C., Lucchetti, D., Vogel, H., et al. (2021). Insect antimicrobial peptides: potential weapons to counteract the antibiotic resistance. Cell. Mol. Life Sci. 78, 4259–4282. doi: 10.1007/s00018-021-03784-z
Mansour, R. (2018). Natural dyes and pigments: Extraction and applications. Handb. Renewable materials coloration finishing 9, 75–102. doi: 10.1038/s41598-018-19669-4
Mant, C. T., Jiang, Z., Gera, L., Davis, T., Nelson, K. L., Bevers, S., et al. (2019). De novo designed amphipathic α-helical antimicrobial peptideAMPss incorporating dab and dap residues on the polar face to treat the gram-negative pathogen, acinetobacter baumannii. J. Medicinal Chem. 62, 3354–3366. doi: 10.1021/acs.jmedchem.8b01785
Margit Mahlapuu, C. B., Ekblom, J. (2020). Antimicrobial peptides as therapeutic agents: opportunities and challenges. Crit. Rev. Biotechnol. 40, 978–992. doi: 10.1080/07388551.2020.1796576
Margolin, E. A., Strasser, R., Chapman, R., Williamson, A.-L., Rybicki, E. P., Meyers, A. E. (2020). Engineering the plant secretory pathway for the production of next-generation pharmaceuticals. Trends Biotechnol. 38, 1034–1044. doi: 10.1016/j.tibtech.2020.03.004
Marsian, J., Lomonossoff, G. P. (2016). Molecular pharming—VLPs made in plants. Curr. Opin. Biotechnol. 37, 201–206. doi: 10.1016/j.copbio.2015.12.007
Mattanovich, D., Branduardi, P., Dato, L., Gasser, B., Sauer, M., Porro, D. (2012b). Recombinant protein production in yeasts. Methods Mol. Biol. (Clifton N.J.), 329–358. doi: 10.1007/978-1-61779-433-9_17
Mazurkiewicz-Pisarek, A., Baran, J., Ciach, T. (2023). Antimicrobial peptides: challenging journey to the pharmaceutical, biomedical, and cosmeceutical use. Int. J. Mol. Sci. 24, 9031. doi: 10.3390/ijms24109031
Medini, K., Harris, P. W. R., Hards, K., Dingley, A. J., Cook, G. M., Brimble, M. A. (2015). Chemical synthesis of A pore-forming antimicrobial protein, caenopore-5, by using native chemical ligation at a glu-cys site. ChemBioChem 16, 328–336. doi: 10.1002/cbic.201402513
Melo, M. N., Ferre, R., Castanho, M. A. R. B. (2009). Antimicrobial peptides: linking partition, activity and high membrane-bound concentrations. Nat. Rev. Microbiol. 7, 245–250. doi: 10.1038/nrmicro2095
Meng, D.-M., Dai, H.-X., Gao, X.-F., Zhao, J.-F., Guo, Y.-J., Ling, X., et al. (2016). Expression, purification and initial characterization of a novel recombinant antimicrobial peptide Mytichitin-A in Pichia pastoris. Protein Expression Purification 127, 35–43. doi: 10.1016/j.pep.2016.07.001
Meng, D.-M., Zhao, J.-F., Ling, X., Dai, H.-X., Guo, Y.-J., Gao, X.-F., et al. (2017a). Recombinant expression, purification and antimicrobial activity of a novel antimicrobial peptide PaDef in Pichia pastoris. Protein Expression Purification 130, 90–99. doi: 10.1016/j.pep.2016.10.003
Meng, S., Zhou, H., Feng, Z., Xu, Z., Tang, Y., Li, P., et al. (2017b). CircRNA: functions and properties of a novel potential biomarker for cancer. Mol. Cancer 16, 1–8. doi: 10.1186/s12943-017-0663-2
Mercx, S., Smargiasso, N., Chaumont, F., De Pauw, E., Boutry, M., Navarre, C. (2017). Inactivation of the β (1, 2)-xylosyltransferase and the α (1, 3)-fucosyltransferase genes in Nicotiana tabacum BY-2 cells by a multiplex CRISPR/Cas9 strategy results in glycoproteins without plant-specific glycans. Front. Plant Sci. 8, 403. doi: 10.3389/fpls.2017.00403
Metlitskaia, L., Cabralda, J. E., Suleman, D., Kerry, C., Brinkman, J., Bartfeld, D., et al. (2004). Recombinant antimicrobial peptides efficiently produced using novel cloning and purification processes. Biotechnol. Appl. Biochem. 39, 339–345. doi: 10.1042/BA20030100
Mitchell, M. J., Billingsley, M. M., Haley, R. M., Wechsler, M. E., Peppas, N. A., Langer, R. (2021). Engineering precision nanoparticles for drug delivery. Nat. Rev. Drug Discovery 20, 101–124. doi: 10.1038/s41573-020-0090-8
Mohammed, E. H. M., Lohan, S., Ghaffari, T., Gupta, S., Tiwari, R. K., Parang, K. (2022). Membrane-active cyclic amphiphilic peptides: broad-spectrum antibacterial activity alone and in combination with antibiotics. J. Medicinal Chem. 65, 15819–15839. doi: 10.1021/acs.jmedchem.2c01469
Molina, A., Hervás-Stubbs, S., Daniell, H., Mingo-Castel, A. M., Veramendi, J. (2004). High-yield expression of a viral peptide animal vaccine in transgenic tobacco chloroplasts. Plant Biotechnol. J. 2, 141–153. doi: 10.1046/j.1467-7652.2004.00057.x
Moon, J.-Y., Henzler-Wildman, K. A., Ramamoorthy, A. (2006). Expression and purification of a recombinant LL-37 from Escherichia coli. Biochim. Biophys. Acta (BBA) - Biomembranes 1758, 1351–1358. doi: 10.1016/j.bbamem.2006.02.003
Moradi, S. V., Hussein, W. M., Varamini, P., Simerska, P., Toth, I. (2016). Glycosylation, an effective synthetic strategy to improve the bioavailability of therapeutic peptides. Chem. Sci. 7, 2492–2500. doi: 10.1039/C5SC04392A
Morassutti, C., De Amicis, F., Skerlavaj, B., Zanetti, M., Marchetti, S. (2002). Production of a recombinant antimicrobial peptide in transgenic plants using a modified VMA intein expression system. FEBS Lett. 519, 141–146. doi: 10.1016/S0014-5793(02)02741-2
Morin, K. M., Arcidiacono, S., Beckwitt, R., Mello, C. M. (2006). Recombinant expression of indolicidin concatamers in Escherichia coli. Appl. Microbiol. Biotechnol. 70, 698–704. doi: 10.1007/s00253-005-0132-5
Moustafa, K., Makhzoum, A., Trémouillaux-Guiller, J. (2016). Molecular farming on rescue of pharma industry for next generations. Crit. Rev. Biotechnol. 36, 840–850. doi: 10.3109/07388551.2015.1049934
Muir, T. W., Sondhi, D., Cole, P. A. (1998). Expressed protein ligation: A general method for protein engineering. Proc. Natl. Acad. Sci. 95, 6705–6710. doi: 10.1073/pnas.95.12.6705
Mukesh Pasupuleti, A. S., Malmsten, M. (2012). Antimicrobial peptides: key components of the innate immune system. Crit. Rev. Biotechnol. 32, 143–171. doi: 10.3109/07388551.2011.594423
Mura, M., Wang, J., Zhou, Y., Pinna, M., Zvelindovsky, A. V., Dennison, S. R., et al. (2016). The effect of amidation on the behaviour of antimicrobial peptides. Eur. Biophysics J. 45, 195–207. doi: 10.1007/s00249-015-1094-x
Murray, C. J. L., Ikuta, K. S., Sharara, F., Swetschinski, L., Robles Aguilar, G., Gray, A., et al. (2022). Global burden of bacterial antimicrobial resistance in 2019: a systematic analysis. Lancet 399, 629–655. doi: 10.1016/S0140-6736(21)02724-0
Murugaiyan, J., Kumar, P. A., Rao, G. S., Iskandar, K., Hawser, S., Hays, J. P., et al. (2022). Progress in alternative strategies to combat antimicrobial resistance: focus on antibiotics. Antibiotics (Basel Switzerland) 11. doi: 10.3390/antibiotics11020200
Muthunayake, N. S., Islam, R., Inutan, E. D., Colangelo, W., Trimpin, S., Cunningham, P. R., et al. (2020a). Expression and in vivo characterization of the antimicrobial peptide oncocin and variants binding to ribosomes. Biochemistry 59, 3380–3391. doi: 10.1021/acs.biochem.0c00600
Mwangi, J., Yin, Y., Wang, G., Yang, M., Li, Y., Zhang, Z., et al. (2019). The antimicrobial peptide ZY4 combats multidrug-resistant Pseudomonas aeruginosa and Acinetobacter baumannii infection. Proc. Natl. Acad. Sci. 116, 26516–26522. doi: 10.1073/pnas.1909585117
Myronovskyi, M., Luzhetskyy, A. (2019). Heterologous production of small molecules in the optimized Streptomyces hosts. Natural product Rep. 36, 1281–1294. doi: 10.1039/C9NP00023B
Nakamura, R., Baumjohann, W., Schödel, R., Brittnacher, M., Sergeev, V., Kubyshkina, M., et al. (2001). Earthward flow bursts, auroral streamers, and small expansions. J. Geophysical Research: Space Phys. 106, 10791–10802. doi: 10.1029/2000JA000306
Nandhini, K., Albericio, F., de la Torre, B. G. (2022). 2-methoxy-4-methylsulfinylbenzyl alcohol as a safety-catch linker for the fmoc/t bu solid-phase peptide synthesis strategy. J. organic Chem. 87, 9433–9442. doi: 10.1021/acs.joc.2c01057
Narh, J. K., Casillas-Vega, N. G., Zarate, X. (2024). LL-37_Renalexin hybrid peptide exhibits antimicrobial activity at lower MICs than its counterpart single peptides. Appl. Microbiol. Biotechnol. 108, 126. doi: 10.1007/s00253-023-12887-5
Nawrot, R., Barylski, J., Nowicki, G., Broniarczyk, J., Buchwald, W., Goździcka-Józefiak, A. (2014). Plant antimicrobial peptides. Folia Microbiologica 59, 181–196. doi: 10.1007/s12223-013-0280-4
Neef, J., Van Dijl, J. M., Buist, G. (2021). Recombinant protein secretion by Bacillus subtilis and Lactococcus lactis: pathways, applications, and innovation potential. Essays Biochem. 65, 187–195. doi: 10.1042/EBC20200171
Ngoc, D. N., Szymczak, B., Krawczyk, A., Drzewiecka, B., Wessely-Szponder, J., Domańska, A., et al. (2023). Nanoparticles associated with antimicrobial peptides (AMPs)–a promising combination for biomedical and veterinary applications. Artykuł przeglądowy 79, 599–609. doi: 10.21521/mw.6835
Nilsson Anna, C., Janson, H., Wold, H., Fugelli, A., Andersson, K., Håkangård, C., et al. (2014). LTX-109 is a novel agent for nasal decolonization of methicillin-resistant and -sensitive staphylococcus aureus. Antimicrobial Agents Chemotherapy 59, 145–151. doi: 10.1128/aac.03513-14
Nishita, M., Park, S.-Y., Nishio, T., Kamizaki, K., Wang, Z., Tamada, K., et al. (2017). Ror2 signaling regulates Golgi structure and transport through IFT20 for tumor invasiveness. Sci. Rep. 7, 1. doi: 10.1038/s41598-016-0028-x
Noki, S., de la Torre, B. G., Albericio, F. (2024). Safety-catch linkers for solid-phase peptide synthesis. Molecules 29, 1429. doi: 10.3390/molecules29071429
Nyembe, P. L., Ntombela, T., Makatini, M. M. (2023). Structure-activity relationship of antimicrobial peptoids. Pharmaceutics 15, 1506. doi: 10.3390/pharmaceutics15051506
Odunitan, T. T., Oyaronbi, A. O., Adebayo, F. A., Adekoyeni, P. A., Apanisile, B. T., Oladunni, T. D., et al. (2023). Antimicrobial peptides: A novel and promising arsenal against methicillin-resistant Staphylococcus aureus (MRSA) infections. Pharm. Sci. Adv., 100034. doi: 10.1016/j.pscia.2023.100034
Offer, J., Boddy, C. N. C., Dawson, P. E. (2002). Extending synthetic access to proteins with a removable acyl transfer auxiliary. J. Am. Chem. Soc. 124, 4642–4646. doi: 10.1021/ja016731w
Oleru, O. O., Akhavan, A. A., Seyidova, N., Ibelli, T., Taub, P. J., Henderson, P. (2023). Did the national ban on bacitracin irrigation affect infection rates in implant-based breast reconstruction? An analysis of a national database. Clin. Breast Cancer 23, e103–e108. doi: 10.1016/j.clbc.2022.12.019
Oliva, R., Chino, M., Pane, K., Pistorio, V., De Santis, A., Pizzo, E., et al. (2018). Exploring the role of unnatural amino acids in antimicrobial peptides. Sci. Rep. 8, 8888. doi: 10.1038/s41598-018-27231-5
Ollivier, N., Desmet, R., Drobecq, H., Blanpain, A., Boll, E., Leclercq, B., et al. (2017). A simple and traceless solid phase method simplifies the assembly of large peptides and the access to challenging proteins. Chem. Sci. 8, 5362–5370. doi: 10.1039/C7SC01912B
Öztürk, S., Ergün, B. G., Çalık, P. (2017). Double promoter expression systems for recombinant protein production by industrial microorganisms. Appl. Microbiol. Biotechnol. 101, 7459–7475. doi: 10.1007/s00253-017-8487-y
Pal, I., Bhattacharyya, D., Kar, R. K., Zarena, D., Bhunia, A., Atreya, H. S. (2019). A peptide-nanoparticle system with improved efficacy against multidrug resistant bacteria. Sci. Rep. 9, 4485. doi: 10.1038/s41598-019-41005-7
Palomares, L. A., Srivastava, I. K., Ramírez, O. T., Cox, M. M. J. (2021). “Glycobiotechnology of the Insect Cell-Baculovirus Expression System Technology,” in Advances in Glycobiotechnology. Eds. Rapp, E., Reichl, U. (Springer International Publishing, Cham), 71–92.
Pandit, K. R., Klauda, J. B. (2012). Membrane models of E. coli containing cyclic moieties in the aliphatic lipid chain. Biochim. Biophys. Acta 1818, 1205–1210. doi: 10.1016/j.bbamem.2012.01.009
Panteleev, P. V., Ovchinnikova, T. V. J. B., Biochemistry, A. (2017). Improved strategy for recombinant production and purification of antimicrobial peptide tachyplesin I and its analogs with high cell selectivity. Biotechnol. Appl. Biochem. 64, 35–42. doi: 10.1002/bab.2017.64.issue-1
Parachin, N. S., Mulder, K. C., Viana, A. A. B., Dias, S. C., Franco, O. L. (2012a). Expression systems for heterologous production of antimicrobial peptides. Peptides 38, 446–456. doi: 10.1016/j.peptides.2012.09.020
Parapouli, M., Vasileiadis, A., Afendra, A.-S., Hatziloukas, E. (2020). Saccharomyces cerevisiae and its industrial applications. AIMS Microbiol. 6, 1. doi: 10.3934/microbiol.2020001
Paremskaia, A. I., Kogan, A. A., Murashkina, A., Naumova, D. A., Satish, A., Abramov, I. S., et al. (2024). Codon-optimization in gene therapy: promises, prospects and challenges. Front. bioengineering Biotechnol. 12, 1371596. doi: 10.3389/fbioe.2024.1371596
Park, A. R., Kim, S. W., Kim, S. Y., Kwon, K.-C. (2021a). Expression of antimicrobial peptide (AMP), cecropin B, in a fused form to SUMO tag with or without three-glycine linker in Escherichia coli and evaluation of bacteriolytic activity of the purified AMP. Probiotics Antimicrobial Proteins 13, 1780–1789. doi: 10.1007/s12602-021-09797-1
Parker, C. C., Clarke, N. W., Cook, A. D., Kynaston, H. G., Petersen, P. M., Catton, C., et al. (2020). Timing of radiotherapy after radical prostatectomy (RADICALS-RT): a randomised, controlled phase 3 trial. Lancet 396, 1413–1421. doi: 10.1016/S0140-6736(20)31553-1
Parks, A. R., Escalante-Semerena, J. C. (2022). Protein N-terminal acylation: An emerging field in bacterial cell physiology. Curr. Trends Microbiol. 16, 1–18. doi: 10.31300/CTMB.16.2022.1-18
Pasrija, P., Jha, P., Upadhyaya, P., Khan, M. S., Chopra, M. (2022). Machine learning and artificial intelligence: a paradigm shift in big data-driven drug design and discovery. Curr. Topics Medicinal Chem. 22, 1692–1727. doi: 10.2174/1568026622666220701091339
Pavelka, A., Vacek, L., Norek, A., Kobzová, Š., Janda, L. (2024). Recombinant production of human antimicrobial peptide LL-37 and its secondary structure. Biologia 79, 263–273. doi: 10.1007/s11756-023-01539-8
Pazgier, M., Hoover, D. M., Yang, D., Lu, W., Lubkowski, J. (2006). Human β-defensins. Cell. Mol. Life Sci. CMLS 63, 1294–1313. doi: 10.1007/s00018-005-5540-2
Pedersen, C. N., Axelsen, K. B., Harper, J. F., Palmgren, M. G. (2012). Evolution of plant P-type ATPases. Front. Plant Sci. 3, 31. doi: 10.3389/fpls.2012.00031
Peng, H., Liu, H.-P., Chen, B., Hao, H., Wang, K.-J. (2012). Optimized production of scygonadin in Pichia pastoris and analysis of its antimicrobial and antiviral activities. Protein Expression purification 82, 37–44. doi: 10.1016/j.pep.2011.11.008
Pereira, A. M., Gomes, D., Da Costa, A., Dias, S. C., Casal, M., MaChado, R. (2021). Protein-engineered polymers functionalized with antimicrobial peptides for the development of active surfaces. Appl. Sci. 11, 5352. doi: 10.3390/app11125352
Pérez De Lastra, J. M., Wardell, S. J., Pal, T., de la Fuente-Núñez, C., Pletzer, D. (2024). From data to decisions: leveraging artificial intelligence and machine learning in combating antimicrobial resistance–a comprehensive review. J Med Systems, 48 (1), 71. doi: 10.1007/s10916-024-02089-5
Pipiya, S. O., Kudzhaev, A. M., Mirzoeva, N. Z., Mokrushina, Y. A., Ziganshin, R. H., Komlev, A. S., et al. (2023). Bioengineering the antimicrobial activity of yeast by recombinant thanatin production. Antibiotics 12, 1719. doi: 10.3390/antibiotics12121719
Plotkin, J. B., Kudla, G. (2011). Synonymous but not the same: the causes and consequences of codon bias. Nat. Rev. Genet. 12, 32–42. doi: 10.1038/nrg2899
Popova, L. G., Khramov, D. E., Nedelyaeva, O. I., Volkov, V. S. (2023). Yeast heterologous expression systems for the Study of Plant Membrane Proteins. Int. J. Mol. Sci. 24, 10768. doi: 10.3390/ijms241310768
Portelinha, J., Duay, S. S., Yu, S. I., Heilemann, K., Libardo, M. D. J., Juliano, S. A., et al. (2021). Antimicrobial peptideAMPss and copper(II) ions: novel therapeutic opportunities. Chem. Rev. 121, 2648–2712. doi: 10.1021/acs.chemrev.0c00921
Pouresmaeil, M., Azizi-Dargahlou, S. (2023). Factors involved in heterologous expression of proteins in E. coli host. Arch. Microbiol. 205, 212. doi: 10.1007/s00203-023-03541-9
Pratama, F., Linton, D., Dixon, N. (2021). Genetic and process engineering strategies for enhanced recombinant N-glycoprotein production in bacteria. Microbial Cell Factories 20, 198. doi: 10.1186/s12934-021-01689-x
Purohit, K., Reddy, N., Sunna, A. (2024). Exploring the potential of bioactive peptides: from natural sources to therapeutics. Int. J. Mol. Sci. 25. doi: 10.3390/ijms25031391
Put, H., Gerstmans, H., Vande Capelle, H., Fauvart, M., Michiels, J., Masschelein, J. (2024). Bacillus subtilis as a host for natural product discovery and engineering of biosynthetic gene clusters. Natural Product Rep. 41, 1113–1151. doi: 10.1039/D3NP00065F
Qiao, D., Yan, Y., Pei, C., Zhang, J., Zhao, X., Jiang, X., et al. (2023). Characterization of hepcidin gene and protection of recombinant hepcidin supplemented in feed against Aeromonas hydrophila infection in Yellow River carp (Cyprinus carpio haematopterus). Fish Shellfish Immunol. 139, 108872. doi: 10.1016/j.fsi.2023.108872
Qu, X.-M., Steiner, H., Engström, Å., Bennich, H., Boman, H. G. (1982). Insect immunity: isolation and structure of cecropins B and D from pupae of the chinese oak silk moth, antheraea pernyi. Eur. J. Biochem. 127, 219–224. doi: 10.1111/j.1432-1033.1982.tb06858.x
Quax, T. E., Claassens, N. J., Söll, D., van der Oost, J. (2015). Codon bias as a means to fine-tune gene expression. Mol. Cell 59, 149–161. doi: 10.1016/j.molcel.2015.05.035
Raibaut, L., El Mahdi, O., Melnyk, O. (2015). “Solid Phase Protein Chemical Synthesis,” in Protein Ligation and Total Synthesis II. Ed. Liu, L. (Springer International Publishing, Cham), 103–154.
Raimondo, T. M., Reed, K., Shi, D., Langer, R., Anderson, D. G. (2023). Delivering the next generation of cancer immunotherapies with RNA. Cell 186, 1535–1540. doi: 10.1016/j.cell.2023.02.031
Rao, X., Hu, J., Li, S., Jin, X., Zhang, C., Cong, Y., et al. (2005). Design and expression of peptide antibiotic hPAB-β as tandem multimers in Escherichia coli. Peptides 26, 721–729. doi: 10.1016/j.peptides.2004.12.016
Raschmanová, H., Weninger, A., Knejzlík, Z., Melzoch, K., Kovar, K. (2021). Engineering of the unfolded protein response pathway in Pichia pastoris: enhancing production of secreted recombinant proteins. Appl. Microbiol. Biotechnol. 105, 4397–4414. doi: 10.1007/s00253-021-11336-5
Reif, A., Siebenhaar, S., Tröster, A., Schmälzlein, M., Lechner, C., Velisetty, P., et al. (2014). Semisynthesis of biologically active glycoforms of the human cytokine interleukin 6. Angewandte Chemie Int. Edition 53, 12125–12131. doi: 10.1002/anie.201407160
Reymond, J.-L., Van Deursen, R., Blum, L. C., Ruddigkeit, L. (2010). Chemical space as a source for new drugs. MedChemComm 1, 30–38. doi: 10.1039/c0md00020e
Rezaei, S., Hadadian, S., Khavari-Nejad, R. A., Norouzian, D. (2020). Recombinant tandem repeated expression of s3 and sδ3 antimicrobial peptides. Rep. Biochem. Mol. Biol. 9, 348. doi: 10.29252/rbmb.9.3.348
Ridyard, K. E., Overhage, J. (2021). The potential of human peptide LL-37 as an antimicrobial and anti-biofilm agent. Antibiotics 10, 650. doi: 10.3390/antibiotics10060650
Romo, A. L., Quirós, R. (2019). Appropriate use of antibiotics: an unmet need. Ther. Adv. Urol. 11, 1756287219832174. doi: 10.1177/1756287219832174
Rosales-Mendoza, S., Angulo, C., Meza, B. (2016). Food-grade organisms as vaccine biofactories and oral delivery vehicles. Trends Biotechnol. 34, 124–136. doi: 10.1016/j.tibtech.2015.11.007
Rosano, G. L., Ceccarelli, E. A. (2014). Recombinant protein expression in Escherichia coli: advances and challenges. Front. Microbiol. 5. doi: 10.3389/fmicb.2014.00172
Rossouw, M., Cripwell, R. A., Vermeulen, R. R., Van Staden, A. D., Van Zyl, W. H., Dicks, L. M. T., et al. (2024). Heterologous Expression of plantaricin 423 and mundticin ST4SA in saccharomyces cerevisiae. Probiotics Antimicrobial Proteins 16, 845–861. doi: 10.1007/s12602-023-10082-6
Saini, S. S., Chopra, A. K., Peterson, J. W. (1999). Melittin activates endogenous phospholipase D during cytolysis of human monocytic leukemia cells. Toxicon 37, 1605–1619. doi: 10.1016/S0041-0101(99)00110-5
Salam, M. A., Al-Amin, M. Y., Salam, M. T., Pawar, J. S., Akhter, N., Rabaan, A. A., et al. Antimicrobial resistance: a growing serious threat for global public health. Healthcare (Basel). 11 (13), 1946. doi: 10.3390/healthcare11131946
Salim, K., Haedens, V., Content, J., Leblon, G., Huygen, K. (1997). Heterologous expression of the Mycobacterium tuberculosis gene encoding antigen 85A in Corynebacterium glutamicum. Appl. Environ. Microbiol. 63, 4392–4400. doi: 10.1128/aem.63.11.4392-4400.1997
Sánchez-López, E., Gomes, D., Esteruelas, G., Bonilla, L., Lopez-MaChado, A. L., Galindo, R., et al. (2020). Metal-based nanoparticles as antimicrobial agents: an overview. Nanomaterials (Basel Switzerland) 10, 292. doi: 10.3390/nano10020292
Sang, M., Wei, H., Zhang, J., Wei, Z., Wu, X., Chen, Y., et al. (2017). Expression and characterization of the antimicrobial peptide ABP-dHC-cecropin A in the methylotrophic yeast Pichia pastoris. Protein Expression Purification 140, 44–51. doi: 10.1016/j.pep.2017.08.001
Santos-Júnior, C. D., Torres, M. D., Duan, Y., Del Río, Á.R., Schmidt, T. S., Chong, H., et al. (2024). Discovery of antimicrobial peptides in the global microbiome with machine learning. Cell. doi: 10.1016/j.cell.2024.05.013
Santry, L. A., Ingrao, J. C., Yu, D. L., De Jong, J. G., Van Lieshout, L. P., Wood, G. A., et al. (2017). AAV vector distribution in the mouse respiratory tract following four different methods of administration. BMC Biotechnol. 17, 43. doi: 10.1186/s12896-017-0365-2
Sarah, R. D., Frederick, H., David, A. P. (2005). Are oblique orientated &945;-helices used by antimicrobial peptides for membrane invasion? Protein Pept. Lett. 12, 27–29. doi: 10.2174/0929866053406039
Sarah, R. D., Leslie, H. G. M., David, A. P. (2012). Effect of amidation on the antimicrobial peptide aurein 2.5 from Australian southern bell frogs. Protein Pept. Lett. 19, 586–591. doi: 10.2174/092986612800494110
Schallmey, M., Singh, A., Ward, O. P. (2004). Developments in the use of Bacillus species for industrial production. Can. J. Microbiol. 50, 1–17. doi: 10.1139/w03-076
Schlegel, J., Denay, G., Wink, R., Pinto, K. G., Stahl, Y., Schmid, J., et al. (2021). Control of Arabidopsis shoot stem cell homeostasis by two antagonistic CLE peptide signalling pathways. Elife 10. doi: 10.7554/eLife.70934.sa2
Schlegel, A. A., Rudelson, J. J., Tse, P. U. (2012). White matter structure changes as adults learn a second language. J. Cogn. Neurosci. 24, 1664–1670. doi: 10.1162/jocn_a_00240
Schreiber, C., Müller, H., Birrenbach, O., Klein, M., Heerd, D., Weidner, T., et al. (2017). A high-throughput expression screening platform to optimize the production of antimicrobial peptides. Microbial Cell Factories 16, 29. doi: 10.1186/s12934-017-0637-5
Schwarz, S., Kehrenberg, C., Doublet, B., Cloeckaert, A. (2004). Molecular basis of bacterial resistance to chloramphenicol and florfenicol. FEMS Microbiol. Rev. 28, 519–542. doi: 10.1016/j.femsre.2004.04.001
Seo, E.-J., Weibel, S., Wehkamp, J., Oelschlaeger, T. A. (2012). Construction of recombinant E. coli Nissle 1917 (EcN) strains for the expression and secretion of defensins. Int. J. Med. microbiology: IJMM 302, 276–287. doi: 10.1016/j.ijmm.2012.05.002
Shameli, K., Izadiyan, Z., Kia, P., Hamrayev, H., Jahangirian, H., Kartouzian, A., et al. (2024). Exploring the potential of gold nanoparticles in nanomedicine: A comprehensive analysis of benefits and limitations. J. Res. Nanoscience Nanotechnology 11, 1–15. doi: 10.37934/jrnn.11.1.115
Shams, M. V., Nazarian-Firouzabadi, F., Ismaili, A., Shirzadian-Khorramabad, R. (2019). Production of a recombinant dermaseptin peptide in nicotiana tabacum hairy roots with enhanced antimicrobial activity. Mol. Biotechnol. 61, 241–252. doi: 10.1007/s12033-019-00153-x
Shanmugaraj, B., Bulaon, C. J. I., Malla, A., Phoolcharoen, W. (2021a). Biotechnological insights on the expression and production of antimicrobial peptides in plants. Molecules (Basel Switzerland) 26. doi: 10.3390/molecules26134032
Shao, C., Zhu, Y., Lai, Z., Tan, P., Shan, A. (2019). Antimicrobial peptides with protease stability: progress and perspective. Future Medicinal Chem. 11, 2047–2050. doi: 10.4155/fmc-2019-0167
Shen, Q., Wu, M., Wang, H.-B., Naranmandura, H., Chen, S.-Q. (2012). The effect of gene copy number and co-expression of chaperone on production of albumin fusion proteins in Pichia pastoris. Appl. Microbiol. Biotechnol. 96, 763–772. doi: 10.1007/s00253-012-4337-0
Shin, J. M., Gwak, J. W., Kamarajan, P., Fenno, J. C., Rickard, A. H., Kapila, Y. L. (2016). Biomedical applications of nisin. J. Appl. Microbiol. 120, 1449–1465. doi: 10.1111/jam.2016.120.issue-6
Shinmyo, A., Kato, K. (2010). Molecular farming: production of drugs and vaccines in higher plants. J. Antibiotics 63, 431–433. doi: 10.1038/ja.2010.63
Silva, O. N., Mulder, K. C., Barbosa, A. A., Otero-Gonzalez, A. J., Lopez-Abarrategui, C., Dias, S. C., et al. (2011). Exploring the pharmacological potential of promiscuous host-defense peptides: from natural screenings to biotechnological applications. Front. Microbiol. 2. doi: 10.3389/fmicb.2011.00232
Simonen, M., Palva, I. (1993). Protein secretion in Bacillus species. Microbiological Rev. 57, 109–137. doi: 10.1128/mr.57.1.109-137.1993
Siriwardena, T. N., Capecchi, A., Gan, B. H., Jin, X., He, R., Wei, D., et al. (2018). Optimizing antimicrobial peptide dendrimers in chemical space. Angewandte Chemie 130, 8619–8623. doi: 10.1002/ange.201802837
Skosyrev, V. S., Kulesskiy, E. A., Yakhnin, A. V., Temirov, Y. V., Vinokurov, L. M. (2003). Expression of the recombinant antibacterial peptide sarcotoxin IA in Escherichia coli cells. Protein Expression purification 28, 350–356. doi: 10.1016/S1046-5928(02)00697-6
Smith, P. J., Friede, M. H., Scott, B. J., Holt, C. V. (1988). Isolation of nuclei from melittin-destabilized cells. Analytical Biochem. 169, 390–394. doi: 10.1016/0003-2697(88)90301-6
Smith, G. E., Summers, M. D., Fraser, M. J. (1983). Production of human beta interferon in insect cells infected with a baculovirus expression vector. Mol. Cell. Biol. 3, 2156–2165. doi: 10.1128/mcb.3.12.2156-2165.1983
Srisangsung, T., Phetphoung, T., Manopwisedjaroen, S., Rattanapisit, K., Bulaon, C. J. I., Thitithanyanont, A., et al. (2024). The impact of N-glycans on the immune response of plant-produced SARS-CoV-2 RBD-Fc proteins. Biotechnol. Rep. (Amsterdam Netherlands) 43, e00847. doi: 10.1016/j.btre.2024.e00847
Stączek, S., Cytryńska, M., Zdybicka-Barabas, A. (2023). Unraveling the role of antimicrobial peptides in insects. Int. J. Mol. Sci. 24, 5753. doi: 10.3390/ijms24065753
Steinstraesser, L., Kraneburg, U., Jacobsen, F., Al-Benna, S. (2011). Host defense peptides and their antimicrobial-immunomodulatory duality. Immunobiology 216, 322–333. doi: 10.1016/j.imbio.2010.07.003
Strasser, R. (2016). Plant protein glycosylation. Glycobiology 26, 926–939. doi: 10.1093/glycob/cww023
Su, Y., Liu, C., Fang, H., Zhang, D. (2020). Bacillus subtilis: a universal cell factory for industry, agriculture, biomaterials and medicine. Microbial Cell factories 19, 1–12. doi: 10.1186/s12934-020-01436-8
Su, W., Xu, M., Radani, Y., Yang, L. (2023). Technological development and application of plant genetic transformation. Int. J. Mol. Sci. 24, 10646. doi: 10.3390/ijms241310646
Sun, H., Brik, A. (2019). The journey for the total chemical synthesis of a 53 kDa protein. Accounts Chem. Res. 52, 3361–3371. doi: 10.1021/acs.accounts.9b00372
Sun, T.-M., Du, J.-Z., Yao, Y.-D., Mao, C.-Q., Dou, S., Huang, S.-Y., et al. (2011). Simultaneous delivery of siRNA and paclitaxel via a “two-in-one” micelleplex promotes synergistic tumor suppression. ACS nano 5, 1483–1494. doi: 10.1021/nn103349h
Sun, X.-J., Wang, D.-N., Zhang, W.-J., Wu, X.-F. (2004). Expression of an antimicrobial peptide identified in the male reproductive system of rats. Mol. Biotechnol. 28, 185–189. doi: 10.1385/MB:28:3:185
Taboureau, O. (2010). Methods for building quantitative structure–activity relationship (QSAR) descriptors and predictive models for computer-aided design of antimicrobial peptides. Antimicrobial Peptides: Methods Protoc., 77–86. doi: 10.1007/978-1-60761-594-1_6
Taguchi, S., Ooi, T., Mizuno, K., Matsusaki, H. (2015). Advances and needs for endotoxin-free production strains. Appl. Microbiol. Biotechnol. 99, 9349–9360. doi: 10.1007/s00253-015-6947-9
Tai, H.-M., You, M.-F., Lin, C.-H., Tsai, T.-Y., Pan, C.-Y., Chen, J.-Y. (2021). Scale-up production of and dietary supplementation with the recombinant antimicrobial peptide tilapia piscidin 4 to improve growth performance in Gallus gallus domesticus. PloS One 16, e0253661. doi: 10.1371/journal.pone.0253661
Tang, S., Tao, J., Li, Y. (2023). Challenges and solutions for the downstream purification of therapeutic proteins. Antibody Ther. 7, 1–12. doi: 10.1093/abt/tbad028
Teixeira, M. C., Carbone, C., Sousa, M. C., Espina, M., Garcia, M. L., Sanchez-Lopez, E., et al. (2020a). Nanomedicines for the delivery of antimicrobial peptides (AMPs). Nanomaterials (Basel Switzerland) 10. doi: 10.3390/nano10030560
Terpe, K. (2006). Overview of bacterial expression systems for heterologous protein production: from molecular and biochemical fundamentals to commercial systems. Appl. Microbiol. Biotechnol. 72, 211–222. doi: 10.1007/s00253-006-0465-8
Thakker, C., Zhu, J., San, K.-Y., Bennett, G. (2011). Heterologous pyc gene expression under various natural and engineered promoters in Escherichia coli for improved succinate production. J. Biotechnol. 155, 236–243. doi: 10.1016/j.jbiotec.2011.05.001
Thomas, A. M., Antony, S. P. (2024). Marine antimicrobial peptides: an emerging nightmare to the life-threatening pathogens. Probiotics Antimicrobial Proteins 16, 552–578. doi: 10.1007/s12602-023-10061-x
Thompson, R. E., Muir, T. W. (2020). Chemoenzymatic semisynthesis of proteins. Chem. Rev. 120, 3051–3126. doi: 10.1021/acs.chemrev.9b00450
Tian, Z.-G., Dong, T.-T., Yang, Y.-L., Teng, D., Wang, J.-H. (2009b). Expression of antimicrobial peptide LH multimers in Escherichia coli C43(DE3). Appl. Microbiol. Biotechnol. 83, 143–149. doi: 10.1007/s00253-009-1893-z
Tian, L., Hires, S. A., Mao, T., Huber, D., Chiappe, M. E., Chalasani, S. H., et al. (2009a). Imaging neural activity in worms, flies and mice with improved GCaMP calcium indicators. Nat. Methods 6, 875–881. doi: 10.1038/nmeth.1398
Tian, J., Li, Y., Ma, B., Tan, Z., Shang, S. (2022). Automated peptide synthesizers and glycoprotein synthesis. Front. Chem. 10, 896098. doi: 10.3389/fchem.2022.896098
Tian, Z.-G., Teng, D., Yang, Y.-L., Luo, J., Feng, X.-J., Fan, Y., et al. (2007). Multimerization and fusion expression of bovine lactoferricin derivative LfcinB15-W4,10 in Escherichia coli. Appl. Microbiol. Biotechnol. 75, 117–124. doi: 10.1007/s00253-006-0806-7
Ting, D. S. J., Beuerman, R. W., Dua, H. S., Lakshminarayanan, R., Mohammed, I. (2020a). Strategies in translating the therapeutic potentials of host defense peptides. Front. Immunol. 11. doi: 10.3389/fimmu.2020.00983
Tomoko, T., Shigenori, I., Hideyuki, G., Kazumi, K., Eiko, O., Morio, I., et al. (1985). Expression of a synthetic human growth hormone gene in yeast. Gene 39, 117–120. doi: 10.1016/0378-1119(85)90117-9
Torres, M. D. T., Sothiselvam, S., Lu, T. K., Fuente-Nunez, C. D. L. (2019). Peptide design principles for antimicrobial applications. J. Mol. Biol. 431, 3547–3567. doi: 10.1016/j.jmb.2018.12.015
Tran, A.-M., Nguyen, T.-T., Nguyen, C.-T., Huynh-Thi, X.-M., Nguyen, C.-T., Trinh, M.-T., et al. (2017). Pichia pastoris versus Saccharomyces cerevisiae: a case study on the recombinant production of human granulocyte-macrophage colony-stimulating factor. BMC Res. Notes 10, 1–8. doi: 10.1186/s13104-017-2471-6
Tripathi, N. K., Shrivastava, A. (2019). Recent developments in bioprocessing of recombinant proteins: expression hosts and process development. Front. Bioengineering Biotechnol. 7. doi: 10.3389/fbioe.2019.00420
Trunschke, S., Piemontese, E., Fuchs, O., Abboud, S., Seitz, O. (2022). Enhancing auxiliary-mediated native chemical ligation at challenging junctions with pyridine scaffolds. 8th EuChemS Chemistry Congress. 28 (68), e202202065. doi: 10.1002/chem.202202065
Tschofen, M., Knopp, D., Hood, E., Stöger, E. (2016). Plant molecular farming: much more than medicines. Annu. Rev. Analytical Chem. 9, 271–294. doi: 10.1146/annurev-anchem-071015-041706
Tucci, P., Veroli, V., Señorale, M., Marín, M. (2016). “Escherichia coli: The Leading Model for the Production of Recombinant Proteins,” in Microbial Models: From Environmental to Industrial Sustainability. Ed. Castro-Sowinski, S. (Springer Singapore, Singapore), 119–147.
Usmani, S. S., Bedi, G., Samuel, J. S., Singh, S., Kalra, S., Kumar, P., et al. (2017). THPdb: Database of FDA-approved peptide and protein therapeutics. PloS One 12, e0181748. doi: 10.1371/journal.pone.0181748
Valore, E. V., Park, C. H., Quayle, A. J., Wiles, K. R., Mccray, P., Ganz, T. (1998). Human beta-defensin-1: an antimicrobial peptide of urogenital tissues. J. Clin. Invest. 101, 1633–1642. doi: 10.1172/JCI1861
Van Oers, M. M., Pijlman, G. P., Vlak, J. M. (2015). Thirty years of baculovirus-insect cell protein expression: from dark horse to mainstream technology. J. Gen. Virol. 96, 6–23. doi: 10.1099/vir.0.067108-0
Varanko, A. K., Su, J. C., Chilkoti, A. J. (2020). Elastin-like polypeptides for biomedical applications. Annu. Rev. Biomed. Eng. 22, 343–369. doi: 10.1146/annurev-bioeng-092419-061127
Viebrock, K., Wilhelm, J., Rölke, B., Pastwa, L., Schrader, S. M., Meinen, S., et al. (2024). PhagoScreener: A novel phagogram platform based on a capillary-wave microbioreactor. New Biotechnol. doi: 10.1016/j.nbt.2024.08.502
Vieira Gomes, A. M., Souza Carmo, T., Silva Carvalho, L., Mendonça Bahia, F., Parachin, N. S. (2018a). Comparison of yeasts as hosts for recombinant protein production. Microorganisms 6. doi: 10.3390/microorganisms6020038
Vincenzi, M., Mercurio, F. A., Leone, M. (2024a). Virtual screening of peptide libraries: the search for peptide-based therapeutics using computational tools. Int. J. Mol. Sci. 25, 1798. doi: 10.3390/ijms25031798
Vinogradov, A. A., Yin, Y., Suga, H. (2019). Macrocyclic peptides as drug candidates: recent progress and remaining challenges. J. Am. Chem. Soc. 141, 4167–4181. doi: 10.1021/jacs.8b13178
Viruly, L., Suhartono, M. T., Nurilmala, M., Saraswati, S., Andarwulan, N. (2023). Identification and characterization of antimicrobial peptide (AMP) candidate from Gonggong Sea Snail (Leavistrombus turturella) extract. J. Food Sci. Technol. 60, 44–52. doi: 10.1007/s13197-022-05585-z
Vogl, T., Glieder, A. (2013). Regulation of Pichia pastoris promoters and its consequences for protein production. New Biotechnol. 30, 385–404. doi: 10.1016/j.nbt.2012.11.010
Waghu, F. H., Gawde, U., Gomatam, A., Coutinho, E., Idicula-Thomas, S. (2020). A QSAR modeling approach for predicting myeloid antimicrobial peptides with high sequence similarity. Chem. Biol. Drug design 96, 1408–1417. doi: 10.1111/cbdd.13749
Wagner, J. M., Alper, H. S. (2016). Synthetic biology and molecular genetics in non-conventional yeasts: Current tools and future advances. Fungal Genet. Biol. 89, 126–136. doi: 10.1016/j.fgb.2015.12.001
Walker, R. S., Pretorius, I. S. (2018). Applications of yeast synthetic biology geared towards the production of biopharmaceuticals. Genes 9, 340. doi: 10.3390/genes9070340
Wan, L., Chen, Z., Shah, N., El-Nezami, H. (2016). Modulation of intestinal epithelial defense responses by probiotic bacteria. Crit. Rev. Food Sci. Nutr. 56, 2628–2641. doi: 10.1080/10408398.2014.905450
Wan, Q., Danishefsky, S. J. (2007). Free-radical-based, specific desulfurization of cysteine: A powerful advance in the synthesis of polypeptides and glycopolypeptides. Angewandte Chemie Int. Edition 46, 9248–9252. doi: 10.1002/anie.200704195
Wang, G. J. (2008). Structures of human host defense cathelicidin LL-37 and its smallest antimicrobial peptide KR-12 in lipid micelles. J. Biol. Chem. 283, 32637–32643. doi: 10.1074/jbc.M805533200
Wang, G. (2012). Post-translational modifications of natural antimicrobial peptides and strategies for peptide engineering. Curr. Biotechnol. 1, 72–79. doi: 10.2174/2211550111201010072
Wang, Y.-Q., Cai, J.-Y. (2007). High-level expression of acidic partner-mediated antimicrobial peptide from tandem genes inEscherichia coli. Appl. Biochem. Biotechnol. 141, 203–213. doi: 10.1007/BF02729062
Wang, Z. A., Cole, P. A. (2020). “Methods and Applications of Expressed Protein Ligation,” in Expressed Protein Ligation: Methods and Protocols. Ed. Vila-Perelló, M. (Springer US, New York, NY), 1–13.
Wang, J.-H., Gessler, D. J., Zhan, W., Gallagher, T. L., Gao, G. (2024). Adeno-associated virus as a delivery vector for gene therapy of human diseases. Signal Transduction Targeted Ther. 9, 78. doi: 10.1038/s41392-024-01780-w
Wang, G., Huang, M., Nielsen, J. (2017). Exploring the potential of Saccharomyces cerevisiae for biopharmaceutical protein production. Curr. Opin. Biotechnol. 48, 77–84. doi: 10.1016/j.copbio.2017.03.017
Wang, A., Su, Y., Wang, S., Shen, M., Chen, F., Chen, M., et al. (2010). High efficiency preparation of bioactive human α-defensin 6 in Escherichia coli Origami(DE3)pLysS by soluble fusion expression. Appl. Microbiol. Biotechnol. 87, 1935–1942. doi: 10.1007/s00253-010-2688-y
Wang, G., Vaisman, I. I., Van Hoek, M. L. (2022a). “Machine Learning Prediction of Antimicrobial Peptides,” in Computational Peptide Science: Methods and Protocols. Ed. Simonson, T. (Springer US, New York, NY), 1–37.
Wang, L., Wang, N., Zhang, W., Cheng, X., Yan, Z., Shao, G., et al. (2022b). Therapeutic peptides: current applications and future directions. Signal transduction targeted Ther. 7, 48. doi: 10.1038/s41392-022-00904-4
Webster, D. E., Thomas, M. C. (2012). Post-translational modification of plant-made foreign proteins; glycosylation and beyond. Biotechnol. Adv. 30, 410–418. doi: 10.1016/j.biotechadv.2011.07.015
Werten, M. W. T., Eggink, G., Cohen Stuart, M. A., De Wolf, F. A. (2019). Production of protein-based polymers in Pichia pastoris. Biotechnol. Adv. 37, 642–666. doi: 10.1016/j.biotechadv.2019.03.012
Westers, L., Westers, H., Quax, W. J. (2004). Bacillus subtilis as cell factory for pharmaceutical proteins: a biotechnological approach to optimize the host organism. Biochim. Biophys. Acta (BBA)-Molecular Cell Res. 1694, 299–310. doi: 10.1016/j.bbamcr.2004.02.011
Wibowo, D., Zhao, C.-X. (2019). Recent achievements and perspectives for large-scale recombinant production of antimicrobial peptides. Appl. Microbiol. Biotechnol. 103, 659–671. doi: 10.1007/s00253-018-9524-1
Wildt, S., Gerngross, T. U. (2005). The humanization of N-glycosylation pathways in yeast. Nat. Rev. Microbiol. 3, 119–128. doi: 10.1038/nrmicro1087
Wood, D. W. (2014). New trends and affinity tag designs for recombinant protein purification. Curr. Opin. Struct. Biol. 26, 54–61. doi: 10.1016/j.sbi.2014.04.006
Wu, Z., Cai, Y., Han, Y., Su, Y., Zhang, T., Wang, X., et al. (2024). Development of α-helical antimicrobial peptides with imperfect amphipathicity for superior activity and selectivity. J. Medicinal Chem. 67, 19561–19572. doi: 10.1021/acs.jmedchem.4c01855
Wu, B., Chen, J., Warren, J. D., Chen, G., Hua, Z., Danishefsky, S. J. (2006). Building complex glycopeptides: development of a cysteine-free native chemical ligation protocol. Angewandte Chemie Int. Edition 45, 4116–4125. doi: 10.1002/anie.200600538
Wu, Q., Ke, H., Li, D., Wang, Q., Fang, J., Zhou, J. (2019). Recent progress in machine learning-based prediction of peptide activity for drug discovery. Curr. topics medicinal Chem. 19, 4–16. doi: 10.2174/1568026619666190122151634
Xu, J., Li, F., Li, C., Guo, X., Landersdorfer, C., Shen, H.-H., et al. (2023). iAMPCN: a deep-learning approach for identifying antimicrobial peptides and their functional activities. Briefings Bioinf. 24, bbad240. doi: 10.1093/bib/bbad240
Xu, Z., Wang, F., Peng, L., Fang, X., Cen, P. (2005). Expression of human β-defensin-2 with multiple joined genes in Escherichia coli. Appl. Biochem. Biotechnol. 120, 1–13. doi: 10.1385/ABAB:120:1:01
Xu, J. Z., Zhang, W. G. (2014). HETEROLOGOUS EXPRESSION OF Escherichia coli FRUCTOSE-1,6-BISPHOSPHATASE IN Corynebacterium glutamicum AND EVALUATING THE EFFECT ON CELL GROWTH AND L-LYSINE PRODUCTION. Preparative Biochem. Biotechnol. 44, 493–509. Q.D.J.J.L.Z.Y.F.G. doi: 10.1080/10826068.2013.833115
Xuan, J., Feng, W., Wang, J., Wang, R., Zhang, B., Bo, L., et al. (2023). Antimicrobial peptides for combating drug-resistant bacterial infections. Drug Resistance Updates 68, 100954. doi: 10.1016/j.drup.2023.100954
Yan, L. Z., Dawson, P. E. (2001). Synthesis of peptides and proteins without cysteine residues by native chemical ligation combined with desulfurization. J. Am. Chem. Soc. 123, 526–533. doi: 10.1021/ja003265m
Yang, C.-H., Chen, Y.-C., Peng, S.-Y., Tsai, A. P.-Y., Lee, T. J.-F., Yen, J.-H., et al. (2018). An engineered arginine-rich α-helical antimicrobial peptides exhibits broad-spectrum bactericidal activity against pathogenic bacteria and reduces bacterial infections in mice. Sci. Rep. 8, 14602. doi: 10.1038/s41598-018-32981-3
Yang, B., Good, D., Mosaiab, T., Liu, W., Ni, G., Kaur, J., et al. (2020). Significance of LL-37 on immunomodulation and disease outcome. BioMed. Res. Int. 2020, 8349712. doi: 10.1155/2020/8349712
Yang, S., Kang, Z., Cao, W., Du, G., Chen, J. (2016). Construction of a novel, stable, food-grade expression system by engineering the endogenous toxin-antitoxin system in Bacillus subtilis. J. Biotechnol. 219, 40–47. doi: 10.1016/j.jbiotec.2015.12.029
Yang, S., Wang, H., Zhao, D., Zhang, S., Hu, C. (2024). Polymyxins: recent advances and challenges. Front. Pharmacol. 15. doi: 10.3389/fphar.2024.1424765
Yang, X., Yousef, A. E. (2018). Antimicrobial peptides produced by Brevibacillus spp.: structure, classification and bioactivity: a mini review. World J. Microbiol. Biotechnol. 34, 57. doi: 10.1007/s11274-018-2437-4
Yi, X., Zhang, Z., Ling, Y., Xu, W., Su, Z. (2015). PNRD: a plant non-coding RNA database. Nucleic Acids Res. 43, D982–D989. doi: 10.1093/nar/gku1162
Yu, X., Conyne, M., Lake, M. R., Walter, K. A., Min, J. (2022). In silico high throughput mutagenesis and screening of signal peptides to mitigate N-terminal heterogeneity of recombinant monoclonal antibodies. mAbs 14, 2044977. doi: 10.1080/19420862.2022.2044977
Yu, X.-B., Jiao, Z., Zhang, C.-H., Dai, Y., Zhou, Z.-Y., Han, L., et al. (2021). Population pharmacokinetic and optimization of polymyxin B dosing in adult patients with various renal functions. Br. J. Clin. Pharmacol. 87, 1869–1877. doi: 10.1111/bcp.14576
Yuan, L., Wu, S., Tian, K., Wang, S., Wu, H., Qiao, J. (2024). Nisin-relevant antimicrobial peptides: synthesis strategies and applications. Food Funct. 15, 9662–9677. doi: 10.1039/D3FO05619H
Zainal Baharin, N. H., Khairil Mokhtar, N. F., Mohd Desa, M. N., Gopalsamy, B., Mohd Zaki, N. N., Yuswan, M. H., et al. (2021). The characteristics and roles of antimicrobial peptides as potential treatment for antibiotic-resistant pathogens: a review. PeerJ 9, e12193. doi: 10.7717/peerj.12193
Zasloff, M. (2002). Antimicrobial peptides of multicellular organisms. Nature 415, 389–395. doi: 10.1038/415389a
Zerbs, S., Frank, A. M., Collart, F. R. (2009). “Chapter 12 Bacterial Systems for Production of Heterologous Proteins,” in Guide to Protein Purification, 2nd Edition. Eds. Burgess, R. R., Deutscher, M. P. (Academic Press), 149–168.
Zhan, N., Zhang, L., Yang, H., Zheng, Y., Wei, X., Wang, J., et al. (2021a). Design and heterologous expression of a novel dimeric LL37 variant in Pichia pastoris. Microbial Cell Factories 20, 143. doi: 10.1186/s12934-021-01635-x
Zhang, L., Li, X., Wei, D., Wang, J., Shan, A., Li, Z. (2015). Expression of plectasin in Bacillus subtilis using SUMO technology by a maltose-inducible vector. J. Ind. Microbiol. Biotechnol. 42, 1369–1376. doi: 10.1007/s10295-015-1673-y
Zhang, J., Movahedi, A., Wei, Z., Sang, M., Wu, X., Wang, M., et al. (2016). High-level SUMO-mediated fusion expression of ABP-dHC-cecropin A from multiple joined genes in Escherichia coli. Analytical Biochem. 509, 15–23. doi: 10.1016/j.ab.2016.06.031
Zhang, Z.-X., Nong, F.-T., Wang, Y.-Z., Yan, C.-X., Gu, Y., Song, P., et al. (2022). Strategies for efficient production of recombinant proteins in Escherichia coli: alleviating the host burden and enhancing protein activity. Microbial Cell Factories 21, 191. doi: 10.1186/s12934-022-01917-y
Zhang, K., Su, L., Wu, J. (2018). Enhanced extracellular pullulanase production in Bacillus subtilis using protease-deficient strains and optimal feeding. Appl. Microbiol. Biotechnol. 102, 5089–5103. doi: 10.1007/s00253-018-8965-x
Zhang, H.-Q., Sun, C., Xu, N., Liu, W. (2024). The current landscape of the antimicrobial peptide melittin and its therapeutic potential. Front. Immunol. 15. doi: 10.3389/fimmu.2024.1326033
Zhang, Y., Teng, D., Mao, R., Wang, X., Xi, D., Hu, X., et al. (2014). High expression of a plectasin-derived peptide NZ2114 in Pichia pastoris and its pharmacodynamics, postantibiotic and synergy against Staphylococcus aureus. Appl. Microbiol. Biotechnol. 98, 681–694. doi: 10.1007/s00253-013-4881-2
Zhang, L., Wei, D., Zhan, N., Sun, T., Shan, B., Shan, A. (2020). Heterologous expression of the novel α-helical hybrid peptide PR-FO in Bacillus subtilis. Bioprocess Biosyst. Eng. 43, 1619–1627. doi: 10.1007/s00449-020-02353-1
Zhang, C., Yang, M., Ericsson, A. C. (2019). Antimicrobial peptides: potential application in liver cancer. Front. Microbiol. 10. doi: 10.3389/fmicb.2019.01257
Zhang, J., Yang, Y., Teng, D., Tian, Z., Wang, S., Wang, J. (2011). Expression of plectasin in Pichia pastoris and its characterization as a new antimicrobial peptide against Staphyloccocus and Streptococcus. Protein Expression purification 78, 189–196. doi: 10.1016/j.pep.2011.04.014
Zhao, L., Li, L., Hu, M., Fang, Y., Dong, N., Shan, A. (2024). Heterologous expression of the novel dimeric antimicrobial peptide LIG in Pichia pastoris. J. Biotechnol. 381, 19–26. doi: 10.1016/j.jbiotec.2023.12.015
Zhao, H., Tang, J., Cao, L., Jia, G., Long, D., Liu, G., et al. (2015). Characterization of bioactive recombinant antimicrobial peptide parasin I fused with human lysozyme expressed in the yeast Pichia pastoris system. Enzyme Microb. Technol. 77, 61–67. doi: 10.1016/j.enzmictec.2015.06.001
Zhao, X., Zhang, M., Muhammad, I., Cui, Q., Zhang, H., Jia, Y., et al. (2021). An antibacterial peptide with high resistance to trypsin obtained by substituting D-amino acids for trypsin cleavage sites. Antibiotics (Basel Switzerland) 10, 1465. doi: 10.3390/antibiotics10121465
Zhong, Z., Xu, Z., Peng, L., Huang, L., Fang, X., Cen, P. (2006). Tandem repeat mhBD2 gene enhance the soluble fusion expression of hBD2 in Escherichia coli. Appl. Microbiol. Biotechnol. 71, 661–667. doi: 10.1007/s00253-005-0212-6
Zhou, Z., Dang, Y., Zhou, M., Li, L., Yu, C.-H., Fu, J., et al. (2016). Codon usage is an important determinant of gene expression levels largely through its effects on transcription. Biol. Sci. 113, E6117–E6125. doi: 10.1073/pnas.1606724113
Zhou, L., Liu, Z., Xu, G., Li, L., Xuan, K., Xu, Y., et al. (2020). Expression of melittin in fusion with GST in Escherichia coli and its purification as a pure peptide with good bacteriostatic efficacy. ACS omega 5, 9251–9258. doi: 10.1021/acsomega.0c00085
Zhou, L., Zhao, Z., Li, B., Cai, Y., Zhang, S. (2009). TrxA mediating fusion expression of antimicrobial peptideAMPs CM4 from multiple joined genes in Escherichia coli. Protein Expression Purification 64, 225–230. doi: 10.1016/j.pep.2008.11.006
Zhu, T., Sun, H., Wang, M., Li, Y. (2019). Pichia pastoris as a versatile cell factory for the production of industrial enzymes and chemicals: current status and future perspectives. Biotechnol. J. 14, 1800694. doi: 10.1002/biot.201800694
Zitzmann, M., Bongers, R., Werler, S., Bogdanova, N., Wistuba, J., Kliesch, S., et al. (2015). Gene expression patterns in relation to the clinical phenotype in Klinefelter syndrome. J. Clin. Endocrinol. Metab. 100, E518–E523. doi: 10.1210/jc.2014-2780
Zitzmann, J., Sprick, G., Weidner, T., Schreiber, C., Czermak, P. (2017). Process optimization for recombinant protein expression in insect cells. New Insights into Cell Culture Technology. 2, 43–98. doi: 10.5772/67849
Zou, F., Tan, C., Shinali, T. S., Zhang, B., Zhang, L., Han, Z., et al. (2023). Plants: a comprehensive review of their classification, production, mode of action, functions, applications, and challenges. Food Funct. 14, 5492–5515. doi: 10.1039/D3FO01119D
Keywords: antimicrobial peptide, AMPs, antibiotic resistance, SPPS, SUMO, Pichia pastoris
Citation: Sadeeq M, Li Y, Wang C, Hou F, Zuo J and Xiong P (2025) Unlocking the power of antimicrobial peptides: advances in production, optimization, and therapeutics. Front. Cell. Infect. Microbiol. 15:1528583. doi: 10.3389/fcimb.2025.1528583
Received: 18 November 2024; Accepted: 19 March 2025;
Published: 28 April 2025.
Edited by:
Giancarlo Ripabelli, University of Molise, ItalyReviewed by:
Giulio Petronio Petronio, University of Molise, ItalyMichel Lopes Leite, University of Brasília, Brazil
Copyright © 2025 Sadeeq, Li, Wang, Hou, Zuo and Xiong. This is an open-access article distributed under the terms of the Creative Commons Attribution License (CC BY). The use, distribution or reproduction in other forums is permitted, provided the original author(s) and the copyright owner(s) are credited and that the original publication in this journal is cited, in accordance with accepted academic practice. No use, distribution or reproduction is permitted which does not comply with these terms.
*Correspondence: Peng Xiong, eGlvbmdwMUBzZHV0LmVkdS5jbg==; Jia Zuo, MDZ6amdvYWxAMTYzLmNvbQ==