- 1School of Basic Medical Science, Zhejiang Chinese Medical University, Hangzhou, China
- 2Key Laboratory of Blood-Stasis-Toxin Syndrome, Zhejiang Chinese Medical University, Hangzhou, China
Urinary tract infection (UTI), a common clinical infectious disease, is marked by high incidence and frequent recurrence. Recurrent UTIs can cause severe complications, negatively affecting health. The emergence and spread of drug-resistant bacteria present significant challenges to UTI treatment. This article systematically reviews the key immune mechanisms in the body’s defense against UTI pathogens. It discusses various immune response components, such as the urinary tract mucosal epithelium, neutrophils, macrophages, dendritic cells, mast cells, innate lymphocytes, T cells, and B cells, with the aim of providing insights for future UTI research.
1 Introduction
Urinary tract infection (UTI) is a prevalent bacterial infectious disease, affecting around 150 million people globally each year (Foxman, 2014). UTI not only has a notably high incidence but also tends to recur. Repeated episodes may lead to severe complications such as pyelonephritis, sepsis, and renal damage (Chugh et al., 2020), which pose significant threats to patients’ health and lives. Reports suggest that 20%-30% of patients with a first infection will experience reinfection (Foxman et al., 2000). Moreover, the increasing prevalence of antibiotic-resistant uropathogens and their transmission in clinical settings complicate UTI treatment, especially in recurrent cases (Halbay, 2023).
The relationship between immune function and recurrent infections is critical. UTIs arise from an imbalance between bacterial virulence and host immune defenses, and alterations in these host mechanisms act as risk factors for UTI onset (Garcia et al., 2018). Recent advances in understanding the urinary immune system and its anti-infection mechanisms has provided significant insights, uncovering new pathways contributing to UTI development. This article systematically reviews the cells and molecules involved in urinary immune defense, using the infection process of Uropathogenic Escherichia coli (UPEC) as a case study to illustrate the mechanisms of urinary anti-infection immunity and recent research advances.
2 Barrier and defensive functions of urothelial mucosal epithelial cells
Bladder epithelial tissues and cells are crucial for maintaining bladder function and defending against pathogenic invasion (Figure 1). They act as a barrier against toxic substances in urine and combat bacteria via various immune mechanisms (Marshall et al., 2019; Garcia et al., 2018). The bladder epithelium is a stratified transitional structure with three layers: basal cells, intermediate cells, and umbrella cells (bladder epithelial cells, BECs). Umbrella cells maintain the impermeability and high-resistance barrier of the urinary mucosal surface through tight junctions (Jaimes-Parra et al., 2015; Winder et al., 2014; Osborn and Kurzrock, 2014). The surface of bladder epithelial cells is coated with a mucosal layer of highly negatively charged proteoglycans and glycosaminoglycans, the glycosaminoglycan (GAG) layer, which protects the epithelium from toxic damage (Terlizzi et al., 2017). The bladder epithelium is also covered with uroplakin protein surface plaques (uroplakins, Ups) (Wu et al., 2017; Hill, 2015). Uroplakin proteins are primary receptors for UPEC adhesion, and their glycosylation changes are linked to urinary epithelium pathologies like UTIs and interstitial cystitis (Taganna et al., 2011).
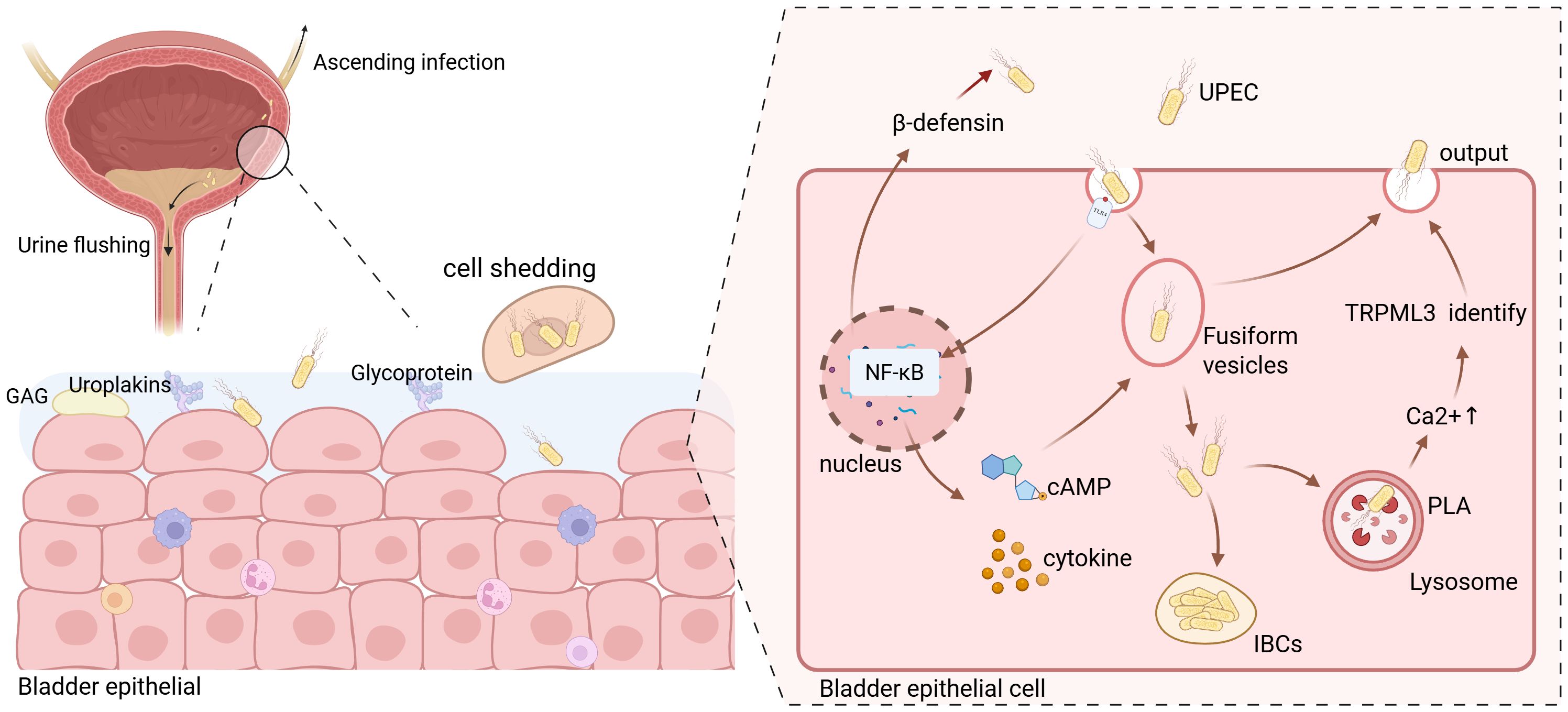
Figure 1. Schematic illustration of Uropathogenic Escherichia coli (UPEC) invasion into bladder epithelial cells. Due to the relatively short length of the female urinary tract, UPEC typically enters the bladder through the urethra and attempts to invade bladder epithelial cells. The urine accumulated in the bladder serves to flush out most bacteria; however, a small fraction of UPEC that adheres to the surface of the bladder epithelial cells proceeds to invade further and may even cause ascending infections. UPEC enters the bladder epithelial cells via endocytosis. Simultaneously, the toll-like receptors (TLRs) on the cell surface transmits signals that activate the nuclear factor kappa-B (NF-κB) signaling pathway. This activation prompts the release of a substantial amount of cytokines and cyclic adenosine monophosphate (cAMP). The produced β-defensin is then secreted into the extracellular space to counteract the invasion of UPEC. Moreover, the released cAMP promotes the exocytosis of vesicles containing UPEC. The cytokines generated primarily combat the invasion of UPEC by recruiting immune cells. Meanwhile, a portion of UPEC within the vesicles can breach the vesicle membrane and enter the cytoplasm. The majority of these intracellular bacteria will be recognized by lysosomes and ultimately be excreted out of the cells. However, a minuscule number of them are capable of forming intracellular bacterial communities (IBCs) within the cells. Created with BioRender.com.
Bladder epithelial cells show morphological plasticity, and fusiform vesicle endocytosis and exocytosis regulate the bladder surface area during urine accumulation (Grasso and Calderón, 2013). During urine voiding, bladder epithelial cell membranes invaginate to form fusiform vesicles that internalize UPEC, leading to bacterial endocytosis (Wu et al., 2017). The fusiform vesicle membrane is similar to the cell membrane and rich in Toll-like receptor 4 (TLR4). TLR4 binding with UPEC activates multiple signaling pathways, including nuclear factor kappa - B (NF - κB), promoting cytokine, inflammatory mediator, and antimicrobial peptide (AMPs) release, exerting a defensive effect. For example, increased intracellular cyclic adenosine monophosphate (cAMP) levels cause Rab27b + fusiform vesicle exocytosis, releasing bacteria into the bladder lumen (Miao et al., 2016). Research shows that within 24 hours of bacterial invasion, over 90% of intracellular bacteria are expelled, demonstrating bladder epithelial cell anti - infection ability (Bishop et al., 2007). However, UPEC expressing phospholipase A (PLA) can penetrate Rab27b + vesicles and enter the cytoplasm, where a few bacteria can replicate to form intracellular bacterial communities (IBCs) (Pang et al., 2022). IBCs allow UPEC to colonize bladder epithelial cells and initiate disease, enhancing antibiotic resistance. After entering bladder epithelial cells, UPEC is recognized by autophagosomes and sent to lysosomes for degradation (Wu et al., 2017). But UPEC can neutralize lysosome pH and disrupt degradation. Abnormal lysosomes are sensed by transient receptor potential mucolipin 3 (TRPML 3), releasing Ca2 + from lysosomes into the cytoplasm and facilitating expulsion of dysfunctional lysosomes with bacteria from the cell.
Bladder epithelial cells release antimicrobial peptides limit pathogen survival in early infection. For example, β - defensin 1 expression increases after TLR4 activation (Hickling et al., 2013). Uromodulin (UrM) can competitively bind to UPEC type 1 fimbriae, preventing UPEC adhesion to uroepithelial cells (Bowyer et al., 2022). If bladder epithelial cell defenses fail to eliminate intracellular bacteria, infected superficial cells undergo caspase - 3 and caspase - 8 - dependent apoptosis, shedding to reduce bacterial load. Basal and intermediate cells then proliferate and regenerate superficial cells. But this exposes intermediate cells to pathogen - containing urine, risking deeper tissue infection and damage.
Uncleared UPEC in the bladder can ascend to the kidneys, causing upper UTI. Renal units consist of principal cells (PCs) and intercalated cells (ICs), with ICs subdivided into type A, type B, and non - type A non - type B (Roy et al., 2015). UPEC adheres to type A intercalated cells, entering via complement internalization or cellular lipid rafts (Neal et al., 2014; Chassin et al., 2006). Renal epithelial cells release inflammatory chemokines and cytokines related to neutrophils, monocytes, or macrophages through pattern recognition receptors, recruiting corresponding immune cells to combat UPEC (Schwartz et al., 2023). Infected intercalated cells increase phagosome maturation - related gene expression, promoting UPEC phagocytosis (Saxena et al., 2021). Intercalated cells also release AMPs, cytokines, and protons into the urine to counteract pathogens (Becknell et al., 2015; Saxena et al., 2018, Saxena et al., 2019). Intercalated cells are associated with UPEC susceptibility. Carbonic anhydrase 2 (CA2) is important for intercalated cell function. Mice lacking CA2 have 50% fewer intercalated cells and impaired UPEC clearance, and transplanting their kidneys into wild - type mice increases pyelonephritis risk (John et al., 2020; Hains et al., 2014). This suggests intercalated cells may prevent renal pyelonephritis, though mechanisms need further study.
The renal tissue extracellular microenvironment and hormone levels affect antibacterial defense. High medullary sodium concentrations enhance kidney antibacterial capacity (Schwartz et al., 2023). During pyelonephritis, the medulla’s hyperosmotic environment promotes monocyte chemotactic protein - 1 (MCP - 1) production by nuclear factor activated T cell 5 (NFAT5) in renal tubular cells, recruiting CD14 + mononuclear phagocytes (MNP) to the medulla (Schwartz et al., 2023). MNP differentiate into M1 macrophages to eliminate UPEC. Medullary epithelial cells and macrophages secrete granulocyte chemokines like IL - 8 and TNF - α, recruiting neutrophils (Naskar and Choi, 2024). Additionally, uromodulin (UrM) released into the renal tubule lumen can competitively bind to type 1 pili expressed by uropathogenic Escherichia coli (UPEC), thereby preventing UPEC adherence to epithelial cells (Mercado-Evans et al., 2025). Changes in the medulla’s sodium concentration gradient can cause abnormal cytokine production, reducing MNP recruitment and increasing pyelonephritis risk (Berry et al., 2017). This shows the renal medulla microenvironment is crucial for immune defense during UTIs. Hormones like antidiuretic hormone, insulin, and sex hormones also play roles in kidney resistance to UPEC infections. Pharmacological antagonism of arginine vasopressin receptor 2 (AVPR2) on renal principal cells stimulates cytokine production and UPEC clearance in infected mice (Zetter et al., 2019). Moreover, insulin might be related to the expression of certain antimicrobial peptides. In the kidneys of rodents with type 1 and type 2 diabetes, the level of Bd1, which encodes the antimicrobial peptide β - defensin 1, is lower compared to that in the kidneys of healthy animals (Froy et al., 2007). A study shows that local insulin receptor deficiency in the bladder urothelium can promote the occurrence of UTI by increasing barrier permeability and inhibiting antimicrobial peptides (Schwartz et al., 2024). In addition, research data indicate that male mice are more susceptible to pyelonephritis and renal abscesses than female mice. Castration of male mice or inhibition of androgen receptor signaling can decrease the risk of urinary tract infections (Hreha et al., 2020a, Hreha et al., 2020b; Olson et al., 2016; Olson et al., 2018). In another study, within 24 hours post - infection, female C57BL/6 and C3H/HeN mice exhibited a more robust cytokine response and a more rapid recruitment of immune cells within the bladder when compared to male mice. This characteristic allows normal female mice to efficiently clear the infectious pathogens present in the bladder within 7 days (Hreha et al., 2024). Although male mice have a higher number of myeloid cells in the circulatory system than female mice, testosterone can attenuate the function of renal neutrophils by impeding neutrophil maturation. This leads to inadequate infiltration of neutrophils in the kidneys of androgen - exposed hosts, rendering them unable to fully control the infection (Hreha et al., 2024). However, at present, the specific mechanism through which androgens inhibit neutrophil maturation remains obscure. Furthermore, it remains to be determined whether androgens can influence the course of urinary tract infections (UTIs) via other pathways.
3 The anti-infection mechanisms of innate immune cells and molecules
3.1 Neutrophils
Upon detecting pathogen invasion, urinary tract epithelial cells promptly mobilize resident tissue cells or circulating immune cells to the infection site via signaling pathways for bacterial clearance (Figure 2). Neutrophils are the primary innate immune cells in fighting urinary tract infections and are vital for controlling early UPEC-induced infections (Hayes and Abraham, 2016; Ashkar et al., 2008). Neutrophil recruitment can be initiated by inflammatory mediators from leukocytes in the infected tissue or cytokines generated through intracellular signaling activated by Toll - like receptor 4 (TLR4) on bladder epithelial cell surfaces and the cytoplasmic Toll/IL - 1 receptor homology domain (TIR) (Yu et al., 2019; Hannan et al., 2010; Billips et al., 2007). The released cytokines like IL - 6, IL - 8, and TNF - α show elevated expression levels correlating with bacterial clearance. Notably, IL - 8 is the main chemokine directing neutrophils to the infection site (Bowyer et al., 2022). After infection, bladder epithelial cells quickly upregulate P - selectin and E - selectin, two adhesion molecules that cooperate to optimize neutrophil recruitment (Phillipson and Kubes, 2011; Petri et al., 2008).
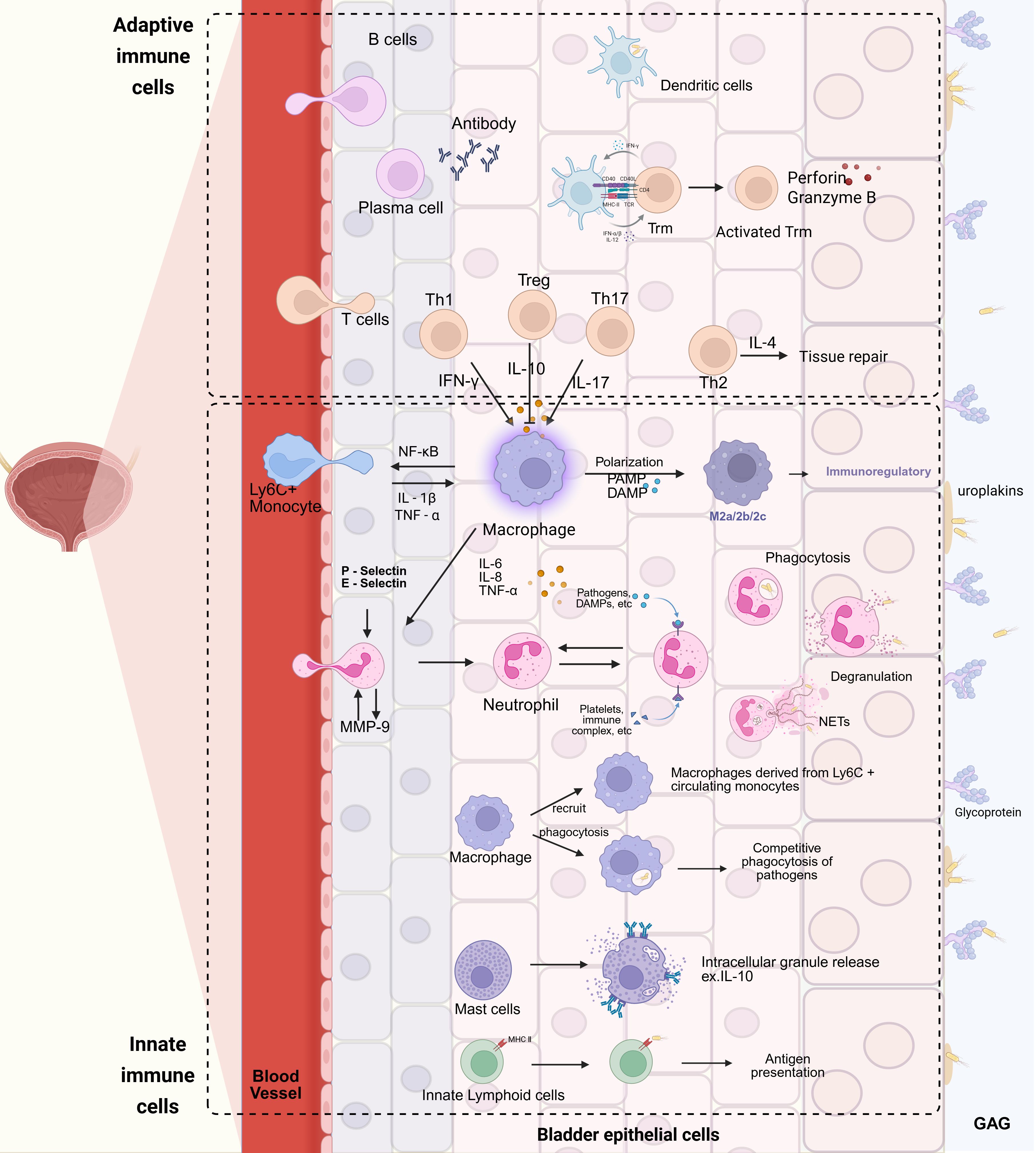
Figure 2. Schematic representation of the immune response to bacterial invasion in the urinary tract. Uropathogenic Escherichia coli (UPEC) initiates the process by penetrating the glycosaminoglycan (GAG) layer and adhering to uroplakins (Ups) on the surface of urinary tract epithelial cells. Upon invasion of the epithelial cells, toll-like receptors (TLRs) are activated, triggering a cascade of cellular immune responses. Neutrophils, in response to chemotactic signals mediated by P-selectin and E-selectin, extravasate from blood vessels and release matrix metalloproteinase-9 (MMP-9), which further enhances neutrophil extravasation and migration to the site of infection. Ly6C+ monocytes, under the stimulation of interleukin-1β (IL-1β) and tumor necrosis factor-α (TNF-α), undergo proliferation and differentiate into macrophages. Subsequently, in the presence of pathogen-associated molecular patterns (PAMPs) and damage-associated molecular patterns (DAMPs), these monocytes can polarize into M2 macrophages. T cells, upon activation, differentiate into distinct subsets, including T helper 1 (Th1), T helper 2 (Th2), T helper 17 (Th17), and regulatory T cells (Tregs). Th17, Treg, and Th1 cells modulate macrophage functions by secreting interleukin-17 (IL-17), interleukin-10 (IL-10), and interferon-γ (IFN-γ), respectively, while Th2 cells promote tissue repair through the release of interleukin-4 (IL-4). Activated B cells differentiate into effector B cells, namely plasma cells, which secrete antibodies to combat pathogen invasion. In addition to the immune cells recruited from the peripheral blood, tissue-resident cells also play pivotal roles. Macrophages residing in tissues can phagocytize pathogens and recruit Ly6C+ macrophages derived from monocytes. Dendritic cells are capable of phagocytosing pathogens and presenting antigens to initiate adaptive immune responses. Innate-like lymphocytes, through MHC class II molecules on their surface, recognize pathogens and participate in antigen presentation. Mast cells release intracellular granules, such as interleukin-10 (IL-10), to counteract UPEC infection. Tissue-resident memory T cells (Trm), once activated, actively engage in the immune response, collaborating with other immune cells to resist UPEC invasion. Created with BioRender.com.
When UPEC invades the kidneys, cytokines secreted by tubular epithelial cells, resident dendritic cells, or tissue - resident macrophages also recruit neutrophil (Bottek et al., 2020). The chemokine receptors CXCR1 and CXCR2, highly expressed on neutrophil surfaces, enable their recruitment to kidney infection sites by CXCL1 and CXCL (Roche et al., 2007). For example, CXCL2 secreted by Ly6C - macrophages can activate MMP - 9 on circulating neutrophil surfaces, allowing them to infiltrate epithelial tissues (Schiwon et al., 2014). Research shows that in children prone to pyelonephritis, CXCR1 expression in neutrophils is lower than in age - matched controls (Gabriela et al., 1997), suggesting susceptibility to pyelonephritis may be related to impaired neutrophil recruitment ability. Additionally, CXCR2 knockout mice have a higher chance of developing pyelonephritis, renal abscesses, and renal scarring when infected with UPEC, potentially leading to sepsis. Neutrophil - deficient mice also show a similar trend, indicating that the absence of CXCR2 is closely related to delayed neutrophil recruitment and reduced bacterial killing efficiency (Svensson et al., 2011). These findings suggest that CXCL1 and CXCL2 are crucial for neutrophil recruitment to the kidneys and that neutrophil recruitment is essential for the kidneys’ anti - infective response.
Neutrophils primarily exert their anti - infection effects through three mechanisms: phagocytosis, degranulation, and the formation of neutrophil extracellular traps (NETs), which consist of DNA released from neutrophils along with proteins that aid in pathogen capture and killing (Rosales, 2018). Their mechanisms are as follows: (1) Neutrophils are potent phagocytic cells that rapidly engulf pathogens, and this phagocytic activity is enhanced by the complement system and immunoglobulin G (IgG) antibodies (Segal, 2005). (2) The cytoplasmic granules of neutrophils are critical for pathogen destruction. These granules are classified into four types: primary, secondary, tertiary, and secretory granules (Sheshachalam et al., 2014; Paige, 2006). Primary granules contain myeloperoxidase (MPO) and neutral protease G (NPG), which directly kill and digest pathogens. Secondary granules (specific granules) contain lactoferrin, which sequesters iron and copper, limiting microbial growth. Tertial granules (gelatinase granules) include matrix metalloproteinase - 9 (MMP - 9), essential for degrading the extracellular matrix and activating interleukin - 1 beta (IL - 1β). Secretory granules contain serum albumin and pre - formed cytokines (Sheshachalam et al., 2014). During degranulation, granules are released into the extracellular environment in a specific order: secretory granules, tertiary granules, secondary granules, and finally, primary granules, contributing to their bactericidal effectsHannan, 2025). However, the efficacy of these mechanisms decreases significantly in blood flow. To address this, neutrophils have evolved NETs. (3) NETs form a web - like structure of DNA released from neutrophils, coated with neutrophil proteases (like elastase), antimicrobial molecules (like histones), and other toxic components for pathogen clearance (Kolaczkowska et al., 2015; Phillipson and Kubes, 2011). These components effectively trap and kill pathogens in the bloodstream. NETs formation begins with nuclear membrane degradation and DNA release triggered by reactive oxygen species (ROS) generated by neutrophils (Lin et al., 2024; Cotzomi-Ortega et al., 2024). NETs play a crucial role in controlling invasive infections. In experiments with mice treated with exogenous DNase to degrade NETs, an increase in bacteremia incidence and bacteria escape from the skin were observed (Yipp et al., 2012). In conclusion, neutrophils, as significant innate immune cells, play a crucial role in combating urinary tract pathogens.
3.2 Macrophages and dendritic cells
In addition to neutrophils, macrophages and dendritic cells are also essential for the body’s defense against urinary tract pathogen invasion (Figure 2). When pathogens invade bladder epithelial cells, macrophages respond rapidly and show strong phagocytic abilities, limiting pathogen proliferation and spread through phagocytosis. Dendritic cells also have the capacity to phagocytose pathogens, and this ability gradually increases within 24 hours post - infection (Mass et al., 2023). However, some studies indicate that in the presence of both macrophages and dendritic cells, the phagocytic function of dendritic cells may be suppressed by macrophage (Bowyer et al., 2022). Moreover, the competition between them for phagocytosing bacteria may lead to a decrease in the antigen - presenting efficiency of dendritic cells, thus weakening the adaptive immune response (Mora-Bau et al., 2015). This implies that their interactions can affect the scale and degree of both innate and adaptive immune responses. The complex network of dendritic cells and macrophages in renal tissue can detect the state of the renal interstitium by sensing self - antigens, danger signals, as well as pathogen - associated molecular patterns (PAMPs) and damage - associated molecular patterns (DAMPs) released by bacteria in the glomeruli and renal tubules (Mariano and Ingersoll, 2020; Hayes and Abraham, 2016a, Weisheit et al., 2015; Sedin et al., 2019; Hayes and Abraham, 2016). This sentinel function enables them to coordinate TLR4 - mediated antimicrobial defense programs.
In the early stages of infection, uropathogenic Escherichia coli (UPEC) releases PAMPs and DAMPs into the urine and surrounding tissues, which can affect the resident macrophage population and the recruited Ly6C + monocyte population, promoting macrophage differentiation toward the “M2 type” (Wang et al., 2014; Davies et al., 2013; Galli et al., 2011). Tissue - resident macrophages recruit Ly6C + monocyte - derived macrophages to the bladder lamina propria by activating the NF - κB signaling pathway (Mariano et al., 2020). These macrophages, through the expression of iNOS and inflammatory cytokines such as TNF and IL - 1β, stimulate the secretion of CXCL2 from Ly6C - macrophages, facilitating the recruitment of dendritic cells and circulating neutrophils into the epithelial tissue to combat infection (Ruiz-Rosado et al., 2021; Berry et al., 2017; Schiwon et al., 2014; Tsou et al., 2007). In studies on mice with pyelonephritis, depleting Ly6C + macrophages and reducing the secretion of inflammatory cytokines and chemokines can stop the increased recruitment of circulating monocytes to the kidneys. Notably, with the reduction of monocytes, the bacterial load in the kidneys decreases, suggesting that Ly6C + macrophages derived from monocytes may have a dual role in promoting inflammation and bacterial dissemination during pyelonephritis (Ruiz-Rosado et al., 2021).
Recently, resident macrophages in the bladders of female C57BL/6 mice have been classified into two functionally distinct populations: muscle layer resident macrophages and lamina propria resident macrophages. Both populations can be supplemented by recruiting monocytes (Mariano et al., 2020). RNA sequencing analysis shows an increased expression of genes related to endocytosis and the formation of phagosomes and lysosomes in muscle layer resident macrophages. This suggests that these macrophages may have enhanced anti - inflammatory and phagocytic activities during early urinary tract infections. In contrast, lamina propria resident macrophages show high expression of chemokines and genes related to TLR signaling pathways, indicating a greater propensity for pro - inflammatory responses (Mariano et al., 2020). Notably, the pro - inflammatory capability of lamina propria resident macrophages is similar to that of recruited Ly6C + circulating monocytes in the bladder lamina propria, suggesting that Ly6C + circulating monocytes could be precursors to lamina propria resident macrophages. The latest research has demonstrated that resident macrophages can release Macrophage Extracellular Traps (METs), which are similar to neutrophil extracellular traps (NETs), for bacterial clearance (Hannan, 2025). However, there is still relatively little research on resident macrophages in the bladder, which may become a new direction for studying the anti-infective immunity of the bladder.
3.3 Other innate immune cells
In addition to neutrophils, macrophages, and dendritic cells, other innate cells in the urinary tract immune network also play significant roles. Mast cells (MCs), as bladder immune sentinel cells, are mainly located in the bladder lamina propria and detrusor muscle (Figure 2). During early infection, mast cells show notable proliferation and migration towards the infection site and can accelerate neutrophil recruitment by releasing pre - stored granule mediators from their cytoplasm (Doener et al., 2013; Siebenhaar et al., 2007; Austen, 2001; Roger et al., 2001). Research shows that mast cell absence impairs neutrophil recruitment and activation, affecting bacterial clearance (Doener et al., 2013). Moreover, inflammatory factors in cytoplasmic granules, like tumor necrosis factor, can also speed up dendritic cell recruitment and adaptive immune response formation (Shelburne et al., 2009). Additionally, mast cells are crucial for promoting homeostasis and tissue recovery after infection resolution (Choi et al., 2016). Recent studies indicate that several hours after bladder infection, mast cells express large amounts of the immunosuppressive cytokine IL - 10, corresponding to bladder epithelial cell shedding and repair (Chan et al., 2013). This suggests that mast cell - derived IL - 10 may facilitate bladder epithelial cell recovery; however, this immunosuppressive phenomenon may lead to premature elimination of adaptive immune responses, affecting the pathological process (Chan et al., 2013). Thus, mast cells have dual “pro - inflammatory” and “anti - inflammatory” roles in UTIs, and regulating these functions influences the UTI course. However, at present, the research regarding the role of mast cells in urinary tract infections (UTIs) is relatively scarce, and numerous aspects remain unresolved. For instance, how do mast cells accomplish the transition between the “pro - inflammatory” and “anti - inflammatory” states during the course of UTIs? Does the interleukin - 10 (IL - 10) secreted by them play a pivotal role? Does it exert its functions in UTIs via alternative pathways? And so forth. All these queries necessitate further in - depth investigation.
Innate lymphoid cells (ILCs) and innate - like T cells also have a role in the response to UTIs. Innate - like lymphoid cells are equivalent effector cells to T cells, residing and integrated within tissues, lacking antigen receptors on their surfaces like T and B cells (Artis and Spits, 2015; Naskar and Choi, 2024). They present and process antigens via major histocompatibility complex class II (MHC II) molecules, indirectly regulating antigen - specific T cells (Vivier et al., 2018; Naskar and Choi, 2024). Functionally, ILCs can be divided into five subgroups: natural killer (NK) cells, ILC1, ILC2, ILC3, and lymphoid tissue inducer (LTi) cells (Vivier et al., 2018; Liu et al., 2025; Huang et al., 2022). Among them, NK cells mainly reflect CD8 + T cell functions, while ILC1, ILC2, and ILC3 represent Th1, Th2, and Th17 type T cells respectively. Notably, NK cells make up about 2% of bladder immune cells in healthy juvenile mice and can kill pathogens by releasing perforin, granzymes, and pro - inflammatory cytokines (Bowyer et al., 2022). However, the relative contributions of NK cell subpopulations in the bladder are unclear. Additionally, recent studies show that ILC3s are highly enriched in mucosal tissues, can regulate Th17 cell responses and participate in innate immune responses against extracellular bacteria (Hepworth et al., 2013). This includes inducing epithelial cells to express antimicrobial peptides via IL - 22 production and enhancing antiviral proteins for INF - λ signaling to resist pathogen invasion (Vivier et al., 2018). Some studies report sex differences in ILC3 populations, with more ILC3s in normal male juvenile bladders than in females, but a more pronounced increase in females after UPEC challenge (Zychlinsky et al., 2019). This may indicate a sex - related distribution and proliferation of ILC3s, but further investigation is needed to explore links to UTI incidence and prognosis.
Mucosal - associated invariant T (MAIT) cells are a unique subset of unconventional T cells, including γδ - T cells, natural killer T (NKT) cells, and MAIT cells, found mainly in barrier tissues like the gut, skin, and lungs, where they express T cell receptors (TCR) (Ribot et al., 2021). Notably, γδ - T cells are important for eliminating bacteria from the bladder, probably due to high IL - 17 levels. Research shows that granulocyte colony - stimulating factor (G - CSF), which regulates neutrophil maturation and release, is influenced by IL - 17 - producing γδ - T cells (Ley et al., 2006). This implies a significant role of γδ - T cells in neutrophil recruitment. However, current studies have not clearly established the role of IL - 17A secreted by γδ - T cells in adaptive immune responses (Chamoun et al., 2020). MAIT cells, associated with the microbiome, are activated by recognizing riboflavin metabolites produced by bacteria, and are abundant in mucosal tissues (Gibbs et al., 2016; Booth et al., 2015; Terpstra et al., 2020). Evidence shows that MAIT cells in mouse UTI models migrate to the bladder during infection, reducing bacterial load. A study on human UTIs also found MAIT cells in patient urine (Cui et al., 2015). These suggest that MAIT cells may play a significant role in human UTIs (Dias et al., 2016). Recent research shows that MAIT cells can reside in renal tissue by expressing CD69 and CD103 (Law et al., 2019), and when activated, secrete cytokines like IL - 2, IL - 17A, and granulocyte - macrophage colony - stimulating factor (GM - CSF) (Terpstra et al., 2020). These cytokines may act as a first line of defense against pathogen invasion in the kidneys. Although the mechanisms involving MAIT cells in UTIs are under - explored, existing evidence suggests they may be critical for the body’s defense against pathogen invasion during UTIs.
4 Antimicrobial mechanisms of adaptive immune cells and molecules
4.1 T cell
Compared with the innate immune system, which rapidly responds to pathogens by recognizing pathogen - associated molecular patterns (PAMPs) or damage - associated molecular patterns (DAMPs) non - specifically, the activation and establishment of the adaptive immune system, characterized by strong specificity and immunological memory, takes a longer time (Neugent et al., 2020) (Figure 2). T cells and B cells, as core components of the adaptive immune system, play important roles in fighting urinary tract infections (UTIs) (Song and Deng, 2020). During a UTI, uropathogenic Escherichia coli (UPEC) adheres to urinary tract epithelial cells and invades tissues, triggering a series of signaling pathways and host defense mechanisms that activate immune cells, including T cells, to enter the infection site and combat pathogen invasion (Isaacson et al., 2017). Research shows that after a UTI begins, the number of CD4 + T cells in the bladders of female mice significantly increases (Scharff et al., 2019), with various subpopulations detected, including Th1, Th2, Th17 cells, and regulatory T cells (Tregs) (Mariano et al., 2020). The emergence of Tregs reflects the host’s downregulation of immune responses to maintain the integrity of the urinary tract epithelium (Yu et al., 2019). Moreover, recent studies have revealed that T cells activated and migrated to the bladder after infection have limited ability to eliminate bacteria (Wu et al., 2020; Li et al., 2019; O’Brien et al., 2018); these T cells are more likely to secrete IL - 4, which promotes tissue repair, rather than IFN - γ, which is associated with bacterial clearance, suggesting a preferential differentiation towards the Th2 phenotype rather than the Th1 phenotype (Wu et al., 2020). Additionally, about 50% of CD11c + CD301b + dendritic cells in the bladder express OX40L, a molecule that preferentially polarizes CD4 + T cells towards the Th2 type (Schuijs et al., 2019; Wu et al., 2020). This cell differentiation tendency helps protect deeper bladder tissues from the harmful effects of salts, urea, and other substances in the urine when the epithelial cell shedding program is activated, but it also reduces the ability to eliminate bacteria in the bladder. This guided T cell differentiation indicates that the host balances antibacterial immune responses and repair processes when pathogens invade, aiming to resist pathogen entry and further infection with minimal damage.
The specific mechanisms of T cell - mediated anti - infective protection during urinary tract infections remain unclear. Activated Th1 and Th17 cells after infection may play a role in bacterial clearance by secreting cytokines IFN - γ and IL - 17, thereby further activating macrophages and CD8 + T cells or accelerating neutrophil recruitment (Mariano et al., 2020; Bowyer et al., 2022). Overall, research on adaptive T cells in the bladder is still in its early stages, and many related mechanisms remain undefined. As research on bladder immunity in urinary tract infections progresses, it is crucial to focus on the roles of different T cell subtypes, especially regulatory T cells, in anti - infective immunity in the bladder. Elucidating the mechanisms that maintain the delicate balance between limiting immune responses and promoting bacterial clearance will be crucial for future research. A deeper understanding of the roles of T cells in resisting urinary tract infections and the differentiation of memory T cells will not only help reveal the diversity of urinary tract immune responses but also assist in developing more effective treatment strategies and preventive vaccines for urinary tract infections.
4.2 Tissue-resident memory T cell
Tissue - resident memory T (Trm) cells, a subset of memory T cells, consist of unique, non - migratory cell populations that permanently reside in organs like the skin, intestines, and lungs (Gebhardt et al., 2009) (Figure 2). They play a crucial role in providing long - term immune protection (Longet and Paul, 2023).
In one study, researchers used lymphocyte - depleting antibodies to deplete systemic circulating T cells to verify the role of tissue - resident memory T cells in resisting recurrent urinary tract infections. The results showed that treated mice had similar anti - infection responses as untreated mice, with a sustained increase in T cell numbers detected in the bladders of treated mice (Rousseau et al., 2023). This finding emphasizes the important protective role of tissue - resident memory T cells in establishing adaptive immunity in the bladder. Moreover, research has found that CD4 + tissue - resident memory T cells are more abundant than CD8 + tissue - resident memory T cells in healthy human kidneys (van der Putten et al., 2021; Krebs et al., 2020). However, in patients with glomerulonephriti, the amounts of CD4 + and CD8 + tissue - resident memory T cells in the kidneys are nearly equal (Lisboa et al., 2020; Krebs et al., 2016). This indicates that during glomerulonephritis, there may be a greater increase in the number of CD8 + tissue - resident memory T cells in the human kidneys, although the reasons for this disproportional proliferation remain unclear. In a mouse model of pyeloneinitis, the number of renal tissue - resident memory T cells also showed a significant increase (Krebs et al., 2020). However, there is currently no data to support whether CD4 + and CD8 + tissue - resident memory T cells show different degrees of increase in this model. It is unclear how renal tissue - resident memory T cells adapt to their environment; however, studies have shown that liver tissue - resident memory T cells upregulate hypoxia - inducible factor pathways to adapt to the hypoxic conditions in the liver (Kim et al., 2020). Similarly, renal tissue - resident memory T cells in hypoxic environments may adapt through similar mechanisms and contribute to anti - infective immunity, providing a new research direction for studying the adaptive immune mechanisms within the bladder during urinary tract infections.
4.3 B cell
After infection onset, dendritic cells and macrophages present antigens to B cell germinal centers by phagocytosing pathogens, leading to B cell activation. Activated B cells can differentiate into either effector B cells or memory B cells (Figure 2). Effector B cells (plasma cells) combat pathogens by producing antibodies, while memory B cells can quickly initiate adaptive immune responses upon re - exposure to the same pathogen (Seifert and Küppers, 2016; Hawas et al., 2023). Additionally, after B cell activation, these cells can influence T cell activation through antigen presentation, provision of co - stimulatory factors, and secretion of cytokine (Petersone et al., 2018; Bishop, 2016). Among these, the co - stimulatory factor OX40 ligand (OX40L) is a crucial regulatory molecule for the proliferation and survival of T cells, promoting the differentiation of CD4 + T cells towards the Th2 phenotype (Rogers et al., 2001; Linton et al., 2003). Furthermore, recent research on tissue - resident B cells has categorized them into two subsets: B - 1 and B - 2 cells. Notably, B - 1 cells, which are enriched in the pleural and peritoneal cavities, have also been found in damaged and infected skin, lungs, and kidneys (Suchanek et al., 2023; Denton et al., 2019; Stark et al., 2018). These B - 1 cells form a significant part of the pool of tissue - resident B cells in these organs [137]. Studies have shown a negative correlation between the number of tissue - resident B cells in the kidneys and susceptibility to urinary tract infections (Suchanek et al., 2023). Moreover, the expression of chemokine transcripts for neutrophil and monocyte recruitment is elevated in the kidneys of B cell - deficient mice (Suchanek et al., 2023), suggesting that tissue - resident B cells may inhibit renal antibacterial immunity and affect the recruitment of circulating immune cells to infected organs.
Single - cell sequencing results also reveal the presence of plasma cells producing secretory IgA in the urethral lamina propria of healthy mice, with secretion levels increasing with age (Ligon et al., 2020). It is unclear whether the observed changes in cell proportion and secretion levels contribute to the increased prevalence of urinary tract infections in the elderly or if they reflect a response to repeated infections. However, the role of secretory IgA in bladder anti - infection remains uncertain. Thus, although some studies have investigated the role of B cells in urinary tract infection defenses, their exact protective roles and mechanisms need further investigation to clarify.
5 Conclusions and perspectives
This review mainly summarizes the functions and mechanisms of innate and adaptive immune cells in the body’s defense against urinary tract infections (UTIs). In the study of urinary tract anti - infective immunity, neutrophils, dendritic cells, macrophages, and other innate immune cells cooperate to form an effective immune network. Although we have obtained initial insights into the crucial role of bladder immunity in UTIs, there are still many research gaps. Current studies by domestic and international scholars mainly focus on the quantity and types of immune cells related to the body’s anti – infective immune mechanisms, paying less attention to changes in the bladder mucosal microenvironment and at the molecular level. Moreover, our understanding of the adaptive immune mechanisms in the bladder is insufficient and requires more detailed studies to clarify the complex regulatory networks and potential mechanisms of action (Table 1).
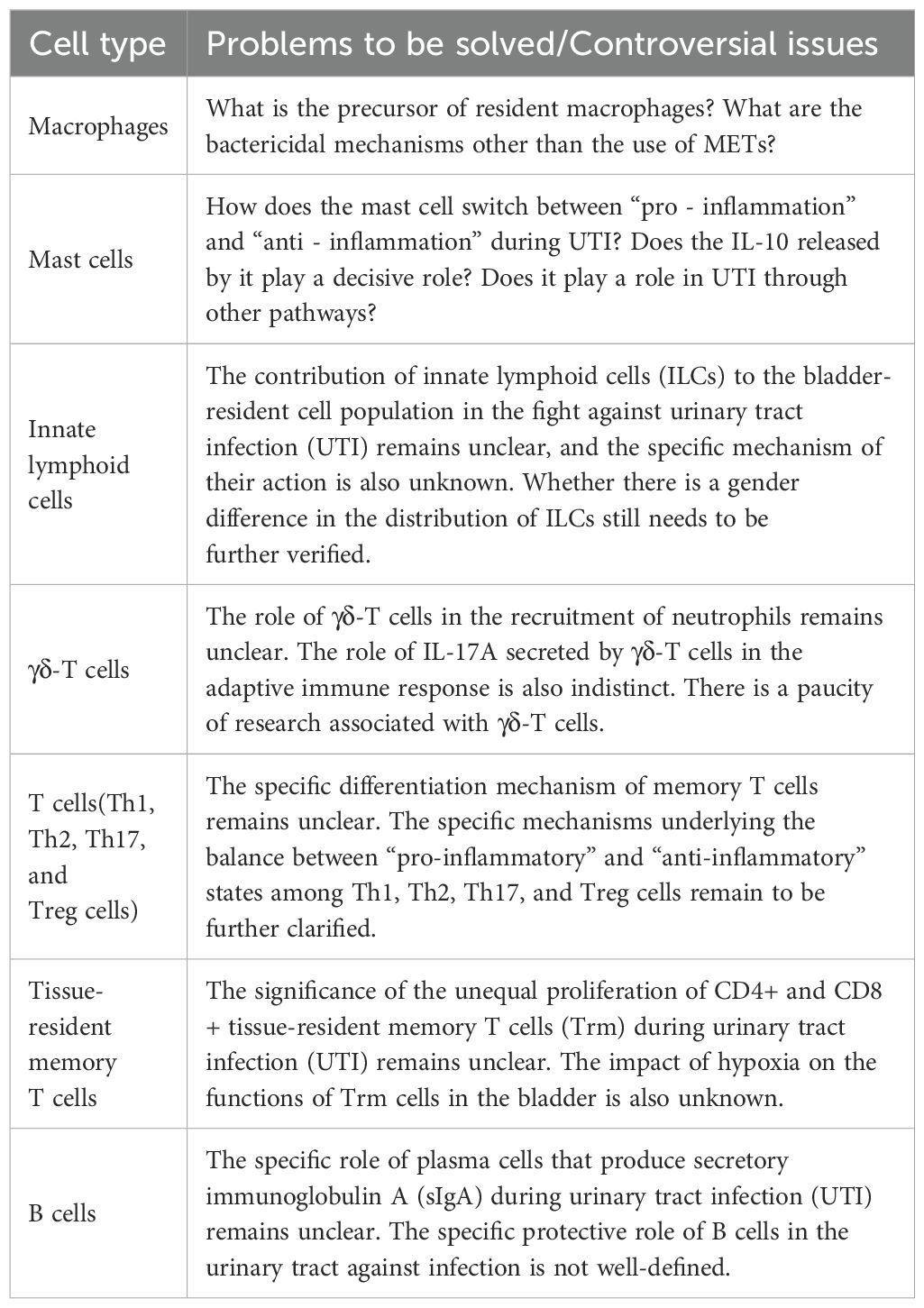
Table 1. Controversies or unresolved questions in the immune mechanisms of the urinary tract against infections.
In exploring the functional mechanisms of adaptive immune cells, tissue - resident memory T cells have become a new focus. There is an urgent need to further understand the mechanisms of how tissue - resident memory T cells are established and maintained on mucosal surfaces and to know about the potential migration of these cells. Additionally, mucosa - associated invariant T cells have been found to have anti - infective effects in the kidneys through cytokine secretion, but their location during bladder inflammation is unclear, which provides a potential new research direction for anti - urinary tract infection immunity. B cells, as a part of adaptive immunity, seem to have bacteriostatic effects different from those of T cells, which requires further investigation. Some evidence also suggests that when initiating anti - infective immunity, the body tends to favor the subtype differentiation of immune cells towards “tissue repair” rather than “bacterial clearance”. This indicates that the body may prioritize minimizing tissue damage over attacking pathogens, aiming to achieve a dynamic balance with pathogens to relieve inflammatory symptoms and ensure normal physiological functions. These studies emphasize the complex relationships among immune cells, as different immune cells usually work together in response to pathogen invasion. A more comprehensive and holistic view is necessary to understand these immune cells.
Exploring novel prevention and treatment methods beyond traditional antibiotics is crucial for managing urinary tract infections (UTIs), particularly those caused by multidrug-resistant bacteria. Recent research has found that T cells in peripheral circulation do not influence the outcomes of urinary tract infections, while tissue-resident memory (TRM) T cells in the bladder play a critical role. This new finding is significant for the development of vaccines and immunotherapeutic strategies for urinary tract infections. Additionally, the role of γδ T cells that produce IL-17 in combating urinary tract infections is also noteworthy. Antibodies IgA and IgG can be detected in the blood and urine of UTI patients; however, the precise role of these antibodies in the risk assessment of UTIs remains uncertain and requires further investigation.
Overall, future research will concentrate on a deeper understanding of the mechanisms of immune cells such as tissue - resident memory T cells, mucosal - associated invariant T cells, and B cells, which are not yet clearly defined in bladder immunity. This understanding will guide the design of innovative vaccines to strengthen the immune system’s defense against urinary tract infections, providing more effective methods for clinical treatment and prevention.
Author contributions
YH: Writing – original draft. ZL: Writing – original draft. QH: Writing – original draft. AZ: Writing – review & editing. HN: Writing – review & editing.
Funding
The author(s) declare that financial support was received for the research and/or publication of this article. This work was supported by Zhejiang Provincial Natural Science Foundation of China (LY24H290004); National Natural Science Foundation of China Youth Foundation (81701969), Natural Science Youth Exploration Program (2022JKZKTS03), and Open fund for the State Key Laboratory of Pathogenic Biology of Animal Diseases (SKLVEB2021KFKT002).
Conflict of interest
The authors declare that the research was conducted in the absence of any commercial or financial relationships that could be construed as a potential conflict of interest.
Generative AI statement
The author(s) declare that no Generative AI was used in the creation of this manuscript.
Publisher’s note
All claims expressed in this article are solely those of the authors and do not necessarily represent those of their affiliated organizations, or those of the publisher, the editors and the reviewers. Any product that may be evaluated in this article, or claim that may be made by its manufacturer, is not guaranteed or endorsed by the publisher.
References
Artis, D., Spits, H. (2015). The biology of innate lymphoid cells. Nature 517, 293–301. doi: 10.1038/nature14189
Ashkar, A. A., Mossman, K. L., Coombes, B. K., Gyles, C. L., Mackenzie, R. (2008). FimH adhesin of type 1 fimbriae is a potent inducer of innate antimicrobial responses which requires TLR4 and type 1 interferon signalling. PloS Pathog. 4, e1000233. doi: 10.1371/journal.ppat.1000233
Austen, M.F.G.a. (2001). The diverse roles of mast cells. J. Exp. Med. 194, , F1–, F5. doi: 10.1084/jem.194.1.f1
Becknell, B., Schwaderer, A., Hains, D. S., Spencer, J. D. (2015). Amplifying renal immunity: the role of antimicrobial peptides in pyelonephritis. Nat. Rev. Nephrol. 11, 642–655. doi: 10.1038/nrneph.2015.105
Berry, M. R. R. J., Ferdinand, J. R., Jing, C. Z., Loudon, K. W., Wlodek, E., Dennison, T. W., et al. (2017). Renal sodium gradient orchestrates a dynamic antibacterial defense zone. Cell , 170, 860–874. doi: 10.1016/j.cell.2017.07.022
Billips, B. K., Forrestal, S. G., Rycyk, M. T., Johnson, J. R., Klumpp, D. J., Schaeffer, A. J. (2007). Modulation of host innate immune response in the bladder by uropathogenicEscherichia coli. Infection Immun. , 75, 5353–5360. doi: 10.1128/iai.00922-07
Bishop, G. A. (2016). B cell-T cell interaction: antigen bridge to antigen presentation. Nat. Rev. Immunol. 16, 467. doi: 10.1038/nri.2016.82
Bishop, B. L. M. J., Song, J., Guojie, L., Zaas, D., Abraham, S. N. (2007). Cyclic AMP–regulated exocytosis of Escherichia coli from infected bladder epithelial cells. Nat. Med. 13, 625–630. doi: 10.1038/nm1572
Booth, J. S., Salerno-Goncalves, R., Blanchard, T. G., Patil, S. A., Kader, H. A., Safta, A. M., et al. (2015). Mucosal-associated invariant T cells in the human gastric mucosa and blood: role in Helicobacter pylori infection. Front. Immunol. 6. doi: 10.3389/fimmu.2015.00466
Bottek, J., Soun, C., Lill, J. K., Dixit, A., Thiebes, S., Beerlage, A. L., et al. (2020). Spatial proteomics revealed a CX(3)CL1-dependent crosstalk between the urothelium and relocated macrophages through IL-6 during an acute bacterial infection in the urinary bladder. Mucosal Immunol. 13, 702–714. doi: 10.1038/s41385-020-0269-7
Bowyer, G. S., Loudon, K. W., Suchanek, O., Clatworthy, M. R. (2022). Tissue immunity in the bladder. Annu. Rev. Immunol. 40, 499–523. doi: 10.1146/annurev-immunol-101220-032117
Chamoun, M. N., Sullivan, M. J., Goh, K. G. K., Acharya, D., Ipe, D. S., Katupitiya, L., et al. (2020). Restriction of chronic Escherichia coli urinary tract infection depends upon T cell-derived interleukin-17, a deficiency of which predisposes to flagella-driven bacterial persistence. FASEB J. 34, 14572–14587. doi: 10.1096/fj.202000760R
Chan, C. Y., St John, A. L., Abraham, S. N. (2013). Mast cell interleukin-10 drives localized tolerance in chronic bladder infection. Immunity 38, 349–359. doi: 10.1016/j.immuni.2012.10.019
Chassin, C., Goujon, J., Darche, S., du Merle, L., Bens, M., Cluzeaud, F., et al. (2006). Renal collecting duct epithelial cells react to pyelonephritis-associated Escherichia coli by activating distinct TLR4-dependent and-independent inflammatory pathways. J. Immunol. 177, 4773–4784. doi: 10.4049/jimmunol.177.7.4773
Choi, H. W., Bowen, S. E., Miao, Y., Chan, C. Y., Miao, E. A., Abrink, M., et al. (2016). Loss of bladder epithelium induced by cytolytic mast cell granules. Immunity , 45, 1258–1269. doi: 10.1016/j.immuni.2016.11.003
Chugh, S., Pietropaolo, A., Montanari, E., Sarica, K., Somani, B. K. (2020). Predictors of urinary infections and urosepsis after ureteroscopy for stone disease: a systematic review from EAU section of urolithiasis (EULIS). Curr. Urol. Rep. 21, 16. doi: 10.1007/s11934-020-0969-2
Cotzomi-Ortega, I., Rosowski, E. E., Wang, X., Sanchez-Zamora, Y. I., Lopez-Torres, J. M., Sanchez-Orellana, G., et al. (2024). Neutrophil NADPH oxidase promotes bacterial eradication and regulates NF-kappaB-Mediated inflammation via NRF2 signaling during urinary tract infections. Mucosal Immunol. 24, 00133–8. doi: 10.1016/j.mucimm.2024.12.010
Cui, Y., Franciszkiewicz, K., Mburu, Y. K., Mondot, S., Le Bourhis, L., Premel, V., et al. (2015). Mucosal-associated invariant T cell-rich congenic mouse strain allows functional evaluation. J. Clin. Invest. 125, 4171–4185. doi: 10.1172/Jci82424
Davies, L. C., Jenkins, S. J., Allen, J. E., Taylor, P. R. (2013). Tissue-resident macrophages. Nat. Immunol. 14, 986–995. doi: 10.1038/ni.2705
Denton, A. E., Innocentin, S., Carr, E. J., Bradford, B. M., Lafouresse, F., Mabbott, N. A., et al. (2019). Type I interferon induces CXCL13 to support ectopic germinal center formation. J. Exp. Med. 216, 621–637. doi: 10.1084/jem.20181216
Dias, J., Sobkowiak, M. J., Sandberg, J. K., Leeansyah, E. (2016). Human MAIT-cell responses to Escherichia coli: activation, cytokine production, proliferation, and cytotoxicity. J. Leucocyte Biol. , 100, 233–240. doi: 10.1189/jlb.4TA0815-391RR
Doener, F., Michel, A., Reuter, S., Friedrich, P., Bohm, L., Relle, M., et al. (2013). Mast cell-derived mediators promote murine neutrophil effector functions. Int. Immunol. , 25, 553–561. doi: 10.1093/intimm/dxt019
Foxman, B. (2014). Urinary tract infection syndromes: occurrence, recurrence, bacteriology, risk factors, and disease burden. Infect. Dis. Clin. North Am. 28, 1–13. doi: 10.1016/j.idc.2013.09.003
Foxman, B., Gillespie, B., Koopman, J., Zhang, L., Palin, K., Tallman, P., et al. (2000). Risk factors for second urinary tract infection among college women. Am. J. Epidemiol. 151, 1194–1205. doi: 10.1093/oxfordjournals.aje.a010170
Froy, O., Hananel, A., Chapnik, N., Madar, Z. (2007). Differential effect of insulin treatment on decreased levels of beta-defensins and Toll-like receptors in diabetic rats. Mol. Immunol. 44, 796–802. doi: 10.1016/j.molimm.2006.04.009
Gabriela, G., Amanda, E. I. P., Catharina, S., Robin, E. O. (1997). Role of epithelial interleukin-8 (IL-8) and neutrophil IL-8 receptor A in escherichia coli-induced transuroepithelial neutrophil migration. Infection And Immun. 65, 3451–3456. doi: 10.1128/iai.65.8.3451-3456.1997
Galli, S. J., Borregaard, N., Wynn, T. A. (2011). Phenotypic and functional plasticity of cells of innate immunity: macrophages, mast cells and neutrophils. Nat. Immunol. 12, 1035–1044. doi: 10.1038/ni.2109
Garcia, M. A., Nelson, W. J., Chavez, N. (2018). Cell-cell junctions organize structural and signaling networks. Cold Spring Harbor Perspect. Biol. 10, a029181. doi: 10.1101/cshperspect.a029181
Gebhardt, T., Wakim, L. M., Eidsmo, L., Reading, P. C., Heath, W. R., Carbone, F. R. (2009). Memory T cells in nonlymphoid tissue that provide enhanced local immunity during infection with herpes simplex virus. Nat. Immunol. 10, 524–530. doi: 10.1038/ni.1718
Gibbs, A., Leeansyah, E., Introini, A., Proulx, D. P., Hasselrot, K., Andersson, E., et al. (2016). MAIT cells reside in the female genital mucosa and are biased towards IL-17 and IL-22 production in response to bacterial stimulation. Mucosal Immunol. , 10, 35–45. doi: 10.1038/mi.2016.30
Grasso, E. J., Calderón, R. O. (2013). Membrane lipids and proteins as modulators of urothelial endocytic vesicles pathways. Histochem. Cell Biol. 140, 507–520. doi: 10.1007/s00418-013-1095-8
Hains, D. S., Chen, X., Saxena, V., Barr-Beare, E., Flemming, W., Easterling, R., et al. (2014). Carbonic anhydrase 2 deficiency leads to increased pyelonephritis susceptibility. Am. J. Physiology-Renal Physiol. 307, F869–F880. doi: 10.1152/ajprenal.00344.2014
Halbay, T. (2023). Whole-genome sequencing-based characteristics of Escherichia coli Rize-53 isolate from Turkey. Adv. Clin. Exp. Med. 32, 91–96. doi: 10.17219/acem/152704
Hannan, T. J. (2025). METs, NETs, and UTIs. Cell Host Microbe 33, 318–320. doi: 10.1016/j.chom.2025.02.014
Hannan, T. J., Mysorekar, I. U., Hung, C. S., Isaacson-Schmid, M. L., Hultgren, S. J. (2010). Early severe inflammatory responses to uropathogenic E.coli predispose to chronic and recurrent urinary tract infection. J. Womens Health 19, 1797–1798. doi: 10.1371/journal.ppat.1001042
Hawas, S., Vagenas, D., Haque, A., Totsika, M. (2023). Bladder-draining lymph nodes support germinal center B cell responses during urinary tract infection in mice. Infect. Immun. 91, e0031723. doi: 10.1128/iai.00317-23
Hayes, B.W., Abraham, S. N. (2016). Innate immune responses to bladder infection. Microbiol. Spectr. 4. doi: 10.1128/microbiolspec.UTI-0024-2016
Hepworth, M. R., Monticelli, L. A., Fung, T. C., Ziegler, C. G. K., Grunberg, S., Sinha, R., et al. (2013). Innate lymphoid cells regulate CD4+T cell responses to intestinal commensal bacteria. Nature 498, 113–117. doi: 10.1038/nature12240
Hickling, D., Liu, Y., Victor, N., Huang, W., Leopr, H., Wu, X. R. (2013). The antimicrobial peptide cathelicidin protects the lower urinary tract against uropathogenic E.Coli. Neurourology Urodynamics 32, 125–126. doi: 10.1038/nm1407
Hill, W. G. (2015). Control of urinary drainage and voiding. Clin. J. Am. Soc. Nephrol. 10, 480–492. doi: 10.2215/Cjn.04520413
Hreha, T. N., Collins, C. A., Cole, E. B., Jin, R. J., Hunstad, D. A. (2024). Androgen exposure impairs neutrophil maturation and function within the infected kidney. mBio 15, e0317023. doi: 10.1128/mbio.03170-23
Hreha, T. N., Collins, C. A., Daugherty, A. L., Griffith, J. M., Hruska, K. A., Hunstad, D. A. (2020a). Androgen-influenced polarization of activin A-producing macrophages accompanies post-pyelonephritic renal scarring. Front. Immunol. 11. doi: 10.3389/fimmu.2020.01641
Hreha, T. N., Collins, C. A., Daugherty, A. L., Twentyman, J., Paluri, N., Hunstad, D. A. (2020b). TGFβ1 orchestrates renal fibrosis following Escherichia coli pyelonephritis. Physiol. Rep. 8, e14401. doi: 10.14814/phy2.14401
Huang, J., Fu, L., Huang, J., Zhao, J., Zhang, X., Wang, W., et al. (2022). Group 3 innate lymphoid cells protect the host from the uropathogenic escherichia coli infection in the bladder. Adv. Sci. (Weinh) 9, e2103303. doi: 10.1002/advs.202103303
Isaacson, B., Hadad, T., Glasner, A., Gur, C., Granot, Z., Bachrach, G., et al. (2017). Stromal cell-derived factor 1 mediates immune cell attraction upon urinary tract infection. Cell Rep. 20, 40–47. doi: 10.1016/j.celrep.2017.06.034
Jaimes-Parra, B. D., Valle-Díaz de la Guardia, F., Arrabal-Polo, M.Á., Herrera-Imbroda, B., Lara, M. F., Machuca-Santa-Cruz, F. J., et al. (2015). Ex vivo construction of a novel model of bioengineered bladder mucosa: A preliminary study. Int. J. Urol. 23, 85–92. doi: 10.1111/iju.12963
John, K., Vijay, S., Samuel, A., Ashley, J., George, J. S., Takafumi, Y., et al. (2020). Developmental loss, but not pharmacological suppression, of renal carbonic anhydrase 2 2 results in pyelonephritis susceptibilit. J. Physiology-Renal Physiol. 318, F1441–F1453. doi: 10.1152/ajprenal.00583.2019
Kim, J. H., Han, J. W., Choi, Y. J., Rha, M. S., Koh, J. Y., Kim, K. H., et al. (2020). Functions of human liver CD69+CD103+CD8+T cells depend on HIF-2α activity in healthy and pathologic livers. J. Hepatol. 72, 1170–1181. doi: 10.1016/j.jhep.2020.01.010
Kolaczkowska, E., Jenne, C. N., Surewaard, B. G. J., Thanabalasuriar, A., Lee, W. Y., Sanz, M. J., et al. (2015). Molecular mechanisms of NET formation and degradation revealed by intravital imaging in the liver vasculature. Nat. Commun. 6, 6673. doi: 10.1038/ncomms7673
Krebs, C. F., Paust, H. J., Krohn, S., Koyro, T., Brix, S. R., Riedel, J. H., et al. (2016). Autoimmune renal disease is exacerbated by S1P-receptor-1-dependent intestinal th17 cell migration to the kidney. Immunity 45, 1078–1092. doi: 10.1016/j.immuni.2016.10.020
Krebs, C. F., Reimers, D., Zhao, Y., Paust, H. J., Bartsch, P., Nuñez, S., et al. (2020). Pathogen-induced tissue-resident memory TH17 (TRM17) cells amplify autoimmune kidney disease. Sci. Immunol. 5, eaba4163. doi: 10.1126/sciimmunol.aba4163
Law, B. M., Wilkinson, R., Xiangju, W., Kildey, K., Giuliani, K., Beagley, K. W., et al. (2019). Human tissue-resident mucosal-associated invariant T (MAIT) cells in renal fibrosis and CKD. J. Am. Soc. Nephrol. 30, 1322–1335. doi: 10.1681/ASN.2018101064
Ley, K., Smith, E., Stark, M. A. (2006). IL-17A-producing neutrophil-regulatory Tn lymphocytes. Immunologic Res. 34, 229–242. doi: 10.1385/IR:34:3:229
Li, Z., Wang, K. E., Zhou, X. L., Zhou, J., Ye, C. H. (2019). Preoperative Th1/Th2 and related cytokines: Prediction value in postoperative febrile UTI after ureteroscopy in patients with ureteral calculi. Adv. Clin. Exp. Med. 28, 125–132. doi: 10.17219/acem/94157
Ligon, M. M., Wang, C., DeJong, E. N., Schulz, C., Bowdish, D. M. E., Mysorekar, I. U. (2020). Single cell and tissue-transcriptomic analysis of murine bladders reveals age- and TNFalpha-dependent but microbiota-independent tertiary lymphoid tissue formation. Mucosal Immunol 13 (6), 908–918. doi: 10.1038/s41385-020-0290-x
Lin, W.-H., Sheu, S.-M., Wu, C.-F., Huang, W.-C., Hsu, L.-J., Yu, K.-C., et al. (2024). O-antigen of uropathogenic Escherichia coli is required for induction of neutrophil extracellular traps. J. Microbiology Immunol. Infection. 58, 209–218. doi: 10.1016/j.jmii.2024.12.007
Linton, P., Bautista, B., Biederman, E., Bradley, E. S., Harbertson, J., Kondrack, R. M., et al. (2003). Costimulation via OX40L expressed by B cells is sufficient to determine the extent of primary CD4 cell expansion and Th2 cytokine secretion. vivo. J. Exp. Med. 197, 875–883. doi: 10.1084/jem.20021290
Lisboa, V. D., Ribeiro-Alves, M., Corrêa, R. D., Lopes, I. R., Mafort, T. T., Santos, A. P., et al. (2020). Predominance of th1 immune response in pleural effusion of patients with tuberculosis among other exudative etiologies. J. Clin. Microbiol. 58, e00927–e00919. doi: 10.1128/JCM.00927-19
Liu, Y., Wang, J., Lin, J., Sun, D., Zhu, K., Diao, T., et al. (2025). The brain-body circuit mediates acute stress-induced anti-inflammatory reflex in bacterial cystitis by suppressing ILC2 activation. JCI Insight. doi: 10.1172/jci.insight.189362
Longet, S., Paul, S. (2023). Pivotal role of tissue-resident memory lymphocytes in the control of mucosal infections: can mucosal vaccination induce protective tissue-resident memory T and B cells? Front. Immunol. , 14. doi: 10.3389/fimmu.2023.1216402
Mariano, L. L., Ingersoll, M. A. (2020). The immune response to infection in the bladder. Nat. Rev. Urol. 17, 439–458. doi: 10.1038/s41585-020-0350-8
Mariano, L. L., Rousseau, M., Varet, H., Legendre, R., Gentek, R., Coronilla, J. S., et al. (2020). Functionally distinct resident macrophage subsets differentially shape responses to infection in the bladder. Sci. Adv. 6, EABC5739. doi: 10.1126/sciadv.abc5739
Marshall, J. S., Portales-Cervantes, L., Leong, E. (2019). Mast cell responses to viruses and pathogen products. Int. J. Mol. Sci. , 20, 4241. doi: 10.3390/ijms20174241
Mass, E., Nimmerjahn, F., Kierdorf, K., Schlitzer, A. (2023). Tissue-specific macrophages: how they develop and choreograph tissue biology. Nat. Rev. Immunol. 23, 563–579. doi: 10.1038/s41577-023-00848-y
Mercado-Evans, V., Branthoover, H., Chew, C., Serchejian, C., Saltzman, A. B., Mejia, M. E., et al. (2025). Tamm-Horsfall protein augments neutrophil NETosis during urinary tract infection. JCI Insight 10, e180024. doi: 10.1172/jci.insight.180024
Miao, Y. X., Wu, J. X., Abraham, S. N. (2016). Ubiquitination of innate immune regulator TRAF3 orchestrates expulsion of intracellular bacteria by exocyst complex. Immunity 45, 94–105. doi: 10.1016/j.immuni.2016.06.023
Mora-Bau, G., Platt, A. M., van Rooijen, N., Randolph, G. J., Albert, M. L., Ingersoll, M. A. (2015). Macrophages subvert adaptive immunity to urinary tract infection. PloS Pathog. 11, e1005044. doi: 10.1371/journal.ppat.1005044
Naskar, M., Choi, H. W. (2024). A dynamic interplay of innate immune responses during urinary tract infection. Immune Network 24, e31. doi: 10.4110/in.2024.24.e31
Neal, P., Ritwij, K., Max, W., Kai, M. S., Catherine, F., Rong, D., et al. (2014). α–Intercalated cells defend the urinary system from bacterial infection. J. Clin. Invest. 124, 2963–2976. doi: 10.1172/JCI71630
Neugent, M. L., Hulyalkar, N. V., Nguyen, V. H., Zimmern, P. E., De Nisco, N. J. (2020). Advances in understanding the human urinary microbiome and its potential role in urinary tract infection. mBio 11, e00218-20. doi: 10.1128/mBio.00218-20
O’Brien, ,. V. P., Dorsey, D. A., Hannan, T. J., Hultgren, S. (2018). Host restriction of Escherichia coli recurrent urinary tract infection occurs in a bacterial strain-specific manner. PloS Pathog. 14, e1007457. doi: 10.1371/journal.ppat.1007457
Olson, P. D., Hruska, K. A., Hunstad, D. A. (2016). Androgens enhance male urinary tract infection severity in a new model. J. Am. Soc. Nephrology. 27 (6), 1625–1634. doi: 10.1681/Asn.2015030327
Olson, P. D., McLellan, L. K., Hreha, T. N., Liu, A., Briden, K. E., Hruska, K. A., et al. (2018). Androgen exposure potentiates formation of intratubular communities and renal abscesses by. Kidney International. 94 (3), 502–513. doi: 10.1016/j.kint.2018.04.023
Osborn, S. L., Kurzrock, E. A. (2014). Production of urothelium from pluripotent stem cells for regenerative applications. Curr. Urol. Rep. 16, 466. doi: 10.1007/s11934-014-0466-6
Paige, L. (2006). Mechanisms of degranulation in neutrophils. Allergy Asthma Clin. Immuno 2, 98–108. doi: 10.2310/7480.2006.00012
Pang, Y., Cheng, Z. H., Zhang, S., Li, S. J., Li, X. P., Li, X. D., et al. (2022). Bladder epithelial cell phosphate transporter inhibition protects mice against uropathogenic infection. Cell Rep. 39, 110698. doi: 10.1016/j.celrep.2022.110698
Petersone, L., Edner, N. M., Ovcinnikovs, V., Heuts, F., Ross, E. M., Ntavli, E., et al. (2018). T cell/B cell collaboration and autoimmunity: an intimate relationship. Front. Immunol. 9. doi: 10.3389/fimmu.2018.01941
Petri, B., Phillipson, M., Kubes, P. (2008). The physiology of leukocyte recruitment: An in vivo perspective. J. Immunol. 180, 6439–6446. doi: 10.4049/jimmunol.180.10.6439
Phillipson, M., Kubes, P. (2011). The neutrophil in vascular inflammation. Nat. Med. , 17, 1381–1390. doi: 10.1038/nm.2514
Ribot, J. C., Lopes, N., Silva-Santos, B. (2021). gammadelta T cells in tissue physiology and surveillance. Nat. Rev. Immunol. 21, 221–232. doi: 10.1038/s41577-020-00452-4
Roche, J. K., Keepers, T. R., Gross, L. K., Seaner, R. M., Obrig, T. G. (2007). CXCL1/KC and CXCL2/MIP-2 are critical effectors and potential targets for therapy of Escherichia coli O157:H7-associated renal inflammation. Am. J. Pathol. 170, 526–537. doi: 10.2353/ajpath.2007.060366
Roger, T. P., Steffen, J., Guiying, C., Wolfgang, W., Yi, L., Martin, D., et al. (2001). Inflammatory chemokine transport and presentation in HEV: A remote control mechanism for monocyte recruitment to lymph nodes in inflamed tissues. J. Exp. Med. ., 194, 1361–1373. doi: 10.1084/jem.194.9.1361
Rogers, P. R., Jianxun, S., Gramaglia, I., Killeen, N., Croft, M. (2001). OX40 promotes Bcl-xL and Bcl-2 expression and is essential for long-term survival of CD4 T cells. Immunity 15, 445–455. doi: 10.1016/s1074-7613(01)00191-1
Rosales, C. (2018). Neutrophil: A cell with many roles in inflammation or several cell types? Front. Physiol. 9. doi: 10.3389/fphys.2018.00113
Rousseau, M., Mariano, L. L., Canton, T., Ingersoll, M. A. (2023). Tissue-resident memory T cells mediate mucosal immunity to recurrent urinary tract infection. Sci. Immunol. 8, eabn4332. doi: 10.1126/sciimmunol.abn4332
Roy, A., Al-bataineh, M. M., Pastor-Soler, N. M. (2015). Collecting duct intercalated cell function and regulation. Clin. J. Am. Soc. Nephrol. 10, 305–324. doi: 10.2215/CJN.08880914
Ruiz-Rosado, J. D., Robledo-Avila, F., Cortado, H., Rangel-Moreno, J., Justice, S. S., Yang, C., et al. (2021). Neutrophil-macrophage imbalance drives the development of renal scarring during experimental pyelonephritis. J. Am. Soc. Nephrol. , 32, 69–85. doi: 10.1681/Asn.2020030362
Saxena, V., Fitch, J., Ketz, J., White, P., Wetzel, A., Chanley, M. A., et al. (2019). Whole transcriptome analysis of renal intercalated cells predicts lipopolysaccharide mediated inhibition of retinoid X receptor alpha function. Sci. Reports 9, 545. doi: 10.1038/s41598-018-36921-z
Saxena, V., Gao, H. Y., Arregui, S., Zollman, A., Kamocka, M. M., Xuei, X. L., et al. (2021). Kidney intercalated cells are phagocytic and acidify internalized uropathogenic. Nat. Commun. 12, 2405. doi: 10.1038/s41467-021-22672-5
Saxena, V., Hains, D. S., Ketz, J., Chanley, M., Spencer, J. D., Becknell, B., et al. (2018). Cell-specific qRT-PCR of renal epithelial cells reveals a novel innate immune signature in murine collecting duct. Am. J. Physiology-Renal Physiol. 315, F812–F823. doi: 10.1152/ajprenal.00512.2016
Scharff, A. Z., Rousseau, M., Mariano, L. L., Canton, T., Consiglio, C. R., Albert, M. L., et al. (2019). Sex differences in IL-17 contribute to chronicity in male versus female urinary tract infection. JCI Insight , 4, e122998. doi: 10.1172/jci.insight.122998
Schiwon, M., Weisheit, C., Franken, L., Gutweiler, S., Dixit, A., Meyer-Schwesinger, C., et al. (2014). Crosstalk between sentinel and helper macrophages permits neutrophil migration into infected uroepithelium. Cell 1 56, 456–468. doi: 10.1016/j.cell.2014.01.006
Schuijs, M. J., Hammad, H., Lambrecht, B. N. (2019). Professional and ‘Amateur’ Antigen-presenting cells in type 2 immunity. Trends Immunol. 40, 22–34. doi: 10.1016/j.it.2018.11.001
Schwartz, L., de Dios Ruiz-Rosado, J., Stonebrook, E., Becknell, B., Spencer, J. D. (2023). Uropathogen and host responses in pyelonephritis. Nat. Rev. Nephrol. 19, 658–671. doi: 10.1038/s41581-023-00737-6
Schwartz, L., Salamon, K., Simoni, A., Eichler, T., Jackson, A. R., Murtha, M., et al. (2024). Insulin receptor signaling engages bladder urothelial defenses that limit urinary tract infection. Cell Rep. 43, 114007. doi: 10.1016/j.celrep.2024.114007
Sedin, J., Giraud, A., Steinr, E. S. E., Ahl, D., Persson, A. E. G., Melican, K., et al. (2019). High resolution intravital imaging of the renal immune response to injury and infection in mice. Front. Immunol. 10. doi: 10.3389/fimmu.2019.02744
Segal, A. W. (2005). How neutrophils kill microbes. Annu. Rev. Immunol. 23, 197–223. doi: 10.1146/annurev.immunol.23.021704.115653
Seifert, M., Küppers, R. (2016). Human memory B cells. Leukemia. 30 (12), 2283–2292. doi: 10.1038/leu.2016.226
Shelburne, C. P., Nakano, H., St. John, A. L., l., C., McLachlan, J. B., Gunn, M. D., et al. (2009). Mast cells augment adaptive immunity by orchestrating dendritic cell trafficking through infected tissues. Cell Host Microbe , 6, 331–342. doi: 10.1016/j.chom.2009.09.004
Sheshachalam, A., Srivastava, N., Mitchell, T., Lacy, P., Eitzen, G. (2014). Granule protein processing and regulated secretion in neutrophils. Front. Immunol. 5. doi: 10.3389/fimmu.2014.00448
Siebenhaar, F., Syska, W., Weller, K., Magerl, M., Zuberbier, T., Metz, M., et al. (2007). Control of pseudomonas aeruginosa skin infections in mice is mast cell-dependent. Am. J. Pathol. 170, 1910–1916. doi: 10.2353/ajpath.2007.060770
Song, J., Deng, T. (2020). The adipocyte and adaptive immunity. Front. Immunol. , 11. doi: 10.3389/fimmu.2020.593058
Stark, A. K., Chandra, A., Chakraborty, K., Alam, R., Carbonaro, V., Clark, J., et al. (2018). PI3Kδ hyper-activation promotes development of B cells that exacerbate Streptococcus pneumoniae infection in an antibody-independent manner. Nat. Commun. 9, 3174. doi: 10.1038/s41467-018-05674-8
Suchanek, O., Ferdinand, J. R., Tuong, Z. K., Wijeyesinghe, S., Chandra, A., Clauder, A., et al. (2023). Tissue-resident B cells orchestrate macrophage polarisation and function. Nat. Commun. , 14, 7081. doi: 10.1038/s41467-023-42625-4
Svensson, M., Yadav, M., Holmqvist, B., Lutay, N., Svanborg, C., Godaly, G. (2011). Acute pyelonephritis and renal scarring are caused by dysfunctional innate immunity in mCxcr2 heterozygous mice. Kidney Int. , 80, 1064–1072. doi: 10.1038/ki.2011.257
Taganna, J., de Boer, A. R., Wuhrer, M., Bouckaert, J. (2011). Glycosylation changes as important factors for the susceptibility to urinary tract infection. Biochem. Soc. Trans. , 39, 349–354. doi: 10.1042/Bst0390349
Terlizzi, M. E., Gribaudo, G., Maffei, M. E. (2017). UroPathogenic escherichia coli (UPEC) infections: virulence factors, bladder responses, antibiotic, and non-antibiotic antimicrobial strategies. Front. Microbiol. , 8. doi: 10.3389/fmicb.2017.01566
Terpstra, M. L., Remmerswaal, E. B., van der Bom-Baylon, N. D., Sinnige, M. J., Kers, J., van Aalderen, M. C., et al. (2020). Tissue-resident mucosal-associated invariant T (MAIT) cells in the human kidney represent a functionally distinct subset. Eur. J. Immunol. , 50, 1783–1797. doi: 10.1002/eji.202048644
Tsou, C., Peters, W., Yue, S., Slaymaker, S., Aslanian, A. M., Weisberg, S. P., et al. (2007). Critical roles for CCR2 and MCP-3 in monocyte mobilization from bone marrow and recruitment to inflammatory sites. J. Clin. Invest. 117, 902–909. doi: 10.1172/jci29919
van der Putten, C., Remmerswaal, E. B. M., Terpstra, M. L., van der Bom, N. D., Kers, J., Ten Berge, I. J. M., et al. (2021). CD8 and CD4 T cell populations in human kidneys. Cells 10, 288. doi: 10.3390/cells10020288
Vivier, E., Artis, D., Colonna, M., Diefenbach, A., Di Santo, J. P., Eberl, G., et al. (2018). Innate lymphoid cells: 10 years on. Cell 174, 1054–1066. doi: 10.1016/j.cell.2018.07.017
Wang, N., Liang, H. W., Zen, K. (2014). Molecular mechanisms that influence the macrophage M1-M2 polarization balance. Front. Immunol. 5. doi: 10.3389/fimmu.2014.00614
Weisheit, C. K., Engel, D. R., Kurts, C. (2015). Dendritic cells and macrophages: sentinels in the kidney. Clin. J. Am. Soc. Nephrol. 10, 1841–1851. doi: 10.2215/Cjn.07100714
Winder, M., Tobin, G., Zupancic, D., Romih, R. (2014). Signalling molecules in the urothelium. BioMed. Res. Int. 2014, 1–14. doi: 10.1155/2014/297295
Wu, J. X., Hayes, B. W., Phoenix, C., Macias, G. S., Miao, Y. X., Choi, H. W., et al. (2020). A highly polarized T2 bladder response to infection promotes epithelial repair at the expense of preventing new infections. Nat. Immunol. 21, 671–683. doi: 10.1038/s41590-020-0688-3
Wu, J. X., Miao, Y. X., Abraham, S. N. (2017). The multiple antibacterial activities of the bladder epithelium. Ann. Trans. Med. 5, 35. doi: 10.21037/atm.2016.12.71
Yipp, B. G., Petri, B., Salina, D., Jenne, C. N., Scott, B. N. V., Zbytnuik, L. D., et al. (2012). Infection-induced NETosis is a dynamic process involving neutrophil multitasking. Nat. Med. 18, 1386–1393. doi: 10.1038/nm.2847
Yu, Z., Liao, J., Chen, Y., Zou, C., Zhang, H., Cheng, J., et al. (2019). Single-cell transcriptomic map of the human and mouse bladders. J. Am. Soc. Nephrol. 30, 2159–2176. doi: 10.1681/ASN.2019040335
Yu, L., O’Brien, V. P., Livny, J., Dorsey, D., Bandyopadhyay, N., Colonna, M., et al. (2019). Mucosal infection rewires TNFα signaling dynamics to skew susceptibility to recurrence. eLife , 8, e46677. doi: 10.7554/eLife.46677
Zetter, M., Barrios-Payan, J., Mata-Espinosa, D., Marquina-Castillo, B., Quintanar-Stephano, A., Hernandez-Pando, R. (2019). Involvement of vasopressin in the pathogenesis of pulmonary tuberculosis: A new therapeutic target? Front. Endocrinol. (Lausanne) 10. doi: 10.3389/fendo.2019.00351
Keywords: urinary tract infection, Uropathogenic Escherichia coli, immune mechanism, urothelial cell, adaptive immunity, innate immunity
Citation: Hou Y, Lv Z, Hu Q, Zhu A and Niu H (2025) The immune mechanisms of the urinary tract against infections. Front. Cell. Infect. Microbiol. 15:1540149. doi: 10.3389/fcimb.2025.1540149
Received: 05 December 2024; Accepted: 28 March 2025;
Published: 16 April 2025.
Edited by:
Diya Binoy Joseph, Institute for Stem Cell Science and Regenerative Medicine (inStem), IndiaReviewed by:
Viplov Kumar Biswas, University of Maryland, College Park, United StatesBrahmchetna Bedi, Emory University, United States
Copyright © 2025 Hou, Lv, Hu, Zhu and Niu. This is an open-access article distributed under the terms of the Creative Commons Attribution License (CC BY). The use, distribution or reproduction in other forums is permitted, provided the original author(s) and the copyright owner(s) are credited and that the original publication in this journal is cited, in accordance with accepted academic practice. No use, distribution or reproduction is permitted which does not comply with these terms.
*Correspondence: Hongxia Niu, bml1aG9uZ3hpYTE5ODVAMTYzLmNvbQ==