- 1Department of Radiobiology and Molecular Genetics, VINČA Institute of Nuclear Sciences - National Institute of the Republic of Serbia, University of Belgrade, Belgrade, Serbia
- 2University Clinical-Hospital Centre Zemun-Belgrade, Clinic of Internal Medicine, Department of Endocrinology and Diabetes, School of Medicine, University of Belgrade, Belgrade, Serbia
- 3King Abdullah University of Science and Technology (KAUST), Computer, Electrical, and Mathematical Sciences and Engineering (CEMSE) Division, Computational Bioscience Research Center (CBRC), Thuwal, Saudi Arabia
Diabetes mellitus (DM) is on the rise, necessitating the development of novel therapeutic and preventive strategies to mitigate the disease’s debilitating effects. Diabetic cardiomyopathy (DCMP) is among the leading causes of morbidity and mortality in diabetic patients globally. DCMP manifests as cardiomyocyte hypertrophy, apoptosis, and myocardial interstitial fibrosis before progressing to heart failure. Evidence suggests that non-coding RNAs, such as long non-coding RNAs (lncRNAs) and microRNAs (miRNAs), regulate diabetic cardiomyopathy-related processes such as insulin resistance, cardiomyocyte apoptosis and inflammation, emphasizing their heart-protective effects. This paper reviewed the literature data from animal and human studies on the non-trivial roles of miRNAs and lncRNAs in the context of DCMP in diabetes and demonstrated their future potential in DCMP treatment in diabetic patients.
1 Introduction
1.1 Diabetes mellitus and diabetes-related cardiomyopathy
Diabetes mellitus (DM) is a group of metabolic disorders characterized by chronic hyperglycemia and perturbed metabolism of carbohydrates, lipids, and proteins, resulting from defects in insulin secretion and action. An estimated 9.3% of the world population (463 million aged 20-79 years) is affected by DM, and this number is projected to reach 10.9% (700 million people) by 2045 (1). Macrovascular complications, such as coronary artery disease (CAD) and ischemic cardiomyopathy, are the leading causes of cardiac death in DM patients. In addition, DM raises the risk of heart failure (HF) and cardiac dysfunction unaided by other risk factors, such as CAD and hypertension (2). Also, microvascular disease and cardiac capillary rarefaction contribute to severe cardiovascular morbidity and mortality in DM patients (3, 4). Diabetes-related cardiomyopathy (DCMP) represents DM-induced morphofunctional cardiac abnormality after the presence of valvular, atherosclerotic, congenital, or hypertensive heart disease is excluded (5–7). Clinically, DCMP can be presented as two distinctive phenotypes, restrictive (heart failure with preserved ejection fraction, HFpEF) and dilated (heart failure with reduced ejection fraction, HFrEF) (8, 9). Transitioning from HFpEF to HFrEF is not mandatory (3, 9). In HFpEF and HFrEF, the presence of DM increases the risk of hospitalization for HF or even death (10, 11). However, the difficulty in identifying HF is due to the asymptomatic presentation in the early stages of DCMP (12). Furthermore, DCMP worsens DM patients’ prognoses and raises their chance for overt HF (13, 14).
The present challenges in the definitive diagnosis of DCMP are the absence of specific circulating or histological biomarkers of the disease (3, 15, 16) and insufficient guidance for managing patients suffering from both DM and HF (15, 17). Currently, DCMP diagnosis is most widely determined using echocardiography; to detect changes in the myocardium structure and function (9, 18–20). However, due to its economic costs, it is not well-suited for routine screening of DCMP. Thus, there is an urgent need to identify and develop novel blood-based biomarkers to identify patients with an increased risk of developing DCMP (18).
1.2 Role of non-coding RNAs in DCMP
Dysregulation of long non-coding RNA (lncRNA) and microRNA (miRNA) regulatory networks is emerging as an important mechanism in the pathophysiology of DCMP (3, 21–23). miRNAs are small, non-coding RNAs (ncRNA) that regulate the expression of numerous genes involved in physiological processes such as metabolism, apoptosis, differentiation, and cell proliferation. Increasing evidence points to miRNAs’ role in the regulation of pathophysiological alterations associated with DCMP, such as cardiac hypertrophy (24), myocardial fibrosis (25), oxidative stress (OS) and apoptosis (26), mitochondrial dysfunction (27), epigenetic modification (28), cardiac electrical remodeling (29). lncRNAs are long, non-translated transcripts with more than 200 nucleotides involved in regulating the activity and abundance of miRNAs through base-pairing interactions (22, 30). lncRNAs mediate numerous physiological processes, such as transcription regulation, RNA splicing, nuclear architecture and compartmentalization, and nuclear-cytoplasmic trafficking (31–34). Recent reports implicate the role of lncRNA in DM pathogenesis and associated cardiovascular complications, such as DCMP (35, 36).
In this review, we provide a systematic overview of DCMP pathogenesis and progression, focusing on the specific roles of miRNAs and lncRNAs in the pathophysiology of DCMP. Also, we discuss novel approaches based on the use of miRNAs and lncRNAs as targets for potential therapeutic interventions.
2 Diabetic cardiomyopathy: Pathogenesis, disease progression, and clinical presentation
2.1 DCMP pathogenesis
The pathogenesis of DCMP is based on joined metabolic conditions (hyperglycemia, hyperinsulinemia, and dyslipidemia) that promote OS, inflammation, the formation and deposition of advanced glycation end products (AGEs), damage and dysfunction of mitochondria, unbalanced Ca2+ homeostasis, endoplasmic reticulum stress (ERS), autonomic neuropathy, the renin-angiotensin system (RAS) activation, microvascular myocardial rarefaction, changes in gene regulation (microRNAs), and cardiomyocyte apoptosis (2, 5, 16, 37–39).
Both types of DM are characterized by decreased insulin signaling and changes in other signaling cascades, such as reduced AMPK and increased PKC and MAPK signaling, with resultant deleterious and maladaptive effects (3). DCMP’s clinical presentation may be preceded by myocardial structure changes and disturbed Ca2+ signaling and metabolism (2, 4, 7, 22). The myocardial structure changes, i.e., myocardial fibrosis, are favoured by increased collagen deposition and variations in extracellular matrix (ECM) protein structure (40). The imbalance between profibrotic factors, such as connective tissue growth factor and transforming growth factor β1, and the inactivity of the ECM-degrading enzyme metalloproteinase can lead to ECM accumulation (41, 42). Among numerous mechanisms that favour DM-induced cardiac fibrosis, the intriguing one is the endothelial-to-mesenchymal transition (EndMT). EndMT is known to be promoted by hyperglycemic conditions, and it evolves gradually, acquiring a fibroblastic phenotype while simultaneously losing the original phenotype of the endothelial cells (ECs). This phenotypic change is accompanied by a progressive decline in EC activity and the cells’ mesenchymal characteristics, such as increased ECM protein production, becoming more pronounced. In injured tissue, the EndMT-derived cells act as immature fibroblasts and promote the fibrosis process (43).
Cardiomyocytes with abnormal metabolism are susceptible to increased free fatty acid (FFA) uptake and oxidation. Increased lipids may promote cardiomyocyte death induced by lipotoxicity due to limited FFA oxidation (3, 44). In addition, reactive oxygen species (ROS) and reactive nitrogen species (RNS) are produced more frequently as a result of increased intracellular fatty acid content and mitochondrial malfunction, which in turn increases OS and ERS and inhibits autophagy (20, 45, 46). The interaction of these effects causes ECM remodeling and fibrosis, along with cardiomyocyte loss, cardiac enlargement, and inflammation (47). Heart stiffness, poor cardiac relaxation, and diastolic dysfunction are early signs of DCMP caused by pathophysiological anomalies (46). In addition, accumulated lipids in ECs may decrease nitric oxide (NO) bioavailability, promoting endothelial dysfunction and accelerating atherosclerosis (9).
Diastolic or systolic dysfunction is encouraged by left ventricular (LV) hypertrophy and perivascular and interstitial cardiac fibrosis (3, 48). On echocardiograms, LV hypertrophy presents increased thickness in the posterior and septal walls (49). Myocyte hypertrophy, thickening of the myocardial capillary basement membrane, and increased interstitial and perivascular fibrosis are confounding factors contributing to the development of LV hypertrophy (50, 51).
2.2 Natural course and diagnostic management of DCMP
DCMP occurs in approximately 12% of patients with DM (52). The prevalence of HF varies between 19 and 26% in both types of DM (3, 4). The link between type 2 DM (DMT2) and HF is bidirectional: HF is highly prevalent in DMT2 patients, and HF increases the risk of DMT2 (3, 53, 54). Sometimes, HF is the first cardiovascular presentation in patients with DMT2 (9, 55). In DM patients, the risk of a negative HF outcome is greater (56). The risks of HF in diabetic patients are in close relation to the quality of retrograde glycemic control, as the patients with type 1 (DMT1) and DMT2 have a 30% and 8% increase in HF risk for each 1% increase in glycated haemoglobin (HbA1c) level, respectively (3, 57).
DCMP’s natural course is determined by phenotype. In DCMP with HFpEF phenotype, the LV is hypertrophied, stiff, and of normal size. In DCMP with HFrEF phenotype, the LV is dilated with reduced ejection fraction (9, 58). In humans, diastolic dysfunction almost always precedes the development of systolic dysfunction (59, 60). Metabolic abnormalities in DMT2 predispose to the development of HFpEF DCMP, while the autoimmune abnormalities in DMT1 favour HFrEF DCMP (9).
At present, echocardiography represents an indicative diagnostic tool for assessing a patient with suspected DCMP (16, 61). The first echocardiographic signs of DCMP are LV diastolic dysfunction and mechanical changes leading to HFpEF and, ultimately, HFrEF (19). In the early stage or the restrictive HFpEF form, the echo findings show normal LV diameters and volumes with concentric hypertrophy, preserved systolic function (EF ≥50%), and indications of diastolic dysfunction (9). Systolic dysfunction is a later manifestation, sometimes misdiagnosed using standard two-dimensional echocardiography (2). Rarely, T1 cardiac MRI mapping is an initial diagnostic procedure in detecting DCMP, as myocardial ECM in DM patients and non-DM controls exhibit significant differences (62). In addition, the increased levels of natriuretic peptide, inflammatory markers, and cardiac fibrosis markers are linked to diastolic dysfunction in DCMP (16, 63, 64). In the advanced stages of DCMP, or the dilated/HFrEF form, systolic dysfunction (ejection fraction <50%) occurs and an increase in LV volume (9).
Continuous inflammatory stimulation appears to be one of the most critical factors of DM pathogenesis (65). In the acute phase of inflammation, cytokines and acute-phase proteins (APPs) mitigate the effects of transient inflammatory processes (66, 67). However, prolonged inflammation results in a chronic condition where immune response leads to tissue damage contributing to the pathogenesis of many diseases, including atherosclerosis, cardiomyopathy, and DM (68). Nevertheless, diagnostics of DCMP based on measurements of circulating markers of inflammation, such as complement compounds, C-reactive protein (CRP) and alpha-macroglobulin (α2M), and amyloid A and P, is not sufficiently reliable, thus requiring identification of more specific biomarkers that enable early detection of DCMP (18, 68).
2.2.1 miRNAs and lncRNAs as potential biomarkers for DCMP
Circulating miRNAs and lncRNAs have been recently proposed as novel type of biomarkers for the diagnosis of cardiovascular disease (CVD), primarily due to their involvement in epigenetic mechanisms that underpin the progression of cardiomyopathies (69–71). Crucial attributes that support their use as potential biomarkers are their abundance and long-term stability in various body fluids (72, 73). In recent years, mounting evidence based on observation of expression patterns of various miRNAs and lncRNAs using high-throughput sequencing methodologies points at their use as reliable and reproducible prognostic and diagnostic biomarkers for various diseases, including DCMP. For instance, numerous clinical and experimental studies proposed various circulating miRNAs as biomarkers for diabetes prognosis (74–76) and the diagnosis of myocardial infarction, cardiac hypertrophy, and myocardial fibrosis (77–80). Similarly, several lncRNAs have been reported to play a crucial role in cardiovascular complications of diabetes and were implicated as potential biomarkers for DCMP (23, 81–83). In the following sections of this review, we provide a more detailed overview of specific miRNAs and lncRNAs emerging as novel, reliable DCMP biomarkers, thus representing valuable addition to existing prognostic and diagnostic tools for DCMP.
2.4 Treatment of DCMP
Stringent control of DM and the treatment of HFpEF or HFrEF is the cornerstone of DCMP management. DCMP is not a rare cardiovascular complication of DM (16). Using tissue Doppler strain analysis and measurements of peak systolic velocity, almost every fifth patient with DM was diagnosed with systolic dysfunction after excluding CAD or hypertension (49). Novel oral agents currently used in DM management (i.e., sodium-glucose cotransporter 2 (SGLT2) inhibitors, glucagon-like peptide 1 receptor agonists (GLP1-RAs)) enable a reduction in hospitalization rates for HF in DM patients independently of the presence of HF at baseline (84, 85). SGLT2 inhibitors exert antioxidative, antiapoptotic, and anti-inflammatory effects and decelerate atherosclerosis (86).
3 miRNAs in diabetic cardiomyopathy
3.1 General characteristics of miRNA
miRNAs represent small (17-25 nucleotides), single-stranded non-coding RNA molecules that regulate gene expression (87). Theoretically, a single miRNA could bind to over 1000 target mRNAs, and various miRNAs could regulate the expression of the same target transcript (88, 89). Since each miRNA may target several mRNAs, it has been estimated that miRNAs may regulate the expression of up to 60% of protein-coding genes in humans (90). Until 2019, the miRBase database (miRBase Release 22.1, https://www.mirbase.org/) reported entries of 38 589 miRNAs in 271 species, including 2654 mature human miRNAs (91). Increasing evidence supports the significant roles of miRNAs in regulating the mechanisms responsible for the pathophysiology of numerous diseases, including cardiovascular diseases, obesity, different types of cancer, and diabetes (73, 92–98).
miRNAs biogenesis is a multistep process that starts with primary miRNA (pri-miRNA) transcription by RNA polymerases II and III in the nucleus, which is subsequently processed by the nuclear endoribonuclease DROSHA or by components of the splicing machinery (99) to approximately 70 nucleotides long precursor (pre-miRNA) molecules that are exported to the cytoplasm by exportin 5 and Ran-GTPase. Additional processing by type III endoribonuclease DICER associated with RNA-binding proteins yields mature double-stranded miRNAs. The guide strand of mature miRNAs associates with Argonaute (AGO) proteins or chaperones HSC70/HSP90 to form the minimal miRNA-induced silencing complex (miRISC) that binds to the target mRNA’s complementary sequences called miRNA response elements (MREs). MiRNAs mainly interact with the target mRNAs’ 3′ untranslated regions (UTR) to induce translational repression and mRNA deadenylation (100–102), but interactions of miRNAs with 5′ UTR, gene promoters, and coding sequences have also been observed (103). It is generally assumed that the interaction of miRNAs with coding regions and 5′ UTR silence gene expression (104, 105), while binding of miRNAs to promoter regions can trigger transcription (106).
3.2 Role of miRNAs in cardiomyocyte hypertrophy and myocardial apoptosis
3.2.1 miRNAs expression and glycemic status in DCMP
The involvement of miRNAs in DM-associated pathophysiological processes in the myocardium is supported by findings that more than 300 different miRNAs have altered expression in DCMP (23). Expression of numerous miRNAs influences cardiomyocyte survival by modulating response to OS and inflammation (107, 108). In addition, levels of different miRNAs correlate with glycemic status, i.e., ‘miRNAs’ synthesis is influenced by high glucose levels (109, 110). This effect is likely mediated by endonucleases DROSHA and DICER, which is supported by a recent study by Lam et al. demonstrating that high glucose reduces DROSHA protein levels (111). Also, Chavali et al. measured the levels of pro-inflammatory tumour necrosis factor-alpha (TNFα), anti-inflammatory interleukin-10 (IL-10), DICER, and miRNAs in hearts of Akita, a genetic mice model for diabetes, and C57BL/6J (WT). The study reported increased mRNA and DICER levels in Akita’s hearts compared to the wild-type ones (112). Subsequent miRNA array analysis showed significant downregulation of several miRNAs, including miR-872, miR-744, miR-542-3p, miR-500, miR-499, miR-494, miR-455, miR-451, miR-450, miR-433, miR-384-3p, miR-345-3p, miR-338, miR-148, miR-142-3p, miR-130, and let-7a. Only one miRNA, miR-295, was found to be upregulated (112), which is in agreement with data from Baseler et al. showing increased levels of miR-295 in DMT1 myocardium (113).
The development of DCMP depends on several mechanisms mediated by mitogen-activated protein kinase (MAPK)-mediated signaling pathways, including inflammation, OS, and extracellular fibrosis. Of particular importance is p38 MAPK which is activated during cardiomyocyte hypertrophy, apoptosis, inflammation, OS, and conditions of metabolic abnormalities (114–117). Increasing evidence demonstrates that p38 MAPK expression is perturbed in the heart in diabetic conditions and that inhibiting p38 MAPK activation with its inhibitor atorvastatin or in a transgenic animal model prevents DCMP development (118, 119). Furthermore, dysregulated miRNAs in the hearts of diabetic mice appear to be primarily associated with the MAPK signaling pathway. For instance, in vitro inhibition of p38 MAPK decreases miR-373 expression, and miR-373 was shown to be significantly downregulated in the cardiac tissue of diabetic mice. Additionally, experiments with rat cardiomyocytes exposed to high glucose in vitro and transfected by miR-373 show miR-373 overexpression accompanied by hypertrophy and decreased transcription factor MEF2C, suggesting that the MEF2C gene is the target of miR-373. Thus, p38 MAPK/miR-373/MEF2C was proposed as a regulatory pathway in glucose-dependent cardiomyocyte hypertrophy (Table 1) (120).
LV miRNA profiling, from streptozotocin-induced diabetic mice, with or without intensive glycaemic control by slow-release insulin implants, demonstrated differential expression of 316 miRNAs. Among the dysregulated miRNAs, downregulation of miR-1 and upregulation of miR-19b, miR-27a, miR-34a, miR-125b, miR-146a, miR-155, miR-210, miR-221 was significant (127). Surprisingly, most dysregulated miRNAs’ expression remained significantly altered after normalization of the glucose levels in diabetic mice. Ingenuity Pathway bioinformatic analysis shows the dysregulated miRNAs were involved in physiological processes such as hypertrophic growth (miR-212, miR-221, miR-125b, miR-29a, miR-214, miR-133a, miR-199a, miR-150, miR-1), apoptosis (miR-320b, miR-378, miR-34a), fibrosis (miR-125b, miR-150, miR-199a, miR-29b, miR30a) (Figure 1), OS (miR-155, miR-27a, miR-125b, miR-19b, miR-221, miR-210, miR-146a, miR-34a), autophagy (miR-133a, miR-221, miR-212, miR30a), and heart failure (miR-423, miR-499, miR-199a). Of particular importance is a set of downregulated miRNAs associated with OS. For instance, miR-221, upregulated in the diabetic myocardium, was suggested to have a key role in the progression of diabetic myocardial damage after restoring normoglycemia, whereas miR-34a may be responsible for cardiac ageing in DM (127). Normalization of glucose levels failed to restore the downregulated miR-1, whose dysregulation is associated with arrhythmias, myocardial hypertrophy, myocardial infarction, and cell reprogramming (126–128). Mir-1 directly targets junctin, a component of the ryanodine receptor Ca2 + release channel complex, and abolishes its expression (Table 1) (126). In high glucose conditions, decreased levels of miR-1 result in an elevated expression of junctin, which is associated with perturbed Ca2 + handling, consequently causing arrhythmia and cardiac hypertrophy (124, 125).
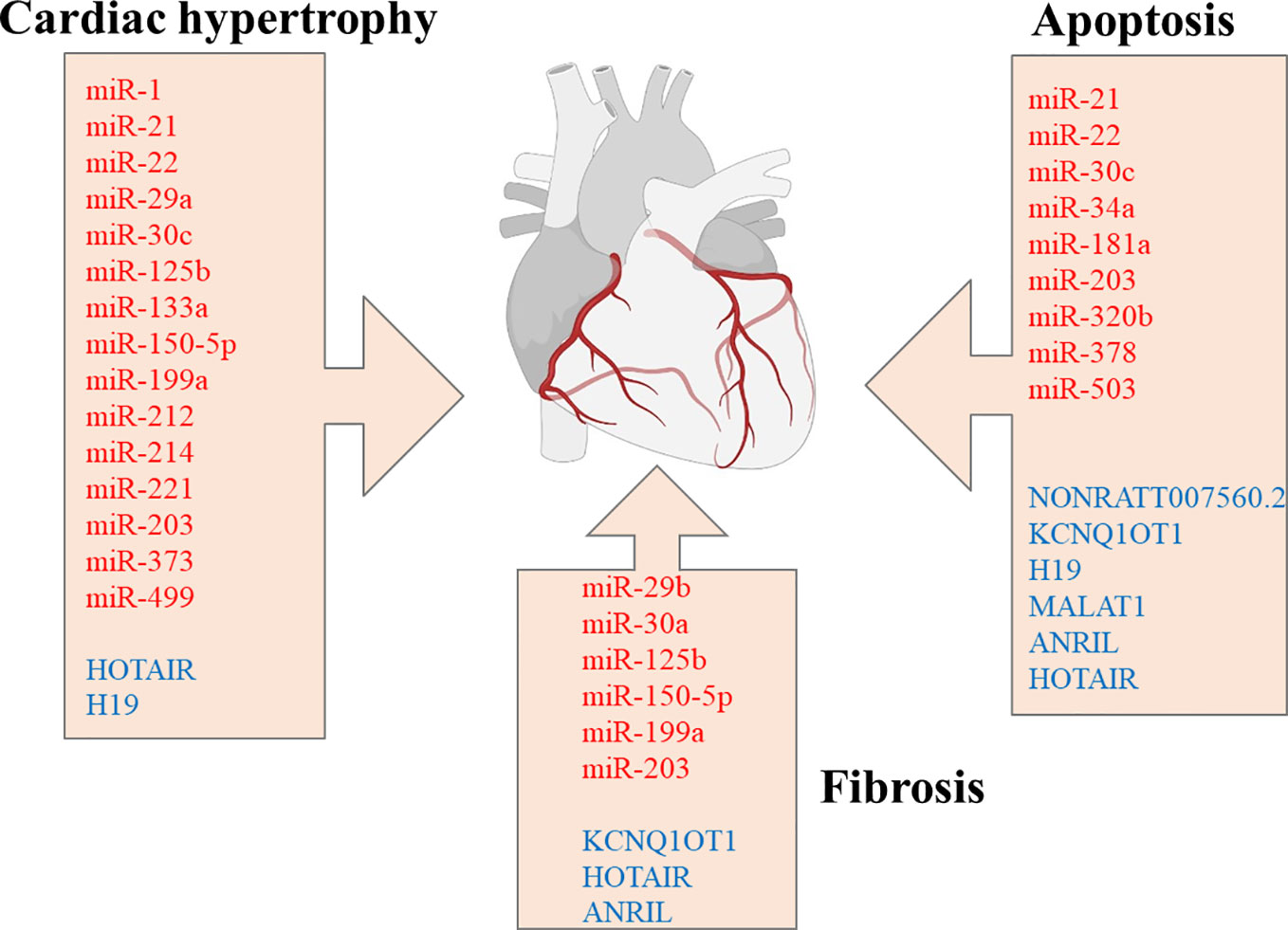
Figure 1 miRNAs and lncRNAs are implicated in regulating cardiac hypertrophy, apoptosis, and fibrosis. miRNAs are marked in red, whereas lncRNAs are marked in blue color. miRNAs, microRNAs; lncRNA, long non-coding RNAs. Created with Biorender.com.
The study by Constatino et al. suggests that failure to restore normal levels of dysregulated miRNAs in diabetic myocardium upon achieving normoglycemia may explain the progression of diabetic cardiovascular complications. It confirms the concept of metabolic memory, which was previously proposed but is insufficiently documented at the molecular level (150). Inhibition of OS-related miRNAs (miR-221, miR-210, miR-155, miR-146a, miR-125b, miR-34a, miR-27a, miR-19b) identified in this study may serve as a potential novel therapeutic strategy, leading to the amelioration of adverse effects of hyperglycaemic memory in diabetic myocardium.
3.2.2 miRNAs involvement in PPAR and Nrf signaling
Sufficient evidence supports the role of miRNAs in modulating cell response to OS, which plays a vital role in the progression of diabetic myocardial dysfunction (122, 150). The activation of Nrf2, a transcriptional factor acting as an essential regulator of OS genes, is increased in DM models due to excessive ROS accumulation (151). Also, transcriptional factors activated by fatty acids, such as PPARα, exhibit anti-inflammatory activity by decreasing the expression of pro-inflammatory genes (152). Several studies report synergistic action of Nrf2 and PPARα signaling pathways (153, 154), where PPARα pathway activation leads to Nrf2 activation via PGC-1α (155). Yin et al. reported that miR-30c has a protective role in diabetic cardiomyopathy via PPARα (122). miR-30c levels were downregulated in the T2D1 diabetic model leading to an increased expression of PGC-1β, a direct target of mir-30c, resulting in metabolic disturbances, cardiac lipotoxicity, and augmented ROS production (122). The overexpression of miR-30c reduced myocardial lipid accumulation and excessive ROS production, improved glucose utilization, and attenuated cardiomyocyte apoptosis and cardiac dysfunction in vitro and db/db mice (122). Another study reported that miR-30c overexpression in rat cardiomyocytes under high-glucose treatment was accompanied by the downregulation of prohypertrophic genes Cdc42 and Pak1, leading to cardiomyocyte hypertrophy attenuation (156). MiR-30c is also linked to the p53-p21 pathway involved in cardiomyocyte hypertrophy and apoptosis in DCM, and its effects may be amplified by miR-181a (121). Cardiomyocyte miR-30c overexpression in the DCM model led to an increased LV ejection fraction and reduced LV mass compared to controls (135). The attenuation of cardiac dysfunction by miR-30c overexpression suggests that miR-30c may be a potential therapeutic target for DCM treatment (122).
Regulation of PPARα and Nrf2 activation is also associated with miR-21 and LAZ3 gene, a transcriptional repressor that interferes with NF-κB signaling, thus regulating inflammation (132). LAZ3 expression is decreased in rat cardiomyocytes and diabetic mouse hearts (Gao, 157). LAZ3 silencing upregulates expression of miR-21, which targets PPARα, consequently downregulating PPARα and Nrf2 signaling pathway and promoting an inadequate response to the OS. Gao et al. proposed that treatments based on miR-21 inhibitors may positively affect DCMP management (Gao, 157). However, the results of other studies conflict with this conclusion and suggest that overexpression of miR-21 may be a promising therapeutic approach for the treatment of DCMP (131). It was found that miR-21 overexpression protects against ROS-induced damage in cardiac myocytes via another target gene, PDCD4, and in cardiac nonmyocyte cells such as fibroblasts, diminished miR-21 expression reduces abnormal heart remodeling (130, 158). Increased levels of cardiac OS biomarkers observed in cardiomyocytes of diabetic mice were significantly decreased upon miR-21 treatment and phospho-Akt and phospho-endothelial Nitric Oxide Synthase (eNOS) overexpression suggesting that miR-21 attenuates cardiac hypertrophy by reducing ROS levels and increasing available NO (131). It appears that miR-21 may have different roles in different cell types and pathophysiological conditions, requiring further studies on human subjects to explain the reported contradictory findings.
Perturbed levels of several other miRNAs in the diabetic myocardium, such as upregulation of miR-503 and downregulation of miR-22, were observed in vivo and in vitro DCMP models (Table 1). Those miRNAs are suggested to impair the ability of Nrf2 to prevent the adverse effects of excessive ROS accumulation observed in DM. miR-503 upregulation is associated with Nrf2 activation that can be further enhanced through the phase II enzyme inducer CPDT, an enzyme complex with a protective role against OS by promoting antioxidative ‘enzymes’ expression (129). Decreased expression of miR-503, accompanied by increased Nrf2 levels and reduced development of cardiomyopathy, was observed in diabetic rats treated with CPTD compared to a control group (129). In the case of miR-22, whose levels were decreased in the myocardium of streptozotocin-induced diabetic mice, it was reported to target 3’- untranslated repeats of Sirt1 and upregulate its expression (Table 1) (159). In a diabetic animal model, overexpression of miR-22 was associated with decreased ROS levels, elevated SOD, and amelioration of blood glucose levels, LV end-diastolic pressure, ejection fraction, and ‘cardiomyocytes’ apoptosis (129).
3.2.3 miRNAs-mediated modulation of PI3K/Akt and NF‐κB signaling pathways
PI3K/Akt signaling pathway has a crucial role in the pathogenesis of insulin resistance and DCMP development, regulating multiple physiological processes, such as cell growth, the proliferation of cardiomyocytes, and apoptosis (160). PI3KT/Akt regulates the nuclear factor-κB (NF‐κB) transcriptional activity that regulates cellular activities related to immune responses and inflammation (161). Also, PI3KT/Akt is involved in platelet activation, which is associated with TGF-β1 release that promotes atrial fibrosis in cell culture and ventricular fibrosis in a mouse model (162, 163). It was reported that upregulation of miR-203 inhibits activation of the PI3KT/Akt pathway by targeting PIK3CA and is associated with reduced cardiac hypertrophy, myocardial apoptosis, fibrosis (Figure 1), and levels of ROS in myocardial tissues of diabetic mice (Table 1) (123). Another study reported that NF‐κB activity and IL‐1β production are significantly increased in cardiac fibroblasts under high glucose conditions and are accompanied by upregulation of miR-150-5p, which negatively regulates Smad7 expression at the post-transcriptional level (137). Since Smad7 was shown to suppress TGF‐β1 signaling (164), miR-150-5p inhibition attenuates ‘cardiomyocytes’ fibrosis and inflammation mediated by NF‐κB and TGF‐β1/Smad pathways. In addition, miR‐150‐5p involvement in the inflammatory cytokine production, the development of T and B lymphocytes, and vascular remodeling and fibrosis are well established (Table 1) (133, 136, 165, 166). It was suggested that miR-150-5p should be considered a promising target for DMCP treatment since its knockdown reverses cardiac remodeling (23, 137).
4 lncRNAs RNA and DCM
4.1 General characteristics on lncRNAs
lncRNAs are heterogenous RNA transcripts with more than 200 nucleotides that are not translated into proteins (167) but can interact with DNA, RNA and proteins via base pairing or chemical interactions, thus exhibiting more versatile roles compared to miRNAs. RNA polymerase II transcribes lncRNAs from exonic, intergenic, or distal protein-coding regions of the genome into pre-mature lncRNAs that are polyadenylated at the 3’-end and capped on the 5’-end with methyl-guanosine (168). The precursor lncRNA undergoes alternative splicing either by interacting with specific splicing factors or forming RNA-RNA duplexes with pre-mRNA molecules (169). lncRNAs regulate gene expression at the transcriptional, translational and post-translational levels (30, 170) by binding to DNA-binding proteins (171), recruiting epigenetic complexes during DNA methylation (172), and serving as precursors of miRNAs (173). Their function depends on the cellular location; lncRNAs expressed in the nucleus regulate gene expression via recruitment of transcription factors or epigenetic complexes (174) whereas cytoplasmic lncRNAs participate in modulation of the mRNA stability and translation and post-translational modifications (175–177). lncRNAs are further classified as signal, guide, decoy, and scaffold lncRNAs depending on their cellular function. Signal lncRNAs respond to specific stimuli at distinct subcellular locations whereas guide lncRNAs direct ribonucleoprotein complexes to specific targets (33). Decoy lncRNAs bind and sequester regulatory proteins such as transcription factors (178), while scaffold lncRNAs have a structural role in chromatin organization as platforms for assembling ribonucleoprotein complexes (179). It has been estimated that the human genome contains over 16000 lncRNAs (Gencode-Human Release 27, https://www.gencodegenes.org/human/) (180). However, despite this remarkable number, the number of functional lncRNAs remains questionable, although they express valuable cellular properties (168, 181).
4.2 Roles of lncRNAs in diabetic cardiomyopathy
Although there are fewer reports in the literature regarding lncRNAs’ connection to DCMP compared to miRNAs, recent evidence strongly supports the equally important emerging role of lncRNAs in DCMP pathophysiology (Figure 1). Levels of several lncRNAs are perturbed in serum and myocardial biopsy of patients with DCMP (182, 183). For instance, the plasma level of the lncRNA, the steroid receptor RNA activator (SRA), is decreased in DM patients with CVDcompared to DM patients without any associated complications and healthy subjects. Furthermore, a 5-year follow-up study demonstrated that perturbed levels of SRA correlate with an increased incidence of cardiovascular disease in DM patients (184). Accumulating evidence shows that lncRNAs participate in the modulation of multiple pathways associated with OS and inflammation, which are implicated as important factors in DCMP development and progression, myocardial injury, cardiac hypertrophy, and diabetic vascular complications (142). HOX transcript antisense RNA (HOTAIR) has a crucial role in the CVD pathophysiology (185), and its expression is significantly downregulated in myocardial tissues and serum of patients with DCMP compared to DM patients and healthy controls (146). HOTAIR expression was also decreased in the hearts of streptozotocin-treated mice, whereas its overexpression decreased OS and inflammation and improved cardiac function (147). HOTAIR was reported to serve as a molecular sponge of miR‐34a, which targets Sirt1 (Table 1) (147, 186). HOTAIR was also shown to ameliorate DCMP by increasing the viability of cardiomyocytes via PI3K/Akt pathway activation (146).
In a study by Yu et al. (145), differentially expressed lncRNAs during cardiomyocytes’ OS and apoptosis induced by high glucose were identified by RNA sequencing. Consequent functional studies showed that inhibition of lncRNA NONRATT007560.2 reduces ROS generation and apoptosis, suggesting its important role in developing cardiomyopathy. In addition, it was observed that NON-RATT007560.2 have binding sites for miR-208a (145), which was previously associated with the perturbed cardiac remodeling in the myocardium of DMT2 patients (144). Xu et al. found that NONRATT021972 siRNA treatment of DM rats decreased the elevated TNF-α expression and abolished serine phosphorylation of IRS-1 in superior cervical ganglion cells, whereas downregulation of NONRATT021972 restored decreased heart rate variability in diabetic rats (Table 1) (143).
Another lncRNA, KCNQ1OT1, whose expression is increased in the serum of diabetic patients, as well as in high glucose-induced cardiomyocytes in vitro, and cardiac tissue of T1DM streptozotocin-induced diabetic mice, has been associated with pathophysiological mechanisms leading to cardiac dysfunction (138, 139, 183). A study by Coto et al. revealed that the increased levels of KCNQ1OT1 induce TGF-β1, p-Smad2 and p-Smad3 expression and are accompanied by collagen deposition, activation of fibrotic formation and cardiac remodeling, ultimately resulting in deterioration of LV function. The inhibition of KCNQ1OT1 expression significantly ameliorated cardiac function and reduced remodeling via TGF-β1/Smads pathway (138). Another study showed that KCNQ1OT1 silencing improves cardiac function by decreasing apoptosis via targeting miR-214-3p and caspase-1 gene, which leads to reduced cell death and abnormalities in cytoskeletal structure as decreased calcium overload (Table 1) (183).
lncRNA H19 also regulated cardiomyocyte apoptosis in diabetic cardiomyopathy (134). Li et al. reported that expression of H19 was significantly downregulated in the myocardium of diabetic rats, whereas its overexpression reduced OS, inflammation and apoptosis, leading to an improvement of LV function (134). In cultured cardiomyocytes transfected with H19 siRNA, decreased expression of H19-derived miR-675 was observed. VDAC1 gene, involved in cardiomyocyte apoptosis and the progression of cardiac muscle dysfunction, was identified as a target of H19/miR-675-mediated downregulation (134). Another study reported that overexpression of H19 epigenetically silences DIRAS3 (DIRAS Family GTPase 3), promotes mTOR (mammalian target of rapamycin) phosphorylation, and inhibits autophagy in cardiomyocytes exposed to high glucose (Table 1) (140).
lncRNAs are also implicated in cardiomyocyte injury via activation of NF-κB and TNF signaling pathways. In obesity, DM and other metabolic disorders, excessive amounts of saturated fatty acids, such as palmitic acid (PA), may be deposited in cardiomyocytes causing lipotoxic damage (2, 187). Upregulation of inflammatory factors TNFα and IL-1β and lncRNA metastasis-associated lung adenocarcinoma transcript 1 (MALAT1), which plays a crucial role in cardiomyocytes ischemia-reperfusion damage, was shown in PA-treated cardiomyocytes (82). MALAT1 knockdown increased the viability of PA-treated cardiomyocytes and reduced TNF-α, IL-1β, myocardial damage markers such as lactate dehydrogenase (LDH) and CK-MB, and apoptosis (142). MALAT1 specifically binds to miR-26a, inhibiting the inflammatory signaling pathway Toll-like receptor 4 (TLR4)/NF-κB by binding to its target gene, HMGB1. Thus, MALAT1 inhibition alleviates lipotoxic myocardial injury via the miR-26a/HMGB1/TLR4/NF-κB axis (142). Downregulation of MALAT-1 also reduces inflammation under high glucose conditions. A study by Puthanveetil et al. reports significant upregulation of MALAT1 in endothelial cells exposed to high glucose levels (141). Increased MALAT1 levels were associated with a parallel increase in TNF-α, interleukin 6 (IL-6) and serum amyloid antigen 3 (SAA3), an inflammatory ligand and target of MALAT1. These findings suggest that MALAT1 regulates glucose-induced upregulation of inflammatory mediators IL-6 and TNF-α by activating SAA3 (141).
The level of lncRNA Antisense Non-coding RNA in the INK4 Locus (ANRIL) is increased in peripheral venous blood from DMT2 patients with acute myocardial infarction (188). ANRIL was shown to regulate the expression of HBEGF and CDH5 genes involved in vascular permeability, leukocyte migration, and associated inflammation (148). ANRIL level is increased in the hearts of diabetic rats, and its silencing is associated with reduced levels of LDH, CK-MB, and inflammatory cytokines TNFα, IL-6, and IL-1β, suggesting that ANRIL inhibition improves cardiac function (Table 1) (149).
5 Therapeutic applications of lncRNAs
RNA-based therapies offer several significant advantages compared to other types of treatments: they allow simultaneous targeting of multiple protein-coding genes, restoration of homeostasis by fine-tuning of ncRNAs expression to their physiological concentrations, targeting of genes that are inaccessible to other therapeutic, and circumvention of drug resistance (189, 190). Manipulation of miRNA levels in vivo is achieved by two main strategies: restoration of downregulated miRNA levels by synthetic double-stranded miRNAs molecules called miRNA mimics or viral vectors expressing miRNA; inhibition of miRNAs activity by anti-miRNA antisense oligonucleotides (ASOs, antimiRs) or competitive miRNA inhibitors (Figure 2). miRNA mimics have the same sequence as an endogenous miRNA and may simultaneously target multiple mRNAs (191). So far, two miRNA mimics, miR-34 mimic MRX34 (192, 193) and the miR-16 mimic MesomiR-1 (194), have been tested in clinical trials for potential cancer treatment. Interestingly, as previously mentioned, miR-34 and mir-16 have been implicated in DCMP physiopathology.
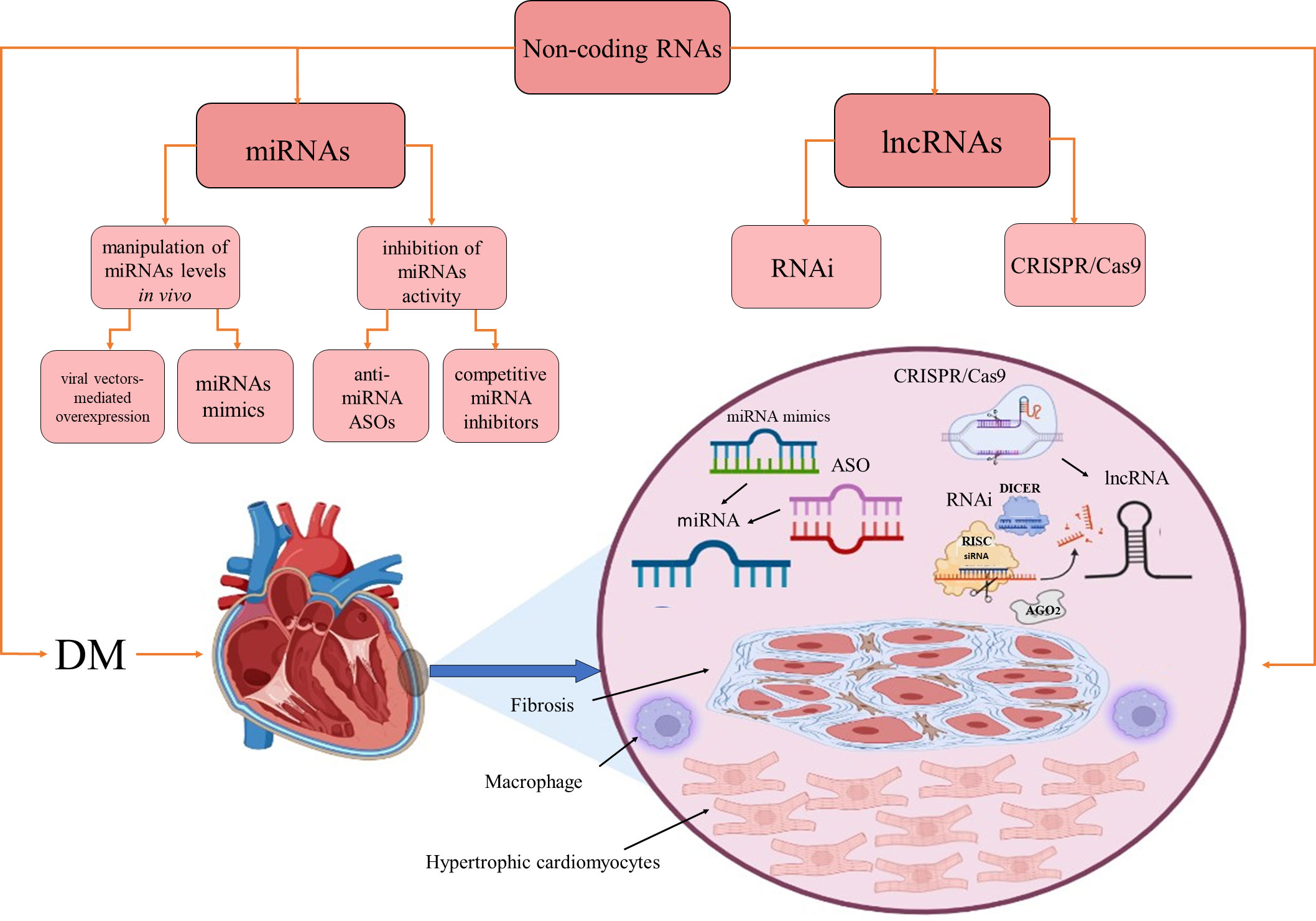
Figure 2 Therapeutic approaches based on miRNAs and lncRNAs. Non-coding RNAs as therapeutics in diabetes-induced cardiomyopathy. AGO2, Argonaute RISC Catalytic Component 2; ASO, antisense oligonucleotide; DM, diabetes mellitus; RISC, RNA-induced silencing complex; RNAi, RNA interference. Created with BioRender.com.
ASOs are single-stranded DNA molecules entirely complementary to one specific target mRNA and may act by arresting protein translation via steric hindrance, causing RNase H-mediated mRNA degradation or altering pre-mRNA splicing by interfering with cis-splicing (195–197). AntimiRs are ASOs with full or partial complementarity to an endogenous miRNA that prevents its interaction with the target genes. When antimiRs are conjugated to cholesterol for improved intracellular delivery, they are called antagomiRs (198). Two miR-122 antimiRs, miravirsen (SPC3649; β-D-oxy-LNA) and RG-101 (N-acetylgalactosamine-conjugated ASO), have been clinically tested in the context of the development of potential hepatitis C virus therapeutics (199). Anti-miR-92a (MRG-110) was clinically tested for its ability to promote angiogenesis and improve wound healing (197). It should be mentioned that the instability of RNA therapeutics, combined with their inability to cross cell membranes due to their negative charge, required various chemical modifications to improve their pharmacokinetics and pharmacodynamics properties (197, 200, 201). First-generation modifications improved stability by replacing phosphodiester with phosphorothioate (PT) backbone linkages. Second-generation modifications improved bioavailability while reducing toxicity and immunostimulation by replacement of the 2′-O-alkyl group of the sugar moieties with 2′-O-Me, 2′-MOE or 2′-F. Third-generation modifications are based on modifications of the furanose ring to create peptide nucleic acids (PNAs), locked nucleic acids (LNAs), and phosphoramidite morpholino oligomers (PMOs). All currently approved RNA therapeutics for clinical investigations have second or third-generation chemical modification (197).
Several antimiRs were tested in experimental animal models in the specific context of DCM. For instance, the administration of antagomiR-155 decreased cardiac infiltration of inflammatory mediators and ameliorated myocardial damage and overall cardiac function (202). However, it was observed that estrogen deficiency in DCM mice increased inflammation due to the excessive infiltration by pro-inflammatory M1 macrophages (203). Estrogen-dependent DCM aggravation was successfully prevented by treatment antagomiR-155 conjugated to gold nanoparticles, improving the heart’s structure and function. It was suggested that a therapeutic approach based on miR-155 inhibition might serve as a promising strategy for ameliorating cardiac function in DCM (203). Also, in the post-infarcted heart of a preclinical animal model, it was shown that an intracoronary injection of antagomiR-92 encapsulated in poly(lactic-co-glycolic acid) stimulated angiogenesis and improved myocardial function (204).
lncRNA-targeting therapeutics have recently become the focus of investigations, but so far, no such therapeutic has entered clinical trials. lncRNAs are currently extensively studied as clinical biomarkers for various diseases, but it could be envisioned that they may serve as novel targets for RNA interference (RNAi) and CRISPR/Cas9 gene-editing interventions. RNAi approach is based on the use of exogenous double-stranded small interfering RNA for specific knockdown of target RNAs by engaging a degradation pathway that involves DICER, a multiprotein RNA induced silencing complex (RISC) and the endonuclease AGO2 (205). Several lncRNAs were successfully knocked down using the RNAi in vitro. However, their silencing in vivo remains challenging, partly due to the lack of efficient delivery methods (190, 206). Clustered Regularly Interspaced Short Palindromic Repeats/associated protein-9 nuclease (CRISPR/Cas9) can be used for editing the whole human genome, including ncRNAs. CRISPR/Cas9 RNA-guided editing platform consists of a Cas9 nuclease that binds to a conserved sequence consisting of three nucleotides, called proto-adjacent motif (PAM), and a short CRISPR RNA (crRNA) that acts as a guide for Cas9, together with an adaptor trans-activating RNA (tracrRNA). The crRNA and tracrRNA can be fused to create the single-guide RNA that can direct Cas9 to any target in the proximity of the PAM sequence (207–209) and create a double-stranded DNA break. The CRISPR/Cas9 platform was used to target the expression of miRNAs implied in various pathophysiological conditions (73, 210, 211), but it can also be employed for lncRNA overexpression or transcriptional repression. CRISPR/Cas9 platform has been used for high-throughput profiling of lncRNAs associated with pathophysiological conditions, especially in oncology (212). Successful use of this editing platform for lncRNA manipulations may require targeting the lncRNA splice acceptor/donor sites (157, 213), precise delivery of CRISPR to specific tissues, and improved control of its off-target effects (214).
Conclusions
miRNA and lncRNA deregulation, in addition to their association with systemic and organ-specific inflammation (via interactions with PPAR and Nrf2, as well as PI3KT/Akt and NF-κB), qualify them as important DCMP diagnostic and treatment tools. Future extensive research must identify miRNAs and lncRNAs as biomarkers and therapeutic targets shared by different components of the metabolic disease cluster.
Author contributions
MM - wrote the article. ZG - wrote the article, JR - wrote the article, ME - wrote the article, XG- wrote the article, and EI - wrote and critically reviewed the article. All authors contributed to the article and approved the submitted version.
Funding
This work is part of the collaboration between the Department of Radiobiology and Molecular Genetics, “VINČA” Institute of Nuclear Sciences - National Institute of the Republic of Serbia, University of Belgrade, Belgrade, Serbia, Clinic for Internal Medicine, Department of Endocrinology and Diabetes, Zemun Clinical Hospital, School of Medicine, University of Belgrade, Belgrade, Serbia, and KAUST. The research was funded by the Ministry of Education, Science and Technological Development of the Republic of Serbia (Contract No# 451-03-47/2023-01/200017) and King Abdullah University of Science and Technology (KAUST) through grant awards Nos. BAS/1/1624-01-01, FCC/1/1976-20-01, FCC/1/1976-26-01, and Contract No#OSR 4129.
Conflict of interest
The authors declare that the research was conducted in the absence of any commercial or financial relationships that could be construed as a potential conflict of interest.
Publisher’s note
All claims expressed in this article are solely those of the authors and do not necessarily represent those of their affiliated organizations, or those of the publisher, the editors and the reviewers. Any product that may be evaluated in this article, or claim that may be made by its manufacturer, is not guaranteed or endorsed by the publisher.
References
1. Saeedi P, Petersohn I, Salpea P, Malanda B, Karuranga S, Unwin N, et al. Global and regional diabetes prevalence estimates for 2019 and projections for 2030 and 2045: Results from the international diabetes federation diabetes atlas, 9(th) edition. Diabetes Res Clin Pract (2019) 157:107843. doi: 10.1016/j.diabres.2019.107843
2. Boudina S, Abel ED. Diabetic cardiomyopathy, causes and effects. Rev Endocr Metab Disord (2010) 11:31–9. doi: 10.1007/s11154-010-9131-7
3. Jia G, Hill MA, Sowers JR. Diabetic cardiomyopathy: An update of mechanisms contributing to this clinical entity. Circ Res (2018) 122:624–38. doi: 10.1161/circresaha.117.311586
4. Dillmann WH. Diabetic cardiomyopathy. Circ Res (2019) 124:1160–2. doi: 10.1161/circresaha.118.314665
5. Borghetti G, von Lewinski D, Eaton DM, Sourij H, Houser SR, Wallner M. Diabetic cardiomyopathy: Current and future therapies. beyond glycemic control. Front Physiol (2018) 9:1514. doi: 10.3389/fphys.2018.01514
6. Seferović PM, Petrie MC, Filippatos GS, Anker SD, Rosano G, Bauersachs J, et al. Type 2 diabetes mellitus and heart failure: a position statement from the heart failure association of the European society of cardiology. Eur J Heart Fail (2018) 20:853–72. doi: 10.1002/ejhf.1170
7. Peng ML, Fu Y, Wu CW, Zhang Y, Ren H, Zhou SS. Signaling pathways related to oxidative stress in diabetic cardiomyopathy. Front Endocrinol (Lausanne) (2022) 13:907757. doi: 10.3389/fendo.2022.907757
8. Forbes JM, Cooper ME. Mechanisms of diabetic complications. Physiol Rev (2013) 93:137–88. doi: 10.1152/physrev.00045.2011
9. Seferović PM, Paulus WJ. Clinical diabetic cardiomyopathy: a two-faced disease with restrictive and dilated phenotypes. Eur Heart J (2015) 36:1718–27. doi: 10.1093/eurheartj/ehv134
10. MacDonald MR, Petrie MC, Varyani F, Ostergren J, Michelson EL, Young JB, et al. Impact of diabetes on outcomes in patients with low and preserved ejection fraction heart failure: an analysis of the candesartan in heart failure: Assessment of reduction in mortality and morbidity (CHARM) programme. Eur Heart J (2008) 29:1377–85. doi: 10.1093/eurheartj/ehn153
11. Cavender MA, Steg PG, Smith SC Jr., Eagle K, Ohman EM, Goto S, et al. Impact of diabetes mellitus on hospitalization for heart failure, cardiovascular events, and death: Outcomes at 4 years from the reduction of atherothrombosis for continued health (REACH) registry. Circulation (2015) 132:923–31. doi: 10.1161/circulationaha.114.014796
12. Zambroski CH, Moser DK, Bhat G, Ziegler C. Impact of symptom prevalence and symptom burden on quality of life in patients with heart failure. Eur J Cardiovasc Nurs (2005) 4:198–206. doi: 10.1016/j.ejcnurse.2005.03.010
13. Qazi MU, Malik S. Diabetes and cardiovascular disease: Original insights from the framingham heart study. Glob Heart (2013) 8:43–8. doi: 10.1016/j.gheart.2012.12.008
14. Marcinkiewicz A, Ostrowski S, Drzewoski J. Can the onset of heart failure be delayed by treating diabetic cardiomyopathy? Diabetol Metab Syndr (2017) 9:21. doi: 10.1186/s13098-017-0219-z
15. Lee MMY, McMurray JJV, Lorenzo-Almorós A, Kristensen SL, Sattar N, Jhund PS, et al. Diabetic cardiomyopathy. Heart (2019) 105:337–45. doi: 10.1136/heartjnl-2016-310342
16. Paolillo S, Marsico F, Prastaro M, Renga F, Esposito L, De Martino F, et al. Diabetic cardiomyopathy: Definition, diagnosis, and therapeutic implications. Heart Fail Clin (2019) 15:341–7. doi: 10.1016/j.hfc.2019.02.003
17. Dunlay SM, Givertz MM, Aguilar D, Allen LA, Chan M, Desai AS, et al. Type 2 diabetes mellitus and heart failure: A scientific statement from the American heart association and the heart failure society of America: This statement does not represent an update of the 2017 ACC/AHA/HFSA heart failure guideline update. Circulation (2019) 140:e294–324. doi: 10.1161/cir.0000000000000691
18. Lorenzo-Almorós A, Tuñón J, Orejas M, Cortés M, Egido J, Lorenzo Ó. Diagnostic approaches for diabetic cardiomyopathy. Cardiovascular Diabetology (2017) 16:28. doi: 10.1186/s12933-017-0506-x
19. Murtaza G, Virk HUH, Khalid M, Lavie CJ, Ventura H, Mukherjee D, et al. Diabetic cardiomyopathy - a comprehensive updated review. Prog Cardiovasc Dis (2019) 62:315–26. doi: 10.1016/j.pcad.2019.03.003
20. Tan Y, Zhang Z. Mechanisms of diabetic cardiomyopathy and potential therapeutic strategies: preclinical and clinical evidence. Nature Reviews Cardiology (2020) 17:585–607. doi: 10.1038/s41569-020-0339-2
21. De Rosa S, Arcidiacono B, Chiefari E, Brunetti A, Indolfi C, Foti DP. Type 2 diabetes mellitus and cardiovascular disease: Genetic and epigenetic links. Front Endocrinol (Lausanne) (2018) 9:2. doi: 10.3389/fendo.2018.00002
22. Pant T, Dhanasekaran A, Fang J, Bai X, Bosnjak ZJ, Liang M, et al. Current status and strategies of long noncoding RNA research for diabetic cardiomyopathy. BMC Cardiovascular Disorders (2018) 18:197. doi: 10.1186/s12872-018-0939-5
23. Jakubik D, Fitas A, Eyileten C. MicroRNAs and long non-coding RNAs in the pathophysiological processes of diabetic cardiomyopathy: emerging biomarkers and potential therapeutics. Cardiovascular Diabetology (2021) 20:55. doi: 10.1186/s12933-021-01245-2
24. Duan Y, Zhou B, Su H, Liu Y, Du C. miR-150 regulates high glucose-induced cardiomyocyte hypertrophy by targeting the transcriptional co-activator p300. Exp Cell Res (2013) 319:173–84. doi: 10.1016/j.yexcr.2012.11.015
25. Chen S, Puthanveetil P, Feng B, Matkovich SJ, Dorn GW, Chakrabarti S. Cardiac miR-133a overexpression prevents early cardiac fibrosis in diabetes. J Cell Mol Med (2014) 18:415–21. doi: 10.1111/jcmm.12218
26. Yu M, Liu Y, Zhang B, Shi Y, Cui L, Zhao X. Inhibiting microRNA-144 abates oxidative stress and reduces apoptosis in hearts of streptozotocin-induced diabetic mice. Cardiovasc Pathol (2015) 24:375–81. doi: 10.1016/j.carpath.2015.06.003
27. Das S, Ferlito M, Kent OA, Fox-Talbot K, Wang R, Liu D, et al. Nuclear miRNA regulates the mitochondrial genome in the heart. Circ Res (2012) 110:1596–603. doi: 10.1161/circresaha.112.267732
28. Chavali V, Tyagi SC, Mishra PK. MicroRNA-133a regulates DNA methylation in diabetic cardiomyocytes. Biochem Biophys Res Commun (2012) 425:668–72. doi: 10.1016/j.bbrc.2012.07.105
29. Panguluri SK, Tur J, Chapalamadugu KC, Katnik C, Cuevas J, Tipparaju SM. MicroRNA-301a mediated regulation of Kv4.2 in diabetes: identification of key modulators. PloS One (2013) 8:e60545. doi: 10.1371/journal.pone.0060545
30. Kopp F, Mendell JT. Functional classification and experimental dissection of long noncoding RNAs. Cell (2018) 172:393–407. doi: 10.1016/j.cell.2018.01.011
31. Mercer TR, Dinger ME, Mattick JS. Long non-coding RNAs: insights into functions. Nat Rev Genet (2009) 10:155–9. doi: 10.1038/nrg2521
32. Clark MB, Mattick JS. Long noncoding RNAs in cell biology. Semin Cell Dev Biol (2011) 22:366–76. doi: 10.1016/j.semcdb.2011.01.001
33. Wang KC, Chang HY. Molecular mechanisms of long noncoding RNAs. Mol Cell (2011) 43:904–14. doi: 10.1016/j.molcel.2011.08.018
34. Moran VA, Perera RJ, Khalil AM. Emerging functional and mechanistic paradigms of mammalian long non-coding RNAs. Nucleic Acids Res (2012) 40:6391–400. doi: 10.1093/nar/gks296
35. Uchida S, Dimmeler S. Long noncoding RNAs in cardiovascular diseases. Circ Res (2015) 116:737–50. doi: 10.1161/circresaha.116.302521
36. Leung A, Natarajan R. Long noncoding RNAs in diabetes and diabetic complications. Antioxid Redox Signal (2018) 29:1064–73. doi: 10.1089/ars.2017.7315
37. Bugger H, Abel ED. ). molecular mechanisms of diabetic cardiomyopathy. Diabetologia (2014) 57:660–71. doi: 10.1007/s00125-014-3171-6
38. Fuentes-Antrás J, Picatoste B, Gómez-Hernández A, Egido J, Tuñón J, Lorenzo Ó. Updating experimental models of diabetic cardiomyopathy. J Diabetes Res (2015) 2015:656795. doi: 10.1155/2015/656795
39. Lee WS, Kim J. Diabetic cardiomyopathy: where we are and where we are going. Korean J Intern Med (2017) 32:404–21. doi: 10.3904/kjim.2016.208
40. Tate M, Grieve DJ, Ritchie RH. Are targeted therapies for diabetic cardiomyopathy on the horizon? Clin Sci (Lond) (2017) 131:897–915. doi: 10.1042/cs20160491
41. Way KJ, Isshiki K, Suzuma K, Yokota T, Zvagelsky D, Schoen FJ, et al. Expression of connective tissue growth factor is increased in injured myocardium associated with protein kinase c beta2 activation and diabetes. Diabetes (2002) 51:2709–18. doi: 10.2337/diabetes.51.9.2709
42. Westermann D, Rutschow S, Jäger S, Linderer A, Anker S, Riad A, et al. Contributions of inflammation and cardiac matrix metalloproteinase activity to cardiac failure in diabetic cardiomyopathy: the role of angiotensin type 1 receptor antagonism. Diabetes (2007) 56:641–6. doi: 10.2337/db06-1163
43. Li A, Peng W, Xia X, Li R, Wang Y, Wei D. Endothelial-to-Mesenchymal transition: A potential mechanism for atherosclerosis plaque progression and destabilization. DNA Cell Biol (2017) 36:883–91. doi: 10.1089/dna.2017.3779
44. Nirengi S, Peres Valgas da Silva C, Stanford KI. Disruption of energy utilization in diabetic cardiomyopathy; a mini review. Curr Opin Pharmacol (2020) 54:82–90. doi: 10.1016/j.coph.2020.08.015
45. Bethel MA, Patel RA, Merrill P, Lokhnygina Y, Buse JB, Mentz RJ, et al. Cardiovascular outcomes with glucagon-like peptide-1 receptor agonists in patients with type 2 diabetes: a meta-analysis. Lancet Diabetes Endocrinol (2018) 6:105–13. doi: 10.1016/s2213-8587(17)30412-6
46. Dabravolski SA, Sadykhov NK, Kartuesov AG, Borisov EE, Sukhorukov VN. The role of mitochondrial abnormalities in diabetic cardiomyopathy. International Journal of Molecular Sciences (2022) 23:7863. doi: 10.3390/ijms23147863
47. Hölscher ME, Bode C, Bugger H. Diabetic cardiomyopathy: Does the type of diabetes matter? Int J Mol Sci (2016) 17:2136. doi: 10.3390/ijms17122136
48. Prandi FR, Evangelista I, Sergi D, Palazzuoli A, Romeo F. Mechanisms of cardiac dysfunction in diabetic cardiomyopathy: molecular abnormalities and phenotypical variants. Heart Fail Rev (2022). doi: 10.1007/s10741-021-10200-y
49. Eguchi K, Boden-Albala B, Jin Z, Rundek T, Sacco RL, Homma S, et al. Association between diabetes mellitus and left ventricular hypertrophy in a multiethnic population. Am J Cardiol (2008) 101:1787–91. doi: 10.1016/j.amjcard.2008.02.082
50. Voulgari C, Papadogiannis D, Tentolouris N. Diabetic cardiomyopathy: from the pathophysiology of the cardiac myocytes to current diagnosis and management strategies. Vasc Health Risk Manag (2010) 6:883–903. doi: 10.2147/vhrm.s11681
51. Velic A, Laturnus D, Chhoun J, Zheng S, Epstein P, Carlson E. Diabetic basement membrane thickening does not occur in myocardial capillaries of transgenic mice when metallothionein is overexpressed in cardiac myocytes. Anat Rec (Hoboken) (2013) 296:480–7. doi: 10.1002/ar.22646
52. Trachanas K, Sideris S, Aggeli C, Poulidakis E, Gatzoulis K, Tousoulis D, et al. Diabetic cardiomyopathy: from pathophysiology to treatment. Hellenic J Cardiol (2014) 55:411–21.
53. Cosentino F, Grant PJ, Aboyans V, Bailey CJ, Ceriello A, Delgado V, et al. 2019 ESC guidelines on diabetes, pre-diabetes, and cardiovascular diseases developed in collaboration with the EASD. Eur Heart J (2020) 41:255–323. doi: 10.1093/eurheartj/ehz486
54. Cai X, Liu X, Sun L, He Y, Zheng S, Zhang Y, et al. Prediabetes Risk Heart failure: A meta-analysis. Diabetes Obes Metab. (2021) 23:1746–53. doi: 10.1111/dom.14388
55. Birkeland KI, Bodegard J. Heart failure and chronic kidney disease manifestation and mortality risk associations in type 2 diabetes. A large multinational cohort study (2020) 22:1607–18. doi: 10.1111/dom.14074
56. Dei Cas A, Spigoni V, Ridolfi V, Metra M. Diabetes and chronic heart failure: from diabetic cardiomyopathy to therapeutic approach. Endocr Metab Immune Disord Drug Targets (2013) 13:38–50. doi: 10.2174/1871530311313010006
57. McMurray JJ, Gerstein HC, Holman RR, Pfeffer MA. Heart failure: a cardiovascular outcome in diabetes that can no longer be ignored. Lancet Diabetes Endocrinol (2014) 2:843–51. doi: 10.1016/s2213-8587(14)70031-2
58. van Heerebeek L, Hamdani N, Handoko ML, Falcao-Pires I, Musters RJ, Kupreishvili K, et al. Diastolic stiffness of the failing diabetic heart: importance of fibrosis, advanced glycation end products, and myocyte resting tension. Circulation (2008) 117:43–51. doi: 10.1161/circulationaha.107.728550
59. Shivalkar B, Dhondt D, Goovaerts I, Van Gaal L, Bartunek J, Van Crombrugge P, et al. Flow mediated dilatation and cardiac function in type 1 diabetes mellitus. Am J Cardiol (2006) 97:77–82. doi: 10.1016/j.amjcard.2005.07.111
60. Brooks BA, Franjic B, Ban CR, Swaraj K, Yue DK, Celermajer DS, et al. Diastolic dysfunction and abnormalities of the microcirculation in type 2 diabetes. Diabetes Obes Metab (2008) 10:739–46. doi: 10.1111/j.1463-1326.2007.00803.x
61. Paulus WJ, Tschöpe C, Sanderson JE, Rusconi C, Flachskampf FA, Rademakers FE, et al. How to diagnose diastolic heart failure: a consensus statement on the diagnosis of heart failure with normal left ventricular ejection fraction by the heart failure and echocardiography associations of the European society of cardiology. Eur Heart J (2007) 28:2539–50. doi: 10.1093/eurheartj/ehm037
62. Shang Y, Zhang X. Assessment of diabetic cardiomyopathy by cardiovascular magnetic resonance T1 mapping: Correlation with left-ventricular diastolic dysfunction and diabetic duration. Journal of Diabetes Research (2017) 2017:9584278. doi: 10.1155/2017/9584278
63. Ban CR, Twigg SM, Franjic B, Brooks BA, Celermajer D, Yue DK, et al. Serum MMP-7 is increased in diabetic renal disease and diabetic diastolic dysfunction. Diabetes Res Clin Pract (2010) 87:335–41. doi: 10.1016/j.diabres.2010.01.004
64. Shaver A, Nichols A, Thompson E, Mallick A, Payne K, Jones C, et al. Role of serum biomarkers in early detection of diabetic cardiomyopathy in the West Virginian population. Int J Med Sci (2016) 13:161–8. doi: 10.7150/ijms.14141
65. Tsalamandris S, Antonopoulos AS, Oikonomou E, Papamikroulis GA, Vogiatzi G, Papaioannou S, et al. The role of inflammation in diabetes: Current concepts and future perspectives. Eur Cardiol (2019) 14:50–9. doi: 10.15420/ecr.2018.33.1
66. Sevaljević L, Macvanin M, Zakula Z, Kanazir DT, Ribarac-Septić N. Adrenalectomy and dexamethasone treatment alter the patterns of basal and acute phase response-induced expression of acute phase protein genes in rat liver. J Steroid Biochem Mol Biol (1998) 66:347–53. doi: 10.1016/s0960-0760(98)00060-0
67. Sevaljević L, Isenović E, Vulović M, Macvanin M, Zakula Z, Kanazir D, et al. The responses of rat liver glucocorticoid receptors and genes for tyrosine aminotransferase, alpha-2-macroglobulin and gamma-fibrinogen to adrenalectomy-, dexamethasone- and inflammation-induced changes in the levels of glucocorticoids and proinflammatory cytokines. Biol Signals Recept (2001) 10:299–309. doi: 10.1159/000046897
68. Germolec DR, Shipkowski KA, Frawley RP, Evans E. Markers Inflammation. Methods Mol Biol (2018) 1803:57–79. doi: 10.1007/978-1-4939-8549-4_5
69. Scheuermann JC, Boyer LA. Getting to the heart of the matter: long non-coding RNAs in cardiac development and disease. EMBO J (2013) 32:1805–16. doi: 10.1038/emboj.2013.134
70. de Gonzalo-Calvo D, Kenneweg F, Bang C, Toro R, van der Meer RW, Rijzewijk LJ, et al. Circulating long-non coding RNAs as biomarkers of left ventricular diastolic function and remodelling in patients with well-controlled type 2 diabetes. Sci Rep (2016) 6:37354. doi: 10.1038/srep37354
71. Copier CU, León L, Fernández M, Contador D, Calligaris SD. Circulating miR-19b and miR-181b are potential biomarkers for diabetic cardiomyopathy. Sci Rep (2017) 7:13514. doi: 10.1038/s41598-017-13875-2
72. Pordzik J, Jakubik D, Jarosz-Popek J, Wicik Z, Eyileten C, De Rosa S, et al. Significance of circulating microRNAs in diabetes mellitus type 2 and platelet reactivity: bioinformatic analysis and review. Cardiovasc Diabetol (2019) 18:113. doi: 10.1186/s12933-019-0918-x
73. Macvanin M, Obradovic M, Zafirovic S, Stanimirovic J, Isenovic ER. The role of miRNAs in metabolic diseases. Curr Med Chem (2022) 30:1922–44. doi: 10.2174/0929867329666220801161536
74. Santovito D, De Nardis V, Marcantonio P, Mandolini C, Paganelli C, Vitale E, et al. Plasma exosome microRNA profiling unravels a new potential modulator of adiponectin pathway in diabetes: effect of glycemic control. J Clin Endocrinol Metab (2014) 99:E1681–1685. doi: 10.1210/jc.2013-3843
75. Zhu H, Leung SW. Identification of microRNA biomarkers in type 2 diabetes: a meta-analysis of controlled profiling studies. Diabetologia (2015) 58:900–11. doi: 10.1007/s00125-015-3510-2
76. Nunez Lopez YO, Garufi G, Seyhan AA. Altered levels of circulating cytokines and microRNAs in lean and obese individuals with prediabetes and type 2 diabetes. Mol Biosyst (2016) 13:106–21. doi: 10.1039/c6mb00596a
77. Matkovich SJ, Hu Y, Eschenbacher WH, Dorn LE, Dorn GW 2nd. Direct and indirect involvement of microRNA-499 in clinical and experimental cardiomyopathy. Circ Res (2012) 111:521–31. doi: 10.1161/circresaha.112.265736
78. Fang L, Ellims AH, Moore XL, White DA, Taylor AJ, Chin-Dusting J, et al. Circulating microRNAs as biomarkers for diffuse myocardial fibrosis in patients with hypertrophic cardiomyopathy. J Transl Med (2015) 13:314. doi: 10.1186/s12967-015-0672-0
79. Zhang L, Chen X, Su T, Li H, Huang Q, Wu D, et al. Circulating miR-499 are novel and sensitive biomarker of acute myocardial infarction. J Thorac Dis (2015) 7:303–8. doi: 10.3978/j.issn.2072-1439.2015.02.05
80. Gholamin S, Pasdar A, Khorrami MS, Mirzaei H, Mirzaei HR, Salehi R, et al. The potential for circulating microRNAs in the diagnosis of myocardial infarction: a novel approach to disease diagnosis and treatment. Curr Pharm Des (2016) 22:397–403. doi: 10.2174/1381612822666151112151924
81. De Rosa S, Chiefari E, Salerno N, Ventura V, D'Ascoli GL, Arcidiacono B, et al. HMGA1 is a novel candidate gene for myocardial infarction susceptibility. Int J Cardiol (2017) 227:331–4. doi: 10.1016/j.ijcard.2016.11.088
82. Zhao ZH, Hao W, Meng QT, Du XB, Lei SQ, Xia ZY. Long non-coding RNA MALAT1 functions as a mediator in cardioprotective effects of fentanyl in myocardial ischemia-reperfusion injury. Cell Biol Int (2017) 41:62–70. doi: 10.1002/cbin.10701
83. Wu QQ, Liu C, Cai Z, Xie Q, Hu T, Duan M, et al. High-mobility group AT-hook 1 promotes cardiac dysfunction in diabetic cardiomyopathy via autophagy inhibition. Cell Death Dis (2020) 11:160. doi: 10.1038/s41419-020-2316-4
84. Zinman B, Wanner C, Lachin JM, Fitchett D, Bluhmki E, Hantel S, et al. Empagliflozin, cardiovascular outcomes, and mortality in type 2 diabetes. N Engl J Med (2015) 373:2117–28. doi: 10.1056/NEJMoa1504720
85. Fitchett D, Zinman B, Wanner C, Lachin JM, Hantel S, Salsali A, et al. Heart failure outcomes with empagliflozin in patients with type 2 diabetes at high cardiovascular risk: results of the EMPA-REG OUTCOME® trial. Eur Heart J (2016) 37:1526–34. doi: 10.1093/eurheartj/ehv728
86. Tahara A, Kurosaki E, Yokono M, Yamajuku D, Kihara R, Hayashizaki Y, et al. Effects of SGLT2 selective inhibitor ipragliflozin on hyperglycemia, hyperlipidemia, hepatic steatosis, oxidative stress, inflammation, and obesity in type 2 diabetic mice. Eur J Pharmacol (2013) 715:246–55. doi: 10.1016/j.ejphar.2013.05.014
87. Bartel DP. MicroRNAs: target recognition and regulatory functions. Cell (2009) 136:215–33. doi: 10.1016/j.cell.2009.01.002
88. Selbach M, Schwanhäusser B, Thierfelder N, Fang Z, Khanin R, Rajewsky N. Widespread changes in protein synthesis induced by microRNAs. Nature (2008) 455:58–63. doi: 10.1038/nature07228
89. O'Brien J, Hayder H, Zayed Y, Peng C. Overview of MicroRNA biogenesis, mechanisms of actions, and circulation. Front Endocrinol (Lausanne) (2018) 9:402. doi: 10.3389/fendo.2018.00402
90. Friedman RC, Farh KK, Burge CB, Bartel DP. Most mammalian mRNAs are conserved targets of microRNAs. Genome Res (2009) 19:92–105. doi: 10.1101/gr.082701.108
91. Kozomara A, Birgaoanu M, Griffiths-Jones S. miRBase: from microRNA sequences to function. Nucleic Acids Res (2019) 47(D1):D155–D162. doi: 10.1093/nar/gky1141
92. Liu B, Li J, Cairns MJ. Identifying miRNAs, targets and functions. Brief Bioinform (2014) 15:1–19. doi: 10.1093/bib/bbs075
93. Peng Y, Croce CM. The role of MicroRNAs in human cancer. Signal Transduct Target Ther (2016) 1:15004. doi: 10.1038/sigtrans.2015.4
94. Samanta S, Balasubramanian S, Rajasingh S, Patel U, Dhanasekaran A, Dawn B, et al. MicroRNA: A new therapeutic strategy for cardiovascular diseases. Trends Cardiovasc Med (2016) 26:407–19. doi: 10.1016/j.tcm.2016.02.004
95. Kim M, Zhang X. The profiling and role of miRNAs in diabetes mellitus. J Diabetes Clin Res (2019) 1:5–23. doi: 10.33696/diabetes.1.003
96. Fu Z, Wang L, Li S, Chen F, Au-Yeung KK, Shi C. MicroRNA as an important target for anticancer drug development. Front Pharmacol (2021) 12:736323. doi: 10.3389/fphar.2021.736323
97. Pirrò S, Matic I, Colizzi V, Galgani A. The microRNA analysis portal is a next-generation tool for exploring and analyzing miRNA-focused data in the literature. Sci Rep (2021) 11:9007. doi: 10.1038/s41598-021-88617-6
98. Tülay Aydın P, Göz M, Kankılıç N. Micro-RNA gene expressions during cardiopulmonary bypass. Journal of Cardiac Surgery (2021) 36:921–7. doi: 10.1111/jocs.15329
99. Treiber T, Treiber N, Meister G. Regulation of microRNA biogenesis and its crosstalk with other cellular pathways. Nat Rev Mol Cell Biol (2019) 20:5–20. doi: 10.1038/s41580-018-0059-1
100. Huntzinger E, Izaurralde E. Gene silencing by microRNAs: contributions of translational repression and mRNA decay. Nat Rev Genet (2011) 12:99–110. doi: 10.1038/nrg2936
101. Ha M, Kim VN. Regulation of microRNA biogenesis. Nat Rev Mol Cell Biol (2014) 15:509–24. doi: 10.1038/nrm3838
102. Ipsaro JJ, Joshua-Tor L. From guide to target: molecular insights into eukaryotic RNA-interference machinery. Nat Struct Mol Biol (2015) 22:20–8. doi: 10.1038/nsmb.2931
103. Broughton JP, Lovci MT, Huang JL, Yeo GW, Pasquinelli AE. Pairing beyond the seed supports MicroRNA targeting specificity. Mol Cell (2016) 64:320–33. doi: 10.1016/j.molcel.2016.09.004
104. Forman JJ, Legesse-Miller A, Coller HA. A search for conserved sequences in coding regions reveals that the let-7 microRNA targets dicer within its coding sequence. Proc Natl Acad Sci U.S.A. (2008) 105:14879–84. doi: 10.1073/pnas.0803230105
105. Zhou H, Rigoutsos I. MiR-103a-3p targets the 5' UTR of GPRC5A in pancreatic cells. Rna (2014) 20:1431–9. doi: 10.1261/rna.045757.114
106. Zhang Y, Fan M, Zhang X, Huang F, Wu K, Zhang J, et al. Cellular microRNAs up-regulate transcription via interaction with promoter TATA-box motifs. Rna (2014) 20:1878–89. doi: 10.1261/rna.045633.114
107. Li W, Ouyang Z, Zhang Q, Wang L, Shen Y, Wu X, et al. SBF-1 exerts strong anticervical cancer effect through inducing endoplasmic reticulum stress-associated cell death via targeting sarco/endoplasmic reticulum Ca(2+)-ATPase 2. Cell Death Dis (2014) 5:e1581. doi: 10.1038/cddis.2014.538
108. Evangelista I, Nuti R, Picchioni T, Dotta F, Palazzuoli A. Molecular dysfunction and phenotypic derangement in diabetic cardiomyopathy. Int J Mol Sci (2019) 20:3264. doi: 10.3390/ijms20133264
109. Mononen N, Lyytikäinen L-P, Seppälä I, Mishra PP, Juonala M, Waldenberger M, et al. Whole blood microRNA levels associate with glycemic status and correlate with target mRNAs in pathways important to type 2 diabetes. Sci Rep (2019) 9:8887. doi: 10.1038/s41598-019-43793-4
110. Greco M, Chiefari E, Accattato F, Corigliano DM, Arcidiacono B, Mirabelli M, et al. MicroRNA-1281 as a novel circulating biomarker in patients with diabetic retinopathy. Front Endocrinol (Lausanne) (2020) 11:528. doi: 10.3389/fendo.2020.00528
111. Lam B, Nwadozi E. High glucose treatment limits drosha protein expression and alters AngiomiR maturation in microvascular primary endothelial cells via an Mdm2-dependent mechanism. (2021) 10:742. doi: 10.3390/cells10040742
112. Chavali V, Tyagi SC, Mishra PK. Differential expression of dicer, miRNAs, and inflammatory markers in diabetic Ins2+/- akita hearts. Cell Biochem Biophys (2014) 68:25–35. doi: 10.1007/s12013-013-9679-4
113. Baseler WA, Thapa D, Jagannathan R, Dabkowski ER, Croston TL, Hollander JM. miR-141 as a regulator of the mitochondrial phosphate carrier (Slc25a3) in the type 1 diabetic heart. Am J Physiol Cell Physiol (2012) 303:C1244–1251. doi: 10.1152/ajpcell.00137.2012
114. Liu Z, Cao W. p38 mitogen-activated protein kinase: a critical node linking insulin resistance and cardiovascular diseases in type 2 diabetes mellitus. Endocr Metab Immune Disord Drug Targets (2009) 9:38–46. doi: 10.2174/187153009787582397
115. Oh CC, Nguy MQ, Schwenke DC, Migrino RQ, Thornburg K, Reaven P. p38α mitogen-activated kinase mediates cardiomyocyte apoptosis induced by palmitate. Biochem Biophys Res Commun (2014) 450:628–33. doi: 10.1016/j.bbrc.2014.06.023
116. Liang Z, Leo S, Wen H, Ouyang M, Jiang W, Yang K. Triptolide improves systolic function and myocardial energy metabolism of diabetic cardiomyopathy in streptozotocin-induced diabetic rats. BMC Cardiovasc Disord (2015) 15:42. doi: 10.1186/s12872-015-0030-4
117. Ruiz M, Coderre L, Lachance D, Houde V, Martel C, Thompson Legault J, et al. MK2 deletion in mice prevents diabetes-induced perturbations in lipid metabolism and cardiac dysfunction. Diabetes (2016) 65:381–92. doi: 10.2337/db15-0238
118. Westermann D, Rutschow S, Van Linthout S, Linderer A, Bücker-Gärtner C, Sobirey M, et al. Inhibition of p38 mitogen-activated protein kinase attenuates left ventricular dysfunction by mediating pro-inflammatory cardiac cytokine levels in a mouse model of diabetes mellitus. Diabetologia (2006) 49:2507–13. doi: 10.1007/s00125-006-0385-2
119. Van Linthout S, Riad A, Dhayat N, Spillmann F, Du J, Dhayat S, et al. Anti-inflammatory effects of atorvastatin improve left ventricular function in experimental diabetic cardiomyopathy. Diabetologia (2007) 50:1977–86. doi: 10.1007/s00125-007-0719-8
120. Shen E, Diao X, Wang X, Chen R, Hu B. MicroRNAs involved in the mitogen-activated protein kinase cascades pathway during glucose-induced cardiomyocyte hypertrophy. Am J Pathol (2011) 179:639–50. doi: 10.1016/j.ajpath.2011.04.034
121. Raut SK, Singh GB, Rastogi B, Saikia UN, Mittal A, Dogra N, et al. miR-30c and miR-181a synergistically modulate p53-p21 pathway in diabetes induced cardiac hypertrophy. Mol Cell Biochem (2016) 417:191–203. doi: 10.1007/s11010-016-2729-7
122. Yin Z, Zhao Y, He M, Li H, Fan J, Nie X, et al. MiR-30c/PGC-1β protects against diabetic cardiomyopathy via PPARα. Cardiovasc Diabetol (2019) 18:7. doi: 10.1186/s12933-019-0811-7
123. Yang X, Li X, Lin Q, Xu Q. Up-regulation of microRNA-203 inhibits myocardial fibrosis and oxidative stress in mice with diabetic cardiomyopathy through the inhibition of PI3K/Akt signaling pathway via PIK3CA. Gene (2019) 715:143995. doi: 10.1016/j.gene.2019.143995
124. Kirchhefer U, Hanske G, Jones LR, Justus I, Kaestner L, Lipp P, et al. Overexpression of junctin causes adaptive changes in cardiac myocyte Ca(2+) signaling. Cell Calcium (2006) 39:131–42. doi: 10.1016/j.ceca.2005.10.004
125. Hong CS, Kwon SJ, Cho MC, Kwak YG, Ha KC, Hong B, et al. Overexpression of junctate induces cardiac hypertrophy and arrhythmia via altered calcium handling. J Mol Cell Cardiol (2008) 44:672–82. doi: 10.1016/j.yjmcc.2008.01.012
126. Yildirim SS, Akman D, Catalucci D, Turan B. Relationship between downregulation of miRNAs and increase of oxidative stress in the development of diabetic cardiac dysfunction: junctin as a target protein of miR-1. Cell Biochem Biophys (2013) 67:1397–408. doi: 10.1007/s12013-013-9672-y
127. Costantino S, Paneni F, Lüscher TF, Cosentino F. MicroRNA profiling unveils hyperglycaemic memory in the diabetic heart. Eur Heart J (2016) 37:572–6. doi: 10.1093/eurheartj/ehv599
128. Song Z, Gao R, Yan B. Potential roles of microRNA-1 and microRNA-133 in cardiovascular disease. Rev Cardiovasc Med (2020) 21:57–64. doi: 10.31083/j.rcm.2020.01.577
129. Miao Y, Wan Q. miR-503 is involved in the protective effect of phase II enzyme inducer (CPDT) in diabetic cardiomyopathy via Nrf2/ARE signaling pathway. BioMed Research International (2017) 2017:9167450. doi: 10.1155/2017/9167450
130. Ramanujam D, Sassi Y, Laggerbauer B, Engelhardt S. Viral vector-based targeting of miR-21 in cardiac nonmyocyte cells reduces pathologic remodeling of the heart. Mol Ther (2016) 24:1939–48. doi: 10.1038/mt.2016.166
131. Dai B, Li H, Fan J, Zhao Y, Yin Z, Nie X, et al. MiR-21 protected against diabetic cardiomyopathy induced diastolic dysfunction by targeting gelsolin. Cardiovasc Diabetol (2018) 17:123. doi: 10.1186/s12933-018-0767-z
132. Gao L, Liu Y, Guo S, Xiao L, Wu L, Wang Z, et al. LAZ3 protects cardiac remodeling in diabetic cardiomyopathy via regulating miR-21/PPARa signaling. Biochim Biophys Acta (BBA)-Molecular Basis Dis (2018) 1864:3322–38. doi: 10.1016/j.bbadis.2018.07.019
133. Li X, Kong M, Jiang D, Qian J, Duan Q, Dong A. MicroRNA-150 aggravates H2O2-induced cardiac myocyte injury by down-regulating c-myb gene. Acta Biochim Biophys Sin (Shanghai) (2013) 45:734–41. doi: 10.1093/abbs/gmt067
134. Li X, Wang H, Yao B, Xu W, Chen J, Zhou X. lncRNA H19/miR-675 axis regulates cardiomyocyte apoptosis by targeting VDAC1 in diabetic cardiomyopathy. Sci Rep (2016) 6:36340. doi: 10.1038/srep36340
135. Chen C, Yang S, Li H, Yin Z, Fan J, Zhao Y, et al. Mir30c is involved in diabetic cardiomyopathy through regulation of cardiac autophagy via BECN1. Mol Ther Nucleic Acids (2017) 7:127–39. doi: 10.1016/j.omtn.2017.03.005
136. Li Y, Ren W, Wang X, Yu X, Cui L, Li X, et al. MicroRNA-150 relieves vascular remodeling and fibrosis in hypoxia-induced pulmonary hypertension. BioMed Pharmacother (2019) 109:1740–9. doi: 10.1016/j.biopha.2018.11.058
137. Che H, Wang Y, Li Y, Lv J, Li H, Liu Y, et al. Inhibition of microRNA-150-5p alleviates cardiac inflammation and fibrosis via targeting Smad7 in high glucose-treated cardiac fibroblasts. Journal of Cellular Physiology (2020) 235:7769–79. doi: 10.1002/jcp.29386
138. Coto E, Calvo D, Reguero JR, Morís C, Rubín JM, Díaz-Corte C, et al. Differential methylation of lncRNA KCNQ1OT1 promoter polymorphism was associated with symptomatic cardiac long QT. Epigenomics (2017) 9:1049–57. doi: 10.2217/epi-2017-0024
139. Li X, Dai Y, Yan S, Shi Y, Han B, Li J, et al. Down-regulation of lncRNA KCNQ1OT1 protects against myocardial ischemia/reperfusion injury following acute myocardial infarction. Biochem Biophys Res Commun (2017) 491:1026–33. doi: 10.1016/j.bbrc.2017.08.005
140. Zhuo C, Jiang R, Lin X, Shao M. LncRNA H19 inhibits autophagy by epigenetically silencing of DIRAS3 in diabetic cardiomyopathy. Oncotarget (2017) 8:1429–37. doi: 10.18632/oncotarget.13637
141. Puthanveetil P, Chen S, Feng B, Gautam A, Chakrabarti S. Long non-coding RNA MALAT1 regulates hyperglycaemia induced inflammatory process in the endothelial cells. J Cell Mol Med (2015) 19:1418–25. doi: 10.1111/jcmm.12576
142. Jia P, Wu N, Jia D, Sun Y. Downregulation of MALAT1 alleviates saturated fatty acid-induced myocardial inflammatory injury via the miR-26a/HMGB1/TLR4/NF-κB axis. Diabetes Metab Syndr Obes (2019) 12:655–65. doi: 10.2147/dmso.s203151
143. Xu H, Liu C, Rao S, He L, Zhang T, Sun S, et al. LncRNA NONRATT021972 siRNA rescued decreased heart rate variability in diabetic rats in superior cervical ganglia. Auton Neurosci (2016) 201:1–7. doi: 10.1016/j.autneu.2016.07.012
144. Rawal S, Nagesh PT, Coffey S, Van Hout I, Galvin IF, Bunton RW, et al. Early dysregulation of cardiac-specific microRNA-208a is linked to maladaptive cardiac remodelling in diabetic myocardium. Cardiovascular Diabetology (2019) 18:13. doi: 10.1186/s12933-019-0814-4
145. Yu M, Shan X, Liu Y, Zhu J, Cao Q, Yang F, et al. RNA-Seq analysis and functional characterization revealed lncRNA NONRATT007560.2 regulated cardiomyocytes oxidative stress and apoptosis induced by high glucose. Journal of Cellular Biochemistry (2019) 120:18278–87. doi: 10.1002/jcb.29134
146. Qi K, Zhong J. LncRNA HOTAIR improves diabetic cardiomyopathy by increasing viability of cardiomyocytes through activation of the PI3K/Akt pathway. Exp Ther Med (2018) 16:4817–23. doi: 10.3892/etm.2018.6755
147. Gao L, Wang X, Guo S, Xiao L, Liang C, Wang Z, et al. LncRNA HOTAIR functions as a competing endogenous RNA to upregulate SIRT1 by sponging miR-34a in diabetic cardiomyopathy. Journal of Cellular Physiology (2019) 234:4944–58. doi: 10.1002/jcp.27296
148. Congrains A, Kamide K, Katsuya T, Yasuda O, Oguro R, Yamamoto K, et al. CVD-associated non-coding RNA, ANRIL, modulates expression of atherogenic pathways in VSMC. Biochem Biophys Res Commun (2012) 419:612–6. doi: 10.1016/j.bbrc.2012.02.050
149. Dai W, Lee D. Interfering with long chain noncoding RNA ANRIL expression reduces heart failure in rats with diabetes by inhibiting myocardial oxidative stress. Journal of Cellular Biochemistry (2019) 120:18446–56. doi: 10.1002/jcb.29162
150. Ceriello A. Hypothesis: the "metabolic memory", the new challenge of diabetes. Diabetes Res Clin Pract (2009) 86 Suppl 1:S2–6. doi: 10.1016/s0168-8227(09)70002-6
151. da Costa RM, Rodrigues D, Pereira CA, Silva JF, Alves JV, Lobato NS, et al. Nrf2 as a potential mediator of cardiovascular risk in metabolic diseases. Front Pharmacol (2019) 10:382. doi: 10.3389/fphar.2019.00382
152. Dubois V, Eeckhoute J, Lefebvre P, Staels B. Distinct but complementary contributions of PPAR isotypes to energy homeostasis. J Clin Invest (2017) 127:1202–14. doi: 10.1172/jci88894
153. Reddy RC, Standiford TJ. Nrf2 and PPAR{gamma}: PPARtnering against oxidant-induced lung injury. Am J Respir Crit Care Med (2010) 182:134–5. doi: 10.1164/rccm.201004-0457ED
154. Polvani S, Tarocchi M, Galli A. PPARγ and oxidative stress: Con(β) catenating NRF2 and FOXO. PPAR Res (2012) 2012:641087. doi: 10.1155/2012/641087
155. Li D, Du Y, Yuan X, Han X, Dong Z, Chen X, et al. Hepatic hypoxia-inducible factors inhibit PPARα expression to exacerbate acetaminophen induced oxidative stress and hepatotoxicity. Free Radic Biol Med (2017) 110:102–16. doi: 10.1016/j.freeradbiomed.2017.06.002
156. Raut SK, Kumar A, Singh GB, Nahar U, Sharma V, Mittal A, et al. miR-30c mediates upregulation of Cdc42 and Pak1 in diabetic cardiomyopathy. Cardiovasc Ther (2015) 33:89–97. doi: 10.1111/1755-5922.12113
157. Liu Y, Cao Z, Wang Y, Guo Y, Xu P, Yuan P, et al. Genome-wide screening for functional long noncoding RNAs in human cells by Cas9 targeting of splice sites. Nat Biotechnol (2018) 36:1203–1210. doi: 10.1038/nbt.4283
158. Cheng Y, Liu X, Zhang S, Lin Y, Yang J, Zhang C. MicroRNA-21 protects against the H(2)O(2)-induced injury on cardiac myocytes via its target gene PDCD4. J Mol Cell Cardiol (2009) 47:5–14. doi: 10.1016/j.yjmcc.2009.01.008
159. Tang Q, Len Q, Liu Z, Wang W. Overexpression of miR-22 attenuates oxidative stress injury in diabetic cardiomyopathy via sirt 1. Cardiovasc Ther (2018) 36. doi: 10.1111/1755-5922.12318
160. Huang X, Liu G, Guo J, Su Z. The PI3K/AKT pathway in obesity and type 2 diabetes. Int J Biol Sci (2018) 14:1483–96. doi: 10.7150/ijbs.27173
161. Li Q, Verma IM. NF-kappaB regulation in the immune system. Nat Rev Immunol (2002) 2:725–34. doi: 10.1038/nri910
162. Meyer A, Wang W, Qu J, Croft L, Degen JL, Coller BS, et al. Platelet TGF-β1 contributions to plasma TGF-β1, cardiac fibrosis, and systolic dysfunction in a mouse model of pressure overload. Blood (2012) 119:1064–74. doi: 10.1182/blood-2011-09-377648
163. Liu Y, Lv H, Tan R, An X, Niu XH, Liu YJ, et al. Platelets promote ang II (Angiotensin II)-induced atrial fibrillation by releasing TGF-β1 (Transforming growth factor-β1) and interacting with fibroblasts. Hypertension (2020) 76:1856–67. doi: 10.1161/hypertensionaha.120.15016
164. Freudlsperger C, Bian Y, Contag Wise S, Burnett J, Coupar J, Yang X, et al. TGF-β and NF-κB signal pathway cross-talk is mediated through TAK1 and SMAD7 in a subset of head and neck cancers. Oncogene (2013) 32:1549–59. doi: 10.1038/onc.2012.171
165. Sang W, Wang Y, Zhang C, Zhang D, Sun C, Niu M, et al. MiR-150 impairs inflammatory cytokine production by targeting ARRB-2 after blocking CD28/B7 costimulatory pathway. Immunol Lett (2016) 172:1–10. doi: 10.1016/j.imlet.2015.11.001
166. Chen Z, Stelekati E, Kurachi M, Yu S, Cai Z, Manne S, et al. miR-150 regulates memory CD8 T cell differentiation via c-myb. Cell Rep (2017) 20:2584–97. doi: 10.1016/j.celrep.2017.08.060
167. Guo X, Gao L, Wang Y, Chiu DK, Wang T, Deng Y. Advances in long noncoding RNAs: identification, structure prediction and function annotation. Brief Funct Genomics (2016) 15:38–46. doi: 10.1093/bfgp/elv022
168. Statello L, Guo C-J, Chen L-L, Huarte M. Gene regulation by long non-coding RNAs and its biological functions. Nat Rev Mol Cell Biol (2021) 22:96–118. doi: 10.1038/s41580-020-00315-9
169. Dhanoa JK, Sethi RS, Verma R, Arora JS, Mukhopadhyay CS. Long non-coding RNA: its evolutionary relics and biological implications in mammals: a review. Journal of Animal Science and Technology (2018) 60:25. doi: 10.1186/s40781-018-0183-7
170. Cabili MN, Trapnell C, Goff L, Koziol M, Tazon-Vega B, Regev A, et al. Integrative annotation of human large intergenic noncoding RNAs reveals global properties and specific subclasses. Genes Dev (2011) 25:1915–27. doi: 10.1101/gad.17446611
171. Hung T, Wang Y, Lin MF, Koegel AK, Kotake Y, Grant GD, et al. Extensive and coordinated transcription of noncoding RNAs within cell-cycle promoters. Nat Genet (2011) 43:621–9. doi: 10.1038/ng.848
172. Yap KL, Li S, Muñoz-Cabello AM, Raguz S, Zeng L, Mujtaba S, et al. Molecular interplay of the noncoding RNA ANRIL and methylated histone H3 lysine 27 by polycomb CBX7 in transcriptional silencing of INK4a. Mol Cell (2010) 38:662–74. doi: 10.1016/j.molcel.2010.03.021
173. Keniry A, Oxley D, Monnier P, Kyba M, Dandolo L, Smits G, et al. The H19 lincRNA is a developmental reservoir of miR-675 that suppresses growth and Igf1r. Nat Cell Biol (2012) 14:659–65. doi: 10.1038/ncb2521
174. Hacisuleyman E, Goff LA, Trapnell C, Williams A, Henao-Mejia J, Sun L, et al. Topological organization of multichromosomal regions by the long intergenic noncoding RNA firre. Nat Struct Mol Biol (2014) 21:198–206. doi: 10.1038/nsmb.2764
175. Andersen AA, Panning B. Epigenetic gene regulation by noncoding RNAs. Curr Opin Cell Biol (2003) 15:281–9. doi: 10.1016/s0955-0674(03)00041-3
176. Tripathi V, Ellis JD, Shen Z, Song DY, Pan Q, Watt AT, et al. The nuclear-retained noncoding RNA MALAT1 regulates alternative splicing by modulating SR splicing factor phosphorylation. Mol Cell (2010) 39:925–38. doi: 10.1016/j.molcel.2010.08.011
177. Patil VS, Zhou R, Rana TM. Gene regulation by non-coding RNAs. Crit Rev Biochem Mol Biol (2014) 49:16–32. doi: 10.3109/10409238.2013.844092
178. Rinn JL, Chang HY. Genome regulation by long noncoding RNAs. Annu Rev Biochem (2012) 81:145–66. doi: 10.1146/annurev-biochem-051410-092902
179. Tsai MC, Manor O, Wan Y, Mosammaparast N, Wang JK, Lan F, et al. Long noncoding RNA as modular scaffold of histone modification complexes. Science (2010) 329:689–93. doi: 10.1126/science.1192002
180. Wu Q, Kim YC, Lu J, Xuan Z, Chen J, Zheng Y, et al. Poly a- transcripts expressed in HeLa cells. PloS One (2008) 3:e2803. doi: 10.1371/journal.pone.0002803
181. Yao R-W, Wang Y, Chen L-L. Cellular functions of long noncoding RNAs. Nat Cell Biol (2019) 21:542–51. doi: 10.1038/s41556-019-0311-8
182. Chen Y, Tan S, Liu M, Li J. LncRNA TINCR is downregulated in diabetic cardiomyopathy and relates to cardiomyocyte apoptosis. Scand Cardiovasc J (2018) 52:335–9. doi: 10.1080/14017431.2018.1546896
183. Yang F, Qin Y, Wang Y, Li A, Lv J, Sun X, et al. LncRNA KCNQ1OT1 mediates pyroptosis in diabetic cardiomyopathy. Cell Physiol Biochem (2018) 50:1230–44. doi: 10.1159/000494576
184. Ren S, Zhang Y, Li B, Bu K, Wu L, Lu Y, et al. Downregulation of lncRNA-SRA participates in the development of cardiovascular disease in type II diabetic patients. Exp Ther Med (2019) 17:3367–72. doi: 10.3892/etm.2019.7362
185. Gao L, Liu Y, Guo S, Yao R, Wu L, Xiao L, et al. Circulating long noncoding RNA HOTAIR is an essential mediator of acute myocardial infarction. Cell Physiol Biochem (2017) 44:1497–508. doi: 10.1159/000485588
186. Yang Y, Cheng HW, Qiu Y, Dupee D, Noonan M, Lin YD, et al. MicroRNA-34a plays a key role in cardiac repair and regeneration following myocardial infarction. Circ Res (2015) 117:450–9. doi: 10.1161/circresaha.117.305962
187. Zlobine I, Gopal K, Ussher JR. Lipotoxicity in obesity and diabetes-related cardiac dysfunction. Biochim Biophys Acta (2016) 1861:1555–68. doi: 10.1016/j.bbalip.2016.02.011
188. Zhang L, Wang YM. Expression and function of lncRNA ANRIL in a mouse model of acute myocardial infarction combined with type 2 diabetes mellitus. J Chin Med Assoc (2019) 82:685–92. doi: 10.1097/jcma.0000000000000182
189. Ling H. Non-coding RNAs: Therapeutic strategies and delivery systems. Adv Exp Med Biol (2016) 937:229–37. doi: 10.1007/978-3-319-42059-2_12
190. Huang CK, Kafert-Kasting S, Thum T. Preclinical and clinical development of noncoding RNA therapeutics for cardiovascular disease. Circ Res (2020) 126:663–78. doi: 10.1161/circresaha.119.315856
191. van Rooij E, Kauppinen S. Development of microRNA therapeutics is coming of age. EMBO Mol Med (2014) 6:851–64. doi: 10.15252/emmm.201100899
192. Beg MS, Brenner AJ, Sachdev J, Borad M, Kang YK, Stoudemire J, et al. Phase I study of MRX34, a liposomal miR-34a mimic, administered twice weekly in patients with advanced solid tumors. Invest New Drugs (2017) 35:180–8. doi: 10.1007/s10637-016-0407-y
193. Hong DS, Kang YK, Borad M, Sachdev J, Ejadi S, Lim HY, et al. Phase 1 study of MRX34, a liposomal miR-34a mimic, in patients with advanced solid tumours. British Journal of Cancer (2020) 122:1630–7. doi: 10.1038/s41416-020-0802-1
194. van Zandwijk N, Pavlakis N, Kao SC, Linton A, Boyer MJ, Clarke S, et al. Safety and activity of microRNA-loaded minicells in patients with recurrent malignant pleural mesothelioma: a first-in-man, phase 1, open-label, dose-escalation study. Lancet Oncol (2017) 18:1386–96. doi: 10.1016/s1470-2045(17)30621-6
195. Crooke ST. Molecular mechanisms of antisense oligonucleotides. Nucleic Acid Ther (2017) 27:70–7. doi: 10.1089/nat.2016.0656
196. Singh NN, Luo D, Singh RN. Pre-mRNA splicing modulation by antisense oligonucleotides. Methods Mol Biol (2018) 1828:415–37. doi: 10.1007/978-1-4939-8651-4_26
197. Winkle M, El-Daly SM, Fabbri M. Noncoding RNA therapeutics - challenges and potential solutions. Nature Reviews Drug Discovery (2021) 20:629–51. doi: 10.1038/s41573-021-00219-z
198. Krützfeldt J, Rajewsky N, Braich R, Rajeev KG, Tuschl T, Manoharan M, et al. Silencing of microRNAs in vivo with 'antagomirs'. Nature (2005) 438:685–9. doi: 10.1038/nature04303
199. Gebert LF, Rebhan MA, Crivelli SE, Denzler R, Stoffel M, Hall J. Miravirsen (SPC3649) can inhibit the biogenesis of miR-122. Nucleic Acids Res (2014) 42:609–21. doi: 10.1093/nar/gkt852
200. Khvorova A, Watts JK. The chemical evolution of oligonucleotide therapies of clinical utility. Nat Biotechnol (2017) 35:238–48. doi: 10.1038/nbt.3765
201. Ochoa S, Milam VT. Modified nucleic acids: Expanding the capabilities of functional oligonucleotides. (2020) 25:4659. doi: 10.3390/molecules25204659
202. Corsten MF, Papageorgiou A, Verhesen W, Carai P, Lindow M, Obad S, et al. MicroRNA profiling identifies microRNA-155 as an adverse mediator of cardiac injury and dysfunction during acute viral myocarditis. Circ Res (2012) 111:415–25. doi: 10.1161/circresaha.112.267443
203. Jia C, Chen H, Wei M, Chen X, Zhang Y, Cao L, et al. Gold nanoparticle-based miR155 antagonist macrophage delivery restores the cardiac function in ovariectomized diabetic mouse model. Int J Nanomed (2017) 12:4963–79. doi: 10.2147/ijn.s138400
204. Bellera N, Barba I, Rodriguez-Sinovas A, Ferret E, Asín MA, Gonzalez-Alujas MT, et al. Single intracoronary injection of encapsulated antagomir-92a promotes angiogenesis and prevents adverse infarct remodeling. J Am Heart Assoc (2014) 3:e000946. doi: 10.1161/jaha.114.000946
205. Hannon GJ, Rossi JJ. Unlocking the potential of the human genome with RNA interference. Nature (2004) 431:371–8. doi: 10.1038/nature02870
206. Arun G, Diermeier SD, Spector DL. Therapeutic targeting of long non-coding RNAs in cancer. Trends Mol Med (2018) 24:257–77. doi: 10.1016/j.molmed.2018.01.001
207. Jinek M, Chylinski K, Fonfara I, Hauer M, Doudna JA, Charpentier E. A programmable dual-RNA-guided DNA endonuclease in adaptive bacterial immunity. Science (2012) 337:816–21. doi: 10.1126/science.1225829
208. Hsu PD, Lander ES, Zhang F. Development and applications of CRISPR-Cas9 for genome engineering. Cell (2014) 157:1262–78. doi: 10.1016/j.cell.2014.05.010
209. Barrangou R, Doudna JA. Applications of CRISPR technologies in research and beyond. Nat Biotechnol (2016) 34:933–41. doi: 10.1038/nbt.3659
210. Chang H, Yi B, Ma R, Zhang X, Zhao H, Xi Y. CRISPR/cas9, a novel genomic tool to knock down microRNA in vitro and in vivo. Sci Rep (2016) 6:22312. doi: 10.1038/srep22312
211. Yoshino H, Yonemori M, Miyamoto K, Tatarano S, Kofuji S, Nohata N, et al. microRNA-210-3p depletion by CRISPR/Cas9 promoted tumorigenesis through revival of TWIST1 in renal cell carcinoma. Oncotarget (2017) 8:20881–94. doi: 10.18632/oncotarget.14930
212. Esposito R, Bosch N, Lanzós A, Polidori T, Pulido-Quetglas C, Johnson R. Hacking the cancer genome: Profiling therapeutically actionable long non-coding RNAs using CRISPR-Cas9 screening. Cancer Cell (2019) 35:545–57. doi: 10.1016/j.ccell.2019.01.019
213. Horlbeck MA, Liu SJ, Chang HY. Fitness effects of CRISPR/Cas9-targeting of long noncoding RNA genes. Nature Biotechnology (2020) 38:573–6. doi: 10.1038/s41587-020-0428-0
Keywords: diabetes, cardiomyopathy, microRNAs, long non-coding RNAs, therapeutic application
Citation: Macvanin MT, Gluvic Z, Radovanovic J, Essack M, Gao X and Isenovic ER (2023) Diabetic cardiomyopathy: The role of microRNAs and long non-coding RNAs. Front. Endocrinol. 14:1124613. doi: 10.3389/fendo.2023.1124613
Received: 15 December 2022; Accepted: 16 February 2023;
Published: 07 March 2023.
Edited by:
Erkan Tuncay, Ankara University, TürkiyeReviewed by:
Zeynep Tokcaer Keskin, Acibadem University, TürkiyeSamarjit Das, Johns Hopkins University, United States
Copyright © 2023 Macvanin, Gluvic, Radovanovic, Essack, Gao and Isenovic. This is an open-access article distributed under the terms of the Creative Commons Attribution License (CC BY). The use, distribution or reproduction in other forums is permitted, provided the original author(s) and the copyright owner(s) are credited and that the original publication in this journal is cited, in accordance with accepted academic practice. No use, distribution or reproduction is permitted which does not comply with these terms.
*Correspondence: Mirjana T. Macvanin, bWlyamFuYS5tYWN2YW5pbkB2aW4uYmcuYWMucnM=