- 1Oxford Academy, Cypress, CA, United States
- 2The Science Academy STEM Magnet., North Hollywood, CA, United States
- 3Crean Lutheran High School, Irvine, CA, United States
- 4Cardiovascular Research Center, Massachusetts General Hospital, Harvard Medical School, Boston, MA, United States
The gut microbiome plays an important role in the protection against various systemic diseases. Its metabolic products profoundly influence a wide range of pathophysiological events, including the regulation of bone health. This review discusses the recently established connections between the gut microbiome and bone metabolism, focusing on the impact of microbiome-derived metabolites such as SCFAs, Bile Acids, and tryptophan to the control of bone remodeling and immunoreactions. Recent advances in metagenomics and microbiome profiling have unveiled new exciting therapeutic opportunities, ranging from the use of probiotics, prebiotics, engineered microbes, and to fecal microbiota transplantation. Understanding of the interplay among diet, microbiota, and bone health provides new avenues for tailored interventions aimed at reducing disease risk in osteoporosis and other related disorders. By drawing knowledge from microbiology, metabolism, and bone biology, this review highlights the potential of microbiome-targeted therapies to transform skeletal health and the management of bone diseases.
1 Introduction
Trillions of microorganisms live in the human gut, and they form a complex but highly balanced ecosystem (1). Accumulating evidence demonstrates that disturbing this complicated ecosystem profoundly affects bone density and may influence the risk of osteoporosis (2, 3). Dysbiosis or gut microbiota imbalance has been strongly associated as the cause of increased bone resorption and reduced bone formation through immune modulation, inflammation, and impaired nutrient absorption. Menopause and aging are particularly to blame for disturbances of microbiota, triggering inflammatory pathways that increase osteoclast activity and bone loss (4–6). Emerging research also links gut microbiota with inflammation that can affect conditions like rheumatoid arthritis (7). Various gut microbiome metabolic products such as short-chain fatty acids (SCFAs), bile acids, and tryptophan metabolites significantly influence bone metabolism and health (8, 9). SCFAs, including acetate, propionate, and butyrate, directly inhibit osteoclastogenesis and enhance osteoblast function, and hence maintain bone structure and bone density. SCFAs facilitate the absorption of calcium by lowering intestinal pH, enhancing calcium solubility, and providing a mechanistic link between diet, microbiome metabolism, and bone mineralization. Recent advances in metagenomics and functional profiling introduce new concepts toward how the gut microbiome may systemically influence overall human health (10). For instance, metagenomics analysis found some microbial gene clusters that are responsible for the production of health-beneficial metabolites including SCFAs, and this identified targeted possibilities of microbiome therapies in osteoporosis (9, 11). Metagenomic sequencing, functional metagenomics, and phylogenetic profiling provide a better understanding of how the gut microbiome regulates bone metabolism through diet, supplementation, or designed microbial therapies. In this review, we discuss various microbiome-targeted interventions such as probiotics, prebiotics, engineered microbes, and fecal microbial transplantation (FMT) in the aspects of bone health. Preclinical and clinical studies affirm the efficacy of these interventions to restore microbiome balance, reduce inflammation, and promote mineral absorption, all of which highlight their therapeutic potential against osteoporosis (12, 13). We aim to bring together different perspectives from the disciplines of microbiology, metabolism, and bone biology with regard to gut microbiome manipulation; it may well translate into new opportunities to optimally manage skeletal health-related diseases (7, 10).
2 Unraveling the gut microbiome
The human gut microbiome consists of hundreds of trillions of microorganisms and plays a crucial role in maintaining human health by supporting digestion, immune function, metabolic balance, and more (14). Disruptions in this huge and intricate microbial network (dysbiosis) are associated with a variety of health conditions, including inflammatory bowel disease (IBD), obesity, diabetes, cardiovascular disease, neurological conditions like Alzheimer’s disease, and autoimmune conditions like rheumatoid arthritis and systemic lupus erythematosus (15–17). Microbiome-focused dietary interventions supplemented with fermented foods significantly increased microbial diversity and reduced markers of inflammation. For instance, a 10-week study demonstrated that subjects who consumed fermented foods experienced notable increases in gut microbiota diversity accompanied by significant reductions in 19 inflammatory cytokines, including IL-6, IL-10, and IL-12b (18). Metagenomic DNA sequencing technologies have been developed to study the diversity of gut microbes (19). Metagenomic sequencing refers to a methodology used to examine genetic material derived directly from microbial populations, enabling researchers to identify microbial genes and their biochemical activities (20, 21). A study showed the alternative ways, through which the gut microbiome has been influencing glycan, amino acid, and xenobiotics metabolisms; such metabolism usually forms a pattern of enrichment in Clusters of Orthologous Groups (22). Other studies applied 16S rRNA gene sequencing to understand how a gut microbiome could promote immune response and absorb food for the liberation of nutrition to the human host (23). However, differences in the efficiency of DNA extraction, sequencing depth, and sample handling can lead to biases with the potential to cause significant errors in the estimation of microbial abundance (24). Separately, simulation studies support this approach because gene functional prediction indicates specific pathways, providing information on the connective chains of reactions expected to occur for gene activity. Because phylogenetic profiling defines gene-gene co-occurrence patterns across species, this method can be used to see in what ways or where the absence or presence of a gene may affect metabolic capabilities in prokaryotes (25). It shows the importance of gene co-occurrence within a microbial network where the interactions go beyond digestion and relate to phenotypic traits (24). One can apply insights from these technologies to work towards therapeutic interventions and diets by the health-promoting properties of the gut microbiome (26). These microbiome-targeted interventions show promising preclinical efficacy and emerging clinical potential in treating neurological disorders such as Alzheimer’s disease, Parkinson’s disease, stroke, and epilepsy by modulating microbial composition and metabolism, thereby influencing disease pathology (27).
The composition of the gut microbiome can predict the susceptibility of a host to some diseases (28). The microbiome uses colonization resistance to prevent infection in the host, where the commensal microbiota of the microbiome competes against invading microorganisms (29, 30). The gut microbiome is known to act to modulate immune homeostasis and serve as a barrier from several diseases at this gut-symbiosis interface (17). SCFAs represent the major products of the gut microbiome and play an essential role in gut homeostasis (30). Butyrate controls the adaptive and innate immune cells, while SCFAs protect against dysbiosis and maintain gut health (29). Finally, antigens are presented through antigen-presenting cells to T cells and afterward may bias the T cells into Th1, Th2, or Th17 cells (17). This interaction downregulates gut macrophage activity and reduces inflammation through modulation of T cell responses in the gut (30). The metabolic activity of the gut microbiota regulates diseases beyond the gut barrier and also serves as an indicator of the local immune system (31). While the activation of the pattern-recognition receptors in the gut controls the appropriate immune response, the barrier function is a multi-step system of the gut that reduces the adhesion of bacteria and regulates nutrition absorption (30). A balanced gut microbiota notably regulates immune mediators in the GIT and profoundly strengthens gut barrier function (29). In fact, studies in mice have shown that proper mucus formation depends on an actively growing, diversely structured microbiota (32). The host gut microbiota regulates the secretion of mucosal digestive enzymes, such as meprin-β, into the small intestine to modulate mucus production (32). Furthermore, on a diet devoid of dietary fibers, the mucus layer is degraded when the bacteria-degrading mucus thrives. On the other hand, leaky gut has recently been associated with pathogenesis leading to several inflammatory ailments (33). It is observed that a healthy gut mucus layer and proper immune system depend on the proper interaction ability of the gut microbes within their host (30). It is, therefore, important to understand the complexity, composition, regulation, and functioning of gut microbiota in order to achieve therapeutic breakthroughs and develop the best microbiome-specific therapies that would promote human health and prevent disease (17).
The diversity of the microbial community in the gut is a direct result of the biochemical profile of food that the host uptakes (34). Dysbiosis is caused by an unstable microbiome leading to changes in composition and diversity of the gut microbiome, which has been associated with a series of circumstances, including the loss of balanced nutrition owing to high sugar and/or low fiber, which leads to disruption to the host’s metabolism. In addition, antibiotic overuse and the consequential antimicrobial resistance of gut bacteria also cause dysbiosis (35, 36). This pathological situation often results in an overgrowth of opportunistic pathogenic microorganisms in conjunction with the loss of beneficial microorganisms (35). Indeed, therapeutic strategies supporting microbiome stability to restore its diversity have been found to play key roles in maintaining proper immune function and long-term health. This form of maintenance requires tailored therapeutic strategies and includes a range of factors, including prebiotics and probiotics, as well as changes in diet that foster microbial diversity and overall health (37, 38). Importantly, currently available microbiome-targeted therapies provide innovative means to suppress dysbiosis and its role in numerous inflammatory diseases (39, 40). Together, understanding the microbiome and its interactions with diverse ranges of different hosts is essential to prevent or intervene in dysbiosis (41).
3 Bone metabolism: foundations and failures
Bone remodeling is a constantly ongoing critical homeostatic process in the body throughout our lives (42). It is tightly regulated by the precise continual resorption of the bone by the osteoclasts and its counteracting, continual formation by the osteoblasts (43, 44). The receptor activator of nuclear factor (RANK) and its ligand, RANKL, are crucial in the promotion of bone resorption by osteoclasts (44). In addition, the balance between estrogen and testosterone properly controls osteoclast activity, promoting bone formation to help maintain bone mass, and also protect against osteoporosis, most notably in postmenopausal women (45). This suggests that an increase in osteoclast activity can be caused by parathyroid hormone (PTH), which acts on osteoblasts and influences the RANKL/Osteoprotegerin (OPG) signaling system (46). Mechanically, following receptor binding of PTH, a signal transduction cascade is initiated and alters the RANKL/OPG-producing profile - with an increase in the RANKL profile and a decrease in the OPG profile (47). This leads to the overall net effect that would favor osteoclast development and productivity levels. On the other hand, calcitonin inhibits osteoclast activity by binding to its cognitive receptors on osteoclasts and triggers the signaling pathways that reduce their bone-resorbing ability. Moreover, hormonal balancing mechanisms would render control over the activity of osteoblasts and osteoclasts and properly maintain the innate homeostasis in the processes essential for the formation and function of bone (43). In the absence of such balance, bone loss will accelerate and predispose to fracture (44). On the other hand, overactivation of osteoblasts may lead to the overproduction of bone and cause abnormalities in bones themselves and marrows therein (48).
Several bone resistance training programs reported the ability to improve bone mineral density (BMD) in postmenopausal women with low bone mass (49–51). The interest in preventive strategies to build bone mass and demand for adequate assessment tools to properly calculate the risk for fractures have grown dramatically in recent years (52). Accurately predicting bone health allows for tailored therapies to address specific weaknesses, improving osteoporosis management and reducing fracture risk (53, 54). Trabeculae, the spongy part of bone, forms thin plates and rod-like structures, enabling it to perform its function of bearing weight and resisting forces more appropriately (55). Also, minerals in the matrix of a healthy bone affect the strength of the bone, while collagen fibers are arranged and cross-linked for the flexibility or bending ability of the bone. Advanced imaging techniques like QCT, High-resolution peripheral computed tomography (HR-pQCT), Trabecular Bone Score (TBS), and Finite Element Analysis (FEA) provide precise and immediate determination of multiple bone strength parameters, such as cortical bone density, cortical thickness, cortical porosity, and density (56). Spectroscopic tools like Fourier Transform Infrared (FTIR) spectroscopy and Raman spectroscopy provide a deeper understanding of the composition of bone with respect to mineral and collagen properties than can be obtained with standard BMD testing (57). These imaging techniques enhance the diagnosis of and guide treatment strategies (58). However, despite these advancements, metabolic failures remain the primary challenge in bone health management.
Bone metabolic failures underlie bone conditions. Rheumatoid arthritis (RA) and osteoarthritis (OA) point to the role of imbalanced metabolism (59). The inflammatory cytokines in RA cause disruption in the balance between RANKL and OPG, triggering bone resorption with enhanced fracture risk (60). In contrast, in OA, there is cartilage degradation and bone spur development, illustrating how mechanical stress and abrasive wear weaken bone (61). Also, hormonal imbalances with reductions in estrogen enhance osteoclast-mediated activity, leading to postmenopausal osteoporosis in women (62). The treatment of these metabolic failures remains paramount despite the availability of advanced diagnostic tools since viable interventions are limited with these advanced diagnostic methods.
In RA, several cytokines have been identified to disrupt the balance between RANKL, an activator of osteoclast activity, and its inhibitor OPG, resulting in excessive bone resorption (63). Despite the recent improvements in treatment, complete recovery of damaged bones in RA remains unlikely, considering that inflammatory cytokines persistently inhibit new bone formation (63). However, in the case of patients with OA, cartilage wears down slowly and results in a loss of elasticity and characteristics as a shock absorber. As the cartilage degrades, it exposes the bone below and results in the formation of bone spurs (64). A study outlined that osteoarthritis is an outcome of physical trauma and day-to-day use and wear of the joints (65). In comparison, the autoimmune response characterizing RA tends to damage the bones. In this process, immune cells invade the tissue membrane that triggers an inflammatory response in the synovium and cause erosion of the neighboring bones.
Numerous genetic factors, including Vitamin D receptor gene polymorphisms, are known to interact with lifestyle choices to affect bone health. Specific polymorphisms of genes of the Vitamin D receptor, such as haplotype A-T-G for rs7975232, rs1544410, and rs73731236, have been associated with low BMD, increased susceptibility to osteoporosis, and other bone diseases (66). Indeed, Vitamin D is a crucial nutrient and promotes calcium absorption, and adequate Vitamin D levels help build strong bones and reduce osteoporosis risk (67). Exercise also reduces bone loss in older age. Regular activities stimulate the formation of bones and likely reduce bone fractures with a significantly diminished chance of developing osteoporosis (68). Smoking negatively affects the flow of hormones and impairs calcium absorption and bone metabolism (69). This leads to low bone mass and density and contributes to a high fracture risk (69). On the other hand, sex hormones, important for osteoblast activation, are negatively affected by smoking. Moderate alcohol consumption has a complex impact on BMD via direct effects on bone mineral metabolism. Moderate alcohol consumption may have varying effects on bone density, especially in postmenopausal women, although its impact on estrogen levels remains complex. Acute alcohol intoxication may lead to the development of transient hypoparathyroidism with consequent hypocalcemia, whereas chronic alcoholism reduces levels of vitamin D metabolites and impairs calcium absorption. Alcohol blunts osteoblast activity, as low levels of osteocalcin, a protein involved in new bone formation, are observed in alcoholics (69). Some influences and risk factors important in determining the state of health of our bones are genetically predetermined - phenomena such as peak bone mass; most of the others will be determined by the accumulation of lifestyle choices that we make over a lifetime (68). Maintaining strong bone structure is essential for mobility, independence, and overall well-being.
Most pathological conditions affecting the bone can benefit from a comprehensive approach to treatment. Traditional treatment methods include bisphosphonates, hormone replacement therapy (HRT), and the supplementation of calcium and vitamin D. Among the newer therapeutic options for osteoporosis are teriparatide and romosozumab. Vitamin D deficiency can delay fracture healing even when surgical procedures are correctly performed (67). Bisphosphonates, including alendronate and zoledronic acid, are used to deal with osteoporosis. Binding to bone minerals inhibits osteoclast activity and thus limits bone resorption. At menopause, reduced estrogen levels disrupt the balance of bone remodeling, which leads to increased bone resorption followed by bone loss. HRT restores estrogen levels, suppresses osteoclast activity, maintains osteoblast function, and thus preserves bone mass in postmenopausal women (70). However, although HRT helps retain bone density, its widespread usage has been limited by its non-trivial adverse effects, such as the occurrence of breast cancer and the potential for stroke and clot formation. Thus, HRT has been very cautiously prescribed to clearly specified patterns of patients in well-controlled medical settings (71). New therapeutic possibilities include romosozumab and anabolic agents such as teriparatide. Romosozumab is a monoclonal antibody that targets a key pathway involved in bone formation. A reduction in the risk of fragility fractures was considerably noted in the romosozumab group compared to that in the control patient population with increased BMD at the lumbar spine, total hip, and femoral neck among the skeletal sites (72). On the other hand, teriparatide is a synthetic form of parathyroid hormone that stimulates new bone formation by enhancing osteoblast activity, resulting in increased BMD and reduced fracture risk (73).
4 Metabolic intersections: microbiome and bone health
SCFAs inhibit osteoclast activity and bone resorption and, thus, indirectly prevent bone loss. Mechanistically, they can also activate GPR41 and GPR43 receptors on osteoclast precursors, preventing their differentiation into mature bone-resorbing osteoclasts (74). In a mouse study, gut dysbiosis lowered butyrate levels. FMT upregulated GPR43 through the elevated levels of butyrate in the bone tissue, leading to the suppression of the osteoclast genesis process and heightened osteoblast function, thus reducing excessive resorption (74). On the other hand, healthy bone promotion by SCFAs is governed through the activation of Tregs. Tregs block the excessive induction of inflammatory responses while polarizing macrophages into an anti-inflammatory M2 phenotype. SCFAs induce M2 macrophage anti-inflammatory polarization through butyrate-mediated STAT6 activation. They also promote regulatory T-cell differentiation, reduce exaggerated inflammatory responses, and support the maintenance of bone mass (75, 76). Fiber-rich diets help prevent bone loss and maintain balanced bone renewal (36, 77–79).
The gut microbiota converts primary bile acids into secondary forms through various enzymatic processes in the intestines. And bile acid signaling is known to play a role in bone metabolism through receptors such as FXR and TGR5 (80). The interaction between blood concentrations of bile salts and bone density suggests that bile acid signaling directly affects bone metabolism. Dietary fibers enhance the growth of beneficial gut microbiota and influence bile acid metabolism, leading to altered bile acid pools. These changes in the bile acid pool may influence bone health by affecting receptor activation and calcium homeostasis. While TGR5 and vitamin D receptor activation could play a role, regulating the gut microbiota through FMT or probiotics may provide therapeutic potential for diseases related to dysregulated bile acid metabolism (81, 82).
The kynurenine pathway metabolizes the major part of total tryptophan into kynurenine in the liver through induction of the rate-limiting enzyme indoleamine-2,3-dioxygenase or, to a lesser extent, by induction of tryptophan 2,3-dioxygenase in other tissues (83). Likewise, the gut microbiota metabolizes tryptophan into various indoles, such as indole-3-acetic acid and indole-3-aldehyde, which might be the ligands of the aryl hydrocarbon receptor pathway in mediating bone health and homeostasis (84). Indole is a potent AhR ligand that influences multiple metabolic pathways relevant to bone health. It translocates into the nucleus, where AhR heterodimerizes with the ARNT protein and binds to the target gene promoter at XRE, and initiates the transcription of XRE. Indole compounds such as indole-3-aldehyde and indole-3-acetic acid increase bone mass by promoting osteoblast differentiation and reducing bone resorption via the AhR pathway (85). In addition, indoles activate microbiota-derived AhR and promote intestinal health by regulating immune responses and epithelial barrier function. AhR activation by microbial indoles reduces the expression of pro-inflammatory cytokines, modulates inflammation-mediated bone resorption and also supports bone health by regulating osteoclast activity (86). A study showed that gut microbiota metabolism of tryptophan-induced AhR signaling acted as a master switch for renal fibrosis (87). This suggested that microbial tryptophan metabolites modulate renal inflammation and fibrosis through the AhR pathway. Moreover, high kynurenine levels promote osteoclastogenesis by increasing the RANKL expression, which is considered to be the major cytokine that induces osteoclast differentiation (86). Therefore, kynurenine resorbs bone and enhances its resorption. However, kynurenine was also known to negatively modulate osteoblast activity and bone formation by suppressing anabolic pathways. Kynurenine induces RANKL expression and promotes osteoclast differentiation while inhibiting osteoblast activity. The imbalance will shift the normal course to age-related osteoporosis and increase the risk of fractures (86). Products of tryptophan metabolism by gut microbiome induce the AhR and pregnane X receptor pathways. This allows them to regulate gut barrier homeostasis and immune modulation (88). Gut microbiota helps maintain gut barrier integrity and regulates inflammatory responses through the metabolite activators of AhR. Indeed, activation of AhR by some indole derivatives showed neuroprotective and anti-inflammatory effects (89). Together, gut microbiota metabolizes tryptophan into various active compounds, including indoles and kynurenine, which play a key role in bone health. Table 1 summarizes the effects of key microbiome-derived metabolites on bone health.
During the past decade, the gut microbiome has emerged as one of the most favorable therapeutic targets for interventions to improve bone health and prevent osteoporosis. More research discovers the intricate association between gut microbiota and bone metabolism. Diet-based interventions, as well as probiotics and prebiotics, proved to regulate the gut microbiome in ways impacting bone density and skeletal health. Fiber, in conjunction with calcium and probiotics, such as Lactobacillus and Bifidobacterium, represent some of the dietary components that can positively influence mineral absorption through the reduction of inflammation (90). Prebiotics, such as primarily inulin-type fructans, modulate calcium absorption and stimulate the proliferation of beneficial bacteria that produce SCFAs (79). Inulin-type fructans from sources such as chicory root and Jerusalem artichokes are fermented by gut microbes into SCFAs, which provide beneficial effects on bone by increasing calcium absorption due to a lower gut pH. This supports conditions that favor increased BMD and strength (91). It is reported that butyrate and propionate have an inhibiting effect on osteoclast differentiation and bone resorption. On the other hand, they stimulate calcium absorption through the colon. Specific bacterial groups known to be SCFA producers have now become targets to harness the gut microbiome for skeletal health (92). Figure 1 summarizes the key ways in which gut microbiota and its metabolites influence bone health.
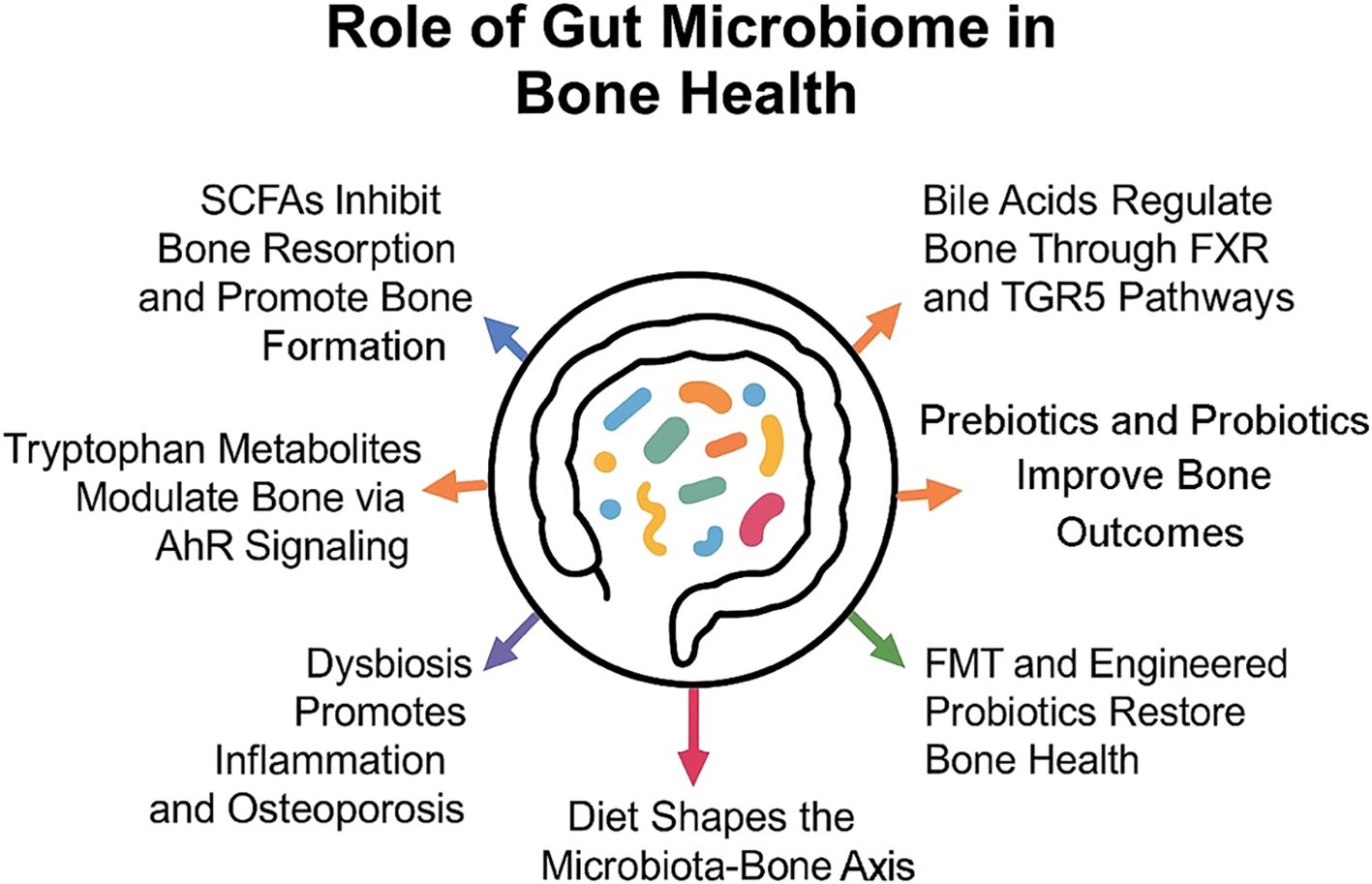
Figure 1. Gut microbiota influences bone health through metabolites and immune modulation, with diet and therapies like probiotics and FMT shaping these effects.
5 Diet, microbiota, and bone: a tripartite alliance
Higher-protein, animal-based diets can shift the gut microbiota composition, as they increase bacteria like Bacteroides, Alistipes, and Bilophilia, and also reduce beneficial microbes such as Lactobacillus and Roseburia (93). Plant-based sources of lipids, nuts, and vegetable oils seemed to favor this gut microbiota diversity (94). In humans, long-term dietary habits are considered to have the maximum impact on microbiota diversity in an individual during neonatal life and thereafter. SCFAs produced within the microbiome reduce the gut pH and have systemic effects that may indirectly support bone health (95). Indeed, the balance between healthy and harmful metabolites is determined by the type of diet that one consumes. Studies showed that diets high in fiber elevate carbohydrate-active enzymes (CAZymes) in the gut and thus reduce inflammation-related proteins (18). Relatively stable and long-term dietary intake is a central determinant for establishing the gut microbiota. However, short-term dietary changes can rapidly alter microbiota, and some enterotypes remain stable over time (93). Long-term dietary interventions seem to modulate bone metabolism, consequently through changes in the gut microbiota (6). Table 2 summarizes key bacterial genera and their roles in bone health. Indeed, the quick changes in gut microbiota owing to alteration in diet could provide an opening for developing dietary interventions against bone disease. For example, calcium supplementation supports bone health and may indirectly influence gut microbiota and inflammation, which may unlock the complex dietary interactions with gut microbiome metabolism and bone health (95). Moving forward, more comprehensive and integrated research is warranted to know the full impact of such processes on bone health and the treatment of bone disease (92).
A diet rich in fruit, vegetables, grain, fish, nuts, and low-fat dairy is generally associated with improved BMD (79). The best way to illustrate the effects of diet on bones is by comparing Western and Mediterranean diets. Long-term intake of Western diets has been shown to lead to an increased risk for bone injuries, specifically hip fractures (96). With a balanced proportion of Bacteroidetes and Firmicutes, the Mediterranean diet helps reduce inflammation. Nutritional intake in the traditional Mediterranean diet is associated with a proper balance of calcium, vitamin D, and protein, which can account for the low rate of osteoporosis in Mediterranean Basin countries (97). However, a Western diet directly increases the risk of fracture and bone resorption and generally leads to osteoporosis (98). Studies have shown that polyphenols used as a prebiotic substrate can stimulate the development of Bifidobacteriaceae and Lactobacillaceae and potentially inhibit the growth of Escherichia coli, Clostridium perfringens, and Helicobacter pylori (99). A good diet supplemented adequately with metabolites is also related to a reduction in inflammatory processes and adequate provision of calcium and magnesium. Finally, modulation of gut microbiota may centrally regulate inflammation. In addition, a leaky gut may be associated with systemic inflammation and osteoporosis; therefore, protection from a leaky gut also ensures a wholesome state of the bone (6). Due to dietary intake and probiotics supplementation, healthy gut microbiota suppression of pro-inflammatory cytokines protects against osteoporosis (2).
Prebiotics modulate the gut microbiota and intestinal function, enormously impacting the gut and bone health (100). Prebiotics trigger some helpful bacteria such as Bifidobacteria and Lactobacilli; their fermentation products are mainly lactic acids which can push away the attack by pathogenic microflora (101). Some studies have shown that gut-derived butyrate led to an expansion of the bone marrow’s regulatory T cells and, thus, participated in the upregulation of bone anabolism (102). Other studies indicate that prebiotic action helps bone strength, bone formation, or even osteoblast/clast activity (103). McCabe et al. showed that pre-/probiotic regulation on bone health and calcium levels in mice (103). In chickens, inulin-induced treatment exhibited increased bone mineralization (104). Several randomized clinical trials present direct evidence of associations between dietary modification and variation in gut microbiome composition and structure. The results showed a significant change in the amount of acetate, increased phyla diversity, an abundance of Firmicutes and Bacteroides, and reduced Actinobacteria (105). This apparently indicated that massive nutrition had a robust effect on the gut microbiota. Changes in metabolic profile support a potential benefit of inulin enriched diet on bone health (106). Notably, So et al. carried out a meta-analysis to investigate the intervention of dietary fiber on gut microbiota. They reported an intervention with fructans and galacto-oligosaccharides that resulted in a significantly higher level of fecal Bifidobacterium and Lactobacillus in their report (101). The increase can be related to the intestine-mineral absorption-enhancing and short-chain fatty acid anti-inflammatory activities (102). These studies have, more importantly, identified a role for dietary ingredients in gut microbiota health for nutritional guidelines concerning bone health.
6 Beyond probiotics: innovating therapeutic strategies
Previous studies evaluated traditionally made doenjang (fermented Korean food), a synbiotic food supplemented with Bacillus subtilis and other beneficial bacteria, in an ovariectomized rat model for its efficacy (107). Rats fed with doenjang for a certain period showed conserved BMD and displayed distinct reduced bone resorption markers compared to the control rat group (108). Another study also found that in humans, a probiotic combination of three Lactobacillus strains significantly reduced lumbar spine bone loss, especially in early postmenopausal women (109). In the same study, however, the combination, composed of Lactobacillus paracasei (DSM 13434) and two Lactobacillus plantarum strains (DSM 15312 and DSM 15313), did not prevent further bone deterioration compared to placebo. Moreover, it was found that certain probiotic strains work only in specific health outcomes, showing rather limited effects (110). Notably, one of the recent studies showed that multistrain probiotics improved ulcerative colitis while uncovering a strong need for site-specific probiotic therapies (111). It was also found that the role of probiotics in inflammatory bowel disease (IBD) is unexpectedly strain-specific. Indeed, when the probiotic mixture was evaluated for its effects on antibiotic-associated diarrhea and gastrointestinal symptoms at different escalating doses, the incidence and severity of antibiotic-associated diarrhea were dose-dependent, with the lowest incidence observed in the highest dose group (110). While this points to a promising therapeutic intervention for bone health, the application of probiotics faces challenges related to strain specificity, viability, and colonization efficiency (112). Table 3 compares therapeutic strategies targeting the gut microbiome.
Synthetic biology holds great promise in therapeutic applications based on genetically modified bacteria to achieve beneficial activities, including targeted production of desired metabolites, modulation of the immune system, and reduction of inflammatory markers (113, 114). If probiotics could be engineered by synthetic biology, they may prove more functional and potent in targeted delivery. In recent years, synthetic biology has been extensively used to create genetically modified bacteria for a broad spectrum of therapeutic applications. For example, exopolysaccharide (EPS) produced by the recombinant Bifidobacterium longum 35624 has been shown to inhibit osteoclast formation and thus increase bone formation in vitro experiments (115). Moreover, orally administered B. longum 35624 could slow down bone loss in an ovariectomized mouse model. However, although the engineered L. plantarum may target bone health, the genes introduced as the result of engineering could influence other gut bacteria in unintended ways (116). For example, if antibiotic resistance genes are passed over to gut bacteria through horizontal gene transfer, this could dangerously increase the chance of the emergence of new multidrug resistance strains (117). Such situations would add antibiotic resistance to the emerging multidrug-resistant bacteria and hence make it difficult to eradicate using the currently available antibiotics (118). Indeed, synthetic biology is a powerful technology that holds great promise in tissue engineering and biomedicine, especially when alluding to the possibility of developing bespoke therapeutic approaches to bone health using engineered probiotics. Realizing this potential requires carefully balanced methods that address related safety and ethical issues. Particular attention should be paid to collateral horizontal gene transfer in food bacteria and the potential induction of antibiotic resistance (119). Bioethical guidelines on synthetic biology regarding the development of technologies that could limit our fight against dangerous pathogens should be properly established and accrue to everybody transparently with public participation and fair access. Discussion on critical concerns and heightened views among participating scientists, ethicists, stockholders of policies, and the public needs to keep going. This collaboration will ensure the development and implementation of probiotics in a responsible and safe manner and reduce their risks (120).
Initially developed for gastrointestinal disorders, FMT is being explored for systemic diseases, including potential impacts on bone health. FMT is a method of introducing healthy fecal material into a recipient to restore a balanced and healthy gut microbiota ecosystem. Recent studies suggest that FMT could be useful to manage bone health disorders. The female rats in an aging model, when obesity was established with accumulated gut alterations, received FMT from young female rats. The control group had no therapy and was analyzed for the small intestines, bone samples, and gut bacteria at weeks 12 and 24 after the intervention by intragastric FMT. At 24 weeks, the bone quantity in terms of volume, fraction, and thickness across the FMT group was noticeably higher than that across the control group (121). These findings indicate that improvements in gut microbiome composition and gut barrier function could be effective means to ameliorate age-related bone loss. Such promising results suggest that FMT could become one of the ways to fight age-related osteoporosis in the future. Separately, the outcomes in formula-fed piglets have shown restored gut health through scaling up the beneficial use of gut bacteria from FMT; such normalization of gut biology and barrier function has suppressed inflammatory responses (122). These findings also emphasize the impact of early-life microbiota modification on systemic health, including bone homeostasis.
FMT influences bone health through complex interactions involving the gut microbiome, immune system, and bone metabolism. Recently, one publication further showed that FMT reconstituted the bone mass in osteoporotic mice, interpreted to relate to changes in gut microbiota composition and metabolic activity (123). A number of its metabolites seem to act directly on the bone cells, while others do by modulating the host immune response to bone resorption and formation (124). Similarly, FMT-mediated changes in the gut microbiome will have an indirect influence on bone metabolism through the regulation of the immune system (125). The gut microbiome is considered to play an important basic role in modulating immune responses and systemic inflammation at levels and along pathways with consequences for bone health (126). Inflammatory processes are involved in bone conditions such as osteoporosis in many ways. A recent review underlined factors in inflammation, namely the modality of action of cytokines and inflammatory osteoclasts (127). In this process of inflammation, a disturbed balance in the balance between the formation and resorption of bone, therefore, predisposes them to frail bones and osteoporosis. As a treatment technique targeting the gut microbiome, one would expect both systemic and local effects of FMT on inflammation, which could be rather important in modulating bone health (128).
A surprising connection, which is being identified between the gut microbiome and health, is becoming more and more linked to the results coming from microbial metabolism studies. This has thus led to therapy that incorporates the direct supplementation of metabolites implicated in bone health, particularly microbial metabolites. As SCFAs and other microbial metabolites present enormous promise for bone health, several challenges must be addressed in order to allow the current potential to manifest in practical life (95). There may be many differences in the individual responses to metabolite supplementation. The crucial aspect is being aware of and recognizing these complexities in order to adjust appropriate treatment options. A proper understanding of the metabolite effects on bone metabolism will thus be central to the development of targeted therapies, such as possible interactions between metabolites, gut microbiota, and bone cells or even more systemic effects of metabolite supplementation across the body (8).
Unlike traditional pharmaceuticals, microbiome-based therapeutics entail living organisms or complex microbial groups, making the regulatory classification quite a challenge (129). This complexity is further increased by the development of standardized manufacturing processes. The establishment of product stability and consistency are critical approval requirements. Variables like diet and lifestyle should be taken into consideration when designing clinical trials for new microbiome-based therapies, which also involve the need for proper control groups and robust analysis methods for acquired data (130). An important obstacle in undertaking such studies is to choose appropriate endpoints and suitable biomarkers. These will better show the therapeutic effects on both the microbiome and the human host. Other variables include the intrinsic variability in the composition of microbiomes among people. Importantly, individual human microbiome varies depending on diet, lifestyle, and antibiotic history (130). These are key variables that need to be controlled and considered for designing clinical trials. Thus, innovative designs of trials and analytic methods would be critical as both the potential therapeutic impacts and their associated risks are rising. New approaches are needed to extract the effects of microbiome-based interventions from the compounding variables (129). Notably, the regulatory and clinical trial landscape for therapeutics developed from the microbiome changes daily, calling for regulatory bodies to work with scientific experts to address these challenges and establish specific guidelines to evaluate therapeutic microorganisms. Precise guidelines, superior analytics, and innovative collaboration between regulators and scientists would establish the continued exploration of microbiome-based therapeutics, enabling these therapeutics as safe and effective treatments for a wide range of bone conditions.
7 Conclusion
The gut microbiota has become a significant determinant of bone health, directly modulating skeletal metabolism through intricate biochemical and immune-regulating mechanisms. Gut microbiota modulation could be an innovative strategy with potential as a treatment option for osteoporosis and other bone disorders. Interventional methods like probiotics, prebiotics, fecal microbiota transplantation (FMT), and synthetic biology are highly prospective measures to improve bone mineral density (BMD) and reduce fracture risk by modulating inflammatory mechanisms, enhancing calcium absorption, and inhibiting osteoclast activity. There is evidence from preclinical and clinical trials that probiotic strains such as Lactobacillus and Bifidobacterium may favorably modulate bone turnover, whereas SCFAs, bile acids, and microbial metabolite-derived tryptophan are able to suppress bone resorption and stimulate osteogenesis. Furthermore, innovative methods such as engineered probiotics and microbiome-specific dietary interventions hold promise as new horizons for individualized therapies. However, to transfer these advances into clinical application, these challenges must first be tackled: individual microbiomes are highly variable, long-term efficacy of interventions remains unproven, and there are issues of regulation over microbiome-based therapies. Future investigation needs to aim to optimize strain-specific effects, improve colonization efficiency, and establish standardized protocols in order to maximize therapeutic benefit. Unlocking the potential of the gut microbiome to manage osteoporosis not only presents a new avenue of therapy but also lays the ground for precision bone health medicine. Combining microbiome science with bone metabolism can transform the prevention and treatment of osteoporosis and hold the promise of more personalized and effective methods in skeletal care.
Author contributions
DH: Conceptualization, Data curation, Formal analysis, Methodology, Writing – original draft. EC: Conceptualization, Data curation, Formal analysis, Methodology, Writing – original draft. YxL: Conceptualization, Data curation, Formal analysis, Methodology, Writing – original draft. YlL: Conceptualization, Data curation, Formal analysis, Methodology, Writing – original draft. KR: Conceptualization, Supervision, Writing – review & editing.
Funding
The author(s) declare that financial support was received for the research and/or publication of this article. KR received funding from RS-2024-00359396, supported by the National Research Foundation of Korea (NRF) grant funded by the Korea government (MSIT).
Conflict of interest
The authors declare that the research was conducted in the absence of any commercial or financial relationships that could be construed as a potential conflict of interest.
Generative AI statement
The author(s) declare that no Generative AI was used in the creation of this manuscript.
Publisher’s note
All claims expressed in this article are solely those of the authors and do not necessarily represent those of their affiliated organizations, or those of the publisher, the editors and the reviewers. Any product that may be evaluated in this article, or claim that may be made by its manufacturer, is not guaranteed or endorsed by the publisher.
References
1. Martens EC, Koropatkin NM, Smith TJ, and Gordon JI. Complex glycan catabolism by the human gut microbiota: the Bacteroidetes Sus-like paradigm. J Biol Chem. (2009) 284:24673–7. doi: 10.1074/jbc.R109.022848
2. de Sire A, de Sire R, Curci C, Castiglione F, and Wahli W. Role of dietary supplements and probiotics in modulating microbiota and bone health: the gut-bone axis. Cells. (2022) 11:743. doi: 10.3390/cells11040743
3. Wei M, Li C, Dai Y, Zhou H, Cui Y, Zeng Y, et al. High-throughput absolute quantification sequencing revealed osteoporosis-related gut microbiota alterations in han chinese elderly. Front Cell Infect Microbiol. (2021) 11:630372. doi: 10.3389/fcimb.2021.630372
4. Waldbaum JDH, Xhumari J, Akinsuyi OS, Arjmandi B, Anton S, and Roesch LFW. Association between dysbiosis in the gut microbiota of primary osteoporosis patients and bone loss. Aging Dis. (2023) 14:2081–95. doi: 10.14336/AD.2023.0425
5. Ahire JJ, Kumar V, and Rohilla A. Understanding osteoporosis: human bone density, genetic mechanisms, gut microbiota, and future prospects. Probiotics Antimicrob Proteins. (2024) 16:875–83. doi: 10.1007/s12602-023-10185-0
6. Zhang YW, Song PR, Wang SC, Liu H, Shi ZM, and Su JC. Diets intervene osteoporosis via gut-bone axis. Gut Microbes. (2024) 16:2295432. doi: 10.1080/19490976.2023.2295432
7. Locantore P, Del Gatto V, Gelli S, Paragliola RM, and Pontecorvi A. The interplay between immune system and microbiota in osteoporosis. Mediators Inflammation. (2020) 2020:3686749. doi: 10.1155/2020/3686749
8. Yan J, Herzog JW, Tsang K, Brennan CA, Bower MA, Garrett WS, et al. Gut microbiota induce IGF-1 and promote bone formation and growth. Proc Natl Acad Sci U S A. (2016) 113:E7554–63. doi: 10.1073/pnas.1607235113
9. Ling CW, Miao Z, Xiao ML, Zhou H, Jiang Z, Fu Y, et al. The association of gut microbiota with osteoporosis is mediated by amino acid metabolism: multiomics in a large cohort. J Clin Endocrinol Metab. (2021) 106:e3852–64. doi: 10.1210/clinem/dgab492
10. Chen S, Zhou G, Han H, Jin J, and Li Z. Causal effects of specific gut microbiota on bone mineral density: a two-sample Mendelian randomization study. Front Endocrinol (Lausanne). (2023) 14:1178831. doi: 10.3389/fendo.2023.1178831
11. Li B, Liu M, Wang Y, Gong S, Yao W, Li W, et al. Puerarin improves the bone micro-environment to inhibit OVX-induced osteoporosis via modulating SCFAs released by the gut microbiota and repairing intestinal mucosal integrity. BioMed Pharmacother. (2020) 132:110923. doi: 10.1016/j.biopha.2020.110923
12. Varvara RA and Vodnar DC. Probiotic-driven advancement: Exploring the intricacies of mineral absorption in the human body. Food Chem X. (2024) 21:101067. doi: 10.1016/j.fochx.2023.101067
13. Zhou J, Cheng J, Liu L, Luo J, and Peng X. Lactobacillus acidophilus (LA) fermenting astragalus polysaccharides (APS) improves calcium absorption and osteoporosis by altering gut microbiota. Foods. (2023) 12:275. doi: 10.3390/foods12020275
14. Gomaa EZ. Human gut microbiota/microbiome in health and diseases: a review. Antonie Van Leeuwenhoek. (2020) 113:2019–40. doi: 10.1007/s10482-020-01474-7
15. Fan Y and Pedersen O. Gut microbiota in human metabolic health and disease. Nat Rev Microbiol. (2021) 19:55–71. doi: 10.1038/s41579-020-0433-9
16. Tilg H, Zmora N, Adolph TE, and Elinav E. The intestinal microbiota fuelling metabolic inflammation. Nat Rev Immunol. (2020) 20:40–54. doi: 10.1038/s41577-019-0198-4
17. Cryan JF, O'Riordan KJ, Sandhu K, Peterson V, and Dinan TG. The gut microbiome in neurological disorders. Lancet Neurol. (2020) 19:179–94. doi: 10.1016/S1474-4422(19)30356-4
18. Wastyk HC, Fragiadakis GK, Perelman D, Dahan D, Merrill BD, Yu FB, et al. Gut-microbiota-targeted diets modulate human immune status. Cell. (2021) 184:4137–4153 e14. doi: 10.1016/j.cell.2021.06.019
19. Turnbaugh PJ and Stintzi A. Human health and disease in a microbial world. Front Microbiol. (2011) 2:190. doi: 10.3389/fmicb.2011.00190
20. Perez-Cobas AE, Gomez-Valero L, and Buchrieser C. Metagenomic approaches in microbial ecology: an update on whole-genome and marker gene sequencing analyses. Microb Genom. (2020) 6. doi: 10.1099/mgen.0.000409
21. Zhang L, Chen F, Zeng Z, Xu M, Sun F, Yang L, et al. Advances in metagenomics and its application in environmental microorganisms. Front Microbiol. (2021) 12:766364. doi: 10.3389/fmicb.2021.766364
22. Gill SR, Pop M, Deboy RT, Eckburg PB, Turnbaugh PJ, Samuel BS, et al. Metagenomic analysis of the human distal gut microbiome. Science. (2006) 312:1355–9. doi: 10.1126/science.1124234
23. Min YW and Rhee PL. The role of microbiota on the gut immunology. Clin Ther. (2015) 37:968–75. doi: 10.1016/j.clinthera.2015.03.009
24. Schroeder BO and Backhed F. Signals from the gut microbiota to distant organs in physiology and disease. Nat Med. (2016) 22:1079–89. doi: 10.1038/nm.4185
25. Ley RE, Peterson DA, and Gordon JI. Ecological and evolutionary forces shaping microbial diversity in the human intestine. Cell. (2006) 124:837–48. doi: 10.1016/j.cell.2006.02.017
26. Selber-Hnatiw S, Rukundo B, Ahmadi M, Akoubi H, Al-Bizri H, Aliu AF, et al. Human gut microbiota: toward an ecology of disease. Front Microbiol. (2017) 8:1265. doi: 10.3389/fmicb.2017.01265
27. Mengoli M, Conti G, Fabbrini M, Candela M, Brigidi P, Turroni S, et al. Microbiota-gut-brain axis and ketogenic diet: how close are we to tackling epilepsy? Microbiome Res Rep. (2023) 2:32. doi: 10.20517/mrr.2023.24
28. Raygoza Garay JA, Turpin W, Lee SH, Smith MI, Goethel A, Griffiths AM, et al. Gut microbiome composition is associated with future onset of crohn’s disease in healthy first-degree relatives. Gastroenterology. (2023) 165:670–81. doi: 10.1053/j.gastro.2023.05.032
29. Schirmer M, Smeekens SP, Vlamakis H, Jaeger M, Oosting M, Franzosa EA, et al. Linking the human gut microbiome to inflammatory cytokine production capacity. Cell. (2016) 167:1125–1136 e8. doi: 10.1016/j.cell.2016.10.020
30. Palm NW, de Zoete MR, and Flavell RA. Immune-microbiota interactions in health and disease. Clin Immunol. (2015) 159:122–7. doi: 10.1016/j.clim.2015.05.014
31. Meng C, Deng P, Miao R, Tang H, Li Y, Wang J, et al. Gut microbiome and risk of ischaemic stroke: a comprehensive Mendelian randomization study. Eur J Prev Cardiol. (2023) 30:613–20. doi: 10.1093/eurjpc/zwad052
32. Paone P and Cani PD. Mucus barrier, mucins and gut microbiota: the expected slimy partners? Gut. (2020) 69:2232–43. doi: 10.1136/gutjnl-2020-322260
33. Zhang YJ, Li S, Gan RY, Zhou T, Xu DP, Li HB, et al. Impacts of gut bacteria on human health and diseases. Int J Mol Sci. (2015) 16:7493–519. doi: 10.3390/ijms16047493
34. Rowland I, Gibson G, Heinken A, Scott K, Swann J, Thiele I, et al. Gut microbiota functions: metabolism of nutrients and other food components. Eur J Nutr. (2018) 57:1–24. doi: 10.1007/s00394-017-1445-8
35. Lozupone CA, Stombaugh JI, Gordon JI, Jansson JK, and Knight R. Diversity, stability and resilience of the human gut microbiota. Nature. (2012) 489:220–30. doi: 10.1038/nature11550
36. Conlon MA and Bird AR. The impact of diet and lifestyle on gut microbiota and human health. Nutrients. (2014) 7:17–44. doi: 10.3390/nu7010017
37. Gagliardi A, Totino V, Cacciotti F, Iebba V, Neroni B, Bonfiglio G, et al. Rebuilding the gut microbiota ecosystem. Int J Environ Res Public Health. (2018) 15:1679. doi: 10.3390/ijerph15081679
38. Vitali B, Ndagijimana M, Cruciani F, Carnevali P, Candela M, Guerzoni ME, et al. Impact of a synbiotic food on the gut microbial ecology and metabolic profiles. BMC Microbiol. (2010) 10:4. doi: 10.1186/1471-2180-10-4
39. Ha CW, Lam YY, and Holmes AJ. Mechanistic links between gut microbial community dynamics, microbial functions and metabolic health. World J Gastroenterol. (2014) 20:16498–517. doi: 10.3748/wjg.v20.i44.16498
40. Koppel N, Maini Rekdal V, and Balskus EP. Chemical transformation of xenobiotics by the human gut microbiota. Science. (2017) 356:eaag2770. doi: 10.1126/science.aag2770
41. Donaldson GP, Lee SM, and Mazmanian SK. Gut biogeography of the bacterial microbiota. Nat Rev Microbiol. (2016) 14:20–32. doi: 10.1038/nrmicro3552
42. Eriksen EF. Cellular mechanisms of bone remodeling. Rev Endocr Metab Disord. (2010) 11:219–27. doi: 10.1007/s11154-010-9153-1
43. Kim JM, Lin C, Stavre Z, Greenblatt MB, and Shim JH. Osteoblast-osteoclast communication and bone homeostasis. Cells. (2020) 9:2073. doi: 10.3390/cells9092073
44. Kearns AE, Khosla S, and Kostenuik PJ. Receptor activator of nuclear factor kappaB ligand and osteoprotegerin regulation of bone remodeling in health and disease. Endocr Rev. (2008) 29:155–92. doi: 10.1210/er.2007-0014
45. Niedzwiedzki T and Filipowska J. Bone remodeling in the context of cellular and systemic regulation: the role of osteocytes and the nervous system. J Mol Endocrinol. (2015) 55:R23–36. doi: 10.1530/JME-15-0067
46. Zuo C, Huang Y, Bajis R, Sahih M, Li YP, Dai K, et al. Osteoblastogenesis regulation signals in bone remodeling. Osteoporos Int. (2012) 23:1653–63. doi: 10.1007/s00198-012-1909-x
47. Sims NA and Martin TJ. Osteoclasts provide coupling signals to osteoblast lineage cells through multiple mechanisms. Annu Rev Physiol. (2020) 82:507–29. doi: 10.1146/annurev-physiol-021119-034425
48. Sims NA and Martin TJ. Coupling signals between the osteoclast and osteoblast: how are messages transmitted between these temporary visitors to the bone surface? Front Endocrinol (Lausanne). (2015) 6:41. doi: 10.1146/annurev-physiol-021119-034425
49. Zhao R, Zhao M, and Xu Z. The effects of differing resistance training modes on the preservation of bone mineral density in postmenopausal women: a meta-analysis. Osteoporos Int. (2015) 26:1605–18. doi: 10.1007/s00198-015-3034-0
50. Holubiac IS, Leuciuc FV, Craciun DM, and Dobrescu T. Effect of strength training protocol on bone mineral density for postmenopausal women with osteopenia/osteoporosis assessed by dual-energy X-ray absorptiometry (DEXA). Sensors (Basel). (2022) 22:1904. doi: 10.3390/s22051904
51. Martyn-St James M and Carroll S. High-intensity resistance training and postmenopausal bone loss: a meta-analysis. Osteoporos Int. (2006) 17:1225–40. doi: 10.1007/s00198-006-0083-4
52. Stengel SV, Kemmler W, Pintag R, Beeskow C, Weineck J, Lauber D, et al. Power training is more effective than strength training for maintaining bone mineral density in postmenopausal women. J Appl Physiol (1985). (2005) 99:181–8. doi: 10.1152/japplphysiol.01260.2004
53. Tanphiriyakun T, Rojanasthien S, and Khumrin P. Bone mineral density response prediction following osteoporosis treatment using machine learning to aid personalized therapy. Sci Rep. (2021) 11:13811. doi: 10.1038/s41598-021-93152-5
54. Wu X and Park S. A prediction model for osteoporosis risk using a machine-learning approach and its validation in a large cohort. J Korean Med Sci. (2023) 38:e162. doi: 10.3346/jkms.2023.38.e162
55. Wang J, Zhou B, Liu XS, Fields AJ, Sanyal A, Shi X, et al. Trabecular plates and rods determine elastic modulus and yield strength of human trabecular bone. Bone. (2015) 72:71–80. doi: 10.1016/j.bone.2014.11.006
56. Carballido-Gamio J. Imaging techniques to study diabetic bone disease. Curr Opin Endocrinol Diabetes Obes. (2022) 29:350–60. doi: 10.1097/MED.0000000000000749
57. Peyrin F, Attali D, Chappard C, and Benhamou CL. Local plate/rod descriptors of 3D trabecular bone micro-CT images from medial axis topologic analysis. Med Phys. (2010) 37:4364–76. doi: 10.1118/1.3447728
58. Wang J, Stein EM, Zhou B, Nishiyama KK, Yu YE, Shane E, et al. Deterioration of trabecular plate-rod and cortical microarchitecture and reduced bone stiffness at distal radius and tibia in postmenopausal women with vertebral fractures. Bone. (2016) 88:39–46. doi: 10.1016/j.bone.2016.04.003
59. Paolino S, Hysa E, Stoian SA, Gotelli E, Casabella A, Clini PV, et al. Metabolic profile and bone status in post-menopausal women with rheumatoid arthritis: A monocentric retrospective survey. Nutrients. (2021) 13:3168. doi: 10.3390/nu13093168
60. Zhong Z, Qian Z, Zhang X, Chen F, Ni S, Kang Z, et al. Tetrandrine prevents bone loss in ovariectomized mice by inhibiting RANKL-induced osteoclastogenesis. Front Pharmacol. (2019) 10:1530. doi: 10.3389/fphar.2019.01530
61. Wang LT, Chen LR, and Chen KH. Hormone-related and drug-induced osteoporosis: A cellular and molecular overview. Int J Mol Sci. (2023) 24:5814. doi: 10.3390/ijms24065814
62. Cheng CH, Chen LR, and Chen KH. Osteoporosis due to hormone imbalance: an overview of the effects of estrogen deficiency and glucocorticoid overuse on bone turnover. Int J Mol Sci. (2022) 23:1376. doi: 10.3390/ijms23031376
63. Schinocca C, Rizzo C, Fasano S, Grasso G, La Barbera L, Ciccia F, et al. Role of the IL-23/IL-17 pathway in rheumatic diseases: an overview. Front Immunol. (2021) 12:637829. doi: 10.3389/fimmu.2021.637829
64. Bondeson J, Blom AB, Wainwright S, Hughes C, Caterson B, and van den Berg WB. The role of synovial macrophages and macrophage-produced mediators in driving inflammatory and destructive responses in osteoarthritis. Arthritis Rheum. (2010) 62:647–57. doi: 10.1002/art.27290
65. Robinson WH, Lepus CM, Wang Q, Raghu H, Mao R, Lindstrom TM, et al. Low-grade inflammation as a key mediator of the pathogenesis of osteoarthritis. Nat Rev Rheumatol. (2016) 12:580–92. doi: 10.1038/nrrheum.2016.136
66. Fang Y, van Meurs JB, d'Alesio A, Jhamai M, Zhao H, Rivadeneira F, et al. Promoter and 3’-untranslated-region haplotypes in the vitamin d receptor gene predispose to osteoporotic fracture: the rotterdam study. Am J Hum Genet. (2005) 77:807–23. doi: 10.1086/497438
68. Borer KT. Physical activity in the prevention and amelioration of osteoporosis in women: interaction of mechanical, hormonal and dietary factors. Sports Med. (2005) 35:779–830. doi: 10.2165/00007256-200535090-00004
69. Zhu K and Prince RL. Lifestyle and osteoporosis. Curr Osteoporos Rep. (2015) 13:52–9. doi: 10.1007/s11914-014-0248-6
70. Gosset A, Pouilles JM, and Tremollieres F. Menopausal hormone therapy for the management of osteoporosis. Best Pract Res Clin Endocrinol Metab. (2021) 35:101551. doi: 10.1016/j.beem.2021.101551
71. Beral V, Banks E, Reeves G, and Appleby P. Use of HRT and the subsequent risk of cancer. J Epidemiol Biostat. (1999) 4:191–210; discussion 210-5.
72. Cosman F, Crittenden DB, Adachi JD, Binkley N, Czerwinski E, Ferrari S, et al. Romosozumab treatment in postmenopausal women with osteoporosis. N Engl J Med. (2016) 375:1532–43. doi: 10.1056/NEJMoa1607948
73. Neer RM, Arnaud CD, Zanchetta JR, Prince R, Gaich GA, Reginster JY, et al. Effect of parathyroid hormone (1-34) on fractures and bone mineral density in postmenopausal women with osteoporosis. N Engl J Med. (2001) 344:1434–41. doi: 10.1056/NEJM200105103441904
74. Xiao L, Zhou Y, Bokoliya S, Lin Q, and Hurley M. Bone loss is ameliorated by fecal microbiota transplantation through SCFA/GPR41/IGF1 pathway in sickle cell disease mice. Sci Rep. (2022) 12:20638. doi: 10.1038/s41598-022-25244-9
75. Terkawi MA, Matsumae G, Shimizu T, Takahashi D, Kadoya K, and Iwasaki N. Interplay between inflammation and pathological bone resorption: insights into recent mechanisms and pathways in related diseases for future perspectives. Int J Mol Sci. (2022) 23:1786. doi: 10.3390/ijms23031786
76. Lucas S, Omata Y, Hofmann J, Bottcher M, Iljazovic A, Sarter K, et al. Short-chain fatty acids regulate systemic bone mass and protect from pathological bone loss. Nat Commun. (2018) 9:55. doi: 10.1038/s41467-017-02490-4
77. Cooney OD, Nagareddy PR, Murphy AJ, and Lee MKS. Healthy gut, healthy bones: targeting the gut microbiome to promote bone health. Front Endocrinol (Lausanne). (2020) 11:620466. doi: 10.3389/fendo.2020.620466
78. Lyu Z, Hu Y, Guo Y, and Liu D. Modulation of bone remodeling by the gut microbiota: a new therapy for osteoporosis. Bone Res. (2023) 11:31. doi: 10.1038/s41413-023-00264-x
79. Weaver CM. Diet, gut microbiome, and bone health. Curr Osteoporos Rep. (2015) 13:125–30. doi: 10.1007/s11914-015-0257-0
80. Xiang T, Deng Z, Yang C, Tan J, Dou C, Luo F, et al. Bile acid metabolism regulatory network orchestrates bone homeostasis. Pharmacol Res. (2023) 196:106943. doi: 10.1016/j.phrs.2023.106943
81. Ramirez-Perez O, Cruz-Ramon V, Chinchilla-Lopez P, and Mendez-Sanchez N. The role of the gut microbiota in bile acid metabolism. Ann Hepatol. (2017) 16:s15–20. doi: 10.5604/01.3001.0010.5672
82. Guzior DV and Quinn RA. Review: microbial transformations of human bile acids. Microbiome. (2021) 9:140. doi: 10.1186/s40168-021-01101-1
83. Vidal C, Li W, Santner-Nanan B, Lim CK, Guillemin GJ, Ball HJ, et al. The kynurenine pathway of tryptophan degradation is activated during osteoblastogenesis. Stem Cells. (2015) 33:111–21. doi: 10.1002/stem.1836
84. Michalowska M, Znorko B, Kaminski T, Oksztulska-Kolanek E, and Pawlak D. New insights into tryptophan and its metabolites in the regulation of bone metabolism. J Physiol Pharmacol. (2015) 66:779–91.
85. Pham HT, Ono M, Hara ES, Nguyen HTT, Dang AT, Do HT, et al. Tryptophan and kynurenine enhances the stemness and osteogenic differentiation of bone marrow-derived mesenchymal stromal cells in vitro and in vivo. Materials (Basel). (2021) 14:208. doi: 10.3390/ma14010208
86. Eisa NH, Reddy SV, Elmansi AM, Kondrikova G, Kondrikov D, Shi XM, et al. Kynurenine promotes RANKL-induced osteoclastogenesis in vitro by activating the aryl hydrocarbon receptor pathway. Int J Mol Sci. (2020) 21:7931. doi: 10.3390/ijms21217931
87. Liu JR, Miao H, Deng DQ, Vaziri ND, Li P, Zhao YY, et al. Gut microbiota-derived tryptophan metabolism mediates renal fibrosis by aryl hydrocarbon receptor signaling activation. Cell Mol Life Sci. (2021) 78:909–22. doi: 10.1007/s00018-020-03645-1
88. Illes P, Krasulova K, Vyhlidalova B, Poulikova K, Marcalikova A, Pecinkova P, et al. Indole microbial intestinal metabolites expand the repertoire of ligands and agonists of the human pregnane X receptor. Toxicol Lett. (2020) 334:87–93. doi: 10.1016/j.toxlet.2020.09.015
89. Zelante T, Iannitti RG, Cunha C, De Luca A, Giovannini G, Pieraccini G, et al. Tryptophan catabolites from microbiota engage aryl hydrocarbon receptor and balance mucosal reactivity via interleukin-22. Immunity. (2013) 39:372–85. doi: 10.1016/j.immuni.2013.08.003
90. Whisner CM and Castillo LF. Prebiotics, bone and mineral metabolism. Calcif Tissue Int. (2018) 102:443–79. doi: 10.1007/s00223-017-0339-3
91. Tanabe K, Nakamura S, Moriyama-Hashiguchi MM, Kitajima M, Ejima H, Imori C, et al. Dietary fructooligosaccharide and glucomannan alter gut microbiota and improve bone metabolism in senescence-accelerated mouse. J Agric Food Chem. (2019) 67:867–74. doi: 10.1021/acs.jafc.8b05164
92. Cronin O, Lanham-New SA, Corfe BM, Gregson CL, Darling AL, Ahmadi KR, et al. Role of the microbiome in regulating bone metabolism and susceptibility to osteoporosis. Calcif Tissue Int. (2022) 110:273–84. doi: 10.1007/s00223-021-00924-2
93. David LA, Maurice CF, Carmody RN, Gootenberg DB, Button JE, Wolfe BE, et al. Diet rapidly and reproducibly alters the human gut microbiome. Nature. (2014) 505:559–63. doi: 10.1038/nature12820
94. Tomova A, Bukovsky I, Rembert E, Yonas W, Alwarith J, Barnard ND, et al. The effects of vegetarian and vegan diets on gut microbiota. Front Nutr. (2019) 6:47. doi: 10.3389/fnut.2019.00047
95. Zaiss MM, Jones RM, Schett G, and Pacifici R. The gut-bone axis: how bacterial metabolites bridge the distance. J Clin Invest. (2019) 129:3018–28. doi: 10.1172/JCI128521
96. Garcia-Montero C, Fraile-Martinez O, Gomez-Lahoz AM, Pekarek L, Castellanos AJ, Noguerales-Fraguas F, et al. Nutritional components in western diet versus mediterranean diet at the gut microbiota-immune system interplay. Implications for health and disease. Nutrients. (2021) 13:699. doi: 10.3390/nu13020699
97. Nagpal R, Shively CA, Register TC, Craft S, and Yadav H. Gut microbiome-Mediterranean diet interactions in improving host health. F1000Res. (2019) 8:699. doi: 10.12688/f1000research
98. Barber TM, Kabisch S, Pfeiffer AFH, and Weickert MO. The effects of the mediterranean diet on health and gut microbiota. Nutrients. (2023) 15:2150. doi: 10.3390/nu15092150
99. Wang K and Hu S. The synergistic effects of polyphenols and intestinal microbiota on osteoporosis. Front Immunol. (2023) 14:1285621. doi: 10.3389/fimmu.2023.1285621
100. Riviere A, Selak M, Lantin D, Leroy F, and Vuyst LD. Bifidobacteria and butyrate-producing colon bacteria: importance and strategies for their stimulation in the human gut. Front Microbiol. (2016) 7:979. doi: 10.3389/fmicb.2016.00979
101. So D, Whelan K, Rossi M, Morrison G, Holtmann G, Kelly JT, et al. Dietary fiber intervention on gut microbiota composition in healthy adults: a systematic review and meta-analysis. Am J Clin Nutr. (2018) 107:965–83. doi: 10.1093/ajcn/nqy041
102. Feng B, Lu J, Han Y, Han Y, Qiu X, and Zeng Z. The role of short-chain fatty acids in the regulation of osteoporosis: new perspectives from gut microbiota to bone health: A review. Med (Baltimore). (2024) 103:e39471. doi: 10.1097/MD.0000000000039471
103. McCabe L, Britton RA, and Parameswaran N. Prebiotic and probiotic regulation of bone health: role of the intestine and its microbiome. Curr Osteoporos Rep. (2015) 13:363–71. doi: 10.1007/s11914-015-0292-x
104. Carlson JL, Erickson JM, Hess JM, Gould TJ, and Slavin JL. Prebiotic dietary fiber and gut health: comparing the in vitro fermentations of beta-glucan, inulin and xylooligosaccharide. Nutrients. (2017) 9:1361. doi: 10.20944/preprints201710.0171.v1
105. Healey G, Murphy R, Butts C, Brough L, Whelan K, and Coad J. Habitual dietary fibre intake influences gut microbiota response to an inulin-type fructan prebiotic: a randomised, double-blind, placebo-controlled, cross-over, human intervention study. Br J Nutr. (2018) 119:176–89. doi: 10.1017/S0007114517003440
106. Salazar N, Dewulf EM, Neyrinck AM, Bindels LB, Cani PD, Mahillon J, et al. Inulin-type fructans modulate intestinal Bifidobacterium species populations and decrease fecal short-chain fatty acids in obese women. Clin Nutr. (2015) 34:501–7. doi: 10.1016/j.clnu.2014.06.001
107. Yang HJ, Zhang T, Yue Y, Jeong SJ, Ryu MS, Wu X, et al. Protective effect of long-term fermented soybeans with abundant bacillus subtilis on glucose and bone metabolism and memory function in ovariectomized rats: modulation of the gut microbiota. Foods. (2023) 12:2958. doi: 10.3390/foods12152958
108. Zhang T, Yue Y, Jeong SJ, Ryu MS, Wu X, Yang HJ, et al. Improvement of estrogen deficiency symptoms by the intake of long-term fermented soybeans (Doenjang) rich in bacillus species through modulating gut microbiota in estrogen-deficient rats. Foods. (2023) 12:1143. doi: 10.3390/foods12061143
109. Chiang SS and Pan TM. Antiosteoporotic effects of Lactobacillus -fermented soy skim milk on bone mineral density and the microstructure of femoral bone in ovariectomized mice. J Agric Food Chem. (2011) 59:7734–42. doi: 10.1021/jf2013716
110. Lenini C, Rodriguez Ayala F, Goni AJ, Rateni L, Nakamura A, Grau RR, et al. Probiotic properties of Bacillus subtilis DG101 isolated from the traditional Japanese fermented food natto. Front Microbiol. (2023) 14:1253480. doi: 10.3389/fmicb.2023.1253480
111. Jeong DY, Daily JW, Lee GH, Ryu MS, Yang HJ, Jeong SY, et al. Short-term fermented soybeans with bacillus amyloliquefaciens potentiated insulin secretion capacity and improved gut microbiome diversity and intestinal integrity to alleviate asian type 2 diabetic symptoms. J Agric Food Chem. (2020) 68:13168–78. doi: 10.1021/acs.jafc.9b07962
112. Choi HJ, Shin D, Shin M, Yun B, Kang M, Yang HJ, et al. Comparative genomic and functional evaluations of bacillus subtilis newly isolated from korean traditional fermented foods. Foods. (2020) 9:1805. doi: 10.3390/foods9121805
113. Liu Y, Feng J, Pan H, Zhang X, and Zhang Y. Genetically engineered bacterium: Principles, practices, and prospects. Front Microbiol. (2022) 13:997587. doi: 10.3389/fmicb.2022.997587
114. Yin T, Diao Z, Blum NT, Qiu L, Ma A, and Huang P. Engineering bacteria and bionic bacterial derivatives with nanoparticles for cancer therapy. Small. (2022) 18:e2104643. doi: 10.1002/smll.202104643
115. Wallimann A, Hildebrand M, Groeger D, Stanic B, Akdis CA, Zeiter S, et al. An Exopolysaccharide Produced by Bifidobacterium longum 35624(R) Inhibits Osteoclast Formation via a TLR2-Dependent Mechanism. Calcif Tissue Int. (2021) 108:654–66. doi: 10.1007/s00223-020-00790-4
116. Yin L, Wang X, Xu H, Yin B, Wang X, Zhang Y, et al. Unrecognized risk of perfluorooctane sulfonate in promoting conjugative transfers of bacterial antibiotic resistance genes. Appl Environ Microbiol. (2023) 89:e0053323. doi: 10.1128/aem.00533-23
117. McInnes RS, McCallum GE, Lamberte LE, and van Schaik W. Horizontal transfer of antibiotic resistance genes in the human gut microbiome. Curr Opin Microbiol. (2020) 53:35–43. doi: 10.1016/j.mib.2020.02.002
118. Wright GD and Sutherland AD. New strategies for combating multidrug-resistant bacteria. Trends Mol Med. (2007) 13:260–7. doi: 10.1016/j.molmed.2007.04.004
119. Lerminiaux NA and Cameron ADS. Horizontal transfer of antibiotic resistance genes in clinical environments. Can J Microbiol. (2019) 65:34–44. doi: 10.1139/cjm-2018-0275
120. Michaelis C and Grohmann E. Horizontal gene transfer of antibiotic resistance genes in biofilms. Antibiotics (Basel). (2023) 12:328. doi: 10.3390/antibiotics12020328
121. Ma S, Wang N, Zhang P, Wu W, and Fu L. Fecal microbiota transplantation mitigates bone loss by improving gut microbiome composition and gut barrier function in aged rats. PeerJ. (2021) 9:e12293. doi: 10.7717/peerj.12293
122. Wang N, Ma S, and Fu L. Gut microbiota dysbiosis as one cause of osteoporosis by impairing intestinal barrier function. Calcif Tissue Int. (2022) 110:225–35. doi: 10.1007/s00223-021-00911-7
123. Seely KD, Kotelko CA, Douglas H, Bealer B, and Brooks AE. The human gut microbiota: A key mediator of osteoporosis and osteogenesis. Int J Mol Sci. (2021) 22:9452. doi: 10.3390/ijms22179452
124. Tilg H, Moschen AR, Kaser A, Pines A, and Dotan I. Gut, inflammation and osteoporosis: basic and clinical concepts. Gut. (2008) 57:684–94. doi: 10.1136/gut.2006.117382
125. Hsu E and Pacifici R. From osteoimmunology to osteomicrobiology: how the microbiota and the immune system regulate bone. Calcif Tissue Int. (2018) 102:512–21. doi: 10.1007/s00223-017-0321-0
126. Ohlsson C and Sjogren K. Effects of the gut microbiota on bone mass. Trends Endocrinol Metab. (2015) 26:69–74. doi: 10.1016/j.tem.2014.11.004
127. Tanaka Y, Nakayamada S, and Okada Y. Osteoblasts and osteoclasts in bone remodeling and inflammation. Curr Drug Targets Inflammation Allergy. (2005) 4:325–8. doi: 10.2174/1568010054022015
128. Ohlsson C and Sjogren K. Osteomicrobiology: A new cross-disciplinary research field. Calcif Tissue Int. (2018) 102:426–32. doi: 10.1007/s00223-017-0336-6
129. Mimee M, Citorik RJ, and Lu TK. Microbiome therapeutics - Advances and challenges. Adv Drug Delivery Rev. (2016) 105:44–54. doi: 10.1016/j.addr.2016.04.032
130. Galloway-Pena JR, Smith DP, Sahasrabhojane P, Wadsworth WD, Fellman BM, Ajami NJ, et al. Characterization of oral and gut microbiome temporal variability in hospitalized cancer patients. Genome Med. (2017) 9:21. doi: 10.1186/s13073-017-0409-1
131. Montalvany-Antonucci CC, Duffles LF, de Arruda JAA, Zicker MC, de Oliveira S, Macari S, et al. Short-chain fatty acids and FFAR2 as suppressors of bone resorption. Bone. (2019) 125:112–21. doi: 10.1016/j.bone.2019.05.016
132. Wu H, Singer J, Kwan TK, Loh YW, Wang C, Tan J, et al. Gut microbial metabolites induce donor-specific tolerance of kidney allografts through induction of T regulatory cells by short-chain fatty acids. J Am Soc Nephrol. (2020) 31:1445–61. doi: 10.1681/ASN.2019080852
133. Li Z, Huang J, Wang F, Li W, Wu X, Zhao C, et al. Dual targeting of bile acid receptor-1 (TGR5) and farnesoid X receptor (FXR) prevents estrogen-dependent bone loss in mice. J Bone Miner Res. (2019) 34:765–76. doi: 10.1002/jbmr.3652
134. Cho SW, An JH, Park H, Yang JY, Choi HJ, Kim SW, et al. Positive regulation of osteogenesis by bile acid through FXR. J Bone Miner Res. (2013) 28:2109–21. doi: 10.1002/jbmr.1961
135. Fiorucci S, Mencarelli A, Palladino G, and Cipriani S. Bile-acid-activated receptors: targeting TGR5 and farnesoid-X-receptor in lipid and glucose disorders. Trends Pharmacol Sci. (2009) 30:570–80. doi: 10.1016/j.tips.2009.08.001
136. Gasaly N, de Vos P, and Hermoso MA. Impact of bacterial metabolites on gut barrier function and host immunity: A focus on bacterial metabolism and its relevance for intestinal inflammation. Front Immunol. (2021) 12:658354. doi: 10.3389/fimmu.2021.658354
137. Zhang J, Zhu S, Ma N, Johnston LJ, Wu C, and Ma X. Metabolites of microbiota response to tryptophan and intestinal mucosal immunity: A therapeutic target to control intestinal inflammation. Med Res Rev. (2021) 41:1061–88. doi: 10.1002/med.21752
138. Roager HM and Licht TR. Microbial tryptophan catabolites in health and disease. Nat Commun. (2018) 9:3294. doi: 10.1038/s41467-018-05470-4
139. Ji J, Shu D, Zheng M, Wang J, Luo C, Wang Y, et al. Microbial metabolite butyrate facilitates M2 macrophage polarization and function. Sci Rep. (2016) 6:24838. doi: 10.1038/srep24838
140. Jin UH, Lee SO, Sridharan G, Lee K, Davidson LA, Jayaraman A, et al. Microbiome-derived tryptophan metabolites and their aryl hydrocarbon receptor-dependent agonist and antagonist activities. Mol Pharmacol. (2014) 85:777–88. doi: 10.1124/mol.113.091165
141. Jia M, Li D, Wang R, Wang A, Strappe P, Wu Q, et al. Gut microbiota derived structural changes of phenolic compounds from colored rice and its corresponding fermentation property. Food Funct. (2022) 13:10759–68. doi: 10.1039/D2FO01678H
142. Zhou T, Liu W, Lai H, Liu Y, Su W, Xu Z, et al. Hydrogen sulfide promotes osteogenesis by modulating macrophage polarization. Int Immunopharmacol. (2023) 115:109564. doi: 10.1016/j.intimp.2022.109564
143. Greenbaum J, Lin X, Su KJ, Gong R, Shen H, Shen J, et al. Integration of the human gut microbiome and serum metabolome reveals novel biological factors involved in the regulation of bone mineral density. Front Cell Infect Microbiol. (2022) 12:853499. doi: 10.3389/fcimb.2022.853499
144. Qi T, Li L, and Weidong T. The role of sphingolipid metabolism in bone remodeling. Front Cell Dev Biol. (2021) 9:752540. doi: 10.3389/fcell.2021.752540
145. Chen C, Cao Z, Lei H, Zhang C, Wu M, Huang S, et al. Microbial tryptophan metabolites ameliorate ovariectomy-induced bone loss by repairing intestinal ahR-mediated gut-bone signaling pathway. Adv Sci (Weinh). (2024) 11:e2404545. doi: 10.1002/advs.202404545
146. Tyagi AM, Yu M, Darby TM, Vaccaro C, Li JY, Owens JA, et al. The microbial metabolite butyrate stimulates bone formation via T regulatory cell-mediated regulation of WNT10B expression. Immunity. (2018) 49:1116–1131 e7. doi: 10.1016/j.immuni.2018.10.013
147. Thomas C, Gioiello A, Noriega L, Strehle A, Oury J, Rizzo G, et al. TGR5-mediated bile acid sensing controls glucose homeostasis. Cell Metab. (2009) 10:167–77. doi: 10.1016/j.cmet.2009.08.001
148. Hiippala K, Jouhten H, Ronkainen A, Hartikainen A, Kainulainen V, Jalanka J, et al. The potential of gut commensals in reinforcing intestinal barrier function and alleviating inflammation. Nutrients. (2018) 10:988. doi: 10.3390/nu10080988
149. Kang X, Liu C, Ding Y, Ni Y, Ji F, Lau HCH, et al. Roseburia intestinalis generated butyrate boosts anti-PD-1 efficacy in colorectal cancer by activating cytotoxic CD8(+) T cells. Gut. (2023) 72:2112–22. doi: 10.1136/gutjnl-2023-330291
150. Maioli TU, Borras-Nogues E, Torres L, Barbosa SC, Martins VD, Langella P, et al. Possible benefits of faecalibacterium prausnitzii for obesity-associated gut disorders. Front Pharmacol. (2021) 12:740636. doi: 10.3389/fphar.2021.740636
151. Roopchand DE, Carmody RN, Kuhn P, Moskal K, Rojas-Silva P, Turnbaugh PJ, et al. Dietary polyphenols promote growth of the gut bacterium akkermansia muciniphila and attenuate high-fat diet-induced metabolic syndrome. Diabetes. (2015) 64:2847–58. doi: 10.2337/db14-1916
152. Martinez-Medina M, Denizot J, Dreux N, Robin F, Billard E, Bonnet R, et al. Western diet induces dysbiosis with increased E coli in CEABAC10 mice, alters host barrier function favouring AIEC colonisation. Gut. (2014) 63:116–24. doi: 10.1136/gutjnl-2012-304119
153. Moens F, Van den Abbeele P, Basit AW, Dodoo C, Chatterjee R, Smith B, et al. A four-strain probiotic exerts positive immunomodulatory effects by enhancing colonic butyrate production in vitro. Int J Pharm. (2019) 555:1–10. doi: 10.1016/j.ijpharm.2018.11.020
154. Wang X, Zhang P, and Zhang X. Probiotics regulate gut microbiota: an effective method to improve immunity. Molecules. (2021) 26:6076. doi: 10.3390/molecules26196076
155. Cristofori F, Dargenio VN, Dargenio C, Miniello VL, Barone M, and Francavilla R. Anti-inflammatory and immunomodulatory effects of probiotics in gut inflammation: A door to the body. Front Immunol. (2021) 12:578386. doi: 10.3389/fimmu.2021.578386
156. McLoughlin RF, Berthon BS, Jensen ME, Baines KJ, and Wood LG. Short-chain fatty acids, prebiotics, synbiotics, and systemic inflammation: a systematic review and meta-analysis. Am J Clin Nutr. (2017) 106:930–45. doi: 10.3945/ajcn.117.156265
157. Zhao L, Zhang F, Ding X, Wu G, Lam YY, Wang X, et al. Gut bacteria selectively promoted by dietary fibers alleviate type 2 diabetes. Science. (2018) 359:1151–6. doi: 10.1126/science.aao5774
158. Li SS, Zhu A, Benes V, Costea PI, Hercog R, Hildebrand F, et al. Durable coexistence of donor and recipient strains after fecal microbiota transplantation. Science. (2016) 352:586–9. doi: 10.1126/science.aad8852
159. Aggarwal N, Breedon AME, Davis CM, Hwang IY, and Chang MW. Engineering probiotics for therapeutic applications: recent examples and translational outlook. Curr Opin Biotechnol. (2020) 65:171–9. doi: 10.1016/j.copbio.2020.02.016
160. Yadav R, Kumar V, Baweja M, and Shukla P. Gene editing and genetic engineering approaches for advanced probiotics: A review. Crit Rev Food Sci Nutr. (2018) 58:1735–46. doi: 10.1080/10408398.2016.1274877
Keywords: gut microbiome, bone health, osteoporosis, microbial metabolites, bone remodeling
Citation: Hwang D, Chong E, Li Y, Li Y and Roh K (2025) Deciphering the gut microbiome’s metabolic code: pathways to bone health and novel therapeutic avenues. Front. Endocrinol. 16:1553655. doi: 10.3389/fendo.2025.1553655
Received: 17 February 2025; Accepted: 25 April 2025;
Published: 22 May 2025.
Edited by:
Jayesh Jagannath Ahire, Dr. Reddy’s Laboratories, IndiaReviewed by:
Vikram Kumar, SRM University (Delhi-NCR), IndiaKunal R More, Nationwide Children’s Hospital, United States
Copyright © 2025 Hwang, Chong, Li, Li and Roh. This is an open-access article distributed under the terms of the Creative Commons Attribution License (CC BY). The use, distribution or reproduction in other forums is permitted, provided the original author(s) and the copyright owner(s) are credited and that the original publication in this journal is cited, in accordance with accepted academic practice. No use, distribution or reproduction is permitted which does not comply with these terms.
*Correspondence: Kangsan Roh, a3JvaEBtZ2guaGFydmFyZC5lZHU=