- 1School of Public Health, University of South China, Hengyang, China
- 2Division of Conservation and Application of Biological Resources, Shenzhen Center for Disease Control and Prevention, Shenzhen, China
- 3Department of Environmental and Occupational Health, School of Public Health, Guangdong Medical University, Dongguan, China
- 4Department of Emergency, The Eighth Affiliated Hospital, Sun Yat-Sen University, Shenzhen, China
- 5Department of Endocrinology, The Eighth Affiliated Hospital, Sun Yat-Sen University, Shenzhen, China
- 6School of Public Health, Shenzhen University Medical School, Shenzhen University, Shenzhen, China
Background: Bisphenol AF (BPAF), an alternative to Bisphenol A (BPA), is increasingly utilized in various industrial applications, yet its toxicological profile remains incompletely understood. This study aims to investigate the impact of BPAF exposure on obesity and lipid metabolism in male mice subjected to either a normal chow diet (ND) or a high-fat diet (HFD).
Methods: Mice were exposed to BPAF at a concentration of 100 μg/kg every other day for five months under different dietary conditions, and body weight, rectal temperature, and food intake were monitored regularly. After the mice were sacrificed, the hepatic lipid metabolism was analyzed by measuring serum, hepatic lipids and performing hepatic metabolomics; energy metabolism was elucidated by assessing thermogenic pathways in brown adipose tissue (BAT) and factors affecting ingestion in the hypothalamus; the development and pathways of obesity were indicated by exploring lipogenesis and lipolysis pathways and fat accumulation in white adipose tissue (WAT).
Results: Histomorphometric analyses indicated that BPAF exposure induced drived fat deposition in white adipose tissue through adipocyte hypertrophy-mediated pathways in eWAT of ND and HFD mice, accompanied by weight gain in HFD mice. Energy metabolism analysis showed that BPAF exposure decreased resting body temperature and reduced thermogenic factor expression in BAT of ND and HFD mice, which may affect energy expenditure. Hepatic metabolomics analysis suggested that BPAF exposure interfered with hepatic lipid metabolism in ND and HFD mice, with elevated levels of hepatic triglycerides, total cholesterol, and free fatty acids in HFD mice. Transcript analysis revealed altered expression levels of genes regulating lipid metabolism in white adipose tissue of ND and HFD mice, with a down-regulation observed in p-HSL protein expression, indicative of a potential inhibition effects of BPAF on lipolysis signaling pathway.
Conclusion: Chronic BPAF exposure differentially exacerbates fat deposition in mice fed normal or high-fat diets via affecting lipid metabolism. Given the widespread prevalence of obesity and the pervasive environmental presence of BPAF, our findings provide valuable insights into the metabolic toxicity of BPAF, thereby raise further concern on the safe utilization and precision prevention of this unique chemical.
1 Introduction
Obesity has become a serious public health problem in the present world, given its rising morbidity, excess mortality, and enormous medical and economic burden (1, 2). It is extensively linked to various chronic diseases, including, but not limited to, diabetes, fatty liver, hyperlipidemia, atherosclerosis, stroke and cancer. In particular, obesity in children and adolescents has evolved into an escalating epidemic, not only interfering with normal growth and metabolism but also persisting into adulthood, with profound long-term health consequences (3). The pathophysiological process of obesity is influenced by genetic, endocrine, behavioral, psychological, and environmental factors, and it is direct result of imbalance between energy intake and expenditure. Among these factors, diets high in fat and sedentary lifestyles are the most widespread causes of obesity (4). However, in recent years, the role of environmental chemicals in promoting metabolic disorders, including obesity, has also received increasingly more attention (5).
Bisphenol A (BPA) is a globally ubiquitous environmental chemical widely used in the production of plastics and resins, and has been detected in a variety of environmental matrices such as water, air, and soil, in human biological samples such as breast milk, plasma, and in other wild animals (6–9). A plethora of deleterious health effects have been demonstrated for BPA, including metabolic disturbance, endocrine disruption, hepatotoxicity, reproductive toxicity and neurotoxicity (10–13). Notably, exposure to BPA during the early stages of life (gestation and/or lactation) can interfere with the normal programming of the body’s systems (14). The epigenetic changes induced by this developmental exposure cause a significant increase in the risk of developing chronic diseases such as abnormalities of glucose and lipid metabolism and cardiovascular disease in adulthood (15, 16). These detrimental effects have prompted the search for safer alternatives. As a result, alternatives such as bisphenol AF (BPAF), bisphenol F (BPF), and bisphenol S (BPS) have been produced and put into use in large quantities. However, these analogs appear to present analogical or even more serious environmental threats and health risks than BPA (17–22).
Most bisphenols (BPs) have the potential to act as obesogens, a property that can be attributed to their capacity to interfere with the equilibrium of the endocrine system and energy metabolism as environmental hormonal pollutants (23). Epidemiologic studies have shown significant associations between BPs exposure and obesity (24–26). For example, a cross-sectional study showed a positive correlation between urinary levels of BPAF and indicators of childhood obesity, particularly in boys (27). Animal studies have shown that BPA and its substitutes (e.g., BPAF and BPF) interfere with hepatic lipid metabolism in male mice fed a high-fat diet, highlighting their potential role in inducing obesity (28). Moreover, perinatal exposure to these chemicals induces disorders of glucose and lipid metabolism, increased body weight and eWAT weight, and hepatic steatosis in offspring during adulthood (29–32). It has also been shown that BPS affects hypothalamic neuropeptides and interferes with feeding behavior, which might induce energy imbalance finally leading to obesity (33).
Although previous studies have revealed a strong association between BPs and obesity, studies on the obesogenic effects of long-term exposure to BPAF are still limited. The aim of this study was to investigate the role of long-term BPs (especially BPAF) exposure in the pathophysiological process of obesity under normal and high-fat dietary conditions. We wished to indicate whether obesity occurs by monitoring body weight changes, blood lipids, and white adipose tissue (WAT) weight (ratio); to explore lipid metabolism disorders by analyzing lipid synthesis and catabolism in epididymal white adipose tissue (eWAT) and subcutaneous white adipose tissue (sWAT) combined with hepatic lipid metabolism profiles; and to elucidate the imbalance of energy homeostasis by investigating thermogenic pathways in brown adipose tissue (BAT), food intake regulatory pathways in the hypothalamus, in conjunction with total energy intake of food and changes in resting body temperature. Our findings are expected to shed light on the health risks associated with BPAF exposure, especially its potential effects on obesity.
2 Materials and methods
2.1 Mice
Male C57BL/6J mice aged 8 weeks were brought from Guangdong Medical Laboratory Animal Center (Guangdong, China). All mice experiments were performed according to the guidelines of the Institutional Animal Care and Use Committee of Shenzhen Center for Disease Control and Prevention (Shenzhen CDC A2018019). Mice were fed either a normal chow diet (ND, 10 kcal% fat, Research Diets, D12450) or a high-fat diet (HFD, 60 kcal% fat, Research Diets, D12492) under controlled conditions of 22-25°C and a 12h light/dark cycle. Mice were provided with ad libitum access to food and water unless specified otherwise for experimental procedures.
2.2 Experimental design and treatment
After acclimatisation feeding, the mice were randomly divided into 8 groups of 10 mice each. Four of the groups (shown as ND-CON, ND-BPAF, ND-BPS, ND-BPF) were given a normal diet, while BPAF, BPF, and BPS were dissolved in olive oil (Macklin, O815211), respectively, and were administered to the mice by oral gavage at a concentration of 100 μg/kg every other day for five months. BPAF, BPF, and BPS were brought form Sigma-Aldrich (≥99% HPLC). Control mice were administered olive oil by gavage only. The other four groups (shown as HFD-CON, HFD-BPAF, HFD-BPS, HFD-BPF) of mice were given a high-fat diet, and other treatments as above.
2.3 Histological analysis of adipose tissue
The eWAT samples from mice were fixed in 4% paraformaldehyde overnight, embedded in paraffin, and sectioned. Sections were then subjected to hematoxylin and eosin staining for histological examination.
2.4 DNA content measurement
DNA was extracted from eWAT and sWAT using a DNA extraction buffer, and DNA concentration was quantified by NanoDrop spectrophotometer using the method of Ying Cheng et al (34).
2.5 TG, TC, FFA measurement
Liver cells lipids were extracted using chloroform-methanol. Serum and liver TG and TC levels were measured using the TG and TC kit (SSUF-C, Shanghai, China). Serum and liver FFA levels were determined using the FFA kit (Wako Pure Chemical Industries, Osaka, Japan).
2.6 Liver metabolites quantification
Targeted quantitative metabolomics analysis of liver tissue was performed on the XporeMET platform (Metabolo - Profile, Shanghai, China). Liver metabolites were quantitated using the ultrahigh-performance liquid chromatography-tandem mass spectrometry (UPLC-MS/MS) system (ACQUITY UPLC-Xevo TQ-S, Waters Corp., Milford, MA, USA). The raw data was performed by QuanMET software (v2.0, Metabo-Profile, Shanghai, China) and metabolites were peak-integrated, calibrated and quantified.
2.7 Western blot
Total proteins were extracted from tissue lysates (eWAT, sWAT, BAT), separated by 10% SDS-PAGE gels, and transferred onto PVDF membranes for western blotting. Primary antibodies were as follows: anti-FAS, anti-phospho-HSL (Ser660), anti-HSL, anti-CPT1A (all obtained from Cell Signaling Technology, Beverly, MA, USA), anti-SCD1, anti-UCP1 (Santa Cruz Biotechnology Inc, CA, USA), anti-β-Actin (Sigma, MO, USA).
2.8 Total RNA extraction and qRT-PCR analysis
Total RNA was extracted from BAT, eWAT, sWAT, and hypothalamus using TRIzol reagent (Invitrogen, USA). Synthesized cDNA from total RNA was used PrimeScript™ RT reagent kit (TaKaRa, Beijing, China). qRT-PCR analysis was performed using PowerUp™ SYBR™ Green Master Mix (ABI, USA) and Prism 7500 system (ABI, USA). Gene expression levels were normalized to GAPDH mRNA. Primers were synthesized by Sangon Biotechnology (Shanghai, China) and are shown in Supplementary Table S1.
2.9 Statistics
All the data are expressed as means ± standard error (SE). A two-tailed Student’s t-test (two groups) or one-way analysis of variance followed by a Student-Newman-Keuls (SNK) test (multiple groups) was used to assess significant differences, when the data conformed to normal distribution with chi-square. Conversely, nonparametric tests were used. P < 0.05 means statistically significant.
3 Results
3.1 BPAF treated mice showed increased body weight under HFD condition
BPA and its analogues have been implicated in adverse health effects and their association with obesity has been documented (35). To explore the effects of BPA analogues (BPAF, BPF, BPS) under varying nutritional conditions, male mice were subjected to 100 μg/kg of the chemicals by gavage every other day for 5 months, while feeding either a normal chow diet (ND) or a high-fat diet (HFD). The experimental setup is illustrated in Figure 1A. Results revealed that, under normal diet condition BPAF, BPF, and BPS treatments exhibited negligible effects on body weight (Figure 1B), with no significant differences observed in relative body weight changes (Figure 1C). However, under a high-fat diet, BPAF and BPS treatments, but not BPF, led to increased body weight (Figure 1D), although the relative body weight remained unchanged (Figure 1E).
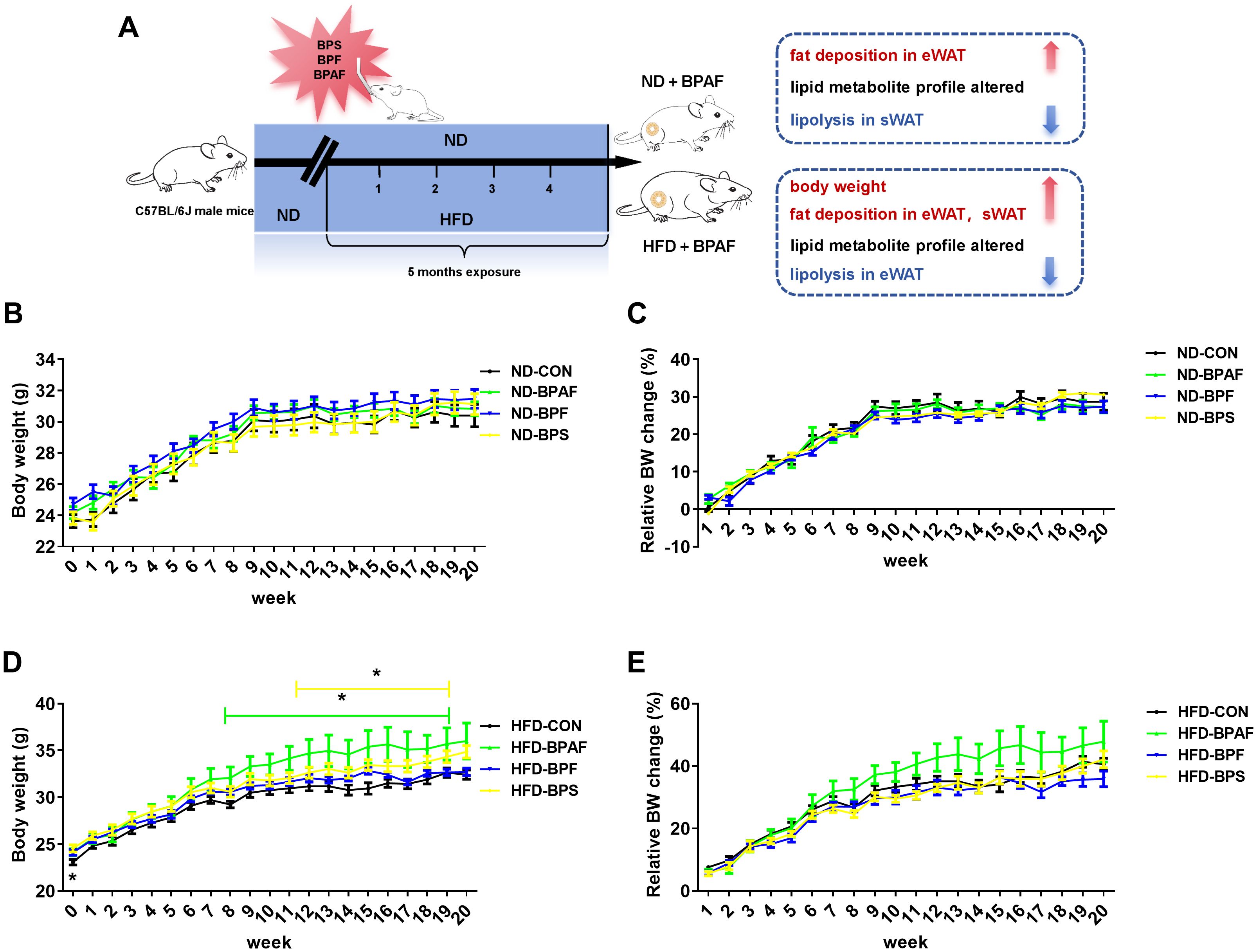
Figure 1. Effects of BPAF, BPF and BPS on body weight under ND and HFD conditions. C57BL/6j mice fed with ND or HFD were gavaged with 100 μg/kg of BPAF, BPF, or BPS for five months every other day, and body weights were measured weekly. (A) design of animal experiments in this study; (B) body weight of ND mice; (C) relative body weight change of ND mice; (D) body weight of HFD mice; (E) relative body weight change of HFD mice. N=8, data were expressed as means ± SE, one-way analysis of variance followed by Student-Newman-Keuls test, * means p <0.05.
3.2 BPAF induced fat accumulation in white adipose tissue
Given the pronounced effects of BPAF on body weight gain, we further investigated its role in fat accumulation. BPAF treatment exhibited a significant increase in eWAT under normal chow diet condition (Figure 2A). Conversely, no discernible effect was observed on the weight of sWAT (Figure 2A). However, mice fed a high-fat diet displayed increased fat deposition in both eWAT and sWAT following BPAF exposure (Figure 2B). Consistently, histological analysis confirmed that adipocytes in eWAT were larger in the BPAF-treated group of mice than in the control mice, both under normal and high-fat diet conditions (Figure 2C). Additionally, DNA content in sWAT and eWAT exhibited no significant differences in mice subjected to either normal diet or high-fat diet with BPAF treatment (Figures 2D, E). This suggests that BPAF exposure did not cause an increase in the number of adipocytes in WAT of mice. Collectively, our mechanistic analyses establish that BPAF exposure drived fat deposition in eWAT through adipocyte hypertrophy-mediated pathways, rather than hyperplastic expansion.
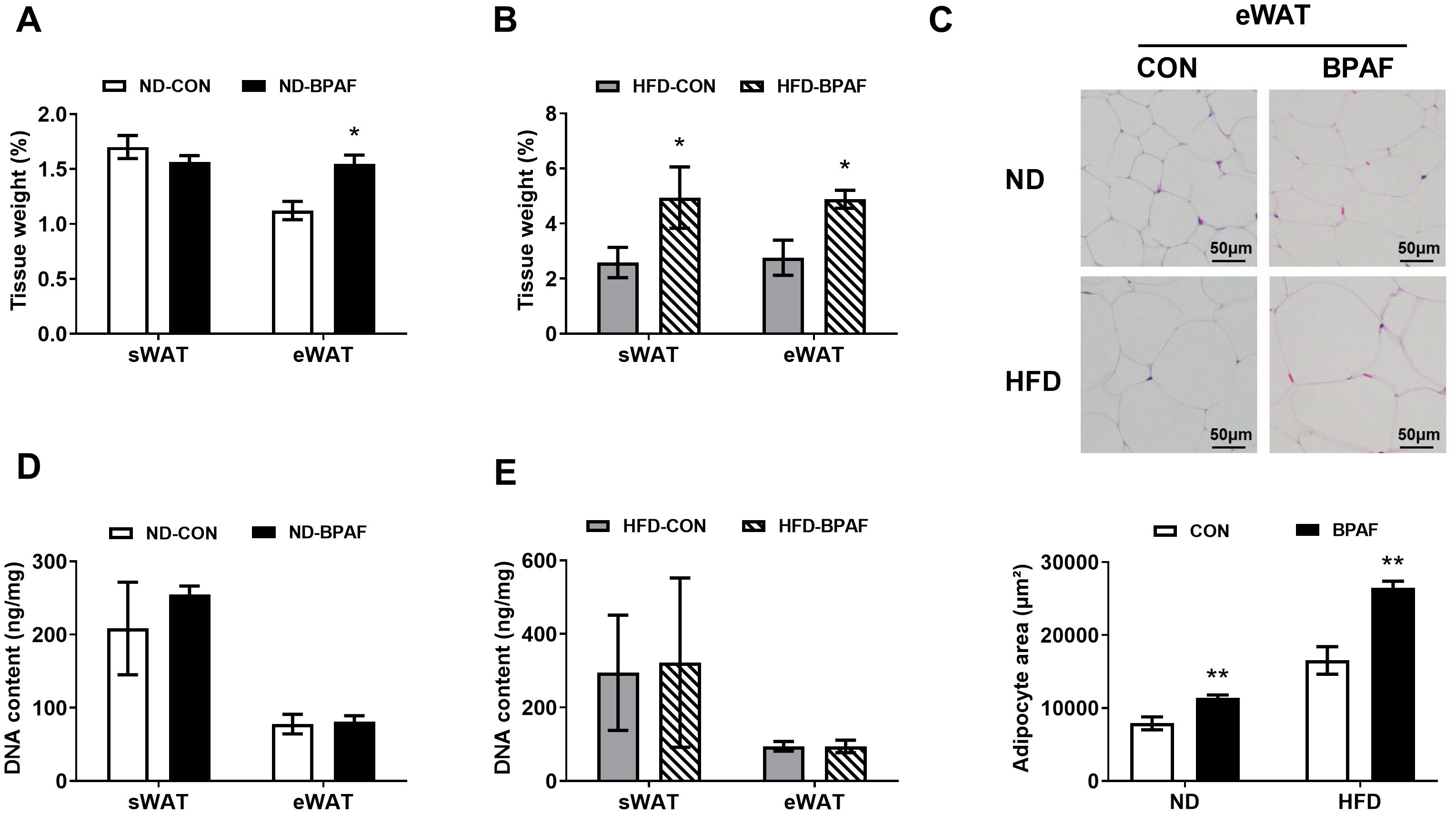
Figure 2. BPAF induced white adipose tissue fat accumulation. C57BL/6j mice fed with ND or HFD were gavaged with 100 μg/kg of BPAF for five months every other day, followed by examination of DNA content in eWAT and sWAT, weighing of eWAT and sWAT, detection of adipocyte size in eWAT. (A) sWAT and eWAT tissue weight (ratio) of ND mice treated with BPAF; (B) sWAT and eWAT tissue weight (ratio) of HFD mice treated with BPAF; (C) adipocyte size in eWAT of BPAF treated ND and HFD mice; top, representative images of H & E staining scale bar is 50 μm; bottom, area statistics of adipocyte; (D) DNA content per unit of tissue in sWAT and eWAT of BPAF treated ND mice; (E) DNA content per unit of tissue in sWAT and eWAT of BPAF treated HFD mice. N=6, data were expressed as means ± SE, two-tailed Student’s t test were used to analyze the difference between CON and BPAF group, * means p <0.05, ** means p <0.01.
3.3 Impact of BPAF exposure on energy homeostasis
Imbalances in energy homeostasis play a pivotal role in fat accumulation (4). To evaluate the effects of BPAF on energy intake, we treated different nutritional mice with BPAF, and results showed that BPAF exposure had no influence on the total energy intake from food (Figure 3A). Rectal temperatures in the resting state were significantly lower in the ND-BPAF group of mice at one month and five months from the start of the experiment compared to the ND-CON group of mice (Figure 3B). Compared with HFD-CON mice, rectal temperatures in the resting state of mice were measured to be lower at 3 and 5 months after BPAF exposure (Figure 3C). The hypothalamus, as a neuroendocrine regulatory center, plays a crucial role in the development of obesity (36). We evaluated the expression levels of key factors regulating feeding behavior and energy metabolism in hypothalamus, including proopiomelanocortin (POMC), neuropeptide (NPY), agouti-related protein (AGRP), cocaine and amphetamine-regulated transcript (CART), thyrotropin releasing hormone (TRH), corticotropin releasing hormone (CRH), and suppressor of cytokine signaling 3 (SOCS3) (36). Under normal diet condition, POMC expression was significantly increased (Figure 3D), while no changes were observed in HFD mice (Figure 3E) after BPAF treatment. The thermogenic function of BAT is not only involved in body temperature maintenance, but also influences obesity by regulating energy distribution balance (37, 38). We found that BPAF exposure decreased the expression levels of genes associated with thermoregulation, including uncoupling protein 1 (UCP1), peroxisome proliferator-activated receptor gamma co-activator-1 alpha (PGC1α) and PR domain containing 16 (PRDM16) (39) in BAT of mice subjected to normal diet (Figure 3F), although UCP1 protein expression was not changed (Figure 3G). Only PGC1α gene expression exhibited a significant decrease, while UCP1 and PRDM16 showed no significant differences in HFD mice after BPAF treatment (Figures 3H, I).
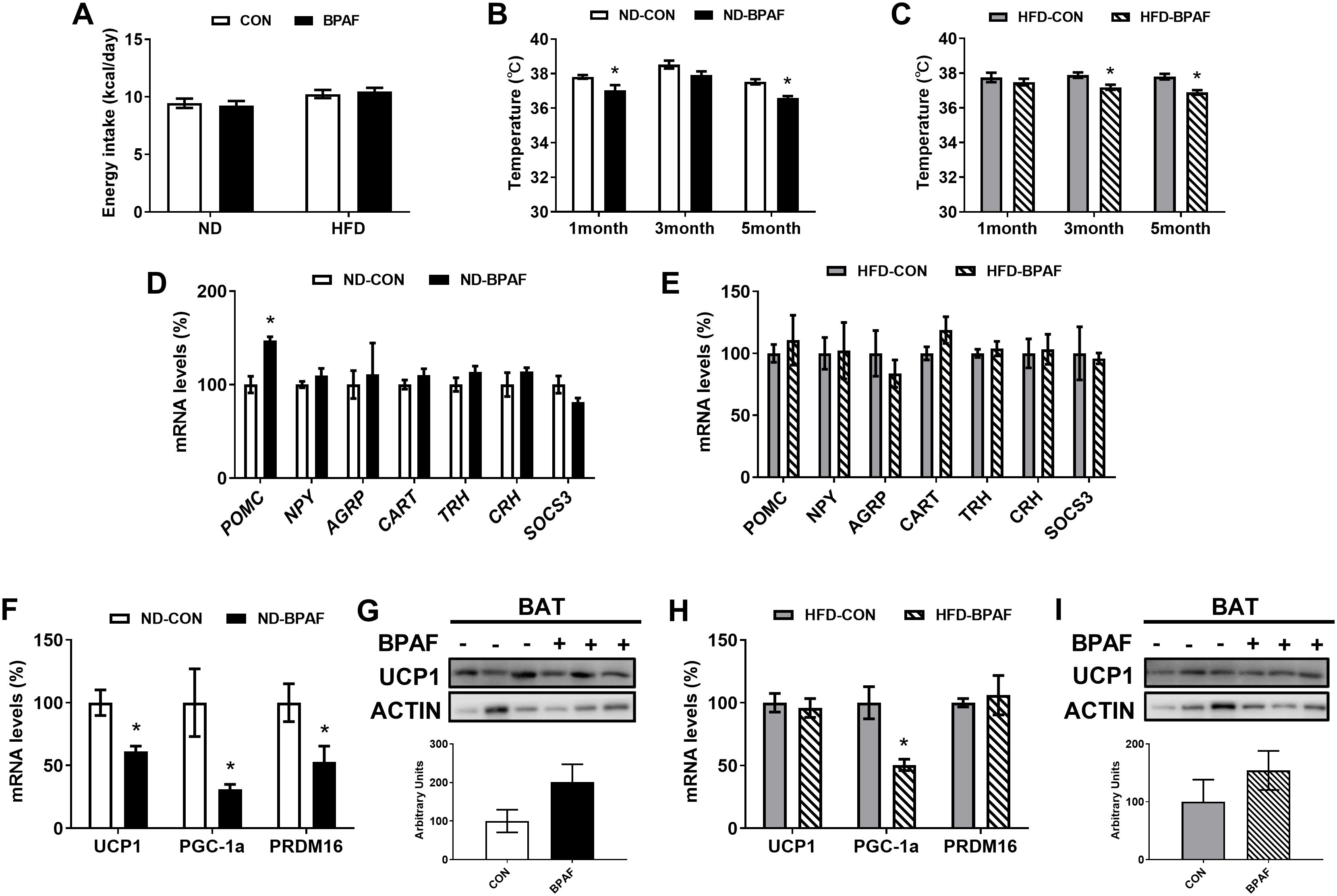
Figure 3. Impact of BPAF exposure on energy homeostasis. C57BL/6j mice fed with ND or HFD were gavaged with 100 μg/kg of BPAF for five months every other day, and monitored for body temperature and energy intake, followed by examination of gene and protein expression of relevant factors in BAT and hypothalamus. (A) changes in daily energy intake in ND and HFD mice; (B) rectal temperature of ND mice at different times of the test; (C) rectal temperature of HFD mice at different times of the test; (D) POMC, NPY, AGRP, CART, TRH, CRH, SOCS3 gene expression in hypothalamus of ND mice; (E) POMC, NPY, AGRP, CART, TRH, CRH, SOCS3 gene expression in hypothalamus of HFD mice; (F) UCP1, PGC1a, PRDM16 gene expression in BAT of ND mice; (G) UCP1 protein expression in BAT of ND mice; (H) UCP1, PGC1a, PRDM16 gene expression in BAT of HFD mice; (I) UCP1 protein expression in BAT of HFD mice. top, western blots; bottom, quantitative measurements of UCP1 protein relative to β-actin protein. N=6, data were expressed as means ± SE, two-tailed Student’s t test were used to analyze the difference between CON and BPAF group, * means p <0.05.
3.4 Impact of BPAF exposure on lipid metabolites
Disorders of lipid metabolism are commonly associated with obesity in a subset of obese patients. To elucidate the role of BPAF in lipid metabolism regulation, we first analyzed liver and serum lipids levels in mice. Under normal diet condition, serum TG levels were decreased while liver TG levels remained unchanged (Figure 4A). Serum TC levels showed no differences, whereas liver TC levels were decreased in BPAF-treated mice (Figure 4C). Serum and liver FFA levels exhibited no significant changes (Figure 4E). Conversely, under high-fat diet condition, BPAF-treated mice displayed elevated liver TG, TC, and FFA levels, as well as increased serum FFA levels, with no differences observed in serum TG and TC levels compared to BPAF untreated mice (Figures 4B, D, F). Additionally, we performed metabolomic analysis of liver tissue, Partial Least Squares Discriminant Analysis (PLS-DA) score plots showed clear separation of control and BPAF, both in ND and HFD mice (Figures 4G, H), suggesting that BPAF exposure disrupted hepatic metabolic profiles. Alterations in liver lipid metabolites were also quantified, with a distinct profile of unregulated and downregulated metabolites in both normal diet and high-fat diet mice following BPAF exposure. In ND mice, there were 1 metabolite up-regulated and 13 metabolites down-regulated (Figure 4G) and in HFD mice, there were 3 metabolites up-regulated and 4 metabolites down-regulated (Figure 4H). Detailed of differential lipid metabolites were presented in Tables 1, 2. These changed molecules indicated the regulated role of BPAF on lipid metabolism homeostasis.
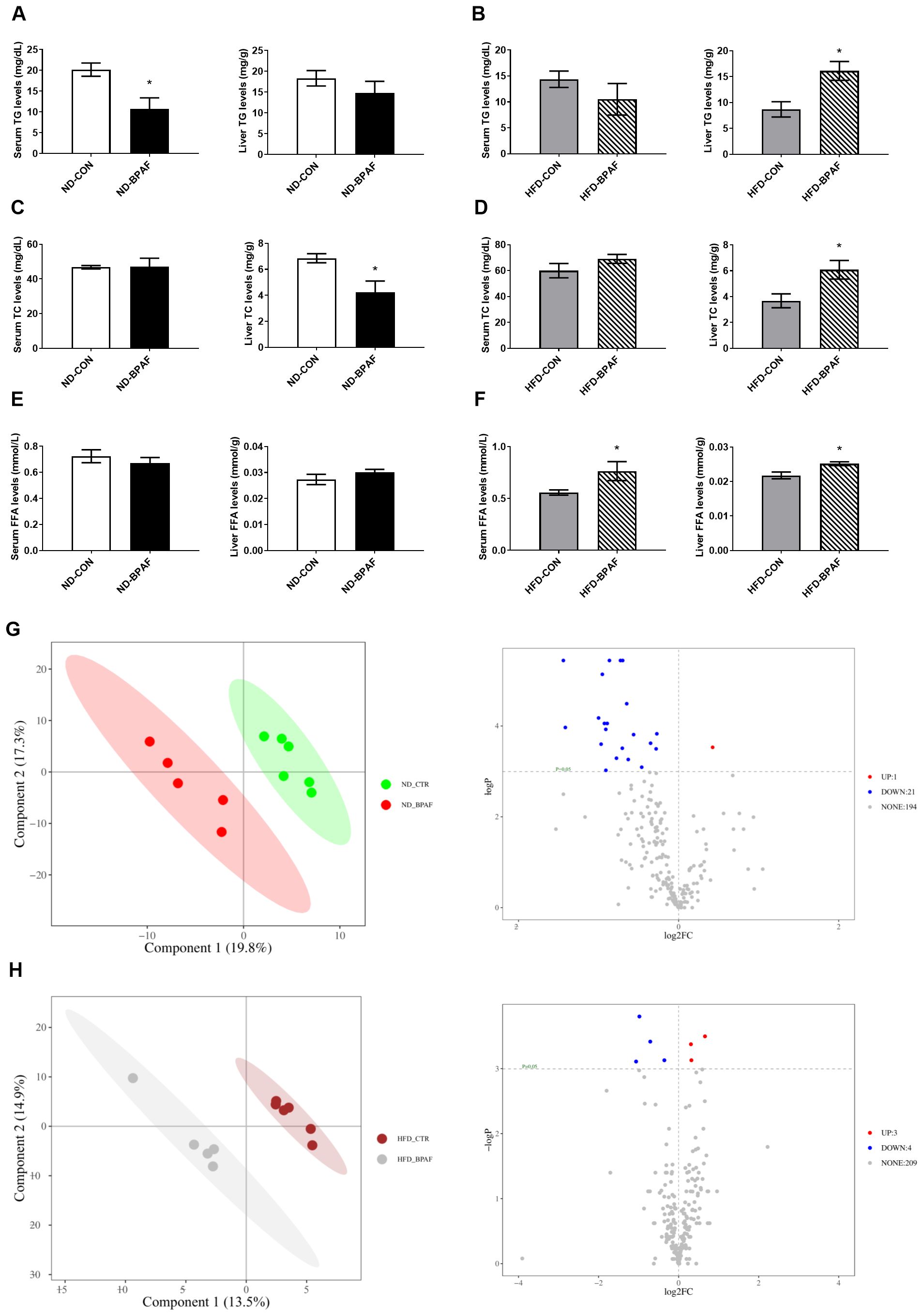
Figure 4. Impact of BPAF exposure on lipid metabolites. C57BL/6j mice fed with ND or HFD were gavaged with 100 μg/kg of BPAF for five months every other day, followed by examination of liver and serum TG, TC, FFA levels and analysis of liver metabolomics. (A) serum and liver TG levels in ND mice; (B) serum and liver TG levels in HFD mice; (C) serum and liver TC levels in ND mice; (D) serum and liver TC levels in HFD mice; (E) serum and liver FFA levels in ND mice; (F) serum and liver FFA levels in HFD mice; (G) analysis of liver metabolomics in ND mice; (H) analysis of liver metabolomics in HFD mice. left, PLS-DA score plots; right, volcano plots. N=6, data were expressed as means ± SE, two-tailed Student’s t test were used to analyze the difference between CON and BPAF group, * means p <0.05.
3.5 Impact of BPAF exposure on lipid metabolism in WAT
Furthermore, we assessed the expression levels of lipid metabolism-related factors in WAT. Under normal diet condition, genes associated with enzymes related to lipogenesis (40), including fatty acid synthase (FAS), stearoyl-Coenzyme A desaturase 1(SCD1) and acetyl-CoA carboxylase (ACC) were upregulated in sWAT following BPAF treatment (Figure 5A). Genes related to fatty acid oxidation and lipolysis (41, 42), peroxisome proliferator-activated receptor alpha (PPARα) and adrenergic receptor bata 3 (ADRB3) was not changed (Figure 5A). However, the gene of adipose triglyceride lipase (ATGL), which is involved in lipolysis, also exhibited increased expression in BPAF exposure mice (Figure 5A). In eWAT, only the gene expression of fatty acid uptake-associatedcluster of differentiation 36 molecule (CD36) was downregulated (Figure 5B). HFD mice displayed no changes in gene expressions in sWAT(Figure 5C), while BPAF treatment resulted in significant decreases in the expression of genes associated with lipid synthesis (SCD1), lipolysis (ATGL, ADRB3 and lipoprotein lipase (LPL)), and fatty acid transport (fatty acid transport protein (FATP)) in eWAT (Figure 5D). Protein expression analysis revealed reduced levels of phosphorylated hormone-sensitive lipase (p-HSL) in sWAT of ND mice following BPAF treatment (Figure 5E), while no changes were observed in HFDmice (Figure 5G). In eWAT, BPAF treatment led to increased CPT1 protein levels (Figure 5F), whereas decreased expression levels of SCD1 and p-HSL were observed in HFD mice (Figure 5H).
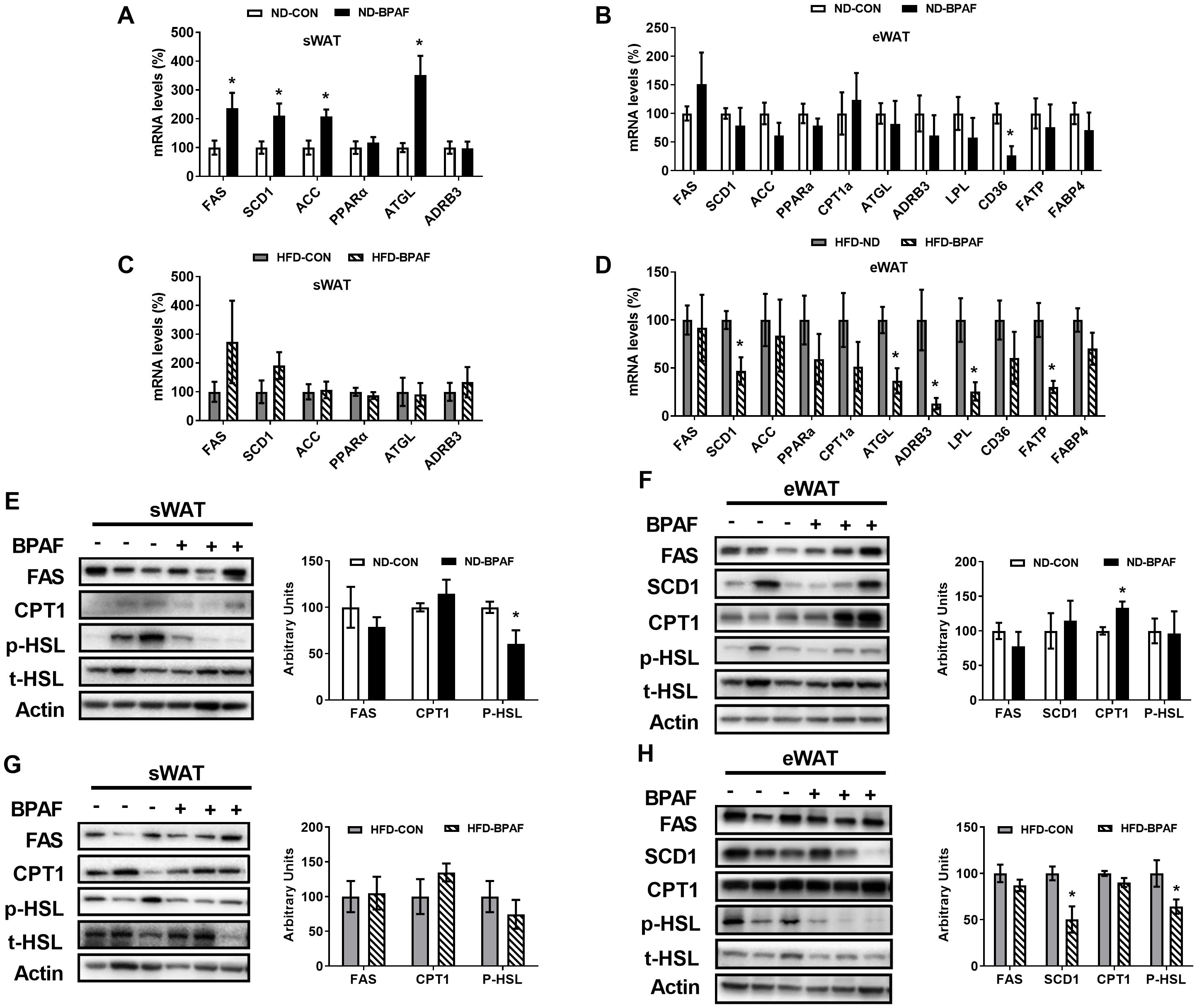
Figure 5. Impact of BPAF exposure on lipid metabolism in WAT. C57BL/6j mice fed with ND or HFD were gavaged with 100 μg/kg of BPAF for five months every other day, followed by examination of gene and protein expression of factors related to lipogenesis and lipolysis in eWAT and sWAT. (A) FAS, SCD1, ACC, PPARα, ATGL, ADRB3 gene expression in sWAT of ND mice; (B) FAS, SCD1, ACC, PPARα, ATGL, ADRB3, LPL, CD36, FATP, FABP4 gene expression in eWAT of ND mice; (C) FAS, SCD1, ACC, PPARα, ATGL, ADRB3 gene expression in sWAT of HFD mice; (D) FAS, SCD1, ACC, PPARα, ATGL, ADRB3, LPL, CD36, FATP, FABP4 gene expression in eWAT of HFD mice; (E) FAS, CPT1, p-HSL (Ser660) protein expression in sWAT of ND mice; (F) FAS, SCD1, CPT1, p-HSL (Ser660) protein expression in eWAT of ND mice; (G) FAS, CPT1, p-HSL(Ser660) protein expression in sWAT of HFD mice; (H) FAS, SCD1, CPT1, p-HSL (Ser660) protein expression in eWAT of HFD mice. left, western blots; right, quantitative measurements of FAS, SCD1, CPT1 protein relative to β-actin protein, quantitative measurements of p-HSL protein relative to t-HSL protein. N=6, data were expressed as means ± SE, two-tailed Student’s t test were used to analyze the difference between CON and BPAF group, * means p <0.05.
4 Discussion
In this study, we firstly examined the effects of BPs exposure on the weight change of male mice subjected to normal diet or high-fat diet. Our findings indicate that a relatively low dose of BPAF and BPS exposure, but not BPF, led to increased body weight in HFD mice, with BPAF exhibiting a more pronounced effect compared to BPS. However, no significant changes were observed in ND mice. Given that the relationship between BPF and BPS with body weight in mice has also been measured in our previous studies (43, 44). The effect of BPAF on obesity, however, remains unclear. Therefore, we discuss and showed only BPAF results in our study. BPAF exposure induced fat deposition in sWAT and eWAT of HFD mice and eWAT of ND mice, accompanied by an increase in adipocyte volume in eWAT. Altered core temperature in the resting state of ND and HFD mice, as well as decreased expression of thermogenic factors in the BAT. Notably, alterations in lipid metabolism were evident in both liver and WAT following BPAF exposure. Specifically, hepatic TG, TC, and FFA levels were elevated in HFD mice after BPAF treatment, accompanied by alterations in hepatic lipid metabolite profiles. Furthermore, BPAF induced changes in the expression of factors related to lipogenesis and hydrolysis that contribute to fat accumulation in eWAT and sWAT. These findings indicate that BPAF exposure disrupts metabolic functions of liver and adipose tissue and outline a possible mechanism that links BPAF to the prevalence of obesity and other metabolic disorders.Based on the dosage range selected in the previous studies and the legal dosage limits released by the government supervision department, we selected the concentration of BPAF at 100 µg/kg every other day in this study.This dosage is close to two equivalents of a temporary tolerable daily intake (t-TDI) of BPA (4 mg/kg bw per day) recommended by European Food Safety Authority in 2015 (7). In previous researches, the exposure dosage of BPAF applied in mice experiments ranged from 0.05mg/kg body weight/day to 5mg/kg body weight/day for days or weeks (28, 32, 45, 46). Some studies investigated the effects of BPAF on liver lipid metabolism and liver damage (28, 30, 32). Others explored the role of BPAF in immune system (22, 46), reproductive system (45) or neurotoxicity (45, 46). Although extensive studies have shown that BPAF increases the risk of obesity, the specific effects of sustained exposure to BPAF on the development of obesity and the mechanism of action remain to be clarified (20, 24, 27, 47). Our current study systematically reveals that chronic exposure to bisphenol AF (BPAF) disrupts lipid metabolism thereby leading to fat accumulation and somewhat reduces energy expenditure, with this effect being significantly amplified under high-fat diet conditions.
BPAF-induced obesity in mice might be influenced by multiple factors, such as the exposure pattern, dosage and duration, and dietary conditions. In our study, 100 µg/kg every other day of BPAF exposure for five months resulted in fat accumulation in both ND and HFD mice, with a significant increase in body weight in HFD mice. However, in a previous study, mice exposed to 50 μg/kg/day of BPAF for 8 weeks under high-fat conditions showed no significant change in body weight (28). In addition, two previous studies have shown that the sex of the subject and the window of exposure (perinatal, adolescent, adulthood) are also key determinants in modulating the hypertrophic effects of BPAF (30, 32). A study using mature human adipocytes also showed that exposure to bisphenol AF mediates inflammatory signaling pathways that disrupt adipocyte metabolism (48).
Energy balance, comprising energy intake and expenditure, plays a crucial role in the development of obesity (4). Previous studies have found that postweaning exposure to BPAF and other bisphenol analogs induced an increase in energy intake in subjects, but this phenomenon was not observed in the present study, which may be related to the specificity of BPAF as well as the different age of exposure (33, 49). Appetite and feeding behavior are controlled by metabolic signaling pathway as well as neuroendocrine system (36). The expression of neuropeptide in the hypothalamus were investigated, including the anorexigenic neuropeptides POMC and CART, the appetite-stimulating neuropeptides AgRP and NPY, two neuroendocrine hormones, TRH and CRH, as well as the leptin-associated factor SOCS3 (36). The results showed that BPAF induced elevated expression of the anorexigenic neuropeptide POMC in ND mice, but no significant changes in other factors. Another study also showed that BPAF upregulates the expression of POMC, which induced sWAT browning, a means of maintaining body temperature and regulating energy metabolism in mice (46). Consequently, it can be concluded that the neuroendocrine disrupting effects of low-dose BPAF exposure should not be disregarded. In addition, the thermogenic function of BAT is critical for energy expenditure and maintenance of resting body temperature (37, 38). However, core body temperature decreased in both ND and HFD mice after BPAF exposure. Meanwhile, the expression of UCP1, PGC-1α, and PRDM16 in the BAT, critical thermogenic tissue, was decreased, indicating that its thermogenic function was inhibited. BPAF may impair energy expenditure in mice to some extent, thereby contributing to the development of fat accumulation and obesity.
Lipids are important biomolecules that play key biophysical roles in material metabolism, energy balance, and physiological signal transduction (50).The effects of BPAF exposure on hepatic and serum lipid homeostasis appear to be inconsistent across dietary conditions. We found that BPAF exposure downregulated hepatic TC and serum TG levels in ND mice but upregulated hepatic TG, TC and FFA levels as well as serum FFA levels in HFD mice. Another study in which mice were treated with BPAF at 0.05 mg/kg bw/day for 8 weeks showed a decrease in hepatic TG and TC levels in HFD mice, but transcriptomics results showed up-regulation of differentiated expressed genes (DEGs) involved in lipogenesis and down-regulation of DEGs involved in the lipolytic pathway (28). This discrepancy might be largely ascribed to different exposure doses, durations and frequencies. However, there is no doubt that BPAF treatment interferes with hepatic lipid metabolism. In vitro experiments have also demonstrated that BPAF interferes with intracellular lipid homeostasis, with lower concentrations of BPAF maximizing intracellular lipid levels during adipocyte differentiation. In contrast, BPAF-glucuronide, the main metabolite of BPAF in vivo, has a different mode of action, increasing lipid accumulation in a dose-dependent manner (51, 52).
Dysregulation of hepatic lipid metabolism is a key factor in distinguishing between healthy and metabolically diseased obesity and suggests a mechanism by which BPAF induces obesity (4, 53). In this study, we examined hepatic metabolites using UPLC-MS/MS, and a total of more than 200 lipid metabolites were detected. The hepatic lipid metabolite profiles in ND and HFD mice were significantly changed after BPAF treatment. Almost all altered hepatic lipid metabolites were down-regulated in BPAF exposed ND mice, whereas altered hepatic lipid metabolites in HFD mice were either up-regulated or down-regulated. Docosapentaenoic acid (22n-6) (DPAn-6) was the lipid metabolite co-reduced in the livers of ND and HFD mice. DPAn-6 is able to increase the activity of lipolysis-related enzymes by inhibiting the activity and expression of lipase-producing enzymes in mouse livers, which in turn attenuated hepatic steatosis (54). At the same time, DPAn-6 has significant anti-inflammatory effects (55). The decrease of DPAn-6 might be a result of the abnormalities in lipid metabolism induced by BPAF.
In addition, we found that several differential hepatic lipid metabolites in BPAF exposed HFD mice were strongly associated with obesity pathogenesis, including L-pipecolic acid, Murocholic acid, Glycylproline and 10,13-Nonadecadienoic acid. In a previous study, serum etabolite pipecolic acid levels were significantly increased in obese mice (56), which is consistent with what we observed in HFD mice treated with BPAF but not in ND mice. This indicates that lipid metabolism disorders are more likely to be a synergistic effect induced by BPAF exposure in combination with high-fat diet consuming. Similarly, it has been shown that murocholic acid has a positive effect on ameliorating obesity and metabolic disorders (57), but we found that BPAF exposure led to a decrease in murocholic acid in HFD mice. A study in male adolescents showed that urinary glycylproline levels were negatively correlated with pubertal body mass index (BMI), and a decrease in this index is a sensitive biomarker of obesity (58). Furthermore, FP fermentation broth of C. leptum (FPF), which was rich in glyceroproline, was able to mediate the AMPK pathway to block hepatic fatty acid synthesis and accelerate the oxidative catabolism of fatty acids, thus effectively reducing body fat in obese mice (59). The above results suggest that simultaneous exposure to HFD and BPAF may aggravate the burden on hepatic lipid metabolism and more easily induce obesity.
WAT is a core storage site for fat (mainly TG) and its quality reflects fat deposition. This energy buffering capacity maintains systemic energy homeostasis by regulating lipid metabolism. Lipid synthesis, lipid hydrolysis and lipid oxidation are the basic processes of lipid metabolism (60). We examined the expression of factors related to lipid metabolism in different adipose tissues. BPAF induced up-regulation of the expression of genes related to fatty acid synthesis (Fas, Scd 1, and Acc) and decreased phosphorylation of HSL, a key enzyme that promotes lipid hydrolysis, in sWAT of ND mice. These results indicate that the risk of fat accumulation was dramatically increased, although the weight of sWAT in ND mice was not significantly elevated. In contrast, the expression of the lipolytic enzyme ATGL was upregulated, which might be an adaptive regulation to maintain lipid homeostasis. Furthermore, the diminished CD36 expression and augmented CPT1 expression in eWAT of ND mice imply that the uptake and transport of long-chain fatty acids is constrained, and that β-oxidation is augmented, consequently leading to a reduction in lipid synthesis and accumulation. Other studies have also observed that perinatal BPAF exposure resulted in up-regulation of CPT1a expression in the adult liver of offspring (30). Concomitantly, in conjunction with the elevated weight of eWAT and the increased adipocyte volume, we conclude that these changes are negative feedback regulated, reducing lipogenesis and accelerating lipolysis. The above suggest that some protective mechanisms exist in ND mice to cope with BPAF-induced lipid metabolism disorders.
In contrast, BPAF elicited a decrease in the expression of ATGL, ADRB3, LPL, and FATP, along with a reduction in HSL phosphorylation (p-HSL) in eWAT of HFD mice, suggesting inhibition of fatty acid catabolism, transport, and utilization. BPAF interfered with lipid depletion processes. Whereas the decreased gene and protein expression of SCD1 was presumably related to a compensatory mechanism that reduces further lipid synthesis. However, the elevated eWAT weight indicated this compensation was not sufficient to completely prevent the occurrence of obesity.
This study has several limitations. First, we did not monitor basal metabolic rate (BMR) by indirect calorimetry, nor did we perform hypothalamic thermoregulatory analyses, which limits our understanding of the neuroendocrine perturbations in the dynamics of BPAF-induced energy expenditure. Second, the study did not assess adipose tissue endocrine function, which is also important for energy intake as well as expenditure. Third, the lack of high-resolution lipidomics precludes a comprehensive description of tissue-specific lipid remodeling. Finally, interspecies differences in the toxicokinetics of BPAF may require more consideration when extrapolating conclusions. In conclusion, our findings demonstrated that BPAF exposure disrupted lipid metabolism homeostasis, leading to fat accumulation in adipose tissue and liver, especially under conditions of high-fat diet feeding. Metabolomics analysis further elucidated the relationship between altered lipid metabolism and changes in lipids levels induced by BPAF exposure. Protein phosphorylation analyses suggested that inhibition of the p-HSL signalling pathway might be responsible for the metabolic disturbances in adipose tissue. Collectively, this study demonstrated the potential obesogenic risk of BPAF via disrupting lipid metabolic homeostasis under both ND and HFD feeding conditions, which would provide scientific evidence for both the development of precision prevention strategy and promotion the safe use of BPAF.
Data availability statement
The original contributions presented in the study are included in the article/Supplementary Material. Further inquiries can be directed to the corresponding authors.
Ethics statement
The animal study was approved by Institutional Animal Care and Use/Ethics Committee, Shenzhen Center for Disease Control and Prevention. The study was conducted in accordance with the local legislation and institutional requirements.
Author contributions
ZL: Data curation, Funding acquisition, Validation, Writing – original draft, Writing – review & editing. XX: Conceptualization, Investigation, Methodology, Writing – original draft, Writing – review & editing. ZT: Conceptualization, Data curation, Investigation, Methodology, Writing – original draft, Writing – review & editing. YL: Conceptualization, Investigation, Methodology, Writing – review & editing. CP: Conceptualization, Investigation, Methodology, Writing – original draft. YW: Conceptualization, Investigation, Methodology, Writing – original draft. DS: Conceptualization, Investigation, Methodology, Writing – review & editing. GJ: Conceptualization, Investigation, Methodology, Writing – review & editing. XH: Conceptualization, Investigation, Writing – original draft. YC: Conceptualization, Investigation, Writing – original draft. GL: Conceptualization, Investigation, Writing – original draft. DW: Conceptualization, Investigation, Writing – original draft. SH: Funding acquisition, Writing – review & editing. YG: Data curation, Funding acquisition, Validation, Writing – original draft, Writing – review & editing.
Funding
The author(s) declare that financial support was received for the research and/or publication of this article. This work was supported by the Noncommunicable Chronic Diseases-National Science and Technology Major Project (2023ZD0510105), Shenzhen Medical Research Fund (Grants C2302001), National Natural Science Foundation of China (81502789, 81973004, 21876116), Natural Science Foundation of Guangdong Province (2023A1515012744), Sanming Project of Medicine in Shenzhen (SZSM202011008), Shenzhen Key Medical Discipline Construction Fund (SZXK066), Futian Healthcare Research Project (No. FTWS2023068), Shenzhen Science and Technology Program (JCYJ20230807111004010, ZDSYS20220606100801004).
Conflict of interest
The authors declare that the research was conducted in the absence of any commercial or financial relationships that could be construed as a potential conflict of interest.
Generative AI statement
The author(s) declared that no Generative AI was used in the creation of this manuscript.
Publisher’s note
All claims expressed in this article are solely those of the authors and do not necessarily represent those of their affiliated organizations, or those of the publisher, the editors and the reviewers. Any product that may be evaluated in this article, or claim that may be made by its manufacturer, is not guaranteed or endorsed by the publisher.
Supplementary material
The Supplementary Material for this article can be found online at: https://www.frontiersin.org/articles/10.3389/fendo.2025.1571076/full#supplementary-material
References
1. Perdomo CM, Cohen RV, Sumithran P, Clément K, and Frühbeck G. Contemporary medical, device, and surgical therapies for obesity in adults. Lancet. (2023) 401:1116–30. doi: 10.1016/S0140-6736(22)02403-5
2. Caballero B. Humans against obesity: who will win? Adv Nutr. (2019) 10:S4–9. doi: 10.1093/advances/nmy055
3. Jebeile H, Kelly AS, O’Malley G, and Baur LA. Obesity in children and adolescents: epidemiology, causes, assessment, and management. Lancet Diabetes Endocrinol. (2022) 10:351–65. doi: 10.1016/S2213-8587(22)00047-X
4. Blüher M. Obesity: global epidemiology and pathogenesis. Nat Rev Endocrinol. (2019) 15:288–98. doi: 10.1038/s41574-019-0176-8
5. Darbre PD. Endocrine disruptors and obesity. Curr Obes Rep. (2017) 6:18–27. doi: 10.1007/s13679-017-0240-4
6. Ehrlich S, Calafat AM, Humblet O, Smith T, and Hauser R. Handling of thermal receipts as a source of exposure to bisphenol A. JAMA. (2014) 311:859–60. doi: 10.1001/jama.2013.283735
7. Huang R-P, Liu Z-H, Yuan S-F, Yin H, Dang Z, and Wu P-X. Worldwide human daily intakes of bisphenol A (BPA) estimated from global urinary concentration data (2000-2016) and its risk analysis. Environ Pollut. (2017) 230:143–52. doi: 10.1016/j.envpol.2017.06.026
8. Barboza LGA, Cunha SC, Monteiro C, Fernandes JO, and Guilhermino L. Bisphenol A and its analogs in muscle and liver of fish from the North East Atlantic Ocean in relation to microplastic contamination. Exposure and risk to human consumers. J Hazard Mater. (2020) 393:122419. doi: 10.1016/j.jhazmat.2020.122419
9. Baralla E, Pasciu V, Varoni MV, Nieddu M, Demuro R, and Demontis MP. Bisphenols’ occurrence in bivalves as sentinel of environmental contamination. Sci Total Environ. (2021) 785:147263. doi: 10.1016/j.scitotenv.2021.147263
10. Ma Y, Liu H, Wu J, Yuan L, Wang Y, Du X, et al. The adverse health effects of bisphenol A and related toxicity mechanisms. Environ Res. (2019) 176:108575. doi: 10.1016/j.envres.2019.108575
11. Ahmad I, Kaur M, Tyagi D, Singh TB, Kaur G, Afzal SM, et al. Exploring novel insights into the molecular mechanisms underlying Bisphenol A-induced toxicity: A persistent threat to human health. Environ Toxicol Pharmacol. (2024) 108:104467. doi: 10.1016/j.etap.2024.104467
12. Della Rocca Y, Traini EM, Diomede F, Fonticoli L, Trubiani O, Paganelli A, et al. Current evidence on bisphenol A exposure and the molecular mechanism involved in related pathological conditions. Pharmaceutics. (2023) 15:908. doi: 10.3390/pharmaceutics15030908
13. Sirasanagandla SR, Al-Mushaiqri M, Al Ghafri F, Al-Abri N, and Al-Huseini I. Protective effects of perinatal resveratrol on bisphenol A exposure-induced cardiovascular alterations and hepatic steatosis in adult offspring mice: A histopathological study. Appl Sci. (2023) 13:13163. doi: 10.3390/app132413163
14. Kundakovic M and Champagne FA. Epigenetic perspective on the developmental effects of bisphenol A. Brain Behav Immun. (2011) 25:1084–93. doi: 10.1016/j.bbi.2011.02.005
15. Sirasanagandla SR, Al-Huseini I, Sofin RGS, and Das S. Perinatal exposure to bisphenol A and developmental programming of the cardiovascular changes in the offspring. Curr Med Chem. (2022) 29:4235–50. doi: 10.2174/0929867328666211206111835
16. Tudurí E, Marroqui L, Dos Santos RS, Quesada I, Fuentes E, and Alonso-Magdalena P. Timing of exposure and bisphenol-A: implications for diabetes development. Front Endocrinol (Lausanne). (2018) 9:648. doi: 10.3389/fendo.2018.00648
17. Liao C, Liu F, Guo Y, Moon H-B, Nakata H, Wu Q, et al. Occurrence of eight bisphenol analogues in indoor dust from the United States and several Asian countries: implications for human exposure. Environ Sci Technol. (2012) 46:9138–45. doi: 10.1021/es302004w
18. Bousoumah R, Leso V, Iavicoli I, Huuskonen P, Viegas S, Porras SP, et al. Biomonitoring of occupational exposure to bisphenol A, bisphenol S and bisphenol F: A systematic review. Sci Total Environ. (2021) 783:146905. doi: 10.1016/j.scitotenv.2021.146905
19. Moreman J, Lee O, Trznadel M, David A, Kudoh T, and Tyler CR. Acute toxicity, teratogenic, and estrogenic effects of bisphenol A and its alternative replacements bisphenol S, bisphenol F, and bisphenol AF in zebrafish embryo-larvae. Environ Sci Technol. (2017) 51:12796–805. doi: 10.1021/acs.est.7b03283
20. Chen D, Kannan K, Tan H, Zheng Z, Feng Y-L, Wu Y, et al. Bisphenol analogues other than BPA: environmental occurrence, human exposure, and toxicity-A review. Environ Sci Technol. (2016) 50:5438–53. doi: 10.1021/acs.est.5b05387
21. Catenza CJ, Farooq A, Shubear NS, and Donkor KK. A targeted review on fate, occurrence, risk and health implications of bisphenol analogues. Chemosphere. (2021) 268:129273. doi: 10.1016/j.chemosphere.2020.129273
22. Vishalakshi GJ, Hemshekhar M, Sandesha VD, Prashanth KS, Jagadish S, Paul M, et al. Bisphenol AF elevates procoagulant platelets by inducing necroptosis via RIPK1-inflammasome axis. Toxicology. (2021) 454:152742. doi: 10.1016/j.tox.2021.152742
23. Heindel JJ and Blumberg B. Environmental obesogens: mechanisms and controversies. Annu Rev Pharmacol Toxicol. (2019) 59:89–106. doi: 10.1146/annurev-pharmtox-010818-021304
24. Liu B, Lehmler H-J, Sun Y, Xu G, Liu Y, Zong G, et al. Bisphenol A substitutes and obesity in US adults: analysis of a population-based, cross-sectional study. Lancet Planet Health. (2017) 1:e114–22. doi: 10.1016/S2542-5196(17)30049-9
25. Liu B, Lehmler HJ, Sun Y, Xu G, Sun Q, Snetselaar LG, et al. Association of bisphenol A and its substitutes, bisphenol F and bisphenol S, with obesity in United States children and adolescents. Diabetes Metab J. (2019) 43:59–75. doi: 10.4093/dmj.2018.0045
26. Zhang Y, Dong T, Hu W, Wang X, Xu B, Lin Z, et al. Association between exposure to a mixture of phenols, pesticides, and phthalates and obesity: Comparison of three statistical models. Environ Int. (2019) 123:325–36. doi: 10.1016/j.envint.2018.11.076
27. Chen M, Lv C, Zhang S, Tse LA, Hong X, Liu X, et al. Bisphenol A substitutes and childhood obesity at 7 years: a cross-sectional study in Shandong, China. Environ Sci Pollut Res Int. (2023) 30:73174–84. doi: 10.1007/s11356-023-27578-x
28. Sun F, Huang Y, Chen H, Huang J, Zhang L, Wei S, et al. BPA and its alternatives BPF and BPAF exaggerate hepatic lipid metabolism disorders in male mice fed a high fat diet. Sci Total Environ. (2023) 867:161521. doi: 10.1016/j.scitotenv.2023.161521
29. Brulport A, Vaiman D, Chagnon M-C, and Le Corre L. Obesogen effect of bisphenol S alters mRNA expression and DNA methylation profiling in male mouse liver. Chemosphere. (2020) 241:125092. doi: 10.1016/j.chemosphere.2019.125092
30. Meng Z, Wang D, Yan S, Li R, Yan J, Teng M, et al. Effects of perinatal exposure to BPA and its alternatives (BPS, BPF and BPAF) on hepatic lipid and glucose homeostasis in female mice adolescent offspring. Chemosphere. (2018) 212:297–306. doi: 10.1016/j.chemosphere.2018.08.076
31. Ivry Del Moral L, Le Corre L, Poirier H, Niot I, Truntzer T, Merlin J-F, et al. Obesogen effects after perinatal exposure of 4,4’-sulfonyldiphenol (Bisphenol S) in C57BL/6 mice. Toxicology. (2016) 357–358:11–20. doi: 10.1016/j.tox.2016.05.023
32. Meng Z, Tian S, Yan J, Jia M, Yan S, Li R, et al. Effects of perinatal exposure to BPA, BPF and BPAF on liver function in male mouse offspring involving in oxidative damage and metabolic disorder. Environ Pollut. (2019) 247:935–43. doi: 10.1016/j.envpol.2019.01.116
33. Rezg R, Abot A, Mornagui B, Aydi S, and Knauf C. Effects of Bisphenol S on hypothalamic neuropeptides regulating feeding behavior and apelin/APJ system in mice. Ecotoxicol Environ Saf. (2018) 161:459–66. doi: 10.1016/j.ecoenv.2018.06.001
34. Cheng Y, Meng Q, Wang C, Li H, Huang Z, Chen S, et al. Leucine deprivation decreases fat mass by stimulation of lipolysis in white adipose tissue and upregulation of uncoupling protein 1 (UCP1) in brown adipose tissue. Diabetes. (2010) 59:17–25. doi: 10.2337/db09-0929
35. Braun JM. Early-life exposure to EDCs: role in childhood obesity and neurodevelopment. Nat Rev Endocrinol. (2017) 13:161–73. doi: 10.1038/nrendo.2016.186
36. Wang L, Yu C-C, Li J, Tian Q, and Du Y-J. Mechanism of action of acupuncture in obesity: A perspective from the hypothalamus. Front Endocrinol (Lausanne). (2021) 12:632324. doi: 10.3389/fendo.2021.632324
37. Chechi K, Nedergaard J, and Richard D. Brown adipose tissue as an anti-obesity tissue in humans. Obes Rev. (2014) 15:92–106. doi: 10.1111/obr.12116
38. Yoneshiro T, Aita S, Matsushita M, Kameya T, Nakada K, Kawai Y, et al. Brown adipose tissue, whole-body energy expenditure, and thermogenesis in healthy adult men. Obesity (Silver Spring). (2011) 19:13–6. doi: 10.1038/oby.2010.105
39. Zhu Y, Cheng P, Peng J, Liu S, Xiang J, Xu D, et al. Cadmium exposure causes transcriptomic dysregulation in adipose tissue and associated shifts in serum metabolites. Environ Int. (2024) 185:108513. doi: 10.1016/j.envint.2024.108513
40. Jeon YG, Kim YY, Lee G, and Kim JB. Physiological and pathological roles of lipogenesis. Nat Metab. (2023) 5:735–59. doi: 10.1038/s42255-023-00786-y
41. Najt CP, Khan SA, Heden TD, Witthuhn BA, Perez M, Heier JL, et al. Lipid droplet-derived monounsaturated fatty acids traffic via PLIN5 to allosterically activate SIRT1. Mol Cell. (2020) 77:810–824.e8. doi: 10.1016/j.molcel.2019.12.003
42. Ding L, Sun W, Balaz M, He A, Klug M, Wieland S, et al. Peroxisomal β-oxidation acts as a sensor for intracellular fatty acids and regulates lipolysis. Nat Metab. (2021) 3:1648–61. doi: 10.1038/s42255-021-00489-2
43. Guo Y, Lv Z, Tang Z, Huang S, Peng C, Wang F, et al. Long-term exposure to low doses of bisphenol S has hypoglycaemic effect in adult male mice by promoting insulin sensitivity and repressing gluconeogenesis. Environ Pollut. (2021) 277:116630. doi: 10.1016/j.envpol.2021.116630
44. Lv Z, Tang Z, Huang S, Hu X, Peng C, Chen Y, et al. In vivo hypoglycemic effects of bisphenol F exposure in high-fat diet mice. Chemosphere. (2023) 311:137066. doi: 10.1016/j.chemosphere.2022.137066
45. Li Y, Xiong Y, Lv L, Li X, and Qin Z. Effects of low-dose bisphenol AF on mammal testis development via complex mechanisms: alterations are detectable in both infancy and adulthood. Arch Toxicol. (2022) 96:3373–83. doi: 10.1007/s00204-022-03377-0
46. Lv L, Li Y, Chen X, and Qin Z. Transcriptomic analysis reveals the effects of maternal exposure to bisphenol AF on hypothalamic development in male neonatal mice. J Environ Sci (China). (2024) 141:304–13. doi: 10.1016/j.jes.2023.05.014
47. Cao L-Y, Ren X-M, Li C-H, Zhang J, Qin W-P, Yang Y, et al. Bisphenol AF and Bisphenol B Exert Higher Estrogenic Effects than Bisphenol A via G Protein-Coupled Estrogen Receptor Pathway. Environ Sci Technol. (2017) 51:11423–30. doi: 10.1021/acs.est.7b03336
48. Chernis N, Masschelin P, Cox AR, and Hartig SM. Bisphenol AF promotes inflammation in human white adipocytes. Am J Physiol Cell Physiol. (2020) 318:C63–72. doi: 10.1152/ajpcell.00175.2019
49. Stoker C, Andreoli MF, Kass L, Bosquiazzo VL, Rossetti MF, Canesini G, et al. Perinatal exposure to bisphenol A (BPA) impairs neuroendocrine mechanisms regulating food intake and kisspetin system in adult male rats. Evidences of metabolic disruptor hypothesis. Mol Cell Endocrinol. (2020) 499:110614. doi: 10.1016/j.mce.2019.110614
50. Lam SM, Wang Z, Li B, and Shui G. High-coverage lipidomics for functional lipid and pathway analyses. Anal Chim Acta. (2021) 1147:199–210. doi: 10.1016/j.aca.2020.11.024
51. Cohen IC, Cohenour ER, Harnett KG, and Schuh SM. BPA, BPAF and TMBPF alter adipogenesis and fat accumulation in human mesenchymal stem cells, with implications for obesity. Int J Mol Sci. (2021) 22:5363. doi: 10.3390/ijms22105363
52. Skledar DG, Carino A, Trontelj J, Troberg J, Distrutti E, Marchianò S, et al. Endocrine activities and adipogenic effects of bisphenol AF and its main metabolite. Chemosphere. (2019) 215:870–80. doi: 10.1016/j.chemosphere.2018.10.129
53. Grabner GF, Xie H, Schweiger M, and Zechner R. Lipolysis: cellular mechanisms for lipid mobilization from fat stores. Nat Metab. (2021) 3:1445–65. doi: 10.1038/s42255-021-00493-6
54. Jiang Y, Qu K, Liu J, Wen Y, and Duan B. Metabolomics study on liver of db/db mice treated with curcumin using UPLC-Q-TOF-MS. J Pharmaceutical Biomed Anal. (2022) 215:114771. doi: 10.1016/j.jpba.2022.114771
55. Weylandt K-H. Docosapentaenoic acid derived metabolites and mediators - The new world of lipid mediator medicine in a nutshell. Eur J Pharmacol. (2016) 785:108–15. doi: 10.1016/j.ejphar.2015.11.002
56. Kim H-J, Kim JH, Noh S, Hur HJ, Sung MJ, Hwang J-T, et al. Metabolomic analysis of livers and serum from high-fat diet induced obese mice. J Proteome Res. (2011) 10:722–31. doi: 10.1021/pr100892r
57. Oteng A-B, Higuchi S, Banks AS, and Haeusler RA. Cyp2c-deficiency depletes muricholic acids and protects against high-fat diet-induced obesity in male mice but promotes liver damage. Mol Metab. (2021) 53:101326. doi: 10.1016/j.molmet.2021.101326
58. Singh A, Kinnebrew G, Hsu P-C, Weng DY, Song M-A, Reisinger SA, et al. Untargeted metabolomics and body mass in adolescents: A cross-sectional and longitudinal analysis. Metabolites. (2023) 13:899. doi: 10.3390/metabo13080899
59. Li T, Liang M, Luo J, and Peng X. Metabolites of Clostridium leptum fermenting flaxseed polysaccharide alleviate obesity in rats. Int J Biol Macromol. (2024) 264:129907. doi: 10.1016/j.ijbiomac.2024.129907
Keywords: BPAF, high-fat diet, fat accumulation, lipid metabolism, obesity
Citation: Lv Z, Xu X, Tang Z, Liang Y, Peng C, Wu Y, Sang D, Jia G, Hu X, Chen Y, Liu G, Wang D, Huang S and Guo Y (2025) Chronic BPAF exposure differentially enhances fat deposition in mice fed normal or high-fat diets via lipid metabolism dysregulation. Front. Endocrinol. 16:1571076. doi: 10.3389/fendo.2025.1571076
Received: 04 February 2025; Accepted: 24 April 2025;
Published: 15 May 2025.
Edited by:
Domenico Sergi, University of Ferrara, ItalyReviewed by:
Ylenia Della Rocca, Università degli Studi G. d’Annunzio Chieti e Pescara, ItalySrinivasa Rao Sirasanagandla, Sultan Qaboos University, Oman
Copyright © 2025 Lv, Xu, Tang, Liang, Peng, Wu, Sang, Jia, Hu, Chen, Liu, Wang, Huang and Guo. This is an open-access article distributed under the terms of the Creative Commons Attribution License (CC BY). The use, distribution or reproduction in other forums is permitted, provided the original author(s) and the copyright owner(s) are credited and that the original publication in this journal is cited, in accordance with accepted academic practice. No use, distribution or reproduction is permitted which does not comply with these terms.
*Correspondence: Suli Huang, aHVhbmdzdWxpNDIwQDE2My5jb20=; Yajie Guo, Z3VveWozNkBtYWlsLnN5c3UuZWR1LmNu
†These authors have contributed equally to this work