- Department of Urology, The 940th Hospital of Joint Service Support Force of Chinese People's Liberation Army, Lanzhou, Gansu, China
An increasing number of people are being exposed to high-altitude environments as they become more important in economic development, resource exploitation, and other areas. This review is focused on the impact of the high-altitude environment on the male reproductive system. In high-altitude areas, the unique conditions lead to complex and significant changes in male reproductive hormone levels. The secretion of GnRH is inhibited, which in turn affects the levels of FSH and LH, ultimately influencing testosterone synthesis and secretion, thus disrupting the normal endocrine regulatory network. Testicular tissue also shows marked morphological changes. The seminiferous tubule structure becomes disordered, and the number and function of spermatogenic and interstitial cells are damaged. These alterations have a direct impact on sperm quality, resulting in a decrease in sperm density and motility, an increase in the deformity rate, and damage to genetic material integrity. Additionally, sexual function is affected, with symptoms such as decreased libido and erectile dysfunction being common. The underlying mechanisms involve oxidative stress damage, an abnormal increase in apoptosis, and enhanced autophagy. Nevertheless, current research, especially human-based studies, is restricted by small sample sizes and insufficiently in-depth exploration of these mechanisms.
1 Introduction
In the progression of global economic and social development, high-altitude areas have assumed increasing importance in mineral exploitation (1), tourism expansion (2), military garrisoning (3), and numerous other fields due to their abundant natural resources and distinctive geographical strategic positions. In recent years, with mineral development projects in high-altitude regions continuously growing, more and more people are settling or frequently traveling in high-altitude areas. The environment in high-altitude regions is characterized by low atmospheric pressure (4), low oxygen partial pressure (5), low temperature (6), and intense ultraviolet radiation (7), posing formidable challenges to human physiological functions.
In high-altitude areas, the atmospheric pressure and the closely related oxygen partial pressure drop significantly with increasing altitude (8). Hypoxia is common in high-altitude regions like the Qinghai-Tibet Plateau and the Andes Mountains, having a profound impact on human physiological functions by affecting energy metabolism, reducing low-temperature tolerance, and interacting with strong ultraviolet radiation (9). There is a highly significant negative correlation between air temperature and altitude in high-altitude areas (10), and this low-temperature environment affects various aspects of the human body including basal metabolism and physiological functions. Also, due to the thin air, ultraviolet radiation attenuation is much lower than in low-altitude regions (11), resulting in a substantial increase in ultraviolet radiation intensity, and excessive ultraviolet irradiation can damage biomacromolecules and skin tissues (12).
The male reproductive system, being a crucial physiological system for maintaining human reproduction and individual quality of life, has attracted significant attention regarding its health status in high-altitude environments (13–15). Male reproductive health is not only directly related to an individual’s fertility but also plays an essential role in family stability and the optimization of the social population structure (16). Therefore, delving deeply into the potential effects and mechanisms of the high-altitude environment on the male reproductive system is of great significance for effectively safeguarding the reproductive health of men in high-altitude areas, scientifically formulating targeted protection strategies, and promoting the advancement of research in related fields.
2 Impacts of high-altitude environment on the male reproductive system
2.1 Impact on reproductive hormone levels
Long-term exposure to the high-altitude hypoxic environment induces intricate and clinically significant changes in male reproductive hormone levels (17). Gonadotropin-releasing hormone (GnRH), a pivotal hormone secreted by the hypothalamus, plays a crucial “directing” role in the reproductive endocrine regulatory network, and its secretion process is notably inhibited by the hypoxic environment (18, 19).
The neurons in the hypothalamus are highly sensitive to oxygen partial pressure. In a hypoxic state, the normal metabolism and signal transduction processes of neurons are severely disrupted, resulting in the suppression of GnRH synthesis and release. Previous studies have demonstrated that in a specific high-altitude environment, such as at an altitude of 4350 meters, the FSH level in 8 healthy male subjects decreased by 17% (19). In another study involving 40 healthy subjects (21 men) within the altitude range of 550 meters to 7050 meters, the results revealed that the male FSH level continuously declined from 5.8 ± 5.9 mU/L at 550 meters (baseline level) with increasing altitude, reaching 3.3 ± 2.1 mU/L at 7050 meters, with a reduction of up to 40%. Simultaneously, LH also decreased significantly from the baseline to 7050 meters, by approximately 50% (20).
The decline in GnRH secretion further affects the pituitary’s secretion of follicle-stimulating hormone (FSH) and luteinizing hormone (LH), and FSH and LH are essential for testicular spermatogenesis and testosterone synthesis and secretion (21, 22). FSH primarily acts on the Sertoli cells in the seminiferous tubules of the testis to promote their maintenance of normal function and provide necessary nutrition and support for the spermatogenic process (23–25). LH mainly acts on the Leydig cells of the testis to stimulate their synthesis and secretion of testosterone (26–29). Hence, when the levels of FSH and LH decrease, the functions of the Sertoli cells and Leydig cells in the seminiferous tubules of the testis are damaged to varying degrees, thereby influencing sperm production and testosterone secretion (22, 30, 31).
Testosterone, as the core hormone of male reproductive function, is also affected by multiple factors in its synthesis and secretion (32, 33). In von Wolff et al.’s study, testosterone concentration remained relatively stable at most altitudes, with minimal fluctuations. However, at 7050 m, testosterone concentration decreased significantly, paralleling the decline in LH concentration (20). Previous research by Benso et al. also reported a significant reduction in testosterone concentrations in eight mountaineers at the 5200-m base camp post-Mount Everest climb (34). Nevertheless, due to the small sample size and confounding effect of excessive exertion, the interpretability of those results was limited. In summary, as altitude rises, particularly at extreme altitudes, testosterone concentration is significantly affected and generally decreases. This change is likely associated with alterations in other hormone levels and altitude-related environmental factors (35).
2.2 Impact on testicular tissue morphology
Animal experiments have provided crucial evidence for elucidating the morphological changes of testicular tissue in a high-altitude environment. For example, in the experiment by Li et al., 3-week-old male Wistar rats were housed in a temperature- and humidity-controlled animal room, and a low-pressure hypoxic environment simulating an altitude of 5000 meters was created using a hypobaric hypoxia chamber (36, 37). The experimental outcomes showed that compared with the control rats in the normal environment, the seminiferous tubule structure of the rats in the low-pressure hypoxic group was significantly disordered, the arrangement of spermatogenic epithelial cells lost its normal order, and the number of spermatogenic cells at all levels decreased sharply, especially the number of spermatocytes and spermatids. Additionally, Li et al.’s study also confirmed that the testosterone levels in the low-pressure hypoxic group were significantly lower than those in the normal environment group (13).
Hypoxia is closely related to the testis and significantly affects testicular function and reproductive health (38, 39). In high-altitude environments, except for certain indigenous populations, human reproductive function is affected (40–42). Animal experiments show that hypoxia can change the morphology of testicular tissue and testosterone levels (43, 44). When the testis is hypoxic, HIF-1α accumulates (45, 46), triggering vascular changes, including angiogenesis. The effect of hypoxia on testicular steroidogenesis is complex, with testosterone levels rising first (47) and then falling (34). Chronic intermittent hypobaric hypoxia damages testicular tissue, reduces sperm quality, and decreases testosterone levels (42). Hypoxia also increases testicular temperature, generates ROS (48), causes oxidative stress, and damages sperm cells (49).
2.3 Impact on sperm quality
The high-altitude environment has multiple adverse effects on sperm quality, encompassing key indicators such as sperm density, motility, deformity rate, and genetic material integrity (15, 50).
Previous research shows sperm density in high-altitude areas is lower. Gasco et al. studied male rats at 4340-meter altitude, finding epididymal sperm count decreased from the 7th day (51). Verratti et al. studied 5 Italian men before and after a 19-day high-altitude trek in the Himalayas, showing sperm concentration decreased (50). Their study has clinical value but needs a larger sample size.
Sperm motility, crucial for fertilization, significantly drops in high-altitude environments, reducing the chance of conception (15). The sperm deformity rate also increases, with head and tail deformities affecting sperm function. Luo et al. found altitude impacts sperm genetic material. Sperm mtDNA copy number and nDNA integrity vary with altitude and residence time (52).
Verratti et al. also noted that high-altitude trekking causes oxidative stress imbalance in semen. This imbalance leads to an increase in ROS production. ROS, with its strong oxidative activity, attacks the unsaturated fatty acids on the sperm cell membrane, triggering lipid peroxidation reactions (53). As a result, not only is the integrity and fluidity of the sperm membrane damaged, impairing sperm membrane function and thus affecting sperm motility and fertilization ability, but additionally, the membrane’s barrier function is weakened. This makes it easier for harmful substances to enter the sperm. Furthermore, ROS directly attacks the DNA in the nucleus, causing damage such as DNA strand breaks and base modifications. This affects the integrity of sperm genetic material and severely damages sperm quality (50).
Figure 1 shows the impact of high-altitude environment on spermatogenesis and sperm quality. In high-altitude conditions, normal spermatogenesis from spermatogonium to sperm is disrupted. Mitochondria are damaged, affecting energy supply. Sperm DNA and cell membranes are also damaged, leading to abnormal sperm vitality and a large number of defective sperm, which seriously impairs sperm quality and male reproductive function.
2.4 Impact on sexual function
Sexual dysfunction problems are relatively prevalent among men residing in high-altitude areas for long periods, primarily manifested as decreased libido and erectile dysfunction (50). This phenomenon is caused by the combined action of multiple factors.
From the perspective of the endocrine and reproductive organs, the fluctuations in reproductive hormone levels and testicular tissue damage play a key role in the occurrence of male sexual dysfunction (54–56). Testosterone, as an important hormone for maintaining male libido and sexual response, the decrease in its level will directly weaken male sexual impulse and sexual ability (57, 58). When men are in a high-altitude environment, due to factors such as hypoxia, the synthesis and secretion of testosterone decrease, resulting in a decline in the level of testosterone in the body, which in turn affects sexual function (35, 59). At the same time, testicular tissue damage caused by the high-altitude environment will disrupt the normal physiological function of the reproductive organs, making it difficult to maintain the erectile function in a normal state (17, 60). The testis is an important part of the male reproductive system, and its normal function is of great significance for maintaining erectile function. Testicular tissue damage may affect multiple aspects such as nerve conduction and vascular function, leading to erectile dysfunction (61–63).
The adaptive changes of the nervous system in the high-altitude environment also have a significant impact on sexual function. Verratti et al. conducted a study on three climbers and found that when the altitude rose above 4450 meters, sleep-related erections (SREs) became abnormal, and there was a lack of erections in the hardness ranges of 80-100% and 60-79%. Moreover, the average hardness percentage and the duration of 80-100% hardness gradually decreased with the increase in altitude (64). The underlying mechanism is that nitric oxide (NO) plays a crucial role in the process of penile erection. Under normal nerve stimulation, nerve endings and endothelial cells release NO, which activates guanylate cyclase and promotes the relaxation of penile smooth muscle, thus achieving erection (65, 66). However, in the high-altitude hypoxic environment, the above process is disrupted (67). Hypoxia significantly inhibits the activity of NO synthase (68), resulting in a significant decrease in NO synthesis, and may also reduce the responsiveness of target cells (smooth muscle) to NO, further hindering the relaxation of trabecular smooth muscle and thus inhibiting erectile function (69, 70). In addition, acute hypoxia enhances sympathetic nerve afferent activation and exacerbates vasoconstriction activity (71). Even after the human body has adapted to the high-altitude environment to a certain extent, the excited state of the sympathetic nerve may still persist (72). This continuous excitement will interfere with the normal nerve regulation function and hinder the conduction of sexual excitement, having an adverse impact on the normal performance of sexual function (64).
The Table 1 presents a comprehensive summary of the specific effects of high-altitude environments on different aspects of the male reproductive system, along with relevant research cases that support these findings.
3 Possible mechanisms of high-altitude environment affecting the male reproductive system
3.1 Oxidative stress damage
The hypoxic environment in high-altitude areas is one of the key inducers of oxidative stress (73). When the body is in a hypoxic state, the function of the mitochondrial respiratory chain in cells is severely damaged, and the electron transfer process is abnormal, resulting in the massive production of reactive oxygen species (ROS) (74). Under normal circumstances, the body maintains the balance of ROS through its own antioxidant defense system. However, in the high-altitude hypoxic environment, when the production of ROS exceeds the limit of the body’s antioxidant defense capacity, oxidative stress occurs (75–77).
In the male reproductive system, oxidative stress causes particularly serious damage to testicular tissue and sperm (78, 79). ROS has strong oxidizing properties and can attack the unsaturated fatty acids on the sperm cell membrane, triggering lipid peroxidation reactions (53). The products of lipid peroxidation will damage the integrity and fluidity of the sperm membrane, resulting in the impairment of sperm membrane function and then affecting sperm motility and fertilization ability (80–82). Because the integrity of the sperm membrane is crucial for maintaining the stability of the internal environment of sperm and protecting DNA, once the sperm membrane is damaged, harmful substances from the outside are more likely to enter the sperm and cause damage to DNA, thus affecting sperm quality and function (83–85).
In addition, oxidative stress may also interfere with the DNA damage repair mechanism of spermatogenic cells, causing the accumulation of DNA damage in cells and then increasing the apoptosis of spermatogenic cells (79, 86). The normal proliferation and differentiation of spermatogenic cells are the basis of sperm production, and excessive apoptosis of spermatogenic cells will seriously affect the quantity and quality of sperm produced (87, 88). In a study of male soldiers in high-altitude areas, it was found that when they were exposed to a hypoxic environment at an altitude of 5380 meters for 6 months, their total sperm count, sperm density, motility, and survival rate all decreased significantly, and the semen liquefaction time was significantly prolonged. After 12 months, the sperm motility, survival rate, and liquefaction time further deteriorated (15). This may be due to the increase in spermatogenic cell damage and apoptosis caused by high-altitude hypoxia-induced oxidative stress, which breaks the balance between the normal proliferation and apoptosis of spermatogenic cells, seriously hinders the sperm production process, and ultimately affects various quality indicators of sperm (89).
Oxidative stress may also affect the secretion of reproductive hormones (90, 91). In the high-altitude environment, the serum testosterone level of male mice may increase in the early stage of hypoxia, which may be a compensatory response of the body to promote adaptation to the hypoxic environment. However, with the continuous extension of hypoxia time, ROS gradually accumulates in testicular cells, leading to damage to Leydig cells and then reducing the secretion of testosterone. This indicates that oxidative stress may affect the function of Leydig cells and interfere with the normal secretion process of reproductive hormones, thus further affecting male reproductive function (92).
Moreover, as shown in Figure 2, hypoxia (93) and ultraviolet radiation (94) can lead to the generation of ROS, which is an important part of the oxidative stress mechanism. Ultraviolet radiation directly inflicts DNA damage (95), activating key signaling molecules ATM and ATR (96). These molecules phosphorylate CHK1 and CHK2 (97), ultimately activating P53 (98). Activated P53 can trigger multiple cellular responses, including apoptosis, cell cycle arrest, and DNA repair (99). In the context of oxidative stress in the male reproductive system, the activation of these pathways may disrupt the normal development and function of spermatogenic cells. Excessive activation of the apoptosis pathway may lead to an increased number of apoptotic spermatogenic cells, reducing sperm production (100). The disruption of the cell cycle arrest and DNA repair pathways may result in the accumulation of damaged DNA in sperm, affecting sperm quality (101).
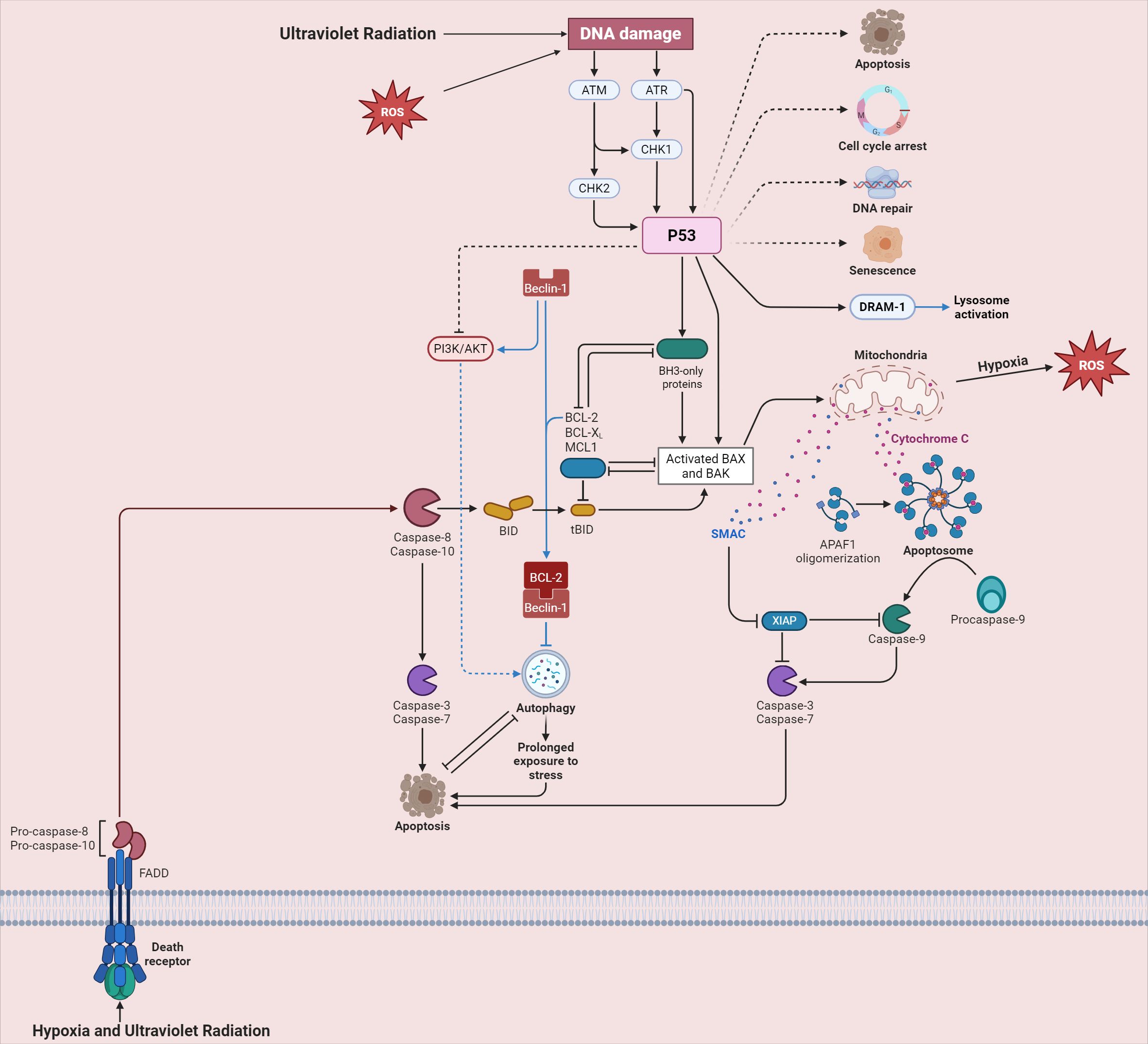
Figure 2. Cellular signaling pathways triggered by ultraviolet radiation and hypoxia in high-altitude areas.
3.2 Apoptosis
The abnormal increase in apoptosis leads to a decrease in the number of spermatogenic cells, seriously disrupting the process of sperm production and development (102). Under normal physiological conditions, the proliferation and apoptosis of spermatogenic cells are in a dynamic balance to maintain the stability of sperm production (103–105). However, in a high-altitude environment, multiple factors lead to an abnormal increase in spermatogenic cell apoptosis, breaking this balance and significantly reducing the number of spermatogenic cells (106, 107). Spermatogenic cells are the basis of sperm production, and the decrease in their number will inevitably affect sperm production and development, resulting in a decrease in sperm quantity and quality and ultimately affecting male reproductive ability (108, 109).
In the testis, apoptosis is an important physiological process, and its functions include maintaining the appropriate ratio of spermatogenic cells to Sertoli cells, clearing abnormal spermatogenic cells, and ensuring quality control of sperm production (110). However, in a high-altitude environment, the abnormal increase in apoptosis caused by factors such as hypoxia greatly disrupts this normal physiological balance (111, 112). Excessive apoptosis of spermatogenic cells leads to a sharp reduction in the number of spermatogenic cells, unable to provide sufficient basal cells for sperm production, resulting in insufficient sperm production. At the same time, a series of biochemical and molecular level changes may occur during apoptosis, which will have a negative impact on the morphology, structure and function of sperm, resulting in a decrease in sperm quality, such as reduced sperm motility and increased deformity rate, and ultimately affecting male reproductive ability (113, 114).
As shown in Figure 2, hypoxia and ultraviolet radiation engage with death receptors, spurring the activation of Pro-caspase-8 and Pro-caspase-10 (115). These then activate downstream Caspase-3 and Caspase-7, driving the process of apoptosis (116). The BCL-2 family proteins play a critical role in regulating mitochondrial outer membrane permeabilization (MOMP). When MOMP occurs, cytochrome C is released, forming an azotosome with APAF-1, which activates Caspase-9 and intensifies apoptosis (117). In spermatogenic cells, the imbalance of these apoptotic regulatory factors in the high-altitude environment leads to excessive apoptosis, further exacerbating the damage to male reproductive function (118, 119).
3.3 Increase in autophagy
Autophagy is a highly conserved catabolic process in cells (120). Its main function is to wrap damaged proteins, organelles and other substances in the cell through the formation of autophagosomes and transport them to lysosomes for degradation, thereby realizing the recycling of intracellular substances and maintaining the stability of the intracellular environment (121). In the high-altitude environment, the change of autophagy is particularly significant (122, 123).
This increase in autophagy is regulated by multiple signaling pathways (124). The AMP-activated protein kinase (AMPK) pathway is one of the key regulatory pathways (125). In a high-altitude environment, the decrease in oxygen availability leads to a decrease in cellular ATP levels. As a result, AMPK is activated, which in turn promotes the initiation of autophagy (126).
Moreover, the mammalian target of rapamycin (mTOR) signaling pathway also plays a crucial role (127). Under normal conditions, mTOR acts as a negative regulator of autophagy (128). However, in high-altitude environments, the mTOR pathway is inhibited, which releases the suppression of autophagy, thus further promoting the increase of autophagy levels (126, 129, 130).
Autophagy in the high-altitude environment is a double-edged sword. Initially, it serves as a self-protective mechanism for cells. By forming autophagosomes to engulf damaged proteins and organelles and transporting them to lysosomes for degradation, it helps maintain the stability of the intracellular environment, which is beneficial for cells to adapt to the harsh high-altitude environment to a certain extent (131, 132). However, excessive autophagy is detrimental. It can lead to the over-degradation of crucial proteins and organelles in spermatogenic cells (133). Moreover, excessive autophagy may trigger apoptosis in spermatogenic cells, ultimately reducing sperm quality and quantity and impairing male reproductive function. Excessive autophagy may lead to the over-degradation of important proteins and organelles in spermatogenic cells. The over-degradation of mitochondria in spermatogenic cells can disrupt the normal energy metabolism of cells, affecting the process of spermatogenesis (134, 135). This complex behavior of autophagy, with its potential for both protection and harm, is closely related to the regulatory pathways that govern it.
Figure 2 shows that the PI3K/AKT pathway and autophagy-related protein Beclin-1 are involved in the cellular stress response and interact with apoptosis-related pathways (136, 137). In high-altitude settings, PI3K/AKT activation may regulate autophagy via Beclin-1, influencing its expression and activity (138). This interaction further complicates autophagy regulation in male reproductive cells, and its dysregulation may harm male reproductive function. Further research is needed to comprehensively understand the molecular mechanisms of this interplay and its impact on male reproduction.
4 Research status and prospects
At present, research on the damage of the high-altitude environment to the male reproductive system has made certain progress in several aspects. In the field of animal experiments, many studies have observed the impact of the high-altitude environment on the animal reproductive system through simulating the high-altitude environment or directly conducting experiments in high-altitude areas, providing important evidence for revealing relevant mechanisms (13).
However, there are still many deficiencies in existing research. In human studies, large-scale epidemiological investigations on the reproductive health of people in high-altitude areas are scarce. Most studies have small sample sizes, usually involving only a limited number of participants. This makes it hard to cover the wide range of genetic, lifestyle, and environmental differences among high-altitude populations. As a result, the findings may not be applicable to the general high-altitude population. Also, the short observation periods in these studies mean they can’t fully assess the long-term effects on the male reproductive system. Regarding mechanism research, although studies on oxidative stress, apoptosis, and autophagy have been done, many molecular mechanisms remain unclear. The intracellular signaling pathways in high-altitude conditions are complex and interconnected. For instance, the relationship between autophagy and the endoplasmic reticulum stress response in spermatogenic cells under high-altitude stress is not well-understood. When cells are exposed to high-altitude stressors, the endoplasmic reticulum may experience stress, which could potentially trigger autophagy as a compensatory mechanism. However, it’s unclear how the autophagy process is precisely regulated during this endoplasmic reticulum stress response and what the long-term consequences are for spermatogenesis. Additionally, individual differences in adaptability to high-altitude environments are often overlooked. Some people may be more resilient due to genetic factors or healthy lifestyles, but research rarely takes these into account. Research models, both animal and cell models, also have limitations. Animal models can’t completely replicate the human body’s response, and cell models lack the complex environment of the whole organism, making it difficult to accurately reflect the real-world situation.
Future research on the male reproductive system in high-altitude environments should be multi-faceted. It should involve large-scale, multi-center longitudinal epidemiological studies across diverse high-altitude regions, using a unified questionnaire and comprehensive detection indicators that cover reproductive hormones, sperm quality, sexual function, and lifestyle/environmental factors. Long-term follow-up of participants will help observe long-term effects on the male reproductive system, identify damage-related factors, and provide reliable data. Meanwhile, modern molecular biology techniques like gene chips, proteomics, and single-cell sequencing should be used to explore the molecular mechanisms of oxidative stress, apoptosis, and autophagy in the male reproductive system. By analyzing the gene and protein expression profiles of relevant cells, key molecular targets can be uncovered, which is essential for understanding the causes of male reproductive damage and developing new therapies. Finally, based on the results of epidemiological and molecular mechanism research, practical protective and treatment methods should be developed. This includes exploring antioxidant-based interventions for oxidative stress damage and designing regulatory drugs or inhibitors for autophagy and apoptosis. These interventions should first be tested in pre-clinical models and then translated into clinical applications to safeguard the male reproductive system in high-altitude areas and enhance men’s reproductive health.
5 Conclusions
In summary, the special environmental factors in high-altitude areas, such as hypoxia, low temperature, and strong ultraviolet radiation, cause extensive and serious damage to the male reproductive system through multiple pathways such as oxidative stress damage, apoptosis, and autophagy. From the disorder of reproductive hormone levels, the change of testicular tissue morphology, to the decline of sperm quality and the appearance of sexual dysfunction, these damages seriously threaten the reproductive health of men in high-altitude areas. Although certain achievements have been made in this field of research, there are still many problems to be solved. In the future, more in-depth and systematic research is needed, strengthen epidemiological investigations of the population, reveal molecular mechanisms, and develop protective and treatment methods to effectively ensure the reproductive health of men in high-altitude areas, promote the sustainable development of high-altitude areas, and provide strong support for human survival and reproduction in high-altitude environments.
Author contributions
D-DM: Conceptualization, Formal analysis, Investigation, Writing – original draft, Writing – review & editing. Y-DK: Investigation, Methodology, Writing – original draft. D-HC: Conceptualization, Writing – review & editing.
Funding
The author(s) declare that no financial support was received for the research and/or publication of this article.
Acknowledgments
We thank BioRender for its valuable contribution. The figures (Figures 1, 2), created with BioRender.com, significantly enhance the clarity of our study. They help illustrate complex concepts related to the impact of high - altitude environments on the male reproductive system.
Conflict of interest
The authors declare that the research was conducted in the absence of any commercial or financial relationships that could be construed as a potential conflict of interest.
Generative AI statement
The author(s) declare that no Generative AI was used in the creation of this manuscript.
Publisher’s note
All claims expressed in this article are solely those of the authors and do not necessarily represent those of their affiliated organizations, or those of the publisher, the editors and the reviewers. Any product that may be evaluated in this article, or claim that may be made by its manufacturer, is not guaranteed or endorsed by the publisher.
References
1. Hao C, Huang Y, Ma D, Fan X, He P, Sun W. Environmental behaviors of PAHs in Ordovician limestone water of Fengfeng coal mining area in China. Environ monitoring Assess. (2018) 190:701. doi: 10.1007/s10661-018-7074-8
2. Chalkias A, Georgiou M, Böttiger B, Monsieurs KG, Svavarsdóttir H, Raffay V, et al. Recommendations for resuscitation after ascent to high altitude and in aircrafts. Int J Cardiol. (2013) 167:1703–11. doi: 10.1016/j.ijcard.2012.11.077
3. Bakker-Dyos J, Vanstone S, Mellor AJ. High altitude adaptation and illness: military implications. J R Naval Med Service. (2016) 102:33–9. doi: 10.1136/jrnms-102-33
4. Lu YZ, Han J, Zhang WJ, Sun J, Li X, Yang ZL, et al. Influence of low air pressure on combined nitritation and anaerobic ammonium oxidation process. Sci total Environ. (2022) 838:156556. doi: 10.1016/j.scitotenv.2022.156556
5. Sharma P, Mohanty S, Ahmad Y. A study of survival strategies for improving acclimatization of lowlanders at high-altitude. Heliyon. (2023) 9:e14929. doi: 10.1016/j.heliyon.2023.e14929
6. Li M, Tang X, Liao Z, Shen C, Cheng R, Fang M, et al. Xie L et al: Hypoxia and low temperature upregulate transferrin to induce hypercoagulability at high altitude. Blood. (2022) 140:2063–75. doi: 10.1182/blood.2022016410
7. Zhang Q, Gou W, Wang X, Zhang Y, Ma J, Zhang H, et al. Genome resequencing identifies unique adaptations of tibetan chickens to hypoxia and high-dose ultraviolet radiation in high-altitude environments. Genome Biol Evol. (2016) 8:765–76. doi: 10.1093/gbe/evw032
8. Hu X, Chen Y, Huo W, Jia W, Ma H, Ma W, et al. Surface oxygen concentration on the Qinghai-Tibet Plateau (2017-2022). Sci Data. (2023) 10:900. doi: 10.1038/s41597-023-02768-x
9. Liu J, Xin Z, Huang Y, Yu J. Climate suitability assessment on the Qinghai-Tibet Plateau. Sci total Environ. (2022) 816:151653. doi: 10.1016/j.scitotenv.2021.151653
10. Wang S, Liu F, Zhou Q, Chen Q, Liu F. Simulation and estimation of future ecological risk on the Qinghai-Tibet Plateau. Sci Rep. (2021) 11:17603. doi: 10.1038/s41598-021-96958-5
11. Guo J, Xie Z, Jiang H, Xu H, Liu B, Meng Q, et al. The molecular mechanism of yellow mushroom (Floccularia luteovirens) response to strong ultraviolet radiation on the qinghai-tibet plateau. Front Microbiol. (2022) 13:918491. doi: 10.3389/fmicb.2022.918491
12. Gromkowska-Kępka KJ, Puścion-Jakubik A, Markiewicz-Żukowska R, Socha K. The impact of ultraviolet radiation on skin photoaging-review of in vitro studies. J cosmetic Dermatol. (2021) 20:3427–31. doi: 10.1111/jocd.14033
13. Li XY, Zhang MH, Chen ZW, Zhang B, Bai G, Wang HF. Male reproductive system and simulated high-altitude environment: preliminary results in rats. Asian J andrology. (2023) 25:426–32. doi: 10.4103/aja202290
14. Zhong Y, Liu F, Zhang X, Guo Q, Wang Z, Wang R. Research progress on reproductive system damage caused by high altitude hypoxia. Endocrine. (2024) 83:559–70. doi: 10.1007/s12020-023-03643-w
15. He J, Cui J, Wang R, Gao L, Gao X, Yang L, et al. Exposure to hypoxia at high altitude (5380 m) for 1 year induces reversible effects on semen quality and serum reproductive hormone levels in young male adults. High altitude Med Biol. (2015) 16:216–22. doi: 10.1089/ham.2014.1046
16. Čegar B, Šipetić Grujičić S, Bjekić J, Vuksanović A, Bojanić N, Bartolović D, et al. Understanding the male perspective: evaluating quality of life and psychological distress in Serbian men undergoing infertility treatment. Life (Basel Switzerland). (2023) 13(9):1894. doi: 10.3390/life13091894
17. Chang D, Kong F, Jiang W, Li F, Zhang C, Ding H, et al. Effects of L-carnitine administration on sperm and sex hormone levels in a male wistar rat reproductive system injury model in a high-Altitude hypobaric hypoxic environment. Reprod Sci (Thousand Oaks Calif). (2023) 30:2231–47. doi: 10.1007/s43032-022-00948-5
18. Keenan DM, Pichler Hefti J, Veldhuis JD, Von Wolff M. Regulation and adaptation of endocrine axes at high altitude. Am J Physiol Endocrinol Metab. (2020) 318:E297–e309. doi: 10.1152/ajpendo.00243.2019
19. Richalet JP, Letournel M, Souberbielle JC. Effects of high-altitude hypoxia on the hormonal response to hypothalamic factors. Am J Physiol Regulatory Integr Comp Physiol. (2010) 299:R1685–1692. doi: 10.1152/ajpregu.00484.2010
20. von Wolff M, Nakas CT, Tobler M, Merz TM, Hilty MP, Veldhuis JD, et al. Adrenal, thyroid and gonadal axes are affected at high altitude. Endocrine connections. (2018) 7:1081–9. doi: 10.1530/EC-18-0242
21. Stamatiades GA, Kaiser UB. Gonadotropin regulation by pulsatile GnRH: Signaling and gene expression. Mol Cell Endocrinol. (2018) 463:131–41. doi: 10.1016/j.mce.2017.10.015
22. Kaprara A, Huhtaniemi IT. The hypothalamus-pituitary-gonad axis: Tales of mice and men. Metabolism: Clin Exp. (2018) 86:3–17. doi: 10.1016/j.metabol.2017.11.018
23. Plant TM, Marshall GR. The functional significance of FSH in spermatogenesis and the control of its secretion in male primates. Endocrine Rev. (2001) 22:764–86. doi: 10.1210/edrv.22.6.0446
24. Simoni M, Brigante G, Rochira V, Santi D, Casarini L. Prospects for FSH treatment of male infertility. J Clin Endocrinol Metab. (2020) 105(7):dgaa243. doi: 10.1210/clinem/dgaa243
25. Sofikitis N, Giotitsas N, Tsounapi P, Baltogiannis D, Giannakis D, Pardalidis N. Hormonal regulation of spermatogenesis and spermiogenesis. J Steroid Biochem Mol Biol. (2008) 109:323–30. doi: 10.1016/j.jsbmb.2008.03.004
26. Anawalt BD, Matsumoto AM. Aging and androgens: Physiology and clinical implications. Rev endocrine Metab Disord. (2022) 23:1123–37. doi: 10.1007/s11154-022-09765-2
27. Wang Z, Wu W, Kim MS, Cai D. GnRH pulse frequency and irregularity play a role in male aging. Nat Aging. (2021) 1:904–18. doi: 10.1038/s43587-021-00116-5
28. Zirkin BR, Papadopoulos V. Leydig cells: formation, function, and regulation. Biol Reprod. (2018) 99:101–11. doi: 10.1093/biolre/ioy059
29. Chen H, Wang Y, Ge R, Zirkin BR. Leydig cell stem cells: Identification, proliferation and differentiation. Mol Cell Endocrinol. (2017) 445:65–73. doi: 10.1016/j.mce.2016.10.010
30. Christin-Maitre S, Young J. Androgens and spermatogenesis. Annales d'endocrinologie. (2022) 83:155–8. doi: 10.1016/j.ando.2022.04.010
31. O'Shaughnessy PJ, Morris ID, Huhtaniemi I, Baker PJ, Abel MH. Role of androgen and gonadotrophins in the development and function of the Sertoli cells and Leydig cells: data from mutant and genetically modified mice. Mol Cell Endocrinol. (2009) 306:2–8. doi: 10.1016/j.mce.2008.11.005
32. Kim SD, Cho KS. Obstructive sleep apnea and testosterone deficiency. World J men's Health. (2019) 37:12–8. doi: 10.5534/wjmh.180017
33. Jiang S, Chen G, Yang Z, Wang D, Lu Y, Zhu L, et al. Testosterone attenuates hypoxia-induced hypertension by affecting NRF1-mediated transcriptional regulation of ET-1 and ACE. Hypertension research: Off J Japanese Soc Hypertension. (2021) 44:1395–405. doi: 10.1038/s41440-021-00703-4
34. Benso A, Broglio F, Aimaretti G, Lucatello B, Lanfranco F, Ghigo E, et al. Endocrine and metabolic responses to extreme altitude and physical exercise in climbers. Eur J Endocrinol. (2007) 157:733–40. doi: 10.1530/EJE-07-0355
35. Gonzales GF. Serum testosterone levels and excessive erythrocytosis during the process of adaptation to high altitudes. Asian J andrology. (2013) 15:368–74. doi: 10.1038/aja.2012.170
36. Liao Y, Chen Z, Yang Y, Shen D, Chai S, Ma Y, et al. Antibiotic intervention exacerbated oxidative stress and inflammatory responses in SD rats under hypobaric hypoxia exposure. Free Radical Biol Med. (2023) 209:70–83. doi: 10.1016/j.freeradbiomed.2023.10.002
37. Brent MB, Emmanuel T, Simonsen U, Brüel A, Thomsen JS. Hypobaric hypoxia deteriorates bone mass and strength in mice. Bone. (2022) 154:116203. doi: 10.1016/j.bone.2021.116203
38. He T, Guo H, Shen X, Wu X, Xia L, Jiang X, et al. Hypoxia-induced alteration of RNA modifications in the mouse testis and sperm†. Biol Reprod. (2021) 105:1171–8. doi: 10.1093/biolre/ioab142
39. Zhang K, Wang Z, Wang H, Fu Q, Zhang H, Cao Q. Hypoxia-induced apoptosis and mechanism of epididymal dysfunction in rats with left-side varicocele. Andrologia. (2016) 48:318–24. doi: 10.1111/and.2016.48.issue-3
40. Gonzales GF. Peruvian contributions to the study on human reproduction at high altitude: from the chronicles of the Spanish conquest to the present. Respiratory Physiol Neurobiol. (2007) 158:172–9. doi: 10.1016/j.resp.2007.03.015
41. Okumura A, Fuse H, Kawauchi Y, Mizuno I, Akashi T. Changes in male reproductive function after high altitude mountaineering. High altitude Med Biol. (2003) 4:349–53. doi: 10.1089/152702903769192304
42. Verratti V, Berardinelli F, Di Giulio C, Bosco G, Cacchio M, Pellicciotta M, et al. Evidence that chronic hypoxia causes reversible impairment on male fertility. Asian J andrology. (2008) 10:602–6. doi: 10.1111/j.1745-7262.2008.00346.x
43. Farias JG, Bustos-Obregón E, Orellana R, Bucarey JL, Quiroz E, Reyes JG. Effects of chronic hypobaric hypoxia on testis histology and round spermatid oxidative metabolism. Andrologia. (2005) 37:47–52. doi: 10.1111/j.1439-0272.2004.00651.x
44. Chai J, Liu S, Tian X, Wang J, Wen J, Xu C. Hypoxia impairs male reproductive functions via inducing rat Leydig cell ferroptosis under simulated environment at altitude of 5000 m. Life Sci. (2024) 357:123076. doi: 10.1016/j.lfs.2024.123076
45. Jewell UR, Kvietikova I, Scheid A, Bauer C, Wenger RH, Gassmann M. Induction of HIF-1alpha in response to hypoxia is instantaneous. FASEB journal: Off Publ Fed Am Societies Exp Biol. (2001) 15:1312–4. doi: 10.1096/fj.00-0732fje
46. Powell JD, Elshtein R, Forest DJ, Palladino MA. Stimulation of hypoxia-inducible factor-1 alpha (HIF-1alpha) protein in the adult rat testis following ischemic injury occurs without an increase in HIF-1alpha messenger RNA expression. Biol Reprod. (2002) 67:995–1002. doi: 10.1095/biolreprod.101.002576
47. Barnholt KE, Hoffman AR, Rock PB, Muza SR, Fulco CS, Braun B, et al. Endocrine responses to acute and chronic high-altitude exposure (4,300 meters): modulating effects of caloric restriction. Am J Physiol Endocrinol Metab. (2006) 290:E1078–1088. doi: 10.1152/ajpendo.00449.2005
48. Dirmeier R, O'Brien KM, Engle M, Dodd A, Spears E, Poyton RO. Exposure of yeast cells to anoxia induces transient oxidative stress. Implications induction hypoxic genes. J Biol Chem. (2002) 277:34773–84. doi: 10.1074/jbc.M203902200
49. Li Y, Zhou Q, Hively R, Yang L, Small C, Griswold MD. Differential gene expression in the testes of different murine strains under normal and hyperthermic conditions. J andrology. (2009) 30:325–37. doi: 10.2164/jandrol.108.005934
50. Verratti V, Mrakic-Sposta S, Fusi J, Sabovic I, Franzoni F, Pietrangelo T, et al. Fertility Impairment after Trekking at High Altitude: A Proof of Mechanisms on Redox and Metabolic Seminal Changes. Int J Mol Sci. (2022) 23(16):9066. doi: 10.3390/ijms23169066
51. Gasco M, Rubio J, Chung A, Villegas L, Gonzales GF. Effect of high altitude exposure on spermatogenesis and epididymal sperm count in male rats. Andrologia. (2003) 35(6):368–74. doi: 10.1046/j.0303-4569.2003.00593.x
52. Luo Y, Liao W, Chen Y, Cui J, Liu F, Jiang C, et al. Altitude can alter the mtDNA copy number and nDNA integrity in sperm. J assisted Reprod Genet. (2011) 28:951–6. doi: 10.1007/s10815-011-9620-y
53. Sharma P, Ghanghas P, Kaushal N, Kaur J, Kaur P. Epigenetics and oxidative stress: A twin-edged sword in spermatogenesis. Andrologia. (2019) 51:e13432. doi: 10.1111/and.v51.11
54. Salonia A, Bettocchi C, Boeri L, Capogrosso P, Carvalho J, Cilesiz NC, et al. European association of urology guidelines on sexual and reproductive health-2021 update: male sexual dysfunction. Eur Urol. (2021) 80:333–57. doi: 10.1016/j.eururo.2021.06.007
55. Kjaergaard AD, Marouli E, Papadopoulou A, Deloukas P, Kuś A, Sterenborg R, et al. Thyroid function, sex hormones and sexual function: a Mendelian randomization study. Eur J Epidemiol. (2021) 36:335–44. doi: 10.1007/s10654-021-00721-z
56. Calabrò RS, Marino S, Bramanti P. Sexual and reproductive dysfunction associated with antiepileptic drug use in men with epilepsy. Expert Rev Neurother. (2011) 11(6):887–95. doi: 10.1586/ern.11.58IF
57. Pencina KM, Travison TG, Cunningham GR, Lincoff AM, Nissen SE, Khera M, et al. Effect of testosterone replacement therapy on sexual function and hypogonadal symptoms in men with hypogonadism. J Clin Endocrinol Metab. (2024) 109:569–80. doi: 10.1210/clinem/dgad484
58. Podlasek CA, Mulhall J, Davies K, Wingard CJ, Hannan JL, Bivalacqua TJ, et al. Translational perspective on the role of testosterone in sexual function and dysfunction. J sexual Med. (2016) 13:1183–98. doi: 10.1016/j.jsxm.2016.06.004
59. Wang S, Wei Y, Hu C, Liu F. Proteomic analysis reveals proteins and pathways associated with declined testosterone production in male obese mice after chronic high-altitude exposure. Front Endocrinol. (2022) 13:1046901. doi: 10.3389/fendo.2022.1046901
60. Chang DH, Kong FY, Jiang W, Li FD, Kang YD, Zhou X, et al. Establishment of a model of reproductive system injury in male Wistar rats in high-altitude hypoxia environment. Zhonghua Nan Ke Xue. (2020) 26(12):1068–73. (In Chinese)
61. Killari KN, Polimati H, Prasanth D, Singh G, Panda SP, Vedula GS, et al. Salazinic acid attenuates male sexual dysfunction and testicular oxidative damage in streptozotocin-induced diabetic albino rats. RSC Adv. (2023) 13:12991–3005. doi: 10.1039/D3RA01542D
62. El Shehaby DM, El-Mahdy RI, Ahmed AM, Hosny A, Abd El-Rady NM. Neurobehavioral, testicular and erectile impairments of chronic ketamine administration: Pathogenesis and ameliorating effect of N-acetyl cysteine. Reprod Toxicol (Elmsford NY). (2020) 96:57–66. doi: 10.1016/j.reprotox.2020.05.016
63. Amaral S, Oliveira PJ, Ramalho-Santos J. Diabetes and the impairment of reproductive function: possible role of mitochondria and reactive oxygen species. Curr Diabetes Rev. (2008) 4:46–54. doi: 10.2174/157339908783502398
64. Verratti V, Di Giulio C, Berardinelli F, Pellicciotta M, Di Francesco S, Iantorno R, et al. The role of hypoxia in erectile dysfunction mechanisms. Int J impotence Res. (2007) 19:496–500. doi: 10.1038/sj.ijir.3901560
65. MacDonald SM, Burnett AL. Physiology of erection and pathophysiology of erectile dysfunction. Urologic Clinics North America. (2021) 48:513–25. doi: 10.1016/j.ucl.2021.06.009
66. Sullivan ME, Mumtaz FH, Dashwood MR, Thompson CS, Naseem KM, Bruckdorfer KR, et al. Enhanced relaxation of diabetic rabbit cavernosal smooth muscle in response to nitric oxide: potential relevance to erectile dysfunction. Int J impotence Res. (2002) 14:523–32. doi: 10.1038/sj.ijir.3900935
67. Ghosh S, Kiyamu M, Contreras P, León-Velarde F, Bigham A, Brutsaert TD. Exhaled nitric oxide in ethnically diverse high-altitude native populations: A comparative study. Am J Phys anthropology. (2019) 170:451–8. doi: 10.1002/ajpa.v170.3
68. Guerby P, Tasta O, Swiader A, Pont F, Bujold E, Parant O, et al. Role of oxidative stress in the dysfunction of the placental endothelial nitric oxide synthase in preeclampsia. Redox Biol. (2021) 40:101861. doi: 10.1016/j.redox.2021.101861
69. Hansen J, Sander M, Hald CF, Victor RG, Thomas GD. Metabolic modulation of sympathetic vasoconstriction in human skeletal muscle: role of tissue hypoxia. J Physiol. (2000) 527 Pt 2:387–96. doi: 10.1111/j.1469-7793.2000.00387.x
70. Xie A, Skatrud JB, Puleo DS, Morgan BJ. Exposure to hypoxia produces long-lasting sympathetic activation in humans. J Appl Physiol (Bethesda Md: 1985). (2001) 91:1555–62. doi: 10.1152/jappl.2001.91.4.1555
71. Tan CO, Tzeng YC, Hamner JW, Tamisier R, Taylor JA. Alterations in sympathetic neurovascular transduction during acute hypoxia in humans. Am J Physiol Regulatory Integr Comp Physiol. (2013) 304:R959–965. doi: 10.1152/ajpregu.00071.2013
72. Peng Y, Kline DD, Dick TE, Prabhakar NR. Chronic intermittent hypoxia enhances carotid body chemoreceptor response to low oxygen. Adv Exp Med Biol. (2001) 499:33–8. doi: 10.1007/978-1-4615-1375-9_5
73. Pena E, El Alam S, Siques P, Brito J. Oxidative stress and diseases associated with high-altitude exposure. Antioxidants (Basel Switzerland). (2022) 11:267. doi: 10.3390/antiox11020267
74. Li X, Zhang J, Liu G, Wu G, Wang R, Zhang J. High altitude hypoxia and oxidative stress: The new hope brought by free radical scavengers. Life Sci. (2024) 336:122319. doi: 10.1016/j.lfs.2023.122319
75. Apel K, Hirt H. Reactive oxygen species: metabolism, oxidative stress, and signal transduction. Annu Rev Plant Biol. (2004) 55:373–99. doi: 10.1146/annurev.arplant.55.031903.141701
76. Zhang B, Pan C, Feng C, Yan C, Yu Y, Chen Z, et al. Role of mitochondrial reactive oxygen species in homeostasis regulation. Redox report: Commun Free Radical Res. (2022) 27:45–52. doi: 10.1080/13510002.2022.2046423
77. Wu Z, Wang H, Fang S, Xu C. Roles of endoplasmic reticulum stress and autophagy on H2O2−induced oxidative stress injury in HepG2 cells. Mol Med Rep. (2018) 18:4163–74. doi: 10.3892/mmr.2018.9443
78. Jing J, Peng Y, Fan W, Han S, Peng Q, Xue C, et al. Obesity-induced oxidative stress and mitochondrial dysfunction negatively affect sperm quality. FEBS Open Bio. (2023) 13:763–78. doi: 10.1002/2211-5463.13589
79. Bisht S, Faiq M, Tolahunase M, Dada R. Oxidative stress and male infertility. Nat Rev Urol. (2017) 14:470–85. doi: 10.1038/nrurol.2017.69
80. Martínez P, Proverbio F, Camejo MI. Sperm lipid peroxidation and pro-inflammatory cytokines. Asian J andrology. (2007) 9:102–7. doi: 10.1111/j.1745-7262.2007.00238.x
81. Palhares PC, Assis IL, MaChado GJ, de Freitas RMP, de Freitas MBD, Paula DAJ, et al. Sperm characteristics, peroxidation lipid and antioxidant enzyme activity changes in milt of Brycon orbignyanus cryopreserved with melatonin in different freezing curves. Theriogenology. (2021) 176:18–25. doi: 10.1016/j.theriogenology.2021.09.013
82. Nichi M, Goovaerts IG, Cortada CN, Barnabe VH, De Clercq JB, Bols PE. Roles of lipid peroxidation and cytoplasmic droplets on in vitro fertilization capacity of sperm collected from bovine epididymides stored at 4 and 34 degrees C. Theriogenology. (2007) 67:334–40. doi: 10.1016/j.theriogenology.2006.08.002
83. García BM, Fernández LG, Ferrusola CO, Salazar-Sandoval C, Rodríguez AM, Martinez HR, et al. Membrane lipids of the stallion spermatozoon in relation to sperm quality and susceptibility to lipid peroxidation. Reprod Domest Anim = Zuchthygiene. (2011) 46:141–8. doi: 10.1111/j.1439-0531.2010.01609.x
84. Komsky-Elbaz A, Roth Z. Fluorimetric techniques for the assessment of sperm membranes. J visualized experiments: JoVE. (2018) 141. doi: 10.3791/58622IF
85. Gholami D, Sharafi M, Esmaeili V, Nadri T, Alaei L, Riazi G, et al. Beneficial effects of trehalose and gentiobiose on human sperm cryopreservation. PloS One. (2023) 18:e0271210. doi: 10.1371/journal.pone.0271210
86. Wright C, Milne S, Leeson H. Sperm DNA damage caused by oxidative stress: modifiable clinical, lifestyle and nutritional factors in male infertility. Reprod biomedicine Online. (2014) 28:684–703. doi: 10.1016/j.rbmo.2014.02.004
87. Li Z, Wang S, Gong C, Hu Y, Liu J, Wang W, et al. Effects of environmental and pathological hypoxia on male fertility. Front Cell Dev Biol. (2021) 9:725933. doi: 10.3389/fcell.2021.725933
88. Kaushal N, Bansal MP. Dietary selenium variation-induced oxidative stress modulates CDC2/cyclin B1 expression and apoptosis of germ cells in mice testis. J Nutr Biochem. (2007) 18:553–64. doi: 10.1016/j.jnutbio.2006.11.003
89. He T, Guo H, Xia L, Shen X, Huang Y, Wu X, et al. Alterations of RNA modification in mouse germ cell-2 spermatids under hypoxic stress. Front Mol Biosci. (2022) 9:871737. doi: 10.3389/fmolb.2022.871737
90. Al-Sawalha NA, Pokkunuri ID, Alzoubi KH, Khabour OF, Almomani BN. Waterpipe tobacco smoke exposure during lactation-susceptibility of reproductive hormones and oxidative stress parameters in male progeny rats. Reprod Sci (Thousand Oaks Calif). (2021) 28:37–42. doi: 10.1007/s43032-020-00282-8
91. Dehdari Ebrahimi N, Sadeghi A, Shojaei-Zarghani S, Shahlaee MA, Taherifard E, Rahimian Z, et al. Protective effects of exogenous melatonin therapy against oxidative stress to male reproductive tissue caused by anti-cancer chemical and radiation therapy: a systematic review and meta-analysis of animal studies. Front Endocrinol. (2023) 14:1184745. doi: 10.3389/fendo.2023.1184745
92. Darbandi M, Darbandi S, Agarwal A, Sengupta P, Durairajanayagam D, Henkel R, et al. Reactive oxygen species and male reproductive hormones. Reprod Biol endocrinology: RB&E. (2018) 16:87. doi: 10.1186/s12958-018-0406-2
93. Okoye CN, Koren SA, Wojtovich AP. Mitochondrial complex I ROS production and redox signaling in hypoxia. Redox Biol. (2023) 67:102926. doi: 10.1016/j.redox.2023.102926
94. Ryšavá A, Vostálová J, Rajnochová Svobodová A. Effect of ultraviolet radiation on the Nrf2 signaling pathway in skin cells. Int J Radiat Biol. (2021) 97:1383–403. doi: 10.1080/09553002.2021.1962566
95. Birch-Machin MA, Russell EV, Latimer JA. Mitochondrial DNA damage as a biomarker for ultraviolet radiation exposure and oxidative stress. Br J Dermatol. (2013) 169 Suppl 2:9–14. doi: 10.1111/bjd.12207IF:11.0 Q1
96. Fedak EA, Adler FR, Abegglen LM, Schiffman JD. ATM and ATR activation through crosstalk between DNA damage response pathways. Bull Math Biol. (2021) 83:38. doi: 10.1007/s11538-021-00868-6
97. Smith J, Tho LM, Xu N, Gillespie DA. The ATM-Chk2 and ATR-Chk1 pathways in DNA damage signaling and cancer. Adv Cancer Res. (2010) 108:73–112. doi: 10.1016/B978-0-12-380888-2.00003-0
98. Rinaldi VD, Bloom JC, Schimenti JC. Oocyte Elimination Through DNA Damage Signaling from CHK1/CHK2 to p53 and p63. Genetics. (2020) 215:373–8. doi: 10.1534/genetics.120.303182
99. Karimian A, Ahmadi Y, Yousefi B. Multiple functions of p21 in cell cycle, apoptosis and transcriptional regulation after DNA damage. DNA Repair. (2016) 42:63–71. doi: 10.1016/j.dnarep.2016.04.008
100. Cheng L, Yi X, Shi Y, Yu S, Zhang L, Wang J, et al. Abnormal lipid metabolism induced apoptosis of spermatogenic cells by increasing testicular HSP60 protein expression. Andrologia. (2020) 52:e13781. doi: 10.1111/and.v52.11
101. Bollwein H, Bittner L. Impacts of oxidative stress on bovine sperm function and subsequent in vitro embryo development. Anim Reprod. (2018) 15:703–10. doi: 10.21451/1984-3143-AR2018-0041
102. Zakariah M, Molele RA, Mahdy MAA, Ibrahim MIA, McGaw LJ. Regulation of spermatogenic cell apoptosis by the pro-apoptotic proteins in the testicular tissues of mammalian and avian species. Anim Reprod Sci. (2022) 247:107158. doi: 10.1016/j.anireprosci.2022.107158
103. Gao J, Xu Z, Song W, Huang J, Liu W, He Z, et al. USP11 regulates proliferation and apoptosis of human spermatogonial stem cells via HOXC5-mediated canonical WNT/β-catenin signaling pathway. Cell Mol Life sciences: CMLS. (2024) 81:211. doi: 10.1007/s00018-024-05248-6
104. Du L, Chen W, Li C, Cui Y, He Z. RNF144B stimulates the proliferation and inhibits the apoptosis of human spermatogonial stem cells via the FCER2/NOTCH2/HES1 pathway and its abnormality is associated with azoospermia. J Cell Physiol. (2022) 237:3565–77. doi: 10.1002/jcp.v237.9
105. Wan C, Chen W, Cui Y, He Z. MAP4K4/JNK signaling pathway stimulates proliferation and suppresses apoptosis of human spermatogonial stem cells and lower level of MAP4K4 is associated with male infertility. Cells. (2022) 11(23):3807. doi: 10.3390/cells11233807
106. Wan L, Wang YM. Impact of high altitude and hypoxia on sperm concentration. Zhonghua Nan Ke Xue. (2012) 18(9):835–9. (In Chinese)
107. Zepeda AB, Figueroa CA, Calaf GM, Farías JG. Male reproductive system and antioxidants in oxidative stress induced by hypobaric hypoxia. Andrologia. (2014) 46:1–8. doi: 10.1111/and.2014.46.issue-1
108. Lord T, Oatley JM. Spermatogenic stem cells: core biology, defining features, and utilities. Mol Reprod Dev. (2024) 91:e23777. doi: 10.1002/mrd.v91.10
109. Yoshida S. Elucidating the identity and behavior of spermatogenic stem cells in the mouse testis. Reprod (Cambridge England). (2012) 144:293–302. doi: 10.1530/REP-11-0320
110. Shukla KK, Mahdi AA, Rajender S. Apoptosis, spermatogenesis and male infertility. Front bioscience (Elite edition). (2012) 4(2):746–54. doi: 10.2741/415
111. Jankovic Velickovic L, Stefanovic V. Hypoxia and spermatogenesis. Int Urol Nephrol. (2014) 46:887–94. doi: 10.1007/s11255-013-0601-1
112. Wang P, Liu H, Zhao S, Yu S, Xie S, Hua S, et al. Hypoxia stress affects the physiological responses, apoptosis and innate immunity of Kuruma shrimp, Marsupenaeus japonicus. Fish shellfish Immunol. (2022) 122:206–14. doi: 10.1016/j.fsi.2022.02.016
113. Ghosh S, Mukherjee S. Testicular germ cell apoptosis and sperm defects in mice upon long-term high fat diet feeding. J Cell Physiol. (2018) 233:6896–909. doi: 10.1002/jcp.v233.10
114. Grunewald S, Fitzl G, Springsguth C. Induction of ultra-morphological features of apoptosis in mature and immature sperm. Asian J andrology. (2017) 19:533–7. doi: 10.4103/1008-682X.180974
115. Zhang T, Xu D, Liu J, Wang M, Duan LJ, Liu M, et al. Prolonged hypoxia alleviates prolyl hydroxylation-mediated suppression of RIPK1 to promote necroptosis and inflammation. Nat Cell Biol. (2023) 25:950–62. doi: 10.1038/s41556-023-01170-4
116. Özdemir A, İbişoğlu B, Şimay Demir YD, Benhür E, Valipour F, Ark M. A novel proteolytic cleavage of ROCK 1 in cell death: Not only by caspases 3 and 7 but also by caspase 2. Biochem Biophys Res Commun. (2021) 547:118–24. doi: 10.1016/j.bbrc.2021.02.024
117. Samraj AK, Sohn D, Schulze-Osthoff K, Schmitz I. Loss of caspase-9 reveals its essential role for caspase-2 activation and mitochondrial membrane depolarization. Mol Biol Cell. (2007) 18:84–93. doi: 10.1091/mbc.e06-04-0263
118. Lei B, Zhou X, Lv D, Wan B, Wu H, Zhong L, et al. Apoptotic and nonapoptotic function of caspase 7 in spermatogenesis. Asian J andrology. (2017) 19:47–51. doi: 10.4103/1008-682X.169563
119. Wang P, Luo C, Li Q, Chen S, Hu Y. Mitochondrion-mediated apoptosis is involved in reproductive damage caused by BPA in male rats. Environ Toxicol Pharmacol. (2014) 38:1025–33. doi: 10.1016/j.etap.2014.10.018
120. Liu S, Yao S, Yang H, Liu S, Wang Y. Autophagy: Regulator of cell death. Cell Death Dis. (2023) 14:648. doi: 10.1038/s41419-023-06154-8
121. Parzych KR, Klionsky DJ. An overview of autophagy: morphology, mechanism, and regulation. Antioxidants Redox Signaling. (2014) 20:460–73. doi: 10.1089/ars.2013.5371
122. Zhao Z, Hou B, Tang L, Wang Y, Zhang Y, Ying Z, et al. High-altitude hypoxia-induced rat alveolar cell injury by increasing autophagy. Int J Exp Pathol. (2022) 103:132–9. doi: 10.1111/iep.v103.4
123. Hu Y, Sun Q, Li Z, Chen J, Shen C, Song Y, et al. High basal level of autophagy in high-altitude residents attenuates myocardial ischemia-reperfusion injury. J thoracic Cardiovasc Surg. (2014) 148:1674–80. doi: 10.1016/j.jtcvs.2014.03.038
124. Sorice M. Crosstalk of autophagy and apoptosis. Cells. (2022) 11(9):1479. doi: 10.3390/cells11091479
125. Kim J, Kundu M, Viollet B, Guan KL. AMPK and mTOR regulate autophagy through direct phosphorylation of Ulk1. Nat Cell Biol. (2011) 13:132–41. doi: 10.1038/ncb2152
126. Chun Y, Kim J. AMPK-mTOR signaling and cellular adaptations in hypoxia. Int J Mol Sci. (2021) 22(18):9765. doi: 10.3390/ijms22189765
127. Ma L, Zhang R, Li D, Qiao T, Guo X. Fluoride regulates chondrocyte proliferation and autophagy via PI3K/AKT/mTOR signaling pathway. Chemico-biological Interact. (2021) 349:109659. doi: 10.1016/j.cbi.2021.109659
128. Wang Y, Liu Z, Shu S, Cai J, Tang C, Dong Z. AMPK/mTOR signaling in autophagy regulation during cisplatin-induced acute kidney injury. Front Physiol. (2020) 11:619730. doi: 10.3389/fphys.2020.619730
129. Cosin-Roger J, Simmen S, Melhem H, Atrott K, Frey-Wagner I, Hausmann M, et al. Hypoxia ameliorates intestinal inflammation through NLRP3/mTOR downregulation and autophagy activation. Nat Commun. (2017) 8:98. doi: 10.1038/s41467-017-00213-3
130. Wang Y, Zhang H. Regulation of autophagy by mTOR signaling pathway. Adv Exp Med Biol. (2019) 1206:67–83. doi: 10.1007/978-981-15-0602-4_3
131. Khedr NF, Werida RH. l-carnitine modulates autophagy, oxidative stress and inflammation in trazodone induced testicular toxicity. Life Sci. (2022) 290:120025. doi: 10.1016/j.lfs.2021.120025
132. Yin J, Ni B, Tian ZQ, Yang F, Liao WG, Gao YQ. Regulatory effects of autophagy on spermatogenesis. Biol Reprod. (2017) 96:525–30. doi: 10.1095/biolreprod.116.144063
133. Zhang X, Chen X, Wang A, Wang L, He C, Shi Z, et al. Yiqi Jiedu decoction attenuates radiation injury of spermatogenic cells via suppressing IκBα/NF-κB pathway-induced excessive autophagy and apoptosis. J ethnopharmacology. (2024) 318:116903. doi: 10.1016/j.jep.2023.116903
134. Zhang YW, Liu J, Ma KH, Tan Y. Effects of autophagy on spermatogenesis and sperm motility. Zhonghua Nan Ke Xue. (2019) 25(1):72–6. (In Chinese)
135. Mu Y, Yan WJ, Yin TL, Zhang Y, Li J, Yang J. Diet-induced obesity impairs spermatogenesis: a potential role for autophagy. Sci Rep. (2017) 7:43475. doi: 10.1038/srep43475
136. Kma L, Baruah TJ. The interplay of ROS and the PI3K/Akt pathway in autophagy regulation. Biotechnol Appl Biochem. (2022) 69:248–64. doi: 10.1002/bab.v69.1
137. Ba L, Gao J, Chen Y, Qi H, Dong C, Pan H, et al. Allicin attenuates pathological cardiac hypertrophy by inhibiting autophagy via activation of PI3K/Akt/mTOR and MAPK/ERK/mTOR signaling pathways. Phytomedicine: Int J phytotherapy phytopharmacology. (2019) 58:152765. doi: 10.1016/j.phymed.2018.11.025
138. Pu Z, Wu L, Guo Y, Li G, Xiang M, Liu L, et al. LncRNA MEG3 contributes to adenosine-induced cytotoxicity in hepatoma HepG2 cells by downregulated ILF3 and autophagy inhibition via regulation PI3K-AKT-mTOR and beclin-1 signaling pathway. J Cell Biochem. (2019) 120:18172–85. doi: 10.1002/jcb.v120.10
Keywords: high-altitude environment, male reproductive system, reproductive hormones, testicular tissue, sperm quality
Citation: Meng D-D, Kang Y-D and Chang D-H (2025) Research progress on the adverse effects of high-altitude environment to the male reproductive system: a review study. Front. Endocrinol. 16:1573502. doi: 10.3389/fendo.2025.1573502
Received: 11 February 2025; Accepted: 22 April 2025;
Published: 14 May 2025.
Edited by:
Hanan H. Abd-ElHafeez, Assiut University, EgyptReviewed by:
Alaa Sayed Abou-Elhamd, Faculty of Veterinary Medicine, EgyptMohamed Abdel Maksoud, Beni-Suef University, Egypt
E. Ahmed Eshrah, Benha University, Egypt
Copyright © 2025 Meng, Kang and Chang. This is an open-access article distributed under the terms of the Creative Commons Attribution License (CC BY). The use, distribution or reproduction in other forums is permitted, provided the original author(s) and the copyright owner(s) are credited and that the original publication in this journal is cited, in accordance with accepted academic practice. No use, distribution or reproduction is permitted which does not comply with these terms.
*Correspondence: Yin-Dong Kang, bWFnaWNib3VsZXZhcmRra0AxNjMuY29t; De-Hui Chang, Y2hkaHVpQDE2My5jb20=