- Conway Institute, School of Medicine, University College Dublin, Dublin, Ireland
Redox medicine is a new therapeutic concept targeting reactive oxygen species (ROS) and secondary reaction products for health benefit. The concomitant function of ROS as intracellular second messengers and extracellular mediators governing physiological redox signaling, and as damaging radicals instigating or perpetuating various pathophysiological conditions will require selective strategies for therapeutic intervention. In addition, the reactivity and quantity of the oxidant species generated, its source and cellular location in a defined disease context need to be considered to achieve the desired outcome. In inflammatory diseases associated with oxidative damage and tissue injury, ROS source specific inhibitors may provide more benefit than generalized removal of ROS. Contemporary approaches in immunity will also include the preservation or even elevation of certain oxygen metabolites to restore or improve ROS driven physiological functions including more effective redox signaling and cell-microenvironment communication, and to induce mucosal barrier integrity, eubiosis and repair processes. Increasing oxidants by host-directed immunomodulation or by exogenous supplementation seems especially promising for improving host defense. Here, we summarize examples of beneficial ROS in immune homeostasis, infection, and acute inflammatory disease, and address emerging therapeutic strategies for ROS augmentation to induce and strengthen protective host immunity.
Introduction
Reactive oxygen species (ROS) is a generic term referring to oxygen-derived compounds capable of reacting with biological molecules through an oxidation-reduction (“redox”) mechanism. ROS include a set of highly reactive radicals (e.g., superoxide anion, hydroxyl radical) as well as non-radical species [e.g., hydrogen peroxide (H2O2)] produced enzymatically or chemically in eukaryotic and prokaryotic cells. In mammals, one of the main intracellular sources of ROS is the mitochondrial electron transport chain (ETC) during the establishment of the proton-motive force. Premature electron leakage to molecular oxygen occurs mainly from complex I and III of the ETC during the serial transfer of electrons, causing superoxide formation that is converted to H2O2 by superoxide dismutase (SOD) (1, 2). In contrast to ROS generation as by-product of aerobic metabolism, of oxidation of fatty acids or proteins, or of enzyme-substrate reactions (e.g., xanthine oxidase, lipoxygenase, cyclooxygenase, monoamine oxidase), the NADPH oxidase family (NOX/DUOX) is solely dedicated to ROS production and catalyzes the reduction of molecular oxygen to superoxide or H2O2. Seven oxidase isoforms have been characterized in humans, differing in their catalytic core (NOX1-5 and DUOX1-2), their requirement for additional components for complex stabilization and/or enzyme activation, their subcellular localization and in tissue specificity (3, 4). The NADPH oxidase prototype is the NOX2 enzyme, an essential superoxide source for pathogen defense by neutrophils and macrophages. The rapid increase of superoxide (‘respiratory burst’) is accompanied by the formation of dismutation and adduct products, including hypochlorite (generated by myeloperoxidase, H2O2 and chloride ion) and peroxynitrite (spontaneous reaction of superoxide with nitric oxide), both strongly oxidizing and nitrating compounds that drive pathogen killing in concert with activated proteases (5). Tight regulation of ROS generation is essential, as ample and uncontrolled production of highly reactive species over an extended time period will cause irreversible redox modifications on biomolecules, thus promoting oxidative damage.
ROS is not only produced during host defense or in proinflammatory situations. Superoxide and H2O2 are continuously generated, converted, and degraded in physiological conditions (6, 7). H2O2 as a key intracellular messenger mediating signal transduction in all cell types maintains homeostasis and physiological host responses (8, 9). H2O2-initiated redox signaling is essential for basic cellular processes (e.g., proliferation, migration, secretion), thereby supporting complex functions in mucosal immunity (e.g., barrier integrity, host-microbiota communication, host defense, chemokine/cytokine generation, wound repair), driving innate immune functions and regulating adaptive immunity. By aquaporin-facilitated diffusion across the plasma membrane or by release from intracellular organelles (e.g., redoxosomes, microvesicles, mitochondria), H2O2 mediates directly or more commonly via redox relays the reversible oxidation of distinct amino acids, modifying the structure and function of targeted proteins and consequently the activation state of the associated signaling pathways. In mammals, thiol oxidation of certain reactive cysteine residues occurs within redox-sensitive proteins, including phosphatases and tyrosine kinases, leading to activation or inactivation of the targeted protein (2, 10). Redox signaling also drives the antioxidant response to prevent excessive ROS production. A notable conduit for transcriptional activation of antioxidant genes is the KEAP1-NRF2 pathway. H2O2-mediated oxidation of the redox-sensitive adaptor KEAP1 disrupts its cytoplasmic complex with NRF2, promoting nuclear translocation of newly synthesized NRF2 which will bind to antioxidant response elements (ARE) located in the promoter region of NRF2 target genes such as NAD(P)H quinone oxidoreductase 1 (NQO1), heme oxygenase 1 (HMOX1), glutamate-cysteine ligase (GCL) and glutathione S transferases (GSTs) (11, 12).
Coupled oxidant-antioxidant pathways have been conserved throughout evolution. For example, in bacteria dedicated transcriptional regulators (e.g., OxyR, OhrR, PerR, SoxR) sense ROS/RNS in order to induce an appropriate detoxification response (e.g., radical scavenging and metal sequestrating systems), and to maintain intracellular oxidant levels within a safe limit (13, 14). The H2O2 sensing transcriptional regulator OxyR is a well-studied example in mainly Gram-negative bacteria. When intracellular H2O2 exceeds a certain threshold in Escherichia coli [~ 0.1-0.2 µM (15, 16)], oxidation of OxyR on two cysteine residues prompts a conformational change due to intramolecular disulfide bond formation, activating the expression of OxyR-dependent antioxidant defense genes (17, 18). At mucosal surfaces physiological H2O2 levels mediate the intricate redox communication between commensal microorganisms and the host barrier tissue. For instance, bacteria can induce or hinder physiological host signaling, either by releasing H2O2 in the vicinity of host epithelia (e.g., lactobacilli) or by altering host enzyme-mediated ROS generation via secreted compounds or physical interaction with epithelial barrier cells (19–21). Likewise, H2O2 released by host cells can alter bacterial signaling in response to a pathogenic insult, particularly in low oxygen environments (22, 23).
It is important to distinguish these beneficial redox-mediated mechanisms supporting health from conditions promoting supraphysiological level of ROS and the resulting oxidative damage. To ensure physiological conditions, the activation of NADPH oxidases is usually regulated by multiple inputs (e.g., transcriptional, posttranslational, metal ions, nucleotides) and ROS generation is coupled with decomposing and scavenging systems (e.g., catalase, peroxidases, antioxidants) (2, 4, 21, 24). Ongoing perturbation of ROS levels, either at high or low range, has been associated with various pathophysiological states. Persistent increase of highly reactive species (e.g., superoxide anion, hydroxyl radical, peroxynitrite) has been associated with chronic inflammatory and hyperglycemic (e.g., rheumatoid arthritis, inflammatory bowel diseases, type 2 diabetes), tumorigenic and neurological (e.g., Parkinson’s, Alzheimer’s) diseases. Therapeutically decreasing ROS levels by non-specific enzyme inhibitors or antioxidants has been pursued for many decades (25, 26). However, the ill-defined and generalized action of these molecules limits their efficacy and, in some cases, exacerbated the underlying condition (27–32). More targeted approaches, namely inhibition of a specific ROS source, may provide more benefit. Conversely, permanently decreased ROS generation due to loss-of-function variants in genes encoding for NADPH oxidases or other ROS sources, or in genes providing an essential upstream trigger for a superoxide/H2O2 generating enzyme, have been linked to various pathologies, including recurrent microbial infections, chronic inflammatory diseases, and autoimmunity (33). In this case, a strategy to restore or enhance ROS may prove beneficial.
Understanding redox regulation of vital physiological processes and how alterations initiate or perpetuate disease is important for developing new therapeutic avenues. In addition to traditional therapeutic strategies aimed at counteracting excessive ROS, contemporary approaches should include preservation or even the elevation of certain oxygen metabolites for physiological purposes. This review will provide selected examples of beneficial ROS in protective host immunity and will discuss emerging therapeutic strategies to preserve or augment ROS with the aim of re-establishing homeostasis and promoting host protection.
Importance of Oxidants in Protective Immunity
Homeostatic Redox Signaling
Physiological ROS levels modulate many immune functions by redox-sensitive signaling. Not only H2O2, but also related second messengers such as nitric oxide and possibly peroxynitrite control signaling responses and thus these short-lived molecules represent an integral part of immune regulation (34–37). All redox-regulated pathways show analogous features, namely inhibitory or activating modifications on proteins and lipids (10, 38, 39), and are conserved across different cell types and organisms. Redox modifications can constrain or stimulate signaling pathways. An example for inhibitory oxidative modifications is dual specificity phosphatase 1 (DUSP1) which will be degraded after oxidation or S-glutathionylation of an active site cysteine, resulting in prolonged MAPK-induced proinflammatory gene transcription (40). Reversible oxidative inactivation of protein tyrosine phosphatase 1B (PTP1B) amplified interleukin (IL)-4 receptor activation (41). On the other hand, redox-dependent oxidation of two cysteine residues in the tyrosine kinase SRC caused structural changes that impacted regulatory tyrosines, enabling SRC kinase activation (42). In airway epithelial cells, epidermal growth factor receptor signaling was linked to activation of the NADPH oxidase DUOX1 and subsequent SRC oxidation (43). Kinase activation can also occur by oxidation-driven dissociation of an inhibitor-protein kinase complex as demonstrated for the complex between thioredoxin and apoptosis signal-regulating kinase 1 (ASK1) (44). The liberated form of ASK1 will then form multimeric complexes with other signaling mediators and positively regulate JNK/p38 MAPK pathways, while itself being further controlled by dephosphorylation and deubiquitination (45). More examples and details on redox regulation of signaling pathways can be found in recent reviews (1, 46).
The initiation of redox signaling occurs mainly after ligand stimulation of receptors (e.g., Toll-like receptors (TLR), G protein-coupled receptors (GPCR), NOD-like receptors (NLR), Fc receptors, chemokine and cytokine receptors, and others) and may include one or several ROS sources concomitantly or consecutively, depending on the context. For instance, redox regulation triggered by TGF-β involves the NADPH oxidase NOX4 as well as mitochondrial ROS (mROS) (47). NADPH oxidases seem to be closely associated with TLR-stimulated pathways where oxidase deficiency either inhibits or enhances host cell responses. Loss of NOX1 in smooth muscle cells increased TLR2-mediated MIP-2 generation but impeded matrix metalloprotease 2 secretion and directed cell migration (48). In epithelial cells NOX4 was reported to associate with TLR4, regulating the transcription factor NF-κB (49). Loss-of-function mutations in any of the five subunits comprising the NOX2 complex cause the inherited immunodeficiency chronic granulomatous disease (CGD) (33, 50). One of the effects of CGD in the immune system is the altered responsiveness to TLR ligands, resulting in augmented cytokine generation and hyperinflammation. Neutrophils, monocytes, and dendritic cells isolated from CGD patients or Nox2 deficient mice generated increased levels of cytokines upon TLR2 or TLR4 stimulation (51–54). A hyperinflammatory phenotype with excessive secretion of pro- and anti-inflammatory cytokines was also observed in CGD B lymphocytes stimulated with TLR7 and TLR9 agonists (55). This cytokine overproduction was associated with hyperactivation of p38 MAPK signaling, which may indicate loss of inhibitory feedback signaling, likely involving phosphatases, when the NOX2 enzyme is inactivated. Increased levels of IFNγ and IL-18 in CGD patient tissues was in part traced to macrophages remaining in a proinflammatory M1 state (56). Innate immune cells derived from CGD patients or mice also showed an exuberant IL-1 response to various inflammasome activating stimuli, while the NRF2-controled antioxidant response was dampened (57–59). The hyperinflammatory CGD phenotype in mice and man is intensified by increased neutrophil recruitment to sites of infection and tissue damage, which was recently linked to feed-forward amplification of neutrophil generated leukotriene B4 upon pulmonary zymosan challenge (60). Hence, deficiency in NOX2 enzyme activity creates a proinflammatory environment that is associated with several chronic inflammatory conditions and can predispose to autoimmune disease (61). The molecular mechanisms underlying various facets of CGD hyperinflammation are still not resolved, but NOX2-derived superoxide seems to be required for the precise regulation of many immune cell functions including gene transcription, autophagy, efferocytosis, and dendritic cell-mediated antigen presentation (61–64).
In recent years, the role of mROS signaling in regulating both innate and adaptive immunity received increased attention (65, 66), although in many cases molecular mechanisms are not well characterized and mROS participation in signaling is mainly inferred by using mitochondria-targeted ROS probes and/or antioxidants. Chandel and coworkers linked early on a TNF receptor-TRAF pathway to an increase in mROS (67), and by now many different stimuli are recognized as triggers of physiological mROS generation (6). Here we summarize recent insights into mROS-mediated immune cell responses. Signaling cascades driven by TLRs stimulate not only NADPH oxidase activity, but also via TRAF6 the mitochondrial matrix protein ECSIT that stimulates superoxide production from ETC complex I (68). Others reported recently that pro-inflammatory cytokine secretion by Listeria monocytogenes-infected macrophages is contingent on mROS-induced disulfide bond formation in the IKK complex regulator NEMO (69). Activation of the NLRP3 inflammasome by pathogen-associated or danger-associated molecular patterns (PAMPS/DAMPS) is controlled by mROS, resulting in release of the proinflammatory cytokines IL-1β and IL-18 (70, 71). The precise sequence of events that may include a transcriptional priming step, oxidized mitochondrial DNA, and mROS-dependent interaction of NLRP3 with thioredoxin-interacting protein is not yet fully established (72). In macrophages NLRP3 inflammasome activation required xanthine oxidase generated superoxide upstream of mROS generation (73), while studies in superoxide deficient peripheral blood mononuclear cells derived from CGD patients revealed that NOX enzymes are not required for NLRP3-mediated IL-1β secretion (74, 75). Cytosolic retinoic acid-inducible gene I (RIG-I)-like receptors such as RIG-I and MDA5 sense and bind distinct features of viral RNA, then oligomerize, and associate with the adaptor protein MAVS at outer mitochondrial membranes and mitochondria-associated membranes. Oligomerization of MAVS recruits several effector proteins to form the MAVS signalosome, driving IRF3/IRF7-mediated transcription of type I/III interferon and NF-κB-induced proinflammatory cytokine expression. MAVS expression has been linked to NOX2 generated superoxide (76), while others reported functional interactions involving MAVS aggregation, mROS generation, and the cytochrome c oxidase component COX5B that couple the antiviral response to autophagy (77). The NLR family member NLRX1 cannot only interact with MAVS at the outer mitochondrial membrane but is also localized within the matrix and inner membrane of mitochondria where interaction with the ETC complex III associated protein UQCRC2 promotes mROS generation, thereby stimulating transcription factors and JNK MAP kinase (78). The signaling function of the immune response-induced mitochondrial interactome is clearly connected to mROS generation, in physiological and pathophysiological conditions, and many more aspects of mitochondrial immune signaling and connections between different ROS sources influencing each other in these processes can be expected in the future.
Mucosal Barriers and Oxidants
The mucosal immune system in lung, gastrointestinal tract (GIT), urogenital tract, oral and nasal passages employs structural, chemical and immunological barriers to control the interaction with the adjacent microorganism- and noxious substance-rich environment. The importance of mucosal barriers as host defense mechanism is reflected in these multiple layers of protection together with the constant renewal of barrier epithelia, specialized protective measures such as secretion of mucins or surfactant, and highly efficient repair mechanisms. Superoxide and H2O2, generated by host enzymes or commensal bacteria, are crucial for various aspects of mucosal barrier maintenance as outlined here in selected examples.
The structural integrity of mucosal epithelial barriers relies on intercellular junctions, in particular tight junctions, and after a breach occurred, on rapid proliferation and migration of epithelial cells for wound repair. ROS regulate cytoskeletal dynamics such as oxidative modification of β-actin and tubulin, RhoA GTPase activation and deactivation depending on the context, redox-induced integrin and focal adhesion kinase (FAK) activation and oxidative modifications on other proteins involved in cytoskeletal rearrangements (79, 80). As many of these physiological processes have not yet been conclusively linked to a particular ROS source or studied in epithelial barriers, we will present here only results that identified the enzyme involved, were obtained by multiple approaches and if possible in vivo. In the GIT, superoxide produced by NOX1 controls colon epithelial cell proliferation and migration, in part by enhancing FAK phosphorylation due to oxidative inactivation of the tyrosine phosphatases LMW-PTP and SHP-2 that will initiate focal adhesion turnover, accelerate cell migration, and improve wound closure (81–85). Others reported NOX1-mediated oxidation of nucleoredoxin that released suppression of the canonical WNT-β-catenin pathway, resulting in transcription of growth promoting genes (86, 87). In airway epithelial cells DUOX was localized at the leading edge of migrating cells, augmenting wound healing (88). DUOX1 generated H2O2 regulated cell migration and epithelial repair in cells and in a naphthalene airway injury model by promoting epidermal growth factor receptor (EGFR)-STAT3 signaling (89, 90).
An important part of the physical gut barrier is the protective mucus layer, either single-layered in the small intestine and pulmonary tract, or 2-layered with a dense, impenetrable layer followed by a loose layer in the colon. Mucus is secreted and continuously renewed by goblet cells and is composed of mainly MUC2 mucin in the intestine, MUC1, MUC5AC and MUC6 mucins in the stomach, and MUC5AC and MUC5B mucins in the lung epithelium (91). NADPH oxidase generated H2O2 has been linked to intestinal mucin granule release by pathways involving endocytosis, autophagy, and NLRP6 signaling (92, 93). A severe colonic phenotype with partial loss of the dense mucus layer was only observed in mice with combined inactivation of Nox1-3 but not in single oxidase knockout mice (94), likely due to in vivo compensation by another oxidase (or ROS source) or by H2O2 producing lactobacilli colonizing the mucus layer. In the airways EGFR and IL-13 signaling promoted mucin gene induction (MUC5AC, CLCA1) via DUOX1 (95, 96), while others reported a TLR5-DUOX2 pathway regulating MUC5AC expression in nasal epithelium (97).
The structural reinforcement of the intestinal barrier is accompanied by chemical and immunological secretions. Immunological secretions are immunoglobulin A (sIgA) and various other antimicrobial factors including lysozyme, regenerating islet-derived proteins 3, and cationic peptides such as cathelicidins or defensins. Cationic peptides range from α-helical (e.g., LL-37) to β-sheet (e.g., β-defensins) conformation or random-coil structures and exert their activity by bacterial membrane disruption and immunomodulatory effects on host cells (98, 99). While their synthesis or secretion has not been linked to a ROS source, the proper folding of some bioactive cysteine-rich peptides such as β-defensin 3 require the oxidative formation of several disulfide bridges (100). Lipocalin-2 (LCN-2), secreted by intestinal epithelial cells or released from neutrophil secondary granules, is often used as a biomarker of inflammatory processes, but also limits bacterial growth by binding iron-loaded siderophores (101, 102). A recent report connected LCN-2 expression via atypical IκBζ-dependent gene transcription to NOX1 activity in colonic epithelial cells and mice (103).
Mucosal barriers generate and release chemical compounds such as superoxide, H2O2, nitric oxide and peroxynitrite when pathogens or danger-associated molecules trigger host cell responses. These chemicals may also be released constitutively at low concentrations as repellent against commensal communities, promoting a mutualistic host-microbiota relationship essential for immunity (21, 104). This is supported by reports that enteric bacteria regulate NADPH oxidase activity by relatively undefined pathways (85, 105, 106), and vice versa that ROS generated by mitochondria or epithelial cells control the microbial population, thereby limiting the access of the microbiota to the immune compartment (19, 107). This scenario likely takes place in the small intestine with its loose, single mucus layer and may involve the oxidase DUOX2. DUOX2 expression is upregulated by microbiota, and its localization at the apical surface of villi is ideally suited for H2O2 release, thereby reinforcing the separation of host and microbial communities (19). Additionally, peroxynitrite generated by the combined activation of NOX1 and NOS2 may participate in the control of ileal homeostasis (108, 109). Similarly, H2O2 release by DUOX1/DUOX2 and subsequent lactoperoxidase-mediated conversion to secondary oxidants (i.e., HOSCN) may repel bacteria in the airways (110). Influenza A virus (IAV) infection stimulated H2O2 release from air-liquid interface cultured primary human airway epithelial cells in a calcium/flavoenzyme dependent manner, suggesting DUOX activation (111).
Chemical messengers are ideally suited to relay signals from the host to the microbiota and vice versa. This interkingdom communication will shape microbiota diversity and composition and provides a stimulus for homeostatic barrier host responses. While aberrant ROS production in inflammatory disease will increase the abundance of certain bacterial communities and may lower overall diversity, decreased levels or loss of NADPH oxidase-generated ROS, as observed in certain patient populations, will also favor dysbiosis, indicating that a tightly balanced mucosa-associated microenvironment is indispensable for gut homeostasis. While CGD is mainly associated with recurrent life-threatening infections, 40-50% of these patients will develop inflammatory bowel disease with some features of Crohn’s disease. When comparing the microbiota of CGD patients to healthy individuals an increased abundance of mucus foraging Ruminococcus gnavus was noted (112). This study included only a limited number of patients (10 individuals) and might be skewed due to the required antibiotic maintenance of these patients. In a CGD mouse model (i.e., p47phox deficiency) the microbiome signature was altered by high abundance of Akkermansia muciniphila (113). This mucolytic bacterium is considered a beneficial probiotic, but can also disturb mucus homeostasis in the host, thereby acting as a pathobiont or aggravating inflammation induced by intestinal pathogens (114–116). In the ileum of Nox1 knockout mice increased abundance of Bifidobacteria and Turicibacter was detected (117). Global inactivation of the oxidases Nox1-3 in mice (Cybanmf333) resulted in increased abundance of Proteobacteria, Ruminococcaceae and Mucispirillum schaedleri (94). An increase of M. schaedleri was also noted in mice with combined Nod2/Nox2 deficiency (118). This mucus dwelling bacterium interfered with Salmonella enterica serovar Typhimurium virulence factor expression and decreased pathogen invasion in wildtype mice (119), but it also promoted inflammation in Nod2/Nox2 double knockout mice. Inactivation of all four murine Nox enzymes by global or intestinal epithelium restricted deletion of the essential Nox partner protein p22phox (Cyba-/-, CybaVil-cre) induced compensatory upregulation of H2O2 producing lactobacilli as a host protective, mutualistically beneficial mechanism (120). These results in mice suggest that H2O2 generation, mucus changes and overgrowth of mucus-dwelling or mucolytic bacteria are closely linked. Thus, ROS maintain and protect the stable microenvironment required for balanced and interconnected host-commensal cooperation and homeostatic barrier function.
Specialized Roles of Oxidants in Innate Immune Cells
Macrophage Polarization
The versatile role of ROS in regulating the intracellular signalosome in a constantly evolving microenvironment underpins their role in promoting polarization of immune cell populations and supporting specialized functions. A pertinent example is altering the polarization and activation state of macrophages in the broad categories of M1 (classical) and M2 (alternative), categorized by a proinflammatory, host defense connected phenotype versus an anti-inflammatory, tissue remodeling phenotype (121). NOX2 is expressed in M1 and M2 macrophages and regulates the transition between phenotypes in a context dependent manner. Nox2 deficient bone marrow derived macrophages (BMDM) upregulated STAT3 activation and anti-inflammatory cytokine expression (i.e., IL-10), while others reported no differences between wildtype and Nox2 knockout M2 macrophages (122, 123). Conflicting results in the literature stem likely from the range of protocols used for macrophage differentiation (e.g., conditioned media versus recombinant GM-CSF or M-CSF for various time periods, LPS content of fetal bovine serum) and the inclusion or omission of further Th1 or Th2 type polarization. M1 polarized BMDM express not only Nox2 but also Nox1 (124). When M-CSF differentiated BMDM or peritoneal macrophages derived from wildtype, Nox1, Nox2 or Nox1/Nox2 knockout mice were further polarized by LPS+IFNγ or IL-4+IL-10, only Nox1/Nox2 deficient macrophages exhibited reduced M2-type polarization while M1 polarization and pro-inflammatory functions were preserved (123). Expression of Nox4 in macrophages and its downstream effects seem to be determined by the microenvironment. Using a similar M1/M2 polarization protocol as described above Helfinger and coworkers observed in Nox4 deficient mouse macrophages amplification of the M1 phenotype, suggesting an anti-inflammatory role for Nox4 (125). On the other hand, NOX4 expression was induced in human monocyte-derived macrophages by low-density lipoprotein (OxLDL) that stimulates a pro-inflammatory response (126), and in profibrotic M2 alveolar macrophages (127). A mechanistically poorly defined link between NOX4 and mROS has been proposed (i.e., ROS-induced ROS) that could play a role in conferring the divergent metabolic signatures observed in M1 versus M2 macrophage polarization (127–131). H2O2-mediated intercellular communication between various innate immune cells can also drive macrophage skewing. In vivo phenotypic conversion of pro-inflammatory to pro-resolving macrophages was dependent on neutrophil Nox2 and H2O2 generation, further confirming that activated neutrophils contribute to resolution of inflammation (132–135). While less characterized, neutrophils can, analogous to macrophages, polarize towards distinct phenotypes. N1 and N2 neutrophil populations are mainly defined by their functional phenotype with N1 considered pro-inflammatory and anti-tumorigenic, and N2 pro-tumorigenic. A role for ROS in this phenotypic conversion has not yet been defined.
Dendritic Cell Function
Dendritic (DC) cells are antigen-presenting cells that are essential for inducing naïve T cell activation and effector differentiation. After taking up antigens and microbes by phagocytosis or endocytosis DCs generate MHC peptide complexes. For activation of CD4 + T helper cells, antigens are degraded in lysosomes for MHC class II presentation, while activation of CD8 + cytotoxic T cells requires that antigens are presented in MHC class I by cross-presentation that occurs via lysosomal and cytosolic pathways. Interaction of mature DCs with T cells takes place in secondary lymphoid organs such as lymph nodes, spleen, and Peyer’s patches, initiating adaptive immune responses (136). Superoxide production by NOX2 is an intricate part of antigen processing, generation of MHC-peptide complexes and cross-presentation. Work by Amigorena and coworkers revealed that active NOX2 regulates the phagosomal pH in DCs by sustained superoxide generation, maintaining it close to the optimal pH 7.4 despite of proton import by V-ATPase. In DCs derived from CGD patients or CGD mice the phagosomal pH acidified and the cross-presentation of antigens was impaired (137, 138). In addition, NOX2 activity preserved antigens from proteolysis by inhibiting endosomal and lysosomal proteases via oxidation (139). Recent work connected NOX2 to antigen release from the endocytic lumen into the cytosol during cross-presentation. NOX2-derived superoxide caused lipid peroxidation, thereby disrupting membranes to enable antigen leakage from endosomes, a process that was impaired in DCs derived from CGD patients (140). Further study of a specialized subset of DCs, plasmacytoid DCs (pDCs), distinguished from conventional DCs by morphology and function, revealed that in this cell type antigen protection and cross-presentation is Nox1- and Nox2-independent. Here, mROS generated by pDCs after TLR7 stimulation promoted antigen cross-presentation and the capacity to trigger CD8+ T cells responses, as well as facilitating additional functions such as IFN-α production (141). Antigen presentation is also linked to macroautophagy that serves not only as mechanism for nutrient recycling and host defense against intracellular bacteria, but also for presenting MHC II molecules to CD4+ T cells. Recruitment of autophagy proteins to phagosomal membranes occurs when antigens are recognized by immune receptors such as TLRs, FcRs or dectin-1, resulting in LC3-associated phagocytosis (LAP) (142, 143). LAP requires NOX2-generated superoxide for recruitment and lipidation of the autophagy protein Atg8/LC3 to phagosomes, and fungal antigen storage in innate immune cells was compromised in CGD patients (144, 145). NOX2 activity was also involved in antimicrobial LAP in endothelial cells (146). Autophagy processes involved in cellular maintenance seem to be coupled to oxidase-derived ROS in many cell types including to NOX4 in cardiomyocytes and to a NOX family member in goblet cells (92, 94, 147), but putative ROS source(s) facilitating pathogen uptake due to LAP or other processes in epithelial cells, an important consideration for mucosal barrier host defense, have not yet been defined.
Host Defense and Oxidants
Respiratory and intestinal barrier epithelia are the first line of defense against airborne or foodborne pathogens. When the initial protective mechanisms (e.g., colonization resistance, antimicrobial secretions, mucus layer) have failed, epithelial sensing pathways will be activated. In the mucosal compartment, sensing of conserved microbial motifs by epithelial cells induces ROS production due to upregulation and/or activation of mucosal NADPH oxidases (e.g., DUOX1/2 in airways, NOX1/DUOX2 in GIT) that participate in the antimicrobial response (97, 110, 148–150). Transient or stable expression of the NOX1 or DUOX2 complex in several epithelial cell lines decreased pathogen attachment and invasion without affecting bacterial viability (151, 152). NOX1 or DUOX2 mediated H2O2 release interfered with the pathogenicity of bacteria by inducing irreversible oxidative modifications in bacterial enzymes and proteins essential for maintaining virulence factor synthesis (22, 23). Duox inactivation in mice, achieved by deleting the essential partner protein Duoxa, increased gastric colonization with Helicobacter felis, reflecting the protective effect of H2O2 in host defense (153). Infection of these mice with Salmonella Typhimurium augmented systemic dissemination of the pathogen, suggesting that Duox is an integral component of the protective mucosal barrier in the small intestine (19). In murine airways Duox silencing increased the Influenza A virus (IAV) load by interfering with viral replication, at least in part by affecting the nuclear splicing machinery and assembly of virions (111). Recent studies in DUOX2 knockdown primary human nasal epithelial cells and in Duox2 inactivated mutant mice linked this oxidase isoform further to IAV host defense (154). Nox1 has also been connected to host protection in IAV infection as Nox1 deficiency resulted in increased chemokine and proinflammatory cytokine generation, and extensive 3-nitrotyrosine modification of murine lung tissue (155). In IAV infected mouse macrophages Nox2-derived endosomal ROS caused suppression of TLR7-dependent antiviral cytokines, suggesting distinct roles of phagocyte Nox2 (i.e. detrimental) in contrast to a beneficial role of Nox1 during IAV infection (156).
Pathogen recognition by epithelial cells induces chemokine production, leading to the recruitment of neutrophils and macrophages to the site of injury. Innate immune cells engage in proteolytic and oxidative killing of pathogens, mainly mediated by secondary reactive species such as hypochlorous acid and peroxynitrite that rely on NOX2, mitochondria, myeloperoxidase and iNOS (157–159). Superoxide generated by NOX2 is essential for host protection against pathogens, as mutational inactivation of NOX2 complex subunits, a characteristic feature of CGD patients, profoundly impedes the ability to clear certain bacterial and fungal infections (160). Studies using murine CGD hosts have advanced our understanding of the mechanisms associated with host protection by Nox2 that seem to depend on the pathogen and the microenvironment encountered. For instance, intestinal colonization by Salmonella Typhimurium was increased in streptomycin pretreated Nox2 deficient mice (i.e., Cybb-/-) (161). Conversely, these mice were not more susceptible to infection with the murine pathogen Citrobacter rodentium (120). Superoxide produced by Nox2 played only a minor role in protection against Mycobacterium tuberculosis (Mtb) infection in contrast to reactive nitrogen species (RNS)-associated immunity, which seems preponderant in the control of murine lung infection with Mtb (162–165). Evasion of ROS-mediated killing (mROS and Nox2) was recently associated with Mtb virulence factor secretion and macrophage fatty acid catabolism, thereby improving mycobacterial survival in macrophages (166, 167).
These observations support the idea of a complex interaction between antimicrobial ROS, environmental factors, and virulence mechanisms employed by pathogens (e.g., detoxification enzymes), all impacting infection outcome and host protection (168). Redox-dependent mechanisms triggered by pathogen recognition in innate immune cells include the pathogen itself (e.g., size, type), and driving superoxide production in the appropriate subcellular compartment (e.g., phagosome, endosome, extracellular environment) by a specific source (e.g., NOX2, mitochondria) (169, 170). Upon pathogen uptake the phagosome is the main compartment where primary and secondary ROS accumulate in high concentrations. Assembly and activation of NOX2 at the phagosome membrane triggers the so-called ‘phagocyte oxidative burst’ that together with proteases supports killing of bacterial and fungal pathogens. In addition to phagosomal NOX2 activity, bactericidal ROS generation in the phagosome is maximized by mitochondria, with both ROS sources cooperating in pathogen killing (171). Mitochondria are recruited to the phagosome of infected macrophages, where they deliver mitochondria-derived effector molecules, including mROS in mitochondria-derived vesicles, to support intraphagosomal killing of pathogens (68, 172–174). Mitochondria mobility to the phagosome is promoted by the protein kinases Mst1/2. Upon phagocytosis, TLR-mediated signaling activates Mst1/2, triggering a TRAF6-Rac signaling axis involved in cytoskeletal rearrangements (172). The NOX2 complex localizes also to the plasma membrane, where it releases superoxide into the extracellular space in response to microbial patterns or large microorganisms that cannot be classically engulfed by phagocytic cells.
Neutrophils employ as additional host defense mechanism the release of nuclear chromatin, forming neutrophil extracellular traps (NETs). NETs act as defense mechanism against pathogens via entrapment and antimicrobial activity (175, 176). The sequence of events for NET formation is not completely defined (177), but it appears that NET release can be triggered in a ROS dependent or independent manner according to the pathogen or stimulus encountered (178–180). Neutrophils derived from CGD patients show an impairment in NET formation and ineffective control of Aspergillus species in the airways, leading to invasive aspergillosis. The importance of a functional NOX2 complex in restricting A. nidulans conidia and hyphae by NET formation was confirmed in a CGD patient undergoing gene therapy (181). The real-life situation of the severely compromised host immunity in CGD emphasizes the importance of ROS in host defense and serves as a reminder that certain disorders or disturbed mucosal environments may greatly benefit from improving oxidant production.
Improving Ros Levels as Therapeutic Intervention
In pathological conditions ROS have been linked to ‘oxidative stress’ for decades. Oxidative stress is often retrospectively inferred when observing oxidatively modified proteins, lipids and DNA, yet without definite identification of the ROS generating enzyme(s) involved or how the damaging sequence of events was initiated. Moreover, several disease-causing genetic variants and disease-associated genetic risk factors have shed light on the harmful consequences of insufficient ROS production for H2O2-induced oxidative modifications, redox signaling and host defense (e.g., CGD, hypothyroidism, inflammatory bowel disease) (33). To date most strategies targeting oxidative stress (i.e., antioxidant therapy) are based on the delivery of ROS scavengers, ROS converting or degrading compounds or on non-selective enzyme inhibitors to protect tissues and organs, but clinical results of antioxidant treatments in inflammatory diseases have so far been disappointing (182–184). More recently, treatments aimed at generating excessive concentrations of ROS or at inhibiting cellular antioxidant defense systems at a designated target location (i.e., prooxidant therapy) have been developed to promote cancer cell death or antimicrobial killing through oxidative damage (185, 186). In contrast, ROS/redox-modulating therapies take a different, nontraditional approach. The goal of this strategy is a middle path intended to restore physiological levels of primary ROS (superoxide, H2O2) or, in certain circumstances, to moderately augment beneficial oxidants. This approach will require innovations in pharmaceutical drug technology such as nanotechnology and smart materials (e.g., liposomes, hydrogels, nanocarriers) enabling controlled delivery of therapeutic agents (e.g., ROS producing or converting systems) to a target site due to utilization of stimulus-sensitive materials (187–190). In some settings ROS deficiencies may be partially compensated by pharmacological manipulation of endogenous oxidant producing enzymes. While still in their infancy science-based fine-tuning and balancing oxidant therapies are poised to deliver prophylactic and remedial benefits, namely improving homeostasis and host defense. This is particularly relevant for primary ROS with their essential role in redox signaling and dedicated immune functions. Here we summarize concepts for restoring physiological levels of beneficial oxygen-derived species in terms of technological approaches and therapeutic applications (Figure 1).
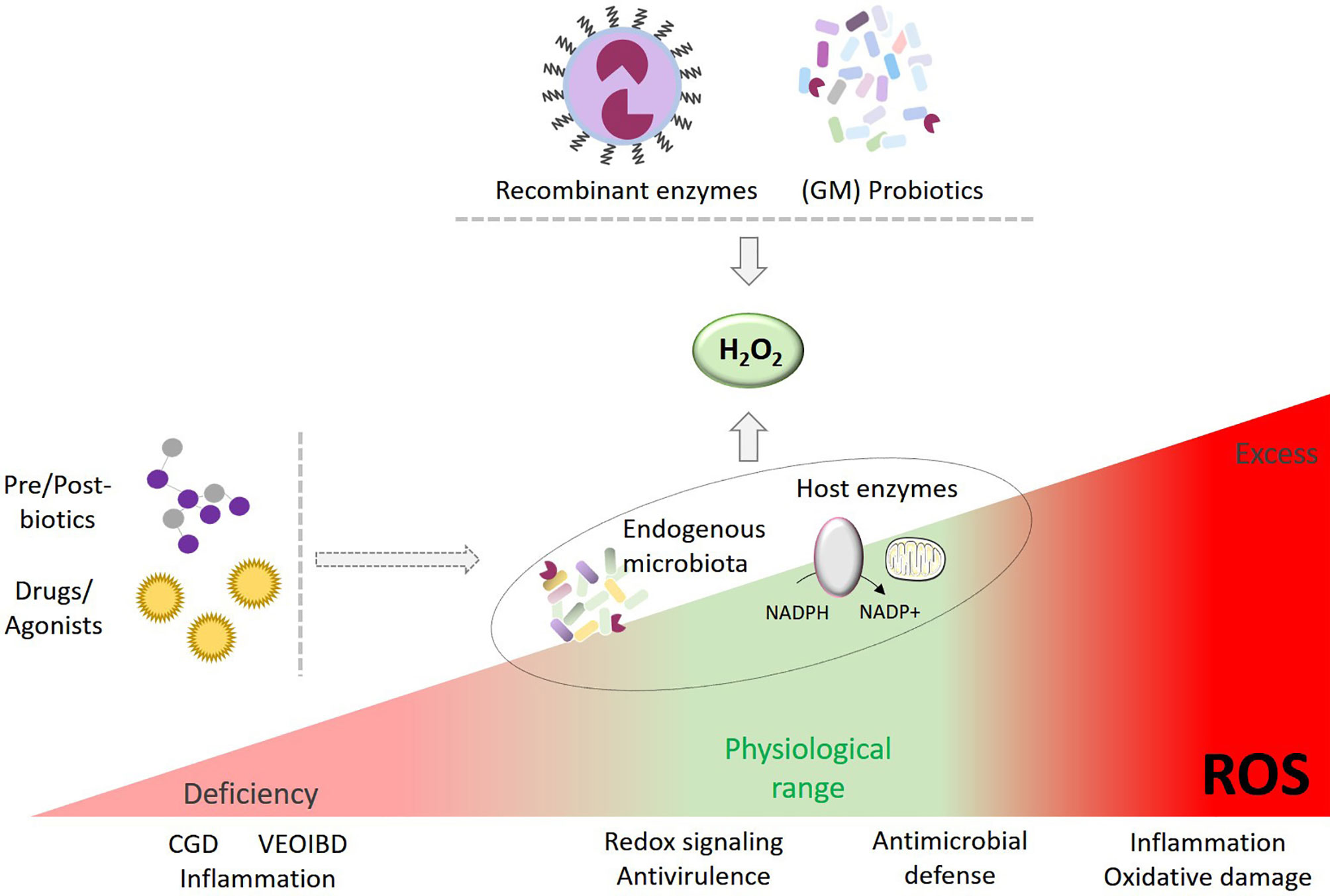
Figure 1 Enhancing H2O2 as therapeutic intervention. Strategies to restore or improve ROS in a controlled manner may include a) administration of selected probiotics or genetically-modified (GM) bacteria producing H2O2, b) innovative drug technology delivering recombinant H2O2 generators to a target area, c) pre-/post-biotics and d) drugs and agonists modulating ROS-generating host enzymes or endogenous microbiota to augment H2O2 production.
Established and Emerging Strategies for Oxidant Generation
Use of Hydrogen Peroxide
In the past, external application of H2O2 has been used for medicinal purposes (191, 192). Although high concentrations of H2O2 have well-known antiseptic properties, tissue damage may occur. On the other hand, application of lower concentrations of H2O2 (0.15-1.25 µmoles/wound) improved the rate of wound closure in wildtype mice and in mice deficient in Nox2-derived superoxide, thereby accelerating tissue regeneration (193, 194). Topical application of micromolar H2O2 promoted the phosphorylation of tyrosine residues in FAK that supported an angiogenic response via vascular endothelial growth factor (VEGF) signaling. Removal of H2O2 by addition of the H2O2 degrading enzyme catalase delayed wound healing and impeded angiogenesis (193). While these experiments may suggest direct exposure of skin to H2O2 as wound healing treatment, the therapeutic window is too narrow for safe and efficient tissue restitution. Further, internal administration of H2O2 solutions poses serious health risks and should not be promoted. To achieve a health benefit, technologies permitting modified release of a standardized dose of nanomolar H2O2 to specific target areas will be required. Strategies promoting controlled H2O2 delivery may include the utilization of nano systems, diffusible molecules, and stimulus-sensitive compounds (195–198). Stimulus-responsive materials are particularly attractive to target specific disease-associated microenvironments such as changes in pH due to inflammation (189, 199, 200). In accord, one can imagine the development of redox-sensitive materials actively releasing appropriate oxidants until a certain threshold is achieved and the system self-inactivates. Moreover, the vehicle strategy is important. For instance, hydrogels are commonly used as drug carriers for clinical use, owing to their tunable mechanistic and physicochemical properties (201). Controlled and continuous release of H2O2 was achieved with an in situ forming hydrogel that can be used as antimicrobial wound dressing (202).
Lactobacillus as H2O2 Source
Lactobacilli, widely used probiotic bacteria that belong to the gastrointestinal and vaginal microbiota (203), are the microbial prototype for controlled release of nanomolar H2O2. Many Lactobacillus strains utilize oxygen to generate H2O2 by various enzymes including flavin reductase (nfr), NADH oxidase (nox) or pyruvate oxidase (pox) (204–207). Lactobacilli colonizing the mucus layer are close enough to the oxygen gradient emanating from host epithelial barrier cells (up to 3% O2) to secrete nanomolar H2O2 continually (208–210). A recent report linked Lactobacillus generated H2O2 to increased colonization resistance, protecting the host from C. rodentium infection (120). A decline in host-derived H2O2 due to genetic deletion or loss-of-function mutations in NADPH oxidases gives rise to microbiota dysbiosis, which is likely niche-driven and tied to changes in the intestinal microenvironment. Thus, manipulation of the microbiota with lactobacilli constitutes a potential strategy to promote microbiota diversity for improved colonization resistance. This approach can be extended to the airways colonized by their unique microbiota. Intranasal administration of probiotics (including various Lactobacillus strains) was recently proposed to modulate local immunity and epithelial barrier function (211, 212). In addition to living lactobacilli, administration of prebiotics to selectively stimulate the growth or activity of resident lactobacilli may improve the barrier redox environment and stimulate host immunity. For example, abundance of lactobacilli seems to increase following the consumption of galacto-oligosaccharides, a common prebiotic generated from the decomposition of lactose, with reported beneficial effects on immune function (203, 213).
Early findings that H2O2 promotes cell migration match with the positive effects of lactobacilli on wound healing. Lactobacilli secrete not only H2O2 but numerous other compounds such as lactic acid and bacteriocins that improve the wound environment. Nevertheless, the ability of L. johnsonii to generate H2O2 was directly linked to accelerated recovery and tissue restitution in murine colitis (210). Transformation of lactobacilli to express exogenous proteins can further improve their wound healing capacity as demonstrated recently for L. reuteri secreting CXCL12 (214). Lactobacilli may also release yet undefined compounds that trigger intracellular ROS generation by host cells, for example by the oxidase Nox1, to improve mucosal repair (85, 105). Bacteria or microbial-derived products can drive expression of ROS-generating enzymes in the host, likely associated with increased H2O2 levels. Ileal colonization with commensal segmented filamentous bacteria (SFB) increased expression of the oxidase Duox2 (19). Intraperitoneal administration of SFB-derived flagellin was sufficient to upregulate Duox2 in the small intestine, suggesting that microbial regulation of Duox2 expression may be TLR5 dependent (215).
Most studies linking lactobacilli to antimicrobial defense, mucosal healing and microbiota modification have been conducted in mice. In clinical trials the outcome of probiotic therapy in IBD has been mixed (216–219). Possible explanations range from poor standardization and viability of bacteria to insufficient colonization due to the human intestinal environment including the mucus layer, as well as to differences between mouse and human physiology such as the presence of a Lactobacillus reservoir in the murine forestomach. Another confining factor is using living organisms, which can lead to systemic bacterial dissemination. Several reports outline the risk for Lactobacillus bacteremia and septicemia in immunocompromised patients, after surgical procedures and in colitis patients (220–223). Even in mice septicemia was observed when either the orally administered dose of L. johnsonii was increased ten-fold or L. johnsonii overproducing H2O2 was administered, indicating that H2O2 production should not exceed an optimal physiological range for health benefit (210). Current developments in the field are focused on standardization, design of consortia and recombinant probiotics, but other inventive strategies as outlined below could address some shortcomings of probiotics as H2O2 generators.
Application of Recombinant Enzymes
Recombinant enzymes involved in ROS generation or conversion can be used to modulate the oxidative microenvironment. The enzyme superoxide dismutase (SOD) catalyzes the dismutation of superoxide into oxygen and H2O2. Several isoforms of this enzyme are expressed in both mammalian and bacterial hosts, where they serve as part of the protective antioxidant system. SOD-mediated superoxide conversion limits the generation of secondary, highly reactive oxygen and nitrogen metabolites (e.g., hydroxyl radical, peroxynitrite) that can cause irreversible modification of proteins, lipids or DNA (224, 225). Therefore, SOD-based applications have been mainly used as antioxidant strategy aiming to decrease secondary ROS levels for prevention of inflammatory diseases. A more universal utilization of SOD as physiological H2O2 source at mucosal surfaces is compromised by its mode of action, including reliance on inflamed conditions, in conjunction with unattainable dosing standardization, and poor enzymatic stability (226).
Many oxidoreductases generate H2O2 as a byproduct of their intended enzymatic reaction including carbohydrate oxidases [e.g., glucose oxidase, galactose oxidase and many others (227)], cholesterol oxidase (228), alcohol oxidase (229) and D-amino acid oxidase (230). We will discuss here as example glucose oxidase (GOx), a prominent representative of carbohydrate oxidases that catalyzes the oxidation of β-D-glucose to D-glucono-1,5-lactone with further hydrolysis to gluconic acid. GOx contains the flavin adenine dinucleotide (FAD) cofactor as initial electron acceptor, which undergoes reduction to FADH2, followed by FADH2 oxidation by molecular oxygen, and reduction of oxygen to H2O2 (231). GOx is found predominantly in fungi (i.e., Aspergillus and Penicillium spp) and its high specificity for glucose renders the enzymatic reaction an attractive tool for monitoring blood glucose levels. Various GOx-based biosensors have been developed for glucose monitoring, sometimes in diabetes therapy in conjunction with insulin release (232, 233). Stability of the enzyme is important for these applications, and thus technology advancements have resulted in genetically engineered GOx modifications in combination with encapsulation strategies for improved long-term enzymatic activity (234). Dermatological applications of GOx as tunable H2O2 generator may hold some promise for therapy. Incorporation of GOx in a collagen dressing improved wound healing and tissue regeneration of rodent skin, presumably due to sustained release of H2O2, although oxidant measurements were not provided. This GOx treatment induced an antioxidant response by the host tissue with upregulation of SOD and catalase (235). GOx is naturally present in honey at low concentrations where it has been studied for its dual function, namely for aiding wound healing and as antimicrobial agent. A medicinal honey (SurgihoneyRO™) with enhanced antimicrobial activity is on the market as a wound antiseptic dressing (236, 237). Due to its GRAS (generally recognized as safe) FDA status GOx is used by the food industry as stabilizing and antibacterial agent (238).
The GRAS status supports considering GOx for internal applications such as hydrogels or oral administration of the enzyme. A gelatin hydrogel incorporating glucose and varying GOx concentrations released micromolar H2O2 for 24-48 hours in a controlled manner and improved the proliferation of cultured endothelial cells. This effect was accompanied by a transient increase of intracellular ROS (measured as DCFH-DA signal) as well as enhanced neovascularization in a CAM model, indicating modulation of redox signaling by GOx-generated H2O2 (239, 240). For oral administration, the protection of GOx’s enzymatic activity can be achieved by microencapsulation. Diet delivered glucose might be sufficient for GOx-mediated H2O2 generation in the small intestine, while supplementation of the GOx drug carrier with compartmentalized glucose will be necessary for affecting the large intestine. Targeted, controlled delivery of GOx/glucose presents a promising opportunity to modulate intestinal redox signaling, mucosal healing and immune defense while limiting oxidative damage. Therapeutic applications of GOx/glucose or similar H2O2 generators will partially mimic the current use of lactobacilli, albeit with superior standardization, more limited dependence on the barrier microenvironment and superior safety in vulnerable patient populations. An additional benefit of the GOx/glucose reaction is the generation of the prebiotic gluconic acid as a secondary reaction product, which stimulates butyrate production in the intestine (241). Examples for potential treatment modalities are gut health improvements (e.g., barrier reinforcement, microbiota diversity), accelerated tissue restitution after injury in patients with intestinal inflammatory diseases, or prophylactic long-term modification of the intestinal environment in patients with insufficient H2O2 generation. Prime examples for the last point are certain patient populations in the categories very early onset IBD (VEO-IBD), CGD-IBD or IBD linked to variants upstream of ROS-generating enzymes (21, 242–244). Variants in genes encoding components of NOX enzymes including NOX1, NOX2 and DUOX2 confer susceptibility to VEO-IBD (152, 245–248), while NOX2 complex variants associated with CGD due to absent or minimal output of superoxide manifest in 40-50% of CGD patients as CGD-associated IBD. Functional evaluation of NOX1 and DUOX2 patient variants in model systems revealed decreased ROS production and impaired antimicrobial defense (152, 246, 247). The compromised immune defense of these hosts in combination with the ensuing dysbiotic microbiota affects not only the overall colonization resistance but also more specialized immune defense mechanisms. Diffusion of nanomolar H2O2 into extracellular pathogens is not bactericidal but can downregulate virulence factors. Nanomolar H2O2 blocked bacterial phosphotyrosine signaling required for virulence factor synthesis by irreversibly modifying tyrosine phosphorylated enzymes and proteins (22, 23). In addition, nanomolar H2O2 inhibited LEE pathogenicity island regulation in enteropathogenic bacteria (C. rodentium) due to impeded expression of the major transcriptional regulator ler, which was associated with lower pathogen colonization and improved recovery of mice (120). While the precise molecular mechanism of LEE downregulation has not yet been resolved, these studies hold great promise for H2O2-mediated interference in enteric bacterial infections. Supplying nanomolar levels of H2O2 via GOx/glucose or similar means may provide multiple benefits including host protection, reinforcement of the intestinal barrier and mucosal healing.
Stimulation of Endogenous ROS Sources
Instead of providing ROS by an exogenous source, agonists or drugs that stimulate expression and/or activation of a ROS-generating enzyme or alter mitochondrial function can be used to improve immune responses. Currently this area is dominated by improving innate immune cell-derived superoxide, but one can envision that future applications will be directed at epithelial superoxide/H2O2-producing enzymes. Neutrophils and macrophages are crucial for pathogen defense with NOX2-derived superoxide playing a key role in this process. Enhancing the neutrophil activation status, and in particular the oxidative burst, will not only be desirable in infections but also in inflammatory disorders (249–251). Activating NOX2 can be achieved by targeting upstream pathways. One approach focusses on developing formyl peptide receptor (FPR1, FPR2) agonists selectively binding to a receptor conformation that will favor one signaling output over another (i.e. biased signaling) without accelerating receptor desensitization (249). In neutrophils FPRs regulate directional migration, secretion of inflammatory mediators and superoxide generation by NOX2. While FPRs are usually triggered by microbial derived formylated peptides, endogenous proteins or lipopeptides, small compounds and peptides acting as exogenous FPR agonists have been identified. Examples are the FPR1 agonist RE-04-001, FPR2 agonists BMS-986235 and Act-389949, and dual FPR1/2 agonists such as compound 17b (252–255). Biased FPR agonists causing functional selectivity in neutrophil responses, namely either inducing chemotaxis or superoxide production, can be used as NOX2 activators (252, 256). Nox2 activity has been associated with the resolution of inflammation in colitis (133), and the pro-resolving Fpr2 agonist Annexin-A1 accelerated wound healing of the murine colonic epithelium via the oxidase Nox1 (81).
Another approach is using the cytokine granulocyte-macrophage colony-stimulating factor (GM-CSF) to augment NOX2 derived superoxide production by acting as priming agent in neutrophils while elevating the phagosomal pH in macrophages (257–259). The efficacy of GM-CSF administration as immunostimulatory adjuvant has been evaluated in clinical trials. In a randomized trial involving critically ill patients, subcutaneous injections with GM-CSF increased neutrophil phagocytic capacity but did not improve superoxide production (EudraCT 2011-005815-10), while an earlier trial using intravenous delivery of GM-CSF had reported statistically significant enhancement of neutrophil superoxide generation (260). GM-CSF has also been assessed in the context of CGD patients. While reconstitution of the oxidative burst in isolated neutrophils was not achieved, as expected for a disease caused by genetic mutations, GM-CSF has shown some promise in treating CGD-IBD by undefined mechanism(s) (261–263). In non-CGD patients biased targeting of NOX2 activating pathways may hold promise, and analogous strategies should be feasible for development of activators of other NOX/DUOX enzymes or of other ROS sources.
Generation of Compensatory ROS
Therapeutically enhancing ROS production in CGD or VEO-IBD patients is imperative for antimicrobial defense, for resolution of inflammation and for repair processes. In these patients gene editing, administration of exogenous ROS generators or stimulation of other endogenous ROS sources are the only options to improve their ROS status. Kuhns and coworkers showed that modest residual superoxide production is sufficient to prevent life-threatening infections in CGD patients (264). Partial restoration of macrophage and dendritic cell superoxide in functional Ncf1 rescue mice was adequate to dampen hyperinflammation (265). These considerations are the basis for screening and identifying compounds capable of compensating partially for NOX2 enzyme activity.
The first compound in this class is the peroxisome proliferator-activated receptor γ (PPARγ) agonist pioglitazone, a drug approved for Type 2 diabetes. As proof of concept, pioglitazone treatment of cultured CGD patient-derived monocytes or of Nox2 deficient mice increased mROS production and subsequently the bactericidal capacity of immune cells (266). Pioglitazone and the related rosiglitazone induced mROS and NETs in CGD neutrophils (267). Monocytes from CGD patients and Nox2 deficient macrophages were impaired in PPARγ signaling, impacting their efferocytosis function which was restored by pioglitazone treatment (268, 269). Increased mROS generation and restoration of efferocytosis were also observed in monocytes isolated from two CGD patients after 30 days of oral pioglitazone treatment, supporting the effectiveness of pioglitazone therapy for superoxide production and improved immune cell function (269). A clinical study reported the effects of daily pioglitazone treatment in an infant with CGD. Using dihydrorhodamine (DHR) fluorescence as readout for ROS an increase in DHR positive granulocytes after 25 days of treatment was observed. The increase in the phorbol ester stimulated DHR signal was relatively low in comparison with healthy donor cells but it was maintained over several weeks, and the clinical condition of the patient progressively improved (270). Efficacy and safety of pioglitazone was assessed in a clinical trial enrolling CGD patients with severe infection. Phase 2 of the study was terminated when the DHR fluorescence signal did not improve in neutrophils after 90 days of treatment with pioglitazone (clinicaltrials.gov NCT03080480). Long-term administration of pioglitazone did not lead to drug-related adverse effects or exacerbation of infection (271). The variation in outcomes might be attributable to different pioglitazone dosage, or to the often required combination therapy with antibiotics and/or interferon IFN-γ. Further studies are necessary to determine if pioglitazone will provide a therapeutic option for CGD patients, for example as adjuvant therapy in severe bacterial infections or as prophylaxis. At this point only hematopoietic stem cell transplantation, gene therapy or gene editing with CRISPR-Cas9 offer a cure for CGD (272).
Future Directions
The field of redox medicine has blossomed over the last decade, but further progress will depend on connecting more tightly distinct oxidant species and their enzymatic sources to physiological or pathophysiological processes. Another important factor will be uncovering and manipulating specific microenvironments. The chemical milieu, in particular oxygen availability, is a limiting factor for ROS production, but the hypoxia developing due to ROS production (e.g., via the neutrophil oxidative burst) in a low oxygen environment can have beneficial effects such as resolution of inflammation and accelerated tissue restitution (133). The presence and concentration of H2O2 and secondary oxidants (hypochlorous acid, peroxynitrite) will modify the barrier environment and the interactions between host and microbiota, leading to changes in microbiota diversity, composition, and the microbial metabolome that can be beneficial or harmful for the host. Case in point is the intestinal dysbiosis and appearance of pathobionts in NADPH oxidase deficient mice (94, 113). The nanomolar H2O2 released from mucosal barriers is inadequate for bactericidal activity, but its interference with the fitness and virulence of certain microorganisms supports its use as a promising alternative to antibiotics (273, 274). However, this antivirulence strategy will require the identification and targeting of specific pathogens to avoid the subversion of these oxidants for their own advantage as reported for some pathogenic bacteria (275–281).
Given the essential role of ROS for basic physiological functions, future therapeutics should include careful modulation of the redox state. Therapeutic interest today focuses on the margins of the ROS continuum, namely anti- or pro-oxidant interventions, but new approaches to modify physiological redox processes will be vital for improving health and well-being. Current limitations are the lack of targeted drugs modulating the activity of specific enzyme isoforms or of drugs that can adapt their mode of action by sensing ROS levels in situ. Sophisticated delivery systems for novel small molecule- or peptide-based activators will help restoring or enhancing beneficial ROS (mainly H2O2) at a predetermined location in order to achieve a positive outcome and to prevent undesired side effects linked to toxicity. For this purpose, exploitation of natural properties of commensals or their genetic modification, discovery of agonists stimulating ROS-generating enzymes, design of functional materials and delivery vehicles releasing H2O2, for example by utilizing recombinant enzyme-substrate pairs, will provide therapeutic options for infections, inflammatory diseases, and regenerative medicine. Next generation treatments will likely include strategies for altering the chemical environment at defined locations in a sustainable manner, with ROS being one of the key targets.
Author Contributions
Concept (UK). Writing and editing (AD, UK). Figure design (AD). Funding (UK). All authors contributed to the article and approved the submitted version.
Conflict of Interest
The authors declare that the research was conducted in the absence of any commercial or financial relationships that could be construed as a potential conflict of interest.
Acknowledgments
We acknowledge the support of Science Foundation Ireland (16/IA/4501) and the National Children's Research Center (K/17/2) (both UK).
References
1. Holmstrom KM, Finkel T. Cellular Mechanisms and Physiological Consequences of Redox-Dependent Signalling. Nat Rev Mol Cell Biol (2014) 15(6):411–21. doi: 10.1038/nrm3801
2. Sies H, Jones DP. Reactive Oxygen Species (ROS) as Pleiotropic Physiological Signalling Agents. Nat Rev Mol Cell Biol (2020) 21(7):363–83. doi: 10.1038/s41580-020-0230-3
3. Lambeth JD, Neish AS. Nox Enzymes and New Thinking on Reactive Oxygen: A Double-Edged Sword Revisited. Annu Rev Pathol (2014) 9:119–45. doi: 10.1146/annurev-pathol-012513-104651
4. Panday A, Sahoo MK, Osorio D, Batra S. NADPH Oxidases: An Overview From Structure to Innate Immunity-Associated Pathologies. Cell Mol Immunol (2015) 12(1):5–23. doi: 10.1038/cmi.2014.89
5. Winterbourn CC, Kettle AJ. Redox Reactions and Microbial Killing in the Neutrophil Phagosome. Antioxid Redox Signal (2013) 18(6):642–60. doi: 10.1089/ars.2012.4827
6. Sena LA, Chandel NS. Physiological Roles of Mitochondrial Reactive Oxygen Species. Mol Cell (2012) 48(2):158–67. doi: 10.1016/j.molcel.2012.09.025
7. Reczek CR, Chandel NS. ROS-Dependent Signal Transduction. Curr Opin Cell Biol (2015) 33:8–13. doi: 10.1016/j.ceb.2014.09.010
8. Veal EA, Day AM, Morgan BA. Hydrogen Peroxide Sensing and Signaling. Mol Cell (2007) 26(1):1–14. doi: 10.1016/j.molcel.2007.03.016
9. Sies H. Hydrogen Peroxide as a Central Redox Signaling Molecule in Physiological Oxidative Stress: Oxidative Eustress. Redox Biol (2017) 11:613–9. doi: 10.1016/j.redox.2016.12.035
10. Stocker S, Van Laer K, Mijuskovic A, Dick TP. The Conundrum of Hydrogen Peroxide Signaling and the Emerging Role of Peroxiredoxins as Redox Relay Hubs. Antioxid Redox Signal (2018) 28(7):558–73. doi: 10.1089/ars.2017.7162
11. Deshmukh P, Unni S, Krishnappa G, Padmanabhan B. The Keap1-Nrf2 Pathway: Promising Therapeutic Target to Counteract ROS-Mediated Damage in Cancers and Neurodegenerative Diseases. Biophys Rev (2017) 9(1):41–56. doi: 10.1007/s12551-016-0244-4
12. Suzuki T, Muramatsu A, Saito R, Iso T, Shibata T, Kuwata K, et al. Molecular Mechanism of Cellular Oxidative Stress Sensing by Keap1. Cell Rep (2019) 28(3):746–58.e4. doi: 10.1016/j.celrep.2019.06.047
13. Imlay JA. Transcription Factors That Defend Bacteria Against Reactive Oxygen Species. Annu Rev Microbiol (2015) 69:93–108. doi: 10.1146/annurev-micro-091014-104322
14. Reniere ML. Reduce, Induce, Thrive: Bacterial Redox Sensing During Pathogenesis. J Bacteriol (2018) 200(17). doi: 10.1128/JB.00128-18
15. Imlay JA. Where in the World do Bacteria Experience Oxidative Stress? Environ Microbiol (2019) 21(2):521–30. doi: 10.1111/1462-2920.14445
16. Li X, Imlay JA. Improved Measurements of Scant Hydrogen Peroxide Enable Experiments That Define Its Threshold of Toxicity for Escherichia Coli. Free Radic Biol Med (2018) 120:217–27. doi: 10.1016/j.freeradbiomed.2018.03.025
17. Lee C, Lee SM, Mukhopadhyay P, Kim SJ, Lee SC, Ahn WS, et al. Redox Regulation of OxyR Requires Specific Disulfide Bond Formation Involving a Rapid Kinetic Reaction Path. Nat Struct Mol Biol (2004) 11(12):1179–85. doi: 10.1038/nsmb856
18. Zheng M, Aslund F, Storz G. Activation of the OxyR Transcription Factor by Reversible Disulfide Bond Formation. Science (1998) 279(5357):1718–21. doi: 10.1126/science.279.5357.1718
19. Grasberger H, Gao J, Nagao-Kitamoto H, Kitamoto S, Zhang M, Kamada N, et al. Increased Expression of DUOX2 Is an Epithelial Response to Mucosal Dysbiosis Required for Immune Homeostasis in Mouse Intestine. Gastroenterology (2015) 149(7):1849–59. doi: 10.1053/j.gastro.2015.07.062
20. Jones RM, Neish AS. Redox Signaling Mediated by the Gut Microbiota. Free Radic Biol Med (2017) 105:41–7. doi: 10.1016/j.freeradbiomed.2016.10.495
21. Aviello G, Knaus UG. NADPH Oxidases and ROS Signaling in the Gastrointestinal Tract. Mucosal Immunol (2018) 11(4):1011–23. doi: 10.1038/s41385-018-0021-8
22. Corcionivoschi N, Alvarez LA, Sharp TH, Strengert M, Alemka A, Mantell J, et al. Mucosal Reactive Oxygen Species Decrease Virulence by Disrupting Campylobacter Jejuni Phosphotyrosine Signaling. Cell Host Microbe (2012) 12(1):47–59. doi: 10.1016/j.chom.2012.05.018
23. Alvarez LA, Kovacic L, Rodriguez J, Gosemann JH, Kubica M, Pircalabioru GG, et al. NADPH Oxidase-Derived H2O2 Subverts Pathogen Signaling by Oxidative Phosphotyrosine Conversion to PB-DOPA. Proc Natl Acad Sci USA (2016) 113(37):10406–11. doi: 10.1073/pnas.1605443113
24. Belambri SA, Rolas L, Raad H, Hurtado-Nedelec M, Dang PM, El-Benna J. NADPH Oxidase Activation in Neutrophils: Role of the Phosphorylation of its Subunits. Eur J Clin Invest (2018) 48(Suppl 2):e12951. doi: 10.1111/eci.12951
25. Rovira-Llopis S, Banuls C, Diaz-Morales N, Hernandez-Mijares A, Rocha M, Victor VM. Mitochondrial Dynamics in Type 2 Diabetes: Pathophysiological Implications. Redox Biol (2017) 11:637–45. doi: 10.1016/j.redox.2017.01.013
26. Teleanu RI, Chircov C, Grumezescu AM, Volceanov A, Teleanu DM. Antioxidant Therapies for Neuroprotection-A Review. J Clin Med (2019) 8(10):1659. doi: 10.3390/jcm8101659
27. Bjelakovic G, Nikolova D, Gluud LL, Simonetti RG, Gluud C. Mortality in Randomized Trials of Antioxidant Supplements for Primary and Secondary Prevention: Systematic Review and Meta-Analysis. JAMA (2007) 297(8):842–57. doi: 10.1001/jama.297.8.842
28. Schmidt HH, Stocker R, Vollbracht C, Paulsen G, Riley D, Daiber A, et al. Antioxidants in Translational Medicine. Antioxid Redox Signal (2015) 23(14):1130–43. doi: 10.1089/ars.2015.6393
29. Szkudlinska MA, von Frankenberg AD, Utzschneider KM. The Antioxidant N-Acetylcysteine Does Not Improve Glucose Tolerance or Beta-Cell Function in Type 2 Diabetes. J Diabetes Complications (2016) 30(4):618–22. doi: 10.1016/j.jdiacomp.2016.02.003
30. Kryscio RJ, Abner EL, Caban-Holt A, Lovell M, Goodman P, Darke AK, et al. Association of Antioxidant Supplement Use and Dementia in the Prevention of Alzheimer’s Disease by Vitamin E and Selenium Trial (Preadvise). JAMA Neurol (2017) 74(5):567–73. doi: 10.1001/jamaneurol.2016.5778
31. Grammatikopoulou MG, Gkiouras K, Theodoridis X, Asteriou E, Forbes A, Bogdanos DP. Oral Adjuvant Curcumin Therapy for Attaining Clinical Remission in Ulcerative Colitis: A Systematic Review and Meta-Analysis of Randomized Controlled Trials. Nutrients (2018) 10(11):1737. doi: 10.3390/nu10111737
32. Shaito A, Posadino AM, Younes N, Hasan H, Halabi S, Alhababi D, et al. Potential Adverse Effects of Resveratrol: A Literature Review. Int J Mol Sci (2020) 21(6):2084. doi: 10.3390/ijms21062084
33. O’Neill S, Brault J, Stasia MJ, Knaus UG. Genetic Disorders Coupled to ROS Deficiency. Redox Biol (2015) 6:135–56. doi: 10.1016/j.redox.2015.07.009
34. Schieber M, Chandel NS. ROS Function in Redox Signaling and Oxidative Stress. Curr Biol (2014) 24(10):R453–62. doi: 10.1016/j.cub.2014.03.034
35. Forman HJ, Ursini F, Maiorino M. An Overview of Mechanisms of Redox Signaling. J Mol Cell Cardiol (2014) 73:2–9. doi: 10.1016/j.yjmcc.2014.01.018
36. Radi R. Oxygen Radicals, Nitric Oxide, and Peroxynitrite: Redox Pathways in Molecular Medicine. Proc Natl Acad Sci USA (2018) 115(23):5839–48. doi: 10.1073/pnas.1804932115
37. Jennings RT, Singh AK, Knaus UG. Redox Regulator Network in Inflammaotry Signaling. Curr Opin Physiol (2019) 9:9–17. doi: 10.1016/j.cophys.2019.03.002
38. Albadri S, Naso F, Thauvin M, Gauron C, Parolin C, Duroure K, et al. Redox Signaling Via Lipid Peroxidation Regulates Retinal Progenitor Cell Differentiation. Dev Cell (2019) 50(1):73–89.e6. doi: 10.1016/j.devcel.2019.05.011
39. Travasso RDM, Sampaio Dos Aidos F, Bayani A, Abranches P, Salvador A. Localized Redox Relays as a Privileged Mode of Cytoplasmic Hydrogen Peroxide Signaling. Redox Biol (2017) 12:233–45. doi: 10.1016/j.redox.2017.01.003
40. Hoppstadter J, Ammit AJ. Role of Dual-Specificity Phosphatase 1 in Glucocorticoid-Driven Anti-Inflammatory Responses. Front Immunol (2019) 10:1446. doi: 10.3389/fimmu.2019.01446
41. Sharma P, Chakraborty R, Wang L, Min B, Tremblay ML, Kawahara T, et al. Redox Regulation of Interleukin-4 Signaling. Immunity (2008) 29(4):551–64. doi: 10.1016/j.immuni.2008.07.019
42. Heppner DE, Dustin CM, Liao C, Hristova M, Veith C, Little AC, et al. Direct Cysteine Sulfenylation Drives Activation of the Src Kinase. Nat Commun (2018) 9(1):4522. doi: 10.1038/s41467-018-06790-1
43. Heppner DE, van der Vliet A. Redox-Dependent Regulation of Epidermal Growth Factor Receptor Signaling. Redox Biol (2016) 8:24–7. doi: 10.1016/j.redox.2015.12.002
44. Psenakova K, Hexnerova R, Srb P, Obsilova V, Veverka V, Obsil T. The Redox-Active Site of Thioredoxin Is Directly Involved in Apoptosis Signal-Regulating Kinase 1 Binding That Is Modulated by Oxidative Stress. FEBS J (2020) 287(8):1626–44. doi: 10.1111/febs.15101
45. Soga M, Matsuzawa A, Ichijo H. Oxidative Stress-Induced Diseases Via the ASK1 Signaling Pathway. Int J Cell Biol (2012) 2012:439587. doi: 10.1155/2012/439587
46. Short JD, Downs K, Tavakoli S, Asmis R. Protein Thiol Redox Signaling in Monocytes and Macrophages. Antioxid Redox Signal (2016) 25(15):816–35. doi: 10.1089/ars.2016.6697
47. Liu RM, Desai LP. Reciprocal Regulation of TGF-beta and Reactive Oxygen Species: A Perverse Cycle for Fibrosis. Redox Biol (2015) 6:565–77. doi: 10.1016/j.redox.2015.09.009
48. Lee JH, Joo JH, Kim J, Lim HJ, Kim S, Curtiss L, et al. Interaction of NADPH Oxidase 1 With Toll-like Receptor 2 Induces Migration of Smooth Muscle Cells. Cardiovasc Res (2013) 99(3):483–93. doi: 10.1093/cvr/cvt107
49. Park HS, Jung HY, Park EY, Kim J, Lee WJ, Bae YS. Cutting Edge: Direct Interaction of TLR4 With NAD(P)H Oxidase 4 Isozyme Is Essential for Lipopolysaccharide-Induced Production of Reactive Oxygen Species and Activation of NF-kappa B. J Immunol (2004) 173(6):3589–93. doi: 10.4049/jimmunol.173.6.3589
51. Bylund J, MacDonald KL, Brown KL, Mydel P, Collins LV, Hancock RE, et al. Enhanced Inflammatory Responses of Chronic Granulomatous Disease Leukocytes Involve ROS-Independent Activation of NF-kappa B. Eur J Immunol (2007) 37(4):1087–96. doi: 10.1002/eji.200636651
52. Kobayashi SD, Voyich JM, Braughton KR, Whitney AR, Nauseef WM, Malech HL, et al. Gene Expression Profiling Provides Insight Into the Pathophysiology of Chronic Granulomatous Disease. J Immunol (2004) 172(1):636–43. doi: 10.4049/jimmunol.172.1.636
53. Hatanaka E, Carvalho BT, Condino-Neto A, Campa A. Hyperresponsiveness of Neutrophils From Gp 91phox Deficient Patients to Lipopolysaccharide and Serum Amyloid A. Immunol Lett (2004) 94(1-2):43–6. doi: 10.1016/j.imlet.2004.04.016
54. Brown KL, Bylund J, MacDonald KL, Song-Zhao GX, Elliott MR, Falsafi R, et al. ROS-Deficient Monocytes Have Aberrant Gene Expression That Correlates With Inflammatory Disorders of Chronic Granulomatous Disease. Clin Immunol (2008) 129(1):90–102. doi: 10.1016/j.clim.2008.06.005
55. McLetchie S, Volpp BD, Dinauer MC, Blum JS. Hyper-Responsive Toll-like Receptor 7 and 9 Activation in NADPH Oxidase-Deficient B Lymphoblasts. Immunology (2015) 146(4):595–606. doi: 10.1111/imm.12530
56. Meda Spaccamela V, Valencia RG, Pastukhov O, Duppenthaler A, Dettmer MS, Erb J, et al. High Levels of IL-18 and IFN-Gamma in Chronically Inflamed Tissue in Chronic Granulomatous Disease. Front Immunol (2019) 10:2236. doi: 10.3389/fimmu.2019.02236
57. Meissner F, Seger RA, Moshous D, Fischer A, Reichenbach J, Zychlinsky A. Inflammasome Activation in NADPH Oxidase Defective Mononuclear Phagocytes From Patients With Chronic Granulomatous Disease. Blood (2010) 116(9):1570–3. doi: 10.1182/blood-2010-01-264218
58. de Luca A, Smeekens SP, Casagrande A, Iannitti R, Conway KL, Gresnigt MS, et al. IL-1 Receptor Blockade Restores Autophagy and Reduces Inflammation in Chronic Granulomatous Disease in Mice and in Humans. Proc Natl Acad Sci USA (2014) 111(9):3526–31. doi: 10.1073/pnas.1322831111
59. Segal BH, Han W, Bushey JJ, Joo M, Bhatti Z, Feminella J, et al. NADPH Oxidase Limits Innate Immune Responses in the Lungs in Mice. PloS One (2010) 5(3):e9631. doi: 10.1371/journal.pone.0009631
60. Song Z, Huang G, Chiquetto Paracatu LC, Grimes D, Gu J, Luke CJ, et al. NADPH Oxidase Controls Pulmonary Neutrophil Infiltration in the Response to Fungal Cell Walls by Limiting LTB4. Blood (2020) 135(12):891–903. doi: 10.1182/blood.2019003525
61. Dinauer MC. Inflammatory Consequences of Inherited Disorders Affecting Neutrophil Function. Blood (2019) 133(20):2130–9. doi: 10.1182/blood-2018-11-844563
62. Rieber N, Hector A, Kuijpers T, Roos D, Hartl D. Current Concepts of Hyperinflammation in Chronic Granulomatous Disease. Clin Dev Immunol (2012) 2012:252460. doi: 10.1155/2012/252460
63. Kuijpers T, Lutter R. Inflammation and Repeated Infections in CGD: Two Sides of a Coin. Cell Mol Life Sci (2012) 69:7–15. doi: 10.1007/s00018-011-0834-z
64. Cachat J, Deffert C, Alessandrini M, Roux-Lombard P, Le Gouellec A, Stasia MJ, et al. Altered Humoral Immune Responses and IgG Subtypes in NOX2-Deficient Mice and Patients: A Key Role for NOX2 in Antigen-Presenting Cells. Front Immunol (2018) 9:1555. doi: 10.3389/fimmu.2018.01555
65. Weinberg SE, Sena LA, Chandel NS. Mitochondria in the Regulation of Innate and Adaptive Immunity. Immunity (2015) 42(3):406–17. doi: 10.1016/j.immuni.2015.02.002
66. Mehta MM, Weinberg SE, Chandel NS. Mitochondrial Control of Immunity: Beyond ATP. Nat Rev Immunol (2017) 17(10):608–20. doi: 10.1038/nri.2017.66
67. Chandel NS, Schumacker PT, Arch RH. Reactive Oxygen Species Are Downstream Products of TRAF-Mediated Signal Transduction. J Biol Chem (2001) 276(46):42728–36. doi: 10.1074/jbc.M103074200
68. West AP, Brodsky IE, Rahner C, Woo DK, Erdjument-Bromage H, Tempst P, et al. TLR Signalling Augments Macrophage Bactericidal Activity Through Mitochondrial ROS. Nature (2011) 472(7344):476–80. doi: 10.1038/nature09973
69. Herb M, Gluschko A, Wiegmann K, Farid A, Wolf A, Utermohlen O, et al. Mitochondrial Reactive Oxygen Species Enable Proinflammatory Signaling Through Disulfide Linkage of NEMO. Sci Signal (2019) 12(568). doi: 10.1126/scisignal.aar5926
70. Zhou R, Yazdi AS, Menu P, Tschopp J. A Role for Mitochondria in NLRP3 Inflammasome Activation. Nature (2011) 469(7329):221–5. doi: 10.1038/nature09663
71. Heid ME, Keyel PA, Kamga C, Shiva S, Watkins SC, Salter RD. Mitochondrial Reactive Oxygen Species Induces NLRP3-Dependent Lysosomal Damage and Inflammasome Activation. J Immunol (2013) 191(10):5230–8. doi: 10.4049/jimmunol.1301490
72. Yang Y, Wang H, Kouadir M, Song H, Shi F. Recent Advances in the Mechanisms of NLRP3 Inflammasome Activation and Its Inhibitors. Cell Death Dis (2019) 10(2):128. doi: 10.1038/s41419-019-1413-8
73. Ives A, Nomura J, Martinon F, Roger T, LeRoy D, Miner JN, et al. Xanthine Oxidoreductase Regulates Macrophage IL1beta Secretion Upon NLRP3 Inflammasome Activation. Nat Commun (2015) 6:6555. doi: 10.1038/ncomms7555
74. van de Veerdonk FL, Smeekens SP, Joosten LA, Kullberg BJ, Dinarello CA, van der Meer JW, et al. Reactive Oxygen Species-Independent Activation of the IL-1beta Inflammasome in Cells From Patients With Chronic Granulomatous Disease. Proc Natl Acad Sci USA (2010) 107(7):3030–3. doi: 10.1073/pnas.0914795107
75. van Bruggen R, Koker MY, Jansen M, van Houdt M, Roos D, Kuijpers TW, et al. Human NLRP3 Inflammasome Activation Is Nox1-4 Independent. Blood (2010) 115(26):5398–400. doi: 10.1182/blood-2009-10-250803
76. Soucy-Faulkner A, Mukawera E, Fink K, Martel A, Jouan L, Nzengue Y, et al. Requirement of NOX2 and Reactive Oxygen Species for Efficient RIG-I-mediated Antiviral Response Through Regulation of MAVS Expression. PloS Pathog (2010) 6(6):e1000930. doi: 10.1371/journal.ppat.1000930
77. Zhao Y, Sun X, Nie X, Sun L, Tang TS, Chen D, et al. COX5B Regulates MAVS-mediated Antiviral Signaling Through Interaction With ATG5 and Repressing ROS Production. PloS Pathog (2012) 8(12):e1003086. doi: 10.1371/journal.ppat.1003086
78. Nagai-Singer MA, Morrison HA, Allen IC. Nlrx1 Is a Multifaceted and Enigmatic Regulator of Immune System Function. Front Immunol (2019) 10:2419. doi: 10.3389/fimmu.2019.02419
79. Hobbs GA, Zhou B, Cox AD, Campbell SL. Rho GTPases, Oxidation, and Cell Redox Control. Small GTPases (2014) 5:e28579. doi: 10.4161/sgtp.28579
80. Valdivia A, Duran C, San Martin A. The Role of Nox-mediated Oxidation in the Regulation of Cytoskeletal Dynamics. Curr Pharm Des (2015) 21(41):6009–22. doi: 10.2174/1381612821666151029112624
81. Leoni G, Alam A, Neumann PA, Lambeth JD, Cheng G, McCoy J, et al. Annexin A1, Formyl Peptide Receptor, and NOX1 Orchestrate Epithelial Repair. J Clin Invest (2013) 123(1):443–54. doi: 10.1172/JCI65831
82. Swanson PA, Kumar A, Samarin S, Vijay-Kumar M, Kundu K, Murthy N, et al. Enteric Commensal Bacteria Potentiate Epithelial Restitution Via Reactive Oxygen Species-Mediated Inactivation of Focal Adhesion Kinase Phosphatases. Proc Natl Acad Sci USA (2011) 108(21):8803–8:1010042108. doi: 10.1073/pnas.1010042108
83. Kato M, Marumo M, Nakayama J, Matsumoto M, Yabe-Nishimura C, Kamata T. The ROS-generating Oxidase Nox1 Is Required for Epithelial Restitution Following Colitis. Exp Anim (2016) 65(3):197–205. doi: 10.1538/expanim.15-0127
84. Moll F, Walter M, Rezende F, Helfinger V, Vasconez E, De Oliveira T, et al. Noxo1 Controls Proliferation of Colon Epithelial Cells. Front Immunol (2018) 9:973. doi: 10.3389/fimmu.2018.00973
85. Alam A, Leoni G, Wentworth CC, Kwal JM, Wu H, Ardita CS, et al. Redox Signaling Regulates Commensal-Mediated Mucosal Homeostasis and Restitution and Requires Formyl Peptide Receptor 1. Mucosal Immunol (2014) 7(3):645–55. doi: 10.1038/mi.2013.84
86. Coant N, Ben Mkaddem S, Pedruzzi E, Guichard C, Treton X, Ducroc R, et al. NADPH Oxidase 1 Modulates WNT and NOTCH1 Signaling to Control the Fate of Proliferative Progenitor Cells in the Colon. Mol Cell Biol (2010) 30(11):2636–50. doi: 10.1128/MCB.01194-09
87. Kajla S, Mondol AS, Nagasawa A, Zhang Y, Kato M, Matsuno K, et al. A Crucial Role for Nox 1 in Redox-Dependent Regulation of Wnt-beta-catenin Signaling. FASEB J (2012) 26(5):2049–59. doi: 10.1096/fj.11-196360
88. Luxen S, Noack D, Frausto M, Davanture S, Torbett BE, Knaus UG. Heterodimerization Controls Localization of Duox-DuoxA NADPH Oxidases in Airway Cells. J Cell Sci (2009) 122(Pt 8):1238–47. doi: 10.1242/jcs.044123. 122/8/1238.
89. Wesley UV, Bove PF, Hristova M, McCarthy S, van der Vliet A. Airway Epithelial Cell Migration and Wound Repair by ATP-Mediated Activation of Dual Oxidase 1. J Biol Chem (2007) 282(5):3213–20. doi: 10.1074/jbc.M606533200
90. Gorissen SH, Hristova M, Habibovic A, Sipsey LM, Spiess PC, Janssen-Heininger YM, et al. Dual Oxidase-1 is Required for Airway Epithelial Cell Migration and Bronchiolar Reepithelialization After Injury. Am J Respir Cell Mol Biol (2013) 48(3):337–45. doi: 10.1165/rcmb.2012-0393OC
91. Wagner CE, Wheeler KM, Ribbeck K. Mucins and Their Role in Shaping the Functions of Mucus Barriers. Annu Rev Cell Dev Biol (2018) 34:189–215. doi: 10.1146/annurev-cellbio-100617-062818
92. Patel KK, Miyoshi H, Beatty WL, Head RD, Malvin NP, Cadwell K, et al. Autophagy Proteins Control Goblet Cell Function by Potentiating Reactive Oxygen Species Production. EMBO J (2013) 32(24):3130–44. doi: 10.1038/emboj.2013.233
93. Birchenough GM, Nystrom EE, Johansson ME, Hansson GC. A Sentinel Goblet Cell Guards the Colonic Crypt by Triggering Nlrp6-Dependent Muc2 Secretion. Science (2016) 352(6293):1535–42. doi: 10.1126/science.aaf7419
94. Aviello G, Singh AK, O’Neill S, Conroy E, Gallagher W, D’Agostino G, et al. Colitis Susceptibility in Mice With Reactive Oxygen Species Deficiency Is Mediated by Mucus Barrier and Immune Defense Defects. Mucosal Immunol (2019) 12(6):1316–26. doi: 10.1038/s41385-019-0205-x
95. Shao MX, Nadel JA. Dual Oxidase 1-Dependent MUC5AC Mucin Expression in Cultured Human Airway Epithelial Cells. Proc Natl Acad Sci USA (2005) 102(3):767–72. doi: 10.1073/pnas.0408932102
96. Habibovic A, Hristova M, Heppner DE, Danyal K, Ather JL, Janssen-Heininger YM, et al. DUOX1 Mediates Persistent Epithelial EGFR Activation, Mucous Cell Metaplasia, and Airway Remodeling During Allergic Asthma. JCI Insight (2016) 1(18):e88811. doi: 10.1172/jci.insight.88811
97. Joo JH, Ryu JH, Kim CH, Kim HJ, Suh MS, Kim JO, et al. Dual Oxidase 2 is Essential for the Toll-Like Receptor 5-Mediated Inflammatory Response in Airway Mucosa. Antioxid Redox Signal (2012) 16(1):57–70. doi: 10.1089/ars.2011.3898
98. Zhang LJ, Gallo RL. Antimicrobial Peptides. Curr Biol (2016) 26(1):R14–9. doi: 10.1016/j.cub.2015.11.017
99. Mahlapuu M, Hakansson J, Ringstad L, Bjorn C. Antimicrobial Peptides: An Emerging Category of Therapeutic Agents. Front Cell Infect Microbiol (2016) 6:194. doi: 10.3389/fcimb.2016.00194
100. Kluver E, Schulz-Maronde S, Scheid S, Meyer B, Forssmann WG, Adermann K. Structure-Activity Relation of Human Beta-Defensin 3: Influence of Disulfide Bonds and Cysteine Substitution on Antimicrobial Activity and Cytotoxicity. Biochemistry (2005) 44(28):9804–16. doi: 10.1021/bi050272k
101. Flo TH, Smith KD, Sato S, Rodriguez DJ, Holmes MA, Strong RK, et al. Lipocalin 2 Mediates an Innate Immune Response to Bacterial Infection by Sequestrating Iron. Nature (2004) 432(7019):917–21. doi: 10.1038/nature03104
102. Chassaing B, Srinivasan G, Delgado MA, Young AN, Gewirtz AT, Vijay-Kumar M. Fecal Lipocalin 2, a Sensitive and Broadly Dynamic Non-Invasive Biomarker for Intestinal Inflammation. PloS One (2012) 7(9):e44328. doi: 10.1371/journal.pone.0044328
103. Makhezer N, Ben Khemis M, Liu D, Khichane Y, Marzaioli V, Tlili A, et al. NOX1-Derived ROS Drive the Expression of Lipocalin-2 in Colonic Epithelial Cells in Inflammatory Conditions. Mucosal Immunol (2019) 12(1):117–31. doi: 10.1038/s41385-018-0086-4
104. Dumont JE, De Deken X, Miot F, Corvilain V, Contempre B, Goyens R, et al. H2O2, Signal, Substrate, Mutagen and Chemorepellent From Physiology to Biochemistry and Disease. Bull Mem Acad R Med Belg (2010) 165(5-6):231–4; discussion 235.
105. Jones RM, Luo L, Ardita CS, Richardson AN, Kwon YM, Mercante JW, et al. Symbiotic Lactobacilli Stimulate Gut Epithelial Proliferation Via Nox-mediated Generation of Reactive Oxygen Species. EMBO J (2013) 32(23):3017–28. doi: 10.1038/emboj.2013.224
106. Sommer F, Backhed F. The Gut Microbiota Engages Different Signaling Pathways to Induce Duox2 Expression in the Ileum and Colon Epithelium. Mucosal Immunol (2015) 8(2):372–9. doi: 10.1038/mi.2014.74
107. Yardeni T, Tanes CE, Bittinger K, Mattei LM, Schaefer PM, Singh LN, et al. Host Mitochondria Influence Gut Microbiome Diversity: A Role for ROS. Sci Signal (2019) 12(588). doi: 10.1126/scisignal.aaw3159
108. Matziouridou C, Rocha SDC, Haabeth OA, Rudi K, Carlsen H, Kielland A. iNOS- and NOX1-Dependent ROS Production Maintains Bacterial Homeostasis in the Ileum of Mice. Mucosal Immunol (2018) 11(3):774–84. doi: 10.1038/mi.2017.106
109. Liu J, Iwata K, Zhu K, Matsumoto M, Matsumoto K, Asaoka N, et al. NOX1/NADPH Oxidase in Bone Marrow-Derived Cells Modulates Intestinal Barrier Function. Free Radic Biol Med (2020) 147:90–101. doi: 10.1016/j.freeradbiomed.2019.12.009
110. De Deken X, Corvilain B, Dumont JE, Miot F. Roles of DUOX-mediated Hydrogen Peroxide in Metabolism, Host Defense, and Signaling. Antioxid Redox Signal (2014) 20(17):2776–93. doi: 10.1089/ars.2013.5602
111. Strengert M, Jennings R, Davanture S, Hayes P, Gabriel G, Knaus UG. Mucosal Reactive Oxygen Species Are Required for Antiviral Response: Role of Duox in Influenza a Virus Infection. Antioxid Redox Signal (2014) 20(17):2695–709. doi: 10.1089/ars.2013.5353
112. Sokol H, Mahlaoui N, Aguilar C, Bach P, Join-Lambert O, Garraffo A, et al. Intestinal Dysbiosis in Inflammatory Bowel Disease Associated With Primary Immunodeficiency. J Allergy Clin Immunol (2019) 143(2):775–778 e6. doi: 10.1016/j.jaci.2018.09.021
113. Falcone EL, Abusleme L, Swamydas M, Lionakis MS, Ding L, Hsu AP, et al. Colitis Susceptibility in p47(phox-/-) Mice Is Mediated by the Microbiome. Microbiome (2016) 4:13. doi: 10.1186/s40168-016-0159-0
114. Ganesh BP, Klopfleisch R, Loh G, Blaut M. Commensal Akkermansia Muciniphila Exacerbates Gut Inflammation in Salmonella Typhimurium-Infected Gnotobiotic Mice. PloS One (2013) 8(9):e74963. doi: 10.1371/journal.pone.0074963
115. Desai MS, Seekatz AM, Koropatkin NM, Kamada N, Hickey CA, Wolter M, et al. A Dietary Fiber-Deprived Gut Microbiota Degrades the Colonic Mucus Barrier and Enhances Pathogen Susceptibility. Cell (2016) 167(5):1339–1353 e21. doi: 10.1016/j.cell.2016.10.043
116. Seregin SS, Golovchenko N, Schaf B, Chen J, Pudlo NA, Mitchell J, et al. Nlrp6 Protects Il10(-/-) Mice From Colitis by Limiting Colonization of Akkermansia Muciniphila. Cell Rep (2017) 19(4):733–45. doi: 10.1016/j.celrep.2017.03.080
117. Matziouridou C, Rocha SDC, Haabeth OA, Rudi K, Carlsen H, Kielland A. iNOS- and NOX1-dependent ROS Production Maintains Bacterial Homeostasis in the Ileum of Mice. Mucosal Immunol (2017) 11(3):774–84. doi: 10.1038/mi.2017.106
118. Caruso R, Mathes T, Martens EC, Kamada N, Nusrat A, Inohara N, et al. A Specific Gene-Microbe Interaction Drives the Development of Crohn’s Disease-Like Colitis in Mice. Sci Immunol (2019) 4(34). doi: 10.1126/sciimmunol.aaw4341
119. Herp S, Brugiroux S, Garzetti D, Ring D, Jochum LM, Beutler M, et al. Mucispirillum Schaedleri Antagonizes Salmonella Virulence to Protect Mice Against Colitis. Cell Host Microbe (2019) 25(5):681–694 e8. doi: 10.1016/j.chom.2019.03.004
120. Pircalabioru G, Aviello G, Kubica M, Zhdanov A, Paclet MH, Brennan L, et al. Defensive Mutualism Rescues NADPH Oxidase Inactivation in Gut Infection. Cell Host Microbe (2016) 19(5):651–63. doi: 10.1016/j.chom.2016.04.007
121. Orecchioni M, Ghosheh Y, Pramod AB, Ley K. Macrophage Polarization: Different Gene Signatures in M1(LPS+) vs. Classically and M2(LPS-) vs. Alternatively Activated Macrophages. Front Immunol (2019) 10:1084. doi: 10.3389/fimmu.2019.01084
122. Barrett JP, Henry RJ, Villapol S, Stoica BA, Kumar A, Burns MP, et al. NOX2 Deficiency Alters Macrophage Phenotype Through an IL-10/STAT3 Dependent Mechanism: Implications for Traumatic Brain Injury. J Neuroinflamm (2017) 14(1):65. doi: 10.1186/s12974-017-0843-4
123. Xu Q, Choksi S, Qu J, Jang J, Choe M, Banfi B, et al. Nadph Oxidases Are Essential for Macrophage Differentiation. J Biol Chem (2016) 291(38):20030–41. doi: 10.1074/jbc.M116.731216
124. Maitra U, Singh N, Gan L, Ringwood L, Li L. IRAK-1 Contributes to Lipopolysaccharide-Induced Reactive Oxygen Species Generation in Macrophages by Inducing NOX-1 Transcription and Rac1 Activation and Suppressing the Expression of Antioxidative Enzymes. J Biol Chem (2009) 284(51):35403–11. doi: 10.1074/jbc.M109.059501
125. Helfinger V, Palfi K, Weigert A, Schroder K. The NADPH Oxidase Nox4 Controls Macrophage Polarization in an NFkappaB-Dependent Manner. Oxid Med Cell Longev (2019) 2019:3264858. doi: 10.1155/2019/3264858
126. Lee CF, Qiao M, Schroder K, Zhao Q, Asmis R. Nox4 is a Novel Inducible Source of Reactive Oxygen Species in Monocytes and Macrophages and Mediates Oxidized Low Density Lipoprotein-Induced Macrophage Death. Circ Res (2010) 106(9):1489–97. doi: 10.1161/CIRCRESAHA.109.215392
127. He C, Larson-Casey JL, Davis D, Hanumanthu VS, Longhini ALF, Thannickal VJ, et al. NOX4 Modulates Macrophage Phenotype and Mitochondrial Biogenesis in Asbestosis. JCI Insight (2019) 4(16). doi: 10.1172/jci.insight.126551
128. Kim YM, Kim SJ, Tatsunami R, Yamamura H, Fukai T, Ushio-Fukai M. ROS-Induced ROS Release Orchestrated by Nox4, Nox2, and Mitochondria in VEGF Signaling and Angiogenesis. Am J Physiol Cell Physiol (2017) 312(6):C749–64. doi: 10.1152/ajpcell.00346.2016
129. Mori K, Uchida T, Yoshie T, Mizote Y, Ishikawa F, Katsuyama M, et al. A Mitochondrial ROS Pathway Controls Matrix Metalloproteinase 9 Levels and Invasive Properties in RAS-activated Cancer Cells. FEBS J (2019) 286(3):459–78. doi: 10.1111/febs.14671
130. Fukai T, Ushio-Fukai M. Cross-Talk Between NADPH Oxidase and Mitochondria: Role in ROS Signaling and Angiogenesis. Cells (2020) 9(8):1849. doi: 10.3390/cells9081849
131. Mills EL, O’Neill LA. Reprogramming Mitochondrial Metabolism in Macrophages as an Anti-Inflammatory Signal. Eur J Immunol (2016) 46(1):13–21. doi: 10.1002/eji.201445427
132. Yang W, Tao Y, Wu Y, Zhao X, Ye W, Zhao D, et al. Neutrophils Promote the Development of Reparative Macrophages Mediated by ROS to Orchestrate Liver Repair. Nat Commun (2019) 10(1):1076. doi: 10.1038/s41467-019-09046-8
133. Campbell EL, Bruyninckx WJ, Kelly CJ, Glover LE, McNamee EN, Bowers BE, et al. Transmigrating Neutrophils Shape the Mucosal Microenvironment Through Localized Oxygen Depletion to Influence Resolution of Inflammation. Immunity (2014) 40(1):66–77. doi: 10.1016/j.immuni.2013.11.020
134. Peiseler M, Kubes P. More Friend Than Foe: The Emerging Role of Neutrophils in Tissue Repair. J Clin Invest (2019) 129(7):2629–39. doi: 10.1172/JCI124616
135. Singel KL, Segal BH. NOX2-Dependent Regulation of Inflammation. Clin Sci (Lond) (2016) 130(7):479–90. doi: 10.1042/CS20150660
136. Eisenbarth SC. Dendritic Cell Subsets in T Cell Programming: Location Dictates Function. Nat Rev Immunol (2019) 19(2):89–103. doi: 10.1038/s41577-018-0088-1
137. Savina A, Jancic C, Hugues S, Guermonprez P, Vargas P, Moura IC, et al. NOX2 Controls Phagosomal Ph to Regulate Antigen Processing During Crosspresentation by Dendritic Cells. Cell (2006) 126(1):205–18. doi: 10.1016/j.cell.2006.05.035
138. Kotsias F, Hoffmann E, Amigorena S, Savina A. Reactive Oxygen Species Production in the Phagosome: Impact on Antigen Presentation in Dendritic Cells. Antioxid Redox Signal (2013) 18(6):714–29. doi: 10.1089/ars.2012.4557
139. Allan ER, Tailor P, Balce DR, Pirzadeh P, McKenna NT, Renaux B, et al. NADPH Oxidase Modifies Patterns of MHC Class II-Restricted Epitopic Repertoires Through Redox Control of Antigen Processing. J Immunol (2014) 192(11):4989–5001. doi: 10.4049/jimmunol.1302896
140. Dingjan I, Verboogen DR, Paardekooper LM, Revelo NH, Sittig SP, Visser LJ, et al. Lipid Peroxidation Causes Endosomal Antigen Release for Cross-Presentation. Sci Rep (2016) 6:22064. doi: 10.1038/srep22064
141. Oberkampf M, Guillerey C, Mouries J, Rosenbaum P, Fayolle C, Bobard A, et al. Mitochondrial Reactive Oxygen Species Regulate the Induction of CD8(+) T Cells by Plasmacytoid Dendritic Cells. Nat Commun (2018) 9(1):2241. doi: 10.1038/s41467-018-04686-8
142. Sanjuan MA, Dillon CP, Tait SW, Moshiach S, Dorsey F, Connell S, et al. Toll-Like Receptor Signalling in Macrophages Links the Autophagy Pathway to Phagocytosis. Nature (2007) 450(7173):1253–7. doi: 10.1038/nature06421
143. Heckmann BL, Boada-Romero E, Cunha LD, Magne J, Green DR. Lc3-Associated Phagocytosis and Inflammation. J Mol Biol (2017) 429(23):3561–76. doi: 10.1016/j.jmb.2017.08.012
144. Huang J, Canadien V, Lam GY, Steinberg BE, Dinauer MC, Magalhaes MA, et al. Activation of Antibacterial Autophagy by NADPH Oxidases. Proc Natl Acad Sci USA (2009) 106(15):6226–31. doi: 10.1073/pnas.0811045106
145. Romao S, Gasser N, Becker AC, Guhl B, Bajagic M, Vanoaica D, et al. Autophagy Proteins Stabilize Pathogen-Containing Phagosomes for Prolonged MHC II Antigen Processing. J Cell Biol (2013) 203(5):757–66. doi: 10.1083/jcb.201308173
146. Cheng YL, Kuo CF, Lu SL, Hiroko O, Wu YN, Hsieh CL, et al. Group A Streptococcus Induces Laposomes Via SLO/beta1 Integrin/Nox2/Ros Pathway in Endothelial Cells That Are Ineffective in Bacterial Killing and Suppress Xenophagy. mBio (2019) 10(5). doi: 10.1128/mBio.02148-19
147. Sciarretta S, Yee D, Ammann P, Nagarajan N, Volpe M, Frati G, et al. Role of NADPH Oxidase in the Regulation of Autophagy in Cardiomyocytes. Clin Sci (Lond) (2015) 128(7):387–403. doi: 10.1042/CS20140336
148. Sarr D, Toth E, Gingerich A, Rada B. Antimicrobial Actions of Dual Oxidases and Lactoperoxidase. J Microbiol (2018) 56(6):373–86. doi: 10.1007/s12275-018-7545-1
149. Boots AW, Hristova M, Kasahara DI, Haenen GR, Bast A, van der Vliet A. ATP-Mediated Activation of the NADPH Oxidase DUOX1 Mediates Airway Epithelial Responses to Bacterial Stimuli. J Biol Chem (2009) 284(26):17858–67. doi: 10.1074/jbc.M809761200
150. Gattas MV, Forteza R, Fragoso MA, Fregien N, Salas P, Salathe M, et al. Oxidative Epithelial Host Defense is Regulated by Infectious and Inflammatory Stimuli. Free Radic Biol Med (2009) 47(10):1450–8. doi: 10.1016/j.freeradbiomed.2009.08.017 S0891-5849(09)00504-8
151. Botteaux A, Hoste C, Dumont JE, Van Sande J, Allaoui A. Potential Role of Noxes in the Protection of Mucosae: H(2)O(2) as a Bacterial Repellent. Microbes Infect (2009) 11(5):537–44. doi: 10.1016/j.micinf.2009.02.009
152. Hayes P, Dhillon S, O’Neill K, Thoeni C, Hui KY, Elkadri A, et al. Defects in NADPH Oxidase Genes NOX1 and DUOX2 in Very Early Onset Inflammatory Bowel Disease. Cell Mol Gastroenterol Hepatol (2015) 1(5):489–502. doi: 10.1016/j.jcmgh.2015.06.005
153. Grasberger H, El-Zaatari M, Dang DT, Merchant JL. Dual Oxidases Control Release of Hydrogen Peroxide by the Gastric Epithelium to Prevent Helicobacter Felis Infection and Inflammation in Mice. Gastroenterology (2013) 145(5):1045–54. doi: 10.1053/j.gastro.2013.07.011
154. Kim HJ, Seo YH, An S, Jo A, Kwon IC, Kim S. Chemiluminescence Imaging of Duox2-Derived Hydrogen Peroxide for Longitudinal Visualization of Biological Response to Viral Infection in Nasal Mucosa. Theranostics (2018) 8(7):1798–807. doi: 10.7150/thno.22481
155. Selemidis S, Seow HJ, Broughton BR, Vinh A, Bozinovski S, Sobey CG, et al. Nox1 Oxidase Suppresses Influenza a Virus-Induced Lung Inflammation and Oxidative Stress. PloS One (2013) 8(4):e60792. doi: 10.1371/journal.pone.0060792
156. To EE, Vlahos R, Luong R, Halls ML, Reading PC, King PT, et al. Selemidis: Endosomal NOX2 Oxidase Exacerbates Virus Pathogenicity and Is a Target for Antiviral Therapy. Nat Commun (2017) 8(1):69. doi: 10.1038/s41467-017-00057-x
157. Winterbourn CC, Kettle AJ, Hampton MB. Reactive Oxygen Species and Neutrophil Function. Annu Rev Biochem (2016) 85:765–92. doi: 10.1146/annurev-biochem-060815-014442
158. Shekhova E. Mitochondrial Reactive Oxygen Species as Major Effectors of Antimicrobial Immunity. PloS Pathog (2020) 16(5):e1008470. doi: 10.1371/journal.ppat.1008470
159. Ware HH, Kulkarni VV, Wang Y, Pantaleon Garcia J, Leiva Juarez M, Kirkpatrick CT, et al. Inducible Lung Epithelial Resistance Requires Multisource Reactive Oxygen Species Generation to Protect Against Bacterial Infections. PloS One (2019) 14(2):e0208216. doi: 10.1371/journal.pone.0208216
160. Marciano BE, Spalding C, Fitzgerald A, Mann D, Brown T, Osgood S, et al. Common Severe Infections in Chronic Granulomatous Disease. Clin Infect Dis (2015) 60(8):1176–83. doi: 10.1093/cid/ciu1154
161. Felmy B, Songhet P, Slack EM, Muller AJ, Kremer M, Van Maele L, et al. NADPH Oxidase Deficient Mice Develop Colitis and Bacteremia Upon Infection With Normally Avirulent, TTSS-1- and TTSS-2-Deficient Salmonella Typhimurium. PloS One (2013) 8(10):e77204. doi: 10.1371/journal.pone.0077204
162. Chan J, Xing Y, Magliozzo RS, Bloom BR. Killing of Virulent Mycobacterium Tuberculosis by Reactive Nitrogen Intermediates Produced by Activated Murine Macrophages. J Exp Med (1992) 175(4):1111–22. doi: 10.1084/jem.175.4.1111
163. Chan J, Tanaka K, Carroll D, Flynn J, Bloom BR. Effects of Nitric Oxide Synthase Inhibitors on Murine Infection With Mycobacterium Tuberculosis. Infect Immun (1995) 63(2):736–40. doi: 10.1128/IAI.63.2.736-740.1995
164. MacMicking JD, North RJ, LaCourse R, Mudgett JS, Shah SK, Nathan CF. Identification of Nitric Oxide Synthase as a Protective Locus Against Tuberculosis. Proc Natl Acad Sci USA (1997) 94(10):5243–8. doi: 10.1073/pnas.94.10.5243
165. Cooper AM, Segal BH, Frank AA, Holland SM, Orme IM. Transient Loss of Resistance to Pulmonary Tuberculosis in p47(phox-/-) Mice. Infect Immun (2000) 68(3):1231–4. doi: 10.1128/iai.68.3.1231-1234.2000
166. Koster S, Upadhyay S, Chandra P, Papavinasasundaram K, Yang G, Hassan A, et al. Mycobacterium Tuberculosis is Protected From NADPH Oxidase and LC3-Associated Phagocytosis by the LCP Protein Cpsa. Proc Natl Acad Sci USA (2017) 114(41):E8711–20. doi: 10.1073/pnas.1707792114
167. Chandra P, He L, Zimmerman M, Yang G, Koster S, Ouimet M, et al. Inhibition of Fatty Acid Oxidation Promotes Macrophage Control of Mycobacterium Tuberculosis. mBio (2020) 11(4). doi: 10.1128/mBio.01139-20
168. Rhen M. Salmonella and Reactive Oxygen Species: A Love-Hate Relationship. J Innate Immun (2019) 11(3):216–26. doi: 10.1159/000496370
169. Warnatsch A, Tsourouktsoglou TD, Branzk N, Wang Q, Reincke S, Herbst S, et al. Reactive Oxygen Species Localization Programs Inflammation to Clear Microbes of Different Size. Immunity (2017) 46(3):421–32. doi: 10.1016/j.immuni.2017.02.013
170. To EE, O’Leary JJ, O’Neill LAJ, Vlahos R, Bozinovski S, Porter CJH, et al. Spatial Properties of Reactive Oxygen Species Govern Pathogen-Specific Immune System Responses. Antioxid Redox Signal (2020) 32(13):982–92. doi: 10.1089/ars.2020.8027
171. Pinegin B, Vorobjeva N, Pashenkov M, Chernyak B. The Role of Mitochondrial ROS in Antibacterial Immunity. J Cell Physiol (2018) 233(5):3745–54. doi: 10.1002/jcp.26117
172. Geng J, Sun X, Wang P, Zhang S, Wang X, Wu H, et al. Kinases Mst1 and Mst2 Positively Regulate Phagocytic Induction of Reactive Oxygen Species and Bactericidal Activity. Nat Immunol (2015) 16(11):1142–52. doi: 10.1038/ni.3268
173. Garaude J, Acin-Perez R, Martinez-Cano S, Enamorado M, Ugolini M, Nistal-Villan E, et al. Mitochondrial Respiratory-Chain Adaptations in Macrophages Contribute to Antibacterial Host Defense. Nat Immunol (2016) 17(9):1037–45. doi: 10.1038/ni.3509
174. Abuaita BH, Schultz TL, O’Riordan MX. Mitochondria-Derived Vesicles Deliver Antimicrobial Reactive Oxygen Species to Control Phagosome-Localized Staphylococcus Aureus. Cell Host Microbe (2018) 24(5):625–636 e5. doi: 10.1016/j.chom.2018.10.005
175. Halverson TW, Wilton M, Poon KK, Petri B, Lewenza S. DNA is an Antimicrobial Component of Neutrophil Extracellular Traps. PloS Pathog (2015) 11(1):e1004593. doi: 10.1371/journal.ppat.1004593
176. Papayannopoulos V. Neutrophil Extracellular Traps in Immunity and Disease. Nat Rev Immunol (2018) 18(2):134–47. doi: 10.1038/nri.2017.105
177. Boeltz S, Amini P, Anders HJ, Andrade F, Bilyy R, Chatfield S, et al. To NET or Not to NET:Current Opinions and State of the Science Regarding the Formation of Neutrophil Extracellular Traps. Cell Death Differ (2019) 26(3):395–408. doi: 10.1038/s41418-018-0261-x
178. Saitoh T, Komano J, Saitoh Y, Misawa T, Takahama M, Kozaki T, et al. Neutrophil Extracellular Traps Mediate a Host Defense Response to Human Immunodeficiency Virus-1. Cell Host Microbe (2012) 12(1):109–16. doi: 10.1016/j.chom.2012.05.015
179. Branzk N, Lubojemska A, Hardison SE, Wang Q, Gutierrez MG, Brown GD, et al. Neutrophils Sense Microbe Size and Selectively Release Neutrophil Extracellular Traps in Response to Large Pathogens. Nat Immunol (2014) 15(11):1017–25. doi: 10.1038/ni.2987
180. Kenny EF, Herzig A, Kruger R, Muth A, Mondal S, Thompson PR, et al. Diverse Stimuli Engage Different Neutrophil Extracellular Trap Pathways. Elife (2017) 6. doi: 10.7554/eLife.24437
181. Bianchi M, Hakkim A, Brinkmann V, Siler U, Seger RA, Zychlinsky A, et al. Restoration of NET Formation by Gene Therapy in CGD Controls Aspergillosis. Blood (2009) 114(13):2619–22. doi: 10.1182/blood-2009-05-221606
182. Moura FA, de Andrade KQ, dos Santos JC, Araujo OR, Goulart MO. Antioxidant Therapy for Treatment of Inflammatory Bowel Disease: Does It Work? Redox Biol (2015) 6:617–39. doi: 10.1016/j.redox.2015.10.006
183. Li CW, Li LL, Chen S, Zhang JX, Lu WL. Antioxidant Nanotherapies for the Treatment of Inflammatory Diseases. Front Bioeng Biotechnol (2020) 8:200. doi: 10.3389/fbioe.2020.00200
184. Bourgonje AR, Feelisch M, Faber KN, Pasch A, Dijkstra G, van Goor H. Oxidative Stress and Redox-Modulating Therapeutics in Inflammatory Bowel Disease. Trends Mol Med (2020) 26(11):1034–46. doi: 10.1016/j.molmed.2020.06.006
185. Kim SJ, Kim HS, Seo YR. Understanding of ROS-Inducing Strategy in Anticancer Therapy. Oxid Med Cell Longev (2019) 2019:5381692. doi: 10.1155/2019/5381692
186. Yang B, Chen Y, Shi J. Reactive Oxygen Species (ROS)-Based Nanomedicine. Chem Rev (2019) 119(8):4881–985. doi: 10.1021/acs.chemrev.8b00626
187. Dunnill C, Patton T, Brennan J, Barrett J, Dryden M, Cooke J, et al. Reactive Oxygen Species (ROS) and Wound Healing: The Functional Role of ROS and Emerging ROS-Modulating Technologies for Augmentation of the Healing Process. Int Wound J (2017) 14(1):89–96. doi: 10.1111/iwj.12557
188. Bertoni S, Machness A, Tiboni M, Bartolo R, Santos HA. Reactive Oxygen Species Responsive Nanoplatforms as Smart Drug Delivery Systems for Gastrointestinal Tract Targeting. Biopolymers (2020) 111(1):e23336. doi: 10.1002/bip.23336
189. Dou Y, Li C, Li L, Guo J, Zhang J. Bioresponsive Drug Delivery Systems for the Treatment of Inflammatory Diseases. J Control Release (2020) 327:641–66. doi: 10.1016/j.jconrel.2020.09.008
190. Mitchell MJ, Billingsley MM, Haley RM, Wechsler ME, Peppas NA, Langer R. Engineering Precision Nanoparticles for Drug Delivery. Nat Rev Drug Discovery (2021) 20(2):101–24. doi: 10.1038/s41573-020-0090-8
191. Tur E, Bolton L, Constantine BE. Topical Hydrogen Peroxide Treatment of Ischemic Ulcers in the Guinea Pig: Blood Recruitment in Multiple Skin Sites. J Am Acad Dermatol (1995) 33(2 Pt 1):217–21. doi: 10.1016/0190-9622(95)90238-4
192. Armogida M, Nistico R, Mercuri NB. Therapeutic Potential of Targeting Hydrogen Peroxide Metabolism in the Treatment of Brain Ischaemia. Br J Pharmacol (2012) 166(4):1211–24. doi: 10.1111/j.1476-5381.2012.01912.x
193. Roy S, Khanna S, Nallu K, Hunt TK, Sen CK. Dermal Wound Healing Is Subject to Redox Control. Mol Ther (2006) 13(1):211–20. doi: 10.1016/j.ymthe.2005.07.684
194. Loo AE, Wong YT, Ho R, Wasser M, Du T, Ng WT, et al. Effects of Hydrogen Peroxide on Wound Healing in Mice in Relation to Oxidative Damage. PloS One (2012) 7(11):e49215. doi: 10.1371/journal.pone.0049215
195. Lavado AS, Chauhan VM, Zen AA, Giuntini F, Jones DR, Boyle RW, et al. Controlled Intracellular Generation of Reactive Oxygen Species in Human Mesenchymal Stem Cells Using Porphyrin Conjugated Nanoparticles. Nanoscale (2015) 7(34):14525–31. doi: 10.1039/c5nr00795j
196. Dharmaraja AT, Chakrapani H. A Small Molecule for Controlled Generation of Reactive Oxygen Species (ROS). Org Lett (2014) 16(2):398–401. doi: 10.1021/ol403300a
197. Wojtovich AP, Foster TH. Optogenetic Control of ROS Production. Redox Biol (2014) 2:368–76. doi: 10.1016/j.redox.2014.01.019
198. Meng X, Zhang X, Lei Y, Cao D, Wang Z. Biodegradable Copper-Metformin Nanoscale Coordination Polymers for Enhanced Chemo/Chemodynamic Synergistic Therapy by Reducing Oxygen Consumption to Promote H2O2 Accumulation. J Mater Chem B (2021) 9(8):1988–2000. doi: 10.1039/d0tb02476g
199. Liu J, Li Y, Chen S, Lin Y, Lai H, Chen B, et al. Biomedical Application of Reactive Oxygen Species-Responsive Nanocarriers in Cancer, Inflammation, and Neurodegenerative Diseases. Front Chem (2020) 8:838. doi: 10.3389/fchem.2020.00838
200. Zhang R, Liu R, Liu C, Pan L, Qi Y, Cheng J, et al. A pH/ROS Dual-Responsive and Targeting Nanotherapy for Vascular Inflammatory Diseases. Biomaterials (2020) 230:119605. doi: 10.1016/j.biomaterials.2019.119605
201. Aswathy SH, Narendrakumar U, Manjubala I. Commercial Hydrogels for Biomedical Applications. Heliyon (2020) 6(4):e03719. doi: 10.1016/j.heliyon.2020.e03719
202. Lee Y, Choi KH, Park KM, Lee JM, Park BJ, Park KD. In Situ Forming and H2O2-Releasing Hydrogels for Treatment of Drug-Resistant Bacterial Infections. ACS Appl Mater Interfaces (2017) 9(20):16890–9. doi: 10.1021/acsami.7b03870
203. Sanders ME, Merenstein DJ, Reid G, Gibson GR, Rastall RA. Probiotics and Prebiotics in Intestinal Health and Disease: From Biology to the Clinic. Nat Rev Gastroenterol Hepatol (2019) 16(10):605–16. doi: 10.1038/s41575-019-0173-3
204. Hertzberger R, Arents J, Dekker HL, Pridmore RD, Gysler C, Kleerebezem M, et al. H(2)O(2) Production in Species of the Lactobacillus Acidophilus Group: A Central Role for a Novel NADH-Dependent Flavin Reductase. Appl Environ Microbiol (2014) 80(7):2229–39. doi: 10.1128/AEM.04272-13
205. Nishiyama Y, Massey V, Takeda K, Kawasaki S, Sato J, Watanabe T, et al. Hydrogen Peroxide-Forming NADH Oxidase Belonging to the Peroxiredoxin Oxidoreductase Family: Existence and Physiological Role in Bacteria. J Bacteriol (2001) 183(8):2431–8. doi: 10.1128/JB.183.8.2431-2438.2001
206. Cornacchione LP, Klein BA, Duncan MJ, Hu LT. Interspecies Inhibition of Porphyromonas Gingivalis by Yogurt-Derived Lactobacillus Delbrueckii Requires Active Pyruvate Oxidase. Appl Environ Microbiol (2019) 85(18). doi: 10.1128/AEM.01271-19
207. Cornacchione LP, Hu LT. Hydrogen Peroxide-Producing Pyruvate Oxidase From Lactobacillus Delbrueckii is Catalytically Activated by Phosphotidylethanolamine. BMC Microbiol (2020) 20(1):128. doi: 10.1186/s12866-020-01788-6
208. Espey MG. Role of Oxygen Gradients in Shaping Redox Relationships Between the Human Intestine and its Microbiota. Free Radic Biol Med (2013) 55:130–40. doi: 10.1016/j.freeradbiomed.2012.10.554
209. Albenberg L, Esipova TV, Judge CP, Bittinger K, Chen J, Laughlin A, et al. Correlation Between Intraluminal Oxygen Gradient and Radial Partitioning of Intestinal Microbiota. Gastroenterology (2014) 147(5):1055–63.e8. doi: 10.1053/j.gastro.2014.07.020
210. Singh AK, Hertzberger RY, Knaus UG. Hydrogen Peroxide Production by Lactobacilli Promotes Epithelial Restitution During Colitis. Redox Biol (2018) 16:11–20. doi: 10.1016/j.redox.2018.02.003
211. Dumas A, Bernard L, Poquet Y, Lugo-Villarino G, Neyrolles O. The Role of the Lung Microbiota and the Gut-Lung Axis in Respiratory Infectious Diseases. Cell Microbiol (2018) 20(12):e12966. doi: 10.1111/cmi.12966
212. Martens K, Pugin B, De Boeck I, Spacova I, Steelant B, Seys SF, et al. Probiotics for the Airways: Potential to Improve Epithelial and Immune Homeostasis. Allergy (2018) 73(10):1954–63. doi: 10.1111/all.13495
213. Vulevic J, Juric A, Walton GE, Claus SP, Tzortzis G, Toward RE, et al. Influence of Galacto-Oligosaccharide Mixture (B-GOS) on Gut Microbiota, Immune Parameters and Metabonomics in Elderly Persons. Br J Nutr (2015) 114(4):586–95. doi: 10.1017/S0007114515001889
214. Vagesjo E, Ohnstedt E, Mortier A, Lofton H, Huss F, Proost P, et al. Accelerated Wound Healing in Mice by On-Site Production and Delivery of CXCL12 by Transformed Lactic Acid Bacteria. Proc Natl Acad Sci USA (2018) 115(8):1895–900. doi: 10.1073/pnas.1716580115
215. Wang Y, Yin Y, Chen X, Zhao Y, Wu Y, Li Y, et al. Induction of Intestinal Th17 Cells by Flagellins From Segmented Filamentous Bacteria. Front Immunol (2019) 10:2750. doi: 10.3389/fimmu.2019.02750
216. Di Cerbo A, Palmieri B, Aponte M, Morales-Medina JC, Iannitti T. Mechanisms and Therapeutic Effectiveness of Lactobacilli. J Clin Pathol (2016) 69(3):187–203. doi: 10.1136/jclinpath-2015-202976
217. Bafeta A, Koh M, Riveros C, Ravaud P. Harms Reporting in Randomized Controlled Trials of Interventions Aimed At Modifying Microbiota: A Systematic Review. Ann Intern Med (2018) 169(4):240–7. doi: 10.7326/M18-0343
218. Jia K, Tong X, Wang R, Song X. The Clinical Effects of Probiotics for Inflammatory Bowel Disease: A Meta-Analysis. Med (Baltimore) (2018) 97(51):e13792. doi: 10.1097/MD.0000000000013792
219. Ghouri YA, Richards DM, Rahimi EF, Krill JT, Jelinek KA, DuPont AW. Systematic Review of Randomized Controlled Trials of Probiotics, Prebiotics, and Synbiotics in Inflammatory Bowel Disease. Clin Exp Gastroenterol (2014) 7:473–87. doi: 10.2147/CEG.S27530
220. Kochan P, Chmielarczyk A, Szymaniak L, Brykczynski M, Galant K, Zych A, et al. Lactobacillus Rhamnosus Administration Causes Sepsis in a Cardiosurgical Patient–Is the Time Right to Revise Probiotic Safety Guidelines? Clin Microbiol Infect (2011) 17(10):1589–92. doi: 10.1111/j.1469-0691.2011.03614.x
221. Kulkarni HS, Khoury CC. Sepsis Associated With Lactobacillus Bacteremia in a Patient With Ischemic Colitis. Indian J Crit Care Med (2014) 18(9):606–8. doi: 10.4103/0972-5229.140152
222. Salminen MK, Rautelin H, Tynkkynen S, Poussa T, Saxelin M, Valtonen V, et al. Lactobacillus Bacteremia, Clinical Significance, and Patient Outcome, With Special Focus on Probiotic L. Rhamnosus GG. Clin Infect Dis (2004) 38(1):62–9. doi: 10.1086/380455
223. Castro-Gonzalez JM, Castro P, Sandoval H, Castro-Sandoval D. Probiotic Lactobacilli Precautions. Front Microbiol (2019) 10:375. doi: 10.3389/fmicb.2019.00375
224. Bartesaghi S, Radi R. Fundamentals on the Biochemistry of Peroxynitrite and Protein Tyrosine Nitration. Redox Biol (2018) 14:618–25. doi: 10.1016/j.redox.2017.09.009
225. Wang Y, Branicky R, Noe A, Hekimi S. Superoxide Dismutases: Dual Roles in Controlling ROS Damage and Regulating ROS Signaling. J Cell Biol (2018) 217(6):1915–28. doi: 10.1083/jcb.201708007
226. Rosa AC, Bruni N, Meineri G, Corsi D, Cavi N, Gastaldi D, et al. Strategies to Expand the Therapeutic Potential of Superoxide Dismutase by Exploiting Delivery Approaches. Int J Biol Macromol (2021) 168:846–65. doi: 10.1016/j.ijbiomac.2020.11.149
227. Savino S, Fraaije MW. The Vast Repertoire of Carbohydrate Oxidases: An Overview. Biotechnol Adv (2020), 107634. doi: 10.1016/j.biotechadv.2020.107634
228. Pollegioni L, Piubelli L, Molla G. Cholesterol Oxidase: Biotechnological Applications. FEBS J (2009) 276(23):6857–70. doi: 10.1111/j.1742-4658.2009.07379.x
229. Goswami P, Chinnadayyala SS, Chakraborty M, Kumar AK, Kakoti A. An Overview on Alcohol Oxidases and Their Potential Applications. Appl Microbiol Biotechnol (2013) 97(10):4259–75. doi: 10.1007/s00253-013-4842-9
230. Sacchi S, Cappelletti P, Murtas G. Biochemical Properties of Human D-Amino Acid Oxidase Variants and Their Potential Significance in Pathologies. Front Mol Biosci (2018) 5:55. doi: 10.3389/fmolb.2018.00055
231. Bankar SB, Bule MV, Singhal RS, Ananthanarayan L. Glucose Oxidase–An Overview. Biotechnol Adv (2009) 27(4):489–501. doi: 10.1016/j.biotechadv.2009.04.003
232. Yoo EH, Lee SY. Glucose Biosensors: An Overview of Use in Clinical Practice. Sensors (Basel) (2010) 10(5):4558–76. doi: 10.3390/s100504558
233. Zhao L, Wang L, Zhang Y, Xiao S, Bi F, Zhao J, et al. Glucose Oxidase-Based Glucose-Sensitive Drug Delivery for Diabetes Treatment. Polymers (Basel) (2017) 9(7):255. doi: 10.3390/polym9070255
234. Harris JM, Reyes C, Lopez GP. Common Causes of Glucose Oxidase Instability in In Vivo Biosensing: A Brief Review. J Diabetes Sci Technol (2013) 7(4):1030–8. doi: 10.1177/193229681300700428
235. Arul V, Masilamoni JG, Jesudason EP, Jaji PJ, Inayathullah M, Dicky John DG, et al. Glucose Oxidase Incorporated Collagen Matrices for Dermal Wound Repair in Diabetic Rat Models: A Biochemical Study. J Biomater Appl (2012) 26(8):917–38. doi: 10.1177/0885328210390402
236. Cooke J, Dryden M, Patton T, Brennan J, Barrett J. The Antimicrobial Activity of Prototype Modified Honeys That Generate Reactive Oxygen Species (ROS) Hydrogen Peroxide. BMC Res Notes (2015) 8:20. doi: 10.1186/s13104-014-0960-4
237. Jull AB, Cullum N, Dumville JC, Westby MJ, Deshpande S, Walker N. Honey as a Topical Treatment for Wounds. Cochrane Database Syst Rev (2015) 3:CD005083. doi: 10.1002/14651858.CD005083.pub4
238. Raveendran S, Parameswaran B, Ummalyma SB, Abraham A, Mathew AK, Madhavan A, et al. Applications of Microbial Enzymes in Food Industry. Food Technol Biotechnol (2018) 56(1):16–30. doi: 10.17113/ftb.56.01.18.5491
239. Kim BY, Lee Y, Son JY, Park KM, Park KD. Dual Enzyme-Triggered in Situ Crosslinkable Gelatin Hydrogels for Artificial Cellular Microenvironments. Macromol Biosci (2016) 16(11):1570–6. doi: 10.1002/mabi.201600312
240. Lee Y, Son JY, Kang JI, Park KM, Park KD. Hydrogen Peroxide-Releasing Hydrogels for Enhanced Endothelial Cell Activities and Neovascularization. ACS Appl Mater Interfaces (2018) 10(21):18372–9. doi: 10.1021/acsami.8b04522
241. Tsukahara T, Koyama H, Okada M, Ushida K. Stimulation of Butyrate Production by Gluconic Acid in Batch Culture of Pig Cecal Digesta and Identification of Butyrate-Producing Bacteria. J Nutr (2002) 132(8):2229–34. doi: 10.1093/jn/132.8.2229
242. Stenke E, Bourke B, Knaus UG. Napdh Oxidases in Inflammatory Bowel Disease. Methods Mol Biol (2019) 1982:695–713. doi: 10.1007/978-1-4939-9424-3_38
243. Pazmandi J, Kalinichenko A, Ardy RC, Boztug K. Early-Onset Inflammatory Bowel Disease as a Model Disease to Identify Key Regulators of Immune Homeostasis Mechanisms. Immunol Rev (2019) 287(1):162–85. doi: 10.1111/imr.12726
244. Dang PM, Rolas L, El-Benna J. The Dual Role of Reactive Oxygen Species-Generating Nicotinamide Adenine Dinucleotide Phosphate Oxidases in Gastrointestinal Inflammation and Therapeutic Perspectives. Antioxid Redox Signal (2020) 33(5):354–73. doi: 10.1089/ars.2020.8018
245. Dhillon SS, Fattouh R, Elkadri A, Xu W, Murchie R, Walters T, et al. Variants in Nicotinamide Adenine Dinucleotide Phosphate Oxidase Complex Components Determine Susceptibility to Very Early Onset Inflammatory Bowel Disease. Gastroenterology (2014) 147(3):680–9.e2. doi: 10.1053/j.gastro.2014.06.005
246. Parlato M, Charbit-Henrion F, Hayes P, Tiberti A, Aloi M, Cucchiara S, et al. First Identification of Biallelic Inherited DUOX2 Inactivating Mutations as a Cause of Very Early Onset Inflammatory Bowel Disease. Gastroenterology (2017) 153(2):609–611 e3. doi: 10.1053/j.gastro.2016.12.053
247. Schwerd T, Bryant RV, Pandey S, Capitani M, Meran L, Cazier JB, et al. NOX1 Loss-of-Function Genetic Variants in Patients With Inflammatory Bowel Disease. Mucosal Immunol (2018) 11(2):562–74. doi: 10.1038/mi.2017.74
248. Lipinski S, Petersen BS, Barann M, Piecyk A, Tran F, Mayr G, et al. Missense Variants in NOX1 and p22phox in a Case of Very-Early-Onset Inflammatory Bowel Disease are Functionally Linked to NOD2. Cold Spring Harb Mol Case Stud (2019) 5(1). doi: 10.1101/mcs.a002428
249. Hultqvist M, Olofsson P, Wallner FK, Holmdahl R. Pharmacological Potential of NOX2 Agonists in Inflammatory Conditions. Antioxid Redox Signal (2015) 23(5):446–59. doi: 10.1089/ars.2013.5788
250. Jones HR, Robb CT, Perretti M, Rossi AG. The Role of Neutrophils in Inflammation Resolution. Semin Immunol (2016) 28(2):137–45. doi: 10.1016/j.smim.2016.03.007
251. Reshetnikov V, Hahn J, Maueroder C, Czegley C, Munoz LE, Herrmann M, et al. Chemical Tools for Targeted Amplification of Reactive Oxygen Species in Neutrophils. Front Immunol (2018) 9:1827. doi: 10.3389/fimmu.2018.01827
252. Lind S, Dahlgren C, Holmdahl R, Olofsson P, Forsman H. Functional Selective FPR1 Signaling in Favor of an Activation of the Neutrophil Superoxide Generating NOX2 Complex. J Leukoc Biol (2020). doi: 10.1002/JLB.2HI0520-317R
253. Asahina Y, Wurtz NR, Arakawa K, Carson N, Fujii K, Fukuchi K, et al. Discovery of BMS-986235/LAR-1219: A Potent Formyl Peptide Receptor 2 (Fpr2) Selective Agonist for the Prevention of Heart Failure. J Med Chem (2020) 63(17):9003–19. doi: 10.1021/acs.jmedchem.9b02101
254. Stalder AK, Lott D, Strasser DS, Cruz HG, Krause A, Groenen PM, et al. Biomarker-Guided Clinical Development of the First-in-Class Anti-Inflammatory FPR2/ALX Agonist ACT-389949. Br J Clin Pharmacol (2017) 83(3):476–86. doi: 10.1111/bcp.13149
255. Qin CX, May LT, Li R, Cao N, Rosli S, Deo M, et al. Small-Molecule-Biased Formyl Peptide Receptor Agonist Compound 17b Protects Against Myocardial Ischaemia-Reperfusion Injury in Mice. Nat Commun (2017) 8:14232. doi: 10.1038/ncomms14232
256. Sundqvist M, Christenson K, Gabl M, Holdfeldt A, Jennbacken K, Moller TC, et al. Staphylococcus Aureus-Derived PSMalpha Peptides Activate Neutrophil FPR2 But Lack the Ability to Mediate Beta-Arrestin Recruitment and Chemotaxis. J Immunol (2019) 203(12):3349–60. doi: 10.4049/jimmunol.1900871
257. Weisbart RH, Kwan L, Golde DW, Gasson JC. Human GM-CSF Primes Neutrophils for Enhanced Oxidative Metabolism in Response to the Major Physiological Chemoattractants. Blood (1987) 69(1):18–21. doi: 10.1182/blood.V69.1.18.18
258. Leino L, Nuutila J, Pelliniemi TT, Rajamaki A. Human Recombinant GM-CSF Selectively Primes Receptor Mediated Respiratory Burst of Neutrophils In Vitro. Immunol Lett (1993) 38(1):26–31. doi: 10.1016/0165-2478(93)90114-h
259. Subramanian Vignesh K, Landero Figueroa JA, Porollo A, Caruso JA, Deepe GS Jr. Granulocyte Macrophage-Colony Stimulating Factor Induced Zn Sequestration Enhances Macrophage Superoxide and Limits Intracellular Pathogen Survival. Immunity (2013) 39(4):697–710. doi: 10.1016/j.immuni.2013.09.006
260. Presneill JJ, Harris T, Stewart AG, Cade JF, Wilson JW. A Randomized Phase II Trial of Granulocyte-Macrophage Colony-Stimulating Factor Therapy in Severe Sepsis With Respiratory Dysfunction. Am J Respir Crit Care Med (2002) 166(2):138–43. doi: 10.1164/rccm.2009005
261. Muhlebach TJ, Feickert HJ, Welte K, Seger RA. Granulocyte-Macrophage Colony Stimulating Factor Does Not Improve Neutrophil Oxidative Metabolism in a Patient With Variant X-Linked Chronic Granulomatous Disease. Eur J Pediatr (1991) 150(8):575–8. doi: 10.1007/BF02072210
262. Oez S, Birkmann J, Kalden JR. Clonal Growth of Functionally Normal and Deficient Neutrophils From the Bone Marrow of a Patient With Variant Chronic Granulomatous Disease. Lack of Reconstitution of Oxidative Burst Defect by G-CSF, Gm-CSF, and IFN-gamma In Vitro. Ann Hematol (1993) 66(1):21–5. doi: 10.1007/BF01737685
263. Wang J, Mayer L, Cunningham-Rundles C. Use of GM-CSF in the Treatment of Colitis Associated With Chronic Granulomatous Disease. J Allergy Clin Immunol (2005) 115(5):1092–4. doi: 10.1016/j.jaci.2005.01.016
264. Kuhns DB, Alvord WG, Heller T, Feld JJ, Pike KM, Marciano BE, et al. Residual NADPH Oxidase and Survival in Chronic Granulomatous Disease. N Engl J Med (2010) 363(27):2600–10. doi: 10.1056/NEJMoa1007097
265. Deffert C, Carnesecchi S, Yuan H, Rougemont AL, Kelkka T, Holmdahl R, et al. Hyperinflammation of Chronic Granulomatous Disease Is Abolished by NOX2 Reconstitution in Macrophages and Dendritic Cells. J Pathol (2012) 228(3):341–50. doi: 10.1002/path.4061
266. Fernandez-Boyanapalli RF, Frasch SC, Thomas SM, Malcolm KC, Nicks M, Harbeck RJ, et al. Pioglitazone Restores Phagocyte Mitochondrial Oxidants and Bactericidal Capacity in Chronic Granulomatous Disease. J Allergy Clin Immunol (2015) 135(2):517–27.e12. doi: 10.1016/j.jaci.2014.10.034
267. Hule GP, Bargir UA, Kulkarni M, Kambli P, Taur P, Desai M, et al. Does Pioglitazone Lead to Neutrophil Extracellular Traps Formation in Chronic Granulomatous Disease Patients? Front Immunol (2019) 10:1739. doi: 10.3389/fimmu.2019.01739
268. Fernandez-Boyanapalli R, Frasch SC, Riches DW, Vandivier RW, Henson PM, Bratton DL. Ppargamma Activation Normalizes Resolution of Acute Sterile Inflammation in Murine Chronic Granulomatous Disease. Blood (2010) 116(22):4512–22. doi: 10.1182/blood-2010-02-272005
269. Fernandez-Boyanapalli RF, Falcone EL, Zerbe CS, Marciano BE, Frasch SC, Henson PM, et al. Impaired Efferocytosis in Human Chronic Granulomatous Disease Is Reversed by Pioglitazone Treatment. J Allergy Clin Immunol (2015) 136(5):1399–401.e3. doi: 10.1016/j.jaci.2015.07.034
270. Migliavacca M, Assanelli A, Ferrua F, Cicalese MP, Biffi A, Frittoli M, et al. Pioglitazone as a Novel Therapeutic Approach in Chronic Granulomatous Disease. J Allergy Clin Immunol (2016) 137(6):1913–5.e2. doi: 10.1016/j.jaci.2016.01.033
271. Hui X, Liu D, Wang W, Hou J, Ying W, Zhou Q, et al. Low-Dose Pioglitazone Does Not Increase Ros Production in Chronic Granulomatous Disease Patients With Severe Infection. J Clin Immunol (2020) 40(1):131–7. doi: 10.1007/s10875-019-00719-z
272. Gennery AR. Progress in Treating Chronic Granulomatous Disease. Br J Haematol (2021) 192(2):251–64. doi: 10.1111/bjh.16939
273. Knaus UG, Hertzberger R, Pircalabioru GG, Yousefi SP, Branco Dos Santos F. Pathogen Control At the Intestinal Mucosa - H2O2 to the Rescue. Gut Microbes (2017) 8(1):67–74. doi: 10.1080/19490976.2017.1279378
274. Allen RC, Popat R, Diggle SP, Brown SP. Targeting Virulence: Can We Make Evolution-Proof Drugs? Nat Rev Microbiol (2014) 12(4):300–8. doi: 10.1038/nrmicro3232
275. Winter SE, Thiennimitr P, Winter MG, Butler BP, Huseby DL, Crawford RW, et al. Gut Inflammation Provides a Respiratory Electron Acceptor for Salmonella. Nature (2010) 467(7314):426–9. doi: 10.1038/nature09415
276. Miller BM, Liou MJ, Zhang LF, Nguyen H, Litvak Y, Schorr EM, et al. Anaerobic Respiration of NOX1-Derived Hydrogen Peroxide Licenses Bacterial Growth At the Colonic Surface. Cell Host Microbe (2020) 28(6):789–797 e5. doi: 10.1016/j.chom.2020.10.009
277. Wagner PL, Acheson DW, Waldor MK. Human Neutrophils and Their Products Induce Shiga Toxin Production by Enterohemorrhagic Escherichia Coli. Infect Immun (2001) 69(3):1934–7. doi: 10.1128/IAI.69.3.1934-1937.2001
278. Los JM, Los M, Wegrzyn A, Wegrzyn G. Hydrogen Peroxide-Mediated Induction of the Shiga Toxin-Converting Lambdoid Prophage ST2-8624 in Escherichia Coli O157:H7. FEMS Immunol Med Microbiol (2010) 58(3):322–9. doi: 10.1111/j.1574-695X.2009.00644.x
279. Gomez SA, Abrey-Recalde MJ, Panek CA, Ferrarotti NF, Repetto MG, Mejias MP, et al. The Oxidative Stress Induced In Vivo by Shiga Toxin-2 Contributes to the Pathogenicity of Haemolytic Uraemic Syndrome. Clin Exp Immunol (2013) 173(3):463–72. doi: 10.1111/cei.12124
280. Rowe SE, Wagner NJ, Li L, Beam JE, Wilkinson AD, Radlinski LC, et al. Reactive Oxygen Species Induce Antibiotic Tolerance During Systemic Staphylococcus Aureus Infection. Nat Microbiol (2020) 5(2):282–90. doi: 10.1038/s41564-019-0627-y
Keywords: reactive oxygen species, NADPH oxidase, microbiota, host defense, immune signaling, redox medicine, lactobacilli, glucose oxidase
Citation: Dumas A and Knaus UG (2021) Raising the ‘Good’ Oxidants for Immune Protection. Front. Immunol. 12:698042. doi: 10.3389/fimmu.2021.698042
Received: 20 April 2021; Accepted: 18 May 2021;
Published: 04 June 2021.
Edited by:
Michael Walch, Université de Fribourg, SwitzerlandReviewed by:
Helmut Sies, Heinrich Heine University of Düsseldorf, GermanyTobias Dallenga, Research Center Borstel (LG), Germany
Copyright © 2021 Dumas and Knaus. This is an open-access article distributed under the terms of the Creative Commons Attribution License (CC BY). The use, distribution or reproduction in other forums is permitted, provided the original author(s) and the copyright owner(s) are credited and that the original publication in this journal is cited, in accordance with accepted academic practice. No use, distribution or reproduction is permitted which does not comply with these terms.
*Correspondence: Ulla G. Knaus, dWxsYS5rbmF1c0B1Y2QuaWU=