- 1Guang’anmen Hospital, China Academy of Chinese Medical Sciences, Beijing, China
- 2China-Japan Friendship Hospital, Institute of Clinical Medical Sciences, Beijing, China
Diabetic nephropathy (DN) often leads to end-stage renal disease. Oxidative stress demonstrates a crucial act in the onset and progression of DN, which triggers various pathological processes while promoting the activation of inflammation and forming a vicious oxidative stress-inflammation cycle that induces podocyte injury, extracellular matrix accumulation, glomerulosclerosis, epithelial-mesenchymal transition, renal tubular atrophy, and proteinuria. Conventional treatments for DN have limited efficacy. Polyphenols, as antioxidants, are widely used in DN with multiple targets and fewer adverse effects. This review reveals the oxidative stress and oxidative stress-associated inflammation in DN that led to pathological damage to renal cells, including podocytes, endothelial cells, mesangial cells, and renal tubular epithelial cells. It demonstrates the potent antioxidant and anti-inflammatory properties by targeting Nrf2, SIRT1, HMGB1, NF-κB, and NLRP3 of polyphenols, including quercetin, resveratrol, curcumin, and phenolic acid. However, there remains a long way to a comprehensive understanding of molecular mechanisms and applications for the clinical therapy of polyphenols.
1 Introduction
Diabetes mellitus (DM) is a complicated chronic disease described by glucose dysregulation that is caused by absolute or relative defects, including type 1 diabetes (T1D) and type 2 diabetes (T2D). The global prevalence indicates that the number of DM is below half a billion individuals and is projected to increase by 25% and 51% in 2030 and 2045, respectively, putting enormous pressure on healthcare systems worldwide (1). As a complication of DM, diabetic nephropathy (DN), also called diabetic kidney disease (DKD), is the primary reason for chronic kidney disease (CKD) and even end-stage renal disease (ESRD), which is related to increased morbidity and mortality in patients with diabetes. Moreover, approximately 30%-40% of diabetic patients with DN (2). Patients with DN require maintenance dialysis or a kidney transplant. However, these therapy approaches bring a considerable economic and psychological burden and consume substantial medical resources. Therefore, promoting the amelioration of DN is of vital clinical significance (3).
The pathogenesis of DN is complicated and still needs to be determined. It has been proven that rigorous management of blood pressure and blood glucose cannot prevent the progression of DN to ESRD, nor can it prevent DN-related deaths. Developing the understanding and research of DN’s pathogenesis is crucial for expanding new methods for DN (4). Multiple pathways and mediators, including oxidative stress, inflammation, and angiotensin II (Ang-II), are involved in the incidence and progression of DN, of which oxidative stress is the most prominent (5, 6). Chronic hyperglycemia induces oxidative stress, promotes excess reactive oxygen species (ROS) production, reduces antioxidant capacity, induces the damage of oxidative stress in DNA and proteins, and stimulates the immune system to release inflammatory mediators and cytokines that affect glomerular capillaries and changes in renal tubular structure and function, thereby exacerbating renal and systemic damage. High glucose (HG)-induced overproduction of ROS is the primary initiator of cell damage in diabetes and its complications. For DN, although several therapies have been developed (7), such as Sodium-glucose cotransporter 2 (SGLT2), which effectively reduces inflammation and oxidative stress and has been shown to reduce the risk of major adverse events and progression of renal disease in patients with T2D, the ultimate treatment effect is not satisfactory (8).
Natural products, primarily from herbal sources, have long been explored as sources of drugs to treat a variety of major diseases. To date, significant efforts have been made to support and validate the potential effectiveness of natural and synthetic products in experimental studies and clinical applications, and indicating the antioxidant effects for kidney (9, 10). In preclinical studies, many natural products have recently been reported to alleviate kidney disease by modulating oxidative stress and inflammation (11). As a natural product, polyphenols are widely distributed in most plants and classified as phenolic acids, flavonoids, stilbenes, and lignans according to their structural properties (12).Nuclear factor E2-related factor 2 (Nrf2) is a significant regulator of antioxidant enzymes that protect the body from oxidative stress and inflammation, and Nrf2/antioxidant response element (ARE) signaling has been suggested as a promising target against oxidative stress-mediated diseases, such as diabetes and fibrosis. Dietary polyphenols, such as resveratrol, curcumin, and quercetin, can modulate Nrf2 signaling by mediating various kinases upstream of Nrf2 and also directly activate the expression of Nrf2 as well as downstream targets, such as heme oxygenase 1(HO-1) superoxide dismutase(SOD), and catalase (CAT), to inhibit oxidative stress and regulate inflammatory mediators (13–15).
Polyphenols exhibit anti-diabetic potential by reducing intestinal glucose absorption, increasing insulin secretion from pancreatic cells, and modulating gut microbiota and its metabolites (16–19). Natural polyphenols have wide-ranging pharmacological activities, particularly antioxidant activity and free radical-scavenging ability; moreover, they have been gradually used in the research of DN in recent years (20). This review provides a comprehensive summary of oxidative stress and oxidative stress-induced inflammation in DN and polyphenols targeting oxidative stress and inflammation to delay the progression of DN, which provides new insights into polyphenols as promising drug candidates for DN.
2 Oxidative stress and inflammation in DN
2.1 Role of oxidative stress
Oxidative stress is defined as an excessive accumulation of ROS caused by an imbalance between oxidants and antioxidants, resulting in oxidative damage to the body. Moderate ROS-mediated damage can usually be reversed, but excessive ROS production beyond the self-regulatory process often leads to irreversible damage to cellular function or death (21).ROS includes superoxide anion, hydrogen peroxide (H2O2), nitric oxide (NO), hydroxyl radical, and peroxynitrite (22). Mitochondria induce the production of ROS through the mitochondrial respiratory chain, which is the primary source of ROS. Other sources include nicotinamide adenine dinucleotide phosphate (NADPH) oxidase (NOX), changes in glucose metabolism, including polyol pathway flux, and changes in hemodynamics, advanced glycation end products (AGEs), and protein kinase C (PKC) (23, 24). Basal ROS levels are critical for maintaining various cellular tissue functions, such as gene expression, molecular transcription, and signaling transduction (25). However, excessive ROS accelerates pathological states, including the progression of DN, whereas the antioxidant defense system is activated to eliminate ROS generated from varied sources. Antioxidant enzymes include SOD, glutathione peroxidase (GSH-Px), CAT, glutathione reductase (GR), and paraoxonase (26). As markers of oxidative damage, malondialdehyde (MDA) and protein carbonyl can exacerbate oxidative damage in the body. Oxidative stress significantly affects the cause, onset, and process of DN, and hyperglycemia triggers the activation of the polyol pathway, AGEs, the receptor for advanced glycation end products (RAGE), and PKC. AGEs-RAGE signaling pathway activation potentiates the action of NOX and stimulates the production of ROS (27). Subsequently, ROS further interacted with NOX, aggravating the production of ROS (28). DN is associated with hypoxia, the generation of Ang II, and the production of ROS, leading to actin cytoskeleton reorganization of the podocyte, which induces podocyte injury, disrupts the glomerular filtration barrier and triggers proteinuria (29). Moreover, Ang II interferes with the formulation of ROS via the PKC/NADPH oxidase pathway, and ROS production is inhibited following the knockdown of PKC (30).
2.2 Oxidative stress and inflammation
Factors such as hyperglycemia and hypoxia induce oxidative stress and inflammatory responses. Additionally, along with inducing tissue oxidative stress damage, the release of ROS triggers the aggregation of inflammatory cells and the formulation of inflammatory cytokines, growth factors, and transcription factors related to the pathological process of DN (31). The infiltration of inflammatory cells, such as lymphocytes, neutrophils, and macrophages, contributes to kidney injury in DN (32). The recruitment and differentiation of immune-inflammatory cells are regulated by numerous inflammatory cytokines, such as nuclear factor-kappaB (NF-κB), NOD-like receptor family pyrin domain containing 3 (NLRP3), interleukin-1β (IL-1β), IL-6, tumor necrosis factor-α (TNF-α), monocyte chemoattractant protein-1 (MCP-1), intercellular adhesion molecule-1 (ICAM-1) and transforming growth factor-β (TGF-β). These factors are expressed in renal vascular endothelial cells (ECs), podocytes, mesangial cells (MCs), fibroblasts, monocytes, macrophages, and renal tubular epithelial cells (RTECs) (33). NF-κB, a key class of nuclear transcription factors, is involved in immune-inflammatory responses, oxidative stress, and apoptosis (34). NF-κB typically exists in the cytoplasm in an inactive form, such as a heterodimer. It is transported to the nucleus when stimulated by various factors, such as ROS. It binds to the NF-κB binding site to activate the transcription of NF-κB and promote the release of adhesion molecules and pro-inflammatory factors, including MCP-1, ICAM-1, TGF-β1, IL-1β, IL-6, and TNF-α, which can also regulate ROS level (35). HG induces the expression of receptor activator of NF-κB in podocytes, which increases the expression of NOX4 and P22phox and mediates the progression of DN (36).
NLRP3 inflammasome is a multimeric protein complex that induces physiological and pathological inflammatory responses by sensing pathogens, mediates cell pyroptosis, and is a critical downstream factor of NF-κB. Moreover, NLRP3 inflammasome can be triggered by ROS, aggravating the inflammatory cascade and cell damage. Additionally, it mediates the release of inflammatory factors, triggers mitochondrial dysfunction, and promotes ROS formation (37). The inhibition of NLRP3 inflammasome improves renal function by markedly inhibiting HG-induced activation of NF-κB p65, the production of mitochondrial ROS (38). In in vivo and in vitro studies, HG induces infiltration of inflammatory cells, including macrophages; stimulates the formulation of inflammatory factors, including IL-1β, IL-6, MCP-1, and TNF-α; triggers oxidative stress; induces MDA expression; inhibits Nrf2 pathway activation; and diminishes the production of downstream enzymes, such as HO-1, NADPH quinone oxidoreductase-1 (NQO-1), GSH-Px, SOD and CAT (39, 40).
High mobility group box 1(HMGB1), a typical intranuclear non-histone protein, reaches the nucleus by both active secretion and passive release. Once transported to the cytoplasm, it is involved in the immune response. In contrast, when released outside the cell, it can act as a potent inflammatory mediator, either alone or as part of a pro-inflammatory cascade response to stimulate the immune system (41). Interestingly, HMGB1 is regulated by ROS and NLRP3, translocates from the nucleus to the cytoplasm, binds toll-like receptor (TLR)4, activates NF-κB, and ultimately induces an inflammatory response (42).Relatively, HMGB1 induces mitochondrial dysfunction, such as increased ROS production and mitochondrial fission (43, 44).Conversely, H2O2 in the mitochondria and nucleus induces the formation and secretion of HMGB1, which promotes inflammatory responses (45).
Oxidative stress and inflammation interact in the pathological mechanism of DN, disrupting the structure and function of the kidney. The foot processes (FP) of podocytes are lost or fused, which leads to podocyte hypertrophy and reduced levels of podocyte-related proteins, such as nephrin and podocin (46) adhesion and platelet thrombosis on ECs, which triggers endothelial dysfunction (47) production of fibronectin (FN), and collagen IV; and accumulation of extracellular matrix (ECM) in MCs, which leads to glomerulosclerosis (48). The inhibition of NF-κB translocation improves mesangial cell fibrosis and subsequently improves ECM accumulation (49). Moreover, Epithelial-mesenchymal transition (EMT) is induced by TGF-β, which is closely related to tubulointerstitial fibrosis (50, 51). Many studies support that oxidative stress and inflammation are interdependent and interconnected processes that coexist in an inflammatory environment. Inflammatory cells release large amounts of ROS at the site of inflammation, leading to increased oxidative damage. In addition, many ROS and oxidative stress products enhance the pro-inflammatory response. Inhibition of inflammatory response and oxidative stress, reduction in the number of renal collagen fibers and glomerulosclerosis, restoration of glomerular barrier function, persistent reduction in proteinuria (52), and reduction in the generation of serum creatinine (Scr) and blood urea nitrogen (BUN), recover renal function and ameliorate the process of DN to ESRD (53, 54).
2.3 Renal impairment
Oxidative stress-induced kidney damage includes direct and indirect mechanisms. ROS may damage the DNA, proteins, and lipids of tissue cells, directly leading to pathological changes in the glomeruli, renal tubules, and renal interstitium, including cellular components, such as decreased levels of nephrin, podocin, and podocyte-related proteins (55), fusion, and disappearance of FP, hypertrophy, and apoptosis of podocyte (56). Oxidative stress exacerbates the release of oxidative stress markers and inflammatory mediators of vascular ECs, such as MDA, TNF-α, and IL-6 (57). These factors reduce NO and endothelial NO synthase expression levels, activating the endothelin-1 signaling pathway (58) and exacerbating vascular smooth muscle cell aging while inducing vascular calcification (59). ROS promotes the expression of collagen IV, FN, and laminin and the accumulation of ECM in HG-induced MCs (60, 61). Oxidative stress affects autophagy (62) and promotes lipid accumulation (63), EMT, and apoptosis in RTECs (64). Ultimately, oxidative stress exacerbates progressive glomerular damage, tubular atrophy, and interstitial fibrosis, leading to decreased renal function and renal failure (65). As the primary source of ROS, the mitochondria are also the organelles that supply energy to the body. The accumulation of ROS can damage the mitochondria, including an imbalance of mitochondrial fission and mitochondrial fusion, mitochondrial mitophagy, downregulation of respiratory chain complexes, insufficient ATP synthesis, interruption of mitochondrial membrane potential, the discharge of cytochrome-c and the generation of caspase-3, leading to mitochondrial damage (66), which are considered a significant factor in glomerular and tubular necrosis and apoptosis (67, 68).
Indirectly, diversified signalling pathways, such as AGE-RAGE (69), Kelch-like ECH-associated protein(Keap1)-Nrf2 (70), AMP-activated protein kinase (AMPK)/Sirtuin-1 (Sirt1) (71), SIRT1-forkhead transcription factor O (FOXO) (72), Sirt1 (73), NF-κB (74) and HMGB1 could be induced by oxidative stress. These pathways not only lead to oxidative stress injury but also stimulate the release of inflammatory and apoptotic factors, which induce inflammation and apoptosis. Primarily, oxidative stress is linked with variations in renal hemodynamics and metabolism, and chronic hyperglycemia-induced oxidative stress can induce elevated levels of Ang-II and activation of PKC (75), glycolipid disorders (76) and insulin resistance (77), which are important stimulants that promote oxidative stress. Ang-II activates NOX, which produces superoxide, thereby promoting renal vascular remodeling and increasing preglomerular resistance (78).PKC promotes significant upregulation of NOX4 and enhances the generation of ROS (79). Additionally, PKC can lead to insulin resistance and exacerbate insulin dysfunction (80). Podocytes contain insulin receptors, maintain insulin signal transduction and podocyte function, specifically knock out insulin receptors on podocytes, decrease autophagy-related proteins, such as Beclin1 and light chain 3 (LC3), cause autophagy disorders and podocyte injury (81). HG can upregulate the expression of AGEs and RAGE; induce the activities of PKCα, PKCβ, and NOX4; promote the production of ROS; destroy the mitochondrial function of human renal MCs, and affect the renal function of db/db mice (79). ROS induces the accumulation of excess lipids and the formulation of growth factors, including TGF-β, vascular endothelial growth factor (VEGF), and inflammatory factors, which are essential factors that lead to renal dysfunction and subsequent nephropathy.ROS mediates HMGB1/mitochondrial DNA signaling, promotes TLRs activation and inflammatory responses, and exacerbates EMT in proximal renal tubular epithelial cells (82) (Figure 1).
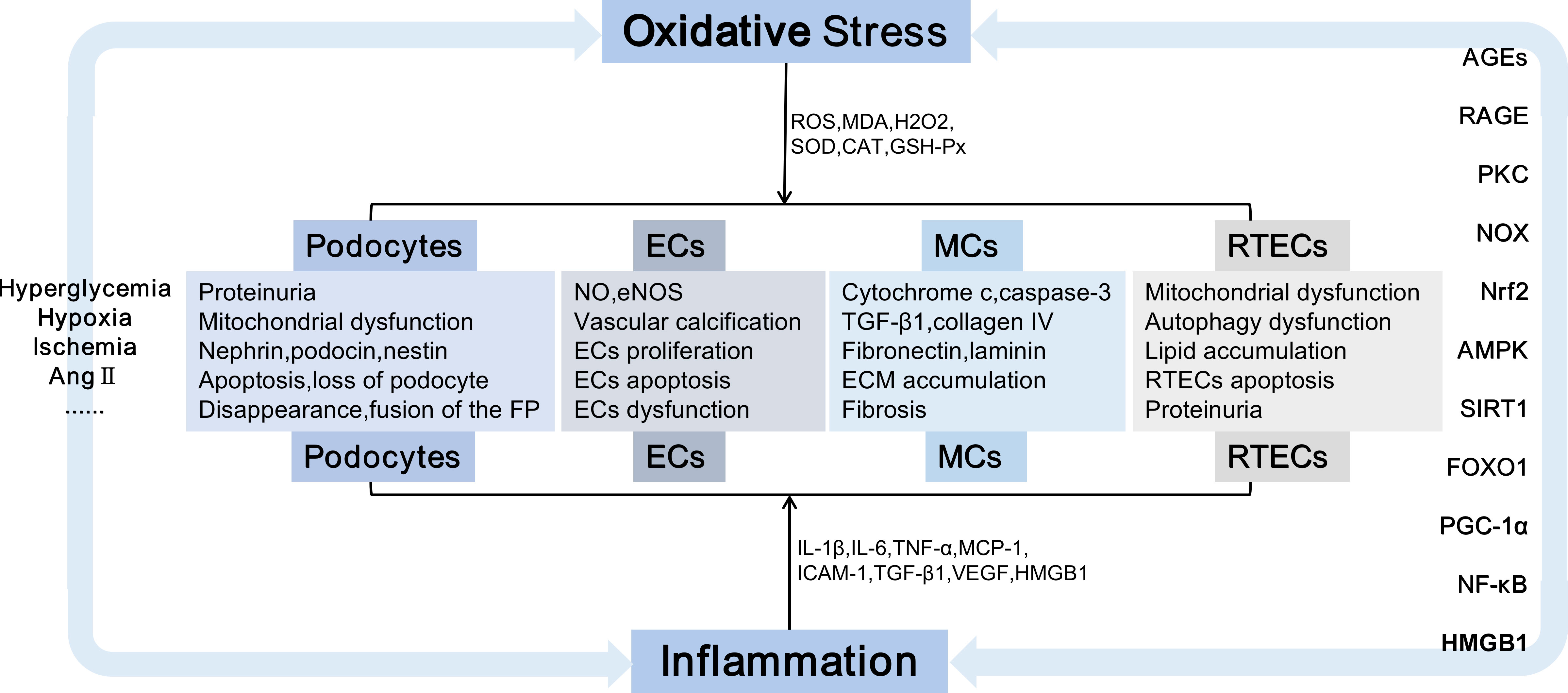
Figure 1 Kidney damage is caused by oxidative stress and inflammation. Damaged cells including podocytes, ECs, MCs, and RTECs, causing podocyte injury, the accumulation of extracellular matrix,epithelial-mesenchymal transition, cell apoptosis, etc., which eventually lead to irreversible glomerular fibrosis and tubular damage, exacerbate the progression of DN.Ang II, Angiotensin II; FP, Foot processes; ECs, endothelial cells; MCs, mesangial cells; RTECs, renal tubular epithelial cells; NO, nitric oxide; eNOS, endothelial nitric oxide synthase; TGF-β, transforming growth factor-β; ECM, extracellular matrix;IL-1, interleukin-1; TNF-α, tumor necrosis factor-α; MCP-1, monocyte chemoattractant protein-1; ICAM-1, intercellular adhesion molecule-1; VEGF,vascular endothelial growth factor; AGEs, advanced glycation end products; RAGE, receptor for advanced glycation end products; NOX, nicotinamide adenine dinucleotide phosphate oxidase; PKC, protein kinase C; Nrf2, nuclear factor E2-related factor 2;AMPK, AMP-activated protein kinase; SIRT1, Sirtuin-1; FOXO, forkhead transcription factor O; PGC-1α, peroxisome proliferator-activated receptor-γ coactivator-1α;NF-κB, nuclear factor-kappaB; DN, diabetic nephropathy;HMGB1,high mobility group box 1.
3 Overview of polyphenols
3.1 Chemistry of polyphenols
Polyphenols are secondary metabolites from various plants and are widely found in foods and beverages of plant origin, and more than 8000 polyphenols have been identified. Due to their antioxidant, anti-inflammatory and immunomodulatory activities, they play an essential role in the management of human health. In recent years, they have attracted much attention from nutritionists and food scientists due to their nutritional and therapeutic value (83). According to chemical structure, polyphenols can be divided into four major groups: Flavonoids, Stilbenes, Phenolic acids, and Lignans. Flavonoids comprise 15 carbon atoms, including two benzene rings (A Ring and B Ring) and a heterocycle (C Ring), abbreviated as C6-C3-C6, with the presence of flavan nucleus. Depending on the oxidation level of the C ring and hydroxylation patterns, flavonoids can be classified as flavonols, flavones, flavanones, anthocyanin, flavan-3-ols, and isoflavones (84). Non-flavonoids, including Stilbenes, Phenolic acids, and Lignans. Phenolic acids are the simplest compounds in the family of polyphenols because they have only one phenolic ring, such as ferulic acid, caffeic acid, and gallic acid (85). Stilbenes is a plant polyphenol with a diphenylethylene backbone. Resveratrol is the most representative compound among the stilbenes (86). Lignans are compounds produced by the oxidative dimerization of two phenyl propane units. Differences in the number of phenol units and how they are combined lead to different physical, chemical, and biological properties (87–89). In particular, the stability of polyphenols is also affected by many structural features, such as The hydrogenation of the C2=C3, hydroxylation, and methoxylation (90) (Figure 2).
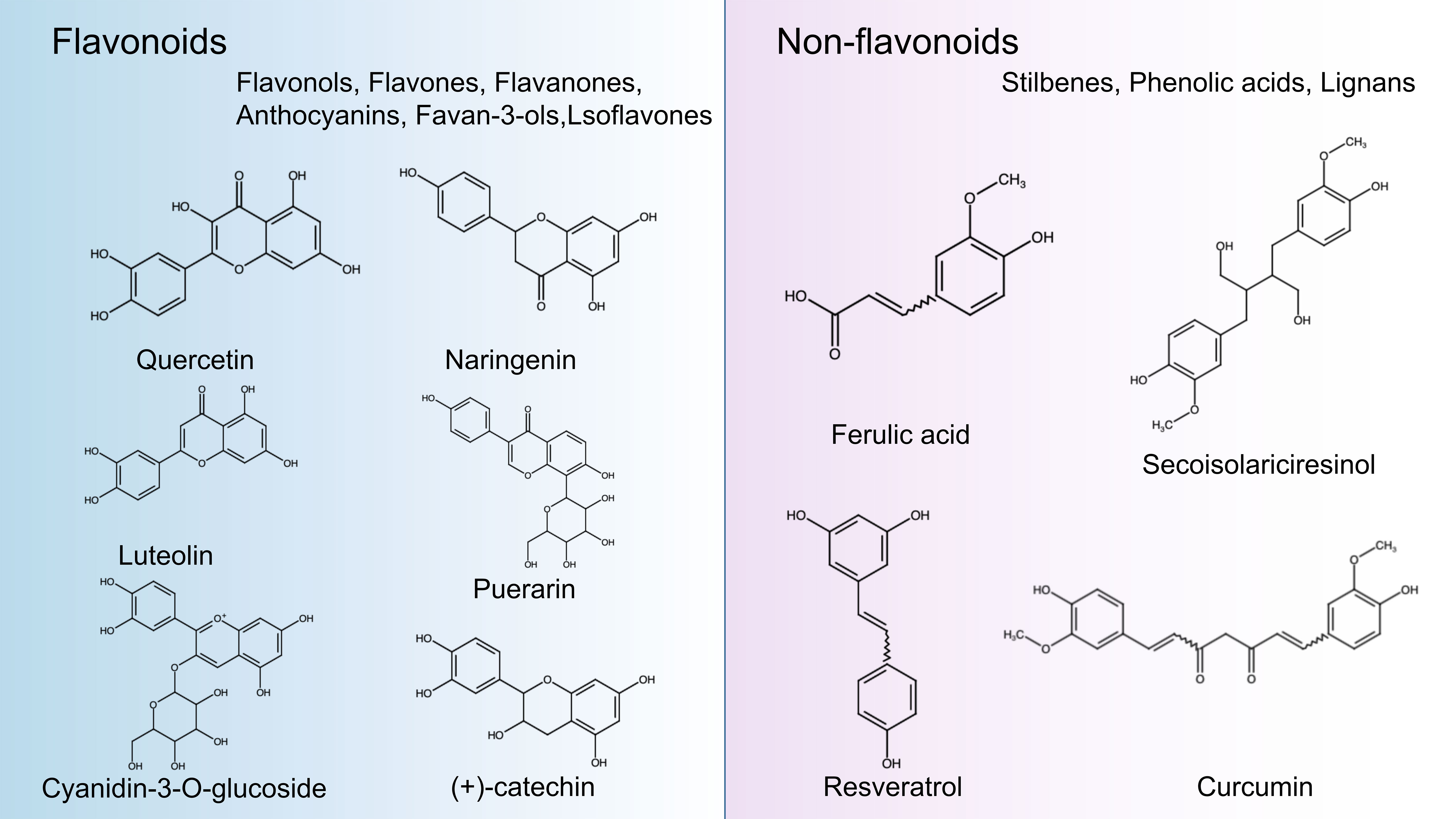
Figure 2 Classification and chemical structure of polyphenols. The structures of common polyphenol representatives (Created with BioRender.com.).
3.2 The action of polyphenols with oxidative stress induced inflammation
Oxidative stress induced by ROS accumulation plays a pro-inflammatory role in various diseases, and NOX is considered a significant source of ROS. Inflammation inducers activate membrane recognition receptors of the innate immune system, such as TLR, which activate NOX and produce superoxide anion, which is rapidly converted to H2O2 by SOD, and H2O2 activates the Nrf2 signaling pathway to promote the release of antioxidant enzymes such as HO-1. More importantly, H2O2 activates inflammatory signaling pathways such as NF-κB, intensifying the release of pro-inflammatory mediators and stimulating inflammatory responses (91) (Figure 3).
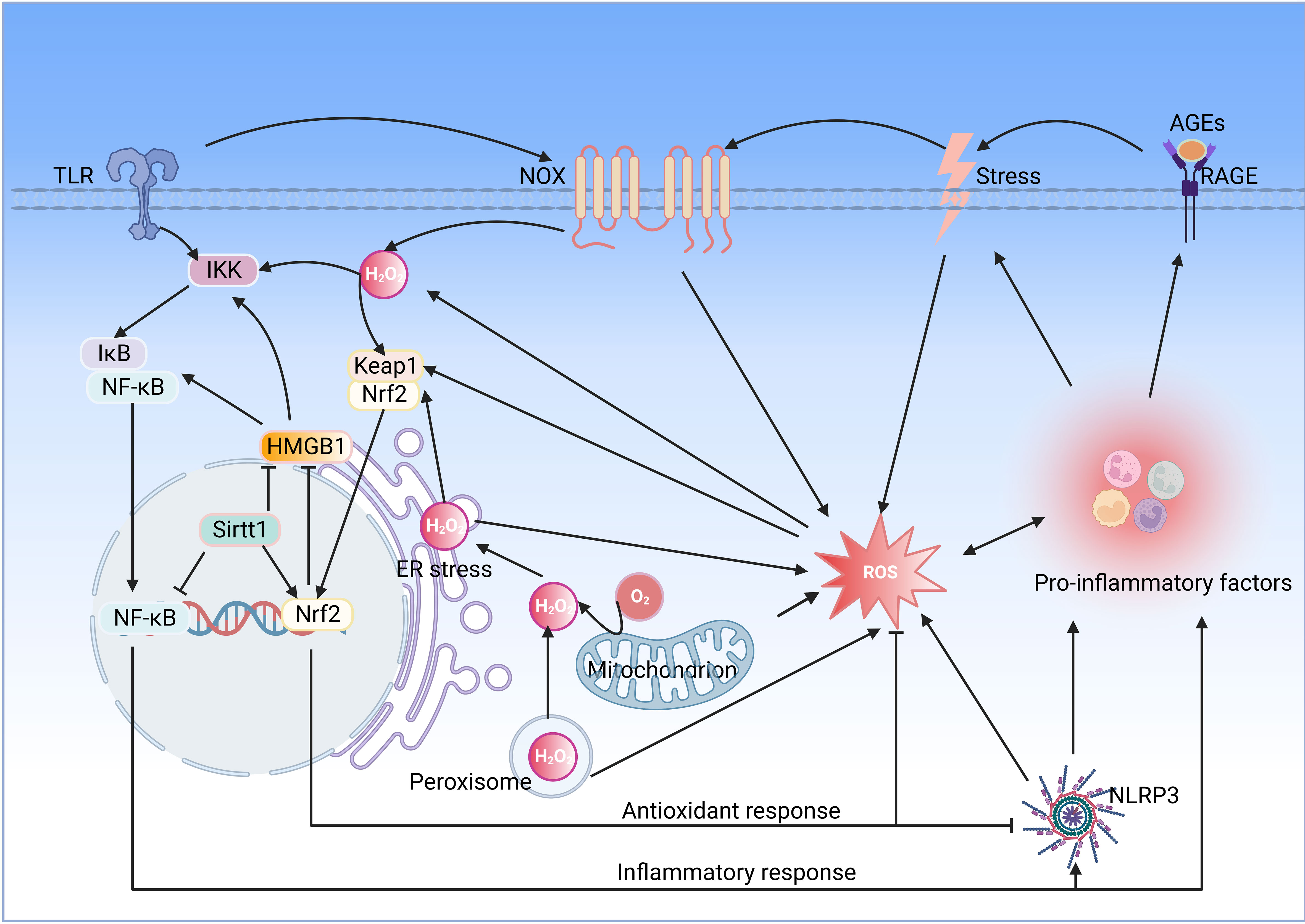
Figure 3 Interaction between oxidative stress and inflammation. Oxidative stress and inflammation interact with each other, and both of which constitute a vicious oxidative stress-inflammation cycle.TLR, toll-like receptor; IKK, IkB kinase; NF-κB, nuclear factor-kappaB; HMGB1, high mobility group box 1; Sirt1, sirtuin 1; Nrf2, nuclear factor E2-related factor 2; Keap, Kelch-like ECH-associated protein; NOX, NADPH oxidase; RAGE, receptor for advanced glycation end products; ER, endoplasmic reticulum; ROS, reactive oxygen species; NLRP3, NOD-like receptor family pyrin domain containing 3(Created with BioRender.com.).
Polyphenols have long been known for their antioxidant power to scavenge ROS. However, the impact of polyphenols goes far beyond this. Polyphenols can be indirectly anti-inflammatory through antioxidant effects or directly alleviate inflammation by modulating signaling pathways. The antioxidant capacity of polyphenols is mainly attributed to the direct scavenging of reactive oxygen species and the inhibition of reactive oxygen species production. Polyphenols inhibit the activity of NOX and significantly inhibit the production of superoxide anion (92). The phenolic ring structure of polyphenols can directly neutralize the free radicals generated by lipid peroxidation (93). Polyphenols can chelate metal ions, such as Fe3+, and prevent the conversion of H2O2 to highly toxic HO• (94). More importantly, polyphenols can promote the expression of antioxidant enzymes by enhancing endogenous antioxidant capacity induced by the Nrf2 pathway (95). Pomegranate polyphenols activate Nrf2 synapse, inhibit NF-κB suppression, reduce cell ROS production, and protect against drug-induced apoptosis (96). Resveratrol may activate the SIRT1/Nrf2 pathway to reduce inflammation and endoplasmic reticulum stress, effectively delaying the structural and functional decline of various tissues and organs due to aging (97). Polyphenols also enhance cellular antioxidant activity, inhibit pyroptosis and alleviate metabolic disorders and inflammatory responses through the Nrf2-NLRP3 axis (98).
While most studies suggest that polyphenols alleviate inflammation thanks to their antioxidant and free radical scavenging abilities, polyphenols can also directly modulate inflammatory signaling pathways. Many polyphenols, such as curcumin and resveratrol, have been reported to be potent inhibitors of NF-κB, inhibiting NF-κB translocation to the nucleus, inhibiting its binding from targeting DNA and subsequent transcription of pro-inflammatory cytokines, inhibiting phosphorylation or ubiquitination of signaling molecules, and inhibiting degradation of IκB (99). Polyphenols also target TLR4, the upstream of NF-κB, mitigating the inflammatory response through the TLR4/NF-κB signaling pathway (100). Resveratrol can suppress excessive inflammatory responses by interfering with HMGB1-mediated activation of the TLR4/NF-κB signaling pathway (101). Resveratrol suppresses HMGB1 expression through upregulation of miR-149, inhibits the ferroptosis formation pathway, and ameliorates injury (102). Also, resveratrol can mitigate downstream inflammatory responses by activating sirt1 and inhibiting the nucleoplasmic translocation and extracellular release of HMGB1 (103). Interestingly, a metabolomic study demonstrated the different roles of polyphenols in lipopolysaccharide-induced mouse embryonic fibroblast cells, with Theobroma cacao exerting mainly antioxidant properties and Lippia citriodora exerting anti-inflammatory effects mainly based on the reduction of pro-inflammatory cytokine and MCP-1 production, it was confirmed that the anti-inflammatory function of polyphenols could not be attributed to one mechanism of action but rather the coordination between different pathways and mechanisms (104) (Figure 4).
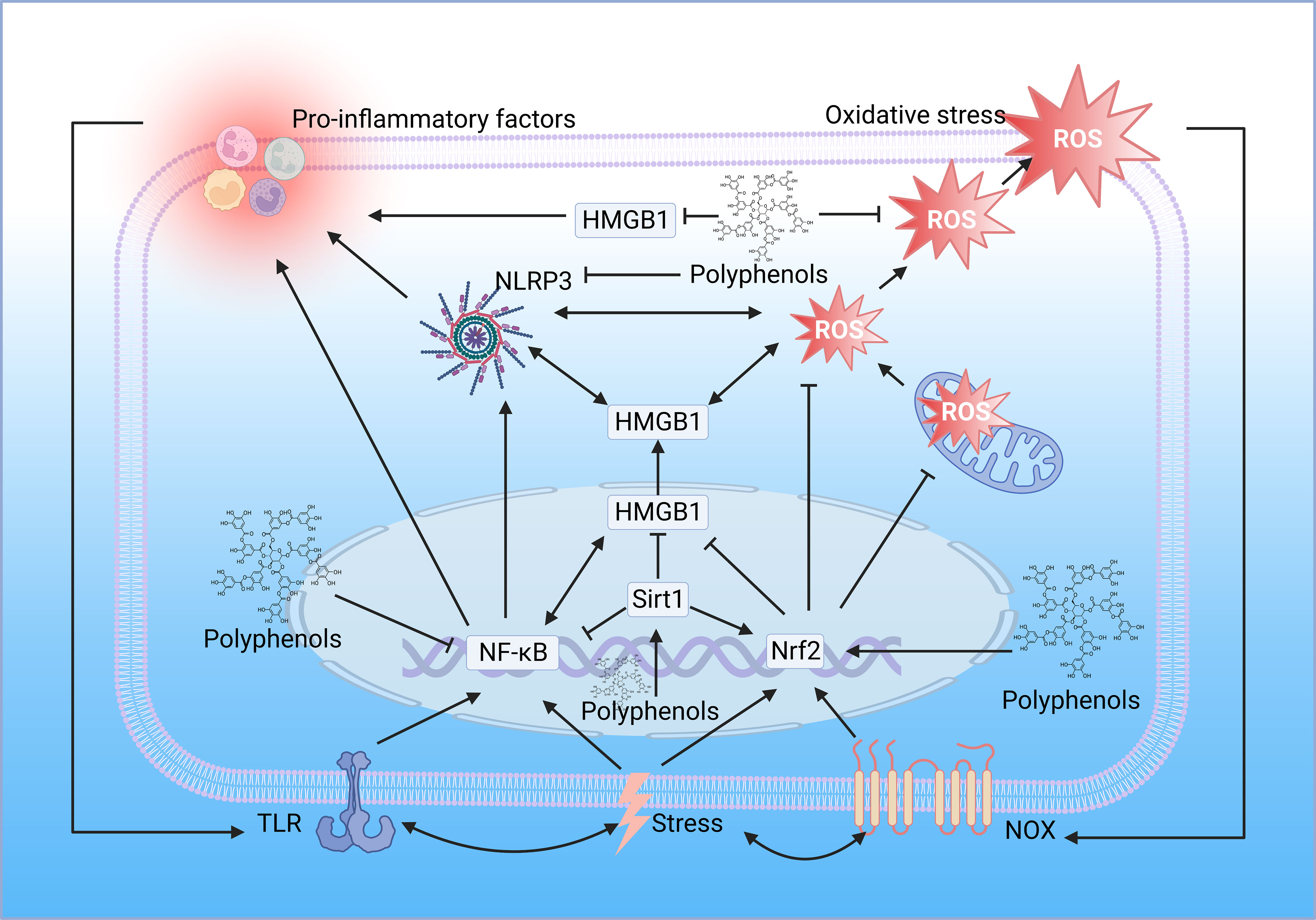
Figure 4 Polyphenols regulate oxidative stress and inflammation. The location of the polyphenol structure diagram in the figure is the critical node of polyphenol regulation. TLR, toll-like receptor; NF-κB, nuclear factor-kappaB; HMGB1, high mobility group box 1; Sirt1, sirtuin 1; Nrf2, nuclear factor E2-related factor 2; NOX, NADPH oxidase; ROS, reactive oxygen species; NLRP3, NOD-like receptor family pyrin domain containing 3 (Created with BioRender.com.).
3.3 Polyphenols in DN
Some polyphenols have been widely used to repair molecules after free radical damage and modulate various dysregulated mediators and pathways, such as directly or indirectly blocking the production of ROS and alleviating the process of oxidative stress and inflammatory response, suggesting that polyphenols are an alternative approach to mitigate DN progression. In this review, “polyphenols”, “flavonoids”, “stilbenes”, “phenolic acids”, “lignans”, “flavonols”, “flavones”, “flavanones”, “anthocyanin”, “flavan-3-ols”, “isoflavones”, “diabetic nephropathy”, “diabetic kidney disease” as search topic words in Web of Science, and combining, de-duplicating to screen polyphenols as the primary intervention preclinical studies. (Table 1) (Figure 5).
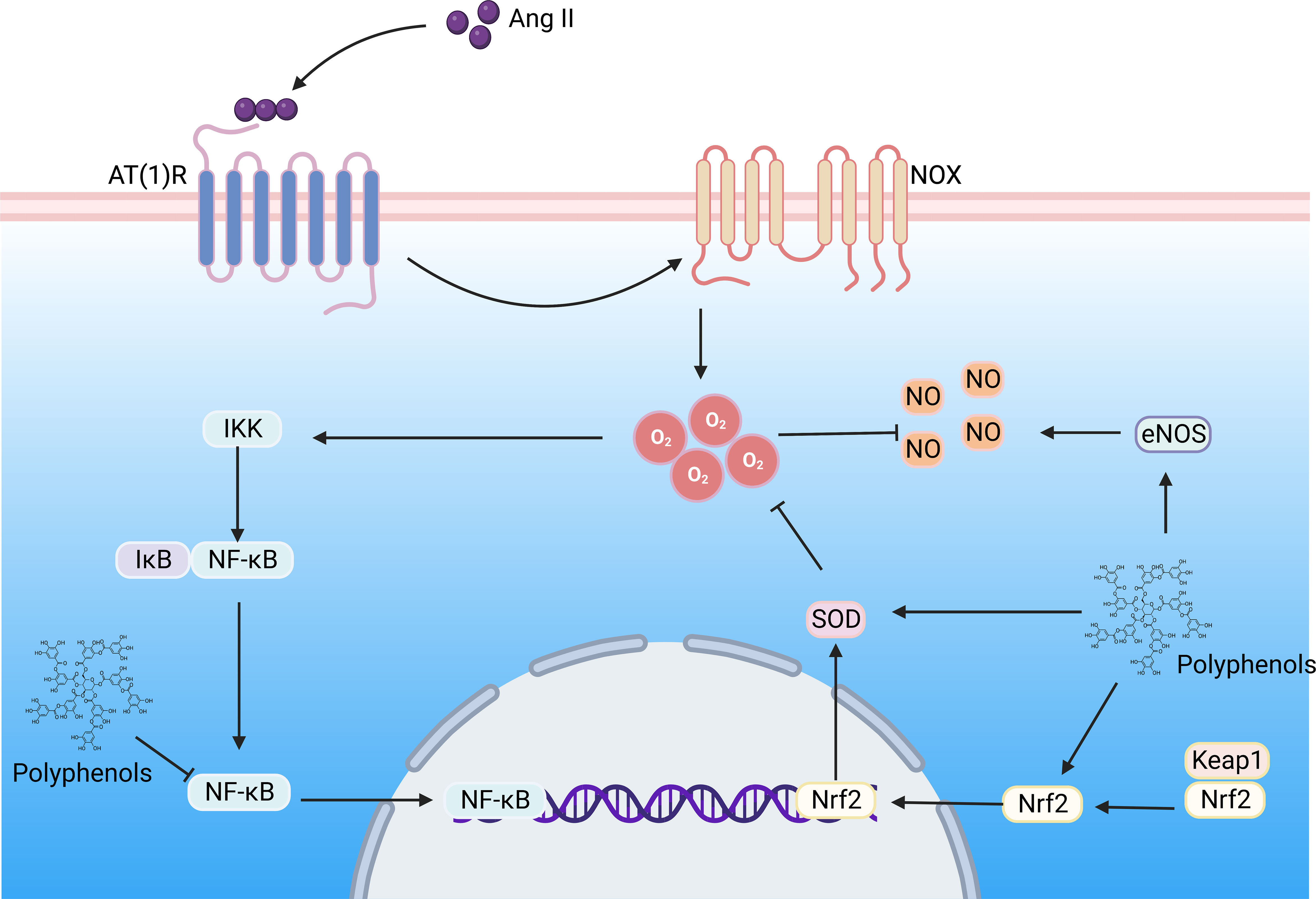
Figure 5 The polyphenols improve DN by regulating the Ang II signaling pathway. Hyperglycemia induces the formation of large amounts of Ang II, which binds to the AT(1)R) in the kidney, activating NOX, contributing to superoxide formation, triggering oxidative stress and inflammation, and resulting in renal vascular remodeling. Ang II, Angiotensin II; AT(1)R, Ang II type 1 receptor; NOX, NADPH oxidase; NF-κB, nuclear factor-kappaB; Nrf2, nuclear factor E2-related factor 2; NLRP3, NOD-like receptor family pyrin domain containing 3; IKK, IkB kinase; Keap, Kelch-like ECH-associated protein; SOD, superoxide dismutase; NO, nitric oxide;eNOS, endothelial nitric oxide synthase. (Created with BioRender.com.).
3.3.1 Flavonoids
3.3.1.1 Quercetin
Quercetin, a polyphenol flavonoid, is ubiquitous in plants. Studies have shown that quercetin has a variety of biological activities, including scavenging free radicals and preventing lipid peroxidation in the body. Furthermore, it has anti-inflammatory properties and prevents diabetes complications (151, 152). Quercetin in streptozotocin (STZ)-induced DN rats can effectively reduce BUN and Scr levels, increase the formulation of nephrin and podocin while decreasing desmin in DN rats, relieve podocyte effacement (125) and improve renal pathological changes, including glomerular volume atrophy, high ECM and glycogen deposition, basement membrane thickening and tubulointerstitial fibrosis (123, 126). Further research illustrated that quercetin upregulated the formulation of SIRT1, activated the Nrf2/HO-1 pathway (128), inhibited the AGE-RAGE pathway (153), enhanced the expression of SOD, GSH-Px, and CAT (127), decreased the MDA, elevated the HDL (high density lipoprotein) content, decreased the expression of triglyceride (TG) and low-density lipoprotein (LDL), and regulated renal lipid accumulation. Additionally, quercetin decreased the expression of NF-κB, TNF-α, IL-1β, ICAM, and ICAM-1 (124). Vitro studies indicated that quercetin promoted the expression of SOD, GSH-Px, CAT, and SIRT1, and reduced the formulation of MDA, TNF-α, IL-1β, IL-6, TGF-β1, Smad 2/3, type IV collagen and laminin (154–156). There is consistent with a recent systematic study that reported that quercetin significantly decreased renal index, Scr, BUN, urine albumin, MDA, TNF-α, and IL-1β and increased the activities of SOD. Furthermore, CAT alleviated the degree of oxidative stress in animal models of DN (157). It is also proposed that the optimal dose for preclinical experiments is 90-150 mg/kg/day, and the administration time is 2-4 months (158).
3.3.1.2 Puerarin
Puerarin is a natural isoflavone of Pueraria lobata (gegen), extensively found in food and Chinese herb medicine in East Asian countries. Puerarin has cardioprotective, antioxidant, and anti-inflammatory properties. Moreover, it exerts anti-diabetic activity by insulin secretion and maintaining metabolic homeostasis in STZ-induced diabetic mice (159). Furthermore, it can improve diabetes and other complications, including DN, by reducing the formation of AGEs and delaying oxidative stress (160). The SIRT1/FOXO1 signaling pathway is firmly related to the progression of DN. Studies have noted that activating the SIRT1/FOXO1 signaling pathway can increase SOD, CAT, and GSH-Px concentration and ameliorate renal injury in DN (161). Additionally, the SIRT1/FOXO1 signaling pathway can induce the expression of mitochondrial PGC-1α and improve mitochondrial function and ROS production (133). SIRT1 inhibited the NF-κB activity through deacetylation, and the expression of IL-6, TNF-α, and NOX4 was decreased, blood glucose, BUN, Scr, albuminuria, and urinary albumin to creatinine ratio (UACR) was ameliorated (134). Moreover, Puerarin directly diminished the formulation of ROS and FP effacement, restored the regular expression of nephrin and podocin of podocytes, decreased the formulation of matrix metalloproteinase-9 (MMP-9) and type IV collagen, and restored podocyte injury (135).
3.3.1.3 Other flavonoids
In DN models, baicalin (162), apigenin, and silybin have been found to promote Nrf2 translocation into the nucleus. Genes induced by ARE are activated, such as HO-1 and NQO-1, accompanied by various antioxidant enzymes, such as GSH-Px, SOD, and CAT. However, the activity of MDA has been inhibited (39). Moreover, the concentration of IL-1β, IL-6, MCP-1, and TNF-α has been decreased, and the levels of FN and TGF-β1 that induced renal fibrosis have been reduced (129). Luteolin (163) promotes the expression of HO-1; reduces the expression of MDA, FN, α-SMA, collagen I, and collagen IV; and decreases the proliferation of MCs and excessive accumulation of ECM (140). The expression of STAT3 is highly correlated with oxidative stress and inflammatory response. Luteolin effectively inhibits the activation of STAT3 (139). Mangiferin inhibits the activation of the AGEs/RAGE axis, and PKCs reduce the phosphorylation of PI3K/Akt and improve the inflammation and oxidative stress in DN (141). Furthermore, similar to naringenin (131), the levels of TC, TG, LDL, and VLDL(very-low-density lipoprotein) were decreased, and the HDL level is raised, which improves lipid peroxidation by targeting oxidative stress and inflammation, cyanidin-3-O-glucoside (C3G) decreases the formulation of Smad2 and Smad3 while enhancing Smad7. It also improves renal pathological and functional changes, including the degree of renal fibrosis, SCr, BUN, urinary albumin, and ACR (137, 164). Eriodictyol can obstruct the production of NOX2, NOX4, directly shorten the production of ROS and MDA and protect MCs from HG stimulation by inhibiting the production of FN, collagen IV, and ECM (165). Notably, phloretin can directly restore nephrin and podocin levels and improve the disappearance of FP of podocytes (166). Furthermore, the inhibition of oxidative stress by apigenin in vitro has demonstrated the activation of Nrf2, HO-1, SOD, and CAT activities; downregulated the generation of TNF-α, IL-1β, and IL-6; inhibited the Nrf2, leading to the disruption of apigenin contrary to HG-induced oxidative damage was disrupted (167).
3.3.2 Stilbenes
3.3.2.1 Resveratrol
Resveratrol is a natural polyphenolic antioxidant. It has various biochemical and physiological properties, including antioxidant, anti-inflammatory, anti-diabetic, anti-obesity, cardiovascular protection, and anti-tumor features (168). Resveratrol exerts its renoprotective effects through multiple mechanisms, including reducing oxidative stress and AGE production, inhibiting endoplasmic reticulum stress and inflammation; improving lipotoxicity; stimulating autophagy (169); and activating a variety of pathways, including Nrf2, AMPK, SIRT1and FOXO3a. A systematic review showed that resveratrol could apply its antioxidant activity by reducing the production of MDA and restoring the action of SOD, CAT, and GSH-Px (170); decreasing 4-hydroxynonenal, an indicator of lipid peroxidation (171); and inhibiting the formation of ROS and lip peroxidation (172). Resveratrol activates antioxidant enzymes and decreases the secretion of superoxide anion, hydroxyl radical, TNF-α, IL-1β, IL-6, and NF-κBp65 by targeting the Nrf2-Keap1 signaling pathway; moreover, it improves the thickened basement membrane, leading to loss of FP (108). AMPK activation also has ameliorating effects on oxidative stress (169).AMPK inhibits HG-induced expression of NOX; decreases the content of FN and the proliferation of MCs; prevents EMT of RTECs (173, 174); restores podocyte-related proteins to normal, including nephrin, podocin, and desmin; and ameliorates mesangial matrix expansion, tubular basement membrane thickening, and glomerular hypertrophy in db/db mice of DN (105). Additionally, AMPK mediates the upregulation of SIRT1; activates the SIRT1/peroxisome proliferator-activated receptor α(PPARα)/FoxO pathway; heightens the generation of CAT, SOD, and GSH-Px; and decreases the expression of MDA, TGF-β1, FN, NF-kBp65, SCr, and urinary protein. In Silencing SIRT1, the protective effects are suppressed (106, 107, 110). Resveratrol elevates the concentration of Mn-SOD. Furthermore, it repairs the expression of SIRT1 and peroxisome proliferator-activated receptor-γ coactivator-1α(PGC-1α) and reestablishes the activity of respiratory chain complexes I and III and mitochondrial membrane potential. Furthermore, it inhibits the transport of Cyto C from the mitochondria to the cytoplasm and delays the progression of apoptosis in glomerular podocyte and tubular epithelial cells (111).
3.3.2.2 Curcumin
Curcumin is an acidic polyphenol with unsaturated aliphatic and aromatic groups as the main chain, which is more common in turmeric (Curcuma longa L.) and Curcumae Rhizoma. The Pharmacological activities of Curcumin include antioxidant, anti-inflammatory, immunomodulatory, and anti-renal fibrosis. A previous study reported that Curcumin mediates the upregulation of GSH-Px, SOD, and other antioxidant factors by activating the antioxidant Nrf2/HO-1 pathway. Furthermore, it downregulates the expression of MDA and ROS effectively promotes improvement of the urinary protein excretion rate (175), elevates the expression of nephrin, and repairs podocyte injury (176). Additionally, Curcumin protects RTECs from HG-induced EMT via Nrf2-mediated upregulation of HO-1, which consequently knocks down Nrf2 and inhibits the upregulation of HO-1 (177). Curcumin activates the phosphorylation of AMPK, significantly increases the protein rate of Nrf2/Keap1, increases the activity of the antioxidant enzyme, and attenuates the expression of MDA, kidney injury molecule-1, and neutrophil gelatinase-associated lipocalin (116, 117). furthermore, it reduces the incidence of lipid accumulation, tubular dilatation, and glomerular sclerosis (178). Curcumin prohibited the activity of PKC and the expression of NF-κB, NADPH oxidase, collagen I/III, and TGF-β1. Also, it increases the protein levels of FOXO-3a, Nrf2, manganese SOD, GSH-Px, and B-cell lymphoma-2(Bcl-2); inhibits the generation of cytochrome-c and the production of caspase-3; and prevents damage to the mitochondria, renal tubules and mesangial cell (179). A systematic review of randomized displayed that Curcumin had some beneficial effects on various parameters, including inflammation or oxidative stress, in patients with renal disease (180). A randomized, double-anonymized trial assessing the redox status of dietary Curcumin supplementation (320 mg/d) in patients with non-diabetic or diabetic proteinuria confirmed that Curcumin enhanced the antioxidant capacity of diabetic proteinuric patients while attenuating lipid peroxidation in non-diabetic patients, suggesting a therapeutic effect of dietary Curcumin supplementation (181). In vitro studies have also found that Curcumin effectively reduces oxidized LDL isolated from human plasma-related markers such as conjugated diene, lipid peroxides, and lysolecithin, preventing oxidation and lipid modification of LDL (182).
3.3.3 Phenolic acid
Multiple phenolic acids have been used, including ferulic, chlorogenic, caffeic, ellagic, etc. Phenolic acids have various functions, such as regulating blood sugar and lipids, anti-oxidation, anti-inflammatory, and anti-fibrosis. Hyperglycemia triggers oxidative stress, leading to the formulation of mitogen-activated protein kinases (MAPK). Ferulic acid inhibits the activation of MAPKs and decreases the expression of ROS, NO, carbonyl, MDA, and inflammatory factors and adhesion molecules, including NF-κB, TNF-α, IL-1β, and IL-6, MCP-1, ICAM-1 and vascular cell adhesion molecule-1. Moreover, it increases the levels of Beclin-1 and LC3-II (144). Long-term treatment with ferulic acid significantly downregulated the expression of p-NF-κB p65, TNF-α, TGF-β1 and type IV collagen protein, and MDA in renal tissue; increased the activity of SOD, CAT, GPx; and upregulated the levels of nephrin and podocin, which improved podocyte injury and reduced the serum levels of BUN, Cr, fasting blood glucose (FBG), TC (total cholesterol), TG by alleviating oxidative stress, inflammation and fibrosis in STZ-induced DN rats (145). Chlorogenic acid and caffeic acid increased renal formulation of Nrf2 and HO-1 and increased the activity of SOD and GSH-Px. Furthermore, they decreased the formulation of NF-ĸB, IL-6, TNF-α, and IL-1β (150, 183). Chlorogenic acid can also alleviate endoplasmic reticulum stress, promote the formulation of anti-apoptosis factors, such as Bcl-2, attenuate the proliferation of MCs and mesangial expansion, and reduce the levels of FBG, BUN, and Cr (149). Caffeic acid reduces the activation of NOX; decreases the generation of ROS; and heightens the action of antioxidant enzymes, including T-SOD, GPx, and GSH (184), thereby reducing the levels of MDA (148). Mainly, ellagic acid significantly inhibits the activation of renal NF-κB, IL-1β, IL-6, and TNF-α. Additionally, it decreases the generation of TGF-β and fibronectin in renal tissue (185).
4 Conclusion and prospect
This review summarizes oxidative stress and oxidative stress-associated inflammation that lead to changes in renal cell structure and function in DN. Oxidative stress is recognized as a powerful mechanism for the occurrence and progression of DN.DN, a common complication of DM, has become a significant cause of end-stage renal disease. Even though the pathogenesis of DKD is complex, oxidative stress has been proposed to be central to the pathogenesis of DKD. Hyperglycemia activates signaling pathways such as AGE/RAGE and Ang II, which induce large production of ROS. Large amounts of ROS activate NF-κB, TGF-β1, AMPK, and other pathways, which induce renal inflammation, autophagy, and fibrosis, triggering abnormal kidney structure and function. In addition, oxidative stress also interacts with other factors in disease progression. ROS are not only messengers of various signaling pathways but also regulators of various cellular metabolism, proliferation, differentiation, and apoptosis. Oxidative stress is accompanied by tissue inflammation, apoptosis, and tissue fibrosis, and in particular, oxidative stress and inflammation are considered to be in a causal relationship, with many factors exacerbating the progression of DKD (186). Oxidative stress induces excessive production of ROS, activates signaling pathways and transcription factors, mediates the infiltration and recruitment of inflammatory cells, such as macrophages and monocytes, and promotes the production of inflammatory factors. Conversely, the formulation of inflammatory mediators can further produce ROS, which aggravates oxidative stress, and oxidative stress and inflammation collaborate to affect the progression of DN. As a natural compound with antioxidant and anti-inflammatory properties, polyphenols can ameliorate or reverse cell damage and pathological changes of DN and are considered to be attractive drugs against DN by targeting signaling pathways, including Nrf2, NF-κB, AMPK, and SIRT1. However, the complex regulatory mechanism of polyphenols in DN has not been thoroughly demonstrated, and inhibition of renal damage and restoration of structure and function should be the core of alternative polyphenol therapy. Therefore, more studies are needed to elucidate polyphenols and how to repair kidney injury and improve kidney function.
Polyphenols exhibit various biological activities, such as antioxidant and anti-inflammatory. Multiple clear experimental evidence suggests that long-term intake of polyphenols can effectively improve the progression of chronic diseases, including DN. However, there are still some limitations, polyphenols are famous for their potent antioxidant properties, but in some cases, such as in reaction systems rich in redox-active metals (e.g., iron, copper), flavonoids have a pro-oxidant effect, reducing the clearance of ROS and inducing mitochondrial toxicity (187). In addition, the lack of pharmacokinetic measurements of ingested polyphenols and the correct dose remains a challenge, so the application should be tailored to the purpose and the selection of the appropriate dose and mode of action. Moreover, the evaluation of the efficacy of polyphenols in combination with other conventional treatments for DN and the specific mechanism of action remains to be investigated. Many natural products did not enter clinical trials or were terminated due to reduced oral utilization or activity, which may limit their widespread clinical application, and further studies are needed to overcome these limitations and to bridge the gap between preclinical and clinical studies of natural products. It is worth noting that the clinical translation of DN therapies from natural products is still a long process.
Author contributions
QJ: Conceptualization, Writing-Original draft, Investigation, Visualization. TL: Conceptualization, Investigation. YQ: Investigation. DL: Investigation. LY: Investigation. HM: Investigation. FM: Investigation. YW: Investigation. LP: Writing-review & editing. YZ: Writing-review & editing.
Funding
This study was supported by the National Nature Science Foundation of China (82074393, 82205094).
Conflict of interest
The authors declare that the research was conducted in the absence of any commercial or financial relationships that could be construed as a potential conflict of interest.
Publisher’s note
All claims expressed in this article are solely those of the authors and do not necessarily represent those of their affiliated organizations, or those of the publisher, the editors and the reviewers. Any product that may be evaluated in this article, or claim that may be made by its manufacturer, is not guaranteed or endorsed by the publisher.
Glossary
References
1. Saeedi P, Petersohn I, Salpea P, Malanda B, Karuranga S, Unwin N, et al. Global and regional diabetes prevalence estimates for 2019 and projections for 2030 and 2045: results from the International Diabetes Federation Diabetes Atlas, 9th edition. Diabetes Res Clin Pract (2019) 157:107843. doi: 10.1016/j.diabres.2019.107843
2. Selby NM, Taal MW. An updated overview of diabetic nephropathy: diagnosis, prognosis, treatment goals and latest guidelines. Diabetes Obes Metab (2020) 22(Suppl 1):3–15. doi: 10.1111/dom.14007
3. Samsu N. Diabetic nephropathy: challenges in pathogenesis, diagnosis, and treatment. BioMed Res Int (2021) 2021:1497449. doi: 10.1155/2021/1497449
4. Kopel J, Pena-Hernandez C, Nugent K. Evolving spectrum of diabetic nephropathy. World J Diabetes (2019) 10(5):269–79. doi: 10.4239/wjd.v10.i5.269
5. Bahreini E, Rezaei-Chianeh Y, Nabi-Afjadi M. Molecular mechanisms involved in intrarenal renin-angiotensin and alternative pathways in diabetic nephropathy - a review. Rev Diabetes Stud (2021) 17(1):1–10. doi: 10.1900/RDS.2021.17.1
6. Dawood AF, Maarouf A, Alzamil NM, Momenah MA, Shati AA, Bayoumy NM, et al. Metformin is associated with the inhibition of renal artery At1r/Et-1/inos axis in a rat model of diabetic nephropathy with suppression of inflammation and oxidative stress and kidney injury. Biomedicines (2022) 10(7):1644. doi: 10.3390/biomedicines10071644
7. Selamoğlu Zeliha AEC. General approaches to the stem cell therapy in diabetes mellitus as innovative researches. J Genet Mutat (2018) 1(1):4–5.
8. Liu H, Sridhar VS, Boulet J, Dharia A, Khan A, Lawler PR, et al. Cardiorenal protection with Sglt2 inhibitors in patients with diabetes mellitus: from biomarkers to clinical outcomes in heart failure and diabetic kidney disease. Metabolism (2022) 126:154918. doi: 10.1016/j.metabol.2021.154918
9. Talas ZS, Ozdemir I, Yilmaz I, Gok Y. Antioxidative effects of novel synthetic organoselenium compound in rat lung and kidney. Ecotoxicol Environ Saf (2009) 72(3):916–21. doi: 10.1016/j.ecoenv.2007.11.012
10. Talas ZS, Ozdemir I, Ciftci O, Cakir O, Gulhan MF, Pasaoglu OM. Role of propolis on biochemical parameters in kidney and heart tissues against L-name induced oxidative injury in rats. Clin Exp Hypertens (2014) 36(7):492–6. doi: 10.3109/10641963.2013.863322
11. Hu Q, Jiang L, Yan Q, Zeng J, Ma X, Zhao Y. A natural products solution to diabetic nephropathy therapy. Pharmacol Ther (2023) 241:108314. doi: 10.1016/j.pharmthera.2022.108314
12. Selamoglu Z. Polyphenolic compounds in human health with pharmacological properties. Journal of Traditional Medicine Clinical Naturopathy (2017) 6(4):137. doi: 10.4172/2573-4555.1000e138
13. Thiruvengadam M, Venkidasamy B, Subramanian U, Samynathan R, Ali Shariati M, Rebezov M, et al. Bioactive compounds in oxidative stress-mediated diseases: targeting the Nrf2/are signaling pathway and epigenetic regulation. Antioxid (Basel) (2021) 10(12):1859. doi: 10.3390/antiox10121859
14. Ozgen S, Kilinc OK, Selamoglu Z. Antioxidant activity of quercetin: A mechanistic review. Turkish J Agriculture-Food Sci Technol (2016) 4(12):1134–8. doi: 10.24925/turjaf.v4i12.1134-1138.1069
15. Sureda A, Tejada S, Mir Khan U, Selamoglu Z. An overview of the biological function of curcumin in the processes of oxidative stress, inflammation, nervous system, and lipid levels. Cent Asian J Med Pharm Sci Innovation (2023) 3(1):1–11. doi: 10.22034/CAJMPSI.2023.01.01
16. Olaokun OO, Manonga SA, Zubair MS, Maulana S, Mkolo NM. Molecular docking and molecular dynamics studies of antidiabetic phenolic compound isolated from leaf extract of Englerophytum magalismontanum (Sond.) T.D.Penn. Molecules (2022) 27(10):3175. doi: 10.3390/molecules27103175
17. Mechchate H, Es-Safi I, Amaghnouje A, Boukhira S, AA A, Al-Zharani M, et al. Antioxidant, anti-inflammatory and antidiabetic proprieties of Lc-Ms/Ms identified polyphenols from coriander seeds. Molecules (2021) 26(2):487. doi: 10.3390/molecules26020487
18. Kandylis P, Bekatorou A, Dimitrellou D, Plioni I, Giannopoulou K. Health promoting properties of cereal vinegars. Foods (2021) 10(2):344. doi: 10.3390/foods10020344
19. Xu S, Wang Y, Wang J, Geng W. Kombucha reduces hyperglycemia in type 2 diabetes of mice by regulating gut microbiota and its metabolites. Foods (2022) 11(5):754. doi: 10.3390/foods11050754
20. Huang DD SG, Jiang Y, Yao C, Zhu C. A review on the potential of resveratrol in prevention and therapy of diabetes and diabetic complications. BioMed Pharmacother (2019) 125:109767. doi: 10.1016/j.biopha.2019.109767
21. Rotariu D, Babes EE, Tit DM, Moisi M, Bustea C, Stoicescu M, et al. Oxidative stress - complex pathological issues concerning the hallmark of cardiovascular and metabolic disorders. BioMed Pharmacother (2022) 152:113238. doi: 10.1016/j.biopha.2022.113238
22. Hernandez LF, Eguchi N, Whaley D, Alexander M, Tantisattamo E, Ichii H. Anti-oxidative therapy in diabetic nephropathy. Front Biosci (Schol Ed) (2022) 14(2):14. doi: 10.31083/j.fbs1402014
23. Darenskaya MA, Kolesnikova LI, Kolesnikov SI. Oxidative stress: pathogenetic role in diabetes mellitus and its complications and therapeutic approaches to correction. Bull Exp Biol Med (2021) 171(2):179–89. doi: 10.1007/s10517-021-05191-7
24. Zhang W, Chen L, Xiong Y, Panayi AC, Abududilibaier A, Hu Y, et al. Antioxidant therapy and antioxidant-related bionanomaterials in diabetic wound healing. Front Bioeng Biotechnol (2021) 9:707479. doi: 10.3389/fbioe.2021.707479
25. Lennicke C, Cochemé HM. Redox metabolism: Ros as specific molecular regulators of cell signaling and function. Mol Cell (2021) 81(18):3691–707. doi: 10.1016/j.molcel.2021.08.018
26. Pisoschi AM, Pop A. The role of antioxidants in the chemistry of oxidative stress: A review. Eur J Med Chem (2015) 97:55–74. doi: 10.1016/j.ejmech.2015.04.040
27. Chen YH, Chen ZW, Li HM, Yan XF, Feng B. Age/Rage-induced Emp release via the Nox-derived Ros pathway. J Diabetes Res (2018) 2018:6823058. doi: 10.1155/2018/6823058
28. Xu X, Chu Q, Chu J, Cai Z, Xuan Y, Luo B, et al. [Effect of dissipating phlegm and blood stasis simultaneously on ages/rage axis and oxidative stress in rats with diabetic myocardial microangiopathy]. Nan Fang Yi Ke Da Xue Xue Bao (2021) 41(10):1527–33. doi: 10.12122/j.issn.1673-4254.2021.10.11
29. Kang JS, Lee SJ, Lee JH, Kim JH, Son SS, Cha SK, et al. Angiotensin II-mediated Myh9 downregulation causes structural and functional podocyte injury in diabetic kidney disease. Sci Rep (2019) 9(1):7679. doi: 10.1038/s41598-019-44194-3
30. Xiao R, Zhao HC, Yan TT, Zhang Q, Huang YS. Angiotensin II and hypoxia induce autophagy in cardiomyocytes via activating specific protein kinase C subtypes. Cardiovasc Diagn Ther (2021) 11(3):744–59. doi: 10.21037/cdt-20-883
31. Mehta MM, Weinberg SE, Chandel NS. Mitochondrial control of immunity: beyond atp. Nat Rev Immunol (2017) 17(10):608–20. doi: 10.1038/nri.2017.66
32. Wang Y, Cui J, Liu M, Shao Y, Dong X. Schisandrin C attenuates renal damage in diabetic nephropathy by regulating macrophage polarization. Am J Transl Res (2021) 13(1):210–22.
33. Sugahara M, Tanaka S, Tanaka T, Saito H, Ishimoto Y, Wakashima T, et al. Prolyl hydroxylase domain inhibitor protects against metabolic disorders and associated kidney disease in obese type 2 diabetic mice. J Am Soc Nephrol (2020) 31(3):560–77. doi: 10.1681/ASN.2019060582
34. Sun HJ, Xiong SP, Cao X, Cao L, Zhu MY, Wu ZY, et al. Polysulfide-mediated sulfhydration of Sirt1 prevents diabetic nephropathy by suppressing phosphorylation and acetylation of p65 Nf-Kappab and Stat3. Redox Biol (2021) 38:101813. doi: 10.1016/j.redox.2020.101813
35. Wang Z, Liu B, Zhu J, Wang D, Wang Y. Nicotine-mediated autophagy of vascular smooth muscle cell accelerates atherosclerosis via Nachrs/Ros/Nf-Kappab signaling pathway. Atherosclerosis (2019) 284:1–10. doi: 10.1016/j.atherosclerosis.2019.02.008
36. Ke G, Chen X, Liao R, Xu L, Zhang L, Zhang H, et al. Receptor activator of Nf-Kappab mediates podocyte injury in diabetic nephropathy. Kidney Int (2021) 100(2):377–90. doi: 10.1016/j.kint.2021.04.036
37. Bai B, Yang Y, Wang Q, Li M, Tian C, Liu Y, et al. Nlrp3 inflammasome in endothelial dysfunction. Cell Death Dis (2020) 11(9):776. doi: 10.1038/s41419-020-02985-x
38. Wu M, Yang Z, Zhang C, Shi Y, Han W, Song S, et al. Inhibition of Nlrp3 inflammasome ameliorates podocyte damage by suppressing lipid accumulation in diabetic nephropathy. Metabolism (2021) 118:154748. doi: 10.1016/j.metabol.2021.154748
39. Ma L, Wu F, Shao Q, Chen G, Xu L, Lu F. Baicalin alleviates oxidative stress and inflammation in diabetic nephropathy via Nrf2 and Mapk signaling pathway. Drug Des Devel Ther (2021) 15:3207–21. doi: 10.2147/DDDT.S319260
40. Sun L, Han Y, Shen C, Luo H, Wang Z. Emodin alleviates high glucose-induced oxidative stress, inflammation and extracellular matrix accumulation of mesangial cells by the circ_0000064/mir-30c-5p/Lmp7 axis. J Recept Signal Transduct Res (2022) 42(3):302–12. doi: 10.1080/10799893.2021.1933028
41. Banks WA, Hansen KM, Erickson MA, Crews FT. High-mobility group box 1 (Hmgb1) crosses the Bbb bidirectionally. Brain Behav Immun (2023) 111:386–94. doi: 10.1016/j.bbi.2023.04.018
42. Liu X, Lu B, Fu J, Zhu X, Song E, Song Y. Amorphous silica nanoparticles induce inflammation via activation of Nlrp3 inflammasome and hmgb1/tlr4/myd88/nf-kb signaling pathway in huvec cells. J Hazard Mater (2021) 404(Pt B):124050. doi: 10.1016/j.jhazmat.2020.124050
43. Dong WW, Liu YJ, Lv Z, Mao YF, Wang YW, Zhu XY, et al. Lung endothelial barrier protection by resveratrol involves inhibition of hmgb1 release and hmgb1-induced mitochondrial oxidative damage via an nrf2-dependent mechanism. Free Radic Biol Med (2015) 88(Pt B):404–16. doi: 10.1016/j.freeradbiomed.2015.05.004
44. Feng W, Wang J, Yan X, Zhang Q, Chai L, Wang Q, et al. Erk/drp1-dependent mitochondrial fission contributes to hmgb1-induced autophagy in pulmonary arterial hypertension. Cell Prolif (2021) 54(6):e13048. doi: 10.1111/cpr.13048
45. Zhao S, Zhou L, Wang Q, Cao JH, Chen Y, Wang W, et al. Elevated branched-chain amino acid promotes atherosclerosis progression by enhancing mitochondrial-to-nuclear H(2)O(2)-disulfide hmgb1 in macrophages. Redox Biol (2023) 62:102696. doi: 10.1016/j.redox.2023.102696
46. Cheng Q, Pan J, Zhou ZL, Yin F, Xie HY, Chen PP, et al. Caspase-11/4 and gasdermin D-mediated pyroptosis contributes to podocyte injury in mouse diabetic nephropathy. Acta Pharmacol Sin (2021) 42(6):954–63. doi: 10.1038/s41401-020-00525-z
47. Zoja C, Buelli S, Morigi M. Shiga toxin triggers endothelial and podocyte injury: the role of complement activation. Pediatr Nephrol (2019) 34(3):379–88. doi: 10.1007/s00467-017-3850-x
48. Xu L, Sharkey D, Cantley LG. Tubular gm-csf promotes late mcp-1/ccr2-mediated fibrosis and inflammation after ischemia/reperfusion injury. J Am Soc Nephrol (2019) 30(10):1825–40. doi: 10.1681/ASN.2019010068
49. Yoon JJ, Lee YJ, Lee SM, Kang DG, Lee HS. Oryeongsan suppressed high glucose-induced mesangial fibrosis. BMC Complement Altern Med (2015) 15:30. doi: 10.1186/s12906-015-0542-6
50. Huang Y, Zhang Z, Huang Y, Mao Z, Yang X, Nakamura Y, et al. Induction of inactive tgf-beta1 monomer formation by hydrogen sulfide contributes to its suppressive effects on ang ii- and tgf-beta1-induced emt in renal tubular epithelial cells. Biochem Biophys Res Commun (2018) 501(2):534–40. doi: 10.1016/j.bbrc.2018.05.032
51. Hong Q, Cai H, Zhang L, Li Z, Zhong F, Ni Z, et al. Modulation of transforming growth factor-beta-induced kidney fibrosis by leucine-rich -2 glycoprotein-1. Kidney Int (2022) 101(2):299–314. doi: 10.1016/j.kint.2021.10.023
52. Boels MGS, Koudijs A, Avramut MC, Sol W, Wang G, van Oeveren-Rietdijk AM, et al. Systemic monocyte chemotactic protein-1 inhibition modifies renal macrophages and restores glomerular endothelial glycocalyx and barrier function in diabetic nephropathy. Am J Pathol (2017) 187(11):2430–40. doi: 10.1016/j.ajpath.2017.07.020
53. Mohany M, Alanazi AZ, Alqahtani F, Belali OM, Ahmed MM, Al-Rejaie SS. Lcz696 mitigates diabetic-induced nephropathy through inhibiting oxidative stress, nf-Kb mediated inflammation and glomerulosclerosis in rats. PeerJ (2020) 8:e9196. doi: 10.7717/peerj.9196
54. Xu J, Wang Y, Wang Z, Guo L, Li X. Fucoidan mitigated diabetic nephropathy through the downregulation of pkc and modulation of nf-Kb signaling pathway: in vitro and in vivo investigations. Phytother Res (2021) 35(4):2133–44. doi: 10.1002/ptr.6966
55. He X, Zeng X. Lncrna Snhg16 Aggravates High Glucose-Induced Podocytes Injury in Diabetic Nephropathy through Targeting Mir-106a and Thereby up-Regulating Klf9. Diabetes Metab Syndr Obes (2020) 13:3551–60. doi: 10.2147/dmso.S271290
56. Han Y, Xu X, Tang C, Gao P, Chen X, Xiong X, et al. Reactive oxygen species promote tubular injury in diabetic nephropathy: the role of the mitochondrial Ros-txnip-Nlrp3 biological axis. Redox Biol (2018) 16:32–46. doi: 10.1016/j.redox.2018.02.013
57. Sun K, Tang X, Song S, Gao Y, Yu H, Sun N, et al. Hyperoxalemia leads to oxidative stress in endothelial cells and mice with chronic kidney disease. Kidney Blood Press Res (2021) 46(3):377–86. doi: 10.1159/000516013
58. van de Lest NA, Bakker AE, Dijkstra KL, Zandbergen M, Heemskerk SAC, Wolterbeek R, et al. Endothelial endothelin receptor a expression is associated with podocyte injury and oxidative stress in patients with focal segmental glomerulosclerosis. Kidney Int Rep (2021) 6(7):1939–48. doi: 10.1016/j.ekir.2021.04.013
59. Lu CL, Liao MT, Hou YC, Fang YW, Zheng CM, Liu WC, et al. Sirtuin-1 and its relevance in vascular calcification. Int J Mol Sci (2020) 21(5):1593. doi: 10.3390/ijms21051593
60. Wei L, Jian P, Erjiong H, Qihan Z. Ginkgetin alleviates high glucose-evoked mesangial cell oxidative stress injury, inflammation, and extracellular matrix (Ecm) deposition in an ampk/mtor-mediated autophagy axis. Chem Biol Drug Des (2021) 98(4):620–30. doi: 10.1111/cbdd.13915
61. Ha KB, Sangartit W, Jeong AR, Lee ES, Kim HM, Shim S, et al. Ew-7197 attenuates the progression of diabetic nephropathy in db/db mice through suppression of fibrogenesis and inflammation. Endocrinol Metab (Seoul) (2022) 37(1):96–111. doi: 10.3803/EnM.2021.1305
62. Fang Y, Xing C, Wang X, Cao H, Zhang C, Guo X, et al. Activation of the Ros/ho-1/nqo1 signaling pathway contributes to the copper-induced oxidative stress and autophagy in duck renal tubular epithelial cells. Sci Total Environ (2021) 757:143753. doi: 10.1016/j.scitotenv.2020.143753
63. Chen H, Jin G. Downregulation of salusin-beta protects renal tubular epithelial cells against high glucose-induced inflammation, oxidative stress, apoptosis and lipid accumulation via suppressing mir-155-5p. Bioengineered (2021) 12(1):6155–65. doi: 10.1080/21655979.2021.1972900
64. Hou Y, Wang Q, Han B, Chen Y, Qiao X, Wang L. Cd36 promotes Nlrp3 inflammasome activation via the mtros pathway in renal tubular epithelial cells of diabetic kidneys. Cell Death Dis (2021) 12(6):523. doi: 10.1038/s41419-021-03813-6
65. Tutun B, Elbe H, Vardi N, Parlakpinar H, Polat A, Gunaltili M, et al. Dexpanthenol reduces diabetic nephropathy and renal oxidative stress in rats. Biotech Histochem (2019) 94(2):84–91. doi: 10.1080/10520295.2018.1508746
66. Sun Y, Ge X, Li X, He J, Wei X, Du J, et al. High-fat diet promotes renal injury by inducing oxidative stress and mitochondrial dysfunction. Cell Death Dis (2020) 11(10):914. doi: 10.1038/s41419-020-03122-4
67. Gong X, Duan Y, Zheng J, Ye Z, Hei TK. Tetramethylpyrazine prevents contrast-induced nephropathy via modulating tubular cell mitophagy and suppressing mitochondrial fragmentation, ccl2/ccr2-mediated inflammation, and intestinal injury. Oxid Med Cell Longev (2019) 2019:7096912. doi: 10.1155/2019/7096912
68. Zhu YT, Wan C, Lin JH, Hammes HP, Zhang C. Mitochondrial oxidative stress and cell death in podocytopathies. Biomolecules (2022) 12(3):403. doi: 10.3390/biom12030403
69. Nabi R, Alvi SS, Shah A, Chaturvedi CP, Faisal M, Alatar AA, et al. Ezetimibe attenuates experimental diabetes and renal pathologies via targeting the advanced glycation, oxidative stress and age-rage signalling in rats. Arch Physiol Biochem (2021), 1–16. doi: 10.1080/13813455.2021.1874996
70. Zhu Z, Liang W, Chen Z, Hu J, Feng J, Cao Y, et al. Mitoquinone protects podocytes from angiotensin ii-induced mitochondrial dysfunction and injury via the keap1-nrf2 signaling pathway. Oxid Med Cell Longev (2021) 2021:1394486. doi: 10.1155/2021/1394486
71. Gao H, Wu H. Maslinic acid activates renal ampk/sirt1 signaling pathway and protects against diabetic nephropathy in mice. BMC Endocr Disord (2022) 22(1):25. doi: 10.1186/s12902-022-00935-6
72. Jalgaonkar MP, Parmar UM, Kulkarni YA, Oza MJ. Sirt1-foxos activity regulates diabetic complications. Pharmacol Res (2022) 175:106014. doi: 10.1016/j.phrs.2021.106014
73. Qiu D, Song S, Wang Y, Bian Y, Wu M, Wu H, et al. Nad(P)H: quinone oxidoreductase 1 attenuates oxidative stress and apoptosis by regulating sirt1 in diabetic nephropathy. J Transl Med (2022) 20(1):44. doi: 10.1186/s12967-021-03197-3
74. Alsuliam SM, Albadr NA, Almaiman SA, Al-Khalifah AS, Alkhaldy NS, Alshammari GM. Fenugreek seed galactomannan aqueous and extract protects against diabetic nephropathy and liver damage by targeting nf-kappab and keap1/nrf2 axis. Toxics (2022) 10(7):362. doi: 10.3390/toxics10070362
75. Zhang D, Jin W, Wu R, Li J, Park SA, Tu E, et al. High glucose intake exacerbates autoimmunity through reactive-oxygen-species-mediated tgf-B Cytokine activation. Immunity (2019) 51(4):671–81.e5. doi: 10.1016/j.immuni.2019.08.001
76. Qin X, Zhao Y, Gong J, Huang W, Su H, Yuan F, et al. Berberine protects glomerular podocytes via inhibiting drp1-mediated mitochondrial fission and dysfunction. Theranostics (2019) 9(6):1698–713. doi: 10.7150/thno.30640
77. Rousseau M, Denhez B, Spino C, Lizotte F, Guay A, Cote AM, et al. Reduction of dusp4 contributes to podocytes oxidative stress, insulin resistance and diabetic nephropathy. Biochem Biophys Res Commun (2022) 624:127–33. doi: 10.1016/j.bbrc.2022.07.067
78. Jiang S, Shui Y, Cui Y, Tang C, Wang X, Qiu X, et al. Gut microbiota dependent trimethylamine N-oxide aggravates angiotensin ii-induced hypertension. Redox Biol (2021) 46:102115. doi: 10.1016/j.redox.2021.102115
79. Qin J, Peng Z, Yuan Q, Li Q, Peng Y, Wen R, et al. Akf-pd alleviates diabetic nephropathy via blocking the rage/ages/Nox and Pkc/Nox pathways. Sci Rep (2019) 9(1):4407. doi: 10.1038/s41598-018-36344-w
80. Singh A, Kukreti R, Saso L, Kukreti S. Mechanistic insight into oxidative stress-triggered signaling pathways and type 2 diabetes. Molecules (2022) 27(3):950. doi: 10.3390/molecules27030950
81. Xu Y, Zhou Q, Xin W, Li Z, Chen L, Wan Q. Autophagy downregulation contributes to insulin resistance mediated injury in insulin receptor knockout podocytes in vitro. PeerJ (2016) 4:e1888. doi: 10.7717/peerj.1888
82. Upadhyay R, Batuman V. Aristolochic acid I induces proximal tubule injury through Ros/hmgb1/mt DNA mediated activation of tlrs. J Cell Mol Med (2022) 26(15):4277–91. doi: 10.1111/jcmm.17451
83. de Araujo FF, de Paulo Farias D, Neri-Numa IA, Pastore GM. Polyphenols and their applications: an approach in food chemistry and innovation potential. Food Chem (2021) 338:127535. doi: 10.1016/j.foodchem.2020.127535
84. Runeberg P, Ryabukhin D, Lagerquist L, Rahkila J, Eklund P. Transformations and antioxidative activities of lignans and stilbenes at high temperatures. Food Chem (2023) 404(Pt B):134641. doi: 10.1016/j.foodchem.2022.134641
85. Caruso G, Torrisi SA, Mogavero MP, Currenti W, Castellano S, Godos J, et al. Polyphenols and neuroprotection: therapeutic implications for cognitive decline. Pharmacol Ther (2022) 232:108013. doi: 10.1016/j.pharmthera.2021.108013
86. Tian X, Zhang S, Zhang Q, Kang L, Ma C, Feng L, et al. Resveratrol inhibits tumor progression by down-regulation of Nlrp3 in renal cell carcinoma. J Nutr Biochem (2020) 85:108489. doi: 10.1016/j.jnutbio.2020.108489
87. Li S, Yin S, Ding H, Shao Y, Zhou S, Pu W, et al. Polyphenols as potential metabolism mechanisms regulators in liver protection and liver cancer prevention. Cell Prolif (2023) 56(1):e13346. doi: 10.1111/cpr.13346
88. Zhu F, Zhang J, Zhong J, Wang T, Li Y, Gu Z. Natural polyphenol-based nanoparticles for the treatment of iron-overload disease. J Control Release (2023) 356:84–92. doi: 10.1016/j.jconrel.2023.02.027
89. Das M, Devi KP, Belwal T, Devkota HP, Tewari D, Sahebnasagh A, et al. Harnessing polyphenol power by targeting enos for vascular diseases. Crit Rev Food Sci Nutr (2023) 63(14):2093–118. doi: 10.1080/10408398.2021.1971153
90. Xiao J, Hogger P. Stability of dietary polyphenols under the cell culture conditions: avoiding erroneous conclusions. J Agric Food Chem (2015) 63(5):1547–57. doi: 10.1021/jf505514d
91. Sies H, Jones DP. Reactive oxygen species (Ros) as pleiotropic physiological signalling agents. Nat Rev Mol Cell Biol (2020) 21(7):363–83. doi: 10.1038/s41580-020-0230-3
92. Trujillo J, Molina-Jijon E, Medina-Campos ON, Rodriguez-Munoz R, Reyes JL, Loredo ML, et al. Curcumin prevents cisplatin-induced decrease in the tight and adherens junctions: relation to oxidative stress. Food Funct (2016) 7(1):279–93. doi: 10.1039/c5fo00624d
93. Teixeira Oliveira J, Machado da Costa F, Goncalvez da Silva T, Dotto Simoes G, Dos Santos Pereira E, Quevedo da Costa P, et al. Green tea and kombucha characterization: phenolic composition, antioxidant capacity and enzymatic inhibition potential. Food Chem (2023) 408:135206. doi: 10.1016/j.foodchem.2022.135206
94. Guo Y, Jia HR, Zhang X, Zhang X, Sun Q, Wang SZ, et al. A glucose/oxygen-exhausting nanoreactor for starvation- and hypoxia-activated sustainable and cascade chemo-chemodynamic therapy. Small (2020) 16(31):e2000897. doi: 10.1002/smll.202000897
95. Bowtell J, Kelly V. Fruit-derived polyphenol supplementation for athlete recovery and performance. Sports Med (2019) 49(Suppl 1):3–23. doi: 10.1007/s40279-018-0998-x
96. Mukherjee S, Ghosh S, Choudhury S, Gupta P, Adhikary A, Chattopadhyay S. Pomegranate polyphenols attenuate inflammation and hepatic damage in tumor-bearing mice: crucial role of nf-kappab and the nrf2/gsh axis. J Nutr Biochem (2021) 97:108812. doi: 10.1016/j.jnutbio.2021.108812
97. Zhu H, Li X, Qiao M, Sun X, Li G. Resveratrol alleviates inflammation and er stress through sirt1/nrf2 to delay ovarian aging in a short-lived fish. J Gerontol A Biol Sci Med Sci (2023) 78(4):596–602. doi: 10.1093/gerona/glad009
98. Chao L, Lin J, Zhou J, Du H, Chen X, Liu M, et al. Polyphenol rich forsythia suspensa extract alleviates dss-induced ulcerative colitis in mice through the nrf2-Nlrp3 pathway. Antioxid (Basel) (2022) 11(3):475. doi: 10.3390/antiox11030475
99. Khan H, Ullah H, Castilho P, Gomila AS, D'Onofrio G, Filosa R, et al. Targeting nf-kappab signaling pathway in cancer by dietary polyphenols. Crit Rev Food Sci Nutr (2020) 60(16):2790–800. doi: 10.1080/10408398.2019.1661827
100. Yu C, Wang D, Yang Z, Wang T. Pharmacological effects of polyphenol phytochemicals on the intestinal inflammation via targeting tlr4/nf-kappab signaling pathway. Int J Mol Sci (2022) 23(13):6939. doi: 10.3390/ijms23136939
101. Lu YN, Shen XY, Lu JM, Jin GN, Lan HW, Xu X, et al. Resveratrol inhibits toxoplasma gondii-induced lung injury, inflammatory cascade and evidences of its mechanism of action. Phytomedicine (2023) 108:154522. doi: 10.1016/j.phymed.2022.154522
102. Wang X, Simayi A, Fu J, Zhao X, Xu G. Resveratrol mediates the mir-149/hmgb1 axis and regulates the ferroptosis pathway to protect myocardium in endotoxemia mice. Am J Physiol Endocrinol Metab (2022) 323(1):E21–32. doi: 10.1152/ajpendo.00227.2021
103. Le K, Chibaatar Daliv E, Wu S, Qian F, Ali AI, Yu D, et al. Sirt1-regulated hmgb1 release is partially involved in tlr4 signal transduction: A possible anti-neuroinflammatory mechanism of resveratrol in neonatal hypoxic-ischemic brain injury. Int Immunopharmacol (2019) 75:105779. doi: 10.1016/j.intimp.2019.105779
104. de la Luz Cadiz-Gurrea M, Micol V, Joven J, Segura-Carretero A, Fernandez-Arroyo S. Different behavior of polyphenols in energy metabolism of lipopolysaccharide-stimulated cells. Food Res Int (2019) 118:96–100. doi: 10.1016/j.foodres.2018.02.027
105. Wang F, Li R, Zhao L, Ma S, Qin G. Resveratrol ameliorates renal damage by inhibiting oxidative stress-mediated apoptosis of podocytes in diabetic nephropathy. Eur J Pharmacol (2020) 885:173387. doi: 10.1016/j.ejphar.2020.173387
106. Hussein MM, Mahfouz MK. Effect of resveratrol and rosuvastatin on experimental diabetic nephropathy in rats. BioMed Pharmacother (2016) 82:685–92. doi: 10.1016/j.biopha.2016.06.004
107. Park HS, Lim JH, Kim MY, Kim Y, Hong YA, Choi SR, et al. Resveratrol increases adipor1 and adipor2 expression in type 2 diabetic nephropathy. J Transl Med (2016) 14(1):176. doi: 10.1186/s12967-016-0922-9
108. Palsamy P, Subramanian S. Resveratrol protects diabetic kidney by attenuating hyperglycemia-mediated oxidative stress and renal inflammatory cytokines via nrf2-keap1 signaling. Biochim Biophys Acta (2011) 1812(7):719–31. doi: 10.1016/j.bbadis.2011.03.008
109. Chang CC, Chang CY, Wu YT, Huang JP, Yen TH, Hung LM. Resveratrol retards progression of diabetic nephropathy through modulations of oxidative stress, proinflammatory cytokines, and amp-activated protein kinase. J BioMed Sci (2011) 18(1):47. doi: 10.1186/1423-0127-18-47
110. Wang X, Meng L, Zhao L, Wang Z, Liu H, Liu G, et al. Resveratrol ameliorates hyperglycemia-induced renal tubular oxidative stress damage via modulating the sirt1/foxo3a pathway. Diabetes Res Clin Pract (2017) 126:172–81. doi: 10.1016/j.diabres.2016.12.005
111. Zhang T, Chi Y, Kang Y, Lu H, Niu H, Liu W, et al. Resveratrol ameliorates podocyte damage in diabetic mice via sirt1/pgc-1alpha mediated attenuation of mitochondrial oxidative stress. J Cell Physiol (2019) 234(4):5033–43. doi: 10.1002/jcp.27306
112. Sharma S, Anjaneyulu M, Kulkarni SK, Chopra K. Resveratrol, a polyphenolic phytoalexin, attenuates diabetic nephropathy in rats. Pharmacology (2006) 76(2):69–75. doi: 10.1159/000089720
113. Bashir SO. Concomitant administration of resveratrol and insulin protects against diabetes mellitus type-1-induced renal damage and impaired function via an antioxidant-mediated mechanism and up-regulation of Na(+)/K(+)-Atpase. Arch Physiol Biochem (2019) 125(2):104–13. doi: 10.1080/13813455.2018.1437752
114. Kim MY, Lim JH, Youn HH, Hong YA, Yang KS, Park HS, et al. Resveratrol prevents renal lipotoxicity and inhibits mesangial cell glucotoxicity in a manner dependent on the ampk-sirt1-pgc1alpha axis in db/db mice. Diabetologia (2013) 56(1):204–17. doi: 10.1007/s00125-012-2747-2
115. Yan C, Xu W, Huang Y, Li M, Shen Y, You H, et al. Hrd1-mediated igf-1r ubiquitination contributes to renal protection of resveratrol in db/db mice. Mol Endocrinol (2016) 30(6):600–13. doi: 10.1210/me.2015-1277
116. Ghasemi H, Einollahi B, Kheiripour N, Hosseini-Zijoud SR, Farhadian Nezhad M. Protective effects of curcumin on diabetic nephropathy via attenuation of kidney injury molecule 1 (Kim-1) and neutrophil gelatinase-associated lipocalin (Ngal) expression and alleviation of oxidative stress in rats with type 1 diabetes. Iran J Basic Med Sci (2019) 22(4):376–83. doi: 10.22038/ijbms.2019.31922.7674
117. Kim BH, Lee ES, Choi R, Nawaboot J, Lee MY, Lee EY, et al. Protective effects of curcumin on renal oxidative stress and lipid metabolism in a rat model of type 2 diabetic nephropathy. Yonsei Med J (2016) 57(3):664–73. doi: 10.3349/ymj.2016.57.3.664
118. Soetikno V, Watanabe K, Sari FR, Harima M, Thandavarayan RA, Veeraveedu PT, et al. Curcumin attenuates diabetic nephropathy by inhibiting pkc-alpha and pkc-beta1 activity in streptozotocin-induced type I diabetic rats. Mol Nutr Food Res (2011) 55(11):1655–65. doi: 10.1002/mnfr.201100080
119. Sharma S, Kulkarni SK, Chopra K. Curcumin, the active principle of turmeric (Curcuma longa), ameliorates diabetic nephropathy in rats. Clin Exp Pharmacol Physiol (2006) 33(10):940–5. doi: 10.1111/j.1440-1681.2006.04468.x
120. Ho C, Hsu YC, Lei CC, Mau SC, Shih YH, Lin CL. Curcumin rescues diabetic renal fibrosis by targeting superoxide-mediated wnt signaling pathways. Am J Med Sci (2016) 351(3):286–95. doi: 10.1016/j.amjms.2015.12.017
121. Sun LN, Liu XC, Chen XJ, Guan GJ, Liu G. Curcumin attenuates high glucose-induced podocyte apoptosis by regulating functional connections between caveolin-1 phosphorylation and Ros. Acta Pharmacol Sin (2016) 37(5):645–55. doi: 10.1038/aps.2015.159
122. AL JZ, AlFaris NA, Al-Farga AM, Alshammari GM, BinMowyna MN, Yahya MA. Curcumin reverses diabetic nephropathy in streptozotocin-induced diabetes in rats by inhibition of pkcbeta/P(66)Shc axis and activation of foxo-3a. J Nutr Biochem (2021) 87:108515. doi: 10.1016/j.jnutbio.2020.108515
123. Tang L, Li K, Zhang Y, Li H, Li A, Xu Y, et al. Quercetin liposomes ameliorate streptozotocin-induced diabetic nephropathy in diabetic rats. Sci Rep (2020) 10(1):2440. doi: 10.1038/s41598-020-59411-7
124. Tong F, Liu S, Yan B, Li X, Ruan S, Yang S. Quercetin nanoparticle complex attenuated diabetic nephropathy via regulating the expression level of icam-1 on endothelium. Int J Nanomed (2017) 12:7799–813. doi: 10.2147/IJN.S146978
125. Gao F, He X, Liang S, Liu S, Liu H, He Q, et al. Quercetin ameliorates podocyte injury via inhibition of oxidative stress and the tgf-beta1/smad pathway in dn rats. RSC Adv (2018) 8(62):35413–21. doi: 10.1039/c8ra07935h
126. Gomes IB, Porto ML, Santos MC, Campagnaro BP, Pereira TM, Meyrelles SS, et al. Renoprotective, anti-oxidative and anti-apoptotic effects of oral low-dose quercetin in the C57bl/6j model of diabetic nephropathy. Lipids Health Dis (2014) 13:184. doi: 10.1186/1476-511X-13-184
127. Abdou HM, Abd Elkader HAE. The potential therapeutic effects of trifolium alexandrinum extract, hesperetin and quercetin against diabetic nephropathy via attenuation of oxidative stress, inflammation, gsk-3beta and apoptosis in male rats. Chem Biol Interact (2022) 352:109781. doi: 10.1016/j.cbi.2021.109781
128. Iskender H, Dokumacioglu E, Sen TM, Ince I, Kanbay Y, Saral S. The effect of hesperidin and quercetin on oxidative stress, nf-kappab and sirt1 levels in a stz-induced experimental diabetes model. BioMed Pharmacother (2017) 90:500–8. doi: 10.1016/j.biopha.2017.03.102
129. Zhang XT, Wang G, Ye LF, Pu Y, Li RT, Liang J, et al. Baicalin reversal of DNA hypermethylation-associated klotho suppression ameliorates renal injury in type 1 diabetic mouse model. Cell Cycle (2020) 19(23):3329–47. doi: 10.1080/15384101.2020.1843815
130. Kulkarni YA, Suryavanshi SV. Combination of naringenin and lisinopril ameliorates nephropathy in type-1 diabetic rats. Endocr Metab Immune Disord Drug Targets (2021) 21(1):173–82. doi: 10.2174/1871530320666200516163919
131. Roy S, Ahmed F, Banerjee S, Saha U. Naringenin ameliorates streptozotocin-induced diabetic rat renal impairment by downregulation of tgf-beta1 and il-1 via modulation of oxidative stress correlates with decreased apoptotic events. Pharm Biol (2016) 54(9):1616–27. doi: 10.3109/13880209.2015.1110599
132. Liu Y, Ye J, Cao Y, Zhang R, Wang Y, Zhang S, et al. Silibinin ameliorates diabetic nephropathy via improving diabetic condition in the mice. Eur J Pharmacol (2019) 845:24–31. doi: 10.1016/j.ejphar.2018.12.031
133. Xu X, Zheng N, Chen Z, Huang W, Liang T, Kuang H. Puerarin, isolated from pueraria lobata (Willd.), protects against diabetic nephropathy by attenuating oxidative stress. Gene (2016) 591(2):411–6. doi: 10.1016/j.gene.2016.06.032
134. Li X, Cai W, Lee K, Liu B, Deng Y, Chen Y, et al. Puerarin attenuates diabetic kidney injury through the suppression of Nox4 expression in podocytes. Sci Rep (2017) 7(1):14603. doi: 10.1038/s41598-017-14906-8
135. Zhong Y, Zhang X, Cai X, Wang K, Chen Y, Deng Y. Puerarin attenuated early diabetic kidney injury through down-regulation of matrix metalloproteinase 9 in streptozotocin-induced diabetic rats. PloS One (2014) 9(1):e85690. doi: 10.1371/journal.pone.0085690
136. Zheng HX, Qi SS, He J, Hu CY, Han H, Jiang H, et al. Cyanidin-3-glucoside from black rice ameliorates diabetic nephropathy via reducing blood glucose, suppressing oxidative stress and inflammation, and regulating transforming growth factor B1/smad expression. J Agric Food Chem (2020) 68(15):4399–410. doi: 10.1021/acs.jafc.0c00680
137. Qin Y, Zhai Q, Li Y, Cao M, Xu Y, Zhao K, et al. Cyanidin-3-O-glucoside ameliorates diabetic nephropathy through regulation of glutathione pool. BioMed Pharmacother (2018) 103:1223–30. doi: 10.1016/j.biopha.2018.04.137
138. Li P, Bukhari SNA, Khan T, Chitti R, Bevoor DB, Hiremath AR, et al. Apigenin-loaded solid lipid nanoparticle attenuates diabetic nephropathy induced by streptozotocin nicotinamide through nrf2/ho-1/nf-kb signalling pathway. Int J Nanomed (2020) 15:9115–24. doi: 10.2147/ijn.S256494
139. Zhang M, He L, Liu J, Zhou L. Luteolin attenuates diabetic nephropathy through suppressing inflammatory response and oxidative stress by inhibiting stat3 pathway. Exp Clin Endocrinol Diabetes (2021) 129(10):729–39. doi: 10.1055/a-0998-7985
140. Wang GG, Lu XH, Li W, Zhao X, Zhang C. Protective effects of luteolin on diabetic nephropathy in stz-induced diabetic rats. Evid Based Complement Alternat Med (2011) 2011:323171. doi: 10.1155/2011/323171
141. Pal PB, Sinha K, Sil PC. Mangiferin attenuates diabetic nephropathy by inhibiting oxidative stress mediated signaling cascade, tnfalpha related and mitochondrial dependent apoptotic pathways in streptozotocin-induced diabetic rats. PloS One (2014) 9(9):e107220. doi: 10.1371/journal.pone.0107220
142. Song Y, Liu W, Tang K, Zang J, Li D, Gao H. Mangiferin alleviates renal interstitial fibrosis in streptozotocin-induced diabetic mice through regulating the pten/pi3k/akt signaling pathway. J Diabetes Res (2020) 2020:9481720. doi: 10.1155/2020/9481720
143. Liu YW, Zhu X, Zhang L, Lu Q, Wang JY, Zhang F, et al. Up-regulation of glyoxalase 1 by mangiferin prevents diabetic nephropathy progression in streptozotocin-induced diabetic rats. Eur J Pharmacol (2013) 721(1-3):355–64. doi: 10.1016/j.ejphar.2013.08.029
144. Chowdhury S, Ghosh S, Das AK, Sil PC. Ferulic acid protects hyperglycemia-induced kidney damage by regulating oxidative insult, inflammation and autophagy. Front Pharmacol (2019) 10:27. doi: 10.3389/fphar.2019.00027
145. Qi MY, Wang XT, Xu HL, Yang ZL, Cheng Y, Zhou B. Protective effect of ferulic acid on stz-induced diabetic nephropathy in rats. Food Funct (2020) 11(4):3706–18. doi: 10.1039/c9fo02398d
146. Choi R, Kim BH, Naowaboot J, Lee MY, Hyun MR, Cho EJ, et al. Effects of ferulic acid on diabetic nephropathy in a rat model of type 2 diabetes. Exp Mol Med (2011) 43(12):676–83. doi: 10.3858/emm.2011.43.12.078
147. Orsolic N, Sirovina D, Odeh D, Gajski G, Balta V, Sver L, et al. Efficacy of caffeic acid on diabetes and its complications in the mouse. Molecules (2021) 26(11):3262. doi: 10.3390/molecules26113262
148. Zhou B, Li Q, Wang J, Chen P, Jiang S. Ellagic acid attenuates streptozocin induced diabetic nephropathy via the regulation of oxidative stress and inflammatory signaling. Food Chem Toxicol (2019) 123:16–27. doi: 10.1016/j.fct.2018.10.036
149. Ye HY, Li ZY, Zheng Y, Chen Y, Zhou ZH, Jin J. The attenuation of chlorogenic acid on oxidative stress for renal injury in streptozotocin-induced diabetic nephropathy rats. Arch Pharm Res (2016) 39(7):989–97. doi: 10.1007/s12272-016-0771-3
150. Bao L, Li J, Zha D, Zhang L, Gao P, Yao T, et al. Chlorogenic acid prevents diabetic nephropathy by inhibiting oxidative stress and inflammation through modulation of the nrf2/ho-1 and nf-ĸb pathways. Int Immunopharmacol (2018) 54:245–53. doi: 10.1016/j.intimp.2017.11.021
151. Tang J, Diao P, Shu X, Li L, Xiong L. Quercetin and quercitrin attenuates the inflammatory response and oxidative stress in lps-induced raw264.7 cells: in vitro assessment and a theoretical model. BioMed Res Int (2019) 2019:7039802. doi: 10.1155/2019/7039802
152. Li D, Jiang C, Mei G, Zhao Y, Chen L, Liu J, et al. Quercetin alleviates ferroptosis of pancreatic beta cells in type 2 diabetes. Nutrients (2020) 12(10):2954. doi: 10.3390/nu12102954
153. Ma X, Hao C, Yu M, Zhang Z, Huang J, Yang W. Investigating the Molecular Mechanism of Quercetin Protecting against Podocyte Injury to Attenuate Diabetic Nephropathy through Network Pharmacology, Microarraydata Analysis, and Molecular Docking. Evid Based Complement Alternat Med (2022) 2022:7291434. doi: 10.1155/2022/7291434
154. Abharzanjani F, Afshar M, Hemmati M, Moossavi M. Short-term high dose of quercetin and resveratrol alters aging markers in human kidney cells. Int J Prev Med (2017) 8:64. doi: 10.4103/ijpvm.IJPVM_139_17
155. Wan H, Wang Y, Pan Q, Chen X, Chen S, Li X, et al. Quercetin attenuates the proliferation, inflammation, and oxidative stress of high glucose-induced human mesangial cells by regulating the mir-485-5p/yap1 pathway. Int J Immunopathol Pharmacol (2022) 36:20587384211066440. doi: 10.1177/20587384211066440
156. Tang DQ, Wei YQ, Yin XX, Lu Q, Hao HH, Zhai YP, et al. In vitro suppression of quercetin on hypertrophy and extracellular matrix accumulation in rat glomerular mesangial cells cultured by high glucose. Fitoterapia (2011) 82(6):920–6. doi: 10.1016/j.fitote.2011.05.001
157. Hu T, Yue J, Tang Q, Cheng KW, Chen F, Peng M, et al. The effect of quercetin on diabetic nephropathy (Dn): A systematic review and meta-analysis of animal studies. Food Funct (2022) 13(9):4789–803. doi: 10.1039/d1fo03958j
158. Li Z, Deng H, Guo X, Yan S, Lu C, Zhao Z, et al. Effective dose/duration of natural flavonoid quercetin for treatment of diabetic nephropathy: A systematic review and meta-analysis of rodent data. Phytomedicine (2022) 105:154348. doi: 10.1016/j.phymed.2022.154348
159. Wu K, Liang T, Duan X, Xu L, Zhang K, Li R. Anti-diabetic effects of puerarin, isolated from pueraria lobata (Willd.), on streptozotocin-diabetogenic mice through promoting insulin expression and ameliorating metabolic function. Food Chem Toxicol (2013) 60:341–7. doi: 10.1016/j.fct.2013.07.077
160. Chen X, Yu J, Shi J. Management of diabetes mellitus with puerarin, a natural isoflavone from pueraria lobata. Am J Chin Med (2018) 46(8):1771–89. doi: 10.1142/S0192415X18500891
161. Ren H, Shao Y, Wu C, Ma X, Lv C, Wang Q. Metformin alleviates oxidative stress and enhances autophagy in diabetic kidney disease via ampk/sirt1-foxo1 pathway. Mol Cell Endocrinol (2020) 500:110628. doi: 10.1016/j.mce.2019.110628
162. Yin F, Liu J, Ji X, Wang Y, Zidichouski J, Zhang J. Baicalin prevents the production of hydrogen peroxide and oxidative stress induced by abeta aggregation in sh-sy5y cells. Neurosci Lett (2011) 492(2):76–9. doi: 10.1016/j.neulet.2011.01.055
163. Gupta G, Tiwari J, Dahiya R, Kumar Sharma R, Mishra A, Dua K. Recent updates on neuropharmacological effects of luteolin. EXCLI J (2018) 17:211–4. doi: 10.17179/excli2018-1041
164. Zheng HX, Qi SS, He J, Hu CY, Han H, Jiang H, et al. Cyanidin-3-glucoside from black rice ameliorates diabetic nephropathy via reducing blood glucose, suppressing oxidative stress and inflammation, and regulating transforming growth factor beta1/smad expression. J Agric Food Chem (2020) 68(15):4399–410. doi: 10.1021/acs.jafc.0c00680
165. Bai J, Wang Y, Zhu X, Shi J. Eriodictyol inhibits high glucose-induced extracellular matrix accumulation, oxidative stress, and inflammation in human glomerular mesangial cells. Phytother Res (2019) 33(10):2775–82. doi: 10.1002/ptr.6463
166. Liu J, Sun M, Xia Y, Cui X, Jiang J. Phloretin ameliorates diabetic nephropathy by inhibiting nephrin and podocin reduction through a non-hypoglycemic effect. Food Funct (2022) 13(12):6613–22. doi: 10.1039/d2fo00570k
167. Zhang J, Zhao X, Zhu H, Wang J, Ma J, Gu M. Apigenin protects against renal tubular epithelial cell injury and oxidative stress by high glucose via regulation of nf-E2-related factor 2 (Nrf2) pathway. Med Sci Monit (2019) 25:5280–8. doi: 10.12659/MSM.915038
168. Huang DD, Shi G, Jiang Y, Yao C, Zhu C. A review on the potential of resveratrol in prevention and therapy of diabetes and diabetic complications. BioMed Pharmacother (2020) 125:109767. doi: 10.1016/j.biopha.2019.109767
169. Gowd V, Kang Q, Wang Q, Wang Q, Chen F, Cheng KW. Resveratrol: evidence for its nephroprotective effect in diabetic nephropathy. Adv Nutr (2020) 11(6):1555–68. doi: 10.1093/advances/nmaa075
170. Hu HC, Lei YH, Zhang WH, Luo XQ. Antioxidant and anti-inflammatory properties of resveratrol in diabetic nephropathy: A systematic review and meta-analysis of animal studies. Front Pharmacol (2022) 13:841818. doi: 10.3389/fphar.2022.841818
171. Al-Hussaini H, Kilarkaje N. Trans-resveratrol mitigates type 1 diabetes-induced oxidative DNA damage and accumulation of advanced glycation end products in glomeruli and tubules of rat kidneys. Toxicol Appl Pharmacol (2018) 339:97–109. doi: 10.1016/j.taap.2017.11.025
172. Hashemzaei M, Tabrizian K, Alizadeh Z, Pasandideh S, Rezaee R, Mamoulakis C, et al. Resveratrol, curcumin and gallic acid attenuate glyoxal-induced damage to rat renal cells. Toxicol Rep (2020) 7:1571–7. doi: 10.1016/j.toxrep.2020.11.008
173. He T, Xiong J, Nie L, Yu Y, Guan X, Xu X, et al. Resveratrol inhibits renal interstitial fibrosis in diabetic nephropathy by regulating ampk/Nox4/Ros pathway. J Mol Med (Berl) (2016) 94(12):1359–71. doi: 10.1007/s00109-016-1451-y
174. He T, Guan X, Wang S, Xiao T, Yang K, Xu X, et al. Resveratrol prevents high glucose-induced epithelial-mesenchymal transition in renal tubular epithelial cells by inhibiting nadph oxidase/Ros/erk pathway. Mol Cell Endocrinol (2015) 402:13–20. doi: 10.1016/j.mce.2014.12.010
175. Yang H, Xu W, Zhou Z, Liu J, Li X, Chen L, et al. Curcumin attenuates urinary excretion of albumin in type ii diabetic patients with enhancing nuclear factor erythroid-derived 2-like 2 (Nrf2) system and repressing inflammatory signaling efficacies. Exp Clin Endocrinol Diabetes (2015) 123(6):360–7. doi: 10.1055/s-0035-1545345
176. Chung H, Lee SW, Hyun M, Kim SY, Cho HG, Lee ES, et al. Curcumin blocks high glucose-induced podocyte injury via ripk3-dependent pathway. Front Cell Dev Biol (2022) 10:800574. doi: 10.3389/fcell.2022.800574
177. Zhang X, Liang D, Guo L, Liang W, Jiang Y, Li H, et al. Curcumin protects renal tubular epithelial cells from high glucose-induced epithelial-to-mesenchymal transition through nrf2-mediated upregulation of heme oxygenase-1. Mol Med Rep (2015) 12(1):1347–55. doi: 10.3892/mmr.2015.3556
178. Ibrahim ZS, Alkafafy ME, Ahmed MM, Soliman MM. Renoprotective effect of curcumin against the combined oxidative stress of diabetes and nicotine in rats. Mol Med Rep (2016) 13(4):3017–26. doi: 10.3892/mmr.2016.4922
179. AL JZ, AlFaris NA, Al-Farga AM, Alshammari GM, BinMowyna MN, Yahya MA. Curcumin reverses diabetic nephropathy in streptozotocin-induced diabetes in rats by inhibition of pkcβ/P(66)Shc axis and activation of foxo-3a. J Nutr Biochem (2021) 87:108515. doi: 10.1016/j.jnutbio.2020.108515
180. Bagherniya M, Soleimani D, Rouhani MH, Askari G, Sathyapalan T, Sahebkar A. The use of curcumin for the treatment of renal disorders: A systematic review of randomized controlled trials. Adv Exp Med Biol (2021) 1291:327–43. doi: 10.1007/978-3-030-56153-6_19
181. Jimenez-Osorio AS, Garcia-Nino WR, Gonzalez-Reyes S, Alvarez-Mejia AE, Guerra-Leon S, Salazar-Segovia J, et al. The effect of dietary supplementation with curcumin on redox status and nrf2 activation in patients with nondiabetic or diabetic proteinuric chronic kidney disease: A pilot study. J Ren Nutr (2016) 26(4):237–44. doi: 10.1053/j.jrn.2016.01.013
182. Mahfouz MM, Zhou SQ, Kummerow FA. Curcumin prevents the oxidation and lipid modification of ldl and its inhibition of prostacyclin generation by endothelial cells in culture. Prostaglandins Other Lipid Mediat (2009) 90(1-2):13–20. doi: 10.1016/j.prostaglandins.2009.06.005
183. Peng X, Wu G, Zhao A, Huang K, Chai L, Natarajan B, et al. Synthesis of novel caffeic acid derivatives and their protective effect against hydrogen peroxide induced oxidative stress via nrf2 pathway. Life Sci (2020) 247:117439. doi: 10.1016/j.lfs.2020.117439
184. Olayinka ET, Ola OS, Ore A, Adeyemo OA. Ameliorative effect of caffeic acid on capecitabine-induced hepatic and renal dysfunction: involvement of the antioxidant defence system. Medicines (Basel) (2017) 4(4):78. doi: 10.3390/medicines4040078
185. Ahad A, Ganai AA, Mujeeb M, Siddiqui WA. Ellagic acid, an nf-kappab inhibitor, ameliorates renal function in experimental diabetic nephropathy. Chem Biol Interact (2014) 219:64–75. doi: 10.1016/j.cbi.2014.05.011
186. Ricciardi CA, Gnudi L. Kidney disease in diabetes: from mechanisms to clinical presentation and treatment strategies. Metabolism (2021) 124:154890. doi: 10.1016/j.metabol.2021.154890
Keywords: polyphenols, quercetin, resveratrol, curcumin, diabetic nephropathy, oxidative stress, inflammation
Citation: Jin Q, Liu T, Qiao Y, Liu D, Yang L, Mao H, Ma F, Wang Y, Peng L and Zhan Y (2023) Oxidative stress and inflammation in diabetic nephropathy: role of polyphenols. Front. Immunol. 14:1185317. doi: 10.3389/fimmu.2023.1185317
Received: 13 March 2023; Accepted: 05 July 2023;
Published: 21 July 2023.
Edited by:
Weici Zhang, University of California, Davis, United StatesReviewed by:
Mithun Rudrapal, Vignan’s Foundation for Science, Technology and Research, IndiaZeliha Selamoglu, Niğde Ömer Halisdemir University, Türkiye
Pritam Sadhukhan, Johns Hopkins University, United States
Copyright © 2023 Jin, Liu, Qiao, Liu, Yang, Mao, Ma, Wang, Peng and Zhan. This is an open-access article distributed under the terms of the Creative Commons Attribution License (CC BY). The use, distribution or reproduction in other forums is permitted, provided the original author(s) and the copyright owner(s) are credited and that the original publication in this journal is cited, in accordance with accepted academic practice. No use, distribution or reproduction is permitted which does not comply with these terms.
*Correspondence: Liang Peng, cGVuZ2xpYW5nODAyOEAxNjMuY29t; Yongli Zhan, emhhbnlvbmdsaTg4QHNpbmEuY29t
†These authors have contributed equally to this work