- 1Department of Colorectal Surgery, Cancer Hospital of China Medical University, Liaoning Cancer Hospital and Institute, Shenyang, China
- 2Department of Bone and Soft Tissue Tumor Surgery, Cancer Hospital of China Medical University, Liaoning Cancer Hospital & Institute, Shenyang, China
Extracellular vesicles (EVs) are small particles secreted by numerous cell types and circulate in almost all body fluids, acting as crucial messengers for cell-to-cell communication. EVs involves multiple physiological and pathological processes, including tumor progression, via their multiple cargoes. Therefore, EVs have become attractive candidates for the treatment of tumor, including melanoma. Notably, due to the crucial role of the tumor microenvironment (TME) in promoting tumor malignant phenotype, and the close intercellular communication in TME, EVs-based therapy by targeting TME has become a cutting-edge and prospective strategy for inhibiting melanoma progression and strengthening the anti-tumor immunity. In this review, we aimed to summarize and discuss the role of therapeutic EVs, which target the components of TME in melanoma, thereby providing insights into these promising clinical strategies for the treatment of melanoma patients.
Introduction
Melanoma is an extremely malignant tumor accounting for approximately 5% of all tumors, which arises from melanocytes (1). Melanoma mainly involves the skin, and can also occur in eyes, meninges and diverse mucosal surfaces (2). Although melanoma is rare, it is responsible for most skin cancer-related death (3), owing to the great metastatic feature of melanoma cells (4). In addition to the tumor-draining sentinel lymph node (SLN) that has been recognized as the most initial metastasis site, melanoma cells also frequently disseminate to the distant regions and organs (5, 6). The tumor microenvironment (TME), a complex environment consists of various cells, including tumor cells, neutrophils, myeloid-derived suppressor cells (MDSC), immune and stromal cells, is vital for the development of melanoma, and exerts a key role in modulating both tumor immunity and the prognosis of melanoma (7–9). Notably, these impacts are mainly mediated by the crosstalk between melanoma tumor cells and other types of TME cells.
In the past decades, the lipid membrane bound nanoparticles extracellular vesicles (EVs), which can be produced by most cells, have been identified as important mediators during the communication between cells (10). EVs have been suggested to be divided into several subtypes according to their size (small and large EVs), origin (exosomes, ectosomes and apoptotic bodies), biochemical components (CD63+/CD81+ EVs) and physiological condition (hypoxic EVs) (11, 12). Although the classification of EVs is distinct, and it is difficult to distinguish them accurately, recent technological advances enable a more refined differentiation of the subset of EVs by diverse markers (13). EVs contain a great variety of biomolecules released from their donor cells, such as proteins, nucleic acids, and lipids. By transmitting their cargo to adjacent or distant recipient cells or tissues, EVs are able to trigger the malignant phenotype changes of the receptor cell, including the augmented tumor cell migration and invasion, enhanced angiogenesis, and impaired tumor immunity (14, 15).
Recently, increasing evidence have shown the close relationship between EVs and TME (Figure 1), which is responsible for the tumor progression, including melanoma (15–17). Therefore, in this review, we aimed to summarize the role of EVs in melanoma by targeting TME, and discuss the potential of EVs to be applied as an alternative option for the clinical treatment of melanoma.
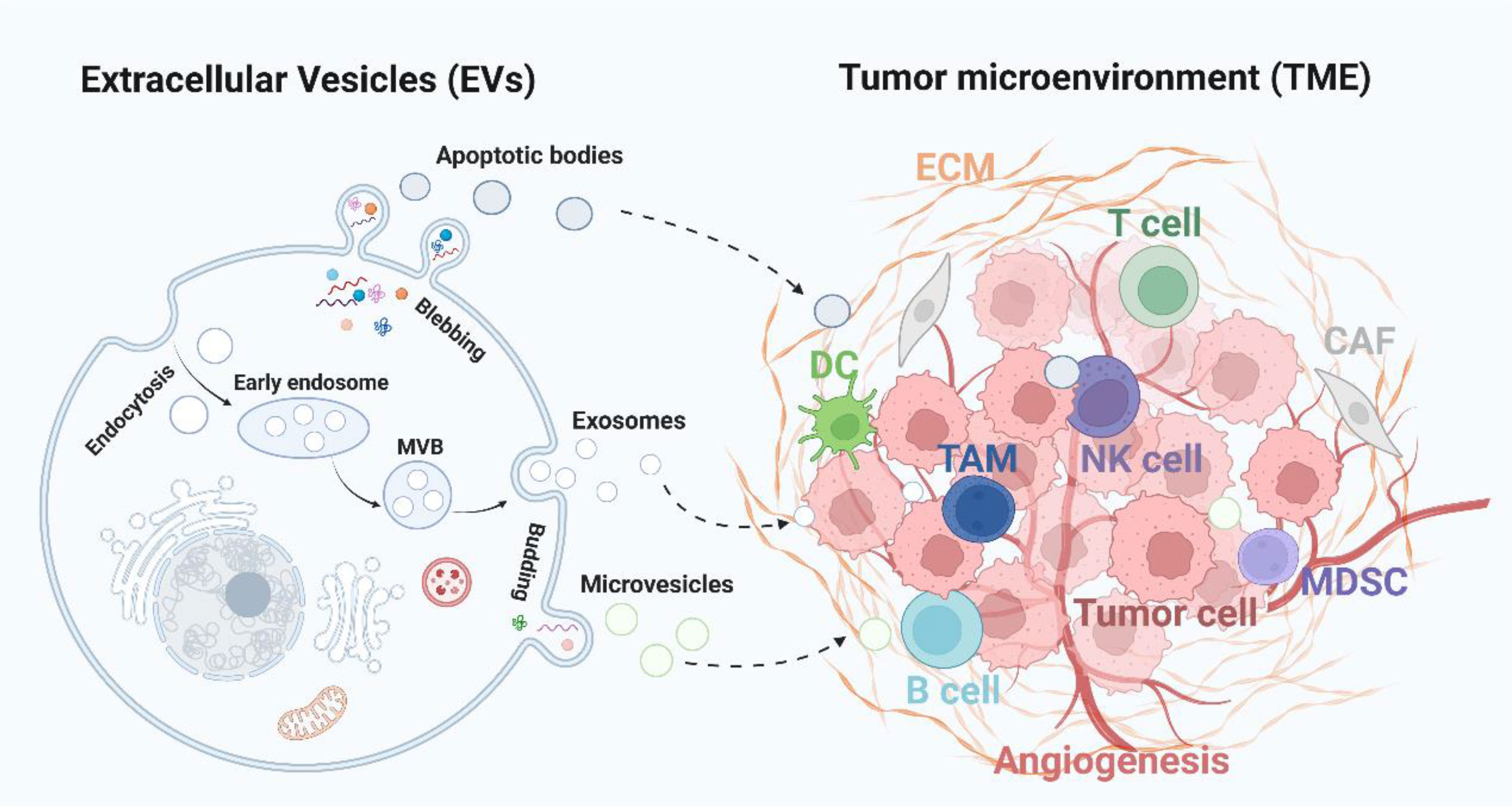
Figure 1 Extracellular vesicles-mediated intercommunication with TME. Tumor cells derived EVs, including exosomes, microvesicles and apoptotic bodies, deliver various cargoes to the TME and influence the immune response (TAM, DC, and T, B, NK cells), angiogenesis, stromal cells activity (CAF and MDSC), and ECM formation. CAF, cancer-associated fibroblasts; ECM, extracellular matrix; MDSC, myeloid-derived suppressor cells; TAM, tumor-associated macrophages; NK cell, natural killer cell.
Crosstalk between EVs and stromal cells in melanoma
In the microenvironment of tumor, stromal cells are vital components and play important roles in the occurrence, development and metastasis of tumor (18, 19). Among them, cancer-associated fibroblasts (CAFs) is the main type of the reactive tumor stroma and contributes to the tumorigenesis, such as melanoma (20, 21). Tumor-related EVs have been demonstrated to be involved in the differentiation of normal fibroblasts (NFs) into CAFs, and then induced a tumor-stimulative stroma (Table 1) (29). Gm26809, a novel lncRNA, was upregulated obviously in cytotoxic T-lymphocytes CTLL2 under the stimulation of EVs derived from B16F0 melanoma cells (30). Moreover, Hu et al. (22) found that the EVs released by B16F0 cell can induce the reprogram of fibroblast NIH/3T3 cells to CAFs, as well as the facilitated Cloundman S91 melanoma cell proliferation and migration, which was mediated by delivering Gm26809 to NIH/3T3 cells. These findings suggest that EVs-Gm26809 might be a potential target for promoting the progression of melanoma. Nevertheless, the potential molecular mechanism about how EVs-Gm26809 regulated the transition of NFs to CAFs remains unclear, and whether melanoma-derived EVs could transfer Gm26809 to other stromal cells required further investigation. The miRNAs with 18–24 nucleotide are major members of the non-coding RNAs, which can control the translation of downstream tumor-associated mRNAs, thus participating the tumor progression (31, 32). The miRNAs secreted by melanoma cells have also been implicated in the transformation of NFs towards CAFs by changing CAFs-related genes expression (33, 34). Similar to EVs, melanosomes with a diameter of 0.5 µm are melanin-containing vesicles that are specifically fabricate by melanocytes (23). As reported before, melanosome-miR-211 released by melanoma cells induced NFs to CAF transition upon absorbed by NFs, whereas the depletion of miR-211 prevented the formation of CAFs. Furthermore, miR-211 was found to target tumor suppressor IGF2R, resulting in the increase of collagen by CAFs so as to promote tumor cell motility (35). Although this study provided an opportunity to attenuate melanoma invasion by blocking the transformation of CAFs, whether melanosomes-miRNA can be absorbed by other cells in the melanoma microenvironment deserves more research. In addition, more efforts to explore EVs-miRNA mediated crosstalk between tumor cells and TME, as well as their potential to act as prognostic biomarkers or novel therapeutic strategy for melanoma should be considered. In addition to the transmission of tumor cell derived EVs to CAFs, EVs secreted by CAFs have also been revealed to exert an essential role in several tumor progression (24, 36). Recent data showed that CAF-released EVs enriched CD9 and CD63, and inhibited the proliferation of melanoma cells remarkably. The patients with CAF-derived CD9-positive EVs displayed a better five-year disease-free survival than patients with CD9-negative, indicating that CD9 expression in CAFs-EVs is a favorable prognostic marker for malignant melanoma patients (25). Furthermore, to develop novel targeted drugs depending on this type of EVs may be beneficial for the clinical treatment of melanoma patients. Moreover, tumor-derived EVs have been revealed to transdifferentiate CAFs by endothelial to mesenchymal transition (EndMT) pathway. By using an in vitro microfluidic model, which enables to observe the synergetic effect of TME in situ, Yeon et al. (37) found that the differentiated CAFs from human umbilical vein endothelial cells (HUVECs) were increased obviously when treated with melanoma-derived EVs, whereas melanoma-secreted EVs could promote EndMT. Predictably, this experimental model is expected to serve as a potent tool in the development of anti-tumor agents by exploring a variety of candidates, not just tumor derived EVs, which can depress the transformation of endothelial cells to CAFs.
Apart from CAFs, mesenchymal stem cells (MSCs) is another essential stromal cells in the TME. EVs secreted by mesenchymal stem cells (MSCs) have shown huge potential for treating tumors because they can precisely locate TME (26). MSC-derived EVs was demonstrated to induce the apoptosis of melanoma cells via transmitting miR-138-5p, and then targeted SOX4. These results indicated the anti-tumor role of miR-138-5p/SOX4 axis during the malignancy of melanoma cells, and validated the potential therapeutic value of MSC-EVs based therapy for melanoma patients (27). In addition, Yang et al. (38) found that NEAT1 loaded in bone marrow mesenchymal stem cell-derived EVs could accelerate the progression of melanoma through inducing macrophages to M2 polarization, providing a novel target for the treatment of melanoma. In melanoma, epithelial-mesenchymal transition (EMT) is usually occurred when epithelial cells transit into mesenchymal cells, thereby promoting tumor metastasis and therapy resistance (28). Chen et al. (39) demonstrated that MSC-EVs carried miR-22-3p could reduce the expression of EMT related gene LGALS1 in melanoma cells so as to inhibit the EMT process of tumor epithelial cells. However, this study is lack of animal studies, and the therapeutic potential of MSC-EVs with miR-22-3p needed further investigation, especially the clinical trials.
Melanoma-secreted EVs targeting angiogenesis
Angiogenesis is a biological process responsible for the formation of new blood vessels, thereby delivering oxygen and nutrients to tissues and organs in human body. Currently, angiogenesis has been considered to be a fundamental procedure in inducing benign tumors to malignant phenotype, such as invasion and metastasis (40). In TME studies, EVs released by tumor cells have been revealed to form the premetastatic niche by accelerating angiogenesis, indicating the vital role of EVs in the communication between tumor and angiogenesis (Table 2) (46, 47). It’s well known that the uPA/uPAR system components (urokinase-type plasminogen activator, uPA; uPA receptor, uPAR) are regarded as critical biomarkers for malignancy, including melanoma (41, 48). By utilizing subcutaneously implanted matrigel plugs containing EVs derived from wild type, uPAR- and uPAR+ melanoma cells, the group treated with uPAR+ EVs displayed more vascularized and micro-vessels, suggesting uPAR expressing melanoma EVs are crucial activators of angiogenesis (49). Therefore, depleting uPAR expression in tumor derived EVs is a promising method for treating melanoma, and the uPAR might be a useful biomarker when obtained EVs from melanoma patients by applying liquid biopsy. Hypoxia is a primary characteristic of solid tumors that is involved in tumor angiogenesis (50, 51). Notably, hypoxia also increases the secretion of EVs from tumor cells and alters the carriers of EVs (42). Tang et al. (52) found that hypoxic melanoma-derived small extracellular vesicles (sEVs) could enhance the angiogenic ability of CAFs by delivering the HSP90/p-IKKα/β complex to activate the IKK/IκB/NF-κB/CXCL1 signaling pathway in CAFs. Despite these finding offered a deeper understanding of the occurrence of angiogenesis in melanoma progression and provided promising targets, more clinical studies are necessary for estimating the therapeutic values of the HSP90/IKK enriched sEVs. The typical M2-like phenotype of TAMs can be induced by cytokines derived from Th2 cell, and IL-13 is the primary cytokine among them. Accordingly, targeting IL-13 receptor and ligand in the TME can block M2 polarization and then inhibit tumor growth (53). As reported, tumor cell-derived EVs are able to recruit and polarize TAMs to activate angiogenic signaling pathways via extensive molecule, including bFGF, TNF-α, and VEGF (43, 54). Given that, Negrea et al. (55) developed a novel nanosystem consists of Il-13-LCL-SIM (Il-13-conjugated long-circulating liposomes with SIM) to target TAMs and PEG-EV-DOX (PEG stabilized EVs with DOX) to target melanoma cells. In this study, it was shown that Il-13-LCL-SIM mainly impaired the pro-angiogenic functions of TAMs and reduced the expression of key proangiogenic proteins (such as VEGF), which further sensitized TME to the killing effects of PEG-EV-DOX. Therefore, combing EVs based therapy with other targeted strategies, including targeted angiogenesis, is promised to be an advantageous method to improve the efficacy of EVs-drugs in melanoma, which may also be applied in other malignant tumors.
Wnt signaling proteins are highly conserved proteins that are tightly involved in the developmental processes, and also multiple diseases, including cancers (56, 57). As an atypical Wnt signaling, WNT5A can promote the spread to distant tissues or organs and the formation of metastatic foci of melanoma, whereas the high expression of WNT5A commonly predicted a poor prognosis in melanoma patients (44, 58). In the endogenous WNT5A low expressed melanoma cells, the stimulation with rWNT5A led to a great release of EVs carrying the immunoregulatory cytokine IL-6, and more important the pro-angiogenic agents, including IL-8, VEGF and MMP2. In particular, these EVs might accelerate the angiogenesis processes and create an immunosuppressive TME of melanoma, thus inducing more rapid tumor growth and distinct metastasis (59). Consequently, targeting WNT5A thereby inhibiting the secretion of related EVs might be a promising strategy for the treatment of melanoma patients. However, more studies are needed to assess the potential side effect caused by the silence of WNT5A. Previous studies have proved that JAK2/STAT3 signaling pathway can regulate proangiogenic modulators expression, such as VEGFa, FGF2, and MMP9 (60, 61). Additionally, SOCS1 (suppressor of cytokine signaling 1) is a powerful inhibitor of JAK2/STAT3 signaling, however, SOCS1 is decreased in a great number of tumors and closely related to tumor angiogenesis (45, 62). Zhou et al. (63) demonstrated that melanoma cell-derived EVs could deliver miR-155 to fibroblasts NIH/3T3 and then elevated the expression of proangiogenic factors, including VEGFa, FGF2, and MMP9, by directly targeting SOCS1, thus triggering the proangiogenic switch of CAFs. Furthermore, in vitro and in vivo assays showed that treatment with EVs with overexpressed miR-155 promoted angiogenesis, whereas the knockdown of miR-155 in melanoma cell-released EVs mitigated angiogenesis obviously. This discovery may provide a new therapeutic target for anti-angiogenic therapy in the treatment of melanoma. Nonetheless, the inhibition of miR-155 in melanoma cell-derived EVs cannot diminish the proangiogenic regulators to the original expression level, combined with other therapies may be a feasible option to obtain better clinical benefits.
EVs regulating immunoresponse in melanoma
The TME is infiltrated by multiple immune cells, including lymphocytes (T cells, B cells, NK cells, and T regulatory cells), dendritic cells (DCs), tumor associated macrophages (TAMs), myeloid-derived suppressor cells (MDSC), as well as granulocytes (neutrophils, basophils, eosinophils, and mast cells). However, it has been recognized that tumor cells can regulate signaling pathways involved these immune cells and switch them to an immunorepressive manner, thus inducing enhanced tumor growth (64, 65). EVs comprise several tumor antigens which may result in the immunosuppression, yet increasing studies have indicated that tumor derived EVs are essential mediators between tumor cells and immune response (Figure 2) by releasing immune-associated factors to the TME (Table 3) (76, 77).
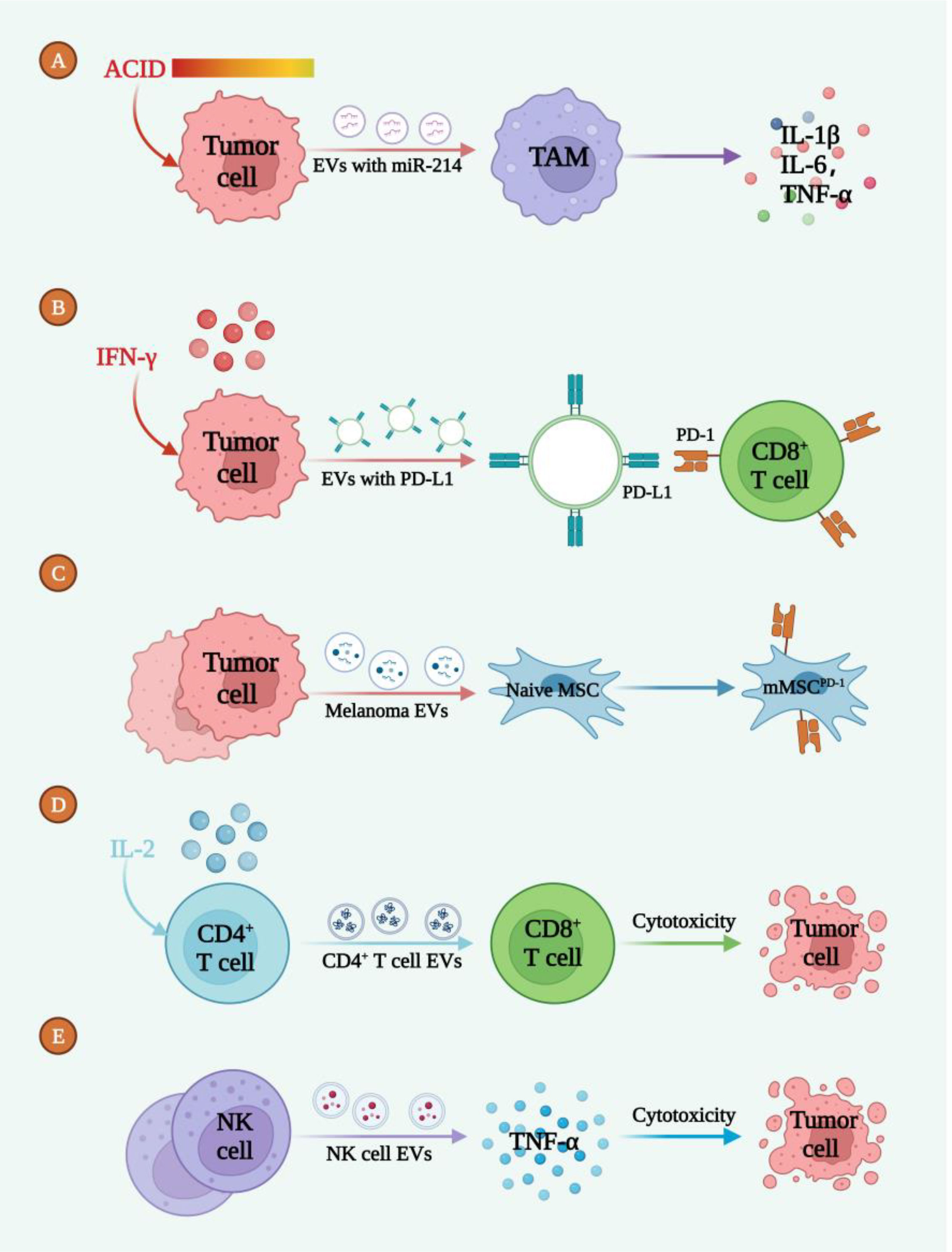
Figure 2 EVs mediated the intercommunication between melanoma cells and immunoresponse. (A) The acidic TME promoted the release of EVs-miR-214 to target TAM, inducing the inflammatory microenvironment. (B) IFN-γ induced tumor cell deriving EVs enriched with PD-L1 to bind with CD8+ T cell. (C) EVs secreted by melanoma cells induced the formation of mMSCPD-1. (D) IL-2 led to the release of CD4+ T cell EVs which caused tumor cell death. (E) NK-cell derived EVs secreted TNF-α to induce tumor cell death.
In addition, T regulatory cells, as immunosuppressive cells, inhibit the antitumor immune response and secrete various immunosuppressive cytokines, promoting the tumor immune escape (78). Melanoma cell derived EVs induce immune suppression by promoting T regulatory cell expansion as well as the demise of antitumor CD8+ effector T cells, thereby enabling tumor cell escape (79). Another study showed, the transfer of miRNA-214 from Melanoma derived EVs to T-cells down-regulates PTEN while favoring the expansion and migration of T regulatory cells in TME (80).
EVs with TAM
The macrophages in TME are mainly divided into two categories: anti-tumor “M1” and tumor supportive “M2” types. In particular, M2 and partial M1 macrophages are considered as TAMs, which is the major immune population in TME and involved in tumor occurrence and development (66, 81, 82). A high TAM infiltration often leads to poor clinical outcomes in a great number of tumors, including melanoma (67, 83, 84). Besides, extracellular acidosis is another crucial aspect of the TME and Andreucci et al. (85) observed that the acidic microenvironment stimulated the secretion of melanoma-EVs enriched with miR-214. Then, these EVs tended to induce a proinflammatory activation of macrophages by increasing the expression of COX-2 and the release of inflammatory cytokines, such as IL-1β, IL-6, TNF-α, and NO, so as to establish an inflammatory TME which in turn facilitated the progression of melanoma. Hence, improving the acidic TME to reduce the release of miR-214-enriched EVs or diminishing the expression of miR-214 in melanoma derived EVs may be potential strategies for the treatment of melanoma. However, this study lacked primary macrophages experiments, and the polarization of macrophages was not detected, further research is urgent. Accumulating evidence showed that natural and modified EVs are capable of inducing a tumor suppressive response in macrophages to attenuate tumor growth (86, 87). From the roots of Panax ginseng C. A. Mey, nanoparticles similar to EVs was isolated and purified successfully, termed as ginseng-derived nanoparticles (GDNPs), which were enriched with digalactosyl monoacylglycerol (DGMG), phosphatidyl ethanolamine (PE), and ceramide (Cer) (88). When being internalized by TAM, GDNPs could promote M1-like polarization via TLR-4/MyD88 signaling pathway and increase the production of total ROS, which then induced the apoptosis of mouse melanoma cells and inhibited tumor growth in vivo. This work demonstrated that GDNPs played an immunoregulatory role on murine macrophages to suppress tumor growth in vivo and provided the valid foundation for further application as nanodrugs to treat melanoma, whereas more studies are needed to clarify the active ingredient in GDNPs, as well as the necessary clinical trials.
EVs with immune checkpoint blockade
Notably, melanoma is a highly immunogenic malignancy characterized by the deeply lymphoid infiltration, thereby providing well foundation for the immunotherapy in melanoma (89, 90). Based on this, some immunotherapeutic strategies, especially the immune checkpoint blockade (ICB), have been demonstrated to be favorable for the refractory melanoma by activating effector T cells (68, 91). Compared with other immunotherapies, even in patients with advanced cancer, ICB therapies can usually illustrate higher response rates and persistent responses (69). Among them, PD-1/PD-L1 and CTLA-4/B7 immune checkpoint pathways are principal targets for ICB, which have been well studied in the past decades (92).
By using reverse phase protein array (RPPA) and western blot assay, Chen et al. (93) found that PD-L1 was significantly higher in EVs, mostly is exosomes, released from metastatic melanoma cells than that from primary melanoma cells. Mechanically, the abundance of PD-L1 on melanoma EVs is raised by IFN-γ, and EVs-PD-L1 chiefly targets PD-1+ CD8 T cells and promotes tumor growth in vivo. Moreover, in patients with advanced melanoma, the expression of circulating EVs-PD-L1 positively related to that of IFN-γ, and changes during the anti-PD-1 therapy with pembrolizumab. High levels of EVs-PD-L1 can reflect the exhaustion of T cells to the limit in melanoma patients, by which the T cells can no longer be re-activated by the anti-PD-1 treatment. Accordingly, to develop EVs-PD-L1 as a biomarker may be attractive. Recently, it has been found that melanoma cells with upregulated PD-1 are highly invasive (94), but it is still not clear how the PD-1 overexpressing subpopulations are generated. As reported, melanoma derived EVs could lead to the formation of a PD-1 overexpressing cell cluster (melanoma-like MSCPD-1+, mMSCPD-1+) from naive MSCs, by carrying a complex reprogramming of carcinogenic molecules (70). Furthermore, EVs and EVs activated mMSCPD-1+ cells are able to facilitate melanoma tumor progression in vivo, because of their highly expression of oncogenes and reduced susceptibility to programmed cell death, highlighting the complexity of EVs communication during the progression of melanoma. Although targeting PD-1/PD-L1 axis can mitigate T-cells exhaustion, it is not effective for all patients with cancer (95). This therapy resistance may be triggered by the deficient primary T-cells activation to tumor antigens, impaired antigen presentation, and decreased T-cell infiltration in the TME (96, 97). In view of this, increasing efforts have been made to explore more effective combination strategies. To investigate whether EVs related therapy could improve the tumor cells susceptibility to anti-PD-1/PD-L1 therapy in a checkpoint-resistant B16 melanoma model, Veerman et al. (98) injected bone marrow dendritic cell (BMDC)–derived EVs, but not checkpoint blockade, into tumor-bearing mice and then induced a strong antigen-specific T-cell response and impaired tumor growth. This demonstrates that the pretreatment with EVs can enhance anti-tumor immune responses in cancers refractory to checkpoint, and sensitize this subset of cancers to anti-PD-1/PD-L1 therapy. Therefore, EVs treatment has the great potential to collaborate with checkpoint blockade therapy, such as PD-1/PD-L1 blockage, in clinical application. Meanwhile, it is expected to be an alternative option for intractable tumors which are reactionless to checkpoint blockade. Myeloid-derived suppressor cells (MDSC) have been confirmed to be a critical role in the TME, and its accumulation in preclinical melanoma mouse models and melanoma patients can promote melanoma tumor progression via inhibiting T and NK cells (71, 72). The mechanism of MDSC-mediated immunosuppression is mainly related to the upregulation of PD-L1 binding with PD-1 expressed on the surface of tumor-infiltrating T cells (99). It has been proved that MDSC could also be derived from immature myeloid cells (IMC) or differentiated myeloid cells by the exposure to EVs released by tumor cells (100). Fleming et al. (101) highlighted that melanoma-derived EVs triggered the conversion of normal myeloid cells into MDSCs by the inducible HSP86 in EVs, which activates TLR4 on myelocytes and lead to the activation of NF-κB as well as the upregulation of PD-L1 expression. Furthermore, the knockdown of HSP86 in melanoma cells prohibited the secreted EVs to upregulate PD-L1. Although it may be primarily owing to the silence of HSP86 on the surface of EVs, whether HSP86 is responsible for the sorting of a variety of compounds into melanoma-EVs requires further exploration. By establishing an experimental pulmonary metastasis model using melanoma cells, Chen et al. (102) discovered melanoma cell-released EVs enriched PD-L1 were responsible for the metastatic progression by inducing the exhaustion of tumor-specific CD8+ T cells, providing a potential target for the treatment of melanoma. However, how to inhibit the release of tumor derived EVs, or reduce the enrichment of PD-L1 into EVs is a great challenge to be resolved.
In addition, the assessment of PD-L1 in tumor tissues has provided a feasible method to identify a patient population sensitive to chemotherapy (103). Nevertheless, PD-L1 levels could be influenced by the activity change of some signaling transduction pathways (104), thus abating its predictive value, whereas EVs-PD-L1 level may be a more appropriate indicators (105, 106). In order to explore other potential biomarkers, a study enrolled in 18 melanoma patients was conducted to evaluate whether PD-L1 mRNA level in plasma-derived EVs could reflect response to the anti-PD-1 agents, such as nivolumab and pembrolizumab. The results showed that PD-L1 expression in plasma-derived EVs decreased obviously in subjects responding to treatment but increased in that with advanced disease, suggesting that PD-L1 expression level in plasma-derived EVs may be a stable and valuable biomarker for the prediction of melanoma patient response to anti-PD-1 antibodies (107). However, it is necessary to collect a large number of clinical samples for further validation. Apart from that, Serrat et al. (108) identified the level of circulating PD-L1+ EVs released from melanoma and CD8+ T cells and that of PD1+ EVs were much higher in unresponsive patients. Besides, the Kaplan-Meier curves showed the higher levels of PD1+ EVs were significantly correlated with poor prognosis. This finding provides the probability for utilizing these circulating EVs, which might be employed as low invasive liquid biopsy to monitor the response of melanoma patients to ICB therapy.
EVs derived from immune cells
In the TME, immune cells released EVs have attracted much attention for cancer immunotherapy, because they also display immunological endogenous features similar to their parental cells (73, 109). Among them, T cell-derived EVs have been reported to exert anti-tumor effects in cancer immunotherapy through mimicking their parental cells (110, 111). As reported, IL2-stimulated CD4+ T cell-derived EVs played certain roles in anti-tumor immune responses via increasing the cytotoxicity of CD8+T cell. Furthermore, in a tumor-bearing mouse model, it was demonstrated that EVs secreted by CD4+ T cell attenuated the growth of melanoma significantly via CD8+ T cell mediated tumor suppression (112). Consequently, EVs originated from CD4+ T cells are promising therapeutic agents to induce potent anti-tumor responses for melanoma patients.
NK cells consist of many granular lymphocytes, and play a leading role in the anti-tumor immune responses by inhibiting tumor growth, and metastasis (74). In the immune microenvironment, NK cells communicate with other immune cells, such as DCs, T and B cells, to modulate innate and adaptive immune responses. This crosstalk is mediated by the release of a wide range of modulators, including cytokines, chemokines, and EVs (75, 113). In particular, NK-cell-derived EVs (NKEVs) are constitutively released and have anti-tumor activities similar to NK cells (114). Moreover, in both NK-derived exosomes and microvesicles, NKEV mass spectrometry and cytokine profile identified the expression of NK cell markers, such as NKG2D, CD94, CD40L, and other cytotoxic molecules, as well as the factors involved in cell adhesion, and immune response, suggesting that NKEVs are potential agents to be applied in cancer therapy. In melanoma patients, it was demonstrated that the quantity of circulating NKEVs is much lower than that in healthy donors, which might result in the low immune activity in melanoma patients. Hence, NKEVs could act as collaborator to coordinate with NK-mediated immunosurveillance, and the supplement of normal NKEVs to enhance the efficacy of immunotherapies might be a favorable option for melanoma patients (115). In addition, after being co-cultured with NK-92 EVs, the melanoma cells exhibited low cell viability but high cell apoptosis in a does dependent manner, which might be mediated by the secretion of TNF-α from NK-92 EVs (116). The inhomogeneity of the TME, especially the acidity distributed in the TME causes the reduction of perforin/granzymes from NK cells and inhibition of Fas/FasL interaction. However, acidity is able to promote the accumulation and release of EVs due to the low pH of TME attracts them and induces membrane fusion (117). Hereby, NK-92 EVs based immunotherapy has obvious advantages over therapy dependent on whole NK cell, and deserves further utilization as a promising immunotherapeutic strategy for melanoma.
EVs remodeling ECM in melanoma
Extracellular matrix (ECM) is a major non-cellular component in TME (118). It has been well demonstrated that ECM is a macromolecular network comprising collagens, elastin, fibronectin, proteoglycans/glycosaminoglycans, and laminins, which acts as a physical scaffold and is exerts essential roles in tumor cell differentiation, migration, and homeostasis (119, 120). The remodeling of ECM arises under physiological and pathological conditions and is regulated by multiple enzymes, such as metalloproteinases. Some EVs have been observed within the matrix and involved in the remodeling of ECM, that will impel the modification of the TME, and the formation of pre-metastatic niches (121, 122). Palmulli et al. (123) found that melanoma-derived sEVs are able to physically interact with collagen I, the primary fibrous component of the ECM, and then enhanced the activity of metalloproteases at the surface of EVs and the remodeling of the ECM, which is involved in the advancement of malignant tumors. Accordingly, blocking the binding between tumor derived EVs with collagen I may be a feasible therapeutic strategy for melanoma patients (Figure 3). However, whether EVs are capable of binding to other collagens or other components of ECM to remodel TME is still a challenge. Matrix metalloproteinases (MMPs) are zinc-dependent proteins, which take part in the tissue remodeling and involve tumor progression (124, 125). The membrane type 1 matrix metalloproteinase (MT1-MMP) is a critical member of metalloproteinases that facilitate tumor progression by remodeling the ECM (126, 127). From the culture medium of melanoma (G361) cells, EVs carrying the full-length and the proteolytically processed forms of MT1-MMP were isolated and identified (128). Interestingly, the EVs could activate pro-MMP-2 and degrade type 1 collagen, indicating EVs-MT1-MMP possessed functional activities. This provided a novel mechanism by which melanoma cells could remodel the ECM in TME, thus targeting MT1-MMP in EVs, or depressing the release of tumor cell derived EVs might be a promising strategy for melanoma treatment.
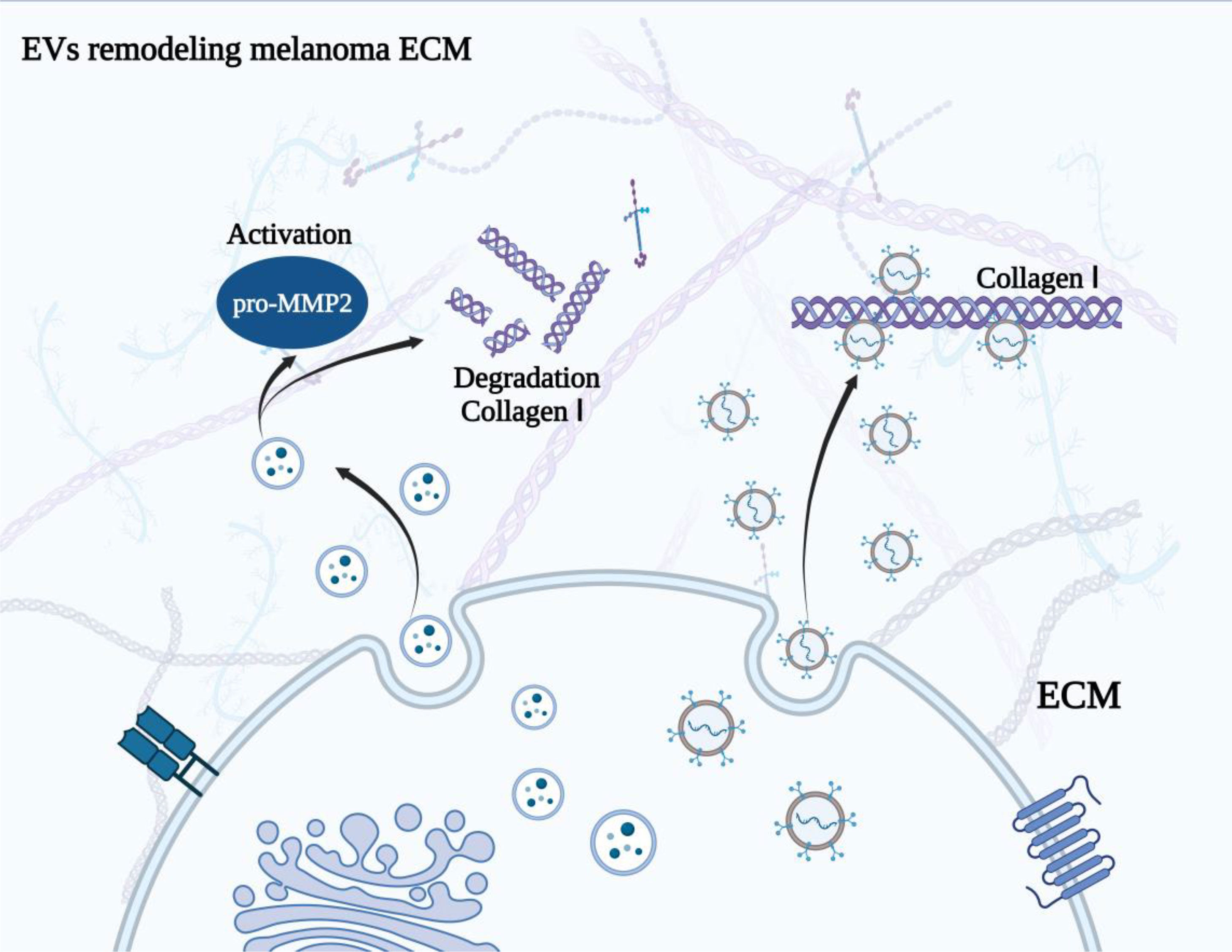
Figure 3 Melanoma derived EVs remodel ECM. The physical interaction between EVs and collagen I enhanced the activity of metalloproteases at the surface of EVs and the remodeling of the ECM. Besides, EVs with MT1-MMP could activate pro-MMP-2 and degrade type 1 collagen, resulting the remodeling of ECM.
Conclusions and perspective
Owing to the essential roles of TME in the occurrence and development of tumors, including melanoma, targeting of the TME has recently been regarded as a great promising therapeutic strategy to improve the anti-cancer efficacy (129, 130). Along with the clinical approval of several drugs and targeted therapies that were designed toward the angiogenesis, immune checkpoints, T and NK cells, additional molecules directly target the cellular or non-cellular components of TME are also under development. In this review, we have highlighted the potential of EVs mediated the crosstalk within the TME to be the therapeutic agents for melanoma patients. Moreover, emerging studies have also revealed that plasma-derived EVs can act as biomarkers for the advancement of melanoma, such as the circulating EVs-PD-1/PD-L1, thus providing a novel direction for the lipid biopsy. Although EVs-related therapies and biomarkers have shown huge application prospects, some shortages still needed to be overcome before its clinical use. For example, the heterogeneity in size, composition and sources of EVs lead to the difficulty to isolate a specific subset with desirable factors, which will reduce the accuracy of targeting therapy and bring some unexpected side effects. Therefore, the isolation and characterization methods should be further optimized to obtain more ‘pure’ EVs. Besides, the natural EVs commonly face problems such as the low production and complex composition. Furthermore, the efficiency of EVs may also be declined due to the low infiltration, insufficient drug delivery, captured by immune cells, and the inadequate immune response (131–133). Recently, a number of engineering methods have been used to obtain generous artificial and modified EVs to improve EVs efficacy and practicability, which have shown encouraging results.
In addition to carry endogenous cargoes, because of the inherent protection of the cargo and capable of enhancing solubility, stability, and specificity, EVs have been proposed as a drug delivery system to load single or multiple drugs to exert anti-tumor effect. In the clinical treatment of melanoma, ICBs are widely used. Currently, several monoclonal antibodies, such as pembrolizumab and nivolumab (anti-PD-1), and ipilimumab (anti-CTLA-4) have been studied and become the appropriate therapy to improve metastatic melanoma survival (134–138). However, there are still around 50% of patients fail to achieve clinical benefits (139). Utilizing EVs to delivery these antibodies to the TME, thus directly targeting tumor cells or T cells may be an alternative method to improve the efficacy of ICBs in melanoma. Moreover, modifying EVs to enhance their melanoma TME targeting will be favorable for increasing the accumulation and sustain of ICIs in the TME. However, few engineered EVs loaded with ICIs or other anti-tumor modulators specially targeting melanoma has been developed, whereas more efforts are needed.
Taken together, although the therapeutic application of EVs in melanoma clinical treatment is still restricted by the deficiency of feasible and standardized methods to obtain moderate EVs from melanoma patients biofluids and tumor cells, the limited loading efficiency of multiple agents, and the difficulty to manufacture sufficient melanoma specific EVs, EVs are still the great promising therapeutic manner for melanoma. With the progress of technology and new findings, EVs will finally shed light on the melanoma diagnosis and treatment in the era of precision medicine.
Author contributions
YL: Conceptualization, Methodology, Software Data curation, Writing- Original draft preparation. FL: Visualization, Investigation, Supervision. Writing- Reviewing and Editing. All authors have read and agreed to the published version of the manuscript.
Funding
This work was financially supported by Natural Grant Program foundation of Liaoning Province (2020-ZLLH-44).
Acknowledgments
This work was supported by the Liaoning Cancer Hospital & Institute (Shenyang).
Conflict of interest
The authors declare that the research was conducted in the absence of any commercial or financial relationships that could be construed as a potential conflict of interest.
Publisher’s note
All claims expressed in this article are solely those of the authors and do not necessarily represent those of their affiliated organizations, or those of the publisher, the editors and the reviewers. Any product that may be evaluated in this article, or claim that may be made by its manufacturer, is not guaranteed or endorsed by the publisher.
References
1. Falcone I, Conciatori F, Bazzichetto C, Ferretti G, Cognetti F, Ciuffreda L, et al. Tumor microenvironment: implications in melanoma resistance to targeted therapy and immunotherapy. Cancers (Basel) (2020) 12(10). doi: 10.3390/cancers12102870
2. Garbe C, Amaral T, Peris K, Hauschild A, Arenberger P, Basset-Seguin N, et al. European consensus-based interdisciplinary guideline for melanoma. Part 1: diagnostics: update 2022. Eur J Cancer (2022) 170:236–55. doi: 10.1016/j.ejca.2022.03.008
3. Siegel RL, Miller KD, Fuchs HE, Jemal A. Cancer statistics, 2021. CA Cancer J Clin (2021) 71(1):7–33. doi: 10.3322/caac.21654
4. Eddy K, Shah R, Chen S. Decoding melanoma development and progression: identification of therapeutic vulnerabilities. Front Oncol (2020) 10:626129. doi: 10.3389/fonc.2020.626129
5. Garcia-Silva S, Benito-Martin A, Nogues L, Hernandez-Barranco A, Mazariegos MS, Santos V, et al. Melanoma-derived small extracellular vesicles induce lymphangiogenesis and metastasis through an ngfr-dependent mechanism. Nat Cancer (2021) 2(12):1387–405. doi: 10.1038/s43018-021-00272-y
6. Falk Delgado A, Zommorodi S, Falk Delgado A. Sentinel lymph node biopsy and complete lymph node dissection for melanoma. Curr Oncol Rep (2019) 21(6):54. doi: 10.1007/s11912-019-0798-y
7. Alvarez-Breckenridge C, Markson SC, Stocking JH, Nayyar N, Lastrapes M, Strickland MR, et al. Microenvironmental landscape of human melanoma brain metastases in response to immune checkpoint inhibition. Cancer Immunol Res (2022) 10(8):996–1012. doi: 10.1158/2326-6066.CIR-21-0870
8. Chelvanambi M, Fecek RJ, Taylor JL, Storkus WJ. Sting agonist-based treatment promotes vascular norMalization and tertiary lymphoid structure formation in the therapeutic melanoma microenvironment. J Immunother Cancer (2021) 9(2). doi: 10.1136/jitc-2020-001906
9. Liu C, Wang M, Zhang H, Li C, Zhang T, Liu H, et al. Tumor microenvironment and immunotherapy of oral cancer. Eur J Med Res (2022) 27(1):198. doi: 10.1186/s40001-022-00835-4
10. Becker A, Thakur BK, Weiss JM, Kim HS, Peinado H, Lyden D. Extracellular vesicles in cancer: cell-to-cell mediators of metastasis. Cancer Cell (2016) 30(6):836–48. doi: 10.1016/j.ccell.2016.10.009
11. Thery C, Witwer KW, Aikawa E, Alcaraz MJ, Anderson JD, Andriantsitohaina R, et al. Minimal information for studies of extracellular vesicles 2018 (Misev2018): A position statement of the international society for extracellular vesicles and update of the misev2014 guidelines. J Extracell Vesicles (2018) 7(1):1535750. doi: 10.1080/20013078.2018.1535750
12. Witwer KW, Thery C. Extracellular vesicles or exosomes? On primacy, precision, and popularity influencing a choice of nomenclature. J Extracell Vesicles (2019) 8(1):1648167. doi: 10.1080/20013078.2019.1648167
13. Hoshino A, Kim HS, Bojmar L, Gyan KE, Cioffi M, Hernandez J, et al. Extracellular vesicle and particle biomarkers define multiple human cancers. Cell (2020) 182(4):1044–61 e18. doi: 10.1016/j.cell.2020.07.009
14. Sheehan C, D'Souza-Schorey C. Tumor-derived extracellular vesicles: molecular parcels that enable regulation of the immune response in cancer. J Cell Sci (2019) 132(20). doi: 10.1242/jcs.235085
15. Marar C, Starich B, Wirtz D. Extracellular vesicles in immunomodulation and tumor progression. Nat Immunol (2021) 22(5):560–70. doi: 10.1038/s41590-021-00899-0
16. Maacha S, Bhat AA, Jimenez L, Raza A, Haris M, Uddin S, et al. Extracellular vesicles-mediated intercellular communication: roles in the tumor microenvironment and anti-cancer drug resistance. Mol Cancer (2019) 18(1):55. doi: 10.1186/s12943-019-0965-7
17. Alia Moosavian S, Hashemi M, Etemad L, Daneshmand S, Salmasi Z. Melanoma-derived exosomes: versatile extracellular vesicles for diagnosis, metastasis, immune modulation, and treatment of melanoma. Int Immunopharmacol (2022) 113(Pt A):109320. doi: 10.1016/j.intimp.2022.109320
18. Lambrechts D, Wauters E, Boeckx B, Aibar S, Nittner D, Burton O, et al. Phenotype molding of stromal cells in the lung tumor microenvironment. Nat Med (2018) 24(8):1277–89. doi: 10.1038/s41591-018-0096-5
19. Zhou Y, Bian S, Zhou X, Cui Y, Wang W, Wen L, et al. Single-cell multiomics sequencing reveals prevalent genomic alterations in tumor stromal cells of human colorectal cancer. Cancer Cell (2020) 38(6):818–28 e5. doi: 10.1016/j.ccell.2020.09.015
20. Mao X, Xu J, Wang W, Liang C, Hua J, Liu J, et al. Crosstalk between cancer-associated fibroblasts and immune cells in the tumor microenvironment: new findings and future perspectives. Mol Cancer (2021) 20(1):131. doi: 10.1186/s12943-021-01428-1
21. Liu T, Zhou L, Xiao Y, Andl T, Zhang Y. Braf inhibitors reprogram cancer-associated fibroblasts to drive matrix remodeling and therapeutic escape in melanoma. Cancer Res (2022) 82(3):419–32. doi: 10.1158/0008-5472.CAN-21-0614
22. Hu T, Hu J. Melanoma-derived exosomes induce reprogramming fibroblasts into cancer-associated fibroblasts via gm26809 delivery. Cell Cycle (2019) 18(22):3085–94. doi: 10.1080/15384101.2019.1669380
23. Mannavola F, D'Oronzo S, Cives M, Stucci LS, Ranieri G, Silvestris F, et al. Extracellular vesicles and epigenetic modifications are hallmarks of melanoma progression. Int J Mol Sci (2019) 21(1). doi: 10.3390/ijms21010052
24. Chen B, Sang Y, Song X, Zhang D, Wang L, Zhao W, et al. Exosomal mir-500a-5p derived from cancer-associated fibroblasts promotes breast cancer cell proliferation and metastasis through targeting usp28. Theranostics (2021) 11(8):3932–47. doi: 10.7150/thno.53412
25. Fujii N, Yashiro M, Hatano T, Fujikawa H, Motomura H. Cd9-positive exosomes derived from cancer-associated fibroblasts might inhibit the proliferation of Malignant melanoma cells. Anticancer Res (2023) 43(1):25–33. doi: 10.21873/anticanres.16130
26. Weng Z, Zhang B, Wu C, Yu F, Han B, Li B, et al. Therapeutic roles of mesenchymal stem cell-derived extracellular vesicles in cancer. J Hematol Oncol (2021) 14(1):136. doi: 10.1186/s13045-021-01141-y
27. Wang X, Cui Z, Zeng B, Qiong Z, Long Z. Human mesenchymal stem cell derived exosomes inhibit the survival of human melanoma cells through modulating mir-138-5p/sox4 pathway. Cancer biomark (2022) 34(4):533–43. doi: 10.3233/CBM-210409
28. Pedri D, Karras P, Landeloos E, Marine JC, Rambow F. Epithelial-to-mesenchymal-like transition events in melanoma. FEBS J (2022) 289(5):1352–68. doi: 10.1111/febs.16021
29. Guo W, Gao Y, Li N, Shao F, Wang C, Wang P, et al. Exosomes: new players in cancer (Review). Oncol Rep (2017) 38(2):665–75. doi: 10.3892/or.2017.5714
30. Bland CL, Byrne-Hoffman CN, Fernandez A, Rellick SL, Deng W, Klinke DJ 2nd. Exosomes derived from B16f0 melanoma cells alter the transcriptome of cytotoxic T cells that impacts mitochondrial respiration. FEBS J (2018) 285(6):1033–50. doi: 10.1111/febs.14396
31. Lee SS, Cheah YK. The interplay between micrornas and cellular components of tumour microenvironment (Tme) on non-small-cell lung cancer (Nsclc) progression. J Immunol Res (2019) 2019:3046379. doi: 10.1155/2019/3046379
32. Su Y, Zhou LL, Zhang YQ, Ni LY. Long noncoding rna hottip is associated with male infertility and promotes testicular embryonal carcinoma cell proliferation. Mol Genet Genomic Med (2019) 7(9):e870. doi: 10.1002/mgg3.870
33. Jorge NAN, Cruz JGV, Pretti MAM, Bonamino MH, Possik PA, Boroni M. Poor clinical outcome in metastatic melanoma is associated with a microrna-modulated immunosuppressive tumor microenvironment. J Transl Med (2020) 18(1):56. doi: 10.1186/s12967-020-02235-w
34. Shelton M, Anene CA, Nsengimana J, Roberts W, Newton-Bishop J, Boyne JR. The role of caf derived exosomal micrornas in the tumour microenvironment of melanoma. Biochim Biophys Acta Rev Cancer (2021) 1875(1):188456. doi: 10.1016/j.bbcan.2020.188456
35. Dror S, Sander L, Schwartz H, Sheinboim D, Barzilai A, Dishon Y, et al. Melanoma mirna trafficking controls tumour primary niche formation. Nat Cell Biol (2016) 18(9):1006–17. doi: 10.1038/ncb3399
36. Ren J, Ding L, Zhang D, Shi G, Xu Q, Shen S, et al. Carcinoma-associated fibroblasts promote the stemness and chemoresistance of colorectal cancer by transferring exosomal lncrna H19. Theranostics (2018) 8(14):3932–48. doi: 10.7150/thno.25541
37. Yeon JH, Jeong HE, Seo H, Cho S, Kim K, Na D, et al. Cancer-derived exosomes trigger endothelial to mesenchymal transition followed by the induction of cancer-associated fibroblasts. Acta Biomater (2018) 76:146–53. doi: 10.1016/j.actbio.2018.07.001
38. Yang Y, Ma S, Ye Z, Zheng Y, Zheng Z, Liu X, et al. Neat1 in bone marrow mesenchymal stem cell-derived extracellular vesicles promotes melanoma by inducing M2 macrophage polarization. Cancer Gene Ther (2022) 29(8-9):1228–39. doi: 10.1038/s41417-021-00392-8
39. Chen Y, Fang Y, Li L, Luo H, Cao T, Tu B. Exosomal mir-22-3p from mesenchymal stem cells inhibits the epithelial-mesenchymal transition (Emt) of melanoma cells by regulating lgals1. Front Biosci (Landmark Ed) (2022) 27(9):275. doi: 10.31083/j.fbl2709275
40. Majidpoor J, Mortezaee K. Angiogenesis as a hallmark of solid tumors - clinical perspectives. Cell Oncol (Dordr) (2021) 44(4):715–37. doi: 10.1007/s13402-021-00602-3
41. Laurenzana A, Chilla A, Luciani C, Peppicelli S, Biagioni A, Bianchini F, et al. Upa/upar system activation drives a glycolytic phenotype in melanoma cells. Int J Cancer (2017) 141(6):1190–200. doi: 10.1002/ijc.30817
42. Bister N, Pistono C, Huremagic B, Jolkkonen J, Giugno R, Malm T. Hypoxia and extracellular vesicles: A review on methods, vesicular cargo and functions. J Extracell Vesicles (2020) 10(1):e12002. doi: 10.1002/jev2.12002
43. SenGupta S, Hein LE, Parent CA. The recruitment of neutrophils to the tumor microenvironment is regulated by multiple mediators. Front Immunol (2021) 12:734188. doi: 10.3389/fimmu.2021.734188
44. Gajos-Michniewicz A, Czyz M. Wnt signaling in melanoma. Int J Mol Sci (2020) 21(14). doi: 10.3390/ijms21144852
45. Meng ZY, Kang HL, Duan W, Zheng J, Li QN, Zhou ZJ. Microrna-210 Promotes Accumulation of Neural Precursor Cells around Ischemic Foci after Cerebral Ischemia by Regulating the Socs1-Stat3-Vegf-C Pathway. J Am Heart Assoc (2018) 7(5). doi: 10.1161/JAHA.116.005052
46. Geng T, Song ZY, Xing JX, Wang BX, Dai SP, Xu ZS. Exosome derived from coronary serum of patients with myocardial infarction promotes angiogenesis through the mirna-143/igf-ir pathway. Int J Nanomed (2020) 15:2647–58. doi: 10.2147/IJN.S242908
47. Wu XG, Zhou CF, Zhang YM, Yan RM, Wei WF, Chen XJ, et al. Cancer-derived exosomal mir-221-3p promotes angiogenesis by targeting thbs2 in cervical squamous cell carcinoma. Angiogenesis (2019) 22(3):397–410. doi: 10.1007/s10456-019-09665-1
48. Schmitt M, Mengele K, Napieralski R, Magdolen V, Reuning U, Gkazepis A, et al. Clinical utility of level-of-evidence-1 disease forecast cancer biomarkers upa and its inhibitor pai-1. Expert Rev Mol Diagn (2010) 10(8):1051–67. doi: 10.1586/erm.10.71
49. Biagioni A, Laurenzana A, Menicacci B, Peppicelli S, Andreucci E, Bianchini F, et al. Upar-expressing melanoma exosomes promote angiogenesis by ve-cadherin, egfr and upar overexpression and rise of erk1,2 signaling in endothelial cells. Cell Mol Life Sci (2021) 78(6):3057–72. doi: 10.1007/s00018-020-03707-4
50. de Heer EC, Jalving M, Harris AL. Hifs, angiogenesis, and metabolism: elusive enemies in breast cancer. J Clin Invest (2020) 130(10):5074–87. doi: 10.1172/JCI137552
51. Wicks EE, Semenza GL. Hypoxia-inducible factors: cancer progression and clinical translation. J Clin Invest (2022) 132(11). doi: 10.1172/JCI159839
52. Tang H, Zhou X, Zhao X, Luo X, Luo T, Chen Y, et al. Hsp90/ikk-rich small extracellular vesicles activate pro-angiogenic melanoma-associated fibroblasts via the nf-kappab/cxcl1 axis. Cancer Sci (2022) 113(4):1168–81. doi: 10.1111/cas.15271
53. Jayasingam SD, Citartan M, Thang TH, Mat Zin AA, Ang KC, Ch'ng ES. Evaluating the polarization of tumor-associated macrophages into M1 and M2 phenotypes in human cancer tissue: technicalities and challenges in routine clinical practice. Front Oncol (2019) 9:1512. doi: 10.3389/fonc.2019.01512
54. Pan Y, Yu Y, Wang X, Zhang T. Tumor-associated macrophages in tumor immunity. Front Immunol (2020) 11:583084. doi: 10.3389/fimmu.2020.583084
55. Negrea G, Rauca VF, Meszaros MS, Patras L, Luput L, Licarete E, et al. Active tumor-targeting nano-formulations containing simvastatin and doxorubicin inhibit melanoma growth and angiogenesis. Front Pharmacol (2022) 13:870347. doi: 10.3389/fphar.2022.870347
56. Chien AJ, Conrad WH, Moon RT. A wnt survival guide: from flies to human disease. J Invest Dermatol (2009) 129(7):1614–27. doi: 10.1038/jid.2008.445
57. Reischl J, Schwenke S, Beekman JM, Mrowietz U, Sturzebecher S, Heubach JF. Increased expression of wnt5a in psoriatic plaques. J Invest Dermatol (2007) 127(1):163–9. doi: 10.1038/sj.jid.5700488
58. Da Forno PD, Pringle JH, Hutchinson P, Osborn J, Huang Q, Potter L, et al. Wnt5a expression increases during melanoma progression and correlates with outcome. Clin Cancer Res (2008) 14(18):5825–32. doi: 10.1158/1078-0432.CCR-07-5104
59. Ekstrom EJ, Bergenfelz C, von Bulow V, Serifler F, Carlemalm E, Jonsson G, et al. Wnt5a induces release of exosomes containing pro-angiogenic and immunosuppressive factors from Malignant melanoma cells. Mol Cancer (2014) 13:88. doi: 10.1186/1476-4598-13-88
60. Xue C, Xie J, Zhao D, Lin S, Zhou T, Shi S, et al. The jak/stat3 signalling pathway regulated angiogenesis in an endothelial cell/adipose-derived stromal cell co-culture, 3d gel model. Cell Prolif (2017) 50(1). doi: 10.1111/cpr.12307
61. Gritsina G, Xiao F, O'Brien SW, Gabbasov R, Maglaty MA, Xu RH, et al. Targeted blockade of jak/stat3 signaling inhibits ovarian carcinoma growth. Mol Cancer Ther (2015) 14(4):1035–47. doi: 10.1158/1535-7163.MCT-14-0800
62. Ying J, Qiu X, Lu Y, Zhang M. Socs1 and its potential clinical role in tumor. Pathol Oncol Res (2019) 25(4):1295–301. doi: 10.1007/s12253-019-00612-5
63. Zhou X, Yan T, Huang C, Xu Z, Wang L, Jiang E, et al. Melanoma cell-secreted exosomal mir-155-5p induce proangiogenic switch of cancer-associated fibroblasts via socs1/jak2/stat3 signaling pathway. J Exp Clin Cancer Res (2018) 37(1):242. doi: 10.1186/s13046-018-0911-3
64. Lei X, Lei Y, Li JK, Du WX, Li RG, Yang J, et al. Immune cells within the tumor microenvironment: biological functions and roles in cancer immunotherapy. Cancer Lett (2020) 470:126–33. doi: 10.1016/j.canlet.2019.11.009
65. Zheng Y, Chen Z, Han Y, Han L, Zou X, Zhou B, et al. Immune suppressive landscape in the human esophageal squamous cell carcinoma microenvironment. Nat Commun (2020) 11(1):6268. doi: 10.1038/s41467-020-20019-0
66. Christofides A, Strauss L, Yeo A, Cao C, Charest A, Boussiotis VA. The complex role of tumor-infiltrating macrophages. Nat Immunol (2022) 23(8):1148–56. doi: 10.1038/s41590-022-01267-2
67. Holtzhausen A, Harris W, Ubil E, Hunter DM, Zhao J, Zhang Y, et al. Tam family receptor kinase inhibition reverses mdsc-mediated suppression and augments anti-pd-1 therapy in melanoma. Cancer Immunol Res (2019) 7(10):1672–86. doi: 10.1158/2326-6066.CIR-19-0008
68. Larkin J, Chiarion-Sileni V, Gonzalez R, Grob JJ, Rutkowski P, Lao CD, et al. Five-year survival with combined nivolumab and ipilimumab in advanced melanoma. N Engl J Med (2019) 381(16):1535–46. doi: 10.1056/NEJMoa1910836
69. Busato D, Mossenta M, Baboci L, Di Cintio F, Toffoli G, Dal Bo M. Novel immunotherapeutic approaches for hepatocellular carcinoma treatment. Expert Rev Clin Pharmacol (2019) 12(5):453–70. doi: 10.1080/17512433.2019.1598859
70. Gyukity-Sebestyen E, Harmati M, Dobra G, Nemeth IB, Mihaly J, Zvara A, et al. Melanoma-derived exosomes induce pd-1 overexpression and tumor progression via mesenchymal stem cell oncogenic reprogramming. Front Immunol (2019) 10:2459. doi: 10.3389/fimmu.2019.02459
71. De Cicco P, Ercolano G, Ianaro A. The new era of cancer immunotherapy: targeting myeloid-derived suppressor cells to overcome immune evasion. Front Immunol (2020) 11:1680. doi: 10.3389/fimmu.2020.01680
72. Zhao H, Teng D, Yang L, Xu X, Chen J, Jiang T, et al. Myeloid-derived itaconate suppresses cytotoxic cd8(+) T cells and promotes tumour growth. Nat Metab (2022) 4(12):1660–73. doi: 10.1038/s42255-022-00676-9
73. Veerman RE, Gucluler Akpinar G, Eldh M, Gabrielsson S. Immune cell-derived extracellular vesicles - functions and therapeutic applications. Trends Mol Med (2019) 25(5):382–94. doi: 10.1016/j.molmed.2019.02.003
74. Melaiu O, Lucarini V, Cifaldi L, Fruci D. Influence of the tumor microenvironment on nk cell function in solid tumors. Front Immunol (2019) 10:3038. doi: 10.3389/fimmu.2019.03038
75. Fauriat C, Long EO, Ljunggren HG, Bryceson YT. Regulation of human nk-cell cytokine and chemokine production by target cell recognition. Blood (2010) 115(11):2167–76. doi: 10.1182/blood-2009-08-238469
76. Zhang Z, Zhou X, Guo J, Zhang F, Qian Y, Wang G, et al. Ta-mscs, ta-mscs-evs, mif: their crosstalk in immunosuppressive tumor microenvironment. J Transl Med (2022) 20(1):320. doi: 10.1186/s12967-022-03528-y
77. Swatler J, Turos-Korgul L, Brewinska-Olchowik M, De Biasi S, Dudka W, Le BV, et al. 4-1bbl-containing leukemic extracellular vesicles promote immunosuppressive effector regulatory T cells. Blood Adv (2022) 6(6):1879–94. doi: 10.1182/bloodadvances.2021006195
78. Dunn GP, Bruce AT, Ikeda H, Old LJ, Schreiber RD. Cancer immunoediting: from immunosurveillance to tumor escape. Nat Immunol (2002) 3(11):991–8. doi: 10.1038/ni1102-991
79. Wieckowski EU, Visus C, Szajnik M, Szczepanski MJ, Storkus WJ, Whiteside TL. Tumor-derived microvesicles promote regulatory T cell expansion and induce apoptosis in tumor-reactive activated cd8+ T lymphocytes. J Immunol (2009) 183(6):3720–30. doi: 10.4049/jimmunol.0900970
80. Yin Y, Cai X, Chen X, Liang H, Zhang Y, Li J, et al. Tumor-secreted mir-214 induces regulatory T cells: A major link between immune evasion and tumor growth. Cell Res (2014) 24(10):1164–80. doi: 10.1038/cr.2014.121
81. Mantovani A, Marchesi F, Malesci A, Laghi L, Allavena P. Tumour-associated macrophages as treatment targets in oncology. Nat Rev Clin Oncol (2017) 14(7):399–416. doi: 10.1038/nrclinonc.2016.217
82. Xiang X, Wang J, Lu D, Xu X. Targeting tumor-associated macrophages to synergize tumor immunotherapy. Signal Transduct Target Ther (2021) 6(1):75. doi: 10.1038/s41392-021-00484-9
83. Zhang Q, He Y, Luo N, Patel SJ, Han Y, Gao R, et al. Landscape and dynamics of single immune cells in hepatocellular carcinoma. Cell (2019) 179(4):829–45 e20. doi: 10.1016/j.cell.2019.10.003
84. Zhou Z, Peng Y, Wu X, Meng S, Yu W, Zhao J, et al. Ccl18 secreted from M2 macrophages promotes migration and invasion via the pi3k/akt pathway in gallbladder cancer. Cell Oncol (Dordr) (2019) 42(1):81–92. doi: 10.1007/s13402-018-0410-8
85. Andreucci E, Ruzzolini J, Bianchini F, Versienti G, Biagioni A, Lulli M, et al. Mir-214-enriched extracellular vesicles released by acid-adapted melanoma cells promote inflammatory macrophage-dependent tumor trans-endothelial migration. Cancers (Basel) (2022) 14(20). doi: 10.3390/cancers14205090
86. Zhao S, Mi Y, Guan B, Zheng B, Wei P, Gu Y, et al. Tumor-derived exosomal mir-934 induces macrophage M2 polarization to promote liver metastasis of colorectal cancer. J Hematol Oncol (2020) 13(1):156. doi: 10.1186/s13045-020-00991-2
87. Gunassekaran GR, Poongkavithai Vadevoo SM, Baek MC, Lee B. M1 macrophage exosomes engineered to foster M1 polarization and target the il-4 receptor inhibit tumor growth by reprogramming tumor-associated macrophages into M1-like macrophages. Biomaterials (2021) 278:121137. doi: 10.1016/j.biomaterials.2021.121137
88. Cao M, Yan H, Han X, Weng L, Wei Q, Sun X, et al. Ginseng-derived nanoparticles alter macrophage polarization to inhibit melanoma growth. J Immunother Cancer (2019) 7(1):326. doi: 10.1186/s40425-019-0817-4
89. Sanlorenzo M, Vujic I, Posch C, Dajee A, Yen A, Kim S, et al. Melanoma immunotherapy. Cancer Biol Ther (2014) 15(6):665–74. doi: 10.4161/cbt.28555
90. Lee AY, Brady MS. Neoadjuvant immunotherapy for melanoma. J Surg Oncol (2021) 123(3):782–8. doi: 10.1002/jso.26229
91. Schachter J, Ribas A, Long GV, Arance A, Grob JJ, Mortier L, et al. Pembrolizumab versus ipilimumab for advanced melanoma: final overall survival results of a multicentre, randomised, open-label phase 3 study (Keynote-006). Lancet (2017) 390(10105):1853–62. doi: 10.1016/S0140-6736(17)31601-X
92. Han X, Li H, Zhou D, Chen Z, Gu Z. Local and targeted delivery of immune checkpoint blockade therapeutics. Acc Chem Res (2020) 53(11):2521–33. doi: 10.1021/acs.accounts.0c00339
93. Chen G, Huang AC, Zhang W, Zhang G, Wu M, Xu W, et al. Exosomal pd-L1 contributes to immunosuppression and is associated with anti-pd-1 response. Nature (2018) 560(7718):382–6. doi: 10.1038/s41586-018-0392-8
94. Kleffel S, Posch C, Barthel SR, Mueller H, Schlapbach C, Guenova E, et al. Melanoma cell-intrinsic pd-1 receptor functions promote tumor growth. Cell (2015) 162(6):1242–56. doi: 10.1016/j.cell.2015.08.052
95. Zhao B, Zhao H, Zhao J. Efficacy of pd-1/pd-L1 blockade monotherapy in clinical trials. Ther Adv Med Oncol (2020) 12:1758835920937612. doi: 10.1177/1758835920937612
96. Liu YT, Sun ZJ. Turning cold tumors into hot tumors by improving T-cell infiltration. Theranostics (2021) 11(11):5365–86. doi: 10.7150/thno.58390
97. Peng W, Chen JQ, Liu C, Malu S, Creasy C, Tetzlaff MT, et al. Loss of pten promotes resistance to T cell-mediated immunotherapy. Cancer Discov (2016) 6(2):202–16. doi: 10.1158/2159-8290.CD-15-0283
98. Veerman RE, Akpinar GG, Offens A, Steiner L, Larssen P, Lundqvist A, et al. Antigen-loaded extracellular vesicles induce responsiveness to anti-pd-1 and anti-pd-L1 treatment in a checkpoint refractory melanoma model. Cancer Immunol Res (2023) 11(2):217–27. doi: 10.1158/2326-6066.CIR-22-0540
99. NOman MZ, Desantis G, Janji B, Hasmim M, Karray S, Dessen P, et al. Pd-L1 is a novel direct target of hif-1alpha, and its blockade under hypoxia enhanced mdsc-mediated T cell activation. J Exp Med (2014) 211(5):781–90. doi: 10.1084/jem.20131916
100. Xiang X, Poliakov A, Liu C, Liu Y, Deng ZB, Wang J, et al. Induction of myeloid-derived suppressor cells by tumor exosomes. Int J Cancer (2009) 124(11):2621–33. doi: 10.1002/ijc.24249
101. Fleming V, Hu X, Weller C, Weber R, Groth C, Riester Z, et al. Melanoma extracellular vesicles generate immunosuppressive myeloid cells by upregulating pd-L1 via tlr4 signaling. Cancer Res (2019) 79(18):4715–28. doi: 10.1158/0008-5472.CAN-19-0053
102. Chen J, Song Y, Miao F, Chen G, Zhu Y, Wu N, et al. Pdl1-positive exosomes suppress antitumor immunity by inducing tumor-specific cd8(+) T cell exhaustion during metastasis. Cancer Sci (2021) 112(9):3437–54. doi: 10.1111/cas.15033
103. Reck M, Rodriguez-Abreu D, Robinson AG, Hui R, Csoszi T, Fulop A, et al. Pembrolizumab versus chemotherapy for pd-L1-positive non-small-cell lung cancer. N Engl J Med (2016) 375(19):1823–33. doi: 10.1056/NEJMoa1606774
104. Jiang X, Zhou J, Giobbie-Hurder A, Wargo J, Hodi FS. The activation of mapk in melanoma cells resistant to braf inhibition promotes pd-L1 expression that is reversible by mek and pi3k inhibition. Clin Cancer Res (2013) 19(3):598–609. doi: 10.1158/1078-0432.CCR-12-2731
105. Zhang J, Zhu Y, Guan M, Liu Y, Lv M, Zhang C, et al. Isolation of circulating exosomes and identification of exosomal pd-L1 for predicting immunotherapy response. Nanoscale (2022) 14(25):8995–9003. doi: 10.1039/d2nr00829g
106. Carretero-Gonzalez A, Hergueta-Redondo M, Sanchez-Redondo S, Ximenez-Embun P, Manso Sanchez L, Gil EC, et al. Characterization of plasma circulating small extracellular vesicles in patients with metastatic solid tumors and newly diagnosed brain metastasis. Oncoimmunology (2022) 11(1):2067944. doi: 10.1080/2162402X.2022.2067944
107. Del Re M, Marconcini R, Pasquini G, Rofi E, Vivaldi C, Bloise F, et al. Pd-L1 mrna expression in plasma-derived exosomes is associated with response to anti-pd-1 antibodies in melanoma and nsclc. Br J Cancer (2018) 118(6):820–4. doi: 10.1038/bjc.2018.9
108. Serrati S, Guida M, Di Fonte R, De Summa S, Strippoli S, Iacobazzi RM, et al. Circulating extracellular vesicles expressing pd1 and pd-L1 predict response and mediate resistance to checkpoint inhibitors immunotherapy in metastatic melanoma. Mol Cancer (2022) 21(1):20. doi: 10.1186/s12943-021-01490-9
109. Mittal S, Gupta P, Chaluvally-Raghavan P, Pradeep S. Emerging role of extracellular vesicles in immune regulation and cancer progression. Cancers (Basel) (2020) 12(12). doi: 10.3390/cancers12123563
110. Fu W, Lei C, Liu S, Cui Y, Wang C, Qian K, et al. Car exosomes derived from effector car-T cells have potent antitumour effects and low toxicity. Nat Commun (2019) 10(1):4355. doi: 10.1038/s41467-019-12321-3
111. Hong J, Kang M, Jung M, Lee YY, Cho Y, Kim C, et al. T-cell-derived nanovesicles for cancer immunotherapy. Adv Mater (2021) 33(33):e2101110. doi: 10.1002/adma.202101110
112. Shin S, Jung I, Jung D, Kim CS, Kang SM, Ryu S, et al. Novel antitumor therapeutic strategy using cd4(+) T cell-derived extracellular vesicles. Biomaterials (2022) 289:121765. doi: 10.1016/j.biomaterials.2022.121765
113. Fitzgerald W, Freeman ML, Lederman MM, Vasilieva E, Romero R, Margolis L. A system of cytokines encapsulated in extracellular vesicles. Sci Rep (2018) 8(1):8973. doi: 10.1038/s41598-018-27190-x
114. Lugini L, Cecchetti S, Huber V, Luciani F, Macchia G, Spadaro F, et al. Immune surveillance properties of human nk cell-derived exosomes. J Immunol (2012) 189(6):2833–42. doi: 10.4049/jimmunol.1101988
115. Federici C, Shahaj E, Cecchetti S, Camerini S, Casella M, Iessi E, et al. Natural-killer-derived extracellular vesicles: immune sensors and interactors. Front Immunol (2020) 11:262. doi: 10.3389/fimmu.2020.00262
116. Zhu L, Kalimuthu S, Gangadaran P, Oh JM, Lee HW, Baek SH, et al. Exosomes derived from natural killer cells exert therapeutic effect in melanoma. Theranostics (2017) 7(10):2732–45. doi: 10.7150/thno.18752
117. Parolini I, Federici C, Raggi C, Lugini L, Palleschi S, De Milito A, et al. Microenvironmental ph is a key factor for exosome traffic in tumor cells. J Biol Chem (2009) 284(49):34211–22. doi: 10.1074/jbc.M109.041152
118. Niland S, Riscanevo AX, Eble JA. Matrix metalloproteinases shape the tumor microenvironment in cancer progression. Int J Mol Sci (2021) 23(1). doi: 10.3390/ijms23010146
119. Mohan V, Das A, Sagi I. Emerging roles of ecm remodeling processes in cancer. Semin Cancer Biol (2020) 62:192–200. doi: 10.1016/j.semcancer.2019.09.004
120. Winkler J, Abisoye-Ogunniyan A, Metcalf KJ, Werb Z. Concepts of extracellular matrix remodelling in tumour progression and metastasis. Nat Commun (2020) 11(1):5120. doi: 10.1038/s41467-020-18794-x
121. Hood JL. Natural melanoma-derived extracellular vesicles. Semin Cancer Biol (2019) 59:251–65. doi: 10.1016/j.semcancer.2019.06.020
122. Al Halawani A, Mithieux SM, Yeo GC, Hosseini-Beheshti E, Weiss AS. Extracellular vesicles: interplay with the extracellular matrix and modulated cell responses. Int J Mol Sci (2022) 23(6). doi: 10.3390/ijms23063389
123. Palmulli R, Bresteau E, Raposo G, Montagnac G, van Niel G. In vitro interaction of melanoma-derived extracellular vesicles with collagen. Int J Mol Sci (2023) 24(4). doi: 10.3390/ijms24043703
124. Carey P, Low E, Harper E, Stack MS. Metalloproteinases in ovarian cancer. Int J Mol Sci (2021) 22(7). doi: 10.3390/ijms22073403
125. Scheau C, Badarau IA, Costache R, Caruntu C, Mihai GL, Didilescu AC, et al. The role of matrix metalloproteinases in the epithelial-mesenchymal transition of hepatocellular carcinoma. Anal Cell Pathol (Amst) (2019) 2019:9423907. doi: 10.1155/2019/9423907
126. Gifford V, Itoh Y. Mt1-mmp-dependent cell migration: proteolytic and non-proteolytic mechanisms. Biochem Soc Trans (2019) 47(3):811–26. doi: 10.1042/BST20180363
127. Yamahana H, Terashima M, Takatsuka R, Asada C, Suzuki T, Uto Y, et al. Tgf-beta1 facilitates mt1-mmp-mediated prommp-9 activation and invasion in oral squamous cell carcinoma cells. Biochem Biophys Rep (2021) 27:101072. doi: 10.1016/j.bbrep.2021.101072
128. Hakulinen J, Sankkila L, Sugiyama N, Lehti K, Keski-Oja J. Secretion of active membrane type 1 matrix metalloproteinase (Mmp-14) into extracellular space in microvesicular exosomes. J Cell Biochem (2008) 105(5):1211–8. doi: 10.1002/jcb.21923
129. Marciscano AE, Anandasabapathy N. The role of dendritic cells in cancer and anti-tumor immunity. Semin Immunol (2021) 52:101481. doi: 10.1016/j.smim.2021.101481
130. Huang TX, Tan XY, Huang HS, Li YT, Liu BL, Liu KS, et al. Targeting cancer-associated fibroblast-secreted wnt2 restores dendritic cell-mediated antitumour immunity. Gut (2022) 71(2):333–44. doi: 10.1136/gutjnl-2020-322924
131. Wiklander OP, Nordin JZ, O'Loughlin A, Gustafsson Y, Corso G, Mager I, et al. Extracellular vesicle in vivo biodistribution is determined by cell source, route of administration and targeting. J Extracell Vesicles (2015) 4:26316. doi: 10.3402/jev.v4.26316
132. Ma J, Zhang H, Tang K, Huang B. Tumor-derived microparticles in tumor immunology and immunotherapy. Eur J Immunol (2020) 50(11):1653–62. doi: 10.1002/eji.202048548
133. Jafari R, Rahbarghazi R, Ahmadi M, Hassanpour M, Rezaie J. Hypoxic exosomes orchestrate tumorigenesis: molecular mechanisms and therapeutic implications. J Transl Med (2020) 18(1):474. doi: 10.1186/s12967-020-02662-9
134. Hodi FS, O'Day SJ, McDermott DF, Weber RW, Sosman JA, Haanen JB, et al. Improved survival with ipilimumab in patients with metastatic melanoma. N Engl J Med (2010) 363(8):711–23. doi: 10.1056/NEJMoa1003466
135. Robert C, Ribas A, Schachter J, Arance A, Grob JJ, Mortier L, et al. Pembrolizumab versus ipilimumab in advanced melanoma (Keynote-006): post-hoc 5-year results from an open-label, multicentre, randomised, controlled, phase 3 study. Lancet Oncol (2019) 20(9):1239–51. doi: 10.1016/S1470-2045(19)30388-2
136. Ascierto PA, Long GV, Robert C, Brady B, Dutriaux C, Di Giacomo AM, et al. Survival outcomes in patients with previously untreated braf wild-type advanced melanoma treated with nivolumab therapy: three-year follow-up of a randomized phase 3 trial. JAMA Oncol (2019) 5(2):187–94. doi: 10.1001/jamaoncol.2018.4514
137. Ralli M, Botticelli A, Visconti IC, Angeletti D, Fiore M, Marchetti P, et al. Immunotherapy in the treatment of metastatic melanoma: current knowledge and future directions. J Immunol Res (2020) 2020:9235638. doi: 10.1155/2020/9235638
138. Simsek M, Tekin SB, Bilici M. Immunological agents used in cancer treatment. Eurasian J Med (2019) 51(1):90–4. doi: 10.5152/eurasianjmed.2018.18194
Keywords: extracellular vesicles, tumor microenvironment, immunotherapy, melanoma, cancer
Citation: Li Y and Liu F (2023) The extracellular vesicles targeting tumor microenvironment: a promising therapeutic strategy for melanoma. Front. Immunol. 14:1200249. doi: 10.3389/fimmu.2023.1200249
Received: 04 April 2023; Accepted: 18 July 2023;
Published: 28 July 2023.
Edited by:
Paola Allavena, Humanitas Clinical and Research Center, University of Milan, ItalyReviewed by:
Mayela Carolina Mendt, University of Texas MD Anderson Cancer Center, United StatesStefano Piatto Clerici, International Center for Research, AC Camargo Cancer Center, Brazil
Copyright © 2023 Li and Liu. This is an open-access article distributed under the terms of the Creative Commons Attribution License (CC BY). The use, distribution or reproduction in other forums is permitted, provided the original author(s) and the copyright owner(s) are credited and that the original publication in this journal is cited, in accordance with accepted academic practice. No use, distribution or reproduction is permitted which does not comply with these terms.
*Correspondence: Fei Liu, bGl1ZmVpMDFAY2FuY2VyaG9zcC1sbi1jbXUuY29t