- 1Lanzhou University Second Hospital, Lanzhou, China
- 2Department of Surgical Oncology, Lanzhou University Second Hospital, Lanzhou, China
- 3Key Laboratory of Environmental Oncology of Gansu Province, Lanzhou, China
Immunotherapy has emerged as a preeminent force in the domain of cancer therapeutics and achieved remarkable breakthroughs. Nevertheless, the high resistance has become the most substantial impediment restricting its clinical efficacy. Beta-2 microglobulin (B2M), the light chain of major histocompatibility complex (MHC) class I, plays an indispensable part by presenting tumor antigens to cytotoxic T lymphocytes (CTLs) for exerting anti-tumor effects. Accumulating evidence indicates that B2M mutation/defect is one of the key mechanisms underlying tumor immunotherapy resistance. Therefore, elucidating the role played by B2M and devising effective strategies to battle against resistance are pressing issues. This review will systematically expound upon them, aiming to provide insight into the potential of B2M as a promising target in anticancer immune response.
1 Introduction
Immunotherapy is a promising therapeutic modality for cancer following surgery, chemotherapy, radiotherapy, and targeted therapy. Distinct from conventional therapies, it exerts anti-tumor efficacy by activating or reconstituting immune defense system, thereby further prolonging the survival of cancer patients and enhancing their quality of life (1, 2). At present, prominent tumor immunotherapies incorporate immune checkpoint inhibitors (ICIs) (3, 4), cancer vaccines (5–7), and chimeric antigen receptor T cells (CAR-T) (8–10), which have revolutionized tumor treatment and represent a significant milestone in the field. Despite the fact that unprecedented durable response has been observed in clinical practice, the majority of patients do not respond to the treatment (primary resistance), and some responders relapse after subsequent treatment (secondary resistance) (11–13). Thus, augmenting the therapeutic potency of immunity and transcending immune resistance constitute the cardinal challenges within the contemporary sphere of tumor therapy. Against this backdrop, in-depth exploration of biomarkers related to the mechanism of tumor immunotherapy resistance is of tremendous significance. Notably, programmed cell death ligand 1 (PD-L1), cytotoxic T lymphocyte-associated protein 4 (CTLA-4), Janus kinase 1/2 (JAK1/2), signal transducer and activator of transcription 3 (STAT3), and phosphatase and tensin homolog (PTEN) have been pinpointed as key biomarkers of immune therapy resistance (14–17). Nevertheless, additional biomarkers are required to provide a scientific basis and directional guidance for enhancing the efficacy of tumor immunotherapy, reversing immune resistance, and resulting in significant amelioration in survival among oncological patients.
B2M, originally discovered as a low molecular protein in the serum of patients with renal tubular lesions (18), is predominantly synthesized by platelets, lymphocytes, and polymorphonuclear leukocytes. It is ubiquitously present in blood, urine, cerebrospinal fluid, saliva, and colostrum, albeit in trace amounts. Notably, its levels are not influenced by gender, age, or the amount of muscle tissue (19). Under physiological conditions, B2M levels in serum remain relatively stable due to constant production and efficient renal clearance. Specifically, B2M is filtered through the glomerulus and nearly completely reabsorbed by the proximal tubules (20). Therefore, abnormal elevation of B2M in serum and urine serve as sensitive indicators of proximal tubule function and glomerular filtration efficiency, making it a valuable surrogate biomarker for renal impairment (21). Moreover, serum B2M has been recognized as a biomarker for a variety of diseases, such as lymphoma, coronary artery, inflammatory, central nervous system and autoimmune diseases (20, 22, 23). Structurally, B2M is a non-glycosylated protein consisting of 119 amino acids with a molecular weight of approximately 12 kDa, encoded on chromosome 15 (15q21.1). It consists of seven antiparallel β-chains that form two β-sheets connected by a single disulfide bond, presenting in a characteristic immunoglobulin (Ig)-like β-sandwich structure (24). As an indispensable subunit (a crucial component of the light chain) of MHC-I molecule, it is entrusted with presenting endogenous tumor antigens to CTLs to elicit immune killing effects. However, emerging evidence suggests that B2M expression is frequently compromised in numerous cancers due to deficiency, mutation and epigenetic suppression (25–27), and B2M alterations are associated with low response rates. B2M mutation/defect can significantly diminish the recognition of cancer cells by lymphocytes, thereby inducing tumor immune evasion and resistance to immunotherapy. A melanoma patient with B2M defeat developed resistance subsequent to receiving PD-1 inhibitor (28), whereas a high level of B2M mRNA was linked to enhanced response to PD-1-based immunotherapy (29). These findings underscore the critical role of B2M in immunotherapy resistance and suggest its potential as a target for overcoming resistance. To date, only one review has delved into the role of B2M in cancer immunotherapy, highlighting that B2M alterations are prevalent across various cancers and are linked to tumor immune escape and immunotherapy resistance, and may serve as a potential biomarker for ICIs treatment (27). Interestingly, this review not only elucidates the biological functions of B2M, its expression regulation, mechanisms B2M-related immunotherapy resistance, and the association between B2M and immunotherapy resistance along with the reversal strategies, but also explores the correlation using emerging technologies such as single-cell RNA sequencing (scRNA-seq), imaging mass cytometry (IMC) and CRISPR/Cas9. Additionally, it was observed that in the context of B2M defect, antigen recognition by CTLs is impaired, while other immune cells (such as CD4+ T lymphocytes, NK cells, and γδ T cells) may still retain their ability to kill tumor, further indicating that the activation of these cells can overcome immunotherapy resistance caused by B2M deficiency.
2 Biological function of B2M
B2M is located on chromosome 15 and encompasses 4 exons. The sequence of B2M gene exhibits certain homology with immunoglobulin (Ig) constant region and MHC class I molecule α3 domain (30). Structurally, B2M consists of two disulfide-linked β-sheets and can form amyloid fibers under specific pathological circumstances (31). B2M is prevalently expressed in considerable nucleated cells, encompassing immune cells and tumor cells. Serum B2M is regarded as a biomarker for the severity of infections, amyloidosis, renal injury, lymphoproliferative diseases, and aging-related disorders (23, 32), while the B2M on the cell membrane surface non-covalently binds to the heavy chain of MHC-I molecule to execute diverse immune functions. The most typical one is to present tumor antigens by participating in the formation of the antigen peptide-MHC class I complex (pMHC-I) to activate CTLs and exerted effects by reinvigorating our immune system to battle against cancer.
B2M, the light chain (β chain) of the MHC-I complex, serves as one of the most salient functions in participating in MHC-I-restricted tumor antigen presentation, including four principal steps (Figure 1): (1) Proteasome-mediated degradation of endogenous protein for the acquisition of antigenic peptides; (2) The conveyance of antigenic peptides to the endoplasmic reticulum (ER) by the transporter associated with antigen processing (TAP); (3) The loading of antigenic peptides into the peptide-binding groove of the MHC-I complex to constitute a stable pMHC-I complex and its translocation to the surface of tumor cells via the Golgi; (4) The recognition of the pMHC-I complex by CTLs to exert immune killing effects. Endogenous protein antigen (tumor antigen) is degraded by proteasome in the cytoplasm for the attainment of antigenic peptides. Next, antigenic peptides are transported to the endoplasmic reticulum by TAP and are pruned by endoplasmic reticulum aminopeptidase 1 (ERAP1) and endoplasmic reticulum aminopeptidase 2 (ERAP2) to acquire mature antigenic peptides consisting of 8-9 amino acids (30). In the ER, human leukocyte antigen-I (HLA-I), B2M, endoplasmic reticulum protein 57 (ERp57), Tapasin, and calreticulin collectively form a peptide-loading complex (PLC), facilitating the folding of the MHC-1α chain and obtaining mature MHC-I complex (33). Subsequently, antigenic peptides are loaded into the peptide-binding groove to form a stable pMHC-I complex, which disengages from the PLC and is transported to the surface of tumor cells through Golgi for recognition by the T cell receptor (TCR) on CTLs to eradicate tumor cells.
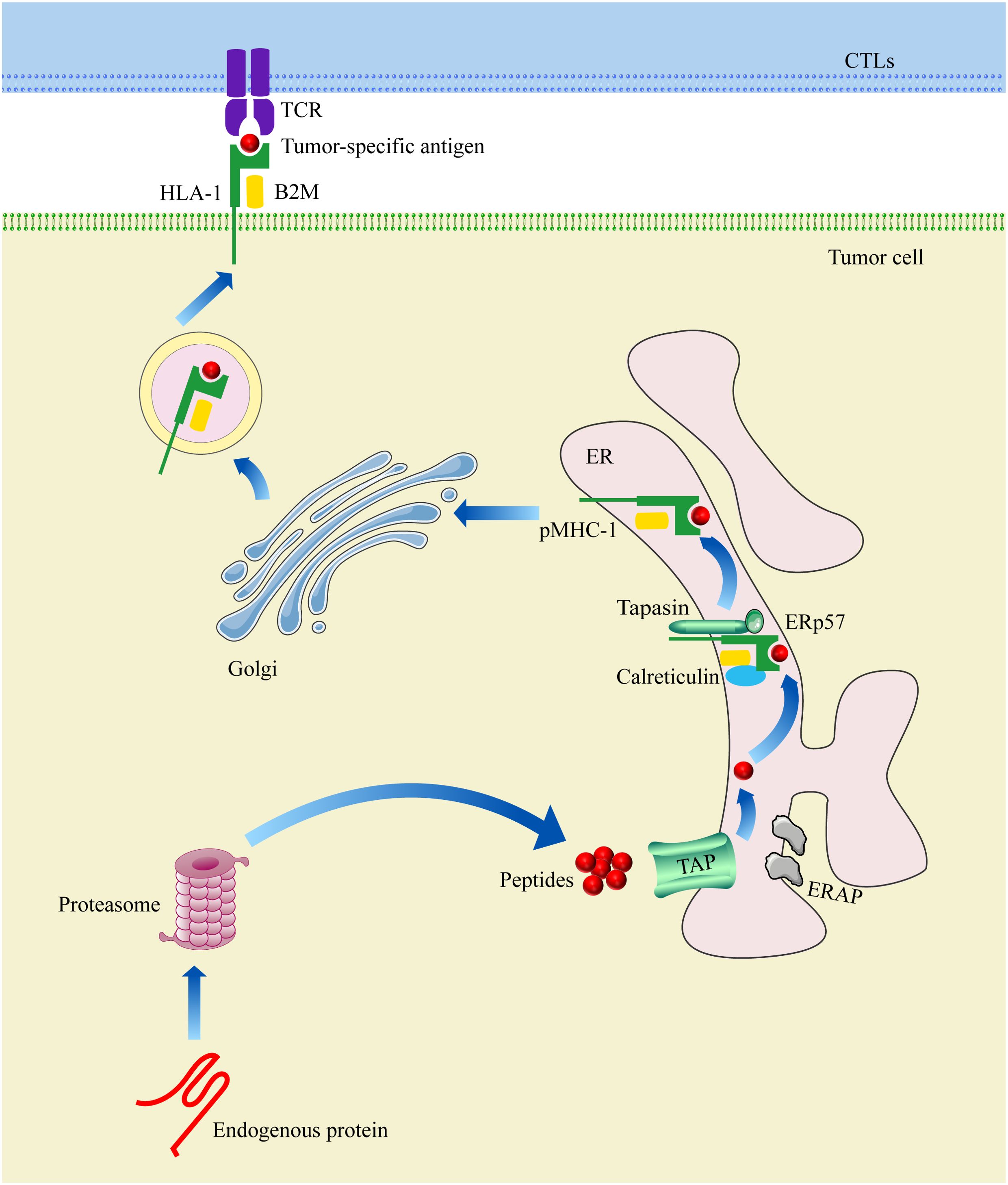
Figure 1. Schematic Illustration of B2M participating in MHC-I restricted tumor antigen presentation.
3 Regulation of B2M expression
The expression of B2M is modulated by multiple mechanisms. At the genetic level, B2M mutation facilitates tumor immune evasion through hampering antigen presentation. Currently, B2M mutation comprises point mutation, frameshift mutation, and loss of heterozygosity (LOH), which have been documented in malignant tumors like gastric cancer, colorectal cancer, renal cancer, and melanoma. In advanced melanoma, it was determined that 29.4% of patients exhibited B2M gene mutation, deletion, or LOH (34); similarly, HLA LOH was detected in 40% of non-small cell lung cancer (NSCLC) patients (35). Nucleotide-binding oligomerization domain-Like Receptor family Caspase recruitment domain containing 5(NLRC5), also known as the Class I transactivator (CITA), constitutes the CITA enhanceosome complex through binding to RFX5 and RFXAP and serves as a transcriptional activator to expedite the expression of MHC class I molecule. Yoshihama et al. (36) divulged that the methylation of the NLRC5 promoter was inversely associated with B2M expression in melanoma and engendered a diminution in MHC -I molecule expression and the advent of tumor immune escape. On the contrary, the Kobayashi team utilized a gene-specific system based on CRISPR/Cas9 technology, designated as TRED-I (Targeted reactivation and demethylation for MHC-I), to accomplish targeted demethylation of the NLRC5 promoter, induce an augmentation in NLRC5 expression, thereby upregulating MHC-I, B2M, TAP1 and genes encoding immune proteasome components (LMP2/PSMB9/β1i, LMP7/PSMB8/β5i), ultimately culminating in enhanced tumor immunogenicity (37). Consequently, NLRC5 contributes to reconstituting the expression and antigen presentation of B2M/MHC-I on tumor cells to potentiate CD8+ T cell dominated anticancer immunity.
In addition, the expression of B2M is also subjected to epigenetic mechanisms, encompassing DNA methylation, histone methylation, and histone deacetylation. In 2019, the research team led by Mark A. Dawson at the Peter MacCallum Cancer Centre in Australia employed whole-genome CRISPR/Cas9 screening to demonstrate that polycomb repressive complex 2 (PRC2) can silence key genes involved in the MHC-I antigen processing pathway, thereby facilitating T cell-mediated immune evasion. Furthermore, they ascertained that histone modifications like H3K4me3 and H3K27me3 enriched in the promoter region of the B2M gene inhibited the expression of MHC class I molecule (38). Grounded on the aforementioned findings, a study (39) revealed that after treating Renca cells with alpha ketoglutarate (the specific substrate of histone demethylase), the abundance of H3K4me1 and the expression level of B2M protein significantly elevated, while the abundance of H3K4me3 decreased, indicating that histone methylation might orchestrate the expression of the B2M gene. Methylation of the B2M gene promoter was observed to interrelate with transcriptional inactivation and down-regulation of B2M expression in colorectal cancer featuring microsatellite instability (MSI) (40), which was up-regulated using the DNA methyltransferase inhibitor, DNMTi. Previous studies have demonstrated that histone deacetylase inhibitor (HDACi) can reverse immune resistance by upregulating the expression of genes pertinent to antigen processing and presentation pathways (including B2M) in melanoma cell lines (41). Analogously, in clinical practice, HDACi can also enhance B2M/MHC-1 expression to address resistance to ICIs therapy (42–44).
Empirical studies have unequivocally demonstrated that the interferon-γ (IFNγ) molecule potentiates the transcriptional functions of NF-κB and IRF via the JAK/STAT signalling cascade, attaches to the enhancer A region and the interferon-stimulated response element (ISRE) of the MHC-I promoter region, upsurges the expression of genes such as MHC-I, B2M, and TAP transporter proteins, to amplify the antigen-presenting capacity. Nevertheless, mutation in genes affiliated with the IFN-γ/JAK/STAT signaling pathway have been identified in a wide spectrum of cancer, giving rise to antigen presentation impairments and resistance to ICIs treatment (45, 46). A plausible explanation for this phenomenon is that the compromised IFN-γ/JAK/STAT signaling axis instigates the inactivation of the histone dimethyltransferase WHSC1, thereby restricting the expression of B2M/MHC-I (47). On the contrary, non-coding RNA (ncRNA) is proficient in curbing the expression of genes associated with the antigen presentation pathway. In colorectal cancer, miR-148a-3p is eminently capable of repressing the expression of calnexin (CANX) and B2M/MHC-I, thereby decidedly restricting the anti-cancer efficacy of CTLs (48). Additionally, in esophageal cancer, miR-148-3p and miR125a markedly depress the expression of MHC-I and B2M, conspicuously curtailing the overall survival of cancer patients (49).
4 Mechanisms of resistance to B2M-related immunotherapy
In 2020, the Society for Immunotherapy of Cancer (SITC) delineated primary and secondary resistance to ICIs therapy for advanced tumors (50). The ascertainment of the mechanism of tumor immune resistance and the sifting of the beneficiary population are among the urgent problems to be tackled in the contemporary medical era. Presently, the mechanisms of tumor immune resistance consist of: (1) intrinsic resistance mechanisms; (2) extrinsic resistance mechanisms; (3) host-related resistance mechanisms. Among them, the mechanism of immunotherapy resistance related to B2M is mainly manifested as antigen presentation impairment (51, 52). that is, B2M reduction/defect impairs the proper assembly and transport of MHC-I molecules to the cell surface. As a result, CD8+ T cells fail to recognize the “danger signals” presented on the tumor cell surface, thereby preventing the initiation of an effective immune response. This phenomenon effectively renders tumor cells “invisible” to the immune system, allowing them to evade immune surveillance and elimination, ultimately promoting tumor growth, metastasis and resistance. Prior investigations have revealed that B2M mutation may aid tumor cells to break away from the normal immune response and attenuate the efficacy of CTLs based immunotherapies by impeding MHC class I-mediated tumor antigen presentation (27, 30). Among melanoma patients undergoing anti-PD-1 treatment, Sade-Feldman et al. (34)ascertained that B2M LOH in non-responders was threefold that in responders. Furthermore, IFN-γ potentiates CTLs activity by inducing upregulation of MHC-I complex and concurrently elicits a robust tumor-killing response via pro-apoptotic and anti-proliferative effects. However, the existence of genetic mutation and defect affiliated with the IFN-γ/IFNGR/JAK/STAT pathway can also give rise to resistance against ICIs. It is worth mentioning that recent research has shown that inhibiting autophagy can restore B2M/MHC-I expression and improve antigen presentation, thereby enhancing anti-tumor T cell responses (53), indicating that autophagy pathway can also promote immune escape by degrading B2M/MHC-I, indirectly contributing to immune resistance.
5 Correlation between B2M and immunotherapy resistance based on scRNA-seq and IMC
scRNA-seq represents an advanced technology that facilitates high-throughput transcriptomic analysis at the single-cell level. This approach not only identifies novel cell types and rare cell populations but also provides precise insights into tumor heterogeneity, the intricate interactions between tumor cells and their microenvironment, and the detailed evolutionary trajectories of individual tumors, so as to further explore the mechanism of tumor immunotherapy resistance (54, 55). IMC compensates for the lack of tissue spatial information in scRNA-seq and effectively resolves the serious cross-color issue among fluorescent groups. Building upon in situ immunohistochemistry (IHC), this technology integrates cytometry by time of flight (CyTOF) and laser ablation techniques, allowing for the simultaneous detection of abundance variations and spatial distribution of up to 50 targets within tissue samples at subcellular resolution, thereby generating single-cell proteome map across temporal and spatial dimensions (56, 57). Owing to its distinctive technical merits, IMC has emerged as a powerful tool in the realm of tumor research. Utilizing scRNA-seq and IMC technologies to unravel the correlation between B2M and immunotherapy resistance offers valuable insights for overcoming resistance and devising innovative therapeutic strategies (Table 1).
In May 2020, the Samsung Medical Center (SMC) team conducted scRNA-seq on tumor samples from 44 patients with lung adenocarcinoma (58). This study not only identified a cancer cell subtype tS2 closely associated with lung adenocarcinoma metastasis but also uncovered that the reprogramming of tumor-derived vascular endothelial cell subsets in lung adenocarcinoma patients impaired antigen presentation (including B2M) and the homing activity of immune cells (58). These findings provide new insights into understanding cell behavior and tumor immune evasion mechanisms within the tumor microenvironment (TME) of lung adenocarcinoma. The team led by Weijian Liu in China utilized scRNA-seq technology to characterize the immunosuppressive tumor microenvironment profile of osteosarcoma (OS) and discovered that MHC-I (HLA-A, HLA-B, and HLA-E)/B2M genes were downregulated, indicating diminished tumor immunogenicity in OS (59). To further investigate whether the downregulation of MHC-I/B2M is prevalent in OS, they assessed the expression of MHC-I/B2M in OS patient samples by IHC. The results confirmed that high-grade OS indeed exhibited downregulation of MHC-I/B2M. Based on these findings, we propose that the downregulation of MHC-I/B2M in high-grade OS may contribute to resistance to immune therapy. What’s more, several scholars conducted a comprehensive analysis of 738 regions with varying degrees of T-cell infiltration from 29 colorectal cancer patients using multiple detection techniques (WES, TCR-seq, RNA-seq, IMC/mIF). They discovered that B2M expression in the tumor parenchyma was lower in non-responsive groups compared to responsive groups, while no significant difference was observed in the tumor stroma (60). Sandra et al. (61) analyzed formalin-fixed, paraffin-embedded (FFPE) tissue microarray (TMA) samples from patients with metastatic melanoma who received PD-1 blockade therapy using IMC technology. Their findings indicated that higher B2M expression correlated with better immune therapy response and longer progression-free survival (PFS) and overall survival (OS). Collectively, these studies implied that silent mutation and loss of B2M are linked to immunotherapy resistance (62, 63), while upregulating B2M may offer a new therapeutic strategy for overcoming resistance. However, Natasja and his colleagues isolated γδT cells from 5 mismatch repair-deficient (MMR-d) colon cancer tissue for scRNA-seq, revealing that PD-1, activation, proliferation and killer genes expressed by γδ1 and γδ3 T cells were significantly upregulated in B2M-deficient tumors (64). Additionally, they performed IMC analysis on 17 MMR-d colon cancer patients who had not undergone ICB treatment. The results showed a notable increase in the infiltration of γδT cells in B2M-mutant tumors, with these cells exhibiting high intraepithelial localization characterized by significant expression of CD103, CD39, GZMB, Ki-67, and PD-1. Subsequently, they established two patient-derived tumor organoid cell lines and generated B2M knockout (B2M KO) cell lines using CRISPR technology. The authors exposed these B2M KO and parental B2M wild-type (B2M WT) counterparts to expanded γδ T cell subsets and quantified γδ T cell activation by measuring IFNγ expression, finding that γδT cells exhibited greater reactivity against the B2M KO cell lines compared to B2M WT cell lines. These findings unveil that γδT cells can serve as crucial effectors in ICIs therapy for combating HLA-I/B2M-deficient cancers, which represent a novel mechanism and therapeutic approach for monitoring tumor immune resistance.
6 Correlation between B2M and immunotherapy resistance based on CRISPR/Cas9
The CRISPR/Cas9 system, hailed as a revolutionary “gene scissors” technology, is renowned for its flexibility, convenience, high throughput, efficiency, and precision. It has found extensive application in various fields, including tumor functional gene screening, signal pathway analysis, and resistance target discovery. The CRISPR-Cas9 system comprises two components: Cas9 nuclease and single guide RNA (sgRNA). The sgRNA plays a critical role in recognizing specific genomic sequences. Upon complementary base pairing with the target DNA sequence, the Cas9 protein, which possesses endonuclease activity, cleaves the DNA at the specified site. Following cleavage, the broken DNA undergoes repair via either non-homologous end joining (NHEJ) or homology-directed repair (HDR), enabling genome editing, insertion, or defect (65). Utilizing CRISPR/Cas9 high-throughput gene screening technology, it is possible to establish stable cell lines with targeted gene knockouts (Table 2), thereby providing an excellent platform for screening resistance targets (66).
Previous studies have successfully utilized CRISPR/Cas9 technology to establish knockout models of B2M, JAK1, and LMP2 in the mouse EMT6 breast cancer cell line, as well as B2M knockout clone model in the mouse MC38 colon cancer cell line. In vivo experiments demonstrated that mice bearing tumors derived from B2M (EMT6 and MC38) or Jak1/LMP2 (EMT6) knockout single-cell clones exhibited no response to αPD-1 or αPD-L1 treatment (67). Similarly, Gettinger et al. (68) employed CRISPR technology to knock out B2M in mouse lung cancer cells. Wild-type and B2M-knockout cells were injected into the right legs of immunocompetent A/J mice. When tumor volumes reached approximately 30 mm³, the mice were randomly assigned to receive anti-PD-1 or isotype control antibodies. The results indicated that mice harboring intact B2M responded to PD-1 antibody treatment, whereas those with B2M KO tumors showed no therapeutic benefit from PD-1 blockade. Furthermore, Kearney et al. (69) performed a series of genome-wide screens using a CRISPR/Cas9-based custom immune escape library in colon cancer mouse models. They identified that the absence of crucial genes (caspase-8, JAK1, STAT1, B2M, TAP1) in TNF signaling, IFN-γ signaling, and antigen presentation pathways conferred resistance to CD8+ T cell and NK cell-mediated cytotoxicity. In conclusion, these findings underscore the significant part of B2M deficiency in mediating immunotherapy resistance. However, several studies have reported that high immunogenic cancer patients with B2M defect can still exhibit durable responses to anti-PD-1 therapy, suggesting the involvement of immune cell subsets beyond CD8+ T cells in these responses (70, 71). The collaborative work of Satu Mustjoki’s team from the University of Helsinki and Constantine S. Mitsiades’ team from Harvard University utilized genome-scale CRISPR knockout (LOF) screens to co-culture transfected tumor cells with expanded NK cells for periods ranging from 24 hours to 2 weeks. They observed that knocking out antigen presentation genes, including MHC-I and B2M, enhanced the sensitivity of all blood cancer cell lines to NK cells, supporting the “missing self” activation mechanism of NK cells (72). Additionally, Torrejon et al. (73) knocked out B2M gene in three mouse tumor cell lines (MC38, YUMM2.1 UV, and B16) with varying baseline MHC-I expression and sensitivities to anti-PD-1 therapy by CRISPR/Cas9 technology. Using the OMIQ platform and CyTOF, they characterized tumor immune infiltration and found that MC38 and YUMMER2.1 cells with B2M KO responded to anti-PD-1 treatment alone or in combination with IL-2 agonists, mediated by CD4+ T cells and NK cells. In contrast, more aggressive B16 cells lacking B2M showed only a partial response to IL-2 agonists, dependent on NK cells. Equally, Germano and his team also used CRISPR-Cas9 to construct B2M KO MMRd colorectal cancer cell lines (MC38-MMRd-B2M-/-and CT26-MMRd-B2M-/-) (74), subcutaneously inoculated the cells into C57BL/6 mice, and administered anti-PD-1 and anti CTLA-4 antibodies and found that B2M-deficient loaded mice treated with ICIs showed significant tumor regression and that in CD8+ T-cell depleted mice, ICIs remained efficacious against MMRd B2M-deficient tumors. Conversely, ICIs failed to induce tumor regression in CD4+ T cell-depleted mice. Additionally, they discovered a correlation between low B2M expression and increased CD4+T cell infiltration in tumors, strongly indicating that CD4+T cells mediate the response to B2M-deficient tumors during ICIs treatment. Evidently, it can be seen that in the context of B2M deficiency, the activation of CD4+ T cells and NK cells can also overcome resistance to exert anti-tumor functions.
7 Association of B2M with different immunotherapy resistance and reversal strategies
Immunotherapy brings new hope to cancer patients, yet it encounters the challenge of resistance. B2M, a pivotal factor in the immune regulatory network, plays a crucial role in immunotherapy resistance. A thorough investigation into the association between B2M and different immunotherapy resistance, along with identifying effective strategies to reverse resistance caused by B2M defect (Figure 2), can not only significantly improve the clinical efficacy of immunotherapy but also potentially pave new avenues for overcoming resistance in cancer immunotherapy.
7.1 ICIs
B2M is a crucial molecule indispensable for the assembly of MHC-I complexes and tumor antigen presentation, and its defect might be a prevailing cause of resistance to ICIs therapy. Relevant research demonstrates that B2M LOH is prevalently observed among cancer patients who exhibit poor or no response to ICIs (75). B2M defect induces resistance in ICIs-treated lung cancer patients, while upregulation of B2M enhances the efficacy of immunotherapy (76). An association could be found in a cohort study between elevated B2M expression and superior immune responses and significantly prolonged survival in metastatic melanoma patients undergoing ICIs treatment (61). Recently, acquired resistance to pembrolizumab was reported in a patient with advanced melanoma, which might be attributed to B2M defect (28). Results from the study showed that B2M truncating mutation occurred in this patient, resulting in antigen presentation defect to induce immune resistance. Similarly, Wei et al. (77) reported a 27-year-old female patient with stage IIIB (pT3N2aM0) colorectal cancer characterized as MSI-H/dMMR who developed resistance after two months of anti-PD-1 therapy. Next-generation sequencing (NGS) analysis revealed an increase in TMB to 60 muts/MB and an MSH2 mutation (p.Q337*) detected in the tumor tissue DNA of the patient. Additionally, INDEL mutation (c.43_44del) was found at the microsatellite site of the B2M gene exon, resulting in the expression of a B2M chimeric protein p.L15Ffs*41. These results suggest that B2M mutation may contribute to ICIs resistance. It is worthy of note that the intensive and persistent T cell selection pressure generated by ICIs can conversely promote B2M mutation. It was observed in lung cancer patients undergoing chemotherapy in combination with ICIs treatment that B2M experienced heterozygous loss during chemotherapy and complete loss subsequent to ICIs (68). Nevertheless, some studies have reported that 85% of B2M mutant colorectal cancer patients can benefit from ICIs, manifested as disease stability or partial remission (78). In a study conducted by Professor Peirong Ding’s team, involving 35 MSI-H CRC patients receiving PD-1 therapy, no statistically significant difference was observed in the efficacy between patients with B2M mutation and those with wild-type B2M (p=0.53). This suggests that MSI-H CRC patients harboring B2M mutation are also potential beneficiaries of PD-1 antibody therapy (79). Considering these findings, we speculate that CTLs antigen recognition becomes compromised due to B2M mutation/deletion, while other immune cells such as CD4+ T lymphocytes, NK cells, and γδ T cells may continue to exert cytotoxic effects on tumor cells (64, 70–74, 80). Unfortunately, there are currently limited studies on B2M, and the precise mechanisms by which these alternative immune cells are activated and mediate immune responses in the setting of B2M deficiency warrant further investigation.
The combination therapeutic strategies have been accorded precedence and undergone extensive exploration aimed at reversing the resistance to ICIs elicited by B2M mutation/defect. Local injection of oncolytic viruses holds the potential to overcome ICIs resistance attributed to B2M mutation and enhances anti-tumor responses through activating the IFN-γ/JAK/STAT signaling axis. B2M frameshift mutation was discerned in a patient with intractable stage IV metastatic melanoma after receiving the combination therapy of ipilimumab and nivolumab. Subsequently, the patient underwent sequential treatment with transgenic oncolytic virus TVCE in conjunction with pembrolizumab and temozolomide, interestingly, the metastatic lesions achieved a protracted (19 months) complete response (81). Furthermore, bempegaldesleukin (NKTR-214, an immunostimulatory IL-2 prodrug), which is competent in activating and continuously expanding CD4+ T and CD8+ T cells, manifests a synergistic anti-tumor effect when combined with ICIs. Systemic administration of bempegaldesleukin can overcome resistance incurred by anti-PD-1 treatment and achieve better survival in knockout B2M melanoma mice (82).
7.2 Cancer vaccines
Cancer vaccines are biological agents used for the prophylaxis or therapeutics of tumors, in which tumor antigens are introduced into the patient’s body in various forms (such as nucleic acids, protein polypeptides, bacterial and viral vectors, DC cells) to activate specific immune responses and establish long-term immune memory. They possess crucial clinical significance on account of the merits of high efficacy, strong specificity, favorable safety, ae well as long-term immune memory. Nevertheless, cancer vaccines based on T cells may develop resistance because of B2M defect. Benitez’s study discovered that two melanoma patients who were refractory to MAGE-peptide tumor vaccine treatment with loss of B2M expression (83). The poor efficacy of cancer vaccination administration with ODN1826 adjuvant in MHC-I negative models may be affiliated with B2M deficiency in TC-1 cell lines (84).
Presently, personalized neoantigen vaccines have inaugurated a novel epoch for cancer vaccines. Neoantigens are exclusively expressed by tumor cells and can evoke a bona fide tumor-specific T-cell response, thereby evading “off-target” damage to non-tumor tissues. Moreover, neoantigens are nascent epitopes originated from somatic mutations and can avoid central immune tolerance, thus possessing a high immunogenicity. Tumor neoantigen vaccines can potentiate immune response elicited by neoantigen-specific T cells, surmount immune escape, and forge new avenues for achieving precise immunotherapy. Cao et al. (85)elaborated the HA-OVA-AuNPs nano-vaccine, which enlisted near-infrared (NIR) irradiation for photothermal regulation of cytoplasmic antigen delivery to potentiate downstream MHC-I antigen presentation. Campo et al. (86) transfected the adenovirus vector harboring B2M gene (AdCMVB2M) into MHC-I negative/B2M-deficient malignant tumor (melanoma, colorectal cancer, prostate cancer) cell lines. It was ascertained that the expression of HLA-I/B2M was positive after 72 hours, escalating the immunogenicity of tumor cells and the capability of T cell immune recognition. Additionally, enhanced clinical responses have been procured in the combined treatment of cancer vaccines and ICIs, which has been validated in clinical studies. In 2020, a phase IIb clinical trial, NCT02897765, was the first to incorporate the personalized neoantigen vaccine NEO-PV-01 with PD-1 inhibitors for NSCLC, advanced melanoma or bladder cancer, which demonstrated that all subjects exhibited neoantigen-specific CD4+ and CD8+ T cell responses with conspicuously higher objective response rate (ORR) than that of monotherapy (87).
7.3 CAR-T therapy
CAR-T therapy, a novel adoptive cell immunotherapy that has garnered considerable attention in recent years, utilizes genetic engineering techniques to activate T cells and empower them to express chimeric antigen receptors (CAR). Through amplifying CAR-T cells ex vivo, it overcomes the local immunosuppressive microenvironment and disrupts the host immune tolerance, targeting and eliminating tumor cells in a non-MHC-restricted manner. Currently, autologous CAR-T therapy has attained remarkable breakthroughs in the realm of immunotherapy for B-cell malignant lymphoma (88). Nevertheless, against the backdrop of autologous T cell therapy that necessitates customization, prolonged preparation cycle (approximately 2-3 weeks), limited quantity, and suboptimal quality, allogeneic CAR-T cell therapy has emerged. The latter, also known as universal CAR-T and off-the-shelf CAR-T, pertains to the procedure where T cells are extracted from healthy donors or obtained through means such as peripheral blood, umbilical cord blood, or pluripotent stem cells, subjected to genetic engineering modification and amplification, and ultimately transferred in vivo to exert the predefined anti-tumor effect. However, graft-versus-host disease (GvHD) and host-versus-graft reaction (HvGA) remain two major obstacles for allogeneic CAR-T therapy.
In recent years, gene editing technologies (CRISPR/Cas9, TALEN, ZFN) have contributed to addressing GvHD and HvGA by knocking out TCR on allogeneic CAR-T along with MHC-I/B2M, thus improving the anti-tumor effectiveness of CAR-T cells (89–94) (Table 2). A study (95) utilized the zinc finger nuclease (ZFN) system, in combination with the Sleeping Beauty transposase/transposition system, to engineer CAR-T cells that are CD19-specific and lack endogenous TCR expression. Eyquem et al. (96) utilized CRISPR/Cas9 technology to target the disruption of endogenous T cell receptor (TRAC) and B2M, thereby minimizing the probability of initiating GvHD and eliciting donor T cell rejection, and subsequently enhancing the efficacy of CAR-T therapy. In 2019, Choi et al. (97) exploited the CRIPSR/Cas9 system to perform multiple gene disruptions of TARC, B2M, and PD-1 (PDCD1), creating allogeneic epidermal growth factor (EGFRvIII) CAR-T cells that can resist PD-1 inhibition. The results demonstrated that the EGFRvIII CAR-T therapy conspicuously augmented the anti-tumor activity in the preclinical model of glioblastoma (GBM) and significantly ameliorated the survival of the mice model. Nevertheless, it is worth noting that B2M/MHC-I deficiency might trigger the activation of natural killer (NK) cells, which would recognize the allogeneic CAR-T cells as “missing self” and exert immune rejection (98). Hence, additional research is requisite restrain or eradicate the reactive NK cells within the host, thereby offering protection to universal CAR-T cells against being killed by NK cells.
7.4 Therapies based on natural killer cells
NK cells, otherwise designated as natural killer cells, can nonspecifically eliminate tumor cells without prerequisite stimulation of tumor antigens. Their killing activity is not circumscribed by MHC and is independent of antibodies, predominantly hinging upon the intricate interaction of activating and inhibitory receptors on their surface. The inhibitory receptors existing on the surface of NK cells, such as killer immunoglobulin-like receptors (KIRs) and Natural Killer Group 2 Member A (NKG2A), are capable of recognizing MHC-I molecule, thereby preventing NK cell activation, polarization, and degranulation. Nevertheless, tumor cells typically exhibit low or no expression of B2M/MHC-I, engendering the deprivation of the B2M/MHC-I/KIRs interaction and subsequently inducing NK cell activation, a process denominated as “missing self-recognition.” A study revealed that, the downregulation of B2M expression (approximately 9.8%) among NSCLC patients was correlated with an augmented infiltration of NK cells (99). Abnormal overexpression of HLA-E/B2M was found to be associated with NKG2A-expressing CD94+ T cells and NK cells in MSI colorectal cancer, and an increased number of NKG2A+CD94+ T cells was interrelated with an unfavorable prognosis (100). These findings imply that the HLA-E/B2M-CD94/NKG2A axis may partake in tumor immune escape, and corresponding strategies to block B2M/NKG2A may usher in novel prospects for NK cell-based immunotherapy for cancer patients.
7.5 Radiotherapy
Radiotherapy can induce immune activation and elicit anti-tumor responses through mediating DNA damage in tumor cells, reshaping tumor immune microenvironment like an in-situ vaccine, and initiating the release of inflammatory factors, which may potentially confer benefits upon cancer patients who are resistant to ICIs again. It has been found that radiotherapy can facilitate the release of interferon-beta (IFNβ) to upregulate B2M/MHC-I expression on resistant tumor cells, enhance antigen presentation, and restore responsiveness to PD-1 therapy (101). It can also significantly increase the transcription of NLRC5, B2M, and TAP1 in a dose-dependent manner to overcome resistance to ICIs (102). Moreover, A synergy is attained when radiotherapy is combined with CAR-T therapy in a mouse glioblastoma model, and the underlying mechanism could be attributed to the circumstance that radiotherapy potentiates recognition and cytotoxicity of CD8+ T cells through upregulating B2M/MHC-I (103). The combinations of Stereotactic radiotherapy (SBRT) with camrelizumab brought pronounced survival benefit in overall survival (OS) and progression-free survival (PFS) for unresectable hepatocellular carcinoma patients, attributed to the “abscopal effect” of radiotherapy, as demonstrated by one prospective single-arm clinical trial (104). However, the optimal radiotherapy dose and fractionation are still under vigorous exploration to evoke systemic anti-tumor immune responses. On the other hand, radiotherapy can also induce the production of neoantigens to circumvent inadequate antigen presentation and promote CTLs infiltration to boost immunogenicity. Collectively, radiotherapy holds the potential to serve as a promising alternative for reversing immune resistance in B2M/MHC-I-deficient cancer patients.
7.6 Other therapies
Mounting evidence suggests that altering the gut microbiota has turned to be a promising approach to reverse resistance across a variety of cancer patients. NCT03772899, a Phase 1 clinical trial, demonstrated that fecal microbiota transplant (FMT) as a latent strategy for overcoming ICIs resistance in patients with advanced melanoma (105). V. Gopalakrishnan’s team discovered that the diversity of gut microbiota was higher in the anti-PD-1 treatment responsive group (R) than in the non-responsive group (NR) (106). It was found that the abundance of fecal bacteria for R group was conspicuously elevated in the FMT experiment of sterile mice, particularly with a marked enrichment of Bacteroides. Moreover, the density of CD8+ T cells in the tumor tissues of mice that received FMT from the R group was higher than that of mice receiving NR transplant. Notably, the role of cytokines can’t be overlooked as immune modulators to markedly ameliorate ICIs efficacy for cancer patients. IFNγ can facilitate CD8+ T cell priming and infiltration by upregulating the expression of B2M/MHC-I on the surface of tumor cells. The Beck research team, by establishing a B2M-deficient mouse tumor model, revealed that MHC-I deficiency would result in severe immune desertification in TME and widespread resistance to ICIs. Long-lasting mRNA-encoded interleukin-2 (IL-2) can boost potent and durable antitumoral immune responses against B2M/MHC-I-deficient tumors, restore immune cell infiltration, and exhibit highly pro-inflammatory TME, thereby overcoming resistance incurred by ICIs treatment (107).
8 Conclusion and prospects
Up to now, immunotherapy has assumed a pivotal role and emerged as a milestone breakthrough in the history of tumor treatment. Nevertheless, immune resistance, to a certain extent, restricts its clinical application and constitutes a “stumbling block” for the long-term benefit for each patient with cancer. It is an urgently pressing and hot issue to elucidate the mechanism of immunotherapy resistance and explore novel therapeutic targets. Among these, B2M mutation/defect, which leads to antigen presentation dysfunction of MHC class I molecule, constitutes an important cause of immunotherapy resistance. Therefore, in-depth investigation into the role of B2M in tumor immunotherapy resistance and the development of corresponding strategies are of paramount importance.
This review systematically elaborates on the role and reversal strategies of B2M in tumor immunotherapy resistance from multiple perspectives. Notably, we have leveraged emerging technologies such as scRNA-seq, IMC, and CRISPR/Cas9 to deeply analyze the association between B2M and tumor immune therapy resistance. As the light chain of MHC-I molecule, B2M plays a pivotal role in tumor antigen presentation, with its expression modulated by diverse mechanisms such as genetics, epigenetics, cytokines, and non-coding RNAs. B2M mutation/defect can impair antigen presentation, which constitutes a significant factor of tumor immunotherapy resistance. Interestingly, during ICIs treatment, immune cells such as CD4+ T cells, NK cells, and γδ T cells mediate responses to B2M-deficient tumors, offering new avenues to overcome the resistance stemming from B2M deficiency. Additionally, combination therapies (such as oncolytic viruses/bempegaldesleukin combined with ICIs), personalized neoantigen tumor vaccines, optimized CAR-T therapy (using gene editing technology to knock out B2M), NK cell therapy, radiotherapy, fecal microbiota transplantation, and cytokine therapy also provide promising approaches to reverse immune resistance induced by B2M mutation.
Looking ahead, several critical fields in B2M-related tumor immunotherapy research warrant further exploration. Firstly, with the rapid development of high-throughput sequencing, single-cell multi-omics technology (including proteomics, transcriptomics, epigenomics, and spatial omics) can be used to comprehensively analyze the expression profiles and functional variations of B2M across diverse cell subpopulations within the tumor microenvironment. It also facilitates the precise mapping of B2M interaction profiles between tumor cells and immune cells, elucidating potential synergistic or antagonistic mechanisms, and providing a more detailed theoretical basis for precision therapy. Secondly, while CD4+ T cells, NK cells, and γδ T cells have been identified as key effector cells for ICB treatment in B2M-deficient cancers, the underlying mechanisms by which these cells mediate their cytotoxic effects remain to be fully elucidated. Lastly, there is a need to develop more targeted and efficacious therapies for B2M mutation/defect, such as small molecule inhibitors or biologics designed to specifically address antigen presentation defects and reverse immune resistance. In conclusion, it is imperative that we make dedicated efforts to translate these issues into practical clinical applications, thereby actualizing the concept of “from bench to bedside” possible.
Author contributions
XWH: Conceptualization, Formal analysis, Methodology, Writing – original draft. JZ: Conceptualization, Writing – original draft, Visualization. WL: Writing – original draft, Formal analysis, Methodology. XDH: Methodology, Writing – original draft, Visualization. XW: Visualization, Writing – original draft. BW: Writing – original draft, Validation. LG: Writing – original draft, Methodology. HC: Conceptualization, Funding acquisition, Resources, Supervision, Writing – review & editing.
Funding
The author(s) declare that financial support was received for the research and/or publication of this article. This research was supported by National Natural Science Foundation of China (No. 82473266); National Natural Science Foundation of China (No. 82160129); General Project of Gansu Province Joint Research Fund (24JRRA929); Key Talents Project of Gansu Province (No.2019RCXM020); Key Project of Science and Technology in Gansu province(No. 22ZD6FA054); Science and technology project of Chengguan District of Lanzhou City (2020SHFZ0039, 2020JSCX0073); Gansu Province Innovation Driven Assistance Project (GXH20230817-14); Cuiying Scientific and Technological Innovation Program of Lanzhou University Second Hospital (No.CY2023-ZD-01); Medical Innovation and Development Project of Lanzhou University (lzuyxcx-2022-160, lzuyxcx-2022-45, lzuyxcx-2022-88).
Conflict of interest
The authors declare that the research was conducted in the absence of any commercial or financial relationships that could be construed as a potential conflict of interest.
Generative AI statement
The author(s) declare that no Generative AI was used in the creation of this manuscript.
Publisher’s note
All claims expressed in this article are solely those of the authors and do not necessarily represent those of their affiliated organizations, or those of the publisher, the editors and the reviewers. Any product that may be evaluated in this article, or claim that may be made by its manufacturer, is not guaranteed or endorsed by the publisher.
References
1. Luri-Rey C, Teijeira Á, Wculek SK, de Andrea C, Herrero C, Lopez-Janeiro A, et al. Cross-priming in cancer immunology and immunotherapy. Nat Rev Cancer. (2025). doi: 10.1038/s41568-024-00785-5
2. Patel L, Kolundzic N, Abedalthagafi M. Progress in personalized immunotherapy for patients with brain metastasis. NPJ Precis Oncol. (2025) 9:31. doi: 10.1038/s41698-025-00812-0
3. Panahizadeh R, Panahi P, Asghariazar V, Makaremi S, Noorkhajavi G, Safarzadeh E. A literature review of recent advances in gastric cancer treatment: exploring the cross-talk between targeted therapies. Cancer Cell Int. (2025) 25:23. doi: 10.1186/s12935-025-03655-8
4. Wei J, Li W, Zhang P, Guo F, Liu M. Current trends in sensitizing immune checkpoint inhibitors for cancer treatment. Mol Cancer. (2024) 23:279. doi: 10.1186/s12943-024-02179-5
5. Eslami SM, Lu X. Recent advances in mRNA-based cancer vaccines encoding immunostimulants and their delivery strategies. J Control Release. (2024) 376:413–28. doi: 10.1016/j.jconrel.2024.10.035
6. Poudel K, Vithiananthan T, Kim JO, Tsao H. Recent progress in cancer vaccines and nanovaccines. Biomater Mar. (2025) 314:122856. doi: 10.1016/j.biomaterials.2024.122856
7. Yao L, Hatami M, Ma W, Skutella T. Vaccine-based immunotherapy and related preclinical models for glioma. Trends Mol Med. (2024) 30:965–81. doi: 10.1016/j.molmed.2024.06.009
8. Buono G, Capozzi M, Caputo R, Lauro VD, Cianniello D, Piezzo M, et al. CAR-T cell therapy for breast cancer: Current status and future perspective. Cancer Treat Rev. (2025) 133:102868. doi: 10.1016/j.ctrv.2024.102868
9. Li Y, Wu Y, Gao S, Sun T, Jiang C. PROTAC delivery in tumor immunotherapy: Where are we and where are we going? J Control Release. (2024) 378:116–44. doi: 10.1016/j.jconrel.2024.11.076
10. Pherez-Farah A, Boncompagni G, Chudnovskiy A, Pasqual G. The bidirectional interplay between T cell-based immunotherapies and the tumor microenvironment. Cancer Immunol Res. (2025). doi: 10.1158/2326-6066.Cir-24-0857
11. Arafat Hossain M. A comprehensive review of immune checkpoint inhibitors for cancer treatment. Int Immunopharmacol. (2024) 143:113365. doi: 10.1016/j.intimp.2024.113365
12. Bell HN, Zou W. Beyond the barrier: unraveling the mechanisms of immunotherapy resistance. Annu Rev Immunol. (2024) 42:521–50. doi: 10.1146/annurev-immunol-101819-024752
13. Icard P, Prieto M, Coquerel A, Fournel L, Gligorov J, Noel J, et al. Why and how citrate may sensitize Malignant tumors to immunotherapy. Drug Resist Update. (2025) 78:101177. doi: 10.1016/j.drup.2024.101177
14. Exposito F, Redrado M, Houry M, Hastings K, Molero-Abraham M, Lozano T, et al. PTEN loss confers resistance to anti-PD-1 therapy in non-small cell lung cancer by increasing tumor infiltration of regulatory T cells. Cancer Res. (2023) 83:2513–26. doi: 10.1158/0008-5472.Can-22-3023
15. Lei Y, Li X, Huang Q, Zheng X, Liu M. Progress and challenges of predictive biomarkers for immune checkpoint blockade. Front Oncol. (2021) 11:617335. doi: 10.3389/fonc.2021.617335
16. Wang L, Bi S, Li Z, Liao A, Li Y, Yang L, et al. Napabucasin deactivates STAT3 and promotes mitoxantrone-mediated cGAS-STING activation for hepatocellular carcinoma chemo-immunotherapy. Biomaterials. (2025) 313:122766. doi: 10.1016/j.biomaterials.2024.122766
17. Zak J, Pratumchai I, Marro BS, Marquardt KL, Zavareh RB, Lairson LL, et al. JAK inhibition enhances checkpoint blockade immunotherapy in patients with Hodgkin lymphoma. Science. (2024) 384:eade8520. doi: 10.1126/science.ade8520
18. Kvist S, Peterson PA. Isolation and partial characterization of a beta2-microglobulin-containing, H-2 antigen-like murine serum protein. Biochemistry. (1978) 17:4794–801. doi: 10.1021/bi00615a029
19. Sivanathan PC, Ooi KS, Mohammad Haniff MAS, Haniff MAS, Ahmadipour M, Dee CF, Mokhtar NM, et al. Lifting the veil: characteristics, clinical significance, and application of β-2-microglobulin as biomarkers and its detection with biosensors. ACS Biomater Sci Eng. (2022) 8:3142–61. doi: 10.1021/acsbiomaterials.2c00036
20. Hu FY, Wu J, Tang Q, Zhang J, Chen Z, Wang X, et al. Serum β2-microglobulin is closely associated with the recurrence risk and 3-month outcome of acute ischemic stroke. Front Neurol. (2019) 10:1334. doi: 10.3389/fneur.2019.01334
21. Assounga AG. Beta 2 microglobulin in kidney failure: A review and an algorithm for renal replacement therapy. Saudi J Kidney Dis Transpl. (2021) 32:1214–20. doi: 10.4103/1319-2442.344740
22. Gao Y, Hong Y, Huang L, Zheng S, Zhang H, Wang S, et al. β2-microglobulin functions as an endogenous NMDAR antagonist to impair synaptic function. Cell. (2023) 186:1026–1038.e20. doi: 10.1016/j.cell.2023.01.021
23. Liu ZY, Tang F, Yang JZ, Chen X, Wang ZF, Li ZQ. The role of beta2-microglobulin in central nervous system disease. Cell Mol Neurobiol. (2024) 44:46. doi: 10.1007/s10571-024-01481-6
24. Li L, Dong M, Wang XG. The implication and significance of beta 2 microglobulin: A conservative multifunctional regulator. Chin Med J (Engl). (2016) 129:448–55. doi: 10.4103/0366-6999.176084
25. Bernal M, Ruiz-Cabello F, Concha A, Paschen A, Garrido F. Implication of the β2-microglobulin gene in the generation of tumor escape phenotypes. Cancer Immunol Immunother. (2012) 61:1359–71. doi: 10.1007/s00262-012-1321-6
26. Barrow P, Richman SD, Wallace AJ, Handley K, Hutchins GGA, Kerr D, et al. Confirmation that somatic mutations of beta-2 microglobulin correlate with a lack of recurrence in a subset of stage II mismatch repair deficient colorectal cancers from the QUASAR trial. Histopathology. (2019) 75:236–46. doi: 10.1111/his.13895
27. Wang H, Liu B, Wei J. Beta2-microglobulin(B2M) in cancer immunotherapies: Biological function, resistance and remedy. Cancer Lett. (2021) 517:96–104. doi: 10.1016/j.canlet.2021.06.008
28. Zaretsky JM, Garcia-Diaz A, Shin DS, Escuin-Ordinas H, Hugo W, Hu-Lieskovan S, et al. Mutations associated with acquired resistance to PD-1 blockade in melanoma. N Engl J Med. (2016) 375:819–29. doi: 10.1056/NEJMoa1604958
29. Zhao Y, Cao Y, Chen Y, Wu L, Hang H, Jiang C, et al. B2M gene expression shapes the immune landscape of lung adenocarcinoma and determines the response to immunotherapy. Immunology. (2021) 164:507–23. doi: 10.1111/imm.13384
30. Wang C, Wang Z, Yao T, Zhou J, Wang Z. The immune-related role of beta-2-microglobulin in melanoma. Front Oncol. (2022) 12:944722. doi: 10.3389/fonc.2022.944722
31. Chiou SJ, Ko HJ, Hwang CC, Hong YR. The double-edged sword of beta2-microglobulin in antibacterial properties and amyloid fibril-mediated cytotoxicity. Int J Mol Sci. (2021) 22(12):6330. doi: 10.3390/ijms22126330
32. Barton KT, Kakajiwala A, Dietzen DJ, Goss CW, Gu H, Dharnidharka VR. Using the newer Kidney Disease: Improving Global Outcomes criteria, beta-2-microglobulin levels associate with severity of acute kidney injury. Clin Kidney J. (2018) 11:797–802. doi: 10.1093/ckj/sfy056
33. Wu X, Li T, Jiang R, Yang X, Guo H, Yang R. Targeting MHC-I molecules for cancer: function, mechanism, and therapeutic prospects. Mol Cancer. (2023) 22:194. doi: 10.1186/s12943-023-01899-4
34. Sade-Feldman M, Jiao YJ, Chen JH, Rooney MS, Barzily-Rokni M, Eliane JP, et al. Resistance to checkpoint blockade therapy through inactivation of antigen presentation. Nat Commun. (2017) 8:1136. doi: 10.1038/s41467-017-01062-w
35. McGranahan N, Rosenthal R, Hiley CT, Rowan AJ, Watkins TBK, Wilson GA, et al. Allele-specific HLA loss and immune escape in lung cancer evolution. Cell. (2017) 171:1259–1271.e11. doi: 10.1016/j.cell.2017.10.001
36. Yoshihama S, Roszik J, Downs I, Meissner TB, Vijayan S, Chapuy B, et al. NLRC5/MHC class I transactivator is a target for immune evasion in cancer. Proc Natl Acad Sci U.S.A. (2016) 113:5999–6004. doi: 10.1073/pnas.1602069113
37. Sun X, Watanabe T, Oda Y, Shen W, Ahmad A, Ouda R, et al. Targeted demethylation and activation of NLRC5 augment cancer immunogenicity through MHC class I. Proc Natl Acad Sci U.S.A. (2024) 121:e2310821121. doi: 10.1073/pnas.2310821121
38. Burr ML, Sparbier CE, Chan KL, Chan YC, Kersbergen A, Lam EYN, et al. An evolutionarily conserved function of polycomb silences the MHC class I antigen presentation pathway and enables immune evasion in cancer. Cancer Cell. (2019) 36:385–401.e8. doi: 10.1016/j.ccell.2019.08.008
39. Li L, Zeng X, Chao Z, Luo J, Guan W, Zhang Q, et al. Targeting alpha-ketoglutarate disruption overcomes immunoevasion and improves PD-1 blockade immunotherapy in renal cell carcinoma. Adv Sci (Weinh). (2023) 10:e2301975. doi: 10.1002/advs.202301975
40. Snahnicanova Z, Kasubova I, Kalman M, Grendar M, Mikolajcik P, Gabonova E, et al. Genetic and epigenetic analysis of the beta-2-microglobulin gene in microsatellite instable colorectal cancer. Clin Exp Med. (2020) 20:87–95. doi: 10.1007/s10238-019-00601-7
41. Woan KV, Lienlaf M, Perez-Villaroel P, Lee C, Cheng F, Knox T, et al. Targeting histone deacetylase 6 mediates a dual anti-melanoma effect: Enhanced antitumor immunity and impaired cell proliferation. Mol Oncol. (2015) 9:1447–57. doi: 10.1016/j.molonc.2015.04.002
42. Ugurel S, Spassova I, Wohlfarth J, Drusio C, Cherouny A, Melior A, et al. MHC class-I downregulation in PD-1/PD-L1 inhibitor refractory Merkel cell carcinoma and its potential reversal by histone deacetylase inhibition: a case series. Cancer Immunol Immunother. (2019) 68:983–90. doi: 10.1007/s00262-019-02341-9
43. Souri Z, Jochemsen AG, Versluis M, Wierenga APA, Nemati F, van der Velden PA, et al. HDAC inhibition increases HLA class I expression in uveal melanoma. Cancers (Basel). (2020) 12(12):3690. doi: 10.3390/cancers12123690
44. Song L, Bretz AC, Gravemeyer J, Spassova I, Muminova S, Gambichler T, et al. The HDAC inhibitor domatinostat promotes cell-cycle arrest, induces apoptosis, and increases immunogenicity of merkel cell carcinoma cells. J Invest Dermatol. (2021) 141:903–912.e4. doi: 10.1016/j.jid.2020.08.023
45. Sucker A, Zhao F, Pieper N, Heeke C, Maltaner R, Stadtler N, et al. Acquired IFNγ resistance impairs anti-tumor immunity and gives rise to T-cell-resistant melanoma lesions. Nat Commun. (2017) 8:15440. doi: 10.1038/ncomms15440
46. Kalbasi A, Tariveranmoshabad M, Hakimi K, Kremer S, Campbell KM, Funes JM, et al. Uncoupling interferon signaling and antigen presentation to overcome immunotherapy resistance due to JAK1 loss in melanoma. Sci Transl Med. (2020) 12(565):eabb0152. doi: 10.1126/scitranslmed.abb0152
47. Ren J, Li N, Pei S, Lian Y, Li L, Peng Y, et al. Histone methyltransferase WHSC1 loss dampens MHC-I antigen presentation pathway to impair IFN-γ-stimulated antitumor immunity. J Clin Invest. (2022) 132(8):e153167. doi: 10.1172/jci153167
48. Zheng J, Yang T, Gao S, Cheng M, Shao Y, Xi Y, et al. miR-148a-3p silences the CANX/MHC-I pathway and impairs CD8(+) T cell-mediated immune attack in colorectal cancer. FASEB J. (2021) 35:e21776. doi: 10.1096/fj.202100235R
49. Mari L, Hoefnagel SJM, Zito D, van de Meent M, van Endert P, Calpe S, et al. microRNA 125a regulates MHC-I expression on esophageal adenocarcinoma cells, associated with suppression of antitumor immune response and poor outcomes of patients. Gastroenterology. (2018) 155:784–98. doi: 10.1053/j.gastro.2018.06.030
50. Kluger HM, Tawbi HA, Ascierto ML, Bowden M, Callahan MK, Cha E, et al. Defining tumor resistance to PD-1 pathway blockade: recommendations from the first meeting of the SITC Immunotherapy Resistance Taskforce. J Immunother Cancer. (2020) 8(1):e000398. doi: 10.1136/jitc-2019-000398
51. Pavelescu LA, Enache RM, Roşu OA, Profir M, Creţoiu SM, Gaspar BS. Predictive biomarkers and resistance mechanisms of checkpoint inhibitors in Malignant solid tumors. Int J Mol Sci. (2024) 25(17):9659. doi: 10.3390/ijms25179659
52. Yang M, Cui M, Sun Y, Liu S, Jiang W. Mechanisms, combination therapy, and biomarkers in cancer immunotherapy resistance. Cell Commun Signal. (2024) 22:338. doi: 10.1186/s12964-024-01711-w
53. Yamamoto K, Venida A, Yano J, Biancur DE, Kakiuchi M, Gupta S, et al. Autophagy promotes immune evasion of pancreatic cancer by degrading MHC-I. Nature. (2020) 581:100–5. doi: 10.1038/s41586-020-2229-5
54. Boldu-Fernández S, Lliberos C, Simon C, Mas A. Mapping human uterine disorders through single-cell transcriptomics. Cells. (2025) 14(3):156. doi: 10.3390/cells14030156
55. Zhang L, Yang Y, Tan J. Applications and emerging challenges of single-cell RNA sequencing technology in tumor drug discovery. Drug Discovery Today. (2025) 30:104290. doi: 10.1016/j.drudis.2025.104290
56. Elaldi R, Hemon P, Petti L, Cosson E, Desrues B, Sudaka A, et al. High dimensional imaging mass cytometry panel to visualize the tumor immune microenvironment contexture. Front Immunol. (2021) 12:666233. doi: 10.3389/fimmu.2021.666233
57. Le Rochais M, Hemon P, Pers JO, Uguen A. Application of high-throughput imaging mass cytometry hyperion in cancer research. Front Immunol. (2022) 13:859414. doi: 10.3389/fimmu.2022.859414
58. Kim N, Kim HK, Lee K, Hong Y, Cho JH, Choi JW, et al. Single-cell RNA sequencing demonstrates the molecular and cellular reprogramming of metastatic lung adenocarcinoma. Nat Commun. (2020) 11:2285. doi: 10.1038/s41467-020-16164-1
59. Liu W, Hu H, Shao Z, Lv X, Zhang Z, Deng X, et al. Characterizing the tumor microenvironment at the single-cell level reveals a novel immune evasion mechanism in osteosarcoma. Bone Res. (2023) 11:4. doi: 10.1038/s41413-022-00237-6
60. Bortolomeazzi M, Keddar MR, Montorsi L, Acha-Sagredo A, Benedetti L, Temelkovski D, et al. Immunogenomics of colorectal cancer response to checkpoint blockade: analysis of the KEYNOTE 177 trial and validation cohorts. Gastroenterology. (2021) 161:1179–93. doi: 10.1053/j.gastro.2021.06.064
61. Martinez-Morilla S, Villarroel-Espindola F, Wong PF, Toki MI, Aung TN, Pelekanou V, et al. Biomarker discovery in patients with immunotherapy-treated melanoma with imaging mass cytometry. Clin Cancer Res. (2021) 27:1987–96. doi: 10.1158/1078-0432.Ccr-20-3340
62. Kypraios A, Bennour J, Imbert V, David L, Calvo J, Pflumio F, et al. Identifying candidate gene drivers associated with relapse in pediatric T-cell acute lymphoblastic leukemia using a gene co-expression network approach. Cancers (Basel). (2024) 16(9):1667. doi: 10.3390/cancers16091667
63. Zhang J, Zhang H, Zhang L, Li D, Qi M, Zhang L, et al. Single-cell transcriptome identifies drug-resistance signature and immunosuppressive microenvironment in metastatic small cell lung cancer. Adv Genet (Hoboken). (2022) 3:2100060. doi: 10.1002/ggn2.202100060
64. de Vries NL, van de Haar J, Veninga V, Chalabi M, Ijsselsteijn ME, van der Ploeg M, et al. γδ T cells are effectors of immunotherapy in cancers with HLA class I defects. Nature. (2023) 613:743–50. doi: 10.1038/s41586-022-05593-1
65. Razeghian E, Nasution MKM, Rahman HS, Gardanova ZR, Abdelbasset WK, Aravindhan S, et al. A deep insight into CRISPR/Cas9 application in CAR-T cell-based tumor immunotherapies. Stem Cell Res Ther. (2021) 12:428. doi: 10.1186/s13287-021-02510-7
66. Wang G, Chow RD, Zhu L, Bai Z, Ye L, Zhang F, et al. CRISPR-GEMM pooled mutagenic screening identifies KMT2D as a major modulator of immune checkpoint blockade. Cancer Discovery. (2020) 10:1912–33. doi: 10.1158/2159-8290.Cd-19-1448
67. Chariou PL, Minnar CM, Tandon M, Guest MR, Chari R, Schlom J, et al. Generation of murine tumor models refractory to αPD-1/-L1 therapies due to defects in antigen processing/presentation or IFNγ signaling using CRISPR/Cas9. PloS One. (2024) 19:e0287733. doi: 10.1371/journal.pone.0287733
68. Gettinger S, Choi J, Hastings K, Truini A, Datar I, Sowell R, et al. Impaired HLA class I antigen processing and presentation as a mechanism of acquired resistance to immune checkpoint inhibitors in lung cancer. Cancer Discovery. (2017) 7:1420–35. doi: 10.1158/2159-8290.Cd-17-0593
69. Kearney CJ, Vervoort SJ, Hogg SJ, Ramsbottom KM, Freeman AJ, Lalaoui N, et al. Tumor immune evasion arises through loss of TNF sensitivity. Sci Immunol. (2018) 3(23):eaar3451. doi: 10.1126/sciimmunol.aar3451
70. Dubrot J, Lane-Reticker SK, Kessler EA, Ayer A, Mishra G, Wolfe CH, et al. In vivo screens using a selective CRISPR antigen removal lentiviral vector system reveal immune dependencies in renal cell carcinoma. Immunity. (2021) 54:571–585.e6. doi: 10.1016/j.immuni.2021.01.001
71. Freeman AJ, Vervoort SJ, Ramsbottom KM, Kelly MJ, Michie J, Pijpers L, et al. Natural killer cells suppress T cell-associated tumor immune evasion. Cell Rep. (2019) 28:2784–2794.e5. doi: 10.1016/j.celrep.2019.08.017
72. Dufva O, Gandolfi S, Huuhtanen J, Dashevsky O, Duàn H, Saeed K, et al. Single-cell functional genomics reveals determinants of sensitivity and resistance to natural killer cells in blood cancers. Immunity. (2023) 56:2816–2835.e13. doi: 10.1016/j.immuni.2023.11.008
73. Torrejon DY, Galvez M, Abril-Rodriguez G, Campbell KM, Medina E, Vega-Crespo A, et al. Antitumor immune responses in B2M-deficient cancers. Cancer Immunol Res. (2023) 11:1642–55. doi: 10.1158/2326-6066.Cir-23-0139
74. Germano G, Lu S, Rospo G, Lamba S, Rousseau B, Fanelli S, et al. CD4 T cell-dependent rejection of beta-2 microglobulin null mismatch repair-deficient tumors. Cancer Discovery. (2021) 11:1844–59. doi: 10.1158/2159-8290.Cd-20-0987
75. Kalbasi A, Ribas A. Tumour-intrinsic resistance to immune checkpoint blockade. Nat Rev Immunol. (2020) 20:25–39. doi: 10.1038/s41577-019-0218-4
76. Pereira C, Gimenez-Xavier P, Pros E, Pajares MJ, Moro M, Gomez A, et al. Genomic profiling of patient-derived xenografts for lung cancer identifies B2M inactivation impairing immunorecognition. Clin Cancer Res. (2017) 23:3203–13. doi: 10.1158/1078-0432.Ccr-16-1946
77. Liu F, Zhong F, Wu H, Che K, Shi J, Wu N, et al. Prevalence and associations of beta2-microglobulin mutations in MSI-H/dMMR cancers. Oncologist. (2023) 28:e136–44. doi: 10.1093/oncolo/oyac268
78. Middha S, Yaeger R, Shia J, Stadler ZK, King S, Guercio S, et al. Majority of B2M-mutant and -deficient colorectal carcinomas achieve clinical benefit from immune checkpoint inhibitor therapy and are microsatellite instability-high. JCO Precis Oncol. (2019) 3:PO.18.00321. doi: 10.1200/po.18.00321
79. Zhang C, Li D, Xiao B, Zhou C, Jiang W, Tang J, et al. B2M and JAK1/2-mutated MSI-H colorectal carcinomas can benefit from anti-PD-1 therapy. J Immunother. (2022) 45:187–93. doi: 10.1097/cji.0000000000000417
80. Gurjao C, Liu D, Hofree M, AlDubayan SH, Wakiro I, Su MJ, et al. Intrinsic resistance to immune checkpoint blockade in a mismatch repair-deficient colorectal cancer. Cancer Immunol Res. (2019) 7:1230–6. doi: 10.1158/2326-6066.Cir-18-0683
81. Khaddour K, Dowling J, Huang J, Council M, Chen D, Cornelius L, et al. Successful administration of sequential TVEC and pembrolizumab followed by Temozolomide in immunotherapy refractory intracranial metastatic melanoma with acquired B2M mutation. Oncotarget. (2020) 11:4836–44. doi: 10.18632/oncotarget.27848
82. Torrejon DY, Abril-Rodriguez G, Champhekar AS, Tsoi J, Campbell KM, Kalbasi A, et al. Overcoming genetically based resistance mechanisms to PD-1 blockade. Cancer Discovery. (2020) 10:1140–57. doi: 10.1158/2159-8290.Cd-19-1409
83. Benitez R, Godelaine D, Lopez-Nevot MA, Brasseur F, Jiménez P, Marchand M, et al. Mutations of the beta2-microglobulin gene result in a lack of HLA class I molecules on melanoma cells of two patients immunized with MAGE peptides. Tissue Antigens. (1998) 52:520–9. doi: 10.1111/j.1399-0039.1998.tb03082.x
84. Lhotakova K, Grzelak A, Polakova I, Vackova J, Smahel M. Establishment and characterization of a mouse tumor cell line with irreversible downregulation of MHC class I molecules. Oncol Rep. (2019) 42:2826–35. doi: 10.3892/or.2019.7356
85. Cao F, Yan M, Liu Y, Liu L, Ma G. Photothermally controlled MHC class I restricted CD8(+) T-cell responses elicited by hyaluronic acid decorated gold nanoparticles as a vaccine for cancer immunotherapy. Adv Healthc Mater. (2018) 7:e1701439. doi: 10.1002/adhm.201701439
86. Del Campo AB, Aptsiauri N, Méndez R, Zinchenko S, Vales A, Paschen A, et al. Efficient recovery of HLA class I expression in human tumor cells after beta2-microglobulin gene transfer using adenoviral vector: implications for cancer immunotherapy. Scand J Immunol. (2009) 70:125–35. doi: 10.1111/j.1365-3083.2009.02276.x
87. Ott PA, Hu-Lieskovan S, Chmielowski B, Govindan R, Naing A, Bhardwaj N, et al. A phase ib trial of personalized neoantigen therapy plus anti-PD-1 in patients with advanced melanoma, non-small cell lung cancer, or bladder cancer. Cell. (2020) 183:347–362.e24. doi: 10.1016/j.cell.2020.08.053
88. Feins S, Kong W, Williams EF, Milone MC, Fraietta JA. An introduction to chimeric antigen receptor (CAR) T-cell immunotherapy for human cancer. Am J Hematol. (2019) 94:S3–s9. doi: 10.1002/ajh.25418
89. Chen X, Tan B, Xing H, Zhao X, Ping Y, Zhang Z, et al. Allogeneic CAR-T cells with of HLA-A/B and TRAC disruption exhibit promising antitumor capacity against B cell Malignancies. Cancer Immunol Immunother. (2024) 73:13. doi: 10.1007/s00262-023-03586-1
90. Guo Y, Tong C, Su L, Zhang W, Jia H, Liu Y, et al. CRISPR/Cas9 genome-edited universal CAR T cells in patients with relapsed/refractory lymphoma. Blood Adv. (2022) 6:2695–9. doi: 10.1182/bloodadvances.2021006232
91. Kagoya Y, Guo T, Yeung B, Saso K, Anczurowski M, Wang CH, et al. Genetic ablation of HLA class I, class II, and the T-cell receptor enables allogeneic T cells to be used for adoptive T-cell therapy. Cancer Immunol Res. (2020) 8:926–36. doi: 10.1158/2326-6066.Cir-18-0508
92. Liu X, Zhang Y, Cheng C, Cheng AW, Zhang X, Li N, et al. CRISPR-Cas9-mediated multiplex gene editing in CAR-T cells. Cell Res. (2017) 27:154–7. doi: 10.1038/cr.2016.142
93. Pavlovic K, Carmona-Luque M, Corsi GI, Maldonado-Pérez N, Molina-Estevez FJ, Peralbo-Santaella E, et al. Generating universal anti-CD19 CAR T cells with a defined memory phenotype by CRISPR/Cas9 editing and safety evaluation of the transcriptome. Front Immunol. (2024) 15:1401683. doi: 10.3389/fimmu.2024.1401683
94. Ren J, Liu X, Fang C, Jiang S, June CH, Zhao Y. Multiplex genome editing to generate universal CAR T cells resistant to PD1 inhibition. Clin Cancer Res. (2017) 23:2255–66. doi: 10.1158/1078-0432.Ccr-16-1300
95. Torikai H, Reik A, Liu PQ, Zhou Y, Zhang L, Maiti S, et al. A foundation for universal T-cell based immunotherapy: T cells engineered to express a CD19-specific chimeric-antigen-receptor and eliminate expression of endogenous TCR. Blood. (2012) 119:5697–705. doi: 10.1182/blood-2012-01-405365
96. Eyquem J, Mansilla-Soto J, Giavridis T, van der Stegen SJ, Hamieh M, Cunanan KM, et al. Targeting a CAR to the TRAC locus with CRISPR/Cas9 enhances tumour rejection. Nature. (2017) 543:113–7. doi: 10.1038/nature21405
97. Choi BD, Yu X, Castano AP, Darr H, Henderson DB, Bouffard AA, et al. CRISPR-Cas9 disruption of PD-1 enhances activity of universal EGFRvIII CAR T cells in a preclinical model of human glioblastoma. J Immunother Cancer. (2019) 7:304. doi: 10.1186/s40425-019-0806-7
98. Depil S, Duchateau P, Grupp SA, Mufti G, Poirot L. ‘Off-the-shelf’ allogeneic CAR T cells: development and challenges. Nat Rev Drug Discovery. (2020) 19:185–99. doi: 10.1038/s41573-019-0051-2
99. Datar IJ, Hauc SC, Desai S, Gianino N, Henick B, Liu Y, et al. Spatial analysis and clinical significance of HLA class-I and class-II subunit expression in non-small cell lung cancer. Clin Cancer Res. (2021) 27:2837–47. doi: 10.1158/1078-0432.Ccr-20-3655
100. Eugène J, Jouand N, Ducoin K, Dansette D, Oger R, Deleine C, et al. The inhibitory receptor CD94/NKG2A on CD8(+) tumor-infiltrating lymphocytes in colorectal cancer: a promising new druggable immune checkpoint in the context of HLAE/β2m overexpression. Mod Pathol. (2020) 33:468–82. doi: 10.1038/s41379-019-0322-9
101. Wang X, Schoenhals JE, Li A, Valdecanas DR, Ye H, Zang F, et al. Suppression of type I IFN signaling in tumors mediates resistance to anti-PD-1 treatment that can be overcome by radiotherapy. Cancer Res. (2017) 77:839–50. doi: 10.1158/0008-5472.Can-15-3142
102. Zebertavage LK, Alice A, Crittenden MR, Gough MJ. Transcriptional upregulation of NLRC5 by radiation drives STING- and interferon-independent MHC-I expression on cancer cells and T cell cytotoxicity. Sci Rep. (2020) 10:7376. doi: 10.1038/s41598-020-64408-3
103. Murty S, Haile ST, Beinat C, Aalipour A, Alam IS, Murty T, et al. Intravital imaging reveals synergistic effect of CAR T-cells and radiation therapy in a preclinical immunocompetent glioblastoma model. Oncoimmunology. (2020) 9:1757360. doi: 10.1080/2162402x.2020.1757360
104. Li JX, Su TS, Gong WF, Zhong JH, Yan LY, Zhang J, et al. Combining stereotactic body radiotherapy with camrelizumab for unresectable hepatocellular carcinoma: a single-arm trial. Hepatol Int. (2022) 16:1179–87. doi: 10.1007/s12072-022-10396-7
105. Routy B, Lenehan JG, Miller WH Jr., Jamal R, Messaoudene M, Daisley BA, et al. Fecal microbiota transplantation plus anti-PD-1 immunotherapy in advanced melanoma: a phase I trial. Nat Med. (2023) 29:2121–32. doi: 10.1038/s41591-023-02453-x
106. Gopalakrishnan V, Spencer CN, Nezi L, Reuben A, Andrews MC, Karpinets TV, et al. Gut microbiome modulates response to anti-PD-1 immunotherapy in melanoma patients. Science. (2018) 359:97–103. doi: 10.1126/science.aan4236
Keywords: B2M, cancer immunotherapy, immune resistance mechanism, expression regulation, reverse resistance strategy
Citation: Han X, Zhang J, Li W, Huang X, Wang X, Wang B, Gao L and Chen H (2025) The role of B2M in cancer immunotherapy resistance: function, resistance mechanism, and reversal strategies. Front. Immunol. 16:1512509. doi: 10.3389/fimmu.2025.1512509
Received: 16 October 2024; Accepted: 03 March 2025;
Published: 21 March 2025.
Edited by:
Elio Gregory Pizzutilo, Grande Ospedale Metropolitano Niguarrda, ItalyReviewed by:
Marcin Cieslik, University of Michigan, United StatesMahnoor Gondal, University of Michigan, Ann Arbor, United States in collaboration with reviewer MC
Weiwei Yu, Yale University, United States
Copyright © 2025 Han, Zhang, Li, Huang, Wang, Wang, Gao and Chen. This is an open-access article distributed under the terms of the Creative Commons Attribution License (CC BY). The use, distribution or reproduction in other forums is permitted, provided the original author(s) and the copyright owner(s) are credited and that the original publication in this journal is cited, in accordance with accepted academic practice. No use, distribution or reproduction is permitted which does not comply with these terms.
*Correspondence: Hao Chen, ZXJ5X2NoZW5oQGx6dS5lZHUuY24=
†These authors have contributed equally to this work