- 1Department of Endoscopy Center, China-Japan Union Hospital of Jilin University, Changchun, China
- 2Department of Medical Imaging, The Third Affiliated Hospital of Changchun University of Chinese Medicine, Changchun, China
- 3Department of Immunology, College of Basic Medical Sciences, Jilin University, Changchun, China
The gut microbiota is a diverse ecosystem that significantly impacts human health and disease. This article focuses on how the gut microbiota interacts with inflammatory bowel diseases and colorectal tumors, especially through immune regulation. The gut microbiota plays a role in immune system development and regulation, while the body’s immune status can also affect the composition of the microbiota. These microorganisms exert pathogenic effects or correct disease states in gastrointestinal diseases through the actions of toxins and secretions, inhibition of immune responses, DNA damage, regulation of gene expression, and protein synthesis. The microbiota and its metabolites are essential in the development and progression of inflammatory bowel diseases and colorectal tumors. The complexity and bidirectionality of this connection with tumors and inflammation might render it a new therapeutic target. Hence, we explore therapeutic strategies for the gut microbiota, highlighting the potential of probiotics and fecal microbiota transplantation to restore or adjust the microbial community. Additionally, we address the challenges and future research directions in this area concerning inflammatory bowel diseases and colorectal tumors.
1 Introduction
The human body harbors a diverse range of microorganisms, encompassing bacteria, viruses, fungi, protozoa and so on, which make up about 1-3% of body weight. The intestinal microbiota is particularly rich, with about 1000 species, consisting mainly of bacteria (1). The number of intestinal microbiota is about equal to the number of human cells in the body, and they encode about 100 times more genes than the body’s own genes (2, 3). As the “second genome”, Intestinal microorganisms fulfill various functions, such as enhancing the host’s immune system, assisting in digestion, regulating intestinal hormone and nerve signals, influencing drug metabolism, detoxifying harmful substances, and affecting the production of metabolic compounds in the host (4). Increasing evidence shows that the gut microbiome directly influences immune regulation, supports the development of the immune system, and helps maintain its normal function. Conversely, the immune status of the body can also affect the composition of the gut microbiota. Thus, the microbiome plays a crucial role in both health and disease (5). Many studies have revealed a strong link between gut microbiota dysbiosis and gastrointestinal diseases, malignant tumors, cardiovascular diseases, neurological disorders, and other disorders in humans, of which tumors are one of the most studied diseases (6–12). The effect of gut microbes on immune function, including immunosuppression and activation of inflammation, has the most direct impact on GI diseases, the most prominent types of which are inflammation and neoplasms, because gut microbes colonize the intestinal tract.
Inflammatory bowel disease (IBD) is a gastrointestinal condition characterized by chronic inflammation, manifested by disorders of mucosal structure, altered intestinal microbial composition, and systemic biochemical abnormalities, and includes ulcerative colitis (UC) and Crohn’s disease (CD) (13). IBD can develop at any age, and is generally common in adolescents at the first onset of disease. Its incidence continues to rise globally, with a growing number of children and elderly individuals (14).The causes and pathogenesis of IBD remain not completely understood, and abnormalities in immunoregulation play a crucial role in the onset of IBD. Many immunosuppressants, biological agents and small molecule substances have been applied to the clinic and have achieved good efficacy in the treatment of IBD and its related complications. Patients with IBD face an increased risk of developing Colorectal cancer (CRC), called colitis-associated cancer, compared to normal subjects (15). Beneficial gut bacteria can exert immunosuppressive effects by modulating host immune cells, while harmful bacteria induce inflammatory cytokines through interactions with immune cells or their metabolites, leading to intestinal damage (16, 17). There are a number of bacteria that can act on both IBD and tumors together, such as a relative increase in Escherichia coli (E. coli) in the patient’s intestinal microbiota, and the abundance of Bacteroides fragilis (Bf) is strongly linked with both active IBD and CRC (18–20). With the substantial development of IBD genetic susceptibility and gut microecology research, future treatments may favor individual precision therapy by targeting the microbiota in order to improve patient symptoms and further enhance quality of life.
The long-term chronic inflammatory response in the intestine is associated with the occurrence of cancer. CRC is a common gastrointestinal tumor and the second leading cause of cancer-related deaths worldwide. Recent years have seen a rise in CRC incidence across various countries, and the incidence and mortality rates vary significantly around the world. According to the National Cancer Center of China, CRC ranks as the second most prevalent cancer in men and the fourth in women (21). As tumor immunology advances quickly, people have gained a more in-depth understanding of the body’s anti-tumor immune response pathway and the immune escape mechanism of tumors, and significant breakthroughs have been made in immunotherapy for tumors, but all of them have certain limitations. Most tumors are insensitive to the various existing immunotherapies, indicating that they have a strong immune tolerance mechanism. Coupled with the high degree of heterogeneity of tumors among patients, it is often difficult for existing single therapies to produce satisfactory results in clinical applications. Current metabolomics and macrogenomics research highlights the gastrointestinal microbiome’s dual role in preventing cancer, promoting tumor development, and influencing the effectiveness of anticancer treatments (22). Some bacteria in the gut microbiome exert pro-cancer effects, while others have tumor suppressor properties (23). Therefore, if the components of gut microbes can be artificially altered, they may not only serve as therapeutic targets, but also exert immunomodulatory functions.
Targeting gut microbes for the treatment of intestinal diseases has a promising future, and we summarize the current mechanisms of gut microbes in IBD and CRC, as well as the present state of targeting gut microbes for managing these diseases.
2 Gut microbiota and inflammatory bowel disease
IBD is a chronic, recurrent inflammatory disorder of the digestive tract linked to autoimmune processes. The composition and diversity of the intestinal microbiota are intimately linked to the onset and progression of IBD (24).
2.1 Anti-inflammatory effects of gut microbiota
In the human gut microbiota, Firmicutes (F) and Bacteroidetes (B) constitute approximately 90% of the total bacterial population (25). IBD patients frequently demonstrate a decline in the F/B ratio, and studies have indicated that the diversity of Firmicutes in the intestinal microbiota of IBD individuals reduces, characterized by a significant decline in anaerobic bacteria of the class Clostridia, particularly the commensal bacterium Faecalibacterium prausnitzii (F. prausnitzii) (26–30). F. prausnitzii is capable of generating butyrate, which suppresses HDAC1, stimulates Foxp3, and blocks the downstream IL-6/STAT3/IL-17 pathway, maintaining the T helper 17 cell/regulatory T cell (Th17/Treg) balance and exerting substantial anti-inflammatory effects (31). Additionally, the abundance of Phascolarctobacterium is significantly decreased, and Phascolarctobacterium, when co-cultured with Paraprevotella, consumes succinate and produces the anti-inflammatory SCFA propionate (32, 33). The reduction of SCFA-producing Phascolarctobacterium in IBD patients implies that the anti-inflammatory impact of SCFA is potentially weakened, potentially exacerbating IBD symptoms (34). There are also some anti-inflammatory bacterial groups, such as non-toxigenic Bacteroides fragilis (NTBF), whose symbiotic factor polysaccharide A (PSA) can stimulate CD4+ T cells and induce the anti-inflammatory effects of Tregs through Toll-like receptor 2 (TLR2), and inhibiting Th17, promoting immune tolerance (Figure 1) (35). Tan et al. confirmed that new strains of Bf HCK-B3 and Bacteroides ovatus ELH-B2 can alleviate LPS-induced inflammation by regulating cytokine production or restoring the Treg/Th-17 balance (36).
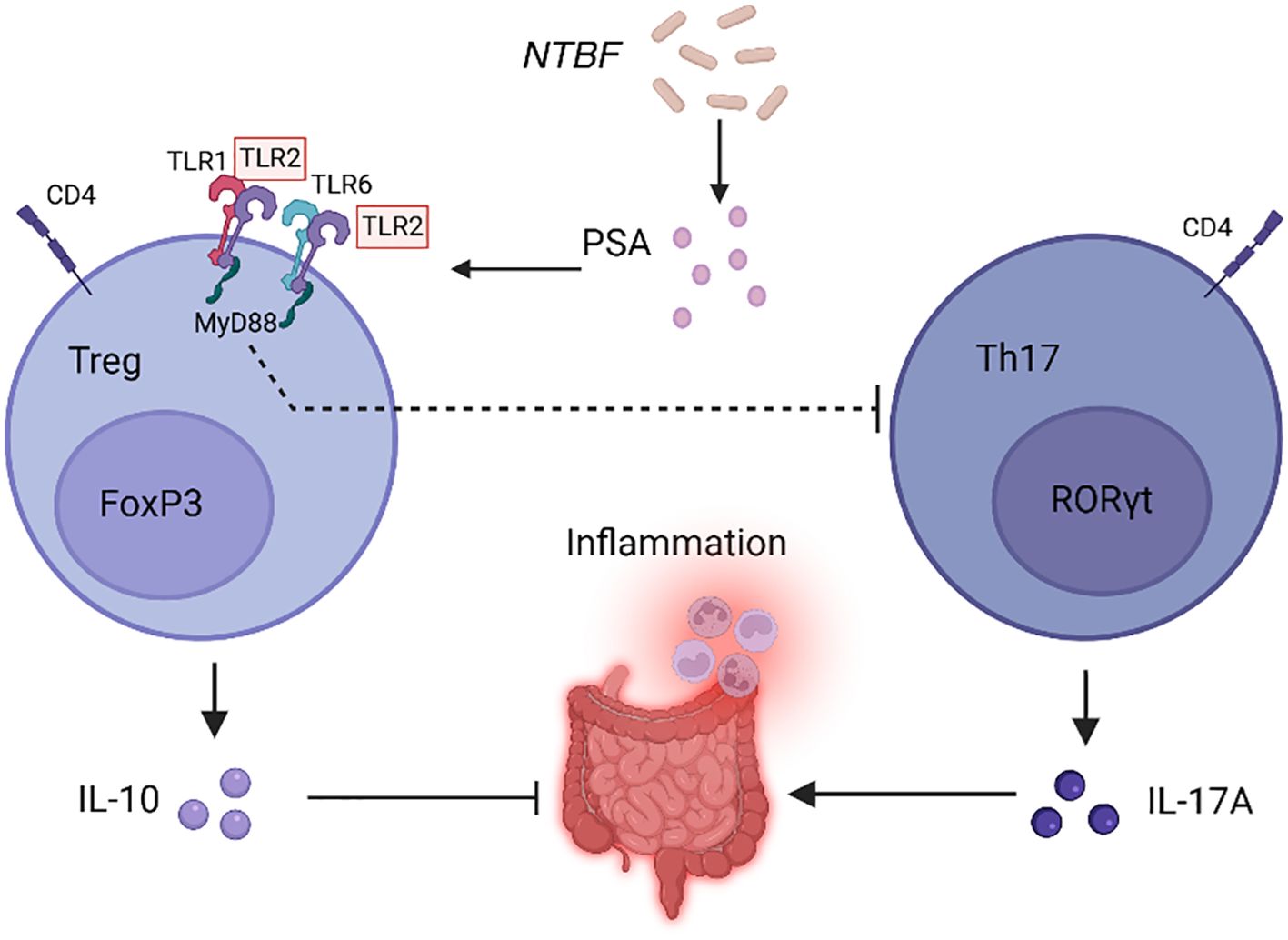
Figure 1. NTBF promotes immunologic tolerance. NTBF releases PSA through outer membrane vesicles, promoting the production of IL-10 through TLR2 signaling on CD4 Foxp3 Tregs, and inhibiting RORγt Th17, reducing the production of IL-17A, thereby promoting immune tolerance. (Foxp3, Forkhead Box P3, a key transcription factor for Treg, which is usually used as the marker for Treg. RORγt, retinoic acid related orphan receptor γt, a key transcription factor for the differentiation and function of Th17).
2.2 Pro-inflammatory effects of gut microbiota
However, certain microbial groups can facilitate inflammatory responses in IBD. Adherent-invasive E. coli can induce epithelial mitochondrial fission, influencing intestinal permeability (37). Enterococcus faecalis (Efa) can enhance colonic cytokine expression and cause colitis (38). ETBF and its secreted zinc-dependent metalloprotease toxin, B.fragilis toxin (BFT), triggers Stat3 activation and Th17 immune responses, promoting mucosal permeability (39, 40). Additionally, Ha et al. discovered that Clostridium innocuum is abundant in the ‘creeping fat’ (CrF) of severe CD individuals, and this bacterial group can stimulate tissue remodeling, resulting in the formation of adipose tissue barriers (41). Henke et al. reported that Ruminococcus gnavus secretes a Toll-like receptor 4 (TLR4)-dependent glucorhamnan polysaccharide, effectively inducing dendritic cells to secrete inflammatory cytokines (TNF-α), thereby facilitating CD inflammatory responses (42).
An investigation on gut microbiota characteristics revealed that within the dataset of genera related to IBD, the number of genera related to CD exceeds that related to UC (43). Additionally, in contrast to healthy individuals, the α-diversity of IBD is diminished, with a more pronounced reduction observed in CD; the microbial community composition (β-diversity) undergoes changes in UC, while the alterations in β-diversity are more conspicuous in CD (Figure 2) (43). Pascal et al. discovered that dysbiosis is notably more pronounced in CD individuals compared to those with UC, manifested as lower diversity and more unstable microbial communities (44). In CD patients, the levels of Actinomyces, Veillonella, and E.coli rose, while the enrichment of Christensenellaceae, Coriobacteriaceae, and particularly Clostridium leptum declined. Similarly, the level of E.coli also rose in UC patients, while the levels of Eubacterium rectale and Akkermansia decreased (45). Intestinibacter abundance increased in both CD and UC, whereas Efa abundance markedly decreased in CD (46).
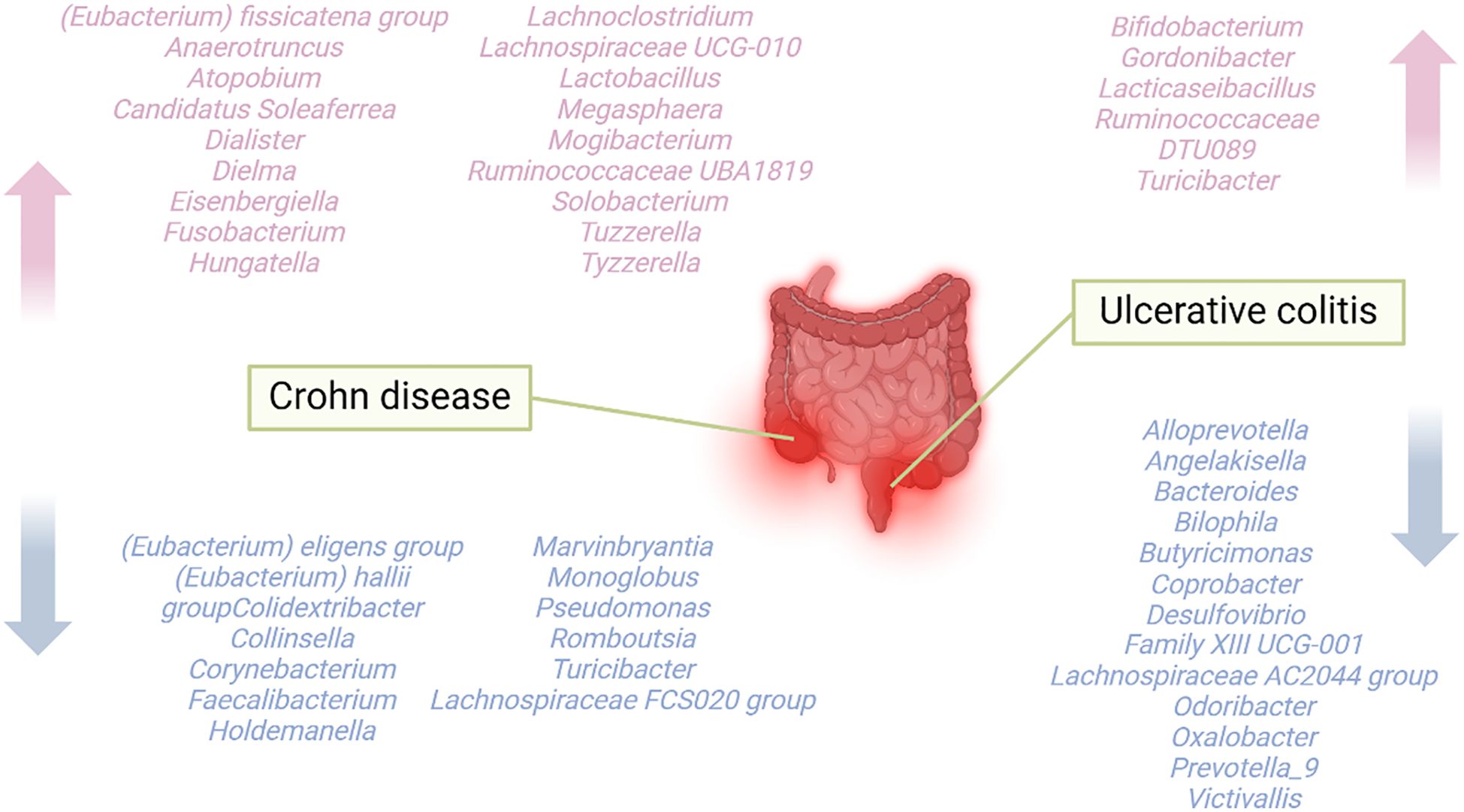
Figure 2. Overview of genera associated with CD or UC. To classify genera based only on their abundance being higher or lower in CD or UC compared to healthy individuals.
2.3 Microbiome therapy in inflammatory bowel disease
Adjusting ecological dysbiosis, using fecal microbiota transplantation (FMT), and supplementing with probiotics, especially butyrate-producing bacteria, can help improve IBD (47). As a research hotspot, FMT therapy is capable of restoring the intestinal mucosal immune homeostasis in IBD patients. The first utilization of FMT in UC patients was documented in 1989 (48).
2.3.1 FMT
FMT is suitable for moderate to severe IBD, particularly for active IBD accompanied by concurrent refractory or recurrent Clostridium difficile infection. Patients might demonstrate more prominent microbiota alterations and generate certain clinical effects. Studies have indicated that a single FMT is relatively safe (49, 50). Nevertheless, the alterations in the intestinal microbiota of the majority of patients are temporary. Chu et al.’s research implies that FMT is a complex mixture of biological entities interacting with the recipient’s microbiota and immune system. By monitoring the recipient’s microbiota, it was discovered that 10 weeks after receiving the treatment, the microbiota had significantly deviated from the donor bacteria (51). Hence, attention ought to be paid to the colonization dynamics of the gut microbiota during FMT treatment, and it is also requisite to conduct repetitive transplantation periodically to maintain the modified microbiota. Research has shown that multi-stage FMT can restore the recipient’s microbiota and induce remission in patients with active UC. This therapeutic approach leads to substantial alterations to the microbiota composition, encompassing an increase in the abundance of Firmicutes both during the FMT period and up to six months afterwards (52). Moayyedi et al. believe that intensified dosing and multi-donor FMT can effectively achieve both clinical and endoscopic remission in patients (53).
Genetic and physiological factors related to both the donor and the recipient will have an impact on the therapeutic effect. Liu et al. emphasized the significance of considering the activity of the gut microbiota in donor samples for FMT. A detailed analysis of the active microbiota is crucial for understanding the mechanisms behind the therapeutic effects of FMT (54). Angelberger et al. identified microbial types associated with disease severity and FMT treatment success, especially related to the enrichment of Enterobacteriaceae and the deficiency of Lachnospiraceae (55). This subset of UC patients is more prone to be an effective subgroup for FMT treatment. Additionally, research has revealed that triple antibiotics (amoxicillin, fosfomycin, and metronidazole [AFM]) can synergize with FMT. Pretreatment with AFM to eliminate the recipient’s dysbiotic Bacteroidetes might facilitate the colonization of the donor’s Bacteroidetes bacteria during FMT, thereby alleviating the intestinal microbiota dysbiosis in UC patients caused by the loss of diversity among Bacteroidetes species (56). Regarding the short-term safety of FMT in IBD, multiple meta-analyses have shown no significant differences compared to the placebo group, with most adverse reactions being mild symptoms such as gastrointestinal discomfort and fever, and a low incidence of severe adverse events (57, 58). There is limited literature on long-term safety. One study mentioned that patients receiving FMT experienced certain adverse reactions in the long term, including rashes and myasthenia gravis (59). Additionally, another study reported that these patients had a comparable risk of developing severe diseases such as autoimmune disorders or tumors compared to the antibiotic group (60). In the future, further improvements in the preparation and delivery methods of fecal microbiota are needed to effectively manage these risks and enhance the safety and efficacy of FMT.
2.3.2 Probiotic
VSL#3 is a probiotic mixture, and several clinical studies have indicated that it can effectively alleviate IBD symptoms (61, 62). VSL#3 encompasses eight types of bacteria, namely Bifidobacterium breve, Bifidobacterium longum(B. longum), Bifidobacterium infantis, Lactobacillus acidophilus, Lactobacillus plantarum, Lactobacillus paracasei, Lactobacillus delbrueckii, and Streptococcus thermophilus (63), which can lower the level of colonic mucosal inflammation by enhancing the intestinal environment, reshaping the microbial composition, and regulating specific bacterial levels, thereby treating or alleviating IBD symptoms (64, 65). Other literature reports that Lactobacillus GG, B. longum/Synergy 1, and Bifidobacteria-fermented milk can effectively ameliorate intestinal inflammation in UC individuals, with the role in maintaining remission (66).
E. coli Nissle 1917 (EcN) is the active strain of the microbial drug Mutaflor®, a non-pathogenic Gram-negative bacterium that is able to inhibit the growth of Salmonella and other pathogenic bacteria. It is the sole probiotic recommended in the ECCO guidelines for UC patients, and its efficacy in maintaining disease remission is comparable to that of mesalazine. The fimbriae F1C of EcN enables it to persistently colonize the intestinal epithelium and directly stimulate cells to produce defensins, preventing pathogens from adhering to and invading intestinal cells. It can also interact with the immune system, decreasing pro-inflammatory cytokines like IL-2, TNF-α, and IFN-γ, while elevating anti-inflammatory cytokines (67).
Clostridium butyricum (CB) is a probiotic utilized clinically for functional gastrointestinal disorders, and the butyrate it produces can ameliorate intestinal mucosal inflammation. Hayashi et al. reported that it can effectively induce colonic mucosal macrophages to generate the anti-inflammatory cytokine IL-10 through TLR2/MyD88 pathway, thereby suppressing intestinal inflammation in a mouse IBD model (68).
2.3.3 Diagnostics and personalized medicine
The onset of IBD can be difficult to predict, and invasive examinations such as colonoscopy are currently commonly used to determine the inflammatory activity of IBD. There is growing interest in diagnosing, monitoring, and treating the disease non-invasively based on the gut microbiota. The rapid progress in synthetic biology offers the possibility of targeted microbial engineering, enabling engineered microorganisms to diagnose IBD by selectively detecting biomarkers. A study has designed an engineered probiotic, EcN, which non-invasively monitors disease activity in patients by detecting the IBD biomarker calprotectin in feces (69). Both nitrate and thiosulfate are biomarkers of intestinal inflammation. Woo et al. introduced a nitrate-responsive genetic circuit into EcN, enabling the biosensor to detect thiosulfate and nitrate, thereby aiding in the diagnosis of colitis (70). Zou et al. developed a smart responsive bacterium (i-ROBOT) composed of EcN, which can monitor thiosulfate and drive the tunable release of the immunomodulator AvCystatin based on its fluctuations (71). By targeting specific microRNAs (miRNAs) in IBD through FMT based on the patient’s microbiome profile, these miRNAs can function in specific intestinal cells, reducing off-target effects and improving stability. This provides a new approach for developing personalized treatment strategies (72).
3 Gut microbiota and colorectal cancer
Chronic inflammation promotes the occurrence and development of tumors and is one of the most common risk factors for cancer. The process by which intestinal inflammation progresses to CRC through dysplasia is faster than the classic adenoma-sequence seen in sporadic CRC (73). IBD patients often exhibit the expression of inflammatory genes and excessive infiltration of inflammatory cells. This mucosal inflammation promotes cell proliferation and ultimately contributes to the development of CRC. Individuals with long-term IBD have a 2–3 times higher risk of developing CRC compared to the general population. The three most common theories regarding the development of CRC from IBD under microbial induction include the alpha-bug hypothesis, driver-passenger hypothesis, and common ground hypothesis (74). Based on genomic mutation diversity, CRC can be categorized into two main types: colitis-associated colorectal cancer (CAC) and sporadic colorectal cancer (SCC). The gut constitutes a complex environment populated by bacteria, fungi, and viruses, with an overall count potentially reaching 100 trillion. The quantity of microbial cells in the gut is projected to be around ten times that of human cells (75). Colorectal cancer can affect the makeup of the intestinal microbiota and may also be affected by the gut microbiota and their secretions. Weisburger and his colleagues released the first report associating the intestinal microbiota with CRC (76). Subsequently, an expanding body of research has confirmed the link between pathogenic bacteria and CRC.
3.1 Pro-carcinogenic effects of gut microbiota
Compared with healthy individuals, the gut microbiota composition of CRC individuals has experienced substantial transformations, featuring enrichment of Firmicutes and Proteobacteria (77). Certain specific bacterial species have also been found to be related to the occurrence and development of CRC, including Fusobacterium nucleatum, Escherichia coli, Enterococcus faecalis, Streptococcus gallolyticus, and Bacteroides fragilis (78, 79). These microorganisms can generate toxins and metabolites that regulate the proliferation and invasion of tumor cells, or contribute to the occurrence and progression of CRC by inhibiting immune responses, promoting the expression of oncogenes, and other means (Table 1).
3.1.1 Fusobacterium nucleatum
Research shows that Fusobacterium nucleatum (Fn) is highly prevalent in CRC, with its abundance in cancerous tissues being over 400 times greater than in adjacent normal tissues (104, 105). The surface adhesion protein FadA, expressed by Fn, is a cell surface virulence factor and a crucial component in regulating bacterial adhesion and invasion (106). FadA attaches to cadherin on intestinal epithelial cells, activating the E-cadherin/β-catenin pathway, which affects cyclin D1, directly influencing the proliferation and growth of epithelial cells and promoting inflammatory responses and tumor formation. The FadA binding site on E-cadherin consists of an 11-amino acid stretch, and a peptide synthesized from this region can eliminate the carcinogenic effects induced by FadA. The expression levels of the FadA gene in the colon tissues of individuals with adenomas and adenocarcinomas are 10 to 100 times greater compared to those in normal individuals (80). The metabolic product of Fn, formate, has been shown to induce tumorigenesis and enhance tumor stemness, promoting glutamine metabolism and driving colorectal cancer progression through the AhR signaling pathway (81). Additionally, research has revealed that short-chain fatty acids (SCFAs) produced by Fn can regulate Th17 responses in an FFAR2-dependent manner, suppressing anti-tumor immune cells and facilitating tumor angiogenesis (82).
3.1.2 Colibactin-producing E.coli
Colibactin-producing E.coli (CoPEC) is strongly associated with CRC (107). One study found that mucosa-associated and internalized E. coli levels are higher in tumors than in normal tissues, and E.coli colonization in the mucosa is linked to a poorer prognosis in CRC (19). E. coli from the B2 phylogenetic group carries a genomic island, the polyketide synthase (pks), which produces the genotoxin colibactin. This toxin can induce DNA damage, cell cycle arrest, mutations, and chromosomal instability in eukaryotic cells. Iftekhar et al.’s research confirmed the in vitro transforming potential of pks+ E.coli, which can undergo malignant transformation by infecting colonoid organoids, causing DNA double-strand breaks, mutations, and promoting tumor development. This emphasizes the relevance of Wnt-independent CNV mutations as early driving factors in colorectal cancer, indicating a clear connection between the mutagenic characteristics of colibactin and its action and the occurrence of colorectal cancer. Additionally, the actual transforming activity of colibactin is likely higher than the estimated activity (90).
3.1.3 Enterococcus faecalis
Efa, similar to E. coli, can contribute to carcinogenesis by damaging the colonic epithelium, augmenting bacterial colonization on the mucosal surface, and facilitating epithelial cell renewal (108, 109). Huycke et al. discovered that Efa generates extracellular superoxide and hydrogen peroxide, leading to DNA damage. These extracellular free radicals are correlated with the advancement of adenomatous polyposis and colorectal cancer (92). Furthermore, its metabolic product biliverdin can modulate the PI3K/AKT/mTOR signaling pathway, prominently elevating the expression levels of IL-8 and VEGFA, fostering tumor cell proliferation and angiogenesis (93).
3.1.4 Streptococcus gallolyticus subspecies gallolyticus
Streptococcus gallolyticus subspecies gallolyticus (Sgg), recognized as Streptococcus bovis biotype I, employs Pil3 pili to adhere to colonic mucus. Literature reports suggest that the abundance of Sgg is significantly heightened in colorectal cancer patients. Sgg can enlist CD11b+TLR4+ cells, promoting the expression of IL-8, COX-2, and IL-1, and escalating the levels of tumor progression-related transcription factors like β-catenin, c-Myc, and PCNA, thereby driving inflammation and facilitating tumor proliferation (95–98). It also possesses the capacity to stimulate CYP1A enzyme activity through an AhR-dependent mechanism, regulating the biotransformation pathways of colonic epithelial cells and further promoting tumor progression (99). On the contrary, the specific environmental conditions of elevated bile acid concentrations in colorectal cancer patients can facilitate Sgg colonization, with secondary bile acids significantly enhancing the activity of gallolysin (110).
3.1.5 Bacteroides fragilis
Bf is another commensal bacterium, which is classified into toxigenic and non-toxigenic types. Research has demonstrated that the existence of enterotoxigenic B. fragilis (ETBF) is strongly linked to active IBD and CRC (Figure 3). The toxin BFT can stimulate c-myc expression and IL-8 release, resulting in DNA oxidation and epithelial barrier damage, and activating STAT3/Th17 immune responses (39, 111, 112). It can also activate NF-κB and upregulate COX-2 in intestinal epithelial cells, generating an inflammatory environment (100). The co-colonization of ETBF and enterotoxigenic E.coli in mice enhances the production of the inflammatory factor IL-17 and causes DNA damage, thereby accelerating the progression of CRC (113). ETBF can upregulate the level of JMJD2B in the TLR4-NFAT5-dependent pathway, inducing the regulation of CRC stemness (101). By reducing miR-149-3p, it additionally promotes the PHF5A-mediated RNA alternative splicing of KAT2A in CRC cells, thereby facilitating intestinal inflammation and tumors (102).
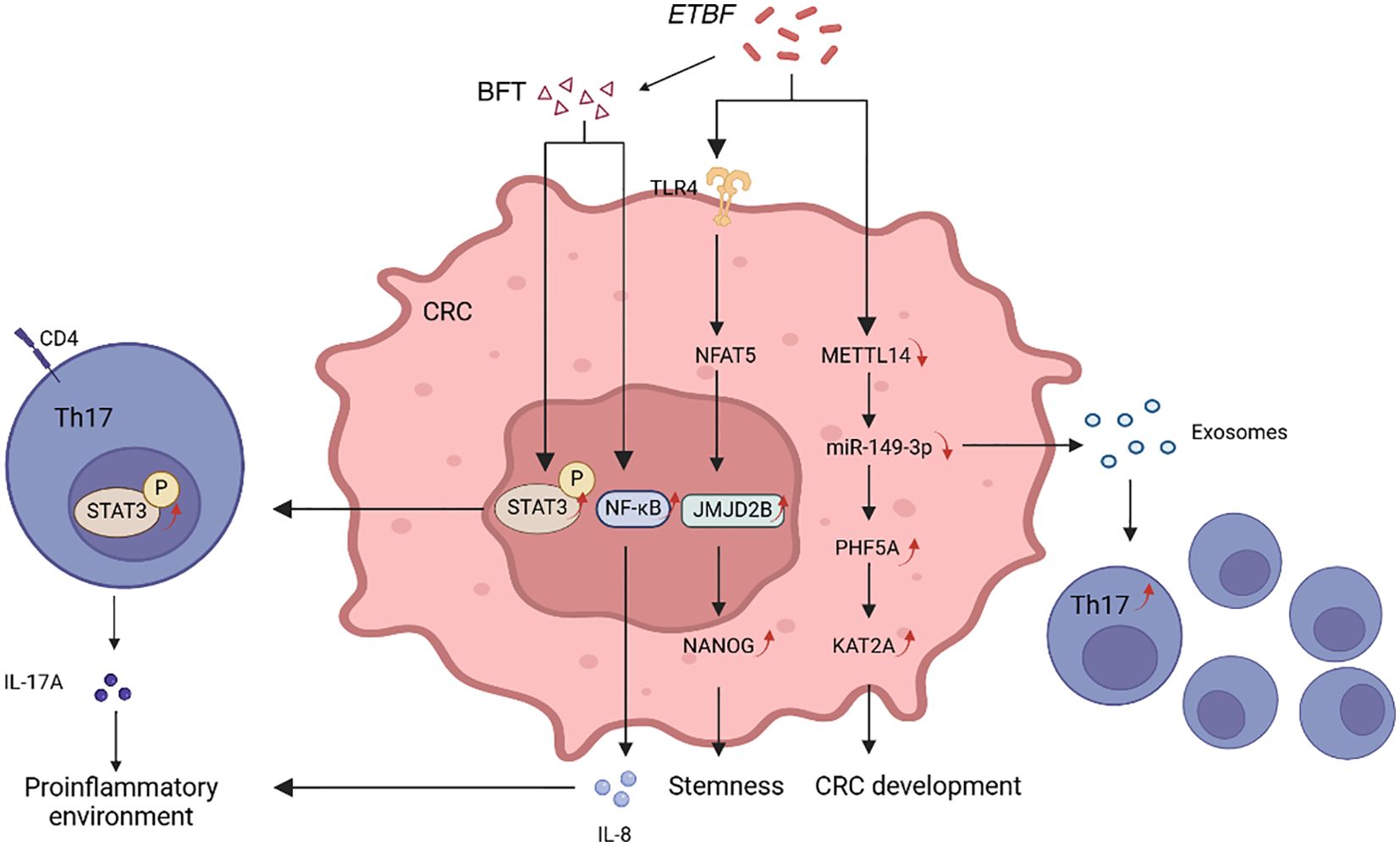
Figure 3. ETBF promotes tumorigenesis by distinct mechanisms. BFT can activate the STAT3 and NF-κB pathways, increasing the production of cytokines IL-17A and IL-8, thereby creating a pro-inflammatory environment. ETBF can upregulate JMJD2B levels in the TLR4-NFAT5-dependent pathway. JMJD2B regulates the stemness of tumor cells by enhancing NANOG expression through demethylation. ETBF can also promote cancer progression by downregulating miR-149-3p through METTL14 methylation. On one hand, it facilitates the differentiation of Th17 cells via exosomes; on the other hand, it further promotes the RNA selective splicing of KAT2A mediated by PHF5A.
3.2 Anti-carcinogenic effects of gut microbiota
However, the connection between the gut microbiota and the host’s immune balance is intricate, and not all microbiota exert a cancer-promoting effect. The metabolic products of certain gut microbial groups also possess the capability to influence the anti-tumor activity of immune cells.
3.2.1 Lactic acid bacteria
Certain beneficial bacteria that contribute to maintaining the ecological balance of the gut are capable of suppressing the growth of opportunistic pathogens, enhancing the barrier function of the intestinal epithelium, and typically exerting a protective role in cancer prevention. Lactic acid bacteria, among the most common beneficial microbes, produce organic acids that have antibacterial effects. Additionally, Lactococcus lactis and Streptococcus lactis secrete nisin, a bacteriocin that blocks the growth of most Gram-positive bacteria and their spores (114). This also implies that the role of the same bacterial group in tumors located in different parts of the digestive tract can be diametrically opposite.
3.2.2 Other SCFA-producing microbiota
As one of the main metabolic products, SCFAs are essential in suppressing intestinal inflammation and serve as key protective factors in preserving the normal immune function of the intestinal mucosa, which can to some extent counteract tumor growth. Luu et al.’s research initially experimentally demonstrated that microbial metabolites like valproic acid and butyric acid boost immune cell anti-tumor activity through metabolic and epigenetic changes. Treating cytotoxic T lymphocytes (CTLs) and chimeric antigen receptor (CAR) T cells with valproic acid and butyric acid in vitro enhances mTOR function as a key metabolic sensor and inhibits class I histone deacetylases. This leads to anti-cancer effects and supports their use in improving cancer immunotherapy (115). Kang et al. discovered that Roseburia intestinalis has tumor-suppressive effects on the MSI-high and MSS subtypes of CRC. This bacterium can directly bind the butyric acid it produces to Toll-like receptor 5 (TLR5) on CD8+ T cells, activating the TLR5-dependent NF-κB pathway to enhance the function of cytotoxic CD8+ T cells, thereby exerting anti-tumor effects and enhancing the efficacy of anti-PD-1 therapy (116).
In summary, the relationship between the gut microbiota and tumors is intricate and bidirectional. Tumor progression can modify the microbiota, and alterations in the microbiota can also influence tumor progression (117). The intestinal microbiota and its metabolites significantly influence the development and progression of CRC, and clarifying the functions and potential mechanisms of the microbiota is essential for delving into more effective treatment strategies for CRC.
3.3 Microbiome therapy in colorectal cancer
Modulating the intestinal microbiota is a potential strategy for treating colorectal cancer. Various methods can alter the gut microbiota composition, improve the intestinal environment, enhance the immune system, and inhibit tumor growth (Figure 4).
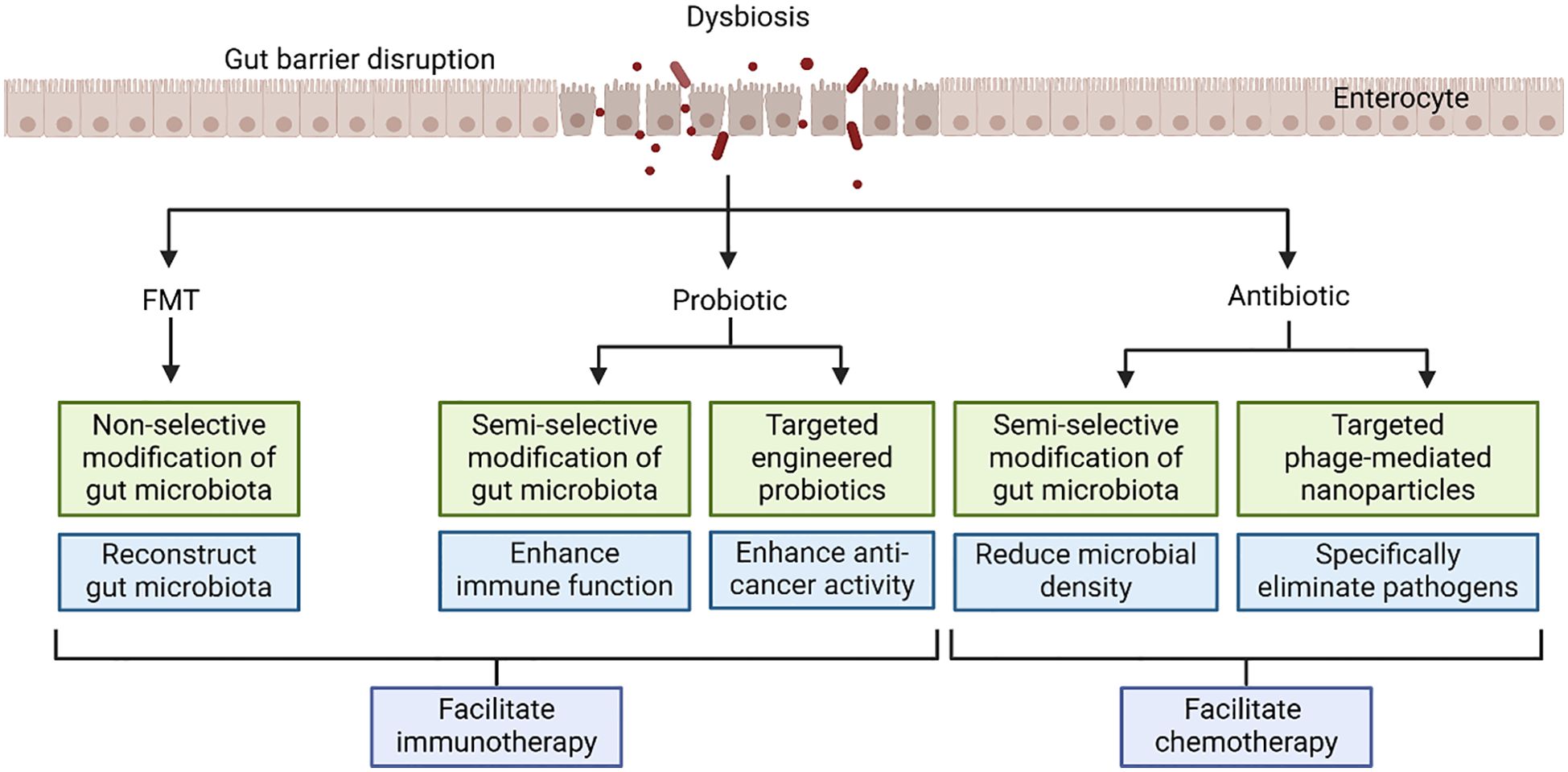
Figure 4. Microbiome therapies correct dysbiosis caused by colorectal cancer. Here are various methods listed from non-selective modification to targeted treatment, and they can enhance the efficacy of other colorectal tumor treatments.
3.3.1 FMT
FMT involves introducing beneficial microorganisms obtained from the feces of healthy donors into the digestive systems of patients. This procedure aims to restore the native microbial community, increase the proportion of regulatory T cells in the mucosa, and promote cooperation among microorganisms, ultimately helping to treat both intestinal and extra-intestinal diseases (118). FMT is FDA-approved for treating refractory Clostridium difficile infections and has a higher cure rate compared to standard therapies (119, 120). FMT preparations can be administered orally using freeze-dried or frozen granules, or through invasive procedures like colonoscopy or gastroscopy (121). FMT has been combined with immune checkpoint inhibitors (ICIs), and a clinical trial by Routy et al. showed that FMT can boost the efficacy of anti-PD-1 therapy (122). Currently, research is predominantly centered on patients with refractory melanoma, but it indicates the potential and safety of FMT in cancer therapy. Additionally, clinical trials are ongoing to assess the utilization of FMT capsules for enhancing the efficacy of anti-PD-1 therapy in gastrointestinal cancer patients (123). The success of FMT relies on both transplanting the microbiota into the recipient’s digestive tract and ensuring long-term colonization to sustain the therapeutic benefit (124). Furthermore, FMT still bears numerous risks, with a 19% incidence of adverse events related to FMT between 2000 and 2020, typically associated with gastrointestinal discomfort, and severe adverse events mainly occur in patients with mucosal barrier damage (125). Clinical research on FMT as an adjuvant therapy for cancer is limited; thus, it is of paramount importance to standardize its application to ensure its safety and long-term efficacy.
3.3.2 Probiotic
Probiotics, being beneficial microorganisms for the host’s health, can inhibit tumor cell proliferation by regulating the gut microbiota and immune response (126, 127). Compared with FMT, they notably reduce the risk of infection for patients. Commonly utilized probiotics such as Bifidobacterium and Lactobacillus have recognized effects in treating various gastrointestinal disorders (128). Research has indicated that Lactobacillus plantarum can suppress the proliferation of colon cancer cells through the cell cycle regulatory ability mediated by butyric acid (129). Many studies regarding the application of probiotics to treat cancer focus on enhancing immune function, which might assist in combating cancer (120). For instance, Bifidobacterium plays a role in strengthening anti-tumor immunity in anti-PD-L1 treatment (130). There are also investigations on engineered probiotics for targeted tumor therapy (131). The substrate of probiotics—prebiotics, has also been demonstrated to participate in tumor treatment. Fructo-oligosaccharides (FOS) and galacto-oligosaccharides (GOS) are two significant groups of prebiotics that selectively encourage the proliferation of beneficial probiotics. They increase the production of SCFAs, which lead to subtle modifications in the intestinal microbiota and potentially enhance the effectiveness of oncology treatments (132–134). Probiotics and prebiotics hold great potential in the domain of cancer treatment.
3.3.3 Antibiotic
Besides augmenting the profusion of probiotics, the application of antibiotics for eradicating pathogenic microorganisms constitutes another strategy for manipulating the gut microbiota. Clinical trials have emerged demonstrating the preventive utilization of rifaximin to curtail infections, gastrointestinal toxicity, and diarrhea associated with cancer treatment (135). Rifaximin proves efficacious in preventing recurrent/refractory Clostridium difficile infections, regulating bacterial metabolism, and enhancing mucosal barrier function. Preventive antibiotic treatment is frequently employed in combination with chemotherapy or immunotherapy; however, studies have discovered that antibiotic administration is correlated with diminished chemotherapy efficacy, decreased responsiveness to immune checkpoint inhibitors (ICIs), and adverse prognosis (136, 137). It can curtail the effectiveness of PD-1 blockade in cancer individuals via the MAdCAM-1-a4b7 axis, adversely influencing prognosis (138). Antibiotics can remove pathogenic microorganisms, though they may also disturb the balance and composition of the body’s microbial community, detrimental to beneficial bacteria, and giving rise to dysregulation of host-microbiota interactions (139). They can reduce the gut microbial load by a factor of 10,000, and some specific microbial species could remain absent for an extended duration after their administration (5). With the progression of nanotechnology, the targeted delivery of antibiotics for selectively eliminating pathogenic microorganisms provides more potentialities for adjusting the gut microbiota to treat digestive disorders. Nanomaterials, serving as carriers, convey therapeutic drugs to target sites, protecting them from degradation and reducing their accumulation in non-target areas, which helps to minimize side effects (140, 141). The targeted action of bacteriophages holds significance for the specific elimination of pathogenic microorganisms (142). Research has devised bacteriophage-mediated nanoparticles targeting nuclear-positive fusobacteria, augmenting the chemotherapeutic effect on CRC (143). Nanomedicine might be an efficacious treatment strategy for regulating the intestinal microbiota in the future.
3.3.4 Diagnostics and personalized medicine
The gut microbiota exhibits unique variability and plasticity among different individuals, making it an important component of personalized medicine. Some studies have already utilized gut microbiota data by integrating multi-omics data to detect CRC. Mulenga et al. proposed a novel feature engineering method that can accurately classify CRC using gut microbiota data through a deep neural network (DNN) model (144). Additionally, research has shown that Raman spectroscopy (RS) can enable real-time analysis of microbial community composition and metabolic activity, further achieving the goals of discovering biomarkers, enhancing diagnostic potential, and enabling personalized treatment (145). Currently, engineered microorganisms are receiving more attention due to their potential applications in the diagnosis and treatment of CRC and IBD (146). A study utilized genetic engineering to enable engineered commensal E. coli to specifically adhere to the surface of CRC cells. By secreting myrosinase, these bacteria convert natural compounds from cruciferous vegetables consumed by the host into organic small molecules with anti-cancer activity, significantly inhibiting tumor proliferation (131). Furthermore, combining gut microbiota with precision medicine is an important approach to enhance drug efficacy and reduce drug toxicity (147). Linking microbiota research with clinical diagnostic and therapeutic strategies holds promise for achieving microbiota-based diagnostics and personalized medicine.
3.4 Gut microbiota modulates other colorectal tumor therapies
Recently, it has been discovered that the intestinal microbiome is strongly associated with the efficacy, toxicity, and side effects of commonly used colorectal cancer treatments. The gut microbiome has different regulatory effects on chemotherapy and immunotherapy; it can improve the effectiveness of immunotherapy while promoting resistance to chemotherapy.
3.4.1 Gut microbiota promotes chemotherapy resistance
The intestinal microbiota can modulate chemotherapeutic drug metabolism, affecting cancer response to treatment and the host’s sensitivity to toxicity (148). Geller et al. are of the opinion that bacteria can metabolize the chemotherapeutic drug gemcitabine into its inactive form, giving rise to gemcitabine resistance. This metabolic process hinges on the expression of a long isoform of the bacterial enzyme cytidine deaminase (CDDL), which is predominantly present in Gammaproteobacteria, and co-treatment with the antibiotic ciprofloxacin can eliminate this resistance (149). The gut microbiota also influences the anticancer activity of cyclophosphamide, cisplatin, and 5-FU (150–152). These effects might be associated with the translocation of Gram-positive bacteria in the course of mucosal inflammation, subsequently triggering cytotoxic ROS and causing pathogenic Th17 cells to invade tumors (148, 151). Translocated bacteria can interact with the immune system and induce inflammation, thereby affecting the efficacy of chemotherapy (153). Cancer treatment demands an intact commensal microbiota to attain the optimal response, which mediates its effects by regulating the function of myeloid-derived cells in the tumor microenvironment (150). Metabolites of the gut microbiota can also modulate the efficacy of chemotherapy. For example, 3-Oxocholic acid, a metabolite associated with Prevotella, reduces the chemotherapeutic effect of FOLFOX in vitro (154). Additionally, after CoPEC infects CRC cells, the production of colibactin induces the accumulation of lipid droplets in cancer cells, limiting genotoxic stress to some extent. CoPEC infection also enhances phosphatidylcholine remodeling through enzymes in the Land’s cycle, providing cancer cells with sufficient energy to sustain survival during chemotherapy, thereby leading to chemoresistance (155). The intestinal microbiota is closely linked to the effectiveness of tumor chemotherapy, but more research is needed to elucidate the mechanisms by which these differential or chemotherapy-adapted bacteria influence chemotherapy responses.
3.4.2 Gut microbiota facilitates tumor immunotherapy
The objective of immune checkpoint blockade is to restore and augment the capacity to assail cancer cells by suppressing the tumor’s immune resistance. In immune therapy-related investigations, the two most targeted immune checkpoint regulatory factors are cytotoxic T lymphocyte-associated antigen-4 (CTLA-4) and programmed cell death protein-1 (PD-1) or its ligand PD-1 ligand-1 (PD-L1). Presently, immune checkpoint inhibitors (ICI) have been sanctioned by the FDA for the treatment of specific CRC. The gut microbiota has been shown to affect how cancer responds to checkpoint inhibitors. Sivan et al. found that the gut microbiota can influence the effectiveness of anti-PD-1/PD-L1 monoclonal antibodies in mice, with Bifidobacterium being correlated with anti-tumor effects by activating dendritic cells, leading to the activation and infiltration of CD8+ T cells in the tumor microenvironment to enhance the efficacy of immunotherapy (130). Oral administration of Bifidobacterium alone yielded anti-cancer effects similar to PD-L1-specific antibody therapy, and the combination of the two nearly completely inhibited tumor growth. Manipulating the microbiota might regulate cancer immunotherapy. Research indicates that Fn may counteract CRC by altering the tumor immune microenvironment. Gao et al. reported that Fn stimulates PD-L1 expression via the STING signaling pathway and boosts the presence of IFN-γ (+) CD8 (+) tumor-infiltrating lymphocytes (TILs), thereby enhancing the tumor’s sensitivity to PD-L1 blockade and exerting anti-tumor effects(Figure 5) (156). In certain cases, the gut microbiome is closely related to the response to ICI and may enhance the anti-cancer effects induced by immunotherapy, including immune responses at the tumor site, although the exact mechanisms remain unclear (157). Recently, Wang et al. elucidated that Fn and its abundant butyrate production can increase the sensitivity of microsatellite stable (MSS) CRC to PD-1 treatment, thereby improving treatment outcomes (158). Additionally, the utilization of antibodies may disrupt the equilibrium of immune tolerance, resulting in the development of autoimmune diseases. Research has shown that Bifidobacterium can alleviate CTLA-4 blockade-induced intestinal mucosal immune reactions without significantly influencing anti-tumor immunity (159). Lo et al. found that colitis induced by anti-CTLA-4 therapy is related to the composition of the gut microbiota, and the inflammation is caused by the unchecked activation of CD4+ T cells and the attenuation of Tregs through their interaction with the Fc domain of the CTLA-4 antibody (160). In summary, the intestinal microbiota can impact the clinical prognosis of cancer immunotherapy.
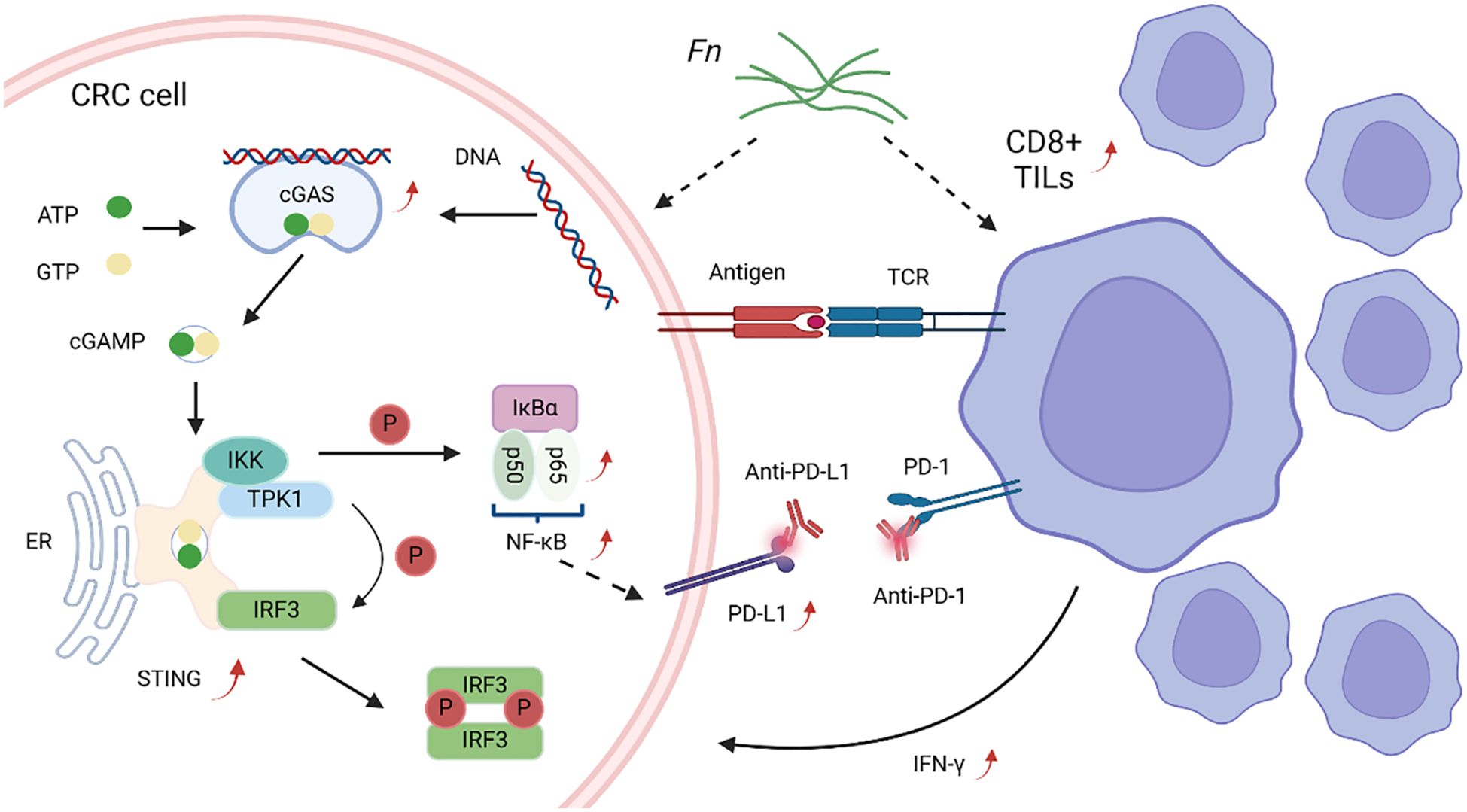
Figure 5. Fn involved in immunotherapy. Fn can activate the cGAS-STING signaling pathway in CRC cells, thereby upregulating PD-L1 expression through NF-κB (p65) transcription and enhancing anti-tumor effects. Fn can also recruit IFN-γ+ CD8+ TILs, increasing IFN-γ production and enhancing the efficacy of anti-PD-L1 therapy, thereby killing tumor cells.
4 Clinical relevant research
Modulating the gut microbiota can play an important role in the treatment of IBD and CRC, and some progress has been made, but extensive research is still needed to determine the efficacy of these treatment options. Several clinical trials have reported that FMT, probiotics, and prebiotics can manipulate the gut microbiota to treat IBD (Table 2) (15). Although the safety and long-term efficacy of FMT require further evaluation, the majority of studies suggest that it has a beneficial impact on achieving clinical, endoscopic, and histological remission (161). VSL#3 has been confirmed by multiple clinical studies to effectively improve IBD with a certain degree of safety. It can reduce endoscopic recurrence after CD surgery, induce remission in patients with mild to moderate active UC, and alleviate inflammation. Its combination with standard therapy is more effective in treating UC than standard therapy alone (15, 162). EcN has also been recognized through double-blind trials as an alternative to mesalazine for the treatment of UC, demonstrating efficacy and safety comparable to the gold standard in maintaining remission (163).
Additionally, there is additional data on the utilization of gut microbiota to assist in the treatment of CRC. For patients undergoing chemotherapy after CRC surgery, the intake of probiotics can effectively mitigate chemotherapy-induced disruptions in gut microbiota and gastrointestinal complications such as diarrhea (164). Moreover, probiotic therapy is beneficial for postoperative recovery, associated with lower individual postoperative mortality rates, shorter hospital stays, and a reduction in surgical site infections and postoperative ileus, among other complications (165, 166). In summary, the application of probiotics can enhance the efficacy of anti-cancer treatments, reduce CRC complications, and improve prognosis. While numerous in vitro and animal experiments have demonstrated the potential of gut microbiota in adjuvant CRC treatment, clinical research remains limited, particularly trials related to FMT. Issues such as how to alleviate adverse reactions when used in conjunction with other anti-cancer drugs still need to be addressed.
5 Conclusion
The composition and quantity of intestinal microbiota vary in different states of the human body. The diversity of Firmicutes exhibits a decreasing tendency in IBD patients but significantly rises in CRC patients. The same microbial community can play diverse roles in different circumstances. Although LAB is a key metabolite in the carcinogenic process of GC, facilitating the colonization of related pathogens in the stomach, while also exerting antibacterial and bactericidal effects in the intestine, playing a protective role in preventing CRC. Fn, a prevalent anaerobic bacterium found in the oral cavity, is an opportunistic pathogen closely related to CRC. Its cell surface virulence factors and metabolic products can promote tumor formation and progression, while also enhancing the sensitivity of tumors to immunotherapy and inducing effective anti-cancer effects. The article also mentions that Efa and ETBF both can boost inflammatory responses in IBD and have cancer-promoting effects on CRC. Nevertheless, NTBF and Bf HCK-B3 have positive impacts on colonic epithelial cells, enhancing barrier function, alleviating inflammation, and promoting immune tolerance.
The heterogeneity of the digestive tract microbiota offers a strong impetus for personalized treatment strategies for IBD and tumors due to its high efficacy and targeted nature. With the progress in detection and identification technologies, the development of targeted therapies based on individual microbiota, such as antibodies or other biological agents, has become more feasible. Currently, common probiotics such as Bifidobacterium and Lactobacillus can be used alongside other anti-tumor treatments, like immunotherapy and chemotherapy, to achieve better synergistic effects. Engineered bacteria and FMT treatments are also being explored, aiming to improve IBD inflammation or normalize tumor immunity by regulating the intestinal microbiota. However, several challenges remain unresolved, such as the lack of standardization in FMT preparation and delivery protocols.
Future research must first further clarify the specific mechanisms by which gut microbiota influence IBD and tumors, particularly how different microbial communities and their metabolites affect host immune responses and the tumor microenvironment. Additionally, the synergistic mechanisms between microbiota and other anti-cancer therapies also require in-depth exploration. Furthermore, how to achieve personalized diagnosis and treatment through precise regulation of microbiota is a critical issue, including the development of engineered bacteria and the optimization of FMT technology. Finally, reducing the risks associated with microbiota-related treatments and improving their safety and long-term efficacy are also key points for future research. The gut microbiota exhibits a “double-edged sword” characteristic in both IBD and CRC, necessitating therapeutic strategies that carefully balance treatment efficacy with safety considerations. With technological advancements and deeper investigations, microbiota-related research is expected to open new avenues for the treatment of IBD and tumors.
Author contributions
XC: Writing – original draft. CFL: Writing – review & editing. JZ: Writing – review & editing. YL: Writing – review & editing. PX: Writing – review & editing. CL: Writing – review & editing. MZ: Writing – review & editing. WY: Writing – review & editing.
Funding
The author(s) declare that financial support was received for the research and/or publication of this article. This work was supported by the Natural Science Foundation of China under Grant (No. 82073299) and Provincial directly affiliated departments of Jilin Province under Grant (2023SCZ25).
Acknowledgments
Figure created in BioRender.com.
Conflict of interest
The authors declare that the research was conducted in the absence of any commercial or financial relationships that could be construed as a potential conflict of interest.
Generative AI statement
The author(s) declare that no Generative AI was used in the creation of this manuscript.
Publisher’s note
All claims expressed in this article are solely those of the authors and do not necessarily represent those of their affiliated organizations, or those of the publisher, the editors and the reviewers. Any product that may be evaluated in this article, or claim that may be made by its manufacturer, is not guaranteed or endorsed by the publisher.
References
1. Xu J-Y, Liu M-T, Tao T, Zhu X, Fei F-Q. The role of gut microbiota in tumorigenesis and treatment. Biomedicine Pharmacotherapy. (2021) 138:111444. doi: 10.1016/j.biopha.2021.111444
2. Sender R, Fuchs S, Milo R. Are we really vastly outnumbered? Revisiting the ratio of bacterial to host cells in humans. Cell. (2016) 164:337–40. doi: 10.1016/j.cell.2016.01.013
3. Qin J, Li R, Raes J, Arumugam M, Burgdorf KS, Manichanh C, et al. A human gut microbial gene catalogue established by metagenomic sequencing. Nature. (2010) 464:59–U70. doi: 10.1038/nature08821
4. Fan Y, Pedersen O. Gut microbiota in human metabolic health and disease. Nat Rev Microbiol. (2021) 19:55–71. doi: 10.1038/s41579-020-0433-9
5. Liu Q, Yang Y, Pan M, Yang F, Yu Y, Qian Z. Role of the gut microbiota in tumorigenesis and treatment. Theranostics. (2024) 14:2304–28. doi: 10.7150/thno.91700
6. Manichanh C, Borruel N, Casellas F, Guarner F. The gut microbiota in ibd. Nat Rev Gastroenterology Hepatology. (2012) 9:599–608. doi: 10.1038/nrgastro.2012.152
7. Helmink BA, Khan MAW, Hermann A, Gopalakrishnan V, Wargo JA. The microbiome, cancer, and cancer therapy. Nat Med. (2019) 25:377–88. doi: 10.1038/s41591-019-0377-7
8. Nemet I, Saha PP, Gupta N, Zhu W, Romano KA, Skye SM, et al. A cardiovascular disease-linked gut microbial metabolite acts via adrenergic receptors. Cell. (2020) 180:862–+. doi: 10.1016/j.cell.2020.02.016
9. Jameson KG, Olson CA, Kazmi SA, Hsiao EY. Toward understanding microbiome-neuronal signaling. Mol Cell. (2020) 78:577–83. doi: 10.1016/j.molcel.2020.03.006
10. Miyauchi E, Shimokawa C, Steimle A, Desai MS, Ohno H. The impact of the gut microbiome on extra-intestinal autoimmune diseases. Nat Rev Immunol. (2023) 23:9–23. doi: 10.1038/s41577-022-00727-y
11. Heijtz RD, Gressens P, Swann JR. Targeting microbial metabolites to treat autism. Nat Med. (2022) 28:448–50. doi: 10.1038/s41591-022-01711-8
12. Vivarelli S, Salemi R, Candido S, Falzone L, Santagati M, Stefani S, et al. Gut microbiota and cancer: from pathogenesis to therapy. Cancers. (2019) 11(1):38. doi: 10.3390/cancers11010038
13. Mulder DJ, Noble AJ, Justinich CJ, Duffin JM. A tale of two diseases: the history of inflammatory bowel disease. J Crohns Colitis. (2014) 8:341–8. doi: 10.1016/j.crohns.2013.09.009
14. Agrawal M, Jess T. Implications of the changing epidemiology of inflammatory bowel disease in a changing world. United Eur Gastroenterology J. (2022) 10:1113–20. doi: 10.1002/ueg2.12317
15. Pandey H, Jain D, Tang DWT, Wong SH, Lal D. Gut microbiota in pathophysiology, diagnosis, and therapeutics of inflammatory bowel disease. Intestinal Res. (2024) 22:15–43. doi: 10.5217/ir.2023.00080
16. Allen-Vercoe E, Coburn B. A microbiota-derived metabolite augments cancer immunotherapy responses in mice. Cancer Cell. (2020) 38:452–3. doi: 10.1016/j.ccell.2020.09.005
17. Stappenbeck TS, Virgin HW. Accounting for reciprocal host-microbiome interactions in experimental science. Nature. (2016) 534:191–9. doi: 10.1038/nature18285
18. Vester-Andersen MK, Mirsepasi-Lauridsen HC, Prosberg MV, Mortensen CO, Traeger C, Skovsen K, et al. Increased abundance of proteobacteria in aggressive crohn's disease seven years after diagnosis. Sci Rep. (2019) 9(1):13473. doi: 10.1038/s41598-019-49833-3
19. Bonnet M, Buc E, Sauvanet P, Darcha C, Dubois D, Pereira B, et al. Colonization of the human gut by E.Coli and colorectal cancer risk. Clin Cancer Res. (2014) 20:859–67. doi: 10.1158/1078-0432.Ccr-13-1343
20. Valencise Quaglio AE, Grillo TG, Souza De Oliveira EC, Di Stasi LC, Sassaki LY. Gut microbiota, inflammatory bowel disease and colorectal cancer. World J Gastroenterology. (2022) 28:4053–60. doi: 10.3748/wjg.v28.i30.4053
21. Han B, Zheng R, Zeng H, Wang S, Sun K, Chen R, et al. Cancer incidence and mortality in China, 2022. J Natl Cancer Center. (2024) 4:47–53. doi: 10.1016/j.jncc.2024.01.006
22. Knight R, Callewaert C, Marotz C, Hyde ER, Debelius JW, McDonald D, et al. The microbiome and human biology. Annu Rev Genomics Hum Genet. (2017) 18:65–86. doi: 10.1146/annurev-genom-083115-022438
24. Schirmer M, Garner A, Vlamakis H, Xavier RJ. Microbial genes and pathways in inflammatory bowel disease. Nat Rev Microbiol. (2019) 17:497–511. doi: 10.1038/s41579-019-0213-6
25. Eckburg PB, Bik EM, Bernstein CN, Purdom E, Dethlefsen L, Sargent M, et al. Diversity of the human intestinal microbial flora. Science. (2005) 308:1635–8. doi: 10.1126/science.1110591
26. Stojanov S, Berlec A, Strukelj B. The influence of probiotics on the firmicutes/bacteroidetes ratio in the treatment of obesity and inflammatory bowel disease. Microorganisms. (2020) 8(11):1715. doi: 10.3390/microorganisms8111715
27. Thomann AK, Mak JWY, Zhang JW, Wuestenberg T, Ebert MP, Sung JJY, et al. Review article: bugs, inflammation and mood-a microbiota-based approach to psychiatric symptoms in inflammatory bowel diseases. Alimentary Pharmacol Ther. (2020) 52:247–66. doi: 10.1111/apt.15787
28. Machiels K, Joossens M, Sabino J, De Preter V, Arijs I, Eeckhaut V, et al. A decrease of the butyrate-producing species roseburia hominis and faecalibacterium prausnitzii defines dysbiosis in patients with ulcerative colitis. Gut. (2014) 63:1275–83. doi: 10.1136/gutjnl-2013-304833
29. Sokol H, Pigneur B, Watterlot L, Lakhdari O, Bermudez-Humaran LG, Gratadoux J-J, et al. Faecalibacterium prausnitzii is an anti-inflammatory commensal bacterium identified by gut microbiota analysis of crohn disease patients. Proc Natl Acad Sci United States America. (2008) 105:16731–6. doi: 10.1073/pnas.0804812105
30. Chen L, Wang J. Gut microbiota and inflammatory bowel disease. Wires Mech Dis. (2022) 14(2):e1540. doi: 10.1002/wsbm.1540
31. Zhou L, Zhang M, Wang Y, Dorfman RG, Liu H, Yu T, et al. Faecalibacterium prausnitzii produces butyrate to maintain th17/treg balance and to ameliorate colorectal colitis by inhibiting histone deacetylase 1. Inflammatory Bowel Dis. (2018) 24:1926–40. doi: 10.1093/ibd/izy182
32. Watanabe Y, Nagai F, Morotomi M. Characterization of phascolarctobacterium succinatutens sp nov., an asaccharolytic, succinate-utilizing bacterium isolated from human feces. Appl Environ Microbiol. (2012) 78:511–8. doi: 10.1128/aem.06035-11
33. Eslick S, Thompson C, Berthon B, Wood L. Short-chain fatty acids as anti-inflammatory agents in overweight and obesity: A systematic review and meta-analysis. Nutr Rev. (2022) 80:838–56. doi: 10.1093/nutrit/nuab059
34. Haneishi Y, Furuya Y, Hasegawa M, Picarelli A, Rossi M, Miyamoto J. Inflammatory bowel diseases and gut microbiota. Int J Mol Sci. (2023) 24(4):3817. doi: 10.3390/ijms24043817
35. Round JL, Lee SM, Li J, Tran G, Jabri B, Chatila TA, et al. The toll-like receptor 2 pathway establishes colonization by a commensal of the human microbiota. Science. (2011) 332:974–7. doi: 10.1126/science.1206095
36. Tan H, Zhao J, Zhang H, Zhai Q, Chen W. Novel strains of bacteroides fragilis and bacteroides ovatus alleviate the lps-induced inflammation in mice. Appl Microbiol Biotechnol. (2019) 103:2353–65. doi: 10.1007/s00253-019-09617-1
37. Mancini NL, Rajeev S, Jayme TS, Wang A, Keita AV, Workentine ML, et al. Crohn's disease pathobiont adherent-invasive E coli disrupts epithelial mitochondrial networks with implications for gut permeability. Cell Mol Gastroenterology Hepatology. (2021) 11:551–71. doi: 10.1016/j.jcmgh.2020.09.013
38. Seishima J, Iida N, Kitamura K, Yutani M, Wang Z, Seki A, et al. Gut-derived enterococcus faecium from ulcerative colitis patients promotes colitis in a genetically susceptible mouse host. Genome Biol. (2019) 20(1):252. doi: 10.1186/s13059-019-1879-9
39. Wu S, Rhee K-J, Albesiano E, Rabizadeh S, Wu X, Yen H-R, et al. A human colonic commensal promotes colon tumorigenesis via activation of T helper type 17 T cell responses. Nat Med. (2009) 15:1016–U64. doi: 10.1038/nm.2015
40. Sears CL, Geis AL, Housseau F. Bacteroides fragilis subverts mucosal biology: from symbiont to colon carcinogenesis. J Clin Invest. (2014) 124:4166–72. doi: 10.1172/jci72334
41. Ha CWY, Martin A, Sepich-Poore GD, Shi B, Wang Y, Gouin K, et al. Translocation of viable gut microbiota to mesenteric adipose drives formation of creeping fat in humans. Cell. (2020) 183:666–+. doi: 10.1016/j.cell.2020.09.009
42. Henke MT, Kenny DJ, Cassilly CD, Vlamakis H, Xavier RJ, Clardy J. Ruminococcus gnavus, a member of the human gut microbiome associated with crohn's disease, produces an inflammatory polysaccharide. Proc Natl Acad Sci United States America. (2019) 116:12672–7. doi: 10.1073/pnas.1904099116
43. Vestergaard MV, Allin KH, Eriksen C, Zakerska-Banaszak O, Arasaradnam RP, Alam MT, et al. Gut microbiota signatures in inflammatory bowel disease. United Eur Gastroenterology J. (2024) 12:22–33. doi: 10.1002/ueg2.12485
44. Pascal V, Pozuelo M, Borruel N, Casellas F, Campos D, Santiago A, et al. A microbial signature for crohn's disease. Gut. (2017) 66:813–22. doi: 10.1136/gutjnl-2016-313235
45. Pittayanon R, Lau JT, Leontiadis GI, Tse F, Yuan Y, Surette M, et al. Differences in gut microbiota in patients with vs without inflammatory bowel diseases: A systematic review. Gastroenterology. (2020) 158:930–+. doi: 10.1053/j.gastro.2019.11.294
46. Forbes JD, Chen C-Y, Knox NC, Marrie R-A, El-Gabalawy H, de Kievit T, et al. A comparative study of the gut microbiota in immune-mediated inflammatory diseasesdoes a common dysbiosis exist? Microbiome. (2018) 6(1):221. doi: 10.1186/s40168-018-0603-4
47. Qiu P, Ishimoto T, Fu L, Zhang J, Zhang Z, Liu Y. The gut microbiota in inflammatory bowel disease. Front Cell Infection Microbiol. (2022) 12:733992. doi: 10.3389/fcimb.2022.733992
48. Bennet JD, Brinkman M. Treatment of ulcerative colitis by implantation of normal colonic flora. Lancet (London England). (1989) 1:164–. doi: 10.1016/S0140-6736(89)91183-5
49. Saha S, Mara K, Pardi DS, Khanna S. Long-term safety of fecal microbiota transplantation for recurrent clostridioides difficile infection. Gastroenterology. (2021) 160:1961–+. doi: 10.1053/j.gastro.2021.01.010
50. Goyal A, Yeh A, Bush BR, Firek BA, Siebold LM, Rogers MB, et al. Safety, clinical response, and microbiome findings following fecal microbiota transplant in children with inflammatory bowel disease. Inflammatory Bowel Dis. (2018) 24:410–21. doi: 10.1093/ibd/izx035
51. Chu ND, Crothers JW, Nguyen LTT, Kearney SM, Smith MB, Kassam Z, et al. Dynamic colonization of microbes and their functions after fecal microbiota transplantation for inflammatory bowel disease. Mbio. (2021) 12(4):e0097521. doi: 10.1128/mBio.00975-21
52. Mankowska-Wierzbicka D, Stelmach-Mardas M, Gabryel M, Tomczak H, Skrzypczak-Zielinska M, Zakerska-Banaszak O, et al. The effectiveness of multi-session fmt treatment in active ulcerative colitis patients: A pilot study. Biomedicines. (2020) 8(8):268. doi: 10.3390/biomedicines8080268
53. Moayyedi P, Surette MG, Kim PT, Libertucci J, Wolfe M, Onischi C, et al. Fecal microbiota transplantation induces remission in patients with active ulcerative colitis in a randomized controlled trial. Gastroenterology. (2015) 149:102–+. doi: 10.1053/j.gastro.2015.04.001
54. Liu J, Lin H, Cao M, Lin T, Lin A, Xu W, et al. Shifts and importance of viable bacteria in treatment of dss-induced ulcerative colitis mice with fmt. Front Cell Infection Microbiol. (2023) 13:1124256. doi: 10.3389/fcimb.2023.1124256
55. Angelberger S, Reinisch W, Makristathis A, Lichtenberger C, Dejaco C, Papay P, et al. Temporal Bacterial Community Dynamics Vary among Ulcerative Colitis Patients after Fecal Microbiota Transplantation. Am J Gastroenterology. (2013) 108:1620–30. doi: 10.1038/ajg.2013.257
56. Ishikawa D, Sasaki T, Takahashi M, Kuwahara-Arai K, Haga K, Ito S, et al. The microbial composition of bacteroidetes species in ulcerative colitis is effectively improved by combination therapy with fecal microbiota transplantation and antibiotics. Inflammatory Bowel Dis. (2018) 24:2590–8. doi: 10.1093/ibd/izy266
57. Paramsothy S, Paramsothy R, Rubin DT, Kamm MA, Kaakoush NO, Mitchell HM, et al. Fecal microbiota transplantation for inflammatory bowel disease: A systematic review and meta-analysis. J Crohns Colitis. (2017) 11:1180–99. doi: 10.1093/ecco-jcc/jjx063
58. Malik S, Naqvi SAA, Shadali AH, Khan H, Christof M, Niu C, et al. Fecal microbiota transplantation (Fmt) and clinical outcomes among inflammatory bowel disease (Ibd) patients: an umbrella review. Digestive Dis Sci. (2025). doi: 10.1007/s10620-025-08946-8
59. Ding X, Li Q, Li P, Zhang T, Cui B, Ji G, et al. Long-term safety and efficacy of fecal microbiota transplant in active ulcerative colitis. Drug Saf. (2019) 42:869–80. doi: 10.1007/s40264-019-00809-2
60. Jalanka J, Hillamaa A, Satokari R, Mattila E, Anttila VJ, Arkkila P. The long-term effects of fecal microbiota transplantation for gastrointestinal symptoms and general health in patients with recurrent clostridium difficile infection. Alimentary Pharmacol Ther. (2018) 47:371–9. doi: 10.1111/apt.14443
61. Kuehbacher T, Ott SJ, Helwig U, Mimura T, Rizzello F, Kleessen B, et al. Bacterial and fungal microbiota in relation to probiotic therapy (Vsl3) in pouchitis. Gut. (2006) 55:833–41. doi: 10.1136/gut.2005.078303
62. Gionchetti P, Rizzello F, Helwig U, Venturi A, Lammers KM, Brigidi P, et al. Prophylaxis of pouchitis onset with probiotic therapy: A double-blind, placebo-controlled trial. Gastroenterology. (2003) 124:1202–9. doi: 10.1016/s0016-5085(03)00171-9
63. Selinger CP, Bell A, Cairns A, Lockett M, Sebastian S, Haslam N. Probiotic vsl3 prevents antibiotic-associated diarrhea in a double-blind, randomized, placebo-controlled clinical trial. J Hosp Infection. (2013) 84:159–65. doi: 10.1016/j.jhin.2013.02.019
64. Rossi G, Pengo G, Caldin M, Piccionello AP, Steiner JM, Cohen ND, et al. Comparison of microbiological, histological, and immunomodulatory parameters in response to treatment with either combination therapy with prednisone and metronidazole or probiotic vsl3 strains in dogs with idiopathic inflammatory bowel disease. PloS One. (2014) 9(4):e94699. doi: 10.1371/journal.pone.0094699
65. Wang C-S-E, Li W-B, Wang H-Y, Ma Y-M, Zhao X-H, Yang H, et al. Vsl3 can prevent ulcerative colitis-associated carcinogenesis in mice. World J Gastroenterology. (2018) 24:4254–62. doi: 10.3748/wjg.v24.i37.4254
66. Matsuoka K, Kanai T. The gut microbiota and inflammatory bowel disease. Semin Immunopathology. (2015) 37:47–55. doi: 10.1007/s00281-014-0454-4
67. Scaldaferri F, Gerardi V, Mangiola F, Lopetuso LR, Pizzoferrato M, Petito V, et al. Role and mechanisms of action of escherichia coli nissle 1917 in the maintenance of remission in ulcerative colitis patients: an update. World J Gastroenterology. (2016) 22:5505–11. doi: 10.3748/wjg.v22.i24.5505
68. Hayashi A, Sato T, Kamada N, Mikami Y, Matsuoka K, Hisamatsu T, et al. A single strain of clostridium butyricum induces intestinal il-10-producing macrophages to suppress acute experimental colitis in mice. Cell Host Microbe. (2013) 13:711–22. doi: 10.1016/j.chom.2013.05.013
69. Xia JY, Hepler C, Tran P, Waldeck NJ, Bass J, Prindle A. Engineered calprotectin-sensing probiotics for ibd surveillance in humans. Proc Natl Acad Sci United States America. (2023) 120(32):e2221121120. doi: 10.1073/pnas.2221121120
70. Woo S-G, Moon S-J, Kim SK, Kim TH, Lim HS, Yeon G-H, et al. A designed whole-cell biosensor for live diagnosis of gut inflammation through nitrate sensing. Biosensors Bioelectronics. (2020) 168:112523. doi: 10.1016/j.bios.2020.112523
71. Zou ZP, Du Y, Fang TT, Zhou Y, Ye BC. Biomarker-responsive engineered probiotic diagnoses, records, and ameliorates inflammatory bowel disease in mice. Cell Host Microbe. (2023) 31:199–+. doi: 10.1016/j.chom.2022.12.004
72. Olga B, Boicean A, Fleaca S-R, Blanca G, Florin S, Corina R-F, et al. Importance of fecal microbiota transplantation and molecular regulation as therapeutic strategies in inflammatory bowel diseases. Nutrients. (2024) 16(24):4411. doi: 10.3390/nu16244411
73. Keller DS, Windsor A, Cohen R, Chand M. Colorectal cancer in inflammatory bowel disease: review of the evidence. Techniques Coloproctology. (2019) 23:3–13. doi: 10.1007/s10151-019-1926-2
74. Popov J, Caputi V, Nandeesha N, Rodriguez DA, Pai N. Microbiota-immune interactions in ulcerative colitis and colitis associated cancer and emerging microbiota-based therapies. Int J Mol Sci. (2021) 22(21):11365. doi: 10.3390/ijms222111365
75. Gao R, Gao Z, Huang L, Qin H. Gut microbiota and colorectal cancer. Eur J Clin Microbiol Infect Dis. (2017) 36:757–69. doi: 10.1007/s10096-016-2881-8
76. Gagniere J, Raisch J, Veziant J, Barnich N, Bonnet R, Buc E, et al. Gut microbiota imbalance and colorectal cancer. World J Gastroenterology. (2016) 22:501–18. doi: 10.3748/wjg.v22.i2.501
77. Silva M, Brunner V, Tschurtschenthaler M. Microbiota and colorectal cancer: from gut to bedside. Front Pharmacol. (2021) 12:760280. doi: 10.3389/fphar.2021.760280
78. Liu K, Yang X, Zeng M, Yuan Y, Sun J, He P, et al. The role of fecal fusobacterium nucleatum and pks+Escherichia coli as early diagnostic markers of colorectal cancer. Dis Markers. (2021) 2021:1171239. doi: 10.1155/2021/1171239
79. Boleij A, Tjalsma H. Gut bacteria in health and disease: A survey on the interface between intestinal microbiology and colorectal cancer. Biol Rev. (2012) 87:701–30. doi: 10.1111/j.1469-185X.2012.00218.x
80. Rubinstein MR, Wang X, Liu W, Hao Y, Cai G, Han YW. Fusobacterium nucleatum promotes colorectal carcinogenesis by modulating E-cadherin/B-catenin signaling via its fada adhesin. Cell Host Microbe. (2013) 14:195–206. doi: 10.1016/j.chom.2013.07.012
81. Ternes D, Tsenkova M, Pozdeev VI, Meyers M, Koncina E, Atatri S, et al. The gut microbial metabolite formate exacerbates colorectal cancer progression. Nat Metab. (2022) 4:458–+. doi: 10.1038/s42255-022-00558-0
82. Brennan CA, Clay SL, Lavoie SL, Bae S, Lang JK, Fonseca-Pereira D, et al. Fusobacterium nucleatum drives a pro-inflammatory intestinal microenvironment through metabolite receptor-dependent modulation of il-17 expression. Gut Microbes. (2021) 13(1):1987780. doi: 10.1080/19490976.2021.1987780
83. Wang M, Wang Z, Lessing DJ, Guo M, Chu W. Fusobacterium nucleatum and its metabolite hydrogen sulfide alter gut microbiota composition and autophagy process and promote colorectal cancer progression. Microbiol Spectr. (2023) 11(6):e0229223. doi: 10.1128/spectrum.02292-23
84. Gur C, Ibrahim Y, Isaacson B, Yamin R, Abed J, Gamliel M, et al. Binding of the fap2 protein of fusobacterium nucleatum to human inhibitory receptor tigit protects tumors from immune cell attack. Immunity. (2015) 42:344–55. doi: 10.1016/j.immuni.2015.01.010
85. Engevik MA, Danhof HA, Ruan W, Engevik AC, Chang-Graham AL, Engevik KA, et al. Fusobacterium nucleatum secretes outer membrane vesicles and promotes intestinal inflammation. Mbio. (2021) 12(2):e02706–20. doi: 10.1128/mBio.02706-20
86. Sayed IM, Chakraborty A, Abd El-Hafeez AA, Sharma A, Sahan AZ, Huang WJM, et al. The DNA glycosylase neil2 suppresses fusobacterium-infection-induced inflammation and DNA damage in colonic epithelial cells. Cells. (2020) 9(9):1980. doi: 10.3390/cells9091980
87. Chen S, Zhang L, Li M, Zhang Y, Sun M, Wang L, et al. Fusobacterium nucleatum reduces mettl3-mediated M6a modification and contributes to colorectal cancer metastasis. Nat Commun. (2022) 13(1):1248. doi: 10.1038/s41467-022-28913-5
88. Zhang Y, Zhang L, Zheng S, Li M, Xu C, Jia D, et al. Fusobacterium nucleatum promotes colorectal cancer cells adhesion to endothelial cells and facilitates extravasation and metastasis by inducing alpk1/nf-Kb/icam1 axis. Gut Microbes. (2022) 14(1):2038852. doi: 10.1080/19490976.2022.2038852
89. Hong J, Guo F, Lu S-Y, Shen C, Ma D, Zhang X, et al. F. Nucleatum targets lncrna eno1-it1 to promote glycolysis and oncogenesis in colorectal cancer. Gut. (2021) 70:2123–37. doi: 10.1136/gutjnl-2020-322780
90. Iftekhar A, Berger H, Bouznad N, Heuberger J, Boccellato F, Dobrindt U, et al. Genomic aberrations after short-term exposure to colibactin-producing E. Coli transform primary colon epithelial cells. Nat Commun. (2021) 12(1):1003. doi: 10.1038/s41467-021-21162-y
91. Dalmasso G, Cougnoux A, Fais T, Bonnin V, Mottet-Auselo B, Nguyen HTT, et al. Colibactin-producing escherichia coli enhance resistance to chemotherapeutic drugs by promoting epithelial to mesenchymal transition and cancer stem cell emergence. Gut Microbes. (2024) 16(1):2310215. doi: 10.1080/19490976.2024.2310215
92. Huycke MM, Abrams V, Moore DR. Enterococcus faecalis produces extracellular superoxide and hydrogen peroxide that damages colonic epithelial cell DNA. Carcinogenesis. (2002) 23:529–36. doi: 10.1093/carcin/23.3.529
93. Zhang L, Liu J, Deng M, Chen X, Jiang L, Zhang J, et al. Enterococcus faecalis promotes the progression of colorectal cancer via its metabolite: biliverdin. J Trans Med. (2023) 21(1):72. doi: 10.1186/s12967-023-03929-7
94. Steck N, Hoffmann M, Sava IG, Kim SC, Hahne H, Tonkonogy SL, et al. Enterococcus faecalis metalloprotease compromises epithelial barrier and contributes to intestinal inflammation. Gastroenterology. (2011) 141:959–71. doi: 10.1053/j.gastro.2011.05.035
95. Martins M, Porrini C, du Merle L, Danne C, Robbe-Masselot C, Trieu-Cuot P, et al. The pil3 pilus of streptococcus gallolyticus binds to intestinal mucins and to fibrinogen. Gut Microbes. (2016) 7:526–32. doi: 10.1080/19490976.2016.1239677
96. Deng Q, Wang C, Yu K, Wang Y, Yang Q, Zhang J, et al. Streptococcus bovis contributes to the development of colorectal cancer via recruiting cd11b+Tlr-4+ Cells. Med Sci Monitor. (2020) 26:e921886. doi: 10.12659/msm.921886
97. Abdulamir AS, Hafidh RR, Abu Bakar F. Molecular detection, quantification, and isolation of streptococcus gallolyticus bacteria colonizing colorectal tumors: inflammation-driven potential of carcinogenesis via il-1, cox-2, and il-8. Mol Cancer. (2010) 9:249. doi: 10.1186/1476-4598-9-249
98. Kumar R, Herold JL, SChady D, Davis J, Kopetz S, Martinez-Moczygemba M, et al. Streptococcus gallolyticus subsp gallolyticus promotes colorectal tumor development. PloS Pathog. (2017) 13(7):e1006440. doi: 10.1371/journal.ppat.1006440
99. Taddese R, Roelofs R, Draper D, Wu X, Wu S, Swinkels DW, et al. Streptococcus gallolyticus increases expression and activity of aryl hydrocarbon receptor-dependent cyp1 biotransformation capacity in colorectal epithelial cells. Front Cell Infection Microbiol. (2021) 11:740704. doi: 10.3389/fcimb.2021.740704
100. Wu SG, Powell J, Mathioudakis N, Kane S, Fernandez E, Sears CL. Bacteroides fragilis enterotoxin induces intestinal epithelial cell secretion of interleukin-8 through mitogen-activated protein kinases and a tyrosine kinase-regulated nuclear factor-Kb pathway. Infection Immun. (2004) 72:5832–9. doi: 10.1128/iai.72.10.5832-5839.2004
101. Liu Q-Q, Li C-M, Fu L-N, Wang H-L, Tan J, Wang Y-Q, et al. Enterotoxigenic bacteroides fragilis induces the stemness in colorectal cancer via upregulating histone demethylase jmjd2b. Gut Microbes. (2020) 12(1):1788900. doi: 10.1080/19490976.2020.1788900
102. Cao Y, Wang Z, Yan Y, Ji L, He J, Xuan B, et al. Enterotoxigenic bacteroides fragilis promotes intestinal inflammation and Malignancy by inhibiting exosome-packaged mir-149-3p. Gastroenterology. (2021) 161:1552–+. doi: 10.1053/j.gastro.2021.08.003
103. Wu X, Yang C, Sun F, Zhang Y, Wang Y, Li X, et al. Enterotoxigenic bacteroides fragilis (Etbf) enhances colorectal cancer cell proliferation and metastasis through hdac3/mir-139-3p pathway. Biochem Genet. (2024) 62(5):3904–19. doi: 10.1007/s10528-023-10621-4
104. Kostic AD, Chun E, Robertson L, Glickman JN, Gallini CA, Michaud M, et al. Fusobacterium nucleatum potentiates intestinal tumorigenesis and modulates the tumor-immune microenvironment. Cell Host Microbe. (2013) 14:207–15. doi: 10.1016/j.chom.2013.07.007
105. Repass J, Maherali N, Owen K. Reproducibility project canc B. Registered report: fusobacterium nucleatum infection is prevalent in human colorectal carcinoma. Elife. (2016) 5:e10012. doi: 10.7554/eLife.10012
106. Meng C, Bai C, Brown TD, Hood LE, Tian Q. Human gut microbiota and gastrointestinal cancer. Genomics Proteomics Bioinf. (2018) 16:33–49. doi: 10.1016/j.gpb.2017.06.002
107. Dejea CM, Fathi P, Craig JM, Boleij A, Taddese R, Geis AL, et al. Patients with familial adenomatous polyposis harbor colonic biofilms containing tumorigenic bacteria. Science. (2018) 359:592–+. doi: 10.1126/science.aah3648
108. Wang X, Sun X, Chu J, Sun W, Yan S, Wang Y. Gut microbiota and microbiota-derived metabolites in colorectal cancer: enemy or friend. World J Microbiol Biotechnol. (2023) 39(11):291. doi: 10.1007/s11274-023-03742-w
109. Peng C, Ouyang Y, Lu N, Li N. The nf-Kb signaling pathway, the microbiota, and gastrointestinal tumorigenesis: recent advances. Front Immunol. (2020) 11:1387. doi: 10.3389/fimmu.2020.01387
110. Aymeric L, Donnadieu F, Mulet C, du Merle L, Nigro G, Saffarian A, et al. Colorectal cancer specific conditions promote streptococcus gallolyticus gut colonization. Proc Natl Acad Sci United States America. (2018) 115:E283–E91. doi: 10.1073/pnas.1715112115
111. Clay SL, Fonseca-Pereira D, Garrett WS. Colorectal cancer: the facts in the case of the microbiota. J Clin Invest. (2022) 132(4):e155101. doi: 10.1172/jci155101
112. Haghi F, Goli E, Mirzaei B, Zeighami H. The association between fecal enterotoxigenic B. Fragilis with colorectal cancer. BMC Cancer. (2019) 19(1):879. doi: 10.1186/s12885-019-6115-1
113. Appunni S, Rubens M, Ramamoorthy V, Tonse R, Saxena A, McGranaghan P, et al. Emerging evidence on the effects of dietary factors on the gut microbiome in colorectal cancer. Front Nutr. (2021) 8:718389. doi: 10.3389/fnut.2021.718389
114. Hols P, Ledesma-Garcia L, Gabant P, Mignolet J. Mobilization of microbiota commensals and their bacteriocins for therapeutics. Trends Microbiol. (2019) 27:690–702. doi: 10.1016/j.tim.2019.03.007
115. Luu M, Riester Z, Baldrich A, Reichardt N, Yuille S, Busetti A, et al. Microbial short-chain fatty acids modulate cd8+ T cell responses and improve adoptive immunotherapy for cancer. Nat Commun. (2021) 12(1):4077. doi: 10.1038/s41467-021-24331-1
116. Kang X, Liu C, Ding Y, Ni Y, Ji F, Lau HCH, et al. Roseburia intestinalis generated butyrate boosts anti-pd-1 efficacy in colorectal cancer by activating cytotoxic cd8+ T cells. Gut. (2023) 72:2112–22. doi: 10.1136/gutjnl-2023-330291
117. Zitvogel L, Daillere R, Roberti MP, Routy B, Kroemer G. Anticancer effects of the microbiome and its products. Nat Rev Microbiol. (2017) 15:465–78. doi: 10.1038/nrmicro.2017.44
118. de Groot PF, Frissen MN, de Clercq NC, Nieuwdorp M. Fecal microbiota transplantation in metabolic syndrome: history, present and future. Gut Microbes. (2017) 8:253–67. doi: 10.1080/19490976.2017.1293224
119. Ting NL-N, Lau HC-H, Yu J. Cancer pharmacomicrobiomics: targeting microbiota to optimize cancer therapy outcomes. Gut. (2022) 71:1412–+. doi: 10.1136/gutjnl-2021-326264
120. McQuade JL, Daniel CR, Helmink BA, Wargo JA. Modulating the microbiome to improve therapeutic response in cancer. Lancet Oncol. (2019) 20:E77–91. doi: 10.1016/s1470-2045(18)30952-5
121. Gopalakrishnan V, Helmink BA, Spencer CN, Reuben A, Wargo JA. The influence of the gut microbiome on cancer, immunity, and cancer immunotherapy. Cancer Cell. (2018) 33:570–80. doi: 10.1016/j.ccell.2018.03.015
122. Routy B, Lenehan JG, Miller WH, Jamal R, Messaoudene M, Daisley BA, et al. Fecal microbiota transplantation plus anti-pd-1 immunotherapy in advanced melanoma: A phase I trial. Nat Med. (2023) 29:2121–+. doi: 10.1038/s41591-023-02453-x
123. Guo Q, Qin H, Liu X, Zhang X, Chen Z, Qin T, et al. The emerging roles of human gut microbiota in gastrointestinal cancer. Front Immunol. (2022) 13:915047. doi: 10.3389/fimmu.2022.915047
124. Danne C, Rolhion N, Sokol H. Recipient factors in fecal microbiota transplantation: one stool does not fit all. Nat Rev Gastroenterology Hepatology. (2021) 18:503–13. doi: 10.1038/s41575-021-00441-5
125. Marcella C, Cui B, Kelly CR, Ianiro G, Cammarota G, Zhang F. Systematic review: the global incidence of fecal microbiota transplantation-related adverse events from 2000 to 2020. Alimentary Pharmacol Ther. (2021) 53:33–42. doi: 10.1111/apt.16148
126. Chen D, Jin D, Huang S, Wu J, Xu M, Liu T, et al. Clostridium butyricum, a butyrate-producing probiotic, inhibits intestinal tumor development through modulating wnt signaling and gut microbiota. Cancer Lett. (2020) 469:456–67. doi: 10.1016/j.canlet.2019.11.019
127. Aindelis G, Tiptiri-Kourpeti A, Lampri E, Spyridopoulou K, Lamprianidou E, Kotsianidis I, et al. Immune responses raised in an experimental colon carcinoma model following oral administration of lactobacillus casei. Cancers. (2020) 12(2):368. doi: 10.3390/cancers12020368
128. Hempel S, Newberry SJ, Maher AR, Wang Z, Miles JNV, Shanman R, et al. Probiotics for the prevention and treatment of antibiotic-associated diarrhea a systematic review and meta-analysis. Jama-Journal Am Med Assoc. (2012) 307:1959–69. doi: 10.1001/jama.2012.3507
129. Botta C, Spyridopoulou K, Bertolino M, Rantsiou K, Chlichlia K, Cocolin L. Lactiplantibacillus plantarum inhibits colon cancer cell proliferation as function of its butyrogenic capability. Biomedicine Pharmacotherapy. (2022) 149:112755. doi: 10.1016/j.biopha.2022.112755
130. Sivan A, Corrales L, Hubert N, Williams JB, Aquino-Michaels K, Earley ZM, et al. Commensal bifidobacterium promotes antitumor immunity and facilitates anti-pd-L1 efficacy. Science. (2015) 350:1084–9. doi: 10.1126/science.aac4255
131. Ho CL, Tan HQ, Chua KJ, Kang A, Lim KH, Ling KL, et al. Engineered commensal microbes for diet-mediated colorectal-cancer chemoprevention. Nat Biomed Eng. (2018) 2:27–37. doi: 10.1038/s41551-017-0181-y
132. Davani-Davari D, Negahdaripour M, Karimzadeh I, Seifan M, Mohkam M, Masoumi SJ, et al. Prebiotics: definition, types, sources, mechanisms, and clinical applications. Foods. (2019) 8(3):92. doi: 10.3390/foods8030092
133. Dewulf EM, Cani PD, Claus SP, Fuentes S, Puylaert PGB, Neyrinck AM, et al. Insight into the prebiotic concept: lessons from an exploratory, double blind intervention study with inulin-type fructans in obese women. Gut. (2013) 62:1112–21. doi: 10.1136/gutjnl-2012-303304
134. Taper HS, Roberfroid MB. Possible adjuvant cancer therapy by two prebioties - inulin or oligofructose. In Vivo. (2005) 19:201–4.
135. Dutta D, Lim SH. Bidirectional interaction between intestinal microbiome and cancer: opportunities for therapeutic interventions. Biomarker Res. (2020) 8:31. doi: 10.1186/s40364-020-00211-6
136. Wu C, Lai R, Li J, Zhang J, Zhao Y, Zhang X, et al. Antibiotics modulate chemotherapy efficacy in patients with esophageal cancer. Cancer Manage Res. (2020) 12:4991–7. doi: 10.2147/cmar.S248130
137. Pinato DJ, Howlett S, Ottaviani D, Urus H, Patel A, Mineo T, et al. Association of prior antibiotic treatment with survival and response to immune checkpoint inhibitor therapy in patients with cancer. JAMA Oncol. (2019) 5:1774–8. doi: 10.1001/jamaoncol.2019.2785
138. Fidelle M, Rauber C, Silva CAC, Tian A-L, Lahmar I, de la Varende A-LM, et al. A microbiota-modulated checkpoint directs immunosuppressive intestinal T cells into cancers. Science. (2023) 380(6649):eabo2296. doi: 10.1126/science.abo2296
139. Nguyen LH, Ortqvist AK, Cao Y, Simon TG, Roelstraete B, Song M, et al. Antibiotic use and the development of inflammatory bowel disease: A national case-control study in Sweden. Lancet Gastroenterology Hepatology. (2020) 5:986–95. doi: 10.1016/s2468-1253(20)30267-3
140. Shi Y, Lammers T. Combining nanomedicine and immunotherapy. Accounts Chem Res. (2019) 52:1543–54. doi: 10.1021/acs.accounts.9b00148
141. Liu Z, Liu H, Cheng J, Wang H, Yang Y, Le J, et al. Strategies and opportunities of micro/nano delivery systems for targeted therapy of ulcerative colitis: focus on underlying mechanisms and future perspectives. Chin Chem Lett. (2024) 35(2):109074. doi: 10.1016/j.cclet.2023.109074
142. Gencay YE, Jasinskyte D, Robert C, Semsey S, Martinez V, Petersen AO, et al. Engineered phage with antibacterial crispr-cas selectively reduce E. Coli burden in mice. Nat Biotechnol. (2024) 42(2):265–74. doi: 10.1038/s41587-023-01759-y
143. Zheng D-W, Dong X, Pan P, Chen K-W, Fan J-X, Cheng S-X, et al. Phage-guided modulation of the gut microbiota of mouse models of colorectal cancer augments their responses to chemotherapy. Nat Biomed Eng. (2019) 3:717–28. doi: 10.1038/s41551-019-0423-2
144. Mulenga M, Rajamanikam A, Kumar S, Muhammad SB, Bhassu S, Samudid C, et al. Revolutionizing colorectal cancer detection: A breakthrough in microbiome data analysis. PloS One. (2025) 20(1):e0316493. doi: 10.1371/journal.pone.0316493
145. Krynicka P, Koulaouzidis G, Skonieczna-Zydecka K, Marlicz W, Koulaouzidis A. Application of raman spectroscopy in non-invasive analysis of the gut microbiota and its impact on gastrointestinal health. Diagnostics. (2025) 15(3):292. doi: 10.3390/diagnostics15030292
146. Mousa WK, Al Ali A. The gut microbiome advances precision medicine and diagnostics for inflammatory bowel diseases. Int J Mol Sci. (2024) 25(20):11259. doi: 10.3390/ijms252011259
147. Feng W, Liu J, Ao H, Yue S, Peng C. Targeting gut microbiota for precision medicine: focusing on the efficacy and toxicity of drugs. Theranostics. (2020) 10:11278–301. doi: 10.7150/thno.47289
148. Gori S, Inno A, Belluomini L, Bocus P, Bisoffi Z, Russo A, et al. Gut microbiota and cancer: how gut microbiota modulates activity, efficacy and toxicity of antitumoral therapy. Crit Rev Oncol Hematol. (2019) 143:139–47. doi: 10.1016/j.critrevonc.2019.09.003
149. Geller LT, Barzily-Rokni M, Danino T, Jonas OH, Shental N, Nejman D, et al. Potential role of intratumor bacteria in mediating tumor resistance to the chemotherapeutic drug gemcitabine. Science. (2017) 357:1156–+. doi: 10.1126/science.aah5043
150. Iida N, Dzutsev A, Stewart CA, Smith L, Bouladoux N, Weingarten RA, et al. Commensal bacteria control cancer response to therapy by modulating the tumor microenvironment. Science. (2013) 342:967–70. doi: 10.1126/science.1240527
151. Pflug N, Kluth S, Vehreschild JJ, Bahlo J, Tacke D, Biehl L, et al. Efficacy of antineoplastic treatment is associated with the use of antibiotics that modulate intestinal microbiota. Oncoimmunology. (2016) 5(6):e1150399. doi: 10.1080/2162402x.2016.1150399
152. Yuan L, Zhang S, Li H, Yang F, Mushtaq N, Ullah S, et al. The influence of gut microbiota dysbiosis to the efficacy of 5-fluorouracil treatment on colorectal cancer. Biomedicine Pharmacotherapy. (2018) 108:184–93. doi: 10.1016/j.biopha.2018.08.165
153. Dahlgren D, Lennernas H. Review on the effect of chemotherapy on the intestinal barrier: epithelial permeability, mucus and bacterial translocation. Biomedicine Pharmacotherapy. (2023) 162:114644. doi: 10.1016/j.biopha.2023.114644
154. Hou X-Y, Zhang P, Du H-Z, Gao Y-Q, Sun R-Q, Qin S-Y, et al. Prevotella contributes to individual response of folfox in colon cancer. Clin Trans Med. (2021) 11(9):e512. doi: 10.1002/ctm2.512
155. Alves NDO, Dalmasso G, Nikitina D, Vaysse A, Ruez R, Ledoux L, et al. The colibactin-producing escherichia coli alters the tumor microenvironment to immunosuppressive lipid overload facilitating colorectal cancer progression and chemoresistance. Gut Microbes. (2024) 16(1):2320291. doi: 10.1080/19490976.2024.2320291
156. Gao Y, Bi D, Xie R, Li M, Guo J, Liu H, et al. Fusobacterium nucleatum enhances the efficacy of pd-L1 blockade in colorectal cancer. Signal Transduction Targeted Ther. (2021) 6(1):398. doi: 10.1038/s41392-021-00795-x
157. Xie Y, Liu F. The role of the gut microbiota in tumor, immunity, and immunotherapy. Front Immunol. (2024) 15:1410928. doi: 10.3389/fimmu.2024.1410928
158. Wang X, Fang Y, Liang W, Wong CC, Qin H, Gao Y, et al. Fusobacterium nucleatum facilitates anti-pd-1 therapy in microsatellite stable colorectal cancer. Cancer Cell. (2024) 43(3):564–74. doi: 10.1016/j.ccell.2024.08.019
159. Wang F, Yin Q, Chen L, Davis MM. Bifidobacterium can mitigate intestinal immunopathology in the context of ctla-4 blockade. Proc Natl Acad Sci United States America. (2018) 115:157–61. doi: 10.1073/pnas.1712901115
160. Lo BC, Kryczek I, Yu J, Vatan L, Caruso R, Matsumoto M, et al. Microbiota-dependent activation of cd4+ T cells induces ctla-4 blockade-associated colitis via fcγ Receptors. Science. (2024) 383:62–70. doi: 10.1126/science.adh8342
161. Haifer C, Paramsothy S, Kaakoush NO, Saikal A, Ghaly S, Yang T, et al. Lyophilized oral fecal microbiota transplantation for ulcerative colitis (Lotus): A randomized, double-blind, placebo-controlled trial. Lancet Gastroenterology Hepatology. (2022) 7:141–51. doi: 10.1016/s2468-1253(21)00400-3
162. Carnero-Gregorio M, Molares-Vila A, Corbalan-Rivas A, Villaverde-Taboada C, Rodriguez-Cerdeira C. Effect of vsl3 probiotic in a patient with glycogen storage disease type ia and irritable bowel disease-like disease. Probiotics Antimicrobial Proteins. (2019) 11:143–9. doi: 10.1007/s12602-017-9372-9
163. Kruis W, Fric P, Pokrotnieks J, Lukas M, Fixa B, Kascák M, et al. Maintaining remission of ulcerative colitis with the probiotic escherichia coli nissle 1917 is as effective as with standard mesalazine. Gut. (2004) 53:1617–23. doi: 10.1136/gut.2003.037747
164. Huang F, Li S, Chen W, Han Y, Yao Y, Yang L, et al. Postoperative probiotics administration attenuates gastrointestinal complications and gut microbiota dysbiosis caused by chemotherapy in colorectal cancer patients. Nutrients. (2023) 15(2):356. doi: 10.3390/nu15020356
165. Carlini M, Grieco M, Spoletini D, Menditto R, Napoleone V, Brachini G, et al. Implementation of the gut microbiota prevents anastomotic leaks in laparoscopic colorectal surgery for cancer: the results of the miracle study. Updates Surg. (2022) 74:1253–62. doi: 10.1007/s13304-022-01305-6
Keywords: gut microbiota, CRC, IBD, immune modulation, SCFAs
Citation: Cui X, Li C, Zhong J, Liu Y, Xiao P, Liu C, Zhao M and Yang W (2025) Gut microbiota — bidirectional modulator: role in inflammatory bowel disease and colorectal cancer. Front. Immunol. 16:1523584. doi: 10.3389/fimmu.2025.1523584
Received: 06 November 2024; Accepted: 08 April 2025;
Published: 30 April 2025.
Edited by:
Lili Sheng, Shanghai University of Traditional Chinese Medicine, ChinaReviewed by:
Sweta Ghosh, University of Louisville, United StatesLia-Mara Ditu, University of Bucharest, Romania
Ji-Xuan Han, Shanghai Jiao Tong University, China
Copyright © 2025 Cui, Li, Zhong, Liu, Xiao, Liu, Zhao and Yang. This is an open-access article distributed under the terms of the Creative Commons Attribution License (CC BY). The use, distribution or reproduction in other forums is permitted, provided the original author(s) and the copyright owner(s) are credited and that the original publication in this journal is cited, in accordance with accepted academic practice. No use, distribution or reproduction is permitted which does not comply with these terms.
*Correspondence: Changfeng Li, Y2ZsaUBqbHUuZWR1LmNu; Wei Yang, eXdlaUBqbHUuZWR1LmNu