- 1Department of Neuroscience and Regenerative Medicine, Medical College of Georgia at Augusta University, Augusta, GA, United States
- 2Center for Biotechnology and Genomic Medicine, Medical College of Georgia at Augusta University, Augusta, GA, United States
Monoamines, including norepinephrine, serotonin, and dopamine, orchestrate a broad spectrum of neurophysiological and homeostatic events. Recent research shows a pivotal role for monoaminergic signaling in modulating neuroinflammation by regulating proinflammatory cytokines and chemokines within the central nervous system. Importantly, this modulation is not unidirectional; released proinflammatory cytokines markedly “feedback” to influence the metabolism of monoamine neurotransmitters, impacting their synthesis, release, and reuptake. This bidirectional interplay significantly links monoaminergic pathways and neuroinflammatory responses. In this review, we summarize current knowledge of the dynamic interactions between monoamine signaling and neuroinflammation, as well as their critical implications for the pathophysiology of neuropsychiatric disorders, including Parkinson’s Disease, Major Depressive Disorder, and Alzheimer’s Disease. By integrating recent findings, we shed light on potential therapeutic targets within these interconnected pathways, providing insights into novel treatment strategies for these devastating disorders.
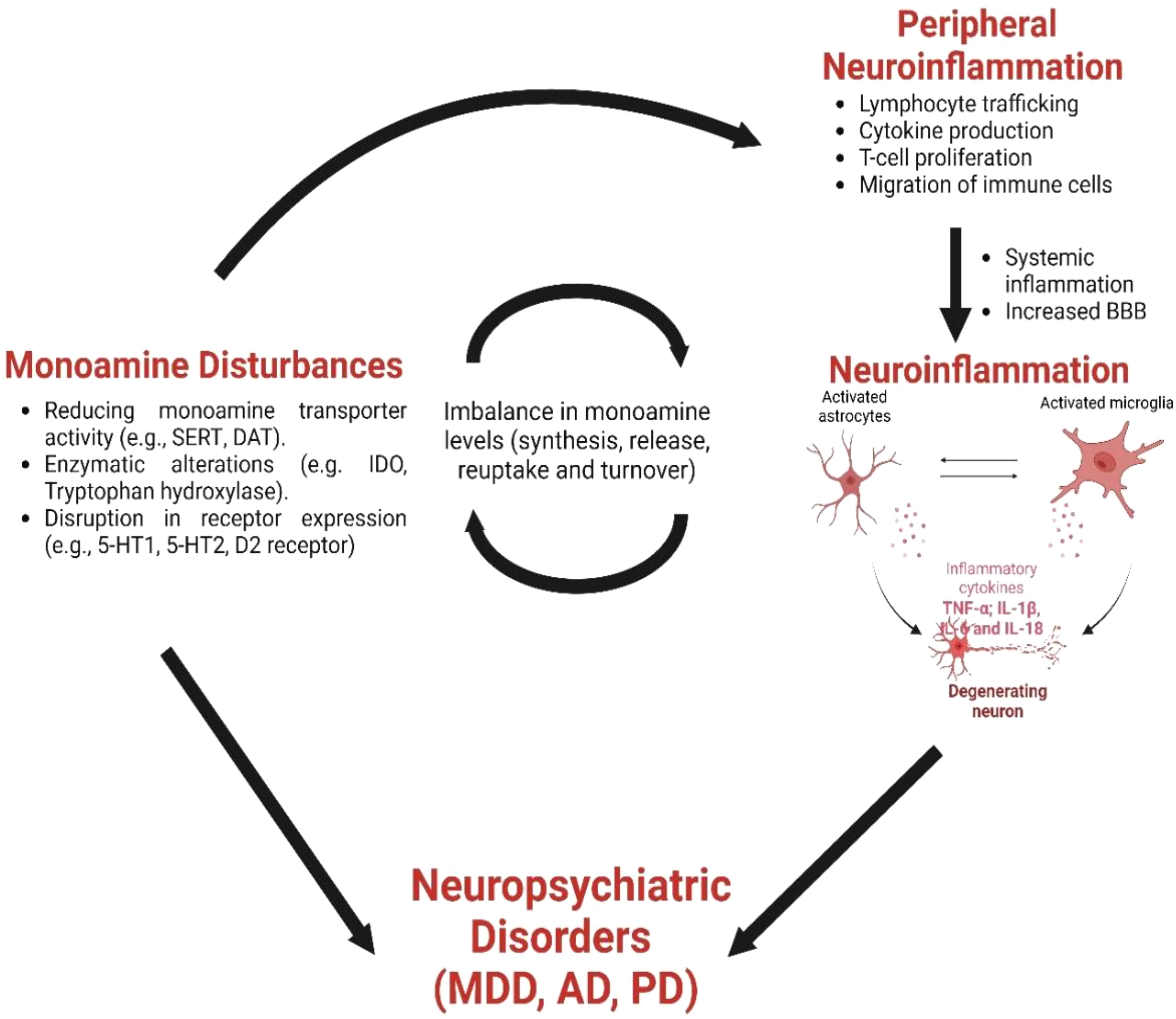
Graphical Abstract. Created with Biorender.com.
1 Introduction
Monoamine neurotransmitters, including norepinephrine (NE), serotonin (5-HT), and dopamine (DA), are important bioactive signaling molecules in the central nervous system (CNS). For example, monoaminergic (MA-ergic) systems regulate the gastrointestinal, respiratory, and cardiovascular systems, as well as modulate mood, cognition, sleep, nociception, temperature, perspiration, and other processes (1). Given their extensive influence, disruptions of these systems produce numerous pathological effects, contributing to the development of neuropsychiatric disorders (2–4).
MA-ergic systems participate in many physiological activities that regulate CNS inflammation (5), which can be initiated in response to various cues such as infection, traumatic brain injury, toxic metabolites, or autoimmune response (5). Neuroinflammation involves local microglial cells, infiltrating immune cells, and cytokines released from these cells and the peripheral nervous system (PNS). Upon infection or neuronal injury, microglia secrete either proinflammatory factors, which enhance cytotoxicity, or anti-inflammatory factors, which aid in wound healing and tissue repair (6). Excessive microglial activation, immune cell infiltration, and the release of proinflammatory cytokines can damage surrounding healthy neural tissue. Additionally, cellular factors released by dying neurons amplify chronic microglial activation and the infiltration of peripheral inflammatory mediators, accelerating further neuronal loss. Proinflammatory cytokines can also dysregulate MA-ergic systems by modulating their synthesis, release, and reuptake, further contributing to neuroinflammation, a common factor in many neurological disorders (7).
In this review, we explore recent findings on the interactions between MA-ergic function and neuroinflammation and briefly discuss the role of these interactions in neurological disorders such as Major Depressive Disorder (MDD), Alzheimer’s Disease (AD), and Parkinson’s Disease (PD). While histamine is also a monoamine involved in CNS function and neuroinflammation (8), this review focuses on NE, 5-HT, and DA due to their direct regulation of mood, cognition, and the pathophysiology of major neuropsychiatric disorders. These monoamine neurotransmitters are also the primary targets of many therapeutic interventions.
While many studies have linked neurological diseases to specific neuroinflammatory changes in the brain, we are continuously discovering that these diseases involve additional neuroinflammatory processes. Neuroinflammation plays a key role in the pathogenesis of depression, AD, and PD (9–11). By delineating the role of MA-ergic systems in regulating neuroinflammation and modulating monoamine neurotransmission by cytokines and chemokines, we endeavor to deepen our comprehension of how these systems contribute to disease pathophysiology. Our goal is to integrate existing knowledge to catalyze future research and the development of novel therapeutic approaches.
2 The monoamine system
The MA-ergic neurotransmitters NE, 5-HT, and DA play important roles in regulating functions within the nervous and immune systems by binding to receptors in the CNS and PNS. Neurochemical and structural changes in brain circuits, particularly in regions like the prefrontal cortex, amygdala, nucleus accumbens, and hippocampus, are linked to neuropsychiatric symptoms, such as depression, which is often associated with dysregulated 5-HT and DA signaling (4, 12, 13). Monoamine receptors, primarily G protein-coupled receptors (GPCRs), except for the 5-HT3 receptor (a ligand-gated ion channel) (14), are involved in cellular responses to neurotransmitters and the modulation of inflammation. GPCR subtypes exhibit distinct affinities for various ligands and influence physiological and inflammatory processes. Many immune cells express multiple GPCRs, regulating various inflammatory pathways (15, 16).
2.1 Norepinephrine
Adrenergic pathways are essential conduits for communication between the nervous and immune systems. NE is synthesized from DA by dopamine β-hydroxylase and is released from the locus coeruleus (LC), acting as a neuromodulator to regulate arousal, stress responses, anxiety, executive control, and memory consolidation. NE also plays a significant role in neuroinflammation, influencing microglia activation and the blood-brain barrier’s integrity (BBB) (17, 18). NE can be converted to epinephrine (EPI) (19), which is involved in the “fight-or-flight” response. Both EPI and NE stimulate adrenergic receptors, common receptors that are classified into three groups: α1 (α1A, α1B, α1D), α2 (α2A, α2B, α2C), and β receptors, all belonging to the G-protein-coupled receptor family.
α1 receptor subtypes activate phospholipase C, through Gαq/11 coupling, elevating levels of inositol triphosphate, Ca2+, and diacylglycerol. In contrast, α2 receptors inhibit adenylyl cyclase, via Gαi/o coupling, decreasing cyclic adenosine monophosphate (cAMP) levels and protein kinase A (PKA) activity (20). Similarly, β receptors, which include three subtypes (β1, β2, and β3), also play an important role in adrenergic signaling. All β receptors associate with Gαs proteins and activate adenylyl cyclase, increasing cAMP levels and PKA signaling. Additionally, the β2 isoform can couple to Gαi/o, allowing for more diverse intracellular effects.
Adrenergic drugs, which target NE and EPI receptors, are commonly used in the treatment of psychiatric conditions. β-blockers like propranolol are sometimes used off-label to manage anxiety, although this is not an FDA-approved indication. Alpha-2 agonists like clonidine are prescribed for anxiety and ADHD, selective NE reuptake inhibitors (SNRIs) like venlafaxine increase NE levels to treat depression, and alpha-1 blockers like prazosin are used to address PTSD-related nightmares. Some of these uses, such as propranolol for anxiety, may not be officially approved but are utilized in clinical practice based on their effects.
2.2 Serotonin
5-HT, a vital neurotransmitter and hormone with diverse functions across various organs, is synthesized from tryptophan, with its rate-limiting step catalyzed by the enzyme tryptophan hydroxylase. 5-HT is primarily produced by enterochromaffin neuroendocrine cells in the gastrointestinal tract (21) and by serotonergic neurons in the raphe nuclei (located in the brainstem) within the CNS (22, 23). These serotonergic neurons are extensively distributed throughout the mammalian brain, making the serotonergic system the CNS’s largest, and perhaps the most complex, efferent system (24). Serotonergic nuclei, such as rostral, dorsal, and medial nuclei, diffuse their projections throughout the CNS, contributing to the regulation of temperature, appetite, sleep cycles, emesis, and sexual behavior. Conversely, caudal nuclei project into the spinal cord, modulating nociception and motor tone (4, 24, 25).
The functions of 5-HT in the CNS are diverse, affecting physiology, cognition, and behavior. Additionally, 5-HT has been implicated in CNS development, acting as a growth factor that guides the proliferation, organization, and maturation of the developing brain (26). It is also stored in blood platelets, highly concentrated in dense granules, and is released during agitation and vasoconstriction (27). Like many other neurotransmitters, 5-HT is usually taken back up by the presynaptic cell (reuptake) or degraded by monoamine oxidase (28). Selective 5-HT reuptake inhibitors (SSRIs), whose mechanism of action is to block 5-HT reuptake, are widely used to treat many psychiatric and mental health conditions (29).
Although only approximately 5% of total bodily 5-HT is found in the CNS, every brain cell is in close proximity to a serotonergic fiber, and all CNS regions express 5-HT receptors (5-HTRs) in a subtype-specific manner (26, 30). Individual neurons have been shown to express multiple 5-HTRs, leading to differential effects of 5-HT on the activity of distinct neurons (30). Furthermore, the serotonergic system interacts with other neurotransmitter systems, such as the catecholaminergic system, to influence numerous physiological processes (31).
5-HT’s actions are mediated by the 15 known receptor subtypes (across 7 mammalian 5-HT receptor classes), its interaction with the 5-HT transporter (SERT), and covalent binding to different effector proteins. Each family of 5-HT receptors has different subtypes, classified by morphology, pharmacologic profiles, and distribution (32). Despite the multitude of CNS functions influenced by 5-HT, its most clinically relevant aspect is its involvement in the pathophysiology of neuropsychiatric disorders.
2.3 Dopamine
DA is a neurotransmitter pivotal to brain function, governing movement control and reward-related behaviors while modulating cognitive functions through molecular substrates linked to plasticity. DA is synthesized from tyrosine through enzymatic reactions that convert it first into L-DOPA and then DA (33). Dysregulated DA signaling is implicated across various neurodegenerative, psychiatric, and autoimmune disorders, often accompanied by CNS neuroinflammation. Dopaminergic neurons, primarily found in specific brain regions such as the substantia nigra pars compacta, the ventral tegmental area (VTA), and the hypothalamus, delineate four principal pathways: the nigrostriatal, the mesolimbic, mesocortical, and the tuberoinfundibular pathways (34). The nigrostriatal pathway is fundamental for motor control, and its dysfunction is associated with PD, characterized by tremors, rigidity, and akinesia (35, 36). The mesocortical pathway, originating from the VTA, influences learning and memory (37) by projecting to various frontal cortex regions. The mesolimbic pathway, also originating from the VTA, regulates motivated behavior (36) through innervation of the ventral striatum, olfactory tubercle, and limbic system. On the other hand, the tuberoinfundibular pathway, originating from the hypothalamic periventricular and arcuate nuclei, modulates prolactin release and milk production.
DA mediates its effects through interactions with five DA receptors (DRs) on target cell membranes, categorized into D1-like (DRD1 and DRD5) and D2-like (DRD2, DRD3, and DRD4) receptor families (38). DRs, belonging to the GPCR class, activate downstream signaling pathways via heterotrimeric G proteins, specifically Gαs/olf, for stimulatory responses and Gαi/o, for inhibitory responses. Activation of D1 class receptors stimulates adenylate cyclase (AC), increasing cAMP levels, while D2-like receptors inhibit AC, decreasing cAMP levels (4, 39). Dopaminergic agonists, such as DA receptor agonists or drugs like pramipexole or ropinirole, mimic DA’s effect by directly activating these receptors (40). These drugs are often used in the treatment of conditions like PD and restless leg syndrome, where dopaminergic signaling is impaired. D1-like receptor agonists tend to increase cAMP levels, whereas D2-like receptor agonists may reduce cAMP levels, influencing neuronal activity and motor control (40, 41).
3 Monoamine signaling in peripheral inflammation
The immune and sympathetic nervous systems are deeply interconnected, with sympathetic nerve fibers innervating primary and secondary lymphoid tissues. These fibers primarily release NE, which regulates immune function by interacting with adrenergic receptors on immune cells, such as the β2-adrenergic receptor. This direct communication enables immune cells to respond to sympathetic signaling, influencing inflammation and immune responses (42). Beyond NE, other monoamines, such as 5-HT and DA, are key regulators of neuroinflammatory processes. Monoamine receptors are expressed on various immune cells such as macrophages, dendritic cells, and T cells, influencing immune function and inflammatory responses. Monoamine signaling influences cytokine secretion and other neuroimmune interactions, which are increasingly recognized as important factors in the development of neuropsychiatric and neurodegenerative diseases.
Further highlighting the overlap between the nervous and immune systems, neurons express pattern-recognition receptors such as Toll-like receptors, along with cytokine receptors. This enables neurons to respond directly to pathogen-associated molecular patterns (PAMPs) and immune-derived signals, further integrating immune and neuronal functions. Additionally, neurotransmitter signaling through monoamine receptors on immune cells helps regulate immune responses, reinforcing the bidirectional communication between these systems (43). This section explores how NE, 5-HT, and DA contribute to peripheral inflammation, with a specific focus on their roles in immune cell activation, cytokine secretion, and neuroinflammatory processes. Understanding these mechanisms provides valuable insight into immune regulation and the pathogenesis of neurological disorders.
3.1 Norepinephrine and peripheral inflammation
NE’s role in regulating peripheral immune responses has been extensively reviewed previously (43–46). NE can be converted into EPI by the enzyme phenylethanolamine N-methyltransferase, and is predominantly released from the adrenal glands, particularly from the adrenal medulla, into the bloodstream. NE and EPI can modulate lymphocyte trafficking, proliferation, and cytokine production, with both pro-inflammatory and anti-inflammatory effects depending on the immune cell type and receptor (45–49). For instance, NE and EPI activate β2 adrenoceptors, which inhibit proinflammatory cytokine production and promote anti-inflammatory cytokine production (45, 46). In contrast, NE and EPI acting on α2 adrenoceptors in monocytes and macrophages promote the production of tumor necrosis factor (TNF) and other cytokines (46, 50, 51). Interestingly, NE’s effects on β2 adrenoceptors can vary. In obese mice, β2 stimulation exerts anti-inflammatory effects, while in lean mice, it induces proinflammatory responses (49). Additionally, β2-adrenergic stimulation inhibits neutrophil functions, such as the formation of extracellular traps in human polymorphonuclear leukocytes (52).
3.2 Serotonin and peripheral inflammation
The role of 5-HT in inflammation and immunity has been widely studied and reviewed (5, 32). 5-HT, primarily synthesized in the gastrointestinal tract and released by platelets during inflammation (53, 54) also modulates immune responses. Notably, peripheral immune cells, including T cells, monocytes, macrophages, and dendritic cells, express one or more 5-HT receptors and possess 5-HT-related machinery transport (e.g. SERT), synthesis (via tryptophan hydroxylase 1), storage (in vesicles), and degradation (via monoamine oxidase). This allows 5HT to influence immune functions, including cellular activation, migration, and cytokine production.
5-HT signaling exerts complex modulatory effects on inflammation and immunity. The role of 5-HT in immune cells, particularly T cells, is of growing interest, as T cells play a central role in immune regulation and inflammation. 5-HT influences T cell responses by modulating T cell proliferation, differentiation, and cytokine production (55–58). It has been shown to promote the differentiation of naive T cells into Th1 or Th17 cells, both of which are involved in inflammatory responses, while its effects on regulatory T cells (Tregs) appear to be more context-dependent, sometimes promoting their differentiation and other times inhibiting it. While serotonergic components are well-documented in general T cell populations, the expression and function of specific 5-HTRs, such as 5-HT1, 5-HT2, 5-HT3, and 5-HT4, as well as SERT and enzymes, such as tryptophan hydroxylase, in T cell subtypes remain limited. The 5-HT1A receptor is known to be expressed on Tregs and has been implicated in promoting their anti-inflammatory functions. In contrast, 5-HT3 receptors, primarily expressed on effector T cells like Th1 and Th17 cells, have been linked to pro-inflammatory responses (5, 26, 59–61).
In addition to its role in regulating T cells, 5-HT regulates macrophage phagocytic function and cytokine secretion (62, 63), and it influences dendritic cell maturation and their ability to promote T-cell activation through modulating chemokine and cytokine release, particularly in response to microbial pathogens (64–67). Additionally, many 5-HT receptors expressed by immune cells have complex and sometimes opposing roles in inflammation. While 5-HT3 receptors are associated with the promotion of inflammation through increased production of pro-inflammatory cytokines, such as IFN-γ and IL-17, 5-HT2A and 5-HT4 receptors may attenuate these responses, possibly by dampening Th1 and Th17 differentiation or promoting Treg function (60, 61, 68). This receptor-specific dichotomy adds to the complexity of 5-HT’s role in modulating immune responses, making it difficult to generalize its pro- or anti-inflammatory effects.
Furthermore, discrepancies in study results and variations in experimental models, species, and even the timing of 5-HT exposure contribute to the ongoing uncertainty surrounding 5-HT’s immune functions. For example, previous research using chemical mitogens like Con A to activate T cells may not fully capture the complexity of in vivo activation, which typically involves interactions with antigen-presenting cells, co-stimulatory signals, and cytokine networks. Discrepancies in the effects of 5-HT on T cells could arise from its differential roles in effector T cells (e.g., Th1, Th17, CD8+ cytotoxic T cells) versus regulatory T cells, as these subsets exhibit distinct signaling pathways, such as IL-2 and mTOR, which are differentially regulated. Its influence on cAMP and mTOR signaling pathways is also likely to vary between effector and regulatory T cells, further complicating its effects. More targeted research is needed to clarify 5-HT’s role in different T cell subtypes, particularly in the context of Th17 and Treg cell interactions (5, 59, 61).
3.3 Dopamine and peripheral inflammation
DA has gained increasing attention for its role in modulating peripheral inflammation, as it both influences and is influenced by inflammatory processes. DA receptors on immune cells regulate the release of pro-inflammatory cytokines, shaping immune responses and contributing to the complex interplay between the nervous and immune systems. Many immune cells, including T cells, B cells, neutrophils, eosinophils, NK cells, dendritic cells, macrophages, microglia, and monocytes, are capable of producing DA at low levels and expressing DA receptors (69, 70). DA acts in an autocrine and paracrine manner, impacting immune cell functions through D1-like and D2-like receptors. These receptors regulate immune cell activation, inhibition, proliferation, and their specific immune functions. For example, DA can reduce reactive oxygen species (ROS) production and migration of human polymorphonuclear leukocytes by activating D1-like receptors, particularly D5R (71). DA also regulates various immune functions, such as cell differentiation, adhesion, migration, cytokine secretion, cytotoxicity, and chemotaxis. Its effects are complex, influencing immune homeostasis and disease, depending on immune cell type, receptor subtype, local concentration, and activation state. For instance, DA can activate normal resting peripheral human T lymphocytes, promoting cell adhesion, trafficking, and cytokine secretion (72–74), but it inhibits activated T cells in functions like cell proliferation, cytokine secretion, and cytotoxicity (75, 76).
DA has been shown to have anti-inflammatory effects, especially in the peripheral dopaminergic system, where activation of DA receptors modulates tissue inflammation and injury in conditions like acute pancreatitis and renal inflammation (77, 78). However, research on DA’s immune regulatory role has shown conflicting results due to variations in experimental models, samples, and methods. DA’s effects depend on factors like immune cell subtype, receptor abundance, and ligand availability, making comparisons across studies difficult (79). While extensive research has explored DA’s role in immune function (79, 80), its complex regulatory mechanisms and potential involvement in peripheral immune responses warrant further investigation.
4 Monoamine regulation of glial cell activation and neuroinflammation
Monoamines are important in regulating glial cell activation and neuroinflammation in the CNS. Glial cells, such as microglia and astrocytes, are essential for maintaining brain homeostasis and responding to stress, injury, or disease. Dysregulated monoamine signaling can lead to excessive activation of these glial cells, triggering neuroinflammation, which is linked to various neurological and psychiatric disorders (81–84). The effects of monoamines on glial function are complex, as they can influence microglial and astrocytic responses in both protective and detrimental ways. In addition, monoamine-induced peripheral inflammation may contribute to neuroinflammation as well. A deeper understanding of how NE, 5-HT, and DA regulate glial cells could open new therapeutic avenues for targeting neuroinflammation in these diseases. This section explores the roles of NE, 5-HT, and DA in glial activation and neuroinflammation, synthesizing key findings on their impact.
4.1 Norepinephrine
Adrenergic receptors are present in varying densities among different types of glial cells (85, 86). Through these receptors, NE mainly exerts anti-inflammatory effects on the CNS. Multiple evidence supporting an anti-inflammatory role of NE come from the neurotoxin N-(2-chloroethyl)-N-ethyl-2-bromobenzylamine (DSP4)-treated animals. DSP4 treatment induces degeneration of central noradrenergic pathways, augments the proinflammatory response to β-amyloid in rat cortex, significantly elevating iNOS, interleukin-beta (IL-1β), and interleukin-6 (IL-6) expression (87). The heightened IL-1β expression in microglia and astrocytes after β-amyloid injection in DSP4-treated rats was mitigated by co-injection with NE or the β-adrenergic receptor agonist isoproterenol. Depletion of NE in aged rats by DSP4, followed by LPS injection to induce systemic inflammation, also increased serum levels of several proinflammatory cytokines, augmented astroglial and microglial activation in the hippocampus, and decreased cognitive performance in a novel object recognition task (88). Similarly, injecting mice with DSP4 induced chronic neuroinflammation and neurodegeneration (89, 90). On the other hand, increasing NE by pharmacological SNRIs, such as desipramine and atomoxetine, enhanced central noradrenergic tone and reduced proinflammatory cytokines IL-1β and TNFα, as well as iNOS and microglial activation markers, in the rat cortex and hippocampus following LPS challenges (91, 92). Desipramine and atomoxetine have also been reported to induce IL-10 and suppressor of cytokine signaling-3 in the rat cortex and hippocampus (93).
β-adrenergic receptors primarily mediate the anti-inflammatory role of NE, and their activation has been shown to reduce proinflammatory responses in microglia and astrocytes induced by inflammatory stimuli such as LPS (92, 94–97). Administration of a β1 adrenergic receptor agonist, xamoterol, suppressed LPS-induced TNF-α production in rat microglia cultures, reducing proinflammatory markers, and attenuating microgliosis and astrogliosis. A β2 adrenergic receptor agonist, clenbuterol, was also able to mitigate LPS-induced expression of TNF-α, IL-6, chemokines RANTES and IP-10, and cell adhesion molecules in rat cortex (98).
NE activation of β-adrenoceptors can exert anti-inflammatory effects by inhibiting the expression of interferon-gamma (IFN-γ)-induced major histocompatibility complex class II molecules (99, 100). Additionally, NE promotes the expression IL-1 receptor antagonist (IL-1ra), and IL-1 type II receptor (IL-1RII), which protect against IL-1β-induced neurotoxicity (101). Administration of the β2-adrenoceptor agonist clenbuterol or the SNRI reboxetine increased the expression of IL-1β, IL-1ra, and IL-1RII in the rat cortex. However, when the rat cortex was activated by systemic LPS, clenbuterol reduced IL-1β expression while maintaining its ability to upregulate IL-1RII and IL-1Ra (98), highlighting the complexity of NE-mediated regulation of the IL-1 system. In addition to IFN-γ and IL-1, NE regulates the NFκB pathway. Pre-treatment with the β2-adrenoceptor agonist clenbuterol suppressed LPS-induced NFκB activation, blocked LPS-induced IκBα phosphorylation and degradation, and downregulated the NFκB-inducible genes TNF-α and ICAM-1 in the cortex and hippocampus (102).
4.2 Serotonin
Unlike NE, which mainly has anti-inflammatory effects, 5-HT plays a dual role in neuroinflammation, with effects that depend on its concentration, receptor subtypes, and cellular contexts. Under basal conditions, 5-HT can promote inflammation, as shown in SERT knockout models, where increased extracellular 5-HT enhances IL-1β and CD11b mRNA expression, indicating elevated microglial activation (103). However, under pathological or injury conditions, 5-HT exerts anti-inflammatory effects, primarily by enhancing microglial neuroprotective functions and promoting astrocytic release of transforming growth factor β (TGF-β1) (104). Different 5-HT receptor subtypes can mediate distinct effects on inflammation. 5-HT1a activation inhibits NF-κB, reducing proinflammatory cytokine release and mediating neuroprotective effects (104). 5-HT7 has also been shown to promote anti-inflammatory and neuroprotective effects in microglia and astrocytes (105–107). On the other hand, 5-HT2a, 5-HT2b and 5-HT2c are linked to microglial activation and increased neuroinflammation by enhancing NF-κB signaling (26, 32). These studies highlight the complexity of 5-HT-induced regulation of neuroinflammation.
5HT receptors are expressed in both microglia and astrocytes. Acting through these receptors, 5-HT influences microglial polarization, shifting their state between proinflammatory (M1) and anti-inflammatory (M2) phenotypes. Activation of 5-HT1a receptors skews microglia toward the M2 phenotype, reducing TNF-α and IL-1β expression, while 5-HT2 receptors are associated with the M1 state, promoting inflammatory responses (108–110). The 5-HT concentration seems to play a decisive role in microglia activation, as high 5-HT concentrations augment TNF-α release in LPS-stimulated microglia (111, 112). Additionally, 5-HT modulates microglial motility, as demonstrated by Krabbe et al., who found that serotonin enhances microglial movement in response to injury, suggesting a role in neuroprotection and tissue repair (113). Astrocytes expressing 5-HT receptors also contribute to the serotonergic regulation of neuroinflammation (113, 114). Pousset et al. found that low-5-HT levels induce both proinflammatory (IL-6, TNF-α) and anti-inflammatory (TGF-β) cytokine expression in rat hippocampal astrocytes (115), again demonstrating the bidirectional regulation by 5-HT. Intriguingly, SSRIs like paroxetine and fluoxetine, which increase extracellular 5-HT, suppress TNF-α, nitric oxide (NO), and NFκB activity, reducing microglial activation and neutrophil infiltration in inflammatory conditions (111, 116–118). More research is needed to fully elucidate the role of 5-HT in neuroinflammation.
4.3 Dopamine
In the CNS, the five DA receptors are differently distributed in brain regions with the relative density: D1 > D2 > D3 > D5 > D4 (119, 120). DA signaling has been found critical for regulating glial cell activation and neuroinflammation. Both astrocytic and microglial cells have been reported to express DA receptors, which may be altered during inflammation (121, 122). Recent studies have revealed multiple mechanisms by which DA regulates neuroinflammation (123).
Using gain-of-function studies, Shao et al. first demonstrated that the astrocytic DRD2 could suppress neuroinflammation through controlled αB-crystallin (CRYAB), a known mediator of neuroinflammation. Analogously, global knockout of Drd2, but not Drd1 or Drd3, pronounced activation of astrocyte and microglia cells, with significant upregulation of proinflammatory mediator genes, including IL-1β, IL-2, IL-6, IL-12β, COX2, and downregulation of the anti-inflammatory cytokine gene, IL-10 (but not TNF-α) (70). In the conditional astrocytic Drd2−knockout mouse, while mesencephalic dopaminergic neurons were maintained, levels of proinflammatory mediators in the substantia nigra were remarkably elevated, indicating the critical role of astrocytic DRD2 in neuroinflammation regulation. The authors further found that DRD2 tightly controlled CRYAB expression, required for Drd2-mediated suppression of proinflammatory response, in astrocytes. Moreover, administration of the DRD2 agonist quinpirole significantly inhibited 1-methyl-4-phenyl-1,2,3,6-tetrahydropyridine (MPTP)-induced activation of astrocytes and microglia, while also suppressing the expression of proinflammatory mediators, and loss of nigral dopaminergic neurons, in control, but not Drd2-null or Cryab-null, mice.
Although DRD2 was found highly expressed in neurons, in vivo selective neuronal deletion of Drd2 only mildly increased levels of proinflammatory mediators. Conversely, despite very low DRD2 levels in resident microglia from healthy mouse brains, DRD2 could be induced in microglia upon activation (122, 124). Likewise, Zhang et al. reported upregulated endogenous DRD2 on astrocytes and microglia following intracerebral hemorrhage (ICH)-induced brain injury (124). They further found that DRD2 knockdown aggravated neurobehavioral deficits, with pronounced expression of cytokines and chemokines (including IL-1β and MCP-1) post-ICH (124). Moreover, the DRD2 agonists, quinpirole and ropinirole, suppressed microglia activation and ameliorated neurobehavioral deficits after ICH, likely mediated by αB-crystallin and enhanced by cytoplasmic binding activity of NF-κB.
DA can also inhibit activation and inflammation of the NLRP3 inflammasome through DRD1 signaling (125), promoting the maturation and release of several proinflammatory cytokines, such as IL-1β and interleukin-18 (IL-18) (126). Yan et al. reported that DA treatment could inhibit IL-1β upregulation by the bacterial neurotoxin nigericin in both microglia and astrocytes, and DA’s inhibitory effects were impaired in Drd1-null cells (125). Moreover, Drd1 knockout mice showed more inflammasome activation, by analyzing IL-1β and IL-18 production or caspase-1 activation, which were impaired in Drd1 and Nlrp3 double-knockout mice. They further found higher NLRP3 expression and less NLRP3 ubiquitination following MPTP neurotoxin treatment (a model for PD) in brains from Drd1-knockout versus control mice (126). Taken together, these results suggest that DRD1 signaling prevents neuroinflammation by inhibiting the NLRP3 inflammasome by promoting NLRP3 ubiquitination. Likewise, Wang et al. reported that DRD1 activation decreased NLRP3-mediated inflammation in ICH-induced mice (127). A DRD1-specific agonist, A68930, inhibited microglia activation, neutrophil infiltration, and expression of NLRP3, caspase 1, and IL-1β, in ICH mice. In contrast to the inhibitory role of DRD1 signaling in neuroinflammation, Wang et al. recently reported that methamphetamine exacerbates an LPS pro-inflammatory response by activating D1-like receptors (128), suggesting complex regulation of neuroinflammation by DA.
Although there is a relatively low expression in the CNS, D3R (DRD3) displays the highest selectivity for DA (Ki ≈ 27 nM), followed by D5R (Ki ≈ 228 nM) and then D4R, D2R, and D1R (129–132). While DRD3 is strongly involved in peripheral and central inflammation, in several experimental systems (133) its role in neuroinflammation regulation remains controversial. For example, Elgueta et al. showed that the D3R-selective antagonist PG01037, when administered intraperitoneally, improves locomotor performance, reduces dopaminergic neuron loss in the nigrostriatal pathway, and promotes astrogliosis and microglial ramification in an MPTP-induced PD mouse model. This study further demonstrates that D3R antagonism protects dopaminergic neurons, enhances motor function, and modulates neuroinflammation by reducing astrocyte reactivity, which may subsequently activate an anti-inflammatory signal to microglia (134). In line with this, IFN-γ, a critical cytokine in stimulating and maintaining glial cell activation, was upregulated in wild-type CD4+ T cells, following DRD3 agonism by PD128907, but decreased by DRD3 deficiency under Th1 differentiation conditions. In contrast, DRD3 deficiency resulted in chronic depression as measured by increased immobility (135–137) and neuroinflammation, as evidenced by increased activation of microglia but not astrocytes, as well as elevated mRNA expression of the proinflammatory cytokines TNF-α, IL-1β, and IL-6, in mesolimbic dopaminergic regions (136). Importantly, microglial inhibition partially ameliorated depressive-like behavior and neuroinflammation in selected mesolimbic reward areas induced by D3R deficiency.
The discrepancy described above could be due to DRD3 cell-specific effects on pro- or anti-inflammatory responses. In Elgueta’s study, CD4+ T cells infiltrated into the CNS after MPTP treatment (134), while low levels of DA selectively activated DRD3 (due to its high affinity), in CD4+ T cells, which preferably differentiated into proinflammatory Th1 cells. Subsequently, IFN-γ and TNF-α, secreted by Th1 CD4+ T cells, enhanced MPTP-induced microglia activation, contributing to neuroinflammation (134). Conversely, in Wang’s studies, microglial DRD3 signaling was proinflammatory, under basal conditions (138). Analogously, DRD3 deficiency resulted in microglia activation and subsequently, chronic inflammation (138).
5 Neuroinflammation effects on monoamine signaling
Various stimuli, including viral and bacterial infections, can disrupt brain neurochemistry, leading to impaired neurotransmission. More specifically, evidence suggests close communication between the immune system and the CNS, as mediated by an immune-neural-synaptic axis. This involves peripheral immune cells infiltrating the brain or activation of glial cells via humoral immune factors that cross the BBB (Figure 1) (36, 37). During neuroinflammation, pro- and anti-inflammatory cytokines, synthesized and released from activated local glial cells and infiltrating immune cells, can in turn regulate monoamine signaling by affecting their synthesis, release, reuptake, and turnover (139–144). Cytokines can also participate in neurogenesis, or mediate cell death of neurons in the CNS, to indirectly affect monoamine synthesis (82, 145).
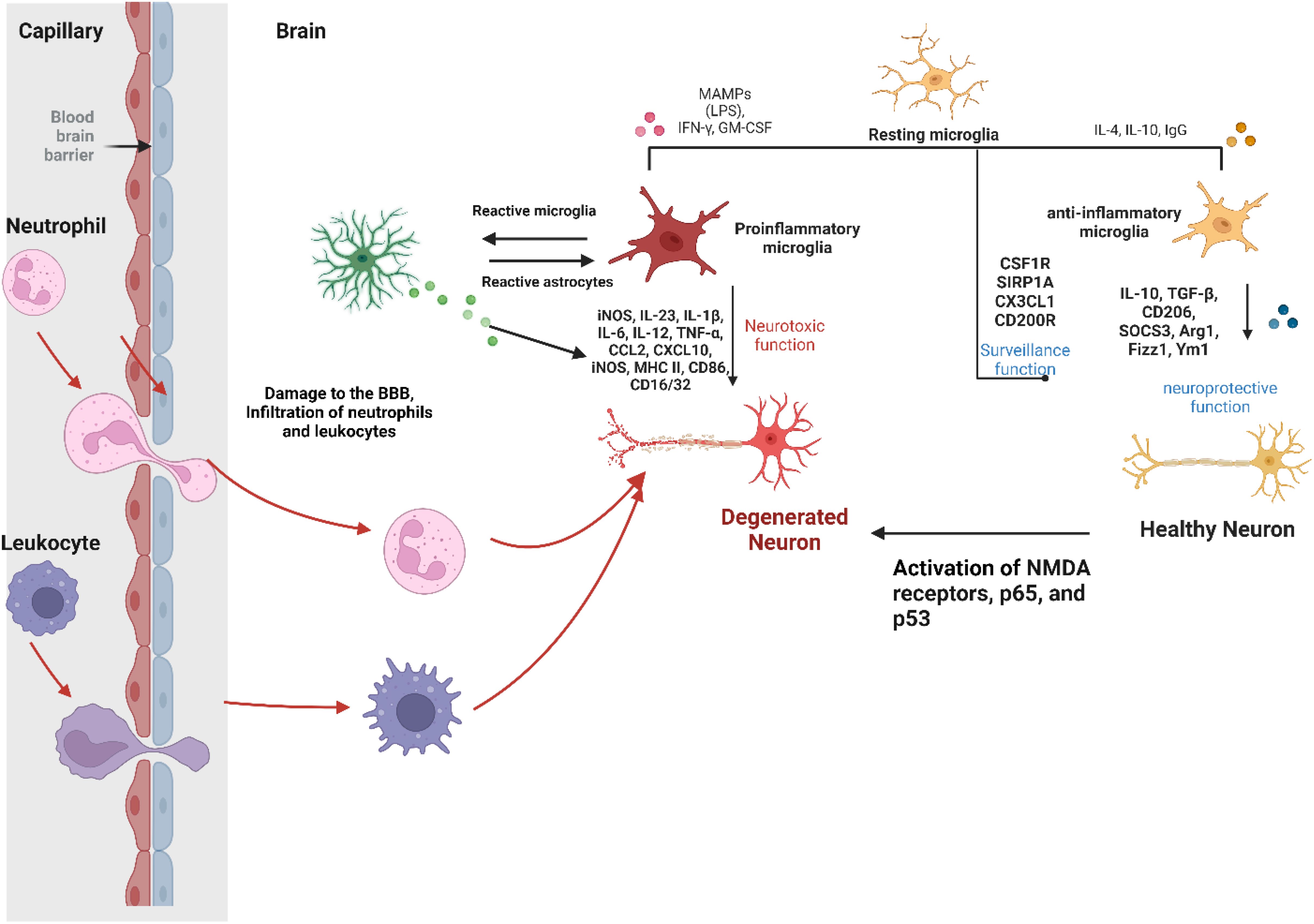
Figure 1. Role of microglia and astrocytes in brain neuroinflammation. Neuroinflammation in the brain, and the role of microglia. Left, damage to the blood-brain barrier (BBB) allows neutrophils and leukocytes into the brain, activating microglia. The central section illustrates microglia in different states: resting, proinflammatory, and anti-inflammatory, withproinflammatory microglia releasing harmful mediators, and anti-inflammatory microglia being protective. Reactive astrocytes interact with activated microglia, affecting inflammatory response. Right, the image contrasts a healthy neuron with a degenerated one, emphasizing how inflammation and receptor activation affect neuronal damage and neurological disorders. Created with Biorender.com.
5.1 Cytokine effects on monoamine synthesis
There are at least two major pathways by which cytokines regulate the synthesis of monoamine neurotransmitters. First, proinflammatory cytokines, including IL-6, IFN‐γ, IL‐1, and TNF-α, may reduce 5-HT synthesis by reducing the availability of its precursor, tryptophan (146–150), via its catabolism into kynurenine by indoleamine-2,3-dioxygenase (IDO), an enzyme present in glial cells and neurons (151). Second, proinflammatory cytokines may interfere with DA synthesis by acting on tetrahydrobiopterin (BH4) (152), a cofactor of three aromatic amino acid hydroxylase enzymes (153) that degrades the amino acid phenylalanine, which is used in the biosynthesis of the neurotransmitters 5-HT, NE, DA, and EPI (154). Although proinflammatory cytokines, including TNFα and IL-1β, can induce BH4 production, they can also stimulate NOS production of NO, a reaction that requires BH4 as a cofactor, decreasing the availability of BH4 for monoamine synthesis (155–157). Proinflammatory cytokines, such as IFN-γ, can also trigger high output of ROS, which can destroy the oxidation-labile BH4, reducing monoamine biosynthesis (158).
5.2 Cytokine effects on monoamine release
Proinflammatory cytokines can also influence monoamine signaling by affecting neurotransmitter release. For instance, it was reported that IL-1α could stimulate DA release by activating type II protein kinase A in PC-12 cells (159), while IL-1β injected directly into the rat anterior hypothalamus, elicited the release of NE, DA, and 5-HT, also increasing their metabolites (160). Moreover, administration of IFN-α to Hepatitis C patients increased reuptake and decreased release of radiolabeled DOPA, the primary precursor of DA (161). This was possibly related to increased cranial production of the tryptophan metabolite kynurenine acid (KA), via activation of IDO, as intrastriatal KA administration (by in vivo micro-dialysis) is known to decrease extracellular DA, in rats (162).
5.3 Cytokine effects on monoamine reuptake
Monoamine transporters (MATs) regulate neurotransmission via reuptake of DA, 5-HT, and NE from extra-neuronal regions and decrease the synaptic availability of monoamine neurotransmitters. There are three main MAT members: the DA transporter (DAT), SERT, and the NE transporter (163–169). Several proinflammatory cytokines, such as IL-1β, TNF-α, IFN-α, and IFN-γ, can upregulate SERT activity (thus reducing availability of extracellular 5-HT) (170–172) while the anti-inflammatory cytokine IL-4 can inhibit SERT activity (173). Similarly, Wu et al. found increased DAT levels following injection of the proinflammatory cytokine TNF-α (174). Many signaling pathways regulate MATs, including mitogen‐activated protein kinase (MAPK) (175) which is activated by increased levels of proinflammatory cytokines such as IL‐1β and TNF‐α, consequently enhancing cell membrane transporter activity and the reuptake of the neurotransmitters 5‐HT, DA, and NE (140, 176, 177).
5.4 Cytokine effects on monoamine turnover
Proinflammatory cytokines may also regulate monoamine signaling through neurotransmitter turnover. For instance, intraperitoneal IL-1 injection increased NE turnover in the whole hypothalamus and several specific hypothalamic nuclei, but not in the medulla oblongata and cerebral cortex (178), while systemic administration of TNF-α into CD-1 mice altered central NE, DA, and 5-HT turnover (179). Likewise, intracerebroventricular infusion of IFN-α into the rat brain promoted 5-HT and DA turnover in the prefrontal cortex and CA2/CA3 hippocampal regions, respectively, as suggested by findings of increased ratios of major metabolites and parental amines (180). A recent study also revealed that significant dopaminergic turnover within the dorsal striatum was induced by IFN-γ treatment (181). Specifically, the effects of proinflammatory cytokines on monoamine turnover largely depend on the specific cytokine, treatment duration, and specific brain region (182).
6 Interconnection of monoamine signaling and neuroinflammation in neuropsychiatric disorders
Research into neuroinflammation and its connection to psychiatric disorders is expanding quickly. A better understanding of this relationship could revolutionize how we treat mental illnesses. This could lead to more targeted and effective therapies that alleviate symptoms and address the underlying causes of these disorders. Classical psychiatric disorders (e.g., schizophrenia, autism, major depression, bipolar disorder, and obsessive-compulsive disorder) and neurological diseases with psychiatric symptoms (e.g. AD and PD) are widely associated with neuroinflammation and immunological abnormalities, in addition to disrupted neurotransmission. Many of these disorders exhibit both chronic neuroinflammation and dysregulated monoamine signaling, suggesting potential interconnections (9, 82, 144). This section discusses the interconnection between each monoamine system and neuroinflammation and how these processes contribute to neurological diseases, particularly MDD, AD, and PD.
6.1 Monoamine signaling and neuroinflammation in major depressive disorder
MDD is a complex and heterogeneous mental health condition characterized by persistent low mood, anhedonia, and cognitive impairments. Its pathophysiology involves disruptions in multiple neurobiological systems, including monoamine neurotransmission and neuroinflammation. A growing body of evidence suggests that neuroinflammation not only contributes to MDD but also interacts with MA-ergic pathways, exacerbating neurotransmitter imbalances and influencing treatment response (183–187). Elevated inflammation in both PNS and CNS, along with glial cell activation, has been consistently linked to MDD (188, 189), highlighting inflammation as a key contributor to disease pathology. Studies suggest that IL-1R/C3/C3aR activation in astrocytes and microglia may contribute to abnormal synaptic pruning in depression, supporting the inflammatory hypothesis of MDD (190). Pro-inflammatory cytokines such as TNF-α, IL-6, and CRP, are consistently elevated in MDD patients (183, 184, 186, 187). These pro-inflammatory cytokines can cross the BBB, influencing brain regions like the insula, which governs emotional processing and cognition (191, 192). Elevated cytokine levels correlate with reduced connectivity in these regions, leading to depressive rumination (193).
Chronic stress, a major environmental risk factor, induces neuroinflammatory processes that disrupt monoamine neurotransmission and modulate monoamine metabolism, reuptake, and receptor function, contributing to imbalances in 5-HT, NE, and DA (194–196). Moreover, chronic stress enhances the kynurenine pathway, leading to higher oxidative stress and neurotoxicity, which in turn disrupts the balance of monoamines and impairs neuronal health (196). In addition to stress, obesity, metabolic dysfunction, genetics, and lifestyle also contribute to inflammation and increase the risk for psychiatric illness (197).
DA, essential for reward processing and motivation, is implicated in anhedonia, a core symptom of MDD (198). Laboratory studies have demonstrated that inflammatory stimuli affect neurotransmitters and reward-processing circuits, including the ventral striatum and ventromedial prefrontal cortex, which are associated with reduced motivation (197). Neuroimaging studies also confirm that increased endogenous inflammation is associated with decreased activation and reduced functional connectivity within reward circuits, reinforcing the association between inflammation and anhedonia (197). Inflammation’s impact on neurotransmitter synthesis, release, and reuptake, particularly of DA and glutamate, appears to drive motivational deficits. For example, inflammation-induced DA dysfunction has been linked to elevated CRP levels, which correlate with reduced DA synthesis and release (197). Chronic stress-induced inflammation further disrupts DA signaling, particularly in the VTA and nucleus accumbens, regions involved in reward processing. These studies collectively suggest that inflammation as a critical factor influencing brain circuits responsible for reward and motivation (197).
In addition to DA signaling, recent findings highlight that inflammation modulates 5-HT signaling (199, 200). Inflammation activates the indoleamine 2,3-dioxygenase (IDO) enzyme, which shifts tryptophan metabolism away from 5-HT production toward the neurotoxic kynurenine pathway (194, 195). This results in 5-HT depletion and increased neurotoxic metabolites, exacerbating depressive symptoms. Inflammation also depletes tryptophan, impairing 5-HT production (201). On the other hand, 5-HT regulates immune responses, and dysregulation of this interaction has been implicated in MDD, particularly in patients with elevated inflammatory markers (200). Genetic variations in the SERT can influence immune system activity, further supporting a bidirectional relationship between 5-HT signaling and neuroinflammation (196).
Given the interplay between inflammation and neurotransmitter systems, it is not surprising that antidepressant responses vary depending on an individual’s inflammatory status. SSRIs increase 5-HT availability but may be less effective in individuals with high inflammation, as cytokines impair 5-HT receptor function (199). In contrast, dopaminergic agents (e.g., bupropion, L-DOPA) may be more effective in patients with elevated CRP or IL-17 levels, as they target inflammation-related DA deficits (200) Indeed, MDD patients with CRP >2 mg/L show increased ventral striatum-ventromedial prefrontal cortex connectivity following L-DOPA administration, suggesting that DA-enhancing treatments may be particularly beneficial in inflammation-associated depression (197). Additionally, elevated IL-17 levels have been identified as a biomarker for antidepressant response, with patients showing better responses to dopaminergic antidepressants like bupropion than to SSRIs alone (200, 202, 203). By contrast, patients with low IL-17 levels respond better to SSRI monotherapy (200). Additionally, TNF-α inhibitors have demonstrated antidepressant effects in high-inflammation patients (204, 205), though some evidence suggests that their combination with SSRIs may reduce efficacy (189, 206). These studies underscore the need for precision medicine in antidepressant selection.
The recognition of both monoamine dysfunction and neuroinflammation has led to the development of treatments targeting these mechanisms. SSRIs and SNRIs aim to increase monoamine availability, and they also support neuroplasticity by increasing brain-derived neurotrophic factor (BDNF) and exhibit modest anti-inflammatory effects, suggesting a connection between inflammation and MDD (207, 208). However, their efficacy can diminish over time due to SERT degradation, particularly when inflammation is involved (209). Some medications can reduce proinflammatory cytokines like TNF-α and IL-6, further emphasizing the role of inflammation in MDD. Combining antidepressants with anti-inflammatory treatments may enhance therapeutic outcomes (210).
Alongside traditional treatments, alternative pharmacological therapies have shown promise. Ketamine, for example, targets NMDA receptors, promotes neuroplasticity, reduces inflammation, and provides rapid relief from suicidal ideation and treatment-resistant depression (211, 212). Cannabinoids, such as THC and CBD, offer both antidepressant and anti-inflammatory effects, with CBD also providing neuroprotective benefits. Psychedelics like psilocybin and ayahuasca, which target the 5-HT2A receptor, have shown potential in reducing neuroinflammation and improving mood, offering long-lasting improvements in mood regulation. However, further research is required to fully understand their mechanisms and long-term effectiveness (212).
The mechanistic links between monoamine signaling and neuroinflammation provide critical insights into the pathophysiology of MDD. Inflammation disrupts 5-HT and DA systems, contributing to treatment resistance in some patients. Identifying biomarkers such as CRP and IL-17 may help guide personalized treatment strategies, optimizing the use of monoaminergic antidepressants, dopaminergic agents, and anti-inflammatory therapies. Future research should focus on refining precision medicine approaches to improve treatment outcomes for individuals with inflammation-associated depression.
6.2 Monoamine signaling and neuroinflammation in Alzheimer’s disease
In AD, pathology often begins with disruptions in monoamine systems: dopaminergic, noradrenergic, and serotonergic signaling (213). Early tau abnormalities target key regions like the locus coeruleus and serotonergic nuclei, initiating the spread of disease to the hippocampus and neocortex. These monoaminergic neurons, with their long, unmyelinated axons and high metabolic demands, are particularly vulnerable to degeneration. This early loss contributes to cognitive decline, neuropsychiatric symptoms (like depression and agitation), and motor deficits (4, 213). For instance, the locus coeruleus, a hub for NE, suffers significant neuronal loss, leading to altered NE transmission and impaired attention and memory (214). Surviving neurons attempt to compensate by sprouting dendrites, ramping up synthesis, and slowing reuptake, which elevates cerebrospinal fluid NE levels (215, 216). This adaptation may cause heightened NE activity via α2A (217, 218), and β2 (219, 220) adrenergic receptors to promote amyloid-beta (Aβ) deposition. Similarly, 5-HT deficits impair cognition and mood regulation, while DA loss affects both motor function and executive abilities. Studies show reduced nucleolar volume and RNA in these neurons, underscoring the profound impact of their dysfunction (213).
As AD progresses, neuroinflammation takes the center stage, driven by microglial activation. Aβ plaques trigger microglia through receptors like toll-like receptors (TLRs) and RAGE, prompting the release of pro-inflammatory cytokines such as TNF-α and IL-1β (10, 164). These cytokines exacerbate neurodegeneration and directly disrupt monoamine signaling (221). Activated microglia increase indoleamine 2,3-dioxygenase (IDO), diverting tryptophan from 5-HT synthesis to the kynurenine pathway, thus depleting 5-HT (222). Inflammation also activates p38 MAPK, accelerating the clearance of DA and NE, further deepening cognitive and mood disturbances (223–225).
Microglial activation is not only involved in neurodegeneration but also plays a critical role in neuropsychiatric symptoms common in AD, including depression, anxiety, agitation, and apathy (226). In regions like the anterior cingulate cortex (part of the default mode network), microglial activation correlates with irritability and negative affect, while elevated cytokine levels track with symptom severity (227). Meanwhile, microglia surrounding Aβ plaques become dysfunctional: they’re meant to phagocytize amyloid, but instead, they fail, perpetuating a cycle of protein accumulation, inflammation, and neurotoxic factor release—ROS, NO, and more. This cascade drives neurodegeneration (228). The kynurenine pathway adds another layer (223), activated microglia convert kynurenine into quinolinic acid, an NMDA receptor agonist. Coupled with impaired glutamate reuptake, this leads to excitotoxicity, reducing BDNF and worsening depressive symptoms—a pattern seen not just in AD but also in diseases like Parkinson’s (229, 230). Some research, like studies in APPswe/PS1ΔE9 mice, suggests that rising cytokines precede drops in SERT activity, with amyloid status further influencing 5-HT function (231).
The interplay among monoamine dysfunction, neuroinflammation, and Aβ accumulation creates a vicious cycle: monoamine deficits fuel early symptoms, neuroinflammation amplifies the damage, and Aβ accumulation keeps the fire burning. Interestingly, disruptions in the default mode network link inflammation to amyloid pathology, suggesting a feedback loop. Some researchers now argue that neuroinflammation may outpace amyloid plaques and tau tangles (232) as a driver of AD progression, with chronic microglial activation accelerating the disease (10, 233). Therapeutically, this opens doors. Targeting microglial activation could break the cycle—post-mortem studies of AD patients treated with an Aβ42 vaccine show reduced microglial activity and plaque burden (234). Recent studies even propose microglial activation as an early diagnostic biomarker, offering a chance to intervene before damage escalates (235, 236). In animal models, deleting receptors like TLR4, TLR6, or CD36 curbs cytokine production and amyloid accumulation, hinting at ways to slow the progression (237–239). In addition, other treatments for neuroinflammation have been explored for AD (240). While not FDA-approved for neurodegenerative diseases, drugs like minocycline, curcumin, vitamin E, and celecoxib, approved for other conditions, target neuroinflammation and may potentially slow disease progression. Pioglitazone, a PPARγ agonist, has shown promise in shifting microglia to a more protective state, which may enhance the clearance of Aβ plaques (240).
Addressing monoamine dysfunction also holds promise: inhibiting IDO could restore 5-HT levels while stabilizing NE might ease cognitive and mood symptoms. Several drugs targeting monoamines and neuroinflammation are being explored in AD (241). Recently, aducanumab (Aduhelm) was approved by the FDA for early-stage AD and mild cognitive impairment, showing promise in reducing amyloid-beta (Aβ) accumulation and halting cognitive decline (4, 241). Lecanemab also demonstrated reduced amyloid markers and slowed cognitive decline in early AD patients. Immunotherapies, mainly targeting tau, are a growing area of research. Some anti-tau vaccines and monoclonal antibodies, like AADvac1, gosuranemab, and tilavonemab, have reached Phase II trials. These treatments utilize the immune system to target Aβ and tau, but their effectiveness and safety are still being evaluated (242). Despite these advances, challenges remain, including recruitment difficulties and high costs of clinical trials. While Aducanumab and Lecanemab provide hope, more targeted therapies are required to address the complex mechanisms of Aβ accumulation, tau aggregation, and neuroinflammation.
6.3 Monoamine signaling and neuroinflammation in Parkinson’s disease
Parkinson’s disease is primarily characterized by the loss of dopaminergic neurons in the substantia nigra, resulting in reduced DA levels and motor symptoms such as tremors, rigidity, and bradykinesia. This neurodegeneration is driven by a complex interplay of genetic, environmental, and immunological factors, contributing to the disease’s multifaceted nature, which shares similarities with autoimmune diseases (243). The pathological mechanisms behind PD are similar to those seen in other neurodegenerative disorders and involve neuron dysfunction, protein aggregation, oxidative stress, mitochondrial dysfunction, and neuroinflammation (244).
Microglia, the resident immune cells of the brain, become activated by the presence of misfolded α-synuclein fibrils, which are a hallmark of PD pathology. While these activated microglia attempt to clear the misfolded proteins, they become chronically activated without success. As dopaminergic neurons die, they release pro-inflammatory mediators such as α-synuclein, MMP-3, and ATP, which further activate microglia and contribute to the release of toxic substances that damage neighboring cells (245). Additionally, pro-inflammatory signals from astrocytes intensify microglial activation, while misfolded proteins drive microglial M1 polarization, which is associated with further neurotoxicity. Studies involving animal models, such as chronic MPTP exposure, show a reduction in the protective M2 phenotype of microglia, which can be reversed by using a mixture of M1 and M2-conditioned media, offering potential therapeutic insights (246).
DA signaling itself plays a significant role in regulating neuroinflammation (247). In PD patients, elevated proinflammatory cytokines, such as IL-1β, TNF-α, and IL-6, have been found in the brain in correlation with dopaminergic neuron loss (248–250). Experimental models, such as the MPTP-induced mouse model, also show a strong association between low DA levels and increased neuroinflammation, including microglial activation and T-cell infiltration. The activated microglia release harmful molecules such as TNFα, INF-γ, ROS, and NO, further contributing to neuronal damage (250–252). Notably, enhancing 5-HT or DA signaling through treatments like the 5-HT1A receptor agonist 8-OH-DPAT and levodopa has been shown to reduce neuroinflammation and alleviate symptoms (253, 254).
Given the critical role of inflammation in PD, immunomodulatory therapies have emerged as a promising avenue for treatment. The inhibition of neuroinflammation has proven to be neuroprotective in various studies, including the use of dominant-negative TNF inhibitors to block soluble TNF signaling, which reduces dopaminergic neuron loss in animal models (255). Anti-inflammatory compounds have also shown promise in restoring DA levels and reversing dopaminergic neuron degeneration in PD models (256). Although nonsteroidal anti-inflammatory drugs (NSAIDs) have yielded inconsistent results in epidemiological studies, likely due to methodological differences (257), targeting glial activation and cytokine shows promise. Drugs like minocycline and dexamethasone, which modulate glial activity, have shown potential benefits, while the NLRP3 inhibitor MCC950 has been demonstrated to reduce microglial activation and alleviate PD symptoms (258). In addition, targeting pro-inflammatory cytokines such as IL-1 and TNF using neutralizing antibodies or activating the GLP1 receptor has demonstrated neuroprotective effects, with clinical trials exploring the use of GLP1 analogs like semaglutide (NCT03659682). Immunomodulation of the peripheral immune system, such as T-cell transfer, has also demonstrated protective effects on dopaminergic neurons (259). Furthermore, immunomodulatory approaches aiming to resolve inflammation, such as the use of resolvin D1, have also been shown to alleviate motor deficits in PD models (260).
While current PD treatments primarily focus on symptom management, typically through maintaining DA levels with inhibitors (monoamine oxidase B and catechol-O-methyl transferase) or DA precursors like levodopa, these therapies do not modify the underlying disease (261). Immunotherapy targeting α-synuclein aggregation, either through active immunization or passive antibody administration, is currently under investigation in clinical trials (262). Research continues to explore potential disease-modifying approaches, with particular attention to how inflammation, neuroinflammation, and monoamine signaling contribute to PD pathophysiology. The complex relationship between DA signaling and neuroinflammation in PD offers opportunities for novel therapeutic strategies. Targeting neuroinflammation, whether through cytokine modulation, glial activation, or α-synuclein aggregation inhibition, holds promise for slowing or halting disease progression. However, more research is needed to clarify the exact causative factors and to identify effective disease-modifying treatments for PD.
7 Conclusion and perspectives
The bidirectional relationship between monoamine signaling and neuroinflammation has emerged as a pivotal factor in the pathophysiology of various neuropsychiatric and neurodegenerative disorders. This interplay, marked by the mutual influence of monoamine neurotransmitters (NE, 5-HT, and DA) and inflammatory processes, is central to understanding diseases like MDD, AD, and PD. Chronic dysregulation of monoamine signaling leads to detrimental consequences for brain function and behavior. Monoamine neurotransmitters also affect immune and glial cells, regulating their migration, proliferation, and inflammasome activation and altering cytokine secretion and various neuroinflammatory processes. Conversely, neuroinflammation disrupts monoamine function by modifying neurotransmitter synthesis, release, reuptake, and turnover, creating a feedback loop that exacerbates the progression of neuropsychiatric and neurodegenerative diseases. Therefore, therapeutic approaches aimed at both normalizing monoamine levels and modulating neuroinflammatory processes offer promising new directions for treatment.
For MDD, the therapeutic landscape is evolving with a focus on combining traditional antidepressants, which primarily target monoamine systems, with anti-inflammatory strategies. Although SSRIs and SNRIs remain the cornerstone of MDD treatment, their anti-inflammatory properties are gaining attention, particularly in treatment-resistant cases. The combination of NSAIDs, cytokine inhibitors, or biologics with conventional antidepressants holds promise in mitigating the neuroinflammation that often accompanies depression (263). In AD, the complex interplay between monoaminergic degeneration, neuroinflammation, Aβ plaque accumulation, and tau pathology requires innovative cocktail therapies. Targeting the monoamine system and neuroinflammation would not only help control neuropsychiatric symptoms, which are prevalent in AD (4), but also hold the potential to slow disease progression. New clinical approaches may combine these approaches with immunotherapies that target Aβ, potentially slowing the progression of the disease and improving patient outcomes. In addition to pharmacological treatments, non-pharmacological interventions such as exercise, dietary modifications, and mindfulness-based therapies are gaining recognition for their ability to modulate neuroinflammatory pathways, potentially enhancing treatment outcomes across all three disorders (264–266). In PD, preclinical studies have highlighted the potential of combining anti-inflammatory treatments with dopaminergic therapies, with NSAIDs, alpha-lipoic acid, and other immunomodulatory agents showing promise in protecting dopaminergic neurons (267). Future therapies will likely focus on neuroprotection while simultaneously addressing inflammation, potentially offering more comprehensive treatment options for PD.
Given the complexity of monoamine signaling and neuroinflammation, advancing therapeutic strategies for diseases involving these systems requires the use of cutting-edge research techniques. Emerging methodologies, such as single-cell RNA sequencing, spatial transcriptomics, and 3D in vitro models, provide unprecedented cellular and molecular insights into the dynamics of glial activation, neurotransmitter signaling, and inflammatory pathways, facilitating the identification of novel therapeutic targets. New organ-on-chip systems and animal models that closely replicate human disease states are available for the preclinical evaluation of novel therapies (268). Imaging techniques like positron emission tomography and magnetic resonance imaging allow monitoring neuroinflammation and assessing the efficacy of treatments in vivo (269). With the advancement of new technologies, researchers are better equipped to address monoamine dysfunction and neuroinflammation for improved treatment strategies.
In conclusion, integrating anti-inflammatory strategies with traditional treatments targeting monoamine dysfunction holds significant promise for improving the management of MDD, AD, and PD. Addressing both systems simultaneously could lead to more effective, holistic therapies that alleviate symptoms, modify disease progression, and enhance overall functional outcomes.
Author contributions
SS: Conceptualization, Supervision, Visualization, Writing – original draft, Writing – review & editing. AP: Conceptualization, Writing – original draft. ED: Investigation, Writing – review & editing. DW: Writing – original draft. KJ: Conceptualization, Project administration, Supervision, Visualization, Writing – review & editing. QW: Conceptualization, Data curation, Formal Analysis, Funding acquisition, Investigation, Methodology, Project administration, Resources, Software, Supervision, Validation, Visualization, Writing – original draft, Writing – review & editing.
Funding
The author(s) declare that financial support was received for the research and/or publication of this article. This work was supported by grants from the National Institutes of Mental Health/National Institute on Aging, MH081917, AG056815, and AG064664 (to QW).
Conflict of interest
QW serves on the Board of Scientific Advisory for Terran Biosciences, Inc. QW and KJ are co-founders of BioQure, LLC.
The remaining authors declare that the research was conducted in the absence of any commercial or financial relationships that could be construed as a potential conflict of interest.
The author(s) declared that they were an editorial board member of Frontiers, at the time of submission. This had no impact on the peer review process and the final decision.
Generative AI statement
The author(s) declare that no Generative AI was used in the creation of this manuscript.
Publisher’s note
All claims expressed in this article are solely those of the authors and do not necessarily represent those of their affiliated organizations, or those of the publisher, the editors and the reviewers. Any product that may be evaluated in this article, or claim that may be made by its manufacturer, is not guaranteed or endorsed by the publisher.
Abbreviations
5-HT, Serotonin; 5-HTR, Serotonin receptor; AC, Adenylate cyclase; AD, Alzheimer’s Disease; BBB, Blood-brain barrier; BH4, Tetrahydrobiopterin; cAMP, Cyclic adenosine monophosphate; CNS, Central nervous system; CRYAB, controlled αB-crystallin; DA, Dopamine; DAT, Dopamine transporters; DMN, Default mode network; DR, Dopamine receptor; DRD2, Astrocytic D2 receptor; DSP4, N-(2-chloroethyl)-N-ethyl-2-bromobenzylamine; EPI, Epinephrine; GPCRs, G protein-coupled receptors; ICH, intracerebral hemorrhage; IDO, Indoleamine-2,3-dioxygenase; IFN-γ, Interferon-gamma; IL-18, Interleukin-18; IL-1Ra, IL-1 receptor antagonist; IL-1RII, IL-1 type II receptor; IL-1β, Interleukin-beta; IL-6, Iinterleukin-6; iNOS, nitric oxide synthase; KA, Kynurenic acid; LC, Locus coeruleus; LPS, Lipopolysaccharide; MA-ergic, Monoaminergic; MAPK, Mitrogen-activated protein kinase; MAT, Monoamine transporters; MDD, Major Depressive Disorder; MPTP, 1-methyl-4-phenyl-1,2,3,6-tetrahydropyridine; NE, Norepinephrine; NO, Nitric oxide; PAMP, Pathogen-associated molecular patterns; PD, Parkinson’s Disease; PKA, Protein Kinase A; PNS, Peripheral nervous system; ROS, Reactive oxygen species; SERT, Serotonin transporter; SNRI, Selective norepinephrine reuptake inhibitor; SSRIs, Selective serotonin reuptake inhibitors; TH, Tyrosine hydroxylase; TNF, Tumor necrosis factor; VTA, Ventral tegmental area.
References
1. Ng J, Papandreou A, Heales SJ, Kurian MA. Monoamine neurotransmitter disorders–clinical advances and future perspectives. Nat Rev Neurol. (2015) 11:567–84. doi: 10.1038/nrneurol.2015.172
2. Jiang Y, Zou D, Li Y, Gu S, Dong J, Ma X, et al. Monoamine neurotransmitters control basic emotions and affect major depressive disorders. Pharm (Basel). (2022) 15(10):1203. doi: 10.3390/ph15101203
3. Doummar D, Moussa F, Nougues MC, Ravelli C, Louha M, Whalen S, et al. Monoamine neurotransmitters and movement disorders in children and adults. Rev Neurol (Paris). (2018) 174:581–8. doi: 10.1016/j.neurol.2018.07.002
4. Saggu S, Bai A, Aida M, Rehman H, Pless A, Ware D, et al. Monoamine alterations in Alzheimer’s disease and their implications in comorbid neuropsychiatric symptoms. Geroscience. (2025) 47(1):457–82. doi: 10.1007/s11357-024-01359-x
5. Wu H, Denna TH, Storkersen JN, Gerriets VA. Beyond a neurotransmitter: The role of serotonin in inflammation and immunity. Pharmacol Res. (2019) 140:100–14. doi: 10.1016/j.phrs.2018.06.015
6. Subhramanyam CS, Wang C, Hu Q, Dheen ST. Microglia-mediated neuroinflammation in neurodegenerative diseases. Semin Cell Dev Biol. (2019) 94:112–20. doi: 10.1016/j.semcdb.2019.05.004
7. Gilhus NE, Deuschl G. Neuroinflammation - a common thread in neurological disorders. Nat Rev Neurol. (2019) 15:429–30. doi: 10.1038/s41582-019-0227-8
8. Carthy E, Ellender T. Histamine, neuroinflammation and neurodevelopment: A review. Front Neurosci. (2021) 15. doi: 10.3389/fnins.2021.680214
9. Wang Q, Liu Y, Zhou J. Neuroinflammation in Parkinson’s disease and its potential as therapeutic target. Transl Neurodegener. (2015) 4:19. doi: 10.1186/s40035-015-0042-0
10. Heneka MT, Carson MJ, El Khoury J, Landreth GE, Brosseron F, Feinstein DL, et al. Neuroinflammation in alzheimer’s disease. Lancet Neurol. (2015) 14(4):388–405. doi: 10.1016/S1474-4422(15)70016-5
11. Troubat R, Barone P, Leman S, Desmidt T, Cressant A, Atanasova B, et al. Neuroinflammation and depression: A review. Eur J Neurosci. (2021) 53:151–71. doi: 10.1111/ejn.14720
12. Duman RS, Aghajanian GK, Sanacora G, Krystal JH. Synaptic plasticity and depression: new insights from stress and rapid-acting antidepressants. Nat Med. (2016) 22:238–49. doi: 10.1038/nm.4050
13. Jesulola E, Micalos P, Baguley IJ. Understanding the pathophysiology of depression: From monoamines to the neurogenesis hypothesis model-are we there yet? Behav Brain Res. (2018) 341:79–90. doi: 10.1016/j.bbr.2017.12.025
14. Rosenbaum DM, Rasmussen SG, Kobilka BK. The structure and function of G-protein-coupled receptors. Nature. (2009) 459:356–63. doi: 10.1038/nature08144
15. Sun L, Ye RD. Role of G protein-coupled receptors in inflammation. Acta Pharmacol Sin. (2012) 33:342–50. doi: 10.1038/aps.2011.200
16. Ge YJ, Liao QW, Xu YC, Zhao Q, Wu BL, Ye RD. Anti-inflammatory signaling through G protein-coupled receptors. Acta Pharmacol Sin. (2020) 41:1531–8. doi: 10.1038/s41401-020-00523-1
17. Zou H-L, Li J, Zhou J-L, Yi X, Cao S. Effects of norepinephrine on microglial neuroinflammation and neuropathic pain. Ibrain. (2021) 7:309–17. doi: 10.1002/ibra.12001
18. Giorgi FS, Saccaro LF, Galgani A, Busceti CL, Biagioni F, Frati A, et al. The role of Locus Coeruleus in neuroinflammation occurring in Alzheimer’s disease. Brain Res Bull. (2019) 153:47–58. doi: 10.1016/j.brainresbull.2019.08.007
19. Kandel ER, Schwartz JH, Jessell TM. Principles of Neural Science. 4th ed. New York: McGraw-Hill, Health Professions Division (2000).
20. Theibert AB. Neurotransmitter systems II: monoamines, purines, neuropeptides, & Unconventional neurotransmitters. In: Amthor FR, Theibert AB, Standaert DG, Roberson ED, editors. Essentials of Modern Neuroscience. McGraw Hill, New York, NY (2020).
21. Racké K, Reimann A, Schwörer H, Kilbinger H. Regulation of 5-HT release from enterochromaffin cells. Behav Brain Res. (1996) 73(1-2):83–7. doi: 10.1016/0166-4328(96)00075-7
22. Hornung JP. The human raphe nuclei and the serotonergic system. J Chem Neuroanat. (2003) 26:331–43. doi: 10.1016/j.jchemneu.2003.10.002
23. Jacobs BL, Azmitia EC. Structure and function of the brain serotonin system. Physiol Rev. (1992) 72:165–229. doi: 10.1152/physrev.1992.72.1.165
24. Berger M, Gray JA, Roth BL. The expanded biology of serotonin. Annu Rev Med. (2009) 60:355–66. doi: 10.1146/annurev.med.60.042307.110802
25. Mohammad-Zadeh L, Moses L, Gwaltney-Brant S. Serotonin: a review. J Vet Pharmacol Ther. (2008) 31:187–99. doi: 10.1111/j.1365-2885.2008.00944.x
26. Shajib MS, Khan WI. The role of serotonin and its receptors in activation of immune responses and inflammation. Acta Physiol. (2015) 213:561–74. doi: 10.1111/apha.2015.213.issue-3
27. Brenner B, Harney JT, Ahmed BA, Jeffus BC, Unal R, Mehta JL, et al. Plasma serotonin levels and the platelet serotonin transporter. J Neurochem. (2007) 102:206–15. doi: 10.1111/j.1471-4159.2007.04542.x
28. Prah A, Purg M, Stare J, Vianello R, Mavri J. How monoamine oxidase A decomposes serotonin: an empirical valence bond simulation of the reactive step. J Phys Chem B. (2020) 124:8259–65. doi: 10.1021/acs.jpcb.0c06502
29. Chu A, Wadhwa R. Selective Serotonin Reuptake Inhibitors. StatPearls. Treasure Island (FL: StatPearls Publishing Copyright © (2023). StatPearls Publishing LLC.; 2023.
30. Kishore S, Stamm S. The snoRNA HBII-52 regulates alternative splicing of the serotonin receptor 2C. science. (2006) 311:230–2. doi: 10.1126/science.1118265
31. D’Souza UM, Craig IW. Functional polymorphisms in dopamine and serotonin pathway genes. Hum Mutation. (2006) 27:1–13. doi: 10.1002/(ISSN)1098-1004
32. Herr N, Bode C, Duerschmied D. The effects of serotonin in immune cells. Front Cardiovasc Med. (2017) 4:48. doi: 10.3389/fcvm.2017.00048
33. Speranza L, di Porzio U, Viggiano D, de Donato A, Volpicelli F. Dopamine: the neuromodulator of long-term synaptic plasticity, reward and movement control. Cells. (2021) 10(4):735. doi: 10.3390/cells10040735
34. Jaber M, Robinson SW, Missale C, Caron MG. Dopamine receptors and brain function. Neuropharmacology. (1996) 35:1503–19. doi: 10.1016/S0028-3908(96)00100-1
35. Lang AE, Lozano AM. Parkinson’s disease. New Engl J Med. (1998) 339:1130–43. doi: 10.1056/NEJM199810153391607
36. Koob GF, Bloom FE. Cellular and molecular mechanisms of drug dependence. Science. (1988) 242:715–23. doi: 10.1126/science.2903550
37. Feldman R, Limbird L, Nadeau J, Fitzgerald G, Robertson D, Wood A. Dynamic regulation of leukocyte beta adrenergic receptor-agonist interactions by physiological changes in circulating catecholamines. J Clin Investigat. (1983) 72:164–70. doi: 10.1172/JCI110954
38. Strange PG. Dopamine receptors: structure and function. Prog Brain Res. (1993) 99:167–79. doi: 10.1016/S0079-6123(08)61345-X
39. Li M, Zhou L, Sun X, Yang Y, Zhang C, Wang T, et al. Dopamine, a co-regulatory component, bridges the central nervous system and the immune system. Biomed Pharmacother. (2022) 145:112458. doi: 10.1016/j.biopha.2021.112458
40. Yaman B. Perspective chapter: the role of dopamine receptors in neuropsychiatric diseases. In: Chandra Yenisetti SC, Koza Z, Kumar D, Singh SK, Ganeshpurkar A, Modi P, Das A, editors. Parkinson’s Disease - Animal Models, Current Therapies and Clinical Trials. IntechOpen, Rijeka (2023).
41. Lee TK, Yankee EL. A review on Parkinson’s disease treatment. Neurosciences. (2021) 8:222. doi: 10.20517/2347-8659.2020.58
42. Sharma D, Farrar JD. Adrenergic regulation of immune cell function and inflammation. Semin Immunopathol. (2020) 42(6):709–17. doi: 10.1007/s00281-020-00829-6
43. Chavan SS, Pavlov VA, Tracey KJ. Mechanisms and therapeutic relevance of neuro-immune communication. Immunity. (2017) 46:927–42. doi: 10.1016/j.immuni.2017.06.008
44. Elenkov I. “Neuroendocrine effects on immune system”. In: Feingold KR, Anawalt B, Boyce A, Chrousos G, Dungan K, Grossman A, et al, editors. Endotext [Internet]. South Dartmouth (MA): MDText.com, Inc. (2000).
45. Elenkov IJ, Wilder RL, Chrousos GP, Vizi ES. The sympathetic nerve–an integrative interface between two supersystems: the brain and the immune system. Pharmacol Rev. (2000) 52:595–638. doi: 10.1016/S0031-6997(24)01470-4
46. Sternberg EM. Neural regulation of innate immunity: a coordinated nonspecific host response to pathogens. Nat Rev Immunol. (2006) 6:318–28. doi: 10.1038/nri1810
47. Pavlov VA, Tracey KJ. Neural regulators of innate immune responses and inflammation. Cell Mol Life Sci. (2004) 61:2322–31. doi: 10.1007/s00018-004-4102-3
48. Marino F, Cosentino M. Adrenergic modulation of immune cells: an update. Amino Acids. (2013) 45:55–71. doi: 10.1007/s00726-011-1186-6
49. Galvez I, Martin-Cordero L, HinChado MD, Alvarez-Barrientos A, Ortega E. Anti-inflammatory effect of beta2 adrenergic stimulation on circulating monocytes with a pro-inflammatory state in high-fat diet-induced obesity. Brain Behav Immun. (2019) 80:564–72. doi: 10.1016/j.bbi.2019.04.042
50. Miksa M, Das P, Zhou M, Wu R, Dong W, Ji Y, et al. Pivotal role of the alpha(2A)-adrenoceptor in producing inflammation and organ injury in a rat model of sepsis. PloS One. (2009) 4(5):e5504. doi: 10.1371/journal.pone.0005504
51. Grisanti LA, Woster AP, Dahlman J, Sauter ER, Combs CK, Porter JE. alpha1-adrenergic receptors positively regulate Toll-like receptor cytokine production from human monocytes and macrophages. J Pharmacol Exp Ther. (2011) 338:648–57. doi: 10.1124/jpet.110.178012
52. Guryanova S, Ferberg A, Sigmatulin I. Inflammatory response modulation by epinephrine and norepinephrine. RUDN J Med. (2023) 27:329–41. doi: 10.22363/2313-0245-2023-27-3-329-341
53. Mossner R, Lesch KP. Role of serotonin in the immune system and in neuroimmune interactions. Brain Behav Immun. (1998) 12:249–71. doi: 10.1006/brbi.1998.0532
54. Duerschmied D, Canault M, Lievens D, Brill A, Cifuni SM, Bader M, et al. Serotonin stimulates platelet receptor shedding by tumor necrosis factor-alpha-converting enzyme (ADAM17). J Thromb Haemost. (2009) 7:1163–71. doi: 10.1111/j.1538-7836.2009.03476.x
55. Fazzino F, Montes C, Urbina M, Carreira I, Lima L. Serotonin transporter is differentially localized in subpopulations of lymphocytes of major depression patients. Effect of fluoxetine on proliferation. J Neuroimmunol. (2008) 196:173–80. doi: 10.1016/j.jneuroim.2008.03.012
56. Edgar VA, Genaro AM, Cremaschi G, Sterin-Borda L. Fluoxetine action on murine T-lymphocyte proliferation: participation of PKC activation and calcium mobilisation. Cell Signal. (1998) 10:721–6. doi: 10.1016/S0898-6568(98)00016-3
57. Fazzino F, Urbina M, Cedeno N, Lima L. Fluoxetine treatment to rats modifies serotonin transporter and cAMP in lymphocytes, CD4+ and CD8+ subpopulations and interleukins 2 and 4. Int Immunopharmacol. (2009) 9:463–7. doi: 10.1016/j.intimp.2009.01.011
58. Sacramento PM, Monteiro C, Dias ASO, Kasahara TM, Ferreira TB, Hygino J, et al. Serotonin decreases the production of Th1/Th17 cytokines and elevates the frequency of regulatory CD4(+) T-cell subsets in multiple sclerosis patients. Eur J Immunol. (2018) 48:1376–88. doi: 10.1002/eji.201847525
59. Kanova M, Kohout P. Serotonin—Its synthesis and roles in the healthy and the critically ill. Int J Mol Sci. (2021) 22:4837. doi: 10.3390/ijms22094837
60. Imamdin A, van der Vorst EPC. Exploring the role of serotonin as an immune modulatory component in cardiovascular diseases. Int J Mol Sci. (2023) 24:1549. doi: 10.3390/ijms24021549
61. Wan M, Ding L, Wang D, Han J, Gao P. Serotonin: A potent immune cell modulator in autoimmune diseases. Front Immunol. (2020) 11:186. doi: 10.3389/fimmu.2020.00186
62. Freire-Garabal M, Nunez MJ, Balboa J, Lopez-Delgado P, Gallego R, Garcia-Caballero T, et al. Serotonin upregulates the activity of phagocytosis through 5-HT1A receptors. Br J Pharmacol. (2003) 139(2):457–63. doi: 10.1038/sj.bjp.0705188
63. de Las Casas-Engel M, Corbi AL. Serotonin modulation of macrophage polarization: inflammation and beyond. Adv Exp Med Biol. (2014) 824:89–115. doi: 10.1007/978-3-319-07320-0_9
64. Idzko M, Panther E, Stratz C, Muller T, Bayer H, Zissel G, et al. The serotoninergic receptors of human dendritic cells: identification and coupling to cytokine release. J Immunol. (2004) 172:6011–9. doi: 10.4049/jimmunol.172.10.6011
65. Kim JJ, Bridle BW, Ghia JE, Wang H, Syed SN, Manocha MM, et al. Targeted inhibition of serotonin type 7 (5-HT7) receptor function modulates immune responses and reduces the severity of intestinal inflammation. J Immunol. (2013) 190:4795–804. doi: 10.4049/jimmunol.1201887
66. Holst K, Guseva D, Schindler S, Sixt M, Braun A, Chopra H, et al. The serotonin receptor 5-HT(7)R regulates the morphology and migratory properties of dendritic cells. J Cell Sci. (2015) 128(15):2866–80. doi: 10.1242/jcs.167999
67. Szabo A, Gogolak P, Koncz G, Foldvari Z, Pazmandi K, Miltner N, et al. Immunomodulatory capacity of the serotonin receptor 5-HT2B in a subset of human dendritic cells. Sci Rep. (2018) 8:1765. doi: 10.1038/s41598-018-20173-y
68. Chen B-R, Wu T, Chen T-H, Wang Y. Neuroimmune interactions and their roles in neurodegenerative diseases. Fundam Res. (2024) 4:251–61. doi: 10.1016/j.fmre.2023.04.002
69. Moore SC, Vaz de Castro PAS, Yaqub D, Jose PA, Armando I. Anti-inflammatory effects of peripheral dopamine. Int J Mol Sci. (2023) 24:13816. doi: 10.3390/ijms241813816
70. Shao W, Zhang S-z, Tang M, Zhang X-h, Zhou Z, Yin Y-q, et al. Suppression of neuroinflammation by astrocytic dopamine D2 receptors via αB-crystallin. Nature. (2013) 494:90–4. doi: 10.1038/nature11748
71. Marino F, Pinoli M, Rasini E, Martini S, Luini A, Pulze L, et al. Dopaminergic inhibition of human neutrophils is exerted through D1-like receptors and affected by bacterial infection. Immunology. (2022) 167:508–27. doi: 10.1111/imm.v167.4
72. Levite M, Chowers Y, Ganor Y, Besser M, Hershkovits R, Cahalon L. Dopamine interacts directly with its D3 and D2 receptors on normal human T cells, and activates beta1 integrin function. Eur J Immunol. (2001) 31:3504–12. doi: 10.1002/1521-4141(200112)31:12<3504::AID-IMMU3504>3.0.CO;2-F
73. Mori M, Oda T, Nishiyama K, Serikawa T, Yamada J, Ichiyama A. A single serine:pyruvate aminotransferase gene on rat chromosome 9q34-q36. Genomics. (1992) 13:686–9. doi: 10.1016/0888-7543(92)90142-F
74. Besser MJ, Ganor Y, Levite M. Dopamine by itself activates either D2, D3 or D1/D5 dopaminergic receptors in normal human T-cells and triggers the selective secretion of either IL-10, TNFalpha or both. J Neuroimmunol. (2005) 169:161–71. doi: 10.1016/j.jneuroim.2005.07.013
75. Saha B, Mondal AC, Basu S, Dasgupta PS. Circulating dopamine level, in lung carcinoma patients, inhibits proliferation and cytotoxicity of CD4+ and CD8+ T cells by D1 dopamine receptors: an in vitro analysis. Int Immunopharmacol. (2001) 1:1363–74. doi: 10.1016/S1567-5769(01)00068-6
76. Ghosh MC, Mondal AC, Basu S, Banerjee S, Majumder J, Bhattacharya D, et al. Dopamine inhibits cytokine release and expression of tyrosine kinases, Lck and Fyn in activated T cells. Int Immunopharmacol. (2003) 3:1019–26. doi: 10.1016/S1567-5769(03)00100-0
77. Xia Q-P, Cheng Z-Y, He L. The modulatory role of dopamine receptors in brain neuroinflammation. Int Immunopharmacol. (2019) 76:105908. doi: 10.1016/j.intimp.2019.105908
78. Han X, Li B, Ye X, Mulatibieke T, Wu J, Dai J, et al. Dopamine D2 receptor signalling controls inflammation in acute pancreatitis via a PP2A-dependent Akt/NF-κB signalling pathway. Br J Pharmacol. (2017) 174:4751–70. doi: 10.1111/bph.v174.24
79. Thomas Broome S, Louangaphay K, Keay KA, Leggio GM, Musumeci G, Castorina A. Dopamine: an immune transmitter. Neural Regen Res. (2020) 15:2173–85. doi: 10.4103/1673-5374.284976
80. Channer B, Matt SM, Nickoloff-Bybel EA, Pappa V, Agarwal Y, Wickman J, et al. Dopamine, immunity, and disease. Pharmacol Rev. (2023) 75:62–158. doi: 10.1124/pharmrev.122.000618
81. Lee J-S, Lee S-B, Kim D-W, Shin N, Jeong S-J, Yang C-H, et al. Social isolation–related depression accelerates ethanol intake via microglia-derived neuroinflammation. Sci Adv. (2021) 7:eabj3400. doi: 10.1126/sciadv.abj3400
82. Leite JA, Orellana AMM, Kinoshita PF, de Mello NP, Scavone C, Kawamoto EM. “Mechanisms of Neuroinflammation”. In: Neuroinflammation and Neurotransmission Mechanisms Involved in Neuropsychiatric Disorders. IntechOpen: London, UK: (2017). https://scholar.google.com/scholar_lookup?title=Mechanisms%20of%20Neuroinflammation&author=J.A.%20Leite&author=A.M.M.%20Orellana&author=P.F.%20Kinoshita&author=N.P.%20de%20Mello&author=C.%20Scavone&publication_year=2017&.
83. Gao C, Jiang J, Tan Y, Chen S. Microglia in neurodegenerative diseases: mechanism and potential therapeutic targets. Signal Transduct Target Ther. (2023) 8:359. doi: 10.1038/s41392-023-01588-0
84. Brisch R, Wojtylak S, Saniotis A, Steiner J, Gos T, Kumaratilake J, et al. The role of microglia in neuropsychiatric disorders and suicide. Eur Arch Psychiatry Clin Neurosci. (2022) 272:929–45. doi: 10.1007/s00406-021-01334-z
85. Hertz L, Lovatt D, Goldman SA, Nedergaard M. Adrenoceptors in brain: cellular gene expression and effects on astrocytic metabolism and [Ca(2+)]i. Neurochem Int. (2010) 57:411–20. doi: 10.1016/j.neuint.2010.03.019
86. Mantyh PW, Rogers SD, Allen CJ, Catton MD, Ghilardi JR, Levin LA, et al. Beta 2-adrenergic receptors are expressed by glia in vivo in the normal and injured central nervous system in the rat, rabbit, and human. J Neurosci. (1995) 15::152–64. doi: 10.1523/JNEUROSCI.15-01-00152.1995
87. Heneka MT, Galea E, Gavriluyk V, Dumitrescu-Ozimek L, Daeschner J, O’Banion MK, et al. Noradrenergic depletion potentiates beta -amyloid-induced cortical inflammation: implications for Alzheimer’s disease. J Neurosci. (2002) 22:2434–42. doi: 10.1523/JNEUROSCI.22-07-02434.2002
88. Bharani KL, Derex R, Granholm AC, Ledreux A. A noradrenergic lesion aggravates the effects of systemic inflammation on the hippocampus of aged rats. PloS One. (2017) 12:e0189821. doi: 10.1371/journal.pone.0189821
89. Pugh PL, Vidgeon-Hart MP, Ashmeade T, Culbert AA, Seymour Z, Perren MJ, et al. Repeated administration of the noradrenergic neurotoxin N-(2-chloroethyl)-N-ethyl-2-bromobenzylamine (DSP-4) modulates neuroinflammation and amyloid plaque load in mice bearing amyloid precursor protein and presenilin-1 mutant transgenes. J Neuroinflamm. (2007) 4:8. doi: 10.1186/1742-2094-4-8
90. Song S, Jiang L, Oyarzabal EA, Wilson B, Li Z, Shih YI, et al. Loss of brain norepinephrine elicits neuroinflammation-mediated oxidative injury and selective caudo-rostral neurodegeneration. Mol Neurobiol. (2019) 56:2653–69. doi: 10.1007/s12035-018-1235-1
91. O’Sullivan JB, Ryan KM, Curtin NM, Harkin A, Connor TJ. Noradrenaline reuptake inhibitors limit neuroinflammation in rat cortex following a systemic inflammatory challenge: implications for depression and neurodegeneration. Int J Neuropsychopharmacol. (2009) 12:687–99. doi: 10.1017/S146114570800967X
92. O’Sullivan JB, Ryan KM, Harkin A, Connor TJ. Noradrenaline reuptake inhibitors inhibit expression of chemokines IP-10 and RANTES and cell adhesion molecules VCAM-1 and ICAM-1 in the CNS following a systemic inflammatory challenge. J Neuroimmunol. (2010) 220:34–42. doi: 10.1016/j.jneuroim.2009.12.007
93. McNamee EN, Ryan KM, Griffin EW, Gonzalez-Reyes RE, Ryan KJ, Harkin A, et al. Noradrenaline acting at central beta-adrenoceptors induces interleukin-10 and suppressor of cytokine signaling-3 expression in rat brain: implications for neurodegeneration. Brain Behav Immun. (2010) 24:660–71. doi: 10.1016/j.bbi.2010.02.005
94. Mori K, Ozaki E, Zhang B, Yang L, Yokoyama A, Takeda I, et al. Effects of norepinephrine on rat cultured microglial cells that express alpha1, alpha2, beta1 and beta2 adrenergic receptors. Neuropharmacology. (2002) 43:1026–34. doi: 10.1016/S0028-3908(02)00211-3
95. Chang JY, Liu LZ. Catecholamines inhibit microglial nitric oxide production. Brain Res Bull. (2000) 52:525–30. doi: 10.1016/S0361-9230(00)00291-4
96. Madrigal JL, Feinstein DL, Dello Russo C. Norepinephrine protects cortical neurons against microglial-induced cell death. J Neurosci Res. (2005) 81:390–6. doi: 10.1002/jnr.20481
97. Ardestani PM, Evans AK, Yi B, Nguyen T, Coutellier L, Shamloo M. Modulation of neuroinflammation and pathology in the 5XFAD mouse model of Alzheimer’s disease using a biased and selective beta-1 adrenergic receptor partial agonist. Neuropharmacology. (2017) 116:371–86. doi: 10.1016/j.neuropharm.2017.01.010
98. McNamee EN, Griffin EW, Ryan KM, Ryan KJ, Heffernan S, Harkin A, et al. Noradrenaline acting at beta-adrenoceptors induces expression of IL-1beta and its negative regulators IL-1ra and IL-1RII, and drives an overall anti-inflammatory phenotype in rat cortex. Neuropharmacology. (2010) 59:37–48. doi: 10.1016/j.neuropharm.2010.03.014
99. Frohman EM, Vayuvegula B, Gupta S, van den Noort S. Norepinephrine inhibits gamma-interferon-induced major histocompatibility class II (Ia) antigen expression on cultured astrocytes via beta-2-adrenergic signal transduction mechanisms. Proc Natl Acad Sci U S A. (1988) 85:1292–6. doi: 10.1073/pnas.85.4.1292
100. Loughlin AJ, Woodroofe MN, Cuzner ML. Modulation of interferon-gamma-induced major histocompatibility complex class II and Fc receptor expression on isolated microglia by transforming growth factor-beta 1, interleukin-4, noradrenaline and glucocorticoids. Immunology. (1993) 79(1):125–30.
101. McNamee EN, Ryan KM, Kilroy D, Connor TJ. Noradrenaline induces IL-1ra and IL-1 type II receptor expression in primary glial cells and protects against IL-1beta-induced neurotoxicity. Eur J Pharmacol. (2010) 626:219–28. doi: 10.1016/j.ejphar.2009.09.054
102. Ryan KJ, Griffin E, Yssel JD, Ryan KM, McNamee EN, Harkin A, et al. Stimulation of central beta2-adrenoceptors suppresses NFkappaB activity in rat brain: a role for IkappaB. Neurochem Int. (2013) 63:368–78. doi: 10.1016/j.neuint.2013.07.006
103. Macchi F, Homberg JR, Calabrese F, Zecchillo C, Racagni G, Riva MA, et al. Altered inflammatory responsiveness in serotonin transporter mutant rats. J Neuroinflamm. (2013) 10:116. doi: 10.1186/1742-2094-10-116
104. Miyazaki I, Asanuma M. Serotonin 1A receptors on astrocytes as a potential target for the treatment of parkinson’s disease. Curr Med Chem. (2016) 23:686–700. doi: 10.2174/0929867323666160122115057
105. Guseva D, Holst K, Kaune B, Meier M, Keubler L, Glage S, et al. Serotonin 5-HT7 receptor is critically involved in acute and chronic inflammation of the gastrointestinal tract. Inflamm Bowel Dis. (2014) 20:1516–29. doi: 10.1097/MIB.0000000000000150
106. Xing C, Chen H, Bi W, Lei T, Hang Z, Du H. Targeting 5-HT is a potential therapeutic strategy for neurodegenerative diseases. Int J Mol Sci. (2024) 25:13446. doi: 10.3390/ijms252413446
107. Quintero-Villegas A, Valdés-Ferrer SI. Central nervous system effects of 5-HT7 receptors: a potential target for neurodegenerative diseases. Mol Med. (2022) 28:70. doi: 10.1186/s10020-022-00497-2
108. Turkin A, TuChina O, Klempin F. Microglia function on precursor cells in the adult hippocampus and their responsiveness to serotonin signaling. Front Cell Dev Biol. (2021) 9:665739. doi: 10.3389/fcell.2021.665739
109. Nakagawa Y, Chiba K. Role of microglial M1/M2 polarization in relapse and remission of psychiatric disorders and diseases. Pharmaceuticals. (2014) 7:1028–48. doi: 10.3390/ph7121028
110. Nagy EE, Frigy A, Szász JA, Horváth E. Neuroinflammation and microglia/macrophage phenotype modulate the molecular background of post-stroke depression: A literature review. Exp Ther Med. (2020) 20:2510–23. doi: 10.3892/etm.2020.8933
111. Tynan RJ, Weidenhofer J, Hinwood M, Cairns MJ, Day TA, Walker FR. A comparative examination of the anti-inflammatory effects of SSRI and SNRI antidepressants on LPS stimulated microglia. Brain Behav Immun. (2012) 26:469–79. doi: 10.1016/j.bbi.2011.12.011
112. Horikawa H, Kato TA, Mizoguchi Y, Monji A, Seki Y, Ohkuri T, et al. Inhibitory effects of SSRIs on IFN-gamma induced microglial activation through the regulation of intracellular calcium. Prog Neuropsychopharmacol Biol Psychiatry. (2010) 34:1306–16. doi: 10.1016/j.pnpbp.2010.07.015
113. Krabbe G, Matyash V, Pannasch U, Mamer L, Boddeke HW, Kettenmann H. Activation of serotonin receptors promotes microglial injury-induced motility but attenuates phagocytic activity. Brain Behav Immun. (2012) 26:419–28. doi: 10.1016/j.bbi.2011.12.002
114. Carson MJ, Thomas EA, Danielson PE, Sutcliffe JG. The 5HT5A serotonin receptor is expressed predominantly by astrocytes in which it inhibits cAMP accumulation: a mechanism for neuronal suppression of reactive astrocytes. Glia. (1996) 17:317–26. doi: 10.1002/(SICI)1098-1136(199608)17:4<317::AID-GLIA6>3.0.CO;2-W
115. Pousset F, Fournier J, Legoux P, Keane P, Shire D, Soubrie P. Effect of serotonin on cytokine mRNA expression in rat hippocampal astrocytes. Brain Res Mol Brain Res. (1996) 38:54–62. doi: 10.1016/0169-328X(95)00324-L
116. Liu RP, Zou M, Wang JY, Zhu JJ, Lai JM, Zhou LL, et al. Paroxetine ameliorates lipopolysaccharide-induced microglia activation via differential regulation of MAPK signaling. J Neuroinflamm. (2014) 11:47. doi: 10.1186/1742-2094-11-47
117. Lim CM, Kim SW, Park JY, Kim C, Yoon SH, Lee JK. Fluoxetine affords robust neuroprotection in the postischemic brain via its anti-inflammatory effect. J Neurosci Res. (2009) 87:1037–45. doi: 10.1002/jnr.21899
118. Chung YC, Kim SR, Jin BK. Paroxetine prevents loss of nigrostriatal dopaminergic neurons by inhibiting brain inflammation and oxidative stress in an experimental model of Parkinson’s disease. J Immunol. (2010) 185:1230–7. doi: 10.4049/jimmunol.1000208
119. Leggio GM, Salomone S, Bucolo C, Platania C, Micale V, Caraci F, et al. Dopamine D(3) receptor as a new pharmacological target for the treatment of depression. Eur J Pharmacol. (2013) 719:25–33. doi: 10.1016/j.ejphar.2013.07.022
120. Mishra A, Singh S, Shukla S. Physiological and functional basis of dopamine receptors and their role in neurogenesis: possible implication for parkinson’s disease. J Exp Neurosci. (2018) 12:1179069518779829. doi: 10.1177/1179069518779829
121. Kuric E, Wieloch T, Ruscher K. Dopamine receptor activation increases glial cell line-derived neurotrophic factor in experimental stroke. Exp Neurol. (2013) 247:202–8. doi: 10.1016/j.expneurol.2013.04.016
122. Huck JH, Freyer D, Bottcher C, Mladinov M, Muselmann-Genschow C, Thielke M, et al. De novo expression of dopamine D2 receptors on microglia after stroke. J Cereb Blood Flow Metab. (2015) 35:1804–11. doi: 10.1038/jcbfm.2015.128
123. Feng Y, Lu Y. Immunomodulatory effects of dopamine in inflammatory diseases. Front Immunol. (2021) 12:663102. doi: 10.3389/fimmu.2021.663102
124. Zhang Y, Chen Y, Wu J, Manaenko A, Yang P, Tang J, et al. Activation of dopamine D2 receptor suppresses neuroinflammation through αB-crystalline by inhibition of NF-κB nuclear translocation in experimental ICH mice model. Stroke. (2015) 46:2637–46. doi: 10.1161/STROKEAHA.115.009792
125. Yan Y, Jiang W, Liu L, Wang X, Ding C, Tian Z, et al. Dopamine controls systemic inflammation through inhibition of NLRP3 inflammasome. Cell. (2015) 160:62–73. doi: 10.1016/j.cell.2014.11.047
126. Schroder K, Tschopp J. The inflammasomes. Cell. (2010) 140:821–32. doi: 10.1016/j.cell.2010.01.040
127. Wang T, Nowrangi D, Yu L, Lu T, Tang J, Han B, et al. Activation of dopamine D1 receptor decreased NLRP3-mediated inflammation in intracerebral hemorrhage mice. J Neuroinflamm. (2018) 15:2. doi: 10.1186/s12974-017-1039-7
128. Wang B, Chen T, Xue L, Wang J, Jia Y, Li G, et al. Methamphetamine exacerbates neuroinflammatory response to lipopolysaccharide by activating dopamine D1-like receptors. Int Immunopharmacol. (2019) 73:1–9. doi: 10.1016/j.intimp.2019.04.053
129. Malmberg A, Jackson DM, Eriksson A, Mohell N. Unique binding characteristics of antipsychotic agents interacting with human dopamine D2A, D2B, and D3 receptors. Mol Pharmacol. (1993) 43:749–54. doi: 10.1016/S0026-895X(25)13652-3
130. Strange PG. Antipsychotic drugs: importance of dopamine receptors for mechanisms of therapeutic actions and side effects. Pharmacol Rev. (2001) 53:119–33. doi: 10.1016/S0031-6997(24)01483-2
131. Sunahara RK, Guan HC, O’Dowd BF, Seeman P, Laurier LG, Ng G, et al. Cloning of the gene for a human dopamine D5 receptor with higher affinity for dopamine than D1. Nature. (1991) 350:614–9. doi: 10.1038/350614a0
132. Wu WL, Burnett DA, Spring R, Greenlee WJ, Smith M, Favreau L, et al. Dopamine D1/D5 receptor antagonists with improved pharmacokinetics: design, synthesis, and biological evaluation of phenol bioisosteric analogues of benzazepine D1/D5 antagonists. J Med Chem. (2005) 48:680–93. doi: 10.1021/jm030614p
133. Pacheco R. Targeting dopamine receptor D3 signalling in inflammation. Oncotarget. (2017) 8:7224–5. doi: 10.18632/oncotarget.14601
134. Gonzalez H, Contreras F, Prado C, Elgueta D, Franz D, Bernales S, et al. Dopamine receptor D3 expressed on CD4+ T cells favors neurodegeneration of dopaminergic neurons during Parkinson’s disease. J Immunol. (2013) 190(10):5048–56. doi: 10.4049/jimmunol.1203121
135. Moraga-Amaro R, Gonzalez H, Pacheco R, Stehberg J. Dopamine receptor D3 deficiency results in chronic depression and anxiety. Behav Brain Res. (2014) 274:186–93. doi: 10.1016/j.bbr.2014.07.055
136. Wang J, Lai S, Li G, Zhou T, Wang B, Cao F, et al. Microglial activation contributes to depressive-like behavior in dopamine D3 receptor knockout mice. Brain Behav Immun. (2020) 83:226–38. doi: 10.1016/j.bbi.2019.10.016
137. Corrigan M, O'Rourke AM, Moran B, Fletcher JM, Harkin A. Inflammation in the pathogenesis of depression: a disorder of neuroimmune origin. Neuronal Signal. (2023) 7(2):NS20220054. doi: 10.1042/NS20220054
138. Wang J, Lai S, Li G, Zhou T, Wang B, Cao F, et al. Microglial activation contributes to depressive-like behavior in dopamine D3 receptor knockout mice. Brain Behav Immun. (2020) 83:226–38. doi: 10.1016/j.bbi.2019.10.016
139. Chan KL, Cathomas F, Russo SJ. Central and peripheral inflammation link metabolic syndrome and major depressive disorder. Physiol (Bethesda). (2019) 34:123–33. doi: 10.1152/physiol.00047.2018
140. Grygiel-Gorniak B, Limphaibool N, Puszczewicz M. Cytokine secretion and the risk of depression development in patients with connective tissue diseases. Psychiatry Clin Neurosci. (2019) 73:302–16. doi: 10.1111/pcn.2019.73.issue-6
141. Pisanu A, Boi L, Mulas G, Spiga S, Fenu S, Carta AR. Neuroinflammation in L-DOPA-induced dyskinesia: beyond the immune function. J Neural Transm (Vienna). (2018) 125:1287–97. doi: 10.1007/s00702-018-1874-4
142. Miller AH, Haroon E, Raison CL, Felger JC. Cytokine targets in the brain: impact on neurotransmitters and neurocircuits. Depress Anxiety. (2013) 30:297–306. doi: 10.1002/da.2013.30.issue-4
143. Dantzer R. Neuroimmune interactions: from the brain to the immune system and vice versa. Physiol Rev. (2018) 98:477–504. doi: 10.1152/physrev.00039.2016
144. Beurel E, Toups M, Nemeroff CB. The bidirectional relationship of depression and inflammation: double trouble. Neuron. (2020) 107:234–56. doi: 10.1016/j.neuron.2020.06.002
145. Felger JC, Lotrich FE. Inflammatory cytokines in depression: neurobiological mechanisms and therapeutic implications. Neuroscience. (2013) 246:199–229. doi: 10.1016/j.neuroscience.2013.04.060
146. Anderson G, Kubera M, Duda W, Lason W, Berk M, Maes M. Increased IL-6 trans-signaling in depression: focus on the tryptophan catabolite pathway, melatonin and neuroprogression. Pharmacol Rep. (2013) 65:1647–54. doi: 10.1016/S1734-1140(13)71526-3
147. Jurgens B, Hainz U, Fuchs D, Felzmann T, Heitger A. Interferon-gamma-triggered indoleamine 2,3-dioxygenase competence in human monocyte-derived dendritic cells induces regulatory activity in allogeneic T cells. Blood. (2009) 114(15):3235–43. doi: 10.1182/blood-2008-12-195073
148. Babcock TA, Carlin JM. Transcriptional activation of indoleamine dioxygenase by interleukin 1 and tumor necrosis factor alpha in interferon-treated epithelial cells. Cytokine. (2000) 12:588–94. doi: 10.1006/cyto.1999.0661
149. Robinson CM, Shirey KA, Carlin JM. Synergistic transcriptional activation of indoleamine dioxygenase by IFN-gamma and tumor necrosis factor-alpha. J Inter Cytokine Res. (2003) 23:413–21. doi: 10.1089/107999003322277829
150. O’Connor JC, Andre C, Wang Y, Lawson MA, Szegedi SS, Lestage J, et al. Interferon-gamma and tumor necrosis factor-alpha mediate the upregulation of indoleamine 2,3-dioxygenase and the induction of depressive-like behavior in mice in response to bacillus Calmette-Guerin. J Neurosci. (2009) 29:4200–9. doi: 10.1523/JNEUROSCI.5032-08
151. Guillemin GJ, Smythe G, Takikawa O, Brew BJ. Expression of indoleamine 2,3-dioxygenase and production of quinolinic acid by human microglia, astrocytes, and neurons. Glia. (2005) 49:15–23. doi: 10.1002/glia.20090
152. Fanet H, Capuron L, Castanon N, Calon F, Vancassel S. Tetrahydrobioterin (BH4) pathway: from metabolism to neuropsychiatry. Curr Neuropharmacol. (2021) 19(5):591–609. doi: 10.2174/1570159X18666200729103529
153. Kappock TJ, Caradonna JP. Pterin-dependent amino acid hydroxylases. Chem Rev. (1996) 96:2659–756. doi: 10.1021/cr9402034
154. Calka J. The role of nitric oxide in the hypothalamic control of LHRH and oxytocin release, sexual behavior and aging of the LHRH and oxytocin neurons. Folia Histochem Cytobiol. (2006) 44(1):3–12.
155. Walter R, Blau N, Kierat L, Schaffner A, Schoedon G. Effects of activating and deactivating cytokines on the functionally linked tetrahydrobiopterin. No pathways in vascular smooth muscle cells. Immunol Lett. (1996) 54:25–9. doi: 10.1016/S0165-2478(96)02638-7
156. Hattori Y, Nakanishi N, Kasai K, Shimoda SI. GTP cyclohydrolase I mRNA induction and tetrahydrobiopterin synthesis in human endothelial cells. Biochim Biophys Acta. (1997) 1358:61–6. doi: 10.1016/S0167-4889(97)00052-9
157. Vann LR, Payne SG, Edsall LC, Twitty S, Spiegel S, Milstien S. Involvement of sphingosine kinase in TNF-alpha-stimulated tetrahydrobiopterin biosynthesis in C6 glioma cells. J Biol Chem. (2002) 277:12649–56. doi: 10.1074/jbc.M109111200
158. Neurauter G, Schrocksnadel K, Scholl-Burgi S, Sperner-Unterweger B, Schubert C, Ledochowski M, et al. Chronic immune stimulation correlates with reduced phenylalanine turnover. Curr Drug Metab. (2008) 9:622–7. doi: 10.2174/138920008785821738
159. Joseph A, Kumar A, O’Connell NA, Agarwal RK, Gwosdow AR. Interleukin-1 alpha stimulates dopamine release by activating type II protein kinase A in PC-12 cells. Am J Physiol. (1995) 269:E1083–8. doi: 10.1152/ajpendo.1995.269.6.E1083
160. Shintani F, Kanba S, Nakaki T, Nibuya M, Kinoshita N, Suzuki E, et al. Interleukin-1 beta augments release of norepinephrine, dopamine, and serotonin in the rat anterior hypothalamus. J Neurosci. (1993) 13:3574–81. doi: 10.1523/JNEUROSCI.13-08-03574.1993
161. Capuron L, Pagnoni G, Drake DF, Woolwine BJ, Spivey JR, Crowe RJ, et al. Dopaminergic mechanisms of reduced basal ganglia responses to hedonic reward during interferon alfa administration. Arch Gen Psychiatry. (2012) 69:1044–53. doi: 10.1001/archgenpsychiatry.2011.2094
162. Wu HQ, Rassoulpour A, Schwarcz R. Kynurenic acid leads, dopamine follows: a new case of volume transmission in the brain? J Neural Transm (Vienna). (2007) 114(1):33–41. doi: 10.1007/s00702-006-0562-y
163. Penmatsa A, Wang KH, Gouaux E. X-ray structure of dopamine transporter elucidates antidepressant mechanism. Nature. (2013) 503:85–90. doi: 10.1038/nature12533
164. Wang KH, Penmatsa A, Gouaux E. Neurotransmitter and psychostimulant recognition by the dopamine transporter. Nature. (2015) 521:322–7. doi: 10.1038/nature14431
165. Penmatsa A, Wang KH, Gouaux E. X-ray structures of Drosophila dopamine transporter in complex with nisoxetine and reboxetine. Nat Struct Mol Biol. (2015) 22:506–8. doi: 10.1038/nsmb.3029
166. Coleman JA, Yang D, Zhao Z, Wen PC, Yoshioka C, Tajkhorshid E, et al. Serotonin transporter-ibogaine complexes illuminate mechanisms of inhibition and transport. Nature. (2019) 569:141–5. doi: 10.1038/s41586-019-1135-1
167. Coleman JA, Gouaux E. Structural basis for recognition of diverse antidepressants by the human serotonin transporter. Nat Struct Mol Biol. (2018) 25:170–5. doi: 10.1038/s41594-018-0026-8
168. Coleman JA, Green EM, Gouaux E. X-ray structures and mechanism of the human serotonin transporter. Nature. (2016) 532:334–9. doi: 10.1038/nature17629
169. Cheng MH, Bahar I. Monoamine transporters: structure, intrinsic dynamics and allosteric regulation. Nat Struct Mol Biol. (2019) 26:545–56. doi: 10.1038/s41594-019-0253-7
170. Ramamoorthy S, Ramamoorthy JD, Prasad PD, Bhat GK, Mahesh VB, Leibach FH, et al. Regulation of the human serotonin transporter by interleukin-1 beta. Biochem Biophys Res Commun. (1995) 216:560–7. doi: 10.1006/bbrc.1995.2659
171. Morikawa O, Sakai N, Obara H, Saito N. Effects of interferon-alpha, interferon-gamma and cAMP on the transcriptional regulation of the serotonin transporter. Eur J Pharmacol. (1998) 349:317–24. doi: 10.1016/S0014-2999(98)00187-3
172. Mossner R, Heils A, Stober G, Okladnova O, Daniel S, Lesch KP. Enhancement of serotonin transporter function by tumor necrosis factor alpha but not by interleukin-6. Neurochem Int. (1998) 33:251–4. doi: 10.1016/S0197-0186(98)00026-6
173. Mossner R, Daniel S, Schmitt A, Albert D, Lesch KP. Modulation of serotonin transporter function by interleukin-4. Life Sci. (2001) 68:873–80. doi: 10.1016/S0024-3205(00)00992-9
174. Wu Y, Na X, Zang Y, Cui Y, Xin W, Pang R, et al. Upregulation of tumor necrosis factor-alpha in nucleus accumbens attenuates morphine-induced rewarding in a neuropathic pain model. Biochem Biophys Res Commun. (2014) 449:502–7. doi: 10.1016/j.bbrc.2014.05.025
175. Bermingham DP, Blakely RD. Kinase-dependent regulation of monoamine neurotransmitter transporters. Pharmacol Rev. (2016) 68:888–953. doi: 10.1124/pr.115.012260
176. Miller AH, Maletic V, Raison CL. Inflammation and its discontents: the role of cytokines in the pathophysiology of major depression. Biol Psychiatry. (2009) 65:732–41. doi: 10.1016/j.biopsych.2008.11.029
177. Zhu CB, Blakely RD, Hewlett WA. The proinflammatory cytokines interleukin-1beta and tumor necrosis factor-alpha activate serotonin transporters. Neuropsychopharmacology. (2006) 31:2121–31. doi: 10.1038/sj.npp.1301029
178. Terao A, Oikawa M, Saito M. Cytokine-induced change in hypothalamic norepinephrine turnover: involvement of corticotropin-releasing hormone and prostaglandins. Brain Res. (1993) 622:257–61. doi: 10.1016/0006-8993(93)90826-9
179. Hayley S, Brebner K, Lacosta S, Merali Z, Anisman H. Sensitization to the effects of tumor necrosis factor-alpha: neuroendocrine, central monoamine, and behavioral variations. J Neurosci. (1999) 19:5654–65. doi: 10.1523/JNEUROSCI.19-13-05654.1999
180. De La Garza R 2nd, Asnis GM. The non-steroidal anti-inflammatory drug diclofenac sodium attenuates IFN-alpha induced alterations to monoamine turnover in prefrontal cortex and hippocampus. Brain Res. (2003) 977:70–9. doi: 10.1016/S0006-8993(03)02757-4
181. Litteljohn D, Rudyk C, Dwyer Z, Farmer K, Fortin T, Hayley S, et al. The impact of murine LRRK2 G2019S transgene overexpression on acute responses to inflammatory challenge. Brain Behav Immun. (2018) 67:246–56. doi: 10.1016/j.bbi.2017.09.002
182. Adzic M, Brkic Z, Mitic M, Francija E, Jovicic MJ, Radulovic J, et al. Therapeutic strategies for treatment of inflammation-related depression. Curr Neuropharmacol. (2018) 16:176–209. doi: 10.2174/1570159X15666170828163048
183. Haroon E, Raison CL, Miller AH. Psychoneuroimmunology meets neuropsychopharmacology: translational implications of the impact of inflammation on behavior. Neuropsychopharmacology. (2012) 37:137–62. doi: 10.1038/npp.2011.205
184. Bhattacharya A, Derecki NC, Lovenberg TW, Drevets WC. Role of neuro-immunological factors in the pathophysiology of mood disorders. Psychopharmacol (Berl). (2016) 233:1623–36. doi: 10.1007/s00213-016-4214-0
185. Krishnadas R, Cavanagh J. Depression: an inflammatory illness? J Neurol Neurosurg Psychiatry. (2012) 83(5):495–502. doi: 10.1136/jnnp-2011-301779
186. Bhattacharya A, Derecki NC, Lovenberg TW, Drevets WC. Role of neuro-immunological factors in the pathophysiology of mood disorders. Psychopharmacology (Berl). (2016) 233(9):1623–36. doi: 10.1007/s00213-016-4214-0
187. Hassamal S. Chronic stress, neuroinflammation, and depression: an overview of pathophysiological mechanisms and emerging anti-inflammatories. Front Psychiatry. (2023) 14:1130989. doi: 10.3389/fpsyt.2023.1130989
188. Roman M, Irwin MR. Novel neuroimmunologic therapeutics in depression: A clinical perspective on what we know so far. Brain Behav Immun. (2020) 83:7–21. doi: 10.1016/j.bbi.2019.09.016
189. Puentes-Orozco M, Albarracin SL, Velásquez MM. Neuroinflammation and major depressive disorder: astrocytes at the crossroads. Front Cell Neurosci. (2024) 18:1504555. doi: 10.3389/fncel.2024.1504555
190. Zhang MM, Guo MX, Zhang QP, Chen XQ, Li NZ, Liu Q, et al. IL-1R/C3aR signaling regulates synaptic pruning in the prefrontal cortex of depression. Cell Biosci. (2022) 12:90. doi: 10.1186/s13578-022-00832-4
191. Gutierrez EG, Banks WA, Kastin AJ. Murine tumor necrosis factor alpha is transported from blood to brain in the mouse. J Neuroimmunol. (1993) 47:169–76. doi: 10.1016/0165-5728(93)90027-V
192. Gasquoine PG. Contributions of the insula to cognition and emotion. Neuropsychol Rev. (2014) 24:77–87. doi: 10.1007/s11065-014-9246-9
193. Chen P, Chen F, Chen G, Zhong S, Gong J, Zhong H, et al. Inflammation is associated with decreased functional connectivity of insula in unmedicated bipolar disorder. Brain Behav Immun. (2020) 89:615–22. doi: 10.1016/j.bbi.2020.07.004
194. Numakawa T, Richards M, Nakajima S, Adachi N, Furuta M, Odaka H, et al. The role of brain-derived neurotrophic factor in comorbid depression: possible linkage with steroid hormones, cytokines, and nutrition. Front Psychiatry. (2014) 5:136. doi: 10.3389/fpsyt.2014.00136
195. Zunszain PA, Anacker C, Cattaneo A, Carvalho LA, Pariante CM. Glucocorticoids, cytokines and brain abnormalities in depression. Prog Neuropsychopharmacol Biol Psychiatry. (2011) 35:722–9. doi: 10.1016/j.pnpbp.2010.04.011
196. Bergamini G, Mechtersheimer J, Azzinnari D, Sigrist H, Buerge M, Dallmann R, et al. Chronic social stress induces peripheral and central immune activation, blunted mesolimbic dopamine function, and reduced reward-directed behaviour in mice. Neurobiol Stress. (2018) 8:42–56. doi: 10.1016/j.ynstr.2018.01.004
197. Bekhbat M, Li Z, Mehta ND, Treadway MT, Lucido MJ, Woolwine BJ, et al. Functional connectivity in reward circuitry and symptoms of anhedonia as therapeutic targets in depression with high inflammation: evidence from a dopamine challenge study. Mol Psychiatry. (2022) 27:4113–21. doi: 10.1038/s41380-022-01715-3
198. Belujon P, Grace AA. Dopamine system dysregulation in major depressive disorders. Int J Neuropsychopharmacol. (2017) 20:1036–46. doi: 10.1093/ijnp/pyx056
199. Robson MJ, Quinlan MA, Blakely RD. Immune system activation and depression: roles of serotonin in the central nervous system and periphery. ACS Chem Neurosci. (2017) 8:932–42. doi: 10.1021/acschemneuro.6b00412
200. Jha MK, Minhajuddin A, Gadad BS, Greer TL, Mayes TL, Trivedi MH. Interleukin 17 selectively predicts better outcomes with bupropion-SSRI combination: Novel T cell biomarker for antidepressant medication selection. Brain Behav Immun. (2017) 66:103–10. doi: 10.1016/j.bbi.2017.07.005
201. Lucido MJ, Dunlop BW. Emerging medications for treatment-resistant depression: A review with perspective on mechanisms and challenges. Brain Sci. (2025) 15(2):161. doi: 10.3390/brainsci15020161
202. Dziedzicka-Wasylewska M, Willner P, Papp M. Changes in dopamine receptor mRNA expression following chronic mild stress and chronic antidepressant treatment. Behav Pharmacol. (1997) 8:607–18. doi: 10.1097/00008877-199711000-00017
203. Kram ML, Kramer GL, Ronan PJ, Steciuk M, Petty F. Dopamine receptors and learned helplessness in the rat: An autoradiographic study. Prog Neuropsychopharmacol Biol Psychiatry. (2002) 26:639–45. doi: 10.1016/S0278-5846(01)00222-6
204. Bai S, Guo W, Feng Y, Deng H, Li G, Nie H, et al. Efficacy and safety of anti-inflammatory agents for the treatment of major depressive disorder: a systematic review and meta-analysis of randomised controlled trials. J Neurol Neurosurg Psychiatry. (2020) 91(1):21–32. doi: 10.1136/jnnp-2019-320912
205. Brymer KJ, Fenton EY, Kalynchuk LE, Caruncho HJ. Peripheral etanercept administration normalizes behavior, hippocampal neurogenesis, and hippocampal reelin and GABAA receptor expression in a preclinical model of depression. Front Pharmacol. (2018) 9:121. doi: 10.3389/fphar.2018.00121
206. Warner-Schmidt JL, Vanover KE, Chen EY, Marshall JJ, Greengard P. Antidepressant effects of selective serotonin reuptake inhibitors (SSRIs) are attenuated by antiinflammatory drugs in mice and humans. Proc Natl Acad Sci U S A. (2011) 108:9262–7. doi: 10.1073/pnas.1104836108
207. Martinowich K, Lu B. Interaction between BDNF and serotonin: role in mood disorders. Neuropsychopharmacology. (2008) 33:73–83. doi: 10.1038/sj.npp.1301571
208. Catena-Dell’Osso M, Rotella F, Dell’Osso A, Fagiolini A, Marazziti D. Inflammation, serotonin and major depression. Curr Drug Targets. (2013) 14:571–7. doi: 10.2174/13894501113149990154
209. Descarries L, Riad M. Effects of the antidepressant fluoxetine on the subcellular localization of 5-HT1A receptors and SERT. Philos Trans R Soc B: Biol Sci. (2012) 367:2416–25. doi: 10.1098/rstb.2011.0361
210. freeman@ roslin. ed. ac. uk MICRTGACCSMBMWPCMHNAHDABETFTCt. Effects of anti-inflammatory drugs on the expression of tryptophan-metabolism genes by human macrophages. J Leukocyte Biol. (2018) 103:681–92. doi: 10.1002/JLB.3A0617-261R
211. Molero P, Ramos-Quiroga J, Martin-Santos R, Calvo-Sánchez E, Gutiérrez-Rojas L, Meana J. Antidepressant efficacy and tolerability of ketamine and esketamine: a critical review. CNS Drugs. (2018) 32:411–20. doi: 10.1007/s40263-018-0519-3
212. Richardson B, MacPherson A, Bambico F. Neuroinflammation and neuroprogression in depression: Effects of alternative drug treatments. Brain Behav Immun Health. (2022) 26:100554. doi: 10.1016/j.bbih.2022.100554
213. Šimić G, Babić Leko M, Wray S, Harrington CR, Delalle I, Jovanov-Milošević N, et al. Monoaminergic neuropathology in Alzheimer’s disease. Prog Neurobiol. (2017) 151:101–38. doi: 10.1016/j.pneurobio.2016.04.001
214. Matthews KL, Chen CP, Esiri MM, Keene J, Minger SL, Francis PT. Noradrenergic changes, aggressive behavior, and cognition in patients with dementia. Biol Psychiatry. (2002) 51:407–16. doi: 10.1016/S0006-3223(01)01235-5
215. Gannon M, Wang Q. Complex noradrenergic dysfunction in Alzheimer’s disease: Low norepinephrine input is not always to blame. Brain Res. (2019) 1702:12–6. doi: 10.1016/j.brainres.2018.01.001
216. Szot P, White SS, Greenup JL, Leverenz JB, Peskind ER, Raskind MA. Changes in adrenoreceptors in the prefrontal cortex of subjects with dementia: evidence of compensatory changes. Neuroscience. (2007) 146:471–80. doi: 10.1016/j.neuroscience.2007.01.031
217. Chen Y, Peng Y, Che P, Gannon M, Liu Y, Li L, et al. α(2A) adrenergic receptor promotes amyloidogenesis through disrupting APP-SorLA interaction. Proc Natl Acad Sci U S A. (2014) 111:17296–301. doi: 10.1073/pnas.1409513111
218. Zhang F, Gannon M, Chen Y, Yan S, Zhang S, Feng W, et al. β-amyloid redirects norepinephrine signaling to activate the pathogenic GSK3β/tau cascade. Sci Transl Med. (2020) 12(526):eaay6931. doi: 10.1126/scitranslmed.aay6931
219. Gutiérrez IL, Dello Russo C, Novellino F, Caso JR, García-Bueno B, Leza JC, et al. Noradrenaline in alzheimer’s disease: A new potential therapeutic target. Int J Mol Sci. (2022) 23(11):6143. doi: 10.3390/ijms23116143
220. Ni Y, Zhao X, Bao G, Zou L, Teng L, Wang Z, et al. Activation of beta2-adrenergic receptor stimulates gamma-secretase activity and accelerates amyloid plaque formation. Nat Med. (2006) 12:1390–6. doi: 10.1038/nm1485
221. Heneka MT, Kummer MP, Latz E. Innate immune activation in neurodegenerative disease. Nat Rev Immunol. (2014) 14:463–77. doi: 10.1038/nri3705
222. Savonije K, Weaver DF. The role of tryptophan metabolism in alzheimer’s disease. Brain Sci. (2023) 13:292. doi: 10.3390/brainsci13020292
223. Capuron L, Neurauter G, Musselman DL, Lawson DH, Nemeroff CB, Fuchs D, et al. Interferon-alpha–induced changes in tryptophan metabolism: relationship to depression and paroxetine treatment. Biol Psychiatry. (2003) 54:906–14. doi: 10.1016/S0006-3223(03)00173-2
224. Miller AH. Mechanisms of cytokine-induced behavioral changes: Psychoneuroimmunology at the translational interface. Brain Behav Immun. (2009) 23:149–58. doi: 10.1016/j.bbi.2008.08.006
225. Rhie SJ, Jung EY, Shim I. The role of neuroinflammation on pathogenesis of affective disorders. J Exerc Rehabil. (2020) 16:2–9. doi: 10.12965/jer.2040016.008
226. Pless A, Ware D, Saggu S, Rehman H, Morgan J, Wang Q. Understanding neuropsychiatric symptoms in Alzheimer’s disease: challenges and advances in diagnosis and treatment. Front Neurosci. (2023) 17. doi: 10.3389/fnins.2023.1263771
227. Scassellati C, Galoforo AC, Esposito C, Ciani M, Ricevuti G, Bonvicini C. Promising intervention approaches to potentially resolve neuroinflammation and steroid hormones alterations in alzheimer’s disease and its neuropsychiatric symptoms. Aging Dis. (2021) 12:1337–57. doi: 10.14336/AD.2021.0122
228. Hansen DV, Hanson JE, Sheng M. Microglia in alzheimer’s disease. J Cell Biol. (2018) 217:459–72. doi: 10.1083/jcb.201709069
229. Harry GJ, Kraft AD. Neuroinflammation and microglia: considerations and approaches for neurotoxicity assessment. Expert Opin Drug Metab Toxicol. (2008) 4:1265–77. doi: 10.1517/17425255.4.10.1265
230. Hashimoto K, Malchow B, Falkai P, Schmitt A. Glutamate modulators as potential therapeutic drugs in schizophrenia and affective disorders. Eur Arch Psychiatry Clin Neurosci. (2013) 263:367–77. doi: 10.1007/s00406-013-0399-y
231. Metaxas A, Anzalone M, Vaitheeswaran R, Petersen S, Landau AM, Finsen B. Neuroinflammation and amyloid-beta 40 are associated with reduced serotonin transporter (SERT) activity in a transgenic model of familial Alzheimer’s disease. Alzheimers Res Ther. (2019) 11:38. doi: 10.1186/s13195-019-0491-2
232. Zhang B, Gaiteri C, Bodea LG, Wang Z, McElwee J, Podtelezhnikov AA, et al. Integrated systems approach identifies genetic nodes and networks in late-onset Alzheimer’s disease. Cell. (2013) 153:707–20. doi: 10.1016/j.cell.2013.03.030
233. Kinney JW, Bemiller SM, Murtishaw AS, Leisgang AM, Salazar AM, Lamb BT. Inflammation as a central mechanism in Alzheimer’s disease. Alzheimers Dement (N Y). (2018) 4:575–90. doi: 10.1016/j.trci.2018.06.014
234. Zotova E, Bharambe V, Cheaveau M, Morgan W, Holmes C, Harris S, et al. Inflammatory components in human Alzheimer’s disease and after active amyloid-β42 immunization. Brain. (2013) 136:2677–96. doi: 10.1093/brain/awt210
235. Schaffer Aguzzoli C, Ferreira PCL, Povala G, Ferrari-Souza JP, Bellaver B, Soares Katz C, et al. Neuropsychiatric symptoms and microglial activation in patients with alzheimer disease. JAMA Netw Open. (2023) 6:e2345175–e. doi: 10.1001/jamanetworkopen.2023.45175
236. Klawonn AM, Fritz M, Castany S, Pignatelli M, Canal C, Similä F, et al. Microglial activation elicits a negative affective state through prostaglandin-mediated modulation of striatal neurons. Immunity. (2021) 54:225–34.e6. doi: 10.1016/j.immuni.2020.12.016
237. El Khoury JB, Moore KJ, Means TK, Leung J, Terada K, Toft M, et al. CD36 mediates the innate host response to beta-amyloid. J Exp Med. (2003) 197:1657–66. doi: 10.1084/jem.20021546
238. Mawuenyega KG, Sigurdson W, Ovod V, Munsell L, Kasten T, Morris JC, et al. Decreased clearance of CNS beta-amyloid in Alzheimer’s disease. Science. (2010) 330:1774. doi: 10.1126/science.1197623
239. Streit WJ, Braak H, Xue QS, Bechmann I. Dystrophic (senescent) rather than activated microglial cells are associated with tau pathology and likely precede neurodegeneration in Alzheimer’s disease. Acta Neuropathol. (2009) 118:475–85. doi: 10.1007/s00401-009-0556-6
240. Dhapola R, Hota SS, Sarma P, Bhattacharyya A, Medhi B, Reddy DH. Recent advances in molecular pathways and therapeutic implications targeting neuroinflammation for Alzheimer’s disease. Inflammopharmacology. (2021) 29:1669–81. doi: 10.1007/s10787-021-00889-6
241. Jadhav D, Saraswat N, Vyawahare N, Shirode D. Targeting the molecular web of Alzheimer’s disease: unveiling pathways for effective pharmacotherapy. Egyptian J Neurol Psychiatry Neurosurg. (2024) 60:7. doi: 10.1186/s41983-023-00775-8
242. Cummings J. Anti-amyloid monoclonal antibodies are transformative treatments that redefine Alzheimer’s disease therapeutics. Drugs. (2023) 83:569–76. doi: 10.1007/s40265-023-01858-9
243. De Virgilio A, Greco A, Fabbrini G, Inghilleri M, Rizzo MI, Gallo A, et al. Parkinson’s disease: Autoimmunity and neuroinflammation. Autoimmun Rev. (2016) 15:1005–11. doi: 10.1016/j.autrev.2016.07.022
244. Jellinger KA. Basic mechanisms of neurodegeneration: a critical update. J Cell Mol Med. (2010) 14:457–87. doi: 10.1111/j.1582-4934.2010.01010.x
245. Block ML, Zecca L, Hong J-S. Microglia-mediated neurotoxicity: uncovering the molecular mechanisms. Nat Rev Neurosci. (2007) 8:57–69. doi: 10.1038/nrn2038
246. Troncoso-Escudero P, Parra A, Nassif M, Vidal RL. Outside in: unraveling the role of neuroinflammation in the progression of parkinson’s disease. Front Neurol. (2018) 9. doi: 10.3389/fneur.2018.00860
247. Sarkar C, Basu B, Chakroborty D, Dasgupta PS, Basu S. The immunoregulatory role of dopamine: an update. Brain Behav Immun. (2010) 24:525–8. doi: 10.1016/j.bbi.2009.10.015
248. Boka G, Anglade P, Wallach D, Javoy-Agid F, Agid Y, Hirsch EC. Immunocytochemical analysis of tumor necrosis factor and its receptors in Parkinson’s disease. Neurosci Lett. (1994) 172:151–4. doi: 10.1016/0304-3940(94)90684-X
249. Mogi M, Harada M, Kondo T, Riederer P, Inagaki H, Minami M, et al. Interleukin-1 beta, interleukin-6, epidermal growth factor and transforming growth factor-alpha are elevated in the brain from parkinsonian patients. Neurosci Lett. (1994) 180:147–50. doi: 10.1016/0304-3940(94)90508-8
250. Isik S, Yeman Kiyak B, Akbayir R, Seyhali R, Arpaci T. Microglia mediated neuroinflammation in parkinson’s disease. Cells. (2023) 12(7):1012. doi: 10.3390/cells12071012
251. Kurkowska-Jastrzebska I, Balkowiec-Iskra E, Ciesielska A, Joniec I, Cudna A, Zaremba MM, et al. Decreased inflammation and augmented expression of trophic factors correlate with MOG-induced neuroprotection of the injured nigrostriatal system in the murine MPTP model of Parkinson’s disease. Int Immunopharmacol. (2009) 9(6):781–91. doi: 10.1016/j.intimp.2009.03.003
252. Banati RB, Gehrmann J, Schubert P, Kreutzberg GW. Cytotoxicity of microglia. Glia. (1993) 7:111–8. doi: 10.1002/glia.440070117
253. Sharifi H, Mohajjel Nayebi A, Farajnia S. 8-OH-DPAT (5-HT1A agonist) Attenuates 6-Hydroxy- dopamine-induced catalepsy and Modulates Inflammatory Cytokines in Rats. Iran J Basic Med Sci. (2013) 16(12):1270–5.
254. Dominguez-Meijide A, Villar-Cheda B, Garrido-Gil P, Sierrra-Paredes G, Guerra MJ, Labandeira-Garcia JL. Effect of chronic treatment with angiotensin type 1 receptor antagonists on striatal dopamine levels in normal rats and in a rat model of Parkinson’s disease treated with L-DOPA. Neuropharmacology. (2014) 76 Pt A:156–68. doi: 10.1016/j.neuropharm.2013.07.016
255. McCoy MK, Martinez TN, Ruhn KA, Szymkowski DE, Smith CG, Botterman BR, et al. Blocking soluble tumor necrosis factor signaling with dominant-negative tumor necrosis factor inhibitor attenuates loss of dopaminergic neurons in models of Parkinson’s disease. J Neurosci. (2006) 26:9365–75. doi: 10.1523/JNEUROSCI.1504-06.2006
256. Wang YD, Bao XQ, Xu S, Yu WW, Cao SN, Hu JP, et al. A novel parkinson’s disease drug candidate with potent anti-neuroinflammatory effects through the src signaling pathway. J Med Chem. (2016) 59:9062–79. doi: 10.1021/acs.jmedchem.6b00976
257. Samii A, Etminan M, Wiens MO, Jafari S. NSAID use and the risk of Parkinson’s disease: systematic review and meta-analysis of observational studies. Drugs Aging. (2009) 26:769–79. doi: 10.2165/11316780-000000000-00000
258. Gordon R, Albornoz EA, Christie DC, Langley MR, Kumar V, Mantovani S, et al. Inflammasome inhibition prevents α-synuclein pathology and dopaminergic neurodegeneration in mice. Sci Trans Med. (2018) 10:eaah4066. doi: 10.1126/scitranslmed.aah4066
259. Benner EJ, Mosley RL, Destache CJ, Lewis TB, Jackson-Lewis V, Gorantla S, et al. Therapeutic immunization protects dopaminergic neurons in a mouse model of Parkinson’s disease. Proc Natl Acad Sci. (2004) 101:9435–40. doi: 10.1073/pnas.0400569101
260. Krashia P, Cordella A, Nobili A, La Barbera L, Federici M, Leuti A, et al. Blunting neuroinflammation with resolvin D1 prevents early pathology in a rat model of Parkinson’s disease. Nat Commun. (2019) 10:3945. doi: 10.1038/s41467-019-11928-w
261. Pajares M, Rojo AI, Manda G, Boscá L, Cuadrado A. Inflammation in parkinson’s disease: mechanisms and therapeutic implications. Cells. (2020) 9(7):1687. doi: 10.3390/cells9071687
262. Zella SM, Metzdorf J, Ciftci E, Ostendorf F, Muhlack S, Gold R, et al. Emerging immunotherapies for Parkinson disease. Neurol Ther. (2019) 8:29–44. doi: 10.1007/s40120-018-0122-z
263. Seo H-J, Wang S-M, Han C, Lee S-J, Patkar AA, Masand PS, et al. Curcumin as a putative antidepressant. Expert Rev Neurotherapeut. (2015) 15:269–80. doi: 10.1586/14737175.2015.1008457
264. Arent SM, Walker AJ, Arent MA. “The effects of exercise on anxiety and depression”. In: Tenenbaum G, Eklund RC, editors. Handbook of Sport Psychology (2020). doi: 10.1002/9781119568124.ch42
265. Lurie DI. An integrative approach to neuroinflammation in psychiatric disorders and neuropathic pain. J Exp Neurosci. (2018) 12:1179069518793639. doi: 10.1177/1179069518793639
266. Ignácio ZM, da Silva RS, Plissari ME, Quevedo J, Réus GZ. Physical exercise and neuroinflammation in major depressive disorder. Mol Neurobiol. (2019) 56:8323–35. doi: 10.1007/s12035-019-01670-1
267. Çınar E, Tel BC, Şahin G. Neuroinflammation in parkinson’s disease and its treatment opportunities. Balkan Med J. (2022) 39(5):318–33. doi: 10.4274/balkanmedj.galenos.2022.2022-7-100
268. Amirifar L, Shamloo A, Nasiri R, de Barros NR, Wang ZZ, Unluturk BD, et al. Brain-on-a-chip: Recent advances in design and techniques for microfluidic models of the brain in health and disease. Biomaterials. (2022) 285:121531. doi: 10.1016/j.biomaterials.2022.121531
Keywords: monoamines, norepinephrine, dopamine, serotonin, intracellular signaling, neuroinflammation, Alzheimer’s disease, major depression
Citation: Saggu S, Pless A, Dew E, Ware D, Jiao K and Wang Q (2025) Monoamine signaling and neuroinflammation: mechanistic connections and implications for neuropsychiatric disorders. Front. Immunol. 16:1543730. doi: 10.3389/fimmu.2025.1543730
Received: 11 December 2024; Accepted: 04 April 2025;
Published: 28 April 2025.
Edited by:
Juan Pablo de Rivero Vaccari, University of Miami, United StatesReviewed by:
Irena Maria Nalepa, Polish Academy of Sciences, PolandStephanie M. Matt, Drexel University, United States
Gilberto Pérez-Sánchez, Instituto Nacional de Psiquiatría Ramón de la Fuente Muñiz, Mexico
Copyright © 2025 Saggu, Pless, Dew, Ware, Jiao and Wang. This is an open-access article distributed under the terms of the Creative Commons Attribution License (CC BY). The use, distribution or reproduction in other forums is permitted, provided the original author(s) and the copyright owner(s) are credited and that the original publication in this journal is cited, in accordance with accepted academic practice. No use, distribution or reproduction is permitted which does not comply with these terms.
*Correspondence: Qin Wang, cWl3YW5nQGF1Z3VzdGEuZWR1