- 1Department of Genetics and Genomics, College of Medicine and Health Sciences, United Arab Emirates University, Al Ain, United Arab Emirates
- 2ASPIRE Precision Medicine Research Institute Abu Dhabi, United Arab Emirates University, Al Ain, United Arab Emirates
- 3Department of Biosciences and Bioinformatics & Suzhou Municipal Key Lab of Biomedical Sciences and Translational Immunology, School of Science, Xi’an Jiaotong-Liverpool University, Suzhou, China
- 4College of Health Sciences, Abu Dhabi University, Abu Dhabi, United Arab Emirates
- 5Biomedical Research Center, School of Science, Engineering and Environment, University of Salford, Manchester, United Kingdom
Over the past few years, immune checkpoint inhibitors resulted in magnificent and durable successes in treating cancer; however, only a minority of patients respond favorably to the treatment due to a broad-spectrum of tumor-intrinsic and tumor-extrinsic factors. With the recent insights gained into the mechanisms of resistance, combination treatment strategies to overcome the resistance and enhance the therapeutic potential of immune checkpoint inhibitors are emerging and showing promising results in both pre-clinical and clinical settings. This has been derived through multiple interconnected mechanisms such as enhancing tumor immunogenicity, improving neoantigen processing and presentation in addition to augmenting T cell infiltration and cytotoxic potentials. In the clinical settings, several avenues of combination treatments involving immune checkpoint inhibitors were associated with considerable improvement in the therapeutic outcome in terms of patient’s survival and tumor growth control. This, in turn, increased the spectrum of cancer patients benefiting from the unprecedented and durable effects of immune checkpoint inhibitors leading to their adoption as a first-line treatment for certain cancers. Moreover, the significance of precision medicine in cancer immunotherapy and the unmet demand to develop more personalized predictive biomarkers and treatment strategies are also highlighted in this review.
1 Introduction
The past decade has witnessed significant advancements with the use of immune checkpoint inhibitors (ICIs) in treating certain cancers (1). Unlike traditional cancer treatment modalities that function through inducing direct tumor cell death, immunotherapies including ICIs boost the immune system to elicit stronger and more specific anti-tumor immune responses and, therefore, control tumor growth (2). However, despite the unprecedented recent breakthroughs reported with the use of ICIs to treat cancer, not all patients respond similarly to the treatment with only 20-40% showing beneficial outcomes (3, 4). Over the past decade, extensive efforts have been employed to understand the lack of beneficial therapeutic outcome among the treated patients. A growing body of knowledge attributes the resistance to ICIs to various interconnected mechanisms, some of which are tumor-intrinsic while others are external to tumor cells (5). Examples of tumor-intrinsic factors include the lack of neoantigens, impaired tumor antigen processing and presentation, altered oncogenic cell signaling pathways in addition to epigenetic changes that promote drug resistance (5–9). On the other hand, the level of intratumoral immune cell infiltration, the compensatory upregulation of alternate immune checkpoint molecules, angiogenesis and gut microbiome are examples of tumor-extrinsic putative mechanisms that could play a role in inducing resistance to ICIs (5, 10–13).
It is evident that the increased understanding of the resistance mechanisms and underlying factors has -in part- directed the wheel of immunotherapy research toward designing strategies to bypass resistance and enhance the therapeutic potential of ICIs through combining them with other anti-cancer treatment approaches. Despite the limitations associated with conventional cancer treatment modalities, the different adjuvant therapies including chemotherapy, radiotherapy, targeted therapy and epigenetic modulators have shown potentials to shape the tumor microenvironment (TME) by increasing tumor immunogenicity, improving antigen presentation capability of antigen-presenting cells (APCs), enhancing the infiltration of immunoreactive cells to the tumor site and promoting their functional activity (14–16). These changes have been shown to play considerable roles in remodeling the TME and rendering it more conducive to deliver the beneficial effects of ICIs (16). Up to date, considerable number of these strategies demonstrated promising and durable results in terms of efficiency, feasibility and safety, and this has been derived from either the additive or synergistic effects of utilizing ICIs in combination with other therapies (17–19). Some of the combinatorial treatment approaches involving ICIs have already been implemented in the clinical settings to treat specific types of cancer while many others are still at early stages. For instance, the last decade has witnessed remarkable success with the use of chemotherapeutic agents in combination with ICIs to ameliorate resistance and enhance the therapeutic outcome in multiple cancer types. Examples of these treatment modalities that have been approved by the Food and Drug Administration (FDA) include pembrolizumab in combination with carboplatin and either paclitaxel or nab-paclitaxel to treat metastatic squamous non-small cell lung carcinoma (NSCLC), in addition to the combination of nivolumab, cisplatin and gemcitabine that was recently approved to treat metastatic or unresectable urothelial cancer (UC) (20, 21). It is worth appreciating that large number of ongoing pre-clinical and clinical studies are still evaluating the safety and efficiency of combining ICIs with other anti-cancer or immunomodulatory agents in order to expand the spectrum of cancer patients benefiting from immunotherapy. In this review, we discuss multiple strategies to overcome resistance to ICIs from the perspective of combining it with other treatments. Furthermore, the significance of personalized cancer immunotherapy and the need to improve the treatment efficacy in light of precision medicine are also highlighted.
2 Potential strategies to overcome resistance by combination therapy
In the last decade, built upon the valuable insights gained into the intrinsic and extrinsic mechanisms of resistance to ICIs, several combination therapeutic approaches were developed in order to overcome resistance and improve the therapeutic efficacy of ICIs. Figure 1 illustrates the different combination modalities involving ICIs and their putative mechanisms of action.
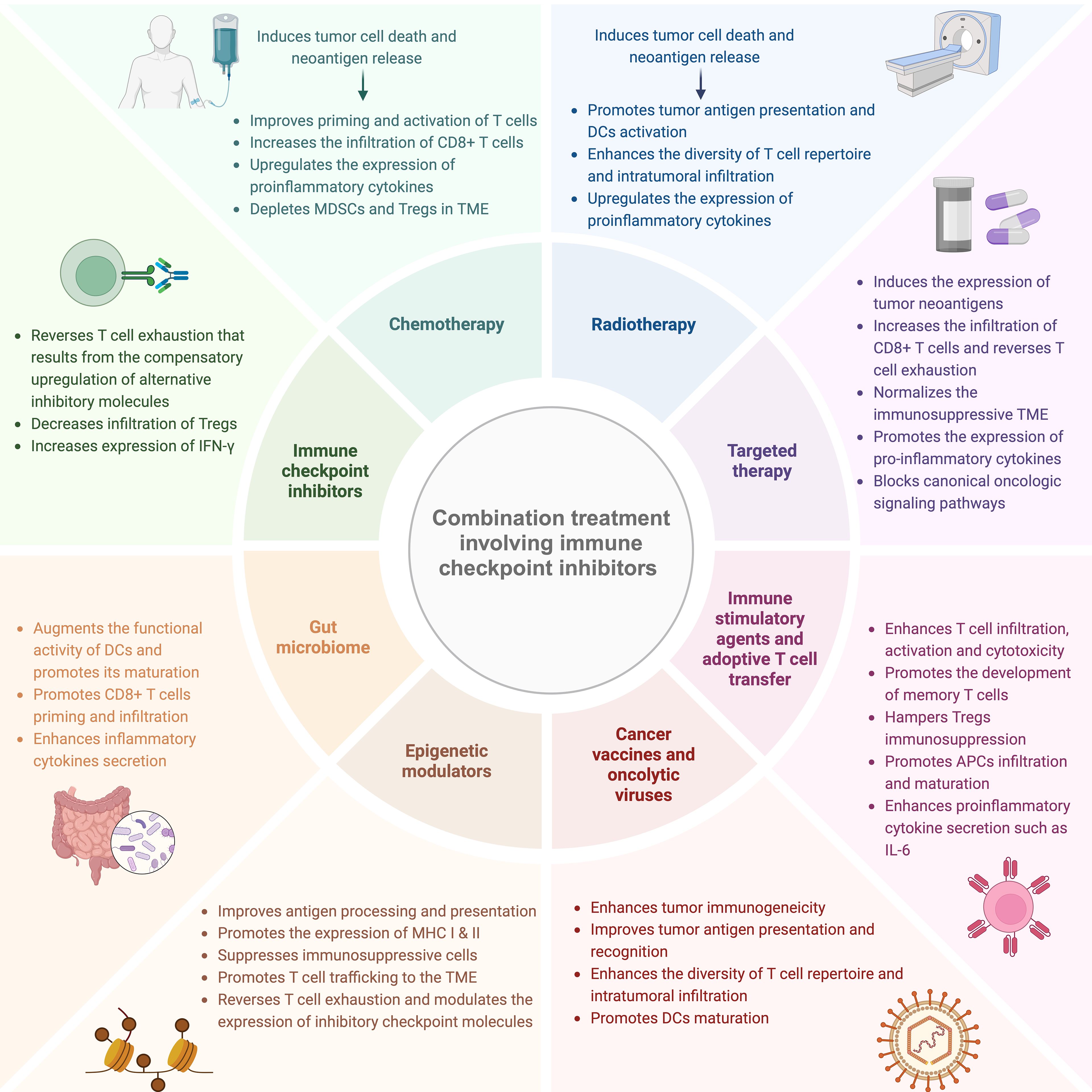
Figure 1. Combination therapeutic strategies to overcome resistance to ICIs and their putative mechanisms of action. Different cancer treatment modalities namely chemotherapy, radiotherapy, dual ICIs, targeted therapy, immune stimulatory agents, epigenetic modulators and gut microbiome manipulation can be employed to ameliorate resistance to ICIs and improve its therapeutic potential through multiple overlapping mechanisms such as enhancing tumor antigenicity, promoting neoantigen processing and presentation in addition to augmenting T cell intratumoral infiltration and functional activity. MDSCs, myeloid-derived suppressor cells; Tregs, regulatory T cells; TME, tumor microenvironment; DCs, dendritic cells; APCs, antigen-presenting cells; MHC, major histocompatibility complex. This figure was created with BioRender.com.
2.1 Combination with chemotherapy
Chemotherapy has been utilized to enhance tumor immunogenicity and overcome resistance to ICIs through inducing tumor cell death and subsequent release of neoantigens (16). This has been shown to improve priming and activation of CD8+ cytotoxic T cells (16). In addition, multiple studies have demonestrated the potential of chemotherapeutic agents to remodel the cellular immune system compartment (22–25). For instance, gemcitabine was shown to dramatically and significantly reduce myeloid-derived suppressor cells (MDSCs) in the spleens of tumor-bearing mice while increasing the anti-tumor potential of CD8+ T cells and activated natural killer cells (22). Other agents namely cisplatin have been shown to enhance the infiltration of cytotoxic T lymphocytes, upregulate the levels of proinflammatory cytokines as well as the expression of immune checkpoint molecules on intratumoral CD8+ T cells (23). Additionally, the role of paclitaxel in modulating the intratumoral immune system has been demonstrated through its capacity to reduce the infiltration of T regulatory cells (Tregs) and stimulate dendritic cells (DC)-mediated antigen presentation (24). Such changes have the potential to alleviate resistance and pave the way to drive the benefits of ICIs among multiple cancer types (26). In a study of advanced melanoma, it was demonstrated that the combination of local chemotherapy (nitrogen mustard alkylating agent melphalan) and anti-CTLA-4 improved response rate and progression-free survival (PFS) through enhancing the infiltration of T cells to the tumor site (27). Other clinical studies of triple-negative breast cancer (TNBC) and NSCLC reported significant improvements in the overall survival (OS) and progression-free survival upon treatment with the combination of chemotherapy (paclitaxel/carboplatin) and ICIs, in comparison to chemotherapy alone (28, 29). In this regard, it is worth noting that not all chemotherapeutic agents are capable to enhance the efficacy of ICIs. For example, vinorelbine and etoposide were not associated with additional benefit when combined with anti-CTLA-4/anti-PD-L1 in murine models of mesothelioma (25). Additionally, the success of chemotherapy and ICIs combination is, in part, dependent on the timing and sequence of chemo immunotherapy administration (30). It is also important to point out that multiple categories of chemotherapy pose different immunomodulatory roles. For instance, fludarabine and cisplatin were shown to interfere with the infiltration and functional capacity of Tregs whereas melphalan and bleomycin were shown to enhance type 1 immunity of Th1 immune response (31, 32). Therefore, it is essential to consider the type and stage of cancer along with the putative resistance mechanism when selecting the chemotherapeutic agent that can be used in combination with ICIs (31, 33). Currently, some combinations of chemotherapy agents and ICIs have become a standard of care to treat some types of cancer in the clinical settings while others are still being investigated in multiple clinical trials (34, 35). A study of NSCLC (KEYNOTE-021) demonstrated that the combination of pembrolizumab (anti-PD1) and chemotherapy (carboplatin and pemetrexed) enhanced the response rate and PFS among treated patients in comparison to those receiving chemotherapy alone (36). This study engaged the FDA to approve the combination of pembrolizumab and standard chemotherapy as a first-line treatment for advanced non-squamous NSCLC (36). Later, other clinical trials examined the potential of combining pembrolizumab and standard chemotherapy to treat squamous NSCLC and documented considerable improvement in patient’s OS and PFS, compared to chemotherapy (37, 38). In 2018, the results of these studies led to the FDA approval of pembrolizumab and chemotherapy for the treatment of squamous NSCLC patients (37, 38). Based on a string of success along with the remarkable improvement in the therapeutic outcome, the indications of chemotherapy in combination with ICIs were extended to include a wider spectrum of cancer patients, for example, the combination of atezolizumab (anti-PD-L1) and chemotherapy was approved to treat metastatic non-squamous NSCLC (atezolizumab plus nab-paclitaxel and carboplatin), unresectable locally advanced or metastatic TNBC (atezolizumab plus nab-paclitaxel) and extensive-stage small cell lung cancer (atezolizumab plus carboplatin and etoposide) (39–41). Table 1 provides a summary for the FDA-approved chemoimmunotherapeutic regimens and their indications.
2.2 Combination with radiotherapy
Radiotherapy is thought to enhance tumor sensitivity to ICIs through inducing tumor cell death and neoantigen release. This, in turn, enhances the diversity of T cell receptor (TCR) repertoire of tumor-infiltrating lymphocytes and promote tumor antigen presentation (14). Other studies have demonstrated that radiotherapy enhances the secretion of proinflammatory cytokines and the activation of DCs, leading to increased accumulation of intratumoral lymphocytes (16). In a study of melanoma, it was reported that the combination of local radiation therapy and anti-CTLA-4 immunotherapy enhanced the therapeutic outcome in comparison to anti-CTLA-4 monotherapy by enhancing the infiltration of CD8+ T cells (80). In line with this study, a combination of radiotherapy, anti-PD-L1 and anti-TIM-3 has been shown to inhibit tumor growth, improve survival, enhance the cytotoxic activity of T cells and decrease infiltration of Tregs in a murine model of head and neck squamous cell carcinoma (HNSCC) (81). Despite this favorable outcome, durable responses were not observed due to the resurgence of Tregs (81). A recent study of metastatic NSCLS reported that the combination of radiotherapy and ICIs was associated with improved outcome only in patients who were PD-L1 negative (82). Moreover, it is worth noting that the total dose, fractionation mode of radiotherapy and sequence of radiotherapy and ICIs administration have impact on modulating the immune response and efficacy of ICIs (82–84). Overall, it is appreciated that radiation therapy plays a role in transforming the TME from non-immunogenic to immunogenic and, therefore, enhance the sensitivity to ICIs (83).
2.3 Combination with targeted therapy
Several lines of evidence documented the potential of different approaches of targeted therapy such as angiogenesis inhibitors, EGFR inhibitors, HER2 inhibitors and hormone receptor inhibitors to overcome the resistance associated with ICIs (17). This was shown to be mediated through different mechanisms that increase tumor sensitivity to immunotherapeutic agents. For example, targeting mitogen-activated protein kinase (MAPK) pathway utilizing EGFR/MEK/BRAF inhibitors was shown to induce the expression of tumor neoantigens, promote the infiltration of CD8+ T cells to the tumor site and block the canonical oncologic signaling pathways (16). In line with these findings, multiple pre-clinical and clinical trials demonstrated that the combination of these inhibitors and PD-1/PD-L1 blockade resulted in improved anti-tumor immunity and overall therapeutic outcomes (85–88). In addition, pre-clinical studies utilizing PI3K inhibitors in combination with ICIs documented the combination’s potential to inhibit tumor growth and improve overall survival (89, 90). This outcome was associated with increased expression of pro-inflammatory cytokines and enhanced T cell cytotoxicity. Moreover, several pre-clinical and clinical studies demonstrated the capacity of other approaches of molecular targeted therapy namely vascular endothelial growth factor (VEGF) inhibitors, indoleamine 2,3-dioxygenase (IDO) inhibitors, A2A receptor blockade and others to reverse the resistance to ICIs through multiple mechanisms such as normalizing the immunosuppressive TME, enhancing the infiltration of intratumoral T cells in addition to reversing T cell exhaustion and promoting its effector function (15–17, 91). For instance, targeting VEGF, an immunosuppressive factor, has been shown to augment endothelial cell activation, inhibit tumor neovascularization and reinforce the anti-cancer immunity (92, 93). Another study demonstrated that bevacizumab treatment, a VEGF inhibitor, resulted in trends towards increase in the expression of genes associated with effector CD8+ T cells, T-helper chemokines and natural killer cells (94). Overall, these changes were shown to enhance tumor sensitivity to ICIs in which the combination treatment was associated with superior outcomes in comparison to monotherapy. Additionally, therapies targeting adenosine axis such as anti-CD38 and anti-CD73 inhibitors succeeded in overcoming resistance associated with ICIs through their potential to increase the infiltration of intratumoral effector CD4+ T cells, enhance the expression of granzyme B and IFN-γ by tumor-infiltrating CD8+ T cells and decrease the accumulation of the immunosuppressive MDSCs and Tregs within the TME (95, 96).
2.4 Combination with other immune checkpoint inhibitors
Given that the compensatory upregulation of alternative inhibitory immune checkpoint molecules is one of the challenges to achieve favorable outcome with ICIs, multiple pre-clinical and clinical trials were directed to utilize multiple blockade as a putative mechanism to bypass resistance (97). The combination of anti-CTLA-4 and anti-PD-1 was associated with improved therapeutic outcomes in perspective to patient’s survival (98). The clinical benefit derived from ICIs combination treatment could be attributed to the complimentary mechanisms in which anti-CTLA-4 functions through promoting T cell priming while anti-PD-1 plays a key role in enhancing the effector function of T cells. Moreover, a recent study in melanoma patients revealed that anti-CTLA-4 induces a robust clonal expansion of progenitor exhausted T cells which, in turn, promotes exhausted T cell reinvigoration when combined with anti-PD-1 treatment (99). Such combination has been approved to treat multiple cancer types including melanoma, hepatocellular carcinoma, renal cell carcinoma and colorectal cancer (100–102). Additionally, various pre-clinical trials have demonstrated the synergistic beneficial outcome of targeting PD-1/PD-L1 axis and other inhibitory immune checkpoint molecules including TIM-3, LAG-3, VISTA, BTLA and TIGIT in terms of tumor growth control and overall survival (103–108). This has been shown to be associated with reversed T cell exhaustion, increased expression of IFN-γ and decreased levels of Tregs. Besides the FDA approved dual ICIs treatment (Table 1), multiple other combinations are under evaluation by a significant number of clinical trials (109–111).
2.5 Combination with immune stimulatory agents
It is well-appreciated that the magnitude of T cell activation and subsequent anti-tumor immune responses is determined by the dynamic interplay between co-stimulatory and inhibitory immune checkpoint molecules. One way to improve T cell activation is through targeting immune stimulatory pathways, and therefore, provide a rationale for immune stimulatory agonist including CD40, ICOS, OX40, GITR and 41BB to be utilized in combination with ICIs to treat cancer patients. Several pre-clinical and clinical trials documented the potential of these immune stimulatory agonists to alter the intratumoral immune system compartment and promote the beneficial response to ICIs by enhancing T cell infiltration, activation and cytotoxicity (15, 16). In addition, other studies reported the capacity of the immune stimulatory ICOS agonist and GITR agonist to improve the therapeutic outcome through promoting the development of memory T cells and hampering Treg immunosuppression, respectively (112, 113). Moreover, the role of other immunostimulatory agents including CD40 agonist to increase infiltration of DCs, reduce MDSCs and promote APC maturation has been documented (114, 115). This, in turn, may contribute to its potential to overcome ICIs resistance.
2.6 Combination with adoptive T cell transfer
Adoptive T cell transfer has led to considerable clinical benefits in treating hematological malignancies; however, this success was limited in solid tumors due to poor infiltration of T cells along with the immunosuppression imposed by the TME. Multiple pre-clinical studies demonstrated that the combination of ICIs with adoptive T cell transfer improved therapeutic outcome in terms of tumor regression and improved survival (116, 117). This was thought to be delivered through enhancing the intratumoral infiltration of T cells as well as the secretion of proinflammatory cytokines such as IL-6. The first-in-human clinical trial that utilized adoptive T cell transfer in combination with ICIs demonstrated that treating melanoma patients with a combination of ipilimumab and adoptive cell transfer is feasible and well-tolerated (118). Another clinical study reported that engineered CD19 chimeric antigen receptor (CAR)-T cells expressing IL-7 and CCL19 in combination with anti-PD-1 improved the anti-tumor immune response and long-term remission rate in relapsed or refractory diffuse large B cell lymphoma patients (119). It is worth noting that IL-7 and CCL9 play a considerable role in promoting the proliferation, infiltration, accumulation and survival of CAR-T cells in lymphoid tissues (120, 121). This, in turn, was shown to be associated with enhanced anti-tumor potentials and improved therapeutic outcome compared to conventional CAR-T cells (121–123). Overall, it can be concluded that the capacity of adoptive T cell transfer to augment the recruitment and functional activity of T cells could act synergistically with ICIs in order to enhance treatment efficacy (117, 118, 124).
2.7 Combination with cancer vaccines and oncolytic viruses
It is known that lack of neoantigens is one of the mechanisms that confer resistance to immunotherapy. Therefore, tumor vaccines have been utilized in order to enhance tumor immunogenicity, augment anti-tumor immune responses, and pave the way to overcome resistance and drive the clinical benefits of ICIs. A significant number of pre-clinical and clinical studies provided a strong evidence that cancer vaccines can significantly improve the therapeutic response to ICIs in multiple cancer types (125–127). This was shown to be mediated by the capacity of cancer vaccines to (a) improve antigen presentation and recognition, (b) expand the repertoire of neoantigen-specific T cells and (c) enhance intratumoral infiltration of T cells (16, 125, 126). Moreover, the first-in-human clinical trials illustrated the safety, feasibility and immunotherapeutic potential of targeting tumor mutations (126–128). In a study of melanoma, it was demonstrated that the combination of nivolumab (anti-PD-1) and tumor neoantigen vaccines was associated with complete tumor regression and enhanced patient’s survival (126, 127). Additionally, durable and complete tumor regression was also documented in metastatic cancer patients following treatment with a combination of neoantigen-loaded monocyte-derived dendritic cell vaccine and anti-PD-1 (129). Given that patients with the same tumor type have distinct mutational signature, the use of personalized cancer vaccines is thought to enhance treatment specificity and effectiveness. On the other hand, cancer vaccines require HLA haplotype compatibility and could result in off-target immune stimulation (130). This, in turn, may limit its application in the clinical use.
In a similar manner to cancer vaccines, oncolytic viruses showed the potential to enhance the therapeutic outcome of ICIs through promoting antigen presentation and T cell priming. The combination of ICIs namely ipilimumab or pembrolizumab and talimogene laherparepvec- a genetically modified oncolytic herpes simplex virus 1 that express granulocyte-macrophage colony-stimulating factor (GM-CSF)-demonstrated improved therapeutic potential in treating unresectable melanoma patients in comparison to monotherapy without any additional safety concerns (131–135). The virus was designed to replicate selectively in cancerous cells and induce lytic cell death and release of tumor-specific antigens (132). This, in turn, was proven to enhance the anti-tumor immune response through promoting DC maturation, IFN-γ expression, T cell activation and infiltration (132). In fact Talimogene laherparepvec is the first oncolytic viral immunotherapy to be approved by the FDA to treat locally advanced or unresectable melanoma patients. Nowadays, multiple pre-clinical and clinical trials are underway in the effort of testing the efficacy of combining ICIs with Talimogene laherparepvec and other novel oncolytic viruses (136).
2.8 Combination with epigenetic modulators
It is known that epigenetic dysregulation plays a crucial role in cancer development and progression. Therefore, targeting this axis has been associated with promising results in multiple malignancies. Beyond their potential as monotherapies, several lines of evidence documented the considerable role of epigenetic modulators such as DNA methyltransferase inhibitors and histone deacetylase inhibitors (HDACi) to improve the sensitivity of cancerous cells to chemotherapy, radiotherapy, targeted therapy and some approaches of immunotherapy (137). The improved efficacy of ICIs when combined with epigenetic modulators was shown to be derived from the potential of combination treatment to modulate the TME (138–142). Multiple studies have demonstrated the capacity of Entinostat- a selective class I HDACi- to enhance the therapeutic potential of PD-1 blockade through inhibiting the immunosuppressive function of MDSCs, suppressing Tregs, enhancing MHC class I expression in addition to promoting the infiltration and functional activity of intratumoral T cells (138–141). Another study of advanced NSCLC showed that a pan HDACi namely Vorinostat is capable to prime the TME and enhance the response to PD-1 inhibitors through enhancing the expression of immune-related genes, inhibiting immunosuppressive cells as well as promoting the production of pro-inflammatory cytokines (142). Moreover, it was reported that DNA methyltransferase inhibitors are capable to reverse the epigenetic silencing of T helper 1 immunostimulatory chemokines including CXCL9 and CXCL10 and, in turn, promote T cell trafficking to the TME and enhance the sensitivity to immunotherapeutic agents such as ICIs and adoptive T cell transfer (9). In a pre-clinical study of colorectal cancer, it was demonstrated that HBI-8000 – a HDACi- augmented the treatment outcome in synergy with CTLA4/PD-1/PD-L1 blockade and resulted in improved tumor growth control (143). This was shown to be mediated by the capacity of HBI to alter the TME from being immunologically cold (nonresponsive) to becoming hot (responsive) through modulating the expression of inhibitory immune checkpoints, enhancing the functional activity of APCs and modulating the expression of MHC I and II (143). Additionally, another pre-clinical study of colorectal and metastatic mammary cancers illustrated that both DNA methyltransferase inhibitors and HDACi are capable to improve the response to CTLA-4 and PD-L1 inhibitors through targeting the immunosuppressive MDSCs (144). Currently, a number of clinical studies are undergoing to assess the translation of these promising results from pre-clinical to clinical settings in perspective to safety and efficacy (145–149).
2.9 Combination with gut microbiome
Given that the composition of gut microbiota plays remarkable roles in shaping the anti-tumor immunity, modulating the microbiome was associated with augmented efficacy of ICIs as demonstrated by multiple pre-clinical and clinical studies. Using a pre-clinical model of melanoma, Sivan et al. demonstrated that the oral administration of Bifidobacterium abolished tumor outgrowth when combined with PD-L1 inhibitors (150). This synergistic effect was driven by the capacity of the combination treatment to augment DC function, promote CD8+ T cell priming and infiltration, in addition to enhancing inflammatory cytokines secretion (150). In line with these findings, it has been reported that the oral administration of Bacteroides fragilis with Burkholderia cepacian or Bacteroides thetaiotaomicron enhanced the efficacy of anti-CTLA-4 through promoting DC maturation and T cell-mediated immune responses (151). Another study demonstrated the improved tumor growth control accompanied with enhanced T cell response and improved efficiency of PD-L1 blockade following reconstitution of germ-free mice with fecal material from patients responding to ICIs (152). Moreover, gut microbiome manipulation was shown to abrogate immune-related adverse events that are associated with ICIs treatment. Wang et al. demonstrated that the oral administration of Lactobacillus reuteri provided a protective effect and inhibited the development of colitis through decreasing the distribution of group 3 innate lymphoid cells that are induced by ICIs-related colitis (153). Such findings have set the stage for clinical trials aiming to assess the safety, feasibility and efficacy of modulating gut microbiome to enhance the therapeutic potential of ICIs among cancer patients (151, 154, 155). Recent clinical trials elucidated insights into the potential of utilizing fecal microbiota transplantation to induce favorable changes in the intratumoral immune cell compartment and gene expression profiles in gut and tumor tissues of patients with PD-1 inhibitors-refractory metastatic melanoma and these were translated into promising clinical benefits (154, 155).
2.10 Other strategies to improve the efficacy of ICIs
Multiple other strategies combining ICIs with other approaches are being investigated with some demonstrating promising results. For example, several lines of evidence documented the potential of cytokines namely IL-2, IL-12 and IL-15 in synergy with ICIs to improve the overall therapeutic outcome (156–158). This was shown to be associated with enhanced effector T cell and natural killer cell responses. Additionally, targeting chemokines plays a considerable role in modulating the TME in favor of tumor inhibition. For instance, utilizing CCR-1/2 antagonists and CXCR-1/2 inhibitors was proven to reduce MDSC infiltration in the TME and inhibit epithelial-mesenchymal transition (EMT) (159–162). Such changes were associated with favorable responses to ICIs in multiple cancer types. Moreover, immunosuppressive cells including Tregs, MDSCs and tumor-associated macrophages (TAMs) could be targeted to bypass resistance to ICIs using different agents such as anti-CCR-4 inhibitors, agonistic TRAIL-R antibody and CSF-1R inhibitor (15, 16). These modulators in synergy with ICIs have shown potential to reverse the immunosuppressive nature of the TME and suppress tumor invasion, metastasis and angiogenesis (16). In addition, some studies demonstrated that bacterial-mediated cancer therapy has the potential to augment the antitumor immune response and ameliorate resistance to ICIs (163–165). Al-Saafeen et al. demonstrated the capacity of attenuated Salmonella typhimurium to improve the efficacy of anti-PD-L1 in a pre-clinical model of colorectal cancer through enhancing intratumoral T cell infiltration, upregulating the expression of MHC II and decreasing the percentage of tumor-associated granulocytic cells (164). Several other studies have also demonestrated the potential of certain bacterial strains and their metabolites to remarkably influence the therapeutic outcome of ICIs through modulating the TME and promoting T cell activation (151, 152, 166–169). In one study using epithelial tumors, Routy et al. showed that Akkermansia muciniphila is capable to enhance DCs maturation, IL-2 secretion and T cell infiltration leading to a better response to anti-PD-1 immunotherapy (167). On the other hand, some bacterial genera, namely Ruminococcus and Roseburia, have been associated with poor response to ICIs due to their role in inducing immunosuppressive cells (166). Despite promising results, implementing this approach in the clinical settings still presents some challenges regarding dosing, safety and other factors. Overall, the favorable outcome associated with the combination treatments is thought to be based on the potential of adjuvant therapy to modulate the TME and transform it from being immunosuppressive to becoming immunogenic and, therefore, pave the way for ICIs to deliver its beneficial therapeutic effect. This comes in addition to utilizing combination treatments in order to target different tumorigenesis promoting axes and abrogate immune evasion fostering a synergistic beneficial outcome. To this point, several pre-clinical and clinical studies are underway in the effort of enhancing the therapeutic potential of ICIs and expanding the spectrum of responsive patients through identifying the mechanisms of resistance and targeting it by combining ICIs with other approaches.
3 Precision cancer immunotherapy
The marked variation in the response to immunotherapy among cancer patients along with the tumor complexity and heterogeneity paved the way for personalized medicine and highlighted its significance for cancer immunotherapy. Precision medicine is a promising approach in oncology that utilizes the intra- and intertumoral genetic and epigenetic variability, the tumor immune microenvironment, in addition to patient’s lifestyle and the surrounding environmental factors to provide the best-fit treatment and prevention strategies for the patient (170). In other words, precision medicine utilizes the comprehensive genomic, transcriptomic and proteomic data to guide treatment choices. Through identifying specific genetic mutations and alterations in signaling pathways along with characterizing the intratumoral immune system compartment, this approach facilitates tailoring the therapy to the unique characteristics of each patient in order to enhance the treatment efficacy while keeping the associated adverse events to the minimal (171). This would expand the fraction of patients who can clinically benefit from ICIs.
Patient’s response to ICIs is a complex trait shaped by several intrinsic and extrinsic factors. Nowadays, three FDA-approved predictive biomarkers are used for routine patient’s selection and those are (a) PD-L1 expression, (b) Microsatellite instability (MSI) and (c) tumor mutational burden (TMB) (172–174). Given that each of these biomarkers has certain limitations and utilizes different assays for various cancer types, there is a lack of a standardized, well-defined framework for implementing these biomarkers in patient selection criteria (174). Consistently, data from 18,792 patients across 100 peer-reviewed studies revealed that patients predicted to respond beneficially to ICIs- based on FDA-approved biomarkers- showed only a small degree of overlap suggesting that each of these biomarkers contribute differently to the overall response (175). Moreover, the use of a single biomarker isn’t feasible to predict the response to immunotherapeutic agents (176–179). Some biomarker-negative patients have shown favorable response to the treatment while other patients with similar levels of these biomarkers showed variable responses to the treatment. For example, the expression of PD-L1 has been associated with beneficial responses to PD-1/PD-L1 blockade across multiple cancer types; however, other studies demonstrated that patients with PD-L1 negative tumors can still achieve favorable outcomes when treated with PD-1/PD-L1 inhibitors (180–183). It is worth noting that the intratumoral heterogeneity and dynamic nature of PD-L1 expression across the tumor-tissue could influence the reliability of PD-L1 as a predicative biomarker for the response to anti-PD-1/PD-L1 immunotherapy (184). Additionally, neoantigen load, immune infiltration status and IFN-γ signaling pathway genes were shown to exhibit heterogeneity among discrete regions within the same tumor in a single patient (185). Therefore, tumor sampling might not precisely reflect the distribution of these parameters which, in turn, compromise their predictive value. In addition, multiple studies have demonstrated the association of high TMB and corresponding neoantigen load with the enhanced sensitivity to ICIs whereas others showed that certain mutations played a role in shaping the overall response to ICIs independently from the tumor mutational load (186–190). Therefore, the limited predictive values of these FDA-approved biomarkers stressed the unmet need to identify highly accurate and more personalized predictive biomarkers to assist patient’s selection in clinical settings. New class of predictive biomarkers related to gene-based expression signature has emerged- though not yet approved by the FDA- including T cell-inflamed gene expression profile (191), T cell dysfunction and exclusion gene signature (192), melanocytic plasticity signature (193, 194), and B cell-focused gene signature (194, 195). These biomarkers were shown to have superior and enhanced predictive value compared to single gene or protein markers. Moreover, recent studies have highlighted the use of differentially expressed non-coding RNA (ncRNA) to predict the response to immunotherapy (196). In a study of NSCLC, specific circulating miRNA namely miR-93, -138-5p, -200, -27a and -424 were shown to be remarkably highly expressed in patients who demonstrated favorable response to anti-PD-1 compared to non-responding patients (197). This emphasizes on the significance of utilizing ncRNAs as crucial biomarkers for the early response to ICIs (197). In line with this, it has been demonstrated that the immune functional long ncRNAs signature is associated with favorable response to ICIs, superior tumor growth control, augmented intratumoral infiltration of cytotoxic T cells and PD-L1 expression (198). An additional study of TNBC documented the association between high levels of long ncRNA LINK-A and resistance to PD-1 blockade (199). This has been attributed to the potential of LINK-A expression to enhance the degradation of the antigen peptide-loading complex and the intrinsic tumor suppressors Rb and p53 interfering with tumor antigenicity and tumor’s intrinsic suppression capacity (199). Overall, it is worth appreciating that the utilization of ncRNAs in patient’s selection would substantially improve the capacity to predict the immunotherapeutic clinical outcome. Over the past decade, multiple molecular tools including PCR-based tests, conventional sequencing, RNA-sequencing and next-generation sequencing were utilized for patient’s enrollment in the clinical trials of targeted cancer therapies and have been approved by the FDA as standard procedures to guide and select the appropriate treatment for cancer patients (171, 200). These technologies help in providing a comprehensive cancer genomic landscape and identifying predictive and prognostic molecular alterations that could result in the resistance to a specific therapeutic agent. However, the efficacy of these methods can be associated with some limitations. For instance, whole exome-sequencing- used in most of the studies- identifies genomic associations with the therapeutic response in the exome regions that represent approximately 1% of the whole genome. However, it does not capture variations in the non-coding regions, which may have predictive value for treatment response or be associated with specific mechanisms of resistance (201, 202). Moreover, the application of these technologies in the clinical settings is still limited due to tumor heterogeneity and the intratumoral variation that could interfere with responsiveness to molecular-targeted therapies (200). It is evident that tumor cells during cancer development acquire genetic mutations and undergo changes in the epigenetic signature resulting in different populations of tumor cells that vary in their morphology, genotype and function (203). The selective pressure allows cancerous cells that acquired mutations with survival and therapy-resistance advantages to dominate tumor tissue. Recent studies assumed that the source of tumor heterogeneity is a population of undifferentiated malignant cells with stem-like phenotype (204, 205). These cells were capable of self-renewal and differentiation into different tumor cells in response to the TME (206). Therefore, more advanced technologies are needed to overcome this obstacle and provide more efficient and personalized cancer treatment.
4 Conclusions and future perspectives
The increased understanding of the resistance mechanisms facilitated efforts to overcome the resistance through targeting the underlying mechanisms using combination treatment strategies. Several lines of evidence demonstrated the potential of combination treatments to reverse the resistance by leveraging the synergistic effects of targeting different immune evasion mechanisms, resulting in improved therapeutic outcome and overall response to the treatment. In addition to combinatorial treatment modalities, the response rate and therapeutic efficacy of ICIs can be enhanced through implementing accurate personalized treatment. Several predicative biomarkers from the tumor, peripheral blood and other sites have been identified to improve patient’s selection for treatment. However, clinical studies have shown that the prognostic value of a single biomarker isn’t sufficient to predict the response to the treatment taking into account that the clinical predictive values of the identified biomarkers don’t apply to all treated patients. These findings paved the path for the personalization of cancer immunotherapy and tailoring the treatment strategy for individual patients. Nowadays, extensive efforts to fine-tune personalized predictive biomarkers using advanced technologies are ongoing. For example, single-cell RNA-sequencing (scRNA-seq) is considered a powerful high-throughput transcriptomics method that uncover tumor complexity and dynamics by profiling the gene expression of each single cell within the tumor tissue (206). It also enables a more refined understanding of the mechanistic details of the multifactorial and dynamic interactions that occur in the TME, in addition to providing insights into the specific immunogenic markers associated with the heterogenous cancerous clones. Overall, scRNA-seq has shown a great promise in improving the efficiency and application of precision cancer immunotherapy. Despite the substantial ongoing efforts, there is an increasing demand to design a multi-omics approach that integrates DNA, RNA, proteomic and metabolomic analyses to provide a better understanding of the tumor biology, tumor tissue heterogeneity, the mechanistic details of the anti-tumor immune responses, in addition to the key drivers of inter-patient variability in response to immunotherapy. Such approach will help in identifying innovative predictive biomarkers, designing novel targeted therapies and optimizing treatment strategies to include combinatorial therapies. This, in turn, should play a remarkable role in ameliorating the resistance to immunotherapeutic agents and improve the overall response rate.
Author contributions
BA: Conceptualization, Investigation, Visualization, Writing – original draft, Writing – review & editing. BA: Conceptualization, Supervision, Writing – review & editing. EE: Conceptualization, Supervision, Writing – review & editing.
Funding
The author(s) declare that financial support was received for the research and/or publication of this article. BHA is supported through a PhD Fellowship from United Arab Emirates University. This research is supported by ASPIRE, the technology program management pillar of Abu Dhabi’s Advanced Technology Research Council (ATRC), via the ASPIRE Precision Medicine Research Institute Abu Dhabi (ASPIREPMRIAD) award grant number VRI-20-10.
Acknowledgments
The authors wish to acknowledge the ASPIRE Precision Medicine Research Institute and the Graduate Studies, College of Medicine and Health Sciences, United Arab Emirates University for the PhD student fellowship awarded to BHA.
Conflict of interest
The authors declare that the research was conducted in the absence of any commercial or financial relationships that could be construed as a potential conflict of interest.
The author(s) declared that they were an editorial board member of Frontiers, at the time of submission. This had no impact on the peer review process and the final decision.
Generative AI statement
The author(s) declare that no Generative AI was used in the creation of this manuscript.
Publisher’s note
All claims expressed in this article are solely those of the authors and do not necessarily represent those of their affiliated organizations, or those of the publisher, the editors and the reviewers. Any product that may be evaluated in this article, or claim that may be made by its manufacturer, is not guaranteed or endorsed by the publisher.
Abbreviations
ICIs, Immune checkpoint inhibitors; TME, tumor microenvironment; APCs, antigen-presenting cells; FDA, Food and Drug Administration; NSCLC, non-small cell lung carcinoma; UC, urothelial cancer; MDSCs, myeloid-derived suppressor cells; Tregs, T regulatory cells; DC, dendritic cell; PFS, progression-free survival; TNBC, triple-negative breast cancer; OS, overall survival; HNSCC, head and neck squamous cell carcinoma; GEJ, gastroesophageal junction; ESCC, esophageal squamous cell carcinoma; HCC, hepatocellular carcinoma; dMMR, mismatch repair deficient; RCC, renal cell carcinoma; MSI, microsatellite instability; CRC, colorectal cancer; TCR, T cell receptor; MAPK, mitogen-activated protein kinase; VEGF, vascular endothelial growth factor; IDO, Indoleamine 2,3-dioxygenase; CAR, Chimeric antigen receptor; GM-CSF, granulocyte-macrophage colony-stimulating factor; HDACi, histone deacetylase inhibitors; EMT, epithelial-mesenchymal transition; TAMs, tumor-associated macrophages; TMB, tumor mutational burden; ncRNA, non-coding RNA; scRNA-seq, single-cell RNA-sequencing.
References
1. Lee JB, Kim HR, Ha SJ. Immune checkpoint inhibitors in 10 years: contribution of basic research and clinical application in cancer immunotherapy. Immune Netw. (2022) 22:e2. doi: 10.4110/IN.2022.22.E2
2. Zhang Y, Zhang Z. The history and advances in cancer immunotherapy: understanding the characteristics of tumor-infiltrating immune cells and their therapeutic implications. Cell Mol Immunol. (2020) 17:807–21. doi: 10.1038/S41423-020-0488-6
3. Xu-Monette ZY, Zhang M, Li J, Young KH. PD-1/PD-L1 blockade: Have we found the key to unleash the antitumor immune response? Front Immunol. (2017) 8:1597. doi: 10.3389/fimmu.2017.01597
4. Brahmer JR, Tykodi SS, Chow LQM, Hwu W-J, Topalian SL, Hwu P, et al. Safety and activity of anti–PD-L1 antibody in patients with advanced cancer. New Engl J Med. (2012) 366:2455–65. doi: 10.1056/nejmoa1200694
5. Alsaafeen BH, Ali BR, Elkord E. Resistance mechanisms to immune checkpoint inhibitors: updated insights. Mol Cancer. (2025) 24:1–20. doi: 10.1186/S12943-024-02212-7
6. Cristescu R, Mogg R, Ayers M, Albright A, Murphy E, Yearley J, et al. Pan-tumor genomic biomarkers for PD-1 checkpoint blockade-based immunotherapy. Science. (2018) 362:eaar3593. doi: 10.1126/SCIENCE.AAR3593
7. Ribas A, Algazi A, Ascierto PA, Butler MO, Chandra S, Gordon M, et al. PD-L1 blockade in combination with inhibition of MAPK oncogenic signaling in patients with advanced melanoma. Nat Commun. (2020) 11:1–10. doi: 10.1038/s41467-020-19810-w
8. Paulson KG, Voillet V, McAfee MS, Hunter DS, Wagener FD, Perdicchio M, et al. Acquired cancer resistance to combination immunotherapy from transcriptional loss of class I HLA. Nat Commun. (2018) 9:1–10. doi: 10.1038/s41467-018-06300-3
9. Peng D, Kryczek I, Nagarsheth N, Zhao L, Wei S, Wang W, et al. Epigenetic silencing of TH1-type chemokines shapes tumour immunity and immunotherapy. Nature. (2015) 527:249–53. doi: 10.1038/nature15520
10. Tiwari A, Trivedi R, Lin SY. Tumor microenvironment: barrier or opportunity towards effective cancer therapy. J Biomed Sci. (2022) 29:1–27. doi: 10.1186/S12929-022-00866-3
11. Koyama S, Akbay EA, Li YY, Herter-Sprie GS, Buczkowski KA, Richards WG, et al. Adaptive resistance to therapeutic PD-1 blockade is associated with upregulation of alternative immune checkpoints. Nat Commun. (2016) 7:10501. doi: 10.1038/NCOMMS10501
12. Borgerding JN, Shang J, Britton GJ, Salmon H, Bigenwald C, Maier B, et al. Human microbial transplant restores T cell cytotoxicity and anti-tumor response to PD-L1 blockade in gnotobiotic mice. bioRxiv. (2020). doi: 10.1101/2020.08.07.242040
13. Sehgal K, Portell A, Ivanova EV, Lizotte PH, Mahadevan NR, Greene JR, et al. Dynamic single-cell RNA sequencing identifies immunotherapy persister cells following PD-1 blockade. J Clin Invest. (2021) 131:e135038. doi: 10.1172/JCI135038
14. Deng L, Liang H, Burnette B, Beckett M, Darga T, Weichselbaum RR, et al. Irradiation and anti-PD-L1 treatment synergistically promote antitumor immunity in mice. J Clin Invest. (2014) 124:687–95. doi: 10.1172/JCI67313
15. Fujiwara Y, Mittra A, Naqash AR, Takebe N. A review of mechanisms of resistance to immune checkpoint inhibitors and potential strategies for therapy. Cancer Drug Resistance. (2020) 3:252. doi: 10.20517/CDR.2020.11
16. Fares CM, Van Allen EM, Drake CG, Allison JP, Hu-Lieskovan S. Mechanisms of resistance to immune checkpoint blockade: why does checkpoint inhibitor immunotherapy not work for all patients? Am Soc Clin Oncol Educ Book. (2019) 39:147–64. doi: 10.1200/EDBK_240837
17. Li B, Jin J, Guo D, Tao Z, Hu X. Immune checkpoint inhibitors combined with targeted therapy: the recent advances and future potentials. Cancers (Basel). (2023) 15:2858. doi: 10.3390/CANCERS15102858/S1
18. Mo DC, Huang JF, Luo PH, Huang SX, Wang HL. The efficacy and safety of combination therapy with immune checkpoint inhibitors in non-small cell lung cancer: A meta-analysis. Int Immunopharmacol. (2021) 96:107594. doi: 10.1016/J.INTIMP.2021.107594
19. Wu Y, Shi H, Jiang M, Qiu M, Jia K, Cao T, et al. The clinical value of combination of immune checkpoint inhibitors in cancer patients: A meta-analysis of efficacy and safety. Int J Cancer. (2017) 141:2562–70. doi: 10.1002/IJC.31012
20. FDA Approves Keytruda (pembrolizumab) in Combination with Carboplatin and Either Paclitaxel or Nab-Paclitaxel for the First-Line Treatment of Patients with Metastatic Squamous Non-Small Cell Lung Cancer (NSCLC). Available online at: https://www.drugs.com/newdrugs/fda-approves-keytruda-pembrolizumab-combination-carboplatin-either-paclitaxel-nab-paclitaxel-first-4853.html (Accessed July 14, 2024).
21. U.S. Food and Drug Administration Approves Opdivo (nivolumab), in Combination with Cisplatin and Gemcitabine, for First-Line Treatment of Adult Patients with Unresectable or Metastatic Urothelial Carcinoma . Available online at: https://www.drugs.com/newdrugs/u-s-food-administration-approves-opdivo-nivolumab-combination-cisplatin-gemcitabine-first-line-6210.html (Accessed July 13, 2024).
22. Suzuki E, Kapoor V, Jassar AS, Kaiser LR, Albelda SM. Gemcitabine selectively eliminates splenic Gr-1+/CD11b+ myeloid suppressor cells in tumor-bearing animals and enhances antitumor immune activity. Clin Cancer Res. (2005) 11:6713–21. doi: 10.1158/1078-0432.CCR-05-0883
23. Wakita D, Iwai T, Harada S, Suzuki M, Yamamoto K, Sugimoto M. Cisplatin augments antitumor T-cell responses leading to a potent therapeutic effect in combination with PD-L1 blockade. Anticancer Res. (2019) 39:1749–60. doi: 10.21873/ANTICANRES.13281
24. Zhu L, Chen L. Progress in research on paclitaxel and tumor immunotherapy. Cell Mol Biol Lett. (2019) 24:40. doi: 10.1186/S11658-019-0164-Y
25. Principe N, Aston WJ, Hope DE, Tilsed CM, Fisher SA, Boon L, et al. Comprehensive testing of chemotherapy and immune checkpoint blockade in preclinical cancer models identifies additive combinations. Front Immunol. (2022) 13:872295. doi: 10.3389/FIMMU.2022.872295
26. Zhang L, Zhou C, Zhang S, Chen X, Liu J, Xu F, et al. Chemotherapy reinforces anti-tumor immune response and enhances clinical efficacy of immune checkpoint inhibitors. Front Oncol. (2022) 12:939249/PDF. doi: 10.3389/FONC.2022.939249/PDF
27. Ariyan CE, Brady MS, Siegelbaum RH, Hu J, Bello DM, Rand J, et al. Robust antitumor responses result from local chemotherapy and CTLA-4 Blockade. Cancer Immunol Res. (2018) 6:189–200. doi: 10.1158/2326-6066.CIR-17-0356/470577/AM/ROBUST-ANTITUMOR-RESPONSES-RESULT-FROM-LOCAL
28. Schmid P, Cortes J, Pusztai L, McArthur H, Kümmel S, Bergh J, et al. Pembrolizumab for early triple-negative breast cancer. New Engl J Med. (2020) 382:810–21. doi: 10.1056/NEJMOA1910549/SUPPL_FILE/NEJMOA1910549_DATA-SHARING.PDF
29. West H, McCleod M, Hussein M, Morabito A, Rittmeyer A, Conter HJ, et al. Atezolizumab in combination with carboplatin plus nab-paclitaxel chemotherapy compared with chemotherapy alone as first-line treatment for metastatic non-squamous non-small-cell lung cancer (IMpower130): a multicentre, randomised, open-label, phase 3 trial. Lancet Oncol. (2019) 20:924–37. doi: 10.1016/S1470-2045(19)30167-6
30. Sordo-Bahamonde C, Lorenzo-Herrero S, Gonzalez-Rodriguez AP, Martínez-Pérez A, Rodrigo JP, García-Pedrero JM, et al. Chemo-immunotherapy: A new trend in cancer treatment. Cancers (Basel). (2023) 15:2912. doi: 10.3390/CANCERS15112912
31. Chen G, Emens LA. Chemoimmunotherapy: reengineering tumor immunity. Cancer Immunol Immunother. (2013) 62:203. doi: 10.1007/S00262-012-1388-0
32. Bracci L, Schiavoni G, Sistigu A, Belardelli F. Immune-based mechanisms of cytotoxic chemotherapy: implications for the design of novel and rationale-based combined treatments against cancer. Cell Death Differentiation. (2013) 21:15–25. doi: 10.1038/cdd.2013.67
33. Liu W, Zhang L, Xiu Z, Guo J, Wang L, Zhou Y, et al. Combination of immune checkpoint inhibitors with chemotherapy in lung cancer. Onco Targets Ther. (2020) 13:7229. doi: 10.2147/OTT.S255491
34. Hayashi H, Nakagawa K. Combination therapy with PD-1 or PD-L1 inhibitors for cancer. Int J Clin Oncol. (2020) 25:818–30. doi: 10.1007/S10147-019-01548-1/METRICS
35. Yi M, Zheng X, Niu M, Zhu S, Ge H, Wu K. Combination strategies with PD-1/PD-L1 blockade: current advances and future directions. Mol Cancer. (2022) 21:1–27. doi: 10.1186/S12943-021-01489-2
36. Langer CJ, Gadgeel SM, Borghaei H, Papadimitrakopoulou VA, Patnaik A, Powell SF, et al. Carboplatin and pemetrexed with or without pembrolizumab for advanced, non-squamous non-small-cell lung cancer: a randomised, phase 2 cohort of the open-label KEYNOTE-021 study. Lancet Oncol. (2016) 17:1497–508. doi: 10.1016/S1470-2045(16)30498-3
37. Paz-Ares L, Luft A, Vicente D, Tafreshi A, Gümüş M, Mazières J, et al. Pembrolizumab plus chemotherapy for squamous non-small-cell lung cancer. N Engl J Med. (2018) 379:2040–51. doi: 10.1056/NEJMOA1810865
38. Gandhi L, Rodríguez-Abreu D, Gadgeel S, Esteban E, Felip E, De Angelis F, et al. Pembrolizumab plus chemotherapy in metastatic non–small-cell lung cancer. New Engl J Med. (2018) 378:2078–92. doi: 10.1056/NEJMOA1801005/SUPPL_FILE/NEJMOA1801005_DISCLOSURES.PDF
39. FDA approves atezolizumab for PD-L1 positive unresectable locally advanced or metastatic triple-negative breast cancer . FDA. Available online at: https://www.fda.gov/drugs/drug-approvals-and-databases/fda-approves-atezolizumab-pd-l1-positive-unresectable-locally-advanced-or-metastatic-triple-negative (Accessed July 7, 2024).
40. FDA Approves Genentech’s Tecentriq Plus Chemotherapy (Abraxane and Carboplatin) for The Initial Treatment of Metastatic Non-Squamous Non-Small Cell Lung Cancer . Available online at: https://www.drugs.com/newdrugs/fda-approves-genentech-s-tecentriq-plus-chemotherapy-abraxane-carboplatin-initial-metastatic-non-5117.html (Accessed July 14, 2024).
41. FDA Approves Genentech’s Tecentriq in Combination With Chemotherapy for the Initial Treatment of Adults With Extensive-Stage Small Cell Lung Cancer . Available online at: https://www.drugs.com/newdrugs/fda-approves-genentech-s-tecentriq-combination-chemotherapy-initial-adults-extensive-stage-small-4932.html (Accessed July 13, 2024).
42. FDA approves atezolizumab with chemotherapy and bevacizumab for first-line treatment of metastatic non-squamous NSCLC . FDA. Available online at: https://www.fda.gov/drugs/fda-approves-atezolizumab-chemotherapy-and-bevacizumab-first-line-treatment-metastatic-non-squamous (Accessed July 7, 2024).
43. FDA Approves Keytruda (pembrolizumab) for First-Line Treatment of Head and Neck Squamous Cell Carcinoma . Available online at: https://www.drugs.com/newdrugs/fda-approves-keytruda-pembrolizumab-first-line-head-neck-squamous-cell-carcinoma-4992.html (Accessed July 14, 2024).
44. FDA Approves Opdivo (nivolumab) + Yervoy (ipilimumab) Combined with Limited Chemotherapy as First-Line Treatment of Metastatic or Recurrent Non-Small Cell Lung Cancer . Available online at: https://www.drugs.com/newdrugs/fda-approves-opdivo-nivolumab-yervoy-ipilimumab-combined-limited-chemotherapy-first-line-metastatic-5256.html (Accessed July 13, 2024).
45. FDA Approves Merck’s Keytruda (pembrolizumab) in Combination With Chemotherapy for Patients With Locally Recurrent Unresectable or Metastatic Triple-Negative Breast Cancer Whose Tumors Express PD-L1 (CPS ≥10) . Available online at: https://www.drugs.com/newdrugs/fda-approves-merck-s-keytruda-pembrolizumab-combination-chemotherapy-patients-locally-recurrent-5381.html (Accessed July 14, 2024).
46. FDA Approves Merck’s Keytruda (pembrolizumab) Plus Platinum- and Fluoropyrimidine-Based Chemotherapy for Treatment of Certain Patients With Locally Advanced or Metastatic Esophageal or Gastroesophageal Junction (GEJ) Carcinoma . Available online at: https://www.drugs.com/newdrugs/fda-approves-merck-s-keytruda-pembrolizumab-plus-platinum-fluoropyrimidine-based-chemotherapy-5468.html (Accessed July 14, 2024).
47. FDA Approves Opdivo (nivolumab) in Combination with Chemotherapy for Patients with Advanced or Metastatic Gastric Cancer, Gastroesophageal Junction Cancer, and Esophageal Adenocarcinoma Regardless of PD-L1 Expression Status. Available online at: https://www.drugs.com/newdrugs/fda-approves-opdivo-nivolumab-combination-chemotherapy-patients-advanced-metastatic-gastric-cancer-5490.html (Accessed July 14, 2024).
48. FDA Approves Merck’s Keytruda (pembrolizumab) Combined With Trastuzumab and Chemotherapy as First-line Treatment in Locally Advanced Unresectable or Metastatic HER2-Positive Gastric or Gastroesophageal Junction Adenocarcinoma . Available online at: https://www.drugs.com/newdrugs/fda-approves-merck-s-keytruda-pembrolizumab-combined-trastuzumab-chemotherapy-first-line-locally-5511.html (Accessed July 14, 2024).
49. FDA Approves Keytruda (pembrolizumab) for Treatment of Patients With High-Risk Early-Stage Triple-Negative Breast Cancer in Combination With Chemotherapy as Neoadjuvant Treatment, Then Continued as Single Agent as Adjuvant Treatment After Surgery . Available online at: https://www.drugs.com/newdrugs/fda-approves-keytruda-pembrolizumab-patients-risk-early-stage-triple-negative-breast-cancer-5611.html (Accessed July 14, 2024).
50. FDA Approves Merck’s Keytruda (pembrolizumab) Plus Chemotherapy, With or Without Bevacizumab, as Treatment for Patients With Persistent, Recurrent or Metastatic Cervical Cancer Whose Tumors Express PD-L1 (CPS ≥1) . Available online at: https://www.drugs.com/newdrugs/fda-approves-merck-s-keytruda-pembrolizumab-plus-chemotherapy-without-bevacizumab-patients-5690.html (Accessed July 14, 2024).
51. U.S. Food and Drug Administration Approves Two Opdivo (nivolumab)-Based Regimens as First-Line Treatments for Unresectable Advanced or Metastatic Esophageal Squamous Cell Carcinoma . Available online at: https://www.drugs.com/newdrugs/u-s-food-administration-approves-two-opdivo-nivolumab-based-regimens-first-line-treatments-5840.html (Accessed July 13, 2024).
52. Imfinzi Plus Chemotherapy Approved in the US as the First Immunotherapy Regimen for Patients with Advanced Biliary Tract Cancer . Available online at: https://www.drugs.com/newdrugs/imfinzi-plus-chemotherapy-approved-us-first-immunotherapy-regimen-patients-advanced-biliary-tract-5891.html (Accessed July 14, 2024).
53. Libtayo (cemiplimab-rwlc) in Combination with Chemotherapy Approved by the FDA as First-line Treatment for Advanced Non-Small Cell Lung Cancer (NSCLC) . Available online at: https://www.drugs.com/newdrugs/libtayo-cemiplimab-rwlc-combination-chemotherapy-approved-fda-first-line-advanced-non-small-cell-5924.html (Accessed July 14, 2024).
54. FDA Approves Imjudo (tremelimumab) in Combination with Imfinzi for Patients with Unresectable Hepatocellular Carcinoma . Available online at: https://www.drugs.com/newdrugs/fda-approves-imjudo-tremelimumab-combination-imfinzi-patients-unresectable-hepatocellular-carcinoma-5919.html (Accessed July 14, 2024).
55. Imfinzi and Imjudo with Chemotherapy Approved in the US for Patients with Metastatic Non-Small Cell Lung Cancer . Available online at: https://www.drugs.com/newdrugs/imfinzi-imjudo-chemotherapy-approved-us-patients-metastatic-non-small-cell-lung-cancer-5926.html (Accessed July 14, 2024).
56. Jemperli (dostarlimab-gxly) Plus Chemotherapy Approved in the US for dMMR/MSI-H Primary Advanced or Recurrent Endometrial Cancer . Available online at: https://www.drugs.com/newdrugs/jemperli-dostarlimab-gxly-plus-chemotherapy-approved-us-dmmr-msi-h-primary-advanced-recurrent-6069.html (Accessed July 14, 2024).
57. FDA Approves Keytruda (pembrolizumab) for Treatment of Patients With Resectable NSCLC in Combination With Chemotherapy as Neoadjuvant Treatment, Then Single Agent as Adjuvant Treatment After Surgery . Available online at: https://www.drugs.com/newdrugs/fda-approves-keytruda-pembrolizumab-patients-resectable-nsclc-combination-chemotherapy-neoadjuvant-6114.html (Accessed July 14, 2024).
58. FDA Approves Merck’s Keytruda (pembrolizumab) Plus Gemcitabine and Cisplatin as Treatment for Patients With Locally Advanced Unresectable or Metastatic Biliary Tract Cancer . Available online at: https://www.drugs.com/newdrugs/fda-approves-merck-s-keytruda-pembrolizumab-plus-gemcitabine-cisplatin-patients-locally-advanced-6135.html (Accessed July 14, 2024).
59. FDA Approves Merck’s Keytruda (pembrolizumab) Plus Chemotherapy as First-Line Treatment for Locally Advanced Unresectable or Metastatic HER2-Negative Gastric or Gastroesophageal Junction (GEJ) Adenocarcinoma . Available online at: https://www.drugs.com/newdrugs/fda-approves-merck-s-keytruda-pembrolizumab-plus-chemotherapy-first-line-locally-advanced-6149.html. (Accessed July 14, 2024).
60. Padcev (enfortumab vedotin-ejfv) with Keytruda (pembrolizumab) Approved by FDA as the First and Only ADC Plus PD-1 to Treat Advanced Bladder Cancer . Available online at: https://www.drugs.com/newdrugs/padcev-enfortumab-vedotin-ejfv-keytruda-pembrolizumab-approved-fda-first-only-adc-plus-pd-1-6161.html (Accessed July 14, 2024).
61. FDA Approves Merck’s Keytruda (pembrolizumab) Plus Carboplatin and Paclitaxel as Treatment for Adult Patients With Primary Advanced or Recurrent Endometrial Carcinoma . Available online at: https://www.drugs.com/newdrugs/fda-approves-merck-s-keytruda-pembrolizumab-plus-carboplatin-paclitaxel-adult-patients-primary-6303.html (Accessed July 14, 2024).
62. FDA Approves Merck’s Keytruda (pembrolizumab) Plus Chemoradiotherapy as Treatment for Patients With FIGO 2014 Stage III-IVA Cervical Cancer . Available online at: https://www.drugs.com/newdrugs/fda-approves-merck-s-keytruda-pembrolizumab-plus-chemoradiotherapy-patients-figo-2014-stage-iii-iva-6181.html (Accessed July 14, 2024).
63. BMS Receives FDA Approval for Opdivo (nivolumab) + Yervoy (ipilimumab) Regimen in BRAF V600 Wild-Type Melanoma . Available online at: https://www.drugs.com/newdrugs/bms-receives-fda-approval-opdivo-nivolumab-yervoy-ipilimumab-regimen-braf-v600-wild-type-melanoma-4271.html (Accessed July 13, 2024).
64. Bristol-Myers Squibb’s Opdivo (nivolumab) + Yervoy (ipilimumab) Regimen Receives Expanded FDA Approval in Unresectable or Metastatic Melanoma Across BRAF Status . Available online at: https://www.drugs.com/newdrugs/bristol-myers-squibb-s-opdivo-nivolumab-yervoy-ipilimumab-regimen-receives-expanded-fda-approval-4332.html (Accessed July 13, 2024).
65. FDA Approves Opdivo (nivolumab) + Yervoy (ipilimumab) Combination as First-Line Treatment for Patients with Intermediate- and Poor-Risk Advanced Renal Cell Carcinoma . Available online at: https://www.drugs.com/newdrugs/fda-approves-opdivo-nivolumab-yervoy-ipilimumab-combination-first-line-patients-intermediate-poor-4731.html (Accessed July 13, 2024).
66. Opdivo (nivolumab) + Low-Dose Yervoy (ipilimumab) Combination Approved for Previously Treated MSI-H/dMMR Metastatic Colorectal Cancer . Available online at: https://www.drugs.com/newdrugs/opdivo-nivolumab-low-yervoy-ipilimumab-combination-approved-previously-treated-msi-h-dmmr-4779.html (Accessed July 13, 2024).
67. FDA Approves Opdivo (nivolumab) + Yervoy (ipilimumab) for Patients with Hepatocellular Carcinoma (HCC) Previously Treated with Sorafenib . Available online at: https://www.drugs.com/newdrugs/fda-approves-opdivo-nivolumab-yervoy-ipilimumab-patients-hepatocellular-carcinoma-hcc-previously-5360.html (Accessed July 13, 2024).
68. FDA Approves Opdivo (nivolumab) + Yervoy (ipilimumab) as First-Line Treatment of Patients with Metastatic Non-Small Cell Lung Cancer Whose Tumors Express PD-L1≥1% . Available online at: https://www.drugs.com/newdrugs/fda-approves-opdivo-nivolumab-yervoy-ipilimumab-first-line-patients-metastatic-non-small-cell-lung-5239.html (Accessed July 13, 2024).
69. FDA Approves Opdivo (nivolumab) + Yervoy (ipilimumab) as the First and Only Immunotherapy Treatment for Previously Untreated Unresectable Malignant Pleural Mesothelioma . Available online at: https://www.drugs.com/newdrugs/fda-approves-opdivo-nivolumab-yervoy-ipilimumab-first-only-immunotherapy-previously-untreated-5359.html (Accessed July 13, 2024).
70. FDA Approves Opdualag (nivolumab and relatlimab-rmbw) for the Treatment of Patients with Unresectable or Metastatic Melanoma . Available online at: https://www.drugs.com/newdrugs/fda-approves-opdualag-nivolumab-relatlimab-rmbw-patients-unresectable-metastatic-melanoma-5804.html (Accessed July 14, 2024).
71. FDA Approves Keytruda (pembrolizumab) in Combination With Inlyta (axitinib) as First-Line Treatment for Patients With Advanced Renal Cell Carcinoma (RCC) . Available online at: https://www.drugs.com/newdrugs/fda-approves-keytruda-pembrolizumab-combination-inlyta-axitinib-first-line-patients-advanced-renal-4956.html (Accessed July 14, 2024).
72. FDA Approves Bavencio (avelumab) Plus Inlyta (axitinib) Combination for Patients with Advanced Renal Cell Carcinoma . Available online at: https://www.drugs.com/newdrugs/fda-approves-bavencio-avelumab-plus-inlyta-axitinib-combination-patients-advanced-renal-cell-4973.html (Accessed July 14, 2024).
73. FDA Approves Keytruda (pembrolizumab) plus Lenvima (lenvatinib) Combination Treatment for Patients with Certain Types of Endometrial Carcinoma . Available online at: https://www.drugs.com/newdrugs/fda-approves-keytruda-pembrolizumab-plus-lenvima-lenvatinib-combination-patients-certain-types-5056.html (Accessed July 14, 2024).
74. FDA Approves Genentech’s Tecentriq in Combination With Avastin for People With Hepatocellular Carcinoma . Available online at: https://www.drugs.com/newdrugs/fda-approves-genentech-s-tecentriq-combination-avastin-hepatocellular-carcinoma-5253.html (Accessed July 14, 2024).
75. FDA Approves Genentech’s Tecentriq plus Cotellic and Zelboraf for People With Advanced Melanoma . Available online at: https://www.drugs.com/newdrugs/fda-approves-genentech-s-tecentriq-plus-cotellic-zelboraf-advanced-melanoma-5311.html (Accessed July 14, 2024).
76. FDA Approves Opdivo (nivolumab) in Combination with Cabometyx (cabozantinib) as First-line Treatment for Patients with Advanced Renal Cell Carcinoma . Available online at: https://www.drugs.com/newdrugs/fda-approves-opdivo-nivolumab-combination-cabometyx-cabozantinib-first-line-patients-advanced-renal-5428.html (Accessed July 13, 2024).
77. FDA Approves Keytruda (pembrolizumab) Plus Lenvima (lenvatinib) Combination for Patients With Certain Types of Advanced Endometrial Carcinoma . Available online at: https://www.drugs.com/newdrugs/fda-approves-keytruda-pembrolizumab-plus-lenvima-lenvatinib-combination-patients-certain-types-5604.html (Accessed July 14, 2024).
78. FDA Approves Keytruda (pembrolizumab) Plus Lenvima (lenvatinib) Combination for First-Line Treatment of Adult Patients With Advanced Renal Cell Carcinoma (RCC) . Available online at: https://www.drugs.com/newdrugs/fda-approves-keytruda-pembrolizumab-plus-lenvima-lenvatinib-combination-first-line-adult-patients-5631.html (Accessed July 14, 2024).
79. FDA Approves Merck’s Keytruda (pembrolizumab) in Combination With Padcev (enfortumab vedotin-ejfv) for First-Line Treatment of Certain Patients With Locally Advanced or Metastatic Urothelial Cancer . Available online at: https://www.drugs.com/newdrugs/fda-approves-merck-s-keytruda-pembrolizumab-combination-padcev-enfortumab-vedotin-ejfv-first-line-5994.html (Accessed July 14, 2024).
80. Hiniker SM, Reddy SA, Maecker HT, Subrahmanyam PB, Rosenberg-Hasson Y, Swetter SM, et al. A prospective clinical trial combining radiation therapy with systemic immunotherapy in metastatic melanoma. Int J Radiat Oncol Biol Phys. (2016) 96:578–88. doi: 10.1016/J.IJROBP.2016.07.005
81. Oweida A, Hararah MK, Phan A, Binder D, Bhatia S, Lennon S, et al. Resistance to radiotherapy and PD-L1 blockade is mediated by TIM-3 upregulation and regulatory T-cell infiltration. Clin Cancer Res. (2018) 24:5368–80. doi: 10.1158/1078-0432.CCR-18-1038
82. Liu Z, Xu T, Chang P, Fu W, Wei J, Xia C, et al. Efficacy and safety of immune checkpoint inhibitors with or without radiotherapy in metastatic non-small cell lung cancer: A systematic review and meta-analysis. Front Pharmacol. (2023) 14:1064227/BIBTEX. doi: 10.3389/FPHAR.2023.1064227/BIBTEX
83. Frak M, Krawczyk P, Kalinka E, Milanowski J. Molecular and clinical premises for the combination therapy consisting of radiochemotherapy and immunotherapy in non-small cell lung cancer patients. Cancers (Basel). (2021) 13:1–15. doi: 10.3390/CANCERS13061222
84. Voronova V, Vislobokova A, Mutig K, Samsonov M, Peskov K, Sekacheva M, et al. Combination of immune checkpoint inhibitors with radiation therapy in cancer: A hammer breaking the wall of resistance. Front Oncol. (2022) 12:1035884. doi: 10.3389/FONC.2022.1035884
85. Liu L, Mayes PA, Eastman S, Shi H, Yadavilli S, Zhang T, et al. The BRAF and MEK inhibitors dabrafenib and trametinib: effects on immune function and in combination with immunomodulatory antibodies targeting PD-1, PD-L1, and CTLA-4. Clin Cancer Res. (2015) 21:1639–51. doi: 10.1158/1078-0432.CCR-14-2339
86. Ribas A, Butler M, Lutzky J, Lawrence DP, Robert C, Miller W, et al. Phase I study combining anti-PD-L1 (MEDI4736) with BRAF (dabrafenib) and/or MEK (trametinib) inhibitors in advanced melanoma. J Clin Oncol. (2015) 33:3003–3. doi: 10.1200/JCO.2015.33.15
87. Dummer R, Long GV, Robert C, Tawbi HA, Flaherty KT, Ascierto PA, et al. Randomized phase III trial evaluating spartalizumab plus dabrafenib and trametinib for BRAF V600-mutant unresectable or metastatic melanoma. J Clin Oncol. (2022) 40:1428–38. doi: 10.1200/JCO.21.01601
88. Hu-Lieskovan S, Mok S, Homet Moreno B, Tsoi J, Robert L, Goedert L, et al. Improved antitumor activity of immunotherapy with BRAF and MEK inhibitors in BRAFV600E melanoma. Sci Transl Med. (2015) 7:279ra41. doi: 10.1126/SCITRANSLMED.AAA4691/SUPPL_FILE/7-279RA41_TABLES_S1_TO_S4.ZIP
89. O’Donnell JS, Massi D, Teng MWL, Mandala M. PI3K-AKT-mTOR inhibition in cancer immunotherapy, redux. Semin Cancer Biol. (2018) 48:91–103. doi: 10.1016/J.SEMCANCER.2017.04.015
90. Kaneda MM, Messer KS, Ralainirina N, Li H, Leem CJ, Gorjestani S, et al. PI3Kγ is a molecular switch that controls immune suppression. Nature. (2016) 539:437–42. doi: 10.1038/nature19834
91. Ren Z, Xu J, Bai Y, Xu A, Cang S, Du C, et al. Sintilimab plus a bevacizumab biosimilar (IBI305) versus sorafenib in unresectable hepatocellular carcinoma (ORIENT-32): a randomised, open-label, phase 2–3 study. Lancet Oncol. (2021) 22:977–90. doi: 10.1016/S1470-2045(21)00252-7
92. Voron T, Colussi O, Marcheteau E, Pernot S, Nizard M, Pointet AL, et al. VEGF-A modulates expression of inhibitory checkpoints on CD8+ T cells in tumors. J Exp Med. (2015) 212:139. doi: 10.1084/JEM.20140559
93. Yasuda S, Sho M, Yamato I, Yoshiji H, Wakatsuki K, Nishiwada S, et al. Simultaneous blockade of programmed death 1 and vascular endothelial growth factor receptor 2 (VEGFR2) induces synergistic anti-tumour effect in vivo. Clin Exp Immunol. (2013) 172:500–6. doi: 10.1111/CEI.12069
94. Wallin JJ, Bendell JC, Funke R, Sznol M, Korski K, Jones S, et al. Atezolizumab in combination with bevacizumab enhances antigen-specific T-cell migration in metastatic renal cell carcinoma. Nat Commun. (2016) 7:12624. doi: 10.1038/NCOMMS12624
95. Beavis PA, Milenkovski N, Henderson MA, John LB, Allard B, Loi S, et al. Adenosine receptor 2A blockade increases the efficacy of anti-PD-1 through enhanced antitumor T-cell responses. Cancer Immunol Res. (2015) 3:506–17. doi: 10.1158/2326-6066.CIR-14-0211
96. Chen L, Diao L, Yang Y, Yi X, Rodriguez BL, Li Y, et al. CD38-mediated immunosuppression as a mechanism of tumor cell escape from PD-1/PD-L1 blockade. Cancer Discov. (2018) 8:1156–75. doi: 10.1158/2159-8290.CD-17-1033
97. Wei SC, Anang NAAS, Sharma R, Andrews MC, Reuben A, Levine JH, et al. Combination anti–CTLA-4 plus anti–PD-1 checkpoint blockade utilizes cellular mechanisms partially distinct from monotherapies. Proc Natl Acad Sci U S A. (2019) 116:22699–709. doi: 10.1073/PNAS.1821218116/SUPPL_FILE/PNAS.1821218116.SAPP.PDF
98. Wolchok JD, Chiarion-Sileni V, Gonzalez R, Rutkowski P, Grob J-J, Cowey CL, et al. Overall survival with combined nivolumab and ipilimumab in advanced melanoma. N Engl J Med. (2017) 377:1345–56. doi: 10.1056/NEJMOA1709684
99. Wang K, Coutifaris P, Brocks D, Wang G, Azar T, Solis S, et al. Combination anti-PD-1 and anti-CTLA-4 therapy generates waves of clonal responses that include progenitor-exhausted CD8+ T cells. Cancer Cell. (2024) 42:1582–1597.e10. doi: 10.1016/J.CCELL.2024.08.007
100. Postow MA, Chesney J, Pavlick AC, Robert C, Grossmann K, McDermott D, et al. Nivolumab and ipilimumab versus ipilimumab in untreated melanoma. N Engl J Med. (2015) 372:2006–17. doi: 10.1056/NEJMOA1414428
101. Motzer RJ, Tannir NM, McDermott DF, Arén Frontera O, Melichar B, Choueiri TK, et al. Nivolumab plus Ipilimumab versus Sunitinib in Advanced Renal-Cell Carcinoma. N Engl J Med. (2018) 378:1277–90. doi: 10.1056/NEJMOA1712126
102. Overman MJ, Lonardi S, Wong KYM, Lenz HJ, Gelsomino F, Aglietta M, et al. Durable clinical benefit with nivolumab plus ipilimumab in DNA mismatch repair-deficient/microsatellite instability-high metastatic colorectal cancer. J Clin Oncol. (2018) 36:773–9. doi: 10.1200/JCO.2017.76.9901
103. Wierz M, Pierson S, Guyonnet L, Viry E, Lequeux A, Oudin A, et al. Dual PD1/LAG3 immune checkpoint blockade limits tumor development in a murine model of chronic lymphocytic leukemia. Blood. (2018) 131:1617–21. doi: 10.1182/BLOOD-2017-06-792267
104. Woo SR, Turnis ME, Goldberg MV, Bankoti J, Selby M, Nirschl CJ, et al. Immune inhibitory molecules LAG-3 and PD-1 synergistically regulate T-cell function to promote tumoral immune escape. Cancer Res. (2012) 72:917–27. doi: 10.1158/0008-5472.CAN-11-1620
105. Hung AL, Maxwell R, Theodros D, Belcaid Z, Mathios D, Luksik AS, et al. TIGIT and PD-1 dual checkpoint blockade enhances antitumor immunity and survival in GBM. Oncoimmunology. (2018) 7:e1466769. doi: 10.1080/2162402X.2018.1466769
106. Kondo Y, Ohno T, Nishii N, Harada K, Yagita H, Azuma M. Differential contribution of three immune checkpoint (VISTA, CTLA-4, PD-1) pathways to antitumor responses against squamous cell carcinoma. Oncol. (2016) 57:54–60. doi: 10.1016/J.ORALONCOLOGY.2016.04.005
107. Choi J, Medikonda R, Saleh L, Kim T, Pant A, Srivastava S, et al. Combination checkpoint therapy with anti-PD-1 and anti-BTLA results in a synergistic therapeutic effect against murine glioblastoma. Oncoimmunology. (2021) 10. doi: 10.1080/2162402X.2021.1956142
108. Limagne E, Richard C, Thibaudin M, Fumet JD, Truntzer C, Lagrange A, et al. Tim-3/galectin-9 pathway and mMDSC control primary and secondary resistances to PD-1 blockade in lung cancer patients. Oncoimmunology. (2019) 8. doi: 10.1080/2162402X.2018.1564505
109. Marin-Acevedo JA, Dholaria B, Soyano AE, Knutson KL, Chumsri S, Lou Y. Next generation of immune checkpoint therapy in cancer: new developments and challenges. J Hematol Oncol. (2018) 11. doi: 10.1186/S13045-018-0582-8
110. Cho BC, Abreu DR, Hussein M, Cobo M, Patel AJ, Secen N, et al. Tiragolumab plus atezolizumab versus placebo plus atezolizumab as a first-line treatment for PD-L1-selected non-small-cell lung cancer (CITYSCAPE): primary and follow-up analyses of a randomised, double-blind, phase 2 study. Lancet Oncol. (2022) 23:781–92. doi: 10.1016/S1470-2045(22)00226-1
111. Schöffski P, Tan DSW, Martín M, Ochoa-De-Olza M, Sarantopoulos J, Carvajal RD, et al. Phase I/II study of the LAG-3 inhibitor ieramilimab (LAG525) ± anti-PD-1 spartalizumab (PDR001) in patients with advanced Malignancies. J Immunother Cancer. (2022) 10. doi: 10.1136/JITC-2021-003776
112. Soldevilla MM, Villanueva H, Meraviglia-Crivelli D, Menon AP, Ruiz M, Cebollero J, et al. ICOS costimulation at the tumor site in combination with CTLA-4 blockade therapy elicits strong tumor immunity. Mol Ther. (2019) 27:1878–91. doi: 10.1016/J.YMTHE.2019.07.013
113. Zappasodi R, Sirard C, Li Y, Budhu S, Abu-Akeel M, Liu C, et al. Rational design of anti-GITR-based combination immunotherapy. Nat Med. (2019) 25:759. doi: 10.1038/S41591-019-0420-8
114. Byrne KT, Vonderheide RH. CD40 stimulation obviates innate sensors and drives T cell immunity in cancer. Cell Rep. (2016) 15:2719–32. doi: 10.1016/J.CELREP.2016.05.058
115. Vonderheide RH, Flaherty KT, Khalil M, Stumacher MS, Bajor DL, Hutnick NA, et al. Clinical activity and immune modulation in cancer patients treated with CP-870,893, a novel CD40 agonist monoclonal antibody. J Clin Oncol. (2007) 25:876–83. doi: 10.1200/JCO.2006.08.3311
116. Shi LZ, Gao J, Allison JP, Sharma P. Combination therapy of adoptive T cell therapy and immune checkpoint blockades engages distinct mechanisms in CD4+ and CD8+ T cells. J Immunol. (2018) 200:122. doi: 10.4049/JIMMUNOL.200.SUPP.122.21
117. Zhang R, Deng Q, Jiang YY, Zhu HB, Wang J, Zhao MF. Effect and changes in PD-1 expression of CD19 CAR-T cells from T cells highly expressing PD-1 combined with reduced-dose PD-1 inhibitor. Oncol Rep. (2019) 41:3455–63. doi: 10.3892/OR.2019.7096
118. Mullinax JE, Hall M, Prabhakaran S, Weber J, Khushalani N, Eroglu Z, et al. Combination of ipilimumab and adoptive cell therapy with tumor-infiltrating lymphocytes for patients with metastatic melanoma. Front Oncol. (2018) 8:44. doi: 10.3389/FONC.2018.00044
119. Ernst M, Oeser A, Besiroglu B, Caro-Valenzuela J, Abd El Aziz M, Monsef I, et al. Chimeric antigen receptor (CAR) T-cell therapy for people with relapsed or refractory diffuse large B-cell lymphoma. Cochrane Database Systematic Rev. (2021) 2021:CD013365. doi: 10.1002/14651858.CD013365.PUB2
120. Pang N, Shi J, Qin L, Chen A, Tang Y, Yang H, et al. IL-7 and CCL19-secreting CAR-T cell therapy for tumors with positive glypican-3 or mesothelin. J Hematol Oncol. (2021) 14:1–6. doi: 10.1186/S13045-021-01128-9/FIGURES/2
121. Adachi K, Kano Y, Nagai T, Okuyama N, Sakoda Y, Tamada K. IL-7 and CCL19 expression in CAR-T cells improves immune cell infiltration and CAR-T cell survival in the tumor. Nat Biotechnol. (2018) 36:346–51. doi: 10.1038/NBT.4086
122. Lei W, Zhao A, Liu H, Yang C, Wei C, Guo S, et al. Safety and feasibility of anti-CD19 CAR T cells expressing inducible IL-7 and CCL19 in patients with relapsed or refractory large B-cell lymphoma. Cell Discov. (2024) 10:1–14. doi: 10.1038/s41421-023-00625-0
123. Goto S, Sakoda Y, Adachi K, Sekido Y, Yano S, Eto M, et al. Enhanced anti-tumor efficacy of IL-7/CCL19-producing human CAR-T cells in orthotopic and patient-derived xenograft tumor models. Cancer Immunol Immunother. (2021) 70:2503–15. doi: 10.1007/S00262-021-02853-3
124. Marotte L, Capitao M, Deleine C, Beauvais T, Cadiou G, Perrin J, et al. Anti-tumor efficacy of a combination therapy with PD-L1 targeted alpha therapy and adoptive cell transfer of PD-1 deficient melanoma-specific human T-lymphocytes. Oncoimmunology. (2021) 10:1940676. doi: 10.1080/2162402X.2021.1940676
125. Ott PA, Hu-Lieskovan S, Chmielowski B, Govindan R, Naing A, Bhardwaj N, et al. A phase ib trial of personalized neoantigen therapy plus anti-PD-1 in patients with advanced melanoma, non-small cell lung cancer, or bladder cancer. Cell. (2020) 183:347–362.e24. doi: 10.1016/J.CELL.2020.08.053
126. Ott PA, Hu Z, Keskin DB, Shukla SA, Sun J, Bozym DJ, et al. An immunogenic personal neoantigen vaccine for patients with melanoma. Nature. (2017) 547:217–21. doi: 10.1038/nature22991
127. Gibney GT, KudChadkar RR, DeConti RC, Thebeau MS, Czupryn MP, Tetteh L, et al. Safety, correlative markers, and clinical results of adjuvant nivolumab in combination with vaccine in resected high-risk metastatic melanoma. Clin Cancer Res. (2015) 21:712–20. doi: 10.1158/1078-0432.CCR-14-2468
128. Sahin U, Türeci Ö. Personalized vaccines for cancer immunotherapy. Sci (1979). (2018) 359:1355–60. doi: 10.1126/SCIENCE.AAR7112
129. Guo Z, Yuan Y, Chen C, Lin J, Ma Q, Liu G, et al. Durable complete response to neoantigen-loaded dendritic-cell vaccine following anti-PD-1 therapy in metastatic gastric cancer. NPJ Precis Oncol. (2022) 6:1–7. doi: 10.1038/s41698-022-00279-3
130. Marabelle A, Tselikas L, de Baere T, Houot R. Intratumoral immunotherapy: using the tumor as the remedy. Ann Oncol. (2017) 28:xii33–43. doi: 10.1093/ANNONC/MDX683
131. Long GV, Dummer R, Ribas A, Puzanov I, VanderWalde A, Andtbacka RHI, et al. Efficacy analysis of MASTERKEY-265 phase 1b study of talimogene laherparepvec (T-VEC) and pembrolizumab (pembro) for unresectable stage IIIB-IV melanoma. J Clin Oncol. (2016) 34:9568–8. doi: 10.1200/JCO.2016.34.15_SUPPL.9568
132. Puzanov I, Milhem MM, Minor D, Hamid O, Li A, Chen L, et al. Talimogene laherparepvec in combination with ipilimumab in previously untreated, unresectable stage IIIB-IV melanoma. J Clin Oncol. (2016) 34:2619. doi: 10.1200/JCO.2016.67.1529
133. Fröhlich A, Niebel D, Fietz S, Egger E, Buchner A, Sirokay J, et al. Talimogene laherparepvec treatment to overcome loco-regional acquired resistance to immune checkpoint blockade in tumor stage IIIB-IV M1c melanoma patients. Cancer Immunol Immunother. (2020) 69:759–69. doi: 10.1007/S00262-020-02487-X
134. Chesney J, Puzanov I, Collichio F, Singh P, Milhem MM, Glaspy J, et al. Randomized, open-label phase II study evaluating the efficacy and safety of talimogene laherparepvec in combination with ipilimumab versus ipilimumab alone in patients with advanced, unresectable melanoma. J Clin Oncol. (2018) 36:1658–67. doi: 10.1200/JCO.2017.73.7379
135. Ribas A, Dummer R, Puzanov I, VanderWalde A, Andtbacka RHI, Michielin O, et al. Oncolytic virotherapy promotes intratumoral T cell infiltration and improves anti-PD-1 immunotherapy. Cell. (2017) 170:1109–1119.e10. doi: 10.1016/J.CELL.2017.08.027
136. Ju F, Luo Y, Lin C, Jia X, Xu Z, Tian R, et al. Oncolytic virus expressing PD-1 inhibitors activates a collaborative intratumoral immune response to control tumor and synergizes with CTLA-4 or TIM-3 blockade. J Immunother Cancer. (2022) 10:e004762. doi: 10.1136/JITC-2022-004762
137. Morel D, Jeffery D, Aspeslagh S, Almouzni G, Postel-Vinay S. Combining epigenetic drugs with other therapies for solid tumours — past lessons and future promise. Nat Rev Clin Oncol. (2019) 17:91–107. doi: 10.1038/s41571-019-0267-4
138. Orillion A, Hashimoto A, Damayanti N, Shen L, Adelaiye-Ogala R, Arisa S, et al. Entinostat neutralizes myeloid-derived suppressor cells and enhances the antitumor effect of PD-1 inhibition in murine models of lung and renal cell carcinoma. Clin Cancer Res. (2017) 23:5187–201. doi: 10.1158/1078-0432.CCR-17-0741/14719/AM/ENTINOSTAT-NEUTRALIZES-MYELOID-DERIVED-SUPPRESSOR
139. Gandhi L, Janne PA, Opyrchal M, Ramalingam SS, Rybkin II, Hafez N, et al. Efficacy and safety of entinostat (ENT) and pembrolizumab (PEMBRO) in patients with non-small cell lung cancer (NSCLC) previously treated with anti-PD-(L)1 therapy. J Clin Oncol. (2018) 36:9036–6. doi: 10.1200/JCO.2018.36.15_SUPPL.9036
140. Hellmann M, Jänne P, Opyrchal M, Hafez N, Raez L, Gabrilovich D, et al. OA05.01 efficacy/safety of entinostat (ENT) and pembrolizumab (PEMBRO) in NSCLC patients previously treated with anti-PD-(L)1 therapy. J Thoracic Oncol. (2018) 13:S330. doi: 10.1016/j.jtho.2018.08.257
141. Shen L, Ciesielski M, Ramakrishnan S, Miles KM, Ellis L, Sotomayor P, et al. Class I histone deacetylase inhibitor entinostat suppresses regulatory T cells and enhances immunotherapies in renal and prostate cancer models. PloS One. (2012) 7:e30815. doi: 10.1371/JOURNAL.PONE.0030815
142. Gray JE, Saltos A, Tanvetyanon T, Haura EB, Creelan B, Antonia SJ, et al. Phase I/ib study of pembrolizumab plus vorinostat in advanced/metastatic non-small cell lung cancer. Clin Cancer Res. (2019) 25:6623–32. doi: 10.1158/1078-0432.CCR-19-1305
143. Bissonnette RP, Cesario RM, Goodenow B, Shojaei F, Gillings M. The epigenetic immunomodulator, HBI-8000, enhances the response and reverses resistance to checkpoint inhibitors. BMC Cancer. (2021) 21:1–17. doi: 10.1186/S12885-021-08702-X/TABLES/1
144. Kim K, Skora AD, Li Z, Liu Q, Tam AJ, Blosser RL, et al. Eradication of metastatic mouse cancers resistant to immune checkpoint blockade by suppression of myeloid-derived cells. Proc Natl Acad Sci U S A. (2014) 111:11774–9. doi: 10.1073/PNAS.1410626111
145. Ny L, Jespersen H, Karlsson J, Alsén S, Filges S, All-Eriksson C, et al. The PEMDAC phase 2 study of pembrolizumab and entinostat in patients with metastatic uveal melanoma. Nat Commun. (2021) 12:1–10. doi: 10.1038/s41467-021-25332-w
146. Mazzone R, Zwergel C, Mai A, Valente S. Epi-drugs in combination with immunotherapy: a new avenue to improve anticancer efficacy. Clin Epigenet. (2017) 9. doi: 10.1186/S13148-017-0358-Y
147. Saltos AN, Tanvetyanon T, Creelan BC, Shafique MR, Antonia SJ, Haura EB, et al. Phase II randomized trial of first-line pembrolizumab and vorinostat in patients with metastatic NSCLC (mNSCLC). J Clin Oncol. (2020) 38:9567–7. doi: 10.1200/JCO.2020.38.15_SUPPL.9567
148. Pili R, Quinn DI, Albany C, Adra N, Logan TF, Greenspan A, et al. Immunomodulation by HDAC inhibition: Results from a phase Ib study with vorinostat and pembrolizumab in metastatic urothelial, renal, and prostate carcinoma patients. J Clin Oncol. (2019) 37:2572–2. doi: 10.1200/JCO.2019.37.15_SUPPL.2572
149. Hellmann MD, Janne PA, Opyrchal M, Hafez N, Raez LE, Gabrilovich DI, et al. Entinostat plus pembrolizumab in patients with metastatic NSCLC previously treated with anti-PD-(L)1 therapy. Clin Cancer Res. (2021) 27:1019–28. doi: 10.1158/1078-0432.CCR-20-3305
150. Sivan A, Corrales L, Hubert N, Williams JB, Aquino-Michaels K, Earley ZM, et al. Commensal Bifidobacterium promotes antitumor immunity and facilitates anti-PD-L1 efficacy. Sci (1979). (2015) 350:1084–9. doi: 10.1126/SCIENCE.AAC4255/SUPPL_FILE/PAPV2.PDF
151. Vétizou M, Pitt JM, Daillère R, Lepage P, Waldschmitt N, Flament C, et al. Anticancer immunotherapy by CTLA-4 blockade relies on the gut microbiota. Sci (1979). (2015) 350:1079–84. doi: 10.1126/SCIENCE.AAD1329/SUPPL_FILE/AAD1329_VETIZOU.SM.PDF
152. Matson V, Fessler J, Bao R, Chongsuwat T, Zha Y, Alegre ML, et al. The commensal microbiome is associated with anti-PD-1 efficacy in metastatic melanoma patients. Science. (2018) 359:104–8. doi: 10.1126/SCIENCE.AAO3290
153. Wang T, Zheng N, Luo Q, Jiang L, He B, Yuan X, et al. Probiotics lactobacillus reuteri abrogates immune checkpoint blockade-associated colitis by inhibiting group 3 innate lymphoid cells. Front Immunol. (2019) 10:1235. doi: 10.3389/FIMMU.2019.01235
154. Davar D, Dzutsev AK, McCulloch JA, Rodrigues RR, Chauvin JM, Morrison RM, et al. Fecal microbiota transplant overcomes resistance to anti-PD-1 therapy in melanoma patients. Science. (2021) 371:595–602. doi: 10.1126/SCIENCE.ABF3363
155. Baruch EN, Youngster I, Ben-Betzalel G, Ortenberg R, Lahat A, Katz L, et al. Fecal microbiota transplant promotes response in immunotherapy-refractory melanoma patients. Science. (2021) 371:602–9. doi: 10.1126/SCIENCE.ABB5920
156. Zhao M, Luo M, Xie Y, Jiang H, Cagliero C, Li N, et al. Development of a recombinant human IL-15·sIL-15Rα/Fc superagonist with improved half-life and its antitumor activity alone or in combination with PD-1 blockade in mouse model. BioMed Pharmacother. (2019) 112:108677. doi: 10.1016/J.BIOPHA.2019.108677
157. Puca E, Probst P, Stringhini M, Murer P, Pellegrini G, Cazzamalli S, et al. The antibody-based delivery of interleukin-12 to solid tumors boosts NK and CD8+ T cell activity and synergizes with immune checkpoint inhibitors. Int J Cancer. (2020) 146:2518–30. doi: 10.1002/IJC.32603
158. Buchbinder EI, Dutcher JP, Daniels GA, Curti BD, Patel SP, Holtan SG, et al. Therapy with high-dose Interleukin-2 (HD IL-2) in metastatic melanoma and renal cell carcinoma following PD1 or PDL1 inhibition. J Immunother Cancer. (2019) 7. doi: 10.1186/S40425-019-0522-3
159. Greene S, Robbins Y, Mydlarz WK, Huynh AP, Schmitt NC, Friedman J, et al. Inhibition of MDSC trafficking with SX-682, a CXCR1/2 inhibitor, enhances NK-cell immunotherapy in head and neck cancer models. Clin Cancer Res. (2020) 26:1420–31. doi: 10.1158/1078-0432.CCR-19-2625
160. Steele CW, Karim SA, Leach JDG, Bailey P, Upstill-Goddard R, Rishi L, et al. CXCR2 inhibition profoundly suppresses metastases and augments immunotherapy in pancreatic ductal adenocarcinoma. Cancer Cell. (2016) 29:832–45. doi: 10.1016/J.CCELL.2016.04.014
161. Flores-Toro JA, Luo D, Gopinath A, Sarkisian MR, Campbell JJ, Charo IF, et al. CCR2 inhibition reduces tumor myeloid cells and unmasks a checkpoint inhibitor effect to slow progression of resistant murine gliomas. Proc Natl Acad Sci U S A. (2020) 117:1129–38. doi: 10.1073/PNAS.1910856117
162. Jung H, Bischof A, Ebsworth K, Ertl L, Schall T, Charo I. Combination therapy of chemokine receptor inhibition plus PDL-1 blockade potentiates anti-tumor effects in a murine model of breast cancer. J ImmunoTherapy Cancer. (2015) 3:1–1. doi: 10.1186/2051-1426-3-S2-P227
163. Ebelt ND, Zuniga E, Marzagalli M, Zamloot V, Blazar BR, Salgia R, et al. Salmonella-based therapy targeting indoleamine 2,3-dioxygenase restructures the immune contexture to improve checkpoint blockade efficacy. Biomedicines. (2020) 8:1–20. doi: 10.3390/biomedicines8120617
164. Al-Saafeen BH, Al-Sbiei A, Bashir G, Mohamed YA, Masad RJ, Fernandez-Cabezudo MJ, et al. Attenuated Salmonella potentiate PD-L1 blockade immunotherapy in a preclinical model of colorectal cancer. Front Immunol. (2022) 13:1017780. doi: 10.3389/FIMMU.2022.1017780
165. Mi Z, Feng ZC, Li C, Yang X, Ma MT, Rong PF. Salmonella-mediated cancer therapy: An innovative therapeutic strategy. J Cancer. (2019) 10:4765–76. doi: 10.7150/jca.32650
166. Gopalakrishnan V, Spencer CN, Nezi L, Reuben A, Andrews MC, Karpinets TV, et al. Gut microbiome modulates response to anti-PD-1 immunotherapy in melanoma patients. Sci (1979). (2018) 359:97–103. doi: 10.1126/SCIENCE.AAN4236/SUPPL_FILE/AAN4236_GOPALAKRISHNAN_SM.PDF
167. Routy B, Le Chatelier E, Derosa L, Duong CPM, Alou MT, Daillère R, et al. Gut microbiome influences efficacy of PD-1-based immunotherapy against epithelial tumors. Science. (2018) 359:91–7. doi: 10.1126/SCIENCE.AAN3706
168. Coutzac C, Jouniaux JM, Paci A, Schmidt J, Mallardo D, Seck A, et al. Systemic short chain fatty acids limit antitumor effect of CTLA-4 blockade in hosts with cancer. Nat Commun. (2020) 11. doi: 10.1038/S41467-020-16079-X
169. Mager LF, Burkhard R, Pett N, Cooke NCA, Brown K, Ramay H, et al. Microbiome-derived inosine modulates response to checkpoint inhibitor immunotherapy. Science. (2020) 369:1481–9. doi: 10.1126/SCIENCE.ABC3421
170. Hoeben A, Joosten EAJ, van den Beuken-Van Everdingen MHJ. Personalized medicine: recent progress in cancer therapy. Cancers (Basel). (2021) 13:1–3. doi: 10.3390/CANCERS13020242
171. Kiyotani K, Toyoshima Y, Nakamura Y. Personalized immunotherapy in cancer precision medicine. Cancer Biol Med. (2021) 18:955–65. doi: 10.20892/J.ISSN.2095-3941.2021.0032
172. Yiong CS, Lin TP, Lim VY, Toh TB, Yang VS. Biomarkers for immune checkpoint inhibition in sarcomas – are we close to clinical implementation? Biomark Res. (2023) 11:1–30. doi: 10.1186/S40364-023-00513-5/FIGURES/3
173. Rizzo A, Ricci AD, Brandi G. PD-L1, TMB, MSI, and other predictors of response to immune checkpoint inhibitors in biliary tract cancer. Cancers (Basel). (2021) 13:1–11. doi: 10.3390/CANCERS13030558
174. Wang Y, Tong Z, Zhang W, Zhang W, Buzdin A, Mu X, et al. FDA-approved and emerging next generation predictive biomarkers for immune checkpoint inhibitors in cancer patients. Front Oncol. (2021) 11:683419/BIBTEX. doi: 10.3389/FONC.2021.683419/BIBTEX
175. Mariam A, Kamath S, Schveder K, McLeod HL, Rotroff DM. Biomarkers for response to anti–PD-1/anti–PD-L1 immune checkpoint inhibitors: A large meta-analysis. Oncol (United States). (2023) 37:210–9. doi: 10.46883/2023.25920995
176. Snyder A, Makarov V, Merghoub T, Yuan J, Zaretsky JM, Desrichard A, et al. Genetic basis for clinical response to CTLA-4 blockade in melanoma. N Engl J Med. (2014) 371:2189–99. doi: 10.1056/NEJMOA1406498
177. Herbst RS, Soria JC, Kowanetz M, Fine GD, Hamid O, Gordon MS, et al. Predictive correlates of response to the anti-PD-L1 antibody MPDL3280A in cancer patients. Nature. (2014) 515:563–7. doi: 10.1038/nature14011
178. Daud AI, Wolchok JD, Robert C, Hwu WJ, Weber JS, Ribas A, et al. Programmed death-ligand 1 expression and response to the anti-programmed death 1 antibody pembrolizumab in melanoma. J Clin Oncol. (2016) 34:4102–9. doi: 10.1200/JCO.2016.67.2477
179. Riaz N, Havel JJ, Makarov V, Desrichard A, Urba WJ, Sims JS, et al. Tumor and microenvironment evolution during immunotherapy with nivolumab. Cell. (2017) 171:934–949.e15. doi: 10.1016/J.CELL.2017.09.028
180. Borghaei H, Paz-Ares L, Horn L, Spigel DR, Steins M, Ready NE, et al. Nivolumab versus docetaxel in advanced nonsquamous non–small-cell lung cancer. New Engl J Med. (2015) 373:1627–39. doi: 10.1056/nejmoa1507643
181. Weber J, Mandala M, Del Vecchio M, Gogas HJ, Arance AM, Cowey CL, et al. Adjuvant nivolumab versus ipilimumab in resected stage III or IV melanoma. New Engl J Med. (2017) 377:1824–35. doi: 10.1056/nejmoa1709030
182. Fehrenbacher L, Spira A, Ballinger M, Kowanetz M, Vansteenkiste J, Mazieres J, et al. Atezolizumab versus docetaxel for patients with previously treated non-small-cell lung cancer (POPLAR): A multicentre, open-label, phase 2 randomised controlled trial. Lancet. (2016) 387:1837–46. doi: 10.1016/S0140-6736(16)00587-0
183. Larkin J, Chiarion-Sileni V, Gonzalez R, Grob JJ, Cowey CL, Lao CD, et al. Combined nivolumab and ipilimumab or monotherapy in untreated melanoma. N Engl J Med. (2015) 373:23–34. doi: 10.1056/NEJMOA1504030
184. Gibney GT, Weiner LM, Atkins MB. Predictive biomarkers for checkpoint inhibitor-based immunotherapy. Lancet Oncol. (2016) 17:e542. doi: 10.1016/S1470-2045(16)30406-5
185. Zhou B, Gao Y, Zhang P, Chu Q. Acquired resistance to immune checkpoint blockades: the underlying mechanisms and potential strategies. Front Immunol. (2021) 12:693609/BIBTEX. doi: 10.3389/FIMMU.2021.693609/BIBTEX
186. Cheng L, Wang Y, Qiu L, Chang Y, Lu H, Liu C, et al. mTOR pathway gene mutations predict response to immune checkpoint inhibitors in multiple cancers. J Transl Med. (2022) 20. doi: 10.1186/S12967-022-03436-1
187. Hsiehchen D, Hsieh A, Samstein RM, Lu T, Beg MS, Gerber DE, et al. DNA repair gene mutations as predictors of immune checkpoint inhibitor response beyond tumor mutation burden. Cell Rep Med. (2020) 1:100034. doi: 10.1016/J.XCRM.2020.100034
188. Goodman AM, Kato S, Bazhenova L, Patel SP, Frampton GM, Miller V, et al. Tumor mutational burden as an independent predictor of response to immunotherapy in diverse cancers. Mol Cancer Ther. (2017) 16:2598–608. doi: 10.1158/1535-7163.MCT-17-0386
189. Rosenberg JE, Hoffman-Censits J, Powles T, van der Heijden MS, Balar AV, Necchi A, et al. Atezolizumab in patients with locally advanced and metastatic urothelial carcinoma who have progressed following treatment with platinum-based chemotherapy: A single-arm, multicentre, phase 2 trial. Lancet. (2016) 387:1909–20. doi: 10.1016/S0140-6736(16)00561-4
190. Rizvi NA, Hellmann MD, Snyder A, Kvistborg P, Makarov V, Havel JJ, et al. Mutational landscape determines sensitivity to PD-1 blockade in non-small cell lung cancer. Sci (1979). (2015) 348:124–8. doi: 10.1126/science.aaa1348
191. Ayers M, Lunceford J, Nebozhyn M, Murphy E, Loboda A, Kaufman DR, et al. IFN-γ-related mRNA profile predicts clinical response to PD-1 blockade. J Clin Invest. (2017) 127:2930–40. doi: 10.1172/JCI91190
192. Jiang P, Gu S, Pan D, Fu J, Sahu A, Hu X, et al. Signatures of T cell dysfunction and exclusion predict cancer immunotherapy response. Nat Med. (2018) 24:1550–8. doi: 10.1038/s41591-018-0136-1
193. Pérez-Guijarro E, Yang HH, Araya RE, El Meskini R, Michael HT, Vodnala SK, et al. Multimodel preclinical platform predicts clinical response of melanoma to immunotherapy. Nat Med. (2020) 26:781–91. doi: 10.1038/S41591-020-0818-3
194. Cabrita R, Lauss M, Sanna A, Donia M, Skaarup Larsen M, Mitra S, et al. Tertiary lymphoid structures improve immunotherapy and survival in melanoma. Nature. (2020) 577:561–5. doi: 10.1038/s41586-019-1914-8
195. Helmink BA, Reddy SM, Gao J, Zhang S, Basar R, Thakur R, et al. B cells and tertiary lymphoid structures promote immunotherapy response. Nature. (2020) 577:549–55. doi: 10.1038/s41586-019-1922-8
196. Alahdal M, Elkord E, Hopkins J. Non-coding RNAs in cancer immunotherapy: Predictive biomarkers and targets. Clin Transl Med. (2023) 13:e1425. doi: 10.1002/CTM2.1425
197. Fan J, Yin Z, Xu J, Wu F, Huang Q, Yang L, et al. Circulating microRNAs predict the response to anti-PD-1 therapy in non-small cell lung cancer. Genomics. (2020) 112:2063–71. doi: 10.1016/J.YGENO.2019.11.019
198. Yu Y, Zhang W, Li A, Chen Y, Ou Q, He Z, et al. Association of long noncoding RNA biomarkers with clinical immune subtype and prediction of immunotherapy response in patients with cancer. JAMA Netw Open. (2020) 3:E202149. doi: 10.1001/JAMANETWORKOPEN.2020.2149
199. Hu Q, Ye Y, Chan LC, Li Y, Liang K, Lin A, et al. Oncogenic lncRNA downregulates cancer cell antigen presentation and intrinsic tumor suppression. Nat Immunol. (2019) 20:835–51. doi: 10.1038/S41590-019-0400-7
200. Gambardella V, Tarazona N, Cejalvo JM, Lombardi P, Huerta M, Roselló S, et al. Personalized medicine: recent progress in cancer therapy. Cancers (Basel). (2020) 12. doi: 10.3390/CANCERS12041009
201. Bullock M, Ren Y, O’Neill C, Gill A, Aniss A, Sywak M, et al. TERT promoter mutations are a major indicator of recurrence and death due to papillary thyroid carcinomas. Clin Endocrinol (Oxf). (2016) 85:283–90. doi: 10.1111/CEN.12999
202. Conway JR, Kofman E, Mo SS, Elmarakeby H, Van Allen E. Genomics of response to immune checkpoint therapies for cancer: implications for precision medicine. Genome Med. (2018) 10:1–18. doi: 10.1186/S13073-018-0605-7
203. Marusyk A, Polyak K. Tumor heterogeneity: Causes and consequences. Biochim Biophys Acta (BBA) - Rev Cancer. (2010) 1805:105–17. doi: 10.1016/J.BBCAN.2009.11.002
204. Marigoudar JB, Sarkar D, Yuguda YM, Abutayeh RF, Kaur A, Pati A, et al. Role of vitamin D in targeting cancer and cancer stem cell populations and its therapeutic implications. Med Oncol. (2022) 40. doi: 10.1007/S12032-022-01855-0
205. Khalighfard S, Khori V, Esmati E, Ahmadi F, Amiriani T, Poorkhani A, et al. Breast tumor metastasis following filgrastim administration due to the SDF-1/CXCR4 pathway. Med Oncol. (2023) 40:1–13. doi: 10.1007/S12032-022-01935-1/METRICS
Keywords: immune checkpoint inhibitors, resistance, combination, precision immunotherapy, tumor mircoenvironment
Citation: Alsaafeen BH, Ali BR and Elkord E (2025) Combinational therapeutic strategies to overcome resistance to immune checkpoint inhibitors. Front. Immunol. 16:1546717. doi: 10.3389/fimmu.2025.1546717
Received: 17 December 2024; Accepted: 31 March 2025;
Published: 24 April 2025.
Edited by:
Andrea Ladányi, National Institute of Oncology (NIO), HungaryReviewed by:
Dipendra Khadka, NADIANBIO Co. Ltd. Wonkwang University School of Medicine, Republic of KoreaSantenna Chenchula, All India Institute of Medical Sciences, India
Copyright © 2025 Alsaafeen, Ali and Elkord. This is an open-access article distributed under the terms of the Creative Commons Attribution License (CC BY). The use, distribution or reproduction in other forums is permitted, provided the original author(s) and the copyright owner(s) are credited and that the original publication in this journal is cited, in accordance with accepted academic practice. No use, distribution or reproduction is permitted which does not comply with these terms.
*Correspondence: Bassam R. Ali, YmFzc2FtLmFsaUB1YWV1LmFjLmFl; Eyad Elkord, RXlhZC5FbGtvcmRAeGp0bHUuZWR1LmNu
†ORCID: Besan H. Alsaafeen, orcid.org/0000-0002-3850-7654 Bassam R. Ali, orcid.org/0000-0003-1306-6618
Eyad Elkord, orcid.org/0000-0002-3868-0318