- 1Department of Thoracic Surgery, The First Affiliated Hospital of Kunming Medical University, Kunming, Yunnan, China
- 2Clinical Medical Research Center, The First Affiliated Hospital of Kunming Medical University, Kunming, Yunnan, China
The rising incidence of cancer has heightened interest in immune cell therapy, particularly the role of natural killer (NK) cells, which are essential components of the immune system. Their applications in tumor treatment have expanded significantly, especially with the incorporation of nanomaterials. This review comprehensively examines NK cell biology, encompassing aspects such as classification, distribution, receptor activation, and mechanisms of cytotoxicity. It also explores various NK cell therapies, including their sources, methods of acquisition, expansion techniques, Chimeric antigen receptor-Natural Killer cell (CAR-NK) technology, gene editing strategies, and combination therapies. Additionally, the review discusses the utilization of nanomaterials in NK cell therapy, focusing on nanoparticle-assisted immune regulation and the modulation of the tumor microenvironment. While NK cell therapy holds promise, CAR-NK technology presents certain limitations. The integration of nanomaterials offers potential strategies to enhance therapeutic efficacy. Future research should prioritize the optimization of NK cell therapy, address the limitations associated with CAR-NK technology, investigate the mechanisms of nanomaterials, and develop more effective nanomaterials to improve clinical outcomes.
1 Introduction
With the rising incidence of cancer-related deaths and newly diagnosed cases, there is renewed focus on immune cells. Natural killer (NK) cells, classified as Innate Lymphoid Cells (ILCs) (1), play a pivotal role in the human immune system (2).They exhibit potent anti-tumor and antiviral effects and also perform additional functions through the production of chemokines (CCL3/CCL4) and cytokines (Interferon γ, tumor necrosis factor α) (3). Moreover, NK cells are actively involved in hypersensitivity reactions and autoimmune diseases (4, 5).
Following promising results in hematological malignancies, there has been increased interest in employing NK cell therapy for the treatment of solid tumors. In recent years, research on NK cell therapy for solid tumors has gradually expanded. Additionally, the integration of nanomaterials into this research area has emerged as a prominent focus, with numerous strategies exploring the combination of NK cell therapy and nanomaterials. Bioactive nanomaterials, which exhibit good biocompatibility and degradability, can address the drawbacks of CAR-NK cells in tumor treatment. Nanoparticles in this therapy assist in immune regulation, act as carriers for enhanced efficacy, and improve NK cell homing. They also play a role in regulating the tumor microenvironment. While extracellular vesicle-related nanomaterials demonstrate anti-tumor effects, challenges remain in their production and delivery. Plant-derived nanomaterials are emerging as a future focus. Furthermore, nanoparticle-modified NK cells and nanomaterial-coated cell membranes can enhance therapy, with different modifications serving distinct functions.
Here we discuss NK cell therapy and its mechanisms as well as the role of related nanomaterials.
2 NK cell biology
2.1 Classification and distribution
Human natural killer (NK) cells are primarily distributed in peripheral blood, liver, spleen, lungs, and lymph nodes (6). Initially, it was believed that NK cells matured solely in the bone marrow. However, advancing research has provided increasing evidence that NK cells can also mature in secondary lymphoid tissues (SLT), such as tonsils and lymph nodes (7). It is important to note that NK cells exhibiting distinct phenotypes display different distribution patterns. CD56dimCD16+ NK cells are more abundant and predominant in peripheral blood; however, they do not truly reside within tissues (8). In contrast, CD56brightCD16− NK cells are more prevalent and widely distributed in both human tissues and lymphoid tissues. Furthermore, functional disparities exist between these two subsets: CD56dimCD16+ NK cells demonstrate high cytotoxic activity even at rest and can produce cytokines while in a quiescent state (9), whereas CD56brightCD16− NK cells require monocyte activation, specifically through the combined action of cytokines such as IL-2, IL-12, IL-15, and IL-18, to acquire cytotoxic activity and produce cytokines (10). Notably, CD56brightCD16− NK cells constitute the primary cell population in inflammatory and cancerous tissues (11).
2.2 Receptors and activation
Natural killer (NK) cells primarily function to eliminate cells that exhibit reduced or absent expression of major histocompatibility complex class I (MHC I) molecules (12). The activation of NK cells is predominantly regulated by a balance between activating and inhibitory receptors (13). Under normal physiological conditions, self-tissue cells typically express MHC class I molecules. In this context, the inhibitory receptors on the surface of NK cells are predominant, thereby suppressing the activity of activating receptors and preventing NK cells from targeting their own healthy tissue (14). However, in the event of viral infection or cancerous transformation, MHC class I molecules may be lost or downregulated (15). (Figure 1).Concurrently, the expression of other non-MHC class I molecules recognized by NK cells may become aberrant or upregulated (16). This alteration can result in the inactivation of NK cell inhibitory receptors, leading to the activation of their activating receptors and, consequently, the activation of NK cells, which enables them to target and kill affected cells (17). This phenomenon is referred to as the ‘missing self’ mechanism (18).
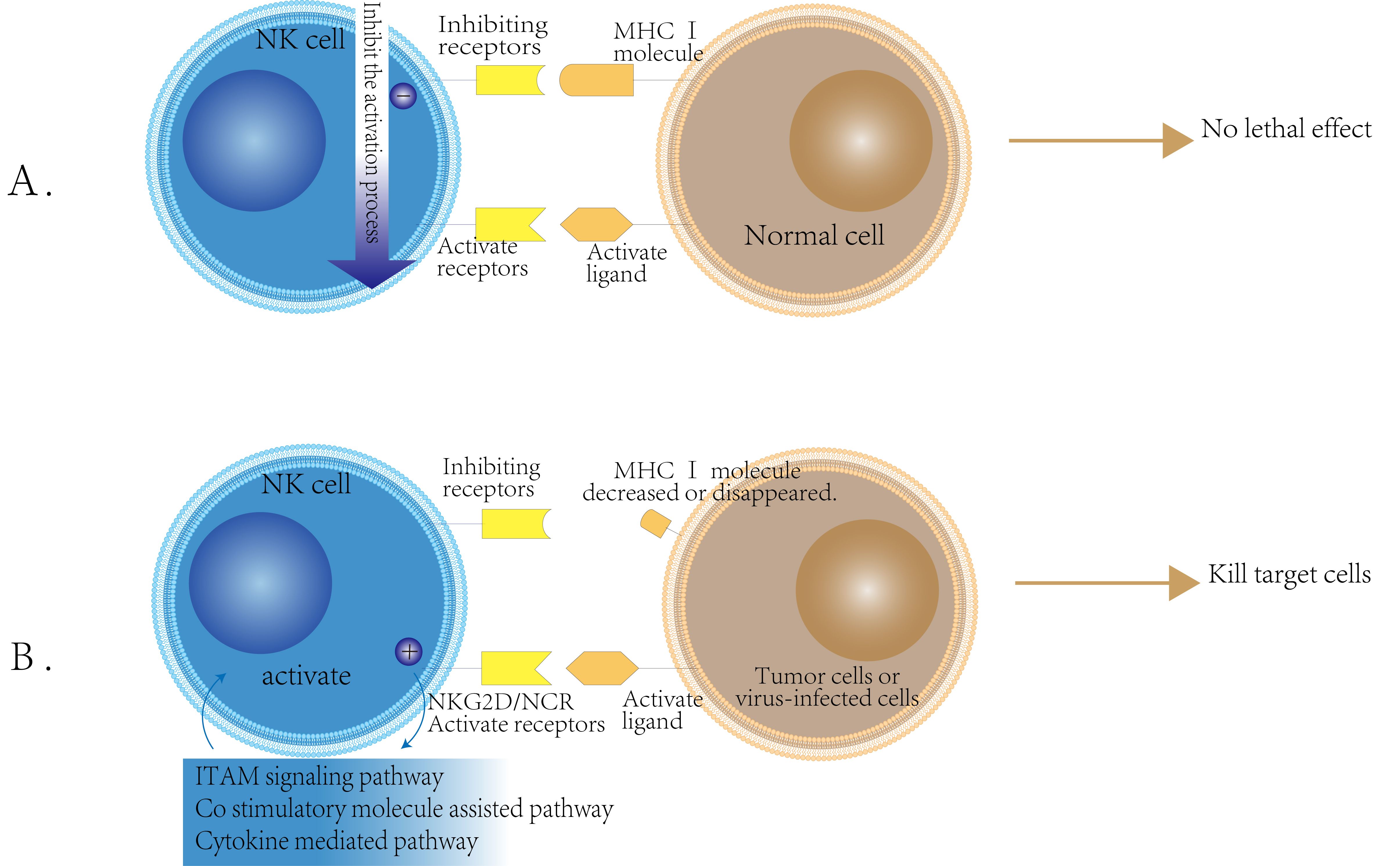
Figure 1. NK cell activity regulation mechanism schematic. (A) Inhibition Mechanism of NK Cells: NK cells possess inhibitory receptors for MHC class I molecules on their surface. When these receptors bind to the normal cell surface MHC class I molecules, they transmit inhibitory signals that prevent NK cell activation. As a result, NK cells do not exert cytotoxic effects on normal cells. (B) Activation Mechanism of NK Cells: The activation of NK cells occurs when the expression of MHC class I molecules on the surface of tumor cells or virus-infected cells is reduced or absent. In this scenario, the inhibitory receptors on NK cells are not engaged, while the expression of ligands for activating receptors, such as NKG2D and NCR, is upregulated on the surface of these target cells. This leads to the activation of NK cells, which then kill target cells through ITAM signaling, costimulatory molecular assistance, and cytokine-mediated pathways.
2.3 The killing function of NK cells against target cells
To evade the cytotoxic activity of CD8+ T cells, infected or cancerous cells downregulate the expression of MHC class I molecules (19). NK cells lyse target cells by two primary mechanisms (20): Firstly, they utilize perforin, a membrane-disrupting protein, and granzyme, a proteolytic enzyme, for specific lysis target cells. Upon recognizing the target cells, NK cells become activated, leading to the degranulation and release of perforin and granzyme through the immune synapse between them (21). Perforin creates pores in the cell membrane of the target cells, granzyme to enter and induce apoptosis (22); Secondly, NK cells can also induce death in target cells by modulating cytokines such as tumor necrosis factors, FAS- FASLG (FAS ligand), and TNF-TRAIL (TNF-related apoptosis-inducing ligand). Activated NK cells express corresponding ligands that bind to death receptors on the surfaces of target cells, triggering caspase-mediated enzymatic cascade reactions that result in apoptosis (23) (Figure 2).
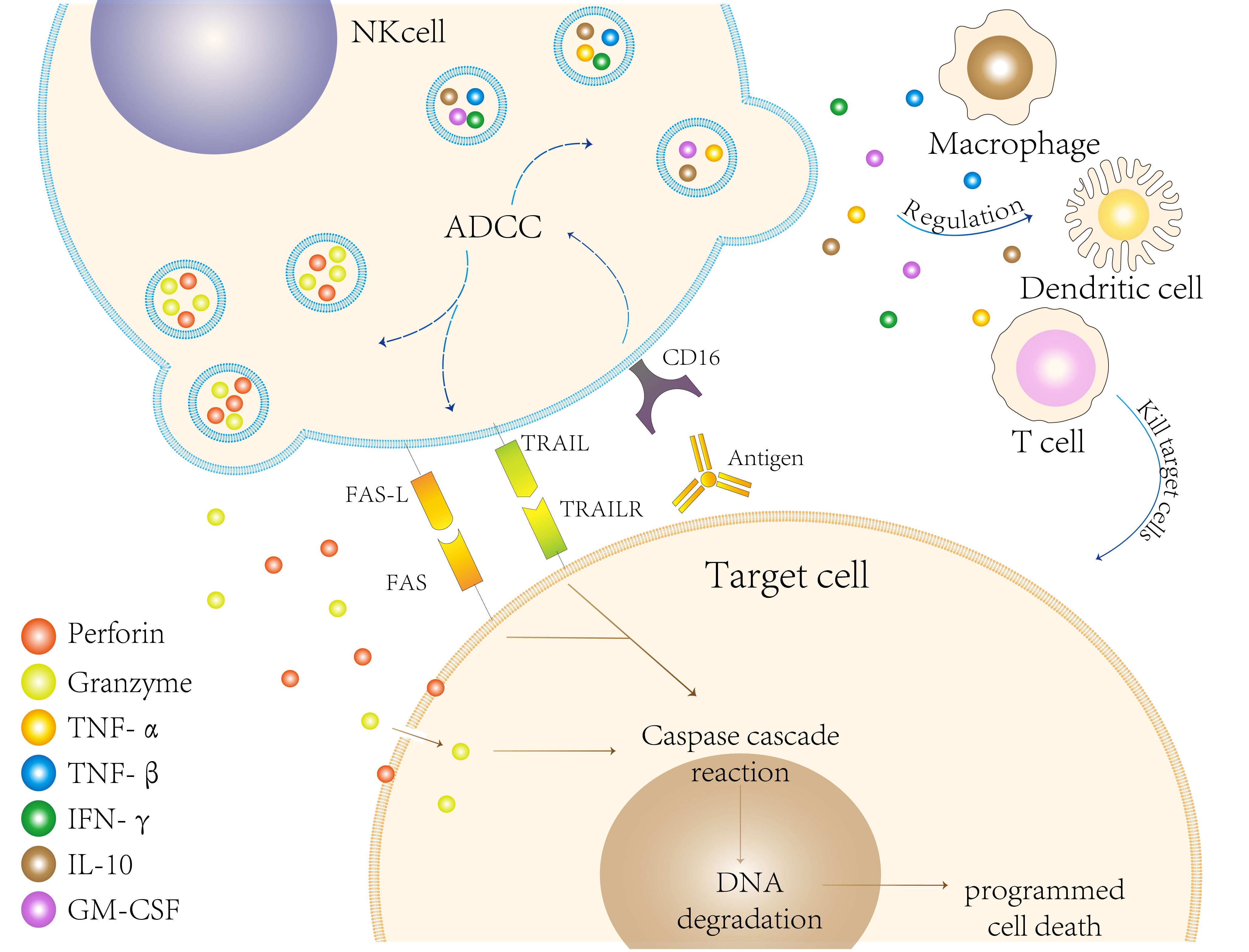
Figure 2. Natural killer (NK) cells eliminate target cells through various mechanisms. First, they can engage in antibody-dependent cellular cytotoxicity (ADCC) by recognizing and binding to the Fc segment of antibodies via CD16 present on their surfaces. Second, NK cells release perforin and granzymes, which induce programmed cell death in target cells. This process activates the caspase cascade, ultimately leading to DNA degradation. Third, FAS ligand (FAS-L) on the surface of NK cells binds to FAS on target cells, while the release of TRAIL binds to TRAIL receptors (TRAILR) on target cells, both of which can induce apoptosis. Collectively, these mechanisms illustrate the effectiveness and diversity of NK cells in targeting and eliminating cells during immune defense. Additionally, NK cells further modulate the immune response by influencing other immune cells, such as macrophages and dendritic cells, as well as regulating cytokine production.
3 NK cell therapy
3.1 Origin of NK cells
In the realm of NK cell therapy research, the source of NK cells is of paramount importance, encompassing peripheral blood, bone marrow, umbilical cord blood, and induced pluripotent stem cells (iPSCs).
Peripheral blood NK cells are frequently utilized in cell therapy due to their ease of collection and capacity for expansion (24). These NK cells primarily originate from the bone marrow and exhibit high cytotoxicity along with rapid responsiveness. Through in vitro culture and stimulation with cytokines such as IL-2 and IL-15, NK cells can be expanded on a large scale for clinical applications (25). Bone marrow serves as the primary site for NK cell production. NK cells derived from umbilical cord blood share similar characteristics with those derived from bone marrow stromal cells or hematopoietic stem cells (26), and they have the capability to induce mature NK cells in vitro (27). Furthermore, NK cells from this source demonstrate robust proliferation and cytotoxic activity, making them suitable candidates for cell therapy (28). iPSCs, with their capacity for unlimited proliferation, can differentiate into various cell lineages, including NK cells, in vitro (29). Advances in gene editing technology hold promise for enhancing the anti-tumor activity of NK cells derived from iPSCs (30). These NK cells not only provide an abundant source but also present opportunities to improve therapeutic efficacy against specific tumors through genetic modifications (31).
In summary, the source of NK cells significantly influences their functionality and therapeutic effectiveness. The success of NK cell therapy relies on the judicious selection of the appropriate NK cell source and the optimization of their amplification and activation conditions (32). Future research is anticipated to explore the characteristics of NK cells from diverse sources and their potential applications in tumor immunotherapy.
3.2 Acquisition and expansion of NK cells
The expansion of natural killer (NK) cells is pivotal in clinical treatment, particularly within the realm of cancer immunotherapy. An effective strategy for NK cell expansion not only increases the overall cell count but also enhances their functional capabilities and specificity, thereby improving the efficacy of immunotherapeutic interventions (33). Current methodologies for NK cell expansion encompass cytokine stimulation, co-culture techniques, and the application of small molecule compounds.
Cytokine stimulation strategies for NK cell expansion typically emphasize interleukin-2 (IL-2) and interleukin-15 (IL-15) as traditional options. IL-2 is widely recognized for its capacity to promote the proliferation and activation of NK cells (34). Furthermore, IL-15 not only improves the survival and functional capacity of NK cells (35) but also significantly enhances their anti-tumor activity (36). Co-culturing NK cells with tumor cells or other immune cells (37), such as dendritic cells, can bolster the proliferation and anti-tumor activity of NK cells through beneficial cell-cell interactions (38). Additionally, certain small molecule compounds, including histone deacetylase (HDAC)inhibitors and transforming growth factor-beta (TGF-β) inhibitors, can also augment the function and proliferation of NK cells by modulating cell signaling pathways (39, 40).
3.3 CAR-NK
3.3.1 What is CAR-NK
Chimeric Antigen Receptor Natural Killer (CAR-NK) cell therapy involves the genetic modification of natural killer (NK) cells using chimeric antigen receptors. This modification enhances the tumor specificity of NK cells and extends their duration of action within the tumor microenvironment (41). Furthermore, CAR-NK engineering can optimize lymphocyte activation signals and utilize specific intracellular signaling molecules to amplify the functions of NK cells, thereby improving their therapeutic efficacy (42).
3.3.2 CAR structure
A chimeric antigen receptor (CAR) typically comprises four structural domains: the extracellular antigen-binding domain, the spacer or hinge region, the transmembrane domain, and the intracellular signaling domain (43). The extracellular antigen-binding domain consists of a single-chain variable fragment (scFv), which is formed by linking the variable heavy chain (VH) and variable light chain (VL) of an antibody through a flexible linker. This domain is crucial for determining the specificity of antigen binding. The spacer region, also referred to as the hinge region, facilitates the exposure of the antigen-binding domain on the surface of CAR-engineered immune cells and serves as an intercellular space between target cells and their antigens. The transmembrane domain anchors the CAR to the cell membrane of immune cells, influencing CAR expression, stability, dimerization, and signal transduction. Finally, the intracellular signaling domain is essential for transmitting signals that activate immune cells to attack target cells (43–45) (Figure 3).
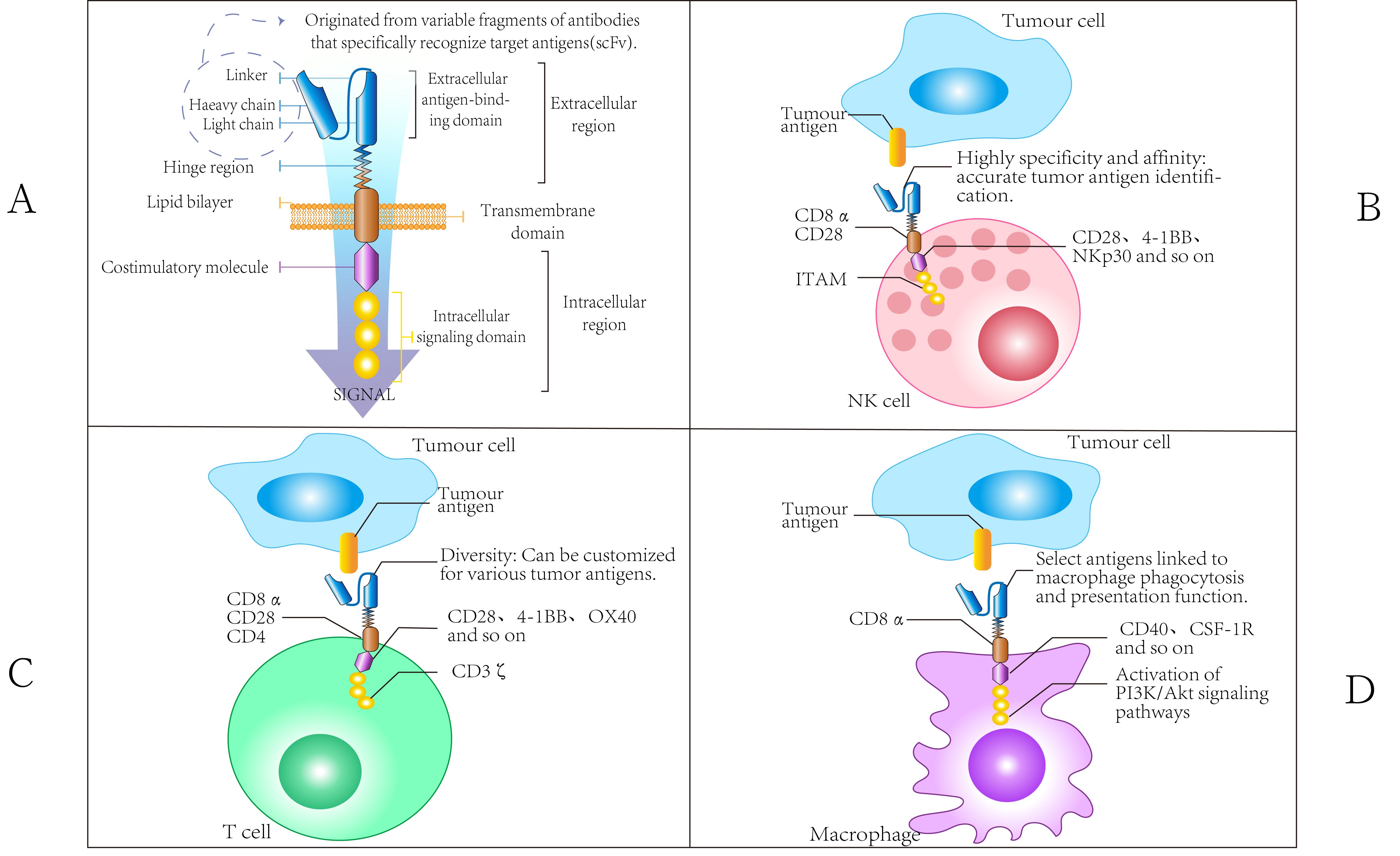
Figure 3. Chimeric Antigen Receptor Domain. (A) The CAR domain comprises an extracellular antigen-binding domain (including both heavy and light chains), a hinge region, a transmembrane domain, and an intracellular region, exhibiting high specificity, affinity, and diversity. Additionally, a variety of costimulatory molecules, such as CD28, play crucial roles in interactions between immune cells and can activate associated signaling pathways. (B) The CAR molecules present on NK cells consist of scFv that specifically recognize tumor antigens, transmembrane domains (such as CD8α), and intracellular signaling domains (including ITAM). The scFv is characterized by high specificity and diversity, allowing for the accurate recognition of various tumor antigens. The transmembrane domain serves to connect the extracellular environment to the intracellular space, with its component characteristics influencing the localization and function of CAR molecules on the cell membrane. The ITAM structure within the intracellular signaling domain is crucial for signaling processes. By interacting with other molecules, it activates the cytotoxic functions of NK cells, enabling them to identify and eliminate tumor cells. (C) The CAR molecules of T cells also incorporate scFv for antigen recognition. The components of the transmembrane domain may differ from those found in NK cells, resulting in distinct molecular compositions that influence their interactions with other cells. When the intracellular signaling domain activates relevant signaling pathways, such as PI3K/Akt, it is linked to the specific functions of T cells. For instance, during the immune response, the activation, proliferation, and differentiation of T cells are intricately regulated by CAR molecular signaling. This process differs from the signaling mechanisms of CAR molecules in NK cells, highlighting the distinct impact on cell function. (D) The scFv component of the CAR molecular structure in macrophages facilitates the specific recognition of tumor antigens. The transmembrane domain may contain macrophage-specific molecular elements that influence the stability of CAR molecules and their interactions with other cell surface proteins. The intracellular region, in conjunction with costimulatory molecules such as CD40 and CSF-1R, primarily regulates the phagocytic function of macrophages, enhancing their ability to engulf tumor cells. This regulatory mechanism is notably distinct from the functional modulation of CAR molecules observed in NK cells and T cells.
3.3.3 Advantages of CAR-NK
Chimeric antigen receptor (CAR) technology initially demonstrated efficacy in T cells and has been employed in the treatment of relapsed/refractory (R/R) B-cell acute lymphocytic leukemia (B-ALL), non-Hodgkin lymphoma (NHL), and multiple myeloma (MM) (46, 47). The application of CAR technology to natural killer (NK) cells holds promise for effectively alleviating the side effects associated with Chimeric Antigen Receptor T-cell (CAR-T) treatment (Table 1).
First, NK cells can target and eliminate cells that downregulate MHC I molecules, which are not recognized by T cells (48). Second, CAR-NK cells are activated independently of the MHC pathway, thereby circumventing the risk of graft-versus-host disease (GVHD) (49) that can arise from allogeneic CAR-T therapies (50). This characteristic enables CAR-NK cells to address the challenges posed by prolonged culture times commonly associated with autologous cell therapies through the use of NK cell lines and induced pluripotent stem cells (iPSCs) (51, 52). Thirdly, T cell activation results in the production of substantial amounts of inflammatory cytokines, including tumor necrosis factor-alpha (TNF-α), interleukin-1 beta (IL-1β), interleukin-2 (IL-2), and interleukin-6 (IL-6). This cascade of cytokine release can lead to cytokine release syndrome (CRS) and neurotoxicity. In contrast, while NK cell activation also produces pro-inflammatory cytokines such as interferon-gamma (IFN-γ), interleukin-3 (IL-3), and TNF-α, the quantities generated are relatively small, which helps to mitigate toxicity. Additionally, the absence of the critical cytokine IL-6 makes it less likely for CRS to occur (53). Finally, CAR-NK cells employ a variety of mechanisms to eliminate target cells, including activating and inhibitory receptors, as well as CD16-mediated antibody-dependent cell cytotoxicity (ADCC) (54, 55).
3.3.4 Limitations of CAR-NK
The selection of target antigens presents significant challenges. Ideal antigens are those that are tumor-specific, rather than those expressed in normal cells; however, most solid tumors lack traditional tumor-specific antigens, complicating target selection (56). Currently, only EGFR III and tumor neoantigens satisfy these criteria (57). Alternatively, when side effects are manageable-such as with CD19 (a B-cell surface marker) and BCMA (a plasma-cell surface marker), which are also expressed in normal tissues-CAR-T therapy can target these antigens, leading to B-cell deficiency or depletion, which can be mitigated through clinical management, such as immunoglobulin replacement therapy. Furthermore, the use of CAR-NK cells in blood cancers benefits from the characteristics of the blood system, allowing for tolerance of on-target, off-tumor toxicity (OTOT) (58). However, solid organ tumor therapies do not enjoy such advantages (59, 60). In the presence of OTOT, non-renewable or difficult-to-regenerate solid organ cells may experience pronounced side effects (61).Killer-cell immunoglobulin-like receptors (KIRs) can limit the ability of NK cells to attack tumor cells that exhibit high levels of MHC class I (62). Additionally, the immunosuppressive tumor microenvironment (TME) can impair the function of CAR-NK cells (63, 64). Lastly, due to the brief lifespan of CAR-NK cells, repeated infusions are necessary to maintain remission. CAR-NK cells expressing IL-12, IL-15, and IL-18 can extend their survival (65).
3.4 NK cell gene editing technology
Five key considerations in cytokine gene editing for enhancing NK cell therapy include: cytokine gene editing, activation of receptor expression, knockout of inhibitory receptors, regulation of immune checkpoints, and anti-apoptotic gene editing.
Cytokine transfection is a technique that utilizes gene editing to introduce or amplify specific cytokines, such as IL-15 and IL-2, to enhance NK cell survival, proliferation, and function, thereby augmenting their anti-tumor effects (64). Upregulating or introducing activating receptors can strengthen activation signals, enhancing NK cell recognition and destruction of tumor cells (65). Gene editing methods, including CRISPR, can be employed to knock out inhibitory receptors, such as NKG2A and PD-1, thereby reducing inhibitory signals and enhancing NK cell activity and anti-tumor potential (66). Additionally, gene editing technology can be utilized to regulate the expression of immune checkpoint molecules, diminishing NK cell inhibition and further amplifying their immune response to tumors (67, 68).
In summary, gene editing technology has opened new avenues for enhancing NK cell function, improving their anti-tumor activity, and developing innovative therapeutic strategies. As research progresses, the clinical translation of engineered NK cells is anticipated to provide significant benefits in the treatment of various cancers and other diseases.
3.5 NK cell combination therapy
Research on the integration of NK cells with other immunotherapies, radiotherapy, and chemotherapy is steadily increasing, demonstrating promising clinical application prospects. The combination of NK cells with immune checkpoint inhibitors has been shown to enhance the immune response within the tumor microenvironment and to counteract the mechanisms by which tumor cells evade the immune system (69). The combination of anti-PD-1/PD-L1 antibodies with NK-cell therapy enhances the inhibition of signaling pathways by blocking PD-1/PD-L1 interactions in NK cells. This strategy also amplifies the antibody-dependent cellular cytotoxicity (ADCC) effect of NK cells, thereby significantly improving outcomes in MHC-deficient tumors characterized by low antigenicity or resistance to T cell recognition and lysis. The results were evident, with a doubling of NK cell counts, a substantial increase in cytokine levels such as IL-2, TNF-β, and IFN-γ, and a marked decrease in various tumor markers, including circulating tumor cells (CTC). Patients receiving the combination therapy exhibited a significantly higher overall response rate (36.5% vs. 18.5%) and improved survival outcomes (overall survival: 15.5 months vs. 13.3 months; progression-free survival: 6.5 months vs. 4.3 months; all p< 0.05) compared to those treated with anti-PD-1 antibodies alone (70). Regarding radiotherapy, it can induce tumor cells to release various immunogenic substances, thereby enhancing NK cell activity and increasing tumor cell sensitivity (71, 72). Kim et al. demonstrated that the long-term migration and infiltration of natural killer (NK) cells to the primary tumor site were significantly enhanced following the combination of local tumor radiotherapy (RT) and NK cell therapy. This combination notably inhibited metastasis to the axillary lymph nodes, liver, and lungs (axillary metastases in the RT group, NK group, and RT + NK group were 85%, 20%, and 0%). Furthermore, the long-term survival rate of the RT + NK group was significantly higher than that of either the RT or NK group alone, with an 80-day survival rate of 0% for the RT group, 40% for the NK group, and 80% for the RT + NK group. This finding is supported by Nguyen et al., whose mouse experiments demonstrated that the combination of natural killer (NK) cells and radiotherapy resulted in increased survival rates and reduced tumor metastasis(p<0.0001 and p=0.0287) (73, 74). Additionally, the strategy of combining chemotherapy with NK cells has garnered considerable attention. Certain chemotherapy agents can bolster NK cell effectiveness by directly inducing apoptosis in tumor cells or by downregulating the expression of inhibitory molecules. Chemotherapy not only reduces tumor burden but may also enhance treatment efficacy by creating a more favorable tumor microenvironment for NK cell infiltration and functional expression (74).
In summary, the strategy of combining NK cells with other immunotherapies, radiotherapy, and chemotherapy offers a novel approach to tumor treatment. Future research should further investigate the underlying mechanisms and optimization strategies to achieve enhanced clinical efficacy.
4 Application of nanomaterials in NK therapy
While CAR-NK therapy has demonstrated considerable advantages in tumor treatment, it also presents certain limitations, as previously discussed (75). Bioactive nanomaterials, known for their excellent biocompatibility and degradability, serve to complement CAR-NK therapy in the treatment of tumors (76). This synergy not only enhances the efficacy of cancer treatment but also improves its safety profile (77). In the subsequent sections, we will provide a comprehensive discussion on nanoparticle-assisted NK cell therapy from various perspectives, including nanoparticle-assisted immune regulation, the regulation of the tumor microenvironment by nanoparticles, exosome-related nanomaterials, nanoparticle-modified NK cells, and the enhancement of therapeutic efficacy through cell membrane-coated nanomaterials.
4.1 Nanoparticles assisted immune regulation
The role of nanoparticles in immune regulation is primarily evident in two key areas. Firstly, they function as carriers for immune modulators, thereby enhancing therapeutic efficacy (78). Due to their extensive delivery range and high efficiency, nanoparticles are capable of encapsulating anticancer drugs, chemokines, and cytokines, facilitating their transport to tumor sites and thereby augmenting the effectiveness of cancer therapies (75, 79). For example, lipid-based nanoparticles loaded with immunomodulatory agents such as TGF-β and IL-2 can enhance immune cell infiltration at tumor locations (80, 81); Alternatively, a dual pH-responsive hydrogel, which contains a tumor acidic neutralizer and neutrophil extracellular trap (NETs) lyase (DNase I), when combined with NK cell infusion, may effectively prevent hepatocellular carcinoma (HCC) recurrence following surgical resection (82). Furthermore, a nanoemulsion system (SSBNMs) designed for the co-delivery of a TGF-β inhibitor and selenocysteine, when co-infused with NK cells, has been shown to improve anti-tumor efficacy. This process relies on the signal transduction of natural killer group 2, member D (NKG2D)/NKG2D ligands (NKG2DLs) and participates in the DNA damage response. Moreover, by inhibiting the TGF-β/TGF-β RI/Smad2/3 signaling pathway, it enhances the expression of NKG2DL on tumor cells, stimulates the surface expression of NKG2D on NK92 cells, and strengthens the immune response (83, 84).
Secondly, nanoparticles significantly enhance the homing of natural killer (NK) cells. The homing process of immune cells is mediated by the release of cytokines and chemokines from tumor cells, which induces inflammation and activates cytotoxic immunity. This dynamic process continuously recruits immune cells into the tumor microenvironment, ultimately leading to the destruction of cancer cells (82). The incorporation of magnetic nanomaterials onto the surface of NK cells facilitates the targeted attraction of these cells to tumor sites, guided by an external magnetic field (85, 86). Magnetic nanomaterials can be categorized into three distinct types (87): firstly, magnetic pure metals (Fe, Co, Ni), which offer the advantage of easy synthesis but are prone to oxidation and combustion in air; secondly, magnetic metal oxides (Fe2O3, Fe3O4) or ferrites (MeFe2O4, me=Fe, Co, Zn) possess excellent magnetic properties and low toxicity (88, 89); and third, multicomponent magnetic nanoparticles, such as core/shell magnetic nanoparticles (MNPs) or magnetic nanoclusters, which overcome the limitations of single-component materials and introduce novel functionalities (90). In a separate study, a therapeutic ‘biohybrid’ (NK: IONP) was developed by conjugating iron oxide nanoparticles (IONPs) to umbilical cord blood-derived NK cells using advanced biological coupling technology, thereby imparting enhanced magnetic guidance capabilities to NK cells (91). Additionally, magnetic nanoparticles not only do not impair NK cell function but also exhibit an activating effect on these cells. A magnetic nanocomposite: hyaluronic acid-protamine-ferumoxytol(HAPF) composed of hyaluronic acid (HA), protamine (P), and ferumoxytol (F) has been formulated, which effectively activates NK cells under the application of an external magnetic field, promotes the secretion of perforin and granzyme, and enhances the efficacy of tumor cell killing (92) (Figure 4).
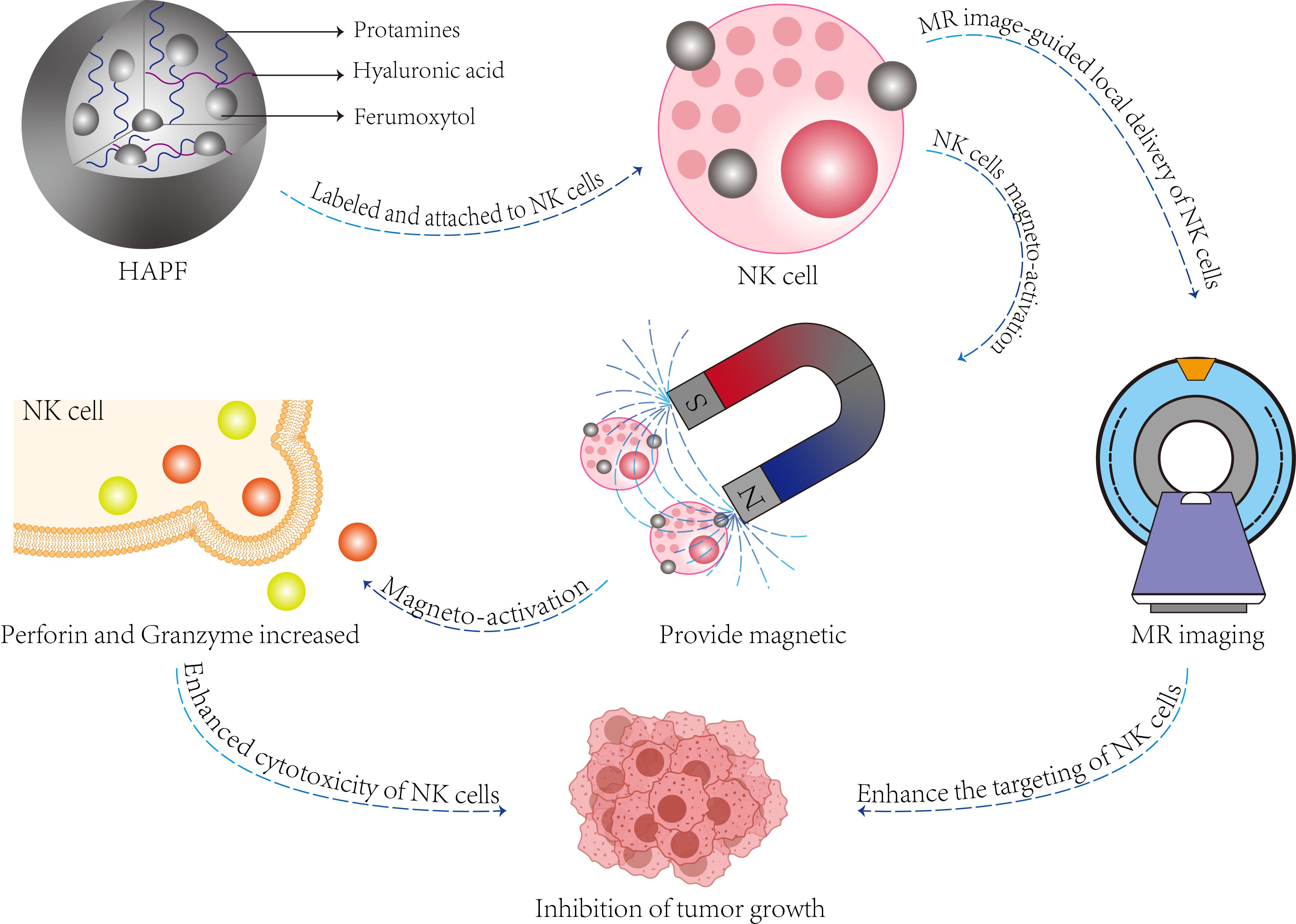
Figure 4. The function and composition of HAPF nanocomplex. The HAPF nanocomplex, composed of hyaluronic acid, protamine, and iron oxide, effectively attaches to natural killer (NK) cells (referred to as HAPF-NK). The application of exogenous magnetic fields facilitates the magnetic activation of NK cells, enhancing the production and secretion of perforin and granzyme within these cells, thereby achieving a cytotoxic effect on target cells. Additionally, magnetically activated HAPF-NK cells enable magnetic resonance (MR) image-guided NK cell therapy for the treatment of solid tumors.
4.2 Nanoparticles regulate tumor microenvironment
As previously mentioned, the accumulation of lactate and adenosine in the tumor microenvironment, along with hypoxia, can create an immunosuppressive milieu that impairs the cytotoxic function of NK cells (93). Therefore, reversing immune suppression within the tumor microenvironment is crucial for the efficacy of NK cell therapy (94). In comparison to conventional treatment modalities such as radiotherapy, chemotherapy, and immunotherapy, nanomaterials present distinct advantages. Research has shown that poly(lactic acid glycolic acid) copolymer (PLGA) - MnO2 nanoparticles alleviate hypoxia and reduce the levels of potent immunosuppressive metabolites like lactate and adenosine upon penetrating cancer spheroids (95); Moreover, vesicular cationic lipid-assisted nanoparticles (CLAN) inhibit the function of the CD47 molecule (96), effectively activate dendritic cells (97), decrease lactate secretion, normalize tumor acidity, enhance immune cell infiltration, and restore the anti-tumor response of T cells and NK cells (98). Additionally, drugs delivered via nanomaterials can also hinder the activation of tumor fibroblasts, reduce extracellular matrix accumulation, and improve the tumor microenvironment (99).
Furthermore, the enhancement of NK cell infiltration at the tumor site can be facilitated by increased release of cytokines and chemokines (100). For instance, These nanowire antibody substrates were able to locate endogenous IL-2 in the skin, increase endogenous IL-2 levels, and activate targeted natural killer cells, enabling tissue-specific and cell-specific immune activation (101).
4.3 Exosome related nanomaterials
Exosomes are spherical nano-vesicles secreted by cells, composed of lipid membranes (102). These subcellular vesicles are encapsulated by a phospholipid bilayer membrane and contain a variety of carriers, including miRNA, mRNA, DNA, and proteins, which play significant roles in the physiological and pathological processes of the body (103). Exosomes exhibit certain anti-tumor activities (104). For instance, NK cell-derived exosomes (NDEs) can clear tumor cells and stimulate adaptive immunity by secreting chemokines and pro-inflammatory cytokines (105, 106); DC cell-derived exosomes (DEXs) can serve as tumor vaccines that kill tumors through T cell-dependent and MHC-restrictive mechanisms (107, 108). Additionally, they can capture tumor-associated antigens (TAAs) and promote immune cell-dependent tumor rejection (109). Intriguingly, tumor cell-derived exosomes (TDES) not only exert immunosuppressive effects and induce M2 polarization of macrophages (110) but also participate in cytotoxic immune responses via antigen presentation or direct activation of NK cells, leading to the elimination of tumor cells (111).
Nucleic acids carried in exosomes can also enhance the efficacy of tumor therapy (112, 113). RNA derivatives such as siRNA, miRNA, and shRNA can silence specific genes, thereby enhancing NK cell activity (114). This approach falls under RNA interference (RNAi)-mediated immunotherapy (115). For example, the in vivo and intracellular transportation of siRNA requires superior carriers to overcome its short half-life and prevent degradation (116). With advancements in technology, lonizable cationic lipid nanoparticles can effectively combine with negatively charged siRNA, resulting in siRNA-lipid nanoparticle (siRNA-LNP) complexes that exhibit remarkable stability, making them the preferred carriers at present (117). Furthermore, NK cell-derived exosomes can be combined with miRNA-loaded biomimetic core-shell nanoparticles (NN) for targeted therapy, which demonstrates a dual inhibitory effect on tumor growth (118).
Exosomes face limitations in large-scale production and are readily captured by the liver in vivo, which significantly diminishes their delivery efficiency (119). Consequently, researchers are exploring alternative approaches, such as Plant-Derived Exosomes-Like Nanoparticles (PELNs), which have shown considerable promise in immune regulation (120), PELNs offer unique advantages, including evasion from immune detection, enhanced bioavailability, and reduced side effects (117). Currently, this technology has demonstrated substantial efficacy in the treatment of inflammatory diseases; however, research in this area remains insufficient, and many underlying mechanisms are not yet fully understood. This represents a promising avenue for future research on nanoparticles in tumor treatment (121).
4.4 Nanoparticle modified NK cells
Nanoparticles can significantly enhance the efficacy of immunotherapy by modifying natural killer (NK) cells. These nanomaterials are capable of delivering chimeric antigen receptor genes to patient-derived NK cells, thereby potentiating the advancement of CAR-NK cell therapy (122). For instance, the multifunctional nanoparticles (MF-NPs) effectively deliver genetic material to immune cells, induce the expression of targeted chimeric antigen receptors (EGFR-CARs) on the surface of NK cells, and improve the anticancer cytotoxicity of the cells in vitro and in vivo (123). Furthermore, nanoparticles serve as a crucial tool for engineering the surface of NK cells (124). Recently, there has been an increasing focus in this area: the core-shell membrane-fusogenic liposome (MFL) can enhance the levels of NK-activated free oligosaccharides while suppressing the immunosuppressive glycans on the surface of tumor cells through membrane fusion (125). Nanobody 7D12, characterized by its high affinity for antigens, low immunogenicity, and improved penetration into tumors, is conjugated with NK cells via orthogonal click chemistry. The resulting 7D12-NK92MI constructs can specifically target and eliminate solid tumor cells that overexpress EGFR (126). In conclusion, the potential of nanomaterial-mediated NK cell modification represents a promising research trajectory in NK cell therapy.
4.5 Cell membrane coated nanomaterials enhance therapeutic efficacy
Cell membrane-coated nanomaterials (CNPs) consist of a synthetic nanoparticle core that is camouflaged by a natural cell membrane. These materials are adept at functioning within complex biological environments and can significantly enhance the efficacy of nanomaterials (127). Nanomaterials enveloped in natural cell membranes exhibit the advantageous properties of both cell membranes and nanomaterials, including improved biocompatibility and targeted cellular delivery (128). Furthermore, the functions derived from membranes of different cellular origins possess unique characteristics. For example, macrophage- or neutrophil-coated nanomaterials can interact with tumor tissue to inhibit cancer progression and metastasis (129). In contrast, nanoparticles modified with red blood cell membranes have a longer half-life and are less likely to be recognized and eliminated by the immune system (130). Additionally, NK cell membrane-coated nanomaterials can greatly enhance targeting capabilities (131),while biomimetic NK cell nanomaterials (DMLN) can overcome multidrug resistance (132). Depending on specific therapeutic needs, various cell membrane modifications can be selected to augment the effects of NK cell therapy, and hybrid cell membrane modifications can also be employed to achieve a range of functions (130).
5 Conclusion and perspectives
NK cell therapy has demonstrated potential in tumor treatment, yet its clinical translation remains challenging. While CAR-NK technology avoids the side effects of CAR-T (e.g., GVHD and CRS), critical issues such as target antigen selection, off-target toxicity in solid tumors, immunosuppressive microenvironments, and short cell lifespan urgently require resolution. Gene editing technologies (e.g., CRISPR) and combination therapies (immune checkpoint inhibitors, chemoradiotherapy) offer new directions for optimizing NK cell function.
The application of nanomaterials has significantly enhanced the efficacy of NK therapy. Nanoparticles can improve treatment by delivering immunomodulators (e.g., TGF-β inhibitors), enhancing NK cell homing (via magnetic nanomaterials), and modulating the tumor microenvironment (e.g., neutralizing lactate/adenosine). Exosome-related nanomaterials exhibit anti-tumor activity, but breakthroughs are still needed in their large-scale production and targeted delivery. Plant-derived nanomaterials (e.g., PELNs), with their low immunogenicity and high biocompatibility, may become a future priority. Additionally, nanomaterial-modified NK cells (e.g., membrane-fused liposomes) and biomimetic membrane coatings (e.g., macrophage membranes) further expand functional diversity (Table 2).
Future research should focus on: 1.Optimizing CAR-NK targeting strategies to reduce off-target toxicity; 2.Elucidating nanomaterial mechanisms, developing low-toxicity and high-efficiency materials, and addressing production/delivery challenges; 3.Exploring synergistic effects of combining nanomaterials with NK therapy to identify more effective regimens; 4.Advancing the clinical translation of plant-derived nanomaterials and engineered exosomes. These efforts will accelerate breakthroughs in NK therapy for solid tumors, providing better options for cancer patients.
Author contributions
XY: Conceptualization, Formal Analysis, Writing – original draft, Writing – review & editing, Methodology. XC: Writing – review & editing, Conceptualization, Data curation, Formal Analysis, Investigation, Methodology, Resources, Supervision, Validation. YY: Conceptualization, Formal Analysis, Methodology, Supervision, Writing – review & editing. YT: Conceptualization, Methodology, Supervision, Writing – original draft, Writing – review & editing. JJ: Formal Analysis, Methodology, Supervision, Writing – review & editing. XT: Writing – review & editing.
Funding
The author(s) declare that financial support was received for the research and/or publication of this article. Clinical key specialty of thoracic surgery in Yunnan Province (Project Number:300067). Health and Wellness Development and Promotion Project – Spark Program Research Project. Project Number: XHJH-0011.
Conflict of interest
The authors declare that the research was conducted in the absence of any commercial or financial relationships that could be construed as a potential conflict of interest.
Generative AI statement
The author(s) declare that no Generative AI was used in the creation of this manuscript.
Publisher’s note
All claims expressed in this article are solely those of the authors and do not necessarily represent those of their affiliated organizations, or those of the publisher, the editors and the reviewers. Any product that may be evaluated in this article, or claim that may be made by its manufacturer, is not guaranteed or endorsed by the publisher.
References
1. Chiossone L, Dumas PY, Vienne M, Vivier E. Natural killer cells and other innate lymphoid cells in cancer. Nat Rev Immunol. (2018) 18:671–88. doi: 10.1038/s41577-018-0061-z
3. Pan R, Ryan J, Pan D, Wucherpfennig KW, Letai A. Augmenting NK cell-based immunotherapy by targeting mitochondrial apoptosis. Cell. (2022) 185:1521–38.e18. doi: 10.1016/j.cell.2022.03.030
4. von Bubnoff D, Andrès E, Hentges F, Bieber T, Michel T, Zimmer J. Natural killer cells in atopic and autoimmune diseases of the skin. J Allergy Clin Immunol. (2010) 125(1):60–8. doi: 10.1016/j.jaci.2009.11.020
5. Caligiuri MA. Human natural killer cells. Blood. (2008) 112:461–9. doi: 10.1182/blood-2007-09-077438
6. Male V, Hughes T, McClory S, Colucci F, Caligiuri MA, Moffett A. Immature NK cells, capable of producing IL-22, are present in human uterine mucosa. J Immunol (Baltimore Md: 1950). (2010) 185:3913–8. doi: 10.4049/jimmunol.1001637
7. Myers JA, Miller JS. Exploring the NK cell platform for cancer immunotherapy. Nat Rev Clin Oncol. (2021) 18:85–100. doi: 10.1038/s41571-020-0426-7
8. Melsen JE, Lugthart G, Lankester AC, Schilham MW. Human circulating and tissue-resident CD56(bright) natural killer cell populations. Front Immunol. (2016) 7:262. doi: 10.3389/fimmu.2016.00262
9. Scoville SD, Freud AG, Caligiuri MA. Modeling human natural killer cell development in the era of innate lymphoid cells. Front Immunol. (2017) 8:360. doi: 10.3389/fimmu.2017.00360
10. Freud AG, Mundy-Bosse BL, Yu J, Caligiuri MA. The broad spectrum of human natural killer cell diversity. Immunity. (2017) 47:820–33. doi: 10.1016/j.immuni.2017.10.008
11. Béziat V, Duffy D, Quoc SN, Le-Garff-Tavernier M, Decocq J, Combadière B, et al. CD56brightCD16+ NK cells: a functional intermediate stage of NK cell differentiation. J Immunol (Baltimore Md: 1950). (2011) 186:6753–61. doi: 10.4049/jimmunol.1100330
12. Shimasaki N, Jain A, Campana D. NK cells for cancer immunotherapy. Nat Rev Drug discov. (2020) 19:200–18. doi: 10.1038/s41573-019-0052-1
13. Islam R, Pupovac A, Evtimov V, Boyd N, Shu R, Boyd R, et al. Enhancing a natural killer: modification of NK cells for cancer immunotherapy. Cells. (2021) 10(5):1058. doi: 10.3390/cells10051058
14. Chen Y, Lu D, Churov A, Fu R. Research progress on NK cell receptors and their signaling pathways. Mediators inflamm. (2020) 2020:6437057. doi: 10.1155/2020/6437057
15. Quatrini L, Della Chiesa M, Sivori S, Mingari MC, Pende D, Moretta L. Human NK cells, their receptors and function. Eur J Immunol. (2021) 51:1566–79. doi: 10.1002/eji.202049028
16. Merino A, Maakaron J, Bachanova V. Advances in NK cell therapy for hematologic Malignancies: NK source, persistence and tumor targeting. Blood Rev. (2023) 60:101073. doi: 10.1016/j.blre.2023.101073
17. Sentman CL, Olsson MY, Kärre K. Missing self recognition by natural killer cells in MHC class I transgenic mice. A ‘receptor calibration’ model for how effector cells adapt to self. Semin Immunol. (1995) 7:109–19. doi: 10.1006/smim.1995.0015
18. Koenig A, Chen CC, Marçais A, Barba T, Mathias V, Sicard A, et al. Missing self triggers NK cell-mediated chronic vascular rejection of solid organ transplants. Nat Commun. (2019) 10:5350. doi: 10.1038/s41467-019-13113-5
19. Vogler M, Shanmugalingam S, Särchen V, Reindl LM, Grèze V, Buchinger L, et al. Unleashing the power of NK cells in anticancer immunotherapy. J Mol Med (Berlin Germany). (2022) 100:337–49. doi: 10.1007/s00109-021-02120-z
20. Prager I, Watzl C. Mechanisms of natural killer cell-mediated cellular cytotoxicity. J leuk Biol. (2019) 105:1319–29. doi: 10.1002/JLB.MR0718-269R
21. Ijaz A, Broere F, Rutten V, Jansen CA, Veldhuizen EJA. Perforin and granzyme A release as novel tool to measure NK cell activation in chickens. Dev Comp Immunol. (2023) 149:105047. doi: 10.1016/j.dci.2023.105047
22. Krzewski K, Coligan JE. Human NK cell lytic granules and regulation of their exocytosis. Front Immunol. (2012) 3:335. doi: 10.3389/fimmu.2012.00335
23. Morvan MG, Lanier LL. NK cells and cancer: you can teach innate cells new tricks. Nat Rev Cancer. (2016) 16:7–19. doi: 10.1038/nrc.2015.5
24. Zhu H, Kaufman DS. Engineered human pluripotent stem cell-derived natural killer cells: the next frontier for cancer immunotherapy. Blood Sci (Baltimore Md). (2019) 1:4–11. doi: 10.1097/BS9.0000000000000023
25. Choi YH, Lim EJ, Kim SW, Moon YW, Park KS, An HJ. IL-27 enhances IL-15/IL-18-mediated activation of human natural killer cells. J immunother cancer. (2019) 7:168. doi: 10.1186/s40425-019-0652-7
26. Hosseini F, Ahmadvand M, Karimi R, Mousavi SA, Ai J, Nikbakht M. Cancer immunotherapy via stem cell-derived NK cells. Immunotherapy. (2023) 15:963–73. doi: 10.2217/imt-2022-0224
27. Wen W, Chen X, Shen XY, Li HY, Zhang F, Fang FQ, et al. Enhancing cord blood stem cell-derived NK cell growth and differentiation through hyperosmosis. Stem Cell Res Ther. (2023) 14:295. doi: 10.1186/s13287-023-03461-x
28. Arias J, Yu J, Varshney M, Inzunza J, Nalvarte I. Hematopoietic stem cell- and induced pluripotent stem cell-derived CAR-NK cells as reliable cell-based therapy solutions. Stem Cells Trans Med. (2021) 10:987–95. doi: 10.1002/sctm.20-0459
29. Goldenson BH, Hor P, Kaufman DS. iPSC-derived natural killer cell therapies - expansion and targeting. Front Immunol. (2022) 13:841107. doi: 10.3389/fimmu.2022.841107
30. Cichocki F, van der Stegen SJC, Miller JS. Engineered and banked iPSCs for advanced NK- and T-cell immunotherapies. Blood. (2023) 141:846–55. doi: 10.1182/blood.2022016205
31. Karagiannis P, Kim SI. iPSC-derived natural killer cells for cancer immunotherapy. Mol Cells. (2021) 44:541–8. doi: 10.14348/molcells.2021.0078
32. Zhu H, Kaufman DS. An improved method to produce clinical-scale natural killer cells from human pluripotent stem cells. Methods Mol Biol (Clifton NJ). (2019) 2048:107–19. doi: 10.1007/978-1-4939-9728-2_12
33. Becker PS, Suck G, Nowakowska P, Ullrich E, Seifried E, Bader P, et al. Selection and expansion of natural killer cells for NK cell-based immunotherapy. Cancer immunol immunother: CII. (2016) 65:477–84. doi: 10.1007/s00262-016-1792-y
34. Koh SK, Park J, Kim SE, Lim Y, Phan MT, Kim J, et al. Natural killer cell expansion and cytotoxicity differ depending on the culture medium used. Ann Lab Med. (2022) 42:638–49. doi: 10.3343/alm.2022.42.6.638
35. Bergamaschi C, Stravokefalou V, Stellas D, Karaliota S, Felber BK, Pavlakis GN. Heterodimeric IL-15 in cancer immunotherapy. Cancers. (2021) 13(4):837. doi: 10.3390/cancers13040837
36. Christodoulou I, Ho WJ, Marple A, Ravich JW, Tam A, Rahnama R, et al. Engineering CAR-NK cells to secrete IL-15 sustains their anti-AML functionality but is associated with systemic toxicities. J immunother Cancer. (2021) 9(12):e003894. doi: 10.1101/2021.09.23.461509
37. Chan IS, Ewald AJ. Organoid co-culture methods to capture cancer cell-natural killer cell interactions. Methods Mol Biol (Clifton NJ). (2022) 2463:235–50. doi: 10.1007/978-1-0716-2160-8_17
38. van Eck van der Sluijs J, van Ens D, Thordardottir S, Vodegel D, Hermens I, van der Waart AB, et al. Clinically applicable CD34(+)-derived blood dendritic cell subsets exhibit key subset-specific features and potently boost anti-tumor T and NK cell responses. Cancer immunol immunother: CII. (2021) 70:3167–81. doi: 10.1007/s00262-021-02899-3
39. Fionda C, Stabile H, Cerboni C, Soriani A, Gismondi A, Cippitelli M, et al. Hitting more birds with a stone: impact of TGF-β on ILC activity in cancer. J Clin Med. (2020) 9(1):143. doi: 10.3390/jcm9010143
40. Karami Z, Mortezaee K, Majidpoor J. Dual anti-PD-(L)1/TGF-β inhibitors in cancer immunotherapy - Updated. Int immunopharmacol. (2023) 122:110648. doi: 10.1016/j.intimp.2023.110648
41. Heipertz EL, Zynda ER, Stav-Noraas TE, Hungler AD, Boucher SE, Kaur N, et al. Current perspectives on “Off-the-shelf” Allogeneic NK and CAR-NK cell therapies. Front Immunol. (2021) 12:732135. doi: 10.3389/fimmu.2021.732135
42. Abel AM, Yang C, Thakar MS, Malarkannan S. Natural killer cells: development, maturation, and clinical utilization. Front Immunol. (2018) 9:1869. doi: 10.3389/fimmu.2018.01869
43. Pan K, Farrukh H, Chittepu V, Xu H, Pan CX, Zhu Z. CAR race to cancer immunotherapy: from CAR T, CAR NK to CAR macrophage therapy. J Exp Clin Cancer res: CR. (2022) 41:119. doi: 10.1186/s13046-022-02327-z
44. Gong Y, Klein Wolterink RGJ, Wang J, Bos GMJ, Germeraad WTV. Chimeric antigen receptor natural killer (CAR-NK) cell design and engineering for cancer therapy. J Hematol Oncol. (2021) 14:73. doi: 10.1186/s13045-021-01083-5
45. Xiao Q, Zhang X, Tu L, Cao J, Hinrichs CS, Su X. Size-dependent activation of CAR-T cells. Sci Immunol. (2022) 7:eabl3995. doi: 10.1126/sciimmunol.abl3995
46. Chohan KL, Siegler EL, Kenderian SS. CAR-T cell therapy: the efficacy and toxicity balance. Curr hematol Malign Rep. (2023) 18:9–18. doi: 10.1007/s11899-023-00687-7
47. Khan AN, Asija S, Pendhari J, Purwar R. CAR-T cell therapy in hematological Malignancies: Where are we now and where are we heading for? Eur J Hematol. (2024) 112:6–18. doi: 10.1111/ejh.14076
48. Chelbi ST, Dang AT, Guarda G. Emerging major histocompatibility complex class I-related functions of NLRC5. Adv Immunol. (2017) 133:89–119. doi: 10.1016/bs.ai.2016.11.003
49. Liu S, Galat V, Galat Y, Lee YKA, Wainwright D, Wu J. NK cell-based cancer immunotherapy: from basic biology to clinical development. J Hematol Oncol. (2021) 14:7. doi: 10.1186/s13045-020-01014-w
50. Zhang X, Zhu L, Zhang H, Chen S, Xiao Y. CAR-T cell therapy in hematological Malignancies: current opportunities and challenges. Front Immunol. (2022) 13:927153. doi: 10.3389/fimmu.2022.927153
51. Chen YJ, Abila B, Mostafa Kamel Y. CAR-T: what is next? Cancers. (2023) 15(3):663. doi: 10.3390/cancers15030663
52. Lin X, Sun Y, Dong X, Liu Z, Sugimura R, Xie G. IPSC-derived CAR-NK cells for cancer immunotherapy. Biomed pharmacother = Biomed pharmacother. (2023) 165:115123. doi: 10.1016/j.biopha.2023.115123
53. Włodarczyk M, Pyrzynska B. CAR-NK as a rapidly developed and efficient immunotherapeutic strategy against cancer. Cancers. (2022) 15(1):117. doi: 10.3390/cancers15010117
54. Chin DS, Lim CSY, Nordin F, Arifin N, Jun TG. Antibody-dependent cell-mediated cytotoxicity through natural killer (NK) cells: unlocking NK cells for future immunotherapy. Curr Pharm Biotechnol. (2022) 23:552–78. doi: 10.2174/1389201022666210820093608
55. Wrona E, Borowiec M, Potemski P. CAR-NK cells in the treatment of solid tumors. Int J Mol Sci. (2021) 22(11):5899. doi: 10.3390/ijms22115899
56. Biederstädt A, Rezvani K. Engineering the next generation of CAR-NK immunotherapies. Int J hematol. (2021) 114:554–71. doi: 10.1007/s12185-021-03209-4
57. Labanieh L, Mackall CL. CAR immune cells: design principles, resistance and the next generation. Nature. (2023) 614:635–48. doi: 10.1038/s41586-023-05707-3
58. Zhang L, Meng Y, Feng X, Han Z. CAR-NK cells for cancer immunotherapy: from bench to bedside. biomark Res. (2022) 10:12. doi: 10.1186/s40364-022-00364-6
59. Alabanza L, Pegues M, Geldres C, Shi V, Wiltzius JJW, Sievers SA, et al. Function of novel anti-CD19 chimeric antigen receptors with human variable regions is affected by hinge and transmembrane domains. Mol Ther. (2017) 25:2452–65. doi: 10.1016/j.ymthe.2017.07.013
60. Flugel CL, Majzner RG, Krenciute G, Dotti G, Riddell SR, Wagner DL, et al. Overcoming on-target, off-tumor toxicity of CAR T cell therapy for solid tumors. Nat Rev Clin Oncol. (2023) 20:49–62. doi: 10.1038/s41571-022-00704-3
61. Hamieh M, Mansilla-Soto J, Rivière I, Sadelain M. Programming CAR T cell tumor recognition: tuned antigen sensing and logic gating. Cancer discov. (2023) 13:829–43. doi: 10.1158/2159-8290.CD-23-0101
62. Dagher OK, Posey AD Jr. Forks in the road for CAR T and CAR NK cell cancer therapies. Nat Immunol. (2023) 24:1994–2007. doi: 10.1038/s41590-023-01659-y
63. Kilgour MK, Bastin DJ, Lee SH, Ardolino M, McComb S, Visram A. Advancements in CAR-NK therapy: lessons to be learned from CAR-T therapy. Front Immunol. (2023) 14:1166038. doi: 10.3389/fimmu.2023.1166038
64. Valeri A, García-Ortiz A, Castellano E, Córdoba L, Maroto-Martín E, Encinas J, et al. Overcoming tumor resistance mechanisms in CAR-NK cell therapy. Front Immunol. (2022) 13:953849. doi: 10.3389/fimmu.2022.953849
65. Hussein BA, Kristenson L, Pesce S, Wöhr A, Tian Y, Hallner A, et al. NKG2A gene variant predicts outcome of immunotherapy in AML and modulates the repertoire and function of NK cells. J immunother Cancer. (2023) 11(8):e007202. doi: 10.1136/jitc-2023-007202
66. Mohammadian Gol T, Kim M, Sinn R, Ureña-Bailén G, Stegmeyer S, Gratz PG, et al. CRISPR-cas9-based gene knockout of immune checkpoints in expanded NK cells. Int J Mol Sci. (2023) 24(22):16065. doi: 10.3390/ijms242216065
67. Bexte T, Alzubi J, Reindl LM, Wendel P, Schubert R, Salzmann-Manrique E, et al. CRISPR-Cas9 based gene editing of the immune checkpoint NKG2A enhances NK cell mediated cytotoxicity against multiple myeloma. Oncoimmunology. (2022) 11:2081415. doi: 10.1080/2162402X.2022.2081415
68. Dubrot J, Du PP, Lane-Reticker SK, Kessler EA, Muscato AJ, Mehta A, et al. In vivo CRISPR screens reveal the landscape of immune evasion pathways across cancer. Nat Immunol. (2022) 23:1495–506. doi: 10.1038/s41590-022-01315-x
69. Sordo-Bahamonde C, Lorenzo-Herrero S, Granda-Díaz R, Martínez-Pérez A, Aguilar-García C, Rodrigo JP, et al. Beyond the anti-PD-1/PD-L1 era: promising role of the BTLA/HVEM axis as a future target for cancer immunotherapy. Mol cancer. (2023) 22:142. doi: 10.1186/s12943-023-01845-4
70. Bai R, Cui J. Burgeoning exploration of the role of natural killer cells in anti-PD-1/PD-L1 therapy. Front Immunol. (2022) 13:886931. doi: 10.3389/fimmu.2022.886931
71. Baude J, Limagne E, Ladjohounlou R, Mirjolet C. Combining radiotherapy and NK cell-based therapies: The time has come. Int Rev Cell Mol Biol. (2023) 378:31–60. doi: 10.1016/bs.ircmb.2023.02.003
72. He J, Yan Y, Zhang J, Wei Z, Li H, Xing L. Synergistic treatment strategy: combining CAR-NK cell therapy and radiotherapy to combat solid tumors. Front Immunol. (2023) 14:1298683. doi: 10.3389/fimmu.2023.1298683
73. Kim KW, Jeong JU, Lee KH, Uong TNT, Rhee JH, Ahn SJ, et al. Combined NK cell therapy and radiation therapy exhibit long-term therapeutic and antimetastatic effects in a human triple negative breast cancer model. Int J Radiat oncol biol phys. (2020) 108:115–25. doi: 10.1016/j.ijrobp.2019.09.041
74. Nguyen HPQ, Bae WK, Park MS, Chung IJ, Nam TK, Jeong JU, et al. Intensified NK cell therapy in combination with low-dose chemoradiotherapy against human colorectal cancer. Cancer immunol immunother: CII. (2023) 72:4089–102. doi: 10.1007/s00262-023-03545-w
75. Al-Shadidi J, Al-Shammari S, Al-Mutairi D, Alkhudhair D, Thu HE, Hussain Z. Chitosan nanoparticles for targeted cancer therapy: A review of stimuli-responsive, passive, and active targeting strategies. Int J nanomed. (2024) 19:8373–400. doi: 10.2147/IJN.S472433
76. Uthaman S, Cutshaw G, Ghazvini S, Bardhan R. Nanomaterials for natural killer cell-based immunoimaging and immunotherapies in cancer. ACS Appl mater interf. (2022) 15(44):50708–20. doi: 10.1021/acsami.2c08619
77. Murugan D, Murugesan V, Panchapakesan B, Rangasamy L. Nanoparticle enhancement of natural killer (NK) cell-based immunotherapy. Cancers. (2022) 14(21):5438. doi: 10.3390/cancers14215438
78. Croitoru GA, Pîrvulescu DC, Niculescu AG, Epistatu D, Rădulescu M, Grumezescu AM, et al. Nanomaterials in immunology: bridging innovative approaches in immune modulation, diagnostics, and therapy. J Funct biomater. (2024) 15(8):225. doi: 10.3390/jfb15080225
79. Nejabat M, Samie A, Khojastehnezhad A, Hadizadeh F, Ramezani M, Alibolandi M, et al. Stimuli-responsive covalent organic frameworks for cancer therapy. ACS Appl mater interf. (2024) 16:51837–59. doi: 10.1021/acsami.4c07040
80. Cheng Z, Fobian SF, Gurrieri E, Amin M, D’Agostino VG, Falahati M, et al. Lipid-based nanosystems: the next generation of cancer immune therapy. J Hematol Oncol. (2024) 17:53. doi: 10.1186/s13045-024-01574-1
81. Papadopoulou P, Arias-Alpizar G, Weeda P, Poppe T, van Klaveren N, Slíva T, et al. Structure-function relationship of phase-separated liposomes containing diacylglycerol analogues. Biomater sci. (2024) 12:5023–35. doi: 10.1039/D4BM00799A
82. Cheng Y, Gong Y, Chen X, Zhang Q, Zhang X, He Y, et al. Injectable adhesive hemostatic gel with tumor acidity neutralizer and neutrophil extracellular traps lyase for enhancing adoptive NK cell therapy prevents post-resection recurrence of hepatocellular carcinoma. Biomaterials. (2022) 284:121506. doi: 10.1016/j.biomaterials.2022.121506
83. Liu C, Lai H, Chen T. Boosting natural killer cell-based cancer immunotherapy with selenocystine/transforming growth factor-beta inhibitor-encapsulated nanoemulsion. ACS nano. (2020) 14:11067–82. doi: 10.1021/acsnano.9b10103
84. Pandey P, Gulati N, Makhija M, Purohit D, Dureja H. Nanoemulsion: A novel drug delivery approach for enhancement of bioavailability. Recent patents nanotechnol. (2020) 14:276–93. doi: 10.2174/1872210514666200604145755
85. Mishra S, Yadav MD. Magnetic nanoparticles: A comprehensive review from synthesis to biomedical frontiers. Langmuir: ACS J surf colloids. (2024) 40:17239–69. doi: 10.1021/acs.langmuir.4c01532
86. Pusta A, Tertis M, Crăciunescu I, Turcu R, Mirel S, Cristea C. Recent advances in the development of drug delivery applications of magnetic nanomaterials. Pharmaceutics. (2023) 15(7):1872. doi: 10.3390/pharmaceutics15071872
87. Singh R, Yadav D, Ingole PG, Ahn YH. Magnetic engineering nanoparticles: Versatile tools revolutionizing biomedical applications. Biomater adv. (2024) 163:213948. doi: 10.1016/j.bioadv.2024.213948
88. Narayana S, Gowda BHJ, Hani U, Shimu SS, Paul K, Das A, et al. Inorganic nanoparticle-based treatment approaches for colorectal cancer: recent advancements and challenges. J nanobiotechnol. (2024) 22:427. doi: 10.1186/s12951-024-02701-3
89. Wu D, Shou X, Zhang Y, Li Z, Wu G, Wu D, et al. Cell membrane-encapsulated magnetic nanoparticles for enhancing natural killer cell-mediated cancer immunotherapy. Nanomed: nanotechnol biol Med. (2021) 32:102333. doi: 10.1016/j.nano.2020.102333
90. Gao J, Gu H, Xu B. Multifunctional magnetic nanoparticles: design, synthesis, and biomedical applications. Accounts Chem Res. (2009) 42:1097–107. doi: 10.1021/ar9000026
91. Burga RA, Khan DH, Agrawal N, Bollard CM, Fernandes R. Designing magnetically responsive biohybrids composed of cord blood-derived natural killer cells and iron oxide nanoparticles. Bioconjugate Chem. (2019) 30:552–60. doi: 10.1021/acs.bioconjchem.9b00048
92. Sim T, Choi B, Kwon SW, Kim KS, Choi H, Ross A, et al. Magneto-activation and magnetic resonance imaging of natural killer cells labeled with magnetic nanocomplexes for the treatment of solid tumors. ACS nano. (2021) 15:12780–93. doi: 10.1021/acsnano.1c01889
93. Wang ZH, Peng WB, Zhang P, Yang XP, Zhou Q. Lactate in the tumour microenvironment: From immune modulation to therapy. EBioMedicine. (2021) 73:103627. doi: 10.1016/j.ebiom.2021.103627
94. Ma C, Cheng Z, Tan H, Wang Y, Sun S, Zhang M, et al. Nanomaterials: leading immunogenic cell death-based cancer therapies. Front Immunol. (2024) 15:1447817. doi: 10.3389/fimmu.2024.1447817
95. Wang L, Li D, Hao Y, Niu M, Hu Y, Zhao H, et al. Gold nanorod-based poly(lactic-co-glycolic acid) with manganese dioxide core-shell structured multifunctional nanoplatform for cancer theranostic applications. Int J nanomed. (2017) 12:3059–75. doi: 10.2147/IJN.S128844
96. Li S, Chen Y, Ma R, Du Y, Han B. Cationic lipid-assisted nanoparticles for simultaneous delivery of CD47 siRNA and R848 to promote antitumor immune responses. Front Pharmacol. (2023) 14:1142374. doi: 10.3389/fphar.2023.1142374
97. Logtenberg MEW, Scheeren FA, Schumacher TN. The CD47-SIRPα Immune checkpoint. Immunity. (2020) 52:742–52. doi: 10.1016/j.immuni.2020.04.011
98. Wang S, Wu Q, Chen T, Su R, Pan C, Qian J, et al. Blocking CD47 promotes antitumor immunity through CD103(+) dendritic cell-NK cell axis in murine hepatocellular carcinoma model. J hepatol. (2022) 77:467–78. doi: 10.1016/j.jhep.2022.03.011
99. Lu Q, Kou D, Lou S, Ashrafizadeh M, Aref AR, Canadas I, et al. Nanoparticles in tumor microenvironment remodeling and cancer immunotherapy. J Hematol Oncol. (2024) 17:16. doi: 10.1186/s13045-024-01535-8
100. Zhang Y, Bi J, Huang J, Tang Y, Du S, Li P. Exosome: A review of its classification, isolation techniques, storage, diagnostic and targeted therapy applications. Int J nanomed. (2020) 15:6917–34. doi: 10.2147/IJN.S264498
101. Zamecnik CR, Lowe MM, Patterson DM, Rosenblum MD, Desai TA. Injectable polymeric cytokine-binding nanowires are effective tissue-specific immunomodulators. ACS nano. (2017) 11:11433–40. doi: 10.1021/acsnano.7b06094
102. Zhou Y, Zhang Y, Gong H, Luo S, Cui Y. The role of exosomes and their applications in cancer. Int J Mol Sci. (2021) 22(22):12204. doi: 10.3390/ijms222212204
103. Propper DJ, Balkwill FR. Harnessing cytokines and chemokines for cancer therapy. Nat Rev Clin Oncol. (2022) 19:237–53. doi: 10.1038/s41571-021-00588-9
104. Kalluri R, LeBleu VS. The biology, function, and biomedical applications of exosomes. Sci (New York NY). (2020) 367(6478):eaau6977. doi: 10.1126/science.aau6977
105. Hatami Z, Hashemi ZS, Eftekhary M, Amiri A, Karpisheh V, Nasrollahi K, et al. Natural killer cell-derived exosomes for cancer immunotherapy: innovative therapeutics art. Cancer Cell Int. (2023) 23:157. doi: 10.1186/s12935-023-02996-6
106. Di Pace AL, Tumino N, Besi F, Alicata C, Conti LA, Munari E, et al. Characterization of human NK cell-derived exosomes: role of DNAM1 receptor in exosome-mediated cytotoxicity against tumor. Cancers. (2020) 12(3):661. doi: 10.3390/cancers12030661
107. Elashiry M, Elsayed R, Cutler CW. Exogenous and endogenous dendritic cell-derived exosomes: lessons learned for immunotherapy and disease pathogenesis. Cells. (2021) 11(1):115. doi: 10.3390/cells11010115
108. Pitt JM, André F, Amigorena S, Soria JC, Eggermont A, Kroemer G, et al. Dendritic cell-derived exosomes for cancer therapy. J Clin Invest. (2016) 126:1224–32. doi: 10.1172/JCI81137
109. Luo S, Chen J, Xu F, Chen H, Li Y, Li W. Dendritic cell-derived exosomes in cancer immunotherapy. Pharmaceutics. (2023) 15(8):2070. doi: 10.3390/pharmaceutics15082070
110. Zhao S, Mi Y, Guan B, Zheng B, Wei P, Gu Y, et al. Tumor-derived exosomal miR-934 induces macrophage M2 polarization to promote liver metastasis of colorectal cancer. J Hematol Oncol. (2020) 13:156. doi: 10.1186/s13045-020-00991-2
111. Baig MS, Roy A, Rajpoot S, Liu D, Savai R, Banerjee S, et al. Tumor-derived exosomes in the regulation of macrophage polarization. Inflammation Res. (2020) 69:435–51. doi: 10.1007/s00011-020-01318-0
112. O’Brien K, Breyne K, Ughetto S, Laurent LC, Breakefield XO. RNA delivery by extracellular vesicles in mammalian cells and its applications. Nat Rev Mol Cell Biol. (2020) 21:585–606. doi: 10.1038/s41580-020-0251-y
113. Zhang J, Li S, Li L, Li M, Guo C, Yao J, et al. Exosome and exosomal microRNA: trafficking, sorting, and function. Genom Proteomics Bioinf. (2015) 13:17–24. doi: 10.1016/j.gpb.2015.02.001
114. Traber GM, Yu AM. RNAi-based therapeutics and novel RNA bioengineering technologies. J Pharmacol Exp Ther. (2023) 384:133–54. doi: 10.1124/jpet.122.001234
115. Setten RL, Rossi JJ, Han SP. The current state and future directions of RNAi-based therapeutics. Nat Rev Drug discov. (2019) 18:421–46. doi: 10.1038/s41573-019-0017-4
116. Kulkarni JA, Witzigmann D, Chen S, Cullis PR, van der Meel R. Lipid nanoparticle technology for clinical translation of siRNA therapeutics. Accounts Chem Res. (2019) 52:2435–44. doi: 10.1021/acs.accounts.9b00368
117. El Moukhtari SH, Garbayo E, Amundarain A, Pascual-Gil S, Carrasco-León A, Prosper F, et al. Lipid nanoparticles for siRNA delivery in cancer treatment. J Controlled release. (2023) 361:130–46. doi: 10.1016/j.jconrel.2023.07.054
118. Wang G, Hu W, Chen H, Shou X, Ye T, Xu Y. Cocktail strategy based on NK cell-derived exosomes and their biomimetic nanoparticles for dual tumor therapy. Cancers. (2019) 11(10):1560. doi: 10.3390/cancers11101560
119. Dad HA, Gu TW, Zhu AQ, Huang LQ, Peng LH. Plant exosome-like nanovesicles: emerging therapeutics and drug delivery nanoplatforms. Mol Ther. (2021) 29:13–31. doi: 10.1016/j.ymthe.2020.11.030
120. Barzin M, Bagheri AM, Ohadi M, Abhaji AM, Salarpour S, Dehghannoudeh G. Application of plant-derived exosome-like nanoparticles in drug delivery. Pharm Dev technol. (2023) 28:383–402. doi: 10.1080/10837450.2023.2202242
121. Zhang Z, Yu Y, Zhu G, Zeng L, Xu S, Cheng H, et al. The emerging role of plant-derived exosomes-like nanoparticles in immune regulation and periodontitis treatment. Front Immunol. (2022) 13:896745. doi: 10.3389/fimmu.2022.896745
122. Han B, Song Y, Park J, Doh J. Nanomaterials to improve cancer immunotherapy based on ex vivo engineered T cells and NK cells. J Controlled release. (2022) 343:379–91. doi: 10.1016/j.jconrel.2022.01.049
123. Kim KS, Han JH, Park JH, Kim HK, Choi SH, Kim GR, et al. Multifunctional nanoparticles for genetic engineering and bioimaging of natural killer (NK) cell therapeutics. Biomaterials. (2019) 221:119418. doi: 10.1016/j.biomaterials.2019.119418
124. Yang H, Yao L, Wang Y, Chen G, Chen H. Advancing cell surface modification in mammalian cells with synthetic molecules. Chem sci. (2023) 14:13325–45. doi: 10.1039/D3SC04597H
125. Zheng C, Zhong Q, Song W, Yi K, Kong H, Wang H, et al. Membrane-fusion-mediated multiplex engineering of tumor cell surface glycans for enhanced NK cell therapy. Adv mater (Deerfield Beach Fla). (2023) 35:e2206989. doi: 10.1002/adma.202206989
126. Gong L, Li Y, Cui K, Chen Y, Hong H, Li J, et al. Nanobody-engineered natural killer cell conjugates for solid tumor adoptive immunotherapy. Small (Weinheim an der Bergstrasse Germany). (2021) 17:e2103463. doi: 10.1002/smll.202103463
127. Fang RH, Gao W, Zhang L. Targeting drugs to tumors using cell membrane-coated nanoparticles. Nat Rev Clin Oncol. (2023) 20:33–48. doi: 10.1038/s41571-022-00699-x
128. Zhu Y, Cui H, Zhang J, Bei Y, Huang Y, Li M, et al. Application of cell membrane-coated nanomaterials for tumor treatment. Mini Rev med Chem. (2023) 23:1535–59. doi: 10.2174/1389557523666230203145645
129. Oroojalian F, Beygi M, Baradaran B, Mokhtarzadeh A, Shahbazi MA. Immune cell membrane-coated biomimetic nanoparticles for targeted cancer therapy. Small (Weinheim an der Bergstrasse Germany). (2021) 17:e2006484. doi: 10.1002/smll.202006484
130. Chen HY, Deng J, Wang Y, Wu CQ, Li X, Dai HW. Hybrid cell membrane-coated nanoparticles: A multifunctional biomimetic platform for cancer diagnosis and therapy. Acta biomater. (2020) 112:1–13. doi: 10.1016/j.actbio.2020.05.028
131. Wang D, Wang S, Zhou Z, Bai D, Zhang Q, Ai X, et al. White blood cell membrane-coated nanoparticles: recent development and medical applications. Adv healthc mater. (2022) 11:e2101349. doi: 10.1002/adhm.202101349
Keywords: natural killer cells, NK cell therapy, CAR-NK, nanometer material, tumor immunotherapy
Citation: Yu X, Chen X, Yang Y, Tian Y, Jia J and Tong X (2025) Nanomaterial assisted natural killer cell therapy. Front. Immunol. 16:1558701. doi: 10.3389/fimmu.2025.1558701
Received: 10 January 2025; Accepted: 24 March 2025;
Published: 05 May 2025.
Edited by:
Nathan Denlinger, The Ohio State University, United StatesReviewed by:
Sara Passos, Century Therapeutics, United StatesKapil Sirohi, National Jewish Health, United States
Copyright © 2025 Yu, Chen, Yang, Tian, Jia and Tong. This is an open-access article distributed under the terms of the Creative Commons Attribution License (CC BY). The use, distribution or reproduction in other forums is permitted, provided the original author(s) and the copyright owner(s) are credited and that the original publication in this journal is cited, in accordance with accepted academic practice. No use, distribution or reproduction is permitted which does not comply with these terms.
*Correspondence: Xiaobo Chen, Y2hlbnhpYW9ibzA0MTZAMTYzLmNvbQ==; Yunping Zhao, emhhb3l1bnBpbmdAeWR5eS5jbg==