- 1Department of Molecular Microbiology and Immunology, School of Medicine, University of Missouri, Columbia, MO, United States
- 2Roy Blunt NextGen Precision Health Building, University of Missouri, Columbia, MO, United States
NF-κB is a crucial transcription factor in lymphocyte signaling. It is activated by environmental cues that drive lymphocyte differentiation to combat infections and cancer. As a key player in inflammation, NF-κB also significantly impacts autoimmunity and transplant rejection, making it an important therapeutic target. While the signaling molecules regulating this pathway are well-studied, the effect of changes in NF-κB signaling levels on T lymphocyte differentiation, fate, and function is not fully understood. Advances in computational biology and new NF-κB-inducible animal models are beginning to clarify these questions. In this review, we highlight recent findings related to T cells, focusing on how environmental cues affecting NF-κB signaling levels determine T cell fate and function.
Introduction
Since its discovery in 1986 (1), the transcription factor NF-κB has been associated with numerous cellular processes, including cell function, differentiation, and stemness (2–5). NF-κB plays a crucial role in opposing cell responses like survival and cell death and influences specific T cell differentiation paths. While subunit composition of NF-κB heterodimers can sometimes explain these outcomes, the regulation of this transcription factor remains complex, involving transcriptional and epigenetic levels. NF-κB is induced via a signaling cascade converging at the IKK complex, composed of catalytic subunits IKKα and IKKβ, and the regulatory subunit NEMO (IKKγ) (6). The upstream signals activating the IKK complex vary with environmental cues (3, 7, 8). Additionally, NF-κB alone is often insufficient for gene regulation; it relies on interactions with other transcription factors, its affinity for binding sites at gene promoters or enhancers, and its influence on chromatin remodeling (9). Modeling studies indicate that the timing and levels of NF-κB signaling are critical in determining T cell responses and fate (10–13). This review examines how environmental cues utilize NF-κB signaling to regulate T cell lymphocytes, highlights unresolved questions, and explores implications for therapeutic interventions.
The NF-κB signaling pathway
NF-κB is a family of transcription factors comprised of several members: RELA (p65/RelA), c-REL (c-Rel), RELB (RelB), NFKB1 (p105/p50), and NFKB2 (p100/p52) (Figure 1A). These members form homo- or heterodimers via an N-terminal Rel homology domain (RHD) (14). Canonical and non-canonical signaling pathways enable different membrane receptors to induce NF-κB nuclear translocation and regulate its transcriptional activity (Figure 2). The canonical NF-κB pathway induces the formation of p50-RelA and p50-c-Rel dimers (15, 16), which in resting cells are sequestered in the cytoplasm by IκB proteins (IκBα, IκBβ, IκBγ, IκBϵ) (17–23) or regulated in the nuclei by the atypical IκB proteins IκBNS (24, 25), IκBζ (26) IkBη (27) and BCL3 (28, 29). Meanwhile, exposure to environmental stimuli induces the activation of the multi-subunit IκB kinase (IKK) complex which drives the phosphorylation, polyubiquitination and degradation of IκB proteins in the 26S proteasome (6, 30). This allows NF-κB heterodimers to translocate to the nucleus to regulate gene expression. In contrast to this, the non-canonical pathway induces p52-RelB heterodimers through the activation of NF-κB-inducing kinase (NIK), which phosphorylates IKKα dimers, leading to p100 processing into p52 (31–33). This pathway is triggered by stimuli such as LIGHT, TWEAK, BAFF, RANKL, and CD40L, while canonical signaling is activated by antigens, CD28, 4-1BB, GITR co-stimulators, TNF, and IL-1 (3, 8, 34), (Figures 1, 2). Notably, while all these receptors share the same downstream signaling steps following IKK complex/IKKc activation (canonical or non-canonical), they differ upstream (Figure 2). Modulation of NF-κB signaling levels lies both in the diversity of intermediates driving IKKc activation and the regulated expression of antigens, TNFRs, and cytokine receptors. It is also important to consider the distinct and overlapping roles of canonical and non-canonical NF-κB signaling. Activation of the canonical pathway has been classically associated with inflammation, while activation of the non-canonical pathway regulates cell development and organogenesis. Both pathways have been considered to signal independently (35). However, extensive overlap exists at the level of p50-RelA, IκBδ and p100 (36, 37). Similarly, canonical control of p52-RelB can occur via the p50-RelA dependent expression of p100 and RelB (36, 37). At the level of NF-κB subunit dimerization, RelA can bind to RelB and prevent its DNA binding (38). Upstream, crosstalk has also been reported at the level of RIPK1 (39, 40) and NIK (41, 42).
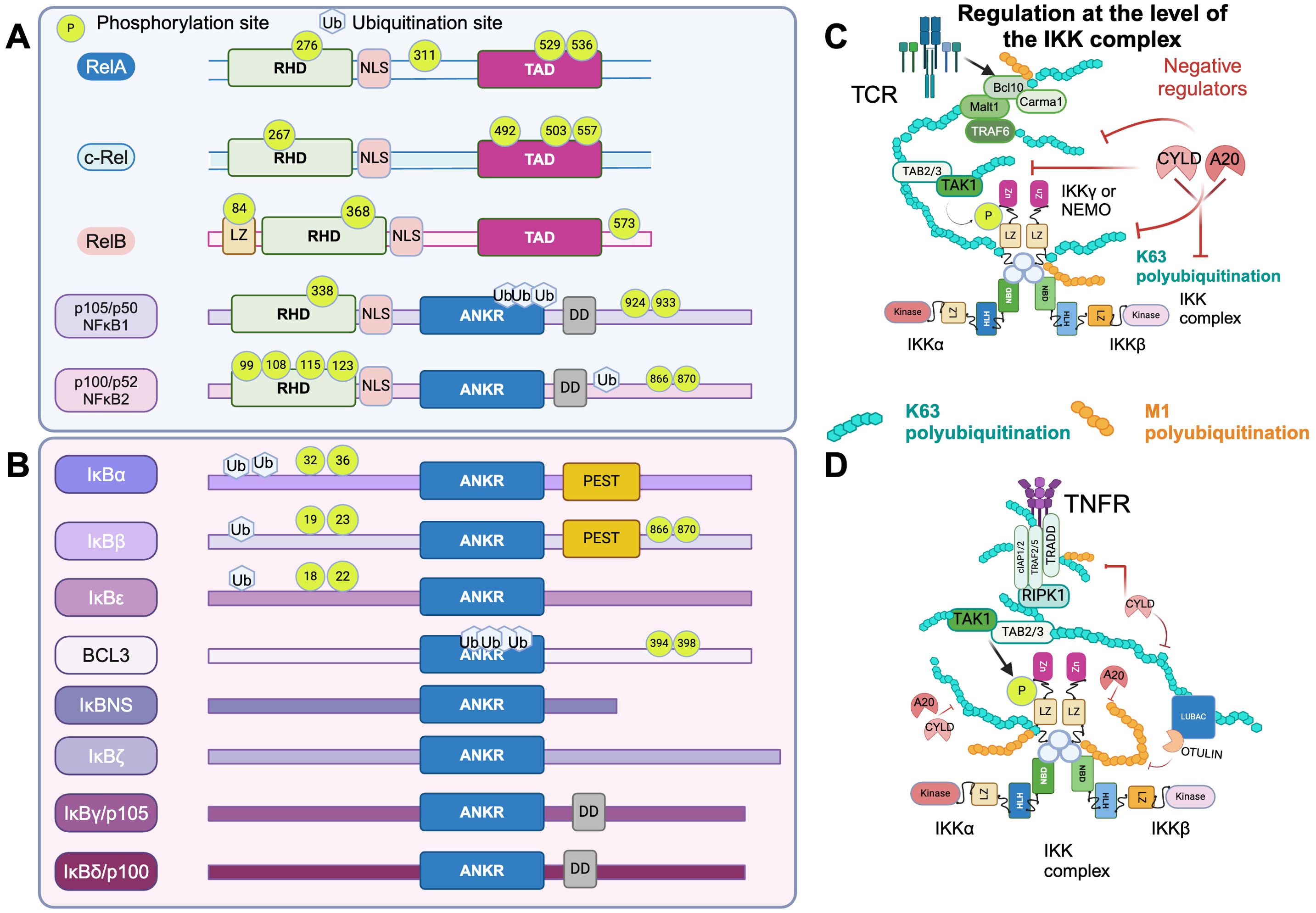
Figure 1. The NF-κB signaling network. (A) NF-κB subunits or Rel Homology domain proteins of the NF-κB family. RHD or Rel homology domain determines dimerization with other members of the NF-κB/Rel protein family; NLS or nuclear localization sequence, which also comprises the site for inhibitory interactions with IkB. TAD, or transactivation domain. LZ or leuzin zipper. AnkR or ankyrin repeats domain. DD or death domain. Regulatory phosphorylation sites are denoted by their position in green. Ubiquitination sites (ub) are also shown. (B) Inhibitors of the NF-κB family. The ankyrin repeats in these proteins mediate the binding to NF-κB dimers. The N terminal part of the IκB proteins contain two Ser residues that when phosphorylated allow for quick ubiquitination and degradation of the protein. This is true for the typical IκB proteins but not for the atypical (IκBNS, IκBζ, IkBη and BCL3). The C-terminal regions of p105 and p100 aside from the ankyrin repeats, they also have a death domain. These regions function as IκB for their RHD domain by forming large complexes with Rel proteins. The death domain can undergo cotranslational processing by the 26S proteosome to produce p50 or p52. DD domains also can allow binding to other proteins with complimentary DD domains enabling the assembly of signaling complexes involved in cell death pathways. Some IκB members also have a PEST domain rich in Proline, glutamic acid, serine and threonine. The PEST domain acts as a signal for rapid degradation. (C) The cartoon illustrates the regulation of the IKK complex by the positive actions of signaling intermediates downstream of the T Cell Receptor (TCR) and the TNF receptor. When the TCR binds to antigen presented in the context of MHC, it leads to the phosphorylation of tyrosines in the CD3 chains of the TCR/CD3 complex, facilitating the recruitment of the kinase ZAP-70. ZAP-70 then phosphorylates its substrates, LAT and SLP76, forming the LAT/SLP76 signalosome. Once phosphorylated, this complex enables the recruitment of Vav, which mediates cytoskeleton reorganization and translocation of PKCθ to the membrane. At the membrane, PKCθ phosphorylates CARMA1, leading to the formation of the CBM complex (CARMA1, BCL10, and MALT1). This complex then oligomerizes and recruits the ligase TRAF6 and the kinase TAK1. A dimer of IKKγ (NEMO) binds to IKKα and IKKβ to form the IKK complex. The linear and K63 polyubiquitination of NEMO allows for the activation of the IKK complex, further supported by phosphorylation by TAK1. IKK activation leads to the phosphorylation of the NF-κB subunit p65 and the inhibitory protein IκBα. K63, K11, and M1 ubiquitination represent key processes in NF-κB signaling that enable the formation of non-degradative ubiquitin chains, which are critical for the recruitment of various components of the NF-κB signaling cascade. (D) Downstream of the TNF receptor, TRAF complexes recruit RIPK1. The LUBAC complex catalyzes the M1 polyubiquitination of NEMO which allows for activation of the IKKc. Negative regulation at the level of the IKKc signalosome can occur through various mechanisms, including dephosphorylation and deubiquitination by phosphatases such as PP2A and PP2C, or by deubiquitinases like A20 and CYLD (K63 polyubiquitin chains), and Otulin (M1 polyubiquitin chains). This figure has been created with BioRender.com.
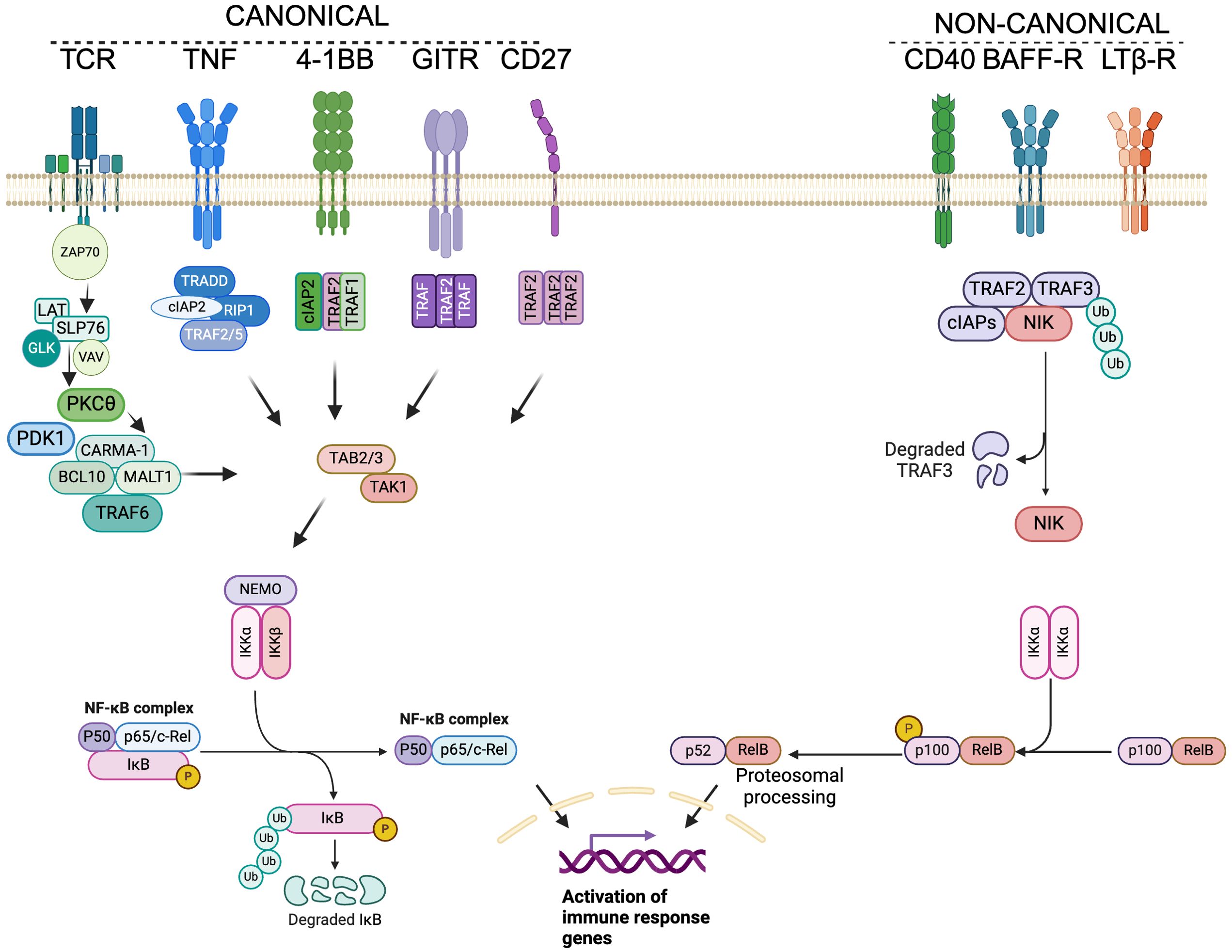
Figure 2. Canonical and non-canonical NF-κB signaling in T cells. Left. This cartoon illustrates the membrane receptors involved in delivering canonical NF-κB signaling in T cells, including the T Cell Receptor (TCR) and members of the TNF receptor superfamily. TCR stimulation leads to the recruitment of the kinase ZAP70 to the phosphorylated ITAMs of the CD3 chains within the TCR/CD3 complex. This recruitment enables ZAP70 to phosphorylate LAT and SLP76, forming the LAT/SLP76 signalosome, which subsequently recruits the guanine exchange factor Vav and the kinase GLK. These components contribute to the membrane translocation and activation of PKCθ. Next, PKCθ phosphorylates CARMA1, resulting in the formation of the CBM complex (comprised of CARMA1, BCL10, and MALT1). The subsequent recruitment of the ligase TRAF6 and the kinase TAK1 into the NF-κB signalosome leads to the ubiquitination of NEMO and the phosphorylation of IKKα and IKKβ. The active IKK complex (NEMO-IKKα-IKKβ) phosphorylates IκBα, which retains NF-κB heterodimers (p50-RelA or p50-c-Rel) in the cytosol, targeting IκBα for degradation. This process allows free NF-κB dimers to translocate to the nucleus and drive gene expression. TNF receptor signaling converges with TCR signaling at the level of TAK1 and the IKK complex; however, each receptor leads to the assembly of specific signalosomes where TRAF proteins play critical roles. Right. Non-canonical NF-κB signaling differs from canonical signaling in terms of the NF-κB dimers (p52-RelB), the IKK complex (which consists of a dimer of IKKα), the kinase activity (NIK), and the membrane receptors depicted in this cartoon. Additionally, while not included in the figure, evidence exists for crosstalk between both pathways. This figure was created using BioRender.com.
There is still a significant gap in understanding how T cells integrate canonical and non-canonical signals from different receptors at the membrane level. Additionally, the ways T cells respond to varying NF-κB signaling levels according to tissue context and how these variations influence T cell outcomes remain unclear. In the following sections, we will discuss how NF-κB signaling regulates T cell development and its resulting effects.
Mechanisms by which NF-κB signaling regulates thymocyte development
In the thymus, immature T cells, or thymocytes, commit to the T cell lineage and develop tolerance to self-antigens during thymic development. T cell progenitors differentiate into CD4 and CD8 double negative thymocytes (43). They rearrange the T cell receptor (TCR) beta chain and express a pre-TCR. During this phase, NF-κB signaling regulates pre-TCR signaling, providing survival signals for thymocytes with productive TCR beta chain rearrangements (44). This is followed by the rearrangement of the TCR alpha chain and the expression of CD4 and CD8 coreceptors, resulting in the development of double-positive (DP) thymocytes (43).
DP thymocytes, in turn, recognize self-antigens and MHC molecules (class I or class II) on the surface of cortical thymic epithelial cells via their TCRs and undergo thymic selection. The affinity of this interaction determines thymocyte fate. DP thymocytes whose TCRs bind to pMHC with insufficient affinity or excessively high affinity undergo death by neglect or are negative selected respectively. Only those with intermediate affinity to self-peptide-MHC (pMHC) interactions are positively selected and further differentiate into single-positive (SP) CD4+ or CD8+ thymocytes (43). The role of NF-κB signaling in thymic development has been investigated using transgenic mice expressing either the super-repressor form of IκBα (Nfkbia-SR) or the constitutively active form of IKKβ (Ikbkb-CA) (45). Data from these studies indicate that increased NF-κB signaling in double-positive (DP) thymocytes recognizing MHC class I, skews them towards the negative selecting threshold, resulting in their death. Conversely, decreased NF-κB signaling prevents DP thymocytes from reaching the positive selecting threshold, leading them to succumb to death by neglect. Interestingly, these studies also reported that DP thymocytes recognizing MHC class II, do not signal through NF-κB and thus, inhibition of NF-κB signaling in these cells do not affect thymic selection (45). Altogether, these studies demonstrate that NF-κB signaling is crucial for establishing the signaling thresholds that determine positive and negative selection, particularly for the selection of CD8 SP thymocytes (45).
Other studies that used transgenic mice expressing mutant IkBα or a conditional deletion of IKKβ or NEMO/IKKγ have also showed that reduced NF-κB signaling affects CD8 SP T cells beyond thymic selection (46–48). Specifically, inhibition of NF-κB leads to a significant decrease in CD8 SP T cells in the thymus. However, it remains unclear whether this defect is due to induction of cell death or a defect in cell survival. Supporting the latter, active NF-kB levels are much higher in CD8 than in CD4 SP thymocytes, while the pro-survival factor Bcl2 is expressed at lower levels in CD8 SP cells (45). These data suggest that CD8 SP survival may require different NF-kB signaling levels than CD4 SP cells, making CD8 SP cells more susceptible to the effects of NF-κB inhibition.
NF-kB signaling intermediates upstream of the IKK complex are also important in thymic development. The kinase TAK1 (Figure 2) specifically regulates the maturation and survival of single-positive (SP) thymocytes, rather than their selection (49). Hogquist’s group demonstrated that TNF-dependent TAK1→ NF-kB signaling is essential for cell survival at the SP stage and late thymic maturation (50). Their findings indicate that TCR-dependent signaling is not involved in this process. This agrees with other studies indicating that TCR-proximal NF-kB intermediates (CARMA1, BCL10, and MALT1) do not affect thymic development (51–53). Notably, TAK1 can also function independently of NF-kB by mediating Type I IFN signals, enhancing thymocyte responsiveness to inflammatory cytokines (50). Additionally, recent work by Seddon’s group has shown that IKK-mediated repression of RIPK1 is a crucial link between TNF receptor signaling and TAK1. This link is necessary to support post-selection SP survival and maturation (54). Thus, environmental factors beyond just antigens tightly control CD8 SP survival/late maturation through mechanisms involving specific NF-kB signaling intermediates, making T cells more responsive to inflammatory signals.
Noteworthy, downstream of the IKK complex in the NF-kB signaling cascade, recent studies using CD4cre-inducible models of RelA and c-Rel deletion have shown that these NF-kB subunits are not necessary for thymic development (55). Hence, while the role of the NF-κB signaling cascade in thymic selection and SP maturation is evident, data suggests that it does not follow conventional transcriptional NF-κB mechanisms. Moreover, depending on the stage of development (thymic selection versus SP maturation) unique NF-κB signaling intermediates play predominant roles.
NF-κB signaling and T cell homeostasis
Single positive T cells migrate to the periphery, where they can persist for months as naive T cells. The homeostasis of these cells relies on their survival and low turnover properties (56). The role of NF-κB signaling in the survival and homeostasis of peripheral T cells has been elucidated through mouse models expressing T cell-restricted conditional transgenes. These models include those with inactive kinase forms (achieved by replacing the serines in the kinase activation loop with alanines to eliminate inducible IKK activity) (57), and those expressing constitutively active forms of IKKβ (where the activation loop serine residues (Ser 177 and 181) are substituted with glutamic acid) (58). Additionally, some models have employed degradation-resistant or truncated forms of IkBα (59–61). Inhibition of canonical NF-κB signaling in any of these models leads to significant reductions in peripheral T cell populations, including both naive and memory T cells (48). However, it has remained unclear whether the extent of this decrease is influenced by defects in thymic selection, inefficient transgene expression, or specific targeting of IKKα, IKKβ, or IkBα (62). Recent investigations have revisited the role of IKKβ, and NF-κB signaling in T cell homeostasis using tamoxifen-inducible and temporally controlled deletion of IKKβ (63). These studies indicated that IKKβ expression is essential for the upregulation of IL-7R on selected T cells as they exit the thymus (63). This report showed that the induced expression of IL-7R via TNF and CD70/CD27-dependent NF-κB signaling is transient but, at the same time, crucial for recent thymic emigrants to fully mature into functional peripheral T cells (63). Interestingly, in contrast to previous reports, these studies found that IKKβ expression did not regulate IL-7R expression or the survival of peripheral naive T cells in immunocompetent mice (63). This suggests that IKKβ regulates recent thymic emigrants transition into naive T cells in an antigen receptor-independent manner, consistent with data from mice deficient in proximal components of TCR-dependent NF-κB induction (48, 64).
Further studies have explored the roles of NF-κB subunits in the survival of mature T cells. Research using p50-c-Rel double-deficient mice found no defects in thymic development, T cell maturation, or naive T cell homeostasis (65). This prompted further investigations into the outcomes observed in c-Rel, p65/RelA and RelB knockout mouse models, where multiorgan inflammation occurs, making it unclear whether defects arise from the stroma or the T cells themselves (66–68). More recent studies using CD4 cre-inducible models for RelA and c-Rel deletion have supported earlier findings seen with IKKβ and IkBα transgenic models (48, 59). These studies revealed a significant reduction in the populations of peripheral naive CD4 and CD8 T cells in the absence of RelA, while c-Rel deficiency did not have the same effect (69). Furthermore, RelA deficiency was linked to lower levels of Ki67-positive proliferating cells and reduced production of key cytokines, such as IFN-γ and IL-2, upon PMA/Ionomycin stimulation (69). This regulation often works in conjunction with AP-1 family members, like Jun and BATF, affecting crucial T cell proteins such as CD83, ICOS, and 4-1BB (69). Overall, these findings highlight the critical role of RelA in T cell homeostasis, as it regulates many genes essential for T cell activation through transcriptional control (Figure 3B) (69).
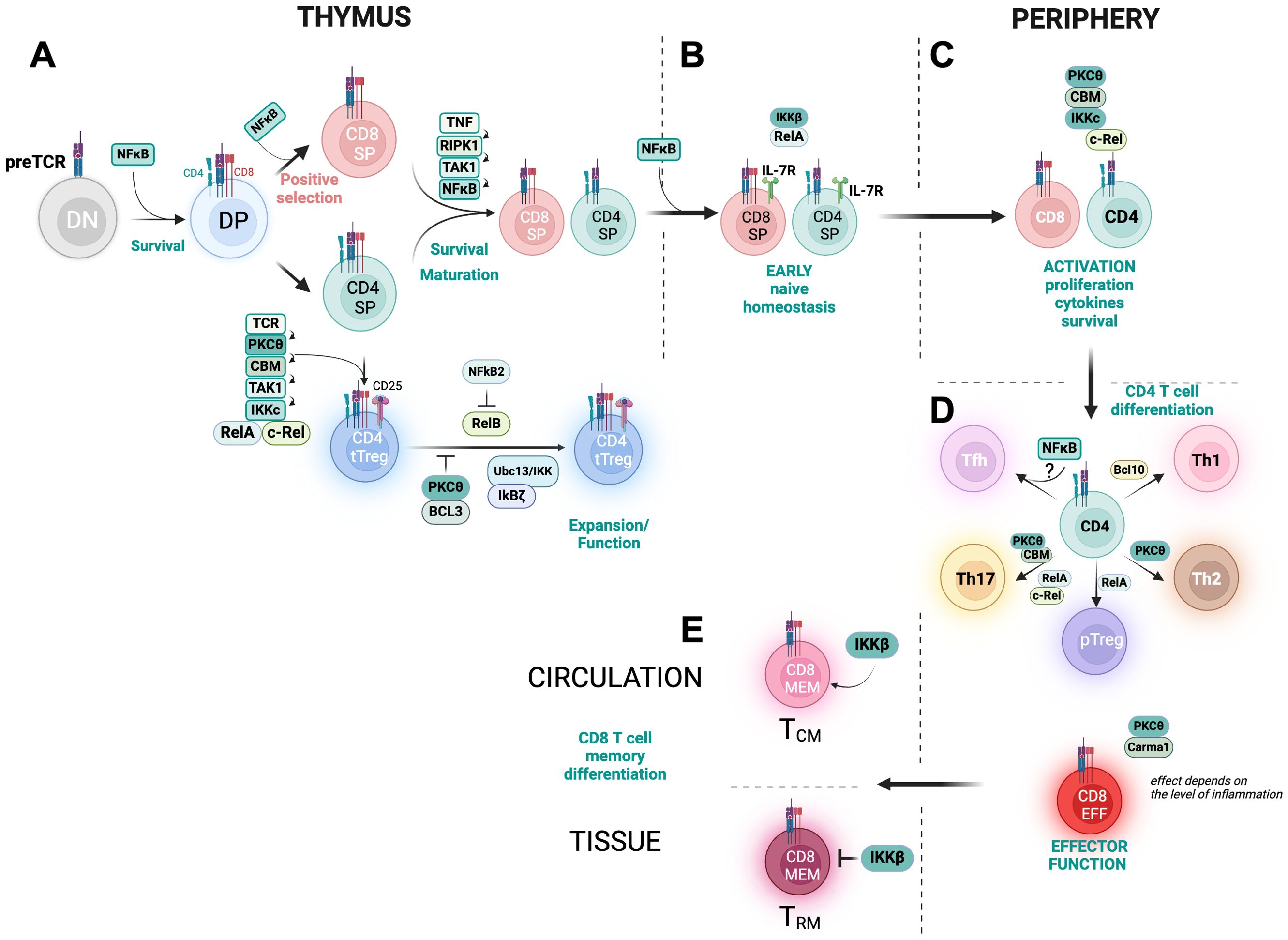
Figure 3. Roles of NF-κB signaling intermediates in T cell differentiation. This cartoon shows the phases of CD4 and CD8 T cell differentiation in the thymus (A, B) and the periphery (C-E) highlighting the roles of different components of the NF-κB signaling network. DN, double negative thymocyte. DP, double positive thymocyte. SP, single positive thymocyte. tTreg refers to thymic T reg while pTreg refers to peripheral Treg. Tfh is follicular helper CD4 T cell, Th is a CD4 T helper cell. CD8EFF is an effector CD8 T cell while CD8MEM is a memory CD8 T cell. TCM is central memory T cell. TRM is tissue resident memory T cell. This figure has been created with BioRender.com.
NF-κB signaling and mature T cell responses: cell division, apoptosis and effector function
Naive CD4 and CD8 T cells recognize cognate antigens and receive costimulatory signals from dendritic cells (DCs) in draining lymph nodes, leading to their activation (70). This activation triggers the early upregulation of CD69, a crucial factor for retaining naive T cells in the lymph node to facilitate continued activation (70). Subsequently, T cells increase the expression of the high-affinity IL-2 receptor alpha chain (CD25) and the pro-survival cytokine IL-2 (70). These processes occur within the first 24 hours post-activation, supporting T cell division and survival, and are influenced by NF-κB signaling (70, 71). Indeed, naive T cells deficient in the NF-κB subunits RelA and c-Rel exhibit impaired proliferation and cytokine production after stimulation (72, 73), (Figure 2). Additionally, defects in upstream signaling components, such as IKKα, IKKβ, and CARMA1 lead to inadequate T cell proliferation (74, 75) and increased apoptosis upon activation (76, 77). The deletion of other members of the CBM complex, including BCL10 and MALT1, as well as PKCθ, similarly hampers T cell proliferation, particularly in CD4 T cells, These CD4 T cell proliferation defects cannot be rescued by exogenous IL-2 (although it enhances their survival). By contrast, in CD8 T cells, deficiency of these NF-κB early signaling intermediates raises the threshold of TCR signaling required for proliferation, resulting in impaired CD8 T cell proliferation even with strong agonistic TCR stimulation (78).
Regarding apoptosis, BCL10 does not have a significant role, whereas PKCθ and CARMA1 are more prominently involved (Figure 2). Specifically, PKCθ is essential for the expression of Fas ligand (FasL), which mediates activation-induced cell death (AICD) (79). Conversely, the role of MALT1 is more complex. It interacts with Caspase-8 during TCR engagement, limiting its autoproteolytic processing and apoptotic activity without affecting c-Flip processing. This suggests that MALT1 contributes to both T cell proliferation and survival through NF-κB signaling (80).
Costimulation, particularly via CD28, is crucial for T cell-dependent NF-κB activation, which occurs through the induced membrane recruitment and enzymatic activity of PKCθ and PDK1 (81) (Figure 2). Depletion of PDK1 disrupts the synergy between TCR and CD28 signals, leading to impaired NF-κB induction. Specifically, the absence of PDK1 hinders PKCθ activation and prevents the formation of the CBM complex (81). However, other functions of PKCθ, such as activating the p38 MAPK, JNK, and Calcium/NFAT pathways, remain unaffected (81). Consequently, while certain aspects of T cell activation—including proliferation, CD25 expression, and IL-2 production—rely on PDK1, neither apoptosis nor survival of CD4 or CD8 T cells is significantly compromised in its absence (81).
Additionally, the MAPK GLK is recruited to the TCR/LAT/SLP76 signalosome, mediating the phosphorylation and activation of PKCθ (S5388), which is necessary for NF-κB induction (82), (Figure 2). Although GLK-deficient T cells demonstrate impaired proliferation and cytokine production, their involvement in survival or apoptosis has not been assessed (82). Furthermore, while GLK does not alter ERK or Calcium/NFAT signaling, the specific role of GLK in JNK and p38 MAPK signaling remains unexamined. Thus, similar to PDK1, GLK may not be critical for T cell survival if these pathways remain active (82) (Figure 2).
Altogether, this body of work supports the notion that TCR and CD28-dependent NF-κB signaling intermediates play a vital role in regulating early T cell activation and responses related to proliferation and cytokine-driven survival (Figure 3C).
NF-κB signaling, T cell effector differentiation and function
CD4 T cell differentiation
As T cells proliferate, they undergo a differentiation process that transforms them into specialized effector T cells and long-lived memory T cells. This differentiation is crucial for establishing long-term protective immunity against pathogens and tumors (83). Naive CD4 T cells initially differentiate into effector precursors in response to antigen recognition, costimulation, and specific cytokine signals. These three inputs drive the expression of unique transcription factors that act as master regulators of T cell fate decisions and guide helper T cell (Th) subset lineage commitment (84). CD4 T cell differentiation is intricately adapted to the type of pathogen and the innate signals it triggers. For example, intracellular pathogens initiate Type I immune responses characterized by the generation of Th1 CD4 effector T cells, which secrete hallmark cytokines IFN-γ, IL-2, and TNF. Naive T cells differentiate into Th1 effectors following initial stimulation through their TCR, CD28, and IL-12 signals (84–89). While TCR and CD28 signaling activate the transcription factors NF-κB, NFAT, and AP-1, IL-12 signaling activates STAT4 (84–89). These transcription factors cooperate to upregulate the expression of T-bet, the master transcription factor of the Th1 subset, which is critical for the expression of IFN-γ and other transcription factors, including Hlx and Runx3 (84–89). Strong TCR signaling also promotes the expression of Blimp-1, IRF4, and the IL-2-dependent transcription factor STAT5, facilitating the re-expression of IL-12R and inducing the chemokine receptor CXCR3. This results in the migration of primed Th1 cells to tissues, where they further differentiate into committed Th1 effectors (84–89). Concurrently, transcriptional networks that promote alternative Th cell fates (e.g., GATA3, RORγt, or Bcl6) are repressed (84–89). In contrast to this, weak TCR signaling drives the expression of the master T follicular helper (Tfh) regulator Bcl6, results in low levels of IL-2R/STAT5 signaling and supports the expression of CXCR5 (84–89). This directs primed CD4 T cells toward the T-B border of the lymph node, where they interact with B cells and specific DCs to receive ICOS/ICOSL signals (84–89). In conjunction with IL-6, IL-21, and IFNγ signals, these interactions enhance Bcl6 levels while inhibiting the expression of master transcription factors associated with other Th subsets (84–89). Ultimately, this results in the generation of differentiated PD-1hi germinal center Tfh cells that provide critical assistance to B cells for isotype switching and affinity maturation (84–89). In contrast to Type I immune responses, Type II responses are elicited in response to extracellular parasites and allergens, driving the generation of Th2 cells through T cell receptor (TCR) engagement, costimulatory signals, and exposure to IL-4 (84–89). IL-4 is essential for the expression of GATA3, the master transcription factor that plays a critical role in the secretion of Th2 signature cytokines, including IL-4, IL-5, and IL-13. Extracellular bacteria and fungi, however, elicit Type III immune responses characterized by the differentiation of Th17 cells (84–89). This process is mediated by antigen recognition, costimulatory signals, and exposure to cytokines such as TGF-β, IL-6, IL-21, and IL-1β. These signals drive the expression of the Th17 master transcription factor RORγt and STAT3, regulating the production of IL-17 and IL-22 (84–89).
Numerous studies conducted in the late 1990s and early 2000s established that both canonical and non-canonical NF-κB signaling pathways were crucial for Th1 and Th2 differentiation, albeit acting at different stages. T cells deficient in IκBα degradation, as well as those lacking c-Rel or RelA expression, display impaired Th1 responses, independent of T-bet expression. Instead, both NF-κB subunits directly regulate IFNγ expression, potentially in cooperation with STAT4. Notably, p50 deficiency did not influence T-bet or IFN-γ expression in CD4 T cells, suggesting that RelA may regulate IFNγ through homo- or heterodimers with other NF-κB subunits (90). RelB, however, is required for T-bet expression, likely through STAT4 induction (91). Other studies have shown that NF-κB signaling was also instrumental in Th2 differentiation (92). Specifically, p50/Rel is necessary for GATA3 induction mediated by IL-4, while RelA/p65 and c-Rel worked in conjunction with NFAT to support IL-4 induction in a TCR-dependent manner (90, 92–95). The roles of NF-κB subunits in Th17 differentiation have been corroborated by various studies, demonstrating the importance of both RelA and c-Rel in IL-17 expression, as well as for c-Rel’s transcriptional activity in promoting RORγt expression (96). Additionally, the atypical IκB protein IκBζ (97) (Figure 1B) is involved in Th17 differentiation. It cooperates with RORγt and RORα to drive IL17A expression, and its deficiency causes a defect in Th17 differentiation and a resistance to EAE (97).
Together these foundational studies established a link between NF-κB and CD4 T cell differentiation. However, they also had technical limitations, such as reliance on germline or conditional gene deletion and in vitro conditions. These factors made it difficult to gain a clear understanding of the timing and specific contributions of each subunit during the differentiation of T helper subsets. To address this, the roles of c-Rel and RelA in CD4 T cell differentiation have recently been revisited using novel tamoxifen-inducible mouse models that specifically delete c-Rel or RelA expression in naive CD4 T cells (69). These studies have demonstrated that c-Rel is more critical than RelA in regulating CD4 T cell proliferation (particularly under conditions of supra-optimal TCR and costimulatory signaling in the absence of IL-2) as well as in the expression of IL-2 and TNF in both human and murine cells (69). Additionally, unlike findings from earlier studies, this recent report shows that neither c-Rel nor RelA are essential for the expression of T-bet, GATA3, IFNγ, or IL-13, and thereby not critical for Th1 and Th2 cell differentiation. By contrast, both NF-κB subunits are essential for the expression of the Th17 master transcription factor RORγt, with RelA also playing a role in regulating IL-17 expression (Figure 3D). Surprisingly, CD4-restricted ablation of RelA (but not c-Rel) provides protection against experimental autoimmune encephalomyelitis (EAE),a model of autoimmune disease that heavily relies on the presence and function of pathogenic Th17 cells (69).
Although these elegant NF-κB -inducible models have not yet been employed to study T follicular helper (Tfh) cell differentiation, recent reports suggest a potential role for NF-κB signaling in this process. For instance, patients with a truncated mutation of NFKB2 exhibit a reduced presence of Tfh and T follicular regulatory T (Tfr) cells, along with impaired germinal center (GC) potential. It remains unclear whether this loss is independent of associated B cell defects caused by the truncated mutation (98). By contrast, non-canonical NF-κB signaling plays an important role in the expression of proteins that facilitate Tfh differentiation, such as ICOS ligand (ICOSL) in BAFF-stimulated B cells (99). Similarly, CD4 T cells deficient in p50 demonstrate defects in CXCR5 expression, which is critical for Tfh cell localization within the germinal center (100). Despite these findings, it is still unknown whether other canonical NF-κB subunits are essential for Tfh development, or whether NF-κB plays a role earlier in Tfh priming and/or later during the differentiation process in a T cell-intrinsic manner. Moreover, significant knowledge gaps persist regarding the impact of T cell-intrinsic NF-κB signaling on affinity maturation and B cell fate or function. Addressing these questions will enhance our understanding of Tfh biology and its implications for immune responses.
While the referenced studies have established a direct correlation between NF-κB epigenetic and transcriptional activity and CD4 T cell effector function and differentiation, the role of upstream intermediates in the NF-κB signaling cascade should not be overlooked. Caution is warranted, however, as upstream components of the NF-κB cascade often engage in significant crosstalk with other signaling pathways (Figure 2). Investigations using CARMA1-deficient T cells have confirmed that TCR-proximal NF-κB signaling is crucial for the Th17 phenotype and function (IL-17, IL-22, IL-21, IL23R, CCR6 expression) but not for Th1 or Th2 differentiation. Interestingly, mice with CARMA1-deficient CD4 T cells exhibit resistance to experimental autoimmune encephalomyelitis (EAE), yet their T cells do not show impaired expression of RORγt, Ahr, IRF4, or STAT3/STAT5 signaling (77). This finding suggests that CARMA1-dependent NF-κB signaling is required following the initial priming and polarization of Th17 cells and is essential for completing Th17 differentiation.
Other studies have also shown that a deficiency in the formation of the CBM complex lead to defects in the induction of Th1 and Th17 subsets. Mechanistically, this did not seem related to IKK and NF-κB but rather to the ability of the CBM complex to facilitate efficient glutamine uptake through ASCT2 and activation of mTORC1 upon TCR/CD28 stimulation (101). On the other hand, T cells expressing constitutively active MALT1 show impaired NF-κB signaling but exhibit continuous degradation of the downstream target Roquin-1, leading to unregulated differentiation of naive T cells into Th1 and Th17 effector cells. Notably, MALT1-mediated degradation of Roquin-1 and regnase-1 appears to regulate Th17 differentiation in a manner dependent on TCR signal strength (102, 103). Roquin-1 naturally represses key proteins involved in CD4 Th17 differentiation (such as IκBNS, ICOS, IRF4, and Regnase-1), but its cleavage by MALT1 is necessary for derepression of IκBNS, which acts on nuclear NF-κB complexes. This cleavage occurs only under strong TCR signaling conditions (102) and similarly applies to Regnase-1 (103). Consistent with these findings, Roquin-deficient T cells are impaired in Th17 differentiation (103), as are mice deficient in the atypical IκB protein IκBNS (104).
As previously mentioned, PKCθ is the most proximal TCR NF-κB signaling intermediate, phosphorylating CARMA1 to facilitate the formation of the CBM complex. Yet, in stark contrast to other downstream NF-κB signaling intermediates, PKCθ deficiency does not affect Th1 differentiation but is linked to defects in Th2 differentiation (105, 106). Some reports also indicate a connection between PKCθ and Th17 differentiation, potentially via STAT3 or by overriding Foxp3 suppression through SRC1 phosphorylation (107, 108). Interestingly, PKCθ-deficient T cells show specific impairment in TCR-dependent NF-κB signaling while retaining functional TNF and IL-1-driven NF-κB signaling (109). This suggests that PKCθ impacts Th differentiation at priming (when antigen is present) either independently of NF-κB or by altering the TCR signaling thresholds required for differentiating into specific Th subsets.
Furthermore, recent studies have explored the role of non-canonical NF-κB signaling in Th differentiation and illuminated the potential roles of upstream NF-κB signaling intermediates that are independent of the NF-κB transcription factors. For example, studies using IkkaAA knock-in mice deficient in IKKα activation found that nuclear IKKα selectively associates with the Il17a locus, promoting histone H3 phosphorylation and transcriptional activation in a NF-κB –independent manner (110). Additionally, mice deficient in NIK or Rag deficient mice reconstituted with NIK deficient CD4 T cells show impaired Th17 differentiation (but not differentiation to other Th subsets) and are resistant to EAE (111). This is in part due to their regulation of RORγt and IL-23R via the cooperation of IL-6R and TCR signals (111). A recent report also indicated that inhibition of IAPs (which leads to NIK stabilization and subsequent induction of p52-RelB) impairs Th17 differentiation and favors Th2 differentiation instead (112). These findings are consistent with a previous study reporting that p100-deficient T cells fail to differentiate into Th17 cells (113). Thus, both canonical and non-canonical NF-κB signaling can regulate Th differentiation. Although it is unclear whether this is related to a crosstalk between both pathways, inhibition of IAPs appear to shift canonical toward non-canonical signaling in CD4 T cell differentiation. This suggests that while non canonical NF-κB signaling is important for the initiation of Th cell differentiation for certain subsets (such as Th17), a shift toward non-canonical signaling may divert CD4 T cells away from Th17 differentiation and inflammatory IL17 or GM-CSF (114) production and toward the generation of more regulatory Th2 and Treg subsets (112).
Regarding Tfh differentiation, there are currently no established links between T cell-intrinsic non-canonical NF-κB signaling intermediates and Tfh development. It is important to note that many studies discussed above did not utilize inducible systems to control non-canonical signaling temporally or to restrict its activation exclusively to CD4 T cells. Given that non-canonical NF-κB signaling regulates lymphoid organogenesis, these studies could not rigorously exclude other non-CD4 T cell-intrinsic defects. This complicates the interpretation of the results. Additionally, in vitro and in vivo findings due to altering non canonical NF-κB signaling in T cells do not often align. This highlights the potential relevance of TNFR members (Figure 2) that are not included in in vitro studies.
In summary, future studies should adopt more rigorous approaches to clarify the T cell-intrinsic interplay of canonical and non-canonical NF-κB signaling and its relationship with TNFR costimulatory receptors in the context of disease.
CD8 T cell differentiation
PKCθ is required for CD8 effector responses in vivo, particularly in the context of weak innate signals (115) (Figure 2). By contrast, CARMA1 deficiency has a more profound impact on CD4 T cells than on CD8 T cells. Supporting this notion, TCR mutant T cells that are impaired in CBM complex formation can still undergo normal CD8 effector differentiation during Listeria monocytogenes infection (64). However, in the context of cancer, one study has indicated that CARMA1 is critical for controlling transplantable tumors (116); although, it remains unclear whether this effect is solely due to the deficiency of CARMA1 in CD8 T cell function or is also influenced by the lack of CARMA1-deficient CD4 T cell help to CD8 T cells, as suggested by a recent investigation (69). Given the limited studies using CD8-restricted conditional or inducible BCL10, MALT1, or any of the NF-κB subunit mouse models, the specific roles and temporal regulation of CD8 effector differentiation remain unresolved in the field (Figure 3D).
γδ T cell differentiation
γδ T cells can differentiate into Th1- or Th17-like γδ T effector cells, with γδ T17 cells playing a crucial role in antibacterial immunity (117–119). Unlike induced NKT cells, NF-κB signaling is dispensable for the generation of γδ T cells; however, it is necessary for IL-17 production by γδ T17 cells in response to LTβR engagement. RelA regulates the expression of LT ligands in accessory thymocytes, while RelB, acting downstream of LTβR, is required for the expression of the transcription factors RORγt and RORα, facilitating the differentiation of thymic precursors into γδ T17 cells (120).
NF-κB signaling, thymic and peripheral Tregs
A substantial body of literature has established the critical role of the NF-κB signaling cascade in the generation and function of regulatory T cells (Tregs). Tregs can be categorized into two types: thymic Tregs (tTregs), which arise during thymic selection (43, 121), and peripheral Tregs (pTregs), generated from conventional CD4 T cells that attain stable Foxp3 expression in tissues such as the gut mucosa. Additionally, a third type known as in vitro-induced Tregs (iTregs) can be generated by differentiating conventional CD4 T cells under specific conditions. Regulatory CD4 T cells are phenotypically characterized by high expression of the IL-2 receptor alpha chain (CD25) and the master transcription factor Foxp3. Foxp3 directly and indirectly regulates a multitude of suppressive mechanisms that control inflammation and autoreactivity. Among these mechanisms are the expression of IL-10, Granzyme B, and CTLA-4, which hampers dendritic cell (DC) activation. Expression of CD25, instead, acts as a sink for IL-2, limiting the expansion and activation of CD8 T cells, natural killer (NK) cells, and innate lymphoid cells (ILCs). Remarkably, Tregs have also recently been recognized for their roles in tissue repair and wound healing, employing mechanisms that are distinct from immunosuppression [recently reviewed in (122, 123)].
The specific role of NF-κB in the generation of thymic Tregs is well established. It predominantly relies on TCR-mediated NF-κB signaling. Tregs that develop in the thymus are selected based on the strength and affinity of their TCR interactions with self-peptide-MHC complexes. Moderately strong interactions guide immature CD4 T cells toward becoming tTregs, endowing them with suppressive competence (43, 121). Interestingly, the selection processes for tTregs and naive T cells do not equally dependent on NF-κB signaling. Deficiencies in various intermediates of TCR-dependent NF-κB signaling (such as PKCθ, CARMA1, BCL10, TAK1, and IKKβ) significantly reduce the number of tTregs without compromising the generation of conventional CD4 or CD8 T cells. The observation that thymic Treg development is restored in TAK1 and Carma-1 deficient mice crossed with transgenic mice expressing a constitutively active form of IKKβ (IKKEE-Tg) further reinforces the idea that NF-κB signaling is sufficient to support Treg development (48, 124–128). However, it is less clear whether other stimuli (aside from antigens) driving NF-κB signaling also support Treg development in the thymus. A study by Mahmud et al. demonstrated that inhibition of TNFRSF members GITR, OX40 and TNFR2 signaling abrogates tTreg development in a TAK1-dependent manner (129). This study also indicated that TCR signaling regulates the levels of these TNFRSF members, allowing thymocytes to respond more efficiently to GITRL, OX40L and TNF signal and thereby facilitating their differentiation into tTregs (129). Importantly, this study did not clarify whether the role of these TNFRSF members in tTreg development is dependent on NF-κB signaling (129). Therefore, it is possible that TAK1 regulates tTreg development in an NF-κB -independent manner, similar to the mechanisms observed in SP thymocytes (50).
Recent reviews underscore the critical roles of both c-Rel and RelA in the development of Tregs within the thymus and their stability in the periphery. RelA deficiency leads to a complete blockade in the development of RORγt+ Treg cells (130, 131). However, studies involving conditional deletion of c-Rel or RelA specifically within developing and mature Tregs have revealed distinct roles in Treg biology. These studies demonstrate that c-Rel is critical for thymic Treg development and the expression of GITR and CD25. In turn, p65/RelA is essential for the differentiation of tTregs and also for peripheral Treg differentiation and maintenance of immune tolerance (131). Mechanistically, the NF-κB subunit c-Rel binds to the TCR-responsive conserved non-coding enhancer sequence 3 (CNS3) in precursor Tregs, significantly contributing to the expression of Foxp3 (128, 132)(Figure 3A). Additional studies indicate that p65/RelA enhances Foxp3 functions through the kinases Stk3 and Stk4. The group of Hongbo Chi found that both kinases are important for Foxp3-mediated peripheral tolerance by regulating the levels and function of peripheral (but not thymic) Tregs (133). These kinases modulate IL-2/STAT5 signaling, which is crucial for CD25 and Foxp3 expression, Treg lineage survival, stability, and function (133). Furthermore, Chatila’s group showed that Stk3 and Stk4 are integral components of a TCR/NF-κB signaling network, wherein TCR signaling leads to the nuclear translocation of Stk4. Stk4 forms a complex with p65/RelA and Foxp3, facilitating the control of the Treg transcriptional program through the phosphorylation of Foxp3 at Ser418 (134). Thus, the canonical NF-κB pathway emerges as a master regulator of Treg development and function.
The non-canonical NF-κB pathway has also been linked to Tregs, albeit in an unexpected manner. Conditional deletion of NFKB2 and/or RelB (in both total T cells and Tregs) has revealed a critical role for NFKB2 in maintaining Treg homeostasis (135). Specifically, NFKB2 limits the formation of RelB complexes that regulate cell-autonomous Treg expansion in the periphery. This uncontrolled Treg expansion is restored upon deletion of RelB (135). In contrast to this, other studies have shown that forced activation of the alternative pathway (through deletion of TRAF3 (Traf3−/− mice) or overexpression of NIK (NIK-transgenic [Tg] mice)) results in an increase in Treg numbers (136, 137). These findings suggest that the primary function of NFKB2 is to prevent aberrant RelB activation, with little or no role in regulation of Tregs under steady-state conditions. Given that NIK and IKKα can also activate the canonical pathway (Figure 2), it is possible that the effects of upstream intermediates in alternative NF-κB signaling on Tregs primarily operate through the canonical pathway (involving p65/c-Rel rather than through the non-canonical NF-κB subunits). Furthermore, the specific roles of the alternative pathway in high-inflammatory contexts, such as chronic infections where TNF receptor superfamily members (e.g., OX40, GITR) are actively engaged, still require further elucidation (138, 139). For instance, unprocessed NFKB2 (p100) may be induced by acute TCR signaling and could function as an inhibitor of RelB, thereby limiting Treg expansion until inflammatory, TNF receptor-mediated signaling activates the processing of p100 into p52. This processing may enable the formation of RelB-containing complexes that promote Treg expansion, potentially affecting their functionality (135, 139).
NF-κB signaling is also essential for the suppressive function of Tregs. For example, conditional deficiency of PDK1 in CD4 T cells results in reduced Treg levels and impaired function (Figure 2). This leads to uncontrolled proliferation of TCRγδ cells and expression of IL-17, ultimately triggering colitis (140). It remains uncertain, however, whether PDK1’s regulation of Treg function depends exclusively on NF-κB signaling or involves other pathways, such as mTOR. Interestingly, the downstream target of PDK1, PKCθ, has been found to inhibit Treg function (141, 142). Research indicates that PKCθ (Figure 2) localizes differently within Tregs compared to conventional CD4 T cells at the immunological synapse, resulting in a bias toward Treg programming over alternative Th effector fates (141, 142). Consistent with this observation, inhibition of PKCθ enhances the suppressive capacity of Tregs, restores the impaired function of Tregs from rheumatoid arthritis patients, and blocks autoimmune responses in mouse models of colitis (143).
Furthermore, the Ubc13/IKK axis plays a positive role in Treg suppressive function as conditional ablation of Ubc13 in Foxp3+ Tregs leads to multi-organ inflammation due to uncontrolled activation of conventional T cells (Figure 2) (144). Additionally, atypical members of the IκB family, such as Bcl3, IκBζ, and IκBNS, also contribute to Treg biology by fine-tuning the transcriptional activity of NF-κB in the nucleus. Unlike classical IkBs, these atypical members are not degraded but are induced upon stimulation (29). They regulate dimer exchange, recruit histone-modifying enzymes, and stabilize DNA-bound NF-κB dimers (29). Specifically, deletion of Bcl3 in Tregs abrogates its interaction with p50, promotes the formation of RORγt+ Tregs, and enhances mice’s resistance to induced colitis (145–147). By contrast, deletion of IκBζ in Tregs results in impaired Treg function, potentially due to the inhibition of Foxp3 promoter activation (97, 148). Taking altogether, while the NF-κB signaling pathway is crucial for the generation and function of Tregs, not all pathway members share identical roles. This suggests that complex, non-redundant mechanisms are involved in fine-tuning this important T cell subset.
NF-κB signaling, T cell memory generation and maintenance
Memory T cells, long-lived plasma cells and memory B cells, are essential components of protective immunity. These memory lymphocytes have a unique ability to survive for extended periods within the body and, respond rapidly upon re-exposure to the same antigen that prompted their generation. This antigen can originate from various sources, including microbes (infections), self-tissues (autoimmunity or cancer), or foreign tissues (transplants). Consequently, memory T cells serve not only as powerful tools for the immune system to prevent disease but also as mediators of autoimmune pathology and transplant rejection. Memory T cells can recognize highly conserved antigens that are not accessible to antibodies. Interestingly, these antigens are often less prone to mutation when compared to epitopes recognized by antibodies or B cell receptors (BCRs). This allows memory T cells to effectively circumvent the immune escape of rapidly mutating pathogens (such as influenza or SARS-CoV-2 viruses). In the context of cancer, the generation of tumor antigen-specific memory CD8 T cells also holds significant promise for preventing metastasis and recurrence. Therefore, eliciting memory T cells is a desirable goal in vaccine development and cancer immune therapies. NF-κB signaling plays a crucial role in the development and maintenance of T cell memory. However, the specific mechanisms and NF-κB-driven signals that regulate these processes during immune responses are still not well understood.
The pool of memory T cells in an individual includes various subsets, such as central memory T cells (TCM), effector memory T cells (TEM), resident memory T cells (TRM), lymphoid tissue-inducible (LIP) memory cells, and virtual memory T cells (149). These subsets can be broadly classified based on their locations. Circulating memory T cells reside in secondary lymphoid organs (TCM) or move in and out of tissues via the bloodstream (TEM). In contrast, TRM cells stay localized within specific tissues and provide immediate protection against infections (150–153) while limiting viral transmission (154). The presence of TRM cells in tumors and draining lymph nodes is often associated with better cancer outcomes (155–157). Although circulating memory T cells are important for protective immunity and play essential roles in certain diseases (158), understanding the generation, function, and maintenance of both TRM and circulating memory T cells is crucial. Elucidating these processes will help develop strategies to enhance immune responses and improve therapeutic outcomes across various diseases.
Early studies using genetic models, alongside analyses of human patients with mutations suggested that NF-κB signaling played a role in establishing long-term protective T cell memory pools (159, 160), However, the lack of inducible T cell-restricted NF-κB models has made it difficult to determine whether the observed defects in memory T cell levels result from early issues with T cell priming or proliferation, or from later problems in differentiation and survival. Likewise, these studies did not identify the environmental cues needed for T cell memory or whether NF-κB signaling is more important for specific memory subsets. In one of our studies, we discovered that T cells with TCRs that poorly activate NF-κB signaling—due to defects in forming the CBM complex—are unable to differentiate into circulating memory CD8 T cells during a systemic bacterial infection, despite normal effector differentiation (64, 161). Further research revealed that NF-κB signaling plays an unexpected role during the late phase of the immune response, particularly during contraction as memory precursors develop into memory cells (162). We used various genetic tools to show that p65/RelA is crucial for generating central memory CD8 T cells (TCM) during this phase (64). NF-κB signaling works in a feed-forward loop with the transcription factor Eomes and the kinase Pim1 (162). Deleting TCR-dependent NF-κB signaling or overexpressing an inactive form of PKCθ led to reduced Pim1 expression, loss of Eomes, and lower TCM levels (162). Additionally, the loss of Pim1 activity resulted in decreased phosphorylated p65 NF-κB levels (162). Collectively, these findings indicate that during the early immune response, TCR signaling through NF-κB induces Pim1 expression. Once the antigen is cleared, Pim1 works with Eomes and NF-κB to sustain the necessary levels of NF-κB required for generation and maintenance of circulating TCM cells.
The role of non-canonical NF-κB signaling in T cell memory has been explored in two studies (163, 164). Rowe et al. utilized NIK-deficient CD4 T cells in the context of LCMV infection and were the first to demonstrate that NIK deficiency impairs both the accumulation of effector and memory CD4 T cells, although it does not affect their function (164). Notably, the memory defect was more severe than the effector defect. Since no difference in the generation of memory precursors was observed, it is possible that NIK play a role in the maturation or survival of CD4 memory cells. Similarly, Li et al. showed that NIK also regulates CD8 T cell memory (163). However, neither study addressed whether the observed memory defects were due to alterations in Treg development, lack of CD4 T cell help, impaired T cell expansion, or differential effects on circulating and resident memory T cell populations. Additionally, these studies did not assess whether the memory defects were NF-κB dependent or identify any TNFRSF members responsible for NIK activation. CD27, 4-1BB, and GITR are TNFR family members known to play important roles in T cell memory. 4-1BB and GITR signal through the canonical NF-κB pathway but they regulate tissue resident memory in an mTOR-dependent manner (165, 166). In contrast, CD27 can activate both the canonical and non-canonical NF-κB pathways (42, 167) and regulates Pim1 expression (168), which is critical for CD8 T cell memory survival via the TCR (162, 168) Thus, it is possible that TCR canonical signaling and CD27 non-canonical signaling converge at the level of Pim1 to regulate CD8 T cell memory.
Recently, we investigated how canonical NF-κB signaling contributes to the development and maintenance of different memory T cell subsets during influenza infection (169). We used two tetON IKKβ inducible mouse models that allow for the expression of both active and inactive forms of the IKKβ kinase (169). This approach enabled us to examine how NF-κB signaling influences CD8 T cells at various stages of the immune response to influenza. Our findings revealed distinct roles for IKKβ/NF-κB signaling in generating influenza-specific circulating memory T cells and resident memory T cells (TRM). Higher levels of IKKβ/NF-κB signaling after day 8 post-infection were associated with an increase in circulating memory CD8 T cells. However, this was accompanied by a decrease in TRM in the lung, which negatively impacted protective immunity against influenza. In contrast, inhibiting IKKβ/NF-κB signaling led to a reduction in circulating memory T cells while increasing TRM populations in the lung (169). We found that NF-κB signaling limits the transcriptional program of TRM cells without affecting their recruitment to the lung, likely due to inhibiting local TGF-β signaling (169). Additionally, TNF acts as a driver of NF-κB signaling during influenza infection and negatively influences TRM cell generation. Blocking TNF increased the number of influenza-specific CD8 TRM cells in the lung and improved TGF-β signaling along with the expression of Runx3 (169). It remains unclear whether high or chronic levels of TNF affect CD8 TRM generation in other contexts, as TNF levels often rise in certain infections and chronic inflammatory diseases. Other cytokines and TNF receptor superfamily members may also modulate NF-κB levels, influencing the size of circulating and resident memory CD8 T cell pools.
While previous studies employing single-cell RNA sequencing have indirectly suggested the importance of NF-κB for tissue-resident memory (151, 170–172), our research demonstrates that NF-κB signaling is crucial for memory diversity and T cell-mediated immunity (169), (Figure 3E). However, the exact mechanisms by which the transcription factor NF-κB differentially regulates circulating and TRM cells remain to be determined, especially regarding the involvement of NF-κB subunits.
Establishing an efficient CD8 T cell memory pool depends on two key factors: effective differentiation and persistent survival. We have discovered that NF-κB signaling also plays a crucial role in maintaining CD8 T cell memory and positively influences both circulating and tissue-resident memory (TRM) cells. When NF-κB signaling is inhibited in CD8 memory T cells, both influenza-specific circulating and lung-resident memory T cells diminish. This loss is likely due to decreased levels of survival factors like Bcl-2 and IL-15R (CD122) (169). On the other hand, increasing IKKβ/NF-κB signaling in memory T cells increases the levels of Bcl-2 and IL-15R, which enhances both TRM and central memory (TCM) populations (169). These findings, altogether, indicate that canonical NF-κB signaling regulates the generation and maintenance of memory CD8 T cells in distinct ways; although, the specific mechanisms still need further investigation.
In summary, NF-κB signaling is critical for forming and maintaining memory T cells. Its role is complex and varies depending on the level of NF-κB activation and the stage of T cell differentiation. The studies we discussed provide insights into how tuning NF-κB signaling can aid to independently regulate the levels of circulating and resident memory CD8 T cells. This approach could be particularly useful in certain diseases, allowing to selectively deplete TRM while preserving circulating memory cells. Such a strategy may help reduce pathogenicity without sacrificing protective immunity.
NF-κB signaling and T cell exhaustion or dysfunction
T cell exhaustion or dysfunction refers to effector T cells that are persistently stimulated by antigens and costimulatory signals, typically in chronic infections, cancer, or autoimmunity (173). Exhausted T cells gradually lose their effector functions, such as cytokine secretion and the ability to kill target cells. This decline is caused by an accumulation of inhibitory signals, marked by high levels of inhibitory costimulatory molecules like PD-1, CTLA-4, Tim-3, LAG-3, TIGIT, and 2B4 (173). Immune checkpoint blockade (ICB) is a therapeutic strategy that uses antibodies to inhibit PD-1 and CTLA-4 signaling. This approach reduces inhibitory signals and helps rejuvenate exhausted T cells, restoring their effector functions (174). However, ICB is not equally effective for all patients. It works best in T cells that have not reached terminal exhaustion, particularly in stem-like or Ly108+ progenitor exhausted T cells located in draining lymph nodes, and CX3CR1 effectors migrating to tumors (175). Recent studies that employed single-cell RNA sequencing have shown that the NF-κB family (including REL, RELB, NFKB1, and NFKB2) is more active in Ly108+ T progenitors of exhausted T cells (176, 177). This suggests that NF-κB signaling is crucial for the development and maintenance of these cells (177). Additionally, different SWI/SNF complexes epigenetically regulate the various stages of T cell exhaustion. The BAF complex promotes the differentiation of exhausted T cell progenitors into an effector-like subset, while the PBAF complex prevents terminal exhaustion, helping to maintain T cell progenitors. Although the exact mechanisms behind the balance between BAF and PBAF are not completely clear, it is likely that NF-κB signaling connects these processes with T progenitors (178, 179). Further research is essential to explore these possibilities and elucidate the complex dynamics of NF-κB signaling in T cell exhaustion.
The potential for NF-κB signaling to regulate T cell exhaustion is significant, but no study has definitively established its role. It’s still unclear which specific components of the NF-κB signaling pathway are critical and how they might function during the differentiation of exhausted T cells. Recent research suggests that 4-1BB-dependent NF-κB signaling can help T progenitors differentiate into effector-like cells and promote their proliferation through RelA and c-Rel signaling. Notably, using 4-1BB therapy alongside anti-PD-1 treatment (but not before treatment or in established tumors) led to reduced tumor burden and longer survival (180). If future studies confirm that NF-κB signaling is essential for maintaining tumor-specific T cells at low exhausted stages (i.e. progenitors of exhausted T cells) this could open new avenues for treatment strategies.
NF-κB signaling, transcriptional, epigenetic and metabolic regulation
NF-κB signaling plays a crucial role in T cell responses at the transcriptional, epigenetic, and metabolic levels (15, 181–183). T cell differentiation involves changes in chromatin structure, which affect gene accessibility. Each transition between naive, effector, and memory T cells includes chromatin remodeling, shifting from compacted (silent) to active states (184–186). This remodeling is controlled by chemical modifications to histones and DNA. Various enzymes act as “writers” (which add acetyl or methyl groups), “erasers” (which remove them), and “readers” (which bind to modified chromatin). Chromatin modifications generally precede gene transcription and are influenced by epigenetic regulators (9, 187–190). Typically, histone acetylation promotes transcription, while methylation is linked to gene silencing.
NF-κB family members are key players in chromatin remodeling. They can recruit and position chromatin modifiers to specific genes (191, 192). For example, GITR-dependent NF-κB signaling helps induce Tregs to become Th9 cells by allowing p50 to recruit deacetylases HDAC1 and Sirt1 to the Foxp3 locus. This process leads to the closure of the Foxp3 locus, enabling induced Tregs to differentiate into inflammatory Th9 cells (193). Research shows that RelB also aids in regulating chromatin in activated T cells. In Th17 conditions, OX40 stimulation represses IL-17 expression by directing RelB to the IL-17 locus, leading to the trimethylation of H3K9 and the closure of the IL-17 locus (192). Conversely, RelB can promote Th19 differentiation by recruiting acetyltransferase p300/CBP to the IL-10 locus (194).
Mounting evidence also suggest that the specific partners of NF-κB subunits can separate their epigenetic and transcriptional tasks (195). However, the mechanisms behind these separations and the factors determining the interaction with specific chromatin modifiers are still unclear. New techniques like ATAC-seq, CUT&RUN, and CUT&Tag offer great promise for advancing our understanding in this area. Recent research using single-cell ATAC-seq has shown that the collaboration of RelA or c-Rel with IRF-3 and MAPK factors during TLR4 responses enhances remodeling selectivity at specific genomic regions (196).
T cell metabolism is essential for T cell differentiation, and NF-κB signaling plays a significant role in this process (197, 198). For example, NIK-deficient tumor-infiltrating CD8 T cells do not shift toward glycolysis, which leads to a loss of effector function and impaired tumor control. Notably, NIK operates independently of NF-κB subunits by regulating levels of intracellular reactive oxygen species (ROS) (199). While there is limited information on how other NF-κB signaling intermediates affect T cell metabolism, some studies suggest a link between NF-κB signaling and mTOR in regulating metabolic changes critical for T cell differentiation (197, 198). mTORC1, which senses nutritional cues, is essential for naive CD8 T cells to transition to glycolysis, ensuring they have enough energy for effector functions. Research by Kane’s group has found that CARMA-1 and MALT1, in addition to activating IKKβ, also contribute to mTORC1 activation, which is vital for CD4 T cell proliferation (200). Moreover, Pearce et al. demonstrated that T cells lacking TRAF6—a ligase important for IKKβ activation in response to antigen, TNF, and IL-1R stimulation—fail to differentiate into memory T cells due to impairments in fatty acid metabolism after IL-2 withdrawal during maturation. They found that treatment with metformin and rapamycin could help recover this memory defect (201). However, it remains unclear whether TRAF6 functions independently of NF-κB signaling in this context. It’s also worth noting that mTOR signaling can influence NF-κB activity (202). Given the significant role of NF-κB signaling in regulating metabolism in other cell types, it is highly likely that NF-κB also plays a crucial role in the metabolic shifts necessary in T cell function and differentiation. Further investigation to fully elucidate the underlying mechanisms (Figure 4).
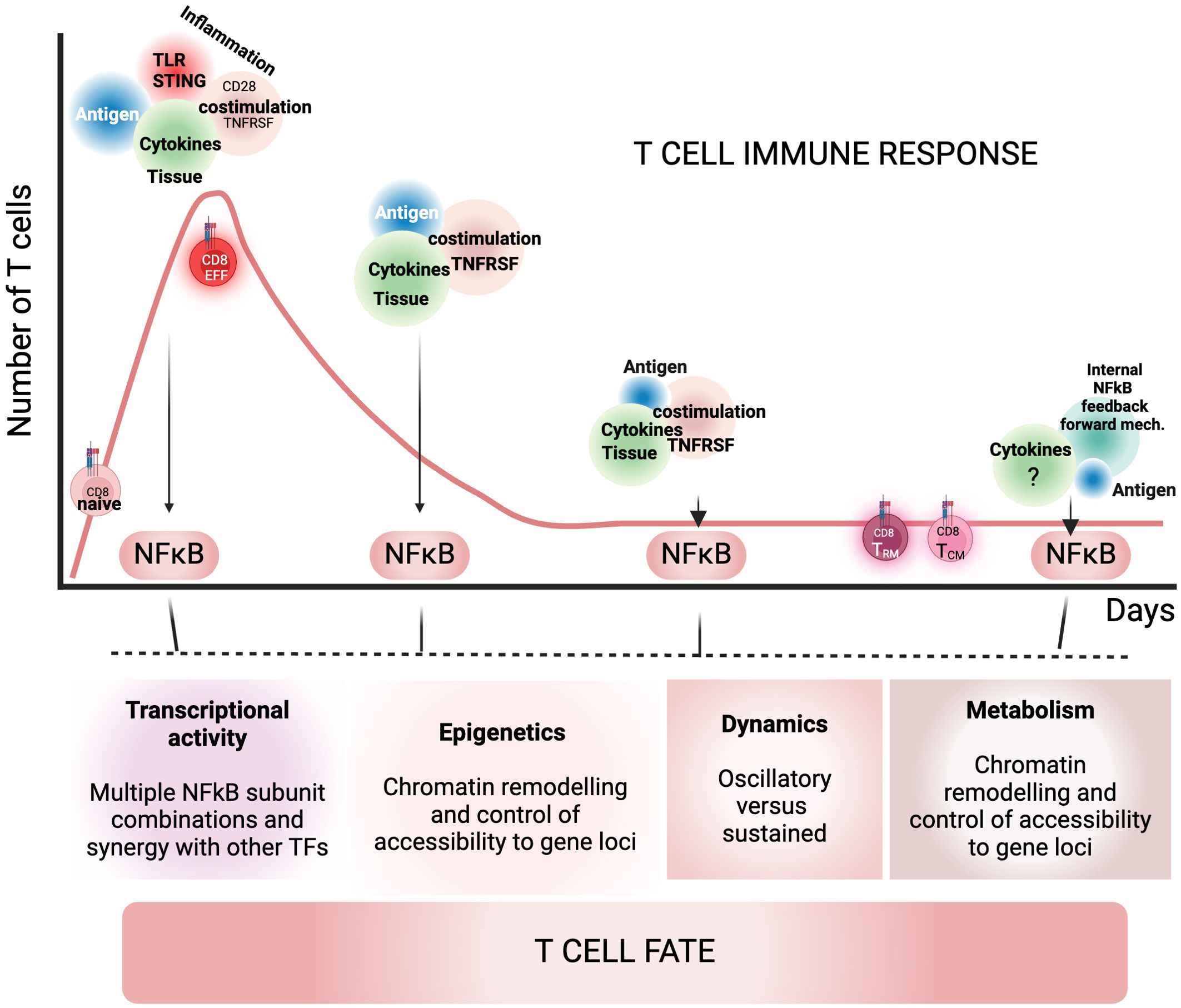
Figure 4. NF-κB signaling triggers and NF-κB mechanisms that regulate CD8 T cell fate during a T cell immune response. This cartoon illustrates the key triggers and mechanisms of NF-κB signaling that influence CD8 T cell fate during a T cell immune response. The primary triggers of NF-κB signaling include antigen recognition, inflammation (including costimulation and pro-inflammatory cytokines), and local cytokines. As levels of antigen and inflammation decrease, other factors—such as tissue cytokines, TNF receptors, and internal NF-κB forward loops established early in the response—assume a more prominent role in determining T cell memory fate during the late phase of the immune response. The functions of NF-κB that operate in T cells are depicted in the cartoon; however, the specific mechanisms by which these factors regulate T cell fate, either independently or synergistically, remain largely unknown. This figure was created using BioRender.com.
Dynamics of NF-κB signaling and their impact in T cell fate decisions
How does the NF-κB signaling network inform a T cell’s decisions, such as committing to a specific fate, secreting certain cytokines over others, or surviving for extended periods? This is a complex question with many unanswered aspects. T cells may encounter a variety of NF-κB signaling triggers some of which may converge on the same canonical or non-canonical pathway. Both the level and timing of these signals, along with other transcriptional and epigenetic factors, are important. Additionally, T cells at different developmental stages may respond differently to the same stimuli (Figure 4).
A foundational study by Hoffman and Baltimore demonstrated that NF-κB signaling operates not as a simple ON/OFF mechanism but rather exhibits a “tidal” pattern (203, 204). This means that NF-κB signaling creates peaks and valleys of activation in an oscillatory manner which the cell interprets for decision-making (203). The IκB family serves as the primary regulator of NF-κB activation and is also induced by NF-κB signaling. Newly synthesized IκBα binds to p50 and p65 in the nucleus, inhibiting their transcriptional activity and shuttling the IκBα-p50-p65 complexes into the cytosol. This process keeps NF-κB inactive until the next signaling burst occurs (205). Other IκB family members also play a role in modulating the dynamics of NF-κB activity (205).The dynamics of NF-κB can vary depending on the ligand, dosage, and duration of cell stimulation, leading to different gene expression profiles and epigenetic changes related to T cell outcomes. This has not been fully demonstrated in T cells. However, studies in other cell types have shown that prolonged TNF stimulation can lead to oscillatory NF-κB responses (203, 204), while prolonged LPS stimulation results in more sustained NF-κB activation with fewer oscillations (206, 207). These differences in NF-κB dynamics impact various epigenetic programs. For instance, sustained non-oscillatory responses in macrophages promote chromatin remodeling and activate latent enhancers that regulate immune response genes. Conversely, oscillatory responses are associated with maturation-related genes in B cells and chemokine production in fibroblasts (208).
Additionally, recent studies suggest that chronic TNF-derived NF-κB signals in the lung inhibit the generation of tissue-resident memory T cells, while sustained signals in the lymph nodes increase the levels of circulating memory T cells (169). This raises the question of whether these effects are linked to different NF-κB dynamics influenced by various triggers.
Growing evidence suggests that the dynamics of NF-κB signaling—whether oscillatory or sustained—play a crucial role in determining the accessibility of NF-κB-dependent genes and influencing cell fate decisions (43, 209). However, the upstream mechanisms that govern these dynamics are not well understood. One key factor is the balance between IKK complex activation and inhibitory mechanisms. Molecules involved in the formation, ubiquitination, and activation of the IKK complex are essential for this balance, along with the activity of inhibitory proteins such as A20. For instance, variations in stimulus dosage, such as TNF, can affect NF-κB signaling dynamics through negative feedback loops involving A20 and IκBα (13). A20, like IκB, is induced by NF-κB signaling and helps degrade upstream intermediates, including RIP1, TRAF6, and MALT1. This degradation inhibits further activation of the IKK complex. Additionally, other negative regulators of the NF-κB pathway, such as CYLD and OTULIN deubiquitinases, may also contribute to the regulation of NF-κB signaling (210, 211) (Figures 1C, D).
Additional mechanisms for controlling NF-κB dynamics include preventing spontaneous activation by regulating the processing of p100 or p105 (212, 213). Temporal separation of activation signals and their receptors can occur in endosomes through the ESCRT pathway (213). Moreover, signaling can be modulated by microRNAs and long non-coding RNAs (214, 215).
Thus, the field of NF-κB biology is approaching a pivotal moment. New inducible NF-κB animal models, along with advances in single-cell RNA sequencing (scRNA-seq) and single-cell ATAC sequencing (scATAC-seq), will significantly enhance our understanding. These technologies, combined with computational and signaling network models, will help elucidate how NF-κB signaling in T cells influences decisions that can either protect against disease or contribute to its progression.
NF-κB, cell type and temporal targeting and therapeutics
NF-κB signaling is vital for T cell biology, especially in the development of T cell subsets that, when deregulated, contribute to various diseases. As discussed throughout this review controlling NF-κB signaling intermediates in a temporal and specific manner presents therapeutic opportunities for treating infections, cancer, autoimmunity, and transplantation. Selectively targeting specific NF-κB subunits could lead to effective treatments that provide benefits without compromising other NF-κB functions. For example, targeting c-Rel may enhance antitumor responses because it is crucial for creating and maintaining activated Tregs that accumulate at tumor sites and impair the function of anti-tumor T cells (216).
However, precise targeting of specific T cell types is essential for effectiveness. Early deletion of c-Rel in conventional CD4 T cells can increase tumor burden and reduce responses to checkpoint blockade (69). In studies, deleting c-Rel during the first five days after tumor implantation reduced CD4 T cell priming, resulting in more naive T cells but impaired effector functions (69). While in this study the later roles of c-Rel and RelA in the antitumor response not explored, it is plausible that inhibiting c-Rel at later stages may not impact conventional T cells but rather affect activated Tregs, potentially enhancing antitumor responses. In related research, the Mempel group found that disrupting the CBM complex—through the genetic deletion of CARMA1 or inhibition of MALT1—promoted Treg activation toward a Th1/IFN-γ producing phenotype. This change, combined with PD-1 blockade, led to the rejection of PD-1 resistant tumors (217). Conversely, other studies suggest that IKKβ or CARMA1 activation can limit tumor growth in a CD8-dependent manner (116, 218, 219). Thus, when evaluating NF-κB inhibitor therapeutics, it is vital to consider their effects on all components of the antitumor response and the timing of their actions. This approach could reveal new opportunities for using multitarget inhibitors effectively.
In autoimmune contexts, early inhibition of p65/RelA (but not other subunits) may be beneficial. Its absence in CD4 T cells has shown complete protection against autoimmunity in an experimental autoimmune encephalomyelitis (EAE) mouse model, which relies on Th17 (69). There is less information on effectively targeting NF-κB signaling to reduce transplant rejection, as current alternatives to broad immunosuppressive treatments often affect multiple immune cells. The potential for selectively activating or deactivating NF-κB subunits to manage diseases with deregulated T cell responses—while preserving T cell memory—also deserves further exploration.
Numerous NF-κB inhibitors are being developed and tested in clinical trials for various treatments. Additionally, existing drugs that affect NF-κB activity are being repurposed, broadening options for targeting this pathway. This topic has been thoroughly reviewed recently, and we will not discuss it further here (55, 220). Nonetheless, it is important to mention that none of the options have achieved considerable success due to limited effectiveness or associated toxicity. As the field continues to clarify the specific roles of NF-κB subunits in disease, it is important to avoid broader inhibitions, such as those affecting proteasome activity or deubiquitination. Optimizing the timing of NF-κB inhibitor administration will enhance T cell response modulation and should consider the specific tissues and T cell subsets involved in disease progression or resolution.
Conclusion and future perspectives
After nearly 40 years of research, the study of NF-κB signaling has not only flourished but continues to yield unexpected discoveries. This pathway has proven crucial in cell biology, regulating essential processes such as cell division, apoptosis, senescence, migration and effector function. NF-κB is classically considered a driver of inflammation and organogenesis but, for T lymphocytes, this signaling pathway is also a ruler of their fate. With the advent of new transgenic inducible animal models, previously contentious issues regarding the role of NF-κB in thymic selection, homeostasis, and CD4 T cell differentiation have been revisited. Recent findings have unveiled surprising roles for NF-κB in antitumor T cell responses, experimental autoimmune encephalomyelitis (EAE), and the development of T cell memory during infections. However, a critical question remains: how does the NF-κB signaling network enable T cells to make specific differentiation choices?
This may depend on how NF-κB signaling influences T cell epigenetics and metabolism. These areas, alongside NF-κB dynamics, are largely unexplored and require further investigation (Figure 4). Gaining a better understanding of how environmental triggers of NF-κB work during the immune response, along with their mechanisms, is essential and could lead to new therapeutic strategies. In addition to this, distinguishing between NF-κB-dependent and independent roles of signaling intermediates in the NF-κB cascade may clarify current knowledge, help identify effective therapeutic targets and anticipate potential side effects in treatments.
While this review does not cover it, existing literature has discussed human mutations in NF-κB pathway components and their disease implications (3, 34, 159, 221–223). Identifying mutations in key NF-κB molecules is an area that offers valuable insights and can enhance the rigor and impact of research using animal models, ultimately contributing to the development of new treatments for human diseases.
In conclusion, although significant progress has been made in understanding NF-κB signaling in T cell biology, many exciting areas remain to be explored. Advances in these fields will deepen our insights into immune responses and pave the way for innovative therapeutic strategies to combat diseases such as cancer, autoimmunity, and transplant rejection.
Author contributions
ET: Conceptualization, Funding acquisition, Writing – original draft, Writing – review & editing. MD: Funding acquisition, Writing – review & editing.
Funding
The author(s) declare that financial support was received for the research and/or publication of this article. This work was supported by grants from the National Institutes of Health (NCI CA244314 and NIAID R01AI181112) and the University of Missouri (Research Council) for ET and by grants from the American Cancer Society (RSG-20-126-01 -CDD), the National Institutes of Health (NIBIB EB026560) and the University of Missouri for MAD.
Acknowledgments
We thank members of the Teixeiro lab (Dezzarae Luera, Elida Lopez, Kara Young and Justine Miller) for critically reading the manuscript. We apologize for the colleagues whose work could not be cited due to space or time limitations.
Conflict of interest
The authors declare that the research was conducted in the absence of any commercial or financial relationships that could be construed as a potential conflict of interest.
The author(s) declared that they were an editorial board member of Frontiers, at the time of submission. This had no impact on the peer review process and the final decision.
Generative AI statement
The author(s) declare that no Generative AI was used in the creation of this manuscript.
Publisher’s note
All claims expressed in this article are solely those of the authors and do not necessarily represent those of their affiliated organizations, or those of the publisher, the editors and the reviewers. Any product that may be evaluated in this article, or claim that may be made by its manufacturer, is not guaranteed or endorsed by the publisher.
References
1. Sen R, Baltimore D. Multiple nuclear factors interact with the immunoglobulin enhancer sequences. Cell. (1986) 46:705–16. doi: 10.1016/0092-8674(86)90346-6
2. Hayden MS, Ghosh S. Nf-kappab, the first quarter-century: remarkable progress and outstanding questions. Genes Dev. (2012) 26:203–34. doi: 10.1101/gad.183434.111
3. Zhang Q, Lenardo MJ, Baltimore D. 30 years of nf-kappab: A blossoming of relevance to human pathobiology. Cell. (2017) 168:37–57. doi: 10.1016/j.cell.2016.12.012
4. Adelaja A, Hoffmann A. Signaling crosstalk mechanisms that may fine-tune pathogen-responsive nfkappab. Front Immunol. (2019) 10:433. doi: 10.3389/fimmu.2019.00433
5. Kaltschmidt C, Greiner JFW, Kaltschmidt B. The transcription factor nf-kappab in stem cells and development. Cells. (2021) 10. doi: 10.3390/cells10082042
6. Karin M, Ben-Neriah Y. Phosphorylation meets ubiquitination: the control of nf-[Kappa]B activity. Annu Rev Immunol. (2000) 18:621–63. doi: 10.1146/annurev.immunol.18.1.621
7. Daniels MA, Luera D, Teixeiro E. Nfkappab signaling in T cell memory. Front Immunol. (2023) 14:1129191. doi: 10.3389/fimmu.2023.1129191
8. Liu T, Zhang L, Joo D, Sun SC. Nf-kappab signaling in inflammation. Signal Transduct Target Ther. (2017) 2:17023–. doi: 10.1038/sigtrans.2017.23
9. Zhang L, Xiao X, Arnold PR, Li XC. Transcriptional and epigenetic regulation of immune tolerance: roles of the nf-kappab family members. Cell Mol Immunol. (2019) 16:315–23. doi: 10.1038/s41423-019-0202-8
10. Sen S, Cheng Z, Sheu KM, Chen YH, Hoffmann A. Gene regulatory strategies that decode the duration of nfkappab dynamics contribute to lps- versus tnf-specific gene expression. Cell Syst. (2020) 10:169–82 e5. doi: 10.1016/j.cels.2019.12.004
11. Tay S, Hughey JJ, Lee TK, Lipniacki T, Quake SR, Covert MW. Single-cell nf-kappab dynamics reveal digital activation and analogue information processing. Nature. (2010) 466:267–71. doi: 10.1038/nature09145
12. Son M, Frank T, Holst-Hansen T, Wang AG, Junkin M, Kashaf SS, et al. Spatiotemporal nf-kappab dynamics encodes the position, amplitude, and duration of local immune inputs. Sci Adv. (2022) 8:eabn6240. doi: 10.1126/sciadv.abn6240
13. Son M, Wang AG, Tu HL, Metzig MO, Patel P, Husain K, et al. Nf-kappab responds to absolute differences in cytokine concentrations. Sci Signal. (2021) 14. doi: 10.1126/scisignal.aaz4382
14. Baldwin AS Jr. The nf-kappa B and I kappa B proteins: new discoveries and insights. Annu Rev Immunol. (1996) 14:649–83. doi: 10.1146/annurev.immunol.14.1.649
15. Hayden MS, Ghosh S. Shared principles in nf-kappab signaling. Cell. (2008) 132:344–62. doi: 10.1016/j.cell.2008.01.020
16. Vallabhapurapu S, Karin M. Regulation and function of nf-kappab transcription factors in the immune system. Annu Rev Immunol. (2009) 27:693–733. doi: 10.1146/annurev.immunol.021908.132641
17. Beg AA, Ruben SM, Scheinman RI, Haskill S, Rosen CA. Baldwin AS, jr. I kappa B interacts with the nuclear localization sequences of the subunits of nf-kappa B: A mechanism for cytoplasmic retention. Genes Dev. (1992) 6:1899–913. doi: 10.1101/gad.6.10.1899
18. Bell S, Matthews JR, Jaffray E, Hay RT. I(Kappa)B(Gamma) inhibits DNA binding of nf-kappab P50 homodimers by interacting with residues that contact DNA. Mol Cell Biol. (1996) 16:6477–85. doi: 10.1128/MCB.16.11.6477
19. Ganchi PA, Sun SC, Greene WC, Ballard DW. I kappa B/mad-3 masks the nuclear localization signal of nf-kappa B P65 and requires the transactivation domain to inhibit nf-kappa B P65 DNA binding. Mol Biol Cell. (1992) 3:1339–52. doi: 10.1091/mbc.3.12.1339
20. Inoue J, Kerr LD, Kakizuka A, Verma IM. I kappa B gamma, a 70 kd protein identical to the C-terminal half of P110 nf-kappa B: A new member of the I kappa B family. Cell. (1992) 68:1109–20. doi: 10.1016/0092-8674(92)90082-n
21. Tran K, Merika M, Thanos D. Distinct functional properties of ikappab alpha and ikappab beta. Mol Cell Biol. (1997) 17:5386–99. doi: 10.1128/MCB.17.9.5386
22. Whiteside ST, Epinat JC, Rice NR, Israel A. I kappa B epsilon, a novel member of the I kappa B family, controls rela and crel nf-kappa B activity. EMBO J. (1997) 16:1413–26. doi: 10.1093/emboj/16.6.1413
23. Zabel U, Baeuerle PA. Purified human I kappa B can rapidly dissociate the complex of the nf-kappa B transcription factor with its cognate DNA. Cell. (1990) 61:255–65. doi: 10.1016/0092-8674(90)90806-p
24. Fiorini E, Schmitz I, Marissen WE, Osborn SL, Touma M, Sasada T, et al. Peptide-induced negative selection of thymocytes activates transcription of an nf-kappa B inhibitor. Mol Cell. (2002) 9:637–48. doi: 10.1016/s1097-2765(02)00469-0
25. Hirotani T, Lee PY, Kuwata H, Yamamoto M, Matsumoto M, Kawase I, et al. The nuclear ikappab protein ikappabns selectively inhibits lipopolysaccharide-induced il-6 production in macrophages of the colonic lamina propria. J Immunol. (2005) 174:3650–7. doi: 10.4049/jimmunol.174.6.3650
26. Yamazaki S, Muta T, Takeshige K. A novel ikappab protein, ikappab-zeta, induced by proinflammatory stimuli, negatively regulates nuclear factor-kappab in the nuclei. J Biol Chem. (2001) 276:27657–62. doi: 10.1074/jbc.M103426200
27. Yamauchi S, Ito H, Miyajima A. Ikappabeta, a nuclear ikappab protein, positively regulates the nf-kappab-mediated expression of proinflammatory cytokines. Proc Natl Acad Sci U S A. (2010) 107:11924–9. doi: 10.1073/pnas.0913179107
28. Zhang Q, Didonato JA, Karin M, McKeithan TW. Bcl3 encodes a nuclear protein which can alter the subcellular location of nf-kappa B proteins. Mol Cell Biol. (1994) 14:3915–26. doi: 10.1128/mcb.14.6.3915-3926.1994
29. Schuster M, Annemann M, Plaza-Sirvent C, Schmitz I. Atypical ikappab proteins - nuclear modulators of nf-kappab signaling. Cell Commun Signal. (2013) 11:23. doi: 10.1186/1478-811X-11-23
30. Sun S, Elwood J, Greene WC. Both amino- and carboxyl-terminal sequences within I kappa B alpha regulate its inducible degradation. Mol Cell Biol. (1996) 16:1058–65. doi: 10.1128/MCB.16.3.1058
31. Solan NJ, Miyoshi H, Carmona EM, Bren GD, Paya CV. Relb cellular regulation and transcriptional activity are regulated by P100. J Biol Chem. (2002) 277:1405–18. doi: 10.1074/jbc.M109619200
32. Sun SC. Controlling the fate of nik: A central stage in noncanonical nf-kappab signaling. Sci Signal. (2010) 3:pe18. doi: 10.1126/scisignal.3123pe18
33. Xiao G, Harhaj EW, Sun SC. Nf-kappab-inducing kinase regulates the processing of nf-kappab2 P100. Mol Cell. (2001) 7:401–9. doi: 10.1016/s1097-2765(01)00187-3
34. Guo Q, Jin Y, Chen X, Ye X, Shen X, Lin M, et al. Nf-kappab in biology and targeted therapy: new insights and translational implications. Signal Transduct Target Ther. (2024) 9:53. doi: 10.1038/s41392-024-01757-9
35. Pomerantz JL, Baltimore D. Two pathways to nf-kappab. Mol Cell. (2002) 10:693–5. doi: 10.1016/s1097-2765(02)00697-4
36. Mitchell S, Vargas J, Hoffmann A. Signaling via the nfkappab system. Wiley Interdiscip Rev Syst Biol Med. (2016) 8:227–41. doi: 10.1002/wsbm.1331
37. Shih VF, Tsui R, Caldwell A, Hoffmann A. A single nfkappab system for both canonical and non-canonical signaling. Cell Res. (2011) 21:86–102. doi: 10.1038/cr.2010.161
38. Jacque E, Tchenio T, Piton G, Romeo PH, Baud V. Rela repression of relb activity induces selective gene activation downstream of tnf receptors. Proc Natl Acad Sci U S A. (2005) 102:14635–40. doi: 10.1073/pnas.0507342102
39. Kim JY, Morgan M, Kim DG, Lee JY, Bai L, Lin Y, et al. Tnfalpha induced noncanonical nf-kappab activation is attenuated by rip1 through stabilization of traf2. J Cell Sci. (2011) 124:647–56. doi: 10.1242/jcs.075770
40. Gentle IE, Wong WW, Evans JM, Bankovacki A, Cook WD, Khan NR, et al. In tnf-stimulated cells, ripk1 promotes cell survival by stabilizing traf2 and ciap1, which limits induction of non-canonical nf-kappab and activation of caspase-8. J Biol Chem. (2011) 286:13282–91. doi: 10.1074/jbc.M110.216226
41. Zarnegar B, Yamazaki S, He JQ, Cheng G. Control of canonical nf-kappab activation through the nik-ikk complex pathway. Proc Natl Acad Sci U S A. (2008) 105:3503–8. doi: 10.1073/pnas.0707959105
42. Ramakrishnan P, Wang W, Wallach D. Receptor-specific signaling for both the alternative and the canonical nf-kappab activation pathways by nf-kappab-inducing kinase. Immunity. (2004) 21:477–89. doi: 10.1016/j.immuni.2004.08.009
43. Ashby KM, Hogquist KA. A guide to thymic selection of T cells. Nat Rev Immunol. (2024) 24:103–17. doi: 10.1038/s41577-023-00911-8
44. Voll RE, Jimi E, Phillips RJ, Barber DF, Rincon M, Hayday AC, et al. Nf-kappa B activation by the pre-T cell receptor serves as a selective survival signal in T lymphocyte development. Immunity. (2000) 13:677–89. doi: 10.1016/s1074-7613(00)00067-4
45. Jimi E, Strickland I, Voll RE, Long M, Ghosh S. Differential role of the transcription factor nf-kappab in selection and survival of cd4+ and cd8+ Thymocytes. Immunity. (2008) 29:523–37. doi: 10.1016/j.immuni.2008.08.010
46. Hettmann T, Leiden JM. Nf-kappa B is required for the positive selection of cd8+ Thymocytes. J Immunol. (2000) 165:5004–10. doi: 10.4049/jimmunol.165.9.5004
47. Mora AL, Chen D, Boothby M, Rubin DH. Lineage-specific differences among cd8+ T cells in their dependence of nf-kappa B/rel signaling. Eur J Immunol. (1999) 29:2968–80. doi: 10.1002/(SICI)1521-4141(199909)29:09<2968::AID-IMMU2968>3.0.CO;2-X
48. Schmidt-Supprian M, Courtois G, Tian J, Coyle AJ, Israel A, Rajewsky K, et al. Mature T cells depend on signaling through the ikk complex. Immunity. (2003) 19:377–89. doi: 10.1016/s1074-7613(03)00237-1
49. Liu HH, Xie M, Schneider MD, Chen ZJ. Essential role of tak1 in thymocyte development and activation. Proc Natl Acad Sci U S A. (2006) 103:11677–82. doi: 10.1073/pnas.0603089103
50. Xing Y, Wang X, Jameson SC, Hogquist KA. Late stages of T cell maturation in the thymus involve nf-kappab and tonic type I interferon signaling. Nat Immunol. (2016) 17:565–73. doi: 10.1038/ni.3419
51. Jost PJ, Weiss S, Ferch U, Gross O, Mak TW, Peschel C, et al. Bcl10/malt1 signaling is essential for tcr-induced nf-kappab activation in thymocytes but dispensable for positive or negative selection. J Immunol. (2007) 178:953–60. doi: 10.4049/jimmunol.178.2.953
52. Molinero LL, Yang J, Gajewski T, Abraham C, Farrar MA, Alegre ML. Carma1 controls an early checkpoint in the thymic development of foxp3+ Regulatory T cells. J Immunol. (2009) 182:6736–43. doi: 10.4049/jimmunol.0900498
53. Barnes MJ, Krebs P, Harris N, Eidenschenk C, Gonzalez-Quintial R, Arnold CN, et al. Commitment to the regulatory T cell lineage requires carma1 in the thymus but not in the periphery. PloS Biol. (2009) 7:e51. doi: 10.1371/journal.pbio.1000051
54. Webb LV, Barbarulo A, Huysentruyt J, Vanden Berghe T, Takahashi N, Ley S, et al. Survival of single positive thymocytes depends upon developmental control of ripk1 kinase signaling by the ikk complex independent of nf-kappab. Immunity. (2019) 50:348–61 e4. doi: 10.1016/j.immuni.2019.01.004
55. Lalle G, Twardowski J, Grinberg-Bleyer Y. Nf-kappab in cancer immunity: friend or foe? Cells. (2021) 10. doi: 10.3390/cells10020355
56. Jameson SC. Maintaining the norm: T-cell homeostasis. Nat Rev Immunol. (2002) 2:547–56. doi: 10.1038/nri853
57. Ren H, Schmalstieg A, van Oers NS, Gaynor RB. I-kappa B kinases alpha and beta have distinct roles in regulating murine T cell function. J Immunol. (2002) 168:3721–31. doi: 10.4049/jimmunol.168.8.3721
58. Attar RM, Macdonald-Bravo H, Raventos-Suarez C, Durham SK, Bravo R. Expression of constitutively active ikappab beta in T cells of transgenic mice: persistent nf-kappab activity is required for T-cell immune responses. Mol Cell Biol. (1998) 18:477–87. doi: 10.1128/MCB.18.1.477
59. Boothby MR, Mora AL, Scherer DC, Brockman JA, Ballard DW. Perturbation of the T lymphocyte lineage in transgenic mice expressing a constitutive repressor of nuclear factor (Nf)-kappab. J Exp Med. (1997) 185:1897–907. doi: 10.1084/jem.185.11.1897
60. Esslinger CW, Wilson A, Sordat B, Beermann F, Jongeneel CV. Abnormal T lymphocyte development induced by targeted overexpression of ikappab alpha. J Immunol. (1997) 158:5075–8. doi: 10.4049/jimmunol.158.11.5075
61. Ferreira V, Sidenius N, Tarantino N, Hubert P, Chatenoud L, Blasi F, et al. In vivo inhibition of nf-kappa B in T-lineage cells leads to a dramatic decrease in cell proliferation and cytokine production and to increased cell apoptosis in response to mitogenic stimuli, but not to abnormal thymopoiesis. J Immunol. (1999) 162:6442–50. doi: 10.4049/jimmunol.162.11.6442
62. Pasparakis M, Luedde T, Schmidt-Supprian M. Dissection of the nf-kappab signalling cascade in transgenic and knockout mice. Cell Death Differ. (2006) 13:861–72. doi: 10.1038/sj.cdd.4401870
63. Silva A, Cornish G, Ley SC, Seddon B. Nf-kappab signaling mediates homeostatic maturation of new T cells. Proc Natl Acad Sci U S A. (2014) 111:E846–55. doi: 10.1073/pnas.1319397111
64. Teixeiro E, Daniels M, Hamilton S, Schrum A, Bragado R, Jameson S, et al. Different T cell receptor signals determine cd8+ Memory versus effector development. Science. (2009) 323:502. doi: 10.1126/science.1163612
65. Zheng Y, Vig M, Lyons J, Van Parijs L, Beg AA. Combined deficiency of P50 and crel in cd4+ T cells reveals an essential requirement for nuclear factor kappab in regulating mature T cell survival and in vivo function. J Exp Med. (2003) 197:861–74. doi: 10.1084/jem.20021610
66. Weih F, Durham SK, Barton DS, Sha WC, Baltimore D, Bravo R. P50-nf-kappab complexes partially compensate for the absence of relb: severely increased pathology in P50(-/-)Relb(-/-) double-knockout mice. J Exp Med. (1997) 185:1359–70. doi: 10.1084/jem.185.7.1359
67. Horwitz BH, Scott ML, Cherry SR, Bronson RT, Baltimore D. Failure of lymphopoiesis after adoptive transfer of nf-kappab-deficient fetal liver cells. Immunity. (1997) 6:765–72. doi: 10.1016/s1074-7613(00)80451-3
68. Ghosh S, May MJ, Kopp EB. Nf-kappa B and rel proteins: evolutionarily conserved mediators of immune responses. Annu Rev Immunol. (1998) 16:225–60. doi: 10.1146/annurev.immunol.16.1.225
69. Lalle G, Lautraite R, Bouherrou K, Plaschka M, Pignata A, Voisin A, et al. Nf-kappab subunits rela and C-rel selectively control cd4+ T cell function in multiple sclerosis and cancer. J Exp Med. (2024) 221. doi: 10.1084/jem.20231348
70. Jenkins MK, Khoruts A, Ingulli E, Mueller DL, McSorley SJ, Reinhardt RL, et al. In vivo activation of antigen-specific cd4 T cells. Annu Rev Immunol. (2001) 19:23–45. doi: 10.1146/annurev.immunol.19.1.23
71. Smith-Garvin J, Koretzky G, Jordan M. T cell activation. Annu Rev Immunol. (2009) 27:591–619. doi: 10.1146/annurev.immunol.021908.132706
72. Doi TS, Takahashi T, Taguchi O, Azuma T, Obata Y. Nf-kappa B rela-deficient lymphocytes: normal development of T cells and B cells, impaired production of iga and igg1 and reduced proliferative responses. J Exp Med. (1997) 185:953–61. doi: 10.1084/jem.185.5.953
73. Liou HC, Jin Z, Tumang J, Andjelic S, Smith KA, Liou ML. C-rel is crucial for lymphocyte proliferation but dispensable for T cell effector function. Int Immunol. (1999) 11:361–71. doi: 10.1093/intimm/11.3.361
74. Newton K, Dixit VM. Mice lacking the card of carma1 exhibit defective B lymphocyte development and impaired proliferation of their B and T lymphocytes. Curr Biol. (2003) 13:1247–51. doi: 10.1016/s0960-9822(03)00458-5
75. Hara H, Bakal C, Wada T, Bouchard D, Rottapel R, Saito T, et al. The molecular adapter carma1 controls entry of ikappab kinase into the central immune synapse. J Exp Med. (2004) 200:1167–77. doi: 10.1084/jem.20032246
76. Egawa T, Albrecht B, Favier B, Sunshine MJ, Mirchandani K, O’Brien W, et al. Requirement for carma1 in antigen receptor-induced nf-kappa B activation and lymphocyte proliferation. Curr Biol. (2003) 13:1252–8. doi: 10.1016/s0960-9822(03)00491-3
77. Molinero LL, Cubre A, Mora-Solano C, Wang Y, Alegre ML. T cell receptor/carma1/nf-kappab signaling controls T-helper (Th) 17 differentiation. Proc Natl Acad Sci U S A. (2012) 109:18529–34. doi: 10.1073/pnas.1204557109
78. Kingeter LM, Schaefer BC. Loss of protein kinase C theta, bcl10, or malt1 selectively impairs proliferation and nf-kappa B activation in the cd4+ T cell subset. J Immunol. (2008) 181:6244–54. doi: 10.4049/jimmunol.181.9.6244
79. Thome M. Carma1, bcl-10 and malt1 in lymphocyte development and activation. Nat Rev Immunol. (2004) 4:348–59. doi: 10.1038/nri1352
80. Kawadler H, Gantz MA, Riley JL, Yang X. The paracaspase malt1 controls caspase-8 activation during lymphocyte proliferation. Mol Cell. (2008) 31:415–21. doi: 10.1016/j.molcel.2008.06.008
81. Park SG, Schulze-Luehrman J, Hayden MS, Hashimoto N, Ogawa W, Kasuga M, et al. The kinase pdk1 integrates T cell antigen receptor and cd28 coreceptor signaling to induce nf-kappab and activate T cells. Nat Immunol. (2009) 10:158–66. doi: 10.1038/ni.1687
82. Chuang HC, Lan JL, Chen DY, Yang CY, Chen YM, Li JP, et al. The kinase glk controls autoimmunity and nf-kappab signaling by activating the kinase pkc-theta in T cells. Nat Immunol. (2011) 12:1113–8. doi: 10.1038/ni.2121
83. Williams MA, Bevan MJ. Effector and memory ctl differentiation. Annu Rev Immunol. (2006) 25:171–92. doi: 10.1146/annurev.immunol.25.022106.141548
84. Zhu J, Yamane H, Paul WE. Differentiation of effector cd4 T cell populations (*). Annu Rev Immunol. (2010) 28:445–89. doi: 10.1146/annurev-immunol-030409-101212
85. O’Shea JJ, Paul WE. Mechanisms underlying lineage commitment and plasticity of helper cd4+ T cells. Science. (2010) 327:1098–102. doi: 10.1126/science.1178334
86. Hermann-Kleiter N, Baier G. Nfat pulls the strings during cd4+ T helper cell effector functions. Blood. (2010) 115:2989–97. doi: 10.1182/blood-2009-10-233585
87. Kanno Y, Vahedi G, Hirahara K, Singleton K, O’Shea JJ. Transcriptional and epigenetic control of T helper cell specification: molecular mechanisms underlying commitment and plasticity. Annu Rev Immunol. (2012) 30:707–31. doi: 10.1146/annurev-immunol-020711-075058
88. Kunzli M, Masopust D. Cd4(+) T cell memory. Nat Immunol. (2023) 24:903–14. doi: 10.1038/s41590-023-01510-4
89. Ruterbusch M, Pruner KB, Shehata L, Pepper M. In vivo cd4(+) T cell differentiation and function: revisiting the th1/th2 paradigm. Annu Rev Immunol. (2020) 38:705–25. doi: 10.1146/annurev-immunol-103019-085803
90. Li-Weber M, Giaisi M, Baumann S, Palfi K, Krammer PH. Nf-kappa B synergizes with nf-at and nf-il6 in activation of the il-4 gene in T cells. Eur J Immunol. (2004) 34:1111–8. doi: 10.1002/eji.200324687
91. Corn RA, Aronica MA, Zhang F, Tong Y, Stanley SA, Kim SR, et al. T cell-intrinsic requirement for nf-kappa B induction in postdifferentiation ifn-gamma production and clonal expansion in a th1 response. J Immunol. (2003) 171:1816–24. doi: 10.4049/jimmunol.171.4.1816
92. Das J, Chen CH, Yang L, Cohn L, Ray P, Ray A. A critical role for nf-kappa B in gata3 expression and th2 differentiation in allergic airway inflammation. Nat Immunol. (2001) 2:45–50. doi: 10.1038/83158
93. Hilliard BA, Mason N, Xu L, Sun J, Lamhamedi-Cherradi SE, Liou HC, et al. Critical roles of C-rel in autoimmune inflammation and helper T cell differentiation. J Clin Invest. (2002) 110:843–50. doi: 10.1172/JCI15254
94. Corn RA, Hunter C, Liou HC, Siebenlist U, Boothby MR. Opposing roles for relb and bcl-3 in regulation of T-box expressed in T cells, gata-3, and th effector differentiation. J Immunol. (2005) 175:2102–10. doi: 10.4049/jimmunol.175.4.2102
95. Balasubramani A, Shibata Y, Crawford GE, Baldwin AS, Hatton RD, Weaver CT. Modular utilization of distal cis-regulatory elements controls ifng gene expression in T cells activated by distinct stimuli. Immunity. (2010) 33:35–47. doi: 10.1016/j.immuni.2010.07.004
96. Ruan Q, Kameswaran V, Zhang Y, Zheng S, Sun J, Wang J, et al. The th17 immune response is controlled by the rel-rorgamma-rorgamma T transcriptional axis. J Exp Med. (2011) 208:2321–33. doi: 10.1084/jem.20110462
97. Okamoto K, Iwai Y, Oh-Hora M, Yamamoto M, Morio T, Aoki K, et al. Ikappabzeta regulates T(H)17 development by cooperating with ror nuclear receptors. Nature. (2010) 464:1381–5. doi: 10.1038/nature08922
98. De Leo P, Gazzurelli L, Baronio M, Montin D, Di Cesare S, Giancotta C, et al. Nfkb2 regulates human tfh and tfr pool formation and germinal center potential. Clin Immunol. (2020) 210:108309. doi: 10.1016/j.clim.2019.108309
99. Hu H, Wu X, Jin W, Chang M, Cheng X, Sun SC. Noncanonical nf-kappab regulates inducible costimulator (Icos) ligand expression and T follicular helper cell development. Proc Natl Acad Sci U S A. (2011) 108:12827–32. doi: 10.1073/pnas.1105774108
100. Serre K, Mohr E, Benezech C, Bird R, Khan M, Caamano JH, et al. Selective effects of nf-kappab1 deficiency in cd4(+) T cells on th2 and tfh induction by alum-precipitated protein vaccines. Eur J Immunol. (2011) 41:1573–82. doi: 10.1002/eji.201041126
101. Nakaya M, Xiao Y, Zhou X, Chang JH, Chang M, Cheng X, et al. Inflammatory T cell responses rely on amino acid transporter asct2 facilitation of glutamine uptake and mtorc1 kinase activation. Immunity. (2014) 40:692–705. doi: 10.1016/j.immuni.2014.04.007
102. Schmidt H, Raj T, O’Neill TJ, Muschaweckh A, Giesert F, Negraschus A, et al. Unrestrained cleavage of roquin-1 by malt1 induces spontaneous T cell activation and the development of autoimmunity. Proc Natl Acad Sci U S A. (2023) 120:e2309205120. doi: 10.1073/pnas.2309205120
103. Jeltsch KM, Hu D, Brenner S, Zoller J, Heinz GA, Nagel D, et al. Cleavage of roquin and regnase-1 by the paracaspase malt1 releases their cooperatively repressed targets to promote T(H)17 differentiation. Nat Immunol. (2014) 15:1079–89. doi: 10.1038/ni.3008
104. Annemann M, Wang Z, Plaza-Sirvent C, Glauben R, Schuster M, Ewald Sander F, et al. Ikappabns regulates murine th17 differentiation during gut inflammation and infection. J Immunol. (2015) 194:2888–98. doi: 10.4049/jimmunol.1401964
105. Marsland BJ, Soos TJ, Spath G, Littman DR, Kopf M. Protein kinase C theta is critical for the development of in vivo T helper (Th)2 cell but not th1 cell responses. J Exp Med. (2004) 200:181–9. doi: 10.1084/jem.20032229
106. Salek-Ardakani S, So T, Halteman BS, Altman A, Croft M. Differential regulation of th2 and th1 lung inflammatory responses by protein kinase C theta. J Immunol. (2004) 173:6440–7. doi: 10.4049/jimmunol.173.10.6440
107. Kwon MJ, Ma J, Ding Y, Wang R, Sun Z. Protein kinase C-theta promotes th17 differentiation via upregulation of stat3. J Immunol. (2012) 188:5887–97. doi: 10.4049/jimmunol.1102941
108. Sen S, Wang F, Zhang J, He Z, Ma J, Gwack Y, et al. Src1 promotes th17 differentiation by overriding foxp3 suppression to stimulate rorgammat activity in a pkc-theta-dependent manner. Proc Natl Acad Sci U S A. (2018) 115:E458–E67. doi: 10.1073/pnas.1717789115
109. Sun Z, Arendt CW, Ellmeier W, Schaeffer EM, Sunshine MJ, Gandhi L, et al. Pkc-theta is required for tcr-induced nf-kappab activation in mature but not immature T lymphocytes. Nature. (2000) 404:402–7. doi: 10.1038/35006090
110. Li L, Ruan Q, Hilliard B, Devirgiliis J, Karin M, Chen YH. Transcriptional regulation of the th17 immune response by ikk(Alpha). J Exp Med. (2011) 208:787–96. doi: 10.1084/jem.20091346
111. Jin W, Zhou XF, Yu J, Cheng X, Sun SC. Regulation of th17 cell differentiation and eae induction by map3k nik. Blood. (2009) 113:6603–10. doi: 10.1182/blood-2008-12-192914
112. Rizk J, Kaplinsky J, Agerholm R, Kadekar D, Ivars F, Agace WW, et al. Smac mimetics promote nik-dependent inhibition of cd4(+) T(H)17 cell differentiation. Sci Signal. (2019) 12. doi: 10.1126/scisignal.aaw3469
113. Koliesnik IO, Andreas N, Romanov VS, Sreekantapuram S, Krljanac B, Haenold R, et al. Relb regulates th17 differentiation in a cell-intrinsic manner. Immunobiology. (2018) 223:191–9. doi: 10.1016/j.imbio.2017.10.026
114. Yu J, Zhou X, Nakaya M, Jin W, Cheng X, Sun SC. T cell-intrinsic function of the noncanonical nf-kappab pathway in the regulation of gm-csf expression and experimental autoimmune encephalomyelitis pathogenesis. J Immunol. (2014) 193:422–30. doi: 10.4049/jimmunol.1303237
115. Marsland BJ, Nembrini C, Schmitz N, Abel B, Krautwald S, Bachmann MF, et al. Innate signals compensate for the absence of pkc-theta during in vivo cd8(+) T cell effector and memory responses. Proc Natl Acad Sci U S A. (2005) 102:14374–9. doi: 10.1073/pnas.0506250102
116. Barnes SE, Wang Y, Chen L, Molinero LL, Gajewski TF, Evaristo C, et al. T cell-nf-kappab activation is required for tumor control in vivo. J Immunother Cancer. (2015) 3:1. doi: 10.1186/s40425-014-0045-x
117. Lockhart E, Green AM, Flynn JL. Il-17 production is dominated by gammadelta T cells rather than cd4 T cells during mycobacterium tuberculosis infection. J Immunol. (2006) 177:4662–9. doi: 10.4049/jimmunol.177.7.4662
118. Shibata K, Yamada H, Hara H, Kishihara K, Yoshikai Y. Resident vdelta1+ Gammadelta T cells control early infiltration of neutrophils after escherichia coli infection via il-17 production. J Immunol. (2007) 178:4466–72. doi: 10.4049/jimmunol.178.7.4466
119. Tagawa T, Nishimura H, Yajima T, Hara H, Kishihara K, Matsuzaki G, et al. Vdelta1+ Gammadelta T cells producing cc chemokines may bridge a gap between neutrophils and macrophages in innate immunity during escherichia coli infection in mice. J Immunol. (2004) 173:5156–64. doi: 10.4049/jimmunol.173.8.5156
120. Powolny-Budnicka I, Riemann M, Tanzer S, Schmid RM, Hehlgans T, Weih F. Rela and relb transcription factors in distinct thymocyte populations control lymphotoxin-dependent interleukin-17 production in gammadelta T cells. Immunity. (2011) 34:364–74. doi: 10.1016/j.immuni.2011.02.019
121. Irla M. Instructive cues of thymic T cell selection. Annu Rev Immunol. (2022) 40:95–119. doi: 10.1146/annurev-immunol-101320-022432
122. Dikiy S, Rudensky AY. Principles of regulatory T cell function. Immunity. (2023) 56:240–55. doi: 10.1016/j.immuni.2023.01.004
123. Sakaguchi S, Mikami N, Wing JB, Tanaka A, Ichiyama K, Ohkura N. Regulatory T cells and human disease. Annu Rev Immunol. (2020) 38:541–66. doi: 10.1146/annurev-immunol-042718-041717
124. Medoff BD, Sandall BP, Landry A, Nagahama K, Mizoguchi A, Luster AD, et al. Differential requirement for carma1 in agonist-selected T-cell development. Eur J Immunol. (2009) 39:78–84. doi: 10.1002/eji.200838734
125. Gupta S, Manicassamy S, Vasu C, Kumar A, Shang W, Sun Z. Differential requirement of pkc-theta in the development and function of natural regulatory T cells. Mol Immunol. (2008) 46:213–24. doi: 10.1016/j.molimm.2008.08.275
126. Schmidt-Supprian M, Tian J, Grant EP, Pasparakis M, Maehr R, Ovaa H, et al. Differential dependence of cd4+Cd25+ Regulatory and natural killer-like T cells on signals leading to nf-kappab activation. Proc Natl Acad Sci U S A. (2004) 101:4566–71. doi: 10.1073/pnas.0400885101
127. Wan YY, Chi H, Xie M, Schneider MD, Flavell RA. The kinase tak1 integrates antigen and cytokine receptor signaling for T cell development, survival and function. Nat Immunol. (2006) 7:851–8. doi: 10.1038/ni1355
128. Long M, Park SG, Strickland I, Hayden MS, Ghosh S. Nuclear factor-kappab modulates regulatory T cell development by directly regulating expression of foxp3 transcription factor. Immunity. (2009) 31:921–31. doi: 10.1016/j.immuni.2009.09.022
129. Mahmud SA, Manlove LS, Schmitz HM, Xing Y, Wang Y, Owen DL, et al. Costimulation via the tumor-necrosis factor receptor superfamily couples tcr signal strength to the thymic differentiation of regulatory T cells. Nat Immunol. (2014) 15:473–81. doi: 10.1038/ni.2849
130. Vasanthakumar A, Liao Y, Teh P, Pascutti MF, Oja AE, Garnham AL, et al. The tnf receptor superfamily-nf-kappab axis is critical to maintain effector regulatory T cells in lymphoid and non-lymphoid tissues. Cell Rep. (2017) 20:2906–20. doi: 10.1016/j.celrep.2017.08.068
131. Hovelmeyer N, Schmidt-Supprian M, Ohnmacht C. Nf-kappab in control of regulatory T cell development, identity, and function. J Mol Med (Berl). (2022) 100:985–95. doi: 10.1007/s00109-022-02215-1
132. Zheng Y, Josefowicz S, Chaudhry A, Peng XP, Forbush K, Rudensky AY. Role of conserved non-coding DNA elements in the foxp3 gene in regulatory T-cell fate. Nature. (2010) 463:808–12. doi: 10.1038/nature08750
133. Shi H, Liu C, Tan H, Li Y, Nguyen TM, Dhungana Y, et al. Hippo kinases mst1 and mst2 sense and amplify il-2r-stat5 signaling in regulatory T cells to establish stable regulatory activity. Immunity. (2018) 49:899–914 e6. doi: 10.1016/j.immuni.2018.10.010
134. Cui Y, Benamar M, Schmitz-Abe K, Poondi-Krishnan V, Chen Q, Jugder BE, et al. A stk4-foxp3-nf-kappab P65 transcriptional complex promotes T(Reg) cell activation and homeostasis. Sci Immunol. (2022) 7:eabl8357. doi: 10.1126/sciimmunol.abl8357
135. Grinberg-Bleyer Y, Caron R, Seeley JJ, De Silva NS, Schindler CW, Hayden MS, et al. The alternative nf-kappab pathway in regulatory T cell homeostasis and suppressive function. J Immunol. (2018) 200:2362–71. doi: 10.4049/jimmunol.1800042
136. Murray SE, Polesso F, Rowe AM, Basak S, Koguchi Y, Toren KG, et al. Nf-kappab-inducing kinase plays an essential T cell-intrinsic role in graft-versus-host disease and lethal autoimmunity in mice. J Clin Invest. (2011) 121:4775–86. doi: 10.1172/JCI44943
137. Yi Z, Lin WW, Stunz LL, Bishop GA. The adaptor traf3 restrains the lineage determination of thymic regulatory T cells by modulating signaling via the receptor for il-2. Nat Immunol. (2014) 15:866–74. doi: 10.1038/ni.2944
138. Lu LF, Gondek DC, Scott ZA, Noelle RJ. Nf kappa B-inducing kinase deficiency results in the development of a subset of regulatory T cells, which shows a hyperproliferative activity upon glucocorticoid-induced tnf receptor family-related gene stimulation. J Immunol. (2005) 175:1651–7. doi: 10.4049/jimmunol.175.3.1651
139. Shimizu J, Yamazaki S, Takahashi T, Ishida Y, Sakaguchi S. Stimulation of cd25(+)Cd4(+) regulatory T cells through gitr breaks immunological self-tolerance. Nat Immunol. (2002) 3:135–42. doi: 10.1038/ni759
140. Park SG, Mathur R, Long M, Hosh N, Hao L, Hayden MS, et al. T regulatory cells maintain intestinal homeostasis by suppressing gammadelta T cells. Immunity. (2010) 33:791–803. doi: 10.1016/j.immuni.2010.10.014
141. Zanin-Zhorov A, Ding Y, Kumari S, Attur M, Hippen KL, Brown M, et al. Protein kinase C-theta mediates negative feedback on regulatory T cell function. Science. (2010) 328:372–6. doi: 10.1126/science.1186068
142. Roybal KT, Wulfing C. Inhibiting the inhibitor of the inhibitor: blocking pkc-theta to enhance regulatory T cell function. Sci Signal. (2010) 3:pe24. doi: 10.1126/scisignal.3132pe24
143. Boschelli DH. Small molecule inhibitors of pkctheta as potential antiinflammatory therapeutics. Curr Top Med Chem. (2009) 9:640–54. doi: 10.2174/156802609789007372
144. Chang JH, Xiao Y, Hu H, Jin J, Yu J, Zhou X, et al. Ubc13 maintains the suppressive function of regulatory T cells and prevents their conversion into effector-like T cells. Nat Immunol. (2012) 13:481–90. doi: 10.1038/ni.2267
145. Collins PE, Kiely PA, Carmody RJ. Inhibition of transcription by B cell leukemia 3 (Bcl-3) protein requires interaction with nuclear factor kappab (Nf-kappab) P50. J Biol Chem. (2014) 289:7059–67. doi: 10.1074/jbc.M114.551986
146. Reissig S, Tang Y, Nikolaev A, Gerlach K, Wolf C, Davari K, et al. Elevated levels of bcl-3 inhibits treg development and function resulting in spontaneous colitis. Nat Commun. (2017) 8:15069. doi: 10.1038/ncomms15069
147. Tang W, Saret S, Tian R, Wang H, Claudio E, Murphy PM, et al. Bcl-3 suppresses differentiation of rorgammat(+) regulatory T cells. Immunol Cell Biol. (2021) 99:586–95. doi: 10.1111/imcb.12441
148. MaruYama T, Kobayashi S, Ogasawara K, Yoshimura A, Chen W, Muta T. Control of ifn-gamma production and regulatory function by the inducible nuclear protein ikappab-zeta in T cells. J Leukoc Biol. (2015) 98:385–93. doi: 10.1189/jlb.2A0814-384R
149. Jameson SC, Masopust D. Diversity in T cell memory: an embarrassment of riches. Immunity. (2009) 31:859–71. doi: 10.1016/j.immuni.2009.11.007
150. Hayward SL, Scharer CD, Cartwright EK, Takamura S, Li Z-RT, Boss JM, et al. Environmental cues regulate epigenetic reprogramming of airway-resident memory cd8+ T cells. Nat Immunol. (2020) 21:309–20. doi: 10.1038/s41590-019-0584-x
151. Slutter B, Pewe LL, Kaech SM, Harty JT. Lung airway-surveilling cxcr3(Hi) memory cd8(+) T cells are critical for protection against influenza a virus. Immunity. (2013) 39:939–48. doi: 10.1016/j.immuni.2013.09.013
152. Wu T, Hu Y, Lee YT, Bouchard KR, Benechet A, Khanna K, et al. Lung-resident memory cd8 T cells (Trm) are indispensable for optimal cross-protection against pulmonary virus infection. J Leukoc Biol. (2014) 95:215–24. doi: 10.1189/jlb.0313180
153. Pizzolla A, Nguyen THO, Smith JM, Brooks AG, Kedzieska K, Heath WR, et al. Resident memory cd8(+) T cells in the upper respiratory tract prevent pulmonary influenza virus infection. Sci Immunol. (2017) 2. doi: 10.1126/sciimmunol.aam6970
154. Uddback I, Michalets SE, Saha A, Mattingly C, Kost KN, Williams ME, et al. Prevention of respiratory virus transmission by resident memory cd8(+) T cells. Nature. (2024) 626:392–400. doi: 10.1038/s41586-023-06937-1
155. Christian LS, Wang L, Lim B, Deng D, Wu H, Wang XF, et al. Resident memory T cells in tumor-distant tissues fortify against metastasis formation. Cell Rep. (2021) 35:109118. doi: 10.1016/j.celrep.2021.109118
156. Okla K, Farber DL, Zou W. Tissue-resident memory T cells in tumor immunity and immunotherapy. J Exp Med. (2021) 218. doi: 10.1084/jem.20201605
157. Molodtsov AK, Khatwani N, Vella JL, Lewis KA, Zhao Y, Han J, et al. Resident memory cd8(+) T cells in regional lymph nodes mediate immunity to metastatic melanoma. Immunity. (2021) 54:2117–32 e7. doi: 10.1016/j.immuni.2021.08.019
158. Han J, Zhao Y, Shirai K, Molodtsov A, Kolling FW, Fisher JL, et al. Resident and circulating memory T cells persist for years in melanoma patients with durable responses to immunotherapy. Nat Cancer. (2021) 2:300–11. doi: 10.1038/s43018-021-00180-1
159. Courtois G, Gilmore TD. Mutations in the nf-kappab signaling pathway: implications for human disease. Oncogene. (2006) 25:6831–43. doi: 10.1038/sj.onc.1209939
160. Dabbah-Krancher G, Snow AL. Mistuned nf-kappab signaling in lymphocytes: lessons from relevant inborn errors of immunity. Clin Exp Immunol. (2023) 212:117–28. doi: 10.1093/cei/uxad006
161. Teixeiro E, Daniels MA, Hausmann B, Schrum AG, Naeher D, Luescher I, et al. T cell division and death are segregated by mutation of tcrbeta chain constant domains. Immunity. (2004) 21:515–26. doi: 10.1016/j.immuni.2004.08.014
162. Knudson KM, Pritzl CJ, Saxena V, Altman A, Daniels MA, Teixeiro E. Nfkappab-pim-1-eomesodermin axis is critical for maintaining cd8 T-cell memory quality. Proc Natl Acad Sci U S A. (2017) 114:E1659–E67. doi: 10.1073/pnas.1608448114
163. Li Y, Wang H, Zhou X, Xie X, Chen X, Jie Z, et al. Cell intrinsic role of nf-kappab-inducing kinase in regulating T cell-mediated immune and autoimmune responses. Sci Rep. (2016) 6:22115. doi: 10.1038/srep22115
164. Rowe AM, Murray SE, Raue HP, Koguchi Y, Slifka MK, Parker DC. A cell-intrinsic requirement for nf-kappab-inducing kinase in cd4 and cd8 T cell memory. J Immunol. (2013) 191:3663–72. doi: 10.4049/jimmunol.1301328
165. Zhou AC, Batista NV, Watts TH. 4-1bb regulates effector cd8 T cell accumulation in the lung tissue through a traf1-, mtor-, and antigen-dependent mechanism to enhance tissue-resident memory T cell formation during respiratory influenza infection. J Immunol. (2019) 202:2482–92. doi: 10.4049/jimmunol.1800795
166. Chu KL, Batista NV, Wang KC, Zhou AC, Watts TH. Gitrl on inflammatory antigen presenting cells in the lung parenchyma provides signal 4 for T-cell accumulation and tissue-resident memory T-cell formation. Mucosal Immunol. (2019) 12:363–77. doi: 10.1038/s41385-018-0105-5
167. Sun SC. The non-canonical nf-kappab pathway in immunity and inflammation. Nat Rev Immunol. (2017) 17:545–58. doi: 10.1038/nri.2017.52
168. Peperzak V, Veraar EA, Keller AM, Xiao Y, Borst J. The pim kinase pathway contributes to survival signaling in primed cd8+ T cells upon cd27 costimulation. J Immunol. (2010) 185:6670–8. doi: 10.4049/jimmunol.1000159
169. Pritzl CJ, Luera D, Knudson KM, Quaney MJ, Calcutt MJ, Daniels MA, et al. Ikk2/nfkb signaling controls lung resident cd8(+) T cell memory during influenza infection. Nat Commun. (2023) 14:4331. doi: 10.1038/s41467-023-40107-1
170. Hombrink P, Helbig C, Backer RA, Piet B, Oja AE, Stark R, et al. Programs for the persistence, vigilance and control of human cd8(+) lung-resident memory T cells. Nat Immunol. (2016) 17:1467–78. doi: 10.1038/ni.3589
171. Kurd NS, He Z, Louis TL, Milner JJ, Omilusik KD, Jin W, et al. Early precursors and molecular determinants of tissue-resident memory cd8(+) T lymphocytes revealed by single-cell rna sequencing. Sci Immunol. (2020) 5. doi: 10.1126/sciimmunol.aaz6894
172. Goplen NP, Wu Y, Son YM, Li C, Wang Z, Cheon IS, et al. Tissue-resident cd8(+) T cells drive age-associated chronic lung sequelae after viral pneumonia. Sci Immunol. (2020) 5. doi: 10.1126/sciimmunol.abc4557
173. Schnell A, Bod L, Madi A, Kuchroo VK. The yin and yang of co-inhibitory receptors: toward anti-tumor immunity without autoimmunity. Cell Res. (2020) 30:285–99. doi: 10.1038/s41422-020-0277-x
174. Schenkel JM, Pauken KE. Localization, tissue biology and T cell state - implications for cancer immunotherapy. Nat Rev Immunol. (2023) 23:807–23. doi: 10.1038/s41577-023-00884-8
175. Zehn D, Thimme R, Lugli E, de Almeida GP, Oxenius A. ‘Stem-like’ Precursors are the fount to sustain persistent cd8(+) T cell responses. Nat Immunol. (2022) 23:836–47. doi: 10.1038/s41590-022-01219-w
176. Jadhav RR, Im SJ, Hu B, Hashimoto M, Li P, Lin JX, et al. Epigenetic signature of pd-1+ Tcf1+ Cd8 T cells that act as resource cells during chronic viral infection and respond to pd-1 blockade. Proc Natl Acad Sci U S A. (2019) 116:14113–8. doi: 10.1073/pnas.1903520116
177. Chen Y, Zander RA, Wu X, Schauder DM, Kasmani MY, Shen J, et al. Batf regulates progenitor to cytolytic effector cd8(+) T cell transition during chronic viral infection. Nat Immunol. (2021) 22:996–1007. doi: 10.1038/s41590-021-00965-7
178. Yao X, Hong JH, Nargund AM, Ng MSW, Heng HL, Li Z, et al. Pbrm1-deficient pbaf complexes target aberrant genomic loci to activate the nf-kappab pathway in clear cell renal cell carcinoma. Nat Cell Biol. (2023) 25:765–77. doi: 10.1038/s41556-023-01122-y
179. Sima J, Yan Z, Chen Y, Lehrmann E, Zhang Y, Nagaraja R, et al. Eda-activated relb recruits an swi/snf (Baf) chromatin-remodeling complex and initiates gene transcription in skin appendage formation. Proc Natl Acad Sci U S A. (2018) 115:8173–8. doi: 10.1073/pnas.1800930115
180. Pichler AC, Carrie N, Cuisinier M, Ghazali S, Voisin A, Axisa PP, et al. Tcr-independent cd137 (4-1bb) signaling promotes cd8(+)-exhausted T cell proliferation and terminal differentiation. Immunity. (2023) 56:1631–48 e10. doi: 10.1016/j.immuni.2023.06.007
181. Mulero MC, Wang VY, Huxford T, Ghosh G. Genome reading by the nf-kappab transcription factors. Nucleic Acids Res. (2019) 47:9967–89. doi: 10.1093/nar/gkz739
182. Ghosh S, Hayden MS. Celebrating 25 years of nf-kappab research. Immunol Rev. (2012) 246:5–13. doi: 10.1111/j.1600-065X.2012.01111.x
183. Brignall R, Moody AT, Mathew S, Gaudet S. Considering abundance, affinity, and binding site availability in the nf-kappab target selection puzzle. Front Immunol. (2019) 10:609. doi: 10.3389/fimmu.2019.00609
184. Pace L, Amigorena S. Epigenetics of T cell fate decision. Curr Opin Immunol. (2020) 63:43–50. doi: 10.1016/j.coi.2020.01.002
185. Dutta A, Venkataganesh H, Love PE. New insights into epigenetic regulation of T cell differentiation. Cells. (2021) 10. doi: 10.3390/cells10123459
186. Frias AB, Boi SK, Lan X, Youngblood B. Epigenetic regulation of T cell adaptive immunity. Immunol Rev. (2021) 300:9–21. doi: 10.1111/imr.12943
187. Dawson MA. The cancer epigenome: concepts, challenges, and therapeutic opportunities. Science. (2017) 355:1147–52. doi: 10.1126/science.aam7304
188. Lim PS, Li J, Holloway AF, Rao S. Epigenetic regulation of inducible gene expression in the immune system. Immunology. (2013) 139:285–93. doi: 10.1111/imm.12100
189. Torres IO, Fujimori DG. Functional coupling between writers, erasers and readers of histone and DNA methylation. Curr Opin Struct Biol. (2015) 35:68–75. doi: 10.1016/j.sbi.2015.09.007
190. Tessarz P, Kouzarides T. Histone core modifications regulating nucleosome structure and dynamics. Nat Rev Mol Cell Biol. (2014) 15:703–8. doi: 10.1038/nrm3890
191. Yang J, Tian B, Brasier AR. Targeting chromatin remodeling in inflammation and fibrosis. Adv Protein Chem Struct Biol. (2017) 107:1–36. doi: 10.1016/bs.apcsb.2016.11.001
192. Xiao X, Shi X, Fan Y, Wu C, Zhang X, Minze L, et al. The costimulatory receptor ox40 inhibits interleukin-17 expression through activation of repressive chromatin remodeling pathways. Immunity. (2016) 44:1271–83. doi: 10.1016/j.immuni.2016.05.013
193. Xiao X, Shi X, Fan Y, Zhang X, Wu M, Lan P, et al. Gitr subverts foxp3(+) tregs to boost th9 immunity through regulation of histone acetylation. Nat Commun. (2015) 6:8266. doi: 10.1038/ncomms9266
194. Xiao X, Fan Y, Li J, Zhang X, Lou X, Dou Y, et al. Guidance of super-enhancers in regulation of il-9 induction and airway inflammation. J Exp Med. (2018) 215:559–74. doi: 10.1084/jem.20170928
195. Nakshatri H, Appaiah HN, Anjanappa M, Gilley D, Tanaka H, Badve S, et al. Nf-kappab-dependent and -independent epigenetic modulation using the novel anti-cancer agent dmapt. Cell Death Dis. (2015) 6:e1608. doi: 10.1038/cddis.2014.569
196. Feng AC, Thomas BJ, Purbey PK, de Melo FM, Liu X, Daly AE, et al. The transcription factor nf-kappab orchestrates nucleosome remodeling during the primary response to toll-like receptor 4 signaling. Immunity. (2024) 57:462–77 e9. doi: 10.1016/j.immuni.2024.02.004
197. Buck MD, Sowell RT, Kaech SM, Pearce EL. Metabolic instruction of immunity. Cell. (2017) 169:570–86. doi: 10.1016/j.cell.2017.04.004
198. Geltink RIK, Kyle RL, Pearce EL. Unraveling the complex interplay between T cell metabolism and function. Annu Rev Immunol. (2018) 36:461–88. doi: 10.1146/annurev-immunol-042617-053019
199. Gu M, Zhou X, Sohn JH, Zhu L, Jie Z, Yang JY, et al. Nf-kappab-inducing kinase maintains T cell metabolic fitness in antitumor immunity. Nat Immunol. (2021) 22:193–204. doi: 10.1038/s41590-020-00829-6
200. Hamilton KS, Phong B, Corey C, Cheng J, Gorentla B, Zhong X, et al. T cell receptor-dependent activation of mtor signaling in T cells is mediated by carma1 and malt1, but not bcl10. Sci Signal. (2014) 7:ra55. doi: 10.1126/scisignal.2005169
201. Pearce EL, Walsh MC, Cejas PJ, Harms GM, Shen H, Wang LS, et al. Enhancing cd8 T-cell memory by modulating fatty acid metabolism. Nature. (2009) 460:103–7. doi: 10.1038/nature08097
202. Dan HC, Cooper MJ, Cogswell PC, Duncan JA, Ting JP, Baldwin AS. Akt-dependent regulation of nf-kappab is controlled by mtor and raptor in association with ikk. Genes Dev. (2008) 22:1490–500. doi: 10.1101/gad.1662308
203. Hoffmann A, Levchenko A, Scott ML, Baltimore D. The ikappab-nf-kappab signaling module: temporal control and selective gene activation. Science. (2002) 298:1241–5. doi: 10.1126/science.1071914
204. Nelson DE, Ihekwaba AE, Elliott M, Johnson JR, Gibney CA, Foreman BE, et al. Oscillations in nf-kappab signaling control the dynamics of gene expression. Science. (2004) 306:704–8. doi: 10.1126/science.1099962
205. O’Dea E, Hoffmann A. The regulatory logic of the nf-kappab signaling system. Cold Spring Harb Perspect Biol. (2010) 2:a000216. doi: 10.1101/cshperspect.a000216
206. Covert MW, Leung TH, Gaston JE, Baltimore D. Achieving stability of lipopolysaccharide-induced nf-kappab activation. Science. (2005) 309:1854–7. doi: 10.1126/science.1112304
207. Lee TK, Denny EM, Sanghvi JC, Gaston JE, Maynard ND, Hughey JJ, et al. A noisy paracrine signal determines the cellular nf-kappab response to lipopolysaccharide. Sci Signal. (2009) 2:ra65. doi: 10.1126/scisignal.2000599
208. Inoue K, Shinohara H, Behar M, Yumoto N, Tanaka G, Hoffmann A, et al. Oscillation dynamics underlie functional switching of nf-kappab for B-cell activation. NPJ Syst Biol Appl. (2016) 2:16024. doi: 10.1038/npjsba.2016.24
209. Chen Y, Shen J, Kasmani MY, Topchyan P, Cui W. Single-cell transcriptomics reveals core regulatory programs that determine the heterogeneity of circulating and tissue-resident memory cd8(+) T cells. Cells. (2021) 10. doi: 10.3390/cells10082143
210. Iwai K, Tokunaga F. Linear polyubiquitination: A new regulator of nf-kappab activation. EMBO Rep. (2009) 10:706–13. doi: 10.1038/embor.2009.144
211. Lork M, Verhelst K, Beyaert R. Cyld, A20 and otulin deubiquitinases in nf-kappab signaling and cell death: so similar, yet so different. Cell Death Differ. (2017) 24:1172–83. doi: 10.1038/cdd.2017.46
212. Fusco AJ, Mazumder A, Wang VY, Tao Z, Ware C, Ghosh G. The nf-kappab subunit relb controls P100 processing by competing with the kinases nik and ikk1 for binding to P100. Sci Signal. (2016) 9:ra96. doi: 10.1126/scisignal.aad9413
213. Maminska A, Bartosik A, Banach-Orlowska M, Pilecka I, Jastrzebski K, Zdzalik-Bielecka D, et al. Escrt proteins restrict constitutive nf-kappab signaling by trafficking cytokine receptors. Sci Signal. (2016) 9:ra8. doi: 10.1126/scisignal.aad0848
214. Ma X, Becker Buscaglia LE, Barker JR, Li Y. Micrornas in nf-kappab signaling. J Mol Cell Biol. (2011) 3:159–66. doi: 10.1093/jmcb/mjr007
215. Mao X, Su Z, Mookhtiar AK. Long non-coding rna: A versatile regulator of the nuclear factor-kappab signalling circuit. Immunology. (2017) 150:379–88. doi: 10.1111/imm.12698
216. Grinberg-Bleyer Y, Oh H, Desrichard A, Bhatt DM, Caron R, Chan TA, et al. Nf-kappab C-rel is crucial for the regulatory T cell immune checkpoint in cancer. Cell. (2017) 170:1096–108 e13. doi: 10.1016/j.cell.2017.08.004
217. Di Pilato M, Kim EY, Cadilha BL, Prussmann JN, Nasrallah MN, Seruggia D, et al. Targeting the cbm complex causes T(Reg) cells to prime tumours for immune checkpoint therapy. Nature. (2019) 570:112–6. doi: 10.1038/s41586-019-1215-2
218. Evaristo C, Spranger S, Barnes SE, Miller ML, Molinero LL, Locke FL, et al. Cutting edge: engineering active ikkbeta in T cells drives tumor rejection. J Immunol. (2016) 196:2933–8. doi: 10.4049/jimmunol.1501144
219. Giordano M, Roncagalli R, Bourdely P, Chasson L, Buferne M, Yamasaki S, et al. The tumor necrosis factor alpha-induced protein 3 (Tnfaip3, A20) imposes a brake on antitumor activity of cd8 T cells. Proc Natl Acad Sci U S A. (2014) 111:11115–20. doi: 10.1073/pnas.1406259111
220. Ramadass V, Vaiyapuri T, Tergaonkar V. Small molecule nf-kappab pathway inhibitors in clinic. Int J Mol Sci. (2020) 21. doi: 10.3390/ijms21145164
221. Aradhya S, Nelson DL. Nf-kappab signaling and human disease. Curr Opin Genet Dev. (2001) 11:300–6. doi: 10.1016/s0959-437x(00)00194-5
222. Fliegauf M, Grimbacher B. Nuclear factor kappab mutations in human subjects: the devil is in the details. J Allergy Clin Immunol. (2018) 142:1062–5. doi: 10.1016/j.jaci.2018.06.050
Keywords: NF-kB, T cell, signaling, differentiation, network
Citation: Daniels MA and Teixeiro E (2025) The NF-κB signaling network in the life of T cells. Front. Immunol. 16:1559494. doi: 10.3389/fimmu.2025.1559494
Received: 12 January 2025; Accepted: 07 April 2025;
Published: 30 April 2025.
Edited by:
Luca Simeoni, University Hospital Magdeburg, GermanyReviewed by:
Yanchuan Li, Nanjing Medical University, ChinaIngo Schmitz, Ruhr University Bochum, Germany
Copyright © 2025 Daniels and Teixeiro. This is an open-access article distributed under the terms of the Creative Commons Attribution License (CC BY). The use, distribution or reproduction in other forums is permitted, provided the original author(s) and the copyright owner(s) are credited and that the original publication in this journal is cited, in accordance with accepted academic practice. No use, distribution or reproduction is permitted which does not comply with these terms.
*Correspondence: Emma Teixeiro, dGVpeGVpcm9wZXJuYXNlQG1pc3NvdXJpLmVkdQ==