- 1Department of Parasitology, Pasteur Institute of Iran, Tehran, Iran
- 2Department of Medical Genetics, Third Faculty of Medicine, Charles University, Prague, Czechia
- 3Institute of Molecular Genetics, Czech Academy of Sciences, Prague, Czechia
- 4Department of Cardiology I-Coronary and Peripheral Vascular Disease, Heart Failure, University Hospital Münster, University of Münster, Münster, Germany
Leishmaniasis is a complex disease caused by protozoan parasites of the genus Leishmania, which are transmitted by phlebotomine sand flies. The clinical manifestations of leishmaniasis are diverse, ranging from self-healing cutaneous lesions to fatal systemic disease. Mouse models are instrumental in advancing our understanding of the immune system against infections, yet their limitations in translating findings to humans are increasingly highlighted. The success rate of translating data from mice to humans remains low, largely due to the complexity of diseases and the numerous factors that influence the disease outcomes. Therefore, for the effective translation of data from murine models of leishmaniasis, it is essential to align experimental conditions with those relevant to human infection. Factors such as parasite characteristics, vector-derived components, host status, and environmental conditions must be carefully considered and adapted to enhance the translational relevance of mouse data. These parameters are potentially modifiable and should be carefully integrated into the design and interpretation of experimental procedures in Leishmania studies. In the current paper, we review the challenges and perspective of using mouse as a model for leishmaniasis. We have particularly emphasized the non-genetic factors that influence experiments and focused on strategies to improve translational value of studies on leishmaniasis using mouse models.
1 Introduction
Leishmaniasis is a complex disease caused by protozoan parasites from more than 20 Leishmania species, which are transmitted by over 90 different species of phlebotomine sand flies (1, 2). Among over 800 species of sand flies recorded, 98 are proven or suspected vectors of human leishmaniases; these include 42 Phlebotomus species in the Old World and 56 Lutzomyia species in the New World (all: Diptera: Psychodidae) (3). The inoculated parasites infect the so-called professional phagocytes (neutrophils, monocytes, and macrophages), as well as dendritic cells and fibroblasts (4–6). Leishmaniasis affects various mammalian hosts, offering diverse opportunities to study immunopathology, genetic control, and host-parasite interactions using animal models. The clinical manifestations of leishmaniasis differ significantly, from self-healing cutaneous lesion to severe systemic disease in humans and asymptomatic infections in many mammals. This variability presents significant challenges in selecting appropriate animal models, which must be carefully aligned with the specific objectives of the study. Various models, including mice, hamsters, dogs, and non-human primates, have been developed to investigate leishmaniasis’ pathology, disease mechanisms, and potential therapeutic or vaccine candidates (7–11). Among these, the mouse has emerged as the most prominent model due to its genetic tractability, short lifespan, and physiological similarities to humans.
Infection with Leishmania species manifests in a wide spectrum of clinical forms, including cutaneous leishmaniasis (CL), mucocutaneous leishmaniasis (MCL), visceral leishmaniasis (VL), and post-kala-azar dermal leishmaniasis (PKDL) types (Table 1). CL is the most common form of disease that typically begins with the formation of a papule at the site of a female sand fly bite, which progressively enlarges and develops into a nodule or probably to a painless open ulcer. While CL is normally a self-healing disease with spontaneous cure without any treatment, the course of infection can vary depending on the host’s immune response and the infecting Leishmania species. MCL arises as a metastatic complication of CL when parasites disseminate through the lymphatic system to the mucosal tissues resulting in destruction and disfigurement. VL is the most severe form of leishmaniasis associated with high fatality rate without proper treatment. Most cases of VL do not show clinical disease and remain asymptomatic, but in active VL the parasites spread to reticuloendothelial system (RES). This leads to systemic disease characterized by prolonged fever, weight loss, hepatosplenomegaly and anemia. PKDL emerges as a complication in some patients who recovered from VL in endemic areas. PKDL is characterized by macular, maculopapular, and nodular rash. PKDL patients serve as a significant reservoir for disease transmission.
It is important to note that the majority of Leishmania infections in humans, particularly in VL cases, remain asymptomatic (12, 13). In endemic regions, most individuals exposed to the parasite are able to control the infection through induction of a robust protective immune response and development of immunological memory (4, 14). Studies in both mice and humans indicate that the outcome of Leishmania infection is influenced by a complex interplay between host factors, parasite-specific characteristics and environmental conditions (15–18). Mice and human share more than 90% of their genome. Mice are easy to breed and maintain, they are useful tools for genetic manipulation and conditional experiments that are usually not possible in human (19). However, despite considerable similarities and numerous advantages, significant differences in their physiology and genetics also exist. These differences, together with environmental factors, influence potential of mouse models to accurately mimic human diseases. For example, mice have a shorter life span and different physiological characteristics, such as hearth beat, body temperature, active/sleeping time, diet, and microbiota composition. In addition, mice are used as a model for some diseases that naturally do not occur in mice such as leishmaniasis. There is a significant overlap in the clinical manifestation of leishmaniasis between mice and human, however, mice and human also exhibit considerable differences in developing the symptoms. For instance, infection with L. major usually does not visceralize in human, while parasite disseminates to visceral organs in susceptible mice. Furthermore, in order to increase the translational capacity of mouse data, influencing parameters should be well characterized, carefully adapted, and considered when designing an experiment and interpreting results in Leishmania studies. Animal models including mouse, golden hamster, dog and monkey have been used in vivo testing of new antileishmanial agent (20–27). To evaluate drug efficacy, choosing an animal with closer evolutionary relatedness might be better. On the other side, mouse model provides fast answer to evaluate some parameters such as toxicity and dose response. According to recent data mouse models are by far the most commonly used animal model for antileishmanial drug discovery (27, 28). Several compounds such as miltefosine, amphotericin B etc. have been tested in mouse models (28–31). Depending on drug formulation, administration route and treatment protocol varies from topical, oral administration or inoculation (30–33). Due to the distinct phylogeny and differences between human and mice, the data may not be predictive of the response in human because compounds may show an effective response in animal model, but has no or very low efficacy in human (34). In addition, in human treatment starts when the clinical symptoms appears, whereas, in mouse animal model particular in mice, treatment usually starts only week or weeks after parasite challenge, which might result in different result than in human (10). The current paper provides comprehensive information of non-genetic influencing factors that limit the translational value of mouse models and offers strategies to improve their relevance to human leishmaniasis.
2 Host genetics
Leishmaniasis is a complex disease with pathogenesis influenced by various factors, including environmental conditions, insect vector, and genetic makeup of both the parasite and the host. Host genetics is especially intriguing because clinical outcomes can differ greatly among patients infected with the same Leishmania species and sharing similar non-genetic factors (35).
Mice are the most widely used model in identifying genetic control of leishmaniasis. The genetic control of susceptibility to various Leishmania species in mouse models has been extensively studied, with several loci linked to disease outcomes. Interestingly, many of the susceptibility genes or loci identified in mice overlap with human genes that play a role in regulating disease severity (36–48). Genome-wide linkage analysis identified more than thirty loci that control susceptibility to Leishmania infection in mice (9), but only two of them have been translated to human. The role of Nramp1/Slc11a1 that is linked to leishmaniasis in mice (49) has also been proved in human (50). Wound healing related gene Fli1 that influences cutaneous leishmaniasis caused by L. major in mouse (51) has impact on susceptibility to L. braziliensis caused CL in human (52).
One of the main reasons for low degree of translational potential of mouse to human concerning genetics studies is the low polymorphic complexity of mouse genome in comparison to highly heterogenic human genome. Genetic studies have been mostly performed on a limited number of inbred strains that do not mimic the high genetic polymorphism observed in the human genome. On one hand, the lower genetic complexity of inbred strains offers an advantage to study mechanism of the diseases; but on the other hand, it fails to show the network of gene-gene interactions in the human genome that play a crucial role in the disease control. Using tools such as crossing two inbreed strains improved the efficiency of mapping of complex quantitative trait loci (QTLs) revealed the network of gene-gene interactions (9, 37, 40, 41, 43, 44, 46, 47, 48). In addition, murine models do not fully recapitulate the complexity of human disease, in part due to intricate interactions between host genetics and environmental factors. This limitation can be partially addressed by employing a broader range of mouse strains with diverse genetic backgrounds (including wild-derived strains), optimization of experimental conditions to reduce limiting factors should be considered. In contrast to mice especially SPF kept mice, human population are heavily encountered with different infections in daily basis, therefore, the genetic polymorphism associated to susceptibility to infectious diseases are under selective pressure (53). The genetic control of leishmaniasis and influence of the host genetic factors in pathogenesis of leishmaniasis has been thoroughly discussed elsewhere (under review).
3 Non-genetic parameters influencing Leishmania infection
A significant proportion of data on the mechanisms of parasite pathogenesis and host immune responses have been collected from animal models. Although the data generated from the experimental models are pivotal, translating the results obtained from experimental studies to human is challenging. Primary goal in developing animal models has often focused on replicating human-like phenotypes, which can differ significantly from their natural forms. Moreover, the infection in experimental models is influenced by various factors such as parasite species (2) and sub-strains (54, 55), dose (56), injection route (57), genetic background of the host (41), sex (58, 59) and hormonal status (60), age (61), microbiome composition (62), as well as presence of other infections (63). In vitro and in vivo experiments show influence of culture conditions (64) and medium composition (65) on parasite infectivity (65) and virulence capabilities (64, 66). These parameters can be controlled and should be described in experimental protocols (Figure 1). In addition, there are differences between infection occurring in the natural cycle of the parasite and those under the experimental conditions. Factors associated with the vector, such as mosquito salivary gland components, must be considered when interpreting the results (67). In addition, there is also an evidence arguing that Leishmania infection in mice by injecting millions of promastigotes subcutaneously in the hind footpad or tail ramp does not reproduce the natural form of the disease, where small number of metacyclic parasites are introduced during a sand fly bite (57).
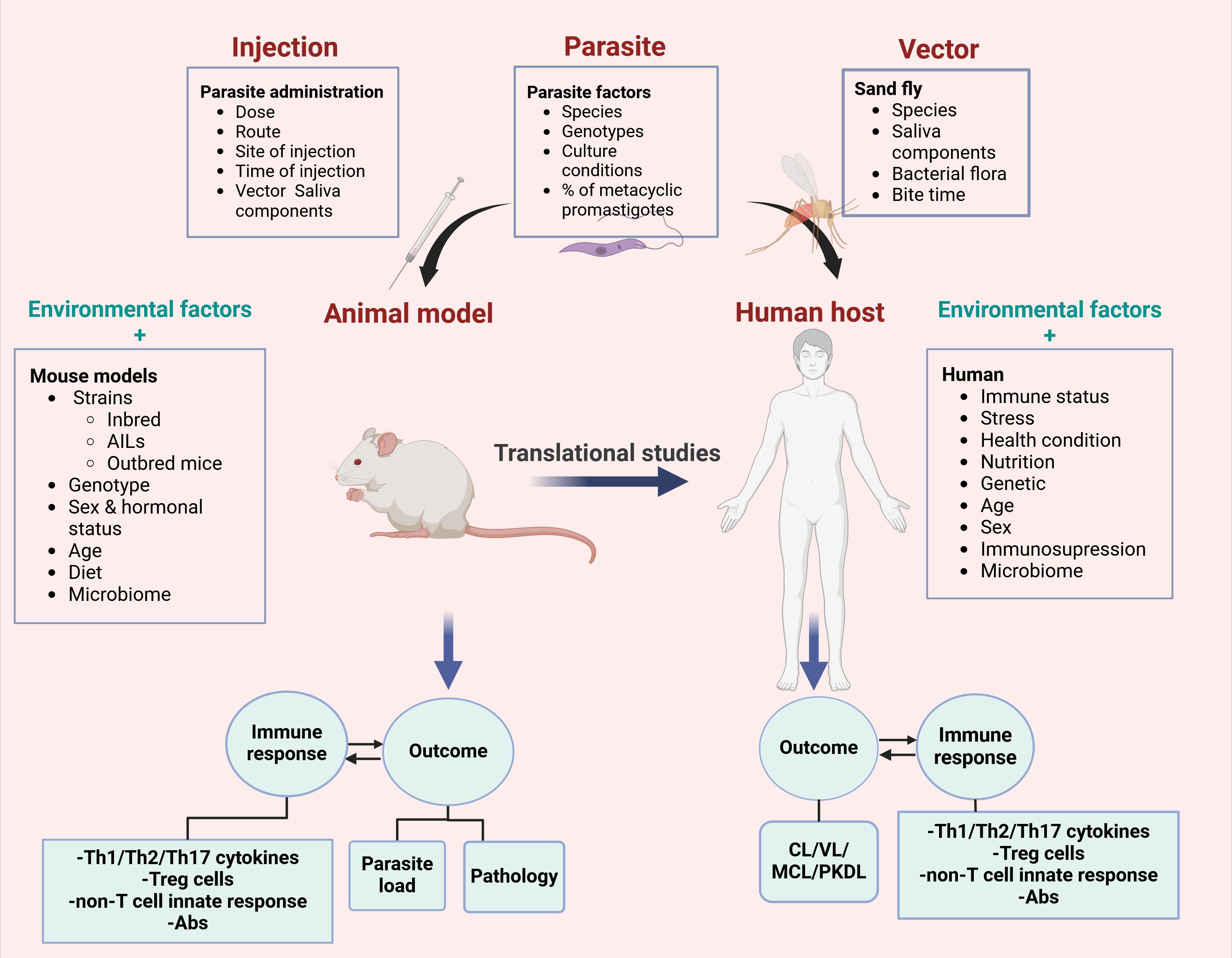
Figure 1. Factors that influence translational potential of mouse models to human leishmaniasis. Many parameters such as parasite, vector and host related factors might have significant impact on the disease outcome in mice and human. In addition to host factors and environment parameters, parasite, vector or inoculation can change the responses to the parasite infection. Therefore, these factors must be adapted and modified in order to increase translational value of mouse results.
3.1 Parasite factors
3.1.1 Cutaneous leishmaniasis: experimental considerations
Different species of Leishmania including L. major, L. tropica, L. mexicana, L. amazonensis, L. braziliensis and L. guyanensis cause CL in the New and Old Worlds (Table 1). Several experimental studies have shown determining role of both parasite dose and site of inoculation in the outcome of cutaneous Leishmania infection in mice with different genetic backgrounds. In addition, type of immune response against the parasites leads to different pathology in the mice.
3.1.1.1 Immune responses in control of the infection
Initial studies using the C57BL/6 and BALB/c mouse strains (Table 2) suggested that resistance (C57BL/6) or susceptibility (BALB/c) to L. major infection is associated with two types of Th1 and Th2 immune responses, respectively (8, 68, 69). It is noteworthy that the BALB/c susceptibility and C57BL/6 resistance model is primarily applicable to L. major infections, which have been the focus of most classical studies on the immune response in leishmaniasis. For other species of Leishmania, including the Viannia subgenus, the outcome of infection might be quite different; for example, typically most commonly used inbred mouse strains, including BALB/c mice, exhibit genetic resistance to L. braziliensis infection, resolving the infection within a few weeks. In contrast, the C57BL/6 strain develops a non-healing infection when infected with L. mexicana (Table 2) (70). For parasites in the Viannia subgenus, hamsters suggested as a more suitable model than mice, for the pathological study of localized and metastatic lesions (71).
Th1 response with production of IFNγ and IL-12 leads to lesion healing in resistant inbred mice (C57BL/6). Th1 type of cytokines particularly IFNγ, induce classically activated (M1) macrophages which initiate parasite killing. Macrophages produce two major anti-Leishmania components; reactive oxygen species (ROS) which is generated by respiratory burst during phagocytosis, and nitric oxide (NO), which is produced by Inducible nitric oxide synthase (iNOS) in response to IFNγ (4, 72, 73). Inhibition of the Th1 cells function by deleting the cytokines genes (IL-12, IFNγ, TNFα), their receptors (IFNγR), transcription factors (T-bet and STAT4) or co-stimulatory molecules (CD40–CD40L) lead to susceptibility to L. major infection (8). The role of ROS in controlling Leishmania infection in murine models varies depending on the parasite species and mouse strain. Unlike L. major infection, where ROS production plays a crucial role, in mouse models of L. braziliensis infection, ROS synthesis does not play a significant role in disease pathogenesis (74). Additionally, NO is a crucial factor in controlling Leishmania infection in mouse models and one of the key mechanisms through which IFN-γ enhances resistance to L. major infection is by stimulating iNOS expression in macrophages (75, 76) The role of iNOS/NO and ROS in human leishmaniasis remains less understood. While ROS production has been implicated in the killing of L. braziliensis by human macrophages, NO alone does not effectively control L. braziliensis infection in monocytes from CL patients in vitro (77). Studies have reported that NO production is undetectable in the supernatants of human macrophages infected with L. infantum; even though, in vitro inhibition of NO involved in parasite growth in these cells (78).
On the contrary, the Th2 response with the production of IL-4 leads to the expansion of the lesion and disseminated visceral infection in susceptible inbred mice (BALB/c). The activation of Th2 type cytokines, such as IL-4 and IL-13, drives the differentiation of alternatively activated macrophages (M2), which are characterized by elevated expression of Arg1 and enhanced polyamine biosynthesis. This metabolic shift creates a favorable environment for amastigote proliferation within macrophages, ultimately contributing to disease progression (79, 80).
In BALB/c mice, lymphocytes of a third group, Th17, play a role in the extension of the lesions by producing cytokines such as IL-17 and IL-22 and infiltrating the polymorphonuclear cells into the infection site (81, 82). IL-17 is a potent pro-inflammatory cytokine that modulates immune responses by stimulating the production of various cytokines, including IL-6, IL-8, and GM-CSF, as well as chemokines such as CXCL1 and CXCL10. Additionally, IL-17 is essential for recruitment and activation of neutrophils at infection sites (83, 84), which are exploited by Leishmania parasites as temporary host cells to evade macrophage-mediated immune mechanisms (85). Th17 cells have also been shown to produce IL-21, IL-22 as well as IL-23, which is essential for the terminal differentiation of IL-17 producing effector T cells (86). In L. major infected BALB/c mice, both Th17 cells and neutrophils produce significantly higher amounts of IL-17 in comparison to cells from resistant C57BL/6 mice (81). Leishmania-infected DCs have been shown to induce IL-23 secretion, which in turn may help in the production of Th17 cells in BALB/c mice (81). In human leishmaniasis, an increased number of IL-17-expressing cells in lesions of L. braziliensis-infected patients has been associated with a higher cellular infiltrate (87). Additionally, elevated IL-17 levels have been observed in PBMC culture supernatants of active American CL cases compared to recovered patients (88).
Although Th1/Th2 paradigm is well established in the resistant strain C57BL/6 and the susceptible strain BALB/c, in human, this paradigm seems to be more complex and might be different in other mice strains (89–91). In murine models of L. major infection, a well-established paradigm suggests that successful healing involved activation of phagocytic cells, expansion of CD4+ Th1 cells, production of key cytokines such as IFN-γ, suppression of the Th2 response, and polarization of M1 macrophages. This ultimately enhances macrophage-mediated parasite-killing mechanisms, including NO synthesis (70). Over the past years, numerous studies have investigated the role of Th1/Th2 responses in human leishmaniasis, characterizing the phenotype of T cells and their polarized cytokines in lesions, cell cultures, or plasma of leishmaniasis patients (91–93). While cytokines like IFNγ are believed to play a role in controlling parasite infection during the healing process of human CL lesions (16), the classic Th1/Th2 polarization and IFN-γ/IL-4 interplays described in murine L. major infections do not fully translate to human disease or to infections caused by other species including the Viannia subgenus (70, 94).
In addition, existence of non-healing phenotypes in spite of a Th1 response (95, 96) along with evidence that some vaccines induce Th1 type cytokines without significantly effecting organ pathology (97, 98), or achieving protection without a strong Th1 response (99) suggests involvement of additional immune mechanisms. On the other hand, a delicate balance between pro- and anti-inflammatory cytokines is essential for an effective wound healing and the resolution of CL/MCL lesions. While a Th1 immune response is generally protective, an overproduction of pro-inflammatory cytokines can drive excessive immune cell recruitment to the infection site, exacerbating inflammation and ultimately leading to tissue destruction and damage. Elevated levels of IL-10 and transforming growth factor (TGF)-β help counteract this effect by suppressing pro-inflammatory cytokines, such as IFNγ, thereby mitigating inflammation and preventing tissue damage (100, 101). Studies have shown that IL-10 levels increase in PBMCs culture from patients with CL, playing a role in preventing immunopathology (102). MCL is typically characterized by an exaggerated inflammatory immune response, driven by an excessive reaction to the parasite, including elevated levels of specific antibodies and high concentrations of pro-inflammatory cytokines such as IFNγ, TNFα, and IL-6 (103). Parasites belonging to the Leishmania (Viannia) subgenus are the primary etiologic agents of human CL in the Americas. but among infected individuals, a small percentage progress to mucosal involvement. In MCL patients caused by L. braziliensis, increased production of IFNγ and TNFα coincides with reduced levels of IL-10 or IL-10 receptor expression in both PBMCs culture and lesion sites compared to patients without mucosal involvement (102, 104–106). CD4+ CD25+ regulatory T (TREG) cells contribute to Th1/Th2 immune modulation by producing cytokines such as TGFβ and IL-10, which suppress macrophage and dendritic cell activity, thereby limiting the release of inflammatory mediators at the Leishmania infection site (96, 107–110). An increased level of IL-17, produced by Th17 cells and polymorphonuclear (PMN) neutrophils, is another key characteristic of MCL lesions and PBMCs (88, 111). IL-17 is a potent pro-inflammatory cytokine contributing to excessive inflammation in the lesions (103).
Genetic studies in the cross between C57BL/6 and BALB/c mice revealed that QTLs (quantitative trait loci) Lmr1 (Leishmania major resistance 1, -2, and -3 control L. major host response and wound healing independent of T helper cell responses and that a vigorous wound healing response was required for lesion resolution during L. major infection (112). Moreover, several studies using other mouse strains have shown that infection with L. major can induce several types of immune responses, which depends on the host genotype (45, 113). This is supported by the analysis of genetically engineered mice showing that some of cytokines (IFNγ, TNFα and IL-12) are necessary for defense against the parasite, whereas the others change their roles depending on genetic background, sub-strain of parasite and experimental design (9).
3.1.1.2 Effect of parasite genotype, dose, and inoculation site
The outcome of the disease is influenced not only by different species of Leishmania (Table 1), but also by the genotypic variation among isolates of one species. Several studies showed that sub-strains of L. major species have different infectivity and virulence capability (64) in mouse model. Consequently, severities of symptoms, including the size and form of the lesions is different in every sub-strains of Leishmania (110, 114–117). Recent study using multilocus sequence typing (MLST) of seven housekeeping genes explored genetic variations of Leishmania strains isolated from atypical vs. typical CL patients in Iran. A high rate of genetic variations and heterozygosity was evident in L. tropica and L. major clinical strains (118). In addition, Leishmania clinical strains isolated from different CL patients demonstrated diverse immune responses and variable pathology in BALB/c mice (114, 116).
Scientists have long been puzzled over the ability of L. major Seidman strain (MHOM/SN/74/SD) to form non-healing cutaneous lesions in the face of a strong Th1 response in C57BL/6. It has been established that this phenomenon is due to ability of L. major sub-strain to infect a population of dermal macrophages in a mannose receptor 1, C-type 1 (MRC1/CD206)-dependent manner (54, 55). These macrophages exhibit an M2-polarized phenotype, making them permissive to infection and unable to effectively control the intracellular multiplication of Leishmania parasites.
In addition, a number of experimental studies have shown determining role of both parasite dose (119–122) and site of inoculation (57) in the outcome of cutaneous Leishmania infection in mice with different genetic backgrounds (57, 94, 123–140). In Table 3 influence of inoculation site on the outcome of L. major infection in different mouse strains is outlined. As it is indicated, in BALB/c mice, subcutaneous (sc) injection of low doses (10^2 to 10^3) L. major did not induce lesion and was accompanied by stimulation of a Th1 type response. However, injecting doses higher than 10^5 caused a significant increase in the lesion size, associated with induction of a Th2 type of immune response (56). Although a low dose (10^2 parasite) initially caused pathology at the infection site in BALB/c mice, the lesion eventually healed (119). In the strain C57BL/6, a broad range of parasite doses from 10^2 to 10^7 elicited an effective Th1 type of immune response, which resulted in lesion healing, and only high doses (>10^6) were associated with lesion onset (120) (Table 2).
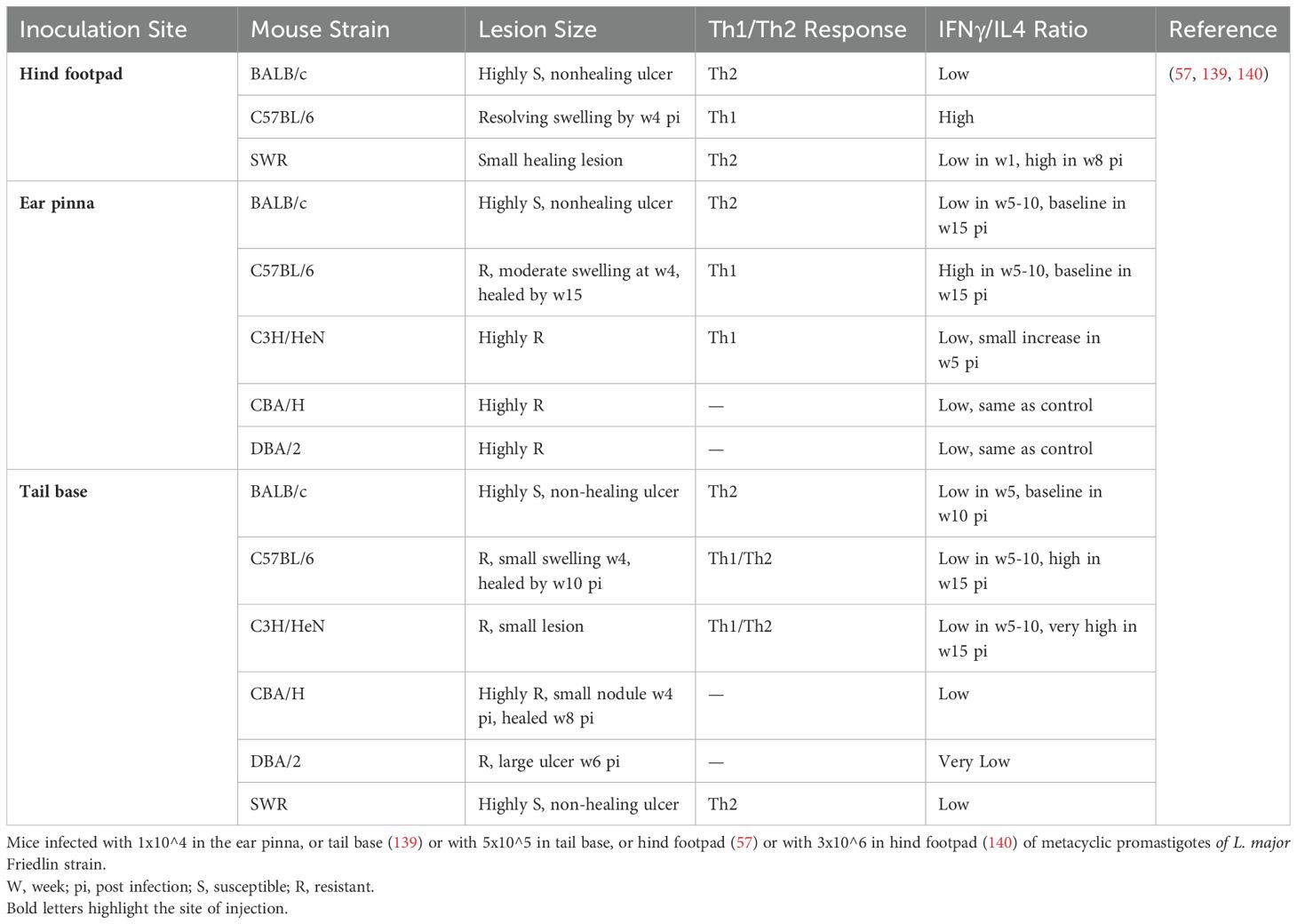
Table 3. Influence of the inoculation site on the output of Leishmania major infection and immune response of mice.
In resistant mice (C57BL/6) intradermal (id) injection of 100 metacyclic promastigotes in the ear can be used to partially mimic natural infection. In this case, two phases in infection course were defined: the first phase at early 4-6 weeks, in which the parasite replicates and the lesion is absent, and the second phase in which the lesion begins to develop while finally the lesion heals due to the predominance of the Th1 immune response (121).
The route of parasite inoculation has also been shown to have an influential role in the disease outcome (Table 3) (57). In the susceptible strain BALB/c, injection at any site induces non-healing lesions associated with an increase in Th2-type cytokine profiles (57). In resistant C57BL/6J mice, injection into the ear pinna induces a Th1 type of immune response with limited and self-healing lesion. However, injection into the tail ramp induces a Th2 type response, although the lesion eventually heals. Albeit, injection into the base of the tail in other resistant strains of mice such as DBA/2 and C3H/HeN caused partial or complete sensitivity to Leishmania infection (57). The clinical outcome of the infection and the severity of the lesions in these studies were not always associated with the type of immune response induced, as it is often seen in BALB/c or C57BL/6J mice (Table 2). Furthermore, the type of culture medium, number of passages, maintenance of the parasites, and percentage of metacyclic promastigotes influence the infectivity potential of the inoculum. That is why preparation of the inoculum need to be characterized and standardized well to reduce experimental variabilities. Together, the dose and the route of the parasite inoculation as well as parasite form determine the outcome of Leishmania infection in the experimental animal models, however, the genetic background of the host (mouse strain) and ultimately expanding of one of the two arms of either Th1 or Th2 immune responses is also of fundamental importance (reviewed in (122).
3.1.2 Visceral leishmaniasis: experimental considerations
3.1.2.1 Immune response in control of the infection
In contrast to a clear dichotomy of immune response against L. major infection in the strains BALB/c and C57BL/6, the Th1/Th2 concept does not explain susceptibility and resistance to visceral leishmaniasis caused by L. infantum (131) and L. donovani (133) in the mouse model.
Initial control and resolution of L. donovani hepatic infection in mice is accomplished within well-formed, mature tissue granulomas, which provide the microenvironment for intracellular Leishmania killing (136). The lack of a Th1 response or the presence of a Th2 response can inhibit granuloma formation in tissues of L. donovani-infected BALB/c (134, 135) and C57BL/6 mice (141). Experimental data indicated that Il12 gene-deficient C57BL/6 mice are susceptible to L. donovani, but have diminished hepatic immunopathology associated with VL (141). The protective role of IL-12 in VL has been attributed to its ability to induce IFNγ production from NK and CD4+ T cells (142).
On the other hand, in the animal model of VL, the immune response is different depending on the infection in target organ (143). When L. infantum is injected intravenously (iv) into BALB/c mice, immune responses with different kinetics occur in the liver and spleen. In spleen, resident macrophages engage in leishmanicidal activity by increasing cytokines and producing nitric oxide (NO), and then parasite load is controlled (144). In the early infection, parasite replication is accompanied by inhibition of IFNγ and IL-2 secretion, and simultaneous increasing production of IL-10 and TGFβ by the spleen tissue inhibits macrophage activity causing further establishment of infection (144). In the later stages after 4 weeks of infection, the production of antigen-induced Th1 cytokines (IL-2 and IFNγ) stimulate leishmanicidal activity of macrophages leading to decrease in parasite burden. However, it seems that activity of CD4+CD25+ TREG cells during the VL period, results in TGFβ production and establishment of a small number of persists in the spleen in L. infantum-infected BALB/c mice (145). In the liver of L. donovani-infected BALB/c and also C57BL/6 mice, the highest level of infection is shown in the 2nd week that were largely eliminated by 4 weeks. It seems that development of immunity is due to formation of parasitized Kupffer cells granuloma leading to restriction of the infection and elimination of amastigotes from granulomas (146). Hence, an organ-specific immunity is suggested in VL: on one hand a protective immune response in the liver, which leads to the parasite elimination and on the other hand an ineffective immune response in the spleen that permits the parasite survival [Reviewed in (122)]. A detailed understanding of difference between these two types of immune responses in VL can be used to formulate new strategies in development of candidate vaccines or effective treatment against human VL.
3.1.2.2 Effect of parasite genotype, dose and route of administration
Similar to L.major, there are intra-strain differences in the virulence of L. infantum isolated from different hosts belonging to the same zymodeme (MON-1) in the mouse model (147, 148). Strains with higher pathogenicity caused an increased parasite load in the spleen and liver of mice, which was associated with an enhanced TGFβ and a decreased IFNγ. Recently, biological differences or the behavior of Old and New World strains of L. infantum (synonym L. chagasi) has been investigated (148). The result showed differences in the infectivity potential of these two parasite strains in mice, the L. infantum Old World strain was more infective in vivo and in vitro than New World strain. The iNOS and arginine activities were also different in infected animals (148). Anyhow, the role of the host in VL virulence diversity is not fully understood and it is difficult to generalize the results from one strain (male BALB/c) to others (Table 2). Increasing numbers of experimental studies on animal model of VL suggest that the severity of the infection is associated with both dose of the parasite and the route of administration (130, 132, 149, 150). Usually, sc injection cause less infectivity than other routes of injection such as intradermal (id), intraperitoneal (ip) and intracardiac (ic). Typically, 10^5 parasite of L. infantum LIVT-1 strain with sc injection in mice was less infectious than iv injection, but this difference was not observed at higher doses (10^6) (122, 151–154) (Table 2).
Ic injection of L. donovani in BALB/c mice promotes development of a Th2 type of immune response associated with increased production of IL-4 and IgG1 as well as increased IL-10 level, which ultimately leads to progressive VL disease and parasite survival, especially in the spleen (149). Ic injection of L. donovani amastigotes causes progressive VL with immunosuppression characterized by defect in proliferative response of the splenic cells to in vitro stimulation with leishmanial antigen or the mitogen (155). Similarly, iv injection, especially in high doses, leads to the establishment of infection and parasite persistence in the liver and spleen (156). Protective immunity, characterized by granuloma formation in the liver and parasite clearance, was observed only in mice injected with a low dose of the parasite.
The usual routes of infection in the hamster model of VL are ic and ip. In experimental studies, L. infantum and L. donovani-infected Syrian hamsters (Mesocricetus auratus) often show typical clinical manifestations and pathological features of progressive VL, which are closely similar to active canine and human disease (152). However, the immunopathology of L. donovani infection in Syrian hamsters is extremely different from that observed in the murine models. Despite a robust Th1-like cytokine response, characterized by mRNA expression of IL-2, IFNγ, and TNFα, the hamster model exhibits increasing parasite replication in the liver, spleen, and bone marrow, indicating a possible dysfunction in macrophage effector activities. Notably, over the course of the infection, there is an absence of detectable inducible NO synthase in liver or spleen tissues, in contrast to what is usually observed in mice infections (153).
Following ic infection with L. infantum, hamsters display severe histopathological changes in both spleen and liver, where higher parasite burden are associated with different stages of granulomas formation with amastigotes in the liver, along with the disruption of the normal splenic architecture (154). Ic infection of BALB/c mice with L. donovani results in higher parasite loads in the liver and higher production of Th2 type cytokines IL-4 and IL-10 in the spleen in comparison to the sc, id or ip inoculation (149).
Overall, it seems that in the animal model of VL, using sc or id injection establishes an infection, which is more similar to natural disease. Moreover, a high dose can cause an effective infection in organs, and a low dose can induce a long-term immune response that might provide protection against Leishmania infection [Reviewed in (122)]. The mouse model of L. infantum infection replicates several features of human and canine VL, but Syrian hamsters exhibit severe clinical manifestations as usually seen in natural Leishmania infections (154). However, BALB/c mice remain the preferred model for studying VL pathogenesis and evaluating vaccine candidates, albeit they reflect self-healing or asymptomatic infections more accurately rather than progressive visceral disease. Unlike human VL, susceptible mouse strains fail to develop the full spectrum of progressive pathology. Moreover, disease severity in BALB/c mice varies based on inoculum size and infection route. In BALB/c mice, iv or id infection with L. infantum triggers organ-specific immune responses that shape disease progression. In the liver, an effective immunity with granuloma formation is formed to parasite elimination, whereas the spleen acts as a reservoir for persistent infection, highlighting its higher susceptibility to L. infantum. Understanding the mechanisms underlying this difference in organ specific immune responses may provide insights for developing targeted treatments for VL (154).
3.2 Host influencing factors
3.2.1 Host sex
Host sex can differentially regulate susceptibility to leishmaniasis by modulating the immune response against the parasite [reviewed in (59)]. Usually, due to their easy handling female mice are used in research experiments, while in order to translate the data to human, both sexes must be equally considered.
Sexual dimorphisms have been observed in susceptibility to many infectious diseases including leishmaniasis. Sex may differentially affect pathology of various organs and its influence is modified by host’s hormonal status and genotype including sex chromosomes X and Y, as well as autosomal genes [reviewed in (59)]. Both DBA/2 female and male mice develop ulcerated lesions after infection with L. major, lesions heals in males, but not in females (157). On the contrary, DBA/2 female mice are highly resistant while males are susceptible to lesion development after infection with L. mexicana (157).
Influence of Leishmania species (L. major and L. tropica), sex and genetic background were analyzed in mouse strains BALB/c, STS, and recombinant congenic strains (RCS) CcS-3, CcS-5, CcS-11, CcS-12, CcS-16, CcS-18, and CcS-20. Each RCS contains a different random set of 12.5% genes from the parental “donor” strain STS and 87.5% genes from the “background” strain BALB/c (158). Infection by L. major induced larger skin lesions in males of strains CcS-3, CcS-5 and CcS-18, whereas no difference between males and females was observed in strains BALB/c, STS, CcS-11, CcS-12, CcS-16 and CcS-20. Females of strains BALB/c, CcS-11, CcS-16 and CcS-20 are more susceptible to development of skin lesions induced by L. tropica, whereas no sex bias was observed in strains STS, CcS-3, CcS-5, CcS-12 and CcS-18. Thus, sex differentially influences infection with L. major and L. tropica, however, observed differences are modified by the host genotype (42).
Interestingly, influence of sex on murine leishmaniasis in some genotypes is organ-specific. Strains BALB/c and CcS-11 did not exhibit any sex influence on lesion size induced by L. major, but males of strain CcS-11 contained more parasites in spleens than females, and males of both strains had much higher parasite load in lymph nodes (37).
3.2.2 Host age
Age is an important factor that must be considered in studying infectious diseases using mice. Mice have a significantly shorter lifespan in comparison to humans; relatively, nine days in mice is almost equal to one year in human terms (159). Therefore, when designing an experiment, corresponding age of mice to human should be carefully estimated. With aging, frequencies of immune cells and expression of various immune receptors such as Toll-like receptors (TLRs) are changed, which can impair the host’s ability to combat infections, because they are part of pattern recognition receptors (PRRs) family that detect molecules from microbes and initiate immune responses (160). Clinical outcomes of diseases, which is dependent on host genetic, host immune response and environmental conditions, become more severe with aging. Several studies have proved that immune responses against Leishmania infection is altered with aging (61, 161). Aged C57BL/6 mice were more susceptible to L. infantum infection compared to young-infected mice, characterized by more parasite load in the spleen and liver (61). In contrast, in L. major infection experiments, macrophages derived from senescent C57BL/6 or BALB/c mice displayed similar anti-leishmanial activities compared to those from young mice. In addition, infection of resistant C57BL/6 mice with L. major revealed a similar course of footpad swelling between senescent and young mice. However, in susceptible BALB/c mice, senescent animals exhibited milder infections than their younger counterparts did, with 40–60% showing healing of lesion, reduced parasite dissemination, and a Th1 cell-mediated response, which was mainly due to spontaneous release of IL-12 by macrophages of aged mice. Interestingly, senescent BALB/c mice raised under specific-pathogen free (SPF) conditions showed neither resistance nor a Th1 response, indicating that exogenous microbial stimulation may also play a role in shaping immune responses during aging (63). Both conventionally kept BALB/c mice and SPF kept mice produced IL-12 cytokine but conventionally kept BALB/c mice were also infected with murine hepatitis virus (MHV). The spontaneous release of IL-12 due to aging and MHV infection induced Th1 response resulted protective response against L. major in the aged BALB/c mice (63). This result very well reflects the role of age and environmental conditions of animal models in experimental researches. In addition, this study highlights the potential impact of previous or co-infection in susceptibility of animal model, which usually is lacking in SPF condition.
3.2.3 Host circadian rhythm
Pathological organism such as Leishmania can significantly alter circadian clock of the host, which can have a significant impact on the development of immune response against the infection. Change in circadian rhythm leads to an elevated inflammatory mediators that are not normally present in healthy individuals (162). In addition, infiltration and homing of circulating immune cells vary during the day/night; therefore, the timing of or the initiation of an experimental infection may lead to different outcomes depending on the time (163). For instance, the number of circulating monocytes and neutrophils are lower during the active (awake) phase while they go back to the peripheral organs such as bone marrow during the resting period (164). Therefore, altered circadian rhythm prior to an infection like Leishmania, can change the level of susceptibility to the disease and the immune responses (162). More importantly, mice and humans have a different circadian rhythm. In contrast to human, mice are nocturnal animals, being active during the night and resting during the day. Due to convenience, most experiments are started during the day, a time when the mice are supposed to be at rest. This situation can induce significant stress in mice that can influence the experiments outcome. Therefore, it might be of an importance to design experiments according to the biological clock of the animal models.
3.2.4 Host microbiota
Increasing evidence shows that the gut microbiome hemostasis plays a crucial role in construction of an effective immune response against a disease. There are studies indicating that the host genetic regulates the host microbiome structure, however, inflammatory or infectious diseases along with environmental conditions can lead to an imbalance in the gut microbiome (165, 166). By analyzing the gut microbiome in mice with different genetic backgrounds, Mrázek et al. showed that the structure of gut microbiota significantly changes according to the genetic background of the host and L. major infection can change these components. Changes in gut microbiome can alter susceptibility to the disease (165, 167, 168). Different studies suggest re-construction of the host microbiota as a tool to create a basis for developing an effective therapeutic or vaccines against infectious diseases (165, 166).
3.2.5 Host nutritional status
Host nutrition plays a major role in building effective immune responses against pathogens. Host diet has a direct effect on gut microbiome structure. In addition, unhealthy diet can enhance predisposition to cardiometabolic diseases such as obesity and diabetes as underlying conditions that make the host more susceptible to infections (169). Obesity influences clinical manifestations cutaneous leishmaniasis caused by L. braziliensis in humans and is associated with greater failure in therapy (170). Obese C57BL/6 mice are more susceptible to L. major, likely due to increased expansion of resident macrophages expressing CD206 (171). Insufficient nutrition intake or malnutrition characterized by deficit in protein, energy, zinc and iron disrupt anti-parasitic immunity during leishmaniasis (169, 172). It was shown that L. donovani disseminate faster from skin to visceral organs in malnourished mice (173). In addition it was reported that VL was significantly higher (more than three times) among malnourished people (174). Malnutrition lowers immunity against an infection by reducing immune cells and decreased inflammatory cytokines and enhanced anti-inflammatory cytokines production (172, 174). It is important to note that in experimental conditions, mice are often fed with chaw diet, which can be different from what they usually receive in the natural life. This artificial condition may have an impact on the Leishmania infection pathology.
3.2.6 Host stress
Increasing evidence shows that mental health, stress and anxiety play an important role in modulating the immune responses against an infection. Leishmaniasis causes social exclusion/isolation, leading to an internalized self-stigma, stress, anxiety and depression (175, 176). A systemic review by Pires et al. showed that CL and PKDL patients and their family experienced high risk of mental illness, psychosocial morbidity and reduced quality of life (177). Moreover, ZCL were correlated with the loss of self-esteem and feelings of inferiority, which negatively correlates with age. Therefore, younger patients are psychologically more affected (178). Intestinally, low quality of life, anxiety and depression was more prevalent in female than male (177). Leishmania infection causes behavioral alterations and anxiety in mice (179). Stress and stigmatization in turn influence leishmaniasis outcomes (180, 181). Scientists are required to design and plan the experiments that have low level of stress. Construction noises, pollution, lack of experience with animal handling and intervention, not paying attention to circadian rhythm etc. can have significant impact on the experimental results.
3.2.7 Vector influence
As it was mentioned earlier, the number of Leishmania parasites transmitted to the site of inoculation during natural transmission is very limited and is not comparable with the inoculum dose typically used in experimental infections. It was shown that salivary components and vector gut microbiota have a considerable role in infectivity and severity of leishmaniasis (67, 166, 182, 183). Salivary cDNA protein libraries has been constructed for 9 species of the genus Phlebotomus and 4 species of the genus Lutzomyia [reviewed in (184)]. More than 20 diverse proteins belonging to the different protein families have been identified in each cDNA library. Protein families that were detected in selected Phlebotomus as well as in Lutzomyia species are: antigen 5–related proteins, apyrases, odorant-binding proteins (D7-related proteins and PpSP15-like proteins), yellow-related proteins (YRPs), silk-related proteins, and lufaxin-like proteins (185). These proteins have anti-hemostatic, anti-inflammatory and immunomodulatory properties (184).
The most efficient way of experimental infection is inducing a natural infection by infected sand flies, however, technically, not every lab has a possibility to breed and maintain sand fly colonies, therefore, formulation of inoculum needs to be standardized and present the most similarity to the natural infection that usually occurs in human. Supplementing salivary gland lysate that contains saliva component and part of the vector microbiome might be a solution to increase the infectivity of Leishmania inoculum (186).
3.2.8 Living conditions
Substantial evidence indicates that variations in laboratory mouse husbandry practices significantly contribute to the discrepancies observed in immune responses against pathogens, not only between mice and humans but also among experiments conducted at different institutes (19).
In the early history of laboratory mouse breeding, preventing contamination of mouse colonies by pathogens was a significant challenge until the filter-equipped cages were developed in the 1980s (187). The term “Specific Pathogen Free” (SPF), first introduced in the late 1950s, refers to mouse colonies that are devoid of specific pathogens, such as particular viruses, bacteria, and parasites (188). Although the list of these pathogens may vary, it typically includes those commonly shared with wild mice. The experimental exposure of SPF mice to specific microorganisms or modifications to their gut microbiome elicited distinct alterations in their immune responses to infection. Therefore, it is argued that manipulating the microbiome-host relationship in SPF models might greatly influence the application of findings to human health (189).
It was suggested that alterations in the living conditions of laboratory mice significantly influence the cellular profile of immune system (19). While the effector and tissue resident memory T cell repertoires were absent in laboratory mice, these cell populations were readily demonstrated in wild and pet store mice, naturally exposed to a broad spectrum of microbial environments. Interestingly, these immune cells were induced in laboratory mice after co-housing with pet store mice (190). It has been recommended that in the study of infectious diseases, colonies of inbred mice in controlled environments with microbiome resembling natural conditions are better suited for translating mouse immunology studies to human contexts (189).
4 Reproducibility of the data
A primary step toward improving the translation of mouse data to humans is to increase the reproducibility of mice experiments. The reproducibility crisis in experimental results remains a significant challenge in biomedical science. Considering aforementioned parameters not only improves the translational value of mouse experiments but also plays an important role in improving the reproducibility of animal experiments across different laboratories or even within the same laboratory. A critical step to increase the probability of data regeneration is the writing of a detailed standard operation procedure (SOP) for an experiment, ensuring it can be consistently followed by different investigators. In addition to the influencing parameters that were comprehensively discussed before, paying attention to the following routine practice should also be taken into consideration:
a. The animal experiments must be performed and repeated by trained staffs.
b. Factors such as the availability of materials and equipment, cleanliness of the facility and adherence to cleaning procedures, proper operation of the equipment, and handling of animals and the performance of interventions and routine checkups need to be standardized in the form of SOPs.
c. The breeding procedure or source of animal colony, along with factors such as age, sex, circadian clock and animal housing condition including bedding, temperature, humidity, food type, cage type (SPF or conventional), number of animals per cage, random distribution of the groups, sample size etc. must be strictly monitored and controlled.
d. Although ethical considerations may not directly influence experimental outcomes, adhering to ethical guidelines can help to unify most of the handling and housing procedures. Investigators must precisely and rigorously follow these ethical guidelines when designing, planning and conducting mouse experiments.
6 Conclusion remarks
Various animal models of CL and VL are employed in experimental studies to investigate the mechanisms of protective immunity and disease pathogenesis in Leishmania infections. The immune response to Leishmania is orchestrated through intricate regulatory networks, but the classic Th1/Th2 polarization observed in murine L. major infections do not fully translate to human disease or to infections caused by other species of Leishmania (70, 94). Therefore, there is no universal consensus on biomarkers of host susceptibility/resistance across human and experimental animal models, as immune responses can vary significantly depending on the host background and parasite factors as discussed above (reviewed in (4)).
Clinical manifestation of a disease such as leishmaniasis is a result of a complex cross talk between host genetic, immune responses and environmental conditions. Although, zoonotic types of leishmania infection naturally occurs in other mammalian hosts, development of mouse models has been an instrumental in furthering our understanding of the disease mechanism. Therefore, optimizing/adapting the influencing parameters in experiments to mimic infection in human is fundamental and will increase translational value of mouse data. Including more strains of mice with distinct genetic background as well as inbred strains to increase the genetic complexity may help to recapitulate the complexity of human genome in mice. In addition, cohosting the laboratory mice with pet mice instead of keeping them in extra clean conditions, optimizing and well characterization of parasite culture, and inclusion of sand fly saliva components in the parasite inoculum will help to induce an infection with closer clinical manifestation of human leishmaniasis. Furthermore, applying the current development in research such multiomics technologies and system biology along with characterizing environmental and host related parameters will help to recapitulate a disease condition closer to human leishmaniasis.
Author contributions
MN-R: Conceptualization, Writing – original draft, Writing – review & editing. ML: Writing – original draft, Writing – review & editing, Conceptualization. YS: Conceptualization, Project administration, Writing – original draft, Writing – review & editing.
Funding
The author(s) declare that financial support was received for the research and/or publication of this article. This work was supported by the Charles University Research Program COOPERATIO: the scientific project “Medical Diagnostics and Basic Medical Sciences” (the field “Medical Genetics”). YS is supported by the University of Münster.
Acknowledgments
The open access was supported by university Münster.
Conflict of interest
The authors declare that the research was conducted in the absence of any commercial or financial relationships that could be construed as a potential conflict of interest.
Generative AI statement
The author(s) declare that no Generative AI was used in the creation of this manuscript.
Publisher’s note
All claims expressed in this article are solely those of the authors and do not necessarily represent those of their affiliated organizations, or those of the publisher, the editors and the reviewers. Any product that may be evaluated in this article, or claim that may be made by its manufacturer, is not guaranteed or endorsed by the publisher.
Abbreviations
Ic, intracardiac; id, intradermal; ip, intraperitoneal; iv, intravenous; sc, subcutaneous; SOP, standard operation procedure.
References
1. Mann S, Frasca K, Scherrer S, Henao-Martinez AF, Newman S, Ramanan P, et al. A review of leishmaniasis: current knowledge and future directions. Curr Trop Med Rep. (2021) 8:121–32. doi: 10.1007/s40475-021-00232-7
2. Burza S, Croft SL, Boelaert M. Leishmaniasis. Lancet. (2018) 392:951–70. doi: 10.1016/S0140-6736(18)31204-2
3. Maroli M, Feliciangeli MD, Bichaud L, Charrel RN, Gradoni L. Phlebotomine sandflies and the spreading of leishmaniases and other diseases of public health concern. Med Vet Entomol. (2013) 27:123–47. doi: 10.1111/j.1365-2915.2012.01034.x
4. Rostami MN, Khamesipour A. Potential biomarkers of immune protection in human leishmaniasis. Med Microbiol Immunol. (2021) 210:81–100. doi: 10.1007/s00430-021-00703-8
5. Bogdan C. Mechanisms and consequences of persistence of intracellular pathogens: leishmaniasis as an example. Cell Microbiol. (2008) 10:1221–34. doi: 10.1111/j.1462-5822.2008.01146.x
6. Terrazas CA, Terrazas LI, Gomez-Garcia L. Modulation of dendritic cell responses by parasites: a common strategy to survive. J BioMed Biotechnol. (2010) 2010:357106. doi: 10.1155/2010/357106
7. Loria-Cervera EN, Andrade-Narvaez FJ. Animal models for the study of leishmaniasis immunology. Rev Inst Med Trop Sao Paulo. (2014) 56:1–11. doi: 10.1590/S0036-46652014000100001
8. Sacks D, Noben-Trauth N. The immunology of susceptibility and resistance to Leishmania major in mice. Nat Rev Immunol. (2002) 2:845–58. doi: 10.1038/nri933
9. Krayem I, Lipoldova M. Role of host genetics and cytokines in Leishmania infection. Cytokine. (2021) 147:155244. doi: 10.1016/j.cyto.2020.155244
10. Kobets T, Grekov I, Lipoldova M. Leishmaniasis: prevention, parasite detection and treatment. Curr Med Chem. (2012) 19:1443–74. doi: 10.2174/092986712799828300
11. Boussoffara T, Labidi I, Trimeche M, Chelbi I, Dachraoui K, Msallem N, et al. LmCen(-/-) based vaccine is protective against canine visceral leishmaniasis following three natural exposures in Tunisia. NPJ Vaccines. (2025) 10:31. doi: 10.1038/s41541-025-01070-8
12. Singh OP, Hasker E, Sacks D, Boelaert M, Sundar S. Asymptomatic Leishmania infection: a new challenge for Leishmania control. Clin Infect Dis. (2014) 58:1424–9. doi: 10.1093/cid/ciu102
13. Pederiva MMC, Santos SMD, Rivarola LGS, Guerreiro VJ, Lopes KS, Lima Junior M, et al. Asymptomatic Leishmania infection in humans: A systematic review. J Infect Public Health. (2023) 16:286–94. doi: 10.1016/j.jiph.2022.12.021
14. Nateghi-Rostami M, Sohrabi Y. Memory T cells: promising biomarkers for evaluating protection and vaccine efficacy against leishmaniasis. Front Immunol. (2024) 15:1304696. doi: 10.3389/fimmu.2024.1304696
15. Nateghi Rostami M, Keshavarz H, Khamesipour A. Immune response of BALB/c mice against an experimental vaccine of Alum precipitated autoclaved Leishmania major (Alum-ALM) mixed with BCG or Mycobacterium vaccae. Trop Biomed. (2010) 27:89–102.
16. Miramin-Mohammadi A, Javadi A, Eskandari SE, Mortazavi H, Rostami MN, Khamesipour A. Immune response in cutaneous leishmaniasis patients with healing vs. non-healing lesions. Iran J Microbiol. (2020) 12:249–55. doi: 10.18502/ijm.v12i3.3243
17. Lera-Nonose D, De Oliveira LF, Brustolin A, Santos TS, Oyama J, Ramos-Milare A, et al. Genetic variations in the human immune system influence susceptibility to tegumentary leishmaniasis: a systematic review and meta-analysis. Expert Rev Clin Immunol. (2021) 17:513–37. doi: 10.1080/1744666X.2021.1906650
18. Bharati K. Human genetic polymorphism and Leishmaniasis. Infection Genet evolution: J Mol Epidemiol evolutionary Genet Infect diseases. (2022) 98:105203. doi: 10.1016/j.meegid.2021.105203
19. Masopust D, Sivula CP, Jameson SC. Of mice, dirty mice, and men: using mice to understand human immunology. J Immunol. (2017) 199:383–8. doi: 10.4049/jimmunol.1700453
20. Mikhail JW, Mansour NS. Leishmania donovani: therapeutic and prophylaciic action of antimony dextran glycoside (RL-712) in the golden hamster. Exp Parasitol. (1975) 37:348–52. doi: 10.1016/0014-4894(75)90002-8
21. Keenan CM, Hendricks LD, Lightner L, Webster HK, Johnson AJ. Visceral leishmaniasis in the German shepherd dog. I. Infection, clinical disease, and clinical pathology. Vet Pathol. (1984) 21:74–9. doi: 10.1177/030098588402100113
22. Chapman WL Jr., Hanson WL, Hendricks LD. Toxicity and efficacy of the antileishmanial drug meglumine antimoniate in the owl monkey (Aotus trivirgatus). J Parasitol. (1983) 69:1176–7. doi: 10.2307/3280894
23. Hommel M, Jaffe CL, Travi B, Milon G. Experimental models for leishmaniasis and for testing anti-leishmanial vaccines. Ann Trop Med Parasitol. (1995) 89 Suppl 1:55–73. doi: 10.1080/00034983.1995.11813015
24. Courret N, Lang T, Milon G, Antoine JC. Intradermal inoculations of low doses of Leishmania major and Leishmania amazonensis metacyclic promastigotes induce different immunoparasitic processes and status of protection in BALB/c mice. Int J Parasitol. (2003) 33:1373–83. doi: 10.1016/S0020-7519(03)00179-6
25. Behforouz NC, Wenger CD, Mathison BA. Prophylactic treatment of BALB/c mice with cyclosporine A and its analog B-5-49 enhances resistance to Leishmania major. J Immunol. (1986) 136:3067–75. doi: 10.4049/jimmunol.136.8.3067
26. Van den Kerkhof M, Mabille D, Chatelain E, Mowbray CE, Braillard S, Hendrickx S, et al. In vitro and in vivo pharmacodynamics of three novel antileishmanial lead series. Int J Parasitol Drugs Drug Resist. (2018) 8:81–6. doi: 10.1016/j.ijpddr.2018.01.006
27. van der Ende J, Schallig H. Leishmania animal models used in drug discovery: A systematic review. Anim (Basel). (2023) 13(10):1650. doi: 10.3390/ani13101650
28. Tegazzini D, Diaz R, Aguilar F, Pena I, Presa JL, Yardley V, et al. A Replicative In Vitro Assay for Drug Discovery against Leishmania donovani. Antimicrob Agents Chemother. (2016) 60:3524–32. doi: 10.1128/AAC.01781-15
29. Fortin A, Caridha DP, Leed S, Ngundam F, Sena J, Bosschaerts T, et al. Direct comparison of the efficacy and safety of oral treatments with oleylphosphocholine (OlPC) and miltefosine in a mouse model of L. major cutaneous leishmaniasis. PloS Negl Trop Dis. (2014) 8:e3144. doi: 10.1371/journal.pntd.0003144
30. Garcia Bustos MF, Barrio A, Prieto GG, de Raspi EM, Cimino RO, Cardozo RM, et al. In vivo antileishmanial efficacy of miltefosine against Leishmania (Leishmania) amazonensis. J Parasitol. (2014) 100:840–7. doi: 10.1645/13-376.1
31. Jaafari MR, Bavarsad N, Bazzaz BS, Samiei A, Soroush D, Ghorbani S, et al. Effect of topical liposomes containing paromomycin sulfate in the course of Leishmania major infection in susceptible BALB/c mice. Antimicrob Agents Chemother. (2009) 53:2259–65. doi: 10.1128/AAC.01319-08
32. Neira LF, Mantilla JC, Escobar P. Anti-leishmanial activity of a topical miltefosine gel in experimental models of New World cutaneous leishmaniasis. J Antimicrob Chemother. (2019) 74:1634–41. doi: 10.1093/jac/dkz049
33. Herrera L, Llanes A, Alvarez J, Degracia K, Restrepo CM, Rivera R, et al. Antileishmanial activity of a new chloroquine analog in an animal model of Leishmania panamensis infection. Int J Parasitol Drugs Drug Resist. (2020) 14:56–61. doi: 10.1016/j.ijpddr.2020.08.002
34. Mears ER, Modabber F, Don R, Johnson GE. A review: the current in vivo models for the discovery and utility of new anti-leishmanial drugs targeting cutaneous leishmaniasis. PloS Negl Trop Dis. (2015) 9:e0003889. doi: 10.1371/journal.pntd.0003889
35. Sakthianandeswaren A, Foote SJ, Handman E. The role of host genetics in leishmaniasis. Trends Parasitol. (2009) 25:383–91. doi: 10.1016/j.pt.2009.05.004
36. Vladimirov V, Badalova J, Svobodova M, Havelkova H, Hart AA, Blazkova H, et al. Different genetic control of cutaneous and visceral disease after Leishmania major infection in mice. Infect Immun. (2003) 71:2041–6. doi: 10.1128/IAI.71.4.2041-2046.2003
37. Kurey I, Kobets T, Havelkova H, Slapnickova M, Quan L, Trtkova K, et al. Distinct genetic control of parasite elimination, dissemination, and disease after Leishmania major infection. Immunogenetics. (2009) 61:619–33. doi: 10.1007/s00251-009-0392-9
38. Krayem I, Sohrabi Y, Havelkova H, Gusareva ES, Strnad H, Cepickova M, et al. Functionally distinct regions of the locus Leishmania major response 15 control IgE or IFNgamma level in addition to skin lesions. Front Immunol. (2023) 14:1145269. doi: 10.3389/fimmu.2023.1145269
39. Krayem I, Sohrabi Y, Javorkova E, Volkova V, Strnad H, Havelkova H, et al. Genetic influence on frequencies of myeloid-derived cell subpopulations in mouse. Front Immunol. (2021) 12:760881. doi: 10.3389/fimmu.2021.760881
40. Sohrabi Y, Volkova V, Kobets T, Havelkova H, Krayem I, Slapnickova M, et al. Genetic regulation of guanylate-binding proteins 2b and 5 during leishmaniasis in mice. Front Immunol. (2018) 9:130. doi: 10.3389/fimmu.2018.00130
41. Lipoldova M, Demant P. Genetic susceptibility to infectious disease: lessons from mouse models of leishmaniasis. Nat Rev Genet. (2006) 7:294–305. doi: 10.1038/nrg1832
42. Kobets T, Havelkova H, Grekov I, Volkova V, Vojtiskova J, Slapnickova M, et al. Genetics of host response to Leishmania tropica in mice - different control of skin pathology, chemokine reaction, and invasion into spleen and liver. PloS Negl Trop Dis. (2012) 6:e1667. doi: 10.1371/journal.pntd.0001667
43. Havelkova H, Badalova J, Svobodova M, Vojtikova J, Kurey I, Vladimirov V, et al. Genetics of susceptibility to leishmaniasis in mice: four novel loci and functional heterogeneity of gene effects. Genes Immun. (2006) 7:220–33. doi: 10.1038/sj.gene.6364290
44. Sohrabi Y, Havelkova H, Kobets T, Sima M, Volkova V, Grekov I, et al. Mapping the genes for susceptibility and response to Leishmania tropica in mouse. PloS Negl Trop Dis. (2013) 7:e2282. doi: 10.1371/journal.pntd.0002282
45. Lipoldova M, Svobodova M, Havelkova H, Krulova M, Badalova J, Nohynkova E, et al. Mouse genetic model for clinical and immunological heterogeneity of leishmaniasis. Immunogenetics. (2002) 54:174–83. doi: 10.1007/s00251-002-0439-7
46. Kobets T, Cepickova M, Volkova V, Sohrabi Y, Havelkova H, Svobodova M, et al. Novel loci controlling parasite load in organs of mice infected with leishmania major, their interactions and sex influence. Front Immunol. (2019) 10:1083. doi: 10.3389/fimmu.2019.01083
47. Badalova J, Svobodova M, Havelkova H, Vladimirov V, Vojtiskova J, Engova J, et al. Separation and mapping of multiple genes that control IgE level in Leishmania major infected mice. Genes Immun. (2002) 3:187–95. doi: 10.1038/sj.gene.6363838
48. Lipoldova M, Svobodova M, Krulova M, Havelkova H, Badalova J, Nohynkova E, et al. Susceptibility to Leishmania major infection in mice: multiple loci and heterogeneity of immunopathological phenotypes. Genes Immun. (2000) 1:200–6. doi: 10.1038/sj.gene.6363660
49. Vidal SM, Malo D, Vogan K, Skamene E, Gros P. Natural resistance to infection with intracellular parasites: isolation of a candidate for Bcg. Cell. (1993) 73:469–85. doi: 10.1016/0092-8674(93)90135-d
50. Bucheton B, Abel L, Kheir MM, Mirgani A, El-Safi SH, Chevillard C, et al. Genetic control of visceral leishmaniasis in a Sudanese population: candidate gene testing indicates a linkage to the NRAMP1 region. Genes Immun. (2003) 4:104–9. doi: 10.1038/sj.gene.6363927
51. Sakthianandeswaren A, Curtis JM, Elso C, Kumar B, Baldwin TM, Lopaticki S, et al. Fine mapping of Leishmania major susceptibility Locus lmr2 and evidence of a role for Fli1 in disease and wound healing. Infect Immun. (2010) 78:2734–44. doi: 10.1128/IAI.00126-10
52. Castellucci L, Jamieson SE, Miller EN, de Almeida LF, Oliveira J, Magalhaes A, et al. FLI1 polymorphism affects susceptibility to cutaneous leishmaniasis in Brazil. Genes Immun. (2011) 12:589–94. doi: 10.1038/gene.2011.37
53. Tao L, Reese TA. Making mouse models that reflect human immune responses. Trends Immunol. (2017) 38:181–93. doi: 10.1016/j.it.2016.12.007
54. Lee SH, Charmoy M, Romano A, Paun A, Chaves MM, Cope FO, et al. Mannose receptor high, M2 dermal macrophages mediate nonhealing Leishmania major infection in a Th1 immune environment. J Exp Med. (2018) 215:357–75. doi: 10.1084/jem.20171389
55. Sohrabi Y, Lipoldova M. Mannose receptor and the mystery of nonhealing leishmania major infection. Trends Parasitol. (2018) 34:354–6. doi: 10.1016/j.pt.2018.03.006
56. Menon JN, Bretscher PA. Parasite dose determines the Th1/Th2 nature of the response to Leishmania major independently of infection route and strain of host or parasite. Eur J Immunol. (1998) 28:4020–8. doi: 10.1002/(SICI)1521-4141(199812)28:12<4020::AID-IMMU4020>3.0.CO;2-3
57. Baldwin TM, Elso C, Curtis J, Buckingham L, Handman E. The site of Leishmania major infection determines disease severity and immune responses. Infect Immun. (2003) 71:6830–4. doi: 10.1128/IAI.71.12.6830-6834.2003
58. Lockard RD, Wilson ME, Rodriguez NE. Sex-related differences in immune response and symptomatic manifestations to infection with leishmania species. J Immunol Res. (2019) 2019:4103819. doi: 10.1155/2019/4103819
59. Lipoldova M, Demant P. Gene-specific sex effects on susceptibility to infectious diseases. Front Immunol. (2021) 12:712688. doi: 10.3389/fimmu.2021.712688
60. Vom Steeg LG, Klein SL. Sex and sex steroids impact influenza pathogenesis across the life course. Semin Immunopathol. (2019) 41:189–94. doi: 10.1007/s00281-018-0718-5
61. Loureiro Salgado C, Mendez Corea AF, Covre LP, De Matos Guedes HL, Falqueto A, Gomes DCO. Ageing impairs protective immunity and promotes susceptibility to murine visceral leishmaniasis. Parasitology. (2022) 149:1249–56. doi: 10.1017/S0031182022000828
62. Oliveira MR, Tafuri WL, Afonso LC, Oliveira MA, Nicoli JR, Vieira EC, et al. Germ-free mice produce high levels of interferon-gamma in response to infection with Leishmania major but fail to heal lesions. Parasitology. (2005) 131:477–88. doi: 10.1017/S0031182005008073
63. Ehrchen J, Sindrilaru A, Grabbe S, Schonlau F, Schlesiger C, Sorg C, et al. Senescent BALB/c mice are able to develop resistance to Leishmania major infection. Infect Immun. (2004) 72:5106–14. doi: 10.1128/IAI.72.9.5106-5114.2004
64. Sadlova J, Svobodova M, Volf P. Leishmania major: effect of repeated passages through sandfly vectors or murine hosts. Ann Trop Med Parasitol. (1999) 93:599–611. doi: 10.1080/00034989958104
65. Grekov I, Svobodova M, Nohynkova E, Lipoldova M. Preparation of highly infective Leishmania promastigotes by cultivation on SNB-9 biphasic medium. J Microbiol Methods. (2011) 87:273–7. doi: 10.1016/j.mimet.2011.08.012
66. Nemati Haravani T, Parvizi P, Hejazi SH, Sedaghat MM, Eskandarian A, Nateghi Rostami M. Evaluation of expression variations in virulence-related genes of Leishmania major after several culture passages compared with Phlebotomus papatasi isolated promastigotes. PloS One. (2023) 18:e0284240. doi: 10.1371/journal.pone.0284240
67. Serafim TD, Coutinho-Abreu IV, Dey R, Kissinger R, Valenzuela JG, Oliveira F, et al. Leishmaniasis: the act of transmission. Trends Parasitol. (2021) 37:976–87. doi: 10.1016/j.pt.2021.07.003
68. Heinzel FP, Sadick MD, Holaday BJ, Coffman RL, Locksley RM. Reciprocal expression of interferon gamma or interleukin 4 during the resolution or progression of murine leishmaniasis. Evidence for expansion of distinct helper T cell subsets. J Exp Med. (1989) 169:59–72. doi: 10.1084/jem.169.1.59
69. Sadick MD, Locksley RM, Tubbs C, Raff HV. Murine cutaneous leishmaniasis: resistance correlates with the capacity to generate interferon-gamma in response to Leishmania antigens in vitro. J Immunol. (1986) 136:655–61. doi: 10.4049/jimmunol.136.2.655
70. Soong L, Henard CA, Melby PC. Immunopathogenesis of non-healing American cutaneous leishmaniasis and progressive visceral leishmaniasis. Semin Immunopathol. (2012) 34:735–51. doi: 10.1007/s00281-012-0350-8
71. Martinez JE, Valderrama L, Gama V, Leiby DA, Saravia NG. Clonal diversity in the expression and stability of the metastatic capability of Leishmania guyanensis in the golden hamster. J Parasitol. (2000) 86:792–9. doi: 10.1645/0022-3395(2000)086[0792:CDITEA]2.0.CO;2
72. Bogdan C, Rollinghoff M, Diefenbach A. Reactive oxygen and reactive nitrogen intermediates in innate and specific immunity. Curr Opin Immunol. (2000) 12:64–76. doi: 10.1016/S0952-7915(99)00052-7
73. Van Assche T, Deschacht M, da Luz RA, Maes L, Cos P. Leishmania-macrophage interactions: insights into the redox biology. Free Radic Biol Med. (2011) 51:337–51. doi: 10.1016/j.freeradbiomed.2011.05.011
74. Rocha FJ, Schleicher U, Mattner J, Alber G, Bogdan C. Cytokines, signaling pathways, and effector molecules required for the control of Leishmania (Viannia) Braziliensis in mice. Infect Immun. (2007) 75:3823–32. doi: 10.1128/IAI.01335-06
75. Mattner J, Schindler H, Diefenbach A, Rollinghoff M, Gresser I, Bogdan C. Regulation of type 2 nitric oxide synthase by type 1 interferons in macrophages infected with Leishmania major. Eur J Immunol. (2000) 30:2257–67. doi: 10.1002/1521-4141(2000)30:8<2257::AID-IMMU2257>3.0.CO;2-U
76. Wei XQ, Charles IG, Smith A, Ure J, Feng GJ, Huang FP, et al. Altered immune responses in mice lacking inducible nitric oxide synthase. Nature. (1995) 375:408–11. doi: 10.1038/375408a0
77. Carneiro PP, Conceicao J, Macedo M, Magalhaes V, Carvalho EM, Bacellar O. The role of nitric oxide and reactive oxygen species in the killing of leishmania Braziliensis by monocytes from patients with cutaneous leishmaniasis. PloS One. (2016) 11:e0148084. doi: 10.1371/journal.pone.0148084
78. Gantt KR, Goldman TL, McCormick ML, Miller MA, Jeronimo SM, Nascimento ET, et al. Oxidative responses of human and murine macrophages during phagocytosis of Leishmania chagasi. J Immunol. (2001) 167:893–901. doi: 10.4049/jimmunol.167.2.893
79. Tomiotto-Pellissier F, Bortoleti B, Assolini JP, Goncalves MD, Carloto ACM, Miranda-Sapla MM, et al. Macrophage polarization in leishmaniasis: broadening horizons. Front Immunol. (2018) 9:2529. doi: 10.3389/fimmu.2018.02529
80. Gaur U, Roberts SC, Dalvi RP, Corraliza I, Ullman B, Wilson ME. An effect of parasite-encoded arginase on the outcome of murine cutaneous leishmaniasis. J Immunol. (2007) 179:8446–53. doi: 10.4049/jimmunol.179.12.8446
81. Lopez Kostka S, Dinges S, Griewank K, Iwakura Y, Udey MC, von Stebut E. IL-17 promotes progression of cutaneous leishmaniasis in susceptible mice. J Immunol. (2009) 182:3039–46. doi: 10.4049/jimmunol.0713598
82. Nateghi Rostami M, Seyyedan Jasbi E, Khamesipour A, Mohammadi AM. Tumour Necrosis Factor-alpha (TNF-alpha) and its soluble receptor type 1 (sTNFR I) in human active and healed leishmaniases. Parasite Immunol. (2016) 38:255–60. doi: 10.1111/pim.2016.38.issue-4
83. Bettelli E, Korn T, Kuchroo VK. Th17: the third member of the effector T cell trilogy. Curr Opin Immunol. (2007) 19:652–7. doi: 10.1016/j.coi.2007.07.020
84. Banerjee A, Bhattacharya P, Joshi AB, Ismail N, Dey R, Nakhasi HL. Role of pro-inflammatory cytokine IL-17 in Leishmania pathogenesis and in protective immunity by Leishmania vaccines. Cell Immunol. (2016) 309:37–41. doi: 10.1016/j.cellimm.2016.07.004
85. van Zandbergen G, Klinger M, Mueller A, Dannenberg S, Gebert A, Solbach W, et al. Cutting edge: neutrophil granulocyte serves as a vector for Leishmania entry into macrophages. J Immunol. (2004) 173:6521–5. doi: 10.4049/jimmunol.173.11.6521
86. McGeachy MJ, Chen Y, Tato CM, Laurence A, Joyce-Shaikh B, Blumenschein WM, et al. The interleukin 23 receptor is essential for the terminal differentiation of interleukin 17-producing effector T helper cells in vivo. Nat Immunol. (2009) 10:314–24. doi: 10.1038/ni.1698
87. Bacellar O, Faria D, Nascimento M, Cardoso TM, Gollob KJ, Dutra WO, et al. Interleukin 17 production among patients with American cutaneous leishmaniasis. J Infect diseases. (2009) 200:75–8. doi: 10.1086/599380
88. Souza MA, Castro MC, Oliveira AP, Almeida AF, Reis LC, Silva CJ, et al. American tegumentary leishmaniasis: cytokines and nitric oxide in active disease and after clinical cure, with or without chemotherapy. Scand J Immunol. (2012) 76:175–80. doi: 10.1111/j.1365-3083.2012.02717.x
89. Darzi F, Davoudian R, Nateghi Rostami M. Differential inflammatory responses associated with Leishmania major and L tropica in culture. Parasite Immunol. (2021) 43:e12841. doi: 10.1111/pim.12841
90. Keshavarz Valian H, Nateghi Rostami M, Tasbihi M, Miramin Mohammadi A, Eskandari SE, Sarrafnejad A, et al. CCR7+ central and CCR7- effector memory CD4+ T cells in human cutaneous leishmaniasis. J Clin Immunol. (2013) 33:220–34. doi: 10.1007/s10875-012-9788-7
91. Khamesipour A, Nateghi Rostami M, Tasbihi M, Miramin Mohammadi A, Shahrestani T, Sarrafnejad A, et al. Phenotyping of circulating CD8(+) T cell subsets in human cutaneous leishmaniasis. Microbes infection/Institut Pasteur. (2012) 14:702–11. doi: 10.1016/j.micinf.2012.02.006
92. Nateghi Rostami M, Seyyedan Jasbi E, Khamesipour A, Miramin Mohammadi A. Plasma levels of tumor necrosis factor-alpha (TNF-alpha), TNF-alpha soluble receptor type 1 (sTNFR I) and IL-22 in human leishmaniasis. Trop Biomed. (2015) 32:478–84.
93. Miramin-Mohammadi A, Javadi A, Eskandari SE, Nateghi-Rostami M, Khamesipour A. Immune Responses in Cutaneous Leishmaniasis: In vitro Thelper1/Thelper2 Cytokine Profiles Using Live Versus Killed Leishmania major. J Arthropod Borne Dis. (2021) 15:126–35. doi: 10.18502/jad.v15i1.6491
94. McMahon-Pratt D, Alexander J. Does the Leishmania major paradigm of pathogenesis and protection hold for New World cutaneous leishmaniases or the visceral disease? Immunol Rev. (2004) 201:206–24. doi: 10.1111/j.0105-2896.2004.00190.x
95. Sacks D, Anderson C. Re-examination of the immunosuppressive mechanisms mediating non-cure of Leishmania infection in mice. Immunol Rev. (2004) 201:225–38. doi: 10.1111/j.0105-2896.2004.00185.x
96. Fromm PD, Kling JC, Remke A, Bogdan C, Korner H. Fatal leishmaniasis in the absence of TNF despite a strong th1 response. Front Microbiol. (2015) 6:1520. doi: 10.3389/fmicb.2015.01520
97. Campos-Neto A. Anti-leishmania vaccine. In: Farrel JP, editor. Leishmania. Kluwer Academic Publishers, Boston (2002). p. 169–90.
98. Sjölander A, Baldwin TM, Curtis JM, Bengtsson KL, Handman E. Vaccination with recombinant Parasite Surface Antigen 2 from Leishmania major induces a Th1 type of immune response but does not protect against infection. Vaccine. (1998) 16:2077–84. doi: 10.1016/S0264-410X(98)00075-9
99. Uzonna JE, Spath GF, Beverley SM, Scott P. Vaccination with phosphoglycan-deficient Leishmania major protects highly susceptible mice from virulent challenge without inducing a strong Th1 response. J Immunol. (2004) 172:3793–7. doi: 10.4049/jimmunol.172.6.3793
100. Rocha PN, Almeida RP, Bacellar O, de Jesus AR, Filho DC, Filho AC, et al. Down-regulation of Th1 type of response in early human American cutaneous leishmaniasis. J Infect diseases. (1999) 180:1731–4. doi: 10.1086/jid.1999.180.issue-5
101. Oliveira WN, Ribeiro LE, Schrieffer A, MaChado P, Carvalho EM, Bacellar O. The role of inflammatory and anti-inflammatory cytokines in the pathogenesis of human tegumentary leishmaniasis. Cytokine. (2014) 66:127–32. doi: 10.1016/j.cyto.2013.12.016
102. Gaze ST, Dutra WO, Lessa M, Lessa H, Guimaraes LH, Jesus AR, et al. Mucosal leishmaniasis patients display an activated inflammatory T-cell phenotype associated with a nonbalanced monocyte population. Scand J Immunol. (2006) 63:70–8. doi: 10.1111/j.1365-3083.2005.01707.x
103. Scott P, Novais FO. Cutaneous leishmaniasis: immune responses in protection and pathogenesis. Nat Rev Immunol. (2016) 16:581–92. doi: 10.1038/nri.2016.72
104. Faria DR, Gollob KJ, Barbosa J Jr., Schriefer A, MaChado PR, Lessa H, et al. Decreased in situ expression of interleukin-10 receptor is correlated with the exacerbated inflammatory and cytotoxic responses observed in mucosal leishmaniasis. Infect Immun. (2005) 73:7853–9. doi: 10.1128/IAI.73.12.7853-7859.2005
105. Bacellar O, Lessa H, Schriefer A, MaChado P, Ribeiro de Jesus A, Dutra WO, et al. Up-regulation of Th1-type responses in mucosal leishmaniasis patients. Infect Immun. (2002) 70:6734–40. doi: 10.1128/IAI.70.12.6734-6740.2002
106. Nylen S, Eidsmo L. Tissue damage and immunity in cutaneous leishmaniasis. Parasite Immunol. (2012) 34:551–61. doi: 10.1111/pim.2012.34.issue-12
107. Dubie T, Mohammed Y. Review on the role of host immune response in protection and immunopathogenesis during cutaneous leishmaniasis infection. J Immunol Res. (2020) 2020:2496713. doi: 10.1155/2020/2496713
108. Belkaid Y, Piccirillo CA, Mendez S, Shevach EM, Sacks DL. CD4+CD25+ regulatory T cells control Leishmania major persistence and immunity. Nature. (2002) 420:502–7. doi: 10.1038/nature01152
109. Belkaid Y. The role of CD4(+)CD25(+) regulatory T cells in Leishmania infection. Expert Opin Biol Ther. (2003) 3:875–85. doi: 10.1517/14712598.3.6.875
110. Attia H, Sghaier MR, Bali A, Guerfali FZ, Chlif S, Atri C, et al. Intra-specific diversity of leishmania major isolates: A key determinant of Tunisian zoonotic cutaneous leishmaniasis clinical polymorphism. Microorganisms. (2022) 10(3):505. doi: 10.3390/microorganisms10030505
111. Boaventura VS, Santos CS, Cardoso CR, de Andrade J, Dos Santos WL, Clarencio J, et al. Human mucosal leishmaniasis: neutrophils infiltrate areas of tissue damage that express high levels of Th17-related cytokines. Eur J Immunol. (2010) 40:2830–6. doi: 10.1002/eji.200940115
112. Elso CM, Roberts LJ, Smyth GK, Thomson RJ, Baldwin TM, Foote SJ, et al. Leishmaniasis host response loci (lmr1-3) modify disease severity through a Th1/Th2-independent pathway. Genes Immun. (2004) 5:93–100. doi: 10.1038/sj.gene.6364042
113. Babay BE, Louzir H, Kebaier C, Boubaker S, Dellagi K, Cazenave PA. Inbred strains derived from feral mice reveal new pathogenic mechanisms of experimental leishmaniasis due to Leishmania major. Infect Immun. (2004) 72:4603–11. doi: 10.1128/IAI.72.8.4603-4611.2004
114. Kebaier C, Louzir H, Chenik M, Ben Salah A, Dellagi K. Heterogeneity of wild Leishmania major isolates in experimental murine pathogenicity and specific immune response. Infect Immun. (2001) 69:4906–15. doi: 10.1128/IAI.69.8.4906-4915.2001
115. Alimohammadian MH, Darabi H, Ajdary S, Khaze V, Torkabadi E. Genotypically distinct strains of Leishmania major display diverse clinical and immunological patterns in BALB/c mice. Infection Genet evolution: J Mol Epidemiol evolutionary Genet Infect diseases. (2010) 10:969–75. doi: 10.1016/j.meegid.2010.06.006
116. Asadpour A, Riazi-Rad F, Khaze V, Ajdary S, Alimohammadian MH. Distinct strains of Leishmania major induce different cytokine mRNA expression in draining lymph node of BALB/c mice. Parasite Immunol. (2013) 35:42–50. doi: 10.1111/pim.2012.35.issue-1
117. Darabi S, Khaze V, Riazi-Rad F, Darabi H, Bahrami F, Ajdary S, et al. Leishmania major strains isolated from distinct endemic areas show diverse cytokine mRNA expression levels in C57BL/6 mice: Toward selecting an ideal strain for the vaccine studies. Cytokine. (2015) 76:303–8. doi: 10.1016/j.cyto.2015.05.022
118. Hosseini M, Nateghi Rostami M, Hosseini Doust R, Khamesipour A. Multilocus sequence typing analysis of Leishmania clinical isolates from cutaneous leishmaniasis patients of Iran. Infection Genet evolution: J Mol Epidemiol evolutionary Genet Infect diseases. (2020) 85:104533. doi: 10.1016/j.meegid.2020.104533
119. Uzonna JE, Wei G, Yurkowski D, Bretscher P. Immune elimination of Leishmania major in mice: implications for immune memory, vaccination, and reactivation disease. J Immunol. (2001) 167:6967–74. doi: 10.4049/jimmunol.167.12.6967
120. Lira R, Doherty M, Modi G, Sacks D. Evolution of lesion formation, parasitic load, immune response, and reservoir potential in C57BL/6 mice following high- and low-dose challenge with Leishmania major. Infect Immun. (2000) 68:5176–82. doi: 10.1128/IAI.68.9.5176-5182.2000
121. Belkaid Y, Mendez S, Lira R, Kadambi N, Milon G, Sacks D. A natural model of Leishmania major infection reveals a prolonged “silent” phase of parasite amplification in the skin before the onset of lesion formation and immunity. J Immunol. (2000) 165:969–77. doi: 10.4049/jimmunol.165.2.969
122. Loeuillet C, Banuls AL, Hide M. Study of Leishmania pathogenesis in mice: experimental considerations. Parasit Vectors. (2016) 9:144. doi: 10.1186/s13071-016-1413-9
123. Ji J, Sun J, Qi H, Soong L. Analysis of T helper cell responses during infection with Leishmania amazonensis. Am J Trop Med hygiene. (2002) 66:338–45. doi: 10.4269/ajtmh.2002.66.338
124. Soong L, Chang CH, Sun J, Longley BJ Jr., Ruddle NH, Flavell RA, et al. Role of CD4+ T cells in pathogenesis associated with Leishmania amazonensis infection. J Immunol. (1997) 158:5374–83. doi: 10.4049/jimmunol.158.11.5374
125. Afonso LC, Scott P. Immune responses associated with susceptibility of C57BL/10 mice to Leishmania amazonensis. Infect Immun. (1993) 61:2952–9. doi: 10.1128/iai.61.7.2952-2959.1993
126. Childs GE, Lightner LK, McKinney L, Groves MG, Price EE, Hendricks LD. Inbred mice as model hosts for cutaneous leishmaniasis. I. Resistance and susceptibility to infection with Leishmania Braziliensis, L. mexicana and L. aethiopica. Ann Trop Med Parasitol. (1984) 78:25–34. doi: 10.1080/00034983.1984.11811769
127. Pereira CG, Silva AL, de Castilhos P, Mastrantonio EC, Souza RA, Romao RP, et al. Different isolates from Leishmania Braziliensis complex induce distinct histopathological features in a murine model of infection. Veterinary Parasitol. (2009) 165:231–40. doi: 10.1016/j.vetpar.2009.07.019
128. DeKrey GK, Lima HC, Titus RG. Analysis of the immune responses of mice to infection with Leishmania Braziliensis. Infect Immun. (1998) 66:827–9. doi: 10.1128/IAI.66.2.827-829.1998
129. de Moura TR, Novais FO, Oliveira F, Clarencio J, Noronha A, Barral A, et al. Toward a novel experimental model of infection to study American cutaneous leishmaniasis caused by Leishmania Braziliensis. Infect Immun. (2005) 73:5827–34. doi: 10.1128/IAI.73.9.5827-5834.2005
130. Oliveira DM, Costa MA, Chavez-Fumagalli MA, Valadares DG, Duarte MC, Costa LE, et al. Evaluation of parasitological and immunological parameters of Leishmania chagasi infection in BALB/c mice using different doses and routes of inoculation of parasites. Parasitol Res. (2012) 110:1277–85. doi: 10.1007/s00436-011-2628-5
131. Honore S, Garin YJ, Sulahian A, Gangneux JP, Derouin F. Influence of the host and parasite strain in a mouse model of visceral Leishmania infantum infection. FEMS Immunol Med Microbiol. (1998) 21:231–9. doi: 10.1016/S0928-8244(98)00079-0
132. Ahmed S, Colmenares M, Soong L, Goldsmith-Pestana K, Munstermann L, Molina R, et al. Intradermal infection model for pathogenesis and vaccine studies of murine visceral leishmaniasis. Infect Immun. (2003) 71:401–10. doi: 10.1128/IAI.71.1.401-410.2003
133. Kaye PM, Curry AJ, Blackwell JM. Differential production of Th1- and Th2-derived cytokines does not determine the genetically controlled or vaccine-induced rate of cure in murine visceral leishmaniasis. J Immunol. (1991) 146:2763–70. doi: 10.4049/jimmunol.146.8.2763
134. Murray HW. Endogenous interleukin-12 regulates acquired resistance in experimental visceral leishmaniasis. J Infect diseases. (1997) 175:1477–9. doi: 10.1086/jid.1997.175.issue-6
135. Murray HW, Hariprashad J, Coffman RL. Behavior of visceral Leishmania donovani in an experimentally induced T helper cell 2 (Th2)-associated response model. J Exp Med. (1997) 185:867–74. doi: 10.1084/jem.185.5.867
136. Murray HW. Tissue granuloma structure-function in experimental visceral leishmaniasis. Int J Exp pathology. (2001) 82:249–67. doi: 10.1046/j.1365-2613.2001.00199.x
137. Neal RA, Hale C. A comparative study of susceptibility of inbred and outbred mouse strains compared with hamsters to infection with New World cutaneous leishmaniases. Parasitology. (1983) 87:7–13. doi: 10.1017/S0031182000052379
138. Munoz-Durango N, Gomez A, Garcia-Valencia N, Roldan M, Ochoa M, Bautista-Erazo DE, et al. A mouse model of ulcerative cutaneous leishmaniasis by leishmania (Viannia) panamensis to investigate infection, pathogenesis, immunity, and therapeutics. Front Microbiol. (2022) 13:907631. doi: 10.3389/fmicb.2022.907631
139. Nabors GS, Farrell JP. Site-specific immunity to Leishmania major in SWR mice: the site of infection influences susceptibility and expression of the antileishmanial immune response. Infect Immun. (1994) 62:3655–62. doi: 10.1128/iai.62.9.3655-3662.1994
140. Krishnan L, Guilbert LJ, Wegmann TG, Belosevic M, Mosmann TR. T helper 1 response against Leishmania major in pregnant C57BL/6 mice increases implantation failure and fetal resorptions. Correlation with increased IFN-gamma and TNF and reduced IL-10 production by placental cells. J Immunol. (1996) 156:653–62. doi: 10.4049/jimmunol.156.2.653
141. Satoskar AR, Rodig S, Telford SR 3rd, Satoskar AA, Ghosh SK, von Lichtenberg F, et al. IL-12 gene-deficient C57BL/6 mice are susceptible to Leishmania donovani but have diminished hepatic immunopathology. Eur J Immunol. (2000) 30:834–9. doi: 10.1002/1521-4141(200003)30:3<834::AID-IMMU834>3.0.CO;2-9
142. Murray HW, Hariprashad J. Interleukin 12 is effective treatment for an established systemic intracellular infection: experimental visceral leishmaniasis. J Exp Med. (1995) 181:387–91. doi: 10.1084/jem.181.1.387
143. Engwerda CR, Kaye PM. Organ-specific immune responses associated with infectious disease. Immunol Today. (2000) 21:73–8. doi: 10.1016/S0167-5699(99)01549-2
144. Melby PC, Tabares A, Restrepo BI, Cardona AE, McGuff HS, Teale JM. Leishmania donovani: evolution and architecture of the splenic cellular immune response related to control of infection. Exp Parasitol. (2001) 99:17–25. doi: 10.1006/expr.2001.4640
145. Rodrigues OR, Marques C, Soares-Clemente M, Ferronha MH, Santos-Gomes GM. Identification of regulatory T cells during experimental Leishmania infantum infection. Immunobiology. (2009) 214:101–11. doi: 10.1016/j.imbio.2008.07.001
146. Squires KE, Kirsch M, Silverstein SC, Acosta A, McElrath MJ, Murray HW. Defect in the tissue cellular immune response: experimental visceral leishmaniasis in euthymic C57BL/6 ep/ep mice. Infect Immun. (1990) 58:3893–8. doi: 10.1128/iai.58.12.3893-3898.1990
147. Baptista-Fernandes T, Marques C, Roos Rodrigues O, Santos-Gomes GM. Intra-specific variability of virulence in Leishmania infantum zymodeme MON-1 strains. Comp immunology Microbiol Infect diseases. (2007) 30:41–53. doi: 10.1016/j.cimid.2006.10.001
148. Ferreira-Paes T, Charret KDS, Ribeiro M, Rodrigues RF, Leon LL. Comparative analysis of biological aspects of Leishmania infantum strains. PloS One. (2020) 15:e0230545. doi: 10.1371/journal.pone.0230545
149. Kaur S, Kaur T, Garg N, Mukherjee S, Raina P, Athokpam V. Effect of dose and route of inoculation on the generation of CD4+ Th1/Th2 type of immune response in murine visceral leishmaniasis. Parasitol Res. (2008) 103:1413–9. doi: 10.1007/s00436-008-1150-x
150. Rolao N, Melo C, Campino L. Influence of the inoculation route in BALB/c mice infected by Leishmania infantum. Acta tropica. (2004) 90:123–6. doi: 10.1016/j.actatropica.2003.09.010
151. Rosypal AC, Zajac AM, Troy GC, Lindsay DS. Infections in immunocompetent and immune-deficient mice with promastigotes of a North American isolate of Leishmania infantum. Veterinary Parasitol. (2005) 130:19–27. doi: 10.1016/j.vetpar.2005.03.017
152. Requena JM, Soto M, Doria MD, Alonso C. Immune and clinical parameters associated with Leishmania infantum infection in the golden hamster model. Veterinary Immunol immunopathology. (2000) 76:269–81. doi: 10.1016/S0165-2427(00)00221-X
153. Melby PC, Chandrasekar B, Zhao W, Coe JE. The hamster as a model of human visceral leishmaniasis: progressive disease and impaired generation of nitric oxide in the face of a prominent Th1-like cytokine response. J Immunol. (2001) 166:1912–20. doi: 10.4049/jimmunol.166.3.1912
154. Nieto A, Dominguez-Bernal G, Orden JA, de la Fuente R, Madrid-Elena N, Carrion J. Mechanisms of resistance and susceptibility to experimental visceral leishmaniosis: BALB/c mouse versus Syrian hamster model. Veterinary Res. (2011) 42:39. doi: 10.1186/1297-9716-42-39
155. Mukherjee P, Ghosh AK, Ghose AC. Infection pattern and immune response in the spleen and liver of BALB/c mice intracardially infected with Leishmania donovani amastigotes. Immunol letters. (2003) 86:131–8. doi: 10.1016/S0165-2478(03)00021-X
156. Carrion J, Nieto A, Iborra S, Iniesta V, Soto M, Folgueira C, et al. Immunohistological features of visceral leishmaniasis in BALB/c mice. Parasite Immunol. (2006) 28:173–83. doi: 10.1111/j.1365-3024.2006.00817.x
157. Alexander J. Sex differences and cross-immunity in DBA/2 mice infected with L. mexicana and L. major. Parasitology. (1988) 96:297–302. doi: 10.1017/S0031182000058303
158. Demant P, Hart AA. Recombinant congenic strains–a new tool for analyzing genetic traits determined by more than one gene. Immunogenetics. (1986) 24:416–22. doi: 10.1007/BF00377961
159. Dutta S, Sengupta P. Men and mice: Relating their ages. Life Sci. (2016) 152:244–8. doi: 10.1016/j.lfs.2015.10.025
160. Sohrabi Y, Reinecke H, Joosten LAB, Netea MG. Deadly COVID-19 among the elderly: Innate immune memory helping those most in need. Med. (2021) 2:378–83. doi: 10.1016/j.medj.2021.02.004
161. Salgado CL, Corea AFM, Covre LP, Fonseca-Martins AMD, Falqueto A, Guedes HLM, et al. Intranasal delivery of LaAg vaccine improves immunity of aged mice against visceral Leishmaniasis. Acta tropica. (2024) 252:107125. doi: 10.1016/j.actatropica.2024.107125
162. Boy-Waxman S, Olivier M, Cermakian N. Clockwork intruders: Do parasites manipulate their hosts’ circadian rhythms? Curr Res Parasitol Vector Borne Dis. (2024) 5:100171. doi: 10.1016/j.crpvbd.2024.100171
163. Dominguez-Andres J, Reinecke H, Sohrabi Y. The immune hunger games: the effects of fasting on monocytes. Cell Mol Immunol. (2023) 20:1098–100. doi: 10.1038/s41423-023-01033-w
164. Pick R, He W, Chen CS, Scheiermann C. Time-of-day-dependent trafficking and function of leukocyte subsets. Trends Immunol. (2019) 40:524–37. doi: 10.1016/j.it.2019.03.010
165. Zhang Q, Schwarz D, Cheng Y, Sohrabi Y. Unraveling host genetics and microbiome genome crosstalk: a novel therapeutic approach. Trends Mol Med. (2024) 30:1007–9. doi: 10.1016/j.molmed.2024.06.007
166. Misra P, Singh S. Site specific microbiome of Leishmania parasite and its cross-talk with immune milieu. Immunol letters. (2019) 216:79–88. doi: 10.1016/j.imlet.2019.10.004
167. Mrazek J, Mrazkova L, Mekadim C, Jarosikova T, Krayem I, Sohrabi Y, et al. Effects of Leishmania major infection on the gut microbiome of resistant and susceptible mice. Appl Microbiol Biotechnol. (2024) 108:145. doi: 10.1007/s00253-024-13002-y
168. Meazzi S, Lauzi S, Martini V, Ferriani R, Peri M, Zanzani SA, et al. Gut microbiota and lymphocyte subsets in canine leishmaniasis. Front Vet Sci. (2022) 9:868967. doi: 10.3389/fvets.2022.868967
169. Sarnaglia GD, Covre LP, Pereira FE, HL DEMG, Faria AM, Dietze R, et al. Diet-induced obesity promotes systemic inflammation and increased susceptibility to murine visceral leishmaniasis. Parasitology. (2016) 143:1647–55. doi: 10.1017/S003118201600127X
170. Lago T, Carvalho LP, Nascimento M, Guimaraes LH, Lago J, Castellucci L, et al. Influence of obesity on clinical manifestations and response to therapy in cutaneous leishmaniasis caused by leishmania Braziliensis. Clin Infect Dis. (2021) 73:1020–6. doi: 10.1093/cid/ciab236
171. Martins VD, Vaz L, Barbosa SC, Paixao PHM, Torres L, de Oliveira MFA, et al. Obesity alters the macrophages’ response to Leishmania major in C57BL/6 mice. J Leukoc Biol. (2024) 116:1372–84. doi: 10.1093/jleuko/qiae171
172. Amorim Sacramento L, Gonzalez-Lombana C, Scott P. Malnutrition disrupts adaptive immunity during visceral leishmaniasis by enhancing IL-10 production. PloS Pathog. (2024) 20:e1012716. doi: 10.1101/2024.06.06.597776
173. Osorio EY, Uscanga-Palomeque A, Patterson GT, Cordova E, Travi BL, Soong L, et al. Malnutrition-related parasite dissemination from the skin in visceral leishmaniasis is driven by PGE2-mediated amplification of CCR7-related trafficking of infected inflammatory monocytes. PloS Negl Trop Dis. (2023) 17:e0011040. doi: 10.1371/journal.pntd.0011040
174. Nweze JA, Nweze EI, Onoja US. Nutrition, malnutrition, and leishmaniasis. Nutrition. (2020) 73:110712. doi: 10.1016/j.nut.2019.110712
175. Bennis I, De Brouwere V, Belrhiti Z, Sahibi H, Boelaert M. Psychosocial burden of localised cutaneous Leishmaniasis: a scoping review. BMC Public Health. (2018) 18:358. doi: 10.1186/s12889-018-5260-9
176. Grifferty G, Shirley H, McGloin J, Kahn J, Orriols A, Wamai R. Vulnerabilities to and the socioeconomic and psychosocial impacts of the leishmaniases: A review. Res Rep Trop Med. (2021) 12:135–51. doi: 10.2147/RRTM.S278138
177. Pires M, Wright B, Kaye PM, da Conceicao V, Churchill RC. The impact of leishmaniasis on mental health and psychosocial well-being: A systematic review. PloS One. (2019) 14:e0223313. doi: 10.1371/journal.pone.0223313
178. Chahed MK, Bellali H, Ben Jemaa S, Bellaj T. Psychological and psychosocial consequences of zoonotic cutaneous leishmaniasis among women in Tunisia: preliminary findings from an exploratory study. PloS Negl Trop Dis. (2016) 10:e0005090. doi: 10.1371/journal.pntd.0005090
179. Portes A, Giestal-de-Araujo E, Fagundes A, Pandolfo P, de Sa Geraldo A, Lira ML, et al. Leishmania amazonensis infection induces behavioral alterations and modulates cytokine and neurotrophin production in the murine cerebral cortex. J Neuroimmunol. (2016) 301:65–73. doi: 10.1016/j.jneuroim.2016.11.003
180. Pal B, Murti K, Siddiqui NA, Das P, Lal CS, Babu R, et al. Assessment of quality of life in patients with post kalaazar dermal leishmaniasis. Health Qual Life Outcomes. (2017) 15:148. doi: 10.1186/s12955-017-0720-y
181. Garapati P, Pal B, Siddiqui NA, Bimal S, Das P, Murti K, et al. Knowledge, stigma, health seeking behaviour and its determinants among patients with post kalaazar dermal leishmaniasis, Bihar, India. PloS One. (2018) 13:e0203407. doi: 10.1371/journal.pone.0203407
182. Amni F, Maleki-Ravasan N, Nateghi-Rostami M, Hadighi R, Karimian F, Meamar AR, et al. Corrigendum: Co-infection of Phlebotomus papatasi (Diptera: Psychodidae) gut bacteria with Leishmania major exacerbates the pathological responses of BALB/c mice. Front Cell Infect Microbiol. (2023) 13:1185912. doi: 10.3389/fcimb.2023.1185912
183. Rohousova I, Volf P, Lipoldova M. Modulation of murine cellular immune response and cytokine production by salivary gland lysate of three sand fly species. Parasite Immunol. (2005) 27:469–73. doi: 10.1111/j.1365-3024.2005.00787.x
184. Lestinova T, Rohousova I, Sima M, de Oliveira CI, Volf P. Insights into the sand fly saliva: Blood-feeding and immune interactions between sand flies, hosts, and Leishmania. PloS Negl Trop Dis. (2017) 11:e0005600. doi: 10.1371/journal.pntd.0005600
185. Abdeladhim M, Kamhawi S, Valenzuela JG. What’s behind a sand fly bite? The profound effect of sand fly saliva on host hemostasis, inflammation and immunity. Infection Genet evolution: J Mol Epidemiol evolutionary Genet Infect diseases. (2014) 28:691–703. doi: 10.1016/j.meegid.2014.07.028
186. Rogers ME, Ilg T, Nikolaev AV, Ferguson MA, Bates PA. Transmission of cutaneous leishmaniasis by sand flies is enhanced by regurgitation of fPPG. Nature. (2004) 430:463–7. doi: 10.1038/nature02675
187. Hessler JR. The history of environmental improvements in laboratory animal science: caging, systems, equipment, and facility design. Fifty Years of Laboratory Animal Science. Memphis, TN: American Association for Laboratory Animal Science (1999).
188. Foster HL. Housing of disease-free vertebrates. Ann N Y Acad Sci. (1959) 78:80–8. doi: 10.1111/j.1749-6632.1959.tb53096.x
189. Dobson GP, Letson HL, Biros E, Morris J. Specific pathogen-free (SPF) animal status as a variable in biomedical research: Have we come full circle? EBioMedicine. (2019) 41:42–3. doi: 10.1016/j.ebiom.2019.02.038
190. Beura LK, Hamilton SE, Bi K, Schenkel JM, Odumade OA, Casey KA, et al. Normalizing the environment recapitulates adult human immune traits in laboratory mice. Nature. (2016) 532:512–6. doi: 10.1038/nature17655
191. Karimkhani C, Wanga V, Coffeng LE, Naghavi P, Dellavalle RP, Naghavi M. Global burden of cutaneous leishmaniasis: a cross-sectional analysis from the Global Burden of Disease Study 2013. Lancet Infect Dis. (2016) 16:584–91. doi: 10.1016/S1473-3099(16)00003-7
192. Pigott DM, Bhatt S, Golding N, Duda KA, Battle KE, Brady OJ, et al. Global distribution maps of the leishmaniases. Elife. (2014) 3:e02851. doi: 10.7554/eLife.02851
193. Alvar J, Velez ID, Bern C, Herrero M, Desjeux P, Cano J, et al. Leishmaniasis worldwide and global estimates of its incidence. PloS One. (2012) 7:e35671. doi: 10.1371/journal.pone.0035671
194. Knight CA, Harris DR, Alshammari SO, Gugssa A, Young T, Lee CM. Leishmaniasis: Recent epidemiological studies in the Middle East. Front Microbiol. (2022) 13:1052478. doi: 10.3389/fmicb.2022.1052478
195. van Henten S, Adriaensen W, Fikre H, Akuffo H, Diro E, Hailu A, et al. Cutaneous leishmaniasis due to leishmania aethiopica. EClinicalMedicine. (2018) 6:69–81. doi: 10.1016/j.eclinm.2018.12.009
196. de Freitas Milagres T, Lopez-de-Felipe M, da Silva WJ, Martin-Martin I, Galvez R, da Silva OS. Same parasite, different outcomes: unraveling the epidemiology of Leishmania infantum infection in Brazil and Spain. Trends Parasitol. (2023) 39:774–85. doi: 10.1016/j.pt.2023.06.008
197. Herrera G, Barragan N, Luna N, Martinez D, De Martino F, Medina J, et al. An interactive database of Leishmania species distribution in the Americas. Sci Data. (2020) 7:110. doi: 10.1038/s41597-020-0451-5
198. Gramiccia M, Gradoni L. The current status of zoonotic leishmaniases and approaches to disease control. Int J Parasitol. (2005) 35:1169–80. doi: 10.1016/j.ijpara.2005.07.001
199. Soares L, Abad-Franch F, Ferraz G. Epidemiology of cutaneous leishmaniasis in central Amazonia: a comparison of sex-biased incidence among rural settlers and field biologists. Trop Med Int Health. (2014) 19:988–95. doi: 10.1111/tmi.2014.19.issue-8
200. Doherty TM, Coffman RL. Leishmania major: effect of infectious dose on T cell subset development in BALB/c mice. Exp Parasitol. (1996) 84:124–35. doi: 10.1006/expr.1996.0098
201. Uzonna JE, Joyce KL, Scott P. Low dose Leishmania major promotes a transient T helper cell type 2 response that is down-regulated by interferon gamma-producing CD8+ T cells. J Exp Med. (2004) 199:1559–66. doi: 10.1084/jem.20040172
202. Bretscher PA, Wei G, Menon JN, Bielefeldt-Ohmann H. Establishment of stable, cell-mediated immunity that makes “susceptible” mice resistant to Leishmania major. Science. (1992) 257:539–42. doi: 10.1126/science.1636090
Keywords: mouse model, human leishmaniasis, translation, influencing factor, experimental analysis, reproducibility of data, experimental conditions
Citation: Nateghi-Rostami M, Lipoldová M and Sohrabi Y (2025) Improving reproducibility and translational potential of mouse models: lessons from studying leishmaniasis. Front. Immunol. 16:1559907. doi: 10.3389/fimmu.2025.1559907
Received: 13 January 2025; Accepted: 24 March 2025;
Published: 22 April 2025.
Edited by:
Hector Mora Montes, University of Guanajuato, MexicoReviewed by:
Silvia Amaral Gonçalves Da-Silva, Rio de Janeiro State University, BrazilSantanu Kar Mahapatra, Midnapore City College, West Bengal, India
Copyright © 2025 Nateghi-Rostami, Lipoldová and Sohrabi. This is an open-access article distributed under the terms of the Creative Commons Attribution License (CC BY). The use, distribution or reproduction in other forums is permitted, provided the original author(s) and the copyright owner(s) are credited and that the original publication in this journal is cited, in accordance with accepted academic practice. No use, distribution or reproduction is permitted which does not comply with these terms.
*Correspondence: Yahya Sohrabi, WWFoeWEuc29ocmFiaUB1a211ZW5zdGVyLmRl
†ORCID: Marie Lipoldová, orcid.org/0000-0002-2101-0334