- 1Department of Cardiology, The Affiliated Hospital of Qingdao University, Qingdao, Shandong, China
- 2Department of Genetics and Cell Biology, Basic Medical College, Qingdao University, Qingdao, China
- 3Department of Cardiology, The Affiliated Cardiovascular Hospital of Qingdao University, Qingdao, China
The outbreak of severe acute respiratory syndrome coronavirus 2 (SARS-CoV-2), which caused the Coronavirus disease 2019 (COVID-19) pandemic, has posed significant healthcare challenges. In addition to respiratory complications, it has led to severe damage in other organs, particularly the cardiovascular system. Of which, myocardial injury is increasingly recognized as a most significant complication, contributing to the high mortality. Recent research indicates the pivotal role of immune dysregulation in mediating myocardial injury in patients infected with SARS-CoV-2. In this review, we provide a comprehensive analysis of the immune mechanisms involved in SARS-CoV-2-induced myocardial damage, focusing on the roles of key immune cells and molecules that contribute to this pathological process. Aiming at mitigating the myocardial injury of COVID-19, we review immune-based treatments under evaluation in preclinical and clinical trials. Along with talking about the similarities and differences in myocardial injury resulting from SARS-CoV-2, the Middle East respiratory syndrome coronavirus (MERS-CoV) and the severe acute respiratory syndrome coronavirus (SARS-CoV). This article provides a unique perspective on using past experiences to prevent myocardial injury in the face of ongoing virus mutations.
1 Introduction
Coronavirus disease 2019 (COVID-19), a highly contagious respiratory illness caused by the severe acute respiratory syndrome coronavirus 2 (SARS-CoV-2), has spread worldwide rapidly and inflicted tremendous harm on the population (1). Different from the two previous pandemics caused by the Middle East respiratory syndrome coronavirus (MERS-CoV) and the severe acute respiratory syndrome coronavirus (SARS-CoV), SARS-CoV-2 has a higher rate of transmission, is extremely contagious, and exhibits frequent mutations (2, 3). SARS-CoV-2 can affect multiple organs in addition to the respiratory system (4), resulting in a variety of extrapulmonary symptoms (5, 6). Among these, cardiac researchers are mainly concerned about myocardial injury induced by the SARS-CoV-2 (7). Infected patients exhibit a variety of cardiovascular symptoms, including myocarditis, pericarditis, heart failure, and arrhythmias (8). Compelling evidence has indicated a compact-connection between the emergence of myocardial injury and adverse events, which lead to a higher risk of mortality (9). As a result, early identification and intervention to avoid myocardial injury plays a key part in determining the outcome and prognosis of COVID-19 patients.
Currently, the pathophysiological process in myocardial injury caused by SARS-CoV-2 infection may be divided into two categories: direct myocardial injury and indirect myocardial injury (10). Some viewpoints speculate that immune dysfunction may participate in the above process and have an obvious impact (11, 12). Immune intervention is thought to be a treatment strategy for myocardial injury related to SARS-CoV-2. This article describes in detail the immune-related factors that contribute to SARS-CoV-2-induced myocardial injury. It also discusses potential strategies for preventing and treating this damage. We undertake a comparison of the myocardial injury induced by SARS-CoV-2, MERS-CoV, and SARS-CoV. Meanwhile, we provide valuable insights into how to learn from past experience to prevent myocardial injury proactively in light of ongoing virus mutations.
2 Excessive inflammation and abnormal immune responses in SARS-CoV-2-induced myocardial injury
The immune system is the first line of defense against virus reproduction and transmission, and is made up of two parts: innate immunity and adaptive immunity (13). During the initial stages of SARS-CoV-2 infection, the pathogen-associated molecular patterns (PAMPs) of SARS-CoV-2 are recognized by pattern recognition receptors (PRRs) of host cells (14). This recognition triggers a series of signaling cascades, ultimately inducing the production of type I and type III interferons (IFNs) along with proinflammatory cytokines and chemokines (15). The IFNs enhance the antiviral state of host cells and limit the replication and spread of the SARS-CoV-2, while cytokines and chemokines recruit innate immune cells, including neutrophils, monocytes, and macrophages, to infection sites (16). Dendritic cells (DCs), a kind of innate immune cell, capture the viral antigen at the site of infection and migrate to secondary lymphoid organs such as lymph nodes (17). DCs present the antigen to T cells, thereby activating T cell-mediated adaptive immune response (18). At the same time, innate immune-derived cytokines such as interleukin-1 (IL-1), interleukin-6 (IL-6), and tumor necrosis factor-α (TNF-α) can serve as warning signals to promote the activation, proliferation and differentiation of T cells and B cells. Virus-specific antibodies from B cells and T cell responses synergistically contain the infection (19). This coordinated response establishes that adaptive immunity requires innate immune priming through both antigen presentation and cytokine signaling (20). However, SARS-CoV-2 employs immune evasion mechanisms that delay innate immune activation (21). This impairment allows uncontrolled viral replication while postponing adaptive immunity initiation (22). If the adaptive immune response is delayed too long, elevated viral loads trigger compensatory hyperactivation of innate immunity, resulting in the production of a large number of cytokines and chemokines (16). The resultant overproduction of inflammatory mediators disrupts the balance between the anti-inflammatory and pro-inflammatory responses (23). A large number of cytokines and chemokines cause cytokine storm, leading to various irreversible tissue damage, including to the lung, heart, and kidney (4). It is worth mentioning that cytokine storm-related hyperinflammatory syndrome is the root cause of many severe COVID-19 symptoms, which is also considered the underlying mechanism of COVID-19-related myocardial injury (24).
Myocardial injury is a common complication of COVID-19. It is an independent risk factor for in-hospital death (9). Infection with SARS-CoV-2 in the host may induce cardiac dysfunction and generate cardiovascular complications (25). In patients with severe and fatal COVID-19, indicators of inflammation and myocardial damage increased dramatically (26, 27). Direct virus infection and the abnormal immune response may be the primary causes of this type of myocardial injury (28–30). Infection with SARS-CoV-2 causes cardiomyocyte dysfunction, inflammation, and cardiac fibrosis, as well as significant aggregation of activated T cells and macrophages (31). The overactive immune response may contribute greatly to the mechanism of myocardial injury. The process of myocardial injury in patients with SARS-CoV-2 has been shown in Figure 1.
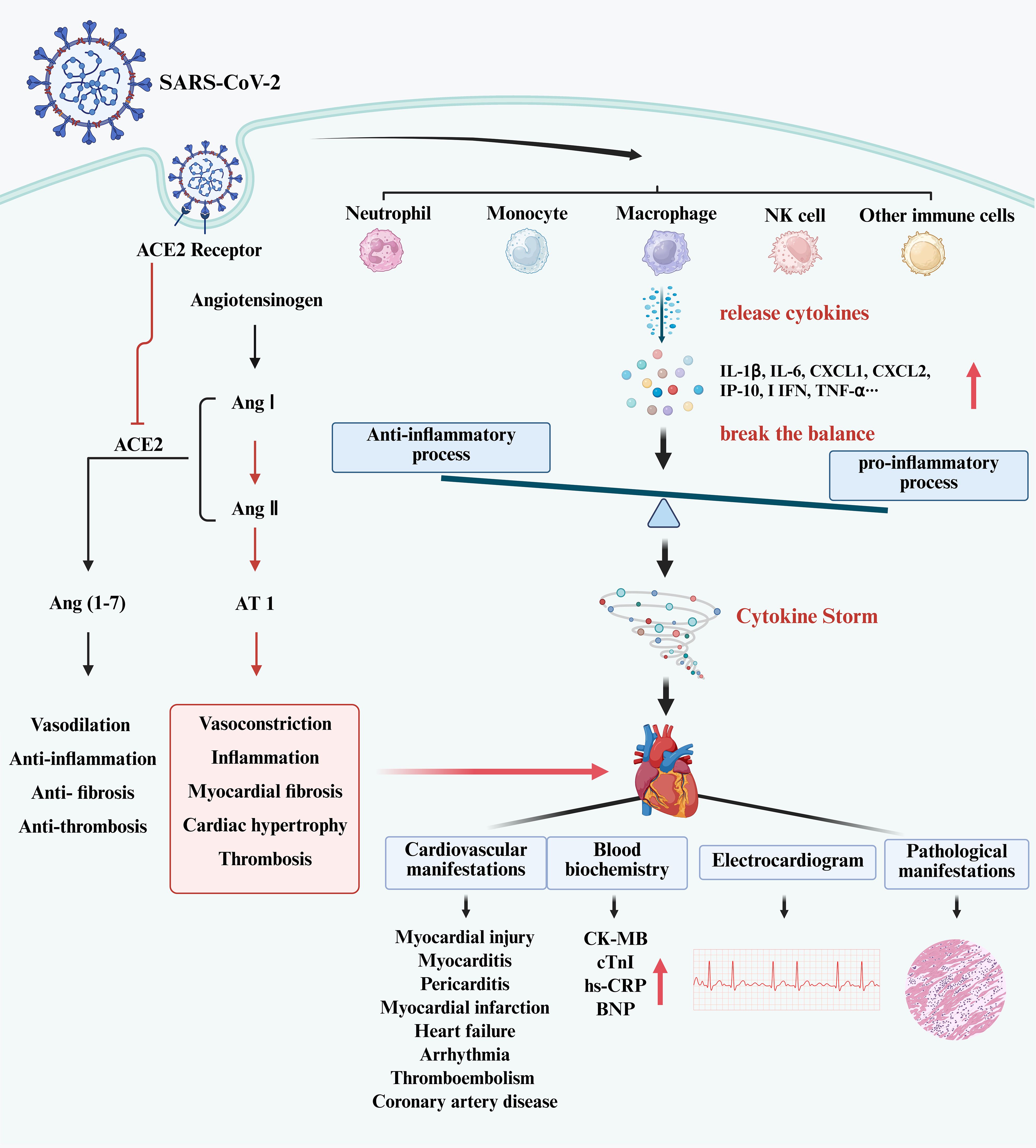
Figure 1. The process of myocardial injury in patients with SARS-CoV-2. (Created in BioRender.com). SARS-CoV-2 infects host cells and activates immune cells, such as neutrophils, monocytes, macrophages, NK cells, T cells, DCs, etc. Those immune cells release cytokines leading to elevated levels of I L-1β, IL-6, CXCL1, CXCL2, I IFN, TNF-α, etc. This breaks the balance between pro-inflammatory and anti-inflammatory processes in the body, produces the cytokine storm, and causes myocardial injury. In clinical practice, myocardial injury may manifest as an increase in CK-MB, cTnI and BNP, with electrocardiographic and pathological changes. In addition, the entry of SARS-CoV-2 into cells has been shown to suppress ACE2 expression. This phenomenon leads to the accumulation of Ang II, resulting in an imbalance between the ACE/Ang II/AT1 axis and the ACE2/Ang1-7/Mas axis. An excessive combination of Ang II and AT1 has the potential to elicit vasoconstriction, inflammation, and myocardial fibrosis. Furthermore, the binding of the S protein of SARS-CoV-2 to the ACE2 receptor will activate the downstream signal cascade, ultimately causing elevated cytokine levels.
2.1 Immune molecules in SARS-CoV-2-induced myocardial injury
2.1.1 Cytokines——the central mediators of cytokine storm
Cytokines are a form of small-molecule protein released by various kinds of immune cells, which are principally engaged in cellular signal transduction. Cytokines encompass interleukins, interferons, chemokines, tumor necrosis factors and so on (23). A previous retrospective study showed that COVID-19 patients exhibit different degrees of elevated levels of inflammatory factors, including IL-1β, IL-2, IL-6, IL-7, IL-8, and TNF-α. The sudden surge in these pro-inflammatory compounds is known as “cytokine storm” (32). Emerging evidence links COVID-19-associated myocardial injury to cytokine storm-mediated hyperinflammation (33). A clinical study indicates that patients with SARS-CoV-2-induced myocardial injury exhibit characteristic laboratory findings. In addition to elevated levels of high-sensitivity C-reactive protein (hs-CRP), COVID-19 patients with myocardial injury also presented with low lymphocyte count, and high levels of IL-6, IL-8, N-terminal pro-B-type natriuretic peptide (NT-proBNP) and TNF-α (34). The above-mentioned biomarker profile implies a potential pathogenic connection between excessive cytokine release and myocardial injury, particularly given the established association between cardiac involvement and adverse clinical outcomes in COVID-19 (26, 35). Therefore, it is necessary to clarify the key role of cytokines in COVID-19-related myocardial injury and, based on this, inhibit cytokine storm to alleviate myocardial injury caused by SARS-CoV-2.
IL-6 is one of the primary mediators of cytokine storm, which is released during infection (36). IL-6 participates in initiating and amplifying the cytokine storm and coordinating the proinflammatory response of immune cells (37). Infection with SARS-CoV-2 may activate T cells, monocytes, macrophages, DCs, and other immune cells, hence producing IL-6 (38). IL-6 has antiviral activity during viral infection, which might promote inflammation resolution and tissue remodeling (39). However, high levels of IL-6 induce T helper 17 (Th17) cells to produce IL-17, which affect the immune defense system and prolong the duration of viral infection collectively (40).
High levels of IL-6 may be the cause of SARS-CoV-2-induced myocardial injury (41). When researchers exposed rat cardiomyocytes to serum from COVID-19 patients, they discovered a significant expression of acute cardiac inhibition a and persistent arrhythmogenic effects on the cardiomyocytes. Serum levels of cytokines including IL-1, IL-6, TNF-α, and others increased significantly (42). Combinatorial inhibition of IL-6 and TNF-α partially restores the viability and function of cardiomyocytes (33). High levels of IL-6 may be associated with cardiac electrophysiological abnormalities in COVID-19 patients. According to several research, an increase in IL-6 is the primary pathogenic factor in COVID-19-related heart rate to repair the QT interval prolongation (43). In patients with severe COVID-19, the activation of systemic inflammatory response can promote QTc prolongation by increasing IL-6, resulting in cardiac electrical remodeling (44). IL-6 influences myocardial stability by regulating of Na+, K+ and Ca2+ currents (45, 46). This regulatory process ultimately contributes to the occurrence of detrimental cardiovascular events, including arrhythmias associated with COVID-19. Specifically, COVID-19-related heart failure showed a positive correlation with IL-6 (47). Injecting IL-6 into mice during animal studies resulted concentric hypertrophy and cardiac fibrosis, which increased myocardial stiffness (48). These findings reveal that IL-6 may both be a biomarker and a possible therapeutic target in cases of heart failure following SARS-CoV-2 infection. Assessing the levels of IL-6 in the circulatory system can serve as a reliable indicator for predicting the likelihood of mortality in COVID-19 patients. Given the key role of IL-6 in triggering cytokine storm and myocardial injury, targeting IL-6 could be a promising approach to alleviate over-activated immune responses and myocardial injury.
2.1.2 The role of chemokines in myocardial injury caused by SARS-CoV-2
Chemokines are a subset of cytokines characterized by their tiny size. They have the ability to attract immune cells to regions of inflammation or infection, acting as chemical mediators in the recruitment of immune cells during an immune response (49). Upon entering the cell, the virus releases its single-stranded RNA molecules into the cytoplasm. The RNA molecules are then identified by the host’s intracellular pattern recognition receptor. As a result, a sequence of cascade signals is activated, eventually leading to the transcription of proinflammatory cytokines and chemokines (21). In the human-induced pluripotent stem cell-derived cardiomyocytes (hiPSC-CMs) model of SARS-Cov-2 infection, inflammatory cytokines like IL-6, IL-8, C-X-C motif chemokine ligand 1 (CXCL1), C-X-C motif chemokine ligand 2 (CXCL2), and TNF-α are increased (50). Newly generated chemokines exhibit chemotactic properties towards many immune cells implicated in innate immune responses, such as monocytes, macrophages, DCs, and NK cells, among others (51). Chemokine ligand 2 (CCL2), alternatively referred to as monocyte chemoattractant protein-1 (MCP-1), is a member of the chemokine family. This family of molecules has a vital function in attracting leukocytes to areas of infection or injury, which aids in immune defense and tissue repair (52). The C-C chemokine receptor type 2 (CCR2), which binds to the chemokine CCL2, is mostly expressed in monocytes. CCL2 is critical in facilitating the recruitment of monocytes to inflamed regions (53). Once recruited, circulating monocytes can induce the production of tissue factor by releasing cytokines derived from activated platelets and endothelial cells. The aforementioned procedure facilitates the formation of thrombus (54). Suppressing the CCL2/CCR2 axis has been proven to impair the aggregation and adherence of arterial platelets to monocytes, hence mitigating plaque development (55). CCL2 levels were shown to rise progressively in severe COVID-19 patients with high D-dimer levels. This observation implies that CCL2 may be involved in the thrombotic inflammatory processes associated with COVID-19 (52). Yang et al. used the hamster model to show that when SARS-CoV-2 infects cardiomyocytes, it releases CCL2, which attracts monocytes (56). A recent animal study discovered that SARS-CoV-2-induced acute respiratory distress syndrome (ARDS) enhanced cardiac inflammation by enlarging the CCR2+ macrophage subset, potentially leading to cardiomyopathy (57). The evidence presented suggests that CCL2 plays an integral part in the development of cardiovascular disease. Suppressing the CCL2/CCR2 axis may mitigate adverse cardiovascular events in COVID-19 patients by restricting the aggregation of monocytes and macrophages at infection sites.
2.1.3 Impaired interferon and immune evasion in myocardial injury caused by SARS-CoV-2
Interferons are one of the important cytokines in innate immune responses (58). Following viral infection of the host, PRRs identify PAMPs and damage-associated molecular patterns (DAMPs), leading to the synthesis of type I and III interferons and proinflammatory cytokines to trigger antiviral responses (14). Type I interferon is mostly composed of IFN-α and IFN-β (59). The IFN-λ family is a subtype of type I interferon, which is also referred to as type III interferon. Following virus infection, all nucleated cells release substantial levels of type I and III interferon. T cells, NK cells, and macrophages produce type II interferon (IFN-γ) (60). Upon entry into the host cell, the viral double-stranded RNA (dsRNA) is detected by the RIG-I/MDA-5 receptors (61). This recognition triggers a series of antiviral signaling events, facilitated by the interaction between RIG-I/MDA-5 and mitochondrial antiviral signals (MAVS). Following this, the MAVS triggers the activation of Iκ B kinase α/β (IKK) and TBK1/IKK ϵ. Then, these kinases turn on the transcription factors NF- κB and IRF3, which causes genes that code for interferon to be transcribed (62). Type I or Type III interferon interact with certain receptors in order to initiate antiviral defense mechanisms via the JAK-STAT signal transduction pathway. The activation of IFN induces the upregulation of gene expression, specifically the expression of interferon-stimulated genes (ISG). This subsequently leads to the production of antiviral effector protein, conferring antiviral capabilities to the host cells (63, 64) (Figure 2).
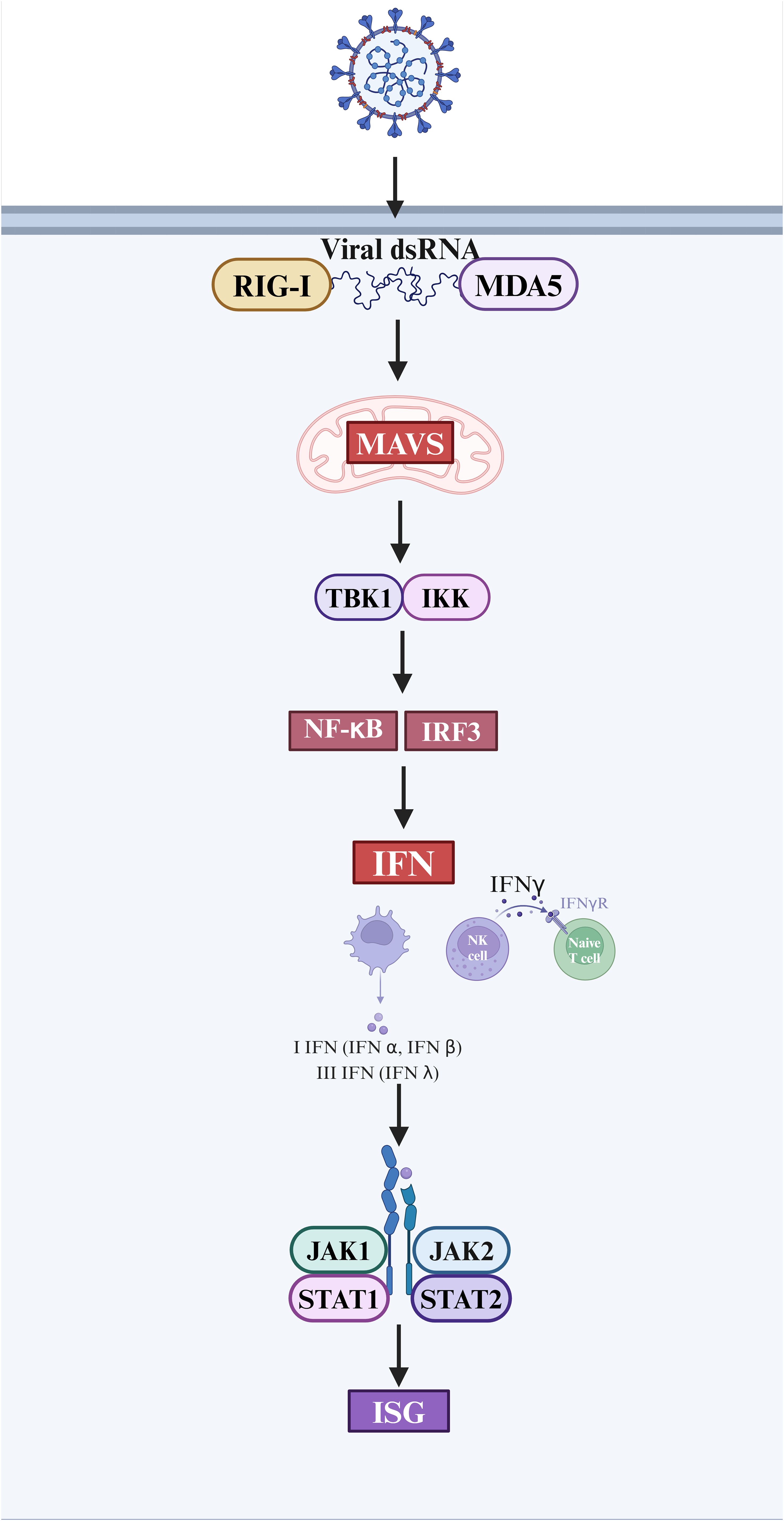
Figure 2. The interferon response and antiviral process after SARS-CoV-2 infection. (Created in BioRender.com).
Type I interferon, such as IFN-α and IFN-β, is essential in fighting against SARS-CoV-2 by effectively regulating the immune response. It is indispensable in regulating viral replication and mitigating the risk of illness exacerbation. Host cells treated with type I interferon significantly inhibit the replication of SARS-CoV (65). However, patients afflicted with COVID-19 frequently exhibit impaired type I interferon response, leading to a protracted elimination of the viral pathogen (66). In the context of influenza infection, the immune response involves the activation of antiviral mechanisms mediated by interferon, which typically precedes the pro-inflammatory response. This temporal sequence seems to enhance host protection while minimizing potential harm to surrounding tissues. But the immunological process described above is not applicable to COVID-19. In hospitalized patients with SARS-CoV-2 infection, we found that the production of IFN- λ and I IFN was reduced or delayed, which could only be produced when a small percentage of patients were in severe condition. Compared to that, pro-inflammatory cytokines, such as TNF-α, IL-6, and IL-8, were observed to be generated prior to the interferon response across all patients (67). During the course of COVID-19, SARS-CoV-2 has the ability to trigger a delayed release of type I interferon during the initial stage of infection. This phenomenon allows the virus to avoid immune system surveillance, leading to its replication within the host’s body and ultimately ending in immunological escape (68). The virus demonstrates the capacity to evade the early innate immune response, specifically the type I interferon response (69), while the activation of the adaptive immunological response is contingent upon the initiation of the innate immune alarm. Consequently, immune escape occurs (70, 71). This reaction attenuates and exacerbates viral replication, leading to excessive activation of Th1 cells. Moreover, the production of INF-γ serves to activate macrophages, triggering the secretion of inflammatory cytokines. This, in turn, leads to a cytokine storm, exacerbating the detrimental effects on the heart.
In the vitro model of manufactured heart tissue, Zhan et al. discovered that the application of IFN-γ may promote a decrease in cardiac contractility and lead to cardiomyocyte dysfunction. The proinflammatory cytokine IFN is linked to an increased risk of cardiac dysfunction. The structural and functional abnormalities generated by IFN are attributed to changes in the balance of pro- and anti-inflammatory cytokines, together with the activation of JAK/STAT signaling pathways. This validates the preceding procedure (72). Furthermore, the investigators conducted a comprehensive examination of the JAK-STAT pathway in primary cardiomyocytes. Their analysis revealed that SARS-CoV-2 has the capacity to selectively affect the proximal constituents of the JAK-STAT pathway. In particular, the virus uses ubiquitin to disrupt the integrity of type I interferon receptors. This makes cells less sensitive to type I interferon (73). Several studies have pointed out that the M protein on the surface of SARS-CoV-2 hinders the generation of type I and III interferons by targeting the RIG-I/MDA-5 signal transduction pathway. Consequently, the disruption weakens the host’s antiviral immune response and facilitates the replication of the virus (62). A group of studies have shown that viruses often use interferon signaling suppression to avoid the body’s natural defenses against viral infections. Targeted therapy that targets immune evasion mechanisms might hinder the virus from replicating in people who have COVID-19, which could lower the risk of serious heart problems.
SARS-CoV-2 is sensitive to IFN treatment, pointing the way for COVID-19 treatment (74). However, several investigations have demonstrated that interferon therapy can increase the expression of host Angiotensin-converting enzyme 2 (ACE2), raising the likelihood that aggravates COVID-19. The study conducted by Busnadiego and colleagues revealed that the use of IFN resulted in an increase in both the transcriptional and cellular expression of ACE2. However, it was shown that the antiviral properties of IFN counteracted the viral infection facilitated by ACE2. This conclusion provides valuable insights for reassessing the therapeutic efficacy of interferon as a pharmaceutical intervention (75).
2.2 Immune cells in SARS-CoV-2-induced myocardial injury
2.2.1 Macrophages
Macrophages are one of the prominent subsets of immune cells in the cardiac tissue. It assumes a pivotal function in the pathophysiological progression of cardiovascular disease. The role of macrophage polarization and macrophage-induced cytokine storm in the development of cardiovascular problems generated by SARS-CoV-2 has sparked significant interest. In the cardiac tissues of individuals who died from COVID-19, there was a rise in the density of CD68 macrophage infiltration in the myocardium. There is an indication that macrophages might play an essential part in myocardial damage among those affected by COVID-19 (76). Interestingly, recent research has revealed that the spike (S) protein of SARS-CoV-2 directly binds to macrophages through the S-protein-angiotensin-converting enzyme 2 interaction (77). The infected macrophages can increase the levels of reactive oxygen species and apoptosis in cardiomyocytes by secreting IL-6 and TNF-α, ultimately resulting in cardiotoxicity (78). The phenotypic and functional properties of macrophages exhibit significant variations as monocytes undergo migration to several tissues and subsequent differentiation. In the course of research, M1 and M2 phenotypic macrophages were discovered in COVID-19 patients and healthy persons (79). M1 macrophages are known to release pro-inflammatory cytokines, like IL-6, whereas M2 macrophages are characterized by their secretion of anti-inflammatory cytokines, such as IL-10 (80, 81). Following entering the host cell, SARS-CoV-2 causes a reduction in ACE2 expression on the cellular surface (82). This leads to a substantial accumulation of Ang II, which promotes the transformation of macrophages into the M1 phenotype and triggers the release of pro-inflammatory cytokines from other immune cells. This exacerbates the process of cytokine storm, therefore aggravating cardiovascular damage (83). Therefore, the potential efficacy of mitigating the cytokine storm induced by macrophage activation in the treatment of myocardial damage associated with COVID-19 is worth considering.
2.2.2 Neutrophils
Neutrophils engage in the host immunological response triggered by SARS-CoV-2 infection and have a distinctive function in post-inflammatory damage. The activation of neutrophils and platelets was seen to be significantly heightened in patients infected with COVID-19 (84). The interplay between platelets and cells of the innate immune system initiates the activation of the coagulation cascade, impeding the dissemination of infections throughout the bloodstream. Excessive inflammation and aberrant immune thrombosis, however, may raise the risk of cardiovascular disease. The increased interaction between neutrophils and platelets results in heightened inflammation and aberrant immunological thrombosis, exacerbating the progression of atherosclerosis and heart failure (85–87). The COVID-19 cohort exhibited a notable presence of thrombosis in both the major and minor blood arteries inside the circulatory system. Evidence of neutrophil-platelet aggregation, neutrophil-rich clusters within significant thrombotic formations, and the creation of neutrophil extracellular traps (NETs) was found in myocardial thrombosis in COVID-19 patients, which is a hallmark of neutrophil activation (88). These data imply that alterations in circulating neutrophils are the root cause of myocardial thrombosis in COVID-19 patients. Furthermore, the autopsy findings of COVID-19 patients with myocarditis revealed a substantial presence of neutrophil infiltration. There was a notable increase in the rates of troponin I, maximal creatine kinase, D-dimer, IL-6, and TNF-α during the patients’ hospitalization, indicating a considerable occurrence of cardiac injury (89). Accordingly, it is vital to direct focus towards the significance of neutrophils in cardiac damage and systemic disorders among COVID-19 individuals.
2.2.3 Lymphocytes
Patients hospitalized with SARS-CoV-2 frequently have heightened neutrophil counts and a decrease in lymphocyte numbers, as indicated by laboratory analyses. Lymphopenia, characterized by a notable decrease in the count of CD4+ and CD8+T cells, B cells, and NK cells, is frequently observed in individuals with severe instances of COVID-19 (5, 90, 91). As a consequence, there is a notable elevation in the neutrophil-lymphocyte ratio (NLR). NLR reflects the dynamic between the innate immune response and the adaptive immune response. The presence of NLR has been identified as an autonomous risk factor for the severity and mortality of patients diagnosed with COVID-19. Several studies have shown a positive correlation between the levels of NLR and the severity and progression of COVID-19. There is a positive correlation between elevated NLR and the severity and duration of a certain disease (92–94). In relation to cardiovascular illness, there is a potential association between NLR and several outcomes, including all-cause mortality, coronary heart disease, and heart failure. The rise in NLR is frequently linked to heightened morbidity and death rates in cardiovascular illness, serving as a significant prognosticator for unfavorable cardiovascular outcomes (95–97).
3 Immune factors in cardiovascular complications from vaccines
Myocarditis or pericarditis is the prevailing cardiovascular complications subsequent to the SARS-CoV-2 vaccination, primarily affecting individuals of the male gender below the age of 40. This occurrence is particularly prominent following the second dose of mRNA vaccines (98, 99). There is evidence that the BNT162b2 and mRNA-1273 vaccines may increase the risk of myocarditis and pericarditis in many studies (100–102). Typically, individuals tend to experience the onset of fever and chest pain within a time frame of 2–4 days after the second dose of the SARS-CoV-2 vaccine. The laboratory tests revealed heightened concentrations of troponin T and creatine kinase. The most common electrocardiogram (ECG) finding was the elevation of the ST segment. There was typical myocarditis in the patients’ cardiac magnetic resonance (CMR) scans, as shown by the presence of late gadolinium enhancement (LGE) and myocardial edema (103–105). Myocarditis typically manifests in persons who possess predisposing conditions. After follow-up, all the myocardial injuries healed. Researchers observed an amplified T cell response in cases of acute myocarditis that occurred four days after vaccination. Meanwhile, endomyocardial biopsy could identify the infiltration of CD4+ cells within the myocardium (106, 107). This observation implies a potential association between vaccine-induced myocarditis and the autoimmune response. The mechanism underlying vaccine-induced myocarditis remains inconclusive. However, ongoing research efforts persist in this area. There is evidence that S protein may evade the recognition of antibodies in the case of people who got myocarditis after being immunized with SARS-CoV-2. These individuals showed a consistent rise in levels of free S protein and did not bind to spike antibodies (108). The potential mechanism by which S protein may induce myocarditis involves its interaction with ACE2, activation of cardiac pericytes, and induction of endothelial cell dysfunction, leading to the mediation of inflammatory processes. Antibody cross-reaction caused by molecular simulation between autoantigen and S protein encoded in vaccines is also considered to be a possible mechanism of vaccine-associated myocarditis. However, after comparing the sequence homology between SARS-CoV-2 stimulating protein-derived peptides and myocarditis-associated antigens, Marrama et al. found that the frequency of spike-derived peptides similar to myocarditis-related antigens was not significantly enriched (109). The empirical findings do not substantiate the perspective that molecular simulation engenders cross-reaction. In addition, Gill et al. highlighted the distinction between some cases and typical myocarditis in their article, specifically noting the presence of catecholamine-mediated stress cardiomyopathy (110). The emotional and physiological response elicited by the SARS-CoV-2 vaccine has the potential to generate an excessive release of catecholamines, initiating an inflammatory response, which may be the cause of vaccine-induced Takotsubo cardiomyopathy (111). In contrast with the adverse events associated with SARS-CoV-2 infection, the incidence of adverse events resulting from vaccination tends to be lower. Furthermore, it is worth noting that some consequences primarily present in individuals with pre-existing medical conditions. Hence, it is imperative to perform a comprehensive physical assessment before administering vaccinations to individuals afflicted with malignancies, cardio-cerebral blood disorders, and other fundamental ailments. Vaccination remains a highly effective and essential strategy for mitigating the COVID-19 pandemic.
4 Genetic susceptibility to SARS-CoV-2-induced myocardial injury
SARS-CoV-2 invades host cells through S protein binding to the host ACE2 receptor. High expression of ACE2 promotes the activation of neutrophils, monocytes/macrophages, NK cells, T-helper-1 (Th 1) cells, Th 2 cells and Th 17 cells to secrete cytokines (112). ACE2 polymorphism may be related to the genetic susceptibility of SARS-CoV-2 (113). Compared to wild-type ACE2, K31R and E37K variants of ACE2 have reduced affinity and the K26R and T92I variants show increased S-protein affinity, which makes the host more susceptible (114). The TT genotype of ACE2 is associated with the severity of COVID-19 (115). ACE2 rs2285666 is greatly associated with both the probability of long-term COVID-19 symptoms and the cumulative incidence of Long COVID (116). Research has shown that variations of ACE2 maybe affect the levels of D-dimer, lactate dehydrogenase (LDH), and CRP. The differences of these biomarkers COVID-19 related give a support for the view that ACE2 rs2285666 may be regarded as a genetic susceptibility marker of COVID19 results (117).
Because of the critical role of genes encoding human leukocyte antigen (HLA) molecules in T cell antigen presentation, HLA has become a major focus of genetic association studies for a variety of infectious and immune-mediated diseases (118). HLA genotypes and related polymorphisms can influence the susceptibility and severity of SARS-CoV-2 infections. Augusto et al. demonstrated a strong and significant association between HLA-B * 15:01 and asymptomatic COVID-19 infection (119). The HLA-C * 01 allele demonstrates a significant association with increased susceptibility to SARS-CoV-2 infection. It serves as a specific ligand for KIR2DL2 and KIR2DL3, which are the receptors that inhibit the activity of NK cells. HLA-C * 01 allele affects the early immune response via its specific interaction with inhibitory NK cell receptors (120). HLA haplotypes may impact the incidence of cytokine storm by interference with immune cell activation. The distinctions among other demographic categories should be taken into account (121). Moreover, variants in cytokine genes, including IL1B, IL1R1, IL1RN, IL6, IL17A, FCGR2A, and TNF may correlate with illness vulnerability and cytokine storm (122). For example, the rs1800629 and rs1800795 variations of proinflammatory cytokines significantly influence the clinical outcomes and systemic inflammatory profiles of COVID-19, elevating TNF-α and IL-6 levels, respectively (123). The effective application of genome-wide association studies (GWASs) and Mendelian randomization can help to identify host genetic variation related to diseases which contribute to explore new mechanisms and therapeutic targets.
5 Long-term cardiovascular sequelae: from PASC to chronic dysfunction
Many patients frequently experience a range of symptoms that are challenging to recover after the improvement of acute covid-19 infection. We refer to it as the post-acute sequelae of COVID-19 (PASC), commonly termed “Long COVID.” Long COVID may impact the cardiovascular system and lead to sequelae, including coronary artery disease, arrhythmias, autonomic dysfunctions, thromboembolic events, and myocarditis (124). Certain individuals endure chronic chest pain and dyspnea after an acute infection, potentially attributable to cardiac injury or persistent inflammation (125). Others may exhibit postural orthostatic tachycardia syndrome (POTS), inappropriate sinus tachycardia (IST), and orthostatic hypotension (OH), which may be related to cardiovascular autonomic dysfunction, with symptoms including tachycardia, orthostatic intolerance, fatigue, and cognitive impairment (126). Thrombotic events described in the context of coronavirus pneumonia are multifactorial and may be associated with platelet activation, leukocyte recruitment, and excessive inflammatory response due to endothelial dysfunction (127). Of note, adverse cardiovascular outcomes may occur in people with no previous history of cardiovascular disease, even in mild or asymptomatic patients. At present, the treatment strategy is limited to symptomatic treatment. Therefore, the establishment of predictive models based on multi-omics technology to develop multi-target intervention schemes for endothelial repair, neuroimmune regulation and coagulation homeostasis is expected to become the key direction of translational medicine research in the future.
6 Strategies for preventing and treating myocardial injury caused by SARS-CoV-2
6.1 Immunomodulatory therapy
SARS-CoV-2 commonly induces damage to several organs beyond the respiratory system, such as the heart. The multi-organ injury observed in COVID-19 patients is a result of the combination of cytokine storm and host immune system dysregulation, ultimately resulting in deteriorated clinical outcomes. Therefore, employing immunomodulatory therapy targeting cytokine storm triggered by excessive inflammatory responses may be beneficial to improving patients’ outcomes.
In COVID-19 patients, elevated levels of IL-6 are crucial in the occurrence of cytokine storm, QT syndrome, and Torsades de Pointes (45, 128). Higher levels of IL-6 are related to severe COVID-19 and adverse prognosis (129). The study has found that using anti-IL-6 receptor monoclonal antibody tocilizumab in COVID-19 patients reduced the risk of inflammation-driven arrhythmias (128). So, using IL-6 inhibitors might ultimately diminish the detrimental consequences of elevated IL-6 levels and also protect the heart. In a prospective analysis conducted on clinical trials including patients who were hospitalized with COVID-19, administration of IL-6 antagonists showed a reduction in 28-day all-cause mortality in comparison to traditional treatment or placebo (130). Tocilizumab, a humanized monoclonal antibody that targets to the IL-6 receptor, has the capability to suppress the physiological activity of IL-6 effectively (131). Treatment with tocilizumab in COVID-19 patients led to a decrease in mortality, lower rates of admission to the intensive care unit (ICU), and reduced reliance on mechanical ventilation compared to patients who did not receive tocilizumab medication (132, 133). Particularly, researchers have found that concurrently administering corticosteroids and tocilizumab enhances clinical benefits, thereby establishing it as a potentially safe and advantageous therapeutic approach (134). In addition to tocilizumab, various additional monoclonal antibodies (mAbs) that have the potential to inhibit the physiological impacts of IL-6 are being evaluated in clinical studies for the treatment of COVID-19, including sarilumab, siltuximab, sirukumab (135, 136).
Cenicriviroc (CVC) functions as an antagonist for both C-C chemokine receptor type 5 (CCR5) and C-C chemokine receptor type 2 (CCR2). Cells within atherosclerotic plaques express both CCR5 and its corresponding ligands (137). CVC demonstrates its anti-inflammatory and immunomodulatory characteristics through the antagonism of CCR2 and CCR5, making it a potentially effective treatment option for myocardial infarction. Research findings have indicated that CVC can impede the replication of the SARS-Cov-2 virus (138). The intervention exhibits the capacity to mitigate the occurrence of respiratory and cardiovascular system dysfunction commonly linked to COVID-19 (139). Current clinical trials are examining the effectiveness of CVC, whether used in conjunction with routine care or in combination with other pharmaceutical agents (140). The purpose of these trials is to utilize the anti-inflammatory properties of CVC to improve the clinical advancement of COVID-19 and mitigate the occurrence of comorbidities.
6.2 Cell -based therapy
Mesenchymal stem cells (MSCs) are a type of stem cell with the ability of self-renewal and multi-lineage differentiation. MSCs perform diverse functions in reducing inflammation, preventing fibrosis, regulating the immune system, and facilitating tissue regeneration (141). Within the realm of cardiac regeneration, MSCs have been shown to enhance cardiac function through various mechanisms, including immune response regulation, promotion of tissue perfusion, inhibition of fibrosis, and stimulation of cardiomyocyte proliferation (142, 143). Multiple groups of clinical trials have found that the application of MSCs can effectively ameliorate the prognosis of moderate and severe COVID-19 patients, as well as increase the survival rate of COVID-19 patients with ARDS (144, 145). Due to the absence of ACE2 and TMPRSS2 expression, MSCs are less susceptible to SARS-CoV-2 infection (146). Thus, MSCs could serve as a potent therapeutic approach for preventing or treating SARS-CoV-2-induced cardiac injury.
The beneficial effects of MSCs are manifold. MSCs regulate immune cell subsets by secreting paracrine substances, which help coordinate the immune response. Research has shown that intravenous infusion of MSCs can regulate B cell subsets and boost CD28 expression on costimulatory T cells (147). Also, MSCs have the potential to alleviate SARS-CoV-2-related cytokine storm (148). Preclinical models of ARDS have indicated that MSCs exert inflammation suppression effects on host tissues through the release of IL-4, IL-10, transforming growth factor β (TGF-β) and prostaglandin E2 (149). Infusion of MSCs in severe and critically ill COVID-19 patients resulted in a considerable reduction in levels of CRP, pro-inflammatory cytokines, and NETs, as evidenced by clinical trials (147). CPR serves as a biomarker for myocardial damage (150). It is worth noting that patients with elevated IL-6 levels have better infusion effects of human umbilical cord-derived mesenchymal stem cells (hUC-MSCs), as seen by a significant reduction in IL-6 levels and a substantial increase in the oxygenation index (145). This suggests that the inflammatory environment may augment the immune regulatory properties of MSCs. On top of that, MSCs treatment also demonstrates a commendable capacity to stimulate tissue differentiation and regeneration. MSCs migrate to areas of injury and release abundant amounts of growth factors, prompting tissue regeneration and diminishing cellular demise (151). At present, studies in humans have confirmed the safety and efficacy of MSCs. Several clinical trials have verified the effectiveness of intravenous infusion of hUC-MSCs in patients with moderate and severe COVID-19, and it has a beneficial impact on patient-related sequelae after infection (152–154). The enduring safety and efficacy of MSCs therapy has been confirmed and are not associated with serious adverse events (155, 156). Autologous stem cells, especially patient-derived ones, pose no danger of immunological rejection (157).
Cardiosphere-derived cells (CDCs) are stem cells originated from heart tissue (158). CDCs, like MSCs, actively contribute to cardiac repair and point out greater myocardial repair potential than MSCs. The beneficial effects of CDCs in promoting cardiomyocyte regeneration, stimulating angiogenesis, inhibiting inflammation and myocardial fibrosis, enhancing cardiac function, and regulating immunity have been confirmed by research (159–162). Several sets of clinical trials have shown the therapeutic efficacy of CDCs in treating various conditions such as myocardial infarction, heart failure with reduced and preserved ejection fraction, non-ischemic cardiomyopathy, Duchenne muscular dystrophy, and others (161, 163–166). CDCs may have the potential to induce the transformation of M1-like macrophages(pro-inflammatory) into M2-type macrophages (anti-inflammatory), and enhance the ability of macrophages to clear cell debris (167, 168). Due to their capacity to suppress excessive inflammation and facilitate the restoration of myocardium, CDCs could potentially be beneficial for COVID-19 patients suffering myocardial injury (169). Clinical study data indicates that intravenous allogeneic CDCs (CAP-1002) is safe in individuals with severe COVID-19. After administration of CAP-1002, the levels of pro-inflammatory biomarkers were reduced in the majority of patients. Additionally, increased levels of cardiac troponin I and D-dimer were dramatically decreased. And the clinical condition of patients showed improvement (170).
6.3 Cell-free therapy based on exosomes
Exosomes are a specific type of nanoparticles with a diameter ranging from 40 to 150 nanometers. Cell-free therapy based on exosomes has shown promise in treating various cardiovascular diseases such as myocardial infarction, myocardial ischemia-reperfusion injury, inflammation of myocardium, and ventricular remodeling (171–173). The therapeutic benefits of MSCs are primarily attributed to exosomes (174). Extracellular vesicles, derived from MSCs, possess comprehensive immunomodulatory and regenerative properties (175). Despite several advantages of MSC-based cell therapy, its potential for causing tumors, the risk of pulmonary embolism, the low in vivo survival rate, and challenges with storage present hurdles. As a result, exosome therapy emerges as a promising option (176). Exosomes exhibit lower immunogenicity in comparison to MSCs, hence diminishing the likelihood of thrombosis and the incidence of adverse cardiovascular events (177). Besides, exosomes exhibit robust sustainability and stability within the body, enabling them to effectively and enduringly mitigate inflammation and modulate the immune response, consequently suppressing the onset of cytokine storm (175). As a kind of nanoparticles, exosomes possess the ability to cross the blood-brain barrier, circumventing the risk of pulmonary embolism, which is associated with MSC transplantation. Significantly, exosomes circumvent the potential hazard of tumor development caused by MSCs (141). Exosomes consist of lipids, proteins, mRNA, LncRNA, microRNA, and various other bioactive compounds. Studies revealed that non-coding RNAs contained in exosomes play a pivotal role in cardiac protection (178). The efficacy and security of exosome-based cell-free treatments for patients with COVID-19 have been validated (142). Similarly, extracellular vesicles derived from CDCs are the key mediators of their therapeutic effects (164, 179). In pig models of acute myocardial infarction, intramyocardial injection of CDCs-exosomes significantly reduced cardiac remodeling and enhanced cardiac functions (180). The study discovered that extracellular vesicles derived from CDCs may be involved in regulating the IL-6/IL-6R axis and suppressing the impacts of diseases mediated by inflammation (181). Currently, exosome treatment is primarily applied by either aerosol inhalation or intravenous injection (182). The precise targeting of the heart is a subject of ongoing research, and its potential for transformation is a topic that warrants further discussion.
6.4 Cardioprotective agents
The invasion of SARS-CoV-2 can result in severe injury to myocardial tissue, necessitating the creation of a novel medication to counteract the myocardial injury induced by SARS-CoV-2. However, the process of developing and implementing novel drugs requires a certain amount of time. Considering the unpredictability and urgency of the novel coronavirus, looking for cardioprotective agents with antiviral effects among existing clinical drugs is a reliable option. Statins are frequently employed in clinical settings as lipid-lowering medications. It serves a crucial function in the regulation of blood lipid abnormalities and cardioprotective therapy (183). Different observational research has shown that the use of statins diminishes mortality and improves outcomes in COVID-19 patients (184, 185). As a possible candidate for adjuvant treatment of COVID-19 patients with myocardial injury, statins have multiple effects. Research analysis speculates that statins may impede the entry of SARS-CoV-2 into host cells as well as hinder the replication and proliferation of the virus in vivo (186, 187). Secondly, statins may have a capacity to reduce the excessive level of pro-inflammatory cytokines and regulate immune responses. A meta-analysis showed that statins have the ability to decrease levels of IL-6 and CRP (188). It exhibits a suppressive effect on cytokine storm and macrophage activation syndrome, which is triggered by raised levels of IL-6. Moreover, statins exhibit remarkable anti-fibrotic potential and accelerate the apoptosis process of fibroblasts against complications induced by SARS-CoV-2 infection (189). In brief, because of its antiviral, anti-inflammatory, anti-fibrotic, immunomodulatory, and cardioprotective properties, statins may be a viable treatment choice for COVID-19 patients with cardiovascular comorbidities. Especially for patients with myocardial damage or dyslipidemia, statins can be used as an auxiliary treatment.
6.5 Phytochemicals
Prior reports indicated that phytochemicals, such as alkaloids, flavonoids, and polyphenols, demonstrated antiviral properties on the MERS-CoV and the SARS-CoV (190). Resveratrol (RES), a phenolic molecule, exhibits inhibitory effects against multiple respiratory viruses, such as influenza virus, MERS-CoV, SARS-CoV, and respiratory syncytial virus (191). RES can drastically counter MERS-CoV infection and enhance the survival of virus-infected cells (192). Similarly, in vitro experiments show that RES can effectively inhibit the replication of SARS-CoV-2, manifested as reduced virus titer and cytotoxicity (193). In the Vero cell model infected with SARS-CoV-2, the cells treated with RES after infection showed a remarkable inhibition rate of 98% against SARS-CoV-2. After incubating the cells with the virus and RES for 1 hour, followed by the removal of RES interference and subsequent culture for a further 48 hours, the inhibition rate remained approximately 64%. The findings point out that RES exhibits a potent inhibitory effect on the replication of SARS-CoV-2 and can impede viral entry into cells (194). RES can activate the immune system, downregulate the production of pro-inflammatory cytokines, and inhibit cytokine storms. It affects T cells, DCs, and macrophages to control immune responses and minimize tissue and organ impairment (195, 196). A randomized, double-blind, placebo-controlled trial showed that RES can decrease the occurrence of hospitalizations, emergency department visits, and pneumonia in outpatients with mild COVID-19 without generating significant adverse events (197). Furthermore, RES is identified as a cardioprotective drug, which means it can alleviate the cardiotoxicity associated with chloroquine/hydroxychloroquine treatment in SARS-CoV-2 patients (198). Therefore, whether to inhibit viral replication during the initial phase of infection or to reduce systemic inflammation-induced tissue damage and its cardioprotective effects during the later phases, RES seems like a good candidate.
6.6 Traditional Chinese medicine
Traditional Chinese medicine (TCM) has been used in previous viral infections, such as SARS-CoV, MERS-CoV and influenza virus (199). After the outbreak of the SARS-CoV-2, the Chinese government quickly adopted a series of prevention and treatment measures, actively promoted the application of TCM, and created three Chinese patent medicines (Jinhua Qinggan Granules, Lianhua Qingwen Capsule and Xuebijing Injection) and three Chinese medicine prescriptions (Qingfei Paidu Decoction, Huashi Paidu Recipe and Xuanfeibaidu Recipe (200). Data from randomized controlled studies of ‘three formulas and three medicines’ indicated that TCM is safe and can mitigate the symptoms of heart damage (201). Whether it is for initial prevention or as an adjuvant treatment for acute myocardial injury induced by SARS-CoV-2, especially for the rehabilitation treatment of post-COVID-19 condition, TCM is a viable option. However, it is necessary to dialectically view the specific conditions of each patient and give a reasonable treatment plan. Strategies for preventing and treating myocardial injury caused by SARS-CoV-2 has been shown in Figure 3.
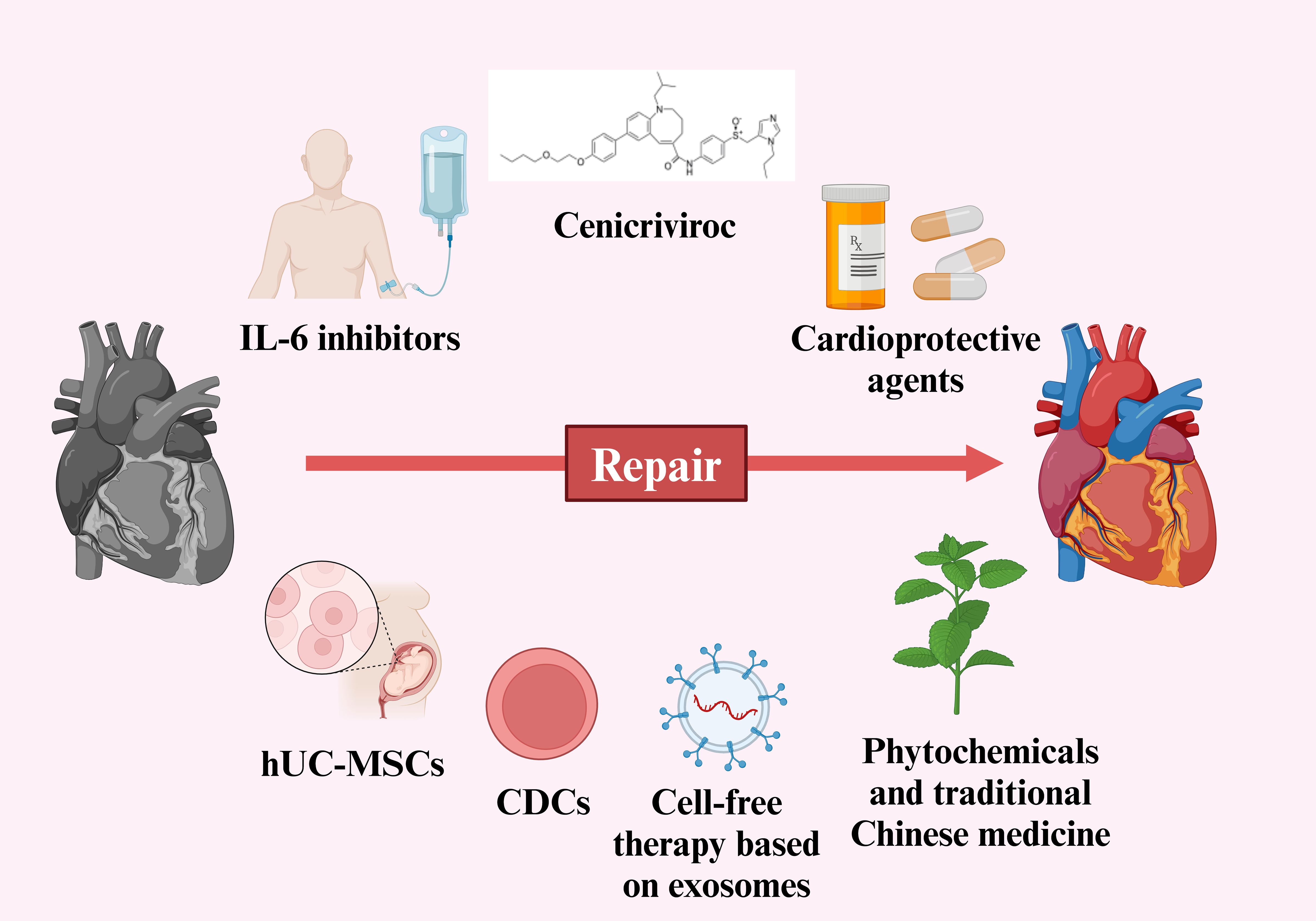
Figure 3. Strategies for preventing and treating myocardial injury caused by SARS-CoV-2. (Created in BioRender.com).
7 Discussion and future perspective
SARS-CoV-2, SARS-CoV, and MERS-CoV are all classified as coronaviruses, which primarily attack the respiratory system of humans and lead to a widespread pandemic (202). Prior studies indicated that infection with SARS-CoV-2, SARS-CoV, and MERS-CoV can impact the human immune system and result in multiple systemic impairments (203). SARS-CoV has the ability to invade alveolar epithelial cells and immune cells; however, it can only replicate in epithelial cells. So its impact on the immune system is indirect (204). The distinction of MERS-CoV is its ability to directly invade and reproduce within a range of immune cells, including macrophages, T cells and DCs. Infected macrophages and DCs secrete pro-inflammatory cytokines and chemokines, leading to inflammation and tissue damage (205). SARS-CoV-2 has a comparable effect on the immune system as the previous two viruses. Acute infection with SARS-CoV-2 results in widespread reductions in various types of immune cells, such as T cells, NK cells, monocytes, and DCs. Following infection with SARS-CoV-2, there is a notable occurrence of immunological dysfunction and cytokine storm. The condition of high inflammation and abnormal immune processes ultimately results in the destruction of multiple tissue and organs (206). Similar to SARS-CoV-2, patients infected with MERS-CoV and SARS-CoV were also complicated with cardiovascular diseases (207, 208). However, there is little research on cardiovascular diseases related to SARS-CoV and MERS-CoV infection. The studies primarily focus on clinical case reports and lack the in-depth exploration and summary of relevant mechanisms (208). Consequently, during the SARS-CoV-2 pandemic, there exists a poor awareness of the prevention and management of myocardial injury caused by coronavirus infection. Therefore, in spite of the last coronavirus pandemic has passed, it is essential to explore and summarize the underlying mechanisms of myocardial injury induced by COVID-19. This exploration aims to deepen the comprehension of myocardial injury due to SARS-CoV-2 as well as provide strategies for subsequent prevention and therapy.
It is inevitable to create novel medicines because there are no new therapeutic drugs for myocardial injury in patients with COVID-19 currently. However, the process of researching and developing novel medications and conducting clinical trials requires a certain amount of time. The existing cardioprotective agents with antiviral and immunomodulatory properties may be an appropriate choice. In recent years, treatments based on MSCs have been extensively studied in many fields (148). MSCs have qualities that reduce inflammation, fight against viruses, modulate the immune system, and facilitate the healing of myocardial damage. Clinical trials utilizing MSCs treatment have been carried out in patients with COVID-19. Considering the regular clinical application of MSCs by atomization or intravenous infusion, there exists a requirement to improve the effectiveness of MSCs in treating heart disease. By integrating stem cells with nanotechnology, combination therapies and additional techniques enhance the delivery efficiency and therapeutic efficacy of MSCs (209, 210). Although, cell-free therapies based on exosomes demonstrate unique advantages by avoiding the immunogenicity and thrombotic risks inherent to stem cells. The clinical translation has faced multifaceted challenges. Globally registered clinical trials investigating exosome therapies remain predominantly in Phase II/III development, with a marked predominance of hUC-MSCs as the cellular source. Notably, the development of embryonic stem cell-derived exosomes remains strictly constrained, primarily stemming from persistent ethical controversies (e.g., legal ambiguities regarding embryonic material procurement) and regulatory deficiencies in quality control standardization.
Given the crucial role of cytokine storm in COVID-19-associated myocardial injury, targeted suppression of SARS-CoV-2-induced inflammatory responses may represent an effective therapeutic strategy. The use of IL-6 inhibitors (e.g., tocilizumab), IL-1 inhibitors (e.g., anakinra and canakinumab), NLRP3 inflammasome inhibitors (e.g., colchicine), and JAK inhibitors (e.g., baricitinib) in COVID-19 patients has shown promising results in suppressing inflammation and reducing clinical progression (133, 211, 212). In particular, the anti-thrombotic effect is observed when targeting the NLRP3/IL-1β axis through canakinumab or colchicine administration. Currently, anakinra has been approved for treating hypoxemic COVID-19 patients exhibiting early signs of hyperinflammation, based on its established safety profile and therapeutic efficacy. The non-selective NLRP3 inhibitor colchicine has demonstrated favorable effects in reducing hospitalization and mortality rates among COVID-19 outpatients, despite lacking formal regulatory approval for this indication (213). It is essential to recognize that since excessive immune activation involves the synergistic actions of multiple proinflammatory cytokines, targeting the single inflammatory factor may not be sufficient to suppress excessive inflammation and improve clinical outcomes (214). Therefore, there is still a need to explore specific myocardial injury biomarkers in COVID-19 patients and develop multi-targeted therapy to address the complex cytokine network dysregulation.
At present, the assessment of myocardial injury mainly depends on cardiac troponin I (cTnI) or high-sensitivity cardiac troponin I (hs-cTnI), with increased inflammatory biomarkers (215). Since the elevation of troponin involves both ischemic and non-ischemic causes, there is a need to find more readily available specific and sensitive markers of myocardial injury (216). Non-coding RNA circulating in the blood may be a good choice. Garg et al. assessed changes in circulating cardiovascular miRNA, and the upregulation of miR-21, miR-155, miR-208a and miR-499 in COVID-19 survivors may be predictors of chronic myocardial injury and inflammation. In particular, myocardial-specific miR-208a, and miR-499 showed higher elevations than troponin (217). This provides possible predictive information for the assessment of SARS-CoV-2-related myocardial injury. RNA biomarkers could be useful in the current COVID-19 situation. Although studies put the immune dysregulation into the potential mechanism underlying myocardial injury, there has been a blank in biomarkers between immune factors and myocardial injury. A research study aimed to assess the relationship between myocardial injury and immunologic profiling found that white blood cell count, neutrophil count, types of lymphocyte count (CD3+, CD4+, CD8+, CD19+, CD16+, CD56+), hs-CRP, and procalcitonin had independent correlations with myocardial injury in COVID-19 patients. The elevated indicators above all may give a clue for considering myocardial injury in patients infected with virus (218). The exploration of novel biomarkers for myocardial injury in COVID-19 patients is a direction that warrants future consideration. This provides the possibility for timely identification of myocardial injury and precise targeted treatment. The levels of some biomarkers can be influenced by a variety of factors, including infection and hypoxia. Therefore, the diagnosis of myocardial injury after COVID-19 infection should not only rely on biomarkers but also consider all relevant clinical parameters. Zhong et al. discovered that a decline in myocardial computed tomography (CT) value indicates the presence of myocardial damage. Chest CT is employed to evaluate pulmonary lesions as well as heart morphology and myocardial tissue characteristics in individuals diagnosed with COVID-19. This utilization aims to enhance the clinical utility of chest CT in cardiovascular diseases and furnish patients with additional valuable information (219). Moreover, CMR can also serve as a supplementary diagnostic tool (220). Combined with inflammatory markers, a variety of myocardial injury markers and imaging examination to assess myocardial injury from multiple perspectives, to provide more reliable support for the diagnosis of myocardial injury.
Since the COVID-19 pandemic, various variants of SARS-CoV-2 and their respective branch subtypes have emerged. These include Alpha, Delta, and Omicron variants, as well as their subtypes, such as the Omicron XBB, BA.2.86 and JN.1 variants (221, 222). These subvariants showed higher immune escape ability. Compared with the early original strain, the Omicron mutant strain is the most heavily modified strain among the numerous SARS-CoV-2 variants that have arisen during the COVID-19 pandemic (223). During the surge in Omicron variants, hospitalized COVID-19 patients exhibited a range of myocardial injury manifestations. As previously mentioned, there exists a strong association between severe myocardial injury and higher rates of morbidity and mortality (224). Therefore, it is of utmost significance to recognize the occurrence of myocardial injury in hospitalized individuals infected with SARS-CoV-2 as early as possible, hence facilitating the categorization of COVID-19 patients into risk strata. This enables the selection of appropriate clinical interventions and subsequent treatment strategies for patients (225).
Despite the conclusion of the COVID-19 pandemic, the coronavirus persists and continues to mutate, perhaps leading to another pandemic in the future. We explore the immunological mechanism of SARS-CoV-2-induced myocardial injury, in order to put forward feasible prevention and treatment measures for patients with COVID-19-complicated myocardial injury, so as to strengthen the preparation for the future reinfection wave of SARS-CoV-2 and its variants.
Author contributions
ZL: Writing – original draft, Visualization, Writing – review & editing. LQ: Writing – review & editing, Visualization. XX: Visualization, Writing – review & editing. RC: Supervision, Writing – review & editing. GZ: Writing – review & editing. BW: Writing – review & editing. BL: Writing – review & editing, Funding acquisition. X-MC: Writing – review & editing, Funding acquisition.
Funding
The author(s) declare that financial support was received for the research and/or publication of this article. This work was supported by The National Natural Science Foundation of China (grant numbers 82172574), The Natural Science Foundation of Shandong Province (grant numbers ZR2024MH083), Grants from the National Natural Science Foundation of China (grant numbers 81871231), Shandong Taishan Scholars Young Experts Program (grant numbers tsqn202103056), Natural Science Foundation project of Shandong Province (grant numbers ZR2023MH082), Qingdao Natural Science Foundation Key Project (grant numbers 24-8-4-zrjj-8-jch).
Conflict of interest
The authors declare that the research was conducted in the absence of any commercial or financial relationships that could be construed as a potential conflict of interest.
Generative AI statement
The author(s) declare that no Generative AI was used in the creation of this manuscript.
Publisher’s note
All claims expressed in this article are solely those of the authors and do not necessarily represent those of their affiliated organizations, or those of the publisher, the editors and the reviewers. Any product that may be evaluated in this article, or claim that may be made by its manufacturer, is not guaranteed or endorsed by the publisher.
Glossary
SARS-CoV-2: Severe acute respiratory syndrome coronavirus 2
COVID-19: Coronavirus disease 2019
MERS-CoV: Middle East respiratory syndrome coronavirus
SARS-CoV: Severe acute respiratory syndrome coronavirus
PAMPs: Pathogen-associated molecular patterns
PRRs: Pattern recognition receptors
IFN: Interferon
DCs: Dendritic cell
IL: Interleukin
TNF-α: Tumor necrosis factor-α
ACE2: Angiotensin-converting enzyme 2
CRP: C-reactive protein
hs-CRP: High-sensitivity C-reactive protein
NT-proBNP: N-terminal pro-B-type natriuretic peptide
Th17: T helper 17
hiPSC-CMs: Human-induced pluripotent stem cell-derived cardiomyocytes
CCL2: Chemokine ligand 2
MCP-1: Monocyte chemoattractant protein-1
NK cells: Natural killer cells
CXCL1: C-X-C motif chemokine ligand 1
CXCL2: C-X-C motif chemokine ligand 2
Ang I: Angiotensin I
AT1: Angiotensin II Receptor Type 1
IP-10: Interferon-gamma-inducible protein
I IFN: Type I interferon
CK-MB: Creatine kinase isoenzymes
cTnI: Cardiac troponin I
BNP: Brain Natriuretic Peptide
DAMPs: Damage-associated molecular patterns
dsRNA: Double-stranded RNA
RIG-I: Retinoic acid-inducible gene-I
MDA-5: Melanoma differentiation related gene 5
MAVS: Mitochondrial antiviral signals
TBK1: TANK-binding kinase 1
IKK ϵ: Inhibitor-kappa B kinase
NF- κB: Nuclear factor kappa-B
IRF3: Interferon regulatory Factor 3
ISG: Interferon-stimulated genes
JAK: Janus Kinase
STAT: Signal transducer and activator of transcription
NETs: Neutrophil extracellular traps
NLR: Neutrophil-lymphocyte ratio
ECG: Electrocardiogram
CMR LGE: Cardiac magnetic resonance Late gadolinium enhancement
NK cells: Natural killer cells
Th 1 cells: T-helper-1 cells
LDH: Lactate dehydrogenase
HLA: Human leukocyte antigen
GWASs: Genome-wide association studies
PASC: Post-acute sequelae of COVID-19
POTS: Postural orthostatic tachycardia syndrome
IST: Inappropriate sinus tachycardia
OH: Orthostatic hypotension
ICU: Intensive care unit
S protein: Spike protein
mAbs: Monoclonal antibodies
CVC CCR2: Cenicriviroc C-C chemokine receptor type 2
CCR5: C-C chemokine receptor type 5
MSCs: Mesenchymal stem cells
ARDS: Acute respiratory distress syndrome
UC-MSCs: Humumbilical cord-derived mesenchymal stem cells
TGF-β: Transforming growth factor β
CDCs: Cardiosphere-derived cells
CAP-1002: Intravenous allogeneic CDCs
RES: Resveratrol
TCM: Traditional Chinese medicine
NLRP3: NOD-like receptor thermal protein domain associated protein 3
hs-cTnI: High-sensitivity cardiac troponin I
CT: Computed tomography
References
1. Zhu N, Zhang D, Wang W, Li X, Yang B, Song J, et al. A novel coronavirus from patients with pneumonia in China, 2019. N Engl J Med. (2020) 382:727–33. doi: 10.1056/NEJMoa2001017
2. Pustake M, Tambolkar I, Giri P, Gandhi C. Sars, Mers and Covid-19: an overview and comparison of clinical, laboratory and radiological features. J Family Med Prim Care. (2022) 11:10–7. doi: 10.4103/jfmpc.jfmpc_839_21
3. Liu J, Xie W, Wang Y, Xiong Y, Chen S, Han J, et al. A comparative overview of Covid-19, Mers and Sars: review article. Int J Surg. (2020) 81:1–8. doi: 10.1016/j.ijsu.2020.07.032
4. Puelles VG, Lutgehetmann M, Lindenmeyer MT, Sperhake JP, Wong MN, Allweiss L, et al. Multiorgan and renal tropism of Sars-Cov-2. N Engl J Med. (2020) 383:590–2. doi: 10.1056/NEJMc2011400
5. Huang C, Wang Y, Li X, Ren L, Zhao J, Hu Y, et al. Clinical features of patients infected with 2019 novel coronavirus in Wuhan, China. Lancet. (2020) 395:497–506. doi: 10.1016/S0140-6736(20)30183-5
6. Durstenfeld MS, Peluso MJ, Kelly JD, Win S, Swaminathan S, Li D, et al. Role of antibodies, inflammatory markers, and echocardiographic findings in postacute cardiopulmonary symptoms after Sars-Cov-2 infection. JCI Insight. (2022) 7:e157053. doi: 10.1172/jci.insight.157053
7. Shiwani H, Artico J, Moon JC, Gorecka M, McCann GP, Roditi G, et al. Clinical significance of myocardial injury in patients hospitalized for Covid-19: A prospective, multicenter, cohort study. JACC Cardiovasc Imaging. (2024) 17:1320–31. doi: 10.1016/j.jcmg.2024.06.008
8. Hendren NS, Drazner MH, Bozkurt B, Cooper LT Jr. Description and proposed management of the acute Covid-19 cardiovascular syndrome. Circulation. (2020) 141:1903–14. doi: 10.1161/CIRCULATIONAHA.120.047349
9. Ali AS, Sheikh D, Chandler TR, Furmanek S, Huang J, Ramirez JA, et al. Cardiovascular complications are the primary drivers of mortality in hospitalized patients with Sars-Cov-2 community-acquired pneumonia. Chest. (2023) 163:1051–60. doi: 10.1016/j.chest.2022.11.013
10. Dou Q, Wei X, Zhou K, Yang S, Jia P. Cardiovascular manifestations and mechanisms in patients with Covid-19. Trends Endocrinol Metab. (2020) 31:893–904. doi: 10.1016/j.tem.2020.10.001
11. Corovic A, Zhao X, Huang Y, Newland S, Gopalan D, Harrison J, et al. Covid-19 related myocardial injury is associated with immune dysregulation in symptomatic patients with cardiac mri abnormalities. Cardiovasc Res. (2024) 120:1752–67. doi: 10.1093/cvr/cvae159
12. Dmytrenko O, Das S, Kovacs A, Cicka M, Liu M, Scheaffer SM, et al. Infiltrating monocytes drive cardiac dysfunction in a cardiomyocyte-restricted mouse model of Sars-Cov-2 infection. J Virol. (2024) 98:e0117924. doi: 10.1128/jvi.01179-24
13. Tay MZ, Poh CM, Renia L, MacAry PA, Ng LFP. The trinity of Covid-19: immunity, inflammation and intervention. Nat Rev Immunol. (2020) 20:363–74. doi: 10.1038/s41577-020-0311-8
14. Yamada T, Takaoka A. Innate immune recognition against Sars-Cov-2. Inflammation Regener. (2023) 43:7. doi: 10.1186/s41232-023-00259-5
15. Samuel CE. Interferon at the crossroads of Sars-Cov-2 infection and Covid-19 disease. J Biol Chem. (2023) 299:104960. doi: 10.1016/j.jbc.2023.104960
16. Sette A, Crotty S. Adaptive immunity to Sars-Cov-2 and Covid-19. Cell. (2021) 184:861–80. doi: 10.1016/j.cell.2021.01.007
17. Roche PA, Furuta K. The ins and outs of mhc class ii-mediated antigen processing and presentation. Nat Rev Immunol. (2015) 15:203–16. doi: 10.1038/nri3818
18. Plantinga M, Affandi AJ. Editorial: novel platform for antigen delivery to dendritic cells for immunotherapy. Front Immunol. (2022) 13:915604. doi: 10.3389/fimmu.2022.915604
19. Du SQ, Yuan W. Mathematical modeling of interaction between innate and adaptive immune responses in Covid-19 and implications for viral pathogenesis. J Med Virol. (2020) 92:1615–28. doi: 10.1002/jmv.25866
20. Blanco-Melo D, Nilsson-Payant BE, Liu WC, Uhl S, Hoagland D, Moller R, et al. Imbalanced host response to Sars-Cov-2 drives development of Covid-19. Cell. (2020) 181:1036–45 e9. doi: 10.1016/j.cell.2020.04.026
21. Diamond MS, Kanneganti TD. Innate immunity: the first line of defense against Sars-Cov-2. Nat Immunol. (2022) 23:165–76. doi: 10.1038/s41590-021-01091-0
22. Unterman A, Sumida TS, Nouri N, Yan X, Zhao AY, Gasque V, et al. Single-cell multi-omics reveals dyssynchrony of the innate and adaptive immune system in progressive Covid-19. Nat Commun. (2022) 13:440. doi: 10.1038/s41467-021-27716-4
23. Fajgenbaum DC, June CH. Cytokine storm. N Engl J Med. (2020) 383:2255–73. doi: 10.1056/NEJMra2026131
24. Pesce M, Agostoni P, Bøtker H-E, Brundel B, Davidson SM, Caterina RD, et al. Covid-19-related cardiac complications from clinical evidences to basic mechanisms: opinion paper of the esc working group on cellular biology of the heart. Cardiovasc Res. (2021) 117:2148–60. doi: 10.1093/cvr/cvab201
25. Chen L, Hu W, Guo X, Zhao P, Tang J, Gu Y, et al. Association of coagulation dysfunction with cardiac injury among hospitalized patients with Covid-19. Sci Rep. (2021) 11:4432. doi: 10.1038/s41598-021-83822-9
26. Ullah R, Khan J, Basharat N, Huo D, Ud Din A, Wang G. Evaluation of cardiac biomarkers and expression analysis of il-1, il-6, il-10, il-17, and il-25 among Covid-19 patients from Pakistan. Viruses. (2022) 14:2149. doi: 10.3390/v14102149
27. Siddiq MM, Chan AT, Miorin L, Yadaw AS, Beaumont KG, Kehrer T, et al. Functional effects of cardiomyocyte injury in Covid-19. J Virol. (2022) 96:e0106321. doi: 10.1128/JVI.01063-21
28. Bavishi C, Bonow RO, Trivedi V, Abbott JD, Messerli FH, Bhatt DL. Special article - acute myocardial injury in patients hospitalized with Covid-19 infection: A review. Prog Cardiovasc Dis. (2020) 63:682–9. doi: 10.1016/j.pcad.2020.05.013
29. Xu SC, Wu W, Zhang SY. Manifestations and mechanism of Sars-Cov2 mediated cardiac injury. Int J Biol Sci. (2022) 18:2703–13. doi: 10.7150/ijbs.69677
30. Siripanthong B, Nazarian S, Muser D, Deo R, Santangeli P, Khanji MY, et al. Recognizing Covid-19-related myocarditis: the possible pathophysiology and proposed guideline for diagnosis and management. Heart Rhythm. (2020) 17:1463–71. doi: 10.1016/j.hrthm.2020.05.001
31. Rabbani MY, Rappaport J, Gupta MK. Activation of immune system may cause pathophysiological changes in the myocardium of Sars-Cov-2 infected monkey model. Cells. (2022) 11:611. doi: 10.3390/cells11040611
32. Cron RQ, Goyal G, Chatham WW. Cytokine storm syndrome. Annu Rev Med. (2023) 74:321–37. doi: 10.1146/annurev-med-042921-112837
33. Colzani M, Bargehr J, Mescia F, Williams EC, Knight-Schrijver V, Lee J, et al. Proinflammatory cytokines driving cardiotoxicity in Covid-19. Cardiovasc Res. (2024) 120:174–87. doi: 10.1093/cvr/cvad174
34. Xia G, Fan D, Ma C, He Y, Wang M, Zhu Y, et al. Hyper-inflammatory response involves in cardiac injury among patients with Coronavirus disease 2019. Am J Med Sci. (2021) 361:718–24. doi: 10.1016/j.amjms.2021.02.007
35. Guo T, Fan Y, Chen M, Wu X, Zhang L, He T, et al. Cardiovascular implications of fatal outcomes of patients with Coronavirus disease 2019 (Covid-19). JAMA Cardiol. (2020) 5:811–8. doi: 10.1001/jamacardio.2020.1017
36. Paranga TG, Mitu I, Pavel-Tanasa M, Rosu MF, Miftode IL, Constantinescu D, et al. Cytokine storm in Covid-19: exploring il-6 signaling and cytokine-microbiome interactions as emerging therapeutic approaches. Int J Mol Sci. (2024) 25:11411. doi: 10.3390/ijms252111411
37. Barnett KC, Xie Y, Asakura T, Song D, Liang K, Taft-Benz SA, et al. An epithelial-immune circuit amplifies inflammasome and il-6 responses to Sars-Cov-2. Cell Host Microbe. (2023) 31:243–59 e6. doi: 10.1016/j.chom.2022.12.005
38. Wang X, Tang G, Liu Y, Zhang L, Chen B, Han Y, et al. The role of il-6 in coronavirus, especially in Covid-19. Front Pharmacol. (2022) 13:1033674. doi: 10.3389/fphar.2022.1033674
39. Li T, Wang D, Wei H, Xu X. Cytokine storm and translating il-6 biology into effective treatments for Covid-19. Front Med. (2023) 17:1080–95. doi: 10.1007/s11684-023-1044-4
40. Hou W, Jin YH, Kang HS, Kim BS. Interleukin-6 (Il-6) and il-17 synergistically promote viral persistence by inhibiting cellular apoptosis and cytotoxic T cell function. J Virol. (2014) 88:8479–89. doi: 10.1128/JVI.00724-14
41. Hayama H, Ide S, Kitami Y, Hara H, Kutsuna S, Hiroi Y. Interleukin-6 is upregulated and may be associated with myocardial injury in some patients who have recovered from Covid-19. Glob Health Med. (2022) 4:61–3. doi: 10.35772/ghm.2021.01090
42. Dimai S, Semmler L, Prabhu A, Stachelscheid H, Huettemeister J, Klaucke SC, et al. Covid19-associated cardiomyocyte dysfunction, arrhythmias and the effect of canakinumab. PloS One. (2021) 16:e0255976. doi: 10.1371/journal.pone.0255976
43. Lazzerini PE, Laghi-Pasini F, Boutjdir M, Capecchi PL. Inflammatory cytokines and cardiac arrhythmias: the lesson from Covid-19. Nat Rev Immunol. (2022) 22:270–2. doi: 10.1038/s41577-022-00714-3
44. Lazzerini PE, Accioli R, Acampa M, Zhang WH, Verrengia D, Cartocci A, et al. Interleukin-6 elevation is a key pathogenic factor underlying Covid-19-associated heart rate-corrected qt interval prolongation. Front Cardiovasc Med. (2022) 9:893681. doi: 10.3389/fcvm.2022.893681
45. Zhu X, Wang Y, Xiao Y, Gao Q, Gao L, Zhang W, et al. Arrhythmogenic mechanisms of interleukin-6 combination with hydroxychloroquine and azithromycin in inflammatory diseases. Sci Rep. (2022) 12:1075. doi: 10.1038/s41598-022-04852-5
46. Lazzerini PE, Laghi-Pasini F, Bertolozzi I, Morozzi G, Lorenzini S, Simpatico A, et al. Systemic inflammation as a novel qt-prolonging risk factor in patients with torsades de pointes. Heart. (2017) 103:1821–9. doi: 10.1136/heartjnl-2016-311079
47. Xi Y, Mao Y, Zhu W, Xi P, Huang F, Tan H, et al. Il-6 is a predictor and potential therapeutic target for Coronavirus disease 2019-related heart failure: A single-center retrospective study. Cytokine. (2024) 176:156514. doi: 10.1016/j.cyto.2024.156514
48. Hunter CA, Jones SA. Il-6 as a keystone cytokine in health and disease. Nat Immunol. (2015) 16:448–57. doi: 10.1038/ni.3153
49. Korobova ZR, Arsentieva NA, Liubimova NE, Dedkov VG, Gladkikh AS, Sharova AA, et al. A comparative study of the plasma chemokine profile in Covid-19 patients infected with different Sars-Cov-2 variants. Int J Mol Sci. (2022) 23:9058. doi: 10.3390/ijms23169058
50. Wong CK, Luk HK, Lai WH, Lau YM, Zhang RR, Wong AC, et al. Human-induced pluripotent stem cell-derived cardiomyocytes platform to study Sars-Cov-2 related myocardial injury. Circ J. (2020) 84:2027–31. doi: 10.1253/circj.CJ-20-0881
51. Karimabad MN, Kounis NG, Hassanshahi G, Hassanshahi F, Mplani V, Koniari I, et al. The involvement of cxc motif chemokine ligand 10 (Cxcl10) and its related chemokines in the pathogenesis of coronary artery disease and in the Covid-19 vaccination: A narrative review. Vaccines (Basel). (2021) 9:1224. doi: 10.3390/vaccines9111224
52. Nieri D, Neri T, Barbieri G, Moneta S, Morelli G, Mingardi D, et al. C-C motive chemokine ligand 2 and thromboinflammation in Covid-19-associated pneumonia: A retrospective study. Thromb Res. (2021) 204:88–94. doi: 10.1016/j.thromres.2021.06.003
53. Liu D, Cao Y, Zhang X, Peng C, Tian X, Yan C, et al. Chemokine cc-motif ligand 2 participates in platelet function and arterial thrombosis by regulating pkcalpha-P38mapk-hsp27 pathway. Biochim Biophys Acta Mol Basis Dis. (2018) 1864:2901–12. doi: 10.1016/j.bbadis.2018.05.025
54. Zhang H, Yang K, Chen F, Liu Q, Ni J, Cao W, et al. Role of the ccl2-ccr2 axis in cardiovascular disease: pathogenesis and clinical implications. Front Immunol. (2022) 13:975367. doi: 10.3389/fimmu.2022.975367
55. Marques P, Collado A, Martinez-Hervas S, Domingo E, Benito E, Piqueras L, et al. Systemic inflammation in metabolic syndrome: increased platelet and leukocyte activation, and key role of cx(3)Cl1/cx(3)Cr1 and ccl2/ccr2 axes in arterial platelet-proinflammatory monocyte adhesion. J Clin Med. (2019) 8:708. doi: 10.3390/jcm8050708
56. Yang L, Nilsson-Payant BE, Han Y, Jaffre F, Zhu J, Wang P, et al. Cardiomyocytes recruit monocytes upon Sars-Cov-2 infection by secreting ccl2. Stem Cell Rep. (2021) 16:2274–88. doi: 10.1016/j.stemcr.2021.07.012
57. Grune J, Bajpai G, Ocak PT, Kaufmann E, Mentkowski K, Pabel S, et al. Virus-induced acute respiratory distress syndrome causes cardiomyopathy through eliciting inflammatory responses in the heart. Circulation. (2024) 150:49–61. doi: 10.1161/CIRCULATIONAHA.123.066433
58. Aliyari SR, Ghaffari AA, Pernet O, Parvatiyar K, Wang Y, Gerami H, et al. Suppressing fatty acid synthase by type I interferon and chemical inhibitors as a broad spectrum anti-viral strategy against Sars-Cov-2. Acta Pharm Sin B. (2022) 12:1624–35. doi: 10.1016/j.apsb.2022.02.019
59. Trinchieri G. Type I interferon: friend or foe? J Exp Med. (2010) 207:2053–63. doi: 10.1084/jem.20101664
60. Park A, Iwasaki A. Type I and type iii interferons - induction, signaling, evasion, and application to combat Covid-19. Cell Host Microbe. (2020) 27:870–8. doi: 10.1016/j.chom.2020.05.008
61. Islamuddin M, Mustfa SA, Ullah SNMN, Omer U, Kato K, Parveen S. Innate immune response and inflammasome activation during Sars-Cov-2 infection. Inflammation. (2022) 45:1849–63. doi: 10.1007/s10753-022-01651-y
62. Zheng Y, Zhuang MW, Han L, Zhang J, Nan ML, Zhan P, et al. Severe acute respiratory syndrome coronavirus 2 (Sars-Cov-2) membrane (M) protein inhibits type I and iii interferon production by targeting rig-I/mda-5 signaling. Signal Transduct Target Ther. (2020) 5:299. doi: 10.1038/s41392-020-00438-7
63. Xia H, Cao Z, Xie X, Zhang X, Chen JY, Wang H, et al. Evasion of type I interferon by Sars-Cov-2. Cell Rep. (2020) 33:108234. doi: 10.1016/j.celrep.2020.108234
64. Platanias LC. Mechanisms of type-I- and type-ii-interferon-mediated signalling. Nat Rev Immunol. (2005) 5:375–86. doi: 10.1038/nri1604
65. Ramasamy S, Subbian S. Critical determinants of cytokine storm and type I interferon response in Covid-19 pathogenesis. Clin Microbiol Rev. (2021) 34:e0016321. doi: 10.1128/CMR.00299-20
66. Hadjadj J, Yatim N, Barnabei L, Corneau A, Boussier J, Smith N, et al. Impaired type I interferon activity and inflammatory responses in severe Covid-19 patients. Science. (2020) 369:718–24. doi: 10.1126/science.abc6027
67. Galani IE, Rovina N, Lampropoulou V, Triantafyllia V, Manioudaki M, Pavlos E, et al. Untuned antiviral immunity in Covid-19 revealed by temporal type I/iii interferon patterns and flu comparison. Nat Immunol. (2021) 22:32–40. doi: 10.1038/s41590-020-00840-x
68. Channappanavar R, Fehr AR, Vijay R, Mack M, Zhao J, Meyerholz DK, et al. Dysregulated type I interferon and inflammatory monocyte-macrophage responses cause lethal pneumonia in Sars-Cov-infected mice. Cell Host Microbe. (2016) 19:181–93. doi: 10.1016/j.chom.2016.01.007
69. Kim YM, Shin EC. Type I and iii interferon responses in Sars-Cov-2 infection. Exp Mol Med. (2021) 53:750–60. doi: 10.1038/s12276-021-00592-0
70. Minkoff JM, tenOever B. Innate immune evasion strategies of Sars-Cov-2. Nat Rev Microbiol. (2023) 21:178–94. doi: 10.1038/s41579-022-00839-1
71. Bouayad A. Innate immune evasion by Sars-Cov-2: comparison with Sars-Cov. Rev Med Virol. (2020) 30:1–9. doi: 10.1002/rmv.2135
72. Zhan RZ, Rao L, Chen Z, Strash N, Bursac N. Loss of sarcomeric proteins via upregulation of jak/stat signaling underlies interferon-gamma-induced contractile deficit in engineered human myocardium. Acta Biomater. (2021) 126:144–53. doi: 10.1016/j.actbio.2021.03.007
73. Chen DY, Khan N, Close BJ, Goel RK, Blum B, Tavares AH, et al. Sars-Cov-2 disrupts proximal elements in the jak-stat pathway. J Virol. (2021) 95:e0086221. doi: 10.1128/JVI.00862-21
74. Lei X, Dong X, Ma R, Wang W, Xiao X, Tian Z, et al. Activation and evasion of type I interferon responses by Sars-Cov-2. Nat Commun. (2020) 11:3810. doi: 10.1038/s41467-020-17665-9
75. Busnadiego I, Fernbach S, Pohl MO, Karakus U, Huber M, Trkola A, et al. Antiviral activity of type I, II, and III interferons counterbalances ace2 inducibility and restricts Sars-Cov-2. mBio. (2020) 11:e01928-20. doi: 10.1128/mBio.01928-20
76. Jum’ah H, Kundrapu S, Jabri A, Kondapaneni M, Tomashefski JF, Loeffler AG. Cardiac macrophage density in Covid-19 infection: relationship to myocyte necrosis and acute lung injury. Cardiovasc Pathol. (2022) 60:107447. doi: 10.1016/j.carpath.2022.107447
77. Wang C, Xie J, Zhao L, Fei X, Zhang H, Tan Y, et al. Alveolar macrophage dysfunction and cytokine storm in the pathogenesis of two severe Covid-19 patients. EBioMedicine. (2020) 57:102833. doi: 10.1016/j.ebiom.2020.102833
78. Yang L, Han Y, Jaffre F, Nilsson-Payant BE, Bram Y, Wang P, et al. An immuno-cardiac model for macrophage-mediated inflammation in Covid-19 hearts. Circ Res. (2021) 129:33–46. doi: 10.1161/CIRCRESAHA.121.319060
79. Liao M, Liu Y, Yuan J, Wen Y, Xu G, Zhao J, et al. Single-cell landscape of bronchoalveolar immune cells in patients with Covid-19. Nat Med. (2020) 26:842–4. doi: 10.1038/s41591-020-0901-9
80. Shapouri-Moghaddam A, Mohammadian S, Vazini H, Taghadosi M, Esmaeili SA, Mardani F, et al. Macrophage plasticity, polarization, and function in health and disease. J Cell Physiol. (2018) 233:6425–40. doi: 10.1002/jcp.26429
81. Jafarzadeh A, Nemati M. Therapeutic potentials of ginger for treatment of multiple sclerosis: A review with emphasis on its immunomodulatory, anti-inflammatory and anti-oxidative properties. J Neuroimmunol. (2018) 324:54–75. doi: 10.1016/j.jneuroim.2018.09.003
82. Cenko E, Badimon L, Bugiardini R, Claeys MJ, De Luca G, de Wit C, et al. Cardiovascular disease and Covid-19: A consensus paper from the esc working group on coronary pathophysiology & microcirculation, esc working group on thrombosis and the association for acute cardiovascular care (Acvc), in collaboration with the European heart rhythm association (Ehra). Cardiovasc Res. (2021) 117:2705–29. doi: 10.1093/cvr/cvab298
83. Xu X, Wang Y, Li Y, Zhang B, Song Q. The future landscape of macrophage research in cardiovascular disease: A bibliometric analysis. Curr Probl Cardiol. (2022) 47:101311. doi: 10.1016/j.cpcardiol.2022.101311
84. Klopf J, Brostjan C, Eilenberg W, Neumayer C. Neutrophil extracellular traps and their implications in cardiovascular and inflammatory disease. Int J Mol Sci. (2021) 22:559. doi: 10.3390/ijms22020559
85. Mereweather LJ, Constantinescu-Bercu A, Crawley JTB, Salles C II. Platelet-neutrophil crosstalk in thrombosis. Int J Mol Sci. (2023) 24:1266. doi: 10.3390/ijms24021266
86. Petzold T, Zhang Z, Ballesteros I, Saleh I, Polzin A, Thienel M, et al. Neutrophil “Plucking” on megakaryocytes drives platelet production and boosts cardiovascular disease. Immunity. (2022) 55:2285–99 e7. doi: 10.1016/j.immuni.2022.10.001
87. Pircher J, Engelmann B, Massberg S, Schulz C. Platelet-neutrophil crosstalk in atherothrombosis. Thromb Haemost. (2019) 119:1274–82. doi: 10.1055/s-0039-1692983
88. Johnson JE, McGuone D, Xu ML, Jane-Wit D, Mitchell RN, Libby P, et al. Coronavirus disease 2019 (Covid-19) coronary vascular thrombosis: correlation with neutrophil but not endothelial activation. Am J Pathol. (2022) 192:112–20. doi: 10.1016/j.ajpath.2021.09.004
89. Zhang Q, Zhang H, Yan X, Ma S, Yao X, Shi Y, et al. Neutrophil infiltration and myocarditis in patients with severe Covid-19: A post-mortem study. Front Cardiovasc Med. (2022) 9:1026866. doi: 10.3389/fcvm.2022.1026866
90. Tan M, Liu Y, Zhou R, Deng X, Li F, Liang K, et al. Immunopathological characteristics of Coronavirus disease 2019 cases in Guangzhou, China. Immunology. (2020) 160:261–8. doi: 10.1111/imm.13223
91. Qin C, Zhou L, Hu Z, Zhang S, Yang S, Tao Y, et al. Dysregulation of immune response in patients with Coronavirus 2019 (Covid-19) in Wuhan, China. Clin Infect Dis. (2020) 71:762–8. doi: 10.1093/cid/ciaa248
92. Wang S, Fu L, Huang K, Han J, Zhang R, Fu Z. Neutrophil-to-lymphocyte ratio on admission is an independent risk factor for the severity and mortality in patients with Coronavirus disease 2019. J Infect. (2021) 82:e16–e8. doi: 10.1016/j.jinf.2020.09.022
93. Fu J, Kong J, Wang W, Wu M, Yao L, Wang Z, et al. The clinical implication of dynamic neutrophil to lymphocyte ratio and D-dimer in Covid-19: A retrospective study in Suzhou China. Thromb Res. (2020) 192:3–8. doi: 10.1016/j.thromres.2020.05.006
94. Ciccullo A, Borghetti A, Zileri Dal Verme L, Tosoni A, Lombardi F, Garcovich M, et al. Neutrophil-to-lymphocyte ratio and clinical outcome in Covid-19: A report from the Italian front line. Int J Antimicrob Agents. (2020) 56:106017. doi: 10.1016/j.ijantimicag.2020.106017
95. Huguet E, Maccallini G, Pardini P, Hidalgo M, Obregon S, Botto F, et al. Reference values for neutrophil to lymphocyte ratio (Nlr), a biomarker of cardiovascular risk, according to age and sex in a Latin American population. Curr Probl Cardiol. (2021) 46:100422. doi: 10.1016/j.cpcardiol.2019.04.002
96. Kim S, Eliot M, Koestler DC, Wu WC, Kelsey KT. Association of neutrophil-to-lymphocyte ratio with mortality and cardiovascular disease in the Jackson heart study and modification by the Duffy antigen variant. JAMA Cardiol. (2018) 3:455–62. doi: 10.1001/jamacardio.2018.1042
97. Saliba W, Barnett-Griness O, Elias M, Rennert G. Neutrophil to lymphocyte ratio and risk of a first episode of stroke in patients with atrial fibrillation: A cohort study. J Thromb Haemost. (2015) 13:1971–9. doi: 10.1111/jth.13006
98. Oster ME, Shay DK, Su JR, Gee J, Creech CB, Broder KR, et al. Myocarditis cases reported after mrna-based Covid-19 vaccination in the us from December 2020 to August 2021. JAMA. (2022) 327:331–40. doi: 10.1001/jama.2021.24110
99. Massari M, Spila Alegiani S, Morciano C, Spuri M, Marchione P, Felicetti P, et al. Postmarketing active surveillance of myocarditis and pericarditis following vaccination with Covid-19 mrna vaccines in persons aged 12 to 39 years in Italy: A multi-database, self-controlled case series study. PloS Med. (2022) 19:e1004056. doi: 10.1371/journal.pmed.1004056
100. Pillay J, Gaudet L, Wingert A, Bialy L, Mackie AS, Paterson DI, et al. Incidence, risk factors, natural history, and hypothesised mechanisms of myocarditis and pericarditis following Covid-19 vaccination: living evidence syntheses and review. BMJ. (2022) 378:e069445. doi: 10.1136/bmj-2021-069445
101. Husby A, Kober L. Covid-19 mrna vaccination and myocarditis or pericarditis. Lancet. (2022) 399:2168–9. doi: 10.1016/S0140-6736(22)00842-X
102. Wong HL, Hu M, Zhou CK, Lloyd PC, Amend KL, Beachler DC, et al. Risk of myocarditis and pericarditis after the covid-19 mrna vaccination in the USA: A cohort study in claims databases. Lancet. (2022) 399:2191–9. doi: 10.1016/S0140-6736(22)00791-7
103. Bozkurt B, Kamat I, Hotez PJ. Myocarditis with Covid-19 mrna vaccines. Circulation. (2021) 144:471–84. doi: 10.1161/CIRCULATIONAHA.121.056135
104. Shiyovich A, Witberg G, Aviv Y, Eisen A, Orvin K, Wiessman M, et al. Myocarditis following Covid-19 vaccination: magnetic resonance imaging study. Eur Heart J Cardiovasc Imaging. (2022) 23:1075–82. doi: 10.1093/ehjci/jeab230
105. Di Dedda EA, Barison A, Aquaro GD, Ismail TF, Hua A, Mantini C, et al. Cardiac magnetic resonance imaging of myocarditis and pericarditis following Covid-19 vaccination: A multicenter collection of 27 cases. Eur Radiol. (2022) 32:4352–60. doi: 10.1007/s00330-022-08566-0
106. Vago H, Szabo L, Szabo Z, Ulakcsai Z, Szogi E, Budai G, et al. Immunological Response and Temporal Associations in Myocarditis after Covid-19 Vaccination Using Cardiac Magnetic Resonance Imaging: An Amplified T-Cell Response at the Heart of It? Front Cardiovasc Med. (2022) 9:961031. doi: 10.3389/fcvm.2022.961031
107. Baumeier C, Aleshcheva G, Harms D, Gross U, Hamm C, Assmus B, et al. Intramyocardial inflammation after Covid-19 vaccination: an endomyocardial biopsy-proven case series. Int J Mol Sci. (2022) 23:6940. doi: 10.3390/ijms23136940
108. Yonker LM, Swank Z, Bartsch YC, Burns MD, Kane A, Boribong BP, et al. Circulating spike protein detected in post-Covid-19 mrna vaccine myocarditis. Circulation. (2023) 147:867–76. doi: 10.1161/CIRCULATIONAHA.122.061025
109. Marrama D, Mahita J, Sette A, Peters B. Lack of evidence of significant homology of Sars-Cov-2 spike sequences to myocarditis-associated antigens. EBioMedicine. (2022) 75:103807. doi: 10.1016/j.ebiom.2021.103807
110. Gill JR, Tashjian R, Duncanson E. Autopsy histopathologic cardiac findings in 2 adolescents following the second Covid-19 vaccine dose. Arch Pathol Lab Med. (2022) 146:925–9. doi: 10.5858/arpa.2021-0435-SA
111. Li YE, Wang S, Reiter RJ, Ren J. Clinical cardiovascular emergencies and the cellular basis of Covid-19 vaccination: from dream to reality? Int J Infect Dis. (2022) 124:1–10. doi: 10.1016/j.ijid.2022.08.026
112. Li G, He X, Zhang L, Ran Q, Wang J, Xiong A, et al. Assessing ace2 expression patterns in lung tissues in the pathogenesis of Covid-19. J Autoimmun. (2020) 112:102463. doi: 10.1016/j.jaut.2020.102463
113. Hou Y, Zhao J, Martin W, Kallianpur A, Chung MK, Jehi L, et al. New insights into genetic susceptibility of Covid-19: an ace2 and tmprss2 polymorphism analysis. BMC Med. (2020) 18:216. doi: 10.1186/s12916-020-01673-z
114. Suryamohan K, Diwanji D, Stawiski EW, Gupta R, Miersch S, Liu J, et al. Human ace2 receptor polymorphisms and altered susceptibility to Sars-Cov-2. Commun Biol. (2021) 4:475. doi: 10.1038/s42003-021-02030-3
115. Keikha M, Karbalaei M. Global distribution of ace1 (Rs4646994) and ace2 (Rs2285666) polymorphisms associated with Covid-19: A systematic review and meta-analysis. Microb Pathog. (2022) 172:105781. doi: 10.1016/j.micpath.2022.105781
116. Udomsinprasert W, Nontawong N, Saengsiwaritt W, Panthan B, Jiaranai P, Thongchompoo N, et al. Host genetic polymorphisms involved in long-term symptoms of Covid-19. Emerg Microbes Infect. (2023) 12:2239952. doi: 10.1080/22221751.2023.2239952
117. Martinez-Gomez LE, Herrera-Lopez B, Martinez-Armenta C, Ortega-Pena S, Camacho-Rea MDC, Suarez-Ahedo C, et al. Ace and ace2 gene variants are associated with severe outcomes of Covid-19 in men. Front Immunol. (2022) 13:812940. doi: 10.3389/fimmu.2022.812940
118. Castro-Santos P, Carracedo A, Diaz-Pena R. Hla alleles: important pieces to the Covid-19 puzzle. Trends Immunol. (2023) 44:754–6. doi: 10.1016/j.it.2023.08.011
119. Augusto DG, Murdolo LD, Chatzileontiadou DSM, Sabatino JJ Jr., Yusufali T, Peyser ND, et al. A common allele of hla is associated with asymptomatic Sars-Cov-2 infection. Nature. (2023) 620:128–36. doi: 10.1038/s41586-023-06331-x
120. Migliorini F, Torsiello E, Spiezia F, Oliva F, Tingart M, Maffulli N. Association between hla genotypes and Covid-19 susceptibility, severity and progression: A comprehensive review of the literature. Eur J Med Res. (2021) 26:84. doi: 10.1186/s40001-021-00563-1
121. Ben Shachar S, Barda N, Manor S, Israeli S, Dagan N, Carmi S, et al. Mhc haplotyping of Sars-Cov-2 patients: hla subtypes are not associated with the presence and severity of Covid-19 in the Israeli population. J Clin Immunol. (2021) 41:1154–61. doi: 10.1007/s10875-021-01071-x
122. Fricke-Galindo I, Falfan-Valencia R. Genetics insight for Covid-19 susceptibility and severity: A review. Front Immunol. (2021) 12:622176. doi: 10.3389/fimmu.2021.622176
123. Deng X, Tang K, Wang Z, He S, Luo Z. Impacts of inflammatory cytokines variants on systemic inflammatory profile and Covid-19 severity. J Epidemiol Glob Health. (2024) 14:363–78. doi: 10.1007/s44197-024-00204-w
124. Al-Aly Z. Long covid and its cardiovascular implications: A call to action. Eur Heart J. (2023) 44:5001–3. doi: 10.1093/eurheartj/ehad723
125. Raman B, Bluemke DA, Luscher TF, Neubauer S. Long covid: post-acute sequelae of Covid-19 with a cardiovascular focus. Eur Heart J. (2022) 43:1157–72. doi: 10.1093/eurheartj/ehac031
126. Hira R, Baker JR, Siddiqui T, Ranada SI, Soroush A, Karalasingham K, et al. Objective hemodynamic cardiovascular autonomic abnormalities in post-acute sequelae of Covid-19. Can J Cardiol. (2023) 39:767–75. doi: 10.1016/j.cjca.2022.12.002
127. Maitz T, Parfianowicz D, Vojtek A, Rajeswaran Y, Vyas AV, Gupta R. Covid-19 cardiovascular connection: A review of cardiac manifestations in Covid-19 infection and treatment modalities. Curr Probl Cardiol. (2023) 48:101186. doi: 10.1016/j.cpcardiol.2022.101186
128. Lazzerini PE, Laghi-Pasini F, Acampa M, Boutjdir M, Leopoldo Capecchi P. Il-6 (Interleukin 6) blockade and heart rate corrected qt interval prolongation in Covid-19. Circ Arrhythm Electrophysiol. (2020) 13:e008791. doi: 10.1161/CIRCEP.120.008791
129. Nguyen N, Nguyen H, Ukoha C, Hoang L, Patel C, Ikram FG, et al. Relation of interleukin-6 levels in Covid-19 patients with major adverse cardiac events. Proc (Bayl Univ Med Cent). (2022) 35:6–9. doi: 10.1080/08998280.2021.1961571
130. Group WHOREAfC-TW, Shankar-Hari M, Vale CL, Godolphin PJ, Fisher D, Higgins JPT, et al. Association between administration of il-6 antagonists and mortality among patients hospitalized for Covid-19: A meta-analysis. JAMA. (2021) 326:499–518. doi: 10.1001/jama.2021.11330
131. Mazzoni A, Salvati L, Maggi L, Capone M, Vanni A, Spinicci M, et al. Impaired immune cell cytotoxicity in severe Covid-19 is il-6 dependent. J Clin Invest. (2020) 130:4694–703. doi: 10.1172/jci138554
132. Guillen L, Padilla S, Fernandez M, Agullo V, Garcia JA, Telenti G, et al. Preemptive interleukin-6 blockade in patients with Covid-19. Sci Rep. (2020) 10:16826. doi: 10.1038/s41598-020-74001-3
133. Patel K, Gooley TA, Bailey N, Bailey M, Hegerova L, Batchelder A, et al. Use of the il-6r antagonist tocilizumab in hospitalized Covid-19 patients. J Intern Med. (2021) 289:430–3. doi: 10.1111/joim.13163
134. Potere N, Di Nisio M, Cibelli D, Scurti R, Frattari A, Porreca E, et al. Response to: ‘Correspondence on ‘Interleukin-6 blockade with subcutaneous tocilizumab in severe Covid-19 pneumonia and hyperinflammation: A case-control study’ by Potere et al’ by Buckley. Ann Rheum Dis. (2022) 81:e195. doi: 10.1136/annrheumdis-2020-218715
135. Coperchini F, Chiovato L, Rotondi M. Interleukin-6, cxcl10 and infiltrating macrophages in Covid-19-related cytokine storm: not one for all but all for one! Front Immunol. (2021) 12:668507. doi: 10.3389/fimmu.2021.668507
136. Potere N, Batticciotto A, Vecchie A, Porreca E, Cappelli A, Abbate A, et al. The role of il-6 and il-6 blockade in Covid-19. Expert Rev Clin Immunol. (2021) 17:601–18. doi: 10.1080/1744666X.2021.1919086
137. Cuesta-Llavona E, Gomez J, Albaiceta GM, Amado-Rodriguez L, Garcia-Clemente M, Gutierrez-Rodriguez J, et al. Variant-genetic and transcript-expression analysis showed a role for the chemokine-receptor ccr5 in Covid-19 severity. Int Immunopharmacol. (2021) 98:107825. doi: 10.1016/j.intimp.2021.107825
138. Okamoto M, Toyama M, Baba M. The chemokine receptor antagonist cenicriviroc inhibits the replication of Sars-Cov-2 in vitro. Antiviral Res. (2020) 182:104902. doi: 10.1016/j.antiviral.2020.104902
139. Gonzalez P, Alvarez R, Batalla A, Reguero JR, Alvarez V, Astudillo A, et al. Genetic variation at the chemokine receptors ccr5/ccr2 in myocardial infarction. Genes Immun. (2001) 2:191–5. doi: 10.1038/sj.gene.6363760
140. Files DC, Tacke F, O’Sullivan A, Dorr P, Ferguson WG, Powderly WG. Rationale of using the dual chemokine receptor ccr2/ccr5 inhibitor cenicriviroc for the treatment of Covid-19. PloS Pathog. (2022) 18:e1010547. doi: 10.1371/journal.ppat.1010547
141. Yin K, Wang S, Zhao RC. Exosomes from mesenchymal stem/stromal cells: A new therapeutic paradigm. Biomark Res. (2019) 7:8. doi: 10.1186/s40364-019-0159-x
142. Lightner AL, Sengupta V, Qian S, Ransom JT, Suzuki S, Park DJ, et al. Bone marrow mesenchymal stem cell-derived extracellular vesicle infusion for the treatment of respiratory failure from Covid-19: A randomized, placebo-controlled dosing clinical trial. Chest. (2023) 164:1444–53. doi: 10.1016/j.chest.2023.06.024
143. Bagno L, Hatzistergos KE, Balkan W, Hare JM. Mesenchymal stem cell-based therapy for cardiovascular disease: progress and challenges. Mol Ther. (2018) 26:1610–23. doi: 10.1016/j.ymthe.2018.05.009
144. Tang JW, Young S, May S, Bird P, Bron J, Mohamedanif T, et al. Comparing hospitalised, community and staff Covid-19 infection rates during the early phase of the evolving Covid-19 epidemic. J Infect. (2020) 81:647–79. doi: 10.1016/j.jinf.2020.05.029
145. Meng F, Xu R, Wang S, Xu Z, Zhang C, Li Y, et al. Human umbilical cord-derived mesenchymal stem cell therapy in patients with Covid-19: A phase 1 clinical trial. Signal Transduct Target Ther. (2020) 5:172. doi: 10.1038/s41392-020-00286-5
146. Leng Z, Zhu R, Hou W, Feng Y, Yang Y, Han Q, et al. Transplantation of ace2(-) mesenchymal stem cells improves the outcome of patients with Covid-19 pneumonia. Aging Dis. (2020) 11:216–28. doi: 10.14336/AD.2020.0228
147. Zhu R, Yan T, Feng Y, Liu Y, Cao H, Peng G, et al. Mesenchymal stem cell treatment improves outcome of Covid-19 patients via multiple immunomodulatory mechanisms. Cell Res. (2021) 31:1244–62. doi: 10.1038/s41422-021-00573-y
148. Shetty AK, Shetty PA, Zanirati G, Jin K. Further validation of the efficacy of mesenchymal stem cell infusions for reducing mortality in Covid-19 patients with ards. NPJ Regener Med. (2021) 6:53. doi: 10.1038/s41536-021-00161-z
149. Walter J, Ware LB, Matthay MA. Mesenchymal stem cells: mechanisms of potential therapeutic benefit in ards and sepsis. Lancet Respir Med. (2014) 2:1016–26. doi: 10.1016/S2213-2600(14)70217-6
150. Bisoendial RJ, Boekholdt SM, Vergeer M, Stroes ES, Kastelein JJ. C-reactive protein is a mediator of cardiovascular disease. Eur Heart J. (2010) 31:2087–91. doi: 10.1093/eurheartj/ehq238
151. Fu X, Liu G, Halim A, Ju Y, Luo Q, Song AG. Mesenchymal stem cell migration and tissue repair. Cells. (2019) 8:784. doi: 10.3390/cells8080784
152. Shi L, Yuan X, Yao W, Wang S, Zhang C, Zhang B, et al. Human mesenchymal stem cells treatment for severe Covid-19: 1-year follow-up results of a randomized, double-blind, placebo-controlled trial. EBioMedicine. (2022) 75:103789. doi: 10.1016/j.ebiom.2021.103789
153. Chen X, Shan Y, Wen Y, Sun J, Du H. Mesenchymal stem cell therapy in severe Covid-19: A retrospective study of short-term treatment efficacy and side effects. J Infect. (2020) 81:647–79. doi: 10.1016/j.jinf.2020.05.020
154. Shi L, Zheng Y, Cheng Z, Ji N, Niu C, Wang Y, et al. One-year follow-up study after patients with severe Covid-19 received human umbilical cord mesenchymal stem cells treatment. Stem Cell Res Ther. (2022) 13:321. doi: 10.1186/s13287-022-02972-3
155. Li TT, Zhang B, Fang H, Shi M, Yao WQ, Li Y, et al. Human mesenchymal stem cell therapy in severe Covid-19 patients: 2-year follow-up results of a randomized, double-blind, placebo-controlled trial. EBioMedicine. (2023) 92:104600. doi: 10.1016/j.ebiom.2023.104600
156. Hashemian SR, Aliannejad R, Zarrabi M, Soleimani M, Vosough M, Hosseini SE, et al. Mesenchymal stem cells derived from perinatal tissues for treatment of critically ill Covid-19-induced ards patients: A case series. Stem Cell Res Ther. (2021) 12:91. doi: 10.1186/s13287-021-02165-4
157. Vij R, Kim H, Park H, Cheng T, Lotfi D, Chang D. Adipose-derived, autologous mesenchymal stem cell therapy for patients with post-Covid-19 syndrome: an intermediate-size expanded access program. Stem Cell Res Ther. (2023) 14:287. doi: 10.1186/s13287-023-03522-1
158. Marban E, Liao K. On the cellular origin of cardiosphere-derived cells (Cdcs). Basic Res Cardiol. (2022) 117:12. doi: 10.1007/s00395-022-00914-x
159. Shiraishi M, Sasaki D, Hibino M, Takeda A, Harashima H, Yamada Y. Human cardiosphere-derived cells with activated mitochondria for better myocardial regenerative therapy. J Control Release. (2024) 367:486–99. doi: 10.1016/j.jconrel.2024.01.058
160. Sano T, Ito T, Ishigami S, Bandaru S, Sano S. Intrinsic activation of cardiosphere-derived cells enhances myocardial repair. J Thorac Cardiovasc Surg. (2022) 163:1479–90 e5. doi: 10.1016/j.jtcvs.2020.05.040
161. Gallet R, Su JB, Corboz D, Chiaroni PM, Bize A, Dai J, et al. Three-vessel coronary infusion of cardiosphere-derived cells for the treatment of heart failure with preserved ejection fraction in a pre-clinical pig model. Basic Res Cardiol. (2023) 118:26. doi: 10.1007/s00395-023-00995-2
162. Kogan PS, Wirth F, Tomar A, Darr J, Teperino R, Lahm H, et al. Uncovering the molecular identity of cardiosphere-derived cells (Cdcs) by single-cell rna sequencing. Basic Res Cardiol. (2022) 117:11. doi: 10.1007/s00395-022-00913-y
163. Chakravarty T, Henry TD, Kittleson M, Lima J, Siegel RJ, Slipczuk L, et al. Allogeneic cardiosphere-derived cells for the treatment of heart failure with reduced ejection fraction: the dilated cardiomyopathy intervention with allogeneic myocardially-regenerative cells (Dynamic) trial. EuroIntervention. (2020) 16:e293–300. doi: 10.4244/EIJ-D-19-00035
164. Lin YN, Mesquita T, Sanchez L, Chen YH, Liu W, Li C, et al. Extracellular vesicles from immortalized cardiosphere-derived cells attenuate arrhythmogenic cardiomyopathy in desmoglein-2 mutant mice. Eur Heart J. (2021) 42:3558–71. doi: 10.1093/eurheartj/ehab419
165. Makkar RR, Smith RR, Cheng K, Malliaras K, Thomson LE, Berman D, et al. Intracoronary cardiosphere-derived cells for heart regeneration after myocardial infarction (Caduceus): A prospective, randomised phase 1 trial. Lancet. (2012) 379:895–904. doi: 10.1016/S0140-6736(12)60195-0
166. Lempriere S. Treatment with cardiosphere-derived cells could slow progress of duchenne muscular dystrophy. Nat Rev Neurol. (2022) 18:251. doi: 10.1038/s41582-022-00657-2
167. de Couto G. Macrophages in cardiac repair: environmental cues and therapeutic strategies. Exp Mol Med. (2019) 51:1–10. doi: 10.1038/s12276-019-0269-4
168. Marban E. A mechanistic roadmap for the clinical application of cardiac cell therapies. Nat BioMed Eng. (2018) 2:353–61. doi: 10.1038/s41551-018-0216-z
169. Pironti G, Andersson DC, Lund LH. Mechanistic and therapeutic implications of extracellular vesicles as a potential link between Covid-19 and cardiovascular disease manifestations. Front Cell Dev Biol. (2021) 9:640723. doi: 10.3389/fcell.2021.640723
170. Singh S, Chakravarty T, Chen P, Akhmerov A, Falk J, Friedman O, et al. Allogeneic cardiosphere-derived cells (Cap-1002) in critically ill Covid-19 patients: compassionate-use case series. Basic Res Cardiol. (2020) 115:36. doi: 10.1007/s00395-020-0795-1
171. Rezaie J, Rahbarghazi R, Pezeshki M, Mazhar M, Yekani F, Khaksar M, et al. Cardioprotective role of extracellular vesicles: A highlight on exosome beneficial effects in cardiovascular diseases. J Cell Physiol. (2019) 234:21732–45. doi: 10.1002/jcp.28894
172. Amirzadeh Gougheri K, Ahmadi A, Ahmadabadi MG, Babajani A, Yazdanpanah G, Bahrami S, et al. Exosomal cargo: pro-angiogeneic, anti-inflammatory, and regenerative effects in ischemic and non-ischemic heart diseases - a comprehensive review. BioMed Pharmacother. (2023) 168:115801. doi: 10.1016/j.biopha.2023.115801
173. Nasser MI, Masood M, Adlat S, Gang D, Zhu S, Li G, et al. Mesenchymal stem cell-derived exosome microrna as therapy for cardiac ischemic injury. BioMed Pharmacother. (2021) 143:112118. doi: 10.1016/j.biopha.2021.112118
174. Burke J, Kolhe R, Hunter M, Isales C, Hamrick M, Fulzele S. Stem cell-derived exosomes: A potential alternative therapeutic agent in orthopaedics. Stem Cells Int. (2016) 2016:5802529. doi: 10.1155/2016/5802529
175. Abdelgawad M, Bakry NS, Farghali AA, Abdel-Latif A, Lotfy A. Mesenchymal stem cell-based therapy and exosomes in Covid-19: current trends and prospects. Stem Cell Res Ther. (2021) 12:469. doi: 10.1186/s13287-021-02542-z
176. Yousefi Dehbidi M, Goodarzi N, Azhdari MH, Doroudian M. Mesenchymal stem cells and their derived exosomes to combat Covid-19. Rev Med Virol. (2022) 32:e2281. doi: 10.1002/rmv.2281
177. Hade MD, Suire CN, Suo Z. Mesenchymal stem cell-derived exosomes: applications in regenerative medicine. Cells. (2021) 10:1959. doi: 10.3390/cells10081959
178. Narang P, Shah M, Beljanski V. Exosomal rnas in diagnosis and therapies. Noncoding RNA Res. (2022) 7:7–15. doi: 10.1016/j.ncrna.2022.01.001
179. Bittle GJ, Morales D, Pietris N, Parchment N, Parsell D, Peck K, et al. Exosomes isolated from human cardiosphere-derived cells attenuate pressure overload-induced right ventricular dysfunction. J Thorac Cardiovasc Surg. (2021) 162:975–86 e6. doi: 10.1016/j.jtcvs.2020.06.154
180. Gallet R, Dawkins J, Valle J, Simsolo E, de Couto G, Middleton R, et al. Exosomes secreted by cardiosphere-derived cells reduce scarring, attenuate adverse remodelling, and improve function in acute and chronic porcine myocardial infarction. Eur Heart J. (2017) 38:201–11. doi: 10.1093/eurheartj/ehw240
181. Lopez E, Marinaro F, de Pedro MLA, Sanchez-Margallo FM, Gomez-Serrano M, Ponath V, et al. The immunomodulatory signature of extracellular vesicles from cardiosphere-derived cells: A proteomic and mirna profiling. Front Cell Dev Biol. (2020) 8:321. doi: 10.3389/fcell.2020.00321
182. Zhao R, Wang L, Wang T, Xian P, Wang H, Long Q. Inhalation of msc-evs is a noninvasive strategy for ameliorating acute lung injury. J Control Release. (2022) 345:214–30. doi: 10.1016/j.jconrel.2022.03.025
183. Castiglione V, Chiriaco M, Emdin M, Taddei S, Vergaro G. Statin therapy in Covid-19 infection. Eur Heart J Cardiovasc Pharmacother. (2020) 6:258–9. doi: 10.1093/ehjcvp/pvaa042
184. Song SL, Hays SB, Panton CE, Mylona EK, Kalligeros M, Shehadeh F, et al. Statin use is associated with decreased risk of invasive mechanical ventilation in Covid-19 patients: A preliminary study. Pathogens. (2020) 9:759. doi: 10.3390/pathogens9090759
185. Kollias A, Kyriakoulis KG, Kyriakoulis IG, Nitsotolis T, Poulakou G, Stergiou GS, et al. Statin use and mortality in Covid-19 patients: updated systematic review and meta-analysis. Atherosclerosis. (2021) 330:114–21. doi: 10.1016/j.atherosclerosis.2021.06.911
186. Reiner Z, Hatamipour M, Banach M, Pirro M, Al-Rasadi K, Jamialahmadi T, et al. Statins and the Covid-19 main protease: in silico evidence on direct interaction. Arch Med Sci. (2020) 16:490–6. doi: 10.5114/aoms.2020.94655
187. Radenkovic D, Chawla S, Pirro M, Sahebkar A, Banach M. Cholesterol in relation to Covid-19: should we care about it? J Clin Med. (2020) 9:1909. doi: 10.3390/jcm9061909
188. Bonsu KO, Reidpath DD, Kadirvelu A. Effects of statin treatment on inflammation and cardiac function in heart failure: an adjusted indirect comparison meta-analysis of randomized trials. Cardiovasc Ther. (2015) 33:338–46. doi: 10.1111/1755-5922.12150
189. Saewong S, Thammasitboon K, Wattanaroonwong N. Simvastatin induces apoptosis and disruption of the actin cytoskeleton in human dental pulp cells and periodontal ligament fibroblasts. Arch Biol. (2013) 58:964–74. doi: 10.1016/j.archoralbio.2013.03.002
190. Swain SS, Panda SK, Luyten W. Phytochemicals against Sars-Cov as potential drug leads. BioMed J. (2021) 44:74–85. doi: 10.1016/j.bj.2020.12.002
191. Filardo S, Di Pietro M, Mastromarino P, Sessa R. Therapeutic potential of resveratrol against emerging respiratory viral infections. Pharmacol Ther. (2020) 214:107613. doi: 10.1016/j.pharmthera.2020.107613
192. Lin SC, Ho CT, Chuo WH, Li S, Wang TT, Lin CC. Effective inhibition of Mers-Cov infection by resveratrol. BMC Infect Dis. (2017) 17:144. doi: 10.1186/s12879-017-2253-8
193. Ojha D, Jessop F, Bosio CM, Peterson KE. Effective inhibition of hcov-oc43 and Sars-Cov-2 by phytochemicals in vitro and in vivo. Int J Antimicrob Agents. (2023) 62:106893. doi: 10.1016/j.ijantimicag.2023.106893
194. Yang M, Wei J, Huang T, Lei L, Shen C, Lai J, et al. Resveratrol inhibits the replication of severe acute respiratory syndrome coronavirus 2 (Sars-Cov-2) in cultured vero cells. Phytother Res. (2021) 35:1127–9. doi: 10.1002/ptr.6916
195. Rossi GA, Sacco O, Capizzi A, Mastromarino P. Can resveratrol-inhaled formulations be considered potential adjunct treatments for Covid-19? Front Immunol. (2021) 12:670955. doi: 10.3389/fimmu.2021.670955
196. Malaguarnera L. Influence of resveratrol on the immune response. Nutrients. (2019) 11:946. doi: 10.3390/nu11050946
197. McCreary MR, Schnell PM, Rhoda DA. Randomized double-blind placebo-controlled proof-of-concept trial of resveratrol for outpatient treatment of mild coronavirus disease (Covid-19). Sci Rep. (2022) 12:10978. doi: 10.1038/s41598-022-13920-9
198. Rotimi DE, Afolabi BL, Adeyemi OS. Covid 19: resveratrol as a potential supplement to mitigate the cardiotoxicity associated with chloroquine and hydroxychloroquine treatment. Biointerface Res Appl Chem. (2020) 11:11172–86. doi: 10.33263/briac114.1117211186
199. Huang K, Zhang P, Zhang Z, Youn JY, Wang C, Zhang H, et al. Traditional chinese medicine (Tcm) in the treatment of Covid-19 and other viral infections: efficacies and mechanisms. Pharmacol Ther. (2021) 225:107843. doi: 10.1016/j.pharmthera.2021.107843
200. Xing D, Liu Z. Effectiveness and safety of traditional Chinese medicine in treating Covid-19: clinical evidence from China. Aging Dis. (2021) 12:1850–6. doi: 10.14336/AD.2021.0906
201. Fan L, Ding X. Potential effects of traditional Chinese medicine on Covid-19 and cardiac injury: mechanisms and clinical evidence. J Multidiscip Healthc. (2023) 16:2863–72. doi: 10.2147/JMDH.S424078
202. Zhang XY, Huang HJ, Zhuang DL, Nasser MI, Yang MH, Zhu P, et al. Biological, clinical and epidemiological features of Covid-19, Sars and Mers and autodock simulation of ace2. Infect Dis Poverty. (2020) 9:99. doi: 10.1186/s40249-020-00691-6
203. Yao Z, Zheng Z, Wu K, Junhua Z. Immune environment modulation in pneumonia patients caused by coronavirus: Sars-Cov, Mers-Cov and Sars-Cov-2. Aging (Albany NY). (2020) 12:7639–51. doi: 10.18632/aging.103101
204. Liang Y, Wang ML, Chien CS, Yarmishyn AA, Yang YP, Lai WY, et al. Highlight of immune pathogenic response and hematopathologic effect in Sars-Cov, Mers-Cov, and Sars-Cov-2 infection. Front Immunol. (2020) 11:1022. doi: 10.3389/fimmu.2020.01022
205. Goyal R, Gautam RK, Chopra H, Dubey AK, Singla RK, Rayan RA, et al. Comparative highlights on Mers-Cov, Sars-Cov-1, Sars-Cov-2, and Neo-Cov. EXCLI J. (2022) 21:1245–72. doi: 10.17179/excli2022-5355
206. Yao T, Foo C, Zheng G, Huang R, Li Q, Shen J, et al. Insight into the mechanisms of coronaviruses evading host innate immunity. Biochim Biophys Acta Mol Basis Dis. (2023) 1869:166671. doi: 10.1016/j.bbadis.2023.166671
207. Chasouraki AM, Violetis OA, Abdelrasoul M, Tsagalou EP. Acute myocarditis related to Covid-19: comparison to Sars and Mers. SN Compr Clin Med. (2020) 2:2684–90. doi: 10.1007/s42399-020-00563-y
208. Lombardi AF, Afsahi AM, Gupta A, Gholamrezanezhad A. Severe acute respiratory syndrome (Sars), middle east respiratory syndrome (Mers), influenza, and Covid-19, beyond the lungs: A review article. Radiol Med. (2021) 126:561–9. doi: 10.1007/s11547-020-01311-x
209. Sengupta V, Sengupta S, Lazo A, Woods P, Nolan A, Bremer N. Exosomes derived from bone marrow mesenchymal stem cells as treatment for severe Covid-19. Stem Cells Dev. (2020) 29:747–54. doi: 10.1089/scd.2020.0080
210. Safari A, Lionetti V, Razeghian-Jahromi I. Combination of mesenchymal stem cells and nicorandil: an emerging therapeutic challenge against Covid-19 infection-induced multiple organ dysfunction. Stem Cell Res Ther. (2021) 12:404. doi: 10.1186/s13287-021-02482-8
211. Cambon A, Guervilly C, Delteil C, Potere N, Bachelier R, Tellier E, et al. Caspase-1 activation, il-1/il-6 signature and ifngamma-induced chemokines in lungs of Covid-19 patients. Front Immunol. (2024) 15:1493306. doi: 10.3389/fimmu.2024.1493306
212. Group RC. Baricitinib in patients admitted to hospital with Covid-19 (Recovery): A randomised, controlled, open-label, platform trial and updated meta-analysis. Lancet. (2022) 400:359–68. doi: 10.1016/S0140-6736(22)01109-6
213. Potere N, Del Buono MG, Caricchio R, Cremer PC, Vecchie A, Porreca E, et al. Interleukin-1 and the nlrp3 inflammasome in Covid-19: pathogenetic and therapeutic implications. EBioMedicine. (2022) 85:104299. doi: 10.1016/j.ebiom.2022.104299
214. Potere N, Garrad E, Kanthi Y, Di Nisio M, Kaplanski G, Bonaventura A, et al. Nlrp3 inflammasome and interleukin-1 contributions to Covid-19-associated coagulopathy and immunothrombosis. Cardiovasc Res. (2023) 119:2046–60. doi: 10.1093/cvr/cvad084
215. Lippi G, Lavie CJ, Sanchis-Gomar F. Cardiac troponin I in patients with Coronavirus disease 2019 (Covid-19): evidence from a meta-analysis. Prog Cardiovasc Dis. (2020) 63:390–1. doi: 10.1016/j.pcad.2020.03.001
216. Imazio M, Klingel K, Kindermann I, Brucato A, De Rosa FG, Adler Y, et al. Covid-19 pandemic and troponin: indirect myocardial injury, myocardial inflammation or myocarditis? Heart. (2020) 106:1127–31. doi: 10.1136/heartjnl-2020-317186
217. Garg A, Seeliger B, Derda AA, Xiao K, Gietz A, Scherf K, et al. Circulating cardiovascular micrornas in critically ill Covid-19 patients. Eur J Heart Fail. (2021) 23:468–75. doi: 10.1002/ejhf.2096
218. Li D, Chen Y, Jia Y, Tong L, Tong J, Wang W, et al. Sars-Cov-2-induced immune dysregulation and myocardial injury risk in China: insights from the Ers-Covid-19 study. Circ Res. (2020) 127:397–9. doi: 10.1161/CIRCRESAHA.120.317070
219. Zhong Y, Sun Z, Xu P, Bai Y, Zhang Z, Wang G. The value of non-contrast chest ct in the prediction of myocardial injury in patients with the Covid-19 omicron variant. Sci Rep. (2023) 13:10321. doi: 10.1038/s41598-023-37335-2
220. Moriwaki K, Fujimoto N, Murakami H, Maruyama K, Ishida M, Tanabe M, et al. Different types of myocardial injury due to the severe acute respiratory syndrome coronavirus 2 (Sars-Cov-2) omicron variant. Int Heart J. (2023) 64:85–9. doi: 10.1536/ihj.22-355
221. Jaumdally S, Tomasicchio M, Pooran A, Esmail A, Kotze A, Meier S, et al. Frequency, kinetics and determinants of viable Sars-Cov-2 in bioaerosols from ambulatory Covid-19 patients infected with the beta, delta or omicron variants. Nat Commun. (2024) 15:2003. doi: 10.1038/s41467-024-45400-1
222. Huiberts AJ, Hoeve CE, de Gier B, Cremer J, van der Veer B, de Melker HE, et al. Effectiveness of omicron xbb.1.5 vaccine against infection with Sars-Cov-2 omicron xbb and jn.1 variants, prospective cohort study, the Netherlands, October 2023 to January 2024. Euro Surveill. (2024) 29:2400109. doi: 10.2807/1560-7917.ES.2024.29.10.2400109
223. Thakur V, Ratho RK. Omicron (B.1.1.529): A new Sars-Cov-2 variant of concern mounting worldwide fear. J Med Virol. (2022) 94:1821–4. doi: 10.1002/jmv.27541
224. Alam L, Omar AMS, Narula J, Argulian E. Echocardiographic findings in patients with Covid-19 with myocardial injury during the omicron variant surge. Am J Cardiol. (2022) 172:168–9. doi: 10.1016/j.amjcard.2022.03.008
225. Yu X, Li X, Xia S, Lu T, Zong M, Suo C, et al. Development and validation of a prognostic model based on clinical laboratory biomarkers to predict admission to icu in omicron variant-infected hospitalized patients complicated with myocardial injury. Front Immunol. (2024) 15:1268213. doi: 10.3389/fimmu.2024.1268213
Keywords: myocardial injury, immunity, SARS-CoV-2, COVID-19, therapeutics, cardiovascular
Citation: Li Z, Qin L, Xu X, Chen R, Zhang G, Wang B, Li B and Chu X-M (2025) Immune modulation: the key to combat SARS-CoV-2 induced myocardial injury. Front. Immunol. 16:1561946. doi: 10.3389/fimmu.2025.1561946
Received: 16 January 2025; Accepted: 23 April 2025;
Published: 14 May 2025.
Edited by:
Aleksandra Piechota-Polanczyk, Medical University of Lodz, PolandReviewed by:
Jianli Jimmy Zhao, University of Alabama at Birmingham, United StatesMichele Golino, Virginia Commonwealth University, United States
Yuhang Wang, The University of Iowa, United States
Mohammad Arish, University of Virginia, United States
Copyright © 2025 Li, Qin, Xu, Chen, Zhang, Wang, Li and Chu. This is an open-access article distributed under the terms of the Creative Commons Attribution License (CC BY). The use, distribution or reproduction in other forums is permitted, provided the original author(s) and the copyright owner(s) are credited and that the original publication in this journal is cited, in accordance with accepted academic practice. No use, distribution or reproduction is permitted which does not comply with these terms.
*Correspondence: Xian-Ming Chu, Y2h1eGlhbm1pbmdAcWR1LmVkdS5jbg==