- 1Department of Infectious Diseases, The University of Melbourne at The Peter Doherty Institute for Infection and Immunity, Melbourne, VIC, Australia
- 2ATRACT Research Centre, Infectious and Inflammatory Diseases Theme, School of Health and Biomedical Sciences, RMIT University, Melbourne, VIC, Australia
- 3Victorian Infectious Diseases Service, The Royal Melbourne Hospital at the Peter Doherty Institute for Infection and Immunity, Melbourne, VIC, Australia
- 4Department of Infectious Diseases, Alfred Hospital and Monash University, Melbourne, VIC, Australia
The ‘shock and kill’ approach to an HIV cure involves the use of latency reversing agents (LRAs) to reactivate latent HIV, with the aim to induce death of infected cells through virus induced cytolysis or immune mediated clearance. Most LRAs tested to date have been unable to overcome the blocks to transcription elongation and splicing that persist in resting CD4+ T cells. Furthermore, most LRAs target host factors and therefore have associated toxicities. Therefore, there remains a high need for HIV-specific LRAs that can also potently upregulate expression of multiply-spliced HIV RNA and viral protein. The HIV Transactivator of Transcription (Tat) protein plays an important role in viral replication - amplifying transcription from the viral promoter - but it is present at low to negligible levels in latently infected cells. As such, it has been hypothesized that providing Tat in trans could result in efficient HIV reactivation from latency. Recent studies exploring different types of Tat-based LRAs have used different nanoparticles for Tat delivery and describe potent, HIV-specific induction of multiply-spliced HIV RNA and protein ex vivo. However, there are several potential challenges to using Tat as a therapeutic, including the ability of Tat to cause systemic toxicities in vivo, limited delivery of Tat to the HIV reservoir due to poor uptake of nucleic acid by resting cells, and challenges in activating truly transcriptionally silent viruses. Identifying ways to mitigate these challenges will be critical to developing effective Tat-based LRA approaches towards an HIV cure.
Introduction
Following cessation of antiretroviral therapy (ART) in people with HIV (PWH), HIV rapidly rebounds from a pool of latently infected cells (1, 2). Latently infected cells contain an integrated intact provirus but express minimal HIV RNA and proteins, resulting in a long-lived persistent reservoir (3). Strategies towards an HIV cure have focused on reducing the number of latently infected cells such that PWH can maintain undetectable viral loads in the absence of ART [reviewed in (4)]. The ‘shock and kill’ approach involves the use of latency reversing agents (LRAs) to upregulate HIV RNA transcription and protein expression, resulting in the death of an infected cell by immune-mediated clearance or virus-mediated cytotoxicity [reviewed in (5)].
Several classes of LRAs with distinct mechanisms of action have been described, and many have demonstrated strong induction of HIV RNA in vitro and ex vivo (6–10). Clinical trials have since been conducted to assess the performance of these in vivo, of which a common primary endpoint for efficacy has been cell-associated, unspliced HIV RNA as a measure of HIV reactivation (see (11) for a systematic review). Although some LRAs both alone and in combination were able to increase unspliced HIV RNA in PWH on ART, few demonstrate a decrease in HIV DNA and/or the replication competent inducible HIV reservoir, indicating a need for more potent LRAs with novel mechanisms of action (11–14).
This lack of potency of current LRAs is thought to be due to two reasons. Firstly, whilst most current LRAs can upregulate transcription initiation, few if any, are able to overcome the subsequent blocks to transcription elongation, completion and splicing (15–17). As splicing is a pre-requisite for HIV protein production, it is considered the best predictor of efficient latency reversal (18), meaning any LRA must be able to potently upregulate multiply-spliced HIV RNA. Secondly, multiple LRAs have been shown to cause various adverse effects as they target host pathways and therefore have effects on cellular transcription and often undesirable effects on immune responses (14, 19, 20). Since PWH can live long, healthy lives on ART, any potential HIV cure approach must minimize toxicities while maximizing efficacy (21, 22). In this review, we focus on the rationale, feasibility and findings of utilizing the HIV Transactivator of Transcription (Tat) protein as a next-generation LRA and discuss strategies for maximizing potency and minimizing toxicities.
Tat structure and function
Tat is a small, basic protein of about 14-16 kDa, which is encoded by two exons that are alternatively spliced to become a full-length protein (23). Tat most commonly spans 101 amino acid residues (Tat101) (24), but a premature stop codon at residue 87 frequently occurs, encoding a truncated Tat86 variant (25). The functional domains of Tat largely reside in the first coding exon (amino acids 1-72; Tat72) such that it can function independently of the second coding exon (26, 27). The proline-rich domain, or N-terminus, mediates Long Terminal Repeat (LTR) transactivation through interactions with positive transcription elongation factor b and is mostly hypervariable except for a highly conserved tryptophan at position 11 (Trp11). In contrast, the cysteine-rich domain contains a highly conserved string of cysteines at positions 22, 25, 27, 30, 31, 34 and 37 and mutations at these sites can significantly affect Tat function (28). The third domain spans residues 38-48 and contains a hydrophobic core sequence. Together, the first three domains compromise the minimal transactivation region which itself is sufficient for transactivation capability (29). The remainder of the first coding exon, referred to as the basic region, is comprised of the Transactivation Response Element (TAR)-binding motif (49RKKRRQRRR57) (30) and a glutamine rich region which is implicated in Tat-mediated apoptosis of T cells (31). Amino acids 31-61 within the first coding exon have also been shown to be related to Tat-related morbidities (32). Although the transactivation domain is localized to Tat72, the second coding exon plays a role in the control of HIV transcription in CD4+ T cells by kappa-light-chain enhancer of activated B cells (NF-κB) (33). The second coding exon largely contributes to viral infectivity and is essential for efficient replication of macrophage-tropic HIV strains (34). This region also contains a 73RGD75 motif which allows for interactions with cell surface molecules, triggering intracellular signaling cascades (35).
HIV transcription is driven by the Tat protein, which amplifies expression from the viral promoter within the HIV LTR (36). By binding to the TAR hairpin in the nascent RNA strand, Tat recruits p-TEFb (37), resulting in the hyperphosphorylation of RNA polymerase II and increased transcriptional processivity from the LTR (Figure 1) (38). This is essential for the production of full-length unspliced HIV RNA, which can be spliced into singly spliced and multiply-spliced HIV RNA which are translated into the various HIV proteins for virion formation. In latently infected cells, Tat levels are reduced, rendering viral transcription low or silent, whilst in productively infected cells, Tat levels are elevated and therefore enhance HIV transcription (39, 40). Even when a cell is in a resting state, stochastic fluctuations in Tat expression can drive a cell to alternate between productive infection or latency (40, 41), which is sufficient to overcome cell-driven silencing of HIV transcription (42).
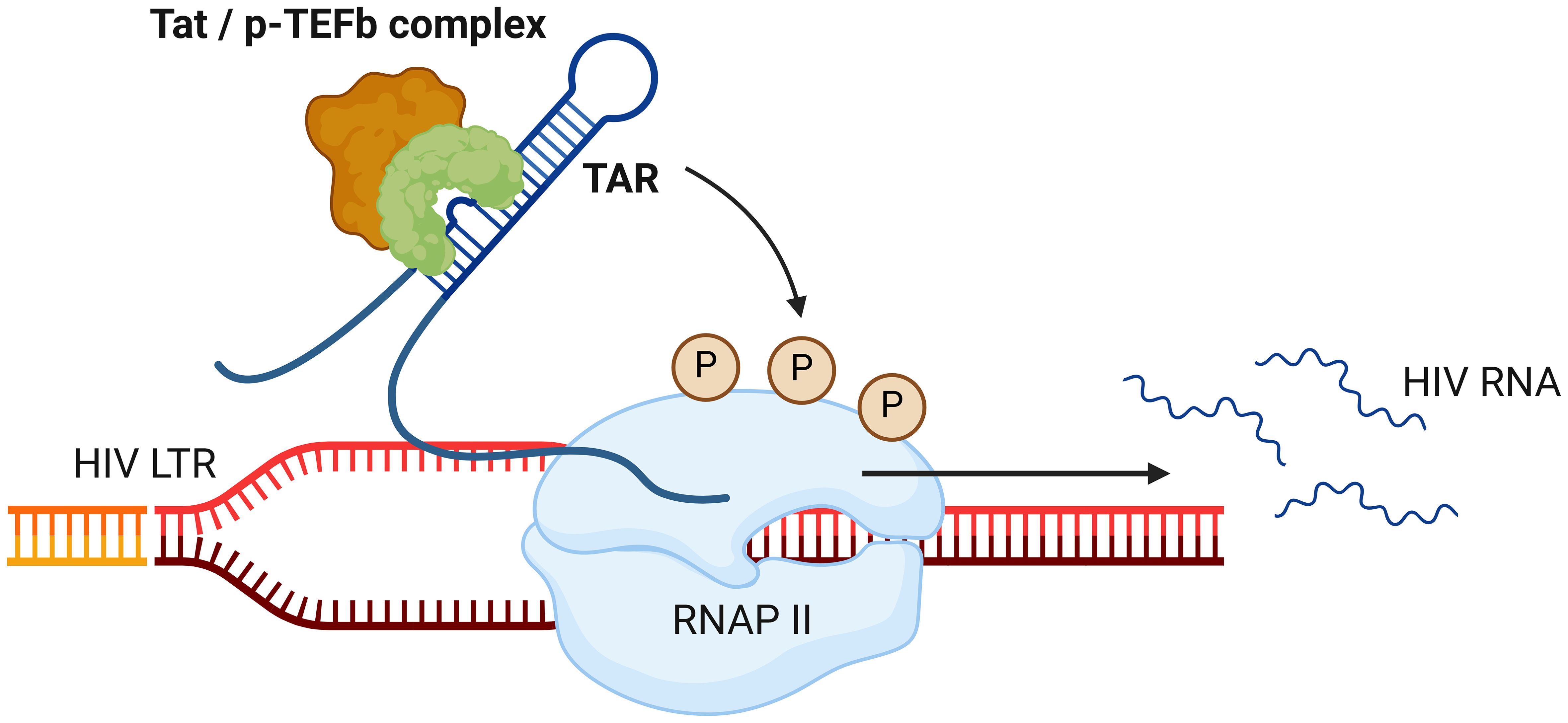
Figure 1. Tat-mediated enhancement of RNA polymerase II processivity. Following its translation, the HIV transactivator of transcription (Tat) protein forms a complex with positive transcription elongation factor b (p-TEFb) and binds to the transactivating-response (TAR) element within the early HIV transcript. This causes the hyperphosphorylation (P) of RNA polymerase II (RNAP II), enhancing the transcription processivity of the enzyme. This process is essential for the production of full-length unspliced HIV RNA; and multiply-spliced HIV RNA and virion production thereafter.
Mechanisms of Tat secretion and uptake
Tat secretion occurs via an unconventional secretion pathway (43), which is initiated by binding of the protein to phosphatidylinositol-4,5-bisphosphate (PtdIns(4,5)P2), a phospholipid component of the inner leaflet of the plasma membrane (Figure 2) (44). These interactions are mediated by the basic domain of Tat and the conserved Trp11 within the first coding exon. Trp11 inserts into the plasma membrane as a pre-requisite for secretion (45).
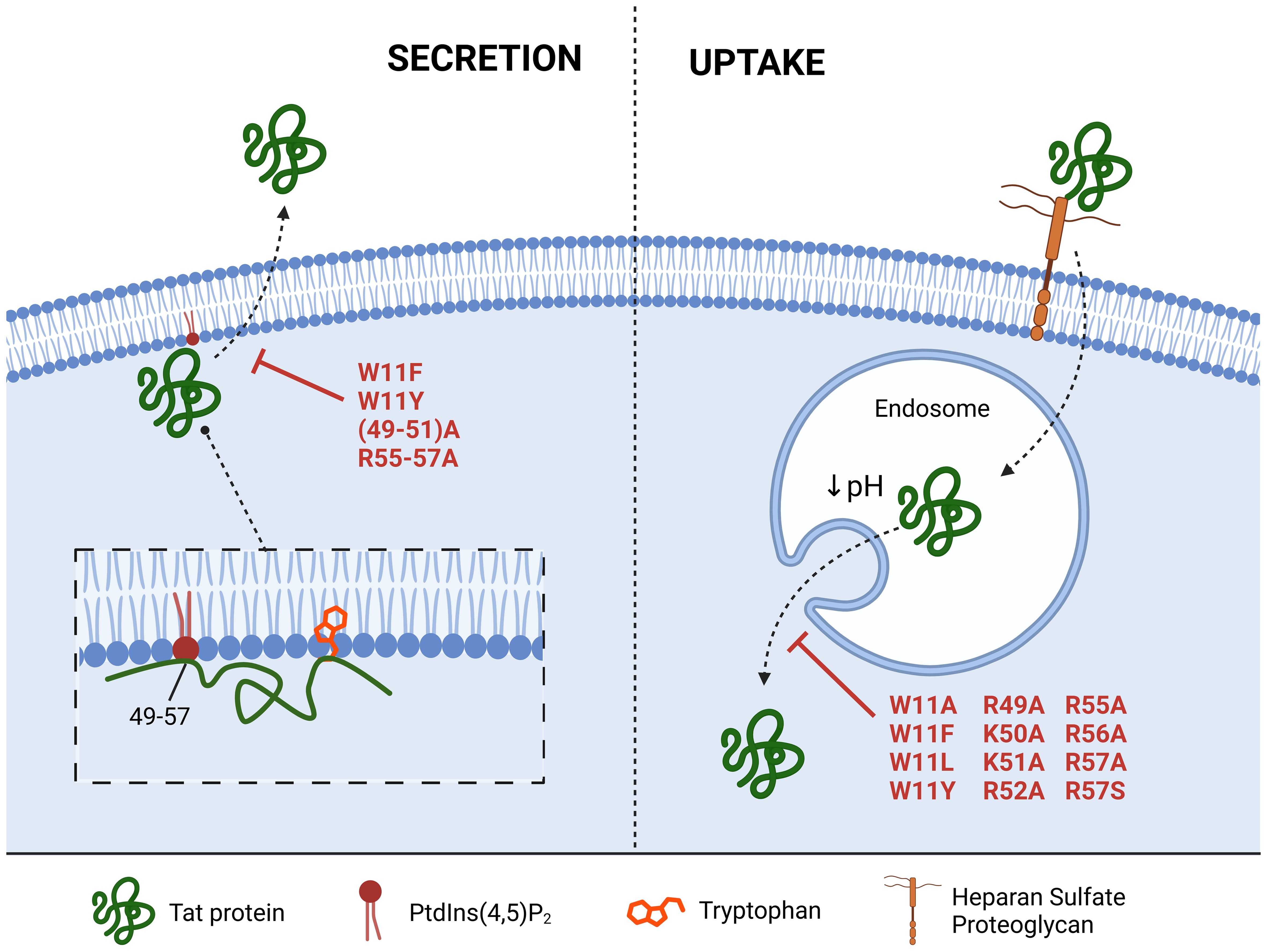
Figure 2. Impact of mutations on Tat secretion and uptake. Tat secretion occurs through interactions between phosphatidylinositol-4,5-bisphosphate (PtdIns(4,5)P2) the Tat basic domain (residues 49-57), and a conserved tryptophan at position 11 (Trp11). Uptake occurs through the binding of Tat to heparan sulfate proteoglycans, followed by uptake into endosomes. Point and combinatorial substitution mutations in both the basic domain and Trp11 reduce secretion and uptake efficiency of Tat, albeit to different degrees.
Tat also contains a protein transduction domain which allows it to penetrate cells from the extracellular environment (46). This mechanism of entry is so efficient that multiple groups have used this cell penetrating peptide sequence to deliver a large variety of cargo to a cell, unrelated to HIV (47–49). Indeed, purified Tat protein is able to transactivate the HIV LTR when added to the extracellular environment, highlighting its ability to cross both the plasma and nuclear membranes (50, 51). This internalisation is dependent on Tat binding to heparan sulfate proteoglycans (HSPGs) on the cell surface, resulting in uptake into endosomes that gradually acidify, leading to protein release (52). The conserved Trp11 residue also plays an essential role in Tat release from the endosome during the engulfment of extracellular Tat (53).
Tat secretion and systemic distribution in vivo
Tat can be secreted from cells, potentially resulting in its dissemination throughout the circulation to multiple cell types and tissues (43, 44, 54). Indeed, secreted Tat has been detected in the cerebrospinal fluid (55, 56) and sera (57–61) of PWH, even in individuals on effective ART with undetectable viral loads.
Tat expression in the central nervous system (CNS) could be from HIV DNA+ cells in the brain, or via the trafficking of Tat protein from the periphery across the blood brain barrier (62). In mice where modifications resulted in Tat being secreted from β cells of the pancreas, Tat was able to distribute widely to the brain, thymus, spleen, heart, lung, kidney, liver and pancreas as well as resting CD4+ T cells (63). Therefore, systemic effects of Tat need to be considered and minimised when using Tat as an LRA.
Tat-related toxicities
Many studies have shown differential expression of genes and proteins in cells after Tat treatment [reviewed in (64)]. Tat is known to modulate the expression of multiple cellular genes including interleukin (IL)-6, tumour necrosis factor (TNF) β, interferon regulatory factor (TRF) 7, IL-2 and cluster of differentiation (CD) 69 by binding to TAR-like sequences and promoter regions or interacting with host transcription factors (65–69). Other studies have shown that expression of HIV Tat can upregulate the expression of human endogenous retroviruses (70–72).
Tat concentrations as low as 10-15 ng/mL in the sera produce significant biological effects such as DNA damage in B cells, contributing to an increased risk of Burkitt’s lymphoma in PWH (57). The presence of Tat can induce dysfunction in microglia, CD4+ T cells, astrocytes, neurons and cardiomyocytes following cellular uptake (73–76). Tat can also act as a chemokine, attracting monocytes and macrophages into areas of productive HIV infection, resulting in localized inflammation (77). The chronic persistence of Tat in the CNS is important as Tat can contribute to the development of HIV-associated neurocognitive disorder [reviewed in (78, 79)].
Tat as a potent, HIV-specific latency reversing agent
Initial studies using transfection of a Tat expression plasmid resulted in weak viral reactivation in a latently infected cell line (80). It was later shown that cells cultured in the presence of purified recombinant Tat protein were unable to establish latency, suggesting that exogenous Tat can enter the nucleus from the extracellular environment to help drive productive infection (50). Since Tat’s activity relies on the expression of the HIV TAR element (37), this approach would likely have a higher degree of HIV-specificity compared to other LRAs which typically target host transcriptional pathways. Given Tat’s important role in overcoming blocks to transcription elongation, it has been hypothesized that providing Tat in trans could result in efficient HIV reactivation, and thus Tat may represent a highly potent and specific latency reversing agent (42).
Recombinant Tat protein
Recent work demonstrated that a truncated Tat variant comprising 66 amino acids (T66) was found to have comparable transactivation activity to both Tat72 and Tat86 in HEK293T cells expressing LTR-driven reporter plasmids (51). T66 also induced HIV RNA expression in an in vitro model of HIV infection and in CD4+ T cells from PWH on ART, similar to positive controls that activated the T-cell, including Phorbol myristate acetate (PMA), phytohemagglutinin (PHA) and anti-CD3/anti-CD28 stimulation (51). Furthermore, T66 protein in CD4+ T cells from PWH on ART did not significantly induce global cellular activation as seen with PHA (51). Only eight genes were identified as being differentially expressed following T66 treatment compared to non-stimulated controls, indicating minimal perturbation to the host cell transcriptome (51).
The ability of T66 protein to work equal to - or better than - the current gold-standards for latency reversal in vitro with few off-target effects highlights its promise as an LRA candidate. However, administering exogenous purified Tat protein in humans is likely problematic due to limited in vivo stability and the concerns of toxicities relating to systemic Tat protein (81). The successful application of Tat as a next-generation LRA will require an efficient delivery mechanism.
Exosomes containing Tat protein
The field of nanotechnology may play an important role in addressing challenges related to efficient Tat delivery ex vivo and in vivo. The first approach describing nanoparticles for the delivery of Tat as an LRA utilized Tat101 protein encapsulated into exosomes (EXO-Tat) (82). Measuring 30 to 150 nm in diameter, exosomes are the smallest type of extracellular vesicle and can be used for various therapeutic purposes due to their ability to encapsulate complex protein, lipid and nucleic acid cargoes (83). EXO-Tat increased unspliced HIV RNA ex vivo in CD4+ T cells from PWH on ART, but at a lower level than T-cell activation using PMA/ionomycin (82). EXO-Tat also upregulated multiply-spliced HIV RNA in 80% of donors, with potency exceeding that of current-generation LRAs panabinostat (30 nM) and disulfiram (500 nM) (82). Treatment with EXO-Tat in combination with either one of these LRAs further increased HIV RNA by 30-fold compared to EXO-Tat alone (82). HIV protein production was also observed in 50% of donors tested, even though all donors expressed p24 following PMA/ionomycin stimulation (82). However, treatment with EXO-Tat resulted in significant perturbations in the host cell proteome, upregulating over 30% of identified cellular proteins associated with translational machinery and metabolic pathways (84). EXO-Tat was also linked to upregulation of proteins associated with oxidative stress and apoptosis (84). These findings agree with multiple other studies showing differential expression of genes and proteins in various cell lines after Tat treatment (85–88). This calls into question the suitability of utilizing EXO-Tat as an LRA.
Lipid nanoparticles encapsulating mRNA encoding Tat
More recently, lipid nanoparticles (LNPs) encapsulating messenger mRNA (mRNA) encoding Tat have been developed. LNPs encapsulating mRNA encoding the T66 protein (T66-LNPs) could upregulate multiply-spliced HIV RNA and protein production in latently infected CD4+ T cells ex vivo (median 188- and 185-fold increase compared to untreated, respectively), with potency similar to that of PMA stimulation (51). T66-LNPs also induced p24 expression, similar to PMA treatment (51). T66-LNPs synergized with classical LRAs including the second mitochondrial-derived activator of caspases (SMAC) mimetic AZD5582 and the histone deacetylase inhibitors panabinostat and vorinostat, leading to a significant increased median fold change in TAR, elongated LTR, polyadenylated and tat-rev transcripts compared to each drug alone (89, 90). Synergistic effects on p24 production were also observed (90). Such findings are important given that it is probable that not all proviruses will be responsive to Tat alone in vivo due to the various cellular factors that maintain latency. However, the continued use of classical LRAs negates the benefits of using an HIV-specific LRA.
Considerations and mitigations strategies pertaining to Tat as an LRA
These studies demonstrate promise for the use of Tat as an LRA, given Tat’s ability to potently increase HIV transcription, upregulate expression of multiply-spliced HIV RNA, and act through an HIV-specific mechanism. However, there remain significant challenges to the use of Tat as an LRA (summarized in Figure 3) relating to the toxicity of Tat, effective delivery of Tat to the latent reservoir, and reservoir diversity impacting Tat-mediated latency reversal and cell death.
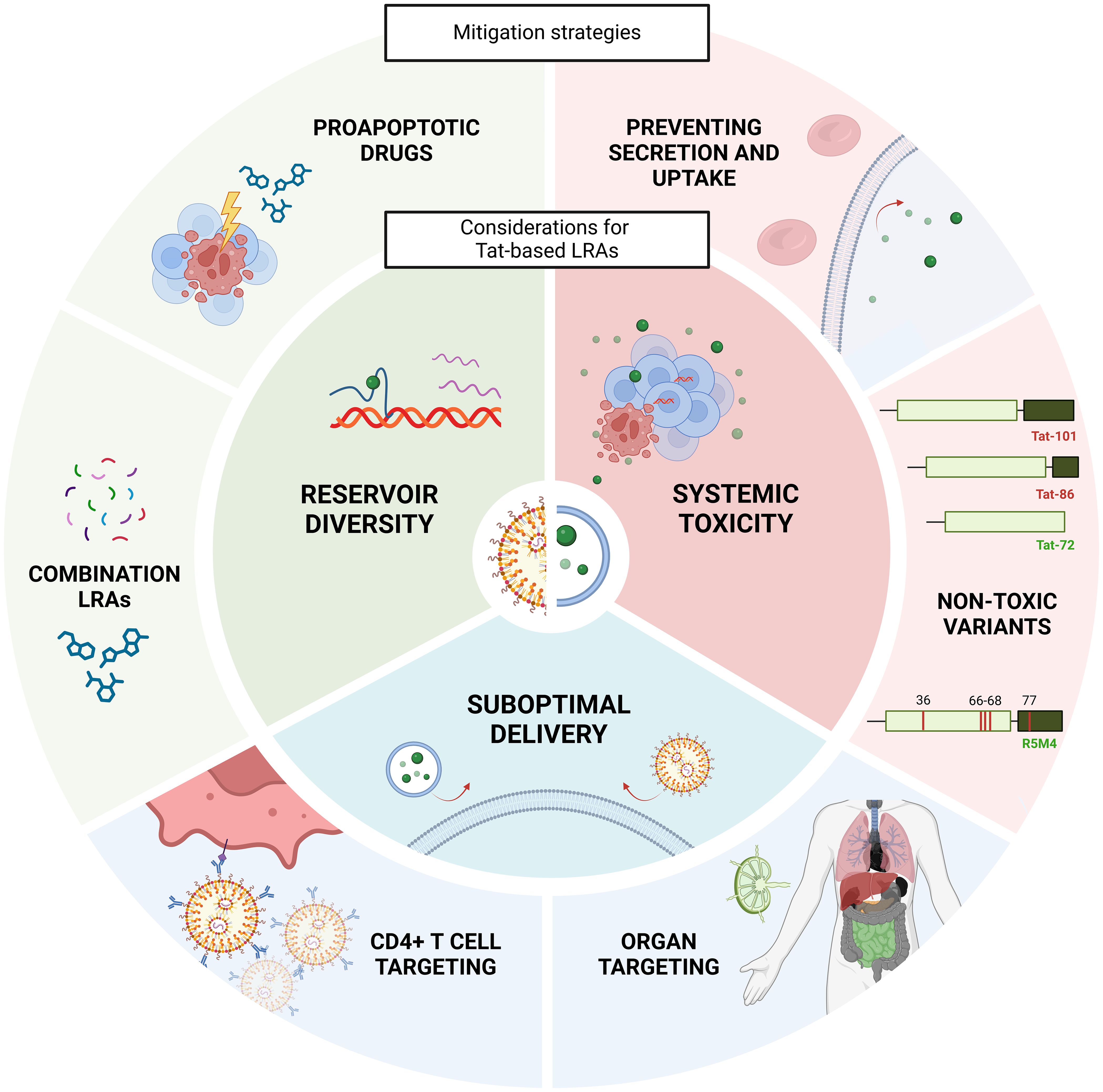
Figure 3. Considerations for the development of Tat-based LRAs. There are multiple potential challenges to the development of Tat-based therapeutics, including systemic toxicities in vivo, suboptimal delivery of Tat to latently infected cells, and reservoir diversity, rendering a proportion of proviruses unresponsive to Tat treatment (inner circle). These could be mitigated in several ways (outer circle) including preventing Tat secretion and uptake, utilizing a non-toxic Tat variant, targeting Tat delivery vehicles to CD4+ T cells and anatomical sites of the HIV reservoir; and combining Tat with other HIV-specific latency reversing agents (LRAs) or pro-apoptotic drugs to drive selective killing of HIV DNA+ cells.
Systemic toxicities may occur after Tat administration
Targeting Tat to CD4+ T cells could potentially minimize Tat-related toxicities, by reducing Tat expression in cells not infected with HIV and reducing circulating systemic levels of Tat. However, direct delivery to T cells may not mitigate the secretion of Tat. For example, using exosomes to deliver Tat protein may inadvertently enhance Tat secretion. Using a cell culture method of producing EXO-Tat, Tat-expressing HEK293T cells released exosomes containing Tat which were then captured for downstream experiments (82). To effectively produce EXO-Tat via this method, the protein needed to be modified to ensure that once expressed, it was targeted towards the intracellular membrane compartment (82). The result was a Tat mutant that favored its own secretion into exosomes, which could then disseminate in vivo. Secretion of unmodified Tat protein could also occur after Tat-LNP treatment, and therefore strategies will be needed to minimize secretion through site directed mutation.
Various mutational studies have identified specific residues of the protein that can prevent Tat secretion and mitigate off-target effects (Figure 2). For example, replacing RKK at positions 49-51 with alanine reduced secretion of Tat to 1% of wildtype (44), but in turn impacted transactivation due to the essential role of these basic residues in TAR-binding (45). Similar mutations on the C-terminal end of the basic domain (55-57A) reduced secretion to 30% of wild type (45). Altering Trp11 also impacted the stability of interactions between Tat and PtdIns(4,5)P2 (91). For example, replacing Trp11 with phenylalanine or tyrosine prevented Tat secretion from Jurkat cells by 80% compared to wildtype (44).
Point mutations within the Tat basic region and Trp11 have also been employed to prevent Tat uptake by bystander cells. Tat mutations at W11A, W11F, W11L and W11Y decreased transactivation capacity when added to the extracellular environment of Jurkat cells, indicating a reduced ability to reach the cytoplasm (53). Furthermore, changing even a single lysine or arginine to alanine within the basic domain reduced Tat uptake as a cell penetrating peptide (92). Tat sequence and function can also vary among HIV subtypes, including effects on uptake. For example, Tat subtype C has a naturally occurring polymorphism, R57S, in its basic domain, which led to a 70% reduction in uptake compared to Tat subtype B (45).
The length of Tat also has varying effects on toxicity. Tat72 lacks the 73RGD75 motif that permits transmigration across the blood brain barrier (93), potentially limiting its dissemination to the CNS in vivo. To reduce Tat-related cytotoxicity and immunogenicity, it is also possible to alter the protein domains associated with such toxicities. Firstly, the R57S substitution reduced the induction of proinflammatory cytokine genes TNFα, IL-6, IL-8, IL-1β and chemokine (C-X-C) motif ligand 1 (CXCL1) in response to reduced efficiency of uptake (45). Secondly, an engineered form of Tat86, R5M4, with point mutations V36A, Q66A, V67A, S68A and S77A reduced both total cell toxicity and the ability to induce inflammatory cytokine production while having no effect on transactivation potency (94). When injected intravenously into wildtype BALB/c mice at 40 mg/kg, Tat-R5M4 caused no change in liver and kidney function (94). It may therefore be advantageous to incorporate these mutations when using Tat as an LRA.
In summary, there are many residues that have been identified that could be mutated to prevent the secretion and uptake of Tat and its ensuing toxicities. However, it will be key to ensure that any mutations made will not impact the transactivation capacity of the protein, ensuring potency is maintained whilst minimizing toxicities.
Suboptimal delivery of Tat to the latent reservoir
A common limitation in exosome and LNP delivery systems is that they are unable to efficiently deliver cargo to resting CD4+ T cells. For example, EXO-Tat could only successfully transfect 13% of resting CD4+ T cells in an in vitro model of infection (82). Therefore, additional modifications will likely be required to deliver nanoparticles to latently infected CD4+ T cells in circulation and tissue.
The major tissue distribution of systemically administered exosomes in mice include the liver, spleen, kidney, lung and gastrointestinal tract, all of which can be altered by various factors such as the cellular origin of the exosomes, the exosomal membrane composition (eg. protein, lipids and glycan), and the pathophysiological conditions of the host (95–101). Furthermore, once exosomes are administered, they are rapidly engulfed by circulating phagocytic cells, which could further impede efficient delivery of Tat to the HIV reservoir in vivo (102).
LNPs can also rapidly accumulate in the liver after administration, thereby reducing their potency in vivo (103). Studies of T66-LNPs have not reported details of the lipid components of the proprietary LNP nor the resulting transfection efficiency in CD4+ T cells (51, 89, 90). However, others have demonstrated poor transfection efficiencies of CD4+ T cell with non-targeted LNP formulations (104–106). Indeed, to ensure successful transfection, activation of the T cells appears to be a pre-requisite (107). As T cell activation should be avoided as an LRA strategy, some form of targeting is likely to be required to ensure effective protein delivery to resting CD4+ T cells.
Targeting Tat to latently infected CD4+ T cells
The targeting of nanomaterials towards particular cells can be achieved via two routes: passive targeting or active targeting. The first approach relies on the physicochemical properties of the LNP, including lipid composition as well as the preparation method, size and surface charge which can alter in vivo biodistribution (108–110). Active targeting instead involves the addition of specific ligands or antibodies to the nanoparticle surface, which can bind to receptors expressed by target tissues or cells, ensuring precise delivery (111–113).
The ability of CD4+ T cells to undergo receptor-mediated endocytosis, even in a resting state, highlights a potential role for active targeting to ensure efficient Tat uptake. To improve protein delivery of EXO-Tat to resting CD4+ T cells, the C terminus of IL-16, the natural ligand for the CD4 receptor, was conjugated to the extracellular domain of the exosomal protein Lamp2b (82). This approach improved Tat protein expression within resting CD4+ T cells ex vivo by 20-fold compared to unmodified EXO-Tat, and induced p24 production in all donors compared to only 50% of donors when using unmodified EXO-Tat, suggesting a previous block to efficient reactivation was due to inefficient Tat protein delivery (82).
Similar targeting approaches have also been utilized by groups employing LNPs for delivery of mRNA to CD4+ T cells. CD4-targeted LNPs, using CD4 antibodies, resulted in a 30-fold higher signal of the reporter mRNA in CD4+ T cells isolated from the spleen in mice, compared to non-targeted LNPs (106). Intravenous injection of the CD4-targeted LNPs into mice resulted in mRNA delivery to 60% and 40% of CD4+ T cells in the spleen and lymph nodes, respectively (106). However, as CD4 is a surface receptor that is not internalised, it is possible that other receptors expressed on the cell surface, such as CD7, transferrin receptor, CD90 and IL-2R may make better candidates for LNP internalisation in T cells (114). CD3 has also been targeted previously to efficiently deliver reporter mRNA to T cells in vitro and in mice, however, this was associated with complex immunological consequences and therefore not suitable for therapeutic application in humans (104).
Targeting Tat to anatomical sites of the latent reservoir
The other approach to optimize Tat as an LRA is to enhance delivery to key tissues, where the majority of latently infected cells reside in PWH on ART, such as lymph nodes or the gastrointestinal tract (115). LNP targeting to peripheral lymph nodes has been extensively explored in different contexts related to vaccination and therapeutic delivery. For example, to optimize uptake of a vaccine in lymph nodes, the chemical structure of the lipids, charge and size have been altered (116), to allow for the most efficient trafficking after subcutaneous injection (117). This route of administration is less relevant for HIV cure, which relies on widespread dissemination of treatment to all lymph nodes. Instead, conjugation of an antibody that binds to a high endothelial venule marker (MECA-79) which recognizes peripheral lymph nodes addressin on the high endothelial venules of lymph nodes, led to active targeting of microparticles to the lymph node after intravenous administration (118). This strategy could potentially be adapted for the delivery of LNPs to the HIV reservoir.
Targeting the gut-associated lymphoid tissue (GALT) with nanoparticles has also been achieved in a variety of ways, including through the use of lipid-polymer nanoparticle hybrids to enhance the association to the Peyer’s patches (119). Unlike in lymph node targeting strategies, most approaches for enhancing GALT delivery rely on oral administration, which is complicated by physiological barriers of the digestive system resulting in nanoparticle degradation or excretion (120).
LNP transport to the CNS has been shown to be even more difficult due to the high-resistance tight junctions within the brain capillaries that restrict brain uptake of small molecules from the periphery (121). Although exosomes have been shown to cross the blood brain barrier from the circulation (122), additional modifications were needed to deliver LNPs to the CNS. These have included intracerebroventricular or intracerebral injection of the LNPs (123, 124) or the use of antibody-conjugated LNPs to ensure that LNPs traverse the endothelial cells lining blood vessels in the brain, rather than simply transfecting the endothelial cells themselves (125). Alternatively, the lack of CNS penetration by LNPs may be beneficial to a Tat-based LRA, avoiding Tat expression and viral reactivation in the brain whilst achieving both in the periphery.
Although Tat is known to be a highly specific and potent reactivator, further targeting it to only the cells or tissues in which it needs to be expressed will further improve HIV-specificity and potency. However, given the large number of requirements for both T cell and organ targeting, it is unlikely that one LNP formulation alone will be able to simultaneously target mRNA encoding Tat to all of the required sites in vivo. Rather, a single formulation which combines various passive and active targeting approaches, or multiple doses of various Tat-LNP formulations, may be advantageous in ensuring widespread HIV reactivation.
Reservoir diversity and its impact on Tat efficacy
The HIV reservoir is highly heterogeneous with the vast majority of proviruses defective, harboring large internal deletions, hypermutations or inversions (126, 127). Furthermore, not all intact proviruses appear to be capable of reactivating (127). HIV cure efforts must therefore be focused on eliminating intact and inducible proviruses as these are the source of viral rebound. Indeed, only 0.1-10 CD4+ T cells per million are estimated to harbor intact and inducible proviruses (128, 129). A further challenge is that infected cells can undergo clonal expansion through homeostatic proliferation or antigen stimulated proliferation, and different clones display diverse responsiveness to T-cell activation (130). Whether there is a need to target all intact proviruses to effectively reduce the rebound-competent reservoir remains unclear, however, recent data suggests that after many years of suppressive ART, there are fewer proviruses capable of reactivation (131).
At a minimum, an LRA must be able to sufficiently reactivate an infected cell that harbors intact proviruses and can be induced to reactivate, and therefore capable of recrudescence following cessation of ART. The ability of the T66-LNP to increase multiply-spliced HIV RNA to higher levels than PMA/ionomycin in CD4+ T cells from PWH on ART is highly encouraging (51). However, it will be important to also understand if activation of virus expression occurred in all infected cells or from a subset of infected cells. This could be addressed using the tat-rev induced limiting dilution assay (TILDA) or quantitative viral outgrowth assay (qVOA), to measure the frequency of cells that can be induced to express multiply-spliced HIV RNA or virions, respectively.
Although the HIV reservoir was typically thought as being transcriptionally silent, cell associated HIV RNA is almost always able to be detected in PWH on ART (17, 18, 132–135), and HIV protein can be occasionally detected (136, 137). As Tat primarily functions by binding to TAR, we hypothesize that this new LRA will be effective in cells that are already transcriptionally active, thereby expressing the TAR stem loop. Consistent with this hypothesis, T66-LNP ex vivo led to no increase in TAR expression, yet potent increase in multiply-spliced HIV RNA (51, 90). More recent studies have shown that only a minority of HIV DNA+ cells express cell associated HIV RNA and this reduces over time on ART with an increased number of proviruses detected in non-genic regions (131, 138, 139). This suggests that Tat as an LRA might be best combined with another LRA that can efficiently initiate HIV transcription, as demonstrated recently using a combination of conventional LRAs and T66-LNP (90).
A further challenge in using latency reversal to reduce the reservoir is that latently infected cells express pro-survival proteins and are resistant to cytotoxic T-cell killing (140–142). Indeed, no studies exploring the efficacy of Tat as an LRA to date have yet investigated whether potent latency reversal by Tat can reduce the size of the HIV reservoir. Studies exploring the use of proapoptotic drugs have shown promise in their ability to selectively kill HIV DNA+ cells (143, 144). Therefore, combining a Tat LRA with a pro-apoptotic drug may also be needed.
Summary and conclusion
The HIV Tat protein is a promising novel agent for the reversal of HIV latency ex vivo. Despite clear advantages over classical LRAs, including a demonstrated ability to potently upregulate multiply-spliced HIV RNA and protein production, and an HIV-specific mechanism of action, several challenges remain in the translation of HIV Tat into a clinically applicable LRA. The use of Tat variants that alter the domains mediating cellular secretion and uptake show potential to avoid inflammation and widespread toxicities in various tissues. Whilst the use of nanoparticles has greatly reduced the concentration of extracellular Tat, methods of efficiently targeting these nanocarriers to CD4+ T cells and diverse tissues which harbour infected cells will need to be developed. Finally, future studies will need to determine whether Tat can reactivate the transcriptionally silent reservoir and whether this is necessary to induce viral remission off-ART.
Author contributions
BF: Conceptualization, Writing – original draft, Writing – review & editing. PC: Conceptualization, Writing – original draft, Writing – review & editing. MR: Conceptualization, Writing – original draft, Writing – review & editing. SL: Conceptualization, Funding acquisition, Writing – original draft, Writing – review & editing.
Funding
The author(s) declare that financial support was received for the research and/or publication of this article. SL is supported by a National Institute of Allergy and Infectious Disease of the National Institutes of Health award (UM1AI164560). SL is supported by grants from the NHMRC including a program grant (APP1149990), practitioner fellowship (APP1135851) and investigator grant (APP2026490). BF is the recipient of an Australian Government Research Training Program Scholarship.
Acknowledgments
All figures were made using Biorender.com.
Conflict of interest
BF, PC, MR and SL are named investigators on a patent related to this work.
The remaining authors declare that the research was conducted in the absence of any commercial or financial relationships that could be construed as a potential conflict of interest.
Generative AI statement
The author(s) declare that no Generative AI was used in the creation of this manuscript.
Publisher’s note
All claims expressed in this article are solely those of the authors and do not necessarily represent those of their affiliated organizations, or those of the publisher, the editors and the reviewers. Any product that may be evaluated in this article, or claim that may be made by its manufacturer, is not guaranteed or endorsed by the publisher.
References
1. Bukrinsky MI, Stanwick TL, Dempsey MP, Stevenson M. Quiescent T lymphocytes as an inducible virus reservoir in HIV-1 infection. Science. (1991) 254:423–7. doi: 10.1126/science.1925601
2. Chun TW, Stuyver L, Mizell SB, Ehler LA, Mican JAM, Baseler M, et al. Presence of an inducible HIV-1 latent reservoir during highly active antiretroviral therapy. Proc Natl Acad Sci United States America. (1997) 94:13193–7. doi: 10.1073/pnas.94.24.13193
3. Finzi D, Hermankova M, Pierson T, Carruth LM, Buck C, Chaisson RE, et al. Identification of a reservoir for HIV-1 in patients on highly active antiretroviral therapy. Science. (1997) 278:1295–300. doi: 10.1126/science.278.5341.1295
4. Deeks SG, Archin N, Cannon P, Collins S, Jones RB, de Jong M, et al. Research priorities for an HIV cure: International AIDS Society Global Scientific Strategy 2021. Nat Med. (2021) 27:2085–98. doi: 10.1038/s41591-021-01590-5
5. Zerbato JM, Purves HV, Lewin SR, Rasmussen TA. Between a shock and a hard place: challenges and developments in HIV latency reversal. Curr Opin Virol. (2019) 38:1–9. doi: 10.1016/j.coviro.2019.03.004
6. Darcis G, Kula A, Bouchat S, Fujinaga K, Corazza F, Ait-Ammar A, et al. An in-depth comparison of latency-reversing agent combinations in various in vitro and ex vivo HIV-1 latency models identified bryostatin-1+JQ1 and ingenol-B+JQ1 to potently reactivate viral gene expression. PloS Pathog. (2015) 11:e1005063. doi: 10.1371/journal.ppat.1005063
7. Huang H, Liu S, Jean M, Simpson S, Huang H, Merkley M, et al. A novel bromodomain inhibitor reverses HIV-1 latency through specific binding with BRD4 to promote tat and P-TEFb association. Front Microbiol. (2017) 8:1035. doi: 10.3389/fmicb.2017.01035
8. Laird GM, Bullen CK, Rosenbloom DI, Martin AR, Hill AL, Durand CM, et al. Ex vivo analysis identifies effective HIV-1 latency-reversing drug combinations. J Clin Invest. (2015) 125:1901–12. doi: 10.1172/JCI80142
9. Nixon CC, Mavigner M, Sampey GC, Brooks AD, Spagnuolo RA, Irlbeck DM, et al. Systemic HIV and SIV latency reversal via non-canonical NF-kappaB signalling in vivo. Nature. (2020) 578:160–5. doi: 10.1038/s41586-020-1951-3
10. Spivak AM, Bosque A, Balch AH, Smyth D, Martins L, Planelles V. Ex vivo bioactivity and HIV-1 latency reversal by ingenol dibenzoate and panobinostat in resting CD4(+) T cells from aviremic patients. Antimicrob Agents Chemother. (2015) 59:5984–91. doi: 10.1128/AAC.01077-15
11. Debrabander Q, Hensley KS, Psomas CK, Bramer W, Mahmoudi T, van Welzen BJ, et al. The efficacy and tolerability of latency-reversing agents in reactivating the HIV-1 reservoir in clinical studies: a systematic review. J Virus Eradication. (2023) 9:100342–2. doi: 10.1016/j.jve.2023.100342
12. Archin NM, Bateson R, Tripathy MK, Crooks AM, Yang KH, Dahl NP, et al. HIV-1 expression within resting CD4+ T cells after multiple doses of vorinostat. J Infect Dis. (2014) 210:728–35. doi: 10.1093/infdis/jiu155
13. Elliott JH, McMahon JH, Chang CC, Lee SA, Hartogensis W, Bumpus N, et al. Short-term administration of disulfiram for reversal of latent HIV infection: a phase 2 dose-escalation study. Lancet HIV. (2015) 2:e520–529. doi: 10.1016/S2352-3018(15)00226-X
14. Elliott JH, Wightman F, Solomon A, Ghneim K, Ahlers J, Cameron MJ, et al. Activation of HIV transcription with short-course vorinostat in HIV-Infected patients on suppressive antiretroviral therapy. PloS Pathog. (2014) 10:e1004473. doi: 10.1371/journal.ppat.1004473
15. Bullen CK, Laird GM, Durand CM, Siliciano JD, Siliciano RF. New ex vivo approaches distinguish effective and ineffective single agents for reversing HIV-1 latency in vivo. Nat Med. (2014) 20:425–9. doi: 10.1038/nm.3489
16. Khoury G, Mota TM, Li S, Tumpach C, Lee MY, Jacobson J, et al. HIV latency reversing agents act through Tat post translational modifications. Retrovirology. (2018) 15:36. doi: 10.1186/s12977-018-0421-6
17. Yukl SA, Kaiser P, Kim P, Telwatte S, Joshi SK, Vu M, et al. HIV latency in isolated patient CD4+ T cells may be due to blocks in HIV transcriptional elongation, completion, and splicing. Sci Trans Med. (2018) 10:eaap9927. doi: 10.1126/scitranslmed.aap9927
18. Zerbato JM, Khoury G, Zhao W, Gartner MJ, Pascoe RD, Rhodes A, et al. Multiply spliced HIV RNA is a predictive measure of virus production ex vivo and in vivo following reversal of HIV latency. EBioMedicine. (2021) 65:103241. doi: 10.1016/j.ebiom.2021.103241
19. Jones RB, O'Connor R, Mueller S, Foley M, Szeto GL, Karel D, et al. Histone deacetylase inhibitors impair the elimination of HIV-infected cells by cytotoxic T-lymphocytes. PloS Pathog. (2014) 10:e1004287. doi: 10.1371/journal.ppat.1004287
20. Wong DJL, Rao A, Avramis E, Matsunaga DR, Komatsubara KM, Atefi MS, et al. Exposure to a histone deacetylase inhibitor has detrimental effects on human lymphocyte viability and function. Cancer Immunol Res. (2014) 2:459–68. doi: 10.1158/2326-6066.CIR-13-0188
21. Lewin SR, Attoye T, Bansbach C, Doehle B, Dubé K, Dybul M, et al. Multi-stakeholder consensus on a target product profile for an HIV cure. Lancet HIV. (2021) 8:e42–50. doi: 10.1016/S2352-3018(20)30234-4
22. Lewin SR, Bansbach C, Kemps D, Mathae L, Das KT, McCune JM, et al. Target product profile for cell-based and gene-based therapies to achieve a cure for HIV. Lancet HIV. (2025) 12:E154–162. doi: 10.1016/S2352-3018(24)00277-7
23. Ratner L, Haseltine W, Patarca R, Livak KJ, Starcich B, Josephs SF, et al. Complete nucleotide sequence of the AIDS virus, HTLV-III. Nature. (1985) 313:277–84. doi: 10.1038/313277a0
24. Lopez-Huertas MR, Callejas S, Abia D, Mateos E, Dopazo A, Alcami J, et al. Modifications in host cell cytoskeleton structure and function mediated by intracellular HIV-1 Tat protein are greatly dependent on the second coding exon. Nucleic Acids Res. (2010) 38:3287–307. doi: 10.1093/nar/gkq037
25. van der Kuyl AC, Vink M, Zorgdrager F, Bakker M, Wymant C, Hall M, et al. The evolution of subtype B HIV-1 tat in the Netherlands during 1985-2012. Virus Res. (2018) 250:51–64. doi: 10.1016/j.virusres.2018.04.008
26. Jeang KT, Xiao H, Rich EA. Multifaceted activities of the HIV-1 transactivator of transcription, Tat. J Biol Chem. (1999) 274:28837–40. doi: 10.1074/jbc.274.41.28837
27. Kuppuswamy M, Subramanian T, Srinivasan A, Chinnadurai G. Multiple functional domains of Tat, the trans-activator of HIV-1, defined by mutational analysis. Nucleic Acids Res. (1989) 17:3551–61. doi: 10.1093/nar/17.9.3551
28. Sadaie MR, Rappaport J, Benter T, Josephs SF, Willis R, Wong-Staal F. Missense mutations in an infectious human immunodeficiency viral genome: functional mapping of tat and identification of the rev splice acceptor. Proc Natl Acad Sci U S A. (1988) 85:9224–8. doi: 10.1073/pnas.85.23.9224
29. Verhoef K, Koper M, Berkhout B. Determination of the minimal amount of Tat activity required for human immunodeficiency virus type 1 replication. Virology. (1997) 237:228–36. doi: 10.1006/viro.1997.8786
30. Hauber J, Malim MH, Cullen BR. Mutational analysis of the conserved basic domain of human immunodeficiency virus tat protein. J Virol. (1989) 63:1181–7. doi: 10.1128/jvi.63.3.1181-1187.1989
31. Campbell GR, Pasquier E, Watkins J, Bourgarel-Rey V, Peyrot V, Esquieu D, et al. The glutamine-rich region of the HIV-1 Tat protein is involved in T-cell apoptosis. J Biol Chem. (2004) 279:48197–204. doi: 10.1074/jbc.M406195200
32. Buscemi L, Ramonet D, Geiger JD. Human immunodeficiency virus type-1 protein Tat induces tumor necrosis factor-alpha-mediated neurotoxicity. Neurobiol Dis. (2007) 26:661–70. doi: 10.1016/j.nbd.2007.03.004
33. Mahlknecht U, Dichamp I, Varin A, Van Lint C, Herbein G. NF-kappaB-dependent control of HIV-1 transcription by the second coding exon of Tat in T cells. J Leukoc Biol. (2008) 83:718–27. doi: 10.1189/jlb.0607405
34. Neuveut C, Scoggins RM, Camerini D, Markham RB, Jeang KT. Requirement for the second coding exon of Tat in the optimal replication of macrophage-tropic HIV-1. J BioMed Sci. (2003) 10:651–60. doi: 10.1159/000073531
35. Barillari G, Gendelman R, Gallo RC, Ensoli B. The Tat protein of human immunodeficiency virus type 1, a growth factor for AIDS Kaposi sarcoma and cytokine-activated vascular cells, induces adhesion of the same cell types by using integrin receptors recognizing the RGD amino acid sequence. Proc Natl Acad Sci U.S.A. (1993) 90:7941–5. doi: 10.1073/pnas.90.17.7941
36. Kashanchi F, Wood C. Human immunodeficiency viral long terminal repeat is functional and can be trans-activated in Escherichia coli. Proc Natl Acad Sci United States America. (1989) 86:2157–61. doi: 10.1073/pnas.86.7.2157
37. Feng S, Holland EC. HIV-1 tat trans-activation requires the loop sequence within tar. Nature. (1988) 334:165–7. doi: 10.1038/334165a0
38. Zhou M, Halanski MA, Radonovich MF, Kashanchi F, Peng J, Price DH, et al. Tat modifies the activity of CDK9 to phosphorylate serine 5 of the RNA polymerase II carboxyl-terminal domain during human immunodeficiency virus type 1 transcription. Mol Cell Biol. (2000) 20:5077–86. doi: 10.1128/mcb.20.14.5077-5086.2000
39. Gomez-Rivera F, Terry VH, Chen C, Painter MM, Virgilio MC, Yaple-Maresh ME, et al. Variation in HIV-1 Tat activity is a key determinant in the establishment of latent infection. JCI Insight. (2024) 10:e184711. doi: 10.1172/jci.insight.184711
40. Tantale K, Garcia-Oliver E, Robert M-C, L'Hostis A, Yang Y, Tsanov N, et al. Stochastic pausing at latent HIV-1 promoters generates transcriptional bursting. Nat Commun. (2021) 12:4503. doi: 10.1038/s41467-021-24462-5
41. Weinberger LS, Burnett JC, Toettcher JE, Arkin AP, Schaffer DV. Stochastic gene expression in a lentiviral positive-feedback loop: HIV-1 Tat fluctuations drive phenotypic diversity. Cell. (2005) 122:169–82. doi: 10.1016/j.cell.2005.06.006
42. Razooky BS, Pai A, Aull K, Rouzine IM, Weinberger LS. A hardwired HIV latency program. Cell. (2015) 160:990–1001. doi: 10.1016/j.cell.2015.02.009
43. Rayne F, Debaisieux S, Bonhoure A, Beaumelle B. HIV-1 Tat is unconventionally secreted through the plasma membrane. Cell Biol Int. (2010) 34:409–13. doi: 10.1042/cbi20090376
44. Rayne F, Debaisieux S, Yezid H, Lin YL, Mettling C, Konate K, et al. Phosphatidylinositol-(4,5)-bisphosphate enables efficient secretion of HIV-1 Tat by infected T-cells. EMBO J. (2010) 29:1348–62. doi: 10.1038/emboj.2010.32
45. Ruiz AP, Ajasin DO, Ramasamy S, DesMarais V, Eugenin EA, Prasad VR. A naturally occurring polymorphism in the HIV-1 tat basic domain inhibits uptake by bystander cells and leads to reduced neuroinflammation. Sci Rep. (2019) 9:3308. doi: 10.1038/s41598-019-39531-5
46. Green M, Loewenstein PM. Autonomous functional domains of chemically synthesized human immunodeficiency virus tat trans-activator protein. Cell. (1988) 55:1179–88. doi: 10.1016/0092-8674(88)90262-0
47. Folini M, Bandiera R, Millo E, Gandellini P, Sozzi G, Gasparini P, et al. Photochemically enhanced delivery of a cell-penetrating peptide nucleic acid conjugate targeting human telomerase reverse transcriptase: Effects on telomere status and proliferative potential of human prostate cancer cells. Cell Proliferation. (2007) 40:905–20. doi: 10.1111/j.1365-2184.2007.00470.x
48. Rajagopalan R, Xavier J, Rangaraj N, Rao NM, Gopal V. Recombinant fusion proteins TAT-Mu, Mu and Mu-Mu mediate efficient non-viral gene delivery. J Gene Med. (2007) 9:275–86. doi: 10.1002/jgm.1014
49. Toro A, Grunebaum E. TAT-mediated intracellular delivery of purine nucleoside phosphorylase corrects its deficiency in mice. J Clin Invest. (2006) 116:2717–26. doi: 10.1172/JCI25052
50. Donahue DA, Kuhl B, Sloan RD, Wainberg MA. The viral protein tat can inhibit the establishment of HIV-1 latency. J Virol. (2012) 86:3253–63. doi: 10.1128/jvi.06648-11
51. Van Gulck E, Pardons M, Nijs E, Verheyen N, Dockx K, Van Den Eynde C, et al. A truncated HIV Tat demonstrates potent and specific latency reversal activity. Antimicrob Agents Chemother. (2023) 67:e0041723. doi: 10.1128/aac.00417-23
52. Tyagi M, Rusnati M, Presta M, Giacca M. Internalization of HIV-1 tat requires cell surface heparan sulfate proteoglycans. J Biol Chem. (2001) 276:3254–61. doi: 10.1074/jbc.M006701200
53. Yezid H, Konate K, Debaisieux S, Bonhoure A, Beaumelle B. Mechanism for HIV-1 tat insertion into the endosome membrane. J Biol Chem. (2009) 284:22736–46. doi: 10.1074/jbc.M109.023705
54. Ensoli B, Barillari G, Salahuddin SZ, Gallo RC, Wong-Staal F. Tat protein of HIV-1 stimulates growth of cells derived from Kaposi's sarcoma lesions of AIDS patients. Nature. (1990) 345:84–6. doi: 10.1038/345084a0
55. Henderson LJ, Johnson TP, Smith BR, Reoma LB, Santamaria UA, Bachani M, et al. Presence of Tat and transactivation response element in spinal fluid despite antiretroviral therapy. AIDS. (2019) 33:S145–57. doi: 10.1097/QAD.0000000000002268
56. Johnson TP, Patel K, Johnson KR, Maric D, Calabresi PA, Hasbun R, et al. Induction of IL-17 and nonclassical T-cell activation by HIV-Tat protein. Proc Natl Acad Sci U.S.A. (2013) 110:13588–93. doi: 10.1073/pnas.1308673110
57. Germini D, Tsfasman T, Klibi M, El-Amine R, Pichugin A, Iarovaia OV, et al. HIV Tat induces a prolonged MYC relocalization next to IGH in circulating B-cells. Leukemia. (2017) 31:2515–22. doi: 10.1038/leu.2017.106
58. Poggi A, Carosio R, Fenoglio D, Brenci S, Murdaca G, Setti M, et al. Migration of V delta 1 and V delta 2 T cells in response to CXCR3 and CXCR4 ligands in healthy donors and HIV-1-infected patients: competition by HIV-1 Tat. Blood. (2004) 103:2205–13. doi: 10.1182/blood-2003-08-2928
59. Shmakova A, Tsimailo I, Kozhevnikova Y, Gérard L, Boutboul D, Oksenhendler E, et al. HIV-1 Tat is present in the serum of people living with HIV-1 despite viral suppression. Int J Infect Dis. (2024) 142:106994. doi: 10.1016/j.ijid.2024.106994
60. Westendorp MO, Frank R, Ochsenbauer C, Stricker K, Dhein J, Walczak H, et al. Sensitization of T cells to CD95-mediated apoptosis by HIV-1 Tat and gp120. Nature. (1995) 375:497–500. doi: 10.1038/375497a0
61. Xiao H, Neuveut C, Tiffany HL, Benkirane M, Rich EA, Murphy PM, et al. Selective CXCR4 antagonism by Tat: Implications for in vivo expansion of coreceptor use by HIV-1. Proc Natl Acad Sci United States America. (2000) 97:11466–71. doi: 10.1073/pnas.97.21.11466
62. Banks WA, Robinson SM, Nath A. Permeability of the blood-brain barrier to HIV-1 Tat. Exp Neurol. (2005) 193:218–27. doi: 10.1016/j.expneurol.2004.11.019
63. Lin X, Irwin D, Kanazawa S, Huang L, Romeo J, Yen TSB, et al. Transcriptional profiles of latent human immunodeficiency virus in infected individuals: effects of tat on the host and reservoir. J Virol. (2003) 77:8227–36. doi: 10.1128/jvi.77.15.8227-8236.2003
64. Clark E, Nava B, Caputi M. Tat is a multifunctional viral protein that modulates cellular gene expression and functions. Oncotarget. (2017) 8:27569–81. doi: 10.18632/oncotarget.15174
65. Ambrosino C, Ruocco MR, Chen X, Mallardo M, Baudi F, Trematerra S, et al. HIV-1 Tat induces the expression of the interleukin-6 (IL6) gene by binding to the IL6 leader RNA and by interacting with CAAT enhancer-binding protein beta (NF-IL6) transcription factors. J Biol Chem. (1997) 272:14883–92. doi: 10.1074/jbc.272.23.14883
66. Buonaguro L, Buonaguro FM, Giraldo G, Ensoli B. The human immunodeficiency virus type 1 Tat protein transactivates tumor necrosis factor beta gene expression through a TAR-like structure. J Virol. (1994) 68:2677–82. doi: 10.1128/jvi.68.4.2677-2682.1994
67. Kim N, Kukkonen S, Martinez-Viedma MDP, Gupta S, Aldovini A. Tat engagement of p38 MAP kinase and IRF7 pathways leads to activation of interferon-stimulated genes in antigen-presenting cells. Blood. (2013) 121:4090–100. doi: 10.1182/blood-2012-10-461566
68. Kumar PP, Purbey PK, Ravi DS, Mitra D, Galande S. Displacement of SATB1-bound histone deacetylase 1 corepressor by the human immunodeficiency virus type 1 transactivator induces expression of interleukin-2 and its receptor in T cells. Mol Cell Biol. (2005) 25:1620–33. doi: 10.1128/mcb.25.5.1620-1633.2005
69. Reeder JE, Kwak YT, McNamara RP, Forst CV, D'Orso I. HIV Tat controls RNA Polymerase II and the epigenetic landscape to transcriptionally reprogram target immune cells. eLife. (2015) 4:e08955. doi: 10.7554/eLife.08955
70. Gonzalez-Hernandez MJ, Swanson MD, Contreras-Galindo R, Cookinham S, King SR, Noel RJ Jr., et al. Expression of human endogenous retrovirus type K (HML-2) is activated by the Tat protein of HIV-1. J Virol. (2012) 86:7790–805. doi: 10.1128/JVI.07215-11
71. Li X, Guo Y, Li H, Huang X, Pei Z, Wang X, et al. Infection by diverse HIV-1 subtypes leads to different elevations in HERV-K transcriptional levels in human T cell lines. Front Microbiol. (2021) 12:662573. doi: 10.3389/fmicb.2021.662573
72. Uleri E, Mei A, Mameli G, Poddighe L, Serra C, Dolei A. HIV Tat acts on endogenous retroviruses of the W family and this occurs via Toll-like receptor 4: inference for neuroAIDS. AIDS. (2014) 28:2659–70. doi: 10.1097/QAD.0000000000000477
73. Nicoli F, Gallerani E, Sforza F, Finessi V, Chachage M, Geldmacher C, et al. The HIV-1 Tat protein affects human CD4+ T-cell programing and activation, and favors the differentiation of naïve CD4+ T cells. AIDS. (2018) 32:575–81. doi: 10.1097/QAD.0000000000001734
74. Qrareya AN, Wise NS, Hodges ER, Mahdi F, Stewart JA, Paris JJ. HIV-1 tat upregulates the receptor for advanced glycation end products and superoxide dismutase-2 in the heart of transgenic mice. Viruses. (2022) 14:2191. doi: 10.3390/v14102191
75. Thangaraj A, Periyasamy P, Liao K, Bendi VS, Callen S, Pendyala G, et al. HIV-1 TAT-mediated microglial activation: role of mitochondrial dysfunction and defective mitophagy. Autophagy. (2018) 14:1596–619. doi: 10.1080/15548627.2018.1476810
76. Zhou BY, Liu Y, Oh Kim B, Xiao Y, He JJ. Astrocyte activation and dysfunction and neuron death by HIV-1 Tat expression in astrocytes. Mol Cell Neurosci. (2004) 27:296–305. doi: 10.1016/j.mcn.2004.07.003
77. Albini A, Ferrini S, Benelli R, Sforzini S, Giunciuglio D, Aluigi MG, et al. HIV-1 Tat protein mimicry of chemokines. Proc Natl Acad Sci United States America. (1998) 95:13153–8. doi: 10.1073/pnas.95.22.13153
78. Marino J, Maubert ME, Mele AR, Spector C, Wigdahl B, Nonnemacher MR. Functional impact of HIV-1 Tat on cells of the CNS and its role in HAND. Cell Mol Life sciences: CMLS. (2020) 77:5079–99. doi: 10.1007/s00018-020-03561-4
79. Marino J, Wigdahl B, Nonnemacher MR. Extracellular HIV-1 tat mediates increased glutamate in the CNS leading to onset of senescence and progression of HAND. Front Aging Neurosci. (2020) 12:168. doi: 10.3389/fnagi.2020.00168
80. Jordan A, Bisgrove D, Verdin E. HIV reproducibly establishes a latent infection after acute infection of T cells in vitro. EMBO J. (2003) 22:1868–77. doi: 10.1093/emboj/cdg188
81. Falahati Z, Mahdavi A, Hassani L. Physicochemical studies on the structural stability of the HIV-1 vaccine candidate recombinant Tat protein. Int J Biol Macromol. (2020) 164:403–14. doi: 10.1016/j.ijbiomac.2020.07.141
82. Tang X, Lu H, Dooner M, Chapman S, Quesenberry PJ, Ramratnam B. Exosomal Tat protein activates latent HIV-1 in primary, resting CD4+ T lymphocytes. JCI Insight. (2018) 3:e95676. doi: 10.1172/jci.insight.95676
83. Dimik M, Abeysinghe P, Logan J, Mitchell M. The exosome: a review of current therapeutic roles and capabilities in human reproduction. Drug Delivery Transl Res. (2023) 13:473–502. doi: 10.1007/s13346-022-01225-3
84. Lu H, Tang X, Sibley M, Coburn J, Rao RSP, Ahsan N, et al. Impact of exosomal HIV-1 Tat expression on the human cellular proteome. Oncotarget. (2019) 10:5632–44. doi: 10.18632/oncotarget.27207
85. Coiras M, Camafeita E, Ureña T, López JA, Caballero F, Fernández B, et al. Modifications in the human T cell proteome induced by intracellular HIV-1 Tat protein expression. Proteomics. (2006) 6 Suppl 1:S63–73. doi: 10.1002/pmic.200500437
86. Ganief T, Gqamana P, Garnett S, Hoare J, Stein DJ, Joska J, et al. Quantitative proteomic analysis of HIV-1 Tat-induced dysregulation in SH-SY5Y neuroblastoma cells. Proteomics. (2017) 17. doi: 10.1002/pmic.201600236
87. Jarboui MA, Bidoia C, Woods E, Roe B, Wynne K, Elia G, et al. Nucleolar protein trafficking in response to HIV-1 tat: rewiring the nucleolus. PloS One. (2012) 7:e48702. doi: 10.1371/journal.pone.0048702
88. Liao W, Tan G, Zhu Z, Chen Q, Lou Z, Dong X, et al. Combined metabonomic and quantitative real-time PCR analyses reveal systems metabolic changes in Jurkat T-cells treated with HIV-1 tat protein. J Proteome Res. (2012) 11:5109–23. doi: 10.1021/pr300173c
89. Pardons M, Cole B, Lambrechts L, van Snippenberg W, Rutsaert S, Noppe Y, et al. Potent latency reversal by Tat RNA-containing nanoparticle enables multi-omic analysis of the HIV-1 reservoir. Nat Commun. (2023) 14:8397. doi: 10.1038/s41467-023-44020-5
90. Raines SLM, Falcinelli SD, Peterson JJ, Van Gulck E, Allard B, Kirchherr J, et al. Nanoparticle delivery of Tat synergizes with classical latency reversal agents to express HIV antigen targets. Antimicrobial Agents Chemotherapy. (2024) 68:e0020124. doi: 10.1128/aac.00201-24
91. Ghanam RH, Eastep GN, Saad JS. Structural insights into the mechanism of HIV-1 tat secretion from the plasma membrane. J Mol Biol. (2023) 435:167880. doi: 10.1016/j.jmb.2022.167880
92. Wender PA, Mitchell DJ, Pattabiraman K, Pelkey ET, Steinman L, Rothbard JB. The design, synthesis, and evaluation of molecules that enable or enhance cellular uptake: Peptoid molecular transporters. Proc Natl Acad Sci United States America. (2000) 97:13003–8. doi: 10.1073/pnas.97.24.13003
93. Mediouni S, Jablonski J, Paris J, Clementz M, Thenin-Houssier S, McLaughlin J, et al. Didehydro-cortistatin A inhibits HIV-1 tat mediated neuroinflammation and prevents potentiation of cocaine reward in tat transgenic mice. Curr HIV Res. (2015) 13:64–79. doi: 10.2174/1570162x13666150121111548
94. Geng G, Liu B, Chen C, Wu K, Liu J, Zhang Y, et al. Development of an attenuated tat protein as a highly-effective agent to specifically activate HIV-1 latency. Mol Ther. (2016) 24:1528–37. doi: 10.1038/mt.2016.117
95. Faruqu FN, Wang JTW, Xu L, McNickle L, Chong EMY, Walters A, et al. Membrane radiolabelling of exosomes for comparative biodistribution analysis in immunocompetent and immunodeficient mice - A novel and universal approach. Theranostics. (2019) 9:1666–82. doi: 10.7150/thno.27891
96. Matsumoto A, Takahashi Y, Nishikawa M, Sano K, Morishita M, Charoenviriyakul C, et al. Role of phosphatidylserine-derived negative surface charges in the recognition and uptake of intravenously injected B16BL6-derived exosomes by macrophages. J Pharm Sci. (2017) 106:168–75. doi: 10.1016/j.xphs.2016.07.022
97. Mirzaaghasi A, Han Y, Ahn SH, Choi C, Park JH. Biodistribution and pharmacokinectics of liposomes and exosomes in a mouse model of sepsis. Pharmaceutics. (2021) 13:427. doi: 10.3390/pharmaceutics13030427
98. Qiao L, Hu S, Huang K, Su T, Li Z, Vandergriff A, et al. Tumor cell-derived exosomes home to their cells of origin and can be used as Trojan horses to deliver cancer drugs. Theranostics. (2020) 10:3474–87. doi: 10.7150/thno.39434
99. Rashid MH, Borin TF, Ara R, Angara K, Cai J, Achyut BR, et al. Differential in vivo biodistribution of 131I-labeled exosomes from diverse cellular origins and its implication for theranostic application. Nanomedicine: Nanotechnology Biology Med. (2019) 21:102072. doi: 10.1016/j.nano.2019.102072
100. Smyth T, Kullberg M, Malik N, Smith-Jones P, Graner MW, Anchordoquy TJ. Biodistribution and delivery efficiency of unmodified tumor-derived exosomes. J Controlled Release. (2015) 199:145–55. doi: 10.1016/j.jconrel.2014.12.013
101. Wiklander OPB, Nordin JZ, O'Loughlin A, Gustafsson Y, Corso G, Mäger I, et al. Extracellular vesicle in vivo biodistribution is determined by cell source, route of administration and targeting. J Extracellular Vesicles. (2015) 4:26316. doi: 10.3402/jev.v4.26316
102. Imai T, Takahashi Y, Nishikawa M, Kato K, Morishita M, Yamashita T, et al. Macrophage-dependent clearance of systemically administered B16BL6-derived exosomes from the blood circulation in mice. J Extracellular Vesicles. (2015) 4:26238. doi: 10.3402/jev.v4.26238
103. Shi B, Keough E, Matter A, Leander K, Young S, Carlini E, et al. Biodistribution of small interfering RNA at the organ and cellular levels after lipid nanoparticle-mediated delivery. J Histochem Cytochem. (2011) 59:727–40. doi: 10.1369/0022155411410885
104. Kheirolomoom A, Kare AJ, Ingham ES, Paulmurugan R, Robinson ER, Baikoghli M, et al. In situ T-cell transfection by anti-CD3-conjugated lipid nanoparticles leads to T-cell activation, migration, and phenotypic shift. Biomaterials. (2022) 281:121339. doi: 10.1016/j.biomaterials.2021.121339
105. Ramishetti S, Kedmi R, Goldsmith M, Leonard F, Sprague AG, Godin B, et al. Systemic gene silencing in primary T lymphocytes using targeted lipid nanoparticles. ACS Nano. (2015) 9:6706–16. doi: 10.1021/acsnano.5b02796
106. Tombácz I, Laczkó D, Shahnawaz H, Muramatsu H, Natesan A, Yadegari A, et al. Highly efficient CD4+ T cell targeting and genetic recombination using engineered CD4+ cell-homing mRNA-LNPs. Mol Ther. (2021) 29:3293–304. doi: 10.1016/j.ymthe.2021.06.004
107. Patel SK, Billingsley MM, Frazee C, Han X, Swingle KL, Qin J, et al. Hydroxycholesterol substitution in ionizable lipid nanoparticles for mRNA delivery to T cells. J Control Release. (2022) 347:521–32. doi: 10.1016/j.jconrel.2022.05.020
108. Cheng Q, Wei T, Farbiak L, Johnson LT, Dilliard SA, Siegwart DJ. Selective organ targeting (SORT) nanoparticles for tissue-specific mRNA delivery and CRISPR-Cas gene editing. Nat Nanotechnology. (2020) 15:313–20. doi: 10.1038/s41565-020-0669-6
109. LoPresti ST, Arral ML, Chaudhary N, Whitehead KA. The replacement of helper lipids with charged alternatives in lipid nanoparticles facilitates targeted mRNA delivery to the spleen and lungs. J Controlled Release. (2022) 345:819–31. doi: 10.1016/j.jconrel.2022.03.046
110. Ongun M, Lokras AG, Baghel S, Shi Z, Schmidt ST, Franzyk H, et al. Lipid nanoparticles for local delivery of mRNA to the respiratory tract: Effect of PEG-lipid content and administration route. Eur J Pharmaceutics Biopharmaceutics. (2024) 198:114266. doi: 10.1016/j.ejpb.2024.114266
111. Billingsley MM, Gong N, Mukalel AJ, Thatte AS, El-Mayta R, Patel SK, et al. In vivo mRNA CAR T Cell Engineering via Targeted Ionizable Lipid Nanoparticles with Extrahepatic Tropism. Small. (2024) 20:e2304378. doi: 10.1002/smll.202304378
112. Herrera-Barrera M, Ryals RC, Gautam M, Jozic A, Landry M, Korzun T, et al. Peptide-guided lipid nanoparticles deliver mRNA to the neural retina of rodents and nonhuman primates. Sci Adv. (2023) 9:eadd4623. doi: 10.1126/sciadv.add4623
113. Rurik JG, Tombácz I, Yadegari A, O Méndez Fernández P, Shewale SV, Li L, et al. CAR T cells produced in vivo to treat cardiac injury. Science. (2022) 375:91–6. doi: 10.1126/science.abm0594
114. Cevaal PM, Ali A, Czuba-Wojnilowicz E, Symons J, Lewin SR, Cortez-Jugo C, et al. In vivo T cell-targeting nanoparticle drug delivery systems: considerations for rational design. ACS Nano. (2021) 15:3736–53. doi: 10.1021/acsnano.0c09514
115. Estes JD, Kityo C, Ssali F, Swainson L, Makamdop KN, Del Prete GQ, et al. Defining total-body AIDS-virus burden with implications for curative strategies. Nat Med. (2017) 23:1271–6. doi: 10.1038/nm.4411
116. Chen B, Chen Y, Li J, Wang C, Song W, Wen Y, et al. A single dose of anti-HBsAg antibody-encoding mRNA-LNPs suppressed HBsAg expression: a potential cure of chronic hepatitis B virus infection. mBio. (2022) 13:e0161222. doi: 10.1128/mbio.01612-22
117. Nakamura T, Kawai M, Sato Y, Maeki M, Tokeshi M, Harashima H. The effect of size and charge of lipid nanoparticles prepared by microfluidic mixing on their lymph node transitivity and distribution. Mol Pharmaceutics. (2020) 17:944–53. doi: 10.1021/acs.molpharmaceut.9b01182
118. Azzi J, Yin Q, Uehara M, Ohori S, Tang L, Cai K, et al. Targeted delivery of immunomodulators to lymph nodes. Cell Rep. (2016) 15:1202–13. doi: 10.1016/j.celrep.2016.04.007
119. Bachhav SS, Dighe VD, Kotak D, Devarajan PV. Rifampicin Lipid-Polymer hybrid nanoparticles (LIPOMER) for enhanced Peyer's patch uptake. Int J Pharmaceutics. (2017) 532:612–22. doi: 10.1016/j.ijpharm.2017.09.040
120. Ren Y, Wu W, Zhang X. The feasibility of oral targeted drug delivery: Gut immune to particulates? Acta Pharm Sin B. (2023) 13:2544–58. doi: 10.1016/j.apsb.2022.10.020
121. Brightman MW, Reese TS. Junctions between intimately apposed cell membranes in the vertebrate brain. J Cell Biol. (1969) 40:648–77. doi: 10.1083/jcb.40.3.648
122. Banks WA, Sharma P, Bullock KM, Hansen KM, Ludwig N, Whiteside TL. Transport of extracellular vesicles across the blood-brain barrier: Brain pharmacokinetics and effects of inflammation. Int J Mol Sci. (2020) 21:4407. doi: 10.3390/ijms21124407
123. Tanaka H, Nakatani T, Furihata T, Tange K, Nakai Y, Yoshioka H, et al. In Vivo Introduction of mRNA Encapsulated in Lipid Nanoparticles to Brain Neuronal Cells and Astrocytes via Intracerebroventricular Administration. Mol Pharmaceutics. (2018) 15:2060–7. doi: 10.1021/acs.molpharmaceut.7b01084
124. Tuma J, Chen YJ, Collins MG, Paul A, Li J, Han H, et al. Lipid Nanoparticles Deliver mRNA to the Brain after an Intracerebral Injection. Biochemistry. (2023) 62:3533–47. doi: 10.1021/acs.biochem.3c00371
125. Kuzminich Y, Shakked A, Calkins R, Rudden S, Jones C, Doan J, et al. Lipid nanoparticles deliver mRNA to the blood-brain barrier. Nano Res. (2024) 62:3533–47. doi: 10.1007/s12274-024-6827-7
126. Hiener B, Horsburgh BA, Eden JS, Barton K, Schlub TE, Lee E, et al. Identification of genetically intact HIV-1 proviruses in specific CD4(+) T cells from effectively treated participants. Cell Rep. (2017) 21:813–22. doi: 10.1016/j.celrep.2017.09.081
127. Ho YC, Shan L, Hosmane NN, Wang J, Laskey SB, Rosenbloom DIS, et al. Replication-competent noninduced proviruses in the latent reservoir increase barrier to HIV-1 cure. Cell. (2013) 155:540–0. doi: 10.1016/j.cell.2013.09.020
128. Chun TW, Carruth L, Finzi D, Shen X, DiGiuseppe JA, Taylor H, et al. Quantification of latent tissue reservoirs and total body viral load in HIV-1 infection. Nature. (1997) 387:183–8. doi: 10.1038/387183a0
129. Eriksson S, Graf EH, Dahl V, Strain MC, Yukl SA, Lysenko ES, et al. Comparative analysis of measures of viral reservoirs in HIV-1 eradication studies. PloS Pathog. (2013) 9:e1003174. doi: 10.1371/journal.ppat.1003174
130. Hosmane NN, Kwon KJ, Bruner KM, Capoferri AA, Beg S, Rosenbloom DIS, et al. Proliferation of latently infected CD4+ T cells carrying replication-competent HIV-1: Potential role in latent reservoir dynamics. J Exp Med. (2017) 214:959–72. doi: 10.1084/jem.20170193
131. Einkauf KB, Osborn MR, Gao C, Sun W, Sun X, Lian X, et al. Parallel analysis of transcription, integration, and sequence of single HIV-1 proviruses. Cell. (2022) 185:266–82. doi: 10.1016/j.cell.2021.12.011
132. Banga R, Procopio FA, Noto A, Pollakis G, Cavassini M, Ohmiti K, et al. PD-1+ and follicular helper T cells are responsible for persistent HIV-1 transcription in treated aviremic individuals. Nat Med. (2016) 22:754–61. doi: 10.1038/nm.4113
133. Furtado MR, Callaway DS, Phair JP, Kunstman KJ, Stanton JL, Macken CA, et al. Persistence of HIV-1 transcription in peripheral-blood mononuclear cells in patients receiving potent antiretroviral therapy. New Engl J Med. (1999) 340:1614–22. doi: 10.1056/nejm199905273402102
134. Lewin SR, Vesanen M, Kostrikis L, Hurley A, Duran M, Zhang L, et al. Use of real-time PCR and molecular beacons to detect virus replication in human immunodeficiency virus type 1-infected individuals on prolonged effective antiretroviral therapy. J Virol. (1999) 73:6099–103. doi: 10.1128/jvi.73.7.6099-6103.1999
135. Pasternak AO, Jurriaans S, Bakker M, Prins JM, Berkhout B, Lukashov VV. Cellular levels of HIV unspliced RNA from patients on combination antiretroviral therapy with undetectable plasma viremia predict the therapy outcome. PloS One. (2009) 4:e8490. doi: 10.1371/journal.pone.0008490
136. Dubé M, Tastet O, Dufour C, Sannier G, Brassard N, Delgado GG, et al. Spontaneous HIV expression during suppressive ART is associated with the magnitude and function of HIV-specific CD4+ and CD8+ T cells. Cell Host Microbe. (2023) 31:1507–22. doi: 10.1016/j.chom.2023.08.006
137. Wu G, Zuck P, Goh SL, Milush JM, Vohra P, Wong JK, et al. Gag p24 is a marker of human immunodeficiency virus expression in tissues and correlates with immune response. J Infect Dis. (2021) 224:1593–8. doi: 10.1093/infdis/jiab121
138. Wei Y, Davenport TC, Collora JA, Ma HK, Pinto-Santini D, Lama J, et al. Single-cell epigenetic, transcriptional, and protein profiling of latent and active HIV-1 reservoir revealed that IKZF3 promotes HIV-1 persistence. Immunity. (2023) 56:2584–601. doi: 10.1016/j.immuni.2023.10.002
139. Wiegand A, Spindler J, Hong FF, Shaoc W, Cyktor JC, Cillo AR, et al. Single-cell analysis of HIV-1 transcriptional activity reveals expression of proviruses in expanded clones during ART. Proc Natl Acad Sci United States America. (2017) 114:e3659–68. doi: 10.1073/pnas.1617961114
140. Clark IC, Mudvari P, Thaploo S, Smith S, Abu-Laban M, Hamouda M, et al. HIV silencing and cell survival signatures in infected T cell reservoirs. Nature. (2023) 614:318–25. doi: 10.1038/s41586-022-05556-6
141. Collora JA, Liu R, Pinto-Santini D, Ravindra N, Ganoza C, Lama JR, et al. Single-cell multiomics reveals persistence of HIV-1 in expanded cytotoxic T cell clones. Immunity. (2022) 55:1013–31. doi: 10.1016/j.immuni.2022.03.004
142. Wu VH, Nordin JML, Nguyen S, Joy J, Mampe F, Del Rio Estrada PM, et al. Profound phenotypic and epigenetic heterogeneity of the HIV-1-infected CD4(+) T cell reservoir. Nat Immunol. (2023) 24:359–70. doi: 10.1038/s41590-022-01371-3
143. Arandjelovic P, Kim Y, Cooney JP, Preston SP, Doerflinger M, McMahon JH, et al. Venetoclax, alone and in combination with the BH3 mimetic S63845, depletes HIV-1 latently infected cells and delays rebound in humanized mice. Cell Rep Med. (2023) 4:101178. doi: 10.1016/j.xcrm.2023.101178
Keywords: HIV, latency, Tat, latency reversal agent, HIV cure
Citation: Fisher BM, Cevaal PM, Roche M and Lewin SR (2025) HIV Tat as a latency reversing agent: turning the tables on viral persistence. Front. Immunol. 16:1571151. doi: 10.3389/fimmu.2025.1571151
Received: 05 February 2025; Accepted: 17 March 2025;
Published: 11 April 2025.
Edited by:
Susana Valente, University of Florida, United StatesReviewed by:
Christina K. Psomas, Hôpital Européen Marseille, FranceTahir Tahirov, University of Nebraska Medical Center, United States
Copyright © 2025 Fisher, Cevaal, Roche and Lewin. This is an open-access article distributed under the terms of the Creative Commons Attribution License (CC BY). The use, distribution or reproduction in other forums is permitted, provided the original author(s) and the copyright owner(s) are credited and that the original publication in this journal is cited, in accordance with accepted academic practice. No use, distribution or reproduction is permitted which does not comply with these terms.
*Correspondence: Sharon R. Lewin, c2hhcm9uLmxld2luQHVuaW1lbGIuZWR1LmF1
†These authors share senior authorship