- 1Institute of Infection, Immunology and Tumor Microenvironment, Hubei Province Key Laboratory of Occupational Hazard Identification and Control, Medical College, Wuhan University of Science and Technology, Wuhan, Hubei, China
- 2Analytical & Testing Center, Wuhan University of Science and Technology, Wuhan, Hubei, China
- 3NHC Key Laboratory of Nuclear Technology Medical Transformation, Mianyang Central Hospital, School of Medicine, University of Electronic Science and Technology of China, Mianyang, Sichuan, China
Outbreaks of emerging and re-emerging infectious diseases have consistently threatened human health. Since vaccinations are a powerful tool for preventing infectious illnesses, developing new vaccines is essential. Compared to traditional injectable vaccines, mucosal vaccines have the potential to offer more effective immune protection at mucosal sites. Mucosal immunization strategies include sublingual, oral, intranasal, genital, and rectal routes, in which intranasal immunization being the most efficient and applicable method for mucosal vaccine delivery. Nevertheless, low antigen availability and weak immunogenicity making it challenging to elicit a potent immune response when administered intranasally, necessitating the incorporation of immune delivery systems. However, there is a notable absence of reviews that summarize the intranasal vaccine delivery system against infectious disease. Therefore, this review summarizes the recent advances in intranasal delivery systems, classified by physical and chemical properties, and proposes potential improvement strategies for clinical translation. This review elucidates the potential and current status of intranasal delivery systems, while also serving as a reference point for the future development of intranasal vaccines.
1 Introduction
Infectious diseases including influenza, acquired immune deficiency syndrome (AIDS), measles, and coronavirus disease 2019 (COVID-19) pose persistent threats to global public health, necessitating urgent advancements in prophylactic strategies (1). While medical advancements over the past two decades have reduced mortality through improved sanitation and healthcare infrastructure (2), the emergence of novel pathogens and antimicrobial resistance underscores the need for innovative immunization approaches. As the cornerstone of infectious disease prevention, vaccines function by priming innate immunity to activate antigen-specific adaptive responses through antibody production and T-cell mediation—a dual mechanism that curbs pathogen transmission while protecting vulnerable populations (3).
Current vaccine delivery modalities can be broadly categorized into systemic injection and mucosal immunization. In contrast to traditional injectable vaccines that primarily elicit systemic immunity, mucosal vaccines offer dual protective advantages: 1) induction of both systemic and mucosal immune responses, 2) generation of secretory IgA and tissue-resident memory T cells at portal-of-entry mucosal sites, and 3) needle-free administration that enhances safety and compliance (4, 5). Given that >90% of pathogens invade through mucosal surfaces, mucosal immunization serves as the primary immunological barrier against infection establishment (6).
Among mucosal delivery routes—including oral, buccal, sublingual, intranasal, and genital approaches—intranasal immunization stands out as a particularly promising candidate. This preference stems from the nasal cavity’s unique immunological architecture: 1) dense networks of microfold (M) cells and antigen-presenting cells (APCs) within nasal-associated lymphoid tissue (NALT), 2) vascularized subepithelial layers facilitating rapid systemic absorption, and 3) interconnected mucosal immunity enabling cross-protection at distal sites such as pulmonary and intestinal mucosa (7). Notably, currently approved intranasal vaccines predominantly target influenza, including FluMist (US) (8) and Nasovac-S (India) (9). The COVID-19 pandemic further accelerated clinical development of intranasal SARS-CoV-2 vaccines (10), with emergency approvals granted for formulations acting as primary immunogens or heterologous boosters to enhance mucosal immunity and tissue-resident memory T cells (11) (Table 1).
The clinical advantages of intranasal vaccines are multifaceted: (i) Non-invasive administration: Intranasal vaccines are an excellent non-invasive vaccination method (7, 12). (ii) Induction of mucosal immunity: In addition to systemic immune response, intranasal vaccines can act on the extensive respiratory mucosa, eliciting mucosal immunity and providing more comprehensive and effective protection for the human body (12). (iii) Improved pediatric compliance: compared to the pain caused by intramuscular injections, nasal drops offer a more comfortable vaccination experience for children and are also suitable for other individuals with needle or pain phobias (13, 14). (iv) Operational and economic benefits: intranasal vaccines offer greater practicality in terms of cost and ease of administration (15). While transient adverse effects (e.g., rhinorrhea, low-grade fever) occur in some recipients, these typically resolve without intervention (16).
The nasal cavity is a complex anatomical structure with superior, inferior, medial, and lateral walls. The two nasal passages have a combined surface area of about 160 cm2 (96 m2 if nasal epithelial microvilli are included), and a combined volume of about 15 mL. The nasal cavity can be divided into three main regions: the nasal vestibule, the respiratory tract, and the olfactory region. The nasal septum, which separates the two nasal passages, forms the medial wall of the nasal cavity. The superior, middle, and inferior turbinates, which delineate the upper, middle, and lower nasal passages respectively, constitute the lateral wall of the nasal cavity (17). The human Waldeyer’s ring, comprising the adenoid, lingual tonsil, two palatine tonsils, and two tubal tonsils, is believed to be analogous to the NALT (nasal-associated lymphoid tissue) observed in rodents (8). The NALT can be defined as an organized mucosal-associated lymphoid tissues within the nasal mucosa, consisting of lymphoid tissue, B cells, T cells, antigen-presenting cells (APCs) and microfold (M) cells. M cells are specifically for antigen uptake (9). NALT plays a critical role in antigen recognition and immune activation following intranasal immunization, serving as a key site for immune induction within the nasal mucosa (Figure 1).
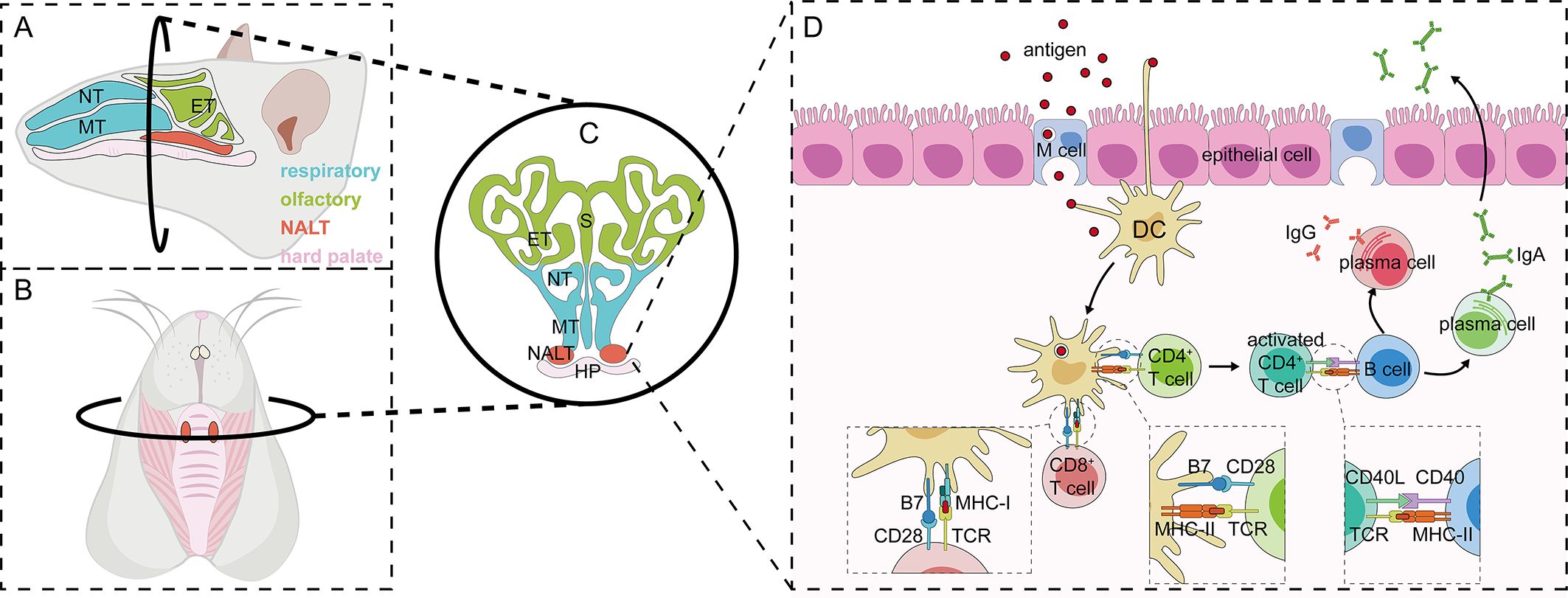
Figure 1. Anatomy of and immune induction in nasal-associated lymphoid tissues in rodents. (A-C) Anatomical location of NALT: The sagittal anatomy of mouse nasal tissues can be divided into two distinct regions—the respiratory and olfactory parts—based on the structure and function of the mucosa (A). A specialized structure in the nasal tissue, the nasal mucosa-associated lymphoid tissue (NALT), consists of two parallel bell-shaped formations located above the hard palate (B, C). Immune response in NALT: The immune response in NALT progresses through three main stages: antigen uptake and presentation, immune cell activation, and the subsequent immune response. Antigens entering the nasal cavity are first internalized by microfold cells (M cells), which then transport them into NALT, where dendritic cells further process and present the antigens. In addition, some dendritic cells are capable of crossing the epithelial barrier by extending their dendrites, allowing them to directly capture antigens. Antigen-loaded dendritic cells activate both CD4+ and CD8+ T cells upon antigen presentation. Activated CD4+ T cells further stimulate B cells via CD40L/CD40 interaction, promoting their differentiation into plasma cells. These fully differentiated plasma cells subsequently initiate an immune response at the effector site through the production of IgA and IgG antibodies (D). NT, nasoturbinate; MT, maxillary turbinate; ET, ethmoid turbinate; S, septum; HP, hard palate.
Low antigen availability and weak immunogenicity, combined with antigen clearance by nasal cilia, the mucous layer barrier, and tight junctions between epithelial cells, significantly reduce vaccine uptake. This hinders the elicitation of a robust immune response, thereby posing challenges for nasal administration (18). The nasal drug delivery system (NDDS) is a needle-free, non-invasive, and efficient method for delivering drugs, vaccines, and other therapeutic agents into the human body via the nasal route which include nanoparticles (Tables 2, 3), nanoemulsions, and some microbial preparations (Table 4) (12, 19). Among them, nanoparticles are the most widely used, which include polysaccharide-based particles, poly lactide-co-glycolide (PLGA), gold nanoparticles, nanogels, lipopeptides, liposomes and others (19, 20). Through boosting antigen penetration, shielding the antigen from degradation, facilitating sustained antigen release, improving nasal retention time, and recruiting/activating APCs, the nasal delivery system can significantly increase the antigen availability and immunogenicity (18). The NDDS offers the advantage of boosting vaccination efficiency against pathogens through the induction of both systemic and mucosal immune responses at respiratory and distal mucosal sites (21).
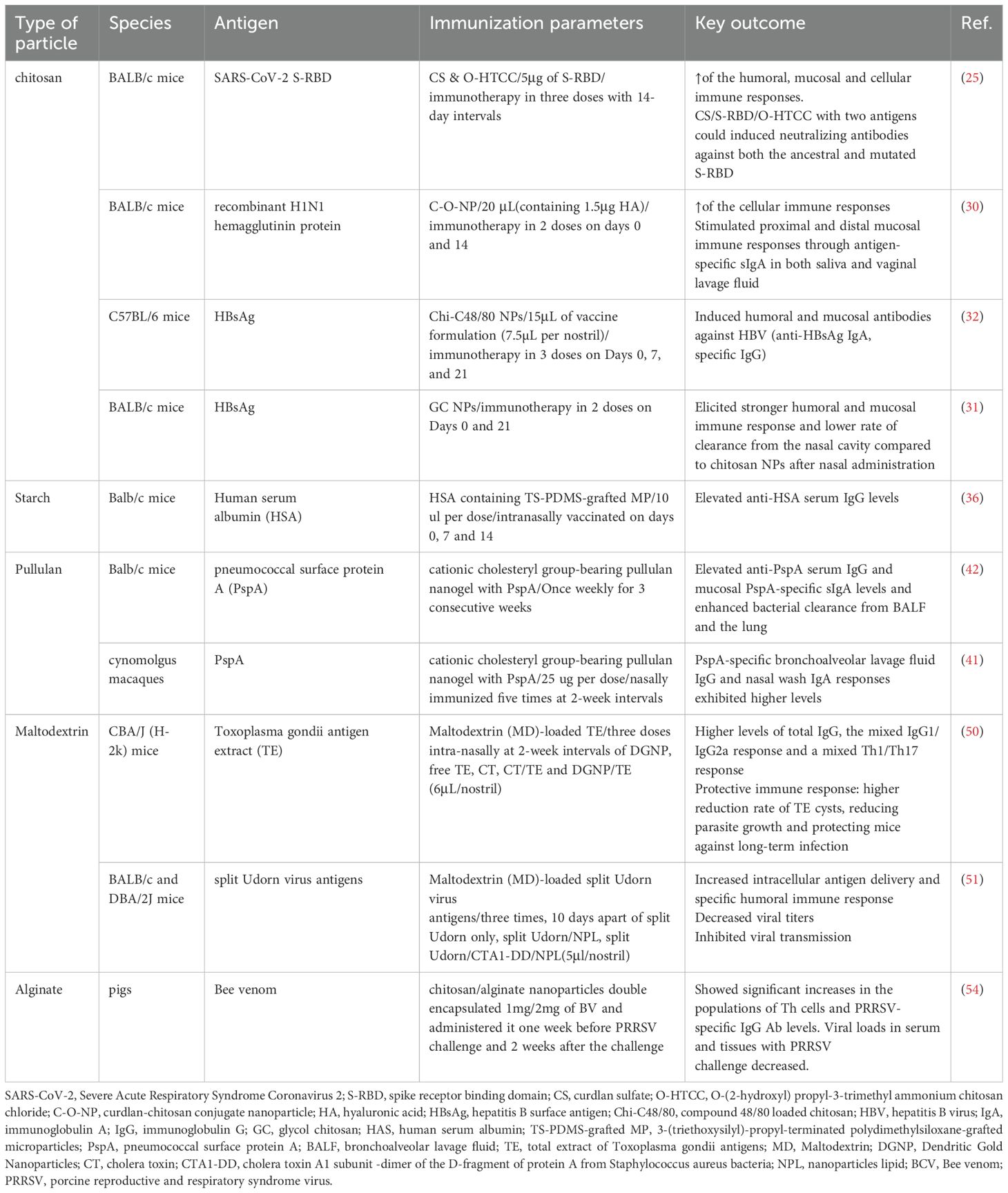
Table 2. Preclinical studies of intranasal vaccines using polysaccharides as an intranasal delivery system.
Currently, the majority of clinical research on intranasal vaccines focuses on respiratory diseases such as influenza, RSV (Respiratory Syncytial Virus), COVID-19, and whooping cough (Table 5). Among these, some vaccines incorporate delivery systems such as nanoemulsions, liposomes, VLPs (Virus-Like Particles), and chitosan to enhance the efficacy of antigens. In these studies, influenza vaccines are the most extensively researched and widely applied intranasal vaccines. The primary type is the live attenuated vaccine, which holds significant research value and market potential, and has also spurred the development of other live attenuated vaccines.
In this review, we focus on NDDS as advanced carriers for optimizing antigen transport and mucosal retention. While the primary emphasis lies in elucidating mechanistic strategies for targeted delivery, we also examine the combination role of adjuvants. To avoid ambiguity, adjuvants (e.g., TLR agonists, cyclic dinucleotides) is restricted to the components enhancing immune responses although certain adjuvants (e.g., particulate carriers like alum or lipid-based nanoparticles) inherently possess dual functionalities as both immunostimulants and delivery vehicles.
2 Nanoparticles types used in nasal vaccines
2.1 Polysaccharide: biocompatible and biodegradable polymers for delivery systems
Polysaccharides represent an exciting class of biomaterials for intranasal vaccine delivery, offering three critical advantages: excellent biocompatibility, predictable biodegradability, and potent immunomodulatory properties (9, 22). The most promising polysaccharide platforms include chitosan, starch, maltodextrin, alginate, etc (Figure 2). These innovative materials effectively solve three major challenges in intranasal vaccination: overcoming mucociliary clearance, enhancing epithelial barrier penetration, and providing sustained antigen release (18), all while maintaining superior safety and stability profiles (8). Ongoing research continues to unlock their full potential for developing next-generation mucosal vaccines.
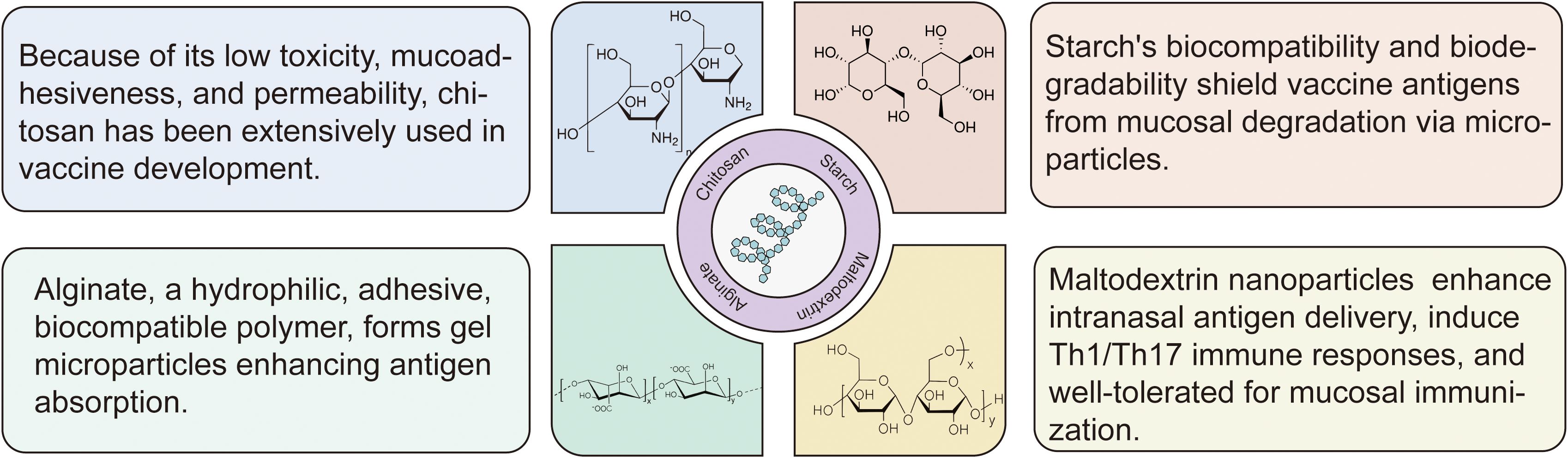
Figure 2. Structural and functional features of polysaccharide-based intranasal delivery systems. The most promising polysaccharide platforms include chitosan, starch, maltodextrin, alginate and others. Low-toxicity and mucoadhesive chitosan improves antigen transport across mucosal barriers; biodegradable starch microparticles protect antigens from enzymatic breakdown; hydrophilic gel microparticles, alginate, aid in the absorption and retention of mucosal antigens; maltodextrin nanoparticles improve intranasal delivery effectiveness, and they induce Th1/Th17 responses with high tolerability.
2.1.1 Chitosan
Chitosan is a naturally occurring, biocompatible linear polysaccharide composed of repeating units of d-glucosamine and N-acetyl-d-glucosamine. It is the product of N-deacetylation of chitin, which is derived from the exoskeletons of crustaceans and insects. Chitosan is a safe and biocompatible cationic polymer that can be degraded by a group of chitinases found in many organisms, including humans (23). In addition to its safety and biocompatibility, chitosan has been extensively researched for its low toxicity, mucoadhesiveness, immunogenicity, and permeability. The U.S. Food and Drug Administration (FDA) has approved the use of chitosan in foods and pharmaceuticals (24).
Nanoparticles (NPs) based on chitosan or chitosan derivatives, ranging in size from 1 to 1000 nanometers have been utilized as promising immune delivery systems, delivering vaccine antigens through mucosal pathways such as the nasal routes. Chitosan nanoparticulate systems are particularly successful as vaccine delivery vehicles due to their positive charge, which can improve antigen absorption and boost cellular uptake. Experiments have revealed that positively charged chitosan nanoparticles tend to adhere to negatively charged mucoproteins, reducing nasal evacuation of the nanoparticles. Additionally, Chitosan and its derivatives have the ability to open tight junctions between epithelial cells, elicit immunological responses, and promote IFN-I production, making them potentially effective immune delivery systems (25–27).
In recent years, vaccines based on chitosan or its derivatives as intranasal immune delivery systems have been extensively studied and have been applied in the development of vaccines for infectious disease. For instance, a lentogenic live-virus vaccine (strain LaSota) against newcastle disease virus encapsulated chitosan nanoparticles can elicit robust mucosal immune responses and show a high level of safety in chickens (28).
Although chitosan has significant potential for application, it is sensitive to pH: chitosan dissolves readily at lower pH levels but is less soluble at higher pH levels. Since many proteins are unstable at low pH levels, chitosan’s pH sensitivity may limit its use in antigen delivery (29). Therefore, chitosan is typically modified, such as by forming nanoparticles or allowing free amino and carboxyl functional groups in chitosan through various chemical reactions, including carboxylation, esterification, hydroxylation, and acetylation, to make chitosan derivatives (24).
Among different chitosan derivatives, quaternization, particularly N-trimethyl chitosan (N-TMC), has received the greatest interest. This is owing to its persistent cationic charge and wide pH solubility range. Even at neutral pH, it exhibits strong mucoadhesive and permeation-enhancing properties (26). Chen et al. developed a nanoparticle delivery system, CS/O-HTCC, using the chitosan derivative O-(2-hydroxy) propyl-3-trimethyl ammonium chitosan chloride (O-HTCC) and the β-glucan derivative, curdlan sulfate (CS). They created a nasal mucosal protein subunit vaccine, CS/S-RBD/O-HTCC, using the SARS-CoV-2 spike receptor binding domain (S-RBD) as the antigen, and confirming its safety in vitro and in vivo. When CS/O-HTCC is loaded with S-RBD mixed antigens (ancestral and omicron), it provoked high levels of ancestral S-RBD specific IgG in serum, sIgA from nasal lavage fluid and antigen-specific IFN-γ from splenic lymphocytes, suggesting that CS/S-RBD/O-HTCC could induce robust humoral, mucosal and cellular immunity. For the neutralizing assay, this vaccination could induce neutralizing antibodies against both ancestral and omicron pseudoviruses than those in the groups using single antigens. This delivery vehicle might be utilized to rapidly prepare vaccines against the continuously mutating SARS-CoV-2 virus (25). In another study, Chen et al. discovered that nanoparticles formed by coupling sulfated lentinan and O-HTCC, as a nasal H1N1 subunit vaccine delivery carrier can stimulate mice macrophage phagocytosis, promote dendritic cell maturation and activation, enhance the production of antigen-specific sIgA in saliva and vaginal lavage fluid and IgG in serum, indicating promising applications (30).
What’s more, numerous recent studies have explored the modification of chitosan nanoparticles with other substances, such as ethylene glycol. Dilip Pawar et al. were the first to synthesize and evaluate glycol chitosan (GC) nanoparticles. In the in vitro Calu-3 cell line model, they found that chitosan and GC exhibited minimal toxicity. In comparison to bare chitosan nanoparticles, intranasal treatment of GC nanoparticles loaded with hepatitis B surface antigen (HBsAg) in mice demonstrated better mucoadhesion, which resulted in a substantially lower rate of clearance as compare to chitosan NPs (31).
In addition to surface modification of chitosan to enhance the immune response elicited by vaccines, studies have also explored the adjuvant combination to develop more effective vaccine formulations. Dulce Bento developed a co-adjuvant delivery system for intranasal administration of the hepatitis B virus vaccine, combining the mast cell activator C40/80 with chitosan nanoparticles. The delivery system was shown to have promising features for vaccine delivery, such as the ability to adsorb high amounts of antigen, internalization by APCs, and stability after lyophilization, which can be important during the development of designing a cold chain-free vaccine. C48/80 loaded chitosan nanoparticles NPs have the ability to enhance the immune response to HBsAg such as inducing mucosal anti-HBsAg IgA and specific IgG titers in the vaccinated mice compared to free HBsAg and chitosan-poly epsilon caprolactone NPs (32).
2.1.2 Starch
Starch is a naturally biocompatible and biodegradable polymer. There are two different types of starch: amylose and amylopectin. Most starch consists of ~20% amylose and ~80% amylopectin. While amylose and amylopectin are readily degraded by α-amylase, starch remains insoluble in water (33). Starch microparticles can be utilized as a vaccine carrier because it prevents the denature of antigen proteins by temporarily protecting antigens from the acidic and enzymatic environment of mucosal surfaces (34). Protein molecules can be encapsulated and coupled into particles made of modified and cross-linked starch. Starch can be shaped in a variety of forms, including Spherex microparticles, polyacryl starch microparticles, silicone-grafted starch microparticles, and more (33). Studies have shown that macrophages stimulated by starch in the form of granules release IL-1 (35). In the meantime, mice immunized intranasally with an antigen and silicone-polymer-grafted starch microparticle system likewise produced a robust circulating IgG responses (34, 36).
Pullulan, a microbial exopolysaccharide generated by Aureobasidium pullulans, is a highly biocompatible and biodegradable polymer that is odorless, tasteless, non-toxic and edible. It has maltotriose repeating units and is a linear, unbranched polymer chain structurally (37). A nanogel particle consisting of a cholesterol-bearing pullulan has been proposed as an intranasal delivery system (38). Charge-based interaction can be employed to make the particles stick to the nasal epithelium and release antigens since the particles have a positive charge while the mucosa has a negative charge (39). This mechanism can slow the release of antigens and prolong the existence time of antigens in the nasal mucosa (40). The antigen can be efficiently delivered to the nasal epithelium, where it is then taken up by dendritic cells. A protective immune response against Streptococcus pneumoniae was induced in mice after they were intranasally immunized with a vaccine conjugated with pneumococcal surface protein A using a cationic cholesteryl-group-bearing pullulan nanogel as a delivery system. This resulted in an increased production of serum PspA-specific IgG1 antibodies, high levels of mucosal antigen-specific sIgA antibodies, Th17 and Th2 responses, and the growth of bacteria in the mice’s lungs and nasal cavities was inhibited (41). Furthermore, the vaccine was administered to rhesus macaques, a non-human primate species. It not only induced systemic and mucosal antibody responses in the macaques, but it also prevented the antigen from being deposited in their brains or olfactory bulbs, making it safe (42). A recent study investigated the utilization of pullulan-bearing cholesterol moieties in nanogel particles as a delivery system. The delivery system induced antigen-specific serum IgG and nasal mucosal IgA by intranasally immunizing mice with pneumococcal surface protein A as an antigen (43).
2.1.3 Maltodextrin
Maltodextrin (MD) is a natural polysaccharide derived from partially hydrolyzed starch. Maltodextrin-based nanoparticles, known as supramolecular biovectors (SMBVs) and cationic porous maltodextrin nanoparticles (NPLs), can be mixed with lipids to be employed as intranasal delivery systems (44).
After intranasal immunization, lipid-coated SMBVs with maltodextrin scaffolds cling to the mucosa continuously, enhancing intranasal antigen delivery and inducing systemic and mucosal immunological responses, such as antibody production and Th1 and Th2 immune responses (45). The efficacy of SMBV-based nasal influenza vaccinations was demonstrated in a dose-escalation clinical trial that was randomized, placebo-controlled, and conducted on healthy individuals. The vaccines produced mucosal immune responses and were well tolerated with moderate immunogenicity (46).
With anionic lipids contained in the maltodextrin scaffold as the core, Maltodextrin nanoparticles (MdNP) is a porous cationic maltodextrin scaffold and has been investigated as nasal route delivery methods for antigens and medications (47, 48). Regarding the safety of maltodextrin, numerous in vivo and in vitro tests have demonstrated that MdNP is safe since it is unable to penetrate the nasal mucosal epithelial barrier (48). When compared to other nanoparticle delivery systems, MdNP also had the longest residence duration in dendritic cells, macrophages, and airway epithelial cells, the highest endocytosis efficiency, and the strongest antigen delivery effect (49). The immune response induced by MdNP is dominated by Th1/Th17 (44). MdNP has been utilized to prevent acute or chronic toxoplasma gondii infection (50). According to a recent study, mice inoculated with the MdNP formulations were not only protected against infection, but also did not transmit the influenza virus (51).
2.1.4 Alginate
Alginate is the basic carbohydrate component of brown algae. It is an unbranched copolymer with the qualities of hydrophilicity, adhesion, biocompatibility and biodegradability (52–54). Gel microparticles are formed when most bivalent cations are added to alginate solutions (52). The resulting microparticles are irregular in shape, have a rough and porous surface, and a high polypoid structure. These particles can develop under more hospitable settings (55). Their benefits include being inexpensive and non-toxic at the same time (52). Alginate has strong adhesion and can extend the duration of contact between microparticles and M cells, hence augmenting antigen absorption (55). By activating macrophage-like cells through the NF-κB pathway, alginate can trigger an innate immune response (56). Alginate microparticles have been employed in the creation of Klebsiella pneumoniae vaccines (55).
Alginate nanoparticles are often negatively charged, and mucus on the mucosal surface is similarly negatively charged, so this makes it unsuitable for substance delivery. Chitosan and alginate are frequently used in combination because the inclusion of chitosan enhances the positive charge on the particle surface while also enhancing the immunostimulatory qualities and stability of chitosan (54, 57). Chitosan/alginate nanoparticle encapsulated bee venom (BV), CH/AL-BV, has slow-releasing properties and mucosal adhesiveness. Nasal delivery of CH/AL-BV in pigs enhanced Th1-related responses, porcine reproductive and respiratory syndrome virus (PRRSV)-specific viral neutralizing antibody and significant reductions in PRRSV load in serum, lung and bronchial lymph nodes (54). Recently, nasal immunization of mice with trimethylchitosan nanoparticles containing influenza virus coated with sodium alginate induced a significant increase in the IgG2a/IgG1 ratio and improved immune protection against the virus (57). The combination of chitosan and alginate nanoparticles has the potential to be a highly effective vaccine delivery system.
Hyaluronic acid and dextran are other polysaccharides that can be also utilized as vaccine delivery platform. As a nanoparticle vaccine delivery system, a biodegradable polymer micellar coated with hyaluronic acid was created. Ovalbumin and adjuvant CpG-DNA were loaded onto the micellar, increasing the expression of MHC-II also IFN-γ and IL-4 mRNA expression in mouse dendritic cells. Correspondingly, nasal immunization resulted in higher antigen-specific serum IgG and nasal IgA levels (58).
2.2 PLGA: a promising and thriving mediators for delivery system
PLGA, poly (lactic-co-glycolic acid), is a biodegradable polymer consisting of lactic acid and glycolic acid linked via ester linkages. PLGA is soluble in common solvents such as acetone, chlorinated solvents, and ethyl acetate, and can be processed into virtually any shape and size, and encapsulated into various molecules (59). PLGA nanoparticles are one of the most widely used particles, having been used in a wide range of cosmetic, food, agricultural, and drug delivery systems (60).
Among vaccine delivery systems, PLGA is commonly used to protect peptide vaccines from degradation, enhance antigen absorption by APCs, and stimulate robust T cell immune responses (61). Furthermore, PLGA nanoparticle vaccines allow for a sustained release of antigen, which is essential for the duration and intensity of the induced vaccine response. Since PLGA nanoparticles are biodegradable, biocompatible, and non-toxic, they have been approved for many biomedical applications, with the U.S. FDA approving them as sustained-release drug delivery systems (62).
Büyükbayraktar et al. developed an anti-tuberculosis vaccine with polycation-coated PLGA nanoparticles as delivery system, a potential vaccine model for long-term pulsatile release of antigenic peptide of ESAT-6 protein of M. tuberculosis, which augment the immunostimulation and also allows to be administered via nasal route. Using in vitro model of murine macrophage cell line (J774) and human macrophage cell line (THP-1), the peptide-loaded nanoparticle enhanced the immunogenicity of the peptides to induce more nitric oxide (NO) than the free peptide and non-coated nanoparticle, demonstrating the immunostimulant activity of the nanoparticle systems. They also discovered that the macrophages exposure to these formulations maintained their intact structure without any cytotoxic effects (63).
Additionally, a number of studies using a model antigen such as tetanus or diphtheria confirmed the potent adjuvant effect of PLGA, which connected with their capacity to be effectively taken up by APCs (61). Many studies also tend to surface-modify it which can help them evade the body’s defense system, and make vaccines safer and more efficient through nasal immunization, a non-invasive route (59).
2.3 Gold nanoparticles (AuNPs) can capture and retain antigenic epitopes
Gold nanoparticles (AuNPs) represent one of the most widely studied metallic nanomaterials, which typically range in size from 1 nm to 100 nm, which may be beneficial in overcoming biological barriers (64). It is available in various types such as gold nanorods, nanocages, nanostars, nanocubes and nanospheres, and the type and size of the nanoparticles affect their physical characteristics and surface functionalization (65). Gold nanoparticles are widely used in the biomedical field due to their small size-to-volume ratio, functionalization, stability, low toxicity, and ease of detection, and they can bind to a variety of functionalized fractions through different types of interactions, including ligands, medicinal agents, DNA, amino acids, proteins, peptides, and oligonucleotides (66, 67).
Gold nanoparticles can capture and retain antigenic epitopes and improve the efficiency of antigen delivery and presentation. By studying different shapes of gold nanoparticles, Hongjuan Zhao et al. found that star-shaped AuNPs captured and retained more repetitive antigenic epitopes and triggered a strong humoral immune response mediated by the cooperation of CD4+ T helper cells and follicular B cells, whereas caged AuNPs caused a strong CD8+ T cell immunity (68).
Immunoreactivity of a DNA vaccine expressing the SARS-CoV-2 spike (S) protein on gold chitosan nanocarriers reveals a sustained surge in antibodies (IgG, IgA, and IgM) (69). In addition, coupling lipopolysaccharide (LPS) from Bacillus thailandensis with AuNPs for intranasal immunization of mice, which promote robust antigen-specific antibody responses (70).
2.4 Nanogels can retain the hydrated nature and shrink-swell properties of hydrogels
Nanogels are drug delivery vehicles with three-dimensional tunable porous structures with particle sizes in the submicron range, from 20 to 250 nm (71). It is formed by a system of chemically or physically cross-linked swellable polymer networks, which help encapsulate small molecules, oligonucleotides and even proteins while maintaining their structural integrity (72). As a hydrogel, nanogels can retain the highly hydrated nature and shrink-swell properties of hydrogels under different conditions, and these unique properties give nanogels the ability to enable drug delivery, diagnostics, and imaging (72, 73). Unlike typical nanoparticles, nanogels exhibit tunable particle size, particle shape, and sensitivity to pH, temperature, ionic strength, redox conditions, and other external stimuli. Nanogels have a pronounced spherical structure, and with the latest advances in their fabrication processes can also be produced in a variety of nanogel types, allowing for effective controlled drug release properties (74, 75).
Nanogel consisting of self-assembled cholesteryl group-bearing pullulan (CHP) has strong advantages as a novel adjuvant-free and safe carrier for mucosal vaccines. For example, nasal administration of nanogels containing subunit types of botulinum antigen (BoHc-nanogel) or pneumococcal surface protein A (PspA-nanogel) enhance antigen-specific systemic and mucosal immune responses, even in the absence of biologically active mucosal adjuvants (e.g., CT) (38).
Gel system consisting of glutamine and benzaldehyde derivatives combined with extracellular vesicles derived from mouse aortic endothelial cells has better absorption efficiency in the nasal cavity and reduces levels of pro-inflammatory Ly6Chi monocytes/macrophages and neutrophils (76). Y Fukuyama et al. established a nanosized nasal vaccine delivery system by using a cationic cholesterol group-bearing pullulan nanogel (cCHP nanogel) in a study examining the central nervous system safety and efficacy of nasal vaccination with their developed cCHP nanogel containing pneumococcal surface protein A (PspA-nanogel) against pneumococcal infection in nonhuman primates, demonstrating that nasal PspA-nanogel vaccination is a safe and effective strategy for the development of a nasal vaccines for the prevention of pneumonia in humans (41).
2.5 Lipopeptides: self-assembly structure and efficient immune response
Lipopeptides are mixtures of lipids and peptides that have the ability to self-assemble into the structures of nanoparticles in an aqueous environment. The interaction or balance between the hydrophilic and lipophilic qualities of lipids and peptides determines the lipopeptides’ ability to self-assemble (77). Lipopeptides are used as delivery systems in nasal vaccines primarily to prevent rheumatic fever and rheumatic heart disease, which are significant respiratory infections caused by Group A streptococcus (GAS), which makes it an ideal delivery system, suitable for both mucosal and systemic immunization (78).
Because of their small nanosize structure and shape, lipopeptide vaccines are well absorbed by APCs and trigger a robust immune response and employed as a nasal vaccination to prevent GAS infection. For instance, the LP-88/30-J14 lipopeptide vaccine (containing both the N-terminal and C-terminal epitope of GAS) has a higher IgG titer and APC uptake of antigen due to its smaller nanosize, which increases the uptake of M protein antigen by DC, in comparison to LP-88/30 (N-terminal epitope) and LP-J14 (C-terminal epitope) alone (79).
2.6 Liposomes enhance the delivery of antigens to antigen-presenting cells
Lipid nanoparticles are submicron capsules with an aqueous core, such as liposomes, micelles, or nanoparticles with oil, solid, or a non-crystalline core surrounded and stabilized by a lipid layer, e.g., nucleic acid-containing lipid nanoparticles (commonly referred to as LNP) (80). Among them, liposomes are safe, biocompatible, biodegradable spherical nanoparticles composed of cholesterol and phospholipids that can be loaded with both hydrophilic and lipophilic molecules, and these properties make liposomes good gene, drug, and vaccine delivery systems (81). Liposomes can be cationic, anionic, or neutral, depending on the phospholipids that make up the liposome. Phospholipids are amphiphilic molecules with hydrophilic heads and lipophilic tails, and the surface charge of liposomes depends on the phospholipid head group (82). Depending on the nature of the loaded substance, liposomes can be loaded with drugs or antigens in two ways, either by encapsulating a water-soluble substance in a hydrophilic core or by containing a lipid-soluble substance in the hydrophobic space of a bilayer lipid membrane (82).
Liposomes enhance the delivery of antigens to antigen-presenting cells and co-deliver antigens with adjuvants, thereby increasing vaccine efficacy and enhancing immunogenicity (83). Cationic liposomes are widely used for gene and protein delivery. Cationic liposomes have been used in nasal immunization (84). Cationic DOTAP/DC-chol liposomal complex OVA drops preferentially induced the Th2 response after nasal immunization and enhanced OVA uptake by CD11c+ DCs in nasal-associated lymphoid tissues (85). In addition, it has also been shown that immunization with cationic liposomes complexed with antigen followed by nasal drops enhances antigen-specific mucosal IgA responses by inducing IL-6 expression. Intranasal liposomal vaccine delivery induces a mucosal response in the respiratory system and a systemic immune response that produces IgA and systemic IgG (86).
In addition, polymeric micelles are considered good carriers for drug delivery due to their stability, nanosize, surface properties, and enhanced permeability and retention effects (87). The hydrophobic core of the micelles consists mainly of polyester, poly (L-amino acids) and polycaprolactone, and the hydrophilic shell consists mainly of polyethylene glycol (PEG), with sizes ranging from 10 to 100 nanometers (88, 89). Polymeric micelle-based antigen delivery systems were developed for bacterial pathogens. Shaobin Shang et al. loaded mycolic acid (MA), a lipid components of Mycobacterium tuberculosis (Mtb) cell wall, into micellar nanocarriers (MA-Mc), which elicited a CD1b-restricted T cell response in the lungs after intranasal immunization of mice, demonstrating that MA-Mc can be explored as subunit vaccines against Mtb infection (90). Kengo Suzuki et al. found that hyaluronic acid (HA)-coated micelles can efficiently deliver antigens and adjuvants to mucosal resident immune cells, and that HA-coated micelles are a promising platform for the development of nasal vaccines against infectious diseases (58).
2.7 VLPs can be easily detected by immune system cells
Virus-like particles (VLPs) are multimeric self-assembled particles made up of one or more structural proteins that lack viral genetic material, making them incapable of infecting the host cell (91). As in the case of virus capsids, the antigenic proteins of VLPs self-assemble into highly symmetrical and strict architectures, usually icosahedral and octahedral, with the most of VLPs’ size ranging from 20-200nm (92). Virus-like particles can be produced using a variety of expression systems (ESs) without the requirement to propagate pathogenic viruses. The ESs include bacteria, yeast, insect cell lines, plants, mammalian cell lines and cell-free ESs (92). VLPs are used for a variety of purposes. Because they contain an internal cavity, they can be used as carriers for the delivery of bio- and nanomaterials, including drugs, vaccines, quantum dots and imaging substances (93). Using VLPs as a delivery strategy has numerous benefits, including precise targeting, biocompatibility, and biodegradability (94).
Vaccines based on virus-like particles have gained popularity due to their high immunogenicity. Because of their underlying geometry, VLPs resemble pathogen-associated structural patterns (PASP), which are easily detected by immune system cells (93). As a result, VLPs are excellent for cellular phagocytosis and antigen presentation of dendritic cells (DCs), eliciting both robust cellular and humoral immune responses even without an adjuvant (91, 95).
To date, numerous perilous viral pathogens have been mimicked by VLPs, including Severe Acute Respiratory Syndrome Coronavirus 2 (SARS-CoV-2); Hand, foot and mouth disease virus. Authorized VLP vaccines against hepatitis B virus (HBV), hepatitis E virus (HEV), and human papillomavirus (HPV) are globally used and many others are in clinical trials (92, 96). VLPs are readily absorbed by APCs, particularly DCs, which improves interactions with NALT. Furthermore, VLP-based intranasal vaccinations can efficiently boost mucosal immune responses, hence reducing viral shedding and local transmission (97). These benefits have made VLP-based vaccinations popular in the development of intranasal vaccines. Rothen et al. described a COVID-19 vaccine based on virus-like particles (VLPs) for intranasal administration. Intranasal delivery of the CuMVTT-RBD vaccine induces robust local and systemic immune responses, generating high-avidity antibodies with broad-spectrum neutralization against SARS-CoV-2 and its variants of concern (VOCs) in a murine model. This work establishes a foundational framework for advancing next-generation mucosal COVID-19 vaccines designed to block viral entry at respiratory portals while curbing transmission (98).
3 Nanoemulsion enhances adhesion and immune response
Nanoemulsion (NE) is composed of water in oil (W/O) or oil in water (O/W), surfactants and cosurfactants, with an average droplet diameter of less than 500nm. Long-chain triglycerides (LCT) and medium-chain triglycerides (MCT) are the two categories of commonly utilized oils (99). Ionic and non-ionic surfactants are the two categories of commonly used surfactants. Non-ionic surfactants include Tween, Span, Poloxamer, etc., while ionic surfactants include Cetylpyridinium Chloride (CPC), Benzalkonium Chloride (BCI), etc (100).
Nanoemulsion with good adhesion enhances the duration of antigen residency in the nasal cavity, hence increasing the capacity of APC to absorb antigens and potently stimulating the immune system. The physical and chemical characteristics of surfactants may be connected to the robust NE adherence to mucosa. The cationic surfactant CPC interacts with the negatively charged mucin and the non-ionic surfactant Tween interacts with the mucin due to its hydrophilicity, both of which can make NE firmly adhere to the surface of nasal mucosa, promote the uptake of antigen by nasal epithelial cells, and thus enhance the specific humoral immune response (100).
NE such as MF59 and W805EC are used for intranasal immunization. MF59, an oil-in-water emulsion (O/W), is primarily used for influenza vaccine (101). A W805EC-based influenza vaccine has been successfully tested in a Phase 1 randomized, controlled, observer blind clinical trial, showing a good safety profile in healthy adult volunteers, and induced systemic and mucosal immunity after a single intranasal vaccination (102). Furthermore, in mice, rats, and guinea pigs, the NE-based hepatitis B vaccine has demonstrated significant immunogenicity and good safety (103). According to research on vaccines against Helicobacter pylori infection, NE vaccine has high delivery efficiency and no obvious cytotoxicity, induces effective specific Th1 response, reduces the colonization of Helicobacter pylori (104). One study has developed an adjuvant integrating NE that activates TLR2/4 and NLRP3 with an RNA agonist of RIG-I (IVTDI). The spike protein vaccine incorporating NE/IVD significantly enhances a Th1-biased cellular immune response and elicits high neutralizing antibody titers (105).
Recently, a new nano-adjuvant, O/ILNE, consisting of an ionic liquid of choline (+) and niacin (–) instead of water. In contrast to MF59 emulsion (O/W), O/IL NE has been shown to increase mucosal IgA, systemic IgG, and cellular immunity. Therefore, the prolonged retention of antigens in the nasal cavity and improved paracellular transport of antigens to the submucosa make it an incredibly effective delivery strategy (106).
4 Probiotics in intranasal delivery system
The WHO defines probiotics as living microorganisms which administered in adequate amounts confer a health benefit on the host (107). Good safety, adherence, and availability make probiotics highly promising for use in vaccine administration (108, 109).
4.1 Bacterial spores: a stable and effective mucosal vaccine delivery system
Bacillus spores can be used as mucosal vaccine carriers (110). Among them, Bacillus subtilis spores have the advantages of thermal stability, and non-pathogenicity and can be used as a vaccine delivery system (111). Bacillus subtilis is a Gram-positive non-pathogenic and endospore-forming bacterium (112). Bacillus subtilis spores can sprout, develop, and revert to mature bacteria when the surrounding conditions are right (113). The resistance and stability of the spore are determined by its unique structure (110). Chromosome copies are found in the spore’s core, which is encased and shielded by a protein shell and peptidoglycan (110). Isticato R et al. reported that mutant spores with modified shells are better at absorbing antigens than spores of the wild type (114). Bacillus subtilis spores can activate innate immunity via TLRs and promote DC maturation and NK cell recruitment. Intranasal immunization of spores absorbed with antigen increased the uptake of antigen, raises cytokine levels and sIgA levels, and conferred protection against viral challenge (114, 115).
Recently, a study using Bacillus subtilis spores coupled with Plasmodium falciparum recombinant protein to intranasally immunize mice showed that the spores increased the immunogenicity of the antigen and induced higher levels of serum IgG (116). Studies have also been conducted to create vaccines against C. difficile (Clostridioides difficile) spores by adsorbing C. difficile spore surface proteins to Bacillus subtilis spores (117, 118). Furthermore, studies that adsorbed tetanus toxin to Bacillus subtilis spores and immunized mice intranasally showed that spore treatment increased tetanus toxin-specific sIgA production and resulted in more rapid serum IgG production (119).
4.2 Others
Apart from Bacillus subtilis spores, mucosal vaccines can also be delivered intranasally via Escherichia coli and Lactic acid bacteria. Some studies have constructed Escherichia coli expressing allergens and demonstrated that intranasal pretreatment with this Escherichia coli before multiple sensitization in mice can significantly reduce allergen-specific serum IgE levels and lung inflammation (120). Lactic acid bacteria are a group of Gram-positive, non-sporogenic bacteria, including Lactobacillus, Lactococcus, etc (121). Pneumococcus and tetanus vaccinations have been developed using lactic acid bacteria as a delivery system to stimulate a favorable immune response (122, 123).
5 Limitations and safety concerns of intranasal vaccine delivery system
While intranasal vaccines offer significant advantages, their development and application are constrained by anatomical, physiological, and technical challenges.
5.1 Anatomical and physiological risks
The nasal cavity’s proximity to the central nervous system poses unique risks. The thin olfactory epithelium-blood-brain barrier raises concerns about neurotoxic effects if vaccine components inadvertently enter the brain. For instance, mild adverse effects such as rhinorrhea and nasal congestion are common, while severe complications—though rare—have been documented (12, 124, 125). A notable example is the NasalFlu vaccine (approved in Switzerland in 2001), which was withdrawn due to reports of Bell’s palsy in immunized individuals (126).
5.2 Technical limitations of delivery systems
Current delivery platforms face three major hurdles: (i) Inefficient Antigen Release: Many carriers, such as PLGA nanoparticles, exhibit incomplete antigen release and cannot undergo sterile filtration, limiting their clinical scalability (127). (ii) Manufacturing Complexity: Materials like chitosan require chemical modification to improve water solubility and biocompatibility, adding to production costs (128). (iii) Carrier-Related Toxicity: Certain formulations, including cationic liposomes, may induce dose-dependent inflammation, genotoxicity, or cell membrane disruption (129, 130).
5.3 Safety evidence from preclinical studies
Emerging preclinical data highlight potential safety issues: (i) Chitosan-based nanoparticles have shown dose-dependent developmental toxicity in zebrafish embryos, including reduced hatching rates, increased malformations, and neurobehavioral abnormalities (131). (ii) Acylated starch nanoparticles exhibit moderate cytotoxicity at high concentrations, though low doses remain tolerable (132). (iii) PLGA particles may trigger local immune overactivation if antigen release kinetics are poorly controlled (127). Despite these challenges, systematic safety evaluations remain limited, underscoring the need for standardized toxicity assays and long-term monitoring in future studies.
6 Conclusions
Intranasal immunization is a safe, effective, and convenient route of mucosal immunization. The nasal cavity itself, with its enormous surface area and specialized immunological tissue (NALT), is an efficient entry channel for vaccinations, making intranasal immunization promising for the mucosal routes. However, intranasal cilia clearance, mucus barrier, and enzyme milieu which make intranasal delivery of antigens challenging. Various intranasal delivery technologies, such as nanoparticles and nanoemulsions, have been created to improve vaccine distribution, increase antigen penetration, and elicit more robust immune responses to solve these problems (Figure 3). Among diverse types of delivery system, nanoparticles especially chitosan nanoparticles are well-studied and offer a promising delivery strategy for promoting antigen uptake and lowering unfavorable vaccine reactions. Despite the promising results in animal models, clinical findings were limited. Therefore, further studies are needed to evaluate the efficiency and safety of these delivery system in human studies. With advances in delivery technologies such as nanoparticles, the challenges of antigen degradation and malabsorption can be mitigated, which may lead to more efficient and broader vaccination strategies. Furthermore, combining advanced delivery systems with adjuvants including STING-activating (e.g., CDNs) could represent a powerful strategy to amplify mucosal immunity. Co-delivery of antigens and adjuvants within nanocarriers may ensure spatiotemporal synchronization of antigen presentation and innate immune activation, thereby enhancing vaccine efficacy. These advances have the potential to increase vaccine efficacy and acceptance, particularly in the prevention of respiratory infections such as influenza, respiratory syncytial virus and coronavirus, and will play an increasingly critical role in global vaccination campaigns and contribute to improved global public health outcomes.
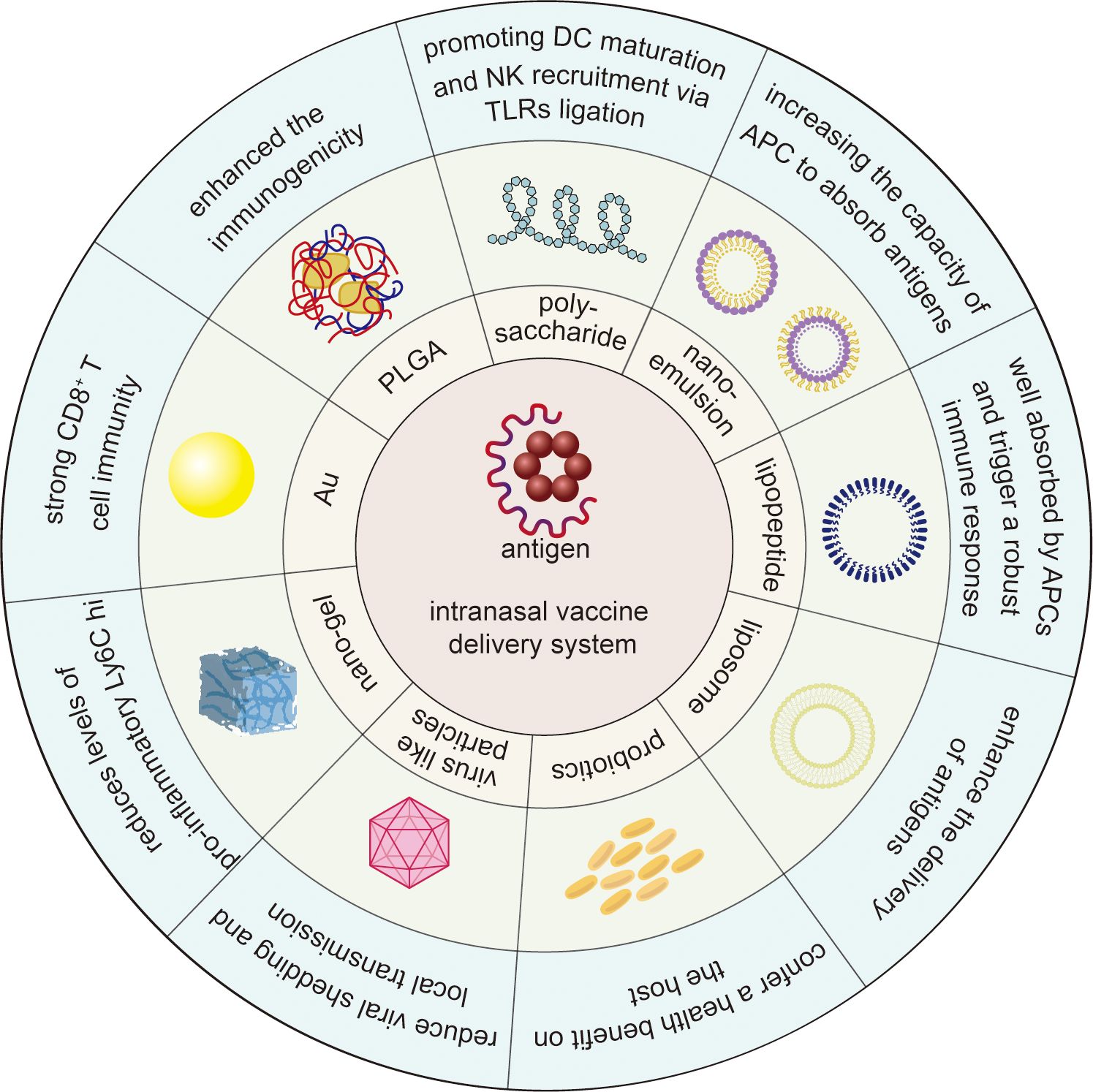
Figure 3. Schematic overview of intranasal vaccine delivery system against infectious disease. The outer blue segment and the green segment in the middle represents the key outcome induced by and the diagram of intranasal delivery system, respectively. Polysaccharides stimulate dendritic cell maturation and natural killer cell recruitment through TLR ligation. Nanoemulsions enhance antigen uptake in antigen-presenting cells. Lipopeptides boost APC absorption and activate potent immune responses. Liposomes optimize antigen delivery efficiency. Probiotics strengthen host health. Virus-like particles inhibit viral shedding and curb local transmission. Nanogels suppress pro-inflammatory Ly6C hi levels. Au nanoparticles elicit robust CD8+ T cell immunity. PLGA nanoparticles amplify antigen immunogenicity.
Author contributions
ZZh: Conceptualization, Writing – original draft, Writing – review & editing, Data curation, Formal Analysis, Investigation, Methodology, Visualization. YY: Conceptualization, Data curation, Formal Analysis, Investigation, Methodology, Visualization, Writing – original draft, Writing – review & editing. LH: Conceptualization, Data curation, Formal Analysis, Investigation, Methodology, Visualization, Writing – original draft, Writing – review & editing. LY: Conceptualization, Data curation, Formal Analysis, Investigation, Methodology, Visualization, Writing – original draft, Writing – review & editing. SH: Investigation, Writing – review & editing. ZZe: Investigation, Writing – review & editing. YC: Investigation, Writing – review & editing. XHW: Investigation, Writing – review & editing. XMW: Writing – review & editing, Conceptualization, Supervision, Validation. MS: Conceptualization, Supervision, Validation, Writing – review & editing. MZ: Conceptualization, Funding acquisition, Project administration, Supervision, Validation, Writing – original draft, Writing – review & editing.
Funding
The author(s) declare financial support was received for the research, and/or publication of this article. This work was supported by NHC Key Laboratory of Nuclear Technology Medical Transformation (MIANYANG CENTRAL HOSPITAL, Nos. 2024HYX027 and 2024HYX001), Undergraduate Innovation and Entrepreneurship Training Program in Hubei Province (No. S202410488174), Hubei Province Key Laboratory of Occupational Hazard Identification and Control (No. OHIC2024G02), the National Natural Science Foundation of China (Nos. 81971568 and 81302600), the Scientific research plan of Education Department of Hubei Province (No. B2023016), “The 14th Five Year Plan” Hubei Provincial advantaged characteristics disciplines (groups) project of Wuhan University of Science and Technology (No. 2023C0307) and Innovation research Fund of Wuchang Hospital (No. WCYY2022G01).
Conflict of interest
The authors declare that the research was conducted in the absence of any commercial or financial relationships that could be construed as a potential conflict of interest.
Generative AI statement
The author(s) declare that no Generative AI was used in the creation of this manuscript.
Publisher’s note
All claims expressed in this article are solely those of the authors and do not necessarily represent those of their affiliated organizations, or those of the publisher, the editors and the reviewers. Any product that may be evaluated in this article, or claim that may be made by its manufacturer, is not guaranteed or endorsed by the publisher.
Glossary
APCs: antigen presenting cells
AuNPs: Gold nanoparticles
BALF: bronchoalveolar lavage fluid
Bm: Burkholderia mallei
BoHc: botulinum type A neurotoxin
BV: Bee venom
cCHP: Cationic-type CHP
Chi-C48/80: compound 48/80 loaded chitosan
Chi-PCL: Chitosan-poly epsilon caprolactone
CHP: cholesteryl group-bearing pullulan
CH/AL-BV: chitosan/alginate nanoparticle encapsulated bee venom
C-O-NP: curdlan-chitosan conjugate nanoparticle
CPC: Cetylpyridinium Chloride
CS: curdlan sulfate
CTA1-DD: cholera toxin A1 subunit -dimer of the D-fragment of protein A from Staphylococcus aureus bacteria
CT: cholera toxin
CTB: cholera toxin B subunit
DGNP: Dendritic Gold Nanoparticles
DOTAP: 1,2-dioleoyl-3-trimethylammonium-propane
DT: diphtheria toxoid
ESs: expression systems
ESAT: Early Secretory Antigenic Target
GAS: Group A Streptococcus
GC: glycol chitosan
HA: hyaluronic acid
HAS: Human serum albumin
HBsAg: hepatitis B surface antigen
HBV: hepatitis B virus
HpaA: Helicobacter pylori adhesin A
HPV: human papillomavirus
IFN-I: type I interferons
IFN-γ: interferon-gamma
IgA: immunoglobulin A
IgG: immunoglobulin G
IL-1: interleukin-1
IL-6: Interleukin-6
IVT DI: an RNA agonist of RIG-I
LNP: Lipid Nano Particle
LP: Lipopeptides
LPS: lipopolysaccharide
MA: mycolic acid
MA-Mc: MA-loaded micellar
MD: Maltodextrin
MdNP: Maltodextrin nanoparticles
Mtb: Mycobacterium tuberculosis
NALT: nasal-associated lymphoid tissue
NE: nanoemulsion
NF-κB: nuclear factor kappa-B
NPL: nanoparticles lipid
O-HTCC: O-(2-hydroxyl) propyl-3-trimethyl ammonium chitosan chloride
OVA: ovalbumin
PASP: pathogen-associated structural patterns
PEG: polyethylene glycol
PLGA: poly (lactic-co-glycolic acid)
PRRSV: porcine reproductive and respiratory syndrome virus
PspA: pneumococcal surface protein A
PVP: poly (4-vinylpyridine)
rPfCSP: recombinant P. falciparum circumsporozoite surface protein
SARS-CoV-2: Severe Acute Respiratory Syndrome Coronavirus 2
SMBVs: supramolecular biovectors
S-RBD: spike receptor binding domain
TE: total extract of Toxoplasma gondii antigens
Th1: type-1 helper T cell
Th2: type-2 helper T cell
Th17: type-17 helper T cell
TS-PDMS-grafted MP: 3-(triethoxysilyl)-propyl-terminated polydimethylsiloxane-grafted microparticles
TTFC: C fragment of the tetanus toxin
VOCs: variants of concern
References
1. Meng QY, Sun Y, Cong HL, Hu H, Xu FJ. An overview of chitosan and its application in infectious diseases. Drug Deliv Trans Res. (2021) 11:1340–51. doi: 10.1007/s13346-021-00913-w
2. Baker RE, Mahmud AS, Miller IF, Rajeev M, Rasambainarivo F, Rice BL, et al. Infectious disease in an era of global change. Nat Rev Microbiol. (2022) 20:193–205. doi: 10.1038/s41579-021-00639-z
3. Vetter V, Denizer G, Friedland LR, Krishnan J, Shapiro M. Understanding modern-day vaccines: what you need to know. Ann Med. (2018) 50:110–20. doi: 10.1080/07853890.2017.1407035
4. Ali Khan A, Munir M, Miraj F, Imran S, Arif Siddiqi D, Altaf A, et al. Examining unsafe injection practices associated with auto-disable (Ad) syringes: A systematic review. Hum Vaccin Immunother. (2021) 17:3247–58. doi: 10.1080/21645515.2021.1911514
5. Khan A, Altaf A, Qureshi H, Orakzai M, Khan A. Reuse of syringes for therapeutic injections in Pakistan: rethinking determinants. East Mediterr Health J. (2020) 26:283–9. doi: 10.26719/emhj.19.028
6. Song Y, Mehl F, Zeichner SL. Vaccine strategies to elicit mucosal immunity. Vaccines (Basel). (2024) 12(2):191. doi: 10.3390/vaccines12020191
7. Xu H, Cai L, Hufnagel S, Cui Z. Intranasal vaccine: factors to consider in research and development. Int J Pharm. (2021) 609:121180. doi: 10.1016/j.ijpharm.2021.121180
8. Mato YL. Nasal route for vaccine and drug delivery: features and current opportunities. Int J Pharm. (2019) 572:118813. doi: 10.1016/j.ijpharm.2019.118813
9. Yusuf H, Kett V. Current prospects and future challenges for nasal vaccine delivery. Hum Vaccin Immunother. (2017) 13:34–45. doi: 10.1080/21645515.2016.1239668
10. Deng S, Liang H, Chen P, Li Y, Li Z, Fan S, et al. Viral vector vaccine development and application during the covid-19 pandemic. Microorganisms. (2022) 10:1450. doi: 10.3390/microorganisms10071450
11. Diallo BK, Ni Chasaide C, Wong TY, Schmitt P, Lee KS, Weaver K, et al. Intranasal covid-19 vaccine induces respiratory memory T cells and protects K18-hace mice against Sars-Cov-2 infection. NPJ Vaccines. (2023) 8:68. doi: 10.1038/s41541-023-00665-3
12. Kehagia E, Papakyriakopoulou P, Valsami G. Advances in intranasal vaccine delivery: A promising non-invasive route of immunization. Vaccine. (2023) 41:3589–603. doi: 10.1016/j.vaccine.2023.05.011
13. Perego G, Vigezzi GP, Cocciolo G, Chiappa F, Salvati S, Balzarini F, et al. Safety and efficacy of spray intranasal live attenuated influenza vaccine: systematic review and meta-analysis. Vaccines (Basel). (2021) 9(9):998. doi: 10.3390/vaccines9090998
14. Shakya AK, Chowdhury MYE, Tao W, Gill HS. Mucosal vaccine delivery: current state and a pediatric perspective. J Control Release. (2016) 240:394–413. doi: 10.1016/j.jconrel.2016.02.014
15. van der Ley PA, Zariri A, van Riet E, Oosterhoff D, Kruiswijk CP. An intranasal omv-based vaccine induces high mucosal and systemic protecting immunity against a Sars-Cov-2 infection. Front Immunol. (2021) 12:781280. doi: 10.3389/fimmu.2021.781280
16. Overton ET, Goepfert PA, Cunningham P, Carter WA, Horvath J, Young D, et al. Intranasal seasonal influenza vaccine and a tlr-3 agonist, rintatolimod, induced cross-reactive Iga antibody formation against Avian H5n1 and H7n9 influenza Ha in humans. Vaccine. (2014) 32:5490–5. doi: 10.1016/j.vaccine.2014.07.078
17. Gizurarson S. Anatomical and histological factors affecting intranasal drug and vaccine delivery. Curr Drug Deliv. (2012) 9:566–82. doi: 10.2174/156720112803529828
18. Teng Z, Meng LY, Yang JK, He Z, Chen XG, Liu Y. Bridging nanoplatform and vaccine delivery, a landscape of strategy to enhance nasal immunity. J Controlled Release. (2022) 351:456–75. doi: 10.1016/j.jconrel.2022.09.044
19. Sadeghi M, Asadirad A, Koushki K, Shahbaz SK, Dehnavi S. Recent advances in improving intranasal allergen-specific immunotherapy; focus on delivery systems and adjuvants. Int Immunopharmacol. (2022) 113(Pt A):109327. doi: 10.1016/j.intimp.2022.109327
20. Bernocchi B, Carpentier R, Betbeder D. Nasal nanovaccines. Int J Pharm. (2017) 530:128–38. doi: 10.1016/j.ijpharm.2017.07.012
21. Nian XX, Zhang JY, Huang SH, Duan K, Li XG, Yang XM. Development of nasal vaccines and the associated challenges. Pharmaceutics. (2022) 14(10):1983. doi: 10.3390/pharmaceutics14101983
22. Popescu R, Ghica MV, Dinu-Pirvu CE, Anuta V, Lupuliasa D, Popa L. New opportunity to formulate intranasal vaccines and drug delivery systems based on chitosan. Int J Mol Sci. (2020) 21(14):5016. doi: 10.3390/ijms21145016
23. Dmour I, Islam N. Recent advances on chitosan as an adjuvant for vaccine delivery. Int J Biol Macromol. (2022) 200:498–519. doi: 10.1016/j.ijbiomac.2021.12.129
24. Wang WQ, Meng QY, Li Q, Liu JB, Zhou M, Jin Z, et al. Chitosan derivatives and their application in biomedicine. Int J Mol Sci. (2020) 21(2):487. doi: 10.3390/ijms21020487
25. Chen YP, Wang Y, Li ZY, Jiang HL, Pan W, Liu MH, et al. Preparation and immunological activity evaluation of an intranasal protein subunit vaccine against ancestral and mutant Sars-Cov-2 with Curdlan Sulfate/O-linked quaternized chitosan nanoparticles as carrier and adjuvant. Int J Biol Macromol. (2024) 276(Pt 1):133733. doi: 10.1016/j.ijbiomac.2024.133733
26. Singh B, Maharjan S, Cho KH, Cui L, Park IK, Choi YJ, et al. Chitosan-based particulate systems for the delivery of mucosal vaccines against infectious diseases. Int J Biol Macromol. (2018) 110:54–64. doi: 10.1016/j.ijbiomac.2017.10.101
27. Dyer AM, Hinchcliffe M, Watts P, Castile J, Jabbal-Gill I, Nankervis R, et al. Nasal delivery of insulin using novel chitosan based formulations: A comparative study in two animal models between simple chitosan formulations and chitosan nanoparticles. Pharm Res. (2002) 19:998–1008. doi: 10.1023/a:1016418523014
28. Zhao K, Chen G, Shi XM, Gao TT, Li W, Zhao Y, et al. Preparation and efficacy of a live newcastle disease virus vaccine encapsulated in chitosan nanoparticles. PloS One. (2012) 7:e53314. doi: 10.1371/journal.pone.0053314
29. Shim S, Yoo HS. The application of mucoadhesive chitosan nanoparticles in nasal drug delivery. Marine Drugs. (2020) 18(12):605. doi: 10.3390/md18120605
30. Jiang HL, Zhang S, Chen YP, Wang FS, Jiang WJ. Preparation and characterization of curdlan-chitosan conjugate nanoparticles as mucosal adjuvants for intranasal influenza H1n1 subunit vaccine. Int J Biol Macromol. (2024) 266(Pt 2):131289. doi: 10.1016/j.ijbiomac.2024.131289
31. Pawar D, Jaganathan KS. Mucoadhesive glycol chitosan nanoparticles for intranasal delivery of hepatitis B vaccine: enhancement of mucosal and systemic immune response. Drug Deliv. (2016) 23:185–94. doi: 10.3109/10717544.2014.908427
32. Bento D, Jesus S, Lebre F, Gonçalves T, Borges O. Chitosan plus compound 48/80: formulation and preliminary evaluation as a hepatitis B vaccine adjuvant. Pharmaceutics. (2019) 11(2):72. doi: 10.3390/pharmaceutics11020072
33. Rydell N, Stertman L, Sjoholm I. Starch microparticles as vaccine adjuvant. Expert Opin Drug Deliv. (2005) 2:807–28. doi: 10.1517/17425247.2.5.807
34. McDermott MR, Heritage PL, Bartzoka V, Brook MA. Polymer-grafted starch microparticles for oral and nasal immunization. Immunol Cell Biol. (1998) 76:256–62. doi: 10.1046/j.1440-1711.1998.00743.x
35. Artursson P, Edman P, Ericsson JL. Macrophage stimulation with some structurally related polysaccharides. Scand J Immunol. (1987) 25:245–54. doi: 10.1111/j.1365-3083.1987.tb01070.x
36. Heritage PL, Brook MA, Underdown BJ, McDermott MR. Intranasal immunization with polymer-grafted microparticles activates the nasal-associated lymphoid tissue and draining lymph nodes. Immunology. (1998) 93:249–56. doi: 10.1046/j.1365-2567.1998.00420.x
37. Singh RS, Kaur N, Hassan M, Kennedy JF. Pullulan in biomedical research and development - a review. Int J Biol Macromol. (2021) 166:694–706. doi: 10.1016/j.ijbiomac.2020.10.227
38. Yuki Y, Nochi T, Kong IG, Takahashi H, Sawada S, Akiyoshi K, et al. Nanogel-based antigen-delivery system for nasal vaccines. Biotechnol Genet Eng Rev. (2013) 29:61–72. doi: 10.1080/02648725.2013.801226
39. Ayame H, Morimoto N, Akiyoshi K. Self-assembled cationic nanogels for intracellular protein delivery. Bioconjug Chem. (2008) 19:882–90. doi: 10.1021/bc700422s
40. Nochi T, Yuki Y, Takahashi H, Sawada S, Mejima M, Kohda T, et al. Nanogel antigenic protein-delivery system for adjuvant-free intranasal vaccines. Nat Mater. (2010) 9:572–8. doi: 10.1038/nmat2784
41. Fukuyama Y, Yuki Y, Katakai Y, Harada N, Takahashi H, Takeda S, et al. Nanogel-based pneumococcal surface protein a nasal vaccine induces microrna-associated th17 cell responses with neutralizing antibodies against streptococcus pneumoniae in macaques. Mucosal Immunol. (2015) 8:1144–53. doi: 10.1038/mi.2015.5
42. Kong IG, Sato A, Yuki Y, Nochi T, Takahashi H, Sawada S, et al. Nanogel-based Pspa intranasal vaccine prevents invasive disease and nasal colonization by streptococcus pneumoniae. Infect Immun. (2013) 81:1625–34. doi: 10.1128/IAI.00240-13
43. Yuki Y, Uchida Y, Sawada SI, Nakahashi-Ouchida R, Sugiura K, Mori H, et al. Characterization and specification of a trivalent protein-based pneumococcal vaccine formulation using an adjuvant-free nanogel nasal delivery system. Mol Pharm. (2021) 18:1582–92. doi: 10.1021/acs.molpharmaceut.0c01003
44. Fasquelle F, Scuotto A, Howsam M, Betbeder D. Maltodextrin-nanoparticles as a delivery system for nasal vaccines: A review article. Pharmaceutics. (2024) 16(2):247. doi: 10.3390/pharmaceutics16020247
45. von Hoegen P. Synthetic biomimetic supra molecular biovector (Smbv) particles for nasal vaccine delivery. Adv Drug Deliv Rev. (2001) 51:113–25. doi: 10.1016/s0169-409x(01)00175-2
46. Halperin SA, Smith B, Clarke K, Treanor J, Mabrouk T, Germain M. Phase I, randomized, controlled trial to study the reactogenicity and immunogenicity of a nasal, inactivated trivalent influenza virus vaccine in healthy adults. Hum Vaccines. (2005) 1:37–42. doi: 10.4161/hv.1.1.1553
47. Jallouli Y, Paillard A, Chang J, Sevin E, Betbeder D. Influence of surface charge and inner composition of porous nanoparticles to cross blood-brain barrier in vitro. Int J Pharm. (2007) 344:103–9. doi: 10.1016/j.ijpharm.2007.06.023
48. Bernocchi B, Carpentier R, Lantier I, Ducournau C, Dimier-Poisson I, Betbeder D. Mechanisms allowing protein delivery in nasal mucosa using Npl nanoparticles. J Controlled Release. (2016) 232:42–50. doi: 10.1016/j.jconrel.2016.04.014
49. Lê MQ, Carpentier R, Lantier I, Ducournau C, Fasquelle F, Dimier-Poisson I, et al. Protein delivery by porous cationic maltodextrin-based nanoparticles into nasal mucosal cells: comparison with cationic or anionic nanoparticles. Int J Pharmaceutics-X. (2019) 1:100001. doi: 10.1016/j.ijpx.2018.100001
50. Dimier-Poisson I, Carpentier R, N’Guyen TT, Dahmani F, Ducournau C, Betbeder D. Porous nanoparticles as delivery system of complex antigens for an effective vaccine against acute and chronic toxoplasma Gondii infection. Biomaterials. (2015) 50:164–75. doi: 10.1016/j.biomaterials.2015.01.056
51. Le MQ, Ye L, Bernasconi V, Carpentier R, Fasquelle F, Lycke N, et al. Prevention of influenza virus infection and transmission by intranasal administration of a porous maltodextrin nanoparticle-formulated vaccine. Int J Pharm. (2020) 582:119348. doi: 10.1016/j.ijpharm.2020.119348
52. Rebelatto MC, Guimond P, Bowersock TL, HogenEsch H. Induction of systemic and mucosal immune response in cattle by intranasal administration of pig serum albumin in alginate microparticles. Vet Immunol Immunopathol. (2001) 83:93–105. doi: 10.1016/s0165-2427(01)00370-1
53. Vasudevan UM, Lee OK, Lee EY. Alginate derived functional oligosaccharides: recent developments, barriers, and future outlooks. Carbohydr Polymers. (2021) 267:118158. doi: 10.1016/j.carbpol.2021.118158
54. Lee J, Kim YM, Kim JH, Cho CW, Jeon JW, Park JK, et al. Nasal delivery of chitosan/alginate nanoparticle encapsulated bee (Apis mellifera) venom promotes antibody production and viral clearance during porcine reproductive and respiratory syndrome virus infection by modulating T cell related responses. Vet Immunol Immunopathol. (2018) 200:40–51. doi: 10.1016/j.vetimm.2018.04.006
55. Jain RR, Mehta MR, Bannalikar AR, Menon MD. Alginate microparticles loaded with lipopolysaccharide subunit antigen for mucosal vaccination against klebsiella pneumoniae. Biologicals. (2015) 43:195–201. doi: 10.1016/j.biologicals.2015.02.001
56. Yang D, Jones KS. Effect of alginate on innate immune activation of macrophages. J Biomed Mater Res Part A. (2009) 90A:411–8. doi: 10.1002/jbm.a.32096
57. Mosafer J, Sabbaghi AH, Badiee A, Dehghan S, Tafaghodi M. Preparation, characterization and in vivo evaluation of alginate-coated chitosan and trimethylchitosan nanoparticles loaded with Pr8 influenza virus for nasal immunization. Asian J Pharm Sci. (2019) 14:216–21. doi: 10.1016/j.ajps.2018.04.005
58. Suzuki K, Yoshizaki Y, Horii K, Murase N, Kuzuya A, Ohya Y. Preparation of hyaluronic acid-coated polymeric micelles for nasal vaccine delivery. Biomater Sci. (2022) 10:1920–8. doi: 10.1039/d1bm01985f
59. Sharma S, Parmar A, Kori S, Sandhir R. Plga-based nanoparticles: A new paradigm in biomedical applications. Trac Trends Anal Chem. (2016) 80:30–40. doi: 10.1016/j.trac.2015.06.014
60. Alsaab HO, Alharbi FD, Alhibs AS, Alanazi NB, Alshehri BY, Saleh MA, et al. Plga-based nanomedicine: history of advancement and development in clinical applications of multiple diseases. Pharmaceutics. (2022) 14(12):2728. doi: 10.3390/pharmaceutics14122728
61. Kabiri M, Sankian M, Sadri K, Tafaghodi M. Robust mucosal and systemic responses against Htlv-1 by delivery of multiepitope vaccine in Plga nanoparticles. Eur J Pharm Biopharm. (2018) 133:321–30. doi: 10.1016/j.ejpb.2018.11.003
62. Shao L, Shen S, Liu H. Recent advances in Plga micro/nanoparticle delivery systems as novel therapeutic approach for drug-resistant tuberculosis. Front Bioeng Biotechnol. (2022) 10:941077. doi: 10.3389/fbioe.2022.941077
63. Büyükbayraktar HK, Araici PP, Ihlamur M, Gökkaya D, Karahan M, Abamor ES, et al. Effect of polycation coating on the long-term pulsatile release of antigenic Esat-61–20 peptide from Plga nanoparticles. Colloids Surf B Biointerf. (2023) 228:113421. doi: 10.1016/j.colsurfb.2023.113421
64. Jeong EH, Jung G, Hong CA, Lee H. Gold nanoparticle (Aunp)-based drug delivery and molecular imaging for biomedical applications. Arch Pharmacal Res. (2014) 37:53–9. doi: 10.1007/s12272-013-0273-5
65. Connor DM, Broome AM. Gold nanoparticles for the delivery of cancer therapeutics. Adv Cancer Res. (2018) 139:163–84. doi: 10.1016/bs.acr.2018.05.001
66. Nicol JR, Dixon D, Coulter JA. Gold nanoparticle surface functionalization: A necessary requirement in the development of novel nanotherapeutics. Nanomedicine. (2015) 10:1315–26. doi: 10.2217/nnm.14.219
67. Amina SJ, Guo B. A review on the synthesis and functionalization of gold nanoparticles as a drug delivery vehicle. Int J Nanomed. (2020) 15:9823–57. doi: 10.2147/ijn.S279094
68. Zhao HJ, Li YT, Zhao BB, Zheng CX, Niu MY, Song QL, et al. Orchestrating antigen delivery and presentation efficiency in lymph node by nanoparticle shape for immune response. Acta Pharm Sin B. (2023) 13:3892–905. doi: 10.1016/j.apsb.2023.02.003
69. Kumar US, Afjei R, Ferrara K, Massoud TF, Paulmurugan R. Gold-nanostar-chitosan-mediated delivery of Sars-Cov-2 DNA vaccine for respiratory mucosal immunization: development and proof-of-principle. ACS Nano. (2021) 15:17582–601. doi: 10.1021/acsnano.1c05002
70. Tapia D, Sanchez-Villamil JI, Torres AG. Multicomponent gold nano-glycoconjugate as a highly immunogenic and protective platform against burkholderia mallei. NPJ Vaccines. (2020) 5:82. doi: 10.1038/s41541-020-00229-9
71. Yin YL, Hu B, Yuan X, Cai L, Gao HL, Yang Q. Nanogel: A versatile nano-delivery system for biomedical applications. Pharmaceutics. (2020) 12:290. doi: 10.3390/pharmaceutics12030290
72. Soni KS, Desale SS, Bronich TK. Nanogels: an overview of properties, biomedical applications and obstacles to clinical translation. J Controlled Release. (2016) 240:109–26. doi: 10.1016/j.jconrel.2015.11.009
73. Li YL, Maciel D, Rodrigues J, Shi XY, Tomás H. Biodegradable polymer nanogels for drug/nucleic acid delivery. Chem Rev. (2015) 115:8564–608. doi: 10.1021/cr500131f
74. Sahiner N, Godbey WT, McPherson GL, John VT. Microgel, nanogel and hydrogel-hydrogel semi-Ipn composites for biomedical applications: synthesis and characterization. Colloid Polymer Sci. (2006) 284:1121–9. doi: 10.1007/s00396-006-1489-4
75. Kabanov AV, Vinogradov SV. Nanogels as pharmaceutical carriers: finite networks of infinite capabilities. Angewandte Chemie International Edition. (2009) 48:5418–29. doi: 10.1002/anie.200900441
76. Wang JZ, Tan Y, Dai Y, Hu K, Tan X, Jiang SL, et al. Intranasal delivery of endothelial cell-derived extracellular vesicles with supramolecular gel attenuates myocardial ischemia-reperfusion injury. Int J Nanomed. (2023) 18:5495–510. doi: 10.2147/ijn.S420301
77. Marasini N, Skwarczynski M, Toth I. Intranasal delivery of nanoparticle-based vaccines. Ther Deliv. (2017) 8:151–67. doi: 10.4155/tde-2016-0068
78. Marasini N, Khalil ZG, Giddam AK, Ghaffar KA, Hussein WM, Capon RJ, et al. Lipid core peptide/poly(Lactic-co-glycolic acid) as a highly potent intranasal vaccine delivery system against group a streptococcus. Int J Pharm. (2016) 513:410–20. doi: 10.1016/j.ijpharm.2016.09.057
79. Zaman M, Chandrudu S, Giddam AK, Reiman J, Skwarczynski M, McPhun V, et al. Group a streptococcal vaccine candidate: contribution of epitope to size, antigen presenting cell interaction and immunogenicity. Nanomedicine. (2014) 9:2613–24. doi: 10.2217/nnm.14.190
80. Fan YC, Marioli M, Zhang K. Analytical characterization of liposomes and other lipid nanoparticles for drug delivery. J Pharm Biomed Anal. (2021) 192:113642. doi: 10.1016/j.jpba.2020.113642
81. Amin M, Seynhaeve ALB, Sharifi M, Falahati M, ten Hagen TLM. Liposomal drug delivery systems for cancer therapy: the Rotterdam experience. Pharmaceutics. (2022) 14:2165. doi: 10.3390/pharmaceutics14102165
82. Nikolova MP, Kumar EM, Chavali MS. Updates on responsive drug delivery based on liposome vehicles for cancer treatment. Pharmaceutics. (2022) 14(10):2195. doi: 10.3390/pharmaceutics14102195
83. Zhou SQ, Luo Y, Lovell JF. Vaccine approaches for antigen capture by liposomes. Expert Rev Vaccines. (2023) 22:1022–40. doi: 10.1080/14760584.2023.2274479
84. Duong VA, Nguyen TTL, Maeng HJ. Recent advances in intranasal liposomes for drug, gene, and vaccine delivery. Pharmaceutics. (2023) 15(1):207. doi: 10.3390/pharmaceutics15010207
85. Tada R, Hidaka A, Iwase N, Takahashi S, Yamakita Y, Iwata T, et al. Intranasal immunization with dotap cationic liposomes combined with dc-cholesterol induces potent antigen-specific mucosal and systemic immune responses in mice. PloS One. (2015) 10(10):e0139785. doi: 10.1371/journal.pone.0139785
86. Sia ZR, Chiem K, Huang WC, Seffouh A, Dereshgi AT, Hogan T, et al. Respiratory vaccination with hemagglutinin nanoliposomes protects mice from homologous and heterologous strains of influenza virus. J Virol. (2022) 96(19):e0100622. doi: 10.1128/jvi.01006-22
87. Ghosh B, Biswas S. Polymeric micelles in cancer therapy: state of the art. J Controlled Release. (2021) 332:127–47. doi: 10.1016/j.jconrel.2021.02.016
88. Chakravarty M, Vora A. Nanotechnology-based antiviral therapeutics. Drug Deliv Trans Res. (2021) 11:748–87. doi: 10.1007/s13346-020-00818-0
89. Perumal S, Atchudan R, Lee W. A review of polymeric micelles and their applications. Polymers. (2022) 14(12):2510. doi: 10.3390/polym14122510
90. Shang S, Kats D, Cao L, Morgun E, Velluto D, He Y, et al. Induction of mycobacterium tuberculosis lipid-specific T cell responses by pulmonary delivery of mycolic acid-loaded polymeric micellar nanocarriers. Front Immunol. (2018) 9:2709. doi: 10.3389/fimmu.2018.02709
91. Zhong L, Krummenacher C, Zhang W, Hong J, Feng Q, Chen Y, et al. Urgency and necessity of Epstein-Barr virus prophylactic vaccines. NPJ Vaccines. (2022) 7:159. doi: 10.1038/s41541-022-00587-6
92. Hadj Hassine I, Ben M’hadheb M, Almalki MA, Gharbi J. Virus-like particles as powerful vaccination strategy against human viruses. Rev Med Virol. (2024) 34:e2498. doi: 10.1002/rmv.2498
93. Nooraei S, Bahrulolum H, Hoseini ZS, Katalani C, Hajizade A, Easton AJ, et al. Virus-like particles: preparation, immunogenicity and their roles as nanovaccines and drug nanocarriers. J Nanobiotechnol. (2021) 19:59. doi: 10.1186/s12951-021-00806-7
94. Steinmetz NF. Viral nanoparticles as platforms for next-generation therapeutics and imaging devices. Nanomedicine. (2010) 6:634–41. doi: 10.1016/j.nano.2010.04.005
95. Qian C, Liu X, Xu Q, Wang Z, Chen J, Li T, et al. Recent progress on the versatility of virus-like particles. Vaccines (Basel). (2020) 8(1):139. doi: 10.3390/vaccines8010139
96. Tsakiri M, Naziris N, Demetzos C. Innovative vaccine platforms against infectious diseases: under the scope of the covid-19 pandemic. Int J Pharm. (2021) 610:121212. doi: 10.1016/j.ijpharm.2021.121212
97. Bai Z, Wan D, Lan T, Hong W, Dong H, Wei Y, et al. Nanoplatform based intranasal vaccines: current progress and clinical challenges. ACS Nano. (2024) 18:24650–81. doi: 10.1021/acsnano.3c10797
98. Rothen DA, Krenger PS, Nonic A, Balke I, Vogt AS, Chang X, et al. Intranasal administration of a virus like particles-based vaccine induces neutralizing antibodies against Sars-Cov-2 and variants of concern. Allergy. (2022) 77:2446–58. doi: 10.1111/all.15311
99. Singh Y, Meher JG, Raval K, Khan FA, Chaurasia M, Jain NK, et al. Nanoemulsion: concepts, development and applications in drug delivery. J Controlled Release. (2017) 252:28–49. doi: 10.1016/j.jconrel.2017.03.008
100. Wong PT, Wang SH, Ciotti S, Makidon PE, Smith DM, Fan YY, et al. Formulation and characterization of nanoemulsion intranasal adjuvants: effects of surfactant composition on mucoadhesion and immunogenicity. Mol Pharm. (2014) 11:531–44. doi: 10.1021/mp4005029
101. Stephenson I, Zambon MC, Rudin A, Colegate A, Podda A, Bugarini R, et al. Phase I evaluation of intranasal trivalent inactivated influenza vaccine with nontoxigenic escherichia coli enterotoxin and novel biovector as mucosal adjuvants, using adult volunteers. J Virol. (2006) 80:4962–70. doi: 10.1128/JVI.80.10.4962-4970.2006
102. Stanberry LR, Simon JK, Johnson C, Robinson PL, Morry J, Flack MR, et al. Safety and immunogenicity of a novel nanoemulsion mucosal adjuvant W805ec combined with approved seasonal influenza antigens. Vaccine. (2012) 30:307–16. doi: 10.1016/j.vaccine.2011.10.094
103. Makidon PE, Bielinska AU, Nigavekar SS, Janczak KW, Knowlton J, Scott AJ, et al. Pre-clinical evaluation of a novel nanoemulsion-based hepatitis B mucosal vaccine. PloS One. (2008) 3(8):e2954. doi: 10.1371/journal.pone.0002954
104. Yang Y, Chen L, Sun HW, Guo H, Song Z, You Y, et al. Epitope-loaded nanoemulsion delivery system with ability of extending antigen release elicits potent Th1 response for intranasal vaccine against helicobacter pylori. J Nanobiotechnol. (2019) 17:6. doi: 10.1186/s12951-019-0441-y
105. Jangra S, Landers JJ, Rathnasinghe R, O’Konek JJ, Janczak KW, Cascalho M, et al. A combination adjuvant for the induction of potent antiviral immune responses for a recombinant Sars-Cov-2 protein vaccine. Front Immunol. (2021) 12:729189. doi: 10.3389/fimmu.2021.729189
106. Lin X, Sheng YA, Zhang X, Li ZJ, Yang YL, Wu J, et al. Oil-in-ionic liquid nanoemulsion-based intranasal delivery system for influenza split-virus vaccine. J Controlled Release. (2022) 346:380–91. doi: 10.1016/j.jconrel.2022.04.036
107. Hill C, Guarner F, Reid G, Gibson GR, Merenstein DJ, Pot B, et al. The international scientific association for probiotics and prebiotics consensus statement on the scope and appropriate use of the term probiotic. Nat Rev Gastroenterol Hepatol. (2014) 11:506–14. doi: 10.1038/nrgastro.2014.66
108. Wyszynska A, Kobierecka P, Bardowski J, Jagusztyn-Krynicka EK. Lactic acid bacteria-20 years exploring their potential as live vectors for mucosal vaccination. Appl Microbiol Biotechnol. (2015) 99:2967–77. doi: 10.1007/s00253-015-6498-0
109. Rosales-Mendoza S, Angulo C, Meza B. Food-grade organisms as vaccine biofactories and oral delivery vehicles. Trends Biotechnol. (2016) 34:124–36. doi: 10.1016/j.tibtech.2015.11.007
110. Saggese A, Baccigalupi L, Donadio G, Ricca E, Isticato R. The bacterial spore as a mucosal vaccine delivery system. Int J Mol Sci. (2023) 24(13):10880. doi: 10.3390/ijms241310880
111. Huang JM, Hong HA, Hoang VT, Hoang TH, Brisson A, Cutting SM. Mucosal delivery of antigens using adsorption to bacterial spores. Vaccine. (2010) 28:1021–30. doi: 10.1016/j.vaccine.2009.10.127
112. de Souza RD, Batista MT, Luiz WB, Cavalcante RC, Amorim JH, Bizerra RS, et al. Bacillus subtilis spores as vaccine adjuvants: further insights into the mechanisms of action. PloS One. (2014) 9:e87454. doi: 10.1371/journal.pone.0087454
113. McKenney PT, Driks A, Eichenberger P. The bacillus subtilis endospore: assembly and functions of the multilayered coat. Nat Rev Microbiol. (2013) 11:33–44. doi: 10.1038/nrmicro2921
114. Isticato R, Sirec T, Treppiccione L, Maurano F, De Felice M, Rossi M, et al. Non-recombinant display of the B subunit of the heat labile toxin of escherichia coli on wild type and mutant spores of bacillus subtilis. Microb Cell Fact. (2013) 12:98. doi: 10.1186/1475-2859-12-98
115. Song M, Hong HA, Huang JM, Colenutt C, Khang DD, Nguyen TV, et al. Killed bacillus subtilis spores as a mucosal adjuvant for an H5n1 vaccine. Vaccine. (2012) 30:3266–77. doi: 10.1016/j.vaccine.2012.03.016
116. de Almeida MEM, Alves KCS, de Vasconcelos MGS, Pinto TS, Gloria JC, Chaves YO, et al. Bacillus subtilis spores as delivery system for nasal plasmodium falciparum circumsporozoite surface protein immunization in a murine model. Sci Rep. (2022) 12:1531. doi: 10.1038/s41598-022-05344-2
117. Maia AR, Reyes-Ramirez R, Pizarro-Guajardo M, Saggese A, Castro-Cordova P, Isticato R, et al. Induction of a specific humoral immune response by nasal delivery of Bcla2(Ctd) of clostridioides difficile. Int J Mol Sci. (2020) 21(4):1277. doi: 10.3390/ijms21041277
118. Maia AR, Reyes-Ramirez R, Pizarro-Guajardo M, Saggese A, Ricca E, Baccigalupi L, et al. Nasal immunization with the C-terminal domain of bcla3 induced specific igg production and attenuated disease symptoms in mice infected with clostridioides difficile spores. Int J Mol Sci. (2020) 21(18):6696. doi: 10.3390/ijms21186696
119. Santos FDS, Mazzoli A, Maia AR, Saggese A, Isticato R, Leite F, et al. A probiotic treatment increases the immune response induced by the nasal delivery of spore-adsorbed ttfc. Microb Cell Factories. (2020) 19(1):42. doi: 10.1186/s12934-020-01308-1
120. Sarate PJ, Heinl S, Poiret S, Drinic M, Zwicker C, Schabussova I, et al. E. Coli Nissle 1917 is a safe mucosal delivery vector for a birch-grass pollen chimera to prevent allergic poly-sensitization. Mucosal Immunol. (2019) 12:132–44. doi: 10.1038/s41385-018-0084-6
121. Wells JM, Mercenier A. Mucosal delivery of therapeutic and prophylactic molecules using lactic acid bacteria. Nat Rev Microbiol. (2008) 6:349–62. doi: 10.1038/nrmicro1840
122. Medina E, Guzman CA. Use of live bacterial vaccine vectors for antigen delivery: potential and limitations. Vaccine. (2001) 19:1573–80. doi: 10.1016/s0264-410x(00)00354-6
123. Grangette C, Muller-Alouf H, Goudercourt D, Geoffroy MC, Turneer M, Mercenier A. Mucosal Immune Responses and Protection against Tetanus Toxin after Intranasal Immunization with Recombinant Lactobacillus Plantarum. Infect Immun. (2001) 69:1547–53. doi: 10.1128/IAI.69.3.1547-1553.2001
124. Riese P, Sakthivel P, Trittel S, Guzman CA. Intranasal formulations: promising strategy to deliver vaccines. Expert Opin Drug Delivery. (2014) 11:1619–34. doi: 10.1517/17425247.2014.931936
125. Pandya T, Joshi P, Pathak R, Shah S. Nano-vaccination strategies: applications and challenges for intranasal immunization. Curr Pharm Biotechnol. (2023) 24:946–69. doi: 10.2174/1389201023666220727105901
126. Mutsch M, Zhou W, Rhodes P, Bopp M, Chen RT, Linder T, et al. Use of the inactivated intranasal influenza vaccine and the risk of Bell’s palsy in Switzerland. N Engl J Med. (2004) 350:896–903. doi: 10.1056/NEJMoa030595
127. Silva AL, Soema PC, Slutter B, Ossendorp F, Jiskoot W. Plga particulate delivery systems for subunit vaccines: linking particle properties to immunogenicity. Hum Vaccin Immunother. (2016) 12:1056–69. doi: 10.1080/21645515.2015.1117714
128. Gong X, Gao Y, Shu J, Zhang C, Zhao K. Chitosan-based nanomaterial as immune adjuvant and delivery carrier for vaccines. Vaccines (Basel). (2022) 10(11):1906. doi: 10.3390/vaccines10111906
129. Knudsen KB, Northeved H, Kumar PE, Permin A, Gjetting T, Andresen TL, et al. In vivo toxicity of cationic micelles and liposomes. Nanomedicine. (2015) 11:467–77. doi: 10.1016/j.nano.2014.08.004
130. Romoren K, Fjeld XT, Poleo AB, Smistad G, Thu BJ, Evensen O. Transfection efficiency and cytotoxicity of cationic liposomes in primary cultures of rainbow trout (Oncorhynchus mykiss) gill cells. Biochim Biophys Acta. (2005) 1717:50–7. doi: 10.1016/j.bbamem.2005.09.011
131. Rizeq BR, Younes NN, Rasool K, Nasrallah GK. Synthesis, bioapplications, and toxicity evaluation of chitosan-based nanoparticles. Int J Mol Sci. (2019) 20(22):5776. doi: 10.3390/ijms20225776
Keywords: infectious disease, vaccine, mucosal vaccination, intranasal immunization, delivery system
Citation: Zhang Z, Yang Y, Huang L, Yuan L, Huang S, Zeng Z, Cao Y, Wei X, Wang X, Shi M and Zhong M (2025) Nanotechnology-driven advances in intranasal vaccine delivery systems against infectious diseases. Front. Immunol. 16:1573037. doi: 10.3389/fimmu.2025.1573037
Received: 10 February 2025; Accepted: 11 April 2025;
Published: 09 May 2025.
Edited by:
Srinivasa Reddy Bonam, Indian Institute of Chemical Technology (CSIR), IndiaReviewed by:
Himanshu Gogoi, Translational Health Science and Technology Institute (THSTI), IndiaPatrícia C. C. Neves, Oswaldo Cruz Foundation, Brazil
Rahmi Anggraeni, National Research and Innovation Agency (BRIN), Indonesia
Copyright © 2025 Zhang, Yang, Huang, Yuan, Huang, Zeng, Cao, Wei, Wang, Shi and Zhong. This is an open-access article distributed under the terms of the Creative Commons Attribution License (CC BY). The use, distribution or reproduction in other forums is permitted, provided the original author(s) and the copyright owner(s) are credited and that the original publication in this journal is cited, in accordance with accepted academic practice. No use, distribution or reproduction is permitted which does not comply with these terms.
*Correspondence: Maohua Zhong, em1oQHd1c3QuZWR1LmNu; Xiaomei Wang, d2FuZ3hpYW9tZWlAd3VzdC5lZHUuY24=; Mingsong Shi, c2hpbXM5MEBzYy1tY2guY24=
†These authors have contributed equally to this work
‡Lead Contact